- 1Department of Psychology, University of Pittsburgh, Pittsburgh, PA, United States
- 2Department of Psychology and Neuroscience Program, Temple University, Philadelphia, PA, United States
- 3Princeton Neuroscience Institute, Princeton University, Princeton, NJ, United States
Early life stress – including experience of child maltreatment, neglect, separation from or loss of a parent, and other forms of adversity – increases lifetime risk of mood, anxiety, and substance use disorders. A major component of this risk may be early life stress-induced alterations in motivation and reward processing, mediated by changes in the nucleus accumbens (NAc) and ventral tegmental area (VTA). Here, we review evidence of the impact of early life stress on reward circuit structure and function from human and animal models, with a focus on the NAc. We then connect these results to emerging theoretical models about the indirect and direct impacts of early life stress on reward circuit development. Through this review and synthesis, we aim to highlight open research questions and suggest avenues of future study in service of basic science, as well as applied insights. Understanding how early life stress alters reward circuit development, function, and motivated behaviors is a critical first step toward developing the ability to predict, prevent, and treat stress-related psychopathology spanning mood, anxiety, and substance use disorders.
Introduction
Early life stress (ELS) increases lifetime risk of depression, suicide, and mood, anxiety, and substance use disorders, and epidemiological studies suggest that approximately 30% of all adult-onset psychiatric disorders are associated with the experience of ELS (1–8). Broadly, ELS includes a range of adverse to traumatic experiences, ranging from socioeconomic disadvantage to loss of a parent, institutionalization, neglect, abuse, or exposure to domestic or community violence. These stressful experiences can be categorized as related to deprivation (an absence of expected age-typical stimuli and experiences) or threat (presence or perceived risk of physical violation or harm) (9, 10). Timing and chronicity of ELS exposure may also impact psychiatric outcomes (11–14). Understanding how different forms, timing, or cumulative ELS exposure alter brain development and function to impart risk is essential in order to develop better interventions for this vulnerable population (15, 16).
The robust impact of ELS on increased risk for mood and substance use disorders implicates enduring alterations within reward and motivation circuitry of the brain, which functions at the intersection of negative and positive valence domains implicated in the pathophysiology of these disorders (17–23). The brain's reward circuitry is classically comprised of the ventral tegmental area (VTA) and its dopaminergic projections to the nucleus accumbens (NAc, part of the ventral striatum; Figure 1) and other forebrain targets including prefrontal cortex (PFC), amygdala, and hippocampus. In addition to dopaminergic modulation from VTA, the NAc also receives dense glutamatergic innervation from these same forebrain regions and serves as a central integration point for cognitive and executive input from PFC, emotional information from amygdala, and contextual and emotional input from hippocampus (24–28). Imbalance of these systems is strongly associated with mood, psychiatric, and substance use disorders (19, 29, 30). GABAergic medium spiny neurons of the NAc project locally as well as to VTA, ventral pallidum, bed nucleus of the stria terminalis, lateral septum, amygdala, and lateral hypothalamus among other regions to ultimately regulate motivated behavior. Neurons of the VTA, NAc and broader reward circuitry undergo protracted development and continue to mature through adolescence which may leave them vulnerable to early environmental insults (31–40). Here, we review the enduring impact of ELS on reward circuit function, connectivity, and molecular development in humans and rodents, with a focus on NAc.
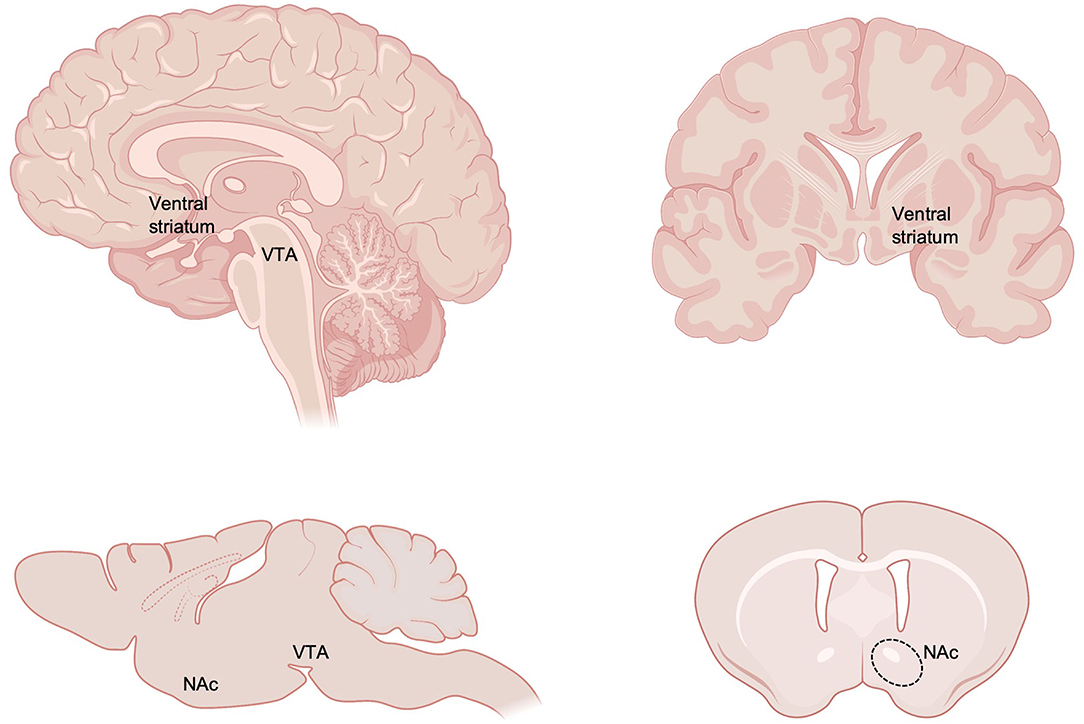
Figure 1. Neuroanatomy of key reward circuitry in humans and rodents. The current review focuses on the impact of early life stress on reward circuitry, with a particular focus on ventral tegmental area (VTA) and nucleus accumbens (NAc), part of the ventral striatum. Created with https://www.BioRender.com.
Impact of Early Life Stress on Human Reward Processing and Connectivity
A growing body of behavioral and neurobiological studies in humans suggest ELS-related alterations in reward-processing and connected brain circuitry. Behaviorally, children and adolescents exposed to ELS show challenges in aspects of reward processing and decision-making. There are multiple reports of lower reward responsivity and approach motivation in youth exposed to physical abuse (41, 42), social neglect (43, 44), and other adverse childhood experiences (42, 45–47). In such work, participants often need to make speeded responses to a target to receive rewards or positive feedback. Looking at different aspects of reward processing and decision-making, data suggests slower learning about rewards after adversity. For example, physical abuse and maltreatment was related to slower reward learning over time and an insensitivity to the expected value of a reward (41, 48); this fits with similar results noting lower reward learning after social neglect (44). Such patterns have also been noted in adult samples where adversity was retrospectively reported. Pechtel and Pizzagalli found women who suffered sexual abuse as children had lower behavioral accuracy when using learned information during a reward task (49). Interestingly, impaired reward learning from childhood institutionalization can be rescued through intervention with high-quality foster care between 6 and 33 months of age, implicating some plasticity through early childhood (44).
ELS-associated reward and decision-making differences are driven by a combination of high levels of risk-taking, insensitivity to different valences of feedback, and other processes. Maltreated children take excessive risks during different decision-making tasks (48, 50). In particular, youth exposed to adversity, such as maltreatment, have difficulty avoiding losses (48, 51) and make more impulsive choices (52–54). In addition, adults who reported high levels of early life adversity showed decreased positive feedback sensitivity during learning (55), and stress-exposed adolescents have challenges in initially learning as well as updating reward contingencies (45). These effects may be mechanistically due to lower feedback-related brain responsivity [measured by event-related potentials; (49)].
Functional MRI (fMRI) has been used to examine neural correlates of reward processing. Early adversity exposure has been found to lower activity levels in portions of the mesocorticolimbic circuit including ventral striatum during different reward-related tasks, such as anticipating monetary gains. For example, adolescents exposed to early social neglect had lower ventral striatal and caudate fMRI responses during the anticipation of rewards (56). These findings are consistent with studies that found lower ventral striatal activity in youths exposed to emotional neglect (57) and youth presenting with attachment issues after maltreatment (58). Moreover, blunted development of reward-related ventral striatum activity partially mediated the association between emotional neglect and greater depressive symptomatology (57). Lower ventral striatal activity has also been found when using rewarding social stimuli (i.e., happy faces) (59). These patterns persist in adults with a history of early adversity: high levels of adversity in childhood and adolescence blunted reward-related ventral striatum responses in adulthood (60–62). In addition to the ventral striatum, higher childhood adversity has been linked to lower activation in the putamen for adults during the anticipation of potential rewards (63), as well as potential losses (64). Other studies, however, reveal a more complex picture. Lower adolescent neighborhood quality, a contextual variable likely correlated with adversity, was associated with greater average fMRI activation in the ventral striatum during the anticipation of monetary gains (65). Early adversity was also found to improve learning from positive outcomes, but also led to impulsive decision-making, both of which were mediated by ventral striatal responses (52).
In addition to task-related activity, ELS has been found to alter resting-state connectivity of reward circuitry. Child maltreatment and early institutional care was associated with increased coupling between ventral striatum and regions of the PFC (66, 67), and socioeconomic disadvantage was associated with increased coupling between ventral striatum and PFC and cerebellum (68). Including broader reward circuitry, early adversity and socioeconomic disadvantage were also recently found to blunt development of VTA-PFC development (69) and reduce VTA-hippocampal connectivity (70).
These functional consequences of ELS may stem from altered brain structure and development, including mesocorticolimbic reward circuitry. For example, in a large cohort of adults (N = 3036), higher childhood adversity exposure was associated with smaller caudate volumes (71). Childhood trauma is also related to lower white matter integrity in fiber tracts connecting the caudate, ventral striatum, and prefrontal cortex (46, 72, 73). However, rodent models of ELS (discussed below) suggest changes in reward circuitry are at the level of excitatory and neuromodulatory signaling rather than gross structural changes. Focusing on connectivity may provide unique information about the impacts of ELS, explaining different aspects of the effects of ELS compared to the task-based activation studies reviewed above.
Of note, there are multiple open questions related to this research area, suggesting many important future research directions in human samples. First, while many projects conceptualize adversity as impacting mesocorticolimbic and reward-related processes, it is possible, instead, that the interaction between adversity and neurobiology could be diagnostic of resilience after stress exposure. Some studies have found childhood adversity was not related to mesocorticolimbic functional activity per se, but rather reduced activity - in the context of high levels of adversity- predicted poorer mental and physical health (74–76). For example, high early adversity in combination with lower ventral striatal responses to reward was related to more depression symptoms (74). Second, it is not yet clear how adversity might impact brain areas outside of the mesocorticolimbic circuit that may be related to reward processing. Conflicting results have noted lower (45, 77), as well as higher activity (78), in portions of the prefrontal cortex including the middle frontal gyrus and subgenual cingulate. Finally, it is still necessary to refine and clarify how reward-related processes are impacted by adversity. As noted above, there is ongoing debate about whether adversity may influence the processing of rewards or punishments, as well as brain activity during the anticipation or the consumption of rewards, although animal studies are providing insights into these questions. Relatedly, research groups have also noted lower mesocorticolimbic reactivity specifically for social, as opposed to monetary, rewards (79, 80). Rich experimental paradigms aimed at decomposing these different processes will be critical to move the field forward.
Impact of Early Life Stress on Motivated Behavior and Reward Circuit Activity From Non-Human Animal Models
Brief Overview of Rodent Models for Studying ELS
Lack of access to brain tissue, experiential and genetic heterogeneity, and ethical constraints on conducting studies with humans have underscored the need for non-human animal models for understanding the neurobiological consequences of ELS. Researchers have modeled ELS in rodents using a variety of paradigms, including separation of pups from their mothers (typically done in the first two wk of life for 3–4 hours/day, with or without early-weaning); a single bout of prolonged (24-h) maternal deprivation; or limited bedding and nesting resources (LBN) provided in the home cage (81, 82). The types of human ELS that these paradigms model are not entirely clear, however. On the surface, maternal separation or deprivation paradigms appear to model neglect (lack of access to or failure of the caregiver to provide adequate care and address the needs of offspring). However, studies have documented that dams increase nursing and grooming time with pups upon reunion, potentially to compensate for lost time nursing during separation (83), and the cognitive and emotional consequences of rodent maternal separation or deprivation are unknown. Limited bedding and nesting paradigms have been described as modeling a lack of resources, erratic, dysfunctional, or fragmented maternal care, or mimicking aspects of abusive behavior – which span dimensions of deprivation and threat (84–87). An important consideration is how human and rodent developmental timing of reward circuitry aligns. Direct comparison of rodent and human reward circuitry development in the neonatal periods is lacking, although it is suggested that rodent brain development is shifted relative to human development such that the first week of rodent life is approximately aligns with the last trimester of human gestation (16, 88). While a majority of rodent models of ELS begin ELS in the first few days after birth (86, 89), some shift stress to start around P9-10 (82, 90, 91). Each of these models of ELS have been documented to alter pups' plasma corticosterone levels acutely (85, 92, 93), although the long-term impact on basal and stress-induced corticosterone appear to depend on type and timing of stress (reviewed in (86). Each of these paradigms has also been shown to alter offspring defensive, depression-like, and reward-related behaviors, although again type and timing of stress have distinct impact, and sex differences have been documented (82, 89, 94–99).
Motivated Behaviors
Rodent models of ELS have identified alterations in motivated and reward-seeking behavior, as well as alterations in physiological functions in the reward circuitry (23, 96, 100–102). ELS impacts motivation for natural rewards, although the literature employing natural rewards in behavioral tasks is scant compared to studies with drugs of abuse (reviewed below), and currently available studies paint a complicated picture, particularly with respect to sex differences in behavior. In one study, maternal separation ELS decreased lever bar pressing for a sucrose solution in male Wistar rats (103), indicating that ELS reduces motivation for palatable rewards. Other studies assessing sucrose preference in a free-choice model confirm that various animal models of ELS reduce sucrose preference in male rats and mice, interpreted as increased anhedonia (82, 94, 96). However, other studies have found either a sex-specific effect of ELS on sucrose preference in female rats (104), no effect in female mice (95), or no effect at all (105, 106). When effects of ELS are found, the general result is that it reduces motivation for food rewards.
