- 1Department of Psychology, University of Rome La Sapienza, Rome, Italy
- 2European Center for Brain Research, Santa Lucia Foundation IRCCS, Rome, Italy
- 3Institute for Complex Systems (ISC), National Council of Research (CNR), Rome, Italy
The occurrence of neuropsychiatric symptoms in the elderly is viewed as an early sign of subsequent cognitive deterioration and conversion from mild cognitive impairment to Alzheimer’s disease. The prognosis in terms of both the severity and progression of clinical dementia is generally aggravated by the comorbidity of neuropsychiatric symptoms and decline in cognitive function. Undeniably, aging and in particular unhealthy aging, is a silent “engine of neuropathology” over which multiple changes take place, including drastic alterations of the gut microbial ecosystem. This narrative review evaluates the role of gut microbiota changes as a possible unifying concept through which the comorbidity of neuropsychiatric symptoms and Alzheimer’s disease can be considered. However, since the heterogeneity of neuropsychiatric symptoms, it is improbable to describe the same type of alterations in the bacteria population observed in patients with Alzheimer’s disease, as well as it is improbable that the variety of drugs used to treat neuropsychiatric symptoms might produce changes in gut bacterial diversity similar to that observed in the pathophysiology of Alzheimer’s disease. Depression seems to be another very intriguing exception, as it is one of the most frequent neuropsychiatric symptoms in dementia and a mood disorder frequently associated with brain aging. Antidepressants (i.e., serotonin reuptake inhibitors) or tryptophan dietary supplementation have been shown to reduce Amyloid β-loading, reinstate microbial diversity and reduce the abundance of bacterial taxa dominant in depression and Alzheimer’s disease. This review briefly examines this trajectory by discussing the dysfunction of gut microbiota composition, selected bacterial taxa, and alteration of tryptophan and serotonin metabolism/neurotransmission as overlapping in-common mechanisms involved with depression, Alzheimer’s disease, and unhealthy aging.
Introduction
In aging populations and due to the increasingly prolonged lifespan of people, “brain aging” has become an increasingly important research subject in recent years. Many studies have explored the expanding gap between chronological age and the estimation of brain age, which is attributed to the acceleration of brain aging processes (1). Brain aging and neurodegeneration are leading constructs for the study of dementia, mild cognitive impairment (MCI), and neurological disorders such as Alzheimer’s disease (AD) in the elderly (2, 3). Brain aging is positively associated with late-life depression (LLD) and anxiety disorders in the elderly, for instance, due to the association between depression, oxidative stress, and neuroinflammation (4–6). Nevertheless, brain aging is more pronounced and accelerated in major depressive disorder (MDD), appearing to be a precipitating factor in defective brain morphology, neuronal senescence, and microglial disease (7–9).
This review focuses on the emergence of neuropsychiatric disorders (NPDs) (e.g., depression, apathy, anxiety, irritability, delusions) in patients with declining brain functions from MCI to early dementia and progression to AD (10, 11). Because of the key importance of affective and neuropsychiatric symptoms (A-NPSs) as early predictors of the progression from MCI to AD (10, 12, 13), the present narrative review will account for the comorbidity between NPDs and cognitive deterioration in older people, taking a different perspective by examining gut microbiota (GM) as a driving force contributing to aging and comorbidity between A-NPS and cognitive decline.
Inflammaging, which includes systemic inflammation, immunosenescence, and neuroinflammation, is a “silent killer” of healthy aging. The GM composition in elderly people undergoes pathophysiological changes in terms of reduced diversity of commensal micro-organisms and decreased abundance of beneficial bacterial phyla (14–16). Hence, gut dysbiosis, intestinal inflammation, and microbiota unbalance in aging are interconnected points that connect inflammaging and intestinal immune response to neuroinflammation and alterations of microglial homeostasis as potential underlying factors in A-NPS and dementia comorbidity.
The gut microbial ecosystem across aging, dementia, and neuropsychiatric symptoms
Crosstalk between intestinal microbial homeostasis and host immunity
The human GM is considered to be a “superorganism” because of the impressive number (1014) of commensal micro-organisms it contains (17). It is composed principally of four main bacterial phyla (i.e., Bacteroidetes, Firmicutes, Proteobacteria, and Actinobacteria) but also by Fusobacteria, Verrucomicrobia, and Cyanobacteria phyla as the remaining bacteria population (18) as well as by Archaea, yeasts, fungi, protozoa, metazoans, and viruses (19). The GM exerts multiple functions such as food digestion, absorption, and nutrient extraction by also generating, after bacterial fermentation, bioactive metabolites in the form of short chain fatty acids (SCFAs), and supervising the energy metabolism of the host (20, 21). The GM contributes to immune system development and regulation of the host’s immune homeostasis by modulating the functioning of both innate and adaptive immunity (22, 23). Alterations of GM stability and diversity, and an imbalance of composition can perturb the microbial ecosystem (i.e., dysbiosis) and may severely affect the immune system response and host immunity. These alterations can generate gut inflammation and disrupt the function of the intestinal epithelial barrier, which means higher permeability and loss of mucosal barrier integrity. Although damage to the intestinal barrier can be triggered by diet-derived metabolites, pathogens, and food antigens (24, 25), dysbiosis is also implicated in the dysfunction of mucosal barrier components and integrity (26). Either in the case of the effects of dietary factors on intestinal permeability (24, 25) or in the case of diet-induced dysbiosis (27, 28), the final result is always an elevated risk of systemic inflammation and metabolic endotoxemia with the associated release of antigens, such as lipopolysaccharide (LPS), both in animal models (27, 28) and in patients (29).
In bidirectional gut-brain communication, there is bidirectional crosstalk and reciprocal influence between the immune system and intestinal microbial homeostasis that affects brain physiology. Such an interplay requires the intervention of gut sensors such as pattern recognition receptors (PRRs) (e.g., Toll-like receptors, TLRs), which detect foreign micro-organisms and pathogens by the intestinal epithelial cells (IECs) and by the cells of the innate immune system (30). The production of specific metabolites (such as SCFAs) by commensal bacteria enables the communication between GM and intestinal macrophages (31). Other examples of innate immunity-GM crosstalk come from the role of TLRs and their expression in the intestinal epithelium. The non-specific response to GM antigens and pathogens-associated molecular pattern (PAMP) mediated by PRRs is also paralleled by the crosstalk between antigen-specific adaptive immune responses and GM (32). A case of crosstalk is given by the immunomodulatory polysaccharide A (PSA) produced by the Bacteroides fragilis (B. fragilis), which can be recognized by TLRs and shape the host immune system (33), also interacting with TLR2 on CD4+ T lymphocytes to stimulate T regulatory (Tregs) cells to secrete the anti-inflammatory IL-10 cytokine and prevent gut inflammation and ulcerative colitis (34, 35). Similarly, PSA administration was also shown to confer neuroprotection against encephalomyelitis in a murine model of multiple sclerosis (36). Interestingly, neuroprotection was suppressed in IL-10-deficient mice (36). PSA-treated mice also develop resilience against experimentally induced viral encephalitis and brainstem inflammation via IL-10 secretion by CD4+ and CD8+ Tregs cells (37).
This excursion into the crosstalk between microbial communities, mucosal immune function, and immune response helps us to convey the impact of dysbiosis on host immune homeostasis and accounts for the development of both autoimmune and neurodegenerative diseases, including AD (38). This link is brightly demonstrated by the alleviation of amyloid-β (Aβ) neuropathology in germ-free AD-like APPPS1 mice (39) as well as by the possibility of converting germ-free mice in AD-like mice by transplanting fecal samples from an AD patient (40).
Connecting gut microbial dysbiosis to Alzheimer’s disease pathogenesis
The role of GM in AD is of particular relevance in accounting for Aβ accumulation in the brain of patients diagnosed with sporadic AD. Indeed, some interesting associations have been established between selected bacterial species in the GM of AD patients (e.g., Escherichia/Shigella), amyloidosis, and expression of pro-inflammatory cytokines in the blood such as IL-6, IL-1β, CXCL2, and NLRP3 inflammasome (41). In AD-like APPPS1 mice a positive correlation between Pseudomonas, Odoribacter, Anaerofustis, and brain Aβ deposition was found (39). AD patients and murine models showed alterations in the gut microbial taxa (39–42). Different studies reported the abnormal increase of Gram-negative Bacteroidetes described in AD patients, which is linked to the production of pro-inflammatory transcription factors and neurotoxins that have a deleterious impact on blood-brain barrier (BBB) permeability, Aβ accumulation and chronic inflammatory signaling (42, 43).
