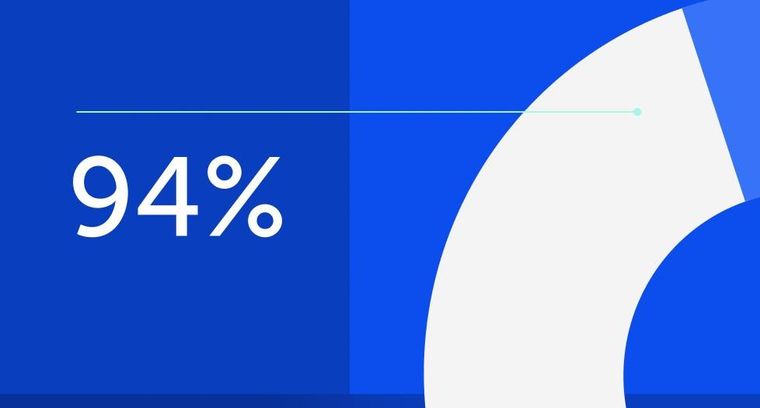
94% of researchers rate our articles as excellent or good
Learn more about the work of our research integrity team to safeguard the quality of each article we publish.
Find out more
ORIGINAL RESEARCH article
Front. Psychiatry, 19 August 2020
Sec. Neuroimaging
Volume 11 - 2020 | https://doi.org/10.3389/fpsyt.2020.00826
This article is part of the Research TopicSensory Information Processing Abnormalities in Schizophrenia and Related Neuropsychiatric DisordersView all 36 articles
Ketamine, an N-methyl-D-aspartate (NMDA) receptor antagonist, exerts broad effects on consciousness and perception. Since NMDA receptor antagonists induce cognitive impairments, ketamine has been used for translational research on several psychiatric diseases, such as schizophrenia. Whereas the effects of ketamine on cognitive functions have been extensively studied, studies on the effects of ketamine on simple sensory information processing remain limited. In this study, we investigated the cortex-wide effects of ketamine administration on auditory information processing in nonhuman primates using whole-cortical electrocorticography (ECoG). We first recorded ECoG from awake monkeys on presenting auditory stimuli of different frequencies or different durations. We observed auditory evoked responses (AERs) across the cortex, including in frontal, parietal, and temporal areas, while feature-specific responses were obtained around the temporal sulcus. Next, we examined the effects of ketamine on cortical auditory information processing. We conducted ECoG recordings from monkeys that had been administered anesthetic doses of ketamine from 10 to 180 min following administration. We observed significant changes in stimulus feature-specific responses. Electrodes showing a frequency preference or offset responses were altered following ketamine administration, while those of the AERs were not strongly influenced. However, the frequency preference of a selected electrode was not significantly altered by ketamine administration over time following administration, while the imbalances in the onset and offset persisted over the course of 150 min following ketamine administration in all three monkeys. These results suggest that ketamine affects the ability to distinguish between sound frequency and duration in different ways. In conclusion, future research on the NMDA sensitivity of cortical wide sensory information processing may provide a new perspective into the development of nonhuman primate models of psychiatric disorders.
Ketamine, an N-methyl-D-aspartate (NMDA) receptor antagonist, exerts broad effects on consciousness and perception. Several reports have documented that ketamine induced dissociative and extracorporeal sensations when patients recovered from ketamine-induced anesthesia (1–3). Furthermore, a subanesthetic dose of ketamine induces illusions and alterations in hearing, vision, or proprioception in healthy human volunteers, as well as psychotomimetic effects, such as hallucinations, paranoia, formal thought disorder, and cognitive impairment [e.g., (4)]. Based on reports where NMDA receptor antagonists induce cognitive impairments in nonhuman primates (NHP) (5–10), a subanesthetic dose of ketamine has been used for translational research on schizophrenia (6, 9, 11), Alzheimer’s disease (12), and autism spectrum disorder (8). These studies suggested that ketamine induces prefrontal cortical dysfunction, while recent studies reported aberrant neuronal oscillations in the prefrontal cortex of NHP (13, 14).
In addition to cognitive impairments, several studies on humans, nonhuman primates, and rodents have examined the effects of a subanesthetic dose of ketamine on neurophysiological indices of sensory information processing. Especially, auditory steady-state response (ASSR) (15, 16) and mismatch negativity (MMN) [e.g. (17, 18)] have been studied as more robust probes of abnormal auditory information processing in schizophrenia. ASSR is an electrophysiological response entrained to the frequency and the phase of periodic auditory stimuli, and is most evident when stimuli are presented in the gamma frequency range 30–50 Hz in humans (19). Alteration of ASSR has reported various neuropsychiatric illness, and is considered to reflect cortical excitation/inhibition imbalance (16). These dysfunctional oscillations may arise owing to anomalies in the networks of gamma-aminobutyric acidergic interneurons and pyramidal neurons (20, 21). Furthermore, recent animal studies suggested that ASSR modulations by ketamine is dose dependent (22, 23). On the other hand, MMN is an event-related potential (ERP) elicited most commonly in the context of auditory oddball paradigms [e.g., (24)], and is considered to reflect neural activity for deviance detections. Gil-da-Costa, Stoner (25) reported reduced MMN in monkeys following the subanesthetic administration of ketamine, as found in humans with schizophrenia (26, 27), and in healthy adult/young subjects having been administered ketamine (4, 28). These results based on electroencephalograms (EEGs) suggest that ketamine induces changes in neural activity related to early auditory information processing in humans and NHPs, like in schizophrenia patients. Furthermore, electrophysiological studies with depth electrodes reported that the primary auditory area of NHPs showed mismatch-related activity, which was inhibited by NMDA receptor antagonists (29, 30). Whereas auditory indices, ASSR and MMN, have been extensively studied, the effects of ketamine on auditory information processing itself have only been studied to a limited degree (28, 31).
