- Center for Alcohol Research in Epigenetics, Department of Psychiatry, University of Illinois Chicago, Chicago, IL, United States
Compulsive alcohol drinking is a key symptom of alcohol use disorder (AUD) that is particularly resistant to treatment. An understanding of the biological factors that underly compulsive drinking will allow for the development of new therapeutic targets for AUD. One animal model of compulsive alcohol drinking involves the addition of bitter-tasting quinine to an ethanol solution and measuring the willingness of the animal to consume ethanol despite the aversive taste. Previous studies have demonstrated that this type of aversion-resistant drinking is modulated in the insular cortex of male mice by specialized condensed extracellular matrix known as perineuronal nets (PNNs), which form a lattice-like structure around parvalbumin-expressing neurons in the cortex. Several laboratories have shown that female mice exhibit higher levels of aversion-resistant ethanol intake, but the role of PNNs in females in this behavior has not been examined. Here we compared PNNs in the insula of male and female mice and determined if disrupting PNNs in female mice would alter aversion-resistant ethanol intake. PNNs were visualized in the insula by fluorescent labeling with Wisteria floribunda agglutinin (WFA) and disrupted in the insula by microinjecting chondroitinase ABC, an enzyme that digests the chondroitin sulfate glycosaminoglycan component of PNNs. Mice were tested for aversion-resistant ethanol consumption by the addition of sequentially increasing concentrations of quinine to the ethanol in a two-bottle choice drinking in the dark procedure. PNN staining intensity was higher in the insula of female compared to male mice, suggesting that PNNs in females might contribute to elevated aversion-resistant drinking. However, disruption of PNNs had limited effect on aversion-resistant drinking in females. In addition, activation of the insula during aversion-resistant drinking, as measured by c-fos immunohistochemistry, was lower in female mice than in males. Taken together, these results suggest that neural mechanisms underlying aversion-resistant ethanol consumption differ in males and females.
1. Introduction
Alcohol use disorder (AUD) is characterized by the inability to stop or limit alcohol use despite adverse effects on one’s health, relationships, and occupation. Although more men than women have historically been diagnosed with AUD, the prevalence of this disorder in women has increased in recent decades. From 2002 to 2012, AUD diagnosis in women increased by 83.7% (1). Socioeconomic factors can account for some of this increase, but biological factors also play a role in the initiation of alcohol use and progression to AUD (2). Clinical and preclinical studies have demonstrated sex differences during different stages of the addiction cycle (3) and suggest that hormonal and genetic factors are involved in sex differences in addiction (4). It is necessary to understand the biological factors that contribute to sex differences in alcohol consumption to develop new treatments that will be effective in reducing alcohol drinking in both men and women.
A key characteristic of AUD is compulsive alcohol use, an inflexible behavior that can persist despite negative consequences (5). This aversion-resistant phenotype is modeled in animals by pairing alcohol delivery with a foot shock or by adding bitter-tasting quinine to the ethanol solution. Animals that are willing to consume alcohol despite the threat of punishment (pain or bitter taste) are considered to exhibit compulsive-like behavior (6). Several studies have shown that female rats and mice exhibit higher levels of aversion-resistant alcohol consumption and alcohol seeking behavior than males (7–11). In terms of pharmacological targets for reducing aversion-resistant ethanol consumption, inhibition of orexin-1 receptor (12), diacylglycerol lipase (13), hyperpolarization-active NMDA receptors (14–16), and alpha-1 noradrenergic receptors (17) can reduce consumption of quinine-adulterated ethanol. However, except for studies examining inhibition of orexin-1 receptor (18), these experiments were conducted in male animals only. Thus, there is a great need to identify whether mechanisms that drive compulsive-like drinking in male rodents are also operational in females.
One brain region involved in compulsive alcohol drinking is the insular cortex (14, 17, 19–23). The insula is a sensory processing area that regulates decision-making, emotion, motivation, and aversion. It integrates information about body states and processes this information to influence emotions and behavior (24). A functional magnetic resonance imaging (fMRI) study in human heavy drinkers demonstrated that the anterior insula was activated when individuals viewed alcohol cues associated with the threat of electric shock (21). In addition, greater connectivity between the anterior insula and nucleus accumbens was observed in heavy vs. light drinkers, which was significantly associated with measures of compulsive alcohol use (21). Similar findings have been observed in rats, demonstrating that projections from the anterior insula to either the nucleus accumbens or brainstem promote aversion-resistant ethanol intake (14, 17). We previously found that disrupting specialized extracellular matrix structures, known as perineuronal nets (PNNs), that surround parvalbumin-expressing interneurons in the insula can render male mice more sensitive to ethanol adulterated with quinine (20), suggesting a role for insular PNNs in maintaining aversion-resistant drinking. It remains to be determined whether manipulating PNNs in female mice has the same effect as in males.
Sex differences in the density and intensity of PNNs have been described in the mammalian basolateral and medial amygdala (25, 26), with male rodents having increased numbers and/or intensity of PNNs in these brain regions. Similarly, juvenile male rats had more PNNs in the CA1 region of the hippocampus compared with juvenile females (27). However, PNNs have not been compared between males and females in the insular cortex. Given that PNNs in male mice regulate aversion-resistant ethanol consumption, that there are sex differences in PNNs, and that female mice exhibit higher levels of aversion-resistant ethanol consumption than males, we hypothesized that there would be sex differences in PNN structure in the insula that could account for increased aversion-resistant ethanol consumption in female mice. Specifically, we hypothesized that there would be increased PNNs intensity in the insula of female mice and decreased activation of the insula, as measured by c-fos immunohistochemistry (IHC) in females during drinking of quinine-adulterated ethanol. Consistent with our hypothesis, we found increased PNN intensity and decreased c-fos levels during aversion-resistant drinking in the insula of female mice compared to males. However, in contrast to males, disrupting PNNs in the insula of female mice did not affect aversion-resistant ethanol consumption. Together, these results indicate that sex-specific mechanisms regulate aversion-resistant ethanol consumption in male and female mice.
2. Materials and methods
2.1. Animals
Adult male and female C57BL/6J mice at the age of 8 weeks were purchased from the Jackson Laboratory (Bar Harbor, ME, USA). All mice were individually housed in a temperature- and humidity-controlled room with a 12-h reversed light/dark cycle (lights off at 10 a.m.) for 2 weeks prior to beginning experiments and tested for alcohol drinking behavior from 10 to 13 weeks of age. Food and water were available ad libitum. Mice were fed Teklad 7912 diet (Envigo, Indianapolis, IN, USA). All procedures with mice were conducted according to the National Institutes of Health Guide for the Care and Use of Laboratory Animals and approved by the UIC Animal Care and Use Committee.
2.2. Ethanol drinking procedures
Mice were tested for consumption of quinine-adulterated ethanol in a modified drinking in the dark (DID) test. Mice received a two-bottle choice between water and 15% ethanol, 3 h into the dark cycle, for 4 h per day over the course of 5 consecutive days. Water and ethanol solutions were provided to mice in 10 ml clear polystyrene serological pipets truncated at the end to accommodate connection to a 2.5-inch stainless steel ball-bearing sipper tube (Ancare Corp., Bellmore, NY, USA). On the first day, mice had access to ethanol without quinine. Over the next 4 days, quinine was added in increasing concentrations each day (50, 100, 250, and 500 μM, respectively) to the ethanol solution. Tube placements were alternated daily to avoid the confound of preference for a particular side. Ethanol consumption was calculated as g ethanol per kg of body weight in 4 h, and % preference for the ethanol solution was calculated as volume of ethanol consumed divided by total fluid consumed × 100. To test consumption of a quinine solution without ethanol, a separate group of mice were presented with a two-bottle choice of water or water plus quinine in the dark cycle for 4 h per day over 5 days. On the first day, both bottles contained water, then over the next 4 consecutive days one bottle of water had quinine added in increasing concentrations daily as described above. Quinine consumption was calculated as ml quinine solution per kg body weight over 4 h and % preference was calculated as volume of quinine solution divided by total fluid consumed × 100.
