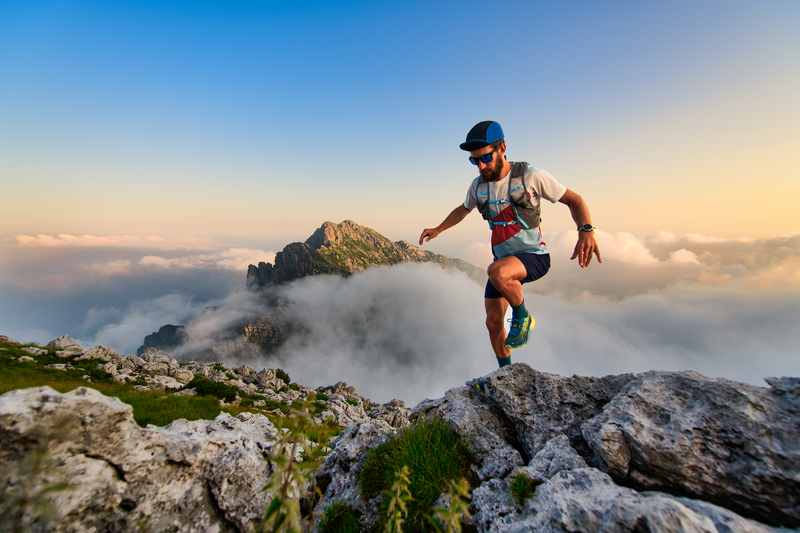
95% of researchers rate our articles as excellent or good
Learn more about the work of our research integrity team to safeguard the quality of each article we publish.
Find out more
REVIEW article
Front. Psychiatry , 03 October 2023
Sec. Molecular Psychiatry
Volume 14 - 2023 | https://doi.org/10.3389/fpsyt.2023.1182536
This article is part of the Research Topic Genome-wide Molecular Mechanisms of Substance Use Disorders View all 5 articles
The second part of this paper builds upon and expands the epigenomic-aging perspective presented in Part 1 to describe the metabolomic and immunomic bases of the epigenomic-aging changes and then considers in some detail the application of these insights to neurotoxicity, neuronal epigenotoxicity, and synaptopathy. Cannabinoids are well-known to have bidirectional immunomodulatory activities on numerous parts of the immune system. Immune perturbations are well-known to impact the aging process, the epigenome, and intermediate metabolism. Cannabinoids also impact metabolism via many pathways. Metabolism directly impacts immune, genetic, and epigenetic processes. Synaptic activity, synaptic pruning, and, thus, the sculpting of neural circuits are based upon metabolic, immune, and epigenomic networks at the synapse, around the synapse, and in the cell body. Many neuropsychiatric disorders including depression, anxiety, schizophrenia, bipolar affective disorder, and autistic spectrum disorder have been linked with cannabis. Therefore, it is important to consider these features and their complex interrelationships in reaching a comprehensive understanding of cannabinoid dependence. Together these findings indicate that cannabinoid perturbations of the immunome and metabolome are important to consider alongside the well-recognized genomic and epigenomic perturbations and it is important to understand their interdependence and interconnectedness in reaching a comprehensive appreciation of the true nature of cannabinoid pathophysiology. For these reasons, a comprehensive appreciation of cannabinoid pathophysiology necessitates a coordinated multiomics investigation of cannabinoid genome-epigenome-transcriptome-metabolome-immunome, chromatin conformation, and 3D nuclear architecture which therefore form the proper mechanistic underpinning for major new and concerning epidemiological findings relating to cannabis exposure.
Part 1 of this paper introduced the salience of an epigenomic and aging perspective in understanding cannabinoid pathophysiology (1). Part 2 presents the metabolomic and immunomic basis and underpinning of these changes and then considers their particular application to neurons and the synapse. Thus, this part should be read in conjunction with Part 1 and is properly considered as an extension of it and has been presented in a separate format in view of space considerations.
Much recent attention has focused on the spatial organization of the cell nucleus and the manner in which three dimensional topologically associated domains and transcription factories are epigenetically coordinated to precisely bring enhancers into close proximity with promoters to control gene expression. Many recent papers make the 3D architecture of the nucleus including chromatin conformation a major and principal focus of interest. This perspective is necessarily interactive between genome-epigenome-transcriptome. However, it is increasingly apparent that nuclear events are based squarely on events occurring in the cytoplasm, particularly as relates to metabolomic and immunomic activity which are not only permissive of nuclear changes but determinative of them. Moreover, neuronal and synaptic activity is a special case of these integrated and interactive levels of control and are clearly of particular relevance to the broad spectrum of neuropsychiatric pathologies linked with cannabis exposure, which now include several major neuropsychiatric syndromes and autistic spectrum disorder.
These features also mechanistically underpin several recent large epidemiological studies linking cannabis to the incidence of several cancers (2–12) and numerous congenital anomalies in both USA and Europe (13–19) and with aging syndromes of various types (12, 20), which were detailed further in Part 1.
The metabolome refers to “the qualitative and the quantitative collection of all low-molecular-weight molecules (metabolites) present in the cell that are participants in general metabolic reactions and that are required for the maintenance, growth, and normal function of a cell” (21). The whole subject of metabolomics is too vast to be covered here. For present purposes, we wish to focus on just three aspects of metabolomics: mitochondriology; code breaking as it relates to the genomic code, histone code, tubulin code, and sugar codes; and the lactylome.
A large and rich literature demonstrates that the numerous inhibitory activities of cannabinoids on mitochondria have been demonstrated in many laboratories and related to essentially all mitochondrial activities (22–42). A long list of cannabinoids is implicated in these activities including Δ8THC, Δ9THC, Δ10THC, cannabidiol, cannabigerol, and cannabichromene as well as full synthetic cannabinoid agonists such as WIN55,212-2 (28).
Functions inhibited by cannabinoids include such activities as mitochondrial bioenergetics, such as the synthesis of the key enzymes of the electron transport chain (ETC) including the F1 ATPase and many key mitochondrial proteins (43), reduction in the flux through the tricarboxylic acid cycle (TCA), inhibition of ETC complexes I, II, IV, and V activity, lowered NAD+/NADH ratio, inhibition of calcium uptake, reduction of the mitochondrial threshold potential for the mitochondrial permeability transition which triggers cellular apoptosis, flux through a new described inner mitochondrial membrane chloride channel, increased reactive oxygen species (ROS) production, especially at complex I, replication of mitochondrial DNA, mitochondrial dynamics (fission and fusion), mitophagy, and biogenesis (28, 43). Most of these actions were triggered through the mitochondrial CB1R and its downstream transduction machinery including a decline in the intracellular cAMP-dependent protein kinase A activity (28). Reduction in the NAD+/NADH ratio impacts gene transcription and expression, cell fate, and differentiation states (28).
Mitochondria also generate and detoxify ROS, synthesize phospholipids, steroids, quinones and haem, perform fatty acid breakdown through β-oxidation, and house the urea cycle (44). Mitochondria have over 1,000 different proteins. The mitochondrial genome is 16,569 bases long and codes for 13 mitochondrial proteins, 22 transfer RNAs, and two ribosomal RNAs (44). Mitochondria are considered major intracellular signaling hubs and coordinate synthesis of nucleotides, lipids, and proteins (45).
Cannabinoids have been shown to impede cellular calcium homeostasis via CB1R, NMDAR, TRPV4, GPR55, and calcium selective Voltage Dependent Anion Channels (VDAC1) of the L-, N-, and P/Q types (28). Release of calcium from mitochondria can occur either through the mitochondrial permeability transition pore or via the Na+/Ca2+ exchanger (NCLX). Calcium homeostasis in turn controls numerous cell functions including neuronal excitability, muscle contraction, exocytosis, synaptic transmission, gene transcription, cell proliferation, and cytoskeletal movements including cell migration (28). Such changes also control stem cell decisions to multiply, stay dormant, or differentiate (28, 46). Indeed, it has been shown that the cellular NAD+/NADH ratio is the key determinant of the rate of embryonic development across multiple species (46).
Many cannabinoids including cannabidiol have been shown to derange mitochondrial structure and cause marked swelling (22, 28, 46).
Importantly, the key master transcription factor for immune activation NF-κB was demonstrated to be sensitive to a buildup of ROS (28).
Both endocannabinoids and phytocannabinoids have been shown to impact mitochondrial bioenergetics, fission and fusion, mitophagy, and mitochondrial transport on intracellular microtubules (28).
Impaired bioenergetic respiratory activity is the initiating event in the caspase-dependent. Cell intrinsic, mitochondrial pathway to cellular apoptosis (28). In neuronal terminals, declining respiratory activity impedes their participation in long-term synaptic depression and potentiation activities, which are key synaptic modulations that underlie the ability to form memories and learn (24, 28).
It should also be underscored that CB1Rs have been identified on astrocytes. This becomes important when it is understood that astrocytes are now known to play an integral and active part in neurotransmission and form, together with endothelial cells, the “quadripartite synapse” (47, 48).
Indeed, the astrocytic CB1R has emerged as a key player in the control of gliotransmission and the metabolic cooperation between astrocytes and neurons. The CB1R has been described as being the key coordinating factor between gliotransmission and metabolic cooperation in the neuronal-astrocytic dyad (48). Astrocytes also express other cannabinoid receptors including TRPV series, GPR55, and GPR18, but their role has not been well-studied at this point (48).
Astrocytes are bound together via gap junctions and signal to each other by calcium waves. These waves have been shown to be critical in the formation of various kinds of memory including short-term, long-term, working, spatial, and aversive memories (47, 48).
NMDAR activation induces an inward calcium current and is key to synaptic plasticity. Astrocytes synthesize and release glycine and D-serine, which are the co-agonists of the NMDAR (48). Astrocytes also release other gliotransmitters including purines and glutamate. Rising extracellular potassium, released by active neurons, mildly depolarizes astrocytes and strongly stimulates the astrocyte Na+/K+ ATPase pump and the electrogenic sodium-bicarbonate transporter, which together alkalinize the astrocytes and stimulate glycolysis and lactate release (48). This response also occurs with other neuronal signals such as ammonium and nitric oxide.
Astrocytes have a low concentration of CB1R but are functionally very important (48). Moreover, astrocytes carry the synthetic and metabolizing enzymes for both anandamide (AEA) and 2-arachidonoyl glycerol (2AG) (48). This production is sensitive to external signals. particularly calcium released as a result of neuronal activity.
The role of lactate has metamorphosed from being conceptually considered as a metabolic waste product, to an energy substrate, to an important metabolic signal (47). Astrocytes provide lactate to neurons via an astrocyte-to-neuron lactate shuttle in order to supplement their very high needs for energy, especially during active firing states (47). Reduction in either the MCT2 or MCT4 (medium chain acyl transporters 2 and 4), which are the main lactate channels in neurons and astrocytes, respectively, impedes memory formation and learning. Loss of neuronal MCT2 causes reduced hippocampal neurogenesis and also reduced maturation of newborn hippocampal neuroblasts (47). Astrocyte glycogen metabolism is also important for neuronal memory formation and this is thought to be acting via lactate signaling. Lactate signals are also transduced by the Hydroxycarboxylic acid 1 (HCA1) receptor (48). In this manner, lactate has been shown to be critical to memory formation in many respects (47).
For these reasons, astrocytes are also involved in long-term synaptic depression and potentiation and thus learning and memory. Cannabinoids disrupt these processes in part by inhibiting mitochondrial processes. Inhibition of astrocytic ETC complex I via mitochondrial membrane CB1Rs slows glycolysis, diminishes ROS production, inhibits HIF1α, and thereby reduces synthesis of glycolytic enzymes and glycolytic flux. This, in turn, reduces lactate production and induces bioenergetic and oxidative stress which then impacts memory, social interactions, and behaviors (48).
Many studies indicate that mild stresses can be good for organisms (44). Calorie restriction and intermittent fasting are thought to work as effective lifespan extension interventions in this manner in aging research (44). This is known as “hormesis”. When it is induced in mitochondria it is known as mitohormesis (44). Mitochondria are major signaling hubs both to the cell nucleus and to other intracellular organelles such as endoplasmic reticulum.
Some of these stress signals are released systemically, where they are known as mitokines. Such mediators that have been identified in mammals include FGF21, humanin, and GDF15 (44). FGF21 signals to the liver, adipose tissue, and brain (44). When damaged components of mitochondria are released into circulation, they become identified by the body as DAMPs (Damage-Associated Molecular Patterns), which stimulate innate immune pattern recognition receptors. These can include components of the ETC, TCA metabolites, mitochondrial DNA, and the mitochondrial transcription machinery proteins (44). Interestingly, all three of these defined mitokines (FGF21, GDF15, and humanin) increase with age (44). They all improve various measures of health. Thus, the suppression of normal mitochondrial function by numerous cannabinoids can be expected to reverse these effects.
For many reasons, the mitochondria and nucleus need to coordinate their activities. Since most mitochondrial proteins are encoded on nuclear DNA and since many nuclear reactions are dependent on mitochondrially derived energy or substrates of intermediate metabolism, there clearly needs to be a close cooperation and coordination of the activities of these organelles. Therefore, much communication occurs in the anterograde direction from nucleus to mitochondria.
However, communication can also occur in the opposite direction and mitochondria can communicate with the nucleus via malate/aspartate exchange, glyceraldehyde-3-phosphate shuttle, nicotinamide mononucleotide transfer via ROS, by SIRT1, AMPK, and ATFS1, and by substrate and energy supply (45, 49). Key metabolites which signal to the nucleus are acetyl-coenzyme A (acCoA), α-ketoglutarate (αKG), nicotinamide adenine dinucleotide (NAD+), succinate, fumarate, and methionine (45). αKG is a required substrate for the major epigenetic demethylation reactions both of DNA—through ten-eleven translocation methylcytosine dioxygenase—and of histones by the Jumonji C(Jmjc) domain containing lysine demethylases (JMJDs) (45). The activity of both TETs and JMJDs is regulated by the ratio of substrates including αKG to inhibitors including succinate, fumarate, and 2-hydroxyglutarate (αHG). In flies, αKG supplementation leads to life span extension by activating AMPK and inhibiting the master anabolic regulator mTOR (45).
NAD+ is a key co-substrate in the core reactions of the TCA, ETC, glycolysis, and β-oxidation of fatty acids (50). It is also a core co-reactant in three groups of key epigenetic enzymes, the sirtuins and HDACs, the PARPs (poly-APR-ribose polymerases), and cADP-ribose synthase (CD38), which control gene expression. In yeast, flies, worms, and mice NAD+ and sirtuins control aging. Pharmacological supplementation of NAD+ in worms extends lifespan via the mitochondria unfolded protein response (UPRmt), which is mediated via ATFS1 (45). PARP is also involved in DNA repair, inflammation, and cell death (45).
Methionine is the chief amino acid source of one carbon group for epigenetic methylation reactions. The cytoplasmic folate cycle is coordinated with the methionine cycle to maintain levels of s-adenosyl methionine, which is the primary one carbon donor for these reactions. It is particularly involved in the generation of H3K4me3, which marks transcriptional start sites and is particularly important during embryogenesis. Thus, anything which slows cytosolic one carbon metabolism will necessarily severely impact epigenetic regulation (45).
It was shown several decades ago that cannabinoids reduce the synthesis of DNA and RNA generally, which necessarily disrupts these key codes (51–53). Moreover, Δ9THC, cannabidiol, and cannabichromene all cause single and double stranded DNA breaks (53–59). Cannabis has long been linked with chromosomal breaks including ring and chain chromosome formation, microchromosomes, and micronuclei (53, 60–63). Broken chromosomes can cause breakage-fusion-bridge cycles that are self-perpetuating (64). Similarly, micronuclei cause chromosomal shattering (“chromothripsis”) which rapidly causes widespread genomic chaos from random repair of the chromosomal fragments to random insertion into other chromosomes (65–70). Cannabis is directly mutagenic and is known to oxidize guanine to oxoguanine, which is a highly mutagenic and oncogenic mutation (54). This has been defined both in vitro (54) and in human cannabis users (71). Indeed, in one clinical study, the percentage of damaged and broken DNA included in the comet tail in a comet assay rose from 4.8% in controls to 66.4% in cannabis smokers (71). Moreover, as shown by the Harvard group, DNA breakages cause re-arrangement of the histone machinery on the genome and relocation of major histone complexes (72). This removes histone suppression from silenced parts of the genome, especially repetitive mobile elements and retrotransposons (73–80). This makes the “jumping genes jump” which is a severe cause of genomic instability, neurodevelopmental defects, cancer, and aging (73, 74, 76, 78, 81–83). Since cannabis impedes mitochondrial and metabolic function generally, and these are the substrates upon which genomic maintenance is built, it follows that metabolic-genomic factors also disrupt the genome. For these reasons it appears that cannabis disrupts the genome in many respects.
From the above remarks in relation to the profound epigenomic disturbances that are induced by many cannabinoids, it is clear that cannabinoids disrupt the epigenomic code in many ways. This effect is itself compounded when it is appreciated that THC actually reduces the synthesis of histones and some of their key post-translational modifications including phosphorylation and acetylation (84, 85). Since histones control the overall genomic architecture and conformation in 3D space, which in turn controls gene transcription, it is clear that the implications of this are very far reaching indeed. They include neurodevelopmental defects, congenital anomalies, cancer, and cell death. Indeed, the North Carolina group noted more than 10,000 differentially methylated regions in cannabis dependence and in excess of 10,000 differentially methylated regions in cannabis withdrawal (86). Cannabis also disrupts the micro-RNA profile of neurons and other cells (87).
“To set these findings in context it may be noted that Schrott et al. performed a profoundly useful longitudinal epigenomic DNA methylation study of human sperm from cannabis users compared to controls before and after an 11 week period of confirmed abstinence from cannabis (86). The authors found thousands of differentially methylated genes altered between cannabis users and controls many of which improved after the period of abstinence. However, after the abstinence period a largely different set of differentially methylated genes emerged in the sperm methylome. The genes affected related to essentially all tissues, to key genes of the epigenomic machinery, to synapse, brain and organ development and to cell growth including many cancers.”
Moreover, as cannabis clearly ages people (see above section) and as aging has now been shown to be a combined genomic-epigenomic disease (72), this also demonstrates that cannabis broadly disrupts the genome and epigenome in several respects.
Cannabis has been shown to reduce the overall synthesis of α-, β-, and γ- tubulin (43). By definition, therefore, it necessarily disrupts the tubulin code. Moreover, it also acts epigenomically to disrupt acetylation of tubulin (86). This greatly reduces the tensile strength of polymerized tubulin in microtubules, which increases their risk of fracture when bent as in mitotic spindles. This is then a cause of chromosomes coming loose from the mitotic spindle at anaphase and a proximate cause of chromosomal missegregation and micronucleus formation which drives genomic instability. Another key posttranslational modification of tubulin is glutaminylation. This is performed by enzymes of the tubulin-tyrosine-like (TTLL) family. Eight members of the TTLL family are epigenomically disrupted by cannabis exposure (86). Thus, cannabis disrupts not only the synthesis of tubulin but also two if its principal posttranslation modifications which govern its intracellular address targeting and final functionality. Similarly, cannabis also disrupts actin synthesis both directly and epigenomically (43, 86, 88). Thus, cannabis disrupts the presence and function of both tubulin and actin, which are the two major components of the cellular cytoskeleton and both of which are intimately involved in genomic (microtubules of the mitotic spindle) and epigenomic (nuclear lamina and gene silencing and heterochromatin formation) stability.
The sugar code has been called the third great alphabet of life after the nucleic acid and protein codes (89–92). The addition of glycan moieties is the commonest posttranslational modification of proteins (89, 92). In the sugar code complex, usually polymerized and branched carbohydrate moieties called glycans are added to DNA, RNA, and protein which greatly changes their function (91–93). Immunoglobulin G (IgG) is one such well-studied molecule (94–102). It is known that in this case glycans can change the IgG activity from immunostimulatory to immunosuppressive. Aging and many diseases, including atherosclerosis, dementia, rheumatoid arthritis, systemic lupus erythematosus, and cancer, are linked with various modifications of this glycan code (94–105). Cannabis has been shown to epigenomically perturb the activity of some of the key enzymes involved with writing this code including O-Linked N-Acetylglucosamine (GlcNAc) Transferase (95 differentially methylated regions, DMRs or “hits” in the Schrott epigenomic database), UDP Glucuronosyltransferase Family 1 Member A1 (9 DMRs), beta-1,4-N-Acetyl-Galactosaminyltransferase 2 (2 DMRs), fucosyltransferase (9 DMRs), various mannosyltransferases (Programmed Cell Death 6, Dpy-19 Like C-Mannosyltransferase 3, Transmembrane O-Mannosyltransferase Targeting Cadherins 1; 16 DMRs in all), and various glucuronosyltransferases (LARGE Xylosyl- And Glucuronyltransferase 1, UDP Glucuronosyltransferase Family 1 Member A1, 13 DMRs in all) (86). Thus, it is seen that the sugar code is also disrupted by cannabis.
Lactate is relevant to cannabis medicine because cellular lactate usually rises when oxidative metabolism is inhibited, as many cannabinoids are known to do. Moreover, high levels of lactate have been documented following human cannabis use (106) and, since extracellular and intracellular levels of lactate are closely correlated (107), it follows that cannabis induces a systemically high lactate environment.
It was shown that at about 20 sites on histone H3 and H4 lactylation can occur as a posttranslational modification (108). It was shown in bone marrow-derived macrophages that during the activation phase of the M1 macrophage response to an infection they switched to anaerobic glycolysis and the use of lactate as a primary metabolic fuel (108). Rising levels of lactate caused the “writing” of the lactylation code on H3 and H4 ε-carbon of the lysine tails, which 16–20 h after activation caused an epigenetically induced switch to an M2 anti-inflammatory type and inflammasome inhibition in order to assist resolving the acute infection (108). This temporal delay is referred to as a “lactate clock” (108). It was subsequently shown that the switch in macrophage metabolism was controlled by B-cell adapter for PI3K (BCAP), which is a signal adapter for toll-like receptors (107) and assists with the formation of H3K9ac and H3K18 lactylation.
Lactate lies at the intersection of aerobic and anaerobic metabolism, is a major metabolic fuel, and has been said to be quantitatively more important than glucose (107). Lactate is a recyclable redox buffer and provides metabolic feedback through direct activities, through intra- and extra- cellular acidification, through active and passive lactylation of proteins including histones, and thereby epigenetic regulation of gene transcription which plays key roles in such major cellular events as inflammation, wound healing, ischaemia-reperfusion injury, immune responses, neuronal function including learning and memory, and tumorigenesis (107). Lactate also functions as a signaling molecule and has its own dedicated receptor GPR81 and can traverse cell membrane via the MCT1-4 (monocarboxylate transporters 1–4). The lactylation code can be passively written non-enzymatically or actively written by P300. The lactylation code is erased by HDACs 1–3 and sirtuins 1–3 with the aid of P53. HDAC3 was the most powerful of these erasers. Lactylation takes its places along other posttranslational acyl modifications of histones including formylation, propionylation, butyrylation, crotonylation, 2-hydroxybutyrylation, β-hydroxybutyrylation, succinylation, malonylation, glutarylation, and benzoylation and indeed there is some evidence for cross-talk between these various acylation modifications (107). Thus, lactylation and the many metabolic functions of lactate itself represents a case of metabolic regulation by metabolites (107). Its involvement in epigenomic regulation represents a key intersection of metabolism and gene regulation.
