- 1Department of Agriculture, Food and Environment, University of Catania, Catania, Italy
- 2Department of Biomedical and Biotechnological Sciences, University of Catania, Catania, Italy
- 3Department of Plant Breeding, Swedish University of Agricultural Sciences, Alnarp, Sweden
Introduction: Despite their adverse environmental effects, modern agriculture relies heavily on agrochemicals to manage diseases and pests and enhance plant growth and productivity. Some of these functions could instead be fulfilled by endophytes from the plant microbiota, which have diverse activities beneficial for plant growth and health.
Methods: We therefore used a microbiome-guided top-down approach to select ten bacterial strains from different taxa in the core microbiome of tomato plants in the production chain for evaluation as potential bioinoculants. High-quality genomes for each strain were obtained using Oxford Nanopore long-read and Illumina short-read sequencing, enabling the dissection of their genetic makeup to identify phyto-beneficial traits.
Results: Bacterial strains included both taxa commonly used as biofertilizers and biocontrol agents (i.e. Pseudomonas and Bacillus) as well as the less studied genera Leclercia, Chryseobacterium, Glutamicibacter, and Paenarthorbacter. When inoculated in the tomato rhizosphere, these strains promoted plant growth and reduced the severity of Fusarium Crown and Root Rot and Bacterial Spot infections. Genome analysis yielded a comprehensive inventory of genes from each strain related to processes including colonization, biofertilization, phytohormones, and plant signaling. Traits directly relevant to fertilization including phosphate solubilization and acquisition of nitrogen and iron were also identified. Moreover, the strains carried several functional genes putatively involved in abiotic stress alleviation and biotic stress management, traits that indirectly foster plant health and growth.
Discussion: This study employs a top-down approach to identify new plant growth-promoting rhizobacteria (PGPRs), offering an alternative to the conventional bottom-up strategy. This method goes beyond the traditional screening of the strains and thus can expand the range of potential bioinoculants available for market application, paving the way to the use of new still underexplored genera.
1 Introduction
Modern farms make extensive use of pesticides to manage diseases and pests as well as chemical fertilizers to enhance productivity (Jacquet et al., 2022). However, the significant negative effects of agrochemicals on both the environment and human health have sparked increasing interest in alternative methods for achieving safe, environmentally sustainable, and eco-friendly crop production (Jacquet et al., 2022). Innovative strategies that minimize reliance on conventional agrochemicals without loss of agricultural productivity and ecological integrity are highly desired.
Current research efforts seeking to reduce agrochemical use are converging towards a prophylactic approach that emphasizes agroecological cropping systems, biodiversity-aware breeding programs, and precision agriculture, with a heavy focus on biological control solutions (Jacquet et al., 2022).
Plant-associated microorganisms play vital roles in protecting plants against abiotic and biotic stress factors (Compant et al., 2005; Singh et al., 2011; Santoyo et al., 2016; Kelbessa et al., 2023). Some constituents of the plant microbiota actively enhance nutrient uptake (Das et al., 2022), improve nutrient utilization efficiency (Kelbessa et al., 2023), and contribute to phytohormone modulation (Backer et al., 2018), biocontrol (Compant et al., 2005), and the induction of systemic resistance (Pieterse et al., 2014), thereby promoting plant growth and health. These microorganisms are known as Plant Growth Promoting Microorganisms (PGPM) (Lugtenberg and Kamilova, 2009; Kumar et al., 2022). Endophytes, i.e. microorganisms capable of residing in the internal tissues of host plants, are major constituents of the plant microbiota (Hardoim et al., 2015). Their beneficial effects often exceed those of many rhizosphere-colonizing bacteria and may be especially pronounced when the plant is growing under stress conditions (Hardoim et al., 2015).
Many studies have sought to evaluate the potential of plant-associated microbiomes as PGPM and Biological Control Agents (BCA), but there remains a need to develop diverse biocontrol solutions that can be effectively applied across various environments and management practices (Das et al., 2022; Ayaz et al., 2023). Many Plant Growth-Promoting Rhizobacteria (PGPR) have been identified using bottom-up approaches based on collections of bacteria that display desirable traits in culture-dependent screenings (Compant et al., 2019; Anzalone et al., 2021). However, relying exclusively on culture-dependent selection methods has proven to be a time-intensive strategy that can yield inconsistent results (Berg et al., 2017; Compant et al., 2019).
