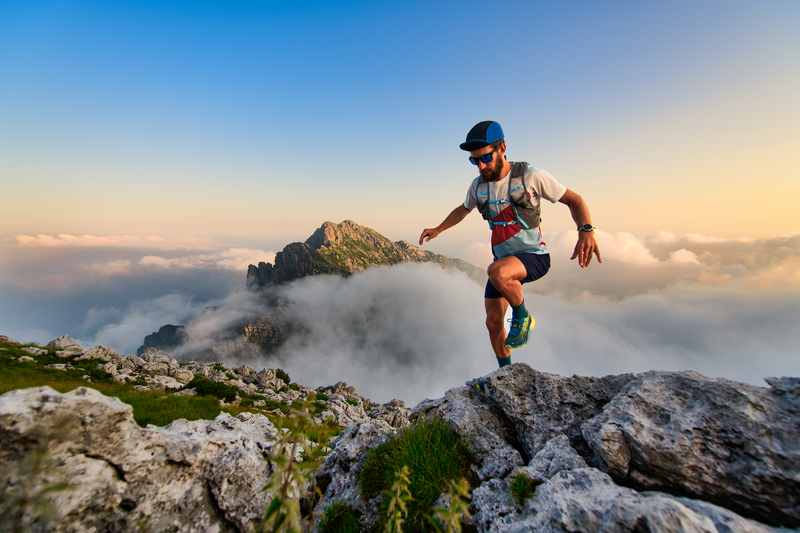
95% of researchers rate our articles as excellent or good
Learn more about the work of our research integrity team to safeguard the quality of each article we publish.
Find out more
SYSTEMATIC REVIEW article
Front. Psychiatry , 08 March 2021
Sec. Molecular Psychiatry
Volume 12 - 2021 | https://doi.org/10.3389/fpsyt.2021.644315
This article is part of the Research Topic Magnetic Resonance Spectroscopy of GABA and Glutamate in Mental Health View all 17 articles
Multimodal neuroimaging studies combining proton magnetic resonance spectroscopy (1H-MRS) to quantify GABA and/or glutamate concentrations and functional magnetic resonance imaging (fMRI) to measure brain activity non-invasively have advanced understanding of how neurochemistry and neurophysiology may be related at a macroscopic level. The present study aimed to perform a systematic review and meta-analysis of available studies examining the relationship between 1H-MRS glutamate and/or GABA levels and task-related fMRI signal in the healthy brain. Ovid (Medline, Embase, and PsycINFO) and Pubmed databases were systematically searched to identify articles published until December 2019. The primary outcome of interest was the association between resting levels of glutamate or GABA and task-related fMRI. Fifty-five papers were identified for inclusion in the systematic review. A further 22 studies were entered into four separate meta-analyses. These meta-analyses found evidence of significant negative associations between local GABA levels and (a) fMRI activation to visual tasks in the occipital lobe, and (b) activation to emotion processing in the medial prefrontal cortex (mPFC)/anterior cingulate cortex (ACC). However, there was no significant association between mPFC/ACC glutamate levels and fMRI activation to cognitive control tasks or to emotional processing, with the relationship to emotion processing related neural activity narrowly missing significance. Moreover, our systematic review also found converging evidence of negative associations between GABA levels and local brain activity, and positive associations between glutamate levels and distal brain activity, outside of the 1H-MRS sampling region. Albeit less consistently, additional relationships between GABA levels and distal brain activity and between glutamate levels and local brain activity were found. It remains unclear if the absence of effects for other brain regions and other cognitive-emotional domains reflects study heterogeneity or potential confounding effects of age, sex, or other unknown factors. Advances in 1H-MRS methodology as well as in the integration of 1H-MRS readouts with other imaging modalities for indexing neural activity hold great potential to reveal key aspects of the pathophysiology of mental health disorders involving aberrant interactions between neurochemistry and neurophysiology such as schizophrenia.
Excitation-inhibition balance plays a major role in determining neural activity (1). At a microscopic level, the influence of the brain's major excitatory and inhibitory neurotransmitters [glutamate and γ-aminobutyric acid (GABA)], on neural activity has been studied in detail [see Isaacson and Scanziani (1), Lauritzen et al. (2)]. At a macroscopic level, this investigation has been achieved through the development and optimisation of neuroimaging techniques enabling quantification of GABA and glutamate concentrations via proton magnetic resonance spectroscopy (1H-MRS), and of neural activation via measurement of blood oxygen level dependent (BOLD) signal with functional magnetic resonance imaging (fMRI). BOLD signal has been linked to local field potentials of dendritic origins, implying the signal is likely to reflect incoming input (3), thereby being closely linked to, and affected by, neurotransmission. However, the relationship between neurotransmitter levels and task-related neural activity remains unclear.
A previous narrative review by Duncan et al. (4) sought to address this question with a comprehensive overview of studies using imaging techniques such as magnetoencephalography, electroencephalography, fMRI, positron emission tomography and 1H-MRS, as well as behavioural measures as proxies for neural activity and pharmaceutical manipulations of neurotransmitter levels. The authors reported that GABA levels were related to reduced positive BOLD response or reduced negative BOLD response (NBR) within the same brain region, while glutamate levels were more commonly related to inter-regional neural responses. The number of publications using multimodal fMRI and 1H-MRS techniques has more than doubled since this review was published, and studies using this type of multimodal combination are expected to keep increasing in the future due to the ease of collecting both types of data within a single scanning session. More recent reports outside of the previous review include studies on regions of interest that had previously been examined too infrequently to permit meta-analysis, providing important insights into the nature of neurochemistry-neurophysiology associations in a variety of brain regions and task domains.
The present study aims to address these issues by conducting a systematic review and meta-analysis of 1H-MRS and task-based fMRI studies in the healthy brain. We then meta-analyse homogeneous studies based on the location of 1H-MRS voxel placement and fMRI paradigm. Finally, we discuss the findings in light of abnormalities in these relationships in psychiatric populations, such as schizophrenia patients, and their potential implications on brain processes in psychiatric disorders.
Systematic searches were performed on Pubmed and Ovid (Medline, Embase and PsycINFO) databases from database inception to 4 December 2019. Search strategies for the individual databases included the terms “functional magnetic resonance imaging” AND “magnetic resonance spectroscopy” AND (“GABA” OR “glutamate”) (for full search syntax see Supplementary Table 1). We included studies that used 1H-MRS to measure GABA, glutamate or Glx (combined glutamate and glutamine) metabolite levels to test associations with task-related fMRI activation. Eligible study designs included observational studies, cohort studies, case-control studies, cross-sectional studies, or experimental studies (for full eligibility criteria see Supplementary Table 2). The population of interest were healthy human participants, or healthy human control groups from case-control studies. Interventional studies were excluded, unless those studies conducted and reported the outcome of interest analysis with baseline measures. Studies assessing only changes in metabolite levels before, during and after a task together with BOLD activation were not included as they do not investigate the relationship between resting-state neurotransmitter levels and neural activity modulated by stimuli. Two reviewers (AK and CB) independently screened search result titles and abstracts of papers for eligibility according to the inclusion and exclusion criteria. All potentially eligible studies were screened full-text for inclusion. Reference lists of eligible studies were hand-searched for further relevant articles.
Two independent reviewers (AK and PBL) extracted data using a standardised template datasheet. The primary outcomes extracted were the metabolite type, 1H-MRS metabolite sampling region, fMRI task paradigm, neural activation region, and relationship between metabolite and neural activity. Studies investigating the relationship between 1H-MRS GABA or glutamate and task-related BOLD signal performed analyses in one or both of two different ways: by including metabolite levels as a covariate in a regression, or by extracting the percentage of BOLD signal change (%SC) from a region of interest (ROI) and using correlation analysis with the metabolite levels. Both types of analysis methods were included in this review. Regressions either covered the whole-brain or a predetermined ROI such as the 1H-MRS voxel, an anatomical ROI or a mask derived from an activation cluster. Correlation analyses used %SC extracted from sources such as the peak voxel within or mean activation across an activated cluster, an anatomically defined ROI, or the 1H-MRS voxel. Additional extracted data for the systematic review included the statistical significance of the relationship, covariates tested in the analysis, sample size, age and sex characteristics of the sample, scanner strength, 1H-MRS sequence, and reference metabolite. In cases where no statistical significance value was reported for the relationship itself and only Pearson's r correlation coefficients were reported [e.g., (5)], p-values were calculated through conversion of r values to test statistic t with the formula
The p-value was then derived using the sampling distribution of Student's t. This calculation of the p-value was used to ascertain the significance of the correlation coefficient.