It is well-known that glycolysis can be triggered by hypoxia or irreversible mitochondrial inhibition. However, it has now been shown that it can also be triggered by metabolic reprogramming as induced in cancer cells by the excessive demand for ATP imposed by the highly proliferative state. Lactate rises in other situations of cellular stress such as myocardial infarction, trauma, infection, and heart failure (107).
Whilst the physiological concentration of lactate is 1.5–3.0 millimolar, it can rise as high as 10–40 millimolar within inflammatory sites (107). Lactate is generated from pyruvate at the end of glycolysis irrespective of the local oxygen tension. Pyruvate is transformed into lactate by lactate dehydrogenase (LDH). Pyruvate is irreversibly removed by pyruvate dehydrogenase (PDH) which transforms pyruvate into acetyl-Coenzyme A (acCoA). Thus, the lactate level is determined by the balance between glycolytic flux and PDH activity (107). In cancer cells lactate also arises from glutamine catabolism (107). Lactate also controls mitochondrial oxidative metabolism and redox balance. When mitochondria are stressed, they generate more ROS, which further impairs oxidative metabolism and can be dangerous to the cell. Extracellular lactate can be converted into pyruvate by extracellular lactate oxidaze and catalase (107).
The lactate shuttle operates in tumor cells which shuttles lactate between glycolytic tumor cells and oxidatively metabolizing tumor cells with the effect of stimulating overall tumor growth. It also occurs between exercising skeletal muscle and the heart, in a paracrine manner between cardiomyocytes and fibroblasts, between astrocytes and neurons, and between the proximal and distal tubules in the kidney (107). Lactate can restore acCoA levels and enhance fatty acid synthesis in part by increasing the activity of the key metabolic enzyme acCoA carboxylase. Thus, lactate can act in either an autocrine, paracrine, or endocrine manner.
During sepsis, the high circulating lactate levels can lactylate HMGB1, which can then make its way from the nucleus to the cytoplasm via GPR81 and MCTs. From the cytoplasm it can be released via exosomes from where it can damage endothelial cells, increase vascular permeability, and exacerbate sepsis (107). Lactylation also plays several key roles in the pathogenesis of systemic lupus erythematosus (SLE). During red blood cell development, a regulatory switch in the ubiquitin proteosomal system (UPS) occurs but the UPS becomes lactylated in SLE. This lactylation inhibits its UPS activity so that it is not completely able to clear the mitochondria from the developing haemoblast precursors. Macrophages engulf the faulty red cells and consume the mitochondrial DNA, which generate an immune response to DNA via the powerful cGAS-STING system (107).
As noted above, lactate is generally anti-inflammatory following the very earliest phases of an inflammatory stimulus. It is worth considering some of these effects by cell type. In general terms, these effects occur either because of lactate as a metabolic substrate, via lactate signaling, or from lactylation of key proteins (107).
In macrophages, lactate activates the ERK-Stat3 pathway, GPR132, Notch signaling, HIF1α stabilization, and histone lactylation. Together these effects induce M2 Polarization and increases IL-6, VEGF (stimulating angiogenesis), ARG1, and CCL5 (107).
In T-cells, lactate induces an acidic environment which completely inactivates T-cell signaling machinery, activates PD-1 and PD-L1 pathway, inhibits P38, JNK, and lactate efflux and thus poisons the T-cell, thereby stopping T-cell activation and cytokine mobilization. Taken together these effects decrease effector function and T-cell proliferation and increase PD-1 expression and immune evasion (107).
In dendritic cells, lactate induces an acidic environment, reduces CD1a and increases CD14 expression, and activates GPR81 signaling and lactate, which is important via SLC16A. Together these effects decrease differentiation, decrease IL-12 production, IL-6 release, and TNFα release, and increase kynurenine release (107).
In NK cells, lactate induces an acidic environment and inhibits HDACs, NFAT, NKp-46, and mTOR signaling. Taken together these effects decrease cytolytic function and interferon γ release and increase apoptosis (107).
In mast cells, lactate induces an acidic environment, targets MAS-associated G protein coupled receptor, and inhibits early calcium mobilization and degranulation and also late chemokine/cytokine phases of activation (107).
In Treg cells, lactate induces FOXP3-mediated repression of Myc and modulation of LDH and sustains fatty acid synthesis through acetyl-CoA carboxylase. Together these effects increase proliferation, differentiation, TGFβ, and IL-10. In combination with effects on the above effector cells these effects further reinforce the immunosuppressive phenotype (107).
Through tissue acidification the effects of lactate are proinflammatory in most chronic inflammation scenarios (107).
Lactate is powerfully protumourigenic in many pathways: as a substrate for energy metabolism; by activating PD-1,/PD-L1 pathway, and T-cell apoptosis; synergizing with oncogene Myc; activating oncogene HIF1α and VEGF /VEGFR2; via lactylation of histone and non-histone proteins; inhibiting NFAT, NKp-46, and mTOR signaling; and increasing tumor growth and metastasis, tumor angiogenesis, and tumor invasion including basement membrane remodeling, metalloproteinase activation by epitranscriptomic m6A methylation mRNA of MTLL3, altered tumor immunity, and induction of immunosuppressive tumor environment (107).
Thus, lactate and lactylation represent key therapeutic targets for the development of future therapies (107).
A detailed dissection of the role of elevated tissue lactate was made in two series of Hepatitis B-related hepatocellular carcinoma (HCC) patients of 52 and 159 cases each from China (109). High lactate is directly and powerfully immunosuppressive to immunocytes (110, 111).
Lactylation was a posttranslational modification made on lysine residues (denoted as Kla). Lactylation itself was found to usually be an active and regulated process. Many proteins were lactylated by P300 and delactylated by HDAC1-3 (109). Indeed, these researchers found 9,275 sites for lactylation on 6,403 proteins. The enzymes most affected were metabolic enzymes (oxidoreductases and dehydrogenases including mitochondrial complex V and F0-F1 ATPase, the powerhouse that actually produces mitochondrial ATP at the end of the ETC) and also translational proteins, translation factors, ribosomal proteins, signal receptors, ligases, and cytoskeletal proteins, indicating that lactylation affects many cellular processes including signal transduction, gene expression, and cellular morphology, shape, and motility (109).
The investigators found that the prognosis of patients with high levels of lactylation was much worse than those with lower levels by about 20% at 8 years follow-up (109). A reciprocal relationship was observed between downregulation of normal liver processes and upregulation of oncogenic signaling. Tumor aggressiveness was directly related to the degree of oncogenic skewing of the lactylome (109). Glutamine metabolism was increased as a result of the lactylation of the β-catenin pathway and glycolysis metabolism was increased as a result of the lactylation of the glycolytic pathway.
Branched chain amino acid (valine, leucine, isoleucine, and BCAA) metabolism was also boosted in HCC (109). Via ACAT1 BCAA are an alternative source of acetyl-CoA two carbon skeleton supply for the citric acid supply and also a source for glutamine synthesis. In mouse models of HCC, higher levels of BCAA were correlated with lower survival. ACAT1 was identified in the Schrott epigenomic cannabis screen by two DMRs (86).
Adenylate kinase (AK2) was studied in detail. Heavy lactylation of AK2 was found to be an adverse prognostic factor in terms of survival and hepatic vein thrombosis. The P53 was downregulated in these tumors, partly explaining the poorer prognosis. AK2 also impacted the apoptotic pathway by inhibiting caspase 9 (a final executioner caspase in the cascade) and thereby conferring an anti-apoptotic protection on tumor cells (109). Lactylation of AK2 at K28 in the enzyme active site abolished enzymic activity. Tumors manifesting AK2 K28Kla had increased proliferation, DNA replication, and nucleotide excision repair (a low fidelity DNA repair pathway). AK2 K28Kla was found to drive cell proliferation and cell migration in various HCC cancer cell lines (109).
For these reasons, protein lactylation was shown to be a key posttranslational modification that transduced HCC metabolism into tumor cell behavior and subsequent adverse patient outcomes.
The immunome may be defined as the set of peptides, proteins, receptors, signaling systems, genes, and cells that together comprise the innate and adaptive immune systems (112, 113). Immune processes are highly relevant in many ways as the strongly oxidizing environment of immune and inflammatory processes can induce DNA breaks. So important is immune activity that chronic immune stimulation is now described as one of the pillars of aging (114) and the immunome has now had its own biological clock developed with which to measure organismal biological age (115). Interestingly, most of the predictive power of this clock is related to CXCL9 (115). Chief among the immune pathways is the recently described innate cytosolic nucleic acid sensing and signaling pathway cGAS-STING.
cGAS-STING is a powerful cytosolic sensor of double stranded DNA (dsDNA) which has been recently described and is of broad relevance to cancer, aging, and genomic stability (116). cGAS also senses R-loops (117–121). Once cGAS binds to dsDNA, its endoplasmic reticulum-bound binding partner STING stimulates a type I interferon response (122). Oligomerization of cGAS, formation of long DNA-protein ladders, and phase separation (in a gel) lead to STING oligomerization and an augmented STING response (122–124). cGAS is activated by DNA in a length-dependent manner and is more powerfully stimulated by longer DNA segments (125). Termination of the cGAS-STING signal occurs by clathrin-associated AP-1 (126). The electron microscopic structure of cGAS-STING has been determined (122, 127–129).
In addition to innate immunity, cGAS-STING also drives autophagy, cell survival, infection, inflammation, cancer, and senescence pathways (123). cGAS can transfer between cells and activate STING nearby in paracrine fashion (123). cGAS-STING drives aging, senescence, anti-tumor immunity, autoimmune disease, and acute and chronic heart failure inflammatory disorders such as pancreatitis, macular degeneration, alcoholic hepatitis, cancer, metastasis, myocardial infarction, sepsis, systemic lupus erythematosus, and Parkinson's disease (116, 130).
cGAS-STING has been shown to be a major driver of organ pathology in COVID-19 infections (131).
cGAS-STING surveils cancer cells. Mice and human cells deficient in cGAS-STING tolerate oncogenic Ras signaling. Neither irradiation nor cancer generate the usual inflammatory responses in cGAS-STING deficient cells. cGAS-STING activity correlates with the inflammatory component of many cancers (116).
cGAS-STING surveillance of micronuclei generates an immune response triggered by genomic instability upon breakdown of the micronuclear envelope (132). Micronuclei can be generated from lagging chromosomes.
cGAS-STING drives the IL-6 dependent survival of chromosomally instable cancers through a chromosomal instability/cGAS-STING/Stat3 /RelB /NF-κB/Stat1/ILK-6/IL-6R/JNK /ASK /cell death pathway (133). This mechanism was found commonly in many cancers that express IL-6R including triple negative breast cancer (133).
cGAS-STING also drives cancer metastasis. Chromosomal missegregation forms micronuclei that trigger cGAS-STING and downstream non-canonical NF-κB signaling that triggers metastasis (134).
In pancreatic cancer cGAS-STING-induced regulatory B-cells compromise NK cells anti-tumor immune response. cGAS-STING promotes immune evasion and metastasis through induction of PD-L1 expression (135). B-cells can also suppress tumor immunity through GABA signaling (136).
During senescence, reduced lamin B synthesis leads to partial breakdown of the nuclear envelope and blebs of nuclear membrane break off carrying chromosomes. These cytoplasmic chromosomes stimulate cGAS-STING (116). Similar nuclear blebs have also been observed from cannabis exposure (53, 55, 60).
Chronic immunostimulation is a prominent hallmark of aging, particularly the elaboration of the well-characterized Senescence Associated Secretory Phenotype (SASP) made up of growth factors and cytokines (114, 137). cGAS-STING recognizes cytosolic DNA fragments in senescence which generates the SASP and drives senescence with autocrine and paracrine effects (138). This was shown experimentally using irradiation and oncogene induction of senescence pathways (138).
cGAS-STING was shown to be essential for senescence induction. When it was inactivated senescence did not occur and mouse fibroblasts became oncogenically transformed more easily (139). cGAS-STING was required for the induction of senescence by irradiation and cytotoxic therapy. DNA damage produces cytoplasmic DNA which is sensed by cGAS. DDR is a key event in senescence induction. Lung cancers with low cGAS-STING expression have a worse prognosis (139).
YAP/TAZ activity in stromal cells controls cGAS-STING activity and this prevents aging (140).
Immune cells produce endocannabinoids (eCB) and also have receptors and metabolic machinery to transduce and extinguish eCB signals. This implies that eCB's signal through autocrine and paracrine routes to immune cells and their neighbors (141).
Toll-like receptors (TLR) are one of the major receptors of innate immunity and respond directly to a wide variety of exogenous DAMPs and PAMPs. Cannabinoids are generally suppressive of TLR signaling albeit there are many exceptions to this (141). Inflammatory signaling can either stimulate or inhibit ECS signaling via CB1R and CB2R signaling or by changing the levels of activity of eCB metabolic enzymes (141).
When THC was administered to homogenized cultures of rat telencephalic brain it was metabolized by mixed cultures of glia along with neurons (142). GABAergic neurons were most sensitive to a single application of 1 μM THC. After repeated applications of 1–2 μM THC markers of GABAergic, cholinergic and astrocytic damage were greatly elevated (142). IL-6 release was also elevated 4-fold after a single application of THC documenting important pro-inflammatory activity for this major cannabinoid (142).
Many other immune actions of cannabinoids are described which have been reviewed (143–156). Both immunosuppressive (143–145, 157, 158) and immunostimulatory (142, 159–161) actions have been described. Cannabidiol appears to be mainly immunosuppressive in its actions (156, 162). CB1R activation is often immunostimulatory (159–161). CB2R activation is frequently immunosuppressive (152, 163–165).
The immunosuppressive activities of cannabinoids are of clinical interest and importance. The immunosuppressive activities of cannabinoids have been shown to be relevant to different human disorders where heightened immune reactivity is problematic and complicate situations where immune compromise may be cause for concern. Experimental studies have explored the application of cannabidiol to skin graft rejection (166), to protect against the dietary and immune dysregulation induced by a high-fat diet (167), to protect against hypothalamic microgliosis and astrogliosis induced by a high-fat diet (168), to protect against experimentally induced oral mucositis induced by chemotherapy (169), to modulate TLR4 co-receptor signaling and thereby improve morphine mediated analgesia (170), and potentially as an adjunctive application in COVID-19-related cytokine storm (171). Contrariwise, cannabinoids have been found to exacerbate experimental sepsis (172) and to suppress anti-tumor immune responses by inhibiting T-cell Jak/Stat signaling (173).
Human studies have explored the use of cannabinoids in multiple sclerosis (156, 174–180). In general terms, cannabinoids have been found to reduce muscle spasticity (176–181). Cannabidiol has been shown to reduce leukocyte recruitment to plaque inflammatory lesions during peak disease activity (182). However, sedation, psychotomimetic, and gastrointestinal symptoms are often problematic side effects of cannabinoids, particularly in patients who are cannabinoid naïve (174, 176–180).
Whilst there are numerous published examples of the epigenomic effects of cannabinoids on immune cells, detailed consideration of a few key examples illustrates the importance of this feature. One of the long-standing mysteries of adaptive immunity has been the way in which long-term memories of past antigenic exposure can be retained despite the fact that most immunocyte effector cells have only short half-lives. This mystery was elucidated recently by the demonstration that the memories are retained epigenetically in the epigenome of the long-lived immune stem cell population (183, 184). Epigenetic immunological memory storage has been demonstrated in macrophages of the bone marrow, tissue resident macrophages and microglia, monocytes, haemopoietic progenitors, natural killer cells, and innate lymphoid cells (183). Moreover, similar nuclear epigenetic storage of past cell experiences including toxic exposures has been demonstrated on cells of the skin, lung, intestine, pancreas, muscle cells, sperm, endothelial cells, nasal epithelia, Schwann cells, and neurons (183). Moreover, these epigenetic memories have been shown to affect the subsequent risk of diseases such as cancer and Alzheimer's disease. Further, encounters with stress (including the nutrient stress of famine) and environmental toxicants can be passed epigenetically from mother to child in utero (183). A broad spectrum of epigenetic machinery is implicated in encoding such memories (183).
One implication of the epigenomic recording of inflammatory memories has been demonstrated in haemopoietic stem cells which become exhausted after recording such encounters (185). This is the mechanistic basis of many of the hematological changes observed in aging including anemias, pancytopaenias, Age-Related Clonal Hematopoiesis (ARCH), leukemogenesis, fatty replacement of the bone marrow, and bone marrow hypocellularity. This study was performed over 1 year in mice, which is equivalent to about 25 years in humans. Thus, these past immune encounters were shown to drive hematological aging (185).
Through blood bank studies, ARCH has recently been shown to be an important risk factor for myocardial infarction, cancer, and all-cause mortality (186). Somatic mutations of DNMT3a are very common in ARCH, although ARCH does not arise for several decades after these are acquired. This has suggested to some investigators that an environmental or inflammatory factor may contribute to oncogenic progression. By studying a mouse line of DNMT3a knockout cells, it was shown that either chronic infection, or just an injection of interferon-γ by itself, was sufficient to cause hypomethylation, reduce cellular differentiation, and lower stress-induced apoptosis rates, which together account for the dominance of the DNMT3a mutant clones amongst myeloid populations during infections (186). Human DNMT3a mutant hemopoietic stem cells exhibit similar defective interferon-γ induced differentiation. These studies demonstrate the manner in which interferon-γ signaling during chronic infection can drive DNMT3a loss of function in ARCH (186).
Immune activity has long been known to suppress stem cell regeneration. In part this can be a useful activity because it helps to maintain stem cells in quiescence and thus protects their genomic material from replication-associated damage. When DNA breaks were introduced into immune cells in mice, changes of accelerated aging were noted in the immune system and also prominently in the liver, lung, and kidney (187). Alterations included increased oxidative levels and hydoxynonenal, oxidized glutathione, and oxidative DNA mutations (187). Thus, immune aging was shown to be causal for systemic aging. Immune activity works partly by inducing oxidative stress which includes the production of DNA breakages and also by induction of the SASP.
Aging and senescent skeletal muscle stem cell niches are known to become inflamed, and this suppresses satellite stem cell regenerative capacity (188). Similarly, obesity also triggers a systemic inflammatory state especially in the abdominal fat. In obese mice, PPARγ is downregulated and its support restores TH2 differentiation of CD4 T-cells and abrogates highly inflammatory TH17 T-cell differentiation which is seen in obese rather than lean mice (189). Interestingly in this study the PPARγ receptor was noted to heterodimerize with the RXRα receptor.
Repeat elements comprise 54% of the human genome and transposable elements (TEs) make up 46% of the total genome length (190). TEs may be either class I retrotransposons, which encode a reverse transcriptase, or class II DNA transposons, which do not. Retrotransposons may be short interspersed repeat elements (SINEs), long interspersed repeat elements (LINEs), or long terminal repeat/endogenous retroviruses (LTR/ERV). Epigenetic remodeling in the cancer landscape can lead to mobilization of the TEs which are also ligands of innate immunity particularly of RNA via TLR3, RIG1, and MDA5 receptors, of DNA via TLR3/7/8, and cGAS (190). cGAS also binds DNA:RNA hybrids as may commonly occur during transcription (190). Low levels of DNA methylation derepress retroelements which then begin to jump through the genome and activate innate immune signaling whilst doing so (190). It has been shown that this process is particularly strongly activated in cancer genome atlas samples from stomach, bladder, liver, and head and neck squamous cancers. However, as mentioned, these mobile elements are immunoreactive and this immunogenicity can be re-purposed to therapeutic advantage for the development of cancer therapeutics (190). These TEs can also be used as a source of adjuvant antigens for CAR T-cell development for personalized cancer treatments. As cannabis is known to hypomethylate the genome and induce DNA breaks, these activities are highly relevant to cannabis medicine.
Class I transposable genomic elements are retrotransposons and include endogenous retroviruses (ERVs) and long interspersed repeat elements (LINE-1) which encode a reverse transcriptase and are thus auto-transcribing and mobilize across the genome through an RNA intermediate (191). One such LINE1 element in mice, known as Lx9c11, controls a Lx9c11-RegoS non-coding RNA which in turn controls hyperstimulated immune responses to viral infection and rescues infective lethality (191). In this case this transposable element is functional and suppresses immune reactivity.
The immune system can either promote or suppress tumourigenesis (192). In either case it is clearly a major force impacting and sculpting tumor development, including selecting for those clones which are able to evade antitumor immunity (192). Chronic inflammation is a well-known precursor to tumor development in many tissue beds (10, 20, 193).
It was shown in pancreatic carcinogenesis that recurrent pancreatic inflammation raises IL-6 released from macrophages, which drives the expression of early growth factor 1 (EGF1) in acinar cells, which is the master transcription factor for neocarcinogenesis and widespread field change (194). The effect of these epigenetic rearrangements is to reduce zymogen secretion with subsequent bouts of pancreatitis and thus the degree of tissue damage of subsequent inflammatory bouts. Induction of oncogenic Kras expression similarly reduced the tissue injury caused by pancreatic inflammation, a finding which suggests that oncogenes might be actively selected due to their anti-inflammatory effect (194). Both EGF and Kras were identified in the epigenomic cannabis screen of Schrott et al. (86).
Other researchers compared gene expression from tumors in mice from two genetic backgrounds with and without a mature immune system. Surprisingly they found that the tumors from the mice in which the immune system was intact were enriched in tumor suppressor genes which was interpreted as indicating that suppression of these genes was important to assist with immune evasion (195). One pathway by which this occurred was CCL2 secretion which attracted immunosuppressive M2 macrophages. CCL2 was positively identified in the epigenomic cannabis screen of Schrott et al. (86).
Age-Related Clonal Hemopoiesis (ARCH) has been described above. It has also been shown that similar effects with clonal dominance happen in nearly all tissue beds (196). It is known to occur often through epigenomic changes that may silence one of the DNA oxidoreductase demethylases TET2/3 or DNMT3A (197). The rate of cancer development in ARCH is greatly elevated and this particularly relates to leukemias, which are 12-times elevated. Of these, acute myeloid leukemia (AML) is a lethal disease carrying a very high mortality rate. Disordered innate immune signaling is common in the development of leukemia and pre-leukemias. TRAF6 is a downstream adapter known for transducing signals from the TNF receptor. It was shown that TRAF6 co-mutation along with TET2 dysfunction caused a highly aggressive and transplantable AML-like disorder in mice (197). TRAF6 was found to function as an E3 ubiquitin ligase which attaches a ubiquitin molecule to K148 of Myc and thereby competes with its activating acetylation at this residue which induces constitutive Myc oncogene activity. TRAF6K148Ub does not affect Myc protein stability. This becomes important as Myc is known to interact with histone acetyltransferases and induce its own acetylation in numerous cancers (197). TRAF6 reduction has been identified in some human AML cases and these cases have a worse prognosis than others. In some cases this is due to increased methylation of the TRAF6 promoter (197). Leukemic blasts and TET2/3 deficient cells are proinflammatory and this may account for the higher incidence of atherosclerotic plaque and myocardial infarction observed in ARCH patients. Thus, ARCH is both fed by and feeds inflammation (196). The effects of inflammation on Myc expression may also be more general (196). Of relevance to cannabinoid medicine, TRAF6 was positively identified in the human sperm cannabis exposure epigenomic screen from Schrott et al. (86).