In this study, we hypothesized ketamine affects even simple auditory feature processing (e.g., frequency or duration), and investigated the cortex-wide effects of ketamine administration by exposing common marmosets to auditory stimuli of different frequencies or durations. The marmoset, a small new-world monkey, has an anatomical organization homologous to that of humans and macaques (32, 33) and has been reported to have MMN-like auditory responses (34). Their lissencephalic brains enable direct access to the core auditory areas. The ECoG array covers a whole hemisphere of the marmoset from the frontal pole to the occipital and temporal poles. The array enables us to observe neuronal activity from an entire hemisphere with high spatiotemporal resolution.
This study was carried out in accordance with the recommendations of the National Institutes of Health Guidelines for the Care and Use of Laboratory Animals. The protocol was approved by the RIKEN Ethical Committee (No. H28-2-221 (3)). All surgical procedures were performed under anesthesia, and all efforts were made to minimize the number of animals used, as well as their discomfort. In so doing, we sought to deliver the most humane care and treatment possible. Parts of the dataset are shared on the public server Brain/MINDS Data Portal (YnJhaW5taW5kcy5yaWtlbi5qcA==).
We used five adult common marmosets (Callithrix jacchus; three males and two females, 320–470 g, 14–53 months). Before the ECoG arrays were implanted into the monkeys, they were familiarized with the experimenter and experimental settings. The animals had ad libitum access to food and water throughout the experimental period. Three of these animals were used for experiments with ketamine administration.
The whole-cortical 96ch ECoG arrays (Cir-Tech Co. Ltd., Japan) (35) were chronically implanted and used for neural recordings. We epidurally implanted the array into the right (monkey O, R, and S) or left (monkey J and M) hemisphere of each monkey. Fifteen and three electrodes from monkeys M and R, respectively, were cut during the implantation. The surgical procedures for electrode implantation have been previously described in detail (35). Following the animals’ recovery, the positions of each electrode contact were identified based on computer tomography, and aligned to pre-acquired T2-weighted anatomical magnetic resonance images using AFNI software (36) (http://afni.nimh.nih.gov). Finally, we estimated the location of each electrode on cortical areas by registering a marmoset brain atlas (37) to the MRI with AFNI and ANTS (38). Based on putative cortical areas, we divided the electrodes into six groups, consisting of the prefrontal, sensorimotor, posterior parietal, visual, temporal, and auditory areas. In all monkeys, the electrode array covered the frontal, parietal, occipital, and temporal cortices (Supplementary Figure S1 and Supplementary Table S1).
We used auditory stimuli of different durations (AD) and frequencies (AF). In AD, 10 types of pure sinusoidal tones (1 ms rise/fall) with different durations (10, 25, 50, 75, 100, 125, 150, 175, 200, and 225 ms; 1,000 Hz; 2,000 stimuli in total) were randomly presented with an equal probability of 10%. In AF, 10 types of tones with different frequencies (700, 800, 900, 1,000, 1,100, 1,200, 1,300, 1,400, 1,500, and 1,600 Hz; 50 ms; 1,000 stimuli in total) were presented with an equal probability of 10%. The stimulus onset asynchrony was 503 ms. Stimulus presentation was controlled by MATLAB (MathWorks Inc., Natick, MA, USA) using the Psychophysics Toolbox extensions (39, 40). Tones were presented through two audio speakers (Fostex, Japan) with an average intensity of 70 dB SPL around the animal’s ear.
ECoG signals were recorded at a sampling rate of 1 kHz using a Grapevine NIP (Ripple Neuro, Salt Lake City, UT). In each recording session, only an auditory stimuli (AD or AF) was used. During the recordings, the monkeys were placed in a sphinx position in a custom bed in a dimly lit, electrically shielded and sound-attenuated chamber with their head fixed. For awake recordings, 5, 4, and 2 sessions were conducted on monkey S, R, and J on separate days. For monkeys M and O, one recording session was conducted. Different numbers of recording sessions were caused by experimental schedules of each animal used in different research projects.
We conducted experiments with ketamine administration on three monkeys (J, M, and O). On each experimental day, a recording session with awake marmosets was first conducted. Next, an anesthetic dose of ketamine (30 mg/kg i.m.) was injected 5 min after an atropine (0.08 mg/kg i.m.) injection. Ten minutes after the injection, ECoG recordings were repeatedly conducted at approximately 30-min intervals for up to 3 h. For monkey J, the AD and AF experiments were conducted on two different days. For monkeys M and O, the AD and AF experiments were conducted on one experimental day. We discarded the data from one experimental day for monkeys J and M since they struggled during the sessions. In the following analysis, we focused on 10, 30, and 150 min following administration (K10, K30, and K150) since increased muscle tone [a common side effect of ketamine (41)] causes artifacts on high-gamma activity during 60–120 min following administration.
Electrophysiological data were analyzed using custom scripts for MATLAB. For signal pre-processing, signals were re-referenced using a common median reference (CMR) montage, and band-pass filtered at 1–30 Hz (LF) and 80–160 Hz (HG). We calculated the envelope of the HG frequency band using the Hilbert transform (Figure 1A). We assumed that the LF mainly reflected a summation of post-synaptic potentials, while HG was related to mean firing activity (14, 42, 43). We segmented datasets from −100 to 450 ms relative to the onsets of the tones. To remove segments that contained outliers, we calculated a standard deviation (SDi, i = 1–nT, in which nT is a number of trials) for each segment and for all segments (SDall) and removed segments with an SDi above 3SDall. After excluding outlier, we applied a baseline correction by subtracting the mean of the 100 ms period prior to stimulus onset. The ERPs were then calculated for each channel.
Figure 1 Cortex-wide auditory evoked responses. (A) An example of an ECoG signal of a single trial from the primary auditory area of monkey R. Left: an example of a low frequency (1–30 Hz) ECoG (LF). Right: an example of a high frequency (80–160 Hz) ECoG (HG). The vertical bars indicate the onset of sound stimuli. (B) The locations of electrodes and putative cortical areas. (C) Auditory evoked potentials of monkey R. LF (left) and HG (right) ECoGs are aligned to sound onset and averaged. The x-axis represents the times and the y-axis represents the labels of the ECoG electrodes in (B). The red dots indicate the electrodes and times that show significant responses (adjusted p < 0.00001). The bottom insets show the cortical map for auditory stimuli. The black dots represent the electrode positions. The blue circles represent the electrodes that showed significant responses. (D) Cortical maps for auditory information processing from the other four monkeys. The black dots represent the electrode positions. The blue and red circles represent the electrodes that showed significant responses in LF and HG, respectively. Individual AEPs are shown in Supplementary Figure 1.
