- 1Department of Pharmacology, Graduate School of Pharmaceutical Sciences, Tohoku University, Sendai, Japan
- 2Division of Neurofunctional Genomics, Department of Immunobiology and Neuroscience, Medical Institute of Bioregulation, Kyushu University, Fukuoka, Japan
Autism spectrum disorder (ASD) is a heterogeneous disorder characterized by repetitive behaviors and social impairments, often accompanied by learning disabilities. It has been documented that the neuropeptide oxytocin (OXT) ameliorates core symptoms in patients with ASD. We recently reported that chronic administration of intranasal OXT reversed social and learning impairments in prenatally valproic acid (VPA)-exposed rats. However, the underlying molecular mechanisms remain unclear. Here, we explored molecular alterations in the hippocampus of rats and the effects of chronic administration of intranasal OXT (12 μg/kg/d). Microarray analyses revealed that prenatal VPA exposure altered gene expression, a part of which is suggested as a candidate in ASD and is involved in key features including memory, developmental processes, and epilepsy. OXT partly improved the expression of these genes, which were predicted to interact with those involved in social behaviors and hippocampal-dependent memory. Collectively, the present study documented molecular profiling in the hippocampus related to ASD and improvement by chronic treatment with OXT.
Introduction
Autism spectrum disorder (ASD) is a neurodevelopmental disorder characterized by social deficits and repetitive behaviors (1). ASD involves heterogeneous and complex causal factors, both genetically and environmentally. While it has been documented that various copy numbers or single nucleotide variations are associated with ASD, multiple environmental factors such as maternal infection, exposure to drugs or toxicants, and immune dysregulation have also been implicated (2). Such diverse causes, their interactions, and the resultant complex symptoms make it difficult to focus on particular targets and therapeutic approaches in ASD.
The neuropeptide oxytocin (OXT) facilitates socio-communicative behaviors in mammals (3). OXT or OXT receptor deficiency impairs multiple social behaviors (4, 5). In addition, plasma levels of OXT are significantly lower in children with autism than in their normal counterparts (6, 7). Clinical studies have documented that the intranasal administration of OXT improves socio-emotional impairments in patients with ASD (8, 9). Thus, OXT could be considered a suitable candidate for treating the core symptoms in ASD.
Maternal use of the anti-epileptic drug valproic acid (VPA) during pregnancy is suggested to increase the risk of teratogenicity and ASD onset in offspring (10, 11). These features are reproducible in animals; they represent defects in the limbs and tail and ASD-like social deficits (12, 13). In addition, transcriptome analyses have identified some key molecular pathways for ASD in the amygdala and prefrontal cortex of the model (12, 14, 15); alteration in signaling of protein kinase A and Rho GTPases in the amygdala and calcium signaling in the prefrontal cortex. These pathways are related to synaptic plasticity, a pathological hallmark in ASD (16–19), suggesting that molecular alterations in these emotion-related regions are involved in the pathogenesis of ASD. Especially, GTPases signaling defects are well documented in neurodevelopmental disorders including ASD (20); Rho GTPase Cdc42, a regulator of neurite outgrowth, is reduced in autistic patients (21). Prenatal VPA exposure decreases mRNA levels of Rho GTPase-activating protein p250GAP (22). In addition, p21-activated protein kinase exchange factor, a Rho GTPase regulatory protein, interacts with SH3 and multiple ankyrin repeat domains (SHANK) proteins in spines to regulate postsynaptic structure (23). Since mutations in SHANK gene have been associated with neurodevelopmental and neuropsychiatric disorders, including ASD (24), it is noteworthy that recent findings show that OXT restores neurite abnormalities in hippocampal cultures with SHANK3 deficiency through amelioration of Rho GTPase levels (25). Thus, prenatally VPA-exposed animals are a valid ASD model in terms of behavioral phenotypes and epigenetic modulation of gene expression as an environmental factor.
In our previous studies, we had reported that prenatal VPA exposure impairs learning and long-term potentiation (LTP) in the hippocampus of rats and that chronic administration of intranasal OXT ameliorates learning disabilities (26, 27). In line with our observations, intranasal administration of OXT blocked the learning disability in prenatally VPA-exposed or restraint stress-exposed animal models (28–30). In contrast, Hara et al. demonstrated that a single dose of OXT was effective for social impairment only for a short period and not for memory impairment (31). These reports suggest that the mechanisms of action of chronic OXT would involve molecular alterations in addition to the acute activation of oxytocinergic signaling. However, it remains unclear which molecular pathways are affected by chronic administration of OXT. Furthermore, molecular profiling in the dorsal hippocampus, a critical region for learning and memory, and partly implicated for social behaviors (32, 33) has not been investigated in a prenatally VPA-exposed model.
In the present study, we explored transcriptome profiling in the hippocampus of prenatally VPA-exposed rats. It was seen that prenatal VPA exposure altered the expression levels of genes involved in multiple behaviors and developmental behaviors, some of which were documented as candidate genes in ASD. We also demonstrated that the chronic administration of intranasal OXT partly ameliorated these alterations.
Materials and Methods
Animals
Animal studies conformed to the Regulations for Animal Experiments and Related Activities at Tohoku University and were approved by the Committee on Animal Experiments at Tohoku University (approval number: 2020PhA-007). Every effort was made to use minimum the number of rats and minimize their discomfort. Animals were bred in a conventional environment (temperature, 21–23°C; humidity, 50–60%; 12-h light-dark cycle) with free access to normal chow and water. Three pregnant Sprague-Dawley rats (Japan SLC, Shizuoka, Japan) received a single administration of oral VPA (600 mg/kg; Sigma-Aldrich, St. Louis, MO, United States) on day 12.5 as described previously (27). Two control rats received water in the same way. Rats gave birth from 7 to 14 pups per litter, of which 3–8 were males (sex ratio of male to female was about 1:1.1). Sizes and body weights of pups were not affected by VPA treatment, as reported by a previous study (26). Only male pups were included in this study because of the higher incidence of ASD in males than in females and even in prenatally VPA-exposed models (34).
Oxytocin Treatment
On day 21 of birth, the rats were randomly divided and subsequently received vehicle or OXT administration: 3 pups from 2 control rats and 6 pups from 3 VPA-treated rats, with the latter further divided into 3 vehicle- or OXT-treated groups of 3 pups each. Male pups received intranasal OXT (Peptide Institute, Osaka, Japan) dissolved in saline at a dose of 12 μg/kg/d, which is in a range of that promoting social behaviors in rodents (35–37), using a pipette tip on postnatal day 21–55. The liquid volume of OXT solution was changed in the range of 2–10 μL according to the growth of rats. The dose and period of treatment were the same as those in a previous study where OXT attenuated autistic behaviors in prenatally VPA-exposed rats (27).
