- 1Department of Geriatric Endocrinology, Anhui Geriatric Institute, The First Affiliated Hospital of Anhui Medical University, Hefei, Anhui, China
- 2Department of Physiology, School of Basic Medical Sciences, Anhui Medical University, Hefei, Anhui, China
- 3Department of Stomatology, The Second Affiliated Hospital of Anhui Medical University, Hefei, China
- 4Department of Otorhinolaryngology Head and Neck Surgery, The First Affiliated Hospital of Anhui Medical University, Hefei, Anhui, China
- 5Department of Human Anatomy, School of Basic Medical Sciences, Anhui Medical University, Hefei, Anhui, China
- 6School of Mental Health and Psychological Sciences, Anhui Medical University, Hefei, Anhui, China
In humans and animals, exposure to changes in internal or external environments causes acute stress, which changes sleep and enhances neurochemical, neuroendocrine, and sympathetic activities. Repeated stress responses play an essential role in the pathogenesis of psychiatric diseases and sleep disorders. However, the underlying mechanism of sleep changes and anxiety disorders in response to acute stress is not well established. In the current study, the effects of restraint stress (RS) on anxiety and sleep–wake cycles in mice were investigated. We found that after RS, the mice showed anxiety-like behavior after RS manipulation and increased the amounts of both non-rapid eye movement (NREM) and rapid eye movement (REM) sleep in the dark period. The increase in sleep time was mainly due to the increased number of episodes of NREM and REM sleep during the dark period. In addition, the mice showed an elevation of the EEG power spectrum of both NREM and REM sleep 2 h after RS manipulation. There was a significant reduction in the EEG power spectrum of both NREM and REM sleep during the darkperiod in the RS condition. The expression of the c-Fos protein was significantly increased in the parabrachial nucleus, bed nucleus of the stria terminalis, central amygdala, and paraventricular hypothalamus by RS manipulation. Altogether, the findings from the present study indicated that neural circuits from the parabrachial nucleus might regulate anxiety and sleep responses to acute stress, and suggest a potential therapeutic target for RS induced anxiety and sleep alterations.
Introduction
Since the COVID-19 pandemic, the prevalence of anxiety and depression has increased, which increases the risk of a wide range of diseases and conditions, including sleep disturbances and cognitive dysfunctions (1–3). In patients with anxiety, sleep disturbance is the most common complaint, with insufficient sleep time, poor sleep quality, or alterations of sleep structure (4). In China, the lifetime prevalence of anxiety disorders is up to 7.6%, and the weighted prevalence of anxiety disorders is 5.0% (5), which indicates the need for innovative approaches to address these mental disorders. However, insomnia with comorbid anxiety is a major obstacle to improving clinical outcomes for treatment. Understanding the risk factors for sleep disorders could benefit the intervention of sleep and the prevention of psychiatric disorders.
A high level of stress is associated with an increased risk of developing several pathologies, including sleep disorders and psychiatric diseases (6, 7). More than 60% of patients with generalized anxiety disorder have insomnia symptoms, with increased wakefulness, reduced sleep amount, and less slow-wave sleep (8, 9). A 3 year follow-up of a community-based study showed that the subjects developed insomnia 1 year later, and the sleep response to stressor exposure was significantly associated with their anxiety symptoms (10). In a group of first-year training physicians, poor sleepers sleep less and tend to experience anxiety when exposed to stressors (11). These studies supported the idea that high levels of stress can lead to insomnia and mental disorders in humans. The effect of stress on emotion and sleep has been reported in many experimental studies in rodents. Restraint stress (RS) induces anxiety-like behavior in both mice and rats, with a dysfunction of the amygdala (12–14). In rats, acute RS has been shown to induce increased of wakefulness for 1 h following the RS session, and then rapid eye movement (REM) sleep and non-REM (NREM) sleep rebound (15, 16). Furthermore, 5 days of 30 min RS increased wakefulness and decreased NREM sleep in rats during the light phase (17). In a chronic stress procedure, mice that suffered from 3 weeks of daily water immersion and RS showed an increased NREM sleep time with low power density of delta band, an increased REM sleep time during the dark period in the first week, and only an increase of REM sleep time during the dark phase in the second and third weeks (18). Social defeat stress has a similar effect. Exposing mice to social stress or foot-shock stress results in increased wakefulness in the initial phase, followed by an altered sleep architecture, including prolonged NREM sleep or REM sleep (19–21). These results indicate that stressors are pathological factors that increase the risk of disturbing sleep and emotion, which may increase the development of dementia and metabolic and cardiovascular diseases (22, 23). Nevertheless, the role and potential regulatory mechanisms of stress-induced mood and sleep alterations have not been fully elucidated.
In the present study, mice received RS for 2 h for 3 consecutive days, and an open field test (OFT) and elevated plus maze (EPM) were performed to assess their anxiety-like behaviors. The sleep–wake recording was used to analyze sleep alteration after the RS treatment. Lastly, immunohistochemistry staining was carried out to estimate the neural activity induced by RS. This study attempted to reveal the effects of RS on anxiety and sleep architecture and the potential neural mechanisms in mice.
Materials and methods
Animals
The Animal Care and Use Committee of Anhui Medical University approved all animal experiments, and all experimental procedures were carried out in accordance with the Anhui Medical University Animal Experiment Guide, as previously described (23, 24). The C57BL/6 male mice (8–10 weeks old) required for the experiment were purchased from the Anhui Provincial Laboratory Animal Center, China (Certificate No. SCXK [WAN] 2022-001). We housed all mice individually in cages and maintained them in the following controlled environment: 22 ± 1°C,55 ± 5% humidity, 100 lux light intensity, 12-h light/dark cycle (Zeitgeber time, ZT 0: beginning of light period; ZT 12: beginning of dark period. Rodents’ chow and water were available ad libitum.
EEG/EMG electrodes implantation
The procedure for EEG/EMG electrodes implantation was as previously described (23, 25). In brief, all mice were anesthetized with isoflurane and fixed on a stereotaxic apparatus (68,043, RWD Life Science, Shenzhen, China), and two stainless steel pins for EEG recording were inserted into the skulls of the right frontal cortex and the right parietal cortex. The EMG electrodes were inserted into the trapezius muscle on both sides. The electrode base was then fixed on the mouse skull with dental cement. Thereafter, the mice recovered for at least 14 days in their home cages.
Restraint stress procedure
The mice used for the behavioral test received the following treatment: the mice in the RS group were put into polypropylene centrifuge tubes with multiple air holes for 2 h for three consecutive days, starting at 1 h after the lights on and lasting for 2 h (ZT 1–3) every day. Similarly, mice in the sham group received gentle handling treatment at ZT 1, similar to the RS mice, and had no access to water and food for 2 h, modified from previous reports (26, 27). All mice used for sleep recording were recovered from EEG/EMG electrode implantation and were placed in a sleep recording chamber for 3 days to acclimate. Sleep recording was started, and the EEG/EMG signals during the first 24 h were used as the data under the baseline condition. During this period, the mice have free access to water and food. The mice received RS manipulation, as described early during the sleep recording. The sleep recording was conducted continuously after the end of the RS protocol for 24 h as data for the post-RS (PRS) condition.
Open field test
The OFT was used to evaluate anxiety-like behaviors and locomotion in mice (28, 29). In a quiet environment, the mouse was placed in the center of the bottom surface of the open field reaction box (50 cm3 × 50 cm3 × 45 cm3), video recording and timing were performed at the same time, and the mouse was allowed to move freely in the box for 5 min. The bottom plane of the open field reaction chamber is divided into nine areas, including the center, perimeter, and corners. The time that the mice passed through each area within 5 min was then recorded and analyzed using a video-tracking system (Shanghai Xinruan Information Technology Co. Ltd., China).
Elevated plus maze test
The EPM test is another specific experimental paradigm for examining the state of anxiety in mice (30, 31). The EPM is composed of a pair of opposite open arms (35 cm × 5 cm) and a pair of opposite closed arms (35 cm × 5 cm × 15 cm) and a central area, which cross each other in a cross shape. At the beginning of the experiment, one mouse was placed in the central area, while videography and recording were started, and it was allowed to explore freely for 5 min. The video-tracking system was used to analyze the number of times the mouse entered the open arm and the closed arm and the residence time within the 5 min.
Sleep–wake cycle recording and scoring
After 14 days of recovery from electrode implantation, the mouse was placed individually in a sleep recording chamber for 3 days to acclimatize before starting to record sleep. The EEG and EMG signals were amplified, filtered, acquired, and digitized at a sampling rate of 128 Hz, and recorded using Vitalrecorder software (Kissei Comtec Co., Ltd., Japan). After the recording was completed, SleepSign software (Kissei Comtec Co., Ltd., Japan) was used to parse the EEG/EMG signals into NREM sleep, REM sleep, and wakefulness phases using an epoch of 4 s, according to the criteria previously reported (23, 32). All phases were manually rechecked and corrected.
Immunohistochemistry
The mice were anesthetized and perfused with 30 ml of 0.1 M phosphate buffer (PBS, PH 7.0) and 30 ml of ice-cold 4% paraformaldehyde via the heart for cervical dissection, and the brains were removed and placed in 4% paraformaldehyde overnight at 4°C. The mouse brains were then transferred to 20% sucrose and stored at 4°C until sunken. Thereafter, they were cut on the coronal surface at a thickness of 30 μm using a frozen sectioning machine (CM1950; Leica, Germany). Immunohistochemistry was performed as described previously (23). Briefly, brain slices were incubated with 0.3% H2O2 for 30 min, rinsed with PBS, and then incubated with primary antibody for c-Fos (1:1000, Abcam, Cambridge, MA, USA) overnight at 4°C. The next day, they were washed 6 times with 0.01 M PBS and incubated with secondary antibody (1,1,000, Jackson Immunoresearch Inc., West Grove, PA, USA) for 90 min at room temperature. After washing 6 times with PBS, all brain slices were incubated with the avidin-biotin complex for 60 min. The sections were then subjected to peroxidase reaction with 3,3-diaminobenzidine and nickel, and the positive cells were stained black. After washing with PBS, the sections were mounted, dried, dehydrated, and cover slipped. Images were taken using a microscope (ECLIPSE Ni-E, Nikon, Japan).
Statistical analysis
All data are expressed as mean ± SEM. Multi-factor ANOVA comparing the changes in the amount of time at each stage and EEG power density in the baseline, RS, and PRS conditions. The correlation between the sleep time and anxiety levels were evaluated by Pearson correlation during PRS condition. Student’s t-test was used to compare changes in anxiety-like behavior over time and c-Fos expression between groups. Statistical significance was achieved at a value of p of 0.05 for all tests.
Results
Restraint stress induces anxiety-like behavior in mice
The behavioral tests of OFT and EPM were used to assess anxiety-like behavior and locomotor activity in mice. The procedure for RS and behavioral testing is shown in Figure 1A. A representative travel path of a mouse in the sham group is shown in Figure 1B. The mouse traversed the center of the maze at a greater frequency than the mouse in the RS group (Figure 1C). However, there was no significant difference in the total distance traveled between the two groups of mice (t = 1.589, p = 0.156, Figure 1D). The traveled distance (t = 5.04, p < 0.01, Figure 1E) and time spent in the central area (t = 8.86, p < 0.01, Figure 1F) was significantly decreased, and the number of entries into the central area was less in the RS group of mice (t = 6.561, p < 0.01, Figure 1G) compared to the sham controls. Representative traces of one mouse in the sham group in Figure 1H and one mouse from the RS group in Figure 1L in the EPM testing are shown. The mouse in the sham group traveled both in the open and closed arms, whereas the RS mouse traveled more in the closed arms. Similarly, there was no significant difference in the total distance between the two groups in the EPM testing (t = 1.103, p = 0.306, Figure 1J). The traveled distance (t = 9.704, p < 0.01, Figure 1K) and time spent in the open arms (t = 6.511, p < 0.01, Figure 1L) were shorter, and the number of entries into the open arms (t = 5.974, p < 0.01, Figure 1M) were significantly reduced in the RS group compared to the control group. These results indicate that RS induces anxiety-like behavior independent of the general locomotor ability of mice.
