- 1Institute for Pediatric Regenerative Medicine (IPRM), Shriners Hospital for Children and UC Davis School of Medicine, Sacramento, CA, United States
- 2Department of Pathology and Laboratory Medicine, UC Davis School of Medicine, Sacramento, CA, United States
- 3MIND Institute, UC Davis School of Medicine, Sacramento, CA, United States
Parvalbumin (PV) is a calcium binding protein expressed by inhibitory fast-spiking interneurons in the cerebral cortex. By generating a fast stream of action potentials, PV+ interneurons provide a quick and stable inhibitory input to pyramidal neurons and contribute to the generation of gamma oscillations in the cortex. Their fast-firing rates, while advantageous for regulating cortical signaling, also leave them vulnerable to metabolic stress. Chandelier (Ch) cells are a type of PV+ interneuron that modulate the output of pyramidal neurons and synchronize spikes within neuron populations by directly innervating the pyramidal axon initial segment. Changes in the morphology and/or function of PV+ interneurons, mostly of Ch cells, are linked to neurological disorders. In ASD, the number of PV+ Ch cells is decreased across several cortical areas. Changes in the morphology and/or function of PV+ interneurons have also been linked to schizophrenia, epilepsy, and bipolar disorder. Herein, we review the role of PV and PV+ Ch cell alterations in ASD and other psychiatric disorders.
Parvalbumin functional properties
Parvalbumin is a high affinity Ca2+ binding protein that acts as a buffer to sequester calcium in the cytoplasm of inhibitory neurons (1). Parvalbumin binds Ca2+ with high affinity (KD, Mg ~ 5–100 uM) and to Mg2+ with medium affinity (KD, Mg ~ 5–500 uM). PV is considered a “slow buffer” molecule due to its binding kinetics to those two ions, along with its slow association and dissociation rate (2). Action potentials in fast-spiking PV+ neurons are characterized by high frequency firing rate with minimal adaptation, narrow AP half width, short membrane time constant, and large afterhyperpolarization (3–5). PV+ interneurons exhibit high depolarized resting membrane potentials and small action potential amplitudes (6). Expression of unique voltage-gated Na+ and K+ channels contribute to the high-frequency repetitive firing patterns in PV+ interneurons (7, 8). By generating such a fast stream of action potentials, PV+ interneurons evoke a quick and stable GABA inhibitory postsynaptic current to pyramidal neurons that is characterized by fast rise and decay phases. This fast synchronization of network activity is responsible for the generation of high frequency gamma oscillations (9–11) that are associated with high level cognitive functions such as attention, memory, and perception (12–17). In-vivo optogenetic manipulation of PV+ interneuron activity is sufficient to produce changes in the network oscillations of the cerebral cortex of rats. Synchronous activity of PV+ cells amplify gamma oscillation and in-vivo inhibition of PV+ cells suppress gamma oscillations (10, 18). During firing, PV buffers intracellular Ca2+ levels back to normal physiological levels (19). The high abundance of mitochondria is the product of the substantial energy input required for PV+ neurons to sustain their characteristic high frequency firing patterns (20, 21). This increases their vulnerability to developing metabolic stress through the release of free radicals (22). Specifically, loss of PV leads to the pathological accumulation of mitochondrial Ca2+ levels that results in disruption of the electron transport chain (23). This leads to a buildup of intracellular reactive oxygen species (ROS) and cytochrome C that renders vulnerability to apoptosis (24). ROS production is highly correlated with PV concentration where alterations in redox homeostasis leads to a cascade of events that contribute to pathophysiological alterations in neurodevelopmental disorders (25, 26).
Parvalbumin is downregulated in various neurological conditions, such as in ASD Spectrum Disorder (ASD), a neurodevelopmental disorder characterized by deficits in social interaction and stereotypical behavior. Alterations of PV in the human ASD brain suggest that PV changes may promote an ASD like phenotype. PV-/- mice that constitutively lack PV expression showed ASD-like behavioral deficits, also suggesting that absent PV expression is sufficient to cause behavioral abnormalities (27). Genes, such as En2, Mecp2, Fmr1, Cntnap2, Shank1 and Shank 3, Ube3, Nlgn3, and KV3.1b, are implicated in ASD development and based on evidence derived from KO models are also believed to contribute to PV+ interneuron dysfunction (28–37). The knockout animal of the Fmr1 gene–responsible for Fragile X Syndrome, the knockout of the Shank3 gene–responsible for the Phelan-Mcdermind Syndrome, and the knockout of the Ube3a–responsible for Angelman syndrome, experience neuronal hyperexcitability, presumably because of impaired PV+ interneuron function (38–40). The Nlgn3 animal model of ASD presents with decreased excitability of fast spiking interneurons and dysfunction of gamma oscillations, which are necessary for memory and attention (41). And the PV+ interneuron voltage-gated potassium channel KV3.1b, responsible for regulating the fast-spiking properties of PV+ interneurons, is reduced in the cortex and thalamus of Valproic Acid (VPA)-exposed mice, a widely used model of ASD (8). These findings indicate that a series of genes that play a direct or indirect role in regulating PV levels and function, are implicated in ASD. In addition, many EEG studies in human show that ASD individuals have abnormal gamma oscillations (41–43). Due to the functional role of PV+ cells in generation of gamma oscillations, and the evidence found in human and animal studies, it has been speculated that gamma oscillations could potentially be a physiological biomarker for abnormal functioning of PV+ neurons.
Parvalbumin+ chandelier cells
Interneurons in the cortex exhibit a wide variety of morphological, physiological, and molecular characteristics. Interneurons can be classified based on the expression of specific molecular markers, among them fast-spiking interneurons can be recognized by their expression of PV. PV+ interneurons are GABAergic and modulate the output of pyramidal neurons by directly innervating their soma or the axon initial segment (AIS). In the cerebral cortex, PV+ interneurons include two distinct types, chandelier (Ch) cells and basket (Bsk) cells (44, 45). In mice, PV+ cells account collectively for approximately 40–50% of all interneurons in the cerebral cortex (46), with Bsk cells being more numerous and Ch cells making up less than 5% of all interneurons in the cortex. In other species, like Rhesus macaque, approximately 75% of all GABAergic neurons in the primary visual cortex are PV+ neurons (47). Ch cells and Bsk cells exhibit substantially different innervation properties and distinct firing properties. Physiologically, Bsk cells have a greater firing latency while Ch cells have a higher firing frequency and adaptation (48, 49). Anatomically, Bsk cells innervate the soma and proximal dendrites of pyramidal neurons, while Ch cells innervate the AIS of pyramidal neurons (44, 45). Basket cells are multipolar PV+ interneurons located throughout layers II–VI, while their prominent basket structures are mostly restricted to layer IV. Bsk cells establish multiple connections with the soma and proximal dendrites of pyramidal neurons in a manner that outlines the pyramidal cell body acquiring a basket-like shape. Bsk cells can also innervate other Bsk cells and non-PV+ GABA cells, like purkinje cells in the cerebellum (49–51). Basket cells are subdivided into small, large, and nest basket cells, that present with differential size, dendritic and axonal projections, firing properties, and the expression of additional molecular markers (52, 53). Ch cells are involved in the generation of gamma oscillations generated by the cortex (45, 54, 55). Specifically, synaptic inhibition from Ch cells controls the firing rate of pyramidal cells, synchronizes spikes within populations of neurons, and participates in cortical executive functions (52, 56, 57). While GABAergic input on dendrites originates from many types of interneurons, the AIS only receives input from Ch cells (58). Therefore, Ch cells play a central role in regulating the final output of excitatory pyramidal neurons. The terminal portions of Ch cell axons form vertical rows of synaptic boutons that resemble candlesticks and are known as cartridges (54, 59) (Figure 1). Ch cartridges express PV but are more easily detected by their expression of GABA transporter 1 (GAT1) (44, 60, 61). Each Ch cell has many cartridges lined with boutons and each cartridge can selectively innervate the AIS of a pyramidal neuron. A single pyramidal cell receives input from 1 to 4 Ch cells, while a single Ch cell innervates up to 250 pyramidal cells (62). Each Ch cell can therefore regulate the output of many pyramidal cells, and the loss of a single Ch cell signifies the loss of inhibitory AIS-mediated regulation of a great number of pyramidal cells belonging to several cortical mini-columns. The loss of a few Ch cells would critically impair pyramidal cell output and cerebral cortex function (58).
