- 1Plant Biochemistry and Physiology, Bielefeld University, Bielefeld, Germany
- 2Fermentation and Formulation of Biologicals and Chemicals, Hochschule Bielefeld - University of Applied Sciences and Arts, Bielefeld, Germany
- 3Computational Biology, Bielefeld University, Bielefeld, Germany
- 4Center of Biotechnology (CeBiTec), Bielefeld University, Bielefeld, Germany
The network of antagonistic, neutral, and synergistic interactions between (micro)organisms has moved into the focus of current research, since in agriculture, this knowledge can help to develop efficient biocontrol strategies. Applying the nematophagous fungus Pochonia chlamydosporia as biocontrol agent to manage the root-knot nematode Meloidogyne hapla is a highly promising strategy. To gain new insight into the systemic response of plants to a plant-parasitic nematode and a nematophagous fungus, Phacelia was inoculated with M. hapla and/or P. chlamydosporia and subjected to transcriptome and metabolome analysis of leaves. While the metabolome proved quite stable except for the early time point of 48 h, comparison of the single P. chlamydosporia with the combined treatment revealed even larger effects after 6 d compared to 48 h, aligning with the later root infestation by P. chlamydosporia compared to M. hapla. Simultaneous exposure to both microorganisms showed a stronger overlap with the single M. hapla treatment than P. chlamydosporia. Changes of transcripts and metabolites were higher in the combined treatment compared to the individual inoculations. The results support the conclusion that P. chlamydosporia induces plant defense in a distinct and beneficial manner if combined with M. hapla although plant defense is partly suppressed by the endophytic growth. The results tentatively suggested that the application of P. chlamydosporia as a biocontrol agent against M. hapla can be more effective by supporting these tritrophic interactions with specific additives, such as phytohormones or amino acids in the formulation.
1 Introduction
Multiple interactions between plants, microbes, nematodes, and other soil organisms determine the rhizosphere (Hunt et al., 1987; Scheu, 2002; Thies and Grossman, 2023). This network of antagonistic, neutral, and synergistic interactions has moved into the focus of current research, since in agriculture, this knowledge can help to develop efficient biocontrol strategies. Biocontrol reduces the application of environmentally harmful pesticides and at the same time assists in ensuring the agricultural food production that is needed for the increasing world population. A well-known example for such a biocontrol agent is the nematophagous fungus Pochonia chlamydosporia (Viaene and Abawi, 2000; Van Damme et al., 2005; Dhawan and Singh, 2010; Askary, 2015; Avelar Monteiro et al., 2017; Manzanilla-López and Lopez-Llorca, 2017). This fungus mainly feeds on nematodes present in the soil but also survives as saprophyte or grows endophytically in a variety of economically important crops (Kerry et al., 1993; Tobin et al., 2008; Hallmann et al., 2009; Manzanilla-López et al., 2011). P. chlamydosporia is applied as biocontrol agent against plant-parasitic nematodes such as root-knot nematodes of the genus Meloidogyne which are one of the most damaging plant-parasitic nematodes infecting a wide range of crops (Carter, 1985; Jones et al., 2013; Askary and Martinelli, 2015).
Meloidogyne penetrates the host root within the first three days after inoculation (Shukla et al., 2018). Secreted effector molecules like proteins and small metabolites initiate and maintain feeding sites and suppress plant defense in host roots (Abad and Williamson, 2010; Mitchum et al., 2013; Shukla et al., 2016). The Meloidogyne life cycle consists of six biological stages starting with the eggs and developing through the juvenile stages J1–J4 to the adult stage. J2 juveniles induce giant root galls by modulating plant metabolism and development 5 to 7 days after inoculation (Shukla et al., 2018).
Plants defend themselves against plant-parasitic nematodes by producing small molecules with anti-nematode activity like phenolic compounds, terpenoids, saponins, or glucosinolates, either before or during nematode infection (Abad and Williamson, 2010; Ghahremani et al., 2022). On the other side, auxin conjugates and cytokinin are important components of the plant machinery that is exploited by the nematode during the establishment of the feeding cell. But the detailed molecular mechanisms are still largely unknown (Abad and Williamson, 2010; Shukla et al., 2018). Shukla et al. (2018) identified 1827 significantly differentially expressed genes (DEGs) in infected tomato roots, with predominantly affected functions varying with infection stage. The majority of these genes are involved in cell wall structure (e.g., plant hydrolases, endo-β-1,4-glucanases, pectin acetyl esterase, β-xylosidases, xyloglucan endotransglucosylases, polygalacturonases, and expansins), cell development (cyclin-dependent kinases, mitotic cyclins, tubulin, and actin), primary and secondary metabolism, and defense signaling pathways (plant hormones like jasmonate and salicylic acid).
Endophytically growing P. chlamydosporia sensitizes the plants by inducing changes in transcription and metabolism and, thereby, functions as a biological control agent as some isolates induce plant defense through cell wall changes in barley and tomato roots (Escudero and Lopez-Llorca, 2012; Larriba et al., 2015; Schouten, 2016). In barley roots, pathogen-induced genes, e.g., encoding chitinases, methyl esterases, germin A-like proteins and late embryogenesis abundant (LEA) protein, are promoted in the plant response during endophytic growth of P. chlamydosporia (Larriba et al., 2015).
P. chlamydosporia colonization of barley roots leads to an enrichment of transcripts involved in abiotic stress response, mainly coding for heat shock proteins and proteins involved in phytohormone biosynthesis, in particular auxin, ethylene, salicylic acid, and jasmonic acid. Other changed transcripts relate to plant defense mechanisms like systemic acquired resistance (SAR) (Larriba et al., 2015; Ghahremani et al., 2019; Tolba et al., 2021).
Since both, P. chlamydosporia and Meloidogyne sp., interact with the plant root, most plant transcriptome analyses focused on infected root tissue and the rhizobiome. In a converse manner, this study aimed to scrutinize the systemic response by exposing the roots of the frequently cultivated cover crop Phacelia tanacetifolia (Hydrophyllaceae) to Meloidogyne hapla, P. chlamydosporia, and a combination of both organisms. Using RNA sequencing and identifying metabolites from P. tanacetifolia leaf tissue, the analysis was designed to focus on leaves 48 h and 6 d after inoculation. These time points mark important steps in the life cycles of P. chlamydosporia and M. hapla in plant root. About 48 h after inoculation M. hapla penetrates the plant roots (Shukla et al., 2018) and the blastospores of P. chlamydosporia have geminated and initiate their endophytic root growth (Escudero and Lopez-Llorca, 2012; Pentimone et al., 2019). After 6 days, the degree of plant root colonization has increased for P. chlamydosporia (Escudero and Lopez-Llorca, 2012; Pentimone et al., 2019) and the M. hapla-induced giant root gall has started to form (Shukla et al., 2018).
As described above P. chlamydosporia mainly feeds on nematodes and some isolates can induce plant defense by endophytic growth. Due to this tri-trophic interaction this study aimed to address two hypotheses for the effect of combined treatment with M. hapla and P. chlamydosporia. The first hypothesis is based on the interaction of P. chlamydosporia and M. hapla. Because P. chlamydosporia feeds on M. hapla and, therefore, reduces the number of nematodes that can enter the plant roots, transcripts that are known as markers for general abiotic and biotic stresses focusing on ROS-related signaling should be less abundant in P. tanacetifolia in the combined treatment compared to the treatment only with M. hapla (H1). In contrast, the second hypothesis is based on the interaction between P. chlamydosporia and P. tanacetifolia. Since some isolates of P. chlamydosporia are described to induce plant defense in different ways, indicators for a plant defense response, like pathogenesis-related transcripts and transcripts related plant hormone signaling, could be stronger regulated by synergistic effects in the combined treatment compared to the single inoculations with either P. chlamydosporia or M. hapla (H2).
2 Material and methods
2.1 Meloidogyne hapla and Pochonia chlamydosporia strains
The nematode M. hapla inoculum was obtained from galled tomato roots (Solanum lycopersicum cv. Moneymaker) grown under greenhouse conditions. The strain is part of the living nematode collection hosted at the Julius Kühn Institute (Braunschweig, Germany), where M. hapla juveniles are routinely tested for purity with the species-specific IGS primers JMV1 and JMVhapla according to the protocol of Adam et al. (2007). The washed tomato roots were placed on a 250 µm-sieve (Ø 12.5 cm) on top of a glass funnel (Ø 15 cm) with a silicone hose attached to the base, which is closed with a hose clamp. This setup was placed in a sprayer chamber with a spraying interval of 30 s and 5 min break. Juveniles hatched from the eggs passed the 250 µm-sieve and sunk into the funnel outlet. Every second day the tube clamp was loosened to collect the freshly hatched nematodes in an Erlenmeyer flask to be stored at 6-8°C. The nematode suspension was adjusted to 350 juveniles/ml tap water.
Pochonia chlamydosporia blastospores were cultivated according to Uthoff et al. (2023). P. chlamydosporia strain Pc001, deposited in the fungal culture collection at the Julius Kühn Institute (Braunschweig, Germany), was originally isolated from surface-sterilized cysts of the sugar beet nematode Heterodera schachtii collected from fields located in North Rhine-Westphalia, Germany (Nuaima et al., 2021). To cultivate blastospores, sterile 250 ml flasks with baffled base were filled with 100 ml sterile potato dextrose broth (PDB, 26.5 g/l, Carl Roth GmbH & Co. KG, Karlsruhe, Germany), inoculated with 106 chlamydospores and incubated at 26°C on a rotary shaker at 150 rpm (IKA KS 4000 ic, Staufen, Germany) for five days. PDB liquid culture was filtered through a 5–10-µm Whatman filter (VWR, Darmstadt, Germany) under sterile conditions to separate blastospores from mycelium. The blastospores were washed twice with 0.9% sterile NaCl solution followed by centrifugation (3600 g, 20°C, 10 min) and resuspension in a 0.9% sterile NaCl solution.
2.2 Experimental setup under greenhouse conditions and Phacelia tanacetifolia harvest
A greenhouse experiment with four treatments was set up to study plant response at transcriptome and metabolome level. To this end, P. tanacetifolia plants (cv. Balo, provided by Feldsaaten Freudenberger GmbH & Co. KG, Krefeld, Germany) were grown in sand and vermiculite (4:1, v:v) substrate fertilized with modified Hoagland solution twice a week (full concentration of the fertilizer solution (pH 6.5) contained 5 mM KNO3, 2.5 mM Ca(NO3)2, 1 mM (NH4)2SO4, 2 mM (NH4)2HPO4, 2 mM MgSO4, 1 mM KCl, 2 mM NaCl, 2 mM Na2SO4, 1 mM FeC6H5O7, 0.025 µM H3BO3, 0.002 µM MnSO4, 0.002 µM ZnSO4, 0.005 µM CuSO4, 0.005 µM MoO3). After four weeks, the plants were separated into four groups namely, A) uninfected substrate as control (C), B) M. hapla-infested substrate (MH); C) P. chlamydosporia-inoculated substrate (PC), and D) in substrate co-inoculated with M. hapla and P. chlamydosporia (MH+PC). For M. hapla infection (treatment B and D), 10 ml tap water with 350 juveniles ml-1 were inoculated per pot through five uniformly distributed holes in the substrate, while for P. chlamydosporia inoculation (treatment C and D), 10 ml blastospore suspension in 0.9% NaCl (with 3.5x107 ± 3.25x106 blastospores per pot) was provided using similar five holes. Each treatment consisted of twelve replicate pots set up randomly in the greenhouse (temperature 22.4 ± 7.2°C; relative humidity 57.5 ± 15.4%) and watered and fertilized every second day or as required. The plant harvest was carried out 48 h, 6 d and 28 d after inoculation.
To harvest the Phacelia plants, the content of a pot was placed in a large bowl and the shoot, and roots were carefully separated from the substrate. The roots were washed quickly in water with as minor damage as possible. Parts of the washed roots and the shoots were separated, packed in aluminum foil and frozen in liquid nitrogen. Two substrate samples were taken to determine (i) the number of colony-forming units (CFU) of P. chlamydosporia and (ii) the density of nematodes. The same procedure was repeated for all harvest time points.
