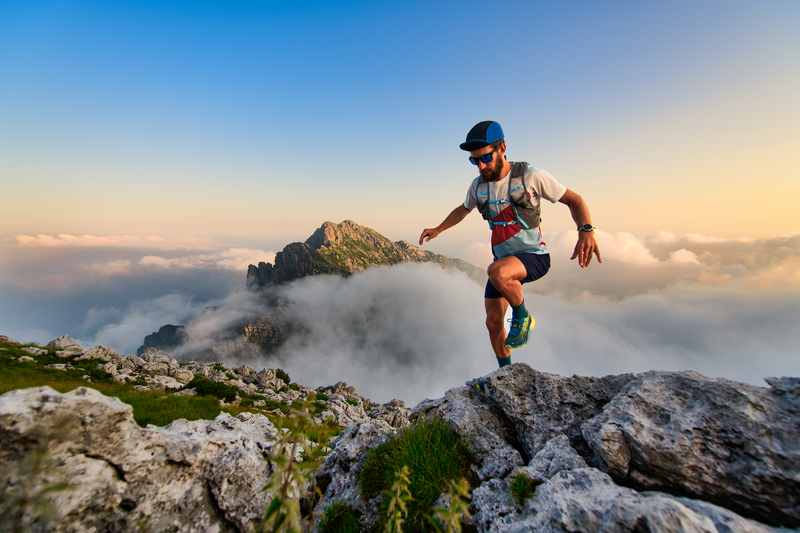
95% of researchers rate our articles as excellent or good
Learn more about the work of our research integrity team to safeguard the quality of each article we publish.
Find out more
REVIEW article
Front. Psychiatry , 09 June 2023
Sec. Anxiety and Stress Disorders
Volume 14 - 2023 | https://doi.org/10.3389/fpsyt.2023.1170625
This article is part of the Research Topic The underlying mechanisms of stress related disorders and potential treatment maneuvers View all 8 articles
Electroconvulsive therapy (ECT) is an important treatment for depression. Although it is known as the most effective acute treatment for severe mood disorders, its therapeutic mechanism is still unclear. With the rapid development of neuroimaging technology, various neuroimaging techniques have been available to explore the alterations of the brain by ECT, such as structural magnetic resonance imaging, functional magnetic resonance imaging, magnetic resonance spectroscopy, positron emission tomography, single photon emission computed tomography, arterial spin labeling, etc. This article reviews studies in neuroimaging on ECT for depression. These findings suggest that the neurobiological mechanism of ECT may regulate the brain functional activity, and neural structural plasticity, as well as balance the brain’s neurotransmitters, which finally achieves a therapeutic effect.
Depression is characterized by persistent low mood and reduced volitional activity, with high incidence, recurrence, and suicide rates, which seriously endanger human physical and mental health (1). According to reports, more than 350 million people worldwide suffer from depression (2). Therefore, it is of great importance to understand the causes of depression and how to combat it. Neuroimaging is now widely used to investigate the pathogenesis of depression, and studies have found that structural or functional alterations in the prefrontal cortex, hippocampus, amygdala, limbic system, and other regions of the brain are significantly associated with the onset of depression.
Electroconvulsive therapy (ECT) is a treatment for mental illness by passing a certain amount of electric current through the brain, causing epileptiform discharges. Since ECT was invented by Italian scientists in 1938, it has now been used in the treatment of mental disorders for more than 80 years (3). As the knowledge of ECT has gradually increased, it has also been found to have good effects on the improvement of depressive symptoms, and the general opinion is now that ECT is one of the most effective methods for depression (4) and has been widely used for severe depressive disorders (5). Some studies have reported that ECT can provide up to 70%–80% remission rate of depressive symptoms (6, 7). Also, its benefits include reduced patient hospitalization rates, and reduced risk of suicide (8–10).
Even though ECT has remarkable efficacy, but how long does ECT affect the brain is unclear, for example, the ECT induced memory loss could last for 2–3 months (11), but some study reported that some still had loss of memory even 3 years after ECT (12). More and more neuroimaging studies are used to understand the effect of ECT. Neuroimaging techniques, as a means of noninvasive observation of brain alterations, can be used to find markers of pathophysiological processes in psychiatric disorders. Previous studies on neuroimaging have found that patients with depression may experience structural or functional abnormalities (13, 14). Neuroimaging studies of depressed patients before/after ECT have also found that ECT can alter the structure and function of specific brain regions. For example, studies have found that ECT can cause changes in hippocampal volume (15, 16). Although there are more and more brain imaging studies trying to explore the therapeutic mechanisms of ECT, there are inconsistencies in the brain regions that cause alterations, so we try to review the brain imaging studies on ECT for depression.
References included in the study were identified through multiple searches of the Embase and PubMed/MEDLINE for articles published until December 2022, using combinations of the following search terms: “Depression”, “Major depressive disorder”, “Gray matter volume”, “GMV”, “Cortical thickness”, “White matter”, “Functional magnetic resonance imaging”, “fMRI”, “Regional homogeneity”, “ReHo”, “Amplitude of low frequency fluctuations”, “ALFF’, “Functional connectivity”, “FC’, “Magnetic Resonance Spectroscopy”, “MRS’, “Positron Emission Tomography”, “PET’, “Single photon emission computed tomography”, “SPECT”, “Arterial spin labeling”, “ASL”, “electroconvulsive therapy” and “ECT”. Due to the breadth of relevant studies, published reviews, meta-analyses, randomised controlled trials and recent studies were prioritised to provide a comprehensive and up-to-date overview of research on ECT in patients with depression.
Previous studies have found that ECT can increase brain gray matter volumes of depressed patients commonly in the hippocampus, amygdala, and temporal lobe (17–19). Among all studies, the most consistent trend of altered brain structures after ECT is to increase the volume of the temporal lobe as well as subcortical areas such as the hippocampus, amygdala, striatum, and anterior cingulate gyrus (20). Some researchers have explored the relationship between ECT and depression, such as Tendolkar et al. (17) found ECT could increase the volumes of bilaterally hippocampus and amygdala, and HAMD scores as well as depressive symptoms significantly reduced after ECT. Gryglewski et al. (21) also found structural changes in hippocampal subregions and amygdala after ECT, and these structural changes were closely related to the pathophysiological mechanisms of depression and the development of stress-related disorders. Cao et al. (22) used the latest hippocampal segmentation method and found that ECT could increase the volume of corpus striatum, granule cell layer, molecular layer, and hypothalamus, while the efficacy of ECT for each patient could be accurately predicted. Jorgensen et al. (23) focused on the volume of hippocampus, amygdala, dorsolateral prefrontal cortex (DLPFC), orbitofrontal cortex, and hypothalamus and found the volume of hippocampus and amygdala in patients increased significantly after ECT, while the volume of DLPFC decreased slightly, but due to the lack of correlation between changes in these brain regions and antidepressant effects, the remodeling of these brain structures cannot directly respond to the antidepressant mechanisms of ECT. There are also some studies based on voxel-based morphometry (VBM) to explore whole brain structural abnormalities, such as Ota et al. (24) found that depressed patients had a significant increase in the volume of bilateral inferior temporal cortex, and the right anterior cingulate gyrus after ECT, and the increased volume correlated with clinical improvement. Sartorius et al. (25) analyzed the changes in structural magnetic resonance pre/post-ECT, found a more pronounced increase in gray matter volume in the bilateral temporal lobe, middle cingulate gyrus, insula, and the putamen. Some studies even found that after unilateral ECT, only the unilateral hippocampus, amygdala, insula, and subgenual cortex volumes increased (16, 26). Changes of hippocampus volume were concerned commonly after ECT, studies have shown a significant reduction in hippocampal volume in depressed patients (27–29). Tendolkar (17) found an increase in bilaterally hippocampal volume after ECT, and Abbott (30) found a significant increase of right hippocampal volume after ECT. All these studies suggest that increased hippocampal volume after ECT may be one of the potential antidepressant mechanisms. However, there are also different opinions, such as Gbyl et al. (31) who argued that current MRI studies have not paid enough attention to the hypothesis that ECT may lead to brain damage, and suggested that future studies should explore the relationship between brain volume changes and cognitive impairment. In addition to focusing on the therapeutic mechanism of ECT, there are also many studies that agree that the increase of hippocampal volume caused by ECT may be related to cognitive side effects, such as Argyelan et al. (32) suggested that hippocampal enlargements are associated with cognitive side effects. van der A et al. (33) found similar change, which is ECT-induced left hippocampal enlargement correlated with decreases in verbal memory functioning. Gbyl et al. (34) found ECT-related transient increases in the volume of major hippocampal subregions within-patients are associated with memory impairment, based on previous studies, ECT-induced volume changes can be due to vascularization (35), inflammation (36) or vasogenic oedema (37) and/or neuroplastic mechanisms including neurogenesis (38), synaptogenesis (39) and gliogenesis (40), but further studies are still needed.