To distinguish spontaneous signals in the baseline period and responses to auditory stimuli, we conducted t-tests at each time point 0 to 450 ms after stimulus onset for every electrode. All p-values from each monkey and each recording session were false discovery rate (FDR)-adjusted (44). The significance level was set to 0.00001 unless otherwise specified.
To examine selective responses for auditory features, frequencies, and durations, we first conducted a one-way analysis of variance (ANOVA) on frequencies or on durations at each time point at 0 to 450 ms for each electrode. All p-values from each monkey and each recording session were FDR-adjusted. The significance level was set to 0.00001 unless otherwise specified. For frequency, we further determined the best frequency for each electrode. We calculated the means of responses for each frequency over statistically significant time points, and defined the frequency showing the maximum value of the means as the best frequency (BF).
To evaluate the effects of ketamine on AERs, we quantified the effects of ketamine administrations as differences in the amplitudes and latencies of the peaks of LF and mean responses of HG. For LF, we identified the first and second peaks of the AERs of electrodes at the significant time points, and obtained their amplitudes and latencies. To statistically compare those values of awake with ketamine conditions, we conducted a two-sample t-test within each electrode group based on putative cortical areas. For visualization purposes, we then normalized the amplitudes by dividing by the value of the awake condition, and calculated the differences in the latencies by subtracting the values obtained from awake conditions. For HG, we calculated the mean values of early (0–30 ms) and late (30–100 ms) responses following tone onset. To investigate the effects of ketamine on the observed responses, we calculated the z-score across conditions (i.e., Awake, K10, K30, K150) at each electrode, then subtracted the z-score of awake conditions from that of ketamine conditions. We determined the significant effects as less than −1.96 or as more than 1.96.
To evaluate the effects of ketamine on auditory feature processing, we quantified the changes in BFs and in onset/offset response ratios of selected electrodes, which showed both significant frequency preferences and offset responses in awake conditions. To calculate onset/offset response ratios, we calculated the means of early (0–200) and late (200–400) HG responses for tones with 200 and 225 ms durations, since neural activity during the early time windows for those two tones do not include offset response. Then we obtained onset/offset response ratios by dividing early HG responses by late HG responses.
The numbers of electrodes showing significant responses following ketamine administration were compared with those of the awake condition using a two-sample t-test with a significance level set to 0.05.
We collected whole-cortical ECoGs from five awake marmosets on presentation of auditory stimuli of different frequencies and durations. First, we calculated event related responses (ERRs) averaged over all AF stimuli for both LF and HG, and then compared the amplitudes at each time point from 0 to 450 ms following stimulus onset with the baseline (100 to 0 prior to onset) activity of each electrode. Figure 1C shows the AERs of LF and HG from monkey R. For LF, significant auditory responses in LF occurred in a large part of the cortex, including frontal and parietal cortices, as well as in temporal cortex (62.5% of the electrodes of monkey R). HG responses appeared in more limited areas (12.5%). Similar tendencies were observed in all animals (Figure 1D and Supplementary Figure S2).
We next examined selective responses to auditory features, frequencies, and durations. For frequency, we conducted a one-way ANOVA on frequencies at each time point from 0 to 450 ms for each electrode. The electrodes around the temporal sulcus showed a tonotopic spatial distributions of electrodes, while other significant electrodes did not show any significant difference in frequencies. For example, in monkey J, electrode 36 showed larger responses to low frequency tones, while electrode 37 showed larger responses for high frequencies (Figure 2A). Furthermore, the frequencies yielding the largest responses varied over time. We calculated the means of responses for each frequency over statistically significant time points, and defined the frequency showing the maximum value as the best frequency (BF). The BFs of electrodes in dorsal auditory cortices tended to be high frequencies for both LF and HG, and the significant electrodes of HG (3.3 ± 1.5%; mean ± SD of all monkeys) showed more limited distributions than those of LF (18.6 ± 9.7%) (Figure 2B).
Figure 2 Frequency preference. (A) Examples of representative ECoG responses to each frequency at selective electrodes of monkey R. Mean responses to each 10 frequencies are represented. The shaded areas correspond to 95% confidence intervals. The red dots represent time points which showed significant different responses to frequencies. The bar plot represents the mean responses to each frequency over time which were significant. The best frequencies of the electrodes are represented as asterisks. (B) Cortical maps of the tonotopy of LF (top insets) and HG (bottom insets). The circles indicate electrodes which show statistical significance, with colors representing the best frequency.
We conducted a one-way ANOVA on durations at each time point from 0 to 450 ms following tone onset for each electrode. The electrodes around the temporal sulcus showed sound offset responses (Figure 3). Unlike frequency-related responses, sound offset responses were found in the same electrodes. The significant electrodes of HG (2.6 ± 1.7%) showed more limited distributions than those of LF (11.3 ± 2.6%) (Figure 3B).
Figure 3 Tone offset responses. (A) Examples of representative ECoG responses to each duration on selective electrodes of monkey R. Mean responses to each 10 duration are represented. The shaded areas correspond to the 95% confidence intervals. The red dots represent the time points which showed significantly different responses to durations. (B) Cortical maps of the tonotopy of LF (blue) and HG (red).