ELS also alters other natural reward-directed behaviors in rodent studies. Overall, ELS (maternal separation or LBN) appears to reduce conditioned place preference for a palatable food reward (107), although there may be a latent impact of ELS where this effect only emerges across development and is stronger in females (108, 109). However, another study reported opposite findings in which female Sprague-Dawley rats exposed to LBN show increased motivation for food reward in a one-h free access task (106). To our knowledge, only one study has assessed the effect of ELS on motivation for access to a social reward (110). In this study, LBN increased sexual motivation in both male and female Long-Evans rats (110). Whether ELS also increases other forms of social motivation is not yet known. Taken together, these findings suggest that the effects of ELS on motivation for natural rewards are dependent on many factors including the type of reward, sex, age, species/strain, and the type of adversity experienced during early life (i.e., forced maternal separation vs. disrupted maternal care due to limited resources). More studies are needed in order to tease apart how these various factors are differentially contributing to the effects of ELS on motivational drive for food and social rewards.
The majority of work assessing the effects of ELS on motivated behavior have focused on motivation for drug rewards such as psychostimulants, ethanol, and opiates. A consistent finding is that ELS increases self-administration of various psychostimulants. For example, maternal separation increases active lever responses as well as the number of infusions of methamphetamine in male Long-Evans rats (111, 112). Studies focused on cocaine largely find that ELS increases cocaine-seeking behaviors. Maternal separation combined with early weaning at P17 increases reward-seeking behavior and self-administration for cocaine in male and female CD1 mice (113). LBN results in higher acquisition of cocaine self-administration in Sprague-Dawley rats without increasing daily cocaine administration (107), suggesting that ELS is altering novelty-seeking behavior for cocaine. ELS from P14-21 in CD1 mice alters cocaine conditioned place preference and relapse behaviors (100). Additionally, LBN rats show a reduced hedonic set point for cocaine, indicating that while they are motivated to lever press for cocaine administration, they do not find it as rewarding as control rats. These differences observed in behavioral responses are likely related to these assays examining different aspects of reward-seeking behavior (i.e., assessing “liking” behavior versus assessing “wanting” behavior) (114, 115).
Many studies report that ELS increases reward-seeking for ethanol. Maternal separation rearing increases both lever pressing and consumption of ethanol in male Swiss ICR mice (116) and male and female Sprague-Dawley rats (117, 118). Additionally, maternal separation increases ethanol consumption in free-choice tasks in both male C57BL/6 mice (105) and male Wistar rats (119). Similarly, increases in ethanol consumption have been reported in maternal deprivation reared male and female Sprague-Dawley rats (120). These reports indicate that ELS models increase both motivated “wanting” and hedonic “liking” behaviors for ethanol. However, inconsistent findings have also been reported. Maternal deprivation reared male and female Wistar rats do not show increased ethanol intake until after two-wk of withdrawal and additional stress in adulthood (121), and LBN rearing decreases ethanol consumption during acquisition in male but not female C57BL/6J mice (122). In this same study, ethanol consumption levels eventually matched between groups, suggesting that ELS reduced the rewarding properties of ethanol only during early exposure and did not cause a long-lasting deficit in preference.
Conflicting results have been reported regarding the impact of ELS on motivation for opioids. In male Wistar rats, maternal separation induced place preference at a lower dose of morphine than in controls (123). A study with female Sprague-Dawley rats reported that LBN did not affect opioid self-administration itself, but rather it increased relapse-like behavior for heroin and remifentanil, and increased motivation for remifentanil (106). These data suggest an ability for LBN to increase vulnerability for opioid misuse following LBN in females. Males were not tested, so it is unclear whether they would also display this vulnerability. Another study including both male and female Long-Evans rats, similarly, found no effect of LBN on acquisition of morphine self-administration in females (124). However, LBN males administered less of a low dose of morphine than control reared males, and administered less morphine on a progressive ratio schedule, suggesting they were less motivated to take morphine at this dose. Consistent with a protective effect of LBN against this addiction-related phenotype in males, LBN reduced impulsive choice, a behavior associated with substance use disorders and mediated, in part, by the NAc (124–126). In contrast, no effects of LBN on motivation for morphine or impulsive choice were observed in females (124). The inconsistent effect of LBN on motivation for opioids in females may be due to the use of different drugs, a focus on different outcome measures, or due to differences in the implementation of the LBN model.
Reward Circuit Activity and Function
Collectively, these behavioral studies reveal that ELS can affect motivated behavior that is mediated by reward circuitry, albeit to varying degrees based on a variety of factors, and suggest that ELS alters reward circuit function. Dopamine release from VTA modulates NAc signal integration and activity and is necessary for the rewarding properties of both natural rewards and drugs of abuse (127, 128). Dopamine signaling has been associated with incentive-salience of rewarding and addicting stimuli (129) and reward prediction-error (130, 131), as well as signaling aversive and stressful stimuli (21, 132, 133). ELS has been found to alter VTA morphology, decrease GABAergic inhibition onto dopamine neurons, increase excitability of dopaminergic neurons in VTA, increase baseline dopamine levels released from VTA into NAc, and alter dopaminergic response to stressors (90, 134–142). However, a meta-analysis evaluated the effects of ELS on biochemical indicators of dopamine signaling and found that while ELS causes a consistent and robust increase in the dopamine metabolites DOPAC and HVA, there may only be a small increase in dopamine itself in the striatum (143). Consistent with these findings, maternal separation increases dopamine turnover (ratio DOPAC/DA) in the NAc of adolescent CD1 mice, an effect increased by exposure to cocaine (144). In addition to dopamine metabolism, dopamine clearance regulates the amount of dopamine in the synapse. Maternal separation decreased the rate of dopamine clearance in the spontaneously hypertensive rat (SHR), a model of attention deficit hyperactivity disorder (145). These studies could suggest ELS impairs dopamine transporter (DAT) function, or this result may be specific to the SHR model. Other studies suggest maternal separation ELS increases cocaine-induced but not baseline DAT levels in the NAc of adolescent CD1 mice, although other aspects of DAT function were not tested (144). Together, these studies indicate that ELS alters tonic and/or stimulus-induced dopamine from VTA to NAc, and that enduring alterations in NAc processing may in part be due to altered dopamine clearance.
Consistent with this idea, there is evidence that ELS alters NAc physiology. LBN decreases presynaptic glutamate transmission, as indexed by a reduction in the frequency of spontaneous excitatory postsynaptic currents (sEPSCs), although only in male rats (124). This effect is consistent with a study that found maternal separation decreased GluA2 AMPA subunit expression in the NAc of males but not female rats (146). The ratio between AMPA and NMDA receptors is a key factor governing glutamatergic plasticity. Morphine increases the AMPA/NMDA ratio in males (147) and LBN prevents this effect (124). Thus, in conditions where ELS reduces opioid self-administration, resilience is linked to a blockade of opioid-induced glutamatergic plasticity in the NAc.
An important question in neuroscience is the cellular specificity of effects. MSNs are the primary neuronal cell type of the striatum. MSNs are GABAergic and subclassified by whether they express D1- (Drd1-expressing) or D2- (Drd2-expressing) type dopamine receptors, which use distinct intracellular signaling cascades and have opposing effects on activity. D1-type receptors are coupled to Gas and Gaolf G-proteins and binding of dopamine leads to increased adenylyl cyclase activity, increased cyclic adenosine monophosphate (CAMP) production, and activation of protein kinase A (PKA) (148, 149). D2-type receptors have 100-fold higher affinity for dopamine and are instead coupled to Gai and Gao proteins, and dopamine binding leads to decreased CAMP and PKA activity. D1-MSNs are thought to be preferentially activated by phasic dopamine bursts, while D2-MSNs — which have greater baseline cellular activity—are thought to have greater sensitivity to tonic dopamine release (150). It is not yet known how ELS alters activity of D1 and D2 MSNs, although differential involvement of these cell types may well mirror their response to adult stress. Chronic stress in adult male mice decreases excitatory transmission onto D1-MSNs, causes D1-MSN long-term depression, and increases the threshold to excite D1-MSN activity (151, 152). Activating D1-MSNs after chronic adult stress alleviates depression-like behavioral changes in male mice. In contrast, chronic adult stress increases excitatory transmission onto D2-MSNs without altering their excitability, and activation of D2-MSNs increases susceptibility to later chronic adult stress (151).
Overall, these findings suggest that reward circuit physiology is particularly vulnerable to the effects of ELS, likely due to the ongoing maturation of dopaminergic reward circuitry in the postnatal period when these ELS manipulations are being implemented (31–40).
Impact of Early Life Stress on Transcription and Epigenetic Regulation Within NAC
Genome-Wide Transcriptional Alterations
The impact of early life stress on functional activity in NAc likely arises from changes in the molecular development of VTA, NAc, and broader reward circuitry. Genome-wide expression changes in reward circuitry resulting from ELS have been surveyed by RNA-sequencing. RNA-seq provides an opportunity not only to look for gene expression changes in an unbiased manner, but also to examine broad patterns of change that would be impossible to observe when sampling only a few candidate genes. ELS consistently induces changes in reward circuitry gene expression that last into adulthood in male and female mice (94, 95, 100, 124, 153). Interestingly, two studies comparing male and female transcriptomic responses to ELS (using different types of stress and at different juvenile states) have found around twice as many genes altered in female than male NAc (95, 124). Indeed, both baseline and stress-induced sex differences in the transcriptome have been described in NAc (95, 154–156). One interesting question is how prior ELS alters gene expression response to future salient experience such as additional stress or drugs of abuse. The transcriptomic response to adult stress is highly dependent upon whether or not a mouse experienced prior ELS: expression of more genes in both male and female NAc were altered after adult stress if mice had previously experienced early life stress. Moreover, the transcriptional response to adult stress showed opposite regulation in ELS vs. standard reared female mice in VTA and NAc (95). Genome-wide analysis also revealed that a subset of genes in male and female VTA and NAc did not have altered expression in response to ELS alone, but instead were primed by ELS such that latent expression changes were only revealed by later adult stress (95). The impact of ELS on genome-wide response to adult stress may be dependent on sex and the timing of stress. The pattern of increased transcriptional changes in response to adult stress given prior ELS from P10-17 was also observed in female (but not male) VTA and PFC which both send inputs to NAc (95). In male PFC, ELS alone predominately down-regulated gene expression, which may blunt response to future stimuli (153). Similarly in hippocampus, early postnatal stress (from P2-12) and adolescent stress (from P38-49) blunted transcriptomic response to acute adult stress in mice and rats (157, 158). Transcriptomic analyses also revealed that one potential mechanism for ELS-induced sensitivity to stress and drugs of abuse in male mice may be through altered plasticity. In VTA, ELS reduced transcriptional programs downstream of the transcription factor OTX2 (94), which has been identified as a key regulator of critical period plasticity (159–161). Transiently reducing Otx2 in VTA in a late postnatal sensitive period for stress exposure mimicked the effects of ELS on sensitivity to future stress experience and depression-like behavior, while restoring Otx2 levels in this sensitive period rescued the effects of ELS (94). In contrast, ELS may inappropriately preserve plasticity in NAc, including changes in genes associated with critical period plasticity and synaptic development (94, 95, 100). In NAc, a second stress exposure reversed such plasticity signatures which may in turn decrease physiological plasticity and lead to behavioral inflexibility and maladaptive coping behavior (95). Reduced plasticity in response to later salient exposures extends beyond stress to response to drugs of abuse as well: cFos (a marker of neuronal and gene expression activity) in NAc was reduced after ELS and cocaine exposure plus reinstatement (100).
Alterations in Target Genes
Examining the effect of ELS on specific candidate genes and proteins has identified a number of key mechanisms through which ELS alters motivated behaviors and stress response. Release of BDNF from VTA and binding to TrkB receptors in NAc is necessary for a depression-like response to adult stress (133) and physiological and behavioral response to reward and drugs of abuse (162–164). Blocking BDNF/TrkB signaling in NAc during exposure to ELS in mice (maternal separation from P3-14) simultaneously restored sucrose preference and sensitized behavioral response to chronic unpredictable adult stress on the open field test (165), indicating that the enduring and opposite consequences of ELS on motivated and anxiety-related behavior are regulated by transient developmental alterations in BDNF-TrkB signaling. ELS also increased expression of cannabinoid 1 receptors (Cb1r) and FK506-binding protein (Fkbp5) in NAc, which was further linked to alcohol consumption in rodents (119, 166). ELS in the form of predator odor exposure from P1-3 decreased expression of mu and kappa opioid receptors (Orpm1 and Oprk1, respectively) in female but not male NAc acutely on P3, although Orpm1 expression rebounded and was significantly higher than control females by postnatal day 33 (167). Mu opioid receptors contribute to euphoric and analgesic properties of opioid use and development of drug tolerance, which could explain a lack of motivation to lever-press for opioids in self-administration tasks (106, 124). Finally, ELS (LBN in male mice) downregulated the alpha-2 subunit of GABAA receptors and reduced frequency of miniature inhibitory postsynaptic currents in NAc, which was associated with both enhanced behavioral response to acute cocaine and blunted sensitization to repeated cocaine exposure (168).
ELS can also alter expression of dopamine D1-type (Drd1) and D2-type (Drd2) receptors themselves, which alters striatal response to stimuli. However, the direction of change is not consistent across reports. In one study, maternal separation ELS reduced NAc levels of DAT, Drd1, Drd2, and Drd3, which were correlated with reduced spatial learning in these rats (169). Other studies have confirmed reduced Drd1 (108) and Drd2 (170) receptor expression in the NAc of female mice which can reduce dopaminergic drive. In contrast, other studies in males found Drd1 expression in the NAc to be unaffected by ELS (108, 170). Maternal separation increased NAc Drd2 in males (119, 144, 171), an effect which was accompanied by a stress-induced increase in dendritic morphology in the NAc (171). Together these studies suggest sex-specific impacts of ELS on dopamine receptors in NAc, although additional research is needed to confirm sex-specific effects directly and determine the contributions of type and timing of ELS.