Research into plant-associated microbial communities has expanded rapidly with the advent of high-throughput sequencing techniques, which have opened up new ways of investigating plant-microbiome and microbe-microbe interactions (Bulgarelli et al., 2012, 2013; Knief, 2014; Du et al., 2020; Kelbessa et al., 2022). For example, top-down approaches were recently used to identify Plant Growth-Promoting (PGP) candidates based on data from microbial community metagenome analyses in an effort to develop biotechnological crop protection strategies (Compant et al., 2019). Microbiome-guided methods for selecting beneficial bacterial strains have mainly focused on the relative abundance and/or enrichment of specific taxa under specific growing conditions (Zhuang et al., 2021), including stress conditions (Kwak et al., 2018; Flemer et al., 2022), or targeting taxa within the ‘core’ microbiome (Tian et al., 2017; Bergna et al., 2018; Penyalver et al., 2022).
The core microbiome consists of a set of microbial taxa associated with a specific host or environment along with their genomic and functional characteristics (Lundberg et al., 2012; Neu et al., 2021). It includes microbial taxa that have become vital for plant health as a result of evolutionary processes that have led to the selection and enrichment of taxa that fulfill critical functions for the fitness of the plant holobiont (Lemanceau et al., 2017; Toju et al., 2018; Risely, 2020). Knowledge of core microbiome components, e.g. microbial communities associated with a plant species across various stages of development or under different growing conditions, can thus provide valuable guidance when selecting beneficial microorganisms that could be used to enhance crop resilience and productivity through strategic application of microbial inoculants or other biocontrol agents (Tian et al., 2017; Bergna et al., 2018; Penyalver et al., 2022; Wang et al., 2023).
To plan future tomato microbiome engineering interventions we previously used amplicon-based metagenomics to perform a comprehensive analysis of tomatoes grown under greenhouse conditions spanning the entire plant production chain (Anzalone et al., 2022). The study involved sampling tomato seeds (Solanum lycopersicum L. cv. ‘Proxy’) and the rhizosphere of seedlings born from those seeds in a commercial nursery. The development of the seedlings’ root microbiomes was then monitored after transplantation into a greenhouse, in agricultural soil and coconut fiber (soilless conditions) (Anzalone et al., 2022). The root-associated bacterial communities differed significantly between the nursery and production stages, and also between conventional and soilless conditions in the greenhouse. These findings suggest that it will be essential to account for the variability of the microbiome when seeking to develop biocontrol solutions that will form stable and effective interactions (Anzalone et al., 2022).
In this study, we sought to advance beyond the traditional bottom-up approach for selecting PGPR strains by employing a top-down strategy informed by microbiome analysis, focusing on representative strains from the tomato core microbiome genera, irrespective of their in vitro performance. The core microbiome data on bacterial communities associated with different tomato compartments during the growing cycle, obtained by Anzalone et al. (2022) was used to guide the selection of new beneficial bacteria from the same samples through in vitro cultivation. This allowed us to expand strain selection to less explored genera, broadly suitable for various application time and methods. Eventually, ten bacterial strains from the tomato ‘core microbiome’ collection were characterized and shown to exhibit plant growth promoting and biocontrol properties in planta, even though some of them showed no antagonistic activity in vitro. Along with strains in the genera Bacillus and Pseudomonas we shed light onto the PGP phenotypes of strains belonging to the Gram-negative genera Leclercia and Chryseobacterium and the Gram-positive Micrococcaceae genera Paenarthrobacter and Glutamicibacter. The construction of high-quality genomes can significantly improve our capacity to investigate and comprehend the intricate mechanisms that make bacterial agents effective in biocontrol.