We examined the effects of ketamine administration on cortex-wide auditory information processing in three marmosets at approximately 10 (K10), 30 (K30), and 150 (K150) min following administration. AERs were observed in temporal, parietal, and frontal areas (Figure 4A and Supplementary Figure S3A). The number of electrodes showing significant AERs did not change significantly across subjects following ketamine administration. Figure 4B and Supplementary Figure S3B show the signal changes in representative electrodes in auditory and frontal areas. We quantified the effects of ketamine administration as the differences in amplitudes and latencies of the peaks of LF and mean responses of HG. For LF, we identified the first and second peaks of the AERs of electrodes at significant time points and obtained their amplitudes and latencies. Figures 4C and S3C show the amplitudes and latencies of the representative electrodes in Figures 4B and S3B. We next calculated the differences from these values obtained from awake conditions (Supplementary Table S2, Figures 4D, E, and Supplementary Figures S3D, E). The amplitudes and the latencies of the first peaks remained relatively unchanged, while most of the amplitudes and latencies of the second peaks increased after 10 and 30 ms following administration. Moreover, the amplitudes of the first peaks did not change much in all monkeys, while the PFC latencies increased after 10 and 30 ms following administration. In the second peaks, both amplitudes and latencies of PFC were significantly increased after 10 and 30 ms following administration (Supplementary Table S2). For HG, we calculated the mean values of early (0–30 ms) and late (30–100 ms) responses. Most of the electrodes showed increased HG responses following ketamine administration (Figure 4F and Supplementary Figure S3). Only early responses in the auditory areas of monkey J decreased 10–30 min following ketamine administration.
Figure 4 The effects of ketamine on auditory evoked neural responses over time following ketamine administration in monkey J. (A) Changes in cortical maps for auditory information processing of LF (blue) and HG (red). Data were acquired form monkey J. (B) Representative waveforms from electrodes in auditory (ch37 and ch36) and prefrontal (ch63) cortices. The top and bottom insets show LF and HG, respectively. The line color indicates the conditions of the recordings, and the dots represent significant time points. (C) The amplitudes and latencies of the 1st and 2nd peaks of LF of the selected electrodes. The marker color indicates the conditions of the recordings. The triangles represent positive peaks, and the inverted triangles represent negative peaks. (D) Normalized amplitudes and latencies of the 1st (left) and 2nd (right) peaks of the selected electrodes. (E) Normalized amplitudes and latencies of the 1st (left) and 2nd (right) peaks of all electrodes. The amplitudes are log scaled. (F) Changes in responses of HG at 0–30 ms (left) and 30–100 ms (right) after the sound onset. The bar plots in (B) show these values of the corresponding electrodes. Asterisks in the bar plots indicate significant (>2) changes.
We next examined the effects on auditory feature processing, and observed changes in all three monkeys. Regarding frequency preference, we observed a significant increase in the numbers of electrodes, which showed a frequency preference for HG in K10 (Figures 5A, B black lines). For HG in K30 and K150, the numbers of significant electrodes also increased but not significantly (paired t-test p = 0.07 and 0.10, respectively). Thereafter, we examined the frequency preference of selected electrodes. The preference for higher or lower tones was not strongly affected by ketamine. Changes in BF were not observed in monkey J, while few (<100 Hz) changes were observed in monkeys M and O (Figure 5C). For sound offset responses, no significant changes were observed in the number of electrodes which showed sound offset responses (Figures 5D, B gray lines), while some of the monkeys showed tendencies similar to the frequency preferences. The number of significant electrodes of HG at 10–150 min and 30–150 min following administration in monkeys M and J, respectively, were increased. Furthermore, we calculated the means of early (0–200) and late (200–400) HG responses for tones of 200- and 225-ms duration (Figure 5E left), and then calculated the onset/offset response ratios. Results demonstrated that onset/offset response ratios increased significantly over time following ketamine administration (Figure 5E right). The onset/offset balance 150 ms following ketamine administration of monkey O returned to its awake value, though the mean offset responses remained larger than the awake responses. Finally, to confirm that the different effects of ketamine on frequency and duration are not contingent on the different experimental days, we compared responses to a 50-ms tone at 1,000 Hz recorded in AF and AD experiments. Even though AF and AD experiments were conducted on different days, we observed similar wave forms (Supplementary Figure S4).
Figure 5 The effects of ketamine on auditory feature processing over time following ketamine administration. (A) Changes in cortical maps for frequency preference of LF (top brain insets) and HG (bottom brain insets). The LF and HG signals of representative electrodes for LF and HG are shown. The dot and line colors represent each frequency. (B) Changes in the number of significant electrodes in terms of frequency preferences (black lines), and offset responses (gray lines). Data from monkey J (circle), M (square), and O (cross). Changes in BF (C). (D) Changes in cortical maps for offset responses of LF (blue) and HG (red) (top). The LF (middle) and HG (bottom) signals of representative electrodes for LF and HG are shown. The dot and line colors indicate each duration. (E) Means of onset (0–200 ms; filled markers) and offset (200–400 ms; open markers) responses for the tones with 200 (solid lines) and 225 ms (dashed lines) (left). On/off response ratio (right).
To the best of our knowledge, this study is the first to demonstrate the effect of ketamine in cortex-wide auditory evoked responses in nonhuman primates. We first recorded ECoGs from awake monkeys on presentation of auditory stimuli of different frequencies or durations. We observed AERs across the cortex, including in frontal, parietal, and temporal areas, while feature specific responses were obtained around the temporal sulcus. Next, we examined the effects of ketamine on cortical auditory information processing. We conducted ECoG recordings from monkeys between 10 and 180 min following ketamine administration, and observed significant changes in stimulus feature-specific responses. The number of electrodes which showed frequency preferences or offset responses changed following ketamine administration, while that of AERs was not largely influenced. However, the frequency preference of a selected electrode was not heavily modulated by ketamine administration over time following administration, while the imbalances in onset and offset persisted over the course of the 150 min following ketamine administration in all three monkeys.
We conducted ECoG recordings from awake marmosets on presentation of auditory stimuli, and found that auditory evoked potentials were observed across the cortex including in frontal, parietal, and temporal areas (Figure 1). Furthermore, feature-specific responses were obtained around the temporal sulcus. Regarding frequency, we observed a tonotopic organization whereby the BFs of electrodes in the dorsal part of the auditory cortices tended to be of high frequencies both in LF and HG (Figure 2). These results are consistent with those of previous studies on tonotopic representations in the primary auditory area of marmosets (45–47). However, the frequency range of the current study was limited to 600–1,700 Hz, while frequency ranges in previous studies varied from 125 to 38,100 Hz. Thus, more broad frequency ranges are required to construct a more complete tonotopic organization. As per duration, we found sound offset responses in the small number of electrodes which showed onset responses (Figure 3), although previous studies have reported that sound offset responses are found throughout the auditory cortex and subcortical structures. This may be due to the fact that we varied the durations of tones by only 1,000 Hz, and that offset responses also have frequency preferences (48).
