- 1Ontario Ministry of Agriculture, Food and Rural Affairs, Guelph, ON, Canada
- 2Department of Plant Agriculture, University of Guelph, Guelph, ON, Canada
Oxidative stress responses of Arabidopsis to reflected low red to far-red signals (R:FR ≈ 0.3) generated by neighboring weeds or an artificial source of FR light were compared with a weed-free control (R:FR ≈1.6). In the low R:FR treatments, induction of the shade avoidance responses (SAR) coincided with increased leaf production of singlet oxygen (1O2). This 1O2 increase was not due to protochlorophyllide accumulation and did not cause cell death. Chemical treatments, however, with 5-aminolevulinic acid (the precursor of tetrapyrrole biosynthesis) and glutathione (a quinone A reductant) enhanced cell death and growth inhibition. RNA sequencing revealed that transcriptome responses to the reflected low R:FR light treatments minimally resembled previously known Arabidopsis 1O2 generating systems that rapidly generate 1O2 following a dark to light transfer. The upregulation of only a few early 1O2 responsive genes (6 out of 1931) in the reflected low R:FR treatments suggested specificity of the 1O2 signaling. Moreover, increased expression of two enzyme genes, the SULFOTRANSFERASE ST2A (ST2a) and the early 1O2-responsive IAA-LEUCINE RESISTANCE (ILR)-LIKE6 (ILL6), which negatively regulate jasmonate level, suggested that repression of bioactive JAs may promote the shade avoidance (versus defense) and 1O2 acclimation (versus cell death) responses to neighboring weeds.
Introduction
Singlet oxygen (1O2) is a potent oxidant that is generated in photosynthetic and non-photosynthetic tissues under multiple stresses (Dmitrieva et al., 2020). Several reports on the lifetime and diffusion distance of 1O2 in cellular environments indicate that 1O2 may diffuse through cell membranes (Dmitrieva et al., 2020; Ogilby, 2010; Hatz et al., 2007; Skovsen et al., 2005). Indeed, in Chlamydomonas reinhardtii, the photosystem II (PSII)-generated 1O2 under high light stress reached cytosol and induced expression of the glutathione reductase homologue GPXH (Fischer et al., 2007). These studies along with the detection of osmotic stress- and drought-induced 1O2 in Arabidopsis roots (Chen and Fluhr, 2018; Mor et al., 2014) indicate the possibility of 1O2 generation in cellular compartments other than chloroplast.
Under severe stress conditions, high levels of 1O2 can damage cellular components and impair plant function through photo-inhibition and uncontrollable cell death (Laloi and Havaux, 2015). At sub-lethal levels, however, 1O2 initiates signaling pathways that trigger disparate stress responses including acclimation to excess light and programmed cell death (op den Camp et al., 2003; Ledford et al., 2007; Laloi and Havaux, 2015; Waszczak et al., 2018; Ambastha et al., 2020).
Several plant systems allow for the study of 1O2 signaling. These include the conditional fluorescent (flu) mutant of Arabidopsis (Meskauskiene et al., 2001; op den Camp et al., 2003), the tigrina (tig-d.12) mutant of barley (Lee et al., 2003), and the chlorina1 (ch1) mutant of Arabidopsis (Ramel et al., 2013a). The flu and tig-d.12 mutants produce 1O2 in the light from dark-accumulated photosensitizer protochlorophyllide (Pchlide), whereas the ch1 mutant of Arabidopsis and the chlorina-f2 mutant of barley are deficient in chlorophyll b (Kim et al., 2009; Havaux et al., 2007; Havaux and Tardy, 1997; Leverenz et al., 1992). In the ch1 mutant of Arabidopsis, PSII is confined to its reaction center due to the inability of PSII light-harvesting antennae to assemble without chlorophyll b. Without light-harvesting complex II (LHCII), PSII lacks photoprotective mechanisms like nonphotochemical quenching. Consequently, increasing photon flux density can lead to PSII overexcitation and the formation of 1O2 (Ramel et al., 2013a; Dall'Osto et al., 2010). While these mutant systems have provided valuable insight into the 1O2 signaling pathways, few plant systems allow the controlled induction of 1O2 and investigation of 1O2 signaling within wild type plants. Recently, 1O2 generation was detected in wild type Arabidopsis leaves following transfer of the FR light-treated seedling to white light (Page et al., 2017). This white light-mediated induction of 1O2 was due to Pchlide accumulation and led to suppression of major chlorophyll synthesis and photosynthetic genes presumably to prevent photo-oxidative damage during de-etiolation (Page et al., 2017).
Despite the detection of 1O2 in wild type Arabidopsis leaves following a short-time (two hours) FR light treatment and exposure to white light (Page et al., 2017), it is not clear whether 1O2 can be induced under low R:FR light environments. Such 1O2 induction may provide an opportunity to explore the involvement of 1O2 signaling as an intermediary between low R:FR light-mediated phytochrome inactivation and modulation of growth-defense trade-offs in response to competition cues. Under low R:FR light environments, phytochrome inactivation promotes growth-related hormonal pathways, while attenuating jasmonic acid (JA)-mediated defense responses (Ballaré, 2014; de Wit et al., 2016; Fernández-Milmanda and Ballaré, 2021). Attenuation of JA synthesis is also indispensable for triggering acclimation response to 1O2 and prevention of cell death, while 1O2-mediated photo-damage and cell death correspond with JA accumulation (Ramel et al., 2013a; Ramel et al., 2013b). Recently, a sulfotransferase (ST2a) has been shown to be up-regulated as a molecular link between low R:FR light environments and attenuation of JA-mediated defense responses through sulfation of bioactive JAs (Fernández-Milmanda et al., 2020). In addition, to a lesser extent, the low R:FR light upregulated the early 1O2-responsive amidohydrolase ILL6 (op den Camp et al., 2003), which catalyzes the amido-hydrolysis of JA-isoleucine (Fernández-Milmanda et al., 2020). Given that Pchlide accumulation in the FR light-adapted plants leads to 1O2 production under white light (Page et al., 2017), a possibility arises that ST2a up-regulation in the low R:FR environments may be linked to 1O2-mediated signaling. In this work, wild-type Arabidopsis was used as a model to distinguish whether increased leaf production of 1O2 in response to competition cues is due to reflected far-red light from neighboring weeds and to gain insights into the 1O2 signaling under low R:FR light environments. Our findings under biological weedy and artificial sources of reflected low R:FR light show that elongation growth responses occur concurrently with 1O2 appearance suggesting its signaling role in response to competition cues. The 1O2 appearance is not a consequence of Pchlide accumulation and not sufficient to cause cell death but chemical treatments that increase Pchlide level or decrease chloroplast electron transport efficiency result in cell death and growth inhibition. The 1O2 signatures under the biological and artificial low R:FR light treatments differ dramatically from those in Arabidopsis mutants that rapidly generate 1O2 after dark to light transfer. Finally, the upregulation of ST2a and ILL6 and the suppression of bioactive JAs may also operate in the acclimation to 1O2 under weed competition.
Materials and methods
Plant material and growth conditions
Wild-type Arabidopsis thaliana (ecotype Columbia) plants were raised in controlled environment growth chambers (Model CMP 3244 Conviron, Winnipeg, Canada) with a 12-hour photoperiod, an irradiance of 160 µmol m-2 s-1, a temperature of 21/18°C, and a relative humidity of 60%. The weed-free control (R:FR ≈ 1.6), biological low R:FR (R:FR ≈ 0.3), and artificial low R:FR (R:FR ≈ 0.3) light treatments were set up by placing plastic tubes (8 × 18 cm, 1 L) in the center of plastic pots (16 × 15 cm, 3.36 L) (Airlite Plastics Company, Omaha, USA). Drainage holes were drilled in the tubes and pots. For the control and the artificial low R:FR light treatments, the area between the plastic pot and plastic tube was filled with Turface MVP (Profile Products LLC, Buffalo Grove, USA). For the biological low R:FR treatment, the area between the plastic pot and plastic tube was filled with Sunshine Mix #4 (Sungro Horticulture, Agawam, MA) and seeded (≈ 200 g m-2) with a commercial mixture of grass seeds (The Scotts Compnay LLC, Marysville, USA). This mixture of grass seeds consisted of perennial ryegrass (Lolium perenne L.), creeping red fescue (Festuca rubra L.), Kentucky bluegrass (Poa pratensis L.), and chewing fescue (Festuca rubra L.) (The Scotts Compnay LLC, Marysville, USA). The grass was watered twice a week and was fertilized every two weeks with a nutrient solution as described previously (Tollenaar, 1989). A dense grass was established within two months and generated a stable biological source of reflected FR light without direct contact between the grass and experimental plants. A simulated FR light environment (artificial low R:FR; R:FR ≈ 0.3) was generated using 13 W, 162 mA far-red LEDs (Phillips Canada). Seeds of Arabidopsis were planted in a mix of PGx (Premier Horticulture LTD, Quebec, Canada) and perlite (Perlite Canada Inc., Quebec, Canada) at a ratio of 3:1 in 355 mL (8×10 cm) plastic cups (Dart Container Corp., Mason, USA). The plastic cups were then placed inside the plastic tubes in each pot in the respective light environments (Figure 1). The central plastic tube acted as a barrier preventing water exchange and nutrient flow between the Arabidopsis plants in the cups and the grass. Further, the empty space between the bottom of the cup and bottom of the central tube prevented secretion of grass metabolites to Arabidopsis roots. Light interference in the growth chambers was eliminated using a white opaque plastic divider between the weed-free control and biological low R:FR while allowing a free upward air flow (1.55 m3 min-1) across treatments. Arabidopsis plants were grown under weed-free control conditions for 21 days and fertilized weekly with a modified Hoagland’s solution (10 mM KNO3, 10 mM Ca(NO3)2.4H2O, and 2.5 mM KH2PO4). Arabidopsis plants were then exposed to either the biological or artificial low R:FR light treatment for 12 hours a day for seven days or kept under control conditions before plants were sampled. The light spectral composition of the weed-free control, biological low R:FR, and artificial low R:FR light treatments were determined at plant height. The incoming and reflected light quantity and quality were measured at nine locations across each treatment using a LI-COR-180 spectrometer (Li-COR Biosciences; Lincoln, NE, USA). For incoming light measurements, the spectrometer was held level at plant height facing the lights. For reflected light measurements, the spectrometer was held level facing the plants. A summary and detailed information about the light spectral composition in the weed-free control, biological low R:FR and artificial low R:FR light treatments are presented in Supplementary Table 1.
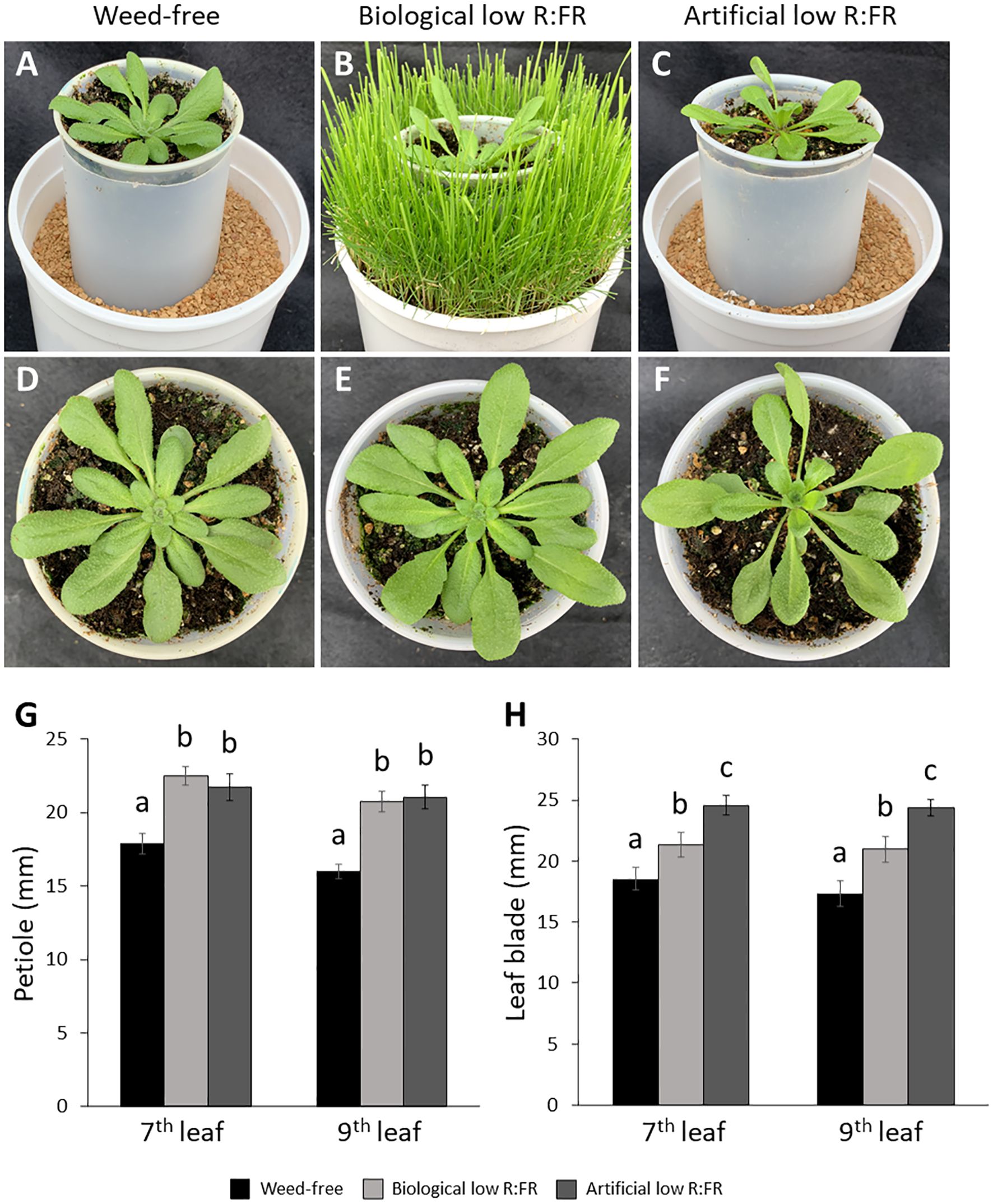
Figure 1. Weed-free control (A), biological low R:FR (B), and artificial low R:FR (C) treatments with reflected R:FR of ≈1.6, ≈0.3, and ≈0.3, respectively. Arabidopsis plants were exposed to the biological and artificial low R:FR light for 12 hours a day for seven days or kept under control (weed-free) condition prior to sampling. Plastic cups containing Arabidopsis seedling were placed in a plastic tube in the center of the pots to prevent direct root contact with neighbouring weeds. Note the hyponastic leaf growth in the biological and artificial low R:FR treatments. Normal leaf growth in the control (D), and petiole elongation in the biological low R:FR (E), and artificial low R:FR (F) treatments. Increases in petiole (G) and leaf blade (H) lengths of 7th and 9th leaf (4-week-old) in the low R:FR treatments. Black bars represent the control treatment while light grey and dark grey bars represent the biological low R:FR and artificial low R:FR light treatments, respectively. Note the similar elongation responses of Arabidopsis in separate growth chambers with the biological and artificial sources of reflected low R:FR light. Data represent means ± SEM for three independent experiments each consisting of five plants per treatment. Means were separated using Tukey’s HSD test (P<0.05). Letters indicate statistical significance of differences across treatments.
