- School of Life Sciences, Tokyo University of Pharmacy and Life Sciences, Hachioji, Tokyo, Japan
Polyphosphate is prevalent in living organisms. To obtain insights into polyphosphate synthesis and its physiological significance in cyanobacteria, we characterize sll0290, a homolog of the polyphosphate-kinase-1 gene, in the freshwater cyanobacterium Synechocystis sp. PCC 6803. The Sll0290 protein structure reveals characteristics of Ppk1. A Synechocystis sll0290 disruptant and sll0290-overexpressing Escherichia coli transformant demonstrated loss and gain of polyphosphate synthesis ability, respectively. Accordingly, sll0290 is identified as ppk1. The disruptant (Δppk1) grows normally with aeration of ordinary air (0.04% CO2), consistent with its photosynthesis comparable to the wild type level, which contrasts with a previously reported high-CO2 (5%) requirement for Δppk1 in an alkaline hot spring cyanobacterium, Synechococcus OS-B’. Synechocystis Δppk1 is defective in polyphosphate hyperaccumulation and survival competence at the stationary phase, and also under sulfur-starvation conditions, implying that sulfur limitation is one of the triggers to induce polyphosphate hyperaccumulation in stationary cells. Furthermore, Δppk1 is defective in the enhancement of total phosphorus contents under sulfur-starvation conditions, a phenomenon that is only partially explained by polyphosphate hyperaccumulation. This study therefore demonstrates that in Synechocystis, ppk1 is not essential for low-CO2 acclimation but plays a crucial role in dynamic P-metabolic regulation, including polyP hyperaccumulation, to maintain physiological fitness under sulfur-starvation conditions.
Introduction
Polyphosphate (polyP) is a linear polymer consisting of three to hundreds of inorganic phosphate residues linked by high-energy phosphoanhydride bonds, and is prevalent in prokaryotes and eukaryotes, serving as reservoirs of phosphorus (P) and energy (Rao et al., 2009). PolyP is stored as granules called polyP bodies, which prokaryotic cells house in the cytoplasm in most cases or acidocalcisomes, acidic organelles rich in calcium, in a few cases, and are usually present in acidocalcisomes in eukaryotic cells (Docampo et al., 2005; Rao et al., 2009; Denoncourt and Downey, 2021). Microorganisms have long been known to hyperaccumulate polyP under stress conditions such as entry into the stationary phase, which accompanies multiple stresses including nutrient depletion (Terry and Hooper, 1970; Kim et al., 1998; Komine et al., 2000; Chen et al., 2002; Zhang et al., 2005; Rao et al., 2009), and specific-nutrient limited conditions, such as starvation for amino acids in non-photosynthetic prokaryotes (Harold, 1966; Ault-Riché et al., 1998; Racki et al., 2017), and nitrogen (N) or sulfur (S) in photosynthetic microbes including cyanobacteria and algae (Harold, 1966; Grillo and Gibson, 1979; Lawry and Jensen, 1979; Kuesel et al., 1989; Aksoy et al., 2014; Ota et al., 2016; Goodenough et al., 2019; Sanz-Luque et al., 2020a). Other typical cases include repletion with Pi after its limitation, which induces polyP hyperaccumulation designated as polyP overplus phenomenon (Sanz-Luque et al., 2020a). Conversely, polyP is degraded in microbes when relived from above stresses that induce polyP hyperaccumulation or exposed simply to P starvation (Goodenough et al., 2019; Li and Dittrich, 2019; Hiyoshi et al., 2021).
PolyP is synthesized by polyP kinase (PPK), which elongates the phosphate chain through successive addition of γ-phosphate groups of ATP, whereas it is degraded by exopolyphosphatase (PPX), which releases the terminal phosphate through hydrolysis (Harold, 1966; Rao et al., 2009). The gene for PPK (ppk1) was initially cloned in E. coli, followed by the discovery of its homologs in many prokaryotes (Akiyama et al., 1992; Rao et al., 2009). In some prokaryotic groups, including Pseudomonas aeruginosa, the ppk2 gene was later found, encoding PPK that is structurally unrelated to Ppk1 and utilizes GTP as well as ATP as the substrate (Zhang et al., 2002; Rao et al., 2009). Ppk1 and Ppk2 can also catalyze reverse reactions for ATP synthesis, and ATP or GTP synthesis, respectively. Ppk1 favors polyP synthesis over ATP synthesis, while Ppk2 shows a preference for ATP/GTP synthesis over polyP synthesis (Rao et al., 2009; Bowlin and Gray, 2021). The ppx gene, first cloned in E. coli, is prevalent in prokaryotes (Akiyama et al., 1993). The ppk1, ppk2, and ppx genes are generally absent in eukaryotes; however, some limited groups of lower eukaryotes exceptionally possess ppk1 homologs (Whitehead et al., 2013). Particularly, in a slime mold, Dictyostelium discoideum, its eukaryotic homolog was functionally identified as ppk1 (Zhang et al., 2005).
Following the identification of ppk and ppx, mutants of these genes have been generated and characterized in a variety of non-photosynthetic microorganisms, deepening the understanding of the commitment of these genes in polyP metabolism in vivo and the physiological roles of polyP metabolism. Regarding polyP synthesis, ppk1 is a main determinant of the cellular polyP content at the steady-state and also at nutrient-stress induced hyperaccumulation level (Chen et al., 2002; Zhang et al., 2005). Above all, ppk1 is essential for cellular acclimation not only to the polyP-hyperaccumulation stress but also other stresses such as heat, oxidation, and high osmosis (Rao and Kornberg, 1996; Kim et al., 1998; Kuroda et al., 1999; Rao et al., 2009). Notably, ppk1 is necessary for motility and biofilm formation, underlying virulence in pathogenic species like P. aeruginosa (Rashid and Kornberg, 2000; Rashid et al., 2000a, 2000b). Meanwhile, ppx as well as ppk1 was crucial for motility and biofilm formation, demonstrating that dynamic regulation of polyP metabolism is crucial for these physiological processes (Gallarato et al., 2014; Denoncourt and Downey, 2021; Tiwari et al., 2022).
Many cyanobacteria possess both ppk1 and ppk2 homologs and ppx one; however, information is limited on their enzymatic and physiological roles. It was first reported in cyanobacteria that a ppk1 homolog could not be completely disrupted in Synechocystis sp. PCC 6803 (hereafter referred to as Synechocystis), implying some essential role of polyP synthesis (Gómez-García et al., 2003). Later, in Synechococcus OS-B’ (hereafter referred to as Synechococcus), a complete disruptant as to its ppk1 homolog was generated to show a defect in polyP hyperaccumulation, thereby, the homolog being functionally identified as ppk1 (Gómez-García et al., 2013). Simultaneously, pleiotropic effects were exerted in the Synechococcus disruptant, Δppk1, including the inability of cells to grow with the aeration of ordinary air containing 0.04% CO2 (low-CO2 conditions) (Gómez-García et al., 2013). Since the Synechococcus Δppk1, similar to the WT, grew vigorously with the aeration of CO2-enriched air (5% CO2, high-CO2 conditions), it might be interpreted that ppk1 was dispensable for cell growth under nutrient-rich conditions (CO2-rich in this case), as is often the case with non-photosynthetic microbes (Crooke et al., 1994; McMeechan et al., 2007; Rashid et al, 2000b; Zhang et al., 2005). Concerning ppx, its Synechocystis disruptant (Δppx) showed normal cell growth under low CO2 conditions but retarded one, specifically under P-starvation (-P) conditions (Hiyoshi et al., 2021). The ppx gene is thus essential in Synechocystis for cellular acclimation to -P stress but not to low-CO2 one. Meanwhile, the expression level of ppk1 peaked in the evening over a diel cycle in Synechococcus whereas the ppx homolog was expressed most strongly in the early morning, which implied the cruciality of the regulatory polyP metabolism for optimized cell growth in the diel cycle (Gómez-García et al., 2013).
To gain deeper insights into polyP metabolism and its physiological significance in cyanobacteria, this study generated a complete disruptant of the ppk1 homolog, sll0290, in Synechocystis. The characterization of the disruptant led us to identify sll0290 as ppk1 and to find that ppk1 is not required for cell growth under low CO2 conditions but is essential for proper maintenance of physiological fitness upon entry into the stationary phase that would accompany nutrient depletion stresses, or during the imposition of -S stress.
Materials and methods
Cyanobacterial strains and growth conditions
The cyanobacterial strains used were Synechocystis and its mutant, in which the genomic copies of ppk1 were completely disrupted (Δppk1, see below). The cells were cultured at 30°C in glass tubes containing BG11 medium, with illumination (60 μmol photons·m-2·s-1) and ordinary-air aeration, as previously described (Hiyoshi et al., 2021). For polyP hyperaccumulation, Synechocystis WT or Δppk1 culture was initially adjusted to OD730 = 0.2 in a modified BG11 medium with sulfate-free (-S) and phosphate (Pi)-surplus (++P: 1 mM Pi, c.f., 0.22 mM Pi normally in BG11 medium). The culture was then grown for 2 days, following the procedure described previously (Lawrence et al., 1998). Otherwise, -S was simply imposed on the cells by culturing in -S BG11 medium, as previously described (Hirai et al., 2019). OD730 in the culture, and chlorophyll (Chl) and phycobilisome (PBS) contents were determined using a spectrophotometer (DU 640, Beckman) for investigation of cell growth, as described previously (Hiyoshi et al., 2021).
