- Institute of Biotechnology, Helsinki Institute of Life Science, University of Helsinki, Helsinki, Finland
Parkinson’s disease (PD) is a progressive neurodegenerative disease characterized by gradual loss of midbrain dopamine neurons, leading to impaired motor function. Preclinical studies have indicated cerebral dopamine neurotrophic factor (CDNF) and mesencephalic astrocyte-derived neurotrophic factor (MANF) to be potential therapeutic molecules for the treatment of PD. CDNF was proven to be safe and well tolerated when tested in Phase I-II clinical trials in PD patients. Neuroprotective and neurorestorative effects of CDNF and MANF were demonstrated in animal models of PD, where they promoted the survival of dopamine neurons and improved motor function. However, biological roles of endogenous CDNF and MANF proteins in the midbrain dopamine system have been less clear. In addition to extracellular trophic activities, CDNF/MANF proteins function intracellularly in the endoplasmic reticulum (ER), where they modulate protein homeostasis and protect cells against ER stress by regulating the unfolded protein response (UPR). Here, our aim is to give an overview of the biology of endogenous CDNF and MANF in the brain dopamine system. We will discuss recent studies on CDNF and MANF knockout animal models, and effects of CDNF and MANF in preclinical models of PD. To elucidate possible roles of CDNF and MANF in human biology, we will review CDNF and MANF tissue expression patterns and regulation of CDNF/MANF levels in human diseases. Finally, we will discuss novel findings related to the molecular mechanism of CDNF and MANF action in ER stress, UPR, and inflammation, all of which are mechanisms potentially involved in the pathophysiology of PD.
Introduction
PD is a progressive neurodegenerative movement disorder where dopamine neurons in the midbrain degenerate and are gradually lost. Aging is the main risk factor for PD, and incidence of PD is markedly increased in people over the age of 65 years (1). Characteristic motor symptoms of PD are resting tremor, slowness of movement, rigidity, and postural instability. The main neuronal population affected in the brain of PD patients is dopamine neurons located in the ventral midbrain, which regulate voluntary movement. Dopamine neurons have cell bodies in the substantia nigra (SN), where they project axons to the striatum, forming the nigrostriatal dopaminergic pathway. Characteristic brain pathology in the vast majority of PD patients is the presence of intraneuronal Lewy body inclusions, which contain aggregated α-synuclein (αSyn) protein (2). Clinical diagnosis of PD is based on motor symptoms, which manifest when degeneration of dopamine neurons is already severe. At the time of clinical diagnosis of PD, there is an estimated 30% reduction of dopamine neurons in the SN, and about 50%–60% of striatal dopamine-releasing axon terminals have been lost (3). Dopamine neurons are also present in the enteric nervous system (ENS), and their function can be affected in PD (4). Indeed, PD is not only a motor disease with patients suffering from various non-motor symptoms, including constipation, REM sleep behavior disorder, hyposmia, and depression etc., which can severely impair the quality of life and often appear years before the actual clinical symptoms (5). Despite extensive research efforts, no therapies are available for PD that would slow down or halt the progressive neurodegeneration.
Neurotrophic factors (NTFs) are small secretory proteins that support neuronal survival and promote formation and maintenance of neuronal contacts. They regulate proliferation, migration, differentiation, and maturation of neurons during development and synaptic plasticity in adults (6–8). Because of these beneficial properties, NTFs are studied as potential therapeutic molecules in PD. Glial cell line-derived neurotrophic factor (GDNF)-family ligands (GFLs), GDNF (9) and neurturin (NRTN) (10), are potent survival factors for midbrain dopamine neurons (7). GFLs function as covalently linked homodimers that bind to GDNF family receptor alpha (GFRα) co-receptors and activate transmembrane protein receptor tyrosine kinase RET on the plasma membrane, leading to activation of signaling cascades that promote neuronal survival and neurite outgrowth. However, when GDNF and NRTN were tested in Phase II clinical trials in PD patients, the outcomes of the trials were modest (11).
CDNF and MANF proteins form a unique family of trophic factors and function in many cell types. They have neurotrophic activity and show protective effects on midbrain dopamine neurons in animal models of PD in vivo (12–17). MANF (alternative name arginine-rich, mutated in early-stage tumors; ARMET) was originally isolated and purified from the culture medium of mesencephalic astrocyte-derived cell line based on its ability to specifically promote the survival of cultured midbrain dopamine neurons (18). CDNF is a vertebrate-specific paralog of MANF (12). In cells, CDNF and MANF localize in the endoplasmic reticulum (ER) (19, 20), where they regulate protein homeostasis and ER stress-induced unfolded protein response (UPR) signaling (21–25). As a potential therapeutic factor for dopamine neurons, CDNF was tested in Phase I-II clinical trials in 17 PD patients, where it was shown to be safe and well tolerated (26, 27).
ER stress and UPR are possible pathogenic mechanisms in PD
The ER is an organelle important for protein synthesis, folding and post-translational modification, lipid synthesis, and calcium storage. Disturbances in the ER function caused by various physiological alterations and pathological conditions can lead to ER stress, a condition where unfolded and misfolded proteins accumulate in the ER (28). Dysregulations in protein homeostasis are associated with aging and can contribute to age-related neurodegenerative protein misfolding disorders, including PD (29).
ER stress activates the unfolded protein response (UPR) signaling pathway that functions as an adaptive mechanism to restore ER protein homeostasis (Figure 1) (28, 30). Activated UPR leads to transient inhibition of general mRNA translation initiation, which decreases protein synthesis and protein folding load in the ER. UPR signaling also leads to increased expression of various chaperones that assist protein folding in the ER and increased degradation of misfolded proteins by ER-associated protein degradation (ERAD) in the cytosol. Although UPR is a homeostatic mechanism, in conditions of severe unresolved ER stress UPR signaling can become chronic, which leads to activation of UPR-mediated apoptotic pathways. Importantly, chronic UPR activation can lead to death of cells and neurons contributing to development of different pathologies, including neurodegenerative diseases (28).
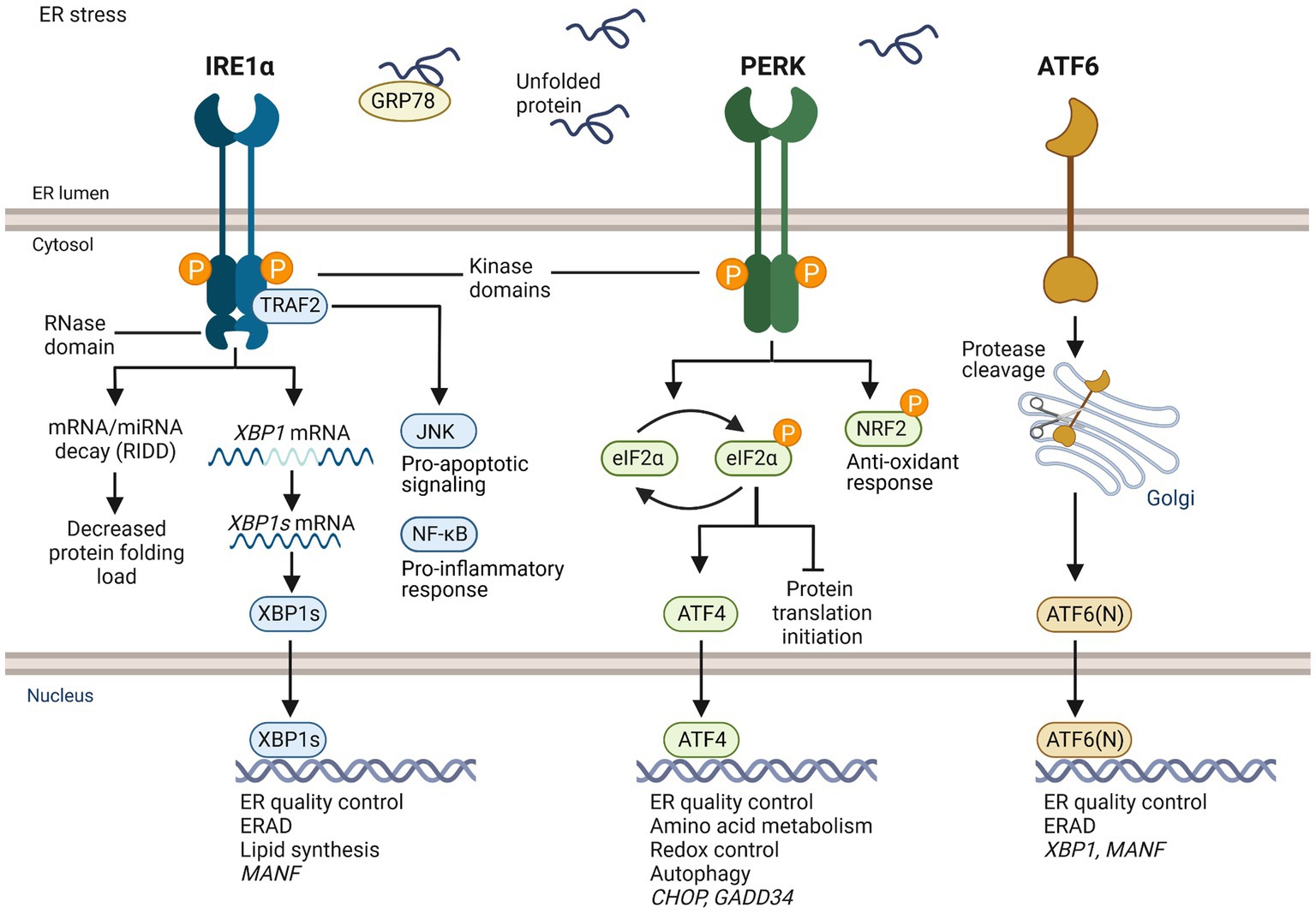
Figure 1. Overview of UPR signaling. IRE1α, PERK, and ATF6 are transmembrane receptors in the ER of mammalian cells that are activated upon ER stress. IRE1α and PERK have ER luminal domains and cytosolic kinase domains. IRE1α has also a cytosolic RNase domain. ATF6 has a luminal and a cytosolic domain, but no kinase activity. GRP78 is thought to be bound to the luminal domain of UPR receptors in homeostatic conditions. When unfolded proteins accumulate in the ER causing ER stress, GRP78 dissociates and binds unfolded client proteins, which allows activation of the UPR. Activated IRE1α forms homodimers leading to activation of cytosolic kinase domain, trans-autophosphorylation, and stimulation of cytosolic RNase domain. The activated RNase domain of IRE1α unconventionally splices XBP1 mRNA to XBP1s, which is translated and functions as a transcription factor. XBP1s induces genes related to ER quality control, ER associated degradation (ERAD), and lipid synthesis. The RNase domain can degrade mRNAs and miRNAs through regulated IRE1α-dependent decay (RIDD). IRE1α adapter TRAF2 mediates c-Jun N-terminal kinase (JNK) activation and apoptotic signaling, and NF-κB activation and inflammatory response. Active PERK phosphorylates an α-subunit of eukaryotic initiation factor 2 (eIF2) leading to transient block of general translation initiation while transcription factor ATF4 is still translated. It induces genes of protein folding, redox control, amino acid metabolism and autophagy. Under unresolved ER stress, ATF4 induces pro-apoptotic transcriptional factor C/EBP homologous protein (CHOP) and growth arrest and DNA damage-inducible 34 (GADD34). GADD34 dephosphorylates elF2α reversing translational inhibition, which can aggravate ER stress and promote apoptosis. PERK also phosphorylates nuclear factor erythroid 2-related factor 2 (NRF2), a transcription factor that regulates antioxidant response. Activated ATF6 moves to the Golgi and is cleaved by endopeptidases, which releases ATF6(N) fragment. ATF6(N) induces expression of XBP1 mRNA and components of ERAD. MANF expression is induced by XBP1s and ATF6(N).
Inositol-requiring enzyme 1 (IRE1; also known as ERN1), protein kinase R-like ER kinase (PERK; also known as EIF2AK3) and activating transcription factor 6 (ATF6) are ER transmembrane receptors in mammalian cells that monitor protein folding homeostasis in the ER and sense the accumulation of misfolded proteins via their luminal domains. IRE1 is the most conserved of the UPR sensors and was originally discovered in yeast (31). IRE1α contains cytosolic kinase and endoribonuclease (RNase) domains. When activated, IRE1α oligomerizes and trans-autophosphorylates leading to the activation of cytosolic RNase domain. The RNase domain of activated IRE1α unconventionally splices XBP1 mRNA to XBP1s in the cytosol (32). XBP1s is translated to a transcription factor that induces expression of genes related to ER protein folding and quality control, ERAD, and lipid synthesis. In addition to XBP1, RNase of IRE1α can degrade other ER-targeted mRNAs and miRNAs through regulated IRE1α-dependent decay (RIDD) activity (33). Upon activation, PERK phosphorylates the α subunit of eukaryotic translation initiation factor 2 (eIF2α), which leads to transient arrest of translation initiation and decrease in the load of newly synthesized proteins in the ER (34). However, activating transcription factor ATF4 mRNA and other mRNAs that have upstream 5′ open reading frames can still be translated (35). ATF4 regulates expression of genes related to protein quality control, amino acid metabolism, antioxidant response, and autophagy. ATF4 can induce expression of CHOP (36), which is an important transcription factor activating apoptosis related genes. ATF4 also induces expression of GADD34, which regulates protein phosphatase 1 and dephosphorylation of eIF2α (37). In unresolved ER stress and sustained UPR, ATF4 and CHOP contribute to increased protein synthesis, which further exacerbates protein folding stress and can lead to cell death. Differently from IRE1α and PERK, activated ATF6 moves to the Golgi, where it is specifically cleaved, releasing transcription factor ATF6(N) (38). ATF6(N) travels to the nucleus and induces expression of genes of protein folding and ERAD (35).
An ER chaperone, GRP78 (BiP) is important in regulating the activation of UPR signaling. Binding of GRP78 to the luminal domain of UPR sensors is thought to keep the sensors inactive (39). When unfolded proteins accumulate in the ER, GRP78 disassociates from the sensors to bind unfolded proteins, enabling dimerization and oligomerization of IRE1α and PERK. IRE1α and PERK may also directly bind unfolded proteins, leading to activation UPR signaling (40, 41).
Studies using human post-mortem brain tissue indicate PD-associated changes in the regulation of UPR (Figure 2). Phosphorylation of PERK (p-PERK) and eIF2α (p-eIF2α) was demonstrated in the nigral dopamine neurons of PD patients using immunohistochemistry (42). Importantly, p-PERK was detected in neurons positive for αSyn, supporting the involvement of ER stress in PD pathology (42). In a post-mortem study of PD patients and cases of incidental Lewy body disease, activation of the PERK pathway was detected in connection to Lewy body pathology in several brain regions, including the midbrain, neocortex, hippocampus, pons, and medulla oblongata (43). In support of UPR activation in PD brain, increased levels of GRP78 and CHOP were detected in samples from the SN region of PD patients (44). In another study, increased levels of GRP78 were also demonstrated in the cingulate gyrus and parietal cortex of patients with dementia with Lewy bodies and Parkinson’s disease dementia relative to patients with Alzheimer disease, suggesting that UPR activation is more pronounced in cases with Lewy body pathology or when Lewy pathology is present with plaque pathologies (45). In the following study, decreased levels of GRP78 were reported in the temporal cortex and cingulate gyrus of PD patients. Although in contrast to the previous report, these results support the idea that impairments in the regulation of GRP78 expression may be related to PD pathophysiology (46). Decreased levels of GRP78 were also detected in the substantia nigra pars compacta (SNpc) and hippocampus of late-stage PD patients (47). Furthermore, detection of αSyn and phosphorylated IRE1α was reported in neuromelanin-containing nigral neurons of PD cases, reflecting the activation of the IRE1α pathway in PD pathology (48). Activated UPR is evidently related to brain pathology in PD, but its role in the onset and pathological progression of PD is completely unclear. Furthermore, it remains unknown how UPR is activated in PD. To the best of our knowledge, there are no published data on CDNF and MANF expression in association with the expression of UPR markers in the human PD brain.
