- 1State Key Laboratory of Crop Gene Exploration and Utilization in Southwest China, Sichuan Agricultural University at Wenjiang, Chengdu, Sichuan, China
- 2Agricultural and Rural Bureau, Hanyuan County Government, Yaan, Sichuan, China
- 3Ecological Security and Protection Key Laboratory of Sichuan Province, Mianyang Teachers’ College, Mianyang, China
Plant spotted leaf (spl) mutants are useful to reveal the regulatory mechanisms of immune responses. Thus, in crop plants, their agronomic traits, especially the grain quality are usually ignored. Here, we characterized a rice spl mutant named spl-A (spotted leaf mutant from A814) that shows autoimmunity, broad-spectrum disease resistance and growth deterioration including decreased rice quality. A single nucleotide mutation of C1144T, which leads to change of the 382nd proline to serine, in the gene encoding the ATPases associated with diverse cellular activities (AAA)-type ATPase LRD6-6 is responsible for the phenotype of the spl-A mutant. Mechanistically, this mutation impairs LRD6-6 ATPase activity and disrupts its interaction with endosomal sorting complex required for transport (ESCRT)-III subunits OsSNF7.1/7.2/7.3. And thus, leading to compromise of multivesicular bodies (MVBs)-mediated vesicle trafficking and accumulation of ubiquitinated proteins in both leaves and seeds of spl-A. Therefore, the immune response of spl-A is activated, and the growth and grain quality are deteriorated. Our study identifies a new amino acid residue that important for LRD6-6 and provides new insight into our understanding of how MVBs-mediated vesicle trafficking regulates plant immunity and growth, including grain quality in rice.
Introduction
During the long-term of plant-pathogen interaction, plants have evolved with a layered immune system to detect and fight with various of pathogens. Pattern recognition receptors (PRRs) localized at the plasm membrane function as the first layer to detect pathogen derived molecular patterns and activate pattern-triggered immunity (PTI) (Jones and Dangl, 2006; Zhao et al., 2022). Intracellular receptors such as nucleotide-binding, leucine-rich repeat receptors (NLRs) function as the second layer to monitor pathogen secreted effectors to activate the more potent immune responses, effector-triggered immunity (ETI) (Jones and Dangl, 2006; Zhao et al., 2022). Once the immune responses are activated, plant will increase the expression of pathogenesis-related (PR) genes, induce the production of reactive oxygen species (ROS) and promote the synthesis of antimicrobial metabolites etc (Tsuda and Katagiri, 2010; Yuan et al., 2021). Finally, the immunity system may launch hypersensitive response (HR) to trigger cell death in and around the infection sites to restrict pathogen invasion and prevent disease development (Mur et al., 2008; Balint-Kurti, 2019).
It’s interesting that in the absence of pathogen infection, insect feeding, stress or mechanical damages, many plant mutants have been found to exhibit spontaneous, HR-like cell death spots on their bodies (Zhu et al., 2020). These mutants are named based on their phenotype as spotted leaf (spl), lesion mimic mutant (lmm), accelerated cell death (acd) and others (Bruggeman et al., 2015). Since these spl mutants usually carry the characteristics of activated immune responses, they are useful to uncover the regulatory mechanisms of plant immune responses. More than 35 genes that responsible for spl phenotype have been identified in rice at present (Zhu et al., 2020; Jiang et al., 2022; Ren et al., 2022; Zheng et al., 2022; Wang et al., 2023). Studies on these genes have revealed many processes or pathways are involved in plant immune responses regulation. These include the gene transcription and protein translation process, post-translational protein modification, the vesicle trafficking pathways, and several cellular metabolic pathways (Zhu et al., 2020). During the study of these genes or mutants, it has also found that these genes are usually involved in the regulation of plant growth as well (Zhu et al., 2020). However, little attention has been paid for their roles in plant growth.
The vesicle trafficking apparatus are critical for the growth and development of eukaryotes. Up to now, at least five vesicle-mediated trafficking pathways have been identified in plant. The coat protein I (COPI)-coated vesicles and the COPII-coated vesicles are involved in endoplasmic reticulum (ER)-Golgi trafficking, the clathrin-coated vesicles (CCVs) mediates endocytosis and post-Golgi trafficking, the exocyst positive organelles (EXPO) regulates exocytosis, and the multivesicular bodies (MVBs) modulates endocytosis, exocytosis, recycling and degradation of cargoes (Aniento et al., 2021; Zhu et al., 2023).
By studying of the spl mutants, several vesicle trafficking pathways have been found to be essential for rice immunity. SPL35 is a CUE domain-containing protein that interacts with the coatomer subunits δ-COP1 and δ-COP2 of the COPI vesicle (Ma et al., 2019). Suppression any of the three genes will lead to spl phenotype (Ma et al., 2019), providing evidence that the COPI trafficking pathway is involved in the regulation of immunity and HR-like cell death in rice. Loss of function of the trans-Golgi network (TGN)-derived CCVs components, SPL28, OsSCYL2 or OsCHC1 results in autoimmunity and cell death of rice (Qiao et al., 2010; Yao et al., 2022). OsSEC3A is a core subunit of the exocyst complex. Knockout of OsSEC3A causes enhanced immune responses and HR-like cell death likely due to the disruption of the EXPO-mediated exocytosis (Ma et al., 2017). The AAA-type ATPase LRD6-6 is a rice homology of VPS4/SKD1 which crucial for MVBs-mediated trafficking of ubiquitinated plasm membrane proteins (Zhu et al., 2016). During the formation of the MVBs, the ESCRT-I, -II, and -III complexes are sequentially recruited to coordinately drive vesicle maturation. At the final step, the VPS4/SKD1 assembles with the ESCRT-III at the neck region of the limiting membrane to hydrolyzes ATP and thus provide energy to facilitate neck scission and disassembly of the ESCRT-III complex (Piper and Katzmann, 2007; Hurley, 2008; Schmidt and Teis, 2012). Mutation of Lrd6-6 in rice leads to autoimmunity and HR-like cell death (Zhu et al., 2016), indicating the important roles of LRD6-6 and MVBs-mediated trafficking in rice immune responses.
Besides their roles in plant immunity, the vesicle trafficking pathways have also been found to regulate rice chalkiness through regulating the transportation of storage proteins in endosperm, thus contributing to grain quality formation (Zhu et al., 2023). Rice endosperm cells contain two types of protein bodies, protein body I (PBI) and PBII, which are responsible for protein storage in seed. PBIs storage prolamins while PBIIs mainly accumulate glutelins and globulins (Li et al., 2022). The delivery of the storage proteins into PBIs and PBIIs are tightly regulated by vesicle trafficking. This is mainly achieved by studying of glutelin precursor accumulation (gpa) mutants with abnormal endosperm and decreased grain quality in rice (Li et al., 2022; Zhu et al., 2023). Interesting, loss of function of an ESCRT-II complex subunit OsVPS22 also leads to chalky endosperm likely for impaired grain filling (Zhang et al., 2013), suggesting that the MVBs-mediated vesicle trafficking pathway may also likely involved in storage protein transportation in rice endosperm cells. However, our knowledges about whether and how MVBs-mediated vesicle trafficking regulate rice endosperm development and grain quality formation are still remain largely unknown.
In this study, we isolated and characterized the rice spotted leaf mutant spl-A, which shows autoimmunity, broad-spectrum disease resistance and growth deterioration including decreased rice quality. By combining map-based cloning strategy and bulked segregant analysis (BSA) sequencing, we found a single nucleotide mutation of C1144T in the gene encoding the AAA-type ATPase LRD6-6 is responsible for the phenotype of the spl-A mutant. This mutation leads to change of the 382nd proline to serine, impairs LRD6-6 ATPase activity and disrupts its interaction with ESCRT-III subunits OsSNF7.1/7.2/7.3. And finally, result in compromise of MVBs-mediated vesicle trafficking and accumulation of ubiquitinated proteins in spl-A. Collectively, our results identify a new amino acid residue that important for LRD6-6 and point to a novel function for MVBs-mediated vesicle trafficking regulates grain quality in rice.
Materials and methods
Plant materials and growth conditions
The spl-A mutant was isolated from the methanesulfonate (EMS) treated japonica rice A814 (XA21-OsSERK2Ri in Kitaake) (Caddell et al., 2017). Two F2 populations derived from the cross Jodan×spl-A and spl-A×Jodan were used for genetic analyses and mapping of the spl-A locus. All plants for mapping were grown in the controlled fields at Sichuan Agricultural University in Wenjiang, Chengdu, China.
For major agronomic traits analysis, plant materials were cultivated in the controlled fields with 6 rows (12 plants per row) at Wenjiang district, Chengdu, China. At maturity, forty plants in the middle row were selected for plant height, tiller number and panicle length determination, and then they were harvested respectively and air-dried. For seed setting rate and 1,000-grain weight, five A814 and spl-A plants were randomly and respectively selected. Scanning electron microscopy observation was performed as described before (Ren et al., 2020). For grain quality determination, the seeds from all the forty plants of A814 and spl-A were mixed respectively, and chalky grain percentage and chalkiness degree were detected using the WSeen SC-E type rice appearance quality detection analyzer (Wanshen Detection, Hangzhou, China) with three technical repetitions. Student’s t-test was used to compare the differences between A814 and spl-A and the data were analyzed by using Microsoft Excel 2019.
Histochemical analysis
Trypan blue staining and DAB (3, 3’-diamiobenzidine) staining were performed on fresh leaves following the method as described previously (Zhu et al., 2016). Briefly, for trypan blue staining, samples were submerged in lactic acid-phenol-trypan blue solution (2.5 mg/ml trypan blue, 25% (w/v) lactic acid, 23% water-saturated phenol and 25% glycerol in H2O) and were boiled in water for 2 min, then de-stained with solution containing 30% (w/v) chloral hydrate for 3 days with multiple exchanges of the solution. After distained completely, the samples were then equilibrated with 50% glycerol for five hours followed by photo-picture taken. For DAB staining, leaf samples were immersed in 1 mg/ml DAB containing10 mM MES (pH 6.5) for 12 h in the dark at 30°C. Then the leaf samples were transferred to solution containing 90% ethanol and 10% glycerol at 90°C until chlorophyll was completely removed. Images were acquired by a microscope (Zeiss imager A2).
