- 1VA RR&D Center for Neurorestoration and Neurotechnology, VA Providence Healthcare System, Providence, RI, United States
- 2Department of Psychiatry and Human Behavior, Alpert Medical School of Brown University, Providence, RI, United States
- 3COBRE Center for Neuromodulation, Butler Hospital, Providence, RI, United States
This article describes an emerging non-invasive neuromodulatory technology, called low intensity focused ultrasound (LIFU). This technology is potentially paradigm shifting as it can deliver non-invasive and reversible deep brain neuromodulation through acoustic sonication, at millimeter precision. Low intensity focused ultrasound's spatial precision, yet non-invasive nature sets it apart from current technologies, such as transcranial magnetic or electrical stimulation and deep brain stimulation. Additionally, its reversible effects allow for the causal study of deep brain regions implicated in psychiatric illness. Studies to date have demonstrated that LIFU can safely modulate human brain activity at cortical and subcortical levels. Due to its novelty, most researchers and clinicians are not aware of the potential applications and promise of this technique, underscoring the need for foundational papers to introduce the community to LIFU. This mini-review and synthesis of recent advances examines several key papers on LIFU administered to humans, describes the population under study, parameters used, and relevant findings that may guide future research. We conclude with a concise overview of some of the more pressing questions to date, considerations when interpreting new data from an emerging field, and highlight the opportunities and challenges in this exciting new area of study.
Introduction
Non-invasive brain stimulation is a rapidly growing area of psychiatric research and clinical practice. As our understanding of the neurocircuitry underlying psychiatric disorders has grown, so too has the development of technologies to modulate disease-relevant brain targets. Though promising, most non-invasive stimulation approaches lack the spatial precision of other invasive techniques, limiting their therapeutic utility.
The most widely used non-invasive brain stimulation modalities are transcranial magnetic simulation (TMS) and transcranial electrical stimulation. Transcranial magnetic simulation has garnered considerable attention following its success in treating pharmacoresistant depression (1, 2) and obsessive compulsive disorder (3), and evidence in treating other psychiatric illnesses (4–7). Transcranial magnetic simulation uses alternating magnetic fields to induce electrical current in the brain. Traditional TMS designs suffer from diffuse induced electric fields that decay exponentially as a function of cortical depth (8). The large extent of TMS-induced neuronal activation limits this technique's ability to directly target and engage deeper brain regions and circuits involved in psychopathology, leading to a reliance on indirect polysynaptic “downstream” modulation from cortical targeting. These limitations have motivated the development of “deep” TMS coils, such as the Hesed (H) and double cone coils, to target deeper brain targets. These two coils have demonstrated efficacy in the treatment of depression (9). While they can directly stimulate targets at depths of up to 4 cm, they still have reduced spatial focality compared to figure-of-eight TMS coils (8, 10). Further, the intensity required to depolarize deeper targets (>4 cm) exceeds the upper limit in current rTMS safety guidelines (i.e., risk of seizure) (10).
Transcranial electrical stimulation also has promise in psychiatric research and treatment (11–13). This technique delivers electrical current between scalp electrodes to produce weak electrical fields in the brain. Several variations of this method exist (e.g., direct currents, alternating currents, or random noise), but all result in a diffuse electric field that is difficult to restrict to a specific brain target (14, 15), thus limiting precise effects. Therefore, both transcranial magnetic and electrical stimulation indirectly target and engage regions involved in psychiatric illnesses, yet lack the spatial precision used in invasive approaches, such as deep brain stimulation.
Low intensity focused ultrasound (LIFU) holds great promise as a novel approach to brain stimulation (16). Unlike transcranial magnetic and electrical stimulation, LIFU can directly modulate activity within deep brain structures with high spatial precision (17), and the effects of even brief sonication may last several hours (18). Low intensity focused ultrasound applies acoustic energy to reversibly modulate neural activity (19). By yielding reversible effects with spatial focality, LIFU distinguishes itself from high intensity focused ultrasound, which is used to thermally ablate tissue in the treatment of Parkinson's and tremor [reviewed in Bachu et al. (20)], as well as transcranial ultrasound, which provides non-focal and reversible sonication. In this mini-review, we describe this emerging and promising new technology, highlighting key papers and other efforts that describe safety and potential efficacy in humans, as well as current limitations and future considerations.