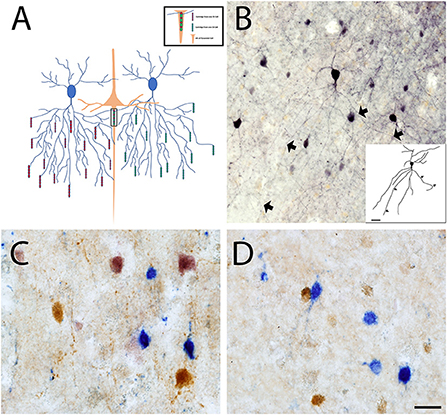
Figure 1. Chandelier cell connectivity and immunostaining. (A) Chandelier cells (blue) have a unique and structurally complex morphology characterized by vertically oriented axon terminal cartridges (red and green), that are perpendicular to the pial surface. Ch cells synapse onto the AIS of pyramidal neurons (orange) and can innervate up to 250 pyramidal cells at once, allowing for fine inhibitory control. (B) Ch cell in primary somatosensory cortex stained with an anti-PV antibody that labels the soma and proximal processes (BA3). Triple enzymatic stain of CR (Blue), CB (Brown) and PV (Pink) labeled interneurons reveals loss of PV+ interneurons in BA3 of both control (C) and ASD cortex (D). Scale Bars = 20 μm.
Ch cells are present in most cortical areas and play an important inhibitory and regulatory role in each of them. However, they are not homogeneously distributed but they differ in density by cortical area and cortical layer (63, 64). PV+ Ch cells are less prominent in deeper layers and some Ch cells co-express calbindin (CB) in human (65). These differences suggests that there is a region specific anatomical and functional organization. In human and other primates there is a higher density of cartridges innervating the AIS in association areas than in primary sensory areas (63, 66, 67). Only one study has systematically looked at the relative abundance of Ch cells in different areas of the human cortex and did so by mapping GAT1+ Ch cartridges (44). The lowest density of GAT1+ cartridges was in primary and secondary visual (BA17 and BA18) and somatosensory areas (BA3b and BA1). In contrast, there was a moderate density in primary motor cortex (BA4) and associative frontolateral areas (BA45 and 46), whereas other associative frontolateral cortex (BA9 and BA10), frontal orbital cortex (BA11, BA12, BA13, BA14, and BA47), associative temporal cortex (BA20, BA21, BA22, and BA38), and cingulate cortex (BA24 and BA32) displayed the highest density of GAT1+ cartridges (44). Despite these differences, the laminar distribution of GAT1+ cartridges was similar in most cortical areas. The highest density was in layer II, followed by layers III, V, VI, and IV. In most cortical areas, the density of GAT1+ cartridges was correlated with the neuronal density (44). PV+ Ch cell interneurons in the cerebral cortex originate subcortically in two waves of proliferation in the medial ganglionic eminence (MGE) during prenatal development. A small pool of radial glial cells that at embryonic day (E)10 are restricted to the caudal MGE generate a small set of layer V-VI and II-III Ch cells. A second and bigger wave at E12 gives rise to Ch cells across layers II-VI and occupies the entire rostral caudal region of the MGE (68). MGE-derived cells then migrate tangentially to the cortex in a spatiotemporal and time sensitive manner, reaching the cortex at E18-P0 (69–71). Once in their final cortical destination, they mature into PV+ Ch cells. Perturbations during the periods of proliferation, migration, and maturation, can result in cell death or malfunction.
While Bsk cells are present in many subcortical regions of the brain, Ch cells outside the cortex have only been identified in the hippocampal formation and the amygdaloid complex. The hippocampal formation is a brain structure involved in the generation, organization, and storage of new memories. Ch cells in the hippocampal formation are similar in morphology, marker expression, and electrophysiological properties to those in the neocortex. In the dentate gyrus (DG), PV+ cells are mostly located within or adjacent to granular zone (GZ) and are larger in size than the surrounding PV- granule cells (72). Most of the PV+ neurons in CA1 and CA3 are in the strata pyramidale and oriens, while a small number are present in stratum lucidum and in stratum radiatum, and rarely in stratum moleculare. In the DG, GAT1+ cartridges are located in the GZ and in the polymorphic layer (72). In the CA, GAT1+ cartridges are located in stratum pyramidale of CA1 and CA3 and are sparse in the stratum oriens. In CA2 and CA4, cartridges are only occasionally present (73–75). Ch cells in CA synapse and inhibit the AIS of pyramidal cells regulating their final output. Ch cells in the DG synapse on to the AIS of the granular cells in the GZ, also regulating their final output (74). The amygdaloid complex (amygdala) is involved in processing emotional responses and affective states (76, 77). Ch cells in the amygdala can be identify based on the morphology of their axonal terminals. Ch cells in the amygdala are similar in morphology, marker expression, and electrophysiological properties to those in the neocortex and hippocampal formation. PV+ interneurons are restricted to the basolateral (BLA) nuclei, with highest density of the cartridges in the lateral (75%), whereas the basal nucleus contains only 20% (76, 78). PV+ Ch cells synapse and inhibit the AIS of the pyramidal cells in the BLA nuclei controlling their final output. Alterations in the PV+ Ch cell population in the cerebral cortex, hippocampal complex, and amygdaloid complex may be a potential characteristic of the ASD brain.
Parvalbumin+ chandelier cells in ASD
To investigate whether there are changes in the number or proportion of interneuron subpopulations in the prefrontal cortex in ASD, our group collected data using a straightforward approach to classify interneurons based on their expression of the calcium-sequestering proteins PV, CB, and calretinin (CR). Previous studies have used this method to comprehensively identify interneuron subpopulations in the cortex of mammalian species, and to identify interneuron subpopulations in human cortical tissue obtained from patients with certain conditions, like ASD (79, 80). We quantified PV+ cells in 10 ASD and 10 CT sex-and age-matched subjects and found that there is a decrease in the ratio of PV+ cells vs. the total number of interneurons, but no change in the ratio of CB+ cells or CR+ cells vs. the total number of interneurons in ASD compared to control cases (Figure 1) (81). Using an exclusion method, in which almost all Ch cells express PV but not the perineural net protein carbohydrate N-acetylgalactosamine, while human Bsk cells express both PV and N-acetylgalactosamine, we found a decrease in the number of PV+ in ASD that is attributable to a decrease in the number of Ch cells (82). Using a different cohort of subjects, we also found a decreased number of GAT1+ cartridges in the prefrontal cortex in ASD that was similar to the decreased number of PV+ cells in ASD, corroborating our previous data (60). In addition, we reported a decreased amount of GABAARα2 in the pyramidal cell AIS (83) - target of Ch cells synapses – and a reduced Ch bouton size, likely indicating a decreased synapsis strength – in the prefrontal cortex in ASD and concluded that Ch cells play an important role in the cortical circuitry dysfunction in ASD. Accordingly, the Barba's group examined PV+ and CB+ neurons in postmortem BA9 tissue from subjects with ASD (n = 2 ASD, 30 and 44 years, and n = 2 controls), and found a decrease in the ratio of PV to CB inhibitory neurons (84). We are not aware of any other data on Ch cells in ASD. We concluded that PV+ Ch cell number is decreased in the prefrontal cortex in ASD.
