- 1Department of Psychiatry, Changxing People's Hospital, Huzhou, China
- 2Department of Psychiatry, Zhejiang Hospital, Hangzhou, China
- 3Department of Radiology, The Second Affiliated Hospital, Zhejiang University School of Medicine, Hangzhou, China
- 4Department of Psychiatry, The Second Affiliated Hospital, Zhejiang University School of Medicine, Hangzhou, China
Mounting evidence demonstrates a close relationship between sleep disturbance and mood disorders, including major depression disorder (MDD) and bipolar disorder (BD). According to the classical two-process model of sleep regulation, circadian rhythms driven by the light–dark cycle, and sleep homeostasis modulated by the sleep–wake cycle are disrupted in mood disorders. However, the exact mechanism of interaction between sleep and mood disorders remains unclear. Recent discovery of the glymphatic system and its dynamic fluctuation with sleep provide a plausible explanation. The diurnal variation of the glymphatic circulation is dependent on the astrocytic activity and polarization of water channel protein aquaporin-4 (AQP4). Both animal and human studies have reported suppressed glymphatic transport, abnormal astrocytes, and depolarized AQP4 in mood disorders. In this study, the “glymphatic dysfunction” hypothesis which suggests that the dysfunctional glymphatic pathway serves as a bridge between sleep disturbance and mood disorders is proposed.
Introduction
Mood disorders are a group of complex debilitating psychiatric diseases identified by symptoms centered on markedly disrupted emotions, including major depressive disorder (MDD) and bipolar disorder (BD) (1). Due to their high prevalence, the risk for recurrence and suicide, they remain a serious health concern worldwide (2, 3). However, the exact neurobiological mechanisms underlying mood disorders remain unclear, resulting in unsatisfactory treatment (2, 3).
Sleep disturbance is a common concomitant and prodromal symptom of mood disorders (1, 4, 5). Specifically, both the two processes of sleep regulation—circadian oscillator and sleep pressure—are disrupted in mood disorders (4, 6). On one hand, circadian rhythms are approximately 24-h patterns in physiology and behavior, which are regulated by molecular clocks in the suprachiasmatic nuclei (SCN) of the hypothalamus (7). Mounting evidence suggests that there are abnormalities of the clock genes in mood disorders, such as single nucleotide polymorphisms (SNPs) (8–13), gene expression (14, 15), and gene–gene interactions (8). Excitingly, antidepressants including fluoxetine (16–18), ketamine (19, 20), and agomelatine (21) can reset the circadian clock along with the amelioration of mood symptoms. On the other hand, sleep pressure fluctuates with the sleep–wake cycle (6). Whereas, disturbance of the sleep–wake cycle has often been reported in mood disorders (22–24). Disturbed sleep architecture, especially decreased percentage of stage 3 non-rapid eye movement sleep (NREM III), represents decreased homeostatic drive for sleep (6). Actually, NREM III serves as a deep and recovery sleep, playing a vital role in the operation of the glymphatic system, and clearance of metabolic wastes (25, 26).
The glymphatic system is considered as an effective waste-removal system in the brain, which facilitates the exchange between the cerebrospinal fluid (CSF) and interstitial fluid (ISF), along with the potentially neurotoxic proteins such as amyloid-β (Aβ) (27), tau protein (28), and α-synuclein (29). Therefore, glymphatic impairment caused by sleep disturbance results in protein aggregation and increased risk for neurological diseases, such as Alzheimer's disease (AD) (30), Parkinson's disease (PD) (31), stroke (32, 33), and idiopathic normal cranial pressure hydrocephalus (iNPH) (34, 35). The water channel protein aquaporin-4 (AQP-4) is highly expressed on astrocytic endfeet and exerts significant influence in glymphatic transport (36). At present, accumulating evidence suggests the presence of abnormal astrocytes (37–43), depolarized AQP-4 (44–46), and dysfunctional glymphatic system (47, 48) in mood disorders. Therefore, we speculated that glymphatic dysfunction serves as an imperative intermediary factor between sleep disturbance and mood disorders.
In this study, we integrated available data from both animal and human studies regarding sleep in mood disorders and highlighted the core role of the glymphatic system. Furthermore, we discussed the glymphatic system dysfunction in mood disorders and identified the potential therapeutic opportunities for mood disorders based on sleep regulation and the glymphatic pathway.
Sleep Disturbance and Mood Disorders
The Model of Sleep Regulation
The classical two-process model of sleep regulation was first proposed by Borbély, and it consists of the process controlled by the circadian oscillator (Process C) and the homeostatic drive for the sleep–wake cycle (Process S). The two processes closely interact with each other but are also relatively independent (6) (Figure 1).
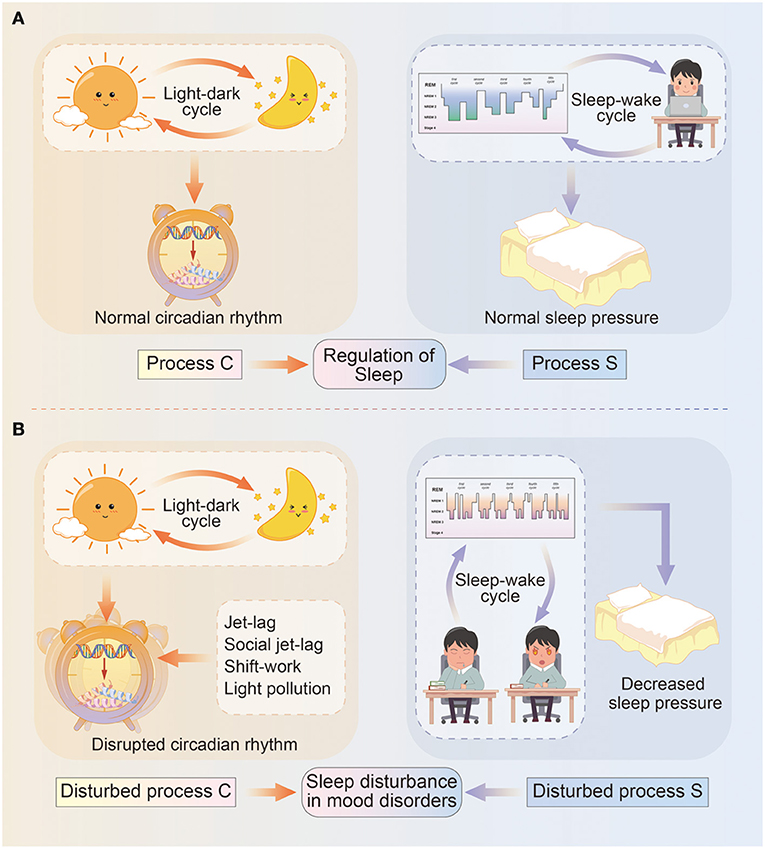
Figure 1. Diagram illustrating the two-process model of sleep regulation. (A) In normal circumstances, sleep regulation depends on the interaction between process C and process S. Specifically, process C represents the circadian rhythm driven by light–dark cycles, and circadian genes deliver circadian information via transcriptional–translational feedback loops and control physical and behavioral states. Process S means sleep pressure influenced by sleep–wake cycles, and include sleep architecture and daytime wakefulness. (B) In mood disorders, circadian rhythms (process C) are misaligned with light–dark cycles due to events such as jet-lag, social jet-lag, shift-work, light pollution, and so on; while sleep pressure (process S) is remarkably decreased due to longer sleep onset latency, a higher percentage of REM sleep, daytime sleepiness, or reduced need for sleep.
Circadian rhythms (Process C) are approximately 24-h rhythms in physiology and behavior, which are primarily driven by a hierarchy of cellular pacemakers located in the SCN (7). The most common measurements of the circadian rhythm are core body temperature and endogenous melatonin, other than the chronotype or morningness-eveningness (49). In fact, circadian rhythms are generated by a molecular clock in a network of positive and negative feedback loops. At the core of SCN timekeeping, the heterodimeric transcription factors CLOCK/BMAL1 translated from CLOCK and Brain and muscle ARNT-like 1 (BMAL1) genes, activate the Period (PER1–3) and Cryptochrome (CRY1–2) genes and initiate the circadian cycle. In turn, the dimer complex protein PER/CRY inhibit the activity of the CLOCK/BMAL1 proteins (50), exerting dominant effect in the negative feedback. As a critical complementary loop, the BMAL1 transcription is activated by the retinoic acid-related orphan receptor (ROR) protein at night, and repressed by the nuclear receptors REV-ERB α/β (encoded by NR1D1/2 genes) at daytime (51), respectively. In addition, other clock genes also participate in the regulation of circadian rhythms. The neuronal PAS domain protein 2 (NPAS2) functions similarly to CLOCK, while albumin gene D-site binding protein (DBP) acts cooperatively with CLOCK/BMAL1 (52, 53). The casein kinase I isoform δ/ε (CSNK1D/E) regulates levels of PER by phosphorylation-mediated degradation, and thus inhibits the activity of CLOCK/BMAL1 (54). The basic helix-loop-helix family 40/41 (BHLHE40/41, also known as DEC1/2) suppresses PER gene transcription via competing with CLOCK-BMAL1 for e-box element binding (55). The TIMELESS gene is also conceived required for circadian rhythmicity, however, the exact role in human clockwork is still unclear (56). These circadian genes expression rise and fall in rhythm, contributing to the regulation of 24-h physical and behavioral cycles (15).
Process S, also referred to as the sleep pressure gradually accumulates during wakefulness and declines during sleep (6). Especially, as deep sleep (NREM III) dominates in the early phases of sleep and dwindles with decreasing sleep pressure in the late phases. Conversely, sleep deficit such as sleep deprivation results in a longer and deeper NREM III to achieve recovery (57), implying greater sleep pressure. Therefore, NREM III sleep is considered as a representation of sleep pressure (6). Sleep electroencephalogram (EEG) and actigraphy are effective assessments of sleep pressure to detect sleep architecture.
