- 1Addiction Psychiatry Research Unit, Research Center, Centre Hospitalier de l’Université de Montréal (CRCHUM), Montreal, QC, Canada
- 2Department of Psychiatry, University of Montreal, Montreal, QC, Canada
- 3Research Center, Institut Universitaire en Santé Mentale de Montréal, Montreal, QC, Canada
Cannabis is one of the most widely used illicit substance among users of stimulants such as cocaine and amphetamines. Interestingly, increasing recent evidence points toward the involvement of the endocannabinoid system (ECBS) in the neurobiological processes related to stimulant addiction. This article presents an up-to-date review with deep insights into the pivotal role of the ECBS in the neurobiology of stimulant addiction and the effects of its modulation on addictive behaviors. This article aims to: (1) review the role of cannabis use and ECBS modulation in the neurobiological substrates of psychostimulant addiction and (2) evaluate the potential of cannabinoid-based pharmacological strategies to treat stimulant addiction. A growing number of studies support a critical role of the ECBS and its modulation by synthetic or natural cannabinoids in various neurobiological and behavioral aspects of stimulants addiction. Thus, cannabinoids modulate brain reward systems closely involved in stimulants addiction, and provide further evidence that the cannabinoid system could be explored as a potential drug discovery target for treating addiction across different classes of stimulants.
Introduction
Addiction to psychostimulants such as cocaine, amphetamine, and its derivatives [i.e., methamphetamine, N-methyl-3,4-methylenedioxymethamphetamine (MDMA)] is a significant global public health problem which affects many aspects of social and economic life. Worldwide, between 16 and 51 million people are users of these types of substances (1). Amphetamines have been identified as the second world’s most widely used illicit drug after cannabis, with an annual prevalence ranging from 0.3 to 1.2% in the adult population. Methamphetamine consumption has increased dramatically the last years, especially in the western and mid-western parts of the United States, although there also appears to be an eastward trend in use (2). Over 15 million people worldwide are cocaine users and 5.9 million of them live in North America (3, 4). Although the prevalence of cocaine consumption has declined in the past decade, cocaine use increased in 2011; dependence to this drug remains a significant issue in North America, Western and Central Europe, particularly in metropolitan areas where crime and violence have increased (4). Given its association with high rates of mental and physical problems as well as premature mortality, cocaine abuse is still an unresolved medical and socio-economic concern which carries a heavy burden for abusers and their families alike (3, 5–7).
In recent decades, development of new treatments for psychostimulant addiction has been a major focus of multidisciplinary research efforts and have included molecular approaches, pre-clinical behavioral studies, and clinical trials. However, in spite of these research endeavors, no specific pharmacological therapy has been found to be truly effective in alleviating psychostimulant cessation symptoms like craving and anxiety, or to prevent relapse (8–11). Neuropharmacological agents such as antidepressants, anticonvulsants, and antipsychotics have been tested as treatments for cocaine dependence but these medications have yielded negative clinical outcomes (12–14). Subsequent attempts at targeting other neurotransmitters such as the dopaminergic and γ-aminobutyric acid (GABA) systems (10, 15, 16), and developing a cocaine vaccine (17) to promote abstinence in cocaine-dependent individuals, have shown promising results but still require further investigation. Importantly, many clinical studies have focused on cocaine addiction rather than other psychostimulants such as amphetamines and methylphenidate. Whether the outcomes related to cocaine addiction can be applied to other psychostimulants remains unclear (18).
Given the need to better understand neurobiological mechanisms that underly psychostimulants addiction and to develop innovative treatment strategies, researchers have explored the involvement of specific neurotransmitter systems and brain structures in the motivational and addictive properties of this class of drugs. Increasing evidence indicates that the endocannabinoid system (ECBS) - a group of neuromodulatory lipids and receptors – plays a central role in various cognitive and physiological processes associated with addiction such as reward, stress responsiveness, and drug-related synaptic plasticity (19–21). The potential of ECBS modulation in treating stimulant addiction has recently been highlighted in human and animal studies investigating its effects on acquisition, maintenance, and relapse of drug-taking behavior. Moreover, endogenous and exogenous cannabinoids such as plant-derived cannabinoid ligands (i.e., Δ9-THC, cannabidiol, CBD) modulate specific neurotransmitter systems which are also pharmacological targets for cocaine. Interestingly, cannabis is widely used by psychostimulant-dependent individuals (22); while it is recognized that components of the ECBS are implicated in psychostimulants, more specifically in cocaine-seeking behaviors, very few studies have focused on an understanding of the neural and behavioral effects of cannabis on psychostimulants use in humans.
The current review will focus on the neurobiological basis of the addictive process from psychostimulant initiation to drug abuse and addiction. The involvement of critical neurotransmitters and neural circuits underlying the pathological modifications at each of these stages will be highlighted with a specific attention given to the ECBS. In turn, this overview will serve as foundations to look at the ECBS as a specific target of pharmacotherapeutic interventions to reduce the addictive effects of psychostimulants.
Neurobiology of Psychostimulants
Occasional use of psychostimulants like cocaine, at low doses, provokes a so-called “rush” (i.e., euphoria) in humans, giving them a sensation of vigilance and increased energy. Higher doses of cocaine induces symptoms described as “cocaine high,” which include enhancement of a euphoric sensation, an increase in motor activity, amplification of sensory perception, talkativeness, and suppression of appetite and thirst (23). Unfortunately, these positive subjective effects (i.e., euphorigenic state) are often followed by repetitive and frequent cocaine abuse which develops into addiction. Drug addiction is a chronically relapsing disorder characterized by loss of control over drug-seeking and the compulsive desire (referred as craving) to use drugs in spite of negative consequences (24). Drug cravings increase with exposure to drug and drug-related-cues, and in the context of emotional stress or negative moods (25, 26). Because addiction is a highly complex disorder, numerous studies have attempted to determine the molecular and cellular factors implicated in the pleasurable effects induced by drug consumption, and their role in the development of addictive behaviors (27–31).
Neurotransmitters Involved in Processes Leading to Psychostimulants Addiction
Psychostimulants affect the central nervous system by modulating the mesocorticolimbic dopamine (DA) system which is involved in several physiological processes such as cognition, memory, and reward-driven learning (32). The mesocorticolimbic system, which has been found to play a role in drug reward and addiction, includes DA projections from cell bodies in the ventral tegmental area (VTA) to limbic structures such as the nucleus accumbens (NAc) (33), amygdala in the forebrain (34, 35), hippocampus (36), and to cortical areas such as the prefontal cortex (PFC), including the orbitofrontal cortex (OFC) and anterior cingulate (AC) (37). Psychostimulants exert their effects on the CNS via a number of mechanisms; cocaine, amphetamines, methamphetamines, and methylphenidate alter normal DA receptor functions by binding the dopamine transporter (DAT) and forming a complex that blocks the transporter’s function. The psychostimulant/DAT complex inhibits DA reuptake into the presynaptic nerve terminal, leading to an excess of DA in the synaptic cleft within the NAc – recognized as the center of the rewarding process (38–40). This phenomenon results in an increased and prolonged post-synaptic effect of dopaminergic signaling at DA receptors on the receiving neuron (30, 31). However, unlike cocaine, which interferes mainly with plasma membrane transporters, other psychostimulants modulate the CNS through a host of mechanisms. First, methamphetamines and amphetamines act as substrate-type releasers (41, 42) to enhance DA efflux. These substrate-type releasers have two modes of action: (i) they reverse the process of transporter-mediated exchange by interacting with specific transporter proteins which are subsequently brought into the cytoplasm of the nerve terminal; (ii) they also increase cytoplasmic levels of DA by interfering with vesicular storage (43, 44). Moreover, these drugs increase cytosolic DA levels by shutting-down the activity of the monoamine oxidase (MAO) – an important enzyme for the catabolism of monoaminergic neurotransmitters. Finally, psychostimulants also enhance the activity and expression of the tyrosine hydroxylase (TH), the DA-synthesizing enzyme [reviewed in Ref. (45)]. However, exactly how these high levels of DA in the NAc mediates drug reward remains partially understood.
Even though DA has been identified as one of the primary mechanisms involved in drug reinforcement initiation, studies reveal that mice lacking the gene expressing the DAT continue to self-administer cocaine (46, 47). Interestingly, several reports have suggested the indirect implication of other neurotransmitter systems [i.e., serotonin (5-HT), norepinephrine (NE), glutamate (GLU), GABA, opioid peptides, and endocannabinoids (48–50)] in the incentive sensitization and reinforcing effects of psychostimulants (29, 51). Indeed, psychostimulants also reduce 5-HT and norepinephrine NE reuptake, which in turn leads to an increase in extracellular monoamines concentrations and contributes to the rewarding subjective feelings mediated by these drugs (42, 52–54). Surprisingly, knock-down of NET, or SERT, or NET/SERT genes does not abolish but rather potentiates the rewarding or aversive effects of cocaine (55, 56). Recently, further lines of evidence have suggested that NE plays a role in the reinstatement of drug seeking, although it does not influence the maintenance phase of cocaine self-administration (SA) (57–59). Blockage of NE cognate receptors – α1-adrenergic receptors (α1ARs) and β-adrenergic receptors (βARs) – in a mice model of addiction diminishes cocaine-primed and foot shock-induced reinstatement respectively, whereas inhibition of both receptors reduces cue-induced reinstatement (60, 61).
Long-term use of psychostimulants leads to homeostatic dysregulation of normal (i.e., without cocaine) dopaminergic signaling. This hypo-dopaminergic state contributes to the appearance of some withdrawal symptoms (i.e., depressive mood disorders), often observed in abstinent psychostimulant addicts, and, also the maintenance of drug-use behaviors. Similarly, withdrawal symptoms from chronic cocaine use have been also associated with cocaine-induced alterations in 5-HT neurotransmission. Interestingly, rodent studies show that enhancement of serotonergic transmission in the NAc through administration of exogenous 5-HT served to offset the DA deficit caused by cocaine withdrawal (62); indeed, accumulating studies suggest that increasing brain 5-HT activity could reduce the behavioral-stimulant and reinforcing properties of psychostimulants (reviewed in Ref. (44)]. Thus, modulation of 5-HT and DA levels might sensitize an important brain reward circuit to the reinforcing effects of psychostimulants contributing to the intractable nature of addiction and relapse.
The glutamatergic system is another important neuronal substrate of behaviors induced by drugs of abuse (63, 64). Indeed, GLU is an excitatory neurotransmitter essential to numerous processes including neuroplasticity, linked to long-term potentiation (LTP), long-term depression (LTD), extinction, and reward-related learning (65–68). Like DA, GLU levels in the NAc core decrease during the early phase of cocaine abstinence (69, 70), whereas both stress and drug-induced reinstatement of cocaine-seeking are associated with an increase of extracellular GLU levels in the NAc in rodents (63, 64, 70–73). Thus, the data suggests that both a decrease in basal GLU transmission and an enhanced GLU response may constitute a neurobiological substrate of cocaine-, cocaine-associated cue-triggered relapse.
While discovery of numerous neurotransmitter systems have yielded significant advances in defining psychostimulants’ effects on the brain neurochemistry, the precise mechanisms underlying their role in addictive behaviors are not as straightforward. While DA and GLU appear to be critical in the development and persistence of stimulant addictive behaviors, a growing body of evidence points toward the impact of other neurotransmission systems, including the ECBS, in various physiological and behavioral processes associated with psychostimulant addiction, through both DA/GLU related and unrelated mechanisms. An overview of this evidence will be presented in Section “The Endocannabinoid System,” with a particular focus on the potential exogenous and endogenous cannabinoids influence on psychostimulant reinforcement, drug-related synaptic plasticity, and drug-seeking behavior.
Neural Regions Involved in Addiction Process to Psychostimulants
A central challenge in addiction research is understanding the neurobiological substrates involved in drug-taking behavior. Over the last two decades, neuroimaging has provided substantial insight into that question by: (i) allowing researchers to investigate the roles of different neural regions in drug-induced euphoria and subsequent craving; (ii) enabling the gathering of tremendous information regarding the neurochemical and physiological adaptations of the brain during the addiction process.
Brain imaging studies of subjects addicted to psychostimulants indicate that the NAc – known to play a fundamental role in goal-directed behaviors (74) – is organized into two functionally distinct sub-compartments termed the shell and core (33). The shell and the VTA are critical in inducing motivational salience and responding to novel rewarding stimuli (75). The core mediates the expression of learned behaviors, and receives glutamatergic afferents from the PFC (33, 75). DA release into the core occurs in response to cues predicting a motivating event (76, 77). The NAc receives information regarding motivationally relevant events from the VTA, amygdala, hippocampus, and PFC, and responds by providing output to brain circuits which modulate the expression of the behavioral response (e.g., to seek the drug or not) (78). Chronic exposure to psychostimulants leads to the dysregulation of the mesolimbic circuitry, which in turn enhances the motivation to take drugs and decreases the ability to regulate the behavioral response to drug cues (33, 74).
The numerous neuroimaging methods used to study the chronic effects of psychostimulants on the brains of drug-addicted individuals have consistently found abnormalities in both cortical and subcortical neural areas (37, 79). More specifically, chronic exposure to psychostimulants causes functional alterations within frontal brain areas, including the dorsolateral prefrontal cortex (DLPFC), the OFC, and the anterior cingulate cortex (ACC) involved in goal identification; selection (80); decision making; impulsivity; behavioral inhibition (81), and assessment of consequences (82), respectively. It has been proposed that abnormalities within these three PFC-striatothalamic circuits play a central role in emotional response to drug cues, craving, compulsive drug-seeking, and relapse (26, 35, 83–85). Moreover, structural magnetic resonance imaging (structural MRI) studies associate chronic use of psychostimulants with alterations in white-matter integrity and gray-matter volume, which are strongly correlated with lower abstinence-based outcomes (86) and drug-induced compulsivity, decision making, and attention impairments in cocaine-dependent subjects, respectively (85). Furthermore, exposure to emotional distress and aversive stimuli also activates the cortico-limbic circuits, including prefrontal, AC, middle frontal, and orbitofrontal regions, limbic and paralimbic structures such as the amygdala, hippocampus, parahippocampal gyrus, fusiform gyrus, and other midbrain areas, but not the ventral striatum (87, 88). Overall, the data shows that chronic use of psychostimulants modulates a set of neural regions implicated in stress, emotions, impulsivity, and reward processing control which precipitate relapse in drug-abstinent individuals.
The Endocannabinoid System
Though the significant role played by various neurotransmitters, genetic factors and specific brain structures in reinforcing the properties of psychostimulants has been established, the common mechanisms underlying the development of addictive behaviors have yet to be fully elucidated. A growing body of evidence points to the involvement of the ECBS in the acquisition and maintenance of drug-taking behaviors and in various physiological, as well as behavioral processes associated with addiction. Interestingly, a characteristic of psychostimulants abuse is the concurrent consumption of other substances including delta-9-tetrahydrocannabinol (Δ9-THC) – the main cannabinoid found in cannabis [reviewed in Ref. (89)]. This poly-substance pattern of use has prompted researchers to investigate the potential interaction with, and effect of, these drug on neuropsycho-biological processes related to addiction. For example, some studies reveal that cannabis consumption enhances the incentive to use cocaine in individuals dependent on both, while others suggested that cannabis reduced withdrawal symptoms in abstinent cocaine-addicted subjects (22, 90, 91). Although it is recognized that components of the ECBS are involved in cocaine-seeking behaviors, very few studies have focused on understanding the neural and behavioral effects of endogenous and exogenous cannabinoids on psychostimulant use. In this section, we will first provide an overview of the ECBS, and then focus on recent findings pointing toward a role of the ECBS in the circuitry underlying psychostimulant addiction.
Overview
The ECBS consists of a family of lipid signaling molecules referred to as endocannabinoids, their cognate receptors and specific metabolic enzymes which are responsible for degradation of the endocannabinoids – anandamide (AEA) and 2-arachidonoylglycerol (2-AG). The neurobiological properties of endocannabinoids are complex, but it is now well established that they modulate a wide diversity of physiological processes including pain and inflammation, immune responses, food intake, synaptic transmission, cognition, reward, and motor activity (92). Endocannabinoids also influence mechanisms involved in addiction and relapse.
Receptors
There are currently two well described subtypes of cannabinoid receptors, termed CB1 and CB2, which differ in their signaling mechanisms and tissue distribution. Even though CB1 receptors are considered the most abundant and widely distributed G-protein-coupled receptors found in the CNS, they are also present in peripheral organs and tissues (i.e., endocrine glands, leukocytes, spleen, heart, and gastrointestinal tracts, etc.) (93–97). CB1 receptors are localized in TH-expressing neurons, probably dopaminergic neurons of the NAc, VTA, striatum, and pyriform cortex, suggesting that the ECBS may directly influence dopaminergic reward mechanisms. In addition, CB1 receptors are expressed in other neural regions related to reward, motivation and memory processing (i.e., basolateral amygdala, hippocampus, and cerebral cortex), movement (i.e., basal ganglia, cerebellum), pain modulation (i.e., certain parts of the spinal cord, periaqueductal gray). Endocannabinoids induce LTD of the inhibitory synapses in the hippocampus, contributing to the synaptic plasticity involved in the learning processes related to addictive behaviors. CB1 receptors are confined at the terminals of central and peripheral nerves, where they inhibit the release of excitatory and inhibitory neurotransmitters (release on command, retrograde signaling) (98–100). Thus, the activation of CB1 receptors protects the nervous system from over-activation or over-inhibition by neurotransmitters and thereby promotes the latter’s prominent role in anxiety, depression, cognition, addiction, motor function, feeding behavior, and pain (101). CB2 receptors are mainly found in immune cells (i.e., spleen, tonsils, and thymus gland) (102–104), although recent experimental data indicate CB2 receptors expression in the cerebellum, brainstem, and cortex (105–107) as well as activated microglial within the CNS (108–110). Simulation of CB2 receptors on microglia modulates the neuro-inflammatory response by regulating cytokines release in the brain (111–113).