Singlet oxygen imaging
Singlet oxygen formation was detected in 4-week-old Arabidopsis thaliana leaves using Singlet Oxygen Sensor Green (SOSG) (ThermoFisher, Waltham, USA). A 500 µM stock solution of SOSG was prepared by dissolving 100 µg of SOSG in 330 µL of methanol and diluted to a working concentration of 10 µM SOSG using 50 mM potassium phosphate buffer (pH 7.5) and 0.01% Tween-20 as a non-ionic surfactant. Plants were manually infiltrated with the 10 µM SOSG solution using a 60 ml needleless syringe and placed back in the treatment for two hours. The SOSG fluorescence (excitation ~450-490 nm; emission ~500-550 nm) was detected using an Axio Zoom V16 fluorescence stereo microscope with a Pan NeoFluor Z 1x/0.25 FWD 56 mm lens and a 38 HE filter set (Zeiss Canada, Toronto, Canada). All leaf images were obtained using the same magnification and an exposure of 500 milliseconds. The SOSG fluorescence signal was quantified using the image analysis application Fiji (Schindelin et al., 2012). Briefly, the fluorescence signal in each leaf image was quantified in five equal (200 × 200) regions of interest (ROIs) and averaged to obtain the mean fluorescence signal. For each treatment, 25 independent images were analyzed.
Pigment analysis
To quantify photosynthetic pigments, leaf discs (nine mm) were taken from the mid-section of fully expanded Arabidopsis leaves (4-week-old) and placed in 1.5 mL of 80% acetone. The leaf discs were incubated in the dark at -20°C for 24 hours until total removal of chlorophyll. Absorbance was measured at 470, 626, 645, 646, 647, 663, and 664 nm. The concentration of Pchlide, chlorophyllide a (Chlide a), chlorophyll a (Chl a), chlorophyll b (Chl b), and total carotenoids were calculated using the previously described equations (Brouers and Michel-Wolwertz, 1983; Lichtenthaler and Wellburn, 1983). A more detailed quantification of individual carotenoids including lutein, β-carotene, violaxanthin, and neoxanthin was performed by high performance liquid chromatography (HPLC). Approximately, 20 mg of fresh ground tissue was used for HPLC analysis. To extract prenyl lipids, 250 µL of acetone:ethyl acetate (3:2; v/v) was added to each sample followed by the addition of 100 µL of ethyl acetate and 200 µL of dH2O. Each sample was then vortexed for five seconds and centrifuged (10 seconds burst) to achieve phase separation. The upper ethyl acetate layer was transferred into a vial and 50 µL of the ethyl acetate layer was separated on a five µm Spherisorb ODS-2 reverse-phase column (250 × 4.6 mm, Supelco) thermostated at 23°C. A linear gradient from 100% acetonitrile:water:trimethylamine (9:1:0.01) to 100% ethyl acetate was used to elute the samples at a flow rate of 1 mL min-1 over 45 minutes. Lutein, β-carotene, violaxanthin, and neoxanthin were detected at A440 with a detection limit of 0.05 nmol and were quantified based on external calibration standards of high purity. For these HPLC standards, lutein was obtained from Cayman Chemicals (Ann Arbor, MI, USA). β-carotene, violaxanthin, and neoxanthin were obtained from Sigma Aldrich (Oakville, ON, Canada).
Chemical treatments
All chemicals were made up in 10 mM MES buffer (pH 6.5) and 0.01% tween-20 as a surfactant. The final concentrations of chemicals were 10 mM ALA (5-aminolevulinic acid; the precursor of tetrapyrrole biosynthesis), 50 mM, 100 mM, 150 mM, and 200 mM GSH (reduced glutathione; a quinone A reductant), and 50 mM, 100 mM, 150 mM, and 200 mM H2O2 (hydrogen peroxide; an oxidizing agent). For the ALA treatment, the upper and lower leaves of 4-week-old Arabidopsis plants were sprayed with a two mL solution of ALA. In total, four experiments were performed and ALA was sprayed on three plants per treatment per experiment. For the GSH and H2O2 treatments, the rosette leaves of 3-week-old Arabidopsis plants were sprayed with a one mL solution of GSH or H2O2. After spraying, plants were allowed to dry for one hour before transfer back to the treatments. The extent of cell death by ALA was monitored and imaged every 24 hours for a total of 72 hours. The extent of cell death by GHS and H2O2 was monitored every 24 hours and imaged 96 hours after spraying. In total, three independent experiments with a similar time course were performed and each chemical was sprayed on five plants per treatment per experiment. In separate experiments, the extent of cell death by GSH was quantified in 18 plants per concentration per treatment using the image analysis application Fiji (Schindelin et al., 2012). Dead leaf area was calculated by subtracting the green leaf area from total leaf area and expressed as percentage of leaf cell death.
RNA-sequencing
RNA extraction from 4-week-old Arabidopsis rosette leaves and subsequent DNase treatment of RNA samples were performed using a RNeasy PowerPlant kit (Qiagen) and an RNase-Free DNase kit (Qiagen) according to the manufacturer’s instructions. In total, nine samples consisting of three treatments (weed-free, biological low R:FR, and artificial low R:FR light) with three replicates, each consisting of a pool of leaves from three individual plants, were analyzed. The RNA quality and quantity were determined using an Agilent 2100 Bioanalyzer (Agilent Technologies). The RNA libraries were constructed using the Illumina TrueSeq RNA kit in three replicates according to the manufacturer’s protocol. The RNA libraries were sequenced on an Illumina sequencer (NovaSeq 6000) at the Genome Quebec Innovation Center (McGill University, Canada) to obtain ≈25 M reads per replicate. Differentially expressed gene (DEG) analysis was performed by Harvest Genomics Inc. (Guelph, Canada), where the quality control of raw.fastq files was performed prior to sequence alignment to the reference genome (https://www.ncbi.nlm.nih.gov/assembly/GCF_000001735.4/) using Bowtie 2 v2.4.3. Count data were retrieved using HTSeq (version 0.13.5) and DEGs were determined using DESeq2 (version 1.30.1). The Benjamini-Hochberg false discovery rate (FDR) correction was used within DESeq2 (version 1.30.1) to obtain adjusted p-values (padj). Tables of log2 fold change (lfc) were generated as described previously (Zhu et al., 2019). The RNA-seq raw reads and expression analysis are available in the NCBI gene expression omnibus (GEO) data repository under accession GSE213185 (https://www.ncbi.nlm.nih.gov/gds/?term=GSE213185).
Real-time quantitative reverse transcription PCR assays
Total RNA was extracted from 4-week-old Arabidopsis leaves using the TRI Reagent (Sigma Aldrich Canada). In total, 16 samples from two independent experiments were analyzed. In each experiment, eight samples were taken from two treatments (weed-free and biological low R:FR light) each consisting of rosette leaves from four individual plants. The DNAse treatment and sample cleanup were performed using the RNAase-Free DNase Set (Qiagen Inc. Canada) and the RNeasy MinElute Cleanup kit (Qiagen Inc. Canada), respectively. Prior to RT-qPCR assays, RNA quality was determined using an Agilent 4150 TapeStation System (Agilent, CA, USA) according to the manufacturer’s instructions. The RNA samples were reverse transcribed using the High Capacity cDNA Reverse Transcription kit following the supplied protocol (Applied Biosystems, Canada). Real-time PCR assays were performed using a QuantaStudio Real Time PCR system (Thermo Fisher Scientific Inc., Canada). Each PCR reaction (20 μl) consisted of 10 μl of 2× SsoAdvanced Universal Inhibitor-Tolerant SYBR supermix (Bio-Rad, Cat No: 172-5017), 0.8 µl of PCR forward and reverse primer mix at 5 µM (final concentration of primer at 200 nM), 4.2 μl of water and 5 µl of 8× diluted cDNA. The thermal cycler conditions were 3 minutes at 98°C polymerase activation step, followed by 40 cycles of a two-step qPCR (10 seconds of 98°C denaturation, 30 seconds of 60°C combined annealing/extension). The amplified PCR products were compared with the PROFILIN1 (PRF1) as a housekeeping gene and relative changes in gene expression were quantified using the 2-ΔΔct equation (Livak and Schmittgen, 2001). Primers were designed using PrimerQuest Tool (Integrated DNA Technologies, Coralville, USA). The primer sequences used for qPCR were: SULFOTRANSFERASE 2A (At5G07010) ST2a fwd 5’-ACCTCAAGCATGAAGAGCATTC-3’ and ST2a rev 5’-CCCTTCATCTTCTTCGGCTTTC-3’; SULFOTRANSFERASE 2B (At5G07000) ST2b fwd 5’-AAGCGAAGGCCAAGAAGAA-3’ and ST2b rev 5’-GTAACGATTTCTCCGTCCTCTC-3’; IAA-LEUCINE RESISTANCE (ILR)-LIKE6 (At1g44350) ILL6 fwd 5’-TCTTGGTGCTGCCCATATTC-3’ and ILL6 rev 5’-AAGCTCCGTCTTCGATCATATTC-3’; U-BOX E3 UBIQUITIN LIGASE (At3g19380) PUB25 fwd 5’-CGACTTCACACTCATCCCTAAC-3’ and PUB25 rev 5’-CAGCTGGTTGTTTAGGAGTAGG-3’; PRF1 (At2G19760) fwd 5’-GGTGAACAAGGAGCTGTGAT-3’ and PRF1 rev 5’-GGTTCATCGTAGAAGCCAAAGA-3’.
Statistical analysis
Statistical analysis was performed using SAS version 9.4 and PROC GLIMMIX. The experiments were arranged as a randomized complete block design. The light treatment was considered the fixed effect, while replication was considered the random effect. Least-square means were generated, and means were separated with a Tukey’s honest significant difference (HSD) test. Unless stated otherwise, each replicate consisted of five Arabidopsis samples per treatment and three replications per experiment. A type I error of 0.05 was used for all tests of significance. A power analysis was performed to ensure adequate power was achieved.
Results
The shade avoidance response is accompanied by an increase of 1O2 level in leaves
We exposed 3-week-old Arabidopsis plants to the biological and artificial low R:FR light for 12 hours a day for seven days or kept them in the weed-free control condition. This exposure to reflected low R:FR light was under resource-independent competition, where incoming light, water and nutrients were not limiting factors. Further, we prevented direct root contact between the Arabidopsis plants and the surrogate weeds by a plastic tube in the center of the pots (Figures 1A–C; Supplementary Table 1). Elongation growth and leaf hyponasty were typical shade avoidance responses (Figures 1A–F). The biological and artificial low R:FR treatments caused similar elongation responses in the petiole lengths (23% and 26%) and leaf blade lengths (14% and 19%) of 4-week-old Arabidopsis plants (Figures 1G–H). These shade avoidance responses were accompanied by increases in leaf production of 1O2 in the biological and artificial low R:FR treatments (Figures 2A–C). Quantification of SOSG fluorescence by the image analysis application Fiji (Schindelin et al., 2012) revealed 2.6× and 3.0× increase in mean fluorescence signal in the biological and artificial low R:FR treatments, respectively, compared with the weed-free control. In addition, mean fluorescence signal in the artificial low R:FR treatments was significantly higher than the biological low R:FR treatment (Figure 2D; Supplementary Table 2). These results not only indicated that the biological low R:FR treatment could elicit the shade avoidance responses in the absence of direct resource competition but also suggested that the 1O2, which was predominantly generated by the reflected FR light from neighboring weeds, might be a molecular component of the shade avoidance response.
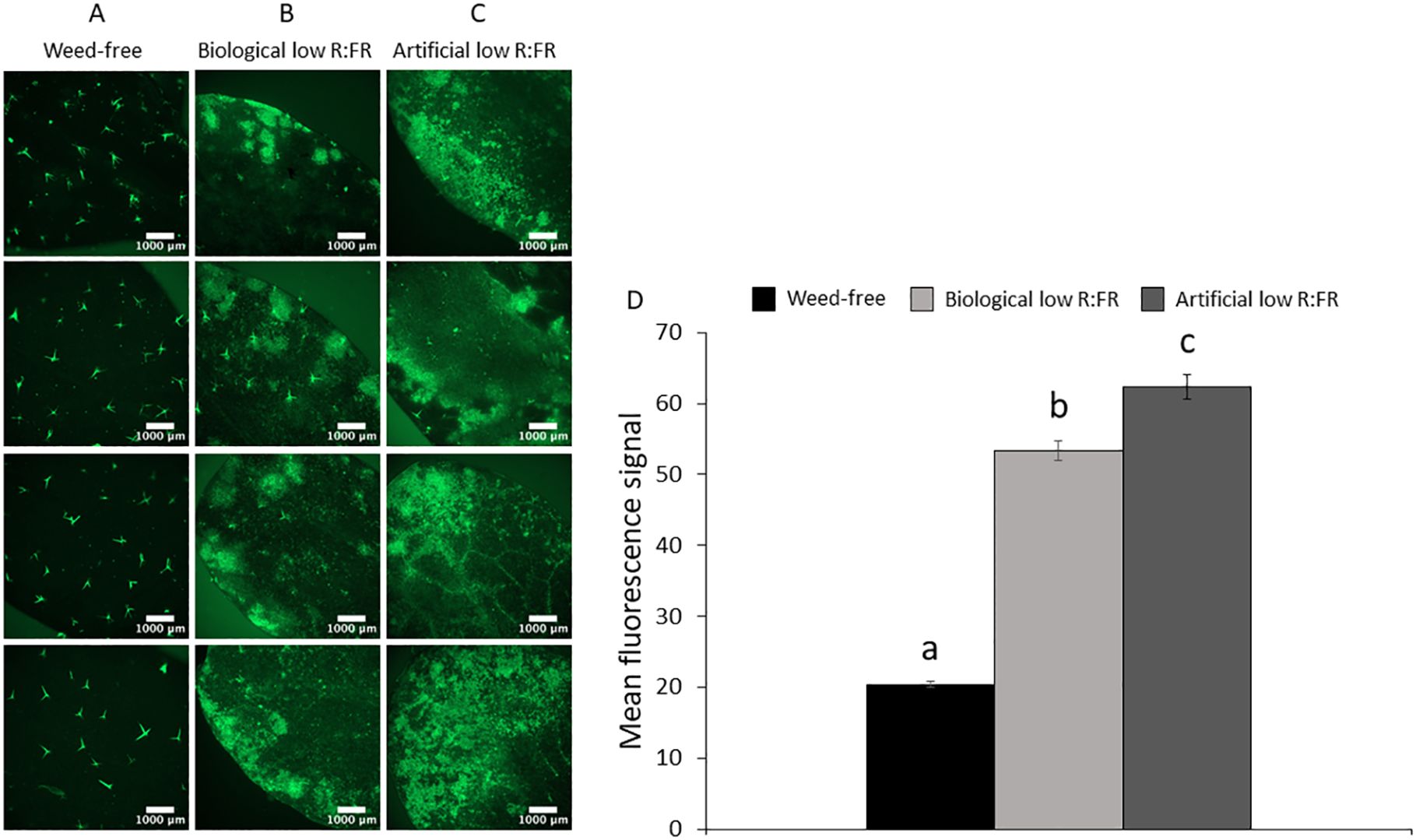
Figure 2. Basal level of 1O2 in the weed-free control (A) and increases in 1O2 levels in the biological low R:FR (B) and artificial low R:FR (C) treatments. Each panel shows four representative images of independent leaf samples. Four-week-old Arabidopsis plants were infiltrated with 10 µM SOSG and the SOSG fluorescence was imaged two hours after infiltration at an exposure time of 500 milliseconds. Scale bar represents 1000 μm. Significant increases in mean fluorescence signal in the biological and artificial low R:FR treatments (D). The SOSG fluorescence was quantified using the image analysis application Fiji (Schindelin et al., 2012). Data represent means ± SEM for 25 independent leaf samples per treatment (Supplementary Table 2). Means were separated using Tukey’s HSD test (P<0.05). Letters indicate statistical significance of differences across treatments.
