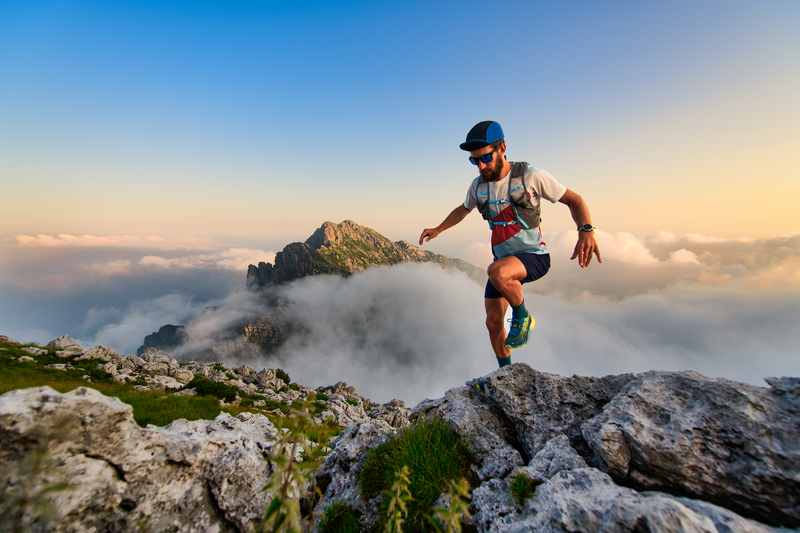
94% of researchers rate our articles as excellent or good
Learn more about the work of our research integrity team to safeguard the quality of each article we publish.
Find out more
SYSTEMATIC REVIEW article
Front. Plant Sci. , 20 February 2025
Sec. Plant Pathogen Interactions
Volume 16 - 2025 | https://doi.org/10.3389/fpls.2025.1425880
Introduction: Glutathione peroxidases (GPXs) are extensively studied for their indispensable roles in eliminating reactive oxygen species by catalyzing the reduction of hydrogen peroxide or lipid peroxides to prevent cell damage. However, knowledge of GPXs in plants still has many gaps to be filled. Thus, we present the first systematic review (SR) aimed at examining the function of GPXs and their protective role against cell death in plants subjected to biotic stress.
Methods: To guide the SR and avoid bias, a protocol was developed that contained inclusion and exclusion criteria based on PRISMA guidelines. Three databases (PubMed, Science Direct, and Springer) were used to identify relevant studies for this research were selected.
Results: A total of 28 articles related to the proposed objective. The results highlight the importance of GPXs in plant defense against biotic stress, including their role in protecting against cell death, similar to the anti-apoptotic GPXs in animals. Data from gene expression and protein accumulation studies in plants under various biotic stresses reveal that GPXs can both increase resistance and susceptibility to pathogens. In addition to their antioxidant functions, GPXs act as sensors and transmitters of H2O2 signals, integrating with the ABA signaling pathway during stress.
Discussion: These findings show that GPXs delay senescence or reinforce physical barriers, thereby modulating resistance or susceptibility to pathogens. Additionally, their functions are linked to their cellular localization, which demonstrates an evolutionary relationship between the studied isoforms and their role in plant defense. This information broadens the understanding of molecular strategies involving GPX isoforms and provides a foundation for discussions and actions aimed at controlling necrotrophic and/or hemibiotrophic pathogens.
Plants are constantly exposed to various stress conditions. Among these, biotic stresses involve significant losses in agricultural yield and food production, affecting the global economy (Pereira, 1989; Blomme et al., 2017; Moreira et al., 2022; Ofori et al., 2022). These problems are caused by various phytopathogenic organisms, such as microorganisms and insects, each with different infection, development, and survival strategies within the host (Meng et al., 2009; Vojnov et al., 2010; Doehlemann et al., 2017; Noman et al., 2021). In most of the responses to stress, the plant promotes an increase in reactive oxygen species (ROS) (Dias et al., 2011; Mittler et al., 2022) that can cause damage such as lipid degradation, protein degradation, nucleic acid damage, and induce programmed cell death (PCD) (Das and Roychoudhury, 2014).
However, plants possess an antioxidant system that protects against damage caused by ROS, composed of enzymatic and non-enzymatic elements (Dumanović et al., 2020). Among the enzymatic components, glutathione peroxidases (GPXs) play a crucial role in resistance to stresses induced by phytopathogens (Rockenbach et al., 2015; Wang et al., 2018). These proteins catalyze the conversion of hydrogen peroxide (H2O2) and organic or lipid hydroperoxides into water or alcohols, using glutathione (GSH) or thioredoxin (Trx) as electron donors (Arthur, 2000; Iqbal et al., 2006; Bela et al., 2022). In addition, they contribute to the regulation of cellular redox homeostasis, maintaining the thiol/disulfide and NADPH/NADP+ balance (Bela et al., 2015).
Generally, proteins of the GPX family are characterized either as selenoproteins or non-selenoproteins depending if they have in their catalytic sites the amino acid residue selenocysteine (SeCys) encoded by the UGA codon or the cysteine (Cys) encoded by the UGU or UGC codon (Trenz et al., 2021). GPXs have three conserved motifs, which although poorly detailed regarding their function, are present in most eukaryotic organisms. In plants, these motifs are VNAS[R/K/Q]CG, LAFPCNQF, and WNF(S/T)KF, wherein three primary catalytic centers are presented as cysteine (Cys) or selenocysteine (Cys)-Glutamine (Gln)-Tryptophan (Trp) (Churin et al., 1999). However, molecular biology techniques of targeted mutations using CRISPR/Cas, as well as functional studies of protein production and characterization, with activity assays, in addition to in silico and in vitro studies of protein-ligand or protein-protein interactions, could clarify the specific roles of these motifs (Létoffé et al., 1998; Scheerer et al., 2007; Shi et al., 2010; Martins Alves et al., 2019; Dolgalev and Poverennaya, 2021; Avery et al., 2022). Furthermore, plant GPXs vary in terms of cellular localization (e.g. chloroplast, mitochondria, cytoplasm, or apoplast) (Navrot et al., 2006; Yang et al., 2006; Attacha et al., 2017), and their function are still poorly understood and explored. The cellular localization of these enzymes is crucial for their protective functions against biotic stress, as it is linked to their effectiveness in providing protection (Mbemba et al., 1985; Navrot et al., 2006). In the chloroplast, during pathogen infections, ROS production can increase significantly, and GPX helps mitigate this stress by protecting photosynthetic components and potentially reducing PCD (Mbemba et al., 1985; Yoshimura et al., 2004; Zhai et al., 2013). Mitochondria, similarly, play a crucial role in preventing oxidative damage to respiratory components. In peroxisomes, where antioxidant enzymes are commonly present, GPXs also help manage H2O2 levels during biotic stress (Rodríguez-Serrano et al., 2016). The apoplast, a critical site for host-pathogen interactions, benefits from extracellular or apoplastic GPXs that reduce ROS levels, thereby helping the plant prevent tissue damage (Foyer and Noctor, 2005). In the nucleus, GPXs protect DNA from oxidative damage and are involved in regulating gene expression during stress responses (Gaber et al., 2012). The localization of these plant enzymes can be investigated using techniques such as localized gene expression studies, cell fractionation, Western blot analysis, immunolocalization with electron microscopy, fusion with fluorescent proteins, as well as transcriptomics and proteomics of cellular compartments (Parish, 1971; Herbette et al., 2004; Zhai et al., 2013; de Paula and Techio, 2014; Chen et al., 2017; Arnaud et al., 2022; Christopher et al., 2022; Wang et al., 2023).
Systematizing published data on GPXs is crucial for understanding their functions and role in mitigating damage induced by pathogens, including cell death, which is vital for advancing future research and potential applications of GPXs in plant responses to biotic stress. Employing a Systematic Review (SR) approach ensures a comprehensive and unbiased compilation of high-quality investigations, akin to its common utilization in the medical field (Morvaridzadeh et al., 2021; Wang et al., 2021; Zhao et al., 2021; Pereira et al., 2022). While traditionally applied in medicine, recent years have seen an increasing exploration of SRs in agronomic studies, particularly in elucidating plant-pathogen interaction mechanisms encompassing molecular, biological, and biochemical responses (Soares et al., 2021; de Novais et al., 2023; dos Santos Lopes et al., 2023; Santos et al., 2023). In this context, our study represents the first SR on plant GPXs concerning biotic stress, revealing their antioxidant activity and diverse functions, including the potential inhibition of cell death, similarly findings in animal systems. This work sets the stage for further research into GPXs, paving the way for hypothesis validations and the discovery of new functionalities crucial for enhancing plant resilience to biotic stresses.
The SR was conducted using R version 4.0.3 (R.C. Team, 2023) with the Bibliometrix package and the StArt (State of the Art through Systematic Review) software version Beta 3.0.3 (Fabbri et al., 2016). This software was developed by the Federal University of São Carlos (UFSCar) and is available for download (http://lapes.dc.ufscar.br/tools/start_tool). The review followed the PRISMA (Preferred Reporting Items for Systematic Reviews and Meta-Analyses) guidelines (Page et al., 2021) and was conducted in three stages: planning, execution, and summarization.
A protocol was developed and discussed by the author team to guide the stages of the SR, incorporating essential information such as the article title, authors, objectives, keywords, research questions, sources of research, inclusion/exclusion criteria, search strings, selection of databases, and quality assessment of collected files. The questions to achieve the objective of the SR (Table 1) were formulated based on Population Intervention Comparison Results (PICOS) criteria (Table 2). The strategy employed was necessary to not only guide the questions but also to seek specific answers in order to exclude any biased responses.
The searches for studies were conducted in the PubMed, Science Direct, and Springer pre-selected databases, which contain peer-reviewed journals aligned with the research theme. The string “glutathione peroxidase” AND “biotic stress” AND “cell death” AND “plant” was used for this search, with the Boolean connector AND used in the string to group keywords and main terms. The obtained files were imported in BIBITEX and MEDLINE formats to StArt and R program. After screening in R program, the files were transferred to StArt (v. Beta 3.0.3), where automated selection occurred based on the reading of titles, abstracts, and keywords. In this selection, inclusion and exclusion criteria were used (Supplementary Table S1).
The accepted studies, according to the inclusion criteria, were read in their entirety. This stage involved the extraction and systematization of data that addressed the questions proposed in the SR, including the creation of tables and figures to represent the findings. Metadata related to the production and dissemination of scientific knowledge (such as collaboration between countries, institutions, authors of the studies, and words from the titles) were analyzed from the selected articles using the R program with the Bibliometrix package. The program was also used to construct a heatmap of differential protein accumulation from Log fold change (LogFC) values provided by articles that used proteomic analysis as a study strategy. Only one of these articles was not included in the heat map because it did not provide the Log fold change (LogFC) value of the differentially accumulated protein (Sharma et al., 2007). The heatmap was constructed using the Complexheatmap package. The subcellular localization of GPX proteins, when not indicated in the studies, was predicted using the DeepLoc 1.0 platform (https://services.healthtech.dtu.dk/services/DeepLoc-1.0/, accessed on August 21, 2023).
The interaction network analysis was conducted to examine the biological functions in which the GPX proteins from the selected studies should be involved. For this purpose, a search for homologs in a model organism (Arabidopsis thaliana) was performed using the STRING server version 11.0 (https://string-db.org/) (Jensen et al., 2009). The protein sequences used and their respective identities with homologs are presented in the Supplementary Table S2. The proteins were analyzed using the following parameters: meaning of network edges - confidence; active interaction sources - text mining, experiments, databases, co-expression, neighborhood, gene fusion, and co-occurrence; minimum required interaction score - high confidence (0.700) in 50 interactions, significance level of 0.7; maximum number of connectors to revel the 1st and 2nd layers and no more than 50 interactions. The data generated in the network were downloaded in TSV format and transferred to Cytoscape version 3.7.2 (https://cytoscape.org/) and BiNGO plugin (https://apps.cytoscape.org/apps/bingo) tools.
The alignment and construction of a phylogenetic tree were performed using amino acid residue sequences of GPXs obtained from the selected studies, to identify similarities and differences between these proteins, as well as evolutionary relationships. For studies that solely provided GPX gene information, BLASTx (https://blast.ncbi.nlm.nih.gov/Blast.cgi, accessed on August 22, 2023) was used to obtain the corresponding or homologous FASTA sequences of amino acid residues (Supplementary Table S3). Alignment was obtained using the Clustal Omega server with the multiple alignment tool (https://www.ebi.ac.uk/Tools/msa/clustalo/, accessed on August 22, 2023). The phylogenetic tree was constructed using the Neighbor-Joining method with 1000 bootstraps (Saitou and Nei, 1987). The method was applied in the MEGA 7 software (Kumar et al., 2016). The Poisson correction method (Zuckerkandl and Pauling, 1965) was employed to calculate evolutionary distances, expressed in units of the number of amino acid substitutions per site. The analysis involved 29 sequences, and all positions containing gaps and missing data were removed.