According to the two-process model, proper alignment of Process C and S is essential for recovery sleep. Otherwise, the daytime sleep fails to fulfill the homeostatic sleep drive, manifesting as lighter and lacking of recovery sleep (NREM III) (58). Moreover, the daytime sleep decreases sleep pressure, causing a negative influence on the more effective nighttime sleep.
Sleep Disturbance in Mood Disorders
Disturbed Circadian Rhythms in Mood Disorders
Disruptions of the circadian rhythms are common in people exposed to jet-lag, social jet-lag, shift-work, as well as light pollution (light exposure at night) (59), and may lead to mood alterations (60, 61). Recently, a large population cross-sectional study (n = 91,105) using a wrist-worn accelerometer reported that lower relative amplitude of the circadian rhythm is associated with the lifetime prevalence of both MDD and BD (4). Individuals with circadian misalignment have higher depressive scores (62, 63). Moreover, a strong correlation between depressive symptoms and advances in dim light melatonin onset (DLMO) has been reported following an adjunctive multimodal chronobiological intervention organically combining psychoeducation, behavioral manipulation, and agomelatine intake (64). Bipolar disorder patients show delayed and decreased melatonin secretion during depressive and euthymic episodes (24, 65), with impaired psychosocial functioning and worse quality of life (24). In addition, manic and mixed episodes present with sustained phase advances, as well as a lower degree of rhythmicity corresponding to the severity of manic symptoms (66, 67). Apart from the daily (solar) cycle mentioned above, the lunar tidal cycles seem to entrain the mood cycles. In patients with rapid cycling BD, the periodicities in mood cycles have been observed to be synchronous with multiples of bi-weekly lunar tidal cycles (68).
The relationship between circadian rhythms and mood disorders is further supported by emerging genomic studies. In depressive cases, genetic association analyses have found SNPs in PER2 (10870), BMAL1 (rs2290035), NPAS2 (S471L), CRY2 (rs10838524), BHLHB2 (rs6442925), CLOCK (rs12504300), CSNK1E (rs135745), and TIMELESS (rs4630333 and rs1082214) (8, 9, 13). Single nucleotide polymorphisms in CSNK1E (rs135745), TIMELESS rs4630333, CRY2 (rs10838524), PER3 (rs707467 and rs10462020), RORB (rs1157358, rs7022435, rs3750420, and rs3903529), REV-ERBA (rs2314339) are strongly related to BD (8, 10–12, 69). In particular, CLOCK SNP rs1801260 contribute to the recurrence of mood episodes, while CRY2 SNP rs10838524 is significantly associated to rapid cycling BD (10, 70). Moreover, the arrhythmic expression of circadian genes including BMAL1, PER1–3, REV-ERBA, DBP, and BHLHE40/41, has been observed in postmortem brain tissues of MDD patients (15). Reduced amplitude of rhythmic expression for BMAL1, REV-ERBα, and DBP has been reported in fibroblast cultures of 12 BD patients (14). Recently, Park et al. have explored gene–gene interactions of clock genes using the non-parametric model-free multifactor-dimensionality reduction (MDR) method, and revealed optimal SNP combination models for predicting mood disorders (8). Specifically, the four-locus model differs between MDD (TIMELESS rs4630333, CSNK1E rs135745, BHLHB2 rs2137947, CSNK1E rs2075984) and BD (TIMELESS rs4630333, CSNK1E rs135745, PER3 rs228669, CLOCK rs12649507), supporting the clinical observation of different circadian characteristics in two disorders.
The Unbalanced Homeostatic Drive of Sleep in Mood Disorders
The sleep–wake cycle is significantly affected by mood disorders. Firstly, a disturbed sleep–wake cycle is one of the most common diagnostic criteria for mood disorders. Individuals suffering from manic or hypomanic episodes often show a reduced demand for the sleep, while depressive patients experience insomnia or hypersomnia (1). Delayed sleep–wake phase and evening chronotype is common in patients with mood disorders (24, 71, 72), and strongly associated with the severity of mood symptoms (73). Sleep deficits predict a poor prognosis with a higher risk of suicide (74). Furthermore, both polysomnography and self-reported studies have revealed longer sleep onset latency, a higher percentage of rapid eye movement (REM) sleep, more fragmentation of the sleep/wake rhythm, and daytime dysfunction in patients with mood disorders during the remission state relative to healthy controls (22, 75, 76). More importantly, sleep disturbance often serves as a prodrome of manic or depressive episodes. Several retrospective studies have revealed that sleep disturbance is the most robust early symptom of manic episodes and the sixth most common prodromal symptom of manic episodes (5, 23). Recently, a 10-year prospective study among adolescents and young adults reported that the sleep problem is a risk factor for the development of BD (77). Sleep abnormalities have also been highly related to subsequent depression (23, 78, 79). Moreover, sleep deprivation is reported to trigger manic-like behavior in animal models (80). Thus, some researchers speculate that a disturbed sleep–wake cycle is probably a causal factor triggering mood episodes. However, because of ethical reasons, sleep generally cannot be manipulated in human research and this weakens the causal evidence between the sleep–wake rhythm and mood disorders.
Chronotherapeutic Treatments in Mood Disorders
In response to the vital roles that Process C and S play in the onset and course of mood disorders, chronotherapeutic interventions have been successfully used. Sleep deprivation combined with bright light therapy has been implicated in improving depressive symptoms (72, 81–83), while virtual darkness therapy via blue-light-blocking increases the regularity of sleep and a rapid decline in manic symptoms (84). These treatments exert great influence on mood recovery by resetting the circadian clock. Also, the hormone melatonin (MT) secreted by the pineal gland acts on the circadian clock via MT1 receptors (85, 86), while the MT agonist agomelatine shows important properties for phase shifts of the clock and anti-depressive effects (21). Additionally, agomelatine functions as an antagonist for 5-HT2c receptors and modulates the master SCN clock via 5-HT innervations (87, 88). Similarly, other antidepressants can regulate the expression of the clock genes and thus affect the circadian rhythms (89). Fluoxetine, a selective serotonin reuptake inhibitor (SSRI) can shift electrical rhythms of the SCN and thus affect the behavior rhythm (16–18). Ketamine results in a rapid increase in glutamate level in the SCN and directly acts on NMDA receptors of the circadian clock in the epi-thalamic lateral habenula (LHb) (19, 20), suggesting that the rapid anti-depressive effects of ketamine might also be through the resetting of the circadian system (90). However, the mood stabilizer lithium is considered a clock-modifying drug in that it delays the sleep–wake cycle in healthy human and increase the length of the circadian period in non-human primates (91, 92). At the molecular level, lithium treatment can not only regulate the rhythm period via increasing PER2 mRNA levels, but also significantly augment the oscillation amplitude PER2 and CRY1 protein rhythms via inhibiting the phosphorylation of glycogen synthase kinase 3β (GSK3B) (93, 94). Furthermore, the lithium efficacy is influenced by two GSK3B SNPs (rs334558 and rs3755557) (95). Considering all the above evidence, more pharmacological manipulations targeting the circadian rhythm and sleep drive are increasingly becoming plausible in the treatment of mood disorders.
Taken together, there seems to be a clear link between sleep disturbance and mood disorders, even though the underlying mechanisms remain unclear. The discovery of the glymphatic system provides researchers with insights into sleep-related diseases.
Sleep and the Glymphatic System
Overview of the Glymphatic System
The lymphatic system accounts for the clearance of ISF and it is also critical to both hydrostatic and homeostatic maintenance (96). With regard to lymphatic system in central nervous system (CNS), it consists of two interacting system, the glymphatic (glia-lymphatic) system and the meningeal lymphatic vessels (97). The glymphatic system is responsible for exchanging between CSF and ISF, and clearing solutes and metabolites from the brain parenchyma through a unique system of perivascular tunnels. More specifically, CSF produced by the choroid plexus and capillary influx is pumped deep into the brain parenchyma via arterial pulsation (36, 98). In the perivascular space (PVS), CSF exchanges with ISF, accompanied by clearance of soluble metabolic waste like Aβ (36). Indeed, large and eccentric PVS provides considerably less hydraulic resistance to CSF-ISF flow compared to concentric annular tunnel (99, 100). During the clearance of solutes, convection coexists with diffusion in the glymphatic system (101–103). It is argued that in the brain interstitium, small molecule transport is best explained by diffusion while convection becomes more predominant with increasing molecular size (104). However, the exact contributions of the two processes are highly dynamic and remain controversial, with one of the reasons being that the glymphatic influx and efflux are influenced by arousal state, pulse, respiration, body position, and more (98, 103, 105, 106). Moreover, CSF–ISF and solutes drain from the CNS via meningeal and cervical lymphatic vessels, as well as the cranial and spinal nerve roots (107, 108). Therefore, interference of the lymphatic system, such as ultraviolet photoablation of meningeal lymphatic vessels and ligation of cervical lymphatics, accounts for the stagnation of glymphatic flow and aggregation of metabolic wastes like Aβ (109, 110).
More importantly, the glymphatic system is supported by the water channel AQP4 which is primarily expressed by the astrocytic endfeet (36). Animals lacking AQP4 exhibit slower CSF influx and less interstitial solute clearance (70% reduction) (36, 111, 112). Deletion of the AQP4 in APP/PS1 transgenic mice results in increased interstitial Aβ plaque accumulation, cerebral amyloid angiopathy, as well as loss of synaptic protein and brain-derived neurotrophic factor in the hippocampus and cortex (113). However, it should be noted that the role of AQP4 in glymphatic clearance function are debated (103, 106). Smith et al. have found that AQP4 gene deletion mice exhibited a similar Aβ distribution as wildtype mice, suggesting that AQP4 gene deletion did not impair clearance of Aβ (114).