Increased leaf production of 1O2 in the low R:FR light environments is not due to the accumulation of chlorophyll precursors
Induction of 1O2 in the flu mutant of Arabidopsis is the result of photosensitization of accumulated Pchlide following transfer from dark to light (Meskauskiene et al., 2001). To investigate whether a similar mechanism is responsible for increased leaf production of 1O2 in the low R:FR treatments, the levels of Pchlide, Chlide a, Chl a, Chl b, and total Chl were compared with that of control plants using spectrophotometry. The levels of Pchlide were not significantly altered by the biological and artificial low R:FR treatments (Figure 3A), while the Chlide a levels were significantly decreased (9.4% and 25.9%, respectively) compared with the control (Figure 3B). In addition, significant decreases were found in the levels of Chl a (10.3% and 8.2%), Chl b (26.9% and 21.9%), and total Chl (9.6% and 25.3%) in the biological and artificial low R:FR treatments compared with the control (Figures 3C–E). Further, exposure of Arabidopsis plants in the biological and artificial low R:FR treatments to dark periods of two, four, and six hours did not affect Pchlide levels (Figure 3A). The Chlide a levels were decreased in the biological and artificial low R:FR treatments (13.9% and 35.7%, respectively) after two hours of dark incubation (Figure 3B). This decrease, however, was significant only in the artificial low R:FR treatment. Further, a significant decrease (34%) was found in the artificial low R:FR treatment after four hours of dark incubation. Although the Chlide a levels in the low R:FR treatments were not significantly different from the weed-free control after six hours of dark incubation, the Chlide a level in the artificial low R:FR treatment was significantly lower (19.3%) than the biological low R:FR treatment (Figure 3B). The Chl a levels were decreased after two hours (13.5% and 39.4%) and four hours (22.1% and 36.1%) of dark incubation compared with the control (Figure 3C). These decreases, however, were significant only in the artificial low R:FR treatment and no significant difference was found between treatments after six hours of dark incubation (Figure 3C). The levels of Chl b were decreased after two hours in the biological and artificial low R:FR treatments (9.2% and 24.9%, respectively). This decrease, however, was significant only in the artificial low R:FR treatment. Further, a significant decrease (23.2%) was found in the artificial low R:FR treatment after four hours of dark incubation (Figure 3D). Although the levels of Chl b in the biological and artificial low R:FR treatments were not different from the weed-free control after six hours of dark incubation, the level of Chl b in the artificial low R:FR treatment was significantly lower (12.2%) compared with the biological low R:FR treatment (Figure 3D). The levels of total Chl were decreased in the biological and artificial low R:FR treatments only after two hours (12.1% and 34.7%) and four hours (20.6% and 31.8%) of dark incubation (Figure 3E). Again, these decreases were significant only in the artificial low R:FR treatment (Figure 3E). In the biological and artificial low R:FR treatments, Arabidopsis plants displayed higher ratios of Pchlide to Chlide a (Pchlide/Chlide a) compared with the control (Figure 3F). The higher Pchlide/Chlide a may be due to decreased conversion of Pchlide to Chlide a. It is not clear whether higher Pchlide/Chlide a may contribute to 1O2 generation. After two and four hours of dark incubation, however, Pchlide/Chlide a were increased in the artificial low R:FR treatment only, whereas six hours of dark incubation did not affect Pchlide/Chlide a (Figure 3F). The lack of accumulation of Pchlide in the dark (Figure 3A), however, suggests that 1O2 generation in the low R:FR treatments occurs via a different mechanism than in the flu mutant of Arabidopsis.
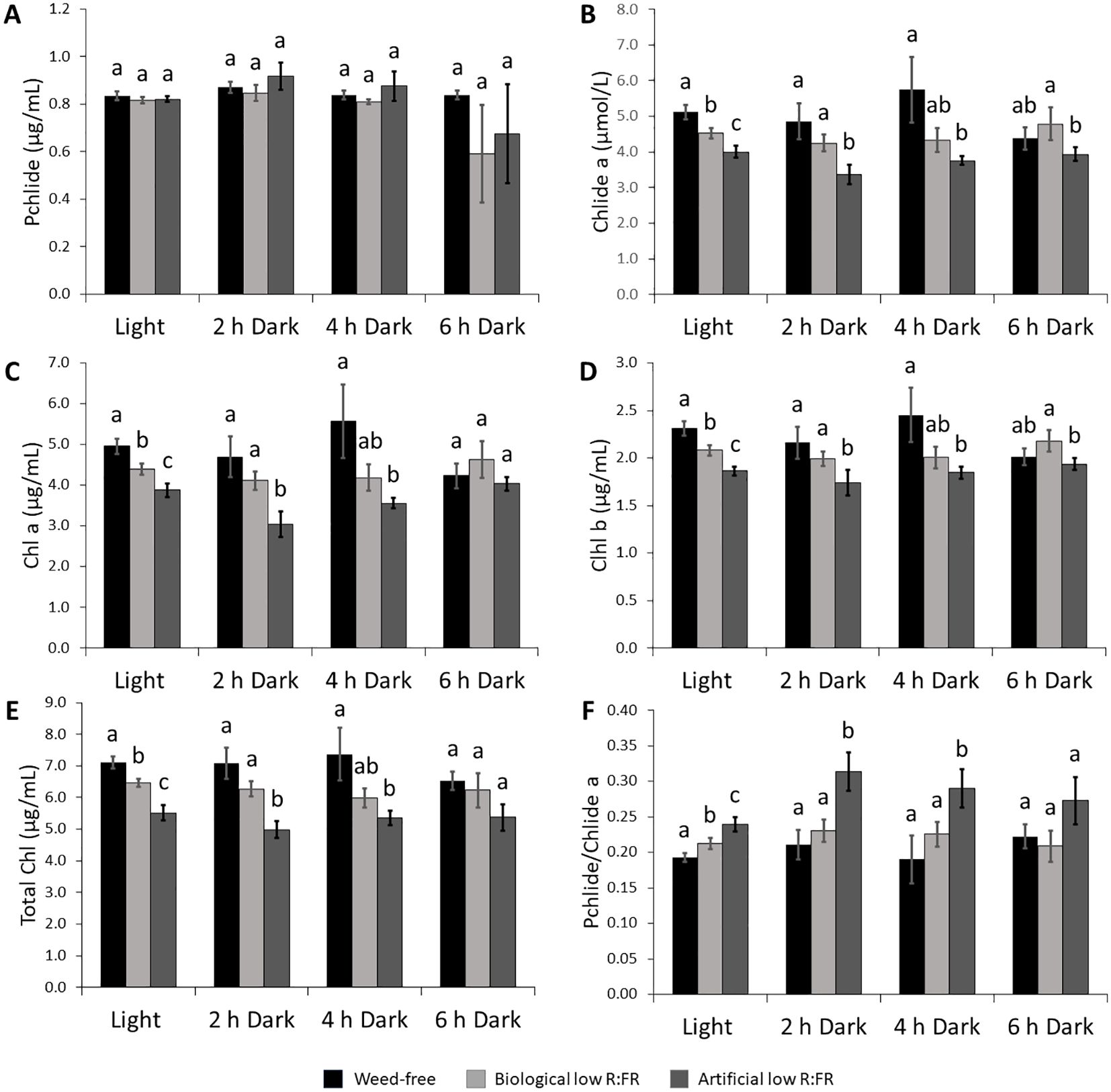
Figure 3. Changes in the levels of photosynthetic pigments in the biological and artificial low R:FR treatments in the light and after two, four, and six hours of dark incubation. Arabidopsis plants were exposed to the biological and artificial low R:FR light for 12 hours a day for seven days or kept under control (weed-free) condition prior to sampling. Black bars represent the control while light grey and dark grey bars represent the biological and artificial low R:FR treatments, respectively. No significant change in Pchlide levels in the reflected low R:FR treatments and after dark incubation for two, four, and six hours (A). Decreases in Chlide a levels in the reflected low R:FR treatments and after dark incubation for two and four hours (B). Decreases in Chl a (C), Chl b (D), and total Chl (E) levels in the reflected low R:FR treatments and after dark incubation for two and four hours. Increases in the ratio of Pchlide to Chlide a in the artificial low R:FR treatment and after dark incubation for two and four hours (F). Data represent means ± SEM for three independent experiments each consisting of three plants per treatment. Means were separated using Tukey’s HSD test (P<0.05). Letters indicate statistical significance of differences across treatments.
Low R:FR light environments decrease total carotenoid content and differentially alter levels of xanthophylls
Since carotenoids are the most efficient physical quenchers of 1O2 that primarily protect photosystems from oxidative damage (Triantaphylidès and Havaux, 2009), we investigated whether increased leaf production 1O2 in the low R:FR light treatments was due to decreases in carotenoid levels. We found that total carotenoid levels were decreased by 7.1% and 37.7% in the biological and artificial low R:FR treatments, respectively (Figure 4A). When individual xanthophyll levels were examined by HPLC, however, there appeared to be no difference between the levels of lutein (Figure 4B), β-carotene (Figure 4C), violaxanthin (Figure 4D), and neoxanthin (Figure 4E) in the biological low R:FR treatment compared with the control. In the artificial low R:FR treatment, however, the levels of lutein, β-carotene, and violaxanthin were deceased by 17.6%, 13.1%, and 17.1%, respectively, compared with the control. The neoxanthin level in this treatment was significantly lower than in the biological low R:FR treatment but did not differ from that of the control. Therefore, the observed decrease in total carotenoids in the biological low R:FR treatment does not appear to be due to decreases in the levels of lutein, β-carotene, violaxanthin, and neoxanthin. In contrast, the decreased level of total carotenoids in the artificial low R:FR treatment may be attributable to decreases in the levels of lutein, β-carotene, and violaxanthin. Further, these results suggest that the 1O2 induction by the artificial low R:FR treatment may arise from decreased levels or the suppression of protective function of these carotenoids. These mechanisms however, do not appear to be behind the 1O2 induction by the biological low R:FR treatment. Unlike the artificial low R:FR treatment, the inability of the biological low R:FR treatment to decrease the levels of the above-mentioned carotenoids may be attributable to the effects associated with the surrogate weed used for the biological low R:FR treatment. We do not, however, rule out the involvement of other carotenoids such as zeaxanthin, which is also a potent physical quencher of 1O2 (Triantaphylidès and Havaux, 2009).
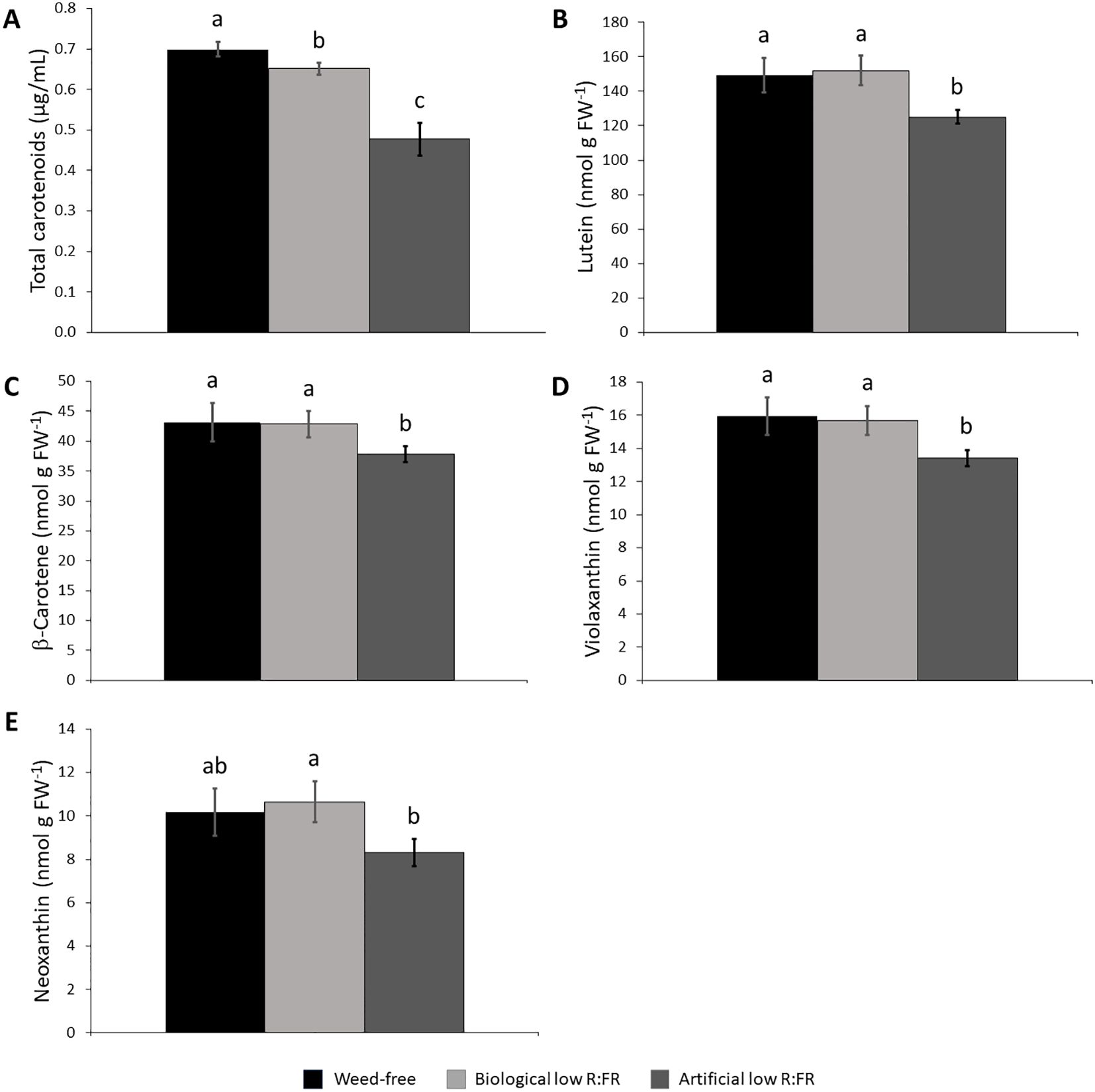
Figure 4. Effect of the reflected low R:FR treatments on carotenoid content. Arabidopsis plants were exposed to the biological and artificial low R:FR light for 12 hours a day for seven days or kept under control (weed-free) condition prior to sampling. Black bars represent the control while light grey and dark grey bars represent the biological and artificial low R:FR treatments, respectively. Decreases in total carotenoid content in the biological and artificial low R:FR treatments (A) and no changes in the levels of lutein (B), β-carotene (C), violaxanthin, (D), and neoxanthin (E) in the biological low R:FR treatment. The levels of lutein, β-carotene, and violaxanthin in the artificial low R:FR treatment are lower than that in the control.Total carotenoid data represent means ± SEM for four replicates consisting of three plants per treatment while individual carotenoid data represent means ± SEM for three independent experiments each consisting of three plants per treatment. Means were separated using Tukey’s HSD test (P<0.05). Letters indicate statistical significance of differences across treatments.