Extraction of polyP bodies, and measurement of their constituent Pi contents
PolyP bodies were isolated from Synechocystis cells cultured under -S/++P or +S/++P conditions, as described above. PolyP bodies were isolated from cells, according to the method described by Shi et al. (2003). The culture (40 mL) was subjected to centrifugation (3,500 g for 15 min at 4°C) to recover cells as a pellet, which was then digested by treating with 1 mL alkaline hypochlorite reagent (5.4%) for 45 min at 25°C. The digested-cell suspension was centrifugated to collect residues, which were washed twice with alkaline hypochlorite reagent. PolyP bodies were extracted from the residues twice with distilled water (0.5 mL) and precipitated by centrifugation (14,000 g for 10 min at 4°C) after the addition of ethanol (9 mL). Isolated polyP bodies were degraded into Pi by hydrolysis, and their constituent Pi contents were spectroscopically measured (Hiyoshi et al., 2021).
31P-NMR measurement
Cells cultured under -S/++P or +S/++P conditions were harvested by centrifugation, and then were resuspended in 0.5 M EDTA with adjustment of Chl concentration to 5 mg·mL-1. 31P-NMR spectra of the cell suspension were measured with a Bruker PDX400 NMR spectrometer, and a 5 mm diameter QNP probe head operating at 162.2 MHz in the pulsed Fourier transform mode, as described previously (Kobayashi et al., 2005). A total of 3,072 scans were accumulated for each spectrum with line broadening of 5.0 Hz. Chemical shifts were measured in ppm relative to external 85% Pi (w/w) (Kobayashi et al., 2005). Signal peaks were assigned to soluble polyP and Pi/phosphate monoesters, according to the report by Yang et al. (1993).
Disruption of sll0290 in Synechocystis
A DNA fragment covering the C-terminal half of Sll0290, a homolog of Ppk1, in Synechocystis was amplified by Ex-taq DNA polymerase (Takara, Japan) with primers 1 (5’- TGACCCTGTACCGCACTTCG-3’) and 2 (5’-TCAAACTGAACGTAGTTCCG-3’), as previously described (Hiyoshi et al., 2021). A product of 1.0 kbp was ligated to the pGEM T-EASY vactor (Promega) to generate the plasmid, which was then cut at two BalI sites present in the center of the DNA region corresponding to the PCR product, followed by insertion of the spectinomycin-resistant gene cassette (SpcR). The resultant plasmid containing the disrupted sll0290 was used to transform wild-type (WT) cells of Synechocystis by homologous recombination, as described previously (Hiyoshi et al., 2021). The disruption of sll0290 was confirmed by PCR with two primer sets: one consists of primers 1 and 2 whereas the other constitutes primers 2 and 3 (5’-CGGACCATTGTTTAAATGGG -3’).
Overexpression of the ppk1 gene in Escherichia coli
For amplification of the coding region of the ppk1 gene in Synechocystis, PCR was performed with primers 4 (5’-ATCCAAGCTATGCCCTCTGC-3’) and 5 (5’-ACTGAACGTAGTTCCGGGTC-3’), as described above, with the change of Ex-Taq DNA polymerase to KOD-plus one (Toyobo, Japan). A product of 2.2 kbp was ligated to the pET15b vector (Promega) that had been blunted after cut by NcoI site. The resultant plasmid was introduced into BL21 competent cells of E. coli, and thereafter the transformants were used for induction of the Synechocystis ppk1 gene expression, through addition of 1 mM IPTG, according to the manufacture’s protocol (Promega).
Microscopic observation of polyP bodies in Synechoystis or E. coli cells
For fluorescence microscopic observations, Synechocystis or E. coli cells were fixed in a culture through addition of glyceraldehyde (1%), thereafter frozen in liquid-nitrogen and thawed in hands. The cells were then stained with 4’, 6-diamidino-2-phenylindole (DAPI, 50 µg/ml) and observed under a fluorescence microscope (BX-FLA, Olympus Optical Co., Tokyo, Japan) with the use of a 340-390 nm excitation filter. Meanwhile, for electron microscopic analysis, Synechocystis cells were fixed in a culture through addition of glutaraldehyde (2.5%) and were harvested by centrifugation (3,500 g for 15 min at 4°C). The cells were washed in 50 mM phosphate buffer (pH 7.0), and were fixed for 1 h in the phosphate buffer containing glutaraldehyde (2.5%). The glutaraldehyde-fixed cells were submitted to Japan Electron Optics Laboratory (JEOL) for contact research, where the cells were subjected to transmission electron microscopy (TEM) with JEM-1010 after post-fixation in 2% OsO4.
Statistics
The significance of differences was evaluated using a two-sided Student’s t-test.
Results
Structural characterization of Sll0290 as an ortholog to known Ppk1 proteins
In general, ppk1 acts as a key player in polyP synthesis, and its homologs are found mainly as a single copy in the genomes of respective cyanobacterial strains (0, 1, and 2-4 copies in 13, 227 and 37 strains, respectively; Supplementary Table 1), the nucleotide sequences of which have been thus far determined (CyanoCyc, https://cyanocyc.org/). In Synechocystis, sll0290 is annotated as a sole ppk1, however, no structural characterization has been reported regarding its protein product. Firstly, we characterized the structure of Sll0290 by aligning its amino acid sequence with those of known Ppk1 proteins from other organisms. The amino acid sequence of Sll0290 showed 37% and 49% identity to Ppk1 proteins of E. coli and Mycobacterium tuberculosis, respectively (Figure 1). Accordingly, Sll0290 consisted of four regions, corresponding to characteristic domains revealed in the X-ray structure of E. coli Ppk1 (Zhu et al., 2005): the N-domain, a highly conserved region located at the N-terminus; the H-domain, with the lowest degree of homology, which interacts with the C1-domain for Ppk1 dimerization; and the C1- and C2-domains, two other highly conserved regions at the C-terminus. Furthermore, amino acid residues responsible for constructing the ATP-binding pocket (Zhu et al., 2005) and/or those found necessary for functioning of PPK and/or polyP: ADP phosphotransferase (Kumble et al., 1996; Tzeng and Kornberg, 2000; Mittal et al., 2011) were well conserved in Sll0290 (Figure 1). These structural features strongly suggested that sll0290 encodes Ppk1.
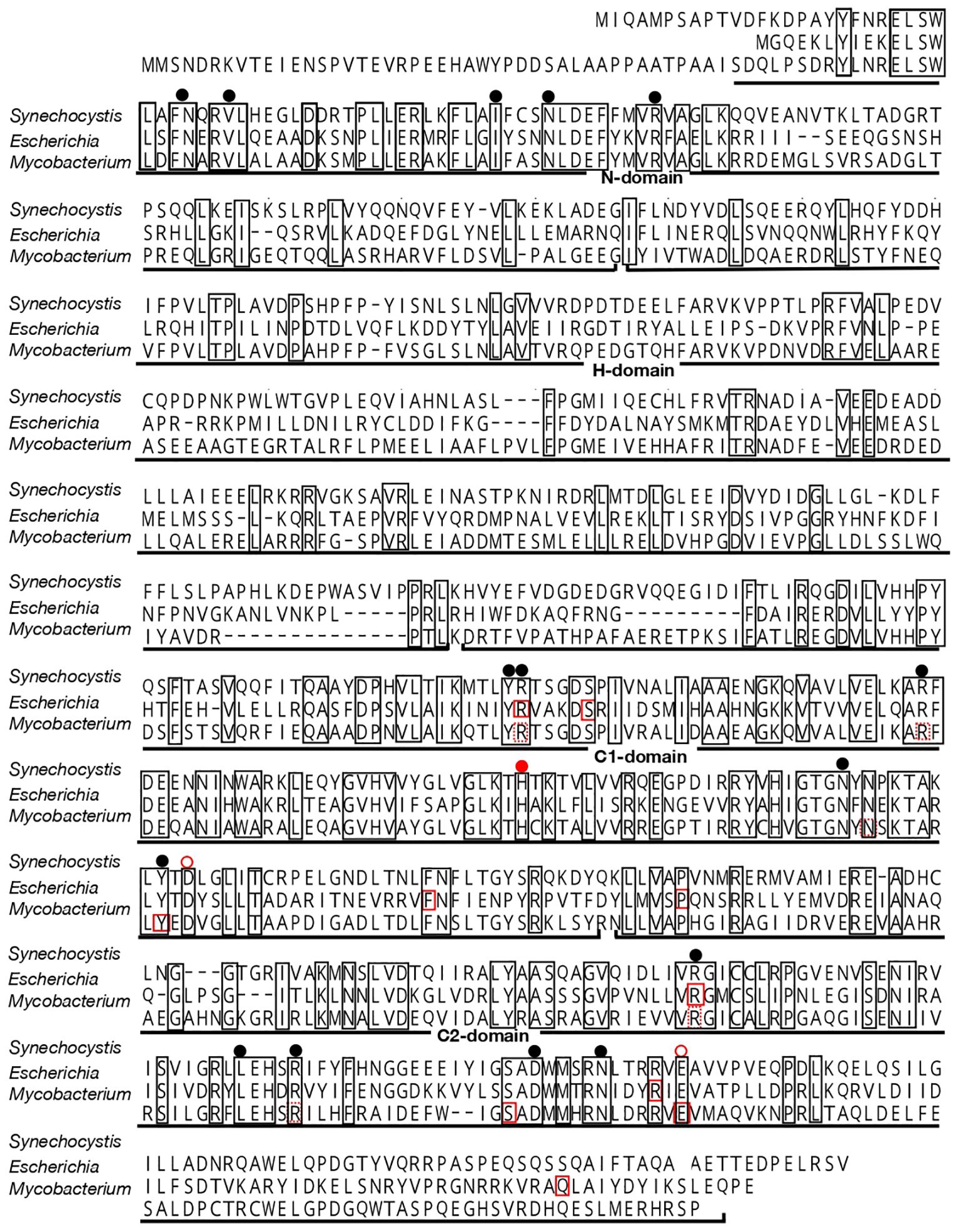
Figure 1 Alignment of the amino acid sequence of Sll0290 with those of Ppk1 proteins from E. coli and M. tuberculosis. Amino acid residues conserved among these three sequences are enclosed in squares. Solid-red rectangles indicate amino acid residues sequences that are responsible for both polyP and ATP syntheses, while dashed-red rectangles denote those for ATP synthesis in E. coli and M. tuberculosis (Tzeng and Kornberg, 2000; Mittal et al., 2011). Black circles indicate positions of amino acid residues involved in ATP binding in polyP synthesis in E. coli (Zhu et al., 2005). A closed red circle represents the His residue that is autophosphorylated for polyP synthesis in E. coli and M. tuberculosis, while open red circles indicate amino acid residues involved in the autophosphorylation in E. coli (Zhu et al., 2005; Mittal et al., 2011).