Identification of Differentially Expressed Genes in Microarray Analysis
Total RNA was extracted from the dorsal hippocampus on postnatal day 56. As with most transcriptome analyses, the number of samples per condition is 2–5 for the analyses using VPA-treated ASD models (12, 14, 15). Therefore, we decided that three samples per condition would be sufficient for this study. Expression profiles were determined using Rat Gene 2.0 ST array systems (Affymetrix, Santa Clara, CA, United States) and analyzed using Transcriptome Analysis Console software (version 4.0; RRID:SCR_018718; Affymetrix). Differentially expressed genes (DEGs) were evaluated as follows: (1) P < 0.05, in a one-way analysis of variance computed by limma (38), (2) more than 1.5 fold-change (both increase and decrease) between control and vehicle-treated VPA groups. (3) Expression levels (in log2 scale) more than 6.6 at least in one group. Among these, significantly different (improved) values between the vehicle-treated VPA and OXT-treated VPA groups were further investigated. Expression levels were normalized to those of the control group. Microarray data were deposited in the GEO database (accession number: GSE196500).
Gene Ontology Enrichment Analysis
Gene ontology (GO) enrichment analysis was performed using Metascape (RRID:SCR_0166201) (39). To obtain the most comprehensive data, gene identifiers were converted from Rattus norvegicus to Homo sapiens orthologs. GO terms classified in biological processes were collected under the following conditions: a minimum count of 3, P < 0.01, and an enrichment factor (the ratio between the observed counts and the counts expected by chance) > 1.5. Cytoscape App (RRID:SCR_003032) was used to create and plot the enrichment network (40).
Protein-Protein Interaction Enrichment Analysis
To investigate protein networks consisting of proteins that form physical interactions each other in DEGs, protein-protein interaction analysis was performed using databases including BioGrid (RRID:SCR_007393) (41), InWeb_IM (42), and OmniPath (43) in Metascape. The molecular complex detection (MCODE) algorithm (RRID:SCR_015828) (44) was applied to identify tightly connected modules in each network. MCODE analysis was performed with default settings; detection when there are at least three genes in a network.
Gene-Disease Association and Regulatory Interaction Analysis
To reveal the involvement of DEGs in diseases and their transcriptional regulation, DisGeNET (RRID:SCR_006178) (45) and TRRUST (46) were performed in Metascape. The algorithm was the same as that of the GO enrichment analysis discussed above.
Overlapping With Autism Spectrum Disorder Risk Genes and Predictive Analysis of Interaction
Two databases were used to explore the overlap between DEGs in the VPA model and candidate genes in ASD patients: SFARI (RRID:SCR_0042612) and Krishnan’s datasets Genome-wide predictions of autism-associated genes3 (47). Gene lists in SFARI were scored as follows: S (syndromic), 1 (high confidence), 2 (strong candidate), and 3 (suggestive evidence). In Krishnan’s dataset, gene list associated with ASD are separated between brain regions and developmental periods, and the hippocampus in middle-late childhood (ID: HIP. 11) was selected to be appropriate for comparison with the results of this study. Additionally, to predict the interactive networks between DEGs improved by OXT and ASD candidate genes, Krishnan’s dataset was used.
Results
Gene Expression Profiles in the Hippocampus of Prenatally Valproic Acid-Exposed Rats
Gene expression profiles of the hippocampus were compared between the control, vehicle-treated VPA, and OXT-treated VPA groups (n = 3 per group). Hierarchical clustering tended to separate the vehicle-treated VPA group from the others, except for one sample from the OXT-treated VPA group (Figure 1A). Microarray analysis revealed that 377 genes differed significantly by more than 1.5-fold between the groups (Figure 1A). Among these, 174 genes were considered DEGs (see section “Materials and Methods”). We then analyzed the biological processes of DEGs between the control and vehicle-treated VPA groups. GO enrichment analysis revealed that DEGs were roughly associated with multiple functions, including signaling, behavior, and developmental processes (Figure 1B). An in-depth analysis further indicated that DEGs are involved in chemical synaptic transmission, short-term memory, brain development, as well as nervous system development (Figure 