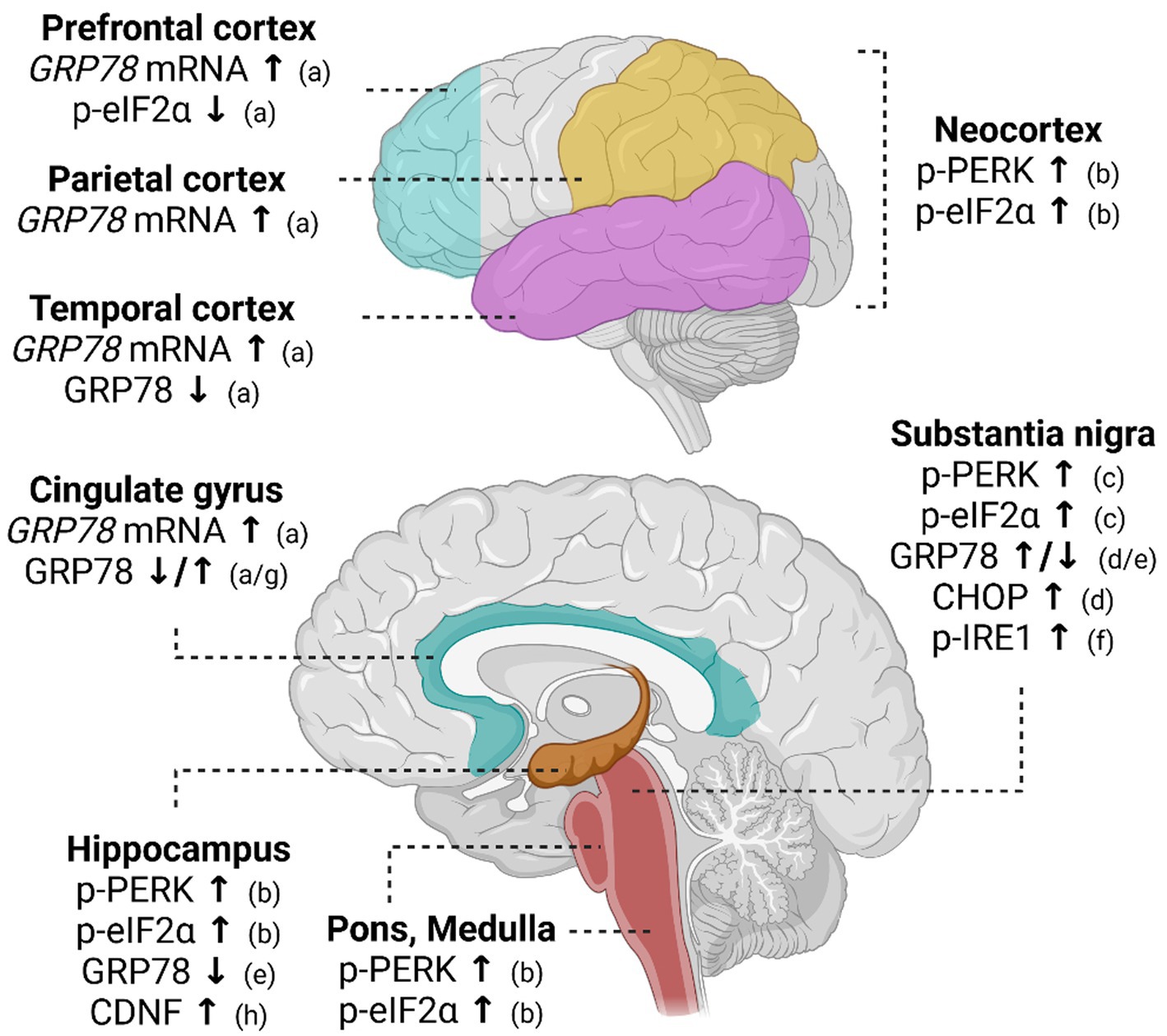
Figure 2. Altered UPR signaling in post-mortem brain of Parkinson’s disease patients. In the post-mortem PD brain, GRP78 mRNA levels are increased in various cortical regions. Expression of GRP78 protein, in turn, has been shown to decrease in the hippocampus and temporal cortex of PD patients. In the substantia nigra and cingulate gyrus, GRP78 is shown to be either increased or decreased. Activation of PERK pathway measured as increased phosphorylation of PERK (p-PERK) and eIF2α (p-eIF2α) is implicated in the neocortex, hippocampus, pons, and medulla. Activation of both IRE1α and PERK pathways has been discovered in the substantia nigra. References indicated in the figure: a (46); b (43); c (42); d (44); e (47); f (48); g (45); h (59). ↑ indicates an increase in expression and ↓ a decrease in expression.
CDNF and MANF tissue expression
Tissue expression of endogenous CDNF and MANF in mammals has mainly been investigated in rodents, while studies reporting CDNF/MANF distribution in human tissues, particularly in the brain, are scant. When CDNF and MANF expression in adult human post-mortem brain tissue was studied by reverse transcription PCR (RT-PCR), CDNF (12) and MANF (49) transcripts were detected in all brain regions examined, including the caudate nucleus, putamen, and SN of the nigrostriatal dopamine pathway.
In the adult mouse brain, Cdnf mRNA and protein expression was detected in neurons of several brain areas (12). Immunohistochemical staining indicated CDNF expression in cortical neurons, hippocampus, striatum, and cerebellar Purkinje cells. CDNF immunosignal was also detected in the SN, although not co-localizing with tyrosine hydroxylase (TH) -positive dopamine neurons (12). MANF expression in the rodent brain was also mainly neuronal and detected in diverse brain regions, including the cerebral cortex, hippocampus, hypothalamus, thalamus, and cerebellum (49, 50). In the midbrain, immunohistochemical signal indicating MANF expression was detected in TH-positive dopamine neurons in the SNpc, but also in nigral TH-negative neurons, indicating that MANF expression is not restricted to dopamine neurons in the SN (50). MANF levels were generally higher in early postnatal stages of rat brain development and lower in adult rats, suggesting that MANF expression is developmentally regulated (50). A similar regulated pattern of MANF expression was also observed in the SN and caudate-putamen brain areas in the rat (50). MANF is also expressed in TH-positive dopamine neurons in the rodent ventral tegmental area, which is part of the mesolimbic pathway involved in reward-related behaviors (51). High expression of MANF was detected especially in brain neurons regulating energy homeostasis and endocrine functions (51).
In non-neuronal tissues, CDNF and MANF are ubiquitously expressed, but their levels differ depending on tissue and cell type. Tissue levels of CDNF are generally lower than those of MANF, except in skeletal muscle (51, 52). Especially high CDNF levels were also detected in the heart and brown adipose tissue (12, 51). MANF expression is particularly high in adult mouse tissues with metabolic and secretory functions, including the pancreas, liver, salivary gland, and testis (49, 51).
MANF and CDNF are present in blood circulation in humans and mice (51, 53–55), and circulating MANF levels were shown to decrease in human aging (56). In human blood cell fractions, MANF levels were high in platelets and lower in white blood cells (54). In the immune system, MANF expression was detected in plasma cells and macrophages in the human spleen, suggesting that MANF functions in plasma cell differentiation and immune system regulation (57).
CDNF and MANF expression in human diseases
Changed levels of endogenous CDNF and MANF have been reported in numerous diseases (Table 1). Expression of endogenous MANF is increased especially in many inflammatory and metabolic diseases. Analyses have mostly focused on changes in protein levels in the serum or plasma, naturally due to availability of these samples. In the serum, MANF levels are increased in conditions such as ischemic stroke, drug-induced liver injury, hyperlipidemia, obesity, and types 1 and 2 diabetes (53, 61, 64, 66, 69, 73). In contrast, decreased levels of MANF in the serum have been reported in adult patients with growth hormone deficiency, major depressive disorder, nonalcoholic steatohepatitis, and polycystic ovary syndrome (56, 62, 68, 70). Expression of CDNF has been investigated in different disease conditions, but not as extensively as MANF. Findings indicate reduced expression of CDNF in the liver of patients with hepatocellular carcinoma and type 2 diabetes and in platelets of male stroke patients (58, 60).
Interestingly, levels of CDNF and MANF are also altered in PD (Table 1). Analysis of post-mortem brain tissue indicates that expression of CDNF was increased in the hippocampus of PD patients (59). Hippocampal levels of CDNF were not associated with levels of total αSyn or phosphorylated αSyn – a modification implicated in PD pathology. In comparison, MANF levels remained unaltered in the hippocampal samples of PD patients (59). Instead, MANF levels were doubled in the serum of PD patients in comparison with the control group (54). Furthermore, circulating MANF levels correlated positively with the depression rating of PD patients. Analysis of whole blood did not show differences in MANF levels between patients and controls, whereas CDNF levels were slightly increased in PD patients (54). The source of MANF and CDNF in the blood is currently unclear, and whether their circulating levels could directly reflect changes in brain tissue or brain function remains to be investigated. Furthermore, it would be worthwhile to characterize possible changes in CDNF and MANF expression levels specifically in dopamine neurons and at different stages of PD progression.
Thus far, there are no known mutations in the CDNF and MANF genes associated with the risk for developing PD. In the CDNF gene, however, there is a single-nucleotide polymorphism (SNP) identified in early onset PD patients (75). This polymorphism was suggested to be associated with susceptibility for PD. Furthermore, another SNP in the CDNF gene was discovered to be a susceptibility locus in schizophrenia (76). Similar to PD, changes in the brain dopamine system play an important role in the pathogenesis of schizophrenia. Dysregulation of the dopamine system and more specifically diminished dopamine D1 receptor function in the prefrontal cortex have been associated with negative symptoms of schizophrenia patients (77). Interestingly, a SNP in the CDNF gene was associated with negative symptoms of schizophrenia patients in the study population (76).
There are two known individuals carrying autosomal recessive loss-of-function mutations in the MANF gene (78, 79). The identified individuals have protein-truncating variants in exon 1 of the MANF gene (78). These subjects presented with childhood onset syndromic diabetes with short stature, deafness, microcephaly, and developmental delay (78, 79). One of these subjects additionally has hypopituitarism, obesity, and partial alopecia (79). Sensorineural hearing loss was diagnosed in another patient already at the age of 11 months (80). Neither of the MANF variants that the patients carry has been detected in any of the tested population databases, indicating that homozygous variants are rare and result in phenotypes too severe to survive in humans (78).
CDNF and MANF mouse models
The first CDNF knockout (KO) mouse model was reported in the year 2020 by Lindahl and co-authors (81). Conventional Cdnf−/− mice were viable and fertile with a normal appearance and normal blood parameters (81). The nigrostriatal dopamine system analyzed in aged mice showed no signs of degeneration. Levels of dopamine and its metabolites were similar between one-year-old Cdnf−/− and wildtype (WT) mice. The relative number of dopamine neurons in the SN was also unaltered in one-year-old Cdnf−/− mice, and still in 2-year-old Cdnf−/− mice. Furthermore, TH- and dopamine transporter (DAT)-positive fiber densities remained similar between Cdnf−/− and WT mice. Spontaneous locomotor activity was also not altered in Cdnf−/− mice, but when dopamine neurons lacking CDNF were induced to secrete high amounts of dopamine in response to amphetamine, their functionality was impaired. Amphetamine releases stored dopamine from vesicles, increasing cytosolic dopamine levels in dopamine neurons, and induces DAT-mediated dopamine efflux (82, 83). Amphetamine administration increased the activity of Cdnf−/− mice compared with control mice, measured as distance traveled in the open field arena (81). This alteration in dopaminergic transmission manifested already at a young age but became more prominent in older Cdnf−/− mice. The effect seemed to be specific to amphetamine, as administration of a cocaine-mimicking compound did not cause a similar effect. The authors hypothesized that the mechanism would relate to DAT activity since amphetamine induces DAT reversal whereas cocaine blocks DAT (84). Therefore, dopamine release at the dorsolateral striatal dopamine terminals of Cdnf−/− mice was analyzed in more detail by fast-scan cyclic-voltammetry (81). Results showed that dopamine is released at similar levels and with similar kinetics in Cdnf−/− and control mice, but adult Cdnf−/− mice had slower dopamine reuptake. Incubation of striatal slices with amphetamine revealed that DAT reversed faster in Cdnf−/− mice, which is in line with observations made in vivo. Furthermore, more dopamine was released through DAT in amphetamine-stimulated striatal slices of Cdnf−/− mice.
While midbrain dopamine neurons maintain normal morphology, enteric neurons present various defects in Cdnf−/− mice. In general, enteric neurons are located in the submucosal and myenteric plexuses, which are interconnected layers in the gut. CDNF is expressed in neuronal subsets of both plexuses along the gut (85). Lack of CDNF caused neuronal hypoplasia in the submucosal plexus but not in the myenteric plexus (81). Lower neuronal density in Cdnf−/− mice was due to increased non-apoptotic neurodegeneration following enhanced autophagy (81). In the ileum, which has the highest dopamine neuron density, DAT transcripts were reduced in Cdnf−/− mice already at 1 week of age, suggesting impaired development of dopamine neurons (85). Together with general neuronal hypoplasia, the density of dopamine neurons was reduced in Cdnf−/− mice already at the age of 1.5 months. Furthermore, an age-dependent decline in the number of dopamine neurons was exacerbated in mice lacking CDNF. However, the defect was not specific to dopamine neurons, also impacting nNOS-, GABA- and CGRP-positive neurons in the submucosal plexus (85). At the functional level, loss of CDNF and consequent loss of dopamine neurons in the submucosal plexus controlling peristaltic activity caused delayed gastric emptying, slower colonic motility, and a longer transit time in 11-month-old mice (85). Late functional deficits observed in the gut of Cdnf−/− mice reflect the importance of CDNF in maintenance of the ENS.
In addition to in vivo observations in embryonic and adult CDNF-deficient mice, in vitro experiments using exogenous recombinant CDNF protein showed a difference in the survival-promoting effect of CDNF between midbrain and enteric dopamine neurons. CDNF did not promote the survival of dopamine neurons isolated from embryonic mouse midbrain floor (20). Instead, CDNF promoted specifically the development/survival of dopamine neurons isolated from mouse enteric neural crest-derived cells (85).
Conventional Manf−/− mice in an outbred ICR strain develop diabetes during the first months of life and die young (25). In contrast, Manf−/− mice in the inbred C57BL/6 strain are perinatal lethal (86–88). The diabetic phenotype of Manf−/− mice in the ICR strain is caused by the decreased proliferation and loss of pancreatic insulin-producing beta cells due to persistent ER stress (25, 51). Postnatal Manf−/− mice have normal glucose levels but start to lose beta cells soon after birth followed by hyperglycemia (25). Manf−/− mice in the ICR strain or in the mixed C57BL/6 J:129SV strain present with a visible growth defect related to disturbances in bone growth and impaired function of the pituitary gland (51, 87). Furthermore, Manf−/− mice are characterized as having hearing loss (89). These phenotypes – diabetes, growth defect, and hearing loss – are noticeably similar to symptoms found in human subjects with MANF loss-of-function mutations (25, 51, 78, 80, 89).
Investigation of the role of MANF removal in the dopamine system was performed with Manffl/fl::NestinCre/+ mice, devoid of loss of pancreatic MANF and consequent hyperglycemia. Nestin Cre-mediated removal of MANF expression applies to neuronal and glial cell precursors. Results showed that MANF deficiency did not affect the relative number of dopamine neurons in the SN, TH- or DAT-positive fiber density in the striatum, or dopamine and its metabolite levels in aged Manffl/fl::NestinCre/+ mice (90). MANF ablation also did not result in changes in expression of TH or DAT in the midbrain. Furthermore, the response to amphetamine or motor behavior was similar between Manffl/fl::NestinCre/+ and control mice measured both at young and old age (90).
As CDNF and MANF are structurally highly similar proteins, the question of possible redundancy was raised. To investigate possible functional compensation between CDNF and MANF, mice lacking both proteins were produced (52). The phenotype of Cdnf−/−::Manf−/− mice is dominated by diabetes and a growth defect, resembling the phenotype of Manf−/− mice. The early onset of diabetes prevented analysis of dopamine neuron maintenance in Cdnf−/−::Manf−/− mice; however, the Cdnf−/−::Manf−/− mouse brain was investigated during development. At embryonic day 13.5, at the time when CDNF and MANF are already expressed (12, 49), the Th levels in the brain were shown to be similar between Cdnf−/−::Manf−/− and control mice despite the already increased UPR activation in the brain of Cdnf−/−::Manf−/− mice (52). At postnatal day 1, TH protein levels were not changed in the Cdnf−/−::Manf−/− mouse brain, remaining unaltered even at 6 weeks of age (52). However, a more extended analysis of the maturation of the midbrain dopamine system would be important since it has been shown that MANF is essential for neuronal migration in the cortex during development (91). The distribution of cortical layer markers is altered in the neocortex of Manf−/− mice, and neuronal migration is delayed in Manf−/− embryos, although the number of cortical neurons is not reduced and MANF is dispensable for neurogenesis (91). Whether similar developmental delays take place during maturation of dopamine neurons remains to be investigated. To examine the effect of CDNF and MANF ablation on the survival of dopamine neurons in the long term, Manffl/fl::NestinCre/+ mice lacking CDNF were bred (52). The simultaneous deficiency of neuronal MANF and CDNF had no effect on dopamine neuron number in the SN or TH-positive fiber density in the striatum of one-year-old mice. Based on these findings, endogenous CDNF and MANF are not significant survival factors for nigrostriatal dopamine neurons in mice, but CDNF regulates their function.
One of the most significant differences in the dopamine system between CDNF and MANF KO mice is the presence of increased UPR activation in MANF KO mice relative to WT mice. Loss of MANF was reported to induce expression of sXbp1 and Grp78 mRNA in the striatum and in the SN of 5-week-old conventional Manf−/− mice (90). In addition, UPR-related genes Atf4, Erdj4, Pdia6, and Grp94 were also upregulated in the striatum of Manf−/− mice (52). Similar to Manf−/− mice, adult Manffl/fl::NestinCre/+ mice had increased expression of sXbp1 and Grp78 in the nigrostriatal pathway (90). Thus, the increase in the activation of the IRE1α pathway and Grp78 expression are persistent through development and aging and in fact, throughout different brain regions of MANF KO models. Aged Manffl/fl::NestinCre/+ mice also had modestly increased mRNA levels of Atf4 and Atf6 in the SN, reflecting activation of other PERK and ATF6 pathways (52). In contrast, lack of CDNF did not affect the UPR signaling in the brain of Cdnf−/− mice (52, 81). Furthermore, lack of CDNF did not aggravate impaired proteostasis caused by loss of MANF alone (52). Instead, CDNF ablation resulted in increased UPR activation in the skeletal muscle and lack of MANF aggravated this increase (52). Thus, loss CDNF and MANF affect UPR status in vivo, but in a tissue-specific manner.
Although MANF deficiency alone does not induce neuronal loss (90, 92), MANF deficiency increases neuronal vulnerability, which was shown to manifest when Manffl/fl::NestinCre/+ mice were subjected to such stressors as ethanol, tunicamycin, or blockage of blood flow in the stroke model (92, 93). When neuronal apoptosis was induced in one-week-old Manffl/fl::NestinCre/+ mice by ethanol or tunicamycin, these exposures resulted in a higher increase in apoptosis and exacerbated ER stress in Manffl/fl::NestinCre/+ mice compared with control mice (92). Inhibiting ER stress by 4-phenylbutyric acid could abrogate increased neuronal apoptosis in Manffl/fl::NestinCre/+ mice subjected to the stressors. Thus, it was suggested that Manffl/fl::NestinCre/+ mice have a lowered buffering capacity for induced neuronal degeneration. In line with these observations, cortical neurons isolated from Manf−/− mice were shown to be more vulnerable to thapsigargin-induced ER stress in culture than neurons extracted from WT mice (90). Thus, it is plausible that dopamine neurons lacking MANF are also highly vulnerable to ER stress. Induction of ischemic infarct by arterial occlusion caused a larger infarction volume in Manffl/fl::NestinCre/+ mice than in control mice (93). When Cdnf−/− mice were subjected to hemorrhagic insult by collagen injection, the lesion volume was significantly larger than in control mice (94). Thus, loss of endogenous CDNF and MANF increases vulnerability to cerebral insults.