Some studies verified their main findings (1H-MRS x fMRI response) in the following ways: (1) substituting the metabolite levels from their primary region of interest with those from a different, control sampling region; (2) substituting the fMRI response from their primary task paradigm with that from a control task; (3) substituting the fMRI response from their primary region of interest with that from a control region during the same task. Such control analyses may be found in Supplementary Tables 7, 8. A formal quality assessment was not conducted due to a lack of standard quality assessment for such types of studies. Imaging and study design parameters were, however, collected (Supplementary Tables 3–6, 9). Review findings [see Figure 1 for PRISMA flowchart (6)] are presented according to the brain region from which metabolite levels were sampled.
Studies were further subdivided by 1H-MRS voxel location, task domain and fMRI activation foci. For quantitative analysis, r correlation coefficients, t-values, z-values and sample sizes were compiled. Although the recommended minimum number of studies for meta-analysis is two, this does not apply to random-effect models (7), which the MetaNSUE method employs (8). Additionally, given the likelihood of a non-statistically significant unreported effect, the minimum number of comparable studies was set at a more conservative number of ≥4. Meta-analysis was conducted using the MetaNSUE package for R (8), where r correlation coefficients were converted into effect sizes for a meta-analytic pooled effect size. This tool was used due to its ability to impute results for unreported correlation coefficients, which can often be the case when correlations are non-significant. Authors were contacted for unreported r correlation coefficients. However, in the case of unreported r correlation coefficients, the number of imputations was kept at the package's default of 500 imputations per study.
In instances where studies reported both t- and z-values of significantly correlated clusters from a regression analysis, t- and z-values were converted into r correlation coefficients as follows:
1. Z-score to p-value calculation:
2. P-value to student t-value: using Microsoft Excel's TINV function
3. T-value to r correlation coefficient conversion:
Significance was determined using two-tailed 95% confidence intervals. Further sensitivity analyses are detailed in the Supplementary Material.
1H-MRS studies sampling GABA levels from the occipital lobe have been largely homogeneous (Table 1): using visual fMRI tasks and focusing only on co-localised neural activity. The first few studies in this domain found negative associations between occipital lobe GABA levels and local BOLD response to visual stimuli (9–12, 14). Muthukumaraswamy et al. (11) not only found a negative association to BOLD response amplitude, but also a positive correlation with BOLD response width. However, this relationship to response amplitude was not replicated in subsequent studies (13, 15, 16, 18). Harris et al. (15) investigated this relationship empirically, and combined their result with prior studies in a meta-analysis, where they found no evidence for a significant association between the two measures. More recently, Duncan et al. (16) attempted to address this issue using improved methodology. A MEGA-PRESS sequence that was adapted to suppress macromolecule signal was used so that both GABA+ and GABA– were measured from the occipital lobe. Although their empirical study found no significant relationship, Duncan et al. (16) added their results to the five studies meta-analysed in Harris et al. (15)—using the same Hunter-Schmidt method employed by the metafor R package—and found evidence in favour of a negative relationship between GABA and BOLD, regardless of macromolecule suppression.
Neural activity in the occipital lobe was also studied in different contexts beyond simple visual perception. Wijtenburg (17) found a positive correlation between GABA and neural activation difference before and after a high frequency visual stimulus, an effect thought to reflect visual plasticity. In agreement with other studies, when only baseline visual stimulation-related activation was used, a negative correlation was found with GABA levels. However, results for this analysis were not published and only mentioned in the discussion. Schallmo et al. (19) used a spatial suppression paradigm where participants had to indicate the direction of moving gratings with varying contrasts. No correlations between GABA levels in the early visual cortex and the more laterally located visual motion complex and neural response in the same regions were found.
Glutamate levels repeatedly did not show a relationship to co-localised neural activity in studies of the occipital lobe (9, 12–14, 20, 21). Some of these studies utilised the same sequence to measure GABA and glutamate levels simultaneously (9, 12–14, 21). Two studies reporting a relationship with GABA levels found no association between neural activity and co-localised Glx (9, 12). Wijtenburg et al. (17) found a relationship between glutamate levels and an activation contrast comparing neural response before and after a high frequency visual stimulus. This relationship was also a positive one, similar to what had been observed with GABA levels. However, in this case, the association between glutamate levels and activity related to baseline visual stimuli was not reported. Finally, within the visual motion complex glutamate levels were positively correlated with neural response during motion perception in a spatial suppression paradigm (21).
Studies sampling metabolite levels in the sensorimotor cortex and adjacent areas (Table 2) mostly utilised a simple paradigm, in this case some form of finger-tapping task. In multiple studies, no significant associations were found between sensorimotor cortex GABA levels and local neural activity (15, 22–24). Using the same finger-tapping task, Draper et al. (24) also found no correlation between GABA levels in a supplementary motor area and BOLD %SC. Only one study (25), using a visually cued reaction time task, indicated a negative relationship between BOLD and GABA concentrations in the sensorimotor cortex. This same study was the only one to look at neural activity with glutamate levels in the sensorimotor cortex area and found no correlated activation clusters in a whole-brain regression.
Due to the involvement of the anterior cingulate cortex (ACC) and medial prefrontal cortex (mPFC) in different processes such as emotion, attention and cognition (26), various task contexts have been used (Table 3). Many studies investigated NBR within this region, due to the close coupling between the ACC/mPFC and the default mode network (DMN). Four studies investigating GABA levels in the mPFC/ACC region found a positive relationship with regional NBR, i.e., a negative relationship with neural activity (27, 30, 31, 35). Northoff et al. (35) found that during conditions that produced little NBR compared to rest, this negative relationship between GABA levels and neural activity was not apparent. In agreement with these findings, using facial expressions Stan et al. (29) observed that mPFC GABA+ concentration was negatively correlated with subgenual ACC (sgACC) fMRI response to sadness. However, neural activity during other emotion categories—happy, fear, or anger—was not correlated with GABA+ concentrations. When using an emotion processing task, Levar et al. (32) only found a negative correlation with GABA+ levels when contrasting neural activity during negative emotional stimuli with response to positive emotional stimuli. Other studies failed to find negative correlations between regional GABA concentrations and neural activity, or positive relationships with NBR, during cognitive control tasks (34, 36), a verbal working memory task (5), a reward-guided decision-making task (28), and a fear conditioning, extinction, and extinction retrieval task (33).
Correlations between GABA levels and distal neural activity were also found in some of these studies (32–34). Neural activity related to fear recovery in the amygdala was positively correlated with dACC GABA levels, while neural activity related to late phases of fear extinction in the right amygdala, cerebellum, middle cingulate gyrus, and right insula were negatively correlated with GABA (33). Levar et al. (32), using non-facial picture stimuli, found that dACC GABA concentrations correlated positively with activity in the left amygdala during positive emotion, negative emotion, all emotion and positive over negative emotion. Right amygdala activity related to positive emotion was also positively correlated with dACC GABA levels. Activity of the right superior frontal gyrus during negative pictures was negatively correlated with GABA levels. BOLD signal related to all picture conditions was positively correlated with GABA levels in the left and right hippocampi. ACC GABA concentrations were negatively associated with cognitive control-related BOLD signal in two clusters. The first was localised mainly to the left parietal cortex, spanning the postcentral gyrus, inferior parietal lobe, supramarginal gyrus, angular gyrus, and the middle occipital lobe. The second cluster was found mainly in the left dlPFC, including regions within the middle frontal lobe, inferior frontal lobe, precentral gyrus, superior temporal lobe, rolandic operculum, and postcentral gyrus.