A total of 872 articles were retrieved from the Pubmed (32.8%), Science Direct (56.5%) and Springer (9.3%) databases. After excluding 5 duplicates in the StArt program, 805 articles were discarded based on title, abstract and keywords (Figure 1; Supplementary Table S1). The main reasons were: reviews (10%); book chapters (6%), animal GPXs (11%), GPXs-only approaches in abiotic stress (20%); duplicates (1%); and other unrelated topics (52%). Sixty-two articles were analyzed in full and 34 were excluded because they met the exclusion criteria, including studies on animal GPXs (6%), abiotic stress only (9%), duplicates (3%), reviews (3%) and other unrelated topics (79%). Finally, 28 articles were aligned with the study objectives and were considered eligible for this SR (Figure 1; Supplementary Table S4). The included studies were grouped according to the bibliometric data of the publishing journal and their respective impact factors (IF) (Figure 2A). They were published in journals with an IF between 2.7 and 10.7, with a focus on Physiological and Molecular Plant Pathology (8), Journal of Proteomics (3) and Plant Science (3), which had the highest number of articles among SR journal (Figure 2A). Most of the studies originated from Brazil (20%), India (18%) and China (16%) (Figure 2B) were published between 2003 and March 2023, with 2020 being the year with the highest number of studies (5) (Figure 2C). The 2020 articles addressed several techniques, such as gene expression, enzymatic activity, biochemistry and functional genomics. However, the number of articles did not show a constant increase, indicating that few studies have explored the potential of GPXs against biotic stresses, and consequently many questions remain unanswered about their role.
Figure 1. PRISMA flowchart depicting the identification and selection of studies on GPX responses to biotic stress and programmed cell death in plants.
Figure 2. Bibliometric indicators of studies related to GPX response to biotic stress. (A) number of articles per journal, with their impact factor (IF). (B) Frequency of studies across countries. (C) Number of articles per year, with their respective study categories. * Searches for publications from the year 2023 were considered until March.
Collaboration and co-occurrence networks were constructed (Figure 3). Clusters were organized in different colors based on co-occurrence, with larger font size highlighting words that occurred more frequently within the studies selected in SR. The word network related to the research titles showed that “Peroxidases” and “Oxidative” appeared consistently associated with “Gluthatione” (in orange, Figure 3A). Collaborations were highlighted between Brazil, France and Japan (red cluster); India, USA and Mexico (blue cluster); China and Singapore (green cluster); and Germany, Poland, Sweden and the United Kingdom (purple cluster). Brazil, India and China represent the countries with the highest frequencies of research involving GPXs in biotic stress in plants (Figure 3B). The networks showing the collaborations between the main scientists of the selected articles and between the institutions were organized into ten clusters. The author “Juarez-Maldonato A” presented the highest number of collaborations (in blue, Figure 3C), while “Universidad Autonoma Agraria Antonio Narro” in Mexico was the most collaborative (in red, Figure 3D). Collaborations may favor more in-depth studies on GPXs in biotic stress, since they may involve different specializations and techniques. However, these collaborations are still limited to specific groups and expanding these partnerships beyond these limits can improve the quality of research and contribute to filling gaps on the role of GPXs in this context.
Figure 3. Networks for dissemination and collaboration of scientific knowledge on GPXs related to biotic stress. (A) Co-occurrence of words contained within journal titles. (B) Collaboration between countries of investigation. (C) Collaboration between authors. (D) Collaboration between institutions. Font sizes in the networks are proportional to the contribution of words, countries, authors, or institutions to the groups.
The types of GPXs addressed in the studies, and their association with different biotic stresses, are presented in Table 3. In 17.8% of the studies, plant GPXs are associated with cell death induced by different pathogens. The silencing of GPX1 and GPX7 genes in transgenic A. thaliana plants showed that these genes were involved in hypersensitive response (HR) during defense against Pseudomonas syringae bacteria (Chang et al., 2009), while the GPX4/PHGPX genes from Nicotiana benthamiana and Lycopersicon esculentum, were associated with defense against ferroptosis-type cell death and PCD induced by viral and fungal (necrotrophic) phytopathogens (Chen et al., 2004; Macharia et al., 2020). Transgenic N. benthamiana with silenced GPX4 exposed to tobacco mosaic virus exhibited increased accelerated ferroptosis-type cell death compared to non-silenced control plants (Macharia et al., 2020). Moreover, transgenic tobacco leaves constitutively over-expressing PHGPX gene from L. esculentum (LePHGPX), inoculated with the necrotrophic fungi Botrytis cinerea and Sclerotinia sclerotiorum, were highly resistant compared to control leaves, which exhibited extended necrotic lesions (Chen et al., 2004). The transgenic tobacco leaves were also subjected to salt stress; the transient expression of LePHGPX in these stress-exposed leaves prevented DNA fragmentation and maintained membrane integrity. LePHGPX thus acted as cytoprotector in plants under different local stress conditions (Chen et al., 2004). Collectively, these data showed functional similarity to antiapoptotic PHGPX genes in animal organisms (Chen et al., 2004). Therefore, the GPX4/PHGPX genes in plants may be involved in defense against cell death induced by pathogens.
Table 3. Types of glutathione peroxidases (GPXs) proteins associated with biotic stress in plants, found in the selected studies.
The evaluation of GPX expression in response to biotic stress was used in 42.9% of the studies (Table 3). GPX genes were upregulated in 50% of the studies in resistant, partially resistant or tolerant plants, and in 11% of the studies in susceptible plants. Downregulation was observed in 22% of the studies with susceptible plants and in 17% of the studies with resistant, partially resistant or tolerant plants (Chen et al., 2004; Yang et al., 2006; Chang et al., 2009; Hajianfar et al., 2016; Koop et al., 2016; Tyagi et al., 2018; Wang et al., 2018; Macharia et al., 2020; Martins Alves et al., 2020; Sa et al., 2020; Zhu et al., 2023). These data indicate that, although resistant or tolerant plants present a higher frequency of upregulation of GPX genes, this induction is not a trend exclusive to them, being also observed in susceptible plants (Figure 4A).
Figure 4. Studies on of plant GPX regulation in response to pathogens. (A) Percentage of studies with GPX genes differentially regulated in resistant or partially resistant or tolerant plants compared to susceptible ones, in the presence of pathogens. (B) Heatmap of the differential accumulation of GPX proteins in plants in interaction with pathogens. The differential accumulation of proteins is represented by the Log fold change (LogFC) value provided by the selected studies; only one of the studies is not included in the heatmap, as this value was not provided in the available information. The following GPXs are represented: VuPHGPX (PHGPX from Vigna unguiculata) in an incompatible interaction with Cowpea severe mosaic virus (CPSMV); MdGPX (GPX from Malus domestica cv. Fuji) in an incompatible interaction with Colletotrichum gloeosporioides; VvGPX (GPX from Vitis vinifera) in a compatible interaction with Plasmopara viticola; PvPHGPX (PHGPX from Phaseolus vulgaris) in a compatible interaction with Uromyces appendiculatus; OsPHGPX (PHGPX from Oryza sativa L.) in a compatible interaction with Magnaporthe oryzae.
GPX accumulation in plants varies according to several factors, including the pathogen’s lifestyle, as demonstrated by a study of this RS (Sharma et al., 2007; Lee et al., 2009; Milli et al., 2012; Rockenbach et al., 2015; Varela et al., 2017; Távora et al., 2021). Pathogen infection induces oxidative stress in cells, increasing ROS levels, which can activate antioxidant pathways, such as increased GPX activity. Depending on the pathogen’s lifestyle, modulation of ROS levels can promote the death of infected tissues, restricting infection or favoring susceptibility. RS studies that used proteomic analysis (25%, Table 3) confirmed the differential accumulation of GPXs in several plant species during infection (Sharma et al., 2007; Lee et al., 2009; Milli et al., 2012; Rockenbach et al., 2015; Varela et al., 2017; Távora et al., 2021). Among these studies, a comparative proteomic analysis in susceptible grapevine leaves (Vitis vinifera) inoculated with the biotrophic oomycete Plasmopora viticola revealed upregulation in all proteins associated with oxidative stress. Among them, GPX (VvGPX) showed a notable increase in expression, particularly 94 hours post inoculation (hpi) (Milli et al., 2012). Another study on compatible interaction between the plant Oryza sativa L. and the hemibiotrophic pathogen Magnaporthe oryzae identified a significant increase in proteins related to oxidative stress. Among them, PHGPX (OsPHGPX) showed marked upregulation in the first 12 hpi (Távora et al., 2021). In a study in common bean leaves (Phaseolus vulgaris) resistant to race 49 and susceptible to race 41 of Uromyces appendiculatus, protein levels were evaluated at 24 and 72 hpi with these biotrophic fungi. At 24 hpi, results showed reduced accumulation of PHGPX (PvPHGPX) in leaves susceptible to race 41. However, at 72 hpi, PHGPX accumulation levels were comparable between leaves inoculated with both races (41 and 49) (Lee et al., 2009). One study investigated Colletotrichum gloeosporioides infection in apple (Malus domestica) leaves of resistant and susceptible cultivars. Proteomic analyses performed at 48 hpi revealed accumulation of MdGPX in the resistant cultivar, indicating an adaptive response to the hemibiotrophic fungus. Another study investigated the proteomic responses in two Brassica napus lines (tolerant and susceptible) challenged by the necrotrophic pathogen Alternaria brassicae. At 48 hpi, the accumulation of GPX (BnGPX) and other proteins related to free radical detoxification was observed, especially in the tolerant line, highlighting its importance to biotic stress. Similarly, a study on cowpea (Vigna unguiculata) in an incompatible interaction with cowpea severe mosaic virus (CPSMV) reported a significant increase in the abundance of two types of chloroplastic PHGPX (VuPHGPX) six days post inoculation (dpi). These results suggest that susceptibility and resistance, related to the greater or lesser accumulation of GPX, are directly influenced by the type of pathogen interacting with the host plant (Figure 4B).
The protein-protein interaction network of plant GPXs obtained from the analyzed studies, was generated from A. thaliana orthologous proteins (Figure 5; Supplementary Table S2). The network resulted in 127 proteins (nodes), with 1017 connectors, forming 5 clusters, with a high level of confidence (0.7). BiNGO analysis revealed the most representative biological processes within the network: catabolic process of methylglyoxal (cluster 1); glutathione metabolic process (cluster 2); cellular response to ROS (cluster 3); regulation of the abscisic acid (ABA)-mediated signaling pathway (cluster 4); and response to temperature (cluster 5) (Figure 5).
Figure 5. Interaction network of GPX proteins extracted from SR studies, using homologous proteins from the model organism Arabidopsis thaliana. Protein-protein interaction network was generated using the String plugin, Cytoscape, and BiNGO. Colors ranging from white to shades of blue represent betweenness values, with more intense colors indicating higher values and representativeness among clusters. Representative biological processes in the network include: methylglyoxal catabolic process (cluster 1), glutathione metabolic process (cluster 2), cellular response to ROS (cluster 3), regulation of the ABA-mediated signaling pathway (cluster 4), response to temperature (cluster 5).
The highest betweenness values – indicating high connectivity between clusters – were represented by darker shades of blue, while the highest degree values (node degree) – reflecting a high number of connections – were represented by thicker node (proteins) borders (Figure 5). Thus, the GPX proteins in the network, especially GPX3, showed high betweenness values, acting as bottlenecks with a high capacity of interaction and/or signaling within the network functions. GPX proteins also showed high degree values, indicating a large number of connections, and characterizing them as hubs with important regulatory functions within the network (Figure 5). The GPX2, GPX3, GPX4, GPX5, GPX7 and GPX8 found in the cluster 2 were directly associated with glutathione metabolic processes, while GPX1 and GPX6, along with other proteins, formed the cluster 3 and were associated with cellular responses to ROS. However, all GPXs were directly and indirectly associated with other processes in the network (Figure 5). GPX3 directly interacted with the ABI1 and ABI2 phosphatases in cluster 4. The GPX1, GPX2, GPX3, GPX4, GPX5, GPX6, GPX7, and GPX8 were linked with a glutathione reductase proteins (EMB2360) in cluster 2, with glutathione transferases (DHAR1 and 2) in clusters 1 and 2, with a heat shock protein (HSP70-4) in cluster 5, and with a superoxide dismutase (CSD1) in cluster 3 (Figure 5). These interactions were related to specific processes such as regulation of glutathione level, ROS elimination, protein folding and translocation into organelles, and degradation of damaged proteins under stress.
The determination of antioxidant enzyme activity is often used to evaluate responses of susceptible and/or resistant plants against pathogens. Among the analyzed studies, 43% employed the strategy of analyzing GPX activity in response to biotic stress (Figure 6A). Among them, 91% of the studies showed increased GPX activity in plant challenged with bacteria, fungi, viruses, nematodes, or aphids (Figure 6B; Supplementary Table S5). Induction of this activity promoted increased tolerance or resistance in 60% of these studies (Figure 6C).
Figure 6. Studies that employed GPX activity analysis as a strategy for defense against pathogens. (A) Percent of studies involving or not GPX activity. (B) Studies containing GPX activity in plants challenged with pathogen. (C) Studies related to GPXs involved in host tolerance or resistance to pathogen. (D) Studies showing increased GPX activity against pathogens with or without inducers. ASM: acibenzolar-S-methyl (benzo (Pereira, 1989; Blomme et al., 2017; Moreira et al., 2022) thiadiazole-7-carbothioic acid S-methyl ester); DPD, 2-amino 4-6-dimethyl pyridine; SA, salicylic acid; Se, selenium.