1B). These results suggest that prenatal VPA exposure affects multiple genes in the hippocampus that are involved in synaptic function, learning and memory, and neurodevelopment, all of which are the key features of ASD. In particular, the enrichment network analysis also indicated that chemical synaptic transmission (#1) serves as a hub function in these biological processes (Figure 1C). Protein-protein interaction analysis further predicted that several interactive networks are formed among the DEGs (Figure 1D). Interestingly, histone deacetylase 1 (HDAC1) and specificity protein 1 (SP1), both of which are regulated by VPA (48, 49), were identified as factors for the transcriptional regulation of DEGs (Figure 1E).
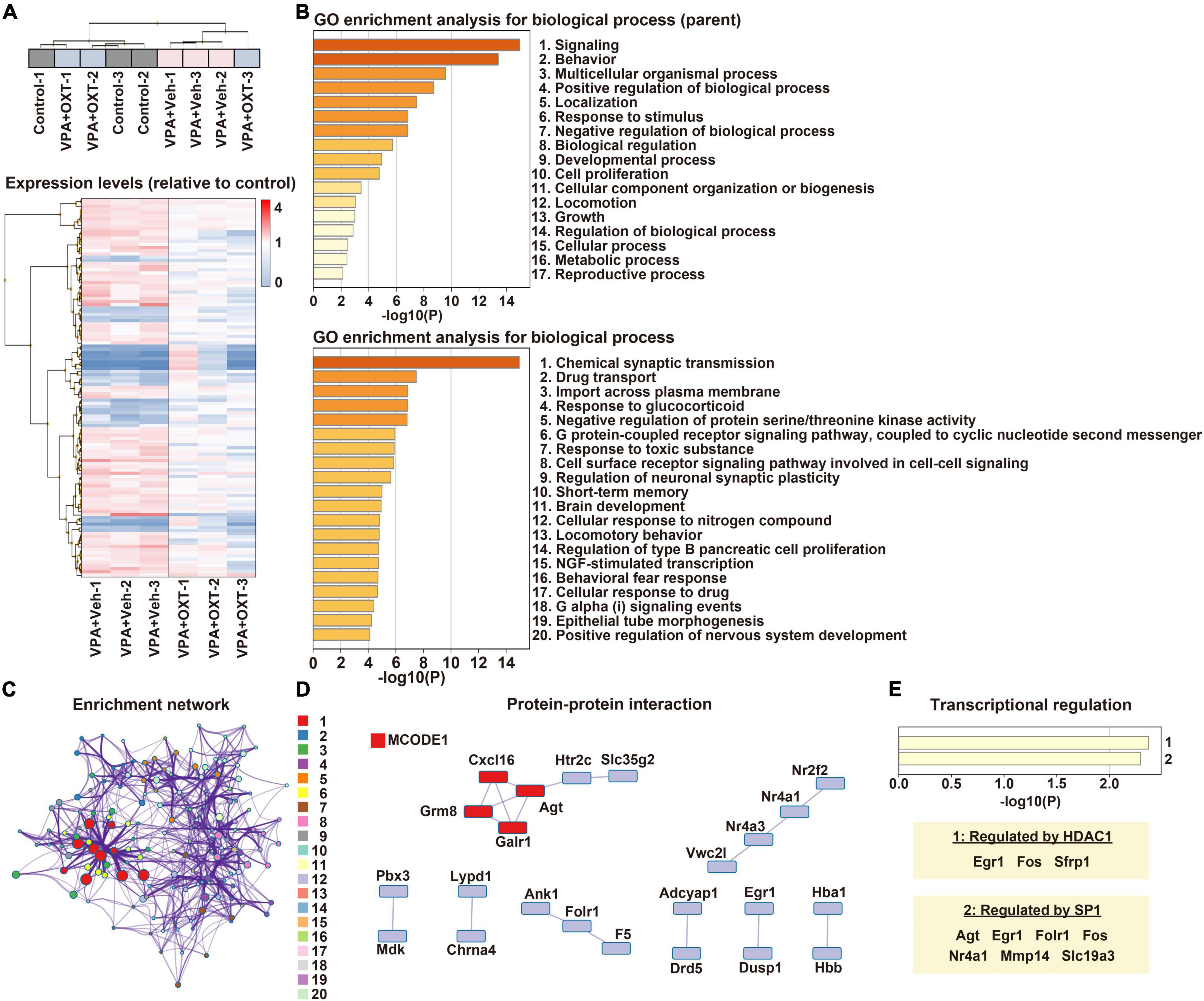
Figure 1. Transcriptome profiling in the hippocampus of prenatally VPA exposed rats. (A) Hierarchical clustering of DEGs between groups. Expression levels were normalized to those of the control. (B) Biological processes of DEGs in GO enrichment analysis. Top, parent; Down, child annotations, respectively. (C) Enrichment networks between clusters of biological processes. Shown numbers are the same with those of child annotations in panel (B). (D) Protein-protein interactive networks between DEGs. Shown by MCODE1 represents a strongly connected network. (E) Analysis of transcriptional regulation of DEGs. HDAC1 and SP1 are detected as significant regulators. VPA, valproic acid; DEG, differentially expressed gene; GO, gene ontology; MCODE1, molecular complex detector; HDAC, histone deacetylase; SP1, specificity protein 1.
Autism Spectrum Disorder-Associated Molecular Changes in the Hippocampus by Prenatal Valproic Acid Exposure
We next addressed which human diseases are associated with DEGs. Notably, DEGs are known to be enriched in ASD-associated [mental disorders, Gilles de la Tourette syndrome (50), and various types of epilepsy] and learning disability-associated (cognition disorders and mental deterioration) diseases (Figure 2A). To further reveal the relationship with human ASD, the overlap between DEGs and ASD candidate genes was investigated using two databases. Eight DEGs were included in the SFARI database, and Ahi1 was indicated as a cause of syndromic ASD (Figure 2B). In addition, 13 DEGs overlapped with gene sets in the hippocampus of ASD in middle-late childhood in Krishnan’s database (Figure 2C). Collectively, these results suggest that molecular profiles in the hippocampus could underlie autistic behaviors, including learning disabilities seen in prenatally VPA-exposed rats.
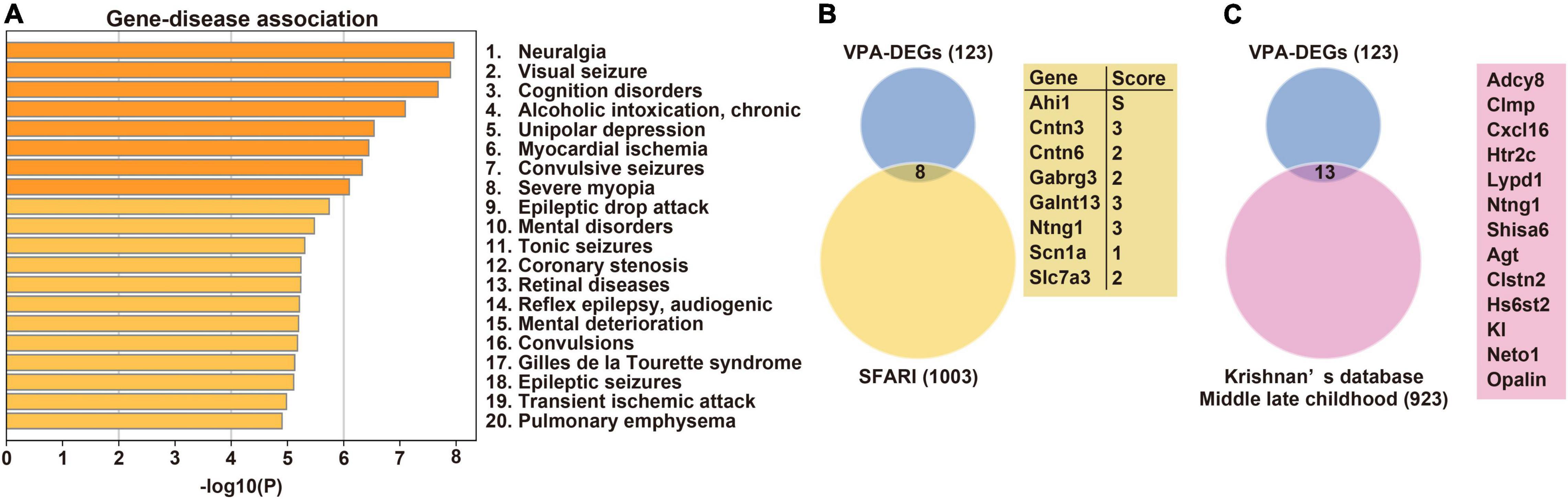
Figure 2. Disease-associated profiling and overlap with ASD candidate genes. (A) Association between DEGs and human diseases. Venn’s diagrams depict overlap between DEGs and candidate genes for ASD registered in SFARI (B) and Krishnan’s database (C), respectively. ASD, Autism spectrum disorder; DEG, differentially expressed gene.
Effects of Chronic Administration of Intranasal Oxytocin on Gene Expression in the Hippocampus of Prenatally Valproic Acid-Exposed Rats
To explore the molecular mechanisms by which chronic OXT treatment improves the prenatal VPA exposure induced autistic behaviors, significantly improved populations by OXT among DEGs were extracted (Figure 3A). Chronic administration of intranasal OXT (12 μg/kg/d) significantly upregulated 13 genes and downregulated two genes among the DEGs (Figure 3B). GO enrichment analysis revealed that these improved genes belonged to partly similar processes with DEGs (Figure 1B), including the developmental process, signaling, and import across the plasma membrane, and different genes such as those involved in epithelial tube morphogenesis and response to steroid hormone (Figure 3C). These GO genes formed networks with each other except for aging, and epithelial tube morphogenesis, and thus would serve as a hub annotation (Figure 3D). Finally, interactive networks were predicted between ASD candidates and DEGs improved by OXT using Krishnan’s database (except for Mt-nd3, which were not registered in the database). The predicted top 10 genes that interacted with 14 of the DEGs improved by OXT are shown in Figure 3E. Of these, three genes were reported to be involved in social behaviors, learning, and memory (Table 1). Chronic administration of intranasal OXT has the potential to partly regulate the molecular pathways in the hippocampus involved in ASD-like behaviors induced by prenatal VPA exposure.
