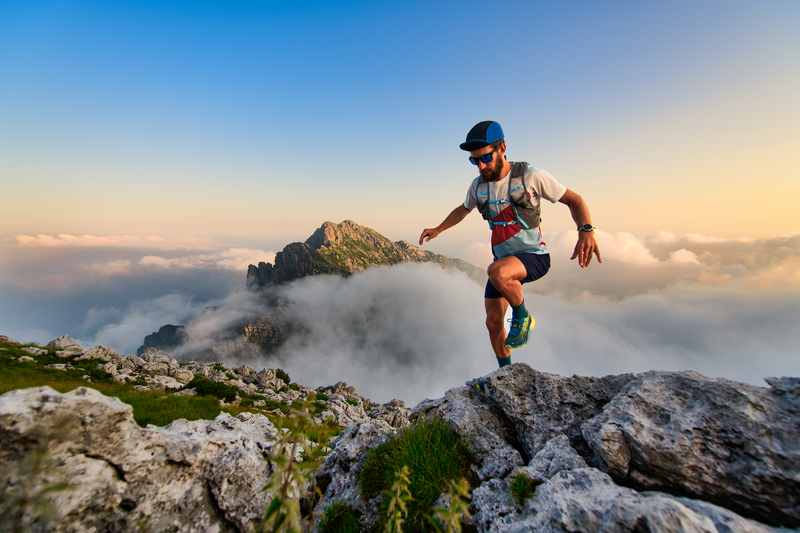
95% of researchers rate our articles as excellent or good
Learn more about the work of our research integrity team to safeguard the quality of each article we publish.
Find out more
ORIGINAL RESEARCH article
Front. Plant Sci. , 10 January 2025
Sec. Functional Plant Ecology
Volume 15 - 2024 | https://doi.org/10.3389/fpls.2024.1471415
The ecophysiological and ecohydrological impacts of climate change and progressively increasing atmospheric carbon dioxide (CO2) concentration on agroecosystems are not well understood compared to the forest ecosystems. In this study, we utilized the presence of old apple and pear trees in the alpine valleys of Northern Italy (maintained for cultural heritage purposes) to investigate climate-scale physiological responses. We developed long-term tree-ring stable isotopic records (δ13C and δ18O) from apple (1976-2021) and pear trees (1943-2021). This allowed the reconstruction of key ecophysiological processes like the variations in intrinsic water use efficiency (iWUE), and we investigated how these trees responded to climate and CO2 changes over decades. Results showed a slight declining trend in carbon discrimination (Δ13C) while intercellular CO2 concentration (Ci) for both species has been increasing since the late 1980s. Concurrently both species exhibited a rising trend in iWUE, with apple trees demonstrating higher efficiency, which appears to be primarily driven by the CO2-fertilization effect. The concomitant trends in tree-ring δ18O suggested a relatively stable local hydroclimate during the study period with some species-specific responses. Analyses further revealed that minimum growing season temperature, not precipitation was the most significant factor influencing the rise in iWUE alongside with CO2 fertilization effect. Analyses of species’ δ13C coupled with their respective δ18O confirmed that the rise in iWUE was due to increased carbon assimilation rather than a decline in evapotranspiration. Moreover, coupled δ13C–δ18O analyses suggested increasing trends in carbon assimilation, with apple trees showing higher inter-decadal variations. These long-term records provide a unique opportunity to test and calibrate how these systems respond to recent and anticipated climate change.
The forest and agroecosystems in the European Alps play a very important role in providing food, goods, and ecosystem services (Stenger et al., 2009). However, concurrent climatic change and continually increasing atmospheric CO2 concentration are expected to strongly affect the ecophysiology of these ecosystems, which could alter their productivity. The fertilizing effects of rising CO2 levels, along with nitrogen depositions and increasing temperatures, have been shown to positively affect the productivity of European forests (Hyvönen et al., 2007; Giammarchi et al., 2017). Important studies that analyzed stable tree-ring isotopes across European forests have revealed valuable insights into forest ecosystem functioning and responses to climate change, including the CO2-fertilization effect (Huang et al., 2024). The intrinsic water use efficiency (iWUE: ratio of photosynthesis to stomatal conductance) along with forest transpiration was found to increase over the 20th century with a consistent south-to-north gradient, which largely depended upon local growth limiting factors (Peñuelas et al., 2011). Across Europe, the strongest increase in iWUE was observed in the water-limited temperate forests in the central region (Saurer et al., 2014). Concerning the magnitude, mechanisms, and spatial patterns, the impact of climate change on European forests is quite diverse depending upon the geography and local growth limiting factors (Saurer et al., 2014; Frank et al., 2015).
Nevertheless, the response of tree physiology to increasing CO2 levels is far from being a straightforward one, indeed it strongly depends on local conditions with a species-specific response (Huang et al., 2024). It could interact with other climate drivers, such as warming-induced soil drying and physiological acclimation to high CO2 levels (Saurer et al., 2014; Frank et al., 2015). Consequently, a global analysis of tree-ring isotope datasets indicates diminishing CO2-driven gains in iWUE, in which deciduous species contributed more than conifers to the recent slowdown (Adams et al., 2020). Particularly, European forests at the northern periphery show a progressively diminishing response to increasing CO2 concentration (Waterhouse et al., 2004). Moreover, the debate on the relative roles of enhanced photosynthesis vs reduced stomatal conductance in the global trends of iWUE has been tried to settle by combining tree‐ring δ13C and δ18O datasets with a water–carbon optimality model (Lin et al., 2022; Walker et al., 2021).
Conversely, the response of climate change including CO2-fertilization effect on the physiological functioning of economically important agroecosystems is less explored. This is primarily because of the lack of old wild or cultivated trees having long-term tree-ring width or isotopic records. Tree-ring stable isotopic records (δ13C and δ18O) differ from classical dendrochronological variables (such as width) as they reflect more directly the plant’s physiological response to climate and environmental variables (Wang et al., 2019). δ13C values depend on factors affecting the photosynthetic uptake of CO2 and are mainly controlled by stomatal conductance and the rate of carboxylation during photosynthesis. Whereas, δ18O values are constrained by the isotopic ratio of the source water and locally integrate the stomatal response to vapor pressure deficit via leaf water enrichment, coupled with transpiration. These factors controlling isotopic fractionation are closely related to the meteorological variables (McCarroll and Loader, 2004; Battipaglia et al., 2013; Gagen et al., 2022). In this context, tree isotopes provide precise, reliable, large-scale, and long-term information to advance our understanding of ecosystem functioning, carbon – water cycling and to reconstruct key metrics and processes for the decades preceding observational data (Babst et al., 2014). Moreover, dual isotope analysis (δ18O – δ13C) provides a physiological basis to understand carbon – water processes including stomatal conductance and the effect of climate warming and CO2 –fertilization (Siegwolf et al., 2023). The physiological interpretation of tree-ring δ18O is indeed complex (Lin et al., 2022). Yet, it remains the only proxy in conjunction with δ13C, which could be used to reconstruct mechanisms through which iWUE changes in response to climatic drivers, including atmospheric CO2 (Siegwolf et al., 2023).