In studies demonstrating increased tolerance or resistance, 83% employed exogenous molecules to stimulate plant resistance, thereby resulting in elevated GPX activity. These molecules acted as inducers of enzyme efficiency in detoxification and cell protection (Figure 6D). The infection of Solanum melongena (eggplant) by the fungus Alternaria alternata led to an inhibition of plant antioxidant protein activities, including GPX one, promoting the plant susceptibility to the disease. However, when eggplants were treated with a pyrimidine derivative (DPDP) and then challenged with A. alternata, the inhibition of antioxidant activities was reversed. Thus, DPDP increased the activities of antioxidant enzymes, including GPX, enhancing the efficiency of ROS elimination by the host, and consequently inhibiting disease progression (Hassan et al., 2013) (Supplementary Table S3). In another study, the inducer acenbenzolar - 5 methyl (ASM) was tested against bacterial canker in tomato: the use of ASM significantly and gradually boosted the activities of peroxidases (POX) and GPX, particularly evident when the treated plants were subjected to Clavibacter michiganensis subsp. michiganensis (Cmm). ASM treatment amplified the GPX activity, thereby fortifying defense against oxidative damage linked to biotic stress induced by Cmm (Supplementary Table S3) (Soylu et al., 2003). The tolerance of tomato plants to Cmm was also assessed under treatment with copper and potassium silicate nanoparticles (Cumplido-Nájera et al., 2019). Treatment with both nanoparticles resulted in a 28% reduction in Cmm disease severity, and when considering enzymatic activity, application of copper nanoparticles (at high concentration) together with low or high doses of silicate, resulted in a notable augmentation of GPX activity by 161% and 191%, respectively, compared to controls. Ascorbate peroxidase (APX) activity was also induced. These associations suggest that nanoparticles collectively stimulate antioxidant activity, thereby enhancing the tomato plants’ ability to tolerate oxidative stress induced by Cmm, resulting in reduced pathogen severity and concurrently, lower yield loss (Cumplido-Nájera et al., 2019) (Supplementary Table S3). The effects of exogenous addition of salicylic acid (SA) were evaluated on the resistance of Neoporphyra haitanensis to Vibrio mediterranei infection, which causes symptoms of yellow spot (Zhu et al., 2023). Antioxidant enzyme activities, such as GPX, increased sharply in proportion to the SA concentration compared to the untreated control. Although it did not inhibit the growth of pathogenic bacteria, SA-treated plants were significantly healthier than the controls. SA may have triggered the antioxidant defense system by inducing high levels of H2O2, signaling the plant to enhance redox regulation efficiency (Zhu et al., 2023) (Supplementary Table S3). Among the studies analyzed, only one associated selenium (Se) interference with GPX enzyme activity in plants subjected to biotic stress. In this study, the effects of nano-Se on Salviae miltiorrhizae growth and defense responses was investigated (Zhang et al., 2023): antioxidant enzyme activities, including GPX, were induced with treatments of 10 mg/l and 20 mg/l of nano-Se. Furthermore, aphid infestation caused chlorosis and cell death in untreated S. miltiorrhizae leaves, whereas these symptoms were absent in plants treated with nano-Se (Zhang et al., 2023) (Supplementary Table S3).
Studies employed different strategies to detect GPX activity (Supplementary Table S3). In 54% of cases, peroxide reduction by GPX was assessed via the glutathione or thioredoxin pathways, based on NADPH oxidation (Soylu et al., 2003; Chang et al., 2009; Hassan et al., 2013; Silveira et al., 2015; Singh et al., 2020; Singh et al., 2022). GPX activity was also measured using the absorbance method for oxidized glutathione (420 nm) (Khajuria and Ohri, 2020), or by the absorbance method at 412 nm using the DTNB reagent (5,5′-dithiobis-(2-nitrobenzoic acid) that binds reduced glutathione; therefore, the decrease in absorbance may reflect GPX activity (Supplementary Table S3) (Cumplido-Nájera et al., 2019; González-García et al., 2022).
The alignment of GPX amino acid residue sequences was performed using sequences from eligible studies of this SR (Figure 7; Supplementary Table S5). The comparison revealed three frequent conserved motifs in plant GPXs (Figure 7). Within these motifs, some sequences showed alterations, such as: in GPX2 homolog of V. unguiculata (HaGPX2), a substitution of an amino acid residue (isoleucine for valine) was identified in motif 1, in GPX8 of Theobroma cacao (TcGPX8), a substitution of an amino acid residue (cysteine for aspartate) occurred in the same motif, and in PHGPX of N. benthamiana (NbPHGPX), a nearly complete alteration in motif 3 was revealed, conserving only one amino acid residue (Figure 7). Conserved amino acid residues among GPXs, outside of the motifs, were detected and are highlighted in gray. Conserved cysteine amino acid residues, characteristic of plant proteins, were demonstrated among the sequences and are marked with red triangles (Figure 7).
Figure 7. Alignment of the amino acid sequence of GPXs obtained from the selected studies of this SR. Black highlights indicate motifs 1, 2, and 3, which are common in plant GPXs. Yellow highlights indicate amino acids that differed within the conserved motifs, and gray highlights indicated the main conserved regions outside the motifs. Red triangles indicate conserved cysteine residues.
The phylogenetic analysis of sequences revealed evolutionary relationships among GPX proteins from T. cacao (PHGPX), Zingiber zerumbet, Cucumis sativus L., and A. thaliana (GPX1 and GPX7) (Chang et al., 2009; Martins Alves et al., 2020; Sa et al., 2020; Alex et al., 2022). These proteins formed the same clade and exhibited chloroplastic subcellular localization (Figure 8; Supplementary Figure S1). The GPX4 of T. cacao and the homologous GPX2 of V. unguiculata also clustered together and displayed the same cytoplasmic localization pattern (Varela et al., 2017; Martins Alves et al., 2020). In another clade, GPX proteins from Solanum tuberosum, GPX8 from A. thaliana, and T. cacao also showed evolutionary relationships, sharing the same chloroplastic localization (Chang et al., 2009; Hajianfar et al., 2016; Martins Alves et al., 2020). The GPX3 of A. thaliana, GPX of Hevea brasiliensis, and GPX2 of T. cacao formed a phylogenetic group with subcellular localization in the endoplasmic reticulum (Chang et al., 2009; Koop et al., 2016; Martins Alves et al., 2020).
Figure 8. Evolutionary relationship between the GPXs obtained from the selected studies of this SR. The phylogenetic tree was generated using the neighbor-joining method with alignment in Clustal W with 1000 bootstraps, conducted in MEGA7. GPXs are highlighted in different colors according to the subcellular localization predicted by DeepLoc-1.0.
Pathogen infection induces oxidative stress in plants, leading to ROS accumulation that disrupts membrane integrity through lipid peroxidation (Keppler et al., 1989; Torres et al., 2006), destabilizing cellular homeostasis and amplifying stress responses (Sharma et al., 2012; Farmer and Mueller, 2013; Kuźniak and Gajewska, 2024). Lipid peroxidation products can activate cell death pathways, influencing pathogen progression (Dey et al., 2020). Antioxidant enzymes like GPXs are crucial in mitigating these damaging effects. GPXs decompose peroxides, preventing their interaction with membrane lipids and averting successive damage (Rouhier and Jacquot, 2005; Dietz, 2011). Among these, phospholipid hydroperoxide glutathione peroxidases (PHGPX), also known as GPX4 in mammals, reduce lipid hydroperoxides to alcohols using glutathione as an electron donor, protecting cell membranes from oxidative damage and cell death (Ursini et al., 1995; Takebe et al., 2002; Imai and Nakagawa, 2003; Nakagawa, 2004). This isoform is conserved in plants, where it exhibits functional similarity to animal GPX4. For instance, LePHGPX in transgenic tobacco plants protected against DNA fragmentation, membrane integrity loss, and oxidative stress induced by necrotrophic fungi, mirroring anti-apoptotic roles observed in animals (Chen et al., 2004).
Ferroptosis, a form of iron-dependent lipid peroxidation-mediated cell death, was first described in animals and is regulated by GPX4 (Dixon et al., 2012; Seibt et al., 2019; Feng et al., 2020; Chen et al., 2021; Ma et al., 2022). In plants, ferroptosis also occurs during pathogen infections (Distéfano et al., 2017), as demonstrated in N. benthamiana infected with the tobacco mosaic virus (TMV 24A/UPD) (Macharia et al., 2020). Silencing GPX4 accelerated this cell death, highlighting its protective role. Interestingly, ferroptosis may function as a plant defense mechanism, enhancing resistance to pathogens such as oomycetes. This parallels the emerging therapeutic potential in animals, where GPX4-regulated ferroptosis is being explored as a strategy for treating diseases such as cancer (Friedmann Angeli et al., 2014; Yang et al., 2014; Viswanathan et al., 2017).
Plant GPXs share homology with mammalian GPX4 (Criqui et al., 1992; Holland et al., 1993; Sugimoto and Sakamoto, 1997; Yang et al., 2005; Navrot et al., 2006), but they generally lack the selenocysteine (SeCys) residue critical for the high catalytic efficiency of animal GPXs, substituting it with cysteine (Figure 7) (Herbette et al., 2007; Bela et al., 2015). Despite this structural difference, plant GPXs exhibit catalytic efficiencies comparable to animal GPXs (i.e. ranging between 10-3 to 106 M-1s-1 in plants and between 10-7 to 108 M-1s-1 in animals) (Dietz, 2011). Recent studies have highlighted their functional relevance. For example, PHGPX from O. sativa efficiently reduces phospholipid hydroperoxides using glutathione or thioredoxin (Wang et al., 2007), while the T. cacao PHGPX protects against H2O2 accumulation, membrane damage, and cell death induced by fungal proteins in N. benthamiana cell suspensions (do Carmo Santos et al., 2024). Although plant GPXs lack the catalytic advantage conferred by SeCys, their protective roles against oxidative stress and regulation of cell death underscore their importance in plant defense (Maiorino et al., 1995; Mannes et al., 2011). The functional similarity to animal GPX4 suggests a conserved evolutionary mechanism linking oxidative stress mitigation and cell death regulation in both kingdoms.
Gene expression analysis has demonstrated that GPX isoforms play distinct roles in plant stress responses, exhibiting differential regulation based on the type of stress encountered (Figure 4A). For instance, in Panax ginseng, GPX1 expression increased 8.6-fold at 48 hpi with the hemibiotrophic fungus C. gloeosporioides. Conversely, GPX2 expression decreased during the same interaction (Kim et al., 2014). This suggests that GPX1 might be critical in mitigating oxidative stress during the early stages of pathogen challenge, while GPX2 might have a reduced role in biotic stress defense. In Camellia sinensis, GPX2 was unresponsive to herbivore attacks but was strongly induced (3.3- to 4.9-fold) following treatments with the phytohormones gibberellic acid (GA) and methyl jasmonate (MeJA). This indicates that CsGPX2 responds primarily to abiotic stresses and developmental signals rather than biotic challenges (Fu, 2014). Additional evidence supports the association of certain GPX isoforms, such as GPX2, with abiotic stress and developmental processes. For instance, HvGPX2 in Hordeum vulgare is induced under salinity, drought, and oxidative herbicide stress, while AtGPX2 in A. thaliana is expressed during key developmental stages, including root elongation, leaf expansion, and flowering (Churin et al., 1999; Chen et al., 2011; Islam et al., 2015; Zhou et al., 2018).
The alignment of GPX amino acid residues under biotic stress revealed conserved cysteine residues essential for catalytic activity within motifs 1 and 2 (red triangles, Figure 7). These residues enable the reduction of peroxides, with key cysteines forming intramolecular disulfide bridges to maintain redox balance. Variations in conserved motifs, such as in T. cacao GPX8 (TcGPX8), V. unguiculata GPX2 (VuGPX2), and N. benthamiana PHGPX (NbPHGPX), suggest potential functional differences. These differences warrant experimental studies to determine how they impact GPX activity in biotic stress (Boschi-Muller et al., 2000; Herbette et al., 2002).
However, global phylogenetic analysis reveals GPXs have diverged across species while retaining their functional core. T. cacao GPXs clustered separately from A. thaliana GPXs, indicating evolutionary divergence despite all GPXs descending from a common ancestor (Figure 8). However, TcGPX shares clades with plant species like C. sinensis and E. grandis, reflecting conserved roles in plant stress responses. Clustering by subcellular localization suggests evolutionary specialization, with GPXs adapting to specific organelle functions to manage oxidative stress effectively (Figure 8; Margis et al., 2008; Martins Alves et al., 2020). In the cytosol, they prevent oxidative damage and ensure metabolic and translational competence. In chloroplasts, GPXs safeguard photosynthetic components and minimize infection-related damage. Mitochondrial GPXs protect against dysfunction, maintaining cellular respiration and delaying PCD. Similarly, GPXs in the endoplasmic reticulum aid in protein folding and stress regulation, while apoplastic GPXs modulate extracellular ROS to activate defense signaling and systemic acquired resistance (SAR) (Figure 9) (Dietz and Hell, 2015; Tripathy and Oelmüller, 2012).
