- 1Department of Psychology, Queens College, City University of New York, Flushing, NY, United States
- 2Psychology Program, The Graduate Center, CUNY, New York, NY, United States
- 3Biology Program, The Graduate Center, City University of New York, New York, NY, United States
- 4Department of Psychology, Hunter College, CUNY, New York, NY, United States
Dopamine has long been implicated as a critical neural substrate mediating anorexia nervosa (AN). Despite nearly 50 years of research, the putative direction of change in dopamine function remains unclear and no consensus on the mechanistic role of dopamine in AN has been achieved. We hypothesize two stages in AN– corresponding to initial development and entrenchment– characterized by opposite changes in dopamine. First, caloric restriction, particularly when combined with exercise, triggers an escalating spiral of increasing dopamine that facilitates the behavioral plasticity necessary to establish and reinforce weight-loss behaviors. Second, chronic self-starvation reverses this escalation to reduce or impair dopamine which, in turn, confers behavioral inflexibility and entrenchment of now established AN behaviors. This pattern of enhanced, followed by impaired dopamine might be a common path to many behavioral disorders characterized by reinforcement learning and subsequent behavioral inflexibility. If correct, our hypothesis has significant clinical and research implications for AN and other disorders, such as addiction and obesity.
Worldwide, millions of people diet and struggle to lose weight, with high rates of relapse and weight rebound. This is not surprising considering that we evolved over millions of years to eat, a behavior that is highly reinforcing. Yet, evolution and a lifetime of reinforcement are seemingly overridden in a subset of dieters who develop anorexia nervosa (AN), an eating disorder characterized by unrelenting self-starvation.
Initially, AN behaviors may be indistinguishable from a successful weight loss routine. AN becomes evident when an inability or unwillingness to stop losing weight emerges, even when it is life threatening. Those with AN develop an obsessive preoccupation with being thin, often engaging in ritualistic, restrictive eating behaviors and vigorous, extended exercise. The nature of these behavioral changes have been compared to compulsions in obesity and addictive disorders [e.g., (1)], where entire patterns of behavior become reorganized around a central focus (i.e., weight-loss, overeating, drug taking) often interfering with other activities and social relationships. As in obesity and addiction, once a behavioral regimen has been established in AN, it can be highly resistant to change, or entrenched (2).
The neuroadaptations underlying AN remain poorly understood. Starvation alone leads to physiological and psychological changes that resemble AN symptoms (3–5) and unintended weight loss has been found to trigger AN in some cases (6). This has led to the suggestion that dieting and exercising – typically motivated by psychosocial and cognitive factors – could lead to weight loss-induced adaptations that trigger AN (7, 8). However, this fails to account for the most crucial aspect of AN, the refusal to eat. After all, a starving person will generally eat when offered food. It is important to understand 1) how weight loss leads to the reorganization of behavior around self-starvation in some individuals and 2) the mechanisms by which these behaviors become compulsive and entrenched.
The midbrain dopamine system has been implicated in the pathophysiology of AN for decades (7, 9, 10). However, consensus on how dopamine mediates AN has yet to emerge. We propose a two-stage model of AN in which opposite changes in dopamine function underlie each stage of the disorder: initial emergence and establishment of AN behaviors, and subsequent entrenchment of an established AN behavioral regimen.
Dopamine in Anorexia Nervosa
The hypothesis that increased dopamine plays a central role in the pathophysiology of AN was first introduced in 1976 by Barry and Klawans (11). This was based on the observation that drugs that increase dopamine, such as amphetamine, lead to changes that resemble AN symptoms. Subsequent studies measuring dopamine or its metabolites reported increased, decreased or unchanged levels in AN (9, 12). Human imaging studies using PET revealed increased D2/3 binding in the ventral striatum of recovered AN patients (13), which could reflect increased receptor expression, decreased dopamine transmission, or both if D2/3 is upregulated in response to diminished basal dopamine. In contrast, Broft et al. (14) found no change in D2 availability in currently ill AN patients. In a subsequent fMRI study using a prediction error task, Frank et al. (15) observed enhanced activity in striatal and insula regions, consistent with enhanced dopaminergic responsivity in AN. Collectively, this work supports a role for abnormal dopamine signaling in AN, but the direction of the abnormality remains unclear.
In the absence of longitudinal, prospective studies, it is difficult to determine whether putative abnormalities in dopamine precede the disorder representing a risk factor or arise as a consequence of starvation. In a widely cited paper, Kaye et al. (16) reported that recovered women with restricting-type AN have reduced homovanillic acid, a major metabolite of dopamine. While one interpretation of this result is that there is trait-like dysfunction in dopamine metabolism, it is also possible that this reflects persistent changes induced by AN. Recovered patients often continue to exhibit AN characteristics even though they no longer fulfill diagnostic criteria (13, 17–20). Therefore, changes in dopamine originally induced by AN could persist after recovery and underlie residual symptomatology. Genetic studies have also not clarified whether pre-existing variation in dopamine constitutes a risk factor for AN. While there have been reports of associations between dopamine related genes and AN, none of these have been consistently replicated and none have been confirmed in large, genome-wide association studies (21–23). Thus, whether altered dopamine represents a risk factor preceding AN or a pathophysiological adaptation arising as a consequence of AN remains unclear.
The results of animal studies have been more consistent with Barry and Klawan's hypothesis that overactive dopamine drives AN. Caloric restriction in rodents has been associated with increased dopamine sensitivity and function (24–26). In activity-based anorexia (ABA), a rodent model of AN that combines food restriction with wheel running (27), antipsychotics that decrease dopamine signaling by blocking D2R have been shown to limit weight loss (28, 29). Unfortunately, the antipsychotic drugs used have effects on activity, motor ability, motivation, and metabolism, confounding interpretation of the results. Furthermore, antipsychotics have not been efficacious in treating AN in humans (30), although there is some evidence that atypical antipsychotics, such as olanzapine, may be effective as a treatment augmentation strategy (31–34). However, a review of randomized controlled trials found insufficient evidence to support atypical antipsychotics as a standard treatment for AN (35).
A role for enhanced dopamine signaling in AN is partially supported by other ABA studies using more targeted approaches. For example, mice that increase dark cycle running across days of food restriction demonstrate an upregulation of D2R expression in the striatum (36), consistent with increased D2/3 binding observed in recovered patients (13). Selective pharmacological blockade of D2R reduces vulnerability to ABA (28), while genetic overexpression of D2R in the nucleus accumbens core increases ABA vulnerability (37). Similarly, we reported that hyperdopaminergia resulting from knockdown of the dopamine transporter in mice also enhances ABA vulnerability (38). Furthermore, the only study to directly measure dopamine during ABA with microdialysis (29) found increased dopamine release in the nucleus accumbens during food intake in rats. However, no changes in dopamine were detected prior to food availability, which is when wheel running progressively increases in some ABA animals (i.e., food anticipatory activity), and dopamine was actually decreased during the light cycle (29). Foldi et al. (39) used chemogenetics to directly target dopamine cells in the mesolimbic pathway during ABA and found that activation of Gq coupled DREADDs in the ventral tegmental area with systemic administration of clozapine-N-oxide (CNO) rescued the ABA phenotype, suggesting that impaired dopamine signaling is a driver of ABA. However, DREADD activation did not exclusively affect dopamine cells and protection against ABA could be attributed to activation of GABAergic projections to the nucleus accumbens. In addition, it is now known that systemic CNO is converted to the antipsychotic clozapine (40), which affects appetite and weight gain via mechanisms that may be independent of the targeted pathway. Notably, the Foldi et al. (11) finding suggests that drugs that increase dopamine, such as amphetamine, could treat AN, which is the opposite of the original Barry and Klawans hypothesis.