Parvalbumin and parvalbumin+ cell alterations in other neuropsychiatric disorders
As in the case of ASD, changes in the morphology and/or function of PV+ interneurons have also been reported in schizophrenia, epilepsy, bipolar disorder, and depression (58, 85–89). During development subjects with schizophrenia exhibit elevated mRNA levels for the transcription factors MafB and Lhx6, expressed in PV+ cell progenitor cells that migrate from the MGE into the developing cortex (90–92). Activation of the Il-6/NOX2 pathway in a mouse model of schizophrenia elevates oxidate stress, perturbs the normal development and maturation of PV+ cortical interneurons, and contributes to the emergence of schizophrenia like behavioral deficits (93). PV+ interneurons in schizophrenic patients present with lower mRNA levels of PV and GAD67 (94–97). GAD67 protein levels have also been shown to be reduced in schizophrenia (98). However, GAD67 protein levels were not altered in the axon cartridges of PV chandelier cells. GAD67 mRNA levels were only reduced in Bsk cells suggesting that GAD67 changes may be cell specific and differentially affected in schizophrenia (98). In addition to mRNA levels, the levels of PV protein are lower in PV+ Bsk boutons and the density of PV+ Ch cell cartridges is decreased in the prefrontal cortex in schizophrenia (89). While a few studies have previously reported deficits in PV neuron density in the dorsolateral prefrontal cortex, it may be possible that lower PV immunoreactivity levels may have rendered these PV+ neurons below the detection threshold (99–101). In addition, Ch cell GABA transmission is altered in schizophrenia at both the pre and post synaptic level. At the presynaptic level there is a reduction of GAT1 immunoreactivity in the axon terminals of Ch cells within the PFC (85, 89). Despite this GAT1 reduction, the levels of vGAT bouton protein were unaltered in schizophrenia (102). These results, combined with the GAD67 alterations highlighted above underlies the importance of assessing multiple markers in a cell specific manner to better understand the extent of GABAergic signaling changes at the presynaptic level.
At the post synaptic level, there is an increase of GABAARα2 at the AIS of pyramidal neurons (88). Moreover, the density of ankyrin G, an adaptor molecule responsible for the recruitment and stabilization of sodium channels at the AIS, is also decreased by 19% in brains with schizophrenia (103, 104). Knockdown of L1CAM in mice, a neural adhesion molecule that is linked to schizophrenia, results in a decrease of pyramidal AIS innervation by Ch cells (105, 106). In line with these findings, transcriptional levels of Erbb4, a known susceptibility gene for schizophrenia that is primarily expressed in PV+ neurons and highly active during early neurodevelopment, is decreased in Ch cells in the prefrontal cortex (107–113). Mice that lack Erbb4 in fast-spiking interneurons, have synaptic defects, while Erbb4 -/- mice are characterized by impaired behavior and GABA release (114, 115). Genetic recovery of the gene in ErbB4 -/- mice at the adult stage ameliorates these deficits demonstrating the role of ErbB4 mediated signaling in establishing and maintaining optimal Ch cell function (107). Activation of the Il-6/NOX2 pathway in a mouse model of schizophrenia elevates oxidate stress, perturbs the normal development and maturation of PV+ cortical interneurons, and contributes to the emergence of schizophrenia like behavioral deficits (93). Together these findings demonstrate that the synaptic integrity of Ch cell pyramidal connection is compromised in schizophrenia.
Half of the cases of ASD also present with epilepsy and the cortex in brains with epilepsy also present significant reductions in mRNA levels of PV. Post-mortem studies in brains of patients with temporal lobe and cryptogenic frontal lobe epilepsy present patches of decreased PV+ immunoreactivity in the neocortex (116), and the human epileptic peritumoral neocortex has a loss of inhibitory synapses on the soma and AIS of pyramidal neurons (117). A more recent human study showed that there was an increased density of PV+ Bsk cell boutons in the dentate gyrus, while the density of PV+ Ch cell boutons increased significantly in subjects with hippocampal sclerosis, a common pathology encountered in mesial temporal love epilepsy (118). Interestingly, bouton densities were not different between epileptic subjects without hippocampal sclerosis and matched controls. In monkeys with cortical focal epilepsy there is a reduction of Ch cell axons at the epileptic foci (119). Moreover, presentation of anxiety like behaviors, coupled with decrease in density of PV+ interneurons, GAD65 containing synaptic terminals and increases in GABAAR β3 subunit are all phenotypic traits observed across multiple pilocarpine-models of temporal lobe epilepsy (120, 121). Accordingly, pharmaco-genetic activation of hippocampal PV interneurons alleviates the severity of seizure onset in chronic epilepsy models (97). These findings suggest that synaptic connectivity is affected in individuals with epilepsy and may contribute to dysregulated inhibitory signaling. Individuals with bipolar disorder also present reduced PV messenger levels in the prefrontal cortex and hippocampus (122, 123), and reduced PV+ interneuron density in the prefrontal and entorhinal cortex, and parasubiculum (124, 125). There is also a decrease in GABA receptor levels in the PFC. The density of neurons containing messenger for GAD65 and GAD67 in the hippocampus is decreased by 45%, and the expression of GAD67 protein is reduced 50% in the prefrontal cortex and cerebellum in bipolar disorder brains (126, 127). Brains from people with depression also present a reduction in PV+ immunostaining in layer VI of the dorsolateral prefrontal cortex (128, 129), and animal models of depression present with chronic stress-induced depressive-like behavior and reduced density of hippocampal PV+ interneurons (99). In addition, ketamine's antidepressant properties are mediated in part through downregulation of NRG1-ErbB4 singling in PV+ interneurons in the rat brain (130). It is well established that stress is a risk factor that exacerbates the development of these disorders, likely in part through the perturbation of normal PV+ interneurons development and function (98–100). Animal models exposed to early stress during development have increased anxiety like behavior coupled with a decrease in PV+ interneuron density (98, 101, 118). Administration of a neurokinin-1 receptor (NK1R) antagonist prior to chronic stress exposure completely prevent the stress-induced reduction of the number of PV interneurons in mice (131). And rearing behavior in an enriched environment augment the number of PV+ interneurons in the basolateral amygdala and decrease anxiety like behavior in young male rats (132). These findings demonstrate that PV function is also impaired in neuropsychiatric disorders like temporal lobe epilepsy, depression and bipolar disorder, at both pre and post synaptic levels.
Overall, there is evidence that parvalbumin and PV+ interneurons in the cortex have a role in ASD, but also in other psychiatric, neurodevelopmental, and mood disorders, including epilepsy, schizophrenia, bipolar disorder, and depression.
Author contributions
VM wrote the manuscript. PJ wrote the manuscript and obtained images for figure. All authors contributed to the article and approved the submitted version.
Funding
This study was funded by the National Institute of Mental Health MH094681 and Shriners Hospitals for Children.
Conflict of interest
The authors declare that the research was conducted in the absence of any commercial or financial relationships that could be construed as a potential conflict of interest.