In this study, we generated long-term stable isotope (δ13C and δ18O) records of unique and old apple (1976-2021) and pear trees (1943-2021), which constitute major agro-economic crops in the Italian Alps (Alto Adige, Northern Italy, Figure 1). The alpine valleys of the region is a major apple production center in the country, where we analyzed δ13C and δ18O chronologies to understand how these trees responded to climate and CO2 changes over decades. This study specifically aims to reconstruct δ13C-based ecophysiological processes and carbon-water coupling process (iWUE) and to investigate long-term climate – carbon responses. This approach lays the foundation for using wood carbon-oxygen stable isotope analysis to understand the meteorological constraints on fruit tree production potential.
Figure 1. Location map of studied apple and pear orchards in the alpine valleys of the Italian Alps from where tree cores have been collected. The meteorological plot indicates regional climatology (Meteorological station: Silandro; Precipitation: 1981-2021; Temperature: 1988-2021).
The study sites are located in valleys of the eastern Alps in South Tyrol, Italy (Figure 1). This region is a major apple producer, boasting around 18,000 hectares of apple orchards that contribute roughly half of Italy’s apple production. In the past, pear trees were also widely cultivated, but this practice declined significantly by the 1970s. Land previously used for pears now primarily houses apple orchards. Current apple yields in the region average around 55 tons per hectare. Due to the relatively short lifespan of apple and pear orchards (around 20-30 years), they are not ideal for studying long-term climate impacts. To address this challenge, we collaborated with experts from South Tyrolean Fruit Tree Cultivation Museum to access two rather unique sites featuring old veteran apple and pear trees maintained for cultural heritage purposes. The apple orchard, within the municipality of Lana (46.60° N, 11.16° E, 310 m asl), featuring trees of the variety “Gravenstein” grafted on seedling rootstocks, was established around 1976. The pear orchard is located in the municipality of Prato allo Stelvio (46.63° N, 10.61° E, 884 m asl). Trees of the variety “Bartlett”, grafted on seedling rootstocks, were planted between 1928 and 1938. The pear site sampling also included a monumental tree of more than 200 years old belonging to the variety “Pala Birne”. Both orchards have received regular management practices since their establishment, including pruning, fertilization, irrigation, pest and disease control, and fruit harvesting. The soil in the sampling area has a sandy loam texture and is relatively fertile due to its high organic matter content.
Meteorological data from the Schlanders-Silandro station (46.62° N, 10.72° E, 698 m asl), located between the two sampling sites (Figure 1), suggests that the sites are energy-limited ecosystems, where temperature remains optimal only during the short growing season (April – September). These records indicate annual precipitation (1981 – 2021) in the range of 400 – 800 mm, of which, the growing season months (April – September) receive about 60% and the rest mostly as snowfall during winter. The mean annual temperature (1988 – 2021) varies between -1.2 and 27.3°C, which remains above 13°C during the growing season (Figure 1). The range of variation of minimum temperature is -3.5 to 14.0°C, which remains above 5°C during the growing season (Figure 1). In recent decades, temperature trends have generally been upward, with an overall warming trend observed across the region. The average temperature in Europe has increased by around 1.5°C since the pre-industrial era, and the warming trend has been more pronounced in winter than in summer. According to the European Environment Agency, overall precipitation has remained relatively stable, with an increase of around 5% since the pre-industrial era.
For each species, five trees were randomly selected and from each tree, two cores were extracted at 0.5 m from the ground using a 10 mm diameter increment borer. These increment cores were air-dried and glued on wooden supports. The cross-sectional surface of the cores was sanded by increasingly fine sandpapers until growth rings were visible and finally digitalized with a high-resolution scanner (2400 d.p.i.; Epson Expression 10000XL, Long Beach). A standard image was created for each sample and all images were saved into a graphic file format for further analysis. Subsequently, the determination of the tree ring width of each sample was performed with the Coo-recorder software (Cybis Elektronik & Data AB, Saltsjöbaden, Sweden) at a precision level of 0.01 mm (García-Hidalgo et al., 2022).
We selected three individuals from each species based on the absence of biotic damages and on the chronological length for the isotopic analyses. Each year’s growth-ring was cut using a sharp razor blade under the binocular microscope and pooling was performed for the individual tree rings of corresponding age. We utilized the whole-wood for the stable isotope analyses (δ13C and δ18O) (Schollaen et al., 2017; Wieser et al., 2016). Stable isotope analyses were carried out at the Università degli studi della Campania “L. Vanvitelli” Dipartimento di Scienze e Tecnologie Ambientali Biologiche e Farmaceutiche, Caserta, Italy. The analytical precision was equal to or better than 0.2‰ for both the isotopes. The isotope ratios are presented in common δ-notation against international standard PDB and VSMOW respectively as:
Where, (13C/12C) sample and (13C/12C) PDB are heavy to light carbon isotope ratios in the wood sample and the standard (Vienna Pee Dee Belemnite), respectively. (18O/16O) sample and (18O/16O) VSMOW are heavy to light oxygen isotope ratios in the wood sample and the international standard (Vienna Standard Mean Ocean water) respectively.
Discrimination against 13C (Δ13C, ‰) during carbon fixation by trees was computed by using atmospheric (δ13Catm) and tree-ring (δ13Cplant) δ13C as:
Where, (δ13Catm) and (δ13Cplant) are fractional differences in isotopic composition (13C/12C) in atmospheric CO2 and that of tree-ring wood. We compiled δ13Catm from McCarroll and Loader (2004) up to the year 2004, and after that was derived from Belmecheri and Lavergne (2020) (https://scrippsco2.ucsd.edu/data/). A widely accepted procedure to correct tree-ring isotope chronology for the incorporation of isotopically light carbon released by the burning of fossil fuels and increasing CO2 concentration was adopted (McCarroll and Loader, 2004). The correction procedure has the advantage of being an objective one as it effectively removes any declining trend in the δ13C series post AD 1850, which is attributed to physiological response to increased atmospheric CO2 concentrations (McCarroll and Loader, 2004).