Ultrasound Neuromodulation
Ultrasound is defined as a sound or acoustic wave higher than 20 kHz (i.e., above human hearing). In a typical neuromodulation experiment, a pulse generator emits an electrical waveform, which is amplified and transferred to a transducer housing a piezoelectric element. This amplified electrical signal excites the element, causing the active face of the transducer to oscillate and produce ultrasound waves. The transducer is affixed to the head, often using a head strap, and with important technical developments (21) can be usable within a magnetic resonance imaging (MRI) scanner, to allow for MR-guided targeting. For example, as illustrated in Figure 1, this approach is accomplished in the scanner itself. Applying the transducer inside the bore provides several important and novel forms of data. First, using built-in fiducials, targeting occurs in real-time using a structural MR scan, with no interpolation of device elements or brain position. Furthermore, in-scanner application of LIFU allows causal estimation of observed deep-brain effects. Lastly, it also facilitates real-time safety assessments of brain structure and function.
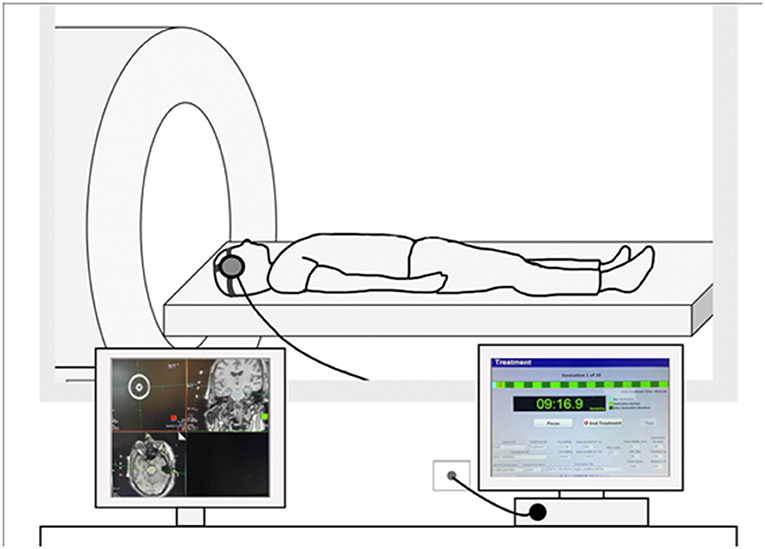
Figure 1. Example of an MRI-guided LIFU setup. Transducer element is affixed to the head using a head strap and connected to a console. (Left) Example of MRI-guided targeting on a structural MRI scan. Transducers include built-in fiducials for targeting via the Siemens MRI interface. (Right) Example image of the sonication console where light green represents active sonication and dark green represents off-line time.
Mechanism of Ultrasound Neuromodulation
Like many other areas of brain stimulation, and more so because of its novelty, the exact mechanism underlying LIFU neuromodulation remains, yet, unknown (19, 22, 23). Research has demonstrated that ultrasound can interact with tissue to induce mechanical and thermal effects. One hypothesis posits that the low amount of acoustic radiation force alters permeability of mechanosensitive ion channels and voltage-gated calcium, sodium, and potassium channels in neuronal membranes (24). Another hypothesis postulates that vibration of extra- and intracellular environments produces mechanical changes in the plasma membrane tension or the lipid bilayer and modulates neuronal activities. Another possible mechanism centers on thermal effects. While an increase in tissue temperature could affect neuronal activity (and is the mechanism of action in irreversible ablative high intensity focused ultrasound), the temperature increase due to LIFU is often <0.1°C (22, 25) and effects are likely negligible (26).
Sonication Parameters
Each transducer is composed of a piezoelectric element(s) that transforms electrical signal into ultrasound waves; the construction of this element also provides focality with a specific associated focal length (measured in mm) that defines a three-dimensional zone of maximum applied ultrasound, called “sonication.” A sonication protocol is defined by five parameters: (1) fundamental frequency, (2) pulse repetition frequency (PRF), (3) duty cycle, (4) sonication duration, and (5) intensity (23). The fundamental frequency refers to the number of oscillations over time and is inversely proportional to wavelength (and often referred to as a carrier wave). The PRF represents the rate at which acoustic pulses are delivered, and duty cycle is the proportion of each pulse filled with cycles of ultrasound at the fundamental frequency (or the ratio of “on time” to total time). The duration is quantified as the length of sonication, i.e., the total time from the onset of the first pulse to the termination of the final pulse. The spatial-peak temporal average (ISPTA) measures the average intensity during an entire sonication and the spatial-peak pulse average (ISPPA) measures the average intensity over a single pulse. The current US Food and Drug Administration safety guidelines for diagnostic ultrasound imaging devices suggest a maximum derated ISPTA of 720 mW/cm2 and a maximum derated ISPPA of 190 W/cm2 (27) to avoid heating and thermal damage. Derating (0.3 dB/cm·MHz; often indicated as a subscript, e.g., ISPTA.3) seeks to account for attenuation that occurs as the LIFU passes through tissue.