Studies have suggested that cortical thickness varies in a variety of psychiatric disorders. For example, a study focusing on bipolar disorder found that the patient group had reduced cortical thickness in the left superior temporal gyrus, bilateral prefrontal compared with healthy controls (HCs) (41), and in a study of cortical thickness in depressed patients, it was found that cortical thickness of right middle frontal gyrus and left anterior cingulate cortex (ACC) were thickened in depressed patients compared with HCs (42). It has been demonstrated that ECT can change cortical thickness in different brain regions (e.g., superior temporal gyrus, inferior temporal gyrus, insula, anterior cingulate gyrus, prefrontal cortex, fusiform gyrus) (25, 43). van Eijndhoven et al. (44) evaluated 19 patients with refractory depression after ECT and found cortical thickness in the left temporal pole, left middle temporal lobe, and right insula increased significantly after ECT, and the average remission rate of depressive symptoms was 57%. A study with a 6-months follow-up after ECT, exploring the cortical thickness in 26 brain regions, showed that significant increased cortical thickness of frontal, temporal, and insula could be seen immediately after ECT, while after 6 months, the thickened cortical thickness returns to baseline (45). Some studies suggested that the cortical thickness of precentral gyrus, lateral occipital lobe can predict relapse rate after ECT for depression (46). Pirnia et al. (43) found that increased cortical thickness of bilateral ACC and superior temporal gyrus cortex after ECT, especially changes in ACC can predict the early efficacy of ECT.
Alterations in the white matter of the brain are also the focus of brain science research, and diffusion tensor imaging (DTI) techniques have an important place in the study of white matter by assessing the diffuse activity of water molecules in neural tissue, which allows noninvasive probing of the direction and intensity of white matter trajectories. One study that included 2,937 psychiatric patients with depression, schizophrenia, bipolar disorder, or autism, focusing on changes in the white matter, found that patients with schizophrenia, bipolar disorder, or autism had similar structural changes in the corpus callosum, but the similarity change was not found in depression patients. The study also found that patients with schizophrenia and bipolar disorder had alterations in the limbic system compared with HCs, but not found in depressed patients (47). Chen et al. (48) conducted a meta-analysis of brain microstructural abnormalities in unmedicated depression patients by DTI, and a reduction of fractional anisotropy (FA) was found in bilateral anterior internal capsule, corpus callosum, right superior frontal gyrus, and right inferior temporal gyrus. Gbyl et al. (31) found ECT could increase the integrity of white matter pathways in the frontal lobe as well as temporal lobe, but the relationship between increased white matter volumes and treatment outcome could not been found. Yrondi et al. (49) used mean diffusivity (MD) to examine patients with refractory depression and found a reduction of MD in the hippocampus and left amygdala after ECT, and considered that ECT could modify microstructural integrity. Gryglewski et al. (50) tried to explore white matter changes in refractory depression patients taking unilateral ECT and found an increased axial diffusivity (AD) in the right posterior limb of internal capsule, but no correlation was found between changed AD and clinical effects. Repple et al. (51) analyzed changes in white matter structure before and after ECT in depressed patients and found increased MD in the right hemisphere after ECT. Kubicki et al. (52) found changed structural connectivity of hippocampal neural circuits after ECT. Lyden et al. (53) observed a significant increase of FA in the dorsal frontal-limbic circuits in depressed patients after ECT. A series of studies using DTI assessment suggest that ECT can effectively modulate white matter structure in depressed patients.
Blood oxygen level-dependent functional magnetic resonance imaging (BOLD-fMRI) has been applied in the field of brain function research since the 1990s and is now widely used as a non-invasive brain imaging assessment technique for psychiatric disorders. fMRI has the following advantages: non-invasive, non-radioactive, and reproducible. It can also analyze the dynamic activity of neurons on an individual and can analyze different response patterns between adjacent cortices. For spontaneous low-frequency activity acquired in the resting state, it is thought to reflect the spontaneous brain functional activity of the central nervous system in the resting state. Therefore, resting-state fMRI (rs-fMRI) has obvious clinical advantages. rs-fMRI is also particularly suitable for brain imaging studies of depressed patients, and the main analysis methods currently available are: regional homogeneity, amplitude of low frequency fluctuations, fractional amplitude of low frequency fluctuations, and functional connectivity.
Regional homogeneity (ReHo) responds to the degree of synchronization of neuronal activity in regional brain regions (54). It has been shown that the brain can have spontaneous neural activity with a high degree of synchrony in BOLD signals (55, 56). Studies on the relationship of depression and ReHo are common, such as Li et al. (57) found that, compared with HCs, depressed patients had lower ReHo in the precuneus. Kong et al. (58) found that there was a significant decreased ReHo in the bilateral superior frontal gyrus after ECT and a significant correlation was found between ReHo in the right superior frontal gyrus and changed HAMD before and after ECT. Mo et al. (59) found that after ECT, ReHo increased in left angular gyrus in depressed patients. Qiu et al. (60) also focused on the changed ReHo in depressed patients after ECT and found that patients showed increased ReHo in the bilateral frontal lobe, bilateral parietal lobe and right caudate nucleus after 8 sessions of ECT, while reduced ReHo was found in the left anterior cerebellar lobe, right cingulate gyrus, and right superior temporal gyrus. Argyelan et al. (61) found ReHo increase significantly in the left angular gyrus in refractory depression patients after ECT. Therefore, ReHo has the potential to be used as a response indicator for ECT used in depressed patients.
Amplitude of low frequency fluctuations (ALFF) as well as fractional amplitude of low frequency fluctuations (fALFF) have been demonstrated valid in the assessment of spontaneous brain activity in different psychiatric disorders (62–64). Studies on depression and ALFF/fALFF involving different ages and different brain regions, such as studies showed abnormal ALFF in the cingulate gyrus in depressed patients (65, 66). Wang et al. (67) found ALFF in the right precuneus and posterior cingulate gyrus decreased significantly in depressed patients compared with HCs. A study focused on depression adolescents showed decreased ALFF in the left somatosensory cortex and increased ALFF in the left insula compared with HCs (68). ECT could change ALFF/fALFF in different brain regions, such as Kong et al. (58) found ALFF in the left middle frontal gyrus increased after ECT, while ALFF in the left middle cingulate gyrus, left precentral gyrus, right superior frontal gyrus, and right middle frontal gyrus decreased. Du et al. (69) found a significant decreased ALFF in the right posterior cerebellar lobe of depressed patients after ECT, and previous studies found that ECT could increase ALFF in the medial prefrontal, orbitofrontal, and perigenual ACC (pgACC) (70, 71). Argyelan et al. (61) compared the differences brain function between refractory depression patients and HCs, and found increased fALFF in the right cingulate cortex in the patient group at baseline, indicating brain function hyperactivity, and after ECT, fALFF in the cingulate gyrus decreased significantly, indicating that ECT could significantly improve abnormal brain functional activity.
Functional connectivity (FC) is a method of calculating the similarity in time of blood oxygen signals between different brain regions. The signal of each brain region changes over time, and for a specific brain region, the sequence of its signal can be extracted from the MRI data, and the correlation of the time series between different anatomical regions of the brain can be obtained (72, 73). FC analysis methods have been widely used to explore the pathogenesis of depression, and analysis methods included: seed-based analysis, graph theory analysis, and brain network connectivity analysis, etc. Previous studies have shown that the hippocampus (74, 75), amygdala (76–78), thalamus (79, 80), insula (76, 81), and cingulate gyrus (74, 82), are related to emotional or cognitive processing in depressed patients. It was found that ECT could increase FC between hippocampus-occipitotemporal area, the dorsal lateral prefrontal-posterior cingulate gyrus, and the prefrontal-limbic system (75–83). Studies showed rs-FC changed in brain regions such as bilateral anterior cingulate gyrus, dorsomedial prefrontal cortex, bilateral superior frontal gyrus, left angular gyrus, left precuneus, bilateral hippocampus, right superior temporal gyrus, right insula, and cerebellum after ECT (84). Mo et al. (59) found FC increased significantly in the left angular gyrus, bilateral inferior temporal gyrus and bilateral middle frontal gyrus after ECT in depressed patients, accompanied by improvement in emotional symptoms. In FC study which used cingulate gyrus as a seed, found that the FC between left pgACC-left parahippocampal gyrus increased after ECT (82). Another study found that the FC of subcallosal cingulate cortex-bilateral hippocampus, subcallosal cingulate cortex-bilateral temporal poles and subcallosal cingulate cortex-ventral prefrontal cortex decreased after ECT (83). Some studies noted rs-FC of subcallosal cingulate cortex-amygdala and subcallosal cingulate cortex-fusiform gyrus changed after ECT. Leaver et al. (84) found that rs-FC of the left DLPFC-subcallosal cingulate cortex may predict the efficacy of ECT for depression.