Carbon isotopic discrimination (Δ13C) is related to intercellular CO2 (Ci) and atmospheric CO2 (Ca) concentration as:
Where, ‘a’ is the fractionation factor during intercellular diffusion (−4.4‰), and ‘b’ is the fractionation factor during carboxylation (−27‰) (Farquhar et al., 1982). The ratio of Ci and Ca was determined as:
or,
Using Ci and Ca values, intrinsic water use efficiency (iWUE) was calculated as:
To substantiate our inferences, we computed a standardized carbon-to-oxygen isotope difference index for all species over the entire observation period, following the model of Scheidegger et al. (2000):
Here, n is the year. Both corrected δ13C and δ18O chronologies were transformed into z-scores (based on the long-term mean and std. dev.) and analyzed in pairs for each crop. This index allows tracking year-by-year changes in trees’ physiological conditions induced by changes in stomatal conductance and photosynthetic capacity. The index assumes values close to 0 when both isotope ratios show similar values, indicating either enhanced stomatal conductance (both isotopic values are negative) or reduced stomatal conductance (both isotopic values are positive). Positive index values indicate high photosynthetic capacity (high δ13C and low δ18O), while negative values indicate low photosynthetic capacity (low δ13C and high δ18O) (Scheidegger et al., 2000; Siegwolf et al., 2023).
To understand relationships between δ13C-based processes (Ci, Δ13C, iWUE) in the two species and the regional climate (Precipitation and Temperature: Mean, Max., Min.), simple Pearson correlations were applied with a response-function approach. The confidence intervals of correlations were analyzed at 95% and 99% levels. This helped to investigate the correlations with monthly climatic averages in the species. To corroborate and stabilize these relations further, we plotted 3-month moving correlation coefficients between physiological processes and monthly hydroclimatic data (precipitation: 1982-2021; temperature: 1988-2021). The response function analysis for the species was plotted from October of the previous growth year to September of the current year (pOct-Sep). To test the relative importance of climate parameters including atmospheric CO2 levels, multiple linear regression models with iWUE as the response variables, and temperature (mean, max, min), precipitation, and atmospheric CO2 as continuous predictor variables, were built.
To test the significance of the slopes (p-values), we first computed Mean Square Error (MSE) as: .
Then, the Standard Error (SE) of the slope was computed as: ; and the t-statistic was calculated as: .
Finally, using the t-distribution, we calculated the two-tailed p-value for the respective t-statistics and degrees of freedom (df = n – 2).
We used ‘lm’ function from the R statistical computing environment (R development Core team, 2015). The relative importance of significant terms was obtained by applying function ‘calc.relimp’ using default options (Supplementary Table S1). The process reconstructions were standardized using Z-scores and smoothed with a 3-year running mean to assess common signals.
Over the study period, raw δ13C in tree-rings of pear (1943-2021) and apple (1976-2021) species exhibited a slight decreasing trend (Figure 2). The break-point analysis identifies the year of change in carbon isotopic composition in the species as the year 1987-88. Therefore, 1990 is assigned as a reference year for the analyses. For the common period, inter-species correlation was moderate at the inter-annual scale (r = 0.398, p< 0.001), which indicates the predominance of both species-specific and site-specific local effects on the assimilation process. The mean δ13C value of apple trees was −25.6‰ (std. dev.: 0.40‰), while for the pear trees, it was ∼1.0‰ lower (−26.6 ± 0.32‰) (Figure 2A; Table 1). This difference suggests a higher level of assimilation rate (isotope discrimination) in pear trees (Figure 2B). However, pear and apple trees have reportedly similar daily radiation use efficiency (Auzmendi et al., 2013). The long-term mean of δ13C (corrected for atmospheric CO2 increase) in apple trees was −23.5 ± 0.6‰, which increased slightly (−23.2 ± 0.52‰) after 1990. The long-term mean for the pear trees (−25.1 ± 0.72‰) increased by ~ 1‰ after 1990 (−24.3 ± 0.42‰) (Supplementary Figure S1). Considering the changes in atmosphere-to-plant 13CO2 discrimination (Δ13C), we found a higher (~2.0‰) level of discrimination in pear trees, which showed a similar temporal pattern to that of apple trees (Figure 2; Table 1). The corrections of the carbon isotope series of the two species for the physiological responses to increasing concentrations of atmospheric CO2 are illustrated in the supplementary figure (Supplementary Figure S1).
Figure 2. (A) δ13C chronologies of pear and apple trees (Pear: 1943 – 2021; Apple: 1976 – 2021). Faded line with dots denote annual values. Colored dark lines represent three year moving mean with three-year moving std. dev. (B) Annual atmosphere-to-plant 13CO2 discrimination (Δ13C) trends in the species with 3-year moving std. dev.
A rising trend in intercellular CO2 (Ci) in the two species was noted during the observation period. The mean Ci value of the apple tree was 223 ± 10.3 µmol mol-1, while for the pear trees, it was 233 ± 13.6 µmol mol-1 (Table 1). Prior to 1990, the mean Ci of apple trees was 207 µmol mol-1 (range: 181 – 230 µmol mol-1), which increased to 227 µmol mol-1 (211 – 247 µmol mol-1). While, for the pear trees, the mean Ci before and after 1990 was 225 µmol mol-1 (211 – 251 µmol mol-1) and 245 µmol mol-1 (231 – 264 µmol mol-1), respectively (Figure 3). The trends in intercellular CO2 (Ci) to atmospheric CO2 (Ca), i.e., Ci/Ca ratio in both species were analogous to the trends in Δ13C. Nonetheless, a declining trend in both species is noticeable after 1990. Prior to 1990, the ratio for the apple trees was 0.62 (0.59 – 0.66), which decreased to 0.60 (0.56 – 0.64). While, in the pear trees the ratio before and after 1990 was 0.69 (0.66 – 0.71) and 0.65 (0.63 – 0.69), respectively. Fitted regression slopes for the Ci/Ca ratio (p< 0.05) in apple and pear trees prior to 1990 were 0.0021 (R2 = 0.2) and -0.0003 (R2 = 0.087), respectively. After 1990, magnitude of the slopes becomes more negative (Apple: -0.0011, R2 = 0.25; Pear: -0.0009, R2 = 0.28). Out of three theoretical scenarios of Ci/Ca ratios to CO2 rise (Panthi et al., 2020), a positive slope in apple trees suggests a ‘Ca – Ci = constant’ scenario prior to 1990 that changed to ‘Ci = constant’ scenario after 1990. Conversely, the response of pear trees appears to be a ‘Ci = constant’ scenario throughout the observation period. These results probably suggest a varying physiological response of tree species to atmospheric CO2 rise (Figure 3).
Figure 3. (A) Trends of Ci/Ca ratios in apple and pear trees during the study period. (B) Long-term Ci trends in the species with respect to increasing atmospheric CO2 (Ca: dashed black line). Light-colored lines with dots indicate annual values, while dark lines indicate 3-year moving average with 3-year moving std. dev.