Low R:FR light environments increase susceptibility to cell death by ALA
It is well recognized that both light-dependent and light-independent induction of 1O2 can trigger cell death responses (Wagner et al., 2004; Ramel et al., 2013a; Mor et al., 2014; Chen and Fluhr, 2018). We sprayed Arabidopsis plants in the control, biological, and artificial low R:FR treatments with ALA (10 mM) to increase the levels of photodynamic tetrapyrrole intermediates in these plants and compare their susceptibility to cell death. After 24 hours, plants in the biological low R:FR treatment wilted, while the control displayed little sign of cellular damage (Figures 5A, B, E, F). After 48 hours, signs of cellular damage were apparent on all the plants, however, it was more severe in the biological low R:FR treatment (Figures 5A, C, E, G, M, O). After 72 hours, the control plants exhibited minor signs of cell death in small localized patches, while in the biological low R:FR treatment, plants displayed total necrosis with the exception of the growing points (Figures 5A, D, E, H). Plants in the artificial low R:FR treatment exhibited cell death more severe than the control treatment, but less so than the biological low R:FR treatment (Figures 5A, D, M, P). Further, after spraying ALA, plants that were transferred from the low R:FR treatments to the control treatment displayed similar cell death symptoms as the plants that had been replaced into their respective treatments pre-spray (Figures 5I–L, Q–T). These results suggest that further increases in 1O2 levels in the low R:FR treatments may tip the 1O2 balance from an acclimation response towards a cell death response.
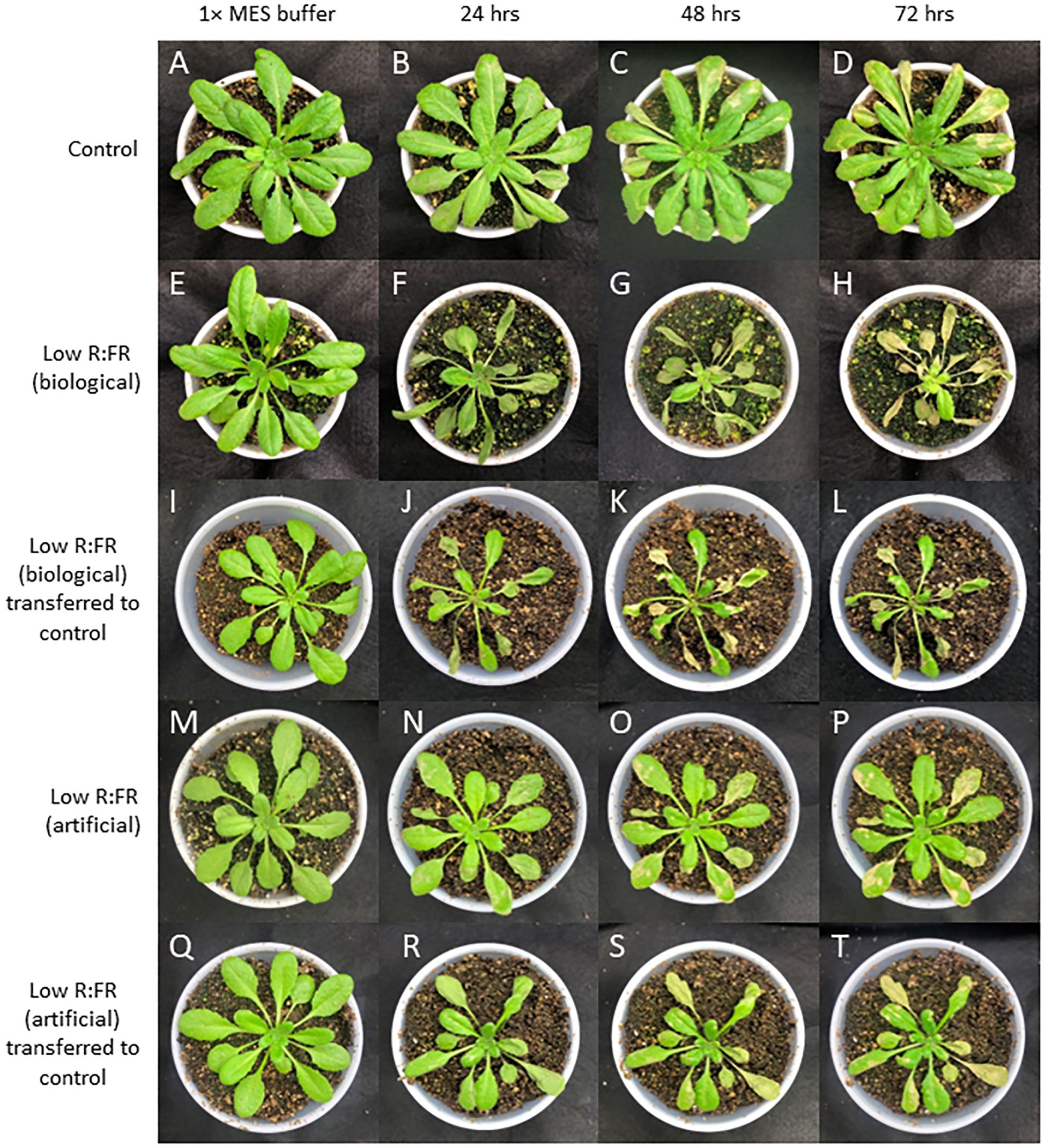
Figure 5. The time course (0-72 h) of Arabidopsis responses to ALA in the control, biological and artificial low R:FR treatments. Arabidopsis plants were exposed to the biological and artificial low R:FR light for 12 hours a day for seven days or kept under control (weed-free) condition prior to sampling. Control plants for each treatment were sprayed with a two mL solution of 1x MES buffer (10 mM; pH 6.5), while treated plants were sprayed with a two mL solution of 10 mM ALA in 1x MES buffer and placed back in their original treatments or transferred from the reflected low R:FR treatments to the control treatment. Experiments were repeated four times and ALA was sprayed on three plants per treatment per experiment. No damage by 1x MES buffer in the control, but minor damage by ALA after 24 h and localized patches of cell death after 48 and 72 hours (A–D). Signs of wilting 24 h after ALA treatment, progression of cellular damage and necrosis after 24 and 48 hours in the biological low R:FR (E–H). A similar trend after transfer of the ALA-treated plants from the biological low R:FR to the control treatment (I–L). Less severe damage by ALA in the artificial low R:FR light (M–P) compared with the biological low R:FR treatment. Persistence of sensitivity to cell death after transfer of the ALA-treated plants from the artificial low R:FR to the control treatment (Q–T).
Low R:FR environments differentially enhance cell death and growth inhibition responses to GSH
It has been established that GSH can increase and H2O2 can decrease photo-oxidative damage through the control of the redox state of the quinone A (QA)-quinone B (QB)-plastoquinone (PQ) pools (Karpinska et al., 2000). We examined susceptibility of Arabidopsis plants to GSH and H2O2 in the low R:FR environments. Treatment of Arabidopsis plants with increasing concentrations of GSH (50 mM to 200 mM) resulted in more severe cell death and growth inhibition in the low R:FR environments compared with the weed-free control (Figure 6). Quantification of leaf cell death by the image analysis application Fiji (Schindelin et al., 2012) revealed significant increases in leaf cell death (%) in the biological low R:FR (65%, 80%, 73%, and 71%) and artificial low R:FR (110%, 55%, 24%, and 31%) treatments relative to the weed-free treatment at 50, 100, 150, and 200 mM GSH, respectively (Figure 7; Supplementary Table 3). In contrast, treatment of Arabidopsis plants with increasing concentrations of H2O2 (50 mM to 200 mM) resulted in small and localized patches of cell death in all environments with no distinguishable differences in susceptibility to H2O2 between the low R:FR light and control environments (Figure 8). These results suggest that the effects of GSH on reduction of the QA-QB-PQ pools, efficiency of chloroplast electron transport, and cell death may be exacerbated under low R:FR light environment.
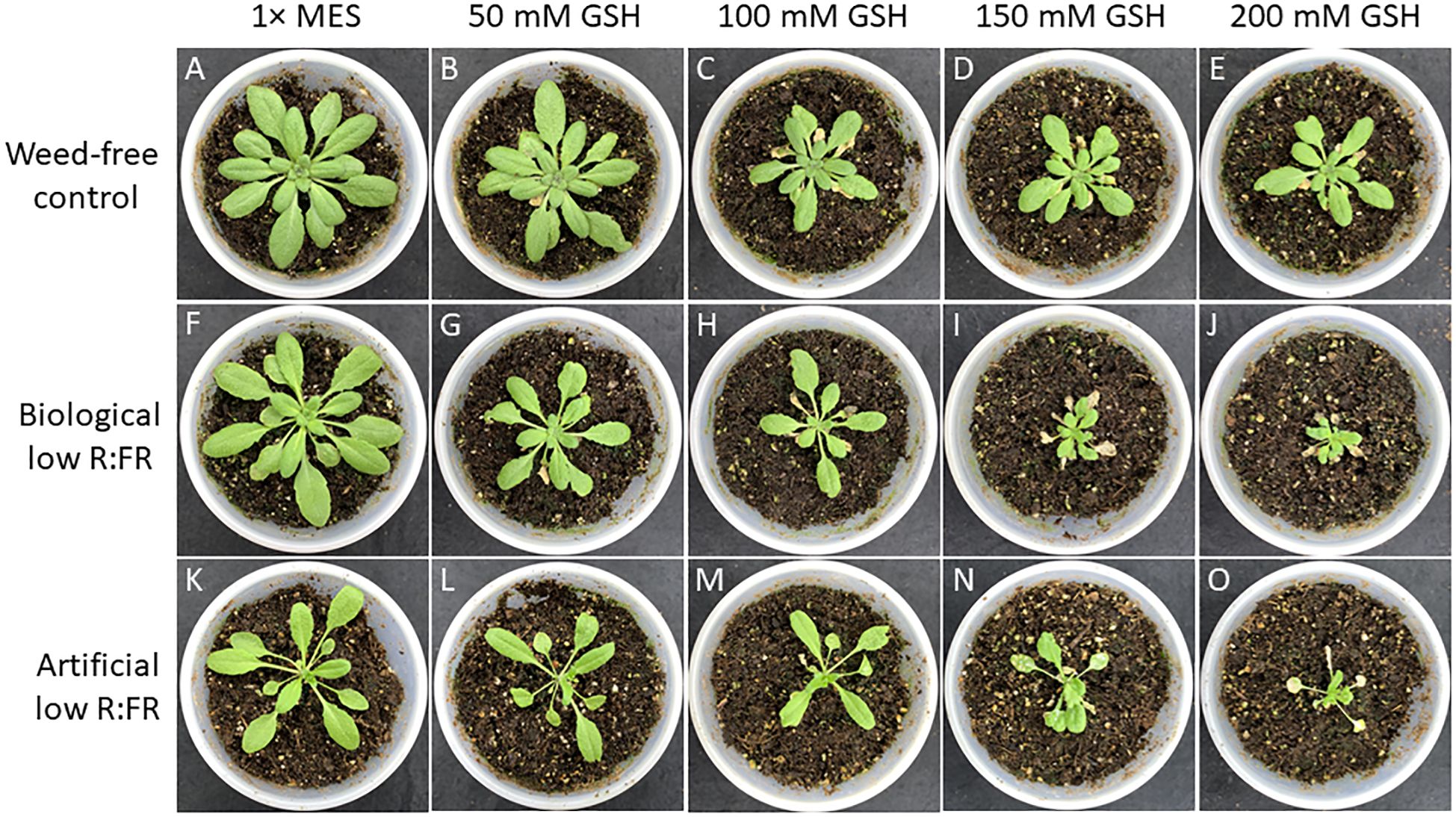
Figure 6. Responses of Arabidopsis plants to increasing concentrations of reduced glutathione (GSH) from 50 mM to 200 mM in the weed-free control (A–E), biological low R:FR light (F–J), and artificial low R:FR (K–O) light treatments. At the 3-week stage, six Arabidopsis plants from each treatment were taken from the growth chambers and all rosette leaves of each plant were sprayed with a one mL solution of each GSH concentration containing 0.01% Tween-20 as a wetting agent. Control plants for each treatment were sprayed with a one mL solution of 1x MES buffer (10 mM, pH 6.5) containing the same concentration of Tween-20. After spraying, the plants were kept outside the growth chambers to dry for one hour and transferred back to the respective treatments. Experiments were repeated three times with five plants per treatment and progression of cell death, and growth inhibition were monitored for one week. Note the dramatic differences in cell death and growth inhibition responses to increasing concentrations of GSH between the control and low R:FR light treatments particularly at 150 mM and 200 mM GSH.
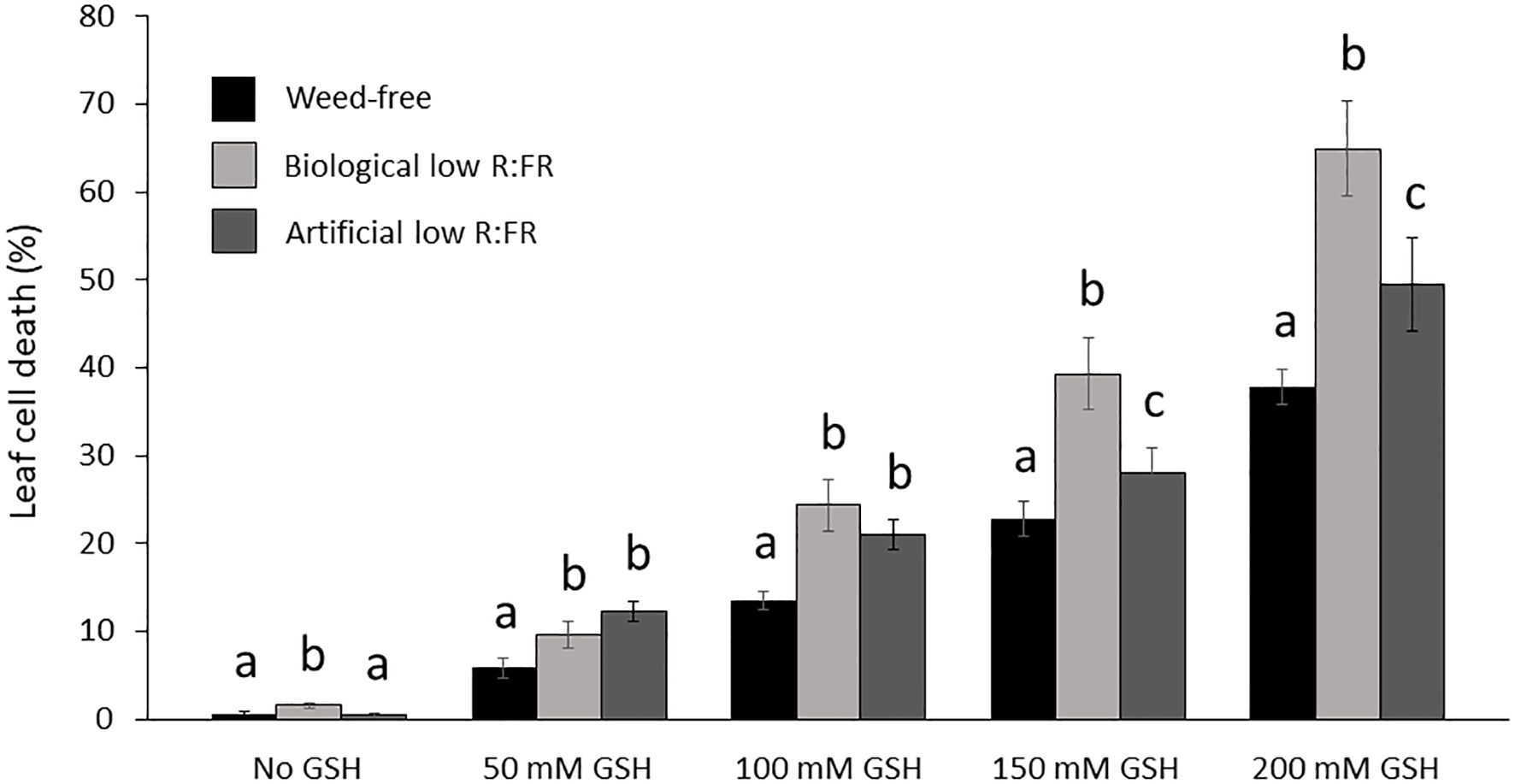
Figure 7. Increases in mean leaf cell death (%) by reduced glutathione (50, 100, 150, and 200 mM GSH) in the weed-free control, biological, and artificial low R:FR light treatments. Plants were treated as described in Figure 6. Note the significant increase in mean cell death (%) at each GSH concentration in the low R:FR light treatments relative to the respective weed-free treatment. Data represent means ± SEM for 18 plants per concentration per treatment. Means were separated using Tukey’s HSD test (P<0.05). Letters indicate statistical significance of differences across treatments. Images of plants were analyzed by the image analysis application Fiji (Schindelin et al., 2012). For each plant, dead leaf area was determined by subtracting green leaf area from total leaf area and expressed as percentage of leaf cell death (Supplementary Table 3).