2 Materials and methods
2.1 Isolation of bacterial endophytes
The endophytes examined in this study were obtained from seed and root endospheres of tomato (Solanum lycopersicum L.) cv. ‘Proxy’ from the same samples used in the metagenomic study in Anzalone et al. (2022). More specifically, during the same production cycle, samples were obtained from the endosphere of tomato seeds (Seeds_T0) and roots of nursery seedlings before commercialization (Plant_T1_Endo) and two months after their transplanting in greenhouse either in agricultural soil (Plant_T2_Soil) or soilless in a coconut fiber substrate (Plant_T2_CF) (Anzalone et al., 2022). Four replicates of 20 seeds and four plant roots bulk samples for each condition were analyzed. Samples were processed according to Anzalone et al. (2021). Cultivable bacterial populations of total, fluorescent, and spore forming bacteria were enumerated in compliance with Anzalone et al. (2021). The root-associated bacteria were selected from plates containing 30–300 colonies, i.e., typically 102 (1:100) dilution (Anzalone et al., 2021). Bacterial strains were selected using a systematically randomized approach in which solid media plates were divided into six equal parts and colonies from one of the six parts were collected as stated in Bergna et al. (2018). Approximately 100 colonies per each biological replicate were harvested, leading to a total of 2000 colonies that after purification were preserved in 96 microwell cell culture plates (Anzalone et al., 2021). Ninety-four representative bacteria were selected for further investigation, based on KOH string test (Halebian et al., 1981) and colony macromorphological diversity (size, color, and morphology of the colony).
2.2 Molecular and phylogenetic identification of bacteria isolated from the tomato endosphere
Partial 16S rRNA gene amplification was obtained by PCR using the universal primer pair 27F-1492R (Edwards et al., 1989; Lane, 1991). The master mixtures consisted of 1 x Taq&Go G2 Hot Start colorless PCR Master Mix (Promega), 0.5 μM of each primer, and 1 µL of template in a total volume of 15 μL. Reactions were performed with a GeneAmp® PCR system 9700 thermal cycler using the thermal protocol described by Anzalone et al. (2021). The DNA amplicons were quantified and sequenced by BMR Genomics (Padova, Italy). The nucleotide sequences were searched against the nucleotide collection database of the National Center for Biotechnology Information (NCBI) using the Basic Local Alignment Search Tool BLASTN (http://www.ncbi.nlm.nih.gov). Sequences were aligned using the Clustal-W algorithm as implemented in MEGA XI and deposited in GenBank; the corresponding accession numbers were obtained. A phylogenetic tree was generated based on the alignment profiles using the Neighbor-Joining method (Kumar et al., 2018) with bootstrap-based branch supports in MEGA XI.
2.3 Culture collection representativeness
To ascertain the representativeness of the strains of our collection in the tomato microbiome in the production chain, we used 16S rRNA sequencing data from the previous study of Anzalone et al. (2022). The 16S rRNA gene sequences of culture collection isolates were compared with the 16S rRNA gene amplicon-based metagenomic data of seeds, root endosphere and rhizosphere samples from Anzalone et al. (2022), using the Basic Local Alignment Search Tool BLASTN (http://www.ncbi.nlm.nih.gov). Sequences with ≥ 97% similarity were assigned to the same OTUs (Carper et al., 2021; Krstić Tomić et al., 2023). In case of multiple matches, the OTU with the highest identity percentage was selected from those exceeding the set threshold.
2.4 Phenotypic characterization of representative bacterial endophytes
In vitro tests were conducted as described by Anzalone et al. (2021) to evaluate three PGP traits - siderophore production, phosphate solubilization, and growth on 8% NaCl – in the selected 94 bacterial endophytes. The production of hydrogen cyanide (HCN) and 1-aminocyclopropane-1-carboxylic acid (ACC) deaminase was assessed using the methods of Strano et al. (2017) and Penrose and Glick (2003), respectively. All experiments were performed twice using three independent replicates.
2.5 Antimicrobial activity of representative bacterial endophytes
Bacterial endophytes were tested on Potato Dextrose Agar (PDA) plates for in vitro antagonistic activity according to Anzalone et al. (2021), against the following pathogens: the bacteria Clavibacter michiganensis subsp. michiganensis strain PVCT 156.1.1 (Cmm), Pseudomonas syringae pv. tomato strain PVCT 28.3.1 (Psto), Xanthomonas euvesicatoria pv. perforans strain NCPPB 4321 (Xep) and the fungi Fusarium oxysporum f. sp. radicis-lycopersici strain PVCT 127 (Forl), and Botrytis cinerea strain Bc5 (Bot). Briefly, bacterial pathogen suspensions were normalized to an OD600 of 0.1 (Anzalone et al., 2021) and inhibition halo radii (in mm) were measured after 48 h of incubation. For fungal pathogens a mycelial plug was placed in the center of the plate (Anzalone et al., 2021) and the antifungal activity was expressed as a Percentage of Growth Inhibition (PGI) according to Vincent (1947). All strains were tested twice using three independent replicates.
