- Brain Language Laboratory, Freie Universtät Berlin, Berlin, Germany
The present work aims at investigating how Toxoplasma gondii (T. gondii) infection may be linked to N-methyl-d-aspartate receptor (NMDAR) dysfunction in schizophrenia and related disorders and puts forward the hypothesis that immune responses against T. gondii may involve NMDARs. Indeed, the analysis of the protozoan proteome and NMDAR subunits for peptide commonalities shows a massive peptide overlap and supports the possibility that anti-T. gondii immune responses raised during active protozoan infection may cross-react with host NMDARs, determining disruption of neural circuits and cognitive deficits. In particular, the NMDA 2D subunit, which is mainly expressed in parvalbumin-positive interneurons, appears to be a hotspot for potential T. gondii-induced cross-reactive immune attacks.
Introduction
Schizophrenia is a multifaceted syndrome characterized by distinctive behavioral symptoms, cognitive deficits, and a complex etiopathogenesis, which seems to involve neurodevelopmental anomalies and a combination of genetic and environmental factors (1, 2). Among the environmental factors, Toxoplasma gondii (T. gondii) is gaining increasing attention, and a causal association between the protozoan infection and schizophrenia has been repeatedly suggested (3–9). Over the last decades, studies on T. gondii antibodies (Abs) in patients with schizophrenia revealed higher levels of anti-T. gondii Abs in the affected persons when compared to controls (8, 10–12). Interestingly, higher anti-T. gondii Ab levels were also found in mothers of offspring who later developed schizophrenia (13) and in newborns who later were diagnosed with the disease, as compared to controls (6, 14). This suggests that toxoplasmosis in early life might affect neurodevelopment and contribute to later onset of schizophrenia. However, the molecular determinants and mechanisms by which T. gondii infection might contribute to the pathophysiology of the disease remain unclear.
One major pathophysiological mechanism underlying development of schizophrenia seems to be N-methyl-d-aspartate glutamate receptor (NMDAR) dysfunction (15–17). The NMDA model of schizophrenia originated from the observation that NMDA antagonists, like ketamine or phencyclidine (PCP), transiently induce symptoms that mimic psychotic episodes (18–21). Following these initial observations, a large body of genetic and molecular evidence has accumulated in the last three decades indicating NMDA dysfunction as a convergence point in the development of schizophrenia (22–27). NMDA dysfunction not only can provide a satisfactory explanation of behavioral and cognitive symptoms of schizophrenia but is also consistent with the neurodevelopmental aspect of the disease, given that early NMDA aberrations/damage can translate into clinical onset later in life (22, 28, 29).
In summary, the NMDA model of schizophrenia seems then to be the common pathway of different etiological factors and is characterized by an early-damage late-onset temporal pattern, which is consistent with findings on increased risk of schizophrenia after early-life T. gondii infection. It is therefore reasonable to hypothesize that T. gondii can affect NMDAR function and glutamatergic neuronal circuits.
On this basis, the present work examines the hypothesis that immune responses to T. gondii may relate to NMDAR dysfunction by way of cross-reactive mechanisms and anti-NMDAR Abs. The rationale is that when a pathogen has sequence/structure similarity with human proteins, then anti-pathogen immune responses may cross-react with human proteins that share sequences/structures with the pathogen, thus triggering autoimmunity (30, 31). Such a hypothesis originates form the observations that (1) anti-T. gondii Ab levels are, as discussed above, higher in schizophrenic patients (8, 10–12), thus suggesting that immune responses following T. gondii active infection might play a role in the association of the parasite with the disease and (2) NMDAR blocking Abs are present in subjects with schizophrenia, schizoaffective, bipolar, and major depressive disorders (32–37), thus suggesting a role of anti-NMDAR immunoreactivity in the genesis of NMDA dysfunction in schizophrenia and other neuropsychiatric disorders. Moreover, a direct effect of early toxoplasmosis on behavioral anomalies and elevation of anti-NMDAR autoantibodies was found in a recent study on mice (38).
In light of this immunologic context, T. gondii proteome and the seven NMDAR subunit proteins (NMDA 1, 2A, 2B, 2C, 2D, 3A, and 3B) were searched for common peptides that might underlie immune cross-reactions between the protozoan and the human host. Data are reported showing that the T. gondii proteome and NMDAR subunits share a vast epitopic peptide platform that is centered on the 2D subunit and appears to be potentially significant to schizophrenia pathogenesis.
Methods
The seven human NMDAR subunit aminoacidic sequence analyzed in this study were retrieved from the UniProt database1 (39), and are listed with their alternative names in parentheses, followed by amino acids (aa) length: NMDA 1 (GluN1, NMDZ1), 938; NMDA 2A (GluN2A, NMDE1), 1,464; NMDA 2B (GluN2B, NMDE2), 1,484; NMDA 2C (GluN2C, NMDE3), 1,233; NMDA 2D (GluN2D, NMDE4), 1,336; NMDA 3A (GluN3A, NMD3A), 1,115; and NMDA 3B (GluN3B, NMD3B), 1,043.
The protein sequence of each NMDA subunit was dissected into sequential hexapeptides that overlapped each other by five aa (for example, MSTMRL, STMRLL, TMRLLT, MRLLTL, and so forth). This procedure produced a library consisting of 8,578 NMDAR subunit hexapeptides. Each NMDAR hexapeptide was used as a probe to search the entire T. gondii proteome for occurrences of the same hexapeptide using the Pir Peptide Match program2 (40).
Toxoplasma gondii (strain VEG, NCBI Tax ID: 432359) was investigated. The T. gondii proteome consists of 8,404 proteins (Uniprot proteome: UP000002226). The protozoan Entamoeba histolytica (NCBI Tax ID: 5759; 7,959 proteins; Uniprot proteome: UP000001926) was used as a control.