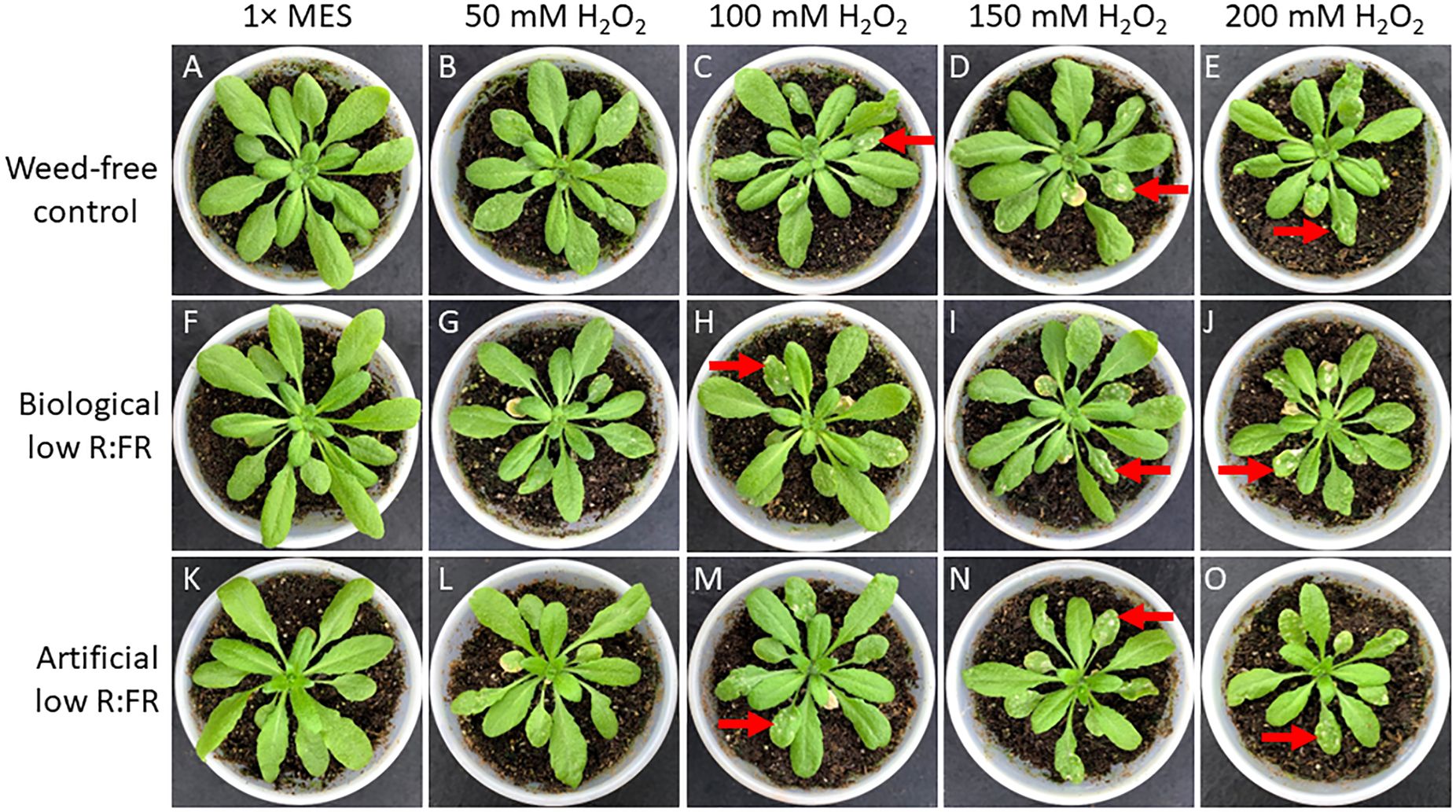
Figure 8. Responses of Arabidopsis plants to increasing concentrations of H2O2 from 50 mM to 200 mM in the weed-free control (A–E), biological low R:FR light (F–J), and artificial low R:FR (K–O) light treatments. At the 3-week stage, six Arabidopsis plants from each treatment were taken from the growth chambers and all rosette leaves of each plant were sprayed with a one mL solution of each H2O2 concentration containing 0.01% Tween-20 as a wetting agent. Control plants for each treatment were sprayed with a one mL solution of 1x MES buffer (10 mM, pH 6.5) containing the same concentration of Tween-20. After spraying, the plants were kept outside the growth chambers to dry for one hour and transferred back to the respective treatments. Experiments were repeated three times with five plants per treatment and progression of cell death was monitored for one week. Note that higher concentrations of H2O2, in contrast to GSH, did not result in a differential cell death in the low R:FR light treatments. Arrows indicate a few patches of cell death across all treatments at higher H2O2 concentrations.
A few early 1O2-responsive genes are induced under low R:FR light environments
RNA-sequencing was performed to compare differentially expressed genes (DEGs) in wild type Arabidopsis under 12-hour low R:FR light per day for one week and 1931 previously reported early 1O2-responsive genes in the mutant backgrounds and rose Bengal-treated wild type Arabidopsis (op den Camp et al., 2003; Gadjev et al., 2006; Alboresi et al., 2011; Mor et al., 2014). A summary of the number of base pairs sequenced and the number of reads mapped is presented in Table 1, which shows an average percentage of mapped reads of 81.7%. Results revealed that 57 of the 1931 1O2-responsive genes were differentially expressed in the low R:FR treatments (Table 2; Supplementary Table 4). Moreover, only six of the 57 1O2-responsive genes were commonly up-regulated in the biological and artificial low R:FR treatments, and four previous studies (Tables 2, 3). The minimal similarity between gene expression profiles in the previous four studies and the present study suggests that disparate modes of 1O2 generation in different genetic backgrounds may elicit unique 1O2 signatures. Further, growth conditions, tissue types and plant age may also affect 1O2 signatures. These results also suggest that the levels of 1O2 in the low R:FR treatments may not be high enough to induce a 1O2 signaling pathway similar to other Arabidopsis systems such as the flu mutant (op den Camp et al., 2003), Alternatively, a 1O2 signaling might have occurred at an earlier time point after the low R:FR light exposure.
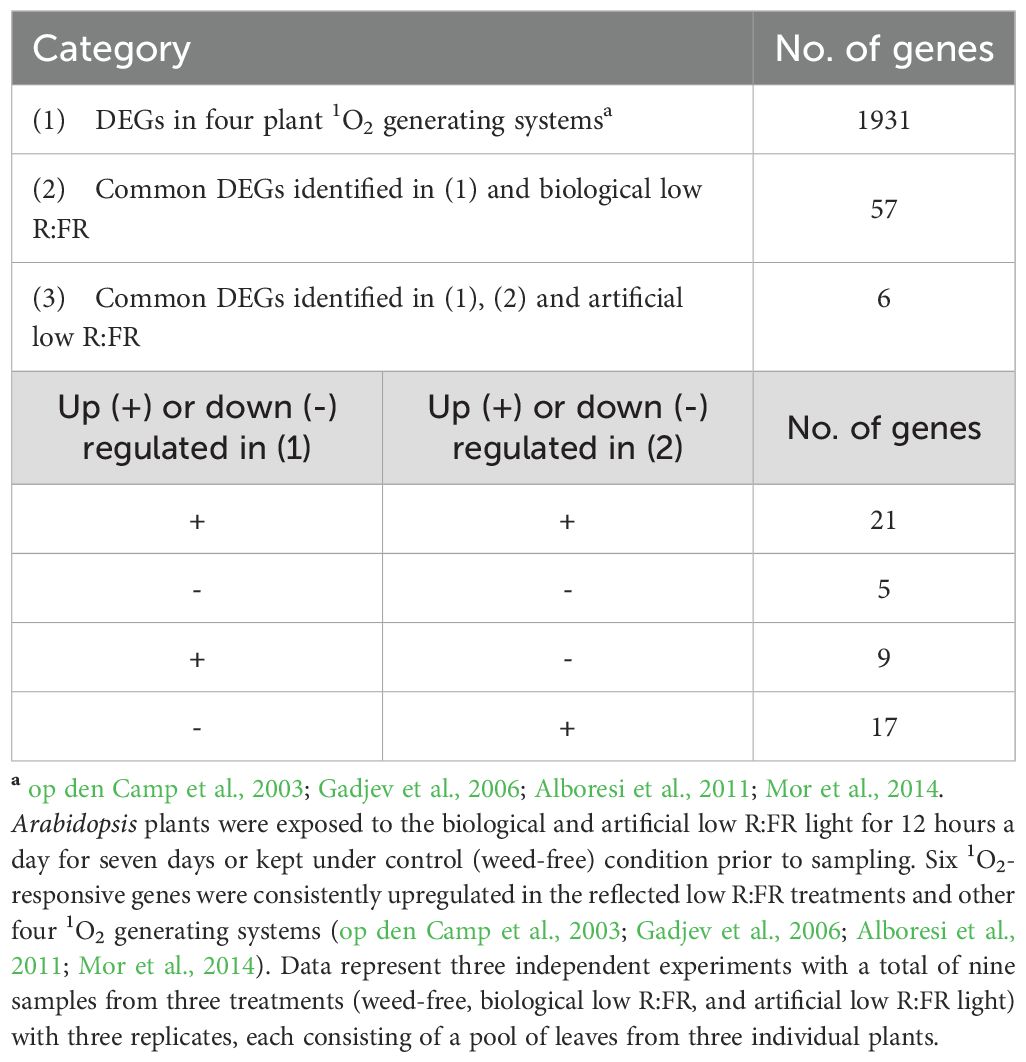
Table 2. Comparison of early 1O2-responsive DEGs in the reflected low R:FR treatments and four previously studied 1O2 generating systems.
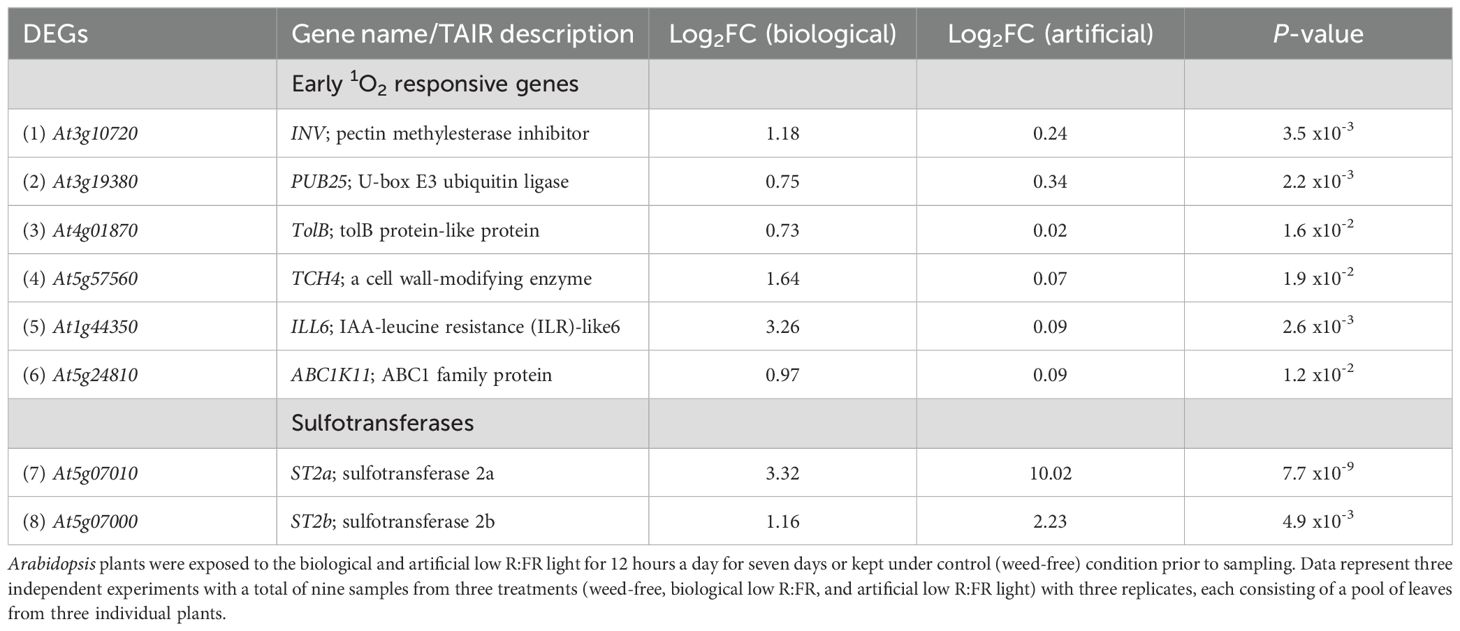
Table 3. Induction of early 1O2-responsive and sulfotransferase genes by the biological and artificial low R:FR light treatments.
Negative regulators of jasmonate accumulation are induced under low R:FR light environments
Increases in 1O2 levels in the low R:FR treatments were below a threshold to induce a cell death response (Figures 1, 2). The lack of a cell death response suggested induction of a 1O2 acclimation response, which is known to be induced by low levels of JA (Ramel et al., 2013b). Therefore, we sought to determine whether genes involved in regulation of bioactive JA levels were up-regulated by the low R:FR treatments. RNA-seq analysis revealed that the early 1O2-responsive gene ILL6 (op den Camp et al., 2003), which is involved in negative regulation of bioactive JA levels (Bhosale et al., 2013), was up-regulated (Table 3). We also found that not only the sulfotransferase ST2a but also the closely related ST2b was up-regulated in the biological and artificial low R:FR treatments (Table 3). Up-regulation of the sulfotransferase ST2a that catalyzes the conversion of 12-hydroxy JA (OH-JA) to JA sulfate (HSO4-JA) (Gidda et al., 2003) is a major mechanism diverting JA precursors from bioactive JA pools thus attenuating JA signaling under low R:FR light (Fernández-Milmanda et al., 2020). Since ST2b lacks sulfotransferase activity (Gidda et al., 2003; Fernández-Milmanda et al., 2020), its function under low R:FR treatments is not clear. The relationships between JA accumulation and 1O2-induced cell death (Przybyla et al., 2008), and between suppression of JA synthesis and photo-tolerance (Ramel et al., 2013a, b) have been well established. Therefore, the up-regulation of ILL6 and ST2a in the low R:FR treatments may represent a possible connection between the low R:FR light, attenuation of bioactive JA levels, and 1O2 acclimation response.
