- Department of Life and Environmental Sciences, New York-Marche Structural Biology Center (NY-MaSBiC), Polytechnic University of Marche, Ancona, Italy
Fragile X Syndrome (FXS) is the most frequent cause of inherited intellectual disabilities and autism spectrum disorders, characterized by cognitive deficits and autistic behaviors. The silencing of the Fmr1 gene and consequent lack of FMRP protein, is the major contribution to FXS pathophysiology. FMRP is an RNA binding protein involved in the maturation and plasticity of synapses and its absence culminates in a range of morphological, synaptic and behavioral phenotypes. Currently, there are no approved medications for the treatment of FXS, with the approaches under study being fairly specific and unsatisfying in human trials. Here we propose peptides/peptidomimetics as candidates in the pharmacotherapy of FXS; in the last years this class of molecules has catalyzed the attention of pharmaceutical research, being highly selective and well-tolerated. Thanks to their ability to target protein-protein interactions (PPIs), they are already being tested for a wide range of diseases, including cancer, diabetes, inflammation, Alzheimer's disease, but this approach has never been applied to FXS. As FXS is at the forefront of efforts to develop new drugs and approaches, we discuss opportunities, challenges and potential issues of peptides/peptidomimetics in FXS drug design and development.
Introduction
Fragile X syndrome (FXS) was first described in 1943 and it is now established as the most common cause of inheritable intellectual disabilities (ID) (1). The molecular cause of FXS is the extensive repeat expansion of a CGG triplet (200 repeats in the full mutation) in the 5′ untranslated region (UTR) and consequential hypermethylation of the Fmr1 gene, finally leading to transcriptional silencing of the fragile X mental retardation protein (FMRP) (2–4). A small proportion of individuals affected by FXS with different levels of severity show deletion or point mutation in the Fmr1 gene, that in turn cause the complete loss of FMRP or the production of a functionally deficient protein (5–7).
This disease affects 1:4,000 males and 1:6,000–8,000 females (8, 9) showing symptoms from moderate to severe ID (10). The clinical picture of the syndrome is complex; FXS phenotype displays characteristics in common with autism spectrum disorder (ASD) and attention deficit hyperactivity disorder (ADHD), with general anxiety, social avoidance and hyperactive behaviors (11–13). Seizures, recurrent otitis media, strabismus and obesity are also often occurring in patients affected by FXS. Besides, about 10% of males with FXS display a Prader-Willi like phenotype (14). The physical features of FXS comprehend elongated face, broad forehead, high palate, prominent ears, hyperextensible finger joints, flat feet and macroorchidism (15). All these behavioral, phenotypical and clinical characteristics of FXS, are due to the lack of FMRP, a well-characterized RNA-binding protein, showing crucial functions mainly related to mRNAs metabolism (16). Its main role is represented by the translational repression of numerous key mRNAs in pre- and postsynaptic neurons (17, 18). The FMRP deficiency results in increased protein synthesis, causing the upregulation of several signaling effectors, such as excitatory metabotropic glutamate receptor (mGluR) (19, 20), α-amino-3-hydroxy-5-methyl-4-isoxazole propionic acid (AMPA) receptor (21), extracellular signal-related kinase (ERK1/2) (22–24), matrix metalloproteinase 9 (MMP-9) (25–27), brain-derived neurotrophic factor (BDNF) (28, 29), and mammalian target of rapamycin (mTOR) (30, 31). Moreover, functional impairment in gamma-aminobutyric acid (GABA) receptor and in the endocannabinoid system have been also documented in FXS (32–34). In healthy conditions all these machineries orchestrate neurotransmission and local protein synthesis that impact synaptic plasticity, learning and memory. Hence, in FXS pathology, the lack of FMRP leads to increased protein synthesis with a direct effect on dendritic spine dysgenesis and cognitive disabilities (9, 17), causing the majority of the FXS symptoms. Evidences from Fmr1 knockout (KO) mice and from human post-mortem brain biopsies showed increased amount and length of dendritic spines, with an immature profile (35–37).
FMRP inhibits translation initiation through its interactions with eIF4E (Eukaryotic translation Initiation Factor 4E) and CYFIP1 (Cytoplasmic FMRP Interacting Protein 1) (4, 7, 38–40). eIF4E is the cap-binding protein known to be activated by the interaction with the scaffold protein eIF4G (Eukaryotic translation Initiation Factor 4G) or inhibited by 4E-binding proteins (4E-BPs), these last being a well-characterized group of proteins that repress protein synthesis (41, 42). The 4E-BPs and eIF4G compete for the same binding site on the eIF4E surface; thus, 4E-BPs inhibit the eIF4E-eIF4G complex formation by sequestering the unbound eIF4E (43, 44). CYFIP1 belongs to the 4E-BPs family and in neurons, mainly at synapses, the FMRP-CYFIP1-eIF4E inhibitory complex regulates protein synthesis during synaptic activity, playing a pivotal role in the modulation of long-term synaptic plasticity at synapses (18, 38). Moreover, the CYFIP1 paralog CYFIP2 is itself able to interact with FMRP and with the FMRP-related proteins FXR1P/2P, which are cytoplasmatic proteins that share with FMRP the functional domains deputed to promote homo- and heteromerization (45, 46).
Current Strategies in FXS Treatment
Recently, strong effort was dedicated to develop specific FXS pharmacological treatment that can lead to a possible cure, or at least alleviate symptoms (47–51). The most promising or studied treatments for FXS are listed in Table 1. However, although several therapeutic approaches are being tested on different FXS animal models (i.e., Fmr1 KO mouse, rat and zebrafish; dFmr null mutant fly) and patients over the years, an approved and successful curative therapy for FXS is missing to date, and the management of the clinical aspects of the syndrome continue to focus on symptomatic treatment of psychiatric and behavioral problems, rather than the molecular causes (49, 50, 52).
One of the first approaches that was suggested for FXS treatment was the Fmr1 gene activity restoration through changes in the DNA methylation levels and epigenetic modifications (53, 54). Although different compounds were tested and successfully achieving in vitro reactivation of the Fmr1 gene, such as 5-azadeoxycytidine (5-azadC) (53), this strategy has not been tested with in vivo studies due to safety problems related to low reactivity and high toxicity of these chromatin-modifying enzymes inhibitors (55). Similarly, another strategy involves the use of non-coding RNAs to affect DNA methylation state and histones modification (56). Based on the promising results obtained in cancer and other diseases (57), several miRNAs and lncRNAs were identified and tested in different FXS models (58–60), but their potential use in clinical therapy is still far away similarly to the modern application of gene therapy methods to restore the Fmr1 gene (61). Indeed, independent groups demonstrated the possibility to use viral-vectors or CRISPR-technology, with encouraging results in preclinical FXS models (62–67); however, the clinical application in patients is being debated for several undisclosed questions, as safety and brain-targeted delivery. Regarding this approach it is also important to consider that the reactivation of the Fmr1 mRNA with the full mutation could be toxic, as it was demonstrated by a correlation detected between the Fmr1 mRNA levels in blood and more severe autism features (68).
However, since several compounds are used to treat behavioral and mental problems, such as stimulants or antipsychotics (69), the majority of pharmacological efforts are employed to compensate the absence of FMRP. Among targeted treatment for FXS, several focused on the neurotransmission imbalance associated with FXS. Particular attention has been dedicated in testing the group 1 metabotropic glutamate receptors 5 (mGluR5) antagonists, such as AFQ056/Mavoglutarant, Fenobam, MPEP, STX107, CTEP, RO4917523 (9, 10, 17, 19), and GABA receptors (GABAa and GABAb) agonists (70). In Fmr1 KO mice these agents showed improvement of several FXS features, including better behavioral abilities, restoration of normal levels of dendritic spines and reduction in protein synthesis (9, 17, 69). Despite these positive results, the transition from animal to human model did not give the same encouraging outcomes, since most of clinical trials failed (71, 72). The high placebo response and the imprecise design and methodology of the trials were the major causes of failure.
