- Department of Neuroscience and Psychology, University of Glasgow, Glasgow, United Kingdom
Schizophrenia is a neuropsychiatric illness with no single definitive aetiology, making its treatment difficult. Antipsychotics are not fully effective because they treat psychosis rather than the cognitive or negative symptoms. Antipsychotics fail to alleviate symptoms when patients enter the chronic stage of illness. Topical application of niacin showed diminished skin flush in the majority of patients with schizophrenia compared to the general population who showed flushing. The niacin skin flush test is useful for identifying patients with schizophrenia at their ultra-high-risk stage, and understanding this pathology may introduce an effective treatment. This review aims to understand the pathology behind the diminished skin flush response, while linking it back to neurons and microglia. First, it suggests that there are altered proteins in the GPR109A-COX-prostaglandin pathway, inflammatory imbalance, and kinase signalling pathway, c-Jun N-terminal kinase (JNK), which are associated with diminished flush. Second, genes from the GPR109A-COX-prostaglandin pathway were matched against the 128-loci genome wide association study (GWAS) for schizophrenia using GeneCards, suggesting that G-coupled receptor-109A (GPR109A) may have a genetic mutation, resulting in diminished flush. This review also suggests that there may be increased pro-inflammatory mediators in the GPR109A-COX-prostaglandin pathway, which contributes to the diminished flush pathology. Increased levels of pro-inflammatory markers may induce microglial-activated neuronal death. Lastly, this review explores the role of JNK on pro-inflammatory mediators, proteins in the GPR109A-COX-prostaglandin pathway, microglial activation, and neuronal death. Inhibiting JNK may reverse the changes observed in the diminished flush response, which might make it a good therapeutic target.
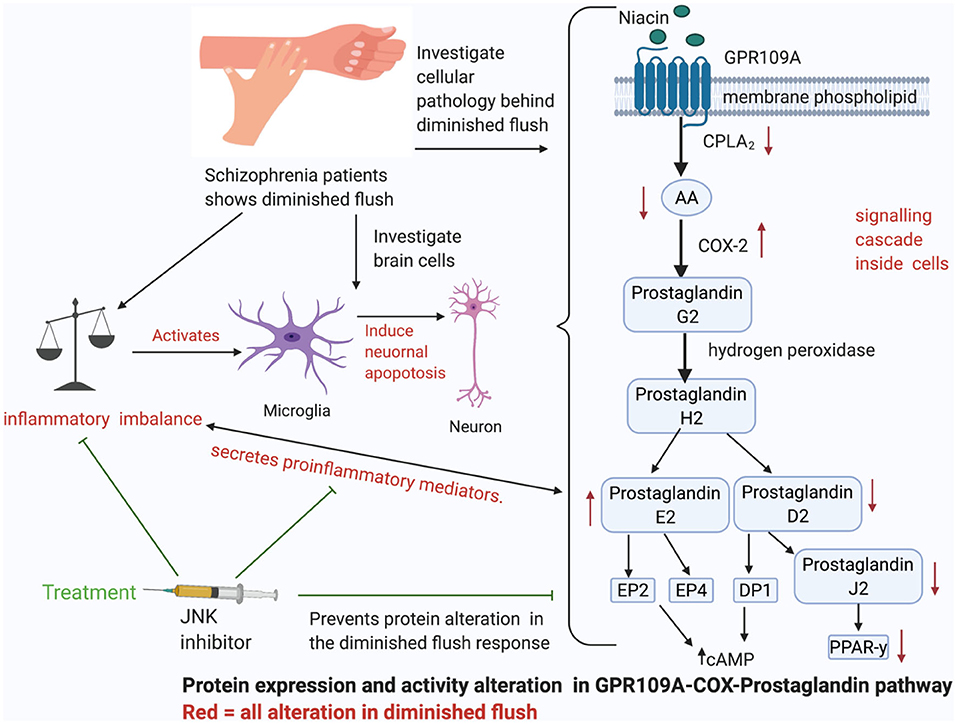
Graphical Abstract. The cellular pathway behind diminished skin flush. The GPR109A-COX-prostaglandin pathways shows how niacin binds to G-coupled receptor-109A (GPR109A) on the cell membrane of leukocytes, and stimulates a cascade of signals, which activates enzymes to release vasodilators, prostaglandin D2 and E2. This pathway induces pro-inflammatory mediators, which can then activate M1 microglia to induce neuronal apoptosis. JNK inhibitors have been observed to act as an anti-inflammatory agent, reduce excessive pro-inflammatory mediators, and prevent microglial induced neuronal apoptosis.
Introduction
Our society has neglected satisfactory categorisation of mental illness for over 2,000 years (1). In the past, schizophrenia had failed to be defined and understood as its own entity (2). The term schizophrenia was coined by Blueler (3, 4). Blueler and Kraepelin described the symptoms and aetiology of the illness (5). Schizophrenia is diagnosed by its symptoms, where positive symptoms include hallucinations, delusions, disorganised thoughts, and speech; negative symptoms include anhedonia, apathy, and social withdrawal; and cognitive symptoms include inattention, impaired working memory, and dysfunctional executive functions, which affect thoughts, intelligence, and ability to plan. Most individuals diagnosed with schizophrenia undergo a prodromal stage before full-blown psychotic symptoms appear, where individuals experience changes in both cognition and behavioural function (6, 7). The early onset of symptoms usually occurs between the ages of 14 to 29 (4); therefore, identifying the ultra-high-risk population is crucial for initiating early intervention.
Schizophrenia represents one percent of the global population and remains one of the top 25 leading disability worldwide (8). The World Health Organisation estimated that the direct cost for schizophrenia ranges from US$94 million to $102 billion (9). However, the substantial burden of the illness has been linked to its early onset and incurable nature with persistent symptoms (10). Heterogeneous illnesses have other problems, where a majority of research focuses on the altered neurotransmitter function of schizophrenia, typically dopamine or glutamate, in which treatments associated with this paradigm (currently dopamine antagonists) fail to alleviate negative and cognitive symptoms in 30–60% of the patients (11–14). Current antipsychotics increase the risk of other comorbidities associated with the heart (15) or diabetes (16).
Alternative approaches should be considered when treating this complex disorder. Both Kraepelin and Bluer identified that the aetiology of schizophrenia is a consequence of gene-environment interactions (5). Dr. Hoffer proposed megavitamin B3 therapy, in which niacin (vitamin B3) intake over time reduces symptoms of schizophrenia (17). The general population exposed to niacin showed skin flush as a side effect (18), whereas, niacin exposure in the majority of schizophrenia patients showed diminished skin flush (19–22). The diminished flush response serves as an endophenotype and separates patients with schizophrenia from other mood disorders such as depression (23), bipolar disorder (24, 25), and social phobia (26).
Prostaglandins in the cyclooxygenase (COX) pathway have been connected to flushing (Figure 1). However, it is unclear how these prostaglandins are deactivated or reduced in patients with schizophrenia. Other factors that have been thought to influence diminished flush include smoking (32, 33), alcohol consumption, caffeine intake, use of medicine (34), and altered chemical mediators such as nitric oxide (NO) (35, 36), histamine, and adrenaline (37). The aberrant immune response observed in these patients may be related to a diminished flush response (38–44). While current studies link the diminished flush in peripheral immune cells, this review aims to investigate possible links between diminished niacin-GPR109A flush responses mediated via the GPR109A-COX-prostaglandin pathway in microglia and neuronal cells. This study aimed to investigate the link between altered protein expression or activity in the GPR109A-COX-prostaglandin pathway with associated inflammatory mediators and the c-Jun N-terminal kinase (JNK) signalling pathway in patients with schizophrenia. Furthermore, GeneCards were used to manually identify chromosome numbers of genes from the GPR109A-COX-prostaglandin pathway with a 128-loci GWAS for schizophrenia (45).
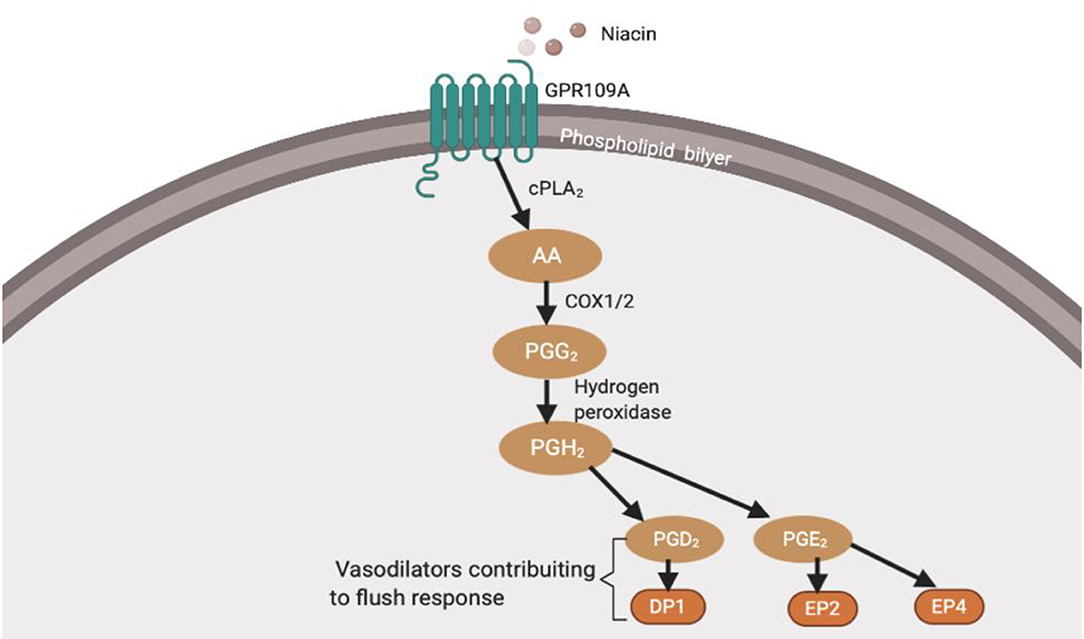
Figure 1. Niacin binds and activates GPR109A receptors on dermal cells, epidermal langerhans cells, and adipose macrophages (27). In langerhans cells, the activated receptor releases intracellular calcium (Ca2+) (28), which triggers phospholipase A2 (PLA2) to catalyse the breakdown of membrane phospholipid to arachidonic acid (AA). Available AA is converted to eicosanoid, prostaglandin G2 (PGG2) and prostaglandin H2 (PGH2) via COX1/2 and hydrogen peroxidase respectively. PGH2 is converted to various prostaglandins, prostacyclins, and leukotrienes. However, because this thesis focuses on the diminished flush observed in schizophrenia, I will focus on prostaglandins (29). Mediators involved in the cutaneous flushing are vasodilators, prostaglandin D2 (PGD2), and E2 (PDE2), which activates prostaglandin D2 receptor 1 (DP1) and prostaglandin E2/4 receptor (EP2/4), respectively (25). Moreover, these are biochemical alteration which may be partially inherited (30, 31). However, the pathophysiology of the attenuated flush response is not fully understood.
Neuroinflammation
A meta-analysis observed a neuroinflammatory imbalance in patients in the early stage of schizophrenia (46, 47). There have been alterations in inflammatory markers such as cytokines, reactive oxygen species (ROS), reactive nitrogen species (RNS), and nitrogen oxygen species (NOS) (47). This section provides evidence for altered neuroinflammatory markers in schizophrenia and links it to neuronal and microglial cells. Inflammatory markers play an important role in regulating flush response and microglial activation. Furthermore, it has been observed that the cytokine subtype released (Figure 2) and oxidants levels (Figure 3) regulates the activation of microglia (80). This may raise questions as to how peripheral cytokines may enter the brain. It may be assumed that patients with schizophrenia have poor blood-brain barrier; however, cytokines can enter the brain in different ways (Figure 4). The brain is vulnerable to oxidative stress, such as ROS, NOS, and superoxide species (75). Oxidative stress is defined as the imbalance between pro-and anti-oxidative processes, and there is an imbalance of oxidative stress throughout the different stages of schizophrenia (74, 89–91). Likewise, there is evidence of abnormal antioxidants in the peripheral blood (92–94), CSF (65) and post-mortem brain tissue (74, 95) of patients with schizophrenia. In conclusion, this evidence suggests that the lack of balance between the pro-oxidant and anti-oxidant may contribute to the neuronal abnormalities observed in schizophrenia patients.
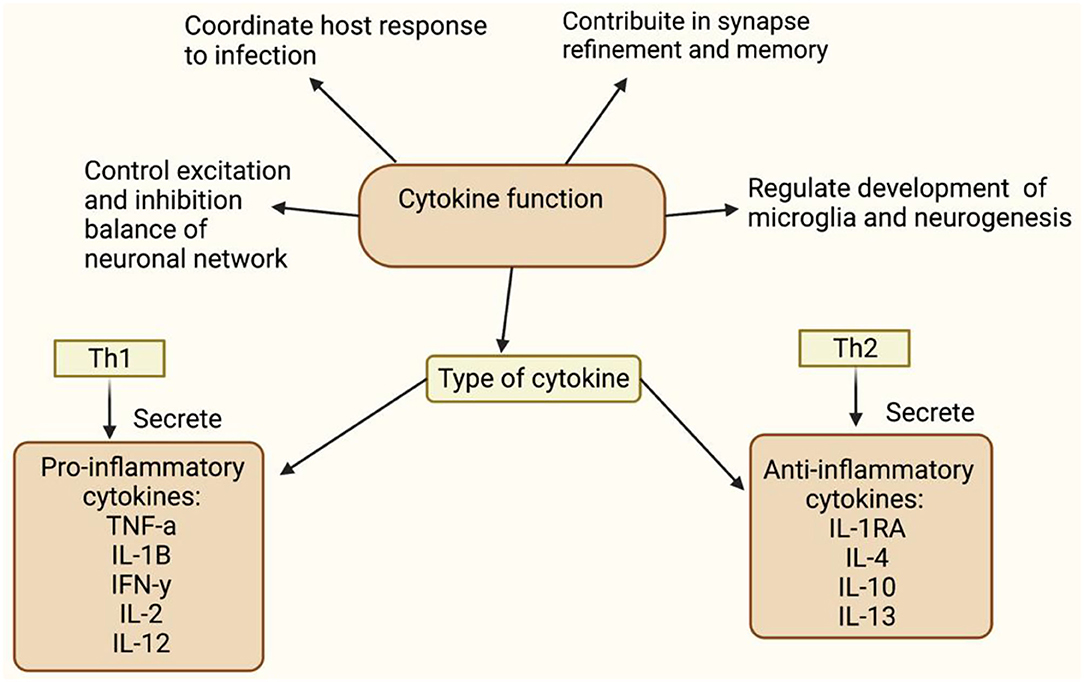
Figure 2. This figure shows function of cytokine (48–51). Tumour necrosis factors-a (TNF-a), Interleukin-1B (IL-1B), Interferon necrosis- y (IFN-y), Interleukin-2 (IL-2), Interleukin-12 (IL-12), Intereukin-4 (IL-4), Interleukin-10 (IL-10), Interleukin-13 (IL-13). Understanding the cytokine function will help to understand inflammation's involvement in diminished flush response and its role in activating microglia, respectively. Elevated pro-inflammatory levels, IL-1B and IL-6, and decreased anti-inflammatory levels have been observed in schizophrenia (52–54). Raison et al. (55) reported increased IL-1B and TNF-a observed in negative and cognitive symptoms of schizophrenia. Goldsmith et al. (56) and Wang and Miller (57) meta-analysis showed consistent upregulated pro-inflammatory cytokine, but variation in anti-inflammatory cytokine levels. Variation in anti-inflammatory markers, may be due to confounding factors, such as stage of illness, gender, age and medication status. Miller et al. (40) and Khandaker et al. (58) showed alternated cytokine levels in different stages of illness, which includes early-onset childhood, acute and relapse phase.
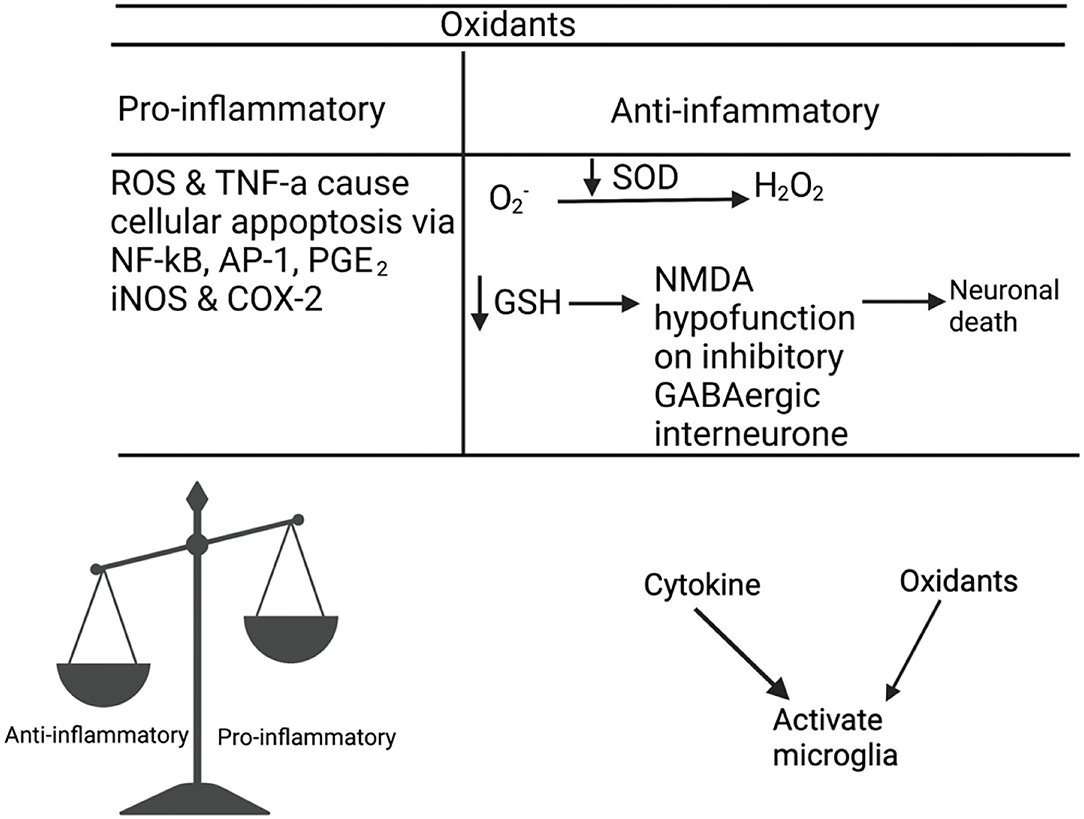
Figure 3. Matsubara and Ziff (59) and Meier et al. (60) reported an interaction between cytokines and oxidants, where they observed the role of TNF-α, IL-1, and IFN-γ in ROS production. Janssen-Heininger et al. (61) Sidoti-de Fraisse et al. (62), and Loukili et al. (63) reported that ROS and TNF-α have a synergistic effect on cell apoptosis via active transcription factors, nuclear factor kappa-light-chain-enhancer of activated B cells (NF-kB), and activator protein-1 (AP-1). The increased pro-inflammatory expression, NF-kB, PGE2, iNOS, and COX-2 are unique in patients with schizophrenia compared to bipolar patients or healthy controls (64). Oxidative stress reduces the levels of antioxidants such as glutathione (GSH) (65–69) and glutamate release from microglia (70). Depleted GSH levels cause NMDA hypofunction in inhibitory GABAergic interneurons (71), which fail to mediate inhibitory and excitatory balance of the microcircuitry, resulting in the loss of synapses or neuronal death (72). Incomplete reduction of oxygen generates superoxide anion (), which is converted to hydrogen peroxide (H202) by superoxide dismutase (SOD). SOD is an antioxidant enzyme that prevents oxidative damage from hydroxyl radicals and lipid peroxidation (73). A meta-analyses (74) confirmed that there is a decrease in SOD activity in patients There is an interaction between cytokines, oxidants, and microglia, as TNF-α and NADPH oxidase have been observed to activate microglia in patients with schizophrenia (75–79).