Epigenetic Alterations
Gene expression is regulated by a complex interaction between transcription factors and epigenetic regulatory mechanisms which fine-tune when and to what extent genes are expressed without altering the genetic sequence itself (172). DNA methylation – the addition of a small methyl group typically to the 5th carbon of cytosine residues (5mC) – is associated with suppression of gene expression in gene promoters, with mixed effects on transcription in other genomic regions (173). De novo DNA methylation is accomplished by the enzyme DNA methyltransferase 3A (DNMT3A) and DNMT3B, while maintenance methylation (across cell division) is accomplished by DNMT1. Maternal separation for three h daily from P2-15 increased overall Dnmt1, Dnmt3a, and Dnmt3b expression in the NAc, increased methylation at several target genes of interest (protein phosphatase catalytic subunit 1c and adenosine receptor 2A, vesicular glutamate transporter 3), and decreased their expression (174, 175). These target genes are involved in neuronal plasticity and response to cocaine, and may be a mechanism for ELA-induced hypersensitized response to cocaine (174). ELS also increased baseline levels of Methyl CpG binding protein 2 (MeCP2) in NAc (112, 175), a protein that binds or “reads” methylated DNA and is involved in gene silencing, although the specific genes differentially bound by MeCP2 after ELS are still unknown. Interestingly, MeCP2 is also elevated in NAc after self-administration of methamphetamine and ELS significantly blunts this effect, which is functionally related to increased methamphetamine self-administration among ELS-exposed rats (111, 112). Alterations in DNA methylation have also been identified as potential mechanisms of ELS-induced changes in gene expression of dopamine receptors and other target genes in NAc, including hypermethylation of the Drd1a promoter region in females (108).
Gene expression is also influenced by interactions between DNA and the histone proteins around which it is wrapped (176). Post-translational modifications to histone protein tails – including acetylation, methylation, and other modifications – can increase or decrease compaction, and repress or allow gene expression, respectively. 24-h maternal deprivation at P9 ELS has been shown to alter histone acetylation in VTA, which is directly linked to altered GABAergic function in VTA and increased dopaminergic excitability, and which is restored by histone deacetylase inhibitor treatment (90, 91, 141, 177). ELS (combined maternal separation and limited nesting material from P10-17) also broadly altered levels of post-translational histone modifications in NAc across postnatal development in a sex-specific manner (178). Most notably, ELS reduced mono- and di- methylation of histone 3 lysine 79 (H3K79) in adult male and female mice. The enzyme that “writes” H3K49 methylation, Dot1l, was also upregulated by ELS in adult male and female NAc, specifically within D2-MSNs (178), consistent with a role for D2-MSN activation increasing susceptibility to subsequent stress (151). H3K79 methylation is generally associated with enhanced transcription and genomic stability, and indeed both ELS and Dot1l overexpression predominately downregulated gene expression in NAc. Moreover, D2-specific overexpression in NAc recapitulated the impact of ELS, while knockdown or small-molecule inhibition of Dot1l ameliorated the impact of ELS on susceptibility to adult stress (178). Finally, ELS alters histone turnover dynamics in NAc. ELS results in faster accumulation of the replication-independent histone H3.3 variant in NAc across development, which is associated with increased susceptibility to adult stress, and reversing this phenomena can rescue depression-like behavior in mice (179). These epigenetic changes in reward circuitry following ELS underlie enduring changes in gene expression, and can also help explain latent changes in gene expression in response to future stressful or rewarding stimuli. These molecular mechanisms ultimately impact physiological functioning of reward circuitry, altered response to stressors and rewards, and risk for psychiatric disease.
Discussion
Looking holistically, there is growing evidence that ELS influences behavior (Table 1) and neurobiology (Table 2) involved with reward processing, with a focus here on NAc. However, additional work is critically needed at multiple “levels of analysis” (e.g., human systems neuroscience; non-human physiology, etc.) to increase understanding regarding the connections between ELS and later negative outcomes. Below, we elaborate on future directions for those working with human and preclinical samples, focused on different constructs connected to the review.
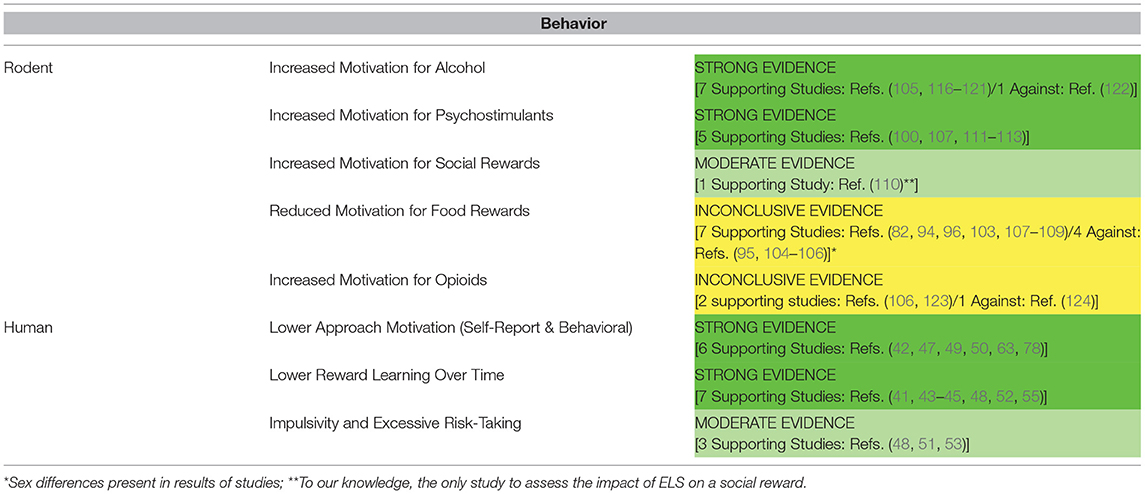
Table 1. Significance of evidence for the effects of ELS on motivated behaviors in human and rodent model studies.
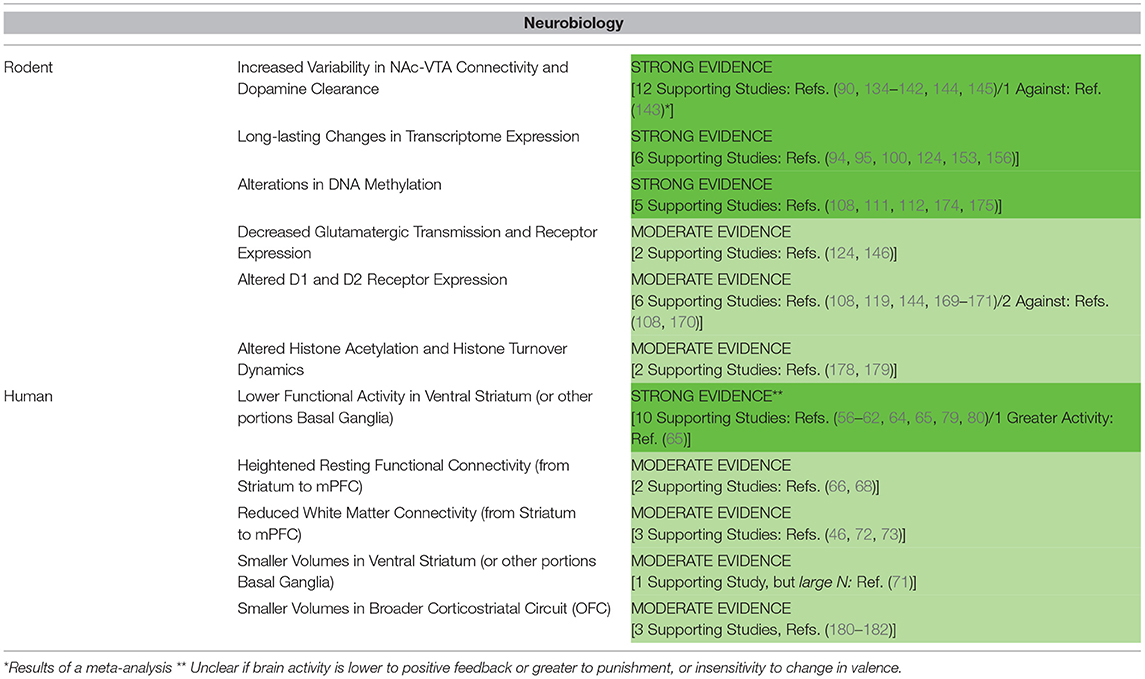
Table 2. Significance of evidence for the effects of ELS on reward circuit neurobiology in human and rodent model studies.
Operationalization and Categorizing Life Stress Across Early Development
In considering human and non-human research focused on ELS, it will be critical to think about rich characterizations of stressful life experiences. Here, we took a more broad and inclusive definition of ELS, but there is a great deal of active inquiry focused on the phenomenology of ELS. For example, in humans, there is an interest in considering the interactions, “lived experiences”, and subjective perceptions of different forms of ELS. Such factors may be important to consider, but difficult to capture in both human and non-human research. Clear from numerous epidemiological studies is that 60% of individuals reporting one form of ELS will experience two or more forms of adversity before reaching adulthood (5–7, 183). Interestingly, regardless of whether objective (court) records suggested maltreatment or ELS, subjective reports of ELS more robustly predicted psychopathology (184). Limited work, to our knowledge, has attempted to richly probe these aspects of ELS, but all of these elements will likely influence neurodevelopment and may impact trajectories of mesocorticolimbic neurobiology.
There are multiple approaches to modeling ELS and the field must strike a balance between more mechanistic approaches and the common co-occurrence of different forms of ELS. Researchers in epidemiology have been drawn to cumulative exposure models that sum up the total number of adversities suffered. This framework has nice connections to allostatic load and other neurobiological frameworks, has high explanatory power, and can deal with the common pattern of co-occurrence between many forms of adversity (185–187). However, these models provide less clarity about potential mediating mechanisms. More recently, starting frameworks (10, 188) argue for the examination of differences between dimensions of adversity (i.e., harshness vs. unpredictability; deprivation vs. threat) to advance mechanistic understanding of the impact of ELS. Further complicating our understanding, while the negative impacts of early life adversity are well-known and well-documented, many people exposed to early life adversity do not develop illnesses, and are, instead, protected from later stressful experiences (189, 190). A burgeoning body of research suggests that adversity, in modest amounts, can lead to the subsequent development of resilience (191–193). For example, non-human primates exposed to challenging, but not overwhelming, stressful events early in life show less anxiety and lower hypothalamic–pituitary–adrenal axis responsivity later in life (194). Similarly, moderate but not extreme levels of early adversity are related to larger volumes and greater activity in the prefrontal cortices of non-human primates (195, 196). Some implementations of LBN in rats leads to resilience after facing adolescent stress (197), adult stress (193) and reduced morphine self-administration (124), consistent with a “stress inoculation” model. However, it is clear that even with controlled rodent models of ELS such as LBN there is wide range in whether studies find evidence of inoculation or sensitivity to additional stress, drugs, or other stimuli. Identifying factors that contribute to vulnerability vs. sensitivity is crucial and will aid in targeted implementation of treatments for stress-related diseases.
Relatedly, one major translational challenge in most preclinical studies is that nearly all of our ELS models do not adequately capture the full “transactional nature” of stress [e.g., (198)]. Put another way– ELS is related to cognitive, affective, physiological, and neurobiological changes, and these alterations may affect how individuals respond to subsequent life stressors (199). In humans, there is rich support for so-called “stress sensitization” or “two-hit models”. For example, women with exposure to one or more childhood adversities (e.g., family violence, parental psychopathology) were more likely to become depressed by a lower “dose” of total stress than women without such adversity (200). This is true for depression, anxiety, and PTSD, and found during childhood, adolescence, and adulthood (7, 200–206). Connected to this, Hanson, Knodt, Brigidi & Hariri (2018) found reward-related functional connectivity between the ventral striatum and the medial prefrontal cortex was heightened after the occurrence of both early and then more contemporaneous life stress (67). These functional changes then linked stress exposure to depressive symptoms. Non-human animal work has likewise found evidence of stress-sensitization behaviorally, within reward circuitry, and in other brain systems (82, 94, 95, 207–210). Moving forward, there is a true need to construct complementary experiments in human and non-human samples, focused on similar neurobiological circuits, with interactive discussion between teams leading work in each species. This could aid in understanding these transactional elements, as well as modeling of ELS.
A number of connected open questions remain on “how” ELS shapes neurobiology, with interest in direct vs. indirect impacts. Direct impacts may be thought of as emerging due specifically to the elements of ELS, vs. indirect influences being related more to cascading influences of ELS. For example, while unlikely to be purely Hebbian in nature, mesocorticolimbic changes in physiology, transcription, or epigenetic expression could be due to the absence or inconsistency of (“direct”) environmental inputs. In contrast, environmental inputs could activate the HPA axis, causing the release of cortisol and related neuroendocrine messengers. Cortisol is known to be neurotoxic in high and chronic conditions (reviewed in reference (211) and this could (“indirectly”) lead to changes in multiple aspects of mesocorticolimbic neurobiology. Similarly, ELS can alter gonadal hormone levels, such as estradiol, which are potent modulators of reward circuitry (212). In line with recommendations from Callaghan et al. (2019), it is and will be critical to consider the developmental ecology and “goals” of an organism (e.g., attachment; independence) and how ELS may impinge upon these elements to create risk for negative life outcomes (213).
Considering Underexplored Moderators: Sex and Developmental Timing
As we continue to focus on the impact of ELS, it will be important to think about underexplored moderators and factors that may be driving inconsistencies in findings to date. Two clear factors are the developmental timing of specific stressors and potential sex differences in stress effects.
