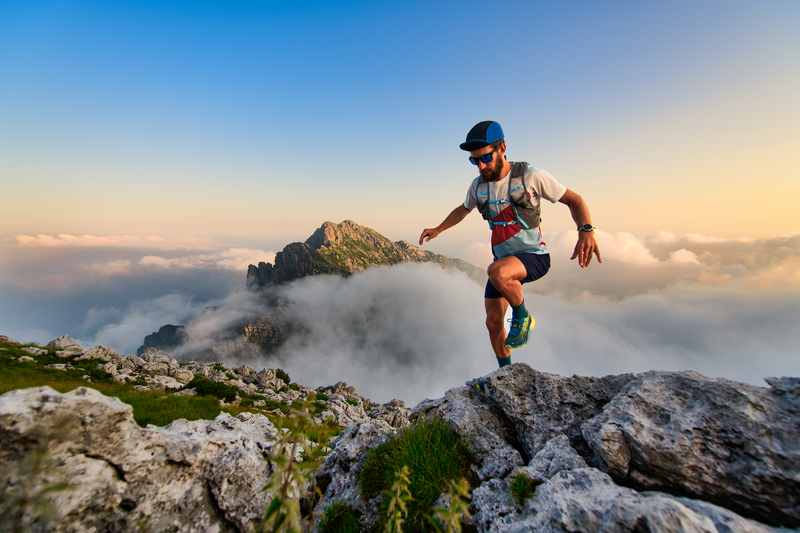
95% of researchers rate our articles as excellent or good
Learn more about the work of our research integrity team to safeguard the quality of each article we publish.
Find out more
MINI REVIEW article
Front. Psychiatry , 28 February 2019
Sec. Molecular Psychiatry
Volume 10 - 2019 | https://doi.org/10.3389/fpsyt.2019.00100
The habenula is a complex nucleus composed of lateral and medial subnuclei, which connect between the limbic forebrain and midbrain. Over the past few years, the lateral habenula has received considerable attention because of its potential roles in cognition and in the pathogenesis of various psychiatric disorders. Unlike extensively studied lateral habenula, anatomically and histologically distinct medial habenula remains largely understudied. The medial habenula can be further subdivided into a dorsal region containing excitatory neurons that express the tachykinin neuropeptide substance P and a ventral region containing dense cholinergic neurons. Although the medial habenula is the source of one of the major cholinergic pathways in the brain, relatively few studies have been conducted to understand its roles. Recently, however, the medial habenula cholinergic system has attracted more attention because of its potential to provide therapeutic targets for the treatment of nicotine withdrawal symptoms, drug addiction, and various mood disorders. Here, we discuss the role of the medial habenula cholinergic system in brain function.
The habenula is part of the epithalamus and is divided into medial and lateral subregions, each with a unique gene-expression profile (1). Over the last few years, studies have shown that neuronal activity in the lateral habenula (LHb) is regulated by factors in the external environment, such as rewards or aversive stimuli, and this altered LHb neuronal activity is associated with the onset of depression (2–4). The medial habenula (MHb) is divided into two subnuclei on the basis of cell type. Cholinergic neurons are located in the ventral two-thirds of the MHb (MHbV), and substance P-ergic neurons are located exclusively in the dorsal part of the MHb (MHbD) (5). Structurally, the MHbD receives input from the bed nucleus of the anterior commissure (BAC) and innervates the outermost portion of the interpeduncular nucleus (IPN). By contrast, the MHbV receives neural input from the triangular septum (TS) and projects to the central part of the IPN (6, 7). The function of substance P-ergic signaling in MHbD neurons is not well known, but MHbD-specific lesion and optogenetic studies have reported that MHbD mediates exercise motivation, regulates the hedonic state, and supports primary reinforcement (8, 9). Signaling from the MHbV cholinergic neurons is involved in drug addiction, including nicotine addiction, nicotine withdrawal symptoms, anxiety, and depression. In this review, we will discuss how cholinergic signaling in the MHbV–IPN pathway affects various brain functions. Table 1 briefly summarizes the results of studies investigating the function of medial habenula cholinergic signaling in addiction and mood-related behaviors.
Habenula cholinergic neurons are the source of a major cholinergic pathway in the central nervous system, and innervate the IPN neurons via the fasciculus retroflexus (fr). Since choline acetyltransferase (ChAT) is the only enzyme responsible for the biosynthesis of acetylcholine (ACh) (23), it is frequently used as a marker of cholinergic neurons. Immunohistochemistry with an anti-ChAT antibody and in situ hybridization against ChAT mRNA both show that habenula cholinergic neurons are restricted to the MHbV (5, 24). Habenula cholinergic neurons release two neurotransmitters, glutamate and ACh, as demonstrated by the colocalization of the vesicular acetylcholine transporter (VAChT) and vesicular glutamate transporter 1 (VGLUT1), visualized with immunogold electron microscopy (25), and by optogenetic stimulation in ChAT-ChR2-EYFP transgenic mice, in which cholinergic neurons express Channelrhodopsoin-2 (ChR2) (26). According to this optogenetic study, the short photostimulation of habenula cholinergic neurons produces ionotropic glutamate receptor-mediated fast excitatory postsynaptic currents, whereas tetanic photostimulation evokes nicotinic acetylcholine receptor (AChR)-mediated slow inward currents in the IPN neurons. Cholinergic habenula neurons exhibit pacemaker activity under the control of circadian rhythms and nicotine withdrawal. Spontaneous firing in the MHb is higher during the day than during the night, probably because of the expression of a circadian gene (27, 28). Although it is not known whether the expression of cholinergic genes is actually involved in generating the circadian rhythm, the MHb is more rhythmic than the LHb (29).