Increasing evidence points toward the existence of additional cannabinoid receptors subtypes in the CNS. Indeed, recent pre-clinical studies suggest the persistence of cannabinoid-like properties after cannabinoid agonists have been administered to mice lacking CB1 and CB2 receptors (CB1−/− and CB2−/−) in neuronal subpopulations. This indicates that these agonists recognize non-CB1/CB2 cannabinoid receptors (114–117). Among these receptors, the orphan G-protein-coupled receptors modulate the ECBS. GPR55 specifically is found in the striatum and to a lesser extent in the hippocampus, the thalamus and the cerebellum (118). GPR55 is phylogenetically different from CB1 and CB2 receptors, in that it is activated by the CB1 antagonists – rimonabant and AM251 – but blocked by the cannabinoid agonist CP55, 940 (119–121). Thus, GPR55 is considered as a non-CB receptor with a binding site for cannabinoid ligands [reviewed in Ref. (122)]. Though a recent study from Rusakov’s group suggests that GPR55 enhances neurotransmitters release at central synapses (123), further studies are required to confirm its neurophysiological function.
The actions of endocannabinoids are not only restricted to the CB1, CB2, and GPR receptors. Transient receptor potential (TRP) receptors have also been identified as sites of endocannabinoid interaction. Exogenous and endogenous cannabinoids interact with at least five TRP receptors (124); AEA binds to the transient receptor vanilloid potential 1 (TRVP1) with low affinity. TRVP1 is found on sensory neurons, where they are partly coexpressed with CB1 receptor, but also in several central nuclei including the hypothalamus and basal ganglia, the hippocampus and cerebellum (125). The efficacy and potency of AEA at TRVP1 is increased when the AEA degrading enzyme FAAH (fatty acid amide hydrolase) is suppressed (126–128). Surprisingly, pharmacological or genetic inhibition of FAAH enhances AEA, but decreases 2-AG levels via TRVP1 receptors (129). Interestingly, both endocannabinoids AEA and 2-AG decrease the excitatory GLU and the inhibitory GABAergic inputs to striatal neurons (130, 131). Therefore, it is likely that the potential of AEA to reduce 2-AG levels by activating TRVP1 receptors might represent a mechanism to integrate excitatory and inhibitory inputs in the basal ganglia.
Endocannabinoids and Their Metabolizing Enzymes
In the CNS, endocannabinoids mediate forms of short-term synaptic plasticity known as depolarization-induced suppression of inhibition (DSI) (132, 133) and depolarization-induced suppression of excitation (DSE) (134). Thus, endocannabinoids are considered as retrograde messengers that neuromodulate diverse physiological processes. AEA and 2-AG are the two most characterized endocannabinoids (135, 136), although other studies have identified of additional endocannabinoids such as 2-arachidonylglyceryl ether (noladin ether) (137), N-arachidonoyl-dopamine (NADA) (128), and O-arachidonoyl-ethanolamine (virodhamine) (138). However, the physiological functions of these endocannabinoids are still being investigated. While 2-AG acts as a full agonist at CB1 and CB2 receptors, AEA behaves as a partial agonist at both receptors subtypes and can also interact with GPR55 and TRVP1 receptors.
Unlike other neurotransmitters, AEA and 2-AG are not synthesized and stored in the nerve cells. Rather, they are produced on an “as needed” basis by their membrane lipid precursors in a Ca2+ dependent fashion (133, 139). Although additional studies are needed to ascertain the exact role of the N-acylphosphatidylethanolamine phospho-lipase D in the ECBS, it has been proposed that this enzyme might play a significant role in the synthesis of AEA (140). The enzyme responsible for 2-AG synthesis is the diacylglycerol lipase alpha (141). Upon depolarization of post-synaptic neurons, the endocannabinoids released into the synaptic cleft bind to and activate the presynaptic CB1 receptors, which in turn suppress the release of both excitatory and inhibitory different neurotransmitters [see for review (142)]. Then, AEA and 2-AG are rapidly deactivated by cellular reuptake into both neurons and glial cells and metabolized by specific enzymes (143). AEA can be metabolized by either the FAAH (144), or monoacylglycerol lipase (MAGL), which degrades specifically 2-AG (145). In addition to MAGL, recent studies have suggested that the enzymes ABHD6 and ABHD12 could also be involved in 2-AG metabolism (146, 147). FAAH is over-expressed in the CNS and FAAH-positive neurons are localized in proximity to CB1 receptor-containing terminals, underlining the role for this enzyme in endocannabinoids inhibition (148). Thus, selective inhibition of FAAH (149) and MAGL (150) can prolong the effects of endocannabinoids. Pre-clinical studies have demonstrated that pharmacological inhibition of FAHH with URB597 (149) or PF-3845 compounds (151) induced-anxiolytic-like effects (152, 153) and anti-nociceptive properties in mice (152, 154). Inhibition of MAGL with JZL184 inhibitor causes analgesia, hypothermia, and hypomotility (155). However, chronic exposure to JZL184 impairs endocannabinoid-mediated synaptic plasticity in mouse hippocampus and cerebellum via 2-AG upregulation. It also induces tolerance to the analgesic effects, physical dependence, and persistent activation as well as desensitization of brain CB1 receptors (156). Surprisingly, MAGL knockout mice show enhanced learning behavior and have normal locomotor activity, suggesting the possible role of MAGL in cognitive function (157, 158).
Exogenous Cannabinoids: Δ9-THC vs. CBD
Cannabis is the world’s most commonly used illicit drug (159, 160). Between 119 and 224 million people are cannabis users worldwide (4). Cannabis contains over 85 different chemical substances unique to the plant and termed phytocannabinoids. Among them, Δ9-tetrahydrocannabinol (Δ9-THC) and CBD are the two main components of cannabis, which has been used for thousands of years for both recreational and medicinal purposes. Most studies regarding cannabis properties have focused on Δ9-THC, which is the main psychoactive constituent in cannabis extracts (161). Although Δ9-THC possesses a number of therapeutic effects (e.g., on pain, spasms, inflammation), its negative impact on the CNS has been highlighted in several clinical studies on subjects smoking cannabis, documenting impulsive behavior, cognitive impairment, consumption of addictive substances, and psychiatric disorders (e.g., schizophrenia, depression, and anxiety) (162– 165). For example, Δ9-THC has been shown to induce psychotic-like and anxiogenic effects when administered intravenously to healthy subjects (166, 167). Other experimental studies revealed that Δ9-THC injection in animal models causes hypolocomotion, catalepsy, antinociception, and hypothermia (168).
Pharmacological studies in animal models suggest that not all therapeutic effects related to cannabis administration can be ascribed to Δ9-THC [reviewed in Ref. (169)]. Indeed, CBD – the second most abundant cannabinoid found in cannabis – acts as an antidepressant and possesses anticonvulsant, antiemetic, anxiolytic, and sleep-promoting as well as neuroprotective properties in humans (160, 170–176). CBD mediates its neuropharmacological properties by acting as an inverse agonist on CB1 and CB2 receptors (177, 178); it also stimulates the TRVP1 and TRVP2 (179) which serve as so-called ionotropic cannabinoid receptors. In addition, CBD inhibits FAAH, the main catabolic enzyme that alters the hydrolysis of the endogenous cannabinoid neurotransmitter AEA (180) (see above section), and is also an antagonist at the putative GPR55 receptor. The clinical association of the modulation of the ECBS by CBD remains to be fully investigated; this effect could arguably be related to DA uptake inhibition (181). Interestingly, ECBS interacts closely with other neurobiological structures which are implicated in the neural adaptations observed during chronic use of drugs and vulnerability to addiction. For example, CBD plays a role in the modulation of extracellular levels of DA (182) as well as μ and δ opioid receptors (183); it increases adenosine signaling through inhibition of uptake (184). Moreover, μ opioid and CB1 receptors colocalized within neural regions are known to modulate reward, goal-directed behavior, and habit formation relevant to addiction including striatal output projection neurons of the NAc and dorsal striatum (185, 186). While further studies are required to better understand the impact of CBD on GLU neurotransmission, its protective effects on GLU toxicity (187) and its psychopharmacologic interaction with ketamine (188), a N-methyl-d-aspartic (NMDA) receptor antagonist, are well documented. CBD activates also the serotoninergic receptors 5-HT1A (5-hydroxytryptamine) (171, 176, 189–193), which in turn diminishes vulnerability to stress and has anxiolytic-like effects in animal models (170, 172, 189, 190, 192–195). Similar results were observed in humans, where CBD administration decreases autonomic arousal and subjective anxiety (196). Interestingly, these anxiolytic effects have been linked to the modulation of core regions involved in the “emotional brain,” including limbic system structures such as the AMG and the ACC (197, 198). CBD’s anxiolytic effects were further confirmed by a study indicating that the effective connectivity between ACC and AMG is attenuated during the emotional processing of fearful faces, while resting activity of the left parahippocampus gyrus is increased. (196, 199). Remarkably, these neural structures are activated during drug craving in cocaine addiction (197, 200). It also decreases compulsive behaviors in rodents, which is hypothesized to be related to CB1-related mechanisms (201, 202).
While CBD has neuroprotective properties (187, 203, 204) and Δ9-THC administration have been shown to cause neurotoxic effects (205), these opposing properties have been highlighted in brain imaging studies where Δ9-THC and CBD activate different brain regions during tasks engaging verbal memory (206, 207), response inhibition (208), and emotional processing (196, 209–211). When given at appropriate doses, CBD counteracts Δ9-THC properties. Thus, CBD can modulate the functional effects of Δ9-THC (177, 178). Pre-clinical studies demonstrate that CBD decreases Δ9-THC-induced conditioned place aversion and social interaction of on operant behavior model (212, 213). In addition, CBD diminishes Δ9-THC-induced anxiety and psychotic-like symptoms in humans (214, 215). Together, this data clearly suggests that CBD limits Δ9-THC adverse effects. Thus, administered together, CBD might increase Δ9-THC clinical efficacy (216, 217). It has been established that unlike, Δ9-THC, CBD possesses therapeutic properties that could reduce withdrawal symptoms often present in individuals with addictive disorders (e.g., anxiety, psychotic, mood symptoms, insomnia, and pain). For example, a recent pre-clinical study from Hurd’s group aimed at assessing the effects of cannabinoids on opioid-seeking behaviors in rats indicates that while Δ9-THC potentiates heroin SA, CBD inhibits cue-induced heroin-seeking behaviors for up to 2 weeks following the last administration (218). In addition, CBD is well tolerated and has no gross effects on motor function (such as locomotor activity). CBD is also protects against damages caused by various substances; it reverses binge ethanol-induced neurotoxicity (219) and mitigates the cardiac effects of Δ9-THC (220, 221). Together this data illustrates the different, and sometimes opposite, neurobiological properties of the two main constituents of cannabis – CBD and Δ9-THC – that are linked to neural circuits which might play significant roles in addiction disorders. However, while numerous studies have highlighted the participation of the ECBS in the rewarding and addictive properties of drugs of abuse such as opioids, nicotine, and alcohol over the last decades, relatively few studies have focus on the impact of this system on addiction to psychostimulants.
Interaction of the eCBS with Biological and Behavioral Correlates of Psychostimulants Addiction
Human Studies
Human studies aimed at understanding the interaction of the ECBS with biological and behavioral correlates of addiction to psychostimulants have mostly focused on ECBS-related risk factors leading to drug dependence. Interestingly, cannabis use is strongly associated with the abuse and/or dependence of several class of drugs including psychostimulants such as cocaine (222). Moreover, exogenous cannabinoids have been shown to modulate the acute rewarding effects of cocaine. These lines of evidence may suggest an association between ECBS and liability to psychostimulant by pointing toward a possible involvement of the ECBS in the motivational effects mediated by psychostimulants (223) [reviewed in Ref. (224)]. Based on these observations, scientific efforts have been devoted to investigate the influence of various genetic (e.g., ECBS-related genes) and environmental characteristics (e.g., previous or current exposure to cannabinoid agonists) in individual progression from occasional use to psychostimulant addiction.
“The gateway theory” and addiction to psychostimulants
Association of prior or concomitant cannabis consumption with other illicit drugs including psychostimulants such as methamphetamine and cocaine, forms the basis of a well-known hypothesis – “ the gateway theory,” which suggests a causal role for cannabis in the development of subsequent drug use and addiction (225). While data indicate that smoking cannabis is positively associated with cocaine consumption, it would be inappropriate to assume that cannabis per se leads to cocaine use. A study from Lynskey et al. in human twins reveals that early cannabis use in life increases the odds of subsequent cocaine use, supporting the causative model of the “gateway theory.” However, results of this study have been refuted by Kandel et al. (226) which argues that several additional genetic, social, and environmental factors, such as life experiences, might link cannabis use with subsequent cocaine consumption (227, 228). Actual neurobiological causal mechanisms underlying this “gateway theory” remain mostly unidentified. Interestingly, Tomasiewicz and colleagues show that Δ9-THC exposure induces epigenetic dysregulation of the endogenous opioid proenkephalin in adolescents; these findings indicate that cannabis exposure, in and of itself, can be considered as a risk factor that acts “above the genome” and can “write” on the existing epigenetic background of adolescent neurodevelopment. Thus, in adolescents, Δ9-THC exposure-mediated epigenetic effects may act in concert with other environmental or social factors to augment future behavioral responses to drugs of abuse via stable and long-term regulation of genes at the transcriptional level. However, while these data establish a direct link between Δ9-THC-induced changes in proenkephalin expression and susceptibility to opiate drugs, no studies have confirmed that this mechanism can be applied to psychostimulants (229).
Genetic determinants of the ECBS and psychostimulant addiction
It is worth mentioning that not every subject who experiences the pleasurable effects of psychostimulants will become a chronic user. Indeed it is more likely that additional factors such as: (1) genetic variabilities (e.g., polymorphisms in the catechol O-methyltransferase gene (Val158met) and in the serotonin transporter gene (5-HTTLPR) (230, 231); (2) monoamine receptors deficiency – either genetically or as a result of their drug excesses – also contribute to the psychostimulants addiction process (232–235) (see Table 1). In the ECBS, different genetic variants of the CB1 receptors – CNR1 – and FAAH genes have been associated with increased susceptibility to drug addiction. Indeed, genetic analyses demonstrate that the CNR1 gene exhibits elevated numbers of (AAT)n triplet repetition in a sample of 192 non-Hispanic Caucasian subjects. Interestingly, this CNR1 polymorphism increases the risk of intravenous drug use in this population, with strongest correlation observed in cocaine, amphetamine, and marijuana dependence (236). Similarly, a study from Ballon and colleagues shows that detection of this CNR1 polymorphism in a sample of 142 African-Caribbean individuals predisposed them to cocaine addiction (237). Unfortunately, while single sequence repetitions can alter transcriptional rates and thereby induced gene overexpression or silencing (238), the functional nature of the microsatellite polymorphism triplet repetition (AAT)n in modulating CNR1 gene expression remains blurred (239). It has been hypothesized that the presence of long alleles with high numbers of AAT triplets alter CNR1 transcriptional gene expression, ultimately leading to low levels of CNR1 protein synthesis (240). A recent meta-analysis of 11 studies aimed at investigating the contribution of three CNR1 polymorphisms (rs1049353, rs806379, and the AAT triplet repetitions) to drug dependence vulnerability confirmed the presence of (AAT)n repeats, but only in the Caucasian population [reviewed in Ref. (239)]. Unfortunately, the effect of the three CNR1 polymorphisms appeared to be insignificant and showed high heterogeneity. Important caveats have to be considered when looking at these studies. First, the ethnicity of the different subjects may prove important, as some studies included several ethnic groups in their samples, and in some cases, these groups were not even mentioned (241, 242). Some reports also examined CNR1 gene polymorphisms in connection with a different phenotype or stage of drug addiction such as craving, drug consumption, dependence, or drug withdrawal (243). Furthermore, a detailed description of the repercussions of CNR1 polymorphisms on CB1 function from a neurobiological standpoint is lacking from the reviewed studies.
Polymorphisms in the gene coding for the endocannabinoid-inactivating enzyme FAAH may constitute another risk factor for problematic drug use, as described by initial reports identifying C385A, a mis-sense single nucleotide polymorphism (SNP) causing reduced FAAH enzymatic activity (245, 246). Indeed, a study from Sipe et al. reveals significant association between C385A SNP and street drug abuse in a sample of 1737 Caucasian subjects with addictive disorders. Neuroimaging studies combined with genetic analysis reveal that low FAAH activity enhances AEA protein expression levels which, in turn, modulate brain regions implicated in drug addiction and reward circuitry such as the OFC, AC gyrus, and NAc (242). Additional neuroimaging studies show that C385A carriers exhibit increased ventral striatal reactivity – a correlate for heightened impulsivity and reward sensitivity. C385A carriers display low threat-related amygdala reactivity – a pattern observed in individuals with high familial risk of alcoholism. Moreover, C385A polymorphism-reduced FAAH functional activity increases risk-taking behavior associated with addiction through abnormal impulsivity and threat perception [reviewed in Ref. (224, 243)]. Contribution of SNPs that modulate FAAH functions to stimulant addiction remain to be explored as the aforementioned data were not obtained in individuals specifically addicted to stimulants.