Publisher's note
All claims expressed in this article are solely those of the authors and do not necessarily represent those of their affiliated organizations, or those of the publisher, the editors and the reviewers. Any product that may be evaluated in this article, or claim that may be made by its manufacturer, is not guaranteed or endorsed by the publisher.
References
1. Lee SH, Rosenmund C, Schwaller B, Neher E. Differences in Ca2+ buffering properties between excitatory and inhibitory hippocampal neurons from the rat. J Physiol. (2000) 525:405–18. doi: 10.1111/j.1469-7793.2000.t01-3-00405.x
2. Schwaller B. The continuing disappearance of “pure” Ca2+ buffers. Cellular Mol Life Sci. (2009) 66:275–300. doi: 10.1007/s00018-008-8564-6
3. Kawaguchi Y. Physiological subgroups of nonpyramidal cells with specific morphological characteristics in layer II/III of rat frontal cortex. J Neurosci Off J Soc. (1995) 15:2638–55. doi: 10.1523/JNEUROSCI.15-04-02638.1995
4. McCormick DA, Connors BW, Lighthall JW, Prince DA. Comparative electrophysiology of pyramidal and sparsely spiny stellate neurons of the neocortex. J Neurophysiol. (1985) 54:782–806. doi: 10.1152/jn.1985.54.4.782
5. Simons DJ. Response properties of vibrissa units in rat SI somatosensory neocortex. J Neurophysiol. (1978) 41:798–820. doi: 10.1152/jn.1978.41.3.798
6. Verret L, Mann EO, Hang GB, Barth AM, Cobos I, Ho K, et al. Inhibitory interneuron deficit links altered network activity and cognitive dysfunction in Alzheimer model. Cell. (2019) 149:708–21. doi: 10.1016/j.cell.2012.02.046
7. Ogiwara I, Miyamoto H, Morita N, Atapour N, Mazaki E, Inoue I, et al. Nav1. 1 localizes to axons of parvalbumin-positive inhibitory interneurons: a circuit basis for epileptic seizures in mice carrying an Scn1a gene mutation J Neurosci 27. (20007) 5903–14. doi: 10.1523/JNEUROSCI.5270-06.2007
8. Chow A, Erisir A, Farb C, Nadal MS, Ozaita A, Lau D, et al. K(+) channel expression distinguishes subpopulations of parvalbumin- and somatostatin-containing neocortical interneurons. J Neurosci Off J Soc Neurosci. (1999) 19:9332–45. doi: 10.1523/JNEUROSCI.19-21-09332.1999
9. Bartos M, Vida I, Frotscher M, Meyer A, Monyer H, Geiger JR, et al. Fast synaptic inhibition promotes synchronized gamma oscillations in hippocampal interneuron networks. Proc Nat Acad Sci USA. (2002) 99:13222–7. doi: 10.1073/pnas.192233099
10. Sohal VS, Zhang F, Yizhar O, Deisseroth K. Parvalbumin neurons and gamma rhythms enhance cortical circuit performance. Nature. (2009) 459:698–702. doi: 10.1038/nature07991
11. Tamas G, Buhl EH, Lorincz A, Somogyi P. Proximally targeted GABAergic synapses and gap junctions synchronize cortical interneurons. Nat Neurosci. (2000) 3:366–71. doi: 10.1038/73936
12. Colgin LL, Denninger T, Fyhn M, Hafting T, Bonnevie T, Jensen O, et al. Frequency of gamma oscillations routes flow of information in the hippocampus. Nature. (2009) 462:353–7. doi: 10.1038/nature08573
13. Fries P, Reynolds JH, Rorie AE, Desimone R. Modulation of oscillatory neuronal synchronization by selective visual attention. Science. (2001) 291:1560–3. doi: 10.1126/science.1055465
14. Gregoriou GG, Gotts SJ, Zhou H, Desimone R. High-frequency, long-range coupling between prefrontal and visual cortex during attention. Science. (2009) 324:1207–10. doi: 10.1126/science.1171402
15. Melloni L, Molina C, Pena M, Torres D, Singer W, Rodriguez E, et al. Synchronization of neural activity across cortical areas correlates with conscious perception. J Neurosci Off J Soc Neurosci. (2007) 27:2858–65. doi: 10.1523/JNEUROSCI.4623-06.2007
16. Pesaran B, Pezaris JS, Sahani M, Mitra PP, Andersen RA. Temporal structure in neuronal activity during working memory in macaque parietal cortex. Nat Neurosci. (2002) 5:805–11. doi: 10.1038/nn890
17. Rodriguez E, George N, Lachaux JP, Martinerie J, Renault B, Varela FJ, et al. Perception's shadow: long-distance synchronization of human brain activity. Nature. (1999) 397:430–3. doi: 10.1038/17120
18. Cardin JA, Carlen M, Meletis K, Knoblich U, Zhang F, Deisseroth K, et al. Driving fast-spiking cells induces gamma rhythm and controls sensory responses. Nature. (2009) 459:663–7. doi: 10.1038/nature08002
19. Chard PS, Bleakman D, Christakos S, Fullmer CS, Miller RJ. Calcium buffering properties of calbindin D28k and parvalbumin in rat sensory neurones. J Physiol. (1993) 472:341–57. doi: 10.1113/jphysiol.1993.sp019950
20. Gulyas AI, Buzsaki G, Freund TF, Hirase H. Populations of hippocampal inhibitory neurons express different levels of cytochrome c. Eur J Neurosci. (2006) 23:2581–94. doi: 10.1111/j.1460-9568.2006.04814.x
21. Kann O, Papageorgiou IE, Draguhn A. Highly energized inhibitory interneurons are a central element for information processing in cortical networks. J Cereb Blood Flow Metab. (2014) 34:1270–82. doi: 10.1038/jcbfm.2014.104
22. Hu H, Gan J, Jonas P. Interneurons. Fast-spiking, parvalbumin(+) GABAergic interneurons: from cellular design to microcircuit function Science. (2014) 345:1255263. doi: 10.1126/science.1255263
23. Liu X, Kim CN, Yang J, Jemmerson R, Wang X. Induction of apoptotic program in cell-free extracts: requirement for dATP and cytochrome c. Cell. (1996) 86:147–57. doi: 10.1016/S0092-8674(00)80085-9
24. Kwak S, Weiss JH. Calcium-permeable AMPA channels in neurodegenerative disease and ischemia. Curr Opin Neurobiol. (2006) 16:281–7. doi: 10.1016/j.conb.2006.05.004
25. Bitanihirwe BK, Woo TU. Oxidative stress in schizophrenia: an integrated approach. Neurosci Biobehav Rev. (2011) 35:878–93. doi: 10.1016/j.neubiorev.2010.10.008
26. Chauhan A, Chauhan V. Oxidative stress in autism. Pathophysiology. (2006) 13:171–81. doi: 10.1016/j.pathophys.2006.05.007
27. Wohr M, Orduz D, Gregory P, Moreno H, Khan U, Vorckel KJ, et al. Lack of parvalbumin in mice leads to behavioral deficits relevant to all human autism core symptoms and related neural morphofunctional abnormalities. Transl Psychiatry. (2015) 5:e525. doi: 10.1038/tp.2015.19
28. Alarcon M, Abrahams BS, Stone JL, Duvall JA, Perederiy JV, Bomar JM, et al. Linkage, association, and gene-expression analyses identify CNTNAP2 as an autism-susceptibility gene. Am J Hum Genet. (2008) 82:150–9. doi: 10.1016/j.ajhg.2007.09.005
29. Benayed R, Gharani N, Rossman I, Mancuso V, Lazar G, Kamdar S, et al. Support for the homeobox transcription factor gene ENGRAILED 2 as an autism spectrum disorder susceptibility locus. Am J Hum Genet. (2005) 77:851–68. doi: 10.1086/497705
30. Beyer KS, Blasi F, Bacchelli E, Klauck SM, Maestrini E, Poustka A, et al. Mutation analysis of the coding sequence of the MECP2 gene in infantile autism. Hum Genet. (2002) 111:305–9. doi: 10.1007/s00439-002-0786-3
31. Carney RM, Wolpert CM, Ravan SA, Shahbazian M, Ashley-Koch A, Cuccaro ML, et al. Identification of MeCP2 mutations in a series of females with autistic disorder. Pediatr Neurol. (2003) 28:205–11. doi: 10.1016/S0887-8994(02)00624-0
32. Cook EH, Lindgren V, Leventhal BL, Courchesne R, Lincoln A, Shulman C, et al. Autism or atypical autism in maternally but not paternally derived proximal 15q duplication. Am J Hum Genetics. (1997) 60:928–34.