The fact that GM dysbiosis can contribute to AD pathogenesis strengthens the idea that sporadic late onset AD (LOAD) may have an infectious etiology is the case for infection driven by neurotropic viruses such as Human herpesvirus 1, bacteria such as Chlamydia pneumoniae, or fungi such as Candida albicans (44–46). However, it is also known that LPS, the major component of a Gram-negative bacteria cell wall, can interact with the TLRs (such as TLR2 and TLR4) expressed on both microglial cells and the intestinal epithelium (47), providing a link between the immune system, PRRs, and the ability to recognize micro-organism-associated molecular patterns. Dysbiosis is responsible for the damage of the mucosal barrier and systemic inflammation, metabolic endotoxemia, and LPS release. Elevated levels of B. fragilis-derived LPS have been found in the hippocampus and neocortex of AD patients (42) as well as overexpression of microglial TLR2, TLR4, and LPS receptor CD14 in both AD patients and AD-like mice models (48, 49). Moreover, neurotoxicity induced by Aβ accumulation is linked to persistent microglia activation that is dependent on the interaction with CD14, TLR2, and TLR4 (50). Aβ interaction with the TLR4/CD14 complex on brain microglia has been suggested as a key mechanism in AD pathogenesis (51).
Another interesting fact is the similar pattern of expression of inflammatory genes triggered by Aβ peptide monomers such as the Aβ42 toxic fibrils or by LPS endotoxin-induced immune response (52). To recognize the link between intestinal bacteria, immune system activation, and AD pathogenesis it is important to understand that amyloid fibrils can be preserved as PAMPs within the biofilms produced by different bacterial phyla such as Bacteroidetes and Proteobacteria (53). For instance, the extracellular matrix of the biofilm of Escherichia coli (E. coli) is largely made for the amyloid curli, which may form amyloid fibrils (54). However, amyloid fibrils can also be generated from other GM members and amyloid-producing bacteria, including Streptococcus, Pseudomonas, Salmonella typhimurium, Mycobacteria, Klebsiella, and Bacillus species (55, 56). Because of dysbiosis and increased permeability of the intestinal barrier, microbial signaling and bacterial amyloidosis can trigger inflammation through the indirect production of pro-inflammatory cytokines and chemokines by immune cells distributed in the lamina propria of the submucosal gut compartment (57). Pro-inflammatory cytokines can access the brain via circulation transport across the BBB (58), which is exacerbated in the case of inflammatory agents or immune-active molecules that contribute to the disruption of BBB tight junctions (59). Moreover, systemic inflammation and peripheral pro-inflammatory cytokine production are processes paralleled by the cytokine production from microglial cells, as demonstrated by the activation of TLR4/CD14 receptors and the resultant increase of NF-κB (50). GM-produced cytokines may reach the brain via the enteric nervous system and the vagus nerve communication pathway (60). In addition, gut bacteria biofilm-derived amyloid fibrils can promote Aβ deposition by cross-seeding with other amyloidogenic proteins. Indeed, E. coli-derived amyloid protein curli was shown to be able to cross-seed with amyloid fibril protein A and aggravate amyloidosis in mice (61).
Dualism between intestinal microbial ecosystem and serotonergic metabolism in the elderly: A “gut- centric” perspective of Alzheimer’s disease
Age progression is a crucial factor determining the composition of the hosted microbiome (62).
The ability to maintain a “healthy microbiota” may determine healthy aging (63). One of the key aspects of the GM transformation across the lifespan is associated with changes occurring during the first 3 years. During this time all the phases of early neurodevelopment can be influenced by GM composition (64, 65), which, together with dietary factors, exogenous stressful events, and exercise, can determine the future pathogenesis of NPDs such as autism spectrum disorder (66, 67). This period is followed by a prolonged phase of stabilization of the GM ecosystem (68). GM diversity decreases with aging, along with a reduction of beneficial species such as Bifidobacteria and the parallel-growing colonies of so-called opportunistic species (e.g., Desulfovibrionaceae) and pathobionts (e.g., Gram-negative Enterobacteriaceae bacteria) contributing to inflammaging (69, 70). Healthy aging is associated with a progressive, but not severe, loss of commensals (e.g., Faecalibacterium, Prevotella, and Bifidobacterium), which are often replaced with the increase of others such as Odoribacter, the family of Christensenellaceae (phylum Firmicutes) and Akkermansia (in particular muciniphila), which are reduced in unhealthy aging (71). Gut colonization by Akkermansia muciniphila (A. muciniphila) may provide anti-inflammatory resilience and maintenance of gut barrier integrity, thus becoming a signature of healthy aging (72). A. muciniphila abundance can be found to be reduced in children with autism spectrum disorder (68, 73). According to some studies, it has emerged that the loss of GM diversity can be balanced by other commensals when individuals become “super-aged,” suggesting that the neuropathological processes underlying neurodegenerative diseases such as AD might be exacerbated when the age-dependent loss of GM diversity is not compensated by beneficial symbiotic bacteria.
An interesting connection between aging, GM senescence, and AD has emerged via investigations of the alterations of the amino acid L-tryptophan (Trp) and its metabolism. GM is essential in the production of different Trp metabolites. Trp is released from some dietary proteins, absorbed by the enterocytes, and made available in the GM as well as in immune and IECs where it exerts regulatory and protective functions against several inflammatory mechanisms (73). In the gut, Trp is responsible for the production of more than 90% of whole body serotonin (5-hydroxytriptamine, 5-HT) in the enterochromaffin cells via the rate-limiting enzyme Trp hydroxylase 1 enzyme (TPH1) (74, 75). Notably, some gut bacteria, and in particular Clostridia, can induce 5-HT production by promoting TPH1 gene expression (76). In addition, other commensals (e.g., Streptococcus thermophilus and Lactobacillus plantarum) are able to use Trp to produce 5-HT (77). To add complexity, 5-HT signaling can affect the immune response and act as an immunomodulator via the 5-HT receptors that have been identified in immune cells (78). The importance of these mechanisms has not only been recognized in different autoimmune disorders (78), but the higher susceptibility of the Aβ peptides released upon multiple challenges such as infection and depression has led to the re-conceptualization of AD itself as an autoimmune disease (79). In this view, Trp metabolites such as 5-HT are identified as effective bioactive molecules against the autoimmune processes triggered by Aβ oligomers (79).
Although peripheral 5-HT cannot cross the BBB, GM can affect central 5-HT synthesis by controlling the availability of Trp and also through different pathways, including microbial metabolites SCFAs (especially butyrate), Trp metabolites (e.g., tryptamine, indolic compounds, L-5-hydroxytryptophan), and by activating 5-HT receptors at terminals of vagal afferents communicating with brainstem neurons (80–82). The reduced 5-HT concentrations in the brains of AD patients (83) support the functional link between Trp metabolism in GM and the role of the brain-gut axis in AD pathogenesis. Decreased fecal Trp metabolites in the 5-HT pathway have been described in MCI and AD patients (84). Interestingly, antidepressants such as the selective 5-HT reuptake inhibitors (SSRIs) citalopram and escitalopram can reduce Aβ aggregates or Aβ plaque load in the cortex and/or hippocampus (85, 86). Remarkably, direct Trp supplementation via dietary intervention, increased 5-HT neurotransmission and 5-HT levels in the hippocampus and frontal cortex, preventing memory decline in aged animals (87). Similarly, high-Trp dietary supplementation was effective in reducing Aβ plaque in transgenic AD-like mice (88). A probiotic dietary supplementation, including S. thermophilus, some bifidobacteria (B. longum, B. breve, B. infantis), and selected lactobacilli (L. acidophilus, L. plantarum, L. paracasei, L. delbrueckii subsp. Bulgaricus, and L. brevis), were demonstrated to reduce Aβ aggregates and partially restore autophagy machinery in AD-like mice (89). Dietary supplementation with the commensal Lactobacillus johnsonii can reduce Trp catabolism and increase 5-HT concentrations in the intestinal ileum (90). In line with age-associated GM alterations and those described in AD patients and AD-like models, long-term dietary supplementation with a probiotic mix containing bifidobacteria (B. lactis and B. bifidum) and lactobacilli (L. casei and L. acidophilus) improved memory deficits and reduced age-associated disruption of BBB and intestinal barrier in a mouse model of accelerated senescence (91). Because of the increased conversion of Trp to kynurenine (Kyn) during immune activation and systemic inflammation, the increase of kynurenine/Trp ratio (KTR) is considered an index of enhanced Trp breakdown, which is increased with age (92) and higher than normal in AD patients (93), whose cognitive performance is inversely correlated to KTR upregulation (94). Along this line, there is to consider the regulatory role exerted by indoleamine 2,3-dioxygenase (IDO), which is the first rate-limiting enzyme initiating Trp catabolism along the Kyn pathway (95). IDO is highly expressed in immune cells and intestinal epithelium, and there is substantial evidence that alterations of Trp metabolism and excessive IDO activity are associated with chronic autoimmune diseases such as inflammatory bowel disease. Of note, there is evidence that the etiology of inflammatory bowel disease is associated with the pathogenetic interaction between the perturbation of GM diversity and the impact of IDO activity on the permeability of the intestinal barrier (96). IDO activity results were abnormally elevated in different chronic inflammatory states, including aging and obesity (97, 98), also producing immunosuppressive effects in different types of cancer and malignant conditions of the gastrointestinal tract (95, 99). A very well-designed study recently provided evidence not only of the association between obesity and an increase in intestinal IDO activity but also that genetic IDO deletion reduced endotoxemia and inflammation (98). In addition to 5-HT, GM bacteria can use Trp to produce indole derivatives or Trp catabolites (e.g., indole lactic acid) that are crucial for the preservation of mucosal barrier integrity via the activation of the aryl hydrocarbon receptor (AhR) (100). Acting as AhR ligands, these Trp-dependent metabolites are fundamental for GM homeostasis and provide higher resilience to intestinal inflammation, including inflammatory bowel disease (101). Notably, the AhR signaling induced by Trp metabolites has been shown to influence astrocyte activity and suppress brain inflammation (102). A decreased abundance of Trp-fermenting intestinal bacteria causing reduced production of Trp-derived indoles has been described in AD patients including, for instance, the decrease of indole-3-propionic acid (IPA)-producing Bacteroides and Firmicutes (103). Interestingly, IPA has shown neuroprotective and anti-oxidative potential against Aβ aggregation (104), and has been causally implicated in AhR upregulation and a parallel decrease of neuroinflammation in AD-like APP/PS1 mice (105).