Functional identification of sll0290 as encoding the polyP kinase
To investigate the catalytic function of Sll0290, we disrupted sll0290 in the genome in Synechocystis by replacing a partial region of sll0290 with the SpcR cassette. Genomic DNA PCR confirmed the disruption of sll0290 in all genomic DNA copies (Figure 2A). The disruptant (Δsll0290), similar to the WT, exhibited vigorous growth in liquid culture with ordinary-air aeration (Figure 2B), with no deleterious effects on the cellular content of Chl or phycobilisome, or photosynthesis (Figure 2C). These results demonstrated that sll0290 is dispensable for the construction and functionality of the photosynthetic machinery, and inevitably for cell growth with ordinary-air aeration. Fluorescence-microscopic observations demonstrated DAPI-stained polyP bodies in logarithmically growing WT cells, contrastingly, no such structure observed in the Δsll0290 counterparts (Figure 2D). Notedly, WT cells exhibited a significant increase in polyP bodies upon entry into the stationary phase (OD730 = 1.0), followed by a subsequent decrease to levels lower than those initially observed as the stationary phase progressed (OD730 = 2.0 or 3.0). In contrast, Δsll0290 cells showed no discernible appearance of polyP bodies throughout the stationary phase (Figure 2D). These results indicated that Synechocystis cells increased polyP-body contents temporarily during the transition from logarithmic to stationary growth phases, and that sll0290 is responsible for polyP-body accumulation at a low steady-state level, and also at an early-stationary-phase induced high level.
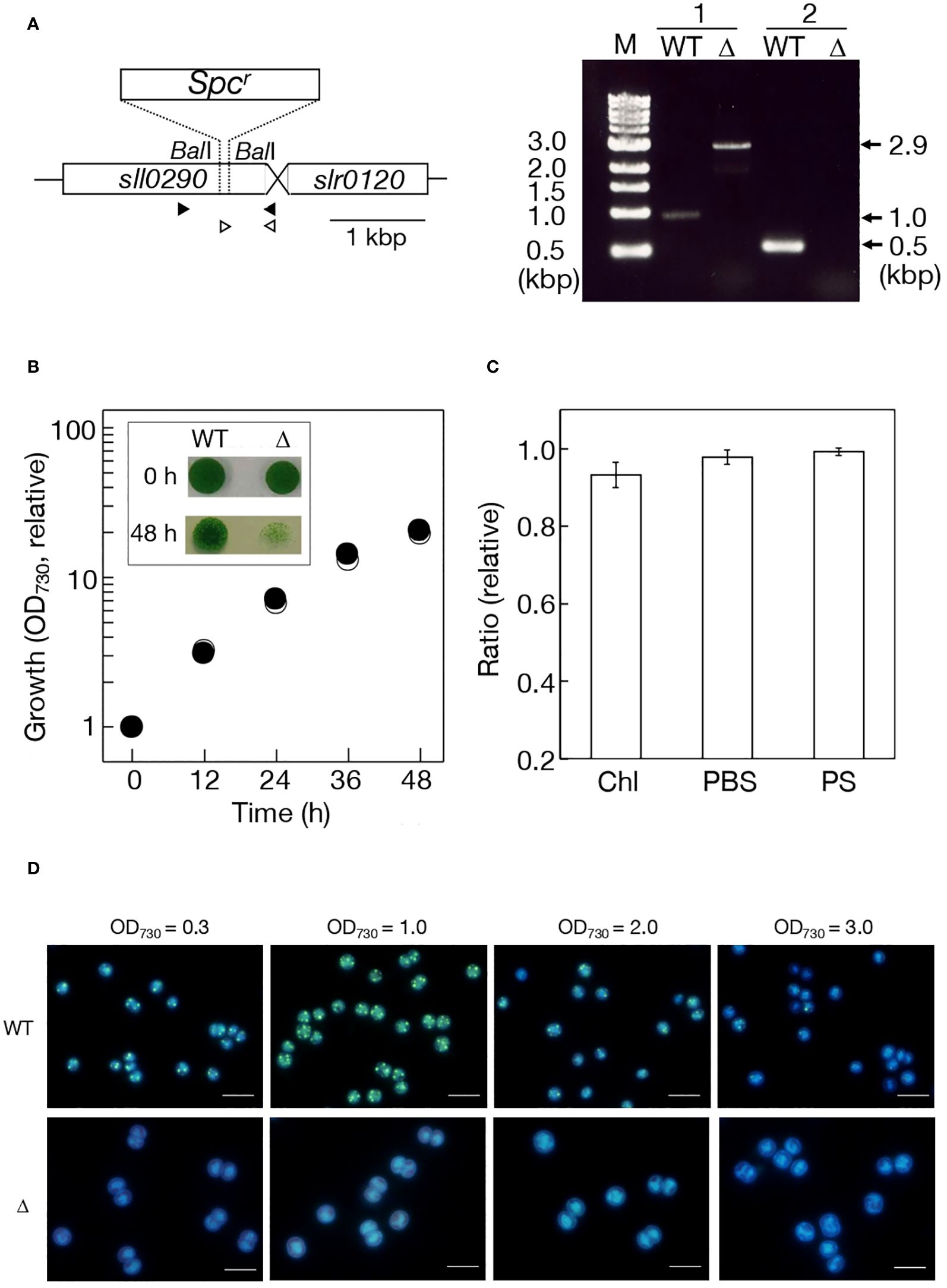
Figure 2 Effects of sll0290 disruption on cell growth, photosynthesis, and polyP body accumulation. (A) Disruption strategy of sll0290 through insertion of the spectinomycin resistant gene cassette (Spcr). Left, a DNA region of 0.2 kbp between two BalI sites in sll0290 was replaced by Spcr. Right, sll0290 disruption was confirmed by genomic DNA PCR. Primer sets 1 (closed arrowheads) and 2 (open arrowheads) were used. Note that only a 2.9-kbp DNA fragment was amplified in Δsll0290 with the primer set 1, corresponding to the disrupted size of sll0290, and that no amplified DNA fragment appeared with the primer set 2 in Δsll0290, compatible with the loss of the 0.2-kbp Ball DNA fragment. (B) Cell growth measured by increases in OD730 values in the culture. Shown are values relative to that in the WT culture at 0 h (0.10). Inset, cell viability on the BG11 agar plate. Liquid cultures at 0 h (precultures at the log phase) and 48 h were respectively diluted to OD730 = 0.1 in the BG11 medium, thereafter, the diluted cultures of 10 μL placed on an agar plate for subsequent cell growth. Open and closed circles indicate WT and Δsll0290, respectively. (C) Effects of sll0290 disruption on photosynthesis. Respective contents of Chl and PBS, and photosynthesis (PS) in Δsll0290 are shown relative to the WT (Chl, 7.08 μg·OD730-1·mL-1; PBS, 0.270 OD730-1·mL-1; PS, 322 μmol O2· mg Chl-1·h-1). (D) Fluorescence-microscopic images of WT and Δsll0290 cells stained with DAPI. Only early-stationary cells in the WT (OD730 = 1.0 corresponding to 10 in relative growth in (B)) exhibited strong green fluorescence emitted from polyP bodies in the background of blue fluorescence from DNA and RNA. Bars indicate 5 μm. The values indicated in (B, C) are the averages ± SD from three biological replicates, with SD bars in (B) hidden within symbols.
-S as well as entry into stationary phase is the well-known stressor for induction of polyP hyperaccumulation in photosynthetic microbes, including cyanobacteria. Here, the conditions of -S with surplus P (++P: 1 mM Pi, c.f., 0.22 mM normally) were used for investigation of polyP-body hyperaccumulation in Synechocystis (Lawrence et al., 1998). Fluorescence-microscopic images exhibited -S-induced polyP-body hyperaccumulation in the WT, and no polyP-body accumulation in Δsll0290 irrespective of +S/++P or -S/++P (Figure 3A). Consistently, transmission electron microscopic images displayed that -S/++P cells in WT contained several large spots of low electron density, which would represent traces of polyP bodies that were lost during ultra-thin sectioning (Lawry and Jensen, 1979; Hackenberg et al., 2012; Moura et al., 2019), whereas such structure was absent in Δsll0290 (Figure 3B). Therefore, it was found that ppk1 is responsible for the -S-induced polyP-body hyperaccumulation by fluorescence- and electron-microscopy.