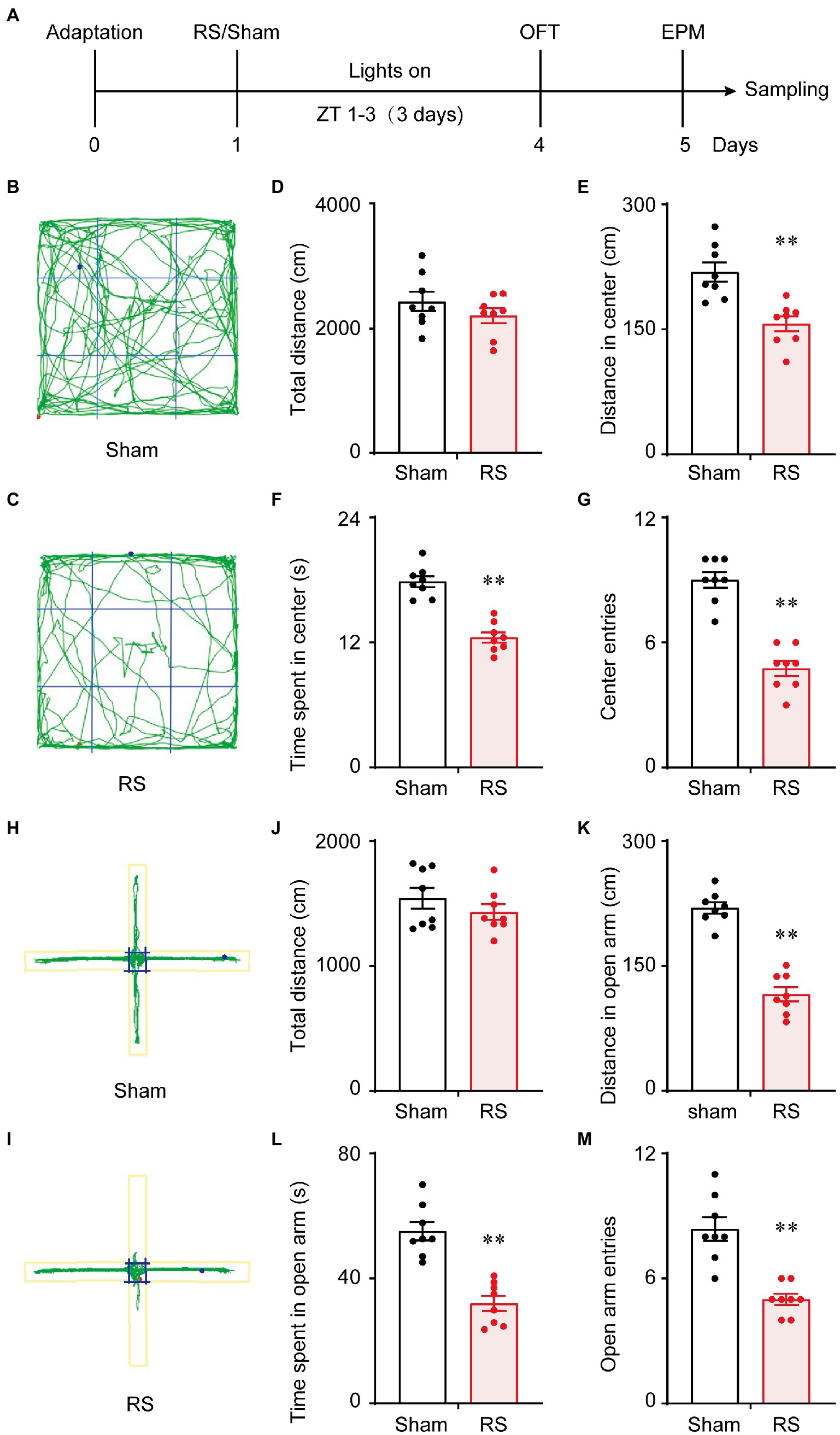
Figure 1. Behavioral experimental schedule and acute restraint stress induced anxiety-like behavior. Schedule for conducting the OFT and EPM (A). OFT trajectory diagram of the sham group (B) and the RS group (C). Total distance traveled for 5 min in the OFT, the distance and time spent in the center, and the number of times the mice entered the center of the OFT (D–G). EPM trajectory diagram of the sham group (H) and the RS group (I). Total distance traveled during 5 min in the EMP, the distance and the time spent in the open arm, and the number of times the mice entered the open arm of the EPM (J–M). **p < 0.01 vs. sham, n = 8. RS, restraint stress; OFT, open field test; EPM, elevated plus maze; ZT, Zeitgeber time.
Restraint stress alters the sleep–wake cycles of mice
Next, we assessed the effect of RS on sleep–wake profiles in mice. After capturing the baseline of sleep–wake rhythms, RS was carried out for 2 h (ZT 1–3) in the mice with the sleep–wake recording simultaneously for 3 days, as shown in Figure 2A. Representative traces of the EEG/EMG and hypnogram are shown in Figure 2B. The RS manipulation induced a significantly increased wakefulness and decreased NREM and REM sleep during the manipulation (*p < 0.05, **p < 0.01, Figures 2C–E) when compared to the baseline. After the RS for 3 days, the mice still tended to show an increase in both NREM and REM sleep amount, with decreased wakefulness during the dark period, even under the drive of circadian rhythm and feeding (#p < 0.05, ##p < 0.01, compared to RS, Figures 2C–E). Furthermore, the cumulated time was calculated during the light and dark periods. During ZT 3–5 (lights on), the time spent in arousals in the RS mice were significantly increased, when compared to baseline (F(2,15) = 38.3, p < 0.01, Figure 3A). After the 3 days of RS, the amount of wakefulness was returned to the baseline (F(2,15) = 38.3, p < 0.01, compared to RS). Correspondingly, both time spent in NREM (F(2,15) = 30.4, p < 0.01, Figure 3B) and REM sleep (F(2,15) = 32.3, p < 0.01, Figure 3C) were significantly decreased in RS mice compared to the baseline, and the time spent in NREM (F(2,15) = 30.4, p < 0.01, compared to RS, Figure 3B) and REM sleep (F(2,15) = 32.3, p < 0.01, compared to RS, Figure 3C) were recovered during the PRS condition. During the ZT 5–12 light period, the time of wakefulness in the RS mice was significantly decreased when compared to baseline (F (2,15) = 5.1, p < 0.05, Figure 3D). No significant differences were found among the time spent on NREM, and REM sleep in the baseline, RS, and PRS conditions (Figures 3E,F). No significant differences were detected among the cumulative time of wakefulness, NREM, and REM sleep in the baseline, RS, and PRS conditions at ZT 3–12 during the light period (Figures 3G–I). By contrast, during the 12 h dark phase (ZT 12–24), the time spent in wakefulness was less in the RS and PRS states than that in the baseline condition (F(2,15) = 50.5, p < 0.01, Figure 3J), although the wake time spent in the PRS state was significantly increased compared to the RS state (F(2,15) = 50.5, p < 0.01, Figure 3J). Conversely, when compared to the baseline condition, both the time spent in NREM (F(2,15) = 35.1, p < 0.01, Figure 3K) and REM sleep (F(2, 15) = 13.2, p < 0.01, Figure 3L) in the RS and PRS conditions dramatically increased. When we compared the total amount of wakefulness, NREM sleep and REM sleep during 24 h, the wakefulness time was significantly decreased (F(2,15) = 7.3, p < 0.01, Figure 3M), whereas the REM sleep time significantly increased (F(2,15) = 4.1, p < 0.05, Figure 3O) in the PRS condition, compared to baseline. These results indicate that RS manipulation resulted in an initial wakefulness and sleep response during the dark period, even after the termination of RS manipulation.
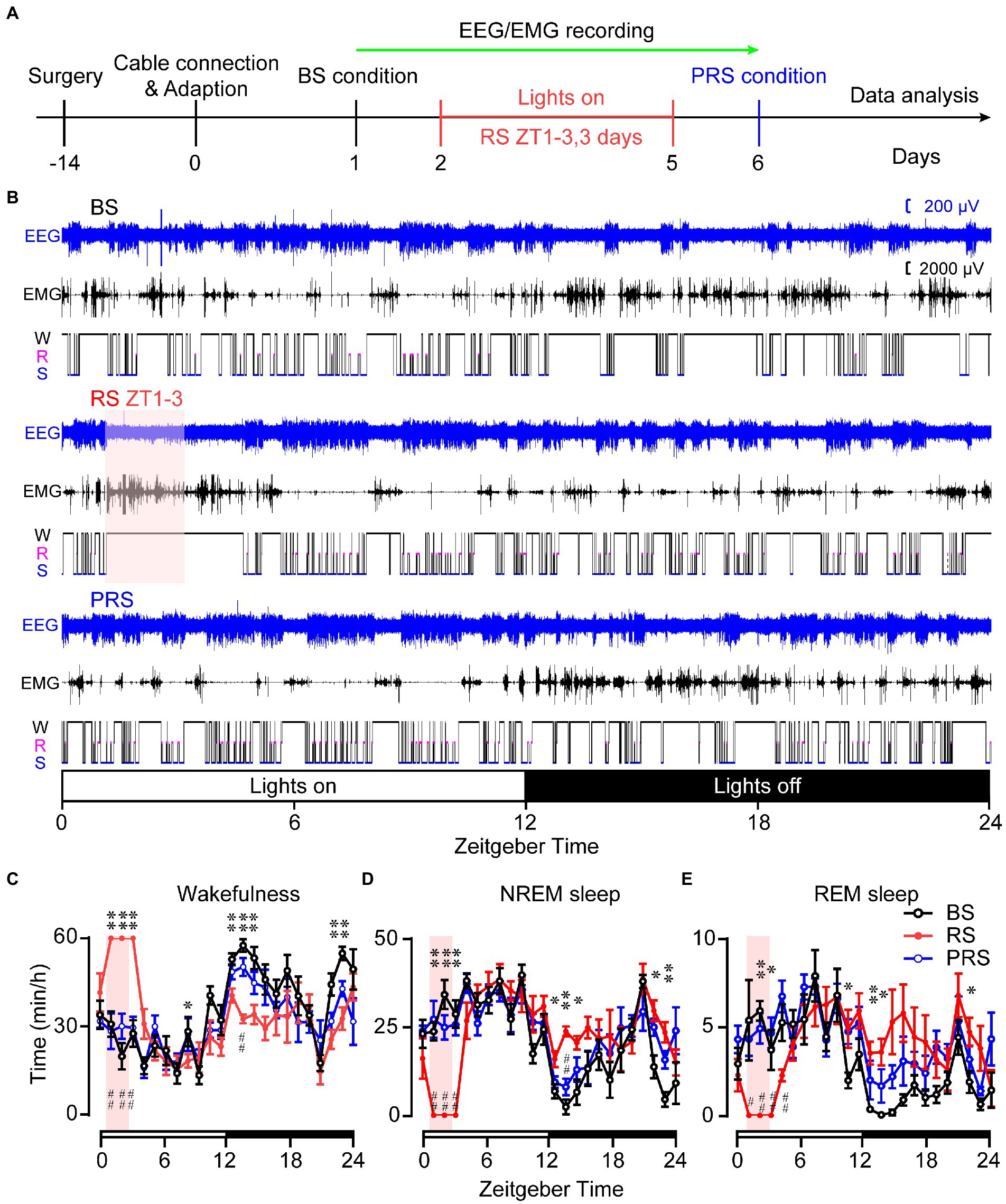
Figure 2. Time spent in the sleep–wake stages of the baseline, RS, and PRS conditions. Timeline and manipulations for conducting the sleep–wake recording. (A) Example of 24 h EEG/EMG traces and the corresponding hypnogram under the BS, RS, and PRS conditions. (B) Time spent in wakefulness (C), NREM sleep (D), and REM sleep (E) during a 24-h period. The pink box indicates restraint stress during 9:00–11:00. The open and closed bars above the X-axis indicate the light and dark periods, respectively. *p < 0.05, **p < 0.01 versus baseline, #p < 0.05, ##p < 0.01 versus RS, n = 6. BS: baseline; RS: restraint stress; PRS: post-restraint stress; ZT: Zeitgeber time.