2.6 Selection of strains for further trials
To identify key bacterial components of the tomato plant-associated samples, the core microbiome of the samples reported by Anzalone et al. (2022) was determined. The samples used in the analysis represented tomato seeds (Seed_T0), the root rhizosphere (Plant_T1_Rhizo) and endorhizosphere (Plant_T1_Endo) of tomato plants ready for sale; and the rhizosphere and endorhizosphere of tomato plants at flowering and fruit set after transplantation into agricultural soil (Plant_T2_Soil_Rhizo; Plant_T2_Soil_Endo) and coconut fiber bags (Plant_T2_CF_Rhizo; Plant_T2_CF _Endo). Five replicates for each sample type were analyzed. Following the selection criteria of Hamonts et al. (2018), bacterial core taxa up to the genus level with prevalences ≥ 75% in the aforementioned samples (i.e. genera that were consistently present across at least the 75% of the samples) were investigated using the microbiome package in R (Shetty and Lahti, 2019). Strains were selected arbitrarily from genera that were present both in the bacterial collection and in the core microbiome. Only bacterial strains that showed growth stability on Nutrient Dextrose Agar (NDA) at 27 ± 1°C were further used.
2.7 In planta bioassays
2.7.1 Microorganisms’ growing conditions and inoculum preparation
Bacterial strains were grown on NDA plates for 24 h at 27 ± 1°C. Single colonies were inoculated in 25 mL of Luria-Bertani (LB) broth and incubated for 24 h at 27 ± 1°C in a rotary shaker (180 rpm). The bacterial cultures were centrifuged at 5,000 rpm for 15 min, and after discarding the supernatant, the pellets containing the bacterial cells were resuspended in sterile water and the density was adjusted to 1·108 colony forming units (cfu)·mL-1.
Xep suspensions were prepared as above, with a final concentration of 1·108 cfu·mL-1 (Anzalone et al., 2021). To produce Forl inoculum, fresh conidia were collected from sporulating colonies grown for 14 days on PDA at 23°C. Petri dishes were flooded with 10 ml of sterile distilled water, then conidia were scraped using sterile spatulas and transferred to sterile 50 ml tubes. After filtration through four layers of cheesecloth, the concentration of the resulting spore suspension was estimated using a hemocytometer under light microscopy and adjusted to 4·106 conidia·mL-1 (Manzo et al., 2016).
2.7.2 Plant material and growing conditions
Tomato plantlets of the variety Moneymaker were produced from seeds in growth chamber. Briefly, seeds were surface-sterilized by immersion in 3% sodium hypochlorite for 5 min followed by three washing steps in sterile water (Ghadamgahi et al., 2022) and dried on sterile filter paper in a laminar flow cabinet. Seed were sown in trays filled with a commercial potting substrate (Krukväxtjord Lera/Kisel, SW Horto). Trays were covered with plastic bags and kept in a growth chamber under controlled conditions (22°C/16 h light, 18°C/8 h dark, 60% relative humidity). After germination, the chamber conditions were changed to 25°C:22°C day:night. The light intensity was set at 300 µmol·m-2s-1 (Fan et al., 2013) and then changed to 225 µmol m-2s-1 when the plants were three weeks old. Plants were transplanted into 2 L volume pots for subsequent experiments.
2.7.3 In vivo biocontrol activity
The biocontrol activity of the selected bacterial endophytes was assessed against the causal agent of Tomato Crown and Root Rot, Fusarium oxysporum f. sp. radicis-lycopersici (strain PVCT 127), and Xanthomonas euvesicatoria pv. perforans (strain NCPPB 4321) one of the causal agents of Tomato Bacterial Spot (Osdaghi et al., 2021). Six plants were used as replicates in each pathogen/endophyte combination. Endophytes were applied by seed soaking (30 minutes) and soil drenching with 50 mL of the bacterial endophyte suspension, three weeks after plant emergence and after approximately three further weeks but exactly 72 or 24 h before Fusarium and Xanthomonas inoculation, respectively.