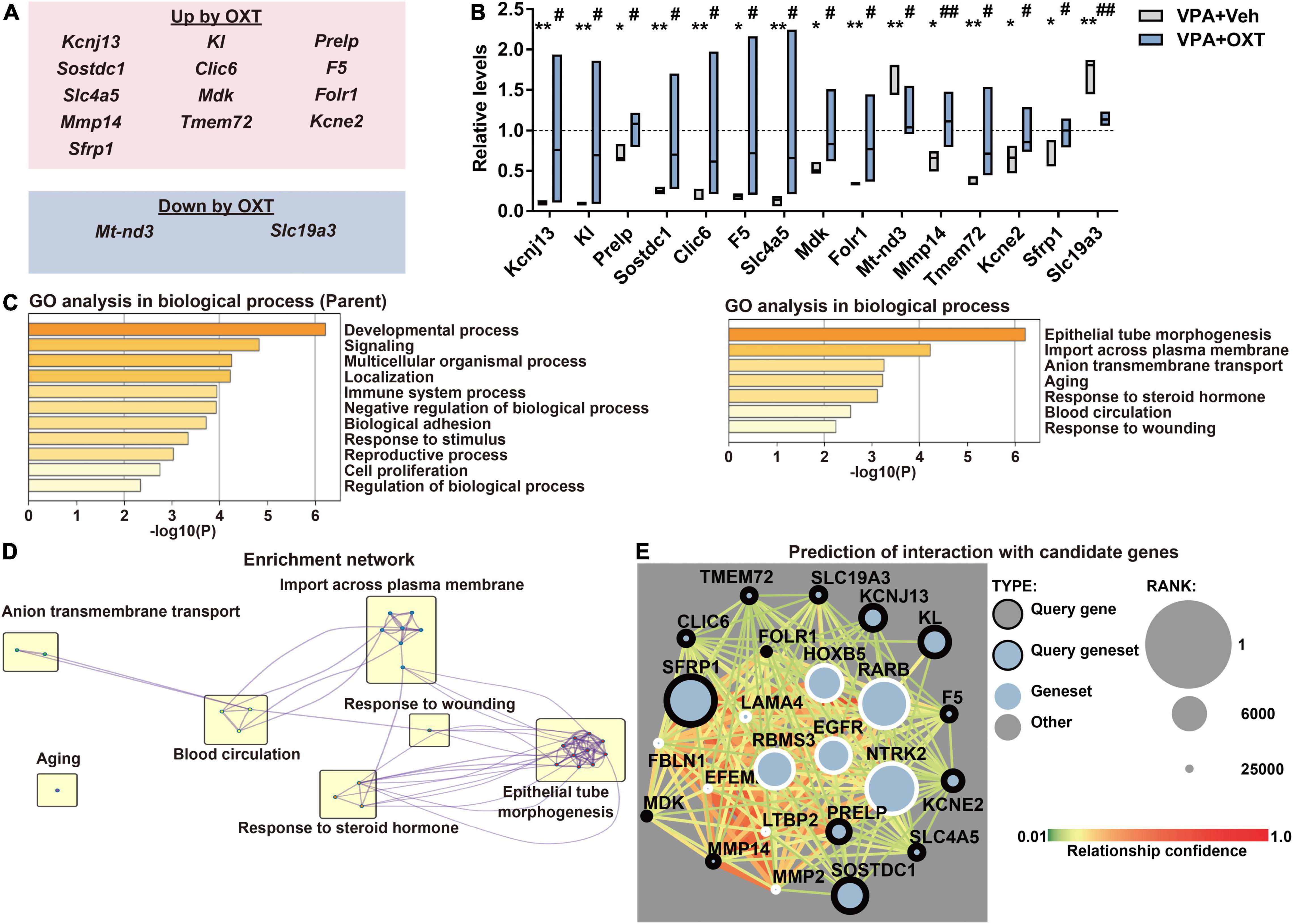
Figure 3. Effect of chronic treatment of intranasal OXT on prenatal VPA exposure-induced molecular changes. (A) Summary of (top) upregulated and (bottom) downregulated DEGs by OXT (12 μg/kg/day). (B) Quantification of expression levels of genes improved by OXT. The levels were normalized to those of the control. The top and bottom of the bar depict the highest and the lowest of values, respectively. The line within the bar shows the median (n = 3 per group). *P < 0.05 and **P < 0.01 vs. control; #P < 0.05 and ##P < 0.01 vs. vehicle-treated VPA. (C) Biological processes of DEGs in GO enrichment analysis. Left, parent; Right, child annotations, respectively. (D) Enrichment networks between clusters of biological processes with child annotations. (E) Predictive interaction between DEGs improved by OXT and ASD candidates in Krishnan’s database. Shown are top 10 of candidates connecting strongly with our gene sets. DEG, differentially expressed gene; OXT, oxytocin; GO, gene ontology.
Discussion
Valproic acid is an inhibitor of HDACs that epigenetically modulates gene expression (48). It has been suggested that VPA-induced teratogenicity results from HDAC inhibition. The effects of HDAC inhibitors on fetal teratogenicity are suppressed by their analogs with lower potency against HDACs (51, 52). Interestingly, it has been reported that the prenatal VPA exposure-induced social deficits were ameliorated by chronic administration of HDAC inhibitors at postnatal days (13), supporting the critical role of epigenetic modulation by HDAC inhibition for both onset and amelioration of ASD. In the present study, HDAC1 and specificity protein 1 were implicated as transcriptional regulators of DEGs, suggesting the relevance of the hippocampus in ASD-like molecular profiling and behaviors induced by prenatal VPA exposure.
The dorsal and ventral hippocampus primarily function in cognition and emotion, respectively; inhibition of protein synthesis in the dorsal hippocampus decreases fear memory consolidation (53). The dorsal hippocampus lesion also impairs spatial memory (54). Optogenetics experiments suggested that activity in the ventral hippocampus is required for social memory recall in mice (55). In terms of emotional behaviors, in contrast, the dorsal and ventral parts are reported to oppositely regulate anxiety in rodents; muscimol infusion into the dorsal hippocampus provokes anxiety, while into the ventral part has anxiolytic effects (56). In addition, dorsal-ventral neural circuits in the hippocampus contribute to social memory (57). These reports suggest that the dorsal hippocampus is also involved in emotion, although the degree of contribution is likely to be less than in the ventral part. It is noteworthy that hippocampal volume is altered in ASD patients compared to typically developed individuals, although there is a discrepancy whether the volume increases or decreases (58, 59). Functional magnetic resonance imaging showed that neural connectivity in the anterior hippocampus (ventral part in rodents) was reduced in ASD patients (60). However, there are few reports on the anatomical and functional findings of the dorsal hippocampus in ASD patients.