Genome-wide association studies suggest that specific single-nucleotide polymorphisms associated with an increased risk of nicotine dependence and nicotine addiction are located within a specific gene cluster on human chromosome 15 that encodes the α5, α3, and β4 nAChR subunits (30–34). Since α3, α5, and β4 nAChR subunits are enriched in the MHbV–IPN pathway, it is has been suggested that this pathway may play a critical role in nicotine withdrawal behaviors (35, 36). The functional nAChR channel forming α3 and β4 subunits are mainly expressed in the MHbV, and α5 subunit is highly expressed in the IPN (37–39). After chronic administration of nicotine in mice, nicotine cessation results in withdrawal symptoms. This behavioral effect can be reproduced by injecting mecamylamine, a non-selective nAChR antagonist, into the IPN of mice chronically exposed to nicotine. Nicotine cessation and mecamylamine administration activate GABAergic neurons in the IPN, leading to physical nicotine withdrawal symptoms (10). Optogenetic stimulation of GAD2-expressing GABAergic neurons in the IPN induces physical withdrawal symptoms in both nicotine-naïve and chronic nicotine-exposed mice, and the affective symptoms are ameliorated by IPN-selective infusion of a NMDA receptor antagonist (10). Therefore, glutamate release from MHb neurons innervating the IPN is necessary for somatic manifestation of nicotine withdrawal. During chronic nicotine exposure, the expression of nAChRs containing the β4-subunit is upregulated in somatostatin-positive GABAergic neurons in the IPN. Selective blockade of these β4-subunit-containing nAChRs induces more dramatic somatic withdrawal signs in nicotine-exposed mice than nicotine-naïve mice (10). Because somatostatin-positive GABAergic neurons in the IPN project principally to the median raphe/paramedian raphe and dorsal tegmental area, two regions rich in serotonergic neurons (39), the activation of these IPN GABAergic neurons by nicotine withdrawal may modulate downstream serotonin release. Zhao-Shea et al. presented a new more complex mechanism for nicotine withdrawal-induced anxiety-related behavior involving corticotropin releasing factor (CRF) signaling from the VTA to the IPN (12). After chronic nicotine administration, CRF synthesis is upregulated in the VTA dopaminergic neurons and the level of the CRF receptor 1 is also increased in a particular subnucleus of the ventral IPN, which appears to receive efferent axons from the VTA. CRF secreted by the VTA may stimulate the neuronal activity of the IPN by promoting glutamate release through the CRF receptor 1 during chronic nicotine withdrawal. Blockade of the CRF receptor by an antagonist in the IPN or CRF knockdown in the VTA was shown to alleviate the anxiety induced by nicotine withdrawal.
IPN is a complex structure composed of several subnuclei (40, 41) and cholinergic signaling in the MHb-IPN pathway has been reported to be mediated by α5 nAChR subunit-expressing GABAergic neurons in the IPN, which project principally to the median/paramedian raphe and dorsal tegmental area (39). Morton et al. demonstrated that acetylcholine and nicotine-evoked responses in the IPN neurons were markedly reduced in α5-null mice (42). However, unlike the result obtained using broad optogenetic stimulation of GAD2-expressing GABAergic neurons in the IPN, selective optogenetic stimulation of only α5-expressing GABAergic neurons did not elicit the somatic signs associated with nicotine withdrawal and had no effect on locomotion or anxiety (42). Rather, after prior stimulation or exposure to nicotine, optogenetic stimulation of α5-expressing IPN neurons produced aversion. The difference in the effect of optogenetic stimulation between the above two mentioned studies may be off-target effect of GAD2-expressing neurons not being limited to the IPN or the function of the neural circuit formed by other GABAergic neurons that do not express α5.
Many studies have shown that nicotine withdrawal reduces levels of dopamine and serotonin (43, 44). In addition, although there is no change in the nicotine-induced firing rate in habenula cholinergic neurons in mice chronically treated with nicotine, re-exposure to nicotine during withdrawal increases the rate of spontaneous firing. This nicotine-sensitive regulation of pacemaker activity in MHb cholinergic neurons may contribute to smoking relapses (11). Recently, Wolfman et al. reported that a high dose of nicotine induces aversive behavior that is mediated by IPN GABAergic inputs to the laterodorsal tegmentum (LDTg) (45). They showed that selective optogenetic stimulation of IPN axon terminals in the LDTg elicits significant behavioral avoidance in a real-time place-preference test. Similarly, optogenetic inhibition of IPN axon terminals in the LDTg blocks conditioned place aversion in mice that receive an aversive nicotine dose (1.5 mg kg−1). Understanding how nicotine cessation and blockade of acetylcholine signaling after chronic nicotine exposure induce activation of GABAergic neurons in the MHb–IPN pathway will require a sophisticated examination of the connections between MHb cholinergic neurons and IPN neurons, the neural circuits within the IPN, and the connections between IPN GABAergic neurons and midbrain serotonergic neurons.