Ultrasound Neuromodulation in Humans
In recent years, researchers have begun to use LIFU in humans, studying how different parameters can induce reversible physiological effects on the nervous system. Several foundational studies examined the effect of LIFU on neural activity using electroencephalography (EEG). The first study demonstrated that LIFU focally applied to the somatosensory cortex (Table 1) attenuated amplitudes of somatosensory-evoked potentials (SEPs) and enhanced sensory discrimination (28). The neuromodulatory effects of LIFU on SEPs were further investigated by leveraging its ability to target deep structures such as the thalamus. Sonication of the ventro-posterior lateral nucleus of the thalamus (Table 1) attenuated amplitudes of SEPs, and worsened the ability to perform difficult tactile threshold judgments (29).
In addition to SEP modulation, recent studies have examined LIFU on the motor cortex. Using a simultaneous ultrasound and TMS paradigm, LIFU to the motor cortex (Table 1) reduced the amplitude of TMS-evoked motor evoked potentials and attenuated intracortical facilitation (31). Conversely, a study using concurrent LIFU-EEG (Table 1), found evidence that LIFU enhanced the movement-related cortical potential (33). A novel theta burst patterned LIFU protocol was also recently introduced that produced a consistent increase in motor cortical excitability (35).
Badran et al. demonstrated that LIFU may be able to modulate pain sensitivity via thalamic sonication (32). Researchers administered MRI-guided LIFU targeting the right anterior thalamus in 19 healthy volunteers (Table 1) and observed that two 10-min sessions produced an antinociceptive effect on pain thresholds compared to sham (32). A first-in-human repetitive, pulsed LIFU platform targeting the hippocampus has also recently been developed and shown to be safe (36).
Presently there are very few reports of LIFU in patients with neurological or psychiatric disorders (Table 1). The first study of clinical application of LIFU was part of a first-in-human case report testing the feasibility, safety, and initial efficacy of thalamic LIFU in a 25-year-old patient with a severe traumatic brain injury and disorder of consciousness (30). Using MRI-guided LIFU, they observed improved alertness, language comprehension, response to commands, and reliable communication (30) following thalamic sonication. In a subsequent unblinded study, three patients with chronic disorder of consciousness received two sessions of MRI-guided LIFU to the left thalamus. Two of the three patients had improved behavioral responsiveness, and one regressed after 3 months (37).
Recently, investigators have investigated whether ultrasound may modulate mood and worry. Several studies have used transcranial ultrasound, a less focal ultrasound technique, to modulate activity in regions associated with mood and anxiety symptoms. In one double-blind pilot study, researchers applied continuous ultrasound over the posterior frontal cortex in 31 healthy participants and observed significant mood improvements at 10- and 40-min post-sonication (38). Another study showed that modulating the right inferior frontal gyrus (rIFG) with transcranial ultrasound could induce positive mood effects (39). In this study, researchers sonicated rIFG in a sample of 51 healthy volunteers and observed that one 30 s exposure of 500 kHz rIFG ultrasound could induce positive mood effects lasting up to 30 min (39).
Similarly, in a double-blind pilot study, researchers investigated the effect of right fronto-temporal cortex non-focal transcranial ultrasound on mood and worry in depressed patients (40). In this study, researchers sonicated the right fronto-temporal cortex in 24 patients with mild to moderate depression as part of a five-session protocol. Ultrasound was administered continuously and without pulsation. Patients received 30 s of sonication for five sessions occurring within 7 days, with sonication sessions separated by at least 1 day. Worry and positive mood scores improved after active group vs. sham stimulation (40).
Taken together, the above work represents initial evidence of the feasibility of ultrasound neuromodulation in humans. Non-focal transcranial ultrasound methods point to this technique's ability to alter mood states and set the stage for more focal LIFU experiments. This work also demonstrates that LIFU can target both cortical and deeper brain structures with lasting neuromodulatory effects and high spatial precision.