Moreover, GM diversity can directly affect Trp metabolism and Trp serum levels, as well as alterations in Trp availability, which may have a drastic impact on bacterial abundance. There is a bidirectional interaction between Trp metabolism and the gut bacteria ecosystem, meaning changes in the composition of dietary macronutrients can reshape the abundance of selected bacteria. This is has been described for the consumption of the Mediterranean diet, which leads to an increase of Lachnospiraceae and Trp metabolites such as IPA (106). As a whole, it should also be noted that the control exerted by gut micro-organisms on Trp production and increase of Trp plasma levels (e.g., by B. infantis) (107) can directly influence 5-HT neurotransmission through the gut-brain axis. Indeed, the increase of Trp plasma levels after supplementation with B. infantis in rats is also accompanied by a decrease of 5-hydroxy indole acetic acid in the frontal cortex, which is an index of reduced 5-HT brain degradation.
The GM alterations in both the elderly and AD, together with the GM regulation of Trp metabolism and 5-HT synthesis, can allow us to view the comorbidity between AD-associated cognitive decline and NPSs from a “gut-centric” perspective. Moreover, the extraordinary capacity of the gut-brain axis in determining 5-HT homeostasis further contributes to linking the GM bionetwork to the emergence of NPSs in AD.
Gut microbiota dysbiosis and serotonin neurotransmission dysfunctions: An essential link between Alzheimer’s disease and neuropsychiatric symptoms
Apathy is the most recurrent NPSs in AD, followed by depression, anxiety, and sleep disturbances, while less frequently considered NPSs include irritability, hallucinations, and delusions (108). Depression is a risk factor that significantly increases the possibility of developing AD (109), including the association between LLD and the risk of dementia (110), and AD neuropathology. Thus, the clinical diagnosis and prognosis of dementia and AD are aggravated by depression (111). The use of SSRIs in AD patients is acknowledged to manage not only depressive symptoms but also contribute to preventing the exacerbation of cognitive decline, slowing down disease progression (112), and reducing conversion from MCI to AD (113). In AD-like mice models, SSRI treatment decreased both Aβ levels and Aβ load (85). Moreover, escitalopram administration reduced Aβ42 levels in the cerebrospinal fluid of healthy elderly subjects (114). The use of SSRIs is associated with a decreased rate of dementia/AD development (115). Notably, if the SSRI-based treatment is administered in non-cognitively impaired subjects who previously received a diagnosis of depression, the risk of AD development is either reduced or neutralized (116). In this view, the improvement of AD, the delay of cognitive aggravation, or the reduced risk of AD diagnosis might be (in part) attributed to the effects of SSRI treatment on GM. Indeed, there is robust evidence that antidepressants can shape GM and revert the disparity in gut bacterial diversity associated with depression. Upon improvement of depressive symptoms after escitalopram treatment, the composition of GM tends to resemble that of control subjects (117). The administration of different SSRIs in mice increased gut microbial diversity and reduced the abundance of Ruminococcus, while Ruminococcus (flavefaciens) dietary supplementation reinstated depressive-like behavior and abolished the effects of duloxetine treatment (118). Lately, a multi-omics study reported an elevated abundance of Actinobacteria and the abundance of Ruminococcus as discriminative bacteria of subjects with major depression (119). As for the increase of Ruminococcus and Actinobacteria species in depression, an increased abundance of Actinobacteria and Ruminococcus occurs in AD patients (Figure 1) (120).
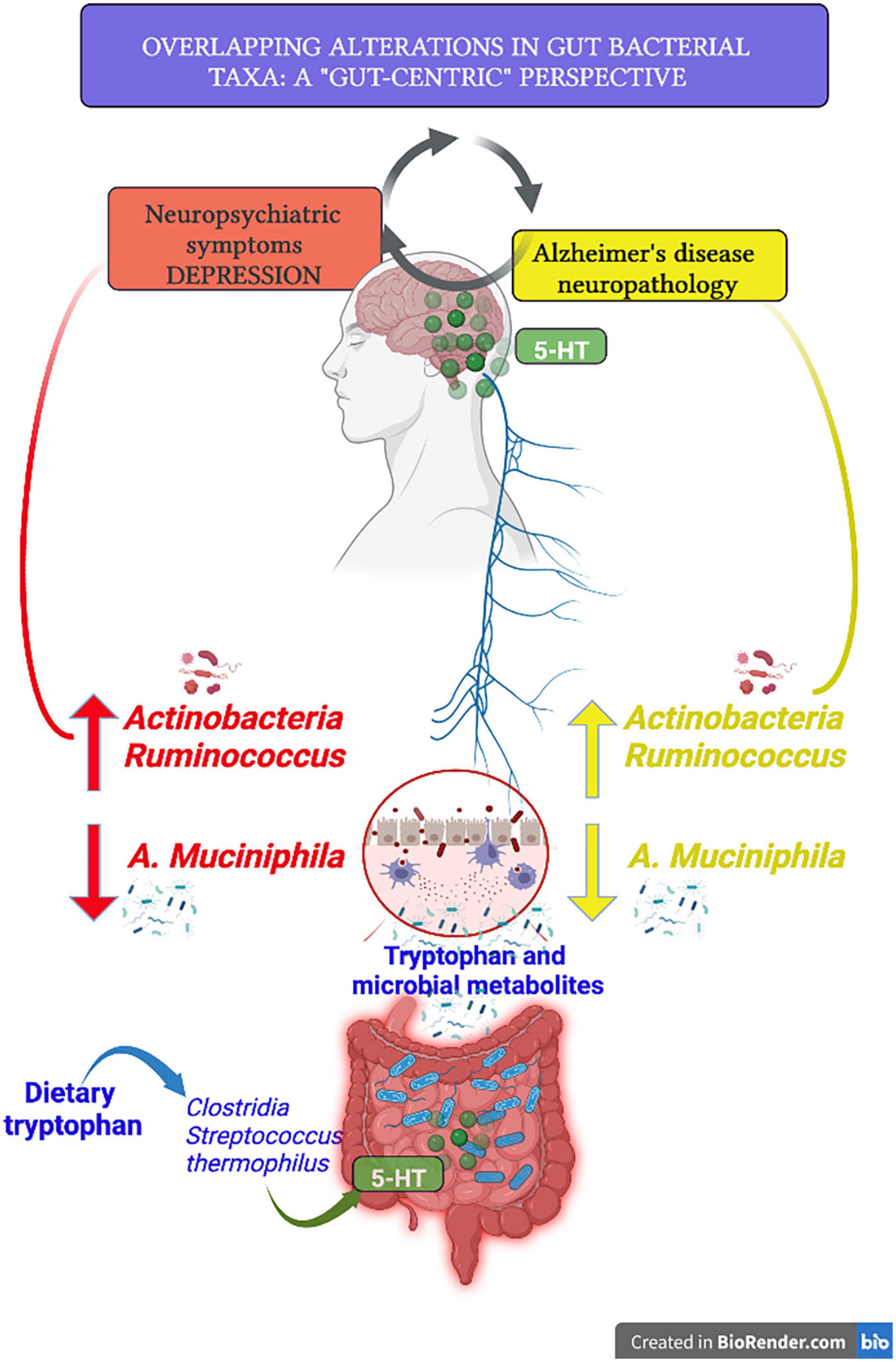
Figure 1. The figure depicts one of the issues reviewed, in particular some selective alterations found in the abundance of gut bacteria taxa in Alzheimer’s disease (AD) patients and in comorbidity with neuropsychiatric symptoms. Both depression and AD have been described as associated with a major increase of Ruminococcus and Actinobacteria and a parallel substantial reduction of gut colonization by Akkermansia Muciniphila. By the use of the dietary source of tryptophan, several gut microbiota (GM) members were recruited for the intestinal production of 5-hydroxytriptamine (5-HT), and multiple microbial (such as butyrate) and tryptophan (such as tryptamine and indolic compounds) metabolites contribute to the modulation of central 5-HT as well as to the activation of 5-HT at terminals of vagal afferents within the brainstem. The image was created by Biorender.com.