Effect of exogenous cannabinoids on psychostimulant reward
As mentioned previously (see The Endocannabinoid System), an intriguing characteristic of psychostimulants abuse is the concurrent consumption of cannabis. Parallel to studies on the long-term effects of cannabis exposure on subsequent psychostimulant use, researchers also examined the acute rewarding effects of cannabis use on concurrent psychostimulant addiction (91, 222). However, studies aimed at investigating such interactions are sparse. Conflicting results from Foltin et al. and Lukas et al. provide evidence that cannabinoids modulate cocaine-mediated euphoric actions. First, data from Foltin and colleagues show that human volunteers who smoked cannabis prior to intravenous cocaine experience a prolongation of the “high” sensation (248). Second, a study from Lukas and colleagues reveals that smoking Δ9-THC, 30 min prior to intranasal cocaine decreases the latency to onset of cocaine-induced euphoria significantly, from 1.87 to 0.53 min, as well as the duration of cocaine-induced dysphoria, from 2.1 to 0.5 min (249). Interestingly, when both drugs are administered concomitantly, no changes are observed in cocaine- and Δ9-THC-induced positive subjective properties. Furthermore, Δ9-THC increases the peak plasma levels and bioavailability of cocaine considerably. This increase might be the result of Δ9-THC-induced vasodilation of the nasal mucosa which, in turn, reduces cocaine-induced vasoconstriction, thereby increasing cocaine’s absorption. In addition, the discrepancies between these two studies might be also due to pharmacodynamic mechanisms including differences in cocaine absorption or in the ratio of CBD/Δ9-THC levels found in the type of cannabis used for each study.
Using fMRI technology combined with script-guided imagery paradigm in which subjects imagined being in a real-life stressful situation, Rajita Sinha’s group found that cannabis abuse contributed to stress-induced blood-oxygen-level-dependent (BOLD) contrast in a group of cocaine-dependent individuals. More specifically, cannabis consumption decreases emotional stress cue-induced frontal and cingulate activation in cocaine-dependent individuals (247). These findings suggest an abnormal cognitive control mechanism during affective processing in association with heavy cannabis use. An important caveat to consider in the latter study is that cocaine-dependent individuals were abstinent for several weeks prior to the neuroimagery session and were not current users of cannabis. Thus the study did not allow to examine the acute effect of cannabis on neural and behavioral responses. However, the fact that this cannabis-induced alteration in stress-response can be translated to cocaine craving and relapse vulnerability has definitely piqued further interest, and initial data on this matter already exists. Indeed, the effects of cannabis consumption on abstinence and relapse to cocaine use have been provided in a study from Labigalini and colleagues, in which 25 cocaine-crack dependent individuals reported to smoke cannabis in order to get relief from abstinence mediated-cocaine-withdrawal symptoms. From this sample, 68% of addicts achieved crack-cocaine cessation while using cannabis during the 9 months duration of the study (90). However, the self-reported nature of this study and its limited duration suggest cautiousness in interpreting its outcome. In a more recent study, Aharonovich et al. drew opposite conclusions on the consequences of smoking cannabis on cocaine relapse. In this study, researchers investigated whether cannabis use after the discharge of 144 drug-addicts from inpatient treatment program could help them to maintain abstinence and thereby preventing relapse to cocaine use. Results from this study suggest that smoking cannabis reduced the achievement of sustained remission and increased relapse to cocaine use (91) (see Table 1). Surprisingly, a study from Jutras-Aswad et al. supports the assumption that irregular cannabis use increases risky behaviors (syringe sharing) of cocaine and opioid users, as opposed to regular cannabis use, suggesting a complex dose-effect relationship between cannabis and addictive behaviors (22). The possibility that cannabis use by recently abstinent cocaine-dependent individuals influences relapse to drug and other related behaviors remains poorly documented.
Animal Studies
Over the last decades, development of animal models have allowed a better understanding of psychostimulant effects and addiction-related behaviors. These studies would not be available through clinical studies for ethical and practical reasons. Notably, invasive measures such as catheter installation for drug administration and surgical brain procedures for assessment of drug-induced neurobiological changes, as well as strictly controlled conditioning protocols involving restrictive environments, have extended the knowledge of psychostimulants effect on neurotransmission. These methods have also allowed observations of specific behavioral aspects of psychostimulant addiction. Thus, studies on animal models of psychostimulants abuse have provided tremendous insights on the role of ECBS in various aspects of psychostimulant addiction, spanning from drug reward, acquisition, and relapse.
Influence of ECBS on psychostimulants-induced behavioral and reinforcing effects
As mentioned above (see Neurobiology of Psychostimulants), substantial evidence indicates that behavioral and addictive properties of psychostimulants come from the interactions of psychostimulants with brain monoamines. Specifically, increase of extracellular levels of DA through promotion of DA release by amphetamine and MDMA, as well as inhibition of DA reuptake by cocaine, represent the primary mechanisms involved in rewarding effects mediated by psychostimulants (224). In animal models of intracranial self-stimulation (ICSS), the rewarding properties of drugs of abuse typically translate into lowering of the so-called reward threshold established after operant training [see Ref. (250) for description]. Initial experiments showed no effect of CB1 antagonist SR141716A on cocaine’s ability to lower ICSS threshold in rats (251), although careful data analysis suggests a tendency toward attenuation. However, different results were obtained when using a more potent antagonist – AM251. This antagonist proves CB1 blockade’s effectiveness in inhibiting cocaine’s action on brain stimulation reward (250). Paradoxically, the non-selective cannabinoid agonist – WIN55, 212-2 – and the endocannabinoid transmission enhancer – AM404 – are also able to abolish cocaine’s reinforcing effects as assessed by ICSS (252). Whether these apparently contradictory findings may indicate an inverse U-shape effect of CB1 stimulation function on rewarding properties of stimulants is not entirely clear. However, these results clearly indicate that cannabinoids might interfere with brain systems responsible for psychostimulants rewarding effects, and the mechanism underlying this phenomenon should be further explored.
Li and colleagues recently found significant reductions in DA levels in striatum of mice lacking the CNR1 gene, when compared to their wildtype counterparts following acute cocaine administration and during the basal state (253). This observation shows consistency with above-cited ICSS studies and with a previous report on the inhibition of cocaine-induced DA release in rats by CB1 antagonist SR141716A (254). In contrast, initial findings suggested that neither basal levels nor cocaine-induced increases in extracellular NAc DA of CB1 knockout mice differed from that of normal mice (255), and that CB1 inactivation by antagonists AM251 and SR141716A failed to alter the increase in extracellular NAc DA responsible for cocaine-mediated rewarding effects in rats (256, 257). Differences in experimental methods used to measure DA levels (voltammetry vs. in vivo microdialysis) and in the genetic background of the knockout animals (C57BL/6J vs. CD1) could account for such discrepancies. Notably, compensatory neurobiological changes due to lack of CB1 receptors could explain the subnormal basal DA levels observed in Li et al. study. This subnormal basal DA levels could also have contributed to apparent attenuation of DA levels enhancement produced by cocaine. Overall, the extent to which ECBS interaction with psychostimulants-mediated reward effects depends on DA transmission seems limited, especially when CB1 is targeted. It remains a controversial issue, with subsequent reports of attenuation of cocaine-enhanced extracellular NAc DA activity by CB2 agonists JWH133 and GW405833 in mice (258), but not by the pharmacological FAAH inhibitor URB597 in rats (259) adding to the complexity of the matter (see Table 2).
The increase of DA neurotransmission in the NAc and other striatal regions responsible for psychostimulant-induced rewarding parallels the stimulation of locomotor activity following acute drug administration. Sensitization to hyperlocomotor responses produced by psychostimulants occurs after chronic treatment, reflecting adaptive changes in DA transmission and potentially correlating with drug-seeking and reinstatement behavior (254, 262). In various studies, neither genetic deletion (262–264) nor pharmacological inhibition of CB1 receptors by SR141716A (265, 266) altered cocaine’s ability to induce acute motor effects or behavioral sensitization in rats and mice. However, a comparable number of reports described contradictory results, showing attenuation of both of these outcomes in CB1 knockout mice (253, 267) (see Table 3) as well as impairment of sensitization in animals pretreated with SR141716A (254, 268) or AM251 (267). Interestingly, although chronic cocaine use still induced sensitization in mice with invalidated CB1 receptors, sensitized response appeared somewhat changed when compared to control animals. Corbille et al. also found that AM251, unlike SR141716A (269), only impaired sensitization to cocaine after a single exposure, but not upon repeated administration. Similar experiments with cannabinoid agonists showed mixed results, as non-selective WIN 55,212-2 reduced cocaine’s motor effects, probably in a non-CB1 mediated fashion (252, 270). Likewise, CB2 agonists JWH133 and GW405833 decreased both acute hyperlocomotion and sensitization in rats (258), which parallels findings observed in mice genetically overexpressing CB2 (271). Δ9-THC failed to alter cocaine’s motor effects in rats (268, 272). Similarly, cannabinoid-amphetamine interactions studies demonstrate that acute cannabinoid exposure antagonizes amphetamine’s locomotion effects in a dose-dependent manner in rats. On the other hand, chronic exposure to Δ9-THC induces sensitization to the psychomotor effects mediated by amphetamine in rats (273). Taken together, these experiments suggest that the acute motor stimulant effects of psychostimulant and the induction of cocaine sensitization may not depend on endocannabinoid tone, even though CB1 receptors could play a minor modulating role in this regard [reviewed in Ref. (274)].
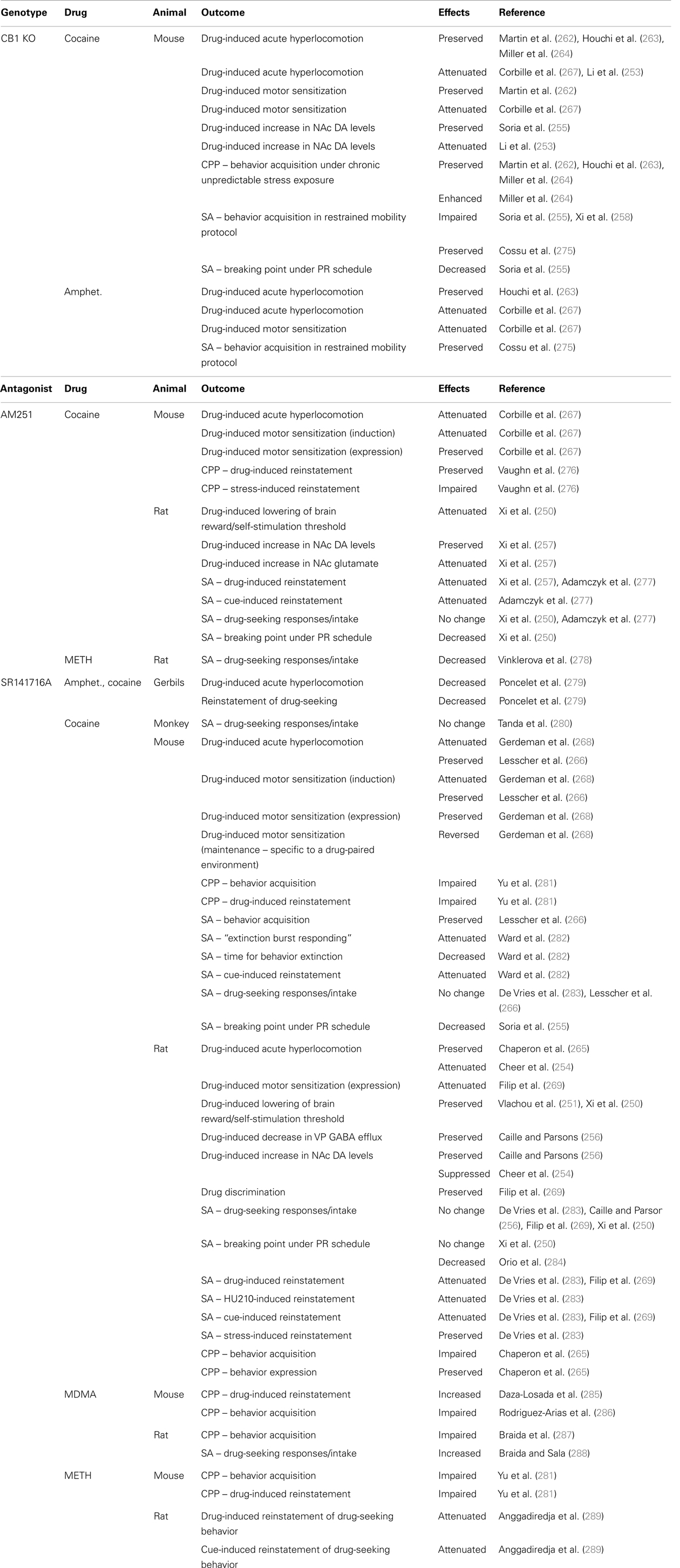
Table 3. Effects of CB1 cannabinoid receptor deletion and properties of psychostimulants; CB1 receptor antagonists and properties of psychostimulants.
Overall, while some evidence of ECBS involvement in the neurobiological and behavioral correlates of psychostimulant reinforcing properties exists, influence of ECBS on acute psychostimulant reward is modest and probably involves a combination of mechanisms which may not directly involve DA activity in the NAc or CB1 receptors.
Influence of ECBS on acquisition and maintenance of psychostimulant-induced seeking behaviors
Models of conditioning such as the SA paradigm and the conditioned place-preference (CPP) procedure illustrate the reinforcing properties of drugs of abuse and demonstrate their ability to induce and maintain drug-seeking behaviors. Consistent with findings showing the limited involvement of the ECBS in the reinforcing properties of psychostimulants (see Influence of ECBS on Psychostimulants-Induced Behavioral and Reinforcing Effects), modulation of the ECBS appears to have a modest influence on acquisition and maintenance of psychostimulant-taking behavior in animals. In CPP experiments, while CB1 receptor deletion did not affect psychostimulant-induced place conditioning in mice, SR141716A impaired cocaine-, methamphetamine-, and MDMA-induced place conditioning in both rats and mice (262–265, 281, 286, 287). Difference in species, compensatory changes in the knockout animals due to the lack of CB1 receptors, as well as use of more intense conditioning and higher doses of drugs in the genetic deletion studies may have contributed to this discrepancy. This suggests that intensity of conditioning could overcome the effects of blocking ECBS signaling [reviewed in Ref. (274)]. It is important to note that the weaker cannabinoid antagonist CBD did not affect establishment of amphetamine-induced CPP in rats (290) and that the genetic overexpression of cannabinoid receptor CB2 impaired acquisition of both cocaine-induced CPP and SA (271). Thus the CPP model indicates that, although not directly interfering with the rewarding properties of psychostimulant drugs, ECBS could play a role in the perception and memory of psychostimulant reward, depending on environment-related factors.
In general, CB1 receptor invalidation does not seem to affect SA of psychostimulants. Experiments with genetic deletion of CB1 show conflicting results, as both knockout and SR141716A-treated mice still acquired amphetamine- and cocaine-taking behavior in restrained mobility conditions (266, 275), whereas knockout mice showed impaired SA behavior in other protocols (255, 258). Overall, results suggest that learning SA behavior might not require extensive ECBS involvement. Maintenance of such behavior may not depend on CB1 either, as drug-taking responses under a fixed-ratio schedule in animals that had already acquired cocaine SA remained unaffected after CB1 blockade by SR141716A in mice (266, 283), monkeys (280), and rats (250, 256, 269, 283) and by AM251 in rats (250, 277). Only one contradicting report exists in which AM251 decreased methamphetamine SA in conditioned rats (278). Cannabinoid signaling enhancement by the pharmacological FAAH inhibitors – URB597 and PMSF – also failed to affect maintenance of fixed-ratio drug-taking behavior in rats (261) and monkeys (260). On the other hand, cannabinoid stimulation by CB1 agonists had significant effects in several studies. Indeed, WIN 55,212-2 increased acquisition of MDMA SA in mice (286) and exposure to CP55,940 enhances development of cocaine SA in female rats (291). THC failed to alter acquisition of cocaine SA and amphetamine SA in monkeys (272) and rats (290), respectively. In regard to maintenance of drug intake in animals with SA behavior, cannabinoid agonists decreased drug-taking responses in rats – CP55,940 diminished MDMA intake (288) and WIN55,212-2 decreased cocaine administration (292) – and in monkeys – Δ9-THC also decreased cocaine intake (272). Fattore et al. first interpreted the shift in psychostimulant intake produced by CB1 agonists as indicative of a synergistic action of CB1 stimulation on reinforcing properties of the drugs, which, incidentally, could account for the frequent use of cannabis among human psychostimulants users (292). Complementary experiments using progressive-ratio schedules also reveal interaction of CB1 receptors with psychostimulant-induced reinforcing properties. In PR schedules, both genetic deletion and antagonist treatment of CB1 receptors produce a decrease in the maximal effort mice provided to self-administer cocaine, as made apparent by decreases in breaking point measures induced by SR141716A (255, 277, 284) and by AM251 (250) (SR141716A producing no effect in this specific report). This adds to the evidence that the CB1 receptors, while not indispensable for acquisition or maintenance of cocaine-seeking behavior, may exert a specific modulation on motivation and reward salience in psychostimulant addiction.