Most of drugs tested in FXS pharmacotherapy are compounds already employed or approved for other disorders. Sertraline is a serotonin reuptake inhibitor approved for treating anxiety and mental disabilities in young children and tested in Fmr1-KO mouse model. Sertraline normalizes serotonin and dopamine levels, with a rescue on synapse and dendritic formation (73, 74). Even in FXS patients, Sertraline showed favorable results, as several studies demonstrated improvements in language, anxiety and social conduct (75, 76). Cannabidiol (CBD), a synthetic molecule active on cannabinoid receptors, has been used for the treatment of neurological disorders, such as Huntington, Parkinson's and Alzheimer's diseases, but also epilepsy, schizophrenia, autoimmune diseases. All these pathologies have in common altered endocannabinoid signaling pathway, condition confirmed to be deregulated also in FXS animal model (77, 78). Clinical studies indicated good results (79–81), albeit with tolerated side effects. The following FDA-approved drugs have been tested in FXS preclinical and clinical studied: acamprosate (for maintenance of alcohol abstinence), lovastatin (for hypercholesterolemia), minocycline (for acne) and metformin (for non-insulin diabetes mellitus). In particular lovastatin targets the RAS-MAPK-ERK1/2 pathway (82, 83) while minocycline inhibits the MMP-9 activity (25). Both compounds showed promising results in preclinic testing using different model systems, with a reduction in protein synthesis and beneficial cognitive and behavioral aspects (25, 82, 84, 85). Nevertheless, these encouraging data were followed by moderate effects in trials on FXS patients, also expressing the need for a more in-depth investigation on the tolerability of these compounds (86–88). To date, the anti-diabetes drug metformin could be considered as one of the most promising treatments for FXS (89, 90). It has different mechanisms of action, depending on dosage and treatment time, including inhibition of mammalian/mechanistic target of rapamycin complex 1 (mTORC1) and mitogen-activated protein kinase/extracellular signal regulated kinase (MAPK/ERK) pathways, both hyperactivated due to the lack of FMRP in FXS humans and mice (91, 92). As a consequence, metformin also affects the proteins downstream to these cascades, reducing specifically the eIF4E phosphorylation and the translation of MMP-9 (93), which in the pathological condition is the cause for the degradation of proteins essential for synaptic maturation and activity (27). Preclinical studies were performed on Fmr1-KO flies and mice models of FXS, showing a rescue of dendritic spine morphology, long-term depression (LTD) of synapses, but also improvement in cognitive, intellectual and social deficits (90, 94). These findings paved the way for treatments in humans, where clinical trials starting in 2018 have been conducted with promising benefits both in terms of behavior and safety of treatment (93, 95–97). Currently 3 trials aimed to evaluate safety, tolerability, and efficacy of metformin in FXS patients are ongoing (ClinicalTrials.gov Identifier: NCT04141163, NCT03862950, NCT03479476). Another interesting therapeutic target in FXS is represented by phosphodiesterases (PDEs), a family of enzymes that regulate the cellular levels of cAMP and cGMP. Among PDEs, PDE1A, PDE2A, and PDE10A have been identified as mRNA targets of FMRP (98). Accordingly, decreased cAMP levels were observed in fly and mouse FXS models and a deregulation of cAMP and cGMP was also identified as a molecular hallmark in FXS patients (99, 100). Hence, several inhibitors have been tested, starting from Drosophila model of fragile X, passing through Fmr1-KO mice, finally to human trials. Interestingly, inhibiting PDE4 (101, 102), PDE2A (103, 104), PDE4D (105, 106), or synergistically PDE2 and PDE4 (107) demonstrated beneficial effects in terms of rescue of social and behavioral impairments and in dendritic spines morphology in fly and mouse models. Cognitive enhancements were pointed out from FXS trials (105, 106), suggesting that PDEs are candidate targets to develop FXS therapeutic strategy.
Peptides/Peptidomimetics: a Feasible Strategy for FXS Treatment
All the strategies mentioned so far target different pathways, whose uncontrolled activity seems to be crucial in the pathology of FXS, but also in other neurological disorders and types of cancer (108, 109), leading to pleiotropic effects. Accordingly, the lack of specificity and selectivity, together with bioavailability and safety problems, could be the main drawbacks of these approaches.
In this scenario, a novel and feasible option in FXS pharmacotherapy could be the use of peptides or peptidomimetics.
Since the last 30 years, and especially in the past decade, severe pathologies are being treated with peptides (110) and this class of molecules have attracted the attention of either academia researchers or pharmaceutical industries. Indeed, the global Peptide Therapeutics market reached USD 25.35 billion in 2018 and is expected to achieve USD 50.60 billion by the year 2026. To date, 400–600 peptides are in the preclinical phase of development and more than 60 peptides are FDA-approved (111). The main fields in which therapeutic peptides are currently in development are oncology, metabolic diseases and inflammation (110). Peptides represent an attractive pharmaceutical source due to their excellent properties, namely high selectivity, safety and tolerability (112). However, this kind of approach has never been applied to FXS to restore the imbalance in protein synthesis. Very recently novel structural information opened new possibilities for developing inhibitors acting on the mRNAs translation initiation complex with high specificity and efficiency.
Among these compounds, the 4EGI-1 is one of the most promising inhibitors of the translation activation complex and it has been already tested in different cancer models (113–116). This molecule was also proved to reduce the eIF4E-eIF4G interaction in a FXS mouse model (117). However, the lack of drug-like characteristics, such as poor target specificity and selectivity, high toxicity, several off-targets, severe side effects, poor metabolic stability, poor membrane permeability and rapid proteolysis, make this molecule fairly unsuitable candidates for therapeutic applications.
Furthermore, the putative molecules effective in disrupting the FMRP-CYFIP1-eIF4E or eIF4E-eIF4G complexes formation, are required to target protein-protein interaction (PPI) interfaces, that are large, flat and hydrophobic binding surfaces considered as “undruggable” by small compounds (118–120). One solution could be represented by antibodies, more powerful in targeting PPI, but anyway scarcely able to cross the cell membrane to perform their specific function. In light of this, peptides are now considered as the most appropriate candidates to regulate disease-associated PPIs. However, peptides have intrinsic weak points, and they did not provide encouraging results in vivo, likely due to their physical, chemical and structural instability and low membrane permeability (112, 119, 121). To overcome these possible limitations several strategies have been developed, such as amino acids substitution with residues mimicry, termini protection or introduction of chemical modifications aimed at stabilizing their active conformation and increasing cellular permeability (119, 121). These advances in the peptides technology results in the development of an alternative class of compounds called peptidomimetics, that is recently emerging as a class of new potential therapeutic molecules able to target PPIs in the treatment of different pathologies (112). Peptidomimetics are organic molecules with physico-chemical features and structural characteristics comparable with classical oligopeptides, but guarantee enhanced protection against peptidases, improved systemic delivery and cellular uptake, high target specificity and poor immune response (122), and for these reasons their use is under investigation for the treatment of cancer, ischemia, Alzheimer's disease (123–127) and other neurodegenerative disorders (128–131).
On the contrary, the use of peptides/peptidomimetics has never been investigated in the FXS context, but it could represent a viable solution as it might result in a compensation of FMRP absence. Indeed, restoring the FMRP-CYFIP1 deficiency via a small chimeric peptide acting on the dysregulation of protein synthesis could be central for the new FXS pharmacological therapy development.
Although the 3D structure of FMRP-CYFIP1 or FMRP with other interacting proteins are still not available, there is a growing number of structures, from different organisms, of complexes belonging to the translation initiation pathway, in particular eIF4G/eIF4E and eIF4E/4E-BPs, and of their regulatory proteins (43, 44, 132, 133). The plethora of structural information, together with the increasing power of computational facilities and refinement of binding prediction tools (110, 112, 119), open the possibility to design peptides/peptidomimetics able to target the translation complexes, with the aim to decrease protein synthesis of specific neuronal mRNAs and rescuing a healthy phenotype in individuals affected by FXS.
