- 1Addictive Substance Project, Tokyo Metropolitan Institute of Medical Science, Setagaya-Ku, Japan
- 2Department of Biosciences, School of Science and Engineering, Teikyo University, Utsunomiya-Shi, Japan
- 3Division of Systems Medical Science, Institute for Comprehensive Medical Science, Fujita Health University, Toyoake-Shi, Japan
- 4Department of Pediatrics, Graduate School of Medicine, The University of Tokyo, Bunkyo-Ku, Japan
- 5Department of Psychiatry, The University of Tokyo Hospital, Bunkyo-Ku, Japan
- 6Department of Obstetrics and Gynecology, Graduate School of Medicine, The University of Tokyo, Bunkyo-Ku, Japan
- 7Center for Basic Technology Research, Tokyo Metropolitan Institute of Medical Science, Setagaya-Ku, Japan
Autism spectrum disorder (ASD) is a neurodevelopmental disorder that is characterized by impairments in social interaction and restricted/repetitive behaviors. The neurotransmitter γ-aminobutyric acid (GABA) through GABAA receptor signaling in the immature brain plays a key role in the development of neuronal circuits. Excitatory/inhibitory imbalance in the mature brain has been investigated as a pathophysiological mechanism of ASD. However, whether and how disturbances of GABA signaling in embryos that are caused by GABAA receptor inhibitors cause ASD-like pathophysiology are poorly understood. The present study examined whether exposure to the GABAA receptor antagonist picrotoxin causes ASD-like pathophysiology in offspring by conducting behavioral tests from the juvenile period to adulthood and performing gene expression analyses in mature mouse brains. Here, we found that male mice that were prenatally exposed to picrotoxin exhibited a reduction of active interaction time in the social interaction test in both adolescence and adulthood. The gene expression analyses showed that picrotoxin-exposed male mice exhibited a significant increase in the gene expression of odorant receptors. Weighted gene co-expression network analysis showed a strong correlation between social interaction and enrichment of the “odorant binding” pathway gene module. Our findings suggest that exposure to a GABAA receptor inhibitor during the embryonic period induces ASD-like behavior, and impairments in odorant function may contribute to social deficits in offspring.
Introduction
Autism spectrum disorder (ASD) is categorized as a neurodevelopmental disorder in the Diagnostic and Statistical Manual of Mental Disorders, 5th edition (1). Although ASD has several peripheral symptoms (e.g., aberrant sensitization and clumsiness of movement), characteristics of ASD are divided into two main categories: impairments in social interaction and communication and restricted and repetitive patterns of behaviors and interests (1). Symptoms of ASD are usually diagnosed during early childhood and remain during an individual's life. The ratio of the prevalence of ASD in males and females is ~4:1 (2). Genetic and environmental causes of ASD have been investigated (3, 4), but the pathophysiology of ASD has not yet been thoroughly defined.
γ-Aminobutyric acid (GABA) is an inhibitory neurotransmitter in the mature brain that hyperpolarizes a membrane through the influx of chloride ions via GABAA receptor channels (5). GABAA receptor activation induces depolarizing membrane responses in the immature central nervous system (CNS), and GABA is important in the development of neuronal circuits, neurogenesis, and synapse formation (5, 6). Secreted GABA increased cell proliferation in the ventricular zone through GABAA receptor activation in mouse fetuses (7). Spontaneous Ca2+ oscillations, which are required for normal neuronal migration, are blocked or their frequency is reduced by the GABAA receptor blocker bicuculine in the cerebral cortex in newborn rats (8). Neuroblast migration in the hippocampus is impaired by treatment with antagonists of GABAA receptors and N-methyl-D-aspartate (NMDA) receptors, and GABAA receptor antagonism is more efficient than NMDA receptor antagonism in reducing cell migration (9). Previous studies showed that perinatal and postnatal GABAA receptor antagonist treatment led to aberrant behaviors in males. Bicuculine treatment during the neonatal period causes aberrant anxiety-like behavior in mature male mice and rats but not in females (10, 11). Male rats that were exposed to picrotoxin as embryos exhibited aberrant heterotypical sexual behaviors compared with control rats (12–14). These findings suggest that GABA signaling through GABAA receptors plays a key role in development of the immature CNS, and the inhibition of GABA signaling during developmental periods causes abnormal behaviors in male offspring.
Tochitani et al. recently reported that prenatal treatment with GABAA receptor agonists or antagonists altered social behaviors and locomotor activity in male offspring (15). They also reported that picrotoxin treatment from embryonic day 10–12 caused a rapid loss of interest in a familiar mouse, decreased locomotor activity, and decreased rearing (15). This study showed that disturbances of GABAA receptor signaling by picrotoxin administration during the embryonic period caused pathophysiological neurodevelopmental abnormalities, including ASD-like symptoms (15). However, unclear are whether and how picrotoxin affects body maturation and behaviors from adolescence to adulthood and gene expression in the mouse brain and whether there are correlations between such behavioral alterations and gene expression.
The present study investigated the effects of prenatal exposure to the GABAA receptor antagonist picrotoxin on body maturation and performance in several behavioral tests, including motor function, social interaction, pain responsiveness, self-grooming, and anxiety-like behavior. We then performed a comprehensive gene expression analysis using microarrays in the whole brain to explore the effects of picrotoxin on ASD-like pathophysiology. We also compared gene expression in the whole brain between picrotoxin-exposed mice and VPA-exposed mice (i.e., an established animal model of ASD). We analyzed data using BaseSpace and weighted gene co-expression network analysis (WGCNA). Here, we present evidence that offspring that are exposed to picrotoxin during the embryonic period exhibit impairments in social interaction in both adolescence and adulthood and that performance in the social interaction is strongly correlated with the odorant pathway in the WGCNA. Our results support the hypothesis that disturbances of GABAA receptor signaling during the embryonic period contributes to the pathophysiology of ASD.
Materials and Methods
Mice and Picrotoxin Administration
Pregnant C57BL/6J mice were purchased from CLEA (Tokyo, Japan) on gestation day 6 and housed individually. All of the mice were housed on a 12/12 h light/dark cycle (lights on 8:00 a.m. to 8:00 p.m.) and had ad libitum access to food and water. Temperature was maintained at 23.0 ± 1.0°C. Picrotoxin was dissolved in saline. Pregnant female mice received a single intraperitoneal injection of 5 mg/kg picrotoxin (Sigma-Aldrich, St. Louis, MO, USA) on gestation day 12.5. The dose of picrotoxin was based on a previous study that reported that the 5.0 mg/kg dose twice daily did not cause malformation or infant death in offspring (16). We also tested 2.5 and 5.0 mg/kg doses in pregnant female mice and observed impairments in social interaction in both adolescence and adulthood only in offspring that were exposed to the 5.0 mg/kg dose (Supplementary Figure 1). Thus, in the present study, we administered 5.0 mg/kg picrotoxin in pregnant mice. Picrotoxin was injected only once on embryonic day 12.5 to avoid possible negative effects of repeated administration in pregnant mice and to avoid the possibility of causing a cleft palate in offspring (17). We chose embryonic day 12.5 for administration to compare gene expression between picrotoxin-exposed mice and mice that were prenatally exposed to VPA on embryonic day 12.5 (i.e., an established animal model of ASD) (18). Control pregnant mice were injected with saline. The volume of injection was 10 ml/kg. All pregnant mice were returned to their home cages immediately after the injection, and their conditions were observed carefully. Eleven pregnant mice were used in this experiment. Five pregnant mice were used as the control group. Six pregnant mice were used as the picrotoxin group. Five minutes after the picrotoxin injection, the movements of pregnant mice were slow, and they mostly lied down on the floor. After ~60 min, the state of pregnant mice became normal (i.e., washing their faces and walking in the cage as usual). On average, 6–10 pups were obtained from picrotoxin- and saline-treated pregnant female mice. As in our previous study (18), the pups were culled to eight animals per litter on postnatal day 4 (P4). For saline-exposed pups, for litters with <8 pups, pups were transferred from litters that had more than nine on P4. We did not observe postnatal mortality, malformation, or stunted pups. The day of birth was defined as day 0. The pups were weaned on P25, and mice of either sex were housed separately. Three to five offspring were housed in one home cage. All of the animal experiments were performed in accordance with the Guidelines for the Care of Laboratory Animals of the Tokyo Metropolitan Institute of Medical Science, and the housing conditions were approved by the Institutional Animal Care and Use Committee (approval no. 12-43).