Role of ECBS in extinction and reinstatement of drug-taking behaviors
Although the mechanism used by endocannabinoid signaling to modulate psychostimulant reward and acquisition or maintenance is still the subject of debate, some form of consensus exists in the literature about the pivotal role of the ECBS in extinction and reinstatement in animal behavioral models of psychostimulant addiction [reviewed in Refs. (89) and (274)]. In conditioning procedures, extinction refers to the learning phase that follows the removal of the reinforcer (i.e., psychostimulant drugs), during which rates of conditioned responses (i.e., SA or CPP) progressively decline back to pre-conditioning levels. After drug-seeking behavior becomes extinct, several behavioral phenomena can reinstate drug-seeking behavior. These include not only re-exposure to the abused drug itself, but also exposure to contextual cues associated with previous drug administration and to environmental stressors (274, 293).
In a recent study from Ward et al. mice treated with SR141716 after removal of cocaine in a SA paradigm altered the burst in cocaine-seeking observed in the initial phase of extinction learning, while decreasing the time required to achieve complete extinction of cocaine-seeking behavior when compared to vehicle-treated mice (282). CB1 blockade by SR141716A also significantly decreases cue-induced reinstatement of cocaine SA behavior following extinction, supporting similar reports of attenuation of cue-induced reinstatement of cocaine-seeking by CB1 antagonism in rats (269, 277, 283). Evaluation of reinstatement of SA behavior induced by drug-priming produced similar results: SR141716A blocks cocaine-induced reinstatement (269, 283), and both AM251 (257, 277) and SR141716A inhibit methamphetamine-induced reinstatement (289). It is worth noting that CB2 antagonism also has an anti-reinstatement effect in cocaine-primed, but not in cue-exposed rats (277). In CPP models, CB1 blockade by SR141716A, but not by AM251 (276), impaired drug-induced reinstatement in cocaine-conditioned mice (281). SR141716A also impaired methamphetamine-induced reinstatement of CPP (281). Few studies focused on stress-induced reinstatement of psychostimulant-seeking. Vaughn et al. recently found that AM251 reverses stress-induced CPP (276). De Vries et al. could not find an impact of SR141716A on stress-induced SA reinstatement (283).
Stimulation of CB1 receptors produced opposite results to those obtained in pharmacological blockade experiments (see Table 4). WIN55,212-2 increases time for extinction of CPP and enhances drug-induced reinstatement in MDMA-conditioned mice (285, 286). Similarly, Δ9-THC increases cue-induced reinstatement of methamphetamine SA in rats (289). However, studies from Parker et al. and Adamczyk et al. complexify the interpretation of these results (290). Indeed, Adamczyk’s group showed that FAAH inhibition impairs cue- and drug-induced reinstatement of cocaine SA. Using the place-preference conditioning paradigm, Parker et al. have assessed the potential of both exogenous cannabinoids – Δ9-THC and CBD – to potentiate the extinction of cocaine- and amphetamine-induced CPP (290). After the establishment of cocaine-induced and amphetamine-induced place preference, rats were given an extinction trial, 30 min prior to which they were injected with a low dose of Δ9-THC, CBD, or vehicle. During conditioning trials, researchers also injected rats with cannabinoids, or vehicle, prior to an amphetamine injection, to determine the effects of Δ9-THC or CBD on the establishment and expression of a place preference. Results indicate that Δ9-THC and CBD potentiate the extinction of stimulant-CPP learning, which is not mediated by an alteration of learning or retrieval. CBD does not have a reinforcing or hedonic property on its own, suggesting that it does not have the addictive potential of Δ9-THC, a significant advantage in terms of therapeutic use. The non-reinforcing aspect of CBD has been replicated in studies looking at the co-administration of CBD and Δ9-THC (212, 294). These discrepancies probably result from the lack of receptor selectivity in the methods used to enhanced cannabinoid signaling. Nonetheless, these experiments support a significant involvement of ECBS in the extinction and reinstatement of behaviors related to psychostimulant addiction. Overall, the positive results of CB1 antagonists on extinction and prevention of reinstatement of psychostimulant SA, combined with their lack of reinforcing properties, suggest a therapeutic potential for CB1 modulation in treatment of psychostimulant addiction.
Conclusion
A growing number of studies have investigated the neurobiological and behavioral mechanisms leading to psychostimulants dependence. A key feature of drug dependence is the relapse to drug use even after long period of abstinence. While greatly improved in recent years, treatment strategies for psychostimulants have yet to address effectively drug-seeking behaviors linked to high rates of relapse, persistent drug use as well as subsequent health, mental, and social problems. There is consequently an urgent need for researchers to identify compounds that might help patients (1) initiate abstinence and (2) avoid relapse. The ECBS appears to play a critical role in dependence to psychostimulants and experimental studies are now providing evidence that while it does not participate in the primary reinforcing properties of psychostimulants, it reliably modulates relapse to drugs. Interestingly, emerging human data supports a role for ECBS modulation in vulnerability to psychostimulant addiction, and more significantly in addictive behaviors among dependent individuals. Accumulating evidence thus points to the ECBS as a critical target for the development of pharmacotherapies for the treatment of addiction to psychostimulants. Given the various neuropharmacological actions of exogenous cannabinoids, and their ability to modulate the acute reinforcing effects of drugs, data on Δ9-THC and CBD is particularly promising as to the potential use of cannabinoids in relapse prevention strategies for psychostimulant-dependent individuals. The effects of these compounds on stimulant use outcomes in humans remains to be clearly established and could be assessed with well-designed controlled trials. The neurobiological correlates of cannabinoids’ impact on stimulant-seeking behaviors could also be examined with neuroimaging studies in stimulants dependent individuals. Among potential barriers, social and scientific acceptability of cannabinoid-based therapy, side effects profiles, as well as addictive potential of certain cannabinoid such as Δ9-THC, have to be taken into consideration.
Conflict of Interest Statement
The authors declare that the research was conducted in the absence of any commercial or financial relationships that could be construed as a potential conflict of interest.
Acknowledgments
Stéphane Potvin is holder of a Junior 1 research award from the Fonds de Recherche du Québec en Santé (FRSQ). This work was supported by the CHUM Department of Psychiatry; Université de Montréal Department of Psychiatry; and the CHUM Research Center (Didier Jutras-Aswad).
References
2. Substance Abuse and Mental Health Services Administration, Office of Applied Studies. The DASIS Report: Geographic Differences in Substance Abuse Treatment Admissions for Methamphetamine/ Amphetamine and Marijuana: 2005. Rockville, MD (2008).
3. Degenhardt L, Hall W. Extent of illicit drug use and dependence, and their contribution to the global burden of disease. Lancet (2012) 379:55–70. doi:10.1016/S0140-6736(11)61138-0
5. Ben Diane MK, Feroni I, Poncet M, Obadia Y. Chief health risks associated with intravenous heroin and cocaine abuse. Presse Med (2000) 29:453–7.
6. Arendt M, Munk-Jorgensen P, Sher L, Jensen SO. Mortality among individuals with cannabis, cocaine, amphetamine, MDMA, and opioid use disorders: a nationwide follow-up study of Danish substance users in treatment. Drug Alcohol Depend (2011) 114(2–3):134–9. doi:10.1016/j.drugalcdep.2010.09.013
7. Pavarin R, Lugoboni F, Mathewson S, Ferrari AM, Guizzardi G, Quaglio G. Cocaine-related medical and trauma problems: a consecutive series of 743 patients from a multicentre study in Italy. Eur J Emerg Med (2011) 18(4):208–14. doi:10.1097/MEJ.0b013e3283440f25
8. Gorelick DA, Gardner EL, Xi ZX. Agents in development for the management of cocaine abuse. Drugs (2004) 64:1547–73. doi:10.2165/00003495-200464140-00004
9. Vocci FJ, Elkashef A. Pharmacotherapy and other treatments for cocaine abuse and dependence. Curr Opin Psychiatry (2005) 18:265–70. doi:10.1097/01.yco.0000165596.98552.02
10. Karila L, Gorelick D, Weinstein A, Noble F, Benyamina A, Coscas S, et al. New treatments for cocaine dependence: a focused review. Int J Neuropsychopharmacol (2008) 11:425–38. doi:10.1017/S1461145707008097
11. Christopher Pierce R, O’Brien CP, Kenny PJ, Vanderschuren LJ. Rational development of addiction pharmacotherapies: successes, failures, and prospects. Cold Spring Harb Perspect Med (2012) 2:a012880. doi:10.1101/cshperspect.a012880
12. Lima MS, Reisser AA, Soares BG, Farrell M. Antidepressants for cocaine dependence. Cochrane Database Syst Rev (2003):CD002950.
13. Amato L, Minozzi S, Pani PP, Davoli M. Antipsychotic medications for cocaine dependence. Cochrane Database Syst Rev (2007):CD006306.
14. Minozzi S, Amato L, Davoli M, Farrell M, Lima Reisser AA, Pani PP, et al. Anticonvulsants for cocaine dependence. Cochrane Database Syst Rev (2008):CD006754. doi:10.1002/14651858.CD006754.pub2
15. Xi ZX, Gardner EL. Hypothesis-driven medication discovery for the treatment of psychostimulant addiction. Curr Drug Abuse Rev (2008) 1:303–27. doi:10.2174/1874473710801030303
16. Perez-Mana C, Castells X, Vidal X, Casas M, Capella D. Efficacy of indirect dopamine agonists for psychostimulant dependence: a systematic review and meta-analysis of randomized controlled trials. J Subst Abuse Treat (2011) 40:109–22. doi:10.1016/j.jsat.2010.08.012
17. Martell BA, Orson FM, Poling J, Mitchell E, Rossen RD, Gardner T, et al. Cocaine vaccine for the treatment of cocaine dependence in methadone-maintained patients: a randomized, double-blind, placebo-controlled efficacy trial. Arch Gen Psychiatry (2009) 66:1116–23. doi:10.1001/archgenpsychiatry.2009.128
18. Ries RK, Fiellin DA, Miller SC, Saitz R. Principles of Addiction Medecine. 4th ed. Philadelphia: Lippincott Williams & Wilkins (2009). p. 707–22.
19. Gardner EL. Endocannabinoid signaling system and brain reward: emphasis on dopamine. Pharmacol Biochem Behav (2005) 81:263–84. doi:10.1016/j.pbb.2005.01.032
20. Heifets BD, Castillo PE. Endocannabinoid signaling and long-term synaptic plasticity. Annu Rev Physiol (2009) 71:283–306. doi:10.1146/annurev.physiol.010908.163149
21. Lutz B. Endocannabinoid signals in the control of emotion. Curr Opin Pharmacol (2009) 9:46–52. doi:10.1016/j.coph.2008.12.001
22. Jutras-Aswad D, Zang G, Bruneau J. Cannabis use correlates of syringe sharing among injection drug users. Am J Addict (2010) 19:231–7. doi:10.1111/j.1521-0391.2010.00031.x
23. WHO. Neuroscience of psychoactive substance use and dependence. Geneva: World Health Organization (2004).
24. Hyman SM, Fox H, Hong KI, Doebrick C, Sinha R. Stress and drug-cue-induced craving in opioid-dependent individuals in naltrexone treatment. Exp Clin Psychopharmacol (2007) 15:134–43. doi:10.1037/1064-1297.15.2.134
25. O’Brien CP, Childress AR, Ehrman R, Robbins SJ. Conditioning factors in drug abuse: can they explain compulsion? J Psychopharmacol (1998) 12:15–22. doi:10.1177/026988119801200103
26. Sinha R, Lacadie C, Skudlarski P, Fulbright RK, Rounsaville BJ, Kosten TR, et al. Neural activity associated with stress-induced cocaine craving: a functional magnetic resonance imaging study. Psychopharmacology (Berl) (2005) 183:171–80. doi:10.1007/s00213-005-0147-8
27. Lingford-Hughes AR, Davies SJ, McIver S, Williams TM, Daglish MR, Nutt DJ. Addiction. Br Med Bull (2003) 65:209–22. doi:10.1093/bmb/65.1.209
28. Robinson TE, Berridge KC. Addiction. Annu Rev Psychol (2003) 54:25–53. doi:10.1146/annurev.psych.54.101601.145237
29. Kalivas PW, Volkow ND. The neural basis of addiction: a pathology of motivation and choice. Am J Psychiatry (2005) 162:1403–13. doi:10.1176/appi.ajp.162.8.1403
30. Everitt BJ, Belin D, Economidou D, Pelloux Y, Dalley JW, Robbins TW. Review. Neural mechanisms underlying the vulnerability to develop compulsive drug-seeking habits and addiction. Philos Trans R Soc Lond B Biol Sci (2008) 363:3125–35. doi:10.1098/rstb.2008.0089
31. Feltenstein MW, See RE. The neurocircuitry of addiction: an overview. Br J Pharmacol (2008) 154:261–74. doi:10.1038/bjp.2008.51
32. Chen PC, Lao CL, Chen JC. The D(3) dopamine receptor inhibits dopamine release in PC-12/hD3 cells by autoreceptor signaling via PP-2B, CK1, and Cdk-5. J Neurochem (2009) 110:1180–90. doi:10.1111/j.1471-4159.2009.06209.x
33. Di Chiara G. Nucleus accumbens shell and core dopamine: differential role in behavior and addiction. Behav Brain Res (2002) 137:75–114. doi:10.1016/S0166-4328(02)00286-3
34. Meil WM, See RE. Lesions of the basolateral amygdala abolish the ability of drug associated cues to reinstate responding during withdrawal from self-administered cocaine. Behav Brain Res (1997) 87:139–48. doi:10.1016/S0166-4328(96)02270-X
35. Childress AR, Mozley PD, McElgin W, Fitzgerald J, Reivich M, O’Brien CP. Limbic activation during cue-induced cocaine craving. Am J Psychiatry (1999) 156:11–8.
36. Robbins TW, Everitt BJ, Nutt DJ. Introduction. The neurobiology of drug addiction: new vistas. Philos Trans R Soc Lond B Biol Sci (2008) 363:3109–11. doi:10.1098/rstb.2008.0108
37. Goldstein RZ, Volkow ND. Drug addiction and its underlying neurobiological basis: neuroimaging evidence for the involvement of the frontal cortex. Am J Psychiatry (2002) 159:1642–52. doi:10.1176/appi.ajp.159.10.1642
38. Koob GF, Swerdlow NR. The functional output of the mesolimbic dopamine system. Ann N Y Acad Sci (1988) 537:216–27. doi:10.1111/j.1749-6632.1988.tb42108.x
39. Volkow ND, Wang GJ, Fischman MW, Foltin RW, Fowler JS, Abumrad NN, et al. Relationship between subjective effects of cocaine and dopamine transporter occupancy. Nature (1997) 386:827–30. doi:10.1038/386827a0
40. Fowler JS, Volkow ND, Wang GJ, Gatley SJ, Logan J. [(11)]Cocaine: PET studies of cocaine pharmacokinetics, dopamine transporter availability and dopamine transporter occupancy. Nucl Med Biol (2001) 28:561–72. doi:10.1016/S0969-8051(01)00211-6
41. Fleckenstein AE, Gibb JW, Hanson GR. Differential effects of stimulants on monoaminergic transporters: pharmacological consequences and implications for neurotoxicity. Eur J Pharmacol (2000) 406:1–13. doi:10.1016/S0014-2999(00)00639-7
42. Rothman RB, Baumann MH. Monoamine transporters and psychostimulant drugs. Eur J Pharmacol (2003) 479:23–40. doi:10.1016/j.ejphar.2003.08.054
43. Rudnick G, Clark J. From synapse to vesicle: the reuptake and storage of biogenic amine neurotransmitters. Biochim Biophys Acta (1993) 1144:249–63. doi:10.1016/0005-2728(93)90109-S
44. Howell LL, Kimmel HL. Monoamine transporters and psychostimulant addiction. Biochem Pharmacol (2008) 75:196–217. doi:10.1016/j.bcp.2007.08.003
45. Barr AM, Panenka WJ, Macewan GW, Thornton AE, Lang DJ, Honer WG, et al. The need for speed: an update on methamphetamine addiction. J Psychiatry Neurosci (2006) 31:301–13.