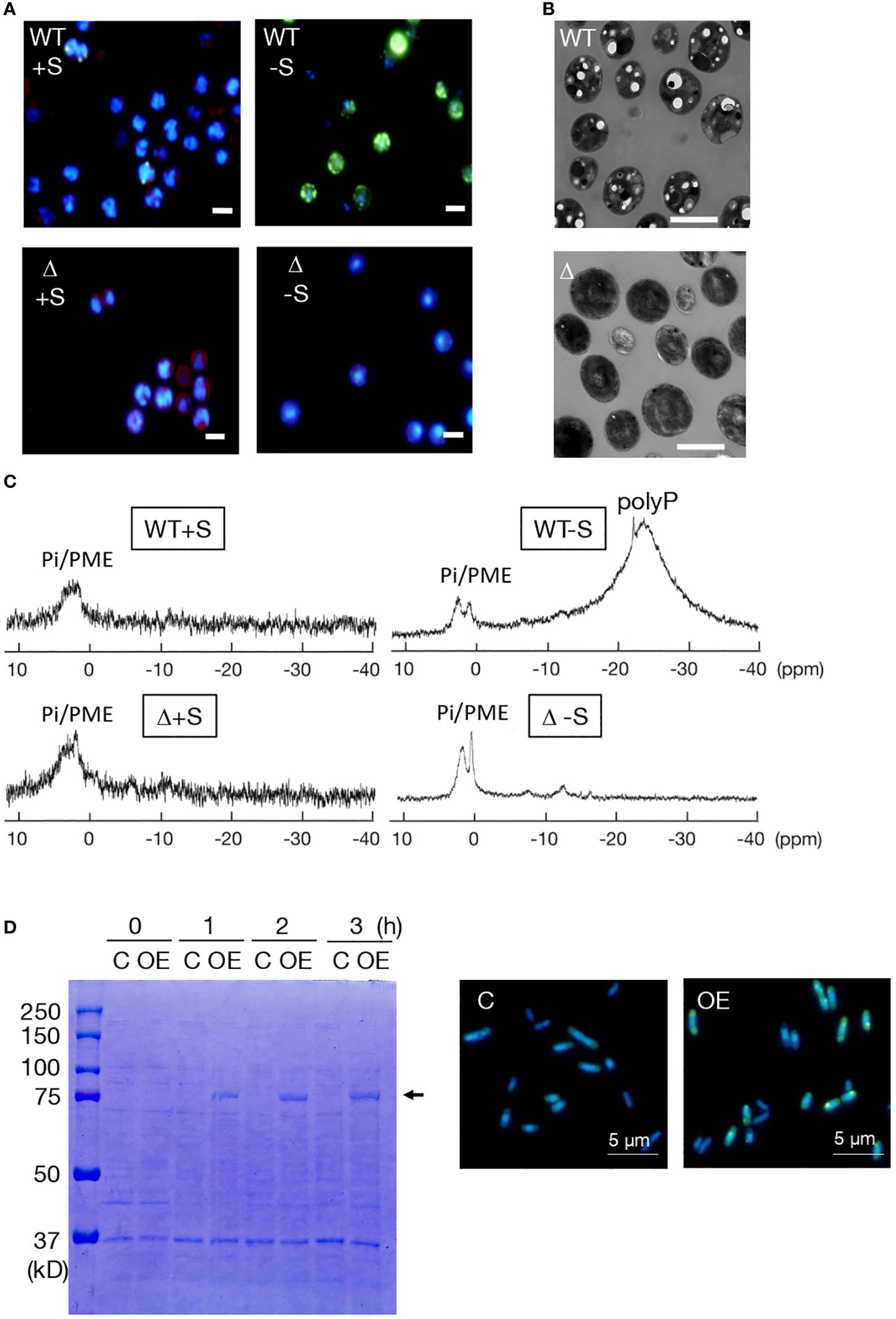
Figure 3 Dependency of polyP accumulation on sll0290 in Synechocystis and E coli. (A) Fluorescence-microscopic images of WT and Δsll0290 cells stained with DAPI. Cells starved for S for 2 days in the presence of surplus Pi (1 mM Pi) hyperaccumulated polyP bodies in the WT but not in Δsll0290; both WT and Δsll0290 showed the lack of polyP body hyperaccumulation when cultured with S repletion in 1 mM Pi. Bars indicate 2 μm. (B) Electron-microscopic images of WT and Δsll0290 cells starved for S for 2 days. Note that only WT cells exhibited several low-electron density holes, which would represent polyP bodies. Bars indicate 2 μm. (C) 31P-NMR spectra of the WT and Δsll0290 cells cultured with or without S, in the presence of 1 mM Pi. Signal peaks were assigned to soluble polyP and Pi/phosphate monoesters, according to the report by Yang et al. (1993). (D) Overexpression of sll0290 in E coli cells. Left, SDS-PAGE of total proteins extracted from E coli cells transformed with the sll0290 overexpression vector (OE) and those with the empty vector (C). The arrow indicates an IPTG-induced protein. Right, fluorescence-microscopic images of OE and C cells stained with DAPI. Note that only OE cells exhibited hyperaccumulation of polyP bodies. Bars indicate 5 μm.
PolyP bodies were then isolated from WT and Δsll0290 cells, subsequently subjected to chemical Pi quantitation (Table 1). Under +S/++P conditions, polyP bodies amounted at 0.60 fmol P·cell-1 in WT, but at a significantly lower level of 0.04 fmol P·cell-1 in Δppk1 (Table 1). Besides, -S caused WT cells to increase the polyP body content by 12.5-fold, consistent with the previous report (Lawrence et al., 1998); in contrast, Δsll0290 exhibited little stimulatory effect of -S on the polyP accumulation. These results, compatible with those obtained through above microscopic observations, strengthened our thought that sll0290 is a key player to maintain the polyP-body level, irrespective of the extent of its accumulation level. Besides polyP bodies or insoluble polyP, soluble polyP was found to accumulate in the WT cells with -S/++P but not in those with +S/++P, through in vivo 31P NMR spectroscopic analysis (Figure 3C), compatible with the previous report (Lawrence et al., 1998). However, Δsll0290 cells showed no apparent signal of soluble polyP under either of these two conditions. Therefore, sll0290 is responsible for -S-inducible accumulation of soluble polyP as well as that of polyP bodies.
We then overexpressed sll0290 in E. coli cells to investigate its function more directly (Figure 3D). SDS-PAGE analysis of total cellular proteins showed that IPTG induced expression of a protein with a molecular weight of 80 kDa in E. coli cells transformed with the sll0290-overexpression vector, but not in those with the empty vector (left panel, Figure 3D). The size of the induced protein coincided well with the postulated molecular weight of the product of introduced sll0290. Fluorescence-microscopic images exhibited abundant DAPI-stained polyP bodies in the sll0290-overexpressing cells, however, polyP body absent in the control, which indicated that sll0290 can force E. coli cells to hyperaccumulate polyP. Collectively, it was proved that sll0290 represents ppk1 by demonstrating the loss of polyP accumulation ability in Synechocystis Δsll0290 and gain of this ability in the E coli sll0290-overexpressing transformant, together with the structural characterization of Sll0290 as Ppk1.
Responsibility of ppk1 for determination of the level of cellular P utilization
Table 1 shows that total P contents were 6.79 and 3.12 fmol P·cell-1 in the WT and Δppk1, respectively, under +S/++P conditions, indicating a 2.2-fold lower level in Δppk1. However, Pi contents were almost equally low for the WT and Δppk1 (0.45 and 0.75 fmol Pi·cell-1, respectively). These results suggested that WT cells require ppk1 not only for polyP accumulation at a steady-state low level but also for the accumulation of large amounts of other P metabolites. Under -S conditions, WT cells increased the total P content by 5.3-fold, while maintaining Pi at a low level. Notedly, polyP bodies explained only a small portion of the -S-induced increase in the total P content in the WT cells (Table 1; 6.9 and 29.2 fmol P·cell-1 increases in polyP and total P, respectively). Relative to WT cells, Δppk1 ones showed repression in the -S-inducible increase in the total P content (only 3.4 fmol P·cell-1 increase, c.f., 29.2 fmol P·cell-1 increase in the WT). These results underscored the significant role of ppk1 in the -S-induced increase in P-metabolite contents, i.e., P-utilization level, including polyP hyperaccumulation as a small portion.
Defects in cell survival in Δppk1 under -S conditions
Corresponding to cell growth in Δppk1 under +S conditions, comparable to that in the WT (Figure 2B), exponentially growing cells showed no obvious defects in ongoing growth after their shift to a fresh BG11 agar plate (see 0 h in Figure 2B inset). However, at the mid-stationary phase (see 48 h in Figure 2B inset, OD730 = 2.0), Δppk1 cells, distinct from WT ones, became seriously impaired in their subsequent growth or survival on the agar plate. These results, together with the inability of Δppk1 cells to hyperaccumulate polyP bodies upon entry into the stationary phase (Figure 2D), demonstrated the crucial role of ppk1-dependent polyP body accumulation for cellular acclimation to ambient stresses imposed during the stationary phase, such as the loss of nutrients like S. Physiological processes for acclimation to -S in place of -S/++P were then investigated in Δppk1 to gain deep insights into the decreased survival ability of its stationary cells. Fluorescence-microscopic observations showed that polyP bodies increased in WT cells with -S at 5 h, followed by continuing increase until at 24 h (Supplementary Figure 1). In contrast, polyP bodies remained undetectable in Δsll0290 cells even under -S conditions (Supplementary Figure 1). It was therefore demonstrated that -S as well as -S/++P induces ppk1-dependent polyP body hyperaccumulation in Synechocystis, and that S-limitation would be one of the triggers for induction of polyP hyperaccumulation in the early stationary cells (Figure 2D).