33. Durand CM, Betancur C, Boeckers TM, Bockmann J, Chaste P, Fauchereau F, et al. Mutations in the gene encoding the synaptic scaffolding protein SHANK3 are associated with autism spectrum disorders. Nat Genet. (2007) 39:25–7. doi: 10.1038/ng1933
34. Gharani N, Benayed R, Mancuso V, Brzustowicz LM, Millonig JH. Association of the homeobox transcription factor, ENGRAILED 2, 3, with autism spectrum disorder. Mol Psychiatr. (2004) 9:474–84. doi: 10.1038/sj.mp.4001498
35. Glessner JT, Wang K, Cai G, Korvatska O, Kim CE, Wood S, et al. Autism genome-wide copy number variation reveals ubiquitin and neuronal genes. Nature. (2009) 459:569–73. doi: 10.1038/nature07953
36. Rogers SJ, Wehner DE, Hagerman R. The behavioral phenotype in fragile X: symptoms of autism in very young children with fragile X syndrome, idiopathic autism, and other developmental disorders. J Dev Behav Pediatr. (2001) 22:409–17. doi: 10.1097/00004703-200112000-00008
37. Sato D, Lionel AC, Leblond CS, Prasad A, Pinto D, Walker S, et al. SHANK1 deletions in males with autism spectrum disorder. Am J Hum Genetics. (2012) 90:879–87. doi: 10.1016/j.ajhg.2012.03.017
38. Chen Q, Deister CA, Gao X, Guo B, Lynn-Jones T, Chen N, et al. Dysfunction of cortical GABAergic neurons leads to sensory hyper-reactivity in a Shank3 mouse model of ASD. Nat Neurosci. (2020) 23:520–32. doi: 10.1038/s41593-020-0598-6
39. Judson MC, Wallace ML, Sidorov MS, Burette AC, Gu B, Van Woerden GM, et al. GABAergic Neuron-specific loss of ube3a causes angelman syndrome-like EEG abnormalities and enhances seizure susceptibility. Neuron. (2016) 90:56–69. doi: 10.1016/j.neuron.2016.02.040
40. Sgado P, Genovesi S, Kalinovsky A, Zunino G, Macchi F, Allegra M, et al. Loss of GABAergic neurons in the hippocampus and cerebral cortex of Engrailed-2 null mutant mice: implications for autism spectrum disorders. Exp Neurol. (2013) 247:496–505. doi: 10.1016/j.expneurol.2013.01.021
41. Tabuchi K, Blundell J, Etherton MR, Hammer RE, Liu X, Powell CM, et al. A neuroligin-3 mutation implicated in autism increases inhibitory synaptic transmission in mice. Science. (2007) 318:71–6. doi: 10.1126/science.1146221
42. Grice SJ, Spratling MW, Karmiloff-Smith A, Halit H, Csibra G, Haan DE, et al. Disordered visual processing and oscillatory brain activity in autism and Williams syndrome. Neuroreport. (2001) 12:2697–700. doi: 10.1097/00001756-200108280-00021
43. Sohal VS. Insights into cortical oscillations arising from optogenetic studies. Biol Psychiatry. (2012) 71:1039–45. doi: 10.1016/j.biopsych.2012.01.024
44. DeFelipe J, Gonzalez-Albo MC. Chandelier cell axons are immunoreactive for GAT-1 in the human neocortex. Neuroreport. (1998) 9:467–70. doi: 10.1097/00001756-199802160-00020
45. Somogyi PA. specific 'axo-axonal' interneuron in the visual cortex of the rat. Brain Res. (1977) 136:345–50. doi: 10.1016/0006-8993(77)90808-3
46. Rudy B, Fishell G, Lee S, Hjerling-Leffler J. Three groups of interneurons account for nearly 100% of neocortical GABAergic neurons. Dev Neurobiol. (2011) 71:45–61. doi: 10.1002/dneu.20853
47. Van Brederode JF, Mulligan KA, Hendrickson AE. Calcium-binding proteins as markers for subpopulations of GABAergic neurons in monkey striate cortex. J Comp Neurol. (1990) 298:1–22. doi: 10.1002/cne.902980102
48. Povysheva NV, Zaitsev AV, Gonzalez-Burgos G, Lewis DA. Electrophysiological heterogeneity of fast-spiking interneurons: chandelier versus basket cells. PLoS ONE. (2013) 8:e70553. doi: 10.1371/journal.pone.0070553
49. Woodruff A, Xu Q, Anderson SA, Yuste R. Depolarizing effect of neocortical chandelier neurons. Front in Neural Circ. (2009) 3:15. doi: 10.3389/neuro.04.015.2009
50. Chan-Palay V, Palay SL. Interrelations of basket cell axons and climbing fibers in the cerebellar cortex of the rat. Z Anat Entwicklungsgesch. (1970) 132:191–227. doi: 10.1007/BF00523377
51. Cobb SR, Halasy K, Vida I, Nyiri G, Tamas G, Buhl EH, et al. Synaptic effects of identified interneurons innervating both interneurons and pyramidal cells in the rat hippocampus. Neuroscience. (1997) 79:629–48. doi: 10.1016/S0306-4522(97)00055-9
52. Kawaguchi Y, Kubota Y. GABAergic cell subtypes and their synaptic connections in rat frontal cortex. Cereb Cortex. (1997) 7:476–86. doi: 10.1093/cercor/7.6.476
53. Wang Y, Gupta A, Toledo-Rodriguez M, Wu CZ, Markram H. Anatomical, physiological, molecular and circuit properties of nest basket cells in the developing somatosensory cortex. Cereb Cortex. (2002) 12:395–410. doi: 10.1093/cercor/12.4.395