Developmentally, expression and activity of neurotransmitter systems centrally involved with the mesocorticolimbic circuit (i.e., dopamine) exhibit major changes during early life. The exact developmental sequencing is still of debate (214), but a number of reports suggest dopamine receptor expression peaks during the peripubertal period (215–218). As such, there may be differential behavioral and neurobiological consequences depending on the timing of ELS exposure. A powerful example of this comes from non-human research on amygdala neurobiology. Multiple studies have focused on this brain region in stress-exposed, young adult rodents (219); however, many paradigms were not sensitive to the differential developmental impacts of stress (cf. work by Regina Sullivan et al.; (220, 221). Interestingly, Rosenkranz et al. completed many commonly-used stress manipulations (e.g., repeated restraint stress) in animals of different ages and found many critical differences (e.g., number of spontaneously firing neurons vs. firing rates) depending on when in development the stress occurred (222–224).
Related to sex differences, there is now a growing emphasis on sex as a biological variable. Impacts of ELS for males and females may not be uniform and there may be evolutionary and developmental mechanisms of altered development in response to adversity (97). Stress during the prenatal and early postnatal period increases risk in males for neurodevelopmental disorders, such as autism spectrum disorder and ADHD (225). In females, the effect of ELS can be precipitated later in life by changes in fluctuating hormones, as occurring during puberty, pregnancy, and perimenopause, and is more likely to manifest as anxiety and depression (225). In addition, different forms of ELS are not equally distributed for males vs. females. For example, females are approximately 3–4 times more likely than males to suffer forms of sexual abuse (226, 227). Sadly, especially in human samples, few investigations have examined if ELS exerts differential impacts on mesocorticolimbic neurobiology for males vs. females. This pattern is surprising given that Andersen and colleagues have reported significant developmental sex differences in the striatum; specifically, males, but not females, over-produce D1 and D2 receptors in the striatum during development. These excessive receptors are then pruned prior to adulthood, only in males (228, 229). Thoughtful examination of both sex and developmental timing, across preclinical and human studies, could significantly advance our understanding of the neurobiological sequelae of ELA and the mediating connections between ELA and later negative outcomes.
Expanding Behavioral and Neurobiology Foci to Further Advance Understanding of ELS
As research continues in this space, increased behavioral and neurobiological precision will aid in understanding the sequelae of ELS, and links with poor mental health. Behaviorally, it will be critical to think about richly decomposing and parsing apart different motivational components connected to the mesocorticolimbic circuit. Basic research indicates that the mesocorticolimbic circuit encodes multiple aspects of reward-learning and decision-making processes such as prediction error, estimation of value, and amount and effort discounting (for review, see (230). However, few studies have examined these types of processes in samples exposed to ELS (77). A richer understanding of how the mesocorticolimbic circuit, and specific connected behavioral processes, may be influenced by ELS – in both human and non-human studies - will be crucial moving forward.
Neurobiologically, the preponderance of work reviewed here is centered on the NAc / ventral striatum (noting that these regions are not defined identically) and VTA, but, this brain region is nested in a larger circuit of motor, cognitive, and limbic brain regions, including portions of the medial prefrontal cortex (mPFC), sub-regions of the anterior cingulate cortex, the thalamus, brain stem, and motor cortex (231–233). As we continue to think about links between stress exposure, neurobiology, and mental health, it will be critical to think about this larger network and how variations in multiple portions of the mesocorticolimbic circuit may give rise to complex behavioral alterations (some of which is reviewed elsewhere (22, 23). For example, several portions of the mPFC support reward responsivity, as well as the processing of self-referential and social information (231, 234). In addition, smaller volumes (180–182) and greater activity in portions of the mPFC were seen for individuals exposed to stress, including child maltreatment or extreme family poverty (235, 236). Interestingly, non-human animal data also supports this idea, as helplessness and stress-vulnerability in rodents was associated with enhanced activity in the mPFC (237, 238). In addition to the mPFC, a few groups have been investigating the VTA and how ELS might impact connectivity of this key node in the mesocorticolimbic circuit in humans. Examined collectively, it is likely that there may be unique neural profiles underlying distinctive deleterious effects of adversity. Individuals exposed to early adversity may show differences in task-based activity in the NAc/ventral straitum, mPFC, or VTA, resting-state connectivity between these areas, or other combinations of these neural phenotypes. A great deal of work is needed to interrogate how individuals with each of these neural patterns may differ in forms of psychopathology, as well as psychosocial risk factors connected to psychopathology (e.g., detrimental emotion processing and regulation strategies). Future research should consider the complex relationships between stress exposure and reward-related brain function in establishing novel strategies to predict, prevent, and treat stress-related psychopathology.
Concluding Remarks
Here, we review multiple connected literatures on the impacts of ELS on mesocorticolimbic neurobiology — spanning human and non-human, and molecular to brain circuits. These bodies of research suggest that ELS may impact critical behavioral and neurobiological processes central to motivation and reward processing. Multiple projects focused on adversity exposure in human and non-human samples suggest decreased responding or inability to use feedback and reward-related cues. Changes in these motivation-related behaviors appear to be mediated by a host of neurobiological changes, including lower functional activity in the ventral striatum, altered glutamatergic signaling, changes in dopaminergic modulation and clearance, and altered molecular signatures of altered brain plasticity that may be regulated by epigenetic mechanisms. While exciting progress is occurring in this space, there are often more open questions about the development and function of mesocorticolimbic neurobiology in relation to ELS. A richer ability to dissect heterogeneity, while integrating stress- and developmental- neurobiology, will be critical to reduce the impacts of ELS and related long-term mental health challenges.
Author Contributions
All authors listed have made a substantial, direct and intellectual contribution to the work, and approved it for publication.
Funding
This work was supported by R00 MH115096 (CP), BBRF Young Investigator Award 28627 (CP), NSF Award IOS-1929829 (DB), R01 DA049837 (DB), and T32DA007237 (AW).
Conflict of Interest
The authors declare that the research was conducted in the absence of any commercial or financial relationships that could be construed as a potential conflict of interest.
Publisher's Note
All claims expressed in this article are solely those of the authors and do not necessarily represent those of their affiliated organizations, or those of the publisher, the editors and the reviewers. Any product that may be evaluated in this article, or claim that may be made by its manufacturer, is not guaranteed or endorsed by the publisher.
References
1. Bernet CZ, Stein MB. Relationship of childhood maltreatment to the onset and course of major depression in adulthood. Depress Anxiety. (1999) 9:169–74.
2. Bensley LS, Van Eenwyk J, Spieker SJ, Schoder J. Self-reported abuse history and adolescent problem behaviors. I Antisocial and suicidal behaviors. J Adolesc Health. (1999) 24:163–72.
3. Heim C, Nemeroff CB. The role of childhood trauma in the neurobiology of mood and anxiety disorders: preclinical and clinical studies. Biol Psychiatry. (2001) 49:1023–39. doi: 10.1016/S0006-3223(01)01157-X
4. Chapman DP, Whitfield CL, Felitti VJ, Dube SR, Edwards VJ, Anda RF. Adverse childhood experiences and the risk of depressive disorders in adulthood. J Affect Disord. (2004) 82:217–25. doi: 10.1016/j.jad.2003.12.013
5. Kessler RC, McLaughlin KA, Green JG, Gruber MJ, Sampson NA, Zaslavsky AM, et al. Childhood adversities and adult psychopathology in the WHO World Mental Health Surveys. Br J Psychiatr. (2010) 197:378–85. doi: 10.1192/bjp.bp.110.080499
6. Green JG, McLaughlin KA, Berglund PA, Gruber MJ, Sampson NA, Zaslavsky AM, et al. Childhood adversities and adult psychiatric disorders in the national comorbidity survey replication I. Arch Gen Psychiatry. (2010) 67:113–23.
7. McLaughlin KA, Green JG, Gruber MJ, Sampson NA, Zaslavsky AM, Kessler RC. Childhood adversities and adult psychiatric disorders in the national comorbidity survey replication II: associations with persistence of DSM-IV disorders. Arch Gen Psychiatry. (2010) 67:124–32. doi: 10.1001/archgenpsychiatry.2009.187
8. Scott KM, McLaughlin KA, Smith DAR, Ellis PM. Childhood maltreatment and DSM-IV adult mental disorders: comparison of prospective and retrospective findings. Br J Psychiatr. (2012) 200:469–75. doi: 10.1192/bjp.bp.111.103267
9. Manly JT, Kim JE, Rogosch FA, Cicchetti D. Dimensions of child maltreatment and children's adjustment: contributions of developmental timing and subtype. Dev Psychopathol. (2001) 13:759–82.
10. McLaughlin KA, Sheridan MA, Lambert HK. Childhood adversity and neural development: Deprivation and threat as distinct dimensions of early experience. Neurosci Biobehav Rev. (2014) 47:578–91. doi: 10.1016/j.neubiorev.2014.10.012
11. Jaffee SR, Maikovich-Fong AK. Effects of chronic maltreatment and maltreatment timing on children's behavior and cognitive abilities. J Child Psychol Psychiatr. (2010) 52:184–94. doi: 10.1111/j.1469-7610.2010.02304.x
12. Dunn EC, Nishimi K, Neumann A, Renaud A, Cecil CAM, Susser ES, Tiemeier H. Time-dependent effects of exposure to physical and sexual violence on psychopathology symptoms in late childhood: in search of sensitive periods in development. J Am Acad Child Adolesc Psychiatr. (2019) 59:283–295. doi: 10.1016/j.jaac.2019.02.022
13. Hambrick EP, Brawner TW, Perry BD. Timing of early-life stress and the development of brain-related capacities. Front Behav Neurosci. (2019) 13:1–14. doi: 10.3389/fnbeh.2019.00183
14. Dubowitz H, Roesch S, Arria AM, Metzger R, Thompson R, Kotch JB, et al. Timing and chronicity of child neglect and substance use in early adulthood. Child Abuse Negl. (2019) 94:104027. doi: 10.1016/j.chiabu.2019.104027
15. Schalinski I, Teicher MH, Nischk D, Hinderer E, Müller O, Rockstroh B. Type and timing of adverse childhood experiences differentially affect severity of PTSD, dissociative and depressive symptoms in adult inpatients. BMC Psychiatry. (2016) 16:295. doi: 10.1186/s12888-016-1004-5
16. Luby JL, Baram TZ, Rogers CE, Barch DM. Neurodevelopmental optimization after early-life adversity: cross-species studies to elucidate sensitive periods and brain mechanisms to inform early intervention. Trends Neurosci. (2020) 43:744–51. doi: 10.1016/j.tins.2020.08.001
17. Insel T, Cuthbert B, Garvey M, Heinssen R, Pine DS, Quinn K, et al. Research domain criteria (RDoC): toward a new classification framework for research on mental disorders. Am J Psychiatry. (2010) 167:748–51. doi: 10.1176/appi.ajp.2010.09091379
18. Trainor BC. Stress responses and the mesolimbic dopamine system: Social contexts and sex differences. Horm Behav. (2011) 60:457–69. doi: 10.1016/j.yhbeh.2011.08.013
19. Russo SJ, Nestler EJ. The brain reward circuitry in mood disorders. Nat Rev Neurosci. (2013) 14:609–25. doi: 10.1038/nrn3381
20. Lammel S, Lim BK, Malenka RC. Reward and aversion in a heterogeneous midbrain dopamine system. Neuropharmacology. (2014) 76:351–9. doi: 10.1016/j.neuropharm.2013.03.019
21. Polter AM, Kauer JA. Stress and VTA synapses: implications for addiction and depression. Eur J Neurosci. (2014) 39:1179–88. doi: 10.1111/ejn.12490
22. Herzberg MP, Gunnar MR. Early life stress and brain function: Activity and connectivity associated with processing emotion and reward. Neuroimage. (2020) 209:116493. doi: 10.1016/j.neuroimage.2019.116493
23. Birnie MT, Kooiker CL, Short AK, Bolton JL, Chen Y, Baram TZ. Plasticity of the reward circuitry after early-life adversity: mechanisms and significance. Biol Psychiatry. (2020) 87:875–84. doi: 10.1016/j.biopsych.2019.12.018
24. French SJ, Totterdell S. Individual nucleus accumbens-projection neurons receive both basolateral amygdala and ventral subicular afferents in rats. Neuroscience. (2003) 119:19–31. doi: 10.1016/s0306-4522(03)00150-7
25. Goto Y, Grace AA. Limbic and cortical information processing in the nucleus accumbens. Trends Neurosci. (2008) 31:552–8. doi: 10.1016/j.tins.2008.08.002
26. Sesack SR, Grace AA. Cortico-basal ganglia reward network: microcircuitry. Neuropsychopharmacol. (2010) 35:27–47. doi: 10.1038/npp.2009.93
27. Britt JPJ, Benaliouad FF, McDevitt RAR, Stuber GDG, Wise RAR, Bonci AA. Synaptic and behavioral profile of multiple glutamatergic inputs to the nucleus accumbens. Neuron. (2012) 76:790–803. doi: 10.1016/j.neuron.2012.09.040
28. Bagot RC, Parise EM, Peña CJ, Zhang H-X, Maze I, Chaudhury D, et al. Ventral hippocampal afferents to the nucleus accumbens regulate susceptibility to depression. Nat Commun. (2015) 6:7062–7062. doi: 10.1038/ncomms8062
29. Hyman SE, Malenka RC, Nestler EJ, Neural Mechanisms of Addiction. The role of reward-related learning and memory. Annu Rev Neurosci. (2006) 29:565–98. doi: 10.1146/annurev.neuro.29.051605.113009
30. Lüscher C. The emergence of a circuit model for addiction. Annu Rev Neurosci. (2016) 39:257–76. doi: 10.1146/annurev-neuro-070815-013920
31. Graybiel AM, Ragsdale CW. Clumping of acetylcholinesterase activity in the developing striatum of the human fetus and young infant. PNAS. (1980) 77:1214–8. doi: 10.1073/pnas.77.2.1214