46. Rocha BA, Fumagalli F, Gainetdinov RR, Jones SR, Ator R, Giros B, et al. Cocaine self-administration in dopamine-transporter knockout mice. Nat Neurosci (1998) 1:132–7. doi:10.1038/381
47. Sora I, Wichems C, Takahashi N, Li XF, Zeng Z, Revay R, et al. Cocaine reward models: conditioned place preference can be established in dopamine- and in serotonin-transporter knockout mice. Proc Natl Acad Sci U S A (1998) 95:7699–704. doi:10.1073/pnas.95.13.7699
48. Lee B, Tiefenbacher S, Platt DM, Spealman RD. Role of the hypothalamic-pituitary-adrenal axis in reinstatement of cocaine-seeking behavior in squirrel monkeys. Psychopharmacology (Berl) (2003) 168:177–83. doi:10.1007/s00213-003-1391-4
49. Arnold JC. The role of endocannabinoid transmission in cocaine addiction. Pharmacol Biochem Behav (2005) 81:396–406. doi:10.1016/j.pbb.2005.02.015
50. Backstrom P, Hyytia P. Ionotropic and metabotropic glutamate receptor antagonism attenuates cue-induced cocaine seeking. Neuropsychopharmacology (2006) 31:778–86. doi:10.1038/sj.npp.1300845
51. Lingford-Hughes A, Nutt D. Neurobiology of addiction and implications for treatment. Br J Psychiatry (2003) 182:97–100. doi:10.1192/bjp.182.2.97
52. Broderick PA, Olabisi OA, Rahni DN, Zhou Y. Cocaine acts on accumbens monoamines and locomotor behavior via a 5-HT2A/2C receptor mechanism as shown by ketanserin: 24-h follow-up studies. Prog Neuropsychopharmacol Biol Psychiatry (2004) 28:547–57. doi:10.1016/j.pnpbp.2004.01.007
53. Filip M. Role of serotonin (5-HT)2 receptors in cocaine self-administration and seeking behavior in rats. Pharmacol Rep (2005) 57:35–46.
54. Wee S, Wang Z, He R, Zhou J, Kozikowski AP, Woolverton WL. Role of the increased noradrenergic neurotransmission in drug self-administration. Drug Alcohol Depend (2006) 82:151–7. doi:10.1016/j.drugalcdep.2005.09.002
55. Sora I, Hall FS, Andrews AM, Itokawa M, Li XF, Wei HB, et al. Molecular mechanisms of cocaine reward: combined dopamine and serotonin transporter knockouts eliminate cocaine place preference. Proc Natl Acad Sci U S A (2001) 98:5300–5. doi:10.1073/pnas.091039298
56. Hall FS, Li XF, Sora I, Xu F, Caron M, Lesch KP, et al. Cocaine mechanisms: enhanced cocaine, fluoxetine and nisoxetine place preferences following monoamine transporter deletions. Neuroscience (2002) 115:153–61. doi:10.1016/S0306-4522(02)00379-2
57. Shaham Y, Erb S, Stewart J. Stress-induced relapse to heroin and cocaine seeking in rats: a review. Brain Res Brain Res Rev (2000) 33:13–33. doi:10.1016/S0165-0173(00)00024-2
58. Weinshenker D, Schroeder JP. There and back again: a tale of norepinephrine and drug addiction. Neuropsychopharmacology (2007) 32:1433–51. doi:10.1038/sj.npp.1301263
59. Gaval-Cruz M, Weinshenker D. Mechanisms of disulfiram-induced cocaine abstinence: antabuse and cocaine relapse. Mol Interv (2009) 9:175–87. doi:10.1124/mi.9.4.6
60. Leri F, Flores J, Rodaros D, Stewart J. Blockade of stress-induced but not cocaine-induced reinstatement by infusion of noradrenergic antagonists into the bed nucleus of the stria terminalis or the central nucleus of the amygdala. J Neurosci (2002) 22:5713–8.
61. Smith RJ, Aston-Jones G. alpha(2) Adrenergic and imidazoline receptor agonists prevent cue-induced cocaine seeking. Biol Psychiatry (2011) 70:712–9. doi:10.1016/j.biopsych.2011.06.010
62. Parsons LH, Weiss F, Koob GF. Serotonin1b receptor stimulation enhances dopamine-mediated reinforcement. Psychopharmacology (Berl) (1996) 128:150–60. doi:10.1007/s002130050120
63. Tzschentke TM, Schmidt WJ. Glutamatergic mechanisms in addiction. Mol Psychiatry (2003) 8:373–82. doi:10.1038/sj.mp.4001269
64. Kalivas PW, Lalumiere RT, Knackstedt L, Shen H. Glutamate transmission in addiction. Neuropharmacology (2009) 56(Suppl 1):169–73. doi:10.1016/j.neuropharm.2008.07.011
65. Sarti F, Borgland SL, Kharazia VN, Bonci A. Acute cocaine exposure alters spine density and long-term potentiation in the ventral tegmental area. Eur J Neurosci (2007) 26:749–56. doi:10.1111/j.1460-9568.2007.05689.x
66. Chen Q, Xiong X, Lee TH, Liu Y, Wetsel WC, Zhang X. Neural plasticity and addiction: integrin-linked kinase and cocaine behavioral sensitization. J Neurochem (2008) 107:679–89. doi:10.1111/j.1471-4159.2008.05619.x
67. Knackstedt LA, Kalivas PW. Glutamate and reinstatement. Curr Opin Pharmacol (2009) 9:59–64. doi:10.1016/j.coph.2008.12.003
68. Novak M, Halbout B, O’Connor EC, Rodriguez Parkitna J, Su T, Chai M, et al. Incentive learning underlying cocaine-seeking requires mGluR5 receptors located on dopamine D1 receptor-expressing neurons. J Neurosci (2010) 30:11973–82. doi:10.1523/JNEUROSCI.2550-10.2010
69. Baker DA, McFarland K, Lake RW, Shen H, Tang XC, Toda S, et al. Neuroadaptation in cystine-glutamate exchange underlie cocaine relapse. Nat Neurosci (2003) 6:743–9. doi:10.1038/nn1069
70. McFarland K, Lapish CC, Kalivas PW. Prefrontal glutamate release into the core of the nucleus accumbens mediates cocaine-induced reinstatement of drug-seeking behavior. J Neurosci (2003) 23:3531–7.
71. Cornish JL, Kalivas PW. Glutamate transmission in the nucleus accumbens mediates relapse in cocaine addiction. J Neurosci (2000) 20:RC89.
72. McFarland K, Davidge SB, Lapish CC, Kalivas PW. Limbic and motor circuitry underlying footshock-induced reinstatement of cocaine-seeking behavior. J Neurosci (2004) 24:1551–60. doi:10.1523/JNEUROSCI.4177-03.2004
73. Backstrom P, Hyytia P. Involvement of AMPA/kainate, NMDA, and mGlu5 receptors in the nucleus accumbens core in cue-induced reinstatement of cocaine seeking in rats. Psychopharmacology (Berl) (2007) 192:571–80. doi:10.1007/s00213-007-0753-8
74. Di Chiara G, Bassareo V, Fenu S, De Luca MA, Spina L, Cadoni C, et al. Dopamine and drug addiction: the nucleus accumbens shell connection. Neuropharmacology (2004) 47(Suppl 1):227–41. doi:10.1016/j.neuropharm.2004.06.032
75. Ito I, Kimura T, Watanabe S, Kirino Y, Ito E. Modulation of two oscillatory networks in the peripheral olfactory system by gamma-aminobutyric acid, glutamate, and acetylcholine in the terrestrial slug Limax marginatus. J Neurobiol (2004) 59:304–18. doi:10.1002/neu.10328
76. Di Ciano P, Cardinal RN, Cowell RA, Little SJ, Everitt BJ. Differential involvement of NMDA, AMPA/kainate, and dopamine receptors in the nucleus accumbens core in the acquisition and performance of pavlovian approach behavior. J Neurosci (2001) 21:9471–7.
77. Kalivas PW, McFarland K. Brain circuitry and the reinstatement of cocaine-seeking behavior. Psychopharmacology (Berl) (2003) 168:44–56. doi:10.1007/s00213-003-1393-2
78. Robbins TW, Everitt BJ. Neurobehavioural mechanisms of reward and motivation. Curr Opin Neurobiol (1996) 6:228–36. doi:10.1016/S0959-4388(96)80077-8
79. Jentsch JD, Taylor JR. Impulsivity resulting from frontostriatal dysfunction in drug abuse: implications for the control of behavior by reward-related stimuli. Psychopharmacology (Berl) (1999) 146:373–90. doi:10.1007/PL00005483
80. Rogers RD, Ramnani N, Mackay C, Wilson JL, Jezzard P, Carter CS, et al. Distinct portions of anterior cingulate cortex and medial prefrontal cortex are activated by reward processing in separable phases of decision-making cognition. Biol Psychiatry (2004) 55:594–602. doi:10.1016/j.biopsych.2003.11.012
81. Dom G, Hulstijn W, Sabbe B. Differences in impulsivity and sensation seeking between early- and late-onsetalcoholics. Addict Behav (2006) 31:298–308. doi:10.1016/j.addbeh.2005.05.009
82. Yucel M, Lubman DI. Neurocognitive and neuroimaging evidence of behavioural dysregulation in human drug addiction: implications for diagnosis, treatment and prevention. Drug Alcohol Rev (2007) 26:33–9. doi:10.1080/09595230601036978
83. Kilts CD, Schweitzer JB, Quinn CK, Gross RE, Faber TL, Muhammad F, et al. Neural activity related to drug craving in cocaine addiction. Arch Gen Psychiatry (2001) 58:334–41. doi:10.1001/archpsyc.58.4.334
84. Sinha R, Lacadie C, Skudlarski P, Wexler BE. Neural circuits underlying emotional distress in humans. Ann N Y Acad Sci (2004) 1032:254–7. doi:10.1196/annals.1314.032
85. Ersche KD, Barnes A, Jones PS, Morein-Zamir S, Robbins TW, Bullmore ET. Abnormal structure of frontostriatal brain systems is associated with aspects of impulsivity and compulsivity in cocaine dependence. Brain (2011) 134:2013–24. doi:10.1093/brain/awr138
86. Xu J, Devito EE, Worhunsky PD, Carroll KM, Rounsaville BJ, Potenza MN. White matter integrity is associated with treatment outcome measures in cocaine dependence. Neuropsychopharmacology (2010) 35:1541–9. doi:10.1038/npp.2010.25
87. Phan KL, Wager T, Taylor SF, Liberzon I. Functional neuroanatomy of emotion: a meta-analysis of emotion activation studies in PET and fMRI. Neuroimage (2002) 16:331–48. doi:10.1006/nimg.2002.1087
88. Sinha R, Rounsaville BJ. Sex differences in depressed substance abusers. J Clin Psychiatry (2002) 63:616–27. doi:10.4088/JCP.v63n0715
89. Tanda G. Modulation of the endocannabinoid system: therapeutic potential against cocaine dependence. Pharmacol Res (2007) 56:406–17. doi:10.1016/j.phrs.2007.09.001
90. Labigalini E Jr, Rodrigues LR, Da Silveira DX. Therapeutic use of cannabis by crack addicts in Brazil. J Psychoactive Drugs (1999) 31:451–5. doi:10.1080/02791072.1999.10471776
91. Aharonovich E, Liu X, Samet S, Nunes E, Waxman R, Hasin D. Postdischarge cannabis use and its relationship to cocaine, alcohol, and heroin use: a prospective study. Am J Psychiatry (2005) 162:1507–14. doi:10.1176/appi.ajp.162.8.1507
92. Pacher P, Batkai S, Kunos G. The endocannabinoid system as an emerging target of pharmacotherapy. Pharmacol Rev (2006) 58:389–462. doi:10.1124/pr.58.3.2
93. Herkenham M, Lynn A, Little M, Johnson M, Melvin L, De Costa B, et al. Cannabinoid receptor localization in brain. Proc Natl Acad Sci U S A (1990) 87:1932. doi:10.1073/pnas.87.5.1932
94. Howlett AC, Bidaut-Russell M, Devane WA, Melvin LS, Johnson MR, Herkenham M. The cannabinoid receptor: biochemical, anatomical and behavioral characterization. Trends Neurosci (1990) 13:420–3. doi:10.1016/0166-2236(90)90124-S
95. Herkenham M, Lynn AB, De Costa BR, Richfield EK. Neuronal localization of cannabinoid receptors in the basal ganglia of the rat. Brain Res (1991) 547:267–74. doi:10.1016/0006-8993(91)90970-7
96. Herkenham M, Lynn AB, Johnson MR, Melvin LS, De Costa BR, Rice KC. Characterization and localization of cannabinoid receptors in rat brain: a quantitative in vitro autoradiographic study. J Neurosci (1991) 11:563–83.
97. Batkai S, Jarai Z, Wagner JA, Goparaju SK, Varga K, Liu J, et al. Endocannabinoids acting at vascular CB1 receptors mediate the vasodilated state in advanced liver cirrhosis. Nat Med (2001) 7:827–32. doi:10.1038/89953
98. Alger BE. Retrograde signaling in the regulation of synaptic transmission: focus on endocannabinoids. Prog Neurobiol (2002) 68:247–86. doi:10.1016/S0301-0082(02)00080-1
99. Szabo B, Schlicker E. Effects of cannabinoids on neurotransmission. Handb Exp Pharmacol (2005) 168:327–65. doi:10.1007/3-540-26573-2_11
100. Vaughan CW, Christie MJ. Retrograde signalling by endocannabinoids. Handb Exp Pharmacol (2005) 168:367–83. doi:10.1007/3-540-26573-2_12
101. Kano H, Niranjan A, Novotny J Jr, Bhatnagar J, Flickinger JC, Lunsford LD. Radiosurgery for desmoplastic melanoma of the head and neck using the Leksell Gamma Knife Perfexion technology: a case report. Stereotact Funct Neurosurg (2009) 87:61–5. doi:10.1159/000195721
102. Munro S, Thomas KL, Abu-Shaar M. Molecular characterization of a peripheral receptor for cannabinoids. Nature (1993) 365:61–5. doi:10.1038/365061a0
103. Galiegue S, Mary S, Marchand J, Dussossoy D, Carriere D, Carayon P, et al. Expression of central and peripheral cannabinoid receptors in human immune tissues and leukocyte subpopulations. Eur J Biochem (1995) 232:54–61. doi:10.1111/j.1432-1033.1995.tb20780.x
104. Fride E, Foox A, Rosenberg E, Faigenboim M, Cohen V, Barda L, et al. Milk intake and survival in newborn cannabinoid CB1 receptor knockout mice: evidence for a “CB3” receptor. Eur J Pharmacol (2003) 461:27–34. doi:10.1016/S0014-2999(03)01295-0
105. Onaivi ES, Ishiguro H, Gong JP, Patel S, Perchuk A, Meozzi PA, et al. Discovery of the presence and functional expression of cannabinoid CB2 receptors in brain. Ann N Y Acad Sci (2006) 1074:514–36. doi:10.1196/annals.1369.052
106. Ashton JC, Glass M. The cannabinoid CB2 receptor as a target for inflammation-dependent neurodegeneration. Curr Neuropharmacol (2007) 5:73–80. doi:10.2174/157015907780866884
107. Onaivi ES, Ishiguro H, Gong JP, Patel S, Meozzi PA, Myers L, et al. Brain neuronal CB2 cannabinoid receptors in drug abuse and depression: from mice to human subjects. PLoS ONE (2008) 3:e1640. doi:10.1371/journal.pone.0001640
108. Witting A, Walter L, Wacker J, Moller T, Stella N. P2X7 receptors control 2-arachidonoylglycerol production by microglial cells. Proc Natl Acad Sci U S A (2004) 101:3214–9. doi:10.1073/pnas.0306707101
109. Maresz K, Pryce G, Ponomarev ED, Marsicano G, Croxford JL, Shriver LP, et al. Direct suppression of CNS autoimmune inflammation via the cannabinoid receptor CB1 on neurons and CB2 on autoreactive T cells. Nat Med (2007) 13:492–7. doi:10.1038/nm1561
110. Cabral GA, Raborn ES, Griffin L, Dennis J, Marciano-Cabral F. CB2 receptors in the brain: role in central immune function. Br J Pharmacol (2008) 153:240–51. doi:10.1038/sj.bjp.0707584
111. Racz I, Bilkei-Gorzo A, Markert A, Stamer F, Gothert M, Zimmer A. Anandamide effects on 5-HT(3) receptors in vivo. Eur J Pharmacol (2008) 596:98–101. doi:10.1016/j.ejphar.2008.08.012
112. Atwood BK, Mackie K. CB2: a cannabinoid receptor with an identity crisis. Br J Pharmacol (2010) 160:467–79. doi:10.1111/j.1476-5381.2010.00729.x
113. Stella N. Cannabinoid and cannabinoid-like receptors in microglia, astrocytes, and astrocytomas. Glia (2010) 58:1017–30. doi:10.1002/glia.20983
114. Marsicano G, Moosmann B, Hermann H, Lutz B, Behl C. Neuroprotective properties of cannabinoids against oxidative stress: role of the cannabinoid receptor CB1. J Neurochem (2002) 80:448–56. doi:10.1046/j.0022-3042.2001.00716.x
115. Begg M, Pacher P, Batkai S, Osei-Hyiaman D, Offertaler L, Mo FM, et al. Evidence for novel cannabinoid receptors. Pharmacol Ther (2005) 106:133–45. doi:10.1016/j.pharmthera.2004.11.005
116. Cristino L, De Petrocellis L, Pryce G, Baker D, Guglielmotti V, Di Marzo V. Immunohistochemical localization of cannabinoid type 1 and vanilloid transient receptor potential vanilloid type 1 receptors in the mouse brain. Neuroscience (2006) 139:1405–15. doi:10.1016/j.neuroscience.2006.02.074
117. Brown AJ. Low-carb diets, fasting and euphoria: is there a link between ketosis and gamma-hydroxybutyrate (GHB)? Med Hypotheses (2007) 68:268–71. doi:10.1016/j.mehy.2006.07.043
118. Godlewski G, Offertaler L, Osei-Hyiaman D, Mo FM, Harvey-White J, Liu J, et al. The endogenous brain constituent N-arachidonoyl L-serine is an activator of large conductance Ca2+-activated K+ channels. J Pharmacol Exp Ther (2009) 328:351–61. doi:10.1124/jpet.108.144717
119. Johns DG, Behm DJ, Walker DJ, Ao Z, Shapland EM, Daniels DA, et al. The novel endocannabinoid receptor GPR55 is activated by atypical cannabinoids but does not mediate their vasodilator effects. Br J Pharmacol (2007) 152:825–31. doi:10.1038/sj.bjp.0707419
120. Kapur A, Hurst DP, Fleischer D, Whitnell R, Thakur GA, Makriyannis A, et al. Mutation studies of Ser7.39 and Ser2.60 in the human CB1 cannabinoid receptor: evidence for a serine-induced bend in CB1 transmembrane helix 7. Mol Pharmacol (2007) 71:1512–24. doi:10.1124/mol.107.034645
121. Ryberg E, Larsson N, Sjogren S, Hjorth S, Hermansson NO, Leonova J, et al. The orphan receptor GPR55 is a novel cannabinoid receptor. Br J Pharmacol (2007) 152:1092–101. doi:10.1038/sj.bjp.0707460
122. Giuffrida A, Seillier A. New insights on endocannabinoid transmission in psychomotor disorders. Prog Neuropsychopharmacol Biol Psychiatry (2012) 38:51–8. doi:10.1016/j.pnpbp.2012.04.002
123. Sylantyev S, Jensen TP, Ross RA, Rusakov DA. Cannabinoid- and lysophosphatidylinositol-sensitive receptor GPR55 boosts neurotransmitter release at central synapses. Proc Natl Acad Sci U S A (2013) 110:5193–8. doi:10.1073/pnas.1211204110
124. Patapoutian A, Tate S, Woolf CJ. Transient receptor potential channels: targeting pain at the source. Nat Rev Drug Discov (2009) 8:55–68. doi:10.1038/nrd2757
125. Mezey E, Toth ZE, Cortright DN, Arzubi MK, Krause JE, Elde R, et al. Distribution of mRNA for vanilloid receptor subtype 1 (VR1), and VR1-like immunoreactivity, in the central nervous system of the rat and human. Proc Natl Acad Sci U S A (2000) 97:3655–60. doi:10.1073/pnas.97.7.3655
126. Szolcsanyi J. Anandamide and the question of its functional role for activation of capsaicin receptors. Trends Pharmacol Sci (2000) 21:203–4. doi:10.1016/S0165-6147(00)01484-X
127. De Petrocellis L, Harrison S, Bisogno T, Tognetto M, Brandi I, Smith GD, et al. The vanilloid receptor (VR1)-mediated effects of anandamide are potently enhanced by the cAMP-dependent protein kinase. J Neurochem (2001) 77:1660–3. doi:10.1046/j.1471-4159.2001.00406.x
128. Huang SM, Bisogno T, Trevisani M, Al-Hayani A, De Petrocellis L, Fezza F, et al. An endogenous capsaicin-like substance with high potency at recombinant and native vanilloid VR1 receptors. Proc Natl Acad Sci U S A (2002) 99:8400–5. doi:10.1073/pnas.122196999
129. Maccarrone M, Rossi S, Bari M, De Chiara V, Fezza F, Musella A, et al. Anandamide inhibits metabolism and physiological actions of 2-arachidonoylglycerol in the striatum. Nat Neurosci (2008) 11:152–9. doi:10.1038/nn2042
130. Gerdeman GL, Ronesi J, Lovinger DM. Postsynaptic endocannabinoid release is critical to long-term depression in the striatum. Nat Neurosci (2002) 5:446–51.
