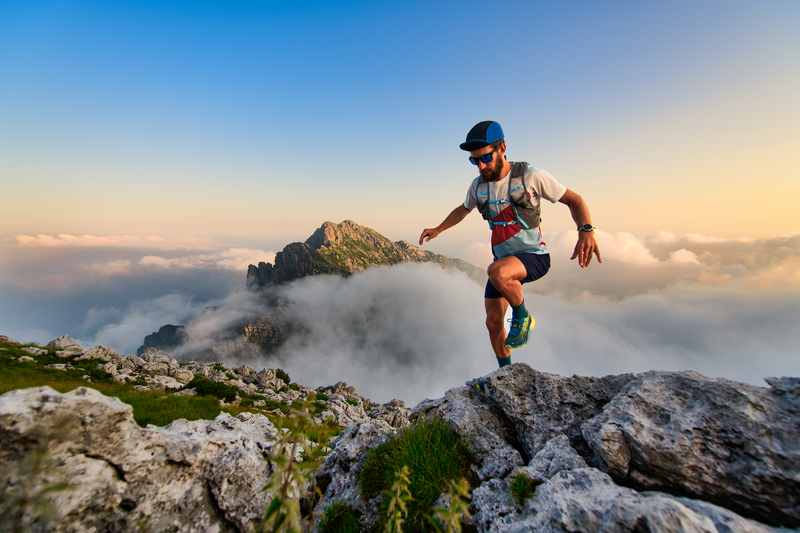
95% of researchers rate our articles as excellent or good
Learn more about the work of our research integrity team to safeguard the quality of each article we publish.
Find out more
ORIGINAL RESEARCH article
Front. Psychiatry , 22 November 2022
Sec. Molecular Psychiatry
Volume 13 - 2022 | https://doi.org/10.3389/fpsyt.2022.996406
This article is part of the Research Topic Behavioral Dysfunctions in Neuropsychiatric Diseases: Exploring New Therapeutic Targets View all 6 articles
Introduction: Cannabis abuse during adolescence is a risk factor for cognitive impairments in psychiatric disorders later in life. To date, the possible causal relationship between cannabinoids, kynurenic acid (KYNA; i.e., a neuroactive metabolite of tryptophan degradation) and cognition has not been investigated in adolescence. Early exposure to delta 9-tetrahydrocannabinol (THC; i.e., the main psychotropic component of cannabis) causes enduring cognitive deficits, which critically involve impaired glutamatergic function in the prefrontal cortex (PFC). In addition, prenatal cannabis exposure results in enduring increases in PFC KYNA levels. Based on these findings, the effects of chronic THC exposure in rats, during another critical period of neurodevelopment particularly sensitive to perturbation by exogenous stimuli, such as adolescence, have been investigated.
Methods: Male Wistar rats were chronically treated with vehicle or ascending intraperitoneal (i.p.) doses of THC starting on postnatal day (PND) 35 until PND 45. In adulthood (PND 75), cognitive assessment (Y-maze) and extracellular KYNA/glutamate levels were measured in the PFC by in vivo microdialysis, before and after a challenge with KYN (5 mg/kg i.p., the biological precursor of KYNA). By using the selective, brain-penetrable KAT II inhibitor PF-04859989, we then examined whether blockade of KYNA neosynthesis prevents the cognitive impairment.
Results: Compared to vehicle-treated controls, extracellular basal KYNA levels were higher in the PFC of adult rats chronically exposed to THC in adolescence (p < 0.01). No changes were observed in extracellular glutamate levels. Following a challenge with KYN, extracellular KYNA levels similarly increased in both groups (i.e., vehicle- and THC-treated; p < 0.001 and p < 0.01, respectively). Chronic adolescent THC exposure negatively affected short-term memory (reduced spontaneous alternation), in adult animals (p < 0.001), while PF-04859989 (30 mg/kg i.p.) restored the cognitive impairment (p < 0.05).
Discussion: We propose that the observed alterations in PFC KYNA signaling might be involved in the cognitive dysfunction induced by the exposure to THC during the adolescence. In the translational realm, these experiments raise the prospect of prevention of KYNA neosynthesis as a possible novel approach to counteract some of the detrimental long-term effects of adolescence cannabis use.
Over the recent past years, changes in the legal status of recreational or/and medical cannabis use have led to increased utilization of the drug among adolescents in the USA and Europe (1, 2). In addition, preliminary data indicate that the frequency of cannabis (as well as alcohol) use has increased during the COVID-19 pandemic (3). Since cannabis availability continues to expand, there is now growing concern about the possible negative effects of the main psychotomimetic component of cannabis, delta 9-tetrahydrocannabinol (THC), particularly during adolescence and other sensitive periods of brain development (4). Specifically, prolonged and heavy use of cannabis is a risk factor for cognitive impairments in psychiatric disorders later in life, especially in subsets of vulnerable adolescents (5).
During adolescence, the brain is characterized by increased plasticity and neuronal circuits refinement, specifically in the prefrontal cortex (PFC), one of the last brain regions to reach full development, and a brain area contributing to behavioral, cognitive and emotional maturity (6, 7). In addition, nowadays cannabis variants contain increasingly higher concentration of THC, multiplying the potency of the psychoactive ingredient and thereby increasing negative effects on several brain areas including the PFC (8). Cannabis abuse in adolescents is linked to cognitive impairments with a greater susceptibility to long-lasting consequences in teens who begin using cannabis at an earlier stage. Specifically, these cognitive impairments are related to the domains of attention, processing speed, verbal learning and memory, and executive functioning (9–11).
Studies in non-human primates have also reported a selective effect of THC for spatial working memory in adolescent monkeys following repeated drug administration (12). Preclinical studies in rodents indicate that adolescent cannabis exposure is associated with long-lasting changes in the structure of the cortical neurons in rats (13, 14) along with cognitive dysfunctions in adulthood (15, 16). Specifically, early exposure to THC in rats, causes enduring cognitive deficits, which critically involve impaired glutamatergic function in the PFC (6, 17).
Interestingly, it has been recently demonstrated the possible role of kynurenic acid (KYNA), an astrocyte-derived neuroactive metabolite of the kynurenine pathway (KP; Figure 1), in the long-term effects of cannabis exposure during brain development (18). Furthermore, the cognitive deficits induced by experimental increases in brain KYNA levels in developing rodents (19–21) are qualitatively very similar to those caused by cannabinoids. KYNA can function as an endogenous antagonist of α7-nicotinic acetylcholine receptors (α7nAChR) and N-methyl-D-aspartate receptors (NMDAR; (22), for review). KYNA is mainly synthesized from its biological precursor kynurenine (KYN), by astrocytic kynurenine aminotransferases (KATs), with KAT-II preferentially responsible for KYNA neosynthesis in mammals (23).
Figure 1. Schematic representation of the kynurenine pathway of tryptophan degradation. Kynurenic acid (KYNA) is synthesized from kynurenine by kynurenine aminotransferases (KATs). In the second ramification of the kynurenine pathway, kynurenine is metabolized by kynurenine 3-monooxygenase (KMO), leading to NAD+ as final product. For a complete representation of the pathway, see Schwarcz et al. (22).
KYNA serves as a functionally significant neuromodulator in the mammalian brain, including the PFC. For example, even modest increases in cerebral KYNA levels induce bi-directional effects on neurotransmitter levels in brain regions of different rodent models. Furthermore, altered brain (including PFC) KYNA levels have been associated with several pathological conditions, as extensively described by Schwarcz et al. (22).