Sleep-Dependent Glymphatic Cycling
Emerging evidence reveals that the function of the glymphatic system fluctuates daily along with the sleep–wake cycle. A two-photon imaging study reported a 60% increase in the interstitial space and two-fold faster clearance of Aβ in natural sleep or anesthesia mice compared with awake mice (27). A coherent pattern of slow-wave activity and CSF influx has been observed during NREM sleep in humans, supporting the exciting possibility of sleep-regulated glymphatic function (25). However, recent evidence using contrast-enhanced MRI has revealed that the glymphatic system is controlled by the circadian rhythm rather than by the sleep–wake cycle (115, 116). The parenchymal redistribution of contrast agent is lowest during the light phase and highest during the dark phase in fully awake rats, regardless of normal or reversed light–dark cycles (115). The diurnal variation of glymphatic cycling persists even under constant light or anesthesia, suggesting the hypothesis that endogenous circadian oscillations determine glymphatic function (116). The discrepancy may be related to the extreme differences in the circadian rhythm between humans and rodents (117). Rodents are nocturnal animals with opposite circadian phase, and they are also poly-phasic sleepers with relatively low sleep drive (118). Presently, the exact contributions of the light–dark cycle, sleep–wake cycle, and other physiological rhythms remain unknown (116). Further studies are warranted to confirm the circadian control of the glymphatic system in humans.
Surprisingly, the deletion of AQP4 effectively eliminates the circadian rhythm in glymphatic fluid transport (116). A recent genomic study reports that AQP4-haplotype influences sleep homeostasis in NREM sleep and response to prolonged wakefulness (119), providing supporting evidence for the sleep-dependent glymphatic pathway. The high polarization of AQP4 in astrocytic endfeet is under the control of the circadian rhythm, and thus, modulates bulk fluid movement, CSF–ISF exchange, and solutes clearance (116). Conversely, there is also evidence that astrocytes repress SCN neurons and regulate circadian timekeeping via glutamate signaling (120). Thus, astrocytes and AQP4 present a checkpoint for the functional glymphatic system during deep sleep.
Considerable evidence suggests a causal relationship between sleep and regulation of the glymphatic flow, thus modulating protein clearance. Sleep disturbance (including shorter total sleep time, sleep fragmentation, and lack of NREM III) causes suppressed glymphatic function and a decline in the clearance of metabolic waste, hence contributing to the development and progression of various neurological diseases including AD (30), PD (31), stroke (32, 33), and iNPH (34, 35).
Taken together, the glymphatic function is considered as a brain fluid transport with astrocyte-regulated mechanisms, while glymphatic dysfunction is intimately associated with neurological diseases, especially neurodegenerative diseases with cognitive decline (30, 31).
Glymphatic Dysfunction in Mood Disorders
Abnormalities of Glymphatic Flow, Astrocytes, and AQP4 in Depression
Individuals suffering from depressive episodes always show diverse cognitive decline (1), including attention, memory, response inhibition, decision speed, and so on. Depression has been considered as a prodrome of dementia (121), with increased Aβ deposition reported in an (18) F-florbetapir positron emission tomography (PET) imaging study (122). These observations raise the exciting possibility that wide-spread disruption of the glymphatic system exists in depression. Recent animal studies using chronic unpredictable mild stress (CUMS) model have provided supporting evidence for the glymphatic dysfunction in depression (47, 48) (Table 1). In the CUMS model, animals were exposed to the various stressors randomly for several weeks and injected with fluorescence tracers from cisterna magna to estimate the glymphatic function (47, 48). The CSF tracer penetration in the brain of CUMS-treated mice was significantly decreased, and recovered to the control level after fluoxetine administration or polyunsaturated fatty acid (PUFA) supplementation (47, 48). In parallel with the impaired glymphatic circulation, the increased deposition of Aβ has been observed (47, 48). Amyloid-β accumulation along the blood vessels, in turn, could impair glymphatic function by reducing PVS and increasing hydraulic resistance, and thus result in a more severe parenchymal build-up of Aβ and neuronal death (134). Another plausible explanation of PVS closure induced by CUMS is the alteration of arterial pulsation and compliance that triggered by neuroinflammation and restored by daily PUFA supplementation (48) (Table 1).
During the neuroinflammatory response, reactive astrocytosis, and AQP4 depolarization have been widely reported in depression (48). Abundant evidence indicated astrocytic abnormalities in patients with depression (Table 2). Golgi-staining of postmortem tissues from depressed suicide cases has revealed reactive astrocytosis within the cingulate cortex (37). Additionally, glial fibrillary acidic protein (GFAP), one of the astrocyte-specific biomarkers, is reduced in depression-associated brain regions including the prefrontal cortex, cingulate cortex (38, 39), hippocampus (40), amygdala (41), locus coeruleus (44), cerebellum (146), thalamus, and caudate nuclei (42). A lower density of S100β-immunopositive astrocytes has been reported in the bilateral hippocampus and locus coeruleus of depressive patients compared to that of healthy controls (44, 135). Downregulated expression of AQP4 has been found in postmortem locus coeruleus and hippocampus in MDD patients (44, 136). More importantly, the reduction in astrocyte density is passed on to offsprings of depressive females via an epigenetic mechanism (123) (Table 1). Nevertheless, there are several contradictory results (Table 2). The density of astrocytes has been observed unchanged in the cingulate cortex and hippocampus of MDD patients (142, 144). A postmortem study using quantitative polymerase chain reaction (qPCR) have observed upregulated expression of GFAP and aldehyde dehydrogenase 1 family member L1 (ALDH1L1) in the basal ganglia of MDD patients (145). Another postmortem study using microarray analysis and qPCR has found upregulated expression of AQP4 in the prefrontal cortex of MDD patients. Obviously, the variety of studied methods involving Glogi-staining, Nissl-staining, qPCR, western blotting, and immunohistochemistry, contributes to the discrepancies.
However, emerging animal studies provide powerful evidence implying the pathological alterations of astrocytes and AQP4 in depression. Decreased astrocytes and downregulated AQP4 expression have been reported in various animal models of depression (47, 48, 123, 124, 130) (Table 1), supporting dysfunctional glymphatic transport in depression. Effective antidepressant therapy, such as fluoxetine (47, 124, 125), escitalopram (48), mirtazapine (126), ketamine (127, 128), and repetitive high-frequency transcranial magnetic stimulation (TMS) (129) could benefit the functioning of both astrocytes and AQP4, and hence alleviate depressive-like behaviors. Additionally, the synergistic agents of antidepressant—lithium—can attenuate the reduction of AQP4 and disruption of the neurovascular unit in the hippocampus of CUMS rats (130), resulting in a functioning glymphatic system. These therapeutic effects can be suppressed by AQP4 knockout. More specifically, AQP4 deficiency abolishes fluoxetine treatment-induced hippocampal neurogenesis and behavioral improvement in depressive mice (133). Recent studies indicate that the therapeutic option for depression is via the restoration of astrocytes function, AQP4, and glymphatic system (131, 132), which provide further supporting evidence for the critical role of glymphatic flow in depression.
Abnormalities of Astrocytes and AQP4 in Bipolar Disorders
To date, the role of the glymphatic function in BD has not been widely studied. However, astrocytic dysfunction has undoubtedly been implicated in the development of BD (43). Different from MDD, pictures from human postmortem studies in BD appear to be highly heterogeneous (Table 2). The density of GFAP-positive astrocytes is reported to be significantly increased in Brodmann area (BA) 9 (137) and reduced in BA10 (138), BA24 (38), BA11, and BA 47 (139), while the level of S100β has been reported to be increased in BA40 and reduced in BA9 (140). Other studies on human postmortem tissues from BD exhibit an unchanged density of astrocytes in the frontal cortex (141), cingulate cortex (142), amygdala (41, 143), hippocampus (144), entorhinal cortex (143), basal ganglia (145), dorsal raphe nucleus, and cerebellum (146). The considerable discrepancy is on account of various confounding factors, including phenotype (depressive episode, manic episode, or remission state) (150), cause of death (depressive suicide or physical diseases) (141, 144), comorbidity (150, 151), the methodology used (137, 144), and the brain regions studied (139, 140). Therefore, additional studies regarding diverse phenotypes of BD are essential to investigate state-related abnormalities of astrocytes (152). In patients with bipolar depression, a reduction in S100β-immunopositive astrocytes has been observe, but with no change in GFAP-immunopositive astrocytes (135, 147). As for manic states, in vivo studies have revealed increased serum levels of S100β, suggesting astrocytic activation (148).
Upregulated expression of AQP4 in the prefrontal cortex has been revealed in BD (149). Evaluation of the qualitative alterations of astrocytes (especially AQP4 function) is far much valuable than quantitative alterations. The apparent diffusion coefficient from ultra-high b-values (ADC uh), a parameter of enhanced diffusion-weighted imaging (eDWI), can reflect the function of AQP4 (45). In individuals suffering from bipolar depression, increased ADC uh values in bilateral superior cerebellar peduncles (SCP) and cerebellar hemisphere is positively associated with depressive scores, implying that a positive correlation exists between the upregulated expression of AQP4 and severity of depression (46). A plausible explanation is that increased and depolarized AQP4 impair water homeostasis and glymphatic transport in BD (149). Lithium is a classical mood-stabilizer, and its effect of regulating AQP4 function is discussed above (130). Additionally, other mood-stabilizers such as valproic acid, topiramate, and lamotrigine have been shown to inhibit AQP4 (153), and hence regulate directed glymphatic flow.
Even though direct evidence for glymphatic impairment in mood disorders is lacking, astrocytes and AQP4 abnormalities provide support to the hypothesis that glymphatic dysfunction functions as a bridge between sleep disturbance and mood disorders. Additionally, treatments for mood improvement, including medicines, light therapy, sleep invention, and TMS can regulate the function of astrocytes and AQP4. Therefore, AQP4-dependent glymphatic system may serve as a new therapeutic target in mood disorders.