32. Murrin LC, Ferrer JR. Ontogeny of the rat striatum: correspondence of dopamine terminals, opiate receptors and acetylcholinesterase. Neurosci Lett. (1984) 47:155–60. doi: 10.1016/0304-3940(84)90422-1
33. Tarazi FIF, Tomasini ECE, Baldessarini RJR. Postnatal development of dopamine and serotonin transporters in rat caudate-putamen and nucleus accumbens septi. Neurosci Lett. (1998) 254:21–4. doi: 10.1016/S0304-3940(98)00644-2
34. Tarazi FI, Tomasini EC, Baldessarini RJ. Postnatal development of dopamine D4-like receptors in rat forebrain regions: comparison with D2-like receptors. Develop Brain Res. (1998) 110:227–33. doi: 10.1016/S0165-3806(98)00111-4
35. Spear LP. The adolescent brain and age-related behavioral manifestations. Neurosci Biobehav Rev. (2000) 24:417–63. doi: 10.1016/S0149-7634(00)00014-2
36. Yetnikoff L, Reichard RA, Schwartz ZM, Parsely KP, Zahm DS. Protracted maturation of forebrain afferent connections of the ventral tegmental area in the rat. J Comp Neurol. (2014) 522:1031–47. doi: 10.1002/cne.23459
37. Burke AR, Miczek KA. Stress in adolescence and drugs of abuse in rodent models: Role of dopamine, CRF, and HPA axis. Psychopharmacology. (2014) 231:1557–80. doi: 10.1007/s00213-013-3369-1
38. Tomasi D, Volkow ND. Functional connectivity of substantia nigra and ventral tegmental area: maturation during adolescence and effects of ADHD. Cerebral Cortex. (2014) 24:935–44. doi: 10.1093/cercor/bhs382
39. Hoops D, Flores C. Making dopamine connections in adolescence. Trends Neurosci. (2017) 40:709–19. doi: 10.1016/j.tins.2017.09.004
40. Lieberman OJ, McGuirt AF, Mosharov EV, Pigulevskiy I, Hobson BD, Choi S, et al. Dopamine triggers the maturation of striatal spiny projection neuron excitability during a critical period. Neuron. (2018) 99:540–54.e4. doi: 10.1016/j.neuron.2018.06.044
41. Hanson JL, Bos W, van den, Roeber BJ, Rudolph KD, Davidson RJ, Pollak SD. Early adversity and learning: implications for typical and atypical behavioral development. J Child Psychol Psychiatr. (2017) 58:770–8. doi: 10.1111/jcpp.12694
42. Kasparek SW, Jenness JL, McLaughlin KA. Reward processing modulates the association between trauma exposure and externalizing psychopathology. Clin Psychol Sci. (2020) 8:989–1006. doi: 10.1177/2167702620933570
43. Wismer Fries AB, Pollak SD. The role of learning in social development: Illustrations from neglected children. Dev Sci. (2017) 20. doi: 10.1111/desc.12431
44. Sheridan MA, McLaughlin KA, Winter W, Fox N, Zeanah C, Nelson CA. Early deprivation disruption of associative learning is a developmental pathway to depression and social problems. Nat Commun. (2018) 9:2216. doi: 10.1038/s41467-018-04381-8
45. Harms MB, Bowen KES, Hanson JL, Pollak SD. Instrumental learning and cognitive flexibility processes are impaired in children exposed to early life stress. Dev Sci. (2018) 21:e12596. doi: 10.1111/desc.12596
46. Dennison MJ, Rosen ML, Sambrook KA, Jenness JL, Sheridan MA, McLaughlin KA. Differential associations of distinct forms of childhood adversity with neurobehavioral measures of reward processing: a developmental pathway to depression. Child Dev. (2019) 90:e96–e113. doi: 10.1111/cdev.13011
47. Kautz MM, Burke TA, Siegel DM, Case J, Alloy LB. The role of reward sensitivity and childhood maltreatment in predicting nonsuicidal self-injury. Suicide Life-Threaten Behav. (2020) 50:1250–63. doi: 10.1111/sltb.12718
48. Weller JA, Fisher PA. Decision-making deficits among maltreated children. Child Maltreat. (2013) 18:184–94. doi: 10.1177/1077559512467846
49. Pechtel P, Pizzagalli DA. Disrupted reinforcement learning and maladaptive behavior in women with a history of childhood sexual abuse: a high-density event-related potential study. JAMA Psychiatry. (2013) 70:499–507. doi: 10.1001/jamapsychiatry.2013.728
50. Guyer AE, Kaufman J, Hodgdon HB, Masten CL, Jazbec S, Pine DS, et al. Behavioral alterations in reward system function: the role of childhood maltreatment and psychopathology. J Am Acad Child Adolesc Psychiat. (2006) 45:1059–67. doi: 10.1097/01.chi.0000227882.50404.11
51. Weller JA, Leve LD, Kim HK, Bhimji J, Fisher PA. Plasticity of decision-making abilities among maltreated adolescents: evidence from a random controlled trial. Dev Psychopathol. (2015) 27:535–51. doi: 10.1017/S0954579415000140
52. Kamkar NH, Lewis DJ, van den Bos W, Morton JB. Ventral striatal activity links adversity and reward processing in children. Dev Cogn Neurosci. (2017) 26:20–7. doi: 10.1016/j.dcn.2017.04.002
53. Oshri A, Kogan SM, Kwon JA, Wickrama KAS, Vanderbroek L, Palmer AA, MacKillop J. Impulsivity as a mechanism linking child abuse and neglect with substance use in adolescence and adulthood. Dev Psychopathol. (2018) 30:417–35. doi: 10.1017/S0954579417000943
54. Hallowell ES, Oshri A, Liebel SW, Liu S, Duda B, Clark US, et al. The mediating role of neural activity on the relationship between childhood maltreatment and impulsivity. Child Maltreat. (2019) 24:389–99. doi: 10.1177/1077559519835975
55. Wilkinson MP, Slaney CL, Mellor JR, Robinson ESJ. Investigation of reward learning and feedback sensitivity in non-clinical participants with a history of early life stress. bioRxiv [Preprint]. (2021) 1–37. doi: 10.1101/2020.11.13.380444
56. Mehta MA, Gore-Langton E, Golembo N, Colvert E, Williams SCR, Sonuga-Barke E. Hyporesponsive reward anticipation in the basal ganglia following severe institutional deprivation early in life. J Cogn Neurosci. (2010) 22:2316–25. doi: 10.1162/jocn.2009.21394
57. Hanson JL, Hariri AR, Williamson DE. Blunted ventral striatum development in adolescence reflects emotional neglect and predicts depressive symptoms. BPS. (2015) 78:598–605. doi: 10.1016/j.biopsych.2015.05.010
58. Takiguchi S, Fujisawa TX, Mizushima S, Saito DN, Okamoto Y, Shimada K, et al. Ventral striatum dysfunction in children and adolescents with reactive attachment disorder: functional MRI study. BJPsych Open. (2015) 1:121–8. doi: 10.1192/bjpo.bp.115.001586
59. Goff B, Gee DG, Telzer EH, Humphreys KL, Gabard-Durnam L, Flannery J, et al. Reduced nucleus accumbens reactivity and adolescent depression following early-life stress. Neuroscience. (2013) 249:129–38. doi: 10.1016/j.neuroscience.2012.12.010
60. Hanson JL, Albert D, Iselin A-MR, Carré JM, Dodge KA, Hariri AR. Cumulative stress in childhood is associated with blunted reward-related brain activity in adulthood. Soc Cogn Affect Neurosci. (2016) 11:405–12. doi: 10.1093/scan/nsv124
61. Holz NE, Boecker-Schlier R, Buchmann AF, Blomeyer D, Jennen-Steinmetz C, Baumeister S, et al. Ventral striatum and amygdala activity as convergence sites for early adversity and conduct disorder. Soc Cogn Affect Neurosci. (2016) 12:261–72. doi: 10.1093/scan/nsw120
62. Richter A, Krämer B, Diekhof EK, Gruber O. Resilience to adversity is associated with increased activity and connectivity in the VTA and hippocampus. NeuroImage: Clinical. (2019) 23:101920. doi: 10.1016/j.nicl.2019.101920
63. Dillon DG, Holmes AJ, Birk JL, Brooks N, Lyons-Ruth K, Pizzagalli DA. Childhood adversity is associated with left basal ganglia dysfunction during reward anticipation in adulthood. Biol Psychiatry. (2009) 66:206–13. doi: 10.1016/j.biopsych.2009.02.019
64. Birn RM, Roeber BJ, Pollak SD. Early childhood stress exposure, reward pathways, and adult decision making. Proc Natl Acad Sci U S A. (2017) 114:13549–54. doi: 10.1073/pnas.1708791114
65. Gonzalez MZ, Allen JP, Coan JA. Lower neighborhood quality in adolescence predicts higher mesolimbic sensitivity to reward anticipation in adulthood. Dev Cogn Neurosci. (2016) 22:48–57. doi: 10.1016/j.dcn.2016.10.003
66. Fareri DS, Gabard-Durnam L, Goff B, Flannery J, Gee DG, Lumian DS, et al. Altered ventral striatal-medial prefrontal cortex resting-state connectivity mediates adolescent social problems after early institutional care. Dev Psychopathol. (2017) 29:1865–76. doi: 10.1017/S0954579417001456
67. Hanson JL, Knodt AR, Brigidi BD, Hariri AR. Heightened connectivity between the ventral striatum and medial prefrontal cortex as a biomarker for stress-related psychopathology: understanding interactive effects of early and more recent stress. Psychol Med. (2018) 23:1–9. doi: 10.1017/S0033291717003348
68. Marshall NA, Marusak HA, Sala-Hamrick KJ, Crespo LM, Rabinak CA, Thomason ME. Socioeconomic disadvantage and altered corticostriatal circuitry in urban youth. Hum Brain Mapp. (2018) 39:1982–94. doi: 10.1002/hbm.23978
69. Park AT, Tooley UA, Leonard JA, Boroshok AL, McDermott CL, Tisdall MD, et al. Early childhood stress is associated with blunted development of ventral tegmental area functional connectivity. Dev Cogn Neurosci. (2021) 47:100909. doi: 10.1016/j.dcn.2020.100909
70. Marusak HA, Hatfield JRB, Thomason ME, Rabinak CA. Reduced ventral tegmental area-hippocampal connectivity in children and adolescents exposed to early threat. Biol Psychiat Cogn Neurosci Neuroimag. (2017) 2:130–7. doi: 10.1016/j.bpsc.2016.11.002
71. Frodl T, Janowitz D, Schmaal L, Tozzi L, Dobrowolny H, Stein DJ, et al. Childhood adversity impacts on brain subcortical structures relevant to depression. J Psychiatr Res. (2017) 86:58–65. doi: 10.1016/j.jpsychires.2016.11.010
72. DeRosse P, Ikuta T, Karlsgodt KH, Szeszko PR, Malhotra AK. History of childhood maltreatment is associated with reduced fractional anisotropy of the accumbofrontal ‘reward' tract in healthy adults. Brain Imaging Behav. (2020) 14:353–61. doi: 10.1007/s11682-020-00265-y
73. Kennedy B, Hanson JL, Buser N, van den Bos W, Rudolph KD, Davidson RJ, et al. Accumbofrontal tract integrity is related to early life adversity and feedback learning. Neuropsychopharmacology. (2021) doi: 10.1038/s41386-021-01129-9. [Epub ahead of print].
74. Corral-Frías NS, Nikolova YS, Michalski LJ, Baranger DAA, Hariri AR, Bogdan R. Stress-related anhedonia is associated with ventral striatum reactivity to reward and transdiagnostic psychiatric symptomatology. Psychol Med. (2015) 45:2605–17. doi: 10.1017/S0033291715000525
75. Dennison MJ, Sheridan MA, Busso DS, Jenness JL, Peverill M, Rosen ML, et al. Neurobehavioral markers of resilience to depression amongst adolescents exposed to child abuse. J Abnorm Psychol. (2016) 125:1201–12. doi: 10.1037/abn0000215
76. Miller GE, White SF, Chen E, Nusslock R. Association of inflammatory activity with larger neural responses to threat and reward among children living in poverty. Am J Psychiatry. (2021) 178:313–20. doi: 10.1176/appi.ajp.2020.20050635
77. Palacios-Barrios EE, Hanson JL, Barry KR, Albert WD, White SF, Skinner AT, et al. Lower neural value signaling in the prefrontal cortex is related to childhood family income and depressive symptomatology during adolescence. Dev Cogn Neurosci. (2021) 48:100920. doi: 10.1016/j.dcn.2021.100920
78. DelDonno SR, Mickey BJ, Pruitt PJ, Stange JP, Hsu DT, Weldon AL, et al. Influence of childhood adversity, approach motivation traits, and depression on individual differences in brain activation during reward anticipation. Biol Psychol. (2019) 146:107709. doi: 10.1016/j.biopsycho.2019.05.009
79. Boecker R, Holz NE, Buchmann AF, Blomeyer D, Plichta MM, Wolf I, et al. Impact of early life adversity on reward processing in young adults: EEG-FMRI results from a prospective study over 25 years. PLoS ONE. (2014) 9:e104185. doi: 10.1371/journal.pone.0104185
80. Boecker-Schlier R, Holz NE, Buchmann AF, Blomeyer D, Plichta MM, Jennen-Steinmetz C, et al. Interaction between COMT Val158Met polymorphism and childhood adversity affects reward processing in adulthood. Neuroimage. (2016) 132:556–70. doi: 10.1016/j.neuroimage.2016.02.006
81. Murthy S, Gould E. Early life stress in rodents: animal models of illness or resilience? Front Behav Neurosci. (2018) 12:320. doi: 10.3389/fnbeh.2018.00157
82. Peña CJ, Nestler EJ, Bagot RC. Environmental programming of susceptibility and resilience to stress in adulthood in male mice. Front Behav Neurosci. (2019) 13:272. doi: 10.3389/fnbeh.2019.00040
83. Orso R, Creutzberg KC, Wearick-Silva LE, Wendt Viola T, Tractenberg SG, Benetti F, et al. How early life stress impact maternal care: a systematic review of rodent studies. Front Behav Neurosci. (2019) 13:197. doi: 10.3389/fnbeh.2019.00197
84. Ivy A, Brunson K, Sandman C, Baram T. Dysfunctional nurturing behavior in rat dams with limited access to nesting material: a clinically relevant model for early-life stress. Neuroscience. (2008) 154:1132–42. doi: 10.1016/j.neuroscience.2008.04.019
85. Rice CJ, Sandman CA, Lenjavi MR, Baram TZ A. novel mouse model for acute and long-lasting consequences of early life stress. Endocrinology. (2008) 149:4892–900. doi: 10.1210/en.2008-0633
86. Molet J, Maras PM, Avishai-Eliner S, Baram TZ. Naturalistic rodent models of chronic early-life stress. Dev Psychobiol. (2014) 56:1675–88. doi: 10.1002/dev.21230
87. Gallo M, Shleifer DG, Godoy LD, Ofray D, Olaniyan A, Campbell T, et al. Limited bedding and nesting induces maternal behavior resembling both hypervigilance and abuse. Front Behav Neurosci. (2019) 13:64. doi: 10.3389/fnbeh.2019.00167
88. Chini M, Hanganu-Opatz IL. Prefrontal cortex development in health and disease: lessons from rodents and humans. Trends Neurosci. (2021) 44:227–40. doi: 10.1016/j.tins.2020.10.017
89. Demaestri C, Pan T, Critz M, Ofray D, Gallo M, Bath KG. Type of early life adversity confers differential, sex-dependent effects on early maturational milestones in mice. Horm Behav. (2020) 124:104763. doi: 10.1016/j.yhbeh.2020.104763
90. Authement ME, Kodangattil JN, Gouty S, Rusnak M, Symes AJ, Cox BM, et al. Histone deacetylase inhibition rescues maternal deprivation-induced GABAergic metaplasticity through restoration of AKAP signaling. Neuron. (2015) 86:1240–52. doi: 10.1016/j.neuron.2015.05.024
91. Shepard RD, Nugent FS. Early life stress- and drug-induced histone modifications within the ventral tegmental area. Front Cell Dev Biol. (2020) 8:1–8. doi: 10.3389/fcell.2020.588476
92. Cirulli F, Santucci D, Laviola G, Alleva E, Levine S. Behavioral and hormonal responses to stress in the newborn mouse: effects of maternal deprivation and chlordiazepoxide. Dev Psychobiol. (1994) 27:301–16. doi: 10.1002/dev.420270505
93. Horii-Hayashi N, Sasagawa T, Matsunaga W, Matsusue Y, Azuma C, Nishi M. Developmental changes in desensitisation of c-Fos expression induced by repeated maternal separation in pre-weaned mice. J Neuroendocrinol. (2013) 25:158–67.