Increased KYNA levels are associated with deficits in cognitive functions, included working memory, sensorimotor gating and attentional processing impairments, in adult rats (19, 24–29). Accordingly, several clinical studies report a pathophysiologically relevant association between increased brain KYNA levels and cognitive dysfunction in individuals with schizophrenia (SZ). In addition, several authors reported that SZ people have increased KYNA levels in the PFC (30, 31) and cerebrospinal fluid (32, 33). This elevation in KYNA levels can also lead to neurotransmission changes, as fluctuations in KYNA normally control aminoacidergic, cholinergic and dopaminergic neurotransmission in the mature brain (34, 35), while the specific inhibition of KYNA synthesis by KAT II inhibitors, improves such cognitive deficits [(22), for review; (21, 36)].
Based on the previous findings demonstrating that the exposure to THC during the prenatal period significantly biased KYNA in the rat PFC at adulthood [see above; (18)], the aim of the present study was to explore the possible consequences, on KP metabolism, of cannabinoid consumption in another critical period of brain development, particularly sensitive to perturbation by exogenous stimuli, i.e., the adolescence. To date, the possible causal relationship between cannabinoids consumption and brain KYNA levels, along with their possible impact on cognitive functions have not been investigated in adolescence.
Delta 9-tetrahydrocannabinol (THC), L-kynurenine sulfate (KYN) and PF-04859989 were purchased from Sigma (Milan, Italy). THC was dissolved in ethanol, cremophor and saline (1:1:18). This solution has been administered to vehicle-treated (i.e., control) rats. KYN and PF-04859989 were dissolved in saline solution.
Male Wistar rats aged 28 days at the time of arrival were obtained from Charles River laboratories (Calco, Italy) and were housed in clear plastic cages on a 12 h light-dark cycle (lights on 08:00 h) and in a temperature (22 ± 2°C) and humidity controlled environment (50 ± 10%). All animals were allowed free access to food and water. All experiments took place during the light phase.
The study was carried out in accordance with current Italian legislation (D.L. 26/2014) that allows experimentation on laboratory animals only after the approval by Ministry of Health (Rome, Italy), and in strict accordance with the European Council Directives on animal use in research (n. 2010/63/EU). Accordingly to the ARRIVE guidelines, all possible efforts were made to minimize animal pain and discomfort and to reduce the number of experimental subjects. 46 rats have been used in this study.
Male rats were housed in pairs, one rat receiving THC and the other one receiving vehicle treatment. Specifically, the animals were injected with increasing doses of THC, or vehicle, twice a day from postnatal day (PND) 35 to PND 45 (2.5 mg/kg, PND 35–37; 5 mg/kg, PND 38–41; 10 mg/kg, PND 42–45), according to our previous published protocol. This protocol mimics a heavy use of marijuana, since, according to the transformation in human equivalent dose proposed by FDA and the average content of THC in a joint, our first dose roughly corresponds to one joint, the second one to two joints, and the higher one to four joints (17). These moderate to high doses were chosen to be within the range known to produce behavioral effects in rats. Animals were then left undisturbed till adulthood. At PND 75 a first cohort of adolescent THC- and vehicle-treated rats was implanted with a microdialysis probe for neurochemical experiments, while a second cohort was used for behavioral and molecular studies (Figure 2).
Figure 2. Schematic representation of adolescent tetrahydrocannabinol treatment protocol and experiment timeline.
On the day of microdialysis probe implantation (PND 75), the animals were kept under isoflurane anesthesia (1.5% mixture of isoflurane and air) and mounted in a David Kopf stereotaxic frame (Tujunga, CA, USA) with the upper incisor bar set at 2.5 mm below the interaural line. A small hole was drilled on one side of the exposed skull. A microdialysis probe of concentric design (CMA12; molecular weight cutoff: 20 kD; outer diameter: 0.5 mm; length of the dialysis membrane: 2 mm; Alfatech S.p.A., Genova, Italy) was implanted vertically in the medial PFC (mPFC) and then secured to the skull with anchor screws and acrylic dental cement. The coordinates used [expressed in millimeters from bregma, according to Paxinos and Watson’s atlas Paxinos and Watson (37)] were: anterior 3.5, lateral 0.8 and vertical – 4.0 for adult rats. After surgery, the animals were housed individually in microdialysis chambers with food and water available.
Experiments were performed in freely moving rats 24 h after the probe implant. The probe was connected to a microperfusion pump (CMA 100; Carnegie Medicin, Stockholm, Sweden) set to a speed of 1.5 μl/min and perfused with Ringer solution containing (in mM): NaCl, 144; KCl, 4.8; MgSO4, 1.2; CaCl2, 1.7; pH 6.7. The collection of dialysate samples commenced 300 min after the onset of perfusion to achieve stable dialysis glutamate levels (18, 38) and perfusates were collected every 30 min thereafter. Following the collection of three stable basal values, KYN (5 mg/kg) or saline was administered i.p. and other nine perfusate samples were then collected (total sample collection time: 360 min).
Following each experiment, the brain was removed from the skull, and the position of the dialysis probe was verified using 30 μm-thick coronal cryostat sections. Only those animals in which the probe was correctly located were included in this study (Figure 3).
Figure 3. Visual representation of microdialysis probe tracks (deeper point) in the medial prefrontal cortex of rats subjected to neurochemical experiments.
The extracellular concentration of KYNA in microdialysates was measured by HPLC (18). Twenty μl of the dialysate were injected onto a 3 μm C18 reverse phase HPLC column (100 mm × 4 mm; Dr. Maisch GmbH, Ammerbuch, Germany), using a mobile phase containing 50 mM sodium acetate and 4% acetonitrile (pH adjusted to 6.2 with glacial acetic acid) at a flow rate of 0.5 ml/min. Zinc acetate (0.5 M, not pH adjusted) was delivered post-column by an auxiliary pump (Dionex AXP, Thermo Fisher, Waltham, MA, USA) at a flow rate of 0.1 ml/min. In the eluate, KYNA was detected fluorimetrically (excitation: 344 nm, emission: 398 nm; Jasco fluorescence spectrophotometer FP2020 Plus, Jasco, Tokyo, Japan). The retention time of KYNA was approximately 14 min. The limit of detection for KYNA was 10 fmol/sample.
In the dialysate, glutamate was measured by HPLC with fluorimetric detection. Briefly, 10 μl were transferred into glass microvials and placed in a temperature-controlled (4°C) Triathlon autosampler (Spark Holland, Emmen, Netherlands). Fifteen μl of o-phthaldialdehyde/mercaptoethanol reagent were added to each sample, and 15 μl of the mixture were injected onto a Chromsep analytical column (3 mm inner diameter, 10 cm length; Chrompack, Middelburg, Netherlands). The column was eluted at a flow rate of 0.48 ml/min (Beckman 125 pump; Beckman Instruments, Fullerton, CA, USA) with a mobile phase containing 0.1 M sodium acetate, 10% methanol and 2.2% tetrahydrofuran (pH 6.5). Glutamate was detected by means of a Jasco fluorescence spectrophotometer FP2020 Plus (Jasco, Tokyo, Japan). The retention times of glutamate was approximately 3.5 min. The limit of detection for glutamate was 20 fmol/sample (18).
The effect of chronic adolescent THC exposure on short-term memory was evaluated by using the Y-maze test. Furthermore, based on the obtained results, the effect of an acute administration of the KAT II inhibitor (PF-04859989; 30 mg/kg i.p., 1 h prior to behavioral testing) has also been tested. Namely, the following 4 groups of rats have been compared: (1) adolescent vehicle-treated rats receiving saline; (2) adolescent THC-treated rats receiving saline; (3) adolescent vehicle-treated rats receiving PF-04859989 and (4) adolescent THC-treated rats receiving PF-04859989.
The maze consisted of three interconnected closed arms, each one of them is 120° from the adjacent. Individual rat was placed into the middle of the maze and was allowed to freely explore the maze for 5 min. The number and order of arm entries were recorded and analyzed using the Any-maze video tracking system (Ugo Basile, Varese, Italy). An alternation was determined as the number of consecutive entries into the three different arms. The number of arm entries was used as a marker of locomotor activity. The number of spontaneous alternations (i.e., the entry into three different arms in sequence (triad), was used as a measure of short-term working memory. The percentage of spontaneous alternations was calculated from the number of triads and arm entries using the following equation: Y = number of triads/(total number of arm entries-2) × 100 (39, 40).