As mentioned above, another way through which GM modulates the gut-brain axis is the production in the colon of transmitters (including 5-HT) and metabolites such as the SCFAs acetate, butyrate, and propionic acid. Upon its transport into blood circulation, butyrate exhibits neuroprotective potential contributing to restoring BBB integrity, reducing depression-like behaviors, and increasing brain 5-HT concentration (121). There might be a complex relationship between specific probiotics, SCFAs production, AD biomarkers, depression, and aging. A. muciniphila results increased in super-centenars (122) exerting a protective role of the gut barrier integrity (72), along with intestinal production of propionate and acetate (123). Recently, an improvement of spatial learning and a reduction of Aβ 40–42 levels in the cerebral cortex of APP/PS1 mice after A. muciniphila dietary intervention has been described (124), although a presymptomatic overexpression of A. muciniphila has also been reported in the same mouse model (125). A. muciniphila has been shown to have a beneficial impact on several inflammatory diseases, including obesity, metabolic syndrome, and type 2 diabetes (126). Considering inflammaging, AD, and depression, it is relevant that alterations of A. muciniphila abundance can be recurrent in these conditions (Figure 1) (127). The inverse relationship between A. muciniphila and depression is substantiated by animal models of adrenocorticotrophic hormone (ACTH)-induced, or social defeat-induced, depression- and anhedonic-like behaviors (128, 129). Analyzing the relationship between GM alterations and depression, nine bacteria genera were found to be increased in depressed patients (130). Among these, Klebsiella, Streptococcus, and Lachnospiraceae are progressively lost during aging.
The existence of cell-secreted particles and cell-released extracellular vesicles (EVs) (e.g., exosomes, microvesicles) acting as mediators for cell-to-cell communication has been recently extended to both gram-negative and gram-positive bacteria (131). These microbiota-secreted EVs (MEVs) can bypass (and regulate) the BBB, thus playing a pivotal role in the communication along the gut-brain axis (132) and, in particular, A. muciniphila-derived MEVs have been shown to protect against LPS-induced gut permeability (133) A. muciniphila probiotic supplementation and A. muciniphila-derived MEVs administration have been shown to increase 5-HT-mediated signaling in both the colon and hippocampus (134). The same authors demonstrated that the administration of A. muciniphila and Faecalibacterium prausnitzii (F. prausnitzii) and their derived MEVs, can increase both 5-HT levels and 5-HT transmission either potentiating the expression of genes involved in 5-HT synthesis in the enterochromaffin cells (e.g., Tph1 gene) or inhibiting genes involved in 5-HT degradation (e.g., MaoA gene) (135).
Despite the robust link between GM homeostasis and 5-HT signaling, several mechanistic explanations underlying AD and depression comorbidity have been suggested in response to the importance of other signaling systems for AD pathogenesis, such as the excitatory glutamatergic system (136) and the remodeling of the inhibitory gamma-aminobutyric acid (GABA) circuits occurring during both the early and later stages of disease progression (137). Within this context, it is important to emphasize that alterations of GABA and glutamate signaling systems are associated with changes in selected GM members. For instance, a specific dietary intervention (i.e., ketogenic diet) was shown to increase the abundance of A. muciniphila and Parabacteroides and create a parallel increase in GABA/glutamate ratios in the hippocampus (138). Some bacterial strains can produce glutamate and convert it to D-glutamate, and these plasma levels are defective in AD patients (139).
Altogether, the dysfunction of Trp metabolism and 5-HT signaling driven by gut microbial dysbiosis and alteration of microbial diversity, or the significant changes in the abundance of selected bacteria, can help to account for the risk of AD neuropathology in the elderly, acting as a unifying concept underlying the incidence of comorbid cognitive decline and A-NPS (e.g., depression) in AD. The recognition of the importance that changes in 5-HT signaling and gut microbial ecosystem may have for the understanding of AD and depression comorbidity can also contribute to refining and potentiating diagnostic tools and the different options of intervention. Intriguingly, irritable bowel syndrome, enteropathies, and dysbiosis are frequent medical co-morbid conditions in patients with A-NPS and depression (140, 141). Hence, significant dietary modifications and personalized probiotic supplementation can be powerful tools of intervention in shaping GM composition. Awareness of this potential should be permanently introduced into clinical practice as a disease-modifying option during the initial stages of AD progression and symptoms of depression.
Author contributions
RC conceived the study. RC and DC wrote the manuscript, which was revised by DD. DC, DD, and GG prepared the images. All authors critically discussed the manuscript.
Funding
This study was supported by research funding to DC from Ateneo 2021 (RG12117A5C2F8800), University “Sapienza” of Rome; Young Researcher Grant, Italian Ministry of Health (GR-2019-12370446).
Acknowledgments
We thank the European Center for Brain Research, Santa Lucia Foundation IRCCS for providing support to GG.
Conflict of interest
The authors declare that the research was conducted in the absence of any commercial or financial relationships that could be construed as a potential conflict of interest.
Publisher’s note
All claims expressed in this article are solely those of the authors and do not necessarily represent those of their affiliated organizations, or those of the publisher, the editors and the reviewers. Any product that may be evaluated in this article, or claim that may be made by its manufacturer, is not guaranteed or endorsed by the publisher.
References
1. Wrigglesworth J, Ward P, Harding IH, Nilaweera D, Wu Z, Woods RL, et al. Factors associated with brain ageing - a systematic review. BMC Neurol. (2021) 21:312. doi: 10.1186/s12883-021-02331-4
2. Azam S, Haque ME, Balakrishnan R, Kim IS, Choi DK. The ageing brain: molecular and cellular basis of neurodegeneration. Front Cell Dev Biol. (2021) 9:683459. doi: 10.3389/fcell.2021.683459
3. Hou Y, Dan X, Babbar M, Wei Y, Hasselbalch SG, Croteau DL, et al. Ageing as a risk factor for neurodegenerative disease. Nat Rev Neurol. (2019) 15:565–81. doi: 10.1038/s41582-019-0244-7
4. Wolkowitz OM, Mellon SH, Epel ES, Lin J, Dhabhar FS, Su Y, et al. Leukocyte telomere length in major depression: correlations with chronicity, inflammation and oxidative stress - preliminary findings. PLoS One. (2011) 6:17837. doi: 10.1371/journal.pone.0017837
5. Bewernick BH, Schlaepfer TE. Chronic depression as a model disease for cerebral aging. Dialogues Clin Neurosci. (2013) 15:77–85. doi: 10.31887/DCNS.2013.15.1/bbewernick
6. Perna G, Iannone G, Alciati A, Caldirola D. Are anxiety disorders associated with accelerated aging? A focus on neuroprogression. Neural Plast. (2016) 2016:8457612. doi: 10.1155/2016/8457612