Figure 9. Plant GPX responses under pathogen-induced stress. (A) Representation of the processes associated with high GPX accumulation or activity. GPX1, GPX2, GPX3, GPX5, GPX6, GPX7, and GPX8 are part of the antioxidant complex, maintaining a balance between the production and reduction of ROS. Heightened stress, such as that triggered by pathogens, can boost GPX activity, maintaining ROS levels adequate for defense signaling without causing damage to organelle function or compromising their structural integrity. Some components of the enzymatic and non-enzymatic antioxidant system are represented: superoxide dismutase (SOD); glutathione peroxidase (GPX); ascorbate peroxidase (APX); dehydroascorbate reductase (DHAR); monodehydroascorbate reductase (MDHAR); NADPH-dependent thioredoxin reductase (NTRC); ascorbate (AsA); monodehydroascorbate (MDHA); dehydroascorbate (DHA); glutathione (GSH); glutathione disulfide (GSSG); reduced (TRX) and oxidized (OX-TRX) forms of thioredoxin. GPX can use both thioredoxin and reduced glutathione as electron donors for antioxidant activity. Cytoplasmic GPX4 is involved in defense by protecting membranes from cell death through ferroptosis or other forms of cell death due to membrane permeability independent of iron. GPX3, in addition to its antioxidant processes, may inhibit phosphatase 2C (PP2C), ABI1 and ABI2, which are inhibitors of ABA signaling. Once inhibited by GPX3, ABA signaling increases, potentially regulating other processes such as GPX3 accumulation itself, which reduces senescence through detoxification, serving as a defense mechanism to keep cells alive, and gene regulation. Additionally, GPX3 can induce the accumulation of phototropin-2, which is key to regulating stomatal opening and closure, potentially acting as a physical barrier to pathogen invasion. Another process that this enzyme may be involved in is the suppression of accumulation and post-translational modification through S-glutathionylation, which controls histone modification and may affect epigenetic regulation. However, maintaining redox balance within the cell can be crucial for preventing infection progression, except for pathogenic organisms that require living cells to develop, such as biotrophs. (B) Representation of the processes associated with low GPX accumulation or activity. Reduced GPX activity or accumulation in response to pathogens may be associated with the progression of lipid peroxidation, leading to destabilization of membrane structure followed by cellular permeability. Furthermore, it can lead to increased senescence due to reduced antioxidant activity. The imbalance between ROS production and GPX detoxification can compromise organelle components, leading to malfunction, such as reduced photosynthesis and respiratory chain activity. Cellular collapse can increase susceptibility to necrotrophic pathogens but deter biotrophic pathogens. The isoforms shown in this figure are GPXs from Arabidopsis thaliana, homologous to those reported in the SR articles (see Supplementary Table S2).
GPXs are integral to redox regulation and pathogen defense, acting across cellular compartments to balance ROS detoxification and signaling. Their evolutionary adaptations underline their versatility and importance in plant immunity. Further studies exploring sequence variations and functional roles could illuminate their contributions to stress resilience and crop improvement.
The direct association of GPX2, GPX3, GPX4, GPX5, GPX7, and GPX8 with glutathione metabolic processes (Figure 5) suggests that the proteins can use glutathione as an electron donor in reducing H2O2 or organic and lipid hydroperoxides to maintain plant cell redox homeostasis (Supplementary Table S2) (Yang et al., 2005). However, plant GPXs are classified in the pyridoxine protein group due to an efficiently reduction of peroxide via the thioredoxin regenerating system (Iqbal et al., 2006; Margis et al., 2008). GPX1 and GPX6 participate in cellular processes responding to ROS, not only maintaining ROS levels’ homeostasis but also detecting and signaling ROS availability and redox imbalance (Supplementary Table S2) (Noctor et al., 2018).
Moreover, GPX3 plays a pivotal role in ABA-mediated stress signaling by interacting with phosphatases ABI1 and ABI2, key regulators of this pathway (Figure 5). It suppresses these phosphatases, acting as both a sensor and transducer of H2O2 signals during stress conditions (Miao et al., 2006; Paiva et al., 2021). While research linking GPX and ABA is limited, studies on rice GPX3 suggest it influences ABA signaling through H2O2-dependent and -independent mechanisms. In the H2O2-dependent pathway, GPX3 induction by ABA reduces oxidative stress by scavenging ROS, delaying senescence, and potentially enhancing resistance to biotrophic pathogens (Paiva et al., 2021). Conversely, this delay may increase susceptibility to necrotrophic pathogens (Apel and Hirt, 2004; Barna, 2022). In the H2O2-independent pathway, GPX3 modulates epigenetic responses by repressing S-glutathionylation of a signal transducer linked to histone modification (Jasmine and Joyous, 2022). It also influences the accumulation of ABA-responsive proteins, including histones, ubiquitin, actin, and vesicle-related proteins, which regulate gene expression and stress responses (Paiva et al., 2021). GPX3 further enhances ABA signaling by suppressing PP2C, a negative ABA regulator, and promoting genes like OsDREB2A, OsABI5, and OsABA8ox3 (Paiva et al., 2021). This regulation impacts stomatal function, with GPX3-mediated ABA signaling inducing stomatal closure to prevent pathogen entry while potentially increasing susceptibility post-invasion by weakening cell wall defenses (Melotto et al., 2006; de Torres-Zabala et al., 2007; Ton et al., 2009; Lim et al., 2015; Hsu et al., 2021). Additionally, GPX3 may contribute indirectly to defense mechanisms through links to heat shock proteins (HSPs). HSP transcription factors activate genes for both HSPs and antioxidant enzymes under oxidative stress triggered by pathogens, underscoring GPX3’s multifaceted role in plant stress adaptation (Yurina, 2023) (Figure 9).
GPX1, GPX2, GPX3, GPX4, GPX5, GPX6, GPX7, and GPX8 associate with chloroplastic glutathione reductase proteins and mitochondrial glutathione transferases (DHAR2) related to the glutathione metabolic process (Figure 5). Regulating glutathione levels protects host plants against various pathogens. This is supported by the negative regulation of glutathione and susceptibility of tomato plants to B. cinereae (Kuźniak and Skłodowska, 2005), and in tomato plants infected with P. syringae, where glutathione levels decreased in susceptible cultivars but not in resistant ones (Kuźniak and SkŁodowska, 2004). GPXs and DHAR2 may cooperate in recycling glutathione and ascorbate during plant detoxification processes (Bobrovskikh et al., 2020) (Figure 9).
This SR highlights the pivotal role of GPXs in modulating plant resistance or susceptibility to pathogens. Key findings include, as first element, a differential GPX expression in resistant vs. susceptible plant varieties as observed in Triticum aestivum infected by barley yellow dwarf virus (BYDV). Resistant plants exhibited significantly higher GPX transcript levels and reduced H2O2 concentrations (4–5 times lower) compared to susceptible varieties. This reflects efficient ROS scavenging as a resistance mechanism (Wang et al., 2018). The second key finding concerns plant resistance increased by GPX mutations. In A. thaliana, loss-of-function mutations in GPX1 and GPX7 enhanced basal resistance to P. syringae. This resistance was linked to ROS- and salicylic acid (SA)-mediated signaling, which are critical for activating HR that confine pathogens to the infection site (Chang et al., 2009). And finally, the increase of susceptibility through GPX downregulation. In C. sativa, Alternaria cucumerina infection downregulated GPX7, causing ROS imbalances and enabling successful fungal colonization in susceptible plants (Sa et al., 2020). Proteomic studies corroborate these findings, showing that plants accumulate GPX proteins differently depending on the pathogen’s lifestyle. Interestingly, the consequences of the ROS-induced PCD depend on the context: in some cases, it facilitates pathogen colonization, increasing susceptibility, while in others, it restricts infection, halting pathogen progression and symptom development (Van Breusegem and Dat, 2006; Gadjev et al., 2008; Coll et al., 2011; Pinto et al., 2012; Daneva et al., 2016). This variation in GPX regulation underpins specific defense responses, as summarized in Figure 4B.
In plants, pathogen infection, including by oomycetes, bacteria, and fungi, typically begins when these organisms penetrate plant tissue through natural openings, wounds, or specialized structures (e.g., haustoria in oomycetes and fungi), and then colonize the apoplast, the intercellular space. Biotrophic pathogens rely on the apoplast for nutrients extracted from living cells (Kemen and Jones, 2012; Mapuranga et al., 2022). In the case of V. vinifera and the oomycete Plasmopara viticola, a compatible interaction leads to increased cytoplasmic GPX (VvGPX) accumulation, triggered by ROS signaling, which activates antioxidant enzymes like GPX to maintain redox balance, ultimately supporting pathogen growth (Milli et al., 2012; Koledenkova et al., 2022). Conversely, in P. vulgaris infected by the fungal pathogen U. appendiculatus, a decrease in the accumulation of chloroplastic and cytoplasmic PHGPX proteins (PvPHGPXs) was observed, which allowed the pathogen to bypass plant defense signaling (Lee et al., 2009). Additionally, biotrophic pathogens can suppress PAMP-induced immunity (PTI) by secreting effectors that inhibit ROS production, weakening plant defense responses. For example, P. syringae strains produce effectors that suppress both PAMP- and chitin-induced ROS, aiding pathogen colonization (Jamir et al., 2004; Fujikawa et al., 2006; Guo et al., 2009; Gimenez-Ibanez et al., 2018).
In an incompatible interaction with a virus, pathogens release viral elicitors that trigger a cascade of signaling pathways, activating plant defense mechanisms. These pathways include an oxidative burst, ROS generation, HR, pathogenesis-related protein activation, and SAR (Mandadi and Scholthof, 2013). However, ROS levels must remain transient to avoid excessive tissue damage, with antioxidant enzymes like GPXs maintaining redox balance (Gill and Tuteja, 2010; Hernández et al., 2016). In V. unguiculata infected by Cowpea severe mosaic virus (CPSMV), an increase in chloroplastic PHGPX proteins was observed at 6 dpi, while APX protein levels were low. The higher PHGPX abundance, coupled with lower APX, led to increased H2O2, promoting HR and resistance to the virus (Varela et al., 2017). In a study comparing non-inoculated (CPU), susceptible (CPI), and induced-resistant (MCPI) cowpeas, MCPI plants exhibited increased SOD activity and reduced CAT activity, causing a transient rise in H2O2 compared to CPI. In contrast, CPI plants showed increased CAT activity, potentially facilitating CPSMV infection. The study also found that in MCPI, elevated GPOX and reduced APX activity contributed to H2O2 buildup, crucial for defense establishment. Further analysis showed that MCPI plants controlled excess H2O2 through SOD and peroxiredoxins but limited viral spread by reducing CAT and APX activity. In susceptible plants, increased CAT and APX activity helped modulate ROS levels, promoting viral replication. These findings highlight the role of antioxidant enzymes in determining disease progression (Souza et al., 2017; Varela et al., 2017; Noronha Souza et al., 2020).
Necrotrophic pathogens survive and develop by obtaining nutrients from dead plant cells, inducing cell death in the infected tissues through toxins and enzymes that cause nutrient leakage (Mengiste, 2012; Cho, 2015). This process triggers oxidative stress, which contributes to susceptibility. For instance, in a study of the necrotrophic fungi Rhynchosporium secalis and Pyrenophora teres infecting barley, ROS production (HO2•/O2-•) was observed in both susceptible and resistant plants. However, in later stages, ROS accumulation was only seen in susceptible plants. Pre-treatment with antioxidants like SOD and Mn(III) desferal reduced fungal growth and phenolic browning in susceptible plants. Additionally, resistant barley exhibited a sixfold increase in SOD activity when challenged with P. teres (Able, 2003). High antioxidant enzyme levels, such as GPXs, help mitigate oxidative stress caused by necrotrophic pathogens, enhancing plant resistance. For example, cytoplasmic GPX accumulation was noted as an induced response during the incompatible interaction of B. napus with A. brassicae (Sharma et al., 2007).
Hemibiotrophic pathogens, which alternate between biotrophic and necrotrophic phases during infection, present unique challenges in plant defense (Glazebrook, 2005). During the biotrophic phase, ROS can inhibit pathogen spread, while in the necrotrophic phase, ROS favor the pathogen by promoting tissue death, which the pathogen uses for nutrient acquisition. The plant’s antioxidant response plays a critical role in this dynamic. For example, infection of Leptosphaeria maculans in B. napus cotyledons triggered H2O2 accumulation, and exogenous elicitors activated antioxidant enzymes, leading to a significant increase in activity of APX, GPX, GR, and SOD (Jindřichová et al., 2011). Similarly, M. oryzae infection in O. sativa resulted in a differential increase in antioxidant proteins, including OsPHGPX, especially in the early stages of a compatible interaction. In these cases, plant susceptibility may be linked to the efficiency of the antioxidant system in controlling ROS to prevent excessive cell death and facilitate pathogen colonization (Távora et al., 2021).
A study comparing antioxidant enzyme activity in susceptible and resistant plants infected with M. oryzae showed that all inoculated plants had increased CAT, APX, GPX, GST, and POX activity compared to controls. However, the level of response varied between species. For example, corn exhibited a greater increase in CAT activity than rice, while rice showed a decrease in GPX after inoculation. Antioxidant enzyme induction occurred earlier in resistant plants, which likely contributes to more effective defense (Gupta et al., 2021). In contrast, in an incompatible interaction with C. gloeosporioides, M. domestica cv. Fuji showed a notable increase in GPX accumulation, highlighting that GPX plays a critical role in detoxifying stressors and varies in its activity depending on the pathogen involved (Rockenbach et al., 2015). This underscores the importance of GPX as a key component of the plant’s antioxidant defense system.
The regulation and accumulation of GPXs in plants vary depending on the type of pathogen, influencing resistance or susceptibility. Increased GPX expression may enhance resistance against necrotrophic pathogens but can also increase susceptibility to biotrophs and viruses. This dynamic reflects the plant’s adaptive response to pathogen pressure in its environment, shaping specialized resistance profiles. However, such specialization might limit adaptability to new biotic challenges, as seen in evolutionary studies linking GPX regulation to altered ROS signaling and pathogen interactions. These changes can drive ecological trade-offs, where plants with high pathogen-specific resistance may underperform in low-pressure environments or against diverse threats (Herbette et al., 2011; Eloy et al., 2015; Camejo et al., 2016; Gupta et al., 2021). Integrated disease management provides a holistic strategy to address these challenges (Atkinson and Urwin, 2012). By combining GPX regulation with cultural management, crop rotation, optimized planting schedules, biological control, and genetic improvement, plants can better navigate the complexities of pathogen pressure and abiotic stress. These approaches reduce pathogen development, disrupt pest-host cycles, and enhance plant resilience, promoting sustainable cultivation and environmental health. Collectively, they ensure more robust, efficient, and adaptive agricultural systems (Mittler, 2006; Peshin and Dhawan, 2009; Bubici et al., 2019; Li et al., 2021; Yao et al., 2023; Ferrucho et al., 2024).