On the day after the behavioral test (PND 76), the animals were sacrificed by decapitation under isoflurane anesthesia. Animals were euthanized using an anesthetic overdose of isoflurane. The brains were rapidly removed and both the PFC dissected out frozen on dry ice and stored at –80°C until the day of the molecular analysis.
Ribonucleic acid (RNA) isolation and reverse transcription was performed as previously described (18). Briefly, total RNA from the PFC was extracted using the Direct-zolTM RNA MiniPrep (Zymo Research, purchased by Euroclone, Milan, Italy) according to manufacturer’s instructions. cDNA was synthesized using the iScript kit (Biorad, Milan, Italy) according to manufacturer’s instructions.
Quantitative real-time PCR (qPCR) analysis was performed on a CFX Connect Real-Time System (Biorad) using the iTaq Universal SYBR Green supermix (Biorad) and the following primers: KAT I Fw: 5′-CAATGATGGCTGGAGGTTG-3′, Rev: 5′-GTTGTTGGGTGTGTTGAGGA-3′; KAT II Fw: 5′-CCCTG TACTTTATCACAGCTC-3′, Rev: 5′ AAACCACGTAACCACT TGTC-3′; KAT III Fw: 5′-TTCAAAAATGCCAAACGAATCG-3′, Rev: 5′-GATGACCAAAGCCCCTTGTGT-3′; KAT IV Fw: 5′-GGACCTCCAGATCCCATCCT-3′, Rev: 5′-GGTTTTCCG TTATCATCCCGGTA-3′; S18: Fw: 5′-CATGCAGAACCCAC GACAAT-3′, Rev.: 5′-CTTCCCATCCTTCACGTCCT-3′. qPCR conditions were: 10 min at 95°C, 40 cycles of 15 s at 95°C and 30 s at 60°C. Relative expression of mRNA was calculated with the comparative Ct (ΔΔCT) method using RPS18 as endogenous control gene. The relative mRNA levels were expressed as fold change. Melting curve analysis was performed to verify the specificity of the PCR products (40).
In vivo microdialysis data were not adjusted for recovery from the dialysis probe. Basal values were calculated as the mean of three consecutive samples (differing by no more than 15%) collected immediately preceding the drug treatment. The area created by the curve (AUC), reflecting the duration of the effect, was determined for each animal by using. Area values (overall effects) were calculated as percentages of changes in baseline value over time by using the trapezoidal rule.
Data are expressed as the mean and SEM. For microdialysis experiment, Student’s t-test was used to analyze the differences in the mean KYNA and glutamate basal levels, while the effects of KYN injection were statistically analyzed by three-way (time-course) or two-way (AUC) analysis of variance (ANOVA) followed by Tukey’s test for multiple comparisons when appropriate. In the Y maze test statistical differences were assessed by two-way ANOVA with THC exposure and PF-04859989 (KAT II inhibitor) as main factors, followed by Tukey’s test for multiple comparisons. For molecular analyses, data were analyzed by Student’s t-test.
In vivo microdialysis experiments indicate that chronic adolescent THC exposure differently affected KYNA and glutamate levels in the mPFC of adult rats. In fact, extracellular KYNA levels were significantly increased (Figure 4A, p < 0.01), while extracellular glutamate levels were not affected (Figure 4B), in adult animals exposed to THC during the adolescence, as compared to vehicle-treated animals.
Figure 4. Basal extracellular kynurenic acid (KYNA; panel A) and glutamate (panel B) levels in the medial prefrontal cortex of adult [post-natal day (PND) 75] male rats chronically exposed to delta 9-tetrahydrocannabinol (THC) or its vehicle (cremophor and saline 1:1:18; Vehicle) during the adolescent period. Ascending doses of THC or its vehicle were administered by i.p. to adolescent rats, from PD 35 to PD 45, wh. Data are the mean ± standard error of the mean (SEM); n = 5/6 male rats/group. **p < 0.01 significantly different from the respective vehicle group based on Student’s t-test.
To further investigate the impact of chronic adolescent-THC treatment on the KP, we evaluated the consequences of an acute challenge with KYN (5 mg/kg, i.p.), the direct bioprecursor of KYNA, on extracellular KYNA and glutamate levels in the mPFC of adult male rats.
In vehicle and THC groups, KYN administration induced a similar increase in extracellular KYNA levels, as assessed by evaluating the nadir of the effect (Figure 5A) or the area under the curve (AUC) results (Figure 5B). In fact, a three-way ANOVA (time-course; Figure 5A) showed significant effects of KYN (F(1,5) = 46.40, p = 0.0010), time (F(11,55) = 30,37, p = 0.0001) and time × KYN (F(11,55) = 20.71, p = 0.0001), but non-significant effects of THC, THC × KYN and THC × KYN × time. The post hoc test showed similar significant increases in KYNA levels 30, 60, 90 and 120 min after KYN injection in vehicle and THC groups. Two-way ANOVA (AUC; Figure 5B) showed significant effects of KYN (F(1,19) = 40.93, p = 0.0001), but non-significant effects of THC and THC × KYN. On the other hand, KYN challenge induced a slight reduction of extracellular glutamate levels in the mPFC of vehicle-treated (Figure 5C). Three-way ANOVA (time-course; Figure 5C) showed significant effects of time (F(11,55) = 3.333, p = 0.0014) and time × KYN (F(11,55) = 11.00, p < 0.0001), but non-significant effects of THC, KYN, THC × KYN, time × THC and time × THC × KYN). The post hoc test showed a significant decrease in glutamate levels 60 and 90 min after KYN injection in the vehicle group. However, the overall AUC results did not show any statistically difference among the groups. In fact, two-way ANOVA (AUC; Figure 5D) showed non-significant effects of THC, KYN and THC × KYN.
Figure 5. Extracellular kynurenic acid (KYNA; panel A,B) and glutamate (panel C,D) levels in the medial prefrontal cortex of adult [post-natal day (PND) 75] male rats chronically exposed to delta 9-tetrahydrocannabinol (THC) or its vehicle (cremophor and saline 1:1:18; vehicle). Ascending doses of THC or its vehicle were administered by i.p. to adolescent rats, from PD 35 to PD 45. (A,C) Data mean ± standard error of the mean (SEM) are expressed as a percentage of the averaged three baseline values prior to treatment. (B,D) Bar graphs of the areas under the curves (AUC), calculated as the percentage of changes in basal values over time. Data were analyzed by three-way (A,C) or two-way (B,D) ANOVA and subsequent Tukey’s post hoc test for multiple comparisons. (A) *p < 0.05, **p < 0.01, ***p < 0.001 Vehicle KYN vs Vehicle-Saline and THC-Saline; #p < 0.05, ##p < 0.01 THC-KYN vs Vehicle-Saline and THC-Salina; (B) ***p < 0.001 Vehicle-KYN vs Vehicle-Saline and THC-Saline; **p < 0.01 THC-KYN vs Vehicle-Saline and THC-Saline. (C) *p < 0.05 Vehicle-Saline vs Vehicle-KYN and THC-Saline; n = 5/6 male rats/group.
