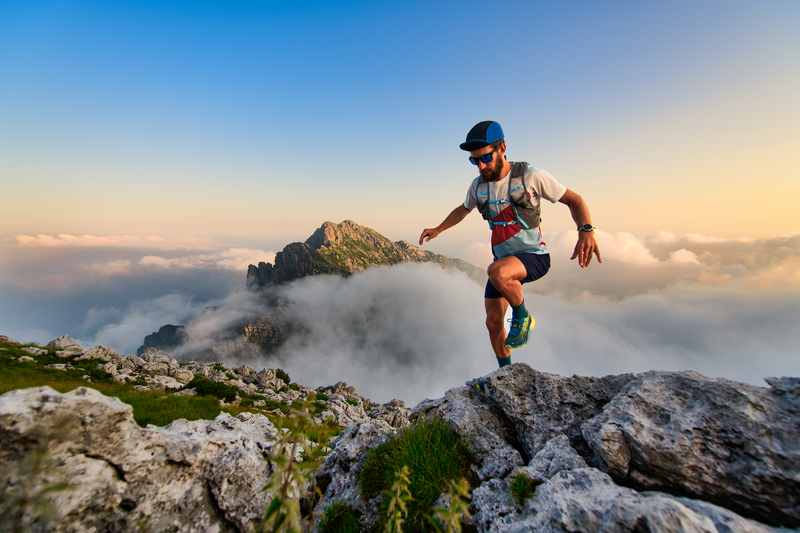
94% of researchers rate our articles as excellent or good
Learn more about the work of our research integrity team to safeguard the quality of each article we publish.
Find out more
REVIEW article
Front. Plant Sci. , 05 February 2025
Sec. Plant Biotechnology
Volume 16 - 2025 | https://doi.org/10.3389/fpls.2025.1540693
Male sterility forms the foundation of hybrid seed production technology in field crops. A variety of genetically controlled male sterility/fertility systems starting with cytoplasmic male sterility (CMS), genic male sterility (GMS) including conditional male sterility and transgenic-based male sterility have been developed and deployed for heterosis breeding over the past century. Here we review environment-sensitive genic male sterility (EGMS) and biotechnology-based male sterility systems and describe the underlying molecular mechanisms. Advances in crop genomics and discovery of a large number of nuclear genes governing anther/pollen development, which are shared across species, are helping design diverse types of male sterile lines suitable for different crop species and situations. In particular, gene editing offers quick and easy route to develop novel male sterility systems for hybrid seed production. We discuss the advantages and challenges of biotechnology-based male sterility systems and present alternative strategies to address concerns of transgenics. Finally, we propose development of functional male sterility systems based on pollen competition as the future area that holds great promise for heterosis breeding.
Plant male sterility is a condition where the plant fails to produce functional pollen. This failure could be due to defects in anther specification and differentiation, abnormal microsporogenesis and pollen development, non-dehiscent anthers, or the inability of pollen to germinate and fertilize the female gametes (Pei et al., 2024). Male-sterile female lines form the foundation of large-scale hybrid seed production in various crop species. Such hybrids with superior agronomic traits such as higher yield, enhanced disease resistance and stress tolerance have greatly contributed to food, feed and nutritional security (Kim and Zhang, 2018). To date, male sterility has been reported in ~617 plant species, including crops like rice, wheat, Indian mustard, cotton, soybean, and Sorghum (Chen and Liu, 2014; Vasupalli et al., 2021; Ren et al., 2022) and tree species like bamboo (De Souza et al., 2020), olive (Besnard et al., 2000), rubber (Shearman et al., 2014). Besides its utility in F1 seed production, male sterility might be an essential feature of plant evolution and adaptation (Budar and Pelletier, 2001).
Various kinds of male sterile lines have been identified/developed to date, such as cytoplasmic male sterility (CMS), genic male sterility (GMS), Environment-sensitive GMS (EGMS), and biotechnology-based male sterile (BBMS) lines. CMS is a maternally inherited trait governed by the mitochondrial genome and rescued by the nuclear genes (Vasupalli et al., 2016). Although CMS has been used commercially in many crops, lack of stable restorer genes and cytoplasmic penalty have severely restricted the expansion of CMS to new crops (Kumar et al., 2012; Chamola et al., 2013). GMS, which is controlled by only nuclear genes, can overcome these disadvantages (Wan et al., 2019). However, the primary disadvantage is that pure male sterile stocks cannot be perpetuated (Anjani, 2005). EGMS is a conditional male sterile system where male sterility/fertility is influenced by environmental conditions such as photoperiod, temperature and humidity. Thus, by growing plants under appropriate environmental conditions, the same stock can be used as male sterile or fertile to produce F1 hybrid seeds or for maintenance, respectively (Peng et al., 2023) (Figure 1). Therefore, EGMS systems are preferred for hybrid seed production and widely adopted especially in China. Further, the discovery of GMS genes and advancements in genetic transformation/gene editing technologies have led to the development of BBMS lines (Wu et al., 2016). In this review, we present a brief introduction to GMS and provide in-depth details of molecular mechanisms of EGMS. Further, we also provide recent developments in BBMS systems for hybrid seed production in crop plants.
Figure 1. Hybrid breeding using two-line genic or environmental genic male sterility system. (A) Genic male sterility (GMS) system comprises of homozygous (ms/ms) male sterile line, hemizygous (Ms/ms) male fertile maintainer line and homozygous (Ms/Ms) wild type line. A cross between the male sterile and the maintainer line yields 50% male sterile and 50% male fertile progenies, from which male sterile plants are used for hybrid seed production by crossing with wild type (Ms/Ms) parent. The other 50% male fertile plants serve as the maintainer line for the next cycle of multiplication of the male sterile line. (B) In the EGMS system, the male sterile line (ms/ms) is multiplied by growing under conditions where it becomes male fertile. For hybrid seed production, the male sterile line is raised under conditions favoring male sterility and crossed with wild type (Ms/Ms) male fertile line. (C) The three-line cytoplasmic male sterility (CMS) system comprises of the male-sterility-inducing line (A line), the maintainer line (B line) and the restorer line (R line). The A line has CMS-inducing mitochondrial genome but lacks the nuclear restorer gene. The B line is isonuclear to the A line, and has normal mitochondria. The R line carries the nuclear Rf gene and is the male parent of the hybrid. A cross between A X B lines generates all male sterile progeny that could be used either to produce hybrid or to multiply the male steriles. A cross between the A X R lines generates the fertile F1 hybrid seeds.
GMS controlled by nuclear genes is widely reported in plants, which is not surprising considering that anther/pollen differentiation and development involve the interplay of many genes (Cheng et al., 2020). Anther/pollen development comprises different phases viz., archesporial cell specification, somatic differentiation, pollen mother cell meiosis, tapetum development and mature pollen development, and involves cell division, differentiation, development, degradation, and maturation (Figure 2). Anther/pollen development by far needs more genes than any other plant organ, and mutations of these genes often lead to complete or partial male sterility (Marchant and Walbot, 2022). Archesporial cells are the premeiotic cells that develop into the plant germline cells, which ultimately develop into gametes. Therefore, defects in archesporial cell development lead to genic male sterility (Zhou et al., 2017). The genes reported to cause GMS in archesporial cell development are OsMIL1 (Hong et al., 2012b), ZmMSCA1 (Chaubal et al., 2003), AtROXY1/2 (Xing and Zachgo, 2008) and AtTGA9/10 (Murmu et al., 2010). After the archesporial cells specification, somatic cell differentiation takes place. During somatic cell division, many genes are reported to be involved in causing GMS. For example, AtEMS1/EXS (Zhao et al., 2002), AtTPD1 (Yang et al., 2005), OsMSP1 (Nonomura et al., 2003), OsMIL2 (Hong et al., 2012a), OsTIP2/bHLH142 (Fu et al., 2014), ZmMAC1 (Wang et al., 2012), ZmOCL4 (Vernoud et al., 2009), and ZmMs23 (Nan et al., 2017). The four somatic layers, epidermis, endothecium, middle layer and tapetum, are formed from the periclinal somatic cell division. The tapetum is the innermost layer that encircles the developing pollen mother cell and provides the nutrients required for pollen development and also supplies important proteins that coat the surface of pollen grains. Thus, any defect in the development of the tapetum layer causes GMS. Further, tapetum differentiation and meiosis occur simultaneously during anther development. Some of the genes reported that cause defects in tapetum development and pollen mother cell meiosis are OsTDF1 (Cai et al., 2015), OsUDT1 (Jung et al., 2005), ZmMS32 (Moon et al., 2013a), AtDYT1 (Zhang et al., 2006), AtMYB33/65 (Millar and Gubler, 2005), OsGAMYB (Liu et al., 2010b), AtNEF1 (Ariizumi et al., 2004) etc. Besides, callose deposition on the newly formed tetrad microspores and timely callose degradation to release the tetrad microspores are crucial in pollen development. Mutations in genes associated with callose metabolism such as OsDMD1 (Ren et al., 2020), OsGSL1, OsGSL5 (Enns et al., 2005), OsLecRK5 (Wang et al., 2020), OsG1 (Wan et al., 2011), AtCDM1 (Lu et al., 2014), AtCalS5 (Dong et al., 2005) have been shown to cause GMS. Further, genes acting at later stages of pollen development, namely, AtMS1/2 (Ito and Shinozaki, 2002; Chen et al., 2011a), AtMYB26 (Steiner-Lange et al., 2003), AtMGP1 (Li et al., 2010), AtSK32 (Dong et al., 2015), OsGT1 (Moon et al., 2013b), OsDTC1 (Yi et al., 2016), and ZmIPE1 (Chen et al., 2017) have been identified whose loss-of-function lead to male sterility. Post anthesis, successful pollen germination on the stigma involves crucial steps such as adhesion, hydration, germination and tube growth. A few genes, such as AtMSL8 (Hamilton and Haswell, 2017), AtAPY1/2 (Wolf et al., 2007), AtSEC8 (Cole et al., 2005), and OsHXK5 (Lee et al., 2020), are reported to be involved in mature pollen interaction with stigma and germination, and mutations in these genes result in male sterility.
Figure 2. Genes causing male sterility identified in different plant species and their expression at various stages of pollen development. Different stages of pollen development indicated include specification of the archesporial cell, anther somatic cell, pollen mother cell, meiosis and tapetum development (microsporocyte, Meiosis I & II), pollen maturation (pollen mitosis I & II) and pollen-stigma interaction and pollen germination. Various male-sterility-causing genes identified at different stages of pollen development are shown in the ash color boxes.
Unlike CMS, GMS requires only two lines for hybrid seed production (Figure 1). Since all normal lines can serve as restorers, GMS permits the testing of a vast pool of germplasm for the exploitation of heterosis. Besides, there is no cytoplasmic effect. The main hindrance, however, is that a complete male sterile population cannot be obtained, and male sterile plants have to be isolated from a 1:1 mixture of sterile and fertile progeny. Further, as in CMS, two separate isolated fields are needed one each for hybrid seed production and multiplication of male steriles. Nevertheless, GMS has been used for hybrid seed production in some crops such as cotton, cowpea, and vegetable Brassica. To circumvent the problem of isolating male steriles from mixture, conditional male sterile lines were identified which could produce fertile pollen under permissible conditions thereby giving rise to all male sterile offspring. Such conditional male sterile systems form the basis of Environment-sensitive GMS (EGMS) systems, and their molecular mechanisms are discussed below.
Genes regulating anther development which respond to environmental stimuli will express male sterility under certain environment and are therefore referred to as EGMS (Fan and Zhang, 2018). EGMS was first identified in rice Nongkeng58S (NK58S) by Shi in 1973 (Peng et al., 2023). So far, four types of EGMS responding to photoperiod, temperature, nitrogen status or humidity have been described (Peng et al., 2023). Generally, photosensitive GMS (PGMS) is characterized by male sterility under long days and male fertility under short days. In contrast, ‘reverse PGMS’ is male sterile during short days and male fertile during long days. Thermo-sensitive GMS (TGMS) is characterized by male sterility under high temperature (exceeding a threshold temperature) and male fertility under low temperatures (below a critical sterility-inducing temperature). On the other hand, ‘reverse TGMS’ exhibits the opposite phenotype. Male sterility caused by nitrogen deficiency is known as nitrogen-sensitive genic male sterility (NGMS), where male fertility is observed under conditions of ample nitrogen supply. Additionally, male sterility under low humidity conditions and male fertility under high humidity conditions is referred to as humidity-sensitive genic male sterility (HGMS). Thus, EGMS behaves as male sterile or male fertile under different conditions and, therefore, could be easily maintained by selfing and used for hybrid seed production under appropriate conditions. Hence, EGMS-based pollination control systems are called two-line systems.
EGMS two-line hybrid system (Figure 1) offers distinct advantages over CMS or GMS, which include ease of maintenance of male steriles, ready availability of restorers, and male sterility being recessive, the F1s will be fertile under all conditions (Chen et al., 2023; Peng et al., 2023). These features allow rapid improvement of parental lines for quality, stress tolerance etc. However, two-line systems have limitations, such as the need for different locations with suitable environmental conditions, and vulnerability to sudden fluctuations in weather patterns. Further, different environmental conditions may also have adverse effects, such as reduced plant vigor, low yield of hybrid or parental seeds (Chen et al., 2023). Despite these limitations, two-line hybrids have been particularly exploited in China for breeding hybrid rice and contributed significantly to enhance rice production and productivity.