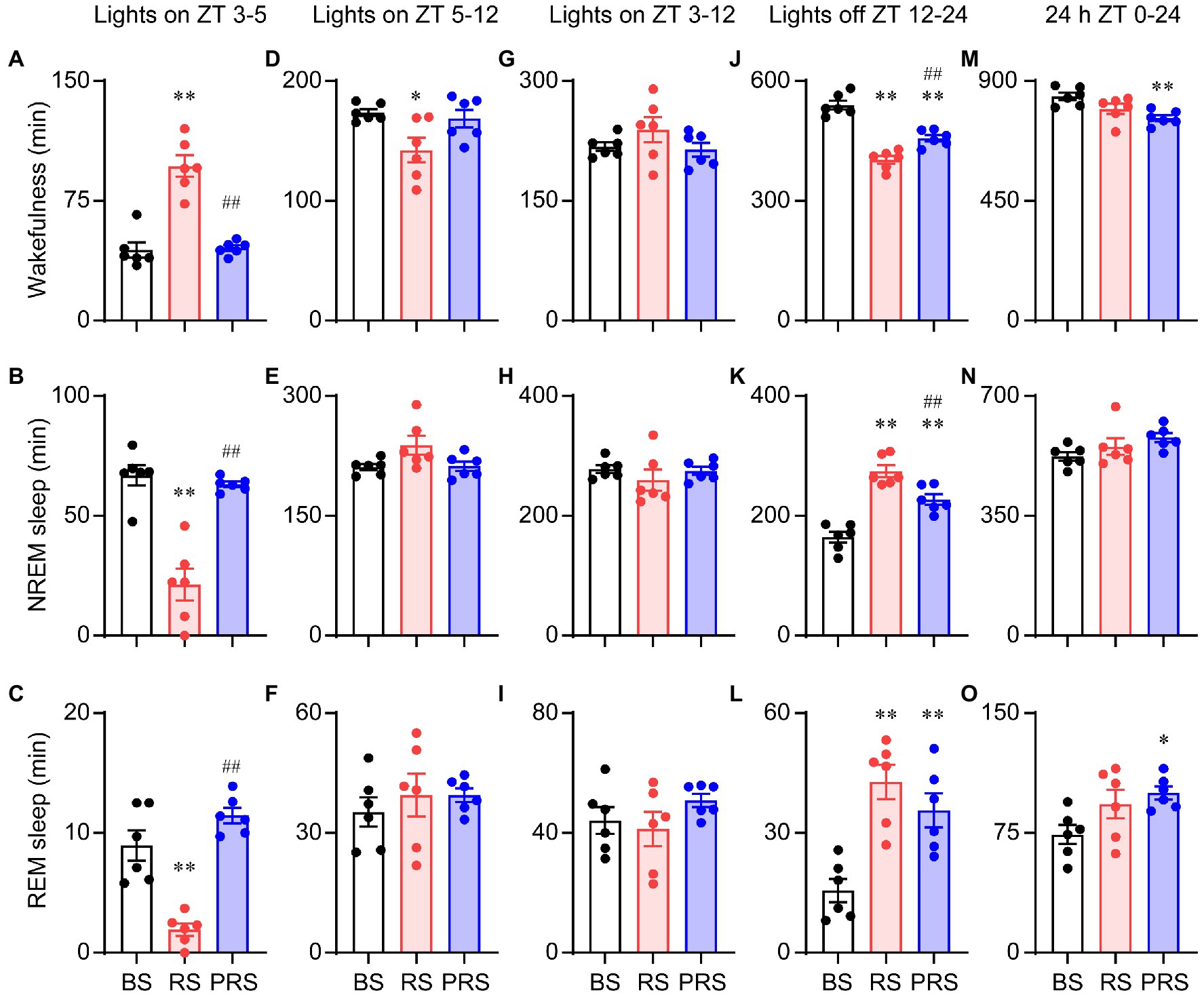
Figure 3. Cumulative time in the sleep–wake stages of the baseline, RS, and PRS conditions. Cumulative time of wakefulness (A), NREM sleep (B), and REM sleep (C) during ZT 3–5 (lights on). Cumulative time of wakefulness (D), NREM sleep (E), and REM sleep (F) during ZT 5–12 (lights on). Cumulative time of wakefulness (G), NREM sleep (H), and REM sleep (I) during ZT 3–12 (lights on). Cumulative time of wakefulness (J), NREM sleep (K), and REM sleep (L) during ZT 12–24 (lights off). Cumulative time of wakefulness (M), NREM sleep (N), and REM sleep (O) during ZT 0–24 (24 h). *p < 0.05, **p < 0.01 versus baseline, ##p < 0.01 versus RS, n = 6. BS, baseline; RS, restraint stress; PRS, post-restraint stress; ZT, Zeitgeber time.
Restraint stress changes the sleep–wake architecture of mice
To assess the sleep–wake architectures of the mice during and after the RS manipulation, we independently calculated the episode number and mean duration of wakefulness, NREM, and REM sleep during the day and night periods. As shown in Figure 4, there was a significant increase in the mean duration of wakefulness in the RS condition at ZT 1–3 (lights on) compared to baseline. A significant decrease in the mean duration of wakefulness was detected in the PRS, compared to RS condition (F(2,15) = 66.0, p < 0.01, Figure 4A). The mean duration of REM sleep was increased in the RS, compared to baseline (F(2,15) = 5.2, p < 0.05, Figure 4C). In the PRS condition, both the mean duration of NREM sleep (F(2,15) = 7.3, p < 0.01, Figure 4B) and REM sleep (F(2,15) = 5.2, p < 0.05, Figure 4C) were decreased when compared to the RS condition. Correspondingly, the number of wakefulness, NREM sleep, and REM sleep episodes significantly decreased in the RS condition compared to baseline and returned to baseline after the RS manipulation (F(2,15) = 41.8, p < 0.01, Figure 4D). In addition, the RS manipulation reduced the sleep–wake transitions compared to baseline (F(2,15) = 55.7, p < 0.01, Figure 4E). Based on these results, the RS resulted in an initial elevated wakefulness by increasing the mean duration and decreasing the number of wakefulness episodes.
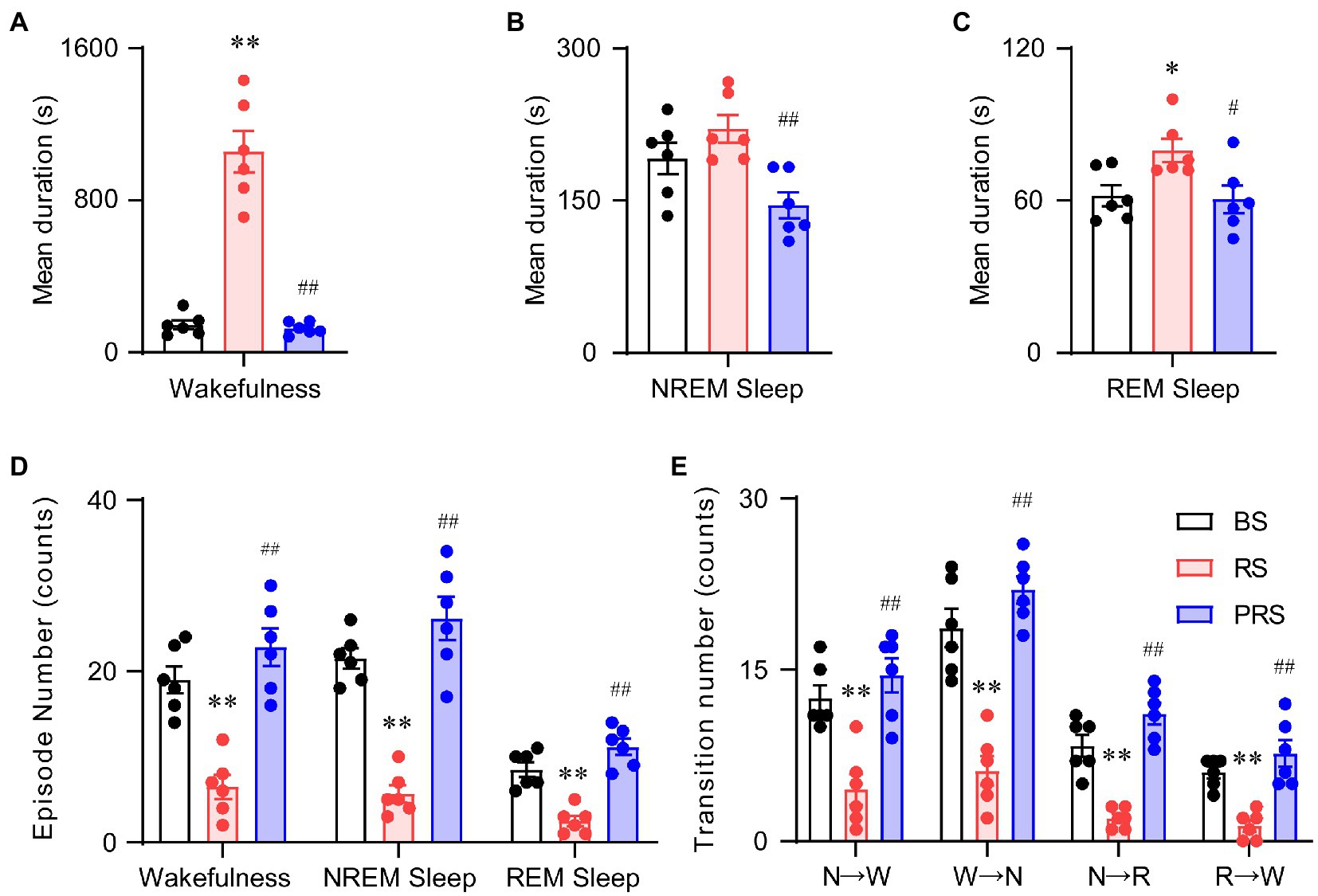
Figure 4. Sleep–wake architectures in the baseline, RS, and PRS conditions during ZT 3–5. Mean durations of wakefulness (A), NREM sleep (B), and REM sleep. (C) Episode numbers of each stage (D) and stage transitions between stages (E) during the 2 h in the lights on period (ZT 3–5) after restraint stress. *p < 0.05, **p < 0.01 versus baseline, #p < 0.05, ##p < 0.01 versus RS, n = 6. BS, baseline; RS, restraint stress; PRS, post-restraint stress. R, REM sleep; S, NREM sleep; W, wakefulness.