In this study, 174 genes were identified as DEGs in the hippocampus of prenatally VPA-exposed rats. Among these, only 32 genes were also identified as DEGs in the amygdala or prefrontal cortex of prenatally VPA-exposed models (12, 14, 15), suggesting a distinct pattern of molecular changes between the brain regions in models such as ASD patients (47). According to the gene-disease analysis, prenatal VPA exposure affects a subset of genes in the hippocampus involved in both ASD- and learning disability-associated diseases (Figure 2A). Using transcriptome profiling, our study thus demonstrated that the hippocampus is a key region in terms of its contribution to ASD phenotypes, including learning disabilities.
We identified some key molecular changes induced by prenatal VPA exposure and upregulated by OXT. Secreted frizzled-related protein 1 is an endogenous inhibitor of Wnt signaling (61), which gets activated in both the brains of ASD patients and the hippocampus of prenatally VPA-exposed rats (62, 63). Interactive networks also revealed a relatively higher connection between Sfrp1 and Rarb (Figure 3E). Transcription of cluster of differentiation 38, which is critical for OXT release (64), is regulated by RARs (65). The severity of ASD has been reported to negatively correlate with serum levels of vitamin A (66). Notably, Lai et al. reported that maternal deficiency of vitamin A in rats induced ASD-like behaviors and decreased the expression levels of cluster of differentiation 38 and retinoic acid receptor β in the hypothalamus and OXT in the serum of the offspring, all of which were rescued by maternal supplementation of vitamin A (67). These reports suggest that the molecular pathways involved in Wnt signaling, including secreted frizzled-related protein and retinoic acid receptor β, play a critical role in ASD pathology and improve following chronic OXT treatment. Epidermal growth factor receptor and tropomyosin receptor kinase B were also predicted to interact with DEGs improved by OXT (Figure 3E and Table 1), and these genes are reported to be involved in social behaviors, learning, and hippocampal LTP (68–70). Oxytocinergic signaling activates epidermal growth factor receptor to promote LTP maintenance (71). Both Ntrk deletion, specifically in oxytocinergic neurons and Bdnf deletion, impair maternal behaviors against offspring in mice (72). These reports support that OXT is involved in the molecular pathways underlying not only social behaviors but also hippocampus-dependent learning and memory. To the best of our knowledge, this is the first report to evaluate effects of OXT on molecular alterations in the dorsal hippocampus of an animal model of ASD. However, a limitation of this study is that it did not evaluate the effects of OXT on molecular alterations in the amygdala and prefrontal cortex, regions related to emotion and well-investigated in ASD. As mentioned above, it is likely that the pattern of molecular alterations is distinct between brain regions in ASD. In order to further confirm the efficacy of OXT for ASD, the effects on molecular alterations in these regions should be investigated in the future.
In this study, chronic administration of OXT was conducted at adolescence just after weaning in order to avoid the risk of parental abandonment. Interestingly, maternal administration of OXT is implicated to suppress postnatal pathogenesis in animal models of ASD through enhancing excitatory/inhibitory switching of γ-aminobutyric acid during development (73). This suggests the possibility of maternal administration of OXT may reduce the risk of postnatal development of ASD, even when epilepsy patients are medicated with anticonvulsants including VPA during pregnancy. Further study is needed in the future to investigate the possibility of maternal OXT medication for restoration of postnatal ASD development and molecular alterations.
In summary, the present study demonstrated that prenatal VPA exposure affects the molecular pathways involved in ASD-like phenotypes in the hippocampus. Chronic administration of intranasal OXT partly ameliorated these alterations, which would underlie the improvement of social and learning disabilities seen in the prenatally VPA-exposed ASD model.
Data Availability Statement
The datasets presented in this study can be found in online repositories. The names of the repository/repositories and accession number(s) can be found below: https://www.ncbi.nlm.nih.gov/geo/query/acc.cgi?acc=GSE196500.
Ethics Statement
The animal study was reviewed and approved by the Committee on Animal Experiments at Tohoku University.
Author Contributions
KM and YS performed the experiments. NA and YN verified the methodology and provided the materials and apparatus. KM and KF wrote the manuscript. KF designed the study. All authors contributed to the article and approved the submitted version.
Funding
This work was partly supported by grants-in-aid for Scientific Research from the Ministry of Education, Science, Sports, and Culture of Japan (Kakenhi 19H03406 to KF), and grants-in-aid for Scientific Research from the Ministry of Education, Science, Sports and Culture of Japan (18J20651 to KM).
Conflict of Interest
The authors declare that the research was conducted in the absence of any commercial or financial relationships that could be construed as a potential conflict of interest.
Publisher’s Note
All claims expressed in this article are solely those of the authors and do not necessarily represent those of their affiliated organizations, or those of the publisher, the editors and the reviewers. Any product that may be evaluated in this article, or claim that may be made by its manufacturer, is not guaranteed or endorsed by the publisher.
Acknowledgments
This work was partly performed in the Cooperative Research Project Program of the Medical Institute of Bioregulation, Kyushu University. We thank E. Koba and M. Oda (Laboratory for Technical Supports Medical Institute of Bioregulation, Kyushu University) for performing the microarray analysis.
Abbreviations
ASD, autism spectrum disorder; DEG, differentially expressed gene; GO, gene ontology; HDAC, histone deacetylase; LTP, long-term potentiation; MCODE, molecular complex detection; OXT, oxytocin; SHANK, SH3 and multiple ankyrin repeat domains; VPA, valproic acid.
Footnotes
References
1. American Psychiatric Association [APA].Diagnostic and Statistical Manual of Mental Disorders. 5th ed. (2013). doi: 10.1176/appi.books.9780890425596.744053
2. Chaste P, Leboyer M. Autism risk factors: genes, environment, and gene-environment interactions. Dialogues Clin Neurosci. (2012) 14:281–92. doi: 10.31887/dcns.2012.14.3/pchaste
3. Gimpl G, Fahrenholz F. The oxytocin receptor system: structure, function, and regulation. Physiol Rev. (2001) 81:629–83. doi: 10.1152/physrev.2001.81.2.629
4. Nishimori K, Young LJ, Guo Q, Wang Z, Insel TR, Matzuk MM. Oxytocin is required for nursing but is not essential for parturition or reproductive behavior. Proc Natl Acad Sci U S A. (1996) 93:11699–704.