2.3 P. chlamydosporia colony-forming units and plant root colonization
To determine the CFU, the substrate was mixed carefully, and 4 g was removed from each pot, mixed with 10 ml of 0.1% Tween 80, and shaken at 50 rpm for 30 min. The samples were stored for 10 min to allow the substrate to sediment. 10 µl supernatant was taken from each sample and plated on selective PDA medium (Strasser et al., 1996). For each sample three technical replicates were incubated at 23°C for 5 d and fungal CFU per plate were counted.
A representative sample was prepared from each washed root system. For this purpose, 1 cm long fragments were cut from different areas of the roots. These root segments were heated for 20 to 60 min (depending on the thickness of the roots) at 90°C in 10% KOH. After heating, the root segments were rinsed with deionized water and stained in ink-vinegar solution (100 ml blue ink, 100 ml 10% acetic acid solution and 800 ml water) at 90°C for 15 to 20 min (Giovannetti and Mosse, 1980). The root pieces were carefully rinsed again in deionized water until no more ink could be washed out. To quantify fungal structures in the roots, stained root fragments were placed on a microscope slide and covered with lactoglycerol. The quantification was carried out microscopically at 200-fold magnification (McGonigle et al., 1990). The slide was passed over vertically (i.e., perpendicular to the roots) with a crosshair ocular. Each time the crosshairs intersected a root, an intersect was counted. The crosshairs were always aligned vertical to the root. At least 100 intersects were counted and the degree of colonization was expressed as the percentage of intersects where hyphae were present relative to the total number of intersects counted.
2.4 Quantification of M. hapla in substrate and plant roots
In order to extract M. hapla from the substrate samples (10 g), a density centrifugation method was used, in which the organic particles were floated on Ludox HS-40 (Pfannkuche and Thiel, 1988). The supernatant containing the nematodes was poured through 10 µm sieves, preserved in 4% formaldehyde and stained with a few drops of Rose Bengal and counted under a stereomicroscope (40-fold magnification).
A representative sample was prepared from each washed root system. The roots were cut into 1–2 cm pieces and transferred to a 500 ml polyethylene bottle. The bottles were filled with 250 ml of 1% NaClO solution (Dan Klorix household bleach diluted 1:1.8 with tap water) and shaken at 450 rpm for 3 min. The suspension was passed through a 250 µm sieve to remove the roots and placed over a 10 µm sieve to collect the nematode eggs. The egg suspension on the 10 µm sieve was rinsed with tap water for a few seconds to remove residual NaClO. Eggs were counted under a microscope (100-fold magnification). The number of eggs/g root dry weight was chosen as most accurate parameter for root-knot nematode reproduction and fecundity, as suggested by Abd-Elgawad (1991).
2.5 RNA isolation and RNAseq
RNA for RNAseq was extracted using RNeasy Plant Mini Kit (Qiagen, Venlo, The Netherlands) following the manufacturer’s instructions. Leaf RNA was extracted and sequenced for each treatment from three replicates 48 h and 6 d after inoculation.
2.5.1 Library preparation and RNAseq
RNA integrity numbers (RIN) were determined with the Agilent 2100 Bioanalyzer using Agilent RNA Nano 6000 Chips to confirm concentration and integrity of isolated RNA samples. Whole transcriptome sequencing was performed as basis for de novo assembly. Therefore, strand-specific, rRNA depleted libraries were constructed based on 500 ng total RNA using the “TruSeq Stranded Total RNA Library Prep Kit with Ribo-Zero Plant” following the manufacturer’s instructions (Illumina, Berlin, Germany) except that enrichment PCR was performed with 11 cycles.
For analysis of differentially expressed genes libraries were constructed based on 800 ng total RNA using the “TruSeq RNA Sample Preparation v2 Kit” following the manufacturers’ instructions (Illumina, Berlin, Germany) except that enrichment PCR was performed with 11 cycles. After equimolar pooling of all libraries (depleted and polyA-enriched), paired end sequencing of 2x100 base pairs was performed in one run on the NextSeq2000 Sequencing System (P2 Flowcell, 400 Mio Reads).
2.5.2 Read data processing, mapping, and gene expression analysis
Raw read data was processed (demultiplexing, adapter masking and main quality score determination) and converted using bcl2fastq conversion tool (Illumina, Berlin, Germany). De novo transcriptome assembly was performed using Trinity v2.11.0 with Trimmomatic plugin (Haas et al., 2013). Trimmed reads from three replicates were quantified using Kallisto (Bray et al., 2016) and functional annotations were identified using BLAST (Altschul et al., 1990) to A. thaliana reference transcriptome (TAIR, file: TAIR10_pep_20110103_representative_gene_model_updated). The obtained transcripts per kilobase million (TPM) values were used for gene expression analysis in R 4.3.3 (R Core Team, 2023). Principal component analysis (PCA) was calculated over all transcript abundance values from all samples for proof of data similarity. Principal components were generated using ‘prcomp’ (Mardia et al., 1981; Becker et al., 1996; Venables and Ripley, 2002) and main components were plotted using ‘ggplot2’ (Wickham, 2009). Mean TPM values were used to calculate total log2-fold changes (Log2FC) for all treatments compared to the control for both harvesting time points separately. R packages “edgeR” (Robinson et al., 2010) and “limma” (Ritchie et al., 2015) were used to conduct statistical analysis based on raw counts. “exactTest” was used to calculate differences in mean expression of treatments and control and using “topTags” attained p-values that were corrected based on Bonferroni-Hochberg correction for multiple testing. Obtained false discovery rates (FDR) were used to identify differentially expressed genes (FDR < 0.05). To identify relevant plant stress markers and plant defense response, pathway analysis was done with Kyoto Encyclopedia of Genes and Genomes (KEGG, Kanehisa and Goto, 2000). To evaluate the mentioned hypotheses the following assumptions for log2FC changes were made (Table 1).
2.6 Analysis of plant metabolites
Leaf metabolites were extracted, derivatized and quantified via GC-MS using tissue harvested and shock-frozen after 48 h, 6 d and 28 d of inoculation. Pulverized plant material (5 mg) from six replicates was freeze-dried and processed according to Wegener et al. (2023). Extraction buffer included 10 μM ribitol as an internal standard. Metabolites were separated on a RTx®-5MS column (30 m, iD 0.25, df 0.25 μm) using a GC-MS-system consisting of a Trace 1310 GC coupled to a TSQ9000 mass spectrometer (Plassmeier et al., 2007). Sample aliquots (1 μL) were injected in splitless mode into the GC-MS column. The initial temperature was kept at 80°C for 3 min, then raised to 325°C at a rate of 5°C min-1 and finally kept at 325°C for 2 min. The MS transfer line and electron impact ion source temperatures were adjusted to 250°C and 220°C, respectively. The spectra were recorded within a scanning range of 50 - 650 mz-1 (mass/charge). All substances were compared with reference substances in terms of retention time and mass spectrum. These reference substances are re-measured each time the column is changed in order to adjust the retention times. Quantification was conducted on peak areas of characteristic compound mass/charge ratio normalized to ribitol (at 217 mz-1) and to the dry weight of the material. Significant changes in metabolite concentrations were calculated in R 4.3.3 (R Core Team, 2023) based on Kruskal–Wallis test with Dunn’s Test for multiple comparisons with Benjamini and Hochberg (1995) correction as post-hoc test.
3 Results
3.1 Determination of M. hapla in substrate and roots
M. hapla was quantified 48 h (Supplementary Figure S1A), 6 d (Supplementary Figure S1B) and 28 d after inoculation. Unexpectedly, nematodes could be found in all substrates 28 d after inoculation (Figure 1C). For example, in substrate of treatments C and PC, 95 ± 53 and 369 ± 163 nematodes in total were found, respectively. Compared to treatments C and PC, 18-fold more nematodes were counted in treatments MH (1709 ± 687 nematodes/pot) and 14-fold (compared to the control) in MH+PC (1293 ± 412 nematodes/pot). The amount of M. hapla in the treatment MH+PC was 24.3% lower compared to the treatment MH. Additionally, M. hapla eggs were extracted from P. tanacetifolia roots and quantified 28 d after inoculation (Figure 1D). In treatment MH and MH+PC, 247 ± 44 and 58 ± 10 eggs/g root dry weight were found, respectively. As expected, no M. hapla eggs were found in C and PC, indicating no nematode reproduction during the experiment. As nematodes were found in the substrate of these treatments, it can be assumed that these were contaminations during the harvest or beginning carry-over from drained irrigation water. Furthermore, replicates with M. hapla contamination in C and PC were excluded from the RNAseq approach.
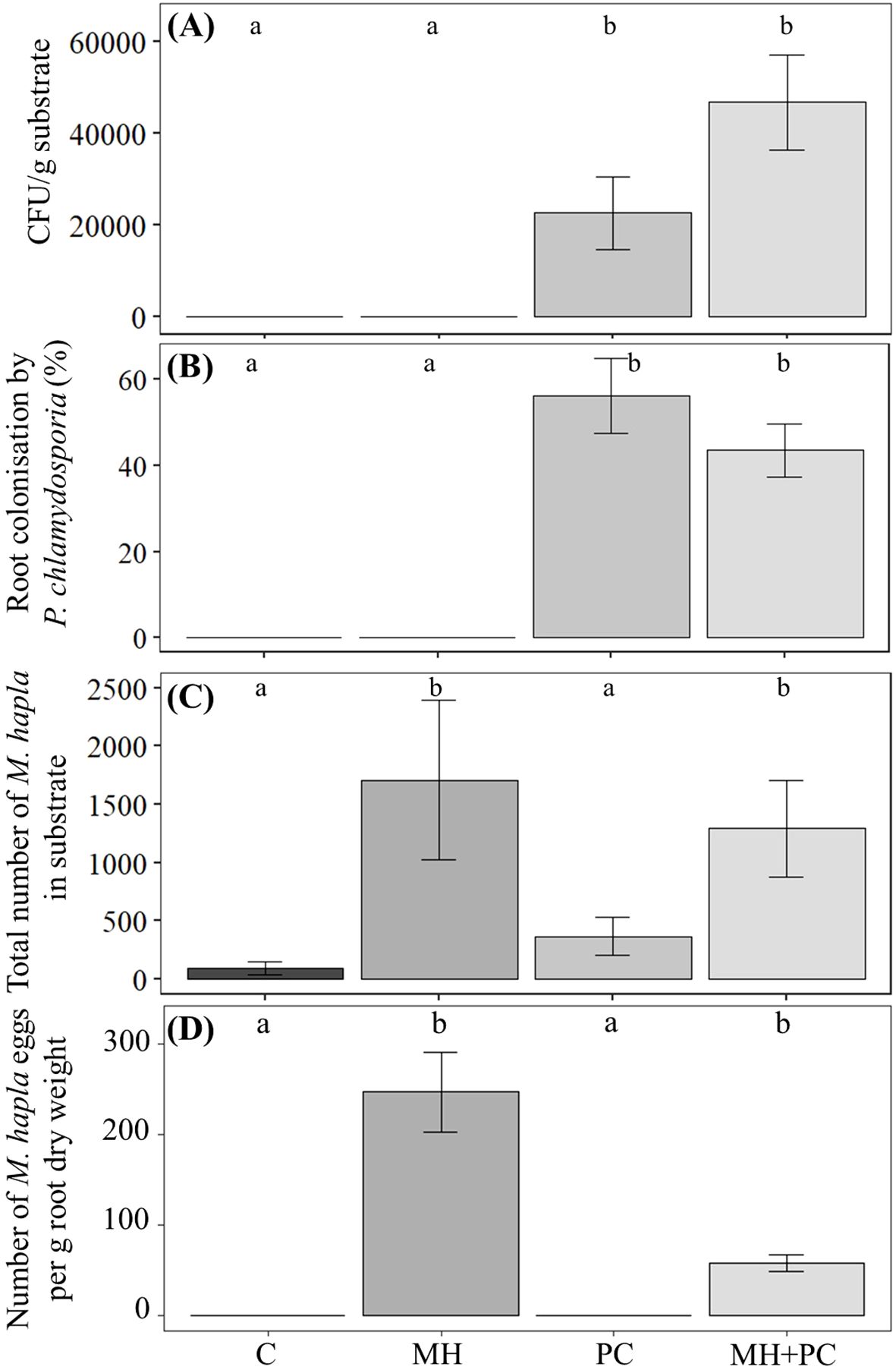
Figure 1. Quantification of Pochonia chlamydosporia and Meloidogyne hapla in substrate, extent of P. chlamydosporia root colonization and amount of M. hapla eggs in Phacelia tanacetifolia 28 d after inoculation with M. hapla (MH), P. chlamydosporia (PC) and their combination (MH+PC). (A) Colony forming units of P. chlamydosporia per g substrate 28 d after inoculation (mean ± SE, n=12). (B) Percentage of intersects where hyphae were present relative to the total number of intersects counted for each treatment 28 d after inoculation (mean ± SD, n=12). (C) Total number of M. hapla in substrate for each treatment 28 d after inoculation (mean ± SE, n=12). (D) Number of M. hapla eggs per g root dry weight for each treatment 28 d after inoculation (mean ± SE, n=12). C, control; MH, inoculated with M. hapla; PC, inoculated with P. chlamydosporia; MH+PC, inoculated with M. hapla and P. chlamydosporia. Different letters indicate significance of difference (Kruskal–Wallis test with Dunn’s post hoc test, p < 0.05).