Twenty-one studies sampled glutamate levels within the mPFC/ACC regions and investigated its relationship to neural activity within the same region as well as in other brain regions. Of those, 15 studies did not find an association between mPFC/ACC glutamate levels and local activation. These studies used a variety of tasks encompassing: emotion (31, 32), cognitive control (34, 37–40), working memory (5, 27), reward (28, 41), intero- and exteroceptive awareness (30), aversion (42), and a verbal fluency task (43). Nonetheless, other studies did find relation between glutamate concentration and regional neural activity. Stan et al. (29) found mPFC glutamate levels were correlated with pregenual ACC (pgACC) response to anger, but not with regional activity during viewing of other emotional categories. Using an empathy task, Duncan et al. (44) sought to investigate the relationship between pgACC glutamate levels and neural activity in the task-activated supragenual ACC, as well as between supragenual ACC glutamate with pgACC activity. A whole-brain regression with pgACC Glx levels showed a relationship to empathy-related BOLD in the supragenual ACC (a cluster overlapping with the supragenual ACC 1H-MRS voxel). supragenual ACC Glx levels, however, were not associated with pgACC activation during the empathy task. ACC glutamate levels were also studied in conjunction to neural activity during a salience processing task using emotional and erotic pictures (45). Activation in the sgACC related to unexpectedness of emotional pictures displayed a negative correlation with glutamate. Dissecting this association, the authors found that a positive relationship between glutamate and expected emotional picture viewing drove this finding. In a separate study, Cadena et al. (46) scanned a control group twice with a 6-week interval (46). ACC Glx levels were positively correlated with ACC activation during cognitive control at the baseline scan, a relationship that was not observed at the second scanning session 6 weeks later. Enzi et al. (47) sampled Glx concentrations from the pgACC, where they found a partial positive correlation to NBR during anticipation of reward and of no outcome. Through further investigation, the authors found that Glx concentrations were correlated to the rest condition and hypothesised that this relationship was largely driving the relationship between glutamate levels and deactivation during reward anticipation.
In terms of associations between glutamate levels and neural activity outside of the 1H-MRS region, three studies reported positive correlations with neural activity outside of the ACC/mPFC. Duncan et al. (42) found mPFC glutamate levels were correlated positively with sensorimotor cortex and left insula activation during the anticipation of aversion. In a different study, Duncan et al. (44) showed, through whole-brain regression with Glx levels in the pgACC, a significant correlation with empathy-related activation in the left precuneus, bilateral amygdala and putamen, right superior frontal gyrus and right supramarginal gyrus. Cadena et al. (46) observed a correlation with cognitive control-related activation within the insula, hippocampus, inferior parietal lobule and precuneus. Notably, the findings in the bilateral insula and left inferior parietal lobule were replicated in the sample 6 weeks later. Interestingly, one study observed different correlations depending on task load. Falkenberg et al. (39) found three activation clusters where there was a significant interaction between glutamate levels and the parameters of a cognitive control task: five auditory intensity difference conditions and the instruction of which ear to pay attention to. Post-hoc tests showed that in individuals with lower levels of glutamate, neural response was higher during high cognitive control conditions, i.e., when attention was directed towards the less salient stimulus, whereas in individuals with high levels of glutamate, neural activity was higher during low cognitive control conditions. Four studies found negative correlations between glutamate or Glx levels and neural activity outside of the ACC/mPFC. Overbeek et al. (34) observed ACC glutamate levels were positively associated with NBR in a cluster spanning from the PCC and precuneus to the occipital cortex and cerebellum. Von During et al. (45) also found that ACC glutamate levels were positively associated with NBR in the PCC, with higher glutamate predicting higher deactivation of this area in response to a variety of stimuli. Neural activity in a variety of other regions were also negatively correlated with ACC glutamate levels in this study (see Table 4). One study investigated ACC glutamate levels with cognitive control-related activation within the striatum only, to which a negative relationship was found in a composite group of ADHD patients, unaffected siblings and healthy controls (48). Similarly, Gleich et al. (41) found a negative relationship with ventral striatum activation in adolescents but not in adults.
All studies sampling GABA or glutamate levels from the dorsolateral prefrontal cortex (dlPFC) used a working memory fMRI task (Table 5). Only one study, comparing children and adolescents with sport-related injuries not involving the head and with no history of concussion, to a group of concussed individuals, found a significant positive correlation between dlPFC GABA levels and local activation (50). Two studies did not find a relationship (15, 27). Similarly, three studies that investigated glutamate levels also found no relationships to regional neural activity (27, 51, 52).
Within studies of the temporal lobe (Table 6), the auditory cortex, anterior temporal lobe and hippocampus were sampled for metabolites and fMRI tasks were largely based on the respective functions of the 1H-MRS sampling regions. Two studies found a negative relationship between GABA levels and local activation during thought retrieval and though suppression (54) and during semantic processing (53). However, Harris et al. (15) found no relationship between fMRI activity in the auditory cortex and local GABA levels.
Unlike GABA, hippocampal glutamate levels were not found to be correlated with local activity in several studies (43, 55, 56). Glutamate levels were, however, positively correlated to neural activity outside of the hippocampus: parahippocampus (55), inferior frontal gyrus (56) and ventral striatum (57).
Three studies (30, 58, 59) measured relationships between metabolites and neural activity in this region (Table 7). Lipp et al. (58) reported a negative association between GABA concentration and insula neural activity only to animal picture stimuli, while pictures of other categories did not elicit such association. Interestingly, another study (30) identified a positive correlation between GABA levels and neural response to interoceptive awareness. Insula GABA levels were additionally correlated to distal neural activity: positively to activation in the amgydala and ventral striatum (58), and negatively to activation in the medial cingulate cortex and supplementary motor area (59). Meanwhile, glutamate levels did not correlate with neural activity in the insular cortex (30, 59).
Two studies investigated both GABA and glutamate levels within the posterior cingulate cortex (PCC)/precuneus (PCu) region (18, 60) (Table 8) that typically deactivate during some cognitive tasks but activate putatively during processing of scenes to support autobiographical memory and imagined events (61). Deactivation of the PCC/PCu region as well as the entire DMN (including the mPFC, PCC/PCu, and bilateral parahippocampus) during a working memory task were investigated separately in relation to glutamate and GABA concentrations (60). Regression models showed that as glutamate increased NBR decreased (i.e., weaker deactivation). Conversely, GABA levels were positively correlated with NBR for all but the lowest cognitive load (1-back condition), i.e., as GABA levels increased, deactivation increased, in both the model with PCC/PCu deactivation and DMN deactivation as dependent variable. In contrast, when using a perceptual discrimination task, Costigan et al. (18) found no association between PCC/PCu activation and local Glx and GABA+ levels.
A few studies have sampled GABA and glutamate levels from less frequently inspected regions combined with neural activity (Table 9). Harris et al. (15) found no relationship between activation related to eye saccades and GABA levels in the frontal eye field. Two studies sampled glutamate from the thalamus, both using a verbal fluency task. Fusar-Poli et al. (43) found no significant effects, while Allen et al. (64) found significant correlations with activation in the right superior frontal gyrus/sulcus and the right cingulate sulcus. Two studies found a positive correlation between Glx levels and local activity in two different brain regions: the substantia nigra (63) and the dorsal striatum (62).
Within the studies sampling GABA, two groups of more than four studies emerged: occipital lobe GABA levels in combination with a visual task, and ACC GABA levels in combination with emotion processing tasks. For illustration of the voxel positions of each study per meta-analysis see Figures 2, 3.
Figure 2. Meta-analysis of studies investigating occipital lobe GABA levels and visual perception-related neural activity in the occipital lobe. (A) Position of occipital voxels per study. (B) Forest plot of meta-analysis results. p < 0.10; *p < 0.05; **p < 0.01; ***p < 0.001.
Figure 3. Meta-analysis of studies investigating GABA levels and emotion related neural activity in the anterior cingulate cortex. (A) Position of ACC voxels per study. (B) Forest plot of meta-analysis results. p < 0.10; *p < 0.05; **p < 0.01.
For the relationship between GABA levels and BOLD within the occipital lobe, the systematic search for articles identified nine eligible studies for quantitative analysis (9–16, 18). The results of the meta-analysis demonstrated a significant relationship between GABA and BOLD in the occipital lobe (r = −0.45 [−0.63, −0.22], p = 0.002, I2 = 47.06%) (Figure 2).