The effect of chronic adolescent THC exposure on short-term memory was evaluated by using the Y-maze test. Furthermore, based on the obtained results, the effect of an acute administration of the KAT II inhibitor (PF-04859989; 30 mg/kg i.p.; 1 h prior to behavioral testing) on adolescent THC-induced cognitive impairment has also been tested. Overall, we observed main effects of chronic THC exposure (F(1,19) = 34.31, p < 0.0001) and PF-04859989 treatment (F(1,19) = 7.34, p < 0.013), and no interaction “chronic THC exposure” × “PF-04859989 treatment” (F(1,19) = 3.69, p = 0.056). As shown in Figure 6, chronic adolescent THC exposure negatively affected (p < 0.0001) short-term memory (reduced spontaneous alternation, Figure 6A), but not locomotor activity (arm entries, Figure 6B), compared to the vehicle-exposed rats. The injection of PF-04859989 in adult rats chronically exposed to THC during adolescence, significantly (p < 0.05) ameliorated the cognitive impairment (Figure 6A), without affecting locomotor activity (Figure 6B). The KAT II inhibitor did not affect cognitive performance or locomotor activity in adult rats chronically exposed to vehicle during adolescence.
Figure 6. Y-maze test showing the percentage of spontaneous alternations (A) and the number of arm entries (B) in adult rats [post-natal day (PND) 75] chronically exposed to delta 9-tetrahydrocannabinol (THC) or its vehicle (cremophor and saline 1:1:18; Vehicle) during the adolescent period. Ascending doses of THC or its vehicle were administered by i.p. to adolescent rats, from PD 35 to PD 45. Bars represent the mean ± standard error of the mean (SEM; n = 5/6 male rats/group). Data were analyzed by two-way ANOVA and subsequent Tukey’s post hoc test for multiple comparisons. ***p < 0.0001 THC-Saline vs Vehicle-Saline; ##p < 0.01 THC-Saline vs Vehicle-PF; *p < 0.05.
We also evaluated the impact of chronic adolescent THC exposure on the expression of the enzymes directly linked to KYNA formation, such as the 4 isoforms of KATs (KAT I, II, III and IV). Unexpectedly, mRNA expression levels of KAT III were significantly elevated in tissues of PFC from adult prenatally THC-exposed rats compared to the vehicle-treated animals (p < 0.01, Figure 7), while no significant differences were observed in the mRNA expression levels of KAT I, II and IV.
Figure 7. Enzyme expression of kynurenine aminotransferases (KATs) isoforms in prefrontal cortex tissues from adult [post-natal day (PND) 75] rats chronically exposed to delta 9-tetrahydrocannabinol (THC) or its vehicle (cremophor and saline 1:1:18; Vehicle) during the adolescent period. Ascending doses of THC or its vehicle were administered by i.p. to adolescent rats, from PD 35 to PD 45. Bars represent the mean ± standard error of the mean (SEM; n = 5/group). Data were analyzed by Student’s t-test. **p < 0.01 THC vs Vehicle.
In the present study we demonstrate for the first time that adolescent THC exposure in rats induces a persistent elevation of mPFC KYNA levels in adulthood. In line with previously published data [(17); see (14), for review], we observed that the adult rats exposed to THC during the adolescence display short-term memory deficits. Furthermore, in contrast to control animals, adult rats which had been treated with THC during adolescence showed a loss of KYNA-induced modulation of PFC glutamate levels when were challenged acutely with a systemic injection of a low dose of KYNA’s immediate bioprecursor KYN. Notably, an acute injection of the specific KAT II inhibitor PF-04859989 reversed the cognitive impairment in THC-exposed rats. Jointly, these results not only further support the relevance of functional links between THC and KYNA, but also suggest a potential new and translationally significant opportunities for therapeutic interventions.
Several lines of evidence support the hypothesis that abnormally elevated brain levels of KYNA, play a pathophysiologically significant role in SZ and other major neurodevelopmental disorders (41, 42). Preclinical studies in rodents suggest that KP impairments in these diseases may originate early in critical periods of neurodevelopment. It has been reported that exposure to KYN during the adolescent period, induced distinct cognitive impairments on spatial, contextual learning and memory in adulthood (19).
Among the several hypotheses on SZ pathophysiology, also a dysfunction of the endocannabinoid system has been postulated (43, 44). KYNA shares multiple physiological functions with the endocannabinoid system in the central nervous system (CNS), including glutamate, acetylcholine, dopamine release regulation and control of learning and memory mechanisms (22, 45). This led to the assumption that the two systems can potentially interact with each other in the CNS, with potential future repercussions for therapeutic interventions. So far, there are limited data describing their actual cross-talk in the CNS, which clearly exist in the adult brain (46–49) although its precise relevance has yet to be ascertained. In a recent study we demonstrated that gestational exposure to THC causes, in rats, long-lasting increases of KYNA levels in the mPFC associated with cognitive deficits in adulthood (18). In the present study, we also demonstrate that cannabinoid consumption in another critical period of brain development, particularly sensitive to perturbation by exogenous stimuli (i.e., adolescence), induces long-term consequences on KP metabolism. Specifically, following in vivo microdialysis experiments, we observed, a significant increase of extracellular KYNA levels in the mPFC of adult male rats exposed to escalating doses of THC in adolescence. On the contrary, and in line with our previous study in female rodents, this treatment did not affect basal extracellular glutamate levels in male rats (17). Based on the well-recognized role of KYNA in reducing mPFC glutamate levels (50–52), the increase in KYNA levels observed in adult rats exposed to THC during the adolescence should be associated with a reduction in mPFC glutamate levels. Accordingly, we recently demonstrated that prenatal THC-induced long-term increase in mPFC KYNA levels was associated with a significant reduction in local glutamate levels (18). These observations suggest in the present study a lack of the tonic control induced by KYNA on mPFC extracellular glutamate levels in adolescent THC-exposed rats. This scenario is also supported by the evidence that, in adolescent THC-exposed rats, the impact of KYN administration on glutamate levels appears lower than that observed in the vehicle treated group (see below). However, due to the very slight effect of KYN on glutamate extracellular levels, this hypothesis remains to be confirmed in further experiments by using higher doses of KYNA precursor. Although the cellular and molecular mechanisms remain to be elucidated, it seems likely that epigenetic processes might be involved in the observed long-term effects of adolescence THC exposure (see Figure 8 for working hypothesis). Epigenetic factors are well-recognized as the principal mechanisms underlying enduring phenotypic changes, mainly due to the evidence that the epigenome provides the cellular context for environmental impacts, including cannabinoid exposure, to modify gene functions and associated behaviors (53). The enduring KYNA increase following adolescent THC treatment, may be associated with astrocyte activation. In fact, KYNA conversion takes places primarily in the astrocytes and previous studies reported, following chronic THC exposure during the adolescence, microglia and astrocyte activation associated with a persistent neuroinflammatory state specifically localized within the adult PFC (54, 55). This hypothesis is supported by the increase in KYNA levels induced by the addition of interleukin 6 to cultured human astrocytes, and the increased astrocytic activity observed in SZ individuals (42). Furthermore, some studies demonstrated that KATs expression/activity is increased under different inflammatory conditions (56–59). Although there are no data linking inflammatory state to the expression/activity of the different isoforms of KATs, it could be speculated that the hypothesized inflammatory state may also explain the small but significant increased expression of KAT III, which does not normally make significant contribution to KYNA neosynthesis in the mammalian brain (23, 60). However, it cannot be ruled out that the other KATs isoforms might be affected in their activity, and not expression, by adolescent THC exposure.
Figure 8. Simplified graphic representation of the kynurenine pathway and the current working hypothesis. Grey box represents what has been analyzed in this study. Hypothesized mechanism: ascending doses of delta 9-tetrahydrocannabinol (THC) administered twice a day (for details see section “Materials and methods”), to adolescent rats (PD35-45), would induce, possibly by epigenetic mechanisms, an increase of KYNA levels which, in turn, leads to neurochemical and cognitive dysfunctions [(22), for review].