During the late light period (ZT 5–12), no significant differences were observed in the mean duration of wakefulness among the three conditions (F(2,15) = 2.5, p = 0.11, Figure 5A). However, the mean duration of both the NREM (F(2,15) = 16.5, p < 0.01, Figure 5B) and REM sleep (F(2,15) = 20.9, p < 0.01, Figure 5C) significantly increased in the RS condition, compared with baseline. Compared to the RS condition, the mean duration of REM sleep decreased in the PRS condition, which was still much longer than that in baseline (F(2,15) = 20.9, p < 0.01, Figure 5C). The episode numbers of wakefulness and REM sleep had no dramatic differences among the three conditions, whereas compared to baseline, the episode number of the NREM sleep was significantly decreased (F(2,15) = 6.3, p < 0.05, Figure 5D). There were no significant changes in the number of sleep–wake transitions during the three conditions (F(2,15) = 3.1, p = 0.07, Figure 5E). The above results indicate that RS manipulation resulted in sleep fragmentation, although there were no significant differences between the time spent in NREM and REM sleep.
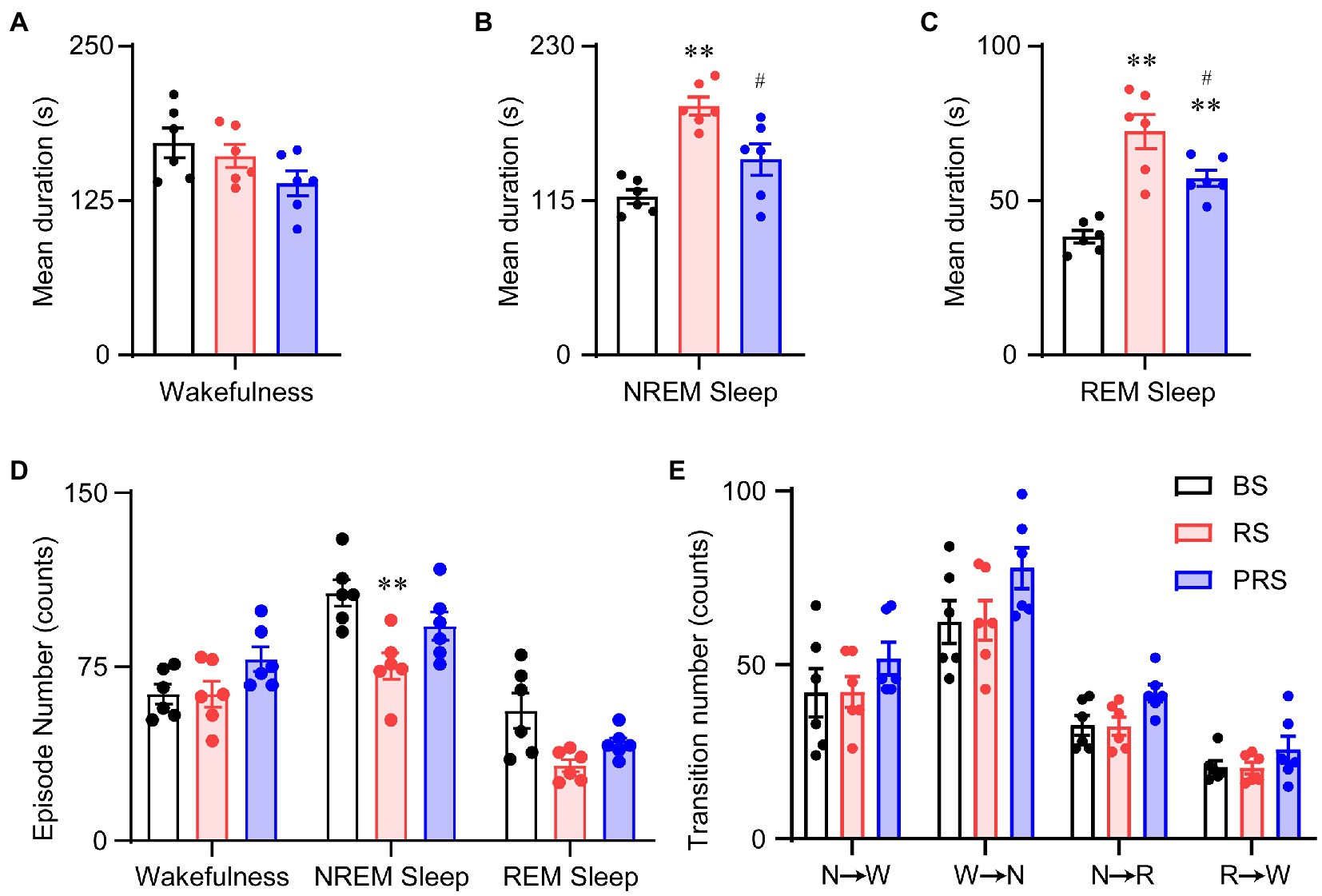
Figure 5. Sleep–wake architectures in the baseline, RS, and PRS groups during ZT 5–12. Mean durations of wakefulness (A), NREM sleep (B), REM sleep (C), and episode numbers for each stage (D) during ZT 5–12. (E) Stage transitions between stages during ZT 5–12. **p < 0.01 versus baseline, #p < 0.05 versus RS, n = 6. BS, baseline; RS, restraint stress; PRS, post-restraint stress; R, REM sleep; S, NREM sleep; W, wakefulness.
In the dark phase at ZT 12–24, the wakefulness duration decreased in both the RS and PRS conditions, compared to the baseline. However, compared to that in the RS, the wakefulness duration in the PRS condition was partially recovered (F(2,15) = 135.4, p < 0.01, Figure 6A). The NREM sleep duration was reduced in the RS condition (F(2,15) = 5.1, p < 0.05, Figure 6B). There was no significant difference in the REM sleep duration among the three conditions (F(2,15) = 0.2, p = 0.8, Figure 6C). In general, the number of episodes of all stages dramatically increased in the RS and PRS conditions at ZT 12–24 compared to the baseline (F(2,15) = 37.5, p < 0.01, Figure 6D). In addition, there was an increase in the transition numbers between the stages of sleep–wake cycles (F(2,15) = 17.5, p < 0.01, Figure 6E). These results suggest that the lethargy of mice under RS and PRS conditions during the dark phase depended on the increasing NREM and REM sleep episodes.
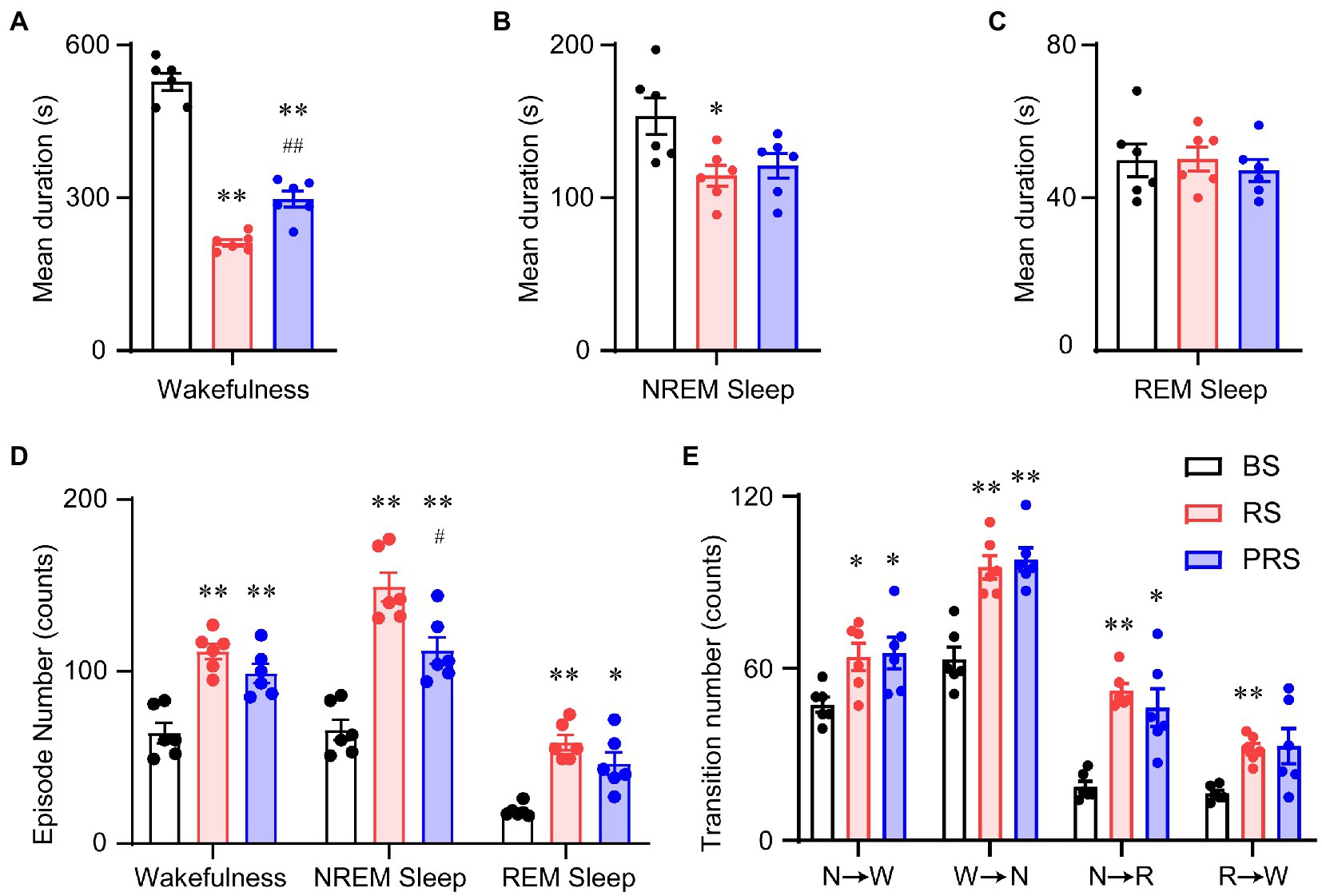
Figure 6. Sleep–wake architectures in the baseline, RS, and PRS groups during ZT 12–24. Mean durations of wakefulness (A), NREM sleep (B), REM sleep (C). Episode numbers of each stage (D) during ZT 12–24. (E) Stage transitions between stages during 12 h at ZT12–24. *p < 0.05, **p < 0.01 versus baseline, #p < 0.05, ##p < 0.01 versus RS, n = 6. BS, baseline; RS, restraint stress; PRS, post-restraint stress; R, REM sleep; S, NREM sleep; W, wakefulness.
Effect of restraint stress manipulation on sleep power density
To assess the effect of RS on sleep quality in mice, EEG power density was calculated among the baseline, RS, and PRS conditions. During the day period at ZT 3–5, the EEG power density of wakefulness decreased of the frequency band from 1.25 Hz to 2.5 Hz, and increased from 4.5 Hz to 5.75 Hz in the RS, compared to the baseline (p < 0.05, Figure 7A). Both the frequency band from 2.25 Hz to 4.25 Hz during NREM sleep (p < 0.05, Figure 7B) and the frequency band from 3.0 Hz to 4.5 Hz and from 6.75 Hz to 9.25 Hz during REM sleep (p < 0.05, Figure 7C) significantly increased in the RS condition compared to the baseline. The EEG power density of wakefulness increased from 4.0 Hz to 5.25 Hz in the RS condition compared to the baseline (p < 0.05, Figure 7D). In contrast, the EEG power density of NREM and REM sleep recovered to baseline levels in the PRS condition. During the light period ZT5–12, the difference in EEG power density among the groups was not significant (Figures 7E,F). However, during the dark period (ZT 12–24), the EEG power density of the frequency band from 1.0 Hz to 2.75 Hz of wakefulness, the frequency band from 2.5 Hz to 4.75 Hz of NREM sleep and the frequency band from 7.0 Hz to 8.25 Hz of REM sleep in the RS group decreased significantly compared to the baseline (p < 0.05, Figures 7G–I). These results indicate that RS stress affected the sleep quality of the mice in both the light and dark periods.