5. Takayanagi Y, Yoshida M, Bielsky IF, Ross HE, Kawamata M, Onaka T, et al. Pervasive social deficits, but normal parturition, in oxytocin receptor-deficient mice. Proc Natl Acad Sci U S A. (2005) 102:16096–101. doi: 10.1073/pnas.0505312102
6. Modahl C, Green LA, Fein D, Morris M, Waterhouse L, Feinstein C, et al. Plasma oxytocin levels in autistic children. Biol Psychiatry. (1998) 43:270–7. doi: 10.1016/S0006-3223(97)00439-3
7. Green LA, Fein D, Modahl C, Feinstein C, Waterhouse L, Morris M. Oxytocin and autistic disorder: alterations in peptide forms. Biol Psychiatry. (2001) 50:609–13. doi: 10.1016/S0006-3223(01)01139-8
8. Guastella AJ, Einfeld SL, Gray KM, Rinehart NJ, Tonge BJ, Lambert TJ, et al. Intranasal oxytocin improves emotion recognition for youth with autism spectrum disorders. Biol Psychiatry. (2010) 67:692–4. doi: 10.1016/j.biopsych.2009.09.020
9. Watanabe T, Abe O, Kuwabara H, Yahata N, Takano Y, Iwashiro N, et al. Mitigation of sociocommunicational deficits of autism through oxytocin-induced recovery of medial prefrontal activity a randomized trial. JAMA Psychiatry. (2014) 71:166–75. doi: 10.1001/jamapsychiatry.2013.3181
10. DiLiberti JH, Farndon PA, Dennis NR, Curry CJR. The fetal valproate syndrome. Am J Med Genet. (1984) 19:473–81. doi: 10.1002/ajmg.1320190308
11. Christensen J, Grønborg TK, Sørensen MJ, Schendel D, Parner ET, Pedersen LH, et al. Prenatal valproate exposure and risk of autism spectrum disorders and childhood autism. JAMA. (2013) 309:1696–703. doi: 10.1001/jama.2013.2270
12. Barrett CE, Hennessey TM, Gordon KM, Ryan SJ, McNair ML, Ressler KJ, et al. Developmental disruption of amygdala transcriptome and socioemotional behavior in rats exposed to valproic acid prenatally. Mol Autism. (2017) 8:42. doi: 10.1186/s13229-017-0160-x
13. Foley AG, Gannon S, Rombach-Mullan N, Prendergast A, Barry C, Cassidy AW, et al. Class I histone deacetylase inhibition ameliorates social cognition and cell adhesion molecule plasticity deficits in a rodent model of autism spectrum disorder. Neuropharmacology. (2012) 63:750–60. doi: 10.1016/j.neuropharm.2012.05.042
14. Oguchi-Katayama A, Monma A, Sekino Y, Moriguchi T, Sato K. Comparative gene expression analysis of the amygdala in autistic rat models produced by pre- and post-natal exposures to valproic acid. J Toxicol Sci. (2013) 38:391–402. doi: 10.2131/jts.38.391
15. Zhao H, Wang Q, Yan T, Zhang Y, Xu H, Yu H, et al. Maternal valproic acid exposure leads to neurogenesis defects and autism-like behaviors in non-human primates. Transl Psychiatry. (2019) 9:267. doi: 10.1038/s41398-019-0608-1
16. Ji L, Chauhan V, Flory MJ, Chauhan A. Brain region-specific decrease in the activity and expression of protein kinase A in the frontal cortex of regressive autism. PLoS One. (2011) 6:e23751. doi: 10.1371/JOURNAL.PONE.0023751
17. Akshoomoff N, Mattson SN, Grossfeld PD. Evidence for autism spectrum disorder in Jacobsen syndrome: identification of a candidate gene in distal 11q. Genet Med. (2015) 17:143–8. doi: 10.1038/GIM.2014.86
18. Nakamura T, Arima-Yoshida F, Sakaue F, Nasu-Nishimura Y, Takeda Y, Matsuura K, et al. PX-RICS-deficient mice mimic autism spectrum disorder in Jacobsen syndrome through impaired GABAA receptor trafficking. Nat Commun. (2016) 7:10861. doi: 10.1038/NCOMMS10861
19. Gilbert J, Man HY. Fundamental elements in autism: from neurogenesis and neurite growth to synaptic plasticity. Front Cell Neurosci. (2017) 11:359. doi: 10.3389/FNCEL.2017.00359
20. Reichova A, Zatkova M, Bacova Z, Bakos J. Abnormalities in interactions of Rho GTPases with scaffolding proteins contribute to neurodevelopmental disorders. J Neurosci Res. (2018) 96:781–8. doi: 10.1002/JNR.24200
21. Bakos J, Bacova Z, Grant SG, Castejon AM, Ostatnikova D. Are molecules involved in neuritogenesis and axon guidance related to autism pathogenesis? Neuromolecular Med. (2015) 17:297–304. doi: 10.1007/S12017-015-8357-7
22. Hara Y, Ago Y, Takano E, Hasebe S, Nakazawa T, Hashimoto H, et al. Prenatal exposure to valproic acid increases miR-132 levels in the mouse embryonic brain. Mol Autism. (2017) 8:33. doi: 10.1186/S13229-017-0149-5
23. Park E, Na M, Choi J, Kim S, Lee JR, Yoon J, et al. The Shank family of postsynaptic density proteins interacts with and promotes synaptic accumulation of the beta PIX guanine nucleotide exchange factor for Rac1 and Cdc42. J Biol Chem. (2003) 278:19220–9. doi: 10.1074/JBC.M301052200
24. Sala C, Vicidomini C, Bigi I, Mossa A, Verpelli C. Shank synaptic scaffold proteins: keys to understanding the pathogenesis of autism and other synaptic disorders. J Neurochem. (2015) 135:849–58. doi: 10.1111/JNC.13232
25. Reichova A, Bacova Z, Bukatova S, Kokavcova M, Meliskova V, Frimmel K, et al. Abnormal neuronal morphology and altered synaptic proteins are restored by oxytocin in autism-related SHANK3 deficient model. Mol Cell Endocrinol. (2020) 518:110924. doi: 10.1016/J.MCE.2020.110924
26. Tian Y, Yabuki Y, Moriguchi S, Fukunaga K, Mao PJ, Hong LJ, et al. Melatonin reverses the decreases in hippocampal protein serine/threonine kinases observed in an animal model of autism. J Pineal Res. (2014) 56:1–11. doi: 10.1111/jpi.12081
27. Matsuo K, Yabuki Y, Fukunaga K. 5-aminolevulinic acid inhibits oxidative stress and ameliorates autistic-like behaviors in prenatal valproic acid-exposed rats. Neuropharmacology. (2020) 168:107975. doi: 10.1016/j.neuropharm.2020.107975
28. Lee SY, Park SH, Chung C, Kim JJ, Choi SY, Han JS. Oxytocin protects hippocampal memory and plasticity from uncontrollable stress. Sci Rep. (2015) 5:18540. doi: 10.1038/srep18540