The Immune Epitope DataBase3 (IEDB) (41) was searched for epitopes containing (or corresponding to) NMDAR hexapeptide(s) shared with T. gondii and experimentally validated as immunopositive in humans. Details, references, and immunoassay type for each epitope reported in the present study are available at http://www.iedb.org/advancedQueryEpitope.php.
Results
Sequence-matching analyses were carried out at the 6-mer level since a grouping of 5–6 aa represents the minimal immune unit able to induce specific Abs and to determine specific antigen–antibody recognition (42–44).
The hexapeptide sharing between NMDAR subunits and T. gondii proteins and its immunological potential is quantified in Table 1 and detailed in Table S1 in the Supplementary Material. Table 1 shows that the seven NMDAR subunit proteins share a high number of hexapeptides with the protozoan proteome. On the whole, 2,215 out of the 8,578 hexapeptides composing the NMDAR library repeatedly occur in the protozoan proteome, for a total of 5,802 multiple occurrences. Theoretically, such an impressive level of peptide sharing equates to a vast source of potential cross-reactions in case of active toxoplasmosis and, indeed, NMDAR hexapeptides shared with the T. gondii proteome are also present in immunopositive epitopes (Table 1, last column).
In order to define the immunologic potential of the hexapeptide commonality between NMDARs and T. gondii, the shared 2,215 hexapeptides were analyzed using the IEDB, an immune epitope catalog resource, in search of epitopes experimentally validated as immunopositive in the human host, and containing (or corresponding to) hexapeptides shared between T. gondii and human NMDAR proteins. One hundred sixty out of the 2,215 hexapeptides shared between the 7 human NMDARs and T. gondii were found to be disseminated through hundreds of IEDB epitopes that have been validated as immunopositive in humans. The 160 epitopic NMDAR hexapeptides and the IEDB epitopes are described in Tables 2 and 3, respectively.
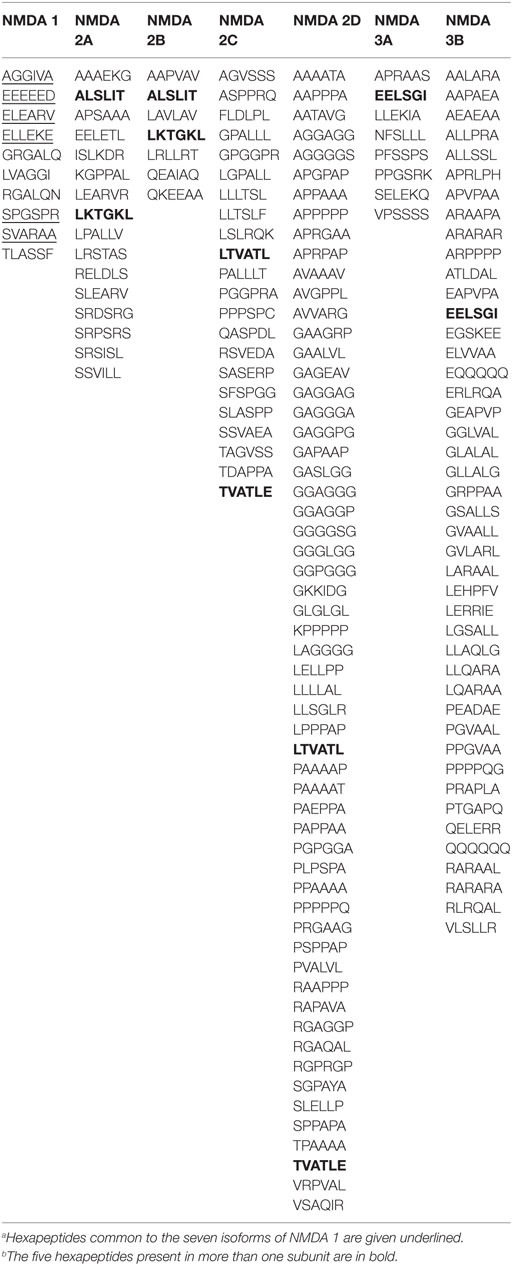
Table 2. Epitopic hexapeptides shared between the seven human N-methyl-d-aspartate receptor (NMDAR) subunit proteins and T. gondii proteome and present in epitopes experimentally validated as immunopositive in humans.a,b
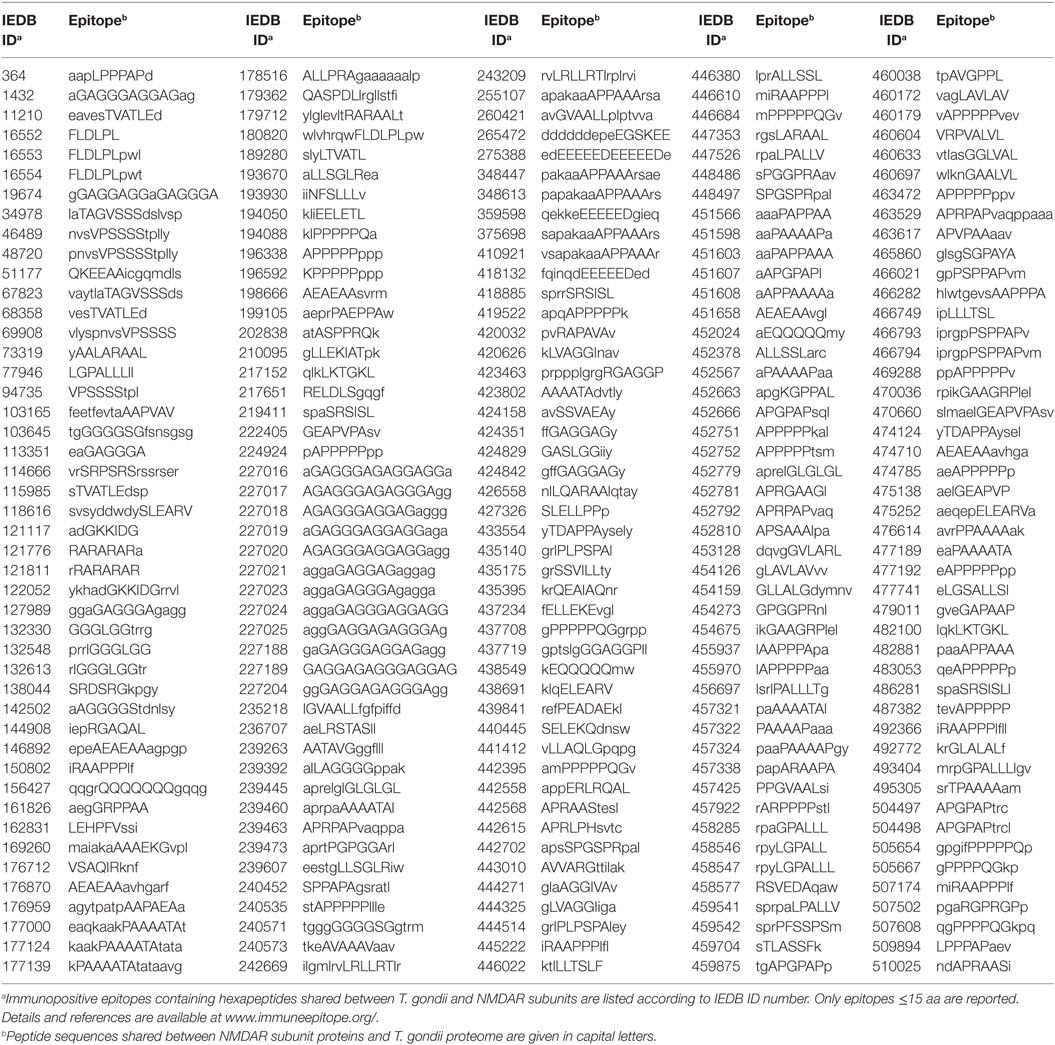
Table 3. Immunopositive epitopes containing hexapeptides shared between the seven human N-methyl-d-aspartate receptor (NMDAR) subunit proteins and T. gondii proteome.