Another four studies investigated ACC GABA in relation to local response to emotion tasks (29, 31, 32, 35). Correlation coefficients were available for three of these, while Levar et al. (32) used a regression with ACC GABA levels as a covariate. The latter found a negatively correlated cluster in the dorsal ACC for negative vs. positive emotion, however, this result was not used for the main meta-analysis for which comparable contrasts were selected only (specific or all emotion vs. baseline). The main meta-analysis of this relationship yielded a significant negative relationship between ACC GABA and regional emotion-related neural activity (r = 0.53 [−0.79, −0.11], p = 0.02, I2 = 34.04%) (Figure 3).
Two meta-analyses were performed. One combined glutamate levels in the ACC and local activation during cognitive control tasks. The other combined glutamate levels in the ACC and local activation during emotional tasks. For illustration of the voxel positions of each study per meta-analysis see Figures 4, 5.
Figure 4. Meta-analysis of studies investigating ACC glutamate levels and regional cognitive control-related neural activity in the anterior cingulate cortex. (A) Position of ACC voxels per study. (B) Forest plot of meta-analysis results. p < 0.10; ***p < 0.001.
Figure 5. Meta-analysis of studies investigating ACC glutamate levels and regional emotion related neural activity in the anterior cingulate cortex. (A) Position of ACC voxels per study. (B) Forest plot of meta-analysis results. p < 0.10; **p < 0.01; ***p < 0.001.
Six studies (34, 37–40, 46) were included in the meta-analysis of the relationship between ACC glutamate levels and local activation during cognitive control. These were a mixture of studies using correlation and regression analysis. When regression analysis had been performed on the whole-brain level and no significant correlation with the ACC was found, this was entered into the meta-analysis as a non-significant result to avoid bias. The meta-analysis did not find support for a relationship between ACC glutamate levels and neural activity during cognitive control [r = 0.19 (−0.24, 0.56), p = 0.39, I2 = 71.62] (Figure 4).
For the meta-analysis investigating the relationship between ACC glutamate levels and local activation during emotion processing, seven studies were included (29, 31, 32, 35, 44, 45, 49). As with the previous meta-analysis on cognitive control, studies utilised a mixture of correlation and regression analyses. This analysis marginally missed significance for a positive relationship between ACC glutamate levels and regional emotion related neural activity [r = 0.41 (−0.04, 0.72), p = 0.07, I2 = 79.69%] (Figure 5).
The main finding of this meta-analysis was that GABA levels were negatively correlated with co-localised neural activity within the ACC during emotion processing and within the occipital lobe during visual stimulation. Further meta-analyses showed that glutamate levels within the ACC were not correlated with co-localised neural activity during either cognitive control or emotion processing, however, the latter meta-analysis narrowly missed significance. These findings were further supported by a systematic review of all fMRI-1H-MRS association studies to date, where increasing resting GABA levels were repeatedly associated with either weaker neural activation or stronger neural deactivation. On the other hand, glutamate levels were largely not correlated with intra-regional neural activity, although a handful of studies did find a positive correlation. An additional finding was that glutamate concentrations were largely positively correlated with regions outside of the 1H-MRS sampling area; however, negative inter-regional associations were also identified in multiple studies. GABA concentrations were less likely to be associated with regions outside of the 1H-MRS sampling area with only a few studies providing evidence of a positive or negative relationship with inter-regional neural activation depending on the metabolite sampling region and the neural activation region.
These findings support and extend prior reported trends. Previously, in a narrative review Duncan et al. (4) highlighted that GABA tended to be negatively correlated with regional neural activity in the visual, auditory, sensorimotor and anterior cingulate cortices, while glutamate was positively correlated with neural activity in regions outside of the sampling region of the metabolite. In the present study, by using a systematic rather than narrative review method and capitalising on the large increase in available studies specifically using 1H-MRS and fMRI, a more region-dependent relationship between these specific measures emerged. The negative relationship between GABA levels and regional neural activation was confirmed within the occipital cortex, ACC/mPFC, temporal lobe, and PCC. However, regions such as the sensorimotor cortex, auditory cortex and dorsolateral prefrontal cortex either did not exhibit this relationship clearly or in a few studies the relationship was in the opposite direction (30, 50). A negative relationship between GABA levels and regional neural activation appears intuitive: increased inhibition as measured through resting state 1H-MRS would be associated with decreased net activation of a region through regulation of resting excitatory activity (4, 65). Prior work in animals found that pharmacological manipulation increasing endogenous GABA concentrations produced lower positive BOLD signal changes (66). In the context of NBR, previous work in rats found hyperpolarisation of neurons through neuronal inhibition to correspond to a decrease in blood oxygenation (67). Findings of a positive relationship between GABA levels and neural activity may be a result of more complex neural processes that require suppression of neural response to irrelevant stimuli to promote concurrent activation to target stimuli (68). A relationship between GABA levels and neural activity in distal regions had not previously been identified in the literature (4). While initial studies suggested that the relationship between GABA levels and neural activity was limited to the same region (4, 68), a handful of recent studies reported both negative and positive relationships with activation outside of the 1H-MRS voxel (32–34, 58–60). Negative relationships with inter-regional neural activity may be the result of local inhibition of excitatory projections that regulate activity in other regions (32–34).
In terms of glutamate, a few studies observed a positive relationship between glutamate and local neural activity in the ACC and PCC (29, 44, 46, 60). Additionally, both positive and negative relationships with distal neural activity were found. These findings add to the simplified positive relationship with inter-regional neural activity reported in Duncan et al. (4). A negative relationship between BOLD activity in DMN regions, such as the PCC/PCu, were speculated to be due to higher ACC glutamate levels supporting facilitation of salience processing, thus resulting in stronger deactivation of DMN regions (39, 45). However, this relationship seems to be region-dependant as glutamate levels measured within the DMN, specifically the PCC, were positively related with DMN neural activation, where higher glutamate levels predicted lower local deactivation (60). The positive relationship between glutamate levels and intra-regional neural activity may stem from an excitatory drive stimulating neural activation within the same region through recurring feedback (46, 69). However, the relationship between glutamate levels and intra-regional neural activity may be difficult to replicate: one study failed to replicate a positive correlation between ACC glutamate and ACC neural activity at a 6-week follow-up scan within the same cohort (46). In fact, Enzi et al. (47) suggested resting glutamate levels may be more closely related to resting-state activation, thus being less intimately linked with task-related activity. As previously observed by Duncan et al. (4), glutamate levels were positively associated with neural activity outside of the sampling regions for glutamate. The long-range projections of glutamatergic neurons (70) are thought to influence neural activity in distal regions, thus giving rise to this relationship. However, a number of studies have shown that these projections may not simply reflect positive relationships with neural activity, but may also indicate that glutamate levels can negatively predict neural activity inter-regionally.
The ACC has been commonly investigated across studies, for which effects have been inconsistent. The discrepancies in 1H-MRS x fMRI relationships may arise from functional segregation within the ACC (71), its involvement in different behaviours (72), and that it hosts both task-positive and task-negative subdivisions (e.g., pgACC and supragenual ACC). The ability of 1H-MRS to discriminate between ACC subregions is still limited due to the 1H-MRS voxel size, especially in the case of GABA. This may be improved by the use of high-field MRI scanners that will allow for smaller voxel sizes (73). An additional consideration is that relationships between metabolite levels and neural activity may also be task-specific. Studies that used an active control task, rather than a low-level baseline condition such as a fixation cross [e.g., (44, 53, 54)], indicate that baseline metabolite levels are not simply correlated with any type of neural response. When neural response was confirmed for both the task of interest and the control task, different relationships between GABA levels and neural response were found indicating that metabolite levels may not be simply associated with all types of neural activity within a region (54).