2.3. PNN labeling and immunohistochemistry
Mice were euthanized during the dark cycle with a lethal dose of a commercial euthanasia solution containing pentobarbital and then transcardially perfused with ice-cold PBS followed by 4% paraformaldehyde. Brains were post-fixed in 4% paraformaldehyde overnight and cryoprotected in 30% sucrose for 48 h. Every fourth section of 50 μm serial coronal sections were collected from the area of the brain containing the insula, spanning 1–2 mm anterior to bregma. Free-floating sections were treated twice with 50% ethanol and then treated with 1% hydrogen peroxide. Sections were blocked in carbo-free blocking solution (Vector Laboratories, Burlingame, CA, USA), and then incubated with biotinylated Wisteria floribunda agglutinin (WFA, Vector Laboratories, 1:1,000) to label PNNs, followed by Dylight 488-conjugated streptavidin secondary antibody (Vector Laboratories). Subsequently, the sections were washed with PBS and blocked with normal donkey serum (Jackson Immunoresearch, West Grove, PA, USA) and then incubated with mouse anti-parvalbumin (PV, #195011, Synaptic Systems, Göttingen, Germany, 1:1,000), followed by Alexa Flour 594-conjugated secondary antibody (#711-585-150, Jackson Immunoresearch, 1:4,000). Fluorescent sections were mounted on slides with Fluoromount-G from SouthernBiotech (Birmingham, AL, USA). For c-fos IHC, 40 μm serial coronal sections were treated with 1% hydrogen peroxide for 10 min and blocked with 5% goat serum plus 0.25% Triton X-100 for 1 h at room temperature, then incubated with rabbit-anti mouse c-fos antibody (Synaptic Systems, #226008, 1:1,000) in blocking solution overnight at 4°C. Then sections were incubated with biotinylated goat anti-rabbit IgG (Vector Laboratories, #PK4001, 1:500). DAB signal was developed by VECTASTAIN® ABC-HRP Kit and DAB Substrate Kit (Vector Laboratories, #PK4001 and SK4100).
2.4. Image acquisition and analysis
Fluorescent images were captured using an Olympus BX51/IX70 fluorescent microscope (Olympus, USA) with a 10× objective. The same exposure time for each fluorescent channel (WFA, 22.2 ms; PV, 83.3 ms) was used for all images so that we could quantify the intensity of staining across images. Cell counts and fluorescence intensity were measured using the ImageJ macro plug-in Pipsqueak AI (Rewire Neuro, Portland, OR, USA) (28). Pipsqueak AI was run in “semi-automatic mode” to select ROIs to identify individual PV+ cells and PNNs. Double-labeled neurons were considered when WFA was located around the perimeter of the PV + staining inside the cell. For reporting PNN and PV intensity, each data point represents the fluorescent WFA or PV intensity in a single section, obtained by calculating the average fluorescent intensity of ∼100 individual cells per section. WFA and PV intensity and cell counts were obtained from 4 mice per sex, with 4 sections (females) and 2–4 sections (males) per mouse, giving an n of 16 for females and 13 for males. For categorizing PNNs and PV neurons into low, medium, and high intensity, quartiles were used as the cutoff based on the intensity of PNNs or PV in female mice. Low intensity PNNs or PV neurons were below the 25th percentile (PNNs <5.23 and PV <2.26 raw intensity units), medium intensity PNNs or PV neurons were between the 25th and 75th percentile (PNNs = 5.23–6.65 and PV = 2.26–3.36 raw intensity units) and high intensity PNNs or PV neurons were in the 75th percentile (PNNs >6.65 and PV >3.36 raw intensity units). For imaging c-fos IHC, brightfield images were taken with a 20× objective using a Zeiss AxioScope A1 microscope and analyzed using ImageJ. Each data point is the average number of c-fos counts per mouse, with 4 sections analyzed per mouse and 6 mice per sex.
2.5. Stereotaxic surgery and intra-cranial injections
For injection of chondroitinase ABC (ChABC), C57BL/6J mice at the age of 8–12 weeks were anesthetized with ketamine [100 mg/kg, intraperitoneal (i.p.)] and xylazine (10 mg/kg, i.p.) and placed into a digital stereotaxic apparatus. A 1 cm incision was made in the scalp and 0.28 mm diameter holes were drilled bilaterally in the skull. ChABC (0.5 μl of a 50 U/ml solution in PBS, Millipore Sigma, St. Louis, MO, USA) or PBS was infused at a rate of 0.2 μl per min into the anterior insular cortex (1.5 mm anterior to bregma, 2.9 mm from the midline, and 2.6 mm ventral from the top of the skull) using 33-gauge stainless steel hypodermic tubing connected to a 1 μl gastight Hamilton syringe with PE20 tubing and an infusion pump. Mice were given a subcutaneous injection of meloxicam (2 mg/kg) for analgesia immediately after the completion of the surgery. Mice recovered for 3 days prior to testing ethanol consumption. After the completion of behavioral testing, WFA staining was performed on insula sections from each mouse spanning 1–2 mm anterior to bregma to confirm that ChABC disrupted PNNs in the anterior insula.
2.6. Experimental design and statistical analysis
Data are presented as the mean ± SEM. Statistical analyses were performed using Prism software (version 9.1, GraphPad, San Diego, CA, USA). Two-way repeated measures (RM) ANOVAs were performed for the results presented in Figure 1 (with sex as the between-subject factor and quinine concentration as the within-subject factor). For Figure 2, an unpaired Student’s t-test was used with sex as the between-subject factor (Figures 2G, I, J, L) and a Chi-squared test was performed to evaluate the distribution between sexes (Figures 2H, K). Three-way RM ANOVAs were performed for the results presented in Figure 3 with sex and treatment as between-subject factors and quinine concentration as the within-subject factor. These were followed by two-way RM ANOVAs within each sex with quinine concentration as the within-subject and treatment as the between-subject factor. Bonferroni’s multiple comparisons tests were done if there was a significant quinine concentration by treatment interaction. The number of mice in each group is indicated in the figure legend for each figure. For the c-fos IHC in Figure 4, data was analyzed by two-way ANOVA with sex and region as between-subject factors.