The habenula has also been linked to drug addiction more generally through a series of rodent experiments and human genome-wide association studies (31–33, 43, 46). Genetic variation in the nAChR subunit CHRNA5 is associated with addiction to drugs such as alcohol and cocaine (47, 48); therefore, a number of studies have investigated the association between nAChR expression and drug addiction in habenula cholinergic neurons. Chronic morphine administration in mice (20–100 mg/kg three times per day) decreases acetylcholinesterase activity in the MHb, but activity returns to baseline levels during morphine withdrawal. Chronic morphine administration reduces c-fos activity in the MHb (13), whereas cocaine promotes c-fos expression in the LHb (49, 50). Ibogaine, which has a therapeutic effect on drug addiction, reduces the self-administration of cocaine, alcohol, and morphine in rodents (16–18). Similarly, 18-methoxycoronaridine (18-MC), a derivative of ibogaine, inhibits abuse of morphine, cocaine, and nicotine but without the severe side effects seen with ibogaine treatment (51, 52). Because 18-MC has antagonistic activity against β4-containing nAChRs in the MHb and IPN (53), MHb cholinergic signaling is thought to play a major role in drug addiction. Habenula cholinergic neurons regulate the self-administration of drugs such as cocaine and methamphetamine, as well as the reinstatement of drug-seeking behaviors (2, 44, 53). Chemogenetic activation of habenula cholinergic neurons by a cre-dependent DREADD (Designer Receptors Exclusively Activated by Designer Drugs) system in ChAT-cre mice induces behaviors that mimic drug-seeking behavior, such as the reinstatement of a cocaine-induced conditioned place preference (54).
Nicotine abuse prompts axonal neurodegeneration in the central region of the fr, and this axonal neurodegeneration is also observed following chronic exposure to various other drugs such as D-amphetamine, methamphetamine, MDMA, and cocaine. Nicotine and other drugs induce neurodegeneration of the central core region and external sheath region of the fr (14, 15). A recent study showed that the sheath region surrounding the fr connects the LHb and the ventral tegmental area (VTA) reciprocally, and that the core region inside the fr is an efferent projection from the MHb to the IPN (55). Thus, if different drugs cause different patterns of fr neurodegeneration, the relative modulation of the LHb–VTA and the MHb–IPN pathways may vary.
The bed nucleus of the anterior commissure (BAC) and the triangular septum (TS) mainly project to the MHbD and MHbV, respectively (6, 7). Using the immunotoxin-mediated cell targeting method, Yamaguchi et al. selectively ablated BAC and TS neurons (6). BAC-ablated mice showed an increased fear response when freezing was measured after presentation of electric shocks, whereas TS-ablated mice did not. These effects of ablation of projection neurons to the MHb suggest that MHbD is associated with the fear response, but not the MHbV. However, direct ablation of MHbD neurons did not change in the fear response (9).
Notably, direct ablation of MHbV cholinergic neurons and genetic inactivation of GABAB receptors in cholinergic neurons increased the expression of conditioned fear (19). GABAB receptors are abundant in habenula cholinergic neurons, which, as noted above, project to the IPN. Notably, MHbV-derived presynaptic GABAB receptors mediate the release of glutamate in the IPN upon activation by GABA released from IPN GABAergic neurons. This retrograde activation of GABAB receptors promotes prolonged enhancement of glutamate release (56). To fully understand the function of the MHbV cholinergic–IPN GABAergic neuronal pathway, it will be necessary to identify the physiological conditions that stimulate MHb cholinergic neurons and develop a systematic understanding of the mechanisms underlying the interactions between acetylcholine and glutamate release from MHb neurons and GABA release from IPN neurons.
Conditional deletion of cannabinoid type 1 (CB1) from MHb neurons by injecting Cre recombinase expressing AAV into the MHb of CB1-floxed mice reduced strongly the freezing response in cued and contextual fear conditioning (20). Pharmacological blockade of the CB1 receptor in the IPN also reduced fear-conditioned freezing in mice. Consistent with the interpretation that downregulation of cholinergic signaling enhances the fear response (19), genetic deletion of MHb-CB1 and pharmacological inhibition of CB1R activity enhances cholinergic neurotransmission. Therefore, synaptic modulation of cholinergic neurotransmission in the MHb-to-IPN synapses by presynaptic CB1 receptor and GABAB receptor activities derived from the MHb cholinergic neurons plays a regulating role in the expression of fear memory.
Cholinergic hyperactivity in the brain has long been associated with depressive phenotypes (57–59). Increased extracellular levels of ACh after administration of acetylcholinesterase inhibitor can lead to depressed mood states in both normal humans and rodents (60, 61). The depression-like behaviors or symptoms are reversed by broad administration of nAChRs or mAChRs antagonists in an established animal model or human patients (62–65). Recent shRNA-mediated knockdown of ChAT in the rat habenula induces anhedonia but not despair-like behavior (21). Downregulation of cholinergic signaling in the habenula, including decreased expression of the genes related to cholinergic signaling, such as ChAT, CHT, VAChT, CHRNA3, and CHRNB4, has been demonstrated in an animal model of depression and in suicide victims diagnosed with major depressive disorder (21). Furthermore, selective pharmacogenetic activation of habenula cholinergic neurons via DREADDs in ChAT-cre mice leads to the excitation of dopamine neurons in the VTA and reduces serotonin immunoreactivity in the DRN (21), suggesting that habenular cholinergic neurons directly or indirectly regulate monoaminergic neurons in the midbrain.