It has also been reported that THC exposure during adolescence alters dynamic changes occurring in glutamatergic and GABAergic signaling and desynchronization of PFC neuronal networks (55). Together with epigenetic modifications strongly affecting synaptic plasticity, these deleterious effects possibly explain the lack of the tonic control induced by KYNA on mPFC extracellular glutamate levels in adolescence THC-exposed rats. Epigenetic differences on specific global chromatin alterations within adult PFC and associated with glutamatergic and GABAergic systems following the same THC protocol in adolescent rats, have been already reported (61). Notably, previous studies, also reported a significant THC-induced attenuation of PFC GABAergic neurotransmission (16, 17, 62). Based on the present results, it can be postulated that the observed increased mPFC KYNA levels, could also contribute to adolescent THC-induced reduction in GABA signaling, since KYNA significantly reduces extracellular GABA levels in the rat PFC (52). This mechanism might be involved in adolescent THC-induced long-lasting cognitive impairment (see below), since increased KYNA levels has been associated with cognitive deficits (63) and an optimal interplay between inhibitory and excitatory neurotransmission is crucial in determining the functional integrity of adult PFC functioning (64–66). In this context, it becomes relevant to evaluate, in further studies, the possible impact of adolescent THC exposure on the PFC inhibitory/excitatory neurotransmission balance.
It is well-known that the negative effects of marijuana during adolescence might be due to disruption of the physiological role of the endocannabinoid system in this brain (including PFC) developmental window (67–69). According to the concept of higher vulnerability of the immature brain to external stimuli, it has been proposed that the exposure to THC during the adolescence may represent a risk factor for the development of a complex psychotic-like behavior in adulthood (16, 17). As previously postulated (18) this putative link is particularly captivating in persons with SZ, displaying elevated KYNA levels in brain and cerebrospinal fluid [see above; (30–33, 63)]. Based on the “two hit” hypothesis of SZ and considering adolescent THC exposure a “first hit,” in a second set of experiments we injected a low dose of KYN (5 mg/kg i.p.), the direct bioprecursor of KYNA to mimic a relevant “second hit.” The THC-exposed animals challenged with KYN, reported a similar quantitative increase on the extracellular KYNA levels as the vehicle exposed animals. This result diverges from our previous findings in prenatally THC exposed animals (18), displaying, when compared to vehicle-treated rats, a significantly greater increase in extracellular KYNA levels in the PFC following KYN injection. This difference might be obviously related to the different developmental period (i.e., prenatal or adolescence) of THC exposure and/or to the different doses of THC used in the present and in the previous study. Interestingly, the expected significant decrease in the extracellular glutamate levels associated to the increased KYNA levels (50, 52) was observed in vehicle-treated, but not in THC-exposed animals. This finding confirms the postulated (see above) lack of KYNA-mediated control on glutamate because of moderate to heavy escalating THC treatment performed in adolescence.
Based on the data obtained we hypothesize that the prolonged increase in PFC KYNA levels may be responsible for the cognitive impairment observed in the THC-exposed group. Since the spontaneous alternation in Y-maze test is strongly controlled by the PFC (70), these findings agree with the hypothesis that a dysfunctional PFC is associated with impairment of the executive functions (71). It has been widely reported that abnormal KYNA elevation is associated with cognitive deficits in pathological conditions like SZ (63). To date, the possible causal relationship between THC, KYNA and cognitive function has not been examined. In a first attempt to investigate this link, we determined the effect of an acute administration of the selective, brain penetrable KAT II inhibitor PF-04859989 (36). This approach has been used to test whether PF-04859989, by blocking KYNA neosynthesis and thus preventing the THC-induced delayed-KYNA increase, would ameliorate the associated impairments observed in the spontaneous alternation task. Administration of PF-04859989 rescued the cognitive impairment observed in the THC-exposed rats. Notably, preliminary in vivo data, also demonstrate that the single dose of the KAT II inhibitor was also associated with a greater reduction (∼40%) of extracellular KYNA levels in THC-exposed rats compared to the vehicle-rats (∼25%).
Although the molecular mechanisms, the role of brain circuits and the clinical relevance need to be still elucidated, the present finding has potential translational relevance and is in line with the fact that a pharmacologic reduction of cortical KYNA levels (36, 72) has evident pro-cognitive effects in several animal models. Thus, inhibition of KYNA neosynthesis by specifically targeting KAT II would constitute a potential new strategy to, at least partially, reduce cannabis-related adverse effects later in life.
Notably, it has been extensively shown that selective genetic or pharmacological interference with KYNA synthesis have pro-cognitive effects in rodents (21, 36, 73, 74).
This study presents some limitations. First, it has been performed only in males. The rationale behind this, was due to the fact that this study aimed at evaluating the possible alterations on KP, following THC exposure during the adolescence and to directly compare the results with the previous findings achieved by prenatally exposing male rats to THC (18). However, considering the well-known sexually dimorphic effects that cannabis consumption during adolescence can induce in cognitive functions, it is possible that adolescent THC exposure differently affects KP metabolism in female rats. This possibility is also corroborated by the fact that sex differences in sleep and arousal have been observed in adult rats born from pregnant dams fed with a diet laced with KYN the last week of gestation (75). Thus, it will be interesting to evaluate, in further studies, the effects of adolescent THC treatment on PFC KYNA levels and cognitive functions in female rats. Second, we tested only one challenge dose of KYN, as well as a single dose of PF-04859989. In addition, the behavioral investigation was performed by using only a single test. This choice is due to the fact that the aim of the behavioral part of the study was to explore, for the first time, the possibility to rescue adolescent THC-induced long-term cognitive impairment with the KAT II inhibitor treatment. Based on the obtained result, it will be interesting to evaluate the effects of different doses of the KAT II inhibitor on THC-induced cognitive impairment by using several behavioral tests, also some relevant to SZ. Finally, in the translational realm, it is relevant to consider that adolescents consuming cannabis are exposed not only to THC, but also to other cannabinoids that could dampen or increase the deleterious consequences of the main psychotropic ingredient of cannabis derivatives.
In summary, the present study revealed that the use of cannabinoids early in life can induce a malfunction of the KP, specifically increased KYNA concentrations, in the adult brain, leading to cognitive impairments in SZ and other major psychiatric disorders. Ongoing experiments are designed to examine this causal connection, and its possible translational significance, in an animal model with abnormal KP metabolism [kynurenine 3-monooxygenase knockout mice; (76, 77)]. Most importantly, the translational relevance of the concept should be carefully assessed in the near future by monitoring the levels of circulating kynurenine, KYNA and other KP metabolites in psychotic patients who consumed marijuana during adolescence.
The raw data supporting the conclusions of this article will be made available by the authors, without undue reservation.
The study was carried out in accordance with current Italian legislation (D.L. 26/2014) that allows experimentation on laboratory animals only after the approval by Ministry of Health (Rome, Italy), and in strict accordance with the European Council Directives on animal use in research (n. 2010/63/EU).
SB: conducted research, formal analysis, writing – original draft, writing – review and editing, visualization, conceptualization, and project administration. AI and MZ: conducted research, methodology, formal analysis, writing – original draft, writing – review and editing, and visualization. PD: conceptualization and visualization. LF and RS: conceptualization, visualization, supervision, and funding acquisition. All authors contributed to the article and approved the submitted version.
This work was funded by the National Institutes of Health Grant No. P50 MH103222 and support from the University of Chieti-Pescara (Ex-60% to SB) and the University of Ferrara (FAR2020 to LF).
The authors declare that the research was conducted in the absence of any commercial or financial relationships that could be construed as a potential conflict of interest.
All claims expressed in this article are solely those of the authors and do not necessarily represent those of their affiliated organizations, or those of the publisher, the editors and the reviewers. Any product that may be evaluated in this article, or claim that may be made by its manufacturer, is not guaranteed or endorsed by the publisher.
1. Substance Abuse and Mental Health Services Administration [SAMHSA]. Key Substance use and Mental Health Indicators in the United States: Results from the 2019 National Survey on Drug Use and Health (HHS Publication No. PEP20-07-01-001, NSDUH Series H-55). Rockville, MD: Substance Abuse and Mental Health Services Administration (2020).