Despite some incongruent findings, a pattern of neurochemical-neurofunctional relationships has emerged, which give valuable insight into psychiatric disorders where this relationship is aberrant. Measurement of metabolite levels at rest with 1H-MRS is thought to reflect individual differences in number of glutamatergic and GABAergic neurons, number of glutamatergic and GABAergic synapses, and glutamate and GABA turnover (68). It is conceivable, then, that abnormalities in metabolite levels would be reflected in differential neurochemical-neurofunctional relationships. In psychiatric disorders such as schizophrenia, case-control studies have suggested an aberrant relationship between ACC glutamate and local neural activity (46) and in regions receiving inputs from the ACC, such as the insula and DMN (34, 46, 74). Differences were found in the relationship between hippocampal Glx and inferior frontal gyrus activation, with medicated schizophrenia patients showing a less robust relationship (56) potentially reflecting differential coupling between the two regions (75). Activation related to prediction error within the substantia nigra was not correlated with regional glutamate levels in schizophrenia patients, which was interpreted as a glutamatergic dysfunction leading to abnormal prediction error coding (38). Furthermore, a relationship between glutamate and working memory activation, both within the dlPFC, was found in unmedicated schizophrenia patients where no such relationship existed in healthy controls—potentially due to compensatory action to glutamatergic insensitivity (52). Given the known and increasingly well-characterised abnormalities in glutamatergic and GABAergic function associated with this psychiatric disorder (76, 77) and the close relationship between metabolites and neurofunction, abnormalities in brain function should arise. The neurochemical-neurofunctional relationship has been investigated less frequently in other psychiatric disorders such as obsessive-compulsive disorder and major depressive disorder. While this line of investigation showed no differences in the relationship in patients with obsessive compulsive disorder (40, 51), patients with major depressive disorder showed stronger coupling between glutamate levels and NBR compared with healthy controls (31). Walter et al. (31) concluded that reduced resting state activity was driven by glutamatergic metabolism in patients with major depressive disorder. Differences in 1H-MRS x fMRI relationships may be the result of variations in neurochemistry, differential abnormal functions regionally and inter-regionally, as well as structural abnormalities. Despite the correlational nature of all the studies identified here, understanding healthy relationships between metabolite and neural activity allows some insight into compensatory action within mental illness and can provide potential pharmaceutical targets for intervention. Targeting GABAergic or glutamatergic neurotransmission may be a promising avenue to treating symptoms of schizophrenia (78) and major depressive disorder (79).
The studies included in this review used two main types of methods to derive relationships. Harris et al. (15) demonstrated that when using the correlational technique with %SC, the source of sampling for %SC could have a large effect on the observed relationship. Harris et al. (15) tested five different BOLD activation sources: peak within the 1H-MRS voxel, mean across the 1H-MRS voxel, mean across significant activation, peak within anatomical ROI and mean across anatomical ROI. From the peak within 1H-MRS voxel to the mean across significant activation, there was a maximum change in effect size of r = 0.47, although it should be noted that these individual correlation coefficients were not statistically significant due to Bonferroni correction. Despite these potential sources of variation, the vast majority of articles cited in our systematic review that used the correlational technique with %SC only tested the relationship between metabolites and neural activation with one source of %SC, which may cause inconsistencies in findings.
Previously published studies controlled for potential confounds in diverse ways. Commonly used covariates were age, voxel tissue composition and sex. Age may have region-specific effects on levels of glutamate (80) and of GABA (81) and thus should be consistently controlled for. Our systematic review was not restricted to a certain age limit and instead attempted to collect data on all age ranges and noted whether studies controlled for age. Similarly, a potentially confounding effect of sex on metabolite levels was not consistently considered in individual studies, although sex has previously been identified to alter levels of glutamate (82) and GABA (83).
A further issue refers to the use of other regions as specificity control (4). In order to confirm that a significant association is not a global effect, metabolite analyses should be conducted both for the main region of interest as well as for a control region. Similarly, to ensure relationships can be specific to the experimental task, researchers should also evaluate the relationship between metabolite levels and a control, unrelated task. However, time constraints and participant fatigue during scanning may not enable such control measures in all studies.
Although it is tempting to suggest that future studies using the correlational approach should test various sources of %SC [e.g., (15)], the multiple testing corrections needed would require strong relationship effects between metabolite and BOLD signal. However, the present systematic review and meta-analyses seem to suggest such effect sizes are moderate at best for the metabolite glutamate. An alternative suggestion would be to consistently utilise %SC derived from the peak voxel within the 1H-MRS voxel as many studies have done, with deviations from a standard source of %SC needing to be specifically addressed and justified.
Other future suggestions for studies of this kind are to include age and sex as confounding covariates more consistently. Similarly, task and region specificity controls should be included wherever possible. One reason for the lack of substantial conclusions to be made from the above listed studies is the heterogeneity of 1H-MRS locations, BOLD %SC sampling locations and region coverage within regression studies. Replication studies would allow more insight into studies that used emotional, working memory, reward and motor tasks. Additionally, combination with additional modalities such as arterial spin labelling as a different measure of brain function may help parse out the less consistent relationships seen here. Future studies should also aim to investigate the 1H-MRS x fMRI relationship in further psychiatric disorders that feature glutamatergic or GABAergic abnormalities, such as autism spectrum disorder (ASD). Abnormalities in excitation-inhibition balance are well-known and characterised in individuals in ASD (84, 85), where reduced neural activity in corticostriatal circuits have been proposed (86, 87) to be linked to the reduction of striatal glutamate (85). However, further investigation of these hypotheses and the nature of the neurochemical-neurofunctional relationship in ASD is required. Finally, capitalising on the findings presented here, future studies in psychiatric populations such as schizophrenia patients may modulate metabolite levels such as glutamate to test whether neurofunction can be modulated as a result.
The significant expansion of studies combining multi-modal 1H-MRS and fMRI in recent years has enabled more detailed examination of the interactions between neurochemistry and neurophysiology in the healthy brain. These studies have shown robust negative correlations between GABA levels and local activation in regions such as the occipital cortex and ACC, and positive correlations between glutamate levels and activation in distal regions. These findings have implications for our understanding of pathophysiology of psychiatric disorders such as schizophrenia, a field in which studies combining 1H-MRS and fMRI are burgeoning in recent years. In this context, findings from multimodal studies may provide key insights into the neurobiology of schizophrenia not only by informing how the interplay between neurochemistry and neurophysiology may be altered prior and across disease stages, but also by their translational potential to elucidate whether pharmacological modulation of glutamate and/or GABA may be a new target for addressing abnormal neurophysiology in this psychiatric disorder.
The original contributions presented in the study are included in the article/Supplementary Material, further inquiries can be directed to the corresponding author/s.
GM, AK, and MJK: concept and design. CB and AK: systematic search. PBL and AK: data extraction. AK: statistical analysis and drafting of the manuscript. MJK and AK: statistical support. CD, JMS, PBL, GM, and AK: critical revisions of the manuscript. CD, JMS, and GM: supervision. All authors contributed to the article and approved the submitted version.
This study was supported by a Sir Henry Dale Fellowship, jointly funded by the Wellcome Trust and the Royal Society to Dr. Gemma Modinos (202397/Z/16/Z).
The authors declare that the research was conducted in the absence of any commercial or financial relationships that could be construed as a potential conflict of interest.
The authors would like to thank Dr. Alice Egerton for kindly providing templates for our figures.
The Supplementary Material for this article can be found online at: https://www.frontiersin.org/articles/10.3389/fpsyt.2021.644315/full#supplementary-material
1. Isaacson JS, Scanziani M. How inhibition shapes cortical activity. Neuron. (2011) 72:231–43. doi: 10.1016/j.neuron.2011.09.027
2. Lauritzen M, Mathiesen C, Schaefer K, Thomsen KJ. Neuronal inhibition and excitation, and the dichotomic control of brain hemodynamic and oxygen responses. Neuroimage. (2012) 62:1040–50. doi: 10.1016/j.neuroimage.2012.01.040
3. Logothetis NK. The underpinnings of the BOLD functional magnetic resonance imaging signal. J Neurosci. (2003) 23:3963–71. doi: 10.1523/JNEUROSCI.23-10-03963.2003
4. Duncan NW, Wiebking C, Northoff G. Associations of regional GABA and glutamate with intrinsic and extrinsic neural activity in humans—a review of multimodal imaging studies. Neurosci Biobehav Rev. (2014) 47:36–52. doi: 10.1016/j.neubiorev.2014.07.016
5. Witt ST, Drissi NM, Tapper S, Wretman A, Szakacs A, Hallbook T, et al. Evidence for cognitive resource imbalance in adolescents with narcolepsy. Brain Imaging Behav. (2018) 12:411–24. doi: 10.1007/s11682-017-9706-y
6. Moher D, Liberati A, Tetzlaff J, Altman DG, The PRISMA Group. preferred reporting items for systematic reviews and meta-analyses: the PRISMA statement. PLoS Med. (2009) 6:e1000097. doi: 10.1371/journal.pmed1000097
7. Borenstein M, Hedges LV, Higgins JP, Rothstein HR. Introduction to Meta-Analysis. Chichester: John Wiley & Sons (2011).