Challenges and Limitations
Safety
Establishing that LIFU can be safely applied to the brain is paramount in enabling this technique to become a viable research and potential clinical tool. The above studies provide preliminary evidence of safety, as no serious adverse events or brain injury were described. Most of these human studies provide safety data in the form of neurological examinations and/or structural MRI before and after sonication. Comprehensive reviews also suggest a favorable human safety profile (19, 26, 41, 42). A recent report (41) described qualitative safety and side effect assessments in 65 participants who previously completed one or more LIFU experiments. No participant, including those undergoing repeated LIFU, reported a serious adverse event. Mild to moderate effects, including neck pain, attention difficulties, muscle twitches, anxiety, and sleepiness were reported by 11% of participants and perceived as “possibly” or “probably” LIFU-related. In contrast, little information has been generated about histological changes in human brain tissue after ultrasound at or exceeding FDA intensity guidelines. As of this writing there is a single example, using tissue from cadavers or resected during neurosurgery. Investigators sonicated tissue at varying intensities and observed no histological changes at intensities up to 11,800 mW/cm2 ISPTA.3 (43), which substantially exceeds FDA guidelines. The safety of LIFU is echoed in animal work. Rodent, large animal, and primate studies have experimentally used a range of ultrasound frequencies (220 kHz−1.9 MHz) to produce effective neuromodulation without neurologic injury [reviewed in Fomenko et al. (23)]. One study in sheep reported that exposure to prolonged sonication incurred cerebral microhemorrhages (44), though this has been the only reported study to date reporting any injury across species.
While prior reports provide evidence for human safety, the majority of studies were conducted in healthy populations. To our knowledge, only three studies have examined safety in clinical patients, and early findings suggest relatively safety in those with brain injury (30, 37) or depression (40). We are currently evaluating safety as part of a series of first-in-human studies (U01 MH123427) utilizing radiological, psychiatric, and neuropsychological assessments in clinical patient populations, as clinical populations often have medical comorbidities (e.g., hypertension, smoking, etc.) associated with more fragile vasculature susceptible to acoustic injury.
Dosing
Low intensity focused ultrasound dose–response curves for desired neurophysiological effects are not established. The parameter space is very large, as multiple parameters can be varied (e.g., fundamental frequency, pulse repetition frequency, duty cycle, sonication duration, and intensity) and the impact of altering parameters awaits systematic examination. Effects of repeated LIFU administration are also in need of evaluation. Furthermore, because LIFU administers low amounts of energy, it is likely that the brain state or context during sonication (e.g., rest vs. task) may affect the dose–response. The ideal way to combine sonication and manipulation of the neurocognitive state remains unclear (45) and may be of particular import in the application of low intensity modalities (46).
Targeting and Delivery
The effect of variable skull anatomy across individuals is another important issue in LIFU research. Skull density and composition, particularly presence of trabecular (porous) bone between the cortical bone inner and outer tables, affect ultrasound conduction, as this porous nature leads to scattering. There are clear examples of this when using high intensity focused ultrasound (47, 48), however, the impact of the skull remains an unknown quantity for LIFU. To date, LIFU has often been applied over the temporal bone (i.e., with its cortical bone composition) to mitigate skull effects (29, 30). One immediate concern is the ability to focally deliver ultrasound when there is substantial variability in skull transmission. To address this issue, modeling (e.g., k-wave) approaches are being developed to determine how to accommodate individual skull variability (19, 49).
Mechanisms of Action and Study Designs
A potential confound with respect to LIFU's ability to modulate deep brain structures is that it may activate intermediate regions like auditory cortex (50, 51). Several studies found that ultrasound-induced activities were eliminated or reduced upon auditory nerve transection or removal of cochlear fluids. These observations motivate careful attention to experimental designs rather than confounding effects.
Whether LIFU settings are more likely to excite, inhibit, or disrupt neural activity remains an important area of inquiry. To this effect, studies have tested whether PRF (52) or duty cycle (53) might determine the directionality of neuromodulation, with conflicting results. More in-depth studies on parameters and protocols are required to understand underlying mechanism(s) of LIFU neuromodulation.
Reflecting the very early nature of the field, there remains no quantifiable metric of LIFU-related physiological effects, with prior work in clinically relevant studies either relying upon behavioral output (30, 32) or indirect measures of perfusion (34). Given this limitation, future studies should include biological measures to provide insight into observed effects. Furthermore, challenges remain to ensure that investigators report parameters that can be easily compared to each other; this harmonization is currently an active area of effort with focused ultrasound experts across the globe (i.e., ITRUSST, https://itrusst.github.io/).
Conclusions
Low intensity focused ultrasound holds appeal for its unique combination of non-invasiveness, likely safety, precision, and broad range of possible targets. Recent studies provide initial evidence of the safety and feasibility. These advances set the stage for pioneering studies to test causal hypotheses in neuropsychiatric research, utilizing focal, and reversible suppression of core brain regions involved in psychiatric illnesses. A virtually infinite parameter space and brain targets remain to be studied, and further prospective studies are needed to determine dose–response effects on neural activity. Yet, for those parametric studies to be successful, research is urgently needed (and currently ongoing) on safety, particularly in patient populations, as well as inquiry into targeting, acoustic confounds, and mechanisms of action. While the field's understanding of neural mechanisms underlying LIFU remain at a very early stage of study, it appears that the clinically relevant LIFU studies are consistently suppressive. Of note, we explicitly do not use the word inhibitory, as this conveys a degree of mechanistic characterization that is not yet supported by the extant literature.