It is known that many infiltrating immunocytes into cancers are functionally downregulated and exhausted. The cause for this immune exhaustion is not well-understood and appears to be multifactorial in origin. A systematic screen of T-cells in mouse and human tumor models found that two remodeling complexes in the SWI/SNF ATP-dependent chromatin remodeling families INO80 and BAF were involved in exhaustion induction (198). The Arid1a complex in the BAF family was particularly implicated in maintaining the exhausted phenotype. Arid1a depletion increased the chromatin accessibility of active T-cell genes and improved anti-tumor immunity (198). Importantly, one of the activities of the INO80 complex is to resolve R-loops (DNA:RNA hybrids) which would otherwise stall DNA polymerase and thereby allow uninterrupted proliferation of cancer cells (199). When R-loops occur in the cytoplasm they are highly immunogenic via cGAS-STING (200). There were 210 DMRs identified in the epigenomic screen of Schrott for Arid1, many hits for members of the BAF complex, and five hits for the INO80 complex (86).
Tumor-infiltrating fibroblasts have also been shown to induce immune exhaustion (201). Irradiation is known to provoke an inflammatory response. In a murine model of rectal cancer, irradiation caused IL-1α release which both polarized fibroblasts toward an inflammatory phenotype and triggered DNA damage thereby predisposing them to P53-mediated senescence which in turn caused chemoradiotherapy resistance and disease progression (201). Blockade of IL-1α, prevention of fibroblast senescence, or senolytic therapy (ablation of senescent cells) rendered the tumors radiosensitive. The main antagonist of IL-1α is IL1RA (IL-1 receptor antagonist). Patients with a lower IL-1RA level had a worse prognosis (201). Thus, this pathway shows both the importance of immune-fibroblast interactions and defines an important therapeutic target for future work. There were eight DMRs identified in the Schrott epigenomic screen for cannabis exposure (86).
Similar findings were made in a mouse model of pancreatic cancer (202). Healthy fibroblasts could be transformed into immunosuppressive cancer-associated LRRC15+ myofibroblasts under the influence of TGFBR2 signaling (202). These cancer-associated fibroblasts (CAFs) suppressed the cytolytic activity of infiltrating CD8 T-cells. Deletion of these CAFs restored tumor sensitivity to checkpoint PD-L1 inhibition (202). There were 152 DMRs identified in the Schrott screen for TGFBRs (86).
It has also been shown that mouse haemopoietic stem cells (which are also lymphopoietic stem cells) present antigens to CD4 T-cells on class II MHC antigens as a means of quality surveillance. Cells that do not pass these checks are induced to differentiate and thereby eliminated from the stem cell pool (203, 204). Similar pathways operate in human haemopoietic stem cells. These findings demonstrate bidirectional signaling between HSCs and surveilling immunocytes and this process is also applied—and perturbed—in cancer (204).
Microglia are known to play an important role in sculpting away unused dendrites and synapses from neurons (205–209) and also in controlling the growth of myelin sheaths laid down by oligodendroglial cells and their progenitors (210). Lung injury including smoking and lung infections are known to exacerbate autoimmune central nervous system disease (211). Using a rat model of experimental allergic encephalitis (EAE), researchers were able to show that prior tracheal insufflation of neomycin completely blocked the effects of subsequent intrathecal vaccination with myelin basic protein and the development of EAE (211). However, if the neomycin was not given EAE predictably developed. Neomycin shifted the pulmonary microbiota toward lipopolysaccharide releasing phyla (211). Changing the flora with polymyxin B removed the lipopolysaccharide releasing microflora and exacerbated EAE. These lung microbiota changes were associated with an alteration of brain macrophage from type I interferon releasing to type II interferon priming. These results demonstrated the existence of a lung-brain axis in the same way as a gut-brain axis has been demonstrated (211).
Documentation relating to an association between poor physical and mental health, somnolence, and unemployability of hashish devotees has long been a major issue in traditional societies and together constitute the reasons cannabis was out of favor to varying degrees in Islamic communities from the 9th to 18th centuries (212). Cannabis was first noted to be linked with insanity in western medical literature as long ago as 1930 (212). More recently, cannabis has been linked with adverse mental health outcomes in diverse psychiatric illnesses including an amotivational state (213–218), anxiety (219–225), depression (226–238), bipolar disorder (239–246), schizophrenia (235, 247–260), and suicidality (222, 230, 240, 243, 261–267). Whilst in previous decades the nature of these associations was somewhat controversial, these matters are now settled in the medical literature. Cannabis is also associated with a dependency and withdrawal syndrome which is more common in daily smokers, those who commence regular use in the early teenage years, and those who use cannabis products with higher THC concentrations where its incidence may rise to 50% of regular users (35, 218). Most recently, cannabis has been linked with mass homicide attacks, especially in the USA (230, 268–276). As has been astutely observed, “cannabis consumption and mental illness in adolescents and young adults are increasing in the United States” (277).
Pediatric autism spectrum disorder (ASD) has also been shown to be growing exponentially across the USA since 2000 (278–280). Increasing evidence supports a link with rising cannabis use, availability, and potency (281–289) and indeed in formal space-time and quantitative causal analyses cannabis has been shown to be the primary driver of this modern autism renaissance (290). Although cannabis or cannabidiol is frequently advocated as potential treatments for ASD, formal clinical trials have only produced mixed and conflicting results (291, 292).
Given that the fundamental units of computation in the brain are variously described as the neuron, the neuronal epigenome, subcellular organellar networks, the synapse, the dendrite, local cortical micronetworks, midbrain internuclear signaling, the machinery of the synaptic boutons, post-synaptic densities, and their associated astroglia, and since cannabinoid signaling disturbs all of these to varying degrees, it is appropriate to consider these issues in the present context to advance understanding of cannabinoid neurological synaptopathies.
The brain has 100 billion neurons and 1015 synapses a number which far surpasses the number of nodes in artificial intelligence networks (293, 294). However, this number can be multiplied by the many astrocytes which are the most numerous cells in the brain and also participate actively in tripartite synaptic trafficking in many ways (295, 296). Dendritic spines are believed to be a key site of much brain computation, learning, and memory, and are involved in essentially all brain functions and numerous neuropsychiatric disorders including depression, schizophrenia, and autism (293, 297, 298). Dendritic spines comprise 70% of the synapses in the cortex and occur with a frequency of 10 per micrometer (293).
Endocannabinoid (eCB)-dependent synaptic plasticity is highly dependent on synaptic activity and traffic. This is referred to as Hebbian learning (299). Synaptic plasticity can be induced by many stimulation protocols, especially those which involve repetitive short latency firing or that are associated with dopamine transients (293, 300, 301). Many neuronal subcompartments are known to be controlled by activity including the dendritic arbors, synaptic spines, axon initial segments, and presynaptic boutons (302). Endocannabinoid (eCB) induction of long-term potentiation has been identified in multiple brain regions including the hippocampus, striatum, amygdala, nucleus accumbens, nucleus of the solitary tract, ventral tegmental area, cerebellum, and the prefrontal, somatosensory, visual, and insular cortices, and spinal cord (303, 304). Synaptic potentiation is normally tightly controlled in both space and time and happens over a range of 0.5–1 micron and over seconds (293). eCBs are also involved in synaptic scaling and metaplasticity which scale the plasticity of the whole system generally (303).
Cannabinoids have long been known to mediate depolarization-induced suppression of inhibition (DSI) at inhibitory synapses (which is excitatory) (305, 306) and have also been shown to induce depolarization-induced excitation (DSE) at excitatory synapses (which is inhibitory) (307). Short- and long-term potentiation and depression of synaptic transmission have now been demonstrated (STE, STL, LTD, and LTP) (305, 308, 309). LTP mediated via CB1R is induced primarily post-synaptically and is demonstrated by increased receptor numbers and spine size, spine head area, and spine volume. It requires extended eCB stimulation (303). It can be induced by many stimulation protocols but is especially sensitive to induction by dopaminergic stimuli (300, 303). LTP is dependent on protein synthesis (300, 310). eCBs induce modifications of the active postsynaptic density zone matrix (303).
Cannabinoids are also known to play a key modulatory role on brain development during the neonatal and postnatal critical periods when GABAergic synapses are sculpting the excitatory and inhibitory brain circuits (307). Projections neurons from striasomes in the striatum form baskets around clusters of neurons in the ventral aspect of the Substantia nigra pars compacta; these structures are known as “striosome-dendron bouquets” (311). CB1Rs are necessary for the proper formation of these bouquets (311). Actin and spectrin form a regular repeating structure on axons (312, 313) which has been shown by super-resolution microscopy to form the framework into which CB1Rs are fitted (314–317). CB1Rs also play a critical role in the stabilization of nascent immature spines (318). All of these GABAergic changes are modulated by eCBs and act on somatostatin- and parvalbumin- positive GABAergic interneurons and also astrocytes (307). eCBs therefore play a critical role in the excitatory/inhibitory balance in both health and disease during development and maturity (307).
The activity of many receptor types drives eCB release and activation including mGluR1, mGluR5, M1/M3 muscarinic, 5HT2 serotoninergic, cholecystokinin (CCK1), orexin (OX1) and oxytocin (OT1), D2R dopaminergic, and α2-adrenergic receptors (319). Reward behavior has also been shown to control the strength of the hippocampus-nucleus accumbens synapses and the strength of these synapses is reduced by stress (320).
Human cannabis use disorder has been shown to reduce brain glucose oxygen consumption by PET scanning in cerebellum, orbitofrontal, and prefrontal cortices and basal ganglia (321). This has been linked with deficits in striatal dopamine release in cannabis dependence (322, 323). Downregulation of brain CB1Rs also occurred to a degree related to the years of cannabis smoking, which reversed after 4 weeks of monitored abstinence (324). This is the molecular representation of cannabinoid tolerance at the nanoscale. Thinning of cortical gray and white matter has also been demonstrated in cannabis dependence (325). Damage to white matter was shown innervating the posterior cingulate and parietal cortex, the basal ganglia, and the temporal cortex. Gray matter in the precuneus was thinned in a causal and dose-response manner (325). Regions with higher MAGL expression (the catabolic enzyme for 2AG) in human postmortem brains had more gray matter damage.
An fMRI study of cannabis use disorder showed increased connectivity between subcortical nuclei in the ventral striatum (housing the nucleus accumbens), the midbrain (accommodating the ventral tegmental area and substantia nigra), and lateral thalamus and brainstem (325). The level of suppression was noted to be related to the years of cannabis use and the degree of negative emotionality, depersonalization, and social alienation and perceived persecution experienced by patients (325). Thus, the heightened subcortical connectivity was believed to generate the negative emotionality which the reduced cortical connectivity was unable to ameliorate (325). It was also shown that cannabis use disorder patients had more recruitment of cerebral cortex to complete neurally demanding tasks together with less discrimination between cognitive and emotional processing (326). Disrupted thalamocortical connectivity in cannabis use disorder was documented in a further study from this group (327). Suppressed nucleus accumbens activity was seen in cannabis use disorder together with depressed corticostriatal and thalamocortical connectivity (327).
Significant structural effects on the brain from cannabis have been found in a meta-analysis of MRI studies and include relative atrophy of the hippocampus and medial and lateral orbitofrontal cortices, which have been found with effect sizes measured as standardized mean differences of 0.14, 0.30, and 0.19, respectively (328).
The clear convergence in this data from the world leading group at Brookhaven National Laboratory's state of the art scanners between negative emotionality, psychological persecutory complexes, social alienation and estrangement, impaired cortical control of subcortical hedonic drives, and confusion between emotional and cognitive processes and difficulty performing neural computation tasks and the above described predisposition to self-directed and other-directed acts of violence is both noteworthy and of serious concern. Data strongly suggest a causal pathway to irrational, ill-considered, and confused psychological states and behaviors.
Whilst eCB release is usually considered to be phasic and eCBs are described as being synthesized on demand, administration of eCB antagonists and genetic studies clearly demonstrates that there is also tonic eCB tone (305, 319). As well as classically described coupling of CB1Rs and CB2Rs to Gi proteins, non-classical coupling with GS and G0 G-proteins and β-arrestins is also documented (305). CB2Rs have also been identified on endoplasmic reticulum (305). When a newer and more sensitive marker for labeling astrocytes was employed, it was shown that 12% of the cerebral CB1R staining occurred on astrocytes (329). Astrocytes also display CB1Rs and eCB release onto astrocytes causes increased release of the gliotransmitters glutamate, D-serine, and adenosine, causing increased LTP (296, 305, 330).
Synaptic spines can be of three types: filipodia, small, and large (293, 302). There is a tight relationship between spine structure and function with LTP increasing and LTD decreasing spine size (302). At the nanoscale, spines fluctuate in size over minutes, hours, and days, with the largest spines being the most stable (331). Filipodia are small thin spines which are usually transient with only 3% lasting for 1 day (293, 297). Spines are rapidly responsive to stimulation within 1 min (293). If they are contacted by an axon, they can become a small spine. Increasing evidence implicates pathology at spines in many diseases including anxiety, depression, autism, intellectual disability, schizophrenia, and bipolar disorder (302). LTP leads to an increase in the size, number, and stability of spines, actin polymerization, an increase in the number of AMPARs and their surface trafficking, and the size of the post-synaptic density matrix (302). NMDA glutamate receptors allow ingress of extracellular calcium and via calcicalmodulin kinase II (CAMK2) are key to the orchestration of the changes of synaptic growth and plasticity (293, 302). Compound synapses also occur of two to six synapses and are more stable than single synapses (293). Large synapses are more stable as they are further from the pruning boundary (293).
Structural studies show an increase in the number and density of spines in the frontal, temporal, and parietal lobes in Layer II in autism and a reduction in schizophrenia (302). GWAS studies of neuropsychiatric disorders most prominently show a reduction in postsynaptic glutamatergic signaling and also changes in cytoskeletal organization, chromatin modifiers, and transcription regulation (302).
Also key to the organization of the postsynpatic bouton are the scaffolding proteins including shank and homer; actin which forms the basic structural protein of the spine, including the “knob” and the neck of the bouton, arrests passing ribosomes toward the bouton, and directs microtubules into it to carry their mitochondria as cargo to supply energy (331); and PSD95/DLG4, which forms the plate-like area subjacent to the synaptic cleft into which AMPARs and NMDARs are inserted and bound (302, 331). Cofilin (CFL1 gene) is a key molecule with severs actin and induces the formation of branches and new actin polymers (331). PICK1 and oligophrenin (OPHN1 gene) are similarly key anchoring and actin controlling proteins (331). Calcium channels such as CACNA1C are clearly key since many of the changes described are calcium-induced, as are the small Ras GTPase family including Ras and RAC1 which induce spine formation, enlargement, maturation, and stabilization, and RhoA and RAP which have the opposite effect (302). RhoA and RAC1 also control the actin cytoskeleton. The Ras family is also a well-known oncogene (302). Neurexin and neuroligin are key transsynaptic scaffolding proteins (331). Shank proteins are highly implicated in autism spectrum disorder (ASD), which highlights ASD in part as a synaptopathy (332). Genes active in neurogenesis, chromatin modification, and synaptic function have also been implicated in autism (333).
It is therefore of interest to observe that many of these key receptors were positively identified in the epigenomic cannabis screen of Schrott et al. (86). including AMPARs (GRIA 132 DMR hits), NMDARs (GRIN 26 hits), metabotropic glutamate receptors (GRM 122 hits), GABA receptors (GABR 143 hits), dopamine receptors (DRD 17 hits), orexin/hypocretin receptors (HCRT 1 hit), μ-opioid (ORPM, 5 hits), and δ-opioid (ORPD, 5 hits). Of the scaffolding and framework molecules, the following were identified: actin (207 hits), tubulin (106 hits), cofilin (CFL1, one hit), PICK1 (one hit), oligophrenin (one hit), DLG/PSD95 (37 hits), SHANK (6 hits), HOMER (2 hits), calcium channel CACNA1 (46 hits), CAMK2 (5 hits), Ras (146 hits), RhoA (1 hit), RAP (438 hits), neurexin (27 hits), and neuroligin (10 hits) (86). When genes involved in depression were intersected with genes modified by cannabis, multiple genes were found in common in mouse, rat, and human studies (334). The autism candidate gene DLGAP2 was found to be altered in rat and human sperm after cannabis exposure (282).
Activities at the presynaptic terminal are generally heavily energy dependent. Terminal depolarization by an action potential causes calcium to flow into the terminal and the exocytosis of neurotransmitters from presynaptic vesicles (294, 335). Restoration of ionic gradients, synaptic cargo transport, synapse assembly and maintenance, pumping out presynaptic calcium, and synaptic vesicle refiling and recycling all require ATP. Indeed, it has been estimated that the recycling of a single glutamate synaptic vesicle requires 20,000 ATP molecules and that maintenance of the terminal at a steady state requires the presence of 1,000,000 ATP molecules (294).
Whilst the human brain is only 2% of body weight, it has been estimated to consume 20% of the energy budget. Glucose may be a major source of brain energy and 55% of the brain's glucose is consumed at axon terminals (294). Running the Na+/K+ and Ca2+ pumps which maintain the ionic gradients is a major energy requirement.
ATP is generated at the nanoscale in cellular microdomains. On demand glycolytic energy can be released from glycolytic enzymes mounted on the plasmalemma, within axonal transport cargos, and on synaptic vesicles to supply motor ATPases and ion transport ATPases with fast on-board refueling. Energy is also supplied from astrocytes by the lactate shuttle. Both mitochondrial oxidative phosphorylation and glycolysis can be upregulated in periods of intense activity (294).
A large calcium influx occurs into the presynaptic terminal with depolarization. This is taken up partly by the mitochondrial voltage-dependent anion selective channels and calcium uniporter of the inner and outer membranes and this has the effect of powerfully upregulating mitochondrial metabolism by stimulating its many calcium sensitive enzymes (294). Calcium also increases the surface area of cristae and cytochrome oxidase activity and induces a proteomic adjustment in favor of increased energy production. The anti-apoptotic protein BAD also stimulates mitochondrial biomass, enhances energy production, and reduces the leak from the F1F0-ATPase, thus enhancing the efficiency of energy production (294).
Mitochondrial biogenesis is a key mechanism by which mitochondria undergo fission and fusion in order to increase energy delivery to the presynapse. Mitochondria are trafficked along dendrites and axons and are typically carried by a kinesin at one end and a dynein at the other, which move the mitochondria toward the plus and minus ends of the microtubule, respectively (294). Mitochondria trafficking along microtubules are arrested by local high calcium levels. Short-range movements of mitochondria within the spine are conducted by myosin motors (294).
Neuronal synaptic terminals possess a master energy sensor called AMP kinase (AMPK) which drives many of the changes to increase energy production for periods of high energy requirements (294). AMPK has multiple presynaptic activities including adapting glycolysis and mitochondrial respiration, sustaining LTP and high frequency stimulation, facilitating mitochondrial transport and presynaptic distribution, and driving the accumulation of mitochondria within the axonal compartment. Importantly, this is the same key molecule that is well-known to sense energy and is a key controller of lifespan in lower organisms (72, 336–348).
Thus, mitochondria play a dual role in the presynaptic terminal by virtue of their interrelated roles for energy supply and calcium buffering. Failure of synaptoenergetics has been shown to play a key role in diseases such as Alzheimer's and Parkinson's disease and in motor neuron disease (294).
Two of the key mitochondrial fusion proteins, OPA1 and MFN1, were identified in the cannabis epigenomic screen of Schrott with three and one DMR identified, respectively (86). There were 217 DMRs in the Schrott screen for kinesins, 16 for dyneins, and four for dynactin, the regulatory partner of dyneins (86). AMPK was identified in the Schrott screen with five DMR hits (86).
Endoplasmic reticulum (ER) also play an important role in presynaptic calcium control as they are able to actively acquire calcium from the cytoplasm and hold the largest cellular stores of this cation (349). Moreover, ER are electrically excitable and can be triggered by action potentials (349). ER are also a major site of protein synthesis as they house ribosomes. Lysosomes also concentrate calcium, and the release of the calcium from just one lysosome has been shown to elevate cytosolic calcium by two orders of magnitude (349).
Extracellular vesicles (EVs) are known to originate in intracellular endosomes.
Brain EVs promote communication between neurons and non-neuronal cells, prune excitatory synapses, and modulate inhibitory synapses the transmission of viruses, and prion protein aggregates in preclinical Alzheimer's disease models (350, 351).
They are also useful for critical events such as during rapid maturation of the neuronal proteome such as in dendrite maturation and growth cone steering (350).
BDNF modulated both eCB LTD and LTP in the hippocampus, neocortex, ventral tegmental area, and striatum via its high affinity TrkB receptor. BDNF stimulates 2-AG release and CB1R activation and DSI causing increased glutamatergic LTP in the neocortex (304). BDNF release leads to the release of neuronal vesicles from the post-synaptic terminal carrying miRNAs (miR-132-5p, miR-218-5p, and miR-690) which induce clustering of the synaptic vesicle in the presynaptic terminal, increased calcium transients in the post-synaptic terminal, regulate the abundance of synaptogenic transcripts, increase BDNF-dependent dendrite outgrowth, increase synapse density, and increase neuronal network bursting and synchronized activity and connectivity (350).
BDNF was necessary and sufficient for the formation of excitatory synapse formation in the hippocampus. EVs induce synchronous neuronal network firing (350).
Of relevance to cannabinoid medicine, THC has been shown to epigenomically downregulate BDNF mRNA and protein expression in the ventral dentate gyrus (352). BDNF was identified by three DMRs in the Schrott cannabis epigenomic database and the high affinity BDNF receptor TrkB (gene NRTK2) was identified by 33 DMRs in this database (86).
From the above discussion, it is clear that whilst topographically the synapse is considered the unit of computation, changes that are localized to the synapse must clearly be coordinated with events in the cell. As mentioned, the apparatus of the synapse involves over 500 proteins which must be synthesized locally or imported and there is a high demand for local energy. Therefore, ribosomes and mitochondria are re-routed as they travel along dendritic microtubules and imported into larger spines (293). It has been convincingly demonstrated that memory formation (involving spine enlargement, consolidation, and maturation) requires protein synthesis and frequently occurs overnight (293, 310). Spine enlargement and maturation involves several signaling cascades including changed distribution of glutamate receptors and synaptic adhesion proteins (18).
From these observations, it becomes clear that epigenomic controls of protein expression must be coordinated with events at the synapse for the whole system to work cohesively. That is, memories which are encoded epigenomically must be coordinated and synergize with local synaptically encoded memories (18). Thus, disorders of DNA methylation, histone methylation, histone acetylation, CBP, CREB1, and HDAC5 have all been linked with impairments of learning and memory (18).