8. Albajes-Eizagirre A, Solanes A, Radua J. Meta-analysis of non-statistically significant unreported effects. Stat Methods Med Res. (2019) 28:3741–54. doi: 10.1177/0962280218811349
9. Muthukumaraswamy SD, Edden RA, Jones DK, Swettenham JB, Singh KD. Resting GABA concentration predicts peak gamma frequency and fMRI amplitude in response to visual stimulation in humans. Proc Natl Acad Sci USA. (2009) 106:8356–61. doi: 10.1073/pnas.0900728106
10. Donahue MJ, Near J, Blicher JU, Jezzard, P. Baseline GABA concentration and fMRI response. NeuroImage. (2010) 53:392–8. doi: 10.1016/j.neuroimage.2010.07.017
11. Muthukumaraswamy SD, Edden RAE, Wise RG, Singh KD. Individual variability in the shape and amplitude of the BOLD-HRF correlates with endogenous GABAergic inhibition. Hum Brain Mapping. (2012) 33:455–65. doi: 10.1002/hbm.21223
12. Violante IR, Ribeiro MJ, Edden RA, Guimaraes P, Bernardino I, Rebola J, et al. GABA deficit in the visual cortex of patients with neurofibromatosis type 1: genotype-phenotype correlations and functional impact. Brain. (2013) 136:918–25. doi: 10.1093/brain/aws368
13. Bridge H, Stagg CJ, Near J, Lau C-i, Zisner A, Cader MZ. Altered neurochemical coupling in the occipital cortex in migraine with visual aura. Cephalalgia. (2015) 35:1025–30. doi: 10.1177/0333102414566860
14. Bednarík P, Tkáč I, Giove F, DiNuzzo M, Deelchand DK, Emir UE, et al. Neurochemical and BOLD responses during neuronal activation measured in the human visual cortex at 7 tesla. J Cerebral Blood Flow Metabolism. (2015) 35:601–10. doi: 10.1038/jcbfm.2014.233
15. Harris AD, Puts NA, Anderson BA, Yantis S, Pekar JJ, Barker PB, Edden RAE. Multi-regional investigation of the relationship between functional MRI blood oxygenation level dependent (BOLD) activation and GABA concentration. PLoS ONE. (2015) 10:e0117531. doi: 10.1371/journal.pone.0117531
16. Duncan NW, Zhang J, Northoff G, Weng X. Investigating GABA concentrations measured with macromolecule suppressed and unsuppressed MEGA-PRESS MR spectroscopy and their relationship with BOLD responses in the occipital cortex. J Magnet Resonance Imag. (2019) 50:1285–94. doi: 10.1002/jmri.26706
17. Wijtenburg SA, West J, Korenic SA, Kuhney F, Gaston FE, Chen H, et al. Glutamatergic metabolites are associated with visual plasticity in humans. Neurosci Let. (2017) 644:30–6. doi: 10.1016/j.neulet.2017.02.020
18. Costigan AG, Umla-Runge K, Evans C, Hodgetts CJ, Lawrence AD, Graham KS. Neurochemical correlates of scene processing in the precuneus/posterior cingulate cortex: a multimodal fMRI and 1H-MRS study. Hum Brain Map. (2019) 40:2884–98. doi: 10.1002/hbm.24566
19. Schallmo MP, Kale AM, Millin R, Flevaris AV, Brkanac Z, Edden RA, Bernier RA, Murray SO. Suppression and facilitation of human neural responses. eLife. (2018) 7:29. doi: 10.7554/eLife.30334
20. Ip IB, Berrington A, Hess AT, Parker AJ, Emir UE, Bridge H. Combined fMRI-MRS acquires simultaneous glutamate and BOLD-fMRI signals in the human brain. NeuroImage. (2017) 155:113–9. doi: 10.1016/j.neuroimage.2017.04.030
21. Schallmo MP, Millin R, Kale AM, Kolodny T, Edden RA, Bernier RA, et al. Glutamatergic facilitation of neural responses in MT enhances motion perception in humans. NeuroImage. (2019) 184:925–31. doi: 10.1016/j.neuroimage.2018.10.001
22. Bhattacharyya PK, Phillips MD, Stone LA, Bermel RA, Lowe MJ. Sensorimotor cortex gamma-aminobutyric acid concentration correlates with impaired performance in patients with MS. AJNR Am J Neuroradiol. (2013) 34:1733–9. doi: 10.3174/ajnr.A3483
23. Bhattacharyya PK, Phillips MD, Stone LA, Lowe MJ. Activation volume vs BOLD signal change as measures of fMRI activation - Its impact on GABA - fMRI activation correlation. Magn Reson Imaging. (2017) 42:123–9. doi: 10.1016/j.mri.2017.06.009
24. Draper A, Stephenson MC, Jackson GM, Pepes S, Morgan PS, Morris PG, Jackson SR. Increased GABA contributes to enhanced control over motor excitability in Tourette syndrome. Curr Biol. (2014) 24:2343–7. doi: 10.1016/j.cub.2014.08.038
25. Stagg CJ, Bachtiar V, Johansen-Berg H. The role of GABA in human motor learning. Curr Biol. (2011) 21:480–4. doi: 10.1016/j.cub.2011.01.069
26. Torta DM, Cauda F. Different functions in the cingulate cortex, a meta-analytic connectivity modeling study. NeuroImage. (2011) 56:2157–72. doi: 10.1016/j.neuroimage.2011.03.066
27. Chen X, Fan X, Hu Y, Zuo C, Whitfield-Gabrieli S, Holt D, et al. Regional GABA concentrations modulate inter-network resting-state functional connectivity. Cereb Cortex. (2019) 29:1607–18. doi: 10.1093/cercor/bhy059
28. Jocham G, Hunt LT, Near J, Behrens TE. A mechanism for value-guided choice based on the excitation-inhibition balance in prefrontal cortex. Nat Neurosci. (2012) 15:960–1. doi: 10.1038/nn.3140
29. Stan AD, Schirda CV, Bertocci MA, Bebko GM, Kronhaus DM, Aslam HA, et al. Glutamate and GABA contributions to medial prefrontal cortical activity to emotion: implications for mood disorders. Psychiatry Res. (2014) 223:253–60. doi: 10.1016/j.pscychresns.2014.05.016
30. Wiebking C, Duncan NW, Tiret B, Hayes DJ, Marjanska M, Doyon J, et al. GABA in the insula - a predictor of the neural response to interoceptive awareness. NeuroImage. (2014) 86:10–8. doi: 10.1016/j.neuroimage.2013.04.042
31. Walter M, Henning A, Grimm S, Schulte RF, Beck J, Dydak U, et al. The relationship between aberrant neuronal activation in the pregenual anterior cingulate, altered glutamatergic metabolism, and anhedonia in major depression. Arch GenPsychiatry. (2009) 66:478–86. doi: 10.1001/archgenpsychiatry.2009.39
32. Levar N, van Leeuwen JM, Denys D, van Wingen GA. Divergent influences of anterior cingulate cortex GABA concentrations on the emotion circuitry. NeuroImage. (2017) 158:136–44. doi: 10.1016/j.neuroimage.2017.06.055
33. Levar N, van Leeuwen JMC, Puts NAJ, Denys D, Van Wingen GA. GABA concentrations in the anterior cingulate cortex are associated with fear network function and fear recovery in humans. Front Hum Neurosci. (2017) 11:202. doi: 10.3389/fnhum.2017.00202
34. Overbeek G, Gawne TJ, Reid MA, Salibi N, Kraguljac NV, White DM, et al. Relationship between cortical excitation and inhibition and task-induced activation and deactivation: a combined magnetic resonance spectroscopy and functional magnetic resonance imaging study at 7T in first-episode psychosis. Biol Psychiatry. (2019) 4:121–30. doi: 10.1016/j.bpsc.2018.10.002
35. Northoff G, Walter M, Schulte RF, Beck J, Dydak U, Henning A, et al. GABA concentrations in the human anterior cingulate cortex predict negative BOLD responses in fMRI. Nat Neurosci. (2007) 10:1515–7. doi: 10.1038/nn2001