94. Peña CJ, Kronman HG, Walker DM, Cates HM, Bagot RC, Purushothaman I, et al. Early life stress confers lifelong stress susceptibility in mice via ventral tegmental area OTX2. Science. (2017) 356:1185–8. doi: 10.1126/science.aan4491
95. Peña CJ, Smith M, Ramakrishnan A, Cates HM, Bagot RC, Kronman HG, et al. Early life stress alters transcriptomic patterning across reward circuitry in male and female mice. Nat Commun. (2019) 10:5098. doi: 10.1038/s41467-019-13085-6
96. Bolton JL, Molet J, Regev L, Chen Y, Rismanchi N, Haddad E, et al. Anhedonia following early-life adversity involves aberrant interaction of reward and anxiety circuits and is reversed by partial silencing of amygdala corticotropin-releasing hormone gene. Biol Psychiatry. (2018) 83:137–47. doi: 10.1016/j.biopsych.2017.08.023
97. Bath KG. Synthesizing views to understand sex differences in response to early life adversity. Trends Neurosci. (2020) 43:300–10. doi: 10.1016/j.tins.2020.02.004
98. Grassi-Oliveira R, Honeycutt JA, Holland FH, Ganguly P, Brenhouse HC. Cognitive impairment effects of early life stress in adolescents can be predicted with early biomarkers: Impacts of sex, experience, and cytokines. Psychoneuroendocrinology. (2016) 71:19–30. doi: 10.1016/j.psyneuen.2016.04.016
99. Honeycutt JA, Demaestri C, Peterzell S, Silveri MM, Cai X, Kulkarni P, et al. Altered corticolimbic connectivity reveals sex-specific adolescent outcomes in a rat model of early life adversity. Elife. (2020) 9:e52651. doi: 10.7554/eLife.52651
100. Lo Iacono L, Valzania A, Visco-Comandini F, Viscomi MT, Felsani A, Puglisi-Allegra S, et al. Regulation of nucleus accumbens transcript levels in mice by early-life social stress and cocaine. Neuropharmacology. (2016) 103:183–94. doi: 10.1016/j.neuropharm.2015.12.011
101. Bolton JL, Short AK, Simeone KA, Daglian J, Baram TZ. Programming of stress-sensitive neurons and circuits by early-life experiences. Front Behav Neurosci. (2019) 13:1–9. doi: 10.3389/fnbeh.2019.00030
102. Levis SC, Mahler SV, Baram TZ. The developmental origins of opioid use disorder and its comorbidities. Front Hum Neurosci. (2021) 15:601905. doi: 10.3389/fnhum.2021.601905
103. Campbell EJ, Mitchell CS, Adams CD, Yeoh JW, Hodgson DM, Graham BA, et al. Chemogenetic activation of the lateral hypothalamus reverses early life stress-induced deficits in motivational drive. Eur J Neurosci. (2017) 46:2285–96. doi: 10.1111/ejn.13674
104. Bai M, Zhang L, Zhu X, Zhang Y, Zhang S, Xue L. Comparison of depressive behaviors induced by three stress paradigms in rats. Physiol Behav. (2014) 131:81–6. doi: 10.1016/j.physbeh.2014.04.019
105. Magalhães T de A, Correia D, Carvalho LM de, Damasceno S, Godard ALB. Maternal separation affects expression of stress response genes and increases vulnerability to ethanol consumption. Brain Behav. (2018) 8:e00841. doi: 10.1002/brb3.841
106. Levis SC, Bentzley BS, Molet J, Bolton JL, Perrone CR, Baram TZ, Mahler SV. On the early life origins of vulnerability to opioid addiction. Mol Psychiatry. (2019). doi: 10.1038/s41380-019-0628-5. [Epub ahead of print].
107. Bolton JL, Ruiz CM, Rismanchi N, Sanchez GA, Castillo E, Huang J, et al. Early-life adversity facilitates acquisition of cocaine self-administration and induces persistent anhedonia. Neurobiology of Stress. (2018) 8:57–67. doi: 10.1016/j.ynstr.2018.01.002
108. Sasagawa T, Horii-Hayashi N, Okuda A, Hashimoto T, Azuma C, Nishi M. Long-term effects of maternal separation coupled with social isolation on reward seeking and changes in dopamine D1 receptor expression in the nucleus accumbens via DNA methylation in mice. Neurosci Lett. (2017) 641:33–9. doi: 10.1016/j.neulet.2017.01.025
109. Goodwill HL, Manzano-Nieves G, Gallo M, Lee H-I, Oyerinde E, Serre T, et al. Early life stress leads to sex differences in development of depressive-like outcomes in a mouse model. Neuropsychopharmacology. (2019) 44:711–20. doi: 10.1038/s41386-018-0195-5
110. Davis LK, Bolton JL, Hanson H, Guarraci FA. Modified limited bedding and nesting is a model of early-life stress that affects reproductive physiology and behavior in female and male Long-Evans rats. Physiol Behav. (2020) 224:113037. doi: 10.1016/j.physbeh.2020.113037
111. Lewis CR, Staudinger K, Scheck L, Olive MF. The effects of maternal separation on adult methamphetamine self-administration, extinction, reinstatement, and mecp2 immunoreactivity in the nucleus accumbens. Front Psychiatry. (2013) 4:1–9. doi: 10.3389/fpsyt.2013.00055
112. Lewis CR, Bastle RM, Manning TB, Himes SM, Fennig P, Conrad PR, et al. Interactions between early life stress, nucleus accumbens MeCP2 expression, and methamphetamine self-administration in male rats. Neuropsychopharmacol. (2016) 41:2851–61. doi: 10.1038/npp.2016.96
113. Castro-Zavala A, Martín-Sánchez A, Luján MÁ, Valverde O. Maternal separation increases cocaine intake through a mechanism involving plasticity in glutamate signalling. Addict Biol. (2021) 26:e12911. https://doi.org/10.1111/adb.12911
114. Berridge KC. The debate over dopamine's role in reward: the case for incentive salience. Psychopharmacology. (2007) 191:391–431. doi: 10.1007/s00213-006-0578-x
115. Berridge KC. From prediction error to incentive salience: mesolimbic computation of reward motivation. Eur J Neurosci. (2012) 35:1124–43. doi: 10.1111/j.1460-9568.2012.07990.x
116. García-Gutiérrez MS, Navarrete F, Aracil A, Bartoll A, Martínez-Gras I, Lanciego JL, et al. Increased vulnerability to ethanol consumption in adolescent maternal separated mice. Addict Biol. (2016) 21:847–58. doi: 10.1111/adb.12266
117. Gondré-Lewis MC, Darius PJ, Wang H, Allard JS. Stereological analyses of reward system nuclei in maternally deprived/separated alcohol drinking rats. J Chem Neuroanat. (2016) 76:122–32. doi: 10.1016/j.jchemneu.2016.02.004
118. Gondré-Lewis MC, Warnock KT, Wang H, Bell KA, Rabe H, Tiruveedhula VVNPB, et al. Early life stress is a risk factor for excessive alcohol drinking and impulsivity in adults and is mediated via a CRF/GABAA mechanism. Stress (Amsterdam, Netherlands). (2016) 19:235–47. doi: 10.3109/10253890.2016.1160280
119. Amancio-Belmont O, Becerril Meléndez AL, Ruiz-Contreras AE, Méndez-Díaz M, Prospéro-García O. Maternal separation plus social isolation during adolescence reprogram brain dopamine and endocannabinoid systems and facilitate alcohol intake in rats. Brain Res Bull. (2020) 164:21–8. doi: 10.1016/j.brainresbull.2020.08.002
120. Bassey RB, Gondré-Lewis MC. Combined early life stressors: prenatal nicotine and maternal deprivation interact to influence behavioral phenotypes in rats. Behav Brain Res. (2018) 359:814–22. doi: 10.1016/j.bbr.2018.07.022
121. Peñasco S, Mela V, López-Moreno JA, Viveros M-P, Marco EM. Early maternal deprivation enhances voluntary alcohol intake induced by exposure to stressful events later in life. Neural Plast. (2015) 2015:342761. doi: 10.1155/2015/342761
122. Okhuarobo A, Bolton JL, Igbe I, Zorrilla EP, Baram TZ, Contet C, et al. novel mouse model for vulnerability to alcohol dependence induced by early-life adversity. Neurobiol Stress. (2020) 13:100269. doi: 10.1016/j.ynstr.2020.100269
123. Pirri F, Akbarabadi A, Sadat-Shirazi M-S, Nouri Zadeh-Tehrani S, Mahboubi S, Karimi Goudarzi A, et al. Comparison and interaction of morphine and CB1 agonist conditioned place preference in the rat model of early life stress. Int J Dev Neurosci. (2021) 81:238–48. doi: 10.1002/jdn.10094
124. Ordoñes Sanchez E, Bavley CC, Deutschmann AU, Carpenter R, Peterson DR, Karbalaei R, et al. Early life adversity promotes resilience to opioid addiction-related phenotypes in male rats and sex-specific transcriptional changes. PNAS. (2021) 118:1–8. doi: 10.1073/pnas.2020173118
125. Basar K, Sesia T, Groenewegen H, Steinbusch HWM, Visser-Vandewalle V, Temel Y. Nucleus accumbens and impulsivity. Prog Neurobiol. (2010) 92:533–57. doi: 10.1016/j.pneurobio.2010.08.007
126. Marino EN, Rosen KD, Gutierrez A, Eckmann M, Ramamurthy S, Potter JS. Impulsivity but not sensation seeking is associated with opioid analgesic misuse risk in patients with chronic pain. Addict Behav. (2013) 38:2154–7. doi: 10.1016/j.addbeh.2013.01.020
127. Kelley AE, Berridge KC. The neuroscience of natural rewards: relevance to addictive drugs. J Neurosci. (2002) 22:3306–11. 20026361
128. Chiara GD, Imperato A. Drugs abused by humans preferentially increase synaptic dopamine concentrations in the mesolimbic system of freely moving rats. PNAS. (1988) 85:5274–8. doi: 10.1073/pnas.85.14.5274
129. Berridge KC, Robinson TE. What is the role of dopamine in reward: hedonic impact, reward learning, or incentive salience? Brain Res Rev. (1998) 28:309–69. doi: 10.1016/S0165-0173(98)00019-8
130. Steinberg EE, Keiflin R, Boivin JR, Witten IB, Deisseroth K, Janak PH, et al. causal link between prediction errors, dopamine neurons and learning. Nat Neurosci. (2013) 16:966–73. doi: 10.1038/nn.3413
131. Parker NF, Cameron CM, Taliaferro JP, Lee J, Choi JY, Davidson TJ, et al. Reward and choice encoding in terminals of midbrain dopamine neurons depends on striatal target. Nat Neurosci. (2016) 19:845–54. doi: 10.1038/nn.4287
132. Tidey JW, Miczek KA. Social defeat stress selectively alters mesocorticolimbic dopamine release: an in vivo microdialysis study. Brain Res. (1996) 721:140–9. doi: 10.1016/0006-8993(96)00159-X
133. Berton O, Mcclung CA, Dileone RJ, Krishnan V, Renthal W, Russo SJ, et al. Essential role of BDNF in the mesolimbic dopamine pathway in social defeat stress. Science. (2006) 311:864–8. doi: 10.1126/science.1120972
134. Cabib S, Puglisi-Allegra S. D'amato FR. Effects of postnatal stress on dopamine mesolimbic system responses to aversive experiences in adult life. Brain Res. (1993) 604:232–9. doi: 10.1016/0006-8993(93)90374-V
135. Matthews K, Wilkinson LS, Robbins TW. Repeated maternal separation of preweanling rats attenuates behavioral responses to primary and conditioned incentives in adulthood. Physiol Behav. (1996) 59:99–107.