7. Han LKM, Dinga R, Hahn T, Ching CRK, Eyler LT, Aftanas L, et al. Brain aging in major depressive disorder: results from the ENIGMA major depressive disorder working group. Mol Psychiatry. (2021) 26:754. doi: 10.1038/s41380-020-0754-0
8. Ballester PL, Suh JS, Nogovitsyn N, Hassel S, Strother SC, Arnott SR, et al. Accelerated brain aging in major depressive disorder and antidepressant treatment response: a CAN-BIND report. NeuroImage Clin. (2021) 32:102864. doi: 10.1016/j.nicl.2021.102864
9. Jia X, Gao Z, Hu H. Microglia in depression: current perspectives. Sci China Life Sci. (2021) 64:911–25. doi: 10.1007/s11427-020-1815-6
10. Spalletta G, Musicco M, Padovani A, Rozzini L, Perri R, Fadda L, et al. Neuropsychiatric symptoms and syndromes in a large cohort of newly diagnosed, untreated patients with Alzheimer disease. Am J Geriatr Psychiatry. (2010) 18:1026–35. doi: 10.1097/JGP.0b013e3181d6b68d
11. Fernandez-Martinez M, Molano A, Castro J, Zarranz JJ. Prevalence of neuropsychiatric symptoms in mild cognitive impairment and Alzheimer’s disease, and its relationship with cognitive impairment. Curr Alzheimer Res. (2010) 999:2050. doi: 10.2174/1567210204558622050
12. Escudero JMS, Beltrán J, Palacios Á, Chimbí CM, Matallana D, Reyes P, et al. Neuropsychiatric symptoms as predictors of clinical course in neurodegeneration. A longitudinal study. Front Aging Neurosci. (2019) 10:176. doi: 10.3389/fnagi.2019.00176
13. Palmer K, Di Iulio F, Varsi AE, Gianni W, Sancesario G, Caltagirone C, et al. Neuropsychiatric predictors of progression from amnestic - mild cognitive impairment to Alzheimer’s disease: the role of depression and apathy. J Alzheimer’s Dis. (2010) 20:1352. doi: 10.3233/JAD-2010-1352
14. Van Tongeren SP, Slaets JPJ, Harmsen HJM, Welling GW. Fecal microbiota composition and frailty. Appl Environ Microbiol. (2005) 71:2005. doi: 10.1128/AEM.71.10.6438-6442.2005
15. Langille MGI, Meehan CJ, Koenig JE, Dhanani AS, Rose RA, Howlett SE, et al. Microbial shifts in the aging mouse gut. Microbiome. (2014) 2:50. doi: 10.1186/s40168-014-0050-9
16. Li H, Ni J, Qing H. Gut microbiota: critical controller and intervention target in brain aging and cognitive impairment. Front Aging Neurosci. (2021) 13:671142. doi: 10.3389/fnagi.2021.671142
17. Thursby E, Juge N. Introduction to the human gut microbiota. Biochem J. (2017) 474:823–1836. doi: 10.1042/BCJ20160510
18. Ghosh S, Pramanik S. Structural diversity, functional aspects and future therapeutic applications of human gut microbiome. Arch Microbiol. (2021) 203:5281–308. doi: 10.1007/s00203-021-02516-y
19. Kodio A, Menu E, Ranque S. Eukaryotic and prokaryotic microbiota interactions. Microorganisms. (2020) 8:2018. doi: 10.3390/microorganisms8122018
20. Rinninella E, Raoul P, Cintoni M, Franceschi F, Miggiano GAD, Gasbarrini A, et al. What is the healthy gut microbiota composition? A changing ecosystem across age, environment, diet, and diseases. Microorganisms. (2019) 7:7010014. doi: 10.3390/microorganisms7010014
21. Heiss CN, Olofsson LE. Gut microbiota-dependent modulation of energy metabolism. J Innate Immun. (2018) 10:163–71. doi: 10.1159/000481519
22. Zheng D, Liwinski T, Elinav E. Interaction between microbiota and immunity in health and disease. Cell Res. (2020) 30:492–506. doi: 10.1038/s41422-020-0332-7
23. Slack E, Hapfelmeier S, Stecher B, Velykoredko Y, Stoel M, Lawson MAE, et al. Innate and adaptive immunity cooperate flexibly to maintain host-microbiota mutualism. Science. (2009) 325:1172747. doi: 10.1126/science.1172747
24. Khoshbin K, Camilleri M. Effects of dietary components on intestinal permeability in health and disease. Am J Physiol Gastrointest Liver Physiol. (2020) 319:G589–608. doi: 10.1152/ajpgi.00245.2020
25. Maurice CF, Haiser HJ, Turnbaugh PJ. Xenobiotics Shape the physiology and gene expression of the active human gut microbiome. Cell. (2013) 152:52. doi: 10.1016/j.cell.2012.10.052
26. Levy M, Kolodziejczyk AA, Thaiss CA, Elinav E. Dysbiosis and the immune system. Nat Rev Immunol. (2017) 2017:17. doi: 10.1038/nri.2017.7
27. Cani PD, Neyrinck AM, Fava F, Knauf C, Burcelin RG, Tuohy KM, et al. Selective increases of bifidobacteria in gut microflora improve high-fat-diet-induced diabetes in mice through a mechanism associated with endotoxaemia. Diabetologia. (2007) 50:791. doi: 10.1007/s00125-007-0791-0
28. Daniel H, Gholami AM, Berry D, Desmarchelier C, Hahne H, Loh G, et al. High-fat diet alters gut microbiota physiology in mice. ISME J. (2014) 8:155. doi: 10.1038/ismej.2013.155
29. Lyte JM, Gabler NK, Hollis JH. Postprandial serum endotoxin in healthy humans is modulated by dietary fat in a randomized, controlled, cross-over study. Lipids Health Dis. (2016) 15:357. doi: 10.1186/s12944-016-0357-6
30. Keogh CE, Rude KM, Gareau MG. Role of pattern recognition receptors and the microbiota in neurological disorders. J Physiol. (2021) 599:1379–89. doi: 10.1113/JP279771
31. Chang PV, Hao L, Offermanns S, Medzhitov R. The microbial metabolite butyrate regulates intestinal macrophage function via histone deacetylase inhibition. Proc Natl Acad Sci U.S.A. (2014) 111:69111. doi: 10.1073/pnas.1322269111
32. Honda K, Littman DR. The microbiota in adaptive immune homeostasis and disease. Nature. (2016) 535:75–84. doi: 10.1038/nature18848
33. Mazmanian SK, Cui HL, Tzianabos AO, Kasper DL. An immunomodulatory molecule of symbiotic bacteria directs maturation of the host immune system. Cell. (2005) 122:7. doi: 10.1016/j.cell.2005.05.007
34. Round JL, Lee SM, Li J, Tran G, Jabri B, Chatila TA, et al. The toll-like receptor 2 pathway establishes colonization by a commensal of the human microbiota. Science. (2011) 2011:1206095. doi: 10.1126/science.1206095
35. Chang YC, Ching YH, Chiu CC, Liu JY, Hung SW, Huang WC, et al. TLR2 and interleukin-10 are involved in bacteroides fragilis-mediated prevention of DSS-induced colitis in gnotobiotic mice. PLoS One. (2017) 12:e180025. doi: 10.1371/journal.pone.0180025
36. Ochoa-Repáraz J, Mielcarz DW, Wang Y, Begum-Haque S, Dasgupta S, Kasper DL, et al. A polysaccharide from the human commensal bacteroides fragilis protects against CNS demyelinating disease. Mucosal Immunol. (2010) 35:487–95. doi: 10.1038/mi.2010.29
37. Ramakrishna C, Kujawski M, Chu H, Li L, Mazmanian SK, Cantin EM. Bacteroides fragilis polysaccharide a induces IL-10 secreting B and T cells that prevent viral encephalitis. Nat Commun. (2019) 10:9884. doi: 10.1038/s41467-019-09884-6
38. Main BS, Minter MR. Microbial immuno-communication in neurodegenerative diseases. Front Neurosci. (2017) 11:151. doi: 10.3389/fnins.2017.00151
39. Harach T, Marungruang N, Duthilleul N, Cheatham V, Mc Coy KD, Frisoni G, et al. Reduction of abeta amyloid pathology in APPPS1 transgenic mice in the absence of gut microbiota. Sci Rep. (2017) 7:1–15. doi: 10.1038/srep41802
40. Fujii Y, Nguyen TTT, Fujimura Y, Kameya N, Nakamura S, Arakawa K, et al. Fecal metabolite of a gnotobiotic mouse transplanted with gut microbiota from a patient with Alzheimer’s disease. OUP. (2019) 83:2144–52. doi: 10.1080/09168451.2019.1644149
41. Cattaneo A, Cattane N, Galluzzi S, Provasi S, Lopizzo N, Festari C, et al. Association of brain amyloidosis with pro-inflammatory gut bacterial taxa and peripheral inflammation markers in cognitively impaired elderly. Neurobiol Aging. (2017) 49:19. doi: 10.1016/j.neurobiolaging.2016.08.019