Control analyses using the protozoan E. histolytica, a human pathogen associated with intestinal and extraintestinal infections (45), highlight a lower extent of peptide sharing (Table 4), thus indicating that the intensity of the hexapeptide sharing between T. gondii and the NMDAR subunits—in particular, NMDA 2D and 3B—is specific (Tables 1 and 2). The detailed description of the peptide sharing between the NMDAR subunits and the protozoan E. histolytica is reported in Tables S2 and S3 in Supplementary Material.
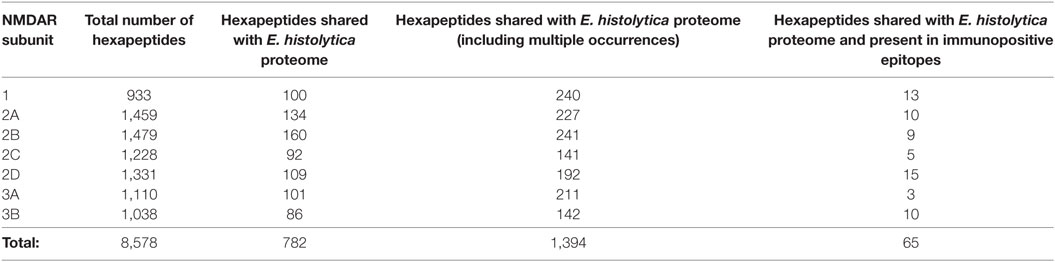
Table 4. N-methyl-d-aspartate receptor (NMDAR) hexapeptide sharing with Entamoeba histolytica proteome.
Discussion
The Spatiotemporal NMDAR Subunit Expression May Shape the Potential Cross-reactions between T. gondii and NMDARs
Tables 2 and 3 indicate that T. gondii active infection might induce immune reactions able to cross-react to different extents with the seven NMDAR subunits. In particular, analysis of Table 2 shows that epitopic hexapeptides shared with T. gondii are mostly allocated in the NMDA 2D, 3B, and 2C subunits (58, 44, and 22 epitopic hexapeptides, respectively), whereas a relatively lower level of hexapeptide sharing characterizes NMDA 2A, 1, 3B, and 3A (16, 10, 8, and 7 epitopic hexapeptides, respectively).
This appears to be relevant in light of the fact that NMDARs consist of four subunits, two of which invariably are NMDA 1 subunits that can associate with NMDA 2 subunits or a combination of NMDA 2 and NMDA 3 subunits. Such a variable composition results in a large number of NMDAR subtypes, which present different spatiotemporal patterns of expression during neurodevelopment and in the young/adult life (46). For instance, NMDA 2B and NMDA 2D are expressed during embryonic development, whereas NMDA 2A and NMDA 2C gene expression starts after birth; NMDA 2A and NMDA 2B are highly expressed in the cortex and hippocampus and NMDA 2C in the cerebellum of adult rodents (47–52). Likewise, NMDA 3A and NMDA 3B genes have specific developmental patterns of expression (53).
Even the NMDA 1 subunit, which is a constitutive component of all NMDARs, presents a heterogenous distribution and a varying immunoreactivity potential when its seven isoforms are analyzed (54). For example, only immune cross-reactions against 6 out of the 10 epitopic hexapeptides present in all NMDA 1 isoforms (Table 2)—namely, AGGIVA, EEEEED, ELEARV, ELLEKE, SPGSPR, and SVARAA—should be able to produce a diffuse immunoreactivity in the brain.