131. Gubellini P, Picconi B, Bari M, Battista N, Calabresi P, Centonze D, et al. Experimental parkinsonism alters endocannabinoid degradation: implications for striatal glutamatergic transmission. J Neurosci (2002) 22:6900–7.
132. Ohno-Shosaku T, Maejima T, Kano M. Endogenous cannabinoids mediate retrograde signals from depolarized postsynaptic neurons to presynaptic terminals. Neuron (2001) 29:729–38. doi:10.1016/S0896-6273(01)00247-1
133. Wilson RI, Nicoll RA. Endogenous cannabinoids mediate retrograde signalling at hippocampal synapses. Nature (2001) 410:588–92. doi:10.1038/35069076
134. Kreitzer AC, Regehr WG. Cerebellar depolarization-induced suppression of inhibition is mediated by endogenous cannabinoids. J Neurosci (2001) 21:RC174.
135. Devane WA, Hanus L, Breuer A, Pertwee RG, Stevenson LA, Griffin G, et al. Isolation and structure of a brain constituent that binds to the cannabinoid receptor. Science (1992) 258:1946–9. doi:10.1126/science.1470919
136. Sugiura T, Kondo S, Sukagawa A, Nakane S, Shinoda A, Itoh K, et al. 2-Arachidonoylglycerol: a possible endogenous cannabinoid receptor ligand in brain. Biochem Biophys Res Commun (1995) 215:89–97. doi:10.1006/bbrc.1995.2437
137. Hanus L, Abu-Lafi S, Fride E, Breuer A, Vogel Z, Shalev DE, et al. 2-Arachidonyl glyceryl ether, an endogenous agonist of the cannabinoid CB1 receptor. Proc Natl Acad Sci U S A (2001) 98:3662–5. doi:10.1073/pnas.061029898
138. Porter AC, Sauer JM, Knierman MD, Becker GW, Berna MJ, Bao J, et al. Characterization of a novel endocannabinoid, virodhamine, with antagonist activity at the CB1 receptor. J Pharmacol Exp Ther (2002) 301:1020–4. doi:10.1124/jpet.301.3.1020
139. Wilson RI, Kunos G, Nicoll RA. Presynaptic specificity of endocannabinoid signaling in the hippocampus. Neuron (2001) 31:453–62. doi:10.1016/S0896-6273(01)00372-5
140. Okamoto Y, Wang J, Morishita J, Ueda N. Biosynthetic pathways of the endocannabinoid anandamide. Chem Biodivers (2007) 4:1842–57. doi:10.1002/cbdv.200790155
141. Tanimura A, Yamazaki M, Hashimotodani Y, Uchigashima M, Kawata S, Abe M, et al. The endocannabinoid 2-arachidonoylglycerol produced by diacylglycerol lipase alpha mediates retrograde suppression of synaptic transmission. Neuron (2010) 65:320–7. doi:10.1016/j.neuron.2010.01.021
142. Fride E. Endocannabinoids in the central nervous system: from neuronal networks to behavior. Curr Drug Targets CNS Neurol Disord (2005) 4:633–42. doi:10.2174/156800705774933069
143. Hillard CJ. Biochemistry and pharmacology of the endocannabinoids arachidonylethanolamide and 2-arachidonylglycerol. Prostaglandins Other Lipid Mediat (2000) 61:3–18. doi:10.1016/S0090-6980(00)00051-4
144. Cravatt BF, Giang DK, Mayfield SP, Boger DL, Lerner RA, Gilula NB. Molecular characterization of an enzyme that degrades neuromodulatory fatty-acid amides. Nature (1996) 384:83–7. doi:10.1038/384083a0
145. Nomura DK, Blankman JL, Simon GM, Fujioka K, Issa RS, Ward AM, et al. Activation of the endocannabinoid system by organophosphorus nerve agents. Nat Chem Biol (2008) 4:373–8. doi:10.1038/nchembio.86
146. Blankman JL, Simon GM, Cravatt BF. A comprehensive profile of brain enzymes that hydrolyze the endocannabinoid 2-arachidonoylglycerol. Chem Biol (2007) 14:1347–56. doi:10.1016/j.chembiol.2007.11.006
147. Marrs WR, Blankman JL, Horne EA, Thomazeau A, Lin YH, Coy J, et al. The serine hydrolase ABHD6 controls the accumulation and efficacy of 2-AG at cannabinoid receptors. Nat Neurosci (2010) 13:951–7. doi:10.1038/nn.2601
148. McKinney MK, Cravatt BF. Structure and function of fatty acid amide hydrolase. Annu Rev Biochem (2005) 74:411–32. doi:10.1146/annurev.biochem.74.082803.133450
149. Fegley D, Gaetani S, Duranti A, Tontini A, Mor M, Tarzia G, et al. Characterization of the fatty acid amide hydrolase inhibitor cyclohexyl carbamic acid 3-carbamoyl-biphenyl-3-yl ester (URB597): effects on anandamide and oleoylethanolamide deactivation. J Pharmacol Exp Ther (2005) 313:352–8. doi:10.1124/jpet.104.078980
150. Straiker A, Hu SS, Long JZ, Arnold A, Wager-Miller J, Cravatt BF, et al. Monoacylglycerol lipase limits the duration of endocannabinoid-mediated depolarization-induced suppression of excitation in autaptic hippocampal neurons. Mol Pharmacol (2009) 76:1220–7. doi:10.1124/mol.109.059030
151. Ahn K, Johnson DS, Mileni M, Beidler D, Long JZ, McKinney MK, et al. Discovery and characterization of a highly selective FAAH inhibitor that reduces inflammatory pain. Chem Biol (2009) 16:411–20. doi:10.1016/j.chembiol.2009.02.013
152. Piomelli D, Tarzia G, Duranti A, Tontini A, Mor M, Compton TR, et al. Pharmacological profile of the selective FAAH inhibitor KDS-4103 (URB597). CNS Drug Rev (2006) 12:21–38. doi:10.1111/j.1527-3458.2006.00021.x
153. Kinsey SG, O’Neal ST, Long JZ, Cravatt BF, Lichtman AH. Inhibition of endocannabinoid catabolic enzymes elicits anxiolytic-like effects in the marble burying assay. Pharmacol Biochem Behav (2011) 98:21–7. doi:10.1016/j.pbb.2010.12.002
154. Naidu PS, Kinsey SG, Guo TL, Cravatt BF, Lichtman AH. Regulation of inflammatory pain by inhibition of fatty acid amide hydrolase. J Pharmacol Exp Ther (2010) 334:182–90. doi:10.1124/jpet.109.164806
155. Long JZ, Nomura DK, Vann RE, Walentiny DM, Booker L, Jin X, et al. Dual blockade of FAAH and MAGL identifies behavioral processes regulated by endocannabinoid crosstalk in vivo. Proc Natl Acad Sci U S A (2009) 106:20270–5. doi:10.1073/pnas.0909411106
156. Schlosburg JE, Blankman JL, Long JZ, Nomura DK, Pan B, Kinsey SG, et al. Chronic monoacylglycerol lipase blockade causes functional antagonism of the endocannabinoid system. Nat Neurosci (2010) 13:1113–9. doi:10.1038/nn.2616
157. Chanda PK, Gao Y, Mark L, Btesh J, Strassle BW, Lu P, et al. Monoacylglycerol lipase activity is a critical modulator of the tone and integrity of the endocannabinoid system. Mol Pharmacol (2010) 78:996–1003. doi:10.1124/mol.110.068304
158. Pan B, Wang W, Zhong P, Blankman JL, Cravatt BF, Liu QS. Alterations of endocannabinoid signaling, synaptic plasticity, learning, and memory in monoacylglycerol lipase knock-out mice. J Neurosci (2011) 31:13420–30. doi:10.1523/JNEUROSCI.2075-11.2011
159. Watson SJ, Benson JA Jr, Joy JE. Marijuana and medicine: assessing the science base: a summary of the 1999 Institute of Medicine report. Arch Gen Psychiatry (2000) 57:547–52. doi:10.1001/archpsyc.57.6.547
160. Zuardi AW, Crippa JA, Hallak JE, Moreira FA, Guimaraes FS. Cannabidiol, a Cannabis sativa constituent, as an antipsychotic drug. Braz J Med Biol Res (2006) 39:421–9. doi:10.1590/S0100-879X2006000400001
161. Curran HV, Brignell C, Fletcher S, Middleton P, Henry J. Cognitive and subjective dose-response effects of acute oral Delta 9-tetrahydrocannabinol (THC) in infrequent cannabis users. Psychopharmacology (Berl) (2002) 164:61–70. doi:10.1007/s00213-002-1169-0
162. Fried PA, Smith AM. A literature review of the consequences of prenatal marihuana exposure. An emerging theme of a deficiency in aspects of executive function. Neurotoxicol Teratol (2001) 23:1–11. doi:10.1016/S0892-0362(00)00119-7
163. Arseneault L, Cannon M, Poulton R, Murray R, Caspi A, Moffitt TE. Cannabis use in adolescence and risk for adult psychosis: longitudinal prospective study. BMJ (2002) 325:1212–3. doi:10.1136/bmj.325.7374.1212
164. Kandel DB. Does marijuana use cause the use of other drugs? JAMA (2003) 289:482–3. doi:10.1001/jama.289.4.482
165. Huizink AC, Mulder EJ. Maternal smoking, drinking or cannabis use during pregnancy and neurobehavioral and cognitive functioning in human offspring. Neurosci Biobehav Rev (2006) 30:24–41. doi:10.1016/j.neubiorev.2005.04.005
166. D’Souza DC, Perry E, Macdougall L, Ammerman Y, Cooper T, Wu YT, et al. The psychotomimetic effects of intravenous delta-9-tetrahydrocannabinol in healthy individuals: implications for psychosis. Neuropsychopharmacology (2004) 29:1558–72. doi:10.1038/sj.npp.1300496
167. D’Souza DC, Ranganathan M, Braley G, Gueorguieva R, Zimolo Z, Cooper T, et al. Blunted psychotomimetic and amnestic effects of delta-9-tetrahydrocannabinol in frequent users of cannabis. Neuropsychopharmacology (2008) 33:2505–16. doi:10.1038/sj.npp.1301643
168. Compton DR, Johnson MR, Melvin LS, Martin BR. Pharmacological profile of a series of bicyclic cannabinoid analogs: classification as cannabimimetic agents. J Pharmacol Exp Ther (1992) 260:201–9.
169. Williamson EM, Evans FJ. Cannabinoids in clinical practice. Drugs (2000) 60:1303–14. doi:10.2165/00003495-200060060-00005
170. Guimaraes FS, De Aguiar JC, Mechoulam R, Breuer A. Anxiolytic effect of cannabidiol derivatives in the elevated plus-maze. Gen Pharmacol (1994) 25:161–4. doi:10.1016/0306-3623(94)90027-2
171. Russo EB, Burnett A, Hall B, Parker KK. Agonistic properties of cannabidiol at 5-HT1a receptors. Neurochem Res (2005) 30:1037–43. doi:10.1007/s11064-005-6978-1
172. Moreira FA, Aguiar DC, Guimaraes FS. Anxiolytic-like effect of cannabidiol in the rat Vogel conflict test. Prog Neuropsychopharmacol Biol Psychiatry (2006) 30:1466–71. doi:10.1016/j.pnpbp.2006.06.004
173. Hermann D, Sartorius A, Welzel H, Walter S, Skopp G, Ende G, et al. Dorsolateral prefrontal cortex N-acetylaspartate/total creatine (NAA/tCr) loss in male recreational cannabis users. Biol Psychiatry (2007) 61:1281–9. doi:10.1016/j.biopsych.2006.08.027
174. El-Alfy AT, Ivey K, Robinson K, Ahmed S, Radwan M, Slade D, et al. Antidepressant-like effect of delta9-tetrahydrocannabinol and other cannabinoids isolated from Cannabis sativa L. Pharmacol Biochem Behav (2010) 95:434–42. doi:10.1016/j.pbb.2010.03.004
175. Long LE, Chesworth R, Huang XF, McGregor IS, Arnold JC, Karl T. A behavioural comparison of acute and chronic Delta9-tetrahydrocannabinol and cannabidiol in C57BL/6JArc mice. Int J Neuropsychopharmacol (2010) 13:861–76. doi:10.1017/S1461145709990605
176. Zanelati TV, Biojone C, Moreira FA, Guimaraes FS, Joca SR. Antidepressant-like effects of cannabidiol in mice: possible involvement of 5-HT1A receptors. Br J Pharmacol (2010) 159:122–8. doi:10.1111/j.1476-5381.2009.00521.x
177. Thomas A, Baillie GL, Phillips AM, Razdan RK, Ross RA, Pertwee RG. Cannabidiol displays unexpectedly high potency as an antagonist of CB1 and CB2 receptor agonists in vitro. Br J Pharmacol (2007) 150:613–23. doi:10.1038/sj.bjp.0707133
178. Pertwee RG. The diverse CB1 and CB2 receptor pharmacology of three plant cannabinoids: delta9-tetrahydrocannabinol, cannabidiol and delta9-tetrahydrocannabivarin. Br J Pharmacol (2008) 153:199–215. doi:10.1038/sj.bjp.0707442
179. Qin N, Neeper MP, Liu Y, Hutchinson TL, Lubin ML, Flores CM. TRPV2 is activated by cannabidiol and mediates CGRP release in cultured rat dorsal root ganglion neurons. J Neurosci (2008) 28:6231–8. doi:10.1523/JNEUROSCI.0504-08.2008
180. Bisogno T, Hanus L, De Petrocellis L, Tchilibon S, Ponde DE, Brandi I, et al. Molecular targets for cannabidiol and its synthetic analogues: effect on vanilloid VR1 receptors and on the cellular uptake and enzymatic hydrolysis of anandamide. Br J Pharmacol (2001) 134:845–52. doi:10.1038/sj.bjp.0704327
181. Pandolfo P, Silveirinha V, Dos Santos-Rodrigues A, Venance L, Ledent C, Takahashi RN, et al. Cannabinoids inhibit the synaptic uptake of adenosine and dopamine in the rat and mouse striatum. Eur J Pharmacol (2011) 655:38–45. doi:10.1016/j.ejphar.2011.01.013
182. Murillo-Rodriguez E, Palomero-Rivero M, Millan-Aldaco D, Mechoulam R, Drucker-Colin R. Effects on sleep and dopamine levels of microdialysis perfusion of cannabidiol into the lateral hypothalamus of rats. Life Sci (2011) 88:504–11. doi:10.1016/j.lfs.2011.01.013
183. Kathmann M, Flau K, Redmer A, Trankle C, Schlicker E. Cannabidiol is an allosteric modulator at mu- and delta-opioid receptors. Naunyn Schmiedebergs Arch Pharmacol (2006) 372:354–61. doi:10.1007/s00210-006-0033-x
184. Carrier EJ, Auchampach JA, Hillard CJ. Inhibition of an equilibrative nucleoside transporter by cannabidiol: a mechanism of cannabinoid immunosuppression. Proc Natl Acad Sci U S A (2006) 103:7895–900. doi:10.1073/pnas.0511232103
185. Rodriguez JJ, Mackie K, Pickel VM. Ultrastructural localization of the CB1 cannabinoid receptor in mu-opioid receptor patches of the rat Caudate putamen nucleus. J Neurosci (2001) 21:823–33.