The SR synthesized information on plant GPXs against biotic stress from 2003 to 2023, providing a better understanding of their role against pathogens and identifying unresolved issues in the literature. While there is substantial knowledge on plant GPXs, specific functions for different isoforms, especially related to biotic stress, remain unclear. For example, clarifying if selenium can directly induce GPX activity against biotic stress or if more specific isoforms mimic animal antiapoptotic GPXs remains crucial. Answering such question could lead to the development of strategies to control necrotrophic or hemibiotrophic pathogens during plant host infection. Plant PHGPXs may function against pathogen-induced cell death, akin to animal antiapoptotic PHGPXs, yet few plant GPXs are explored for this. The susceptibility or resistance associated with plant GPXs depends on the lifestyle of the pathogen, but it’s not a strict rule, as plants possess various other mechanisms and antioxidant proteins that can be regulated and/or bypassed during pathogen attacks. Boosting the antioxidant activity of GPXs provides enhanced protection against pathogens, and employing strategies with exogenous inducers such as nano-Se, SA, pyrimidine derivatives, ASM, copper, and silica nanoparticles can aid in bolstering the defense provided by these proteins. GPXs not only act as antioxidants but also participate in glutathione metabolism, inhibit phosphatases, and detect/transduce H2O2 signals via ABA. While plant GPXs associated with biotic stress maintain characteristic motifs, notable changes occur, highlighting evolutionary relationships among them. This review underscores existing gaps in understanding plant GPXs against biotic stress, encouraging further experimental studies for strategic and biotechnological utilization in agriculture.
The datasets presented in this study can be found in the article/Supplementary Material.
MLCS: Conceptualization, Writing – original draft, Writing – review & editing, Formal analysis, Investigation. ASS: Conceptualization, Formal analysis, Visualization, Writing – original draft. DPSN: Formal analysis, Visualization, Writing – original draft. NSL: Formal analysis, Investigation, Writing – original draft. CPP: Conceptualization, Writing – original draft. FM: Conceptualization, Funding acquisition, Supervision, Writing – original draft, Writing – review & editing.
The author(s) declare financial support was received for the research, authorship, and/or publication of this article. MLCS was funded by Fundação de Amparo a Pesquisas do Estado da Bahia (FAPESB). ASS was funded by the Coordenação de Aperfeiçoamento de Pessoal de Nível Superior (CAPES). NSL was funded by the Conselho Nacional de Desenvolvimento Científico e Tecnológico (CNPq). FM and CPP received a Productivity Grant from the CNPq.
The authors declare that the research was conducted in the absence of any commercial or financial relationships that could be construed as a potential conflict of interest.
All claims expressed in this article are solely those of the authors and do not necessarily represent those of their affiliated organizations, or those of the publisher, the editors and the reviewers. Any product that may be evaluated in this article, or claim that may be made by its manufacturer, is not guaranteed or endorsed by the publisher.
The Supplementary Material for this article can be found online at: https://www.frontiersin.org/articles/10.3389/fpls.2025.1425880/full#supplementary-material
Able, A. J. (2003). Role of reactive oxygen species in the response of barley to necrotrophic pathogens. Protoplasma 221, 137–143. doi: 10.1007/s00709-002-0064-1
Alex, T. E., Nath, V. S., Varghese, L., Geetha, K. A., Augustine, L., Ramaswamy, V. M., et al. (2022). Transcriptome-wide identification and transcriptional profiling reveal remarkable expression modulation of redox genes in Zingiber zerumbet against Pythium myriotylum. Physiol. Mol. Plant Pathol. 121, 101885. doi: 10.1016/j.pmpp.2022.101885
Apel, K., Hirt, H. (2004). Reactive oxygen species: metabolism, oxidative stress, and signal transduction. Annu. Rev. Plant Biol. 55, 373–399. doi: 10.1146/annurev.arplant.55.031903.141701
Arnaud, D., Deeks, M. J., Smirnoff, N. (2022). Organelle-targeted biosensors reveal distinct oxidative events during pattern-triggered immune responses. Plant Physiol. 191, 2551–2569. doi: 10.1093/plphys/kiac603
Arthur, J. R. (2000). The glutathione peroxidases. Cell. Mol. Life sciences: CMLS 57, 1825–1835. doi: 10.1007/PL00000664
Atkinson, N. J., Urwin, P. E. (2012). The interaction of plant biotic and abiotic stresses: from genes to the field. J. Exp. Bot. 63, 3523–3543. doi: 10.1093/jxb/ers100
Attacha, S., Solbach, D., Bela, K., Moseler, A., Wagner, S., Schwarzländer, M., et al. (2017). Glutathione peroxidase-like enzymes cover five distinct cell compartments and membrane surfaces in Arabidopsis thaliana. Plant Cell Environ. 40, 1281–1295. doi: 10.1111/pce.12919
Avery, C., Patterson, J., Grear, T., Frater, T., Jacobs, D. J. (2022). Protein function analysis through machine learning. Biomolecules 12, 1246. doi: 10.3390/biom12091246
Barna, B. (2022). Manipulation of senescence of plants to improve biotic stress resistance. Life 12, 1496. doi: 10.3390/life12101496
Bela, K., Horváth, E., Gallé, Á., Szabados, L., Tari, I., Csiszár, J. (2015). Plant glutathione peroxidases: emerging role of the antioxidant enzymes in plant development and stress responses. J. Plant Physiol. 176, 192–201. doi: 10.1016/j.jplph.2014.12.014
Bela, K., Riyazuddin, R., Csiszár, J. (2022). Plant glutathione peroxidases: non-heme peroxidases with large functional flexibility as a core component of ROS-processing mechanisms and signalling. Antioxidants 11, 1624. doi: 10.3390/antiox11081624
Blomme, G., Dita, M., Jacobsen, K. S., Pérez Vicente, L., Molina, A., Ocimati, W., et al. (2017). Bacterial diseases of bananas and enset: current state of knowledge and integrated approaches toward sustainable management. Front. Plant Sci. 8. doi: 10.3389/fpls.2017.01290
Bobrovskikh, A., Zubairova, U., Kolodkin, A., Doroshkov, A. (2020). Subcellular compartmentalization of the plant antioxidant system: an integrated overview. PeerJ 8, e9451. doi: 10.7717/peerj.9451
Boschi-Muller, S., Azza, S., Sanglier-Cianferani, S., Talfournier, F., Van Dorsselear, A., Branlant, G. (2000). A Sulfenic Acid Enzyme Intermediate Is Involved in the Catalytic Mechanism of Peptide Methionine Sulfoxide Reductase from Escherichia coli. Journal of Biological Chemistry (2000) 275, 35908–35913.
Bubici, G., Kaushal, M., Prigigallo, M. I., Gómez-Lama Cabanás, C., Mercado-Blanco, J. (2019). Biological control agents against fusarium wilt of banana. Front. Microbiol. 10. doi: 10.3389/fmicb.2019.00616
Camejo, D., Guzmán-Cedeño, Á., Moreno, A. (2016). Reactive oxygen species, essential molecules, during plant–pathogen interactions. Plant Physiol. Biochem. 103, 10–23. doi: 10.1016/j.plaphy.2016.02.035
Chang, C. C. C., Slesak, I., Jordá, L., Sotnikov, A., Melzer, M., Miszalski, Z., et al. (2009). Arabidopsis chloroplastic glutathione peroxidases play a role in cross talk between photooxidative stress and immune responses. Plant Physiol. 150, 670–683. doi: 10.1104/pp.109.135566
Chen, M., Li, K., Li, H., Song, C.-P., Miao, Y. (2017). The glutathione peroxidase gene family in gossypium hirsutum: genome-wide identification, classification, gene expression and functional analysis. Sci. Rep. 7, 44743. doi: 10.1038/srep44743
Chen, S., Vaghchhipawala, Z., Li, W., Asard, H., Dickman, M. B. (2004). Tomato phospholipid hydroperoxide glutathione peroxidase inhibits cell death induced by Bax and oxidative stresses in yeast and plants. Plant Physiol. 135, 1630–1641. doi: 10.1104/pp.103.038091
Chen, S., Zimei, L., Cui, J., Jiangang, D., Xia, X., Liu, D., et al. (2011). Alleviation of chilling-induced oxidative damage by salicylic acid pretreatment and related gene expression in eggplant seedlings. Plant Growth Regul. 65, 101–108. doi: 10.1007/s10725-011-9579-9
Chen, X., Kang, R., Kroemer, G., Tang, D. (2021). Broadening horizons: the role of ferroptosis in cancer. Nat. Rev. Clin. Oncol. 18, 280–296. doi: 10.1038/s41571-020-00462-0
Cho, Y. (2015). How the necrotrophic fungus alternaria brassicicola kills plant cells remains an enigma. Eukaryotic Cell 14, 335–344. doi: 10.1128/EC.00226-14
Christopher, J. A., Geladaki, A., Dawson, C. S., Vennard, O. L., Lilley, K. S. (2022). Subcellular transcriptomics and proteomics: A comparative methods review. Mol. Cell. Proteomics 21, 100186. doi: 10.1016/j.mcpro.2021.100186
Churin, Y., Schilling, S., Börner, T. (1999). A gene family encoding glutathione peroxidase homologues in Hordeum vulgare (barley). FEBS Lett. 459, 33–38. doi: 10.1016/S0014-5793(99)01208-9
Coll, N. S., Epple, P., Dangl, J. L. (2011). Programmed cell death in the plant immune system. Cell Death Differentiation 18, 1247–1256. doi: 10.1038/cdd.2011.37
Criqui, M. C., Jamet, E., Parmentier, Y., Marbach, J., Durr, A., Fleck, J. (1992). Isolation and characterization of a plant cDNA showing homology to animal glutathione peroxidases. Plant Mol. Biol. 18, 623–627. doi: 10.1007/BF00040684
Cumplido-Nájera, C. F., González-Morales, S., Ortega-Ortíz, H., Cadenas-Pliego, G., Benavides-Mendoza, A., Juárez-Maldonado, A. (2019). The application of copper nanoparticles and potassium silicate stimulate the tolerance to Clavibacter michiganensis in tomato plants. Sci. Hortic. 245, 82–89. doi: 10.1016/j.scienta.2018.10.007
Daneva, A., Gao, Z., Van Durme, M., Nowack, M. K. (2016). Functions and regulation of programmed cell death in plant development. Annu. Rev. Cell Dev. Biol. 32, 441–468. doi: 10.1146/annurev-cellbio-111315-124915
Das, K., Roychoudhury, A. (2014). Reactive oxygen species (ROS) and response of antioxidants as ROS-scavengers during environmental stress in plants. Front. Environ. Sci. 2. doi: 10.3389/fenvs.2014.00053
Dietz, K.-J., Hell, R. (2015). Thiol switches in redox regulation of chloroplasts: balancing redox state, metabolism and oxidative stress. Biological Chemistry 396, 483–494.
de Novais, D. P. S., Batista, T. M., Costa, E. A., Pirovani, C. P. (2023). Genomic and pathogenicity mechanisms of the main theobroma cacao L. Eukaryotic Pathogens: A Systematic Review Microorganisms 11, 1567. doi: 10.3390/microorganisms11061567
de Paula, C. M. P., Techio, V. H. (2014). Immunolocalization of chromosome-associated proteins in plants – principles and applications. Botanical Stud. 55, 63. doi: 10.1186/s40529-014-0063-5
de Torres-Zabala, M., Truman, W., Bennett, M. H., Lafforgue, G., Mansfield, J. W., Rodriguez Egea, P., et al. (2007). Pseudomonas syringae pv. tomato hijacks the Arabidopsis abscisic acid signalling pathway to cause disease. EMBO J. 26, 1434–1443. doi: 10.1038/sj.emboj.7601575
Dey, N., Roy, U. K., Aditya, M., Bhattacharjee, S. (2020). Defensive strategies of ROS in Programmed Cell Death associated with hypertensive response in plant pathogenesis. Ann. Syst. Biol. 3, 001–009. doi: 10.17352/asb.000004
Dias, C. V., Mendes, J. S., dos Santos, A. C., Pirovani, C. P., da Silva Gesteira, A., Micheli, F., et al. (2011). Hydrogen peroxide formation in cacao tissues infected by the hemibiotrophic fungus Moniliophthora perniciosa. Plant Physiol. Biochem. 49, 917–922. doi: 10.1016/j.plaphy.2011.05.004
Dietz, K. J. (2011). Peroxiredoxins in plants and cyanobacteria. Antioxidants Redox Signaling 15, 1129–1159. doi: 10.1089/ars.2010.3657
Distéfano, A. M., Martin, M. V., Córdoba, J. P., Bellido, A. M., D’Ippólito, S., Colman, S. L., et al. (2017). Heat stress induces ferroptosis-like cell death in plants. J. Cell Biol. 216, 463–476. doi: 10.1083/jcb.201605110
Dixon, S. J., Lemberg, K. M., Lamprecht, M. R., Skouta, R., Zaitsev, E. M., Gleason, C. E., et al. (2012). Ferroptosis: an iron-dependent form of nonapoptotic cell death. Cell 149, 1060–1072. doi: 10.1016/j.cell.2012.03.042
do Carmo Santos, M. L., Santos, T. A., dos Santos Lopes, N., Macedo Ferreira, M., Martins Alves, A. M., Pirovani, C. P., et al. (2024). The selenium-independent phospholipid hydroperoxide glutathione peroxidase from Theobroma cacao (TcPHGPX) protects plant cells against damages and cell death. Plant Physiol. Biochem. 207, 108332. doi: 10.1016/j.plaphy.2023.108332
Doehlemann, G., Ökmen, B., Zhu, W., Sharon, A. (2017). Plant pathogenic fungi. Microbiol. Spectr. 5, 1–23. doi: 10.1128/microbiolspec.FUNK-0023-2016
Dolgalev, G., Poverennaya, E. (2021). Applications of CRISPR-cas technologies to proteomics. Genes 12, 1790. doi: 10.3390/genes12111790
dos Santos Lopes, N., Silva Santos, A., de Novais, D. P. S., Pirovani, C. P., Micheli, F. (2023). Pathogenesis-related protein 10 in resistance to biotic stress: progress in elucidating functions, regulation and modes of action. Front. Plant Sci. 14. doi: 10.3389/fpls.2023.1193873
Dumanović, J., Nepovimova, E., Natić, M., Kuča, K., Jaćević, V. (2020). The significance of reactive oxygen species and antioxidant defense system in plants: A concise overview. Front. Plant Sci. 11, 552969. doi: 10.3389/fpls.2020.552969
Eloy, Y. R. G., Vasconcelos, I. M., Barreto, A. L. H., Freire-Filho, F. R., Oliveira, J. T. A. (2015). H2O2 plays an important role in the lifestyle of Colletotrichum gloeosporioides during interaction with cowpea [Vigna unguiculata (L.) Walp. Fungal Biol. 119, 747–757. doi: 10.1016/j.funbio.2015.05.001
Fabbri, S., Silva, C., Hernandes, E., Octaviano, F., Thommazo, A. D., Belgamo, A. (2016). “Improvements in the StArt tool to better support the systematic review process,” in Proceedings of the 20th International Conference on Evaluation and Assessment in Software Engineering, Limerick, Ireland. Article 21 (Association for Computing Machinery).