36. Wang GY, van Eijk J, Demirakca T, Sack M, Krause-Utz A, Cackowski S, et al. ACC GABA levels are associated with functional activation and connectivity in the fronto-striatal network during interference inhibition in patients with borderline personality disorder. NeuroImage. (2017) 147:164–74. doi: 10.1016/j.neuroimage.2016.12.013
37. Yücel M, Lubman DI, Harrison BJ, Fornito A, Allen NB, Wellard RM, et al. A combined spectroscopic and functional MRI investigation of the dorsal anterior cingulate region in opiate addiction. Mol Psychiatry. (2007) 12:691–702. doi: 10.1038/sj.mp.4001955
38. Reid MA, Stoeckel LE, White DM, Avsar KB, Bolding MS, Akella NS, et al. Assessments of function and biochemistry of the anterior cingulate cortex in schizophrenia. Biol Psychiatry. (2010) 68:625–33. doi: 10.1016/j.biopsych.2010.04.013
39. Falkenberg LE, Westerhausen R, Specht K, Hugdahl K. Resting-state glutamate level in the anterior cingulate predicts blood-oxygen level-dependent response to cognitive control. Proc Natl Acad Sci USA. (2012) 109:5069–73. doi: 10.1073/pnas.1115628109
40. Brennan BP, Tkachenko O, Schwab ZJ, Juelich RJ, Ryan EM, Athey AJ, et al. An examination of rostral anterior cingulate cortex function and neurochemistry in obsessive-compulsive disorder. Neuropsychopharmacology. (2015) 40:1866–76. doi: 10.1038/npp.2015.36
41. Gleich T, Lorenz RC, Pöhland L, Raufelder D, Deserno L, Beck A, et al. Frontal glutamate and reward processing in adolescence and adulthood. Brain Struct Funct. (2015) 220:3087–99. doi: 10.1007/s00429-014-0844-3
42. Duncan NW, Hayes DJ, Wiebking C, Tiret B, Pietruska K, Chen DQ, et al. Negative childhood experiences alter a prefrontal-insular-motor cortical network in healthy adults: a preliminary multimodal rsfMRI-fMRI-MRS-dMRI study. Hum Brain Mapp. (2015) 36:4622–37. doi: 10.1002/hbm.22941
43. Fusar-Poli P, Stone JM, Broome MR, Valli I, Mechelli A, McLean MA, et al. Thalamic glutamate levels as a predictor of cortical response during executive functioning in subjects at high risk for psychosis. Arch Gen Psychiatry. (2011) 68:881–90. doi: 10.1001/archgenpsychiatry.2011.46
44. Duncan NW, Enzi B, Wiebking C, Northoff, G. Involvement of glutamate in rest-stimulus interaction between perigenual and supragenual anterior cingulate cortex: a combined fMRI-MRS study. Hum Brain Mapp. (2011) 32:2172–82. doi: 10.1002/hbm.21179
45. von Düring F, Ristow I, Li M, Denzel D, Colic L, Demenescu LR, et al. Glutamate in salience network predicts BOLD response in default mode network during salience processing. Front Behav Neurosci. (2019) 13:2172–82. doi: 10.3389/fnbeh.2019.00232
46. Cadena EJ, White DM, Kraguljac NV, Reid MA, Maximo JO, Nelson EA, et al. A longitudinal multimodal neuroimaging study to examine relationships between resting state glutamate and task related BOLD response in schizophrenia. Front Psychiatry. (2018) 9:632. doi: 10.3389/fpsyt.2018.00632
47. Enzi B, Duncan NW, Kaufmann J, Tempelmann C, Wiebking C, Northoff G. Glutamate modulates resting state activity in the perigenual anterior cingulate cortex - a combined fMRI-MRS study. Neuroscience. (2012) 227:102–9. doi: 10.1016/j.neuroscience.2012.09.039
48. Naaijen J, Lythgoe DJ, Zwiers MP, Hartman CA, Hoekstra PJ, Buitelaar JK, et al. Anterior cingulate cortex glutamate and its association with striatal functioning during cognitive control. Eur Neuropsychopharmacol. (2018) 28:381–91. doi: 10.1016/j.euroneuro.2018.01.002
49. Modinos G, McLaughlin A, Egerton A, McMullen K, Kumari V, Barker GJ, et al. Corticolimbic hyper-response to emotion and glutamatergic function in people with high schizotypy: a multimodal fMRI-MRS study. Transl Psychiatry Psychiatry. (2017) 7:e1083. doi: 10.1038/tp.2017.53
50. Friedman SD, Poliakov AV, Budech C, Shaw DWW, Breiger D, Jinguji T, et al. GABA alterations in pediatric sport concussion. Neurology. (2017) 89:2151–6. doi: 10.1212/WNL.0000000000004666
51. Moon CM, Jeong GW. Associations of neurofunctional, morphometric and metabolic abnormalities with clinical symptom severity and recognition deficit in obsessive-compulsive disorder. J Affect Disord. (2018) 227:603–12. doi: 10.1016/j.jad.2017.11.059
52. Kaminski J, Gleich T, Fukuda Y, Katthagen T, Gallinat J, Heinz A, et al. Association of cortical glutamate and working memory activation in patients with schizophrenia: a multimodal proton magnetic resonance spectroscopy and functional magnetic resonance imaging study. Biol Psychiatry. (2020) 87:225–33. doi: 10.1016/j.biopsych.2019.07.011
53. Jung J, Williams SR, Sanaei Nezhad F, Lambon Ralph MA. GABA concentrations in the anterior temporal lobe predict human semantic processing. Sci Rep. (2017) 7:15748. doi: 10.1038/s41598-017-15981-7
54. Schmitz TW, Correia MM, Ferreira CS, Prescot AP, Anderson MC. Hippocampal GABA enables inhibitory control over unwanted thoughts. Nat Commun. (2017) 8:1311. doi: 10.1038/s41467-017-00956-z
55. Valli I, Stone J, Mechelli A, Bhattacharyya S, Raffin M, Allen P, et al. Altered medial temporal activation related to local glutamate levels in subjects with prodromal signs of psychosis. Biol Psychiatry. (2011) 69:97–9. doi: 10.1016/j.biopsych.2010.08.033
56. Hutcheson NL, Reid MA, White DM, Kraguljac NV, Avsar KB, Bolding MS, et al. Multimodal analysis of the hippocampus in schizophrenia using proton magnetic resonance spectroscopy and functional magnetic resonance imaging. Schizophr Res. (2012) 140:136–42. doi: 10.1016/j.schres.2012.06.039
57. Bossong MG, Wilson R, Appiah-Kusi E, McGuire P, Bhattacharyya S. Human striatal response to reward anticipation linked to hippocampal glutamate levels. Int J Neuropsychopharmacol. (2018) 21:623–30. doi: 10.1093/ijnp/pyy011
58. Lipp I, Evans CJ, Lewis C, Murphy K, Wise RG, Caseras X. The relationship between fearfulness, GABA+, and fear-related BOLD responses in the insula. PLoS ONE. (2015) 10:e0120101. doi: 10.1371/journal.pone.0120101
59. Cleve M, Gussew A, Wagner G, Bär KJ, Reichenbach JR. Assessment of intra- and inter-regional interrelations between GABA+, Glx and BOLD during pain perception in the human brain – a combined 1H fMRS and fMRI study. Neuroscience. (2017) 365:125–36. doi: 10.1016/j.neuroscience.2017.09.037
60. Hu Y, Chen X, Gu H, Yang Y. Resting-state glutamate and GABA concentrations predict task-induced deactivation in the default mode network. J Neurosci. (2013) 33:18566–73. doi: 10.1523/JNEUROSCI.1973-13.2013
61. Robin J, Buchsbaum BR, Moscovitch M. The primacy of spatial context in the neural representation of events. J Neurosci. (2018) 2018:1638–17. doi: 10.1523/JNEUROSCI.1638-17.2018
62. Lorenz RC, Gleich T, Buchert R, Schlagenhauf F, Kühn S, Gallinat J. Interactions between glutamate, dopamine, and the neuronal signature of response inhibition in the human striatum. Hum Brain Mapp. (2015) 36:4031–40. doi: 10.1002/hbm.22895