Signaling from the synapse to the nucleus can occur via activation-induced CREB-regulated transcriptional coactivator 1 (CRTC1). Strong training also induces the brain-specific FGF1B, which is required for CA3-CA1 learning in the hippocampus (18). FGF1B transcription in the nucleus is induced by CRTC1, which displaces the transcriptional co-repressor HDAC3 nuclear receptor corepressor (NCOR) complex, leaving phosphorylated CREB-CBP to bind in its place, thereby activating FGF1B transcription (18). Strong training-induced continued CRTC1 expression displaced CBP and drove the insertion of the HAT KAT5, which induces sustained FGF1B expression. KAT5 substitution was required for hippocampal synaptic plasticity and memory enhancement (18). Afadin is another protein that shuttles from the synapse to the nucleus and induces epigenetic change, which in this case is H2S10 which opens heterochromatin to allow a more permissive chromatin state for gene transcription (18). From remarks made above, it is also likely that extrasynaptic vesicles also traffic information into the dendrite and neuronal soma.
It is noted that, in the Schrott cannabis epigenomic screen, there were two hits for CRTC1 and six hits for NCOR (86).
Signaling from the nucleus to the synapse occurs by virtue of control of AMPAR synthesis during critical periods, stress, and drug exposure. Stress-induced cortisol release which increased HDAC2 occupancy of the G9a promoter and impacts on E3 ubiquitin ligase leads to increased ubiquitinoylation of AMPARs and their down regulation. Similarly, an epigenetic cascade of histone acetylation and H3K9 trimethylation can control the alternate splicing of neurexin-1 which controls its affinity for post-synaptic binding partners and thus synaptic activity and remodeling (18).
In this way detailed, complex, and profound coordination between the epigenomic machinery of the nucleus and the structural machinery of the synapse is orchestrated.
One elegant example of these changes is with PRKCZ. PRKCZ has been well-characterized as being involved in long-term potentiation and synaptic strengthening (353). PRKCZ abnormalities have been implicated in alcoholism, depression, and Alzheimer's disease. DNA methylation of the internal intronic promoter carrying a CREB -binding site of the human brain-specific PRKCZ gene was noted to be controlled by DNA methylation (353). Methylation of this site caused reduced CREB binding and downregulation of PRKCZ transcription (353). CREB has been implicated in both short- and long-term synaptic potentiation and adult neurogenesis and controls cassettes of genes that are involved in these processes (353). DNA methylation prevents CREB docking at the site (353). Similar control mechanisms were found in three other genes involved in long-term potentiation and neuronal differentiation from stem cells (LRRTM2, NEUROD2, and FAM163B) (353). PRKCZ recruits AMPARs to the post-synaptic density and LRRTM2 stabilizes them there.
PRKCZ was identified by two DMRs in the Schrott cannabis epigenomic screen (86).
It is thus apparent that cannabinoids can modulate brain synaptic signaling at many levels including neuronal, neuronal mitochondria, neuronal and astroglial epigenomic, subcellular trafficking, and pre- and post- synaptic levels. and modulate neurotransmission in several subtle and classical directions including impacting both long-term synaptic depression and potentiation. Therefore, these various and interrelated mechanisms form the neurobiological substrate for the now documented widely diverse clinical syndromic phenomenology of adult and inheritable pediatric neurotoxicity described in the opening paragraphs.
With this widespread disruption of neural activity at many levels, it becomes clear how cannabis exposure might be related to so many diverse neuropsychiatric syndromes including depression (227, 229, 230), anxiety (224, 225, 230), schizophrenia (354–358), bipolar affective disorder (239, 240, 245), and autistic spectrum disorder (278, 279, 290, 359).
The putatively causal pathway from confused thinking, heightened subcortical hedonic drive, impaired cortical control of subcortical activity, and underlying highly negative emotional state with advanced social alienation and persecutory perseverative thinking is of particular concern in terms of contemporary issues with cannabis-related violence, suicides, and homicides. In that this pathway has been well-described in reports from the Brookhaven National Laboratories an internationally pathfinding group in the brain scanning of neuropsychiatric disorders (323, 325–328, 360–363), this evidence can only be considered state of the art.
It is also of great interest that AMPK, which is one of the best established molecules with an important role in aging medicine (364–366), is also a key and central regulator of synaptoenergetics at the presynapse (294).
A real concern has been expressed that overwhelming this delicate synaptic and epigenomic machinery of DNA methylation, histone modifications, and microRNAs with exogenous phytocannabinoids will disrupt the delicate spatiotemporally defined patterns of eCB-controlled systems which maintain normal brain function and development (367). Given that mitochondria play such a key role in synaptoenergetics, it is clear that the general disruption of mitochondrial metabolism will necessarily perturb both neuronal and synaptic activity and epigenomic regulation. Given that synaptic dysregulation is a key component of most neuropsychiatric disorders, these disruptions are likely to have far reaching and protean manifestations (293, 297, 298). In view of the fact that both the brain and mitochondria also control systemic aging and disease (364, 365) these impacts are likely to ramify beyond even psychiatry to the general domain of whole-body health.
This wide-ranging overview considered in Parts 1 and 2 of this review has considered the manner in which interrelated, interlocking, and interdependent deleterious changes have been shown to be induced by cannabinoids in multiple mechanistic layers including the genome, epigenome, metabolome, and immunome. Changes in synaptic plasticity illustrate and highlight these metabolic, proteomic, and epigenomic alterations. It is emphasized that these changes do not occur in isolation but in a coordinated cross-platform manner. A striking feature of the data is its cross-disciplinary concordance so that, for example, CB1R downregulation occurring in cannabis dependence and first demonstrated by autoradiography in brain slices has now been confirmed by super-resolution microscopy and has been shown to be reflected in blunted hedonic drive in the midbrain and ventral tegmental area and in inhibited cortical connectivity from the frontal lobes. Synaptic alterations are necessarily supported by metabolic and epigenomic alterations and synergize with altered dopaminergic drive, all of which impact on learning, emotionality, behavior, and memory. The evidence for altered and confused behavior including persecutory and emotively driven thinking from the Brookhaven scans is relevant to modern considerations of cannabis-associated self- and other- directed violence.
There is an impressive synergy between the altered immunome, metabolome, and epigenome that fits with the altered gene expression seen in clinical syndromes such as diverse cancers and numerous congenital anomalies identified in modern large-scale epidemiological studies (2–20, 69, 368–385) and the genomic, epigenomic, metabolomic, and immunomic changes of aging (386–391) which together reflect altered and disrupted epigenomic-metabolomic regulation. Clearly, the well-established inhibition of mitochondrial oxidation is compounded by the immunostimulatory CB1R-mediated cannabinoid actions and together they have greatly amplified downstream effects.
Thus, it is important to hold a proper appreciation of the epigenomically mediated alterations of three-dimensional genomic architecture, gene expression, and epitranscriptome in mind in order to foster a comprehensive appreciation of multifaceted and diverse cannabinoid pathophysiology. Moreover, given the rapid growth of the cannabis industry worldwide, there is a pressing and urgent need to complete single cell three dimensional chromosomal conformation capture (low input Hi-C methods) and associated mass spectrometry histone modification, DNA methylation, whole genome, whole epigenome, enhancer, superenhancer, superanchor, and m6A epitranscriptome studies in many brain areas, and in the heart, liver, respiratory tract, immune, muscle, testicular, and ovarian tissues in a timely manner.
AR conceived the idea, performed the literature review, and wrote the first draft. GH added meaningful intellectual input, edited the first draft, provided project supervision and support, curated resources, and supervised the conduct of the project. All authors contributed to the article and approved the submitted version.
The authors declare that the research was conducted in the absence of any commercial or financial relationships that could be construed as a potential conflict of interest.
All claims expressed in this article are solely those of the authors and do not necessarily represent those of their affiliated organizations, or those of the publisher, the editors and the reviewers. Any product that may be evaluated in this article, or claim that may be made by its manufacturer, is not guaranteed or endorsed by the publisher.
1. Reece AS, Hulse GK. Perturbation of 3D nuclear architecture, epigenomic aging and dysregulation and cannabinoid synaptopathy reconfigures conceptualization of cannabinoid pathophysiology: part 1 – aging and epigenomics. Front Psychiatry. (2023) 14:1182535. doi: 10.3389/fpsyt.2023.1182535
2. Reece AS, Hulse GK. Geotemporospatial and causal inferential epidemiological overview and survey of USA cannabis, cannabidiol and cannabinoid genotoxicity expressed in cancer incidence 2003–2017: part 1 – Continuous bivariate analysis. Arch Public Health. (2022) 80:99–133. doi: 10.1186/s13690-022-00811-8
3. Reece AS, Hulse GK. Geotemporospatial and causal inferential epidemiological overview and Survey of USA cannabis, cannabidiol and cannabinoid genotoxicity expressed in cancer incidence 2003–2017: part 2 – Categorical bivariate analysis and attributable fractions. Arch Public Health. (2022) 80:100–35. doi: 10.1186/s13690-022-00812-7
4. Reece AS, Hulse GK. Geotemporospatial and causal inferential epidemiological overview and survey of USA cannabis, cannabidiol and cannabinoid genotoxicity expressed in cancer incidence 2003–2017: part 3 – Spatiotemporal, multivariable and causal inferential pathfinding and exploratory analyses of prostate and ovarian cancers. Arch Public Health. (2022) 80:100–36. doi: 10.1186/s13690-022-00813-6
5. Reece AS, Hulse GK. A geospatiotemporal and causal inference epidemiological exploration of substance and cannabinoid exposure as drivers of rising US pediatric cancer rates. BMC Cancer. (2021) 21:197–230. doi: 10.1186/s12885-021-07924-3
6. Reece AS, Hulse GK. Causal inference multiple imputation investigation of the impact of cannabinoids and other substances on ethnic differentials in US testicular cancer incidence. BMC Pharmacol Toxicol. (2021) 22:40–71. doi: 10.1186/s40360-021-00505-x
7. Reece AS, Hulse GK. Cannabis genotoxicity and cancer incidence: a highly concordant synthesis of european and USA datasets. In:Preedy V, Patel V, editors. Cannabis, Cannabinoids and Endocannabinoids. London: Elsevier (2023). p. 93–112.
8. Reece AS, Hulse GK. Geospatiotemporal and causal inference study of cannabis and other drugs as risk factors for female breast cancer USA 2003-2017. Environ Epigenet. (2022) 8:1–22. doi: 10.1093/eep/dvac006
9. Reece AS, Hulse GK. Sociodemographically stratified exploration of pancreatic cancer incidence in younger US patients: implication of cannabis exposure as a risk factor. Gastroenterol Insights. (2023) 14:204–35. doi: 10.3390/gastroent14020016
10. Reece AS, Hulse GK. Novel insights into potential cannabis-related cancerogenesis from recent key whole epigenome screen of cannabis dependence and withdrawal: epidemiological comment and explication of Schrott et al. Genes. (2022) 14:1–14. doi: 10.3390/genes14010032
11. Reece AS, Hulse GK. State trends of cannabis liberalization as a causal driver of increasing testicular cancer rates across the USA. Int J Environ Res Public Health. (2022) 19:12759–96. doi: 10.3390/ijerph191912759
12. Reece AS, Hulse GK. Clinical epigenomic explanation of the epidemiology of cannabinoid genotoxicity manifesting as transgenerational teratogenesis, cancerogenesis and aging acceleration. Int J Environ Res Public Health. (2023) 20:3360–83. doi: 10.3390/ijerph20043360
13. Reece AS, Hulse GK. Geotemporospatial and causal inference epidemiological analysis of US survey and overview of cannabis, cannabidiol and cannabinoid genotoxicity in relation to congenital anomalies 2001–2015. BMC Pediatr. (2022) 22:47–124. doi: 10.1186/s12887-021-02996-3
14. Reece AS, Hulse GK. Cannabinoid genotoxicity and congenital anomalies: a convergent synthesis of european and USA datasets. In:Preedy V, Patel V, editors. Cannabis, Cannabinoids and Endocannabinoids. London: Elsevier (2023). p. 71–92.
15. Reece AS, Hulse GK. Cannabinoid- and substance- relationships of european congenital anomaly patterns: a space-time panel regression and causal inferential study. Environ Epigenet. (2022) 8:1–40. doi: 10.1093/eep/dvab015
16. Reece AS, Hulse GK. Congenital anomaly epidemiological correlates of Δ8THC across USA 2003-2016: panel regression and causal inferential study. Environ Epigenet. (2022) 8:1–17. doi: 10.1093/eep/dvac012
17. Reece AS, Hulse GK. European epidemiological patterns of cannabis- and substance- related congenital cardiovascular anomalies: geospatiotemporal and causal inferential study. Environ Epigenet. (2022) 8:1–55. doi: 10.1093/eep/dvac015
18. Reece AS, Hulse GK. Patterns of cannabis- and substance- related congenital general anomalies in Europe: a geospatiotemporal and causal inferential study. Pediatr Rep. (2023) 15:69–121. doi: 10.3390/pediatric15010009
19. Reece AS, Hulse GK. Epidemiological overview of multidimensional chromosomal and genome toxicity of cannabis exposure in congenital anomalies and cancer development. Sci Rep. (2021) 11:13892–912. doi: 10.1038/s41598-021-93411-5
20. Reece AS, Hulse GK. Epigenomic and other evidence for cannabis-induced aging contextualized in a synthetic epidemiologic overview of cannabinoid-related teratogenesis and cannabinoid-related carcinogenesis. Int J Environ Res Public Health. (2022) 19:16721–76. doi: 10.3390/ijerph192416721
21. Færgestad EM, Langsrud Ø, Høy M, Hollung K. 4.08 - analysis of megavariate data in functional genomics. In:Brown SD, Tauler R, Walczak B, editors. Comprehensive Chemometrics. Oxford: Elsevier (2009). p. 221–78.
22. Bino T, Chari-Bitron A, Shahar A. Biochemical effects and morphological changes in rat liver mitochondria exposed to 1 -tetrahydrocannabinol. Biochim Biophys Acta. (1972) 288:195–202. doi: 10.1016/0005-2736(72)90238-6
23. Chan JZ, Duncan RE. Regulatory effects of cannabidiol on mitochondrial functions: a review. Cells. (2021) 10:1251. doi: 10.3390/cells10051251
24. Drummond-Main CD, Ahn Y, Kesler M, Gavrilovici C, Kim DY, Kiroski I, et al. Cannabidiol impairs brain mitochondrial metabolism and neuronal integrity. Cannabis Cannabinoid Res. (2022). doi: 10.1089/can.2022.0011
25. Lamanna-Rama N, MacDowell KS, López G, Leza JC, Desco M, Ambrosio E, et al. Neuroimaging revealed long-lasting glucose metabolism changes to morphine withdrawal in rats pretreated with the cannabinoid agonist CP-55,940 during periadolescence. Eur Neuropsychopharmacol. (2023) 69:60–76. doi: 10.1016/j.euroneuro.2023.01.005
26. Ma L, Zhang H, Liu C, Liu M, Shangguan F, Liu Y, et al. A novel mechanism of cannabidiol in suppressing ovarian cancer through LAIR-1 mediated mitochondrial dysfunction and apoptosis. Environ Toxicol. (2023) 38:1118–1132. doi: 10.1002/tox.23752
27. Mahmoud AM, Kostrzewa M, Marolda V, Cerasuolo M, Maccarinelli F, Coltrini D, et al. Cannabidiol alters mitochondrial bioenergetics via VDAC1 and triggers cell death in hormone-refractory prostate cancer. Pharmacol Res. (2023) 189:106683. doi: 10.1016/j.phrs.2023.106683
28. Malheiro RF, Carmo H, Carvalho F, Silva JP. Cannabinoid-mediated targeting of mitochondria on the modulation of mitochondrial function and dynamics. Pharmacol Res. (2023) 187:106603. doi: 10.1016/j.phrs.2022.106603
29. Olivas-Aguirre M, Torres-López L, Pottosin I, Dobrovinskaya O. Phenolic compounds cannabidiol, curcumin and quercetin cause mitochondrial dysfunction and suppress acute lymphoblastic leukemia cells. Int J Mol Sci. (2020) 22:204. doi: 10.3390/ijms22010204
30. Zottola ACP, Severi I, Cannich A, Ciofi P, Cota D, Marsicano G, et al. Expression of functional cannabinoid type-1 (CB(1)) receptor in mitochondria of white adipocytes. Cells. (2022) 11:2582. doi: 10.3390/cells11162582
31. Rupprecht A, Theisen U, Wendt F, Frank M, Hinz B. The combination of Δ(9)-tetrahydrocannabinol and cannabidiol suppresses mitochondrial respiration of human glioblastoma cells via downregulation of specific respiratory chain proteins. Cancers. (2022) 14:3129. doi: 10.3390/cancers14133129
32. Ryan D, Drysdale AJ, Lafourcade C, Pertwee RG, Platt B. Cannabidiol targets mitochondria to regulate intracellular Ca2+ levels. J Neurosci. (2009) 29:2053–63. doi: 10.1523/JNEUROSCI.4212-08.2009
33. Winklmayr M, Gaisberger M, Kittl M, Fuchs J, Ritter M, Jakab M. Dose-dependent cannabidiol-induced elevation of intracellular calcium and apoptosis in human articular chondrocytes. J Orthop Res. (2019) 37:2540–9. doi: 10.1002/jor.24430
34. Wu HY, Huang CH, Lin YH, Wang CC, Jan TR. Cannabidiol induced apoptosis in human monocytes through mitochondrial permeability transition pore-mediated ROS production. Free Radic Biol Med. (2018) 124:311–8. doi: 10.1016/j.freeradbiomed.2018.06.023
35. Wolff V, Rouyer O, Geny B. Adverse health effects of marijuana use. N Engl J Med. (2014) 371:878–9. doi: 10.1056/NEJMc1407928
36. Badawy ZS, Chohan KR, Whyte DA, Penefsky HS, Brown OM, Souid AK. Cannabinoids inhibit the respiration of human sperm. Fertil Steril. (2009) 91:2471–6. doi: 10.1016/j.fertnstert.2008.03.075
37. Costa B, Colleoni M. Changes in rat brain energetic metabolism after exposure to anandamide or Delta(9)-tetrahydrocannabinol. Eur J Pharmacol. (2000) 395:1–7. doi: 10.1016/S0014-2999(00)00170-9
38. Rossato M, Ion Popa F, Ferigo M, Clari G, Foresta C. Human sperm express cannabinoid receptor Cb1, the activation of which inhibits motility, acrosome reaction, and mitochondrial function. J Clin Endocrinol Metab. (2005) 90:984–91. doi: 10.1210/jc.2004-1287
39. Sarafian TA, Habib N, Oldham M, Seeram N, Lee R-P, Lin L, et al. Inhaled marijuana smoke disrupts mitochondrial energetics in pulmonary epithelial cells in vivo. Am J Physiol. (2006) 290:L1202–9. doi: 10.1152/ajplung.00371.2005
40. Sarafian TA, Kouyoumjian S, Khoshaghideh F, Tashkin DP, Roth MD. Delta 9-tetrahydrocannabinol disrupts mitochondrial function and cell energetics. Am J Physiol. (2003) 284:L298–306. doi: 10.1152/ajplung.00157.2002
41. Wolff V, Rouyer O, Schlagowski A, Zoll J, Raul JS, Marescaux C. Study of the effect of THC on mitochondrial respiration of the rat brain. One line of thought to explain the link between cannabis use and the occurrence of cerebral infarction in men. Neurol Rev. (2014) 170:A19–20. doi: 10.1016/j.neurol.2014.01.081
42. Wolff V, Schlagowski A-I, Rouyer O, Charles A-L, Singh F, Auger C, et al. Tetrahydrocannabinol induces brain mitochondrial respiratory chain dysfunction and increases oxidative stress: a potential mechanism involved in cannabis-related stroke. Biomed Res Int. (2015) 2015:323706. doi: 10.1155/2015/323706
43. Wang J, Yuan W, Li MD. Genes and pathways co-associated with the exposure to multiple drugs of abuse, including alcohol, amphetamine/methamphetamine, cocaine, marijuana, morphine, and/or nicotine: a review of proteomics analyses. Mol Neurobiol. (2011) 44:269–86. doi: 10.1007/s12035-011-8202-4
44. Bar-Ziv R, Bolas T, Dillin A. Systemic effects of mitochondrial stress. EMBO Rep. (2020) 21:e50094. doi: 10.15252/embr.202050094
45. Zhu D, Li X, Tian Y. Mitochondrial-to-nuclear communication in aging: an epigenetic perspective. Trends Biochem Sci. (2022) 47:645–59. doi: 10.1016/j.tibs.2022.03.008
46. Singlár Z, Ganbat N, Szentesi P, Osgonsandag N, Szabó L, Telek A, et al. Genetic manipulation of CB1 cannabinoid receptors reveals a role in maintaining proper skeletal muscle morphology and function in mice. Int J Mol Sci. (2022) 23:15653. doi: 10.3390/ijms232415653
47. Bohmbach K, Henneberger C, Hirrlinger J. Astrocytes in memory formation and maintenance. Essays Biochem. (2022) 67:107–117. doi: 10.1042/EBC20220091
48. Fernández-Moncada I, Marsicano G. Astroglial CB1 receptors, energy metabolism, and gliotransmission: an integrated signaling system? Essays Biochem. (2023) 67:49–61. doi: 10.1042/EBC20220089
49. Canto C, Menzies KJ, Auwerx J. NAD(+) metabolism and the control of energy homeostasis: a balancing act between mitochondria and the nucleus. Cell Metab. (2015) 22:31–53. doi: 10.1016/j.cmet.2015.05.023
50. Alberts B, Johnson A, Lewis J, Raff M, Roberts K, Walter P, (editors). Molecular Biology of the Cell. 6th ed. New York, NY: Garland Science (2014).
51. Blevins RD, Regan JD. delta-9-Tetrahydrocannabinol: effect on macromolecular synthesis in human and other mammalian cells. Arch Toxicol. (1976) 35:127–35. doi: 10.1007/BF00372766
52. McClean DK, Zimmerman AM. Action of delta 9-tetrahydrocannabinol on cell division and macromolecular synthesis in division-synchronized protozoa. Pharmacology. (1976) 14:307–21. doi: 10.1159/000136610
53. Nahas GG, Morishima A, Desoize B. Effects of cannabinoids on macromolecular synthesis and replication of cultured lymphocytes. Fed Proc. (1977) 36:1748–52.