EGMS has been reported in numerous plant species, such as Arabidopsis (P/TGMS Acos5-2, TGMS Res1 Rpg1, HGMS Cer1-1, Cer1-m) (Aarts et al., 1995; Zhang et al., 2020a; Zhu et al., 2020), rice (PGMS NK58S, TGMS AnS-1, Zhu1S, HGMS hms1) (Zhou et al., 2014; Fan et al., 2016; Chen et al., 2020; Wu et al., 2022), maize (TGMS TMS5, MAGOKD) (Li et al., 2017; Lee et al., 2021), soybean (rPGMS MS3‐KO) (Hou et al., 2022), wheat (TGMS YanZhan 4110S, P/TGMS K78S and K456S) (Li et al., 2020; Yang et al., 2021), millet (P/TGMS A2) (Wei et al., 2021), tomato (TGMS Da107) (Yu et al., 2015), sorghum (TGMS Ji 130A) (Ma et al., 2012), rapeseed (TGMS TE5A) (Yan et al., 2016), cotton (PGMS CCRI9106) (Zhang et al., 2020b) and barley (rTGMS HvMS1OEx) (Fernandez-Gomez et al., 2020) (Table 1). The EGMS genes were identified by fine mapping, map-based cloning, or transcriptome analysis. At least 18 TGMS, five rTGMS, six PGMS, three rPGMS, three P/TGMS, one NGMS and four HGMS genes have been identified in rice (Table 1). Similarly, in other plant species, such as Arabidopsis, maize, wheat, soybean, barley, millet and rapeseed, numerous EGMS genes have been identified. The characterization of EGMS genes not only helps devise two-line hybrid systems but also provides a better understanding of plant-environment interactions influencing male reproductive development (Liu et al., 2023). Molecular analyses of various EGMS lines have revealed differential gene regulation operating at different levels such as transcription, translation or post translation, and involving non-coding RNA as responsible for male sterility/fertility transition. Here we focus on the molecular mechanisms of various EGMS systems.
Non-coding RNAs (ncRNAs) play a pivotal role in governing P/TGMS in rice. The first PGMS rice line, NK58S, has a highly complex genetic mechanism. Both photoperiod and temperature act in a complementary manner to induce male sterility in NK58S line. Male sterility expression in NKS58S requires >13.75 h day length and >29°C temperature. In contrast, in indica background, PA64S, male sterility is predominantly governed by temperature which highlights the role of genetic background on expression of EGMS (Zhou et al., 2012; Fan and Zhang, 2018). PGMS in NK58S is governed by pms1 and pms3 (Zhang et al., 1994; Mei et al., 1999). pms1 is a semi-dominant allele that codes for a long non-coding RNA (lncRNA) named PMS1T (Figure 3A). Under long-day conditions, PMS1 transcript is targeted by miR2118, leading to the generation of 21-nt phased secondary small interfering RNAs (phasiRNAs). A single nucleotide mutation G to A in pms1 near the miR2118 recognition site alters the secondary structure of pms1 transcript, leading to a decrease in the accumulation of phasiRNAs. Thus, miR2118 seems to effectively cleave PMS1T under long daylight, increasing the production of 21-nt phasiRNAs. These phasiRNAs, in turn, target downstream anther-related genes leading to pre-mature programmed cell death of the tapetum (Fan et al., 2016).
Figure 3. Molecular mechanisms of various EGMS systems used in two-line hybrid breeding. (A) Molecular mechanism of Photosensitive GMS (PGMS) involving male sterility genes pms1, pms3 and csa. Under long-day conditions, an SNP in the pms1 leads to differential expression of phasiRNAs, leading to male sterility (left). An SNP in the siRNA gene ldmar/pms3 leads to methylation of its promoter region under long-day conditions, thereby reducing siRNA production, which in turn leads to male sterility (middle). Under short days, a csa mutant (a transcription factor) leads to reduced OsMST8 expression, causing abnormal sugar partition and thereby disrupting pollen development (right). (B) Molecular mechanism of thermo-sensitive GMS (TGMS) caused by tms5 and ugp1 genes. An SNP mutation in the tms5 gene coding for an RNase ZS1 disrupts the processing of its cognate UbL40 mRNAs under high-temperature conditions, leading to male sterile phenotype (left). UGP1 gene is involved in callose deposition during pollen development. ugp1 mutant plants are male sterile at high temperatures due to failed processing of primary transcript containing introns. They become male fertile under low temperatures where primary transcripts are processed to remove introns (middle). (C) Molecular mechanism of humidity-sensitive GMS (HGMS) involving the male sterility gene hms1. The hms1 mutant shows abnormal pollen coat development at low humid conditions, leading to male sterility phenotype, which can be rescued at high humid conditions.
The PMS3 locus also encodes a lncRNA, Long-day-specific male-fertility-associated RNA (LDMAR) (Ding et al., 2012a; Ding et al., 2012b). The pms3 allele has a single nucleotide substitution C to G. Under long-day conditions, effective pollen development requires elevated LDMAR expression. However, under long-day conditions, the single nucleotide mutation in pms3 alters the secondary structure of LDMAR. This in turn increases LDMAR promoter methylation, consequently decreasing the production of LDMAR and causing pollen abortion (Figure 3A) (Ding et al., 2012b). Another gene p/tms12 gene is also present at the PMS3 locus. The P/TMS12-1 encodes a lncRNA, which generates a 21 nt small RNA named osa-smR5864w. A single nucleotide substitution in p/tms12 leads to TGMS and PGMS in indica and japonica rice, respectively (Zhou et al., 2012). These discoveries underscore the role of the non-coding RNA in modulating PGMS/TGMS in rice. Despite cloning and examining their molecular mechanisms, how pms1 and pms3 (p/tms121) govern pollen mother cell and tapetum growth and their potential genetic interplay in indica and japonica lines remain to be elucidated.
Besides the lncRNA, 24-nt phasiRNAs were also identified to regulate P/TGMS. The monocot-specific DICER-LIKE 5 (DCL5) is involved in generating 24-nt phasiRNAs. In maize, the dcl5 mutants show differential accumulation of the 24 nt phasiRNAs in tapetal cells under high- and low-temperature environments. At temperatures ≥28°C, dcl5 mutants show delayed/arrested tapetum development, leading to abnormal meiosis, which results in pollen abortion. However, at temperatures ≤26°C, dcl5 mutants are partially or entirely fertile (Teng et al., 2020). Moreover, anther wall specific ARGONAUTE 1d (AGO1d) mediates phasiRNA biosynthesis and function by interacting with miR2118, miR2275, and miR2118-triggered 21-nt phasiRNAs through a 5′ uridine. In rice, the ago1d mutants show reduced levels of 21- and 24-nt phasiRNAs and rTGMS phenotype. At ~22°C, ago1d mutants show faulty tapetal cell PCD and meiosis, which leads to male sterility. However, at ~28°C, ago1d mutants could restore fertility (Shi et al., 2022; Si et al., 2023). Similarly, in maize, MALE ASSOCIATED ARGONAUTE-1 and -2 (MAGO1 and MAGO2) are associated with 21-nt heat-activated phasiRNA (Hphasi), which are vital for normal pollen development under heat stress. To heat response, a large number of 21-nt Hphasi RNAs are accumulated in the wild type in comparison to RNA interference lines of MAGO1 and MAGO2, causing male fertility at low temperatures (28°C/25°C day/night) and sterility under heat stress (35°C/25°C day/night) in RNAi lines (Lee et al., 2021).
A network of transcription factors operating at different stages control anther development. Several conserved transcription factors across plant species have been shown to be involved in P/TGMS. TGMS trait in commercially used rice lines Hengnong S‐1 and Tian1S is caused by alleles tms9-1 and OsMS1wenmin1, respectively. The candidate gene identified for tms9-1 is OsMS1 (Qi et al., 2014). The OsMS1 is a PHD-finger transcription factor that contains the nuclear localization signal, LXXLL motif (Qi et al., 2014; Wu et al., 2022). The T‐to‐C substitution within the LXXLL motif of the OsMS1wenmin1 leads to Leu‐to‐Pro amino acid change that confers the TGMS trait (Wu et al., 2022). As a consequence, the mutant protein OsMS1wenmin1 is distributed in both the nucleus and cytoplasm, unlike the OsMS1 protein, which is restricted to the nucleus, thereby causing TGMS. At high temperatures (30°C), OsMS1wenmin1 is less abundant than OsMS1 in the nucleus. Further, OsMS1 and OsMS1wenmin1 proteins can bind in a temperature-dependent manner to the promoter of the ETERNAL TAPETUM 1 (EAT1) gene and by interacting with TAPETUM DEGENERATION RETARDATION (TDR) regulate downstream genes (Wu et al., 2022). In soybean, a spontaneous mutation in MS3, an Ostms9-1/AtMS1 ortholog, has intriguingly led to reverse PGMS, expressing male sterility under short days and fertility restoration under long days (Hou et al., 2022). Similarly, overexpression of the barley ortholog HvMS1 exhibits a reverse TGMS, being sterile at <15°C and fertile at >20°C (Fernandez-Gomez et al., 2020). However, mutant alleles of ms1 that lack the LXXLL motif and PHD domain exhibit male sterility independent of temperature and photoperiod in diverse plant species (Ito et al., 2007; Li et al., 2011; Fernandez Gomez and Wilson, 2014; Yang et al., 2019; An et al., 2020). These studies clearly demonstrate the conserved functions of these genes across monocot and dicot species and highlight the role of LXXLL and PHD domain in temperature/photoperiod sensitive male sterility/fertility. This also points to opportunity to engineer similar male sterility in other species.
In rice, the carbon-starved anther (CSA) and its paralog CSA2 encoding, respectively, R2R3 MYB and MYB transcription factors control sugar partition during development of male reproductive organs in response to photoperiod (Zhang et al., 2010, Zhang et al., 2013; Wang et al., 2021). Mutations in either of these genes display PGMS. The csa mutant, a reverse PGMS line, exhibits male sterility during short days and male fertility during long days (Figure 3A) (Zhang et al., 2010; Zhang et al., 2013). In contrast, csa2 mutant displays partial sterility during extended daylight periods and complete fertility under shorter day period (Wang et al., 2021). Besides this, under long-day conditions, the csa-csa2 double mutant exhibits semi-sterility, suggesting dominant epistasis of csa2. Under short-day conditions, the double mutant is entirely sterile, mirroring the characteristics of csa. The CSA exerts direct regulation over the monosaccharide transporter gene OsMST8, an essential gene in the apoplastic sugar transport pathway, whereas CSA2 exerts indirect regulation over OsMST8 (Zhang et al., 2010; Wang et al., 2021). In short-day scenario, the csa mutant experiences a notable reduction in the expression of OsMST8, coupled with impaired carbohydrate transport from flag leaves to anthers, culminating in male sterility. Whereas during long-day conditions, csa2 mutants exhibit suboptimal sugar transport from flag leaves to anthers, resulting in partial male sterility (Zhang et al., 2010; Wang et al., 2021). These outcomes underscore the shared molecular functions of CSA and CSA2 in influencing pollen development through orchestrated regulation of sugar transport from leaves to anthers, responsive to varying photoperiods.
Temperature-dependent mRNA splicing and degradation have also been identified as molecular mechanisms controlling TGMS. The AnnongS‐1 (AnS‐1) is the pioneer indica line that shows male fertility at <24°C and male sterility at >26°C. A single recessive gene, tms5, governs this TGMS trait. The TMS5 gene encodes RNase ZS1, which cleaves three ubiquitin-ribosomal L40 (UbL40) mRNAs in the pollen mother cell. A nucleotide substitution from C to A at 71st position in the tms5 gene leads to male sterility at high temperatures due to the lack of function of RNase ZS1, leading to excessive accumulation of UbL40 mRNAs. However, under permissive temperature, RNase ZS1 is able to process the UbL40 mRNAs like in the TMS5 line, so its pollen fertility is normal (Figure 3B) (Zhou et al., 2014). Further, UDP-Glucose Pyrophosphorylase1 (Ugp1) expresses during anther development and catalyzes the production of UDP-glucose and pyrophosphate. The Ugp1-silenced, or -overexpression rice plants are male sterile. The Ugp1 overexpressing plants are male sterile because of the impaired splicing of endogenous Ugp1 primary mRNA. However, these plants revert to fertility at low temperatures (≤21°C) and under short day conditions (<12.5 h) due to more efficient splicing of Ugp1 primary mRNA (Figure 3B) (Chen et al., 2007).
Rice TGMS gene OsTMS15 encodes a Leucine‐rich repeat receptor‐like kinase (LRR–RLK) protein named MULTIPLE SPOROCYTE1 (MSP1). The TIR motif in the LRR region of MSP1 protein interacts with its ligand OsTDL1A to initiate tapetum development to support pollen formation. However, a point mutation in the TIR motif leads to TGMS phenotype in the ostms15 mutant. At temperatures >29°C, the interaction of MSP1 and OsTDL1A proteins is reduced, causing male sterility. Whereas at lower temperatures <23°C, slow developmental rate and partial recovery of MSP1 and OsTDL1A interaction restore fertility to ostms15 mutant (Yang et al., 2016; Han et al., 2023). Similar to OsTMS15, another two LRR–RLK proteins TMS10, and its homolog TMS10L, control pollen and postmeiotic tapetum development. The kinase activity of TMS10 plays a crucial role at higher temperatures in maintaining pollen viability. The tms10 mutants exhibit male sterility at high temperatures (25-32°C) and male fertility at low temperatures (22-24°C) (Yu et al., 2017). Conversely, the tms10l mutant displays normal fertility at both high and low temperatures. However, double mutants (tms10 tms10l) exhibit male sterility at both temperatures, suggesting their functional redundancy in pollen development under low temperatures (Yu et al., 2017).