54. Szentagothai J, Arbib MA. Conceptual models of neural organization. Neurosci Res Program Bull. (1974) 12:305–510.
55. Jones EG. Varieties and distribution of non-pyramidal cells in the somatic sensory cortex of the squirrel monkey. The J Comp Neurol. (1975) 160:205–67. doi: 10.1002/cne.901600204
56. Goldman-Rakic PS. The “psychic” neuron of the cerebral cortex. Annal NY Acad Sci. (1999) 868:13–26. doi: 10.1111/j.1749-6632.1999.tb11270.x
57. Markram H, Toledo-Rodriguez M, Wang Y, Gupta A, Silberberg G, Wu C, et al. Interneurons of the neocortical inhibitory system. Nat Rev Neurosci. (2004) 5:793–807. doi: 10.1038/nrn1519
58. De Felipe J. Chandelier cells and epilepsy Brain. J Neurol. (1999) 122:1807–22. doi: 10.1093/brain/122.10.1807
59. De Felipe J, Hendry SH, Jones EG, Schmechel D. Variability in the terminations of GABAergic chandelier cell axons on initial segments of pyramidal cell axons in the monkey sensory-motor cortex. J Comp Neurol. (1985) 231:364–84. doi: 10.1002/cne.902310307
60. Amina S, Falcone C, Hong T, Wolf-Ochoa MW, Vakilzadeh G, Allen E, et al. Chandelier cartridge density is reduced in the prefrontal cortex in autism. Cereb Cortex. (2021) 31:2944–51. doi: 10.1093/cercor/bhaa402
61. Hardwick C, French SJ, Southam E, Totterdell SA. Comparison of possible markers for chandelier cartridges in rat medial prefrontal cortex and hippocampus. Brain Res. (2005) 1031:238–44. doi: 10.1016/j.brainres.2004.10.047
62. Inan M, Blazquez-Llorca L, Merchan-Perez A, Anderson SA, DeFelipe J, Yuste R, et al. Dense and overlapping innervation of pyramidal neurons by chandelier cells. J Neurosci Off J Soc Neurosci. (2013) 33:1907–14. doi: 10.1523/JNEUROSCI.4049-12.2013
63. Inda MC, Defelipe J, Munoz A. The distribution of chandelier cell axon terminals that express the GABA plasma membrane transporter GAT-1 in the human neocortex. Cereb Cortex. (2007) 17:2060–71. doi: 10.1093/cercor/bhl114
64. Inda MC, DeFelipe J, Munoz A. Morphology and distribution of chandelier cell axon terminals in the mouse cerebral cortex and claustroamygdaloid complex. Cereb Cortex. (2009) 19:41–54. doi: 10.1093/cercor/bhn057
65. Boccara CN, Kjonigsen LJ, Hammer IM, Bjaalie JG, Leergaard TB, Witter MPA, et al. three-plane architectonic atlas of the rat hippocampal region. Hippocampus. (2015) 25:838–57. doi: 10.1002/hipo.22407
66. Akil M, Lewis DA. Postnatal development of parvalbumin immunoreactivity in axon terminals of basket and chandelier neurons in monkey neocortex. Prog Neuropsychopharmacol Biol Psychiatry. (1992) 16:329–37. doi: 10.1016/0278-5846(92)90084-R
67. Conde F, Lund JS, Lewis DA. The hierarchical development of monkey visual cortical regions as revealed by the maturation of parvalbumin-immunoreactive neurons. Brain Res Dev Brain Res. (1996) 96:261–76. doi: 10.1016/0165-3806(96)00126-5
68. Gallo NB, Paul A, Van Aelst L. Shedding light on chandelier cell development, connectivity, and contribution to neural disorders. Trends Neurosci. (2020) 43:565–80. doi: 10.1016/j.tins.2020.05.003
69. Guo J, Anton ES. Decision making during interneuron migration in the developing cerebral cortex. Trends Cell Biol. (2014) 24:342–51. doi: 10.1016/j.tcb.2013.12.001
70. Hu JS, Vogt D, Sandberg M, Rubenstein JL. Cortical interneuron development: a tale of time and space. Development. (2017) 144:3867–78. doi: 10.1242/dev.132852
71. Lim L, Mi D, Llorca A, Marin O. Development and functional diversification of cortical interneurons. Neuron. (2018) 100:294–313. doi: 10.1016/j.neuron.2018.10.009
72. Lawrence YA, Kemper TL, Bauman ML, Blatt GJ. Parvalbumin-, calbindin-, and calretinin-immunoreactive hippocampal interneuron density in autism. Acta Neurol Scand. (2010) 121:99–108. doi: 10.1111/j.1600-0404.2009.01234.x
73. Seress L, Gulyas AI, Ferrer I, Tunon T, Soriano E, Freund TF, et al. Distribution, morphological features, and synaptic connections of parvalbumin- and calbindin D28k-immunoreactive neurons in the human hippocampal formation. J Comp Neurol. (1993) 337:208–30. doi: 10.1002/cne.903370204
74. Arellano JI, Munoz A, Ballesteros-Yanez I, Sola RG, De Felipe J. Histopathology and reorganization of chandelier cells in the human epileptic sclerotic hippocampus. Brain J Neurol. (2004) 127:45–64. doi: 10.1093/brain/awh004
75. Wittner L, Magloczky Z, Borhegyi Z, Halasz P, Toth S, Eross L, et al. Preservation of perisomatic inhibitory input of granule cells in the epileptic human dentate gyrus. Neuroscience. (2001) 108:587–600. doi: 10.1016/S0306-4522(01)00446-8
76. Garcia-Amado M, Prensa L. Neurons expressing parvalbumin and calretinin in the human amygdaloid complex: a quantitative and qualitative analysis in every nucleus and nuclear subdivision. Neuroscience. (2021) 452:153–68. doi: 10.1016/j.neuroscience.2020.11.001
77. Schumann CM, Amaral DG. Stereological estimation of the number of neurons in the human amygdaloid complex. J Comp Neurol. (2005) 491:320–9. doi: 10.1002/cne.20704
78. Pantazopoulos H, Lange N, Hassinger L, Berretta S. Subpopulations of neurons expressing parvalbumin in the human amygdala. J Comp Neurol. (2006) 496:706–22. doi: 10.1002/cne.20961
79. Hof PR, Glezer II, Conde F, Flagg RA, Rubin MB, Nimchinsky EA, et al. Cellular distribution of the calcium-binding proteins parvalbumin, calbindin, and calretinin in the neocortex of mammals: phylogenetic and developmental patterns. J Chem Neuroanat. (1999) 16:77–116. doi: 10.1016/S0891-0618(98)00065-9
80. Kuchukhidze G, Wieselthaler-Holzl A, Drexel M, Unterberger I, Luef G, Ortler M, et al. Calcium-binding proteins in focal cortical dysplasia. Epilepsia. (2015) 56:1207–16. doi: 10.1111/epi.13053
81. Hashemi E, Ariza J, Rogers H, Noctor SC, Martinez-Cerdeno V. The number of parvalbumin-expressing interneurons is decreased in the medial prefrontal cortex in autism. Cereb Cortex. (2017) 27:1931–43. doi: 10.1093/cercor/bhw021
82. Ariza J, Rogers H, Hashemi E, Noctor SC, Martinez-Cerdeno V. The number of chandelier and basket cells are differentially decreased in prefrontal cortex in autism. Cereb Cortex. (2018) 28:411–20. doi: 10.1093/cercor/bhw349
83. Hong T, Falcone C, Dufour B, Amina S, Castro RP, Regalado J, et al. GABAARalpha2 is decreased in the axon initial segment of pyramidal cells in specific areas of the prefrontal cortex in autism. Neuroscience. (2020) 437:76–86. doi: 10.1016/j.neuroscience.2020.04.025