For instance, peptides could target and affect the assembly of the eukaryotic Initiation Factor 4F (eIF4F) complex (composed by eIF4E, the DEAD-box helicase eIF4A and the scaffold protein eIF4G) (41, 42), and the formation of 43S pre-initiation complex (43S PIC), composed by small ribosomal subunit 40S and the eukaryotic translation initiation factors (eIFs): eIF1, eIF1A, eIF3, eIF5 (41, 42) (Figure 1). In addition to those already mentioned, the PPIs that could be targeted and disrupted by peptides, could be for instance, eIF4E/cap, eIF4A/eIF4G or eIF4G/eIF3 dimers (Figure 1). Alternatively, peptides/peptidomimetics could be designed to target the eIF4E-upstream regulators, as the PI3K–mTOR pathway, likely inhibiting the interactions of mTORC1 with Raptor and other partners, affecting the activity of the downstream S6K or 4E-BP proteins (Figure 1) (134). Furthermore, not only protein synthesis but also actin dynamic imbalance concurs to the pathophysiology of FXS, leading to defects in dendritic spines morphology (135, 136). Although much information is still lacking, there are indications that Rac1–PAK pathway and FMRP are linked (137, 138). Active Rac1, the Rho-family of small GTPases, activates p21-activated kinases (PAKs) which in turn phosphorylates Cofilin, an actin-binding protein that regulates actin turnover. Rac1 also activates the Wave Regulatory Complex (WRC, composed by five proteins: CYFIP1, NCKAP1, Abi2, HSPC300, and WAVE1) by directly binding CYFIP1 and leading to Arp2/3 complex-mediated actin polymerization (139–141). The release of the X-ray structure of WRC (140), together with data coming from computational analyses that highlighted structural-dynamical features of CYFIP1 (39, 40), may provide useful details to be exploited for the CYFIP1-based peptidomimetics design (Figure 1). To offer some realistic examples, using different regions of CYFIP1 as templates, CYFIP1-derived peptidomimetics could interfere with the eIF4F complex formation by sequestering eIF4E from the binding with eIF4G. Similarly, impeding the CYFIP1/Rac1 interaction or the CYFIP1/NCKAP1 dimer formation, could have a dual beneficial effect in concomitantly restoring normal levels of protein synthesis and actin dynamics, both processes being dysregulated in FXS. Aside from CYFIP1, other 4E-BPs structures in complex with eIF4E are available and represent an attractive template for peptides design. For example, 4E-BP1, 4E-BP2, and Angel1-based peptides were developed and tested in different cancer cell lines (142–144). Furthermore, Lama et al. developed a set of peptides with chemical modifications that increase the pharmacological properties and binding affinity to eIF4E, providing a strong starting point for future oncological preclinical studies (145–147).
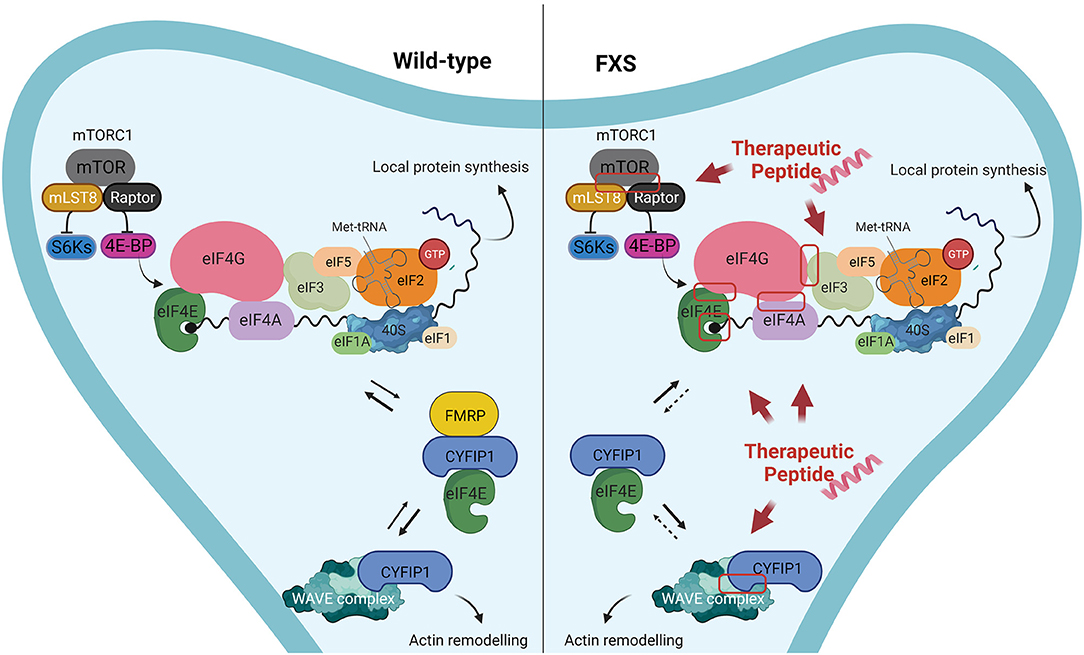
Figure 1. Proposed examples of protein-protein interactions that could be targeted by peptides/peptidomimetics in Fragile X Syndrome. Left panel: In wild-type neurons FMRP plays a key role in down-regulating the translation of FMRP targets, by forming a complex with CYFIP1 and the eukaryotic initiation factor 4E (eIF4E). Furthermore, CYFIP1 can bind and inhibit the WAVE regulatory complex, thereby regulating actin remodeling. Right panel: In FXS neurons the absence of FMRP leads to increased local protein synthesis in neurons, due to the lack of the formation CYFIP1-FMRP-eIF4E complex, that represses the translation initiation. Consequently, a bigger amount of eIF4E is bound to eIF4G, while CYFIP1 interacts mostly with WRC-Rac1-GTP, resulting in higher levels of protein synthesis an altered actin remodeling at dendritic spines. Examples of macromolecular complexes that could be disrupted by peptides/peptidomimetics with the aim to restore the FMRP activity are highlighted by a red box.
On the basis of the knowledge acquired in cancer research, we assume that the peptides/peptidomimetics approach could also be applied to other diseases, in particular in the FXS pharmacotherapeutic.
Discussion
In the last decade many efforts were employed in research and development of new pharmacological treatments of FXS and simultaneously great advances were made in developing peptides therapeutics against several diseases. With this perspective we speculate that these two roads might cross, starting a new era of the pharmacotherapeutic approach for patients with FXS. To date, chemical compounds that inhibit several pathways deregulated in FXS represent the most studied approaches for FXS management. However, the cause of FXS is a genetic defect (the Fmr1 silencing) and to effectively correct the absence of FMRP protein is still a challenge. Moreover, although several available treatments are effective in animal models, many clinical trials testified lack of success of these approaches. The emerging peptides technology, in combination with increasingly advanced computational approaches and number of proteins structures deposited in databases, provide an alternative and powerful method to develop a more specific and safe molecules targeting those protein complexes that could be considered as the major players in the FXS pathology. The main point of strength of the use of peptides include selectivity, tolerability, predictable metabolism, ability to target PPIs and lower synthesis complexity that in turn leads to lower production costs compared to others pharmaceutical molecules (119, 121). However, as mentioned above, peptides in general, and thus their possible application against FXS, have several weaknesses that is necessary to discuss. Poor in vivo stability, membrane impermeability, and toxicity are widely accounted as major drawbacks in peptides technology. Nevertheless, several of these aspects have been successfully sorted out over recent years through the new technologies available in peptides design field: new bioinformatics tools in combination with other approaches such as virtual screening, structure-based drug design, high throughput screening (HTS) and chemical strategies, provide a comprehensive pharmacological description of putative peptides, improving their chemical and physical features (119, 121, 148). Moreover, the treatment of neurodevelopmental disorders, as FXS, require the delivery of molecules to the brain, accounting for the crossing of the blood–brain barrier (BBB), that could limit the access of peptides to the central nervous system (CNS). In this respect, a strategy to overcome these issues could be the exploration of other drugs administration methods, e.g., the non-invasive intranasal delivery (129, 131). The direct access to CNS allows to overcome limitations linked to the degradation, bioavailability problems and also to possible systemic side effects onset that occur if peptides are present in blood vessels after intravenous administration. Additionally, several strategies have been developed to specifically deliver peptides to target regions of the CNS, such as cyclodextrins, PEI or others (129), resulting in a lower dosage of peptides, also decreasing the toxicity issues which have been demonstrated toward eukaryotic cells (149, 150).
Furthermore, peptides therapy would not be a chronic intervention, but these molecules would be administered only in a limited period of time during the 1st years of life of FXS children, when brain is still remodeling, to allow proper formation of the synaptic network.
Hence, we propose that peptides/peptidomimetics could compensate the FMRP deficiency restoring the imbalance of protein synthesis and actin dynamics, suggesting a new and promising strategy for treating FXS.
Data Availability Statement
The original contributions presented in the study are included in the article/supplementary material, further inquiries can be directed to the corresponding author/s.
Author Contributions
This perspective was conceived by DD and AR. DD and AR wrote the manuscript. Table and figure were prepared by AR. All authors contributed to the article and approved the submitted version.
Funding
AR was supported by FRAXA Foundation.
Conflict of Interest
The authors declare that the research was conducted in the absence of any commercial or financial relationships that could be construed as a potential conflict of interest.
Publisher's Note
All claims expressed in this article are solely those of the authors and do not necessarily represent those of their affiliated organizations, or those of the publisher, the editors and the reviewers. Any product that may be evaluated in this article, or claim that may be made by its manufacturer, is not guaranteed or endorsed by the publisher.