The pollen wall is the most complex of any plant cell wall, protecting the pollen from damage and desiccation during its dispersal from anther to stigma (Blackmore et al., 2007; Xue et al., 2018). The pollen wall contains the inner intine and the outer exine layers with the exine coated with sporopollenin, a major biopolymer containing very-long-chain fatty acids (VLCFAs). Disruption of genes involved in sporopollenin synthesis leads to TGMS phenotype. For example, the rice OsTMS18 gene encoding a glucose-methanol-choline (GMC) oxidoreductase is required pollen wall formation. Further, OsTMS18 is regulated by tapetal transcription factor OsMS188 (Zhang et al., 2022b). Whereas the upstream tapetum development regulator OsTDR regulates the OSMS188 through direct interaction with it (Han et al., 2021). A point mutation in ostms18 leading to Gly to Ser amino acid change, confers TGMS phenotype. The ostms18 mutant exhibits defective pollen exine wall at high temperatures (≥ 28°C). Under low temperatures (≤ 24°C), pollen double exine layer forms, leading to viable pollen and eventually fertility restoration (Zhang et al., 2022b). While the knocked out osms188 lines exhibit altered PCD mechanism of the tapetum layer, defective anther cuticle and also absence of the sexine layer cause complete male sterility (Han et al., 2021). Similarly, a wheat rTGMS line BS366 is fertile at ≥20°C, and exposure to ≤10°C for five days at the PMC stage leads to male sterility (Tang et al., 2011). A TaSCULP1 gene is expressed during sporopollenin assembly and plays a crucial role in sporopollenin p-coumaroylation and maturing exine. The overexpression of the TaSCULP1 gene in the BS366 line restores the integrity of exine and fertility at low temperatures (Xu et al., 2023).
Pollination process begins with the adhesion of pollen exine to the stigma, followed by hydration and germination of the pollen grain (Wheeler et al., 2001). Therefore, mutations in genes involved in pollen adhesion and hydration can cause male sterility. Further, in some cases, high humidity conditions can rescue the male fertility, generating HGMS lines. For example, rice OsOSC12 encodes a grass-species-specific triterpene synthase that catalyzes the cyclization of 2,3-oxidosqualene into poaceatapetol (Xue et al., 2018). Pollen grains of ososc12 mutant at ambient or low humid conditions (<60%) show rapid dehydration, leading to male sterility. This desiccation is reduced at high humidity (>80%) thereby make pollen fertile. The male sterility is attributed to reduced synthesis of C16 and C18 fatty acids, triterpene esters and sterols, leading to ineffective adhesion and hydration (Xue et al., 2018). Similarly, OsCER1/OsGL1-4 synthesizes the C25 and C27 long-chain alkanes. The oscer1/osgl1-4 mutants anthers show defective adhesion and hydration leading to male sterility under ambient humidity conditions. In contrast, high humid conditions rescue male fertility by increasing pollen adhesion and hydration (Yu et al., 2019). The OsCER2/OsHMS1 encoding a β-ketoacyl CoA synthase interacts with HMS1I and catalyzes the biosynthesis of C26 and C28 VLCFAs, eventually helps in preventing pollen dehydration. Pollen produced on oshms1 plants show impaired pollen grain adherence, hydration, and germination on the stigma under low humid conditions (<60% RH), leading to male sterility, which could be rescued at higher humid conditions (>80% RH)(Figure 3C) (Chen et al., 2020). Similarly, mutations in Arabidopsis genes CER1 (Aarts et al., 1995), CER2, CER2-LIKE (Haslam et al., 2015; Zhan et al., 2018), CER3, CER6, CER8, and CER10 (Koornneef et al., 1989) also induce HGMS under different humid conditions.
Recently, a slow pollen development rate under low temperature, short photoperiod, or low light intensity is identified as a general mechanism of male fertility restoration in P/TGMS lines. The slow pollen development rate was first identified in the Arabidopsis EMS mutant line reversible male sterile (rvms), which is male fertile at 17°C and sterile at 24°C. The RVMS gene encodes a GDSL lipase/hydrolase protein, and an SNP mutation/T-DNA insertion led to the TGMS phenotype, due to cytoplasmic leakage and defective nexine development. Zhu et al. (2020) identified that decrease in the microspore development rate might be the primary reason for the development of functional pollen at low temperatures. Further, when a slow microspore development rate mutant, restorer of rvms 1 (res1), a weak allele encoding A-TYPE CYCLIN-DEPENDENT KINASE;1 (CDKA;1), is crossed with rvms mutant, the generated double mutant is fertile at 24°C, confirming the slow growth rate of pollen rescues the pollen fertility. Shi et al. (2021) identified res2 (or qrt3) as an additional mutant that can rescue rvms mutation. It encodes a polygalacturonase and exhibits delayed tetrad pectin wall degradation. Double mutants res2 rvms-2 were fertile and displayed delay in degradation of the tetrad pectin wall (Shi et al., 2021). Similarly, the res3, encoding UPEX1, is also able to restore the fertility of the rvms-2 TGMS line by delaying the degradation of tetrad callose wall and callose A6 secretion from tapetum to locule (Wang et al., 2022).
Further, res1, res2 and res3 alleles are also able to rescue male sterility of other TGMS mutants, such as abcg26, acos5, cals5, cyp703a2, and rpg1, which are sterile at 24°C and fertile at 17°C (Zhu et al., 2020; Shi et al., 2021). The rpg1 is defective in primeexine development and is partially restored under low temperatures. However, the fertility restoration in the double mutant rpg1 rpg2 is significantly reduced at low temperatures compared to the rpg1 mutant, indicating the gene redundancy function plays a vital role in the fertility restoration of TGMS lines (Zhang et al., 2022a). Further, although these mutants acos5, cals5, cyp703a2, npu and rvms have been identified as TGMS lines, they also exhibit PGMS features, i.e., sterile at 16 h LD and fertile at 8 h SD (Zhang et al., 2020a). The fertility of these mutants has been restored at the SD or low-intensity light conditions due to slow development (Zhang et al., 2020a; Zhu et al., 2020; Shi et al., 2021). Besides Arabidopsis, the rice OsTMS15 male sterility can be rescued by growing at low temperature which indicates that slow developmental rate at low temperatures recovers the OsTMS15 protein interaction with its ligand, thereby restoring pollen fertility (Han et al., 2023). Likewise, B. napus rTGMS lines 9012AB and male-sterility Lembke (MSL) share a similar male sterile chloroplast localized protein, BnChimera. BnChimera interacts with its fertility restorer BnaC9-Tic40, which restores the pollen fertility at high temperatures by slowing the flower development and synthesis of cell wall precursors (Schuhmann et al., 2022). These studies indicate that slow pollen development is a general mechanism to restore the fertility of P/TGMS lines to rectify pollen developmental defects.
Among all plant nutrients, nitrogen constitutes 1-5% of the dry matter of plant tissues, and is integral to growth, metabolism and physiology, and also plays a vital role in various developmental processes (Muratore et al., 2021). In rice, Yang et al. (2022) recently identified a mutant defective in the gene encoding electron transfer flavoprotein subunit β (etfβ). This mutant exhibited both male and female sterility under N-starvation condition due to failure of sporogenous cells to enter into meiosis. Under N-deficient conditions, ETFβ helps in N reutilization and remobilization through its role in the catabolism of branched-chain amino acids (BCAA), and thereby helps meet the N requirements of spikelets, ensuring sexual reproduction. The etfβ mutant, under N-starvation conditions, exhibits excessive BCAA accumulation and reduced total N content, leading to male sterility. However, the etfβ mutant meiotic disorders can be rescued by exogenous N supply, thereby allowing the recovery of male fertility (Yang et al., 2022). As etfβ mutation causes both male and female sterility under N-deficient condition, this NGMS system cannot be used for hybrid seed production.
Although many of the genes described have not yet been put to practical use, these examples show how basic studies on pollen development can help us in identifying candidate genes and necessary mutations therein that can be harnessed to engineer EGMS in new crop species.
EGMS systems have been mostly restricted to rice. Although many GMS mutants have been identified in several crop species, their utility in commercial seed production is restricted due to issues such as unstable sterility or unintended side effects, the complexity of producing pure male sterile progenies on a large scale, labor-intensive and time-consuming plant breeding methods to transfer recessive male sterility traits into elite lines. Persistent challenges, like false selection and linkage drag appear unavoidable (Zhou et al., 2023). The advent of genetic engineering offered alternative ways to quickly generate male steriles in elite varieties in a single generation avoiding linkage drag (Gao, 2021).
The first transgenic male sterility/fertility system was developed in tobacco and rape seed through tapetum-specific expression of barnase and barstar genes sourced from Bacillus amyloliquefaciens strain H (IAM1521) (Mariani et al., 1990, Mariani et al., 1992). barnase gene codes for an extracellular ribonuclease enzyme with two cysteine residues, which is explicitly inhibited by a small 89 amino acids intracellular protein coded by the barstar gene. Male sterile plants were obtained by anther specific expression of the barnase gene (Mariani et al., 1990) whereas fertility restoration was achieved by anther-specific expression of the barstar gene (Mariani et al., 1992). A bar gene conferring tolerance to glufosinate herbicide was linked to barnase and barstar genes. Barnase-barstar system has been demonstrated to work in several crop species like rapeseed, Indian mustard, soybean, rice, vegetable species (Jagannath et al., 2002; Colombo and Galmarini, 2017). This system is comparable to CMS system and involves three lines for hybrid seed production. Kriete et al. (1996) engineered conditional male sterility system by anther-specific expression of a bacterial gene argE coding for N-acetyl L-ornithine deacetylase. When transgenic tobacco plants are sprayed with non-phytotoxic herbicide conjugate N-acetyl phosphinothricin, expression of argE gene in anthers produces toxic phosphinothricin in anthers causing their destruction. This conditional male sterility system is a two-line system. Ruiz and Daniell (2005) engineered cytoplasmic male sterility through plastid transformation. Transplastomic tobacco plants carrying phA gene coding for ß-ketothiolase driven by psbA promoter showed complete male sterility. Male fertility restoration was achieved when such plants were grown under continuous illumination. However, a practical male fertility restoration system for transplastomic male sterility has not been devised so far. Subsequently, several transgenic male sterility-fertility restoration systems have been reported in model plants (Shukla et al., 2017; Gautam et al., 2023). So far, only the Barnase-barstar system has been commercialized in a few countries such as USA, Canada and Australia. Objections to genetically modified crops in Europe and many other countries have prevented commercialization of transgenic male sterility systems for hybrid seed production.
To bypass the use of transgenic lines in hybrid seed production, Perez-Prat and Van Lookeren Campagne (2002) proposed generation of transgenic male fertile maintainer lines for recessive GMS lines. Using this concept, DuPont Pioneer developed the Seed Production Technology (SPT) system in maize utilising ms45 male sterility gene (Wu et al., 2016). This system consists of a transgenic hemizygous SPT maintainer line (in ms45/ms45 background) and carries wild-type Ms45 transgene linked to a chimeric α-amylase gene containing amyloplast targeting signal peptide to disrupt the pollen germination, and a fluorescent seed color marker gene (DsRed2) (Figure 4A). The wild-type Ms45 allele in transgenic plant rescues male sterility, producing 50% non-transgenic viable pollen. The rest 50% transgenic pollen are rendered non-viable due to breakdown of starch by the action of α-amylase transgene. Therefore, the cross between male-sterile line and hemizygous SPT maintainer line yields only non-transgenic male-sterile seeds for use in hybrid seed production. Further, the SPT maintainer line could be propagated through self-pollination where 50% seeds containing the SPT construct can be separated from non-transgenic seeds by fluorescent seed color sorting (Wu et al., 2016).
Figure 4. Examples of the third-generation hybrid seed production technologies. (A) Schematic diagram of DuPont-Pioneer propriety Seed Production Technology (SPT). This system uses the maintainer line developed from the male sterile line to produce non-transgenic hybrid seeds using transgenic technology. Male sterile lines with mutations in genes expressed in the sporophyte are obtained from natural mutants or through gene editing. The male fertile maintainer line is developed by transformation of the male sterile line with a triple gene construct containing i) the corresponding wild-type male fertility gene, ii) a pollen disruption gene operating at the gametophyte stage, and iii) a gene imparting seed color. The maintainer line produces only non-transgenic pollen because transgenic pollen is non-viable due to the presence of the pollen disruption gene. Therefore, a cross between the male sterile line and the maintainer line generates 100% male sterile progeny, which can be used for F1 hybrid seed production. Self-pollination of the maintainer line generates 50% maintainer line seeds and 50% male sterile seeds, which can be sorted through the seed-sorting marker. (B) Schematic diagram of dominant male sterility system (DMS). In this system, the transgenic DMS maintainer line is developed through transformation of triple gene construct containing i) tapetum specific dominant male sterility causing gene ZmMs7, ii) fluorescent marker gene mcherry, iii) herbicide tolerance bar gene, into the wild type plants. Male sterile seeds can be maintained through a cross between transgenic male sterile line and a wild type generates 50% non-transgenic male fertile seeds and 50% transgenic fluorescent sterile seeds, which can be sorting by fluorescence and also through bar gene. A cross between transgenic male sterile line and a fertile non-transgenic combiner line generates hybrid seeds, in which 50% non-transgenic hybrid seeds can be sorted through fluorescence.