2. Benedetti E, Resce G, Brunori P, Molinaro S. Cannabis policy changes and adolescent cannabis use: evidence from Europe. Int J Environ Res Public Health. (2021) 18:5174. doi: 10.3390/ijerph18105174
3. Dumas TM, Ellis W, Litt DM. What does adolescent substance use look like during the COVID-19 pandemic? Examining changes in frequency, social contexts, and pandemic-related predictors. J Adolesc Health. (2020) 67:354–61. doi: 10.1016/j.jadohealth.2020.06.018
4. Shen H. News feature: cannabis and the adolescent brain. Proc Natl Acad Sci U S A. (2020) 117:7–11. doi: 10.1073/pnas.1920325116
5. D’Souza DC, Ganesh S, Cortes-Briones J, Campbell MH, Emmanuel MK. Characterizing psychosis-relevant phenomena and cognitive function in a unique population with isolated, chronic and very heavy cannabis exposure. Psychol Med. (2020) 50:2452–9. doi: 10.1017/S0033291719002721
6. Rubino T, Parolaro D. The impact of exposure to cannabinoids in adolescence: insights from animal models. Biol Psychiatry. (2016) 79:578–85. doi: 10.1016/j.biopsych.2015.07.024
7. Peters KZ, Zlebnik NE, Cheer JF. Cannabis exposure during adolescence: a uniquely sensitive period for neurobiological effects. Int. Rev. Neurobiol. (2022) 161:95–120. doi: 10.1016/bs.irn.2021.07.002
8. Lafaye G, Karila L, Blecha L, Benyamina A. Cannabis, cannabinoids, and health. Dialogues Clin Neurosci. (2017) 19:309–16. doi: 10.31887/DCNS.2017.19.3/glafaye
9. Dougherty DM, Mathias CW, Dawes MA, Furr RM, Charles NE, Liguori A, et al. Impulsivity, attention, memory, and decision-making among adolescent marijuana users. Psychopharmacology. (2013) 226:307–19. doi: 10.1007/s00213-012-2908-5
10. Broyd SJ, Van Hell HH, Beale C, Yücel M, Solowij N. Acute and chronic effects of cannabinoids on human cognition – a systematic review. Biol Psychiatry. (2016) 79:557–67. doi: 10.1016/j.biopsych.2015.12.002
11. Fischer AS, Tapert SF, Lee Louie D, Schatzberg AF, Singh MK. Cannabis and the developing adolescent brain. Curr Treat Options Psychiatry. (2020) 7:144–61. doi: 10.1007/s40501-020-00202-2
12. Verrico CD, Gu H, Peterson ML, Sampson AR, Lewis DA. Repeated Δ9-tetrahydrocannabinol exposure in adolescent monkeys: persistent effects selective for spatial working memory. Am J Psychiatry. (2014) 171:416–25. doi: 10.1176/appi.ajp.2013.13030335
13. Antonelli T, Tomasini MC, Tattoli M, Cassano T, Tanganelli S, Finetti S, et al. Prenatal exposure to the CB1 receptor agonist WIN 55,212-2 causes learning disruption associated with impaired cortical NMDA receptor function and emotional reactivity changes in rat offspring. Cereb Cortex. (2005) 15:2013–20. doi: 10.1093/cercor/bhi076
14. Ginder DE, Wright HR, McLaughlin RJ. The stoned age: sex differences in the effects of adolescent cannabinoid exposure on prefrontal cortex structure and function in animal models. Int Rev Neurobiol. (2022) 161:121–45. doi: 10.1016/bs.irn.2021.07.005
15. Renard J, Rushlow WJ, Laviolette SR. What can rats tell us about adolescent cannabis exposure? Insights from preclinical research. Can J Psychiatry. (2016) 61:328–34. doi: 10.1177/0706743716645288
16. Renard J, Szkudlarek HJ, Kramar CP, Jobson CEL, Moura K, Rushlow WJ, et al. Adolescent THC exposure causes enduring prefrontal cortical disruption of GABAergic inhibition and dysregulation of sub-cortical dopamine function. Sci Rep. (2017) 7:11420. doi: 10.1038/s41598-017-11645-8
17. Zamberletti E, Beggiato S, Steardo L Jr., Prini P, Antonelli T, Ferraro L, et al. Alterations of prefrontal cortex GABAergic transmission in the complex psychotic-like phenotype induced by adolescent delta-9-tetrahydrocannabinol exposure in rats. Neurobiol Dis. (2014) 63:35–47. doi: 10.1016/j.nbd.2013.10.028
18. Beggiato S, Ieraci A, Tomasini MC, Schwarcz R, Ferraro L. Prenatal THC exposure raises kynurenic acid levels in the prefrontal cortex of adult rats. Prog Neuropsychopharmacol Biol Psychiatry. (2020) 100:109883. doi: 10.1016/j.pnpbp.2020.109883
19. Akagbosu CO, Evans GC, Gulick D, Suckow RF, Bucci DJ. Exposure to kynurenic acid during adolescence produces memory deficits in adulthood. Schizophr Bull. (2012) 38:769–78. doi: 10.1093/schbul/sbq151
20. Pocivavsek A, Thomas MA, Elmer GI, Bruno JP, Schwarcz R. Continuous kynurenine administration during the prenatal period, but not during adolescence, causes learning and memory deficits in adult rats. Psychopharmacology. (2014) 231:2799–809. doi: 10.1007/s00213-014-3452-2
21. Pocivavsek A, Elmer GI, Schwarcz R. Inhibition of kynurenine aminotransferase II attenuates hippocampus-dependent memory deficit in adult rats treated prenatally with kynurenine. Hippocampus. (2019) 29:73–7. doi: 10.1002/hipo.23040
22. Schwarcz R, Bruno JP, Muchowski PJ, Wu HQ. Kynurenines in the mammalian brain: when physiology meets pathology. Nat Rev Neurosci. (2012) 13:465–77. doi: 10.1038/nrn3257
23. Guidetti P, Okuno E, Schwarcz R. Characterization of rat brain kynurenine aminotransferases I and II. J Neurosci Res. (1997) 50:457–65. doi: 10.1002/(SICI)1097-4547(19971101)50:33.0.CO;2-3
24. Shepard PD, Joy B, Clerkin L, Schwarcz R. Micromolar brain levels of kynurenic acid are associated with a disruption of auditory sensory gating in the rat. Neuropsychopharmacology. (2003) 28:1454–62. doi: 10.1038/sj.npp.1300188
25. Erhardt S, Schwieler L, Emanuelsson C, Geyer M. Endogenous kynurenic acid disrupts prepulse inhibition. Biol. Psychiatry. (2004) 56:255–60. doi: 10.1016/j.biopsych.2004.06.006
26. Chess AC, Bucci DJ. Increased concentration of cerebral kynurenic acid alters stimulus processing and conditioned responding. Behav Brain Res. (2006) 170:326–32. doi: 10.1016/j.bbr.2006.03.006
27. Chess AC, Simoni MK, Alling TE, Bucci DJ. Elevations of endogenous kynurenic acid produce spatial working memory deficits. Schizophr Bull. (2007) 33:797–804. doi: 10.1093/schbul/sbl033
28. DeAngeli NE, Todd TP, Chang SE, Yeh HH, Yeh PW, Bucci DJ. Exposure to kynurenic acid during adolescence increases sign-tracking and impairs long-term potentiation in adulthood. Front Behav Neurosci. (2015) 8:451. doi: 10.3389/fnbeh.2014.00451
29. Pershing ML, Bortz DM, Pocivavsek A, Fredericks PJ, Jørgensen CV, Vunck SA, et al. Elevated levels of kynurenic acid during gestation produce neurochemical, morphological, and cognitive deficits in adulthood: implications for schizophrenia. Neuropharmacology. (2015) 90:33–41. doi: 10.1016/j.neuropharm.2014.10.017
30. Schwarcz R, Rassoulpour A, Wu HQ, Medoff D, Tamminga CA, Roberts RC. Increased cortical kynurenate content in schizophrenia. Biol. Psychiatry. (2001) 50:521–30. doi: 10.1016/s0006-3223(01)01078-2
31. Sathyasaikumar KV, Stachowski EK, Wonodi I, Roberts RC, Rassoulpour A, McMahon RP, et al. Impaired kynurenine pathway metabolism in the prefrontal cortex of individuals with schizophrenia. Schizophr Bull. (2011) 37:1147–56. doi: 10.1093/schbul/sbq112
32. Erhardt S, Blennow K, Nordin C, Skogh E, Lindstrom LH, Engberg G. Kynurenic acid levels are elevated in the cerebrospinal fluid of patients with schizophrenia. Neurosci. Lett. (2001) 313:96–8. doi: 10.1016/s0304-3940(01)02242-x
33. Linderholm KR, Skogh E, Olsson SK, Dahl ML, Holtze M, Engberg G, et al. Increased levels of kynurenine and kynurenic acid in the CSF of patients with schizophrenia. Schizophr Bull. (2012) 38:426–32. doi: 10.1093/schbul/sbq086
34. Amori L, Wu HQ, Marinozzi M, Pellicciari R, Guidetti P, Schwarcz R. Specific inhibition of kynurenate synthesis enhances extracellular dopamine levels in the rodent striatum. Neuroscience. (2009) 159:196–203. doi: 10.1016/j.neuroscience.2008.11.055
35. Pocivavsek A, Wu HQ, Potter MC, Elmer GI, Pellicciari R, Schwarcz R. Fluctuations in endogenous kynurenic acid control hippocampal glutamate and memory. Neuropsychopharmacology. (2011) 36:2357–67. doi: 10.1038/npp.2011.127
36. Kozak R, Campbell BM, Strick CA, Horner W, Hoffmann WE, Kiss T, et al. Reduction of brain kynurenic acid improves cognitive function. J Neurosci. (2014) 34:10592–602. doi: 10.1523/JNEUROSCI.1107-14.2014