In sum, accumulated evidence in both humans and animal models indicates that dopamine is altered in AN, but characterizing this abnormality and its contribution to AN symptomology remains an unresolved challenge.
Hypothesis
Dopamine has been studied extensively in addiction research, where there is also a question of whether the core problem is increased or decreased dopamine. In that debate, a critical distinction can be made between the acute effects of drugs, which are known to cause increased dopamine release, and the more complex, progressive changes in dopamine that occur over time as the brain adapts to chronic drug use. The nature of these progressive changes is controversial, with evidence for both impaired, diminished dopamine function and sensitized dopamine responses to drugs and drug-related stimuli (41).
Here, we incorporate this idea that dopamine changes progressively over time into our hypothesis of AN. We propose a pattern of first enhanced and then diminished dopamine function, corresponding to a gain and then a loss of behavioral flexibility. This results in two stages in the development of AN, each mediated by different underlying neural mechanisms.
Stage 1: Initial Development of Anorexia Nervosa
We propose that in stage 1 of the disorder, weight loss resulting from caloric restriction triggers an increase in midbrain dopamine signaling, particularly when combined with high levels of physical activity. This increase could be mediated by stress-induced activation of the HPA axis (42), increased insulin sensitivity (43), decreased leptin (44), altered ghrelin (45), and/or other mechanisms (Table 1). As originally suggested by Barry and Klawans (11), the resulting increase in dopamine acts like a psychostimulant fueling both caloric restriction and exercise, which further augments dopamine signaling in an escalating spiral, creating a ‘dopamine storm' (Figure 1A). This escalation in dopamine facilitates reinforcement learning and behavioral plasticity necessary for establishing AN behaviors, as originally suggested by Södersten et al. (100). As a result, eating and activity routines are reorganized around achieving persistent weight loss. Individuals in stage 1 might be difficult to distinguish from non-anorexic dieters. As problematic behavior emerges, some individuals may receive EDNOS diagnoses during this earlier stage of development.
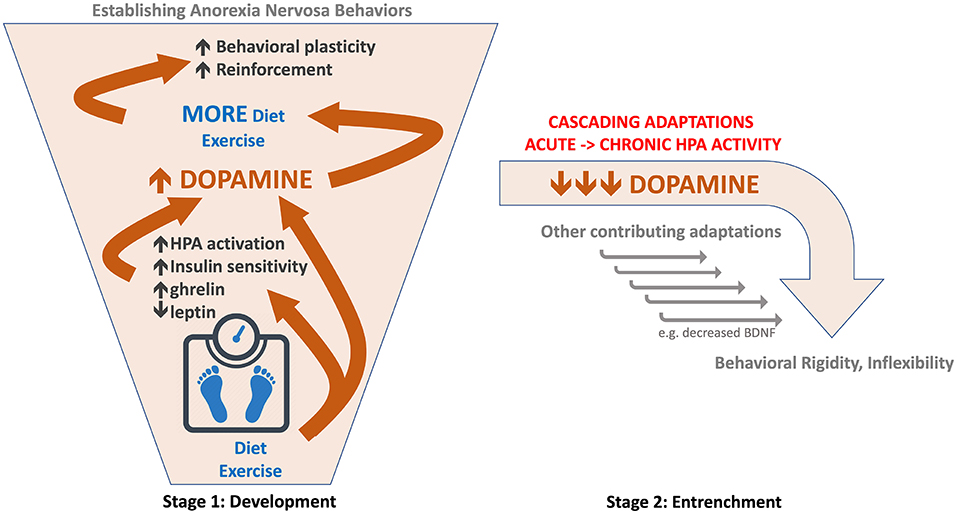
Figure 1. Schematic of hypothesized role of dopamine in two-stage model of anorexia nervosa. (Left) Stage 1: Development of AN. Diet and exercise trigger an escalating spiral of increased dopamine function (orange arrows and bounding box). This facilitates behavioral plasticity and reinforcement required for establishing a consistent self-starvation weight loss behavioral regimen. (Right) Stage 2: Entrenchment of AN behaviors. Persistent caloric deficit leads to a reversal in dopamine (orange) from augmented to reduced or impaired function; hypothesized as part of a cascade of adaptations resulting from chronic HPA activation.
Increased physical activity was recognized as a characteristic of AN in its earliest description (101) and is observed in up to 80% of patients (102, 103). Adolescent girls who develop AN tend to exhibit higher premorbid activity levels (104) and athletes are at higher risk for developing AN (105). Vigorous exercise may contribute to the development of AN by driving dopamine escalation, thereby accelerating development of the disorder (38).
Midbrain dopamine projects to and receives afferents from several brain regions implicated in AN, including the prefrontal cortex, insula, hippocampus, amygdala, and hypothalamus (106). Weight loss-induced changes in these regions could contribute to the proposed escalation in dopamine through their afferent projections to the midbrain. Conversely, as targets of dopamine, escalating dopamine activity could affect plasticity and processing in these same regions. Dopamine is thus situated to be an engine driving a cascade of neuroadaptations across the brain [e.g., (107)].
Stage 2: Entrenchment of Anorexia Nervosa
Stimuli effective at releasing dopamine, such as drugs of abuse and palatable food, can paradoxically reduce dopamine function with chronic, repeated exposure (108, 109). We propose a similar pattern in AN where the escalating dopamine spiral 'collapses' following long-term caloric restriction and dopamine becomes impaired (Table 1). In contrast to the behavioral flexibility associated with hyperdopaminergia in stage 1, hypodopaminergic function decreases behavioral plasticity, driving inflexibility and compulsivity. This gives rise to stage 2, when established AN behaviors are “locked in” and rendered resistant to change (2).
In this hypodopaminergic state, dopamine receptors likely upregulate expression and sensitivity (110–114), creating a physiological state of low basal dopamine concomitant with sensitized responses to phasic dopamine activity. Reduced basal/tonic dopamine coupled with enhanced receptor sensitivity has been described with chronic food restriction by Carr and colleagues [reviewed in Carr (50)] and proposed by Frank et al. (115) to play an important role in AN. Sensitization of D2R in particular has received attention in AN. Interestingly, the stimulation of D2R differentially affects cognitive flexibility based on levels of basal dopamine (116, 117), such that D2R activation improves cognitive flexibility when dopamine is low but impairs flexibility when dopamine is high. Consequently, differential D2R effects arising from different basal dopamine conditions may contribute importantly to changes in behavioral flexibility as individuals progress from stage 1 to stage 2 of AN.
The hypothesized dopamine reversal is likely driven by chronic HPA activation. Acute stress increases but chronic stress decreases dopamine function (42), possibly causing dopamine cell loss (70). As the HPA axis is a master orchestrator, these contrasting effects of acute and chronic stress can mediate reversals in other systems as well. For example, BDNF is increased with acute and decreased with chronic stress (118). Thus, chronic HPA activation resulting from persistent caloric restriction and low body weight may reverse neuroadaptations driving stage 1 and initiate a cascade of long-term adaptations, generating an entirely different profile of changes in stage 2.