Graph theory analysis is applied to complex network functions of human brain, which focuses on the relationship between nodes and edges. The application of graph theory in the FC analysis of the brain can be characterized by different metrics to demonstrate different aspects of connectivity. Such as (i) average path length; (ii) clustering coefficient; (iii) degree of node; (iv) centrality measures; (v) level of modularity, etc. The clustering coefficient reflects the local connectivity of the network. The node degree is the simplest metric to quantify the total number of connected nodes. A node with a higher degree indicates that it plays an important role in the information flow of a particular network. Path length is another graph theoretic metric that represents the global level of efficiency of the network. Thus, the shortest path length represents the minimum number of edges required to connect one node to another in the network. Degree centrality (DC) has been widely studied in depressed patients, some studies found that depressed patients with suicidal ideation had elevated DC in the inferior frontal gyrus, orbital part, the supplementary motor area compared with HCs (85, 86). Wagner et al. (87) found that reduced DC in the frontoparietal network can distinguish suicidal ideation and suicide attempts in depressed patients. A study on DC alterations after ECT, which focused on anhedonia in depressed patients, found that ECT significantly increased DC in bilateral dorsomedial prefrontal cortex, right DLPFC, and bilateral orbitofrontal cortex, and alterations of anhedonia were positively correlated with DC in the abovementioned regions (88).
Brain networks change in depressed patients are commonly observed, and a number of studies have also suggested that ECT may play a role by modulating brain networks in depressed patients. Wang et al. (89) observed the default mode network (DMN), dorsal attention network (DAN), executive control network (CON), salience network (SN), and sensory-motor network (SMN) and tried to explore the FC within each network and between networks, and found that within the network, the FC within CON of depressed patients increased after ECT, and between different networks, DMN-SN, CON-DMN, CON-DAN, and CON-SN increased after ECT. Pang et al. (90) also focused on changes of brain networks in depressed patients after ECT, and found FC within DMN, and FC of DMN-CEN significantly increased after ECT, and could predict the efficacy of depression.
Magnetic resonance spectroscopy (MRS) is used to identify abnormal metabolism by measuring changes in tissue concentrations of metabolites in humans and observing different peaks and ratios in the spectral profile. It is a non-invasive technique that measures specific functional areas of the brain and analyzes neuro-biochemicals. These compounds include γ-aminobutyric acid (GABA), glutamate (Glu), N-acetyl-L-aspartate (NAA), glutamine (Gln), creatine (Cr), etc.
Glu plays a key role in the pathophysiology of depression (91). There is evidence that the levels of Glu and Gln are reduced in pgACC (92, 93), whereas the levels of Glu in DLPFC are unchanged (94, 95). ECT induces changes in glutamatergic neurotransmitters that may be closely related to their antidepressant effects (96, 97). Njau et al. (98) found depressed patients treated with ECT showed that Glx (Glu and Gln) increased in the subgenual ACC (sgACC) but decreased in the left hippocampus, and these changes were correlated with clinical improvement. Some studies found elevated levels of Glx in the DLPFC and ACC after ECT (99, 100), but these results could not reproducible (96), Overall, the metabolism of Glu is an important component of ECT efficacy for depression, but the exact mechanism still needs further research. The reduction of GABA in cerebrospinal fluid and the frontal cortex has been frequently reported in patients with depression (101). Meanwhile, some studies have confirmed an increased GABA in serum levels and occipital lobe after ECT (102, 103). However, Knudsen et al. (104) measured changes of GABA in the prefrontal and occipital cortex pre/post-ECT and found compared with HCs, no significant differences were detected by GABA/Cr levels in the prefrontal or occipital lobes, while the patient group did not show significant differences in GABA, Glu and Gln after ECT, so it was speculated that GABA alterations should not be considered as a key factor of ECT for depression. NAA is a marker of neurons and axons, and it can reflect the number of neurons as well as their function. Proton MRS (H-MRS) showed that ECT could increase NAA levels in the ACC and amygdala, suggested that ECT may have a neuromodulation effect. Njau et al. (98) examined depressed patients who underwent ECT measured by 1H MRS and found decreased NAA in the left hippocampus of the patient group compared with HCs. At the same time, reduction of NAA in the dorsal ACC (dACC) and right hippocampus were found after ECT. Overall, the antidepressant effects of ECT may involve different neuro-transporter systems, and the mechanisms of neuro-transporter alterations between them are not fully understood, which is one of the reasons for the inconsistent findings.
Positron emission tomography (PET) and Single photon emission computed tomography (SPECT) monitor local cerebral blood flow (CBF) by injecting radionuclides into the body and then imaging γ-rays emitted from the body. Studies on CBF with ECT are common, but the findings are inconsistent. Mervaala et al. (105) found ECT could reduce CBF of temporal lobe and bilateral parietal lobe by SPECT, while Milo et al. (106) suggested that increased CBF in frontal lobe was significant of ECT for antidepressant effects. However, Schmidt et al. (107) found ECT decreased brain metabolism in the anterior and posterior frontal lobes assessed by PET. Suwa et al. (108) also found that ECT could reduce brain metabolism in the frontal and lateral temporal lobes, and the reduced brain metabolism was correlated with the antidepressant effect of ECT (109). On the other hand, previous studies on PET probing neurotransmitter changes after ECT, Masuoka et al. (110) used [18F] FE-PE2I PET to detect neurotransmitter levels in depressed patients before, during and after ECT and found that all patients showed reduced dopamine transporters in the striatum, and the dopamine system has been shown to be one of the mechanisms of ECT. Tiger et al. (111) used PET and [11C] raclopride to detect neurotransmitter levels in patients with depression before and after ECT, as well as HCs, and found that depressed patients had decreased [11C] raclopride binding in all three striatal regions compared to HCs. Yatham et al. (112) used [18F] setoperone PET and found that depressed patients treated with ECT showed significant reduced 5-HT2 receptors in all cortical regions of the brain and the decreased 5-HT2 receptors in the right parahippocampal gyrus, right lingual gyrus and right medial superior frontal gyrus were correlated with improvements in depressive symptoms, this result is also consistent with previous studies on antidepressant drugs (113–115).
PET is also widely used to assess ECT-induced changes of [18F]-fluorodeoxyglucose (FDG). One of the most consistent findings was decreased glucose metabolism in the bilateral medial and inferior frontal regions, and increased glucose metabolism in the hippocampus, middle temporal lobe, left occipital lobe, and parietal lobe (116). Bak et al. (117) used [18F]-FDG PET to study the efficacy of ECT in a 55 years-old woman with late-onset depression and found that PET/CT images of the patient’s brain showed decreased brain metabolism, whereas after ECT, the PET imaging returned to normal brain metabolism. Hassama et al. (118) found decreased metabolic in the frontal, parietal and temporal cortices before ECT assessed by 18F-FDG-PET/CT, and after 8 ECT sessions, hypometabolism revealed significant improvements in the left parietal cortex, the left temporal/occipital cortex, and bilateral frontal lobe. These improvements in brain glucose hypometabolism levels may represent a neurobiological mechanism of ECT for psychiatric disorders. However, inconsistent results were also reported by Reininghaus et al. (119). They used FDG-PET to assess changes of brain glucose metabolism before and after ECT in patients with depression and found the patients did not have significant changes of glucose metabolism levels before and after ECT; therefore, they did not believe that FDG-PET could assess changes in brain function after ECT.