The SPT system has many advantages, such as the availability of a broad range of germplasm for parental selection, an increase in hybrid seed purity and yield, and the elimination of public concerns about GM crops. However, the SPT constructs show pollen transmission ranging from 0.002% to 0.518% depending on the SPT construct, genetic background and transformation event (Wu et al., 2016). To reduce transgene escape, multicontrol sterility (MCS) system carrying two pollen disruption genes (α-amylase and Dam) has been developed (Zhang et al., 2018). Further, the MCS system also includes a herbicide-resistant gene (Bar) for selecting transgenic seeds (Zhang et al., 2018).
Similar to SPT and MCS systems, a nuclear male sterile rice maintainer line was developed by cloning OsNP1, α-amylase and DsRed2 genes into the EMS mutant osnp1 (Chang et al., 2016). Likewise, Du et al. (2020) created transgenic maintainer line in tomato by introducing the wild type fertility allele linked to seedling color marker in male sterile mutant. The offspring of homozygous male sterile plants and hemizygous maintainers produce 50% non-transgenic male sterile and 50% transgenic fertile seedlings, which can be sorted out through the seedling color before transplantation and obtain a large quantity of recessive genic male-sterile plants.
The above SPT systems are based on recessive male sterility and require prior knowledge of male sterility causing genes and male sterile mutants. If the mutants are not available, two different stocks have to be generated through transformation; the recessive male sterile line through genome editing (followed by selection of transgene-free lines) and transgenic male fertile maintainer line. To circumvent this major bottleneck, An et al. (2020) developed a dominant male sterility system (DMS) in maize, which would require development of only one transgenic line for hybrid seed production. They demonstrated that premature expression of a tapetum specific gene ZmMs7 causes dominant male sterility through delayed PCD of tapetum leading to defects in pollen exine deposition. By linking male sterility construct (p5126::ZmMs7) with the fluorescent marker gene mcherry (expressed in aleurone layer of seed) and constitutively expressed bar gene (conferring Basta herbicide tolerance), they developed a system to track the male sterile individuals at the seed stage. The dominant male sterility-based hybrid seed production system is depicted in Figure 4B. The male sterile line could be multiplied by crossing with its non-transgenic counterpart, which will yield 50% transgenic (male sterile) seeds that could be sorted by fluorescence screening. The presence of bar gene in male sterile lines provides additional opportunity to eliminate any non-transgenic plants in hybrid seed production field. Similarly, hybrid seeds could be produced by crossing with non-transgenic combiner line where non-transgenic hybrid seeds could be separated for commercial use by fluorescence screening. The Ms7 gene is highly conserved among diverse plant species such as rice, barley, Arabidopsis, Brassica etc. Further, transformation of Arabidopsis with the above construct gave dominant male sterility as observed in maize (An et al., 2020) thereby demonstrating that this is a versatile hybrid seed production system. Thus, DMS system provides a robust, widely applicable approach to engineer pollination control system across crop species.
In a further attempt to avoid the use of transgenics altogether, Zhou et al. (2023) identified a male sterility gene (TM6) in tomato which is closely linked to a seedling marker gene Anthocyanin Without (AW) coding for DFR enzyme. Male sterile TM6 mutants (ms-15, ms-26 and ms-47) can thus be identified at the seedling stage by hypocotyl color. They employed CRISPR/Cas9 system to knock out these two genes to obtain male sterile line lacking anthocyanin pigment in the seedling hypocotyls. This system exhibited male sterility along with a distinctive marker (green hypocotyls), facilitating the identification of sterile plants at the seedling stage. However, it still gives rise to a few recombinants between these linked genes which need physical rouging (Figure 5). Likewise, mutant alleles of tomato MS-10 gene, ms-10, ms-24, ms-35 and ms-36, cause male sterility and MS-10 is linked to anthocyanin absent (aa) gene (Zhang et al., 2024). Thus ms-10 and aa gene pair provides another opportunity to use linked seedling marker for selecting male sterile progenies. However, finding a closely linked morphological marker to the male sterility gene in each crop species will be a challenge.
Figure 5. Schematic diagram of use of CRISPR/Cas double knockout mutant in hybrid seed production. Here, a male sterile line developed through the knockout of two closely linked genes, a male sterility-causing gene and a morphological seedling marker gene. A cross between the double knockout mutant and the wild type generates the 1:1 ratio of male sterile and male fertile progenies and a few recombinants, which can be sorted based on the color at the seedling stage.
EGMS has demonstrated significant value in hybrid seed production due to its ability to exploit environmental conditions to induce male sterility and fertility phenotype. However, the molecular mechanisms underlying EGMS systems have been extensively studied only in rice, leaving a major gap in understanding their mode of function in other major crops. Investigations into EGMS systems of other major crops are crucial to maximize their utility. Additionally, studies exploring the impact of climate change on EGMS systems are also needed to ensure their stability and adaptability in hybrid seed production. Meanwhile, SPT, MCS and DMS systems offer innovative solutions to overcome the challenges of conventional seed production methods such as manual emasculation and dependence on environmental conditions. Despite their advantages, these systems have limitations such as the need for sophisticated seed sorting machinery and increased cost of seed production associated with 50% unused seeds. Addressing these challenges through technological advancements and cost-effective solutions will be crucial for optimizing their utilization in hybrid seed production. Seedling marker linked male sterility as demonstrated in tomato (Zhou et al., 2023) is a very good approach. However, detecting and removing low frequency recombinant male fertile plants is a problem in seed production. In recent years gene editing technologies have been employed to create chromosomal inversions at target locations (Zhang et al., 2017; Schmidt et al., 2019; Ronspies et al., 2021; Hu et al., 2024). Thus, creating a small inversion between the seedling marker gene and male sterility gene can overcome the problem of recombination and thereby make this a perfect pollination control system.
Heterosis benefits have largely been restricted to a few major global crops. In particular, lack of reliable male sterility/fertility control systems has discouraged heterosis breeding in many crops such as pulses, crops bearing small, hermaphrodite flowers such as millets (e.g. finger millet, foxtail millet etc.), seed spices, amaranth. The accumulated knowledge of genes causing male sterility in crops, availability of whole genome sequences, conserved genes and genetic pathways of male gamete development offer great scope for developing male sterility systems in these crops. Although the third-generation DMS system presents the best promise, CMS and EGMS systems will continue to serve the hybrid seed industry, especially in countries where transgenics are not accepted.
Pollen competition is a phenomenon where pollen of different genetic constitutions display variation in the rate of pollen tube growth, leading to one type of pollen showing preferential transmission through the male side. For example, Arabidopsis Cals5 gene mutants produce pollen whose tubes lack callose, a major component in the pollen wall. The cals5-3 mutant produces seeds upon selfing (no pollen competition) but mutant pollen competes poorly against wild type pollen (Nishikawa et al., 2005). Hence, in heterozygous plants mutant allele is poorly transmitted from the male side. Similarly, in rice, loss-of-function mutants of pollen expressed hexokinase gene Hxk5, produce viable pollen and give 5-10% seed set upon selfing. However, hkx5 mutant pollen grow slowly and fail to compete with wild type pollen. Hence, in heterozygous (+/hkx5) plants, the mutant allele is not transmitted from the male side (Lee et al., 2020). A similar slow pollen germination mutant has been identified in ragi (Eleucine coracana), which shows promise for heterosis breeding (Manjappa et al., 2015). Such slow pollen germination mutants offer unique opportunity for hybrid seed production because homozygous mutant stocks can serve as male sterile line for hybrid seed production and can also be multiplied through selfing. Considering that starch metabolism pathway in pollen grains is highly conserved, slow pollen germination feature is particularly attractive. In crops where seedlings are transplanted, genic male sterility combined with seedling/herbicide marker can be effectively used for heterosis breeding.
NV: Conceptualization, Funding acquisition, Project administration, Supervision, Writing – original draft, Writing – review & editing. KM: Writing – original draft. VS: Writing – original draft. SR: Writing – original draft. SB: Writing – review & editing. XL: Project administration, Supervision, Writing – review & editing.
The author(s) declare that financial support was received for the research, authorship, and/or publication of this article. This work is supported by a grant from the Research Fund for International Young Scientists by the National Natural Science Foundation of China (32150410354) to Naresh Vasupalli and the Department of Biotechnology, Government of India, for the Ramalingaswami re-entry grant (BT/RLF/Re-entry/11/2023) to Kanakachari Mogilicherla.
The authors declare that the research was conducted in the absence of any commercial or financial relationships that could be construed as a potential conflict of interest.
The author(s) declare that no Generative AI was used in the creation of this manuscript.
All claims expressed in this article are solely those of the authors and do not necessarily represent those of their affiliated organizations, or those of the publisher, the editors and the reviewers. Any product that may be evaluated in this article, or claim that may be made by its manufacturer, is not guaranteed or endorsed by the publisher.
Aarts, M., Keijzer, C. J., Stiekema, W. J., Pereira, A. (1995). Molecular characterization of the CER1 gene of arabidopsis involved in epicuticular wax biosynthesis and pollen fertility. Plant Cell 7, 2115–2127. doi: 10.1105/tpc.7.12.2115
An, X., Ma, B., Duan, M., Dong, Z., Liu, R., Yuan, D., et al. (2020). Molecular regulation of ZmMs7 required for maize male fertility and development of a dominant male-sterility system in multiple species. Proc. Natl. Acad. Sci. U.S.A. 117, 23499–23509. doi: 10.1073/pnas.2010255117
Anjani, K. (2005). Stabilization and maintenance of male sterility percent in recessive genetic male sterile line. s of safflower (Carthamus tinctorius L.). Indian J. Genet. Plant Breed. 65, 141–142.