CDNF, MANF, and other model organisms
A single homologous gene to vertebrate CDNF/MANF is found in invertebrates. The invertebrate homolog of CDNF/MANF, first discovered in the fruit fly, shows higher sequence similarity to MANF than CDNF (95). During development DmManf expression localizes specifically to glial cells, while adult flies express DmManf in both glia and neurons (95, 96). DmManf null mutants were reported to be late embryonic lethal with severe cuticular defects (95). DmManf mutants appeared to have a massive loss of dopamine neurites and low levels of dopamine, although, interestingly, neuronal somas persisted. In fruit flies, dopamine is required for cuticle formation, including cuticle pigmentation and sclerotization (97). Therefore, extremely low levels of dopamine might at least partially explain disorganization of cuticle layers in DmManf mutant flies. In contrast to DmManf mutant flies, removal of DmManf from neurons or glia did not affect morphology of dopamine neurons in the brain, indicating unaltered neuronal differentiation during development (96). Thus, DmManf was concluded to not be required in a cell-autonomous fashion for the survival of dopamine neurons in Drosophila. Furthermore, overexpression of DmManf in neurons or glia had no impact on the dopamine system of the fly (96).
MANF ortholog in Caenorhabditis elegans nematodes, manf-1, has a wide expression pattern, including the intestine and central nervous system (CNS) (98). Nematodes with mutated manf-1 were reported to have no clear morphological defects but had a slower growth rate (99). Regarding the dopamine system, these manf-1 mutant nematodes had a normal number of dopamine neuron somas and neurites in the first days of adulthood. However, from day 3 onwards, manf-1 mutants started to lose dopamine neurons, accruing a loss of almost 30% by day 9 (99). In agreements with these results, Hartman and others (100) showed that their manf-1 mutants had normal development in dopamine neurons, regular locomotion, and even similar sensitivity to the 6-OHDA toxin as controls in early adulthood. However, they observed that tunicamycin caused modulation of the innate immune system in manf-1 mutants, making them resistant to the growth arrest observed in tunicamycin-treated WT nematodes. An increase in the expression of hsp-4 (an ortholog of human GRP78) was documented in the manf-1 mutant nematodes (98, 99) and upregulation of UPR genes in DmManf mutant fruit flies (101), in line with observations of increased UPR activation in response to loss of MANF in mice.
Expression of both CDNF and MANF is present in zebrafish. In the zebrafish brain, MANF is expressed in neurons (102). Knocking down MANF expression by antisense splice-blocking morpholino oligonucleotides produced manf mutants with a rather normal appearance and normal swimming behavior (102). However, a detailed analysis of the manf mutant zebrafish revealed reduced dopamine levels and decreased expression of th1 and th2 transcripts, both of the duplicated th genes in zebrafish. Moreover, manf mutant fish had region-specific alterations in the TH1 and TH2 cell populations, now in the prethalamus and periventricular nucleus of the posterior tuberculum. The decrease in TH1-positive cell number and reduced dopamine levels could be rescued by manf mRNA injections to the embryos. Overexpression of manf in WT fish embryos, by contrast, had no effect on dopamine neuron development.
Zebrafish express CDNF predominantly in the eye and the brain, which differs from the expression pattern seen in mice. The physiological function of CDNF in zebrafish was addressed using CRISPR-Cas9-mediated removal of CDNF expression. Zebrafish lacking CDNF were discovered to have elevated levels of th2 and normal th1 levels in the brain (103). Furthermore, they had normal dopamine levels but a misbalance in dopamine neuron clusters; more TH2-positive neurons were present in the caudal hypothalamus and less TH1-positive neurons in the prethalamus. However, dopamine neurons were not the only neuron population affected by loss of CDNF, as both histamine and GABA neurons were reduced in the brain. Thus, lack of CDNF affects several neurotransmitter circuits in zebrafish. Furthermore, cdnf mutant zebrafish had increased swimming speed and altered shoaling pattern, indicating reduced sociability.
CDNF, MANF, and in vivo PD models
Toxin-based animal models of PD are extensively used in preclinical studies to investigate possible treatments for the disease. Neurotoxins are used as tools to induce degeneration of the nigrostriatal pathway to mimic the dopamine neuron loss present in patients with PD. The widely used method is a unilateral lesion model caused by intrastriatal injections of 6-hydroxydopamine (6-OHDA), which destroys dopamine nerve terminals in the striatum and cell somas in the SN. Lesion-induced deficits are normally evaluated by drug-induced rotational behavior and quantification of the survival of dopamine neurons and their striatal fiber integrity. Compared with intrastriatal injections, 6-OHDA injections to the medial forebrain bundle (MFB) cause a more severe loss of dopamine neurons in the SN (104), modeling a late-stage PD. Another commonly used neurotoxin, 1-methyl-4-phenyl-1,2,3,4-tetrahydropyridine (MPTP), can be administered systemically and outcome measures include behavioral assessment in addition to the analysis of the dopamine neuron survival. Moreover, exposure to rotenone, an inhibitor of complex I, also causes neuropathological features of PD in rats (105). Instead of toxin, αSyn preformed fibril (PFF)-induced PD model involves injection of PFFs into the brain, which leads to formation of Lewy body-like aggregates (106). This model has become a valuable tool to examine the effect of potential therapeutic compounds on αSyn pathology.
At the time of its discovery, CDNF was tested in the rat 6-OHDA model of PD (Table 2). When CDNF protein was administered before the 6-OHDA-induced lesion, it could protect dopamine neurons from degeneration. The amphetamine-induced rotations were decreased and TH-positive fiber density in the striatum and the amount of dopamine neurons were higher in the SN than in vehicle-treated rats (12). Intrastriatal administration of CDNF after 6-OHDA lesion induction could also improve rotational asymmetry and survival of dopamine neurons in the SN. Thus, CDNF exhibited both protective and restorative activity for dopamine neurons. Two-week chronic delivery of CDNF also demonstrated similar restorative activity in the 6-OHDA model of PD by reducing amphetamine-induced rotational asymmetry and improving TH-positive cell survival (14). In a later study, a smaller dose of CDNF provided only modest protection of dopamine neurons (107). In the abovementioned studies, CDNF has mainly been delivered to the striatum. From the striatum, injected CDNF has been shown to diffuse well, reach the SN, and localize into dopamine neurons (14, 113). The half-life of CDNF in brain tissue is 5.5 h when injected intrastriatally (113).
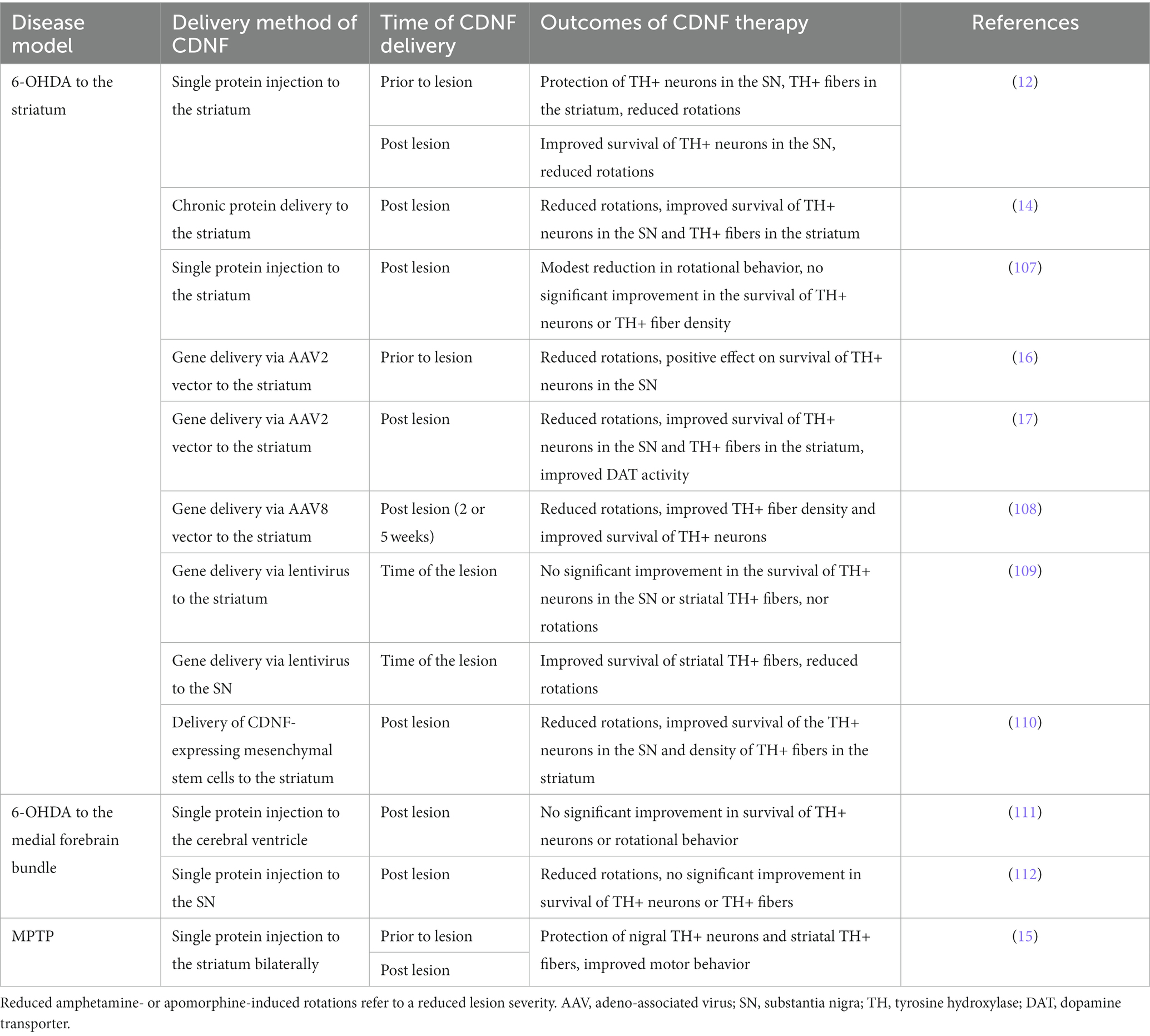
Table 2. Summary of studies examining the effect of CDNF in the rodent models of Parkinson’s disease.
Several studies have investigated the effect of CDNF overexpression via virus vector-mediated delivery in the 6-OHDA model (Table 2). In general, enhancement of intracellular CDNF expression has been shown to be an efficient way to protect dopamine neurons in PD models. Adeno-associated virus 2 (AAV2)-mediated overexpression of CDNF in the striatum produced different levels of neuroprotection depending on the time of CDNF induction in relation to the 6-OHDA lesion. When CDNF was expressed before the 6-OHDA lesion, it had only a modest protective effect (16), but when delivered after 6-OHDA, CDNF was able to reduce amphetamine-induced rotations, improve the survival of the dopamine neurons in the SN, and even improve DAT activity (17). In another study, CDNF expression was induced via lentivirus vector in either the striatum or the SN. CDNF improved rotational asymmetry and dopamine fiber density only when it was expressed in the striatum (109). In the study of Wang et al. (108), AAV8-CDNF reduced amphetamine-induced rotational behavior and improved survival of TH-positive neurons more efficiently when administered 2 weeks after 6-OHDA than 5 weeks after lesion induction, reflecting mild and severe lesions, respectively. CDNF-expressing mesenchymal stem cells injected to the striatum was also shown to improve motor behavior and neuronal survival (110). In the mouse MPTP model of PD, CDNF—applied either before or after toxin—improved motor behavior and survival of nigral dopamine neurons (15).
Therapeutic efficacy of CDNF may depend on several factors such as the severity of PD model and the route of administration. After injections of 6-OHDA into the MFB, CDNF has not achieved neuronal restoration in rodent PD models (111, 112). CDNF could improve only apomorphine-induced rotational asymmetry (112). It is plausible that this model of late-stage PD is too severe for CDNF to rescue dopamine neurons. These data suggest that CDNF therapy would be more suitable for early-stage PD patients. While in most preclinical studies CDNF is delivered intrastriatally, these two studies used different routes for the delivery: the intracerebral ventricle (111) and the SN (112). In a naïve rat brain, CDNF injected to the SN is not transported to the striatum, indicating that anterograde transport of CDNF is not active, in contrast to the observed active retrograde CDNF transport (114). However, injected CDNF localizes into TH-positive neurons in the SN, enabling intracellular protective activity (114).
Interestingly, a recent study indicated that CDNF can bind αSyn in vitro, and CDNF injected into brain tissue was shown to interact with αSyn using proximity ligation assay (115). Furthermore, CDNF was shown to decrease αSyn internalization into primary hippocampal neurons (115). Neuroprotective effects of CDNF were also demonstrated against toxicity of αSyn oligomers in primary cultures of mesencephalic neurons and in dopamine neurons differentiated from the Neuro 2a cell line (116). Based on these findings, CDNF was tested in the PFF-induced rodent model of PD. Despite no loss of TH-positive neurons or degeneration of TH-positive fibers, CDNF improved paw use in the cylinder test, measuring asymmetry of paw use (115).
Many studies have reported of significantly improved outcomes when CDNF protein is administered with another protein or with another therapeutic strategy. For instance, CDNF treatment has been tested in combination with GDNF in the rat 6-OHDA model of PD (107). Co-administration of CDNF and GDNF reduced amphetamine-induced ipsilateral turns and protected TH-positive neurons in the SN more efficiently than proteins alone. CDNF overexpression has also been boosted with a simultaneous gene delivery of aromatic L-amino acid decarboxylase (AADC), which is an enzyme involved in dopamine synthesis and has been shown to alleviate symptoms of PD patients in clinical trials (117). Overexpression of AADC on top of CDNF increased striatal dopamine levels more efficiently than gene delivery of CDNF alone (108). Enhancing the effect of CDNF gene therapy by simultaneous MANF delivery promoted survival of TH-positive neurons in the SN and fibers in the striatum in addition to reduced rotational turns, suggesting a synergistic effect of CDNF and MANF on neurorestoration (109). An alternative strategy was presented in a study where CDNF injections were combined with a deep brain stimulation of the subthalamic nucleus (STN) in a rat MFB 6-OHDA model (112). Intranigral delivery of CDNF improved efficacy of subthalamic stimulation through implanted electrodes in a cylinder test and in the survival of TH-positive fibers. Delivery of CDNF was also combined with a STN lesion, modeling chronic stimulation of the nucleus, and results showed amelioration of motor deficits (112). More recently, CDNF was administered with ventral mesencephalic (VM) grafted tissue to rats with 6-OHDA injections to the MFB. When CDNF was co-administered with VM grafted tissue, it improved the outcome of cell transplantation (111).
Given that CDNF provided positive effects in preclinical studies of PD in rodents, the efficacy of CDNF was also tested in nonhuman primates with a parkinsonian model to validate its translational potential. Although CDNF did not increase the survival of TH-positive neurons in 6-OHDA-lesioned marmoset monkeys, there was an increase in DAT binding activity in 6-OHDA-lesioned animals treated with CDNF (118).
Neuroprotective activity of MANF has been tested in different PD models through protein and gene delivery, as summarized in Table 3. A single injection of MANF protein to the striatum reduced amphetamine-induced rotations and improved the survival of TH-positive neurons in the SN when applied before 6-OHDA in unilaterally 6-OHDA-lesioned rats (13). Furthermore, MANF delivery after 6-OHDA improved amphetamine-induced turning behavior in two independent studies (13, 119). Chronic delivery of MANF protein for 2 weeks, by contrast, did not alleviate rotational behavior or the survival of dopamine neurons compared with vehicle-treated animals (14). In the same study, chronic delivery of GDNF also did not promote neuroprotection (14). Inspection of intrastriatally injected iodinated MANF showed transport to the cortex but not to the SN, indicating effective retrograde and inefficient anterograde transport (14). While lentivirus vector-mediated MANF overexpression in the striatum did not promote dopamine neuron function or survival in 6-OHDA model (109), intrastriatal AAV9-mediated gene delivery of MANF, in turn, showed a significant improvement in 6-OHDA-treated rats (120). Hao and others (120) reported an increase in the survival of midbrain dopamine neurons and an increase in striatal dopamine levels in addition to reduced amphetamine-induced rotations. Similarly, AAV8-vector-mediated MANF expression in the striatum resulted in improved motor behavior and survival of TH-positive neurons in the SN in a 6-OHDA rat model of PD (121). Improved motor behavior, increased survival of TH-positive cells in the SN, and increased striatal dopamine levels were also observed in the mouse MPTP model of PD in response to MANF delivery to the striatum (122). In the rotenone model of PD, MANF gene transfer resulted in improved motor behavior and enhanced survival of TH-positive neurons in the SN (123).
Regarding the mechanism of action, it is important to note that increasing CDNF and MANF levels in a naïve rodent brain by protein injections has no or very modest effect on the dopamine system. Administration of CDNF or MANF does not affect TH-positive cell number in the SN, TH-positive fiber density in the striatum, or TH activity in the intact rat brain (15, 107, 124). In addition, CDNF injection does not alter TH or DAT expression or amphetamine-induced locomotor activity in naïve mice or rats (15, 107, 124). One finding indicates that MANF, but not CDNF, increases potassium- and amphetamine-evoked dopamine release and dopamine turnover, reflecting increased dopamine metabolism (124). Overall, these findings emphasize that the mechanism of action of CDNF and MANF warrants investigation in stressed cells.
Molecular structure of CDNF/MANF proteins
CDNF/MANF family members are small, soluble proteins with a molecular weight of about 18 kDa that consist of two domains and a flexible linker region, allowing free movement of the domains (116, 125, 126). A characteristic feature in the primary structure of CDNF/MANF proteins is eight conserved cysteine residues, which form four cysteine bridges that are important for protein folding and biological activity (Figure 3A). The three-dimensional structure of human CDNF and MANF was resolved using x-ray crystallography and nuclear magnetic resonance (NMR) spectroscopy (116, 125–127). The amino (N)-terminal domain of CDNF/MANF contains three disulfide bridges and is conformationally similar to saposin-like proteins, which commonly interact with lipids and membranes (125, 126, 128) (Figure 3B). In accord with this, MANF was shown to interact with sulfoglycolipid 3-O-sulfogalactosylceramide (sulfatide), which is present in the outer leaflet of the plasma membrane of various cell types (98). In the nervous system, sulfatide is abundant in the myelin sheath of oligodendrocytes and Schwann cells and it is also present in neurons and astrocytes (129). Bai and others (98) suggested that sulfatide is associated with MANF during its secretion, and sulfatide binding facilitates MANF uptake to cells. MANF together with sulfatide alleviated ER stress of manf-1 null mutants in C. elegans and protected mammalian cell lines under ER stress and hypoxia-glucose deprivation. Unlike MANF, CDNF does not bind sulfatide (98), and lipid partners of CDNF have not been described to date. MANF was also reported to interact with lipid kinase phosphatidylinositol 5-phosphate 4-kinase type-2 beta (PIP4K2b), which phosphorylates phosphatidylinositol-5-phosphate to phosphatidylinositol-4,5-bisphosphate in the ER (130), thus connecting MANF to the biology of phosphoinositide lipids. MANF interaction with PIP4K2b was suggested to enhance PIP4K2b activity and regulate insulin signaling in the hypothalamus (130).