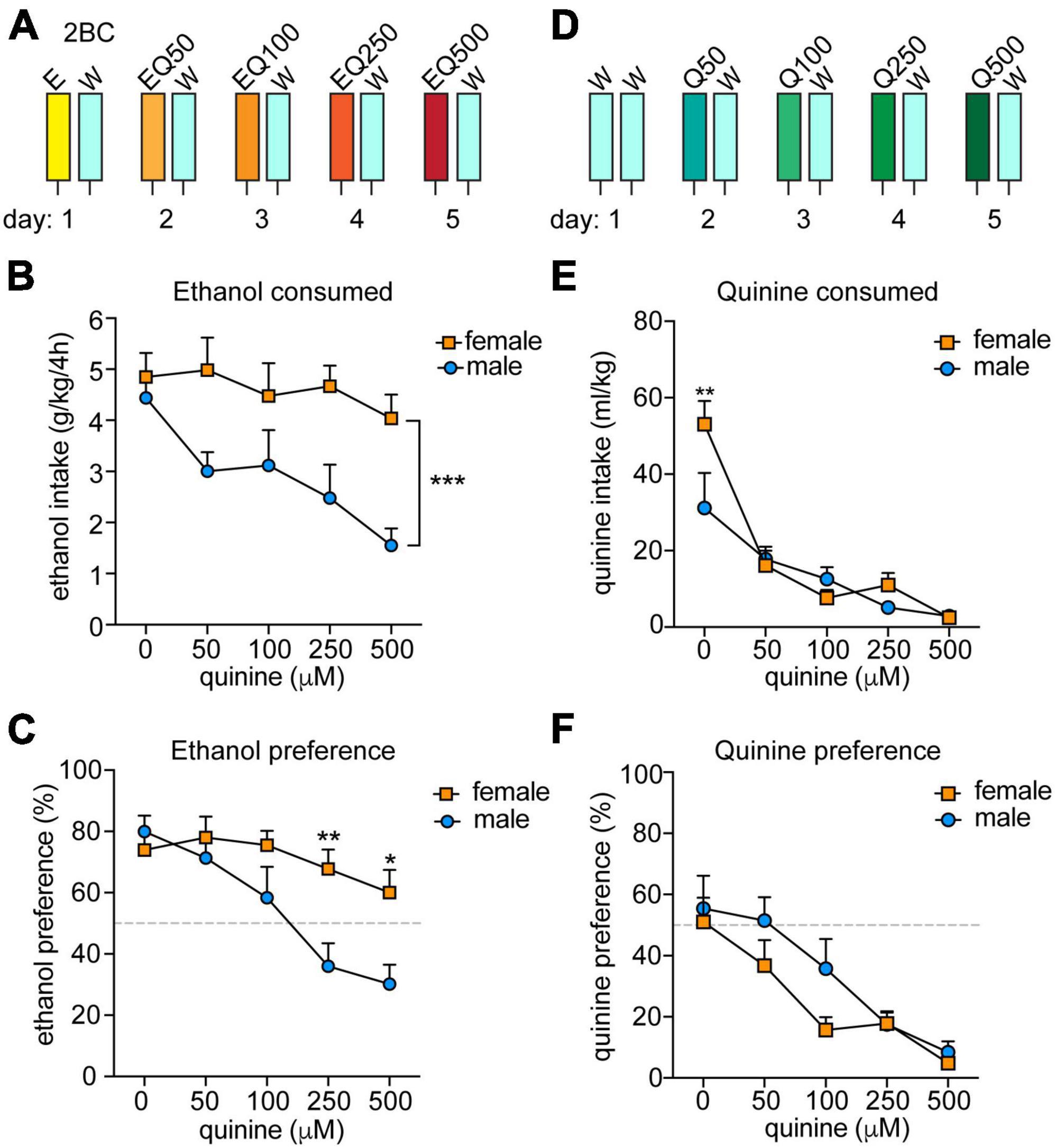
Figure 1. Sex differences in aversion-resistant ethanol consumption. (A) Experimental design for measuring aversion-resistant ethanol consumption. Mice (n = 11 males and 12 females) were given a two-bottle choice (2BC) ethanol drinking in the dark test with 15% ethanol (E) and water (W) on the first day. Each day, the quinine (Q) concentration was increased in the ethanol from 50 to 500 μM (EQ50, EQ100, EQ250, and EQ500). (B) Daily ethanol consumed in g per kg body weight over 4 h. ***P < 0.001, effect of sex by two-way RM ANOVA. (C) Daily ethanol preference, expressed as ethanol consumed divided by total fluid consumed × 100. **P < 0.01 and *P < 0.05 when comparing females to males at EQ250 and EQ500, respectively, by Bonferroni’s post-hoc multiple comparisons testing. (D) Experimental setup for measuring quinine consumption. Mice (n = 8 males and 8 females) underwent 2BC in the dark on the first day with water only and then with quinine doses increasing daily from 50 to 500 μM (Q50, Q100, Q250, and Q500). (E) Daily quinine consumed in ml quinine per kg body weight over 4 h. **P < 0.01 when comparing females to males in water consumption by Bonferroni’s post-hoc multiple comparisons testing. (F) Daily quinine preference, expressed as quinine consumed divided by total fluid consumed × 100.
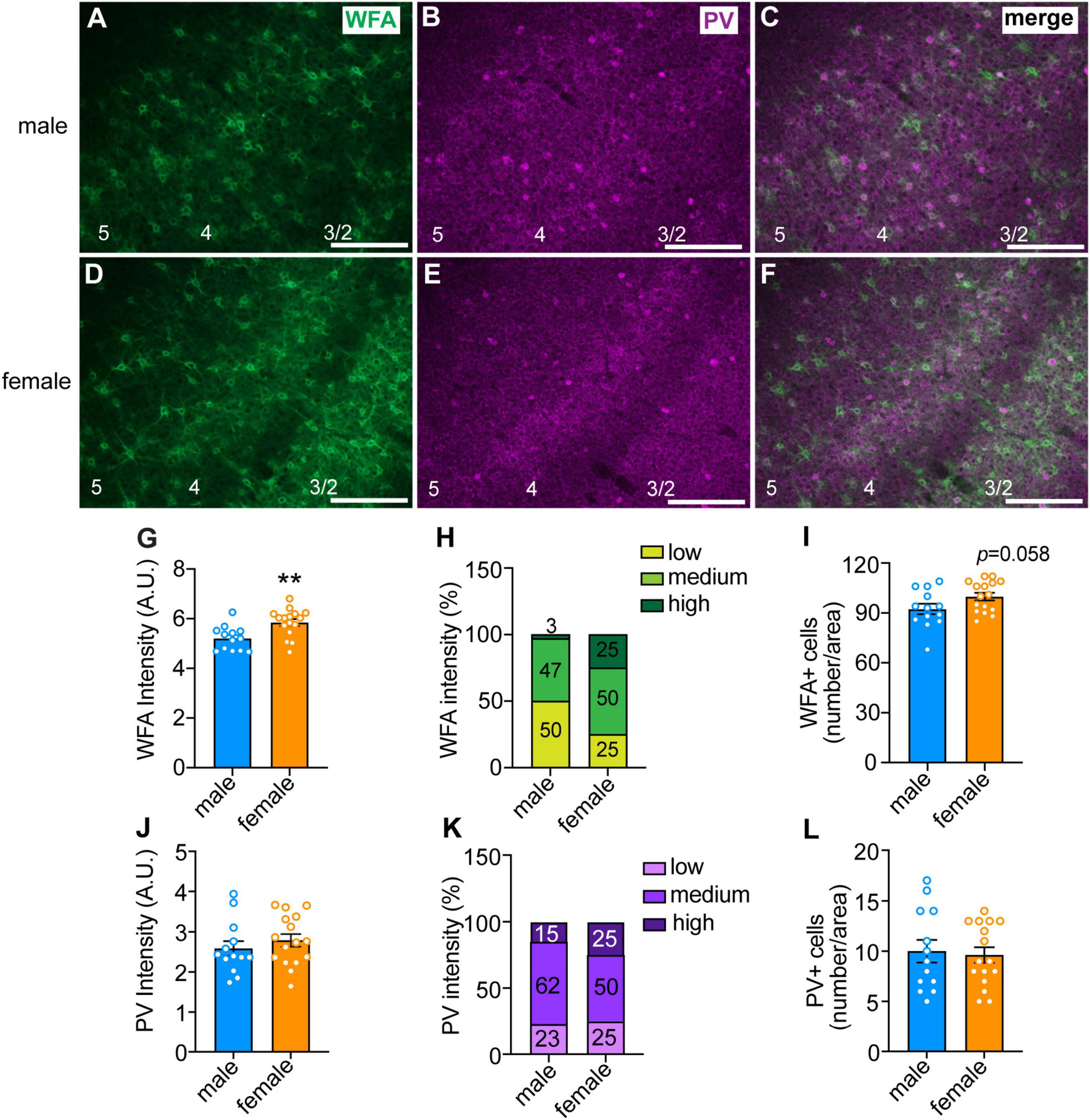
Figure 2. Sex differences in perineuronal net (PNN) intensity in the anterior insula. (A–F) Representative images of male (top panels) and female (bottom panels) insula sections that were fluorescently labeled with Wisteria floribunda agglutinin (WFA, in green), which binds to PNNs, and parvalbumin (PV, in magenta). Scale bar, 200 μm. Cortical layers are indicated at the bottom of the images. Quantified WFA (G) and PV (J) fluorescence intensity. Each data point represents the average intensity surrounding neurons in a single section, with ∼100 cells per section analyzed in 2–4 sections per mouse from 4 mice per sex. **P < 0.01. Percentage of neurons with low, medium, and high intensity PNNs (H) and PV staining (K). There was a significant sex difference in PNN intensity, with females having more high intensity and less low intensity PNNs than males, X2 = 9.77 × 10– 37. Average number of PNNs (I) and PV (L) neurons per section in males and females.