Postnatal Body Maturation and Behavioral Analyses
The body weights and eye opening of the mice were monitored to assess postnatal body maturation. Body weight was recorded on P7, P9, P11, P14, P21, and P25. Eye opening was observed once daily from P12 to P18. The eye-opening score was the following: 0 = both eyes closed, 1 = one eye open, and 2 = both eyes open. All of the behavioral tests were performed from 9:00 a.m. to 6:00 p.m. The mice were given 60 min to habituate to the behavioral test room before the start of each test. Motor function during from P7 to P25 was assessed by the negative-geotaxis, righting reflex, cliff avoidance, and hanging wire tests. The social interaction test was conducted in both adolescence (5–6 weeks of age) and adulthood (10–11 weeks of age). The mice underwent the hot plate test (6–7 weeks of age), grooming test (7–8 weeks of age), open field test (8–9 weeks of age), and elevated plus maze test (9–10 weeks of age).
Negative Geotaxis Test
Negative geotaxis was tested on P7, P9, and P11. Each mouse was placed on a board that was tilted at 40°, facing downward. We assessed the latency to turn 180° (i.e., the tip of the nose faced upward). The cutoff time was a maximum of 20 s.
Righting Reflex Test
The righting reflex was tested on P7, P9, and P11. Each mouse was placed in the supine position, and the latency to return to the prone position was assessed. The cutoff time was a maximum of 15 s.
Cliff Avoidance Test
Cliff avoidance was tested on P7, P9, and P11. Each mouse was set on a desk at a height of 1 m, with its nose positioned outward at the edge of the desk. The latency to avoid the cliff was assessed. The cutoff time was a maximum of 20 s.
Hanging Wire Test
The hanging wire test (O'Hara & Co., Tokyo, Japan) was conducted on P25. The mice were placed on a grid wire surface (150 × 150 mm, divided into 10 mm grid squares), and the plane was inverted. The latency to fall was recorded. The cutoff time was a maximum of 600 s.
Social Interaction Test
The social interaction test was conducted during both adolescence (5–6 weeks of age) and adulthood (10–11 weeks of age) as previously described (18, 19). For habituation, each mouse was left alone in its home cage in a sound-attenuating chamber for 15 min. One unfamiliar C57BL/6J mouse of the same sex and age was then introduced to the cage. The behavior of the test mouse was video-recorded for 10 min and blindly scored for active social interaction, consisting of sniffing, allo-grooming, mounting, and following. One mouse that went out of its home cage during the 15 min habituation period was excluded from the analysis. Body weight was also recorded when each mouse performed the social interaction test. The number of mice per group was the following: 5–6 weeks of age (n = 18 control male mice, n = 26 picrotoxin-exposed male mice, n = 22 control female mice, n = 22 picrotoxin-exposed female mice) and 10–11 weeks of age (n = 18 control male mice, n = 26 picrotoxin-exposed male mice, n = 22 control female mice, n = 21 picrotoxin-exposed female mice).
Hot Plate Test
The hot plate test (Muromachi, Tokyo, Japan) was conducted at 6–7 weeks of age. Each mouse was set on a hot plate (55.0 ± 0.5°C), and the latency to flicking, jumping, and licking its paws was recorded.
Grooming Test
The grooming test was conducted at 7–8 weeks of age and consisted of a 2-day sequence. The first day was the habituation phase and the tested mice were placed in the experimental room for 60 min in their home cage. After 60 min, the mice were placed in a sound-attenuating chamber, and their movements were recorded for 30 min. In this phase, the mice were habituated to the experimental room and apparatus. The second day of the grooming test was the recording day. As on the first day, the mice were habituated to the experimental room, and their movements were recorded in a sound-attenuating chamber for 30 min. Grooming involved wiping the face, nose, ears, and head with forepaws and licking the body other than the face. We counted the number and seconds of grooming for 30 min.
Open Field Test
The open field test was conducted at 8–9 weeks of age. The apparatus (Muromachi) consisted of an open field (500 × 500 × 500 mm). Each mouse was placed in the center of the open field and allowed to explore it for 20 min under dim light. Behaviors were automatically recorded by a video tracking system (Muromachi).
Elevated Plus Maze Test
The elevated plus maze test (Muromachi) was conducted at 9–10 weeks of age. The apparatus consisted of two closed arms (300 × 60 mm, with 150-mm-high walls) and two open arms (297 × 54 mm). The apparatus was raised 40 cm above the floor. A video tracking system (Muromachi) automatically recorded behaviors.
RNA Extraction From Whole Brains
We conducted brain collection and RNA extraction according to a previous study (18). After the end of the social interaction test (10–11 weeks of age), whole brains were collected. We examined the whole brain in the present study because the precise brain regions that are associated with ASD have not yet been clearly defined. Total RNA was extracted from the whole brain and homogenized in Ambion TRIzol reagent (Thermo Fisher Scientific, Waltham, MA, USA) using a homogenizer. RNA was isolated using chloroform and precipitated using isopropyl alcohol. The quality of RNA was assessed with Nanodrop 1000 (Thermo Fisher Scientific). All of the RNA samples had an A260/280 ratio between 2.01 and 2.02 and A230/260 ratio between 2.26 and 2.31.
Analyses of Whole-Genome Gene Expression
We conducted whole-genome gene expression using a microarray analysis according to a previous study (18). cRNA targets were synthesized and hybridized using the Whole Mouse Genome Microarray according to the manufacturer's instructions (Agilent Technologies, Santa Clara, CA, USA). The array slides were scanned using a SureScan Microarray Scanner (Agilent Technologies, Santa Clara, CA, USA). Before analyzing gene expression, microarray data were normalized and sorted using GeneSpring 14.5 software (Agilent Technologies, Santa Clara, CA, USA). Each sample was normalized by a 75% percentile shift. Compromised probes were removed. Each group comparison was performed using t-tests (p < 0.05). Each group consisted of five male mice (control mice and picrotoxin-exposed mice). Gene ontology, pathway enrichment analysis, and comparisons with curated studies were conducted using BaseSpace (Illumine, San Diego, CA, USA; https://login.illumina.com/platform-services-manager/?rURL=https://accounts.public. basespace.illumina.com/b/authentication/login.nb;jsessionid=69E78F49406EDD33C6DE7D3C1A2F8FD8&clientId=NBR-Public #/) (20).
Weighted Gene Co-expression Network Analysis
The WGCNA was performed using the WGCNA package in R software and conducted for step-by-step block-wise network construction and module detection using the package implemented in R version 3.5.2 with the code provided by Langfelder and Horvath (21, 22). To focus on differentially expressed genes, genes with p < 0.05 were used for the WGCNA. In accordance with WGCNA default preprocessing steps, any obvious outliers in our sample were checked with an average linkage hierarchical cluster analysis of expression levels. Pearson correlation coefficients for all transcript pairs were then calculated to determine connection strengths between two transcripts. The connection strength between transcript m and transcript n was defined as αmn = [correlation (m, n)], where the β value is set as the weighting coefficient. Power of beta = 30 was chosen based on the scale-free topology criterion (the linear regression model fitting index, R2, was ~0.9).
Statistical Analysis
The results of the behavioral tests were analyzed using Prism 9.2.0 software (GraphPad, San Diego, CA, USA). The data were analyzed using unpaired t-tests, the Mann-Whitney U-test, and two-way repeated-measures analysis of variance (ANOVA) followed by the Bonferroni post-hoc test. All of the data are presented as mean ± standard error of the mean (SEM). Values of p < 0.05 were considered statistically significant.
Results
Effects of Prenatal Exposure to Picrotoxin on Postnatal Development and Motor Function
No significant difference in body weight was found between control and picrotoxin-exposed male mice from P7 to P25 (Figure 1A). The two-way repeated-measures ANOVA showed no significant main effect of picrotoxin treatment [F(1, 42) = 0.281, p = 0.599; n = 18 control mice, n = 26 picrotoxin-exposed mice] and no picrotoxin treatment × day of testing interaction [F(5, 210) = 1.035, p = 0.398] but a significant effect of day of testing [F(1.916, 80.46) = 2,034, p < 0.0001]. Eye-opening scores in picrotoxin-exposed male mice tended to be low compared with control male mice on P13 (U = 106.500, p = 0.059; n = 18 control mice, n = 22 picrotoxin-exposed mice; Figure 1B), with no significant difference between control and picrotoxin-exposed mice on P12 or P14-18 (p > 0.9999). In the negative-geotaxis test, the two-way repeated-measures ANOVA showed no main effect of picrotoxin treatment [F(1, 42) = 0.298, p = 0.588], no picrotoxin treatment × postnatal day interaction [F(2, 84) = 0.805, p = 0.450; n = 18 control mice, n = 26 picrotoxin-exposed mice; Figure 2A], and a significant effect of day of testing [F(1.970, 82.73) = 5.361, p = 0.007]. In the righting reflex test, the two-way repeated-measures ANOVA showed a trend toward an effect of picrotoxin treatment [F(1, 42) = 3.216, p = 0.080], no picrotoxin treatment × day of testing interaction [F(2, 84) = 1.415, p = 0.249; n = 18 control mice, n = 26 picrotoxin-exposed mice; Figure 2B], and a significant effect of day of testing [F(1.424, 59.80) = 39.04, p < 0.0001]. In the cliff avoidance test, the two-way repeated-measures ANOVA showed a main effect of picrotoxin treatment [F(1, 42) = 12.10, p = 0.001], a trend toward a picrotoxin treatment × day of testing interaction [F(2, 84) = 3.080, p = 0.051; n = 18 control mice, n = 26 picrotoxin-exposed mice; Figure 2C], and a significant effect of day of testing [F(1.612, 67.72) = 10.71, p = 0.0003]. The Bonferroni post-hoc test revealed a significant difference on P7 (p = 0.005). The Bonferroni post-hoc test did not reveal a significant difference on P9 (p = 0.401) or P11 (p = 0.248). No difference in the latency to fall was found between control and picrotoxin-exposed male mice on P25 [t(42) = 0.836, p = 0.408; n = 18 control mice, n = 26 picrotoxin-exposed mice; Figure 2D]. The results of these tests indicated that picrotoxin-exposed male mice exhibited a delay in body maturation and poor motor performance compared with control male mice.