54. Russo C, Ferk F, Mišík M, Ropek N, Nersesyan A, Mejri D, et al. Low doses of widely consumed cannabinoids (cannabidiol and cannabidivarin) cause DNA damage and chromosomal aberrations in human-derived cells. Arch Toxicol. (2019) 93:179–88. doi: 10.1007/s00204-018-2322-9
55. Dalterio S, Badr F, Bartke A, Mayfield D. Cannabinoids in male mice: effects on fertility and spermatogenesis. Science. (1982) 216:315–6. doi: 10.1126/science.6801767
56. Dalterio SL, deRooij DG. Maternal cannabinoid exposure. Effects on spermatogenesis in male offspring. Int J Androl. (1986) 9:250–8. doi: 10.1111/j.1365-2605.1986.tb00888.x
57. Leuchtenberger C, Leuchtenberger R. Morphological and cytochemical effects of marijuana cigarette smoke on epithelioid cells of lung explants from mice. Nature. (1971) 234:227–9. doi: 10.1038/234227a0
58. Leuchtenberger C, Leuchtenberger R, Schneider A. Effects of marijuana and tobacco smoke on human lung physiology. Nature. (1973) 241:137–9. doi: 10.1038/241137a0
59. Stenchever MA, Kunysz TJ, Allen MA. Chromosome breakage in users of marihuana. Am J Obstet Gynecol. (1974) 118:106–13. doi: 10.1016/S0002-9378(16)33653-5
60. Huang H.FS, Nahas GG, Hembree WC. Effects of marijuana inhalation on spermatogenesis of the rat. In:Nahas GG, Sutin KM, Harvey DJ, Agurell S, editors. Marijuana in Medicine. Totowa, NY: Human Press (1999). p. 359–66.
61. Hall W, Degenhardt L. Adverse health effects of non-medical cannabis use. Lancet. (2009) 374:1383–91. doi: 10.1016/S0140-6736(09)61037-0
62. Cozens DD, Nahas GG, Harvey D. Prenatal exposure to cannabis and fetal development. In:Nahas GG, Sutin KM, Harvey DJ, Agurell S, editors. Marijuana in Medicine. Totowa, NY: Humana Press (1999). p. 431–40.
63. Nahas GG, Suciu-Foca N, Armand JP, Morishima A. Inhibition of cellular mediated immunity in marihuana smokers. Science. (1974) 183:419–20. doi: 10.1126/science.183.4123.419
64. McClintock B. The stability of broken ends of chromosomes in zea mays. Genetics. (1941) 26:234–82. doi: 10.1093/genetics/26.2.234
65. de Pagter MS, van Roosmalen MJ, Baas AF, Renkens I, Duran KJ, van Binsbergen E, et al. Chromothripsis in healthy individuals affects multiple protein-coding genes and can result in severe congenital abnormalities in offspring. Am J Hum Genet. (2015) 96:651–6. doi: 10.1016/j.ajhg.2015.02.005
66. Forment JV, Kaidi A, Jackson SP. Chromothripsis and cancer: causes and consequences of chromosome shattering. Nat Rev Cancer. (2012) 12:663–70. doi: 10.1038/nrc3352
67. Kloosterman WP, Guryev V, van Roosmalen M, Duran KJ, de Bruijn E, Bakker SCM, et al. Chromothripsis as a mechanism driving complex de novo structural rearrangements in the germline. Hum Mol Genet. (2011) 20:1916–24. doi: 10.1093/hmg/ddr073
68. Kloosterman WP, Hoogstraat M, Paling O, Tavakoli-Yaraki M, Renkens I, Vermaat JS, et al. Chromothripsis is a common mechanism driving genomic rearrangements in primary and metastatic colorectal cancer. Genome Biol. (2011) 12:R103. doi: 10.1186/gb-2011-12-10-r103
69. Reece AS, Hulse GK. Chromothripsis and epigenomics complete causality criteria for cannabis- and addiction-connected carcinogenicity, congenital toxicity and heritable genotoxicity. Mutat Res. (2016) 789:15–25. doi: 10.1016/j.mrfmmm.2016.05.002
70. Zhang C-Z, Spektor A, Cornils H, Francis JM, Jackson EK, Liu S, et al. Chromothripsis from DNA damage in micronuclei. Nature. (2015) 522:179–84. doi: 10.1038/nature14493
71. Guler EM, Bektay MY, Akyildiz AG, Sisman BH, Izzettin FV, Kocyigit A. Investigation of DNA damage, oxidative stress, and inflammation in synthetic cannabinoid users. Hum Exp Toxicol. (2020) 39:1454–62. doi: 10.1177/0960327120930057
72. Yang J-H, Hayano M, Griffin PT, Amorim JA, Bonkowski MS, Apostolides JK, et al. Loss of epigenetic information as a cause of mammalian aging. Cell. (2023) 186:305–26.e27. doi: 10.1016/j.cell.2022.12.027
73. De Cecco M, Ito T, Petrashen AP, Elias AE, Skvir NJ, Criscione SW, et al. L1 drives IFN in senescent cells and promotes age-associated inflammation. Nature. (2019) 566:73–8. doi: 10.1038/s41586-018-0784-9
74. Gorbunova V, Seluanov A, Mita P, McKerrow W, Fenyö D, Boeke JD, et al. The role of retrotransposable elements in ageing and age-associated diseases. Nature. (2021) 596:43–53. doi: 10.1038/s41586-021-03542-y
75. Sakamoto K, Ohmido N, Fukui K, Kamada H, Satoh S. Site-specific accumulation of a LINE-like retrotransposon in a sex chromosome of the dioecious plant Cannabis sativa. Plant Mol Biol. (2000) 44:723–32. doi: 10.1023/A:1026574405717
76. Shpyleva S, Melnyk S, Pavliv O, Pogribny I, Jill James S. Overexpression of LINE-1 Retrotransposons in Autism Brain. Mol Neurobiol. (2018) 55:1740–9. doi: 10.1007/s12035-017-0421-x
77. Strom AR, Emelyanov AV, Mir M, Fyodorov DV, Darzacq X, Karpen GH. Phase separation drives heterochromatin domain formation. Nature. (2017) 547:241–5. doi: 10.1038/nature22989
78. Su Y, Davies S, Davis M, Lu H, Giller R, Krailo M, et al. Expression of LINE-1 p40 protein in pediatric malignant germ cell tumors and its association with clinicopathological parameters: a report from the Children's Oncology Group. Cancer Lett. (2007) 247:204–12. doi: 10.1016/j.canlet.2006.04.010
79. Xu R, Li S, Wu Q, Li C, Jiang M, Guo L, et al. Stage-specific H3K9me3 occupancy ensures retrotransposon silencing in human pre-implantation embryos. Cell Stem Cell. (2022) 29:1051–66.e8. doi: 10.1016/j.stem.2022.06.001
80. Yu H, Chen M, Hu Y, Ou S, Yu X, Liang S, et al. Dynamic reprogramming of H3K9me3 at hominoid-specific retrotransposons during human preimplantation development. Cell Stem Cell. (2022) 29:1031–50.e12. doi: 10.1016/j.stem.2022.06.006
81. Childs B, van Deursen J. Inhibition of 'jumping genes' promotes healthy ageing. Nature. (2019) 566:46–8. doi: 10.1038/d41586-018-07553-0
82. Kloosterman WP, Francioli LC, Hormozdiari F, Marschall T, Hehir-Kwa JY, Abdellaoui A, et al. Characteristics of de novo structural changes in the human genome. Genome Res. (2015) 25:792–801. doi: 10.1101/gr.185041.114
83. Nohara K, Nakabayashi K, Okamura K, Suzuki T, Suzuki S, Hata K. Gestational arsenic exposure induces site-specific DNA hypomethylation in active retrotransposon subfamilies in offspring sperm in mice. Epigenet Chromat. (2020) 13:53. doi: 10.1186/s13072-020-00375-3
84. Mon MJ, Haas AE, Stein JL, Stein GS. Influence of psychoactive and nonpsychoactive cannabinoids on cell proliferation and macromolecular biosynthesis in human cells. Biochem Pharmacol. (1981) 30:31–43. doi: 10.1016/0006-2952(81)90282-3
85. Mon MJ, Jansing RL, Doggett S, Stein JL, Stein GS. Influence of delta9-tetrahydrocannabinol on cell proliferation and macromolecular biosynthesis in human cells. Biochem Pharmacol. (1978) 27:1759–65. doi: 10.1016/0006-2952(78)90553-1
86. Schrott R, Murphy SK, Modliszewski JL, King DE, Hill B, Itchon-Ramos N, et al. Refraining from use diminishes cannabis-associated epigenetic changes in human sperm. Environ Epigenet. (2021) 7:1–10. doi: 10.1093/eep/dvab009
87. Bright U, Akirav I. Cannabidiol modulates alterations in PFC microRNAs in a rat model of depression. Int J Mol Sci. (2023) 24:2052. doi: 10.3390/ijms24032052
88. Tahir SK, Zimmerman AM. Influence of marihuana on cellular structures and biochemical activities. Pharmacol Biochem Behav. (1991) 40:617–23. doi: 10.1016/0091-3057(91)90372-9
89. Spiro RG. Protein glycosylation: nature, distribution, enzymatic formation, and disease implications of glycopeptide bonds. Glycobiology. (2002) 12:43r−56r. doi: 10.1093/glycob/12.4.43R
90. Alavi A, Axford JS. The pivotal nature of sugars in normal physiology and disease. Wien Med Wochenschr. (2006) 156:19–33. doi: 10.1007/s10354-005-0247-4
91. Booklet. Precision medicine in China. Science. (2016) 354:1601. doi: 10.1126/science.2016.354.6319.354_1601b
92. Gabius HJ. The sugar code: why glycans are so important. Biosystems. (2018) 164:102–11. doi: 10.1016/j.biosystems.2017.07.003
93. Wang W. Glycomics research in China: the current state of the art. Omics. (2019) 23:601–2. doi: 10.1089/omi.2019.0163
94. Wang H. Next-generation (glycomic) biomarkers for cardiometabolic health: a community-based study of immunoglobulin G N-glycans in a Chinese Han population. OMICS. (2019) 23:649–59. doi: 10.1089/omi.2019.0099
95. Liu D, Li Q, Dong J, Li D, Xu X, Xing W, et al. The association between normal BMI with central adiposity and proinflammatory potential immunoglobulin G N-glycosylation. Diabetes Metab Syndr Obes. (2019) 12:2373–85. doi: 10.2147/DMSO.S216318
96. Russell AC, Kepka A, Trbojević-Akmačić I, Ugrina I, Song M, Hui J, et al. Increased central adiposity is associated with pro-inflammatory immunoglobulin G N-glycans. Immunobiology. (2019) 224:110–5. doi: 10.1016/j.imbio.2018.10.002
97. Meng Z, Li C, Ding G, Cao W, Xu X, Heng Y, et al. Glycomics: immunoglobulin G N-glycosylation associated with mammary gland hyperplasia in women. OMICS. (2020) 24:551–8. doi: 10.1089/omi.2020.0091
98. Hou H, Yang H, Liu P, Huang C, Wang M, Li Y, et al. Profile of immunoglobulin G N-glycome in COVID-19 patients: a case-control study. Front Immunol. (2021) 12:748566. (In English). doi: 10.3389/fimmu.2021.748566
99. Li X, Wang H, Zhu Y, Cao W, Song M, Wang Y, et al. Heritability enrichment of immunoglobulin G N-glycosylation in specific tissues. Fronti Immunol. (2021) 12:741705. (In English). doi: 10.3389/fimmu.2021.741705
100. Russell A, Wang W. The Rapidly expanding nexus of immunoglobulin G N-glycomics, suboptimal health status, and precision medicine. Exp Suppl. (2021) 112:545–64. doi: 10.1007/978-3-030-76912-3_17
101. Zhang X, Yuan H, Lyu J, Meng X, Tian Q, Li Y, et al. Association of dementia with immunoglobulin G N-glycans in a Chinese Han Population. npj Aging Mech Dis. (2021) 7:3. doi: 10.1038/s41514-021-00055-w
102. Wang B, Liu D, Song M, Wang W, Guo B, Wang Y. Immunoglobulin G N-glycan, inflammation and type 2 diabetes in East Asian and European populations: a Mendelian randomization study. Mol Med. (2022) 28:114. doi: 10.1186/s10020-022-00543-z
103. Sebastian A, Borkhoff CM, Wahi G, Giglia L, Bayliss A, Kanani R, et al. Glycan biomarkers for rheumatoid arthritis and its remission status in Han Chinese patients. OMICS. (2016) 20:343–51. doi: 10.1089/omi.2016.0050
104. Liu D, Dong J, Zhang J, Xu X, Tian Q, Meng X, et al. Genome-wide mapping of plasma IgG N-glycan quantitative trait loci identifies a potentially causal association between IgG N-glycans and rheumatoid arthritis. J Immunol. (2022) 208:2508–14. doi: 10.4049/jimmunol.2100080
105. Vučković F, Krištić J, Gudelj I, Teruel M, Keser T, Pezer M, et al. Association of systemic lupus erythematosus with decreased immunosuppressive potential of the IgG glycome. Arthrit Rheumatol. (2015) 67:2978–89. doi: 10.1002/art.39273
106. Papadakis DP, Michael CM, Kephalas TA, Miras CJ. Effects of cannabis smoking in blood lactic acid and glucose in humans. Experientia. (1974) 30:1183–4. doi: 10.1007/BF01923679
107. Li X, Yang Y, Zhang B, Lin X, Fu X, An Y, et al. Lactate metabolism in human health and disease. Signal Transduct Target Therapy. (2022) 7:305. doi: 10.1038/s41392-022-01151-3
108. Zhang D, Tang Z, Huang H, Zhou G, Cui C, Weng Y, et al. Metabolic regulation of gene expression by histone lactylation. Nature. (2019) 574:575–80. doi: 10.1038/s41586-019-1678-1
109. Yang Z, Yan C, Ma J, Peng P, Ren X, Cai S, et al. Lactylome analysis suggests lactylation-dependent mechanisms of metabolic adaptation in hepatocellular carcinoma. Nat Metab. (2023) 5:61–79. doi: 10.1038/s42255-022-00710-w
110. Valvona CJ, Fillmore HL, Nunn PB, Pilkington GJ. The regulation and function of lactate dehydrogenase a: therapeutic potential in brain tumor. Brain Pathol. (2016) 26:3–17. doi: 10.1111/bpa.12299
111. Wishart DS. Emerging applications of metabolomics in drug discovery and precision medicine. Nat Rev. (2016) 15:473–84. doi: 10.1038/nrd.2016.32
112. Ortutay C, Vihinen M. Immunome Knowledge Base (IKB): an integrated service for immunome research. BMC Immunol. (2009) 10:3. doi: 10.1186/1471-2172-10-3
113. Arnaout RA, Prak ETL, Schwab N, Rubelt F. The future of blood testing is the immunome. Front Immunol. (2021) 12:626793. doi: 10.3389/fimmu.2021.626793
114. López-Otín C, Blasco MA, Partridge L, Serrano M, Kroemer G. Hallmarks of aging: an expanding universe. Cell. (2023) 186:243–78. doi: 10.1016/j.cell.2022.11.001
115. Sayed N, Huang Y, Nguyen K, Krejciova-Rajaniemi Z, Grawe AP, Gao T, et al. An inflammatory aging clock (iAge) based on deep learning tracks multimorbidity, immunosenescence, frailty and cardiovascular aging. Nat Aging. (2021) 1:598–615. doi: 10.1038/s43587-021-00082-y
116. Dou Z, Ghosh K, Vizioli MG, Zhu J, Sen P, Wangensteen KJ, et al. Cytoplasmic chromatin triggers inflammation in senescence and cancer. Nature. (2017) 550:402–6. doi: 10.1038/nature24050
117. Marinello J, Arleo A, Russo M, Delcuratolo M, Ciccarelli F, Pommier Y, et al. Topoisomerase I poison-triggered immune gene activation is markedly reduced in human small-cell lung cancers by impairment of the cGAS/STING pathway. Br J Cancer. (2022) 127:1214–25. doi: 10.1038/s41416-022-01894-4
118. McLemore AF, Hou H-A, Meyer BS, Lam NB, Ward GA, Aldrich AL, et al. Somatic gene mutations expose cytoplasmic DNA to co-opt the cGAS/STING/NLRP3 axis in myelodysplastic syndromes. JCI Insight. (2022) 7:e159430. doi: 10.1172/jci.insight.159430
119. Schwab N, Taskina D, Leung E, Innes BT, Bader GD, Hazrati LN. Neurons and glial cells acquire a senescent signature after repeated mild traumatic brain injury in a sex-dependent manner. Front Neurosci. (2022) 16:1027116. doi: 10.3389/fnins.2022.1027116
120. Weinreb JT, Ghazale N, Pradhan K, Gupta V, Potts KS, Tricomi B, et al. Excessive R-loops trigger an inflammatory cascade leading to increased HSPC production. Dev Cell. (2021) 56:627–40.e5. doi: 10.1016/j.devcel.2021.02.006
121. Zhao Y, Simon M, Seluanov A, Gorbunova V. DNA damage and repair in age-related inflammation. Nat Rev Immunol. (2023) 23:75–89. doi: 10.1038/s41577-022-00751-y
122. Zhang X, Bai XC, Chen ZJ. Structures and mechanisms in the cGAS-STING innate immunity pathway. Immunity. (2020) 53:43–53. doi: 10.1016/j.immuni.2020.05.013
123. Wan D, Jiang W, Hao J. Research advances in how the cGAS-STING pathway controls the cellular inflammatory response. Front Immunol. (2020) 11:615. doi: 10.3389/fimmu.2020.00615
124. Andreeva L, Hiller B, Kostrewa D, Lässig C, de Oliveira Mann C, Drexler DJ, et al. cGAS senses long and HMGB/TFAM-bound U-turn DNA by forming protein-DNA ladders. Nature. (2017) 549:394–8. doi: 10.1038/nature23890
125. Luecke S, Holleufer A, Christensen MH, Jønsson KL, Boni GA, Sørensen LK, et al. cGAS is activated by DNA in a length-dependent manner. EMBO Rep. (2017) 18:1707–15. doi: 10.15252/embr.201744017
126. Liu Y, Xu P, Rivara S, Liu C, Ricci J, Ren X, et al. Clathrin-associated AP-1 controls termination of STING signalling. Nature. (2022) 610:761–7. doi: 10.1038/s41586-022-05354-0
127. Ablasser A. Structures of STING protein illuminate this key regulator of inflammation. Nature. (2019) 567:321–2. doi: 10.1038/d41586-019-00707-8
128. Shang G, Zhang C, Chen ZJ, Bai XC, Zhang X. Cryo-EM structures of STING reveal its mechanism of activation by cyclic GMP-AMP. Nature. (2019) 567:389–93. doi: 10.1038/s41586-019-0998-5
129. Morehouse BR, Yip MCJ, Keszei AFA, McNamara-Bordewick NK, Shao S, Kranzusch PJ. Cryo-EM structure of an active bacterial TIR-STING filament complex. Nature. (2022) 608:803–7. doi: 10.1038/s41586-022-04999-1
130. Ablasser A, Chen ZJ. cGAS in action: expanding roles in immunity and inflammation. Science. (2019) 363:eaat8657. doi: 10.1126/science.aat8657
131. Di Domizio J, Gulen MF, Saidoune F, Thacker VV, Yatim A, Sharma K, et al. The cGAS-STING pathway drives type I IFN immunopathology in COVID-19. Nature. (2022) 603:145–51. doi: 10.1038/s41586-022-04421-w
132. Mackenzie KJ, Carroll P, Martin CA, Murina O, Fluteau A, Simpson DJ, et al. cGAS surveillance of micronuclei links genome instability to innate immunity. Nature. (2017) 548:461–5. doi: 10.1038/nature23449
133. Hong C, Schubert M, Tijhuis AE, Requesens M, Roorda M, van den Brink A, et al. cGAS-STING drives the IL-6-dependent survival of chromosomally instable cancers. Nature. (2022) 607:366–73. doi: 10.1038/s41586-022-04847-2
134. Bakhoum SF, Ngo B, Laughney AM, Cavallo J-A, Murphy CJ, Ly P, et al. Chromosomal instability drives metastasis through a cytosolic DNA response. Nature. (2018) 553:467–72. doi: 10.1038/nature25432
135. Li S, Mirlekar B, Johnson BM, Brickey WJ, Wrobel JA, Yang N, et al. STING-induced regulatory B cells compromise NK function in cancer immunity. Nature. (2022) 610:373–80. doi: 10.1038/s41586-022-05254-3
136. Zhang B, Vogelzang A, Miyajima M, Sugiura Y, Wu Y, Chamoto K, et al. B cell-derived GABA elicits IL-10(+) macrophages to limit anti-tumour immunity. Nature. (2021) 599:471–6. doi: 10.1038/s41586-021-04082-1
137. Lopez-Otin C, Blasco MA, Partridge L, Serrano M, Kroemer G. The hallmarks of aging. Cell. (2013) 153:1194–217. doi: 10.1016/j.cell.2013.05.039
138. Glück S, Guey B, Gulen MF, Wolter K, Kang T-W, Schmacke NA, et al. Innate immune sensing of cytosolic chromatin fragments through cGAS promotes senescence. Nat Cell Biol. (2017) 19:1061–70. doi: 10.1038/ncb3586