RNA isolation and RT-qPCR
For determination of gene expression levels, the desired samples were harvested and total mRNA were respectively extracted using TRIzol agent (15596018CN, Invitrogen, Carlsbad, USA) following the procedures described by the manufacturer. The mRNA was then subject to reverse transcription to synthesize first-strand cDNA by using the PrimeScript™ RT reagent Kit with gDNA Eraser (RR047A, Takara, Beijing, China) for genomic DNA removal.
The reverse transcription-quantitative PCR (RT-qPCR) was conducted using a Bio-Rad CFX96 Real-Time System coupled to a C1000 Thermal Cycler (Bio-Rad, Hercules, USA). The reference gene Ubiquitin (Ubq) was used as reference (Zhu et al., 2016). Primer sequences are listed in Supplementary Table 1.
Disease resistance evaluation
For blast resistance, rice leaves from three-week-old seedlings of A814 and spl-A plants were used. The inoculation was performed as described by Li et al (Li et al., 2020). Briefly, the spore concentration of the blast was adjusted to 5×105 conidia mL-1. 4 μL of the spore suspension was dipped onto the leaves with three spots per leaf. Then the leaves were kept in a culture dish contain 0.1% 6-benzylaminopurin (6-BA) for 5 days. The lesion length was measured and the relative fungi biomass was detected and calculated according to the method reported before (Li et al., 2020). The fungal isolates Zhong10-8-14, ZE-1, 0755-1-1 and 99-20-2 that are compatible with A814 were respectively used for blast resistance evaluation.
For bacterial blight disease, the Xoo strains P2, P6 and Xoo-4, which are compatible with A814 were used for inoculation. Xoo bacterial suspensions of OD600 = 0.5 were used to inoculate by using the scissors-dip method as described previously (Zhu et al., 2016). The lesion lengths and bacterial populations were determined respectively at day 0, 7, 14 post inoculation.
Genetic analysis and cloning of the locus Spl-A
Two F2 populations derived from the cross Jodan×spl-A and spl-A×Jodan were used for genetic analyses and mapping of the spl-A locus as described before (Zhu et al., 2016). The SSR primers were synthesized according to the information from Gramene database (http://www.gramene.org/microsat).
For BSA sequencing, leaf samples of 30 F2 individuals with the spl-A phenotype and the wild-type phenotype were respectively mixed at an equal ratio and subjected to whole-genome resequencing and analysis at Biomarker Technologies Corporation (Beijing, China).
For candidate gene validation, the DNA segments containing the mutation sites were amplified using gene-specific primers and sequenced at Tsingke Biotech (Chengdu, China). The genotypes of the lrd6-6/spl-A F1 plants were also confirmed by sequencing of spl-A and lrd6-6-specific PCR-based agarose gel electrophoresis respectively. All the primers used for gene mapping and genotyping are listed in Supplementary Table 1.
Plasmid construction
The desired DNA segments were amplified by using the Phanta Super-Fidelity DNA Polymerase (P505-d1, Vazyme Biotech, Nanjing, China). The segments were then inserted into the vectors to produce the final plasmids by using the ClonExpress II One Step Cloning Kit (C112-02, Vazyme Biotech, Nanjing, China). All the plasmids were then confirmed by sequencing at Tsingke Biotech (Chengdu, China) subsequently. The primers and vectors used were listed in Supplementary Table 1.
ATPase activity assay
ATPase activity determination assay was performed as described previously (Zhu et al., 2016). The truncated protein His-LRD6-6(125-487)P382S (covering the ATPase domain) was purified and subjected to this analysis. His-LRD6-6(125-487) and His-LRD6-6(125-487)E315Q were included as positive and negative control respectively. ATPase activity of the recombinant proteins were measured by the malachite green-based colorimetric method (Zhu et al., 2016). The relative ATPase activity was calculated by setting the activity of His-LRD6-6(125-487) as 100%.
Protein interaction detection and subcellular localization
Yeast two-hybrid (Y2H) assay and bimolecular fluorescence complementation (BiFC) were performed as described previously (Zhu et al., 2016).
For subcellular localization, plasmids were introduced into Nicotiana benthamiana leaf by agroinfiltration. Fluorescence was examined under a confocal microscopy (Leica STELLARIS STED, Germany) 48 h post transformation. The fluorescence intensity were measured by using ImageJ (National Institutes of Health, USA).
Protein extraction and immunoblot analyses
For protein extraction from plant leaf samples, the denaturing buffer (50 mM Tris-HCl pH=7.5, 150 mM NaCl, 0.1% NP-40, 4 M urea and 1 mM PMSF) was used (Liu et al., 2010). For the dry seeds, the extraction buffer (125 mM Tris-HCl pH=6.8, 4% SDS, 4 M urea and β-mercaptoethanol) described before was used (Pan et al., 2021). The total protein content was determined by the Protein Content Assay Kit (BC3185, Solarbio, Beijing, China).
The proteins were resolved by sodium dodecyl sulfate polyacrylamide gel electrophoresis (SDS-PAGE) analysis and followed by Coomassie brilliant blue staining or electro transfer to nitrocellulose membranes to perform immunoblot analyses using anti-His antibody (ab18184, Abcam, Shanghai, China), anti-Ub (P4D1) antibody (ab303664, Abcam, Shanghai, China) and anti-HSP82 antibody (AbM51099-31-PU, Beijing Protein Innovation, Beijing, China).
Results
Characterization of the spl-A mutant in rice
The spotted leaf mutant which we named spl-A (spotted leaf mutant from A814) was isolated from ethyl methanesulfonate (EMS) treated japonica rice A814 for its visible lesion mimic phenotype on the leaves when growing in the field. The spl-A mutant exhibited reddish-brown lesion spots on the leaves about 20 days after sowing and the lesion spots expand through the entire plants along with development (Figures 1A, B). To examine whether the agronomic traits including grain quality were affected in spl-A, we compared the chalky grain percentage, chalky grain degree, plant height, tiller number, panicle length, seed setting rate, 1,000-grain weight, and grain size between A814 and spl-A. It was found that the chalky grain percentage and chalky grain degree were significantly increased (Figures 1C–E), indicating that the grain quality of spl-A was seriously deteriorated. The plant height, seed setting rate, 1,000-grain weight and grain length of spl-A were also decreased prominently when compared to those of the wild-type A814, while the tiller number, panicle length and grain width of spl-A were not affected (Supplementary Figure 1).
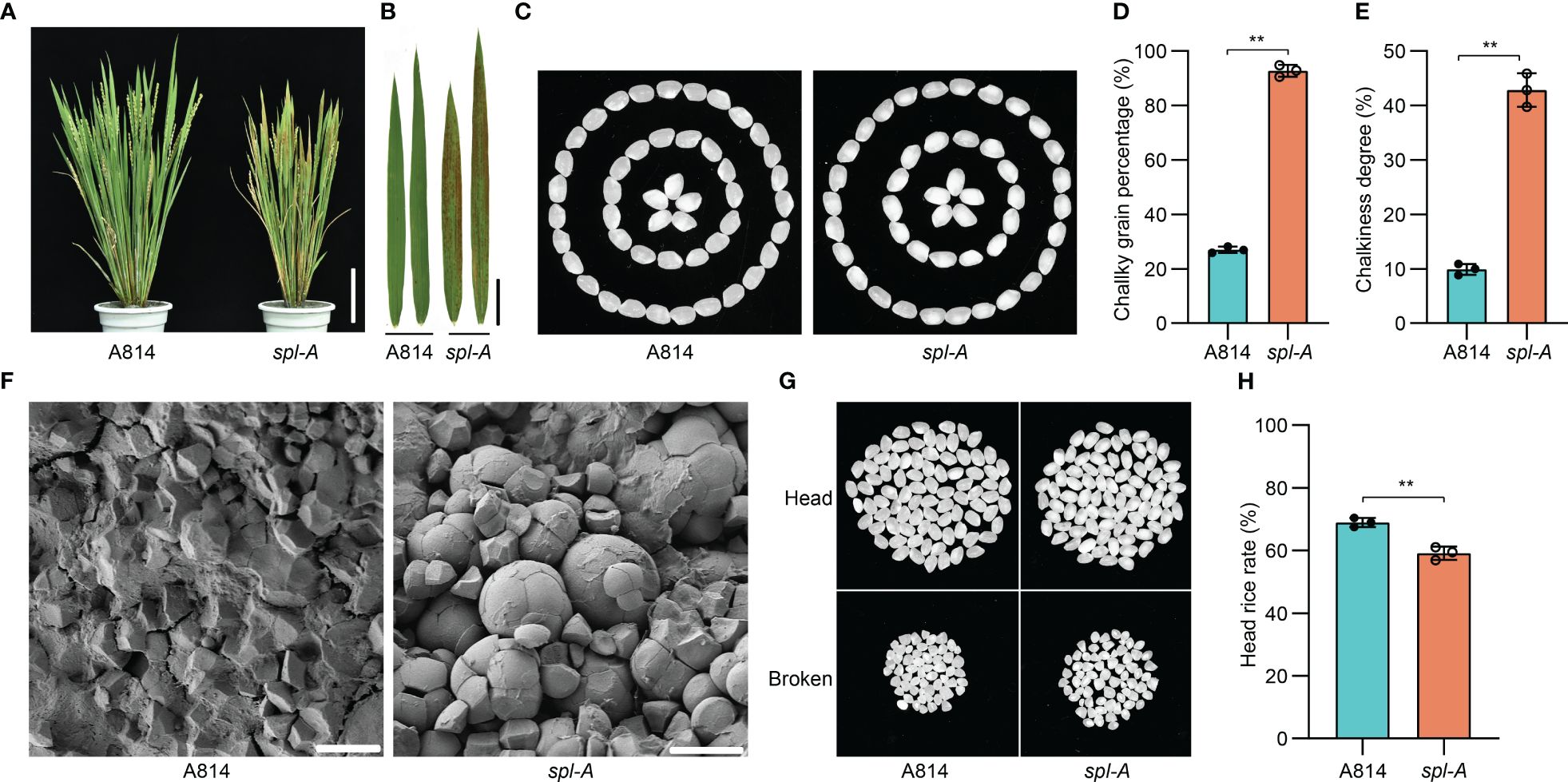
Figure 1 Phenotypic characterization of the spl-A mutant. (A, B) Photographs of the wild-type A814 and the spl-A mutant plants (A), and their leaves (B) at the filling stage. Bar = 10 cm in (A), Bar = 2 cm (B). (C) The grain appearance of A814 and spl-A. (D, E) Comparison on the chalky grain percentage (D) and chalky grain degree (E) between A814 and spl-A (mean ± s.d.). (F) Scanning electron microscopy images of transverse sections on the wild-type A814 and the spl-A mutant dry seeds. Bars = 10 μm. (G, H) The head rice rate of the spl-A mutant. Representative images of 100 dehulled and milled rice grains per genotype were separated into head and broken grains (G). (H) Statistical comparison of head rice rate between A814 and spl-A seeds (mean ± s.d.). All statistics were analyzed by Student’s t-test for P values (**, P <= 0.01; *, P <= 0.05; ns, no significant differences).