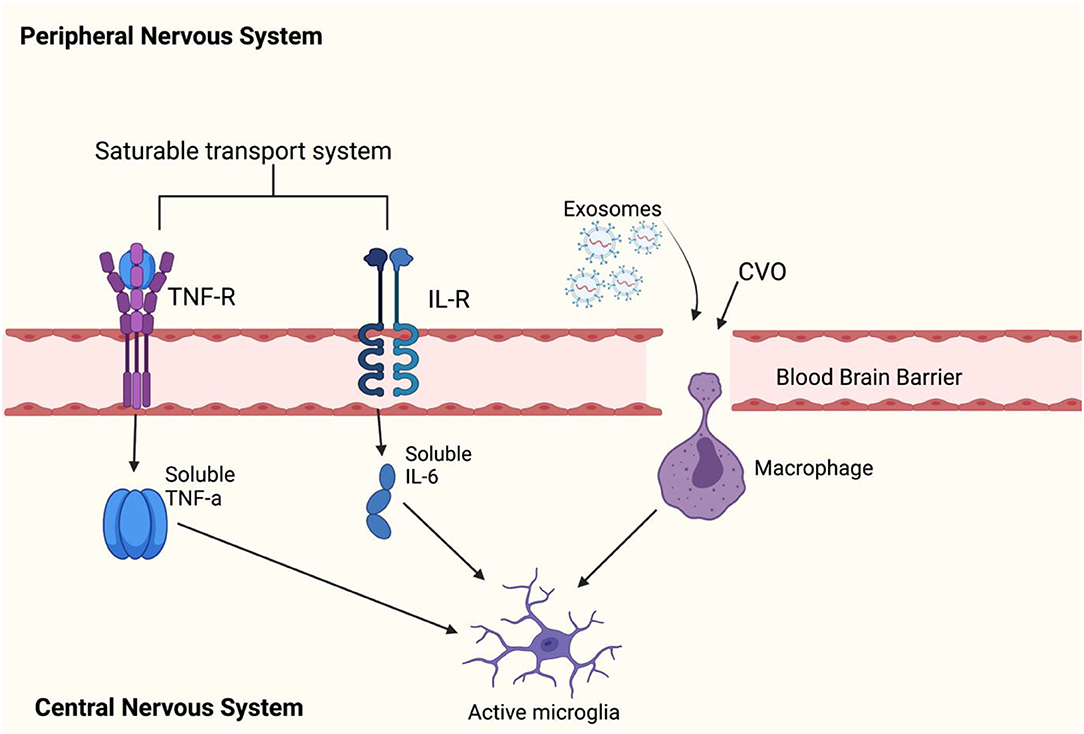
Figure 4. This figure shows how peripheral cytokines can enter the blood brain barrier (BBB) to activate microglia. Peripheral cytokines may activate microglial cells in the brain by passing through the BBB. Previous reports have found that TNF-α, IL-1α, and IL-1B can enter the brain through saturable transport systems (81, 82) or through areas of the brain where the BBB is incomplete such as circumventricular organs (CVOs) (83), and lastly, circulating cytokines can activate IL-1 receptors located on perivascular macrophages and endothelial cells of brain venules (84), which may allow entry of IL-1B cytokines to the brain. There are reports of exosomes easily crossing the BBB (85). However, it is not known whether exosomes directly influence inflammation by activating microglia. Leukocytes can cross the BBB and secrete exosomes, but a (86) has debunked this theory. The (86) study has showed that exosomes can be carried by hematopoietic cells into the blood circulation and released into the brain cells. This can be taken up by membrane receptor-mediator (87) or by phagocytosis (88). However, it is not known whether exosomes directly influence inflammation by activating microglia. Leukocytes can cross the BBB and secrete exosomes; however, a study has debunked this theory.
Microglia Activation
The microglia hypothesis (43, 44) suggests that activated microglia are present from prenatal infection to adolescence. When the immune system is challenged, microglial cells are exacerbated, and therefore, prolonged microglial hyperactivity causes cellular or neuronal apoptosis (96). The two-hit process supported by this hypothesis may explain why people who may have exposure to infection in childhood may not go to develop the illness.
Microglia have been shown to be more activated in schizophrenia than in control subjects (97). Studies using positron emission tomography (PET) and peripheral benzodiazepine receptor ligand, (11)C-(R)-PK11195, detected microglial activation in the hippocampus (38) and grey matter (97) of patients with schizophrenia. Bloomfield et al. (98) observed that ultra-high-risk individuals showed increased microglial activation.
Neurons
Patients with schizophrenia have a selective loss of grey matter volume, including the left superior temporal gyrus (STG), left Heschl gyrus (HG), left planum temporale (PT), and reduced spine density in the frontal cortex and hippocampus (99–103). The frontal cortex and hippocampus are associated with cognitive functions and reduction in neurons in brain regions, resulting in the cognitive deficits observed in schizophrenia (104). In the cellular pathology of diminished flush response, there are elevated levels of IL-1B and TNF-α, which might mean that microglia are activated. Active microglia and increased pro-inflammatory levels alter the functioning role of LTP, and AMPA and GABA receptors result in neuronal damage. Cognitive deficits may be due to impaired microglia-neuronal function, as microglia and neurons share bidirectional communication (Figure 5).
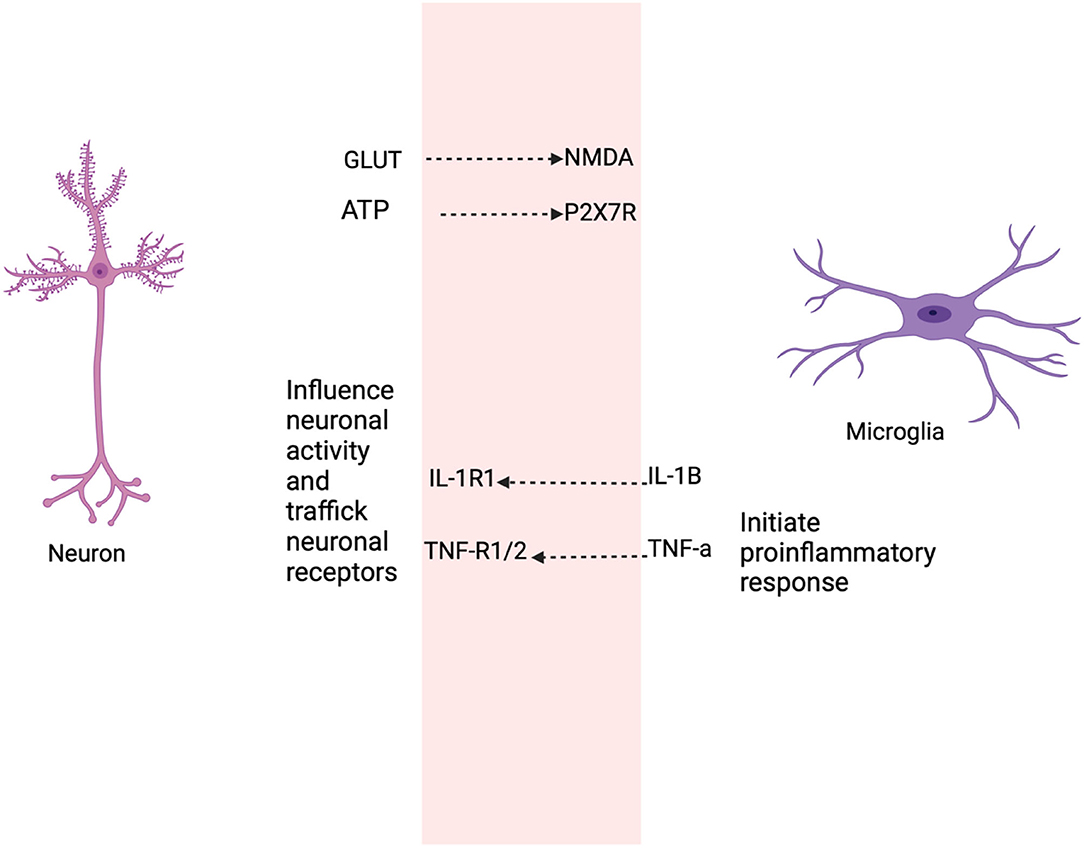
Figure 5. This figure shows the selective roles that neurons and microglia share as a result of the diminished GPR109A-COX pathway. Neurons secrete soluble factors such as cytokines to regulate or maintain microglia activation sites (105–107), the release of neurotransmitter, such as glutamate from neurons influence microglial motility (108, 109). Adenosine triphosphate (ATP) in microglia mediates through P2X7 receptor and produce pro-inflammatory cytokine (110). Likewise, active microglia secrete cytokine, prostaglandin which modulate neuronal function. For example, low levels of IL-1B are required for long term potentiation (LTP) (111, 112), while basal levels of TNF-α are necessary for AMPA and GABAA receptor trafficking (111). IL-1B and TNF induce neurotoxicity through elevated glutamate production resulting in neuronal excitotoxic death (113, 114).
JNK
Schizophrenia is a complex disorder that involves disruption of metabolism, neurotransmission, and cell signalling, and requires the coordination of kinase-mediated signalling events. There has been a signalling imbalance, which may be associated with diminished flush in schizophrenia. MAPKs are a family of serine/threonine protein kinases that are directly modified by ROS/RNS. MAPK can be activated by its upstream MAPKK, MAPKKK, or ROS/RNS (115). The MAPK pathway links inflammation and microglial activation (116). The MAPK family consists of the ERK, JNK, and p38 pathways. JNK has been the most affected kinase in the anterior cingulate circuit (ACC) of patients with chronic schizophrenia (117). This review focuses on JNK, and (Figure 6) shows the characteristic profile of JNK. JNK interacts with both microglia and neurons (Figure 7) through inflammatory mediators.
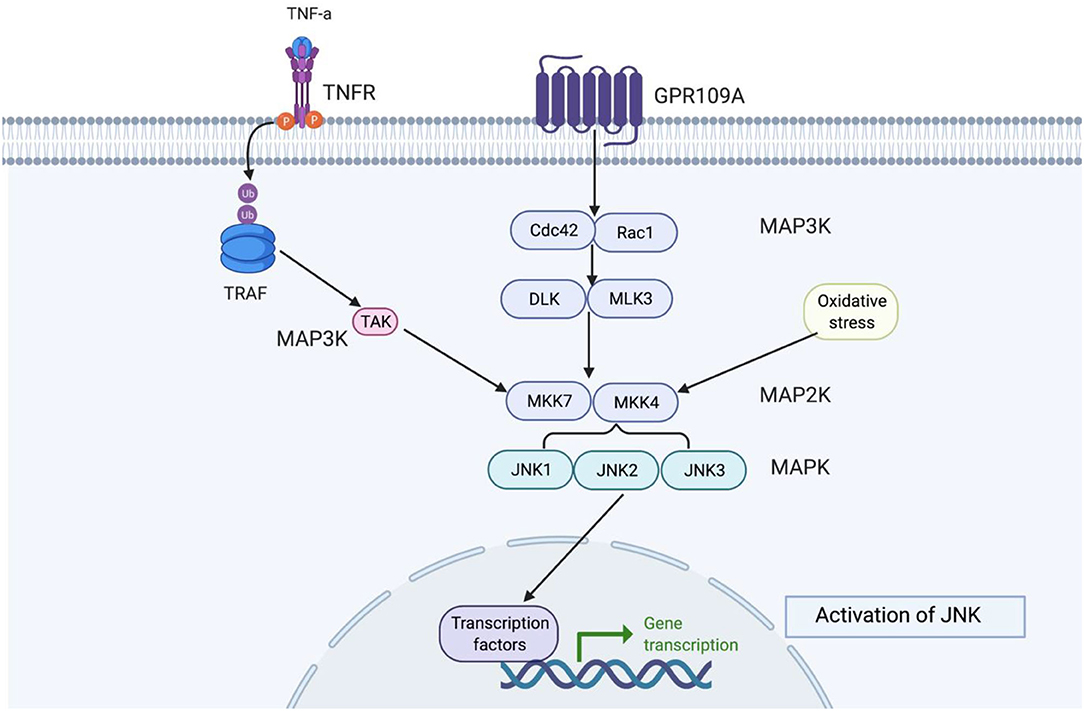
Figure 6. This figure shows how JNK functions in the signalling cascade. Depending on the extracellular signal, there are different MAPKKK proteins, but MAKK and MAPK remain constant. MAPKKK is always a precursor to MAPKK and MAPK, respectively. c-Jun N-terminal kinases (JNKs) belong to stress-activated protein kinases (SAPKs) and are part of the MAPK signalling cascade group, which are involved in signal amplification (118). JNK are activated by stress signals such as hyperosmolarity and heat shock, oxidative stress, UV and ionising radiation, pro-inflammatory cytokines, TNF-α, and IL-1B (119, 120). JNK activity is highest in the brain compared to other non-neural tissues (121, 122). JNK protein are encoded by three genes, JNK1 (Mapk8), JNK2 (Mapk9), and JNK3 (Mapk10). Where JNK1 and JNK2 are expressed ubiquitously in all mammalian tissues and JNK3 is restricted to the heart, testis, and brain (123). The highest level of JNK1-3 mRNA are found in the neocortex, followed by the hippocampus, thalamus, and midbrain (124). Downstream JNK are transcription factors such as c-Jun, c-fos JunD, ATF-2, and ELK-1, which can become activated when exposed to stress signals (125).
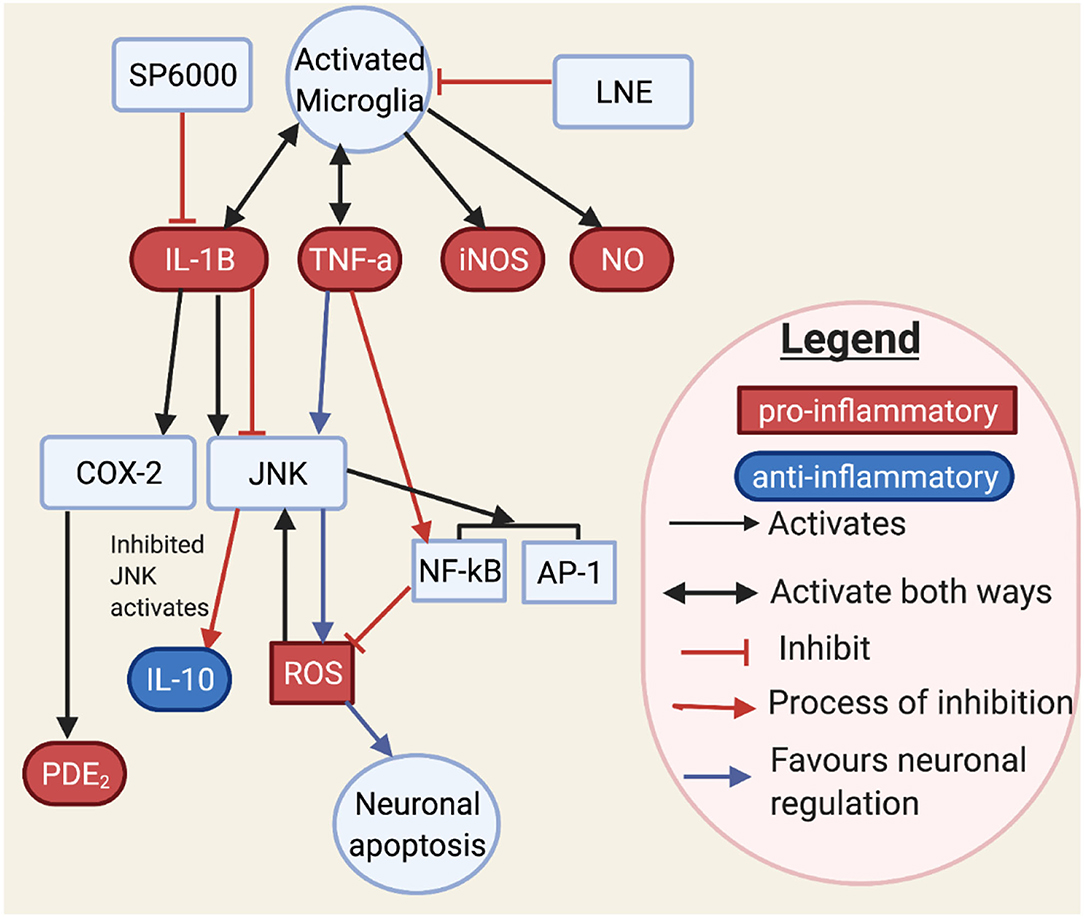
Figure 7. This figure shows how JNK may interact with neurons and microglia through cytokines and transcription factors. JNK controls inflammatory mediators such as IL-1B, TNF-α, iNOS, and NO (126–128). Activated JNK has been involved with cytokine, oxidative species, and transcription factors. TNF-α stimulates JNK, which in turn stimulates ROS. However, ROS may in turn stimulate JNK. It is known that TNF-α stimulating JNK would result in neuronal apoptosis. Moreover, NF-kB when stimulated by TNF would inhibit ROS (127). An aromatic herb, lindera neesiana kurz (LNE), used as an anti-inflammatory substance, reduces pro-inflammatory expression in LPS stimulated microglia cells, such as JNK, p-38, NO, iNOS, COX-2 production and pro-inflammatory cytokine related neuronal injury to JNK phosphorylation in microglia cells (116, 129) and suggested that JNK activation, triggers pro-inflammatory mediators such as TNF-α, IL-6, IL-1β, COX-2, iNOS, NO and PGE2, and transcription factors such as AP-1 and NF-κB. SP600125 is a JNK inhibitor which inhibits COX-2 activity through IL-1B. Conversely, IL-1B induces both COX-2 and JNK activation (126). This makes IL-1B a main target for JNK. JNK inhibition has also been observed to increase anti-inflammatory markers (116), which may restore the inflammatory imbalance observed in flush response and prevent microglial activated neuronal death (130).
Niacin-GPR109A Flush Response
PGD2 and PGE2 are potent vasodilators, and studies have linked them to diminished flush responses (27, 131). However, it is not fully understood how they are reduced in patients with schizophrenia. In addition, niacin is an antioxidant in many diseases and has a high affinity for its receptor, GPR109A (132–134). It is not well understood why niacin binding to GPR109A is ineffective in lowering the levels of pro-inflammatory mediators observed in schizophrenia. This indicates that there are other potential mediators associated with this aberrant response. This section will discuss (Figure 8) and explore inflammation involvement with the cellular mechanism behind the diminished flush response. It explores the link between the GPR109A-COX-prostaglandin pathway and inflammatory mediators, all of which are relevant to cellular biology behind diminished flush, signal transduction, and inflammation.
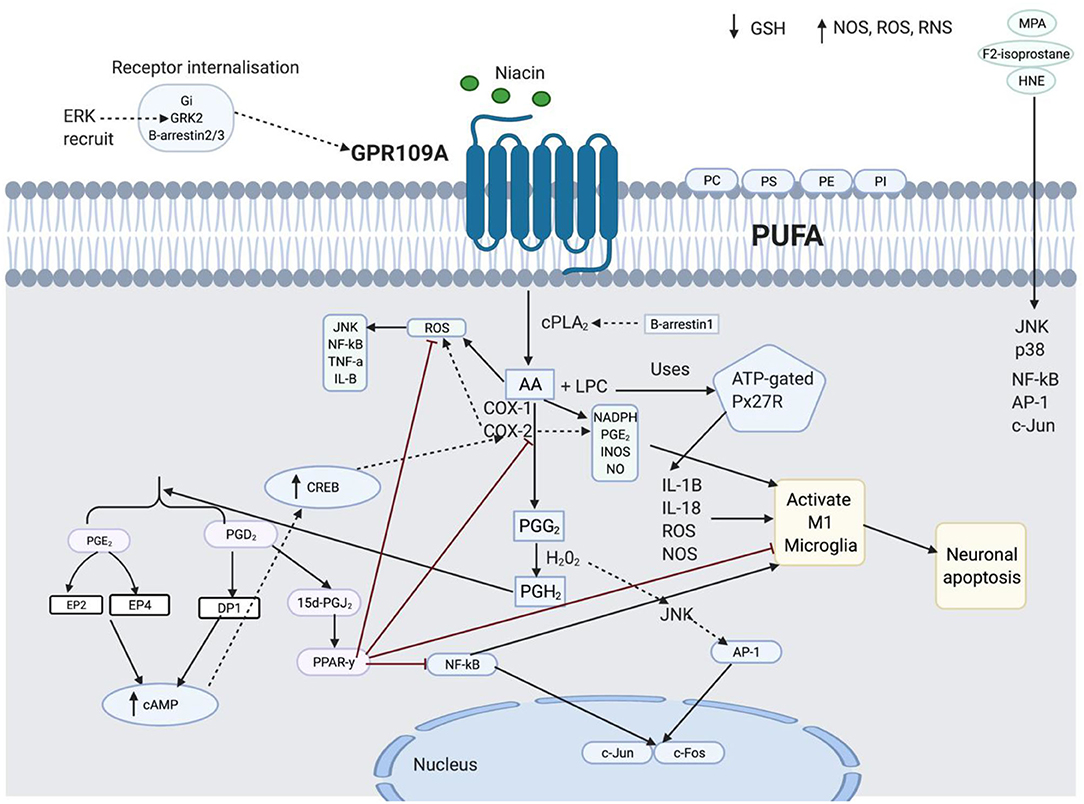
Figure 8. This figure shows the spider-web of inflammation involved in diminished flush response. The details are separated into four subheadings: lipid peroxidation and inflammation, the role of 15d-prostaglandin J2 (15d-PGJ2) and peroxisome proliferator-activated receptor-y (PPARγ) in anti-inflammation, Transduction Signal role in GPR109A components, and Function of enzymes in diminished flush response and inflammation.
Lipid Peroxidation and Inflammation
Membrane phospholipids contain polysaturated fatty acids (PUFAs), which have a high content of n-6 arachidonic acid (AA) (135). PUFAs contain phosphatidylethanolamine (PE), phosphatidylserine (PS), phosphatidylcholine (PC), and phosphatidylinositol (PI) (136). An inflammatory phospholipid, lysophosphatidylcholine (LPC), is generated when cPLA2 cleaves the acyl ester bond of PC (137). LPC uses ATP-gated P2X7 receptor (P2X7R), which is abundant in microglia, to induce IL-1B, IL-18, ROS, and NOS and activate microglia (138). PUFA exposure to oxidative stress is called lipid peroxidation, which induces ROS (139). NOS, ROS, and RNS activate pro-inflammatory mediators, NF-kB, and AP-1 (180) and mediate GSH deficiency (72). The inflammatory imbalance activates NF-κB. Active NF-κB can activate AP-1, which regulates the transcription of Jun and Fos, which are responsible for cell growth and differentiation (140, 141). In contrast, transcription factors may be regulated by ROS-stimulated MAPK (142). This means that the transcription factor may be controlled by MAPK independent of the oxidative species. HNE, a biomarker of lipid peroxidation, has been observed to activate both NF-κB, AP-1, and c-Jun expression, and cell signalling pathways, JNK and p38, when exposed to ROS (143, 144, 180). Therefore, pro-oxidants activate HNE-induced activation of the cell signalling pathway.