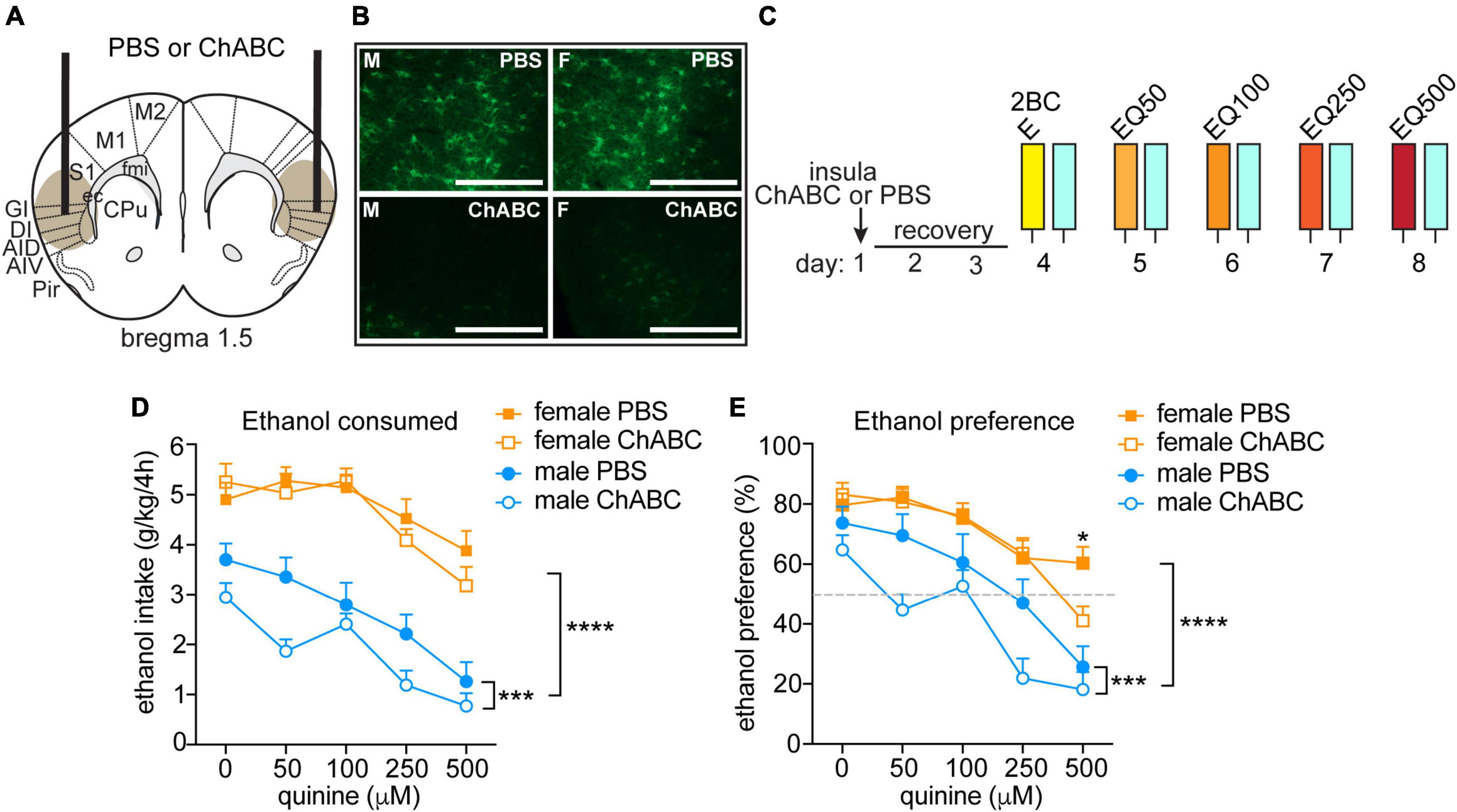
Figure 3. Disrupting PNNs only in male mice increases sensitivity to quinine-adulterated ethanol. (A) Illustration of mouse brain coronal section indicating sites of chondroitinase ABC (ChABC) or PBS injection into the anterior insula. Brown oval shows approximate spread of ChABC, as determined by reduced fluorescent WFA staining. (B) Representative images of fluorescent WFA staining in male (M, left panels) and female (F, right panel) mice 3 days after PBS (top panels) or ChABC injections into the insula. Scale bar, 200 μm. (C) Experimental design. Mice were injected with ChABC (n = 16 males and 16 females) or PBS (n = 16 males and 16 females) in the insula and recovered from surgery for 3 days, followed by two-bottle choice (2BC) ethanol drinking in the dark. Quinine was added in increasing concentrations from 50 to 500 μM each day to the ethanol (EQ50, EQ100, EQ250, and EQ500). (D) Daily ethanol consumed in g per kg body weight in 4 h. (E) Daily ethanol preference, expressed as ethanol consumed divided by total fluid consumed × 100. ****P < 0.0001, main effect of sex by three-way ANOVA; ***P < 0.001, main effect of ChABC in males by two-way ANOVA; *P < 0.05, PBS vs. ChABC in females at 500 μM quinine.
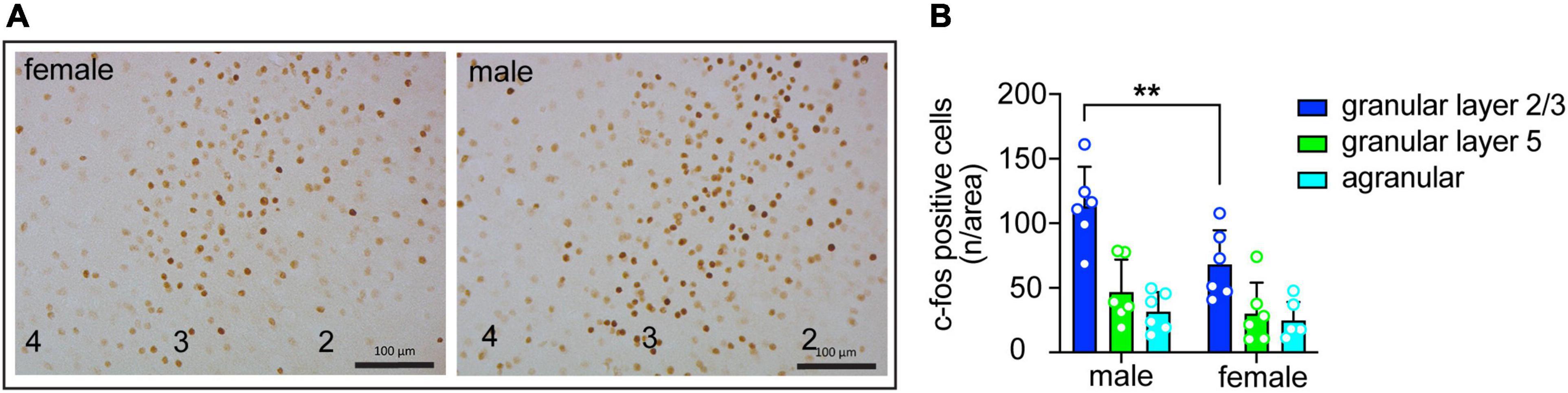
Figure 4. Sex differences in insula activation during consumption of quinine-adulterated ethanol. (A) Representative images of c-fos immunohistochemistry in the granular layer of the insula of male and female mice after drinking ethanol with 250 μM quinine. Cortical layers are indicated at the bottom. Scale bars, 100 μm. (B) Quantification of c-fos immunohistochemistry (n = 6 males and 6 females) in the granular and agranular layers of the insula of mice after drinking ethanol with 250 μM quinine. **P < 0.05, effect of sex in granular layer 2/3 by Bonferroni’s multiple comparisons test after two-way ANOVA.