Grain chalkiness is the opaque part of translucent endosperm in grains that usually caused by altered package of the starch granules (Yao et al., 2020). We then analyzed the starch granules organization of spl-A seed. Scanning electron microscopy revealed that the spl-A mutant endosperm was composed of loosely arranged and round-shaped compound starch granules, while that of the wild-type A814 was tightly packed and polyhedral shaped (Figure 1F). Grain chalkiness often affects processing quality (Li et al., 2022). Indeed, the head rice rate of spl-A was prominently decreased when compared to A814 (Figures 1G, H). Taken together, these results indicate that Spl-A regulates multiple aspects of rice development including grain quality.
The spl-A mutant presents autoimmunity and broad-spectrum disease resistance
The spl mutants usually exhibit excessive accumulation of hydrogen peroxide (H2O2) and autoimmunity which then lead to programmed cell death (Li et al., 2017; Yao et al., 2022). We thus employed the DAB (3, 3’-diamiobenzidine) staining and trypan blue staining methods to examine the accumulation of H2O2 and the existence of cell death in spl-A leaves respectively at 15 days after sowing, before visible lesions appeared. DAB staining analysis detected extensive stains in leaves of spl-A when compared to A814 (Figure 2A). Trypan blue staining also detected many blue staining spots on the leaves of spl-A (Figure 2B). To determinate whether the immune responses of the spl-A mutant was activated, we then compared the expression levels of immunity related genes, such as the pathogenesis-related (PR) genes, OsNAC4, OsPBZ1, OsPR1a and OsPR10 between spl-A and A814 at 15 days after sowing (Li et al., 2017; Tao et al., 2021). We found that the expression levels of all the PR genes analyzed were largely increased in spl-A when compared to A814 (Figure 2C). These results suggest that the spl-A mutant accumulates an excess of H2O2 and high levels of PR genes which may cause cell death and enhance plant basal disease resistance.
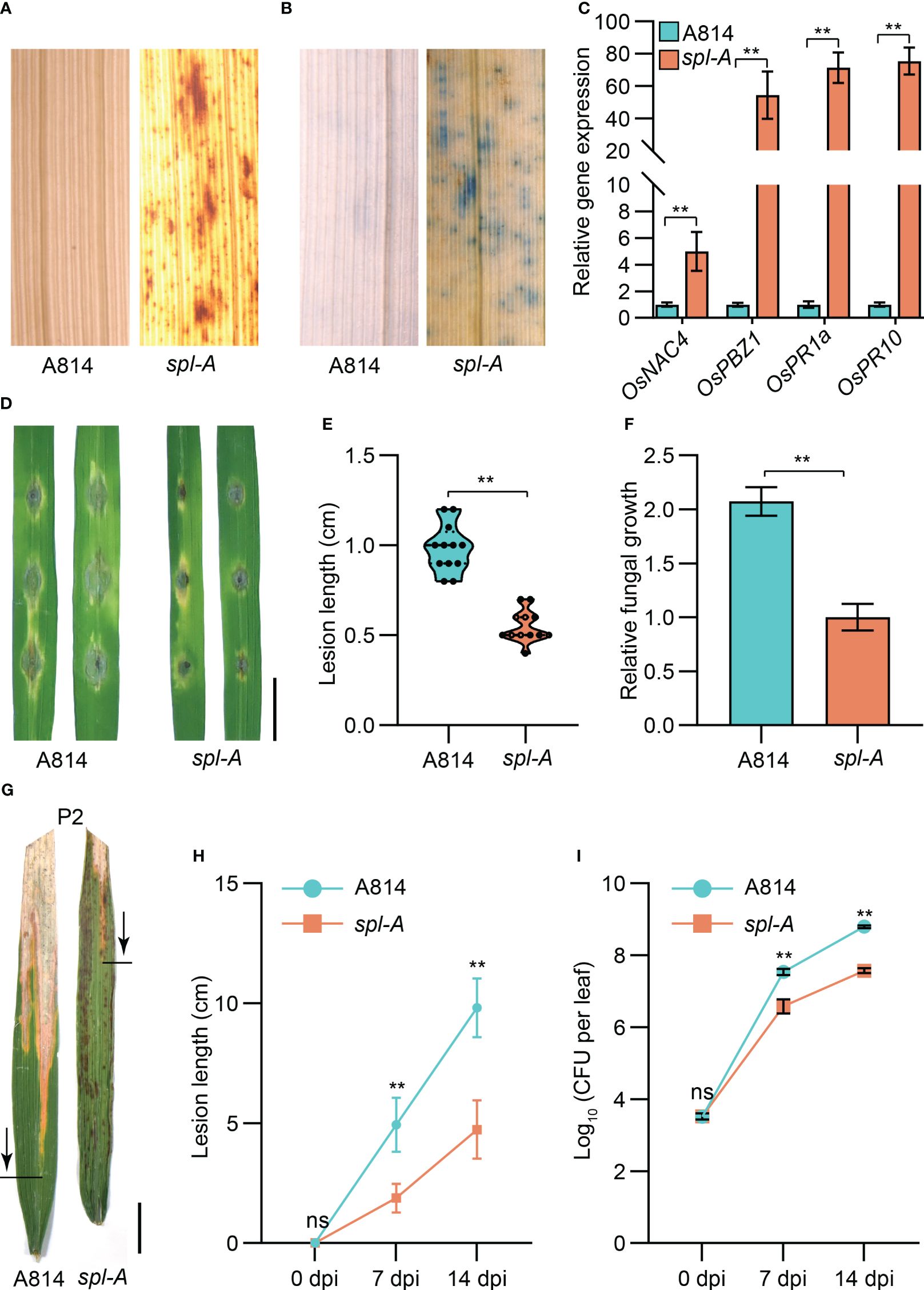
Figure 2 Determination on the immunity and disease resistance of the spl-A mutant. (A, B) The DAB staining (A) and trypan blue staining (B) analyses on A814 and spl-A at 15 days after sowing. (C) Comparison the expression levels of the pathogenesis-related (PR) genes between A814 and spl-A at 15 days after sowing. The expression levels of the PR genes were normalized to the reference gene Ubiquitin (mean ± s.d., n = 3 technical repetitions). (D–F) Evaluation on the blast resistance of the spl-A mutant. Punch inoculation method was employed. The blast fungal isolate Zhong10-8-14 that is compatible with A814 was used. Photograph of representative lesions (D) were shown. Bar = 1 cm. Lesion length (E) and the relative fungal growth (F) were measured at 5 day-post-inoculation (dpi). The relative fungal growth was determinated as fungi MoPot2 DNA to rice OsUbq DNA by qPCR (mean ± s.d., n = 3 technical repetitions). (G–I) Evaluation on the resistance of the spl-A mutant to bacterial blight disease. Photographs of representative leaves were taken at 14 dpi with Xanthomonas oryzae pv oryzae (Xoo) strain P2, which is compatible with A814 (G). Bar = 1 cm. Disease lesion lengths (H) and bacterial populations (I) of the spl-A mutant and A814 were measured at 0, 7 and 14 dpi respectively (mean ± s.d., n = 12 for lesion lengths and n = 3 for bacterial populations). All statistics were analyzed by Student’s t-test for P values (**, P <= 0.01; ns, no significant differences).
We then challenged the spl-A mutant with the fungal pathogen Magnaporthe oryzae (M. oryzae) and the bacterial pathogen Xanthomonas oryzae pv oryzae (Xoo) which cause rice blast and bacterial blight diseases respectively. As expected, the lesion length and the relative fungal growth of leaves inoculated with M. oryzae strains (Zhong10-8-14, ZE-1, 0755-1-1 and 99-20-2) which compatible with A814 were all dramatically reduced in the spl-A mutant when compared with those of A814 (Figures 2D–F and Supplementary Figure 2). When inoculated with compatible Xoo strains (P2, P6 and Xoo-4), the spl-A mutant also exhibited enhanced bacterial resistance, showing much shorter lesion length and less bacteria than A814 (Figures 2G–I and Supplementary Figure 3). Collectively, these results indicate that the spl-A mutant possesses enhanced immunity and broad-spectrum disease resistance.
Cloning of the Spl-A gene by combining map-based cloning strategy and BSA sequencing
In order to isolate the Spl-A gene, we reciprocally crossed the indica rice Jodan with the spl-A mutant and developed two F2 populations. Then, genetic analysis on the spl-A locus was carried out. We found the phenotype of spl-A was controlled by a single recessive nuclear locus through genetic analysis (Supplementary Table 2). Next, map-based cloning strategy and the bulked segregant analysis (BSA) sequencing method were simultaneously adopted to accelerate the cloning of Spl-A gene. The candidate gene was mapped in the interval with a physical distance of 3 Mb between the SSR markers RM19274 and RM19472 on chromosome 6 through map-based cloning strategy (Figure 3A). Combining with BSA sequencing analysis, we then identified the gene LOC_Os06g03940 which harbored two single nucleotide mutations in the spl-A mutant was likely the candidate of Spl-A gene (Figures 3B, C). The C147T mutation in the first exon of LOC_Os06g03940 is a nonsense mutation, while the C1144T mutation in the 10th exon leads to change of 382nd proline (P) to Serine (S) (Figures 3B, C).