The Δ13C discrimination at the plant level is controlled by Ci/Ca ratio. This ratio could decline either because of low stomatal/mesophyll conductance to CO2 associated with water stress or low temperatures, or due to a high assimilation rate (McCarroll and Loader, 2004). At our sites, in the alpine valleys with frequent irrigation, water cannot be assumed to be a limiting factor. Growth at higher latitudes is generally limited by suboptimal temperatures for xylogenesis (i.e., formation of water conductive tissue), which remains almost optimal during the growing season. In energy-limited ecosystems at higher latitudes, climate warming may improve tree-water status where xylogenesis is temperature-limited and given that sufficient water is available. Therefore, increasing assimilation rates due to rising CO2 levels could be another reason for the observed declining Ci/Ca ratio in recent decades. The effect of CO2 fertilization has been observed globally, which is quite pervasive in European forests, particularly over the northern ecosystems (Mathias and Thomas, 2021; Waterhouse et al., 2004; Saurer et al., 2014; Frank et al., 2015).
Hydro-climate response function: the Pearson correlation with a response function approach demonstrates the relationship between species’ physiological processes (δ13C, Δ13C, and Ci) and monthly hydroclimatic data (precipitation and temperature: mean, maximum, and minimum). To corroborate and stabilize these monthly relations, we plotted 3-month moving correlation coefficients (precipitation: 1982-2021; temperature: 1988-2021) (Figure 4). The response function analysis for the δ13C – precipitation relationship from October of the previous growth year to September of the current year (pOct-Sep) revealed non-significant correlations. The relationship confirms that water is not a limiting factor in these orchards, having provision of irrigation, especially for the apple trees. For this reason, at the beginning of the growing season (March-May) we observed an enhanced positive correlation for the pear trees. In contrast and irrespective of the seasons, δ13C – precipitation relationship for the apple trees was non-significant (Figure 4A). Likewise, Δ13C – and Ci – precipitation relationship during peak growing season (June-October) was significant for the pear trees but non-significant for the apple trees having inverse correlations (Figures 4B, C). Yet, Pearson correlations and 3-month moving correlations with temperature indicated that the latter has a major control on the species’ ecophysiological processes (Figures 4D–F). The δ13C – temperature (mean) relationship was non-significant for both species (Figure 4D). Nevertheless, we observed significant, Δ13C – and Ci – mean temperature correlations across the months for both species (Figures 4E, F). Particularly, the influence of minimum temperature on Δ13C and Ci was prominent during the entire growing season (March – October) (Supplementary Figures S2A–C). Whereas, maximum temperature appears to affect these processes during the start of the growing season (April-June) (Supplementary Figures S2D–F). The relationships between temperature (Min., Max.) and δ13C, Δ13C, and Ci have been detailed in the supplementary figure (Supplementary Figures S2A–F).
Figure 4. Hydro-climatic response function for Apple (orange) and Pear (green). (A) Monthly (left panel) correlations between δ13C time series of species and precipitation (1981-2021). The corresponding right panel indicates three-month moving correlation coefficients between δ13C and precipitation. (B) Monthly (left panel) and three-month moving (right panel) correlations between Δ13C and precipitation. (C) Monthly (left panel) and three-month moving (right panel) correlations between species’ Ci chronologies and precipitation. (D) Monthly (left panel) correlations between δ13C chronologies of species and mean temperature (1988-2021). The corresponding right panel indicates three-month moving correlation coefficients between δ13C and mean temperature. (E) Monthly (left panel) and three-month moving (right panel) correlations between species Δ13C values and mean temperature. (F) Monthly (left panel) and three-month moving (right panel) correlations between species Ci chronologies and mean temperature. The dotted horizontal line indicates a 95% confidence level. The dashed vertical line delimits months with seasonal aggregates. Prefix “p” before the months denotes the months of the previous growth year. The hydro-climatic response of species’ δ13C, Δ13C and Ci to minimum and maximum temperature has been illustrated in Supplementary Figure S2.
Oxygen isotope in the tree-rings complemented with respective δ13C values, remains the only proxy with proven potential to decipher a comprehensive picture of past and current ecophysiological status (Battipaglia et al., 2013; Nock et al., 2011; Siegwolf et al., 2023). Consequently, we have taken into account the δ18O chronologies of the trees (Figure 5). The mean δ18O value of pear trees (1943 – 2021) was 25.9 ± 0.99 ‰ (Coefficient of Variation (CV): 3.8%), while for the apple trees (1976 – 2021), it was ∼1.0 ‰ higher (26.3 ± 1.25 ‰) with a higher CV (5.6%) (Table 1). Prior to 1990, the mean δ18O of apple trees was 25.8 ‰ (range: 24.3 – 28.0 ‰), which showed a rising trend after 1990 with a similar range of variation. While, for the pear trees, the mean δ18O before 1990 was 26.3 ‰ (24.5 – 28.4 ‰), which dropped to 25.2 ‰ (23.5 – 26.3 ‰) since then (Figure 5A). The mean difference between species indicates a higher level of oxygen isotope discrimination and evapotranspiration in pear trees relative to the apple trees. At the same vapor pressure deficit, leaves with high transpiration rates are known to become isotopically enriched in heavy isotopes as compared to leaves having low transpiration (McCarroll and Loader, 2004; Battipaglia et al., 2013). The optimal transpiration rate coupled with the use of enriched surface irrigated water by the apple trees and differences in the stomatal conductance could be responsible for such an 18O enrichment offset. Moreover, because of limited evaporation, groundwater is less enriched (compared to surface water) and the probable use of this less enriched groundwater by the pear trees (having greater tree height and rooting depth) is reflected in its stable time series having lower CV (Figure 5A) and δ13C – precipitation relationship (Figure 4A). However, a decline (after 1990) in 18O enrichment (~1.0 ‰) in pear trees could be linked to increasing precipitation trend and irrigation provisioning.
Figure 5. (A) δ18O isotope chronologies of apple (orange line) and pear (green line) tree species. (B) Monthly (left panel) correlations between δ18O chronologies of species and precipitation (1981-2021). The corresponding right panel indicates three-month moving correlation coefficients between δ18O and precipitation. (C) Monthly (left panel) and three-month moving (right panel) correlations between species’ δ18O chronologies and mean temperature (1988-2021). The dotted horizontal line indicates a 95% confidence level. The dashed vertical line delimits months with seasonal aggregates. Prefix “p” before the months denotes the months of the previous growth year. The response of species’ δ18O to minimum and maximum temperature has been illustrated in Supplementary Figure S3.