Conclusion and Outlook
Mood symptoms often occur with the onset of sleep disturbance and ameliorate with improved sleep disturbance. Moreover, early-life sleep problems due to jet-lag, social jet-lag, shift-work, or light pollution can significantly increase the lifetime risk of mood disorders (60). In addition, sleep deprivation can directly trigger mania-like symptoms (80). Based on considerable evidence, a causal relationship between sleep disturbance and mood disorders is hypothesized (154). Therefore, how does disrupted sleep affect the development and phenotype of mood disorders? An intriguing possibility has emerged that glymphatic dysfunction serves as a bridge between sleep disturbance and mood disorders. Adequate sleep, especially deep sleep (NREM III), is a key factor in the functioning of the glymphatic system which accounts for the clearance of metabolic wastes. The effects of sleep on the glymphatic system are mainly dependent on the dynamic alterations of astrocytic function and AQP4 distribution (113, 119, 155). Significantly, suppressed glymphatic circulation, astrocytic abnormalities, and AQP4 depolarization are consistently reported in mood disorders, providing support for the posited hypothesis.
However, several limitations exist in this study. First, much of the existing evidence on the glymphatic system has been conducted in rodents and only a few in humans. Although sleep is an evolutionarily conserved physiological behavior, the reversed circadian rhythms and polyphasic sleep which reduces sleep pressure in rodents make it less representative. Most of the current human studies use invasive methods such as intrathecal injection of contrast agents, while the ADCuh value obtained from the emerging eDWI fails to identify the distribution of AQP4. Therefore, non-invasive methods to explore the glymphatic system in humans are necessary for future studies. Secondly, there is a lack of evidence of known metabolic wastes that fail to be cleared by the glymphatic system and trigger or exacerbate mood symptoms, such as Aβ in AD and α-synuclein in PD. Exploring the excessive metabolic wastes in mood disorders is warranted, and can provide promising biomarkers for indicating the occurrence and severity of mood disorders.
Data Availability Statement
The original contributions presented in the study are included in the article/supplementary material, further inquiries can be directed to the corresponding author/s.
Author Contributions
TY, YQ, and LY defined the research questions and aims of the study. TY and YQ carried out the literature search, selected and interpreted relevant articles, and wrote the first draft of the manuscript. XY made the original figure and tables. LY and XY critically appraised the texts, figure and tables, corrected them, and made suggestions for further improvement. All authors contributed to the article and approved the submitted version.
Funding
This work was funded by National Natural Science Foundation of China (81901319 and 81901706).
Conflict of Interest
The authors declare that the research was conducted in the absence of any commercial or financial relationships that could be construed as a potential conflict of interest.
Abbreviations
MDD, major depression disorder; BD, bipolar disorder; AQP4, aquaporin-4; SCN, suprachiasmatic nuclei; SNP, single nucleotide polymorphisms; NREM III, stage 3 non-rapid eye movement sleep; CSF, cerebrospinal fluid; ISF, interstitial fluid; Aβ, amyloid-β; AD, Alzheimer's disease; PD, Parkinson's disease; iNPH, idiopathic normal cranial pressure hydrocephalus; EEG, electroencephalogram; DLMO, dim light melatonin onset; MDR, multifactor-dimensionality reduction; REM, rapid eye movement sleep; MT, melatonin; SSRI, selective serotonin reuptake inhibitor; LHb, lateral habenula; ROS, reactive oxygen species; CNS, central nervous system; PET, positron emission tomography; RBD, REM sleep behavior disorder; DTI, diffusion tensor imaging; ALPS, analysis along the perivascular space; CUMS, chronic unpredictable mild stress; PUFA, polyunsaturated fatty acid; GFAP, glial fibrillary acidic protein; TMS, transcranial magnetic stimulation; BA, Brodmann area; ADC uh, the apparent diffusion coefficient from ultra-high b-values; eDWI, enhanced diffusion-weighted imaging; SCP, superior cerebellar peduncles; PVS, perivascular space; ALDH1L1, aldehyde dehydrogenase 1 family member L1; qPCR, quantitative polymerase chain reaction.
References
1. American Psychiatric Association. Diagnostic and Statistical Manual of Mental Disorders (DSM). 5th edition. Washington, DC: American Psychiatric Publishing (2013).
2. Grande I, Berk M, Birmaher B, Vieta E. Bipolar disorder. Lancet (London, England). (2016) 387:1561–72. doi: 10.1016/S0140-6736(15)00241-X
3. O'Leary OF Dinan TG Cryan JF. Faster, better, stronger: towards new antidepressant therapeutic strategies. Eur J Pharmacol. (2015) 753:32–50. doi: 10.1016/j.ejphar.2014.07.046
4. Lyall LM, Wyse CA, Graham N, Ferguson A, Lyall DM, Cullen B, et al. Association of disrupted circadian rhythmicity with mood disorders, subjective wellbeing, and cognitive function: a cross-sectional study of 91 105 participants from the UK Biobank. Lancet Psychiatry. (2018) 5:507–14. doi: 10.1016/S2215-0366(18)30139-1
5. Jackson A, Cavanagh J, Scott J. A systematic review of manic and depressive prodromes. J Affect Disord. (2003) 74:209–17. doi: 10.1016/S0165-0327(02)00266-5
6. Borbély AA, Daan S, Wirz-Justice A, Deboer T. The two-process model of sleep regulation: a reappraisal. J Sleep Res. (2016) 25:131–43. doi: 10.1111/jsr.12371
7. Yamazaki S, Numano R, Abe M, Hida A, Takahashi R, Ueda M, et al. Resetting central and peripheral circadian oscillators in transgenic rats. Science. (2000) 288:682–5. doi: 10.1126/science.288.5466.682
8. Park M, Kim SA, Yee J, Shin J, Lee KY, Joo EJ. Significant role of gene-gene interactions of clock genes in mood disorder. J Affect Disord. (2019) 257:510–7. doi: 10.1016/j.jad.2019.06.056
9. Lavebratt C, Sjöholm LK, Soronen P, Paunio T, Vawter MP, Bunney WE, et al. CRY2 is associated with depression. PLoS ONE. (2010) 5:e9407. doi: 10.1371/journal.pone.0009407
10. Sjöholm LK, Backlund L, Cheteh EH, Ek IR, Frisén L, Schalling M, et al. CRY2 is associated with rapid cycling in bipolar disorder patients. PLoS ONE. (2010) 5:e12632. doi: 10.1371/journal.pone.0012632
11. Brasil Rocha PM, Campos SB, Neves FS, da Silva Filho HC. Genetic association of the PERIOD3 (Per3) clock gene with bipolar disorder. Psychiatry Investig. (2017) 14:674–80. doi: 10.4306/pi.2017.14.5.674
12. McGrath CL, Glatt SJ, Sklar P, Le-Niculescu H, Kuczenski R, Doyle AE, et al. Evidence for genetic association of RORB with bipolar disorder. BMC Psychiatry. (2009) 9:70. doi: 10.1186/1471-244X-9-70
13. Partonen T, Treutlein J, Alpman A, Frank J, Johansson C, Depner M, et al. Three circadian clock genes Per2, Arntl, and Npas2 contribute to winter depression. Ann Med. (2007) 39:229–38. doi: 10.1080/07853890701278795
14. Yang S, Van Dongen HP, Wang K, Berrettini W, Bućan M. Assessment of circadian function in fibroblasts of patients with bipolar disorder. Mol Psychiatry. (2009) 14:143–55. doi: 10.1038/mp.2008.10
15. Li JZ, Bunney BG, Meng F, Hagenauer MH, Walsh DM, Vawter MP, et al. Circadian patterns of gene expression in the human brain and disruption in major depressive disorder. Proc Natl Acad Sci USA. (2013) 110:9950–5. doi: 10.1073/pnas.1305814110
16. Horikawa K, Yokota S, Fuji K, Akiyama M, Moriya T, Okamura H, et al. Nonphotic entrainment by 5-HT1A/7 receptor agonists accompanied by reduced Per1 and Per2 mRNA levels in the suprachiasmatic nuclei. J Neurosci. (2000) 20:5867–73. doi: 10.1523/JNEUROSCI.20-15-05867.2000
17. Horikawa K, Shibata S. Phase-resetting response to (+)8-OH-DPAT, a serotonin 1A/7 receptor agonist, in the mouse in vivo. Neurosci Lett. (2004) 368:130–4. doi: 10.1016/j.neulet.2004.06.072
18. Shibata S, Tsuneyoshi A, Hamada T, Tominaga K, Watanabe S. Phase-resetting effect of 8-OH-DPAT, a serotonin1A receptor agonist, on the circadian rhythm of firing rate in the rat suprachiasmatic nuclei in vitro. Brain Res. (1992) 582:353–6. doi: 10.1016/0006-8993(92)90156-4