42. Zhao Y, Jaber V, Lukiw WJ. Secretory products of the human gi tract microbiome and their potential impact on Alzheimer’s disease (AD): detection of lipopolysaccharide (LPS) in AD hippocampus. Front Cell Infect Microbiol. (2017) 7:318. doi: 10.3389/fcimb.2017.00318
43. Jiang C, Li G, Huang P, Liu Z, Zhao B. The gut microbiota and Alzheimer’s disease. J. Alzheimer’s Dis. (2017) 58:1–15. doi: 10.3233/JAD-161141
44. Sochocka M, Zwolińska K, Leszek J. The infectious etiology of Alzheimer’s disease. Curr Neuropharmacol. (2017) 15:996–1009. doi: 10.2174/1570159X15666170313122937
45. Ashraf GM, Tarasov VV, Makhmutovà A, Chubarev VN, Avila-Rodriguez M, Bachurin SO, et al. The possibility of an infectious etiology of Alzheimer disease. Mol Neurobiol. (2019) 56:4479–91. doi: 10.1007/s12035-018-1388-y
46. Chacko A, Delbaz A, Walkden H, Basu S, Armitage CW, Eindorf T, et al. Chlamydia pneumoniae can infect the central nervous system via the olfactory and trigeminal nerves and contributes to Alzheimer’s disease risk. Sci Rep. (2022) 12:749. doi: 10.1038/s41598-022-06749-9
47. Abreu MT. Toll-like receptor signalling in the intestinal epithelium: how bacterial recognition shapes intestinal function. Nat Rev Immunol. (2010) 10:131–44. doi: 10.1038/nri2707
48. Letiembre M, Liu Y, Walter S, Hao W, Pfander T, Wrede A, et al. Screening of innate immune receptors in neurodegenerative diseases: a similar pattern. Neurobiol Aging. (2009) 30:18. doi: 10.1016/j.neurobiolaging.2007.08.018
49. Dallas ML, Widera D. TLR2 and TLR4-mediated inflammation in Alzheimer’s disease: self-defense or sabotage? Neural Regen Res. (2021) 16:1552–3. doi: 10.4103/1673-5374.303016
50. Reed-Geaghan EG, Savage JC, Hise AG, Landreth GE. CD14 and toll-like receptors 2 and 4 are required for fibrillar Aβ-stimulated microglial activation. J. Neurosci. (2009) 29:2009. doi: 10.1523/JNEUROSCI.3158-09.2009
51. Banks WA, Gray AM, Erickson MA, Salameh TS, Damodarasamy M, Sheibani N, et al. Lipopolysaccharide-induced blood-brain barrier disruption: roles of cyclooxygenase, oxidative stress, neuroinflammation, and elements of the neurovascular unit. J Neuroinflam. (2015) 15:434. doi: 10.1186/s12974-015-0434-1
52. Ferrera D, Mazzaro N, Canale C, Gasparini L. Resting microglia react to Aβ42 fibrils but do not detect oligomers or oligomer-induced neuronal damage. Neurobiol Aging. (2014) 35:23. doi: 10.1016/j.neurobiolaging.2014.05.023
53. Larsen P, Nielsen JL, Dueholm MS, Wetzel R, Otzen D, Nielsen PH. Amyloid adhesins are abundant in natural biofilms. Environ Microbiol. (2007) 9:1418. doi: 10.1111/j.1462-2920.2007.01418.x
54. Chapman MR, Robinson LS, Pinkner JS, Roth R, Heuser J, Hammar M, et al. Role of Escherichia Coli curli operons in directing amyloid fiber formation. Science. (2002) 295:1067484. doi: 10.1126/science.1067484
55. Dueholm MS, Petersen SV, Sønderkær M, Larsen P, Christiansen G, Hein KL, et al. Functional amyloid in Pseudomonas. Mol Microbiol. (2010) 77:7269. doi: 10.1111/j.1365-2958.2010.07269.x
56. Friedland RP, Chapman MR. The role of microbial amyloid in neurodegeneration. PLoS Pathog. (2017) 13:e1006654. doi: 10.1371/journal.ppat.1006654
57. Hine AM, Loke P. Intestinal macrophages in resolving inflammation. J Immunol. (2019) 203:345. doi: 10.4049/jimmunol.1900345
58. Banks W. Blood-brain barrier transport of cytokines: a mechanism for neuropathology. Curr Pharm Des. (2005) 11:1684. doi: 10.2174/1381612053381684
59. Banks WA. The blood-brain barrier in neuroimmunology: tales of separation and assimilation. Brain Behav Immun. (2015) 44:1–8. doi: 10.1016/j.bbi.2014.08.007
60. Zanos TP, Silverman HA, Levy T, Tsaava T, Battinelli E, Lorraine PW, et al. Identification of cytokine-specific sensory neural signals by decoding murine vagus nerve activity. Proc Natl Acad Sci U.S.A. (2018) 115:3115. doi: 10.1073/pnas.1719083115
61. Lundmark K, Westermark GT, Olsén A, Westermark P. Protein fibrils in nature can enhance amyloid protein a amyloidosis in mice: cross-seeding as a disease mechanism. Proc Natl Acad Sci U.S.A. (2005) 102:4102. doi: 10.1073/pnas.0501814102
62. Xu C, Zhu H, Qiu P. Aging progression of human gut microbiota. BMC Microbiol. (2019) 19:1616. doi: 10.1186/s12866-019-1616-2
63. Kim S, Jazwinski SM. The gut microbiota and healthy aging: a mini-review. Gerontology. (2018) 64:513–20. doi: 10.1159/000490615
64. Schächtle MA, Rosshart SP. The microbiota-gut-brain axis in health and disease and its implications for translational research. Front Cell Neurosci. (2021) 15:698172. doi: 10.3389/fncel.2021.698172
65. Laue HE, Coker MO, Madan JC. The developing microbiome from birth to 3 years: the gut-brain axis and neurodevelopmental outcomes. Front Pediatr. (2022) 10:254. doi: 10.3389/fped.2022.815885
66. Marrone MC, Coccurello R. Dietary fatty acids and microbiota-brain communication in neuropsychiatric diseases. Biomolecules. (2020) 10:10012. doi: 10.3390/biom10010012
67. Warner BB. The contribution of the gut microbiome to neurodevelopment and neuropsychiatric disorders. Pediatr Res. (2019) 85:216–24. doi: 10.1038/s41390-018-0191-9
68. Faith JJ, Guruge JL, Charbonneau M, Subramanian S, Seedorf H, Goodman AL, et al. The long-term stability of the human gut microbiota. Science. (2013) 341:439. doi: 10.1126/science.1237439
69. Kumar M, Babaei P, Ji B, Nielsen J. Human gut microbiota and healthy aging: recent developments and future prospective. Nutr Heal Aging. (2016) 4:150002. doi: 10.3233/NHA-150002
70. Bosco N, Noti M. The aging gut microbiome and its impact on host immunity. Genes Immun. (2021) 22:289–303. doi: 10.1038/s41435-021-00126-8
71. Ghosh TS, Shanahan F, O’Toole PW. The gut microbiome as a modulator of healthy ageing. Nat Rev Gastroenterol Hepatol. (2022) 2022:1–20. doi: 10.1038/s41575-022-00605-x
72. Everard A, Belzer C, Geurts L, Ouwerkerk JP, Druart C, Bindels LB, et al. Cross-talk between akkermansia muciniphila and intestinal epithelium controls diet-induced obesity. Proc Natl Acad Sci U.S.A. (2013) 110:9066–71. doi: 10.1073/pnas.1219451110
73. Ma N, Ma X. Dietary amino acids and the gut-microbiome-immune axis: physiological metabolism and therapeutic prospects. Compr Rev Food Sci Food Saf. (2019) 18:221–42. doi: 10.1111/1541-4337.12401
74. Kennedy PJ, Cryan JF, Dinan TG, Clarke G. Kynurenine pathway metabolism and the microbiota-gut-brain axis. Neuropharmacology. (2017) 112:399–412. doi: 10.1016/j.neuropharm.2016.07.002
75. Agus A, Planchais J, Sokol H. Gut microbiota regulation of tryptophan metabolism in health and disease. Cell Host Microbe. (2018) 23:716–24. doi: 10.1016/j.chom.2018.05.003
76. Yano JM, Yu K, Donaldson GP, Shastri GG, Ann P, Ma L, et al. Indigenous bacteria from the gut microbiota regulate host serotonin biosynthesis. Cell. (2015) 161:47. doi: 10.1016/j.cell.2015.02.047
77. Özoǧul F, Kuley E, Özoǧul Y, Özoǧul I. The function of lactic acid bacteria on biogenic amines production by food-borne pathogens in arginine decarboxylase broth. Food Sci Technol Res. (2012) 18:795. doi: 10.3136/fstr.18.795
78. Wan M, Ding L, Wang D, Han J, Gao P. Serotonin: a potent immune cell modulator in autoimmune diseases. Front Immunol. (2020) 11:716–24.