Mechanisms
Data supporting our proposed escalating spiral in dopamine in stage 1 is strong. Caloric restriction, exercise, stress, enhanced insulin sensitivity, decreased leptin, increased ghrelin and increased orexin can all enhance dopamine function, as outlined in Table 1. Furthermore, there is evidence demonstrating synergy between these modulators; for example, both exercise and caloric restriction enhance insulin sensitivity. Progressive adaptations over time are more difficult to characterize, as is observed in the literature on obesity (108, 119), addiction (41) and stress (42, 68). In each field, there are differences between acute and chronic conditions, often suggesting a reversal from enhanced to diminished dopamine function, as proposed here for AN. Studying progressive changes requires looking across longer periods of time, which can be challenging in research studies, including determining what amount of time constitutes ‘chronic.' This issue of time course is compounded by the fact that many adaptations not only interact, but potentially undergo long-term changes at different rates and induce compensatory adaptations, which may themselves arise at different times. In our hypothesis, we suggest that in stage 2, chronic caloric deficits induce a cascade of neuroadaptations (Figure 1B), but do not speculate on the detailed order of these adaptations, their interactions or compensatory changes, as this is beyond the scope of the current perspective. Instead, in Table 1 we include data supporting the notion that there is a reversal of adaptations in chronic conditions that may underlie stage 2. With the exception of altered D2 binding observed by Frank and colleagues (13), specific mechanisms affecting dopamine (i.e., synthesis, reuptake, storage, synaptic plasticity of inputs, burst activity) have not been characterized in AN patients.
Implications
If each stage is mediated by different underlying neuroadaptations, then pharmacological treatments might differ by stage. In stage 1, drugs that prevent dopamine escalation (e.g., tetrabenazine) may slow development of the disorder, facilitating preventative cognitive-behavioral interventions. In stage 2, drugs that enhance dopamine might promote the behavioral flexibility needed to change entrenched behaviors. Conversely, treatments that modulate dopamine in the wrong direction would be predicted to be ineffective and could even be detrimental and facilitate the disorder. Patients are most likely to receive an AN diagnosis in our stage 2 when dopamine is low, potentially explaining the lack of efficacy of dopamine antagonists in AN treatment (30, 120, 121). In contrast, Frank and colleagues proposed using dopamine agonists to treat AN (115), arguing receptor activation would downregulate receptor hypersensitivity arising from diminished dopamine. As a partial D2R agonist, aripiprazole would remediate low basal DA through its agonist properties while the reduced (partial) activation would mitigate super sensitized responses to phasic DA, putatively normalizing the dynamic range of dopamine signaling. Consistent with these ideas, aripiprazole has been shown to promote weight gain in AN (122, 123). Interestingly, aripiprazole may have utility in stage 1 as well where its partial agonist properties may counteract escalating increases in dopamine. Notably, the finding that D2R acting drugs impair cognitive flexibility when dopamine is high (116, 117) calls into question whether decreasing plasticity during stage 1 would be advantageous (slowing development of AN behaviors) or detrimental (reducing impact of CBT intervention), possibilities that need to be investigated.
Given that few prospective studies have been conducted, most of what is known about AN is based on studying individuals in our putative stage 2, while stage 1 remains relatively uncharted territory. If our hypothesis is correct, stage 1 reflects a period of high behavioral plasticity providing a window of opportunity where interventions may be more successful, even preventative. The challenge is identifying those in stage 1 where evidence of AN may not yet fulfill diagnostic requirements; that is, differentiating individuals who are simply successful dieters from those who will develop AN. Prospective studies of dieters that identify factors predictive of AN could lead to diagnostic tools, ideally biomarkers, for early detection of AN in our proposed stage 1 [e.g., (122)]. These predictive factors may also apply to those who develop AN following unintentional weight loss. Furthermore, such prospective studies in dieters could provide insight into factors predicting (non-AN) success vs. failure in establishing sustained weight loss behaviors.
Our hypothesis would suggest any gene variants that regulate how the dopamine system responds to weight loss, exercise or chronic stress may in turn modify AN risk. This might include dopamine-related genes or genes of other systems– such as leptin, ghrelin, HPA axis, insulin– that modulate dopamine. Risk modification may be stage specific such that some variants may render an individual more likely to develop AN (stage 1) or more likely to progress to severe, persisting AN (stage 2).
Testing the Hypothesis
Our hypothesis can be tested in humans by measuring dopamine (e.g., PET) prior to and at timepoints following initiation of dieting in a prospective study. We predict that weight loss resulting from dieting, particularly in combination with exercise, will increase dopamine function in most participants, but those who develop AN will show a more pronounced dopamine increase. We predict that those with AN who develop behavioral rigidity and treatment resistance will subsequently exhibit impaired dopamine, while those who are successfully treated will not. Moreover, we predict that behavioral change in successful, non-AN dieters might be associated with a more modest, time-limited increase in dopamine, while unsuccessful dieters will exhibit a minimal change in dopamine during diet adherence. Impaired dopamine found in obese individuals (108) may render them less capable of upregulating dopamine and establishing new weight loss behaviors. Though conceptually straightforward, such human studies can be challenging. Alternatively, in preclinical studies dopamine can be measured in awake-behaving rodents in the activity-based anorexia model (38). If our hypothesis is correct, we expect vulnerable mice to show dopamine escalation followed by impairment, while resilient mice may show a modest, time-limited increase in dopamine, as proposed in successful dieters.
Conclusions
Our hypothesis is specific to AN but reflects a broader pattern common to disorders marked by compulsive behavior1, including addiction, obesity, and possibly other eating disorders (130). When an individual repeatedly engages in behavior that releases dopamine, this dopamine activation enhances behavioral plasticity, that in turn facilitates the reorganization of behavior around those dopamine releasing activities. Over time, these behaviors induce neuroadaptations that impair dopamine, reducing behavioral plasticity and entrenching the reorganized behaviors. If correct, our hypothesis would have broad implications for understanding and treating many behavioral disorders that incur profound social and economic costs.
Data Availability Statement
The original contributions presented in the study are included in the article/supplementary material, further inquiries can be directed to the corresponding author/s.
Author Contributions
All authors listed have made a substantial, direct, and intellectual contribution to the work and approved it for publication.
Funding
This work was supported by NIDA, DA046058 (JB), NIMH, R21MH114182 (NB), US National Institute on Minority Health and Health Disparities of the NIH, G12MD007599 (NB), PSC-CUNY Awards jointly funded by the Professional Staff Congress and The City University of New York (JB and NB) and a Klarman Family Foundation Eating Disorders Grant (JB and NB).
Conflict of Interest
The authors declare that the research was conducted in the absence of any commercial or financial relationships that could be construed as a potential conflict of interest.
Publisher's Note
All claims expressed in this article are solely those of the authors and do not necessarily represent those of their affiliated organizations, or those of the publisher, the editors and the reviewers. Any product that may be evaluated in this article, or claim that may be made by its manufacturer, is not guaranteed or endorsed by the publisher.