Ariizumi, T., Hatakeyama, K., Hinata, K., Inatsugi, R., Nishida, I., Sato, S., et al. (2004). Disruption of the novel plant protein NEF1 affects lipid accumulation in the plastids of the tapetum and exine formation of pollen, resulting in male sterility in Arabidopsis thaliana. Plant J. 39, 170–181. doi: 10.1111/j.1365-313X.2004.02118.x
Besnard, G., Khadari, B., Villemur, P., Bervillé, A. (2000). Cytoplasmic male sterility in the olive (Olea europaea L.). Theor. Appl. Genet. 100, 1018–1024. doi: 10.1007/s001220051383
Blackmore, S., Wortley, A. H., Skvarla, J. J., Rowley, J. R. (2007). Pollen wall development in flowering plants. New Phytol. 174, 483–498. doi: 10.1111/j.1469-8137.2007.02060.x
Budar, F., Pelletier, G. (2001). Male sterility in plants: occurrence, determinism, significance and use. C. R. Acad. Sci. III. 324, 543–550. doi: 10.1016/S0764-4469(01)01324-5
Cai, C.-F., Zhu, J., Lou, Y., Guo, Z.-L., Xiong, S.-X., Wang, K., et al. (2015). The functional analysis of OsTDF1 reveals a conserved genetic pathway for tapetal development between rice and Arabidopsis. Sci. Bull. 60, 1073–1082. doi: 10.1007/s11434-015-0810-3
Chamola, R., Balyan, H. S., Bhat, S. R. (2013). Effect of alien cytoplasm and fertility restorer genes on agronomic and physiological traits of Brassica juncea (L.) C zern. Plant Breed. 132, 681–687. doi: 10.1111/pbr.2013.132.issue-6
Chang, Z., Chen, Z., Wang, N., Xie, G., Lu, J., Yan, W., et al. (2016). Construction of a male sterility system for hybrid rice breeding and seed production using a nuclear male sterility gene. Proc. Natl. Acad. Sci. U.S.A. 113, 14145–14150. doi: 10.1073/pnas.1613792113
Chaubal, R., Anderson, J. R., Trimnell, M. R., Fox, T. W., Albertsen, M. C., Bedinger, P. (2003). The transformation of anthers in the msca1 mutant of maize. Planta 216, 778–788. doi: 10.1007/s00425-002-0929-8
Chen, L., Liu, Y. G. (2014). Male sterility and fertility restoration in crops. Annu. Rev. Plant Biol. 65, 579–606. doi: 10.1146/annurev-arplant-050213-040119
Chen, X. D., Sun, D. F., Rong, D. F., Peng, J. H., Li, C. D. (2011b). A recessive gene controlling male sterility sensitive to short daylength/low temperature in wheat (Triticum aestivum L.). J. Zhejiang Univ. Sci. B. 12, 943–950. doi: 10.1631/jzus.B1000371
Chen, Z., Wu, J., Deng, X. W., Tang, X. (2023). Establishment and advances of third-generation hybrid rice technology: a review. Rice 16, 56. doi: 10.1186/s12284-023-00670-z
Chen, W., Yu, X.-H., Zhang, K., Shi, J., De Oliveira, S., Schreiber, L., et al. (2011a). Male Sterile2 encodes a plastid-localized fatty acyl carrier protein reductase required for pollen exine development in Arabidopsis. Plant Physiol. 157, 842–853. doi: 10.1104/pp.111.181693
Chen, H., Zhang, Z., Ni, E., Lin, J., Peng, G., Huang, J., et al. (2020). HMS1 interacts with HMS1I to regulate very-long-chain fatty acid biosynthesis and the humidity-sensitive genic male sterility in rice (Oryza sativa). New Phytol. 225, 2077–2093. doi: 10.1111/nph.v225.5
Chen, X., Zhang, H., Sun, H., Luo, H., Zhao, L., Dong, Z., et al. (2017). IRREGULAR POLLEN EXINE1 Is a novel factor in anther cuticle and pollen exine formation. Plant Physiol. 173, 307–325. doi: 10.1104/pp.16.00629
Chen, R., Zhao, X., Shao, Z., Wei, Z., Wang, Y., Zhu, L., et al. (2007). Rice UDP-glucose pyrophosphorylase1 is essential for pollen callose deposition and its cosuppression results in a new type of thermosensitive genic male sterility. Plant Cell 19, 847–861. doi: 10.1105/tpc.106.044123
Cheng, X.-Q., Zhang, X.-Y., Xue, F., Zhu, S.-H., Li, Y.-J., Zhu, Q.-H., et al. (2020). Characterization and transcriptome analysis of a dominant genic male sterile cotton mutant. BMC Plant Biol. 20, 1–14. doi: 10.1186/s12870-020-02522-0
Chueasiri, C., Chunthong, K., Pitnjam, K., Chakhonkaen, S., Sangarwut, N., Sangsawang, K., et al. (2014). Rice ORMDL controls sphingolipid homeostasis affecting fertility resulting from abnormal pollen development. PloS One 9, e106386. doi: 10.1371/journal.pone.0106386
Cole, R. A., Synek, L., Zarsky, V., Fowler, J. E. (2005). SEC8, a subunit of the putative Arabidopsis exocyst complex, facilitates pollen germination and competitive pollen tube growth. Plant Physiol. 138, 2005–2018. doi: 10.1104/pp.105.062273
Colombo, N., Galmarini, C. R. (2017). The use of genetic, manual and chemical methods to control pollination in vegetable hybrid seed production: a review. Plant Breed. 136, 287–299. doi: 10.1111/pbr.2017.136.issue-3
Deng, Y., Srivastava, R., Quilichini, T. D., Dong, H., Bao, Y., Horner, H. T., et al. (2016). IRE1, a component of the unfolded protein response signaling pathway, protects pollen development in Arabidopsis from heat stress. Plant J. 88, 193–204. doi: 10.1111/tpj.2016.88.issue-2
De Souza, P. F., Dos Santos, C. M. R., Ree, J., Guerra, M. P., Pescador, R. (2020). Male sterility in Bambusa tuldoides Munro. Protoplasma 257, 911–920. doi: 10.1007/s00709-019-01479-8
Ding, J., Lu, Q., Ouyang, Y., Mao, H., Zhang, P., Yao, J., et al. (2012a). A long noncoding RNA regulates photoperiod-sensitive male sterility, an essential component of hybrid rice. Proc. Natl. Acad. Sci. U.S.A. 109, 2654–2659. doi: 10.1073/pnas.1121374109
Ding, J., Shen, J., Mao, H., Xie, W., Li, X., Zhang, Q. (2012b). RNA-directed DNA methylation is involved in regulating photoperiod-sensitive male sterility in rice. Mol. Plant 5, 1210–1216. doi: 10.1093/mp/sss095
Dong, X., Hong, Z., Sivaramakrishnan, M., Mahfouz, M., Verma, D. P. (2005). Callose synthase (CalS5) is required for exine formation during microgametogenesis and for pollen viability in Arabidopsis. Plant J. 42, 315–328. doi: 10.1111/j.1365-313X.2005.02379.x
Dong, X., Nou, I. S., Yi, H., Hur, Y. (2015). Suppression of ASKβ (AtSK32), a Clade III Arabidopsis GSK3, leads to the pollen defect during late pollen development. Mol. Cells 38, 506–517. doi: 10.14348/molcells.2015.2323
Dong, N., Subudhi, P., Luong, P., Quang, V., Quy, T., Zheng, H., et al. (2000). Molecular mapping of a rice gene conditioning thermosensitive genic male sterility using AFLP, RFLP and SSR techniques. Theor. Appl. Genet. 100, 727–734. doi: 10.1007/s001220051345
Du, M., Zhou, K., Liu, Y., Deng, L., Zhang, X., Lin, L., et al. (2020). A biotechnology-based male-sterility system for hybrid seed production in tomato. Plant J. 102, 1090–1100. doi: 10.1111/tpj.v102.5
Enns, L. C., Kanaoka, M. M., Torii, K. U., Comai, L., Okada, K., Cleland, R. E. (2005). Two callose synthases, GSL1 and GSL5, play an essential and redundant role in plant and pollen development and in fertility. Plant Mol. Biol. 58, 333–349. doi: 10.1007/s11103-005-4526-7
Fan, Y., Yang, J., Mathioni, S. M., Yu, J., Shen, J., Yang, X., et al. (2016). PMS1T, producing phased small-interfering RNAs, regulates photoperiod-sensitive male sterility in rice. Proc. Natl. Acad. Sci. U.S.A. 113, 15144–15149. doi: 10.1073/pnas.1619159114
Fan, Y., Zhang, Q. (2018). Genetic and molecular characterization of photoperiod and thermo-sensitive male sterility in rice. Plant Reprod. 31, 3–14. doi: 10.1007/s00497-017-0310-5
Fang, C., Li, L., He, R., Wang, D., Wang, M., Hu, Q., et al. (2019). Identification of S23 causing both interspecific hybrid male sterility and environment-conditioned male sterility in rice. Rice 12, 10. doi: 10.1186/s12284-019-0271-4
Fernandez-Gomez, J., Talle, B., Wilson, Z. A. (2020). Increased expression of the MALE STERILITY1 transcription factor gene results in temperature-sensitive male sterility in barley. J. Exp. Bot. 71, 6328–6339. doi: 10.1093/jxb/eraa382
Fernandez Gomez, J., Wilson, Z. A. (2014). A barley PHD finger transcription factor that confers male sterility by affecting tapetal development. Plant Biotechnol. J. 12, 765–777. doi: 10.1111/pbi.12181
Fiebig, A., Mayfield, J. A., Miley, N. L., Chau, S., Fischer, R. L., Preuss, D. (2000). Alterations in CER6, a gene identical to CUT1, differentially affect long-chain lipid content on the surface of pollen and stems. Plant Cell 12, 2001–2008. doi: 10.1105/tpc.12.10.2001
Fu, Z., Yu, J., Cheng, X., Zong, X., Xu, J., Chen, M., et al. (2014). The rice basic helix-loop-helix transcription factor TDR INTERACTING PROTEIN2 is a central switch in early anther development. Plant Cell 26, 1512–1524. doi: 10.1105/tpc.114.123745
Gao, C. (2021). Genome engineering for crop improvement and future agriculture. Cell 184, 1621–1635. doi: 10.1016/j.cell.2021.01.005
Gautam, R., Shukla, P., Kirti, P. B. (2023). Male sterility in plants: an overview of advancements from natural CMS to genetically manipulated systems for hybrid seed production. Theor. Appl. Genet. 136, 195. doi: 10.1007/s00122-023-04444-5
Guo, R. X., Sun, D. F., Tan, Z. B., Rong, D. F., Li, C. D. (2006). Two recessive genes controlling thermophotoperiod-sensitive male sterility in wheat. Theor. Appl. Genet. 112, 1271–1276. doi: 10.1007/s00122-006-0228-z
Hamilton, E. S., Haswell, E. S. (2017). The tension-sensitive ion transport activity of MSL8 is critical for its function in pollen hydration and germination. Plant Cell Physiol. 58, 1222–1237. doi: 10.1093/pcp/pcw230
Han, Y., Jiang, S. Z., Zhong, X., Chen, X., Ma, C. K., Yang, Y. M., et al. (2023). Low temperature compensates for defective tapetum initiation to restore the fertility of the novel TGMS line ostms15. Plant Biotechnol. J. 21, 1659–1670. doi: 10.1111/pbi.14066
Han, Y., Zhou, S.-D., Fan, J.-J., Zhou, L., Shi, Q.-S., Zhang, Y.-F., et al. (2021). OsMS188 is a key regulator of tapetum development and sporopollenin synthesis in rice. Rice 14, 1–14. doi: 10.1186/s12284-020-00451-y
Haslam, T. M., Haslam, R., Thoraval, D., Pascal, S., Delude, C., Domergue, F., et al. (2015). ECERIFERUM2-LIKE proteins have unique biochemical and physiological functions in very-long-chain fatty acid elongation. Plant Physiol. 167, 682–692. doi: 10.1104/pp.114.253195
Hong, L., Tang, D., Shen, Y., Hu, Q., Wang, K., Li, M., et al. (2012a). MIL 2 (MICROSPORELESS 2) regulates early cell differentiation in the rice anther. New Phytol. 196, 402–413. doi: 10.1111/j.1469-8137.2012.04270.x
Hong, L., Tang, D., Zhu, K., Wang, K., Li, M., Cheng, Z. (2012b). Somatic and reproductive cell development in rice anther is regulated by a putative glutaredoxin. Plant Cell 24, 577–588. doi: 10.1105/tpc.111.093740
Hou, J., Fan, W., Ma, R., Li, B., Yuan, Z., Huang, W., et al. (2022). MALE STERILITY 3 encodes a plant homeodomain-finger protein for male fertility in soybean. J. Integr. Plant Biol. 64, 1076–1086. doi: 10.1111/jipb.13242
Hu, H., Scheben, A., Wang, J., Li, F., Li, C., Edwards, D., et al. (2024). Unravelling inversions: Technological advances, challenges, and potential impact on crop breeding. Plant Biotechnol. J. 22, 544–554. doi: 10.1111/pbi.14224
Huang, W., Li, Y., Du, Y., Pan, L., Huang, Y., Liu, H., et al. (2022). Maize cytosolic invertase INVAN6 ensures faithful meiotic progression under heat stress. New Phytol. 236, 2172–2188. doi: 10.1111/nph.v236.6
Huang, T.-Y., Wang, Z., Hu, Y.-G., Shi, S.-P., Peng, T., Chu, X.-D., et al. (2008). Genetic analysis and primary mapping of pms4, a photoperiod-sensitive genic male sterility gene in rice (Oryza sativa). Rice Sci. 15, 153–156. doi: 10.1016/S1672-6308(08)60035-9
Huang, H., Wang, C., Tian, H., Sun, Y., Xie, D., Song, S. (2014). Amino acid substitutions of GLY98, LEU245 and GLU543 in COI1 distinctively affect jasmonate-regulated male fertility in Arabidopsis. Sci. China Life Sci. 57, 145–154. doi: 10.1007/s11427-013-4590-1
Hussain, A. J., Ali, J., Siddiq, E. A., Gupta, V. S., Reddy, U. K., Ranjekar, P. K. (2011). Mapping of tms8 gene for temperature-sensitive genic male sterility (TGMS) in rice (Oryza sativa L.). Plant Breed. 131, 42–47. doi: 10.1111/j.1439-0523.2011.01897.x
Ishiguro, S., Nishimori, Y., Yamada, M., Saito, H., Suzuki, T., Nakagawa, T., et al. (2010). The Arabidopsis FLAKY POLLEN1 gene encodes a 3-hydroxy-3-methylglutaryl-coenzyme A synthase required for development of tapetum-specific organelles and fertility of pollen grains. Plant Cell Physiol. 51, 896–911. doi: 10.1093/pcp/pcq068
Ito, T., Nagata, N., Yoshiba, Y., Ohme-Takagi, M., Ma, H., Shinozaki, K. (2007). Arabidopsis MALE STERILITY1 encodes a PHD-type transcription factor and regulates pollen and tapetum development. Plant Cell 19, 3549–3562. doi: 10.1105/tpc.107.054536
Ito, T., Shinozaki, K. (2002). The MALE STERILITY1 gene of Arabidopsis, encoding a nuclear protein with a PHD-finger motif, is expressed in tapetal cells and is required for pollen maturation. Plant Cell Physiol. 43, 1285–1292. doi: 10.1093/pcp/pcf154
Iwata, Y., Fedoroff, N. V., Koizumi, N. (2008). Arabidopsis bZIP60 Is a proteolysis-activated transcription factor involved in the endoplasmic reticulum stress response. Plant Cell 20, 3107–3121. doi: 10.1105/tpc.108.061002
Jagannath, A., Arumugam, N., Gupta, V., Pradhan, A., Burma, P. K., Pental, D. (2002). Development of transgenic barstar lines and identification of a male sterile (barnase)/restorer (barstar) combination for heterosis breeding in Indian oilseed mustard (Brassica juncea). Curr. Sci. 82, 46–52.