136. Matthews K, Dalley JW, Matthews C, Tsai TH, Robbins TW. Periodic maternal separation of neonatal rats produces region- and gender-specific effects on biogenic amine content in postmortem adult brain. Synapse. (2001) 40:1–10. doi: 10.1002/1098-2396(200104)40:1<1::AID-SYN1020>3.0.CO;2-E
137. Brake WG, Zhang TY, Diorio J, Meaney MJ, Gratton A. Influence of early postnatal rearing conditions on mesocorticolimbic dopamine and behavioural responses to psychostimulants and stressors in adult rats. Eur J Neurosci. (2004) 19:1863–74. doi: 10.1111/j.1460-9568.2004.03286.x
138. Arborelius L, Eklund M. Both long and brief maternal separation produces persistent changes in tissue levels of brain monoamines in middle-aged female rats. Neuroscience. (2007) 145:738–50. doi: 10.1016/j.neuroscience.2006.12.007
139. Jahng JW, Ryu V, Yoo SB, Noh SJ, Kim JY, Lee JH. Mesolimbic dopaminergic activity responding to acute stress is blunted in adolescent rats that experienced neonatal maternal separation. Neuroscience. (2010) 171:144–52. doi: 10.1016/j.neuroscience.2010.08.063
140. Afonso VM, King SJ, Novakov M, Burton CL, Fleming AS. Accumbal dopamine function in postpartum rats that were raised without their mothers. Horm Behav. (2011) 60:632–43. doi: 10.1016/j.yhbeh.2011.08.016
141. Shepard RD, Langlois LD, Authement ME, Nugent FS. Histone deacetylase inhibition reduces ventral tegmental area dopamine neuronal hyperexcitability involving AKAP150 signaling following maternal deprivation in juvenile male rats. J Neurosci Res. (2020) 98:1457–67. doi: 10.1002/jnr.24613
142. Spyrka J, Gugula A, Rak A, Tylko G, Hess G, Blasiak A. Early life stress-induced alterations in the activity and morphology of ventral tegmental area neurons in female rats. Neurobiol Stress. (2020) 13:100250. doi: 10.1016/j.ynstr.2020.100250
143. Bonapersona V, Joëls M, Sarabdjitsingh RA. Effects of early life stress on biochemical indicators of the dopaminergic system: a 3 level meta-analysis of rodent studies. Neurosci Biobehav Rev. (2018) 95:1–16. doi: 10.1016/j.neubiorev.2018.09.003
144. Gracia-Rubio I, Valverde O, Martinez-Laorden E, Moscoso-Castro M, Milanés MV. Laorden ML maternal separation impairs cocaine-induced behavioural sensitization in adolescent. Mice. (2016) 11:e0167483. doi: 10.1371/journal.pone.0167483
145. Womersley JS, Hsieh JH, Kellaway LA, Gerhardt GA, Russell VA. Maternal separation affects dopamine transporter function in the spontaneously hypertensive rat: an in vivo electrochemical study. Behav Brain Funct. (2011) 7:49. doi: 10.1186/1744-9081-7-49
146. Ganguly P, Honeycutt JA, Rowe JR, Demaestri C, Brenhouse HC. Effects of early life stress on cocaine conditioning and AMPA receptor composition are sex-specific and driven by TNF. Brain Behav Immun. (2019) 78:41–51. doi: 10.1016/j.bbi.2019.01.006
147. Hearing MC, Jedynak J, Ebner SR, Ingebretson A, Asp AJ, Fischer RA, et al. Reversal of morphine-induced cell-type–specific synaptic plasticity in the nucleus accumbens shell blocks reinstatement. PNAS. (2016) 113:757–62. doi: 10.1073/pnas.1519248113
148. Sibley DR, Monsma FJ. Molecular biology of dopamine receptors. Trends Pharmacol Sci. (1992) 13:61–9. doi: 10.1016/0165-6147(92)90025-2
149. Gingrich JA, Caron MG. Recent Advances in the Molecular Biology of Dopamine Receptors. Annu Rev Neurosci. (1993) 16:299–321. doi: 10.1146/annurev.ne.16.030193.001503
150. Baik J-H. Dopamine Signaling in reward-related behaviors. Front Neural Circ. (2013) 7:1–16. doi: 10.3389/fncir.2013.00152
151. Francis TC, Chandra R, Friend DM, Finkel E, Dayrit G, Miranda J, et al. Nucleus accumbens medium spiny neuron subtypes mediate depression-related outcomes to social defeat stress. Biol Psychiat. (2014) 7:212–22 doi: 10.1016/j.biopsych.2014.07.021
152. Peña CJ. D1 and D2 type medium spiny neuron contributions to depression. Biol Psychiatry. (2017) 81:636–8. doi: 10.1016/j.biopsych.2017.02.010
153. Green NFO, Maniam J, Riese J, Morris MJ, Voineagu I. Transcriptomic signature of early life stress in male rat prefrontal cortex. Neurobiol Stress. (2021) 14:100316. doi: 10.1016/j.ynstr.2021.100316
154. Hodes GE, Pfau ML, Purushothaman I, Ahn HF, Golden SA, Christoffel DJ, et al. Sex differences in nucleus accumbens transcriptome profiles associated with susceptibility versus resilience to subchronic variable stress. J Neurosci. (2015) 35:16362–76. doi: 10.1523/JNEUROSCI.1392-15.2015
155. Labonté B, Engmann O, Purushothaman I, Menard C, Wang J, Tan C, et al. Sex-specific transcriptional signatures in human depression. Nat Med. (2017) 23:1102–11. doi: 10.1038/nm.4386
156. Parel ST, Peña CJ. Genome-wide signatures of early life stress: influence of sex. Biol Psychiat. (2020) doi: 10.1016/j.biopsych.2020.12.010. [Epub ahead of print].
157. Marrocco J, Gray JD, Kogan JF, Einhorn NR, O'Cinneide EM, Rubin TG, et al. Early life stress restricts translational reactivity in CA3 neurons associated with altered stress responses in adulthood. Front Behav Neurosci. (2019) 13:321. doi: 10.3389/fnbeh.2019.00157
158. Rowson SA, Bekhbat M, Kelly SD, Binder EB, Hyer MM, Shaw G, et al. Chronic adolescent stress sex-specifically alters the hippocampal transcriptome in adulthood. Neuropsychopharmacology. (2019) 44:1207–15. doi: 10.1038/s41386-019-0321-z
159. Sugiyama S, Di Nardo AA, Aizawa S, Matsuo I, Volovitch M, Prochiantz A, et al. Experience-dependent transfer of Otx2 Homeoprotein into the visual cortex activates postnatal plasticity. Cell. (2008) 134:508–20. doi: 10.1016/j.cell.2008.05.054
160. Beurdeley M, Spatazza J, Lee HHC, Sugiyama S, Bernard C, Di Nardo AA, et al. Otx2 binding to perineuronal nets persistently regulates plasticity in the mature visual cortex. J Neurosci. (2012) 32:9429–37. doi: 10.1523/JNEUROSCI.0394-12.2012
161. Apulei J, Kim N, Testa D, Ribot J, Morizet D, Bernard C, et al. Non-cell autonomous OTX2 homeoprotein regulates visual cortex plasticity through Gadd45b/g. Cerebral Cortex. (2018) 17:3165. doi: 10.1093/cercor/bhy108
162. Lobo MK, Covington HE, Chaudhury D, Friedman AK, Sun H, Damez-Werno D, et al. Cell type-specific loss of BDNF signaling mimics optogenetic control of cocaine reward. Science. (2010) 330:385–90. doi: 10.1126/science.1188472
163. Koo JW, Mazei-Robison MS, Chaudhury D, Juarez B, Laplant Q, Ferguson D, et al. BDNF is a negative modulator of morphine action. Science. (2012) 338:124–8. doi: 10.1126/science.1222265
164. Bath KG, Schilit A, Lee FS. Stress effects on BDNF expression: Effects of age, sex, and form of stress. Neuroscience. (2013) 239:149–56. doi: 10.1016/j.neuroscience.2013.01.074
165. Prowse N, Dwyer Z, Thompson A, Fortin T, Elson K, Robeson H, et al. Early life selective knockdown of the TrkB receptor and maternal separation modulates adult stress phenotype. Behav Brain Res. (2020) 378:112260. doi: 10.1016/j.bbr.2019.112260
166. Nylander I, Todkar A, Granholm L, Vrettou M, Bendre M, Boon W, et al. Evidence for a link between Fkbp5/FKBP5, early life social relations and alcohol drinking in young adult rats and humans. Mol Neurobiol. (2017) 54:6225–34. doi: 10.1007/s12035-016-0157-z
167. Chang L, Kigar SL, Ho JH, Cuarenta A, Gunderson HC, Baldo BA, et al. Early life stress alters opioid receptor mRNA levels within the nucleus accumbens in a sex-dependent manner. Brain Res. (2019) 1710:102–8. doi: 10.1016/j.brainres.2018.12.040
168. Mitchell SJ, Maguire EP, Cunningham L, Gunn BG, Linke M, Zechner U, et al. Early-life adversity selectively impairs α2-GABAA receptor expression in the mouse nucleus accumbens and influences the behavioral effects of cocaine. Neuropharmacology. (2018) 141:98–112. doi: 10.1016/j.neuropharm.2018.08.021
169. Zhu X, Li T, Peng S, Ma X, Chen X, Zhang X. Maternal deprivation-caused behavioral abnormalities in adult rats relate to a non-methylation-regulated D2 receptor levels in the nucleus accumbens. Behav Brain Res. (2010) 209:281–8. doi: 10.1016/j.bbr.2010.02.005
170. Majcher-Maślanka I, Solarz A, Wedzony K, Chocyk A. The effects of early-life stress on dopamine system function in adolescent female rats. Int J Dev Neurosci. (2017) 57:24–33. doi: 10.1016/j.ijdevneu.2017.01.001
171. Romano-López A, Méndez-Díaz M, García FG, Regalado-Santiago C, Ruiz-Contreras AE, Prospéro-García O. Maternal separation and early stress cause long-lasting effects on dopaminergic and endocannabinergic systems and alters dendritic morphology in the nucleus accumbens and frontal cortex in rats. Dev Neurobiol. (2016) 76:819–31. doi: 10.1002/dneu.22361
172. Jaenisch R, Bird A. Epigenetic regulation of gene expression: how the genome integrates intrinsic and environmental signals. Nat Genet. (2003) 33:245–54. doi: 10.1038/ng1089
173. Jin B, Li Y, Robertson KD, DNA. Methylation: Superior or Subordinate in the Epigenetic Hierarchy? Genes Cancer. (2011) 2:607–17. doi: 10.1177/1947601910393957
174. Anier K, Malinovskaja K, Pruus K, Aonurm-Helm A, Zharkovsky A, Kalda A. Maternal separation is associated with DNA methylation and behavioural changes in adult rats. Eur Neuropsychopharmacol. (2013) 24:459–68. doi: 10.1016/j.euroneuro.2013.07.012
175. Vrettou M, Granholm L, Todkar A, Nilsson KW, Wallén-Mackenzie Å, Nylander I, et al. Ethanol affects limbic and striatal presynaptic glutamatergic and DNA methylation gene expression in outbred rats exposed to early-life stress. Addict Biol. (2017) 22:369–80. doi: 10.1111/adb.12331
176. Jenuwein T, Allis CD. Translating the histone code. Science. (2001) 293:1074–80. doi: 10.1126/science.1063127
177. Shepard RD, Gouty S, Kassis H, Berenji A, Zhu W, Cox BM, et al. Targeting histone deacetylation for recovery of maternal deprivation-induced changes in BDNF and AKAP150 expression in the VTA. Exp Neurol. (2018) 309:160–8. doi: 10.1016/j.expneurol.2018.08.002
178. Kronman H, Torres-Berrío A, Sidoli S, Issler O, Godino A, Ramakrishnan A, et al. Long-term behavioral and cell-type-specific molecular effects of early life stress are mediated by H3K79me2 dynamics in medium spiny neurons. Nat Neurosci. (2021) 24:667–76. doi: 10.1038/s41593-021-00814-8
179. Lepack AE, Bagot RC, Peña CJ, Loh Y-HE, Farrelly LA, Lu Y, et al. Aberrant H33 dynamics in NAc promote vulnerability to depressive-like behavior. Proc Natl Acad Sci USA. (2016) 113:12562–7. doi: 10.1073/pnas.1608270113
180. Hanson JL, Chung MK, Avants BB, Shirtcliff EA, Gee JC, Davidson RJ, et al. Early stress is associated with alterations in the orbitofrontal cortex: a tensor-based morphometry investigation of brain structure and behavioral risk. J Neurosci. (2010) 30:7466–72. doi: 10.1523/JNEUROSCI.0859-10.2010
181. Brito SAD, Viding E, Sebastian CL, Kelly PA, Mechelli A, Maris H, et al. Reduced orbitofrontal and temporal grey matter in a community sample of maltreated children. J Child Psychol Psychiat. (2013) 54:105–12. doi: 10.1111/j.1469-7610.2012.02597.x
182. Holz NE, Boecker R, Hohm E, Zohsel K, Buchmann AF, Blomeyer D, et al. The long-term impact of early life poverty on orbitofrontal cortex volume in adulthood: results from a prospective study over 25 years. Neuropsychopharmacol. (2015) 40:996–1004. doi: 10.1038/npp.2014.277
183. McLaughlin KA, Green JG, Gruber MJ, Sampson NA, Zaslavsky AM, Kessler RC. Childhood adversities and first onset of psychiatric disorders in a national sample of US adolescents. Arch Gen Psychiatry. (2012) 69:1151–60. doi: 10.1001/archgenpsychiatry.2011.2277
184. Danese A, Widom CS. Objective and subjective experiences of child maltreatment and their relationships with psychopathology. Nat Hum Behav. (2020) 4:811–8. doi: 10.1038/s41562-020-0880-3
185. Appel AE, Holden GW. The co-occurrence of spouse and physical child abuse: A review and appraisal. J Family Psychol. (1998) 12:578–99. doi: 10.1037/0893-3200.12.4.578
186. Emery RE, Laumann-Billings L. An overview of the nature, causes, and consequences of abusive family relationships. Toward differentiating maltreatment and violence. Am Psychol. (1998) 53:121–35. doi: 10.1037//0003-066x.53.2.121
187. Kellogg ND, Menard SW. Violence among family members of children and adolescents evaluated for sexual abuse. Child Abuse Negl. (2003) 27:1367–76. doi: 10.1016/j.chiabu.2003.10.008
188. Belsky J, Schlomer GL, Ellis BJ. Beyond cumulative risk: distinguishing harshness and unpredictability as determinants of parenting and early life history strategy. Dev Psychol. (2012) 48:662–73. doi: 10.1037/a0024454
189. Lyons DM, Parker KJ, Schatzberg AF. Animal models of early life stress: implications for understanding resilience. Dev Psychobiol. (2010) 52:616–24. doi: 10.1002/dev.20500
190. Masten AS. Ordinary magic. Resilience processes in development. Am Psychol. (2001) 56:227–38. doi: 10.1037//0003-066x.56.3.227
191. Lyons DM, Parker KJ. Stress inoculation-induced indications of resilience in monkeys. J Trauma Stress. (2007) 20:423–33. doi: 10.1002/jts.20265
192. Santarelli S, Lesuis SL, Wang X-D, Wagner KV, Hartmann J, Labermaier C, Scharf SH, Müller MB, Holsboer F, Schmidt MV. Evidence supporting the match/mismatch hypothesis of psychiatric disorders. Eur Neuropsychopharmacol. (2014) 24:907–18. doi: 10.1016/j.euroneuro.2014.02.002
193. Santarelli S, Zimmermann C, Kalideris G, Lesuis SL, Arloth J, Uribe A, et al. An adverse early life environment can enhance stress resilience in adulthood. Psychoneuroendocrinology. (2017) 78:213–21. doi: 10.1016/j.psyneuen.2017.01.021
194. Parker KJ, Buckmaster CL, Schatzberg AF, Lyons DM. Prospective investigation of stress inoculation in young monkeys. Arch Gen Psychiatry. (2004) 61:933–41. doi: 10.1001/archpsyc.61.9.933
195. Lyons DM, Afarian H, Schatzberg AF, Sawyer-Glover A, Moseley ME. Experience-dependent asymmetric variation in primate prefrontal morphology. Behav Brain Res. (2002) 136:51–9. doi: 10.1016/s0166-4328(02)00100-6
196. Parker KJ, Buckmaster CL, Justus KR, Schatzberg AF, Lyons DM. Mild early life stress enhances prefrontal-dependent response inhibition in monkeys. Biol Psychiatry. (2005) 57:848–55. doi: 10.1016/j.biopsych.2004.12.024
197. Hsiao Y-M, Tsai T-C, Lin Y-T, Chen C-C, Huang C-C, Hsu K-S. Early life stress dampens stress responsiveness in adolescence: evaluation of neuroendocrine reactivity and coping behavior. Psychoneuroendocrinology. (2016) 67:86–99. doi: 10.1016/j.psyneuen.2016.02.004
198. Lazarus RS, Folkman S. Transactional theory and research on emotions and coping. Eur J Pers. (1987) 1:141–69. doi: 10.1002/per.2410010304
199. Monroe SM, Harkness KL. Life stress, the “kindling” hypothesis, and the recurrence of depression: considerations from a life stress perspective. Psychol Rev. (2005) 112:417–45. doi: 10.1037/0033-295X.112.2.417
200. Hammen C, Henry R, Daley SE. Depression and sensitization to stressors among young women as a function of childhood adversity. J Consult Clin Psychol. (2000) 68:782. doi: 10.1037/0022-006X.68.5.782
201. Dougherty LR, Klein DN, Davila J A. growth curve analysis of the course of dysthymic disorder: the effects of chronic stress and moderation by adverse parent-child relationships and family history. J Consult Clin Psychol. (2004) 72:1012–21. doi: 10.1037/0022-006X.72.6.1012
202. Kendler KS, Kuhn JW, Prescott CA. Childhood sexual abuse, stressful life events and risk for major depression in women. Psychol Med. (2004) 34:1475–82.