Cell growth under -S stress in aeration-liquid culture was then compared between the WT and Δppk1 (Figure 4A). Consistent with our previous report (Hirai et al., 2019), WT cells showed only a two-fold increase in the first 12 h of -S, and thereafter, the increased level remained almost unaltered until at 96 h. Δppk1, like the WT, achieved a two-fold elevation in cell growth at 12 h, which, however, was followed by a slight but definite decrease, distinct from the WT (Figure 4A). Importantly, Δppk1 cells grown under -S conditions for 12 h, compared to the WT counterparts, were impaired in survival after their shift to an agar plate containing normal +S medium (see Figure 4A inset). The impairment in cell survival became more serious in Δppk1 with the -S-culturing period extended to 24 h. The defect of Δppk1 in cell survival at the early-stationary phase might be triggered at least partially by a loss of external S-source in the culture.
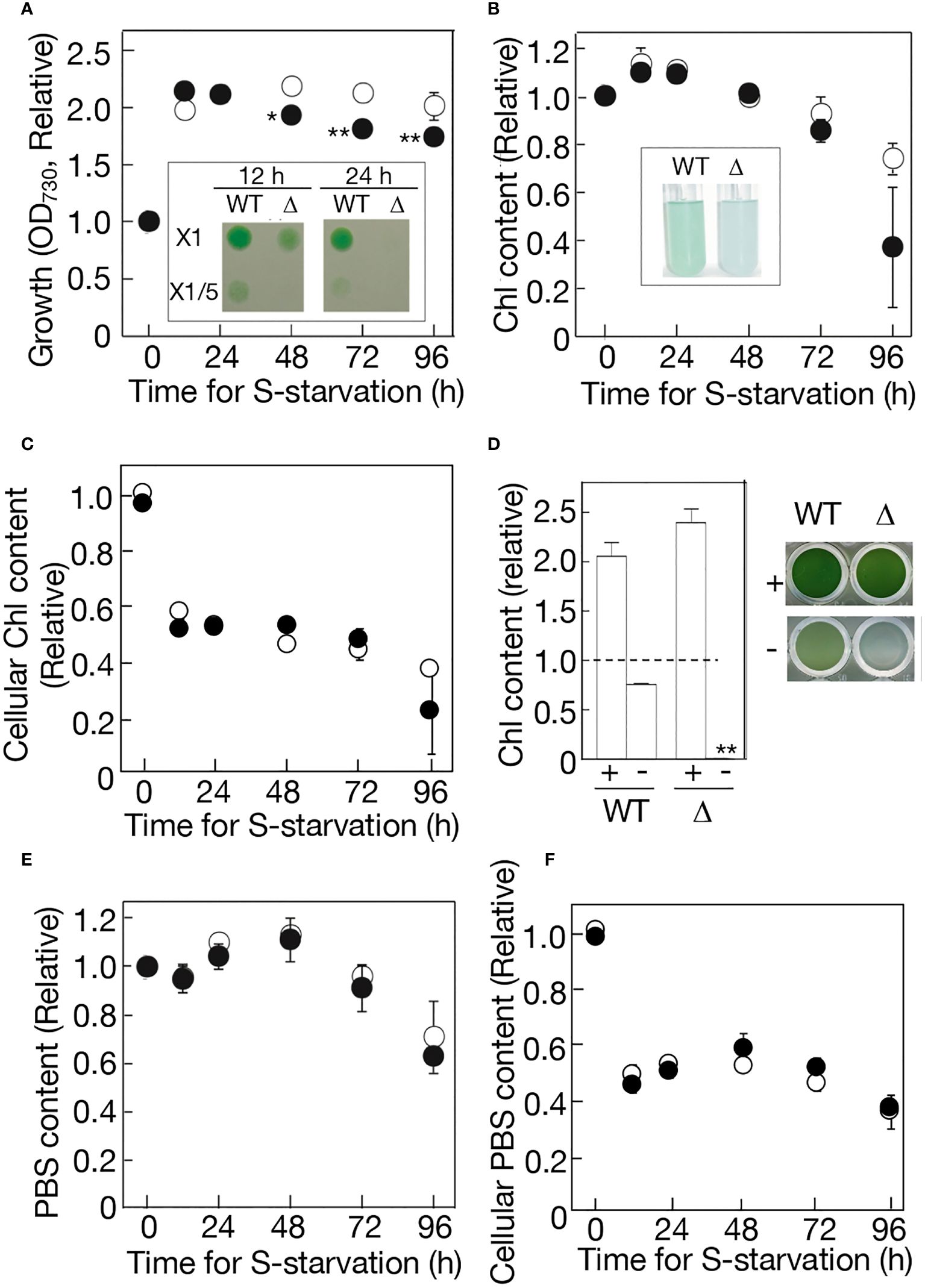
Figure 4 Effects of ppk1 disruption on physiological responses to -S. (A) Cell growth with aeration of ordinary air, which was measured by OD730 values in the culture. WT and Δppk1 values are shown relative to that at 0 h in the WT (0.293). Inset, cell viability on the BG11 agar plate. The liquid cultures at 12 h and 24 h were diluted to OD730 = 0.1 in the BG11 medium, thereafter, and 10 μL of the diluted cultures were placed on an agar plate for subsequent cell growth. (B) Chl contents in the batch culture. WT and Δppk1 values are shown relative to that at 0 h in the WT (2.13 μg Chl·mL-1). Inset, the photograph of -S cultures at 96 (h) (C) Chl contents per OD730·mL culture, which were estimated through division of values in (B) by those in (A). WT and Δppk1 values are shown relative to that at 0 h in the WT (7.27 μg Chl· OD730-1·mL-1). (D) Quantitative behavior of Chl in the standing culture in a titer plate under ordinary air. Left, Chl contents in 7-day standing culture of the WT and Δppk1. + and - indicate culturing with and without external S-source, respectively. WT and Δppk1 values are shown relative to those at 0 h in the WT and Δppk1, respectively (2.09 and 1.88 μg Chl·mL-1 in WT and Δppk1, respectively). Right, the photograph of the standing-culture in the titer plate. (E) PBS contents in the batch culture. WT and Δppk1 values are shown relative to that at 0 h in the WT (0.0821). (F) PBS contents per OD730·mL culture, which was estimated through division of values in (E) by those in (A). WT and Δppk1 values are shown relative to that at 0 h in the WT (0.280 OD730-1·mL-1). Open circles represent WT, while closed circles represent Δppk1. The values indicated in (A–F) are the averages ± SD from three biological replicates, with some SD bars hidden within symbols. The significance of differences in (A) regarding the Δppk1 values at 48-96 h relative to that at 0 h, and that in (D) regarding the Δppk1 value relative to WT one under -S conditions, was evaluated by means of two-sided Student’s t-test. **P<0.01. *P<0.05.
Regarding photosynthetic pigments, the WT kept Chl at an almost unaltered level in the first 72 h, and then degraded it mildly to 74% of the initial level at 96 h (Figure 4B). Δppk1 also kept Chl at almost the same level in the first 72 h, thereafter, showing a trend to degrade it more drastically than the WT to as low as 37% at 96 h. As a result, the cellular content of Chl decreased similarly for WT and Δppk1 in the first 12 h (Figure 4C), mainly through cell-growth dependent Chl dilution (Figure 4A), thereafter kept at the decreased level until at 72 h. However, in the next 24 h, the accelerated Chl degradation in Δppk1, relative to in the WT, resulted in a steeper decrease in the cellular Chl content on average in Δppk1 than in WT (Figure 4C). The Chl degradation in Δppk1 at later times was obvious when the cells were standing-liquid cultured in a microtiter plate: -S culturing for 7 days caused Chl in the culture to be degraded to 76% of the initial level in the WT, and to almost zero in Δppk1, although +S culturing enabled the Chl content to increase by ca. 2-fold in both the WT and Δppk1 (Figure 4D).
Meanwhile, PBS demonstrated almost indistinguishable quantitative behavior for the WT and Δppk1 in the aeration-liquid culture: PBS was maintained almost at the initial level in the first 72 h, followed by a decrease to 63-71% of the initial levels in the next 24 h (Figure 4E). Accordingly, WT and Δppk1 showed a similar decreasing pattern in the cellular content of PBS (Figure 4F). It therefore seemed that the functioning of ppk1 for polyP hyperaccumulation is necessary for -S cells at the early phase to properly maintain physiological fitness, and also for -S cells at the later phase to avoid intensive degradation of the photosystem complexes I and/or II.
Discussion
Identification of ppk1 in Synechocystis
A substantial amount of information has accumulated regarding the cyanobacterial homologs of ppk and ppx over the past nearly three decades through the determination of the genomic DNA sequences in more than 200 cyanobacterial strains (CyanoCyc, https://cyanocyc.org/). However, little progress has been made in the functional identification of genes involved in polyP metabolism or their physiological significance. This lack of progress can be attributed largely to only a few successful reports in genetic manipulation of polyP metabolism in cyanobacteria, such as Δppk1 in Synechococcus (Gómez-García et al., 2013) and Δppx in Synechocystis (Hiyoshi et al., 2021).