139. Yang H, Wang H, Ren J, Chen Q, Chen ZJ. cGAS is essential for cellular senescence. Proc Natl Acad Sci USA. (2017) 114:E4612–20. doi: 10.1073/pnas.1705499114
140. Sladitschek-Martens HL, Guarnieri A, Brumana G, Zanconato F, Battilana G, Xiccato RL, et al. YAP/TAZ activity in stromal cells prevents ageing by controlling cGAS-STING. Nature. (2022) 607:790–8. doi: 10.1038/s41586-022-04924-6
141. McCoy KL. Interaction between cannabinoid system and toll-like receptors controls inflammation. Mediators Inflamm. (2016) 2016:5831315. doi: 10.1155/2016/5831315
142. Monnet-Tschudi F, Hazekamp A, Perret N, Zurich MG, Mangin P, Giroud C, et al. Delta-9-tetrahydrocannabinol accumulation, metabolism and cell-type-specific adverse effects in aggregating brain cell cultures. Toxicol Appl Pharmacol. (2008) 228:8–16. doi: 10.1016/j.taap.2007.11.007
143. Klein TW, Newton C, Larsen K, Lu L, Perkins I, Nong L, et al. The cannabinoid system and immune modulation. J Leukoc Biol. (2003) 74:486–96. doi: 10.1189/jlb.0303101
144. Eisenstein TK, Meissler JJ. Effects of cannabinoids on T-cell function and resistance to infection. J Neuroimmune Pharmacol. (2015) 10:204–16. doi: 10.1007/s11481-015-9603-3
145. Chiurchiu V. Endocannabinoids and Immunity. Cannabis Cannabinoid Res. (2016) 1:59–66. doi: 10.1089/can.2016.0002
146. van den Hoogen NJ, Harding EK, Davidson CED, Trang T. Cannabinoids in chronic pain: therapeutic potential through microglia modulation. Front Neural Circuits. (2021) 15:816747. doi: 10.3389/fncir.2021.816747
147. Young AP, Denovan-Wright EM. The dynamic role of microglia and the endocannabinoid system in neuroinflammation. Front Pharmacol. (2021) 12:806417. doi: 10.3389/fphar.2021.806417
148. Anil SM, Peeri H, Koltai H. Medical cannabis activity against inflammation: active compounds and modes of action. Front Pharmacol. (2022) 13:908198. doi: 10.3389/fphar.2022.908198
149. Khan H, Ghori FK, Ghani U, Javed A, Zahid S. Cannabinoid and endocannabinoid system: a promising therapeutic intervention for multiple sclerosis. Mol Biol Rep. (2022) 49:5117–31. doi: 10.1007/s11033-022-07223-5
150. Khoury M, Cohen I, Bar-Sela G. “The two sides of the same coin”-medical cannabis, cannabinoids and immunity: pros and cons explained. Pharmaceutics. (2022) 14:389. doi: 10.3390/pharmaceutics14020389
151. López-Gómez L, Szymaszkiewicz A, Zielińska M, Abalo R. The enteric glia and its modulation by the endocannabinoid system, a new target for cannabinoid-based nutraceuticals? Molecules. (2022) 27:6773. doi: 10.3390/molecules27196773
152. Morcuende A, García-Gutiérrez MS, Tambaro S, Nieto E, Manzanares J, Femenia T. Immunomodulatory role of CB2 receptors in emotional and cognitive disorders. Front Psychiatry. (2022) 13:866052. doi: 10.3389/fpsyt.2022.866052
153. Morris G, Sominsky L, Walder KR, Berk M, Marx W, Carvalho AF, et al. Inflammation and nitro-oxidative stress as drivers of endocannabinoid system aberrations in mood disorders and schizophrenia. Mol Neurobiol. (2022) 59:3485–503. doi: 10.1007/s12035-022-02800-y
154. Shade RD, Ross JA, Van Bockstaele EJ. Targeting the cannabinoid system to counteract the deleterious effects of stress in Alzheimer's disease. Front Aging Neurosci. (2022) 14:949361. doi: 10.3389/fnagi.2022.949361
155. Marinelli S, Marrone MC, Di Domenico M, Marinelli S. Endocannabinoid signaling in microglia. Glia. (2023) 71:71–90. doi: 10.1002/glia.24281
156. Martini S, Gemma A, Ferrari M, Cosentino M, Marino F. Effects of cannabidiol on innate immunity: experimental evidence and clinical relevance. Int J Mol Sci. (2023) 24:3125. doi: 10.3390/ijms24043125
157. Rieder SA, Chauhan A, Singh U, Nagarkatti M, Nagarkatti P. Cannabinoid-induced apoptosis in immune cells as a pathway to immunosuppression. Immunobiology. (2010) 215:598–605. doi: 10.1016/j.imbio.2009.04.001
158. Zumbrun EE, Sido JM, Nagarkatti PS, Nagarkatti M. Epigenetic regulation of immunological alterations following prenatal exposure to marijuana cannabinoids and its long term consequences in offspring. J Neuroimmune Pharmacol. (2015) 10:245–54. doi: 10.1007/s11481-015-9586-0
159. Callén L, Moreno E, Barroso-Chinea P, Moreno-Delgado D, Cortés A, Mallol J, et al. Cannabinoid receptors CB1 and CB2 form functional heteromers in brain. J Biol Chem. (2012) 287:20851–65. doi: 10.1074/jbc.M111.335273
160. Ge Q, Maury E, Rycken L, Gérard J, Noël L, Detry R, et al. Endocannabinoids regulate adipokine production and the immune balance of omental adipose tissue in human obesity. Int J Obesity. (2013) 37:874–80. doi: 10.1038/ijo.2012.123
161. Mukhopadhyay B, Schuebel K, Mukhopadhyay P, Cinar R, Godlewski G, Xiong K, et al. Cannabinoid receptor 1 promotes hepatocellular carcinoma initiation and progression through multiple mechanisms. Hepatology. (2015) 61:1615–26. doi: 10.1002/hep.27686
162. Pagano C, Savarese B, Coppola L, Navarra G, Avilia G, Laezza C, et al. Cannabinoids in the modulation of oxidative signaling. Int J Mol Sci. (2023) 24:2513. doi: 10.3390/ijms24032513
163. Julien B, Grenard P, Teixeira-Clerc F, Van Nhieu JT Li L, Karsak M, et al. Antifibrogenic role of the cannabinoid receptor CB2 in the liver. Gastroenterology. (2005) 128:742–55. doi: 10.1053/j.gastro.2004.12.050
164. Bagavandoss P, Grimshaw S. Temporal and spatial distribution of the cannabinoid receptors (CB1, CB2) and fatty acid amide hydroxylase in the rat ovary. Anat Rec (Hoboken). (2010) 293:1425–32. doi: 10.1002/ar.21181
165. Hong H, Sloan L, Saxena D, Scott DA. The antimicrobial properties of cannabis and cannabis-derived compounds and relevance to CB2-targeted neurodegenerative therapeutics. Biomedicines. (2022) 10:1959. doi: 10.3390/biomedicines10081959
166. Jayarajan S, Meissler JJ, Adler MW, Eisenstein TK. A Cannabinoid 2-selective agonist inhibits allogeneic skin graft rejection in vivo. Front Pharmacol. (2021) 12:804950. doi: 10.3389/fphar.2021.804950
167. Franco-Arroyo NN, Viveros-Paredes JM, Zepeda-Morales ASM, et al. β-Caryophyllene, a dietary cannabinoid, protects against metabolic and immune dysregulation in a diet-induced obesity mouse model. J Med Food. (2022) 25:993–1002. doi: 10.1089/jmf.2021.0166
168. Rorato R, Ferreira NL, Oliveira FP, Fideles HJ, Camilo TA, Antunes-Rodrigues J, et al. Prolonged activation of brain CB2 signaling modulates hypothalamic microgliosis and astrogliosis in high fat diet-fed mice. Int J Mol Sci. (2022) 23:5527. doi: 10.3390/ijms23105527
169. Li L, Xuan Y, Zhu B, Wang X, Tian X, Zhao L, et al. Protective effects of cannabidiol on chemotherapy-induced oral mucositis via the Nrf2/Keap1/ARE signaling pathways. Oxid Med Cell Longev. (2022) 2022:4619760. doi: 10.1155/2022/4619760
170. Wang X, Lin C, Wu S, Zhang T, Wang Y, Jiang Y, et al. Cannabidivarin alleviates neuroinflammation by targeting TLR4 co-receptor MD2 and improves morphine-mediated analgesia. Front Immunol. (2022) 13:929222. doi: 10.3389/fimmu.2022.929222
171. Zielińska A, Eder P, Karczewski J, Szalata M, Hryhorowicz S, Wielgus K, et al. Tocilizumab-coated solid lipid nanoparticles loaded with cannabidiol as a novel drug delivery strategy for treating COVID-19: a review. Front Immunol. (2023) 14:1147991. doi: 10.3389/fimmu.2023.1147991
172. Chen J, Wang F, Zhang S, Lin Q, Xu H, Zhu T, et al. Activation of CD4(+) T cell-derived cannabinoid receptor 2 signaling exacerbates sepsis via inhibiting IL-10. J Immunol. (2022) 208:2515–22. doi: 10.4049/jimmunol.2101015
173. Xiong X, Chen S, Shen J, You H, Yang H, Yan C, et al. Cannabis suppresses antitumor immunity by inhibiting JAK/STAT signaling in T cells through CNR2. Signal Transduct Target Ther. (2022) 7:99. doi: 10.1038/s41392-022-00918-y
174. Abd-Nikfarjam B, Dolati-Somarin A, Baradaran Rahimi V, Askari VR. Cannabinoids in neuroinflammatory disorders: Focusing on multiple sclerosis, Parkinsons, and Alzheimers diseases. Biofactors. (2023) 49:560–83. doi: 10.1002/biof.1936
175. Gajofatto A, Cardobi N, Gobbin F, Calabrese M, Turatti M, Benedetti MD. Resting-state functional connectivity in multiple sclerosis patients receiving nabiximols for spasticity. BMC Neurol. (2023) 23:128. doi: 10.1186/s12883-023-03171-0
176. Martinez-Paz C, García-Cabrera E, Vilches-Arenas Á. Effectiveness and safety of cannabinoids as an add-on therapy in the treatment of resistant spasticity in multiple sclerosis: a systematic review. Cannabis Cannabinoid Res. (2023) 8:580–8. doi: 10.1089/can.2022.0254
177. Nicholas J, Lublin F, Klineova S, Berwaerts J, Chinnapongse R, Checketts D, et al. Efficacy of nabiximols oromucosal spray on spasticity in people with multiple sclerosis: treatment effects on Spasticity Numeric Rating Scale, muscle spasm count, and spastic muscle tone in two randomized clinical trials. Mult Scler Relat Disord. (2023) 75:104745. doi: 10.1016/j.msard.2023.104745
178. Nouh RA, Kamal A, Abdelnaser A. Cannabinoids and multiple sclerosis: a critical analysis of therapeutic potentials and safety concerns. Pharmaceutics. (2023) 15:1151. doi: 10.3390/pharmaceutics15041151
179. Sirbu C-A, Georgescu R, Pleşa FC, Paunescu A, Tântu MM, Nicolae AC, et al. Cannabis and cannabinoids in multiple sclerosis: from experimental models to clinical practice-a review. Am J Ther. (2023) 30:e220–31. doi: 10.1097/MJT.0000000000001568
180. Solmi M, De Toffol M, Kim JY, Choi MJ, Stubbs B, Thompson T, et al. Balancing risks and benefits of cannabis use: umbrella review of meta-analyses of randomised controlled trials and observational studies. BMJ. (2023) 382:e072348. doi: 10.1136/bmj-2022-072348
181. Longoria V, Parcel H, Toma B, Minhas A, Zeine R. Neurological benefits, clinical challenges, and neuropathologic promise of medical marijuana: a systematic review of cannabinoid effects in multiple sclerosis and experimental models of demyelination. Biomedicines. (2022) 10:539. doi: 10.3390/biomedicines10030539
182. da Silva TV, Bernardes D, Oliveira-Lima OC, Pinto BF, Filho ML, Faraco CCF, et al. Cannabidiol attenuates in vivo leukocyte recruitment to the spinal cord microvasculature at peak disease of experimental autoimmune encephalomyelitis. Cannabis Cannabinoid Res. (2023). doi: 10.1089/can.2022.0103
183. Naik S, Fuchs E. Inflammatory memory and tissue adaptation in sickness and in health. Nature. (2022) 607:249–55. doi: 10.1038/s41586-022-04919-3
184. de Laval B, Maurizio J, Kandalla PK, Brisou G, Simonnet L, Huber C, et al. C/EBPβ-dependent epigenetic memory induces trained immunity in hematopoietic stem cells. Cell Stem Cell. (2020) 26:657–74.e8. doi: 10.1016/j.stem.2020.01.017
185. Bogeska R, Mikecin A-M, Kaschutnig P, Fawaz M, Büchler-Schäff M, Le D, et al. Inflammatory exposure drives long-lived impairment of hematopoietic stem cell self-renewal activity and accelerated aging. Cell Stem Cell. (2022) 29:1273–84.e8. doi: 10.1016/j.stem.2022.06.012
186. Hormaechea-Agulla D, Matatall KA, Le DT, Kain B, Long X, Kus P, et al. Chronic infection drives Dnmt3a-loss-of-function clonal hematopoiesis via IFNγ signaling. Cell Stem Cell. (2021) 28:1428–42.e6. doi: 10.1016/j.stem.2021.03.002
187. Yousefzadeh MJ, Flores RR, Zhu Y, Schmiechen ZC, Brooks RW, Trussoni CE, et al. An aged immune system drives senescence and ageing of solid organs. Nature. (2021) 594:100–5. doi: 10.1038/s41586-021-03547-7
188. Moiseeva V, Cisneros A, Sica V, Deryagin O, Lai Y, Jung S, et al. Senescence atlas reveals an aged-like inflamed niche that blunts muscle regeneration. Nature. (2023) 613:169–78. doi: 10.1038/s41586-022-05535-x
189. Bapat SP, Whitty C, Mowery CT, Liang Y, Yoo A, Jiang Z, et al. Obesity alters pathology and treatment response in inflammatory disease. Nature. (2022) 604:337–42. doi: 10.1038/s41586-022-04536-0
190. Reid Cahn A, Bhardwaj N, Vabret N. Dark genome, bright ideas: recent approaches to harness transposable elements in immunotherapies. Cancer Cell. (2022) 40:792–7. doi: 10.1016/j.ccell.2022.07.003
191. Bartonicek N, Rouet R, Warren J, Loetsch C, Rodriguez GS, Walters S, et al. The retroelement Lx9 puts a brake on the immune response to virus infection. Nature. (2022) 608:757–65. doi: 10.1038/s41586-022-05054-9
192. Ho WJ, Wood LD. Opposing roles of the immune system in tumors. Science. (2021) 373:1306–7. doi: 10.1126/science.abl5376
193. Jameson JL, Fauci AS, Hauser SL, Longo DL, Jameson JL, Loscalzo J, (editors). Harrison's Principles of Internal Medicine. 20th ed. New York, NY: McGraw Hill (2018).
194. Poggetto ED, Ho I-L, Balestrieri C, Yen E-Y, Zhang S, Citron F, et al. Epithelial memory of inflammation limits tissue damage while promoting pancreatic tumorigenesis. Science. (2021) 373:eabj0486. doi: 10.1126/science.abj0486
195. Martin TD, Patel RS, Cook DR, Choi MY, Patil A, Liang AC, et al. The adaptive immune system is a major driver of selection for tumor suppressor gene inactivation. Science. (2021) 373:1327–35. doi: 10.1126/science.abg5784
196. Vyas P. Genetic and non-genetic mechanisms of inflammation may promote transformation in leukemia. Cell Stem Cell. (2022) 29:184–6. doi: 10.1016/j.stem.2022.01.006
197. Muto T, Guillamot M, Yeung J, Fang J, Bennett J, Nadorp B, et al. TRAF6 functions as a tumor suppressor in myeloid malignancies by directly targeting MYC oncogenic activity. Cell Stem Cell. (2022) 29:298–314.e9. doi: 10.1016/j.stem.2021.12.007
198. Belk JA, Yao W, Ly N, Freitas KA, Chen YT, Shi Q, et al. Genome-wide CRISPR screens of T cell exhaustion identify chromatin remodeling factors that limit T cell persistence. Cancer Cell. (2022) 40:768–86.e7. doi: 10.1016/j.ccell.2022.06.001
199. Prendergast L, McClurg UL, Hristova R, Berlinguer-Palmini R, Greener S, Veitch K, et al. Resolution of R-loops by INO80 promotes DNA replication and maintains cancer cell proliferation and viability. Nat Commun. (2020) 11:4534. doi: 10.1038/s41467-020-18306-x
200. Crossley MP, Song C, Bocek MJ, Choi JH, Kousorous J, Sathirachinda A, et al. R-loop-derived cytoplasmic RNA-DNA hybrids activate an immune response. Nature. (2023) 613:187–94. doi: 10.1038/s41586-022-05545-9
201. Nicolas AM, Pesic M, Engel E, Ziegler PK, Diefenhardt M, Kennel KB, et al. Inflammatory fibroblasts mediate resistance to neoadjuvant therapy in rectal cancer. Cancer Cell. (2022) 40:168–84.e13. doi: 10.1016/j.ccell.2022.01.004
202. Krishnamurty AT, Shyer JA, Thai M, Gandham V, Buechler MB, Yang YA, et al. LRRC15(+) myofibroblasts dictate the stromal setpoint to suppress tumour immunity. Nature. (2022) 611:148–54. doi: 10.1038/s41586-022-05272-1
203. Tomei S, Audiger C, Naik SH. Death by differentiation: CD4(+) T cells kick out suspicious stem cells. Cell Stem Cell. (2022) 29:655–6. doi: 10.1016/j.stem.2022.04.013
204. Hernández-Malmierca P, Vonficht D, Schnell A, Uckelmann HJ, Bollhagen A, Mahmoud MAA, et al. Antigen presentation safeguards the integrity of the hematopoietic stem cell pool. Cell Stem Cell. (2022) 29:760–75.e10. doi: 10.1016/j.stem.2022.04.007
205. Iweka CA, Seigneur E, Hernandez AL, Paredes SH, Cabrera M, Blacher E, et al. Myeloid deficiency of the intrinsic clock protein BMAL1 accelerates cognitive aging by disrupting microglial synaptic pruning. J Neuroinflamm. (2023) 20:48. doi: 10.1186/s12974-023-02727-8
206. Li H, McLaurin KA, Mactutus CF, Booze RM. Microglia proliferation underlies synaptic dysfunction in the prefrontal cortex: implications for the pathogenesis of HIV-1-associated neurocognitive and affective alterations. bioRxiv. (2023). doi: 10.1101/2023.01.20.524942
207. Mordelt A, de Witte LD. Microglia-mediated synaptic pruning as a key deficit in neurodevelopmental disorders: hype or hope? Curr Opin Neurobiol. (2023) 79:102674. doi: 10.1016/j.conb.2022.102674
208. Nebeling FC, Poll S, Justus LC, Steffen J, Keppler K, Mittag M, et al. Microglial motility is modulated by neuronal activity and correlates with dendritic spine plasticity in the hippocampus of awake mice. Elife. (2023) 12. doi: 10.7554/eLife.83176
209. Pinosanu LR, Capitanescu B, Glavan D, Godeanu S, Cadenas IFN, Doeppner TR, et al. Neuroglia cells transcriptomic in brain development, aging and neurodegenerative diseases. Aging Dis. (2023) 14:63–83. doi: 10.14336/AD.2022.0621
210. McNamara NB, Munro DAD, Bestard-Cuche N, Uyeda A, Bogie JFJ, Hoffmann A, et al. Microglia regulate central nervous system myelin growth and integrity. Nature. (2023) 613:120–9. doi: 10.1038/s41586-022-05534-y
211. Hosang L, Canals RC, van der Flier FJ, Hollensteiner J, Daniel R, Flügel A, et al. The lung microbiome regulates brain autoimmunity. Nature. (2022) 603:138–44. doi: 10.1038/s41586-022-04427-4
214. Lynskey M, Hall W. The effects of adolescent cannabis use on educational attainment: a review. Addiction. (2000) 95:1621–30. doi: 10.1046/j.1360-0443.2000.951116213.x
215. Reece AS. Chronic toxicology of cannabis. Clin Toxicol (Phila). (2009) 47:517–24. doi: 10.1080/15563650903074507
216. Reece AS, Hulse GK. Chapter 1: Close Parallels between Cannabis Use and Deteriorating US Mental Health at Four Levels Supports and Extends the Epidemiological Salience of Demonstrated Causal Mental Health Relationships: A Geospatiotemporal Study. Epidemiology of Cannabis: Genotoxicity and Neurotoxicity, Epigenomics and Aging. New York, NY: Elsevier (2023).