Hence, T. gondii-induced immune cross-reactions might have different outcomes depending on the targeted NMDAR subunit and might target different subunits depending on the timing of exposure to the protozoan. Indeed, the timing of exposure to T. gondii is a crucial factor in generating different specific anti-NMDAR Abs and, consequently, different associated neurobehavioral disorders (38).
NMDA 2D Is the Main Potential Target of Anti-T. gondii Immune Response
NMDA 2D exemplifies the potential relationship between toxoplasmosis-induced immunoreactivity, the spatiotemporal expression profile of NMDAR subunits, and different potential outcomes. Indeed, NMDA 2D contains 496 hexapeptides in common with the protozoan (Table 1), 58 of which are present in validated immunopositive epitopes (Table 1). Therefore, NMDA 2D might be the main target of the immune cross-reactivity potentially associated with T. gondii infection. Studies in animals [(48–52) and more references therein] showed that NMDA 2D gene expression:
– is high in the midbrain, the diencephalon, and the spinal cord before birth;
– is abundant around birth in thalamic and hypothalamic nuclei and in the brainstem;
– reaches a peak around 1 week after birth; and
– subsequently declines and persists mainly in the hippocampal interneurons, most of which are somatostatin (SOM)- and parvalbumin (PV)-positive cells.
Translating data from animal models to the human brain, it is logical to presume that immune cross-reactions involving NMDA 2D in the fetus and the newborn can extensively occur throughout the brain, whereas secondary immune responses after early sensitivization might target hippocampal PV- and SOM-positive interneurons in the young/adult. Indeed, alterations in the hippocampal PV- and SOM-positive interneurons have been repeatedly related to the hippocampal hyperactivity that characterizes schizophrenia (55–57).
NMDARs vs T. gondii Epitopic Peptide Overlap and the NMDA Model of Schizophrenia
The vast epitopic peptide sharing between T. gondii and the seven NMDAR subunits (see Tables 2 and 3) suggests that anti-T. gondii immune responses cross-reacting with NMDARs might lead to NMDAR damage and dysfunction, targeting in particular interneurons expressing NMDA 2D. Crucially, this hypothesis, which links mechanistically toxoplasmosis and schizophrenia by way of peptide sharing with NMDARs, is consistent with the well-established NMDA dys/hypofunction model of schizophrenia (15–17). As mentioned in the Introduction, the NMDA model is based on the observation that NMDA antagonists, like PCP, Ketamine, and MK801, induce symptoms that resemble schizophrenia (2, 17), and it seems to be able to provide a good account of some aspects of the complex symptomatology of the disease, including cognitive symptoms (17). Remarkably, both of these two fundamental aspects of the NMDA model are related to the NMDA 2D subunit type. First, targeting NMDA 2D appears to be a major mechanism in the pharmacodynamics of MK801 (58), ketamine (59, 60), and PCP (61, 62). Second, 2D-containing NMDARs are typically expressed in GABAergic interneurons, where they largely contribute to excitatory post-synaptic potentials (49, 51, 63). Consequently, NMDAR dysfunction in these cells would translate into reduced GABAergic activity and consequent reduced inhibitory control of pyramidal cell activity (2, 64). The excitatory–inhibitory balance in cortical networks is crucial for generating high-frequency (gamma) oscillatory activity (65, 66), and it appears that the disruption of gamma-band oscillations (GBOs) might indeed underlie cognitive deficits in schizophrenia (67–70). On the one hand, GBOs are well known to be physiologically related to higher cognitive functions (71–74), on the other hand, they are typically altered in schizophrenic patients (75–77). Moreover, ketamine alters gamma oscillatory activity by targeting NMDA 2D (59). It appears then that NMDA 2D damage can be directly related to cognitive deficits in schizophrenia.
In summary, in the complex and articulated picture that connects PV interneurons, brain oscillation, and cognition [Ref. (78, 79) for review], a large body of evidence from pharmacological, genetic, electrophysiological, and clinical research converges on a critical role of NMDA 2D in cognition within the context of the NMDA model of schizophrenia. It is likely then that T. gondii-induced anti-NMDA 2D cross-reactivity might, among other different mechanisms triggered by both genetic and environmental factors, play a role in contributing to NMDA dysfunction and GABA hypofunction, thus resulting in cortical circuitry disequilibrium and, potentially, in disruption of brain oscillation and cognitive processes.
Conclusion
This work presents and examines the hypothesis that the relationship between T. gondii and schizophrenia might be explained by way of shared peptides (as molecular determinants) and immune cross-reactivity (as biological mechanism) between T. gondii proteins and the NMDAR subunits. The high and specific peptide commonality with the NMDARs shown by T. gondii, as compared to the control, supports the possibility that the infection might induce anti-NMDAR immune responses in the human host through cross-reactivity (Table 1) and more so in light of the epitopic nature of many of the shared peptides (Tables 2 and 3). Such a hypothesis is consistent, on the one hand, with previous studies describing the potential neuropsychiatric relevance of the vast peptide commonality existing between infectious agents and the human host (80–82) and, on the other hand, with the well-established NMDA dysfunction model of schizophrenia. Hence, a possible scenario unfolds, where the differential spatiotemporal patterns of expression of the NMDAR subunits might generate the diversity of neuropathological outcomes. In this regard, immune attacks on NMDA 2D, a main potential target of T. gondii-induced cross-reactions, may represent a mechanistic link between T. gondii infection and NMDAR dysfunction in neuropsychiatric disorders.