Induction of jasmonate repressors connects reflected far-red light cues from neighboring weeds with 1O2 acclimation response
We performed two independent RT-qPCR assays to investigate whether the reflected far-red light from neighboring weeds was indeed responsible for the induction of JA repressors and 1O2 responsive genes. We included in our experiments ST2a (a negative regulator of JA) and the closely related ST2b, as well as the early 1O2 responsive genes ILL6, which is also a negative regulator of JA, and PUB25, which encodes a U-box E3 ligase involved in plant organ growth. We found that all of these transcripts in Arabidopsis leaves were at higher levels in the biological low R:FR treatment relative to weed-free control (Figure 9) confirming the expression profile of the selected genes in RNA-seq results (Table 3). These results further suggested that hydrolysis of bioactive JA conjugates (JA-isoleucine) by the 1O2 responsive ILL6 and sulfation of JA metabolites by ST2a may act to promote both the shade avoidance response and the 1O2 acclimation response under resource-independent weed competition.
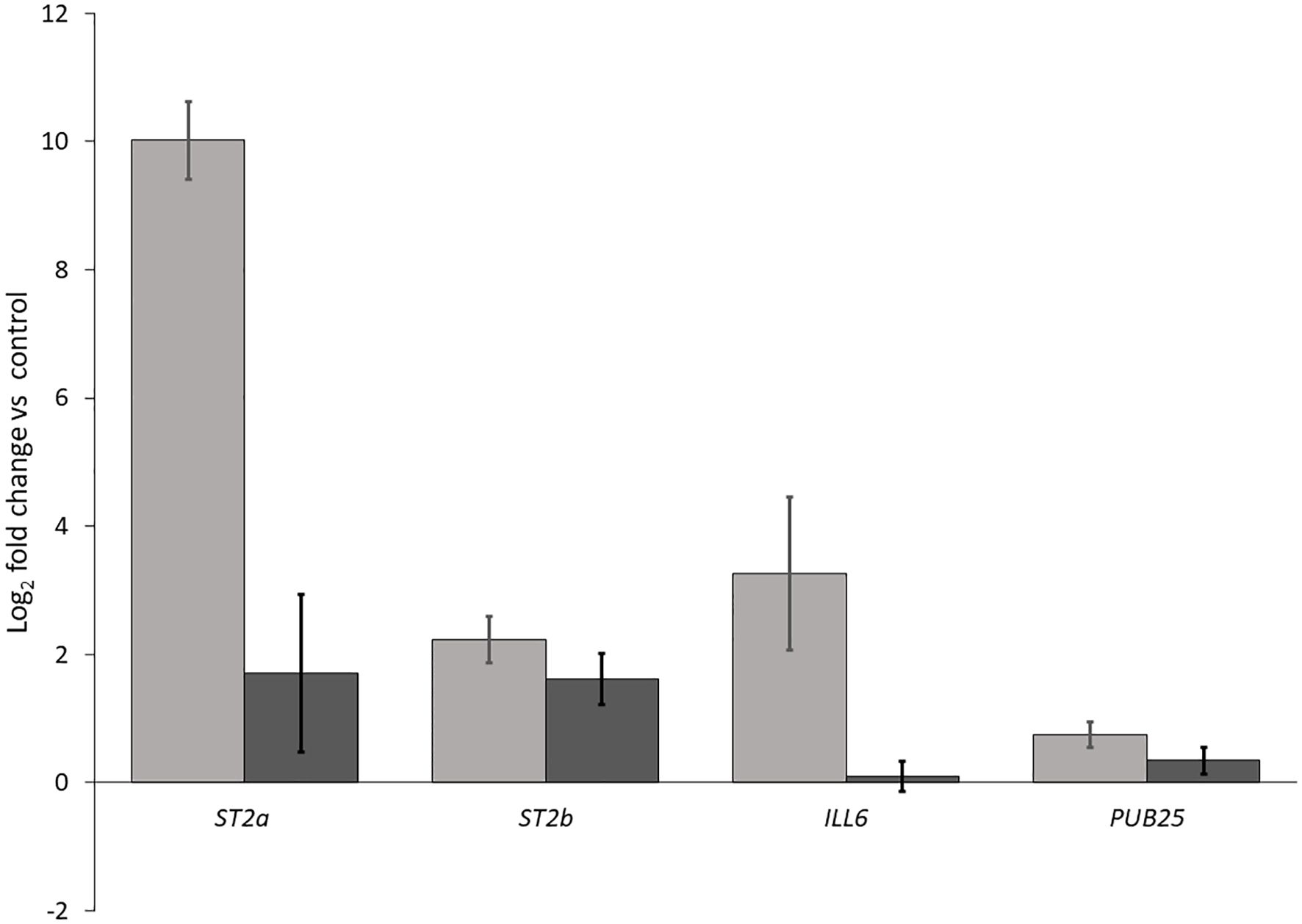
Figure 9. Increases in transcript levels of ST2a, ST2b, ILL6, and PUB25 in the biological low R:FR treatment relative to the weed-free control. Data represents fold change gene expression in the biological low R:FR light relative to the weed-free control treatment ± SEM for two independent experiments each consisting of leaves from four plants per treatment (P ≤ 0.05).
Discussion
Our results indicate that induction of SAR in Arabidopsis due to proximity to neighboring weeds coincides with increased leaf production of 1O2 (Figures 1, 2). Some of the elongation responses are modulated by the low R:FR light-mediated elevation of the volatile hormone ethylene in plants or in the canopy environment (Pierik et al., 2004a, b). Other reports have shown the suppression of terpenoids and other green leaf volatiles under low R:FR light (Kegge et al., 2013) and suggested the dominant effect of light signaling over volatile cues during weed-crop interactions (Pierik and de Wit, 2014). We are not, however, aware of any previous work describing 1O2 induction in plants by volatile compounds emanating from neighboring weeds. Moreover, a similar 1O2 increase along with induction of the SAR in the artificial low R:FR treatment (Figures 1, 2) suggests that the low R:FR light emanating from neighboring weeds may be the main signal responsible for increased 1O2 production.
Environmental stress factors elevate reactive oxygen species (ROS) levels in plants (Fryer et al., 2002; Hideg et al., 2002; Xiong et al., 2002; Apel and Hirt, 2004). Disparate ROS such as 1O2 and O2-/H2O2, which are elevated under disparate physiological conditions (Apel and Hirt, 2004), may also exhibit antagonistic interactions under certain conditions. This antagonistic interaction was exemplified in the flu mutant of Arabidopsis in which suppression of H2O2 by overexpression of a thylakoid-bound ascorbate peroxidase resulted in enhanced 1O2-mediated cell death and growth inhibition (Laloi et al., 2007). Also, the simultaneous induction of 1O2 and reduction of O2- in the etiolated phytochrome interacting factor 3 and phytochrome interacting factor quadruple mutants of Arabidopsis upon transfer to light has been attributed to the antagonistic effect of 1O2 on O2- and H2O2 (Chen et al., 2013). Given the established specificity of 1O2- and O2-/H2O2-dependent signaling in the flu mutant of Arabidopsis (Laloi et al., 2007), the 1O2 induction in the low R:FR light treatments may give rise to a distinct stress signaling response. A recent study found no increase in the intensity of SOSG fluorescence in Arabidopsis leaf discs following a two-hour exposure to supplemental FR light (Dmitrieva et al., 2021). This discrepancy may be due to small size of leaf discs (0.5 × 0.5 cm), lower concentration of SOSG (5 µM), and the short duration of exposure to FR light (two hours). Several lines of evidence indicate that a FR light pre-treatment of Arabidopsis results in Pchlide accumulation (Sperling et al., 1997; McCormac and Terry, 2002). Further, photo-excitation of Pchlide following transfer of Arabidopsis from FR light to white light resulted in rapid generation of 1O2 (Page et al., 2017). In the absence of FR light, Pchlide accumulation in the dark and photo-excitation following transfer to light is the mechanism behind rapid 1O2 generation in the flu mutant (op den Camp et al., 2003). Also, differential effects of several components of the Arabidopsis light signaling pathway (PHYTOCHROMES, PHYTOCHROME INTERACTING FACTORS, ELONGATED HYPOCOTYL 5, ELONGATED HYPOCOTYL 5 HOMOLOG, and CONSTITUTIVE PHOTOMORPHOGENIC 1) on 1O2 production and cell death following transfer to light corresponded closely to the Pchlide levels formed during seedling de-etiolation (Chen et al., 2013). Accumulation of Pchlide, however, does not appear to be the main cause of 1O2 generation in the wild-type Arabidopsis under the low R:FR light treatments as Pchlide levels were not significantly different from the control, nor did Pchlide levels increase following dark incubations for up to six hours (Figure 3A). Although the mechanism of 1O2 generation under our low R:FR treatments is not clear, decreased levels of Chlide a (Figure 3B), and therefore, increases in Pchlide to Chlide ratios (Figure 3F) may be interpreted as slower conversion rates of Pchlide to Chlide allowing for photo-excitation of a part of free Pchlide and thus 1O2 generation.
Carotenoids (car) including lutein, zeaxanthin, and β-carotene are potent physical and chemical quenchers of 1O2 (Trebst, 2003; Triantaphylidès and Havaux, 2009; Dogra and Kim, 2020). The lack of zeaxanthin and lutein in the lycopene-ε-cyclase and violaxanthin de-epoxidase double mutant (npq1 lut2) of Arabidopsis resulted in 1O2 generation under a combination of low temperature and high light stress (Alboresi et al., 2011). In addition, 1O2 generation in the npq1 lut2 mutant was accompanied by an increase in Chl a/Chl b and a decrease in Chl/Car reflecting the reduction of PSII/PSI and induction of carotenoid biosynthetic genes as 1O2-mediated acclimation responses (Alboresi et al., 2011). Under the low R:FR treatments, however, 1O2 induction did not coincide with such increases (data not shown), indicating differences in 1O2 acclimation responses between these 1O2 generating systems under two different stresses. In addition, the levels of lutein, β-carotene, violaxanthin, and neoxanthin were not affected by the biological low R:FR treatment, while total carotenoid levels were decreased in the biological and artificial low R:FR treatments (Figure 4). Decreases in chlorophylls and carotenoids are among universal responses to low R:FR light (Meng et al., 2019; Zhen and Bugbee, 2020; Kong and Nemali, 2021; Frosch and Mohr, 1980; Li and Kubota, 2009) and are regulated by phytochrome in a coordinated and co-localized manner (Meier et al., 2011; Rodríguez-Villalón et al., 2009; Welsch et al., 2000; Von Lintig et al., 1997). Although carotenoid deficiency may lead to 1O2 generation (Krieger-Liszkay, 2005), it is not clear whether decreases in chlorophylls (Figure 3) and total carotenoids (Figure 4) in the low R:FR treatments contribute to 1O2 generation.
It is known that ALA is the first committed compound in the synthesis of tetrapyrrole pigments (Tanaka and Tanaka, 2007; Beale, 1990). Earlier studies have shown that plants fed with exogenous ALA accumulate tetrapyrrole intermediates including protoporphyrin IX and Pchlide (Gough, 1972; Mascia, 1978). These intermediates are highly photodynamic and generate 1O2 as the major ROS in the light (Becerril and Duke, 1989; op den Camp et al., 2003; Shao et al., 2007; Rebeiz et al., 1988). Similarly, exogenous treatment of Arabidopsis leaves with ALA resulted in a cell death response, which was exaggerated under the low R:FR treatments (Figure 5). This differential response cannot be attributed to higher Pchlide level prior to ALA feeding as Pchlide levels in the low R:FR treatments were not significantly different from the control (Figure 3A). Given the direct relationship between the 1O2 levels and the extent of cell death in Arabidopsis leaves (op den Camp et al., 2003; Laloi et al., 2007), the differential cell death response under the low R:FR treatments (Figure 5) may be due to higher 1O2 levels prior to the ALA treatment. This may increase susceptibility to cell death following ALA feeding. Such increased susceptibility to cell death due to higher steady-state cellular levels of ROS has been shown in tobacco plants treated with the signaling molecules salicylic acid and nitric oxide (Amirsadeghi et al., 2006). This 1O2-mediated susceptibility to cell death by ALA may provide an explanation for the differential cell death responses of Arabidopsis plants under low R:FR light treated with ALA and transferred to the control (Figures 5I–L, Q–T). This, to some extent, is reminiscent of the increased sensitivity to oxidative stress and cell death due to a salicylic acid-dependent accumulation of O2- and H2O2 under short days in the fhy3 far1 double mutant of Arabidopsis lacking two light signaling components FAR-RED ELONGATED HYPOCOTYL3 (FHY3) and FAR-RED IMPAIRED RESPONSE1 (FAR1) (Ma et al., 2016). On the other hand, the cell death response in the AtIPS1 mutant of Arabidopsis lacking 1L-myo-inositol-1-phosphate synthase activity under long days did not result in increased ROS sensitivity (Meng et al., 2009). Therefore, we do not exclude the possibility that factors other than higher steady state 1O2 levels in the low R:FR treatments may contribute to increased susceptibility to cell death by ALA.
A recent study indicated that the complexity of leaf tissue including the cuticle layer shielding the upper and lower epidermis could limit SOSG penetration into Arabidopsis leaves (Prasad et al., 2018). Force infiltration with a syringe led to nonuniform penetration and distribution of SOSG in the leaf spaces while the resulting mechanical injury induced a strong SOSG fluorescence signal (Prasad et al., 2018). In contrast, pressure infiltration of Arabidopsis leaf pieces with a shut syringe resulted in uniform delivery of SOSG to the leaf tissue without 1O2 induction (Prasad et al., 2018). In our experiments, we used pressure infiltration with a 60 mL syringe allowing for complete infiltration of whole plant tissue while avoiding mechanical injury that could trigger 1O2 production. Given the complexity of leaf tissue and the uneven occurrence of SOSG fluorescence signal in the leaf samples (Figures 2B, C), we do not rule out the possibility of nonuniform generation of SOSG in the leaf cell compartments such as chloroplasts in the cells across the leaf tissue. This uneven occurrence of SOSG fluorescence signal is to some extent similar to that in Arabidopsis leaves following transfer from FR light to white light (Page et al., 2017). In addition, increases in mean fluorescence signal in the biological low R:FR (2.6×) and artificial low R:FR (3.0×) (Figure 2D) were similar to that reported one hour after the transfer of FR light-treated Arabidopsis to white light (Page et al., 2017).
High concentrations of H2O2 and GSH in the chloroplast can increase the degree of oxidation and reduction of quinone A (QA), respectively. While higher degrees of QA oxidation by H2O2 enhance the efficiency of electron transport in PS II, higher degrees of QA reduction by GSH have the opposite effect. Therefore, the H2O2 action can decrease and the GSH action can increase the extent of photo-oxidative stress and photoinhibition (Karpinska et al., 2000). Based on the opposing effects of H2O2 and GSH on the redox state of QA and efficiency of electron transport in PS II, it was hypothesized that treatment of the flu mutant of Arbabidopsis with H2O2 may reduce and treatment with GSH may increase 1O2 production and growth inhibition (Laloi et al., 2007). Given that our experimental low R:FR environments enhanced 1O2 production, we examined whether GSH treatment could enhance cell death. Although it was not clear to what extent GSH could reach the chloroplasts, our observations indicated that higher concentrations of GSH could result in differential cell death and growth inhibition responses in the low R:FR environments (Figures 6, 7). This was, however, not the case in the H2O2-treated plants. Increasing concentrations of H2O2 resulted in a few localized patches of cell death in all treatments (Figure 8). The development of these patches of cell death in all treatments may be due to inability of the H2O2 detoxifying systems to scavenge excess H2O2 at higher concentrations. While in some cases, FR light supplementation has enhanced photosynthetic efficiency of shorter wavelength light (Zhen and van Iersel, 2017), in other cases it has led to overproduction of ROS and damage to PSII and PSI (Tjus et al., 2001). Preferential excitation of PSI over PSII by FR light would enhance electron transfer from the PQ pools to PSI, which would be supplied by GSH. This would mitigate overreduction of PQ pools. Therefore, the observed increases in cell death by GHS in the low R:FR treatments (Figure 6; Supplementary Table 3) may be due to factors other than over-reduction of PQ pools. Alternatively, the damage to PSI and to a greater extent to PSII by FR light-induced ROS (Tjus et al., 2001) would affect the efficiency of electron transport, in which case, addition of excess electron to PQ pools via GSH may exacerbate ROS damage leading to increased cell death.