79. Meier-Stephenson FS, Meier-Stephenson VC, Carter MD, Meek AR, Wang Y, Pan L, et al. Alzheimer’s disease as an autoimmune disorder of innate immunity endogenously modulated by tryptophan metabolites. Alzheimer’s dement. Transl Res Clin Interv. (2022) 8:e12283. doi: 10.1002/trc2.12283
80. Gao K, Mu CL, Farzi A, Zhu WY. Tryptophan metabolism: a link between the gut microbiota and brain. Adv Nutr. (2020) 11:709–23. doi: 10.1093/advances/nmz127
81. Lukiæ I, Ivkoviæ S, Mitiæ M, Adžiæ M. Tryptophan metabolites in depression: modulation by gut microbiota. Front Behav Neurosci. (2022) 16:367. doi: 10.3389/fnbeh.2022.987697
82. Roth W, Zadeh K, Vekariya R, Ge Y, Mohamadzadeh M. Tryptophan metabolism and gut-brain homeostasis. Int J Mol Sci. (2021) 22:2973. doi: 10.3390/ijms22062973
83. Palmer AM, Wilcock GK, Esiri MM, Francis PT, Bowen DM. Monoaminergic innervation of the frontal and temporal lobes in Alzheimer’s disease. Brain Res. (1987) 401:408. doi: 10.1016/0006-8993(87)91408-9
84. Wu L, Han Y, Zheng Z, Peng G, Liu P, Yue S, et al. Altered gut microbial metabolites in amnestic mild cognitive impairment and alzheimer’s disease: signals in host–microbe interplay. Nutrients. (2021) 13:228. doi: 10.3390/nu13010228
85. Cirrito JR, Wallace CE, Yan P, Davis TA, Gardiner WD, Doherty BM, et al. Effect of escitalopram on Aβ levels and plaque load in an alzheimer mouse model. Neurology. (2020) 95:10733. doi: 10.1212/WNL.0000000000010733
86. Reddy AP, Sawant N, Morton H, Kshirsagar S, Bunquin LE, Yin X, et al. Selective serotonin reuptake inhibitor citalopram ameliorates cognitive decline and protects against amyloid beta-induced mitochondrial dynamics, biogenesis, autophagy, mitophagy and synaptic toxicities in a mouse model of Alzheimer’s disease. Hum Mol Genet. (2021) 30:91. doi: 10.1093/hmg/ddab091
87. Musumeci G, Castrogiovanni P, Castorina S, Imbesi R, Szychlinska MA, Scuderi S, et al. Changes in serotonin (5-HT) and brain-derived neurotrophic factor (BDFN) expression in frontal cortex and hippocampus of aged rat treated with high tryptophan diet. Brain Res Bull. (2015) 119:10. doi: 10.1016/j.brainresbull.2015.09.010
88. Noristani HN, Verkhratsky A, Rodríguez JJ. High tryptophan diet reduces CA1 intraneuronal β-amyloid in the triple transgenic mouse model of Alzheimer’s disease. Aging Cell. (2012) 11:845. doi: 10.1111/j.1474-9726.2012.00845.x
89. Bonfili L, Cecarini V, Berardi S, Scarpona S, Suchodolski JS, Nasuti C, et al. Microbiota modulation counteracts Alzheimer’s disease progression influencing neuronal proteolysis and gut hormones plasma levels. Sci. Rep. (2017) 7:587. doi: 10.1038/s41598-017-02587-2
90. Valladares R, Bojilova L, Potts AH, Cameron E, Gardner C, Lorca G, et al. Lactobacillus johnsonii inhibits indoleamine 2,3-dioxygenase and alters tryptophan metabolite levels in biobreeding rats. FASEB J. (2013) 27:339. doi: 10.1096/fj.12-223339
91. Yang X, Yu D, Xue L, Li H, Du J. Probiotics modulate the microbiota–gut–brain axis and improve memory deficits in aged SAMP8 mice. Acta Pharm Sin B. (2020) 10:475–87. doi: 10.1016/j.apsb.2019.07.001
92. Theofylaktopoulou D, Midttun Ø, Ulvik A, Ueland PM, Tell GS, Vollset SE. A community-based study on determinants of circulating markers of cellular immune activation and kynurenines: the hordaland health study. Clin Exp Immunol. (2013) 173:121–30. doi: 10.1111/cei.12092
93. Almulla AF, Supasitthumrong T, Amrapala A, Tunvirachaisakul C, Jaleel AK, Oxenkrug G, et al. The tryptophan catabolite or kynurenine pathway in Alzheimer’s disease: a systematic review and meta-analysis. medRxiv [preprint]. (2022): doi: 10.1101/2022.03.18.22272608
94. Widner B, Leblhuber F, Walli J, Tilz GP, Demel U, Fuchs D. Tryptophan degradation and immune activation in Alzheimer’s disease. J Neural Transm. (2000) 107:29. doi: 10.1007/s007020050029
95. Bilir C, Sarisozen C. Indoleamine 2,3-dioxygenase (IDO): only an enzyme or a checkpoint controller? J Oncol Sci. (2017) 32:52–6. doi: 10.1016/j.jons.2017.04.001
96. Matsuoka K, Kanai T. The gut microbiota and inflammatory bowel disease. Semin Immunopathol. (2015) 37:47–55. doi: 10.1007/s00281-014-0454-4
97. Salminen A. Role of indoleamine 2,3-dioxygenase 1 (IDO1) and kynurenine pathway in the regulation of the aging process. Ageing Res Rev. (2022) 75:101573. doi: 10.1016/j.arr.2022.101573
98. Laurans L, Venteclef N, Haddad Y, Chajadine M, Alzaid F, Metghalchi S, et al. Genetic deficiency of indoleamine 2,3-dioxygenase promotes gut microbiota-mediated metabolic health. Nat Med. (2018) 24:60. doi: 10.1038/s41591-018-0060-4
99. Acovic A, Gazdic M, Jovicic N, Harrell CR, Fellabaum C, Arsenijevic N, et al. Role of indoleamine 2,3-dioxygenase in pathology of the gastrointestinal tract. Therap. Adv Gastroenterol. (2018) 11:1756284818815334. doi: 10.1177/1756284818815334
100. Roager HM, Licht TR. Microbial tryptophan catabolites in health and disease. Nat Commun. (2018) 9:3294. doi: 10.1038/s41467-018-05470-4
101. Dong F, Perdew GH. The aryl hydrocarbon receptor as a mediator of host-microbiota interplay. Gut Microbes. (2020) 12:1859812. doi: 10.1080/19490976.2020.1859812
102. Rothhammer V, Mascanfroni ID, Bunse L, Takenaka MC, Kenison JE, Mayo L, et al. Type i interferons and microbial metabolites of tryptophan modulate astrocyte activity and central nervous system inflammation via the aryl hydrocarbon receptor. Nat Med. (2016) 22:4106. doi: 10.1038/nm.4106
103. Vogt NM, Kerby RL, Dill-McFarland KA, Harding SJ, Merluzzi AP, Johnson SC, et al. Gut microbiome alterations in Alzheimer’s disease. Sci. Rep. (2017) 7:13601. doi: 10.1038/s41598-017-13601-y
104. Bendheim PE, Poeggeler B, Neria E, Ziv V, Pappolla MA, Chain DG. Development of indole-3-propionic acid (OXIGONTM) for Alzheimer’s disease. J Mol Neurosci. (2002) 19:36. doi: 10.1007/s12031-002-0036-0
105. Sun J, Zhang Y, Kong Y, Ye T, Yu Q, Kumaran Satyanarayanan S, et al. Microbiota-derived metabolite indoles induced aryl hydrocarbon receptor activation and inhibited neuroinflammation in APP/PS1 mice. Brain Behav Immun. (2022) 106:76–88. doi: 10.1016/j.bbi.2022.08.003
106. Zhu C, Sawrey-Kubicek L, Beals E, Rhodes CH, Houts HE, Sacchi R, et al. Human gut microbiome composition and tryptophan metabolites were changed differently by fast food and mediterranean diet in 4 days: a pilot study. Nutr Res. (2020) 77:5. doi: 10.1016/j.nutres.2020.03.005
107. Desbonnet L, Garrett L, Clarke G, Bienenstock J, Dinan TG. The probiotic bifidobacteria infantis: an assessment of potential antidepressant properties in the rat. J Psychiatr Res. (2008) 43:164–74. doi: 10.1016/j.jpsychires.2008.03.009
108. Zhao QF, Tan L, Wang HF, Jiang T, Tan MS, Tan L, et al. The prevalence of neuropsychiatric symptoms in Alzheimer’s disease: systematic review and meta-analysis. J Affect Disord. (2016) 190:69. doi: 10.1016/j.jad.2015.09.069
109. Sáiz-Vázquez O, Gracia-García P, Ubillos-Landa S, Puente-Martínez A, Casado-Yusta S, Olaya B, et al. Depression as a risk factor for Alzheimer’s disease: a systematic review of longitudinal meta-analyses. J Clin Med. (2021) 10:1809. doi: 10.3390/jcm10091809
110. Wu JJ, Wang HX, Yao W, Yan Z, Pei JJ. Late-life depression and the risk of dementia in 14 countries: a 10-year follow-up study from the survey of health, ageing and retirement in europe. J Affect Disord. (2020) 274:59. doi: 10.1016/j.jad.2020.05.059
111. Zahodne LB, Ornstein K, Cosentino S, Devanand DP, Stern Y. Longitudinal relationships between Alzheimer disease progression and psychosis, depressed mood, and agitation/aggression. Am J Geriatr Psychiatry. (2015) 23:14. doi: 10.1016/j.jagp.2013.03.014