However, Xu et al. reported that electrically-induced lesions of the MHb in rats attenuated the lower sucrose consumption caused by chronic unpredictable mild stress (CUMS), but did not attenuate the increased immobility time in the FST, in accord with the cholinergic hyperactivity theory of depression (22). The release of substance P derived from MHbD was increased in the IPN of CUMS-exposed rats and the resulting higher SP levels increased the neuronal activity of the IPN. This study suggests that hyperactivity of the MHb-IPN pathway triggers the anhedonia-like behavior and higher SP signaling mediates a lower hedonic state. The increased hedonic state caused by the MHb-lesion in the CUMS-exposed rats is contradictory in that both the reduced cholinergic signaling following ChAT knockdown in the MHbV (21) and genetic ablation of MHbD (9) resulted in anhedonia-like behavior. Considering that SP-expressing neurons are present in the MHbD and that the SP receptor Tacr1 is mainly expressed in the MHbV (5), the close correlation between neuronal signaling in the MHbD and MHbV in the MHb-IPN pathway will require further study.
The MHbV, which contains the cholinergic neurons of the habenula, can be further subdivided longitudinally into inferior, central, and lateral subnuclei according to various anatomical, gene expression, and electrophysiological studies (5). This suggests that the cholinergic neurons present in these subnuclei may have distinct roles depending on their respective characteristics. Therefore, it will be necessary to identify the connections between neurons in the MHbV subnuclei and neurons in the various subnuclei of the IPN. In addition, Shih et al. revealed the precise localizations of nAChRs subunits in specific regions of MHb and IPN using immunohistochemistry and electrophysiology in the knock-in mouse strains expressing α3, α4, α6, β2, β3, and β4 subunit fused GFP, suggesting that despite their small size, neurons of the MHb have distinct neurophysiology, nAChR subunit expression, and sensitivity to nicotine (66). This study also indicated that nAChR-expressing cells of inferior, central, and lateral neurons in the MHbV project preferentially to ventral, central, and dorsal subnuclei of the IPN, respectively (Figure 1). Recently, Lima et al. also showed that the rostral part of the IPN connects with the central and lateral MHbV and the caudal part of the IPN connects with the central and inferior MHbV using retrograde tracing from the IPN to the MHb (67). Lima et al. also traced the efferent projections of the rat IPN using the anterograde tracing method and showed that the IPN makes a large number of connections with a variety of brain regions including the supramammillary nucleus, the septum/diagonal band complex, the temporal part of the hippocampus, the nucleus incertus, the laterodorsal tegmental nucleus (LDTg), and the caudal dorsal/median raphe nucleus (67). However, the results of this study were not confirmed by the retrograde labeling of IPN projecting sites. Quina et al. traced the afferent and efferent neural circuits of the IPN more finely using region-specific cre-expressing transgenic mice, anterograde viral tracer, and retrograde cholera toxin subunit b (CTb) tracer (68). After injecting the retrograde tracer CTb into several IPN projecting areas revealed by IPN anterograde tracing, such as hippocampus, septal nuclei, lateral hypothalamus, and lateral preoptic area, a comparison of CTb labeling with that of major immunohistochemical markers revealed that CTb labeling was not present in any IPN subnuclei receiving MHbD (SP) and MHbV (CHAT) inputs. Because there is no IPN-specific cre-transgenic mouse that can completely exclude the presence of neurons in surrounding structures, IPN descending sites cannot be easily observed with anterograde tracing without performing retrograde tracing. In addition, Ables et al. dissected the α5 nAChR subunit-expressing IPN neurons into two non-overlapping the α5+ cell populations containing highly enriched genes, Amigo1 and Epyc, both of which encode cell-surface adhesion proteins. α5-Epyc neurons project locally whereas α5-Amigo1 neurons send long projections to raphe and LDTg nuclei. Neuron-specific silencing via prevention of neurotransmitter release by cre-dependent membrane-tethered Ca2+-channel toxin showed that silencing Amigo1-cells prevented place preference for nicotine, whereas silencing Epyc-cells had no effect (69). This result suggests that although both α5-Amigo1 cells and α5-Epyc cells receive major inputs from the MHbV, only Amigo1 cells contribute the majority of projections from the IPN to the raphe and LDTg nuclei and functionally modulate the circuit responsible for behavioral changes to nicotine. In conclusion, more work will be required to elucidate each neural network that constitutes the cholinergic MHb–IPN–other brain regions axis and to study in detail the functions of each neural network and the axis as a whole.
Figure 1. Schematic summary of nAChR subunit expression and the MHb-IPN pathway. (A) nAChR subunits are differentially expressed in the MHbV neurons, which project to central regions (IPDM, IPR, IPC, and IPI) excluding lateral regions (IPL and IPDL) of the IPN through the fasciculus retroflexus (fr). MHbV is composed of three subregions, such as inferior (MHbVI), central (MHbVC), and lateral (MHbVL). α6 nAChR subunit (CHRNA6) is exclusively found in the MHbVI. In contrast to CHRNA6, α4 nAChR subunit (CHRNA4) is mainly expressed in the MHbVL. IPN is composed of various subregions: rostral (IPR), dorsomedial (IPDM), dorsolateral (IPDL), caudal (IPC), and intermediate (IPI). (B) MHbVI, MHbVC, and MHbVL preferentially project to ventral, central, and dorsal regions of the IPN, respectively.
As described above, habenula cholinergic neurons are closely tied to a variety of brain functions. Pharmacological approaches are needed to control the activity of these neurons. Interestingly, habenula cholinergic neurons express pharmacologically controllable GPCR proteins such as the GABAB receptor and Tachykinin receptor 1 (TacR1). These ligand-dependent activity-modulating receptors may be useful targets for the treatment of various psychiatric disorders associated with malfunctioning habenula cholinergic neurons. For example, anhedonia-like behavior due to reduced habenular cholinergic signaling is not reversed by chronic administration of the standard antidepressant fluoxetine (21). Thus, enhancement of cholinergic signaling by direct activation of habenula cholinergic neurons may open the way for a new drug therapy for depression.