3.2 Colony-forming units in the substrate and colonization of plant roots by P. chlamydosporia
To evaluate establishment of P. chlamydosporia in the substrate, we counted colony-forming units (CFU) at 48 h (Supplementary Figure S1C), 6 d (Supplementary Figure S1D) and 28 d after inoculation. As expected, P. chlamydosporia was absent in treatment C and MH after 28 d. On the other hand, in treatment PC 3.26×104 ± 7.97×103 CFU/g substrate and in MH+PC 4.68×104 ± 1.05×104 CFU/g substrate were counted with no significant difference (Figure 1A).
The percentage of intersects where hyphae were present relative to the total number of intersects was determined 28 d after inoculation. Complementary to the CFU results, no hyphae were found in P. tanacetifolia roots in treatments C and MH, while roots were colonized by P. chlamydosporia in treatments PC and MH+PC by 56.3 ± 8.7% and 43.6 ± 6.2%, respectively, with no significant difference of colonization (Figure 1B).
3.3 Shoot and root dry weight and nutrient state of P. tanacetifolia
After 28 d, the inoculation with MH and/or PC had no effect on P. tanacetifolia above ground dry weight (Supplementary Figure S2A). In contrast, inoculation of M. hapla alone significantly reduced root dry weight by 42% (Supplementary Figure S2B). Additionally, there existed no differences for nitrogen, phosphate, and carbon content in P. tanacetifolia tissue (Supplementary Figure S3).
3.4 P. tanacetifolia transcriptome changes induced by nematode and fungal inoculation
Trinity assembled a total of 105,493 transcripts and 69,473 genes derived from these transcripts. Functional annotations based on the Arabidopsis thaliana reference transcriptome were identified for 21,227 of these genes.
3.4.1 Principal component analysis and differential gene expression
For an overview, TPM values were subjected to principal component analysis (PCA). The PCA for both time points showed a reliable clustering of the replicates, with an obvious treatment-dependent pattern. This clear difference between the control (C) and all other treatments reflects the impact of M. hapla and P. chlamydosporia on the transcriptome of P. tanacetifolia. After 48 h, MH and PC conditions were not entirely separated from each other, but they clearly differed from C and especially from the combination MH+PC (Figure 2A). A similar treatment-specific clustering is visible 6 d after inoculation. An overlap appears between C and PC due to high variance for PC, while treatments MH and MH+PC lack an overlap and clearly separated from C and PC (Figure 2C).
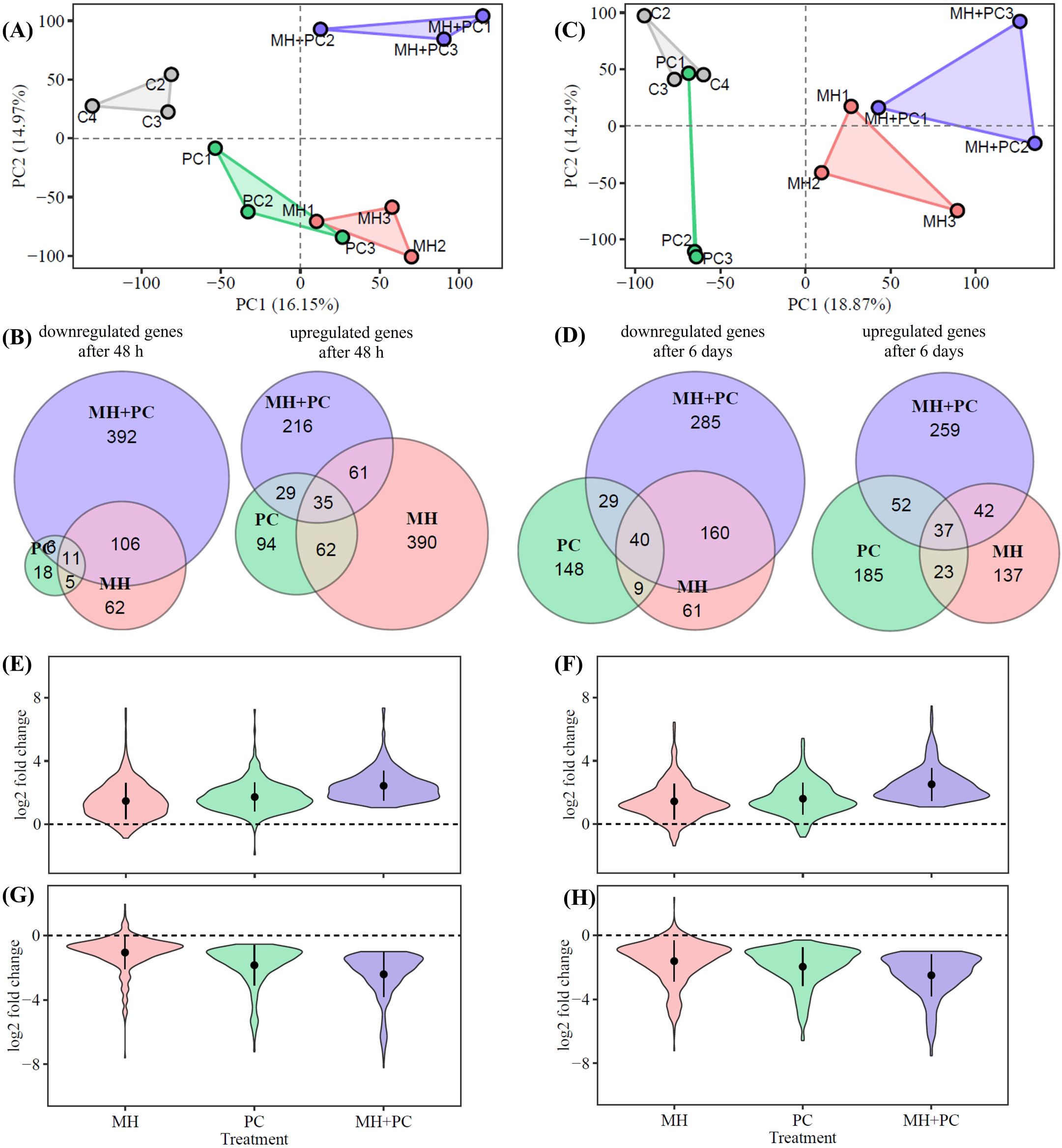
Figure 2. Transcriptome analysis of Phacelia tanacetifolia for the systemic effects after inoculation with Meloidogyne hapla (MH), Pochonia chlamydosporia (PC) and a combination of both (MH+PC). Total leaf RNA was subjected to RNAseq. Principal component analysis (PCA) (A) 48 h or (C) 6 d after inoculation displays the clustering of the three replicates for each treatment. Specificity and overlap of differentially expressed genes (DEGs) in P. tanacetifolia (B) 48 h and (D) 6 d after inoculation are shown in Venn diagrams (FDR < 0.05, upregulated: log2FC > 1 or downregulated log2FC < -1, n = 3). To globally investigate the additive effect of the combined inoculation (hypothesis 2), upregulated transcripts of MH+PC (FDR < 0.05, log2FC > 1) were compared to single inoculated treatments (Kruskal–Wallis test with Dunn’s post hoc test, p < 0.001), (E) 48 h (341) or (F) 6 d (390) after inoculation and downregulated transcripts of MH+PC (FDR < 0.05, log2FC < -1) were compared to single inoculated treatments (G) 48 h (515) or (H) 6 d (514) after inoculation.
For the 48 h and 6 d time points, 1539 and 1573 transcripts showed differential accumulation, respectively (Figures 2B, D). The largest portion of differentially expressed genes (DEGs) occurred with MH+PC, namely 392 decreased and 216 increased transcripts after 48 h, and 285 decreased and 259 increased transcripts after 6 d. However, MH had a stronger effect on the shoot transcriptome at the early time point of 48 h than after 6 d, since 548 transcripts revealed increased abundance after 48 h and 239 transcripts after 6 d. In contrast, PC-treatment effects become more apparent with increasing treatment duration (Figures 2B, D). A transcript comparison of the MH+PC exposure to the individual exposure regimes revealed a stronger overlap between MH and MH+PC, especially for transcripts with reduced expression for both treatments. But the most surprising observation was that the combined treatment MH+PC altered a very large set of unique transcripts, and this was already apparent at the early time point of 48 h when the P. chlamydosporia growth was still very low.
Furthermore, the comparison between MH+PC and the single inoculated treatments underlines the additive effect of the combined inoculation. Transcripts that were regulated in MH+PC were less altered in MH and PC. Overall, this effect applied to 37.9% downregulated and 30.3% upregulated transcripts for both harvest times (Figures 2E–H; Supplementary Table S1 for downregulated transcripts and Supplementary Table S2 for upregulated transcripts).
About 40% of the P. tanacetifolia DEGs could be functionally annotated with Arabidopsis thaliana as reference (Figure 3). Repeating the analysis with the functionally annotated transcripts did not change the proportions of DEGs and overlap of DEGs between the treatments to any major extent. Therefore, the following in-depth analysis of individual transcripts was carried out with functionally annotated transcripts to assign functional dependencies.
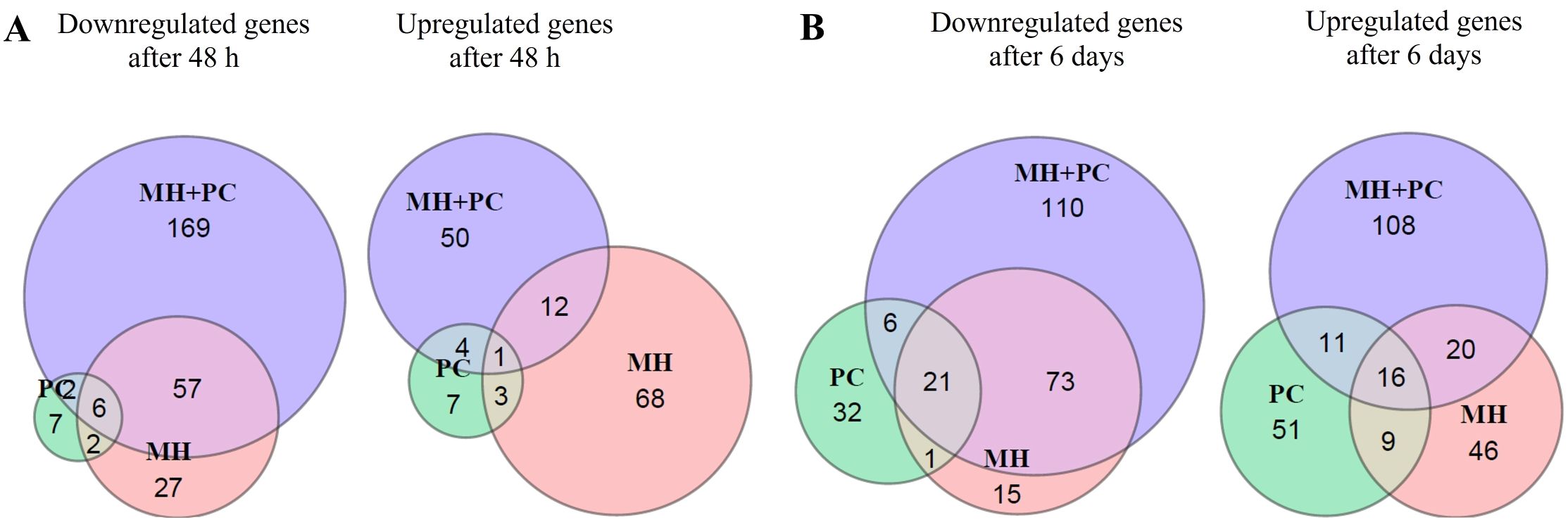
Figure 3. Analysis of functionally annotated transcripts of Phacelia tanacetifolia for the systemic effects after inoculation with Meloidogyne hapla (MH), Pochonia chlamydosporia (PC) and a combination of both (MH+PC). Leaf RNA was subjected to RNAseq. Specificity and overlap of differentially expressed genes (DEGs) in P. tanacetifolia (A) 48 h and (B) 6 d after inoculation are shown in Venn diagrams (FDR < 0.05, upregulated: log2FC > 1 or downregulated log2FC < -1, n = 3).