Due to the above reasons, response function analysis for the monthly δ18O – precipitation relationship (pOct-Sep) showed opposite correlations in the species (Figures 5B, C). Cross-correlations of δ18O chronology of pear trees with precipitation revealed a strong positive relationship during the start of the growing season (March-May), which becomes negative during peak growing season (June-October). The δ18O – precipitation relationship for the apple trees, on the contrary, was opposite to that of the pear trees and insignificant throughout the growing season (March-October) (Figure 5B). Similarly, correlations with mean temperature indicated opposite but a major control on species’ ecophysiological processes associated with 18O enrichment, particularly during peak growing season (June-October). For this period, the correlation was significantly positive and negative for apple and pear trees, respectively (Figure 5C). In contrast, during March-May, correlation was negative in both species but remained statistically insignificant. Both Pearson and 3-month moving correlations between minimum temperature and δ18O have been detailed in the supplementary figure (Supplementary Figures S3A, B), which reflects greater but opposite response for the two species during peak growing season (June-October). On the contrary, the impact of maximum temperature was minimal. Modelling studies indicate that tree-ring δ18O values integrate signals from three primary factors: source water δ18O, evaporative enrichment of 18O in leaf water, and biochemical fractionation during organic matter synthesis. Consequently, tree-ring δ18O signals vary as a function of temperature, relative humidity, precipitation, water sources, and regional climate conditions (Barbour, 2007; Gessler et al., 2014; Kahmen et al., 2011). Based on the premise that tree roots take up soil water without fractionation, a major part of the isotopic signature in tree rings should reflect the variation in precipitation or hydroclimatic conditions (Farquhar et al., 2007; Lehmann et al., 2018; Roden et al., 2005). However, depending upon the local humidity condition, species-specific ecophysiological processes and responses (e.g.: isotopic composition of soil water, leaf-water enrichment, and oxygen isotope exchange reactions of photosynthates with water) may have a considerable effect on tree ring δ18O values (Baker et al., 2016; Lehmann et al., 2018), as revealed in our study.
Broadly, an increasing trend in iWUE was observed during the study period in both species that raised sharply after the 1990s (Figure 6). The mean iWUE of the apple tree was 88.5 ± 9.0 µmol mol-1, while for the pear trees, it was lower at 70.3 ± 10.5 µmol mol-1. Prior to 1990, the mean iWUE of apple trees was 80.2 µmol mol-1 (72 – 86 µmol mol-1), which increased to 93.6 µmol mol-1 (80 – 108 µmol mol-1). In contrast, for the pear trees, the mean iWUE before and after 1990 was 62.6 µmol mol-1 (55 – 73 µmol mol-1) and 81.8 µmol mol-1 (69 – 91 µmol mol-1), respectively. During the observation period, mean iWUE of the apple trees was higher than that of the pear trees (~18 µmol mol-1), which after 1990 increased by 15.5 and 30.6% respectively for apple and pear trees (Table 1). For the common period, inter-species correlation was significantly high (r = 0.82, p< 0.001), which probably indicates the predominant control of climate on species’ ecophysiological process.
Figure 6. (A) iWUE time series of Apple and Pear. Light-colored lines with dots indicate annual values, while dark lines indicate 3-year moving average with std. dev. (B) Monthly (left panel) correlations between iWUE time series of species and precipitation (1981-2021). The corresponding right panel indicates three-month moving correlation coefficients between them. (C) Monthly (left panel) and three-month moving (right panel) correlations between species’ iWUE time series and mean temperature (1988-2021). The dotted horizontal line indicates a 95% confidence level. The dashed vertical line delimits months with seasonal aggregates. Prefix “p” before the months denotes the months of the previous growth year. The response of species’ iWUE to minimum and maximum temperature has been illustrated in Supplementary Figure S4. (D) Linear regression between iWUE and atmospheric CO2 concentration (Ca) for apple and pear trees. Apple is slightly more sensitive to changes in Ca (steeper slope), but Pear’s iWUE is more tightly correlated with atmospheric CO2 levels (higher R2).
Moreover, it would be informative to note that long-term gains in iWUE by vegetation are usually overestimated (Gong et al., 2022). To provide a more accurate assessment, it is crucial to account for post-photosynthetic fractionations and mesophyll conductance, which influence CO2 diffusion to carboxylation sites (Gimeno et al., 2021; Gong et al., 2022; Ma et al., 2021). Our iWUE calculations, based on a linear model of photosynthetic ¹³C discrimination (Δ¹³C), do not fully capture long-term structural and physiological acclimations. Therefore, we advocate for the adoption of advanced models that should incorporate post-photosynthetic fractionations and mesophyll conductance as well as photorespiration to mitigate errors in estimating iWUE from Δ¹³C across vegetation types.
Nevertheless, to elaborate on the climatic controls of iWUE, we performed response function analysis on iWUE chronologies and monthly hydroclimatic datasets. For both species, major hydroclimatic variables (temperature and precipitation) showed positive relations with iWUE (Figures 6B, C). During the beginning of the growing season (March-May), iWUE – precipitation relationship was negative (p > 0.05) for the species that turned to positive correlations during the peak growing season (June-October) (Figure 6B). Cross-correlations with mean temperature revealed a strong positive correlation during peak growing season in both species, which was insignificantly low during March to May (Figure 6C). Similarly, with respect to minimum and maximum temperature, we noted a higher influence of minimum temperature on iWUE during peak growing season in both species (Supplementary Figures S4A, B).
Furthermore, stepwise regression between annual and 5-year mean iWUE (as dependent variable) and mean temperature (Tmean), precipitation (Ppt), and atmospheric CO2 concentrations (CO2) (as independent variables) were employed to access the explanatory power of climate variables. In both species, annual CO2 explained more than 70% of the variability. Whereas, 5-year mean CO2 explained more than 90% of the variability in iWUE. Analyses further reveal that Tmean is the second most important variable, while precipitation has a minimal impact on species’ iWUE (Supplementary Table S1). Several studies have inferred that rising atmospheric CO2 levels do not always imply enhanced photosynthetic rate and tree growth (Nock et al., 2011; Rahman et al., 2020; Van Der Sleen et al., 2015), with species-specific responses. We also observed species-specific responses and noted a differential response of species’ iWUE to atmospheric CO2 (Ca) (Figure 6D). The regression coefficient in apple trees (R2 = 0.76) was lower than that of the pear trees (R2 = 0.91). Results further suggest a slightly higher sensitivity of apple trees to rising Ca (slope: 0.38). However, a lower regression coefficient in apple trees indicates the possible role of other environmental factors particularly soil moisture (via stomatal regulation) in influencing their iWUE response. On the other hand, pear trees’ iWUE appears more tightly correlated with atmospheric CO2 levels (higher R2) possibly due to their access to relatively invariable source water (groundwater), but having a slightly lower slope (0.35). Despite this, both species have similar intercepts (Apple: -50.89 and Pear: -50.64), suggesting comparable baseline iWUE in the context of CO2 response. This implies that both species started from a similar baseline, but their responses to rising CO2 levels have diverged.
In conclusion, both species have responded to rising CO2 levels, with apple trees showing higher sensitivity as well as variability to environmental changes. Pear trees, on the other hand, have exhibited a more predictable and consistent response. It’s possible that apple trees may have reached a threshold in their ability to increase WUE as CO2 rises (Figure 6D). This information is valuable for predicting how these species may adapt to future environmental conditions, especially in regions with fluctuating water availability.
Tree-ring δ18O, in combination with δ13C, is a powerful proxy to decipher a comprehensive picture of past and current ecophysiological status (Battipaglia et al., 2013; Nock et al., 2011; Siegwolf et al., 2023). Therefore, we computed carbon-to-oxygen isotope difference index for both species (Figure 7A). This difference serves as an indicator of the trees’ physiological conditions related to changes in stomatal conductance and photosynthetic capacity (Scheidegger et al., 2000).