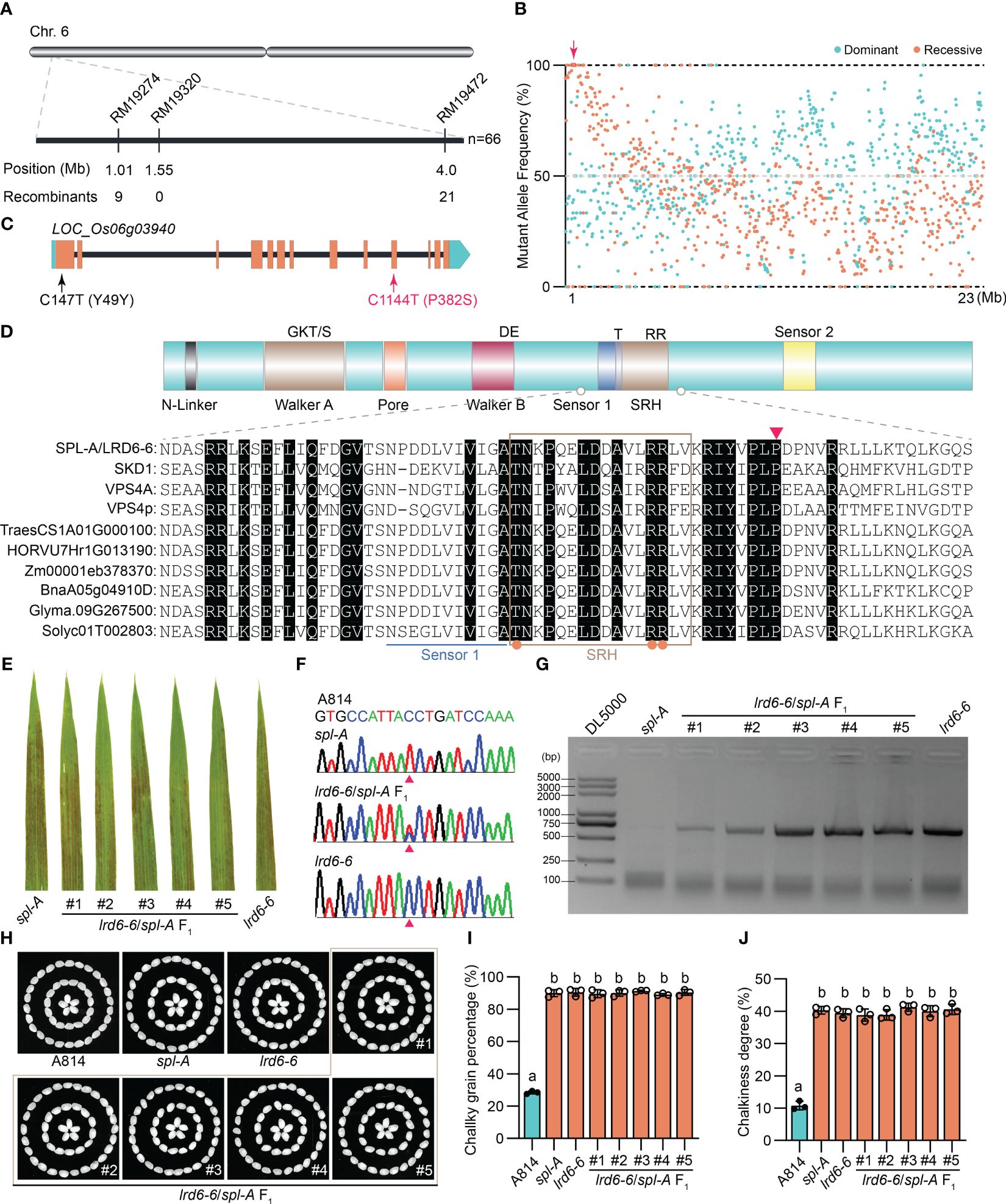
Figure 3 Molecular cloning and functional identification of Spl-A gene. (A) Preliminary mapping of the Spl-A locus. The Spl-A locus was delimited to a 3 Mb interval between SSR markers RM19274 and RM19472 on chromosome 6. The molecular markers and the number of recombinants is respectively shown. (B) Bulked segregant analysis (BSA) of the Spl-A locus. The candidate gene in the preliminary mapping interval is boxed and indicated by red arrow. (C) The schematic diagram of the Spl-A candidate gene identified by our combined strategy. The gene LOC_Os06g03940 harbors two mutation sites in the spl-A mutant. One mutation is a nonsense mutation (C147T) in the first exon and another one is missense mutation (C1144T) in the 10th exon that leads to change of Proline (P) to Serine (S). (D) Conserved structure of the LRD6-6 protein. The characteristics of typical AAA ATPase (upper panel) and partial sequence alignment analysis on LRD6-6 with some known AAA ATPases (lower panel) are respectively shown. The key elements of Walker A and B motifs, the Pore, Sensors 1 and 2, and the second region of homology (SRH) are respectively marked. The conserved residues in the SRH motif are indicated by orange dots. The 382th amino acid P that mutated to S in spl-A is indicated by red triangle. (E–J) Allelic analysis between the spl-A and lrd6-6 mutants. The leaves of spl-A, lrd6-6 and five F1 progenies from the crossing of lrd6-6 and spl-A were respectively shown (A). The genotypes of the five F1 progenies were detected (F, G). PCR-based sequencing was employed to determinate the C1144T mutant in spl-A (F). The representative DNA sequencing chromatograms for the wild-type A814, the mutants spl-A and lrd6-6, and the F1 progenies are respectively shown. PCR-based agarose gel electrophoresis was used to distinguish the genotype of lrd6-6. The grain appearance of spl-A, lrd6-6 and five F1 progenies from the crossing of lrd6-6 and spl-A were also determined (H–J). The photographs (H) and the chalky grain percentage (I), chalky grain degree (J) are respectively shown. Statistical significance was determined by one-way ANOVA, where different letters indicate significant differences (P <= 0.01), while the same letter indicates no significant differences (P > 0.05).
The gene LOC_Os06g03940 encoding an AAA-type ATPase has been previously reported by us as Lesion resembling disease 6-6 (Lrd6-6). The lrd6-6 mutant harbors a 1446-nt tandem repeat in LOC_Os06g03940 gene, resulting in autoimmunity and broad-spectrum disease resistance (Zhu et al., 2016). We then found that the amino acid mutation P382S caused by the C1144T mutation in spl-A was conserved among the AAA-type ATPase family (Figure 3D). To further test whether spl-A and lrd6-6 were allelic mutants caused by mutation of LOC_Os06g03940, we crossed lrd6-6 with spl-A and investigated the phenotype of the F1 progenies. We found that all the F1 progenies from lrd6-6×spl-A exhibited lesion spots on the leaves as that of the lrd6-6 and spl-A mutants (Figure 3E). The heterozygous genotypes (spl-A/lrd6-6) of the F1 progenies were confirmed by PCR-based sequencing and PCR-based agarose gel electrophoresis analysis respectively using specific primers (Figures 3F, G and Supplementary Table 1). Moreover, like the lrd6-6 and spl-A mutants, the grain quality of all the F1 progenies were found to deteriorate as indicated by increased chalky grain percentage and chalky grain degree (Figures 3H–J). These results suggest that spl-A and lrd6-6 are allelic mutants and the C1144T mutation in LOC_Os06g03940 is responsible for the autoimmunity and decreased grain quality of the spl-A mutant. In order to maintain the consistency of the gene name, Lrd6-6 was adopted to represent the Spl-A gene hereafter.
The P382S mutation of LRD6-6 in spl-A impairs its ATPase activity and disrupts its interaction with ESCRT-III subunits OsSNF7.1/7.2/7.3
LRD6-6 is an active ATPase that can interact with itself and the ESCRT-III subunits OsSNF7.1/7.2/7.3 and OsVPS2 to regulate the MVBs-mediated vesicular trafficking pathway (Zhu et al., 2016). To determinate the effects of the P382S mutation in LRD6-6, we examined the ATPase activity of LRD6-6P382S, the interaction of LRD6-6P382S with itself and the ESCRT-III subunits OsSNF7.1/7.2/7.3 and OsVPS2, and the MVBs localization of LRD6-6P382S.
We found that the P382S mutation in LRD6-6 impaired its ATPase activity because the His–LRD6-6(125–487)P382S recombinant protein purified from E. coli showed significantly reduced ATPase activity when compared to the wild-type protein His–LRD6-6(125–487) (Figure 4). The self-interaction of LRD6-6 was not affected by the P382S mutation as detected by using the yeast two hybrid (Y2H) and bimolecular fluorescence complementation (BiFC) assays (Supplementary Figure 4). The interactions between LRD6-6P382S and OsSNF7.1/7.2/7.3, LRD6-6P382S and OsVPS2 were also examined using these two methods. And it was found that the interactions between LRD6-6P382S and OsSNF7.1/7.2/7.3 were abolished while the interaction between LRD6-6P382S and OsVPS2 was not affected (Figure 5). We also checked the MVBs localization of the protein LRD6-6P382S by using RabF1/ARA6-RFP as the MVBs marker (Šamaj et al., 2005; Ebine et al., 2011). The co-localization of LRD6-6P382S-GFP punctate green fluorescence with RabF1/ARA6-RFP punctate red fluorescence was observed in Nicotiana benthamiana (N. benthamiana) cells, similar to that of the wild-type protein LRD6-6-GFP (Supplementary Figure 5), suggesting the P382S mutation in LRD6-6 not affects its MVBs localization.