Footnotes
1. ^Here we refer to disorders that arise from behaviors that induce dopamine release, such as drug taking, overeating, and gambling. We do not include obsessive compulsive disorder (OCD). Compulsive behaviors in OCD may be reinforced by providing relief from anxiety surrounding intrusive, obsessive thoughts. However, the root of the disorder likely lies in failure of executive inhibitory control to stop the obsessive thoughts that generate the compulsive behavior rather than in the reinforcement of the behavior per se (124). While there is evidence suggesting linkage between OCD and AN (125), pharmacological treatments for OCD have not been effective in AN (126, 127) and some have argued against this OCD-AN linkage (128, 129).
References
1. O'Hara CB, Campbell IC, Schmidt U. A reward-centred model of anorexia nervosa: A focussed narrative review of the neurological and psychophysiological literature. Neurosci Biobehav Rev. (2015) 52:131–52. doi: 10.1016/j.neubiorev.2015.02.012
2. Ambwani S, Cardi V, Albano G, Cao L, Crosby RD, Macdonald P, et al. A multicenter audit of outpatient care for adult anorexia nervosa: Symptom trajectory, service use, and evidence in support of “early stage” versus “severe and enduring” classification. Int J Eat Disord. (2020) 53:1337–48. doi: 10.1002/eat.23246
3. Bergh C, Callmar M, Danemar S, Hölcke M, Isberg S, Leon M, et al. Effective treatment of eating disorders: Results at multiple sites. Behav Neurosci. (2013) 127:878–89. doi: 10.1037/a0034921
4. Casper RC. Restless activation and drive for activity in anorexia nervosa may reflect a disorder of energy homeostasis: Restless activation in anorexia nervosa. Int J Eat Disord. (2016) 49:750–2. doi: 10.1002/eat.22575
5. Keys A, Brozek J, Henschel A, Michelsen O, Taylor H. The Biology of Human Starvation. Minneapolis, MN: University of Minnesota Press (1950). doi: 10.5749/j.ctv9b2tqv
6. Brandenburg BMP, Andersen AE. Unintentional onset of anorexia nervosa. Eat Weight Disord. (2007) 12:97–100. doi: 10.1007/BF03327584
7. Södersten P, Bergh C, Leon M, Zandian M. Dopamine and anorexia nervosa. Neurosci Biobehav Rev. (2016) 60:26–30. doi: 10.1016/j.neubiorev.2015.11.003
8. Casper RC. Not the function of eating, but spontaneous activity and energy expenditure, reflected in “restlessness” and a “drive for activity” appear to be dysregulated in anorexia nervosa: treatment implications. Front Psychol. (2018) 9:2303. doi: 10.3389/fpsyg.2018.02303
9. Kontis D, Theochari E. Dopamine in anorexia nervosa: a systematic review. Behav Pharmacol. (2012) 23:496–515. doi: 10.1097/FBP.0b013e328357e115
10. Kaye WH, Wierenga CE, Bailer UF, Simmons AN, Bischoff-Grethe A. Nothing tastes as good as skinny feels: the neurobiology of anorexia nervosa. Trends Neurosci. (2013) 36:110–20. doi: 10.1016/j.tins.2013.01.003
11. Barry VC, Klawans HL. On the role of dopamine in the pathophysiology of anorexia nervosa. J Neural Transmission. (1976) 38:107–22. doi: 10.1007/BF01262969
12. Van Binsbergen CJ, Odink J, Van der Beek EJ, Westenberg HM, Bennink HJ. Biogenic amines in anorexia nervosa: circadian rhythm in urinary excretion and influence of posture and physical task load on plasma catecholamines. Psychosomatic Med. (1991) 53:440–52. doi: 10.1097/00006842-199107000-00009
13. Frank GK, Bailer UF, Henry SE, Drevets W, Meltzer CC, Price JC, et al. Increased dopamine D2/D3 receptor binding after recovery from anorexia nervosa measured by positron emission tomography and [11C]Raclopride. Biol Psychiatry. (2005) 58:908–12. doi: 10.1016/j.biopsych.2005.05.003
14. Broft A, Slifstein M, Osborne J, Kothari P, Morim S, Shingleton R, et al. Striatal dopamine type 2 receptor availability in anorexia nervosa. Psychiatry Res. (2015) 233:380–7. doi: 10.1016/j.pscychresns.2015.06.013
15. Frank GKW, DeGuzman MC, Shott ME, Laudenslager ML, Rossi B, Pryor T. Association of brain reward learning response with harm avoidance, weight gain, and hypothalamic effective connectivity in adolescent anorexia nervosa. JAMA Psychiatry. (2018) 75:1071. doi: 10.1001/jamapsychiatry.2018.2151
16. Kaye W. Altered dopamine activity after recovery from restricting-type anorexia nervosa. Neuropsychopharmacology. (1999) 21:503–6. doi: 10.1016/S0893-133X(99)00053-6
17. Srinivasagam NM, Kaye WH, Plotnicov KH, Greeno C, Weltzin TE, Rao R. Persistent perfectionism, symmetry, and exactness after long-term recovery from anorexia nervosa. AJP. (1995) 152:1630–4. doi: 10.1176/ajp.152.11.1630
18. Wagner A, Barbarich-Marsteller NC, Frank GK, Bailer UF, Wonderlich SA, Crosby RD, et al. Personality traits after recovery from eating disorders: Do subtypes differ? Int J Eat Disord. (2006) 39:276–84. doi: 10.1002/eat.20251
19. Cowdrey FA, Park RJ, Harmer CJ, McCabe C. Increased neural processing of rewarding and aversive food stimuli in recovered anorexia nervosa. Biol Psychiatry. (2011) 70:736–43. doi: 10.1016/j.biopsych.2011.05.028
20. Scaife JC, Godier LR, Reinecke A, Harmer CJ, Park RJ. Differential activation of the frontal pole to high vs low calorie foods: The neural basis of food preference in Anorexia Nervosa? Psychiatry Res. (2016) 258:44–53. doi: 10.1016/j.pscychresns.2016.10.004
21. Duncan L, Yilmaz Z, Walters R, Goldstein J, Anttila V, Bulik-Sullivan B, et al. Genome-wide association study reveals first locus for anorexia nervosa and metabolic correlations. Genomics. (2016) 174:850–858. doi: 10.1101/088815
22. Himmerich H, Bentley J, Kan C, Treasure J. Genetic risk factors for eating disorders: an update and insights into pathophysiology. Therap Adv Psychopharmacol. (2019) 9:204512531881473. doi: 10.1177/2045125318814734
23. Shih PB, Woodside DB. Contemporary views on the genetics of anorexia nervosa. Eur Neuropsychopharmacol. (2016) 26:663–73. doi: 10.1016/j.euroneuro.2016.02.008
24. Branch SY, Goertz RB, Sharpe AL, Pierce J, Roy S, Ko D, et al. Food restriction increases glutamate receptor-mediated burst firing of dopamine neurons. J Neurosci. (2013) 33:13861–72. doi: 10.1523/JNEUROSCI.5099-12.2013
25. Carr KD. Food scarcity, neuroadaptations, and the pathogenic potential of dieting in an unnatural ecology: Binge eating and drug abuse. Physiol Behav. (2011) 104:162–7. doi: 10.1016/j.physbeh.2011.04.023
26. Carr KD, Tsimberg Y, Berman Y, Yamamoto N. Evidence of increased dopamine receptor signaling in food-restricted rats. Neuroscience. (2003) 119:1157–67. doi: 10.1016/S0306-4522(03)00227-6