In recent years, the development of magnetic arterial spin-labeled perfusion fMRI (ASL-fMRI) has provided a new method to study CBF. Like PET and SPECT, ASL-fMRI can explore CBF, and without radiation. Now ASL-fMRI has been widely used to study the neural mechanisms of depression, but there are few ASL-fMRI studies on ECT. Shi et al. (120) focused on ASL differences in depressed patients by frontotemporal ECT and found significantly reduced rCBF in the bilateral frontal lobes before ECT, and after ECT, decreased rCBF was found in the left amygdala, parahippocampal gyrus, olfactory cortex, right occipital lobe, while increased rCBF was found in the bilateral frontal lobe. More studies using ASL-fMRI mainly focused on alterations in the hippocampus. Recently, Leaver et al. (121) tried to predict the clinical outcome of ECT measured by ASL, and found lower whole-brain CBF levels in the patient group at baseline predicted a better ECT outcome, while after acute treatment of ECT and 4 weeks follow-up, it showed elevated rCBF in the right anterior hippocampus, regardless of clinical outcome. On the other hand, this study found an elevated CBF in the dorsal thalamus as well as in the motor cortex and a decreased CBF in the frontotemporal areas in patients with a clinical response to ECT. Another study focused on the difference in cerebral perfusion in the hippocampus between responding group and non-responding group after ECT assessed by ASL and found that patients who did not respond to ECT showed increased CBF in the bilaterally anterior hippocampus, while patients who responded to ECT showed increased CBF in the bilateral posterior part of hippocampus (122). However, in a recent study, Bracht et al. (123) also focused on the CBF changed in the hippocampus, and found CBF increase in the hippocampus were observed in the ECT-group but not in treatment response group, showing that CBF in the hippocampus was not associated with antidepressant response.
The development of brain imaging provide an important approach to the study of depression and ECT, Table 1 gives a summary of relatively consistent findings. However, there are still some limitations, and also should consider the directions for future research, mainly in the following aspects: (1) there are many choices of statistical methods, together with the different subjects, different MRI equipment parameters, resulted in a large number of inconsistent findings, so future research with more samples in multi-center is needed; (2) there are more studies focusing on the efficacy of ECT, but fewer studies on its side effects, such as cognitive impairment or delirium after ECT, and this is needed to be further investigated; (3) the efficacy of ECT may be related to multiple mechanisms, but there are few animal MRI studies on ECT, and it is difficult to study the mechanism, so in the future, more animal studies on ECT is needed.
XC: investigation, methodology, writing—original draft, and writing—review and editing. HY: conceptualization, investigation, methodology, supervision, and writing—review and editing. L-BC: conceptualization, investigation, methodology, project administration, supervision, and writing—review and editing. XL: conceptualization, investigation, methodology, project administration, resources, supervision, writing—original draft, and writing—review and editing. All authors contributed to the article and approved the submitted version.
The authors declare that the research was conducted in the absence of any commercial or financial relationships that could be construed as a potential conflict of interest.
All claims expressed in this article are solely those of the authors and do not necessarily represent those of their affiliated organizations, or those of the publisher, the editors and the reviewers. Any product that may be evaluated in this article, or claim that may be made by its manufacturer, is not guaranteed or endorsed by the publisher.
1. Kupfer, DJ, Frank, E, and Phillips, ML. Major depressive disorder: new clinical, neurobiological, and treatment perspectives. Lancet. (2012) 379:1045–55. doi: 10.1016/S0140-6736(11)60602-8
2. World Health Organization. Depression [OB/OL]. (2019). Available at: https://www.who.int/mental_health/management/depression/en/. (Accessed April 07, 2017) (Retrieved June 06, 2019).
3. Gazdag, G, and Ungvari, GS. Electroconvulsive therapy: 80 years old and still going strong. World J Psychiatry. (2019) 9:1–6. doi: 10.5498/wjp.v9.i1.1
4. Sackeim, HA. Modern electroconvulsive therapy: vastly improved yet greatly underused. JAMA Psychiatry. (2017) 74:779–80. doi: 10.1001/jamapsychiatry.2017.1670
5. Payne, NA, and Prudic, J. Electroconvulsive therapy: part I. a perspective on the evolution and current practice of ECT. J Psychiatr Pract. (2009) 15:346–68. doi: 10.1097/01.pra.0000361277.65468.ef
6. Husain, MM, Rush, AJ, Fink, M, Knapp, R, Petrides, G, Rummans, T, et al. Speed of response and remission in major depressive disorder with acute electroconvulsive therapy (ECT): a consortium for research in ECT (CORE) report. J Clin Psychiatry. (2004) 65:485–91. doi: 10.4088/JCP.v65n0406
7. Rush, AJ, Trivedi, MH, Wisniewski, SR, Nierenberg, AA, Stewart, JW, Warden, D, et al. Acute and longer-term outcomes in depressed outpatients requiring one or several treatment steps: a STAR*D report. Am J Psychiatry. (2006) 163:1905–17. doi: 10.1176/ajp.2006.163.11.1905
8. Slade, EP, Jahn, DR, Regenold, WT, and Case, BG. Association of electroconvulsive therapy with psychiatric readmissions in US hospitals. JAMA Psychiatry. (2017) 74:798–804. doi: 10.1001/jamapsychiatry.2017.1378
9. Olfson, M, Marcus, S, Sackeim, HA, Thompson, J, and Pincus, HA. Use of ECT for the inpatient treatment of recurrent major depression. Am J Psychiatry. (1998) 155:22–9. doi: 10.1176/ajp.155.1.22
10. Fink, M, Kellner, CH, and McCall, WV. The role of ECT in suicide prevention. J ECT. (2014) 30:5–9. doi: 10.1097/YCT.0b013e3182a6ad0d
11. Semkovska, M, and McLoughlin, DM. Objective cognitive performance associated with electroconvulsive therapy for depression: a systematic review and meta-analysis. Biol Psychiatry. (2010) 68:568–77. doi: 10.1016/j.biopsych.2010.06.009
12. Squire, LR, and Slater, PC. Electroconvulsive therapy and complaints of memory dysfunction: a prospective three-year follow-up study. Br J Psychiatry. (1983) 142:1–8. doi: 10.1192/bjp.142.1.1
13. Li, H, Zhang, H, Yin, L, Zhang, F, Chen, Z, Chen, T, et al. Altered cortical morphology in major depression disorder patients with suicidality. Psychoradiology. (2021) 1:13–22. doi: 10.1093/psyrad/kkaa002
14. Zhang, L, Hu, X, Hu, Y, Tang, M, Qiu, H, Zhu, Z, et al. Structural covariance network of the hippocampus-amygdala complex in medication-naïve patients with first-episode major depressive disorder. Psychoradiology. (2022) 2:190–8. doi: 10.1093/psyrad/kkac023
15. Nordanskog, P, Dahlstrand, U, Larsson, MR, Larsson, EM, Knutsson, L, and Johanson, A. Increase in hippocampal volume after electroconvulsive therapy in patients with depression: a volumetric magnetic resonance imaging study. J ECT. (2010) 26:62–7. doi: 10.1097/YCT.0b013e3181a95da8