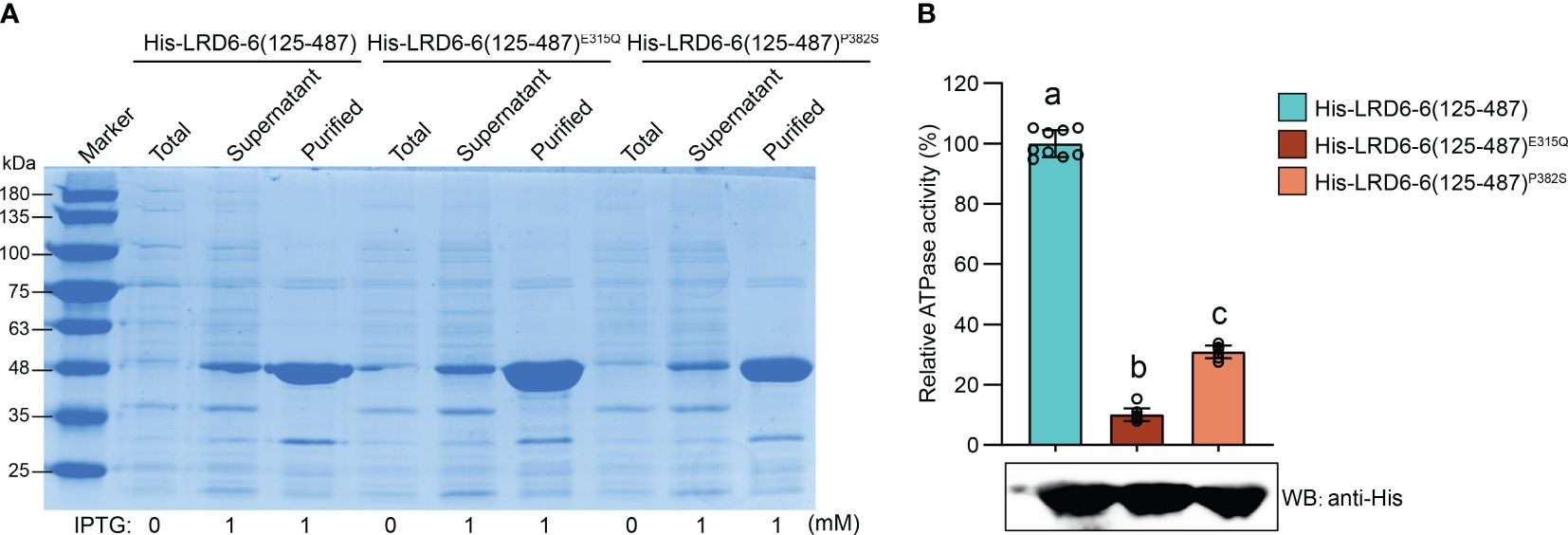
Figure 4 Determination of the ATPase activity on LRD6-6P382S protein in vitro. (A) Expression and purification of the N-terminal truncated recombinant proteins His–LRD6-6(125–487), His–LRD6-6(125–487)E315Q and His–LRD6-6(125–487)P382S from E. coli. Coomassie brilliant blue staining was used to detect the total proteins from E. coli before IPTG addition, and proteins in supernatant and the purified proteins after IPTG induction. (B) In vitro ATPase assay on recombinant proteins His–LRD6-6(125–487), His–LRD6-6(125–487)E315Q and His–LRD6-6(125–487)P382S. The proteins His–LRD6-6(125–487) and His–LRD6-6(125–487)E315Q were used as positive and negative control respectively. ATPase activities were measured using a malachite green-based colorimetric approach (mean ± s.d., n = 9 technical repetitions). The purified proteins used for ATPase activity determination were detected by anti-His antibody. Statistical significance was determined by one-way ANOVA, where different letters indicate significant differences (P <= 0.01).
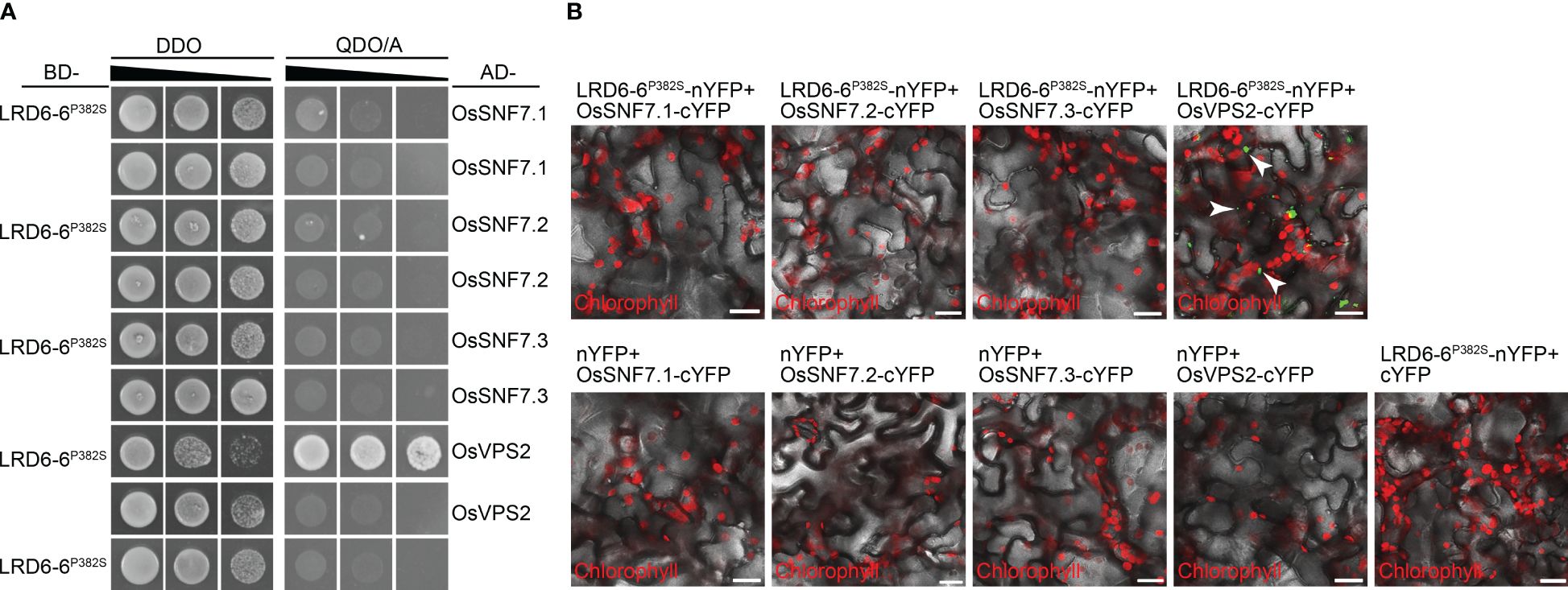
Figure 5 Detection the interaction between LRD6-6P382S with OsSNF7 and OsVPS2. The interaction between LRD6-6P382S with OsSNF7.1/7.2/7.3 and OsVPS2 were detected by using both yeast two hybrid (Y2H) (A) and bimolecular fluorescence complementation (BiFC) (B) approaches. BD, pGBKT7; AD, pGADT7; DDO, double dropout medium ((SD/–Leu/–Trp); QDO/A, quadruple dropout medium supplemented with Aureobasidin A (SD/–Ade/–His/–Leu/–Trp/AbA). BiFC assay was performed in N. benthamiana. The green fluorescence signals present the interaction between the tested proteins, the red signals represent the auto-fluorescence of chlorophyll. Some of the green fluorescence signals are indicated by white arrows to clearly shown the interaction. Bar = 20 μm.
Taken together, these results indicating that the conserved amino acid residue proline at 382nd of LRD6-6 is essential for its ATPase activity and is also pivotal for interacting with the ESCRT-III subunits OsSNF7.1/7.2/7.3. These results further confirming that the C1144T mutation in spl-A which leads to P382S mutation of LRD6-6 is responsible for the autoimmunity, broad-spectrum disease resistance and decreased grain quality of the spl-A mutant.
The MVBs-mediated vesicle trafficking is compromised in the spl-A mutant
To dissect the mechanism on how LRD6-6P382S affects rice growth and immune responses, we then collected leaf samples from A814 and spl-A respectively before the emergence of the spotted lesions on spl-A and analyzed the mRNA transcriptional level of genes associated with MVBs-mediated vesicle trafficking pathway firstly (Zhu et al., 2016). We found genes encoding possible MVBs-pathway components and MVBs-trafficking cargoes were largely dysregulated in the spl-A mutant when compared to the wild-type A814 (Figure 6A and Supplementary Table 3), suggesting that the MVBs-mediated vesicle trafficking pathway is defective in spl-A. Deficient in MVBs-mediated vesicle trafficking will lead to ROS burst and antimicrobial metabolites accumulation (Zhu et al., 2016). We thus also detected the mRNA expression levels of the genes associated with these processes (Zhu et al., 2016). In accordance with previous report, the expression of genes involved in ROS metabolism and biosynthesis of antimicrobial metabolites serotonin and diterpenoid phytoalexin were found to increase significantly in spl-A (Figure 6A and Supplementary Table 3), suggesting spl-A may accumulate excess of ROS and antimicrobial metabolites which can activate immune responses and increase disease resistance.