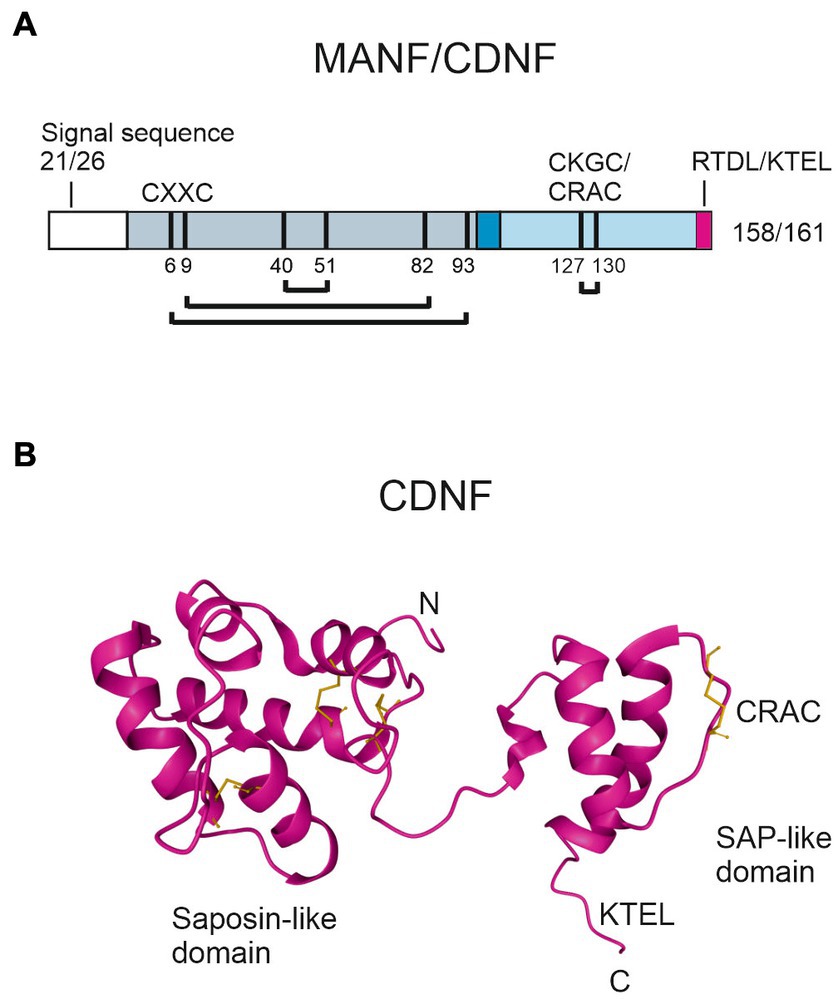
Figure 3. Structure of human MANF and CDNF proteins. (A) Schematic presentation of the primary structure of MANF/CDNF. After predicted cleavage of the signal peptide directing newly synthetized MANF/CDNF to the ER, mature MANF/CDNF consists of 158/161 amino acid residues, respectively. N-terminal saposin-like domain of mature protein is indicated in gray, linker region connecting the two domains in dark blue, and C-terminal SAP-like domain in light blue. A KDEL-like ER retention signal (pink) is located at the C-terminus. Eight conserved cysteines are indicated as black bars and numbered according to MANF. The cysteines can form four disulfide bridges, three in the N-terminal domain and one in the C-terminal domain (connecting lines). The C-terminal cysteine bridge is in CXXC motif (CKGC/CRAC in MANF/CDNF, respectively), which is crucial for MANF activity. (B) NMR solution structure of CDNF (PDB ID: 4BIT) (116). Disulfide bridges (in yellow) connect α-helices in the N-terminal domain, stabilizing the saposin-like fold. Characteristic for SAP-like proteins is the helix–loop–helix structure, which is a putative DNA binding motif.
The carboxy (C)-terminal domain of CDNF/MANF proteins has a helix–loop–helix arrangement and is structurally similar to members of SAF-A/B, Acinus, and PIAS protein superfamily (SAP), which are putative DNA binding proteins (131) (Figure 3B). The SAP-like domain of CDNF/MANF is important for the neuroprotective activity. Recombinant C-terminal MANF protein lacking the N-terminal domain, which was microinjected to mouse superior cervical ganglion (SCG) sympathetic neurons, promoted neuron survival against etoposide-induced apoptosis in vitro (126). An important functional motif in the SAP-domain of CDNF/MANF is a cysteine-X-X-cysteine (CXXC) sequence that can be found in the catalytic center of thiol-disulfide oxidoreductases (132). Microinjected, intracellular MANF protein with a mutated CXXC motif CKGS in the SAP domain was inactive against etoposide- or thapsigargin-induced apoptosis in SCG or dorsal root ganglion (DRG) sensory neurons in vitro as well as in vivo in a rat model of stroke, where mutated MANF CKGS was injected into brain parenchyma (133). Another potential CXXC motif is located in the N-terminal domain of CDNF/MANF (Figure 3A). However, studies did not show oxidoreductase activity in MANF (133–135).
ER resident proteins have a characteristic lysine-aspartic acid-glutamic acid-leucine (KDEL) sequence that binds to KDEL receptor (KDELR), which functions in retrieving proteins from the Golgi back to the ER, preventing their secretion and maintaining ER homeostasis. KDEL-like sequence is located at the very end of the C-terminal domain of CDNF/MANF proteins (134, 136, 137) (Figures 3A,B). Deletion of the KDEL-like sequence, i.e., KTEL in CDNF and RTDL in MANF, respectively, increased CDNF and MANF secretion (136, 138, 139). Interestingly, it has been suggested that KDELR can translocate to the cell surface particularly during ER stress (136). In the plasma membrane, KDELR could bind CDNF and MANF via the C-terminal KDEL-like sequences (136, 140). The presence of KDEL-like sequence was required for the cardioprotective activity of recombinant CDNF in a rat model of myocardial ischemia (140). However, recombinant MANF lacking the RTDL sequence was neuroprotective in a rat model of stroke in vivo (133), suggesting that KDELR binding is not essential for the cytoprotective activity of extracellularly delivered MANF.
CDNF and MANF molecular interactions
In addition to extracellular neurotrophic activities, CDNF and MANF are intracellular ER stress-regulated proteins that function in the ER. MANF promoter contains ER stress response elements (ERSE) I and ERSE II, and UPR transcription factors XBP1s and ATF6 can induce MANF expression (134, 141, 142). An ER stressor tunicamycin increased CDNF expression in cardiomyocytes in vitro (140) and in mouse kidney and liver in vivo (20), indicating that CDNF expression is also regulated by ER stress.
Mechanistic studies on CDNF/MANF activity in ER stress and UPR indicate that an important functional partner for MANF, and also for CDNF, is the ER chaperone GRP78. Protein–protein interaction studies have demonstrated that both MANF (143–145) and CDNF (20) bind GRP78. In the first study, calcium-dependent interaction of MANF with GRP78 was shown to regulate MANF secretion (143). Later, it was suggested that MANF functions as a nucleotide exchange inhibitor (NEI) of GRP78 in the ER (Figure 4A). MANF could prolong GRP78 interaction with its client proteins, which would help to maintain protein folding homeostasis in the ER (144). Interaction of MANF and GRP78 was localized between the C-terminal domain of MANF and the nucleotide-binding domain (NBD) of GRP78, indicating that MANF is indeed a cofactor and not a client of GRP78 (144, 145). Interestingly, MANF can bind ATP, and the presence of ATP prevents the interaction of MANF with GRP78, as tested with purified proteins (145). However, the functional importance of the MANF-ATP interaction is not clear and warrants further studies. Differently from what one might expect, interaction with GRP78 was not needed for the neuroprotective activity of MANF. MANF mutants unable to bind GRP78 (144) still promoted the survival of dopamine and SCG neurons under ER stress in vitro (119, 145), and a MANF mutant unable to protect neurons perfectly bound GRP78 (119).
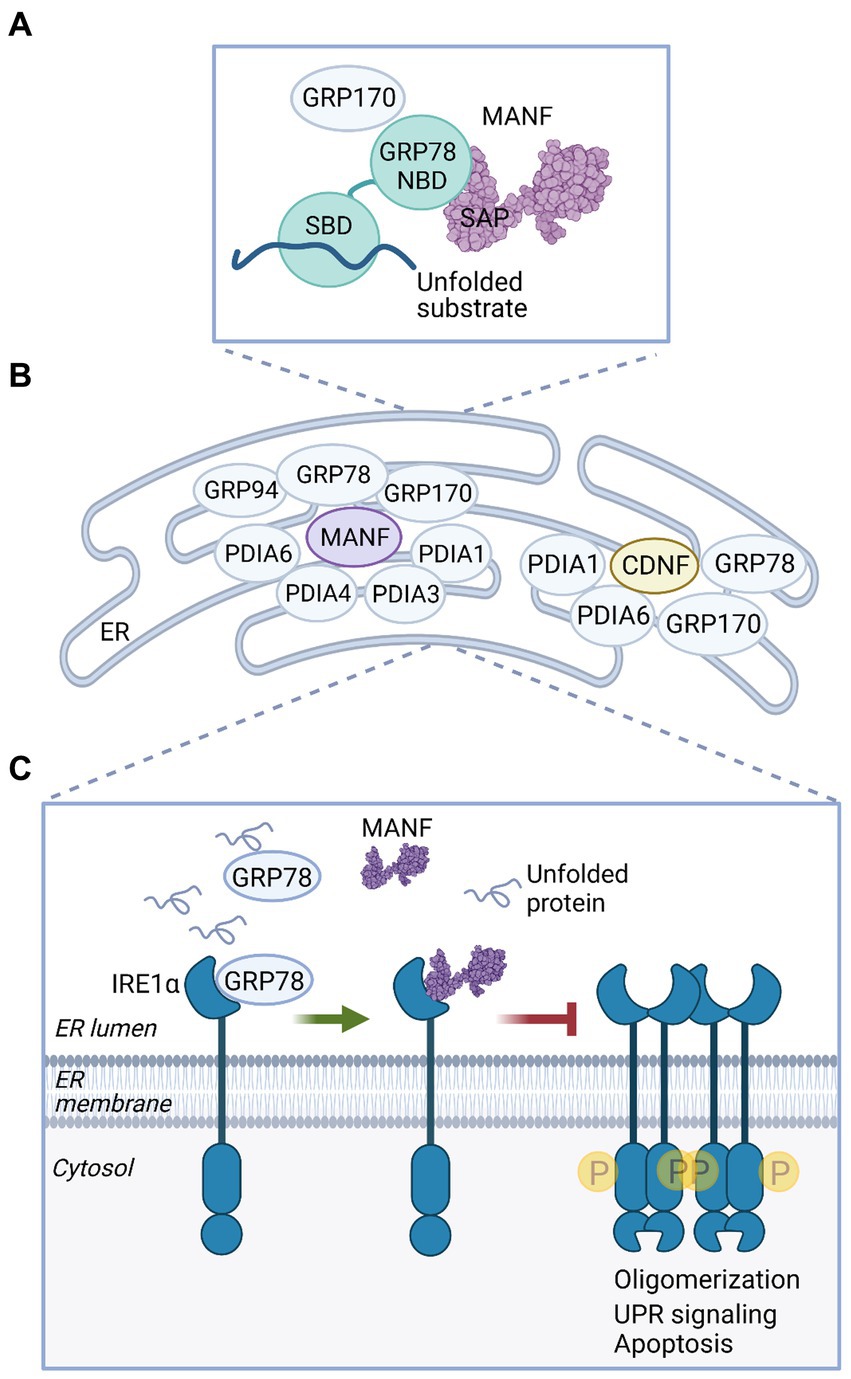
Figure 4. MANF and CDNF activities in the ER. (A) MANF functions as a nucleotide exchange inhibitor for GRP78. MANF (PDB ID: 2KVD) (126) interacts with the nucleotide-binding domain (NBD) of GRP78 via the C-terminal SAP-like domain and prolongs GRP78 interaction with a substrate protein, thus promoting protein folding homeostasis in the ER (144). GRP170 is a nucleotide exchange factor of GRP78 and was identified as an interaction partner of MANF and CDNF (20, 87, 145). SBD, substrate binding domain. (B) MANF and CDNF are members of the ER-localized multiprotein complex of chaperones, as demonstrated in affinity-purification mass spectrometry experiments (20, 145). (C) MANF regulates IRE1α signaling under ER stress. When GRP78 is dissociated from IRE1α to bind unfolded proteins, MANF is able to bind IRE1α. MANF binding inhibits IRE1α phosphorylation, oligomerization, and downstream signaling, preventing cell death (119).
MANF and CDNF protein–protein interactomes were characterized using affinity purification-mass spectrometry (AP-MS) in human embryonic kidney HEK293 and rat insulinoma INS1 cell lines induced to overexpress MANF or CDNF (20, 145). From both HEK293 and INS1 cell lines, GRP78, GRP170, PDIA1, and PDIA6 were identified as ER-localized interaction partners for MANF (Figure 4B), and MANF interaction with GRP78, GRP170, and PDIA6 in the ER was also verified by bimolecular fluorescence complementation assay (BiFC) in cells (145). This finding was in accordance with other studies reporting GRP170 and PDIA6 as MANF interacting proteins (87, 144). GRP170 functions as a nucleotide exchange factor for GRP78 (146), and it is possible that MANF interaction with GRP170 is mediated by GRP78. PDIA1 and PDIA6 are members of the large family of protein disulfide isomerases (PDIs) that catalyze oxidative protein folding in the ER, a process where disulfide bonds are formed and proteins acquire native conformation (147). In addition to the abovementioned partners, GRP94, PDIA3, and PDIA4 were identified as potential MANF interacting chaperones and isomerases in HEK293 cells (145) (Figure 4B). These data suggest that MANF is a component of an ER-localized multiprotein complex containing several chaperones (145). Although MANF apparently does not have oxidoreductase activity (133), it may still have chaperone activity. MANF was shown to function as a chaperone specifically in reductive stress in vitro, and it was suggested that the cysteine residues of MANF are important for the chaperone activity (148).
Resembling results from MANF interactome analysis (145), characterization of ER-localized interactome of CDNF based on AP-MS analysis of HEK293 and INS1 cells identified GRP78, GRP170, PDIA1, and PDIA6 as potential ER luminal binding partners for CDNF (20) (Figure 4B). CDNF interaction with GRP78 and GRP170 in the ER was also verified using BiFC in vitro. Further analysis suggested that the interaction between CDNF and GRP78 takes place between the C-terminal domain of CDNF and the NBD of GRP78, which indicates that CDNF may function as a cofactor of GRP78 (20). However, the exact interaction interface between CDNF and GRP78 proteins remains to be defined. Based on these studies CDNF and MANF have common interaction partners in the ER, suggesting that they share similar activity mechanisms in cells.
A recent mechanistic study proposed a novel function for MANF as a direct regulator of UPR receptor IRE1α activation during ER stress (119) (Figure 4C). MANF was shown to directly bind to the luminal domain of IRE1α, suggesting that IRE1α functions as an ER-located receptor of MANF. MANF was shown to decrease ER stress-induced IRE1α phosphorylation, oligomerization, and downstream signaling. In cultured primary dopamine neurons, extracellularly added MANF decreased levels of sXbp1 and Atf6 under thapsigargin-induced ER stress. Importantly, the results suggested that MANF ability to interact with IRE1α was necessary for the neuroprotective activity of both extracellularly delivered MANF and intracellular, microinjected MANF during ER stress. Unlike WT MANF, mutant MANF unable to bind IRE1α neither promoted the survival of cultured primary dopamine neurons under thapsigargin-induced stress when added to the media nor promoted the survival of SCG neurons under tunicamycin-induced ER stress when MANF was microinjected into the cytoplasm. Interestingly, GRP78 prevented MANF binding to IRE1α, indicating that MANF and GRP78 binding sites may overlap in IRE1α. Thus, the authors suggested that MANF binds IRE1α under ER stress when GRP78 is dissociated from IRE1α and regulates IRE1α signaling to prevent irreversible ER stress, thus promoting cell survival. In addition to IRE1α, MANF also binds luminal domains of PERK and ATF6, albeit with lower affinity (119). However, the functional role of these interactions remains to be elucidated. Whether CDNF can interact with the UPR sensors similarly to MANF is unknown.
Neuroprotection of CDNF and MANF by regulating UPR
Unlike classical NTFs, survival-promoting effects of exogenous CDNF and MANF on naïve dopamine neurons and other types of naïve neurons seem to be very low or possibly even non-existent (12, 20, 145). When CDNF was added to the culture media, it did not promote the survival of naïve primary SCG neurons, motoneurons, or DRG neurons in vitro, differently from the respective control NTFs, i.e., nerve growth factor, ciliary neurotrophic factor, and GDNF (12). However, under ER stress, CDNF and MANF show neuroprotective effects, suggesting that stress conditions are required for their activity. CDNF and MANF added to the culture media of mouse embryonic dopamine neurons increased neuron survival under thapsigargin-induced ER stress (20, 145). Furthermore, CDNF and MANF treatment significantly decreased the expression of UPR genes sXBP1, Atf6 and Grp78, implying that CDNF and MANF can both regulate IRE1α and ATF6 UPR branches in primary dopamine neurons under ER stress (20, 145). Additionally, protective effects of recombinant MANF against ER stress-induced apoptosis were demonstrated in tunicamycin-treated primary cortical neurons in culture (149).