References
1. Martin JP, Bell J. A pedigree of mental defect showing sex-linkage. J Neurol Psychiatry. (1943) 6:154–7. doi: 10.1136/jnnp.6.3-4.154
2. Verkerk AJ, Pieretti M, Sutcliffe JS, Fu YH, Kuhl DP, Pizzuti A, et al. Identification of a gene (FMR-1) containing a CGG repeat coincident with a breakpoint cluster region exhibiting length variation in fragile X syndrome. Cell. (1991) 65:905–14. doi: 10.1016/0092-8674(91)90397-H
3. Oberlé I, Rousseau F, Heitz D, Kretz C, Devys D, Hanauer A, et al. Instability of a 550-base pair DNA segment and abnormal methylation in fragile X syndrome. Science. (1991) 252:1097–102. doi: 10.1126/science.252.5009.1097
4. D'Annessa I, Cicconardi F, Di Marino D. Handling FMRP and its molecular partners: structural insights into Fragile X Syndrome. Prog Biophys Mol Biol. (2019) 141:3–14. doi: 10.1016/j.pbiomolbio.2018.07.001
5. Myrick LK, Nakamoto-Kinoshita M, Lindor NM, Kirmani S, Cheng X, Warren ST. Fragile X syndrome due to a missense mutation. Eur J Hum Genet. (2014) 22:1185–9. doi: 10.1038/ejhg.2013.311
6. Quartier A, Poquet H, Gilbert-Dussardier B, Rossi M, Casteleyn AS, Portes V des, et al. Intragenic FMR1 disease-causing variants: a significant mutational mechanism leading to Fragile-X syndrome. Eur J Hum Genet. (2017) 25:423–31. doi: 10.1038/ejhg.2016.204
7. Di Marino D, Achsel T, Lacoux C, Falconi M, Bagni C. Molecular dynamics simulations show how the FMRP Ile304Asn mutation destabilizes the KH2 domain structure and affects its function. J Biomol Struct Dyn. (2014) 32:337–50. doi: 10.1080/07391102.2013.768552
8. Pugin A, Faundes V, Santa María L, Curotto B, Aliaga S, Salas I, et al. Clinical, molecular, and pharmacological aspects of FMR1-related disorders. Neurología. (2017) 32:241–52. doi: 10.1016/j.nrleng.2014.10.018
9. Hagerman RJ, Berry-Kravis E, Hazlett HC, Bailey DBJ, Moine H, Kooy RF, et al. Fragile X syndrome. Nat Rev Dis Prim. (2017) 3:17065. doi: 10.1038/nrdp.2017.65
10. Richter JD, Bassell GJ, Klann E. Dysregulation and restoration of translational homeostasis in fragile X syndrome. Nat Rev Neurosci. (2015) 16:595–605. doi: 10.1038/nrn4001
11. Thurman AJ, McDuffie A, Hagerman R, Abbeduto L. Psychiatric symptoms in boys with fragile X syndrome: a comparison with nonsyndromic autism spectrum disorder. Res Dev Disabil. (2014) 35:1072–86. doi: 10.1016/j.ridd.2014.01.032
12. McDuffie A, Thurman AJ, Hagerman RJ, Abbeduto L. Symptoms of autism in males with fragile X syndrome: a comparison to nonsyndromic ASD using current ADI-R scores. J Autism Dev Disord. (2015) 45:1925–37. doi: 10.1007/s10803-013-2013-6
13. Berry-Kravis E, Raspa M, Loggin-Hester L, Bishop E, Holiday D, Bailey DB. Seizures in fragile X syndrome: characteristics and comorbid diagnoses. Am J Intellect Dev Disabil. (2010) 115:461–72. doi: 10.1352/1944-7558-115.6.461
14. Nowicki ST, Tassone F, Ono MY, Ferranti J, Croquette MF, Goodlin-Jones B, et al. The Prader-Willi phenotype of fragile X syndrome. J Dev Behav Pediatr. (2007) 28:133–8. doi: 10.1097/01.DBP.0000267563.18952.c9
15. Salcedo-Arellano MJ, Dufour B, McLennan Y, Martinez-Cerdeno V, Hagerman R. Fragile X syndrome and associated disorders: clinical aspects and pathology. Neurobiol Dis. (2020) 136:104740. doi: 10.1016/j.nbd.2020.104740
16. Richter JD, Zhao X. The molecular biology of FMRP: new insights into fragile X syndrome. Nat Rev Neurosci. (2021) 22:209–22. doi: 10.1038/s41583-021-00432-0
17. Bagni C, Neri G, Hagerman R. Fragile X syndrome: causes, diagnosis, mechanisms, and therapeutics. J Clin Invest. (2012) 122:4314–22. doi: 10.1172/JCI63141
18. De Rubeis S, Bagni C. Fragile X mental retardation protein control of neuronal mRNA metabolism: insights into mRNA stability. Mol Cell Neurosci. (2010) 43:43–50. doi: 10.1016/j.mcn.2009.09.013
19. Bear MF, Huber KM, Warren ST. The mGluR theory of fragile X mental retardation. Trends Neurosci. (2004) 27:370–7. doi: 10.1016/j.tins.2004.04.009
20. Gross C, Berry-Kravis EM, Bassell GJ. Therapeutic strategies in fragile X syndrome: dysregulated mGluR signaling and beyond. Neuropsychopharmacology. (2012) 37:178–95. doi: 10.1038/npp.2011.137
21. Cheng GR, Li XY, Xiang YD, Liu D, Mcclintock SM, Zeng Y. The implication of AMPA receptor in synaptic plasticity impairment and intellectual disability in fragile X syndrome. Physiol Res. (2017) 66:715–27. doi: 10.33549/physiolres.933473
22. Kim SH, Markham JA, Weiler IJ, Greenough WT. Aberrant early-phase ERK inactivation impedes neuronal function in fragile X syndrome. Proc Natl Acad Sci USA. (2008) 105:4429–34. doi: 10.1073/pnas.0800257105
23. Matic K, Eninger T, Bardoni B, Davidovic L, Macek B. Quantitative phosphoproteomics of Murine Fmr1 -KO cell lines provides new insights into FMRP-dependent signal transduction mechanisms. J Proteome Res. (2014) 13:4388–97. doi: 10.1021/pr5006372
24. Curia G, Gualtieri F, Bartolomeo R, Vezzali R, Biagini G. Resilience to audiogenic seizures is associated with p-ERK1/2 dephosphorylation in the subiculum of Fmr1 knockout mice. Front Cell Neurosci. (2013) 7:46. doi: 10.3389/fncel.2013.00046
25. Bilousova TV, Dansie L, Ngo M, Aye J, Charles JR, Ethell DW, et al. Minocycline promotes dendritic spine maturation and improves behavioural performance in the fragile X mouse model. J Med Genet. (2009) 46:94–102. doi: 10.1136/jmg.2008.061796
26. Dansie LE, Phommahaxay K, Okusanya AG, Uwadia J, Huang M, Rotschafer SE, et al. Long-lasting effects of minocycline on behavior in young but not adult fragile X mice. Neuroscience. (2013) 246:186–98. doi: 10.1016/j.neuroscience.2013.04.058
27. Michaluk P, Wawrzyniak M, Alot P, Szczot M, Wyrembek P, Mercik K, et al. Influence of matrix metalloproteinase MMP-9 on dendritic spine morphology. J Cell Sci. (2011) 124:3369–80. doi: 10.1242/jcs.090852
28. Kim SW, Cho KJ. Activity-dependent alterations in the sensitivity to BDNF-TrkB signaling may promote excessive dendritic arborization and spinogenesis in fragile X syndrome in order to compensate for compromised postsynaptic activity. Med Hypotheses. (2014) 83:429–35. doi: 10.1016/j.mehy.2014.07.007
29. Castrén M, Lampinen KE, Miettinen R, Koponen E, Sipola I, Bakker CE, et al. BDNF regulates the expression of fragile X mental retardation protein mRNA in the hippocampus. Neurobiol Dis. (2002) 11:221–9. doi: 10.1006/nbdi.2002.0544
30. Sharma A, Hoeffer CA, Takayasu Y, Miyawaki T, McBride SM, Klann E, et al. Dysregulation of mTOR signaling in fragile X syndrome. J Neurosci. (2010) 30:694–702. doi: 10.1523/JNEUROSCI.3696-09.2010
31. Price TJ, Rashid MH, Millecamps M, Sanoja R, Entrena JM, Cervero F. Decreased nociceptive sensitization in mice lacking the Fragile X mental retardation protein: role of mGluR1/5 and mTOR. J Neurosci. (2007) 27:13958–67. doi: 10.1523/JNEUROSCI.4383-07.2007