Figure 7. (A) Standardized carbon-to-oxygen isotope difference index for apple (orange) and pear (green) for the corresponding observation periods. Right panel plots the C-to-O difference index with atmospheric CO2 levels (Ca) indicating physiological responses (through changes in stomatal conductance and photosynthetic capacity) to rising Ca. Slopes of both species are significant (**p< 0.0001). (B) Monthly (left panel) correlations between index time series of species and precipitation (1981-2021). The corresponding right panel indicates three-month moving correlation coefficients between them. (C) Monthly (left panel) and three-month moving (right panel) correlations between species’ index time series and mean temperature (1988-2021). The dotted horizontal line indicates a 95% confidence level. The dashed vertical line delimits months with seasonal aggregates. Prefix “p” before the months denotes the months of the previous growth year. The response of species’ index time series to minimum and maximum temperature has been illustrated in Supplementary Figure S5.
The C-to-O difference index for apple trees exhibits an increasing trend from the late 1970s onwards, with a more pronounced positive shift after the year 2000. The index also shows considerable year-to-year variability. In the early years (1976-1980), values fluctuate around the zero line, indicating a balance between low stomatal conductance and high photosynthetic capacity. From 1980 onwards, periods of high photosynthetic capacity (positive index values) appear more frequent and sustained, likely reflecting the irrigation effect. In recent years (2000-2021), the index mostly remained positive, indicating a generally high photosynthetic capacity for apple trees, despite occasional dips around 2010 and 2015.
The index for pear trees shows an overall increasing trend over the entire period. There is a clear positive shift around the late 1980s, aligning with the apple tree index. However, the species’ pattern briefly diverged during the 2010s when δ13C levels were stable and δ18O values were high in apple trees (Figures 2, 5). Similar to apple trees, pear trees exhibit considerable inter-annual variability. The period before 1980 shows more frequent negative values, indicating periods of low photosynthesis, likely induced by stomatal limitations given limited irrigation provisioning. Post-1980, positive values become more dominant, suggesting an improvement in photosynthetic assimilation. In recent decades (2000-2021), the overall trend remained positive, with a few dips indicating brief periods of moisture stress and reduced photosynthetic efficiency. The analysis suggests an overall improvement in photosynthetic capacity over the studied periods, with more frequent and sustained positive index values in recent decades in both species. This trend reflects adaptive physiological responses to changing environmental conditions including the CO2 fertilization effect in the Italian Alps. However, both species exhibit notable year-to-year variability, highlighting the influence of inter-annual climatic variations on tree physiology and productivity.
Previously, we observed species-specific responses of iWUE to atmospheric CO2 (Ca), with pear trees exhibiting lower iWUE and a higher regression coefficient (R² = 0.91) compared to apple trees (R² = 0.76) (Figures 6A, D). To further explore these differences, we plotted species’ C-to-O difference indices against Ca (Figure 7A). The slope of pear trees (0.056, p< 0.001) with respect to apple trees (0.019, p< 0.001) suggests a stronger physiological response to increased CO2, with clear changes in stomatal conductance and photosynthetic activity (Figure 7A). Further, a much higher R² value for pear trees (69%) indicates that a significant portion of the changes in stomatal conductance and photosynthetic activity can be explained by changes in CO2 concentration.
This suggests that pear trees’ physiological conditions, as indicated by the index, are more closely tied to rising CO2 levels compared to apple trees. The weak slope and low regression coefficient suggest that the physiological processes in the apple tree are primarily related to the stomatal regulation via soil moisture. Overall, our analysis revealed a significantly higher correlation for pear trees (r = 0.83, p< 0.0001) than for apple trees (r = 0.32, p< 0.05). These results suggest that apple trees may have reached a threshold in their ability to increase water use efficiency as CO2 levels rise, while pear trees continue to show a stronger response. This could explain the differential responses of these two tree species to changing environmental conditions, including the CO2 fertilization effect.
Cross-correlations with hydroclimatic variables were further performed to gain insights into the combined responses for the index series of the species. Response analyses clearly indicate that precipitation has a minimal impact on isotope-inferred ecophysiological processes, except for the pear trees which show a significant positive correlation during peak growing season (Figure 7B). Similarly, index – mean temperature relationship was significant only for the pear trees that only during peak growing season (Figure 7C). Both Pearson and 3-month moving correlations between minimum/maximum temperature and species index series have been detailed in the supplementary figure (Supplementary Figures S5A, B). These results demonstrate hydroclimate and ecophysiological relationships in these energy-limited ecosystems.
This study utilized unique old growth veteran apple and pear trees from energy-limited alpine valleys of the Italian Alps (a major national production center) to investigate climate-scale physiological responses. Results broadly indicate a species-coherent behavior and trends in δ18O and processes such as iWUE, carbon discrimination (Δ13C) and intercellular CO2 concentration (Ci). Importantly, results suggest a similar physiological response of tree species to atmospheric CO2 rise. A significant increase in iWUE has been observed in recent decades, primarily driven by the CO2-fertilization effect. Dual isotope analyses (δ18O–δ13C) confirmed that the recent rise in iWUE is due to increased carbon assimilation rather than reduced evapotranspiration. The analyses also highlight common inter-annual variability in carbon assimilation across both species, with some site- and species-specific responses. Among the major climatic controls on ecophysiological processes, precipitation has minimal impact in this moist, energy-limited ecosystem. Statistical and climate response function analyses further revealed that, besides CO2-fertilization, the second most important environmental driver of ecophysiological processes is the minimum temperature during the growing season. We believe that such long-term records could be valuable for fine-tuning land surface models to account for the combined effects of CO2-fertilization and climate impact.
The raw data supporting the conclusions of this article will be made available by the authors, without undue reservation.
NS: Formal analysis, Investigation, Methodology, Conceptualization, Data curation, Writing – original draft. MT: Data curation, Investigation, Supervision, Validation, Visualization, Writing – review & editing. ET: Data curation, Investigation, Validation, Visualization, Writing – review & editing, Methodology. LM: Investigation, Methodology, Visualization, Writing – review & editing, Formal analysis, Supervision.
The author(s) declare financial support was received for the research, authorship, and/or publication of this article. The study received main support from the EUREGIO funded ASTER project, EGTC European Region Tyrol-South Tyrol Trentino—IPN 101-32 and Austrian Science Fund (FWF) and was partially supported by the CarboST project funded by the Autonomous Province of Bolzano-Bozen.
The authors thank the Department of Innovation, Research, University and Museums of the Autonomous Province of Bozen/Bolzano for covering the Open Access publication costs. We are grateful to G. Battipaglia for her support for the stable isotope analyses that were carried out at the Università degli studi della Campania “L. Vanvitelli” Dipartimento di Scienze e Tecnologie Ambientali Biologiche e Farmaceutiche, Caserta, Italy. The authors also thanks W. Drahorad and R. Stainer for making possible the sampling of the old apple and pear trees.