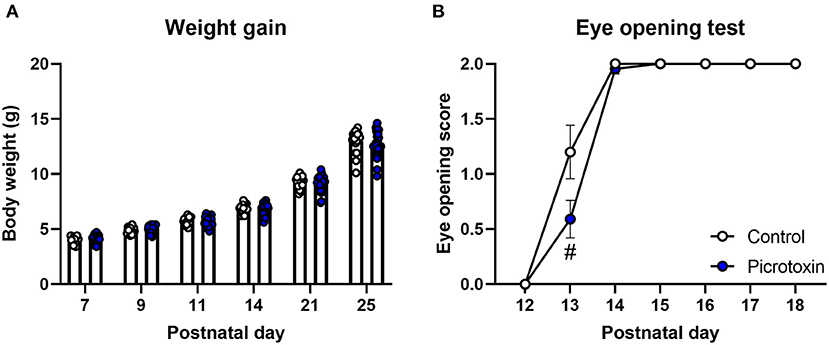
Figure 1. Body maturation and eye-opening scores. (A) No significant difference in body weight was found between control and picrotoxin-exposed male mice. (B) A trend toward a difference in eye opening was observed between control and picrotoxin-exposed male mice on P13. The data are expressed as mean ± SEM. #p < 0.1 [two-way repeated-measures ANOVA in (A), Mann-Whitney U-test in (B)].
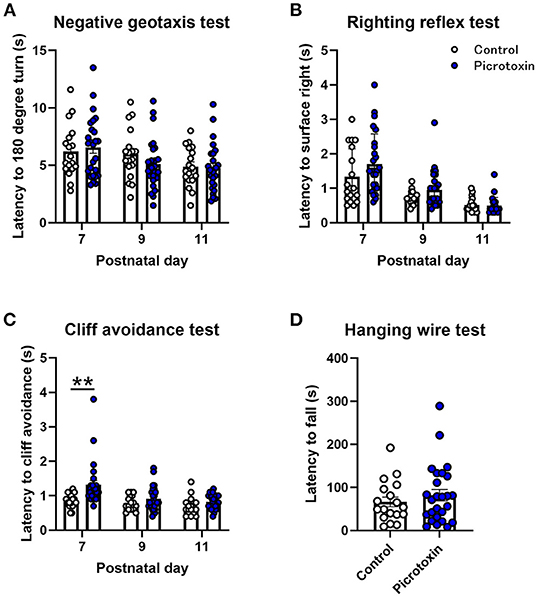
Figure 2. Motor function tests. (A) No significant difference in the negative-geotaxis test was found between control and picrotoxin-exposed male mice. (B) No significant difference in the righting reflex test was found between control and picrotoxin-exposed male mice. (C) Picrotoxin-exposed male mice exhibited a significantly longer latency to cliff avoidance. (D) No significant difference in the hanging-wire test was found between control and picrotoxin-exposed mice. The data expressed as mean ± SEM. **p < 0.01 (two-way repeated-measures ANOVA).
Effects of Prenatal Exposure to Picrotoxin on Affective-Like Behaviors
We assessed the effects of picrotoxin on social interaction during both 5–6 weeks of age (adolescence) and 10–11 weeks of age (adulthood). In adolescence, picrotoxin-exposed male mice exhibited a decrease in active interaction time compared with control male mice [t(42) = 3.378, p = 0.002; n = 18 control mice, n = 26 picrotoxin-exposed mice; Figure 3A]. In adulthood, picrotoxin-exposed male mice exhibited a decrease in active interaction time compared with control male mice [t(42) = 2.723, p = 0.009; n = 18 control mice, n = 26 picrotoxin-exposed mice; Figure 3A]. No significant difference in the latency to flicking, jumping, and licking paws in the hot plate test was found between the control and picrotoxin-exposed male mice at 6–7 weeks of age [t(42) = 0.547, p = 0.587; n = 18 control mice, n = 26 picrotoxin-exposed mice; Supplementary Figure 2A]. Patients with ASD typically engage in repetitive behaviors (1). The self-grooming test has been used to monitor repetitive behaviors in mouse models of ASD (23). Picrotoxin-exposed male mice exhibited a significant difference in the total number of self-grooming episodes in the grooming test compared with control male mice [t(35) = 2.221, p = 0.032; n = 20 control mice, n = 17 picrotoxin-exposed mice; Figure 3B]. No significant difference in the total time of self-grooming in the grooming test was found between control and picrotoxin-exposed male mice [t(35) = −1.350, p = 0.186; n = 20 control mice, n = 17 picrotoxin-exposed mice; Supplementary Figure 1B]. No significant difference in the total distance traveled in the open field test was found between control and picrotoxin-exposed male mice [t(42) = 0.412, p = 0.682; n = 18 control mice, n = 26 picrotoxin-exposed mice; Figure 3C]. No significant difference in the time spent in the peripheral area was found between control and picrotoxin-exposed male mice [t(42) = 0.214, p = 0.832; n = 18 control mice, n = 26 picrotoxin-exposed mice; Figure 3D]. Picrotoxin-exposed male mice exhibited an increase in the number of turning episodes compared with control male mice [t(42) = −2.218, p = 0.032; n = 18 control mice, n = 26 picrotoxin-exposed mice; Figure 3E]. No significant difference in the time spent on the open arms of the elevated plus maze was found between control and picrotoxin-exposed male mice [t(42) = 0.253, p = 0.801; n = 18 control mice, n = 26 picrotoxin-exposed mice; Figure 3F]. Body weight was recorded at both 5–6 and 10–11 weeks of age in the social interaction test. No significant difference was found between control and picrotoxin-exposed male mice in either adolescence or adulthood (Supplementary Results and Supplementary Table 1). After the social interaction test at 10–11 weeks of age, we collected the whole mouse brain and recorded brain weights to confirm the effects of picrotoxin treatment. No significant difference in brain weight was found between control and picrotoxin-exposed male mice (Supplementary Results and Supplementary Table 1).
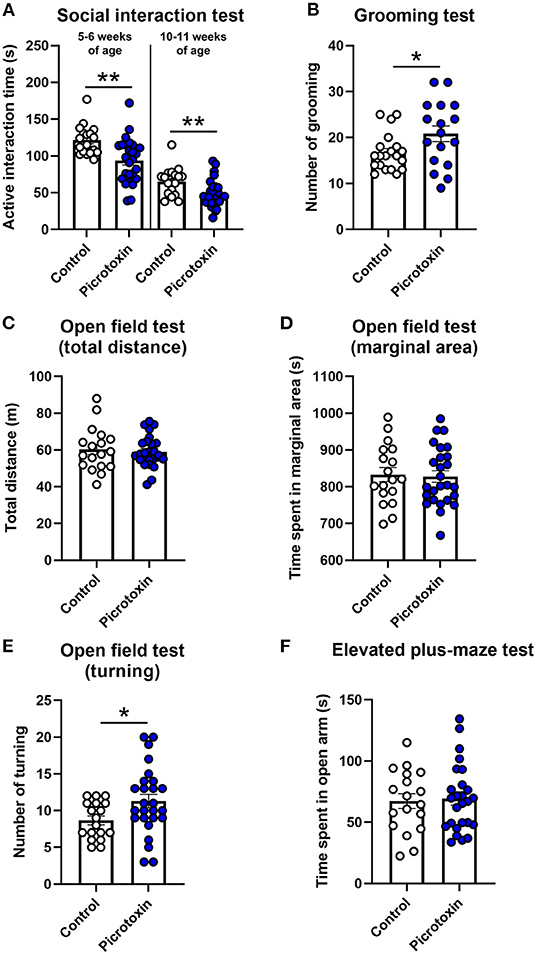
Figure 3. Social interaction, hot plate, grooming, open field, and elevated plus maze tests. (A) Social interaction test. Picrotoxin-exposed male mice exhibited a decrease in active social interaction time compared with control male mice at 5–6 and 10–11 weeks of age. (B) Grooming test. Picrotoxin-exposed male mice exhibited an increase in the number of grooming episodes compared with control male mice. (C,D) Open field test. No significant difference was found between control and picrotoxin-exposed male mice in the total distance traveled (C) or time spent in the peripheral area (D). (E) Open field test (turning behavior). Picrotoxin-exposed male mice exhibited an increase in the number of turning episodes compared with control male mice. (F) Elevated plus maze test. No significant difference in the time spent on the open arms was found between control and picrotoxin-exposed male mice. The data are expressed as mean ± SEM. **p < 0.01, *p < 0.05 (unpaired t-test).