Next, we examined the effects of an anesthetic dose of ketamine on auditory responses, and observed a significant increase in the auditory evoked responses and latencies for both LF and HG signals from many electrodes. These observations are inconsistent with those of previous studies. Schwender, Klasing (31) reported that there was no change in the latencies or amplitudes of the mid-latency peaks of AEPs after induction of general anesthesia with ketamine according to human electroencephalography (EEG) data. Umbricht, Schmid (28) also reported that there were significant changes in N1 amplitude, though its latencies were not significant. Furthermore, Javitt and colleagues reported that PCP administration inhibits auditory N1 responses in the primary auditory area of macaque monkeys as shown using cranial electrodes on Cz (49) or depth electrodes on A1 (17). There are several possible reasons for these varied reports with regard to the effects of ketamine. First, these three studies used different doses and administration routes, which are known to strongly influence the effects of ketamine (50). Second, the nature of the sound stimuli was another modulating factor. The amplitude of N1 depends on the inter stimulus interval (ISI) of the tone sequence, and PCP enhances monkey N1 responses to tone sequences with short ISIs, while it decreases monkey N1 responses to tone sequences with long ISIs (49). Thus, the effects of ketamine may vary depending on the sound stimuli. Third, the timing of the recordings relative to the administration of ketamine also had an influence. In our study, increased amplitudes diminished over time. Thus, if we were to conduct observations for longer periods of time, there is a possibility that there may be a time zone during which auditory responses are less strong than those of awake conditions. Despite these inconsistencies, current results were consistent between all three subjects and appeared not to contradict the fact that ketamine induces auditory hallucinations.
Finally, we investigated the effects of ketamine on feature-specific responses. The numbers of electrodes that showed frequency preferences or offset responses were altered following the administration of ketamine. However, the BF of a selected electrode was not modulated much by ketamine administration over time following the administration, while the imbalances in onset and offset persisted over the course of 150 min following the administration of ketamine in all three monkeys. These results suggest that ketamine affects the ability to distinguish between sound frequency and duration in different ways. Despite the difficulty in specifying the precise molecular mechanism of the observed ketamine effects, different influences of NMDA receptors blockade on sound duration and frequency may be able to explain the alterations in duration and frequency MMN at different stages of schizophrenia. Friedman, Sehatpour (51) reported that the deficits in duration MMN processing may be specifically related to impaired premorbid function, whereas deficits in MMN to frequency deviants may continue to worsen even during early disease stages (52, 53). Furthermore, a similar imbalance in the onset and offset responses in K10 has been reported in an electrophysiological study on the primary auditory area of aged monkeys (54). This study also showed that the increase in firing rates of auditory cortex neurons in aged monkeys was significantly higher than that in young monkeys, a result consistent with those of previous studies (55–57). The authors argued that this result was caused by weakened inhibition in the ascending auditory pathway. In addition to these experiments on aged monkeys, Scholl, Gao (58) reported that sound offset responses of rat auditory cortex are not only products of local inhibitory circuits but also of the auditory ascending pathway. Therefore, ketamine may suppress the auditory ascending pathway at least immediately following administration. Kopp-Scheinpflug, Sinclair (59) argued that specific abnormalities in sound offset responses may, rather than reflect problems in tone-detection thresholds, reflect impairments in temporal processing and the ability to rapidly perceive varying signals, such as auditory scene analysis and speech perception. As such, understanding the cellular and circuit origins of sound offset responses in the auditory brain may be key to advancing the treatment of auditory temporal processing deficits in aging and disease.
In this study, we focused on high-gamma band ECoG power as well as low-frequency ECoG. Aberrant gamma-band oscillations have been ubiquitously observed in schizophrenia and in animal models of ketamine administrations [e.g., (20, 60)]. Several studies have reported excessive gamma oscillatory activity in schizophrenia (61) and in animal with ketamine administrations (14). Skoblenick, Womelsdorf (14) argued that the increased gamma-band activity may reduce the signal-to-noise ratio of task related neurons. It may be that the increased significant electrodes observed in the auditory feature specific responses may be another manifestation of an increase in background noise and dysfunctions of auditory feature discriminations. Further investigation is required to confirm this hypothesis.
There are some limitations to this study. First, the variations in the frequencies and durations of sound stimuli were limited in scope. These current results need to be confirmed using a broader range of different parameters since onset/offset balance is affected by stimulus intensity and frequency. Second, the study was based on a small number of subjects. In this study, three monkeys showed several different results in response to the administration of ketamine. This was to be expected since psychotomimetic effects are reported in only 43% of human subjects (62). It is essential to acquire and accumulate larger dataset from animal models in order to compare these results with reports from human subjects. Third, we examined data from limited time frames. During 60–120 min following ketamine administration, increased muscle tone was observed and caused artifacts on high-gamma activity.
In conclusion, whole cortex ECoG allows us to investigate cortex-wide information processing. Recent results indicate that frontal and parietal areas are involved in auditory information processing, as are temporal areas. However, stimulus feature-specific responses were primarily observed around the temporal sulcus. The administration of ketamine affected these stimulus-feature specific processes. Future research on the NMDA sensitivity of cortex-wide auditory information processing may provide a new perspective which will help to develop NHP models to study psychiatric disorders.
The raw data supporting the conclusions of this article are available in online repositories, Brain/MINDS Data Portal (Data ID: 4924).
The animal study was reviewed and approved by RIKEN Ethical Committee (No. H28-2-221(3)).
MK designed and conducted the experiments, and analyzed the data. NI supervised the project. All authors contributed to the article and approved the submitted version.
This study was supported by the Brain Mapping by Integrated Neurotechnologies for Disease Studies (Brain/MINDS) project of the Japan Agency for Medical Research and Development (AMED) (JP20dm0207069, JP20dm0207001) and by the Japan Society for the Promotion of Science (JSPS) KAKENHI (JP19H04993, JP17H06034).
The authors declare that the research was conducted in the absence of any commercial or financial relationships that could be construed as potential conflict of interest.