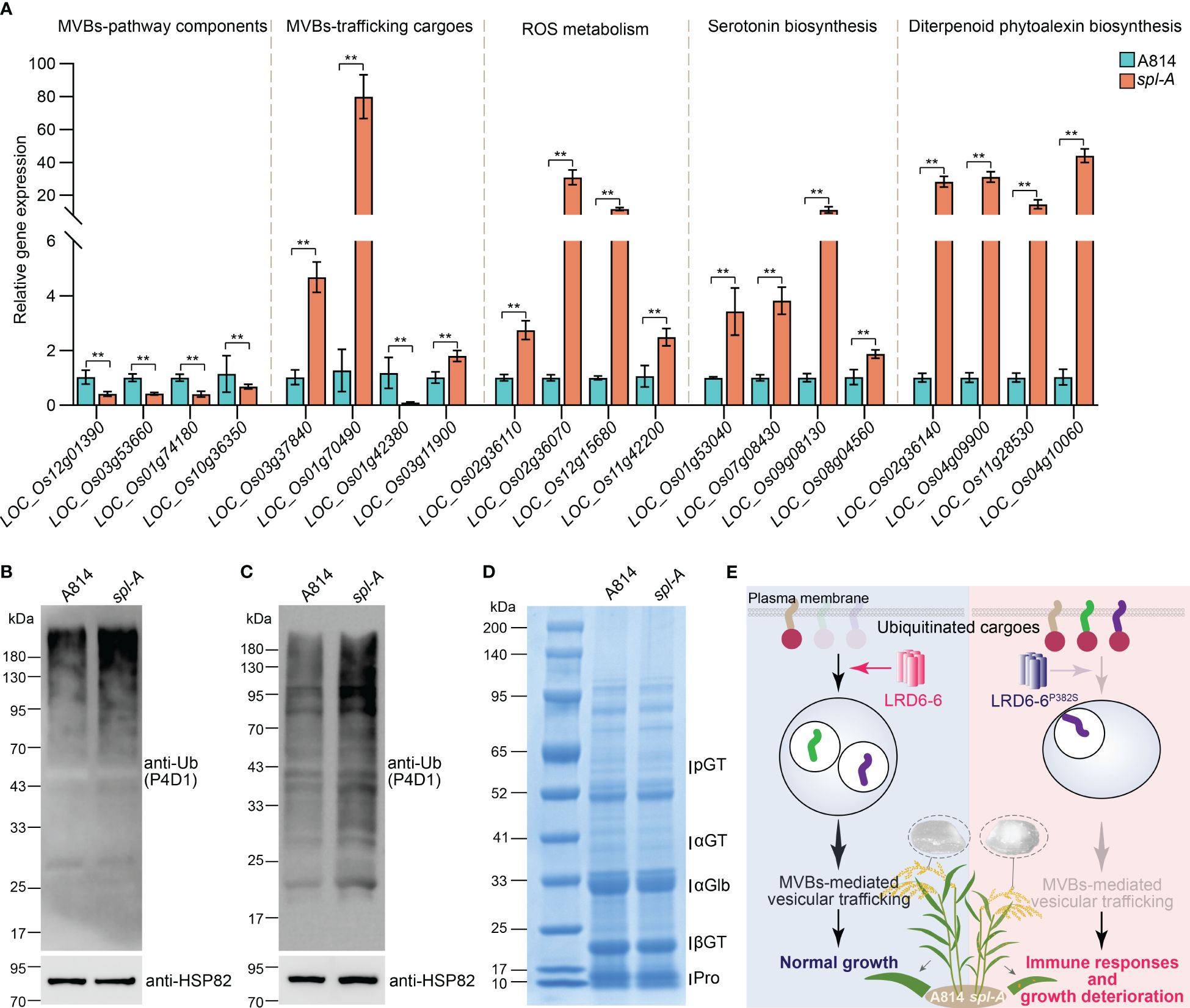
Figure 6 Characterization of the MVBs-mediated vesicle trafficking pathway in spl-A. (A) The mRNA transcriptional level of genes associated with MVBs-mediated vesicle trafficking pathway. The expression of genes that encoding possible MVBs-pathway components, possible MVBs-mediated trafficking cargoes, regulators involved in ROS metabolism, and key transcriptional factor and enzymes which regulate the biosynthesis of antimicrobial metabolites serotonin and diterpenoid phytoalexin was analyzed by RT-qPCR. The expression levels of the genes were normalized to the reference gene Ubiquitin and then normalized to the expression level of the genes in A814 (mean ± s.d., n = 3 technical repetitions). The detailed information of these genes was listed in Supplementary Table S2. All statistics were analyzed by Student’s t-test for P values (**, P <= 0.01). (B) Detection of the ubiquitinated conjugates in A814 and spl-A leaves. (C) Detection of the ubiquitinated conjugates in A814 and spl-A dry seeds. Total protein from A814 and spl-A leaves (C) or dry seeds (C) were respectively extracted and subjected to immunoblot analysis using the anti-Ub antibody (P4D1). The protein levels of HSP82 were detected to indicate the loading amount of total protein in each lane. (D) Comparison the protein profiles between A814 and spl-A dry seeds on SDS–PAGE gels stained by Coomassie blue. 20 μg of total protein for each sample was loaded. blue. pGT, 57-kDa proglutelins; αGT, 40-kDa glutelin acidic subunits; αGlb, 26-kDa a-globulin; βGT, 20-kDa glutelin basic subunits; Pro, prolamins. (E) An illustration summarizes how spl-A mutant activates immune responses and deteriorates plant growth in rice. The AAA ATPase LRD6-6 regulates the maturation of MVBs and promotes MVBs-mediated transportation of ubiquitinated proteins to guarantee normal growth of rice plant (left panel). The P382S mutation of LRD6-6 in spl-A impairs its ATPase activity and disrupts its interaction with the ESCRT-III subunits OsSNF7.1/7.2/7.3, thus compromise MVBs-mediated transportation process and leading to immune responses activation and growth deterioration including decreased rice quality (right panel).
The MVBs are well-known for their function in sorting ubiquitinated plasma membrane proteins (Hurley, 2008; Gao et al., 2014). To further characterize the effects of LRD6-6 P382S mutation in spl-A, we then detected the ubiquitination level of total protein from the spl-A mutant leaf. More ubiquitinated proteins were detected in spl-A by using the anti-Ub (P4D1) antibody when compared to A814 (Figure 6B), indicating P382S mutation of LRD6-6 leading to accumulate of more ubiquitinated proteins in plant. Together, our current results suggest that the enhanced immune responses and deteriorated growth of the spl-A mutant is likely caused by the compromise of MVBs-mediated vesicle trafficking which leading to ROS burst and antimicrobial metabolites accumulation.
Since spl-A exhibited increased chalky grain percentage and chalky grain degree (Figures 1C–E), we speculated that the MVBs-mediated vesicle trafficking of ubiquitinated proteins in seeds were also inhibited. Thus, we also explored the ubiquitination level of total protein from spl-A seeds. It was found that the ubiquitinated proteins accumulated in spl-A seeds were obviously more than that of A814 (Figure 6C), suggesting the MVBs-mediated vesicle trafficking is also inhibited in seed. The contents of storage proteins in rice seeds are important for establishment of grain quality including chalkiness (Li et al., 2022). We then compared the contents of major types of rice seeds storage proteins, glutelins, α-globulin and prolamins between spl-A and A814. The SDS-PAGE based Coomassie blue staining showed that there were no obvious differences in the content of storage proteins between spl-A and A814 seeds (Figure 6D). These results suggest that the compromised MVBs-mediated vesicle trafficking in spl-A seed deteriorate grain chalkiness likely through a pathway independent of storage proteins contents.
Discussion
The spl-A mutant exhibits activated immune responses and decreased grain quality in rice
The mutation of the Spl-A locus has been previously reported as lesion mimic resembling (lmr), lesion resembling disease 6-6 (lrd6-6) and spotted leaf 4 (spl4) in rice (Fekih et al., 2015; Zhu et al., 2016; Song et al., 2019). Like most of spl mutants, the lmr and lrd6-6 mutants accumulated an excess of H2O2 and death cells, exhibited enhanced disease resistance to both fungal and bacterial diseases (Lorrain et al., 2003; Fekih et al., 2015; Zhu et al., 2016, 2020). Consistently with previous reports, the spl-A mutant also presented constitutive activated immune responses and enhanced broad-spectrum disease resistance (Figure 2, Supplementary Figure 2 and Supplementary Figure 3). Besides immune responses, the Spl-A locus also regulates other aspects of plant phenotypes, such as leaf senescence and agronomic traits including grain weight, plant height and seed setting rate etc. It’s interesting that the lrd6-6 and spl-A mutants exhibited decreased grain weight, while the spl4 mutant showed increased grain weight (Fekih et al., 2015; Zhu et al., 2016; Song et al., 2019) (Supplementary Figure 1E). These results suggest that the Spl-A locus may regulate grain weight or even other traits depends on the genetic background of rice.
Although the spl mutants are broadly studied for their roles in immune responses, senescence, and plant growth or development (Zhu et al., 2020). It still remains unknown whether these mutants/genes regulate grain quality. In our study, we examined the chalkiness of the spl-A mutant and found that the spl-A mutant showed increased chalky grain percentage and chalky grain degree which deteriorates grain quality (Figures 1C–E and Figure 3H–J). Thus, our current study shed light on the new role of the spl mutants in grain quality regulation.
The conserved 382nd amino acid residue proline is essential for the full function of the AAA-type ATPase LRD6-6
The AAA-type ATPase family proteins contain conserved ATPase domains spanning 200–250 residues which cover the Walker A, Walker B and the SRH (Second Region of Homology) motifs that distinguish them from classic p-loop NTPases (Ogura and Wilkinson, 2001; Hanson and Whiteheart, 2005). VPS4 is a member of the AAA-type ATPase family which has been found to form hexamer and assemble with the ESCRT-III complex subunits to drive the maturation of MVBs (Babst et al., 1997; Schmidt and Teis, 2012). SKD1 and LRD6-6 are homologs of VPS4 from Arabidopsis and rice respectively, and they may function in MVBs maturation like that of VPS4 (Zhu et al., 2016). Several amino acid residues that essential for the function of VPS4/SKD1/LRD6-6 have been identified. For example, the 261st (LRD6-6 as reference) lysine in Walker A motif is essential for nucleotide binding, the 315th glutamic acid in Walker B is responsible for ATP hydrolysis, and the 372nd arginine in SRH is vital for both ATP hydrolysis and oligomerization (Babst et al., 1998; Ogura et al., 2004; Zhu et al., 2016). The spl-A mutant in this study was caused by a single nucleotide mutation (C1144T) which led to a single amino acid substitution (P382S) in the AAA-type ATPase LRD6-6 (Figures 3A–C). Unlike the mutations in lmr, lrd6-6 and spl4 whose genes were destructed (Fekih et al., 2015; Zhu et al., 2016; Song et al., 2019), the mutation of spl-A provides us a unique allele to study the function of the 382nd amino acid residue proline for LRD6-6.
The 382nd amino acid residue proline locates in neither of the above-mentioned motifs of LRD6-6, but it’s conserved among this AAA-type ATPase family (Figure 3D). We then assayed the ATPase activity, the interaction with the ESCRT-III subunits OsSNF7.1/7.2/7.3 and OsVPS2, and the MVBs localization of the mutation protein LRD6-6P382S from the spl-A mutant. Our results indicate that the P382S mutation of LRD6-6 impairs its ATPase activity and disrupts the interaction with the ESCRT-III subunits OsSNF7.1/7.2/7.3, while not affects its MVBs localization (Figures 4, 5 and Supplementary Figures 4, 5). Thus, in this study, we have characterized a new conserved amino acid residue that is important for the full function of LRD6-6 and likely, for all the AAA-type ATPase family proteins since this site is conserved among them.
LRD6-6 regulates MVBs-mediated vesicle trafficking to modulate rice immunity and grain quality
MVBs are single membrane bound organelles that serve as core converging station of the secretory and endocytic pathways for cargo trafficking in eukaryotic cells (Piper and Katzmann, 2007; Cui et al., 2016). Generally, ubiquitinated cargo proteins on the plasm membrane will be recognized and packaged into the intralumenal vesicles (ILVs) of MVBs by the ESCRT complexes. Then, these cargo proteins are transported by the MVBs to different destinations for proper functions (Piper and Katzmann, 2007; Cui et al., 2016; Zhu et al., 2023). Impairment of the MVBs-mediated vesicle trafficking pathway will lead to accumulate of ubiquitinated proteins in cells as reported in Yeast and Arabidopsis (Mizuno et al., 2003; Stringer and Piper, 2011; Gao et al., 2014; Katsiarimpa et al., 2014). However, whether rice cells will accumulate ubiquitinated proteins when this pathway is inhibited still remain unknown. In this study, we found ubiquitinated proteins accumulated in both the leaves and seeds of the spl-A mutant in which the MVBs-mediated vesicle trafficking was inhibited by mutation of the AAA-type ATPase LRD6-6 (Figures 6B, C). Thus, our discovery not only reveals the conserved roles of MVBs-mediated vesicle trafficking on the transportation of ubiquitinated proteins, but also expands its function in grain quality formation.