In total, 20 transcripts related to general stress markers fitted the parameters for H1 and were less regulated in MH+PC compared to MH (Figure 4; Supplementary Table S3). After 48 h, CINNAMYL ALCOHOL DEHYDROGENASE 8, ALANINE: GLYOXYLATE AMINOTRANSFERASE and GUTATHIONE-S-TRANSFERASE L3 (GSTL3) were less downregulated in MH+PC compared to MH, while among other peroxidases (PER44, 52, 71 and 72) and MYO-INOSITOL OXYGENASE 4 (MIOX4) were less upregulated. After 6 d, ACYL-CoA SYNTHETASE 5, PER44 and 6-PHOSPHOGLUCONATE DEHYDROGENASE were less downregulated in MH+PC compared to MH, while CINNAMYL ALCOHOL DEHYDROGENASE 5 and 9, PER64 and CATALASE 2 (CAT2) were less upregulated. Contrary to H1, 29 transcripts were stronger regulated in MH+PC compared to MH. In more detail, 9 transcripts decreased more in MH+PC compared to MH, including for example GSTU7, 8, 25, and GSTF2 and ASCORBATE PEROXIDASE 1 and 2 (APX1 and 2), while 20 transcripts were stronger upregulated, including for example PER 2, 16, 56, GSTF3 and 9 and MIOX1 (Supplementary Table S4).
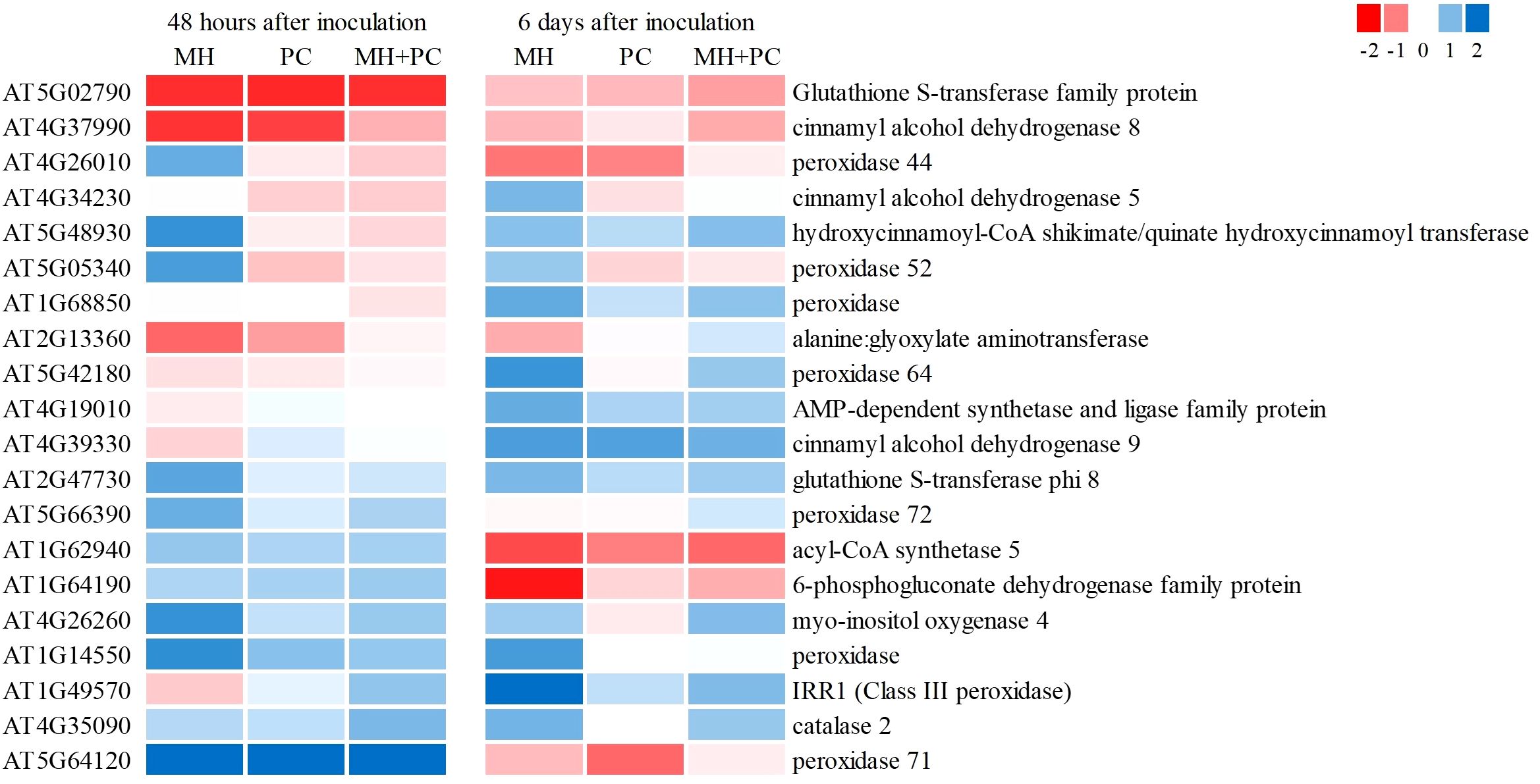
Figure 4. Heatmap of stress markers in Phacelia tanacetifolia in response to Meloidogyne hapla (MH), Pochonia chlamydosporia (PC) and a combination of both (MH+PC). Heat maps with log2-fold changes of transcripts that were less regulated in MH+PC compared to MH (hypothesis 1: MH > 1 and MH+PC < MH or MH < -1 and MH+PC > MH) for one or both harvesting time points (Supplementary Table S3). KEGG pathways: phenylpropanoid biosynthesis (ath00940), glutathione metabolism (ath00480), ascorbate and aldarate metabolism (ath00053), and peroxisomes (ath04146).
As assumed in H2, the combined inoculation MH+PC could enhance the plant defense response due to synergistic regulation of defense-related transcripts. Several transcripts with a role in abscisic acid (ABA) and jasmonic acid (JA) signaling, as well as transcripts involved in plant-pathogen interactions and MAPK signaling responded stronger in the combined treatment than in the single inoculation. Examples for this type of response were PYRABACTIN RESISTANCE 1-LIKE 2 (PYL2), 12-OXOPHYTODIENOIC ACID REDUCTASE 2 (OPR2), LIPOXYGENASE 2 (LOX2), CAT2 and MITOGEN ACTIVATED PROTEIN KINASE 4 (MPK4) (Figure 5; Supplementary Table S5).
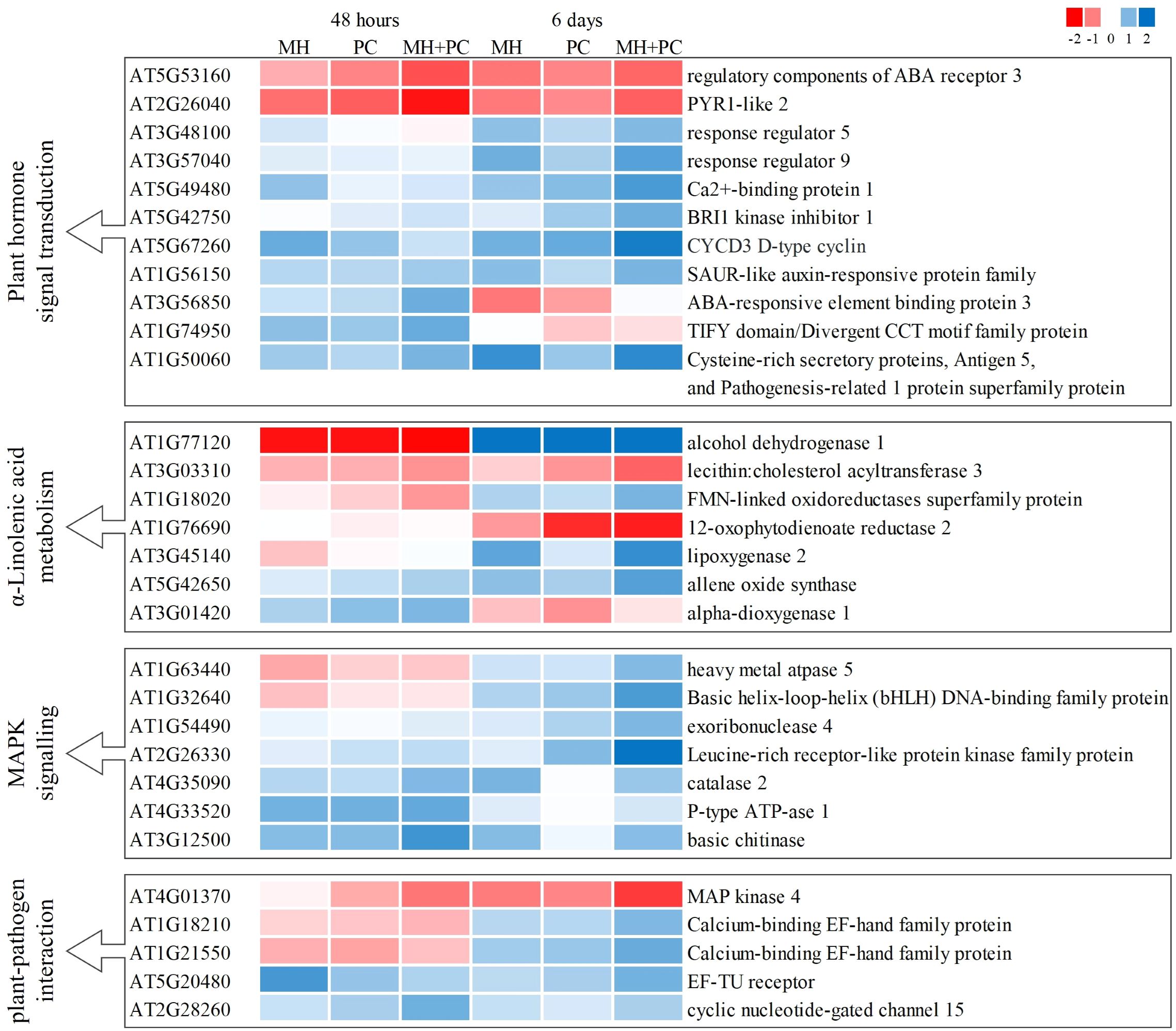
Figure 5. Heatmap of transcripts related to plant defense signaling in Phacelia tanacetifolia in response to Meloidogyne hapla (MH), Pochonia chlamydosporia (PC) and a combination of both (MH+PC). Heat maps with DEGs (FDR < 0.05) for plants inoculated with M. hapla (MH) or P. chlamydosporia (PC) or with a combination of both organisms (MH+PC) in comparison to uninoculated control plants. Heatmaps show log2-fold changes for treatments compared to the control for harvest 48 h and 6 d after inoculation fitting the parameters of hypothesis 2. KEGG pathways: Plant hormone signal transduction (ath04075), α-Linolenic acid metabolism (ath00592), MAPK signaling pathway (ath04016), Plant-pathogen interaction (ath04626).