We thank Junichi Hata for correcting the MRI data, Takaaki Kaneko and Ken Nakae for the annotations of brain regions to electrodes, and Yuri Shinomoto for the recordings of a part of data. We also would like to thank Editage (www.editage.com) for English language editing.
The Supplementary Material for this article can be found online at: https://www.frontiersin.org/articles/10.3389/fpsyt.2020.00826/full#supplementary-material
1. Garfield JM, Garfield FB, Stone JG, Hopkins D, Johns LA. A comparison of psychologic responses to ketamine and thiopental–nitrous oxide–halothane anesthesia. Anesthesiology (1972) 36(4):329–38. doi: 10.1097/00000542-197204000-00006
2. White PF, Ham J, Way WL, Trevor AJ. Pharmacology of ketamine isomers in surgical patients. Anesthesiology (1980) 52(3):231–9. doi: 10.1097/00000542-198003000-00008
3. White PF, Way WL, Trevor AJ. Ketamine–its pharmacology and therapeutic uses. Anesthesiology (1982) 56(2):119–36. doi: 10.1097/00000542-198202000-00007
4. Krystal JHK, Laurence P, Seiby L, John P. Subanesthetic Effects of the Noncompetitive NMDA Antagonist, Ketamine, in Humans. Arch Gen Psychiatry (1994) 51(3):199. doi: 10.1001/archpsyc.1994.03950030035004
5. Elsworth JD, Groman SM, Jentsch JD, Valles R, Shahid M, Wong E, et al. Asenapine effects on cognitive and monoamine dysfunction elicited by subchronic phencyclidine administration. Neuropharmacology (2012) 62(3):1442–52. doi: 10.1016/j.neuropharm.2011.08.026
6. Buccafusco JJ, Terry AV Jr. A reversible model of the cognitive impairment associated with schizophrenia in monkeys: potential therapeutic effects of two nicotinic acetylcholine receptor agonists. Biochem Pharmacol (2009) 78(7):852–62. doi: 10.1016/j.bcp.2009.06.102
7. Buffalo EA, Gillam MP, Allen RR, Paule MG. Acute behavioral effects of MK-801 in rhesus monkeys: Assessment using an operant test battery. Pharmacol Biochem Behav (1994) 48(4):935–40. doi: 10.1016/0091-3057(94)90203-8
8. Nakako T, Murai T, Ikejiri M, Hashimoto T, Kotani M, Matsumoto K, et al. Effects of lurasidone on ketamine-induced joint visual attention dysfunction as a possible disease model of autism spectrum disorders in common marmosets. Behav Brain Res (2014) 274:349–54. doi: 10.1016/j.bbr.2014.08.032
9. Nakako T, Murai T, Ikejiri M, Ishiyama T, Taiji M, Ikeda K. Effects of a dopamine D1 agonist on ketamine-induced spatial working memory dysfunction in common marmosets. Behav Brain Res (2013) 249:109–15. doi: 10.1016/j.bbr.2013.04.012
10. Taffe MA, Davis SA, Gutierrez T, Gold LH. Ketamine impairs multiple cognitive domains in rhesus monkeys. Drug Alcohol Depend (2002) 68(2):175–87. doi: 10.1016/S0376-8716(02)00194-1
11. Roberts BM, Holden DE, Shaffer CL, Seymour PA, Menniti FS, Schmidt CJ, et al. Prevention of ketamine-induced working memory impairments by AMPA potentiators in a nonhuman primate model of cognitive dysfunction. Behav Brain Res (2010) 212(1):41–8. doi: 10.1016/j.bbr.2010.03.039
12. Taffe MA, Weed MR, Gutierrez T, Davis SA, Gold LH. Differential muscarinic and NMDA contributions to visuo-spatial paired-associate learning in rhesus monkeys. Psychopharmacology (2002) 160(3):253–62. doi: 10.1007/s00213-001-0954-5
13. Ma L, Skoblenick K, Johnston K, Everling S. Ketamine Alters Lateral Prefrontal Oscillations in a Rule-Based Working Memory Task. J Neurosci (2018) 38(10):2482–94. doi: 10.1523/JNEUROSCI.2659-17.2018
14. Skoblenick KJ, Womelsdorf T, Everling S. Ketamine Alters Outcome-Related Local Field Potentials in Monkey Prefrontal Cortex. Cereb Cortex (2016) 26(6):2743–52. doi: 10.1093/cercor/bhv128
15. Thune H, Recasens M, Uhlhaas PJ. The 40-Hz Auditory Steady-State Response in Patients With Schizophrenia: A Meta-analysis. JAMA Psychiatry (2016) 73(11):1145–53. doi: 10.1001/jamapsychiatry.2016.2619
16. Tada M, Kirihara K, Koshiyama D, Fujioka M, Usui K, Uka T, et al. Gamma-Band Auditory Steady-State Response as a Neurophysiological Marker for Excitation and Inhibition Balance: A Review for Understanding Schizophrenia and Other Neuropsychiatric Disorders. Clin EEG Neurosci (2019) 51(4):234–43. doi: 10.1177/1550059419868872
17. Javitt DC. Neurophysiological models for new treatment development in schizophrenia: early sensory approaches. Ann N Y Acad Sci (2015) 1344:92–104. doi: 10.1111/nyas.12689
18. Tada M, Kirihara K, Mizutani S, Uka T, Kunii N, Koshiyama D, et al. Mismatch negativity (MMN) as a tool for translational investigations into early psychosis: A review. Int J Psychophysiol (2019) 145:5–14. doi: 10.1016/j.ijpsycho.2019.02.009