In summary, immune cross-reactions with NMDARs following T. gondii infection might be one of the factors contributing to the pathophysiology of schizophrenia and associated disorders, and NMDAR subunit composition could relate to the timing and the targets of the neuropathologic sequela of the exposure to T. gondii. The hypothesis presented here might help to address aspects of the complex and multifactorial etiopathogenesis of schizophrenia in future clinical and basic research.
Author Contributions
GL designed the study, performed the analyses, and wrote the manuscript.
Conflict of Interest Statement
The author declares that the research was conducted in the absence of any commercial or financial relationships that could be construed as a potential conflict of interest.
Funding
GL gratefully acknowledges support from the Deutscher Akademischer Austauschdienst (DAAD), the Deutsche Forschungsgemeinschaft (DFG), and the Freie Universität Berlin, Germany.
Supplementary Material
The Supplementary Material for this article can be found online at http://journal.frontiersin.org/article/10.3389/fpsyt.2017.00037/full#supplementary-material.
Footnotes
References
1. Owen MJ, Sawa A, Mortensen PB. Schizophrenia. Lancet (2016) 388:86–97. doi:10.1016/S0140-6736(15)01121-6
2. Lisman JE, Coyle JT, Green RW, Javitt DC, Benes FM, Heckers S, et al. Circuit-based framework for understanding neurotransmitter and risk gene interactions in schizophrenia. Trends Neurosci (2008) 31:234–42. doi:10.1016/j.tins.2008.02.005
3. Yolken RH, Dickerson FB, Fuller Torrey E. Toxoplasma and schizophrenia. Parasite Immunol (2009) 31:706–15. doi:10.1111/j.1365-3024.2009.01131.x
4. Tomasik J, Schultz TL, Kluge W, Yolken RH, Bahn S, Carruthers VB. Shared immune and repair markers during experimental Toxoplasma chronic brain infection and schizophrenia. Schizophr Bull (2016) 42:386–95. doi:10.1093/schbul/sbv134
5. Dickerson F, Boronow J, Stallings C, Origoni A, Yolken R. Toxoplasma gondii in individuals with schizophrenia: association with clinical and demographic factors and with mortality. Schizophr Bull (2007) 33:737–40. doi:10.1093/schbul/sbm005
6. Mortensen P, Norgaard-Pedersen B, Waltoft B, Sorensen T, Hougaard D, Yolken R. Early infections of Toxoplasma gondii and the later development of schizophrenia. Schizophr Bull (2007) 33:741–4. doi:10.1093/schbul/sbm009
7. Wang HL, Wang GH, Li QY, Shu C, Jiang MS, Guo Y. Prevalence of Toxoplasma infection in first-episode schizophrenia and comparison between Toxoplasma-seropositive and Toxoplasma-seronegative schizophrenia. Acta Psychiatr Scand (2006) 114:40–8. doi:10.1111/j.1600-0447.2006.00780.x
8. Torrey EF, Bartko JJ, Lun ZR, Yolken RH. Antibodies to Toxoplasma gondii in patients with schizophrenia: a meta-analysis. Schizophr Bull (2007) 33:729–36. doi:10.1093/schbul/sbl050
9. Hamidinejat H, Ghorbanpoor M, Hosseini H, Alavi SM, Nabavi L, Jalali MHR, et al. Toxoplasma gondii infection in first-episode and inpatient individuals with schizophrenia. Int J Infect Dis (2010) 14:e978–81. doi:10.1016/j.ijid.2010.05.018
10. Sutterland AL, Fond G, Kuin A, Koeter MW, Lutter R, van Gool T, et al. Beyond the association. Toxoplasma gondii in schizophrenia, bipolar disorder, and addiction: systematic review and meta-analysis. Acta Psychiatr Scand (2015) 132:161–79. doi:10.1111/acps.12423
11. Torrey EF, Bartko JJ, Yolken RH. Toxoplasma gondii and other risk factors for schizophrenia: an update. Schizophr Bull (2012) 38:642–7. doi:10.1093/schbul/sbs043
12. Monroe JM, Buckley PF, Miller BJ. Meta-analysis of anti-Toxoplasma gondii IgM antibodies in acute psychosis. Schizophr Bull (2015) 41:989–98. doi:10.1093/schbul/sbu159
13. Brown AS, Schaefer CA, Quesenberry CP Jr, Liu L, Babulas VP, Susser ES. Maternal exposure to toxoplasmosis and risk of schizophrenia in adult offspring. Am J Psychiatry (2005) 162:767–73. doi:10.1176/appi.ajp.162.4.767
14. Mortensen PB, Nørgaard-Pedersen B, Waltoft BL, Sørensen TL, Hougaard D, Torrey EF, et al. Toxoplasma gondii as a risk factor for schizophrenia: analysis of filter paper blood samples obtained at birth. Biol Psychiatry (2007) 61:688–93. doi:10.1016/j.biopsych.2006.05.024
15. Olney JW, Newcomer JW, Farber NB. NMDA receptor hypofunction model of schizophrenia. J Psychiatr Res (1999) 33:523–33. doi:10.1016/S0022-3956(99)00029-1
16. Javitt DC. Glutamate and schizophrenia: phencyclidine, N-methyl-d-aspartate receptors, and dopamine-glutamate interactions. Int Rev Neurobiol (2007) 78:69–108. doi:10.1016/S0074-7742(06)78003-5
18. Javitt DC. Negative schizophrenic symptomatology and the PCP (phencyclidine) model of schizophrenia. Hillside J Clin Psychiatry (1987) 9:12–35.