Regarding polyP synthesis, here, Sll0290 in Synechocystis exhibited primary structural features characteristic of known Ppk1 proteins (Figure 1). Consistently, sll0290 was functionally identified as ppk1 through loss-of-function analysis in Synechocystis using chemical-quantitative, fluorescence and electron microscopy, and in vivo NMR techniques (Table 1; Figures 3A–C), and also through gain-of-function analysis in E. coli (Figure 3D). It was demonstrated that Synechocystis ppk1 is responsible for the main part of polyP body accumulation at a low level under +S conditions (Table 1; Figure 2D), and also for -S or stationary-phase induced hyperaccumulation of polyP bodies and/or soluble polyP (Table 1; Figures 2D, 3; Supplementary Figure 1). The low levels of polyP detected in Δppk1 (Table 1) might be synthesized by the action of other polyP metabolic genes, including ppk2 slr1363 (Wang et al., 2018).
Gómez-García et al. (2013) identified ppk1 in Synechococcus through its protein structural and functional characterization. Particularly, the functional identification of ppk1 relied on observations of disruptant defects in polyP accumulation at a low steady-state level and an elevated level during the logarithmic and stationary phases, respectively, using techniques of enzymatic polyP quantitation and/or electron microscopy. During the preparation of our manuscript, Sebesta et al. (2024) recently reported the functioning of sll0290 as ppk1 through the observations of Δppk1 defects in polyP overplus using polyP quantitation. Therefore, we have identified ppk1 in Synechocystis not only through stationary-phase-inducible but also through a distinctive -S-inducible polyP hyperaccumulation phenomenon and by obtaining more cumulative evidence using diverse techniques.
Non-essentiality of ppk1 for cell growth in Synechocystis under normal conditions
This study demonstrated that ppk1 is dispensable for cell growth in Synechocystis under ordinary-air aeration (Figure 2B). Similar results were presented by Sebesta et al. (2024); we further concluded that the dispensability of ppk1 stems from its lack of involvement in the construction or functionality of the photosynthetic machinery (Figure 2C). These results, combined with the lack of requirement for ppx in Synechocystis (Hiyoshi et al., 2021), suggested that both ppk and ppx, i.e., main players in polyP metabolism, are not necessary for cell growth in Synechocystis under low-CO2 conditions. However, it should be emphasized that this lack of requirement holds true in logarithmic cells but not in stationary cells (Figure 2B inset, see below). Meanwhile, Gomez-Garcia et al. (2003) previously proposed the responsibility of ppk1 for carboxysome biogenesis in Synechococcus, based on the high-CO2 requiring phenotype and increased levels of mRNAs of the genes for carboxysome construction in Synechococcus Δppk1. The Synechococcus strain was isolated from microbial mats in extreme and nutrient-limited environments of an alkaline hot spring (Allewalt et al., 2006). The physiological roles of ppk1 might have developed in species-dependent manners in cyanobacteria through their evolutional diversification. PolyP bodies were often positioned in the vicinity of carboxysomes in some cyanobacteria (Iancu et al., 2010; Moura et al., 2019). Future studies will aim to elucidate what determines whether or not ppk1 is responsible for carboxysome biogenesis in cyanobacteria: specific properties of polyP bodies and/or Ppk1, or other factors associated with them?
Essentiality of ppk1 for cellular acclimation to ambient stresses in Synechocystis
In non-photosynthetic microorganisms, it has been demonstrated, through genetic manipulation of ppk1, that ppk1-dependent polyP synthesis is crucial for cellular acclimation to ambient stresses, such as entry into the stationary phase, nutrient depletion, heat, oxidants, and hyperosmosis (Rao and Kornberg, 1996; Kim et al., 1998; Kuroda et al., 1999; Rao et al., 2009). Among these polyP-synthesis-dependent stress-acclimation responses, those that accompanying polyP hyperaccumulation would convincingly represent the importance of polyP synthesis. In cyanobacteria, polyP hyperaccumulation can be observed under stationary-phase, -S, or -N conditions, or as an overplus phenomenon (Harold, 1966; Grillo and Gibson, 1979; Lawry and Jensen, 1979; Lawrence et al., 1998; Gómez-García et al., 2013 Sebesta et al., 2024). However, information is limited on the gene(s) responsible for polyP hyperaccumulation and its physiological significance in cyanobacteria: polyP hyperaccumulation, as mentioned above, was demonstrated to depend on ppk1 in only Synechococcus stationary cells (Gómez-García et al., 2013) and Synechocystis overplus ones (Sebesta et al., 2024), whereas the physiological significance of polyP hyperaccumulation, including these two cases, remains unclear. This study strongly suggested that ppk1 is the gene responsible for polyP hyperaccumulation not only temporarily in early-stationary cells but also in -S cells in Synechocystis (Table 1, Figures 2D and 3A–C, Supplementary Figure 1), and that ppk1 is essential for physiological fitness in both stationary and -S cells (Figures 2B inset and 4A inset, 4B, D). It thus seems probable that ppk1-dependent polyP hyperaccumulation in early-stationary cells are triggered, at least partially, by -S stress. Considering the polyP hyperaccumulation in -S/++P cells as well as in -S cells, it would be improbable that polyP hyperaccumulation in -S cells was facilitated by a decrease in external-P source dependent on cell growth. This strengthens the hypothesis that -S triggers polyP hyperaccumulation in early stationary phase cells.
It was previously reported that prokaryotes lacking both ppk1 and ppk2 include vector-borne or obligate intracellular pathogens that rarely encounter ambient stresses, distinct from free-living prokaryotes (Zhang et al., 2002; Wang et al., 2018). Wang et al. (2018) further observed that the presence of polyP-metabolism genes, including ppk1, correlated positively with bacterial proteome size and the number of virulence genes, suggesting a potential relationship of polyP in bacterial life style and environmental durability. Consistently, among cyanobacteria lacking both ppk1 and ppk2 are a sponge symbiont Candidatus Synechococcus spongiarum and diatom symbionts Richelia intracellularis HH 01 and Richelia intracellularis HM 01 (Supplementary Table 1; Usher, 2008; Hilton et al., 2013). Together, these observations suggested that ppk1-containing cyanobacteria, as well as non-photosynthetic microorganisms containing ppk1, may utilize polyP for cellular acclimation to ambient stresses such as nutrient limitation. In this context, it is plausible that in Synechococcus as well as in Synechocystis, ppk1-dependent polyP hyperaccumulation in stationary phase cells could be induced by nutrient loss stresses, including -S. Comparative studies using Synechocystis and Synechococcus Δppk1 mutants to assess responses to other stresses such as heat, oxidants, and hyperosmosis would help elucidate the roles of ppk1 further.
Future studies will investigate how physiological fitness is properly maintained through polyP hyperaccumulation in -S cells. Additionally, they will explore what stresses, including the possibility of -S, trigger ppk1-dependent polyP hyperaccumulation in early-stationary cells, and how physiological fitness is properly maintained in stationary cells where the hyperaccumulated polyP is degraded. In this context, it is important to understand what physiological disadvantages manifest in Δppk1, relative to the WT, under -S conditions. As a physiological response to -S, WT and Δppk1 showed a similar quantitative decrease in Chl or PBS in the first 72 h (Figures 4C, F). The decrease would indicate that the size of the photosynthetic machinery was properly downregulated in Δppk1 as well as in WT, to repress the generation of photosynthesis-derived reactive oxygen species (ROS) (Schwarz and Forchhammer, 2005; Latifi et al., 2009). Physiological unfitness began to appear in Δppk1 as early as at 12 h under -S conditions (Figure 4A inset), reflecting injuries in some other physiological process than the photosynthetic size reduction. In a green alga, Chlamydomonas reinhardtii, a polyP-deficient mutant was found to have a disruption in the gene for polyP-synthesis catalytic subunit of the vacuolar transporter chaperon (VTC) (Sanz-Luque et al., 2020b). The mutant characterization then revealed that -S-induced polyP accumulation is vital for controlling ATP homeostasis to repress disordered photosynthetic and respiratory electron flows, and accordingly is essential for cellular acclimation to -S conditions (Sanz-Luque et al., 2020b). Our study revealed that in Synechocystis cells, ppk1 is necessary not only for determination of the polyP content but also for promoting the accumulation of total P metabolites or enhancing P-utilization level under +S conditions, with this trend particularly strengthened under -S conditions (Table 1). P-metabolites that require ppk1 for high P-utilization levels would include RNA, in particular, and DNA, which represented 62 and 15% of the total P in Synechocystis +S cells (Hiyoshi et al., 2024). Meanwhile, -S-inducible P-metabolic regulation, including that of cellular energization, might become so malfunctional in Δppk1 as to cause early-phase defects in physiological fitness. However, this hypothesis awaits experimental validation.
In summary, this study demonstrated that ppk1 contributes to the generation of the majority of polyP in Synechocystis cells, regardless of whether they are polyP-hyperaccumulating under -S or early-stationary conditions or have low polyP accumulation under +S conditions. Additionally, it showed that ppk1 is essential for physiological fitness in -S or stationary cells. It was therefore likely that polyP hyperaccumulation in early-stationary cells are at least partially triggered by concurrent S limitation. Besides, it appeared that ppk1 promotes P utilization in Synechocystis cells to maintain its proper levels not only through polyP synthesis but also through extensive involvement in the regulation of other P metabolism pathways. Our study contributes to the construction of a fundamental framework for a comprehensive understanding of the ppk1-dependent mechanism by which cyanobacterial cells acclimate to nutrient-depletion stresses, including those encountered during the stationary phase of culture.
Data availability statement
The raw data supporting the conclusions of this article will be made available by the authors, without undue reservation.