32. Centonze D, Rossi S, Mercaldo V, Napoli I, Ciotti MT, De Chiara V, et al. Abnormal striatal GABA transmission in the mouse model for the fragile X syndrome. Biol Psychiatry. (2008) 63:963–73. doi: 10.1016/j.biopsych.2007.09.008
33. Curia G, Papouin T, Séguéla P, Avoli M. Downregulation of tonic GABAergic inhibition in a mouse model of fragile X syndrome. Cereb Cortex. (2009) 19:1515–20. doi: 10.1093/cercor/bhn159
34. D'Hulst C, De Geest N, Reeve SP, Van Dam D, De Deyn PP, Hassan BA, et al. Decreased expression of the GABAA receptor in fragile X syndrome. Brain Res. (2006) 1121:238–45. doi: 10.1016/j.brainres.2006.08.115
35. Pan F, Aldridge GM, Greenough WT, Gan WB. Dendritic spine instability and insensitivity to modulation by sensory experience in a mouse model of fragile X syndrome. Proc Natl Acad Sci USA. (2010) 107:17768–73. doi: 10.1073/pnas.1012496107
36. Hinton VJ, Brown WT, Wisniewski K, Rudelli RD. Analysis of neocortex in three males with the fragile X syndrome. Am J Med Genet. (1991) 41:289–94. doi: 10.1002/ajmg.1320410306
37. Comery TA, Harris JB, Willems PJ, Oostra BA, Irwin SA, Weiler IJ, et al. Abnormal dendritic spines in fragile X knockout mice: maturation and pruning deficits. Proc Natl Acad Sci USA. (1997) 94:5401–4. doi: 10.1073/pnas.94.10.5401
38. Napoli I, Mercaldo V, Boyl PP, Eleuteri B, Zalfa F, De Rubeis S, et al. The fragile X syndrome protein represses activity-dependent translation through CYFIP1, a new 4E-BP. Cell. (2008) 134:1042–54. doi: 10.1016/j.cell.2008.07.031
39. Di Marino D, Chillemi G, De Rubeis S, Tramontano A, Achsel T, Bagni C. MD and docking studies reveal that the functional switch of CYFIP1 is mediated by a butterfly-like motion. J Chem Theory Comput. (2015) 11:3401–10. doi: 10.1021/ct500431h
40. Di Marino D, D'Annessa I, Tancredi H, Bagni C, Gallicchio E. A unique binding mode of the eukaryotic translation initiation factor 4E for guiding the design of novel peptide inhibitors. Protein Sci. (2015) 24:1370–82. doi: 10.1002/pro.2708
41. Sonenberg N, Hinnebusch AG. Regulation of translation initiation in eukaryotes. Cell. (2009) 136:731–45. doi: 10.1016/j.cell.2009.01.042
42. Merrick WC, Pavitt GD. Protein synthesis initiation in eukaryotic cells. Cold Spring Harb Perspect Biol. (2018) 10:1–22. doi: 10.1101/cshperspect.a033092
43. Grüner S, Weber R, Peter D, Chung MY, Igreja C, Valkov E, et al. Structural motifs in eIF4G and 4E-BPs modulate their binding to eIF4E to regulate translation initiation in yeast. Nucleic Acids Res. (2018) 46:6893–908. doi: 10.1093/nar/gky542
44. Igreja C, Peter D, Weiler C, Izaurralde E. 4E-BPs require non-canonical 4E-binding motifs and a lateral surface of eIF4E to repress translation. Nat Commun. (2014) 5:1–14. doi: 10.1038/ncomms5790
45. Siomi MC, Siomi H, Sauer WH, Srinivasan S, Nussbaum RL, Dreyfuss G. FXR1, an autosomal homolog of the fragile X mental retardation gene. EMBO J. (1995) 14:2401–8. doi: 10.1002/j.1460-2075.1995.tb07237.x
46. Schenck A, Bardoni B, Moro A, Bagni C, Mandel JL. A highly conserved protein family interacting with the fragile X mental retardation protein (FMRP) and displaying selective interactions with FMRP-related proteins FXR1P and FXR2P. Proc Natl Acad Sci USA. (2001) 98:8844–9. doi: 10.1073/pnas.151231598
47. Erickson CA, Kaufmann WE, Budimirovic DB, Lachiewicz A, Haas-Givler B, Miller RM, et al. Best practices in fragile X syndrome treatment development. Brain Sci. (2018) 8:1–9. doi: 10.3390/brainsci8120224
48. Davenport MH, Schaefer TL, Friedmann KJ, Fitzpatrick SE, Erickson CA. Pharmacotherapy for fragile X syndrome: progress to date. Drugs. (2016) 76:431–45. doi: 10.1007/s40265-016-0542-y
49. Bagni C, Oostra BA. Fragile X syndrome: from protein function to therapy. Am J Med Genet Part A. (2013) 161A:2809–21. doi: 10.1002/ajmg.a.36241
50. Berry-Kravis EM, Lindemann L, Jønch AE, Apostol G, Bear MF, Carpenter RL, et al. Drug development for neurodevelopmental disorders: lessons learned from fragile X syndrome. Nat Rev Drug Discov. (2018) 17:280–99. doi: 10.1038/nrd.2017.221
51. Protic D, Salcedo-Arellano MJ, Dy JB, Potter LA, Hagerman RJ. New targeted treatments for fragile X syndrome. Curr Pediatr Rev. (2019) 15:251–8. doi: 10.2174/1573396315666190625110748
52. Castagnola S, Bardoni B, Maurin T. The search for an effective therapy to treat fragile X syndrome : dream or reality ? Front Synaptic Neurosci. (2017) 9:15. doi: 10.3389/fnsyn.2017.00015
53. Chiurazzi P, Pomponi MG, Willemsen R, Oostra BA, Neri G. In vitro reactivation of the FMR1 gene involved in fragile X syndrome. Hum Mol Genet. (1998) 7:109–13. doi: 10.1093/hmg/7.1.109
54. Chiurazzi P, Pomponi MG, Pietrobono R, Bakker CE, Neri G, Oostra BA. Synergistic effect of histone hyperacetylation and DNA demethylation in the reactivation of the FMR1 gene. Hum Mol Genet. (1999) 8:2317–23. doi: 10.1093/hmg/8.12.2317
55. Tabolacci E, Palumbo F, Nobile V, Neri G. Transcriptional reactivation of the FMR1 gene. A possible approach to the treatment of the fragile X syndrome. Genes. (2016) 7:49. doi: 10.3390/genes7080049
56. Holoch D, Moazed D. RNA-mediated epigenetic regulation of gene expression. Nat Rev Genet. (2015) 16:71–84. doi: 10.1038/nrg3863
57. Rupaimoole R, Slack FJ. MicroRNA therapeutics: towards a new era for the management of cancer and other diseases. Nat Rev Drug Discov. (2017) 16:203–22. doi: 10.1038/nrd.2016.246
58. Suhl JA, Muddashetty RS, Anderson BR, Ifrim MF, Visootsak J, Bassell GJ, et al. A 3' untranslated region variant in FMR1 eliminates neuronal activity-dependent translation of FMRP by disrupting binding of the RNA-binding protein HuR. Proc Natl Acad Sci USA. (2015) 112:E6553–61. doi: 10.1073/pnas.1514260112
59. Wang C, Ge L, Wu J, Wang X, Yuan L. MiR-219 represses expression of dFMR1 in Drosophila melanogaster. Life Sci. (2019) 218:31–7. doi: 10.1016/j.lfs.2018.12.008
60. Pastori C, Peschansky VJ, Barbouth D, Mehta A, Silva JP, Wahlestedt C. Comprehensive analysis of the transcriptional landscape of the human FMR1 gene reveals two new long noncoding RNAs differentially expressed in Fragile X syndrome and Fragile X-associated tremor/ataxia syndrome. Hum Genet. (2014) 133:59–67. doi: 10.1007/s00439-013-1356-6
61. Shitik EM, Velmiskina AA, Dolskiy AA, Yudkin DV. Reactivation of FMR1 gene expression is a promising strategy for fragile X syndrome therapy. Gene Ther. (2020) 27:247–53. doi: 10.1038/s41434-020-0141-0
62. Gantois I, Bakker CE, Reyniers E, Willemsen R, D'Hooge R, De Deyn PP, et al. Restoring the phenotype of fragile X syndrome: insight from the mouse model. Curr Mol Med. (2001) 1:447–55. doi: 10.2174/1566524013363492
63. Zeier Z, Kumar A, Bodhinathan K, Feller JA, Foster TC, Bloom DC. Fragile X mental retardation protein replacement restores hippocampal synaptic function in a mouse model of fragile X syndrome. Gene Ther. (2009) 16:1122–9. doi: 10.1038/gt.2009.83