19. Galambos R, Makeig S, Talmachoff PJ. A 40-Hz auditory potential recorded from the human scalp. Proc Natl Acad Sci U S A (1981) 78(4):2643–7. doi: 10.1073/pnas.78.4.2643
20. Uhlhaas PJ, Singer W. Abnormal neural oscillations and synchrony in schizophrenia. Nat Rev Neurosci (2010) 11(2):100–13. doi: 10.1038/nrn2774
21. Sohal VS, Zhang F, Yizhar O, Deisseroth K. Parvalbumin neurons and gamma rhythms enhance cortical circuit performance. Nature (2009) 459(7247):698–702. doi: 10.1038/nature07991
22. Sivarao DV, Chen P, Senapati A, Yang Y, Fernandes A, Benitex Y, et al. 40 Hz Auditory Steady-State Response Is a Pharmacodynamic Biomarker for Cortical NMDA Receptors. Neuropsychopharmacology (2016) 41(9):2232–40. doi: 10.1038/npp.2016.17
23. Kozono N, Honda S, Tada M, Kirihara K, Zhao Z, Jinde S, et al. Auditory Steady State Response; nature and utility as a translational science tool. Sci Rep (2019) 9(1):8454. doi: 10.1038/s41598-019-44936-3
24. Naatanen R, Paavilainen P, Rinne T, Alho K. The mismatch negativity (MMN) in basic research of central auditory processing: a review. Clin Neurophysiol (2007) 118(12):2544–90. doi: 10.1016/j.clinph.2007.04.026
25. Gil-da-Costa R, Stoner GR, Fung R, Albright TD. Nonhuman primate model of schizophrenia using a noninvasive EEG method. Proc Natl Acad Sci U S A (2013) 110(38):15425–30. doi: 10.1073/pnas.1312264110
26. Koshiyama D, Kirihara K, Tada M, Nagai T, Fujioka M, Usui K, et al. Reduced Auditory Mismatch Negativity Reflects Impaired Deviance Detection in Schizophrenia. Schizophr Bull (2020) 46(4):937–46. doi: 10.1093/schbul/sbaa006
27. Koshiyama D, Kirihara K, Tada M, Nagai T, Koike S, Suga M, et al. Duration and frequency mismatch negativity shows no progressive reduction in early stages of psychosis. Schizophr Res (2017) 190:32–8. doi: 10.1016/j.schres.2017.03.015
28. Umbricht D, Schmid L, Koller R, Vollenweider FX, Hell D, Javitt DC. Ketamine-induced deficits in auditory and visual context-dependent processing in healthy volunteers: implications for models of cognitive deficits in schizophrenia. Arch Gen Psychiatry (2000) 57(12):1139–47. doi: 10.1001/archpsyc.57.12.1139
29. Javitt DC, Steinschneider M, Schroeder CE, Arezzo JC. Role of cortical N-methyl-D-aspartate receptors in auditory sensory memory and mismatch negativity generation: implications for schizophrenia. Proc Natl Acad Sci U S A (1996) 93(21):11962–7. doi: 10.1073/pnas.93.21.11962
30. Lakatos P, O’Connell MN, Barczak A, McGinnis T, Neymotin S, Schroeder CE, et al. The Thalamocortical Circuit of Auditory Mismatch Negativity. Biol Psychiatry (2019) 87(8):770–80. doi: 10.1016/j.biopsych.2019.10.029
31. Schwender D, Klasing S, Madler C, Poppel E, Peter K. Mid-latency auditory evoked potentials during ketamine anaesthesia in humans. Br J Anaesth (1993) 71(5):629–32. doi: 10.1093/bja/71.5.629
32. de la Mothe LA, Blumell S, Kajikawa Y, Hackett TA. Cortical connections of auditory cortex in marmoset monkeys: lateral belt and parabelt regions. Anat Rec (2012) 295(5):800–21. doi: 10.1002/ar.22451
33. Kajikawa Y, de La Mothe L, Blumell S, Hackett TA. A comparison of neuron response properties in areas A1 and CM of the marmoset monkey auditory cortex: tones and broadband noise. J Neurophysiol (2005) 93(1):22–34. doi: 10.1152/jn.00248.2004
34. Komatsu M, Takaura K, Fujii N. Mismatch negativity in common marmosets: Whole-cortical recordings with multi-channel electrocorticograms. Sci Rep (2015) 5:15006. doi: 10.1038/srep15006
35. Komatsu M, Kaneko T, Okano H, Ichinohe N. Chronic Implantation of Whole-cortical Electrocorticographic Array in the Common Marmoset. J Vis Exp (2019) 144). doi: 10.3791/58980
36. Cox RW. AFNI: software for analysis and visualization of functional magnetic resonance neuroimages. Comput BioMed Res (1996) 29(3):162–73. doi: 10.1006/cbmr.1996.0014
37. Hashikawa T, Nakatomi R, Iriki A. Current models of the marmoset brain. Neurosci Res (2015) 93:116–27. doi: 10.1016/j.neures.2015.01.009
38. Avants BB, Tustison NJ, Song G, Cook PA, Klein A, Gee JC. A reproducible evaluation of ANTs similarity metric performance in brain image registration. Neuroimage (2011) 54(3):2033–44. doi: 10.1016/j.neuroimage.2010.09.025
39. Pelli DG. The VideoToolbox software for visual psychophysics: transforming numbers into movies. Spatial Vision (1997) 10(4):437–42. doi: 10.1163/156856897x00366
40. Brainard DH. The Psychophysics Toolbox. Spatial Vision (1997) 10(4):433–6. doi: 10.1163/156856897x00357
41. Best W, Bodenshatz C, Beran D. World Health Organisation Critical Review of Ketamine. In: 36th WHO Expert Committee on Drug Dependence report. Geneva, Switzerland: World Health Organisation (2014).