19. Orozco-Solis R, Montellier E, Aguilar-Arnal L, Sato S, Vawter MP, Bunney BG, et al. A circadian genomic signature common to ketamine and sleep deprivation in the anterior cingulate cortex. Biol Psychiatry. (2017) 82:351–60. doi: 10.1016/j.biopsych.2017.02.1176
20. Cui Y, Hu S, Hu H. Lateral habenular burst firing as a target of the rapid antidepressant effects of ketamine. Trends Neurosci. (2019) 42:179–91. doi: 10.1016/j.tins.2018.12.002
21. de Bodinat C, Guardiola-Lemaitre B, Mocaër E, Renard P, Muñoz C, Millan MJ. Agomelatine, the first melatonergic antidepressant: discovery, characterization and development. Nat Rev Drug Discov. (2010) 9:628–42. doi: 10.1038/nrd3140
22. Jones SH, Hare DJ, Evershed K. Actigraphic assessment of circadian activity and sleep patterns in bipolar disorder. Bipolar Disord. (2005) 7:176–86. doi: 10.1111/j.1399-5618.2005.00187.x
23. Jaussent I, Bouyer J, Ancelin ML, Akbaraly T, Pérès K, Ritchie K, et al. Insomnia and daytime sleepiness are risk factors for depressive symptoms in the elderly. Sleep. (2011) 34:1103–10. doi: 10.5665/SLEEP.1170
24. Bradley AJ, Webb-Mitchell R, Hazu A, Slater N, Middleton B, Gallagher P, et al. Sleep and circadian rhythm disturbance in bipolar disorder. Psychol Med. (2017) 47:1678–89. doi: 10.1017/S0033291717000186
25. Fultz NE, Bonmassar G, Setsompop K, Stickgold RA, Rosen BR, Polimeni JR, et al. Coupled electrophysiological, hemodynamic, and cerebrospinal fluid oscillations in human sleep. Science. (2019) 366:628–31. doi: 10.1126/science.aax5440
26. Hablitz LM, Vinitsky HS, Sun Q, Stæger FF, Sigurdsson B, Mortensen KN, et al. Increased glymphatic influx is correlated with high EEG delta power and low heart rate in mice under anesthesia. Sci Adv. (2019) 5:eaav5447. doi: 10.1126/sciadv.aav5447
27. Xie L, Kang H, Xu Q, Chen MJ, Liao Y, Thiyagarajan M, et al. Sleep drives metabolite clearance from the adult brain. Science. (2013) 342:373–7. doi: 10.1126/science.1241224
28. Simon MJ, Wang MX, Murchison CF, Roese NE, Boespflug EL, Woltjer RL, et al. Transcriptional network analysis of human astrocytic endfoot genes reveals region-specific associations with dementia status and tau pathology. Sci Rep. (2018) 8:12389. doi: 10.1038/s41598-018-30779-x
29. van Dijk KD, Bidinosti M, Weiss A, Raijmakers P, Berendse HW, van de Berg WD. Reduced α-synuclein levels in cerebrospinal fluid in Parkinson's disease are unrelated to clinical and imaging measures of disease severity. Eur J Neurol. (2014) 21:388–94. doi: 10.1111/ene.12176
30. Roh JH, Huang Y, Bero AW, Kasten T, Stewart FR, Bateman RJ, et al. Disruption of the sleep-wake cycle and diurnal fluctuation of β-amyloid in mice with Alzheimer's disease pathology. Sci Transl Med. (2012) 4:150ra122. doi: 10.1126/scitranslmed.3004291
31. Sundaram S, Hughes RL, Peterson E, Müller-Oehring EM, Brontë-Stewart HM, Poston KL, et al. Establishing a framework for neuropathological correlates and glymphatic system functioning in Parkinson's disease. Neurosci Biobehav Rev. (2019) 103:305–15. doi: 10.1016/j.neubiorev.2019.05.016
32. Gaberel T, Gakuba C, Goulay R, Martinez De Lizarrondo S, Hanouz JL, Emery E, et al. Impaired glymphatic perfusion after strokes revealed by contrast-enhanced MRI: a new target for fibrinolysis? Stroke. (2014) 45:3092–6. doi: 10.1161/STROKEAHA.114.006617
33. Pu T, Zou W, Feng W, Zhang Y, Wang L, Wang H, et al. Persistent malfunction of glymphatic and meningeal lymphatic drainage in a mouse model of subarachnoid hemorrhage. Exp Neurobiol. (2019) 28:104–18. doi: 10.5607/en.2019.28.1.104
34. Ringstad G, Vatnehol SAS, Eide PK. Glymphatic MRI in idiopathic normal pressure hydrocephalus. Brain. (2017) 140:2691–705. doi: 10.1093/brain/awx191
35. Ringstad G, Valnes LM, Dale AM, Pripp AH, Vatnehol SS, Emblem KE, et al. Brain-wide glymphatic enhancement and clearance in humans assessed with MRI. JCI Insight. (2018) 3:e121537. doi: 10.1172/jci.insight.121537
36. Iliff JJ, Wang M, Liao Y, Plogg BA, Peng W, Gundersen GA, et al. A paravascular pathway facilitates CSF flow through the brain parenchyma and the clearance of interstitial solutes, including amyloid β. Sci Transl Med. (2012) 4:147ra111. doi: 10.1126/scitranslmed.3003748
37. Torres-Platas SG, Hercher C, Davoli MA, Maussion G, Labonté B, Turecki G, et al. Astrocytic hypertrophy in anterior cingulate white matter of depressed suicides. Neuropsychopharmacology. (2011) 36:2650–8. doi: 10.1038/npp.2011.154
38. Webster MJ, O'Grady J, Kleinman JE, Weickert CS. Glial fibrillary acidic protein mRNA levels in the cingulate cortex of individuals with depression, bipolar disorder and schizophrenia. Neuroscience. (2005) 133:453–61. doi: 10.1016/j.neuroscience.2005.02.037
39. Gittins RA, Harrison PJ. A morphometric study of glia and neurons in the anterior cingulate cortex in mood disorder. J. Affect. Disord. (2011) 133:328–32. doi: 10.1016/j.jad.2011.03.042
40. Cobb JA, O'Neill K, Milner J, Mahajan GJ, Lawrence TJ, May WL, et al. Density of GFAP-immunoreactive astrocytes is decreased in left hippocampi in major depressive disorder. Neuroscience. (2016) 316:209–20. doi: 10.1016/j.neuroscience.2015.12.044
41. Altshuler LL, Abulseoud OA, Foland-Ross L, Bartzokis G, Chang S, Mintz J, et al. Amygdala astrocyte reduction in subjects with major depressive disorder but not bipolar disorder. Bipolar Disord. (2010) 12:541–9. doi: 10.1111/j.1399-5618.2010.00838.x
42. Torres-Platas SG, Nagy C, Wakid M, Turecki G, Mechawar N. Glial fibrillary acidic protein is differentially expressed across cortical and subcortical regions in healthy brains and downregulated in the thalamus and caudate nucleus of depressed suicides. Mol Psychiatry. (2016) 21:509–15. doi: 10.1038/mp.2015.65
43. Peng L, Li B, Verkhratsky A. Targeting astrocytes in bipolar disorder. Exp Rev. Neurotherap. (2016) 16:649–57. doi: 10.1586/14737175.2016.1171144
44. Bernard R, Kerman IA, Thompson RC, Jones EG, Bunney WE, Barchas JD, et al. Altered expression of glutamate signaling, growth factor, and glia genes in the locus coeruleus of patients with major depression. Mol Psychiatry. (2011) 16:634–46. doi: 10.1038/mp.2010.44
45. Xueying L, Zhongping Z, Zhoushe Z, Li G, Yongjin T, Changzheng S, et al. Investigation of apparent diffusion coefficient from ultra-high b-values in Parkinson's disease. Eur Radiol. (2015) 25:2593–600. doi: 10.1007/s00330-015-3678-3
46. Zhao L, Luo Z, Qiu S, Jia Y, Zhong S, Chen G, et al. Abnormalities of aquaporin-4 in the cerebellum in bipolar II disorder: an ultra-high b-values diffusion weighted imaging study. J Affect Disord. (2020) 274:136–43. doi: 10.1016/j.jad.2020.05.035
47. Xia M, Yang L, Sun G, Qi S, Li B. Mechanism of depression as a risk factor in the development of Alzheimer's disease: the function of AQP4 and the glymphatic system. Psychopharmacology (Berl). (2017) 234:365–79. doi: 10.1007/s00213-016-4473-9
48. Liu X, Hao J, Yao E, Cao J, Zheng X, Yao D, et al. Polyunsaturated fatty acid supplement alleviates depression-incident cognitive dysfunction by protecting the cerebrovascular and glymphatic systems. Brain Behav Immun. (2020) 89:357–70. doi: 10.1016/j.bbi.2020.07.022
49. Bauducco S, Richardson C, Gradisar M. Chronotype, circadian rhythms and mood. Curr Opin Psychol. (2020) 34:77–83. doi: 10.1016/j.copsyc.2019.09.002
50. Takahashi JS. Molecular components of the circadian clock in mammals. Diabetes Obes Metab. (2015) 17(Suppl. 1):6–11. doi: 10.1111/dom.12514
51. Preitner N, Damiola F, Lopez-Molina L, Zakany J, Duboule D, Albrecht U, et al. The orphan nuclear receptor REV-ERBalpha controls circadian transcription within the positive limb of the mammalian circadian oscillator. Cell. (2002) 110:251–60. doi: 10.1016/S0092-8674(02)00825-5
52. DeBruyne JP, Weaver DR, Reppert SM. CLOCK and NPAS2 have overlapping roles in the suprachiasmatic circadian clock. Nat Neurosci. (2007) 10:543–5. doi: 10.1038/nn1884
53. Yamaguchi S, Mitsui S, Yan L, Yagita K, Miyake S, Okamura H. Role of DBP in the circadian oscillatory mechanism. Mol Cell Biol. (2000) 20:4773–81. doi: 10.1128/MCB.20.13.4773-4781.2000
54. Aryal RP, Kwak PB, Tamayo AG, Gebert M, Chiu PL, Walz T, et al. Macromolecular assemblies of the mammalian circadian clock. Mol Cell. (2017) 67:770.e6–82.e6. doi: 10.1016/j.molcel.2017.07.017
