- 1Department of Computational Biology, Cornell University, Ithaca, NY, United States
- 2MAPS Public Benefit Corporation, San Jose, CA, United States
- 3Department of Psychiatry and Behavioral Sciences, Medical University of South Carolina, Charleston, SC, United States
- 4Wake Forest School of Medicine, Winston-Salem, NC, United States
- 5Ralph H. Johnson VA Medical Center, Charleston, SC, United States
- 6Department of Neurology, University of California, San Francisco, San Francisco, CA, United States
- 7Multidisciplinary Association for Psychedelic Studies, San Jose, CA, United States
- 8Department of Radiology, Weill Cornell Medicine, New York, NY, United States
Introduction: 3,4-methylenedioxymethamphetamine-assisted therapy (MDMA-AT) for post-traumatic stress disorder (PTSD) has demonstrated promise in multiple clinical trials. MDMA is hypothesized to facilitate the therapeutic process, in part, by decreasing fear response during fear memory processing while increasing extinction learning. The acute administration of MDMA in healthy controls modifies recruitment of brain regions involved in the hyperactive fear response in PTSD such as the amygdala, hippocampus, and insula. However, to date there have been no neuroimaging studies aimed at directly elucidating the neural impact of MDMA-AT in PTSD patients.
Methods: We analyzed brain activity and connectivity via functional MRI during both rest and autobiographical memory (trauma and neutral) response before and two-months after MDMA-AT in nine veterans and first-responders with chronic PTSD of 6 months or more.
Results: We hypothesized that MDMA-AT would increase amygdala-hippocampus resting-state functional connectivity, however we only found evidence of a trend in the left amygdala—left hippocampus (t = –2.91, uncorrected p = 0.0225, corrected p = 0.0901). We also found reduced activation contrast (trauma > neutral) after MDMA-AT in the cuneus. Finally, the amount of recovery from PTSD after MDMA-AT correlated with changes in four functional connections during autobiographical memory recall: the left amygdala—left posterior cingulate cortex (PCC), left amygdala—right PCC, left amygdala—left insula, and left isthmus cingulate—left posterior hippocampus.
Discussion: Amygdala—insular functional connectivity is reliably implicated in PTSD and anxiety, and both regions are impacted by MDMA administration. These findings compliment previous research indicating that amygdala, hippocampus, and insula functional connectivity is a potential target of MDMA-AT, and highlights other regions of interest related to memory processes. More research is necessary to determine if these findings are specific to MDMA-AT compared to other types of treatment for PTSD.
Clinical trial registration: https://clinicaltrials.gov/ct2/show/NCT02102802, identifier NCT02102802.
1. Introduction
Post-traumatic stress disorder (PTSD), which can arise following exposure to a traumatic event or repeated stressful events and is a debilitating social and economic burden on individuals and their families (1, 2). PTSD is associated with an increased fear response (3) and distressing and intrusive re-experiencing of traumatic memories (4) that often serves as a barrier to the therapeutic process. This may explain why individuals with the most severe PTSD symptoms after trauma are more likely to end up with chronic PTSD durations (5, 6). Lifetime occurrence of PTSD in the general population is around 8%, and, prevalence is significantly higher in military personnel (17.1%) and first responders (10–32%), individuals on the front lines of societal trauma (7, 8). A meta-analysis of trials for military-related PTSD found that cognitive behavioral therapy and prolonged exposure therapy delivered clinically meaningful symptom improvements in 49–70% of patients, however 60–72% of veterans receiving these therapies still retained their PTSD diagnosis (9). Adverse outcomes such as increased symptoms and disengagement from treatment cause many current psychological therapies for PTSD to have high dropout rates (10), especially trauma focused therapies (11, 12).
One approach to developing more effective psychotherapies for PTSD is to administer a drug alongside psychotherapy to aid the therapeutic process (13). 3,4-methylenedioxymethamphetamine-assisted therapy (MDMA-AT) is hypothesized to reduce the fear response associated with re-experiencing traumatic memories, and therefore may facilitate tolerable processing of traumatic content in patients with PTSD (14). Phase 2 and 3 trials have demonstrated promise for MDMA-AT as a viable treatment for PTSD (15–18). MDMA, particularly the R-enantiomer, increases pro-social behavior and enhances fear extinction in mice and this effect appears to be mediated by serotonergic mechanisms (19, 20). In healthy humans, acute administration of MDMA has been shown to enhance positive and reduce negative affect during the recollection of autobiographical memories, while preserving vividness and emotional intensity (21). In another study, MDMA was found to preserve the memory accuracy when administered during both encoding and retrieval phases, while attenuating the recollection of salient details for both positive and negative memories, suggesting that MDMA alters emotional memory representations (22). Again in healthy controls, MDMA was found to enhance fear extinction learning/retention rates compared to placebo when administered during extinction training phases (23, 24). These findings suggest that MDMA may aid the therapeutic process, in part, by enabling patient access to emotionally challenging material and facilitating memory reconsolidation/fear extinction processes (25).
Functional magnetic resonance imaging (fMRI) measures changes in regional blood oxygenation over time and is thus used as a proxy for fluctuating neuronal activity. In-scanner environments can be absent of stimuli (resting-state fMRI—thought to measure intrinsic brain activity), or tasks or stimuli may be presented to study the regional brain dynamics underlying specific cognitive processes (26). In addition to the study of isolated regional activation changes, functional connectivity (FC) can be assessed to infer interaction between two or more brain regions. FC is defined as the statistical relationship (Pearson correlation in the case of the present study) between two brain regions’ activity over time. These tools have been used to study functioning of brain regions and their networks in a wide range of neuropathology and psychiatric disorders (27–29). PTSD patients have shown altered functioning of the precuneus, posterior cingulate cortex (PCC), anterior cingulate cortex (ACC), insula, prefrontal and frontoparietal regions, as well as the hippocampus and amygdala (30–43), suggesting augmented recruitment of brain regions involved in self-referential processing (44), salient autobiographical memory (45–49), and fear and emotion (50).
The specific effects of MDMA-AT on brain function in individuals with PTSD have not been characterized, but several studies suggest the amygdala and hippocampus may play an important role. The amygdala is broadly associated with fear response, and the hippocampus, associated with learning and memory, may provide contextual information necessary for cognitive-affect during memory recall (31, 36, 50). Sripada et al. (43) found combat veterans with PTSD have decreased amygdala-hippocampal resting-state functional connectivity (RSFC) compared to combat veterans without PTSD, which the authors speculate may represent an inability to contextualize affective information in PTSD. Increased amygdala-hippocampal RSFC following stress/trauma exposure has been shown to correlate with recovery from stress or trauma symptoms (51–53), suggesting a possible adaptive mechanism to threat exposure. In healthy volunteers, the acute administration of MDMA increases amygdala-hippocampal RSFC (54). Intranasal oxytocin also increases amygdala-hippocampal RSFC (53), and this effect appears to be mediated by serotonin system signaling (55)—two neuromodulators that MDMA significantly increases in the extracellular/plasma concentration of, and that play a crucial role in its effects on pro-social behavior and fear extinction (20, 56–61). Despite evidence that RSFC between the amygdala and hippocampus is implicated in PTSD and that this connection may be modulated by MDMA, no study to date has shown relationships between changes in these regions’ functional connectivity and the therapeutic effects of MDMA-AT.
Herein, we describe results from a study of combat veterans and first-responders undergoing MDMA-AT for PTSD in a randomized, double-blind, dose-response phase 2 clinical trial (62). The Clinician-Administered PTSD Scale (CAPS-IV) (an hour-long, semi-structured interview centered around an index trauma) (63) was assessed throughout the study to track PTSD severity. Enrolled individuals had moderate-to-severe PTSD with a chronic PTSD duration of 6 months or more. Both resting-state and task-fMRI data, acquired while individuals listened to trauma-related and neutral audio scripts, were collected before and two months after MDMA-AT (follow-up scans were collected after the blind was broken).
Prior to analysis, we hypothesized that MDMA-AT would increase RSFC between the amygdala and hippocampus (43, 52–55, 64). We further hypothesized that, at baseline, brain activity would be higher during the trauma-related listening task compared with the neutral listening task in regions associated with autobiographical memory, fear, and emotion, and that this effect would be reduced post-treatment (30). In a final set of analyses, we assessed pre-to-post treatment change in the FC of several regions of interest contained within the limbic, salience, and default mode networks known to be hyperactive in PTSD (65) during the trauma and neutral autobiographical memory task-fMRI scans. FC changes were then correlated with the pre-to-post treatment recovery in overall PTSD symptomatology—as measured by decreases in CAPS-IV total severity scores.
2. Methods
2.1. Trial design
The present study analyzed data from a sub-study (NCT02102802) of a Phase 2 randomized, double-blind, dose-response trial of MDMA-AT in veterans and first responders with severe and chronic PTSD (NCT01211405) (62). A detailed study description of the parent study can be found in (62), and we summarize the study design in the Supplementary material. Here, we provide a description of the MRI-based sub-study design.
Participants in the parent study were able to opt into the MRI-based sub-study after which they provided written informed consent approved by the Medical University of South Carolina Institutional Review Board. They were screened for additional neuroimaging related eligibility criteria and were excluded for any conditions that could render MRI unsafe. A script-driven autobiographical memory paradigm was used to assess brain activity during symptom provocation (66–68). Following baseline CAPS-IV assessment in the parent study, sub-study participants worked with investigators to create two scripts: one describing a personally traumatic event and one reflecting their typical morning routine at home. Two audio recordings, each six minutes in length, were created from the participant’s reading of each script. Each audio recording was divided into two 3-minute blocks for the task-fMRI. All participants, in all arms, were imaged at baseline, prior to therapy, and again at the follow-up visit two months after their final dosing session (Figure 1). LD (N = 2) and MD (N = 2) participants were additionally imaged after the primary endpoint visit in Stage 1 (one month following their second dosing session), however the small sample sizes prevented any meaningful analysis with these scans. The present analysis focuses on the pre- and post-therapy effects of MDMA-AT on fMRI biomarkers, and thus uses the scans collected at pre-treatment (baseline) and at least 2 months after the largest dose of MDMA (follow-up).