(15d-PGJ2) and (PPARγ) in Anti-inflammation
15d-PGJ2 increased the transcriptional activity of PPARγ. This downregulates the pro-inflammatory markers, COX-2, iNOS, AP-1, Stat-1, NF-kB, TNF-α, IL-1β, and PGE2, and increases antioxidant enzymes, hemeoxygenase-1 (HO-1) and GSH by PPARγ and 15d-PGJ2, respectively. PPAR-y and 15d-PGJ2 negatively regulate microglial activation and prevent neuronal apoptosis (145–153). NF-κB may be activated by ROS, cytokines, JNK (154), AP-1, and COX-2 (155). 15d-PGJ2 participates in the feedback mechanism (156) by PPARy, which inhibits activated NF-κB by increasing IKB expression (157, 158). PPAR-y activates antioxidant enzymes such as SOD, HO-1, and GSH to reduce ROS.
Transduction Signal Role in GPR109A Components
Gi, GRK2, and B-arrestin 3 are important for receptor internalisation (159). Upon activation of the GPR109A receptor by niacin, the Gi subunit is released from the GBY subunit, followed by desensitisation, which catalyses and phosphorylates the activated receptor by G protein-coupled receptor kinase (GRK2). Activated GPR109A promotes translocation and binding of B-arrestin 3 to the plasma membrane, resulting in receptor internalisation (159). Gi is involved in GRK2 recruitment to phosphorylate the C-terminus of GPR109A and subsequent ERK1/2 activation (160, 161). Phosphorylated ERK1/2 has been observed to potentiate GRK2 activity, resulting in the inhibition of leukocyte migration. In comparison, p38 blocks GRK2 function and facilitates cell migration (162, 163). The ERK pathway uses GRK2 to activate GPR109A; conversely, the ERK pathway is GPR109A independent, when activating B-arrestin 1. B-arrestin 2 phosphorylates and activates JNK3 in endosomes (164). It has also been observed that disrupted, ubiquitinated B-arrestin 2 promotes NF-κB signalling (165). ERK has been associated with B-Arestin 1, whereas it may be inferred that B-arrestin 2 may be associated with the JNK pathway, as it is both a precursor for c-Jun and an activator of NF-kB.
Function of Enzymes in Diminished Flush Response and Inflammation
Phosphorylated cPLA2a releases AA to induce pro-inflammatory markers, NADPH oxidase, superoxide, PGE2, INOS expression, and NO production, which activate microglia cells (166, 167). AA release produces ROS as a by-product, which activates JNK, NF-κB, TNF-α, and IL-1 to further activate COX-2 (168–171). Overactive COX-2 increases pro-inflammatory, iNOS, PGE2, nitric oxide, and peroxynitrite anions, which attack membrane phospholipids and lower their antioxidant defence (172). There was a synergistic effect between COX-2 and PGE2 expression; an increase in one would increase the expression of the other. PGE2 acts as a pro-inflammatory mediator and increases M1 microglial activation by increasing COX-2, IL-1B, and IL-6 levels (173–176). Active PGE2 activates the EP2 receptor, which increases cAMP production and activates cAMP response element-binding protein (CREB), which is responsible for increasing COX-2 expression (177–179). Different receptors induce different functions; for example, EP2 receptors regulate TNF-α, whereas EP4 receptors mediate IL-1B secretion (173). JNK inhibitor is known to reduce COX-2 expression, mediated by IL-1B, and it may be questioned whether this is also mediated through the EP4 receptor. H202 partially activates JNK (180) and AP-1 protein (181) to increase c-Jun and c-Fos (182) and resulting in cell apoptosis.
Phospholipid Abnormality
The membrane phospholipid hypothesis suggests that the abnormality observed in schizophrenia may be due to altered phospholipid metabolism (183, 184). LPC levels are disrupted in schizophrenia (185–187). LPC inflammatory activity is controlled by NLRP3 and NLRC4 genes (110).
Fatty Acid Abnormality
Fusar-Poli and Berger (188) showed reduced PUFA levels in patients with schizophrenia. PUFA is responsible for both membrane fluidity and its ligand-receptor interaction; it increases the concentration of receptors in the membrane and allows the ligand to interact with the receptor (189). Disrupted ligand-receptor interaction might be a reason for the reduced binding between GPR109A and its ligand, niacin, and therefore, its inability to release PGD2 and PGE2, resulting in a diminished flush response. Niacin has anti-inflammatory properties, and less exposure to niacin may contribute to the inflammatory imbalance observed in schizophrenia. Smesny et al. (190) suggested that structural changes observed in grey matter may be due to lipid membrane alterations, and that antipsychotics may influence lipid metabolism. A meta-analysis (191) showed that PUFA supplement intake and omega-3 or 6 reduced TNF-α levels and delayed onset of illness in ultra-high-risk patients with schizophrenia (192).
Biomarkers of Lipid Peroxidation
Lipid peroxidation is described as an oxidant that attacks PUFAs by inserting oxygen into the carbon-carbon double bond and altering the membrane structure (193). Lipid peroxidation can form secondary products such as malondialdehyde (MDA), propanal, and 4-hydroxynonenal (4-HNE) (91, 194). It has been observed that 4-HNE at low levels is metabolised, and therefore maintains a homeostatic environment, but at high levels, it can cause cell death and damage cell signalling proteins (195). HNE increases intracellular calcium levels in neurons (196), which may activate MAPK proteins, activate the COX pathway, or induce neuronal toxicity (197). Uchida et al. (180) confirmed that JNK is an important signalling mediator in cellular defence against toxic products generated from lipid peroxidation. MDA is a specific biomarker for lipid peroxidation in omega-6 fatty acids (198). MDA exposure alters membrane fluidity, resulting in the loss of membrane integrity (199). However, there is a heterogeneous distribution of MDA in schizophrenia, which may be due to confounders such as antipsychotics, which were not separated in the study (200). The sensitivity of biomarkers can also be an issue when measuring lipid peroxidation. There have been reports of increased F2-isoprostane (201) and microRNAs (miRNAs) in schizophrenia (202–205), which are more sensitive biomarkers of lipid peroxidation (201, 206–208).
Arachidonic Acid
Glen et al. (209), McNamara et al. (210), and Yao et al. (211) reported AA depletion in red blood cells (RBCs) in patients with schizophrenia. There is a controversy about the cause of the depleted AA; some researchers suggest that it may be due to niacin blunted response (212), whereas others would argue that niacin blunted response has been observed at normal AA levels, and instead may be due to disrupted AA metabolism (213). Skosnik and Yao (11), Horrobin (214), and du Bois et al. (215) suggested that oxidative stress reduces AA levels and modifies the signal transduction pathways to cause neuronal damage, as observed in schizophrenia. Cao et al. (216) and Covault et al. (217) reported that increased long-chain fatty acid-CoA ligase, type 4 (FACL4) activity as a result of genetic mutation leads to more rapid sequestration of free AA, resulting in reduced AA.
AA and JNK
In phagocytic cells, AA translocates activated rac from the cytosol to the membrane to activate NADPH oxidase and activate JNK, respectively (218–220). However, it has been observed that JNK activation is independent of AA metabolism. Minden et al. (221) showed that the antioxidant N-acetylcysteine blocked two-thirds of AA-induced JNK activation. It may be inferred that activated JNK is more dependent on oxidative species than AA.
Prostaglandin
A systemic imbalance of pro-inflammatory and anti-inflammatory prostaglandin levels has been reported in patients with schizophrenia (222). This imbalance may be associated with altered mediators involved in the niacin-GPR109A-COX pathway. The degradation of phospholipid membranes into eicosanoids results in the production of free radicals, which may contribute to the imbalance (223).
PGD2 and PGE2
Morrow et al. (224) used gas chromatography-mass spectrometry to detect large levels of PGD2 and its metabolite 9a,11 β-PGF2 following oral niacin. However, (225, 226) suggested that flushing is strictly related to PGE2. Furthermore, (227) suggested that increased cAMP production by their receptors, DP1, EP2, and EP4, contributes to flushing. However, Wise et al. (228) countered earlier studies by showing that DP1 and EP2 receptor knockout showed 40 and 20% reduced flushing, respectively. In addition, laropiprant, which is an antagonist with high selectivity for DP1, showed reduced flushing when compared to placebo, but 70% of the time, the participants still had flushes (229). This suggests that PGD2, PGE2, and their receptors are important in the flushing response, but partially contribute to its effect.
Moreover, PGE2 is synergistic with COX-2 to activate microglia (173–176), and active microglia can damage neurons. COX-2 inhibitors serve as neuroprotectants by reducing PGE2 levels (230). High concentrations of PGD2 have also been observed to be neurotoxic (231, 232). This is interesting because the diminished flush effect resulted in low PGD2 levels. PGD2 exerts anti-inflammatory properties through PPAR-Y; therefore, it may be suggested that high PGD2 would be beneficial for cells. Furthermore, Liang et al. (233) cleared our understanding by stating PGD2 concentration of 1 nM-1 μM, and PGE2 at concentrations of 0.01–1 μM are neuroprotective.
PGE2 Level Controversy
Cytosolic PGE2 levels were observed to be reduced in the temporal cortex of patients (234). Other studies have suggested that PGE2 levels (64, 235–238). Pierre et al. (239) and Quraishi et al. (240) showed that PDE2 can be modulated by peroxisome proliferator-activated receptor γ (PPARy), a nuclear receptor stimulated by prostaglandin J2 (PGJ2). As PGE2 is a pro-inflammatory mediator, this may suggest that PPARy may regulate both pro- and anti-inflammatory properties based on its interaction with the prostaglandin type. A recent study, which considered the acute phase of schizophrenia, eliminated potential confounders such as drug dependency, alcohol consumption, development delay, and dementia, and matched patients based on their age, sex, marital status, education, and onset of illness, confirmed that there are lower serum levels of PGE2, 15d-PGJ2, and PPARy levels in patients (241). In contrast, Martínez-Gras et al. (222) showed reduced levels of 15d-PGJ2, PPARy, and IkBa, but increased levels of PGE2. However, participants in the study had been using antipsychotic drugs and did not match the severity of the illness. The variation in PGE2 levels may depend on the severity of illness and the use of antipsychotic drugs.
PPARy and 15d-PGJ2 Role
PGD2 can be degraded non-enzymatically to form a J-series, 15-deoxy-Δ12, 14-PGJ2 (15d-PGJ2), which binds to PPAR-γ (242, 243). 15d-PGJ2 is a cyclopentenone prostaglandin, which reportedly exerts anti-inflammatory effects on microglia (150). 15d-PGJ2 is the first endogenous ligand of PPAR-γ. PPAR-γ plays an important role in lipid metabolism, inflammation, proliferation, and differentiation of cells. Furthermore, PPARy is considered a negative regulator of activated macrophages, and can stimulate or inhibit 15d-PGJ2 gene expression by altering transcription factors, AP-1, STAT, and NF-kB (148, 158). To reverse macrophage activation, transcription factors are downregulated by PPARγ. PPARy regulates the relationship between microglia and neurons by modulating cytokines IL-18 expression in microglia, which has an inhibitory effect on LTP. PPARy agonist reverses IL-18 mediated attenuation of LTP by enhancing synaptic plasticity (148, 244). JNK inhibitors are also known to act as PPARy agonists, supporting their anti-inflammatory role (245–248).
G-Coupled Receptor
PUMA-G in mice is an orthologue of the human GPR109A receptor. Mice lacking PUMA-G did not release PGD2 or PGE2, and therefore, did not show flushing (35). The alteration of receptor components has been associated with diminished flush. B-arrestin is used for cell signalling, receptor desensitisation, and internalisation (249). Internalisation is involved in receptor desensitisation and signalling, and contributes to the diversity of GPCR-dependent signalling (250). B-arrestin1 is a biassed agonist because it may induce a flushing response independent of the GPR109A receptor by increasing cPLA2 phosphorylation, while depletion of B-arrestin1 reduces activated cPLA2 (249). B-arrestin2/3 was significantly reduced in the schizophrenia group compared to that in the control group. Furthermore, reduced GRK in the frontal cortex was observed in both younger and older patients with schizophrenia. However, Bychkov et al. (251) observed a difference in GRK levels in both young and older patients with schizophrenia compared to controls. In young patients with schizophrenia, GRK3 had been reduced, whereas in the older schizophrenia group, GRK6 showed the greatest reduction. It may be inferred that disrupted B-arrestin or GRKs may result in diminished flush response, and confirmed that age is an important factor in schizophrenia.
Enzymes
Enzymes are biological catalysts that convert essential fatty acids to prostaglandins in the GPR109A-COX-prostaglandin pathway. Horrobin (252) suggested that one of the factors behind diminished flush was dysfunctional enzyme activity, which contributes to reduced prostaglandin levels. Furthermore, the GPR109A flushing response can be ablated by inhibiting PLA2 and COX-1/COX-2 activity (253). Figures 9, 10 shows the profiles for the PLA2 and COX families, respectively.
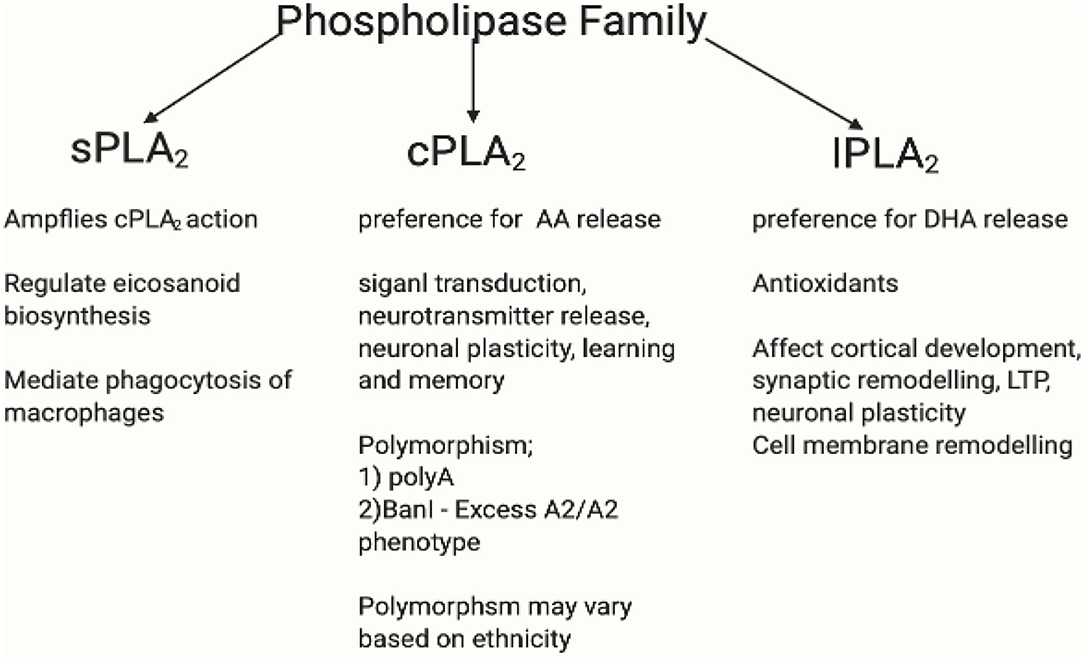
Figure 9. This figure shows the PLA2 family profile. The phospholipase A2 superfamily consists of enzymes that catalyse the hydrolysis of (sn-2) ester bond of phospholipid to liberate free fatty acids such as AA (254). The family of PLA2 consists of secretory calcium dependent PLA2 (sPLA2), intracellular calcium independent PLA2 (iPLA2), and the intracellular calcium dependent PLA2 (cPLA2) (255). iPLA2 possesses antioxidant and anti-inflammatory properties and have preference for DHA release. Likewise, cPLA2 has preference for AA release (256, 257). iPLA2 affects cortical development, synaptic remodelling, long-term potentiation (LTP), neuronal plasticity, and cell membrane remodelling (258). Whereas, cPLA2 participates in signal transaction, neurotransmitter release, neuronal plasticity, and learning and memory (259–261). Overexpressed iPLA2 did not induce COX-2-dependent PGE2 release, but instead mediated PGE2 release by COX-1 (262–264). sPLA2 amplifies cPLA2 action by regulating eicosanoid biosynthesis and mediate phagocytosis of macrophages (265). cPLA2 mutation varies in different ethnicities. There is an existing association between niacin flush response and PLA2G4A and PTGS2 gene polymorphism. The PLA2G4A gene encodes a calcium dependent form of cPLA2 (266, 267), whereas the PLA2G4C encodes a calcium independent form (268). There had been two polymorphisms of PLA2G4A: polyA and BanI polymorphism occur near the first intron and promoter region, respectively (269). Association between PLA2G4A polymorphisms and disease have been reported (270–272). The difference in BanI alleles between A1 (cut) and A2 (uncut) showed that cPLA2 activity with A2A2 genotype was higher than that with A1A2 and A1A1 (273). Excess A2/A2 homozygote has been associated with BanI polymorphism in schizophrenia (272, 274). A Korean study replicated those in western countries which supported cPLA2 gene Ban I polymorphism in schizophrenia (275). In a Brazilian population, higher cPLA2 activity correlated significantly with G allele of BanI polymorphic site and was associated with a higher risk of developing schizophrenia (273). However, some studies contradict this by reporting the lack of association between cPLA2 gene and schizophrenia (276–278). It may be inferred here that while there is disruptive cPLA2 gene for schizophrenia in different ethnicity, its polymorphism mutation may vary. Created in BioRender.com.
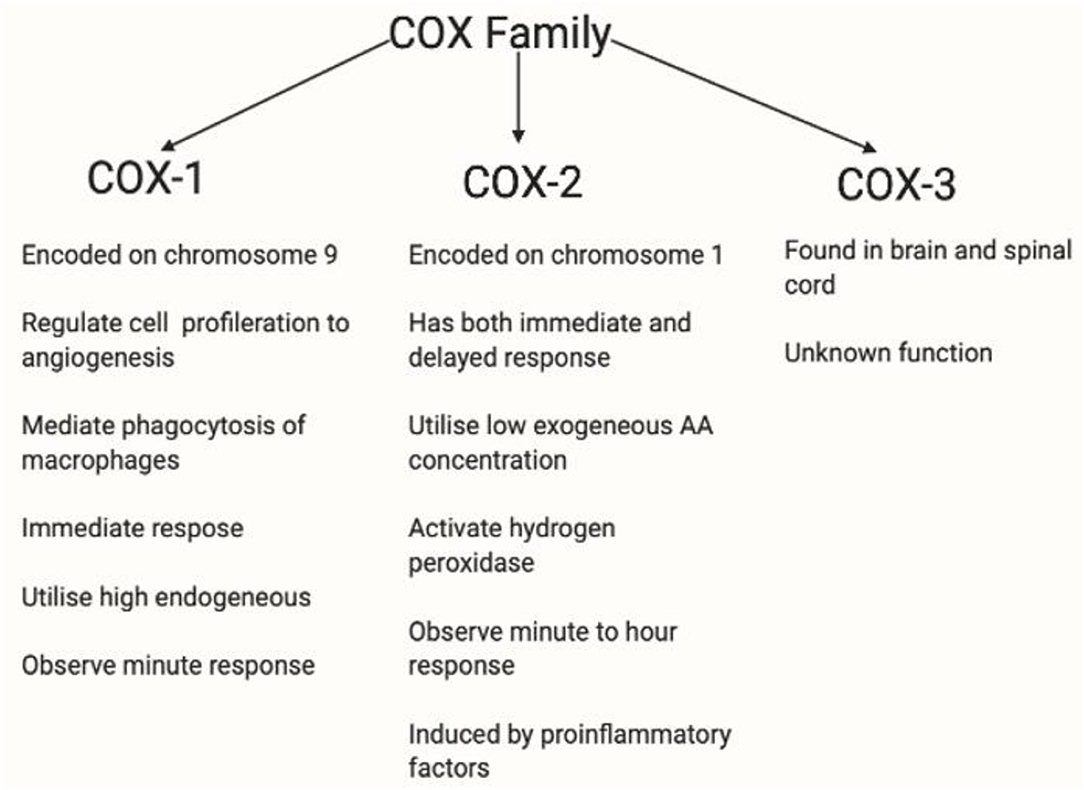
Figure 10. This figure shows the COX profile. There are three isoforms of COX. COX-1 plays a role in homeostasis (279). COX-2 is regulated by growth factors and cytokines such as IL1B, IL6, or TNF-α (280) and is overexpressed in inflammation, and therefore is relevant in this thesis. COX-3 has been used in the brain and spinal cord. However, the functions of COX-3 are currently unknown (281). COX-1 and COX-2 are rate-limiting enzymes in AA-derived prostaglandin production, COX-1 is expressed in most tissues whereas COX-2 is induced by pathophysiological responses by inflammatory stimuli such as IL-1, IL-1B, iNOS and growth factor, and EGF (36, 175, 282, 283). COX-1 is restricted to immediate response, whereas COX-2 is active in both immediate and delayed response. Also, low exogeneous AA concentration been observed and are utilised by COX-2 (284), whereas high endogenous AA concentration are utilised by COX-1 (285). COX-2 has a lower threshold for hydrogen peroxidase activation at low AA concentration (286, 287). Depleted AA is observed in diminished flushing response, and hydrogen peroxidase is relevant in stimulating microglia cells, thus, making COX-2 a relevant enzyme in diminished flush.