For the artificial inoculations with Forl, 30 ml of conidial suspension was poured into the soil near each tomato plant and a wound in the crown was made by a razor blade to assist pathogen penetration. Control plants were wounded in the same way but inoculated with sterile water. The growth chamber was set at 22°C/16 h light and 20°C/18 h dark, with 80% relative humidity. Disease evaluation was performed 45 days after Forl inoculation (Vitale et al., 2014). All seedlings were gently uprooted and their crowns and stems were examined. To determine disease incidence, all plants were sectioned to ascertain the presence of disease symptoms and the percentage of infected tomato plants was determined. Disease severity was assessed by measuring the length (cm) of vascular discoloration in each tomato stem.
For the bacterial spot biocontrol assay, Xep cell suspensions (1·108 cfu·mL-1) or water as a negative control, supplemented with 0.01% tween 20, were spray-inoculated on tomato plants. Plants were covered with plastic bags 24 h before pathogen inoculation and remained covered for the following 72 h to maintain a relative humidity of close to 100%. The growth chamber was set at 26°C/16 h light and 24°C/18 h dark, with 80% relative humidity. Six days after inoculation, the disease incidence was recorded and the disease severity was calculated by estimating the percentage of the leaf area affected (necrotic tissue) by bacterial spot in approximately 10 leaflets using the ImageJ software (https://imagej.nih.gov/ij/).
2.7.4 Tomato growth promotion assay
Growth promotion activity was evaluated using a completely randomized block experimental design. After transplanting, 20 mL of the appropriate bacterial suspension (or water as a negative control) was added to each pot by soil drenching (Anzalone et al., 2021). Pots were observed regularly and watered daily as needed. Shoot height was recorded at five different time points: T0 (treatment) and T1-4 (from 1 to 4 weeks after treatment). After one month, the seedlings were uprooted and the fresh and dry shoot and root weights were determined. For dry weight measurements, plant shoots and roots were oven-dried at 70°C for three days before weighing. Seven replicates were used for each treatment.
2.8 DNA extraction and whole genome sequencing
Bacterial strains were grown in LB broth inoculated with a single bacterial colony from a 24-h-old culture on NDA and incubated overnight at 27 ± 1°C under continuous shaking (180 rpm). Total genomic DNA was extracted from bacterial cultures using the Wizard® HMW DNA Extraction Kit (Promega) according to the manufacturer’s instructions. Complete bacterial genome sequences were determined by a combination of long and short reads. Long and short read sequencing were performed with an Oxford Nanopore GridION X5 platform and an Illumina NovaSeq 6000 platform (paired-end read length, 150 bp), respectively.
2.9 Pre-processing of reads, genome assembly and annotation
Illumina raw reads were pre-processed (adapter trimming, quality filtering [>Q30] and quality checking) with fastp v 0.23.4 (Chen et al., 2018).
The quality of raw Nanopore reads was checked with NanoPlot v1.42.0 (De Coster and Rademakers, 2023). Adapters were trimmed with Porechop_ABIv0.5.0 (Bonenfant et al., 2022). Seqkit v2.8.1 (Shen et al., 2016) was used for quality filtering with 1,000 bp read length and Q10 quality cutoffs. Filtered nanopore reads were assembled using Flye v.2.9.4 (Kolmogorov et al., 2019). PILON v1.24 (Walker et al., 2014) was used for polishing with Illumina reads.
CheckM v1.1.6 (Parks et al., 2015) was used to determine the completeness and contamination of the assemblies. Assembly statistics were computed with QUAST v5.2.0 (Gurevich et al., 2013). Plasmer (Zhu et al., 2023) was used to identify plasmid sequences. General annotation of genomes was performed using Prokka v1.14.5 (Seemann, 2014).