Author contributions
NS: Writing – review & editing, Writing – original draft, Validation, Supervision, Methodology, Investigation, Funding acquisition, Conceptualization. ME: Writing – review & editing, Validation, Methodology, Investigation. HN: Writing – review & editing, Validation, Methodology, Investigation. SF: Writing – review & editing, Validation, Methodology, Investigation, Conceptualization. MT: Writing – review & editing, Validation, Methodology, Investigation, Conceptualization.
Funding
The author(s) declare financial support was received for the research, authorship, and/or publication of this article. This work was financially supported by the SEI Group CSR Foundation (NS) and Grants-in-Aid for Scientific Research (C) from the Japan Society for the Promotion of Science (19K12384, 23K11481).
Acknowledgments
The authors would like to extend their sincere appreciation to Ms. Megumi Haga for her invaluable technical assistance.
Conflict of interest
The authors declare that the research was conducted in the absence of any commercial or financial relationships that could be construed as a potential conflict of interest.
Publisher’s note
All claims expressed in this article are solely those of the authors and do not necessarily represent those of their affiliated organizations, or those of the publisher, the editors and the reviewers. Any product that may be evaluated in this article, or claim that may be made by its manufacturer, is not guaranteed or endorsed by the publisher.
Supplementary material
The Supplementary Material for this article can be found online at: https://www.frontiersin.org/articles/10.3389/fpls.2024.1441626/full#supplementary-material
References
Akiyama, M., Crooke, E., Kornberg, A. (1992). The polyphosphate kinase gene of Escherichia coli. Isolation and sequence of the ppk gene and membrane location of the protein. J. Biol. Chem. 267, 22556–22561. doi: 10.1016/S0021-9258(18)41708-5
Akiyama, M., Crooke, E., Kornberg, A. (1993). An exopolyphosphatase of Escherichia coli. The enzyme and its ppx gene in a polyphosphate operon. J. Biol. Chem. 268, 633–639. doi: 10.1016/S0021-9258(18)54198-3
Aksoy, M., Pootakham, W., Grossman, A. R. (2014). Critical function of a Chlamydomonas reinhardtii putative polyphosphate polymerase subunit during nutrient deprivation. Plant Cell. 26, 4214–4229. doi: 10.1105/tpc.114.129270
Allewalt, J. P., Bateson, M. M., Revsbech, N. P., Slack, K., Ward, D. M. (2006). Effect of temperature and light on growth of and photosynthesis by Synechococcus isolates typical of those predominating in the octopus spring microbial mat community of Yellowstone National Park. Appl. Environ. Microbiol. 72, 544–550. doi: 10.1128/AEM.72.1.544-550.2006
Ault-Riché, D., Fraley, C. D., Tzeng, C. M., Kornberg, A. (1998). Novel assay reveals multiple pathways regulating stress-induced accumulations of inorganic polyphosphate in Escherichia coli. J. Bacteriol. 180, 1841–1847. doi: 10.1128/JB.180.7.1841-1847.1998
Bowlin, M. Q., Gray, M. J. (2021). Inorganic polyphosphate in host and microbe biology. Trends Microbiol. 29, 1013–1023. doi: 10.1016/j.tim.2021.02.002
Chen, W., Palmer, R. J., Kuramitsu, H. K. (2002). Role of polyphosphate kinase in biofilm formation by Porphyromonas gingivalis. Infect. Immun. 70, 4708–4715. doi: 10.1128/IAI.70.8.4708-4715.2002
Crooke, E., Akiyama, M., Rao, N. N., Kornberg, A. (1994). Genetically altered levels of inorganic polyphosphate in Escherichia coli. J. Biol. Chem. 269, 6290–6295. doi: 10.1016/S0021-9258(17)37370-2
Denoncourt, A., Downey, M. (2021). Model systems for studying polyphosphate biology: a focus on microorganisms. Curr. Genet. 67, 331–346. doi: 10.1007/s00294-020-01148-x
Docampo, R., de Souza, W., Miranda, K., Rohloff, P., Moreno, S. N. (2005). Acidocalcisomes - conserved from bacteria to man. Nat. Rev. Microbiol. 3, 251–261. doi: 10.1038/nrmicro1097
Gallarato, L. A., Sánchez, D. G., Olvera, L., Primo, E. D., Garrido, M. N., Beassoni, P. R., et al. (2014). Exopolyphosphatase of Pseudomonas aeruginosa is essential for the production of virulence factors, and its expression is controlled by NtrC and PhoB acting at two interspaced promoters. Microbiol. (Reading). 160, 406–417. doi: 10.1099/mic.0.074773-0
Gómez-García, M. R., Fazeli, F., Grote, A., Grossman, A. R., Bhaya, D. (2013). Role of polyphosphate in thermophilic Synechococcus sp. microbial mats. J. Bacteriol. 195, 3309–3319. doi: 10.1128/JB.00207-13
Gómez-García, M. R., Losada, M., Serrano, A. (2003). Concurrent transcriptional activation of ppa and ppx genes by phosphate deprivation in the cyanobacterium Synechocystis sp. strain PCC 6803. Biochem. Biophys. Res. Commun. 302, 601–609. doi: 10.1016/S0006-291X(03)00162-1
Goodenough, U., Heiss, A. A., Roth, R., Rusch, J., Lee, J. H. (2019). Acidocalcisomes: ultrastructure, biogenesis, and distribution in microbial eukaryotes. Protist. 170, 287–313. doi: 10.1016/j.protis.2019.05.001
Grillo, J. F., Gibson, J. (1979). Regulation of phosphate accumulation in the unicellular cyanobacterium Synechococcus. J. Bacteriol. 140, 508–517. doi: 10.1128/jb.140.2.508-517.1979
Hackenberg, C., Huege, J., Engelhardt, A., Wittink, F., Laue, M., Matthijs, H. C. P., et al. (2012). Low-carbon acclimation in carboxysome-less and photorespiratory mutants of the cyanobacterium Synechocystis sp. strain PCC 6803. Microbiol. (Reading). 158, 398–413. doi: 10.1099/mic.0.054544-0
Harold, F. M. (1966). Inorganic polyphosphates in biology: structure, metabolism, and function. Bacteriol Rev. 30, 772–794. doi: 10.1128/br.30.4.772-794.1966
Hilton, J. A., Foster, R. A., Tripp, H. J., Carter, B. J., Zehr, J. P., Villareal, T. A. (2013). Genomic deletions disrupt nitrogen metabolism pathways of a cyanobacterial diatom symbiont. Nat. Commun. 4, 1767. doi: 10.1038/ncomms2748
Hirai, K., Nojo, M., Sato, Y., Tsuzuki, M., Sato, N. (2019). Contribution of protein synthesis depression to poly-β-hydroxybutyrate accumulation in Synechocystis sp. PCC 6803 under nutrient-starved conditions. Sci. Rep. 9, 19944. doi: 10.1038/srep25825
Hiyoshi, T., Oyanagi, K., Niki, T., Fujiwara, S., Sato, N. (2021). Requirement of the exopolyphosphatase gene for cellular acclimation to phosphorus starvation in a cyanobacterium, Synechocystis sp. PCC 6803. Biochem. Biophys. Res. Commun. 540, 16–21. doi: 10.1016/j.bbrc.2020.12.095
Hiyoshi, T., Haga, M., Sato, N. (2024). Preferential phosphatidylglycerol synthesis via phosphorus supply through rRNA degradation in the cyanobacterium, Synechocystis sp. PCC 6803, under phosphate-starved conditions. Front. Plant Sci. 15, 1335085.