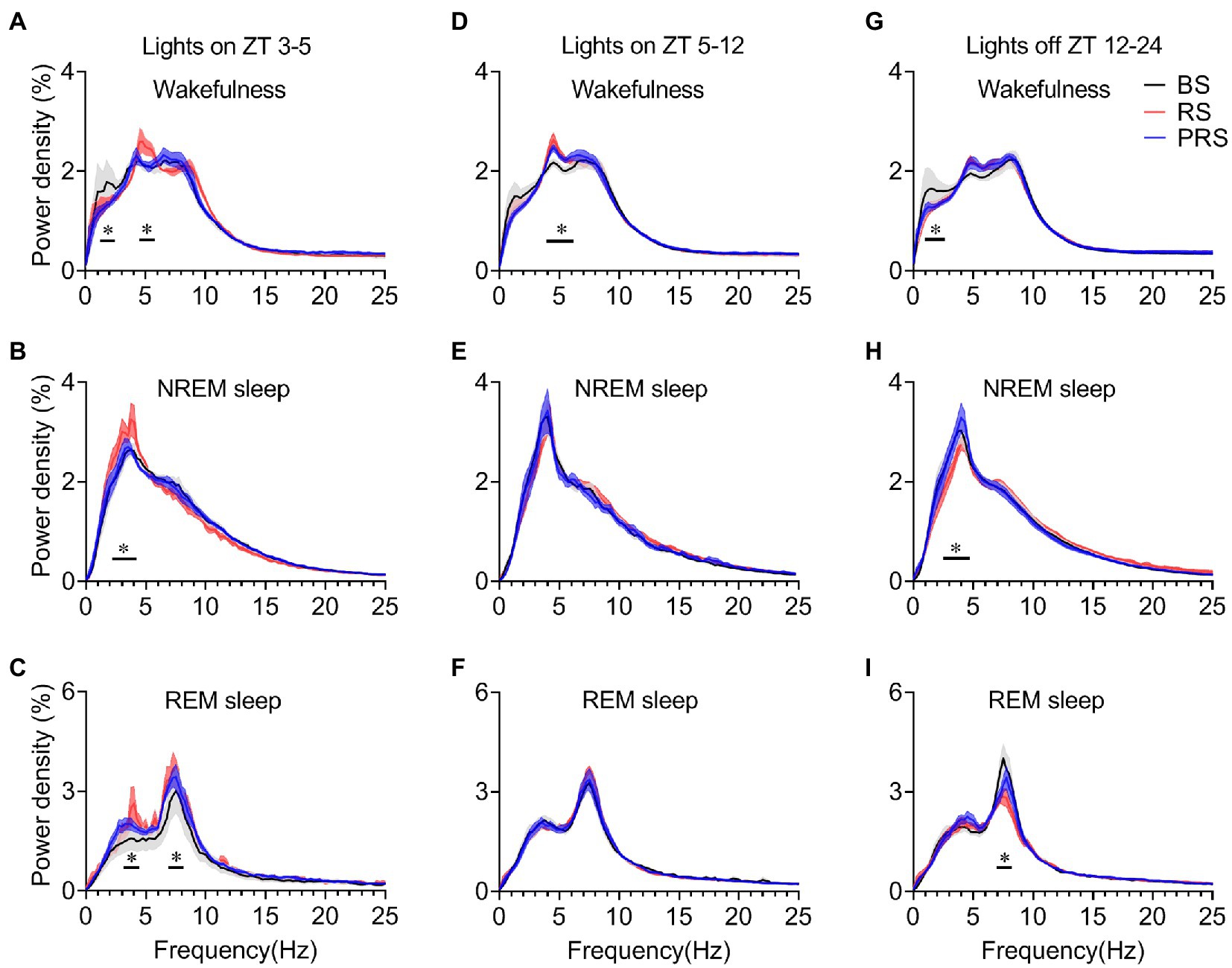
Figure 7. Changes in EEG power density during wakefulness, NREM sleep and REM sleep in the three time periods. EEG power density of wakefulness (A), NREM sleep (B) and REM sleep (C) during ZT 3–5. EEG power density of wakefulness (D), NREM sleep (E) and REM sleep (F) during ZT 5–12. EEG power density of wakefulness (G), NREM sleep (H), and REM sleep (I) during ZT 12–24. *p < 0.05, compared with baseline, n = 6. BS, baseline; RS, restraint stress; PRS, post-restraint stress; ZT, Zeitgeber time.
Relationship between sleep amount and anxiety levels after RS manipulation
Given that anxiety and depression are often preceded by sleep disorders in humans, we next investigated the relationship between sleep amount and anxiety levels after the RS for 3 days (during the PRS condition). The higher anxiety levels of rodents were indicated by the shorter distance traveled in the center arena in the OFT and the less time spent in the open arms of the EPM (33). Both the time of NREM and REM sleep during the dark period was negatively correlated with the distance in the center in OFT and time spent in open arms in the EPM (Figures 8A–D). In other words, sleep time during the night period was positively correlated with anxiety levels after RS treatment. However, there was no correlation between sleep time during the light period and anxiety-like phenotypes (Figures 8E–H). Thus, these results suggest that the increased sleep time during the dark period might reflect anxiety levels after RS manipulation.
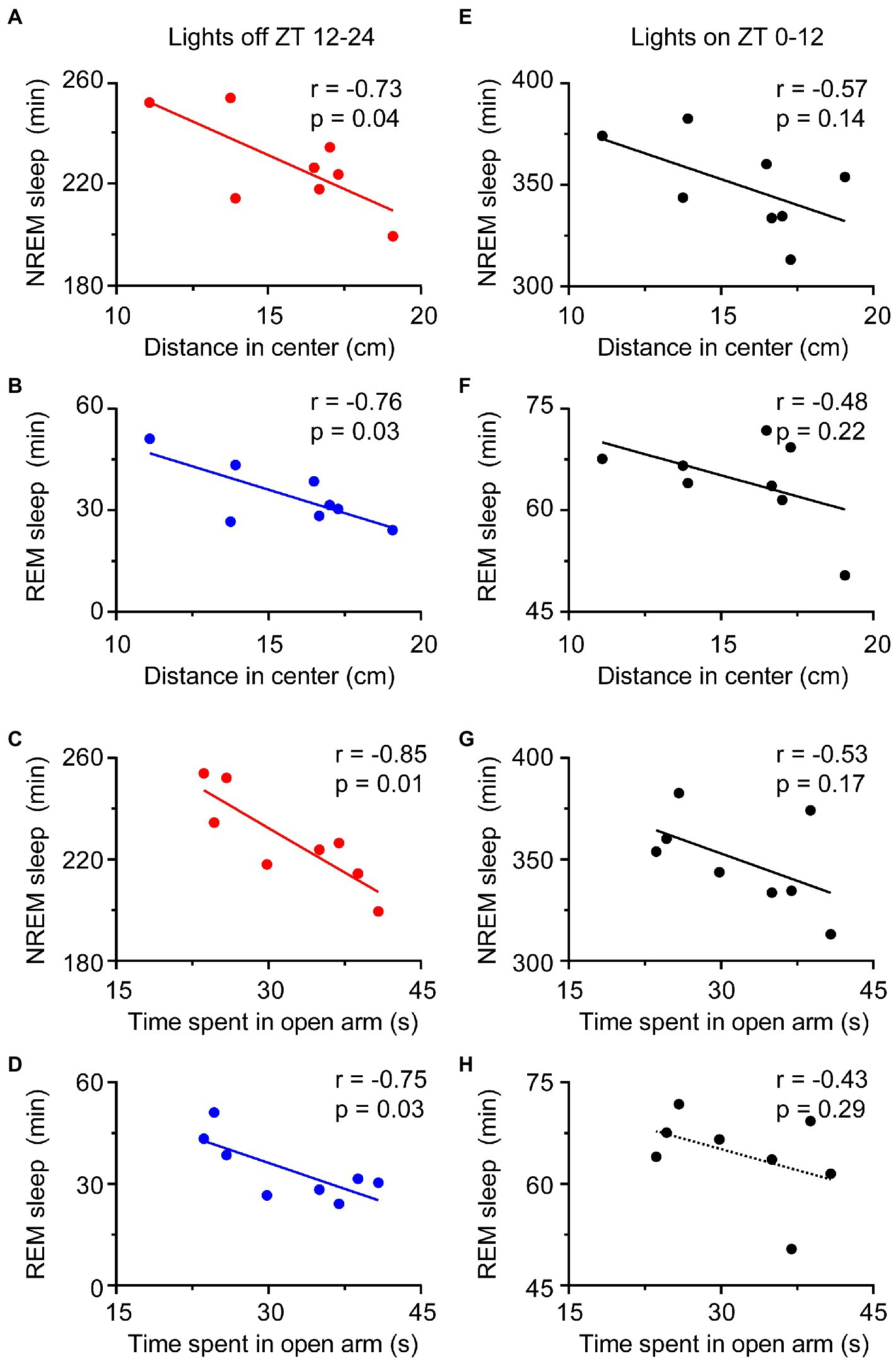
Figure 8. Relationship of sleep amount and anxiety-like behaviors during the PRS condition. The amount of NREM and REM sleep during the night period correlated with anxiety-like behaviors (A–D), but the amount of NREM and REM sleep during the day period was not correlated with anxiety-like behaviors (E–H). n = 8. ZT, Zeitgeber time.
Restraint stress drives the expression of the c-Fos protein in mouse brain
To further investigate the potential mechanism of RS-induced anxiety and sleep, we detected the c-Fos protein by immunohistochemical staining in the brains of the RS mice and sham controls. As shown in Figure 9, very few neurons expressed c-Fos in the parabrachial nucleus (PB, Figure 9A), the bed nucleus of the stria terminalis (BNST, Figure 9C), the central amygdala (CEA, Figure 9E), and the paraventricular hypothalamus (PVH, Figure 9G) in the sham group. In contrast, robust expression of c-Fos was observed in the PB (Figure 9B), BNST (Figure 9D), CEA (Figure 9F), and PVH (Figure 9H) of the RS mice. A quantitative analysis of the number of c-Fos-positive neurons revealed a significant increase in the PB (t = 20.5, p < 0.01, Figure 9I), BNST (t = 11.9, p < 0.01, Figure 9J), CEA (t = 20.1, p < 0.01, Figure 9K), and PVH (t = 25.7, p < 0.01, Figure 9L) of RS mice, compared to the sham group. These results suggest that the nuclei, including PB, BNST, CEA, and PVH, may contribute to RS-induced changes in sleep with anxiety-like behavior.