Intracellular CDNF or MANF, delivered by microinjection of recombinant protein or plasmid cDNA, promoted survival of SCG neurons under tunicamycin-induced ER stress (20, 145). Similarly, microinjection of MANF encoding cDNA plasmid promoted survival of DRG neurons under thapsigargin-induced ER stress (133). The survival-promoting effects of CDNF and MANF in SCG neurons were dependent on the activity of the PERK and IRE1α pathways (20, 145). These in vitro studies suggest that CDNF and MANF can promote neuron survival both as extracellular paracrine factors and as intracellular proteins, and both the extracellular and intracellular activities may engage UPR pathways. The survival-promoting activity of CDNF and MANF is possibly related to IRE1α and ATF6 pathways in dopamine neurons and IRE1α and PERK pathways in SCG neurons, suggesting that CDNF and MANF may regulate multiple UPR pathways in parallel (20, 145), and the regulatory activity may differ between different types of neurons and conditions of ER stress.
Alleviation of ER stress has been proposed to account for neuroprotective activity of CDNF and MANF also in animal models of PD. This possible mechanism was investigated in the study of Voutilainen and others (107) in a rat 6-OHDA model of PD, where GRP78 expression and phosphorylation of eIF2α were analyzed at 4 weeks post lesion and 4 h post-CDNF delivery. The results indicated that CDNF had a trend for decreasing both GRP78 expression and p-eIF2α levels relative to vehicle-treated rats (107). In a rodent model of intracerebral hemorrhage, by contrast, CDNF administration was shown to efficiently reduce expression of GRP78, ATF6α, ATF4, and CHOP in the hemorrhage area of the striatum (94). Furthermore, a recent study demonstrated that CDNF administration attenuated activation of all three UPR pathways in motoneurons isolated from the superoxide dismutase (SOD)-G93A mice model of amyotrophic lateral sclerosis (ALS) (150). Thus, CDNF is capable of downregulating UPR signaling in vivo. The involvement of ER stress in MANF-mediated neuroprotection of dopamine neurons has been investigated in C. elegans. The neuroprotective effect of MANF was lost when UPR genes were blocked by RNAi in the mutant αSyn overexpression model in nematodes, suggesting that MANF protects neurons via affecting UPR signaling (151).
Striatal administration of MANF was shown to increase SOD activity and glutathione production in the MPTP model of PD, demonstrating antioxidative activity of MANF (122). MANF was also shown to increase levels of antioxidant regulator NRF2 and promote the translocation of NRF2 to the nucleus (152). Moreover, MANF increased activation of the AKT/GSK3β-Nrf-2 signaling axis in the 6-OHDA model of PD in vivo, suggesting that this would serve as a potential neuroprotective mechanism (121). Furthermore, a recent study revealed that CDNF could also increase transcriptional activity of NRF2 in an experimental model of intracerebral hemorrhage (94).
MANF and CDNF in inflammation
Microglia is a major immune cell type that functions in inflammatory response in the CNS. Resident microglia are constantly monitoring the tissue environment in the brain parenchyma in search of damage. In response to injury, microglia are activated and acquire macrophage morphology. Activated microglia release various factors including pro-inflammatory and anti-inflammatory cytokines, produce reactive oxygen and nitrogen species, and function in phagocytosis (153). Inflammatory cytokines produced by activated microglia participate in the progression of PD pathogenesis (154).
Increasing evidence suggests that CDNF and MANF possess immunomodulatory functions that contribute to their cytoprotective and neuroprotective properties (56, 88, 94, 111, 155). Although MANF was originally isolated as a secreted factor from the culture medium of a mesencephalic astrocyte cell line (18), it was noted that in normal conditions MANF expression is low in brain glial cells but can be induced upon glial cell activation in stress conditions (156). In vitro, an increase in MANF expression was shown in cultured primary microglia, astrocytes, and oligodendrocytes upon treatment with ER stressor tunicamycin (156).
Evidence indicates that MANF can attenuate inflammatory response by repressing NF-κB signaling in ER stress and inflammatory conditions (71, 157, 158). A recent study identified neuroplastin (NPTN) as a receptor of MANF on the cell surface (159). In the presence of NPTN, MANF was shown to repress ER stress-induced NF-kB activation and expression of pro-inflammatory IL-6 and Cxcl-1, and pro-apoptotic Chop in vitro. The authors suggested that MANF binding to NPTN reduces inflammation, ER stress, and subsequent cell death (159). It was also suggested that MANF could directly regulate NF-κB signaling in cells. MANF may enter the cell nucleus upon ER stress and interact via the C-terminal SAP domain with the DNA binding domain of p65 subunit of NF-κB, and thus, prevent transcription of NF-κB target genes (71). In a subsequent study, the authors suggested that SUMO1 promotes nuclear import of MANF and regulates MANF interaction with p65 (158).
Unlike MANF, CDNF does not bind to NPTN (159) but shows anti-inflammatory effects. Lentivirus vector-mediated overexpression of CDNF in cultured primary astrocytes was demonstrated to decrease ER stressor tunicamycin-induced astrocyte damage and secretion of inflammatory cytokines IL-1β, IL-6, and TNFα (160). CDNF treatment decreased secretion of pro-inflammatory prostaglandin E2 and IL-1β from LPS-induced primary microglia and prevented LPS-induced inflammatory injury in the microglia by inhibiting phosphorylation of JNK (161). CDNF treatment was also reported to decrease inflammatory response in LPS-induced primary microglia via inhibiting Akt phosphorylation and FoxO1/mTOR signaling (162). In a study by Nadella and others (155), the effect of CDNF on inflammation levels was investigated in vivo in the 6-OHDA model of PD. CDNF overexpression via a plasmid vector reduced activated microglia and IL-6 levels in the SN. As mentioned before, CDNF co-administration was shown to attenuate neuroinflammation and promote survival of dopamine neurons in grafted fetal ventral mesencephalic tissue that was transplanted into the striatum of 6-OHDA-treated hemiparkinsonian rats (111). In addition, CDNF administration decreased recruitment of microglia/macrophages to the graft and decreased production of pro-inflammatory cytokine IL-1β and TNFα levels in the grafted striatum. In vitro studies using 6-OHDA-stimulated BV2 microglial cell line indicated that CDNF treatment decreased pro-inflammatory markers iNOS, CD11b, and IL-6, while increasing expression of IL-10 and Arg-1, suggesting that CDNF treatment enhances microglial polarization toward pro-repair state macrophages (111). The ability of CDNF to attenuate pro-inflammatory cytokines was also demonstrated in the intracerebral hemorrhage model, where administration of CDNF reduced levels of IL-6, TNF-α, IL-1β, and IFN-γ, while increasing the level of IL-10 in the peri-hematoma striatum on days 1 and 3 after hematoma induction (94). Similarly, intracerebroventricular delivery of CDNF after ischemic stroke induction in mice decreased production of TNF-α and IL-1β (163). Thus, CDNF may have immune modulatory effects in the CNS, but the exact mechanisms need to be identified.
Discussion
The biology of endogenous CDNF and MANF proteins in the mammalian dopamine system has remained largely unknown until recent years when papers describing the effects of CDNF and MANF depletion in the mouse dopaminergic system on the CNS and ENS were published (52, 81, 85, 90).
Collectively, the results from different animal models show that the role of endogenous CDNF and MANF in the brain and specifically in the dopaminergic system varies between model organisms. In mice, loss of endogenous CDNF, MANF, or both does not impair the survival of the nigrostriatal dopamine neurons (52, 81, 90). However, loss of mouse CDNF does lead to a functional defect in the nigral dopamine neurons and to specific degeneration of enteric dopamine neurons (81, 85). Differently from Manf−/− mice, in the fruit fly and zebrafish, MANF deletion leads to defects in development of dopamine neurons (95, 102). Dopaminergic phenotype was also detected in zebrafish lacking CDNF (103). These differences in observed dopaminergic phenotypes can be partially explained by different tissue expression patterns of CDNF and MANF in the model organisms. In the developing fruit fly, DmMANF is expressed by glial cells (95), while in the two-week-old mouse brain, MANF is already highly expressed in neurons (51).
The main brain phenotype of Cdnf−/− mice associates CDNF with DAT activity (81). Amphetamine-induced hyperactivity response of Cdnf−/− mice is notable and ex vivo experiments explain them partially. However, understanding of the mechanism for how CDNF regulates the reverse activity of DAT is lacking. Whether CDNF induces post-translational modifications in DAT in response to amphetamine, altering its activity or plasma membrane localization, is not known. Moreover, the effect of CDNF on dopamine synapses should be studied in detail.
The indispensability of CDNF in the development and maintenance of enteric dopamine neurons vs. the dispensability of CDNF in the maintenance of midbrain dopamine neurons in mice is a paradox warranting further investigations. Midbrain dopamine neurons originate from the mesodiencephalic floor plate and are formed earlier during development than the enteric dopamine neurons that originate from the neural crest in mammals (4). However, these neurons share a similar dopamine-related gene expression pattern and requirement of similar factors, such as GDNF, TGF-β and BMP, for development, function, or survival in the long term (4). Comparison of transcriptomes between nigral and enteric dopamine neurons of Cdnf−/− mice has not been performed but could assist in the analysis of increased vulnerability of enteric dopamine neurons lacking CDNF. It is important to note that CDNF is expressed in most enteric dopamine neurons (85), while no studies have identified co-localization of CDNF in nigral dopamine neurons. There are no reports about alterations in the number of enteric dopamine neurons of PD patients (4). Furthermore, expression of CDNF in enteric neurons of PD patients has not been evaluated; however, colonic biopsies could be informative regarding potential changes in CDNF levels. Since loss of CDNF impairs gut functionality (81, 85), one might ask whether CDNF administration would improve functionality and alleviate constipation in PD patients.
It would be interesting to ascertain the degree of similarity/difference in the biological roles of CDNF and MANF. CDNF is present only in vertebrates, while MANF is highly evolutionarily conserved and described even in sponges belonging to the phylum Porifera (164). The phenotypes of Cdnf−/− and Manf−/− mice are strikingly different (25, 81), and the phenotype of Cdnf−/−::Manf−/− mice resembles Manf−/− mice, indicating no significant redundancy between CDNF and MANF (52). In the skeletal muscle, there seems to be functional compensation between CDNF and MANF since Cdnf−/−::Manf−/− mice have exacerbated UPR activation compared with Cdnf−/− and Manf−/− mice (52). While Cordero-Llana and others (109) suggested a synergistic neurorestorative effect of virally delivered CDNF and MANF on nigral dopamine neurons in a 6-OHDA model of PD, simultaneous deletion of endogenous CDNF and MANF did not result in loss of nigral dopamine neurons in aged mice (52). To further elucidate roles of CDNF and MANF in the midbrain dopamine system, studies using CDNF- and MANF-ablated mice in PD models could be informative. Possibly, loss of CDNF, MANF, or both can increase the vulnerability of midbrain dopamine neurons to toxin-induced stress in PD models similarly to that demonstrated using ER stressors to neurons lacking MANF (90). Thus far, the role of CDNF and MANF in the maintenance of midbrain dopamine neurons in rodents has been studied using models of embryonic gene deletion, which might result in compensatory mechanisms during mouse development, masking the direct effects of the gene deletion. Therefore, it would be interesting to generate mouse models where CDNF and MANF are ablated in adult mice.
Based on studies using human post-mortem brain tissue, UPR activation seems to be linked to pathophysiology of PD. However, it is not known whether changes in endogenous MANF or CDNF expression in the brain tissue are associated with UPR activation in PD patients. Therefore, it would be informative to assess MANF and CDNF expression in human brain tissue in relation to αSyn pathology and UPR markers. An interesting question is whether MANF and CDNF could be used as biomarkers for PD since both proteins can be detected in blood circulation, which is an easily accessible matrix. Increased levels of circulating MANF were detected in PD (54) and acute ischemic stroke (61), conditions related to the brain. When ER stress-regulated proteins in the blood were studied as potential biomarkers for PD, it was found that MANF together with three other proteins, PDIA1, PDIA3, and clusterin in combination with age and gender confounders were able to discriminate PD patients from the non-PD group (165). These studies suggest that the biomarker potential of MANF in PD should be investigated further. An open question is, of course, whether MANF levels in the blood can directly reflect brain physiology or is the supposed association of MANF with PD pathophysiology indirect. It might be useful to investigate MANF and CDNF levels in cerebrospinal fluid, which should better reflect biochemical changes in the brain of PD patients.
Resolving the mechanism of CDNF and MANF neuroprotective action is important for the development of efficient CDNF/MANF-based therapies for PD. CDNF and MANF can function both inside the cells in the ER and extracellularly, possibly in a paracrine or autocrine manner in vivo. Both modes of action, extracellular and intracellular, can alleviate ER stress in neurons. Furthermore, in vitro studies in neurons suggest that MANF and CDNF can decrease the activity of two or even three UPR pathways in parallel to promote neuron survival under ER stress (20, 119, 145). Characterization of molecular mechanism of CDNF/MANF in UPR regulation and its timing in relation to acute or chronic ER stress events would be informative and could help to develop targeted MANF/CDNF-based interventions to modulate activity of UPR pathways and enhance neuronal survival, possibly also in PD patients in the future.
After many years of speculation about possible lipid binding (166), MANF interaction with sulfatide lipid was reported in 2018 (98). However, elucidation of the role of sulfatide binding in MANF uptake and cytoprotective activity against ER stress necessitates further investigations. Mechanistic information about how MANF-sulfatide is internalized to cells, where it is localized in the cells, and how it alleviates ER stress is currently lacking. CDNF ability to bind lipids is completely unknown. Moreover, NPTN, the novel plasma membrane receptor for MANF, does not bind CDNF (159), suggesting that CDNF has other receptors on the cell surface. Structural features that determine MANF binding to NPTN have not yet been fully characterized.
In the ER lumen, two important mechanistic roles for MANF were recently reported. Firstly, it was suggested that MANF interacts with GRP78 as a collaborator that functions as a NEI of GRP78, thus helping to maintain protein homeostasis (144). Secondly, MANF was shown to directly interact with IRE1α and decrease IRE1α activity in chronic ER stress to promote cell survival (119). Since the MANF binding site to IRE1α overlapped with that of GRP78, and GRP78 binding to IRE1α was stronger than that of MANF, the authors suggested that MANF could bind to IRE1α only when GRP78 is dissociated, as is the case under severe ER stress (119). An additional feature of MANF is that it can interact either directly or indirectly with a set of chaperones and isomerases in the ER (145), being involved in regulation of protein folding homeostasis. How these additional interactions affect the above-mentioned functions of MANF remains unknown. For example, MANF partners PDIA1 and PDIA6 also regulate IRE1α activity (167, 168). The potential chaperone activity of MANF itself (148) also requires further investigations.
Increasing evidence indicates that the cytoprotective activities of MANF and CDNF are related to their ability to dampen inflammatory response in microglia and astrocytes by reducing expression and secretion of proinflammatory cytokines. MANF was reported to regulate activity of the NF-κB pathway (71, 157, 158), and NPTN was shown to mediate the anti-inflammatory effect of MANF (159). Immune modulatory effects of MANF on macrophage polarization toward pro-repair phenotype in tissue injury have been described (88), and CDNF may also affect polarization of microglia/macrophages in vitro (111). Aging is related to impairment in the regulation of immune system function, leading to chronic, non-resolved low-grade inflammation. Dysregulated immune response can contribute to the gradual development of PD years before the start of clinical motor symptoms (154). Moreover, alterations in MANF levels have been reported in autoimmune and inflammatory diseases (53, 67, 71) (Table 1). Investigations of the roles of CDNF and MANF in the immune system, inflammatory response, and in association with UPR would be useful for developing future therapies also for PD.
When evaluating the therapeutic potential of CDNF in PD, it is important to consider possible side effects. CDNF and MANF delivered to brain parenchyma do not have any obvious impact on naïve rodent brains, suggesting a low risk for therapeutic side effects. Interestingly, CDNF has been shown to improve long-term memory in both WT mice and APP/PS1 mice, modeling Alzheimer’s disease (169). The mechanism behind improved memory was not identified, but no association emerged between CDNF and neurogenesis in the hippocampus or amyloid pathology. Recently, CDNF administration was demonstrated to increase Bdnf levels in the hippocampus in a N171-82Q mouse model of Huntington’s disease (170). Thus, the effects of CDNF on memory and cognitive functions as well as on BDNF signaling certainly warrant further investigations.
Recently, MANF was associated with rejuvenating effects in chronic parabiosis experiments in mice, suggesting that MANF has anti-aging properties (56). Thus, one could consider how to increase endogenous levels of CDNF and MANF in humans to promote healthy aging and possibly prevent or delay the development of neurodegenerative diseases. Dietary restriction and exercise are potential ways to increase health span (171, 172). Circulating MANF levels were shown to increase in therapeutic fasting in humans, suggesting that MANF is associated with fasting-induced beneficial effects (55). Interestingly, exercise was reported to induce endogenous CDNF expression in the cerebral cortex of naïve rats and in the striatum of 6-OHDA-lesioned rats (173, 174). Exercise was also shown to increase CDNF expression in the rat spinal cord in a 6-OHDA model of PD (175), indicating that exercise might have a broader effect on CDNF regulation in the CNS. These findings highlight the potential of exercise as a non-pharmacological approach to increase CDNF expression.
In conclusion, CDNF and MANF are neuroprotective proteins that can modulate UPR signaling and inhibit inflammatory processes, both of which are potentially relevant to PD pathogenesis. Since ER stress and inflammation are interconnected processes, deeper understanding of their complex interplay and the potential roles of CDNF and MANF in these processes, could benefit development of new therapeutic strategies for PD.
Author contributions
PL drafted the manuscript. EP and PL wrote the paper, prepared figures, edited the manuscript, and accepted the final version. All authors contributed to the article and approved the submitted version.
Funding
This work was financially supported by the Academy of Finland (grant 343299), the Jane and Aatos Erkko Foundation, and Cure Parkinson’s Trust UK. Open access funding was provided by University of Helsinki Library.
Acknowledgments
The authors thank Maria Lindahl and Mart Saarma for helpful comments on the manuscript. Figures 1, 2, 4 were created with BioRender.com. Figure 1 was adapted from BioRender (2019). UPR Signaling (ATF6, PERK, IRE1) (https://app.biorender.com/biorender-templates/figures/all/t-5dd9a632e8baa5007f62f161-upr-signaling-atf6-perk-ire1). The image in Figure 3B was created from PDB ID: 4BIT (116) using Mol* 3D Viewer (176) on RCSB PDB (RCSB.org).