Jessen, D., Olbrich, A., Knufer, J., Kruger, A., Hoppert, M., Polle, A., et al. (2011). Combined activity of LACS1 and LACS4 is required for proper pollen coat formation in Arabidopsis. Plant J. 68, 715–726. doi: 10.1111/j.1365-313X.2011.04722.x
Jia, J., Zhang, D., Li, C., Qu, X., Wang, S., Chamarerk, V., et al. (2001). Molecular mapping of the reverse thermo-sensitive genic male-sterile gene (rtms1) in rice. Theor. Appl. Genet. 103, 607–612. doi: 10.1007/PL00002916
Jiang, S. Y., Cai, M., Ramachandran, S. (2007). ORYZA SATIVA MYOSIN XI B controls pollen development by photoperiod-sensitive protein localizations. Dev. Biol. 304, 579–592. doi: 10.1016/j.ydbio.2007.01.008
Jin-Long, N. I., De-Zheng, W., Da-Hu, N. I., Feng-Shun, S., Jian-Bo, Y., Da-Nian, Y. (2022). Characterization and fine mapping of RTMS10, a semi-dominant reverse thermo-sensitive genic male sterile locus in rice. J. Integr. Agric. 21, 316–325. doi: 10.1016/S2095-3119(20)63563-8
Jung, K.-H., Han, M.-J., Lee, Y.-S., Kim, Y.-W., Hwang, I., Kim, M.-J., et al. (2005). Rice Undeveloped Tapetum1 is a major regulator of early tapetum development. Plant Cell 17, 2705–2722. doi: 10.1105/tpc.105.034090
Kim, Y. J., Zhang, D. (2018). Molecular control of male fertility for crop hybrid breeding. Trends Plant Sci. 23, 53–65. doi: 10.1016/j.tplants.2017.10.001
Koornneef, M., Hanhart, C., Thiel, F. (1989). A genetic and phenotypic description of Eceriferum (cer) mutants in Arabidopsis thaliana. J. Hered. 80, 118–122. doi: 10.1093/oxfordjournals.jhered.a110808
Kriete, G., Niehaus, K., Perlick, A., Pühler, A., Broer, I. (1996). Male sterility in transgenic tobacco plants induced by tapetum-specific deacetylation of the externally applied non-toxic compound N-acetyl-l-phosphinothricin. Plant J. 9, 809–818. doi: 10.1046/j.1365-313X.1996.9060809.x
Kumar, P., Vasupalli, N., Srinivasan, R., Bhat, S. R. (2012). An evolutionarily conserved mitochondrial orf108 is associated with cytoplasmic male sterility in different alloplasmic lines of Brassica juncea and induces male sterility in transgenic Arabidopsis thaliana. J. Exp. Bot. 63, 2921–2932. doi: 10.1093/jxb/err459
Lee, D. S., Chen, L. J., Suh, H. S. (2005). Genetic characterization and fine mapping of a novel thermo-sensitive genic male-sterile gene tms6 in rice (Oryza sativa L.). Theor. Appl. Genet. 111, 1271–1277. doi: 10.1007/s00122-005-0044-x
Lee, S. K., Kim, H., Cho, J. I., Nguyen, C. D., Moon, S., Park, J. E., et al. (2020). Deficiency of rice hexokinase HXK5 impairs synthesis and utilization of starch in pollen grains and causes male sterility. J. Exp. Bot. 71, 116–125. doi: 10.1093/jxb/erz436
Lee, Y. S., Maple, R., Durr, J., Dawson, A., Tamim, S., Del Genio, C., et al. (2021). A transposon surveillance mechanism that safeguards plant male fertility during stress. Nat. Plants 7, 34–41. doi: 10.1038/s41477-020-00818-5
Li, H., Li, S., Abdelkhalik, S., Shahzad, A., Gu, J., Yang, Z., et al. (2020). Development of thermo-photo sensitive genic male sterile lines in wheat using doubled haploid breeding. BMC Plant Biol. 20, 246. doi: 10.1186/s12870-020-02458-5
Li, H., Yuan, Z., Vizcay-Barrena, G., Yang, C., Liang, W., Zong, J., et al. (2011). PERSISTENT TAPETAL CELL1 encodes a PHD-finger protein that is required for tapetal cell death and pollen development in rice. Plant Physiol. 156, 615–630. doi: 10.1104/pp.111.175760
Li, J., Zhang, H., Si, X., Tian, Y., Chen, K., Liu, J., et al. (2017). Generation of thermosensitive male-sterile maize by targeted knockout of the ZmTMS5 gene. J. Genet. Genom. 44, 465–468. doi: 10.1016/j.jgg.2017.02.002
Li, W.-Q., Zhang, X.-Q., Xia, C., Deng, Y., Ye, D. (2010). MALE GAMETOPHYTE DEFECTIVE 1, encoding the FAd subunit of mitochondrial F1F0-ATP synthase, is essential for pollen formation in Arabidopsis thaliana. Plant Cell Physiol. 51, 923–935. doi: 10.1093/pcp/pcq066
Li, Y. F., Zhao, C. P., Zhang, F. T., Sun, H., Sun, D. F. (2006). Fertility alteration in the photo-thermo-sensitive male sterile line BS20 of wheat (Triticum aestivum L.). Euphytica 151, 207–213. doi: 10.1007/s10681-006-9141-4
Liang-Bi, C., Guang-Qia, Z., Yu-Xiang, H. (1994). Effects of temperature and photoperiod on fertility and physiological activities of rice Annong S-1 and Hengnong S-1. J. Integr. Plant Biol. 36, 119.
Lin, S., Liu, Z., Sun, S., Xue, F., Li, H., Tursun, A., et al. (2023). Rice HEAT SHOCK PROTEIN60-3B maintains male fertility under high temperature by starch granule biogenesis. Plant Physiol. 192, 2301–2317. doi: 10.1093/plphys/kiad136
Lin, X., Xie, H., Xi, Z., Hu, Y., Zhao, G., Duan, L., et al. (2009). Identification and mapping of a thermo-sensitive genic self-incompatibility gene in maize. Genes Genom. 31, 227–234. doi: 10.1007/BF03191194
Liping, Z., Changping, Z., Fuhua, S., Fengting, Z., Zhijie, Y. (2009). The mixed genetic analysis of photoperiod-temperature sensitive male sterility of BS210 in wheat. Zuowu Xuebao 33, 1553–1557.
Liu, Z., Bao, W., Liang, W., Yin, J., Zhang, D. (2010b). Identification of gamyb-4 and analysis of the regulatory role of GAMYB in rice anther development. J. Integr. Plant Biol. 52, 670–678. doi: 10.1111/j.1744-7909.2010.00959.x
Liu, Y. J., Li, D., Gong, J., Wang, Y. B., Chen, Z. B., Pang, B. S., et al. (2021). Comparative transcriptome and DNA methylation analysis in temperature-sensitive genic male sterile wheat BS366. BMC Genom. 22, 911. doi: 10.1186/s12864-021-08163-3
Liu, X., Li, X., Zhang, X., Wang, S. (2010a). Genetic analysis and mapping of a thermosensitive genic male sterility gene, tms6(t), in rice (Oryza sativa L.). Genome 53, 119–124. doi: 10.1139/G09-092
Liu, D., Shi, J., Liang, W., Zhang, D. (2023). Molecular mechanisms underlying plant environment-sensitive genic male sterility and fertility restoration. Seed Biol. 2, 13. doi: 10.48130/SeedBio-2023-0013
Lu, P., Chai, M., Yang, J., Ning, G., Wang, G., Ma, H. (2014). The Arabidopsis CALLOSE DEFECTIVE MICROSPORE1 gene is required for male fertility through regulating callose metabolism during microsporogenesis. Plant Physiol. 164, 1893–1904. doi: 10.1104/pp.113.233387
Ma, X.-D., Wang, J.-S., Lu, Y.-Q., Li, S.-Y., Liu, G.-Q., Hou, S.-L., et al. (2012). Characterization of fertility and pollen abortion of sorghum sterile line Ji 130A under different temperature conditions. J. Plant Genet. Res. 13, 212–218.
Ma, J., Wei, H., Liu, J., Song, M., Pang, C., Wang, L., et al. (2013). Selection and characterization of a novel photoperiod-sensitive male sterile line in upland cotton. J. Integr. Plant Biol. 55, 608–618. doi: 10.1111/jipb.12067
Manjappa, M., Rangaiah, S., Gowda, M. (2015). Assessment of heterotic potential of hybrids using a novel partial male sterile mutant (PS 1) in finger millet (Eleusine coracana (L.) Geartn.). Mysore J. Agric. Sci. 49, 266–269.
Marchant, D. B., Walbot, V. (2022). Anther development—The long road to making pollen. Plant Cell 34, 4677–4695. doi: 10.1093/plcell/koac287
Mariani, C., Beuckeleer, M. D., Truettner, J., Leemans, J., Goldberg, R. B. (1990). Induction of male sterility in plants by a chimaeric ribonuclease gene. nature 347, 737–741. doi: 10.1038/347737a0
Mariani, C., Gossele, V., Beuckeleer, M. D., Block, M. D., Goldberg, R. B., Greef, W. D., et al. (1992). A chimaeric ribonuclease-inhibitor gene restores fertility to male sterile plants. Nature 357, 384–387. doi: 10.1038/357384a0
Mei, M., Chen, L., Zhang, Z., Li, Z., Xu, C., Zhang, Q. (1999). pms3 is the locus causing the original photoperiod-sensitive male sterility mutation of’Nongken 58S’. Sci. China Life Sci. 42, 316–322. doi: 10.1007/BF03183609
Millar, A. A., Gubler, F. (2005). The Arabidopsis GAMYB-like genes, MYB33 and MYB65, are microRNA-regulated genes that redundantly facilitate anther development. Plant Cell 17, 705–721. doi: 10.1105/tpc.104.027920
Mitterreiter, M. J., Bosch, F. A., Brylok, T., Schwenkert, S. (2020). The ER luminal C-terminus of AtSec62 is critical for male fertility and plant growth in Arabidopsis thaliana. Plant J. 101, 5–17. doi: 10.1111/tpj.v101.1
Moon, S., Kim, S. R., Zhao, G., Yi, J., Yoo, Y., Jin, P., et al. (2013b). Rice glycosyltransferase1 encodes a glycosyltransferase essential for pollen wall formation. Plant Physiol. 161, 663–675. doi: 10.1104/pp.112.210948
Moon, J., Skibbe, D., Timofejeva, L., Wang, C. J. R., Kelliher, T., Kremling, K., et al. (2013a). Regulation of cell divisions and differentiation by MALE STERILITY 32 is required for anther development in maize. Plant J. 76, 592–602. doi: 10.1111/tpj.2013.76.issue-4
Mou, Z., Wang, X., Fu, Z., Dai, Y., Han, C., Ouyang, J., et al. (2002). Silencing of phosphoethanolamine N-methyltransferase results in temperature-sensitive male sterility and salt hypersensitivity in Arabidopsis. Plant Cell 14, 2031–2043. doi: 10.1105/tpc.001701
Muratore, C., Espen, L., Prinsi, B. (2021). Nitrogen uptake in plants: The plasma membrane root transport systems from a physiological and proteomic perspective. Plants 10, 681. doi: 10.3390/plants10040681
Murmu, J., Bush, M. J., Delong, C., Li, S., Xu, M., Khan, M., et al. (2010). Arabidopsis basic leucine-zipper transcription factors TGA9 and TGA10 interact with floral glutaredoxins ROXY1 and ROXY2 and are redundantly required for anther development. Plant Physiol. 154, 1492–1504. doi: 10.1104/pp.110.159111
Nan, G.-L., Zhai, J., Arikit, S., Morrow, D., Fernandes, J., Mai, L., et al. (2017). MS23, a master basic helix-loop-helix factor, regulates the specification and development of the tapetum in maize. Development 144, 163–172. doi: 10.1242/dev.140673
Ni, E., Deng, L., Chen, H., Lin, J., Ruan, J., Liu, Z., et al. (2021). OsCER1 regulates humidity-sensitive genic male sterility through very-long-chain (VLC) alkane metabolism of tryphine in rice. Funct. Plant Biol. 48, 461–468. doi: 10.1071/FP20168
Nishikawa, S., Zinkl, G. M., Swanson, R. J., Maruyama, D., Preuss, D. (2005). Callose (beta-1,3 glucan) is essential for Arabidopsis pollen wall patterning, but not tube growth. BMC Plant Biol. 5, 22. doi: 10.1186/1471-2229-5-22
Nonomura, K.-I., Miyoshi, K., Eiguchi, M., Suzuki, T., Miyao, A., Hirochika, H., et al. (2003). The MSP1 gene is necessary to restrict the number of cells entering into male and female sporogenesis and to initiate anther wall formation in rice. Plant Cell 15, 1728–1739. doi: 10.1105/tpc.012401
Omidvar, V., Mohorianu, I., Dalmay, T., Zheng, Y., Fei, Z., Pucci, A., et al. (2017). Transcriptional regulation of male-sterility in 7B-1 male-sterile tomato mutant. PloS One 12, e0170715. doi: 10.1371/journal.pone.0170715
Palve, S., Santhy, V., Bhat, S., Laxman, S., Rajesh Patil, R. P., Khadi, B., et al. (2011). Thermosensitive genetic male Sterility system in cotton (G. arboreum L.). World Cotton Res. Conf. Technol. Prosperity, 62–68.