19. Javitt DC, Zukin SR. Recent advances in the phencyclidine model of schizophrenia. Am J Psychiatry (1991) 148:1301–8. doi:10.1176/ajp.148.10.1301
20. Krystal JH, Karper LP, Seibyl JP, Freeman GK, Delaney R, Bremner JD, et al. Subanesthetic effects of the noncompetitive NMDA antagonist, ketamine, in humans. Psychotomimetic, perceptual, cognitive, and neuroendocrine responses. Arch Gen Psychiatry (1994) 51:199–214. doi:10.1001/archpsyc.1994.03950030035004
21. Lahti AC, Holcomb HH, Medoff DR, Tamminga CA. Ketamine activates psychosis and alters limbic blood flow in schizophrenia. Neuroreport (1995) 6:869–72. doi:10.1097/00001756-199504190-00011
22. Kantrowitz JT, Javitt DC. N-methyl-d-aspartate (NMDA) receptor dysfunction or dysregulation: The final common pathway on the road to schizophrenia? Brain Res Bull (2010) 83:108–21. doi:10.1016/j.brainresbull.2010.04.006
23. Coyle JT. NMDA receptor and schizophrenia: a brief history. Schizophr Bull (2012) 38:920–6. doi:10.1093/schbul/sbs076
24. Hall J, Trent S, Thomas KL, O’Donovan MC, Owen MJ. Genetic risk for schizophrenia: convergence on synaptic pathways involved in plasticity. Biol Psychiatry (2015) 77:52–8. doi:10.1016/j.biopsych.2014.07.011
25. Veerman SR, Schulte PF, de Haan L. The glutamate hypothesis: a pathogenic pathway from which pharmacological interventions have emerged. Pharmacopsychiatry (2014) 47:121–30. doi:10.1055/s-0034-1383657
26. Howes O, McCutcheon R, Stone J. Glutamate and dopamine in schizophrenia: an update for the 21st century. J Psychopharmacol (2015) 29:97–115. doi:10.1177/0269881114563634
27. Snyder MA, Gao WJ. NMDA hypofunction as a convergence point for progression and symptoms of schizophrenia. Front Cell Neurosci (2013) 7:31. doi:10.3389/fncel.2013.00031
28. Harris LW, Sharp T, Gartlon J, Jones DN, Harrison PJ. Long-term behavioural, molecular and morphological effects of neonatal NMDA receptor antagonism. Eur J Neurosci (2003) 18:1706–10. doi:10.1046/j.1460-9568.2003.02902.x
29. Frohlich J, Van Horn JD. Reviewing the Ketamine Model for schizophrenia. J Psychopharmacol (2014) 28:287–302. doi:10.1177/0269881113512909
31. Kohm A, Fuller K, Miller S. Mimicking the way to autoimmunity: an evolving theory of sequence and structural homology. Trends Microbiol (2003) 11:101–5. doi:10.1016/S0966-842X(03)00006-4
32. Pearlman DM, Najjar S. Meta-analysis of the association between N-methyl-d-aspartate receptor antibodies and schizophrenia, schizoaffective disorder, bipolar disorder, and major depressive disorder. Schizophr Res (2014) 157:249–58. doi:10.1016/j.schres.2014.05.001
33. Tsutsui K, Kanbayashi T, Tanaka K, Boku S, Ito W, Tokunaga J, et al. Anti-NMDA-receptor antibody detected in encephalitis, schizophrenia, and narcolepsy with psychotic features. BMC Psychiatry (2012) 12:37. doi:10.1186/1471-244X-12-37
34. Zandi MS, Irani SR, Lang B, Waters P, Jones PB, McKenna P, et al. Disease-relevant autoantibodies in first episode schizophrenia. J Neurol (2011) 258:686–8. doi:10.1007/s00415-010-5788-9
35. Steiner J, Walter M, Glanz W, Sarnyai Z, Bernstein HG, Vielhaber S, et al. Increased prevalence of diverse N-methyl-d-aspartate glutamate receptor antibodies in patients with an initial diagnosis of schizophrenia: specific relevance of IgG NR1a antibodies for distinction from N-methyl-d-aspartate glutamate receptor encephalitis. JAMA Psychiatry (2013) 70:271–8. doi:10.1001/2013.jamapsychiatry.86
36. Dickerson F, Stallings C, Vaughan C, Origoni A, Khushalani S, Yolken RH. Antibodies to the glutamate receptor in mania. Bipolar Disord (2012) 14:547–53. doi:10.1111/j.1399-5618.2012.01028.x
37. Deakin J, Lennox BR, Zandi MS. Antibodies to the N-methyl-d-aspartate receptor and other synaptic proteins in psychosis. Biol Psychiatry (2014) 75:284–91. doi:10.1016/j.biopsych.2013.07.018
38. Kannan G, Crawford JA, Yang C, Gressitt KL, Ihenatu C, Krasnova IN, et al. Anti-NMDA receptor autoantibodies and associated neurobehavioral pathology in mice are dependent on age of first exposure to Toxoplasma gondii. Neurobiol Dis (2016) 91:307–14. doi:10.1016/j.nbd.2016.03.005
39. UniProt Consortium. The universal protein resource (UniProt) 2009. Nucleic Acids Res (2009) 37:D169–74. doi:10.1093/nar/gkn664
40. Chen C, Li Z, Huang H, Suzek BE, Wu CH, UniProt Consortium. A fast Peptide Match service for UniProt knowledgebase. Bioinformatics (2013) 29:2808–9. doi:10.1093/bioinformatics/btt484
41. Vita R, Zarebski L, Greenbaum JA, Emami H, Hoof I, Salimi N, et al. The immune epitope database 2.0. Nucleic Acids Res (2010) 38:D854–62. doi:10.1093/nar/gkp1004