29. Park SH, Kim YJ, Park JC, Han JS, Choi SY. Intranasal oxytocin following uncontrollable stress blocks impairments in hippocampal plasticity and recognition memory in stressed rats. Int J Neuropsychopharmacol. (2017) 20:861–6. doi: 10.1093/ijnp/pyx061
30. Lefter R, Ciobica A, Antioch I, Ababei DC, Hritcu L, Luca AC. Oxytocin differentiated effects according to the administration route in a prenatal valproic acid-induced rat model of autism. Medicina (Kaunas). (2020) 56:267. doi: 10.3390/medicina56060267
31. Hara Y, Ago Y, Higuchi M, Hasebe S, Nakazawa T, Hashimoto H, et al. Oxytocin attenuates deficits in social interaction but not recognition memory in a prenatal valproic acid-induced mouse model of autism. Horm Behav. (2017) 96:130–6. doi: 10.1016/j.yhbeh.2017.09.013
32. Stevenson EL, Caldwell HK. Lesions to the CA2 region of the hippocampus impair social memory in mice. Eur J Neurosci. (2014) 40:3294–301. doi: 10.1111/ejn.12689
33. Zinn CG, Clairis N, Cavalcante LES, Furini CRG, De Carvalho Myskiw J, Izquierdo I. Major neurotransmitter systems in dorsal hippocampus and basolateral amygdala control social recognition memory. Proc Natl Acad Sci U S A. (2016) 113:E4914–9. doi: 10.1073/pnas.1609883113
34. Nicolini C, Fahnestock M. The valproic acid-induced rodent model of autism. Exp Neurol. (2018) 299:217–27. doi: 10.1016/j.expneurol.2017.04.017
35. Gigliucci V, Leonzino M, Busnelli M, Luchetti A, Palladino VS, D’Amato FR, et al. Region specific up-regulation of oxytocin receptors in the opioid oprm1 (-/-) mouse model of autism. Front Pediatr. (2014) 2:91. doi: 10.3389/FPED.2014.00091
36. Bales KL, Perkeybile AM, Conley OG, Lee MH, Guoynes CD, Downing GM, et al. Chronic intranasal oxytocin causes long-term impairments in partner preference formation in male prairie voles. Biol Psychiatry. (2013) 74:180–8. doi: 10.1016/J.BIOPSYCH.2012.08.025
37. Huang H, Michetti C, Busnelli M, Managò F, Sannino S, Scheggia D, et al. Chronic and acute intranasal oxytocin produce divergent social effects in mice. Neuropsychopharmacology. (2014) 39:1102–14. doi: 10.1038/NPP.2013.310
38. Ritchie ME, Phipson B, Wu D, Hu Y, Law CW, Shi W, et al. Limma powers differential expression analyses for RNA-sequencing and microarray studies. Nucleic Acids Res. (2015) 43:e47. doi: 10.1093/nar/gkv007
39. Zhou Y, Zhou B, Pache L, Chang M, Khodabakhshi AH, Tanaseichuk O, et al. Metascape provides a biologist-oriented resource for the analysis of systems-level datasets. Nat Commun. (2019) 10:1523. doi: 10.1038/s41467-019-09234-6
40. Shannon P, Markiel A, Ozier O, Baliga NS, Wang JT, Ramage D, et al. Cytoscape: a software environment for integrated models of biomolecular interaction networks. Genome Res. (2003) 13:2498–504. doi: 10.1101/gr.1239303
41. Stark C, Breitkreutz BJ, Reguly T, Boucher L, Breitkreutz A, Tyers M. BioGRID: a general repository for interaction datasets. Nucleic Acids Res. (2006) 34:D535–9. doi: 10.1093/nar/gkj109
42. Li T, Wernersson R, Hansen RB, Horn H, Mercer J, Slodkowicz G, et al. A scored human protein-protein interaction network to catalyze genomic interpretation. Nat Methods. (2016) 14:61–4. doi: 10.1038/nmeth.4083
43. Türei D, Korcsmáros T, Saez-Rodriguez J. OmniPath: guidelines and gateway for literature-curated signaling pathway resources. Nat Methods. (2016) 13:966–7. doi: 10.1038/nmeth.4077
44. Bader GD, Hogue CWV. An automated method for finding molecular complexes in large protein interaction networks. BMC Bioinformatics. (2003) 4:2. doi: 10.1186/1471-2105-4-2
45. Piñero J, Bravo Á, Queralt-Rosinach N, Gutiérrez-Sacristán A, Deu-Pons J, Centeno E, et al. DisGeNET: a comprehensive platform integrating information on human disease-associated genes and variants. Nucleic Acids Res. (2017) 45:D833–9. doi: 10.1093/nar/gkw943
46. Han H, Shim H, Shin D, Shim JE, Ko Y, Shin J, et al. TRRUST: a reference database of human transcriptional regulatory interactions. Sci Rep. (2015) 5:11432. doi: 10.1038/srep11432
47. Krishnan A, Zhang R, Yao V, Theesfeld CL, Wong AK, Tadych A, et al. Genome-wide prediction and functional characterization of the genetic basis of autism spectrum disorder. Nat Neurosci. (2016) 19:1454–62. doi: 10.1038/nn.4353
48. Phiel CJ, Zhang F, Huang EY, Guenther MG, Lazar MA, Klein PS. Histone deacetylase is a direct target of valproic acid, a potent anticonvulsant, mood stabilizer, and teratogen. J Biol Chem. (2001) 276:36734–41. doi: 10.1074/jbc.M101287200
49. Arinze IJ, Kawai Y. Sp family of transcription factors is involved in valproic acid-induced expression of Gαi2. J Biol Chem. (2003) 278:17785–91. doi: 10.1074/jbc.M209430200
50. Baron-Cohen S, Scahill VL, Izaguirre J, Hornsey H, Robertson MM. The prevalence of Gilles de la Tourette syndrome in children and adolescents with autism: a large scale study. Psychol Med. (1999) 29:1151–9. doi: 10.1017/S003329179900896X
51. Radatz M, Ehlers K, Yagen B, Bialer M, Nau H. Valnoctamide, valpromide and valnoctic acid are much less teratogenic in mice than valproic acid. Epilepsy Res. (1998) 30:41–8. doi: 10.1016/S0920-1211(97)00095-8
52. Gurvich N, Berman MG, Wittner BS, Gentleman RC, Klein PS, Green JBA. Association of valproate-induced teratogenesis with histone deacetylase inhibition in vivo. FASEB J. (2005) 19:1166–8. doi: 10.1096/fj.04-3425fje
53. Barrientos RM, Higgins EA, Sprunger DB, Watkins LR, Rudy JW, Maier SF. Memory for context is impaired by injecting anisomycin into dorsal hippocampus following context exploration. Behav Brain Res. (2002) 134:299–306. doi: 10.1016/S0166-4328(02)00045-1
54. Moser MB, Moser EI, Forrest E, Andersen P, Morris RGM. Spatial learning with a minislab in the dorsal hippocampus. Proc Natl Acad Sci U S A. (1995) 92:9697–701. doi: 10.1073/PNAS.92.21.9697