Additionally, as stated above, it appeared interesting that the total number of DEGs was in all cases higher in MH+PC compared to MH and/or PC, except of upregulated transcripts 48 h after inoculation, where MH treatment had a higher impact on plant transcriptomic changes (Figure 3). DEGs mainly regulated in MH treatment were for example disease resistance proteins, LACCASEs, and ACC OXIDASE 5 but also membrane proteins, cell wall localized proteins, peroxidases and proteins involved in inositol metabolism (Supplementary Table S6) and WALL-ASSOCIATED KINASE 2 and 5 (WAK2 und 5) were downregulated in PC and MH+PC (6 d) but not in MH. A detailed list of downregulated and upregulated transcripts mainly for MH, PC or MH+PC is available in the supplements (Supplementary Table S6 for MH, Supplementary Table S7 for PC and Supplementary Table S8 for MH+PC).
3.5 Treatment effects on plant metabolites
In order to assess changes in metabolism as ultimate process in response to the treatments, a metabolome analysis complemented the transcriptome assessment. The GC-MS-based analysis covered metabolites from central and specialized metabolism. The most conspicuous observation concerned the fact that significant differences between the treatments almost only occurred 48 h after inoculation (Figure 6). The metabolite contents at the later time points of 6 d and 28 d were usually lower than in the younger developmental stage.
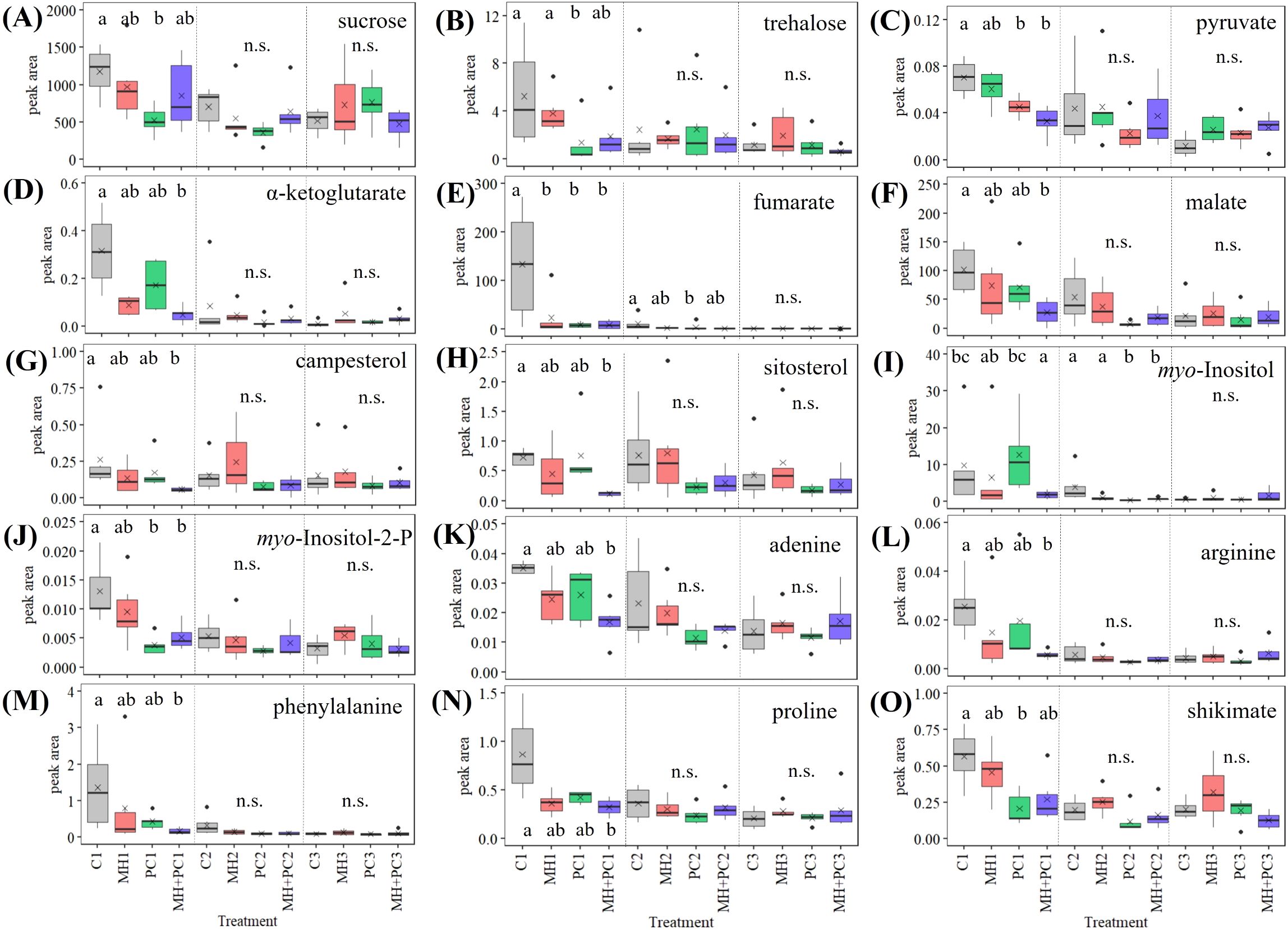
Figure 6. Relative amounts of selected Phacelia tanacetifolia metabolites from central sugar metabolism (A–C), citric acid cycle (D–F), phytosterols (G, H) and myo-inositol (I, J), amino acids (K–N) and shikimate (O) in response to Meloidogyne hapla (MH), Pochonia chlamydosporia (PC) and a combination of both (MH+PC). Box plots show the peak areas of characteristic compound mass/charge ratio of the metabolites normalized to ribitol (217 mz-1) and leaf dry weight. The treatments with M. hapla (MH), with P. chlamydosporia (PC) or with a combination of both organisms (MH+PC) were compared with the uninoculated control plants (48 h after inoculation = C1, MH1, PC1, MH+PC1; 6 d after inoculation = C2, MH2, PC2, MH+PC2; 28 d after inoculation = C3, MH3, PC3, MH+PC3). The horizontal line in the boxes indicates the median and x the mean (n=6). Values with no letter in common are significantly different (Kruskal–Wallis test with Dunn’s Test for multiple comparisons with Benjamini and Hochberg (1995) correction as post-hoc test, p < 0.05). n.s. indicates no significant differences between the treatments for the respective harvesting time point.
PC treatment lowered the contents of the disaccharides sucrose (α, β-1,2-Glc-Fru) and trehalose (α-1,1-Glc-Glc) compared to control plants. The trend of decreased amounts of sucrose and trehalose in the treated plants after 48 h was mimicked by several other metabolites of the central energy pathways, namely pyruvate, α-ketoglutarate, and malate. And also, the amino acids arginine, phenylalanine and proline, and shikimate, the intermediate for synthesis of aromatic amino acids and diverse specialized metabolites, followed this pattern.
The analysis of phytosterols showed significant differences between the treatments exclusively after 48 h in P. tanacetifolia leaf tissue (Figures 6F, G). Treatment MH+PC led to significantly lower campesterol and sitosterol content in P. tanacetifolia leaves compared to control plants after 48 h.
The in part severe and differentiated effect of treatments on metabolite contents at the early time point of inoculation was not seen at the later time points of 6 d and 28 d, with the exception of myo-inositol and fumarate (Figure 6). Overall, the pronounced treatment-induced perturbation of metabolism after 48 h was followed by a homeostatic state of leaf metabolism irrespective of the treatment at later time points.
4 Discussion
This study on the commonly used cover crop P. tanacetifolia explores the systemic response of the shoot to infection of the roots with M. hapla or P. chlamydosporia, and the combination of both. The ultimate goal of this research and possible application in the field is to establish a P. chlamydosporia population in the cover crop to reduce the number of the root-knot nematode M. hapla in the soil. The hypothesis is that following ploughing under of the cover crop, the presence of P. chlamydosporia will lower the nematode pressure during cultivation of the main crop (Uthoff et al., 2023). Apart from testing this system in field trials, there is the need to understand the interference between M. hapla, P. chlamydosporia and P. tanacetifolia. Therefore, we set up this study to sensitively monitor changes in P. tanacetifolia following the single or combined inoculation. We sought answers to two questions, namely whether P. chlamydosporia inoculation poses a stress to the plant and whether and how the combined treatment affects metabolism and gene expression? Plant responses to stress combinations have moved into the focus of current research on abiotic and biotic plant-environment interactions in order to bridge lab experiments and field experiments (Dietz and Vogelsang, 2024; Kumar et al., 2024). To this end we chose the systemic response as appropriate readout.
Growth data revealed that after 28 d, the shoot biomass was indistinguishable between all treatments including the control. This kind of no-effect was observed before for example upon inoculating six parsley genotypes irrespective whether classified as good or no host plants (Noskov et al., 2024). However more commonly, strong prevalence of root nematodes in the field drastically reduces crop yield, for example in soybeans (Saha et al., 2023). In a converse manner to the no-effect on shoots, the root biomass dropped to 41% in the M. hapla-infested plants. Presumably, the soil was a rich substrate, and the reduced root growth had no effect on the shoot. This explanation could be tested by decreased fertilization. The P. chlamydosporia-treatment insignificantly lowered the root biomass to 91% of the control. P. chlamydosporia inoculation often has no effect or even a beneficial effect on biomass production of plants including cover crops (Coutinho et al., 2021).
Simultaneous addition of P. chlamydosporia reduced the M. hapla-induced root growth inhibition to 67% after 28 d. In order to avoid this late stage of infection and progressed damage, we opted for an early time point of 48 h and a later time point of 6 d for the analysis of transcripts and metabolites where still the nematodes are in the second juvenile stage (J2) (Dávila-Negrón and Dickson, 2013). Nevertheless, after 28 d the tendency of the beneficial effect of P. chlamydosporia on root health was shown in the combined treatment. Furthermore, in treatments inoculated with P. chlamydosporia and M. hapla the CFU/g substrate were higher while root colonization was lower compared to treatments inoculated with P. chlamydosporia alone. This indicates that P. chlamydosporia as nematophagous fungus prefers to feed on nematodes instead of growing endophytically in plant roots and, therefore, supports plants by reducing the number of nematodes in the soil. Uthoff et al. (2023) already confirmed the potential of P. chlamydosporia isolate P001 as a biocontrol agent against M. hapla if inoculated to P. tanacetifolia. In addition, they found that plants inoculated simultaneously with P. chlamydosporia and M. hapla had a higher root dry weight 50 d after inoculation compared to plants only inoculated with M. hapla. In this study, the amount of M. hapla and the root dry weight was measured 28 d after inoculation. This indicates that significant effects on the M. hapla population and the resulting effects on root weight might only be found after a longer period of time. Furthermore, it is likely that studies on multiple interacting organisms will not result in strongly significant differences as the establishment and growth of the individual organisms depends on several factors that additionally interact with each other, resulting in a highly complex system.
4.1 Less response of ROS-related general stress markers in leaf tissue of the combined treatment
As starting point for this study, we hypothesized that P. tanacetifolia exposed to the combination of the nematode M. hapla and the fungus P. chlamydosporia encounters less stress even in the early stages of infestation due to the antagonistic interaction between P. chlamydosporia and M. hapla. We focused on transcripts coding for markers of general abiotic and biotic stress related to phenylpropanoid biosynthesis, glutathione metabolism, ascorbate and aldarate metabolism, and peroxisomes. Indeed, these transcripts accumulated less in the shoot of plants exposed to the combined treatment compared to the single treatment with M. hapla (H1). Nematode infestation triggers ROS- and redox-related responses in the roots of the host plants (Denjalli et al., 2024). Both stimulatory and inhibitory effects at the site of infestation have been described, in particular recent research identified the inhibitor Mj-NEROS that interacts with the RIESKE center of the photosynthetic electron transport chain and suppresses ROS generation in the plastids of roots and leaves (Stojilković et al., 2024). Published research mostly concerned the local response of the infestation site. Here we focused on the systemic effect of root infestation on the shoot.
Both microorganisms, M. hapla and P. chlamydosporia, induce ROS production while interacting with the plant root (Baker and Orlandi, 1995; Lamb and Dixon, 1997; Mata-Pérez and Spoel, 2019). Heme peroxidases (PER) exist as a huge gene family and the responsive PER transcripts showed no clear pattern regarding the treatments and harvesting time points. As the nature of the electron donor for PER depends on the structure of the enzyme (Apel and Hirt, 2004) and class III PER are known to be involved in abiotic and biotic stress responses (Kidwai et al., 2020), it can be assumed that during infection a variety of systemic ROS-related reactions take place.