SY and JK led the literature search and HL drafted the manuscript. HK provided substantial contributions to the intellectual content of the manuscript. All authors approved the final version of manuscript to be published.
This work was supported by the Brain Program through the National Research Foundation of Korea (NRF), funded by the Ministry of Science, ICT, & Future Planning (NRF-2017M3C7A1079692 to HK; NRF-2017R1D1A1B06032730 to HL).
The authors declare that the research was conducted in the absence of any commercial or financial relationships that could be construed as a potential conflict of interest.
We gratefully acknowledge Ciel Lee (Taegang Samyook elementary school) for the schematic drawing of Figure 1.
1. Quina LA, Wang S, Ng L, Turner EE. Brn3a and Nurr1 mediate a gene regulatory pathway for habenula development. J Neurosci. (2009) 29:14309–22. doi: 10.1523/JNEUROSCI.2430-09.2009
2. Yang Y, Wang H, Hu J, Hu H. Lateral habenula in the pathophysiology of depression. Curr Opin Neurobiol. (2018) 48:90–6. doi: 10.1016/j.conb.2017.10.024
3. Li B, Piriz J, Mirrione M, Chung C, Proulx CD, Schulz D. et al. Synaptic potentiation onto habenula neurons in the learned helplessness model of depression. Nature. (2011) 470:535–9. doi: 10.1038/nature09742
4. Lawson RP, Nord CL, Seymour B, Thomas DL, Dayan P, Pilling S. et al. Disrupted habenula function in major depression. Mol Psychiatry. (2017) 22:202–8. doi: 10.1038/mp.2016.81
5. Aizawa H, Kobayashi M, Tanaka S, Fukai T, Okamoto H. Molecular characterization of the subnuclei in rat habenula. J Comp Neurol. (2012) 520:4051–66. doi: 10.1002/cne.23167
6. Yamaguchi T, Danjo T, Pastan I, Hikida T, Nakanishi S. Distinct roles of segregated transmission of the septo-habenular pathway in anxiety and fear. Neuron. (2013) 78:537–44. doi: 10.1016/j.neuron.2013.02.035
7. Herkenham M, Nauta WJ. Afferent connections of the habenular nuclei in the rat. a horseradish peroxidase study, with a note on the fiber-of-passage problem. J Comp Neurol. (1977) 173:123–46. doi: 10.1002/cne.901730107
8. Hsu YW, Wang SD, Wang S, Morton G, Zariwala HA, de la Iglesia HO. et al. Role of the dorsal medial habenula in the regulation of voluntary activity, motor function, hedonic state, and primary reinforcement. J Neurosci. (2014) 34:11366–84. doi: 10.1523/JNEUROSCI.1861-14.2014
9. Hsu YW, Morton G, Guy EG, Wang SD, Turner EE. Dorsal medial habenula regulation of mood-related behaviors and primary reinforcement by tachykinin-expressing habenula neurons. eNeuro. (2016) 3. doi: 10.1523/ENEURO.0109-16.2016
10. Zhao-Shea R, Liu L, Pang X, Gardner PD, Tapper AR. Activation of GABAergic neurons in the interpeduncular nucleus triggers physical nicotine withdrawal symptoms. Curr Biol. (2013) 23:2327–35. doi: 10.1016/j.cub.2013.09.041
11. Gorlich A, Antolin-Fontes B, Ables JL, Frahm S, Slimak MA, Dougherty JD, et al Reexposure to nicotine during withdrawal increases the pacemaking activity of cholinergic habenular neurons. Proc Natl Acad Sci U.S.A. (2013) 110:17077–82. doi: 10.1073/pnas.1313103110
12. Zhao-Shea R, DeGroot SR, Liu L, Vallaster M, Pang X, Su Q, et al. Increased CRF signalling in a ventral tegmental area-interpeduncular nucleus-medial habenula circuit induces anxiety during nicotine withdrawal. Nat Commun. (2015) 6:6770. doi: 10.1038/ncomms7770
13. Neugebauer NM, Einstein EB, Lopez MB, McClure-Begley TD, Mineur YS, Picciotto MR. Morphine dependence and withdrawal induced changes in cholinergic signaling. Pharmacol Biochem Behav. (2013) 109:77–83. doi: 10.1016/j.pbb.2013.04.015
14. Ellison G. Neural degeneration following chronic stimulant abuse reveals a weak link in brain, fasciculus retroflexus, implying the loss of forebrain control circuitry. Eur Neuropsychopharmacol. (2002) 12:287–97. doi: 10.1016/S0924-977X(02)00020-2
15. Ciani E, Severi S, Bartesaghi R, Contestabile A. Neurochemical correlates of nicotine neurotoxicity on rat habenulo-interpeduncular cholinergic neurons. Neurotoxicology. (2005) 26:467–74. doi: 10.1016/j.neuro.2005.04.001
16. Cappendijk SL, Dzoljic MR. Inhibitory effects of ibogaine on cocaine self-administration in rats. Eur J Pharmacol. (1993) 241:261–5. doi: 10.1016/0014-2999(93)90212-Z
17. Rezvani AH, Overstreet DH, Lee YW. Attenuation of alcohol intake by ibogaine in three strains of alcohol-preferring rats. Pharmacol Biochem Behav. (1995) 52:615–20. doi: 10.1016/0091-3057(95)00152-M