Caveats aside, this technology has tremendous potential for clinical applications. Focal, non-invasive, deep-brain stimulation is currently impossible with available technologies. If the promise of LIFU-induced neuromodulation is realized, it may become not only a powerful tool to test causal relationships between brain activity and function, and transform clinical therapeutics.
Author Contributions
AA wrote the original draft. All authors reviewed, edited, and approved the final version of the manuscript.
Funding
This work was supported in part by the National Institutes of Health grants U01MH123427 and P20 GM130452, and the US Department of Veterans Affairs RR&D Center for Neurorestoration and Neurotechnology (I50 RX002864) and a CSR&D Career Development Award (IK2 CX001824). The contents do not represent the views of the National Institutes of Health, U.S. Department of Veterans Affairs, or the United States Government.
Conflict of Interest
The authors declare that the research was conducted in the absence of any commercial or financial relationships that could be construed as a potential conflict of interest.
Publisher's Note
All claims expressed in this article are solely those of the authors and do not necessarily represent those of their affiliated organizations, or those of the publisher, the editors and the reviewers. Any product that may be evaluated in this article, or claim that may be made by its manufacturer, is not guaranteed or endorsed by the publisher.
Acknowledgments
We wish to thank McKenna Brennan for her assistance developing the figure.
Abbreviations
SEP, somatosensory evoked potential; MEP, motor evoked potential; PRF, pulse repetition frequency; DC, duty cycle; ISPPA, intensity spatial peak pulse average; ISPTA.3, derated intensity spatial peak temporal average; SD, sonication duration; np, number of pulses.
References
1. O'Reardon JP, Solvason HB, Janicak PG, Sampson S, Isenberg KE, Nahas Z, et al. Efficacy and safety of transcranial magnetic stimulation in the acute treatment of major depression: a multisite randomized controlled trial. Biol Psychiatry. (2007) 62:1208–16. doi: 10.1016/j.biopsych.2007.01.018
2. George MS, Lisanby SH, Avery D, McDonald WM, Durkalski V, Pavlicova M, et al. Daily left prefrontal transcranial magnetic stimulation therapy for major depressive disorder: a sham-controlled randomized trial. Arch Gen Psychiatry. (2010) 67:507–16. doi: 10.1001/archgenpsychiatry.2010.46
3. Carmi L, Tendler A, Bystritsky A, Hollander E, Blumberger DM, Daskalakis J, et al. Efficacy and safety of deep transcranial magnetic stimulation for obsessive-compulsive disorder: a prospective multicenter randomized double-blind placebo-controlled trial. Am J Psychiatry. (2019) 176:931–8. doi: 10.1176/appi.ajp.2019.18101180
4. Philip NS, Ridout SJ, Albright SE, Sanchez G, Carpenter LL. 5-Hz transcranial magnetic stimulation for comorbid posttraumatic stress disorder and major depression. J Trauma Stress. (2016) 29:93–6. doi: 10.1002/jts.22065
5. Kozel FA, Motes MA, Didehbani N, DeLaRosa B, Bass C, Schraufnagel CD, et al. Repetitive TMS to augment cognitive processing therapy in combat veterans of recent conflicts with PTSD: a randomized clinical trial. J Affect Disord. (2018) 229:506–14. doi: 10.1016/j.jad.2017.12.046
6. Koek RJ, Roach J, Athanasiou N, van 't Wout-Frank M, Philip NS. Neuromodulatory treatments for post-traumatic stress disorder, (PTSD). Prog Neuropsychopharmacol Biol Psychiatry. (2019) 92:148–60. doi: 10.1016/j.pnpbp.2019.01.004
7. Madore MR, Kozel FA, Williams LM, Green LC, George MS, Holtzheimer PE, et al. Prefrontal transcranial magnetic stimulation for depression in US military veterans - a naturalistic cohort study in the veterans health administration. J Affect Disord. (2021) 297:671–8. doi: 10.1016/j.jad.2021.10.025
8. Deng ZD, Lisanby SH, Peterchev AV. Electric field depth-focality tradeoff in transcranial magnetic stimulation: simulation comparison of 50 coil designs. Brain Stimul. (2013) 6:1–13. doi: 10.1016/j.brs.2012.02.005