27. Routtenberg A, Kuznesof AW. Self-starvation of rats living in activity wheels on a restricted feeding schedule. J Comparative Physiol Psychol. (1967) 64:414–21. doi: 10.1037/h0025205
28. Klenotich SJ, Ho EV, McMurray MS, Server CH, Dulawa SC. Dopamine D2/3 receptor antagonism reduces activity-based anorexia. Translational Psychiatry. (2015) 5:e613. doi: 10.1038/tp.2015.109
29. Verhagen LAW, Luijendijk MCM, Hillebrand JJG, Adan RAH. Dopamine antagonism inhibits anorectic behavior in an animal model for anorexia nervosa. Eur Neuropsychopharmacol. (2009) 19:153–60. doi: 10.1016/j.euroneuro.2008.09.005
30. Frank GKW, Shott ME. The role of psychotropic medications in the management of anorexia nervosa: rationale, evidence and future prospects. CNS Drugs. (2016) 30:419–42. doi: 10.1007/s40263-016-0335-6
31. Alonso-Pedrero L, Bes-Rastrollo M, Marti A. Effects of antidepressant and antipsychotic use on weight gain: A systematic review. Obesity Rev. (2019) 20:1680–90. doi: 10.1111/obr.12934
32. Grajales D, Ferreira V, Valverde ÁM. Second-Generation Antipsychotics and Dysregulation of Glucose Metabolism: Beyond Weight Gain. Cells. (2019) 8:1336. doi: 10.3390/cells8111336
33. Li H, Peng S, Li S, Liu S, Lv Y, Yang N, et al. Chronic olanzapine administration causes metabolic syndrome through inflammatory cytokines in rodent models of insulin resistance. Sci Rep. (2019) 9:1582. doi: 10.1038/s41598-018-36930-y
34. Xu H, Zhuang X. Atypical antipsychotics-induced metabolic syndrome and nonalcoholic fatty liver disease: a critical review. NDT. (2019) 15:2087–99. doi: 10.2147/NDT.S208061
35. Dold M, Aigner M, Klabunde M, Treasure J, Kasper S. Second-generation antipsychotic drugs in anorexia nervosa: a meta-analysis of randomized controlled trials. Psychother Psychosomatics. (2015) 84:110–6. doi: 10.1159/000369978
36. Gelegen C, van den Heuvel J, Collier DA, Campbell IC, Oppelaar H, Hessel E, et al. Dopaminergic and brain-derived neurotrophic factor signalling in inbred mice exposed to a restricted feeding schedule. Genes Brain Behav. (2008) 7:552–9. doi: 10.1111/j.1601-183X.2008.00394.x
37. Welch AC, Zhang J, Lyu J, McMurray MS, Javitch JA, Kellendonk C, et al. Dopamine D2 receptor overexpression in the nucleus accumbens core induces robust weight loss during scheduled fasting selectively in female mice. Mol Psychiatry. (2019) 26:3765–77. doi: 10.1038/s41380-019-0633-8
38. Beeler JA, Mourra D, Zanca RM, Kalmbach A, Gellman C, Klein BY, et al. Vulnerable and resilient phenotypes in a mouse model of anorexia nervosa. Biol Psychiatry. (2020) 2020:17443. doi: 10.1016/j.biopsych.2020.06.030
39. Foldi CJ, Milton LK, Oldfield BJ. The role of mesolimbic reward neurocircuitry in prevention and rescue of the Activity-Based Anorexia (ABA) phenotype in rats. Neuropsychopharmacology. (2017) 42:2292–2300. doi: 10.26226/morressier.5b31ec552afeeb001345b8f8
40. Gomez JL, Bonaventura J, Lesniak W, Mathews WB, Sysa-Shah P, Rodriguez LA, et al. Chemogenetics revealed: DREADD occupancy and activation via converted clozapine. Science. (2017) 357:503–7. doi: 10.1126/science.aan2475
41. Samaha A-N, Khoo SY-S, Ferrario CR, Robinson TE. Dopamine 'ups and downs' in addiction revisited. Trends Neurosci. (2021) 44:516–526. doi: 10.31234/osf.io/8vch5
42. Douma EH, de Kloet ER. Stress-induced plasticity and functioning of ventral tegmental dopamine neurons. Neurosci Biobehav Rev. (2020) 108:48–77. doi: 10.1016/j.neubiorev.2019.10.015
43. Chen W, Li J, Liu J, Wang D, Hou L. Aerobic exercise improves food reward systems in obese rats via insulin signalling regulation of dopamine levels in the nucleus accumbens. ACS Chem Neurosci. (2019) 10:2801–8. doi: 10.1021/acschemneuro.9b00022
44. Fernandes MFA, Matthys D, Hryhorczuk C, Sharma S, Mogra S, Alquier T, et al. Leptin suppresses the rewarding effects of running via STAT3 signaling in dopamine neurons. Cell Metabolism. (2015) 22:741–9. doi: 10.1016/j.cmet.2015.08.003
45. Cone JJ, Roitman JD, Roitman MF. Ghrelin regulates phasic dopamine and nucleus accumbens signaling evoked by food-predictive stimuli. J Neurochem. (2015) 133:844–56. doi: 10.1111/jnc.13080
46. Stouffer MA, Woods CA, Patel JC, Lee CR, Witkovsky P, Bao L, et al. Insulin enhances striatal dopamine release by activating cholinergic interneurons and thereby signals reward. Nat Commun. (2015) 6:8543. doi: 10.1038/ncomms9543
47. Carr KD. Nucleus accumbens AMPA receptor trafficking upregulated by food restriction: an unintended target for drugs of abuse and forbidden foods. Curr Opin Behav Sci. (2016) 9:32–9. doi: 10.1016/j.cobeha.2015.11.019
48. Pothos E, Creese I, Hoebel B. Restricted eating with weight loss selectively decreases extracellular dopamine in the nucleus accumbens and alters dopamine response to amphetamine, morphine, and food intake. J Neurosci. (1995) 15:6640–50. doi: 10.1523/JNEUROSCI.15-10-06640.1995
49. Pan Y, Chau L, Liu S, Avshalumov MV, Rice ME, Carr KD. A food restriction protocol that increases drug reward decreases tropomyosin receptor kinase B in the ventral tegmental area, with no effect on brain-derived neurotrophic factor or tropomyosin receptor kinase B protein levels in dopaminergic forebrain regions. Neuroscience. (2011) 197:330–8. doi: 10.1016/j.neuroscience.2011.08.065
50. Carr KD. Homeostatic regulation of reward via synaptic insertion of calcium-permeable AMPA receptors in nucleus accumbens. Physiol Behav. (2020) 2020:112850. doi: 10.1016/j.physbeh.2020.112850
51. Fisher BE, Li Q, Nacca A, Salem GJ, Song J, Yip J, et al. Treadmill exercise elevates striatal dopamine D2 receptor binding potential in patients with early Parkinson's disease. Neuroreport. (2013) 24:509–14. doi: 10.1097/WNR.0b013e328361dc13
52. MacRae PG, Spirduso WW, Cartee GD, Farrar RP, Wilcox RE. Endurance training effects on striatal D2 dopamine receptor binding and striatal dopamine metabolite levels. Neurosci Lett. (1987) 79:138–44. doi: 10.1016/0304-3940(87)90686-0
53. Foley TE, Fleshner M. Neuroplasticity of dopamine circuits after exercise: implications for central fatigue. NeuroMolecular Med. (2008) 10:67–80. doi: 10.1007/s12017-008-8032-3
54. Robertson CL, Ishibashi K, Chudzynski J, Mooney LJ, Rawson RA, Dolezal BA, et al. Effect of exercise training on striatal dopamine D2/D3 receptors in methamphetamine users during behavioral treatment. Neuropsychopharmacology. (2016) 41:1629–36. doi: 10.1038/npp.2015.331
55. Smith MA, Gergans SR, Iordanou JC, Lyle MA. Chronic exercise increases sensitivity to the conditioned rewarding effects of cocaine. Pharmacol Rep. (2008) 60:561–5.