42. Frank A. Immunology and Evolution of Infectious Disease. Princeton, NJ: Princeton University Press (2002).
43. Zeng W, Pagnon J, Jackson DC. The C-terminal pentapeptide of LHRH is a dominant B cell epitope with antigenic and biological function. Mol Immunol (2007) 44:3724–31. doi:10.1016/j.molimm.2007.04.004
44. Kanduc D. Pentapeptides as minimal functional units in cell biology and immunology. Curr Protein Pept Sci (2013) 14:111–20. doi:10.2174/1389203711314020003
45. Ralston KS. Taking a bite: amoebic trogocytosis in Entamoeba histolytica and beyond. Curr Opin Microbiol (2015) 28:26–35. doi:10.1016/j.mib.2015.07.009
46. Paoletti P, Bellone C, Zhou Q. NMDA receptor subunit diversity: impact on receptor properties, synaptic plasticity and disease. Nat Rev Neurosci (2013) 14:383–400. doi:10.1038/nrn3504
47. Watanabe M, Inoue Y, Sakimura K, Mishina M. Developmental changes in distribution of NMDA receptor channel subunit mRNAs. Neuroreport (1992) 3:1138–40. doi:10.1097/00001756-199212000-00027
48. Monyer H, Burnashev N, Laurie DJ, Sakmann B, Seeburg PH. Developmental and regional expression in the rat brain and functional properties of four NMDA receptors. Neuron (1994) 12:529–40. doi:10.1016/0896-6273(94)90210-0
49. Standaert DG, Landwehrmeyer GB, Kerner JA, Penney JB Jr, Young AB. Expression of NMDAR2D glutamate receptor subunit mRNA in neurochemically identified interneurons in the rat neostriatum, neocortex and hippocampus. Brain Res Mol Brain Res (1996) 42:89–102. doi:10.1016/S0169-328X(96)00117-9
50. Thompson CL, Drewery DL, Atkins HD, Stephenson FA, Chazot PL. Immunohistochemical localization of N-methyl-d-aspartate receptor subunits in the adult murine hippocampal formation: evidence for a unique role of the NR2D subunit. Brain Res Mol Brain Res (2002) 102:55–61. doi:10.1016/S0169-328X(02)00183-3
51. von Engelhardt J, Bocklisch C, Tönges L, Herb A, Mishina M, Monyer H. GluN2D-containing NMDA receptors-mediate synaptic currents in hippocampal interneurons and pyramidal cells in juvenile mice. Front Cell Neurosci (2015) 9:95. doi:10.3389/fncel.2015.00095
52. Ewald RC, Cline HT. NMDA receptors and brain development. In: Van Dongen AM, editor. Biology of the NMDA Receptor. Boca Raton, FL: CRC Press/Taylor & Francis (2009). p. 1–15.
53. Kehoe LA, Bernardinelli Y, Muller D. GluN3A: an NMDA receptor subunit with exquisite properties and functions. Neural Plast (2013) 2013:145387. doi:10.1155/2013/145387
54. Laurie DJ, Seeburg PH. Regional and developmental heterogeneity in splicing of the rat brain NMDAR1 mRNA. J Neurosci (1994) 14:3180–94.
55. Heckers S, Konradi C. GABAergic mechanisms of hippocampal hyperactivity in schizophrenia. Schizophr Res (2015) 167:4–11. doi:10.1016/j.schres.2014.09.041
56. Wang AY, Lohmann KM, Yang CK, Zimmerman EI, Pantazopoulos H, Herring N, et al. Bipolar disorder type 1 and schizophrenia are accompanied by decreased density of parvalbumin- and somatostatin-positive interneurons in the parahippocampal region. Acta Neuropathol (2011) 122:615–26. doi:10.1007/s00401-011-0881-4
57. Konradi C, Yang CK, Zimmerman EI, Lohmann KM, Gresch P, Pantazopoulos H, et al. Hippocampal interneurons are abnormal in schizophrenia. Schizophr Res (2011) 131:165–73. doi:10.1016/j.schres.2011.06.007
58. Suryavanshi PS, Ugale RR, Yilmazer-Hanke D, Stairs DJ, Dravid SM. GluN2C/GluN2D subunit-selective NMDA receptor potentiator CIQ reverses MK-801-induced impairment in prepulse inhibition and working memory in Y-maze test in mice. Br J Pharmacol (2014) 171:799–809. doi:10.1111/bph.12518
59. Sapkota K, Mao Z, Synowicki P, Lieber D, Liu M, Ikezu T, et al. GluN2D N-methyl-d-aspartate receptor subunit contribution to the stimulation of brain activity and gamma oscillations by ketamine: implications for schizophrenia. J Pharmacol Exp Ther (2016) 356:702–11. doi:10.1124/jpet.115.230391
60. Yamamoto T, Nakayama T, Yamaguchi J, Matsuzawa M, Mishina M, Ikeda K, et al. Role of the NMDA receptor GluN2D subunit in the expression of ketamine-induced behavioral sensitization and region-specific activation of neuronal nitric oxide synthase. Neurosci Lett (2016) 610:48–53. doi:10.1016/j.neulet.2015.10.049
61. Yamamoto H, Kamegaya E, Sawada W, Hasegawa R, Yamamoto Y, Hagino Y, et al. Involvement of the N-methyl-d-aspartate receptor GluN2D subunit in phencyclidine-induced motor impairment, gene expression, and increased Fos immunoreactivity. Mol Brain (2013) 6:56. doi:10.1186/1756-6606-6-56
62. Hagino Y, Kasai S, Han W, Yamamoto H, Nabeshima T, Mishina M, et al. Essential role of NMDA receptor channel ε4 subunit (GluN2D) in the effects of phencyclidine, but not methamphetamine. PLoS One (2010) 5:e13722. doi:10.1371/journal.pone.0013722