84. Zikopoulos B, Barbas H. Altered neural connectivity in excitatory and inhibitory cortical circuits in autism. Front Hum Neurosci. (2013) 7:609. doi: 10.3389/fnhum.2013.00609
85. Pierri JN, Chaudry AS, Woo TU, Lewis DA. Alterations in chandelier neuron axon terminals in the prefrontal cortex of schizophrenic subjects. Am J Psychiatry. (1999) 156:1709–19. doi: 10.1176/ajp.156.11.1709
86. Lewis DA, Curley AA, Glausier JR, Volk DW. Cortical parvalbumin interneurons and cognitive dysfunction in schizophrenia. Trends Neurosci. (2012) 35:57–67. doi: 10.1016/j.tins.2011.10.004
87. Volk DW, Lewis DA. Impaired prefrontal inhibition in schizophrenia: relevance for cognitive dysfunction. Physiol Behav. (2002) 77:501–5. doi: 10.1016/S0031-9384(02)00936-8
88. Volk DW, Pierri JN, Fritschy JM, Auh S, Sampson AR, Lewis DA, et al. Reciprocal alterations in pre- and postsynaptic inhibitory markers at chandelier cell inputs to pyramidal neurons in schizophrenia. Cereb Cortex. (2002) 12:1063–70. doi: 10.1093/cercor/12.10.1063
89. Woo TU, Whitehead RE, Melchitzky DS, Lewis DAA. subclass of prefrontal gamma-aminobutyric acid axon terminals are selectively altered in schizophrenia. Proc Nat Acad Sci USA. (1998) 95:5341–6. doi: 10.1073/pnas.95.9.5341
90. Flandin P, Zhao Y, Vogt D, Jeong J, Long J, Potter G, et al. Lhx6 and Lhx8 coordinately induce neuronal expression of Shh that controls the generation of interneuron progenitors. Neuron. (2011) 70:939–50. doi: 10.1016/j.neuron.2011.04.020
91. Lewis DA, Hashimoto T, Volk DW. Cortical inhibitory neurons and schizophrenia. Nat Rev Neurosci. (2005) 6:312–24. doi: 10.1038/nrn1648
92. Volk DW, Matsubara T, Li S, Sengupta EJ, Georgiev D, Minabe Y, et al. Deficits in transcriptional regulators of cortical parvalbumin neurons in schizophrenia. Am J Psychiatry. (2012) 169:1082–91. doi: 10.1176/appi.ajp.2012.12030305
93. Powell SB, Sejnowski TJ, Behrens MM. Behavioral and neurochemical consequences of cortical oxidative stress on parvalbumin-interneuron maturation in rodent models of schizophrenia. Neuropharmacology. (2012) 62:1322–31. doi: 10.1016/j.neuropharm.2011.01.049
94. Enwright JF Iii, Huo Z, Arion D, Corradi JP, Tseng G, Lewis DA, et al. Transcriptome alterations of prefrontal cortical parvalbumin neurons in schizophrenia. Mol Psychiatr. (2018) 23:1606–13. doi: 10.1038/mp.2017.216
95. Hashimoto T, Volk DW, Eggan SM, Mirnics K, Pierri JN, Sun Z, et al. Gene expression deficits in a subclass of GABA neurons in the prefrontal cortex of subjects with schizophrenia. J Neurosci. (2003) 23:6315–26. doi: 10.1523/JNEUROSCI.23-15-06315.2003
96. Volk DW, Austin MC, Pierri JN, Sampson AR, Lewis DA. Decreased glutamic acid decarboxylase67 messenger RNA expression in a subset of prefrontal cortical gamma-aminobutyric acid neurons in subjects with schizophrenia. Arch Gen Psychiatry. (2000) 57:237–45. doi: 10.1001/archpsyc.57.3.237
97. Wang Y, Liang J, Chen L, Shen Y, Zhao J, Xu C, et al. Pharmaco-genetic therapeutics targeting parvalbumin neurons attenuate temporal lobe epilepsy. Neurobiol Dis. (2018) 117:149–60. doi: 10.1016/j.nbd.2018.06.006
98. Hu W, Zhang M, Czeh B, Flugge G, Zhang W. Stress impairs GABAergic network function in the hippocampus by activating nongenomic glucocorticoid receptors and affecting the integrity of the parvalbumin-expressing neuronal network. Am Neuropsychopharmacol. (2010) 35:1693–707. doi: 10.1038/npp.2010.31
99. Magarinos AM, Verdugo JM, McEwen BS. Chronic stress alters synaptic terminal structure in hippocampus. Proc Nat Acad Sci USA. (1997) 94:14002–8. doi: 10.1073/pnas.94.25.14002
100. Xie X, Chen Y, Ma L, Shen Q, Huang L, Zhao B, et al. Major depressive disorder mediates accelerated aging in rats subjected to chronic mild stress. Behav Brain Res. (2017) 329:96–103. doi: 10.1016/j.bbr.2017.04.022
101. Lussier SJ, Stevens HE. Delays in GABAergic interneuron development and behavioral inhibition after prenatal stress. Dev Neurobiol. (2016) 76:1078–91. doi: 10.1002/dneu.22376
102. Rocco BR, Lewis DA, Fish KN. Markedly lower glutamic acid decarboxylase 67 protein levels in a subset of boutons in schizophrenia. Biol Psychiatry. (2016) 79:1006–15. doi: 10.1016/j.biopsych.2015.07.022
103. Cruz DA, Weaver CL, Lovallo EM, Melchitzky DS, Lewis DA. Selective alterations in postsynaptic markers of chandelier cell inputs to cortical pyramidal neurons in subjects with schizophrenia. Neuropsychopharmacol Off Pub Am College Neuropsychopharmacol. (2009) 34:2112–24. doi: 10.1038/npp.2009.36
104. Zhou D, Lambert S, Malen PL, Carpenter S, Boland LM, Bennett V, et al. AnkyrinG is required for clustering of voltage-gated Na channels at axon initial segments and for normal action potential firing. J Cell Biol. (1998) 143:1295–304. doi: 10.1083/jcb.143.5.1295
105. Kurumaji A, Nomoto H, Okano T, Toru M. An association study between polymorphism of L1CAM gene and schizophrenia in a Japanese sample. Am J Med Genetics. (2001) 105:99–104. doi: 10.1002/1096-8628(20010108)105:1andlt;99::AID-AJMG1071andgt;3.0.CO;2-U
106. Tai Y, Gallo NB, Wang M, Yu JR, Van Aelst L. Axo-axonic innervation of neocortical pyramidal neurons by gabaergic chandelier cells requires ankyring-associated L1CAM. Neuron. (2019) 102:358–72. doi: 10.1016/j.neuron.2019.02.009
107. Wang H, Liu F, Chen W, Sun X, Cui W, Dong Z, et al. Genetic recovery of ErbB4 in adulthood partially restores brain functions in null mice. Proc Nat Acad Sci USA. (2018) 115:13105–10. doi: 10.1073/pnas.1811287115
108. Yau HJ, Wang HF, Lai C, Liu FC. Neural development of the neuregulin receptor ErbB4 in the cerebral cortex and the hippocampus: preferential expression by interneurons tangentially migrating from the ganglionic eminences. Cereb Cortex. (2003) 13:252–64. doi: 10.1093/cercor/13.3.252
109. Wen L, Lu YS, Zhu XH, Li XM, Woo RS, Chen YJ, et al. Neuregulin 1 regulates pyramidal neuron activity via ErbB4 in parvalbumin-positive interneurons. Proc Nat Acad Sci USA. (2010) 107:1211–6. doi: 10.1073/pnas.0910302107
110. Vullhorst D, Neddens J, Karavanova I, Tricoire L, Petralia RS, McBain CJ, et al. Selective expression of ErbB4 in interneurons, but not pyramidal cells, of the rodent hippocampus. J Neurosci. (2009) 29:12255–64. doi: 10.1523/JNEUROSCI.2454-09.2009