Within the redox regulatory network, the cytosolic APX1 and APX2 were stronger downregulated in treatments with a combined inoculation of P. chlamydosporia and M. hapla compared to control at both harvesting time points. APXs efficiently detoxifies H2O2 using ascorbate as electron donor and is a central component of the ROS gene network in different subcellular compartment. Its expression is often enhanced in response to abiotic and biotic stress such as pathogen attack (Davletova et al., 2005). The lower amounts of cytosolic APX in the combined treatment indicates, contrary to H1, an antagonistic effects of P. chlamydosporia on M. hapla-induced plant damage and suppression of plant defense response with systemic effects on the shoot.
Another example is MIOX4 that was stronger upregulated in M. hapla compared to both treatments inoculated with P. chlamydosporia. MIOX4 is the first enzyme in the inositol route to ascorbate and important for syncytium development of sugar beet nematode Heterodera schachtii (Siddique et al., 2009). An A. thaliana quadruple myo-inositol oxygenase mutant showed a significant reduction in susceptibility to H. schachtii and the primary function of myo-inositol oxygenase for syncytium development is assumed to be the reduction of myo-inositol levels and thereby a decrease in the galactinol level to avoid the induction of defense-related genes (Siddique et al., 2014). This is in line with this study as M. hapla and the combined treatment with P. chlamydosporia and M. hapla decreased myo-inositol content, while P. chlamydosporia alone did not affect the myo-inositol content. Liu and Chen (2003) described that excluding myo-inositol from the medium increased P. chlamydosporia conidia formation which could be a reason MIOX4 is less upregulated in plants with potentially endophytic fungal growth. Here it remains unclear, if the slight upregulation of MIOX4 is actively caused by the fungus or if it is a plant response to support fungal endophytic growth.
4.2 Synergistic effects of P. chlamydosporia and M. hapla intensify plant defense
Previous studies categorize P. chlamydosporia as endophyte with neutral or positive effects on plants like tomato, Arabidopsis, or barley (Escudero and Lopez-Llorca, 2012; Larriba et al., 2015; Schouten, 2016; de Souza Gouveia et al., 2019). In line with these findings, the second hypothesis addressed the presumed stimulatory effect of P. chlamydosporia on activating defense responses, helping the plant to better defend against the nematode in the combined treatment with M. hapla and P. chlamydosporia.
Defense responses of plants are often regulated on long distance by phytohormones, namely salicylic acid (SA), jasmonic acid (JA), ethylene (ET) and abscisic acid (ABA). For example, LOX2 that is part of the JA-pathway and α-Dioxygenase 1 (DOX1) that is induced in response to SA, and different transcripts related to the ABA pathway were stronger regulated in the combined treatment compared to single inoculated treatments (Supplementary Table S5). ABA plays an important role in the preinfectional defense of A. thaliana against M. paranaensis as their population was reduced by over 50% with the exogenous application of ABA 24 h before the nematode inoculation even though ABA had no toxic effect on the nematodes in vitro (Yop et al., 2023).
Besides ABA, JA is well described to be involved in pathogen defense (Halim et al., 2006) and the resistance against root-knot nematode of the genus Meloidogyne (Fan et al., 2015; Medeirosa et al., 2015; Bali et al., 2018; Gouveia et al., 2023). Furthermore, JA signaling is crucial for the establishment of mutualistic interactions between plants and microorganisms, including bacteria and fungi (Hause and Schaarschmidt, 2009), also shown for P. chlamydosporia (Zavala-Gonzalez et al., 2017). Our study underlines the systemic importance of phytohormone signaling, as several transcripts related to their metabolism were systemically regulated in leaves.
4.3 The phenomenon of early metabolic disturbance
Most metabolic effects were exclusively detected 48 h after inoculation and also in the combined treatment with M. hapla and P chlamydosporia (Figure 6). Only the combined inoculation with P. chlamydosporia and M. hapla led to significantly lower adenine, arginine, phenylalanine, and proline as well as campesterol and sitosterol contents compared to control plants. It is important to note here again, that we focused on systemic effects caused either by long distance signaling from the infested root to the shoot or by increased sink strength (Zhou et al., 2023).
Pyruvate is metabolized to ethanol and serves as precursor of acetyl-CoA, the acetyl moiety of which enters the citric acid cycle. The combined treatment significantly decreased the content of α-ketoglutarate and malate compared to the control plants after 48 h, while fumarate was significantly decreased in all treatments after 48 h, and also in plants treated with P. chlamydosporia 6 days after inoculation. Fumarate and malate have a variety of functions in biochemical pathways in plants and fumarate acts as an alternative carbon sink for photosynthates similar to starch (Araújo et al., 2011). The reduction of fumarate and malate content in P. tanacetifolia leaves by P. chlamydosporia and M. hapla could be caused due to consumption of carbohydrates by both inoculated organisms which establish additional sinks in the plant root.
Additionally, several amino acids function as C- and N-source and are metabolized by P. chlamydosporia. However, isolates differ in their effectiveness of carbon source utilization (Vieira dos Santos et al., 2019). The here studied P. chlamydosporia isolate P001 remains unexplored regarding its utilization of carbon sources. This metabolome analysis may give a first hint, but detailed investigations are needed. On the one hand, external application of amino acids, especially phenylalanine, can reduce the number of galls caused by root knot nematode M. javanica in tomato roots (Amdadul Hoque et al., 2014). On the other hand, low amino acid availability to the nematode reduces susceptibility of roots to M. incognita infestation (Dutta et al., 2024). The reduction of amino acids in plant leaf tissue could also be a plant response to suppress M. hapla growth, or amino acids could be metabolized by P. chlamydosporia, or most probably a combination thereof that lead to a synergistic effect of the combined inoculation. The metabolome data (Figure 6) suggest that the bipartite and tripartite interaction challenge plant homeostasis systemically only at an early stage. Later on, the plant returns to a homeostatic metabolism. This conclusion is supported by the observation that above-ground biomass was indifferent between the various treatments.
M. hapla and P. chlamydosporia invade the root cells of plants and secrete different molecules and proteins to support this process by destabilizing the root cell wall and membrane, and suppressing the plant defense (Abad and Williamson, 2010; Larriba et al., 2014; Shukla et al., 2018; Pentimone et al., 2019). Additionally, P. chlamydosporia secrets chitinases, chitin deacetylases and a chitosanase during nematode egg parasitism (Aranda-Martinez et al., 2016), which may also interact with nearby plant roots and target cell wall-related processes. The WAK2 and WAK5 were downregulated in the combined treatment and the single inoculation with P. chlamydosporia. WAKs are candidates as polygalacturonic acid receptors and are induced by SA. M. hapla releases polygalacturonases during infection (Jaubert et al., 2002), but for P. chlamydosporia it is not described if it releases polygalacturonases while different plant pathogenic fungi do (Nakamura and Iwai, 2019).
The effector proteins of P. chlamydosporia and M. hapla trigger local responses and likely initiate systemic plant signaling processes that explain the transcriptional and metabolic responses of the leaves.
5 Conclusions
In general, the plant response to plant-parasitic nematodes and endophytic fungi differs strongly. In our experimental setup, P. chlamydosporia had neither a negative nor a positive effect on shoot or root growth. In the combined treatment with M. hapla, however, a positive effect of P. chlamydosporia on root growth was detected. It is noteworthy that in many cases the regulation of transcripts is stronger in the combined treatment compared to the individually inoculated treatments. Therefore, the combined treatment with M. hapla and P. chlamydosporia had a partially additive effect on the transcriptome and metabolome of the plant as suggested in hypotheses 2. This effect is beneficial if it concerns relevant defense genes. Hypotheses 1 was supported by 20 transcripts related to general stress markers as these transcripts were less abundant in P. tanacetifolia in the combined treatment compared to the treatment only with M. hapla. Therefore, both hypotheses seem to be at least partly confirmed. But, the exact mechanism of interaction between P. chlamydosporia, M. hapla and P. tanacetifolia remains largely unknown and needs further research. The co-inoculation of P. chlamydosporia and M. hapla in this study and the resulting variation in expression patterns and regulations underline the complexity of trophic interaction. Especially the here demonstrated systemic response including phytohormones and ROS as long-distance signaling offers interesting research perspectives as the cross-talk between different phytohormones as well as the specific function of peroxidases still leaves many questions unanswered. Even though potentially contaminated replicates were excluded from RNA sequencing, further studies are warranted with strict regime that avoid any nematode contaminations with consequently proper analyses of the RNAseq in control and P. chlamydosporia treatments.
In an agricultural context, the understanding of these interactions could improve the application of P. chlamydosporia as a biocontrol agent against M. hapla. To improve the establishment of the biocontrol agent, several ingredients can be added. For example, amino acids, phytohormones and/or nutrients that support P. chlamydosporia performance as well as ingredients that suppress M. hapla growth such as chitinases, chitin deacetylases and chitosanases which naturally decompose the nematodes’ cuticula. Nonetheless, abiotic parameters in the field must be taken into account since they affect the conditional expression of the plant (defense) response. In conclusion, this study underlines the systemic response of plants to microorganisms entering the plant roots.
Data availability statement
The raw data supporting the conclusions of this article will be made available by the authors, without undue reservation. RNA-seq data will be available after acceptance at NCBI Sequence Read Archive SRA - NCBI (nih.gov), BioProject ID: PRJNA1205911, available online at http://www.ncbi.nlm.nih.gov/bioproject/1205911.
Author contributions
JK: Conceptualization, Data curation, Formal analysis, Investigation, Methodology, Visualization, Writing – original draft, Writing – review & editing. SZ: Data curation, Writing – review & editing, Formal analysis. AM: Data curation, Writing – review & editing, Formal analysis. AP: Conceptualization, Funding acquisition, Supervision, Writing – review & editing, Resources. K-JD: Conceptualization, Data curation, Validation, Writing – review & editing, Resources.
Funding
The author(s) declare financial support was received for the research, authorship, and/or publication of this article. This work is part of the FORK project funded by the Federal Ministry of Education and Research (BMBF, FKZ: 13FH118PA8). We acknowledge the financial support of the German Research Foundation (DFG) and the Open Access Publication Fund of Bielefeld University for the article processing charge.
Acknowledgments
The authors thank Dr. Rasha Haj Nuaima (Julius Kühn-Institut, Braunschweig, Germany) for providing Pochonia chlamydosporia and Dr. Jan Henrik Schmidt (Julius Kühn-Institut, Braunschweig, Germany) for providing Meloidogyne hapla juveniles. The authors further thank Dr. Marcus Persicke (Centre for Biotechnology, Bielefeld University, Germany) for primary metabolite analysis, Prisca Viehöver (sequencing core facility, Centre for Biotechnology, Bielefeld University, Germany) for RNA quality checks, preparation of cDNA libraries and RNA sequencing, and Prof. Dr. Andrea Bräutigam for her support during data processing.
Conflict of interest
The authors declare that the research was conducted in the absence of any commercial or financial relationships that could be construed as a potential conflict of interest.
Publisher’s note
All claims expressed in this article are solely those of the authors and do not necessarily represent those of their affiliated organizations, or those of the publisher, the editors and the reviewers. Any product that may be evaluated in this article, or claim that may be made by its manufacturer, is not guaranteed or endorsed by the publisher.