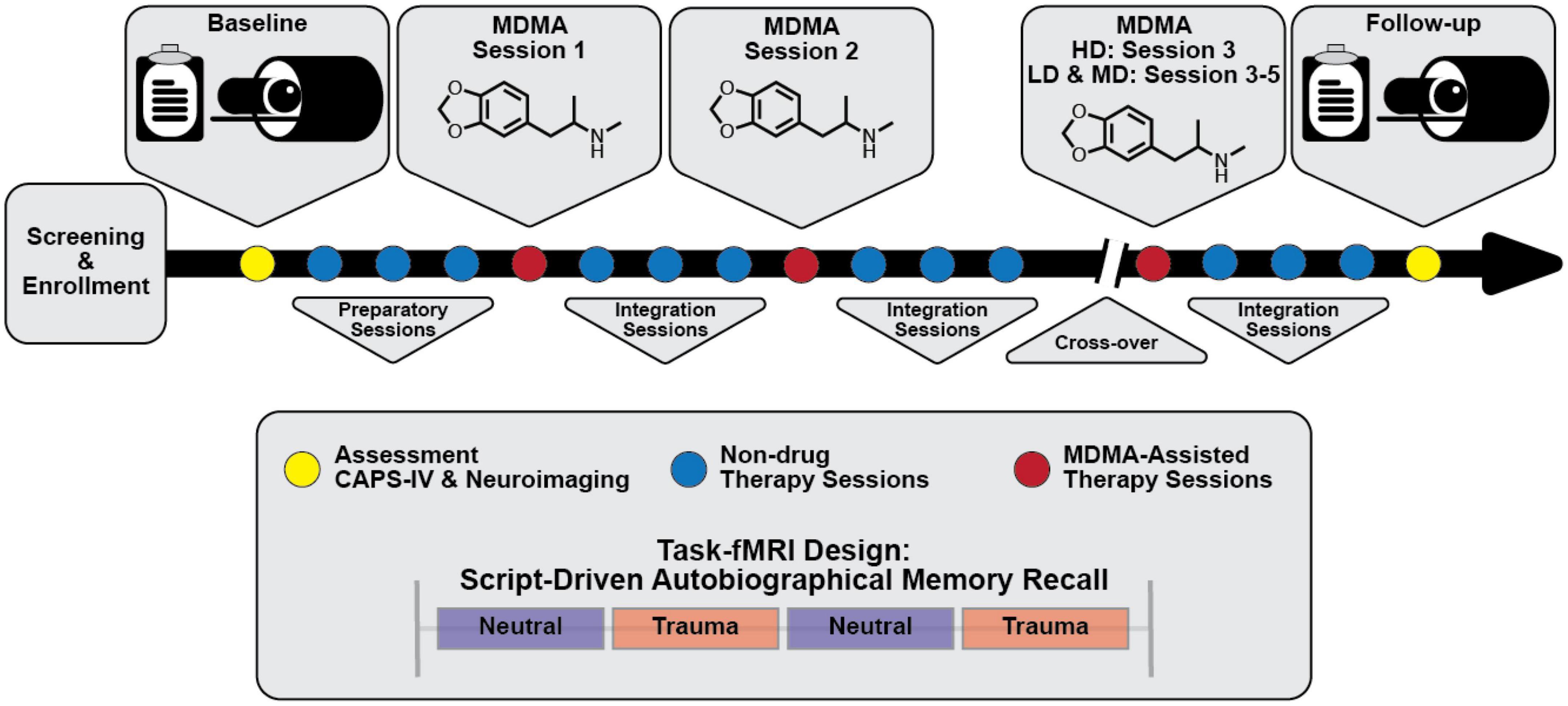
Figure 1. Simplified study design. Subjects were assessed and imaged at the start of the study (baseline). All subjects [low dose (LD; 30 mg MDMA), medium dose (MD; 75 mg MDMA), and high dose (HD; 125 mg MDMA)] underwent three non-drug preparatory therapy sessions prior to their first MDMA dosing session. Each MDMA session was followed by three non-drug integration therapy sessions. After MDMA session 2 and the subsequent integration sessions, subjects were assessed and the dosing blind was broken. HD subjects completed their final set of drug and non-drug therapy sessions unblinded, and LD/MD subjects crossed over into the HD arm where they completed three sets of drug and non-drug sessions, now with the higher dose and unblinded. All subjects were assessed and underwent MRI approximately two months following their last HD MDMA session. See “2. Methods” section for a full description of study design and scanning protocols.
2.2. MRI acquisition
At each scanning session, participants underwent MRI on a 32 channel 3T Siemens system. T1 anatomical scans with TR/TE = 1,900/2.34 ms and 0.9 mm × 0.9 mm × 1.0 mm voxel size were collected, followed by two identical task fMRI (design described below) (TR/TE = 2,200/35 ms, 3.0 mm isotropic voxel size, length of each scan = 14:25 min) and one resting state fMRI (TR/TE = 2,000/30 ms, 3.3 mm × 3.3 mm × 3.0 mm voxel size, length = 5:00 min).
Participants’ 6-minute trauma and neutral audio scripts were divided into two three-minute trauma and neutral blocks each (see “2.1 Trial design” for description of audio recordings). During fMRI, participants were presented with the visual cue “allow” and instructed to allow themselves to experience the scripts as their audio recordings were played for both neutral and trauma blocks. Each task scan had an alternating block design (neutral 1, trauma 1, neutral 2, trauma 2) with an 18 second “rest” period at the start of the scan and between each block, and about a minute of rest at the end of the scan. The precise length of each audio block was 2.95 min.
2.3. Image preprocessing
FreeSurfer (69) was applied to the T1s to create white matter (WM), gray matter (GM) and cerebrospinal fluid (CSF) segmentations. FMRIB Software Library (FSL) (70) was used for (1) brain extraction, (2) registration between T1s and fMRI’s (brain-boundary registration, non-linear, full-search), (3) high-pass filtering (4) slice-time and (5) motion correction.
2.4. Motion
High-motion frames [defined as >0.9 mm relative framewise displacement (FD); CONN Toolbox standard parameter (71)] were counted as outlier volumes and scrubbed from functional connectivity and activation analyses. The percentage of scrubbed volumes for resting-state scans ranged from 0 to 6.8% (mean = 2.1%) of total volumes. The percentage of scrubbed volumes for task scans ranged from 0 to 11% (mean = 1.7%) of total volumes. The mean composite FD (72) for each condition (rest and task) was calculated and compared across time-points using two-sided, paired t-tests. Mean FD during resting-state fMRI scans at baseline and follow-up were 0.12 (±0.06 s.d.) mm and 0.12 (±0.07 s.d.) mm, respectively, and were not significantly different from one another (t-statistic = –0.02; p = 0.99). Mean FD during task fMRI scans at baseline and follow-up were 0.22 (±0.16 s.d.) mm and 0.17 (±0.09 s.d.) mm, respectively, and were not significantly different from one another (t-statistic = 1.23, p = 0.25).
2.5. Activation analysis: Brain response to trauma versus neutral audio listening
FSL’s fMRI Expert Analysis Tool (FEAT) (73) was used for fitting a general linear model (GLM) to the voxelwise timeseries for each task scan after spatial smoothing using a Gaussian kernel function [6 mm full width at half maximum (FWHM)]. For 1st- level analysis, models were generated for the neutral block, the trauma block, and a contrast of the two (trauma > neutral). Confound explanatory variables (EVs) included the temporal derivative of each block, 5 nuisance regressors each for WM and CSF signal, outlier volumes [spikes in global signal (>5 standard deviations) and motion (>0.9 mm FD); CONN Toolbox standard parameters (71)], and 24 motion confounds (74). Second-level analysis averaged the models from each of the two task scans performed at each time point. Third-level analyses, using a two-sided, one-sample t-test [FSL randomize; (75)] identified group-level response for the contrast model (i) at baseline, and (ii) at the two-month follow-up. A final third-level analysis (iii) compared the group-level responses to the contrast model at baseline and follow-up using a two-sample, two-sided, paired t-test (FSL randomize; (75)). Third-level results were corrected for multiple comparisons using threshold-free cluster enhancement (TFCE; alpha = 0.05) (76) which identifies significant clusters based on the extent of local support from surrounding voxels.
2.6. RSFC analysis
Prior to extraction of RSFC, in addition to the preprocessing steps taken in ‘‘2.3 Image preprocessing,’’ fMRI data were further denoised using an in-house pipeline.1 FMRIs were bandpass filtered and regressed for 24 motion confounds (74), 5 nuisance regressors each for WM and CSF, and one for global GM signal. The first five frames (scanner start-up noise) and confound frames (spikes in global signal and motion) were discarded. RSFC (Fisher Z-transformed Pearson correlation values) between the right and left amygdala and right and left hippocampus (77) was calculated for each resting-state scan. For the supplemental RSFC analysis, each hippocampus was further segmented into head and tail portions using FreeSurfer’s hippocampal subregion segmentation tool (78). Two-tailed, paired t-tests were used to compare the 4 RSFC measures before and after MDMA-AT. Baseline to follow-up changes in these 4 measures were also correlated (Pearson’s) with individual level reductions in CAPS-IV using participant’s age and mean FD changes (follow-up—baseline) as covariates of non-interest. All statistical tests were performed at an alpha level of 0.05. P-values were corrected for multiple comparisons using the Benjamini-Hochberg algorithm (79) where indicated (pFDR).
2.7. Task FC analysis
Prior to extraction of task FC, the preprocessed residuals from the task activation analysis (section “2.5 Activation analysis: Brain response to trauma versus neutral audio listening”) were further denoised with bandpass filtering and regressed for global GM signal. The first five frames (scanner start-up noise) and confound frames [spikes in global signal (>5 standard deviations) and motion (>0.9 mm FD); CONN Toolbox standard parameters (71)] were ignored. Functional connectivity (Fisher Z-transformed Pearson correlation values) during each task fMRI scan (task FC) was calculated between 18 regions of interest (ROIs): the right and left hippocampus head, hippocampus tail, amygdala, precuneus, caudal anterior cingulate cortex (ACC), rostral ACC, posterior cingulate cortex (PCC), isthmus cingulate, and insula. All ROIs were extracted from the Disikan–Killiany cortical atlas (see Supplementary Figures 7, 8 for ROI definitions) (77), and head and tail portions of the hippocampus were created using FreeSurfer’s hippocampal subregion segmentation tool (78). Two identical task scans were collected at each time point, thus FC values obtained from both scans were averaged to give a single value for each connection per subject. Group-level changes from pre- to post-therapy in the strength of functional connections were assessed using two-tailed, paired t-tests. Pearson correlations were calculated between individuals’ changes in functional connection strength and change in CAPS-IV total severity scores (follow-up—baseline) using participant age and mean FD changes between baseline and follow-up as covariates of non-interest. All statistical tests were performed at an alpha level of 0.05. P-values were corrected for multiple comparisons using the Benjamini-Hochberg algorithm (79) where indicated (pFDR).
3. Results
3.1. CAPS-IV total severity scores significantly decreased after HD MDMA-AT
Ten participants enrolled in the sub-study, and one withdrew consent after baseline due to anxiety in the MRI scanner, leaving nine participants with MRI data at both time points (6 male, 3 female, aged 41.3; standard deviation (SD) = ±10.9 years; 8 veterans and 1 first-responder; see Supplementary Table 1 for additional demographic information). All participants had chronic PTSD (mean duration = 84 (±45) months). One participant’s baseline resting-state fMRI was truncated due to technical issues, leaving eight participants for resting-state analysis and nine for the task fMRI analysis. One participant began the trial with moderate PTSD (CAPS-IV > 39), while the remaining eight presented with severe PTSD (CAPS-IV > 59). Mean (SD) CAPS-IV total severity scores of the nine individuals pre- and post-MDMA-AT were 86 (±16) and 39 (±25), respectively, representing a significant decrease in PTSD symptom severity between the two time points (Figure 2; N = 9, t = 6.36, p = 0.00022). The average percent decrease in CAPS was 57 (±26)%. Results on all participants enrolled in the Phase 2 parent trial have been previously reported (62).