Pei, Q., Liu, J., Guo, C., Ma, X., Liu, X., You, C., et al. (2024). Morphological and cytological assessments reveal pollen degradation causes pollen abortion in cotton cytoplasmic male sterility lines. J. Cotton Sci. 7, 26. doi: 10.1186/s42397-024-00189-8
Peng, G., Liu, Z., Zhuang, C., Zhou, H. (2023). Environment-sensitive genic male sterility in rice and other plants. Plant Cell Environ. 46, 1120–1142. doi: 10.1111/pce.14503
Peng, H. F., Zhang, Z. F., Wu, B., Chen, X. H., Zhang, G. Q., Zhang, Z. M., et al. (2008). Molecular mapping of two reverse photoperiod-sensitive genic male sterility genes (rpms1 and rpms2) in rice (Oryza sativa L.). Theor. Appl. Genet. 118, 77–83. doi: 10.1007/s00122-008-0877-1
Perez-Prat, E., Van Lookeren Campagne, M. M. (2002). Hybrid seed production and the challenge of propagating male-sterile plants. Trends Plant Sci. 7, 199–203. doi: 10.1016/S1360-1385(02)02252-5
Qi, Y., Liu, Q., Zhang, L., Mao, B., Yan, D., Jin, Q., et al. (2014). Fine mapping and candidate gene analysis of the novel thermo-sensitive genic male sterility tms9-1 gene in rice. Theor. Appl. Genet. 127, 1173–1182. doi: 10.1007/s00122-014-2289-8
Reddy, O., Siddiq, E., Sarma, N., Ali, J., Hussain, A., Nimmakayala, P., et al. (2000). Genetic analysis of temperature-sensitive male sterilty in rice. Theor. Appl. Genet. 100, 794–801. doi: 10.1007/s001220051354
Ren, W., Si, J., Chen, L., Fang, Z., Zhuang, M., Lv, H., et al. (2022). Mechanism and utilization of ogura cytoplasmic male sterility in cruciferae crops. Int. J. Mol. Sci. 23, 9099. doi: 10.3390/ijms23169099
Ren, L., Zhao, T., Zhang, L., Du, G., Shen, Y., Tang, D., et al. (2020). Defective microspore development 1 is required for microspore cell integrity and pollen wall formation in rice. Plant J. 103, 1446–1459. doi: 10.1111/tpj.v103.4
Rick, C. M., Boynton, J. E. (1967). A temperature-sensitive male-sterile mutant of the tomato. Am. J. Bot. 54, 601–611. doi: 10.1002/j.1537-2197.1967.tb10683.x
Ronspies, M., Dorn, A., Schindele, P., Puchta, H. (2021). CRISPR-Cas-mediated chromosome engineering for crop improvement and synthetic biology. Nat. Plants 7, 566–573. doi: 10.1038/s41477-021-00910-4
Ru, Z.-G., Zhang, L.-P., Hu, T.-Z., Liu, H.-Y., Yang, Q.-K., Weng, M.-L., et al. (2014). Genetic analysis and chromosome mapping of a thermo-sensitive genic male sterile gene in wheat. Euphytica 201, 321–327. doi: 10.1007/s10681-014-1218-x
Ruiz, O. N., Daniell, H. (2005). Engineering cytoplasmic male sterility via the chloroplast genome by expression of β-ketothiolase. Plant Physiol. 138, 1232–1246. doi: 10.1104/pp.104.057729
Schmidt, C., Pacher, M., Puchta, H. (2019). Efficient induction of heritable inversions in plant genomes using the CRISPR/Cas system. Plant J. 98, 577–589. doi: 10.1111/tpj.2019.98.issue-4
Schuhmann, P., Engstler, C., Klopfer, K., Gugel, I. L., Abbadi, A., Dreyer, F., et al. (2022). Two wrongs make a right: heat stress reversion of a male-sterile Brassica napus line. J. Exp. Bot. 73, 3531–3551. doi: 10.1093/jxb/erac082
Shearman, J. R., Sangsrakru, D., Ruang-Areerate, P., Sonthirod, C., Uthaipaisanwong, P., Yoocha, T., et al. (2014). Assembly and analysis of a male sterile rubber tree mitochondrial genome reveals DNA rearrangement events and a novel transcript. BMC Plant Biol. 14, 1–11. doi: 10.1186/1471-2229-14-45
Sheng, Z., Wei, X., Shao, G., Chen, M., Song, J., Tang, S., et al. (2013). Genetic analysis and fine mapping of tms9, a novel thermosensitive genic male-sterile gene in rice (Oryza sativa L.). Plant Breed. 132, 159–164. doi: 10.1111/pbr.2013.132.issue-2
Shi, Q. S., Lou, Y., Shen, S. Y., Wang, S. H., Zhou, L., Wang, J. J., et al. (2021). A cellular mechanism underlying the restoration of thermo/photoperiod-sensitive genic male sterility. Mol. Plant 14, 2104–2114. doi: 10.1016/j.molp.2021.08.019
Shi, C., Zhang, J., Wu, B., Jouni, R., Yu, C., Meyers, B. C., et al. (2022). Temperature-sensitive male sterility in rice determined by the roles of AGO1d in reproductive phasiRNA biogenesis and function. New Phytol. 236, 1529–1544. doi: 10.1111/nph.v236.4
Shukla, P., Singh, N. K., Gautam, R., Ahmed, I., Yadav, D., Sharma, A., et al. (2017). Molecular approaches for manipulating male sterility and strategies for fertility restoration in plants. Mol. Biotechnol. 59, 445–457. doi: 10.1007/s12033-017-0027-6
Si, F., Luo, H., Yang, C., Gong, J., Yan, B., Liu, C., et al. (2023). Mobile ARGONAUTE 1d binds 22-nt miRNAs to generate phasiRNAs important for low-temperature male fertility in rice. Sci. China Life Sci. 66, 197–208. doi: 10.1007/s11427-022-2204-y
Song, X. Y., Qian, H. H., Zhang, L. L. (2013). Cytogenetic analysis of cytoplasmic male sterility in wheat line KTP116A and molecular mapping of two thermo-sensitive restoration genes. Euphytica 196, 129–136. doi: 10.1007/s10681-013-1020-1
Steiner-Lange, S., Unte, U. S., Eckstein, L., Yang, C., Wilson, Z. A., Schmelzer, E., et al. (2003). Disruption of Arabidopsis thaliana MYB26 results in male sterility due to non-dehiscent anthers. Plant J. 34, 519–528. doi: 10.1046/j.1365-313x.2003.01745.x
Subudhi, P., Borkakati, R., Virmani, S., Huang, N. (1997). Molecular mapping of a thermosensitive genetic male sterility gene in rice using bulked segregant analysis. Genome 40, 188–194. doi: 10.1139/g97-027
Tang, J. H., Fu, Z. Y., Hu, Y. M., Li, J. S., Sun, L. L., Ji, H. Q. (2006). Genetic analyses and mapping of a new thermo-sensitive genic male sterile gene in maize. Theor. Appl. Genet. 113, 11–15. doi: 10.1007/s00122-006-0262-x
Tang, Z., Zhang, L., Yang, D., Zhao, C., Zheng, Y. (2011). Cold stress contributes to aberrant cytokinesis during male meiosis I in a wheat thermosensitive genic male sterile line. Plant Cell Environ. 34, 389–405. doi: 10.1111/j.1365-3040.2010.02250.x
Teng, C., Zhang, H., Hammond, R., Huang, K., Meyers, B. C., Walbot, V. (2020). Dicer-like 5 deficiency confers temperature-sensitive male sterility in maize. Nat. Commun. 11, 2912. doi: 10.1038/s41467-020-16634-6
Vasupalli, N., Kumar, V., Bhattacharya, R., Bhat, S. R. (2021). Analysis of mitochondrial recombination in the male sterile Brassica juncea cybrid Og1 and identification of the molecular basis of fertility reversion. Plant Mol. Biol. 106, 109–122. doi: 10.1007/s11103-021-01132-0
Vasupalli, N., Singh, S. K., Watts, A., Kumar, P., Kumar, V., Rao, K. R. S. S., et al. (2016). Mutations in the mitochondrial orf108 render Moricandia arvensis restorer ineffective in restoring male fertility to Brassica oxyrrhina-based cytoplasmic male sterile line of B. juncea. Mol. Breed. 36, 67. doi: 10.1007/s11032-016-0489-4
Vernoud, V., Laigle, G., Rozier, F., Meeley, R. B., Perez, P., Rogowsky, P. M. (2009). The HD-ZIP IV transcription factor OCL4 is necessary for trichome patterning and anther development in maize. Plant J. 59, 883–894. doi: 10.1111/j.1365-313X.2009.03916.x
Wan, X., Wu, S., Li, Z., Dong, Z., An, X., Ma, B., et al. (2019). Maize genic male-sterility genes and their applications in hybrid breeding: Progress and perspectives. Mol. Plant 12, 321–342. doi: 10.1016/j.molp.2019.01.014
Wan, L., Zha, W., Cheng, X., Liu, C., Lv, L., Liu, C., et al. (2011). A rice β-1, 3-glucanase gene Osg1 is required for callose degradation in pollen development. Planta 233, 309–323. doi: 10.1007/s00425-010-1301-z
Wang, B., Fang, R., Zhang, J., Han, J., Chen, F., He, F., et al. (2020). Rice LecRK5 phosphorylates a UGPase to regulate callose biosynthesis during pollen development. J. Exp. Bot. 71, 4033–4041. doi: 10.1093/jxb/eraa180
Wang, D., Li, J., Sun, L., Hu, Y., Yu, J., Wang, C., et al. (2021). Two rice MYB transcription factors maintain male fertility in response to photoperiod by modulating sugar partitioning. New Phytol. 231, 1612–1629. doi: 10.1111/nph.v231.4
Wang, H., Lu, Y., Jiang, T., Berg, H., Li, C., Xia, Y. (2013). The Arabidopsis U-box/ARM repeat E3 ligase AtPUB4 influences growth and degeneration of tapetal cells, and its mutation leads to conditional male sterility. Plant J. 74, 511–523. doi: 10.1111/tpj.2013.74.issue-3
Wang, C.-J. R., Nan, G.-L., Kelliher, T., Timofejeva, L., Vernoud, V., Golubovskaya, I. N., et al. (2012). Maize multiple archesporial cells 1 (mac1), an ortholog of rice TDL1A, modulates cell proliferation and identity in early anther development. Development 139, 2594–2603. doi: 10.1242/dev.077891
Wang, B., Xu, W., Wang, J., Wu, W., Zheng, H., Yang, Z., et al. (1995). Tagging and mapping the thermo-sensitive genic male-sterile gene in rice (Oryza sativa L.) with molecular markers. Theor. Appl. Genet. 91, 1111–1114. doi: 10.1007/BF00223928
Wang, K. Q., Yu, Y. H., Jia, X. L., Zhou, S. D., Zhang, F., Zhao, X., et al. (2022). Delayed callose degradation restores the fertility of multiple P/TGMS lines in Arabidopsis. J. Integr. Plant Biol. 64, 717–730. doi: 10.1111/jipb.13205
Wang, Y., Zha, X., Zhang, S., Qian, X., Dong, X., Sun, F., et al. (2010). Down-regulation of the OsPDCD5 gene induced photoperiod-sensitive male sterility in rice. Plant Sci. 178, 221–228. doi: 10.1016/j.plantsci.2009.12.001
Wei, D., Liu, M., Chen, H., Zheng, Y., Liu, Y., Wang, X., et al. (2018). INDUCER OF CBF EXPRESSION 1 is a male fertility regulator impacting anther dehydration in Arabidopsis. PloS Genet. 14, e1007695. doi: 10.1371/journal.pgen.1007695
Wei, W., Wang, P., Li, S., Fan, G., Zhao, F., Zhang, X., et al. (2021). Rapid identification of candidate genes controlling male-sterility in Foxtail millet (Setaria italica). Mol. Breed. 41, 73. doi: 10.1007/s11032-021-01269-2
Wen, J., Zeng, Y., Chen, Y., Fan, F., Li, S. (2021). Genic male sterility increases rice drought tolerance. Plant Sci. 312, 111057. doi: 10.1016/j.plantsci.2021.111057
Wheeler, M. J., Franklin-Tong, V. E., Franklin, F. C. H. (2001). The molecular and genetic basis of pollen-pistil interactions. New Phytol. 151, 565–584. doi: 10.1046/j.0028-646x.2001.00229.x
Wolf, C., Hennig, M., Romanovicz, D., Steinebrunner, I. (2007). Developmental defects and seedling lethality in apyrase AtAPY1 and AtAPY2 double knockout mutants. Plant Mol. Biol. 64, 657–672. doi: 10.1007/s11103-007-9184-5
Wu, Y., Fox, T. W., Trimnell, M. R., Wang, L., Xu, R. J., Cigan, A. M., et al. (2016). Development of a novel recessive genetic male sterility system for hybrid seed production in maize and other cross-pollinating crops. Plant Biotechnol. J. 14, 1046–1054. doi: 10.1111/pbi.2016.14.issue-3
Wu, L., Jing, X., Zhang, B., Chen, S., Xu, R., Duan, P., et al. (2022). A natural allele of OsMS1 responds to temperature changes and confers thermosensitive genic male sterility. Nat. Commun. 13, 2055. doi: 10.1038/s41467-022-29648-z
Xing, S., Zachgo, S. (2008). ROXY1 and ROXY2, two Arabidopsis glutaredoxin genes, are required for anther development. Plant J. 53, 790–801. doi: 10.1111/j.1365-313X.2007.03375.x