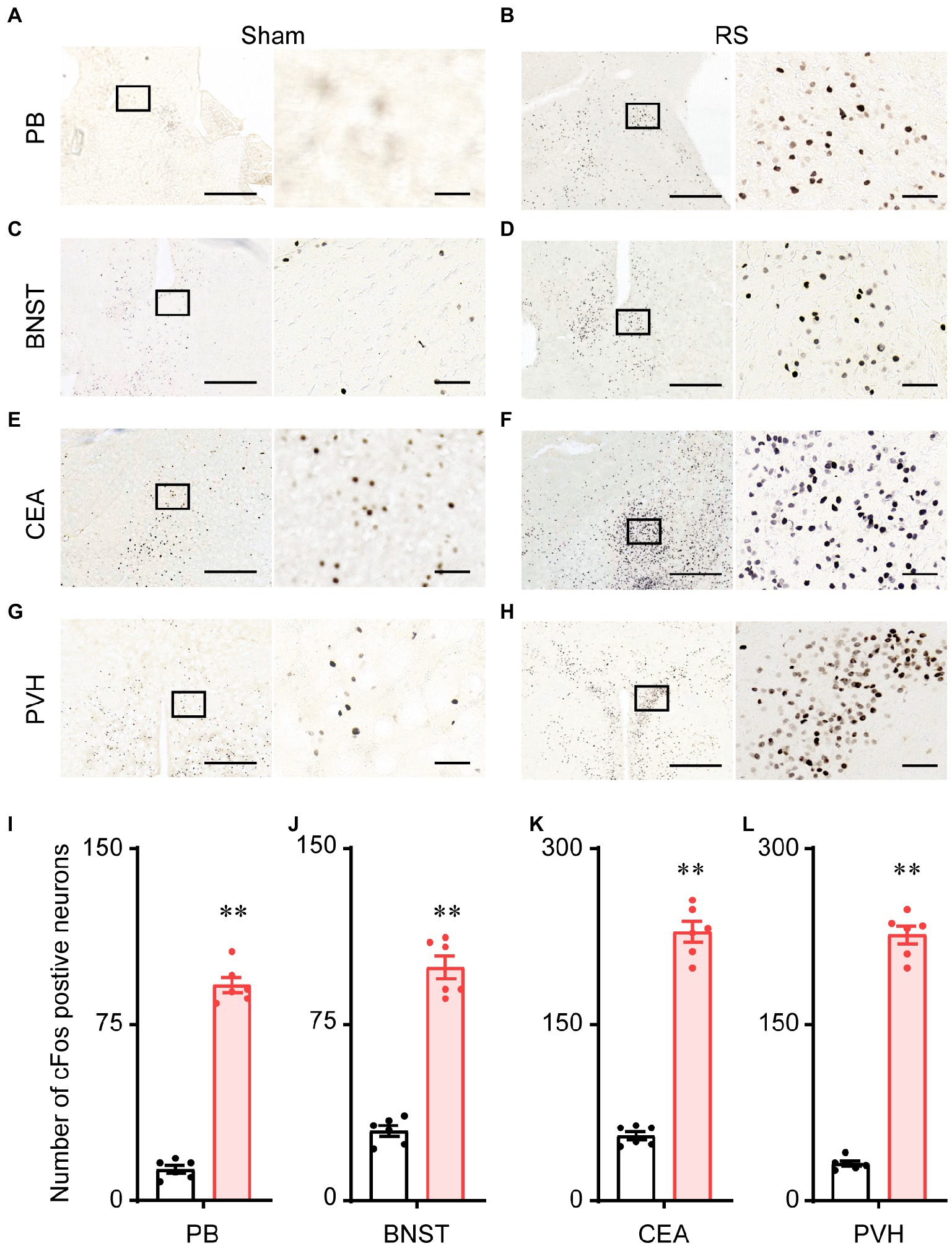
Figure 9. Expression of the c-Fos protein in the mouse brain. Representative images of c-Fos expression in the PB (A,B), BNST (C,D), CEA (E,F), and PVH (G,H) after sham and RS manipulation in mice. (I–L). Mean numbers of c-Fos-positive neurons in PB (I), BNST (J), CEA (K), and PVH (L). The right panel shows an enlargement of the box in the left panel. Scale bar: 500 μm in the left panels and 50 μm in the right panels. **p < 0.01, compared with sham, n = 6. RS, restraint stress; PB, parabrachial nucleus; BNST, bed nucleus of the stria terminalis; CEA, central amygdala; PVH, paraventricular hypothalamus.
Discussion
In the present study, we performed restraint stress on mice to analyze its effects on anxiety-like behavior and sleep–wake cycles. The results showed that 2 h of RS manipulation for 3 days led to anxiety-like behavior and sleep disturbance. RS also led to a sleep response during the subsequent dark period, when the mice were more active at baseline. In particular, the increased episode numbers of NREM and REM sleep resulted in hypersomnia of RS mice during the dark period, even when the 3 days of RS were terminated. Acute restraint stress-induced sleep fragmentation may affect neural activity in the central nervous system, and thereby contribute to mental disorders. The results of c-Fos expression in the anxiety-and sleep-related nuclei suggested a potential neural network that mediated these effects of restraint stress in mice.
Anxiety disorders are a very common mental illness in the general population (34). Then are the most prevalent class of lifetime psychiatric diseases, with a prevalence of up to 5.0% in China over the last 12 months (5). Here, acute restraint stress for 3 days resulted in anxiety behaviors in mice without leading to impaired mobilization capacity (Figure 1). Numerous studies have shown that acute or chronic restraint stress induces anxiety-like behavior in rodents, which is caused by dysfunction of the amygdala or BNST (13, 14, 35, 36). Similarly, a previous study observed anxiety-like behaviors in mice that underwent immobilization stress with increasing corticosterone levels in the blood and the activation of nuclear factor-κB and microglia in the hippocampus (37). These results indicate that restraint stress comprises both physical stimuli and psychological components that lead to dysfunction of the central nervous system and cause mental disorders. Hospital intensive care units are stressful environments for both patients and their families. When the patients were admitted to the intensive care unit, 32% of their family members experienced anxiety disorders, 16% of family members experienced depression, and 15% of family members experienced posttraumatic stress symptoms during the 3-month follow-up (38). These results suggest that acute or traumatic events can have long-term consequences for the brain and behavior. Therefore, the prevention of acute stress may prohibit such effects from developing into chronic and irreversible pathological states.
Anxiety disorders and depression are accompanied by sleep disturbances (7, 39). Sleep disorders affect more than 50% of patients with anxiety disorders and can lead to increased anxiety (40, 41). Compared to healthy subjects, patients with anxiety disorders showed prolonged sleep latency and a significant reduction in total sleep time and sleep efficiency (42). Here, the mice that suffered from RS showed an immediate loss of both NREM and REM sleep, which was followed by a strong sleep rebound during the dark period (Figures 3J–L), when the mice had to overcome the drive of circadian rhythm and food seeking during the dark period (43). NREM and REM sleep rebounds were still observed during the recovery night, indicating the long-term effect of restraint on sleep in mice. The elevation in NREM and REM sleep times was consistent with previous reports in mice that experienced chronic restraint stress with water immersion (18), or acute social defeat stress (21, 44). Although one of the most characteristic features of sleep in depressive patients is an increase in REM sleep (45, 46), whether increased sleep after restraint is beneficial or detrimental to anxiety is unclear. Our correlation analysis showed that anxiety levels were negatively correlated with sleep time during the post-restraint condition (Figure 8), suggesting that sleep recovery may help relieve anxiety in mice. When people are exposed to trauma, a longer duration of REM sleep episodes is associated with a lower likelihood of developing posttraumatic stress disorder (47, 48). A shorter latency to sleep onset was predictive of lower levels of anxiety-like behaviors in social defeat stressed mice (44). These findings support the idea that sleep plays a beneficial role in treating anxiety. Antidepressants, including serotonin and norepinephrine reuptake inhibitors, monoamine oxidase inhibitors, and selective serotonin reuptake inhibitors, decrease the amount of REM sleep in patients with psychiatric disease (49). REM sleep deprivation or serotonin and noradrenaline re-uptake inhibitors reduced anxiety-like behavior in rodents (50, 51), indicating that the increased REM is detrimental for emotion. In addition, patients with mood disorders often experience sleep fragmentation (52). The mice after restraint showed a decreased mean duration of wakefulness and NREM sleep with increased episode numbers and transitions between each stage (Figures 4–6). These findings demonstrate overnight sleep disruption in restrained mice with anxiety-like behaviors. In mice, a chronic mild stress paradigm can also lead to fragmented sleep, with changes in neurochemistry and neuroendocrine factors (53). Other mechanisms, such as stress-induced corticotropin-releasing hormones and prolactin, may be involved in sleep regulation (20, 54). However, further studies are needed to elucidate the relationship between stress induced sleep and anxiety-like behaviors.
Stress also induces alterations in EEG power density in humans and animals (21, 55). Slow-wave sleep is thought to correlate with changes in the levels of stress hormones (56). Patients with depression often show decreased slow-wave sleep (57). Thus, changes in sleep amount and EEG power density may contribute to the emergence of anxiety and depression-like behaviors in restraint mice. Here, we found that during ZT 3–5 of the light period, the delta power density of NREM sleep increased in RS mice, whereas the delta power density of NREM sleep in PRS conditions returned to the baseline. Similar results were observed in REM sleep across the baseline, RS, and PRS conditions. The increased EEG power density during NREM and REM sleep in RS conditions is consistent with previous reports in mice and rats with acute social defeat stress (21, 58). However, during the dark period, the power density of both the NREM and REM sleep decreased in RS conditions (Figure 7), with the amount of sleep increasing (Figure 3). Our results support the idea that more anxious individuals experience severe sleep disturbances.
The exact neuronal mechanisms of the restraint stress-induced behavior response are unknown. Restraint stress induces anxiety-like behavior with dysfunction of the amygdala (12–14, 35), including connectivity with the medial prefrontal cortex (12) and changes in dendritic spine density and spine type (14). The PB is located in the dorsolateral pons, which plays an essential role in the development of anxiety-related behaviors (59). The PB relays external information, such as pain, itch, and threat to the hypothalamus, CEA, and BNST, which are critical for modulating affective states (60, 61). Consistent with these previous findings, the c-Fos mapping results from the present study showed robust expression of c-Fos in the lateral PB, CEA, and BNST after restraint stress (Figure 9). Yu et al. showed that a subset of neurons in the ventral tegmental area, which project to the lateral hypothalamus, are activated and induce sleep by social defeat. However, the release of corticotrophin-releasing hormone in the PVH can be inhibited by the activation of the same group of neurons in the midbrain (62). The corticotrophin-releasing hormone secreted from the PVH, which initiates the hypothalamic–pituitary adrenal axis, drives corticosterone synthesis and mediates the stress response (63, 64). These results provide insights into the neural mechanisms that link stress and sleep. In addition, the PB plays an important role in sleep–wake regulation (65, 66). The activation of neurotensinergic neurons in the PB induces anxiety-like behavior and increased wakefulness, which largely project to the emotional control nuclei, such as the CEA and BNST (67). Thus, the neural circuits from PB may regulate anxiety-like behavior and sleep responses to restraint stress. Future studies employing cutting-edge technologies, such as optogenetics and chemogenetics may provide more clues to elucidate the neural mechanisms of stress-induced anxiety and sleep.
There has been abundant research confirming that women are more likely than men to have anxiety and mood disorders (68–70). According to a meta-analysis of 29 studies, women are 41% more likely to have insomnia than men. Furthermore, women have a significantly greater risk of sleep disorders than men, especially with increasing age (71). The high prevalence of anxiety and sleep disorders in women may be due to hormonal changes during menstruation, pregnancy, and menopause (72, 73). However, only male mice were used in the present study, which might be a limitation of our study. Thus, we could not compare gender differences in stress-induced anxiety and sleep.
Conclusion
The present study revealed anxiety-like behaviors and sleep responses related to acute restraint stress in mice. Sleep amount, architecture, and intensity were affected by restraint stress, especially during the dark period. The robust expression of the c-Fos protein in the brain after RS suggests that PB networks may play an important role in coping with stress.
Data availability statement
The raw data supporting the conclusions of this article will be made available by the authors, without undue reservation.
Ethics statement
The animal study was reviewed and approved by The Animal Care and Use Committee of Anhui Medical University.
Author contributions
QX, C-BG, and M-KZ conceived and designed the study. Y-XX, Z-ZJ, Y-YL, and J-LL carried out the experiments. Y-XX, G-YL, Y-LW, and D-XM analyzed the data. C-BG, M-KZ, X-YW, and QX wrote the manuscript. All authors contributed to the study and approved the final version.