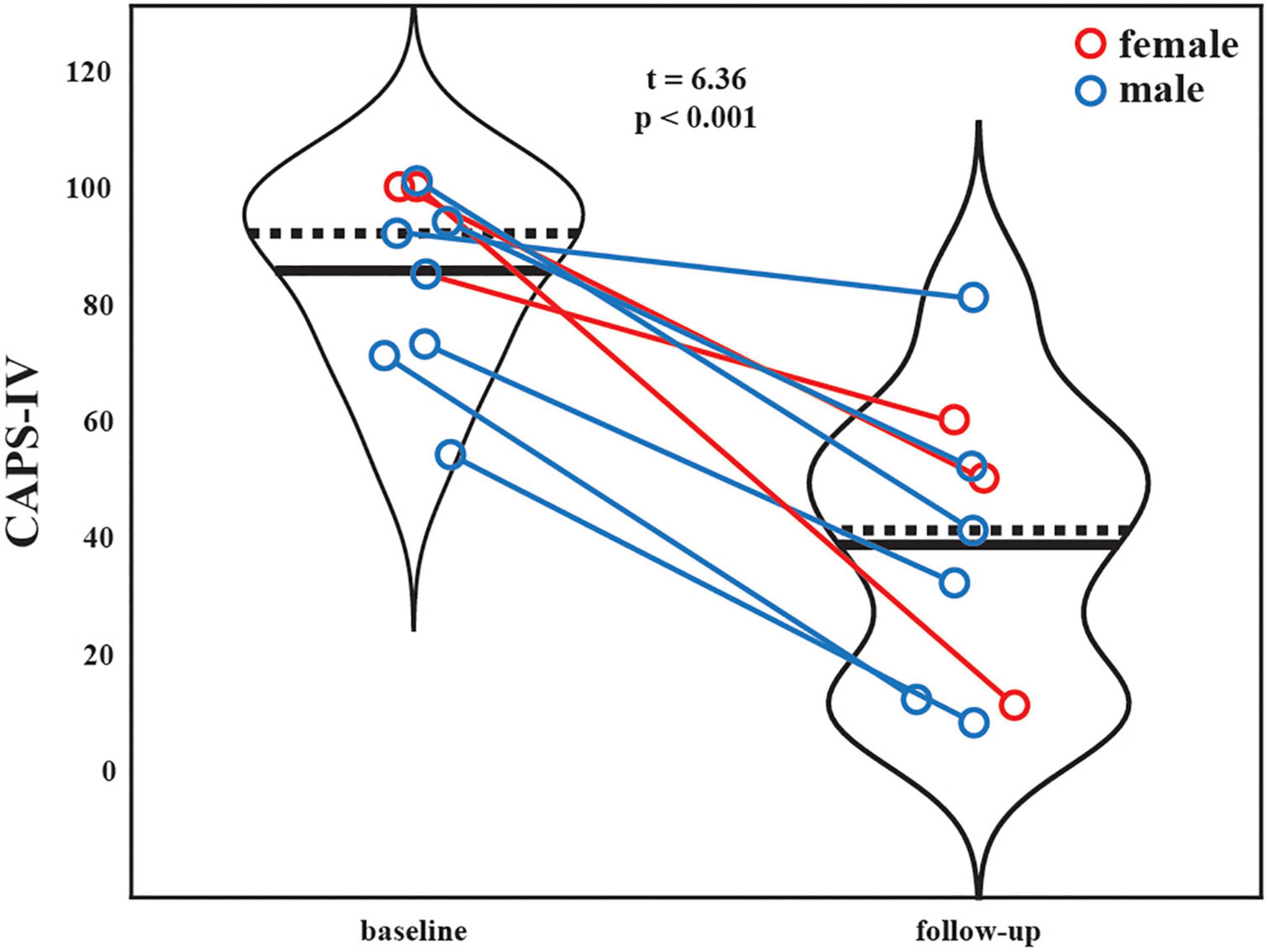
Figure 2. Patient CAPS-IV total severity scores at the baseline (pre-therapy) and two-month follow-up (post-therapy) scanning sessions. Black solid and dashed lines indicate group means and medians, respectively. Blue marker = male. Red marker = female. A significant reduction is PTSD severity was observed after MDMA-AT (baseline > follow-up; N = 9, t = 6.36, p = 0.00022).
3.2. Baseline versus two-month follow-up amygdala-hippocampal RSFC
The RSFC was assessed between the amygdala and hippocampus before and after MDMA-AT and the strengths of these connections are illustrated in Figure 3. All connections trended toward increased RSFC after therapy compared to before therapy (using a two-sided paired t-test), with left amygdala to left hippocampus having a significant increase prior to (but not after) corrections for multiple comparisons (N = 8, t = –2.91, uncorrected p = 0.0225, pFDR = 0.0901).
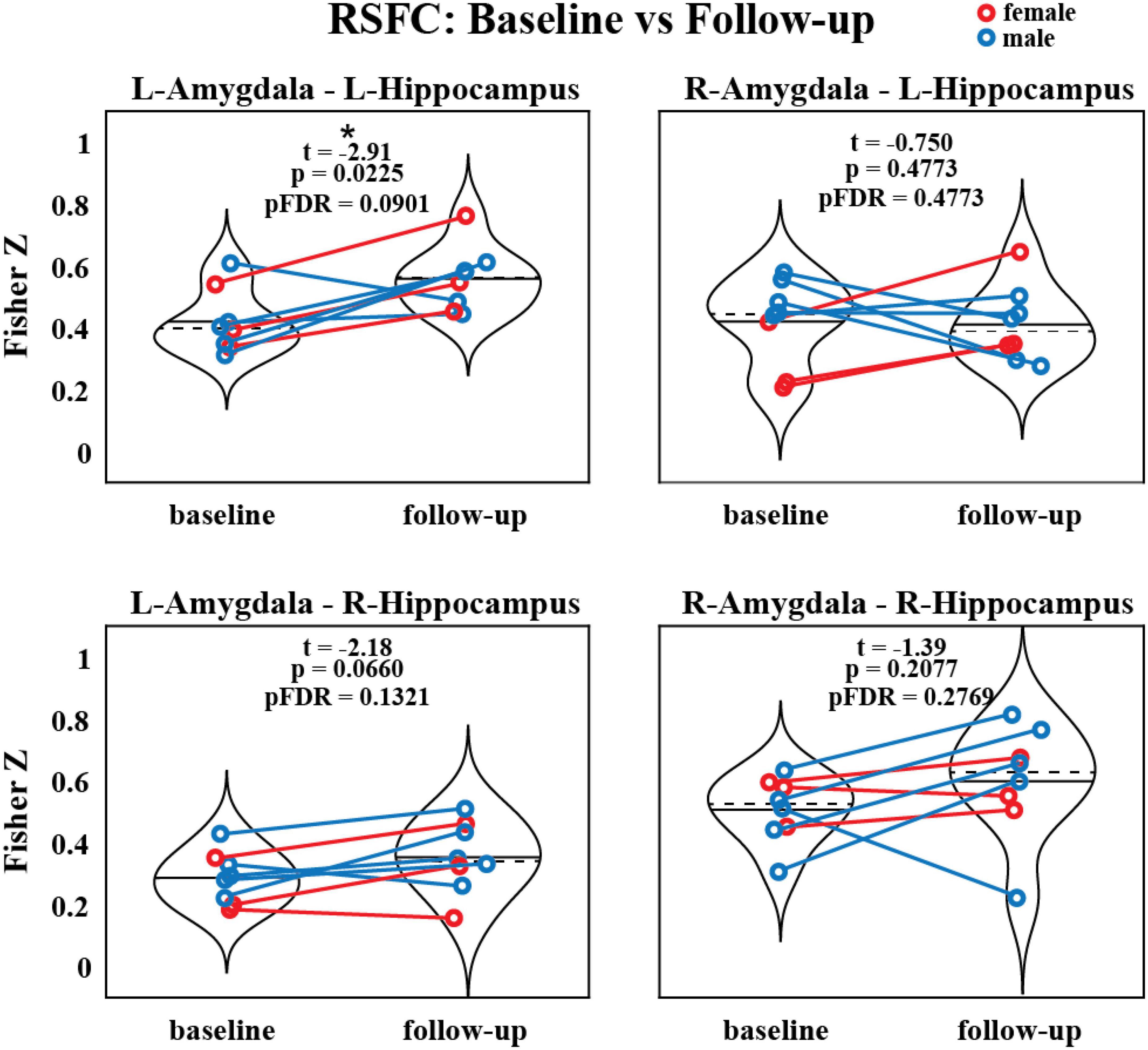
Figure 3. Resting-state functional connectivity between the amygdala and hippocampus before and after MDMA-AT. P-values from a two-sided, paired t-test. Blue = male. Red = female. Black solid and dashed lines indicate group means and medians, respectively (N = 8; t-statistics indicate baseline > follow-up; *uncorrected p < 0.05).
Individual-level pre-to-post-therapy changes between the strength of these functional connections were then correlated (two-sided Pearson’s) with changes in CAPS scores (Supplementary Figure 1). Only one of these correlations (right amygdala to left hippocampus FC versus CAPS) was significant before correction (N = 8; R = –0.820, uncorrected p = 0.0460, pFDR = 0.183).
3.3. Brain activation during symptom provocation
A script-driven autobiographical memory paradigm was used to assess brain activity during symptom provocation (66–68). We compared whole-brain, script-driven activations (trauma > neutral) at baseline and follow-up (Figure 4). Before therapy (baseline), there tended to be larger magnitude activation in response to the trauma script versus the neutral script, as evidenced by the generally positive t-statistics (Figures 4A–D). After correction using threshold free cluster enhancement (TFCE), there was significantly greater activation during the trauma scripts compared to the neutral scripts in four separate areas (see Figure 4 caption for details of each). After therapy, there were smaller magnitude differences between brain activity in response to the two scripts, with no significant clusters (Figure 4E). Finally, we assessed the differences in the contrast before and after therapy (follow-up > baseline). There was generally greater contrast between the trauma and neutral scripts at baseline compared to at follow-up, with one cluster in the bilateral cuneus and lingual gyrus demonstrating significance after correction using TFCE (Figure 4F).
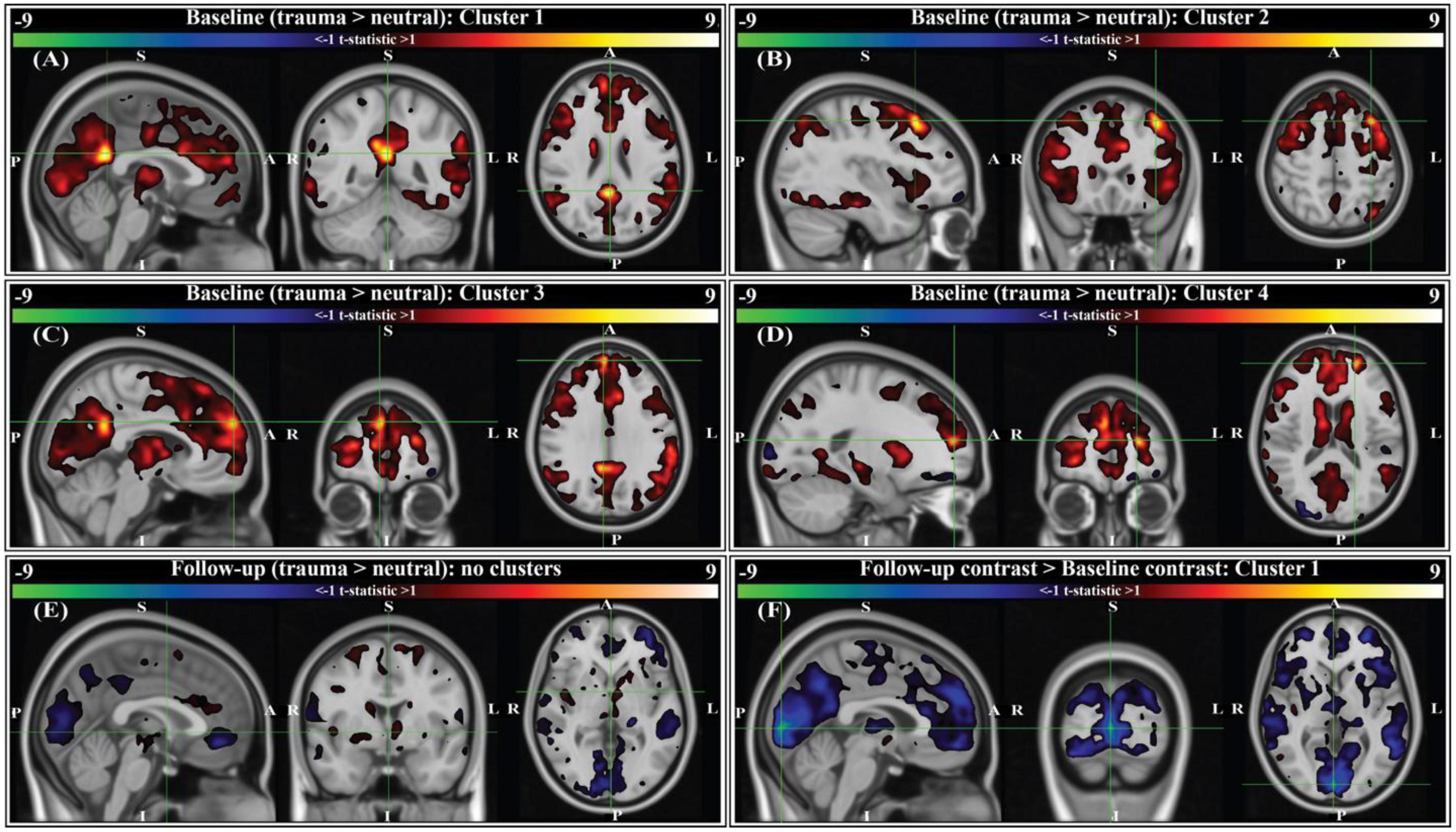
Figure 4. Group-level activation contrasts for trauma versus neutral script listening tasks (N = 9). All panels show t-statistics for corresponding contrasts. Analyses were performed in 3 mm MNI standard space, however results here are interpolated into 1 mm MNI standard space and clipped to only show t-statistics greater than ± 1 for visualization purposes. Statistics reported below (voxels, volume, t-statistic, corrected p-value) were calculated from original 3 mm results. P-values were corrected using threshold-free cluster enhancement (TFCE; see section “2. Methods”). For panels (A–E), positive t-statistics indicate greater activation to trauma scripts compared to neutral. For panel (F), sign indicates the direction of change in trauma > neutral contrast from baseline (i.e., negative t-statistics indicate the contrast between trauma and neutral scripts was decreased at the two-month follow-up compared to baseline). Crosshairs are located on the center of gravity (c.o.g.) of significant clusters. (A) Cluster 1 for the baseline contrast is located primarily in the right and left isthmus cingulate, with some overlap into the right and left precuneus (c.o.g. MNI152 [0, –48, 24]; 6 voxels (162 mm3); c.o.g. t = 9.61, p(TFCE) = 0.0293). (B) Cluster 2 for the baseline contrast is located in the left caudal middle-frontal gyrus (c.o.g. MNI152 [-36, 21, 51]; 3 voxels (81 mm3); c.o.g. t = 9.01, p(TFCE) = 0.0234). (C) Cluster 3 for the baseline contrast is located in the right medial prefrontal cortex (c.o.g. MNI152 [6, 57, 30]; 2 voxels (54 mm3); c.o.g. t = 7.41, p(TFCE) = 0.0488). (D) Cluster 4 for the baseline contrast is located in the left rostral middle frontal gyrus (c.o.g. MNI152 [-21, 54, 15]; 1 voxel (27 mm3); c.o.g. t = 9.45, p(TFCE) = 0.0312). (E) There were no significant activation contrasts at the two-month follow-up (crosshairs shown at MNI152 [0, 0, 0]). (F) Comparing the group-level contrasts between time points (follow-up > baseline), there exists one significant cluster located primarily in the right and left cuneus, with some overlap into the right and left lingual gyrus (c.o.g. MNI152 [3, –90, 3]; 47 voxels (1,269 mm3); c.o.g t = –9.31, p(TFCE) = 0.0391).