63. White DM, Kraguljac NV, Reid MA, Lahti AC. Contribution of substantia nigra glutamate to prediction error signals in schizophrenia: a combined magnetic resonance spectroscopy/functional imaging study. NPJ Schizophr. (2015) 1:14001. doi: 10.1038/npjschz.2014.1
64. Allen P, Chaddock CA, Egerton A, Howes OD, Barker G, Bonoldi I, et al. Functional outcome in people at high risk for psychosis predicted by thalamic glutamate levels and prefronto-striatal activation. Schizophr Bull. (2015) 41:429–39. doi: 10.1093/schbul/sbu115
65. Hyder F, Fulbright RK, Shulman RG, Rothman DL. Glutamatergic function in the resting awake human brain is supported by uniformly high oxidative energy. J Cerebral Blood Flow Metab. (2013) 33:339–47. doi: 10.1038/jcbfm.2012.207
66. Chen Z, Silva AC, Yang J, Shen J. Elevated endogenous GABA level correlates with decreased fMRI signals in the rat brain during acute inhibition of GABA transaminase. J Neurosci Res. (2005) 79:383–91. doi: 10.1002/jnr.20364
67. Devor A, Tian P, Nishimura N, Teng IC, Hillman EMC, Narayanan SN, et al. Suppressed neuronal activity and concurrent arteriolar vasoconstriction may explain negative blood oxygenation level-dependent signal. J Neurosci. (2007) 27:4452–9. doi: 10.1523/JNEUROSCI.0134-07.2007
68. Sumner P, Edden RA, Bompas A, Evans CJ, Singh KD. More GABA, less distraction: a neurochemical predictor of motor decision speed. Nat Neurosci. (2010) 13:825–7. doi: 10.1038/nn.2559
69. Logothetis NK. What we can do and what we cannot do with fMRI. Nature. (2008) 453:869–78. doi: 10.1038/nature06976
70. Rockland KS. Elements of cortical architecture. In: Extrastriate Cortex in Primates. Boston, MA: Springer (1997). p. 243-93. doi: 10.1007/978-1-4757-9625-4_6
71. Stevens FL, Hurley RA, Taber KH. Anterior cingulate cortex: unique role in cognition and emotion. J Neuropsychiatry Clin Neurosci. (2011) 23:121–5. doi: 10.1176/jnp.23.2.jnp121
72. Devinsky O, Morrell MJ, Vogt BA. Contributions of anterior cingulate cortex to behavior. Brain. (1995) 118:279–306. doi: 10.1093/brain/118.1.279
73. Fleysher R, Fleysher L, Liu S, Gonen O. On the voxel size and magnetic field strength dependence of spectral resolution in magnetic resonance spectroscopy. Magn Reson Imaging. (2009) 27:222–32. doi: 10.1016/j.mri.2008.06.009
74. Falkenberg LE, Westerhausen R, Craven AR, Johnsen E, Kroken RA, Loerg EM, et al. Impact of glutamate levels on neuronal response and cognitive abilities in schizophrenia. NeuroImage Clin. (2014) 4:576–84. doi: 10.1016/j.nicl.2014.03.014
75. Gallinat J, Kunz D, Senkowski D, Kienast T, Seifert F, Schubert F, et al. Hippocampal glutamate concentration predicts cerebral theta oscillations during cognitive processing. Psychopharmacology. (2006) 187:103–11. doi: 10.1007/s00213-006-0397-0
76. Merritt K, Egerton A, Kempton MJ, Taylor MJ, McGuire PK. Nature of glutamate alterations in schizophrenia: a meta-analysis of proton magnetic resonance spectroscopy studies. JAMA Psychiatry. (2016) 73:665–74. doi: 10.1001/jamapsychiatry.2016.0442
77. Hashimoto T, Volk DW, Eggan SM, Mirnics K, Pierri JN, Sun Z, et al. Gene expression deficits in a subclass of GABA neurons in the prefrontal cortex of subjects with schizophrenia. J Neurosci. (2003) 23:6315–26. doi: 10.1523/JNEUROSCI.23-15-06315.2003
78. Yang AC, Tsai SJ. New targets for schizophrenia treatment beyond the dopamine hypothesis. Int J Mol Sci. (2017) 18:1689. doi: 10.3390/ijms18081689
79. Sanacora G, Zarate CA, Krystal JH, Manji HK. Targeting the glutamatergic system to develop novel, improved therapeutics for mood disorders. Nat Rev Drug Discov. (2008) 7:426–37. doi: 10.1038/nrd2462
80. Chang L, Jiang CS, Ernst T. Effects of age and sex on brain glutamate and other metabolites. Magnetic Resonance Imaging. (2009) 27:142–5. doi: 10.1016/j.mri.2008.06.002
81. Gao F, Edden RAE, Li M, Puts NAJ, Wang G, Liu C, et al. Edited magnetic resonance spectroscopy detects an age-related decline in brain GABA levels. NeuroImage. (2013) 78:75–82. doi: 10.1016/j.neuroimage.2013.04.012
82. Sailasuta N, Ernst T, Chang L. Regional variations and the effects of age and gender on glutamate concentrations in the human brain. Magnetic Resonance Imaging. (2008) 26:667–75. doi: 10.1016/j.mri.2007.06.007
83. O'Gorman RL, Michels L, Edden RA, Murdoch JB, Martin E. In vivo detection of GABA and glutamate with MEGA-PRESS: reproducibility and gender effects. J Magnetic Resonance Imaging. (2011) 33:1262–7. doi: 10.1002/jmri.22520
84. Canitano R, Pallagrosi M. Autism spectrum disorders and schizophrenia spectrum disorders: excitation/inhibition imbalance and developmental trajectories. Front Psychiatry. (2017) 8:69. doi: 10.3389/fpsyt.2017.00069
85. Horder J, Petrinovic MM, Mendez MA, Bruns A, Takumi T, Spooren W, et al. Glutamate and GABA in autism spectrum disorder—a translational magnetic resonance spectroscopy study in man and rodent models. Translational Psychiatry. (2018) 8:106. doi: 10.1038/s41398-018-0155-1
86. Delmonte S, Balsters JH, McGrath J, Fitzgerald J, Brennan S, Fagan AJ, et al. Social and monetary reward processing in autism spectrum disorders. Mol Autism. (2012) 3:1–13. doi: 10.1186/2040-2392-3-7
Keywords: glutamate, GABA, magnetic resonance spectroscopy, fMRI, multimodal neuroimaging
Citation: Kiemes A, Davies C, Kempton MJ, Lukow PB, Bennallick C, Stone JM and Modinos G (2021) GABA, Glutamate and Neural Activity: A Systematic Review With Meta-Analysis of Multimodal 1H-MRS-fMRI Studies. Front. Psychiatry 12:644315. doi: 10.3389/fpsyt.2021.644315
Received: 20 December 2020; Accepted: 15 February 2021;
Published: 08 March 2021.
Edited by:
Richard Edden, Johns Hopkins University, United StatesReviewed by:
Yoshihiko Matsumoto, Yamagata University, JapanCopyright © 2021 Kiemes, Davies, Kempton, Lukow, Bennallick, Stone and Modinos. This is an open-access article distributed under the terms of the Creative Commons Attribution License (CC BY). The use, distribution or reproduction in other forums is permitted, provided the original author(s) and the copyright owner(s) are credited and that the original publication in this journal is cited, in accordance with accepted academic practice. No use, distribution or reproduction is permitted which does not comply with these terms.
*Correspondence: Amanda Kiemes, YW1hbmRhLnMua2llbWVzQGtjbC5hYy51aw==
Disclaimer: All claims expressed in this article are solely those of the authors and do not necessarily represent those of their affiliated organizations, or those of the publisher, the editors and the reviewers. Any product that may be evaluated in this article or claim that may be made by its manufacturer is not guaranteed or endorsed by the publisher.
Research integrity at Frontiers
Learn more about the work of our research integrity team to safeguard the quality of each article we publish.