56. Clark PJ, Amat J, McConnell SO, Ghasem PR, Greenwood BN, Maier SF, et al. Running reduces uncontrollable stress-evoked serotonin and potentiates stress-evoked dopamine concentrations in the rat dorsal striatum. PLoS ONE. (2015) 10:e0141898. doi: 10.1371/journal.pone.0141898
57. Augustin SM, Loewinger GC, O'Neal TJ, Kravitz AV, Lovinger DM. Dopamine D2 receptor signaling on iMSNs is required for initiation and vigor of learned actions. Neuropsychopharmacol. (2020) 45:2087–97. doi: 10.1038/s41386-020-00799-1
58. Kravitz AV, O'Neal TJ, Friend DM. Do dopaminergic impairments underlie physical inactivity in people with obesity? Front Human Neurosci. (2016) 10:514. doi: 10.3389/fnhum.2016.00514
59. Lovinger DM. Neurotransmitter roles in synaptic modulation, plasticity and learning in the dorsal striatum. Neuropharmacology. (2010) 58:951–61. doi: 10.1016/j.neuropharm.2010.01.008
60. Lerner TN, Kreitzer AC. Neuromodulatory control of striatal plasticity and behavior. Curr Opin Neurobiol. (2011) 21:322–7. doi: 10.1016/j.conb.2011.01.005
61. Surmeier DJ, Carrillo-Reid L, Bargas J. Dopaminergic modulation of striatal neurons, circuits, and assemblies. Neuroscience. (2011) 198:3–18. doi: 10.1016/j.neuroscience.2011.08.051
62. Graf EN, Wheeler RA, Baker DA, Ebben AL, Hill JE, McReynolds JR, et al. Corticosterone acts in the nucleus accumbens to enhance dopamine signaling and potentiate reinstatement of cocaine seeking. J Neurosci. (2013) 33:11800–10. doi: 10.1523/JNEUROSCI.1969-13.2013
63. Holly EN, DeBold JF, Miczek KA. Increased mesocorticolimbic dopamine during acute and repeated social defeat stress: modulation by corticotropin releasing factor receptors in the ventral tegmental area. Psychopharmacology. (2015) 232:4469–79. doi: 10.1007/s00213-015-4082-z
64. Daftary SS, Panksepp J, Dong Y, Saal DB. Stress-induced, glucocorticoid-dependent strengthening of glutamatergic synaptic transmission in midbrain dopamine neurons. Neurosci Lett. (2009) 452:273–6. doi: 10.1016/j.neulet.2009.01.070
65. Saal D, Dong Y, Bonci A, Malenka RC. Drugs of abuse and stress trigger a common synaptic adaptation in dopamine neurons. Neuron. (2003) 37:577–82. doi: 10.1016/S0896-6273(03)00021-7
66. Wanat MJ, Hopf FW, Stuber GD, Phillips PEM, Bonci A. Corticotropin-releasing factor increases mouse ventral tegmental area dopamine neuron firing through a protein kinase C-dependent enhancement of I h: CRF increases VTA dopamine neuron firing. J Physiol. (2008) 586:2157–70. doi: 10.1113/jphysiol.2007.150078
67. Overton PG, Tong Z-Y, Clark D. Preferential occupation of mineralocorticoid receptors by corticosterone enhancesgluatmate-induced burst firing in rat midbrain dopaminergic neurons. Brain Res. (1996) 737:146–54. doi: 10.1016/0006-8993(96)00722-6
68. Ironside M, Kumar P, Kang M-S, Pizzagalli DA. Brain mechanisms mediating effects of stress on reward sensitivity. Curr Opin Behav Sci. (2018) 22:106–13. doi: 10.1016/j.cobeha.2018.01.016
69. Shimamoto A, DeBold JF, Holly EN, Miczek KA. Blunted accumbal dopamine response to cocaine following chronic social stress in female rats: exploring a link between depression and drug abuse. Psychopharmacology. (2011) 218:271–9. doi: 10.1007/s00213-011-2364-7
70. Sugama S, Sekiyama K, Kodama T, Takamatsu Y, Takenouchi T, Hashimoto M, et al. Chronic restraint stress triggers dopaminergic and noradrenergic neurodegeneration: Possible role of chronic stress in the onset of Parkinson's disease. Brain Behav Immunity. (2016) 51:39–46. doi: 10.1016/j.bbi.2015.08.015
71. Wei N-L, Quan Z-F, Zhao T, Yu X-D, Xie Q, Zeng J, et al. Chronic stress increases susceptibility to food addiction by increasing the levels of DR2 and MOR in the nucleus accumbens. NDT. (2019) 15:1211–29. doi: 10.2147/NDT.S204818
72. Carr KD, Kim G-Y, Cabeza de Vaca S. Hypoinsulinemia may mediate the lowering of self-stimulation thresholds by food restriction and streptozotocin-induced diabetes. Brain Res. (2000) 863:160–8. doi: 10.1016/S0006-8993(00)02143-0
73. Cai W, Xue C, Sakaguchi M, Konishi M, Shirazian A, Ferris HA, et al. Insulin regulates astrocyte gliotransmission and modulates behavior. J Clin Investigation. (2018) 128:2914–26. doi: 10.1172/JCI99366
74. Könner AC, Hess S, Tovar S, Mesaros A, Sánchez-Lasheras C, Evers N, et al. Role for insulin signaling in catecholaminergic neurons in control of energy homeostasis. Cell Metabolism. (2011) 13:720–8. doi: 10.1016/j.cmet.2011.03.021
75. Sallam NA, Borgland SL. Insulin and endocannabinoids in the mesolimbic system. J Neuroendocrinol. (2021) 33:e12965. doi: 10.1111/jne.12965
76. Caravaggio F, Borlido C, Hahn M, Feng Z, Fervaha G, Gerretsen P, et al. Reduced insulin sensitivity is related to less endogenous dopamine at D2/3 receptors in the ventral striatum of healthy nonobese humans. Int J Neuropsychopharmacol. (2015) 18:pyv014. doi: 10.1093/ijnp/pyv014
77. Hommel JD, Trinko R, Sears RM, Georgescu D, Liu ZW, Gau XB, et al. Leptin receptor signaling in midbrain dopamine neurons regulates feeding. Neuron. (2006) 51:678–80. doi: 10.1016/j.neuron.2006.08.023
78. Thompson JL, Borgland SL. Presynaptic leptin action suppresses excitatory synaptic transmission onto ventral tegmental area dopamine neurons. Biol Psychiatry. (2013) 73:860–8. doi: 10.1016/j.biopsych.2012.10.026
79. Fulton S, Pissios P, Manchon RP, Stiles L, Frank L, Pothos EN, et al. Leptin regulation of the mesoaccumbens dopamine pathway. Neuron. (2006) 51:811–22. doi: 10.1016/j.neuron.2006.09.006
80. Jerlhag E, Egecioglu E, Dickson SL, Douhan A, Svensson L, Engel JA. Ghrelin administration into tegmental areas stimulates locomotor activity and increases extracellular concentration of dopamine in the nucleus accumbens. Addiction Biol. (2007) 12:6–16. doi: 10.1111/j.1369-1600.2006.00041.x