3.4. Baseline versus two-month follow-up changes in task FC
We compared the pre- and post-therapy FC strength between 18 brain regions of interest (ROIs) during the task fMRI scans involving neutral and traumatic autobiographical audio recordings (Figure 5A). The ROIs are as follows: the right and left hippocampus head, hippocampus tail, amygdala, precuneus, caudal anterior cingulate cortex (ACC), rostral ACC, posterior cingulate cortex (PCC), isthmus cingulate, and the insula. Only one functional connection was significantly modified at follow-up compared to baseline: the right amygdala to left caudal ACC (N = 9; t-statistic = 3.04, p = 0.0148). This finding was no longer significant after corrections for multiple comparisons, however (pFDR = 0.9875).
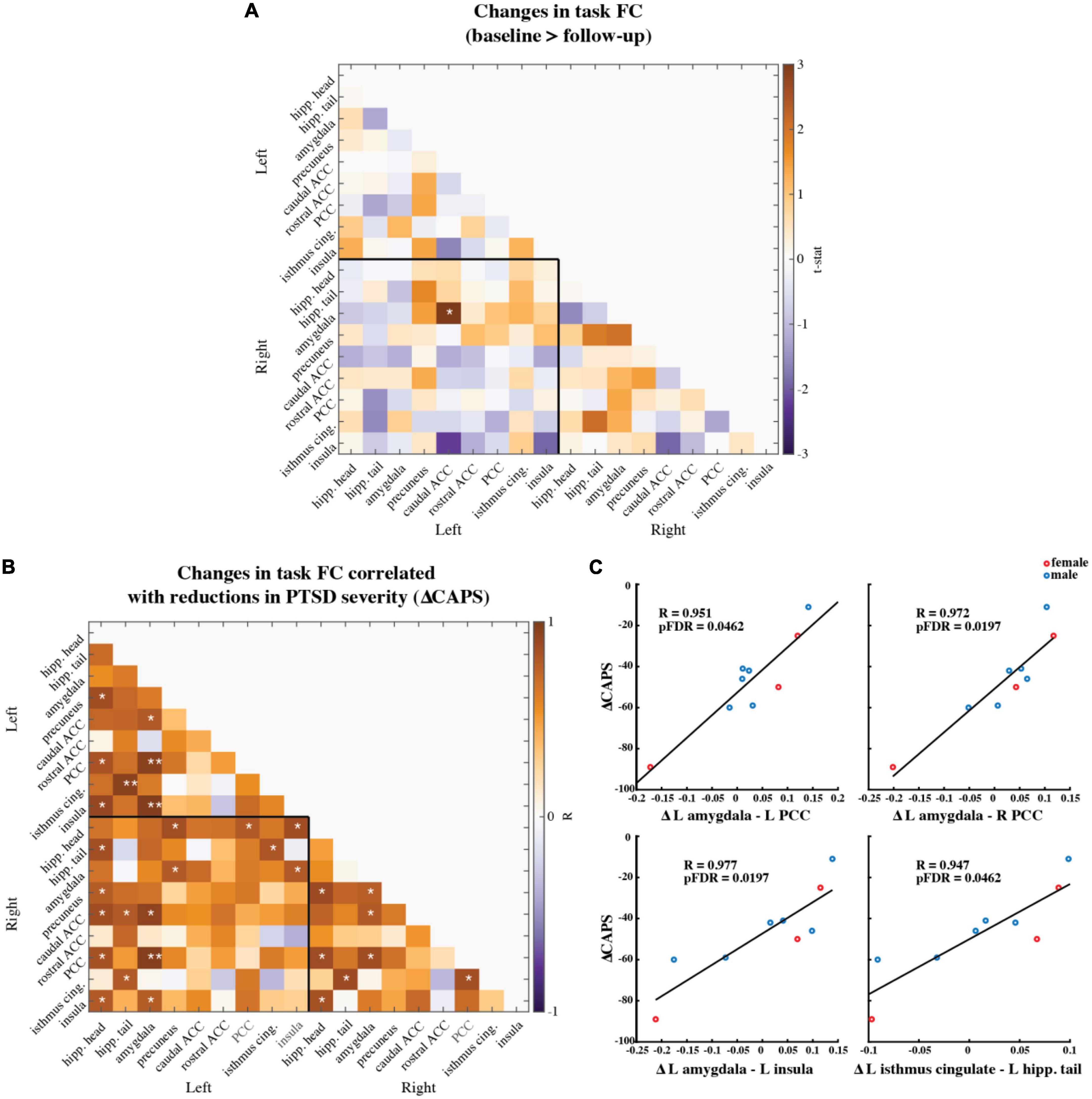
Figure 5. (A) Paired t-statistics shown for differences in functional connectivity between all brain regions of interest during the task fMRI scan involving neutral and trauma memory audio listening (N = 9; baseline > follow-up; *two-sided uncorrected p < 0.05; **pFDR < 0.05, corrected). (B) Pearson correlation values between changes in ROI functional connectivity and reduction in CAPS scores. Changes were calculated as follow-up values minus baseline values. (N = 9; *uncorrected p < 0.05; **pFDR < 0.05) Age and mean FD difference between baseline and follow-up were included as covariates of non-interest. (C) Scatter plots of the three correlations that remained significant after corrections for multiple comparisons (i.e., pFDR < 0.05). Red marker = female. Blue marker = male.
Individual-level pre-to-post therapy changes in these functional connections were then correlated (two-sided, Pearson’s) with the individual-level reductions in CAPS-IV scores (Figure 5B). Most correlations were positive, meaning that larger reductions in connectivity from pre- to post-therapy corresponded to larger improvements in PTSD symptoms. Four correlations between FC and CAPS-IV changes were significant following multiple comparisons correction: the left amygdala and left PCC (N = 9; Pearson’s R = 0.951, pFDR = 0.0462), the left amygdala and right PCC (N = 9; Pearson’s R = 0.972, pFDR = 0.0197), the left amygdala and left insula (N = 9; Pearson’s R = 0.977, pFDR = 0.0197), and the left isthmus cingulate and left hippocampal tail (N = 9; Pearson’s R = 0.947, pFDR = 0.0462) (Figure 5C).
3.5. Supplemental analyses
Although not the primary focus of our analysis, we also repeated the previous correlations using other secondary outcome measures in place of CAPS-IV total severity scores. Namely, changes between baseline and follow-up in the BDI-II (depression symptoms), the PSQI (sleep quality), the PTGI (perceived growth following trauma), the DES-II (symptoms of dissociation), and the GAF (general psychological function) were used (Supplementary Figure 2). Following correction for multiple comparisons, none of these correlations were significant.
We also replicated our a priori analyses of amygdala-hippocampal RSFC using head (anterior) and tail (posterior) sub-regions of the hippocampus (Supplementary Figure 3). The left hippocampal head to left amygdala RSFC was increased at follow-up compared to baseline (N = 8; t = –2.593, uncorrected p = 0.0358), as was the RSFC between the left hippocampal tail and right amygdala (N = 8; t = –3.00, uncorrected p = 0.0199). Neither of these effects were significant after corrections for multiple comparisons.
Lastly, we replicate our main functional connectivity analyses without the use of global signal regression and find that these results largely show the same trends, however there is less significance in some cases (Supplementary Figures 4–6). The correlation between the left amygdala and left insula task functional connectivity change and CAPS reductions was significant both with and without the use of global signal regression (Supplementary Figure 6; N = 9; R = 0.971, pFDR = 0.0229).
4. Discussion
We report signatures of brain response during rest and audio listening task in eight veterans and one first-responder with clinically diagnosed chronic and severe PTSD before and two-months after MDMA-assisted therapy. We found a significant reduction in CAPS-IV total severity scores after therapy (Figure 2), indicating our sub-study participants mirrored the results from the parent study (62). We found a trend suggesting that RSFC between the amygdala and hippocampus was strengthened post-therapy, particularly in the left hemisphere (Figure 3). Prior work suggests that modulation of amygdalae-hippocampal RSFC may be an important component of MDMA-AT for PTSD (43, 51–55), thus investigating this connection in future studies is warranted. We also found participants had increased activation in areas involved with self-referential processing and autobiographical memory while listening to traumatic versus neutral memory narrations pre-therapy (Figures 4A–D), and that no significant contrast existed after MDMA-AT (Figure 4E). Comparing trauma versus neutral contrasts between baseline and follow-up revealed a significant decrease in cuneus contrast after MDMA-AT (Figure 4F). Finally, the pre- to post-therapy reductions in FC during the script listening task between the left amygdala and right PCC, left PCC, and left insula, as well as FC between the left isthmus cingulate and left hippocampal tail strongly and significantly correlated with PTSD symptom improvement (Figure 5C). These results begin to shed light on the neurological mechanisms that may drive MDMA-AT for PTSD.