16. Bouckaert, F, de Winter, FL, Emsell, L, Dols, A, Rhebergen, D, Wampers, M, et al. Grey matter volume increase following electroconvulsive therapy in patients with late life depression: a longitudinal MRI study. J Psychiatry Neurosci. (2016) 41:105–14. doi: 10.1503/jpn.140322
17. Tendolkar, I, van Beek, M, van Oostrom, I, Mulder, M, Janzing, J, Voshaar, RO, et al. Electroconvulsive therapy increases hippocampal and amygdala volume in therapy refractory depression: a longitudinal pilot study. Psychiatry Res. (2013) 214:197–203. doi: 10.1016/j.pscychresns.2013.09.004
18. Nordanskog, P, Larsson, MR, Larsson, EM, and Johanson, A. Hippocampal volume in relation to clinical and cognitive outcome after electroconvulsive therapy in depression. Acta Psychiatr Scand. (2014) 129:303–11. doi: 10.1111/acps.12150
19. Gyger, L, Ramponi, C, Mall, JF, Swierkosz-Lenart, K, Stoyanov, D, Lutti, A, et al. Temporal trajectory of brain tissue property changes induced by electroconvulsive therapy. NeuroImage. (2021) 232:117895. doi: 10.1016/j.neuroimage.2021.117895
20. Enneking, V, Dzvonyar, F, Dück, K, Dohm, K, Grotegerd, D, Förster, K, et al. Brain functional effects of electroconvulsive therapy during emotional processing in major depressive disorder. Brain Stimul. (2020) 13:1051–8. doi: 10.1016/j.brs.2020.03.018
21. Gryglewski, G, Baldinger-Melich, P, Seiger, R, Godbersen, GM, Michenthaler, P, Klöbl, M, et al. Structural changes in amygdala nuclei, hippocampal subfields and cortical thickness following electroconvulsive therapy in treatmentresistant depression: longitudinal analysis. Br J Psychiatry. (2019) 214:159–67. doi: 10.1192/bjp.2018.224
22. Cao, B, Luo, Q, Fu, Y, du, L, Qiu, T, Yang, X, et al. Predicting individual responses to the electroconvulsive therapy with hippocampal subfield volumes in major depression disorder. Sci Rep. (2018) 8:5434. doi: 10.1038/s41598-018-23685-9
23. Jorgensen, A, Magnusson, P, Hanson, LG, Kirkegaard, T, Benveniste, H, Lee, H, et al. Regional brain volumes, diffusivity, and metabolite changes after electroconvulsive therapy for severe depression. Acta Psychiatr Scand. (2016) 133:154–64. doi: 10.1111/acps.12462
24. Ota, M, Noda, T, Sato, N, Okazaki, M, Ishikawa, M, Hattori, K, et al. Effect of electroconvulsive therapy on gray matter volume in major depressive disorder. J Affect Disord. (2015) 186:186–91. doi: 10.1016/j.jad.2015.06.051
25. Sartorius, A, Demirakca, T, Böhringer, A, Clemm von Hohenberg, C, Aksay, SS, Bumb, JM, et al. Electroconvulsive therapy increases temporal gray matter volume and cortical thickness. Eur Neuropsychopharmacol. (2016) 26:506–17. doi: 10.1016/j.euroneuro.2015.12.036
26. Dukart, J, Regen, F, Kherif, F, Colla, M, Bajbouj, M, Heuser, I, et al. Electroconvulsive therapy-induced brain plasticity determines therapeutic outcome in mood disorders. Proc Natl Acad Sci U S A. (2014) 111:1156–61. doi: 10.1073/pnas.1321399111
27. Arnone, D, Job, D, Selvaraj, S, Abe, O, Amico, F, Cheng, Y, et al. Computational meta-analysis of statistical parametric maps in major depression. Hum Brain Mapp. (2016) 37:1393–404. doi: 10.1002/hbm.23108
28. Boccia, M, Acierno, M, and Piccardi, L. Neuroanatomy of Alzheimer’s disease and late life depression: a coordinate-based meta-analysis of MRI studies. J Alzheimers Dis. (2015) 46:963–70. doi: 10.3233/JAD-142955
29. Peng, W, Chen, ZQ, Yin, L, Jia, Z, and Gong, Q. Essential brain structural alterations in major depressive disorder: a voxel-wise meta-analysis on first episode, medication-naïve patients. J Affect Disord. (2016) 199:114–23. doi: 10.1016/j.jad.2016.04.001
30. Abbott, CC, Jones, T, Lemke, NT, Gallegos, P, McClintock, SM, Mayer, AR, et al. Hippocampal structural and functional changes associated with electroconvulsive therapy response. Transl Psychiatry. (2014) 4:e483. doi: 10.1038/tp.2014.124
31. Gbyl, K, and Videbech, P. Electroconvulsive therapy increases brain volume in major depression: a systematic review and meta-analysis. Acta Psychiatr Scand. (2018) 138:180–95. doi: 10.1111/acps.12884
32. Argyelan, M, Lencz, T, Kang, S, Ali, S, Masi, PJ, Moyett, E, et al. ECT-induced cognitive side effects are associated with hippocampal enlargement. Transl Psychiatry. (2021) 11:516. doi: 10.1038/s41398-021-01641-y
33. van der A, J, de Jager, JE, van Dellen, E, Mandl, RCW, Somers, M, Boks, MPM, et al. Changes in perfusion, and structure of hippocampal subfields related to cognitive impairment after ECT: a pilot study using ultra high field MRI. J Affect Disord. (2023) 325:321–8. doi: 10.1016/j.jad.2023.01.016
34. Gbyl, K, Støttrup, MM, Mitta Raghava, J, Xue Jie, S, and Videbech, P. Hippocampal volume and memory impairment after electroconvulsive therapy in patients with depression. Acta Psychiatr Scand. (2021) 143:238–52. doi: 10.1111/acps.13259
35. Hellsten, J, Wennström, M, Bengzon, J, Mohapel, P, and Tingström, A. Electroconvulsive seizures induce endothelial cell proliferation in adult rat hippocampus. Biol Psychiatry. (2004) 55:420–7. doi: 10.1016/j.biopsych.2003.08.013
36. Jansson, L, Wennström, M, Johanson, A, and Tingström, A. Glial cell activation in response to electroconvulsive seizures. Prog Neuro-Psychopharmacol Biol Psychiatry. (2009) 33:1119–28. doi: 10.1016/j.pnpbp.2009.06.007
37. Kunigiri, G, Jayakumar, PN, Janakiramaiah, N, and Gangadhar, BN. MRI T2 relaxometry of brain regions and cognitive dysfunction following electroconvulsive therapy. Indian J Psychiatry. (2007) 49:195–9. doi: 10.4103/0019-5545.37321
38. Perera, TD, Coplan, JD, Lisanby, SH, Lipira, CM, Arif, M, Carpio, C, et al. Antidepressant-induced neurogenesis in the hippocampus of adult nonhuman primates. J Neurosci. (2007) 27:4894–901. doi: 10.1523/JNEUROSCI.0237-07.2007
39. Okada-Tsuchioka, M, Segawa, M, Kajitani, N, Hisaoka-Nakashima, K, Shibasaki, C, Morinobu, S, et al. Electroconvulsive seizure induces thrombospondin-1 in the adult rat hippocampus. Prog Neuro-Psychopharmacol Biol Psychiatry. (2014) 48:236–44. doi: 10.1016/j.pnpbp.2013.10.001
40. Wennström, M, Hellsten, J, and Tingström, A. Electroconvulsive seizures induce proliferation of NG2-expressing glial cells in adult rat amygdala. Biol Psychiatry. (2004) 55:464–71. doi: 10.1016/j.biopsych.2003.11.011
41. Hanford, LC, Nazarov, A, and Hall, GB. Cortical thickness in bipolar disorder: a systematic review. Bipolar Disord. (2016) 18:4–18. doi: 10.1111/bdi.12362
42. Reynolds, S, Carrey, N, Jaworska, N, Langevin, LM, Yang, XR, and MacMaster, FP. Cortical thickness in youth with major depressive disorder. BMC Psychiatry. (2014) 14:83. doi: 10.1186/1471-244X-14-83
43. Pirnia, T, Joshi, SH, Leaver, AM, Vasavada, M, Njau, S, Woods, RP, et al. Electroconvulsive therapy and structural neuroplasticity in neocortical, limbic and paralimbic cortex. Transl Psychiatry. (2016) 6:e832. doi: 10.1038/tp.2016.102
44. van Eijndhoven, P, Mulders, P, Kwekkeboom, L, van Oostrom, I, van Beek, M, Janzing, J, et al. Bilateral ECT induces bilateral increases in regional cortical thickness. Transl Psychiatry. (2016) 6:e874. doi: 10.1038/tp.2016.139
45. Gbyl, K, Rostrup, E, Raghava, JM, Carlsen, JF, Schmidt, LS, Lindberg, U, et al. Cortical thickness following electroconvulsive therapy in patients with depression: a longitudinal MRI study. Acta Psychiatr Scand. (2019) 140:205–16. doi: 10.1111/acps.13068