186. Schoffelmeer AN, Hogenboom F, Wardeh G, De Vries TJ. Interactions between CB1 cannabinoid and mu opioid receptors mediating inhibition of neurotransmitter release in rat nucleus accumbens core. Neuropharmacology (2006) 51:773–81. doi:10.1016/j.neuropharm.2006.05.019
187. Hampson AJ, Grimaldi M, Axelrod J, Wink D. Cannabidiol and (−)Delta 9-tetrahydrocannabinol are neuroprotective antioxidants. Proc Natl Acad Sci U S A (1998) 95:8268–73. doi:10.1073/pnas.95.14.8268
188. Hallak JE, Dursun SM, Bosi DC, De Macedo LR, Machado-De-Sousa JP, Abrao J, et al. The interplay of cannabinoid and NMDA glutamate receptor systems in humans: preliminary evidence of interactive effects of cannabidiol and ketamine in healthy human subjects. Prog Neuropsychopharmacol Biol Psychiatry (2011) 35:198–202. doi:10.1016/j.pnpbp.2010.11.002
189. Campos AC, Guimaraes FS. Involvement of 5HT1A receptors in the anxiolytic-like effects of cannabidiol injected into the dorsolateral periaqueductal gray of rats. Psychopharmacology (Berl) (2008) 199:223–30. doi:10.1007/s00213-008-1168-x
190. Alves FH, Crestani CC, Gomes FV, Guimaraes FS, Correa FM, Resstel LB. Cannabidiol injected into the bed nucleus of the stria terminalis modulates baroreflex activity through 5-HT1A receptors. Pharmacol Res (2010) 62:228–36. doi:10.1016/j.phrs.2010.05.003
191. Magen I, Avraham Y, Ackerman Z, Vorobiev L, Mechoulam R, Berry EM. Cannabidiol ameliorates cognitive and motor impairments in bile-duct ligated mice via 5-HT1A receptor activation. Br J Pharmacol (2010) 159:950–7. doi:10.1111/j.1476-5381.2009.00589.x
192. Soares Vde P, Campos AC, Bortoli VC, Zangrossi H Jr, Guimaraes FS, Zuardi AW. Intra-dorsal periaqueductal gray administration of cannabidiol blocks panic-like response by activating 5-HT1A receptors. Behav Brain Res (2010) 213:225–9. doi:10.1016/j.bbr.2010.05.004
193. Gomes FV, Reis DG, Alves FH, Correa FM, Guimaraes FS, Resstel LB. Cannabidiol injected into the bed nucleus of the stria terminalis reduces the expression of contextual fear conditioning via 5-HT1A receptors. J Psychopharmacol (2012) 26:104–13. doi:10.1177/0269881110389095
194. Guimaraes FS, Chiaretti TM, Graeff FG, Zuardi AW. Antianxiety effect of cannabidiol in the elevated plus-maze. Psychopharmacology (Berl) (1990) 100:558–9. doi:10.1007/BF02244012
195. Granjeiro EM, Gomes FV, Guimaraes FS, Correa FM, Resstel LB. Effects of intracisternal administration of cannabidiol on the cardiovascular and behavioral responses to acute restraint stress. Pharmacol Biochem Behav (2011) 99:743–8. doi:10.1016/j.pbb.2011.06.027
196. Fusar-Poli P, Crippa JA, Bhattacharyya S, Borgwardt SJ, Allen P, Martin-Santos R, et al. Distinct effects of {delta}9-tetrahydrocannabinol and cannabidiol on neural activation during emotional processing. Arch Gen Psychiatry (2009) 66:95–105. doi:10.1001/archgenpsychiatry.2008.519
197. Crippa JA, Zuardi AW, Garrido GE, Wichert-Ana L, Guarnieri R, Ferrari L, et al. Effects of cannabidiol (CBD) on regional cerebral blood flow. Neuropsychopharmacology (2004) 29:417–26. doi:10.1038/sj.npp.1300340
198. Crippa JA, Derenusson GN, Ferrari TB, Wichert-Ana L, Duran FL, Martin-Santos R, et al. Neural basis of anxiolytic effects of cannabidiol (CBD) in generalized social anxiety disorder: a preliminary report. J Psychopharmacol (2011) 25:121–30. doi:10.1177/0269881110379283
199. Fusar-Poli P, Allen P, Bhattacharyya S, Crippa JA, Mechelli A, Borgwardt S, et al. Modulation of effective connectivity during emotional processing by Delta 9-tetrahydrocannabinol and cannabidiol. Int J Neuropsychopharmacol (2010) 13(4): 421–32. doi:10.1017/S1461145709990617
200. Pessoa L. On the relationship between emotion and cognition. Nat Rev Neurosci (2008) 9:148–58. doi:10.1038/nrn2317
201. Casarotto PC, Gomes FV, Resstel LB, Guimaraes FS. Cannabidiol inhibitory effect on marble-burying behaviour: involvement of CB1 receptors. Behav Pharmacol (2010) 21:353–8. doi:10.1097/FBP.0b013e32833b33c5
202. Deiana S, Watanabe A, Yamasaki Y, Amada N, Arthur M, Fleming S, et al. Plasma and brain pharmacokinetic profile of cannabidiol (CBD), cannabidivarine (CBDV), Delta(9)-tetrahydrocannabivarin (THCV) and cannabigerol (CBG) in rats and mice following oral and intraperitoneal administration and CBD action on obsessive-compulsive behaviour. Psychopharmacology (Berl) (2012) 219(3):859–73.
203. Mechoulam R, Parker LA, Gallily R. Cannabidiol: an overview of some pharmacological aspects. J Clin Pharmacol (2002) 42:11S–9S.
204. Lastres-Becker I, Molina-Holgado F, Ramos JA, Mechoulam R, Fernandez-Ruiz J. Cannabinoids provide neuroprotection against 6-hydroxydopamine toxicity in vivo and in vitro: relevance to Parkinson’s disease. Neurobiol Dis (2005) 19:96–107. doi:10.1016/j.nbd.2004.11.009
205. Sarne Y, Mechoulam R. Cannabinoids: between neuroprotection and neurotoxicity. Curr Drug Targets CNS Neurol Disord (2005) 4:677–84. doi:10.2174/156800705774933005
206. Bhattacharyya S, Fusar-Poli P, Borgwardt S, Martin-Santos R, Nosarti C, O’Carroll C, et al. Modulation of mediotemporal and ventrostriatal function in humans by Delta9-tetrahydrocannabinol: a neural basis for the effects of Cannabis sativa on learning and psychosis. Arch Gen Psychiatry (2009) 66:442–51. doi:10.1001/archgenpsychiatry.2009.17
207. Bhattacharyya S, Morrison PD, Fusar-Poli P, Martin-Santos R, Borgwardt S, Winton-Brown T, et al. Opposite effects of delta-9-tetrahydrocannabinol and cannabidiol on human brain function and psychopathology. Neuropsychopharmacology (2010) 35:764–74. doi:10.1038/npp.2009.184
208. Borgwardt SJ, Allen P, Bhattacharyya S, Fusar-Poli P, Crippa JA, Seal ML, et al. Neural basis of delta-9-tetrahydrocannabinol and cannabidiol: effects during response inhibition. Biol Psychiatry (2008) 64:966–73. doi:10.1016/j.biopsych.2008.05.011
209. Fox HC, Hong KI, Siedlarz K, Sinha R. Enhanced sensitivity to stress and drug/alcohol craving in abstinent cocaine-dependent individuals compared to social drinkers. Neuropsychopharmacology (2008) 33:796–805. doi:10.1038/sj.npp.1301470
210. Katona I, Freund TF. Endocannabinoid signaling as a synaptic circuit breaker in neurological disease. Nat Med (2008) 14:923–30. doi:10.1038/nm.f.1869
211. Lalumiere RT, Kalivas PW. Glutamate release in the nucleus accumbens core is necessary for heroin seeking. J Neurosci (2008) 28:3170–7. doi:10.1523/JNEUROSCI.5129-07.2008
212. Vann RE, Gamage TF, Warner JA, Marshall EM, Taylor NL, Martin BR, et al. Divergent effects of cannabidiol on the discriminative stimulus and place conditioning effects of Delta(9)-tetrahydrocannabinol. Drug Alcohol Depend (2008) 94:191–8. doi:10.1016/j.drugalcdep.2007.11.017
213. Malone DT, Jongejan D, Taylor DA. Cannabidiol reverses the reduction in social interaction produced by low dose Delta(9)-tetrahydrocannabinol in rats. Pharmacol Biochem Behav (2009) 93:91–6. doi:10.1016/j.pbb.2009.04.010
214. Karniol IG, Takahashi RN, Musty RE. Effects of delta9-tetrahydrocannabinol and cannabinol on operant performance in rats. Arch Int Pharmacodyn Ther (1974) 212:230–7.
215. Zuardi AW, Shirakawa I, Finkelfarb E, Karniol IG. Action of cannabidiol on the anxiety and other effects produced by delta 9-THC in normal subjects. Psychopharmacology (Berl) (1982) 76:245–50. doi:10.1007/BF00432554
216. Pertwee RG. Inverse agonism and neutral antagonism at cannabinoid CB1 receptors. Life Sci (2005) 76:1307–24. doi:10.1016/j.lfs.2004.10.025
217. Russo E, Guy GW. A tale of two cannabinoids: the therapeutic rationale for combining tetrahydrocannabinol and cannabidiol. Med Hypotheses (2006) 66:234–46. doi:10.1016/j.mehy.2005.08.026
218. Ren Y, Whittard J, Higuera-Matas A, Morris CV, Hurd YL. Cannabidiol, a nonpsychotropic component of cannabis, inhibits cue-induced heroin seeking and normalizes discrete mesolimbic neuronal disturbances. J Neurosci (2009) 29:14764–9. doi:10.1523/JNEUROSCI.4291-09.2009
219. Hamelink C, Hampson A, Wink DA, Eiden LE, Eskay RL. Comparison of cannabidiol, antioxidants, and diuretics in reversing binge ethanol-induced neurotoxicity. J Pharmacol Exp Ther (2005) 314:780–8. doi:10.1124/jpet.105.085779
220. Trouve R, Nahas G, Stuker O, Latour C. Antagonistic effects of two natural cannabinoids on the isolated heart. C R Seances Acad Sci III (1983) 297:191–4.
221. Nahas G, Trouve R. Effects and interactions of natural cannabinoids on the isolated heart. Proc Soc Exp Biol Med (1985) 180:312–6. doi:10.3181/00379727-180-42181
222. Agrawal A, Neale MC, Prescott CA, Kendler KS. Cannabis and other illicit drugs: comorbid use and abuse/dependence in males and females. Behav Genet (2004) 34:217–28. doi:10.1023/B:BEGE.0000017868.07829.45
223. Miller NS, Klahr AL, Gold MS, Sweeney K, Cocores JA. The prevalence of marijuana (cannabis) use and dependence in cocaine dependence. N Y State J Med (1990) 90:491–2.
224. Serrano A, Parsons LH. Endocannabinoid influence in drug reinforcement, dependence and addiction-related behaviors. Pharmacol Ther (2011) 132:215–41. doi:10.1016/j.pharmthera.2011.06.005
225. Fergusson DM, Boden JM, Horwood LJ. Cannabis use and other illicit drug use: testing the cannabis gateway hypothesis. Addiction (2006) 101:556–69. doi:10.1111/j.1360-0443.2005.01322.x
226. Kandel DB, Yamaguchi K, Klein LC. Testing the gateway hypothesis. Addiction (2006) 101:470–2. doi:10.1111/j.1360-0443.2006.01426.x discussion 474-476.
227. Lynskey MT, Vink JM, Boomsma DI. Early onset cannabis use and progression to other drug use in a sample of Dutch twins. Behav Genet (2006) 36:195–200. doi:10.1007/s10519-005-9023-x
228. Agrawal A, Lynskey MT, Bucholz KK, Martin NG, Madden PA, Heath AC. Contrasting models of genetic co-morbidity for cannabis and other illicit drugs in adult Australian twins. Psychol Med (2007) 37:49–60. doi:10.1017/S0033291706009287
229. Tomasiewicz HC, Jacobs MM, Wilkinson MB, Wilson SP, Nestler EJ, Hurd YL. Proenkephalin mediates the enduring effects of adolescent Cannabis exposure associated with adult opiate vulnerability. Biol Psychiatry (2012) 72(10):803–10. doi:10.1016/j.biopsych.2012.04.026
230. Mattay VS, Goldberg TE, Fera F, Hariri AR, Tessitore A, Egan MF, et al. Catechol O-methyltransferase val158-met genotype and individual variation in the brain response to amphetamine. Proc Natl Acad Sci U S A (2003) 100:6186–91. doi:10.1073/pnas.0931309100
231. Hariri AR, Tessitore A, Mattay VS, Fera F, Weinberger DR. The amygdala response to emotional stimuli: a comparison of faces and scenes. Neuroimage (2002) 17:317–23. doi:10.1006/nimg.2002.1179
232. Volkow ND, Fowler JS, Wolf AP, Schlyer D, Shiue CY, Alpert R, et al. Effects of chronic cocaine abuse on postsynaptic dopamine receptors. Am J Psychiatry (1990) 147:719–24.