Funding
This study was supported in part by grants-in-aid for scientific research from the National Natural Science Foundation of China (81401100), Natural Science Foundation of Anhui Province (2208085MH225, 2108085MH282, and 1508085QH155), The Key Program in the Youth Elite Support Plan in Universities of Anhui Province (gxyqZD2018020), Natural Science Foundation of Anhui Education Department (KJ2018A0174), Foundational and Clinical Collaborative Research Project from Anhui Medical University (2019xkjT013 and 2022xkjT026), Scientific Research Platform and Base Upgrading Plan of Anhui Medical University (2021xkjT048), the doctoral research fund project of the First Affiliated Hospital of Anhui Medical University (1545), and the National College Students’ Innovation and Entrepreneurship Training Program (202210366039).
Conflict of interest
The authors declare that the research was conducted in the absence of any commercial or financial relationships that could be construed as a potential conflict of interest.
Publisher’s note
All claims expressed in this article are solely those of the authors and do not necessarily represent those of their affiliated organizations, or those of the publisher, the editors and the reviewers. Any product that may be evaluated in this article, or claim that may be made by its manufacturer, is not guaranteed or endorsed by the publisher.
References
1. Caycho-Rodriguez, T, Tomas, JM, Vilca, LW, Carbajal-Leon, C, Cervigni, M, Gallegos, M, et al. Socio-demographic variables, fear of Covid-19, anxiety, and depression: prevalence, relationships and explanatory model in the general population of seven latin american countries. Front Psychol. (2021) 12:695989. doi: 10.3389/fpsyg.2021.695989
2. Radfar, A, Ferreira, MM, Sosa, JP, and Filip, I. Emergent crisis of Covid-19 pandemic: mental health challenges and opportunities. Front Psych. (2021) 12:631008. doi: 10.3389/fpsyt.2021.631008
3. Wang, W, Guo, Y, Du, X, Li, W, Wu, R, Guo, L, et al. Associations between poor sleep quality, anxiety symptoms, and depressive symptoms among Chinese adolescents before and during Covid-19: a longitudinal study. Front Psych. (2021) 12:786640. doi: 10.3389/fpsyt.2021.786640
4. Chellappa, SL, and Aeschbach, D. Sleep and anxiety: from mechanisms to interventions. Sleep Med Rev. (2022) 61:101583. doi: 10.1016/j.smrv.2021.101583
5. Huang, Y, Wang, Y, Wang, H, Liu, Z, Yu, X, Yan, J, et al. Prevalence of mental disorders in China: a cross-sectional epidemiological study. Lancet Psychiatry. (2019) 6:211–24. doi: 10.1016/S2215-0366(18)30511-X
6. Kalmbach, DA, Anderson, JR, and Drake, CL. The impact of stress on sleep: pathogenic sleep reactivity as a vulnerability to insomnia and circadian disorders. J Sleep Res. (2018) 27:e12710. doi: 10.1111/jsr.12710
7. Manzar, MD, Alghadir, AH, Khan, M, Salahuddin, M, Albougami, A, Maniago, JD, et al. Anxiety symptoms are associated with higher psychological stress, poor sleep, and inadequate sleep hygiene in collegiate young adults-a cross-sectional study. Front Psych. (2021) 12:677136. doi: 10.3389/fpsyt.2021.677136
8. Monti, JM, and Monti, D. Sleep disturbance in generalized anxiety disorder and its treatment. Sleep Med Rev. (2000) 4:263–76. doi: 10.1053/smrv.1999.0096
9. Johnson, EO, Roth, T, and Breslau, N. The association of insomnia with anxiety disorders and depression: exploration of the direction of risk. J Psychiatr Res. (2006) 40:700–8. doi: 10.1016/j.jpsychires.2006.07.008
10. Kalmbach, DA, Pillai, V, Arnedt, JT, Anderson, JR, and Drake, CL. Sleep system sensitization: evidence for changing roles of etiological factors in insomnia. Sleep Med. (2016) 21:63–9. doi: 10.1016/j.sleep.2016.02.005
11. Kalmbach, DA, Abelson, JL, Arnedt, JT, Zhao, Z, Schubert, JR, and Sen, S. Insomnia symptoms and short sleep predict anxiety and worry in response to stress exposure: a prospective cohort study of medical interns. Sleep Med. (2019) 55:40–7. doi: 10.1016/j.sleep.2018.12.001
12. Liu, WZ, Zhang, WH, Zheng, ZH, Zou, JX, Liu, XX, Huang, SH, et al. Identification of a prefrontal cortex-to-amygdala pathway for chronic stress-induced anxiety. Nat Commun. (2020) 11:2221. doi: 10.1038/s41467-020-15920-7
13. Luo, ZY, Huang, L, Lin, S, Yin, YN, Jie, W, Hu, NY, et al. Erbin in amygdala parvalbumin-positive neurons modulates anxiety-like behaviors. Biol Psychiatry. (2020) 87:926–36. doi: 10.1016/j.biopsych.2019.10.021
14. Moreno-Martinez, S, Tendilla-Beltran, H, Sandoval, V, Flores, G, and Terron, JA. Chronic restraint stress induces anxiety-like behavior and remodeling of dendritic spines in the central nucleus of the amygdala. Behav Brain Res. (2022) 416:113523. doi: 10.1016/j.bbr.2021.113523
15. Koehl, M, Bouyer, JJ, Darnaudery, M, Le Moal, M, and Mayo, W. The effect of restraint stress on paradoxical sleep is influenced by the circadian cycle. Brain Res. (2002) 937:45–50. doi: 10.1016/s0006-8993(02)02463-0
16. Bonnet, C, Leger, L, Baubet, V, Debilly, G, and Cespuglio, R. Influence of a 1 H immobilization stress on sleep states and corticotropin-like intermediate lobe peptide (clip or Acth18-39, Ph-Acth18-39) brain contents in the rat. Brain Res. (1997) 751:54–63. doi: 10.1016/s0006-8993(96)01390-x
17. Gargiulo, AT, Jasodanand, V, Luz, S, O'Mara, L, Kubin, L, Ross, RJ, et al. Sex differences in stress-induced sleep deficits. Stress. (2021) 24:541–50. doi: 10.1080/10253890.2021.1879788
18. Yasugaki, S, Liu, CY, Kashiwagi, M, Kanuka, M, Honda, T, Miyata, S, et al. Effects of 3 weeks of water immersion and restraint stress on sleep in mice. Front Neurosci. (2019) 13:1072. doi: 10.3389/fnins.2019.01072
19. Sanford, LD, Yang, L, and Tang, X. Influence of contextual fear on sleep in mice: a strain comparison. Sleep. (2003) 26:527–40. doi: 10.1093/sleep/26.5.527
20. Meerlo, P, Easton, A, Bergmann, BM, and Turek, FW. Restraint increases prolactin and rem sleep in C57bl/6j mice but not in Balb/Cj mice. Am J Physiol Regul Integr Comp Physiol. (2001) 281:R846–54. doi: 10.1152/ajpregu.2001.281.3.R846
21. Fujii, S, Kaushik, MK, Zhou, X, Korkutata, M, and Lazarus, M. Acute social defeat stress increases sleep in mice. Front Neurosci. (2019) 13:322. doi: 10.3389/fnins.2019.00322
22. Ba, L, Huang, L, He, Z, Deng, S, Xie, Y, Zhang, M, et al. Does chronic sleep fragmentation Lead to Alzheimer's disease in young wild-type mice? Front Aging Neurosci. (2021) 13:759983. doi: 10.3389/fnagi.2021.759983
23. Xu, YX, Liu, GY, Jiang, Q, Bi, HQ, Wang, SC, Zhang, PP, et al. Effect of restricted feeding on metabolic health and sleep-wake rhythms in aging mice. Front Neurosci. (2021) 15:745227. doi: 10.3389/fnins.2021.745227
24. Xu, YX, Wang, C, Li, XD, Guo, WL, Liu, GY, Zhang, HB, et al. Activation of cholinergic basal forebrain neurons improved cognitive functions in adult-onset hypothyroid mice. Biomed Pharmacother. (2022) 153:113495. doi: 10.1016/j.biopha.2022.113495
25. Xu, Q, Xu, XH, Qu, WM, Lazarus, M, Urade, Y, and Huang, ZL. A mouse model mimicking human first night effect for the evaluation of hypnotics. Pharmacol Biochem Behav. (2014) 116:129–36. doi: 10.1016/j.pbb.2013.11.029
26. Tang, H, Hao, S, Chen, X, Li, Y, Yin, Z, Zou, Y, et al. Epigallocatechin-3-gallate protects immunity and liver drug-metabolism function in mice loaded with restraint stress. Biomed Pharmacother. (2020) 129:110418. doi: 10.1016/j.biopha.2020.110418
27. Zhang, Z, Shao, S, Zhang, Y, Jia, R, Hu, X, Liu, H, et al. Xiaoyaosan slows cancer progression and ameliorates gut Dysbiosis in mice with chronic restraint stress and colorectal cancer xenografts. Biomed Pharmacother. (2020) 132:110916. doi: 10.1016/j.biopha.2020.110916
28. Zhang, J, Xie, J, Liang, Y, Li, Y, Zhang, Y, Wang, C, et al. Anxiolytic effects, metabolism and plasma pharmacokinetics of 3, 6′ -Disinapoylsucrose. Biomed Pharmacother. (2022) 149:112913. doi: 10.1016/j.biopha.2022.112913
29. Ngoupaye, GT, Yassi, FB, Bahane, DAN, Pahaye, DB, and Ngo, BE. Antidepressant and anti-amnesic effects of the aqueous Lyophilisate of the leaves of Leptadenia Arborea on an animal model of cognitive deficit associated depression. Biomed Pharmacother. (2020) 130:110603. doi: 10.1016/j.biopha.2020.110603
30. Yi, SS, Zou, JJ, Meng, L, Chen, HM, Hong, ZQ, Liu, XF, et al. Ultrasound stimulation of prefrontal cortex improves lipopolysaccharide-induced depressive-like behaviors in mice. Front Psych. (2022) 13:864481. doi: 10.3389/fpsyt.2022.864481
31. Coelho, AA, Vila-Verde, C, Sartim, AG, Uliana, DL, Braga, LA, Guimaraes, FS, et al. Inducible nitric oxide synthase inhibition in the medial prefrontal cortex attenuates the Anxiogenic-like effect of acute restraint stress via Cb1 receptors. Front Psych. (2022) 13:923177. doi: 10.3389/fpsyt.2022.923177
32. Oishi, Y, Xu, Q, Wang, L, Zhang, BJ, Takahashi, K, Takata, Y, et al. Slow-wave sleep is controlled by a subset of nucleus accumbens core neurons in mice. Nat Commun. (2017) 8:734. doi: 10.1038/s41467-017-00781-4
33. Sudakov, SK, Nazarova, GA, Alekseeva, EV, and Bashkatova, VG. Estimation of the level of anxiety in rats: differences in results of open-field test, elevated plus-maze test, and vogel's conflict test. Bull Exp Biol Med. (2013) 155:295–7. doi: 10.1007/s10517-013-2136-y
34. Kandola, A, Vancampfort, D, Herring, M, Rebar, A, Hallgren, M, Firth, J, et al. Moving to beat anxiety: epidemiology and therapeutic issues with physical activity for anxiety. Curr Psychiatry Rep. (2018) 20:63. doi: 10.1007/s11920-018-0923-x
35. Ciccocioppo, R, de Guglielmo, G, Hansson, AC, Ubaldi, M, Kallupi, M, Cruz, MT, et al. Restraint stress alters nociceptin/orphanin FQ and CRF systems in the rat central amygdala: significance for anxiety-like behaviors. J Neurosci. (2014) 34:363–72. doi: 10.1523/JNEUROSCI.2400-13.2014