PLA2
Controversy in Phospholipase Activity
There is increased PLA2 activity in the cortex and thalamus in patients with first-onset schizophrenia (190). This study provides an insight that PLA2 activity is dependent on the stage of illness, and its activity may vary based on the brain region and use of medication. However, the study has limitations, as it did not indicate which PLA2 activity is being measured, as different PLA2 have different functions and activities.
Dr. Horrobin's membrane hypothesis suggests that elevated levels of calcium-dependent cytosolic group IVA PLA2a (cPLA2a) observed in schizophrenia are responsible for the depletion of AA (183). Messamore et al. (288) and Kim et al. (289) reported an increase in intracellular calcium concentration, which may result in increased cPLA2 activity. Instead, (290–293) suggested that there is increased iPLA2 and decreased cPLA2 activity in patients with schizophrenia. The iPLA2 may be increased as negative feedback by producing an antioxidant that mediates increased oxidative stress, as observed in schizophrenia patients (294–296). The reduced cPLA2 has a higher preference for cleaving AA, which may explain the reduced AA levels in patients with schizophrenia. It may be concluded that the variation in cPLA2 activity may be due to confounders such as age, medication, disease stage, ethnicity, and other medical status which induce pro-inflammatory and anti-inflammatory imbalances.
cPLA2, JNK and Its Effect on Cells
Phosphorylation of Ser505, Ser515, and Ser727 activates cPLA2 (297, 298). Activated cPLA2 cleaves AA and induces the production of inflammatory mediators such as eicosanoids (299–301). There are insufficient studies regarding Ser515 and Ser727 and their effect on cPLA2. However, phosphorylation of ser505 on cPLA2a increases phospholipase binding to membrane phospholipids at low calcium concentrations, altering the PLA2 conformation to ensure a better fit to the catalytic domain of membrane phospholipids (298). Some studies have suggested a relationship between MAPK and cPLA2 activity. However, in macrophages, there have been inconsistent reports of ERK1/2 and p38 links in phosphorylating cPLA2 at ser505 (302–304). Casas et al. (305) used MAPK inhibitors for ERK, p38, and JNK and found that only the JNK inhibitor effectively blocked cPLA2a phosphorylation in macrophages. This advances our understanding of the prominent role of JNK in cPLA2a phosphorylation in macrophages. Microglia are resident macrophages of the brain, which share similar functional and morphological properties to macrophages (306), therefore it may be inferred that microglia would have similar effects. However, there is no study linking MAPK and cPLA2a to neurons, although from our understanding of how microglia and neurons influence each other, there is a possibility that alteration in cPLA2 activity in microglia might affect neuronal functions. Furthermore, it has been observed that cPLA2 and dopamine are inversely related (307), where increased dopamine levels reduced cPLA2. The mechanism is not understood properly, but studies have shown that dopamine and glutamate alternation have specifically affected cPLA2 mediated AA release, but not mediators downstream of AA (289, 308).
COX
Activators of COX
PGD2, PDE2 mediated by COX-1 and COX-2, play an important role in the flushing response (35, 228). COX-2 knockout reduces both pro-inflammatory, PGE2, and NF-kB (309–311). Deng et al. (312) suggests that overexpression of COX-2 activity has been associated with increased histone acetyl transferase (HAT) and p300 gene, which is located near the NF-kB promoter, deletion or suppression of these transcriptional activators, and reduced COX-2 expression. Future studies need to investigate the link between HAT, p300, and COX-2 overexpression in schizophrenia. Ultimately, IL-1B is a potent inducer of COX and induces the synthesis and activity of PLA2 in cells (313). Therefore, it may be used as a target to control both the activation levels of COX and PLA2 by JNK.
COX in Microglia
COX-2 is important for producing inflammatory responses, which can activate microglia (314). During prostaglandin production via the COX pathway, ROS are generated as a by-product, along with the production of inflammatory agents such as cytokines and oxidative stress (282), all of which contribute to microglial activation.
Inhibitors of COX-2 Expression
When there is a high inflammation level, antipsychotics are less effective in reducing psychosis (315, 316). COX-2 overexpression has been linked to cognitive deficits in schizophrenia; COX-2 inhibition has been shown to have therapeutic effects, particularly when administered in the early stage of the disease (317–324). Mattson et al. (325) Weggen et al. (326), and Morihara et al. (327) suggested that nonsteroidal anti-inflammatory drugs (NSAIDs) regulate NF-κB and can serve as a therapeutic target for several psychiatric disorders. Nitta et al. (319) observed that NSAID celecoxib and risperidone are more beneficial in patients than the administration of antipsychotic risperidone alone. Niederberger et al. (328) and Tegeder et al. (329) showed that patients who used both NSAIDs and antipsychotic drugs had a higher psychotic relapse rate. These reports suggest that NSAIDs may play a controversial role in upregulating COX-2 expression, instead of downregulating COX-2. Harris et al. (330) theory on COX-2 as a double agent may influence the role of NSAIDs or COX-2 inhibitors. COX-2 can also participate in both pro-inflammatory and anti-inflammatory effects. During the development of inflammation, pro-inflammatory (via PGE2), but anti-inflammatory (via PGD2 and 15d-PGJ2) during resolution. Therefore, there is a chance that COX-2 inhibitors may instead inhibit anti-inflammatory properties, therefore, exacerbating schizophrenic symptoms. Therefore, alternative methods should be explored to ensure the selective downregulation of overactive COX-2 expression.
Increased COX-2 expression is dependent on MAPK activation (331). Yang et al. (332) showed that IL-1B induction is responsible for elevated COX-2 expression in hippocampal neurons. Rösch et al. (331) showed fibroblasts released PGE2 when stimulated with IL-1B, were also found to have overexpressed COX-2 and defective JNK signalling. To confirm this finding, the JNK inhibitor, SP600125, along with IL-1B, lowered both PGE2 and COX-2 expression (333–336). It may be inferred that schizophrenia patients with overexpressed COX-2 may present with increased levels of pro-inflammatory mediators. Therefore, to maintain inflammatory balance, the JNK inhibitor SP600125 may be administered, which may downregulate pro-inflammatory mediators. Other inhibitors such as glucocorticoids and minocycline have been shown to downregulate AP-1 or NF-κB in microglial cells and protect against neurotoxicity, while improving cognitive and negative symptoms of schizophrenia (337, 338).
Hydroperoxide
Stimulated hydrogen peroxide produces NADPH oxidase, otherwise known as phagocytic oxidase (PHOX), which converts microglia to an activated or cytotoxic state (339).
Exosomes
Exosomes transmit genetic information between cells, and miRNAs are found inside exosomes. These exosomes can be secreted by neurons or astrocytes (340). These exosomes circulate around the body to nearby and distant cells (341). Exosomal miRNAs have also been shown to be involved in the inflammatory response (342). A recent study found an association between dysregulated exosomes and schizophrenia (343). Du et al. reported a pattern between dysregulated exosomes and glycerophospholipid metabolism. The relationship between exosomes and GPR109A receptor should be investigated in future studies.
Genes
Schizophrenia is caused by the cumulative effects of risk variants in over 100 genes (45, 344). Most these genes are associated with neurons, neurotransmitters or synaptic plasticity (345–347). Table 1 attempts to match the current GWAS for schizophrenia with genes which may be involved in the diminished flush response. A negative result may be a false negative, whereas a positive match may be false positive. As observed in the table, GPR109A has been a match, which may suggest that risk variants in GPR109A may contribute to the aetiology of schizophrenia, as well as to an abnormal flushing response. GPR109A showed a positive match, indicating genetic mutation. This matched with the review analysis which suggested that there is an alteration in the receptor protein conformation and components, B-Arestin and GRK. We would have expected alternation in cPLA2 and COX-2, as there had been strong evidence in this review suggesting alterations in its genetic, protein expression, and activity. The dual role of PPARG in inflammation and its reduced expression in patients with schizophrenia would make it a good target. We would not expect much alteration in prostaglandin enzymes and receptors, as strong evidence suggests that they do not significantly contribute to the flushing response.
Conclusion
This review shows altered cellular pathology behind a diminished flush response. First, diminished flush is not only caused by vasodilators, but also by altered protein expression, protein activity, and inflammatory imbalance. Altered protein levels in the GPR109A-COX-prostaglandin pathways include membrane phospholipids, GPR109A, enzymes, cPLA2 and COX-2, and prostaglandins with their receptors and downstream products, such as PGD2, PGE2, DP1, EP2, EP4, 15d-PGJ2, and PPAR-y. Furthermore, we found that there was an inflammatory imbalance in the flush response. Although there is a possibility of genetic alteration in GPR109A, it is possible that environmental factors, such as oxidative stress, may alter receptor conformation, causing reduced receptor-ligand bonds, resulting in diminished flush. Second, as patient demographics interfere with the flush effect, future studies should consider the age, illness stage, ethnicity, use of antipsychotics, and presence of health comorbidities in their participants. The niacin skin flush test is essentially used to diagnose patients at their prodromal stage; however, this review contains limited research on the altered cell pathology at the prodromal stage. This review well supports the evidence for M1 microglia activation; however, evidence on neurons is weak, as there is no direct evidence linking diminished flush response to neurons. Given that microglia and neurons share a bidirectional relationship, it is likely that M1 activation may indirectly influence neuronal apoptosis. Lastly, JNK inhibition can inhibit M1 activation, neuronal apoptosis, and reduce inflammatory mediators, NF-κB, IL-1B, and TNF-α, and influence protein phosphorylation or expression, cPLA2, COX-2, and PPAR-y, respectively. Although further investigation is required to understand whether ROS-mediated JNK may influence GPR109A, we believe that the ability of JNK to control multiple targets in the diminished flush response would make it a good therapeutic target for schizophrenia. Future research should investigate whether stimulation of GPR109A results in PGD2 or PGE2 release from microglial cells and whether this is mediated by the JNK pathway. Future research should also bear in mind that Table 1 has established a match with 128 GWAS, which may be essential for the updated GWAS for schizophrenia in the future.
Author Contributions
The author confirms being the sole contributor of this work and has approved it for publication.
Acknowledgements
I would like to thank my Supervisor, Dr. Brian Morris for his support and guidance which has enabled me to complete this review.
Conflict of Interest
The author declares that the research was conducted in the absence of any commercial or financial relationships that could be construed as a potential conflict of interest.
Publisher's Note
All claims expressed in this article are solely those of the authors and do not necessarily represent those of their affiliated organizations, or those of the publisher, the editors and the reviewers. Any product that may be evaluated in this article, or claim that may be made by its manufacturer, is not guaranteed or endorsed by the publisher.
Supplementary Material
The Supplementary Material for this article can be found online at: https://www.frontiersin.org/articles/10.3389/fpsyt.2021.771144/full#supplementary-material
References
1. Ellard J. Did schizophrenia exist before the eighteenth century? Aust N Z J Psychiatry. (1987) 21:306–18. doi: 10.1080/00048678709160925
2. Jeste DV. del Carmen R, Lohr JB, Wyatt RJ. Did schizophrenia exist before the eighteenth century? Comprehensive Psychiat. (1985) 26:493–503. doi: 10.1016/0010-440X(85)90016-1
3. JC DAaC. The Neuropathology of Schizophrenia. Lawrence Erlbaum Associates. East Sussex: Lawrence Erlbaum Associates, Inc. (1994).
4. Larson MK, Walker EF, Compton MT. Early signs, diagnosis and therapeutics of the prodromal phase of schizophrenia and related psychotic disorders. Expert Rev Neurother. (2010) 10:1347–59. doi: 10.1586/ern.10.93
5. Dean K, Murray RM. Environmental risk factors for psychosis. Dialogues Clin Neurosci. (2005) 7:69–80. doi: 10.31887/DCNS.2005.7.1/kdean
6. Yung AR, Stanford C, Cosgrave E, Killackey E, Phillips L, Nelson B, et al. Testing the ultra high risk (prodromal) criteria for the prediction of psychosis in a clinical sample of young people. Schizophr Res. (2006) 84:57–66. doi: 10.1016/j.schres.2006.03.014
7. Yung AR, McGorry PD, McFarlane CA, Jackson HJ, Patton GC, Rakkar A. Monitoring and care of young people at incipient risk of psychosis. Schizophr Bull. (1996) 22:283–303. doi: 10.1093/schbul/22.2.283
8. Vos T, Barber RM, Bell B, Bertozzi-Villa A, Biryukov S, Bolliger I, et al. Global, regional, and national incidence, prevalence, and years lived with disability for 301 acute and chronic diseases and injuries in 188 countries, 1990–2013: a systematic analysis for the Global Burden of Disease Study 2013. Lancet. (2015) 386:743–800. doi: 10.1016/S0140-6736(15)60692-4
9. Chong HY, Teoh SL, Wu DB-C, Kotirum S, Chiou C-F, Chaiyakunapruk N. Global economic burden of schizophrenia: a systematic review. Neuropsychiatr Dis Treat. (2016) 12:357–73. doi: 10.2147/NDT.S96649
11. Skosnik PD, Yao JK. From membrane phospholipid defects to altered neurotransmission: is arachidonic acid a nexus in the pathophysiology of schizophrenia? Prostaglandins, Leukotrienes and Essential Fatty Acids. (2003) 69:367–84. doi: 10.1016/j.plefa.2003.08.008
12. Carlsson A, Lindqvist M. Effect of chlorpromazine or haloperidol on formation of 3-methoxytyramine and normetanephrine in mouse brain. Acta Pharmacol Toxicol. (1963) 20:140–4. doi: 10.1111/j.1600-0773.1963.tb01730.x
13. Van Rossum J. The significance of dopamine-receptor blockade for the mechanism of action of neuroleptic drugs. Arch Int Pharmacodyn Ther. (1966) 160:492.
14. Lieberman JA, Stroup TS, McEvoy JP, Swartz MS, Rosenheck RA, Perkins DO, et al. Effectiveness of antipsychotic drugs in patients with chronic schizophrenia. New England journal of medicine. (2005) 353:1209–23. doi: 10.1056/NEJMoa051688
15. Ray WA, Chung CP, Murray KT, Hall K, Stein CM. Atypical antipsychotic drugs and the risk of sudden cardiac death. N Engl J Med. (2009) 360:225–35. doi: 10.1056/NEJMoa0806994
16. McIntyre RS, McCann SM, Kennedy SH. Antipsychotic metabolic effects: weight gain, diabetes mellitus, and lipid abnormalities. Can J Psychiatry. (2001) 46:273–81. doi: 10.1177/070674370104600308
18. Nadalin S, Buretić-Tomljanović A, Rubesa G, Tomljanović D, Gudelj L. Niacin skin flush test: a research tool for studying schizophrenia. Psychiatr Danub. (2010) 22:14–27.
19. Fiedler P, Wolkin A, Rotrosen J. Niacin-induced flush as a measure of prostaglandin activity in alcoholics and schizophrenics. Biol Psychiatry. (1986) 21:1347–50. doi: 10.1016/0006-3223(86)90321-5
20. Rybakowski J, Weterle R. Niacin test in schizophrenia and affective illness. Biol Psychiatry. (1991) 29:834–6. doi: 10.1016/0006-3223(91)90202-W
22. Hudson C, Gotowiec A, Seeman M, Warsh J, Ross BM. Clinical subtyping reveals significant differences in calcium-dependent phospholipase A2 activity in schizophrenia. Biol Psychiatry. (1999) 46:401–5. doi: 10.1016/S0006-3223(99)00010-4
23. Bosveld-van Haandel L, Knegtering R, Kluiter H, van den Bosch RJ. Niacin skin flushing in schizophrenic and depressed patients and healthy controls. Psychiat Res. (2006) 143:303–6. doi: 10.1016/j.psychres.2005.10.010
24. Yao JK, Dougherty GG Jr, Gautier CH, Haas GL, Condray R, Kasckow JW, et al. Prevalence and Specificity of the Abnormal Niacin Response: A Potential Endophenotype Marker in Schizophrenia. Schizophrenia Bulletin. (2015) 42:369–76. doi: 10.1093/schbul/sbv130
25. Hudson CJ, Lin A, Cogan S, Cashman F, Warsh JJ. The niacin challenge test: Clinical manifestation of altered transmembrane signal transduction in schizophrenia? Biol Psychiatry. (1997) 41:507–13. doi: 10.1016/S0006-3223(96)00112-6
26. Bouwer C, Stein DJ. Hyperresponsivity to nicotinic acid challenge in generalized social phobia: a pilot study. Eur Neuropsychopharmacol. (1998) 8:311–3. doi: 10.1016/S0924-977X(97)00089-8
27. Pike NB. Flushing out the role of GPR109A (HM74A) in the clinical efficacy of nicotinic acid. J Clin Invest. (2005) 115:3400–3. doi: 10.1172/JCI27160
28. Benyó Z, Gille A, Bennett CL, Clausen BE, Offermanns S. Nicotinic acid-induced flushing is mediated by activation of epidermal langerhans cells. Mol Pharmacol. (2006) 70:1844–9. doi: 10.1124/mol.106.030833
29. Lin LL, Lin AY, Knopf JL. Cytosolic phospholipase A2 is coupled to hormonally regulated release of arachidonic acid. Proc Natl Acad Sci U S A. (1992) 89:6147–51. doi: 10.1073/pnas.89.13.6147
30. Waldo MC. Co-distribution of sensory gating and impaired niacin flush response in the parents of schizophrenics. Schizophr Res. (1999) 40:49–53. doi: 10.1016/S0920-9964(99)00031-6
31. Lin S-H, Liu C-M, Chang S-S, Hwu H-G, Liu SK, Hwang TJ, et al. Familial Aggregation in Skin Flush Response to Niacin Patch Among Schizophrenic Patients and Their Nonpsychotic Relatives. Schizophr Bull. (2006) 33:174–82. doi: 10.1093/schbul/sbl038
32. Liu CM, Chang SS, Liao SC, Hwang TJ, Shieh MH, Liu SK, et al. Absent response to niacin skin patch is specific to schizophrenia and independent of smoking. Psychiatry Res. (2007) 152:181–7. doi: 10.1016/j.psychres.2006.10.002
33. Ross BM, Hughes B, Turenne S, Seeman M, Warsh JJ. Reduced vasodilatory response to methylnicotinate in schizophrenia as assessed by laser Doppler flowmetry. Eur Neuropsychopharmacol. (2004) 14:191–7. doi: 10.1016/S0924-977X(03)00145-7
34. Shah S, Vankar G, Peet M, Ramchand C. Unmedicated schizophrenic patients have a reduced skin flush in response to topical niacin. Schizophr Res. (2000) 43:163.