Previous work quantifying functional connectivity in PTSD and in stress exposure (43, 51–53) and acute MDMA administration in controls (54) suggests one mechanism of MDMA-AT may be to increase pathologically lowered amygdala-hippocampal RSFC (25). The amygdala is associated with fear expression, threat recognition, and heightened response to emotional memories and is often dysregulated in patients with PTSD (31, 36, 50, 65, 80, 81). The hippocampus also plays a central role in PTSD as it is thought to provide contextual information important for cognitive-affect during memory recollection (31, 36). Sripada et al. (43) found combat veterans with PTSD had reduced amygdala-hippocampal RSFC compared to combat-exposed controls, leading them to speculate that this may relate to an inability to contextualize affective information in PTSD. Carhart-Harris et al. (54) demonstrated that amygdala-hippocampal RSFC is increased acutely in MDMA administration compared to placebo and this increase occurred in a manner that correlated with the drug’s subjective effects at a near-significant level, leading these researchers to propose that this functional connection was a primary target of MDMA-AT. Increased amygdala-hippocampal RSFC has also been linked to intranasal oxytocin administration after stress exposure (53) and this effect was mediated by serotonin signaling (55)—two neuromodulators that play a significant role in the pro-social and fear extinction effects of MDMA (20, 56–61). Prior to our analysis (although after the study was designed and the data collected), we hypothesized that the RSFC between the amygdala and hippocampus would be higher after MDMA-AT compared to pre-therapy levels. Only the RSFC between the left amygdala and left hippocampus was significantly increased (Figure 3) however, this finding no longer met thresholds for significance after multiple comparisons correction (pFDR = 0.09). We also found that the amount of increased right amygdala—left hippocampal RSFC after MDMA-AT positively correlated with PTSD symptom improvement at a near-significant level (Supplementary Figure 1; R = –0.820, uncorrected p = 0.046, pFDR = 0.183). Our current findings, though inconclusive, are suggestive of a trend toward moderate increases in amygdala—hippocampal RSFC two-months after MDMA-AT. It is possible that more significant changes would have been observed with a larger sample size, longer resting-state scans, or imaging performed closer to MDMA administration. These findings justify the continued investigation of amygdala-hippocampal RSFC in the therapeutic mechanisms of MDMA-AT in future studies.
We next sought to study brain response during autobiographical memory listening to draw additional conclusions about MDMA-AT’s effects in individuals with PTSD. Before therapy, participants had larger activation in four areas during an individualized trauma script listening task compared to neutral script listening: the right and left isthmus cingulate and precuneus, the left caudal middle frontal gyrus, the right medial prefrontal cortex, and the left rostral middle frontal gyrus (Figures 4A–D). These regions are broadly involved in self-processing operations (e.g., first-person perspective taking), episodic memory retrieval, visual-spatial imagery, auto-biographical memory recollection, and are included in or interact with the default mode network (44, 46, 49, 82–85). The retrosplenial cortex—located within the isthmus cingulate—is also found to be consistently activated by emotionally salient stimuli, and has been proposed to play a role in the interaction between emotion and memory (48). We conjecture that increased activation in these regions during traumatic compared to neutral audio listening (Figures 4A–D) could be related to an increased intensity of the recollection or re-experiencing of traumatic memories compared to neutral ones for patients before therapy. At 2-month follow-up to MDMA-AT, there was no significant difference in the trauma vs neutral script activation (Figure 4E). The longitudinal comparison of these two time points indicated that the contrast between trauma and neutral was larger at baseline, particularly in a significant cluster in the right and left cuneus/lingual gyrus (Figure 4F). Cuneus activity during autobiographical memory tasks often coincides with activity in the frontal regions highlighted by the baseline contrast, and has been found to correlate with memory recall accuracy (49, 83, 84). Cuneus activity is thought to enhance the visual imagery of autobiographical memory recollection (86), therefore decreased contrast in this area at follow-up suggests that intensity of visual imagery contrast between trauma and neutral memories may be decreased after MDMA-AT. Larger studies may allow more statistical power to identify additional longitudinal differences. Other longitudinal studies of individuals with PTSD have found that decreases in precuneus, isthmus cingulate, and middle frontal gyrus activation during symptom provocation is correlated with reductions in PTSD symptom severity (87, 88).
PTSD is often associated with hyperactivity in the amygdala (36); the acute administration of MDMA in healthy volunteers decreases blood flow to the amygdala during rest (54) and attenuates its response to angry faces (89). We had hypothesized that we would observe hyperactivity of the amygdala to trauma versus neutral scripts at baseline and that MDMA-AT would attenuate this response, however we observed neither. It is important to note inconsistencies in the literature here. Amygdala hyperactivity in PTSD is not always observed, possibly due to differences in subtypes, sex, cultural representations, or choice of paradigm (66, 90–94). Additionally, while MDMA did suppress amygdala activity during rest and in response to angry faces as previously mentioned, there was no observed impact on its response to autobiographical memories (21). While activation-based analyses deserve continued attention in future studies to rectify these inconsistencies, functional connectivity is a complimentary approach we can use to extract additional information from fMRI.
Functional connectivity analyses in individuals with PTSD have revealed aberrant connectivity between several regions within default mode, limbic, and salience networks, and more broadly, regions involved in emotional and self-referential processing (32–35, 37–41, 43, 67), and, further, the administration of MDMA in healthy volunteers has been shown to disrupt the functional integrity of these networks (54, 95–98). Here, we measured functional connectivity during script-driven autobiographical memory recall between the right and left hippocampus head, hippocampus tail, amygdala, precuneus, caudal ACC, rostral ACC, PCC, isthmus cingulate, and the insula. Our ROIs were defined and labeled based on the Desikan-Killiany (DK) brain atlas (Supplementary Figures 7, 8) (77). We chose to segment the hippocampus into anterior (head) and posterior (tail) ROIs based on recent work showing that the two portions’ FC are differentially effected by PTSD (34). We assessed group-level changes in the strength of these functional connections and found no significant differences between baseline and follow-up after corrections for multiple comparisons (Figure 5A). However, we did find that greater recovery (larger decreases in CAPS-IV at follow-up) was associated with reductions in FC between the left amygdala and the right and left PCC, as well as the left insula (Figure 5C). The acute effects of MDMA in healthy volunteers has been shown to decrease the FC of the PCC (96, 97) and insula (95), and alter amygdala and hippocampus FC (54), highlighting the potential relevance of our current findings. Amygdala to posterior and mid-cingulate cortex FC has been shown to be associated with PTSD severity at different stages of disease progression, although differing patient populations and assessment time-lines lead to conflicting results (40, 99–102). One finding in healthy adults shows increased amygdala—PCC FC following the acute exposure to stress (103), thus the association between recovery and reduced amygdala—PCC task FC at follow-up possibly relates to reduced stress response to trauma memories (although the finding by Veer and colleagues is more posterior to the ROI used here). Amygdala and insula RSFC is increased in PTSD (39, 43) [except in one study which finds the opposite (38)], and reduced amygdala-insula FC during negative image reappraisal is associated with larger improvements in PTSD symptoms (101). The strength of left amygdala-insula FC also positively correlates with the amount of acute anxiety measured in participants just before scanning (104). Attenuated functional connectivity of these two regions at follow-up in the present study possibly suggests a decreased intensity of recalled events, less “re-experiencing,” or reduced anxiety during the script-driven memory recall (65). Lastly, we found that reductions in CAPS-IV at follow-up were associated with reduced FC between the left isthmus cingulate and left hippocampal tail (Figure 5C). The isthmus cingulate labeled here consists of the most posterior potions of the PCC (Supplementary Figure 7). Increased FC between these two regions has previously been reported in PTSD patients compared to trauma-exposed health controls (33, 34), and the present finding is possibly indicative of changes in memory contextualization and reduced threat sensitivity at two-month follow-up to MDMA-AT compared to baseline (100).
PTSD is characterized by decreased fear extinction in response to trauma-related stimuli. One possible mechanism through which MDMA-AT operates is enhanced reconsolidation and/or fear extinction processes (25). Several studies with MDMA implicate reconsolidation or fear extinction processes, and while it is currently unclear whether MDMA acts on only one or both, it is important to note that the two interact (105). Rodent models have demonstrated that the administration of MDMA prior to extinction learning enhances extinction retention (tested 48 h after learning) and this effect is blocked by acute and chronic treatment with a serotonin transporter inhibitor (20, 106). Hake et al. (107) found that MDMA administered during extinction learning phases did not enhance fear extinction memory, while MDMA administration during reconsolidation phases resulted in prolonged reductions in conditioned fear. In addition, MDMA administered prior to trauma-cue exposure (reconsolidation phase) in rodents resulted in reduced stress-related behavioral responses 7 days later (108). Two recent trials in healthy humans found that MDMA (100 and 125 mg, respectively) administered prior to extinction learning resulted in improved extinction learning at extinction recall phases (48 and 24 h later, respectively) compared to the placebo group (23, 24). Doss et al. (22) found that 1 mg/kg of MDMA in healthy humans attenuated the encoding and retrieval of salient details from positive and negative stimuli (but not neutral stimuli), suggesting an ability for MDMA to alter emotional memory representation. Interestingly, a fMRI study in healthy humans found decreased activation in the precuneus/PCC during fear extinction learning (109), regions highlighted by our present study and others in PTSD (87, 88).
5. Limitations
The small sample size of the present study and the lack of a control population (e.g., trauma-exposed healthy controls) may decrease the generalizability of these findings. The trial design was placebo-controlled for dose-response (low, medium, and high), however, the follow-up scans used in this study were after the breaking of the blind and dose cross-over (low/medium to high) had occurred. For neuroimaging studies, comparisons with control populations are helpful for contextualizing longitudinal changes in brain response and provide information about whether changes in patient populations represent an abnormal response being restored to normality or a compensatory mechanism. In addition, multi-point imaging of healthy control or non-treatment (placebo) groups allow for the quantification of test-retest variability. Lastly, had we imaged a cohort that received therapy without MDMA, we would have been able to assess longitudinal brain changes that were unique to or enhanced by MDMA.
Here it must be discussed that PTSD is a disorder exhibiting at least two major sub-types (dissociative and non-dissociative) with characteristically opposing phenomenological and physiological responses to symptom provocation, which may explain inconsistencies in the PTSD neurobiology literature (90). PTSD sub-type information was not collected in the present study. In addition to sub-type heterogeneity, males and females may also differ in their adaptive neural responses to trauma (92). Limited by our sample size, we did not investigate differences between males and females in this study.
The accepted standard for assessing PTSD severity is the Clinician-Administered PTSD Scale (63). Specifically, CAPS-IV was used in this study. CAPS-IV involves an hour-long semi-structured interview with a clinician and, though comprehensive, faces limitations. In their baseline CAPS-IV assessment, and subsequently thereafter, patients were asked to refer to an index trauma that was measured throughout the study. This may present an issue in accurately assessing global PTSD severity if an adjacent or un-related trauma surfaces during therapy and becomes the prominent driver of their symptoms. These issues, combined with difficulty in blinding and expectancy effects, present additional challenges in accurately mapping fMRI metrics to clinical outcomes.
The task design used in this study examined differences in brain response to personalized audio scripts generated from narrations of traumatic and neutral memories. Many different stimuli have been used in fMRI studies of PTSD (30, 42, 110), each providing its own unique advantages and disadvantages. Our present design optimizes personal relevance of the stimuli; however, this has the consequence of presenting each subject with a different set of stimuli, whereby brain responses within each block are not time-locked across participants. Also, it has previously been shown that PTSD survivors take longer to retrieve unrelated autobiographical information when listening to taped imagery scripts of their traumatic memories (111). This suggests the possibility that those with the most severe PTSD will take the longest to cognitively transition to the neutral block from the trauma block. If this is true, then there would perhaps be an inverse-“U” relationship between PTSD severity and contrast between the trauma and neutral conditions, if the blocks are not spaced far enough apart to allow adequate time for patients to return to a baseline level of cognitive functioning. Additionally, because our repeated task fMRI scans were identical (rather than counterbalanced for condition), there could be primacy effects in the neutral condition (which was always first) and/or fatigue effects in the trauma condition (which was always last).
We did not aim to characterize lateralization in our findings, and though most of our significant FC results were found in the left amygdala, we did not test for statistical interaction effects between hemispheres. While lateralization of the amygdala remains debated, it has been suggested by early work that the left amygdala is more strongly related to conscious (versus unconscious) perception and emotional regulation (112).
Finally, the pre-specified aim of this study was to estimate longitudinal (baseline to 2-months after final MDMA session) changes in ROI response to traumatic audio scripts. Between the start of data collection and analysis, new literature emerged (54, 55) implicating amygdala-hippocampus RSFC as a potential target of MDMA-AT, compelling us to expand our analysis beyond the pre-specified aims. Functional connectivity estimates from shorter scans (e.g., five minutes in the case of our resting-state data) can have lower reliability (113) and therefore the trends in increased RSFC between amygdala and hippocampal regions reported here should be considered preliminary.