Supplementary material
The Supplementary Material for this article can be found online at: https://www.frontiersin.org/articles/10.3389/fpls.2024.1497575/full#supplementary-material
References
Abad, P., Williamson, V. M. (2010). “Plant nematode interaction: A sophisticated dialogue,” in Advances in Botanical Research (United Kingdom: Academic Press), 147–192. doi: 10.1016/S0065-2296(10)53005-2
Abd-Elgawad, M. M. (1991). A new rating scale for screening plant genotypes against root-knot and reniform nematodes. Anz. Schadlingskde, Pflanzenschutz, Umweltschutz 64, 37–39. doi: 10.1111/j.1365-3059.2006.01455.x
Adam, M. A. M., Phillips, M. S., Blok, V. C. (2007). Molecular diagnostic key for identification of single juveniles of seven common and economically important species of root-knot nematode (Meloidogyne spp.). Plant Pathol. 56, 190–197. doi: 10.1111/j.1365-3059.2006.01455.x
Altschul, S. F., Gish, W., Miller, W., Myers, E. W., Lipman, D. J. (1990). Basic local alignment search tool. J. Mol. Biol. 215, 403–410. doi: 10.1016/S0022-2836(05)80360-2
Amdadul Hoque, A. K. M., Bhuiyan, R.M.D., Ashik Iqbal Khan, M., Mahmud, A., Uddin Ahmad, M. (2014). Effect of amino acids on root-knot nematode (Meloidogyne javanica) infecting tomato plant. Arch. Phytopathol. Plant Prot. 47, 1921–1928. doi: 10.1080/03235408.2013.862039
Apel, K., Hirt, H. (2004). Reactive oxygen species: Metabolism, Oxidative Stress, and Signal Transduction. Annu. Rev. Plant Biol. 55, 373–399. doi: 10.1146/annurev.arplant.55.031903.141701
Aranda-Martinez, A., Lenfant, N., Escudero, N., Zavala-Gonzalez, E. A., Henrissat, B., Lopez-Llorca, L. V. (2016). CAZyme content of Pochonia chlamydosporia reflects that chitin and chitosan modification are involved in nematode parasitism. Environ. Microbiol. 18, 4200–4215. doi: 10.1111/1462-2920.13544
Araújo, W. L., Nunes-Nesi, A., Fernie, A. R. (2011). Fumarate: Multiple functions of a simple metabolite. Phytochemistry 72, 838–843. doi: 10.1016/j.phytochem.2011.02.028
Askary, T. H. (2015). “Nematophagous fungi as biocontrol agents of phytonematodes,” in Biocontrol Agents of Phytonematodes. Eds. Askary, T. H., Martinelli, P. R. P. (CAB International, Wallingford, UK), 81–125. doi: 10.1079/9781780643755.0081
Askary, T. H., Martinelli, P. R. P. (2015). Biocontrol Agents of Phytonematodes (Wallingford, UK: CAB International), 470, ISBN: 978-1-78064-375-5.
Avelar Monteiro, T. S., Lopes, E. A., Evans, H. C., Grassi de Freitas, L. (2017). “Interactions between Pochonia chlamydosporia and nematodes,” in Perspectives in Sustainable Nematode Management Through Pochonia chlamydosporia Applications for Root and Rhizosphere Health. Eds. Manzanilla-López, R. H., Lopez-Llorca, L. V. (Springer International Publishing, Cham). doi: 10.1007/978-3-319-59224-4
Baker, C. J., Orlandi, E. W. (1995). Active oxygen in plant pathogenesis. Annul. Rev. Phytopathol. 33, 299–321. doi: 10.1146/annurev.py.33.090195.001503
Bali, S., Kaur, P., Sharma, A., Ohri, P., Bhardwaj, R., AlYemeni, M. N., et al. (2018). Jasmonic acid-induced tolerance to root-knot nematodes in tomato plants through altered photosynthetic and antioxidative defense mechanisms. Protoplasma 255, 471–484. doi: 10.1007/s00709-017-1160-6
Becker, R. A., Chambers, J. M., Wilks, A. R., Wilks, A. R. (1996). The new S language: a programming environment for data analysis and graphics. (Boca Raton: Chapman & Hall).
Benjamini, Y., Hochberg, Y. (1995). Controlling the false discovery rate: a practical and powerful approach to multiple testing. J. R. Stat. Soc. Ser. B 57, 289–300. doi: 10.1111/j.2517-6161.1995.tb02031.x
Bray, N. L., Pimentel, H., Melsted, P., Pachter, L. (2016). Near-optimal probabilistic RNA-seq quantification. Nat. Biotechnol. 34, 525–527. doi: 10.1038/nbt.3519
Carter, C. (1985). Literature search: host range of Meloidogyne hapla. Int. Nematol. Network Newslett. 2, 16–24.
Coutinho, R. R., Pacheco, P. V. M., Monteiro, T. S. A., Balbino, H. M., Moreira, B. C., Freitas, L. G. D. (2021). Root colonization and growth promotion of cover crops by Pochonia chlamydosporia. Rhizosphere 20, 100432. doi: 10.1016/j.rhisph.2021.100432
Dávila-Negrón, M., Dickson, D. W. (2013). Comparative thermal-time requirements for development of Meloidogyne arenria, M. incognita and M. javanica at constant temperatures. Nematropica 43, 152–163.
Davletova, S., Rizhsky, L., Liang, H., Shengqiang, Z., Oliver, D. J., Coutu, J., et al. (2005). Cytosolic ascorbate peroxidase 1 is a central component of the reactive oxygen gene network of arabidopsis. Plant Cell 17, 268–281. doi: 10.1105/tpc.104.026971
Denjalli, I., Knieper, M., Uthoff, J., Vogelsang, L., Kumar, V., Seidel, T., et al. (2024). The centrality of redox regulation and sensing of reactive oxygen species in abiotic and biotic stress acclimatization. J. Exp. Bot. 75, 4494–4511. doi: 10.1093/jxb/erae041
de Souza Gouveia, A., Monteiro, T. S. A., Valadares, S. V., Sufiate, B. L., De Freitas, L. G., Ramos, H. J. D. O., et al. (2019). Understanding how pochonia chlamydosporia increases phosphorus availability. Geomicrobiol. J. 36, 747–751. doi: 10.1080/01490451.2019.1616857
Dhawan, S. C., Singh, S. (2010). Management of root-knot nematode, Meloidogyne incognita using Pochonia chlamydosporia on okra. Indian J. Nematol. 40, 171–178. doi: 10.1016/j.sjbs.2021.07.081
Dietz, K.-J., Vogelsang, L. (2024). A general concept of quantitative abiotic stress sensing. Trends Plant Sci. 29, 319–328. doi: 10.1016/j.tplants.2023.07.006
Dutta, T. K., Rupinikrishna, K., Akhil, V. S., Vashisth, N., Phani, V., Pankaj, et al. (2024). CRISPR/Cas9-induced knockout of an amino acid permease gene (AAP6) reduced Arabidopsis thaliana susceptibility to Meloidogyne incognita. BMC Plant Biol. 24, 515. doi: 10.1186/s12870-024-05175-5
Escudero, N., Lopez-Llorca, L. V. (2012). Effects on plant growth and root-knot nematode infection of an endophytic GFP transformant of the nematophagous fungus Pochonia chlamydosporia. Symbiosis 57, 33–42. doi: 10.1007/s13199-012-0173-3
Fan, J. W., Hu, C. L., Zhang, L. N., Li, Z. L., Zhao, F. K., Wang, S. H. (2015). Jasmonic acid mediates tomato’s response to root knot nematodes. J. Plant Growth Regul. 34, 196–205. doi: 10.1007/s00344-014-9457-6
Ghahremani, Z., Escudero, N., Marín, I., Sanz, A., García, S., Expósito, A., et al. (2022). Pochonia chlamydosporia is the most prevalent fungal species responsible for meloidogyne suppression in sustainable vegetable production systems. Sustainability 14, 16941. doi: 10.3390/su142416941
Ghahremani, Z., Escudero, N., Saus, E., Gabaldón, T., Sorribas, F. J. (2019). Pochonia chlamydosporia Induces Plant-Dependent Systemic Resistance to Meloidogyne incognita. Front. Plant Sci. 10. doi: 10.3389/fpls.2019.00945
Giovannetti, M., Mosse, B. (1980). An evaluation of techniques for measuring vesicular arbuscular mycorrhizal infection in roots. New Phytol. 84, 489–500. doi: 10.1111/j.1469-8137.1980.tb04556.x
Gouveia, A. D. S., Monteiro, T. S. A., Balbino, H. M., Magalhães, F. C. D., Ramos, M. E. S., Moura, V. A. S., et al. (2023). Inoculation of Pochonia chlamydosporia triggers a defense response in tomato roots, affecting parasitism by Meloidogyne javanica. Microbiol. Res. 266, 127242. doi: 10.1016/j.micres.2022.127242
Haas, B. J., Papanicolaou, A., Yassour, M., Grabherr, M., Blood, P. D., Bowden, J., et al. (2013). De novo transcript sequence reconstruction from RNA-seq using the Trinity platform for reference generation and analysis. Nat. Protoc. 8, 1494–1512. doi: 10.1038/nprot.2013.084
Halim, V. A., Vess, A., Scheel, D., Rosahl, S. (2006). The role of salicylic acid and jasmonic acid in pathogen defence. Plant Biol. 8, 307–313. doi: 10.1055/s-2006-924025
Hallmann, J., Davies, K. G., Sikora, R. (2009). “Biological control using microbial pathogens, endophytes and antagonists,” in Root-knot Nematodes. Eds. Perry, R. N., Moens, M., Starr, J. L. (CABI, UK), 380–411. doi: 10.1079/9781845934927.0380
Hause, B., Schaarschmidt, S. (2009). The role of jasmonates in mutualistic symbioses between plants and soil-born microorganisms. Phytochemistry 70, 1589–1599. doi: 10.1016/j.phytochem.2009.07.003
Hunt, H. W., Coleman, D. C., Ingham, E. R., Ingham, R. E., Elliott, E. T., Moore, J. C., et al. (1987). The detrital food web in a shortgrass prairie. Biol. Fertil. Soils 3, 57–68. doi: 10.1007/BF00260580
Jaubert, S., Laffaire, J.-B., Abad, P., Rosso, M.-N. (2002). A polygalacturonase of animal origin isolated from the root-knot nematode Meloidogyne incognita. FEBS Lett. 522, 109–112. doi: 10.1016/s0014-5793(02)02906-x
Jones, J. T., Haegeman, A., Danchin, E. G. J., Gaur, H. S., Helder, J., Jones, M. G. K., et al. (2013). Top 10 plant-parasitic nematodes in molecular plant pathology. Mol. Plant Pathol. 14, 946–961. doi: 10.1111/mpp.12057
Kanehisa, M., Goto, S. (2000). KEGG: kyoto encyclopedia of genes and genomes. Nucleic Acids Res. 28, 27–30. doi: 10.1093/nar/28.1.27
Kerry, B. R., Kirkwood, I. A., de Leij, F. A. A. M., Barba, J., Leijdens, M. B., Brookes, P. C. (1993). Growth and survival of Verticillium chlamydosporium goddard, a parasite of nematodes, in soil. Biocontrol Sci. Technol. 3, 355–365. doi: 10.1080/09583159309355290
Kidwai, M., Ahmad, I. Z., Chakrabarty, D. (2020). Class III peroxidase: an indispensable enzyme for biotic/abiotic stress tolerance and a potent candidate for crop improvement. Plant Cell Rep. 39, 1381–1393. doi: 10.1007/s00299-020-02588-y
Kumar, V., Wegener, M., Knieper, M., Kaya, A., Viehhauser, A., Dietz, K.-J. (2024). “Strategies of molecular signal integration for optimized plant acclimation to stress combinations. Methods in molecular biology,” in Plant Stress Tolerance: Methods and Protocols. Ed. Sunkar, R. (Springer US, New York, NY). doi: 10.1007/978-1-0716-3973-3
Lamb, C., Dixon, R. A. (1997). The oxidative burst in plant disease resistance. Annu. Rev. Plant Physiol. Plant Mol. Biol. 48, 251–275. doi: 10.1146/annurev.arplant.48.1.251
Larriba, E., Jaime, M. D. L. A., Carbonell-Caballero, J., Conesa, A., Dopazo, J., Nislow, C., et al. (2014). Sequencing and functional analysis of the genome of a nematode egg-parasitic fungus, Pochonia chlamydosporia. Fungal Genet. Biol. 65, 69–80. doi: 10.1016/j.fgb.2014.02.002
Larriba, E., Jaime, M. D. L. A., Nislow, C., Martín-Nieto, J., Lopez-Llorca, L. V. (2015). Endophytic colonization of barley (Hordeum vulgare) roots by the nematophagous fungus Pochonia chlamydosporia reveals plant growth promotion and a general defense and stress transcriptomic response. J. Plant Res. 128, 665–678. doi: 10.1007/s10265-015-0731-x
Liu, X. Z., Chen, S. Y. (2003). Nutritional requirements of Pochonia chlamydosporia and ARF18, fungal parasites of nematode eggs. J. Invertebr. Pathol. 83, 10–15. doi: 10.1016/S0022-2011(03)00037-5
Manzanilla-López, R. H., Esteves, I., Powers, S. J., Kerry, B. R. (2011). Effects of crop plants on abundance of Pochonia chlamydosporia and other fungal parasites of root-knot and potato cyst nematodes. Ann. Appl. Biol. 159, 118–129. doi: 10.1111/j.1744-7348.2011.00479.x
Manzanilla-López, R. H., Lopez-Llorca, L. V. (Eds.) (2017). Perspectives in Sustainable Nematode Management Through Pochonia chlamydosporia Applications for Root and Rhizosphere Health (Cham, Switzerland: Springer International Publishing). doi: 10.1007/978-3-319-59224-4
Mardia, K. V., Kent, J. T., Bibby, J. M. (1981). Multivariate analysis, Probability and mathematical statistics. Z Angew Math Mech. 61, 206–206. doi: 10.1002/zamm.19810610315
Mata-Pérez, C., Spoel, S. H. (2019). Thioredoxin-mediated redox signalling in plant immunity. Plant Sci. 279, 27–33. doi: 10.1016/j.plantsci.2018.05.001
McGonigle, T. P., Miller, M. H., Evans, D. G., Fairchild, G. L., Swan, J. A. (1990). A new method which gives an objective measure of colonization of roots by vesicular—arbuscular mycorrhizal fungi. New Phytol. 115, 495–501. doi: 10.1111/j.1469-8137.1990.tb00476.x
Medeirosa, H. A. D., Resende, R. S., Ferreira, F. C., Freitas, L. G., Rodrigues, F.Á. (2015). Induction of resistance in tomato against Meloidogyne javanica by Pochonia chlamydosporia. Nematoda 2, e10015. doi: 10.4322/nematoda.10015
Mitchum, M. G., Hussey, R. S., Baum, T. J., Wang, X., Elling, A. A., Wubben, M., et al. (2013). Nematode effector proteins: an emerging paradigm of parasitism. New Phytol. 199, 879–894. doi: 10.1111/nph.12323
Nakamura, M., Iwai, H. (2019). Functions and mechanisms: polygalacturonases from plant pathogenic fungi as pathogenicity and virulence factors. J. Gen. Plant Pathol. 85, 243–250. doi: 10.1007/s10327-019-00856-8
Noskov, I., Blum, H., Komnik, H., Hallmann, J. (2024). Host Status and Response Differences of Flat-Leaf and Curly-Leaf Parsley to Meloidogyne hapla, M. chitwoodi, M. fallax, and M. incognita Infestation. Plants 13, 1730. doi: 10.3390/plants13131730
Nuaima, R. H., Ashrafi, S., Maier, W., Heuer, H. (2021). Fungi isolated from cysts of the beet cyst nematode parasitized its eggs and counterbalanced root damages. J. Pest Sci. 94, 563–572. doi: 10.1007/s10340-020-01254-2
Pentimone, I., Colagiero, M., Ferrara, M., Nigro, F., Rosso, L. C., Ciancio, A. (2019). Time-dependent effects of Pochonia chlamydosporia endophytism on gene expression profiles of colonized tomato roots. Appl. Microbiol. Biotechnol. 103, 8511–8527. doi: 10.1007/s00253-019-10058-z
Pfannkuche, O., Thiel, H. (1988). “Sample processing,” in Introduction to the study of meiofauna. Eds. Higgins, R. P., Thiel, H. (Smithsonian Institution Press, Washington, D.C., USA; London, UK), 134–145.