Our results suggest that under reflected low R:FR light, wild type Arabidopsis generates 1O2 via a mechanism different from those reported in four plant 1O2 generating systems (Table 2), allowing the identification of the transcriptomic signature of 1O2 signaling. Differential expression of only 3.2% of the previously reported early 1O2-responsive genes (57 out of 1931) suggests specificity of the 1O2 signaling under reflected low R:FR light stress (Table 2; Supplementary Table 4). This specificity is further highlighted by the up-regulation of only six previously reported 1O2-responsive genes in the biological and artificial low R:FR light treatments (Table 3). Among these, ILL6, which is also known to be rapidly induced within two hours following 1O2 release (op den Camp et al., 2003), encodes an amidohydrolase that catalyzes the cleavage of isoleucine (Ile) from JA-Ile thus making JA biologically inactive (Bhosale et al., 2013). Recently, the up-regulation of ILL6 was reported under low R:FR light as part of a mechanism that attenuates the JA signaling pathway (Fernández-Milmanda et al., 2020). Our results suggest that the up-regulation of ILL6 in the reflected low R:FR treatments may be an 1O2 response, which raises the intriguing possibility that attenuation of the JA signaling pathway may be partly mediated via a 1O2 signaling pathway. This attenuation is a major process that generates a trade-off between defense and growth in favor of the SAR under plant competition (Fernández-Milmanda et al., 2020). It has been demonstrated that attenuation of the JA signaling pathway under a 10-hour low R:FR light treatment occurs via the up-regulation of the sulfotransferase ST2a. The ST2a encoded protein exclusively catalyzes the conversion of JA to sulfated JA (HSO4-JA), while the closely related ST2b fails to respond to the above FR light treatment and is dispensable for the accumulation of HSO4-JA (Fernández-Milmanda et al., 2020). We found that both ST2a and ST2b were up-regulated under a 12-hour reflected low R:FR light treatment for seven days (Table 3; Figure 9) and cannot exclude the possibility that ST2a and ST2b may be 1O2-responsive. The discrepancy in the ST2b response may be due to differences in experimental FR light conditions.
Upregulation of ST2a and ST2b have been shown in a transgenic Arabidopsis line with suppressed mitochondrial serine acetyltransferase (SAT3) level (Haas et al., 2008). It is noteworthy that upregulation of ST2a and ST2b in the SAT3 line coincided with the downregulation of disease or pathogen response genes whose responses are coordinated by methyl jasmonate (Haas et al., 2008). Although the upregulation of ST2b (Table 3; Figure 9) suggests that it may play a role under certain low R:FR light conditions, it is not feasible to predict the function of a plant sulfotransferase solely based on its sequence similarity to sulfotransferases with known functions (Hirschmann et al., 2014). So far, there is no evidence for the involvement of ST2b in the JA pathway. Initial screens for ST2b substrates found no activity against hydroxyjasmonate compounds (Gidda et al., 2003). Further, a recent study found no evidence for the JA sulfation by ST2b under low R:FR light (Fernández-Milmanda et al., 2020).
An important question regarding 1O2 induction under reflected low R:FR light is whether 1O2 serves a physiological function under plant competition. Under this condition, generation of high levels of 1O2 can place the rapidly growing plants at a disadvantage as high levels of 1O2 can steer the cell fate towards cell death (Danon et al., 2005; Kim et al., 2012). We envision a possibility that the low R:FR-mediated attenuation of JA accumulation by ST2a and ILL6 may result in an acclimation response to 1O2. This acclimation response, in contrast to a cell death response (Ramel et al., 2013b), may have a growth inhibitory effect similar to that in the flu mutant (Laloi et al., 2006, 2007). This growth inhibitory effect of 1O2 may be exploited by plants under competition to modulate elongation growth to avoid excess elongation in anticipation of oncoming competition. The growth inhibitory effect of 1O2 may also be exerted via the action of other 1O2-responsive genes. In this regard, overexpression of the early 1O2-responsive PUB25, which encodes a U-box E3 ubiquitin ligase (Table 3), is known to cause growth inhibition in Arabidopsis (Li et al., 2021). Interestingly, PUB25 was among the 1O2-responsive genes that were upregulated under the biological and artificial low R:FR treatments (Table 3), and may play a role in modulation of growth by 1O2.
In closing, our results indicate that 1O2 production is an early response to reflected FR light from neighboring weeds. Our biological low R:FR light treatment allows the investigation of 1O2 acclimation responses under resource-independent competition. Under this condition, 1O2 itself may contribute to the acclimation response by up-regulating genes such as ILL6, which acts to reduce the pool of bioactive JAs. Further, the demonstration of up-regulation of ST2b under artificial and biological low R:FR light environments allows further investigations into the role of ST2b in plant competition.
Data availability statement
The datasets presented in this study can be found in online repositories. The names of the repository/repositories and accession number(s) can be found in the article/Supplementary Material.
Author contributions
NB and SA carried out the experimental work and wrote the manuscript. NB performed data analysis. CS contributed to the experimental design and revised the manuscript. All authors contributed to the article and approved the submitted version.
Funding
The author(s) declare financial support was received for the research, authorship, and/or publication of this article. Financial support for this work was partially provided by the Natural Sciences and Engineering Research Council of Canada, File RGPIN-2022-03454 (CJS). This research was undertaken thanks in part to the University of Guelph’s Food from Thought research program, funded by the Canada First Research Excellence Fund.
Acknowledgments
The authors would like to thank Harvest Genomics Inc. for technical guidance and analysis of RNA sequencing data. We extend our sincere thanks to Dr. Michaela Strüder-Kypke (Molecular and Cellular Imaging Facility, University of Guelph) for providing expertise and assistance on fluorescence microscopy. We thank Dr. Tariq Akhtar and Kevin Rea for their technical expertise and assistance on HPLC. We also thank Jing Zhang for her expertise and assistance on RT-qPCR.
Conflict of interest
The authors declare that the research was conducted in the absence of any commercial or financial relationships that could be construed as a potential conflict of interest.
Publisher’s note
All claims expressed in this article are solely those of the authors and do not necessarily represent those of their affiliated organizations, or those of the publisher, the editors and the reviewers. Any product that may be evaluated in this article, or claim that may be made by its manufacturer, is not guaranteed or endorsed by the publisher.
Supplementary material
The Supplementary Material for this article can be found online at: https://www.frontiersin.org/articles/10.3389/fpls.2024.964476/full#supplementary-material
Supplementary Table 1 | Incoming and reflected light spectral composition in the weed-free control, biological low R:FR and artificial low R:FR treatments.
Supplementary Table 2 | Fluorescence intensity in leaf images of SOSG-treated Arabidopsis plants in the weed-free control, biological low R:FR and artificial low R:FR treatments.
Supplementary Table 3 | Image analysis of leaf cell death in GSH-treated Arabidopsis plants in the weed-free control, biological low R:FR and artificial low R:FR treatments.
Supplementary Table 4 | Expression profiles of 1O2-responsive genes in the biological and artificial low R:FR treatments.
References
Alboresi, A., Dall'osto, L., Aprile, A., Carillo, P., Roncaglia, E., Cattivelli, L., et al. (2011). Reactive oxygen species and transcript analysis upon excess light treatment in wild-type Arabidopsis thaliana vs a photosensitive mutant lacking zeaxanthin and lutein. BMC Plant Biol. 11, 62. doi: 10.1186/1471-2229-11-62
Ambastha, V., Chauhan, G., Tiwari, B. S., Tripathy, B. C. (2020). Execution of programmed cell death by singlet oxygen generated inside the chloroplasts of Arabidopsis thaliana. Protoplasma 257, 841–851. doi: 10.1007/s00709-019-01467-y
Amirsadeghi, S., Robson, C. A., McDonald, A. E., Vanlerberghe, G. C. (2006). Changes in plant mitochondrial electron transport alter cellular levels of reactive oxygen species and susceptibility to cell death signaling molecules. Plant Cell Physiol. 47, 1509–1519. doi: 10.1093/pcp/pcl016
Apel, K., Hirt, H. (2004). Reactive oxygen species: metabolism, oxidative stress, and signal transduction. Annu. Rev. Plant Biol. 55, 373–399. doi: 10.1146/annurev.arplant.55.031903.141701
Ballaré, C. L. (2014). Light regulation of plant defense. Annu. Rev. Plant Biol. 65, 335–363. doi: 10.1146/annurev-arplant-050213-040145
Beale, S. I. (1990). Biosynthesis of the tetrapyrrole pigment precursor, delta-aminolevulinic acid, from glutamate. Plant Physiol. 93, 1273–1279. doi: 10.1104/pp.93.4.1273
Becerril, J. M., Duke, S. O. (1989). Protoporphyrin IX content correlates with activity of photobleaching herbicides. Plant Physiol. 90, 1175–1181. doi: 10.1104/pp.90.3.1175
Bhosale, R., Jewell, J. B., Hollunder, J., Koo, A. J., Vuylsteke, M., Michoel, T., et al. (2013). Predicting gene function from uncontrolled expression variation among individual wild-type Arabidopsis plants. Plant Cell 25, 2865–2877. doi: 10.1105/tpc.113.112268
Brouers, M., Michel-Wolwertz, M. R. (1983). Estimation of protochlorophyll(ide) contents in plant extracts; re-evaluation of the molar absorption coefficient of protochlorophyll(ide). Photosynth. Res. 4, 265–270. doi: 10.1007/BF00052130
Chen, T., Fluhr, R. (2018). Singlet Oxygen plays an essential role in the root's response to osmotic stress. Plant Physiol. 177, 1717–1727. doi: 10.1104/pp.18.00634
Chen, D., Xu, G., Tang, W., Jing, Y., Ji, Q., Fei, Z., et al. (2013). Antagonistic basic helix-loop-helix/bZIP transcription factors form transcriptional modules that integrate light and reactive oxygen species signaling in Arabidopsis. Plant Cell 25, 1657–1673. doi: 10.1105/tpc.112.104869
Dall'Osto, L., Cazzaniga, S., Havaux, M., Bassi, R. (2010). Enhanced photoprotection by protein-bound vs free xanthophyll pools: a comparative analysis of chlorophyll b and xanthophyll biosynthesis mutants. Mol. Plant 3, 576–593. doi: 10.1093/mp/ssp117
Danon, A., Miersch, O., Felix, G., op den Camp, R. G., Apel, K. (2005). Concurrent activation of cell death-regulating signaling pathways by singlet oxygen in Arabidopsis thaliana. Plant J. 41, 68–80. doi: 10.1111/j.1365-313X.2004.02276.x
de Wit, M., Galvão, V. C., Fankhauser, C. (2016). Light-mediated hormonal regulation of plant growth and development. Annu. Rev. Plant Biol. 67, 513–537. doi: 10.1146/annurev-arplant-043015-112252
Dmitrieva, V. A., Domashkina, V. V., Ivanova, A. N., Sukhov, V. S., Tyutereva, E. V., Voitsekhovskaja, O. V. (2021). Regulation of plasmodesmata in Arabidopsis leaves: ATP, NADPH and chlorophyll b levels matter. J. Exp. Bot. 72, 5534–5552. doi: 10.1093/jxb/erab205
Dmitrieva, V. A., Tyutereva, E. V., Voitsekhovskaja, O. V. (2020). Singlet oxygen in plants: generation, detection, and signaling roles. Int. J. Mol. Sci. 21, 3237. doi: 10.3390/ijms21093237
Dogra, V., Kim, C. (2020). Singlet oxygen metabolism: from genesis to signaling. Front. Plant Sci. 10. doi: 10.3389/fpls.2019.01640
Fernández-Milmanda, G. L., Ballaré, C. L. (2021). Shade avoidance: Expanding the color and hormone palette. Trends Plant Sci. 26, 509–523. doi: 10.1016/j.tplants.2020.12.006
Fernández-Milmanda, G. L., Crocco, C. D., Reichelt, M., Mazza, C. A., Köllner, T. G., Zhang, T., et al. (2020). A light-dependent molecular link between competition cues and defense responses in plants. Nat. Plants 6, 223–230. doi: 10.1038/s41477-020-0604-8
Fischer, B. B., Krieger-Liszkay, A., Hideg, E., Snyrychová, I., Wiesendanger, M., Eggen, R. I. (2007). Role of singlet oxygen in chloroplast to nucleus retrograde signaling in Chlamydomonas reinhardtii. FEBS Lett. 581, 5555–5560. doi: 10.1016/j.febslet.2007.11.003
Frosch, S., Mohr, H. (1980). Analysis of light-controlled accumulation of carotenoids in mustard (Sinapis alba L.) seedlings. Planta 148, 279–286. doi: 10.1007/BF00380039
Fryer, M. J., Oxborough, K., Mullineaux, P. M., Baker, N. R. (2002). Imaging of photo-oxidative stress responses in leaves. J. Exp. Bot. 53, 1249–1254. doi: 10.1093/jexbot/53.372.1249
Gadjev, I., Vanderauwera, S., Gechev, T. S., Laloi, C., Minkov, I. N., Shulaev, V., et al. (2006). Transcriptomic footprints disclose specificity of reactive oxygen species signaling in Arabidopsis. Plant Physiol. 141, 436–445. doi: 10.1104/pp.106.078717
Gidda, S. K., Miersch, O., Levitin, A., Schmidt, J., Wasternack, C., Varin, L. (2003). Biochemical and molecular characterization of a hydroxyjasmonate sulfotransferase from Arabidopsis thaliana. J. Biol. Chem. 278, 17895–17900. doi: 10.1074/jbc.M211943200
Gough, S. (1972). Defective synthesis of porphyrins in barley plastids caused by mutation in nuclear genes. Biochim. Biophys. Acta 286, 36–54. doi: 10.1016/0304-4165(72)90086-4
Haas, F. H., Heeg, C., Queiroz, R., Bauer, A., Wirtz, M., Hell, R. (2008). Mitochondrial serine acetyltransferase functions as a pacemaker of cysteine synthesis in plant cells. Plant Physiol. 148, 1055–1067. doi: 10.1104/pp.108.125237
Hatz, S., Lambert, J. D. C., Ogilby, P. R. (2007). Measuring the lifetime of singlet oxygen in a single cell: addressing the issue of cell viability. Photochem. Photobiol. Sci. 6, 1106–1116. doi: 10.1039/b707313e