6. Conclusion
We report functional brain changes associated with MDMA-AT in veterans and first responders with moderate-to-severe and chronic PTSD. We had hypothesized that MDMA-AT may act through strengthening the RSFC between the amygdala and hippocampus, a connection which is weaker in PTSD populations (43) and increased acutely by MDMA in healthy volunteers (54). The trends found here are suggestive of such in the left amygdala—left hippocampus, however larger studies are needed. We also provide preliminary evidence that MDMA-AT alters brain response during symptom provocation in regions associated with fear response, anxiety, self-referential processing, and salient autobiographical memory, and are commonly found to be hyperactive in PTSD patients (30, 110). Finally, the reduction of several functional connections during autobiographical memory audio co-varied with symptom reduction in PTSD. Of these connections, the left amygdala—left insula is perhaps the most interesting, due to the role of amygdala-insular FC in anxiety and PTSD symptomatology (39, 43, 101, 104). More research is necessary to confirm these results and to disentangle effects specific to MDMA and its combination with psychotherapy.
Data availability statement
The raw data supporting the conclusions of this article will be made available by the authors, without undue reservation.
Ethics statement
The studies involving human participants were reviewed and approved by Medical University of South Carolina Institutional Review Board. The patients/participants provided their written informed consent to participate in this study.
Author contributions
SPS and AK had full access to all the data in the study and take responsibility for the integrity of the data and for the accuracy of the data analysis. BY-K, AE, MM, and RD conceived and designed the clinical trial part of the study. MM and AM carried out experimental treatments and recorded participant scripts. AE carried out sponsor oversight of data collection. CH and MG designed the imaging tasks and acquisition. CH, MG, and OM oversaw the scanning and data collection. SPS, AK, JW, and AC drafted the manuscript. SPS carried out preprocessing, analysis, and interpretation of data. RD obtained funding for the study. All authors contributed to the critical review and final version of the manuscript.
Funding
The clinical trial was sponsored by the Multidisciplinary Association for Psychedelic Studies (MAPS), a 501(c)(3) non-profit organization. MAPS provided the MDMA and fully funded this study from private and foundation donations. MAPS Public Benefit Corporation (MAPS PBC), wholly owned by MAPS, was the trial organizer. SPS was supported by the National Science Foundation Graduate Research Fellowship (Grant No. DGE-1650441). AK was supported by the National Institutes of Health (RF1MH123232, R01NS102646, and R21NS104634).
Acknowledgments
The authors thank the candidates who were willing to be screened for eligibility; the participants in the trial who contributed their data; S. Sadler for her dedication as the Study Coordinator; and S. Braswell for serving as night attendant, M. Wagner and J. Wymer for Independent Rater assessments. The authors thank J. Holland who supported this trial as a Medical Monitor; L. Jerome for her numerous and varied contributions to this Clinical Development Program since prior to its inception with global systematic literature reviews and medical coding; C. Hennigan for data management; R. Matthews and B. Shechet for clinical operations and monitoring; J. Sonstroem and A. Seltzer for randomization support and system programming; E. Sola, Y. Gelfand, and B. Cohen for conducting adherence ratings to facilitate standardization of therapy; I. Gorman for development of adherence ratings; and A. Wilens for supporting video recording. The authors also thank Dr. Edmund Higgins for catalyzing the first discussions about acquiring imaging data in the MAPS trial.
Conflict of interest
MM was paid as a contractor by MAPS PBC. AE, AC, and JW received salary support for full-time employment with MAPS PBC. BY-K and RD received salary support for full-time employment with MAPS. MM is on the Clinical Advisory Board of Awakn Life Sciences.
The remaining authors declare that the research was conducted in the absence of any commercial or financial relationships that could be construed as a potential conflict of interest.
Publisher’s note
All claims expressed in this article are solely those of the authors and do not necessarily represent those of their affiliated organizations, or those of the publisher, the editors and the reviewers. Any product that may be evaluated in this article, or claim that may be made by its manufacturer, is not guaranteed or endorsed by the publisher.
Supplementary material
The Supplementary Material for this article can be found online at: https://www.frontiersin.org/articles/10.3389/fpsyt.2022.947622/full#supplementary-material
Footnotes
References
1. Davidson JRT. Trauma: the impact of post-traumatic stress disorder. J Psychopharmacol. (2000) 14:S5–12. doi: 10.1177/02698811000142S102
2. Gradus J. Prevalence and prognosis of stress disorders: a review of the epidemiologic literature. Clin Epidemiol. (2017) 9:251–60. doi: 10.2147/CLEP.S106250
3. VanElzakker MB, Lindsay K, Bradley S, Lisa MS. The neurocircuitry of fear and PTSD. In: E Vermetten, A Germain, TC Neylan editors. Sleep and Combat-Related Post Traumatic Stress Disorder. New York, NY: Springer (2018). p. 111–25. doi: 10.1007/978-1-4939-7148-0_10
4. Ehlers A. Understanding and treating unwanted trauma memories in posttraumatic stress disorder. Z Psychol. (2010) 218:141–5.
5. Shalev A, Gevonden M, Ratanatharathorn A, Laska E, van der MW, Qi W, et al. Estimating the risk of PTSD in recent trauma survivors: results of the international consortium to predict PTSD (ICPP). World Psychiatry. (2019) 18:77–87. doi: 10.1002/wps.20608
6. van der Mei W, Barbano A, Ratanatharathorn A, Bryant R, Delahanty D, deRoon-Cassini T, et al. Evaluating a screener to quantify PTSD risk using emergency care information: a proof of concept study. BMC Emerg Med. (2020) 20:16. doi: 10.1186/s12873-020-00308-z
7. Hoge C, Castro C, Messer S, McGurk D, Cotting D, Koffman R. Combat duty in Iraq and Afghanistan, mental health problems, and barriers to care. N Engl J Med. (2004) 351:13–22. doi: 10.1056/NEJMoa040603
9. Steenkamp M, Litz B, Hoge C, Marmar C. Psychotherapy for military-related PTSD: a review of randomized clinical trials. JAMA. (2015) 314:489–500. doi: 10.1001/jama.2015.8370
10. Goetter E, Bui E, Ojserkis R, Zakarian R, Brendel R, Simon N. A systematic review of dropout from psychotherapy for posttraumatic stress disorder among iraq and afghanistan combat veterans. J Trauma Stress. (2015) 28:401–9. doi: 10.1002/jts.22038
11. Lewis C, Roberts N, Gibson S, Bisson J. Dropout from psychological therapies for post-traumatic stress disorder (PTSD) in adults: systematic review and meta-analysis. Eur J Psychotraumatol. (2020) 11:1709709. doi: 10.1080/20008198.2019.1709709
12. Mott J, Mondragon S, Hundt N, Beason-Smith M, Grady R, Teng E. Characteristics of U.S. veterans who begin and complete prolonged exposure and cognitive processing therapy for PTSD. J Trauma Stress. (2014) 27:265–73. doi: 10.1002/jts.21927
13. Feduccia A, Mithoefer M, Jerome L, Holland J, Emerson A, Doblin R. Response to the consensus statement of the PTSD psychopharmacology working group. Biol Psychiatry. (2018) 84:e21–2. doi: 10.1016/j.biopsych.2017.11.023
14. Mithoefer M, Wagner M, Mithoefer A, Jerome L, Doblin R. The safety and efficacy of {+/-}3,4-methylenedioxymethamphetamine-assisted psychotherapy in subjects with chronic, treatment-resistant posttraumatic stress disorder: the first randomized controlled pilot study. J Psychopharmacol. (2011) 25:439–52. doi: 10.1177/0269881110378371
15. Mitchell J, Bogenschutz M, Lilienstein A, Harrison C, Kleiman S, Parker-Guilbert K, et al. MDMA-assisted therapy for severe PTSD: a randomized, double-blind, placebo-controlled phase 3 study. Nat Med. (2021) 27:1025–33. doi: 10.1038/s41591-021-01336-3
16. Mithoefer M, Feduccia A, Jerome L, Mithoefer A, Wagner M, Walsh Z, et al. MDMA-assisted psychotherapy for treatment of PTSD: study design and rationale for phase 3 trials based on pooled analysis of six phase 2 randomized controlled trials. Psychopharmacology. (2019) 236:2735–45. doi: 10.1007/s00213-019-05249-5
17. Wang J, Lin J, Bedrosian L, Coker A, Jerome I, Feduccia A, et al. Scaling up: multisite open-label clinical trials of MDMA-assisted therapy for severe posttraumatic stress disorder. J Humanist Psychol. (2021). doi: 10.1177/00221678211023663
18. Jerome L, Feduccia A, Wang J, Hamilton S, Yazar-Klosinski B, Emerson A, et al. Long-term follow-up outcomes of MDMA-assisted psychotherapy for treatment of PTSD: a longitudinal pooled analysis of six phase 2 trials. Psychopharmacology. (2020) 237:2485–97. doi: 10.1007/s00213-020-05548-2
19. Curry D, Young M, Tran A, Daoud G, Howell L. Separating the agony from ecstasy: R(–)-3,4-methylenedioxymethamphetamine has prosocial and therapeutic-like effects without signs of neurotoxicity in mice. Neuropharmacology. (2018) 128:196–206. doi: 10.1016/j.neuropharm.2017.10.003
20. Young M, Norrholm S, Khoury L, Jovanovic T, Rauch S, Reiff C, et al. Inhibition of serotonin transporters disrupts the enhancement of fear memory extinction by 3,4-methylenedioxymethamphetamine (MDMA). Psychopharmacology. (2017) 234:2883–95. doi: 10.1007/s00213-017-4684-8
21. Carhart-Harris RL, Wall MB, Erritzoe D, Kaelen M, Ferguson B, Meer ID, et al. The effect of acutely administered MDMA on subjective and BOLD-FMRI responses to favourite and worst autobiographical memories. Int J Neuropsychopharmacol. (2014) 17:527–40. doi: 10.1017/S1461145713001405
22. Doss M, Weafer J, Gallo D, de Wit H. MDMA impairs both the encoding and retrieval of emotional recollections. Neuropsychopharmacology. (2018) 43:791–800. doi: 10.1038/npp.2017.171
23. Vizeli P, Straumann I, Duthaler U, Varghese N, Eckert A, Paulus M, et al. Effects of 3,4-methylenedioxymethamphetamine on conditioned fear extinction and retention in a crossover study in healthy subjects. Front Pharmacol. (2022) 13:906639. doi: 10.3389/fphar.2022.906639
24. Maples-Keller J, Norrholm S, Burton M, Reiff C, Coghlan C, Jovanovic T, et al. A randomized controlled trial of 3,4-methylenedioxymethamphetamine (MDMA) and fear extinction retention in healthy adults. J Psychopharmacol. (2022) 36:368–77. doi: 10.1177/02698811211069124
25. Feduccia A, Mithoefer M. MDMA-assisted psychotherapy for PTSD: are memory reconsolidation and fear extinction underlying mechanisms? Prog Neuropsychopharmacol Biol Psychiatry. (2018) 84:221–8. doi: 10.1016/J.PNPBP.2018.03.003
26. Glover G. Overview of functional magnetic resonance imaging. Neurosurg Clin N Am. (2011) 22:133–9. doi: 10.1016/j.nec.2010.11.001
27. Zhou J, Liu S, Ng K, Wang J. Applications of resting-state functional connectivity to neurodegenerative disease. Neuroimaging Clin. (2017) 27:663–83. doi: 10.1016/j.nic.2017.06.007
28. Lucassen P, Pruessner J, Sousa N, Almeida O, Van DA. Neuropathology of stress. Acta Neuropathol. (2014) 127:109–35. doi: 10.1007/s00401-013-1223-5
29. Woodward N, Cascio C. Resting-state functional connectivity in psychiatric disorders. JAMA Psychiatry. (2015) 72:743–4. doi: 10.1001/jamapsychiatry.2015.0484