35. Benyó Z, Gille A, Kero J, Csiky M, Suchánková MC, Nüsing RM, et al. GPR109A (PUMA-G/HM74A) mediates nicotinic acid–induced flushing. J Clin Invest. (2005) 115:3634–40. doi: 10.1172/JCI23626
36. Kim EJ, Kwon KJ, Park J-Y, Lee SH, Moon C-H, Baik EJ. Effects of peroxisome proliferator-activated receptor agonists on LPS-induced neuronal death in mixed cortical neurons: associated with iNOS and COX-2. Brain Res. (2002) 941:1–10. doi: 10.1016/S0006-8993(02)02480-0
37. Svedmyr N, Harthon L, Lundholm L. The relationship between the plasma concentration of free nicotinic acid and some of its pharmacologic effects in man. Clin Pharmacol Ther. (1969) 10:559–70. doi: 10.1002/cpt1969104559
38. Doorduin J, de Vries EF, Willemsen AT, de Groot JC, Dierckx RA, Klein HC. Neuroinflammation in schizophrenia-related psychosis: a PET study. J Nucl Med. (2009) 50:1801–7. doi: 10.2967/jnumed.109.066647
39. Micó JA, Rojas-Corrales MO, Gibert-Rahola J, Parellada M, Moreno D, Fraguas D, et al. Reduced antioxidant defense in early onset first-episode psychosis: a case-control study. BMC Psychiatry. (2011) 11:26. doi: 10.1186/1471-244X-11-26
40. Miller BJ, Buckley P, Seabolt W, Mellor A, Kirkpatrick B. Meta-analysis of cytokine alterations in schizophrenia: clinical status and antipsychotic effects. Biol Psychiatry. (2011) 70:663–71. doi: 10.1016/j.biopsych.2011.04.013
41. García-Bueno B, Bioque M, Mac-Dowell KS, Barcones MF, Martínez-Cengotitabengoa M, Pina-Camacho L, et al. Pro-/anti-inflammatory dysregulation in patients with first episode of psychosis: toward an integrative inflammatory hypothesis of schizophrenia. Schizophr Bull. (2014) 40:376–87. doi: 10.1093/schbul/sbt001
42. Kenk M, Selvanathan T, Rao N, Suridjan I, Rusjan P, Remington G, et al. Imaging neuroinflammation in gray and white matter in schizophrenia: an in-vivo PET study with [18F]-FEPPA. Schizophr Bull. (2015) 41:85–93. doi: 10.1093/schbul/sbu157
43. Monji A, Kato T, Kanba S. Cytokines and schizophrenia: Microglia hypothesis of schizophrenia. Psychiatry Clin Neurosci. (2009) 63:257–65. doi: 10.1111/j.1440-1819.2009.01945.x
44. Monji A, Kato TA, Mizoguchi Y, Horikawa H, Seki Y, Kasai M, et al. Neuroinflammation in schizophrenia especially focused on the role of microglia. Prog Neuropsychopharmacol Biol Psychiatry. (2013) 42:115–21. doi: 10.1016/j.pnpbp.2011.12.002
45. Ripke S, Neale BM, Corvin A, Walters JTR, Farh K-H, Holmans PA, et al. Biological insights from 108 schizophrenia-associated genetic loci. Nature. (2014) 511:421–7. doi: 10.1038/nature13595
46. Feigenson KA, Kusnecov AW, Silverstein SM. Inflammation and the two-hit hypothesis of schizophrenia. Neurosci Biobehav Rev. (2014) 38:72–93. doi: 10.1016/j.neubiorev.2013.11.006
47. Najjar S, Pearlman DM. Neuroinflammation and white matter pathology in schizophrenia: systematic review. Schizophr Res. (2015) 161:102–12. doi: 10.1016/j.schres.2014.04.041
48. Pelidou SH, Kostulas N, Matusevicius D, Kivisäkk P, Kostulas V, Link H. High levels of IL-10 secreting cells are present in blood in cerebrovascular diseases. Eur J Neurol. (1999) 6:437–42. doi: 10.1046/j.1468-1331.1999.640437.x
49. Kronfol Z, Daniel G. Remick, M. D. Cytokines and the brain: implications for clinical psychiatry. Am J Psychiatry. (2000) 157:683–94. doi: 10.1176/appi.ajp.157.5.683
50. Yilmaz G, Arumugam TV, Stokes KY, Granger DN. Role of T lymphocytes and interferon-in ischemic stroke. Circulation. (2006) 113:2105–12. doi: 10.1161/CIRCULATIONAHA.105.593046
52. Potvin S, Stip E, Sepehry AA, Gendron A, Bah R, Kouassi E. Inflammatory cytokine alterations in schizophrenia: a systematic quantitative review. Biol Psychiatry. (2008) 63:801–8. doi: 10.1016/j.biopsych.2007.09.024
53. Müller N, Riedel M, Ackenheil M, Schwarz MJ. The role of immune function in schizophrenia: an overview. Eur Arch Psychiatry Clin Neurosci. (1999) 249:S62–S8. doi: 10.1007/PL00014187
54. Söderlund J, Schröder J, Nordin C, Samuelsson M, Walther-Jallow L, Karlsson H, et al. Activation of brain interleukin-1beta in schizophrenia. Mol Psychiatry. (2009) 14:1069–71. doi: 10.1038/mp.2009.52
55. Raison CL, Capuron L, Miller AH. Cytokines sing the blues: inflammation and the pathogenesis of depression. Trends Immunol. (2006) 27:24–31. doi: 10.1016/j.it.2005.11.006
56. Goldsmith DR, Rapaport MH, Miller BJ A meta-analysis of blood cytokine network alterations in psychiatric patients: comparisons between schizophrenia bipolar disorder and depression. Mol Psychiatry. (2016) 21:1696–709. doi: 10.1038/mp.2016.3
57. Wang AK, Miller BJ. Meta-analysis of Cerebrospinal Fluid Cytokine and Tryptophan Catabolite Alterations in Psychiatric Patients: Comparisons Between Schizophrenia, Bipolar Disorder, and Depression. Schizophr Bull. (2018) 44:75–83. doi: 10.1093/schbul/sbx035
58. Khandaker GM, Pearson RM, Zammit S, Lewis G, Jones PB. Association of serum interleukin 6 and C-reactive protein in childhood with depression and psychosis in young adult life: a population-based longitudinal study. JAMA psychiatry. (2014) 71:1121–8. doi: 10.1001/jamapsychiatry.2014.1332
59. Matsubara T, Ziff M. Increased superoxide anion release from human endothelial cells in response to cytokines. J Immunol. (1986) 137:3295–8.
60. Meier B, Cross AR, Hancock JT, Kaup FJ, Jones OTG. Identification of a superoxide-generating NADPH oxidase system in human fibroblasts. Biochemical Journal. (1991) 275:241–5. doi: 10.1042/bj2750241
61. Janssen-Heininger YM, Macara I, Mossman BT. Cooperativity between oxidants and tumor necrosis factor in the activation of nuclear factor (NF)-κ b: requirement of ras/mitogen-activated protein kinases in the activation of Nf-κ B by oxidants. Am J Respir Cell Mol Biol. (1999) 20:942–52. doi: 10.1165/ajrcmb.20.5.3452
62. Sidoti-de Fraisse C, Rincheval V, Risler Y, Mignotte B, Vayssière J-L. TNF-α activates at least two apoptotic signaling cascades. Oncogene. (1998) 17:1639–51. doi: 10.1038/sj.onc.1202094
63. Loukili N, Rosenblatt-Velin N, Rolli J, Levrand S, Feihl F, Waeber B, et al. Oxidants positively or negatively regulate nuclear factor kappaB in a context-dependent manner. J Biol Chem. (2010) 285:15746–52. doi: 10.1074/jbc.M110.103259
64. García-Álvarez L, Caso JR, García-Portilla MP, de la Fuente-Tomás L, González-Blanco L, Sáiz Martínez P, et al. Regulation of inflammatory pathways in schizophrenia: A comparative study with bipolar disorder and healthy controls. Eur Psychiatry. (2018) 47:50–9. doi: 10.1016/j.eurpsy.2017.09.007
65. Do K, Trabesinger A, Kirsten-Krüger M, Lauer C, Dydak U, Hell D, et al. Schizophrenia: glutathione deficit in cerebrospinal fluid and prefrontal cortex in vivo. Eur J Neurosci. (2000) 12:3721–8. doi: 10.1046/j.1460-9568.2000.00229.x
66. Yao JK, Leonard S, Reddy R. Altered glutathione redox state in schizophrenia. Disease Markers. (2006) 22:83–93. doi: 10.1155/2006/248387
67. Gawryluk JW, Wang J-F, Andreazza AC, Shao L, Young LT. Decreased levels of glutathione, the major brain antioxidant, in post-mortem prefrontal cortex from patients with psychiatric disorders. Int J Neuropsychopharmacol. (2011) 14:123–30. doi: 10.1017/S1461145710000805
68. Altuntas I, Aksoy H, Coskun I, Çayköylü A, Akçay F. Erythrocyte superoxide dismutase and glutathione peroxidase activities, and malondialdehyde and reduced glutathione levels in schizophrenic patients. Clin Chem Lab Med. (2000) 38:1277. doi: 10.1515/CCLM.2000.201
69. Woo T-UW, Kim AM, Viscidi E. Disease-specific alterations in glutamatergic neurotransmission on inhibitory interneurons in the prefrontal cortex in schizophrenia. Brain Res. (2008) 1218:267–77. doi: 10.1016/j.brainres.2008.03.092
70. Barger SW, Goodwin ME, Porter MM, Beggs ML. Glutamate release from activated microglia requires the oxidative burst and lipid peroxidation. J Neurochem. (2007) 101:1205–13. doi: 10.1111/j.1471-4159.2007.04487.x
71. Bains JS, Shaw CA. Neurodegenerative disorders in humans: the role of glutathione in oxidative stress-mediated neuronal death. Brain Res Rev. (1997) 25:335–58. doi: 10.1016/S0165-0173(97)00045-3
72. Grima G, Benz B, Parpura V, Cuénod M, Do KQ. Dopamine-induced oxidative stress in neurons with glutathione deficit: implication for schizophrenia. Schizophr Res. (2003) 62:213–24. doi: 10.1016/S0920-9964(02)00405-X
73. Vendemiale G, Grattagliano I, Altomare E. An update on the role of free radicals and antioxidant defense in human disease. Int J Clin Lab Res. (1999) 29:49. doi: 10.1007/s005990050063
74. Flatow J, Buckley P, Miller BJ. Meta-Analysis of Oxidative Stress in Schizophrenia. Biol Psychiatry. (2013) 74:400–9. doi: 10.1016/j.biopsych.2013.03.018
75. Halliwell B. Reactive oxygen species and the central nervous system. J Neurochem. (1992) 59:1609–23. doi: 10.1111/j.1471-4159.1992.tb10990.x
76. Choi S-H, Aid S, Kim H-W, Jackson SH, Bosetti F. Inhibition of NADPH oxidase promotes alternative and anti-inflammatory microglial activation during neuroinflammation. J Neurochem. (2012) 120:292–301. doi: 10.1111/j.1471-4159.2011.07572.x
77. Taylor JM, Main BS, Crack PJ. Neuroinflammation and oxidative stress: Co-conspirators in the pathology of Parkinson's disease. Neurochem Int. (2013) 62:803–19. doi: 10.1016/j.neuint.2012.12.016
78. Zeng K-W, Zhao M-B, Ma Z-Z, Jiang Y, Tu P-F. Protosappanin A inhibits oxidative and nitrative stress via interfering the interaction of transmembrane protein CD14 with Toll-like receptor-4 in lipopolysaccharide-induced BV-2 microglia. Int Immunopharmacol. (2012) 14:558–69. doi: 10.1016/j.intimp.2012.09.004
79. Riazi K, Galic MA, Kuzmiski JB, Ho W, Sharkey KA, Pittman QJ. Microglial activation and TNFα production mediate altered CNS excitability following peripheral inflammation. Proc Nat Acad Sci. (2008) 105:17151–6. doi: 10.1073/pnas.0806682105
80. Schwartz M. Macrophages and microglia in central nervous system injury: are they helpful or harmful? J Cereb Blood Flow Metab. (2003) 23:385–94. doi: 10.1097/01.WCB.0000061881.75234.5E
81. Gutierrez EG, Banks WA, Kastin AJ. Murine tumor necrosis factor alpha is transported from blood to brain in the mouse. J Neuroimmunol. (1993) 47:169–76. doi: 10.1016/0165-5728(93)90027-V
82. Banks WA, Ortiz L, Plotkin SR, Kastin AJ. Human interleukin (IL) 1 alpha, murine IL-1 alpha and murine IL-1 beta are transported from blood to brain in the mouse by a shared saturable mechanism. J Pharmacol Exp Ther. (1991) 259:988–96.
83. Bentivoglio M, Kristensson K, Rottenberg ME. Circumventricular Organs and Parasite Neurotropism: Neglected Gates to the Brain? Front Immunol. (2018) 9:2877. doi: 10.3389/fimmu.2018.02877
84. Banks WA. The blood–brain barrier in psychoneuroimmunology. Immunol Allergy Clin North Am. (2009) 29:223–8. doi: 10.1016/j.iac.2009.02.001
85. Qu M, Lin Q, Huang L, Fu Y, Wang L, He S, et al. Dopamine-loaded blood exosomes targeted to brain for better treatment of Parkinson's disease. J Control Release. (2018) 287:156–66. doi: 10.1016/j.jconrel.2018.08.035
86. Ridder K, Keller S, Dams M, Rupp A-K, Schlaudraff J, Del Turco D, et al. Extracellular Vesicle-Mediated Transfer of Genetic Information between the Hematopoietic System and the Brain in Response to Inflammation. PLoS Biol. (2014) 12:e1001874. doi: 10.1371/journal.pbio.1001874
87. El-Andaloussi S, Lee Y, Lakhal-Littleton S, Li J, Seow Y, Gardiner C, et al. Exosome-mediated delivery of siRNA in vitro and in vivo. Nat Protoc. (2012) 7:2112–26. doi: 10.1038/nprot.2012.131
88. Feng D, Zhao WL, Ye YY, Bai XC, Liu RQ, Chang LF, et al. Cellular internalization of exosomes occurs through phagocytosis. Traffic. (2010) 11:675–87. doi: 10.1111/j.1600-0854.2010.01041.x
89. Ng F, Berk M, Dean O, Bush AI. Oxidative stress in psychiatric disorders: evidence base and therapeutic implications. Int J Neuropsychopharmacol. (2008) 11:851–76. doi: 10.1017/S1461145707008401
90. Pedrini M, Massuda R, Fries GR, de Bittencourt Pasquali MA, Schnorr CE, Moreira JCF, et al. Similarities in serum oxidative stress markers and inflammatory cytokines in patients with overt schizophrenia at early and late stages of chronicity. J Psychiatr Res. (2012) 46:819–24. doi: 10.1016/j.jpsychires.2012.03.019
91. Dietrich-Muszalska A. Oxidative Stress in Schizophrenia. In: Dietrich-Muszalska A, Chauhan V, Grignon S, editors. Studies on Psychiatric Disorders. Springer: New York. (2015). p. 43–72 doi: 10.1007/978-1-4939-0440-2_2
92. Michelson A. Biological role of the superoxide anion radical and of superoxyde-dismutase in cellular metabolism. C R Seances Soc Biol Fil. (1976) 170:1137–46.
93. Okusaga OO. Accelerated aging in schizophrenia patients: the potential role of oxidative stress. Aging Dis. (2014) 5:256. doi: 10.14336/AD.2014.0500256
94. Sirota P, Gavrieli R, Wolach B. Overproduction of neutrophil radical oxygen species correlates with negative symptoms in schizophrenic patients: parallel studies on neutrophil chemotaxis, superoxide production and bactericidal activity. Psychiatry Res. (2003) 121:123–32. doi: 10.1016/S0165-1781(03)00222-1
95. Do KQ, Cabungcal JH, Frank A, Steullet P, Cuenod M. Redox dysregulation, neurodevelopment, and schizophrenia. Curr Opin Neurobiol. (2009) 19:220–30. doi: 10.1016/j.conb.2009.05.001
96. Bilbo SD, Schwarz JM. Early-life programming of later-life brain and behavior: a critical role for the immune system. Front Behav Neurosci. (2009) 3:14. doi: 10.3389/neuro.08.014.2009
97. Van Berckel BN, Bossong MG, Boellaard R, Kloet R, Schuitemaker A, Caspers E, et al. Microglia activation in recent-onset schizophrenia: a quantitative (R)-[11C] PK11195 positron emission tomography study. Biol Psychiatry. (2008) 64:820–2. doi: 10.1016/j.biopsych.2008.04.025
98. Bloomfield PS, Selvaraj S, Veronese M, Rizzo G, Bertoldo A, Owen DR, et al. Microglial activity in people at ultra high risk of psychosis and in schizophrenia: an [11C] PBR28 PET brain imaging study. Am J Psychiatry. (2016) 173:44–52. doi: 10.1176/appi.ajp.2015.14101358
99. Vita A, De Peri L, Deste G, Sacchetti E. Progressive loss of cortical gray matter in schizophrenia: a meta-analysis and meta-regression of longitudinal MRI studies. Transl Psychiatry. (2012) 2:e190. doi: 10.1038/tp.2012.116
100. Garey L, Ong W, Patel T, Kanani M, Davis A, Mortimer A, et al. Reduced dendritic spine density on cerebral cortical pyramidal neurons in schizophrenia. J Neurol Neurosurg Psychiatry. (1998) 65:446–53. doi: 10.1136/jnnp.65.4.446
101. Konopaske GT, Lange N, Coyle JT, Benes FM. Prefrontal cortical dendritic spine pathology in schizophrenia and bipolar disorder. JAMA Psychiat. (2014) 71:1323–31. doi: 10.1001/jamapsychiatry.2014.1582
102. Cannon TD, Chung Y, He G, Sun D, Jacobson A, van Erp TGM, et al. Progressive reduction in cortical thickness as psychosis develops: a multisite longitudinal neuroimaging study of youth at elevated clinical risk. Biol Psychiatry. (2015) 77:147–57. doi: 10.1016/j.biopsych.2014.05.023
103. Osimo EF, Beck K, Reis Marques T, Howes OD. Synaptic loss in schizophrenia: a meta-analysis and systematic review of synaptic protein and mRNA measures. Mol Psychiatry. (2019) 24:549–61. doi: 10.1038/s41380-018-0041-5
104. Sigurdsson T, Duvarci S. Hippocampal-prefrontal interactions in cognition, behavior and psychiatric disease. Front Syst Neurosci. (2016) 9:190. doi: 10.3389/fnsys.2015.00190
105. Ponomarev ED, Veremeyko T, Barteneva N, Krichevsky AM. Weiner HL. MicroRNA-124 promotes microglia quiescence and suppresses EAE by deactivating macrophages via the C/EBP-α-PU1 pathway. Nat Med. (2011) 17:64–70. doi: 10.1038/nm.2266
106. Hoek RM, Ruuls SR, Murphy CA, Wright GJ, Goddard R, Zurawski SM, et al. Down-regulation of the macrophage lineage through interaction with OX2 (CD200). Science. (2000) 290:1768–71. doi: 10.1126/science.290.5497.1768
107. Akio S. Neuron-microglia interaction in neuroinflammation. Curr Protein Pept Sci. (2013) 14:16–20. doi: 10.2174/1389203711314010004
108. Wake H, Moorhouse AJ, Jinno S, Kohsaka S, Nabekura J. Resting microglia directly monitor the functional state of synapses in vivo and determine the fate of ischemic terminals. J Neurosci. (2009) 29:3974–80. doi: 10.1523/JNEUROSCI.4363-08.2009
109. Li Y. Du X-f, Liu C-s, Wen Z-l, Du J-l. Reciprocal regulation between resting microglial dynamics and neuronal activity in vivo developmental. Cell. (2012) 23:1189–202. doi: 10.1016/j.devcel.2012.10.027
110. Freeman L, Guo H, David CN, Brickey WJ, Jha S, Ting JPY, et al. members NLRC4 and NLRP3 mediate sterile inflammasome activation in microglia and astrocytes. J Exp Med. (2017) 214:1351–70. doi: 10.1084/jem.20150237
111. Stellwagen D, Beattie EC, Seo JY, Malenka RC. Differential regulation of AMPA receptor and GABA receptor trafficking by tumor necrosis factor-alpha. J Neurosci. (2005) 25:3219–28. doi: 10.1523/JNEUROSCI.4486-04.2005