9. Kaster TS, Daskalakis ZJ, Noda Y, Knyahnytska Y, Downar J, Rajji TK, et al. Efficacy, tolerability, and cognitive effects of deep transcranial magnetic stimulation for late-life depression: a prospective randomized controlled trial. Neuropsychopharmacology. (2018) 43:2231–8. doi: 10.1038/s41386-018-0121-x
10. Deng ZD, Lisanby SH, Peterchev AV. Coil design considerations for deep transcranial magnetic stimulation. Clin Neurophysiol. (2014) 125:1202–12. doi: 10.1016/j.clinph.2013.11.038
11. Nitsche MA, Boggio PS, Fregni F, Pascual-Leone A. Treatment of depression with transcranial direct current stimulation, (tDCS): a review. Exp Neurol. (2009) 219:14–19. doi: 10.1016/j.expneurol.2009.03.038
12. Kekic M, Boysen E, Campbell IC, Schmidt U. A systematic review of the clinical efficacy of transcranial direct current stimulation, (tDCS) in psychiatric disorders. J Psychiatr Res. (2016) 74:70–86. doi: 10.1016/j.jpsychires.2015.12.018
13. Palm U, Hasan A, Strube W, Padberg F. tDCS for the treatment of depression: a comprehensive review. Eur Arch Psychiatry Clin Neurosci. (2016) 266:681–94. doi: 10.1007/s00406-016-0674-9
14. Datta A, Bansal V, Diaz J, Patel J, Reato D, Bikson M. Gyri-precise head model of transcranial direct current stimulation: improved spatial focality using a ring electrode versus conventional rectangular pad. Brain Stimul. (2009) 2:201.e1–7.e1. doi: 10.1016/j.brs.2009.03.005
15. Reinhart RM, Woodman GF. Enhancing long-term memory with stimulation tunes visual attention in one trial. Proc Natl Acad Sci USA. (2015) 112:625–30. doi: 10.1073/pnas.1417259112
16. Bowary P, Greenberg BD. Noninvasive focused ultrasound for neuromodulation: a review. Psychiatr Clin North Am. (2018) 41:505–14. doi: 10.1016/j.psc.2018.04.010
17. Bystritsky A, Korb AS, Douglas PK, Cohen MS, Melega WP, Mulgaonkar AP, et al. A review of low-intensity focused ultrasound pulsation. Brain Stimul. (2011) 4:125–36. doi: 10.1016/j.brs.2011.03.007
18. Folloni D, Verhagen L, Mars RB, Fouragnan E, Constans C, Aubry JF, et al. Manipulation of subcortical and deep cortical activity in the primate brain using transcranial focused ultrasound stimulation. Neuron. (2019) 101:1109.e5–16.e5. doi: 10.1016/j.neuron.2019.01.019
19. Blackmore J, Shrivastava S, Sallet J, Butler CR, Cleveland RO. Ultrasound neuromodulation: a review of results, mechanisms and safety. Ultrasound Med Biol. (2019) 45:1509–36. doi: 10.1016/j.ultrasmedbio.2018.12.015
20. Bachu VS, Kedda J, Suk I, Green JJ, Tyler B. High-intensity focused ultrasound: a review of mechanisms and clinical applications. Ann Biomed Eng. (2021) 49:1975–91. doi: 10.1007/s10439-021-02833-9
21. Schafer ME, Spivak NM, Korb AS, Bystritsky A. Design, development, and operation of a low-intensity focused ultrasound pulsation, (LIFUP) system for clinical use. IEEE Trans Ultrason Ferroelectr Freq Control. (2021) 68:54–64. doi: 10.1109/TUFFC.2020.3006781
22. Baek H, Pahk KJ, Kim H. A review of low-intensity focused ultrasound for neuromodulation. Biomed Eng Lett. (2017) 7:135–42. doi: 10.1007/s13534-016-0007-y
23. Fomenko A, Neudorfer C, Dallapiazza RF, Kalia SK, Lozano AM. Low-intensity ultrasound neuromodulation: an overview of mechanisms and emerging human applications. Brain Stimul. (2018) 11:1209–17. doi: 10.1016/j.brs.2018.08.013
24. Tyler WJ. Noninvasive neuromodulation with ultrasound? A continuum mechanics hypothesis. Neuroscientist. (2011) 17:25–36. doi: 10.1177/1073858409348066
25. Yoo SS, Bystritsky A, Lee JH, Zhang Y, Fischer K, Min BK, et al. Focused ultrasound modulates region-specific brain activity. Neuroimage. (2011) 56:1267–75. doi: 10.1016/j.neuroimage.2011.02.058
26. Pasquinelli C, Hanson LG, Siebner HR, Lee HJ, Thielscher A. Safety of transcranial focused ultrasound stimulation: a systematic review of the state of knowledge from both human and animal studies. Brain Stimul. (2019) 12:1367–80. doi: 10.1016/j.brs.2019.07.024
27. FDA. Marketing Clearance of Diagnostic Ultrasound Systems and Transducers. Draft Guidance for Industry and Food and Drug Administration Staff (2017).