46. Wade, BSC, Sui, J, Hellemann, G, Leaver, AM, Espinoza, RT, Woods, RP, et al. Inter and intra-hemispheric structural imaging markers predict depression relapse after electroconvulsive therapy: a multisite study. Transl Psychiatry. (2017) 7:1270. doi: 10.1038/s41398-017-0020-7
47. Koshiyama, D, Fukunaga, M, Okada, N, Morita, K, Nemoto, K, Usui, K, et al. White matter microstructural alterations across four major psychiatric disorders: mega-analysis study in 2937 individuals. Mol Psychiatry. (2020) 25:883–95. doi: 10.1038/s41380-019-0553-7
48. Chen, G, Guo, Y, Zhu, H, Kuang, W, Bi, F, Ai, H, et al. Intrinsic disruption of white matter microarchitecture in first-episode, drug-naive major depressive disorder: a voxel-based meta-analysis of diffusion tensor imaging. Prog Neuro-Psychopharmacol Biol Psychiatry. (2017) 76:179–87. doi: 10.1016/j.pnpbp.2017.03.011
49. Yrondi, A, Nemmi, F, Billoux, S, Giron, A, Sporer, M, Taib, S, et al. Significant decrease in hippocampus and amygdala mean diffusivity in treatment-resistant depression patients who respond to electroconvulsive therapy. Front Psychiatry. (2019) 10:694. doi: 10.3389/fpsyt.2019.00694
50. Gryglewski, G, Seiger, R, Baldinger-Melich, P, Unterholzner, J, Spurny, B, Vanicek, T, et al. Changes in white matter microstructure after electroconvulsive therapy for treatment-resistant depression. Int J Neuropsychopharmacol. (2020) 23:20–5. doi: 10.1093/ijnp/pyz059
51. Repple, J, Meinert, S, Bollettini, I, Grotegerd, D, Redlich, R, Zaremba, D, et al. Influence of electroconvulsive therapy on white matter structure in a diffusion tensor imaging study. Psychol Med. (2020) 50:849–56. doi: 10.1017/S0033291719000758
52. Kubicki, A, Leaver, AM, Vasavada, M, Njau, S, Wade, B, Joshi, SH, et al. Variations in hippocampal white matter diffusivity differentiate response to electroconvulsive therapy in major depression. Biol Psychiatry Cogn Neurosci Neuroimaging. (2019) 4:300–9. doi: 10.1016/j.bpsc.2018.11.003
53. Lyden, H, Espinoza, RT, Pirnia, T, Clark, K, Joshi, SH, Leaver, AM, et al. Electroconvulsive therapy mediates neuroplasticity of white matter microstructure in major depression. Transl Psychiatry. (2014) 4:e380. doi: 10.1038/tp.2014.21
54. Zang, Y, Jiang, T, Lu, Y, He, Y, and Tian, L. Regional homogeneity approach to fMRI data analysis. NeuroImage. (2004) 22:394–400. doi: 10.1016/j.neuroimage.2003.12.030
55. Biswal, B, Zerrin Yetkin, F, Haughton, VM, and Hyde, JS. Functional connectivity in the motor cortex of resting human brain using echo-planar MRI. Magn Reson Med. (1995) 34:537–41. doi: 10.1002/mrm.1910340409
56. Mandeville, JB, and Marota, JJ. Vascular filters of functional MRI: spatial localization using BOLD and CBV contrast. Magn Reson Med. (1999) 42:591–8. doi: 10.1002/(SICI)1522-2594(199909)42:3<591::AID-MRM23>3.0.CO;2-8
57. Li, GZ, Rossbach, K, Zhang, AX, Liu, P, and Zhang, K. Resting-state functional changes in the precuneus within first-episode drug-naive patients with MDD. Neuropsychiatr Dis Treat. (2018) 14:1991–8. doi: 10.2147/NDT.S168060
58. Kong, XM, Xu, SX, Sun, Y, Wang, KY, Wang, C, Zhang, J, et al. Electroconvulsive therapy changes the regional resting state function measured by regional homogeneity (ReHo) and amplitude of low frequency fluctuations (ALFF) in elderly major depressive disorder patients: an exploratory study. Psychiatry Res Neuroimaging. (2017) 264:13–21. doi: 10.1016/j.pscychresns.2017.04.001
59. Mo, YT, Wei, Q, Bai, TJ, Zhang, T, Lv, H, Zhang, L, et al. Bifrontal electroconvulsive therapy changed regional homogeneity and functional connectivity of left angular gyrus in major depressive disorder. Psychiatry Res. (2020) 294:113461. doi: 10.1016/j.psychres.2020.113461
60. Qiu, H, Li, X, Zhao, W, du, L, Huang, P, Fu, Y, et al. Electroconvulsive therapy-induced brain structural and functional changes in major depressive disorders: a longitudinal study. Med Sci Monit. (2016) 22:4577–86. doi: 10.12659/MSM.898081
61. Argyelan, M, Lencz, T, Kaliora, S, Sarpal, DK, Weissman, N, Kingsley, PB, et al. Subgenual cingulate cortical activity predicts the efficacy of electroconvulsive therapy. Transl Psychiatry. (2016) 6:e789. doi: 10.1038/tp.2016.54
62. Shen, Y, Yao, J, Jiang, X, Zhang, L, Xu, L, Feng, R, et al. Sub-hubs of baseline functional brain networks are related to early improvement following two-week pharmacological therapy for major depressive disorder. Hum Brain Mapp. (2015) 36:2915–27. doi: 10.1002/hbm.22817
63. Hueston, CM, Cryan, JF, and Nolan, YM. Stress and adolescent hippocampal neurogenesis: diet and exercise as cognitive modulators. Transl Psychiatry. (2017) 7:e1081. doi: 10.1038/tp.2017.48
64. Redlich, R, Opel, N, Grotegerd, D, Dohm, K, Zaremba, D, Bürger, C, et al. Prediction of individual response to electroconvulsive therapy via machine learning on structural magnetic resonance imaging data. JAMA Psychiatry. (2016) 73:557–64. doi: 10.1001/jamapsychiatry.2016.0316
65. Liu, Y, Du, L, Li, YM, Liu, H, Zhao, W, Liu, D, et al. First-episode depression resting state of brain function low frequency amplitude research. Medicine. (2015) 94:e2033. doi: 10.1097/MD.0000000000002033
66. Jacobs, RH, Barba, A, Gowins, JR, Klumpp, H, Jenkins, LM, Mickey, BJ, et al. Resting-state functional connectivity of subgenual anterior cingulate cortex in depressed adolescents. Psychol Med. (2016) 46:1055–67. doi: 10.1017/S0033291715002615
67. Wang, M, Ju, YM, Lu, XW, Sun, J, Dong, Q, Liu, J, et al. Longitudinal changes of amplitude of low-frequency fluctuations in MDD patients: a 6-month follow-up resting-state functional magnetic resonance imaging study. J Affect Disord. (2020) 276:411–7. doi: 10.1016/j.jad.2020.07.067
68. Wu, ZY, Luo, QY, Wu, HW, Wu, Z, Zheng, Y, Yang, Y, et al. Amplitude of low-frequency oscillations in major depressive disorder with childhood trauma. Front Psychiatry. (2021) 11:596337. doi: 10.3389/fpsyt.2020.596337
69. du, L, Qiu, HT, Liu, HX, Zhao, W, Tang, Y, Fu, Y, et al. Changes in problem-solving capacity and association with spontaneous brain activity after a single electroconvulsive treatment in major depressive disorder. J ECT. (2016) 32:49–54. doi: 10.1097/YCT.0000000000000269
70. Bai, T, Wei, Q, Zu, M, Xie, W, Wang, J, Gong-Jun, J, et al. Functional plasticity of the dorsomedial prefrontal cortex in depression reorganized by electroconvulsive therapy: validation in two independent samples. Hum Brain Mapp. (2019) 40:465–73. doi: 10.1002/hbm.24387
71. Fransson, P. Spontaneous low-frequency BOLD signal fluctuations: an fMRI investigation of the resting-state default mode of brain function hypothesis. Hum Brain Mapp. (2005) 26:15–29. doi: 10.1002/hbm.20113
72. Fox, MD, and Greicius, M. Clinical applications of resting state functional connectivity. Front Syst Neurosci. (2010) 4:19. doi: 10.3389/fnsys.2010.00019
73. Lui, S, Wu, QZ, Qiu, LH, Yang, X, Kuang, W, Chan, RCK, et al. Resting-state functional connectivity in treatment-resistant depression. Am J Psychiatry. (2011) 168:642–8. doi: 10.1176/appi.ajp.2010.10101419