55. Honma S, Kawamoto T, Takagi Y, Fujimoto K, Sato F, Noshiro M, et al. Dec1 and Dec2 are regulators of the mammalian molecular clock. Nature. (2002) 419:841–4. doi: 10.1038/nature01123
56. Mazzoccoli G, Laukkanen MO, Vinciguerra M, Colangelo T, Colantuoni V. A timeless link between circadian patterns and disease. Trends Mol Med. (2016) 22:68–81. doi: 10.1016/j.molmed.2015.11.007
57. Rodriguez AV, Funk CM, Vyazovskiy VV, Nir Y, Tononi G, Cirelli C. Why does sleep slow-wave activity increase after extended wake? Assessing the effects of increased cortical firing during wake and sleep. J. Neurosci. (2016) 36:12436–47. doi: 10.1523/JNEUROSCI.1614-16.2016
58. Nedergaard M, Goldman SA. Glymphatic failure as a final common pathway to dementia. Science. (2020) 370:50–6. doi: 10.1126/science.abb8739
59. Foster RG, Peirson SN, Wulff K, Winnebeck E, Vetter C, Roenneberg T. Sleep and circadian rhythm disruption in social jetlag and mental illness. Prog Mol Biol Transl Sci. (2013) 119:325–46. doi: 10.1016/B978-0-12-396971-2.00011-7
60. Inder ML, Crowe MT, Porter R. Effect of transmeridian travel and jetlag on mood disorders: evidence and implications. Aust N Z J Psychiatry. (2016) 50:220–7. doi: 10.1177/0004867415598844
61. Kalmbach DA, Pillai V, Cheng P, Arnedt JT, Drake CL. Shift work disorder, depression, and anxiety in the transition to rotating shifts: the role of sleep reactivity. Sleep Med. (2015) 16:1532–8. doi: 10.1016/j.sleep.2015.09.007
62. Nguyen C, Murray G, Anderson S, Filipowicz A, Ingram KK. In vivo molecular chronotyping, circadian misalignment, and high rates of depression in young adults. J Affect Disord. (2019) 250:425–31. doi: 10.1016/j.jad.2019.03.050
63. Murray JM, Sletten TL, Magee M, Gordon C, Lovato N, Bartlett DJ, et al. Prevalence of circadian misalignment and its association with depressive symptoms in delayed sleep phase disorder. Sleep. (2017) 40:zsw002. doi: 10.1093/sleep/zsw002
64. Robillard R, Carpenter JS, Feilds KL, Hermens DF, White D, Naismith SL, et al. Parallel changes in mood and melatonin rhythm following an adjunctive multimodal chronobiological intervention with agomelatine in people with depression: a proof of concept open label study. Front Psychiatry. (2018) 9:624. doi: 10.3389/fpsyt.2018.00624
65. Robillard R, Naismith SL, Rogers NL, Scott EM, Ip TK, Hermens DF, et al. Sleep-wake cycle and melatonin rhythms in adolescents and young adults with mood disorders: comparison of unipolar and bipolar phenotypes. Eur Psychiatry. (2013) 28:412–6. doi: 10.1016/j.eurpsy.2013.04.001
66. Salvatore P, Ghidini S, Zita G, De Panfilis C, Lambertino S, Maggini C, et al. Circadian activity rhythm abnormalities in ill and recovered bipolar I disorder patients. Bipolar Disord. (2008) 10:256–65. doi: 10.1111/j.1399-5618.2007.00505.x
67. Gonzalez R, Tamminga CA, Tohen M, Suppes T. The relationship between affective state and the rhythmicity of activity in bipolar disorder. J Clin Psychiatry. (2014) 75:e317–22. doi: 10.4088/JCP.13m08506
68. Wehr TA. Bipolar mood cycles and lunar tidal cycles. Mol Psychiatry. (2018) 23:923–31. doi: 10.1038/mp.2016.263
69. Kripke DF, Nievergelt CM, Joo E, Shekhtman T, Kelsoe JR. Circadian polymorphisms associated with affective disorders. J Circadian Rhythms. (2009) 7:2. doi: 10.1186/1740-3391-7-2
70. Lee KY, Song JY, Kim SH, Kim SC, Joo EJ, Ahn YM, et al. Association between CLOCK 3111T/C and preferred circadian phase in Korean patients with bipolar disorder. Prog Neuropsychopharmacol Biol Psychiatry. (2010) 34:1196–201. doi: 10.1016/j.pnpbp.2010.06.010
71. Drennan MD, Klauber MR, Kripke DF, Goyette LM. The effects of depression and age on the Horne-Ostberg morningness-eveningness score. J Affect Disord. (1991) 23:93–8. doi: 10.1016/0165-0327(91)90096-B
72. Maruani J, Geoffroy PA. Bright light as a personalized precision treatment of mood disorders. Front Psychiatry. (2019) 10:85. doi: 10.3389/fpsyt.2019.00085
73. Park H, Lee HK, Lee K. Chronotype and suicide: the mediating effect of depressive symptoms. Psychiatry Res. (2018) 269:316–20. doi: 10.1016/j.psychres.2018.08.046
74. Stange JP, Kleiman EM, Sylvia LG, Magalhães PV, Berk M, Nierenberg AA, et al. Specific mood symptoms confer risk for subsequent suicidal ideation in bipolar disorder with and without suicide attempt history: multi-wave data from step-BD. Depress Anxiety. (2016) 33:464–72. doi: 10.1002/da.22464
75. Cretu JB, Culver JL, Goffin KC, Shah S, Ketter TA. Sleep, residual mood symptoms, and time to relapse in recovered patients with bipolar disorder. J Affect Disord. (2016) 190:162–6. doi: 10.1016/j.jad.2015.09.076
76. Wichniak A, Wierzbicka A, Jernajczyk W. Sleep as a biomarker for depression. Int Rev Psychiatry. (2013) 25:632–45. doi: 10.3109/09540261.2013.812067
77. Ritter PS, Höfler M, Wittchen HU, Lieb R, Bauer M, Pfennig A, et al. Disturbed sleep as risk factor for the subsequent onset of bipolar disorder–data from a 10-year prospective-longitudinal study among adolescents and young adults. J Psychiatr Res. (2015) 68:76–82. doi: 10.1016/j.jpsychires.2015.06.005
78. Chang PP, Ford DE, Mead LA, Cooper-Patrick L, Klag MJ. Insomnia in young men and subsequent depression. The Johns Hopkins Precursors Study. Am J Epidemiol. (1997) 146:105–14. doi: 10.1093/oxfordjournals.aje.a009241
79. Buysse DJ, Angst J, Gamma A, Ajdacic V, Eich D, Rössler W. Prevalence, course, and comorbidity of insomnia and depression in young adults. Sleep. (2008) 31:473–80. doi: 10.1093/sleep/31.4.473
80. Arent CO, Valvassori SS, Steckert AV, Resende WR, Dal-Pont GC, Lopes-Borges J, et al. The effects of n-acetylcysteine and/or deferoxamine on manic-like behavior and brain oxidative damage in mice submitted to the paradoxal sleep deprivation model of mania. J Psychiatr Res. (2015) 65:71–9. doi: 10.1016/j.jpsychires.2015.04.011
81. Lopez-Rodriguez F, Kim J, Poland RE. Total sleep deprivation decreases immobility in the forced-swim test. Neuropsychopharmacology. (2004) 29:1105–11. doi: 10.1038/sj.npp.1300406
82. Zhang B, Gao Y, Li Y, Yang J, Zhao H. Sleep deprivation influences circadian gene expression in the lateral habenula. Behav Neurol. (2016) 2016:7919534. doi: 10.1155/2016/7919534
83. Terman M, Terman JS. Light therapy for seasonal and nonseasonal depression: efficacy, protocol, safety, and side effects. CNS Spectr. (2005) 10:647–63;quiz 672. doi: 10.1017/S1092852900019611
84. Henriksen TE, Skrede S, Fasmer OB, Hamre B, Grønli J, Lund A. Blocking blue light during mania - markedly increased regularity of sleep and rapid improvement of symptoms: a case report. Bipolar Disord. (2014) 16:894–8. doi: 10.1111/bdi.12265
85. Yokota H, Vijayasarathi A, Cekic M, Hirata Y, Linetsky M, Ho M, et al. Diagnostic performance of glymphatic system evaluation using diffusion tensor imaging in idiopathic normal pressure hydrocephalus and mimickers. Curr Gerontol Geriatr Res. (2019) 2019:5675014. doi: 10.1155/2019/5675014
86. Scott FF, Belle MD, Delagrange P, Piggins HD. Electrophysiological effects of melatonin on mouse Per1 and non-Per1 suprachiasmatic nuclei neurones in vitro. J Neuroendocrinol. (2010) 22:1148–56. doi: 10.1111/j.1365-2826.2010.02063.x
87. Antle MC, Ogilvie MD, Pickard GE, Mistlberger RE. Response of the mouse circadian system to serotonin 1A/2/7 agonists in vivo: surprisingly little. J Biol Rhythms. (2003) 18:145–58. doi: 10.1177/0748730403251805
88. Dudley TE, DiNardo LA, Glass JD. Endogenous regulation of serotonin release in the hamster suprachiasmatic nucleus. J Neurosci. (1998) 18:5045–52. doi: 10.1523/JNEUROSCI.18-13-05045.1998
89. Spencer S, Falcon E, Kumar J, Krishnan V, Mukherjee S, Birnbaum SG, et al. Circadian genes Period 1 and Period 2 in the nucleus accumbens regulate anxiety-related behavior. Eur J Neurosci. (2013) 37:242–50. doi: 10.1111/ejn.12010
90. Duncan WC Jr., Slonena E, Hejazi NS, Brutsche N, Yu KC, Park L, et al. Motor-activity markers of circadian timekeeping are related to ketamine's rapid antidepressant properties. Biol Psychiatry. (2017) 82:361–9. doi: 10.1016/j.biopsych.2017.03.011
91. Kripke DF, Judd LL, Hubbard B, Janowsky DS, Huey LY. The effect of lithium carbonate on the circadian rhythm of sleep in normal human subjects. Biol Psychiatry. (1979) 14:545–8.