Plassmeier, J., Barsch, A., Persicke, M., Niehaus, K., Kalinowski, J. (2007). Investigation of central carbon metabolism and the 2-methylcitrate cycle in Corynebacterium glutamicum by metabolic profiling using gas chromatography–mass spectrometry. J. Biotechnol. 130, 354–363. doi: 10.1016/j.jbiotec.2007.04.026
R Core Team (2023). R: A language and environment for statistical computing (Vienna, Austria: R Foundation for Statistical Computing). Available at: https://www.R-project.org/ (Accessed August 9, 2022).
Ritchie, M. E., Phipson, B., Wu, D., Hu, Y., Law, C. W., Shi, W., et al. (2015). limma powers differential expression analyses for RNA-sequencing and microarray studies. Nucleic Acids Res. 43, e47–e47. doi: 10.1093/nar/gkv007
Robinson, M. D., McCarthy, D. J., Smyth, G. K. (2010). edgeR: a Bioconductor package for differential expression analysis of digital gene expression data. Bioinformatics 26, 139–140. doi: 10.1093/bioinformatics/btp616
Saha, N., Schwarz, T., Mowery, S., Gorny, A. M. (2023). Reaction of Winter Cover Crops to Meloidogyne enterolobii and Glasshouse Bioassay for Evaluating Utility in Managing M. enterolobii in Soybeans. J. Nematol. 55, 20230014. doi: 10.2478/jofnem-2023-0014
Scheu, S. (2002). The soil food web: structure and perspectives. Eur. J. Soil Biol. 38, 11–20. doi: 10.1016/S1164-5563(01)01117-7
Schouten, A. (2016). Mechanisms involved in nematode control by endophytic fungi. Annu. Rev. Phytopathol. 54, 121–142. doi: 10.1146/annurev-phyto-080615-100114
Shukla, N., Kaur, P., Kumar, A. (2016). Molecular aspects of plant-nematode interactions. Indian J. Plant Physiol. 21, 477–488. doi: 10.1007/s40502-016-0263-y
Shukla, N., Yadav, R., Kaur, P., Rassmussen, S., Goel, S., Agarwal, M., et al. (2018). Transcriptome analysis of root-knot nematode (Meloidogyne incognita)-infected tomato (Solanum lycopersicum) roots reveals complex gene expression profiles and metabolic networks of both host and nematode during susceptible and resistance responses. Mol. Plant Pathol. 19, 615–633. doi: 10.1111/mpp.12547
Siddique, S., Endres, S., Atkins, J. M., Szakasits, D., Wieczorek, K., Hofmann, J., et al. (2009). Myo-inositol oxygenase genes are involved in the development of syncytia induced by Heterodera schachtii in Arabidopsis roots. New Phytol. 184, 457–472. doi: 10.1111/j.1469-8137.2009.02981.x
Siddique, S., Endres, S., Sobczak, M., Radakovic, Z. S., Fragner, L., Grundler, F. M. W., et al. (2014). Myo-inositol oxygenase is important for the removal of excess myo-inositol from syncytia induced by Heterodera schachtii in Arabidopsis roots. New Phytol. 201, 476–485. doi: 10.1111/nph.12535
Stojilković, B., Xiang, H., Chen, Y., Maulana, M. I., Bauters, L., Van de Put, H., et al. (2024). The nematode effector Mj-NEROSs interacts with Rieske’s iron–sulfur protein influencing plastid ROS production to suppress plant immunity. New Phytol. 242, 2787–2802. doi: 10.1111/nph.19781
Strasser, H., Forer, A., Schinner, F. (1996). “Development of media for the selective isolation and maintenance of virulence of Beauveria brongniartii” in Proceedings of the 3rd International Workshop on Microbial Control of Soil Dwelling Pests, eds. Glare, T., Jackson, T. (Lincoln, New Zealand: Canterbury Agriculture & Science Centre), 125–130.
Thies, J. E., Grossman, J. M. (2023). “The soil habitat and soil ecology,” in Biological Approaches to Regenerative Soil Systems, 2nd ed. eds. Uphoff, N., Thies, J. (Boca Raton: CRC press). doi: 10.1201/9781003093718-9
Tobin, J. D., Haydock, P. P. J., Hare, M. C., Woods, S. R., Crump, D. H. (2008). Effect of the fungus Pochonia chlamydosporia and fosthiazate on the multiplication rate of potato cyst nematodes (Globodera pallida and G. rostochiensis) in potato crops grown under UK field conditions. Biol. Control 46, 194–201. doi: 10.1016/j.biocontrol.2008.03.014
Tolba, S. R. T., Rosso, L. C., Pentimone, I., Colagiero, M., Moustafa, M. M. A., Elshawaf, I. I. S., et al. (2021). Root Endophytism by Pochonia chlamydosporia Affects Defense-Gene Expression in Leaves of Monocot and Dicot Hosts under Multiple Biotic Interactions. Plants 10, 718. doi: 10.3390/plants10040718
Uthoff, J., Jakobs-Schönwandt, D., Schmidt, J. H., Hallmann, J., Dietz, K.-J., Patel, A. (2023). Biological enhancement of the cover crop Phacelia tanacetifolia (Boraginaceae) with the nematophagous fungus Pochonia chlamydosporia to control the root-knot nematode Meloidogyne hapla in a succeeding tomato plant. BioControl 69, 77–90. doi: 10.1007/s10526-023-10222-5
Van Damme, V., Hoedekie, A., Viaene, N. M. (2005). Long-term efficacy of Pochonia chlamydosporia for management of Meloidogyne javanica in glasshouse crops. Nematology 7, 727–736. doi: 10.1163/156854105775142973
Venables, W. N., Ripley, B. D. (2002). Modern Applied Statistics with S (New York: Springer-Verlag). doi: 10.1007/978-0-387-21706-2
Viaene, N. M., Abawi, G. S. (2000). Hirsutella rhossiliensis and Verticillium chlamydosporium as biocontrol agents of the root-knot nematode Melodiogyne hapla on lettuce. J. Nematol 32, 85–100.
Vieira dos Santos, M. C., Horta, J., Moura, L., Pires, D. V., Conceição, I., Abrantes, I., et al. (2019). An integrative approach for the selection of Pochonia chlamydosporia isolates for biocontrol of potato cyst and root knot nematodes. Phytopathol. Mediterr. 1, 187–199. doi: 10.13128/Phytopathol_Mediterr-23780
Wegener, M., Persicke, M., Dietz, K.-J. (2023). Reprogramming the translatome during daily light transitions as affected by cytosolic glyceraldehyde-3-phosphate dehydrogenases GAPC1/C2. J. Exp. Bot. 75, 2494–2509. doi: 10.1093/jxb/erad509
Wickham, H. (2009). ggplot2: Elegant Graphics for Data Analysis (New York: Springer-Verlag). Available at: https://ggplot2.tidyverse.org (Accessed August 21, 2022).
Yop, G., de, S., Gair, L. H. V., da Silva, V. S., MaChado, A. C. Z., Santiago, D. C., et al. (2023). Abscisic Acid Is Involved in the Resistance Response of Arabidopsis thaliana Against Meloidogyne paranaensis. Plant Dis. 107, 2778–2783. doi: 10.1094/PDIS-07-22-1726-RE
Zavala-Gonzalez, E. A., Rodríguez-Cazorla, E., Escudero, N., Aranda-Martinez, A., Martínez-Laborda, A., Ramírez-Lepe, M., et al. (2017). Arabidopsis thaliana root colonization by the nematophagous fungus Pochonia chlamydosporia is modulated by jasmonate signaling and leads to accelerated flowering and improved yield. New Phytol. 213, 351–364. doi: 10.1111/nph.14106
Keywords: plant defense, biotic stress, synergistic effects, systemic plant response, biological control, plant-parasitic nematodes
Citation: Könker J, Zenker S, Meierhenrich A, Patel A and Dietz K-J (2025) Pochonia chlamydosporia synergistically supports systemic plant defense response in Phacelia tanacetifolia against Meloidogyne hapla. Front. Plant Sci. 15:1497575. doi: 10.3389/fpls.2024.1497575
Received: 17 September 2024; Accepted: 17 December 2024;
Published: 16 January 2025.
Edited by:
Mahfouz M. M. Abd-Elgawad, National Research Centre, EgyptReviewed by:
Justyna Nawrocka, University of Łódź, PolandHeriksen Higashi Puerari, Federal University of Piauí, Brazil
Tarique Hassan Askary, Sher-e-Kashmir University of Agricultural Sciences and Technology, India
Copyright © 2025 Könker, Zenker, Meierhenrich, Patel and Dietz. This is an open-access article distributed under the terms of the Creative Commons Attribution License (CC BY). The use, distribution or reproduction in other forums is permitted, provided the original author(s) and the copyright owner(s) are credited and that the original publication in this journal is cited, in accordance with accepted academic practice. No use, distribution or reproduction is permitted which does not comply with these terms.
*Correspondence: Jana Könker, amFuYS51dGhvZmZAdW5pLWJpZWxlZmVsZC5kZQ==