18. Glick SD, Rossman K, Steindorf S, Maisonneuve IM, Carlson JN. Effects and aftereffects of ibogaine on morphine self-administration in rats. Eur J Pharmacol. (1991) 195:341–5. doi: 10.1016/0014-2999(91)90474-5
19. Zhang J, Tan L, Ren Y, Liang J, Lin R, Feng Q, et al. Presynaptic excitation via GABAB receptors in habenula cholinergic neurons regulates fear memory expression. Cell. (2016) 166:716–28. doi: 10.1016/j.cell.2016.06.026
20. Soria-Gomez E, Busquets-Garcia A, Hu F, Mehidi A, Cannich A, Roux L, et al. Habenular CB1 receptors control the expression of aversive memories. Neuron. (2015) 88:306–13. doi: 10.1016/j.neuron.2015.08.035
21. Han S, Yang SH, Kim JY, Mo S, Yang E, Song KM, et al. Down-regulation of cholinergic signaling in the habenula induces anhedonia-like behavior. Sci Rep. (2017) 7:900. doi: 10.1038/s41598-017-01088-6
22. Xu C, Sun Y, Cai X, You T, Zhao H, Li Y, et al. Medial habenula-interpeduncular nucleus circuit contributes to anhedonia-like behavior in a rat model of depression. Front Behav Neurosci. (2018) 12:238. doi: 10.3389/fnbeh.2018.00238
23. Misgeld T, Burgess RW, Lewis RM, Cunningham JM, Lichtman JW, Sanes JR. Roles of neurotransmitter in synapse formation: development of neuromuscular junctions lacking choline acetyltransferase. Neuron. (2002) 36:635–48. doi: 10.1016/S0896-6273(02)01020-6
24. Renier N, Wu Z, Simon DJ, Yang J, Ariel P, Tessier-Lavigne M. iDISCO: a simple, rapid method to immunolabel large tissue samples for volume imaging. Cell. (2014) 159:896–910. doi: 10.1016/j.cell.2014.10.010
25. Frahm S, Antolin-Fontes B, Gorlich A, Zander JF, Ahnert-Hilger G, Ibanez-Tallon I. An essential role of acetylcholine-glutamate synergy at habenular synapses in nicotine dependence. Elife. (2015) 4:e11396. doi: 10.7554/eLife.11396
26. Ren J, Qin C, Hu F, Tan J, Qiu L, Zhao S, et al. Habenula “cholinergic” neurons co-release glutamate and acetylcholine and activate postsynaptic neurons via distinct transmission modes. Neuron. (2011) 69:445–52. doi: 10.1016/j.neuron.2010.12.038
27. Zhao H, Rusak B. Circadian firing-rate rhythms and light responses of rat habenular nucleus neurons in vivo and in vitro. Neuroscience. (2005) 132:519–28. doi: 10.1016/j.neuroscience.2005.01.012
28. Sakhi K, Belle MD, Gossan N, Delagrange P, Piggins HD. Daily variation in the electrophysiological activity of mouse medial habenula neurones. J Physiol. (2014) 592:587–603. doi: 10.1113/jphysiol.2013.263319
29. Landgraf D, Long JE, Welsh DK. Depression-like behaviour in mice is associated with disrupted circadian rhythms in nucleus accumbens and periaqueductal grey. Eur J Neurosci. (2016) 43:1309–20. doi: 10.1111/ejn.13085
30. Saccone SF, Hinrichs AL, Saccone NL, Chase GA, Konvicka K, Madden PA, et al. Cholinergic nicotinic receptor genes implicated in a nicotine dependence association study targeting 348 candidate genes with 3713 SNPs. Hum Mol Genet. (2007) 16:36–49. doi: 10.1093/hmg/ddl438
31. Berrettini W, Yuan X, Tozzi F, Song K, Francks C, Chilcoat H, et al. Alpha-5/alpha-3 nicotinic receptor subunit alleles increase risk for heavy smoking. Mol Psychiatry. (2008) 13:368–73. doi: 10.1038/sj.mp.4002154
32. Bierut LJ, Stitzel JA, Wang JC, Hinrichs AL, Grucza RA, Xuei X, et al. Variants in nicotinic receptors and risk for nicotine dependence. Am J Psychiatry. (2008) 165:1163–71. doi: 10.1176/appi.ajp.2008.07111711
33. Thorgeirsson TE, Geller F, Sulem P, Rafnar T, Wiste A, Magnusson KP, et al. variant associated with nicotine dependence, lung cancer and peripheral arterial disease. Nature. (2008) 452:638–42. doi: 10.1038/nature06846
34. Berrettini WH, Doyle GA. The CHRNA5-A3-B4 gene cluster in nicotine addiction. Mol Psychiatry. (2012) 17:856–66. doi: 10.1038/mp.2011.122
35. Salas R, Sturm R, Boulter J, De Biasi M. Nicotinic receptors in the habenulo-interpeduncular system are necessary for nicotine withdrawal in mice. J Neurosci. (2009) 29:3014–8. doi: 10.1523/JNEUROSCI.4934-08.2009
36. Fowler CD, Lu Q, Johnson PM, Marks MJ, Kenny PJ. Habenular alpha5 nicotinic receptor subunit signalling controls nicotine intake. Nature. (2011) 471:597–601. doi: 10.1038/nature09797