92. Welsh DK, Moore-Ede MC. Lithium lengthens circadian period in a diurnal primate, Saimiri sciureus. Biol Psychiatry. (1990) 28:117–26. doi: 10.1016/0006-3223(90)90629-G
93. Li J, Lu WQ, Beesley S, Loudon AS, Meng QJ. Lithium impacts on the amplitude and period of the molecular circadian clockwork. PLoS ONE. (2012) 7:e33292. doi: 10.1371/journal.pone.0033292
94. Schnell A, Sandrelli F, Ranc V, Ripperger JA, Brai E, Alberi L, et al. Mice lacking circadian clock components display different mood-related behaviors and do not respond uniformly to chronic lithium treatment. Chronobiol Int. (2015) 32:1075–89. doi: 10.3109/07420528.2015.1062024
95. Iwahashi K, Nishizawa D, Narita S, Numajiri M, Murayama O, Yoshihara E, et al. Haplotype analysis of GSK-3β gene polymorphisms in bipolar disorder lithium responders and nonresponders. Clin Neuropharmacol. (2014) 37:108–10. doi: 10.1097/WNF.0000000000000039
96. Aukland K, Reed RK. Interstitial-lymphatic mechanisms in the control of extracellular fluid volume. Physiol Rev. (1993) 73:1–78. doi: 10.1152/physrev.1993.73.1.1
97. Louveau A, Plog BA, Antila S, Alitalo K, Nedergaard M, Kipnis J. Understanding the functions and relationships of the glymphatic system and meningeal lymphatics. J Clin Invest. (2017) 127:3210–9. doi: 10.1172/JCI90603
98. Mestre H, Tithof J, Du T, Song W, Peng W, Sweeney AM, et al. Flow of cerebrospinal fluid is driven by arterial pulsations and is reduced in hypertension. Nat Commun. (2018) 9:4878. doi: 10.1038/s41467-018-07318-3
99. Rey J, Sarntinoranont M. Pulsatile flow drivers in brain parenchyma and perivascular spaces: a resistance network model study. Fluids Barriers CNS. (2018) 15:20. doi: 10.1186/s12987-018-0105-6
100. Tithof J, Kelley DH, Mestre H, Nedergaard M, Thomas JH. Hydraulic resistance of periarterial spaces in the brain. Fluids Barriers CNS. (2019) 16:19. doi: 10.1186/s12987-019-0140-y
101. Koundal S, Elkin R, Nadeem S, Xue Y, Constantinou S, Sanggaard S, et al. Optimal mass transport with Lagrangian workflow reveals advective and diffusion driven solute transport in the glymphatic system. Sci Rep. (2020) 10:1990. doi: 10.1038/s41598-020-59045-9
102. Thomas JH. Fluid dynamics of cerebrospinal fluid flow in perivascular spaces. J R Soc Interface. (2019) 16:20190572. doi: 10.1098/rsif.2019.0572
103. Mestre H, Mori Y, Nedergaard M. The brain's glymphatic system: current controversies. Trends Neurosci. (2020) 43:458–66. doi: 10.1016/j.tins.2020.04.003
104. Ray L, Iliff JJ, Heys JJ. Analysis of convective and diffusive transport in the brain interstitium. Fluids Barriers CNS. (2019) 16:6. doi: 10.1186/s12987-019-0126-9
105. Kiviniemi V, Wang X, Korhonen V, Keinänen T, Tuovinen T, Autio J, et al. Ultra-fast magnetic resonance encephalography of physiological brain activity - glymphatic pulsation mechanisms? J Cereb Blood Flow Metab. (2016) 36:1033–45. doi: 10.1177/0271678X15622047
106. Christensen J, Yamakawa GR, Shultz SR, Mychasiuk R. Is the glymphatic system the missing link between sleep impairments and neurological disorders? Examining the implications and uncertainties. Prog Neurobiol. (2021) 198:101917. doi: 10.1016/j.pneurobio.2020.101917
107. Ma Q, Ries M, Decker Y, Müller A, Riner C, Bücker A, et al. Rapid lymphatic efflux limits cerebrospinal fluid flow to the brain. Acta Neuropathol. (2019) 137:151–65. doi: 10.1007/s00401-018-1916-x
108. Ahn JH, Cho H, Kim JH, Kim SH, Ham JS, Park I, et al. Meningeal lymphatic vessels at the skull base drain cerebrospinal fluid. Nature. (2019) 572:62–6. doi: 10.1038/s41586-019-1419-5
109. Wang L, Zhang Y, Zhao Y, Marshall C, Wu T, Xiao M. Deep cervical lymph node ligation aggravates AD-like pathology of APP/PS1 mice. Brain Pathol. (2019) 29:176–92. doi: 10.1111/bpa.12656
110. Da Mesquita S, Louveau A, Vaccari A, Smirnov I, Cornelison RC, Kingsmore KM, et al. Functional aspects of meningeal lymphatics in ageing and Alzheimer's disease. Nature. (2018) 560:185–91. doi: 10.1038/s41586-018-0368-8
111. Mestre H, Hablitz LM, Xavier AL, Feng W, Zou W, Pu T, et al. Aquaporin-4-dependent glymphatic solute transport in the rodent brain. Elife. (2018) 7:e40070. doi: 10.7554/eLife.40070
112. Tarasoff-Conway JM, Carare RO, Osorio RS, Glodzik L, Butler T, et al. Clearance systems in the brain-implications for Alzheimer disease. Nat Rev Neurol. (2015) 11:457–70. doi: 10.1038/nrneurol.2015.119
113. Xu Z, Xiao N, Chen Y, Huang H, Marshall C, Gao J, et al. Deletion of aquaporin-4 in APP/PS1 mice exacerbates brain Aβ accumulation and memory deficits. Mol Neurodegener. (2015) 10:58. doi: 10.1186/s13024-015-0056-1
114. Smith AJ, Yao X, Dix JA, Jin BJ, Verkman AS. Test of the 'glymphatic' hypothesis demonstrates diffusive and aquaporin-4-independent solute transport in rodent brain parenchyma. Elife. (2017) 6:e27679. doi: 10.7554/eLife.27679
115. Cai X, Qiao J, Kulkarni P, Harding IC, Ebong E, Ferris CF. Imaging the effect of the circadian light-dark cycle on the glymphatic system in awake rats. Proc. Natl. Acad. Sci. USA. (2020) 117:668–76. doi: 10.1073/pnas.1914017117
116. Hablitz LM, Plá V, Giannetto M, Vinitsky HS, Stæger FF, Metcalfe T, et al. Circadian control of brain glymphatic and lymphatic fluid flow. Nat Commun. (2020) 11:4411. doi: 10.1038/s41467-020-18115-2
117. Cederroth CR, Albrecht U, Bass J, Brown SA, Dyhrfjeld-Johnsen J, Gachon F, et al. Medicine in the fourth dimension. Cell Metab. (2019) 30:238–50. doi: 10.1016/j.cmet.2019.06.019
118. Simasko SM, Mukherjee S. Novel analysis of sleep patterns in rats separates periods of vigilance cycling from long-duration wake events. Behav Brain Res. (2009) 196:228–36. doi: 10.1016/j.bbr.2008.09.003
119. Ulv Larsen SM, Landolt HP, Berger W, Nedergaard M, Knudsen GM, Holst SC. Haplotype of the astrocytic water channel AQP4 is associated with slow wave energy regulation in human NREM sleep. PLoS Biol. (2020) 18:e3000623. doi: 10.1371/journal.pbio.3000623
120. Brancaccio M, Patton AP, Chesham JE, Maywood ES, Hastings MH. Astrocytes control circadian timekeeping in the suprachiasmatic nucleus via glutamatergic signaling. Neuron. (2017) 93:1420.e5–35.e5. doi: 10.1016/j.neuron.2017.02.030
121. Mirza SS, Wolters FJ, Swanson SA, Koudstaal PJ, Hofman A, Tiemeier H, et al. 10-year trajectories of depressive symptoms and risk of dementia: a population-based study. Lancet Psychiatry. (2016) 3:628–35. doi: 10.1016/S2215-0366(16)00097-3
122. Wu KY, Liu CY, Chen CS, Chen CH, Hsiao IT, Hsieh CJ, et al. Beta-amyloid deposition and cognitive function in patients with major depressive disorder with different subtypes of mild cognitive impairment: (18)F-florbetapir (AV-45/Amyvid) PET study. Eur J Nucl Med Mol Imaging. (2016) 43:1067–76. doi: 10.1007/s00259-015-3291-3
123. Gong Y, Sun X-L, Wu F-F, Su C-J, Ding J-H, Hu G. Female early adult depression results in detrimental impacts on the behavioral performance and brain development in offspring. CNS Neurosci Ther. (2012) 18:461–70. doi: 10.1111/j.1755-5949.2012.00324.x
124. Czéh B, Simon M, Schmelting B, Hiemke C, Fuchs E. Astroglial plasticity in the hippocampus is affected by chronic psychosocial stress and concomitant fluoxetine treatment. Neuropsychopharmacology. (2006) 31:1616–26. doi: 10.1038/sj.npp.1300982
125. Kinoshita M, Hirayama Y, Fujishita K, Shibata K, Shinozaki Y, Shigetomi E. Anti-depressant fluoxetine reveals its therapeutic effect via astrocytes. EBioMedicine. (2018) 32:72–83. doi: 10.1016/j.ebiom.2018.05.036
126. Hisaoka-Nakashima K, Taki S, Watanabe S, Nakamura Y, Nakata Y, Morioka N. Mirtazapine increases glial cell line-derived neurotrophic factor production through lysophosphatidic acid 1 receptor-mediated extracellular signal-regulated kinase signaling in astrocytes. Eur J Pharmacol. (2019) 860:172539. doi: 10.1016/j.ejphar.2019.172539
127. Wang Y, Xie L, Gao C, Zhai L, Zhang N, Guo L. Astrocytes activation contributes to the antidepressant-like effect of ketamine but not scopolamine. Pharmacol Biochem Behav. (2018) 170:1–8. doi: 10.1016/j.pbb.2018.05.001