3. Results
3.1. Sex differences in aversion-resistant ethanol consumption
Previous reports indicated that female mice exhibit greater aversion-resistant ethanol consumption than males when tested for quinine-adulterated ethanol drinking (7, 10). To confirm these results, we performed a modified two-bottle choice DID procedure in which quinine was added to the ethanol solution consecutively each day in sequentially increasing concentrations (Figure 1A; 50, 100, 250, and 500 μM quinine). We observed significant sex differences in both ethanol consumption and preference, with male mice reducing ethanol intake and preference as the quinine concentration increased, whereas females did not significantly alter their ethanol consumption or preference at any of the tested quinine concentrations (Figure 1B, ethanol consumption: sex, F(1,21) = 15.16, P = 0.0008; quinine concentration, F(4,84) = 3.97, P = 0.0054; Figure 1C, ethanol preference: sex, F(1,21) = 6.66, P = 0.017; quinine concentration, F(4,84) = 11.32, P < 0.0001). There was also a significant interaction between sex and quinine concentration for ethanol preference (F(4,84) = 3.63, P = 0.0089; post-hoc Bonferroni’s multiple comparisons test, P = 0.0071 and P = 0.013 when comparing males and females at 250 and 500 μM quinine, respectively). These results confirm that female mice demonstrate higher levels of aversion-resistant ethanol drinking.
To determine if female mice are simply less sensitive than males to the bitter taste of quinine, we tested them in a modified two-bottle choice DID procedure with quinine added to water (Figure 1D). Both sexes similarly decreased their consumption and preference for the quinine-adulterated water as the quinine concentration increased (Figure 1E, consumption: quinine concentration, F(4,56) = 27.94, P < 0.0001; Figure 1F, preference: quinine concentration, F(4,56) = 17.46, P < 0.0001). There were no significant effects of sex on water consumption or preference, although there was a sex by quinine concentration interaction for water consumption on the first day, which was due to females drinking more water relative to body weight than males (F(4,56) = 3.32, P = 0.017; post-hoc test comparing males to females at 0 μM quinine, P = 0.002). Thus, male and female mice appear to be equally sensitive to the taste of quinine, indicating that the increased aversion-resistant ethanol drinking by female mice is not simply due to deficient bitter taste perception.
3.2. Sex differences in PNN intensity in the anterior insula
We previously demonstrated a role for PNNs in the insular cortex of mice in modulating aversion-resistant ethanol drinking (20). One possible reason for greater aversion-resistant ethanol consumption in females could be due to altered PNN structure in the insula. To test this, we fluorescently labeled PNNs using WFA in the insula of mice that were euthanized during the dark phase because this is when we found sex differences in aversion-resistant drinking and PNN intensity has been shown to vary throughout the diurnal cycle (29, 30). The mean WFA fluorescence intensity around individual neurons was greater in females than in males (Figure 2G; t27 = 3.26, P = 0.003; female mean: 5.84 ± 0.14, male mean: 5.20 ± 0.13), which was primarily seen in cortical layers 2/3 (Figures 2A–F). Additionally, when PNNs were categorized as high, medium, or low intensity, only 3% of the PNNs in males were high intensity compared to 25% in females, while 50% of PNNs in males were categorized as low intensity compared to 25% in females (Figure 2H, χ2 = 22.71, P < 0.0001). No significant difference was found between males and females in the number of neurons surrounded by PNNs, although there was a trend toward an increase in females (Figure 2I, t27 = 1.97, P = 0.058; female mean: 99.88 ± 2.29, male mean: 92.38 ± 3.12). There were no significant sex differences in the intensity of PV immunofluorescence or number of PV-immunoreactive neurons (Figures 2J–L). Consistent with the WFA fluorescence staining, we also found by Western blotting increased levels in the insula of females of the chondroitin sulfate proteoglycans aggrecan and brevican that are components of PNNs (Supplementary Figure 1). Together, these results suggest that females have innately higher PNN deposition around insular neurons than males during the dark phase of the diurnal cycle.
3.3. Disrupting PNNs in male mice increases sensitivity to quinine-adulterated ethanol
To determine if disrupting PNNs in female mice alters aversion-resistant ethanol drinking, we injected ChABC, a bacterial enzyme that digests chondroitin sulfate glycosaminoglycans, into the anterior insula and compared the results to males injected intra-insula with ChABC (Figures 3A, B). Mice were tested 3 days after injection for consumption and preference of quinine-adulterated ethanol as described above (Figure 3C). A three-way RM ANOVA indicated significant main effects of treatment (F(1,60) = 5.29, P = 0.025), quinine concentration (F(4,240) = 34.17, P < 0.0001) and sex (F(1,60) = 120.9, P < 0.0001) on ethanol consumption (Figure 3D). Similar results were found for ethanol preference (Figure 3E, treatment, F(1,60) = 5.74, P = 0.0197; quinine concentration, F(4,240) = 46.2, P < 0.0001; sex, F(1,60) = 36.45, P < 0.0001; treatment by quinine concentration by sex interaction, F(4,240) = 2.54, P = 0.041). Since we observed sex differences in ethanol drinking, we next performed focused two-way RM ANOVAs within each sex to determine if ChABC treatment significantly altered ethanol consumption and preference. In males, ChABC treatment resulted in reduced ethanol intake and preference when compared to the PBS control (ethanol consumption: treatment, F(1,30) = 5.81, P = 0.022; quinine concentration, F(4,120) = 25.66, P < 0.0001; ethanol preference: treatment, F(1,30) = 5.78, P = 0.023; quinine concentration, F(4,120) = 23.97, P < 0.0001), whereas in females, ChABC had no significant effect on ethanol consumption and only reduced ethanol preference at 500 μM quinine when compared to the PBS control (ethanol consumption: treatment, F(1,30) = 0.43, P = 0.52; quinine concentration, F(4,120) = 12.38, P < 0.0001; interaction, F(4,120) = 1.05, P = 0.39; ethanol preference: treatment, F(1,30) = 0.52, P = 0.48; quinine concentration, F(4,120) = 24.67, P < 0.0001; interaction, F(4,120) = 2.96, P = 0.023, post-hoc Bonferroni’s, PBS vs. ChABC at 500 μM quinine, P = 0.015). These results show that digesting chondroitin sulfate glycosaminoglycans and thus disrupting PNNs in the insula of male mice renders them sensitive to quinine-adulterated ethanol, while the same manipulation in the insula of female mice alters their sensitivity to quinine-adulterated ethanol only at a very high quinine concentration.