The authors declare that the research was conducted in the absence of any commercial or financial relationships that could be construed as a potential conflict of interest.
The author(s) declared that they were an editorial board member of Frontiers, at the time of submission. This had no impact on the peer review process and the final decision.
All claims expressed in this article are solely those of the authors and do not necessarily represent those of their affiliated organizations, or those of the publisher, the editors and the reviewers. Any product that may be evaluated in this article, or claim that may be made by its manufacturer, is not guaranteed or endorsed by the publisher.
The Supplementary Material for this article can be found online at: https://www.frontiersin.org/articles/10.3389/fpls.2024.1471415/full#supplementary-material
Adams, M. A., Buckley, T. N., Turnbull, T. L. (2020). Diminishing CO2-driven gains in water-use efficiency of global forests. Nat. Climate Change 10, 466–471. doi: 10.1038/s41558-020-0747-7
Auzmendi, I., Marsal, J., Girona, J., Lopez, G. (2013). Daily photosynthetic radiation use efficiency for apple and pear leaves: seasonal changes and estimation of canopy net carbon exchange rate. Eur. J. Agron. 51, 1–8. doi: 10.1016/j.eja.2013.05.007
Babst, F., Alexander, M. R., Szejner, P., Bouriaud, O., Klesse, S., Roden, J., et al. (2014). A tree-ring perspective on the terrestrial carbon cycle. Oecologia 176, 307–322. doi: 10.1007/s00442-014-3031-6
Baker, J. C. A., Gloor, M., Spracklen, D. V., Arnold, S. R., Tindall, J. C., Clerici, S. J., et al. (2016). What drives interannual variation in tree ring oxygen isotopes in the Amazon? Geophysical Res. Lett. 43, 11–831. doi: 10.1002/2016GL071507
Barbour, M. M. (2007). Stable oxygen isotope composition of plant tissue: a review. Funct. Plant Biol. 34, 83–94. doi: 10.1071/FP06228
Battipaglia, G., Saurer, M., Cherubini, P., Calfapietra, C., McCarthy, H. R., Norby, R. J., et al. (2013). Elevated CO2 increases tree-level intrinsic water use efficiency: Insights from carbon and oxygen isotope analyses in tree rings across three forest FACE sites. New Phytol. 197, 544–554. doi: 10.1111/nph.2012.197.issue-2
Belmecheri, S., Lavergne, A. (2020). Compiled records of atmospheric CO2 concentrations and stable carbon isotopes to reconstruct climate and derive plant ecophysiological indices from tree rings. Dendrochronologia 63, 125748. doi: 10.1016/j.dendro.2020.125748
Core, R. (2015). Team. R: a language and environment for statistical computing. R Foundation for Statistical Computing. Vienna.
Farquhar, G. D., Cernusak, L. A., Barnes, B. (2007). Heavy water fractionation during transpiration. Plant Physiol. 143, 11–18. doi: 10.1104/pp.106.093278
Farquhar, G. D., O’Leary, M. H., Berry, J. A. (1982). On the relationship between carbon isotope discrimination and the intercellular carbon dioxide concentration in leaves. Funct. Plant Biol. 9, 121–137. doi: 10.1071/PP9820121
Frank, D. C., Poulter, B. T., Saurer, M. A., Esper, J. A., Huntingford, C. T., Helle, G. F., et al. (2015). Water-use efficiency and transpiration across European forests during the Anthropocene. Nature Climate Change 5(6), 579–583.
Gagen, M., Battipaglia, G., Daux, V., Duffy, J., Dorado-Liñán, I., Hayles, L. A., et al. (2022). “Climate signals in stable isotope tree-ring records,” in Stable Isotopes in Tree Rings: Inferring Physiological, Climatic and Environmental Responses (Springer International Publishing, Cham), 537–579.
García-Hidalgo, M., García-Pedrero, Á., Colón, D., Sangüesa-Barreda, G., García-Cervigón, A. I., López-Molina, J., et al. (2022). CaptuRING: A do-it-yourself tool for wood sample digitization. Methods Ecol. Evol. 13, 1185–1191. doi: 10.1111/2041-210X.13847
Gessler, A., Ferrio, J. P., Hommel, R., Treydte, K., Werner, R. A., Monson, R. K. (2014). Stable isotopes in tree rings: towards a mechanistic understanding of isotope fractionation and mixing processes from the leaves to the wood. Tree Physiol. 34, 796–818. doi: 10.1093/treephys/tpu040
Giammarchi, F., Cherubini, P., Pretzsch, H., Tonon, G. (2017). The increase of atmospheric CO2 affects growth potential and intrinsic water-use efficiency of Norway spruce forests: insights from a multi-stable isotope analysis in tree rings of two Alpine chronosequences. Trees 31, 503–515. doi: 10.1007/s00468-016-1478-2
Gimeno, T. E., Campany, C. E., Drake, J. E., Barton, C. V., Tjoelker, M. G., Ubierna, N., et al. (2021). Whole-tree mesophyll conductance reconciles isotopic and gas-exchange estimates of water-use efficiency. New Phytol. 229, 2535–2547. doi: 10.1111/nph.v229.5
Gong, X. Y., Ma, W. T., Yu, Y. Z., Fang, K., Yang, Y., Tcherkez, G., et al. (2022). Overestimated gains in water-use efficiency by global forests. Global Change Biol. 28, 4923–4934. doi: 10.1111/gcb.v28.16
Huang, R., Xu, C., Grießinger, J., Feng, X., Zhu, H., Bräuning, A. (2024). Rising utilization of stable isotopes in tree rings for climate change and forest ecology. J. Forestry Res. 35, 13. doi: 10.1007/s11676-023-01668-5
Hyvönen, R., Ågren, G. I., Linder, S., Persson, T., Cotrufo, M. F., Ekblad, A., et al. (2007). The likely impact of elevated [CO2], nitrogen deposition, increased temperature and management on carbon sequestration in temperate and boreal forest ecosystems: a literature review. New Phytol. 173 (3), 463–480. doi: 10.1111/j.1469-8137.2007.01967.x
Kahmen, A., Sachse, D., Arndt, S. K., Tu, K. P., Farrington, H., Vitousek, P. M., et al. (2011). Cellulose δ18O is an index of leaf-to-air vapor pressure difference (VPD) in tropical plants. Proc. Natl. Acad. Sci. 108, 1981–1986. doi: 10.1073/pnas.1018906108
Lehmann, M. M., Goldsmith, G. R., Schmid, L., Gessler, A., Saurer, M., Siegwolf, R. T. (2018). The effect of 18O-labelled water vapour on the oxygen isotope ratio of water and assimilates in plants at high humidity. New Phytol. 217, 105–116. doi: 10.1111/nph.2018.217.issue-1