128. Lasič E, Lisjak M, Horvat A, BoŽić M, Šakanović A, Anderluh G, et al. Astrocyte specific remodeling of plasmalemmal cholesterol composition by ketamine indicates a new mechanism of antidepressant action. Sci Rep. (2019) 9:10957. doi: 10.1038/s41598-019-47459-z
129. Xue S-S, Xue F, Ma Q-R, Wang S-Q, Wang Y, Tan Q-R, et al. Repetitive high-frequency transcranial magnetic stimulation reverses depressive-like behaviors and protein expression at hippocampal synapses in chronic unpredictable stress-treated rats by enhancing endocannabinoid signaling. Pharmacol Biochem Behav. (2019) 184:172738. doi: 10.1016/j.pbb.2019.172738
130. Taler M, Aronovich R, Henry Hornfeld S, Dar S, Sasson E, Weizman A, et al. Regulatory effect of lithium on hippocampal blood-brain barrier integrity in a rat model of depressive-like behavior. Bipolar Disord. (2020) 23:55–65. doi: 10.1111/bdi.12962
131. Wang Y, Ni J, Zhai L, Gao C, Xie L, Zhao L, et al. Inhibition of activated astrocyte ameliorates lipopolysaccharide- induced depressive-like behaviors. J Affect Disord. (2019) 242:52–9. doi: 10.1016/j.jad.2018.08.015
132. Portal B, Delcourte S, Rovera R, Lejards C, Bullich S, Malnou CE, et al. Genetic and pharmacological inactivation of astroglial connexin 43 differentially influences the acute response of antidepressant and anxiolytic drugs. Acta Physiol. (Oxf). 2020:e13440. doi: 10.1111/apha.13440
133. Kong H, Sha LL, Fan Y, Xiao M, Ding JH, Wu J, Hu G. Requirement of AQP4 for antidepressive efficiency of fluoxetine: implication in adult hippocampal neurogenesis. Neuropsychopharmacology. (2009) 34:1263–76. doi: 10.1038/npp.2008.185
134. Hughes TM, Kuller LH, Barinas-Mitchell EJ, Mackey RH, McDade EM, Klunk WE, et al. Pulse wave velocity is associated with β-amyloid deposition in the brains of very elderly adults. Neurology. (2013) 81:1711–8. doi: 10.1212/01.wnl.0000435301.64776.37
135. Gos T, Schroeter ML, Lessel W, Bernstein H-G, Dobrowolny H, Schiltz K, et al. S100B-immunopositive astrocytes and oligodendrocytes in the hippocampus are differentially afflicted in unipolar and bipolar depression: a postmortem study. J Psychiatr Res. (2013) 47:1694–9. doi: 10.1016/j.jpsychires.2013.07.005
136. Medina A, Watson SJ, Bunney W Jr, Myers RM, Schatzberg A, Barchas J, et al. Evidence for alterations of the glial syncytial function in major depressive disorder. J Psychiatr Res. (2016) 72:15–21. doi: 10.1016/j.jpsychires.2015.10.010
137. Feresten AH, Barakauskas V, Ypsilanti A, Barr AM, Beasley CL. Increased expression of glial fibrillary acidic protein in prefrontal cortex in psychotic illness. Schizophr Res. (2013) 150:252–7. doi: 10.1016/j.schres.2013.07.024
138. Johnston-Wilson NL, Sims CD, Hofmann JP, Anderson L, Shore AD, Torrey EF, et al. Disease-specific alterations in frontal cortex brain proteins in schizophrenia, bipolar disorder, and major depressive disorder. The Stanley Neuropathology Consortium. Mol Psychiatry. (2000) 5:142–9. doi: 10.1038/sj.mp.4000696
139. Toro CT, Hallak JE, Dunham JS, Deakin JF. Glial fibrillary acidic protein and glutamine synthetase in subregions of prefrontal cortex in schizophrenia and mood disorder. Neurosci Lett. (2006) 404:276–81. doi: 10.1016/j.neulet.2006.05.067
140. Dean B, Gray L, Scarr E. Regionally specific changes in levels of cortical S100beta in bipolar 1 disorder but not schizophrenia. Aust N Z J Psychiatry. (2006) 40:217–24. doi: 10.1111/j.1440-1614.2006.01777.x
141. Hercher C, Chopra V, Beasley CL. Evidence for morphological alterations in prefrontal white matter glia in schizophrenia and bipolar disorder. J Psychiatry Neurosci. (2014) 39:376–85. doi: 10.1503/jpn.130277
142. Williams MR, Hampton T, Pearce RKB, Hirsch SR, Ansorge O, Thom M, et al. Astrocyte decrease in the subgenual cingulate and callosal genu in schizophrenia. Eur Arch Psychiatry Clin Neurosci. (2013) 263:41–52. doi: 10.1007/s00406-012-0328-5
143. Pantazopoulos H, Woo T-UW, Lim MP, Lange N, Berretta S. Extracellular matrix-glial abnormalities in the amygdala and entorhinal cortex of subjects diagnosed with schizophrenia. Arch Gen Psychiatry. (2010) 67:155–66. doi: 10.1001/archgenpsychiatry.2009.196
144. Malchow B, Strocka S, Frank F, Bernstein H-G, Steiner J, Schneider-Axmann T, et al. Stereological investigation of the posterior hippocampus in affective disorders. J Neural Transm (Vienna). (2015) 122:1019–33. doi: 10.1007/s00702-014-1316-x
145. Barley K, Dracheva S, Byne W. Subcortical oligodendrocyte- and astrocyte-associated gene expression in subjects with schizophrenia, major depression and bipolar disorder. Schizophr Res. (2009) 112:54–64. doi: 10.1016/j.schres.2009.04.019
146. Fatemi SH, Laurence JA, Araghi-Niknam M, Stary JM, Schulz SC, Lee S, et al. Glial fibrillary acidic protein is reduced in cerebellum of subjects with major depression, but not schizophrenia. Schizophr Res. (2004) 69:317–23. doi: 10.1016/j.schres.2003.08.014
147. Steiner J, Bielau H, Brisch R, Danos P, Ullrich O, Mawrin C, et al. Immunological aspects in the neurobiology of suicide: elevated microglial density in schizophrenia and depression is associated with suicide. J Psychiatr Res. (2008) 42:151–7. doi: 10.1016/j.jpsychires.2006.10.013
148. da Rosa MI, Simon C, Grande AJ, Barichello T, Oses JP, Quevedo J. Serum S100B in manic bipolar disorder patients: systematic review and meta-analysis. J Affect Disord. (2016) 206:210–5. doi: 10.1016/j.jad.2016.07.030
149. Iwamoto K, Kakiuchi C, Bundo M, Ikeda K, Kato T. Molecular characterization of bipolar disorder by comparing gene expression profiles of postmortem brains of major mental disorders. Mol Psychiatry. (2004) 9:406–6. doi: 10.1038/sj.mp.4001437
150. Woo T-UW, Walsh JP, Benes FM. Density of glutamic acid decarboxylase 67 messenger RNA-containing neurons that express the N-methyl-D-aspartate receptor subunit NR2A in the anterior cingulate cortex in schizophrenia and bipolar disorder. Arch Gen Psychiatry. (2004) 61:649–57. doi: 10.1001/archpsyc.61.7.649
151. Seredenina T, Sorce S, Herrmann FR, Ma Mulone XJ, Plastre O, Aguzzi A, et al. Decreased NOX2 expression in the brain of patients with bipolar disorder: association with valproic acid prescription and substance abuse. Transl Psychiatry. (2017) 7:e1206. doi: 10.1038/tp.2017.175
152. Harrison PJ, Geddes JR, Tunbridge EM. The emerging neurobiology of bipolar disorder. Trends Neurosci. (2018) 41:18–30. doi: 10.1016/j.tins.2017.10.006
153. Huber VJ, Tsujita M, Kwee IL, Nakada T. Inhibition of aquaporin 4 by antiepileptic drugs. Bioorg Med Chem. (2009) 17:418–24. doi: 10.1016/j.bmc.2007.12.038
154. Morton E, Murray G. An update on sleep in bipolar disorders: presentation, comorbidities, temporal relationships and treatment. Curr Opin Psychol. (2020) 34:1–6. doi: 10.1016/j.copsyc.2019.08.022
Keywords: glymphatic system, depression, sleep, bipolar disorder, astrocyte, aquaporin-4
Citation: Yan T, Qiu Y, Yu X and Yang L (2021) Glymphatic Dysfunction: A Bridge Between Sleep Disturbance and Mood Disorders. Front. Psychiatry 12:658340. doi: 10.3389/fpsyt.2021.658340
Received: 25 January 2021; Accepted: 12 April 2021;
Published: 07 May 2021.
Edited by:
Sarah Jane Baracz, University of New South Wales, AustraliaReviewed by:
Casimiro Cabrera Abreu, Queens University, CanadaJennaya Christensen, Monash University, Australia
Copyright © 2021 Yan, Qiu, Yu and Yang. This is an open-access article distributed under the terms of the Creative Commons Attribution License (CC BY). The use, distribution or reproduction in other forums is permitted, provided the original author(s) and the copyright owner(s) are credited and that the original publication in this journal is cited, in accordance with accepted academic practice. No use, distribution or reproduction is permitted which does not comply with these terms.
*Correspondence: Xinfeng Yu, eXV4aW5mZW5nJiN4MDAwNDA7emp1LmVkdS5jbg==; Linglin Yang, MjUxNzEwNiYjeDAwMDQwO3pqdS5lZHUuY24=; eWFuZ19saW5nbGluJiN4MDAwNDA7MTYzLmNvbQ==