217. Volkow ND, Baler RD, Compton WM, Weiss SRB. Adverse health effects of marijuana use. N Engl J Med. (2014) 370:2219–27. doi: 10.1056/NEJMra1402309
218. Zehra A, Burns J, Liu CK, Manza P, Wiers CE, Volkow ND, et al. Cannabis addiction and the brain: a review. Focus. (2019) 17:169–82. doi: 10.1176/appi.focus.17204
219. Hser Y-I, Mooney LJ, Huang D, Zhu Y, Tomko RL, McClure E, et al. Reductions in cannabis use are associated with improvements in anxiety, depression, and sleep quality, but not quality of life. J Subst Abuse Treat. (2017) 81:53–8. doi: 10.1016/j.jsat.2017.07.012
220. Lisboa SF, Gomes FV, Terzian AL, Aguiar DC, Moreira FA, Resstel LB, et al. The endocannabinoid system and anxiety. Vitam Horm. (2017) 103:193–279. doi: 10.1016/bs.vh.2016.09.006
221. Otten R, Huizink AC, Monshouwer K, Creemers HE, Onrust S. Cannabis use and symptoms of anxiety in adolescence and the moderating effect of the serotonin transporter gene. Addict Biol. (2017) 22:1081–9. doi: 10.1111/adb.12372
222. Karila L, Roux P, Rolland B, Benyamina A, Reynaud M, Aubin H-J, et al. Acute and long-term effects of cannabis use: a review. Curr Pharm Des. (2014) 20:4112–8. doi: 10.2174/13816128113199990620
223. Dean KE, Ecker AH, Buckner JD. Anxiety sensitivity and cannabis use-related problems: the impact of race. Am J Addict. (2017) 26:209–14. doi: 10.1111/ajad.12511
224. Ecker AH, Buckner JD. Cannabis-related problems and social anxiety: the mediational role of post-event processing. Subst Use Misuse. (2018) 53:36–41. doi: 10.1080/10826084.2017.1322984
225. Duperrouzel J, Hawes SW, Lopez-Quintero C, Pacheco-Colon I, Comer J, Gonzalez R. The association between adolescent cannabis use and anxiety: a parallel process analysis. Addict Behav. (2018) 78:107–13. doi: 10.1016/j.addbeh.2017.11.005
226. Bahorik AL, Leibowitz A, Sterling SA, Travis A, Weisner C, Satre DD. Patterns of marijuana use among psychiatry patients with depression and its impact on recovery. J Affect Disord. (2017) 213:168–71. doi: 10.1016/j.jad.2017.02.016
227. Dierker L, Selya A, Lanza S, Li R, Rose J. Depression and marijuana use disorder symptoms among current marijuana users. Addict Behav. (2018) 76:161–8. doi: 10.1016/j.addbeh.2017.08.013
228. Feingold D, Brill S, Goor-Aryeh I, Delayahu Y, Lev-Ran S. Depression and anxiety among chronic pain patients receiving prescription opioids and medical marijuana. J Affect Disord. (2017) 218:1–7. doi: 10.1016/j.jad.2017.04.026
229. Filbey FM, Aslan S, Lu H, Peng SL. Residual effects of THC via novel measures of brain perfusion and metabolism in a large group of chronic cannabis users. Neuropsychopharmacology. (2018) 43:700–7. doi: 10.1038/npp.2017.44
230. Gobbi G, Atkin T, Zytynski T, Wang S, Askari S, Boruff J, et al. Association of cannabis use in adolescence and risk of depression, anxiety, and suicidality in young adulthood: a systematic review and meta-analysis. JAMA Psychiatry. (2019) 76:426–34. doi: 10.1001/jamapsychiatry.2018.4500
231. Hodgson K, Almasy L, Knowles EEM, Jr JWK, Curran JE, Dyer TD, et al. The genetic basis of the comorbidity between cannabis use and major depression. Addiction. (2017) 112:113–23. doi: 10.1111/add.13558
232. Huang WJ, Chen WW, Zhang X. Endocannabinoid system: role in depression, reward and pain control (review). Mol Med Rep. (2016) 14:2899–903. doi: 10.3892/mmr.2016.5585
233. Huckins LM. Linking cannabis use to depression and suicidal thoughts and behaviours. Lancet Psychiatry. (2017) 4:654–6. doi: 10.1016/S2215-0366(17)30311-5
234. Lev-Ran S, Roerecke M, Le Foll B, George TP, McKenzie K, Rehm J. The association between cannabis use and depression: a systematic review and meta-analysis of longitudinal studies. Psychol Med. (2014) 44:797–810. doi: 10.1017/S0033291713001438
235. Lubman DI, Cheetham A, Yucel M. Cannabis and adolescent brain development. Pharmacol Ther. (2015) 148:1–16. doi: 10.1016/j.pharmthera.2014.11.009
236. Smolkina M, Morley KI, Rijsdijk F, Agrawal A, Bergin JE, Nelson EC, et al. Cannabis and depression: a twin model approach to co-morbidity. Behav Genet. (2017) 47:394–404. doi: 10.1007/s10519-017-9848-0
237. Spechler PA, Orr CA, Chaarani B, Kan K-J, Mackey S, Morton A, et al. Cannabis use in early adolescence: evidence of amygdala hypersensitivity to signals of threat. Dev Cogn Neurosci. (2015) 16:63–70. doi: 10.1016/j.dcn.2015.08.007
238. Troup LJ, Torrence RD, Andrzejewski JA, Braunwalder JT. Effects of cannabis use and subclinical depression on the P3 event-related potential in an emotion processing task. Medicine. (2017) 96:e6385. doi: 10.1097/MD.0000000000006385
239. Hanna RC, Perez JM, Ghose S. Cannabis and development of dual diagnoses: a literature review. Am J Drug Alcohol Abuse. (2017) 43:442–55. doi: 10.1080/00952990.2016.1213273
240. Bartoli F, Crocamo C, Carra G. Cannabis use disorder and suicide attempts in bipolar disorder: a meta-analysis. Neurosci Biobehav Rev. (2019) 103:14–20. doi: 10.1016/j.neubiorev.2019.05.017
241. Tyler E, Jones S, Black N, Carter LA, Barrowclough C. The relationship between bipolar disorder and cannabis use in daily life: an experience sampling study. PLoS ONE. (2015) 10:e0118916. doi: 10.1371/journal.pone.0118916
242. Zorrilla I, Aguado J, Haro JM, Barbeito S, Zurbano SL, Ortiz A, et al. Cannabis and bipolar disorder: does quitting cannabis use during manic/mixed episode improve clinical/functional outcomes? Acta Psychiatr Scand. (2015) 131:100–10. doi: 10.1111/acps.12366
243. Leite RTP, Nogueira SD, Nascimento JPR, de Lima LS, da Nóbrega TB, Virgínio MD, et al. The use of cannabis as a predictor of early onset of bipolar disorder and suicide attempts. Neural Plast. (2015) 2015:434127. doi: 10.1155/2015/434127
244. Weinstock LM, Gaudiano BA, Wenze SJ, Epstein-Lubow G, Miller IW. Demographic and clinical characteristics associated with comorbid cannabis use disorders (CUDs) in hospitalized patients with bipolar I disorder. Compr Psychiatry. (2016) 65:57–62. doi: 10.1016/j.comppsych.2015.10.003
245. Marwaha S, Winsper C, Bebbington P, Smith D. Cannabis use and hypomania in young people: a prospective analysis. Schizophr Bull. (2018) 44:1267–74. doi: 10.1093/schbul/sbx158
246. Kvitland LR, Ringen PA, Aminoff SR, Demmo C, Hellvin T, Lagerberg TV, et al. Duration of untreated illness in first-treatment bipolar I disorder in relation to clinical outcome and cannabis use. Psychiatry Res. (2016) 246:762–8. doi: 10.1016/j.psychres.2016.07.064
247. Andreasson S, Allebeck P, Engstrom A, Rydberg U. Cannabis and schizophrenia. A longitudinal study of Swedish conscripts. Lancet. (1987) 2:1483–6. doi: 10.1016/S0140-6736(87)92620-1
248. D'Souza DC, Sewell RA, Ranganathan M. Cannabis and psychosis/schizophrenia: human studies. Eur Arch Psychiatry Clin Neurosci. (2009) 259:413–31. doi: 10.1007/s00406-009-0024-2
249. Gage SH, Hickman M, Zammit S. Association between cannabis and psychosis: epidemiologic evidence. Biol Psychiatry. (2016) 79:549–56. doi: 10.1016/j.biopsych.2015.08.001
250. Ksir C, Hart CL. Cannabis and psychosis: a critical overview of the relationship. Curr Psychiatry Rep. (2016) 18:12. doi: 10.1007/s11920-015-0657-y
251. Manrique-Garcia E, Zammit S, Dalman C, Hemmingsson T, Andreasson S, Allebeck P. Prognosis of schizophrenia in persons with and without a history of cannabis use. Psychol Med. (2014) 44:2513–21. doi: 10.1017/S0033291714000191
252. Marconi A, Di Forti M, Lewis CM, Murray RM, Vassos E. Meta-analysis of the association between the level of cannabis use and risk of psychosis. Schizophr Bull. (2016) 42:1262–9. doi: 10.1093/schbul/sbw003
253. Moustafa AA, Salama M, Peak R, Tindle R, Salem A, Keri S, et al. Interactions between cannabis and schizophrenia in humans and rodents. Rev Neurosci. (2017) 28:811–23. doi: 10.1515/revneuro-2016-0083
254. Nielsen SM, Toftdahl NG, Nordentoft M, Hjorthoj C. Association between alcohol, cannabis, and other illicit substance abuse and risk of developing schizophrenia: a nationwide population based register study. Psychol Med. (2017) 47:1668–77. doi: 10.1017/S0033291717000162
255. Ortiz-Medina MB, Perea M, Torales J, Ventriglio A, Vitrani G, Aguilar L, et al. Cannabis consumption and psychosis or schizophrenia development. Int J Soc Psychiatry. (2018) 64:690–704. doi: 10.1177/0020764018801690
256. Pushpa-Rajah JA, McLoughlin BC, Gillies D, et al. Cannabis and schizophrenia. Schizophr Bull. (2015) 41:336–7. doi: 10.1093/schbul/sbu168
257. Schnell T, Gliese R, Schroter R, Kasten E, Gouzoulis-Mayfrank E. Motivational changes of cannabis use prior to and during the course of schizophrenia. Am J Addict. (2017) 26:122–8. doi: 10.1111/ajad.12494
258. Starzer MSK, Nordentoft M, Hjorthoj C. Rates and predictors of conversion to schizophrenia or bipolar disorder following substance-induced psychosis. Am J Psychiatry. (2018) 175:343–50. doi: 10.1176/appi.ajp.2017.17020223
259. Vaucher J, Keating BJ, Lasserre AM, Gan W, Lyall DM, Ward J, et al. Cannabis use and risk of schizophrenia: a Mendelian randomization study. Mol Psychiatry. (2018) 23:1287–92. doi: 10.1038/mp.2016.252
260. Verweij KJ, Abdellaoui A, Nivard MG, Sainz Cort A, Ligthart L, Draisma HH, et al. Short communication: genetic association between schizophrenia and cannabis use. Drug Alcohol Depend. (2017) 171:117–21. doi: 10.1016/j.drugalcdep.2016.09.022
261. Bohnert KM, Ilgen MA, Louzon S, McCarthy JF, Katz IR. Substance use disorders and the risk of suicide mortality among men and women in the US Veterans Health Administration. Addiction. (2017) 112:1193–201. doi: 10.1111/add.13774
262. Borges G, Bagge CL, Orozco R. A literature review and meta-analyses of cannabis use and suicidality. J Affect Disord. (2016) 195:63–74. doi: 10.1016/j.jad.2016.02.007
263. Borges G, Benjet C, Orozco R, Medina-Mora ME, Menendez D. Alcohol, cannabis and other drugs and subsequent suicide ideation and attempt among young Mexicans. J Psychiatr Res. (2017) 91:74–82. doi: 10.1016/j.jpsychires.2017.02.025
264. Feingold D, Rehm J, Lev-Ran S. Cannabis use and the course and outcome of major depressive disorder: a population based longitudinal study. Psychiatry Res. (2017) 251:225–34. doi: 10.1016/j.psychres.2017.02.027
265. Kimbrel NA, Newins AR, Dedert EA, Van Voorhees EE, Elbogen EB, Naylor JC, et al. Cannabis use disorder and suicide attempts in Iraq/Afghanistan-era veterans. J Psychiatr Res. (2017) 89:1–5. doi: 10.1016/j.jpsychires.2017.01.002
266. Waterreus A, Di Prinzio P, Badcock JC, Martin-Iverson M, Jablensky A, Morgan VA. Is cannabis a risk factor for suicide attempts in men and women with psychotic illness? Psychopharmacology. (2018) 235:2275–85. doi: 10.1007/s00213-018-4924-6
267. Han B, Compton WM, Einstein EB, Volkow ND. Associations of suicidality trends with cannabis use as a function of sex and depression status. JAMA Netw Open. (2021) 4:e2113025. doi: 10.1001/jamanetworkopen.2021.13025
268. Devine, M,. The Link Between Pot and Mass Shootings May Be Closer Than We Think. Citizens Against Legalizing Marijuana. Available online at: https://calmca.org/calmcablog/2019/8/8/the-link-between-pot-and-massshootings-may-be-closer-than-we-think?ss_source=sscampaigns&ss_campaign_id=5d4c3f20755775398d1df26d&ss_email_id=5d4c4edcdf4ba44ca217c8db&ss_campaign_name=The$+$link$+$between$+$pot$+$and$+$mass$+$shootings$+$may$+$be$+$closer$+$than$+$we$+$think&ss_campaign_sent_date=2019-08-08T16%3A33%3A47Z (accessed February 1, 2023).
269. Radio.US WtP. Shooters, Terrorists and Mass Killers whoUsed Marijuana (Month / Year). We the People Radio.US. Available online at: https://www.wethepeopleradio.us/pot—shooter-list.html (accessed February 1, 2023).
271. Service USS,. US Secret Service Report on Mass Attacks in Public Places. (2019). US Secret Service. Available online at: https://www.secretservice.gov/sites/default/files/reports/2020-09/MAPS2019.pdf (accessed February 1, 2023).
272. Miller NS, Ipeku R, Oberbarnscheidt T. A review of cases of marijuana and violence. Int J Environ Res Public Health. (2020) 17:1578. doi: 10.3390/ijerph17051578
273. Mok PLH, Pedersen CB, Springate D, Astrup A, Kapur N, Antonsen S, et al. Parental psychiatric disease and risks of attempted suicide and violent criminal offending in offspring: a population-based cohort study. JAMA Psychiatry. (2016) 73:1015–22. doi: 10.1001/jamapsychiatry.2016.1728
274. Miller NS, Oberbarnscheidt T. Marijuana violence and law. J Addict Res Ther. (2017) 2017:S11–6. doi: 10.4172/2155-6105.S11-014
275. Dellazizzo L, Potvin S, Beaudoin M, Luigi M, Dou BY, Giguère C-É, et al. Cannabis use and violence in patients with severe mental illnesses: A meta-analytical investigation. Psychiatry Res. (2019) 274:42–8. doi: 10.1016/j.psychres.2019.02.010
276. Shorey RC, Haynes E, Brem M, Florimbio AR, Grigorian H, Stuart GL. Marijuana use is associated with intimate partner violence perpetration among men arrested for domestic violence. Transl Issues Psychol Sci. (2018) 4:108–18. doi: 10.1037/tps0000140
277. Smith A, Kaufman F, Sandy MS, Cardenas A. Cannabis exposure during critical windows of development: epigenetic and molecular pathways implicated in neuropsychiatric disease. Curr Environ Health Rep. (2020) 7:325–42. doi: 10.1007/s40572-020-00275-4
278. Reece AS, Hulse GK. Epidemiological associations of various substances and multiple cannabinoids with autism in USA. Clin Pediatr. (2019) 4:1–20. doi: 10.35248/2572-0775.19.4.155
279. Reece AS, Hulse GK. Effect of cannabis legalization on US autism incidence and medium term projections. Clin Pediatr. (2019) 4:1–17. doi: 10.35248/2572-0775.19.4.154
280. Nevison C, Blaxill M, Zahorodny W. California autism prevalence trends from 1931 to 2014 and comparison to National ASD Data from IDEA and ADDM. J Autism Dev Disord. (2018) 48:4103–17. doi: 10.1007/s10803-018-3670-2
281. Roux AM, Tao S, Marcus S, Lushin V, Shea LL. A national profile of substance use disorder among Medicaid enrollees on the autism spectrum or with intellectual disability. Disabil Health J. (2022) 15:101289. doi: 10.1016/j.dhjo.2022.101289
282. Schrott R, Acharya K, Itchon-Ramos N, Hawkey AB, Pippen E, Mitchell JT, et al. Cannabis use is associated with potentially heritable widespread changes in autism candidate gene DLGAP2 DNA methylation in sperm. Epigenetics. (2020) 15:161–73. doi: 10.1080/15592294.2019.1656158
283. Bortoletto R, Colizzi M. Cannabis use in autism: reasons for concern about risk for psychosis. Healthcare. (2022) 10:1553. doi: 10.3390/healthcare10081553
284. Colizzi M, Bortoletto R, Costa R, Bhattacharyya S, Balestrieri M. The autism-psychosis continuum conundrum: exploring the role of the endocannabinoid system. Int J Environ Res Public Health. (2022) 19:5616. doi: 10.3390/ijerph19095616
285. Garrison-Desany HM, Hong X, Maher BS, Beaty TH, Wang G, Pearson C, et al. Individual and combined association between prenatal polysubstance exposure and childhood risk of attention-deficit/hyperactivity disorder. JAMA Netw Open. (2022) 5:e221957. doi: 10.1001/jamanetworkopen.2022.1957
286. Reece AS, Hulse GK. Gastroschisis and autism-dual canaries in the californian coalmine. JAMA Surg. (2019) 154:366–7. doi: 10.1001/jamasurg.2018.4694
287. Rees E, Creeth HDJ, Hwu H-G, Chen WJ, Tsuang M, Glatt SJ, et al. Schizophrenia, autism spectrum disorders and developmental disorders share specific disruptive coding mutations. Nat Commun. (2021) 12:5353. doi: 10.1038/s41467-021-25532-4
288. Foldy C, Malenka RC, Sudhof TC. Autism-associated neuroligin-3 mutations commonly disrupt tonic endocannabinoid signaling. Neuron. (2013) 78:498–509. doi: 10.1016/j.neuron.2013.05.033
289. Schrott R, Greeson KW, King D, Symosko Crow KM, Easley CA, et al. Cannabis alters DNA methylation at maternally imprinted and autism candidate genes in spermatogenic cells. Syst Biol Reprod Med. (2022) 68:1–13. doi: 10.1080/19396368.2022.2073292
290. Reece AS, Hulse GK. Impact of converging sociocultural and substance-related trends on US autism rates: combined geospatiotemporal and causal inferential analysis. Eur Arch Psychiatry Clin Neurosci. (2022). 19:7726–52. doi: 10.1007/s00406-022-01446-0
291. Schnapp A, Harel M, Cayam-Rand D, Cassuto H, Polyansky L, Aran A. A placebo-controlled trial of cannabinoid treatment for disruptive behavior in children and adolescents with autism spectrum disorder: effects on sleep parameters as measured by the CSHQ. Biomedicines. (2022) 10:1685. doi: 10.3390/biomedicines10071685
292. Junior EA, Medeiros WMB, Dos Santos JPM, de Sousa JMM, da Costa FB, Pontes KM, et al. Evaluation of the efficacy and safety of cannabidiol-rich cannabis extract in children with autism spectrum disorder: randomized, double-blind and controlled placebo clinical trial. Trends Psychiatry Psychother. (2022) 44. doi: 10.47626/2237-6089-2021-0396
293. Kasai H, Ziv NE, Okazaki H, Yagishita S, Toyoizumi T. Spine dynamics in the brain, mental disorders and artificial neural networks. Nat Rev Neurosci. (2021) 22:407–22. doi: 10.1038/s41583-021-00467-3
294. Li S, Sheng ZH. Energy matters: presynaptic metabolism and the maintenance of synaptic transmission. Nat Rev Neurosci. (2022) 23:4–22. doi: 10.1038/s41583-021-00535-8
295. Halassa MM, Fellin T, Haydon PG. Tripartite synapses: roles for astrocytic purines in the control of synaptic physiology and behavior. Neuropharmacology. (2009) 57:343–6. doi: 10.1016/j.neuropharm.2009.06.031
296. Oliveira da Cruz JF, Robin LM, Drago F, Marsicano G, Metna-Laurent M. Astroglial type-1 cannabinoid receptor (CB1): a new player in the tripartite synapse. Neuroscience. (2016) 323:35–42. doi: 10.1016/j.neuroscience.2015.05.002
297. Moyer CE, Zuo Y. Cortical dendritic spine development and plasticity: insights from in vivo imaging. Curr Opin Neurobiol. (2018) 53:76–82. doi: 10.1016/j.conb.2018.06.002
298. Morrison PD, Murray RM. Cannabis points to the synaptic pathology of mental disorders: how aberrant synaptic components disrupt the highest psychological functions. Dialog Clin Neurosci. (2020) 22:251–8. doi: 10.31887/DCNS.2020.22.3/pmorrison
300. Song S, Miller KD, Abbott LF. Competitive Hebbian learning through spike-timing-dependent synaptic plasticity. Nat Neurosci. (2000) 3:919–26. doi: 10.1038/78829
301. Bloomfield MA, Ashok AH, Volkow ND, Howes OD. The effects of Delta9-tetrahydrocannabinol on the dopamine system. Nature. (2016) 539:369–77. doi: 10.1038/nature20153
302. Forrest MP, Parnell E, Penzes P. Dendritic structural plasticity and neuropsychiatric disease. Nat Rev Neurosci. (2018) 19:215–34. doi: 10.1038/nrn.2018.16
303. Winters BL, Vaughan CW. Mechanisms of endocannabinoid control of synaptic plasticity. Neuropharmacology. (2021) 197:108736. doi: 10.1016/j.neuropharm.2021.108736
304. Piette C, Cui Y, Gervasi N, Venance L. Lights on endocannabinoid-mediated synaptic potentiation. Front Mol Neurosci. (2020) 13:132. doi: 10.3389/fnmol.2020.00132
305. Balezina OP, Tarasova EO, Gaydukov AE. Noncanonical activity of endocannabinoids and their receptors in central and peripheral synapses. Biochemistry. (2021) 86:818–32. doi: 10.1134/S0006297921070038
306. Wilson RI, Nicoll RA. Endogenous cannabinoids mediate retrograde signalling at hippocampal synapses. Nature. (2001) 410:588–92. doi: 10.1038/35069076
307. Durieux LJA, Gilissen SRJ, Arckens L. Endocannabinoids and cortical plasticity: CB1R as a possible regulator of the excitation/inhibition balance in health and disease. Eur J Neurosci. (2022) 55:971–88. doi: 10.1111/ejn.15110
308. Lemtiri-Chlieh F, Levine ES. 2-AG and anandamide enhance hippocampal long-term potentiation via suppression of inhibition. Front Cell Neurosci. (2022) 16:1023541. doi: 10.3389/fncel.2022.1023541
309. Busquets-Garcia A, Bains J, Marsicano G. CB(1) receptor signaling in the brain: extracting specificity from ubiquity. Neuropsychopharmacology. (2018) 43:4–20. doi: 10.1038/npp.2017.206
310. Campbell RR, Wood MA. How the epigenome integrates information and reshapes the synapse. Nat Rev Neurosci. (2019) 20:133–47. doi: 10.1038/s41583-019-0121-9
311. Crittenden JR, Yoshida T, Venu S, Mahar A, Graybiel AM. Cannabinoid receptor 1 is required for neurodevelopment of striosome-dendron bouquets. eNeuro. (2022) 9. doi: 10.1523/ENEURO.0318-21.2022
312. Xu K, Zhong G, Zhuang X. Actin, spectrin, and associated proteins form a periodic cytoskeletal structure in axons. Science. (2013) 339:452–6. doi: 10.1126/science.1232251
313. Zhou R, Han B, Xia C, Zhuang X. Membrane-associated periodic skeleton is a signaling platform for RTK transactivation in neurons. Science. (2019) 365:929–34. doi: 10.1126/science.aaw5937
314. Li H, Yang J, Tian C, Diao M, Wang Q, Zhao S, et al. Organized cannabinoid receptor distribution in neurons revealed by super-resolution fluorescence imaging. Nat Commun. (2020) 11:5699. doi: 10.1038/s41467-020-19510-5
315. Dudok B, Barna L, Ledri M, Szabó SI, Szabadits E, Pintér B, et al. Cell-specific STORM super-resolution imaging reveals nanoscale organization of cannabinoid signaling. Nat Neurosci. (2015) 18:75–86. doi: 10.1038/nn.3892
316. Dudok B, Soltesz I. Imaging the endocannabinoid signaling system. J Neurosci Methods. (2022) 367:109451. doi: 10.1016/j.jneumeth.2021.109451
317. Zöldi M, Katona I. STORM super-resolution imaging of CB(1) receptors in tissue preparations. Methods Mol Biol. (2023) 2576:437–51. doi: 10.1007/978-1-0716-2728-0_36
318. Cheng J, Chen X, Xia H, Kong F, Wang L, Zhong L, et al. Endogenous cannabinoid receptors modulate plasticity at immature synapses. J Integr Neurosci. (2022) 21:121. doi: 10.31083/j.jin2104121