Lin, W., Barbour, M. M., Song, X. (2022). Do changes in tree-ring δ18O indicate changes in stomatal conductance? New Phytol. 236 (3), 803–808. doi: 10.1111/nph.18431
Ma, W. T., Tcherkez, G., Wang, X. M., Schäufele, R., Schnyder, H., Yang, Y., et al. (2021). Accounting for mesophyll conductance substantially improves 13C-based estimates of intrinsic water-use efficiency. New Phytol. 229, 1326–1338. doi: 10.1111/nph.v229.3
Mathias, J. M., Thomas, R. B. (2021). Global tree intrinsic water use efficiency is enhanced by increased atmospheric CO2 and modulated by climate and plant functional types. Proc. Natl. Acad. Sci. 118, e2014286118. doi: 10.1073/pnas.2014286118
McCarroll, D., Loader, N. J. (2004). Stable isotopes in tree rings. Quaternary Sci. Rev. 23, 771–801. doi: 10.1016/j.quascirev.2003.06.017
Nock, C. A., Baker, P. J., Wanek, W., Leis, A., Grabner, M., Bunyavejchewin, S., et al. (2011). Long-term increases in intrinsic water-use efficiency do not lead to increased stem growth in a tropical monsoon forest in western Thailand. Global Change Biol. 17, 1049–1063. doi: 10.1111/j.1365-2486.2010.02222.x
Panthi, S., Fan, Z. X., van der Sleen, P., Zuidema, P. A. (2020). Long-term physiological and growth responses of Himalayan fir to environmental change are mediated by mean climate. Global Change Biol. 26, 1778–1794. doi: 10.1111/gcb.14910
Peñuelas, J., Canadell, J. G., Ogaya, R. (2011). Increased water-use efficiency during the 20th century did not translate into enhanced tree growth. Global Ecol. Biogeogr. 20, 597–608. doi: 10.1111/j.1466-8238.2010.00608.x
Rahman, M., Islam, M., Gebrekirstos, A., Bräuning, A. (2020). Disentangling the effects of atmospheric CO2 and climate on intrinsic water-use efficiency in South Asian tropical moist forest trees. Tree Physiol. 40, 904–916. doi: 10.1093/treephys/tpaa043
Roden, J. S., Bowling, D. R., McDowell, N. G., Bond, B. J., Ehleringer, J. R. (2005). Carbon and oxygen isotope ratios of tree ring cellulose along a precipitation transect in Oregon, United States. J. Geophysical Res.: Biogeosci. 110. doi: 10.1029/2005JG000033
Saurer, M. A., Spahni, R. F., Frank, D. C., Joos, F. L., Leuenberger, M. L., Loader, N. J., et al. (2014). Spatial variability and temporal trends in water-use efficiency of European forests. Global Change Biology 20 (12), 3700–3712.
Scheidegger, Y., Saurer, M., Bahn, M., Siegwolf, R. (2000). Linking stable oxygen and carbon isotopes with stomatal conductance and photosynthetic capacity: a conceptual model. Oecologia 125, 350–357. doi: 10.1007/s004420000466
Schollaen, K., Baschek, H., Heinrich, I., Slotta, F., Pauly, M., Helle, G. (2017). A guideline for sample preparation in modern tree-ring stable isotope research. Dendrochronologia 44, 133–145. doi: 10.1016/j.dendro.2017.05.002
Siegwolf, R. T., Lehmann, M. M., Goldsmith, G. R., Churakova, O. V., Mirande-Ney, C., Timoveeva, G., et al. (2023). Updating the dual C and O isotope-Gas-exchange model: A concept to understand plant responses to the environment and its implications for tree rings. Plant Cell Environ. 46, 2606–2627. doi: 10.1111/pce.14630
Stenger, A., Harou, P., Navrud, S. (2009). Valuing environmental goods and services derived from the forests. J. For. Economics 15, 1–14. doi: 10.1016/j.jfe.2008.03.001
Van Der Sleen, P., Groenendijk, P., Vlam, M., Anten, N. P., Boom, A., Bongers, F., et al. (2015). No growth stimulation of tropical trees by 150 years of CO2 fertilization but water-use efficiency increased. Nat. Geosci. 8, 24–28. doi: 10.1038/ngeo2313
Walker, A. P., De Kauwe, M. G., Bastos, A., Belmecheri, S., Georgiou, K., Keeling, R. F., et al. (2021). Integrating the evidence for a terrestrial carbon sink caused by increasing atmospheric CO2. New Phytol. 229, 2413–2445. doi: 10.1111/nph.v229.5
Wang, W., Liu, X., Xu, G., Treydte, K., Shao, X., Qin, D., et al. (2019). CO2 fertilization confounds tree-ring records of regional hydroclimate at northeastern Qinghai-Tibetan Plateau. Earth Space Sci. 6, 730–740. doi: 10.1029/2018EA000529
Waterhouse, J. S., Switsur, V. R., Barker, A. C., Carter, A. H. C., Hemming, D. L., Loader, N. J., et al. (2004). Northern European trees show a progressively diminishing response to increasing atmospheric carbon dioxide concentrations. Quaternary Sci. Rev. 23, 803–810. doi: 10.1016/j.quascirev.2003.06.011
Keywords: dendrochronology, ecophysiology, WUE, climate-carbon response, Italian Alps
Citation: Singh N, Tagliavini M, Tomelleri E and Montagnani L (2025) Multi-decadal tree-ring stable isotope records of apple and pear trees indicate coherent ecophysiological responses to environmental changes in alpine valleys. Front. Plant Sci. 15:1471415. doi: 10.3389/fpls.2024.1471415
Received: 27 July 2024; Accepted: 27 November 2024;
Published: 10 January 2025.
Edited by:
Lucian Copolovici, Aurel Vlaicu University of Arad, RomaniaReviewed by:
Xiaoying Gong, Fujian Normal University, ChinaCopyright © 2025 Singh, Tagliavini, Tomelleri and Montagnani. This is an open-access article distributed under the terms of the Creative Commons Attribution License (CC BY). The use, distribution or reproduction in other forums is permitted, provided the original author(s) and the copyright owner(s) are credited and that the original publication in this journal is cited, in accordance with accepted academic practice. No use, distribution or reproduction is permitted which does not comply with these terms.
*Correspondence: Leonardo Montagnani, bGVvbmFyZG8ubW9udGFnbmFuaUB1bmliei5pdA==
Disclaimer: All claims expressed in this article are solely those of the authors and do not necessarily represent those of their affiliated organizations, or those of the publisher, the editors and the reviewers. Any product that may be evaluated in this article or claim that may be made by its manufacturer is not guaranteed or endorsed by the publisher.
Research integrity at Frontiers
Learn more about the work of our research integrity team to safeguard the quality of each article we publish.