Farmer, E. E., Mueller, M. J. (2013). ROS-mediated lipid peroxidation and RES-activated signaling. Annu. Rev. Plant Biol. 64, 429–450. doi: 10.1146/annurev-arplant-050312-120132
Feng, H., Schorpp, K., Jin, J., Yozwiak, C. E., Hoffstrom, B. G., Decker, A. M., et al. (2020). Transferrin receptor is a specific ferroptosis marker. Cell Rep. 30, 3411–3423.e7. doi: 10.1016/j.celrep.2020.02.049
Ferrucho, R. L., Marín-Ramírez, G. A., Gaitan, A. (2024). Integrated disease management for the sustainable production of Colombian coffee. Agronomy 14, 1286. doi: 10.3390/agronomy14061286
Foyer, C. H., Noctor, G. (2005). Redox homeostasis and antioxidant signaling: A metabolic interface between stress perception and physiological responses. Plant Cell 17, 1866–1875. doi: 10.1105/tpc.105.033589
Friedmann Angeli, J. P., Schneider, M., Proneth, B., Tyurina, Y. Y., Tyurin, V. A., Hammond, V. J., et al. (2014). Inactivation of the ferroptosis regulator Gpx4 triggers acute renal failure in mice. Nat. Cell Biol. 16, 1180–1191. doi: 10.1038/ncb3064
Fu, J.-Y. (2014). Cloning of a new glutathione peroxidase gene from tea plant (Camellia sinensis) and expression analysis under biotic and abiotic stresses. Botanical Stud. 55, 7. doi: 10.1186/1999-3110-55-7
Fujikawa, T., Ishihara, H., Leach, J. E., Tsuyumu, S. (2006). Suppression of Defense Response in Plants by the avrBs3/pthA Gene Family of Xanthomonas spp. Mol. Plant-Microbe Interactions® 19, 342–349. doi: 10.1094/MPMI-19-0342
Gaber, A., Ogata, T., Maruta, T., Yoshimura, K., Tamoi, M., Shigeoka, S. (2012). The involvement of Arabidopsis glutathione peroxidase 8 in the suppression of oxidative damage in the nucleus and cytosol. Plant Cell Physiol. 53, 1596–1606. doi: 10.1093/pcp/pcs100
Gadjev, I., Stone, J. M., Gechev, T. S. (2008). Programmed cell death in plants: new insights into redox regulation and the role of hydrogen peroxide. Int. Rev. Cell Mol. Biol. 270, 87–144. doi: 10.1016/S1937-6448(08)01403-2
Gill, S. S., Tuteja, N. (2010). Reactive oxygen species and antioxidant machinery in abiotic stress tolerance in crop plants. Plant Physiol. Biochem. 48, 909–930. doi: 10.1016/j.plaphy.2010.08.016
Gimenez-Ibanez, S., Hann, D. R., Chang, J. H., Segonzac, C., Boller, T., Rathjen, J. P. (2018). Differential Suppression of Nicotiana benthamiana Innate Immune Responses by Transiently Expressed Pseudomonas syringae Type III Effectors. Front. Plant Sci. 9. doi: 10.3389/fpls.2018.00688
Glazebrook, J. (2005). Contrasting mechanisms of defense against biotrophic and necrotrophic pathogens. Annu. Rev. Phytopathol. 43, 205–227. doi: 10.1146/annurev.phyto.43.040204.135923
González-García, Y., Cadenas-Pliego, G., Alpuche-Solís, Á.G., Cabrera, R. I., Juárez-Maldonado, A. (2022). Effect of carbon-based nanomaterials on Fusarium wilt in tomato. Sci. Hortic. 291, 110586. doi: 10.1016/j.scienta.2021.110586
Guo, M., Tian, F., Wamboldt, Y., Alfano, J. R. (2009). The Majority of the Type III Effector Inventory of Pseudomonas syringae pv. tomato DC3000 Can Suppress Plant Immunity. Mol. Plant-Microbe Interactions® 22, 1069–1080. doi: 10.1094/MPMI-22-9-1069
Gupta, D. R., Khanom, S., Rohman, M. M., Hasanuzzaman, M., Surovy, M. Z., Mahmud, N. U., et al. (2021). Hydrogen peroxide detoxifying enzymes show different activity patterns in host and non-host plant interactions with Magnaporthe oryzae Triticum pathotype. Physiol. Mol. Biol. Plants 27, 2127–2139. doi: 10.1007/s12298-021-01057-4
Hajianfar, R., Kolics, B., Cernák, I., Wolf, I., Polgár, Z., Taller, J. (2016). Expression of biotic stress response genes to Phytophthora infestans inoculation in White Lady, a potato cultivar with race-specific resistance to late blight. Physiol. Mol. Plant Pathol. 93, 22–28. doi: 10.1016/j.pmpp.2015.12.001
Hassan, N. M., Abu-Doubara, M. I., Waly, M. A., Nemat Alla, M. M. (2013). Efficacy of a pyrimidine derivative to control spot disease on Solanum melongena caused by Alternaria alternata. J. advanced Res. 4, 393–401. doi: 10.1016/j.jare.2012.07.008
Herbette, S., Brunel, N., Prensier, G., Julien, J.-L., Drevet, J. R., Roeckel-Drevet, P. (2004). Immunolocalization of a plant glutathione peroxidase-like protein. Planta 219, 784–789. doi: 10.1007/s00425-004-1284-8
Herbette, S., Lenne, C., Leblanc, N., Julien, J.L., Drevet, J.R., Roeckel-Drevet, P. (2002). Two GPX-like proteins from Lycopersicon esculentum and Helianthus annuus are antioxidant enzymes with phospholipid hydroperoxide glutathione peroxidase and thioredoxin peroxidase activities. Eur J Biochem 269, 2414–2420.
Herbette, S., Roeckel-Drevet, P., Drevet, J. R. (2007). Seleno-independent glutathione peroxidases. More than simple antioxidant scavengers. FEBS J. 274, 2163–2180. doi: 10.1111/j.1742-4658.2007.05774.x
Herbette, S., Tourvieille de Labrouhe, D., Drevet, J. R., Roeckel-Drevet, P. (2011). Transgenic tomatoes showing higher glutathione peroxydase antioxidant activity are more resistant to an abiotic stress but more susceptible to biotic stresses. Plant Sci. 180, 548–553. doi: 10.1016/j.plantsci.2010.12.002
Hernández, J. A., Gullner, G., Clemente-Moreno, M. J., Künstler, A., Juhász, C., Díaz-Vivancos, P., et al. (2016). Oxidative stress and antioxidative responses in plant–virus interactions. Physiol. Mol. Plant Pathol. 94, 134–148. doi: 10.1016/j.pmpp.2015.09.001
Holland, D., Ben-Hayyim, G., Faltin, Z., Camoin, L., Strosberg, A. D., Eshdat, Y. (1993). Molecular characterization of salt-stress-associated protein in citrus: protein and cDNA sequence homology to mammalian glutathione peroxidases. Plant Mol. Biol. 21, 923–927. doi: 10.1007/BF00027124
Hsu, P. K., Dubeaux, G., Takahashi, Y., Schroeder, J. I. (2021). Signaling mechanisms in abscisic acid-mediated stomatal closure. Plant J. 105, 307–321. doi: 10.1111/tpj.v105.2
Imai, H., Nakagawa, Y. (2003). Biological significance of phospholipid hydroperoxide glutathione peroxidase (PHGPx, GPx4) in mammalian cells. Free Radical Biol. Med. 34, 145–169. doi: 10.1016/S0891-5849(02)01197-8
Iqbal, A., Yabuta, Y., Takeda, T., Nakano, Y., Shigeoka, S. (2006). Hydroperoxide reduction by thioredoxin-specific glutathione peroxidase isoenzymes of Arabidopsis thaliana. FEBS J. 273, 5589–5597. doi: 10.1111/j.1742-4658.2006.05548.x
Islam, T., Manna, M., Reddy, M. K. (2015). Glutathione Peroxidase of Pennisetum glaucum (PgGPx) Is a Functional Cd2+ Dependent Peroxiredoxin that Enhances Tolerance against Salinity and Drought Stress. PloS One 10, e0143344. doi: 10.1371/journal.pone.0143344
Jamir, Y., Guo, M., Oh, H.-S., Petnicki-Ocwieja, T., Chen, S., Tang, X., et al. (2004). Identification of Pseudomonas syringae type III effectors that can suppress programmed cell death in plants and yeast. Plant J. 37, 554–565. doi: 10.1046/j.1365-313X.2003.01982.x
Jasmine, M. S., Joyous, T. J. (2022). “Involvement of epigenetic regulation in plant defence during biotic stress,” in Plant defense mechanisms. Ed. Josphert Ngui, K. (IntechOpen, Rijeka), Ch. 4.