Havaux, M., Dall’Osto, L., Bassi, R. (2007). Zeaxanthin has enhanced antioxidant capacity with respect to all other xanthophylls in Arabidopsis leaves and functions independent of binding to PSII antennae. Plant Physiol. 145, 1506–1520. doi: 10.1104/pp.107.108480
Havaux, M., Tardy, F. (1997). Thermostability and photostability of photosystem II in leaves of the Chlorina-f2 barley mutant deficient in light-harvesting chlorophyll a/b protein complexes. Plant Physiol. 113, 913–923. doi: 10.1104/pp.113.3.913
Hideg, E., Barta, C., Kálai, T., Vass, I., Hideg, K., Asada, K. (2002). Detection of singlet oxygen and superoxide with fluorescent sensors in leaves under stress by photoinhibition or UV radiation. Plant Cell Physiol. 43, 1154–1164. doi: 10.1093/pcp/pcf145
Hirschmann, F., Krause, F., Papenbrock, J. (2014). The multi-protein family of sulfotransferases in plants: composition, occurrence, substrate specificity, and functions. Front. Plant Sci. 5. doi: 10.3389/fpls.2014.00556
Karpinska, B., Wingsle, G., Karpinski, S. (2000). Antagonistic effects of hydrogen peroxide and glutathione on acclimation to excess excitation energy in Arabidopsis. IUBMB Life 50, 21–26. doi: 10.1080/15216540050176548
Kegge, W., Weldegergis, B. T., Soler, R., Eijk, M. V., Dicke, M., Voesenek, L. A. C. J., et al. (2013). Canopy light cues affect emission of constitutive and methyl jasmonate-induced volatile organic compounds in Arabidopsis thaliana. New Phytol. 200, 861–874. doi: 10.1111/nph.12407
Kim, E. H., Li, X. P., Razeghifard, R., Anderson, J. M., Niyogi, K. K., Pogson, B. J., et al. (2009). The multiple roles of light-harvesting chlorophyll a/b-protein complexes define structure and optimize function of Arabidopsis chloroplasts: a study using two chlorophyll b-less mutants. Biochim. Biophys. Acta 1787, 973–984. doi: 10.1016/j.bbabio.2009.04.009
Kim, C., Meskauskiene, R., Zhang, S., Lee, K. P., Ashok, M. L., Blajecka, K., et al. (2012). Chloroplasts of Arabidopsis are the source and a primary target of a plant-specific programmed cell death signaling pathway. Plant Cell 24, 3026–3039. doi: 10.1105/tpc.112.100479
Kong, Y., Nemali, K. (2021). Blue and far-red light affect area and number of individual leaves to influence vegetative growth and pigment synthesis in lettuce. Front. Plant Sci. 12. doi: 10.3389/fpls.2021.667407
Krieger-Liszkay, A. (2005). Singlet oxygen production in photosynthesis. J. Exp. Bot. 56, 337–346. doi: 10.1093/jxb/erh237
Laloi, C., Havaux, M. (2015). Key players of singlet oxygen-induced cell death in plants. Front. Plant Sci. 6. doi: 10.3389/fpls.2015.00039
Laloi, C., Przybyla, D., Apel, K. (2006). A genetic approach towards elucidating the biological activity of different reactive oxygen species in Arabidopsis thaliana. J. Exp. Bot. 57, 1719–1724. doi: 10.1093/jxb/erj183
Laloi, C., Stachowiak, M., Pers-Kamczyc, E., Warzych, E., Murgia, I., Apel, K. (2007). Cross-talk between singlet oxygen- and hydrogen peroxide-dependent signaling of stress responses in Arabidopsis thaliana. Proc. Natl. Acad. Sci. U.S.A. 104, 672–677. doi: 10.1073/pnas.0609063103
Ledford, H. K., Chin, B. L., Niyogi, K. K. (2007). Acclimation to singlet oxygen stress in Chlamydomonas reinhardtii. Eukaryot. Cell 6, 919–930. doi: 10.1128/EC.00207-06
Lee, K. P., Kim, C., Lee, D. W., Apel, K. (2003). TIGRINA d, required for regulating the biosynthesis of tetrapyrroles in barley, is an ortholog of the FLU gene of Arabidopsis thaliana. FEBS Lett. 553, 119–124. doi: 10.1016/S0014-5793(03)00983-9
Leverenz, J. W., Öquist, G., Wingsle, G. (1992). Photosynthesis and photoinhibition in leaves of chlorophyll b-less barley in relation to absorbed light. Physiol. Plant 85, 495–502. doi: 10.1111/j.1399-3054.1992.tb05817.x
Li, Q., Kubota, C. (2009). Effects of supplemental light quality on growth and phytochemicals of baby leaf lettuce. Environ. Exp. Bot. 67, 59–64. doi: 10.1016/j.envexpbot.2009.06.011
Li, J., Zhang, Y., Gao, Z., Xu, X., Wang, Y., Lin, Y., et al. (2021). Plant U-box E3 ligases PUB25 and PUB26 control organ growth in Arabidopsis. New Phytol. 229, 403–413. doi: 10.1111/nph.16885
Lichtenthaler, H. K., Wellburn, A. R. (1983). Determinations of total carotenoids and chlorophylls a and b of leaf extracts in different solvents. Biochem. Soc. Transact. 11, 591–592. doi: 10.1042/bst0110591
Livak, K. J., Schmittgen, T. D. (2001). Analysis of relative gene expression data using real-time quantitative PCR and the 2(-Delta Delta C(T)) method. Methods 25, 402–408. doi: 10.1006/meth.2001.1262
Ma, L., Tian, T., Lin, R., Deng, X. W., Wang, H., Li, G. (2016). Arabidopsis FHY3 and FAR1 regulate light-induced myo-Inositol biosynthesis and oxidative stress responses by transcriptional activation of MIPS1. Mol. Plant 9, 541–557. doi: 10.1016/j.molp.2015.12.013
Mascia, P. (1978). An analysis of precursors accumulated by several chlorophyll biosynthetic mutants of maize. Mol. Gen. Genet. 161, 237–244. doi: 10.1007/BF00330996
McCormac, A. C., Terry, M. J. (2002). Loss of nuclear gene expression during the phytochrome A-mediated far-red block of greening response. Plant Physiol. 130, 402–414. doi: 10.1104/pp.003806
Meier, S., Tzfadia, O., Vallabhaneni, R., Gehring, C., Wurtzel, E. T. (2011). A transcriptional analysis of carotenoid, chlorophyll and plastidial isoprenoid biosynthesis genes during development and osmotic stress responses in Arabidopsis thaliana. BMC Syst. Biol. 5, 77. doi: 10.1186/1752-0509-5-77
Meng, Q., Kelly, N., Runkle, E. S. (2019). Substituting green or far-red radiation for blue radiation induces shade avoidance and promotes growth in lettuce and kale. Environ. Exp. Bot. 162, 383–391. doi: 10.1016/j.envexpbot.2019.03.016
Meng, P. H., Raynaud, C., Tcherkez, G., Blanchet, S., Massoud, K., Domenichini, S., et al. (2009). Crosstalks between myo-inositol metabolism, programmed cell death and basal immunity in Arabidopsis. PloS One 4, e7364. doi: 10.1371/journal.pone.0007364
Meskauskiene, R., Nater, M., Goslings, D., Kessler, F., op den Camp, R., Apel, K. (2001). FLU: a negative regulator of chlorophyll biosynthesis in Arabidopsis thaliana. Proc. Natl. Acad. Sci. U.S.A. 98, 12826–12831. doi: 10.1073/pnas.221252798
Mor, A., Koh, E., Weiner, L., Rosenwasser, S., Sibony-Benyamini, H., Fluhr, R. (2014). Singlet oxygen signatures are detected independent of light or chloroplasts in response to multiple stresses. Plant Physiol. 165, 249–261. doi: 10.1104/pp.114.236380
Ogilby, P. R. (2010). Singlet oxygen: there is indeed something new under the sun. Chem. Soc. Rev. 39, 3181–3209. doi: 10.1039/b926014p
op den Camp, R. G., Przybyla, D., Ochsenbein, C., Laloi, C., Kim, C., Danon, A., et al. (2003). Rapid induction of distinct stress responses after the release of singlet oxygen in Arabidopsis. Plant Cell 15, 2320–2332. doi: 10.1105/tpc.014662
Page, M. T., McCormac, A. C., Smith, A. G., Terry, M. J. (2017). Singlet oxygen initiates a plastid signal controlling photosynthetic gene expression. New Phytol. 213, 1168–1180. doi: 10.1111/nph.14223
Pierik, R., Cuppens, M. L., Voesenek, L. A., Visser, E. J. (2004a). Interactions between ethylene and gibberellins in phytochrome-mediated shade avoidance responses in tobacco. Plant Physiol. 136, 2928–2936. doi: 10.1104/pp.104.045120
Pierik, R., de Wit, M. (2014). Shade avoidance: phytochrome signalling and other aboveground neighbour detection cues. J. Exp. Bot. 65, 2815–2824. doi: 10.1093/jxb/ert389
Pierik, R., Whitelam, G. C., Voesenek, L. A., de Kroon, H., Visser, E. J. (2004b). Canopy studies on ethylene-insensitive tobacco identify ethylene as a novel element in blue light and plant-plant signaling. Plant J. 38, 310–319. doi: 10.1111/j.1365-313X.2004.02044.x
Prasad, A., Sedlářová, M., Pospíšil, P. (2018). Singlet oxygen imaging using fluorescent probe Singlet Oxygen Sensor Green in photosynthetic organisms. Sci. Rep. 8, 13685. doi: 10.1038/s41598-018-31638-5
Przybyla, D., Göbel, C., Imboden, A., Hamberg, M., Feussner, I., Apel, K. (2008). Enzymatic, but not non-enzymatic, 1O2-mediated peroxidation of polyunsaturated fatty acids forms part of the EXECUTER1-dependent stress response program in the flu mutant of Arabidopsis thaliana. Plant J. 54, 236–248. doi: 10.1111/j.1365-313X.2008.03409.x
Ramel, F., Ksas, B., Akkari, E., Mialoundama, A. S., Monnet, F., Krieger-Liszkay, A., et al. (2013a). Light-induced acclimation of the Arabidopsis chlorina1 mutant to singlet oxygen. Plant Cell 25, 1445–1462. doi: 10.1105/tpc.113.109827
Ramel, F., Ksas, B., Havaux, M. (2013b). Jasmonate: A decision maker between cell death and acclimation in the response of plants to singlet oxygen. Plant Signal. Behav. 8, e26655. doi: 10.4161/psb.26655
Rebeiz, C. A., Montazerzouhoor, A., Mayasich, J. M., Tripathy, B. C., Wu, S. M., Rebeiz, C. C., et al. (1988). Photodynamic herbicides. Recent developments and molecular basis of selectivity. Crit. Rev. Plant Sci. 6, 385–436. doi: 10.1080/07352688809382256
Rodríguez-Villalón, A., Gas, E., Rodríguez-Concepción, M. (2009). Phytoene synthase activity controls the biosynthesis of carotenoids and the supply of their metabolic precursors in dark-grown Arabidopsis seedlings. Plant J. 60, 424–435. doi: 10.1111/j.1365-313X.2009.03966.x
Schindelin, J., Arganda-Carreras, I., Frise, E., Kaynig, V., Longair, M., Pietzsch, T., et al. (2012). Fiji: an open-source platform for biological-image analysis. Nat. Methods 9, 676–682. doi: 10.1038/nmeth.2019
Shao, N., Krieger-Liszkay, A., Schroda, M., Beck, C. F. (2007). A reporter system for the individual detection of hydrogen peroxide and singlet oxygen: its use for the assay of reactive oxygen species produced in vivo. Plant J. 50, 475–487. doi: 10.1111/j.1365-313X.2007.03065.x
Skovsen, E., Snyder, J. W., Lambert, J. D. C., Ogilby, P. R. (2005). Lifetime and diffusion of singlet oxygen in a cell. J. Phys. Chem. B. 109, 8570–8573. doi: 10.1021/jp051163i
Sperling, U., van Cleve, B., Frick, G., Apel, K., Armstrong, G. A. (1997). Overexpression of light-dependent PORA or PORB in plants depleted of endogenous POR by far-red light enhances seedlings survival in white light and protects against photooxidative damage. Plant J. 12, 649–658. doi: 10.1046/j.1365-313X.1997.00649.x
Tanaka, R., Tanaka, A. (2007). Tetrapyrrole biosynthesis in higher plants. Annu. Rev. Plant Biol. 58, 321–346. doi: 10.1146/annurev.arplant.57.032905.105448
Tjus, S. E., Scheller, H. V., Andersson, B., Møller, B. L. (2001). Active oxygen produced during selective excitation of photosystem I is damaging not only to photosystem I, but also to photosystem II. Plant Physiol. 125, 2007–2015. doi: 10.1104/pp.125.4.2007
Tollenaar, M. (1989). Response of dry matter accumulation in maize to temperature: I. Dry matter partitioning. Crop Sci. 29, 1239–1246. doi: 10.2135/cropsci1989.0011183X002900050030x
Trebst, A. (2003). Function of beta-carotene and tocopherol in photosystem II. Z Naturforsch. C J. Biosci. 58, 609–620. doi: 10.1515/znc-2003-9-1001
Triantaphylidès, C., Havaux, M. (2009). Singlet oxygen in plants: production, detoxification and signaling. Trends Plant Sci. 14, 219–228. doi: 10.1016/j.tplants.2009.01.008
Von Lintig, J., Welsch, R., Bonk, M., Giuliano, G., Batschauer, A., Kleinig, H. (1997). Light-dependent regulation of carotenoid biosynthesis occurs at the level of phytoene synthase expression and is mediated by phytochrome in Sinapis alba and Arabidopsis thaliana seedlings. Plant J. 12, 625–634. doi: 10.1046/j.1365-313X.1997.00625.x
Wagner, D., Przybyla, D., Op den Camp, R., Kim, C., Landgraf, F., Lee, K. P., et al. (2004). The genetic basis of singlet oxygen-induced stress responses of Arabidopsis thaliana. Science 306, 1183–1185. doi: 10.1126/science.1103178
Waszczak, C., Carmody, M., Kangasjärvi, J. (2018). Reactive oxygen species in plant signaling. Annu. Rev. Plant Biol. 69, 209–236. doi: 10.1146/annurev-arplant-042817-040322
Welsch, R., Beyer, P., Hugueney, P., Kleinig, H., Von Lintig, J. (2000). Regulation and activation of phytoene synthase, a key enzyme in carotenoid biosynthesis, during photomorphogenesis. Planta 211, 846–854. doi: 10.1007/s004250000352
Xiong, L., Schumaker, K. S., Zhu, J. K. (2002). Cell signaling during cold, drought, and salt stress. Plant Cell 14, S165–S183. doi: 10.1105/tpc.000596
Zhen, S., Bugbee, B. (2020). Substituting far-red for traditionally defined photosynthetic photons results in equal canopy quantum yield for CO2 fixation and increased photon capture during long-term studies: Implications for re-defining PAR. Front. Plant Sci. 11. doi: 10.3389/fpls.2020.581156
Zhen, S., van Iersel, M. W. (2017). Far-red light is needed for efficient photochemistry and photosynthesis. J. Plant Physiol. 209, 115–122. doi: 10.1016/j.jplph.2016.12.004
Keywords: acclimation response, Arabidopsis thaliana, low red to far-red ratio, plant competition, RNA sequencing, shade avoidance response, singlet oxygen signaling, jasmonate-dependent defenses
Citation: Berardi N, Amirsadeghi S and Swanton CJ (2024) Plant competition cues activate a singlet oxygen signaling pathway in Arabidopsis thaliana. Front. Plant Sci. 15:964476. doi: 10.3389/fpls.2024.964476
Received: 08 June 2022; Accepted: 30 July 2024;
Published: 20 August 2024.
Edited by:
Anna N. Stepanova, North Carolina State University, United StatesReviewed by:
Guadalupe Fernandez Milmanda, Flemish Institute for Biotechnology, BelgiumOlga V. Voitsekhovskaja, Komarov Botanical Institute (RAS), Russia
Copyright © 2024 Berardi, Amirsadeghi and Swanton. This is an open-access article distributed under the terms of the Creative Commons Attribution License (CC BY). The use, distribution or reproduction in other forums is permitted, provided the original author(s) and the copyright owner(s) are credited and that the original publication in this journal is cited, in accordance with accepted academic practice. No use, distribution or reproduction is permitted which does not comply with these terms.
*Correspondence: Clarence J. Swanton, Y3N3YW50b25AdW9ndWVscGguY2E=
†These authors have contributed equally to this work