203. Harkness KL, Bruce AE, Lumley MN. The role of childhood abuse and neglect in the sensitization to stressful life events in adolescent depression. J Abnorm Psychol. (2006) 115:730–41. doi: 10.1037/0021-843X.115.4.730
204. Espejo EP, Hammen CL, Connolly NP, Brennan PA, Najman JM, Bor W. Stress sensitization and adolescent depressive severity as a function of childhood adversity: a link to anxiety disorders. J Abnorm Child Psychol. (2007) 35:287–99. doi: 10.1007/s10802-006-9090-3
205. Saxton K, Chyu L. Early life adversity increases the salience of later life stress: an investigation of interactive effects in the PSID. J Dev Orig Health Dis. (2019) 147:1–12. doi: 10.1017/S2040174419000308
206. Wade M, Zeanah CH, Fox NA, Tibu F, Ciolan LE, Nelson CA. Stress sensitization among severely neglected children and protection by social enrichment. Nat Commun. (2019) 10:5771. doi: 10.1038/s41467-019-13622-3
207. Gray JD, Rubin TG, Hunter RG, McEwen BS. Hippocampal gene expression changes underlying stress sensitization and recovery. Mol Psychiatry. (2014) 19:1171–8. doi: 10.1038/mp.2013.175
208. Franco AJ, Chen C, Scullen T, Zsombok A, Salahudeen AA, Di S, Herman JP, et al. Sensitization of the Hypothalamic-Pituitary-Adrenal Axis in a Male Rat Chronic Stress Model. Endocrinology. (2016) 157:2346–55. doi: 10.1210/en.2015-1641
209. Ferle V, Repouskou A, Aspiotis G, Raftogianni A, Chrousos G, Stylianopoulou F, et al. Synergistic effects of early life mild adversity and chronic social defeat on rat brain microglia and cytokines. Physiol Behav. (2020) 215:112791. doi: 10.1016/j.physbeh.2019.112791
210. Huang J, Shen C, Ye R, Shi Y, Li W. The effect of early maternal separation combined with adolescent chronic unpredictable mild stress on behavior and synaptic plasticity in adult female rats. Front Psychiat. (2021) 12:1–11. doi: 10.3389/fpsyt.2021.539299
211. Lupien SJ, Mcewen BS, Gunnar MR, Heim C. Effects of stress throughout the lifespan on the brain, behaviour and cognition. Nat Rev Neurosci. (2009) 10:434–45. doi: 10.1038/nrn2639
212. Eck SR, Ardekani CS, Salvatore M, Luz S, Kim ED, Rogers CM, et al. The effects of early life adversity on growth, maturation, and steroid hormones in male and female rats. Eur J Neurosci. (2020) 52:2664–80. doi: 10.1111/ejn.14609
213. Callaghan B, Meyer H, Opendak M, Van Tieghem M, Harmon C, Li A, et al. Using a developmental ecology framework to align fear neurobiology across species. Annu Rev Clin Psychol. (2019) 15:345–69. doi: 10.1146/annurev-clinpsy-050718-095727
214. Galván A. The teenage brain: sensitivity to rewards. Curr Dir Psychol Sci. (2013) 22:88–93. doi: 10.1177/0963721413480859
215. Andersen SL, Thompson AT, Rutstein M, Hostetter JC, Teicher MH. Dopamine receptor pruning in prefrontal cortex during the periadolescent period in rats. Synapse. (2000) 37:167–9. doi: 10.1002/1098-2396(200008)37:2<167::AID-SYN11>3.0.CO;2-B
216. Brenhouse HC, Sonntag KC, Andersen SL. Transient D1 dopamine receptor expression on prefrontal cortex projection neurons: relationship to enhanced motivational salience of drug cues in adolescence. J Neurosci. (2008) 28:2375–82. doi: 10.1523/JNEUROSCI.5064-07.2008
217. Tarazi FI, Baldessarini RJ. Comparative postnatal development of dopamine D(1), D(2) and D(4) receptors in rat forebrain. Int J Dev Neurosci. (2000) 18:29–37. doi: 10.1016/s0736-5748(99)00108-2
218. Teicher MH, Andersen SL, Hostetter JC. Evidence for dopamine receptor pruning between adolescence and adulthood in striatum but not nucleus accumbens. Brain Res Develop Brain Res. (1995) 89:167–72.
219. Vyas A, Mitra R, Rao BSS, Chattarji S. Chronic stress induces contrasting patterns of dendritic remodeling in hippocampal and amygdaloid neurons. J Neurosci. (2002) 22:6810–8. doi: 10.1523/JNEUROSCI.22-15-06810.2002
220. Rincón-Cortés M, Sullivan RM. Early life trauma and attachment: immediate and enduring effects on neurobehavioral and stress axis development. Front Endocrinol. (2014) 5:33. doi: 10.3389/fendo.2014.00033
221. Sullivan RM, Opendak M. Neurobiology of infant fear and anxiety: impacts of delayed amygdala development and attachment figure quality. Biol Psychiatry. (2021) 89:641–50. doi: 10.1016/j.biopsych.2020.08.020
222. Zhang W, Rosenkranz JA. Repeated restraint stress increases basolateral amygdala neuronal activity in an age-dependent manner. Neuroscience. (2012) 226:459–74. doi: 10.1016/j.neuroscience.2012.08.051
223. Padival M, Quinette D, Rosenkranz JA. Effects of repeated stress on excitatory drive of basal amygdala neurons in vivo. Neuropsychopharmacology. (2013) 38:1748–62. doi: 10.1038/npp.2013.74
224. Hanson JL, Nacewicz BM. Amygdala allostasis and early life adversity: considering excitotoxicity and inescapability in the sequelae of stress. Front Hum Neurosci. (2021) 15:624705. doi: 10.3389/fnhum.2021.624705
225. Hodes GE, Epperson CN. Sex Differences in vulnerability and resilience to stress across the life span. Biol Psychiatry. (2019) 86:421–32. doi: 10.1016/j.biopsych.2019.04.028
226. Boney-McCoy S, Finkelhor D. Prior victimization: a risk factor for child sexual abuse and for PTSD-related symptomatology among sexually abused youth. Child Abuse Negl. (1995) 19:1401–21. doi: 10.1016/0145-2134(95)00104-9
227. Harrison PA, Fulkerson JA, Beebe TJ. Multiple substance use among adolescent physical and sexual abuse victims. Child Abuse Negl. (1997) 21:529–39. doi: 10.1016/s0145-2134(97)00013-6
228. Andersen SL, Dumont NL. Teicher MH. Developmental differences in dopamine synthesis inhibition by (±)-7-OH-DPAT: Naunyn-Schmiedeberg's. Arch Pharmacol. (1997) 356:173–81. doi: 10.1007/PL00005038
229. Andersen SL, Teicher MH. Sex differences in dopamine receptors and their relevance to ADHD. Neurosci Biobehav Rev. (2000) 24:137–41.
230. Huys QJM, Browning M, Paulus MP, Frank MJ. Advances in the computational understanding of mental illness. Neuropsychopharmacol. (2021) 46:3–19. doi: 10.1038/s41386-020-0746-4
231. Haber SN, Knutson B. The reward circuit: linking primate anatomy and human imaging. Neuropsychopharmacol. (2010) 35:4–26. doi: 10.1038/npp.2009.129
232. Shepherd GMG. Corticostriatal connectivity and its role in disease. Nat Rev Neurosci. (2013) 14:278–91. doi: 10.1038/nrn3469
233. Becker S, Bräscher A-K, Bannister S, Bensafi M, Calma-Birling D, Chan RCK, et al. The role of hedonics in the human affectome. Neurosci Biobehav Rev. (2019) 102:221–41. doi: 10.1016/j.neubiorev.2019.05.003
234. Amodio DM, Frith CD. Meeting of minds: the medial frontal cortex and social cognition. Nat Rev Neurosci. (2006) 7:268–77. doi: 10.1038/nrn1884
235. van Harmelen A-L, Hauber K, Gunther Moor B, Spinhoven P, Boon AE, Crone EA, et al. Childhood emotional maltreatment severity is associated with dorsal medial prefrontal cortex responsivity to social exclusion in young adults. PLoS One. (2014) 9:e85107. doi: 10.1371/journal.pone.0085107
236. Romens SE, Casement MD, McAloon R, Keenan K, Hipwell AE, Guyer AE, et al. Adolescent girls' neural response to reward mediates the relation between childhood financial disadvantage and depression. J Child Psychol Psychiatry. (2015) 56:1177–84. doi: 10.1111/jcpp.12410
237. Kumar S, Hultman R, Hughes D, Michel N, Katz BM, Dzirasa K. Prefrontal cortex reactivity underlies trait vulnerability to chronic social defeat stress. Nat Commun. (2014) 5:4537. doi: 10.1038/ncomms5537
Keywords: early life stress (ELS), reward, nucleus accumbens (NAc), ventral tegmental area (VTA), development, ventral striatum
Citation: Hanson JL, Williams AV, Bangasser DA and Peña CJ (2021) Impact of Early Life Stress on Reward Circuit Function and Regulation. Front. Psychiatry 12:744690. doi: 10.3389/fpsyt.2021.744690
Received: 20 July 2021; Accepted: 21 September 2021;
Published: 20 October 2021.
Edited by:
Julia C. Lemos, University of Minnesota Twin Cities, United StatesReviewed by:
Matthew Wanat, University of Texas at San Antonio, United StatesAndrew Burke, Indiana University School of Medicine, United States
Copyright © 2021 Hanson, Williams, Bangasser and Peña. This is an open-access article distributed under the terms of the Creative Commons Attribution License (CC BY). The use, distribution or reproduction in other forums is permitted, provided the original author(s) and the copyright owner(s) are credited and that the original publication in this journal is cited, in accordance with accepted academic practice. No use, distribution or reproduction is permitted which does not comply with these terms.
*Correspondence: Catherine J. Peña, Y3BlbmFAcHJpbmNldG9uLmVkdQ==