Jensen, L. J., Kuhn, M., Stark, M., Chaffron, S., Creevey, C., Muller, J., et al. (2009). STRING 8–a global view on proteins and their functional interactions in 630 organisms. Nucleic Acids Res. 37, D412–D416. doi: 10.1093/nar/gkn760
Jindřichová, B., Fodor, J., Šindelářová, M., Burketová, L., Valentová, O. (2011). Role of hydrogen peroxide and antioxidant enzymes in the interaction between a hemibiotrophic fungal pathogen, Leptosphaeria maculans, and oilseed rape. Environ. Exp. Bot. 72, 149–156. doi: 10.1016/j.envexpbot.2011.02.018
Kemen, E., Jones, J. D. G. (2012). Obligate biotroph parasitism: can we link genomes to lifestyles? Trends Plant Sci. 17, 448–457. doi: 10.1016/j.tplants.2012.04.005
Keppler, L. D., Atkinson, M. M., Baker, C. J. (1989). Active oxygen production during a bacteria-induced hypersensitive reaction in tobacco suspension cells. Phytopathol. Res. 79, 974–978. doi: 10.1094/Phyto-79-974
Khajuria, A., Ohri, P. (2020). Polyamines induced nematode stress tolerance in Solanum lycopersicum through altered physico-chemical attributes. Physiol. Mol. Plant Pathol. 112, 101544. doi: 10.1016/j.pmpp.2020.101544
Kim, Y.-J., Jang, M.-G., Noh, H.-Y., Lee, H.-J., Sukweenadhi, J., Kim, J.-H., et al. (2014). Molecular characterization of two glutathione peroxidase genes of Panax ginseng and their expression analysis against environmental stresses. Gene 535, 33–41. doi: 10.1016/j.gene.2013.10.071
Koledenkova, K., Esmaeel, Q., Jacquard, C., Nowak, J., Clément, C., Ait Barka, E. (2022). Plasmopara viticola the causal agent of downy mildew of grapevine: from its taxonomy to disease management. Front. Microbiol. 13. doi: 10.3389/fmicb.2022.889472
Koop, D. M., Rio, M., Sabau, X., Almeida Cardoso, S. E., Cazevieille, C., Leclercq, J., et al. (2016). Expression analysis of ROS producing and scavenging enzyme-encoding genes in rubber tree infected by Pseudocercospora ulei. Plant Physiol. Biochem. 104, 188–199. doi: 10.1016/j.plaphy.2016.03.022
Kumar, S., Stecher, G., Tamura, K. (2016). MEGA7: molecular evolutionary genetics analysis version 7. 0 Bigger Datasets Mol. Biol. Evol. 33, 1870–1874. doi: 10.1093/molbev/msw054
Kuźniak, E., Gajewska, E. (2024). Lipids and lipid-mediated signaling in plant–pathogen interactions. Int. J. Mol. Sci. 25, 7255. doi: 10.3390/ijms25137255
Kuźniak, E., SkŁodowska, M. (2004). Differential implication of glutathione, glutathione-metabolizing enzymes and ascorbate in tomato resistance to pseudomonas syringae. J. Phytopathol. 152, 529–536. doi: 10.1111/j.1439-0434.2004.00884.x
Kuźniak, E., Skłodowska, M. (2005). Compartment-specific role of the ascorbate–glutathione cycle in the response of tomato leaf cells to Botrytis cinerea infection. J. Exp. Bot. 56, 921–933. doi: 10.1093/jxb/eri086
Lee, J., Feng, J., Campbell, K. B., Scheffler, B. E., Garrett, W. M., Thibivilliers, S., et al. (2009). Quantitative proteomic analysis of bean plants infected by a virulent and avirulent obligate rust fungus. Mol. Cell Proteomics 8, 19–31. doi: 10.1074/mcp.M800156-MCP200
Létoffé, S., Redeker, V., Wandersman, C. (1998). Isolation and characterization of an extracellular haem-binding protein from Pseudomonas aeruginosa that shares function and sequence similarities with the Serratia marcescens HasA haemophore. Mol. Microbiol. 28, 1223–1234. doi: 10.1046/j.1365-2958.1998.00885.x
Li, J., Wang, C., Liang, W., Liu, S. (2021). Rhizosphere microbiome: the emerging barrier in plant-pathogen interactions. Front. Microbiol. 12, 772420. doi: 10.3389/fmicb.2021.772420
Lim, C. W., Baek, W., Jung, J., Kim, J. H., Lee, S. C. (2015). Function of ABA in stomatal defense against biotic and drought stresses. Int. J. Mol. Sci. 16, 15251–15270. doi: 10.3390/ijms160715251
Ma, T., Du, J., Zhang, Y., Wang, Y., Wang, B., Zhang, T. (2022). GPX4-independent ferroptosis—a new strategy in disease’s therapy. Cell Death Discovery 8, 434. doi: 10.1038/s41420-022-01212-0
Macharia, M., Das, P. P., Naqvi, N. I., Wong, S.-M. (2020). iTRAQ-based quantitative proteomics reveals a ferroptosis-like programmed cell death in plants infected by a highly virulent tobacco mosaic virus mutant 24A+UPD. Phytopathol. Res. 2, 1. doi: 10.1186/s42483-019-0043-5
Mahesh, H. M., Murali, M., Anup Chandra Pal, M., Melvin, P., Sharada, M. S. (2017). Salicylic acid seed priming instigates defense mechanism by inducing PR-Proteins in Solanum melongena L. upon infection with Verticillium dahliae Kleb. Plant Physiol. Biochem. 117, 12–23. doi: 10.1016/j.plaphy.2017.05.012
Maiorino, M., Aumann, K. D., Brigelius-Flohé, R., Doria, D., van den Heuvel, J., McCarthy, J., et al. (1995). Probing the presumed catalytic triad of selenium-containing peroxidases by mutational analysis of phospholipid hydroperoxide glutathione peroxidase (PHGPx). Biol. Chem. Hoppe-Seyler 376, 651–660. doi: 10.1515/bchm3.1995.376.11.651
Mandadi, K. K., Scholthof, K.-B. G. (2013). Plant immune responses against viruses: how does a virus cause disease? Plant Cell 25, 1489–1505. doi: 10.1105/tpc.113.111658
Mannes, A. M., Seiler, A., Bosello, V., Maiorino, M., Conrad, M. (2011). Cysteine mutant of mammalian GPx4 rescues cell death induced by disruption of the wild-type selenoenzyme. FASEB J. 25, 2135–2144. doi: 10.1096/fj.10-177147
Mapuranga, J., Zhang, N., Zhang, L., Chang, J., Yang, W. (2022). Infection strategies and pathogenicity of biotrophic plant fungal pathogens. Front. Microbiol. 13. doi: 10.3389/fmicb.2022.799396
Margis, R., Dunand, C., Teixeira, F. K., Margis-Pinheiro, M. (2008). Glutathione peroxidase family – an evolutionary overview. FEBS J. 275, 3959–3970. doi: 10.1111/j.1742-4658.2008.06542.x
Martins Alves, A. M., Pereira Menezes, S., Matos Lima, E., Peres Gramacho, K., Silva Andrade, B., Macêdo Ferreira, M., et al. (2019). The selenium-binding protein of Theobroma cacao: A thermostable protein involved in the witches’ broom disease resistance. Plant Physiol. Biochem. 142, 472–481. doi: 10.1016/j.plaphy.2019.08.005
Martins Alves, A. M., Pereira Menezes Reis, S., Peres Gramacho, K., Micheli, F. (2020). The glutathione peroxidase family of Theobroma cacao: Involvement in the oxidative stress during witches’ broom disease. Int. J. Biol. Macromol. 164, 3698–3708. doi: 10.1016/j.ijbiomac.2020.08.222
Mbemba, F., Houbion, A., Raes, M., Remacle, J. (1985). Subcellular localization and modification with ageing of glutathione, glutathione peroxidase and glutathione reductase activities in human fibroblasts. Biochim. Biophys. Acta (BBA) - Gen. Subj. 838, 211–220. doi: 10.1016/0304-4165(85)90081-9
Melotto, M., Underwood, W., Koczan, J., Nomura, K., He, S. Y. (2006). Plant stomata function in innate immunity against bacterial invasion. Cell 126, 969–980. doi: 10.1016/j.cell.2006.06.054
Meng, S., Torto-Alalibo, T., Chibucos, M. C., Tyler, B. M., Dean, R. A. (2009). Common processes in pathogenesis by fungal and oomycete plant pathogens, described with Gene Ontology terms. BMC Microbiol. 9 Suppl 1, S7. doi: 10.1186/1471-2180-9-S1-S7
Mengiste, T. (2012). Plant immunity to necrotrophs. Annu. Rev. Phytopathol. 50, 267–294. doi: 10.1146/annurev-phyto-081211-172955
Miao, Y., Lv, D., Wang, P., Wang, X. C., Chen, J., Miao, C., et al. (2006). An Arabidopsis glutathione peroxidase functions as both a redox transducer and a scavenger in abscisic acid and drought stress responses. Plant Cell 18, 2749–2766. doi: 10.1105/tpc.106.044230
Milli, A., Cecconi, D., Bortesi, L., Persi, A., Rinalducci, S., Zamboni, A., et al. (2012). Proteomic analysis of the compatible interaction between Vitis vinifera and Plasmopara viticola. J. Proteomics 75, 1284–1302. doi: 10.1016/j.jprot.2011.11.006
Mittler, R. (2006). Abiotic stress, the field environment and stress combination. Trends Plant Sci. 11, 15–19. doi: 10.1016/j.tplants.2005.11.002
Mittler, R., Zandalinas, S. I., Fichman, Y., Van Breusegem, F. (2022). Reactive oxygen species signalling in plant stress responses. Nat. Rev. Mol. Cell Biol. 23, 663–679. doi: 10.1038/s41580-022-00499-2
Moreira, R. R., MaChado, F. J., Lanza, F. E., Trombin, V. G., Bassanezi, R. B., de Miranda, M. P., et al. (2022). Impact of diseases and pests on premature fruit drop in sweet orange orchards in São Paulo state citrus belt, Brazil. Pest Manage. Sci. 78, 2643–2656. doi: 10.1002/ps.v78.6
Morvaridzadeh, M., Sadeghi, E., Agah, S., Fazelian, S., Rahimlou, M., Kern, F. G., et al. (2021). Effect of ginger (Zingiber officinale) supplementation on oxidative stress parameters: A systematic review and meta-analysis. J. Food Biochem. 45, e13612. doi: 10.1111/jfbc.13612
Nakagawa, Y. (2004). Role of mitochondrial phospholipid hydroperoxide glutathione peroxidase (PHGPx) as an antiapoptotic factor. Biol. Pharm. Bull. 27, 956–960. doi: 10.1248/bpb.27.956
Navrot, N., Collin, V., Gualberto, J., Gelhaye, E., Hirasawa, M., Rey, P., et al. (2006). Plant glutathione peroxidases are functional peroxiredoxins distributed in several subcellular compartments and regulated during biotic and abiotic stresses. Plant Physiol. 142, 1364–1379. doi: 10.1104/pp.106.089458
Noctor, G., Reichheld, J. P., Foyer, C. H. (2018). ROS-related redox regulation and signaling in plants. Semin. Cell Dev. Biol. 80, 3–12. doi: 10.1016/j.semcdb.2017.07.013
Noman, A., Aqeel, M., Islam, W., Khalid, N., Akhtar, N., Qasim, M., et al. (2021). Insects-plants-pathogens: Toxicity, dependence and defense dynamics. Toxicon 197, 87–98. doi: 10.1016/j.toxicon.2021.04.004
Noronha Souza, P. F., Abreu Oliveira, J. T., Vasconcelos, I. M., Magalhães, V. G., Albuquerque Silva, F. D., Guedes Silva, R. G., et al. (2020). H2O2Accumulation, host cell death and differential levels of proteins related to photosynthesis, redox homeostasis, and required for viral replication explain the resistance of EMS-mutagenized cowpea to cowpea severe mosaic virus. J. Plant Physiol. 245, 153110. doi: 10.1016/j.jplph.2019.153110
Ofori, A., Padi, F. K., Ameyaw, G. A., Dadzie, A. M., Opoku-Agyeman, M., Domfeh, O., et al. (2022). Field evaluation of the impact of cocoa swollen shoot virus disease infection on yield traits of different cocoa (Theobroma cacao L.) clones in Ghana. PloS One 17, e0262461. doi: 10.1371/journal.pone.0262461
Page, M. J., McKenzie, J. E., Bossuyt, P. M., Boutron, I., Hoffmann, T. C., Mulrow, C. D., et al. (2021). The PRISMA 2020 statement: an updated guideline for reporting systematic reviews. BMJ (Clinical Res. ed.) 372, n71. doi: 10.1136/bmj.n71
Paiva, A. L. S., Passaia, G., Jardim-Messeder, D., Nogueira, F. C. S., Domont, G. B., Margis-Pinheiro, M. (2021). The mitochondrial isoform glutathione peroxidase 3 (OsGPX3) is involved in ABA responses in rice plants. J. Proteomics 232, 104029. doi: 10.1016/j.jprot.2020.104029
Parish, R. W. (1971). The Isolation of Peroxisomes, Mitochondria and Chloroplasts from Leaves of Spinach Beet (Beta vulgaris L. ssp. vulgaris). Eur. J. Biochem. 22, 423–429. doi: 10.1111/j.1432-1033.1971.tb01560.x
Pereira, J. L. (1989). Primeira ocorrência de vassoura-de-bruxa na principal região produtora de cacau do Brasil. Agrotrópica 1, 79–81.
Pereira, M. E., Souza, J. V., Galiciolli, M. E. A., Sare, F., Vieira, G. S., Kruk, I. L., et al. (2022). Effects of selenium supplementation in patients with mild cognitive impairment or alzheimer’s disease: A systematic review and meta-analysis. Nutrients 14, 3205. doi: 10.3390/nu14153205
Peshin, R., Dhawan, A. K. (2009). Integrated pest management: volume 1: innovation-development process Vol. 1 (Springer Dordrecht: Springer Science & Business Media).