37. Wada E, McKinnon D, Heinemann S, Patrick J, Swanson LW. The distribution of mRNA encoded by a new member of the neuronal nicotinic acetylcholine receptor gene family (alpha 5) in the rat central nervous system. Brain Res. (1990) 526:45–53 doi: 10.1016/0006-8993(90)90248-A
38. Marks MJ, Pauly JR, Gross SD, Deneris ES, Hermans-Borgmeyer I, Heinemann SF, et a. Nicotine binding and nicotinic receptor subunit RNA after chronic nicotine treatment. J Neurosci. (1992) 12:2765–84. doi: 10.1523/JNEUROSCI.12-07-02765.1992
39. Hsu YW, Tempest L, Quina LA, Wei AD, Zeng H, Turner EE. Medial habenula output circuit mediated by alpha5 nicotinic receptor-expressing GABAergic neurons in the interpeduncular nucleus. J Neurosci. (2013) 33:18022–35. doi: 10.1523/JNEUROSCI.2927-13.2013
40. Groenewegen HJ, Ahlenius S, Haber SN, Kowall NW, Nauta WJ. Cytoarchitecture, fiber connections, and some histochemical aspects of the interpeduncular nucleus in the rat. J Comp Neurol. (1986) 249:65–102. doi: 10.1002/cne.902490107
41. Lenn NJ, Hamill GS. Subdivisions of the interpeduncular nucleus: a proposed nomenclature. Brain Res Bull. (1984) 13:203–4. doi: 10.1016/0361-9230(84)90023-6
42. Morton G, Nasirova N, Sparks DW, Brodsky M, Sivakumaran S, Lambe EK, et al. Chrna5-expressing neurons in the interpeduncular nucleus mediate aversion primed by prior stimulation or nicotine exposure. J Neurosci. (2018) 38:6900–920. doi: 10.1523/JNEUROSCI.0023-18.2018
43. Staley JK, Krishnan-Sarin S, Zoghbi S, Tamagnan G, Fujita M, Seibyl JP, et al. Sex differences in [123I]beta-CIT SPECT measures of dopamine and serotonin transporter availability in healthy smokers and nonsmokers. Synapse. (2001) 41:275–84. doi: 10.1002/syn.1084
44. Rao TS, Correa LD, Adams P, Santori EM, Sacaan AI. Pharmacological characterization of dopamine, norepinephrine and serotonin release in the rat prefrontal cortex by neuronal nicotinic acetylcholine receptor agonists. Brain Res. (2003) 990:203–8. doi: 10.1016/S0006-8993(03)03532-7
45. Wolfman SL, Gill DF, Bogdanic F, Long K, Al-Hasani R, McCall JG, et al. Nicotine aversion is mediated by GABAergic interpeduncular nucleus inputs to laterodorsal tegmentum. Nat Commun. (2018) 9:2710. doi: 10.1038/s41467-018-04654-2
46. Amos CI, Wu X, Broderick P, Gorlov IP, Gu J, Eisen T, et al. Genome-wide association scan of tag SNPs identifies a susceptibility locus for lung cancer at 15q25.1. Nat Genet. (2008) 40:616–22. doi: 10.1038/ng.109
47. Grucza RA, Wang JC, Stitzel JA, Hinrichs AL, Saccone SF, Saccone NL, et al. A risk allele for nicotine dependence in CHRNA5 is a protective allele for cocaine dependence. Biol Psychiatry. (2008) 64:922–9. doi: 10.1016/j.biopsych.2008.04.018
48. Wang JC, Grucza R, Cruchaga C, Hinrichs AL, Bertelsen S, Budde JP, et al. Genetic variation in the CHRNA5 gene affects mRNA levels and is associated with risk for alcohol dependence. Mol Psychiatry. (2009) 14:501–10. doi: 10.1038/mp.2008.42
49. Brown RM, Short JL, Lawrence AJ. Identification of brain nuclei implicated in cocaine-primed reinstatement of conditioned place preference: a behaviour dissociable from sensitization. PLoS ONE. (2010) 5:e15889. doi: 10.1371/journal.pone.0015889
50. James MH, Charnley JL, Flynn JR, Smith DW, Dayas CV. Propensity to 'relapse' following exposure to cocaine cues is associated with the recruitment of specific thalamic and epithalamic nuclei. Neuroscience. (2011) 199:235–42. doi: 10.1016/j.neuroscience.2011.09.047
51. Glick SD, Maisonneuve IM, Szumlinski KK. 18-Methoxycoronaridine (18-MC) and ibogaine: comparison of antiaddictive efficacy, toxicity, and mechanisms of action. Ann N Y Acad Sci. (2000) 914:369–86. doi: 10.1111/j.1749-6632.2000.tb05211.x
52. Taraschenko OD, Shulan JM, Maisonneuve IM, Glick SD. 18-MC acts in the medial habenula and interpeduncular nucleus to attenuate dopamine sensitization to morphine in the nucleus accumbens. Synapse. (2007) 61:547–60. doi: 10.1002/syn.20396
53. Glick SD, Ramirez RL, Livi JM, Maisonneuve IM. 18-Methoxycoronaridine acts in the medial habenula and/or interpeduncular nucleus to decrease morphine self-administration in rats. Eur J Pharmacol. (2006) 537:94–8. doi: 10.1016/j.ejphar.2006.03.045
54. Lopez AJ, Jia Y, White AO, Kwapis JL, Espinoza M, Hwang P, et al. Medial habenula cholinergic signaling regulates cocaine-associated relapse-like behavior. Addict Biol. (2018) doi: 10.1111/adb.12605. [Epub ahead of print].