In summary, our study reveals that the conserved amino acid residue proline at the 382th of the AAA-type ATPase LRD6-6 is essential for its ATPase activity and its interaction with the ESCRT-III subunits OsSNF7.1/7.2/7.3. Once this site is mutated, the MVBs-mediated vesicle trafficking pathway governed by LRD6-6 is compromised and rice plant harboring this mutation will accumulate excessive ubiquitinated cargo proteins in both leaves and seeds. Then, the immune responses of this mutant plant will be activated, and the growth and grain quality of this plant will be deteriorated (Figure 6E).
Data availability statement
The original contributions presented in the study are included in the article/Supplementary Material. Further inquiries can be directed to the corresponding author/s.
Author contributions
JY: Funding acquisition, Methodology, Resources, Writing – original draft, Data curation, Investigation, Validation. CZ: Investigation, Methodology, Validation, Writing – review & editing. QZ: Investigation, Methodology, Validation, Writing – review & editing. FL: Investigation, Methodology, Validation, Writing – review & editing. WH: Investigation, Formal analysis, Writing – review & editing. YiZ: Formal analysis, Investigation, Writing – review & editing. FM: Formal analysis, Investigation, Writing – review & editing. YuZ: Investigation, Writing – review & editing. BW: Investigation, Writing – review & editing. MZ: Investigation, Writing – review & editing. LZ: Formal analysis, Funding acquisition, Writing – review & editing. XZ: Formal analysis, Funding acquisition, Conceptualization, Methodology, Project administration, Resources, Supervision, Visualization, Writing – original draft, Writing – review & editing.
Funding
The author(s) declare financial support was received for the research, authorship, and/or publication of this article. This work was funded by the National Key Research and Development Program of China for young scientists (2022YFD1401400), the National Natural Science Foundation of China (32072407, 32072041 and 32272560), and the Sichuan Science and Technology Program (2024NSFSC0326, 2023NSFSC1937 and 2022NSFSC0156).
Conflict of interest
The authors declare that the research was conducted in the absence of any commercial or financial relationships that could be construed as a potential conflict of interest.
Publisher’s note
All claims expressed in this article are solely those of the authors and do not necessarily represent those of their affiliated organizations, or those of the publisher, the editors and the reviewers. Any product that may be evaluated in this article, or claim that may be made by its manufacturer, is not guaranteed or endorsed by the publisher.
Supplementary material
The Supplementary Material for this article can be found online at: https://www.frontiersin.org/articles/10.3389/fpls.2024.1451897/full#supplementary-material
Supplementary Figure 1 | Comparison on the main agronomic traits between and spl-A. The plant height (A), tiller number (B), panicle length (C), seed setting rate (D) and 1,000-grain weight (E) of the spl-A mutant were respectively investigated and analyzed (mean ± s.d.). (F–H) Comparison on grain length (G) and grain width (H) between A814 and spl-A (mean ± s.d.). Representative photograph of ten grains were respectively shown. Bar = 1 cm. All statistics were analyzed by Student’s t-test for P values (**, P <= 0.01; ns, no significant differences).
Supplementary Figure 2 | Evaluation the blast resistance of spl-A to different blast fungal isolates. Punch inoculation method was employed. The blast fungal isolates ZE-1 (A–C), 0755-1-1 (D–F) and 99-20-2 (G–I) that are compatible with A814 was respectively used. Photograph of representative lesions (A, D, G) were shown. Lesion length (B, E, H) and the relative fungal growth (C, F, I) were measured at 5 day-post-inoculation (dpi). The relative fungal growth was determinated as fungi MoPot2 DNA to rice OsUbq DNA by qPCR (mean ± s.d., n = 3 technical repetitions). Bars = 1 cm. All statistics were analyzed by Student’s t-test for P values (**, P <= 0.01).
Supplementary Figure 3 | Evaluation the bacterial blight disease resistance of spl-A to different Xoo isolates. The resistance of spl-A to Xoo isolates P6 (A–C) and Xoo-4 (D–F) were tested. Photographs of representative leaves were taken at 14 dpi (A, D). Disease lesion lengths (B, E) and bacterial populations (C, F) of A814 and the spl-A mutant were measured at 0, 7 and 14 dpi respectively (mean ± s.d., n = 12 for lesion lengths and n = 3 for bacterial populations). Bars = 1 cm. All statistics were analyzed by Student’s t-test for P values (**, P <= 0.01).
Supplementary Figure 4 | Detection the self-interaction of LRD6-6P382S protein. The self-interaction of LRD6-6P382S was detected by using both Y2H (A) and BiFC (B) approaches. BD, pGBKT7; AD, pGADT7; DDO, double dropout medium ((SD/–Leu/–Trp); QDO/A, quadruple dropout medium supplemented with Aureobasidin A (SD/–Ade/–His/–Leu/–Trp/AbA). BiFC assay was performed in N. benthamiana. The green fluorescence signals present the interaction between the tested proteins, the red signals represent the auto-fluorescence of chlorophyll. Some of the green fluorescence signals are indicated by white arrows to clearly shown the interaction. Bar = 20 μm.
Supplementary Figure 5 | Subcellular localization of the protein LRD6-6P382S. (A) Determination the subcellular localization of protein LRD6-6P382S fused with green fluorescence protein (LRD6-6P382S-GFP) in N. benthamiana. The RabF1/ARA6 protein that fused with red fluorescence protein (RabF1/ARA6-RFP) was used as the multivesicular bodies (MVBs) marker. Some of the overlapped punctate fluorescence signals were indicated by white arrows to clearly shown the co-localization. The fluorescence intensity along the white line was also measured and shown to indicate the co-localization (B). The co-localization of the wild-type LRD6-6-GFP with RabF1/ARA6-RFP was included as control (C, D). Bar = 20 μm.
References
Aniento, F., Sánchez De Medina Hernández, V., Dagdas, Y., Rojas-Pierce, M., Russinova, E. (2021). Molecular mechanisms of endomembrane trafficking in plants. Plant Cell 34, 146–173. doi: 10.1093/plcell/koab235
Babst, M., Sato, T. K., Banta, L. M., Emr, S. D. (1997). Endosomal transport function in yeast requires a novel AAA-type ATPase, Vps4p. EMBO J. 16, 1820–1831. doi: 10.1093/emboj/16.8.1820
Babst, M., Wendland, B., Estepa, E. J., Emr, S. D. (1998). The Vps4p AAA ATPase regulates membrane association of a Vps protein complex required for normal endosome function. EMBO J. 17, 2982–2993. doi: 10.1093/emboj/17.11.2982
Balint-Kurti, P. (2019). The plant hypersensitive response: concepts, control and consequences. Mol. Plant Pathol. 20, 1163–1178. doi: 10.1111/mpp.12821
Bruggeman, Q., Raynaud, C., Benhamed, M., Delarue, M. (2015). To die or not to die? Lessons from lesion mimic mutants. Front. Plant Sci. 6. doi: 10.3389/fpls.2015.00024
Caddell, D. F., Park, C.-J., Thomas, N. C., Canlas, P. E., Ronald, P. C. (2017). Silencing of the rice gene LRR1 compromises rice Xa21 transcript accumulation and XA21-mediated immunity. Rice 10, 23. doi: 10.1186/s12284-017-0162-5
Cui, Y., Shen, J., Gao, C., Zhuang, X., Wang, J., Jiang, L. (2016). Biogenesis of plant prevacuolar multivesicular bodies. Mol. Plant 9, 774–786. doi: 10.1016/j.molp.2016.01.011
Ebine, K., Fujimoto, M., Okatani, Y., Nishiyama, T., Goh, T., Ito, E., et al. (2011). A membrane trafficking pathway regulated by the plant-specific RAB GTPase ARA6. Nat. Cell Biol. 13, 853–859. doi: 10.1038/ncb2270
Fekih, R., Tamiru, M., Kanzaki, H., Abe, A., Yoshida, K., Kanzaki, E., et al. (2015). The rice (Oryza sativa L.) LESION MIMIC RESEMBLING, which encodes an AAA-type ATPase, is implicated in defense response. Mol. Genet. Genomics 290, 611–622. doi: 10.1007/s00438-014-0944-z
Gao, C., Luo, M., Zhao, Q., Yang, R., Cui, Y., Zeng, Y., et al. (2014). A unique plant ESCRT component, FREE1, regulates multivesicular body protein sorting and plant growth. Curr. Biol. 24, 2556–2563. doi: 10.1016/j.cub.2014.09.014
Hanson, P. I., Whiteheart, S. W. (2005). AAA+ proteins: have engine, will work. Nat. Rev. Mol. Cell Biol. 6, 519–529. doi: 10.1038/nrm1684
Hurley, J. H. (2008). ESCRT complexes and the biogenesis of multivesicular bodies. Curr. Opin. Cell Biol. 20, 4–11. doi: 10.1016/j.ceb.2007.12.002
Jiang, M., Yu, N., Zhang, Y., Liu, L., Li, Z., Wang, C., et al. (2022). Deletion of diterpenoid biosynthetic genes CYP76M7 and CYP76M8 induces cell death and enhances bacterial blight resistance in indica rice ‘9311’. Int. J. Mol. Sci. 23, 7234. doi: 10.3390/ijms23137234