55. Okuyama T, Kitamura T, Roy DS, Itohara S, Tonegawa S. Ventral CA1 neurons store social memory. Science. (2016) 353:1536–41. doi: 10.1126/SCIENCE.AAF7003
56. Zhang WN, Bast T, Xu Y, Feldon J. Temporary inhibition of dorsal or ventral hippocampus by muscimol: distinct effects on measures of innate anxiety on the elevated plus maze, but similar disruption of contextual fear conditioning. Behav Brain Res. (2014) 262:47–56. doi: 10.1016/J.BBR.2013.10.044
57. Meira T, Leroy F, Buss EW, Oliva A, Park J, Siegelbaum SA. A hippocampal circuit linking dorsal CA2 to ventral CA1 critical for social memory dynamics. Nat Commun. (2018) 9:4163. doi: 10.1038/S41467-018-06501-W
58. Aylward EH, Minshew NJ, Goldstein G, Honeycutt NA, Augustine AM, Yates KO, et al. MRI volumes of amygdala and hippocampus in non-mentally retarded autistic adolescents and adults. Neurology. (1999) 53:2145–50. doi: 10.1212/WNL.53.9.2145
59. Schumann CM, Hamstra J, Goodlin-Jones BL, Lotspeich LJ, Kwon H, Buonocore MH, et al. The amygdala is enlarged in children but not adolescents with autism; the hippocampus is enlarged at all ages. J Neurosci. (2004) 24:6392–401. doi: 10.1523/JNEUROSCI.1297-04.2004
60. Gotts SJ, Simmons WK, Milbury LA, Wallace GL, Cox RW, Martin A. Fractionation of social brain circuits in autism spectrum disorders. Brain. (2012) 135:2711–25. doi: 10.1093/BRAIN/AWS160
61. Üren A, Reichsman F, Anest V, Taylor WG, Muraiso K, Bottaro DP, et al. Secreted frizzled-related protein-1 binds directly to wingless and is a biphasic modulator of Wnt signaling. J Biol Chem. (2000) 275:4374–82. doi: 10.1074/jbc.275.6.4374
62. Chow ML, Pramparo T, Winn ME, Barnes CC, Li HR, Weiss L, et al. Age-dependent brain gene expression and copy number anomalies in autism suggest distinct pathological processes at young versus mature ages. PLoS Genet. (2012) 8:e1002592. doi: 10.1371/journal.pgen.1002592
63. Qin L, Dai X, Yin Y. Valproic acid exposure sequentially activates Wnt and mTOR pathways in rats. Mol Cell Neurosci. (2016) 75:27–35. doi: 10.1016/j.mcn.2016.06.004
64. Jin D, Liu HX, Hirai H, Torashima T, Nagai T, Lopatina O, et al. CD38 is critical for social behaviour by regulating oxytocin secretion. Nature. (2007) 446:41–5. doi: 10.1038/nature05526
65. Mehta K, McQueen T, Manshouri T, Andreeff M, Collins S, Albitar M. Involvement of retinoic acid receptor-α-mediated signaling pathway in induction of CD38 cell-surface antigen. Blood. (1997) 89:3607–14. doi: 10.1182/blood.v89.10.3607
66. Liu X, Liu J, Xiong X, Yang T, Hou N, Liang X, et al. Correlation between nutrition and symptoms: nutritional survey of children with autism spectrum disorder in Chongqing, China. Nutrients. (2016) 8:294. doi: 10.3390/nu8050294
67. Lai X, Wu X, Hou N, Liu S, Li Q, Yang T, et al. Vitamin A deficiency induces autistic-like behaviors in rats by regulating the RARβ-CD38-Oxytocin axis in the hypothalamus. Mol Nutr Food Res. (2018) 62:1700754. doi: 10.1002/mnfr.201700754
68. Tang Y, Ye M, Du Y, Qiu X, Lv X, Yang W, et al. EGFR signaling upregulates surface expression of the GluN2B-containing NMDA receptor and contributes to long-term potentiation in the hippocampus. Neuroscience. (2015) 304:109–21. doi: 10.1016/j.neuroscience.2015.07.021
69. Minichiello L, Korte M, Wolfer D, Kühn R, Unsicker K, Cestari V, et al. Essential role for TrkB receptors in hippocampus-mediated learning. Neuron. (1999) 24:401–14. doi: 10.1016/S0896-6273(00)80853-3
70. Scattoni ML, Martire A, Cartocci G, Ferrante A, Ricceri L. Reduced social interaction, behavioural flexibility and BDNF signalling in the BTBR T+tf/J strain, a mouse model of autism. Behav Brain Res. (2013) 251:35–40. doi: 10.1016/j.bbr.2012.12.028
71. Lin YT, Huang CC, Hsu K. Oxytocin promotes long-term potentiation by enhancing epidermal growth factor receptor-mediated local translation of protein kinase Mζ. J Neurosci. (2012) 32:15476–88. doi: 10.1523/JNEUROSCI.2429-12.2012
72. Maynard KR, Hobbs JW, Phan BN, Gupta A, Rajpurohit S, Williams C, et al. BDNF-TrkB signaling in oxytocin neurons contributes to maternal behavior. Elife. (2018) 7:e33676. doi: 10.7554/eLife.33676
73. Tyzio R, Nardou R, Ferrari DC, Tsintsadze T, Shahrokhi A, Eftekhari S, et al. Oxytocin-mediated GABA inhibition during delivery attenuates autism pathogenesis in rodent offspring. Science. (2014) 343:675–9. doi: 10.1126/SCIENCE.1247190
Keywords: autism spectrum disorders, hippocampus, oxytocin, transcriptome analysis, valproic acid
Citation: Matsuo K, Shinoda Y, Abolhassani N, Nakabeppu Y and Fukunaga K (2022) Transcriptome Analysis in Hippocampus of Rats Prenatally Exposed to Valproic Acid and Effects of Intranasal Treatment of Oxytocin. Front. Psychiatry 13:859198. doi: 10.3389/fpsyt.2022.859198
Received: 21 January 2022; Accepted: 04 March 2022;
Published: 30 March 2022.
Edited by:
Juehua Yu, The First Affiliated Hospital of Kunming Medical University, ChinaReviewed by:
Jan Bakos, Biomedical Research Center, Slovak Academy of Sciences, SlovakiaChan Young Shin, Konkuk University, South Korea
Copyright © 2022 Matsuo, Shinoda, Abolhassani, Nakabeppu and Fukunaga. This is an open-access article distributed under the terms of the Creative Commons Attribution License (CC BY). The use, distribution or reproduction in other forums is permitted, provided the original author(s) and the copyright owner(s) are credited and that the original publication in this journal is cited, in accordance with accepted academic practice. No use, distribution or reproduction is permitted which does not comply with these terms.
*Correspondence: Kohji Fukunaga, a2Z1a3VuYWdhQHRvaG9rdS5hYy5qcA==