55. Schmidt ERE, Brignani S, Adolfs Y, Lemstra S, Demmers J, Vidaki M, et al. Subdomain-mediated axon-axon signaling and chemoattraction cooperate to regulate afferent innervation of the lateral habenula. Neuron. (2014) 83:372–87. doi: 10.1016/j.neuron.2014.05.036
56. Koppensteiner P, Melani R, Ninan IA. Cooperative mechanism involving Ca2+-permeable AMPA receptors and retrograde activation of GABAB receptors in interpeduncular nucleus plasticity. Cell Rep. (2017) 20:1111–22. doi: 10.1016/j.celrep.2017.07.013
57. Dilsaver SC. Cholinergic mechanisms in depression. Brain Res. (1986) 396:285–316. doi: 10.1016/0165-0173(86)90016-0
58. Janowsky DS, el-Yousef MK, Davis JM, Sekerke HJ. A cholinergic-adrenergic hypothesis of mania and depression. Lancet. (1972) 2:632–5. doi: 10.1016/S0140-6736(72)93021-8
59. Janowsky DS, Risch SC, Gillin JC. Adrenergic-cholinergic balance and the treatment of affective disorders. Prog Neuropsychopharmacol Biol Psychiatry. (1983) 7:297–307. doi: 10.1016/0278-5846(83)90119-7
60. Risch SC, Cohen RM, Janowsky DS, Kalin NH, Murphy DL. Mood and behavioral effects of physostigmine on humans are accompanied by elevations in plasma beta-endorphin and cortisol. Science. (1980) 209:1545–6. doi: 10.1126/science.7433977
61. Mineur YS, Obayemi A, Wigestrand MB, Fote GM, Calarco CA, Li AM, et al. Cholinergic signaling in the hippocampus regulates social stress resilience and anxiety- and depression-like behavior. Proc Natl Acad Sci USA. (2013) 110:3573–8. doi: 10.1073/pnas.1219731110
62. Drevets WC, Furey ML. Replication of scopolamine's antidepressant efficacy in major depressive disorder: a randomized, placebo-controlled clinical trial. Biol Psychiatry. (2010) 67:432–8. doi: 10.1016/j.biopsych.2009.11.021
63. Navarria A, Wohleb ES, Voleti B, Ota KT, Dutheil S, Lepack AE, et al. Rapid antidepressant actions of scopolamine: role of medial prefrontal cortex and M1-subtype muscarinic acetylcholine receptors. Neurobiol Dis. (2015) 82:254–61. doi: 10.1016/j.nbd.2015.06.012
64. Shytle RD, Silver AA, Lukas RJ, Newman MB, Sheehan DV, Sanberg PR. Nicotinic acetylcholine receptors as targets for antidepressants. Mol Psychiatry. (2002) 7:525–35. doi: 10.1038/sj.mp.4001035
65. Drevets WC, Zarate CA Jr, Furey ML. Antidepressant effects of the muscarinic cholinergic receptor antagonist scopolamine: a review. Biol Psychiatry. (2013) 73:1156–63. doi: 10.1016/j.biopsych.2012.09.031
66. Shih PY, Engle SE, Oh G, Deshpande P, Puskar NL, Lester HA, et al. Differential expression and function of nicotinic acetylcholine receptors in subdivisions of medial habenula. J Neurosci. (2014) 34:9789–802. doi: 10.1523/JNEUROSCI.0476-14.2014
67. Lima LB, Bueno D, Leite F, Souza S, Goncalves L, Furigo IC, et al. Afferent and efferent connections of the interpeduncular nucleus with special reference to circuits involving the habenula and raphe nuclei. J Comp Neurol. (2017) 525:2411–42. doi: 10.1002/cne.24217
68. Quina LA, Harris J, Zeng H, Turner EE. Specific connections of the interpeduncular subnuclei reveal distinct components of the habenulopeduncular pathway. J Comp Neurol. (2017) 525:2632–56. doi: 10.1002/cne.24221
Keywords: habenula, cholinergic system, nicotine addiction and withdrawal, drug addiction, fear, depression
Citation: Lee HW, Yang SH, Kim JY and Kim H (2019) The Role of the Medial Habenula Cholinergic System in Addiction and Emotion-Associated Behaviors. Front. Psychiatry 10:100. doi: 10.3389/fpsyt.2019.00100
Received: 21 November 2018; Accepted: 11 February 2019;
Published: 28 February 2019.
Edited by:
Todd Denton Gould, University of Maryland, Baltimore, United StatesReviewed by:
Andrew Tapper, University of Massachusetts Medical School, United StatesCopyright © 2019 Lee, Yang, Kim and Kim. This is an open-access article distributed under the terms of the Creative Commons Attribution License (CC BY). The use, distribution or reproduction in other forums is permitted, provided the original author(s) and the copyright owner(s) are credited and that the original publication in this journal is cited, in accordance with accepted academic practice. No use, distribution or reproduction is permitted which does not comply with these terms.
*Correspondence: Hyun Woo Lee, YmlvY2Fpc0Brb3JlYS5hYy5rcg==
Hyun Kim, a2ltaHl1bkBrb3JlYS5hYy5rcg==
Disclaimer: All claims expressed in this article are solely those of the authors and do not necessarily represent those of their affiliated organizations, or those of the publisher, the editors and the reviewers. Any product that may be evaluated in this article or claim that may be made by its manufacturer is not guaranteed or endorsed by the publisher.
Research integrity at Frontiers
Learn more about the work of our research integrity team to safeguard the quality of each article we publish.