28. Legon W, Sato TF, Opitz A, Mueller J, Barbour A, Williams A, et al. Transcranial focused ultrasound modulates the activity of primary somatosensory cortex in humans. Nat Neurosci. (2014) 17:322–9. doi: 10.1038/nn.3620
29. Legon W, Ai L, Bansal P, Mueller JK. Neuromodulation with single-element transcranial focused ultrasound in human thalamus. Hum Brain Mapp. (2018) 39:1995–2006. doi: 10.1002/hbm.23981
30. Monti MM, Schnakers C, Korb AS, Bystritsky A, Vespa PM. Non-invasive ultrasonic thalamic stimulation in disorders of consciousness after severe brain injury: a first-in-man report 2016. Brain Stimul. (2016) 9:940–1. doi: 10.1016/j.brs.2016.07.008
31. Legon W, Bansal P, Tyshynsky R, Ai L, Mueller JK. Transcranial focused ultrasound neuromodulation of the human primary motor cortex. Sci Rep. (2018) 8:10007. doi: 10.1038/s41598-018-28320-1
32. Badran BW, Caulfield KA, Stomberg-Firestein S, Summers PM, Dowdle LT, Savoca M, et al. Sonication of the anterior thalamus with MRI-guided transcranial focused ultrasound, (tFUS) alters pain thresholds in healthy adults: a double-blind, sham-controlled study. Brain Stimul. (2020) 13:1805–12. doi: 10.1016/j.brs.2020.10.007
33. Yu K, Liu C, Niu X, He B. Transcranial focused ultrasound neuromodulation of voluntary movement-related cortical activity in humans. IEEE Trans Biomed Eng. (2021) 68:1923–31. doi: 10.1109/TBME.2020.3030892
34. Cain JA, Visagan S, Johnson MA, Crone J, Blades R, Spivak NM, et al. Real time and delayed effects of subcortical low intensity focused ultrasound. Sci Rep. (2021) 11:6100. doi: 10.1038/s41598-021-85504-y
35. Zeng K, Darmani G, Fomenko A, Xia X, Tran S, Nankoo JF, et al. Induction of human motor cortex plasticity by theta burst transcranial ultrasound stimulation. Ann Neurol. (2022) 91:238–52. doi: 10.1002/ana.26294
36. Brinker ST, Preiswerk F, White PJ, Mariano TY, McDannold NJ, Bubrick EJ. Focused ultrasound platform for investigating therapeutic neuromodulation across the human hippocampus. Ultrasound Med Biol. (2020) 46:1270–4. doi: 10.1016/j.ultrasmedbio.2020.01.007
37. Cain JA, Spivak NM, Coetzee JP, Crone JS, Johnson MA, Lutkenhoff ES, et al. Ultrasonic thalamic stimulation in chronic disorders of consciousness. Brain Stimul. (2021) 14:301–3. doi: 10.1016/j.brs.2021.01.008
38. Hameroff S, Trakas M, Duffield C, Annabi E, Gerace MB, Boyle P, et al. Transcranial ultrasound, (TUS) effects on mental states: a pilot study. Brain Stimul. (2013) 6:409–15. doi: 10.1016/j.brs.2012.05.002
39. Sanguinetti JL, Hameroff S, Smith EE, Sato T, Daft CMW, Tyler WJ, et al. Transcranial focused ultrasound to the right prefrontal cortex improves mood and alters functional connectivity in humans. Front Hum Neurosci. (2020) 14:52. doi: 10.3389/fnhum.2020.00052
40. Reznik SJ, Sanguinetti JL, Tyler WJ, Daft C, Allen JJB. A double-blind pilot study of transcranial ultrasound, (TUS) as a five-day intervention: TUS mitigates worry among depressed patients. Neurol. Psychiatry Brain Res. (2020) 37:60–6. doi: 10.1016/j.npbr.2020.06.004
41. Legon W, Adams S, Bansal P, Patel PD, Hobbs L, Ai L, et al. A retrospective qualitative report of symptoms and safety from transcranial focused ultrasound for neuromodulation in humans. Sci Rep. (2020) 10:5573. doi: 10.1038/s41598-020-62265-8
42. Stern JM, Spivak NM, Becerra SA, Kuhn TP, Korb AS, Kronemyer D, et al. Safety of focused ultrasound neuromodulation in humans with temporal lobe epilepsy. Brain Stimul. (2021) 14:1022–31. doi: 10.1016/j.brs.2021.06.003