74. Wang, Z, Yuan, YG, Bai, F, Shu, H, You, J, Li, L, et al. Altered functional connectivity networks of hippocampal subregions in remitted late-onset depression: a longitudinal resting-state study. Neurosci Bull. (2015) 31:13–21. doi: 10.1007/s12264-014-1489-1
75. Ambrosi, E, Arciniegas, DB, Madan, A, Curtis, KN, Patriquin, MA, Jorge, RE, et al. Insula and amygdala resting-state functional connectivity differentiate bipolar from unipolar depression. Acta Psychiatr Scand. (2017) 136:129–39. doi: 10.1111/acps.12724
76. Cheng, W, Rolls, ET, Qiu, J, Xie, X, Lyu, W, Li, Y, et al. Functional connectivity of the human amygdala in health and in depression. Soc Cogn Affect Neurosci. (2018) 13:557–68. doi: 10.1093/scan/nsy032
77. Cullen, KR, Westlund, MK, Klimes-Dougan, B, Mueller, BA, Houri, A, Eberly, LE, et al. Abnormal amygdala resting-state functional connectivity in adolescent depression. JAMA Psychiatry. (2014) 71:1138–47. doi: 10.1001/jamapsychiatry.2014.1087
78. Greicius, MD, Flores, BH, Menon, V, Glover, GH, Solvason, HB, Kenna, H, et al. Resting-state functional connectivity in major depression: abnormally increased contributions from subgenual cingulate cortex and thalamus. Biol Psychiatry. (2007) 62:429–37. doi: 10.1016/j.biopsych.2006.09.020
79. Peng, DH, Shen, T, Zhang, J, Huang, J, Liu, J, Liu, SY, et al. Abnormal functional connectivity with mood regulating circuit in unmedicated individual with major depression: a resting-state functional magnetic resonance study. Chin Med J. (2012) 125:3701–6. doi: 10.3760/cma.j.issn.0366-6999.2012.20.019
80. Cullen, KR, Gee, DG, Klimes-Dougan, B, Gabbay, V, Hulvershorn, L, Mueller, BA, et al. A preliminary study of functional connectivity in comorbid adolescent depression. Neurosci Lett. (2009) 460:227–31. doi: 10.1016/j.neulet.2009.05.022
81. Horn, DI, Yu, CS, Steiner, J, Buchmann, J, Kaufmann, J, Osoba, A, et al. Glutamatergic and resting-state functional connectivity correlates of severity in major depression—the role of pregenual anterior cingulate cortex and anterior insula. Front Syst Neurosci. (2010) 15:33. doi: 10.3389/fnsys.2010.00033
82. Cano, M, Cardoner, N, Urretavizcaya, M, Martínez-Zalacaín, I, Goldberg, X, Via, E, et al. Modulation of limbic and prefrontal connectivity by electroconvulsive therapy in treatment-resistant depression: a preliminary study. Brain Stimul. (2016) 9:65–71. doi: 10.1016/j.brs.2015.08.016
83. Sinha, P, Joshi, H, and Ithal, D. Resting state functional connectivity of brain with electroconvulsive therapy in depression: meta-analysis to understand its mechanisms. Front Hum Neurosci. (2020) 14:616054. doi: 10.3389/fnhum.2020.616054
84. Leaver, AM, Wade, B, Vasavada, M, Hellemann, G, Joshi, SH, Espinoza, R, et al. Fronto-temporal connectivity predicts ECT outcome in major depression. Front Psychiatry. (2018) 9:92. doi: 10.3389/fpsyt.2018.00092
85. Gao, CH, Wenhua, L, Liu, YL, Ruan, X, Chen, X, Liu, L, et al. Decreased subcortical and increased cortical degree centrality in a nonclinical college student sample with subclinical depressive symptoms: a resting-state fMRI study. Front Hum Neurosci. (2016) 10:617. doi: 10.3389/fnhum.2016.00617
86. Guo, WB, Liu, F, Xue, ZM, Xu, XJ, Wu, RR, Ma, CQ, et al. Alterations of the amplitude of low-frequency fluctuations in treatment-resistant and treatment-response depression: a resting-state fMRI study. Prog Neuro-Psychopharmacol Biol Psychiatry. (2012) 37:153–60. doi: 10.1016/j.pnpbp.2012.01.011
87. Wagner, G, Li, M, Sacchet, MD, Richard-Devantoy, S, Turecki, G, Bär, KJ, et al. Functional network alterations differently associated with suicidal ideas and acts in depressed patients: an indirect support to the transition model. Transl Psychiatry. (2021) 11:100. doi: 10.1038/s41398-021-01232-x
88. Zhang, T, He, KL, Bai, TJ, Lv, H, Xie, X, Nie, J, et al. Altered neural activity in the reward-related circuit and executive control network associated with amelioration of anhedonia in major depressive disorder by electroconvulsive therapy. Prog Neuro-Psychopharmacol Biol Psychiatry. (2021) 109:110193. doi: 10.1016/j.pnpbp.2020.110193
89. Wang, J, Wei, Q, Wang, L, Zhang, H, Bai, T, Cheng, L, et al. Functional reorganization of intra-and internetwork connectivity in major depressive disorder after electroconvulsive therapy. Hum Brain Mapp. (2018) 39:1403–11. doi: 10.1002/hbm.23928
90. Pang, Y, Wei, Q, Zhao, S, Li, N, Li, Z, Lu, F, et al. Enhanced default mode network functional connectivity links with electroconvulsive therapy response in major depressive disorder. J Affect Disord. (2022) 306:47–54. doi: 10.1016/j.jad.2022.03.035
91. Sanacora, G, Treccani, G, and Popoli, M. Towards a glutamate hypothesis of depression: an emerging frontier of neuropsychopharmacology for mood disorders. Neuropharmacology. (2012) 62:63–77. doi: 10.1016/j.neuropharm.2011.07.036
92. Walter, M, Henning, A, Grimm, S, Schulte, RF, Beck, J, Dydak, U, et al. The relationship between aberrant neuronal activation in the pregenual anterior cingulate, altered glutamatergic metabolism, and anhedonia in major depression. Arch Gen Psychiatry. (2009) 66:478–86. doi: 10.1001/archgenpsychiatry.2009.39
93. Arnone, D, Mumuni, AN, Jauhar, S, Condon, B, and Cavanagh, J. Indirect evidence of selective glial involvement in glutamate-based mechanisms of mood regulation in depression: meta-analysis of absolute prefrontal neuro-metabolic concentrations. Eur Neuropsychopharmacol. (2015) 25:1109–17. doi: 10.1016/j.euroneuro.2015.04.016
94. Grimm, S, Ernst, J, Boesiger, P, Schuepbach, D, Boeker, H, and Northoff, G. Reduced negative BOLD responses in the default-mode network and increased self-focus in depression. World J Biol Psychiatry. (2011) 12:627–37. doi: 10.3109/15622975.2010.545145
95. Merkl, A, Schubert, F, Quante, A, Luborzewski, A, Brakemeier, EL, Grimm, S, et al. Abnormal cingulate and prefrontal cortical neurochemistry in major depression after electroconvulsive therapy. Biol Psychiatry. (2011) 69:772–9. doi: 10.1016/j.biopsych.2010.08.009
96. Pfleiderer, B, Michael, N, Erfurth, A, Ohrmann, P, Hohmann, U, Wolgast, M, et al. Effective electroconvulsive therapy reverses glutamate/glutamine deficit in the left anterior cingulum of unipolar depressed patients. Psychiatry Res. (2003) 122:185–92. doi: 10.1016/S0925-4927(03)00003-9
97. Baldinger, P, Lotan, A, Frey, R, Kasper, S, Lerer, B, and Lanzenberger, R. Neurotransmitters and electroconvulsive therapy. J ECT. (2014) 30:116–21. doi: 10.1097/YCT.0000000000000138
98. Njau, S, Joshi, SH, Espinoza, R, Leaver, AM, Vasavada, M, Marquina, A, et al. Neurochemical correlates of rapid treatment response to electroconvulsive therapy in patients with major depression. J Psychiatry Neurosci. (2017) 42:6–16. doi: 10.1503/jpn.150177
99. Michael, N, Erfurth, A, Ohrmann, P, Arolt, V, Heindel, W, and Pfleiderer, B. Metabolic changes within the left dorsolateral prefrontal cortex occurring with electroconvulsive therapy in patients with treatment resistant unipolar depression. Psychol Med. (2003) 33:1277–84. doi: 10.1017/S0033291703007931
100. Kobayashi, K, Imoto, Y, Yamamoto, F, Kawasaki, M, Ueno, M, Segi-Nishida, E, et al. Rapid and lasting enhancement of dopaminergic modulation at the hippocampal mossy fiber synapse by electroconvulsive treatment. J Neurophysiol. (2017) 117:284–9. doi: 10.1152/jn.00740.2016