We also analyzed body maturation, motor function, social interaction, behavior in the hot plate test, grooming behavior, activity in the open field test, and anxiety-like behavior in the elevated plus maze test in control female mice and picrotoxin-exposed female mice. No significant difference was found between control and picrotoxin-exposed female mice in the social interaction test. All behavioral data in female mice are presented in the Supplementary Results and Supplementary Table 2.
Effects of Picrotoxin Exposure in utero on Gene Expression
Picrotoxin-exposed male mice (n = 5) exhibited the differential expression of 465 genes (438 upregulated genes, 27 downregulated genes) compared with control male mice (n = 5; Table 1). To further analyze the functional significance of these genes, enrichment pathway analysis was performed for each upregulated and downregulated gene using BaseSpace. The enrichment pathways for each regulated gene are described in Table 2, Supplementary Tables 4, 5. The top five pathways for upregulated genes were odorant binding, neuropeptide receptor activity, positive regulation of neutrophil migration, coenzyme A (CoA)-ligase activity, and acid-thiol ligase activity (Table 2). The top five pathways for downregulated genes were protein N-terminus binding, regulation of myeloid cell differentiation, carbohydrate binding, regulation of hemopoiesis, and negative regulation of hemopoiesis (Table 2). We also identified common genes whose expression was altered in whole brains between picrotoxin-exposed male mice and VPA-exposed male mice [which have been used as an animal model of ASD and were reported in our previous study (18)]. The common genes whose expression was altered were Camk1d, Platr26, Zfp599, Fyb, and Cdc7 (Table 3). These genes were upregulated in both picrotoxin-exposed male mice and VPA-exposed male mice. Of these altered genes, Fyb expression was recovered by rapamycin treatment in VPA-exposed mice (18). These results suggest that exposure to picrotoxin in utero alters gene expression in the offspring brain, and the genes whose expression is altered are common to an existing mouse model of ASD.
Weighted Gene Co-expression Network Analysis
We next constructed a WGCNA to explore relationships between behavioral and gene traits in picrotoxin-exposed mice. The dendrogram represents a single tight-clustering branch of each sample (Figure 4). Gene expression in the whole brain in both control and picrotoxin-exposed male mice was discriminated into two clusters, indicating that there were no outliers in the samples. A total of four distinct gene modules were identified from the expression of 1,867 genes using a dynamic tree cutting algorithm (Figure 5). The brown, blue, turquoise, and gray gene modules included 18, 28, 1,776, and 45 genes, respectively. The 45 uncorrelated genes were grouped into in the gray module. To find modules of interest, correlations between the color modules and performance in the behavioral tests were computed (Figure 6A). Performance in the grooming test was excluded in this correlation analysis because we could not find a consistent result with regard to the number and time of self-grooming. Picrotoxin treatment significantly correlated with four modules (brown module: r = −0.78, p = 0.008; blue module: r = 0.82, p = 0.004; turquoise module: r = 0.96, p = 1e-05; gray module: r = 0.88, p = 7e-04). Performance in the social interaction test at 5–6 weeks of age was significantly associated with picrotoxin treatment (gray module: r = −0.75, p = 0.01). All four modules significantly correlated with performance in the social interaction test at 10–11 weeks of age. Among these four modules, the turquoise module showed the highest negative correlation (brown module: r = 0.73, p = 0.02; blue module: r = −0.77, p = 0.11; turquoise module: r = −0.91, p = 2e-04; gray module: r = −0.79, p = 0.006). The number of turning episodes in the open field test was significantly associated with the blue module (r = 0.85, p = 0.002). The enrichment pathways for each module are listed in Supplementary Tables 6–9. The top three pathways for each module are described in Figure 6B. The turquoise module that was most associated with performance in the social interaction test at 10–11 weeks of age was enriched for the odorant binding and tissue differentiation and development pathways (Figure 6B, Supplementary Table 6). The blue module that was most associated with performance in the open field test at 8–9 weeks of age was enriched for synapse-associated pathways (Figure 6B, Supplementary Table 7).
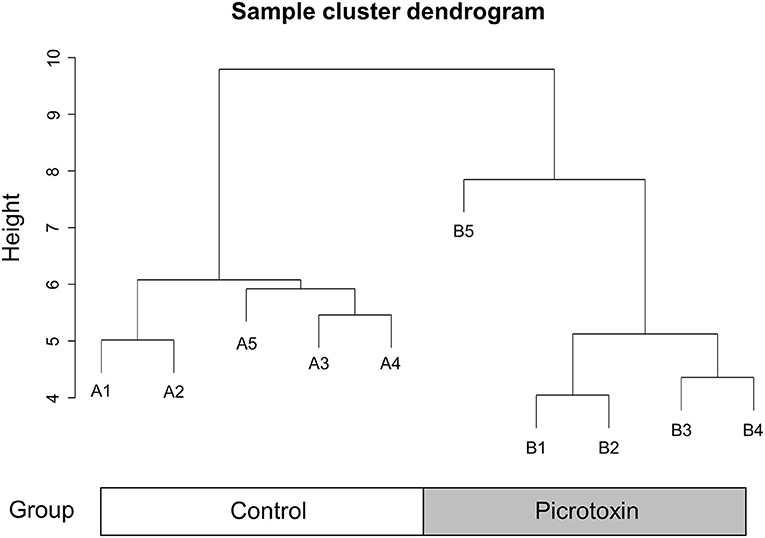
Figure 4. Sample cluster dendrogram. Hierarchical clustering of samples of 1,867 genes in the mouse whole brain.
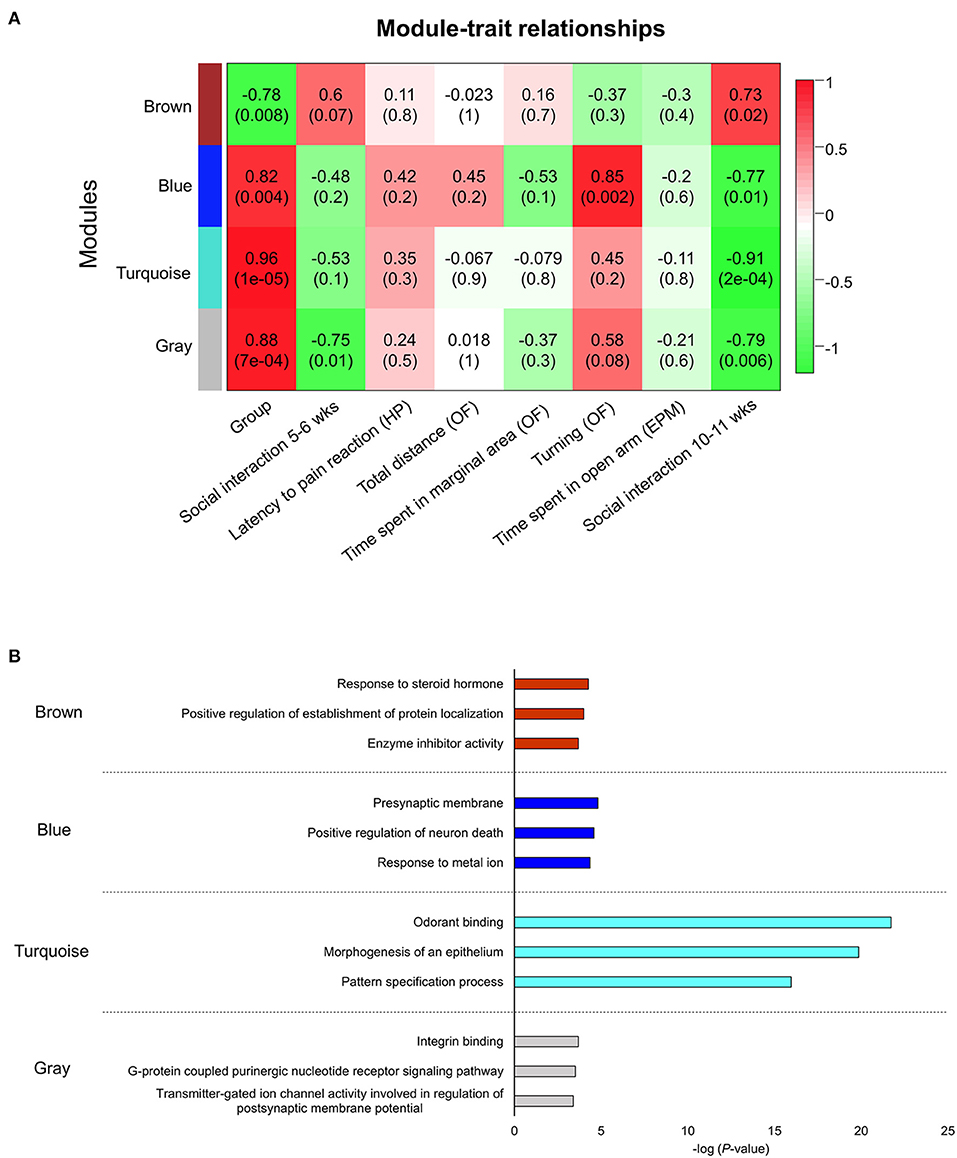
Figure 6. Network construction of co-expressed genes that correlated with performance in the behavioral tests and gene ontology pathway enrichment analysis of each module. (A) Module trait correlation for treatment, social interaction (5–6 weeks of age), latency to flicking, jumping, licking paws (hot plate test), total distance traveled (open field test), time spent in the peripheral area (open field test), turning episodes (open field test), time spent on the open arms (elevated plus maze test), and social interaction (10–11 weeks of age). HP, hot plate test; OF, open field test; EPM, elevated plus maze test. (B) The blue module was the most correlated with turning episodes in the open field test. The turquoise module was the most correlated with social interaction (10–11 weeks of age).