64. Gholizadeh S, Arsenault J, Xuan ICY, Pacey LK, Hampson DR. Reduced phenotypic severity following adeno-associated virus-mediated Fmr1 gene delivery in fragile X mice. Neuropsychopharmacol. (2014) 39:3100–11. doi: 10.1038/npp.2014.167
65. Xie N, Gong H, Suhl JA, Chopra P, Wang T, Warren ST. Reactivation of FMR1 by CRISPR/Cas9-mediated deletion of the expanded CGG-repeat of the fragile X chromosome. PLoS ONE. (2016) 11:e0165499. doi: 10.1371/journal.pone.0165499
66. Park CY, Halevy T, Lee DR, Sung JJ, Lee JS, Yanuka O, et al. Reversion of FMR1 methylation and silencing by editing the triplet repeats in fragile X iPSC-derived neurons. Cell Rep. (2015) 13:234–41. doi: 10.1016/j.celrep.2015.08.084
67. Lee B, Lee K, Panda S, Gonzales-Rojas R, Chong A, Bugay V, et al. Nanoparticle delivery of CRISPR into the brain rescues a mouse model of fragile X syndrome from exaggerated repetitive behaviours. Nat Biomed Eng. (2018) 2:497–507. doi: 10.1038/s41551-018-0252-8
68. Baker EK, Arpone M, Aliaga SM, Bretherton L, Kraan CM, Bui M, et al. Incomplete silencing of full mutation alleles in males with fragile X syndrome is associated with autistic features. Mol Autism. (2019) 10:21. doi: 10.1186/s13229-019-0271-7
69. Berry-Kravis E, Potanos K. Psychopharmacology in fragile X syndrome—present and future. Ment Retard Dev Disabil Res Rev. (2004) 10:42–8. doi: 10.1002/mrdd.20007
70. Schaefer TL, Davenport MH, Erickson CA. Emerging pharmacologic treatment options for fragile X syndrome. Appl Clin Genet. (2015) 8:75–93. doi: 10.2147/TACG.S35673
71. Berry-Kravis E, Des Portes V, Hagerman R, Jacquemont S, Charles P, Visootsak J, et al. Mavoglurant in fragile X syndrome: results of two randomized, double-blind, placebo-controlled trials. Sci Transl Med. (2016) 8:321ra5. doi: 10.1126/scitranslmed.aab4109
72. Youssef EA, Berry-Kravis E, Czech C, Hagerman RJ, Hessl D, Wong CY, et al. Effect of the mGluR5-NAM basimglurant on behavior in adolescents and adults with fragile X syndrome in a randomized, double-blind, placebo-controlled trial: fragXis phase 2 results. Neuropsychopharmacol. (2018) 43:503–12. doi: 10.1038/npp.2017.177
73. Hanson AC, Hagerman RJ. Serotonin dysregulation in fragile X syndrome: implications for treatment. Intractable Rare Dis Res. (2014) 3:110–7. doi: 10.5582/irdr.2014.01027
74. Lim C, Hoang ET, Viar KE, Stornetta RL, Scott MM, Zhu JJ. Pharmacological rescue of ras signaling, GluA1-dependent synaptic plasticity, and learning deficits in a fragile X model. Genes Dev. (2014) 28:273–89. doi: 10.1101/gad.232470.113
75. Greiss Hess L, Fitzpatrick SE, Nguyen DV, Chen Y, Gaul KN, Schneider A, et al. A randomized, double-blind, placebo-controlled trial of low-dose sertraline in young children with fragile X syndrome. J Dev Behav Pediatr. (2016) 37:619–28. doi: 10.1097/DBP.0000000000000334
76. Yoo KH, Burris JL, Gaul KN, Hagerman RJ, Rivera SM. Low-dose sertraline improves receptive language in children with fragile X syndrome when eye tracking methodology is used to measure treatment outcome. Psychiatry Clin. (2017) 1:1–8. doi: 10.15406/jpcpy.2017.07.00465
77. Maccarrone M, Rossi S, Bari M, De Chiara V, Rapino C, Musella A, et al. Abnormal mGlu 5 receptor/endocannabinoid coupling in mice lacking FMRP and BC1 RNA. Neuropsychopharmacology. (2010) 35:1500–9. doi: 10.1038/npp.2010.19
78. Jung KM, Sepers M, Henstridge CM, Lassalle O, Neuhofer D, Martin H, et al. Uncoupling of the endocannabinoid signalling complex in a mouse model of fragile X syndrome. Nat Commun. (2012) 3:1080. doi: 10.1038/ncomms2045
79. Heussler H, Cohen J, Silove N, Tich N, Bonn-Miller MO, Du W, et al. A phase 1/2, open-label assessment of the safety, tolerability, and efficacy of transdermal cannabidiol (ZYN002) for the treatment of pediatric fragile X syndrome. J Neurodev Disord. (2019) 11:16. doi: 10.1186/s11689-019-9277-x
80. Bergamaschi MM, Queiroz RHC, Chagas MHN, de Oliveira DCG, De Martinis BS, Kapczinski F, et al. Cannabidiol reduces the anxiety induced by simulated public speaking in treatment-naïve social phobia patients. Neuropsychopharmacol. (2011) 36:1219–26. doi: 10.1038/npp.2011.6
81. Busquets-Garcia A, Gomis-González M, Guegan T, Agustín-Pavón C, Pastor A, Mato S, et al. Targeting the endocannabinoid system in the treatment of fragile X syndrome. Nat Med. (2013) 19:603–7. doi: 10.1038/nm.3127
82. Osterweil EK, Chuang SC, Chubykin AA, Sidorov M, Bianchi R, Wong RKS, et al. Lovastatin corrects excess protein synthesis and prevents epileptogenesis in a mouse model of fragile X syndrome. Neuron. (2013) 77:243–50. doi: 10.1016/j.neuron.2012.01.034
83. Muscas M, Louros SR, Osterweil EK. Lovastatin, not simvastatin, corrects core phenotypes in the fragile X mouse model. eNeuro. (2019) 6:2019. doi: 10.1523/ENEURO.0097-19.2019
84. Cerezo-Guisado MI, García-Román N, García-Marín LJ, Alvarez-Barrientos A, Bragado MJ, Lorenzo MJ. Lovastatin inhibits the extracellular-signal-regulated kinase pathway in immortalized rat brain neuroblasts. Biochem J. (2007) 401:175–83. doi: 10.1042/BJ20060731
85. Siller SS, Broadie K. Neural circuit architecture defects in a Drosophila model of fragile X syndrome are alleviated by minocycline treatment and genetic removal of matrix metalloproteinase. Dis Model Mech. (2011) 4:673–85. doi: 10.1242/dmm.008045
86. Çaku A, Pellerin D, Bouvier P, Riou E, Corbin F. Effect of lovastatin on behavior in children and adults with fragile X syndrome: an open-label study. Am J Med Genet Part A. (2014) 164:2834–42. doi: 10.1002/ajmg.a.36750
87. Utari A, Chonchaiya W, Rivera SM, Schneider A, Hagerman RJ, Faradz SMH, et al. Side effects of minocycline treatment in patients with fragile X syndrome and exploration of outcome measures. Am J Intellect Dev Disabil. (2010) 115:433–43. doi: 10.1352/1944-7558-115.5.433
88. Leigh MJS, Nguyen DV, Mu Y, Winarni TI, Schneider A, Chechi T, et al. A randomized double-blind, placebo-controlled trial of minocycline in children and adolescents with fragile x syndrome. J Dev Behav Pediatr. (2013) 34:147–55. doi: 10.1097/DBP.0b013e318287cd17
89. Gantois I, Khoutorsky A, Popic J, Aguilar-Valles A, Freemantle E, Cao R, et al. Metformin ameliorates core deficits in a mouse model of fragile X syndrome. Nat Med. (2017) 23:674–7. doi: 10.1038/nm.4335
90. Gantois I, Popic J, Khoutorsky A, Sonenberg N. Metformin for treatment of fragile X syndrome and other neurological disorders. Annu Rev Med. (2019) 70:167–81. doi: 10.1146/annurev-med-081117-041238
91. Gkogkas CG, Khoutorsky A, Cao R, Jafarnejad SM, Prager-Khoutorsky M, Giannakas N, et al. Pharmacogenetic inhibition of eIF4E-dependent Mmp9 mRNA translation reverses fragile X syndrome-like phenotypes. Cell Rep. (2014) 9:1742–55. doi: 10.1016/j.celrep.2014.10.064