81. Kim M-S, Yoon C-Y, Park K-H, Shin C-S, Park K-S, Kim S-Y, et al. Changes in ghrelin and ghrelin receptor expression according to feeding status. NeuroReport. (2003) 14:1317–20. doi: 10.1097/00001756-200307180-00006
82. Harmatz ES, Stone L, Lim SH, Lee G, McGrath A, Gisabella B, et al. Central ghrelin resistance permits the overconsolidation of fear memory. Biol Psychiatry. (2017) 81:1003–13. doi: 10.1016/j.biopsych.2016.11.009
83. Stone LA, Harmatz ES, Goosens KA. Ghrelin as a stress hormone: implications for psychiatric illness. Biol Psychiatry. (2020) 88:531–40. doi: 10.1016/j.biopsych.2020.05.013
84. Narita M. Direct involvement of orexinergic systems in the activation of the mesolimbic dopamine pathway and related behaviors induced by morphine. J Neurosci. (2006) 26:398–405. doi: 10.1523/JNEUROSCI.2761-05.2006
85. Moorman DE, Aston-Jones G. Orexin/hypocretin modulates response of ventral tegmental dopamine neurons to prefrontal activation: diurnal influences. J Neurosci. (2010) 30:15585–99. doi: 10.1523/JNEUROSCI.2871-10.2010
86. Borgland SL, Taha SA, Sarti F, Fields HL, Bonci A. Orexin A in the VTA Is critical for the induction of synaptic plasticity and behavioral sensitization to cocaine. Neuron. (2006) 49:589–601. doi: 10.1016/j.neuron.2006.01.016
87. Godfrey N, Borgland SL. Sex differences in the effect of acute fasting on excitatory and inhibitory synapses onto ventral tegmental area dopamine neurons. J Physiol. (2020) 2020:JP280412. doi: 10.1101/2020.06.25.172585
88. Teske JA, Mavanji V. Energy Expenditure, in Vitamins & Hormones. Elsevier (2012). doi: 10.1016/B978-0-12-394623-2.00006-8
89. Zink AN, Perez-Leighton CE, Kotz CM. The orexin neuropeptide system: physical activity and hypothalamic function throughout the aging process. Front Syst Neurosci. (2014) 8:211. doi: 10.3389/fnsys.2014.00211
90. Borgland SL, Ungless MA, Bonci A. Convergent actions of orexin/hypocretin and CRF on dopamine neurons: Emerging players in addiction. Brain Res. (2010) 1314:139–44. doi: 10.1016/j.brainres.2009.10.068
91. Burdakov D, Luckman SM, Verkhratsky A. Glucose-sensing neurons of the hypothalamus. Philos Trans R Soc Lond B Biol Sci. (2005) 360:2227–35. doi: 10.1098/rstb.2005.1763
92. Griffond B, Risold P-Y, Jacquemard C, Colard C, Fellmann D. Insulin-induced hypoglycemia increases preprohypocretin (orexin) mRNA in the rat lateral hypothalamic area. Neurosci Lett. (1999) 262:77–80. doi: 10.1016/S0304-3940(98)00976-8
93. Ouedraogo R, Naslund E, Kirchgessner AL. Glucose regulates the release of Orexin-A from the endocrine pancreas. Diabetes. (2003) 52:111–7. doi: 10.2337/diabetes.52.1.111
94. Thorpe AJ, Mullett MA, Wang C, Kotz CM. Regional, metabolic, and circadian specificity of lateral hypothalamic orexin A feeding stimulation. Am J Physiol Regulat Integrative Comparative Physiol. (2003) 284:R1409–7. doi: 10.1152/ajpregu.00344.2002
95. Venner A, Karnani MM, Gonzalez JA, Jensen LT, Fugger L, Burdakov D. Orexin neurons as conditional glucosensors: paradoxical regulation of sugar sensing by intracellular fuels. J Physiol. (2011) 8:217000. doi: 10.1113/jphysiol.2011.217000
96. Shams WM, Cossette M-P, Shizgal P, Brake WG. 17β-estradiol locally increases phasic dopamine release in the dorsal striatum. Neurosci Lett. (2018) 665:29–32. doi: 10.1016/j.neulet.2017.11.039
97. Song Z, Yang H, Peckham EM, Becker JB. Estradiol-induced potentiation of dopamine release in dorsal striatum following amphetamine administration requires estradiol receptors and mGlu5. eNeuro. (2019) 6:ENEURO.0446-18.2019. doi: 10.1523/ENEURO.0446-18.2019
98. Vandegrift BJ, Hilderbrand ER, Satta R, Tai R, He D, You C, et al. Estrogen receptor α regulates ethanol excitation of ventral tegmental area neurons and binge drinking in female mice. J Neurosci. (2020) 2020:JN-RM-2364-19. doi: 10.1101/800052
99. Thomas J, Météreau E, Déchaud H, Pugeat M, Dreher J-C. Hormonal treatment increases the response of the reward system at the menopause transition: A counterbalanced randomized placebo-controlled fMRI study. Psychoneuroendocrinology. (2014) 50:167–80. doi: 10.1016/j.psyneuen.2014.08.012
100. Bergh C, Södersten P. Anorexia nervosa, self-starvation and the reward of stress. Nat Med. (1996) 2:21–22. doi: 10.1038/nm0196-21
102. Rizk M, Lalanne C, Berthoz S, Kern L, EVHAN Group, Godart N. Problematic exercise in anorexia nervosa: testing potential risk factors against different definitions. PLoS ONE. (2015) 10:e0143352. doi: 10.1371/journal.pone.0143352
103. Casper RC, Voderholzer U, Naab S, Schlegl S. Increased urge for movement, physical and mental restlessness, fundamental symptoms of restricting anorexia nervosa? Brain Behav. (2020) 10:1556. doi: 10.1002/brb3.1556
104. Kostrzewa E, Eijkemans MJC, Kas MJ. The expression of excessive exercise co-segregates with the risk of developing an eating disorder in women. Psychiatry Res. (2013) 210:1123–8. doi: 10.1016/j.psychres.2013.08.050
105. Bratland-Sanda S, Sundgot-Borgen J. Eating disorders in athletes: Overview of prevalence, risk factors and recommendations for prevention and treatment. Eur J Sport Sci. (2013) 13:499–508. doi: 10.1080/17461391.2012.740504
106. Frank GKW, DeGuzman MC, Shott ME. Motivation to eat and not to eat – The psycho-biological conflict in anorexia nervosa. Physiol Behav. (2019) 206:185–90. doi: 10.1016/j.physbeh.2019.04.007
107. Kahnt T, Tobler PN. Dopamine modulates the functional organization of the orbitofrontal cortex. J Neurosci. (2017) 37:1493–504. doi: 10.1523/JNEUROSCI.2827-16.2016
108. Naef L, Pitman KA, Borgland SL. Mesolimbic dopamine and its neuromodulators in obesity and binge eating. CNS Spectrums. (2015) 20:574–83. doi: 10.1017/S1092852915000693
109. Lüscher C, Robbins TW, Everitt BJ. The transition to compulsion in addiction. Nat Rev Neurosci. (2020) 21:247–63. doi: 10.1038/s41583-020-0289-z