233. Volkow ND, Fowler JS, Wang GJ, Hitzemann R, Logan J, Schlyer DJ, et al. Decreased dopamine D2 receptor availability is associated with reduced frontal metabolism in cocaine abusers. Synapse (1993) 14:169–77. doi:10.1002/syn.890140210
234. Volkow ND, Chang L, Wang GJ, Fowler JS, Ding YS, Sedler M, et al. Low level of brain dopamine D2 receptors in methamphetamine abusers: association with metabolism in the orbitofrontal cortex. Am J Psychiatry (2001) 158:2015–21. doi:10.1176/appi.ajp.158.12.2015
235. Andersen SL, Napierata L, Brenhouse HC, Sonntag KC. Juvenile methylphenidate modulates reward-related behaviors and cerebral blood flow by decreasing cortical D3 receptors. Eur J Neurosci (2008) 27:2962–72. doi:10.1111/j.1460-9568.2008.06254.x
236. Comings DE, Muhleman D, Gade R, Johnson P, Verde R, Saucier G, et al. Cannabinoid receptor gene (CNR1): association with i.v. drug use. Mol Psychiatry (1997) 2:161–8. doi:10.1038/sj.mp.4000247
237. Ballon N, Leroy S, Roy C, Bourdel MC, Charles-Nicolas A, Krebs MO, et al. (AAT)n repeat in the cannabinoid receptor gene (CNR1): association with cocaine addiction in an African-Caribbean population. Pharmacogenomics J (2006) 6:126–30. doi:10.1038/sj.tpj.6500352
238. Li YC, Korol AB, Fahima T, Nevo E. Microsatellites within genes: structure, function, and evolution. Mol Biol Evol (2004) 21:991–1007. doi:10.1093/molbev/msh073
239. Benyamina A, Kebir O, Blecha L, Reynaud M, Krebs MO. CNR1 gene polymorphisms in addictive disorders: a systematic review and a meta-analysis. Addict Biol (2011) 16:1–6. doi:10.1111/j.1369-1600.2009.00198.x
240. Barrero FJ, Ampuero I, Morales B, Vives F, De Dios Luna Del Castillo J, Hoenicka J, et al. Depression in Parkinson’s disease is related to a genetic polymorphism of the cannabinoid receptor gene (CNR1). Pharmacogenomics J (2005) 5:135–41. doi:10.1038/sj.tpj.6500301
241. Haughey HM, Marshall E, Schacht JP, Louis A, Hutchison KE. Marijuana withdrawal and craving: influence of the cannabinoid receptor 1 (CNR1) and fatty acid amide hydrolase (FAAH) genes. Addiction (2008) 103:1678–86. doi:10.1111/j.1360-0443.2008.02292.x
242. Filbey FM, Schacht JP, Myers US, Chavez RS, Hutchison KE. Individual and additive effects of the CNR1 and FAAH genes on brain response to marijuana cues. Neuropsychopharmacology (2010) 35:967–75. doi:10.1038/npp.2009.200
243. Lopez-Moreno JA, Echeverry-Alzate V, Buhler KM. The genetic basis of the endocannabinoid system and drug addiction in humans. J Psychopharmacol (2012) 26:133–43. doi:10.1177/0269881111416689
244. Clarke TK, Bloch PJ, Ambrose-Lanci LM, Ferraro TN, Berrettini WH, Kampman KM, et al. Further evidence for association of polymorphisms in the CNR1 gene with cocaine addiction: confirmation in an independent sample and meta-analysis. Addict Biol (2013) 18(4):702–8. doi:10.1111/j.1369-1600.2011.00346.x
245. Sipe JC, Chiang K, Gerber AL, Beutler E, Cravatt BF. A missense mutation in human fatty acid amide hydrolase associated with problem drug use. Proc Natl Acad Sci U S A (2002) 99:8394–9. doi:10.1073/pnas.082235799
246. Flanagan JM, Gerber AL, Cadet JL, Beutler E, Sipe JC. The fatty acid amide hydrolase 385 A/A (P129T) variant: haplotype analysis of an ancient missense mutation and validation of risk for drug addiction. Hum Genet (2006) 120:581–8. doi:10.1007/s00439-006-0250-x
247. Li CS, Milivojevic V, Constable RT, Sinha R. Recent cannabis abuse decreased stress-induced BOLD signals in the frontal and cingulate cortices of cocaine dependent individuals. Psychiatry Res (2005) 140:271–80. doi:10.1016/j.pscychresns.2005.09.002
248. Foltin RW, Fischman MW, Pippen PA, Kelly TH. Behavioral effects of cocaine alone and in combination with ethanol or marijuana in humans. Drug Alcohol Depend (1993) 32:93–106. doi:10.1016/0376-8716(93)80001-U
249. Lukas SE, Sholar M, Kouri E, Fukuzako H, Mendelson JH. Marihuana smoking increases plasma cocaine levels and subjective reports of euphoria in male volunteers. Pharmacol Biochem Behav (1994) 48:715–21. doi:10.1016/0091-3057(94)90338-7
250. Xi ZX, Spiller K, Pak AC, Gilbert J, Dillon C, Li X, et al. Cannabinoid CB1 receptor antagonists attenuate cocaine’s rewarding effects: experiments with self-administration and brain-stimulation reward in rats. Neuropsychopharmacology (2008) 33:1735–45. doi:10.1038/sj.npp.1301552
251. Vlachou S, Nomikos GG, Panagis G. WIN 55,212-2 decreases the reinforcing actions of cocaine through CB1 cannabinoid receptor stimulation. Behav Brain Res (2003) 141:215–22. doi:10.1016/S0166-4328(02)00370-4
252. Vlachou S, Stamatopoulou F, Nomikos GG, Panagis G. Enhancement of endocannabinoid neurotransmission through CB1 cannabinoid receptors counteracts the reinforcing and psychostimulant effects of cocaine. Int J Neuropsychopharmacol (2008) 11:905–23. doi:10.1017/S1461145708008717
253. Li X, Hoffman AF, Peng XQ, Lupica CR, Gardner EL, Xi ZX. Attenuation of basal and cocaine-enhanced locomotion and nucleus accumbens dopamine in cannabinoid CB1-receptor-knockout mice. Psychopharmacology (Berl) (2009) 204:1–11. doi:10.1007/s00213-008-1432-0
254. Cheer JF, Wassum KM, Sombers LA, Heien ML, Ariansen JL, Aragona BJ, et al. Phasic dopamine release evoked by abused substances requires cannabinoid receptor activation. J Neurosci (2007) 27:791–5. doi:10.1523/JNEUROSCI.4152-06.2007
255. Soria G, Mendizabal V, Tourino C, Robledo P, Ledent C, Parmentier M, et al. Lack of CB1 cannabinoid receptor impairs cocaine self-administration. Neuropsychopharmacology (2005) 30:1670–80. doi:10.1038/sj.npp.1300707
256. Caille S, Parsons LH. Cannabinoid modulation of opiate reinforcement through the ventral striatopallidal pathway. Neuropsychopharmacology (2006) 31:804–13. doi:10.1038/sj.npp.1300848
257. Xi ZX, Gilbert JG, Peng XQ, Pak AC, Li X, Gardner EL. Cannabinoid CB1 receptor antagonist AM251 inhibits cocaine-primed relapse in rats: role of glutamate in the nucleus accumbens. J Neurosci (2006) 26:8531–6. doi:10.1523/JNEUROSCI.0726-06.2006
258. Xi ZX, Peng XQ, Li X, Song R, Zhang HY, Liu QR, et al. Brain cannabinoid CB(2) receptors modulate cocaine’s actions in mice. Nat Neurosci (2011) 14:1160–6. doi:10.1038/nn.2874
259. Luchicchi A, Lecca S, Carta S, Pillolla G, Muntoni AL, Yasar S, et al. Effects of fatty acid amide hydrolase inhibition on neuronal responses to nicotine, cocaine and morphine in the nucleus accumbens shell and ventral tegmental area: involvement of PPAR-alpha nuclear receptors. Addict Biol (2010) 15:277–88. doi:10.1111/j.1369-1600.2010.00222.x
260. Justinova Z, Mangieri RA, Bortolato M, Chefer SI, Mukhin AG, Clapper JR, et al. Fatty acid amide hydrolase inhibition heightens anandamide signaling without producing reinforcing effects in primates. Biol Psychiatry (2008) 64:930–7. doi:10.1016/j.biopsych.2008.08.008
261. Adamczyk P, McCreary AC, Przegalinski E, Mierzejewski P, Bienkowski P, Filip M. The effects of fatty acid amide hydrolase inhibitors on maintenance of cocaine and food self-administration and on reinstatement of cocaine-seeking and food-taking behavior in rats. J Physiol Pharmacol (2009) 60:119–25.
262. Martin M, Ledent C, Parmentier M, Maldonado R, Valverde O. Cocaine, but not morphine, induces conditioned place preference and sensitization to locomotor responses in CB1 knockout mice. Eur J Neurosci (2000) 12:4038–46. doi:10.1046/j.1460-9568.2000.00287.x
263. Houchi H, Babovic D, Pierrefiche O, Ledent C, Daoust M, Naassila M. CB1 receptor knockout mice display reduced ethanol-induced conditioned place preference and increased striatal dopamine D2 receptors. Neuropsychopharmacology (2005) 30:339–49. doi:10.1038/sj.npp.1300568
264. Miller LL, Ward SJ, Dykstra LA. Chronic unpredictable stress enhances cocaine-conditioned place preference in type 1 cannabinoid receptor knockout mice. Behav Pharmacol (2008) 19:575–81. doi:10.1097/FBP.0b013e32830ded11
265. Chaperon F, Soubrie P, Puech AJ, Thiebot MH. Involvement of central cannabinoid (CB1) receptors in the establishment of place conditioning in rats. Psychopharmacology (Berl) (1998) 135:324–32. doi:10.1007/s002130050518
266. Lesscher HM, Hoogveld E, Burbach JP, Van Ree JM, Gerrits MA. Endogenous cannabinoids are not involved in cocaine reinforcement and development of cocaine-induced behavioural sensitization. Eur Neuropsychopharmacol (2005) 15:31–7. doi:10.1016/j.euroneuro.2004.04.003
267. Corbille AG, Valjent E, Marsicano G, Ledent C, Lutz B, Herve D, et al. Role of cannabinoid type 1 receptors in locomotor activity and striatal signaling in response to psychostimulants. J Neurosci (2007) 27:6937–47. doi:10.1523/JNEUROSCI.3936-06.2007
268. Gerdeman GL, Schechter JB, French ED. Context-specific reversal of cocaine sensitization by the CB1 cannabinoid receptor antagonist rimonabant. Neuropsychopharmacology (2008) 33:2747–59. doi:10.1038/sj.npp.1301648
269. Filip M, Golda A, Zaniewska M, McCreary AC, Nowak E, Kolasiewicz W, et al. Involvement of cannabinoid CB1 receptors in drug addiction: effects of rimonabant on behavioral responses induced by cocaine. Pharmacol Rep (2006) 58:806–19.
270. Przegalinski E, Gothert M, Frankowska M, Filip M. WIN 55,212-2-induced reduction of cocaine hyperlocomotion: possible inhibition of 5-HT(3) receptor function. Eur J Pharmacol (2005) 517:68–73. doi:10.1016/j.ejphar.2005.05.014
271. Aracil-Fernandez A, Trigo JM, Garcia-Gutierrez MS, Ortega-Alvaro A, Ternianov A, Navarro D, et al. Decreased cocaine motor sensitization and self-administration in mice overexpressing cannabinoid CB(2) receptors. Neuropsychopharmacology (2012) 37:1749–63. doi:10.1038/npp.2012.22
272. Panlilio LV, Solinas M, Matthews SA, Goldberg SR. Previous exposure to THC alters the reinforcing efficacy and anxiety-related effects of cocaine in rats. Neuropsychopharmacology (2007) 32:646–57. doi:10.1038/sj.npp.1301109
273. Gorriti MA, Rodriguez De Fonseca F, Navarro M, Palomo T. Chronic (-)-delta9-tetrahydrocannabinol treatment induces sensitization to the psychomotor effects of amphetamine in rats. Eur J Pharmacol (1999) 365:133–42. doi:10.1016/S0014-2999(98)00851-6
274. Wiskerke J, Pattij T, Schoffelmeer AN, De Vries TJ. The role of CB1 receptors in psychostimulant addiction. Addict Biol (2008) 13:225–38. doi:10.1111/j.1369-1600.2008.00109.x
275. Cossu G, Ledent C, Fattore L, Imperato A, Bohme GA, Parmentier M, et al. Cannabinoid CB1 receptor knockout mice fail to self-administer morphine but not other drugs of abuse. Behav Brain Res (2001) 118:61–5. doi:10.1016/S0166-4328(00)00311-9
276. Vaughn LK, Mantsch JR, Vranjkovic O, Stroh G, Lacourt M, Kreutter M, et al. Cannabinoid receptor involvement in stress-induced cocaine reinstatement: potential interaction with noradrenergic pathways. Neuroscience (2012) 204:117–24. doi:10.1016/j.neuroscience.2011.08.021
277. Adamczyk P, Miszkiel J, McCreary AC, Filip M, Papp M, Przegalinski E. The effects of cannabinoid CB1, CB2 and vanilloid TRPV1 receptor antagonists on cocaine addictive behavior in rats. Brain Res (2012) 1444:45–54. doi:10.1016/j.brainres.2012.01.030
278. Vinklerova J, Novakova J, Sulcova A. Inhibition of methamphetamine self-administration in rats by cannabinoid receptor antagonist AM 251. J Psychopharmacol (2002) 16:139–43. doi:10.1177/026988110201600204
279. Poncelet M, Barnouin MC, Breliere JC, Le Fur G, Soubrie P. Blockade of cannabinoid (CB1) receptors by 141716 selectively antagonizes drug-induced reinstatement of exploratory behaviour in gerbils. Psychopharmacology (Berl) (1999) 144:144–50. doi:10.1007/s002130050987
280. Tanda G, Munzar P, Goldberg SR. Self-administration behavior is maintained by the psychoactive ingredient of marijuana in squirrel monkeys. Nat Neurosci (2000) 3:1073–4. doi:10.1038/80577
281. Yu LL, Zhou SJ, Wang XY, Liu JF, Xue YX, Jiang W, et al. Effects of cannabinoid CB(1) receptor antagonist rimonabant on acquisition and reinstatement of psychostimulant reward memory in mice. Behav Brain Res (2011) 217:111–6. doi:10.1016/j.bbr.2010.10.008
282. Ward SJ, Rosenberg M, Dykstra LA, Walker EA. The CB1 antagonist rimonabant (SR141716) blocks cue-induced reinstatement of cocaine seeking and other context and extinction phenomena predictive of relapse. Drug Alcohol Depend (2009) 105:248–55. doi:10.1016/j.drugalcdep.2009.07.002
283. De Vries TJ, Shaham Y, Homberg JR, Crombag H, Schuurman K, Dieben J, et al. A cannabinoid mechanism in relapse to cocaine seeking. Nat Med (2001) 7:1151–4. doi:10.1038/nm1001-1151
284. Orio L, Edwards S, George O, Parsons LH, Koob GF. A role for the endocannabinoid system in the increased motivation for cocaine in extended-access conditions. J Neurosci (2009) 29:4846–57. doi:10.1523/JNEUROSCI.0563-09.2009
285. Daza-Losada M, Minarro J, Aguilar MA, Valverde O, Rodriguez-Arias M. Acute blockade of CB1 receptor leads to reinstatement of MDMA-induced conditioned place preference. Pharmacol Biochem Behav (2011) 100:33–9. doi:10.1016/j.pbb.2011.07.011
286. Rodriguez-Arias M, Manzanedo C, Roger-Sanchez C, Do Couto BR, Aguilar MA, Minarro J. Effect of adolescent exposure to WIN 55212-2 on the acquisition and reinstatement of MDMA-induced conditioned place preference. Prog Neuropsychopharmacol Biol Psychiatry (2010) 34:166–71. doi:10.1016/j.pnpbp.2009.10.019
287. Braida D, Iosue S, Pegorini S, Sala M. 3,4 Methylenedioxymethamphetamine-induced conditioned place preference (CPP) is mediated by endocannabinoid system. Pharmacol Res (2005) 51:177–82. doi:10.1016/j.phrs.2004.07.009
288. Braida D, Sala M. Role of the endocannabinoid system in MDMA intracerebral self-administration in rats. Br J Pharmacol (2002) 136:1089–92. doi:10.1038/sj.bjp.0704825
289. Anggadiredja K, Nakamichi M, Hiranita T, Tanaka H, Shoyama Y, Watanabe S, et al. Endocannabinoid system modulates relapse to methamphetamine seeking: possible mediation by the arachidonic acid cascade. Neuropsychopharmacology (2004) 29:1470–8. doi:10.1038/sj.npp.1300454
290. Parker LA, Burton P, Sorge RE, Yakiwchuk C, Mechoulam R. Effect of low doses of delta9-tetrahydrocannabinol and cannabidiol on the extinction of cocaine-induced and amphetamine-induced conditioned place preference learning in rats. Psychopharmacology (Berl) (2004) 175:360–6. doi:10.1007/s00213-004-1825-7
291. Higuera-Matas A, Soto-Montenegro ML, Del Olmo N, Miguens M, Torres I, Vaquero JJ, et al. Augmented acquisition of cocaine self-administration and altered brain glucose metabolism in adult female but not male rats exposed to a cannabinoid agonist during adolescence. Neuropsychopharmacology (2008) 33:806–13. doi:10.1038/sj.npp.1301467
292. Fattore L, Martellotta MC, Cossu G, Mascia MS, Fratta W. CB1 cannabinoid receptor agonist WIN 55,212-2 decreases intravenous cocaine self-administration in rats. Behav Brain Res (1999) 104:141–6. doi:10.1016/S0166-4328(99)00059-5
293. Sinha R, Fuse T, Aubin LR, O’Malley SS. Psychological stress, drug-related cues and cocaine craving. Psychopharmacology (Berl) (2000) 152:140–8. doi:10.1007/s002130000499
Keywords: addiction, stimulants, psychostimulants, cocaine, cannabis, cannabinoids or endocannabinoids
Citation: Olière S, Jolette-Riopel A, Potvin S and Jutras-Aswad D (2013) Modulation of the endocannabinoid system: vulnerability factor and new treatment target for stimulant addiction. Front. Psychiatry 4:109. doi: 10.3389/fpsyt.2013.00109
Received: 30 May 2013; Accepted: 02 September 2013;
Published online: 23 September 2013.
Edited by:
Elizabeth Clare Temple, University of Ballarat, AustraliaReviewed by:
Elizabeth Clare Temple, University of Ballarat, AustraliaLuigi Janiri, Università Cattolica del S. Cuore, Italy
Copyright: © 2013 Olière, Jolette-Riopel, Potvin and Jutras-Aswad. This is an open-access article distributed under the terms of the Creative Commons Attribution License (CC BY). The use, distribution or reproduction in other forums is permitted, provided the original author(s) or licensor are credited and that the original publication in this journal is cited, in accordance with accepted academic practice. No use, distribution or reproduction is permitted which does not comply with these terms.
*Correspondence: Didier Jutras-Aswad, CRCHUM, St-Luc Hospital, Édouard-Asselin Pavilion, 264 René-Lévesque Blvd. East, Montreal, QC H2X 1P1, Canada e-mail:ZGlkaWVyLmp1dHJhcy1hc3dhZEB1bW9udHJlYWwuY2E=