112. Rizzo FR, Musella A, De Vito F, Fresegna D, Bullitta S, Vanni V, et al. Tumor necrosis factor and interleukin-1β modulate synaptic plasticity during neuroinflammation. Neural Plasticity. (2018) 2018:8430123. doi: 10.1155/2018/8430123
113. Ye L, Huang Y, Zhao L, Li Y, Sun L, Zhou Y, et al. IL-1β and TNF-α induce neurotoxicity through glutamate production: a potential role for neuronal glutaminase. J Neurochem. (2013) 125:897–908. doi: 10.1111/jnc.12263
114. Pascual O, Ben Achour S, Rostaing P, Triller A, Bessis A. Microglia activation triggers astrocyte-mediated modulation of excitatory neurotransmission. Proc Natl Acad Sci U S A. (2012) 109:E197–205. doi: 10.1073/pnas.1111098109
115. Zarubin T, Han J. Activation and signaling of the p38 MAP kinase pathway. Cell Res. (2005) 15:11–8. doi: 10.1038/sj.cr.7290257
116. Subedi L, Lee JH, Yumnam S, Ji E, Kim SY. Anti-inflammatory effect of sulforaphane on LPS-Activated Microglia Potentially through JNK/AP-1/NF-κB Inhibition and Nrf2/HO-1 Activation. Cells. (2019) 8:194. doi: 10.3390/cells8020194
117. McGuire JL, Depasquale EA, Funk AJ, O'Donnovan SM, Hasselfeld K, Marwaha S, et al. Abnormalities of signal transduction networks in chronic schizophrenia. NPJ Schizophrenia. (2017) 3:30. doi: 10.1038/s41537-017-0032-6
118. Kyriakis JM, Avruch J. Mammalian MAPK signal transduction pathways activated by stress and inflammation: a 10-year update. Physiol Rev. (2012). doi: 10.1152/physrev.00028.2011
119. Kyriakis JM, Avruch J. Protein kinase cascades activated by stress and inflammatory cytokines. Bioessays. (1996) 18:567–77. doi: 10.1002/bies.950180708
120. Ip YT, Davis RJ. Signal transduction by the c-Jun N-terminal kinase (JNK) — from inflammation to development. Curr Opin Cell Biol. (1998) 10:205–19. doi: 10.1016/S0955-0674(98)80143-9
121. Hu Y, Metzler B, Xu Q. Discordant activation of stress-activated protein kinases or c-Jun NH2-terminal protein kinases in tissues of heat-stressed mice. J Biol Chem. (1997) 272:9113–9. doi: 10.1074/jbc.272.14.9113
122. Coffey ET, Hongisto V, Dickens M, Davis RJ, Courtney MJ. Dual roles for c-Jun N-terminal kinase in developmental and stress responses in cerebellar granule neurons. J Neurosci. (2000) 20:7602–13. doi: 10.1523/JNEUROSCI.20-20-07602.2000
123. Gupta S, Barrett T, Whitmarsh AJ, Cavanagh J, Sluss HK, Derijard B, et al. Selective interaction of JNK protein kinase isoforms with transcription factors. EMBO J. (1996) 15:2760–70. doi: 10.1002/j.1460-2075.1996.tb00636.x
124. Carboni L, Carletti R, Tacconi S, Corti C, Ferraguti F. Differential expression of SAPK isoforms in the rat brain. An in situ hybridisation study in the adult rat brain and during post-natal development. Mol Brain Res. (1998) 60:57–68. doi: 10.1016/S0169-328X(98)00166-1
125. Livingstone C, Patel G, Jones N. ATF-2 contains a phosphorylation-dependent transcriptional activation domain. EMBO J. (1995) 14:1785–97. doi: 10.1002/j.1460-2075.1995.tb07167.x
126. Ishikawa T, Morris PL. Interleukin-1beta signals through a c-Jun N-terminal kinase-dependent inducible nitric oxide synthase and nitric oxide production pathway in Sertoli epithelial cells. Endocrinology. (2006) 147:5424–30. doi: 10.1210/en.2006-0643
127. Ventura JJ, Cogswell P, Flavell RA, Baldwin AS Jr, Davis RJ JNK potentiates TNF-stimulated necrosis by increasing the production of cytotoxic reactive oxygen species. Genes Dev. (2004) 18:2905–15. doi: 10.1101/gad.1223004
128. Neniskyte U, Vilalta A, Brown GC. Tumour necrosis factor alpha-induced neuronal loss is mediated by microglial phagocytosis. FEBS Lett. (2014) 588:2952–6. doi: 10.1016/j.febslet.2014.05.046
129. Subedi L, Gaire BP, Do MH, Lee TH, Kim SY. Anti-neuroinflammatory and neuroprotective effects of the Lindera neesiana fruit in vitro. Phytomedicine. (2016) 23:872–81. doi: 10.1016/j.phymed.2016.05.002
130. Xie Z, Smith CJ, Van Eldik LJ. Activated glia induce neuron death via MAP kinase signaling pathways involving JNK and p38. Glia. (2004) 45:170–9. doi: 10.1002/glia.10314
131. Nilsson BM, Hultman CM, Wiesel FA. Niacin skin-flush response and electrodermal activity in patients with schizophrenia and healthy controls. Prostaglandins, Leukotrienes and Essential Fatty Acids. (2006) 74:339–46. doi: 10.1016/j.plefa.2006.02.002
132. Rahman M, Muhammad S, Khan MA, Chen H, Ridder DA, Müller-Fielitz H, et al. The β-hydroxybutyrate receptor HCA2 activates a neuroprotective subset of macrophages. Nat Commun. (2014) 5:3944. doi: 10.1038/ncomms4944
133. Kwon WY, Suh GJ, Kim KS, Kwak YH. Niacin attenuates lung inflammation and improves survival during sepsis by downregulating the nuclear factor-κB pathway. Crit Care Med. (2011) 39:328–34. doi: 10.1097/CCM.0b013e3181feeae4
134. Cho KH, Kim HJ, Rodriguez-Iturbe B, Vaziri ND. Niacin ameliorates oxidative stress, inflammation, proteinuria, and hypertension in rats with chronic renal failure. Am J Physiol Renal Physiol. (2009) 297:F106–13. doi: 10.1152/ajprenal.00126.2009
135. Diau G-Y, Hsieh AT, Sarkadi-Nagy EA, Wijendran V, Nathanielsz PW, Brenna JT. The influence of long chain polyunsaturate supplementation on docosahexaenoic acid and arachidonic acid in baboon neonate central nervous system. BMC Med. (2005) 3:11. doi: 10.1186/1741-7015-3-11
136. Ghioni C, Bell JG, Sargent JR. Polyunsaturated fatty acids in neutral lipids and phospholipids of some freshwater insects. Comparative Biochem Physiol Part B Biochem Mol Biol. (1996) 114:161–70. doi: 10.1016/0305-0491(96)00019-3
137. Masuda S, Murakami M, Takanezawa Y, Aoki J, Arai H, Ishikawa Y, et al. Neuronal expression and neuritogenic action of group X secreted phospholipase A2. J Biol Chem. (2005) 280:23203–14. doi: 10.1074/jbc.M500985200
138. Takenouchi T, Sato M, Kitani H. Lysophosphatidylcholine potentiates Ca2+ influx, pore formation and p44/42 MAP kinase phosphorylation mediated by P2X7 receptor activation in mouse microglial cells. J Neurochem. (2007) 102:1518–32. doi: 10.1111/j.1471-4159.2007.04570.x
139. Halliwell B, Gutteridge JM. Free Radicals in Biology and Medicine. Oxford University Press (2015). doi: 10.1093/acprof:oso/9780198717478.001.0001
140. Radler-Pohl A, Gebel S, Sachsenmaier C, König H, Krämer M, Oehler T, et al. The activation and activity control of AP-1 (fos/jun). Ann N Y Acad Sci. (1993) 684:127–48. doi: 10.1111/j.1749-6632.1993.tb32277.x
141. Yamamoto Y, Gaynor RB. Therapeutic potential of inhibition of the NF-kappaB pathway in the treatment of inflammation and cancer. J Clin Invest. (2001) 107:135–42. doi: 10.1172/JCI11914
142. Rao GN, Katki KA, Madamanchi NR, Wu Y, Birrer MJ. JunB forms the majority of the AP-1 complex and is a target for redox regulation by receptor tyrosine kinase and G protein-coupled receptor agonists in smooth muscle cells*. J Biol Chem. (1999) 274:6003–10. doi: 10.1074/jbc.274.9.6003
143. Rao GN, Glasgow WC, Eling TE, Runge MS. Role of hydroperoxyeicosatetraenoic acids in oxidative stress-induced activating protein 1 (AP-1) activity. J Biol Chem. (1996) 271:27760–4. doi: 10.1074/jbc.271.44.27760
144. Zarkovic N. 4-Hydroxynonenal as a bioactive marker of pathophysiological processes. Mol Aspects Med. (2003). 24:281–91. doi: 10.1016/S0098-2997(03)00023-2
145. Koppal T, Petrova TV, Van Eldik LJ. Cyclopentenone prostaglandin 15-deoxy-Δ12,14-prostaglandin J2 acts as a general inhibitor of inflammatory responses in activated BV-2 microglial cells. Brain Res. (2000) 867:115–21. doi: 10.1016/S0006-8993(00)02270-8
146. Rossi A, Kapahi P, Natoli G, Takahashi T, Chen Y, Karin M, et al. Anti-inflammatory cyclopentenone prostaglandins are direct inhibitors of IκB kinase. Nature. (2000) 403:103–8. doi: 10.1038/47520
147. Fine SM, Maggirwar SB, Elliott PR, Epstein LG, Gelbard HA, Dewhurst S. Proteasome blockers inhibit TNF-α release by lipopolysaccharide stimulated macrophages and microglia: implications for HIV-1 dementia. J Neuroimmunol. (1999) 95:55–64. doi: 10.1016/S0165-5728(98)00267-7
148. Ricote M, Li AC, Willson TM, Kelly CJ, Glass CK. The peroxisome proliferator-activated receptor-γ is a negative regulator of macrophage activation. Nature. (1998) 391:79–82. doi: 10.1038/34178
149. Petrova TV, Akama KT, Van Eldik LJ. Cyclopentenone prostaglandins suppress activation of microglia: down-regulation of inducible nitric-oxide synthase by 15-deoxy-Δ12, 14-prostaglandin J2. Proc Nat Acad Sci. (1999) 96:4668–73. doi: 10.1073/pnas.96.8.4668
150. Bernardo A, Levi G, Minghetti L. Role of the peroxisome proliferator-activated receptor-γ (PPAR-γ) and its natural ligand 15-deoxy-Δ12, 14-prostaglandin J2 in the regulation of microglial functions. Eur J Neurosci. (2000) 12:2215–23. doi: 10.1046/j.1460-9568.2000.00110.x
151. Heneka MT, Klockgether T, Feinstein DL. Peroxisome proliferator-activated receptor-gamma ligands reduce neuronal inducible nitric oxide synthase expression and cell death in vivo. J Neurosci. (2000) 20:6862–7. doi: 10.1523/JNEUROSCI.20-18-06862.2000
152. Kapadia R, Yi JH, Vemuganti R. Mechanisms of anti-inflammatory and neuroprotective actions of PPAR-gamma agonists. Front Biosci. (2008) 13:1813–26. doi: 10.2741/2802
153. Landreth GE, Heneka MT. Anti-inflammatory actions of peroxisome proliferator-activated receptor gamma agonists in Alzheimer's disease. Neurobiol Aging. (2001) 22:937–44. doi: 10.1016/S0197-4580(01)00296-2
154. Schulze-Osthoff K, Ferrari D, Riehemann K, Wesselborg S. Regulation of NF-kappa B activation by MAP kinase cascades. Immunobiology. (1997) 198:35–49. doi: 10.1016/S0171-2985(97)80025-3
155. Allport VC, Slater DM, Newton R, Bennett PR. NF-κB and AP-1 are required for cyclo-oxygenase 2 gene expression in amnion epithelial cell line (WISH). Mol Hum Reprod. (2000) 6:561–5. doi: 10.1093/molehr/6.6.561
156. Kondo M, Shibata T, Kumagai T, Osawa T, Shibata N, Kobayashi M, et al. 15-Deoxy-Delta(12,14)-prostaglandin J(2): the endogenous electrophile that induces neuronal apoptosis. Proc Natl Acad Sci U S A. (2002) 99:7367–72. doi: 10.1073/pnas.112212599
157. Vanden Berghe W, Vermeulen L, Delerive P, De Bosscher K, Staels B, Haegeman G, et al. Paradigm for gene regulation: inflammation, NF-κB and PPAR. US: Springer. (2003). 181–96. doi: 10.1007/978-1-4419-9072-3_22
158. Korbecki J, Bobiński R, Dutka M. Self-regulation of the inflammatory response by peroxisome proliferator-activated receptors. Inflammat Res. (2019) 68:443–58. doi: 10.1007/s00011-019-01231-1
159. Li G, Shi Y, Huang H, Zhang Y, Wu K, Luo J, et al. Internalization of the human nicotinic acid receptor GPR109A is regulated by G(i), GRK2, and arrestin3. J Biol Chem. (2010) 285:22605–18. doi: 10.1074/jbc.M109.087213
160. Jin T, Zhang N, Long Y, Parent CA, Devreotes PN. Localization of the G protein betagamma complex in living cells during chemotaxis. Science. (2000) 287:1034–6. doi: 10.1126/science.287.5455.1034
161. English J, Pearson G, Wilsbacher J, Swantek J, Karandikar M, Xu S, et al. New insights into the control of MAP kinase pathways. Exp Cell Res. (1999) 253:255–70. doi: 10.1006/excr.1999.4687
162. Liu X, Ma B, Malik AB, Tang H, Yang T, Sun B, et al. Bidirectional regulation of neutrophil migration by mitogen-activated protein kinases. Nat Immunol. (2012) 13:457–64. doi: 10.1038/ni.2258
163. Liu Z, Jiang Y, Li Y, Wang J, Fan L, Scott MJ, et al. TLR4 Signaling augments monocyte chemotaxis by regulating G protein–coupled receptor kinase 2 translocation. J Immunol. (2013) 191:857–64. doi: 10.4049/jimmunol.1390044
164. McDonald PH, Chow C-W, Miller WE, Laporte SA, Field ME, Lin F-T, et al. β-arrestin 2: A receptor-regulated MAPK scaffold for the activation of JNK3. Science. (2000) 290:1574–7. doi: 10.1126/science.290.5496.1574
165. Jean-Charles P-Y, Zhang L, Wu J-H, Han S-o, Brian L, Freedman NJ, et al. Ubiquitin-specific protease 20 regulates the reciprocal functions of β-arrestin2 in toll-like receptor 4-promoted nuclear factor κB (NFκB) activation. J Biol Chem. (2016) 291:7450–64. doi: 10.1074/jbc.M115.687129
166. Szaingurten-Solodkin I, Hadad N, Levy R. Regulatory role of cytosolic phospholipase A2alpha in NADPH oxidase activity and in inducible nitric oxide synthase induction by aggregated Abeta1-42 in microglia. Glia. (2009) 57:1727–40. doi: 10.1002/glia.20886
167. Shmelzer Z, Haddad N, Admon E, Pessach I, Leto TL, Eitan-Hazan Z, et al. Unique targeting of cytosolic phospholipase A2 to plasma membranes mediated by the NADPH oxidase in phagocytes. J Cell Biol. (2003) 162:683–92. doi: 10.1083/jcb.200211056
168. Cui X-L, Douglas JG. Arachidonic acid activates c-jun N-terminal kinase through NADPH oxidase in rabbit proximal tubular epithelial cells. Proc Nat Acad Sci. (1997) 94:3771–6. doi: 10.1073/pnas.94.8.3771
169. Lee SH, Soyoola E, Chanmugam P, Hart S, Sun W, Zhong H, et al. Selective expression of mitogen-inducible cyclooxygenase in macrophages stimulated with lipopolysaccharide. J Biol Chem. (1992) 267:25934–8. doi: 10.1016/S0021-9258(18)35698-9
170. Schwenger P, Bellosta P, Vietor I, Basilico C, Skolnik EY, Vilček J. Sodium salicylate induces apoptosis via p38 mitogen-activated protein kinase but inhibits tumor necrosis factor-induced c-Jun N-terminal kinase/stress-activated protein kinase activation. Proc Nat Acad Sci. (1997) 94:2869–73. doi: 10.1073/pnas.94.7.2869
171. Marnett LJ, Rowlinson SW, Goodwin DC, Kalgutkar AS, Lanzo CA. Arachidonic acid oxygenation by COX-1 and COX-2. Mechanisms of catalysis and inhibition. J Biol Chem. (1999) 274:22903–6. doi: 10.1074/jbc.274.33.22903
172. García-Bueno B, Caso JR, Leza JC. Stress as a neuroinflammatory condition in brain: damaging and protective mechanisms. Neurosci Biobehav Rev. (2008) 32:1136–51. doi: 10.1016/j.neubiorev.2008.04.001
173. Sheppe AEF, Kummari E, Walker A, Richards A, Hui WW, Lee JH, et al. PGE2 Augments Inflammasome Activation and M1 Polarization in Macrophages Infected With Salmonella Typhimurium and Yersinia enterocolitica. Front Microbiol. (2018) 9:2447. doi: 10.3389/fmicb.2018.02447
174. Stolina M, Sharma S, Lin Y, Dohadwala M, Gardner B, Luo J, et al. Specific inhibition of cyclooxygenase 2 restores antitumor reactivity by altering the balance of IL-10 and IL-12 synthesis. J Immunol. (2000) 164:361–70. doi: 10.4049/jimmunol.164.1.361
175. Konsman JP, Vigues S, Mackerlova L, Bristow A, Blomqvist A. Rat brain vascular distribution of interleukin-1 type-1 receptor immunoreactivity: Relationship to patterns of inducible cyclooxygenase expression by peripheral inflammatory stimuli. J Comparat Neurol. (2004) 472:113–29. doi: 10.1002/cne.20052
176. Holmes DR, Wester W, Thompson RW, Reilly JM. Prostaglandin E2 synthesis and cyclooxygenase expression in abdominal aortic aneurysms. J Vasc Surg. (1997) 25:810–5. doi: 10.1016/S0741-5214(97)70210-6
177. Bradbury DA, Newton R, Zhu YM, El-Haroun H, Corbett L, Knox AJ. Cyclooxygenase-2 induction by bradykinin in human pulmonary artery smooth muscle cells is mediated by the cyclic AMP response element through a novel autocrine loop involving endogenous prostaglandin E2, E-prostanoid 2 (EP2), and EP4 receptors. J Biol Chem. (2003) 278:49954–64. doi: 10.1074/jbc.M307964200
178. Díaz-Muñoz MD, Osma-García IC, Fresno M, Iñiguez MA. Involvement of PGE2 and the cAMP signalling pathway in the up-regulation of COX-2 and mPGES-1 expression in LPS-activated macrophages. Biochem J. (2012) 443:451–61. doi: 10.1042/BJ20111052
179. Harada Y, Hatanaka K, Kawamura M, Saito M, Ogino M, Majima M, et al. Role of prostaglandin H synthase-2 in prostaglandin E2 formation in rat carrageenin-induced pleurisy. Prostaglandins. (1996) 51:19–33. doi: 10.1016/0090-6980(95)00168-9
180. Uchida K, Shiraishi M, Naito Y, Torii Y, Nakamura Y, Osawa T. Activation of stress signaling pathways by the end product of lipid peroxidation. 4-hydroxy-2-nonenal is a potential inducer of intracellular peroxide production. J Biol Chem. (1999) 274:2234–42. doi: 10.1074/jbc.274.4.2234
181. Pinkus R, Weiner LM, Daniel V. Role of oxidants and antioxidants in the induction of AP-1, NF-kappaB, and glutathione S-transferase gene expression. J Biol Chem. (1996) 271:13422–9. doi: 10.1074/jbc.271.23.13422
182. Whitmarsh AJ, Davis RJ. Transcription factor AP-1 regulation by mitogen-activated protein kinase signal transduction pathways. J Mol Med (Berl). (1996) 74:589–607. doi: 10.1007/s001090050063
183. Horrobin DF, Glen AIM, Vaddadi K. The membrane hypothesis of schizophrenia. Schizophr Res. (1994) 13:195–207. doi: 10.1016/0920-9964(94)90043-4
184. Rotrosen J, Wolkin A. Phospholipid and prostaglandin hypotheses of schizophrenia. In: Psychopharmacology: The third generation of progress. New York: Raven Press. (1987). p. 759–64.