37. Paxinos G, Watson C. The Rat Brain in Stereotaxic Coordinates. 7th ed. London: Elsevier Academic Press (2007).
38. Beggiato S, Antonelli T, Tomasini MC, Tanganelli S, Fuxe K, Schwarcz R, et al. Kynurenic acid, by targeting α7 nicotinic acetylcholine receptors, modulates extracellular GABA levels in the rat striatum in vivo. Eur. J. Neurosci. (2013) 37:1470–7. doi: 10.1111/ejn.12160
39. Nookala AR, Schwartz DC, Chaudhari NS, Glazyrin A, Stephens EB, Berman NEJ, et al. Methamphetamine augment HIV-1 Tat mediated memory deficits by altering the expression of synaptic proteins and neurotrophic factors. Brain Behav. Immun. (2018) 71:37–51. doi: 10.1016/j.bbi.2018.04.018
40. Ieraci A, Beggiato S, Ferraro L, Barbieri SS, Popoli M. Kynurenine pathway is altered in BDNF Val66Met knock-in mice: effect of physical exercise. Brain Behav Immun. (2020) 89:440–50. doi: 10.1016/j.bbi.2020.07.031
41. Maddison DC, Giorgini F. The kynurenine pathway and neurodegenerative disease. Semin Cell Dev Biol. (2015) 40:134–41. doi: 10.1016/j.semcdb.2015.03.002
42. Plitman E, Iwata Y, Caravaggio F, Nakajima S, Chung JK, Gerretsen P, et al. Kynurenic acid in schizophrenia: a systematic review and meta-analysis. Schizophr Bull. (2017) 43:764–77. doi: 10.1093/schbul/sbw221
43. Seabra G, Falvella ACB, Guest PC, Martins-de-Souza D, de Almeida V. Proteomics and lipidomics in the elucidation of endocannabinoid signaling in healthy and schizophrenia brains. Proteomics. (2018) 18:e1700270. doi: 10.1002/pmic.201700270
44. Ibarra-Lecue I, Pilar-Cuéllar F, Muguruza C, Florensa-Zanuy E, Díaz Á, Urigüen L, et al. The endocannabinoid system in mental disorders: evidence from human brain studies. Biochem Pharmacol. (2018) 157:97–107. doi: 10.1016/j.bcp.2018.07.009
45. Zou S, Kumar U. Cannabinoid receptors and the endocannabinoid system: signaling and function in the central nervous system. Int J Mol Sci. (2018) 19:833. doi: 10.3390/ijms19030833
46. Justinova Z, Mascia P, Wu HQ, Secci ME, Redhi GH, Panlilio LV, et al. Reducing cannabinoid abuse and preventing relapse by enhancing endogenous brain levels of kynurenic acid. Nat Neurosci. (2013) 16:1652–61. doi: 10.1038/nn.3540
47. Colín-González AL, Aguilera G, Santamaría A. Cannabinoids: glutamatergic transmission and kynurenines. Adv Neurobiol. (2016) 12:173–98. doi: 10.1007/978-3-319-28383-8_10
48. Nagy-Grócz G, Zádor F, Dvorácskó S, Bohár Z, Benyhe S, Tömböly C, et al. Interactions between the kynurenine and the endocannabinoid system with special emphasis on migraine. Int. J. Mol. Sci. (2017) 18:1617. doi: 10.3390/ijms18081617
49. Secci ME, Mascia P, Sagheddu C, Beggiato S, Melis M, Borelli AC, et al. Astrocytic mechanisms involving kynurenic acid control Δ9-tetrahydrocannabinol-induced increases in glutamate release in brain reward-processing areas. Mol Neurobiol. (2019) 56:3563–75. doi: 10.1007/s12035-018-1319-y
50. Konradsson-Geuken A, Wu HQ, Gash CR, Alexander KS, Campbell A, Sozeri Y, et al. Cortical kynurenic acid bi-directionally modulates prefrontal glutamate levels as assessed by microdialysis and rapid electrochemistry. Neuroscience. (2010) 169:1848–59. doi: 10.1016/j.neuroscience.2010.05.052
51. Wu HQ, Pereira EF, Bruno JP, Pellicciari R, Albuquerque EX, Schwarcz R. The astrocyte-derived alpha7 nicotinic receptor antagonist kynurenic acid controls extracellular glutamate levels in the prefrontal cortex. J Mol Neurosci. (2010) 40:204–10. doi: 10.1007/s12031-009-9235-2
52. Beggiato S, Tanganelli S, Fuxe K, Antonelli T, Schwarcz R, Ferraro L. Endogenous kynurenic acid regulates extracellular GABA levels in the rat prefrontal cortex. Neuropharmacology. (2014) 82:11–8. doi: 10.1016/j.neuropharm.2014.02.019
53. Bara A, Ferland JN, Rompala G, Szutorisz H, Hurd YL. Cannabis and synaptic reprogramming of the developing brain. Nat Rev Neurosci. (2021) 22:423–38. doi: 10.1038/s41583-021-00465-5
54. Zamberletti E, Gabaglio M, Prini P, Rubino T, Parolaro D. Cortical neuroinflammation contributes to long-term cognitive dysfunctions following adolescent delta-9-tetrahydrocannabinol treatment in female rats. Eur Neuropsychopharmacol. (2015) 25:2404–15. doi: 10.1016/j.euroneuro.2015.09.021
55. Testai FD, Gorelick PB, Aparicio HJ, Filbey FM, Gonzalez R, Gottesman RF, et al. American Heart Association stroke brain health science subcommittee of the stroke council; council on arteriosclerosis, thrombosis and vascular biology; council on cardiovascular and stroke nursing; council on lifestyle and cardiometabolic health; and council on peripheral vascular disease. use of marijuana: effect on brain health: a scientific statement from the American Heart Association. Stroke. (2022) 53:e176–87. doi: 10.1161/STR.0000000000000396
56. Ishida Y, Fujita H, Aratani S, Chijiiwa M, Taniguchi N, Yokota M, et al. The NRF2-PGC-1β pathway activates kynurenine aminotransferase 4 via attenuation of an E3 ubiquitin ligase, synoviolin, in a cecal ligation/perforation-induced septic mouse model. Mol Med Rep. (2018) 18:2467–75. doi: 10.3892/mmr.2018.9175
57. Kindler J, Lim CK, Weickert CS, Boerrigter D, Galletly C, Liu D, et al. Dysregulation of kynurenine metabolism is related to proinflammatory cytokines, attention, and prefrontal cortex volume in schizophrenia. Mol Psychiatry. (2020) 25:2860–72. doi: 10.1038/s41380-019-0401-9
58. Cai Y, Kim DJ, Takahashi T, Broadhurst DI, Yan H, Ma S, et al. Kynurenic acid may underlie sex-specific immune responses to COVID-19. Sci Signal. (2021) 14:eabf8483. doi: 10.1126/scisignal.abf8483