319. Benarroch EE. Synaptic effects of cannabinoids: complexity, behavioral effects, and potential clinical implications. Neurology. (2014) 83:1958–67. doi: 10.1212/WNL.0000000000001013
320. LeGates TA, Kvarta MD, Tooley JR, Francis TC, Lobo MK, Creed MC, et al. Reward behaviour is regulated by the strength of hippocampus-nucleus accumbens synapses. Nature. (2018) 564:258–62. doi: 10.1038/s41586-018-0740-8
321. Volkow ND, Gillespie H, Mullani N, Tancredi L, Grant C, Valentine A, et al. Brain glucose metabolism in chronic marijuana users at baseline and during marijuana intoxication. Psychiatry Res. (1996) 67:29–38. doi: 10.1016/0925-4927(96)02817-X
322. Volkow ND, Wang G-J, Telang F, Fowler JS, Alexoff D, Logan J, et al. Decreased dopamine brain reactivity in marijuana abusers is associated with negative emotionality and addiction severity. Proc Natl Acad Sci USA. (2014) 111:E3149–56. doi: 10.1073/pnas.1411228111
323. van de Giessen E, Weinstein JJ, Cassidy CM, Haney M, Dong Z, Ghazzaoui R, et al. Deficits in striatal dopamine release in cannabis dependence. Mol Psychiatry. (2017) 22:68–75. doi: 10.1038/mp.2016.21
324. Hirvonen J, Goodwin RS Li C-T, Terry GE, Zoghbi SS, Morse C, et al. Reversible and regionally selective downregulation of brain cannabinoid CB1 receptors in chronic daily cannabis smokers. Mol Psychiatry. (2012) 17:642–9. doi: 10.1038/mp.2011.82
325. Manza P, Yuan K, Shokri-Kojori E, Tomasi D, Volkow ND. Brain structural changes in cannabis dependence: association with MAGL. Mol Psychiatry. (2020) 25:3256–66. doi: 10.1038/s41380-019-0577-z
326. Manza P, Shokri-Kojori E, Volkow ND. Reduced segregation between cognitive and emotional processes in cannabis dependence. Cereb Cortex. (2020) 30:628–39. doi: 10.1093/cercor/bhz113
327. Demiral S B, Tomasi D, Wiers CE, Manza P, Shokri-Kojori E, Studentsova Y, et al. Methylphenidate's effects on thalamic metabolism and functional connectivity in cannabis abusers and healthy controls. Neuropsychopharmacology. (2019) 44:1389–97. doi: 10.1038/s41386-018-0287-2
328. Lorenzetti V, Chye Y, Silva P, Solowij N, Roberts CA. Does regular cannabis use affect neuroanatomy? An updated systematic review and meta-analysis of structural neuroimaging studies. Eur Arch Psychiatry Clin Neurosci. (2019) 269:59–71. doi: 10.1007/s00406-019-00979-1
329. Achicallende S, Río IB-D, Serrano M, Mimenza A, Lekunberri L, Anaut-Lusar I, et al. GLAST versus GFAP as astroglial marker for the subcellular study of cannabinoid CB(1) receptors in astrocytes. Histochem Cell Biol. (2022) 158:561–9. doi: 10.1007/s00418-022-02139-4
330. Metna-Laurent M, Marsicano G. Rising stars: modulation of brain functions by astroglial type-1 cannabinoid receptors. Glia. (2015) 63:353–64. doi: 10.1002/glia.22773
331. Okabe S. Regulation of actin dynamics in dendritic spines: Nanostructure, molecular mobility, and signaling mechanisms. Mol Cell Neurosci. (2020) 109:103564. doi: 10.1016/j.mcn.2020.103564
332. Monteiro P, Feng G. SHANK proteins: roles at the synapse and in autism spectrum disorder. Nat Rev Neurosci. (2017) 18:147–57. doi: 10.1038/nrn.2016.183
333. Willsey HR, Willsey AJ, Wang B, State MW. Genomics, convergent neuroscience and progress in understanding autism spectrum disorder. Nat Rev Neurosci. (2022) 23:323–41. doi: 10.1038/s41583-022-00576-7
334. Mohammad GS, Joca S, Starnawska A. The cannabis-induced epigenetic regulation of genes associated with major depressive disorder. Genes. (2022) 13:1435. doi: 10.3390/genes13081435
335. Kushnireva L, Basnayake K, Holcman D, Segal M, Korkotian E. Dynamic regulation of mitochondrial [Ca(2+)] in hippocampal neurons. Int J Mol Sci. (2022) 23:12321. doi: 10.3390/ijms232012321
336. Thandapilly SJ, Louis XL, Yang T, Stringer DM Yu L, Zhang S, et al. Resveratrol prevents norepinephrine induced hypertrophy in adult rat cardiomyocytes, by activating NO-AMPK pathway. Eur J Pharmacol. (2011) 668:217–24. doi: 10.1016/j.ejphar.2011.06.042
337. Wang A, Liu M, Liu X, Dong LQ, Glickman RD, Slaga TJ, et al. Up-regulation of adiponectin by resveratrol: the essential roles of the Akt/FOXO1 and AMP-activated protein kinase signaling pathways and DsbA-L. J Biol Chem. (2011) 286:60–6. doi: 10.1074/jbc.M110.188144
338. Wu Y, Li X, Zhu JX, Xie W, Le W, Fan Z, et al. Resveratrol-activated AMPK/SIRT1/autophagy in cellular models of Parkinson's disease. Neurosignals. (2011) 19:163–74. doi: 10.1159/000328516
339. Yi C-O, Jeon BT, Shin HJ, Jeong EA, Chang KC, Lee JE, et al. Resveratrol activates AMPK and suppresses LPS-induced NF-kappaB-dependent COX-2 activation in RAW 2647 macrophage cells. Anat Cell Biol. (2011) 44:194–203. doi: 10.5115/acb.2011.44.3.194
340. Fei W, Tian DR, Tso P, Han JS. Diet-induced obese rats exhibit impaired LKB1-AMPK signaling in hypothalamus and adipose tissue. Peptides. (2012) 35:23–30. doi: 10.1016/j.peptides.2012.02.003
341. de Morentin PBM, Whittle AJ, Fernø J, Nogueiras R, Diéguez C, Vidal-Puig A, et al. Nicotine induces negative energy balance through hypothalamic AMP-activated protein kinase. Diabetes. (2012) 61:807–17. doi: 10.2337/db11-1079
342. Song Z, Liu L, Yue Y, Jiao H, Lin H, Sheikhahmadi A, et al. Fasting alters protein expression of AMP-activated protein kinase in the hypothalamus of broiler chicks (Gallus gallus domesticus). Gen Comp Endocrinol. (2012) 178:546–55. doi: 10.1016/j.ygcen.2012.06.026
343. Yu W, Liu-Bryan R, Stevens S, Damanahalli JK, Terkeltaub R. RAGE signaling mediates post-injury arterial neointima formation by suppression of liver kinase B1 and AMPK activity. Atherosclerosis. (2012) 222:417–25. doi: 10.1016/j.atherosclerosis.2012.04.001
344. Crane JD, MacNeil LG, Lally JS, Ford RJ, Bujak AL, Brar IK, et al. Exercise-stimulated interleukin-15 is controlled by AMPK and regulates skin metabolism and aging. Aging Cell. (2015). doi: 10.1111/acel.12341
345. Han X, Tai H, Wang X, Wang Z, Zhou J, Wei X, et al. AMPK activation protects cells from oxidative stress-induced senescence via autophagic flux restoration and intracellular NAD+ elevation. Aging Cell. (2016) 15:416–427. doi: 10.1111/acel.12446
346. Toyama EQ, Herzig S, Courchet J, Lewis TL, Jr., Loson OC, Hellberg K, et al. Metabolism. AMP-activated protein kinase mediates mitochondrial fission in response to energy stress. Science. (2016) 351:275–81. doi: 10.1126/science.aab4138
347. Wang X, Xue G-X, Liu W-C, Shu H, Wang M, Sun Y, et al. Melatonin alleviates lipopolysaccharide-compromised integrity of blood–brain barrier through activating AMP-activated protein kinase in old mice. Aging Cell. (2017) 16:414–21. doi: 10.1111/acel.12572
348. Ma T, Tian X, Zhang B, Li M, Wang Y, Yang C, et al. Low-dose metformin targets the lysosomal AMPK pathway through PEN2. Nature. (2022) 603:159–65. doi: 10.1038/s41586-022-04431-8
349. Plotegher N. Physiological roles of organelles at the pre-synapse in neurons. Int J Biochem Cell Biol. (2023) 154:106345. doi: 10.1016/j.biocel.2022.106345
350. Antoniou A, Auderset L, Kaurani L, Sebastian E, Zeng Y, Allahham M, et al. Neuronal extracellular vesicles and associated microRNAs induce circuit connectivity downstream BDNF. Cell Rep. (2023) 42:112063. doi: 10.1016/j.celrep.2023.112063
351. Xylaki M, Paiva I, Al-Azzani M, Gerhardt E, Jain G, Islam MR, et al. miR-101a-3p impairs synaptic plasticity and contributes to synucleinopathy. J Parkinsons Dis. (2023). doi: 10.3233/JPD-225055
352. Winstone J, Shafique H, Clemmer ME, Mackie K, Wager-Miller J. Effects of tetrahydrocannabinol and cannabidiol on brain-derived neurotrophic factor and tropomyosin receptor kinase B expression in the adolescent hippocampus. Cannabis Cannabinoid Res. (2022). doi: 10.1089/can.2021.0025
353. Pramio DT, Vieceli FM, Varella-Branco E, Goes CP, Kobayashi GS, da Silva Pelegrina DV, et al. DNA methylation of the promoter region at the CREB1 binding site is a mechanism for the epigenetic regulation of brain-specific PKMζ. Biochim Biophys Acta Gene Regul Mech. (2023) 1866:194909. doi: 10.1016/j.bbagrm.2023.194909
355. Del Re EC. Emerging themes in schizophrenia research at SIRS 2022: inclusivity, equality and Cannabis impact on mental health. Schizophr Res. (2022) 250:39–40. doi: 10.1016/j.schres.2022.10.003
356. Greco LA, Reay WR, Dayas CV, Cairns MJ. Pairwise genetic meta-analyses between schizophrenia and substance dependence phenotypes reveals novel association signals with pharmacological significance. Transl Psychiatry. (2022) 12:403. doi: 10.1038/s41398-022-02186-4
357. Ibarra-Lecue I, Unzueta-Larrinaga P, Barrena-Barbadillo R, Villate A, Horrillo I, Mendivil B, et al. Cannabis use selectively modulates circulating biomarkers in the blood of schizophrenia patients. Addict Biol. (2022) 27:e13233. doi: 10.1111/adb.13233
358. Kayir H, Ruffolo J, McCunn P, Khokhar JY. The relationship between cannabis, cognition, and schizophrenia: it's complicated. Curr Top Behav Neurosci. (2022). doi: 10.1007/7854_2022_396
359. Corsi DJ, Donelle J, Sucha E, Hawken S, Hsu H, El-Chaâr D, et al. Maternal cannabis use in pregnancy and child neurodevelopmental outcomes. Nat Med. (2020) 26:1536–40. doi: 10.1038/s41591-020-1002-5
360. Manza P, Tomasi D, Volkow ND. Subcortical local functional hyperconnectivity in cannabis dependence. Biol Psychiatry Cogn Neurosci Neuroimaging. (2018) 3:285–93. doi: 10.1016/j.bpsc.2017.11.004
361. Zehra A, Burns J, Liu CK, Manza P, Wiers CE, Volkow ND, et al. Cannabis addiction and the brain: a review. J Neuroimmune Pharmacol. (2018) 13:438–52. doi: 10.1007/s11481-018-9782-9
362. McPherson KL, Tomasi DG, Wang GJ, Manza P, Volkow ND. Cannabis affects cerebellar volume and sleep differently in men and women. Front Psychiatry. (2021) 12:643193. doi: 10.3389/fpsyt.2021.643193
363. Lorenzetti V, Kowalczyk M, Duehlmeyer L, Greenwood L-M, Chye Y, Yücel M, et al. brain anatomical alterations in young cannabis users: is it all hype? A meta-analysis of structural neuroimaging studies. Cannabis Cannabinoid Res. (2022). doi: 10.1089/can.2021.0099
364. Hadley EC, Lakatta EG, Morrison-Bogorad M, Warner HR, Hodes RJ. The future of aging therapies. Cell. (2005) 120:557–67. doi: 10.1016/j.cell.2005.01.030
365. Kirkwood TB. Understanding the odd science of aging. Cell. (2005) 120:437–47. doi: 10.1016/j.cell.2005.01.027
366. Balaban RS, Nemoto S, Finkel T. Mitochondria, oxidants, and aging. Cell. (2005) 120:483–95. doi: 10.1016/j.cell.2005.02.001
367. Bara A, Ferland JN, Rompala G, Szutorisz H, Hurd YL. Cannabis and synaptic reprogramming of the developing brain. Nat Rev Neurosci. (2021) 22:423–38. doi: 10.1038/s41583-021-00465-5
368. Reece AS. Rapid response: cannabinoid genotoxic trifecta - cancerogenesis, clinical teratogenesis and cellular ageing. Br Med J. (2022) 376:n3114. doi: 10.1136/bmj.n3114
369. Reece AS. Disruption of interlocking synchrony between metabolome and epigenome key to understanding widespread embyrotoxicity and carcinogenicity of diverse cannabinoids. Br Med J. (2022). Available online at: https://www.bmj.com/content/377/bmj.o1567/rr-0
370. Reece AS, Hulse GK. Epidemiology of Δ8THC-related carcinogenesis in USA: a panel regression and causal inferential study. Int J Environ Res Public Health. (2022) 19:7726–52. doi: 10.3390/ijerph19137726
371. Reece AS, Hulse GK. Cannabis- and substance-related carcinogenesis in europe: a lagged causal inferential panel regression modelling study. J Xenobiot. (2023) 13:323–85. doi: 10.3390/jox13030024
372. Reece AS, Hulse GK. Cannabis could be the missing environmental pancreatic carcinogen hiding in plain view. Gastroenterology. (2023) 23:1092–1093. doi: 10.1053/j.gastro.2023.02.050
373. Reece AS, Hulse GK. Chapter 4: Geospatiotemporal and Causal Inferential Epidemiological Survey and Exploration of Cannabinoid- and Substance- Related Carcinogenesis in USA 2003-2017. Epidemiology of Cannabis: Genotoxicity and Neurotoxicity, Epigenomics and Aging. New York, NY: Elsevier (2023).
374. Reece AS, Hulse GK. Cannabinoid exposure as a major driver of pediatric acute lymphoid Leukaemia rates across the USA: combined geospatial, multiple imputation and causal inference study. BMC Cancer. (2021) 21:1:984–1017. doi: 10.1186/s12885-021-08598-7
375. Reece AS, Hulse GK. Cannabis teratology explains current patterns of coloradan congenital defects: the contribution of increased cannabinoid exposure to rising teratological trends. Clin Pediatr. (2019) 58:1085–123. doi: 10.1177/0009922819861281
376. Reece AS, Hulse GK. Canadian cannabis consumption and patterns of congenital anomalies: an ecological geospatial analysis. J Addict Med. (2020) 14:e195–210. doi: 10.1097/ADM.0000000000000638
377. Reece AS, Hulse GK. European epidemiological patterns of cannabis- and substance- related congenital body wall anomalies: geospatiotemporal and causal inferential study. Int J Environ Res Public Health. (2022) 19:9027–64. doi: 10.3390/ijerph19159027
378. Reece AS, Hulse GK. European epidemiological patterns of cannabis- and substance- related congenital chromosomal anomalies: geospatiotemporal and causal inferential study. Int J Environ Res Public Health. (2022) 19:11208–58. doi: 10.3390/ijerph191811208
379. Reece AS, Hulse GK. European epidemiological patterns of cannabis- and substance- related congenital neurological anomalies: geospatiotemporal and causal inferential study. Int J Environ Res Public Health. (2022) 20:441–75. doi: 10.3390/ijerph20010441
380. Reece AS, Hulse GK. Effects of cannabis on congenital limb anomalies in 14 European nations: a geospatiotemporal and causal inferential study. Environ Epigenet. (2022) 8:1–34. doi: 10.1093/eep/dvac016
381. Reece AS, Hulse GK. European epidemiological patterns of cannabis- and substance- related congenital uronephrological anomalies: geospatiotemporal and causal inferential study. Int J Environ Res Public Health. (2022) 19:13769–828. doi: 10.3390/ijerph192113769
382. Reece AS, Hulse GK. Geospatiotemporal and causal inferential study of european epidemiological patterns of cannabis- and substance- related congenital orofacial anomalies. J Xenobiot. (2023) 13:42–74. doi: 10.3390/jox13010006
383. Reece AS, Hulse GK. Congenital gastrointestinal anomalies in Europe 2010-2019: a geospatiotemporal and causal inferential study of epidemiological patterns in relationship to cannabis- and substance- exposure Gastrointestinal Insights. (2023) 14:64–109. doi: 10.3390/gastroent14010007
384. Reece AS, Hulse GK. Chapter 3: Geospatiotemporal and Causal Inferential Analysis of United States Congenital Anomalies as a Function of Multiple Cannabinoid- and Substance- Exposures: Phenocopying Thalidomide and Hundred Megabase-Scale Genotoxicity. Epidemiology of Cannabis: Genotoxicity and Neurotoxicity, Epigenomics and Aging. New York, NY: Elsevier (2023).
385. Forrester MB, Merz RD. Risk of selected birth defects with prenatal illicit drug use, Hawaii, 1986-2002. J Toxicol Environ Health. (2007) 70:7–18. doi: 10.1080/15287390600748799
386. Phillips KT, Pedula KL, Choi NG, Tawara K-AK, Simiola V, Satre DD, et al. Chronic health conditions, acute health events, and healthcare utilization among adults over age 50 in Hawai'i who use cannabis: a matched cohort study. Drug Alcohol Depend. (2022) 234:109387. doi: 10.1016/j.drugalcdep.2022.109387
387. Allen JP, Danoff JS, Costello MA, Hunt GL, Hellwig AF, Krol KM, et al. Lifetime marijuana use and epigenetic age acceleration: a 17-year prospective examination. Drug Alcohol Depend. (2022) 233:109363. doi: 10.1016/j.drugalcdep.2022.109363
388. Reece AS, Norman A, Hulse GK. Cannabis exposure as an interactive cardiovascular risk factor and accelerant of organismal ageing: a longitudinal study. BMJ Open. (2016) 6:e011891–901. doi: 10.1136/bmjopen-2016-011891
389. Pavarin RM, Berardi D. Mortality risk in a cohort of subjects reported by authorities for cannabis possession for personal use. Results of a longitudinal study. Epidemiol Prev. (2011) 35:89–93.
390. Dwyer-Lindgren L, Bertozzi-Villa A, Stubbs RW, Morozoff C, Shirude S, Unützer J, et al. Trends and patterns of geographic variation in mortality from substance use disorders and intentional injuries among US counties, 1980-2014. JAMA. (2018) 319:1013–23. doi: 10.1001/jama.2018.0900
391. von Greiff N, Skogens L, Berlin M, Bergmark A. Mortality and cause of death-a 30-year follow-up of substance misusers in sweden. Subst Use Misuse. (2018) 53:1–9. doi: 10.1080/10826084.2018.1452261
NF-κB, Nuclear Factor kappaB, the key master transcription factor of the immune system; ROS, Reactive Oxygen Species; also known as oxygen radical; includes hydrogen peroxide, superoxide anion, and the hydroxyl radical; HIF1α, Hypoxia Inducible Factor 1; FGF21, Fibroblast Growth Factor 21; GDF15, Growth Differentiation Factor 15; SIRT1, Silencer of Information Transfer 1; AMPK, Adenosine Monophosphate Kinase; TFS1, Stress-activated transcription factor ATFS-1; ATFS-1, Activating Transcription Factor Associated With Stress-1; GPR, G-Protein Receptor; HDAC, Histone Deacetylase; HMGB1, High Molecular Weight Group Box protein 1; ARG1, Arginase 1—catalyzes the hydrolysis of arginine to ornithine and urea; CCL5, C-C Motif Chemokine Ligand 5; SLC16A, Solute Carrier Family 16, Member 1 (Monocarboxylic Acid Transporter 1); NFAT, Nuclear Factor of Activated T Cells; VEGF, Vascular Endothelial Growth Factor; Kla, K is the abbreviation for lysine and la stands for lactate; ACAT1, Acetyl-CoA Aceteyltransferase 1; CXCL9, C-X-C Motif Chemokine Ligand 9; cGAS-STING, cyclic AMP—cyclic GMP—Stimulator of INterferon Gamma (STING); R-loops are DNA, RNA hybrids and can arise during DNA transcription; Stat, Signal Transducer and Activator of Transcription; RelB, RELB Proto-Oncogene, NF-KB Subunit; NF-κB, Nuclear Factor kappa B; JNK, c-Jun—N-terminal kinase; ASK, Apoptosis Signal Regulating Kinase 1/Mitogen-Activated Protein Kinase Kinase Kinase 5/MAPKKK5/MAP3K5; ABA, Gamma Amino-Butyric Acid; DAMP, Damage-Associated Molecular Pattern; PAMPm, Pattern-Associated Molecular Pattern; ARCH, Age Related Clonal Haematopoiesis; SASP, Senescence-Associated Secretory Phenotype; LR, Toll-Like Receptor; RIG1, Retinoic Acid Inducible Gene 1; MDA5, Melanoma Differentiation-Associated Protein 5; cGAS, cyclic GMP – cyclic AMP Synthase; CAR T-cells, Chimeric Antigen Receptor T-cell lymphocytes; CCL2, C-C Motif Chemokine ligand/MCP-1, Macrophage Chemotactic Protein 1; TRAF6, TNF Receptor-Associated Factor 6; SWI/SNF, SWItch/Sucrose Non-Fermentable chromatin remodeling complexes; INO80, INO80 Complex ATPase Subunit; BAF complex, the mammalian equivalent of SWI/SNF in yeast; Includes BANF1, BAF Nuclear Assembly Factor 1; ARID1a, AT-Rich Interaction Domain 1A; LRRC15, Leucine-Rich Repeat Containing 15; TGFBR2, Transforming Growth Factor Beta Receptor 2; mGlu1, Glutamate Metabotropic Receptor 1/GRM1; MAGL, MonoAcyl Glycerol Lipase the enzyme which metabolizes 2-arachidonyl glycerol; AMPAR, α-Amino-3-hydroxy-5-Methyl-4-isoxazole Propionic Acid glutamate Receptor; PSD95, Post-Synaptic Density 95; DLG4, Discs Large MAGUK Scaffold Protein 4; PICK1, Protein Interacting With PRKCA 1; CACNA1C, Calcium Voltage-Gated Channel Subunit Alpha1 C; Ras, KRAS Proto-Oncogene, GTPase; RAC1, Rac Family Small GTPase 1; RhoA, Ras Homolog Family Member A; RAP - RAP, Member of RAS Oncogene Family; AMPK, AMP kinase/PRKAA1, Protein Kinase AMP-Activated Catalytic Subunit Alpha 1; OPA1, OPA1 Mitochondrial Dynamin Like GTPase / Optic Atrophy 1 (Autosomal Dominant); MFN1, Mitofusin 1; BDNF, Brain-Derived Neurotrophic Factor; CBP, CREB Binding Protein; HDAC5, Histone DeACetylase 5; FGF1B, Fibroblast Growth Factor 1B; PRKCZ, Protein Kinase C Zeta; CREB1, cAMP Response Element Binding Protein 1; LRRTM2, Leucine Rich Repeat Transmembrane Neuronal 2; NEUROD2, Neuronal Differentiation 2; FAM163B, Family With Sequence Similarity 163 Member B.
Keywords: cannabis, cannabinoid, genotoxicity, epigenotoxicity, transgenerational inheritance
Citation: Reece AS and Hulse GK (2023) Perturbation of 3D nuclear architecture, epigenomic aging and dysregulation, and cannabinoid synaptopathy reconfigures conceptualization of cannabinoid pathophysiology: part 2—Metabolome, immunome, synaptome. Front. Psychiatry 14:1182536. doi: 10.3389/fpsyt.2023.1182536
Received: 08 March 2023; Accepted: 11 September 2023;
Published: 03 October 2023.
Edited by:
Ilya Blokhin, Jackson Memorial Hospital, United StatesReviewed by:
Bruno Miguel Fonseca, University of Porto, PortugalCopyright © 2023 Reece and Hulse. This is an open-access article distributed under the terms of the Creative Commons Attribution License (CC BY). The use, distribution or reproduction in other forums is permitted, provided the original author(s) and the copyright owner(s) are credited and that the original publication in this journal is cited, in accordance with accepted academic practice. No use, distribution or reproduction is permitted which does not comply with these terms.
*Correspondence: Albert Stuart Reece, c3R1YXJ0LnJlZWNlQHV3YS5lZHUuYXU=
Disclaimer: All claims expressed in this article are solely those of the authors and do not necessarily represent those of their affiliated organizations, or those of the publisher, the editors and the reviewers. Any product that may be evaluated in this article or claim that may be made by its manufacturer is not guaranteed or endorsed by the publisher.
Research integrity at Frontiers
Learn more about the work of our research integrity team to safeguard the quality of each article we publish.