Conflict of interest
PL is an inventor in the CDNF- and MANF-related patents (7,452,969; 9,592,270) owned by Herantis Pharma Plc.
The remaining author declares that the research was conducted in the absence of any commercial or financial relationships that could be construed as a potential conflict of interest.
Publisher’s note
All claims expressed in this article are solely those of the authors and do not necessarily represent those of their affiliated organizations, or those of the publisher, the editors and the reviewers. Any product that may be evaluated in this article, or claim that may be made by its manufacturer, is not guaranteed or endorsed by the publisher.
References
1. Dorsey, ER, Sherer, T, Okun, MS, and Bloem, BR. The emerging evidence of the Parkinson pandemic. J Parkinsons Dis. (2018) 8:S3–8. doi: 10.3233/JPD-181474
2. Spillantini, MG, Schmidt, ML, Lee, VMY, Trojanowski, JQ, Jakes, R, and Goedert, M. Alpha-synuclein in Lewy bodies. Nature. (1997) 388:839–40. doi: 10.1038/42166
3. Cheng, HC, Ulane, CM, and Burke, RE. Clinical progression in Parkinson disease and the neurobiology of axons. Ann Neurol. (2010) 67:715–25. doi: 10.1002/ana.21995
4. Chalazonitis, A, Rao, M, and Sulzer, D. Similarities and differences between nigral and enteric dopaminergic neurons unravel distinctive involvement in Parkinson’s disease. NPJ Parkinsons Dis. (2022) 8:50. doi: 10.1038/s41531-022-00308-9
5. Kalia, LV, and Lang, AE. Parkinson’s disease. Lancet. (2015) 386:896–912. doi: 10.1016/S0140-6736(14)61393-3
6. Huang, EJ, and Reichardt, LF. Neurotrophins: roles in neuronal development and function. Annu Rev Neurosci. (2001) 24:677–736. doi: 10.1146/annurev.neuro.24.1.677
7. Airaksinen, MS, and Saarma, M. The GDNF family: signalling, biological functions and therapeutic value. Nat Rev Neurosci. (2002) 3:383–94. doi: 10.1038/nrn812
8. Poo, MM. Neurotrophins as synaptic modulators. Nat Rev Neurosci. (2001) 2:24–32. doi: 10.1038/35049004
9. Lin, LF, Doherty, DH, Lile, JD, Bektesh, S, and Collins, F. GDNF: a glial cell line-derived neurotrophic factor for midbrain dopaminergic neurons. Science. (1993) 260:1130–2. doi: 10.1126/science.8493557
10. Kotzbauer, PT, Lampe, PA, Heuckeroth, RO, Golden, JP, Creedon, DJ, Johnson Jr, EM, et al. Neurturin, a relative of glial-cell-line-derived neurotrophic factor. Nature. (1996) 384:467–70. doi: 10.1038/384467a0
11. Sidorova, YA, and Saarma, M. Can growth factors cure Parkinson’s disease? Trends Pharmacol Sci. (2020) 41:909–22. doi: 10.1016/j.tips.2020.09.010
12. Lindholm, P, Voutilainen, MH, Laurén, J, Peränen, J, Leppänen, VM, Andressoo, JO, et al. Novel neurotrophic factor CDNF protects and rescues midbrain dopamine neurons in vivo. Nature. (2007) 448:73–7. doi: 10.1038/nature05957
13. Voutilainen, MH, Bäck, S, Pörsti, E, Toppinen, L, Lindgren, L, Lindholm, P, et al. Mesencephalic astrocyte-derived neurotrophic factor is neurorestorative in rat model of Parkinson’s disease. J Neurosci. (2009) 29:9651–9. doi: 10.1523/JNEUROSCI.0833-09.2009
14. Voutilainen, MH, Bäck, S, Peränen, J, Lindholm, P, Raasmaja, A, Männistö, PT, et al. Chronic infusion of CDNF prevents 6-OHDA-induced deficits in a rat model of Parkinson’s disease. Exp Neurol. (2011) 228:99–108. doi: 10.1016/j.expneurol.2010.12.013
15. Airavaara, M, Harvey, BK, Voutilainen, MH, Shen, H, Chou, J, Lindholm, P, et al. CDNF protects the nigrostriatal dopamine system and promotes recovery after MPTP treatment in mice. Cell Transplant. (2012) 21:1213–23. doi: 10.3727/096368911X600948
16. Bäck, S, Peränen, J, Galli, E, Pulkkila, P, Lonka-Nevalaita, L, Tamminen, T, et al. Gene therapy with AAV2-CDNF provides functional benefits in a rat model of Parkinson’s disease. Brain Behav. (2013) 3:75–88. doi: 10.1002/brb3.117
17. Ren, X, Zhang, T, Gong, X, Hu, G, Ding, W, and Wang, X. AAV2-mediated striatum delivery of human CDNF prevents the deterioration of midbrain dopamine neurons in a 6-hydroxydopamine induced parkinsonian rat model. Exp Neurol. (2013) 248:148–56. doi: 10.1016/j.expneurol.2013.06.002
18. Petrova, P, Raibekas, A, Pevsner, J, Vigo, N, Anafi, M, Moore, MK, et al. MANF: a new mesencephalic, astrocyte-derived neurotrophic factor with selectivity for dopaminergic neurons. J Mol Neurosci. (2003) 20:173–88. doi: 10.1385/JMN:20:2:173
19. Apostolou, A, Shen, Y, Liang, Y, Luo, J, and Fang, S. Armet, a UPR-upregulated protein, inhibits cell proliferation and ER stress-induced cell death. Exp Cell Res. (2008) 314:2454–67. doi: 10.1016/j.yexcr.2008.05.001
20. Eesmaa, A, Yu, LY, Goos, H, Danilova, T, Noges, K, Pakarinen, E, et al. CDNF interacts with ER chaperones and requires UPR sensors to promote neuronal survival. Int J Mol Sci. (2022) 23:9489. doi: 10.3390/ijms23169489
21. Lindahl, M, Saarma, M, and Lindholm, P. Unconventional neurotrophic factors CDNF and MANF: structure, physiological functions and therapeutic potential. Neurobiol Dis. (2017) 97:90–102. doi: 10.1016/j.nbd.2016.07.009
22. Jntti, M, and Harvey, BK. Trophic activities of endoplasmic reticulum proteins CDNF and MANF. Cell Tissue Res. (2020) 382:83–100. doi: 10.1007/s00441-020-03263-0
23. Kim, Y, Park, SJ, and Chen, YM. Mesencephalic astrocyte-derived neurotrophic factor (MANF), a new player in endoplasmic reticulum diseases: structure, biology, and therapeutic roles. Transl Res. (2017) 188:1–9. doi: 10.1016/j.trsl.2017.06.010
24. Lõhelaid, H, Anttila, JE, Liew, HK, Tseng, KY, Teppo, J, Stratoulias, V, et al. UPR responsive genes Manf and Xbp1 in stroke. Front Cell Neurosci. (2022) 16:900725. doi: 10.3389/fncel.2022.900725
25. Lindahl, M, Danilova, T, Palm, E, Lindholm, P, Võikar, V, Hakonen, E, et al. MANF is indispensable for the proliferation and survival of pancreatic β cells. Cell Rep. (2014) 7:366–75. doi: 10.1016/j.celrep.2014.03.023
26. Huttunen, HJ, Booms, S, Sjogren, M, Kerstens, V, Johansson, J, Holmnas, R, et al. Intraputamenal cerebral dopamine Neurotrophic factor in Parkinson’s disease: a randomized, Double-blind, multicenter phase 1 trial. Mov Disord. (2023). doi: 10.1002/mds.29426 [Epub ahead of print].
27. Lindholm, P, and Saarma, M. Cerebral dopamine neurotrophic factor protects and repairs dopamine neurons by novel mechanism. Mol Psychiatry. (2022) 27:1310–21. doi: 10.1038/s41380-021-01394-6
28. Walter, P, and Ron, D. The unfolded protein response: from stress pathway to homeostatic regulation. Science. (2011) 334:1081–6. doi: 10.1126/science.1209038
29. Hetz, C, and Saxena, S. ER stress and the unfolded protein response in neurodegeneration. Nat Rev Neurol. (2017) 13:477–91. doi: 10.1038/nrneurol.2017.99
30. Oakes, SA, and Papa, FR. The role of endoplasmic reticulum stress in human pathology. Annu Rev Pathol. (2015) 10:173–94. doi: 10.1146/annurev-pathol-012513-104649
31. Cox, JS, Shamu, CE, and Walter, P. Transcriptional induction of genes encoding endoplasmic reticulum resident proteins requires a transmembrane protein kinase. Cells. (1993) 73:1197–206. doi: 10.1016/0092-8674(93)90648-A
32. Yoshida, H, Matsui, T, Yamamoto, A, Okada, T, and Mori, K. XBP1 mRNA is induced by ATF6 and spliced by IRE1 in response to ER stress to produce a highly active transcription factor. Cells. (2001) 107:881–91. doi: 10.1016/S0092-8674(01)00611-0
33. Maurel, M, Chevet, E, Tavernier, J, and Gerlo, S. Getting RIDD of RNA: IRE1 in cell fate regulation. Trends Biochem Sci. (2014) 39:245–54. doi: 10.1016/j.tibs.2014.02.008
34. Harding, HP, Zhang, Y, Bertolotti, A, Zeng, H, and Ron, D. Perk is essential for translational regulation and cell survival during the unfolded protein response. Mol Cell. (2000) 5:897–904. doi: 10.1016/S1097-2765(00)80330-5
35. Hetz, C, Zhang, K, and Kaufman, RJ. Mechanisms, regulation and functions of the unfolded protein response. Nat Rev Mol Cell Biol. (2020) 21:421–38. doi: 10.1038/s41580-020-0250-z
36. Harding, HP, Novoa, I, Zhang, YH, Zeng, HQ, Wek, R, Schapira, M, et al. Regulated translation initiation controls stress-induced gene expression in mammalian cells. Mol Cell. (2000) 6:1099–108. doi: 10.1016/S1097-2765(00)00108-8
37. Novoa, I, Zeng, HQ, Harding, HP, and Ron, D. Feedback inhibition of the unfolded protein response by GADD34-mediated dephosphorylation of eIF2 alpha. J Cell Biol. (2001) 153:1011–21. doi: 10.1083/jcb.153.5.1011
38. Haze, K, Yoshida, H, Yanagi, H, Yura, T, and Mori, K. Mammalian transcription factor ATF6 is synthesized as a transmembrane protein and activated by proteolysis in response to endoplasmic reticulum stress. Mol Biol Cell. (1999) 10:3787–99. doi: 10.1091/mbc.10.11.3787
39. Bertolotti, A, Zhang, Y, Hendershot, LM, Harding, HP, and Ron, D. Dynamic interaction of BiP and ER stress transducers in the unfolded-protein response. Nat Cell Biol. (2000) 2:326–32. doi: 10.1038/35014014
40. Karagoz, GE, Acosta-Alvear, D, Nguyen, HT, Lee, CP, Chu, F, and Walter, P. An unfolded protein-induced conformational switch activates mammalian IRE1. elife. (2017) 6:e30700. doi: 10.7554/eLife.30700
41. Wang, P, Li, J, Tao, J, and Sha, B. The luminal domain of the ER stress sensor protein PERK binds misfolded proteins and thereby triggers PERK oligomerization. J Biol Chem. (2018) 293:4110–21. doi: 10.1074/jbc.RA117.001294
42. Hoozemans, JJ, van Haastert, ES, Eikelenboom, P, de Vos, RA, Rozemuller, JM, and Scheper, W. Activation of the unfolded protein response in Parkinson’s disease. Biochem Biophys Res Commun. (2007) 354:707–11. doi: 10.1016/j.bbrc.2007.01.043
43. Mercado, G, Castillo, V, Soto, P, Lopez, N, Axten, JM, Sardi, SP, et al. Targeting PERK signaling with the small molecule GSK2606414 prevents neurodegeneration in a model of Parkinson’s disease. Neurobiol Dis. (2018) 112:136–48. doi: 10.1016/j.nbd.2018.01.004
44. Selvaraj, S, Sun, Y, Watt, JA, Wang, S, Lei, S, Birnbaumer, L, et al. Neurotoxin-induced ER stress in mouse dopaminergic neurons involves downregulation of TRPC1 and inhibition of AKT/mTOR signaling. J Clin Invest. (2012) 122:1354–67. doi: 10.1172/JCI61332
45. Baek, JH, Whitfield, D, Howlett, D, Francis, P, Bereczki, E, Ballard, C, et al. Unfolded protein response is activated in Lewy body dementias. Neuropathol Appl Neurobiol. (2016) 42:352–65. doi: 10.1111/nan.12260
46. Baek, JH, Mamula, D, Tingstam, B, Pereira, M, He, Y, and Svenningsson, P. GRP78 level is altered in the brain, but not in plasma or cerebrospinal fluid in Parkinson’s disease patients. Front Neurosci. (2019) 13:697. doi: 10.3389/fnins.2019.00697
47. Esteves, AR, and Cardoso, SM. Differential protein expression in diverse brain areas of Parkinson’s and Alzheimer’s disease patients. Sci Rep. (2020) 10:13149. doi: 10.1038/s41598-020-70174-z
48. Heman-Ackah, SM, Manzano, R, Hoozemans, JJM, Scheper, W, Flynn, R, Haerty, W, et al. Alpha-synuclein induces the unfolded protein response in Parkinson’s disease SNCA triplication iPSC-derived neurons. Hum Mol Genet. (2017) 26:4441–50. doi: 10.1093/hmg/ddx331
49. Lindholm, P, Peränen, J, Andressoo, JO, Kalkkinen, N, Kokaia, Z, Lindvall, O, et al. MANF is widely expressed in mammalian tissues and differently regulated after ischemic and epileptic insults in rodent brain. Mol Cell Neurosci. (2008) 39:356–71. doi: 10.1016/j.mcn.2008.07.016
50. Wang, H, Ke, Z, Alimov, A, Xu, M, Frank, JA, Fang, S, et al. Spatiotemporal expression of MANF in the developing rat brain. PLoS One. (2014) 9:e90433. doi: 10.1371/journal.pone.0090433
51. Danilova, T, Galli, E, Pakarinen, E, Palm, E, Lindholm, P, Saarma, M, et al. Mesencephalic astrocyte-derived Neurotrophic factor (MANF) is highly expressed in mouse tissues with metabolic function. Front Endocrinol. (2019) 10:765. doi: 10.3389/fendo.2019.00765
52. Pakarinen, E, Lindholm, P, Saarma, M, and Lindahl, M. CDNF and MANF regulate ER stress in a tissue-specific manner. Cell Mol Life Sci. (2022) 79:124. doi: 10.1007/s00018-022-04157-w
53. Galli, E, Härkönen, T, Sainio, MT, Ustav, M, Toots, U, Urtti, A, et al. Increased circulating concentrations of mesencephalic astrocyte-derived neurotrophic factor in children with type 1 diabetes. Sci Rep. (2016) 6:29058. doi: 10.1038/srep29058
54. Galli, E, Planken, A, Kadastik-Eerme, L, Saarma, M, Taba, P, and Lindholm, P. Increased serum levels of Mesencephalic astrocyte-derived Neurotrophic factor in subjects with Parkinson’s disease. Front Neurosci. (2019) 13:929. doi: 10.3389/fnins.2019.00929
55. Galli, E, Rossi, J, Neumann, T, Andressoo, JO, Drinda, S, and Lindholm, P. Mesencephalic astrocyte-derived Neurotrophic factor is Upregulated with therapeutic fasting in humans and diet fat withdrawal in obese mice. Sci Rep. (2019) 9:14318. doi: 10.3389/fendo.2019.00765
56. Sousa-Victor, P, Neves, J, Cedron-Craft, W, Ventura, PB, Liao, CY, Riley, RR, et al. MANF regulates metabolic and immune homeostasis in ageing and protects against liver damage. Nat Metab. (2019) 1:276–90. doi: 10.1038/s42255-018-0023-6
57. Liu, J, Zhou, C, Tao, X, Feng, L, Wang, X, Chen, L, et al. ER stress-inducible protein MANF selectively expresses in human spleen. Hum Immunol. (2015) 76:823–30. doi: 10.1016/j.humimm.2015.09.043
58. Liu, GM, Zeng, HD, Zhang, CY, and Xu, JW. Key genes associated with diabetes mellitus and hepatocellular carcinoma. Pathol Res Pract. (2019) 215:152510. doi: 10.1016/j.prp.2019.152510
59. Virachit, S, Mathews, KJ, Cottam, V, Werry, E, Galli, E, Rappou, E, et al. Levels of glial cell line-derived neurotrophic factor are decreased, but fibroblast growth factor 2 and cerebral dopamine neurotrophic factor are increased in the hippocampus in Parkinson’s disease. Brain Pathol. (2019) 29:813–25. doi: 10.1111/bpa.12730
60. Joshi, H, McIntyre, WB, Kooner, S, Rathbone, M, Gabriele, S, Gabriele, J, et al. Decreased expression of cerebral dopamine Neurotrophic factor in platelets of stroke patients. J Stroke Cerebrovasc Dis. (2020) 29:104502. doi: 10.1016/j.jstrokecerebrovasdis.2019.104502
61. Yang, F, Qu, YW, Yan, ZZ, Wang, DJ, Li, WB, and Yao, LF. Increased serum concentrations of Mesencephalic astrocyte-derived neurotrophic factor in patients and rats with ischemic stroke. J Stroke Cerebrovasc. (2022) 31:106752. doi: 10.1016/j.jstrokecerebrovasdis.2022.106752
62. Ren, ZY, Wang, YT, Chen, Q, Long, JC, Zhang, R, Wu, X, et al. Correlation of significantly decreased serum circulating Mesencephalic astrocyte-derived Neurotrophic factor level with an increased risk of future cardiovascular disease in adult patients with growth hormone deficiency. Front Endocrinol. (2021) 12:671126. doi: 10.3389/fendo.2021.671126
63. Liu, XC, Qi, XH, Fang, H, Zhou, KQ, Wang, QS, and Chen, GH. Increased MANF expression in the inferior temporal Gyrus in patients with Alzheimer disease. Frontiers in aging. Neuroscience. (2021):13. doi: 10.3389/fnagi.2021.639318