110. Cai G, Wang H-Y, Friedman E. Increased dopamine receptor signaling and dopamine receptor-g protein coupling in denervated striatum. J Pharmacol Exp Ther. (2002) 302:1105–12. doi: 10.1124/jpet.102.036673
111. Calon F, Tahar AH, Blanchet PJ, Morissette M, Grondin R, Goulet M, et al. Dopamine-receptor stimulation: biobehavioral and biochemical consequences. Trends Neurosci. (2000) 23:S92–100. doi: 10.1016/S1471-1931(00)00026-4
112. Fauchey V, Jaber M, Caron MG, Bloch B, Le Moine C. Differential regulation of the dopamine D1, D2 and D3 receptor gene expression and changes in the phenotype of the striatal neurons in mice lacking the dopamine transporter: Dopamine receptor gene regulation in DAT-KO mice. Eur J Neurosci. (2000) 12:19–26. doi: 10.1046/j.1460-9568.2000.00876.x
113. Gerfen CR, Miyachi S, Paletzki R, Brown P. D1 dopamine receptor supersensitivity in the dopamine-depleted striatum results from a switch in the regulation of ERK1/2/MAP Kinase. J Neurosci. (2002) 22:5042–54. doi: 10.1523/JNEUROSCI.22-12-05042.2002
114. Kostrzewa RM, Kostrzewa JP, Brown RW, Nowak P, Brus R. Dopamine receptor supersensitivity: Development, mechanisms, presentation, and clinical applicability. Neurotox Res. (2008) 14:121–8. doi: 10.1007/BF03033804
115. Frank GKW. Could dopamine agonists aid in drug development for anorexia nervosa? Front Nutr. (2014) 1:19. doi: 10.3389/fnut.2014.00019
116. van Holstein M, Aarts E, van der Schaaf ME, Geurts DEM, Verkes RJ, Franke B, et al. Human cognitive flexibility depends on dopamine D2 receptor signaling. Psychopharmacology. (2011) 218:567–78. doi: 10.1007/s00213-011-2340-2
117. Cools R, Frank MJ, Gibbs SE, Miyakawa A, Jagust W, D'Esposito M. Striatal dopamine predicts outcome-specific reversal learning and its sensitivity to dopaminergic drug administration. J Neurosci. (2009) 29:1538–43. doi: 10.1523/JNEUROSCI.4467-08.2009
118. Schaaf MJ, De Kloet ER, Vreugdenhil E. Corticosterone effects on BDNF expression in the hippocampus. Implications for memory formation. Stress. (2000) 3:201–8. doi: 10.3109/10253890009001124
119. Beeler JA, Frazier CRM, Zhuang X. Putting desire on a budget: dopamine and energy expenditure, reconciling reward and resources. Front Integrative Neurosci. (2012) 6:49. doi: 10.3389/fnint.2012.00049
120. de Vos J, Houtzager L, Katsaragaki G, van de Berg E, Cuijpers P, Dekker J. Meta analysis on the efficacy of pharmacotherapy versus placebo on anorexia nervosa. J Eat Disord. (2014) 2:27. doi: 10.1186/s40337-014-0027-x
121. Lebow J, Sim LA, Erwin PJ, Murad MH. The effect of atypical antipsychotic medications in individuals with anorexia nervosa: A systematic review and meta-analysis. Int J Eating Disord. (2013) 46:332–9. doi: 10.1002/eat.22059
122. Frank GKW, Shott ME, Hagman JO, Schiel MA, DeGuzman MC, Rossi B. The partial dopamine D2 receptor agonist aripiprazole is associated with weight gain in adolescent anorexia nervosa: FRANK et al. Int J Eat Disord. (2017) 50:447–50. doi: 10.1002/eat.22704
123. Tahillioglu A, Özcan T, Yüksel G, Majroh N, Köse S, Özbaran B. Is aripiprazole a key to unlock anorexia nervosa?: A case series. Clin Case Rep. (2020) 8:2826–33. doi: 10.1002/ccr3.3271
124. Pauls DL, Abramovitch A, Rauch SL, Geller DA. Obsessive-compulsive disorder: an integrative genetic and neurobiological perspective. Nat Rev Neurosci. (2014) 15:410–24. doi: 10.1038/nrn3746
125. Yilmaz Z, Halvorsen M, Bryois J, Yu D, Thornton LM, Zerwas S, et al. Examination of the shared genetic basis of anorexia nervosa and obsessive-compulsive disorder. Mol Psychiatry. (2019) 25:2036–46. doi: 10.1038/s41380-018-0115-4
126. Attia E, Steinglass JE, Walsh BT, Wang Y, Wu P, Schreyer C, et al. Olanzapine versus placebo in adult outpatients with anorexia nervosa: a randomized clinical trial. AJP. (2019) 176:449–56. doi: 10.1176/appi.ajp.2018.18101125
127. Walsh BT, Kaplan AS, Attia E, Olmsted M, Parides M, Carter JC, et al. Fluoxetine after weight restoration in anorexia nervosa: a randomized controlled trial. JAMA. (2006) 295:2605–12. doi: 10.1001/jama.295.22.2605
128. Wu KD. Eating disorders and obsessive-compulsive disorder: A dimensional approach to purported relations. J Anxiety Disord. (2008) 22:1412–20. doi: 10.1016/j.janxdis.2008.02.003
129. Bohon C, Weinbach N, Lock J. Performance and brain activity during the Wisconsin Card Sorting Test in adolescents with obsessive-compulsive disorder and adolescents with weight-restored anorexia nervosa. Eur Child Adolesc Psychiatry. (2020) 29:217–26. doi: 10.1007/s00787-019-01350-4
Keywords: anorexia nervosa, compulsive behavioral disorders, dopamine, chronic stress, behavioral plasticity
Citation: Beeler JA and Burghardt NS (2022) The Rise and Fall of Dopamine: A Two-Stage Model of the Development and Entrenchment of Anorexia Nervosa. Front. Psychiatry 12:799548. doi: 10.3389/fpsyt.2021.799548
Received: 21 October 2021; Accepted: 14 December 2021;
Published: 11 January 2022.
Edited by:
Michael Kluge, University Hospital Leipzig, Leipzig, GermanyReviewed by:
Per Sodersten, Karolinska Institutet (KI), SwedenBarbara Scolnick, Boston University, United States
Copyright © 2022 Beeler and Burghardt. This is an open-access article distributed under the terms of the Creative Commons Attribution License (CC BY). The use, distribution or reproduction in other forums is permitted, provided the original author(s) and the copyright owner(s) are credited and that the original publication in this journal is cited, in accordance with accepted academic practice. No use, distribution or reproduction is permitted which does not comply with these terms.
*Correspondence: Jeff A. Beeler, amJlZWxlckBxYy5jdW55LmVkdQ==; Nesha S. Burghardt, bmI4NDRAaHVudGVyLmN1bnkuZWR1