30. Sartory G, Cwik J, Knuppertz H, Schürholt B, Lebens M, Seitz R, et al. In search of the trauma memory: a meta-analysis of functional neuroimaging studies of symptom provocation in posttraumatic stress disorder (PTSD). PLoS One. (2013) 8:e58150. doi: 10.1371/journal.pone.0058150
31. Harnett N, Goodman A, Knight D. PTSD-related neuroimaging abnormalities in brain function, structure, and biochemistry. Exp Neurol. (2020) 330:113331. doi: 10.1016/j.expneurol.2020.113331
32. Abdallah CG, Wrocklage KM, Averill CL, Akiki T, Schweinsburg B, Roy A, et al. Anterior hippocampal dysconnectivity in posttraumatic stress disorder: a dimensional and multimodal approach. Transl Psychiatry. (2017) 7:e1045. doi: 10.1038/tp.2017.12
33. Lazarov A, Zhu X, Suarez-Jimenez B, Rutherford B, Neria Y. Resting-state functional connectivity of anterior and posterior hippocampus in posttraumatic stress disorder. J Psychiatr Res. (2017) 94:15–22. doi: 10.1016/j.jpsychires.2017.06.003
34. Malivoire B, Girard T, Patel R, Monson C. Functional connectivity of hippocampal subregions in PTSD: relations with symptoms. BMC Psychiatry. (2018) 18:129. doi: 10.1186/s12888-018-1716-9
35. Brown V, LaBar K, Haswell C, Gold A. Altered resting-state functional connectivity of basolateral and centromedial amygdala complexes in posttraumatic stress disorder. Neuropsychopharmacology. (2014) 39:351–9. doi: 10.1038/npp.2013.197
36. Pitman R, Rasmusson A, Koenen K, Shin L, Orr S, Gilbertson M, et al. Biological studies of post-traumatic stress disorder. Nat Rev Neurosci. (2012) 13:769–87. doi: 10.1038/nrn3339
37. Gilboa A, Shalev A, Laor L, Lester H, Louzoun Y, Chisin R, et al. Functional connectivity of the prefrontal cortex and the amygdala in posttraumatic stress disorder. Biol Psychiatry. (2004) 55:263–72. doi: 10.1016/j.biopsych.2003.08.004
38. Fonzo G, Goodkind M, Oathes D, Zaiko Y, Harvey M, Peng K, et al. Amygdala and insula connectivity changes following psychotherapy for posttraumatic stress disorder: a randomized clinical trial. Biol Psychiatry. (2021) 89:857–67. doi: 10.1016/j.biopsych.2020.11.021
39. Rabinak C, Angstadt M, Welsh R, Kenndy A, Lyubkin M, Martis B, et al. Altered amygdala resting-state functional connectivity in post-traumatic stress disorder. Front Psychiatry. (2011) 2:62. doi: 10.3389/fpsyt.2011.00062
40. Belleau E, Ehret L, Hanson J, Brasel K, Larson C, deRoon-Cassini T. Amygdala functional connectivity in the acute aftermath of trauma prospectively predicts severity of posttraumatic stress symptoms. Neurobiol Stress. (2020) 12:100217. doi: 10.1016/j.ynstr.2020.100217
41. Thome J, Terpou B, McKinnon M, Lanius R. The neural correlates of trauma-related autobiographical memory in posttraumatic stress disorder: a meta-analysis. Depress Anxiety. (2020) 37:321–45. doi: 10.1002/da.22977
42. Lanius RA, Bluhm R, Lanius U, Pain C. A review of neuroimaging studies in PTSD: heterogeneity of response to symptom provocation. J Psychiatr Res. (2006) 40:709–29. doi: 10.1016/j.jpsychires.2005.07.007
43. Sripada R, King A, Garfinkel S, Wang X, Sripada C, Welsh R, et al. Altered resting-state amygdala functional connectivity in men with posttraumatic stress disorder. J Psychiatry Neurosci. (2012) 37:241. doi: 10.1503/JPN.110069
44. Cavanna A, Trimble M. The precuneus: a review of its functional anatomy and behavioural correlates. Brain. (2006) 129(Pt 3):564–83. doi: 10.1093/brain/awl004
45. Svoboda E, McKinnon M, Levine B. The functional neuroanatomy of autobiographical memory: a meta-analysis. Neuropsychologia. (2006) 44:2189–208. doi: 10.1016/j.neuropsychologia.2006.05.023
46. Spreng R, Mar R, Kim A. The common neural basis of autobiographical memory, prospection, navigation, theory of mind, and the default mode: a quantitative meta-analysis. J Cogn Neurosci. (2009) 21:489–510. doi: 10.1162/jocn.2008.21029
47. Sestieri C, Corbetta M, Romani G, Shulman G. Episodic memory retrieval, parietal cortex, and the default mode network: functional and topographic analyses. J Neurosci. (2011) 31:4407–20. doi: 10.1523/JNEUROSCI.3335-10.2011
48. Maddock RJ. The retrosplenial cortex and emotion: new insights from functional neuroimaging of the human brain. Trends Neurosci. (1999) 22:310–6. doi: 10.1016/S0166-2236(98)01374-5
49. Spreng R, Grady C. Patterns of brain activity supporting autobiographical memory, prospection, and theory of mind, and their relationship to the default mode network. J Cogn Neurosci. (2010) 22:1112–23. doi: 10.1162/jocn.2009.21282
50. LeDoux J. The emotional brain, fear, and the amygdala. Cell Mol Neurobiol. (2003) 23:727–38. doi: 10.1023/A:1025048802629
51. Ben-Zion Z, Keynan NJ, Admon R, Sharon H, Halpern P, Liberzon I, et al. Hippocampal-amygdala resting state functional connectivity serves as resilience factor for short- and long-term stress exposure. Biol Psychiatry. (2020) 87(Suppl. 9):S88–9. doi: 10.1016/j.biopsych.2020.02.248
52. Vaisvaser S, Lin T, Admon R, Podlipsky I, Greenman Y, Stern N, et al. Neural traces of stress: cortisol related sustained enhancement of amygdala-hippocampal functional connectivity. Front Hum Neurosci. (2013) 7:313. doi: 10.3389/fnhum.2013.00313
53. Fan Y, Pestke K, Feeser M, Aust S, Pruessner J, Böker H, et al. Amygdala–hippocampal connectivity changes during acute psychosocial stress: joint effect of early life stress and oxytocin. Neuropsychopharmacology. (2015) 40:2736–44. doi: 10.1038/npp.2015.123
54. Carhart-Harris R, Murphy K, Leech R, Erritzoe D, Wall M, Ferguson B, et al. The effects of acutely administered 3,4-methylenedioxymethamphetamine on spontaneous brain function in healthy volunteers measured with arterial spin labeling and blood oxygen level-dependent resting state functional connectivity. Biol Psychiatry. (2015) 78:15. doi: 10.1016/J.BIOPSYCH.2013.12.015
55. Lan C, Liu C, Li K, Zhao Z, Yang J, Ma Y, et al. Oxytocinergic modulation of stress-associated amygdala-hippocampus pathways in humans is mediated by serotonergic mechanisms. Int J Neuropsychopharmacol. (2022) 25:807–17. doi: 10.1093/ijnp/pyac037
56. Dumont GJH, Sweep FCGJ, van der Steen R, Hermsen R, Donders ART, Touw DJ, et al. Increased oxytocin concentrations and prosocial feelings in humans after ecstasy (3,4-methylenedioxymethamphetamine) administration. Soc Neurosci. (2009) 4:359–66. doi: 10.1080/17470910802649470
57. Feduccia A, Duvauchelle C. Auditory stimuli enhance MDMA-conditioned reward and MDMA-induced nucleus accumbens dopamine, serotonin and locomotor responses. Brain Res Bull. (2008) 77:189–96. doi: 10.1016/j.brainresbull.2008.07.007
58. de la Torre R, Farré M, Roset P, Pizarro N, Abanades S, Segura M, et al. Human pharmacology of MDMA: pharmacokinetics, metabolism, and disposition. Ther Drug Monit. (2004) 26:137–44.
59. Green A, Mechan A, Elliott J, O’Shea E, Colado M. The pharmacology and clinical pharmacology of 3,4-methylenedioxymethamphetamine (MDMA, ‘Ecstasy’). Pharmacol Rev. (2003) 55:463–508. doi: 10.1124/pr.55.3.3
60. Thompson MR, Callaghan PD, Hunt GE, Cornish JL, McGregor IS. A role for oxytocin and 5-HT1A receptors in the prosocial effects of 3,4 methylenedioxymethamphetamine (‘Ecstasy’). Neuroscience. (2007) 146:509–14. doi: 10.1016/j.neuroscience.2007.02.032
61. Nardou R, Lewis E, Rothhaas R, Xu R, Yang A, Boyden E, et al. Oxytocin-dependent reopening of a social reward learning critical period with MDMA. Nature. (2019) 569:116–20. doi: 10.1038/s41586-019-1075-9
62. Mithoefer M, Mithoefer A, Feduccia A, Jerome L, Wagner M, Wymer J, et al. 3,4-Methylenedioxymethamphetamine (MDMA)-assisted psychotherapy for post-traumatic stress disorder in military veterans, firefighters, and police officers: a randomised, double-blind, dose-response, phase 2 clinical trial. Lancet Psychiatry. (2018) 5:486–97. doi: 10.1016/S2215-0366(18)30135-4
63. Blake D, Weathers F, Nagy L, Kaloupek G, Charney D, Keane T. A clinician rating scale for assessing current and lifetime PTSD: the CAPS-1. Behav Ther. (1990) 18:75–90. doi: 10.1007/BF02105408
64. Ben-Zion Z, Zeevi Y, Keynan N, Admon R, Kozlovski T, Sharon H, et al. Multi-domain potential biomarkers for post-traumatic stress disorder (PTSD) severity in recent trauma survivors. Transl Psychiatry. (2020) 10:208. doi: 10.1038/s41398-020-00898-z
65. Etkin A, Wager T. Functional neuroimaging of anxiety: a meta-analysis of emotional processing in PTSD, social anxiety disorder, and specific phobia. Am J Psychiatry. (2007) 164:1476–88. doi: 10.1176/appi.ajp.2007.07030504
66. Lanius R, Williamson P, Boksman K, Densmore M, Gupta M. Brain activation during script-driven imagery induced dissociative responses in PTSD: a functional magnetic resonance imaging investigation. Biol Psychiatry. (2002) 52:305–11. doi: 10.1016/S0006-3223(02)01367-7
67. Lanius R, Williamson P, Densmore M, Boksman K, Neufeld R, Gati J. The nature of traumatic memories: a 4-T FMRI functional connectivity analysis. Am J Psychiatry. (2004) 161:36–44. doi: 10.1176/appi.ajp.161.1.36
68. Mertens Y, Manthey A, Sierk A, Walter H, Daniels J. Neural correlates of acute post-traumatic dissociation: a functional neuroimaging script-driven imagery study. BJPsych Open. (2022) 8:e109. doi: 10.1192/bjo.2022.65
69. Dale AM, Fischl B, Sereno MI. Cortical surface-based analysis. I. Segmentation and surface reconstruction. Neuroimage. (1999) 9:179–94. doi: 10.1006/nimg.1998.0395
70. Smith S, Jenkinson M, Woolrich M, Beckmann C, Behrens T, Johansen-Berg H, et al. Advances in functional and structural mr image analysis and implementation as FSL. Neuroimage. (2004) 23(Suppl. 1):S208–19. doi: 10.1016/j.neuroimage.2004.07.051
71. Whitfield-Gabrieli S, Nieto-Castanon A. Conn: a functional connectivity toolbox for correlated and anticorrelated brain networks. Brain Connect. (2012) 2:125–41. doi: 10.1089/brain.2012.0073
72. Power J, Barnes K, Snyder A, Schlaggar B, Petersen S. Spurious but systematic correlations in functional connectivity MRI networks arise from subject motion. Neuroimage. (2012) 59:2142–54. doi: 10.1016/j.neuroimage.2011.10.018
73. Woolrich M, Behrens T, Beckmann C, Jenkinson M, Smith S. Multilevel linear modelling for FMRI group analysis using bayesian inference. Neuroimage. (2004) 21:1732–47. doi: 10.1016/j.neuroimage.2003.12.023
74. Friston K, Williams S, Howard R, Frackowiak R, Turner R. Movement-related effects in FMRI time-series. Magn Reson Med. (1996) 35:346–55. doi: 10.1002/mrm.1910350312
75. Winkler A, Ridgway G, Webster M, Smith S, Nichols T. Permutation inference for the general linear model. Neuroimage. (2014) 92:381–97. doi: 10.1016/j.neuroimage.2014.01.060
76. Smith S, Nichols T. Threshold-free cluster enhancement: addressing problems of smoothing, threshold dependence and localisation in cluster inference. Neuroimage. (2009) 44:83–98. doi: 10.1016/j.neuroimage.2008.03.061
77. Desikan R, Ségonne F, Fischl B, Quinn B, Dickerson B, Blacker D, et al. An automated labeling system for subdividing the human cerebral cortex on MRI scans into gyral based regions of interest. Neuroimage. (2006) 31:968–80. doi: 10.1016/j.neuroimage.2006.01.021
78. Iglesias J, Augustinack J, Nguyen K, Player C, Player A, Wright M, et al. A computational atlas of the hippocampal formation using ex vivo, ultra-high resolution MRI: application to adaptive segmentation of in vivo MRI. Neuroimage. (2015) 115:117–37. doi: 10.1016/j.neuroimage.2015.04.042
79. Benjamini Y, Hochberg Y. Controlling the false discovery rate: a practical and powerful approach to multiple testing. J R Stat Soc Ser B. (1995) 57:289–300.