64. Hou, X, Liu, Q, Gao, YM, Yong, L, Xie, HY, Li, WT, et al. Mesencephalic astrocyte-derived neurotrophic factor reprograms macrophages to ameliorate acetaminophen-induced acute liver injury via p38 MAPK pathway. Cell Death Dis. (2022) 13:100. doi: 10.1038/s41419-022-04555-9
65. Wang, D, Hou, C, Cao, Y, Cheng, Q, Zhang, L, Li, H, et al. XBP1 activation enhances MANF expression via binding to endoplasmic reticulum stress response elements within MANF promoter region in hepatitis B. Int J Biochem Cell Biol. (2018) 99:140–6. doi: 10.1016/j.biocel.2018.04.007
66. Fu, JL, Nchambi, KM, Wu, H, Luo, X, An, XZ, and Liu, DF. Liraglutide protects pancreatic beta cells from endoplasmic reticulum stress by upregulating MANF to promote autophagy turnover. Life Sci. (2020) 252:117648. doi: 10.1016/j.lfs.2020.117648
67. Yang, L, Shen, WW, Shao, W, Zhao, Q, Pang, GZ, Yang, Y, et al. MANF ameliorates DSS-induced mouse colitis via restricting Ly6C(hi)CX3CR1(int) macrophage transformation and suppressing CHOP-BATF2 signaling pathway. Acta Pharmacol Sin. (2023) 44:1175–90. doi: 10.1038/s41401-022-01045-8
68. Xu, K, Zheng, P, Zhao, S, Wang, MY, Tu, DJ, Wei, Q, et al. MANF/EWSR1/ANXA6 pathway might as the bridge between hypolipidemia and major depressive disorder. Transl Psychiatry. (2022) 12:527. doi: 10.1038/s41398-022-02287-0
69. Wu, T, Liu, QH, Li, YP, Li, H, Chen, L, Yang, XP, et al. Feeding-induced hepatokine, Manf, ameliorates diet-induced obesity by promoting adipose browning via p38 MAPK pathway. J Exp Med. (2021) 218:e20201203. doi: 10.1084/jem.20201203
70. Wei, J, Wang, C, Yang, GY, Jia, YJ, Li, Y, Deng, WZ, et al. Decreased circulating MANF in women with PCOS is elevated by metformin therapy and is inversely correlated with insulin resistance and Hyperandrogenism. Horm Metab Res. (2020) 52:109–16. doi: 10.1055/a-1082-1080
71. Chen, L, Feng, L, Wang, X, du, J, Chen, Y, Yang, W, et al. Mesencephalic astrocyte-derived Neurotrophic factor is involved in inflammation by negatively regulating the NF-κB pathway. Sci Rep. (2015) 5:8133. doi: 10.1038/srep08133
72. de Seny, D, Baiwir, D, Bianchi, E, Cobraiville, G, Deroyer, C, Poulet, C, et al. New proteins contributing to immune cell infiltration and Pannus formation of synovial membrane from arthritis diseases. Int J Mol Sci. (2022) 23:434. doi: 10.3390/ijms23010434
73. Wu, T, Zhang, F, Yang, Q, Zhang, Y, Liu, Q, Jiang, W, et al. Circulating mesencephalic astrocyte-derived neurotrophic factor is increased in newly diagnosed prediabetic and diabetic patients, and is associated with insulin resistance. Endocr J. (2017) 64:403–10. doi: 10.1507/endocrj.EJ16-0472
74. Wang, C, Peng, JJ, Miao, H, Liu, DF, and Zhang, LL. Decreased plasma MANF levels are associated with type 2 diabetes. Biomed Environ Sci. (2021) 34:236–40. doi: 10.3967/bes2021.030
75. Choi, JM, Hong, JH, Chae, MJ, Hung, NP, Kang, HS, Ma, HI, et al. Analysis of mutations and the association between polymorphisms in the cerebral dopamine neurotrophic factor (CDNF) gene and Parkinson disease. Neurosci Lett. (2011) 493:97–101. doi: 10.1016/j.neulet.2011.02.013
76. Yang, Y, Yu, H, Li, W, Liu, B, Zhang, H, Ding, S, et al. Association between cerebral dopamine neurotrophic factor (CDNF) 2 polymorphisms and schizophrenia susceptibility and symptoms in the Han Chinese population. Behav Brain Funct. (2018) 14:1. doi: 10.1186/s12993-017-0133-4
77. Laruelle, M. Schizophrenia: from dopaminergic to glutamatergic interventions. Curr Opin Pharmacol. (2014) 14:97–102. doi: 10.1016/j.coph.2014.01.001
78. Montaser, H, Patel, KA, Balboa, D, Ibrahim, H, Lithovius, V, Näätänen, A, et al. Loss of MANF causes childhood-onset Syndromic diabetes due to increased endoplasmic reticulum stress. Diabetes. (2021) 70:1006–18. doi: 10.2337/db20-1174
79. Yavarna, T, al-Dewik, N, al-Mureikhi, M, Ali, R, al-Mesaifri, F, Mahmoud, L, et al. High diagnostic yield of clinical exome sequencing in middle eastern patients with Mendelian disorders. Hum Genet. (2015) 134:967–80. doi: 10.1007/s00439-015-1575-0
80. Ikäheimo, K, Herranen, A, Iivanainen, V, Lankinen, T, Aarnisalo, AA, Sivonen, V, et al. MANF supports the inner hair cell synapse and the outer hair cell stereocilia bundle in the cochlea. Life Sci Alliance. (2022) 5:e202101068. doi: 10.26508/lsa.202101068
81. Lindahl, M, Chalazonitis, A, Palm, E, Pakarinen, E, Danilova, T, Pham, TD, et al. Cerebral dopamine neurotrophic factor-deficiency leads to degeneration of enteric neurons and altered brain dopamine neuronal function in mice. Neurobiol Dis. (2020) 134:104696. doi: 10.1016/j.nbd.2019.104696
82. Kahlig, KM, Binda, F, Khoshbouei, H, Blakely, RD, McMahon, DG, Javitch, JA, et al. Amphetamine induces dopamine efflux through a dopamine transporter channel. Proc Natl Acad Sci U S A. (2005) 102:3495–500. doi: 10.1073/pnas.0407737102
83. Sulzer, D, Sonders, MS, Poulsen, NW, and Galli, A. Mechanisms of neurotransmitter release by amphetamines: a review. Prog Neurobiol. (2005) 75:406–33. doi: 10.1016/j.pneurobio.2005.04.003
84. Zhu, J, and Reith, MEA. Role of the dopamine transporter in the action of Psychostimulants, nicotine, and other drugs of abuse. Cns Neurol Disord. (2008) 7:393–409. doi: 10.2174/187152708786927877
85. Chalazonitis, A, Li, Z, Pham, TD, Chen, J, Rao, M, Lindholm, P, et al. Cerebral dopamine neurotrophic factor is essential for enteric neuronal development, maintenance, and regulation of gastrointestinal transit. J Comp Neurol. (2020) 528:2420–44. doi: 10.1002/cne.24901
86. Yang, S, Huang, S, Gaertig, MA, Li, XJ, and Li, S. Age-dependent decrease in chaperone activity impairs MANF expression, leading to Purkinje cell degeneration in inducible SCA17 mice. Neuron. (2014) 81:349–65. doi: 10.1016/j.neuron.2013.12.002
87. Bell, PA, Dennis, EP, Hartley, CL, Jackson, RM, Porter, A, Boot-Handford, RP, et al. Mesencephalic astrocyte-derived neurotropic factor is an important factor in chondrocyte ER homeostasis. Cell Stress Chaperones. (2019) 24:159–73. doi: 10.1007/s12192-018-0953-7
88. Neves, J, Zhu, J, Sousa-Victor, P, Konjikusic, M, Riley, R, Chew, S, et al. Immune modulation by MANF promotes tissue repair and regenerative success in the retina. Science. (2016) 353:aaf3646. doi: 10.1126/science.aaf3646
89. Herranen, A, Ikäheimo, K, Lankinen, T, Pakarinen, E, Fritzsch, B, Saarma, M, et al. Deficiency of the ER-stress-regulator MANF triggers progressive outer hair cell death and hearing loss. Cell Death Dis. (2020) 11:100. doi: 10.1038/s41419-020-2286-6
90. Pakarinen, E, Danilova, T, Võikar, V, Chmielarz, P, Piepponen, P, Airavaara, M, et al. MANF ablation causes prolonged activation of the UPR without Neurodegeneration in the mouse midbrain dopamine system. eNeuro. (2020) 7:ENEURO.0477–19.2019. doi: 10.1523/ENEURO.0477-19.2019
91. Tseng, KY, Danilova, T, Domanskyi, A, Saarma, M, Lindahl, M, and Airavaara, M. MANF is essential for Neurite extension and neuronal migration in the developing cortex. eNeuro. (2017) 4:ENEURO.0214-17.2017. doi: 10.1523/ENEURO.0214-17.2017
92. Wang, YC, Wen, W, Li, H, Clementino, M, Xu, H, Xu, M, et al. MANF is neuroprotective against ethanol-induced neurodegeneration through ameliorating ER stress. Neurobiol Dis. (2021) 148:105216. doi: 10.1016/j.nbd.2020.105216
93. Matlik, K, Anttila, JE, Kuan-Yin, T, Smolander, OP, Pakarinen, E, Lehtonen, L, et al. Poststroke delivery of MANF promotes functional recovery in rats. Sci Adv. (2018) 4:eaap8957. doi: 10.1126/sciadv.aap8957
94. Tseng, KY, Stratoulias, V, Hu, WF, Wu, JS, Wang, V, Chen, YH, et al. Augmenting hematoma-scavenging capacity of innate immune cells by CDNF reduces brain injury and promotes functional recovery after intracerebral hemorrhage. Cell Death Dis. (2023) 14:128. doi: 10.1038/s41419-022-05520-2
95. Palgi, M, Lindstrom, R, Peranen, J, Piepponen, TP, Saarma, M, and Heino, TI. Evidence that DmMANF is an invertebrate neurotrophic factor supporting dopaminergic neurons. Proc Natl Acad Sci U S A. (2009) 106:2429–34. doi: 10.1073/pnas.0810996106
96. Stratoulias, V, and Heino, TI. Analysis of the conserved neurotrophic factor MANF in the drosophila adult brain. Gene Expr Patterns. (2015) 18:8–15. doi: 10.1016/j.gep.2015.04.002
97. Yamamoto, S, and Seto, ES. Dopamine dynamics and signaling in drosophila: An overview of genes, drugs and behavioral paradigms. Exp Anim Tokyo. (2014) 63:107–19. doi: 10.1538/expanim.63.107
98. Bai, M, Vozdek, R, Hnizda, A, Jiang, C, Wang, B, Kuchar, L, et al. Conserved roles of C. elegans and human MANFs in sulfatide binding and cytoprotection. Nat Commun. (2018) 9:897. doi: 10.1038/s41467-018-03355-0
99. Richman, C, Rashid, S, Prashar, S, Mishra, R, Selvaganapathy, PR, and Gupta, BP. C. elegans MANF homolog is necessary for the protection of dopaminergic neurons and ER unfolded protein response. Front Neurosci. (2018) 12:544. doi: 10.3389/fnins.2018.00544
100. Hartman, JH, Richie, CT, Gordon, KL, Mello, DF, Castillo, P, Zhu, A, et al. MANF deletion abrogates early larval Caenorhabditis elegans stress response to tunicamycin and Pseudomonas aeruginosa. Eur J Cell Biol. (2019) 98:151043. doi: 10.1016/j.ejcb.2019.05.002
101. Palgi, M, Greco, D, Lindstrom, R, Auvinen, P, and Heino, TI. Gene expression analysis of Drosophilaa Manf mutants reveals perturbations in membrane traffic and major metabolic changes. BMC Genomics. (2012) 13:134. doi: 10.1186/1471-2164-13-134
102. Chen, YC, Sundvik, M, Rozov, S, Priyadarshini, M, and Panula, P. MANF regulates dopaminergic neuron development in larval zebrafish. Dev Biol. (2012) 370:237–49. doi: 10.1016/j.ydbio.2012.07.030
103. Chen, YC, Baronio, D, Semenova, S, Abdurakhmanova, S, and Panula, P. Cerebral dopamine Neurotrophic factor regulates multiple neuronal subtypes and behavior. J Neurosci. (2020) 40:6146–64. doi: 10.1523/JNEUROSCI.2636-19.2020
104. Zuch, CL, Nordstroem, VK, Briedrick, LA, Hoernig, GR, Granholm, AC, and Bickford, PC. Time course of degenerative alterations in nigral dopaminergic neurons following a 6-hydroxydopamine lesion. J Comp Neurol. (2000) 427:440–54. doi: 10.1002/1096-9861(20001120)427:3<440::AID-CNE10>3.0.CO;2-7
105. Betarbet, R, Sherer, TB, MacKenzie, G, Garcia-Osuna, M, Panov, AV, and Greenamyre, JT. Chronic systemic pesticide exposure reproduces features of Parkinson’s disease. Nat Neurosci. (2000) 3:1301–6. doi: 10.1038/81834
106. Luk, KC, Kehm, V, Carroll, J, Zhang, B, O’Brien, P, Trojanowski, JQ, et al. Pathological α-Synuclein transmission initiates Parkinson-like Neurodegeneration in nontransgenic mice. Science. (2012) 338:949–53. doi: 10.1126/science.1227157
107. Voutilainen, MH, de Lorenzo, F, Stepanova, P, Bäck, S, Yu, LY, Lindholm, P, et al. Evidence for an additive Neurorestorative effect of simultaneously administered CDNF and GDNF in Hemiparkinsonian rats: implications for different mechanism of action. eNeuro. (2017) 4:ENEURO.0117–16.2017. doi: 10.1523/ENEURO.0117-16.2017
108. Wang, L, Wang, Z, Xu, X, Zhu, R, Bi, J, Liu, W, et al. Recombinant AAV8-mediated intrastriatal gene delivery of CDNF protects rats against methamphetamine neurotoxicity. Int J Med Sci. (2017) 14:340–7. doi: 10.7150/ijms.18623
109. Cordero-Llana, O, Houghton, BC, Rinaldi, F, Taylor, H, Yáñez-Muñoz, RJ, Uney, JB, et al. Enhanced efficacy of the CDNF/MANF family by combined intranigral overexpression in the 6-OHDA rat model of Parkinson’s disease. Mol Ther. (2015) 23:244–54. doi: 10.1038/mt.2014.206
110. Jiaming, M, and Niu, C. Comparing neuroprotective effects of CDNF-expressing bone marrow derived mesenchymal stem cells via differing routes of administration utilizing an in vivo model of Parkinson’s disease. Neurol Sci. (2015) 36:281–7. doi: 10.1007/s10072-014-1929-8
111. Tseng, KY, Wu, JS, Chen, YH, Airavaara, M, Cheng, CY, and Ma, KH. Modulating microglia/macrophage activation by CDNF promotes transplantation of fetal ventral Mesencephalic graft survival and function in a Hemiparkinsonian rat model. Biomedicine. (2022) 10:1446. doi: 10.3390/biomedicines10061446
112. Huotarinen, A, Penttinen, AM, Bäck, S, Voutilainen, MH, Julku, U, Piepponen, TP, et al. Combination of CDNF and deep brain stimulation decreases neurological deficits in late-stage model Parkinson’s disease. Neuroscience. (2018) 374:250–63. doi: 10.1016/j.neuroscience.2018.01.052
113. Mätlik, K, Vihinen, H, Bienemann, A, Palgi, J, Voutilainen, MH, Booms, S, et al. Intrastriatally infused exogenous CDNF is Endocytosed and Retrogradely transported to Substantia Nigra. eNeuro. (2017) 4:ENEURO.0128–16.2017. doi: 10.1523/ENEURO.0128-16.2017
114. Albert, K, Renko, JM, Matlik, K, Airavaara, M, and Voutilainen, MH. Cerebral dopamine Neurotrophic factor diffuses around the brainstem and does not undergo anterograde transport after injection to the Substantia Nigra. Front Neurosci. (2019) 13:590. doi: 10.3389/fnins.2019.00590
115. Albert, K, Raymundo, DP, Panhelainen, A, Eesmaa, A, Shvachiy, L, Araújo, GR, et al. Cerebral dopamine neurotrophic factor reduces α-synuclein aggregation and propagation and alleviates behavioral alterations in vivo. Mol Ther. (2021) 29:2821–40. doi: 10.1016/j.ymthe.2021.04.035
116. Latge, C, Cabral, KM, de Oliveira, GA, Raymundo, DP, Freitas, JA, Johanson, L, et al. The solution structure and dynamics of full-length human cerebral dopamine Neurotrophic factor and its Neuroprotective role against α-Synuclein oligomers. J Biol Chem. (2015) 290:20527–40. doi: 10.1074/jbc.M115.662254
117. Muramatsu, S, Fujimoto, K, Kato, S, Mizukami, H, Asari, S, Ikeguchi, K, et al. A phase I study of aromatic L-amino acid decarboxylase gene therapy for Parkinson’s disease. Mol Ther. (2010) 18:1731–5. doi: 10.1038/mt.2010.135
118. Garea-Rodríguez, E, Eesmaa, A, Lindholm, P, Schlumbohm, C, König, J, Meller, B, et al. Comparative analysis of the effects of Neurotrophic factors CDNF and GDNF in a nonhuman primate model of Parkinson’s disease. PLoS One. (2016) 11:e0149776. doi: 10.1371/journal.pone.0149776
119. Kovaleva, V, Yu, LY, Ivanova, L, Shpironok, O, Nam, J, Eesmaa, A, et al. MANF regulates neuronal survival and UPR through its ER-located receptor IRE1α. Cell Rep. (2023) 42:112066. doi: 10.1016/j.celrep.2023.112066
120. Hao, F, Yang, C, Chen, SS, Wang, YY, Zhou, W, Hao, Q, et al. Long-term protective effects of AAV9-mesencephalic astrocyte-derived neurotrophic factor gene transfer in parkinsonian rats. Exp Neurol. (2017) 291:120–33. doi: 10.1016/j.expneurol.2017.01.008
121. Zhang, JX, Zhou, KG, Yin, YX, Jin, LJ, Tong, WF, Guo, J, et al. Mesencephalic astrocyte-derived neurotrophic factor (MANF) prevents the neuroinflammation induced dopaminergic neurodegeneration. Exp Gerontol. (2023) 171:112037. doi: 10.1016/j.exger.2022.112037
122. Liu, Y, Zhang, J, Jiang, M, Cai, Q, Fang, J, and Jin, L. MANF improves the MPP(+)/MPTP-induced Parkinson’s disease via improvement of mitochondrial function and inhibition of oxidative stress. Am J Transl Res. (2018) 10:1284–94.