3.4. Sex differences in insula activation during consumption of quinine-adulterated ethanol
Excitatory neurons in the insula are activated during drinking of ethanol containing quinine (20). To determine if the extent of insular neuron activation differs by sex, we measured c-fos expression by IHC in male and female mice after an ethanol plus quinine drinking session. c-fos expression was higher in granular layers 2/3 compared to granular layer 5 and agranular regions of the insula in both sexes. In addition, c-fos expression was elevated in males compared to females (Figure 4; region, F(2,30) = 24.84, P < 0.0001; sex, F(1,30) = 8.69, P = 0.0061; post-hoc Bonferroni, P = 0.0064 when comparing males and females within granular layers 2/3). These results suggest greater activation of the insula of male mice compared to female mice during quinine-adulterated ethanol drinking.
4. Discussion
This study confirms previous observations that female mice are more resistant than males to the suppressive effects of quinine on ethanol consumption and provides new evidence that mechanisms regulating aversion-resistant ethanol consumption differ in male and female mice. We previously demonstrated that disrupting PNNs by injecting ChABC into the insula of male mice decreased aversion-resistant ethanol consumption. We show here that females have a higher intensity of PNN staining in the insula, suggesting that this might be a factor that contributes to increased aversion-resistant drinking in females. However, disrupting PNNs in females did not result in reduced intake of quinine-adulterated ethanol as it did in males.
The observation that female mice are less sensitive to the addition of quinine to ethanol than male mice is consistent with other studies. For example, Fulenwider et al. found that ethanol consumption was suppressed in male mice when 30, 100, and 300 μM quinine was added to the ethanol, but 300 μM quinine was required to suppress ethanol intake by females (7). Sneddon et al. showed that female mice self-administered more ethanol in an operant procedure and were able to tolerate higher quinine concentrations in the ethanol solution than males (10). However, others have reported no sex differences in quinine-adulterated ethanol intake, but testing was done after a few weeks of pre-exposure to ethanol in limited-access procedures (31, 32), which has been shown to induce aversion-resistant ethanol intake and increased insular PNN intensity (33) in male mice (34). Thus, innate sex differences in aversion-resistant ethanol consumption may disappear after repeated bouts of ethanol drinking but this could depend on the exposure method. We found that males and females were equally sensitive to the suppressive effect of quinine on water intake, indicating that differences in bitter taste perception are not the cause of increased aversion-resistant ethanol drinking in female mice.
We initially hypothesized that sex differences in PNNs in the insula might be driving the higher levels of aversion-resistant ethanol drinking in female mice. We found that female mice had more PNN deposition around neurons and increased aggrecan and brevican protein levels in the insula. Several groups have examined PNNs in different brain regions of male and female rats and mice. The number of PNNs in the cornu Ammonis-1 (CA1) region of the rat hippocampus was higher in juvenile males than females, but no significant sex differences were observed in the CA3 region or neocortex, and the sex differences in CA1 PNNs were no longer apparent in adult rats (27). Similarly, in adult rats and mice, no sex differences in PNNs in the prefrontal cortex (PFC) were observed under standard housing and rearing conditions (35–37). In the basolateral amygdala (BLA), juvenile male rats had more cells surrounded by PNNs in the left BLA and a greater percentage of PV cells with PNNs in the right BLA than females (26). The intensity of PNN staining was also higher in the medial amygdala and medial tuberal nucleus of adult male compared to female mice (25). These results are the opposite of what we observed in the insula, in which females had increased PNN staining. This discrepancy could be due to differences in the brain region examined.
To add another layer of complexity, recent reports have shown that PNNs are regulated by hormones in female rats and mice (38, 39). For example, in the CA1 region of the mouse hippocampus, PV neurons express aromatase, the enzyme that converts testosterone to estradiol (39). Treatment with letrozole, an aromatase inhibitor, increased WFA staining intensity in females, but not in males (39), suggesting that local hippocampal 17β-estradiol production in females reduces PNN intensity. In addition, ventral dentate gyrus PNNs change throughout the estrous cycle in mice, with more PV cells enveloped by PNNs during estrus than diestrus, indicating that ovarian hormones modulate PNNs (40). Interestingly, estrous cycle phase had no effect on ventral hippocampal CA1 PNNs (40). Together, these results indicate that hormones can regulate the number of cells or intensity of PNN staining in the hippocampus. It will be important in future studies to determine whether the sex differences in PNNs in the insula are due to hormonal (such as gonadal or brain-derived 17β-estradiol) or chromosomal factors.
Sex differences in the intensity of PNN staining might also be affected by the timing of brain tissue collection. We examined PNNs during the dark phase of the diurnal cycle, whereas the aforementioned studies measured PNNs during the light phase. The intensity of PNN staining by WFA has been reported to fluctuate throughout the diurnal cycle (29, 30). PNN staining was higher in the medial PFC of rats (29) and the PFC, hippocampus, amygdala, and other brain regions in mice (30) during the dark phase, when rodents are more active. We have not directly compared PNNs between light and dark phases but we have observed that there is no longer a sex difference in PNNs in the insula when mice are euthanized during the light phase of the cycle (data not shown). However, it is important to note that our alcohol drinking experiments were done in the dark so we collected tissue for PNNs analysis in the dark phase.
To our surprise, injection of ChABC into the insula of female mice had no effect on aversion-resistant ethanol consumption. A caveat of the use of ChABC is that it does not selectively disrupt PNNs and will remove all chondroitin sulfate glycosaminoglycan modifications on extracellular matrix proteins. Nonetheless, disruption of PNNs in the insula did not decrease aversion-resistant drinking in females as it did in males. One possibility is that this behavior may be differentially encoded anatomically in female brain. We injected ChABC into the anterior insula, but other insular subregions are involved in processing of negative information (41). The posterior insula plays a role in processing aversive states (42) and sex differences in posterior insula cortex connectivity with the bed nucleus of the stria terminalis (BNST) have been observed in mice (43) and humans (44). In addition, sexually dimorphic effects of ethanol on the posterior insula to BNST circuit have also been described (43). The PFC is another possibility, since a PFC to nucleus accumbens circuit promotes aversion-resistant ethanol intake in rats (14). Finally, other brain regions involved in aversion should be considered, such as the habenula and rostral medial tegmental area (45).
The other explanation for the lack of effect of intra-insular ChABC in female mice on aversion-resistant ethanol consumption could be due to hormonal influences on PV interneurons. 17β-estradiol increases the excitability of cortical PV interneurons in rats through actions at estrogen receptor β in PV neurons (46). In the rat agranular insula, approximately 32% of all PV+ neurons express estrogen receptor β (47). In addition, as mentioned above, in the mouse hippocampus, PV interneurons express aromatase, and blocking aromatase activity in females increased the intensity of PNNs surrounding PV interneurons and increased inhibitory neurotransmission onto CA1 pyramidal neurons (39). ChABC injection into the hippocampus of female mice did not affect inhibitory neurotransmission onto CA1 pyramidal cells, but was able to block the effect of an aromatase inhibitor on inhibitory currents, suggesting that when local 17β-estradiol synthesis is intact, disruption of PNNs does not alter inhibitory activity in the female hippocampus (39). We hypothesize that estrogen in female insula could mask the effects of PNN removal on PV interneuron excitability and thus behavioral output. The increase in PNN staining intensity in female mouse insula might be a consequence of higher estrogen levels than in males, but this remains to be determined.