Discussion
In the present study, we found that prenatal exposure to picrotoxin in mice on day 12.5 of gestation had long-term and selective effects on postnatal behaviors and gene expression in male offspring. In the behavioral tests, prenatal exposure to picrotoxin (i) tended to delay body maturation and motor function, (ii) induce impairments in social interaction in both adolescence and adulthood, and (iii) increase the number of turning episodes in the open field test. The gene expression analysis showed that picrotoxin-exposed mice exhibited alterations of the expression of 465 genes, including five genes that were in common with alterations in VPA-exposed male mice. The WGCNA showed that social interaction in adulthood had the strongest negative correlation with the turquoise gene module, and turning episodes in the open field test had a strongest positive correlation with the blue gene module. “Odorant binding” and “presynaptic membrane” were the top pathways for the turquoise and blue gene modules, respectively.
The pathophysiology of ASD has not been well-defined. Many hypotheses suggest causal explanations of ASD. An E/I imbalance in the mature brain is theorized to be a key pathophysiological mechanism of ASD (24–28). The influence of GABA during prenatal development is excitatory, and GABA/GABAA receptor signaling plays a key role in cellular processes during development. Previous studies reported that disturbances of GABA/GABAA receptor signaling affect postnatal behaviors in offspring. Perinatal exposure to picrotoxin in rat dams affected sexual behavior in male offspring by increasing the latency to the first mount and intromission (12). The neonatal blockade of GABAA receptors with bicuculline produced abnormal passive avoidance memory and increased brain-derived neurotrophic factor levels in the brain on P61-70 (11). Prenatal exposure to ethanol, a GABAA receptor agonist, dysregulated the vertical and horizontal cleavage planes of neural progenitors in the developing neocortex, and picrotoxin treatment prior to ethanol administration restored regulation of the cleavage plane to control levels (16). Tyzio et al. reported that a rat model of VPA-induced ASD and mouse model of Fragile X syndrome abolished the switch of GABA from excitatory to inhibitory transmission. Maternal pretreatment with bumetanide, an antagonist of Na-K-Cl cotransporter 1 chloride importers, before delivery improved this switch in GABA activity and aberrant ultrasonic vocalizations in offspring (29). Tochitani et al. recently reported that the modulation of GABAA receptors by agonists and antagonists did not cause social deficits but resulted in the rapid loss of interest in a stranger mouse compared with control mice (15). The different findings between their study and the present study might be attributable to the treatment duration, dose, social interaction test that was conducted, or mouse strain. Nonetheless, the present results support the findings of Tochitani et al. and further our understanding of correlations between disturbances of GABAA receptor signaling during the embryonic period and the pathophysiology of ASD. Tochitani et al. also reported that the administration of 5.0 mg/kg picrotoxin twice daily on embryonic days 10–12 affected neuronal progenitor cells in the neocortex on embryonic day 13, decreased Tbr2-positive cells, increased Pax 6-positive cells, and decreased Doublecortin (Dcx; a marker for immature and migrating neurons)-positive layers (15). Picrotoxin administration during the embryonic period disturbs development of the neocortex and might contribute to the pathophysiology of ASD. In the present study, we found that prenatal GABAA receptor blockade with picrotoxin affected behavior in male offspring in both adolescence and adulthood and caused ASD-like behaviors, including deficits of social interaction.
Picrotoxin-exposed male mice exhibited alterations of genes, including 438 upregulated genes and 27 downregulated genes, compared with control male mice. We conducted gene ontology pathway enrichment analysis to understand the function of these genes. We found that genes whose expression was upregulated are related to odorant binding, neuropeptide receptor activity, neutrophil migration, and catalysis (Table 2). The downregulated genes are related to protein-N-terminus binding, myeloid cell differentiation, carbohydrate binding, and hemopoiesis (Table 2). Upregulated and downregulated genes with normalized scores of 100 were related to odorant binding and protein-N-terminus binding, respectively. A previous clinical study reported that patients with ASD exhibited aberrant olfactory responses, with a lower discrimination score and higher bias score in an olfactory test (30). One of the identified genes in the protein-N-terminus binding pathway was Hesx1, which is required to program human embryonic stem cell neural fate (31).
We also detected alterations of the expression of genes that were regulated in the same direction (i.e., upregulated) in picrotoxin-exposed male mice and VPA-exposed male mice. Exposure to VPA has been used as an animal model of ASD (18, 32–34). We detected five genes whose expression was commonly altered between picrotoxin-exposed male mice and VPA-exposed male mice: Camk1d, Platr26, Zfp599, Fyb, and Cdc7 (Table 3). Camk1d and Cdc7 were reportedly associated with brain and embryonic development (35, 36). Platr26 is a long non-coding RNA, and Platr1-32 were suggested to be functionally integrated into the mouse embryonic stem cell gene expression program (37). Zinc finger protein plays a key role in tissue development and differentiation (38). De novo deletions in the 19q.13.11 region that encompasses four KRAB-ZNFs, including Zfp 599, were identified in two unrelated cases of microcephaly (39). In our previous study (18), Fyb expression was recovered by the mammalian target of rapamycin complex 1 inhibitor rapamycin in VPA-exposed male mice. Fyb is broadly expressed in the mouse brain and strongly expressed in the olfactory bulb (40). Valproic acid is clinically used as an anti-epileptic drug and increases GABA release (41). We speculate that these five genes are key molecules in the pathophysiology of ASD-like behavior in mice, in which GABA signaling is disturbed during embryonic periods.