42. Ray S, Crone NE, Niebur E, Franaszczuk PJ, Hsiao SS. Neural correlates of high-gamma oscillations (60-200 Hz) in macaque local field potentials and their potential implications in electrocorticography. J Neurosci (2008) 28(45):11526–36. doi: 10.1523/JNEUROSCI.2848-08.2008
43. Whittingstall K, Logothetis NK. Frequency-band coupling in surface EEG reflects spiking activity in monkey visual cortex. Neuron (2009) 64(2):281–9. doi: 10.1016/j.neuron.2009.08.016
44. Benjamini Y, Hochberg Y. Controlling the False Discovery Rate - a Practical and Powerful Approach to Multiple Testing. J R Stat Soc Ser B-Methodol (1995) 57(1):289–300. doi: 10.1111/j.2517-6161.1995.tb02031.x
45. Tani T, Abe H, Hayami T, Banno T, Miyakawa N, Kitamura N, et al. Sound Frequency Representation in the Auditory Cortex of the Common Marmoset Visualized Using Optical Intrinsic Signal Imaging. eNeuro (2018) 5(2). doi: 10.1523/ENEURO.0078-18.2018
46. Nishimura M, Takemoto M, Song WJ. Organization of auditory areas in the superior temporal gyrus of marmoset monkeys revealed by real-time optical imaging. Brain Struct Funct (2018) 223(4):1599–614. doi: 10.1007/s00429-017-1574-0
47. Bendor D, Wang X. Neural coding of periodicity in marmoset auditory cortex. J Neurophysiol (2010) 103(4):1809–22. doi: 10.1152/jn.00281.2009
48. Ramamurthy DL, Recanzone GH. Spectral and spatial tuning of onset and offset response functions in auditory cortical fields A1 and CL of rhesus macaques. J Neurophysiol (2017) 117(3):966–86. doi: 10.1152/jn.00534.2016
49. Javitt DC, Jayachandra M, Lindsley RW, Specht CM, Schroeder CE. Schizophrenia-like deficits in auditory P1 and N1 refractoriness induced by the psychomimetic agent phencyclidine (PCP). Clin Neurophysiol (2000) 111(5):833–6. doi: 10.1016/s1388-2457(99)00313-2
50. Zanos P, Moaddel R, Morris PJ, Riggs LM, Highland JN, Georgiou P, et al. Ketamine and Ketamine Metabolite Pharmacology: Insights into Therapeutic Mechanisms. Pharmacol Rev (2018) 70(3):621–60. doi: 10.1124/pr.117.015198
51. Friedman T, Sehatpour P, Dias E, Perrin M, Javitt DC. Differential relationships of mismatch negativity and visual p1 deficits to premorbid characteristics and functional outcome in schizophrenia. Biol Psychiatry (2012) 71(6):521–9. doi: 10.1016/j.biopsych.2011.10.037
52. Umbricht DS, Bates JA, Lieberman JA, Kane JM, Javitt DC. Electrophysiological indices of automatic and controlled auditory information processing in first-episode, recent-onset and chronic schizophrenia. Biol Psychiatry (2006) 59(8):762–72. doi: 10.1016/j.biopsych.2005.08.030
53. Salisbury DF, Kuroki N, Kasai K, Shenton ME, McCarley RW. Progressive and interrelated functional and structural evidence of post-onset brain reduction in schizophrenia. Arch Gen Psychiatry (2007) 64(5):521–9. doi: 10.1001/archpsyc.64.5.521
54. Ramamurthy DL, Recanzone GH. Age-related changes in sound onset and offset intensity coding in auditory cortical fields A1 and CL of rhesus macaques. J Neurophysiol (2020) 123(3):1015–25. doi: 10.1152/jn.00373.2019
55. Engle JR, Recanzone GH. Characterizing spatial tuning functions of neurons in the auditory cortex of young and aged monkeys: a new perspective on old data. Front Aging Neurosci (2012) 4:36. doi: 10.3389/fnagi.2012.00036
56. Ng CW, Recanzone GH. Age-Related Changes in Temporal Processing of Rapidly-Presented Sound Sequences in the Macaque Auditory Cortex. Cereb Cortex (2018) 28(11):3775–96. doi: 10.1093/cercor/bhx240
57. Overton JA, Recanzone GH. Effects of aging on the response of single neurons to amplitude-modulated noise in primary auditory cortex of rhesus macaque. J Neurophysiol (2016) 115(6):2911–23. doi: 10.1152/jn.01098.2015
58. Scholl B, Gao X, Wehr M. Nonoverlapping sets of synapses drive on responses and off responses in auditory cortex. Neuron (2010) 65(3):412–21. doi: 10.1016/j.neuron.2010.01.020
59. Kopp-Scheinpflug C, Sinclair JL, Linden JF. When Sound Stops: Offset Responses in the Auditory System. Trends Neurosci (2018) 41(10):712–28. doi: 10.1016/j.tins.2018.08.009
60. Kocsis B, Brown RE, McCarley RW, Hajos M. Impact of ketamine on neuronal network dynamics: translational modeling of schizophrenia-relevant deficits. CNS Neurosci Ther (2013) 19(6):437–47. doi: 10.1111/cns.12081
61. Barr MS, Farzan F, Tran LC, Chen R, Fitzgerald PB, Daskalakis ZJ. Evidence for excessive frontal evoked gamma oscillatory activity in schizophrenia during working memory. Schizophr Res (2010) 121(1-3):146–52. doi: 10.1016/j.schres.2010.05.023
Keywords: electrocorticography, common marmoset, monkey, tonotopy, offset response, ECoG
Citation: Komatsu M and Ichinohe N (2020) Effects of Ketamine Administration on Auditory Information Processing in the Neocortex of Nonhuman Primates. Front. Psychiatry 11:826. doi: 10.3389/fpsyt.2020.00826
Received: 24 April 2020; Accepted: 30 July 2020;
Published: 19 August 2020.
Edited by:
Neal R. Swerdlow, University of California, San Diego, United StatesReviewed by:
Gregor Leicht, University Medical Center Hamburg-Eppendorf, GermanyCopyright © 2020 Komatsu and Ichinohe. This is an open-access article distributed under the terms of the Creative Commons Attribution License (CC BY). The use, distribution or reproduction in other forums is permitted, provided the original author(s) and the copyright owner(s) are credited and that the original publication in this journal is cited, in accordance with accepted academic practice. No use, distribution or reproduction is permitted which does not comply with these terms.
*Correspondence: Misako Komatsu, bWtvbWF0c3VAYnJhaW4ucmlrZW4uanA=
Disclaimer: All claims expressed in this article are solely those of the authors and do not necessarily represent those of their affiliated organizations, or those of the publisher, the editors and the reviewers. Any product that may be evaluated in this article or claim that may be made by its manufacturer is not guaranteed or endorsed by the publisher.
Research integrity at Frontiers
Learn more about the work of our research integrity team to safeguard the quality of each article we publish.