111. Norton N, Moskvina V, Morris DW, Bray NJ, Zammit S, Williams NM, et al. Evidence that interaction between neuregulin 1 and its receptor erbB4 increases susceptibility to schizophrenia. Am J Med Genetics. (2006) 141:96–101. doi: 10.1002/ajmg.b.30236
112. Silberberg G, Darvasi A, Pinkas-Kramarski R, Navon R. The involvement of ErbB4 with schizophrenia: association and expression studies. Am J Med Genetics. (2006) 141:142–8. doi: 10.1002/ajmg.b.30275
113. Law AJ, Kleinman JE, Weinberger DR, Weickert CS. Disease-associated intronic variants in the ErbB4 gene are related to altered ErbB4 splice-variant expression in the brain in schizophrenia. Hum Mol Genetics. (2007) 16:129–41. doi: 10.1093/hmg/ddl449
114. Del Pino I, Garcia-Frigola C, Dehorter N, Brotons-Mas JR, Alvarez-Salvado E, Martinez DE, et al. Erbb4 deletion from fast-spiking interneurons causes schizophrenia-like phenotypes. Neuron. (2013) 79:1152–68. doi: 10.1016/j.neuron.2013.07.010
115. Yang JM, Shen CJ, Chen XJ, Kong Y, Liu YS, Li XW, et al. erbb4 deficits in chandelier cells of the medial prefrontal cortex confer cognitive dysfunctions: implications for schizophrenia. Cereb Cortex. (2019) 29:4334–46. doi: 10.1093/cercor/bhy316
116. Ferrer I, Oliver B, Russi A, Casas R, Rivera R. Parvalbumin and calbindin-D28k immunocytochemistry in human neocortical epileptic foci. J Neurol Sci. (1994) 123:18–25. doi: 10.1016/0022-510X(94)90198-8
117. Marco P, Sola RG, Ramony Cajal S, De Felipe J. Loss of inhibitory synapses on the soma and axon initial segment of pyramidal cells in human epileptic peritumoural neocortex: implications for epilepsy. Brain Res Bull. (1997) 44:47–66. doi: 10.1016/S0361-9230(97)00090-7
118. Scott H, Phillips TJ, Sze Y, Alfieri A, Rogers MF, Volpato V, et al. Maternal antioxidant treatment prevents the adverse effects of prenatal stress on the offspring's brain and behavior. Neurobiol Stress. (2020) 13:100281. doi: 10.1016/j.ynstr.2020.100281
119. Ribak CE. Axon terminals of GABAergic chandelier cells are lost at epileptic foci. Brain Res. (1985) 326:251–60. doi: 10.1016/0006-8993(85)90034-4
120. Knopp A, Frahm C, Fidzinski P, Witte OW, Behr J. Loss of GABAergic neurons in the subiculum and its functional implications in temporal lobe epilepsy. Brain J Neurol. (2008) 131:1516–27. doi: 10.1093/brain/awn095
121. Zhu X, Yao Y, Li X, Dong J, Zhang A. Alteration of GABAergic signaling is associated with anxiety-like behavior in temporal lobe epilepsy mice. Prog Neuropsychopharmacol Biol Psychiatry. (2019) 93:141–8. doi: 10.1016/j.pnpbp.2019.03.020
122. Konradi C, Zimmerman EI, Yang CK, Lohmann KM, Gresch P, Pantazopoulos H, et al. Hippocampal interneurons in bipolar disorder. Arch Gen Psychiatry. (2011) 68:340–50. doi: 10.1001/archgenpsychiatry.2010.175
123. Sibille E, Morris HM, Kota RS, Lewis DA. GABA-related transcripts in the dorsolateral prefrontal cortex in mood disorders. Int J Neuropsychopharmacol. (2011) 14:721–34. doi: 10.1017/S1461145710001616
124. Wang AY, Lohmann KM, Yang CK, Zimmerman EI, Pantazopoulos H, Herring N, et al. Bipolar disorder type 1 and schizophrenia are accompanied by decreased density of parvalbumin- and somatostatin-positive interneurons in the parahippocampal region. Acta Neuropathol. (2011) 122:615–26. doi: 10.1007/s00401-011-0881-4
125. Beasley CL, Zhang ZJ, Patten I, Reynolds GP. Selective deficits in prefrontal cortical GABAergic neurons in schizophrenia defined by the presence of calcium-binding proteins. Biol Psychiatry. (2002) 52:708–15. doi: 10.1016/S0006-3223(02)01360-4
126. Heckers S, Stone D, Walsh J, Shick J, Koul P, Benes FM, et al. Differential hippocampal expression of glutamic acid decarboxylase 65 and 67 messenger RNA in bipolar disorder and schizophrenia. Arch Gen Psychiatry. (2002) 59:521–9. doi: 10.1001/archpsyc.59.6.521
127. Ishikawa M, Mizukami K, Iwakiri M, Asada T. Immunohistochemical and immunoblot analysis of gamma-aminobutyric acid B receptor in the prefrontal cortex of subjects with schizophrenia and bipolar disorder. Neurosci Letters. (2005) 383:272–7. doi: 10.1016/j.neulet.2005.04.025
128. Khundakar A, Morris C, Thomas AJ. The immunohistochemical examination of GABAergic interneuron markers in the dorsolateral prefrontal cortex of patients with late-life depression. Int Psychogeriatr. (2011) 23:644–53. doi: 10.1017/S1041610210001444
129. Rajkowska G, O'Dwyer G, Teleki Z, Stockmeier CA, Miguel-Hidalgo JJ. GABAergic neurons immunoreactive for calcium binding proteins are reduced in the prefrontal cortex in major depression. Am Neuropsychopharmacol. (2007) 32:471–82. doi: 10.1038/sj.npp.1301234
130. Wang N, Zhang GF, Liu XY, Sun HL, Wang XM, Qiu LL, et al. Downregulation of neuregulin 1-ErbB4 signaling in parvalbumin interneurons in the rat brain may contribute to the antidepressant properties of ketamine. J Mol Neurosci. (2014) 54:211–8. doi: 10.1007/s12031-014-0277-8
131. Czeh B, Simon M, van der Hart MG, Schmelting B, Hesselink MB, Fuchs E, et al. Chronic stress decreases the number of parvalbumin-immunoreactive interneurons in the hippocampus: prevention by treatment with a substance P receptor (NK1) antagonist. Am Colleg Neuropsychopharmacol. (2005) 30:67–79. doi: 10.1038/sj.npp.1300581
Keywords: chandelier cell, parvalbumin, autism, schizophrenia, interneuron
Citation: Juarez P and Martínez Cerdeño V (2022) Parvalbumin and parvalbumin chandelier interneurons in autism and other psychiatric disorders. Front. Psychiatry 13:913550. doi: 10.3389/fpsyt.2022.913550
Received: 05 April 2022; Accepted: 22 September 2022;
Published: 12 October 2022.
Edited by:
Daniel Vogt, Michigan State University, United StatesReviewed by:
Bart Christiaan Jongbloets, Utrecht University, NetherlandsGuillermo Gonzalez-Burgos, University of Pittsburgh, United States
Copyright © 2022 Juarez and Martínez Cerdeño. This is an open-access article distributed under the terms of the Creative Commons Attribution License (CC BY). The use, distribution or reproduction in other forums is permitted, provided the original author(s) and the copyright owner(s) are credited and that the original publication in this journal is cited, in accordance with accepted academic practice. No use, distribution or reproduction is permitted which does not comply with these terms.
*Correspondence: Verónica Martínez Cerdeño, dm1hcnRpbmV6Y2VyZGVubyYjeDAwMDQwO3VjZGF2aXMuZWR1