Xu, L., Tang, Y., Yang, Y., Wang, D., Wang, H., Du, J., et al. (2023). Microspore-expressed SCULP1 is required for p-coumaroylation of sporopollenin, exine integrity, and pollen development in wheat. New Phytol. 239, 102–115. doi: 10.1111/nph.v239.1
Xu, F., Zheng, L., Yang, Z., Zhang, S. (2020). Arabidopsis ECERIFERUM3 (CER3) functions to maintain hydration for pollen–stigma recognition during fertilization. J. Plant Biol. 63, 347–359. doi: 10.1007/s12374-020-09257-3
Xue, Z., Xu, X., Zhou, Y., Wang, X., Zhang, Y., Liu, D., et al. (2018). Deficiency of a triterpene pathway results in humidity-sensitive genic male sterility in rice. Nat. Commun. 9, 604. doi: 10.1038/s41467-018-03048-8
Yamaguchi, Y., Hirasawa, H., Minami, M., Ujihara, A. (1997). ). Linkage analysis of thermosensitive genic male sterility gene, tms-2 in rice (Oryza sativa L.). Japanese J. Breed. 47, 371–373. doi: 10.1270/jsbbs1951.47.371
Yan, J., Li, H., Li, S., Yao, R., Deng, H., Xie, Q., et al. (2013). The Arabidopsis F-box protein CORONATINE INSENSITIVE1 is stabilized by SCFCOI1 and degraded via the 26S proteasome pathway. Plant Cell 25, 486–498. doi: 10.1105/tpc.112.105486
Yan, W., Yuan, S., Zu, Y., Chang, Z., Li, Y., Chen, Z., et al. (2023). Ornithine δ-aminotransferase OsOAT is critical for male fertility and cold tolerance during rice plant development. Plant J. 114, 1301–1318. doi: 10.1111/tpj.v114.6
Yan, X., Zeng, X., Wang, S., Li, K., Yuan, R., Gao, H., et al. (2016). Aberrant meiotic prophase i leads to genic male sterility in the novel TE5A mutant of Brassica napus. Sci. Rep. 6, 33955. doi: 10.1038/srep33955
Yang, S.-L., Jiang, L., Puah, C. S., Xie, L.-F., Zhang, X.-Q., Chen, L.-Q., et al. (2005). Overexpression of TAPETUM DETERMINANT1 alters the cell fates in the Arabidopsis carpel and tapetum via genetic interaction with excess EXCESS ICROSPOROCYTES1/EXTRA SPOROGENOUS CELLS. Plant Physiol. 139, 186–191. doi: 10.1104/pp.105.063529
Yang, H., Li, Y., Cao, Y., Shi, W., Xie, E., Mu, N., et al. (2022). Nitrogen nutrition contributes to plant fertility by affecting meiosis initiation. Nat. Commun. 13, 485. doi: 10.1038/s41467-022-28173-3
Yang, Z., Liu, L., Sun, L., Yu, P., Zhang, P., Abbas, A., et al. (2019). OsMS1 functions as a transcriptional activator to regulate programmed tapetum development and pollen exine formation in rice. Plant Mol. Biol. 99, 175–191. doi: 10.1007/s11103-018-0811-0
Yang, L., Qian, X., Chen, M., Fei, Q., Meyers, B. C., Liang, W., et al. (2016). Regulatory role of a receptor-like kinase in specifying anther cell identity. Plant Physiol. 171, 2085–2100. doi: 10.1104/pp.16.00016
Yang, K. Z., Xia, C., Liu, X. L., Dou, X. Y., Wang, W., Chen, L. Q., et al. (2009). A mutation in Thermosensitive Male Sterile 1, encoding a heat shock protein with DnaJ and PDI domains, leads to thermosensitive gametophytic male sterility in Arabidopsis. Plant J. 57, 870–882. doi: 10.1111/j.1365-313X.2008.03732.x
Yang, X., Ye, J., Niu, F., Feng, Y., Song, X. (2021). Identification and verification of genes related to pollen development and male sterility induced by high temperature in the thermo-sensitive genic male sterile wheat line. Planta 253, 83. doi: 10.1007/s00425-021-03601-8
Yi, J., Moon, S., Lee, Y. S., Zhu, L., Liang, W., Zhang, D., et al. (2016). Defective Tapetum Cell Death 1 (DTC1) regulates ros levels by binding to metallothionein during tapetum degeneration. Plant Physiol. 170, 1611–1623. doi: 10.1104/pp.15.01561
Yu, J., Han, J., Kim, Y. J., Song, M., Yang, Z., He, Y., et al. (2017). Two rice receptor-like kinases maintain male fertility under changing temperatures. Proc. Natl. Acad. Sci. U.S.A. 114, 12327–12332. doi: 10.1073/pnas.1705189114
Yu, Q., Liang, C., Wang, X., Du, Y., Masuda, M., Murakami, K. (2015). Study on the pollen thermo-sensitive and stigma exsertion male sterile line tomato (Lycopersicon esculentum Mill.) cv. Da107. Am. J. Plant Sci. 06, 2535–2539. doi: 10.4236/ajps.2015.616255
Yu, B., Liu, L., Wang, T. (2019). Deficiency of very long chain alkanes biosynthesis causes humidity-sensitive male sterility via affecting pollen adhesion and hydration in rice. Plant Cell Environ. 42, 3340–3354. doi: 10.1111/pce.v42.12
Yu, C., Xu, X., Ge, J., Guo, Y., Dong, J., Dong, Z. (2016). Premature breakdown of tapetum associated with reverse thermo-sensitive genic male-sterile line Huiyou50S in rapeseed (Brassica napus). Acta Physiol. Plant 38, 54. doi: 10.1007/s11738-015-2039-9
Zhan, H., Xiong, H., Wang, S., Yang, Z. N. (2018). Anther endothecium-derived very-long-chain fatty acids facilitate pollen hydration in Arabidopsis. Mol. Plant 11, 1101–1104. doi: 10.1016/j.molp.2018.05.002
Zhang, J.-K., Dong, J., Zong, X.-F., Yu, G.-D., Dai, X.-M., Ruan, R.-W. (2009). Fertility alternation of thermo-photo-sensitive genic male sterile (TGMS) wheat line C412S and its association with adenine phosphoribosyltransferase gene expression. Acta Phys. Sin. 35, 662–671. doi: 10.1016/S1875-2780(08)60077-2
Zhang, Y. F., Li, Y. L., Zhong, X., Wang, J. J., Zhou, L., Han, Y., et al. (2022b). Mutation of glucose-methanol-choline oxidoreductase leads to thermosensitive genic male sterility in rice and Arabidopsis. Plant Biotechnol. J. 20, 2023–2035. doi: 10.1111/pbi.v20.10
Zhang, H., Liang, W., Yang, X., Luo, X., Jiang, N., Ma, H., et al. (2010). Carbon starved anther encodes a MYB domain protein that regulates sugar partitioning required for rice pollen development. Plant Cell 22, 672–689. doi: 10.1105/tpc.109.073668
Zhang, M., Liu, J., Ma, Q., Qin, Y., Wang, H., Chen, P., et al. (2020b). Deficiencies in the formation and regulation of anther cuticle and tryphine contribute to male sterility in cotton PGMS line. BMC Genom. 21, 825. doi: 10.1186/s12864-020-07250-1
Zhang, C., Liu, C., Weng, J., Cheng, B., Liu, F., Li, X., et al. (2017). Creation of targeted inversion mutations in plants using an RNA-guided endonuclease. Crop J. 5, 83–88. doi: 10.1016/j.cj.2016.08.001
Zhang, C., Ren, M. Y., Han, W. J., Zhang, Y. F., Huang, M. J., Wu, S. Y., et al. (2022a). Slow development allows redundant genes to restore the fertility of rpg1, a TGMS line in Arabidopsis. Plant J. 109, 1375–1385. doi: 10.1111/tpj.v109.6
Zhang, Q., Shen, B., Dai, X., Mei, M., Saghai Maroof, M., Li, Z. (1994). Using bulked extremes and recessive class to map genes for photoperiod-sensitive genic male sterility in rice. Proc. Natl. Acad. Sci. U.S.A. 91, 8675–8679. doi: 10.1073/pnas.91.18.8675
Zhang, W., Sun, Y., Timofejeva, L., Chen, C., Grossniklaus, U., Ma, H. (2006). Regulation of Arabidopsis tapetum development and function by DYSFUNCTIONAL TAPETUM1 (DYT1) encoding a putative bHLH transcription factor. Development 133, 3085–3095. doi: 10.1242/dev.02463
Zhang, D., Wu, S., An, X., Xie, K., Dong, Z., Zhou, Y., et al. (2018). Construction of a multicontrol sterility system for a maize male-sterile line and hybrid seed production based on the ZmMs7 gene encoding a PHD-finger transcription factor. Plant Biotechnol. J. 16, 459–471. doi: 10.1111/pbi.2018.16.issue-2
Zhang, H., Xu, C., He, Y., Zong, J., Yang, X., Si, H., et al. (2013). Mutation in CSA creates a new photoperiod-sensitive genic male sterile line applicable for hybrid rice seed production. Proc. Natl. Acad. Sci. U.S.A. 110, 76–81. doi: 10.1073/pnas.1213041110
Zhang, C., Xu, T., Ren, M. Y., Zhu, J., Shi, Q. S., Zhang, Y. F., et al. (2020a). Slow development restores the fertility of photoperiod-sensitive male-sterile plant lines. Plant Physiol. 184, 923–932. doi: 10.1104/pp.20.00951
Zhang, L., Yang, M., Wei, K., Yang, W., Li, S., Wang, X., et al. (2024). Fine-Mapping of Tomato male sterile-24 Locus and Marker Development for ms-24 and Its Alleles, ms-10, ms-35, and ms-36. Horticulturae 10, 1322. doi: 10.3390/horticulturae10121322
Zhao, D.-Z., Wang, G.-F., Speal, B., Ma, H. (2002). The excess microsporocytes1 gene encodes a putative leucine-rich repeat receptor protein kinase that controls somatic and reproductive cell fates in the Arabidopsis anther. Genes Dev. 16, 2021–2031. doi: 10.1101/gad.997902
Zhou, M., Deng, L., Yuan, G., Zhao, W., Ma, M., Sun, C., et al. (2023). Rapid generation of a tomato male sterility system and its feasible application in hybrid seed production. Theor. Appl. Genet. 136, 197. doi: 10.1007/s00122-023-04428-5
Zhou, L.-Z., Juranić, M., Dresselhaus, T. (2017). Germline development and fertilization mechanisms in maize. Mol. Plant 10, 389–401. doi: 10.1016/j.molp.2017.01.012
Zhou, H., Liu, Q., Li, J., Jiang, D., Zhou, L., Wu, P., et al. (2012). Photoperiod- and thermo-sensitive genic male sterility in rice are caused by a point mutation in a novel noncoding RNA that produces a small RNA. Cell Res. 22, 649–660. doi: 10.1038/cr.2012.28
Zhou, H., Zhou, M., Yang, Y., Li, J., Zhu, L., Jiang, D., et al. (2014). RNase ZS1 processes UbL40 mRNAs and controls thermosensitive genic male sterility in rice. Nat. Commun. 5, 4884. doi: 10.1038/ncomms5884
Keywords: genic male sterility (GMS), biotechnology-based male sterile (BBMS) systems, environment-sensitive genic male sterility (EGMS), long non-coding RNAs (lncRNAs), phased secondary small interfering RNAs (phasiRNAs)
Citation: Vasupalli N, Mogilicherla K, Shaik V, Rao KRSS, Bhat SR and Lin X (2025) Advances in plant male sterility for hybrid seed production: an overview of conditional nuclear male sterile lines and biotechnology-based male sterile systems. Front. Plant Sci. 16:1540693. doi: 10.3389/fpls.2025.1540693
Received: 06 December 2024; Accepted: 20 January 2025;
Published: 05 February 2025.
Edited by:
Karthikeyan Adhimoolam, Jeju National University, Republic of KoreaReviewed by:
Taras P. Pasternak, Miguel Hernández University of Elche, SpainCopyright © 2025 Vasupalli, Mogilicherla, Shaik, Rao, Bhat and Lin. This is an open-access article distributed under the terms of the Creative Commons Attribution License (CC BY). The use, distribution or reproduction in other forums is permitted, provided the original author(s) and the copyright owner(s) are credited and that the original publication in this journal is cited, in accordance with accepted academic practice. No use, distribution or reproduction is permitted which does not comply with these terms.
*Correspondence: Xinchun Lin, bGlueGN4QDE2My5jb20=
†These authors have contributed equally to this work
Disclaimer: All claims expressed in this article are solely those of the authors and do not necessarily represent those of their affiliated organizations, or those of the publisher, the editors and the reviewers. Any product that may be evaluated in this article or claim that may be made by its manufacturer is not guaranteed or endorsed by the publisher.
Research integrity at Frontiers
Learn more about the work of our research integrity team to safeguard the quality of each article we publish.