185. Cai HL Li HD, Yan XZ, Sun B, Zhang Q, Yan M, et al. Metabolomic analysis of biochemical changes in the plasma and urine of first-episode neuroleptic-naïve schizophrenia patients after treatment with risperidone. J Proteome Res. (2012) 11:4338–50. doi: 10.1021/pr300459d
186. Pangerl AM, Steudle A, Jaroni HW, Rüfer R, Gattaz WF. Increased platelet membrane lysophosphatidylcholine in schizophrenia. Biol Psychiatry. (1991) 30:837–40. doi: 10.1016/0006-3223(91)90239-I
187. Orešič M, Seppänen-Laakso T, Sun D, Tang J, Therman S, Viehman R, et al. Phospholipids and insulin resistance in psychosis: a lipidomics study of twin pairs discordant for schizophrenia. Genome Med. (2012) 4:1. doi: 10.1186/gm300
188. Fusar-Poli P, Berger G. Eicosapentaenoic acid interventions in schizophrenia: meta-analysis of randomized, placebo-controlled studies. J Clin Psychopharmacol. (2012) 32:179–85. doi: 10.1097/JCP.0b013e318248b7bb
189. Farkas E, De Wilde MC, Kiliaan AJ, Meijer J, Keijser JN, Luiten PG. Dietary long chain PUFAs differentially affect hippocampal muscarinic 1 and serotonergic 1A receptors in experimental cerebral hypoperfusion. Brain Res. (2002) 954:32–41. doi: 10.1016/S0006-8993(02)03300-0
190. Smesny S, Milleit B, Nenadic I, Preul C, Kinder D, Lasch J, et al. Phospholipase A2 activity is associated with structural brain changes in schizophrenia. Neuroimage. (2010) 52:1314–27. doi: 10.1016/j.neuroimage.2010.05.009
191. Preti A, Cella M. Randomized-controlled trials in people at ultra high risk of psychosis: a review of treatment effectiveness. Schizophr Res. (2010) 123:30–6. doi: 10.1016/j.schres.2010.07.026
192. López-Alarcón M, Martínez-Coronado A, Velarde-Castro O, Rendón-Macías E, Fernández J. Supplementation of n3 long-chain polyunsaturated fatty acid synergistically decreases insulin resistance with weight loss of obese prepubertal and pubertal children. Arch Med Res. (2011) 42:502–8. doi: 10.1016/j.arcmed.2011.06.010
193. Yin H, Xu L, Porter NA. Free radical lipid peroxidation: mechanisms and analysis. Chem Rev. (2011) 111:5944–72. doi: 10.1021/cr200084z
194. Esterbauer H, Schaur RJ, Zollner H. Chemistry and biochemistry of 4-hydroxynonenal, malonaldehyde and related aldehydes. Free Radic Biol Med. (1991) 11:81–128. doi: 10.1016/0891-5849(91)90192-6
195. Ayala A, Muñoz MF, Argüelles S. Lipid peroxidation: production, metabolism, and signaling mechanisms of malondialdehyde and 4-hydroxy-2-nonenal. Oxid Med Cell Longev. (2014) 2014:360438. doi: 10.1155/2014/360438
196. Mark RJ, Lovell MA, Markesbery WR, Uchida K, Mattson MP. A Role for 4-hydroxynonenal, an aldehydic product of lipid peroxidation, in disruption of ion homeostasis and neuronal death induced by amyloid β-peptide. J Neurochem. (1997) 68:255–64. doi: 10.1046/j.1471-4159.1997.68010255.x
197. Camandola S, Poli G, Mattson MP. The lipid peroxidation product 4-hydroxy-2,3-nonenal increases AP-1-binding activity through caspase activation in neurons. J Neurochem. (2000) 74:159–68. doi: 10.1046/j.1471-4159.2000.0740159.x
198. Pryor WA. On the detection of lipid hydroperoxides in biological samples. Free Radic Biol Med. (1989) 7:177–8. doi: 10.1016/0891-5849(89)90010-5
199. Halliwell B, Gutteridge JM. Oxygen toxicity, oxygen radicals, transition metals and disease. Biochem J. (1984) 219:1–14. doi: 10.1042/bj2190001
200. Grignon S, Chianetta JM. Assessment of malondialdehyde levels in schizophrenia: a meta-analysis and some methodological considerations. Prog Neuropsychopharmacol Biol. (2007) 31:365–9. doi: 10.1016/j.pnpbp.2006.09.012
201. Dietrich-Muszalska A, Olas B. Isoprostenes as indicators of oxidative stress in schizophrenia. World J Biol Psychiatry. (2009) 10:27–33. doi: 10.1080/15622970701361263
202. Moret-Tatay I, Iborra M, Cerrillo E, Tortosa L, Nos P, Beltrán B. Possible biomarkers in blood for crohn's disease: oxidative stress and micrornas-current evidences and further aspects to unravel. Oxid Med Cell Longev. (2016) 2016:2325162. doi: 10.1155/2016/2325162
203. Camkurt MA, Karababa F, Erdal ME, Bayazit H, Kandemir SB, Ay ME, et al. Investigation of dysregulation of several micrornas in peripheral blood of schizophrenia patients. Clin Psychopharmacol Neurosci. (2016) 14:256–60. doi: 10.9758/cpn.2016.14.3.256
204. Lai CY, Lee SY, Scarr E, Yu YH, Lin YT, Liu CM, et al. Aberrant expression of microRNAs as biomarker for schizophrenia: from acute state to partial remission, and from peripheral blood to cortical tissue. Transl Psychiatry. (2016) 6:e717. doi: 10.1038/tp.2015.213
205. Wei H, Yuan Y, Liu S, Wang C, Yang F, Lu Z, et al. Detection of circulating miRNA levels in schizophrenia. Am J Psychiatry. (2015) 172:1141–7. doi: 10.1176/appi.ajp.2015.14030273
206. Morrow JD, Hill KE, Burk RF, Nammour TM, Badr KF, Roberts LJ, et al. series of prostaglandin F2-like compounds are produced in vivo in humans by a non-cyclooxygenase, free radical-catalyzed mechanism. Proc Nat Acad Sci. (1990) 87:9383–7. doi: 10.1073/pnas.87.23.9383
207. Cracowski J-L, Durand T, Bessard G. Isoprostanes as a biomarker of lipid peroxidation in humans: physiology, pharmacology and clinical implications. Trends Pharmacol Sci. (2002) 23:360–6. doi: 10.1016/S0165-6147(02)02053-9
208. Montuschi P, Barnes PJ, Roberts LJ. Isoprostanes: markers and mediators of oxidative stress. The FASEB J. (2004) 18:1791–800. doi: 10.1096/fj.04-2330rev
209. Glen A, Glen E, Horrobin D, Vaddadi K, Spellman M, Morse-Fisher N, et al. A red cell membrane abnormality in a subgroup of schizophrenic patients: evidence for two diseases. Schizophr Res. (1994) 12:53–61. doi: 10.1016/0920-9964(94)90084-1
210. McNamara RK, Jandacek R, Rider T, Tso P, Hahn C-G, Richtand NM, et al. Abnormalities in the fatty acid composition of the postmortem orbitofrontal cortex of schizophrenic patients: gender differences and partial normalization with antipsychotic medications. Schizophr Res. (2007) 91:37–50. doi: 10.1016/j.schres.2006.11.027
211. Yao JK, Leonard S, Reddy RD. Membrane phospholipid abnormalities in postmortem brains from schizophrenic patients. Schizophr Res. (2000) 42:7–17. doi: 10.1016/S0920-9964(99)00095-X
212. Maclean R, Ward PE, Glen I, Roberts SJ, Ross BM. On the relationship between methylnicotinate-induced skin flush and fatty acids levels in acute psychosis. Prog Neuropsychopharmacol Biol. (2003) 27:927–33. doi: 10.1016/S0278-5846(03)00152-0
213. Messamore E, Hoffman WF, Yao JK. Niacin sensitivity and the arachidonic acid pathway in schizophrenia. Schizophr Res. (2010) 122:248–56. doi: 10.1016/j.schres.2010.03.025
214. Horrobin DF. The membrane phospholipid hypothesis as a biochemical basis for the neurodevelopmental concept of schizophrenia. Schizophr Res. (1998) 30:193–208. doi: 10.1016/S0920-9964(97)00151-5
215. du Bois TM, Deng C, Huang X-F. Membrane phospholipid composition, alterations in neurotransmitter systems and schizophrenia. Prog Neuropsychopharmacol Biol. (2005) 29:878–88. doi: 10.1016/j.pnpbp.2005.04.034
216. Cao Y, Murphy KJ, McIntyre TM, Zimmerman GA, Prescott SM. Expression of fatty acid-CoA ligase 4 during development and in brain. FEBS Lett. (2000) 467:263–7. doi: 10.1016/S0014-5793(00)01159-5
217. Covault J, Pettinati H, Moak D, Mueller T, Kranzler HR. Association of a long-chain fatty acid-CoA ligase 4 gene polymorphism with depression and with enhanced niacin-induced dermal erythema. Am J Med Genet B Neuropsychiatr Genet. (2004) 127:42–7. doi: 10.1002/ajmg.b.20156
218. Sakata A, Ida E, Tominaga M, Onoue K. Arachidonic acid acts as an intracellular activator of NADPH-oxidase in Fc gamma receptor-mediated superoxide generation in macrophages. J Immunol. (1987) 138:4353–9.
219. Sawai T, Asada M, Nunoi H, Matsuda I, Ando S, Sasaki T, et al. Combination of Arachidonic Acid and Guanosine 5′-O-(3-Thiotriphosphate) Induces Translocation of rac p21s to Membrane and Activation of NADPH Oxidase in a Cell-Free System. Biochem Biophys Res Commun. (1993) 195:264–9. doi: 10.1006/bbrc.1993.2039
220. Abo A, Webb MR, Grogan A, Segal AW. Activation of NADPH oxidase involves the dissociation of p21rac from its inhibitory GDP/GTP exchange protein (rhoGDI) followed by its translocation to the plasma membrane. Biochemical Journal. (1994) 298:585–91. doi: 10.1042/bj2980585
221. Minden A, Lin A, Claret F-X, Abo A, Karin M. Selective activation of the JNK signaling cascadeand c-Jun transcriptional activity by the small GTPases Rac and Cdc42Hs. Cell. (1995) 81:1147–57. doi: 10.1016/S0092-8674(05)80019-4
222. Martínez-Gras I, Pérez-Nievas BG, García-Bueno B, Madrigal JL, Andrés-Esteban E, Rodríguez-Jiménez R, et al. The anti-inflammatory prostaglandin 15d-PGJ2 and its nuclear receptor PPARgamma are decreased in schizophrenia. Schizophr Res. (2011) 128:15–22. doi: 10.1016/j.schres.2011.01.018
223. Cheng Y, Sun AY. Oxidative mechanisms involved in kainate-induced cytotoxicity in cortical neurons. Neurochem Res. (1994) 19:1557–64. doi: 10.1007/BF00969006
224. Morrow JD, Awad JA, Oates JA, Roberts LJ II. Identification of skin as a major site on prostaglandin D2 release following oral administration of niacin in humans. J Investigat Dermatol. (1992) 98:812–5. doi: 10.1111/1523-1747.ep12499963
225. Aberg G. Inhibition of flush induced by nicotinic acid. IRCS (General Pharmacology). (1973) 10:13.
226. Andersson RGG, Åberg G, Brattsand R, Ericsson E, Lundholm L. Studies on the Mechanism of Flush Induced by Nicotinic Acid. Acta Pharmacol Toxicol. (1977) 41:1–10. doi: 10.1111/j.1600-0773.1977.tb02116.x
227. D'Mello SR, Galli C, Ciotti T, Calissano P. Induction of apoptosis in cerebellar granule neurons by low potassium: inhibition of death by insulin-like growth factor I and cAMP. Proc Nat Acad Sci. (1993) 90:10989–93. doi: 10.1073/pnas.90.23.10989
228. Wise A, Foord SM, Fraser NJ, Barnes AA, Elshourbagy N, Eilert M, et al. Molecular identification of high and low affinity receptors for nicotinic acid. J Biol Chem. (2003) 278:9869–74. doi: 10.1074/jbc.M210695200
229. Lai E, De Lepeleire I, Crumley TM, Liu F, Wenning LA, Michiels N, et al. Suppression of niacin-induced vasodilation with an antagonist to prostaglandin D2 receptor subtype 1. Clin Pharmacol Ther. (2007) 81:849–57. doi: 10.1038/sj.clpt.6100180
230. Nogawa S, Zhang F, Ross ME, Iadecola C. Cyclo-oxygenase-2 gene expression in neurons contributes to ischemic brain damage. J Neurosci. (1997) 17:2746–55. doi: 10.1523/JNEUROSCI.17-08-02746.1997
231. Yagami T, Ueda K, Asakura K, Sakaeda T, Kuroda T, Hata S, et al. Effects of S-2474, a novel nonsteroidal anti-inflammatory drug, on amyloid β protein-induced neuronal cell death. Br J Pharmacol. (2001) 134:673–81. doi: 10.1038/sj.bjp.0704261
232. Yagami T, Ueda K, Asakura K, Nakazato H, Hata S, Kuroda T, et al. Human group IIA secretory phospholipase A2 potentiates Ca2+ influx through L-type voltage-sensitive Ca2+ channels in cultured rat cortical neurons. J Neurochem. (2003) 85:749–58. doi: 10.1046/j.1471-4159.2003.01712.x
233. Liang X, Wu L, Hand T, Andreasson K. Prostaglandin D2 mediates neuronal protection via the DP1 receptor. J Neurochem. (2005) 92:477–86. doi: 10.1111/j.1471-4159.2004.02870.x
234. Maida ME, Hurley SD, Daeschner JA, Moore AH, O'Banion MK. Cytosolic prostaglandin E2 synthase (cPGES) expression is decreased in discrete cortical regions in psychiatric disease. Brain Res. (2006) 1103:164–72. doi: 10.1016/j.brainres.2006.05.048
235. Kaiya H, Uematsu M, Ofuji M, Nishida A, Takeuchi K, Nozaki M, et al. Elevated plasma prostaglandin E2 levels in schizophrenia. J Neural Transm. (1989) 77:39–46. doi: 10.1007/BF01255817
236. Muller N, Schwarz MJ. COX-2 inhibition in schizophrenia and major depression. Curr Pharm Des. (2008) 14:1452–65. doi: 10.2174/138161208784480243
237. Das I, Khan N. Increased arachidonic acid induced platelet chemiluminescence indicates cyclooxygenase overactivity in schizophrenic subjects. Prostaglandins, Leukotrienes and Essential Fatty Acids. (1998) 58:165–8. doi: 10.1016/S0952-3278(98)90109-0
238. Müller N, Myint AM, Schwarz MJ. Immunological treatment options for schizophrenia. Curr Pharm Biotechnol. (2012) 13:1606–13. doi: 10.2174/138920112800784826
239. Pierre S-R, Lemmens MA, Figueiredo-Pereira ME. Subchronic infusion of the product of inflammation prostaglandin J2 models sporadic Parkinson's disease in mice. J Neuroinflammation. (2009) 6:1–12. doi: 10.1186/1742-2094-6-18
240. Quraishi O, Mancini JA, Riendeau D. Inhibition of inducible prostaglandin E2 synthase by 15-deoxy-Δ12, 14-prostaglandin J2 and polyunsaturated fatty acids. Biochem Pharmacol. (2002) 63:1183–9. doi: 10.1016/S0006-2952(02)00844-4
241. Yüksel RN, Titiz AP, Yaylaci ET, Ünal K, Turhan T, Erzin G, et al. Serum PGE2, 15d-PGJ, PPARγ and CRP levels in patients with schizophrenia. Asian J Psychiatr. (2019) 46:24–8. doi: 10.1016/j.ajp.2019.09.026
242. Kliewer SA, Lenhard JM, Willson TM, Patel I, Morris DC, Lehmann JM, et al. prostaglandin J2 metabolite binds peroxisome proliferator-activated receptor γ and promotes adipocyte differentiation. Cell. (1995) 83:813–9. doi: 10.1016/0092-8674(95)90194-9
243. Fitzpatrick FA, Wynalda MA. Albumin-catalyzed metabolism of prostaglandin D2. Identification of products formed in vitro. J Biol Chem. (1983) 258:11713–8. doi: 10.1016/S0021-9258(17)44287-6
244. Heneka MT, Dumitrescu L, Löschmann P-A, Wüllner U, Klockgether T. Temporal, regional, and cell-specific changes of iNOS expression after intrastriatal microinjection of interferon gamma and bacterial lipopolysaccharide. J Chem Neuroanat. (2000) 18:167–79. doi: 10.1016/S0891-0618(00)00041-7
245. Yin R. Dong Y-g, Li H-l. PPARγ phosphorylation mediated by JNK MAPK: a potential role in macrophage-derived foam cell formation. Acta Pharmacologica Sinica. (2006) 27:1146–52. doi: 10.1111/j.1745-7254.2006.00359.x
246. Chistyakov DV, Aleshin SE, Astakhova AA, Sergeeva MG, Reiser G. Regulation of peroxisome proliferator-activated receptors (PPAR) α and -γ of rat brain astrocytes in the course of activation by toll-like receptor agonists. J Neurochem. (2015) 134:113–24. doi: 10.1111/jnc.13101
247. Camp HS, Tafuri SR, Leff T. c-Jun N-terminal kinase phosphorylates peroxisome proliferator-activated receptor-γ1 and negatively regulates its transcriptional activity. Endocrinology. (1999) 140:392–7. doi: 10.1210/endo.140.1.6457
248. Song J, Choi S-M, Kim BC. Adiponectin Regulates the polarization and function of microglia via PPAR-γ signaling under amyloid β toxicity. Front Cellular Neurosci. (2017) 11:64. doi: 10.3389/fncel.2017.00064
249. Walters RW, Shukla AK, Kovacs JJ, Violin JD, DeWire SM, Lam CM, et al. beta-Arrestin1 mediates nicotinic acid-induced flushing, but not its antilipolytic effect, in mice. J Clin Invest. (2009) 119:1312–21. doi: 10.1172/JCI36806
250. Levoye A, Zwier JM, Jaracz-Ros A, Klipfel L, Cottet M, Maurel D, et al. A broad G protein-coupled receptor internalization assay that combines SNAP-tag labeling, diffusion-enhanced resonance energy transfer, and a highly emissive terbium cryptate. Front Endocrinol. (2015) 6:167. doi: 10.3389/fendo.2015.00167
251. Bychkov ER, Ahmed MR, Gurevich VV, Benovic JL, Gurevich EV. Reduced expression of G protein-coupled receptor kinases in schizophrenia but not in schizoaffective disorder. Neurobiol Dis. (2011) 44:248–58. doi: 10.1016/j.nbd.2011.07.009
252. Horrobin DF. Schizophrenia as a prostaglandin deficiency disease. Lancet. (1977) 1:936–7. doi: 10.1016/S0140-6736(77)92228-0
253. Choudhury QG, Mckay DT, Flower RJ, Croxtall JD. Investigation into the involvement of phospholipases A2 and MAP kinases in modulation of AA release and cell growth in A549 cells. Br J Pharmacol. (2000) 131:255–65. doi: 10.1038/sj.bjp.0703573
254. Kramer RM, Roberts EF, Manetta J, Putnam JE. The Ca2(+)-sensitive cytosolic phospholipase A2 is a 100-kDa protein in human monoblast U937 cells. J Biol Chem. (1991) 266:5268–72. doi: 10.1016/S0021-9258(19)67782-3
255. Schaloske RH, Dennis EA. The phospholipase A2 superfamily and its group numbering system. Biochim Biophys Acta. (2006) 1761:1246–59. doi: 10.1016/j.bbalip.2006.07.011
256. Bazan NG. Cellular and molecular events mediated by docosahexaenoic acid-derived neuroprotectin D1 signaling in photoreceptor cell survival and brain protection. Prostaglandins, Leukotrienes and Essential Fatty Acids. (2009) 81:205–11. doi: 10.1016/j.plefa.2009.05.024
257. A Farooqui A. n-3 fatty acid-derived lipid mediators in the brain: new weapons against oxidative stress and inflammation. Current Med Chem. (2012) 19:532–43. doi: 10.2174/092986712798918851
258. Balsinde J, Balboa MA, Dennis EA. Antisense inhibition of group VI Ca2+-independent phospholipase A2 blocks phospholipid fatty acid remodeling in murine P388D1 macrophages. J Biol Chem. (1997) 272:29317–21. doi: 10.1074/jbc.272.46.29317
259. Fitzpatrick JS, Baudry M. Blockade of long-term depression in neonatal hippocampal slices by a phospholipase A2 inhibitor. Dev Brain Res. (1994) 78:81–6. doi: 10.1016/0165-3806(94)90012-4
260. Allyson J, Bi X, Baudry M, Massicotte G. Maintenance of synaptic stability requires calcium-independent phospholipase A2 activity. Neural Plasticity. (2012) 2012:987–6. doi: 10.1155/2012/569149
261. Wolf MJ, Tachibana H, Rockman HA. Methods for the detection of altered beta-adrenergic receptor signaling pathways in hypertrophied hearts. Methods Mol Med. (2005) 112:353–62. doi: 10.1385/1-59259-879-X:353
262. Lehman JJ, Brown KA, Ramanadham S, Turk J, Gross RW. Arachidonic acid release from aortic smooth muscle cells induced by [Arg8]vasopressin is largely mediated by calcium-independent phospholipase A2. J Biol Chem. (1993) 268:20713–6. doi: 10.1016/S0021-9258(19)36837-1
263. Murakami M, Kambe T, Shimbara S, Kudo I. Functional coupling between various phospholipase A2s and cyclooxygenases in immediate and delayed prostanoid biosynthetic pathways. J Biol Chem. (1999) 274:3103–15. doi: 10.1074/jbc.274.5.3103
264. Gupta S, Campbell D, Derijard B, Davis R. Transcription factor ATF2 regulation by the JNK signal transduction pathway. Science. (1995) 267:389–93. doi: 10.1126/science.7824938
265. Balestrieri B, Hsu VW, Gilbert H, Leslie CC, Han WK, Bonventre JV, et al. Group V secretory phospholipase A2 translocates to the phagosome after zymosan stimulation of mouse peritoneal macrophages and regulates phagocytosis. J Biol Chem. (2006) 281:6691–8. doi: 10.1074/jbc.M508314200
266. Dennis EA. Diversity of group types, regulation, and function of phospholipase A2. J Biol Chem. (1994) 269:13057–60. doi: 10.1016/S0021-9258(17)36794-7
267. Pickard RT, Strifler BA, Kramer RM, Sharp JD. Molecular cloning of two new human paralogs of 85-kDa cytosolic phospholipase A2. J Biol Chem. (1999) 274:8823–31. doi: 10.1074/jbc.274.13.8823
268. Underwood KW, Song C, Kriz RW, Chang XJ, Knopf JL, Lin L-L, et al. novel calcium-independent phospholipase A2, cPLA2-γ, that is prenylated and contains homology to cPLA2. J Biol Chem. (1998) 273:21926–32. doi: 10.1074/jbc.273.34.21926
269. Tay A, Simon JS, Squire J, Hamel K, Jacob HJ, Skorecki K. Cytosolic phospholipase A2 gene in human and rat: chromosomal localization and polymorphic markers. Genomics. (1995) 26:138–41. doi: 10.1016/0888-7543(95)80093-2
270. Hudson CJ, Kennedy JL, Gotowiec A, Lin A, King N, Gojtan K, et al. Genetic variant near cytosolic phospholipase A2 associated with schizophrenia. Schizophr Res. (1996) 21:111–6. doi: 10.1016/0920-9964(96)00031-X
271. Bjerkenstedt L, Edman G, Wiesel F. Membrane abnormalities in schizophrenia as revealed by tyrosine transport. In: Phospholid Spectrum Disorder in Psychiatry. Springer nature, (1999).