92. Hou L, Antion MD, Hu D, Spencer CM, Paylor R, Klann E. Dynamic translational and proteasomal regulation of fragile X mental retardation protein controls mGluR-dependent long-term depression. Neuron. (2006) 51:441–54. doi: 10.1016/j.neuron.2006.07.005
93. Dy ABC, Tassone F, Eldeeb M, Salcedo-Arellano MJ, Tartaglia N, Hagerman R. Metformin as targeted treatment in fragile X syndrome. Clin Genet. (2018) 93:216–22. doi: 10.1111/cge.13039
94. Monyak RE, Emerson D, Schoenfeld BP, Zheng X, Chambers DB, Rosenfelt C, et al. Insulin signaling misregulation underlies circadian and cognitive deficits in a Drosophila fragile X model. Mol Psychiatry. (2017) 22:1140–8. doi: 10.1038/mp.2016.51
95. Biag HMB, Potter LA, Wilkins V, Afzal S, Rosvall A, Salcedo-Arellano MJ, et al. Metformin treatment in young children with fragile X syndrome. Mol Genet Genomic Med. (2019) 7:e956. doi: 10.1002/mgg3.956
96. Protic D, Aydin EY, Tassone F, Tan MM, Hagerman RJ, Schneider A. Cognitive and behavioral improvement in adults with fragile X syndrome treated with metformin-two cases. Mol Genet Genomic Med. (2019) 7:e00745. doi: 10.1002/mgg3.745
97. Proteau-Lemieux M, Lacroix A, Galarneau L, Corbin F, Lepage JF, Çaku A. The safety and efficacy of metformin in fragile X syndrome: an open-label study. Prog Neuro-Psychopharmacol Biol Psychiatry. (2021) 110:110307. doi: 10.1016/j.pnpbp.2021.110307
98. Maurin T, Lebrigand K, Castagnola S, Paquet A, Jarjat M, Popa A, et al. HITS-CLIP in various brain areas reveals new targets and new modalities of RNA binding by fragile X mental retardation protein. Nucleic Acids Res. (2018) 46:6344–55. doi: 10.1093/nar/gky267
99. Androschuk A, He RX, Weber S, Rosenfelt C, Bolduc FV. Stress odorant sensory response dysfunction in drosophila fragile X syndrome mutants. Front. Mol. Neurosci. (2018) 11:242. doi: 10.3389/fnmol.2018.00242
100. Delhaye S, Bardoni B. Role of phosphodiesterases in the pathophysiology of neurodevelopmental disorders. Mol Psychiatry. (2021). doi: 10.1038/s41380-020-00997-9 [Epub ahead of print].
101. Choi CH, Schoenfeld BP, Weisz ED, Bell AJ, Chambers DB, Hinchey J, et al. PDE-4 inhibition rescues aberrant synaptic plasticity in Drosophila and mouse models of fragile X syndrome. J Neurosci. (2015) 35:396–408. doi: 10.1523/JNEUROSCI.1356-12.2015
102. Gurney ME, Nugent RA, Mo X, Sindac JA, Hagen TJ, Fox D 3rd, et al. Design and synthesis of selective phosphodiesterase 4D (PDE4D) allosteric inhibitors for the treatment of fragile X syndrome and other brain disorders. J Med Chem. (2019) 62:4884–901. doi: 10.1021/acs.jmedchem.9b00193
103. Maurin T, Melancia F, Jarjat M, Castro L, Costa L, Delhaye S, et al. Involvement of phosphodiesterase 2A activity in the pathophysiology of fragile X syndrome. Cereb Cortex. (2019) 29:3241–52. doi: 10.1093/cercor/bhy192
104. García-Font N, Martín R, Torres M, Oset-Gasque MJ, Sánchez-Prieto J. The loss of β adrenergic receptor mediated release potentiation in a mouse model of fragile X syndrome. Neurobiol Dis. (2019) 130:104482. doi: 10.1016/j.nbd.2019.104482
105. Gurney ME, Cogram P, Deacon RM, Rex C, Tranfaglia M. Multiple behavior phenotypes of the fragile-X syndrome mouse model respond to chronic inhibition of phosphodiesterase-4D (PDE4D). Sci Rep. (2017) 7:14653. doi: 10.1038/s41598-017-15028-x
106. Berry-Kravis EM, Harnett MD, Reines SA, Reese MA, Ethridge LE, Outterson AH, et al. Inhibition of phosphodiesterase-4D in adults with fragile X syndrome: a randomized, placebo-controlled, phase 2 clinical trial. Nat Med. (2021) 27:862–70. doi: 10.1038/s41591-021-01321-w
107. Paes D, Xie K, Wheeler DG, Zook D, Prickaerts J, Peters M. Inhibition of PDE2 and PDE4 synergistically improves memory consolidation processes. Neuropharmacology. (2021) 184:108414. doi: 10.1016/j.neuropharm.2020.108414
108. Bhat M, Robichaud N, Hulea L, Sonenberg N, Pelletier J, Topisirovic I. Targeting the translation machinery in cancer. Nat Rev Drug Discov. (2015) 14:261–78. doi: 10.1038/nrd4505
109. Laplante M, Sabatini DM. mTOR signaling in growth control and disease. Cell. (2012) 149:274–93. doi: 10.1016/j.cell.2012.03.017
110. Kaspar AA, Reichert JM. Future directions for peptide therapeutics development. Drug Discov Today. (2013) 18:807–17. doi: 10.1016/j.drudis.2013.05.011
111. Muttenthaler M, King GF, Adams DJ, Alewood PF. Trends in peptide drug discovery. Nat Rev Drug Discov. (2021) 20:309–25. doi: 10.1038/s41573-020-00135-8
112. D'Annessa I, Di Leva FS, La Teana A, Novellino E, Limongelli V, Di Marino D. Bioinformatics and biosimulations as toolbox for peptides and peptidomimetics design: where are we? Front Mol Biosci. (2020) 7:66. doi: 10.3389/fmolb.2020.00066
113. Moerke NJ, Aktas H, Chen H, Cantel S, Reibarkh MY, Fahmy A, et al. Small-molecule inhibition of the interaction between the translation initiation factors eIF4E and eIF4G. Cell. (2007) 128:257–67. doi: 10.1016/j.cell.2006.11.046
114. Wu M, Zhang C, Li XJ, Liu Q, Wanggou S. Anti-cancer effect of cap-translation inhibitor 4EGI-1 in human glioma U87 cells: involvement of mitochondrial dysfunction and ER stress. Cell Physiol Biochem. (2016) 40:1013–28. doi: 10.1159/000453158
115. Descamps G, Gomez-Bougie P, Tamburini J, Green A, Bouscary D, Maïga S, et al. The cap-translation inhibitor 4EGI-1 induces apoptosis in multiple myeloma through Noxa induction. Br J Cancer. (2012) 106:1660–7. doi: 10.1038/bjc.2012.139
116. Romagnoli A, Maracci C, D'Agostino M, Teana A La, Di Marino D. Targeting mTOR and eIF4E: a feasible scenario in ovarian cancer therapy. Cancer Drug Resist. (2021) 4:596–606. doi: 10.20517/cdr.2021.20
117. Santini E, Huynh TN, Longo F, Koo SY, Mojica E, D'Andrea L, et al. Reducing eIF4E-eIF4G interactions restores the balance between protein synthesis and actin dynamics in fragile X syndrome model mice. Sci Signal. (2018) 10:1–28. doi: 10.1126/scisignal.aan0665
118. Tan YS, Lane DP, Verma CS. Stapled peptide design: principles and roles of computation. Drug Discov Today. (2016) 21:1642–53. doi: 10.1016/j.drudis.2016.06.012
119. Lee ACL, Harris JL, Khanna KK, Hong JH. A comprehensive review on current advances in peptide drug development and design. Int J Mol Sci. (2019) 20:2383. doi: 10.3390/ijms20102383
120. Wells JA, McClendon CL. Reaching for high-hanging fruit in drug discovery at protein–protein interfaces. Nature. (2007) 450:1001–9. doi: 10.1038/nature06526
121. Fosgerau K, Hoffmann T. Peptide therapeutics: current status and future directions. Drug Discov Today. (2015) 20:122–8. doi: 10.1016/j.drudis.2014.10.003
122. Lenci E, Trabocchi A. Peptidomimetic toolbox for drug discovery. Chem Soc Rev. (2020) 49:3262–77. doi: 10.1039/D0CS00102C