101. Lloyd, KG, Morselli, PL, and Bartholini, G. GABA and affective disorders. Med Biol. (1987) 65:159–65.
102. Esel, E, Kose, K, Hacimusalar, Y, Ozsoy, S, Kula, M, Candan, Z, et al. The effects of electroconvulsive therapy on GABAergic function in major depressive patients. J ECT. (2008) 24:224–8. doi: 10.1097/YCT.0b013e31815cbaa1
103. Sanacora, G, Mason, GF, Rothman, DL, Hyder, F, Ciarcia, JJ, Ostroff, RB, et al. Increased cortical GABA concentrations in depressed patients receiving ECT. Am J Psychiatry. (2003) 160:577–9. doi: 10.1176/appi.ajp.160.3.577
104. Knudsen, MK, Near, J, Blicher, AB, Videbech, P, and Blicher, JU. Magnetic resonance (MR) spectroscopic measurement of γ-aminobutyric acid (GABA) in major depression before and after electroconvulsive therapy. Acta Neuropsychiatr. (2019) 31:17–26. doi: 10.1017/neu.2018.22
105. Mervaala, E, Könönen, M, Föhr, J, Husso-Saastamoinen, M, Valkonen-Korhonen, M, Kuikka, JT, et al. SPECT and neuropsychological performance in severe depression treated with ECT. J Affect Disord. (2001) 66:47–58. doi: 10.1016/S0165-0327(00)00288-3
106. Milo, TJ, Kaufman, GE, Barnes, WE, Konopka, LM, Crayton, JW, Ringelstein, JG, et al. Changes in regional cerebral blood flow after electroconvulsive therapy for depression. J ECT. (2001) 17:15–21. doi: 10.1097/00124509-200103000-00004
107. Schmidt, EZ, Reininghaus, B, Enzinger, C, Ebner, C, Hofmann, P, and Kapfhammer, HP. Changes in brain metabolism after ECT–positron emission tomography in the assessment of changes in glucose metabolism subsequent to electroconvulsive therapy—lessons, limitations and future applications. J Affect Disord. (2008) 106:203–8. doi: 10.1016/j.jad.2007.06.009
108. Suwa, T, Namiki, C, Takaya, S, Oshita, A, Ishizu, K, Fukuyama, H, et al. Corticolimbic balance shift of regional glucose metabolism in depressed patients treated with ECT. J Affect Disord. (2012) 136:1039–46. doi: 10.1016/j.jad.2011.11.040
109. Henry, ME, Schmidt, ME, Matochik, JA, Stoddard, EP, and Potter, WZ. The effects of ECT on brain glucose: a pilot FDG PET study. J ECT. (2001) 17:33–40. doi: 10.1097/00124509-200103000-00007
110. Masuoka, T, Tateno, A, Sakayori, T, Tiger, M, Kim, WC, Moriya, H, et al. Electroconvulsive therapy decreases striatal dopamine transporter binding in patients with depression: a positron emission tomography study with [18F] FE-PE2I. Psychiatry Res Neuroimaging. (2020) 301:111086. doi: 10.1016/j.pscychresns.2020.111086
111. Tiger, M, Svensson, J, Liberg, B, Saijo, T, Schain, M, Halldin, C, et al. [11C] raclopride positron emission tomography study of dopamine-D2/3 receptor binding in patients with severe major depressive episodes before and after electroconvulsive therapy and compared to control subjects. Psychiatry Clin Neurosci. (2020) 74:263–9. doi: 10.1111/pcn.12980
112. Yatham, LN, Liddle, PF, Lam, RW, Zis, AP, Stoessl, AJ, Sossi, V, et al. Effect of electroconvulsive therapy on brain 5-HT(2) receptors in major depression. Br J Psychiatry. (2010) 196:474–9. doi: 10.1192/bjp.bp.109.069567
113. Meyer, JH, Kapur, S, Eisfeld, B, Brown, GM, Houle, S, DaSilva, J, et al. The effect of paroxetine on 5-HT(2A) receptors in depression: an [18F] setoperone PET imaging study. Am J Psychiatry. (2001) 158:78–85. doi: 10.1176/appi.ajp.158.1.78
114. Mischoulon, D, Dougherty, DD, Bottonari, KA, Gresham, RL, Sonawalla, SB, Fischman, AJ, et al. An open pilot study of nefazodone in depression with anger attacks: relationship between clinical response and receptor binding. Psychiatry Res. (2002) 116:151–61. doi: 10.1016/S0925-4927(02)00082-3
115. Yatham, LN, Liddle, PF, Dennie, J, Shiah, IS, Adam, MJ, Lane, CJ, et al. Decrease in brain serotonin 2 receptor binding in patients with major depression following desipramine treatment: a positron emission tomography study with fluorine-18-labeled setoperone. Arch Gen Psychiatry. (1999) 56:705–11. doi: 10.1001/archpsyc.56.8.705
116. Abbott, CC, Gallegos, P, Rediske, N, Lemke, NT, and Quinn, DK. A review of longitudinal electroconvulsive therapy: neuroimaging investigations. J Geriatr Psychiatry Neurol. (2014) 27:33–46. doi: 10.1177/0891988713516542
117. Bak, J, Lee, SM, Kwon, YJ, Shim, SH, and Kim, JI. The normalization of brain 18F-fluorodeoxy-D-glucose positron emission tomography hypometabolism following electroconvulsive therapy in a 55-yearold woman with treatment-resistant late onset depression: a case report. Clin Psychopharmacol Neurosci. (2017) 15:82–6. doi: 10.9758/cpn.2017.15.1.82
118. Hassamal, S, Jolles, P, and Pandurangi, A. Reversal of cerebral glucose hypometabolism on positron emission tomography with electroconvulsive therapy in an elderly patient with a psychotic episode. Psychogeriatrics. (2016) 16:376–81. doi: 10.1111/psyg.12174
119. Reininghaus, EZ, Reininghaus, B, Ille, R, Fitz, W, Lassnig, RM, Ebner, C, et al. Clinical effects of electroconvulsive therapy in severe depression and concomitant changes in cerebral glucose metabolism—an exploratory study. J Affect Disord. (2013) 146:290–4. doi: 10.1016/j.jad.2012.07.034
120. Shi, Y, Li, J, Tong, P, Yang, J, Zhang, H, and Dong, L. Regional cerebral blood flow in major depression treated with electroconvulsive therapy: an arterial spin labeling magnetic resonance study. Neurocase. (2022) 28:246–50. doi: 10.1080/13554794.2022.2044861
121. Leaver, AM, Vasavada, M, Joshi, SH, Wade, B, Woods, RP, Espinoza, R, et al. Mechanisms of antidepressant response to electroconvulsive therapy studied with perfusion magnetic resonance imaging. Biol Psychiatry. (2018) 85:466–76. doi: 10.1016/j.biopsych.2018.09.021
122. Leaver, AM, Vasavada, M, Kubicki, A, Wade, B, Loureiro, J, Hellemann, G, et al. Hippocampal subregions and networks linked with antidepressant response to electroconvulsive therapy. Mol Psychiatry. (2021) 26:4288–99. doi: 10.1038/s41380-020-0666-z
123. Bracht, T, Walther, S, Breit, S, Mertse, N, Federspiel, A, Meyer, A, et al. Distinct and shared patterns of brain plasticity during electroconvulsive therapy and treatment as usual in depression: an observational multimodal MRI-study. Transl Psychiatry. (2023) 13:6. doi: 10.1038/s41398-022-02304-2
Keywords: depression, electroconvulsive therapy, magnetic resonance imaging, positron emission tomography, mechanism, arterial spin labeling
Citation: Chen X, Yang H, Cui L-B and Li X (2023) Neuroimaging study of electroconvulsive therapy for depression. Front. Psychiatry. 14:1170625. doi: 10.3389/fpsyt.2023.1170625
Received: 21 February 2023; Accepted: 23 May 2023;
Published: 09 June 2023.
Edited by:
Christopher C. Abbott, University of New Mexico Health Sciences Center, United StatesReviewed by:
Maolin Hu, Renmin Hospital of Wuhan University, ChinaCopyright © 2023 Chen, Yang, Cui and Li. This is an open-access article distributed under the terms of the Creative Commons Attribution License (CC BY). The use, distribution or reproduction in other forums is permitted, provided the original author(s) and the copyright owner(s) are credited and that the original publication in this journal is cited, in accordance with accepted academic practice. No use, distribution or reproduction is permitted which does not comply with these terms.
*Correspondence: Long-Biao Cui, bGJjdWlAZm1tdS5lZHUuY24=; Xiao Li, bGl4aWFvQGhvc3BpdGFsLmNxbXUuZWR1LmNu
†These authors have contributed equally to this work and share first authorship
Disclaimer: All claims expressed in this article are solely those of the authors and do not necessarily represent those of their affiliated organizations, or those of the publisher, the editors and the reviewers. Any product that may be evaluated in this article or claim that may be made by its manufacturer is not guaranteed or endorsed by the publisher.
Research integrity at Frontiers
Learn more about the work of our research integrity team to safeguard the quality of each article we publish.