These findings may also be consistent with our c-fos results. We found that induction of c-fos expression in the insula was reduced in females compared to males during quinine-adulterated ethanol consumption, and we previously demonstrated that c-fos is induced almost exclusively in excitatory neurons in the insula during this behavior (20). If females have increased activity of insular PV interneurons, one might expect increased inhibition on pyramidal cells and reduced c-fos expression during aversion-resistant ethanol consumption. More experiments are needed to determine if 17β-estradiol modulation of PV interneurons in the insula is involved in PNN structure, the regulation of PV and pyramidal neuron excitability, and aversion-resistant ethanol consumption.
This study demonstrates that the mechanisms contributing to aversion-resistant ethanol consumption differ between males and females. When considering potential therapeutic targets to reduce compulsive alcohol drinking, sex differences need to be taken into account. Pharmacotherapies that reduce compulsive alcohol drinking in males may be ineffective in females. A greater understanding of the biology of sex differences in AUD will help in determine the most effective therapies for both sexes.
Data availability statement
The raw data supporting the conclusions of this article will be made available by the authors, without undue reservation.
Ethics statement
The animal study was reviewed and approved by the Animal Care and Use Committee of the University of Illinois Chicago.
Author contributions
LM, HC, and AL contributed to the conception and design of the study. LM, HC, and MS conducted the experiments. LM, HC, MS, and AL performed the statistical analysis. LM and AL wrote the first draft of the manuscript. All authors contributed to manuscript revision, read, and approved the submitted version.
Funding
This work was supported by grants from the National Institute on Alcohol Abuse and Alcoholism (U01 AA020912 and R01 AA027231 to AL).
Conflict of interest
The authors declare that the research was conducted in the absence of any commercial or financial relationships that could be construed as a potential conflict of interest.
Publisher’s note
All claims expressed in this article are solely those of the authors and do not necessarily represent those of their affiliated organizations, or those of the publisher, the editors and the reviewers. Any product that may be evaluated in this article, or claim that may be made by its manufacturer, is not guaranteed or endorsed by the publisher.
Author disclaimer
The content is solely the responsibility of the authors and does not represent the official views of the National Institutes of Health.
Supplementary material
The Supplementary Material for this article can be found online at: https://www.frontiersin.org/articles/10.3389/fpsyt.2023.1122423/full#supplementary-material
References
1. Grant B, Chou S, Saha T, Pickering R, Kerridge B, Ruan W, et al. Prevalence of 12-month alcohol use, high-risk drinking, and DSM-IV alcohol use disorder in the United States, 2001-2002 to 2012-2013: results from the national epidemiologic survey on alcohol and related conditions. JAMA Psychiatry. (2017) 74:911–23. doi: 10.1001/jamapsychiatry.2017.2161
2. Finn D. The endocrine system and alcohol drinking in females. Alcohol Res. (2020) 40:2. doi: 10.35946/arcr.v40.2.02
3. Flores-Bonilla A, Richardson H. Sex differences in the neurobiology of alcohol use disorder. Alcohol Res. (2020) 40:4. doi: 10.35946/arcr.v40.2.04
4. Becker J, Chartoff E. Sex differences in neural mechanisms mediating reward and addiction. Neuropsychopharmacology. (2019) 44:166–83. doi: 10.1038/s41386-018-0125-6
5. Luscher C, Robbins T, Everitt B. The transition to compulsion in addiction. Nat Rev Neurosci. (2020) 21:247–63. doi: 10.1038/s41583-020-0289-z
6. Hopf F, Lesscher H. Rodent models for compulsive alcohol intake. Alcohol. (2014) 48:253–64. doi: 10.1016/j.alcohol.2014.03.001
7. Fulenwider H, Nennig S, Price M, Hafeez H, Schank J. Sex differences in aversion-resistant ethanol intake in mice. Alcohol Alcohol. (2019) 54:345–52. doi: 10.1093/alcalc/agz022
8. Radke A, Held I, Sneddon E, Riddle C, Quinn J. Additive influences of acute early life stress and sex on vulnerability for aversion-resistant alcohol drinking. Addict Biol. (2020) 25:e12829. doi: 10.1111/adb.12829
9. Radke A, Sneddon E, Frasier R, Hopf F. Recent perspectives on sex differences in compulsion-like and binge alcohol drinking. Int J Mol Sci. (2021) 22:3788. doi: 10.3390/ijms22073788
10. Sneddon E, Ramsey O, Thomas A, Radke A. Increased responding for alcohol and resistance to aversion in female mice. Alcohol Clin Exp Res. (2020) 44:1400–9. doi: 10.1111/acer.14384
11. Xie Q, Buck L, Bryant K, Barker J. Sex differences in ethanol reward seeking under conflict in mice. Alcohol Clin Exp Res. (2019) 43:1556–66. doi: 10.1111/acer.14070
12. Lei K, Wegner S, Yu J, Hopf F. Orexin-1 receptor blockade suppresses compulsive-like alcohol drinking in mice. Neuropharmacology. (2016) 110(Pt A):431–7. doi: 10.1016/j.neuropharm.2016.08.008
13. Winters N, Bedse G, Astafyev A, Patrick T, Altemus M, Morgan A, et al. Targeting diacylglycerol lipase reduces alcohol consumption in preclinical models. J Clin Invest. (2021) 131:e146861. doi: 10.1172/JCI146861
14. Seif T, Chang S, Simms J, Gibb S, Dadgar J, Chen B, et al. Cortical activation of accumbens hyperpolarization-active NMDARs mediates aversion-resistant alcohol intake. Nature Neurosci. (2013) 16:1094–100. doi: 10.1038/nn.3445
15. Seif T, Simms J, Lei K, Wegner S, Bonci A, Messing R, et al. Serine and D-cycloserine reduce compulsive alcohol intake in rats. Neuropsychopharmacology. (2015) 40:2357–67. doi: 10.1038/npp.2015.84
16. Wegner S, Hu B, De Oliveira Sergio T, Darevsky D, Kwok C, Lei K, et al. A novel NMDA receptor-based intervention to suppress compulsion-like alcohol drinking. Neuropharmacology. (2019) 157:107681. doi: 10.1016/j.neuropharm.2019.107681
17. De Oliveira Sergio T, Lei K, Kwok C, Ghotra S, Wegner S, Walsh M, et al. The role of anterior insula-brainstem projections and alpha-1 noradrenergic receptors for compulsion-like and alcohol-only drinking. Neuropsychopharmacology. (2021) 46:1918–26. doi: 10.1038/s41386-021-01071-w
18. Kwok C, Lei K, Pedrozo V, Anderson L, Ghotra S, Walsh M, et al. Differential importance of nucleus accumbens Ox1Rs and AMPARs for female and male mouse binge alcohol drinking. Sci Rep. (2021) 11:231. doi: 10.1038/s41598-020-79935-2
19. Betka S, Harris L, Rae C, Palfi B, Pfeifer G, Sequeira H, et al. Signatures of alcohol use in the structure and neurochemistry of insular cortex: a correlational study. Psychopharmacology. (2019) 236:2579–91. doi: 10.1007/s00213-019-05228-w
20. Chen H, Lasek A. Perineuronal nets in the insula regulate aversion-resistant alcohol drinking. Addict Biol. (2020) 25:e12821. doi: 10.1111/adb.12821