Jones, J. D. G., Dangl, J. L. (2006). The plant immune system. Nature 444, 323–329. doi: 10.1038/nature05286
Katsiarimpa, A., Muñoz, A., Kalinowska, K., Uemura, T., Rojo, E., Isono, E. (2014). The ESCRT-III-interacting deubiquitinating enzyme AMSH3 is essential for degradation of ubiquitinated membrane proteins in Arabidopsis thaliana. Plant Cell Physiol. 55, 727–736. doi: 10.1093/pcp/pcu019
Li, P., Chen, Y.-H., Lu, J., Zhang, C.-Q., Liu, Q.-Q., Li, Q.-F. (2022). Genes and their molecular functions determining seed structure, components, and quality of rice. Rice 15, 18. doi: 10.1186/s12284-022-00562-8
Li, W., Wang, K., Chern, M., Liu, Y., Zhu, Z., Liu, J., et al. (2020). Sclerenchyma cell thickening through enhanced lignification induced by OsMYB30 prevents fungal penetration of rice leaves. New Phytol. 226, 1850–1863. doi: 10.1111/nph.16505
Li, Z., Ding, B., Zhou, X., Wang, G.-L. (2017). The rice dynamin-related protein OsDRP1E negatively regulates programmed cell death by controlling the release of cytochrome c from mitochondria. PloS Pathog. 13, e1006157. doi: 10.1371/journal.ppat.1006157
Liu, L., Zhang, Y., Tang, S., Zhao, Q., Zhang, Z., Zhang, H., et al. (2010). An efficient system to detect protein ubiquitination by agroinfiltration in Nicotiana benthamiana. Plant J. 61, 893–903. doi: 10.1111/tpj.2010.61.issue-5
Lorrain, S., Vailleau, F., Balagué, C., Roby, D. (2003). Lesion mimic mutants: keys for deciphering cell death and defense pathways in plants? Trends Plant Sci. 8, 263–271. doi: 10.1016/S1360-1385(03)00108-0
Ma, J., Chen, J., Wang, M., Ren, Y., Wang, S., Lei, C., et al. (2017). Disruption of OsSEC3A increases the content of salicylic acid and induces plant defense responses in rice. J. Exp. Bot. 69, 1051–1064. doi: 10.1093/jxb/erx458
Ma, J., Wang, Y., Ma, X., Meng, L., Jing, R., Wang, F., et al. (2019). Disruption of gene SPL35, encoding a novel CUE domain-containing protein, leads to cell death and enhanced disease response in rice. Plant Biotechnol. J. 17, 1679–1693. doi: 10.1111/pbi.13093
Mizuno, E., Kawahata, K., Kato, M., Kitamura, N., Komada, M. (2003). STAM proteins bind ubiquitinated proteins on the early endosome via the VHS domain and ubiquitin-interacting motif. Mol. Biol. Cell 14, 3675–3689. doi: 10.1091/mbc.e02-12-0823
Mur, L., Kenton, P., Lloyd, A. J., Ougham, H., Prats, E. (2008). The hypersensitive response; the centenary is upon us but how much do we know? J. Exp. Bot. 59, 501–520. doi: 10.1093/jxb/erm239
Ogura, T., Whiteheart, S. W., Wilkinson, A. J. (2004). Conserved arginine residues implicated in ATP hydrolysis, nucleotide-sensing, and inter-subunit interactions in AAA and AAA+ ATPases. J. Struct. Biol. 146, 106–112. doi: 10.1016/j.jsb.2003.11.008
Ogura, T., Wilkinson, A. J. (2001). AAA+ superfamily ATPases: common structure–diverse function. Genes to Cells 6, 575–597. doi: 10.1046/j.1365-2443.2001.00447.x
Pan, T., Wang, Y., Jing, R., Wang, Y., Wei, Z., Zhang, B., et al. (2021). Post-Golgi trafficking of rice storage proteins requires the small GTPase Rab7 activation complex MON1–CCZ1. Plant Physiol. 187, 2174–2191. doi: 10.1093/plphys/kiab175
Piper, R. C., Katzmann, D. J. (2007). Biogenesis and function of multivesicular bodies. Annu. Rev. Cell Dev. Biol. 23, 519–547. doi: 10.1146/annurev.cellbio.23.090506.123319
Qiao, Y., Jiang, W., Lee, J., Park, B., Choi, M.-S., Piao, R., et al. (2010). SPL28 encodes a clathrin-associated adaptor protein complex 1, medium subunit μ1 (AP1M1) and is responsible for spotted leaf and early senescence in rice (Oryza sativa). New Phytol. 185, 258–274. doi: 10.1111/j.1469-8137.2009.03047.x
Ren, Y., Wang, Y., Pan, T., Wang, Y., Wang, Y., Gan, L., et al. (2020). GPA5 encodes a Rab5a effector required for post-Golgi trafficking of rice storage proteins. Plant Cell 32, 758–777. doi: 10.1105/tpc.19.00863
Ren, D., Xie, W., Xu, Q., Hu, J., Zhu, L., Zhang, G., et al. (2022). LSL1 controls cell death and grain production by stabilizing chloroplast in rice. Sci. China Life Sci. 65, 2148–2161. doi: 10.1007/s11427-022-2152-6
Šamaj, J., Read, N. D., Volkmann, D., Menzel, D., Baluška, F. (2005). The endocytic network in plants. Trends Cell Biol. 15, 425–433. doi: 10.1016/j.tcb.2005.06.006
Schmidt, O., Teis, D. (2012). The ESCRT machinery. Curr. Biol. 22, R116–R120. doi: 10.1016/j.cub.2012.01.028
Song, G., Kwon, C.-T., Kim, S.-H., Shim, Y., Lim, C., Koh, H.-J., et al. (2019). The Rice SPOTTED LEAF4 (SPL4) encodes a plant spastin that inhibits ROS accumulation in leaf development and functions in leaf senescence. Front. Plant Sci. 9. doi: 10.3389/fpls.2018.01925
Stringer, D. K., Piper, R. C. (2011). A single ubiquitin is sufficient for cargo protein entry into MVBs in the absence of ESCRT ubiquitination. J. Cell Biol. 192, 229–242. doi: 10.1083/jcb.201008121
Tao, H., Shi, X., He, F., Wang, D., Xiao, N., Fang, H., et al. (2021). Engineering broad-spectrum disease-resistant rice by editing multiple susceptibility genes. J. Integr. Plant Biol. 63, 1639–1648. doi: 10.1111/jipb.13145
Tsuda, K., Katagiri, F. (2010). Comparing signaling mechanisms engaged in pattern-triggered and effector-triggered immunity. Curr. Opin. Plant Biol. 13, 459–465. doi: 10.1016/j.pbi.2010.04.006
Wang, Y., Teng, Z., Li, H., Wang, W., Xu, F., Sun, K., et al. (2023). An activated form of NB-ARC protein RLS1 functions with cysteine-rich receptor-like protein RMC to trigger cell death in rice. Plant Commun. 4, 100459. doi: 10.1016/j.xplc.2022.100459
Yao, D., Wu, J., Luo, Q., Li, J., Zhuang, W., Xiao, G., et al. (2020). Influence of high natural field temperature during grain filling stage on the morphological structure and physicochemical properties of rice (Oryza sativa L.) starch. Food Chem. 310, 125817. doi: 10.1016/j.foodchem.2019.125817
Yao, Y., Zhou, J., Cheng, C., Niu, F., Zhang, A., Sun, B., et al. (2022). A conserved clathrin-coated vesicle component, OsSCYL2, regulates plant innate immunity in rice. Plant Cell Environ. 45, 542–555. doi: 10.1111/pce.14240
Yuan, M., Ngou, B. P. M., Ding, P., Xin, X.-F. (2021). PTI-ETI crosstalk: an integrative view of plant immunity. Curr. Opin. Plant Biol. 62, 102030. doi: 10.1016/j.pbi.2021.102030
Zhang, X.-Q., Hou, P., Zhu, H.-T., Li, G.-D., Liu, X.-G., Xie, X.-M. (2013). Knockout of the VPS22 component of the ESCRT-II complex in rice (Oryza sativa L.) causes chalky endosperm and early seedling lethality. Mol. Biol. Rep. 40, 3475–3481. doi: 10.1007/s11033-012-2422-1
Zhao, Y., Zhu, X., Chen, X., Zhou, J.-M. (2022). From plant immunity to crop disease resistance. J. Genet. Genomics 49, 693–703. doi: 10.1016/j.jgg.2022.06.003
Zheng, Y., Zhu, Y., Mao, X., Jiang, M., Wei, Y., Lian, L., et al. (2022). SDR7-6, a short-chain alcohol dehydrogenase/reductase family protein, regulates light-dependent cell death and defence responses in rice. Mol. Plant Pathol. 23, 78–91. doi: 10.1111/mpp.13144
Zhu, X., Yin, J., Guo, H., Wang, Y., Ma, B. (2023). Vesicle trafficking in rice: too little is known. Front. Plant Sci. 14. doi: 10.3389/fpls.2023.1263966
Zhu, X., Yin, J., Liang, S., Liang, R., Zhou, X., Chen, Z., et al. (2016). The multivesicular bodies (MVBs)-localized AAA ATPase LRD6-6 inhibits immunity and cell death likely through regulating MVBs-mediated vesicular trafficking in rice. PloS Genet. 12, e1006311. doi: 10.1371/journal.pgen.1006311
Keywords: AAA-type ATPase, multivesicular bodies, vesicle trafficking, immune response, grain quality, rice
Citation: Yin J, Zhang C, Zhang Q, Long F, Hu W, Zhou Y, Mou F, Zhong Y, Wu B, Zhu M, Zou L and Zhu X (2024) A single amino acid substitution in the AAA-type ATPase LRD6-6 activates immune responses but decreases grain quality in rice. Front. Plant Sci. 15:1451897. doi: 10.3389/fpls.2024.1451897
Received: 20 June 2024; Accepted: 22 July 2024;
Published: 06 August 2024.
Edited by:
Hao Zhou, Sichuan Agricultural University, ChinaReviewed by:
Zhiqiang Li, Chinese Academy of Agricultural Sciences, ChinaXiaofeng Su, Chinese Academy of Agricultural Sciences, China
Copyright © 2024 Yin, Zhang, Zhang, Long, Hu, Zhou, Mou, Zhong, Wu, Zhu, Zou and Zhu. This is an open-access article distributed under the terms of the Creative Commons Attribution License (CC BY). The use, distribution or reproduction in other forums is permitted, provided the original author(s) and the copyright owner(s) are credited and that the original publication in this journal is cited, in accordance with accepted academic practice. No use, distribution or reproduction is permitted which does not comply with these terms.
*Correspondence: Xiaobo Zhu, bXlzZWxmMTk4NjEwMjVAMTYzLmNvbQ==
†These authors have contributed equally to this work and share first authorship