112. Dafsari FS, Jessen F. Depression—an underrecognized target for prevention of dementia in Alzheimer’s disease. Transl. Psychiatry. (2020) 10:160. doi: 10.1038/s41398-020-0839-1
113. Bartels C, Wagner M, Wolfsgruber S, Ehrenreich H, Schneider A. Impact of SSRI therapy on risk of conversion from mild cognitive impairment to alzheimer’s dementia in individuals with previous depression. Am J Psychiatry. (2018) 175:404. doi: 10.1176/appi.ajp.2017.17040404
114. Sheline YI, Snider BJ, Beer JC, Seok D, Fagan AM, Suckow RF, et al. Effect of escitalopram dose and treatment duration on CSF Aβ levels in healthy older adults: a controlled clinical trial. Neurology. (2020) 95:10725. doi: 10.1212/WNL.0000000000010725
115. Kessing LV, Søndergård L, Forman JL, Andersen PK. Antidepressants and dementia. J Affect Disord. (2009) 117:20. doi: 10.1016/j.jad.2008.11.020
116. Burke SL, Maramaldi P, Cadet T, Kukull W. Decreasing hazards of Alzheimer’s disease with the use of antidepressants: mitigating the risk of depression and apolipoprotein E. Int J Geriatr Psychiatry. (2018) 33:4709. doi: 10.1002/gps.4709
117. Shen Y, Yang X, Li G, Gao J, Liang Y. The change of gut microbiota in MDD patients under SSRIs treatment. Sci Rep. (2021) 11:94481. doi: 10.1038/s41598-021-94481-1
118. Lukić I, Getselter D, Ziv O, Oron O, Reuveni E, Koren O, et al. Antidepressants affect gut microbiota and ruminococcus flavefaciens is able to abolish their effects on depressive-like behavior. Transl Psychiatry. (2019) 9:466. doi: 10.1038/s41398-019-0466-x
119. Zhao H, Jin K, Jiang C, Pan F, Wu J, Luan H, et al. A pilot exploration of multi-omics research of gut microbiome in major depressive disorders. Transl Psychiatry. (2022) 12:1769. doi: 10.1038/s41398-021-01769-x
120. Zhuang ZQ, Shen LL, Li WW, Fu X, Zeng F, Gui L, et al. Gut microbiota is altered in patients with Alzheimer’s disease. J Alzheimer’s Dis. (2018) 63:180176. doi: 10.3233/JAD-180176
121. Sun J, Wang F, Hong G, Pang M, Xu H, Li H, et al. Antidepressant-like effects of sodium butyrate and its possible mechanisms of action in mice exposed to chronic unpredictable mild stress. Neurosci. Lett. (2016) 618:159–66. doi: 10.1016/j.neulet.2016.03.003
122. Biagi E, Franceschi C, Rampelli S, Severgnini M, Ostan R, Turroni S, et al. Gut microbiota and extreme longevity. Curr Biol. (2016) 26:16. doi: 10.1016/j.cub.2016.04.016
123. Ottman N, Davids M, Suarez-Diez M, Boeren S, Schaap PJ, dos Santos VAPM, et al. Genomescale model and omics analysis of metabolic capacities of akkermansia muciniphila reveal a preferential mucin-degrading lifestyle. Appl Environ Microbiol. (2017) 83:17. doi: 10.1128/AEM.01014-17
124. Ou Z, Deng L, Lu Z, Wu F, Liu W, Huang D, et al. Protective effects of akkermansia muciniphila on cognitive deficits and amyloid pathology in a mouse model of Alzheimer’s disease. Nutr Diabet. (2020) 10:115. doi: 10.1038/s41387-020-0115-8
125. Chen Y, Fang L, Chen S, Zhou H, Fan Y, Lin L, et al. Gut microbiome alterations precede cerebral amyloidosis and microglial pathology in a mouse model of alzheimer’s disease. Biomed Res Int. (2020) 2020:596. doi: 10.1155/2020/8456596
126. Xu Y, Wang N, Tan HY, Li S, Zhang C, Feng Y. Function of akkermansia muciniphila in obesity: interactions with lipid metabolism, immune response and gut systems. Front Microbiol. (2020) 11:219. doi: 10.3389/fmicb.2020.00219
127. Troubat R, Barone P, Leman S, Desmidt T, Cressant A, Atanasova B, et al. Neuroinflammation and depression: a review. Eur J Neurosci. (2021) 53:14720. doi: 10.1111/ejn.14720
128. Song J, Ma W, Gu X, Zhao L, Jiang J, Xu Y, et al. Metabolomic signatures and microbial community profiling of depressive rat model induced by adrenocorticotrophic hormone. J Transl Med. (2019) 17:1970. doi: 10.1186/s12967-019-1970-8
129. McGaughey KD, Yilmaz-Swenson T, Elsayed NM, Cruz DA, Rodriguiz RM, Kritzer MD, et al. Relative abundance of akkermansia spp. and other bacterial phylotypes correlates with anxiety- and depressive-like behavior following social defeat in mice. Sci Rep. (2019) 9:140. doi: 10.1038/s41598-019-40140-5
130. Cheung SG, Goldenthal AR, Uhlemann AC, Mann JJ, Miller JM, Sublette ME. Systematic review of gut microbiota and major depression. Front Psychiatry. (2019) 10:34. doi: 10.3389/fpsyt.2019.00034
131. Ñahui Palomino RA, Vanpouille C, Costantini PE, Margolis L. Microbiota–host communications: bacterial extracellular vesicles as a common language. PLoS Pathog. (2021) 17:e1009508. doi: 10.1371/journal.ppat.1009508
132. Busatto S, Morad G, Guo P, Moses MA. The role of extracellular vesicles in the physiological and pathological regulation of the blood–brain barrier. FASEB BioAdv. (2021) 3:665–75. doi: 10.1096/fba.2021-00045
133. Chelakkot C, Choi Y, Kim DK, Park HT, Ghim J, Kwon Y, et al. Akkermansia muciniphila-derived extracellular vesicles influence gut permeability through the regulation of tight junctions. Exp Mol Med. (2018) 50:282. doi: 10.1038/emm.2017.282
134. Yaghoubfar R, Behrouzi A, Ashrafian F, Shahryari A, Moradi HR, Choopani S, et al. Modulation of serotonin signaling/metabolism by akkermansia muciniphila and its extracellular vesicles through the gut-brain axis in mice. Sci Rep. (2020) 10:171. doi: 10.1038/s41598-020-79171-8
135. Yaghoubfar R, Behrouzi A, Zare Banadkoki E, Ashrafian F, Lari A, Vaziri F, et al. Effect of akkermansia muciniphila, faecalibacterium prausnitzii, and their extracellular vesicles on the serotonin system in intestinal epithelial cells. Probiotics Antimicrob Proteins (2021) 13:9786. doi: 10.1007/s12602-021-09786-4
136. Wang R, Reddy PH. Role of glutamate and NMDA receptors in Alzheimer’s disease. J Alzheimer’s Dis. (2017) 57:1041–8. doi: 10.3233/JAD-160763
137. Govindpani K, Guzmán BCF, Vinnakota C, Waldvogel HJ, Faull RL, Kwakowsky A. Towards a better understanding of gabaergic remodeling in Alzheimer’s disease. Int J Mol Sci. (2017) 18:1813. doi: 10.3390/ijms18081813
138. Olson CA, Vuong HE, Yano JM, Liang QY, Nusbaum DJ, Hsiao EY. The gut microbiota mediates the anti-seizure effects of the ketogenic diet. Cell. (2018) 173:27. doi: 10.1016/j.cell.2018.04.027
139. Chang CH, Lin CH, Lane HY. D-glutamate and gut microbiota in Alzheimer’s disease. Int J Mol Sci. (2020) 21:2676. doi: 10.3390/ijms21082676
140. Marrone MC, Coccurello R. Dietary fatty acids and microbiota-brain communication in neuropsychiatric diseases. Biomolecules. (2019) 10:12.
Keywords: dementia, Alzheimer’s disease, aging, neuropsychiatric symptoms, depression, gut microbiota, tryptophan, serotonin
Citation: Cutuli D, Giacovazzo G, Decandia D and Coccurello R (2022) Alzheimer’s disease and depression in the elderly: A trajectory linking gut microbiota and serotonin signaling. Front. Psychiatry 13:1010169. doi: 10.3389/fpsyt.2022.1010169
Received: 02 August 2022; Accepted: 28 October 2022;
Published: 30 November 2022.
Edited by:
Nerisa Banaj, Santa Lucia Foundation IRCCS, ItalyReviewed by:
Ashok Aiyar, Louisiana State University, United StatesLouise Madeleine Ince, The University of Texas at Austin, United States
Copyright © 2022 Cutuli, Giacovazzo, Decandia and Coccurello. This is an open-access article distributed under the terms of the Creative Commons Attribution License (CC BY). The use, distribution or reproduction in other forums is permitted, provided the original author(s) and the copyright owner(s) are credited and that the original publication in this journal is cited, in accordance with accepted academic practice. No use, distribution or reproduction is permitted which does not comply with these terms.
*Correspondence: Roberto Coccurello, cm9iZXJ0by5jb2NjdXJlbGxvQGNuci5pdA==