63. Jones RS, Buhl EH. Basket-like interneurones in layer II of the entorhinal cortex exhibit a powerful NMDA-mediated synaptic excitation. Neurosci Lett (1993) 149:35–9. doi:10.1016/0304-3940(93)90341-H
64. Cohen SM, Tsien RW, Goff DC, Halassa MH. The impact of NMDA receptor hypofunction on GABAergic neurons in the pathophysiology of schizophrenia. Schizophr Res (2015) 167:8–107. doi:10.1016/j.schres.2014.12.026
65. Wang XJ. Neurophysiological and computational principles of cortical rhythms in cognition. Physiol Rev (2010) 90:1195–268. doi:10.1152/physrev.00035.2008
66. Buzsáki G, Wang XJ. Mechanisms of gamma oscillations. Annu Rev Neurosci (2012) 35:203–25. doi:10.1146/annurev-neuro-062111-150444
67. Jadi MP, Behrens MM, Sejnowski TJ. Abnormal gamma oscillations in N-methyl-d-aspartate receptor hypofunction models of schizophrenia. Biol Psychiatry (2016) 79:716–26. doi:10.1016/j.biopsych.2015.07.005
68. Gonzalez-Burgos G, Lewis DA. NMDA receptor hypofunction, parvalbumin-positive neurons, and cortical gamma oscillations in schizophrenia. Schizophr Bull (2012) 38:950–7. doi:10.1093/schbul/sbs010
69. McNally JM, McCarley RW. Gamma band oscillations: a key to understanding schizophrenia symptoms and neural circuit abnormalities. Curr Opin Psychiatry (2016) 29:202–10. doi:10.1097/YCO.0000000000000244
70. Ford JM, Krystal JH, Mathalon DH. Neural synchrony in schizophrenia: from networks to new treatments. Schizophr Bull (2007) 33:848–52. doi:10.1093/schbul/sbm062
71. Ward LM. Synchronous neural oscillations and cognitive processes. Trends Cogn Sci (2003) 7:553–9. doi:10.1016/j.tics.2003.10.012
72. Fries P. Neuronal gamma-band synchronisation as a fundamental process in cortical computation. Annu Rev Neurosci (2009) 32:209–24. doi:10.1146/annurev.neuro.051508.135603
73. Pulvermüller F, Birbaumer N, Lutzenberger W, Mohr B. High-frequency brain activity: its possible role in attention, perception and language processing. Prog Neurobiol (1997) 52:427–45. doi:10.1016/S0301-0082(97)00023-3
74. Garagnani M, Lucchese G, Tomasello R, Wennekers T, Pulvermüller F. A spiking neurocomputational model of high-frequency oscillatory brain responses to words and pseudowords. Front Comput Neurosci (2017) 10:145. doi:10.3389/fncom.2016.00145
75. Gandal MJ, Edgar JC, Klook K, Siegel SJ. Gamma synchrony: towards a translational biomarker for the treatment resistant symptoms of schizophrenia. Neuropharmacology (2012) 62:1504–18. doi:10.1016/j.neuropharm.2011.02.007
76. Uhlhaas PJ, Singer W. Abnormal neural oscillations and synchrony in schizophrenia. Nat Rev Neurosci (2010) 11:100–13. doi:10.1038/nrn2774
77. Hirano Y, Oribe N, Kanba S, Onitsuka T, Nestor PG, Spencer KM. Spontaneous gamma activity in schizophrenia. JAMA Psychiatry (2015) 72(8):813–21. doi:10.1001/jamapsychiatry.2014.2642
78. Castelnovo A, Ferrarelli F, D’Agostino A. Schizophrenia: from neurophysiological abnormalities to clinical symptoms. Front Psychol (2015) 6:478. doi:10.3389/fpsyg.2015.00478
79. Lewis DA, Curley AA, Glausier JR, Volk DW. Cortical parvalbumin interneurons and cognitive dysfunction in schizophrenia. Trends Neurosci (2012) 35(1):57–67. doi:10.1016/j.tins.2011.10.004
80. Lucchese G. Confronting JC virus and Homo sapiens biological signatures. Front Biosci (2013) 18:716–24. doi:10.2741/4133
81. Lucchese G, Capone G, Kanduc D. Peptide sharing between influenza A H1N1 hemagglutinin and human axon guidance proteins. Schizophr Bull (2014) 40:362–75. doi:10.1093/schbul/sbs197
Keywords: Toxoplasma gondii, N-methyl-d-aspartate receptors, NMDA 2D, peptide commonality, immune cross-reactivity, schizophrenia, parvalbumin-positive interneurons, gamma oscillations
Citation: Lucchese G (2017) From Toxoplasmosis to Schizophrenia via NMDA Dysfunction: Peptide Overlap between Toxoplasma gondii and N-Methyl-d-Aspartate Receptors As a Potential Mechanistic Link. Front. Psychiatry 8:37. doi: 10.3389/fpsyt.2017.00037
Received: 03 August 2016; Accepted: 27 February 2017;
Published: 15 March 2017
Edited by:
Stefan Borgwardt, University of Basel, SwitzerlandReviewed by:
Bernhard J. Mitterauer, Volitronics-Institute for Basic Research Psychopathology and Brain Philosophy, AustriaArmando D’Agostino, University of Milan, Italy
Copyright: © 2017 Lucchese. This is an open-access article distributed under the terms of the Creative Commons Attribution License (CC BY). The use, distribution or reproduction in other forums is permitted, provided the original author(s) or licensor are credited and that the original publication in this journal is cited, in accordance with accepted academic practice. No use, distribution or reproduction is permitted which does not comply with these terms.
*Correspondence: Guglielmo Lucchese, Z3VnbGllbG1vLmx1Y2NoZXNlJiN4MDAwNDA7ZnUtYmVybGluLmRl