A WGCNA was used to detect correlations between co-expression genes and performance in various behavioral tests. We identified four modules based on the WGCNA. All four gene modules (brown, blue, turquoise, and gray) had a significant correlation with picrotoxin treatment (Group) and social interaction at 10–11 weeks of age, and the blue gene module had a significant correlation with turning episodes in the open field test. The turquoise module had the strongest correlation with performance in the social interaction test at 10–11 weeks of age. The top three enrichment pathways for the turquoise module were odorant binding, epithelium morphogenesis, and pattern specification process. Overall, the turquoise module included pathways that are related to tissue differentiation and development (Figure 6B, Supplementary Table 6). Interestingly, the most significantly enriched pathway was “odorant binding.” Active interaction time in the social interaction test consisted of sniffing, allo-grooming, mounting, and following. The sniffing ratio was the most active social interaction behavior (Supplementary Table 3). Aberrant olfactory function may have altered social interaction in picrotoxin-exposed male mice. Patients with ASD also exhibit sensory issues, including aberrant olfactory function (42). A previous clinical study reported that patients with ASD exhibited aberrant odor awareness (43). A significant correlation was found between olfactory threshold and social problems, determined by the Child Behavior Checklist, in male children with ASD (44). The sniffing duration ratio correlated with the social affect component of the Autism Diagnostic Observation Schedule but not the restricted/repetitive behavior component (45). Impairments in social interaction in ASD are often caused by the misreading of emotional cues. Endevelt-Shapira et al. investigated social chemosignals in adult patients with ASD. When typically developing participants sniffed an undetectable scent of fear (i.e., skydiver sweat) or control sweat, they presented enhanced autonomic arousal responses (i.e., electrodermal activity) in response to the skydiver sweat compared with control sweat, whereas patients with ASD did not exhibit such changes in the autonomic arousal response (46). These clinical findings suggest that the olfactory system plays a key role in sociability in patients with ASD. Franco et al. reported that a reduction of lateral inhibition in the brain impaired odor discrimination and social behavior in a Drosophila model of Fragile X syndrome that exhibited ASD-like behaviors (47). Scn1a+/− mice also exhibit ASD-like behaviors, including social deficits and the avoidance of social odors (e.g., male urine odors), in a Y-maze olfactory choice test (48). Mitral cells in the olfactory bulb are generated on embryonic days 9–13. The olfactory tubercle receives a robust axonal projection from mitral cells that are generated on embryonic day 12 (49). The present study did not investigate specific changes in the olfactory bulb or olfactory cortex, but our findings suggest that picrotoxin administration during the embryonic period may affect development of the olfactory system and its connections with other brain regions. The blue module was directory correlated with turning behavior in the open field test at 8–9 weeks of age. The blue module included pathways that are related to synapses as the most enriched pathways (Figure 6B, Supplementary Table 7). The top network in the blue module was “presynaptic membrane.” Presynaptic function has been investigated in neurodevelopmental disorders, including ASD (50). Patients with ASD and animal models of ASD exhibit different behaviors in a novel environment. Patients with ASD exhibited higher stress responses to novel stimuli compared with controls (51, 52). The ablation of metabotropic glutamate receptor 5 in mice resulted in synaptic deficiency and an increase in novelty-induced locomotion compared with wildtype mice (53). We speculate that the high number of turning episodes is an aberrant response to a novel environment. Patients with ASD often exhibit stimming behavior (e.g., hand-flapping, body rocking, and spinning in circles), also known as repetitive/restricted behavior, to manage their emotions and overwhelming sensory inputs (54). A previous study reported that prenatal zinc deficiency in mice, which is an animal model of ASD, resulted in a side preference of rotational behavior in a round arena (a 360° turn was considered a rotation) (55), but we did not observe differences between right and left turning episodes in the present study (P8, Supplementary Table 10).
In the present study, the detected pathways in the turquoise and blue modules are helpful for clarifying the mechanisms of ASD-like behaviors, which may aid the identification of possible treatment targets. The uncorrelated genes were assigned to the gray module and was thus normally excluded from further analysis. However, the gray module negatively correlated with performance in the social interaction test in both adolescence and adulthood in picrotoxin-exposed male mice. The key pathways that caused social deficits in both adolescence and adulthood were in the gray module. Social interaction at 10–11 weeks of age was the most correlated with the turquoise module. Social interaction at 5–6 weeks of age was not correlated with the turquoise module. We speculate that adults and adolescents may recruit different pathways that contribute to social behaviors. The present findings may help clarify differences in neural mechanisms that are involved in sociability between adults and adolescents.
We observed sex differences in picrotoxin-exposed mice. Exposure to picrotoxin during the embryonic period caused social deficits in males but not females. The ratio of males to females is higher in the ASD patient population (2). A previous study reported that treatment with the GABAA receptor inhibitor bicuculine during the neonatal period elicited aberrant anxiety-like behavior in male mice but not in female mice (10, 11). Tracosis et al. reported that the autistic male mice showed reduced the Hurst exponent in resting state fMRI in the medial prefrontal cortex, indicating increased excitation but female mice did not show the reduced the Hurst exponent (56). Valproic acid-exposed female mice did not exhibit impairments in social interaction (33) but exhibited a decrease in Nissl-positive cells in the somatosensory cortex compared with male mice (57). Female mice also did not exhibit ASD-like behaviors despite having the Nlgn3/Cyfip1 risk allele (58). A recent study reported that low placenta levels of allopregnanolone (ALLO), a progesterone-derived GABAA receptor modulator, resulted in sex-dependent alterations of neurodevelopment and behavior in offspring (59). Male plKO mice that were derived from pregnant plKO mice that have specific placental ALLO reduction exhibited ASD-like behaviors, including social deficits, and an increase in cerebellar myelin proteins on P30, whereas female plKO mice did not exhibit these changes (59). Similar results were found in human preterm infants that were characterized by premature loss of the placenta (59). This study suggests that GABAA receptor function in female mice might not be disrupted by low ALLO levels or that female mice may recruit a compensatory mechanism in response to impairments in GABAA receptor function (59). Sex bias in ASD may be related to chromosomal and hormonal mechanisms, immune activation, or interactions among sex, genetics, and environmental factors (60). These previous studies and the present study suggest that female sex may be a protective factor against ASD, making males more susceptible to ASD. Further studies are warranted to verify possible sex differences in animal models of ASD.
We suggest that exposure to picrotoxin during the embryonic period contributes to the pathophysiology of ASD via GABAA receptor signaling. Picrotoxin also blocks homomeric glycine receptor (GlyR) subtypes (61, 62). Glycine receptors are ligand-gated chloride channels that are expressed in the brain and spinal cord (63). Pilorge et al. reported that male patients with ASD had a de novo missense mutation of GLRA2, which encodes the GlyRα2 subunit. These authors also found that Glra2 knockout mice did not exhibit social behavior and spent less time exploring a novel object (64). In brain slices that were prepared on embryonic day 11, pretreatment with picrotoxin inhibited the response of neuronal progenitors to the application of taurine, which is a ligand of GlyRs and GABAA receptors (15). We did not investigate the specific mechanisms of action of picrotoxin during the embryonic period in the present study, but GABAA receptor or GlyR inhibition by picrotoxin is suggested to contribute to the pathophysiology of ASD.
The present study found ASD-like behaviors, gene aberrations, and correlations between gene expression and behavior in mice that were exposed to picrotoxin during the embryonic period. Our results provide a better understanding of the pathophysiology of ASD. Our study suggests that prenatal exposure to an GABAA receptor inhibitor induces ASD-like behaviors in offspring. The prenatal inhibition of GABAA signaling may a mechanism that contributes to ASD.
Data Availability Statement
The original contributions presented in the study are included in the article/Supplementary Materials, further inquiries can be directed to the corresponding author/s.
Ethics Statement
The animal study was reviewed and approved by Animal Experimentation Ethics Committee of Tokyo Metropolitan Institute of Medical Science.
Author Contributions
HK-M and KI designed the experiments and wrote the paper. HK-M performed the mouse behavioral testing and analyzed the behavioral data. HK-M and HH analyzed the gene expression data. HH conducted the WGCNA and wrote the methods of WGCNA. YN and YT performed the microarray analysis. HK-M and YH generated the picrotoxin mouse model of ASD and were responsible for breeding management. AS, MT, YK, SU, and TM participated in refinements of the experiments and discussion. All authors read and approved the final manuscript to be published.
Funding
This research was supported by Grants-in-Aid for Scientific Research from the Japan Society for the Promotion of Science, KAKENHI [17K15765, JP16H06276 (AdAMS)].
Conflict of Interest
The authors declare that the research was conducted in the absence of any commercial or financial relationships that could be construed as a potential conflict of interest.
Publisher's Note
All claims expressed in this article are solely those of the authors and do not necessarily represent those of their affiliated organizations, or those of the publisher, the editors and the reviewers. Any product that may be evaluated in this article, or claim that may be made by its manufacturer, is not guaranteed or endorsed by the publisher.
Acknowledgments
We thank Michael Arends for editing the manuscript and Etsuko Kamegaya and Yukiko Matsushima for assistance with breeding mice.
Supplementary Material
The Supplementary Material for this article can be found online at: https://www.frontiersin.org/articles/10.3389/fpsyt.2022.821354/full#supplementary-material
References
1. American Psychiatric Association. Diagnostic and Statistical Manual of Mental Disorders, 5th edition. Washington, DC: American Psychiatric Publishing (2013). p. 50–9. doi: 10.1176/appi.books.9780890425596
2. Lai MC, Lombardo MV, Baron-Cohen S. Autism. Lancet. (2014) 383:896–910. doi: 10.1016/S0140-6736(13)61539-1
3. Sato A, Ikeda K. Genetic and environmental contributions to autism spectrum disorder through mechanistic target of rapamycin. Biol Psychiatry Glob Open Sci. (in press). doi: 10.1016/j.bpsgos.2021.08.005
4. Sato A, Kotajima-Murakami H, Tanaka M, Katoh Y, Ikeda K. Influence of prenatal drug exposure, maternal inflammation, and parental aging on the development of autism spectrum disorder. Front Psychiatry. (in press).