123. Ryan P, Patel B, Makwana V, Jadhav HR, Kiefel M, Davey A, et al. Peptides, peptidomimetics, and carbohydrate–peptide conjugates as amyloidogenic aggregation inhibitors for Alzheimer's disease. ACS Chem Neurosci. (2018) 9:1530–51. doi: 10.1021/acschemneuro.8b00185
124. Ellert-Miklaszewska A, Poleszak K, Kaminska B. Short peptides interfering with signaling pathways as new therapeutic tools for cancer treatment. Future Med Chem. (2017) 9:199–221. doi: 10.4155/fmc-2016-0189
125. Marqus S, Pirogova E, Piva TJ. Evaluation of the use of therapeutic peptides for cancer treatment. J Biomed Sci. (2017) 24:21. doi: 10.1186/s12929-017-0328-x
126. Mabonga L, Kappo AP. Peptidomimetics: a synthetic tool for inhibiting protein–protein interactions in cancer. Int J Pept Res Ther. (2020) 26:225–41. doi: 10.1007/s10989-019-09831-5
127. Vercelli A, Biggi S, Sclip A, Repetto IE, Cimini S, Falleroni F, et al. Exploring the role of MKK7 in excitotoxicity and cerebral ischemia: a novel pharmacological strategy against brain injury. Cell Death Dis. (2015) 6:e1854. doi: 10.1038/cddis.2015.226
128. Baig MH, Ahmad K, Saeed M, Alharbi AM, Barreto GE, Ashraf GM, et al. Peptide based therapeutics and their use for the treatment of neurodegenerative and other diseases. Biomed Pharmacother. (2018) 103:574–81. doi: 10.1016/j.biopha.2018.04.025
129. Meredith ME, Salameh TS, Banks WA. Intranasal delivery of proteins and peptides in the treatment of neurodegenerative diseases. AAPS J. (2015) 17:780–7. doi: 10.1208/s12248-015-9719-7
130. De la Torre BG, Albericio F. Peptide therapeutics 2.0. Molecules. (2020) 25:2293. doi: 10.3390/molecules25102293
131. Chen RPY. From nose to brain: the promise of peptide therapy for Alzheimer's disease and other neurodegenerative diseases. J Alzheimers Dis Parkinsonism. (2017) 7:2–4. doi: 10.4172/2161-0460.1000314
132. Grüner S, Peter D, Weber R, Wohlbold L, Chung MY, Weichenrieder O, et al. The structures of eIF4E-eIF4G complexes reveal an extended interface to regulate translation initiation. Mol Cell. (2016) 64:467–79. doi: 10.1016/j.molcel.2016.09.020
133. Peter D, Weber R, Köne C, Chung MY, Ebertsch L, Truffault V, et al. Mextli proteins use both canonical bipartite and novel tripartite binding modes to form eIF4E complexes that display differential sensitivity to 4E-BP regulation. Genes Dev. (2015) 29:1835–49. doi: 10.1101/gad.269068.115
134. Saxton RA, Sabatini DM. mTOR signaling in growth, metabolism, and disease. Cell. (2017) 168:960–76. doi: 10.1016/j.cell.2017.02.004
135. Cingolani LA, Goda Y. Actin in action: the interplay between the actin cytoskeleton and synaptic efficacy. Nat Rev Neurosci. (2008) 9:344–56. doi: 10.1038/nrn2373
136. Irwin SA, Galvez R, Greenough WT. Dendritic spine structural anomalies in fragile-X mental retardation syndrome. Cereb Cortex. (2000) 10:1038–44. doi: 10.1093/cercor/10.10.1038
137. Chen LY, Rex CS, Babayan AH, Kramár EA, Lynch G, Gall CM, et al. Physiological activation of synaptic Rac>PAK (p-21 Activated Kinase) signaling is defective in a mouse model of fragile X syndrome. J Neurosci. (2010) 30:10977. doi: 10.1523/JNEUROSCI.1077-10.2010
138. Castets M, Schaeffer C, Bechara E, Schenck A, Khandjian EW, Luche S, et al. FMRP interferes with the Rac1 pathway and controls actin cytoskeleton dynamics in murine fibroblasts. Hum Mol Genet. (2005) 14:835–44. doi: 10.1093/hmg/ddi077
139. Chen B, Chou HT, Brautigam CA, Xing W, Yang S, Henry L, et al. Rac1 GTPase activates the WAVE regulatory complex through two distinct binding sites. Elife. (2017) 6:e29795. doi: 10.7554/eLife.29795
140. Chen Z, Borek D, Padrick SB, Gomez TS, Metlagel Z, Ismail AM, et al. Structure and control of the actin regulatory WAVE complex. Nature. (2010) 468:533–8. doi: 10.1038/nature09623
141. Rottner K. WAVE regulatory complex. Curr Biol. (2021) 31:R496–552. doi: 10.1016/j.cub.2021.01.086
142. Song YK, Guo H, Barengo N, Naora H. Inhibition of ovarian cancer growth by a tumor-targeting peptide that binds eukaryotic translation initiation factor 4E. Clin Cancer Res. (2009) 15:4336–47. doi: 10.1158/1078-0432.CCR-08-2924
143. Masse M, Glippa V, Saad H, Le Bloas R, Gauffeny I, Berthou C, et al. An eIF4E-interacting peptide induces cell death in cancer cell lines. Cell Death Dis. (2014) 5:e1500–8. doi: 10.1038/cddis.2014.457
144. Gallagher EE, Menon A, Chmiel AF, Deprey K, Kritzer JA, Garner AL. A cell-penetrant lactam-stapled peptide for targeting eIF4E protein-protein interactions. Eur J Med Chem. (2020) 205:112655. doi: 10.1016/j.ejmech.2020.112655
145. Lama D, Liberatore AM, Frosi Y, Nakhle J, Tsomaia N, Bashir T, et al. Structural insights reveal a recognition feature for tailoring hydrocarbon stapled-peptides against the eukaryotic translation initiation factor 4E protein. Chem Sci. (2019) 10:2489–500. doi: 10.1039/C8SC03759K
146. Lama D, Quah ST, Verma CS, Lakshminarayanan R, Beuerman RW, Lane DP, et al. Rational optimization of conformational effects induced by hydrocarbon staples. Sci Rep. (2013) 3:1–10. doi: 10.1038/srep03451
147. Lama D, Verma CS. Deciphering the mechanistic effects of eIF4E phosphorylation on mRNA-cap recognition. Protein Sci. (2020) 29:1373–86. doi: 10.1002/pro.3798
148. Gentilucci L, Marco R De, Cerisoli L. Chemical modifications designed to improve peptide stability : incorporation of non-natural amino acids, pseudo-peptide bonds, and cyclization. Curr Pharm Des. (2010) 16:3185–203. doi: 10.2174/138161210793292555
149. Greco I, Molchanova N, Holmedal E, Jenssen H, Hummel BD, Watts JL, et al. Correlation between hemolytic activity, cytotoxicity and systemic in vivo toxicity of synthetic antimicrobial peptides. Sci Rep. (2020) 10:13206. doi: 10.1038/s41598-020-69995-9
Keywords: Fragile X syndrome, targeted therapy, peptides, peptidomimetics, drug development
Citation: Romagnoli A and Di Marino D (2021) The Use of Peptides in the Treatment of Fragile X Syndrome: Challenges and Opportunities. Front. Psychiatry 12:754485. doi: 10.3389/fpsyt.2021.754485
Received: 06 August 2021; Accepted: 11 October 2021;
Published: 04 November 2021.
Edited by:
Randi Jenssen Hagerman, University of California, Davis, United StatesReviewed by:
Maija Liisa Castrén, University of Helsinki, FinlandBarbara Bardoni, UMR7275 Institut de Pharmacologie Moléculaire et Cellulaire (IPMC), France
Copyright © 2021 Romagnoli and Di Marino. This is an open-access article distributed under the terms of the Creative Commons Attribution License (CC BY). The use, distribution or reproduction in other forums is permitted, provided the original author(s) and the copyright owner(s) are credited and that the original publication in this journal is cited, in accordance with accepted academic practice. No use, distribution or reproduction is permitted which does not comply with these terms.
*Correspondence: Daniele Di Marino, ZC5kaW1hcmlub0B1bml2cG0uaXQ=