272. Peet M, Ramchand C, Lee J, Telang S, Vankar G, Shah S, et al. Association of the Ban I dimorphic site at the human cytosolic phospholipase A2 gene with schizophrenia. Psychiatr Genet. (1998) 8:191–2. doi: 10.1097/00041444-199800830-00010
273. Barbosa NR, Junqueira RM, Vallada HP, Gattaz WF. Association between BanI genotype and increased phospholipase A2 activity in schizophrenia. Eur Arch Psychiatry Clin Neurosci. (2007) 257:340–3. doi: 10.1007/s00406-007-0736-0
274. Wei J, Lee K, Hemmings G. Is the cPLA2 gene associated with schizophrenia. Mol Psychiatry. (1998) 3:480–1. doi: 10.1038/sj.mp.4000445
275. Pae C-U, Yu H-S, Lee K-U, Kim J-J, Lee C-U, Lee S-J, et al. BanI polymorphism of the cytosolic phospholipase A2 gene may confer susceptibility to the development of schizophrenia. Prog Neuropsychopharmacol Biol. (2004) 28:739–41. doi: 10.1016/j.pnpbp.2004.05.009
276. Price S, Fox H, St DC, Shaw D. Lack of association between schizophrenia and a polymorphism close to the cytosolic phospholipase A2 gene. Psychiatr Genet. (1997) 7:111–4. doi: 10.1097/00041444-199723000-00004
277. Chowdari KV, Brandstaetter B, Semwal P, Bhatia T, Deshpande S, Reddy R, et al. Association studies of cytosolic phospholipase A2 polymorphisms and schizophrenia among two independent family-based samples. Psychiatr Genet. (2001) 11:207. doi: 10.1097/00041444-200112000-00005
278. Frieboes RM, Moises HW, Gattaz WF, Yang L, Li T, Liu X, et al. Lack of association between schizophrenia and the phospholipase-A2 genes cPLA2 and sPLA2. Am J Med Genet. (2001) 105:246–9. doi: 10.1002/ajmg.1262
279. Chandrasekharan N, Simmons DL. The cyclooxygenases. Genome Biol. (2004) 5:241. doi: 10.1186/gb-2004-5-9-241
280. Ramsay R, Ciznadija D, Vanevski M, Mantamadiotis T. Transcriptional regulation of cyclo-oxygenase expression: three pillars of control. Int J Immunopathol Pharmacol. (2003) 16:59–67.
281. Kurumbail RG, Stevens AM, Gierse JK, McDonald JJ, Stegeman RA, Pak JY, et al. Structural basis for selective inhibition of cyclooxygenase-2 by anti-inflammatory agents. Nature. (1996) 384:644–8. doi: 10.1038/384644a0
282. Vane JR, Botting RM. Mechanism of action of anti-inflammatory drugs: an overview. In: Vane J, Botting J. Selective COX-2 Inhibitors: Pharmacology, Clinical Effects and Therapeutic Potential. Netherlands: Springer. p.1–17 (1998). doi: 10.1007/978-94-011-4872-6
283. Cavigelli M, Dolfi F, Claret F-X, Karin M. Induction of c-fos expression through JNK-mediated TCF/Elk-1 phosphorylation. EMBO J. (1995) 14:5957–64. doi: 10.1002/j.1460-2075.1995.tb00284.x
284. Shitashige M, Morita I, Murota S-i. Different substrate utilization between prostaglandin endoperoxide H synthase-1 and-2 in NIH3T3 fibroblasts. Biochimica et Biophysica Acta (BBA)-Lipids and Lipid Metabolism. (1998) 1389:57–66. doi: 10.1016/S0005-2760(97)00129-X
285. Reddy ST, Herschman HR. Ligand-induced prostaglandin synthesis requires expression of the TIS10/PGS-2 prostaglandin synthase gene in murine fibroblasts and macrophages. J Biol Chem. (1994) 269:15473–80. doi: 10.1016/S0021-9258(17)40703-4
286. Kulmacz RJ, Wang LH. Comparison of hydroperoxide initiator requirements for the cyclooxygenase activities of prostaglandin H synthase-1 and−2. J Biol Chem. (1995) 270:24019–23. doi: 10.1074/jbc.270.41.24019
287. Capdevila JH, Wei S, Helvig C, Falck JR, Belosludtsev Y, Truan G, et al. The highly stereoselective oxidation of polyunsaturated fatty acids by cytochrome P450BM-3. J Biol Chem. (1996) 271:22663–71. doi: 10.1074/jbc.271.37.22663
288. Messamore E, Hoffman WF, Janowsky A. The niacin skin flush abnormality in schizophrenia: a quantitative dose–response study. Schizophr Res. (2003) 62:251–8. doi: 10.1016/S0920-9964(02)00311-0
289. Kim DK, Rordorf G, Nemenoff RA, Koroshetz WJ, Bonventre JV. Glutamate stably enhances the activity of two cytosolic forms of phospholipase A2 in brain cortical cultures. Biochem J. (1995) 310:83–90. doi: 10.1042/bj3100083
290. Ross BM, Turenne S, Moszczynska A, Warsh JJ, Kish SJ. Differential alteration of phospholipase A2 activities in brain of patients with schizophrenia. Brain Res. (1999) 821:407–13. doi: 10.1016/S0006-8993(99)01123-3
291. Xu C, Yang X, Sun L, Yang T, Cai C, Wang P, et al. An investigation of calcium-independent phospholipase A2 (iPLA2) and cytosolic phospholipase A2 (cPLA2) in schizophrenia. Psychiatry Res. (2019) 273:782–7. doi: 10.1016/j.psychres.2019.01.095
292. Pettegrew JW, Keshavan MS, Minshew NJ. 31P nuclear magnetic resonance spectroscopy: neurodevelopment and schizophrenia. Schizophr Bull. (1993) 19:35–53. doi: 10.1093/schbul/19.1.35
293. Tavares H Jr, Yacubian J, Talib LL, Barbosa NR, Gattaz WF. Increased phospholipase A2 activity in schizophrenia with absent response to niacin. Schizophr Res. (2003) 61:1–6. doi: 10.1016/S0920-9964(02)00281-5
294. Kuo C-F, Cheng S, Burgess JR. Deficiency of vitamin E and selenium enhances calcium-independent phospholipase A2 activity in rat lung and liver. J Nutr. (1995) 125:1419–29.
295. Bošković M, Grabnar I, Terzič T, Plesničar BK, Vovk T. Oxidative stress in schizophrenia patients treated with long-acting haloperidol decanoate. Psychiatry Res. (2013) 210:761–8. doi: 10.1016/j.psychres.2013.08.035
296. Prabakaran S, Swatton J, Ryan M, Huffaker S, Huang J-J, Griffin J, et al. Mitochondrial dysfunction in schizophrenia: evidence for compromised brain metabolism and oxidative stress. Mol Psychiatry. (2004) 9:684–97. doi: 10.1038/sj.mp.4001511
297. Pavicevic Z, Leslie CC, Malik KU. cPLA2 phosphorylation at serine-515 and serine-505 is required for arachidonic acid release in vascular smooth muscle cells. J Lipid Res. (2008) 49:724–37. doi: 10.1194/jlr.M700419-JLR200
298. Börsch-Haubold AG, Bartoli F, Asselin J, Dudler T, Kramer RM, Apitz-Castro R, et al. Identification of the phosphorylation sites of cytosolic phospholipase A2 in agonist-stimulated human platelets and HeLa cells. J Biol Chem. (1998) 273:4449–58. doi: 10.1074/jbc.273.8.4449
299. Gijón MA, Leslie CC. Regulation of arachidonic acid release and cytosolic phospholipase A2 activation. J Leukoc Biol. (1999) 65:330–6. doi: 10.1002/jlb.65.3.330
300. Law M, Cotton R, Berger G. The role of phospholipases A2 in schizophrenia. Mol Psychiatry. (2006) 11:547–56. doi: 10.1038/sj.mp.4001819
301. Bonventre JV. The 85-kD Cytosolic Phospholipase A Knockout Mouse. A New Tool for Physiology and Cell Biology. J Am Soc Nephrol. (1999) 10:404–12. doi: 10.1681/ASN.V102404
302. Balboa MA, Balsinde J, Dennis EA. Phosphorylation of cytosolic group IV phospholipase A2 is necessary but not sufficient for arachidonic acid release in P388D1 macrophages. Biochem Biophys Res Commun. (2000) 267:145–8. doi: 10.1006/bbrc.1999.1964
303. Balboa MaA, Balsinde J, Dillon DA, Carman GM, Dennis EA. Proinflammatory macrophage-activating properties of the novel phospholipid diacylglycerol pyrophosphate. J Biol Chem. (1999) 274:522–6. doi: 10.1074/jbc.274.1.522
304. Ruipérez V, Astudillo AM, Balboa MA, Balsinde J. Coordinate regulation of TLR-mediated arachidonic acid mobilization in macrophages by group IVA and group V phospholipase A2s. J Immunol. (2009) 182:3877–83. doi: 10.4049/jimmunol.0804003
305. Casas J, Meana C, Esquinas E, Valdearcos M, Pindado J, Balsinde J, et al. Requirement of JNK-Mediated Phosphorylation for Translocation of Group IVA Phospholipase A to Phagosomes in Human Macrophages. J Immunol. (2009) 183:2767–74. doi: 10.4049/jimmunol.0901530
306. Sedgwick JD, Schwender S, Imrich H, Dörries R, Butcher GW, ter Meulen V. Isolation and direct characterization of resident microglial cells from the normal and inflamed central nervous system. Proc Natl Acad Sci. (1991) 88:7438–42. doi: 10.1073/pnas.88.16.7438
307. Breier A, Su T-P, Saunders R, Carson R, Kolachana B, De Bartolomeis A, et al. Schizophrenia is associated with elevated amphetamine-induced synaptic dopamine concentrations: evidence from a novel positron emission tomography method. Proc Nat Acad Sci. (1997) 94:2569–74. doi: 10.1073/pnas.94.6.2569
308. Vial D, Piomelli D. Dopamine D2 Receptors Potentiate Arachidonate Release via Activation of Cytosolic, Arachidonate-Specific Phospholipase A2. J Neurochem. (1995) 64:2765–72. doi: 10.1046/j.1471-4159.1995.64062765.x
309. Sales KJ, Grant V, Jabbour HN. Prostaglandin E2 and F2α activate the FP receptor and up-regulate cyclooxygenase-2 expression via the cyclic AMP response element. Mol Cell Endocrinol. (2008) 285:51–61. doi: 10.1016/j.mce.2008.01.016
310. Choi SH, Langenbach R, Bosetti F. Cyclooxygenase-1 and-2 enzymes differentially regulate the brain upstream NF-κB pathway and downstream enzymes involved in prostaglandin biosynthesis. J Neurochem. (2006) 98:801–11. doi: 10.1111/j.1471-4159.2006.03926.x
311. Rao JS, Langenbach R, Bosetti F. Down-regulation of brain nuclear factor-kappa B pathway in the cyclooxygenase-2 knockout mouse. Mol Brain Res. (2005) 139:217–24. doi: 10.1016/j.molbrainres.2005.05.008
312. Deng WG, Zhu Y, Wu KK. Up-regulation of p300 binding and p50 acetylation in tumor necrosis factor-alpha-induced cyclooxygenase-2 promoter activation. J Biol Chem. (2003) 278:4770–7. doi: 10.1074/jbc.M209286200
313. Coyne DW, Nickols M, Bertrand W, Morrison AR. Regulation of mesangial cell cyclooxygenase synthesis by cytokines and glucocorticoids. Am J Physiol. (1992) 263:F97–102. doi: 10.1152/ajprenal.1992.263.1.F97
314. O'Banion MK. COX-2 and Alzheimer's disease: potential roles in inflammation and neurodegeneration. Expert Opin Investig Drugs. (1999) 8:1521–36. doi: 10.1517/13543784.8.10.1521
315. Zhang XY, Zhou DF, Cao LY, Wu GY, Shen YC. Cortisol and cytokines in chronic and treatment-resistant patients with schizophrenia: association with psychopathology and response to antipsychotics. Neuropsychopharmacology. (2005) 30:1532–8. doi: 10.1038/sj.npp.1300756
316. Mondelli V, Ciufolini S, Belvederi Murri M, Bonaccorso S, Di Forti M, Giordano A, et al. Cortisol and inflammatory biomarkers predict poor treatment response in first episode psychosis. Schizophr Bull. (2015) 41:1162–70. doi: 10.1093/schbul/sbv028
317. Sommer IE, de Witte L, Begemann M, Kahn RS. Nonsteroidal anti-inflammatory drugs in schizophrenia: ready for practice or a good start? A meta-analysis. J Clinical Psychiat. (2011) 73:414–9. doi: 10.4088/JCP.10r06823
318. Nitta M, Kishimoto T, Müller N, Weiser M, Davidson M, Kane JM, et al. Adjunctive use of nonsteroidal anti-inflammatory drugs for schizophrenia: a meta-analytic investigation of randomized controlled trials. Schizophr Bull. (2013) 39:1230–41. doi: 10.1093/schbul/sbt070
319. Müller N, Riedel M, Scheppach C, Brandstätter B, Sokullu S, Krampe K, et al. Beneficial antipsychotic effects of celecoxib add-on therapy compared to risperidone alone in schizophrenia. Am J Psychiatry. (2002) 159:1029–34. doi: 10.1176/appi.ajp.159.6.1029
320. Müller N, Ulmschneider M, Scheppach C, Schwarz MJ, Ackenheil M, Möller HJ, et al. COX-2 inhibition as a treatment approach in schizophrenia: Immunological considerations and clinical effects of celecoxib add-on therapy. Eur Arch Psychiatry Clin Neurosci. (2004) 254:14–22. doi: 10.1007/s00406-004-0478-1
321. Müller N, Krause D, Dehning S, Musil R, Schennach-Wolff R, Obermeier M, et al. Celecoxib treatment in an early stage of schizophrenia: results of a randomized, double-blind, placebo-controlled trial of celecoxib augmentation of amisulpride treatment. Schizophr Res. (2010) 121:118–24. doi: 10.1016/j.schres.2010.04.015
322. Hata AN, Breyer RM. Pharmacology and signaling of prostaglandin receptors: multiple roles in inflammation and immune modulation. Pharmacol Ther. (2004) 103:147–66. doi: 10.1016/j.pharmthera.2004.06.003
323. Murakami M, Kudo I. Recent advances in molecular biology and physiology of the prostaglandin E2-biosynthetic pathway. Prog Lipid Res. (2004) 43:3–35. doi: 10.1016/S0163-7827(03)00037-7
324. Melnikova T, Savonenko A, Wang Q, Liang X, Hand T, Wu L, et al. Cycloxygenase-2 activity promotes cognitive deficits but not increased amyloid burden in a model of Alzheimer's disease in a sex-dimorphic pattern. Neuroscience. (2006) 141:1149–62. doi: 10.1016/j.neuroscience.2006.05.001
325. Mattson MP, Culmsee C, Yu Z, Camandola S. Roles of nuclear factor κB in neuronal survival and plasticity. J Neurochem. (2000) 74:443–56. doi: 10.1046/j.1471-4159.2000.740443.x
326. Weggen S, Eriksen JL, Das P, Sagi SA, Wang R, Pietrzik CU, et al. A subset of NSAIDs lower amyloidogenic Aβ42 independently of cyclooxygenase activity. Nature. (2001) 414:212–6. doi: 10.1038/35102591
327. Morihara T, Chu T, Ubeda O, Beech W, Cole G. Selective inhibition of Aβ42 production by NSAID R-enantiomers. J Neurochem. (2002) 83:1009–12. doi: 10.1046/j.1471-4159.2002.01195.x
328. Niederberger E, Tegeder I, Vetter G, Schmidtko A, Schmidt H, Euchenhofer C, et al. Celecoxib loses its anti-inflammatory efficacy at high doses through activation of NF-κB. The FASEB Journal. (2001) 15:1622–4. doi: 10.1096/fj.00-0716fje
329. Tegeder I, Pfeilschifter J, Geisslinger G. Cyclooxygenase-independent actions of cyclooxygenase inhibitors. The FASEB Journal. (2001) 15:2057–72. doi: 10.1096/fj.01-0390rev
330. Harris SG, Padilla J, Koumas L, Ray D, Phipps RP. Prostaglandins as modulators of immunity. Trends Immunol. (2002) 23:144–50. doi: 10.1016/S1471-4906(01)02154-8
331. Rösch S, Ramer R, Brune K, Hinz B. Prostaglandin E2 induces cyclooxygenase-2 expression in human non-pigmented ciliary epithelial cells through activation of p38 and p42/44 mitogen-activated protein kinases. Biochem Biophys Res Commun. (2005) 338:1171–8. doi: 10.1016/j.bbrc.2005.10.051
332. Yang H, Zhang J, Andreasson K, Chen C. COX-2 oxidative metabolism of endocannabinoids augments hippocampal synaptic plasticity. Mol Cell Neurosci. (2008) 37:682–95. doi: 10.1016/j.mcn.2007.12.019
333. Nieminen R, Lahti A, Jalonen U, Kankaanranta H, Moilanen E. JNK inhibitor SP600125 reduces COX-2 expression by attenuating mRNA in activated murine J774 macrophages. Int Immunopharmacol. (2006) 6:987–96. doi: 10.1016/j.intimp.2006.01.009
334. Nieminen R, Leinonen S, Lahti A, Vuolteenaho K, Jalonen U, Kankaanranta H, et al. Inhibitors of mitogen-activated protein kinases downregulate COX-2 expression in human chondrocytes. Mediators Inflamm. (2005) 2005:249–55. doi: 10.1155/MI.2005.249
335. Arasa J, Terencio MC, Andrés RM, Marín-Castejón A, Valcuende-Cavero F, Payá M, et al. Defective Induction of COX-2 Expression by Psoriatic Fibroblasts Promotes Pro-inflammatory Activation of Macrophages. Front Immunol. (2019) 10:536. doi: 10.3389/fimmu.2019.00536
336. Deva R, Shankaranarayanan P, Ciccoli R, Nigam S. Candida albicans induces selectively transcriptional activation of cyclooxygenase-2 in HeLa cells: pivotal roles of Toll-like receptors, p38 mitogen-activated protein kinase, and NF-kappa B. J Immunol. (2003) 171:3047–55. doi: 10.4049/jimmunol.171.6.3047
337. Vijitruth R. Roles Of Cyclooxygenase-2 In Microglial Activation And Dopaminergic Cell Death. In: College of Medicine. Univeristy of Kentucky. New York: Oxford Academic (2006). p. 124.
338. Chaudhry IB, Hallak J, Husain N, Minhas F, Stirling J, Richardson P, et al. Minocycline benefits negative symptoms in early schizophrenia: a randomised double-blind placebo-controlled clinical trial in patients on standard treatment. Journal of psychopharmacology. (2012) 26:1185–93. doi: 10.1177/0269881112444941
339. Zekry D, Epperson TK, Krause K-HA. Role for NOX NADPH Oxidases in Alzheimer's Disease and Other Types of Dementia? IUBMB Life. (2003) 55:307–13. doi: 10.1080/1521654031000153049
340. Zhang J, Li S, Li L, Li M, Guo C, Yao J, et al. Exosome and exosomal microRNA: trafficking, sorting, and function. Genom Proteom Bioinform. (2015) 13:17–24. doi: 10.1016/j.gpb.2015.02.001
341. Fries GR, Quevedo J. Exosomal microRNAs as potential biomarkers in neuropsychiatric disorders. MicroRNA Protocols. (2018) 79–85. doi: 10.1007/978-1-4939-7601-0_6
342. Alexander M, Hu R, Runtsch M, Kagele D, Mosbruger T, Tolmachova T, et al. Exosomedelivered microRNAs modulate the inflammatory response to endotoxin. Nat Commun. (2015) 6:7321. doi: 10.1038/ncomms8321
343. Du Y, Chen L, Li X-S, Li X-L, Xu X-D, Tai S-B, et al. Metabolomic Identification of Exosome-Derived Biomarkers for Schizophrenia: A Large Multicenter Study. Schizophr Bull. (2020) 47:615–23. doi: 10.1093/schbul/sbaa166
344. Pardiñas AF, Holmans P, Pocklington AJ, Escott-Price V, Ripke S, Carrera N, et al. Common schizophrenia alleles are enriched in mutation-intolerant genes and in regions under strong background selection. Nat Genet. (2018) 50:381–9. doi: 10.1038/s41588-018-0059-2
345. Finucane HK, Reshef YA, Anttila V, Slowikowski K, Gusev A, Byrnes A, et al. Heritability enrichment of specifically expressed genes identifies disease-relevant tissues and cell types. Nat Genet. (2018) 50:621–9. doi: 10.1038/s41588-018-0081-4
346. Skene NG, Bryois J, Bakken TE, Breen G, Crowley JJ, Gaspar HA, et al. Genetic identification of brain cell types underlying schizophrenia. Nat Genet. (2018) 50:825–33. doi: 10.1038/s41588-018-0129-5
Keywords: diminished GPR109A-flushed effect, niacin, microglia, JNK treatment, schizophrenia, c-Jun N-terminal kinase (JNK) pathway, neuron
Citation: Ansarey SH (2021) Inflammation and JNK's Role in Niacin-GPR109A Diminished Flushed Effect in Microglial and Neuronal Cells With Relevance to Schizophrenia. Front. Psychiatry 12:771144. doi: 10.3389/fpsyt.2021.771144
Received: 05 September 2021; Accepted: 02 November 2021;
Published: 30 November 2021.
Edited by:
Błażej Misiak, Wroclaw Medical University, PolandReviewed by:
Yong Cheng, Minzu University of China, ChinaMichael Wheeler, Brigham and Women's Hospital and Harvard Medical School, United States
Copyright © 2021 Ansarey. This is an open-access article distributed under the terms of the Creative Commons Attribution License (CC BY). The use, distribution or reproduction in other forums is permitted, provided the original author(s) and the copyright owner(s) are credited and that the original publication in this journal is cited, in accordance with accepted academic practice. No use, distribution or reproduction is permitted which does not comply with these terms.
*Correspondence: Sabrina H. Ansarey, c2FicmluYWFuc2FyZXkmI3gwMDA0MDtnbWFpbC5jb20=