36. Zhao, D, Wang, D, Wang, W, Dai, J, Cui, M, Wu, M, et al. The altered sensitivity of acute stress induced anxiety-related behaviors by modulating insular cortex-paraventricular thalamus-bed nucleus of the stria terminalis neural circuit. Neurobiol Dis. (2022) 174:105890. doi: 10.1016/j.nbd.2022.105890
37. Jang, HM, Lee, KE, Lee, HJ, and Kim, DH. Immobilization stress-induced Escherichia coli causes anxiety by inducing Nf-Kappab activation through gut microbiota disturbance. Sci Rep. (2018) 8:13897. doi: 10.1038/s41598-018-31764-0
38. Beesley, SJ, Hopkins, RO, Holt-Lunstad, J, Wilson, EL, Butler, J, Kuttler, KG, et al. Acute physiologic stress and subsequent anxiety among family members of ICU patients. Crit Care Med. (2018) 46:229–35. doi: 10.1097/CCM.0000000000002835
39. Li, Q, Zhang, H, Zhang, M, Li, T, Ma, W, An, C, et al. Prevalence and risk factors of anxiety, depression, and sleep problems among caregivers of people living with neurocognitive disorders during the Covid-19 pandemic. Front Psych. (2020) 11:590343. doi: 10.3389/fpsyt.2020.590343
40. Blake, MJ, Trinder, JA, and Allen, NB. Mechanisms underlying the association between insomnia, anxiety, and depression in adolescence: implications for behavioral sleep interventions. Clin Psychol Rev. (2018) 63:25–40. doi: 10.1016/j.cpr.2018.05.006
41. Oh, CM, Kim, HY, Na, HK, Cho, KH, and Chu, MK. The effect of anxiety and depression on sleep quality of individuals with high risk for insomnia: a population-based study. Front Neurol. (2019) 10:849. doi: 10.3389/fneur.2019.00849
42. Papadimitriou, GN, and Linkowski, P. Sleep disturbance in anxiety disorders. Int Rev Psychiatry. (2005) 17:229–36. doi: 10.1080/09540260500104524
43. Cano, G, Mochizuki, T, and Saper, CB. Neural circuitry of stress-induced insomnia in rats. J Neurosci. (2008) 28:10167–84. doi: 10.1523/JNEUROSCI.1809-08.2008
44. Feng, X, Zhao, HY, Shao, YJ, Lou, HF, Zhu, LY, Duan, S, et al. Anxiolytic effect of increased NREM sleep after acute social defeat stress in mice. Neurosci Bull. (2020) 36:1137–46. doi: 10.1007/s12264-020-00473-y
45. Wang, YQ, Li, R, Zhang, MQ, Zhang, Z, Qu, WM, and Huang, ZL. The neurobiological mechanisms and treatments of REM sleep disturbances in depression. Curr Neuropharmacol. (2015) 13:543–53. doi: 10.2174/1570159x13666150310002540
46. Li, Z, Zhao, X, Feng, L, Zhao, Y, Pan, W, Liu, Y, et al. Can daytime transcranial direct current stimulation treatment change the sleep electroencephalogram complexity of REM sleep in depressed patients? A double-blinded, randomized, placebo-controlled trial. Front Psych. (2022) 13:851908. doi: 10.3389/fpsyt.2022.851908
47. Mellman, TA, Bustamante, V, Fins, AI, Pigeon, WR, and Nolan, B. Rem sleep and the early development of posttraumatic stress disorder. Am J Psychiatry. (2002) 159:1696–701. doi: 10.1176/appi.ajp.159.10.1696
48. Mellman, TA, Kobayashi, I, Lavela, J, Wilson, B, and Hall Brown, TS. A relationship between rem sleep measures and the duration of posttraumatic stress disorder in a young adult urban minority population. Sleep. (2014) 37:1321–6. doi: 10.5665/sleep.3922
49. Wichniak, A, Wierzbicka, A, Walecka, M, and Jernajczyk, W. Effects of antidepressants on sleep. Curr Psychiatry Rep. (2017) 19:63. doi: 10.1007/s11920-017-0816-4
50. Suchecki, D, Tiba, PA, and Tufik, S. Hormonal and behavioural responses of paradoxical sleep-deprived rats to the elevated plus maze. J Neuroendocrinol. (2002) 14:549–54. doi: 10.1046/j.1365-2826.2002.00812.x
51. de Oliveira, RA, Cunha, GM, Borges, KD, de Bruin, GS, dos Santos-Filho, EA, Viana, GS, et al. The effect of venlafaxine on behaviour, body weight and striatal monoamine levels on sleep-deprived female rats. Pharmacol Biochem Behav. (2004) 79:499–506. doi: 10.1016/j.pbb.2004.09.001
52. Lopes, MC, Quera-Salva, MA, and Guilleminault, C. Non-rem sleep instability in patients with major depressive disorder: subjective improvement and improvement of non-rem sleep instability with treatment (Agomelatine). Sleep Med. (2007) 9:33–41. doi: 10.1016/j.sleep.2007.01.011
53. Nollet, M, Hicks, H, McCarthy, AP, Wu, H, Moller-Levet, CS, Laing, EE, et al. Rem Sleep's unique associations with corticosterone regulation, apoptotic pathways, and behavior in chronic stress in mice. Proc Natl Acad Sci U S A. (2019) 116:2733–42. doi: 10.1073/pnas.1816456116
54. Kimura, M, Muller-Preuss, P, Lu, A, Wiesner, E, Flachskamm, C, Wurst, W, et al. Conditional corticotropin-releasing hormone overexpression in the mouse forebrain enhances rapid eye movement sleep. Mol Psychiatry. (2010) 15:154–65. doi: 10.1038/mp.2009.46
55. Zhang, Y, Wu, W, Toll, RT, Naparstek, S, Maron-Katz, A, Watts, M, et al. Identification of psychiatric disorder subtypes from functional connectivity patterns in resting-state electroencephalography. Nat Biomed Eng. (2021) 5:309–23. doi: 10.1038/s41551-020-00614-8
56. Chapotot, F, Buguet, A, Gronfier, C, and Brandenberger, G. Hypothalamo-pituitary-adrenal Axis activity is related to the level of central arousal: effect of sleep deprivation on the association of high-frequency waking electroencephalogram with cortisol release. Neuroendocrinology. (2001) 73:312–21. doi: 10.1159/000054648
57. Medina, AB, Lechuga, DA, Escandon, OS, and Moctezuma, JV. Update of sleep alterations in depression. Sleep Sci. (2014) 7:165–9. doi: 10.1016/j.slsci.2014.09.015
58. Kamphuis, J, Lancel, M, Koolhaas, JM, and Meerlo, P. Deep sleep after social stress: NREM sleep slow-wave activity is enhanced in both winners and losers of a conflict. Brain Behav Immun. (2015) 47:149–54. doi: 10.1016/j.bbi.2014.12.022
59. Kim, SY, Adhikari, A, Lee, SY, Marshel, JH, Kim, CK, Mallory, CS, et al. Diverging neural pathways assemble a behavioural state from separable features in anxiety. Nature. (2013) 496:219–23. doi: 10.1038/nature12018
60. Jimenez, JC, Su, K, Goldberg, AR, Luna, VM, Biane, JS, Ordek, G, et al. Anxiety cells in a hippocampal-hypothalamic circuit. Neuron. (2018) 97:670–683.e6 e6. doi: 10.1016/j.neuron.2018.01.016
61. Li, JN, and Sheets, PL. Spared nerve injury differentially alters parabrachial monosynaptic excitatory inputs to molecularly specific neurons in distinct subregions of the central amygdala. Pain. (2020) 161:166–76. doi: 10.1097/j.pain.0000000000001691
62. Yu, X, Zhao, G, Wang, D, Wang, S, Li, R, Li, A, et al. A specific circuit in the midbrain detects stress and induces restorative sleep. Science. (2022) 377:63–72. doi: 10.1126/science.abn0853
63. Qin, C, Li, J, and Tang, K. The paraventricular nucleus of the hypothalamus: development, function, and human diseases. Endocrinology. (2018) 159:3458–72. doi: 10.1210/en.2018-00453
64. Yuan, Y, Wu, W, Chen, M, Cai, F, Fan, C, Shen, W, et al. Reward inhibits paraventricular CRH neurons to relieve stress. Curr Biol. (2019) 29:1243–1251.e4 e4. doi: 10.1016/j.cub.2019.02.048
65. Kaur, S, and Saper, CB. Neural circuitry underlying waking up to hypercapnia. Front Neurosci. (2019) 13:401. doi: 10.3389/fnins.2019.00401
66. Xu, Q, Wang, DR, Dong, H, Chen, L, Lu, J, Lazarus, M, et al. Medial parabrachial nucleus is essential in controlling wakefulness in rats. Front Neurosci. (2021) 15:645877. doi: 10.3389/fnins.2021.645877
67. Chen, J, Gannot, N, Li, X, Zhu, R, Zhang, C, and Li, P. Control of emotion and wakefulness by Neurotensinergic neurons in the parabrachial nucleus. Neurosci Bull. (2022):1–13. doi: 10.1007/s12264-022-00994-8
68. Hankin, BL. Development of sex differences in depressive and co-occurring anxious symptoms during adolescence: descriptive trajectories and potential explanations in a multiwave prospective study. J Clin Child Adolesc Psychol. (2009) 38:460–72. doi: 10.1080/15374410902976288
69. Altemus, M, Sarvaiya, N, and Neill, EC. Sex differences in anxiety and depression clinical perspectives. Front Neuroendocrinol. (2014) 35:320–30. doi: 10.1016/j.yfrne.2014.05.004
70. Wensu, Z, Xidi, Z, Shaojie, L, Baohua, Z, Yunhan, Y, Huilan, X, et al. Does the presence of anxiety and depression symptoms mediate the association between family functions and self-efficacy in pregnant women in the third trimester?: a community-based cross-sectional survey. Front Psych. (2021) 12:726093. doi: 10.3389/fpsyt.2021.726093
71. Zhang, B, and Wing, YK. Sex differences in insomnia: a meta-analysis. Sleep. (2006) 29:85–93. doi: 10.1093/sleep/29.1.85
72. Harrington, YA, Parisi, JM, Duan, D, Rojo-Wissar, DM, Holingue, C, and Spira, AP. Sex hormones, sleep, and memory: interrelationships across the adult female lifespan. Front Aging Neurosci. (2022) 14:800278. doi: 10.3389/fnagi.2022.800278
Keywords: anxiety, mouse, sleep disorder, restraint stress, parabrachial nucleus
Citation: Xu Y-X, Liu G-Y, Ji Z-Z, Li Y-Y, Wang Y-L, Wu X-Y, Liu J-L, Ma D-X, Zhong M-K, Gao C-B and Xu Q (2023) Restraint stress induced anxiety and sleep in mice. Front. Psychiatry. 14:1090420. doi: 10.3389/fpsyt.2023.1090420
Edited by:
Agata Gabryelska, Medical University of Lodz, PolandReviewed by:
Ya-Dong Li, Shanghai Jiao Tong University, ChinaWilliam Wisden, Imperial College London, United Kingdom
Copyright © 2023 Xu, Liu, Ji, Li, Wang, Wu, Liu, Ma, Zhong, Gao and Xu. This is an open-access article distributed under the terms of the Creative Commons Attribution License (CC BY). The use, distribution or reproduction in other forums is permitted, provided the original author(s) and the copyright owner(s) are credited and that the original publication in this journal is cited, in accordance with accepted academic practice. No use, distribution or reproduction is permitted which does not comply with these terms.
*Correspondence: Ming-Kui Zhong, bWluZ2t1aXpob25nQGFobXUuZWR1LmNu; Chao-Bing Gao, Z2NiMTEwMDExQDE2My5jb20=; Qi Xu, cWl4dUBhaG11LmVkdS5jbg==
†These authors have contributed equally to this work