21. Grodin E, Sussman L, Sundby K, Brennan G, Diazgranados N, Heilig M, et al. Neural correlates of compulsive alcohol seeking in heavy drinkers. Biol Psychiatry Cogn Neurosci Neuroimaging. (2018) 3:1022–31. doi: 10.1016/j.bpsc.2018.06.009
22. Liu J, Claus ED, Calhoun V, Hutchison K. Brain regions affected by impaired control modulate responses to alcohol and smoking cues. J Stud Alcohol Drugs. (2014) 75:808–16. doi: 10.15288/jsad.2014.75.808
23. Sommer W, Canals S, Bifone A, Heilig M, Hyytia P. From a systems view to spotting a hidden island: a narrative review implicating insula function in alcoholism. Neuropharmacology. (2022) 209:108989. doi: 10.1016/j.neuropharm.2022.108989
25. Ciccarelli A, Weijers D, Kwan W, Warner C, Bourne J, Gross C. Sexually dimorphic perineuronal nets in the rodent and primate reproductive circuit. J Comp Neurol. (2021) 529:3274–91. doi: 10.1002/cne.25167
26. Guadagno A, Verlezza S, Long H, Wong T, Walker C. It is all in the right amygdala: increased synaptic plasticity and perineuronal nets in male, but not female, juvenile rat pups after exposure to early-life stress. J Neurosci. (2020) 40:8276–91. doi: 10.1523/JNEUROSCI.1029-20.2020
27. Griffiths B, Madden A, Edwards K, Zup S, Stary C. Age-dependent sexual dimorphism in hippocampal cornu ammonis-1 perineuronal net expression in rats. Brain Behav. (2019) 9:e01265. doi: 10.1002/brb3.1265
28. Slaker ML, Harkness JH, Sorg BA. A standardized and automated method of perineuronal net analysis using Wisteria floribunda agglutinin staining intensity. IBRO Rep. (2016) 1:54–60. doi: 10.1016/j.ibror.2016.10.001
29. Harkness J, Gonzalez A, Bushana P, Jorgensen E, Hegarty D, Di Nardo A, et al. Diurnal changes in perineuronal nets and parvalbumin neurons in the rat medial prefrontal cortex. Brain Struct Funct. (2021) 226:1135–53. doi: 10.1007/s00429-021-02229-4
30. Pantazopoulos H, Gisabella B, Rexrode L, Benefield D, Yildiz E, Seltzer P, et al. Circadian rhythms of perineuronal net composition. eNeuro. (2020) 7:ENEURO.0034-19.2020. doi: 10.1523/ENEURO.0034-19.2020
31. Bauer M, McVey M, Boehm S II. Three weeks of binge alcohol drinking generates increased alcohol front-loading and robust compulsive-like alcohol drinking in male and female C57BL/6J mice. Alcohol Clin Exp Res. (2021) 45:650–60. doi: 10.1111/acer.14563
32. Sneddon E, White R, Radke A. Sex differences in binge-like and aversion-resistant alcohol drinking in C57BL/6J mice. Alcohol Clin Exp Res. (2019) 43:243–9. doi: 10.1111/acer.13923
33. Chen H, He D, Lasek A. Repeated binge drinking increases perineuronal nets in the insular cortex. Alcohol Clin Exp Res. (2015) 39:1930–8. doi: 10.1111/acer.12847
34. Lesscher H, van Kerkhof L, Vanderschuren L. Inflexible and indifferent alcohol drinking in male mice. Alcohol Clin Exp Res. (2010) 34:1219–25. doi: 10.1111/j.1530-0277.2010.01199.x
35. Gildawie K, Ryll L, Hexter J, Peterzell S, Valentine A, Brenhouse HC. A two-hit adversity model in developing rats reveals sex-specific impacts on prefrontal cortex structure and behavior. Dev Cogn Neurosci. (2021) 48:100924. doi: 10.1016/j.dcn.2021.100924
36. Gildawie K, Honeycutt J, Brenhouse H. Region-specific effects of maternal separation on perineuronal net and parvalbumin-expressing interneuron formation in male and female rats. Neuroscience. (2020) 428:23–37. doi: 10.1016/j.neuroscience.2019.12.010
37. Page C, Coutellier L. Adolescent stress disrupts the maturation of anxiety-related behaviors and alters the developmental trajectory of the prefrontal cortex in a sex- and age-specific manner. Neuroscience. (2018) 390:265–77. doi: 10.1016/j.neuroscience.2018.08.030
38. Uriarte N, Ferreno M, Mendez D, Nogueira J. Reorganization of perineuronal nets in the medial Preoptic Area during the reproductive cycle in female rats. Sci Rep. (2020) 10:5479. doi: 10.1038/s41598-020-62163-z
39. Hernandez-Vivanco A, Cano-Adamuz N, Sanchez-Aguilera A, Gonzalez-Alonso A, Rodriguez-Fernandez A, Azcoitia I. Sex-specific regulation of inhibition and network activity by local aromatase in the mouse hippocampus. Nat Commun. (2022) 13:3913. doi: 10.1038/s41467-022-31635-3
40. Laham B, Murthy S, Hanani M, Clappier M, Boyer S, Vasquez B, et al. The estrous cycle modulates early-life adversity effects on mouse avoidance behavior through progesterone signaling. Nat Commun. (2022) 13:7537. doi: 10.1038/s41467-022-35068-w
41. Centanni S, Janes A, Haggerty D, Atwood B, Hopf F. Better living through understanding the insula: why subregions can make all the difference. Neuropharmacology. (2021) 198:108765. doi: 10.1016/j.neuropharm.2021.108765
42. Gehrlach D, Dolensek N, Klein A, Roy Chowdhury R, Matthys A, Junghanel M, et al. Aversive state processing in the posterior insular cortex. Nat Neurosci. (2019) 22:1424–37. doi: 10.1038/s41593-019-0469-1
43. Marino R, Girven K, Figueiredo A, Navarrete J, Doty C, Sparta D. Binge ethanol drinking associated with sex-dependent plasticity of neurons in the insula that project to the bed nucleus of the stria terminalis. Neuropharmacology. (2021) 196:108695. doi: 10.1016/j.neuropharm.2021.108695
44. Flook E, Feola B, Avery S, Winder D, Woodward N, Heckers S, et al. BNST-insula structural connectivity in humans. Neuroimage. (2020) 210:116555. doi: 10.1016/j.neuroimage.2020.116555
45. Batalla A, Homberg J, Lipina T, Sescousse G, Luijten M, Ivanova S, et al. The role of the habenula in the transition from reward to misery in substance use and mood disorders. Neurosci Biobehav Rev. (2017) 80: 276–85.
46. Clemens A, Lenschow C, Beed P, Li L, Sammons R, Naumann R, et al. Estrus-cycle regulation of cortical inhibition. Curr Biol. (2019) 29:605–15. doi: 10.1016/j.cub.2019.01.045
Keywords: ethanol, sex differences, insula, compulsive, parvalbumin, extracellular matrix
Citation: Martins de Carvalho L, Chen H, Sutter M and Lasek AW (2023) Sexually dimorphic role for insular perineuronal nets in aversion-resistant alcohol consumption. Front. Psychiatry 14:1122423. doi: 10.3389/fpsyt.2023.1122423
Received: 12 December 2022; Accepted: 08 February 2023;
Published: 28 February 2023.
Edited by:
James K. Rowlett, University of Mississippi Medical Center, United StatesReviewed by:
Harry Pantazopoulos, University of Mississippi Medical Center, United StatesMarina Guizzetti, Oregon Health and Science University, United States
Copyright © 2023 Martins de Carvalho, Chen, Sutter and Lasek. This is an open-access article distributed under the terms of the Creative Commons Attribution License (CC BY). The use, distribution or reproduction in other forums is permitted, provided the original author(s) and the copyright owner(s) are credited and that the original publication in this journal is cited, in accordance with accepted academic practice. No use, distribution or reproduction is permitted which does not comply with these terms.
*Correspondence: Amy W. Lasek, YW15Lmxhc2VrQHZjdWhlYWx0aC5vcmc=
†Present address: Luana Martins de Carvalho and Amy W. Lasek, Department of Pharmacology and Toxicology, Virginia Commonwealth University, Richmond, VA, United States