59. Simonato M, Dall’Acqua S, Zilli C, Sut S, Tenconi R, Gallo N, et al. Tryptophan metabolites, cytokines, and fatty acid binding protein 2 in myalgic encephalomyelitis/chronic fatigue syndrome. Biomedicines. (2021) 9:1724. doi: 10.3390/biomedicines9111724
60. Han Q, Robinson H, Cai T, Tagle DA, Li J. Biochemical and structural properties of mouse kynurenine aminotransferase III. Mol Cell Biol. (2009) 29:784–93. doi: 10.1128/MCB.01272-08
61. Prini P, Rusconi F, Zamberletti E, Gabaglio M, Penna F, Fasano M, et al. Adolescent THC exposure in female rats leads to cognitive deficits through a mechanism involving chromatin modifications in the prefrontal cortex. J Psychiatry Neurosci. (2018) 43:87–101. doi: 10.1503/jpn.170082
62. Lee SE, Lee Y, Lee GH. The regulation of glutamic acid decarboxylases in GABA neurotransmission in the brain. Arch Pharm Res. (2019) 42:1031–9. doi: 10.1007/s12272-019-01196-z
63. Wonodi I, Schwarcz R. Cortical kynurenine pathway metabolism: a novel target for cognitive enhancement in Schizophrenia. Schizophr Bull. (2010) 36:211–8. doi: 10.1093/schbul/sbq002
64. Constantinidis C, Williams GV, Goldman-Rakic PS. A role for inhibition in shaping the temporal flow of information in prefrontal cortex. Nat. Neurosci. (2002) 5:175–80. doi: 10.1038/nn799
65. Lisman JE, Coyle JT, Green RW, Javitt DC, Benes FM, Heckers S, et al. Circuit-based framework for understanding neurotransmitter and risk gene interactions in schizophrenia. Trends Neurosci. (2008) 31:234–42. doi: 10.1016/j.tins.2008.02.005
66. Benes FM. Amygdalocortical circuitry in schizophrenia: from circuits to molecules. Neuropsychopharmacology. (2010) 35:239–57. doi: 10.1038/npp.2009.116
67. Keimpema E, Mackie K, Harkany T. Molecular model of cannabis sensitivity in developing neuronal circuits. Trends Pharmacol Sci. (2011) 32:551–61. doi: 10.1016/j.tips.2011.05.004
68. Meyer HC, Lee FS, Gee DG. The role of the endocannabinoid system and genetic variation in adolescent brain development. Neuropsychopharmacology. (2018) 43:21–33. doi: 10.1038/npp.2017.143
69. Cass DK, Flores-Barrera E, Thomases DR, Vital WF, Caballero A, Tseng KY. CB1 cannabinoid receptor stimulation during adolescence impairs the maturation of GABA function in the adult rat prefrontal cortex. Mol Psychiatry. (2014) 19:536–43. doi: 10.1038/mp.2014.14
70. Kraeuter AK, Guest PC, Sarnyai Z. The Y-maze for assessment of spatial working and reference memory in mice. Methods Mol Biol. (2019) 1916:105–11. doi: 10.1007/978-1-4939-8994-2_10
71. Watanabe M. The Prefrontal Cortex as an Executive, Emotional, and Social Brain. Tokyo: Springer (2017). doi: 10.1007/978-4-431-56508-6
72. Wu HQ, Okuyama M, Kajii Y, Pocivavsek A, Bruno JP, Schwarcz R. Targeting kynurenine aminotransferase II in psychiatric diseases: promising effects of an orally active enzyme inhibitor. Schizophr Bull. (2014) 40:S152–8. doi: 10.1093/schbul/sbt157
73. Potter MC, Elmer GI, Bergeron R, Albuquerque EX, Guidetti P, Wu HQ, et al. Reduction of endogenous kynurenic acid formation enhances extracellular glutamate, hippocampal plasticity, and cognitive behavior. Neuropsychopharmacology. (2010) 35:1734–42. doi: 10.1038/npp.2010.39
74. Rossi F, Casazza V, Garavaglia S, Sathyasaikumar KV, Schwarcz R, Kojima SI, et al. Crystal structure-based selective targeting of the Pyridoxal 5’-phosphate dependent enzyme kynurenine aminotransferase II for cognitive enhancement. J Med. Chem. (2010) 53:5684–9. doi: 10.1021/jm100464k
75. Rentschler KM, Baratta AM, Ditty AL, Wagner NTJ, Wright CJ, Milosavljevic S, et al. Prenatal kynurenine elevation elicits sex-dependent changes in sleep and arousal during adulthood: implications for psychotic disorders. Schizophr Bull. (2021) 47:1320–30. doi: 10.1093/schbul/sbab029
76. Giorgini F, Huang SY, Sathyasaikumar KV, Notarangelo FM, Thomas MA, Tararina M, et al. Targeted deletion of kynurenine 3-monooxygenase in mice: a new tool for studying kynurenine pathway metabolism in periphery and brain. J Biol Chem. (2013) 288:36554–66. doi: 10.1074/jbc.M113.503813
Keywords: schizophrenia, kynurenine pathway, KAT II inhibitor, glutamate, THC
Citation: Beggiato S, Ieraci A, Zuccarini M, Di Iorio P, Schwarcz R and Ferraro L (2022) Alterations in rat prefrontal cortex kynurenic acid levels are involved in the enduring cognitive dysfunctions induced by tetrahydrocannabinol exposure during the adolescence. Front. Psychiatry 13:996406. doi: 10.3389/fpsyt.2022.996406
Received: 17 July 2022; Accepted: 01 November 2022;
Published: 22 November 2022.
Edited by:
Antonia Manduca, Roma Tre University, ItalyReviewed by:
Roberto Frau, University of Cagliari, ItalyCopyright © 2022 Beggiato, Ieraci, Zuccarini, Di Iorio, Schwarcz and Ferraro. This is an open-access article distributed under the terms of the Creative Commons Attribution License (CC BY). The use, distribution or reproduction in other forums is permitted, provided the original author(s) and the copyright owner(s) are credited and that the original publication in this journal is cited, in accordance with accepted academic practice. No use, distribution or reproduction is permitted which does not comply with these terms.
*Correspondence: Sarah Beggiato, c2FyYWguYmVnZ2lhdG9AdW5pZmUuaXQ=
Disclaimer: All claims expressed in this article are solely those of the authors and do not necessarily represent those of their affiliated organizations, or those of the publisher, the editors and the reviewers. Any product that may be evaluated in this article or claim that may be made by its manufacturer is not guaranteed or endorsed by the publisher.
Research integrity at Frontiers
Learn more about the work of our research integrity team to safeguard the quality of each article we publish.