5. Ben-Ari Y, Gaiarsa JL, Tyzio R, Khazipov R. GABA: a pioneer transmitter that excites immature neurons and generates primitive oscillations. Physiol Rev. (2007) 87:1215–84. doi: 10.1152/physrev.00017.2006
6. Ben-Ari Y. The GABA excitatory/inhibitory developmental sequence: a personal journey. Neuroscience. (2014) 279:187–219. doi: 10.1016/j.neuroscience.2014.08.001
7. Haydar TF, Wang F, Schwartz ML, Rakic P. Differential modulation of proliferation in the neocortical ventricular and subventricular zones. J Neurosci. (2000) 20:5764–74. doi: 10.1523/JNEUROSCI.20-15-05764.2000
8. Heck N, Kilb W, Reiprich P, Kubota H, Furukawa T, Fukuda A, et al. GABA-A receptors regulate neocortical neuronal migration in vitro and in vivo. Cereb Cortex. (2007) 17:138–48. doi: 10.1093/cercor/bhj135
9. Manent JB, Demarque M, Jorquera I, Pellegrino C, Ben-Ari Y, Aniksztejn L, et al. A noncanonical release of GABA and glutamate modulates neuronal migration. J Neurosci. (2005) 25:4755–65. doi: 10.1523/JNEUROSCI.0553-05.2005
10. Salari AA, Amani M. Neonatal blockade of GABA-A receptors alters behavioral and physiological phenotypes in adult mice. Int J Dev Neurosci. (2017) 57:62–71. doi: 10.1016/j.ijdevneu.2017.01.007
11. Naderipoor P, Amani M, Abedi A, Sakhaie N, Sadegzadeh F, Saadati H. Alterations in the behavior, cognitive function, and BDNF level in adult male rats following neonatal blockade of GABA-A receptors. Brain Res Bull. (2021) 169:35–42. doi: 10.1016/j.brainresbull.2021.01.006
12. Silva MR, Oliveira CA, Felicio LF, Nasello AG, Bernardi MM. Perinatal treatment with picrotoxin induces sexual, behavioral, and neuroendocrine changes in male rats. Pharmacol Biochem Behav. (1998) 60:203–8. doi: 10.1016/S0091-3057(97)00582-0
13. Teodorov E, Habr-Alencar SF, Sider LH, Felicio LF, Varoli FM, Bernardi MM. Prenatal treatment with picrotoxin promotes heterotypical sexual behavioral and neurochemical changes in male rat offspring. Brain Res. (2006) 1069:113–9. doi: 10.1016/j.brainres.2005.11.006
14. Bernardi MM, Kirsten TB, Teodorov E, Baso AC, Prosdocimi FC, Felicio LF. Maternal exposure to picrotoxin modifies the response of the GABA[[sb]]A[[/S]] receptor during sexual behavior of adult male rat offspring. Behav Pharmacol. (2012) 23:703–9. doi: 10.1097/FBP.0b013e3283586072
15. Tochitani S, Furukawa T, Bando R, Kondo S, Ito T, Matsushima Y, et al. GABA[[sb]]A[[/S]] receptors and maternally derived taurine regulate the temporal specification of progenitors of excitatory glutamatergic neurons in the mouse developing cortex. Cereb Cortex. (2021) 31:4554–75. doi: 10.1093/cercor/bhab106
16. Tochitani S, Sakata-Haga H, Fukui Y. Embryonic exposure to ethanol disturbs regulation of mitotic spindle orientation via GABA[[sb]]A[[/S]] receptors in neural progenitors in ventricular zone of developing neocortex. Neurosci Lett. (2010) 472:128–32. doi: 10.1016/j.neulet.2010.01.071
17. Ding R, Tsunekawa N, Obata K. Cleft palate by picrotoxin or 3-MP and palatal shelf elevation in GABA-deficient mice. Neurotoxicol Teratol. (2004) 26:587–92. doi: 10.1016/j.ntt.2004.04.002
18. Kotajima-Murakami H, Kobayashi T, Kashii H, Sato A, Hagino Y, Tanaka M, et al. Effects of rapamycin on social interaction deficits and gene expression in mice exposed to valproic acid in utero. Mol Brain. (2019) 12:3. doi: 10.1186/s13041-018-0423-2
19. Sato A, Kasai S, Kobayashi T, Takamatsu Y, Hino O, Ikeda K, et al. Rapamycin reverses impaired social interaction in mouse models of tuberous sclerosis complex. Nat Commun. (2012) 3:1292. doi: 10.1038/ncomms2295
20. Kupershmidt I, Su QJ, Grewal A, Sundaresh S, Halperin I, Flynn J, et al. Ontology-based meta-analysis of global collections of high-throughput public data. PLoS ONE. (2010) 5:e13066. doi: 10.1371/journal.pone.0013066
21. Langfelder P, Horvath S. WGCNA: an R package for weighted correlation network analysis. BMC Bioinformatics. (2008) 9:559. doi: 10.1186/1471-2105-9-559
22. Hagihara H, Ohira K, Miyakawa T. Transcriptomic evidence for immaturity induced by antidepressant fluoxetine in the hippocampus and prefrontal cortex. Neuropsychopharmacol Rep. (2019) 39:78–89. doi: 10.1002/npr2.12048
23. Peça J, Feliciano C, Ting JT, Wang W, Wells MF, Venkatraman TN, et al. Shank3 mutant mice display autistic-like behaviours and striatal dysfunction. Nature. (2011) 472:437–42. doi: 10.1038/nature09965
24. Sohal VS, Rubenstein JLR. Excitation-inhibition balance as a framework for investigating mechanisms in neuropsychiatric disorders. Mol Psychiatry. (2019) 24:1248–57. doi: 10.1038/s41380-019-0426-0
25. Yizhar O, Fenno LE, Prigge M, Schneider F, Davidson TJ, O'Shea DJ, et al. Neocortical excitation/inhibition balance in information processing and social dysfunction. Nature. (2011) 477:171–8. doi: 10.1038/nature10360
26. Gogolla N, Leblanc JJ, Quast KB, Südhof TC, Fagiolini M, Hensch TK. Common circuit defect of excitatory-inhibitory balance in mouse models of autism. J Neurodev Disord. (2009) 1:172–81. doi: 10.1007/s11689-009-9023-x
27. Cao W, Lin S, Xia QQ, Du YL, Yang Q, Zhang MY, Lu YQ, et al. Gamma oscillation dysfunction in mPFC leads to social deficits in neuroligin 3 R451C knockin mice. Neuron. (2018) 97:1253–60. doi: 10.1016/j.neuron.2018.02.001
28. Nakamura T, Arima-Yoshida F, Sakaue F, Nasu-Nishimura Y, Takeda Y, Matsuura K, et al. PX-RICS-deficient mice mimic autism spectrum disorder in Jacobsen syndrome through impaired GABA[[sb]]A[[/S]] receptor trafficking. Nat Commun. (2016) 7:10861. doi: 10.1038/ncomms10861
29. Tyzio R, Nardou R, Ferrari DC, Tsintsadze T, Shahrokhi A, Eftekhari S, et al. Oxytocin-mediated GABA inhibition during delivery attenuates autism pathogenesis in rodent offspring. Science. (2014) 343:675–9. doi: 10.1126/science.1247190
30. Wicker B, Monfardini E, Royet JP. Olfactory processing in adults with autism spectrum disorders. Mol Autism. (2016) 7:4. doi: 10.1186/s13229-016-0070-3
31. Li Y, Wang R, Qiao N, Peng G, Zhang K, Tang K, et al. Transcriptome analysis reveals determinant stages controlling human embryonic stem cell commitment to neuronal cells. J Biol Chem. (2017) 292:19590–604. doi: 10.1074/jbc.M117.796383
32. Schneider T, Przewtocki R. Behavioral alterations in rats prenatally exposed to valproic acid: animal model of autism. Neuropsychopharmacology. (2005) 30:80–9. doi: 10.1038/sj.npp.1300518
33. Kataoka S, Takuma K, Hara Y, Maeda Y, Ago Y, Matsuda T. Autism-like behaviours with transient histone hyperacetylation in mice treated prenatally with valproic acid. Int J Neuropsychopharmacol. (2013) 16:91–103. doi: 10.1017/S1461145711001714
34. Sanagi T, Sasaki T, Nakagaki K, Minamimoto T, Kohsaka S, Ichinohe N. Segmented Iba1-positive processes of microglia in autism model marmosets. Front Cell Neurosci. (2019) 13:344. doi: 10.3389/fncel.2019.00344
35. Takemoto-Kimura S, Suzuki K, Horigane SI, Kamijo S, Inoue M, Sakamoto M, et al. Calmodulin kinases: essential regulators in health and disease. J Neurochem. (2017) 141:808–18. doi: 10.1111/jnc.14020
36. Durak O, Gao F, Kaeser-Woo YJ, Rueda R, Martorell AJ, Nott A, et al. Chd8 mediates cortical neurogenesis via transcriptional regulation of cell cycle and wnt signaling. Nat Neurosci. (2016) 19:1477–88. doi: 10.1038/nn.4400
37. Bergmann JH Li J, Eckersley-Maslin MA, Rigo F, Freier SM, Spector DL. Regulation of the ESC transcriptome by nuclear long noncoding RNAs. Genome Res. (2015) 25:1336–46. doi: 10.1101/gr.189027.114
38. Cassandri M, Smirnov A, Novelli F, Pitolli C, Agostini M, Malewicz M, et al. Zinc-finger proteins in health and disease. Cell Death Discov. (2017) 3:17071. doi: 10.1038/cddiscovery.2017.71
39. Gana S, Veggiotti P, Sciacca G, Fedeli C, Bersano A, Micieli G, et al. 19q1311 cryptic deletion: description of two new cases and indication for a role of WTIP haploinsufficiency in hypospadias. Eur J Hum Genet. (2012) 20:852–6. doi: 10.1038/ejhg.2012.19
40. The Human Protein Atlas. Available online at: https://www.proteinatlas.org/ENSG00000082074-FYB1/brain (accessed October 28, 2021).