80. Liberzon I, Taylor S, Amdur R, Jung T, Chamberlain K, Minoshima S, et al. Brain activation in PTSD in response to trauma-related stimuli. Biol Psychiatry. (1999) 45:817–26. doi: 10.1016/S0006-3223(98)00246-7
81. Bremner J, Vermetten E, Schmahl C, Vaccarino V, Vythilingam M, Afzal N, et al. Positron emission tomographic imaging of neural correlates of a fear acquisition and extinction paradigm in women with childhood sexual-abuse-related post-traumatic stress disorder. Psychol Med. (2005) 35:791–806. doi: 10.1017/S0033291704003290
82. Cauda F, Geminiani G, D’Agata F, Sacco K, Duca S, Bagshaw A, et al. Functional connectivity of the posteromedial cortex. PLoS One. (2010) 5:e13107. doi: 10.1371/journal.pone.0013107
83. Kalenzaga S, Sperduti M, Anssens A, Martinelli P, Devauchelle A, Gallarda T, et al. Episodic memory and self-reference via semantic autobiographical memory: insights from an FMRI study in younger and older adults. Front Behav Neurosci. (2015) 8:449. doi: 10.3389/fnbeh.2014.00449
84. Demblon J, Bahri M, D’Argembeau A. Neural correlates of event clusters in past and future thoughts: how the brain integrates specific episodes with autobiographical knowledge. Neuroimage. (2016) 127:257–66. doi: 10.1016/j.neuroimage.2015.11.062
85. Raichle ME. The brain’s default mode network. Annu Rev Neurosci. (2015) 38:433–47. doi: 10.1146/annurev-neuro-071013-014030
86. Cabeza R, St Jacques P. Functional neuroimaging of autobiographical memory. Trends Cogn Sci. (2007) 11:219–27. doi: 10.1016/j.tics.2007.02.005
87. Garrett A, Cohen J, Zack S, Carrion V, Jo B, Blader J, et al. Longitudinal changes in brain function associated with symptom improvement in youth with PTSD. J Psychiatr Res. (2019) 114:161–9. doi: 10.1016/j.jpsychires.2019.04.021
88. Ke J, Zhang L, Qi R, Li W, Hou C, Zhong Y, et al. A longitudinal FMRI investigation in acute post-traumatic stress disorder (PTSD). Acta Radiol. (2016) 57:1387–95. doi: 10.1177/0284185115585848
89. Bedi G, Phan K, Angstadt M, de Wit H. Effects of MDMA on sociability and neural response to social threat and social reward. Psychopharmacology. (2009) 207:73–83. doi: 10.1007/s00213-009-1635-z
90. van Huijstee J, Vermetten E. The dissociative subtype of post-traumatic stress disorder: research update on clinical and neurobiological features. Curr Top Behav Neurosci. (2018) 38:229–48. doi: 10.1007/7854_2017_33
91. Lanius RA, Williamson PC, Densmore M, Boksman K, Gupta MA, Neufeld RW, et al. Neural correlates of traumatic memories in posttraumatic stress disorder: a functional MRI investigation. Am J Psychiatry. (2001) 158:1920–2. doi: 10.1176/appi.ajp.158.11.1920
92. Helpman L, Zhu X, Zilcha-Mano S, Suarez-Jimenez B, Lazarov A, Rutherford B, et al. Reversed patterns of resting state functional connectivity for females vs. males in posttraumatic stress disorder. Neurobiol Stress. (2021) 15:100389. doi: 10.1016/J.YNSTR.2021.100389
93. Chiao J, Iidaka T, Gordon H, Nogawa J, Bar M, Aminoff E, et al. Cultural specificity in amygdala response to fear faces. J Cogn Neurosci. (2008) 20:2167–74. doi: 10.1162/jocn.2008.20151
94. Liddell B, Jobson L. The impact of cultural differences in self-representation on the neural substrates of posttraumatic stress disorder. Eur J Psychotraumatol. (2016) 7:30464. doi: 10.3402/ejpt.v7.30464
95. Walpola I, Nest T, Roseman L, Erritzoe D, Feilding A, Nutt D, et al. Altered insula connectivity under MDMA. Neuropsychopharmacology. (2017) 42:2152–62. doi: 10.1038/npp.2017.35
96. Müller F, Holze F, Dolder P, Ley L, Vizeli P, Soltermann A, et al. MDMA-induced changes in within-network connectivity contradict the specificity of these alterations for the effects of serotonergic hallucinogens. Neuropsychopharmacology. (2021) 46:545–53. doi: 10.1038/s41386-020-00906-2
97. Dipasquale O, Selvaggi P, Veronese M, Gabay A, Turkheimer F, Mehta M. Receptor-enriched analysis of functional connectivity by targets (REACT): a novel, multimodal analytical approach informed by pet to study the pharmacodynamic response of the brain under MDMA. Neuroimage. (2019) 195:252–60. doi: 10.1016/j.neuroimage.2019.04.007
98. Avram M, Müller F, Rogg H, Korda A, Andreou C, Holze F, et al. Characterizing thalamocortical (Dys)connectivity following D-amphetamine, LSD, and MDMA administration. Biol Psychiatry. (2022) 7:885–94. doi: 10.1016/j.bpsc.2022.04.003
99. Lanius RA, Bluhm RL, Coupland NJ, Hegadoren KM, Rowe B, Théberge J, et al. Default mode network connectivity as a predictor of post-traumatic stress disorder symptom severity in acutely traumatized subjects. Acta Psychiatr Scand. (2010) 121:33–40. doi: 10.1111/j.1600-0447.2009.01391.x
100. Bluhm R, Williamson P, Osuch E, Frewen P, Stevens T, Boksman K, et al. Alterations in default network connectivity in posttraumatic stress disorder related to early-life trauma. J Psychiatry Neurosci. (2009) 34:187–94.
101. Cisler JM, Sigel BA, Steele JS, Smitherman S, Vanderzee K, Pemberton J, et al. Changes in functional connectivity of the amygdala during cognitive reappraisal predict symptom reduction during trauma-focused cognitive-behavioral therapy among adolescent girls with post-traumatic stress disorder. Psychol Med. (2016) 46:3013–23. doi: 10.1017/S0033291716001847
102. Shou H, Yang Z, Satterthwaite T, Cook P, Bruce S, Shinohara R, et al. Cognitive behavioral therapy increases amygdala connectivity with the cognitive control network in both MDD and PTSD. Neuroimage. (2017) 14:464–70. doi: 10.1016/J.NICL.2017.01.030
103. Veer I, Oei N, Spinhoven P, van Buchem M, Elzinga B, Rombouts S. Beyond acute social stress: increased functional connectivity between amygdala and cortical midline structures. Neuroimage. (2011) 57:1534–41. doi: 10.1016/j.neuroimage.2011.05.074
104. Baur V, Hänggi J, Langer N, Jäncke L. Resting-state functional and structural connectivity within an insula-amygdala route specifically index state and trait anxiety. Biol Psychiatry. (2013) 73:85–92. doi: 10.1016/j.biopsych.2012.06.003
105. Suzuki A, Josselyn S, Frankland P, Masushige S, Silva A, Kida S. Memory reconsolidation and extinction have distinct temporal and biochemical signatures. J Neurosci. (2004) 24:4787–95. doi: 10.1523/JNEUROSCI.5491-03.2004
106. Young MB, Andero R, Ressler KJ, Howell LL. 3,4-Methylenedioxymethamphetamine facilitates fear extinction learning. Transl Psychiatry. (2015) 5:e634. doi: 10.1038/tp.2015.138
107. Hake H, Davis J, Wood R, Tanner M, Loetz E, Sanchez A, et al. 3,4-Methylenedioxymethamphetamine (MDMA) impairs the extinction and reconsolidation of fear memory in rats. Physiol Behav. (2019) 199:343–50. doi: 10.1016/j.physbeh.2018.12.007
108. Arluk S, Matar M, Carmi L, Arbel O, Zohar J, Todder D, et al. MDMA treatment paired with a trauma-cue promotes adaptive stress responses in a translational model of PTSD in rats. Transl Psychiatry. (2022) 12:181. doi: 10.1038/s41398-022-01952-8
109. Ridderbusch I, Wroblewski A, Yang Y, Richter J, Hollandt M, Hamm A, et al. Neural adaptation of cingulate and insular activity during delayed fear extinction: a replicable pattern across assessment sites and repeated measurements. Neuroimage. (2021) 237:118157. doi: 10.1016/j.neuroimage.2021.118157
110. Patel R, Spreng R, Shin L, Girard T. Neurocircuitry models of posttraumatic stress disorder and beyond: a meta-analysis of functional neuroimaging studies. Neurosci Biobehav Rev. (2012) 36:2130–42. doi: 10.1016/j.neubiorev.2012.06.003
111. Kleim B, Wallott F, Ehlers A. Are trauma memories disjointed from other autobiographical memories in posttraumatic stress disorder? An experimental investigation. Behav Cogn Psychother. (2008) 36:221–34. doi: 10.1017/S1352465807004080
112. Morris JS, Öhman A, Dolan RJ. Conscious and unconscious emotional learning in the human amygdala. Nature. (1998) 393:467–70. doi: 10.1038/30976
Keywords: PTSD, MDMA, amygdala, hippocampus, insula, fMRI, functional connectivity, autobiographical memory
Citation: Singleton SP, Wang JB, Mithoefer M, Hanlon C, George MS, Mithoefer A, Mithoefer O, Coker AR, Yazar-Klosinski B, Emerson A, Doblin R and Kuceyeski A (2023) Altered brain activity and functional connectivity after MDMA-assisted therapy for post-traumatic stress disorder. Front. Psychiatry 13:947622. doi: 10.3389/fpsyt.2022.947622
Received: 18 May 2022; Accepted: 19 December 2022;
Published: 12 January 2023.
Edited by:
Peter Schuyler Hendricks, University of Alabama at Birmingham, United StatesReviewed by:
Andrew Burke, Indiana University Bloomington, United StatesManoj Doss, Johns Hopkins University, United States
Haley Dourron, University of Alabama at Birmingham, United States
Copyright © 2023 Singleton, Wang, Mithoefer, Hanlon, George, Mithoefer, Mithoefer, Coker, Yazar-Klosinski, Emerson, Doblin and Kuceyeski. This is an open-access article distributed under the terms of the Creative Commons Attribution License (CC BY). The use, distribution or reproduction in other forums is permitted, provided the original author(s) and the copyright owner(s) are credited and that the original publication in this journal is cited, in accordance with accepted academic practice. No use, distribution or reproduction is permitted which does not comply with these terms.
*Correspondence: S. Parker Singleton, c3BzMjUzQGNvcm5lbGwuZWR1