Iancu, C. V., Morris, D. M., Dou, Z., Heinhorst, S., Cannon, G. C., Jensen, G. J. (2010). Organization, structure, and assembly of alpha-carboxysomes determined by electron cryotomography of intact cells. J. Mol. Biol. 396, 105–117. doi: 10.1016/j.jmb.2009.11.019
Kim, H. Y., Schlictman, D., Shankar, S., Xie, Z., Chakrabarty, A. M., Kornberg, A. (1998). Alginate, inorganic polyphosphate, GTP and ppGpp synthesis co-regulated in Pseudomonas aeruginosa: implications for stationary phase survival and synthesis of RNA/DNA precursors. Mol. Microbiol. 27, 717–725. doi: 10.1046/j.1365-2958.1998.00702.x
Kobayashi, I., Fujiwara, S., Shimogawara, K., Sakuma, C., Shida, Y., Kaise, T., et al. (2005). High intracellular phosphorus contents exhibit a correlation with arsenate resistance in Chlamydomonas mutants. Plant Cell Physiol. 46, 489–496. doi: 10.1093/pcp/pci047
Komine, Y., Eggink, L. L., Park, H., Hoober, J. K. (2000). Vacuolar granules in Chlamydomonas reinhardtii: polyphosphate and a 70-kDa polypeptide as major components. Planta. 210, 897–905. doi: 10.1007/s004250050695
Kuesel, A. C., Sianoudis, J., Leibfritz, D., Grimme, L. H., Mayer, A. (1989). P-31 in-vivo NMR investigation on the function of polyphosphates as phosphate-and energysource during the regreening of the green alga Chlorella fusca. Arch. Microbiol. 152, 167–171. doi: 10.1007/BF00456096
Kumble, K. D., Ahn, K., Kornberg, A. (1996). Phosphohistidyl active sites in polyphosphate kinase of Escherichia coli. Proc. Natl. Acad. Sci. U. S. A. 93, 14391–14395. doi: 10.1073/pnas.93.25.14391
Kuroda, A., Tanaka, S., Ikeda, T., Kato, J., Takiguchi, N., Ohtake, H. (1999). Inorganic polyphosphate kinase is required to stimulate protein degradation and for adaptation to amino acid starvation in Escherichia coli. Proc. Natl. Acad. Sci. U. S. A. 96, 14264–14269. doi: 10.1073/pnas.96.25.14264
Latifi, A., Ruiz, M., Zhang, C. C. (2009). Oxidative stress in cyanobacteria. FEMS Microbiol. Rev. 33, 258–278. doi: 10.1111/j.1574-6976.2008.00134.x
Lawrence, B. A., Suarez, C., DePina, A., Click, E., Kolodny, N. H., Allen, M. M. (1998). Two internal pools of soluble polyphosphate in the cyanobacterium Synechocystis sp. strain PCC 6308: an in vivo31P NMR spectroscopic study. Arch. Microbiol. 169, 195–200. doi: 10.1007/s002030050560
Lawry, N. H., Jensen, T. E. (1979). Deposition of condensed phosphate as an effect of varying sulfur deficiency in the cyanobacterium Synechococcus sp. (Anacystis nidulans). Arch. Microbiol. 120, 1–7. doi: 10.1007/BF00413264
Li, J., Dittrich, M. (2019). Dynamic polyphosphate metabolism in cyanobacteria responding to phosphorus availability. Environ. Microbiol. 21, 572–583. doi: 10.1111/1462-2920.14488
McMeechan, A., Lovell, M. A., Cogan, T. A., Marston, K. L., Humphrey, T. J., Barrow, P. A. (2007). Inactivation of ppk differentially affects virulence and disrupts ATP homeostasis in Salmonella enterica serovars Typhimurium and Gallinarum. Res. Microbiol. 158, 79–85. doi: 10.1016/j.resmic.2006.10.008
Mittal, P., Karthikeyan, S., Chakraborti, P. K. (2011). Amino acids involved in polyphosphate synthesis and its mobilization are distinct in polyphosphate kinase-1 from Mycobacterium tuberculosis. PloS One 6, e27398. doi: 10.1371/journal.pone.0027398
Moura, K. A. F., Lizieri, C., Wittig Franco, M., Vaz, M. G. M. V., Araújo, W. L., Convey, P., et al. (2019). Physiological and thylakoid ultrastructural changes in cyanobacteria in response to toxic manganese concentrations. Ecotoxicology. 28, 1009–1021. doi: 10.1007/s10646-019-02098-y
Ota, S., Yoshihara, M., Yamazaki, T., Takeshita, T., Hirata, A., Konomi, M., et al. (2016). Deciphering the relationship among phosphate dynamics, electron-dense body and lipid accumulation in the green alga Parachlorella kessleri. Sci. Rep. 6, 25731. doi: 10.1038/srep25731
Racki, L. R., Tocheva, E. I., Dieterle, M. G., Sullivan, M. C., Jensen, G. J., Newman, D. K. (2017). Polyphosphate granule biogenesis is temporally and functionally tied to cell cycle exit during starvation in Pseudomonas aeruginosa. Proc. Natl. Acad. Sci. U.S.A. 114, E2440–E2449. doi: 10.1073/pnas.1615575114
Rao, N. N., Gómez-García, M. R., Kornberg, A. (2009). Inorganic polyphosphate: essential for growth and survival. Annu. Rev. Biochem. 78, 605–647. doi: 10.1146/annurev.biochem.77.083007.093039
Rao, N. N., Kornberg, A. (1996). Inorganic polyphosphate supports resistance and survival of stationary-phase Escherichia coli. J. Bacteriol. 178, 1394–1400. doi: 10.1128/jb.178.5.1394-1400.1996
Rashid, M. H., Kornberg, A. (2000). Inorganic polyphosphate is needed for swimming, swarming, and twitching motilities of Pseudomonas aeruginosa. Proc. Natl. Acad. Sci. U. S. A. 97, 4885–4890. doi: 10.1073/pnas.060030097
Rashid, M. H., Rao, N. N., Kornberg, A. (2000b). Inorganic polyphosphate is required for motility of bacterial pathogens. J. Bacteriol 182, 225–227. doi: 10.1128/JB.182.1.225-227.2000
Rashid, M. H., Rumbaugh, K., Passador, L., Davies, D. G., Hamood, A. N., Iglewski, B. H., et al. (2000a). Polyphosphate kinase is essential for biofilm development, quorum sensing, and virulence of Pseudomonas aeruginosa. Proc. Natl. Acad. Sci. U. S. A. 97, 9636–9641. doi: 10.1073/pnas.170283397
Sanz-Luque, E., Bhaya, D., Grossman, A. R. (2020a). Polyphosphate: A multifunctional metabolite in cyanobacteria and algae. Front. Plant Sci. 11, 938. doi: 10.3389/fpls.2020.00938
Sanz-Luque, E., Saroussi, S., Huang, W., Akkawi, N., Grossman, A. R. (2020b). Metabolic control of acclimation to nutrient deprivation dependent on polyphosphate synthesis. Sci. Adv. 6, eabb5351. doi: 10.1126/sciadv.abb5351
Schwarz, R., Forchhammer, K. (2005). Acclimation of unicellular cyanobacteria to macronutrient deficiency: emergence of a complex network of cellular responses. Microbiol. (Reading). 151, 2503–2514. doi: 10.1099/mic.0.27883-0
Sebesta, J., Cantrell, M., Schaedig, E., Hou, H. J. M., Pastore, C., Chou, K. J., et al. (2024). Polyphosphate kinase deletion increases laboratory productivity in cyanobacteria. Front. Plant Sci. 15, 1342496. doi: 10.3389/fpls.2024.1342496
Shi, X., Yang, L., Niu, X., Xiao, L., Kong, Z., Qin, B., et al. (2003). Intracellular phosphorus metabolism of Microcystis aeruginosa under various redox potential in darkness. Microbiol. Res. 158, 345–352. doi: 10.1078/0944-5013-00214
Terry, K. R., Hooper, A. B. (1970). Polyphosphate and orthophosphate content of Nitrosomonas europaea as a function of growth. J. Bacteriol. 103, 199–206. doi: 10.1128/jb.103.1.199-206.1970
Tiwari, P., Gosain, T. P., Chugh, S., Singh, M., Sankhe, G. D., Arora, G., et al. (2022). Exopolyphosphatases PPX1 and PPX2 from Mycobacterium tuberculosis regulate dormancy response and pathogenesis. Microb. Pathog. 173, 105885. doi: 10.1016/j.micpath.2022.105885
Tzeng, C. M., Kornberg, A. (2000). The multiple activities of polyphosphate kinase of Escherichia coli and their subunit structure determined by radiation target analysis. J. Biol. Chem. 275, 3977–3983. doi: 10.1074/jbc.275.6.3977
Usher, K. M. (2008). The ecology and phylogeny of cyanobacterial symbionts in sponges. Mar. Ecol. 29, 178–192. doi: 10.1111/j.1439-0485.2008.00245.x
Wang, L., Yan, J., Wise, M. J., Liu, Q., Asenso, J., Huang, Y., et al. (2018). Distribution patterns of polyphosphate metabolism pathway and its relationships with bacterial durability and virulence. Front. Microbiol. 9, 782. doi: 10.3389/fmicb.2018.00782
Whitehead, M. P., Hooley P, W., Brown, M. R. (2013). Horizontal transfer of bacterial polyphosphate kinases to eukaryotes: implications for the ice age and land colonisation. BMC Res. Notes. 6, 221. doi: 10.1186/1756-0500-6-221
Yang, Y. C., Bastos, M., Chen, K. Y. (1993). Effects of osmotic stress and growth stage on cellular pH and polyphosphate metabolism in Neurospora crassa as studied by 31P nuclear magnetic resonance spectroscopy. Biochim. Biophys. Acta 1179, 141–147. doi: 10.1016/0167-4889(93)90135-C
Zhang, H., Gómez-García, M. R., Brown, M. R., Kornberg, A. (2005). Inorganic polyphosphate in Dictyostelium discoideum: influence on development, sporulation, and predation. Proc. Natl. Acad. Sci. U. S. A. 102, 2731–2735. doi: 10.1073/pnas.0500023102
Zhang, H., Ishige, K., Kornberg, A. (2002). A polyphosphate kinase (PPK2) widely conserved in bacteria. Proc. Natl. Acad. Sci. U. S. A. 99, 16678–16683. doi: 10.1073/pnas.262655199
Keywords: cyanobacteria, polyphosphate, polyphosphate kinase1, PPK1, sulfur starvation, Synechocystis
Citation: Sato N, Endo M, Nishi H, Fujiwara S and Tsuzuki M (2024) Polyphosphate-kinase-1 dependent polyphosphate hyperaccumulation for acclimation to nutrient loss in the cyanobacterium, Synechocystis sp. PCC 6803. Front. Plant Sci. 15:1441626. doi: 10.3389/fpls.2024.1441626
Received: 31 May 2024; Accepted: 15 July 2024;
Published: 31 July 2024.
Edited by:
Rei Narikawa, Shizuoka University, JapanReviewed by:
Martin Hagemann, University of Rostock, GermanyJiangxin Wang, Shenzhen University, China
Copyright © 2024 Sato, Endo, Nishi, Fujiwara and Tsuzuki. This is an open-access article distributed under the terms of the Creative Commons Attribution License (CC BY). The use, distribution or reproduction in other forums is permitted, provided the original author(s) and the copyright owner(s) are credited and that the original publication in this journal is cited, in accordance with accepted academic practice. No use, distribution or reproduction is permitted which does not comply with these terms.
*Correspondence: Norihiro Sato, bnNhdG9AbHMudG95YWt1LmFjLmpw