123. Zhang, JX, Tong, WF, Jiang, M, Zhou, KG, Xiang, XR, He, YJ, et al. MANF inhibits alpha-Synuclein accumulation through activation of Autophagic pathways. Oxidative Med Cell Longev. (2022) 2022:7925686. doi: 10.1155/2022/7925686
124. Renko, JM, Bäck, S, Voutilainen, MH, Piepponen, TP, Reenilä, I, Saarma, M, et al. Mesencephalic astrocyte-derived Neurotrophic factor (MANF) elevates stimulus-evoked release of dopamine in freely-moving rats. Mol Neurobiol. (2018) 55:6755–68. doi: 10.1007/s12035-018-0872-8
125. Parkash, V, Lindholm, P, Peränen, J, Kalkkinen, N, Oksanen, E, Saarma, M, et al. The structure of the conserved neurotrophic factors MANF and CDNF explains why they are bifunctional. Protein Engineer Design Select. (2009) 22:233–41. doi: 10.1093/protein/gzn080
126. Hellman, M, Arumäe, U, Yu, LY, Lindholm, P, Peränen, J, Saarma, M, et al. Mesencephalic astrocyte-derived neurotrophic factor (MANF) has a unique mechanism to rescue apoptotic neurons. J Biol Chem. (2011) 286:2675–80. doi: 10.1074/jbc.M110.146738
127. Hoseki, J, Sasakawa, H, Yamaguchi, Y, Maeda, M, Kubota, H, Kato, K, et al. Solution structure and dynamics of mouse ARMET. FEBS Lett. (2010) 584:1536–42. doi: 10.1016/j.febslet.2010.03.008
128. Bruhn, H. A short guided tour through functional and structural features of saposin-like proteins. Biochem J. (2005) 389:249–57. doi: 10.1042/BJ20050051
129. Eckhardt, M. The role and metabolism of sulfatide in the nervous system. Mol Neurobiol. (2008) 37:93–103. doi: 10.1007/s12035-008-8022-3
130. Yang, S, Yang, HM, Chang, RB, Yin, P, Yang, Y, Yang, WL, et al. MANF regulates hypothalamic control of food intake and body weight. Communications. (2017) 8:579. doi: 10.1038/s41467-017-00750-x
131. Aravind, L, and Koonin, EV. SAP—a putative DNA-binding motif involved in chromosomal organization. Trends Biochem Sci. (2000) 25:112–4. doi: 10.1016/S0968-0004(99)01537-6
132. Berndt, C, Lillig, CH, and Holmgren, A. Thioredoxins and glutaredoxins as facilitators of protein folding. BBA-Mol Cell Res. (2008) 1783:641–50. doi: 10.1016/j.bbamcr.2008.02.003
133. Mätlik, K, Yu, LY, Eesmaa, A, Hellman, M, Lindholm, P, Peränen, J, et al. Role of two sequence motifs of mesencephalic astrocyte-derived neurotrophic factor in its survival-promoting activity. Cell Death Dis. (2015) 6:e2032. doi: 10.1038/cddis.2015.371
134. Mizobuchi, N, Hoseki, J, Kubota, H, Toyokuni, S, Nozaki, J, Naitoh, M, et al. ARMET is a soluble ER protein induced by the unfolded protein response via ERSE-II element. Cell Struct Funct. (2007) 32:41–50. doi: 10.1247/csf.07001
135. Hartley, CL, Edwards, S, Mullan, L, Bell, PA, Fresquet, M, Boot-Handford, RP, et al. Armet/Manf and Creld2 are components of a specialized ER stress response provoked by inappropriate formation of disulphide bonds: implications for genetic skeletal diseases. Hum Mol Genet. (2013) 22:5262–75. doi: 10.1093/hmg/ddt383
136. Henderson, MJ, Richie, CT, Airavaara, M, Wang, Y, and Harvey, BK. Mesencephalic astrocyte-derived neurotrophic factor (MANF) secretion and cell surface binding are modulated by KDEL receptors. J Biol Chem. (2013) 288:4209–25. doi: 10.1074/jbc.M112.400648
137. Liu, H, Zhao, CL, Zhong, L, Liu, J, Zhang, SX, Cheng, BH, et al. Key subdomains in the C-terminal of cerebral dopamine neurotrophic factor regulate the protein secretion. Biochem Biophys Res Commun. (2015) 465:427–32. doi: 10.1016/j.bbrc.2015.08.029
138. Norisada, J, Hirata, Y, Amaya, F, Kiuchi, K, and Oh-hashi, K. A comparative analysis of the molecular features of MANF and CDNF. PLoS One. (2016) 11:e0146923. doi: 10.1371/journal.pone.0146923
139. Galli, E, Lindholm, P, Kontturi, LS, Saarma, M, Urtti, A, and Yliperttula, M. Characterization of CDNF-secreting ARPE-19 cell clones for encapsulated cell therapy. Cell Transplant. (2019) 28:4213. doi: 10.1177/0963689719827943
140. Maciel, L, de Oliveira, DF, Mesquita, F, Souza, H, Oliveira, L, Christie, MLA, et al. New Cardiomyokine reduces myocardial ischemia/reperfusion injury by PI3K-AKT pathway via a putative KDEL-receptor binding. J Am Heart Assoc. (2021) 10:e019685. doi: 10.1161/JAHA.120.019685
141. Lee, AH, Iwakoshi, NN, and Glimcher, LH. XBP-1 regulates a subset of endoplasmic reticulum resident chaperone genes in the unfolded protein response. Mol Cell Biol. (2003) 23:7448–59. doi: 10.1128/MCB.23.21.7448-7459.2003
142. Oh-Hashi, K, Hirata, Y, and Kiuchi, K. Transcriptional regulation of mouse mesencephalic astrocyte-derived neurotrophic factor in Neuro2a cells. Cell Mol Biol Lett. (2013) 18:398–415. doi: 10.2478/s11658-013-0096-x
143. Glembotski, CC, Thuerauf, DJ, Huang, C, Vekich, JA, Gottlieb, RA, and Doroudgar, S. Mesencephalic astrocyte-derived neurotrophic factor protects the heart from ischemic damage and is selectively secreted upon sarco/endoplasmic reticulum calcium depletion. J Biol Chem. (2012) 287:25893–904. doi: 10.1074/jbc.M112.356345
144. Yan, Y, Rato, C, Rohland, L, Preissler, S, and Ron, D. MANF antagonizes nucleotide exchange by the endoplasmic reticulum chaperone BiP. Nat Commun. (2019) 10:541. doi: 10.1038/s41467-019-08450-4
145. Eesmaa, A, Yu, LY, Goos, H, Noges, K, Kovaleva, V, Hellman, M, et al. The cytoprotective protein MANF promotes neuronal survival independently from its role as a GRP78 cofactor. J Biol Chem. (2021) 100295. doi: 10.1016/j.jbc.2021.100295
146. Behnke, J, Feige, MJ, and Hendershot, LM. BiP and its nucleotide exchange factors Grp170 and Sil1: mechanisms of action and biological functions. J Mol Biol. (2015) 427:1589–608. doi: 10.1016/j.jmb.2015.02.011
147. Wang, L, and Wang, CC. Oxidative protein folding fidelity and redoxtasis in the endoplasmic reticulum. Trends Biochem Sci. (2023) 48:40–52. doi: 10.1016/j.tibs.2022.06.011
148. Arrieta, A, Blackwood, EA, Stauffer, WT, Santo Domingo, M, Bilal, AS, Thuerauf, DJ, et al. Mesencephalic astrocyte-derived neurotrophic factor is an ER-resident chaperone that protects against reductive stress in the heart. J Biol Chem. (2020) 295:7566–83. doi: 10.1074/jbc.RA120.013345
149. Yu, YQ, Liu, LC, Wang, FC, Liang, Y, Cha, DQ, Zhang, JJ, et al. Induction profile of MANF/ARMET by cerebral ischemia and its implication for neuron protection. J Cereb Blood Flow Metab. (2010) 30:79–91. doi: 10.1038/jcbfm.2009.181
150. De Lorenzo, F, Pl, JN, Beckett, L, Pilotto, F, Galli, E, Lindholm, P, et al. CDNF rescues motor neurons in three animal models of amyotrophic lateral sclerosis by targeting ER stress. Brain. (2023):awad087. doi: 10.1093/brain/awad087 [Epub ahead of print].
151. Zhang, ZY, Shen, YJ, Luo, H, Zhang, F, Peng, D, Jing, L, et al. MANF protects dopamine neurons and locomotion defects from a human α-synuclein induced Parkinson’s disease model in C. elegans by regulating ER stress and autophagy pathways. Exp Neurol. (2018) 308:59–71. doi: 10.1016/j.expneurol.2018.06.016
152. Zhang, J, Tong, W, Sun, H, Jiang, M, Shen, Y, Liu, Y, et al. Nrf2-mediated neuroprotection by MANF against 6-OHDA-induced cell damage via PI3K/AKT/GSK3β pathway. Exp Gerontol. (2017) 100:77–86. doi: 10.1016/j.exger.2017.10.021
153. Ransohoff, RM, and Cardona, AE. The myeloid cells of the central nervous system parenchyma. Nature. (2010) 468:253–62. doi: 10.1038/nature09615
154. Tansey, MG, Wallings, RL, Houser, MC, Herrick, MK, Keating, CE, and Joers, V. Inflammation and immune dysfunction in Parkinson disease. Nat Rev Immunol. (2022) 22:657–73. doi: 10.1038/s41577-022-00684-6
155. Nadella, R, Voutilainen, MH, Saarma, M, Gonzalez-Barrios, JA, Leon-Chavez, BA, Jiménez, JMD, et al. Transient transfection of human CDNF gene reduces the 6-hydroxydopamine-induced neuroinflammation in the rat substantia nigra. J Neuroinflammation. (2014) 11:209. doi: 10.1186/s12974-014-0209-0
156. Shen, Y, Sun, A, Wang, Y, Cha, D, Wang, H, Wang, F, et al. Upregulation of mesencephalic astrocyte-derived neurotrophic factor in glial cells is associated with ischemia-induced glial activation. J Neuroinflammation. (2012) 9:254. doi: 10.1186/1742-2094-9-254
157. Hakonen, E, Chandra, V, Fogarty, CL, Yu, NY, Ustinov, J, Katayama, S, et al. MANF protects human pancreatic beta cells against stress-induced cell death. Diabetologia. (2018) 61:2202–14. doi: 10.1007/s00125-018-4687-y
158. Liu, J, Wu, Z, Han, D, Wei, C, Liang, Y, Jiang, T, et al. Mesencephalic astrocyte‐derived Neurotrophic factor inhibits liver cancer through small ubiquitin‐related modifier (SUMO)ylation‐related suppression of NF‐κB/snail signaling pathway and epithelial‐Mesenchymal transition. Hepatology. (2020) 71:1262–78. doi: 10.1002/hep.30917
159. Yagi, T, Asada, R, Kanekura, K, Eesmaa, A, Lindahl, M, Saarma, M, et al. Neuroplastin modulates anti-inflammatory effects of MANF. iScience. (2020) 23:101810. doi: 10.1016/j.isci.2020.101810
160. Cheng, L, Zhao, H, Zhang, W, Liu, B, Liu, Y, Guo, Y, et al. Overexpression of conserved dopamine neurotrophic factor (CDNF) in astrocytes alleviates endoplasmic reticulum stress-induced cell damage and inflammatory cytokine secretion. Biochem Biophys Res Commun. (2013) 435:34–9. doi: 10.1016/j.bbrc.2013.04.029
161. Zhao, H, Cheng, L, Liu, Y, Zhang, W, Maharjan, S, Cui, Z, et al. Mechanisms of anti-inflammatory property of conserved dopamine neurotrophic factor: inhibition of JNK signaling in lipopolysaccharide-induced microglia. J Mol Neurosci. (2014) 52:186–92. doi: 10.1007/s12031-013-0120-7
162. Zhang, Y, Xiang, Y, Wang, X, Zhu, L, Li, H, Wang, S, et al. Cerebral dopamine neurotrophic factor protects microglia by combining with AKT and by regulating FoxO1/mTOR signaling during neuroinflammation. Biomed Pharmacother. (2019) 109:2278–84. doi: 10.1016/j.biopha.2018.11.028
163. Caglayan, AB, Beker, MC, Sertel Evren, E, Caglayan, B, Kilic, U, Ates, N, et al. The unconventional growth factors cerebral dopamine Neurotrophic factor and Mesencephalic astrocyte-derived Neurotrophic factor promote post-ischemic neurological recovery, Perilesional brain remodeling, and lesion-remote axonal plasticity. Transl Stroke Res. (2023) 14:263–77. doi: 10.1007/s12975-022-01035-2
164. Sereno, D, Muller, WEG, Bausen, M, Elkhooly, TA, Markl, JS, and Wiens, M. An evolutionary perspective on the role of mesencephalic astrocyte-derived neurotrophic factor (MANF): at the crossroads of poriferan innate immune and apoptotic pathways. Biochem Biophys Rep. (2017) 11:161–73. doi: 10.1016/j.bbrep.2017.02.009
165. Mnich, K, Moghaddam, S, Browne, P, Counihan, T, Fitzgerald, SP, Martin, K, et al. Endoplasmic reticulum stress-regulated chaperones as a serum biomarker panel for Parkinson’s disease. Mol Neurobiol. (2023) 60:1476–85. doi: 10.1007/s12035-022-03139-0
166. Lindholm, P, and Saarma, M. Novel CDNF/MANF family of neurotrophic factors. Dev Neurobiol. (2010) 70:360–71. doi: 10.1002/dneu.20760
167. Eletto, D, Eletto, D, Dersh, D, Gidalevitz, T, and Argon, Y. Protein disulfide Isomerase A6 controls the decay of IRE1 alpha signaling via disulfide-dependent association. Mol Cell. (2014) 53:562–76. doi: 10.1016/j.molcel.2014.01.004
168. Yu, J, Li, T, Liu, Y, Wang, X, Zhang, J, Wang, X, et al. Phosphorylation switches protein disulfide isomerase activity to maintain proteostasis and attenuate ER stress. EMBO J. (2020) 39:e103841. doi: 10.15252/embj.2019103841
169. Kemppainen, S, Lindholm, P, Galli, E, Lahtinen, HM, Koivisto, H, Hämäläinen, E, et al. Cerebral dopamine neurotrophic factor improves long-term memory in APP/PS1 transgenic mice modeling Alzheimer’s disease as well as in wild-type mice. Behav Brain Res. (2015) 291:1–11. doi: 10.1016/j.bbr.2015.05.002
170. Stepanova, P, Kumar, D, Cavonius, K, Korpikoski, J, Sirjala, J, Lindholm, D, et al. Beneficial behavioral effects of chronic cerebral dopamine neurotrophic factor (CDNF) infusion in the N171-82Q transgenic model of Huntington’s disease. Sci Rep. (2023) 13:2953. doi: 10.1038/s41598-023-28798-4
171. Longo, VD, and Mattson, MP. Fasting: molecular mechanisms and clinical applications. Cell Metab. (2014) 19:181–92. doi: 10.1016/j.cmet.2013.12.008
172. Rebelo-Marques, A, de Sousa Lages, A, Andrade, R, Ribeiro, CF, Mota-Pinto, A, Carrilho, F, et al. Aging hallmarks: the benefits of physical exercise. Front Endocrinol. (2018) 9:258. doi: 10.3389/fendo.2018.00258
173. Shirvani, H, Aslani, J, Mohammadi, F, and Arabzadeh, E. Short-term effect of low-, moderate-, and high-intensity exercise training on cerebral dopamine neurotrophic factor (CDNF) and oxidative stress biomarkers in brain male Wistar rats. Comp Clin Pathol. (2019) 28:369–76. doi: 10.1007/s00580-018-2885-0
174. Fallah Mohammadi, Z, Falah Mohammadi, H, and Patel, DI. Comparing the effects of progressive and mild intensity treadmill running protocols on neuroprotection of parkinsonian rats. Life Sci. (2019) 229:219–24. doi: 10.1016/j.lfs.2019.05.036
175. da Silva, WAB, Ferreira Oliveira, K, Caroline Vitorino, L, Ferreira Romao, L, Allodi, S, and Lourenco, CC. Physical exercise increases the production of tyrosine hydroxylase and CDNF in the spinal cord of a Parkinson’s disease mouse model. Neurosci Lett. (2021) 760:136089. doi: 10.1016/j.neulet.2021.136089
Keywords: CDNF, MANF, dopamine, Parkinson’s disease, unfolded protein response
Citation: Pakarinen E and Lindholm P (2023) CDNF and MANF in the brain dopamine system and their potential as treatment for Parkinson’s disease. Front. Psychiatry. 14:1188697. doi: 10.3389/fpsyt.2023.1188697
Edited by:
P. Hemachandra Reddy, Texas Tech University Health Sciences Center, United StatesReviewed by:
Thyagarajan Subramanian, Independent Researcher, Hummelstown, PA, United StatesNicola B. Mercuri, University of Rome Tor Vergata, Italy
Brandon K. Harvey, National Institute on Drug Abuse (NIH), United States
Copyright © 2023 Pakarinen and Lindholm. This is an open-access article distributed under the terms of the Creative Commons Attribution License (CC BY). The use, distribution or reproduction in other forums is permitted, provided the original author(s) and the copyright owner(s) are credited and that the original publication in this journal is cited, in accordance with accepted academic practice. No use, distribution or reproduction is permitted which does not comply with these terms.
*Correspondence: Päivi Lindholm, cGFpdmkucHVsa2tpbGFAaGVsc2lua2kuZmk=