Pinto, M. C. De, Locato, V., De Gara, L. (2012). Redox regulation in plant programmed cell death. Plant Cell Environ. 35, 234–244. doi: 10.1111/j.1365-3040.2011.02387.x
Rockenbach, M. F., Boneti, J. I., Cangahuala-Inocente, G. C., Gavioli-Nascimento, M. C. A., Guerra, M. P. (2015). Histological and proteomics analysis of apple defense responses to the development of Colletotrichum gloeosporioides on leaves. Physiol. Mol. Plant Pathol. 89, 97–107. doi: 10.1016/j.pmpp.2015.01.003
Rodríguez-Serrano, M., Romero-Puertas, M. C., Sanz-Fernández, M., Hu, J., Sandalio, L. M. (2016). Peroxisomes extend peroxules in a fast response to stress via a reactive oxygen species-mediated induction of the peroxin PEX11a. Plant Physiol. 171, 1665–1674. doi: 10.1104/pp.16.00648
Rouhier, N., Jacquot, J. P. (2005). The plant multigenic family of thiol peroxidases. Free Radical Biol. Med. 38, 1413–1421. doi: 10.1016/j.freeradbiomed.2004.07.037
Sa, R., Liu, D., Chen, L., Liu, L., Zhang, Y., Zhang, X., et al. (2020). Transcriptome analysis of mechanisms and candidate genes associated with cucumber response to cucumber alternaria leaf spot infection. Physiol. Mol. Plant Pathol. 111, 101490. doi: 10.1016/j.pmpp.2020.101490
Saitou, N., Nei, M. (1987). The neighbor-joining method: a new method for reconstructing phylogenetic trees. Mol. Biol. Evol. 4, 406–425. doi: .1093/oxfordjournals.molbev.a040454
Santos, A. S., Mora-Ocampo, I. Y., de Novais, D. P. S., Aguiar, E. R. G. R., Pirovani, C. P. (2023). State of the art of the molecular biology of the interaction between cocoa and witches’ broom disease: A systematic review. Int. J. Mol. Sci. 24, 5684. doi: 10.3390/ijms24065684
Scheerer, P., Borchert, A., Krauss, N., Wessner, H., Gerth, C., Höhne, W., et al. (2007). Structural basis for catalytic activity and enzyme polymerization of phospholipid hydroperoxide glutathione peroxidase-4 (GPx4). Biochemistry 46, 9041–9049. doi: 10.1021/bi700840d
Seibt, T. M., Proneth, B., Conrad, M. (2019). Role of GPX4 in ferroptosis and its pharmacological implication. Free Radical Biol. Med. 133, 144–152. doi: 10.1016/j.freeradbiomed.2018.09.014
Sharma, P., Jha, A. B., Dubey, R. S., Pessarakli, M. (2012). Reactive oxygen species, oxidative damage, and antioxidative defense mechanism in plants under stressful conditions. J. Bot. 2012, 217037. doi: 10.1155/2012/217037
Sharma, N., Rahman, M. H., Strelkov, S., Thiagarajah, M., Bansal, V. K., Kav, N. N. V. (2007). Proteome-level changes in two Brassica napus lines exhibiting differential responses to the fungal pathogen Alternaria brassicae. Plant Sci. 172, 95–110. doi: 10.1016/j.plantsci.2006.07.016
Shi, L.-g., Xun, W.-j., Yue, W.-b., Zhang, C.-x., Ren, Y.-s., Wang, Q., et al. (2010). Cloning, characterization, and expression analysis of goat (Capra hircus) phospholipid hydroperoxide glutathione peroxidase (PHGPx). Int. J. Biol. Sci. 6, 316–326. doi: 10.7150/ijbs.6.316
Silveira, P. R., Nascimento, K. J. T., Andrade, C. C. L., Bispo, W. M. S., Oliveira, J. R., Rodrigues, F. A. (2015). Physiological changes in tomato leaves arising from Xanthomonas gardneri infection. Physiol. Mol. Plant Pathol. 92, 130–138. doi: 10.1016/j.pmpp.2015.10.001
Singh, M., Avtar, R., Lakra, N., Pal, A., Singh, V. K., Punia, R., et al. (2022). Early oxidative burst and anthocyanin-mediated antioxidant defense mechanism impart resistance against Sclerotinia sclerotiorum in Indian mustard. Physiol. Mol. Plant Pathol. 120, 101847. doi: 10.1016/j.pmpp.2022.101847
Singh, Y. J., Grewal, S. K., Gill, R. K. (2020). Role of glutathione in methylglyoxal detoxification pathway during yellow mosaic virus (YMV) infection in black gram (Vigna mungo (L.) Hepper). Physiol. Mol. Plant Pathol. 111, 101513. doi: 10.1016/j.pmpp.2020.101513
Soares, J. M. S., Rocha, A. J., Nascimento, F. S., Santos, A. S., Miller, R. N. G., Ferreira, C. F., et al. (2021). Genetic improvement for resistance to black sigatoka in bananas: A systematic review. Front. Plant Sci. 12. doi: 10.3389/fpls.2021.657916
Souza, P. F. N., Silva, F. D. A., Carvalho, F. E. L., Silveira, J. A. G., Vasconcelos, I. M., Oliveira, J. T. A. (2017). Photosynthetic and biochemical mechanisms of an EMS-mutagenized cowpea associated with its resistance to cowpea severe mosaic virus. Plant Cell Rep. 36, 219–234. doi: 10.1007/s00299-016-2074-z
Soylu, S., Baysal, Ö., Soylu, E. M. (2003). Induction of disease resistance by the plant activator, acibenzolar-S-methyl (ASM), against bacterial canker (Clavibacter michiganensis subsp. michiganensis) tomato seedlings Plant Sci. 165, 1069–1075. doi: 10.1016/S0168-9452(03)00302-9
Sugimoto, M., Sakamoto, W. (1997). Putative phospholipid hydroperoxide glutathione peroxidase gene from Arabidopsis thaliana induced by oxidative stress. Genes Genet. Syst. 72, 311–316. doi: 10.1266/ggs.72.311
Takebe, G., Yarimizu, J., Saito, Y., Hayashi, T., Nakamura, H., Yodoi, J., et al. (2002). A comparative study on the hydroperoxide and thiol specificity of the glutathione peroxidase family and selenoprotein P. J. Biol. Chem. 277, 41254–41258. doi: 10.1074/jbc.M202773200
Távora, F., Bevitori, R., Mello, R. N., Cintra, M., Oliveira-Neto, O. B., Fontes, W., et al. (2021). Shotgun proteomics coupled to transient-inducible gene silencing reveal rice susceptibility genes as new sources for blast disease resistance. J. Proteomics 241, 104223. doi: 10.1016/j.jprot.2021.104223
Ton, J., Flors, V., Mauch-Mani, B. (2009). The multifaceted role of ABA in disease resistance. Trends Plant Sci. 14, 310–317. doi: 10.1016/j.tplants.2009.03.006
Torres, M. A., Jones, J. D. G., Dangl, J. L. (2006). Reactive oxygen species signaling in response to pathogens. Plant Physiol. 141, 373–378. doi: 10.1104/pp.106.079467
Trenz, T. S., Delaix, C. L., Turchetto-Zolet, A. C., Zamocky, M., Lazzarotto, F., Margis-Pinheiro, M. (2021). Going forward and back: the complex evolutionary history of the GPx. Biol. 10, 1–12. doi: 10.3390/biology10111165
Tripathy, B.C., Oelmüller, R. (2012). Reactive oxygen species generation and signaling in plants. Plant Signaling & Behavior 7, 1621–1633.
Tyagi, S., Himani, Sembi, J. K., Upadhyay, S. K. (2018). Gene architecture and expression analyses provide insights into the role of glutathione peroxidases (GPXs) in bread wheat (Triticum aestivum L.). J. Plant Physiol. 223, 19–31. doi: 10.1016/j.jplph.2018.02.006
Ursini, F., Maiorino, M., Brigelius-Flohé, R., Aumann, K. D., Roveri, A., Schomburg, D., et al. (1995). Diversity of glutathione peroxidases. Methods Enzymol. 252, 38–53. doi: 10.1016/0076-6879(95)52007-4
Van Breusegem, F., Dat, J. F. (2006). Reactive oxygen species in plant cell death. Plant Physiol. 141, 384–390. doi: 10.1104/pp.106.078295
Varela, A. L. N., Komatsu, S., Wang, X., Silva, R. G. G., Souza, P. F. N., Lobo, A. K. M., et al. (2017). Gel-free/label-free proteomic, photosynthetic, and biochemical analysis of cowpea (Vigna unguiculata [L.] Walp.) resistance against Cowpea severe mosaic virus (CPSMV). J. Proteomics 163, 76–91. doi: 10.1016/j.jprot.2017.05.003
Viswanathan, V. S., Ryan, M. J., Dhruv, H. D., Gill, S., Eichhoff, O. M., Seashore-Ludlow, B., et al. (2017). Dependency of a therapy-resistant state of cancer cells on a lipid peroxidase pathway. Nature 547, 453–457. doi: 10.1038/nature23007
Vojnov, A. A., do Amaral, A. M., Dow, J. M., Castagnaro, A. P., Marano, M. R. (2010). Bacteria causing important diseases of citrus utilise distinct modes of pathogenesis to attack a common host. Appl. Microbiol. Biotechnol. 87, 467–477. doi: 10.1007/s00253-010-2631-2
Wang, Y., Cao, S., Sui, X., Wang, J., Geng, Y., Gao, F., et al. (2023). Genome-wide characterization, evolution, and expression analysis of the ascorbate peroxidase and glutathione peroxidase gene families in response to cold and osmotic stress in ammopiptanthus nanus. J. Plant Growth Regul. 42, 502–522. doi: 10.1007/s00344-021-10570-5
Wang, X., Rong, W., Liu, Y., Wang, X., Zhang, Z. (2018). Investigation of the mechanism of adult-stage resistance to barley yellow dwarf virus associated with a wheat–Thinopyrum intermedium translocation. Crop J. 6, 394–405. doi: 10.1016/j.cj.2018.02.002
Wang, Z., Wang, F., Duan, R., Liu, J. Y. (2007). Purification and physicochemical characterization of a recombinant phospholipid hydroperoxide glutathione peroxidase from Oryza sativa. J. Biochem. Mol. Biol. 40, 412–418. doi: 10.5483/BMBRep.2007.40.3.412
Wang, J., Yang, J., Wang, C., Zhao, Z., Fan, Y. (2021). Systematic review and meta-analysis of oxidative stress and antioxidant markers in oral lichen planus. Oxid. Med. Cell. Longevity 2021, 9914652. doi: 10.1155/2021/9914652
Yang, X. D., Dong, C. J., Liu, J. Y. (2006). A plant mitochondrial phospholipid hydroperoxide glutathione peroxidase: its precise localization and higher enzymatic activity. Plant Mol. Biol. 62, 951–962. doi: 10.1007/s11103-006-9068-0
Yang, X. D., Li, W. J., Liu, J. Y. (2005). Isolation and characterization of a novel PHGPx gene in Raphanus sativus. Biochim. Biophys. Acta 1728, 199–205. doi: 10.1016/j.bbaexp.2005.02.003
Yang, W. S., SriRamaratnam, R., Welsch, M. E., Shimada, K., Skouta, R., Viswanathan, V. S., et al. (2014). Regulation of ferroptotic cancer cell death by GPX4. Cell 156, 317–331. doi: 10.1016/j.cell.2013.12.010
Yang, B., Srivastava, S., Deyholos, M. K., Kav, N. N. V. (2007). Transcriptional profiling of canola (Brassica napus L.) responses to the fungal pathogen Sclerotinia sclerotiorum. Plant Sci. 173, 156–171. doi: 10.1016/j.plantsci.2007.04.012
Yao, X., Guo, H., Zhang, K., Zhao, M., Ruan, J., Chen, J. (2023). Trichoderma and its role in biological control of plant fungal and nematode disease. Front. Microbiol. 14. doi: 10.3389/fmicb.2023.1160551
Yoshimura, K., Miyao, K., Gaber, A., Takeda, T., Kanaboshi, H., Miyasaka, H., et al. (2004). Enhancement of stress tolerance in transgenic tobacco plants overexpressing Chlamydomonas glutathione peroxidase in chloroplasts or cytosol. Plant J. 37, 21–33. doi: 10.1046/j.1365-313X.2003.01930.x
Yurina, N. P. (2023). Heat shock proteins in plant protection from oxidative stress. Molekuliarnaia biologiia 57, 949–964. doi: 10.31857/S0026898423060228
Zhai, C.-Z., Zhao, L., Yin, L.-J., Chen, M., Wang, Q.-Y., Li, L.-C., et al. (2013). Two wheat glutathione peroxidase genes whose products are located in chloroplasts improve salt and H2O2 tolerances in arabidopsis. PloS One 8, e73989. doi: 10.1371/journal.pone.0073989
Zhang, Y., Zhang, T., Pan, Y., Ma, L., Fang, Y., Pan, C., et al. (2023). Nano-selenium promotes the product quality and plant defense of Salvia miltiorrhiza by inducing tanshinones and salvianolic acids accumulation. Ind. Crops Prod. 195, 116436. doi: 10.1016/j.indcrop.2023.116436
Zhao, F., Guo, L., Wang, X., Zhang, Y. (2021). Correlation of oxidative stress-related biomarkers with postmenopausal osteoporosis: a systematic review and meta-analysis. Arch. osteoporosis 16, 4. doi: 10.1007/s11657-020-00854-w
Zhou, Y., Li, J., Wang, J., Yang, W., Yang, Y. (2018). Identification and characterization of the glutathione peroxidase (GPX) gene family in watermelon and its expression under various abiotic stresses. Agronomy 8, 206. doi: 10.3390/agronomy8100206
Zhu, S., Jin, Z., Chen, J., Yang, R., Luo, Q., Wang, T., et al. (2023). Salicylic acid enhances the resistance of Neoporphyra haitanensis to pathogen Vibrio mediterranei 117-T6. Aquaculture 562, 738773. doi: 10.1016/j.aquaculture.2022.738773
Keywords: antioxidant action, defense responses, pathogens, cell death, plant disease
Citation: do Carmo Santos ML, Silva Santos A, Pereira Silva de Novais D, dos Santos Lopes N, Pirovani CP and Micheli F (2025) The family of glutathione peroxidase proteins and their role against biotic stress in plants: a systematic review. Front. Plant Sci. 16:1425880. doi: 10.3389/fpls.2025.1425880
Received: 30 April 2024; Accepted: 09 January 2025;
Published: 20 February 2025.
Edited by:
Maria Raffaella Ercolano, University of Naples Federico II, ItalyReviewed by:
Eva Nouri, Université de Fribourg, SwitzerlandCopyright © 2025 do Carmo Santos, Silva Santos, Pereira Silva de Novais, dos Santos Lopes, Pirovani and Micheli. This is an open-access article distributed under the terms of the Creative Commons Attribution License (CC BY). The use, distribution or reproduction in other forums is permitted, provided the original author(s) and the copyright owner(s) are credited and that the original publication in this journal is cited, in accordance with accepted academic practice. No use, distribution or reproduction is permitted which does not comply with these terms.
*Correspondence: Fabienne Micheli, ZmFiaWVubmUubWljaGVsaUBjaXJhZC5mcg==
Disclaimer: All claims expressed in this article are solely those of the authors and do not necessarily represent those of their affiliated organizations, or those of the publisher, the editors and the reviewers. Any product that may be evaluated in this article or claim that may be made by its manufacturer is not guaranteed or endorsed by the publisher.
Research integrity at Frontiers
Learn more about the work of our research integrity team to safeguard the quality of each article we publish.