43. Spivak NM, Korb AS, Reyes SD, Bych BP, Schafer SF, Khanlou N, et al. Histological examination of focused ultrasound effects on human brain tissue. Brain Stimul. (2021) 14:1486–8. doi: 10.1016/j.brs.2021.09.015
44. Lee W, Lee SD, Park MY, Foley L, Purcell-Estabrook E, Kim H, et al. Image-guided focused ultrasound-mediated regional brain stimulation in sheep. Ultrasound Med Biol. (2016) 42:459–70. doi: 10.1016/j.ultrasmedbio.2015.10.001
45. Sathappan AV, Luber BM, Lisanby SH. The dynamic duo: combining noninvasive brain stimulation with cognitive interventions. Prog Neuropsychopharmacol Biol Psychiatry. (2019) 89:347–60. doi: 10.1016/j.pnpbp.2018.10.006
46. Philip NS, Nelson BG, Frohlich F, Lim KO, Widge AS, Carpenter LL. Low-intensity transcranial current stimulation in psychiatry. Am J Psychiatry. (2017). 174:628–39. doi: 10.1176/appi.ajp.2017.16090996
47. Boutet A, Gwun D, Gramer R, Ranjan M, Elias GJB, Tilden D, et al. The relevance of skull density ratio in selecting candidates for transcranial MR-guided focused ultrasound. J Neurosurg. (2019) 132:1785–91. doi: 10.3171/2019.2.JNS182571
48. D'Souza M, Chen KS, Rosenberg J, Elias WJ, Eisenberg HM, Gwinn R, et al. Impact of skull density ratio on efficacy and safety of magnetic resonance-guided focused ultrasound treatment of essential tremor. J Neurosurg. (2019) 132:1392–7. doi: 10.3171/2019.2.JNS183517
49. Mueller JK, Ai L, Bansal P, Legon W. Numerical evaluation of the skull for human neuromodulation with transcranial focused ultrasound. J Neural Eng. (2017) 14:066012. doi: 10.1088/1741-2552/aa843e
50. Guo H, Hamilton Ii M, Offutt SJ, Gloeckner CD, Li T, Kim Y, et al. Ultrasound produces extensive brain activation via a cochlear pathway. Neuron. (2018) 99:866. doi: 10.1016/j.neuron.2018.07.049
51. Sato T, Shapiro MG, Tsao DY. Ultrasonic neuromodulation causes widespread cortical activation via an indirect auditory mechanism. Neuron. (2018) 98:1031.e5–41.e5. doi: 10.1016/j.neuron.2018.05.009
52. Kim H, Chiu A, Lee SD, Fischer K, Yoo SS. Focused ultrasound-mediated non-invasive brain stimulation: examination of sonication parameters. Brain Stimul. (2014) 7:748–56. doi: 10.1016/j.brs.2014.06.011
Keywords: non-invasive brain stimulation, acoustic stimulation, low intensity focused ultrasound, neuromodulation, brain stimulation
Citation: Arulpragasam AR, van 't Wout-Frank M, Barredo J, Faucher CR, Greenberg BD and Philip NS (2022) Low Intensity Focused Ultrasound for Non-invasive and Reversible Deep Brain Neuromodulation—A Paradigm Shift in Psychiatric Research. Front. Psychiatry 13:825802. doi: 10.3389/fpsyt.2022.825802
Received: 30 November 2021; Accepted: 18 January 2022;
Published: 24 February 2022.
Edited by:
Stefan Borgwardt, University of Lübeck, GermanyReviewed by:
Xiaodan Niu, Carnegie Mellon University, United StatesCopyright © 2022 Arulpragasam, van 't Wout-Frank, Barredo, Faucher, Greenberg and Philip. This is an open-access article distributed under the terms of the Creative Commons Attribution License (CC BY). The use, distribution or reproduction in other forums is permitted, provided the original author(s) and the copyright owner(s) are credited and that the original publication in this journal is cited, in accordance with accepted academic practice. No use, distribution or reproduction is permitted which does not comply with these terms.
*Correspondence: Noah S. Philip, bm9haF9waGlsaXAmI3gwMDA0MDticm93bi5lZHU=