41. Macdonald RL, Kelly KM. Antiepileptic drug mechanisms of action. Epilepsia. (1995) 36(Suppl. 2):S2–12. doi: 10.1111/j.1528-1157.1995.tb05996.x
42. Robertson CE, Baron-Cohen S. Sensory perception in autism. Nat Rev Neurosci. (2017) 18:671–84. doi: 10.1038/nrn.2017.112
43. Kumazaki H, Okamoto M, Yoshimura Y, Ikeda T, Hasegawa C, Saito DN, et al. Brief report: odour awareness in young children with autism spectrum disorders. J Autism Dev Disord. (2020) 50:1809–15. doi: 10.1007/s10803-018-3710-y
44. Muratori F, Tonacci A, Billeci L, Catalucci T, Igliozzi R, Calderoni S, et al. Olfactory processing in male children with autism: atypical odor threshold and identification. J Autism Dev Disord. (2017) 47:3243–51. doi: 10.1007/s10803-017-3250-x
45. Rozenkrantz L, Zachor D, Heller I, Plotkin A, Weissbrod A, Snitz K, et al. A mechanistic link between olfaction and autism spectrum disorder. Curr Biol. (2015) 25:1904–10. doi: 10.1016/j.cub.2015.05.048
46. Endevelt-Shapira Y, Perl O, Ravia A, Amir D, Eisen A, Bezalel V, et al. Altered responses to social chemosignals in autism spectrum disorder. Nat Neurosci. (2018) 21:111–9. doi: 10.1038/s41593-017-0024-x
47. Franco LM, Okray Z, Linneweber GA, Hassan BA, Yaksi E. Reduced lateral inhibition impairs olfactory computations and behaviors in a Drosophila model of Fragile X syndrome. Curr Biol. (2017) 27:1111–23. doi: 10.1016/j.cub.2017.02.065
48. Han S, Tai C, Westenbroek RE Yu FH, Cheah CS, Potter GB, et al. Autistic-like behaviour in Scn1a+/- mice and rescue by enhanced GABA-mediated neurotransmission. Nature. (2012) 489:385–90. doi: 10.1038/nature11356
49. Imamura F, Ayoub AE, Rakic P, Greer CA. Timing of neurogenesis is a determinant of olfactory circuitry. Nat Neurosci. (2011) 14:331–7. doi: 10.1038/nn.2754
50. Bonnycastle K, Davenport EC, Cousin MA. Presynaptic dysfunction in neurodevelopmental disorders: insights from the synaptic vesicle life cycle. J Neurochem. (2021) 157:179–207. doi: 10.1111/jnc.15035
51. Spratt EG, Nicholas JS, Brady KT, Carpenter LA, Hatcher CR, Meekins KA, et al. Enhanced cortisol response to stress in children in autism. J Autism Dev Disord. (2012) 42:75–81. doi: 10.1007/s10803-011-1214-0
52. Taylor JL, Corbett BA. A review of rhythm and responsiveness of cortisol in individuals with autism spectrum disorders. Psychoneuroendocrinology. (2014) 49:207–28. doi: 10.1016/j.psyneuen.2014.07.015
53. Jew CP, Wu CS, Sun H, Zhu J, Huang JY, Yu D, et al. mGlur5 ablation in cortical glutamatergic neurons increases novelty-induced locomotion. PLoS ONE. (2013) 8:e70415. doi: 10.1371/journal.pone.0070415
54. Masiran R. Stimming behaviour in a 4-year-old girl with autism spectrum disorder. BMJ Case Rep. (2018) 23: 223671. doi: 10.1136/bcr-2017-223671
55. Grabrucker S, Haderspeck JC, Sauer AK, Kittelberger N, Asoglu H, Abaei A, et al. Brain lateralization in mice is associated with zinc signaling and altered in prenatal zinc deficient mice that display features of autism spectrum disorder. Front Mol Neurosci. (2018) 10:450. doi: 10.3389/fnmol.2017.00450
56. Trakoshis S, Martinez-Canada P, Rocchi F, Canella C, You W, Chakrabarti B, et al. Intrinsic excitation-inhibition imbalance affects medial prefrontal cortex differently in autistic men versus women. Elife. (2020) 9:e55684. doi: 10.7554/eLife.55684
57. Hara Y, Maeda Y, Kataoka S, Ago Y, Takuma K, Matsuda T. Effect of prenatal valproic acid exposure on cortical morphology in female mice. J Pharmacol Sci. (2012) 118:543–6. doi: 10.1254/jphs.12025SC
58. Sledziowska M, Kalbassi S, Baudouin SJ. Complex interactions between genes and social environment cause phenotypes associated with autism spectrum disorders in mice. eNeuro (2020) 7:ENEURO.0124-20.2020. doi: 10.1523/ENEURO.0124-20.2020
59. Vacher CM, Lacaille H, O'Reilly JJ, Salzbank J, Bakalar D, Sebaoui S, et al. Placental endocrine function shapes cerebellar development and social behavior. Nat Neurosci. (2021) 24:1392–401. doi: 10.1038/s41593-021-00896-4
60. Ferri SL, Abel T, Brodkin ES. Sex differences in autism spectrum disorder: a review. Curr Psychiatry Rep. (2018) 20:9. doi: 10.1007/s11920-018-0874-2
61. Pribilla I, Takagi T, Langosch D, Bormann J, Betz H. The atypical M2 segment of the beta subunit confers picrotoxinin resistance to inhibitory glycine receptor channels. EMBO J. (1992) 11:4305–11. doi: 10.1002/j.1460-2075.1992.tb05529.x
62. Wang DS, Mangin JM, Moonen G, Rigo JM, Legendre P. Mechanisms for picrotoxin block of alpha2 homomeric glycine receptors. J Biol Chem. (2006) 281:3841–55. doi: 10.1074/jbc.M511022200
63. Malosio ML, Marquèze-Pouey B, Kuhse J, Betz H. Widespread expression of glycine receptor subunit mRNAs in the adult and developing rat brain. EMBO J. (1991) 10:2401–9. doi: 10.1002/j.1460-2075.1991.tb07779.x
Keywords: autism spectrum disorder, picrotoxin, GABAA receptor, social interaction, odorant binding, gene expression, microarray, WGCNA
Citation: Kotajima-Murakami H, Hagihara H, Sato A, Hagino Y, Tanaka M, Katoh Y, Nishito Y, Takamatsu Y, Uchino S, Miyakawa T and Ikeda K (2022) Exposure to GABAA Receptor Antagonist Picrotoxin in Pregnant Mice Causes Autism-Like Behaviors and Aberrant Gene Expression in Offspring. Front. Psychiatry 13:821354. doi: 10.3389/fpsyt.2022.821354
Received: 24 November 2021; Accepted: 11 January 2022;
Published: 03 February 2022.
Edited by:
Hideo Matsuzaki, University of Fukui, JapanReviewed by:
Yukio Ago, Hiroshima University, JapanShiro Tochitani, Suzuka University of Medical Science, Japan
Copyright © 2022 Kotajima-Murakami, Hagihara, Sato, Hagino, Tanaka, Katoh, Nishito, Takamatsu, Uchino, Miyakawa and Ikeda. This is an open-access article distributed under the terms of the Creative Commons Attribution License (CC BY). The use, distribution or reproduction in other forums is permitted, provided the original author(s) and the copyright owner(s) are credited and that the original publication in this journal is cited, in accordance with accepted academic practice. No use, distribution or reproduction is permitted which does not comply with these terms.
*Correspondence: Kazutaka Ikeda, aWtlZGEta3pAaWdha3VrZW4ub3IuanA=