GTDB-Tk v2.3.0 (Chaumeil et al., 2022) was used for taxonomic annotation of each genome. Genome sequence data were uploaded to the Type (Strain) Genome Server (TYGS), a free bioinformatics platform available at https://tygs.dsmz.de, to perform whole genome-based taxonomic analyses (Meier-Kolthoff and Göker, 2019). Genomic relatedness was determined using average nucleotide identity (ANI) values computed with EzBioCloud (Yoon et al., 2017). Two genomes belonging to the same species should have a dDDH of at least 70%, corresponding to an ANI of at least 95% (Goris et al., 2007; Auch et al., 2010; Meier-Kolthoff et al., 2013). Plant growth promoting traits (PGPT) were predicted using the PGPT-Pred module of PLaBAse v1.01 (Patz et al., 2021). PIFAR-BASE was used to identify ‘plant bacterial only interaction factors’ from the annotated protein files for each strain using the BlastP+HMMER Aligner/Mapper (Patz et al., 2021). The bacterial version of antiSMASH 7.0 (Blin et al., 2023) was used to screen for secondary metabolites.
2.10 Statistical analysis
Data from the PGP and biocontrol experiments were analyzed by analysis of variance (ANOVA) using Minitab 20 statistical software (Minitab, Inc., State College, PA). Means were separated using Tukey’s post-hoc HSD test.
2.11 Data availability
16S rRNA gene sequences of the strains used in this work were submitted to the GenBank database under accession numbers from MZ066824 to MZ066917.
All of the assembled genomes and respective raw reads are available under BioProject ID: PRJNA1096641.
3 Results
3.1 Isolation and identification of bacterial endophytes
The bacterial endophytes examined in this study were obtained from samples that were prepared for metagenomic analysis of microbial communities in tomato plants at multiple stages in the cultivation chain from nursery to greenhouse (Anzalone et al., 2022). Bacteria (total, fluorescent, and spore-forming) were enumerated on different media from samples obtained from the seed endosphere as well as from the endorhizosphere of nursery plantlets and two months after transplanting into either agricultural soil or coconut fiber bags. The total, spore forming, and fluorescent bacterial population sizes in the seeds were 1.46, 0.4, and 0.8 log CFU per gram of seed, respectively. The root endosphere bacterial concentrations of adult plants grown in agricultural soil and coconut fiber substrate were similar, and both were higher than the bacterial titers of plantlets in the nursery (Supplementary Figure S1). From a collection of approximately 2000 bacterial colonies isolated across the entire experiment, ninety-four representative bacteria from the endospheric (root or seed) compartments were selected for further investigation. Based on BLASTN sequence identities of partial 16S rRNA gene sequences, the 94 bacterial isolates were assigned to genera spanning seven orders (Supplementary Table S1): the Gram-positive Bacillales and Micrococcales, and the Gram-negative Pseudomonadales, Enterobacteriales, Flavobacteriales, Burkholderiales, and Xanthomonadales. Identities ranging from 97 to 100% were observed (Supplementary Table S1). More specifically, the Bacillales strains were assigned to the genera Bacillus, Paenibacillus, Staphylococcus, and Priestia; the Micrococcales strains belonged to the genera Glutamicibacter, Microbacterium, Curtobacterium, Paenarthrobacter, and Arthrobacter; the Pseudomonadales strains belonged to the genus Pseudomonas; the Enterobacteriales strains belonged to the genera Enterobacter, Ewingella, and Serratia; Flavobacteriales was represented by the genera Flavobacterium and Chryseobacterium; Burkholderiales was represented by a single strain of the genus Delftia; and Xanthomonadales was represented by multiple strains in the genus Stenotrophomonas. Sequences were deposited at GenBank under accession numbers from MZ066824 to MZ066917 (Supplementary Table S1). The majority of the strains in all samples belonged to the order Bacillales and/or Pseudomonadales (Figure 1A). A dendrogram showing the phylogenetic relationships of the selected bacterial strains is shown in Figure 1B. Comparing the 16S rRNA gene sequences of our culture collection isolates with 16S rRNA gene amplicon-based metagenomic data from seeds, root endosphere, and rhizosphere samples within the tomato growth chain (Anzalone et al., 2022), we found that 88% of the isolates had representatives within the amplicon-based metagenomic sequences at 97% identity (Supplementary Table S2). The strains in culture collection represented 12.77% of the total in seeds and 26.48%, 22.38%, and 27.40% of the total in the root endosphere of nursery plantlets and plants in coconut fiber substrate or agricultural soil, respectively (Figure 2A). Lower values were observed in the respective rhizosphere samples (Figure 2A).
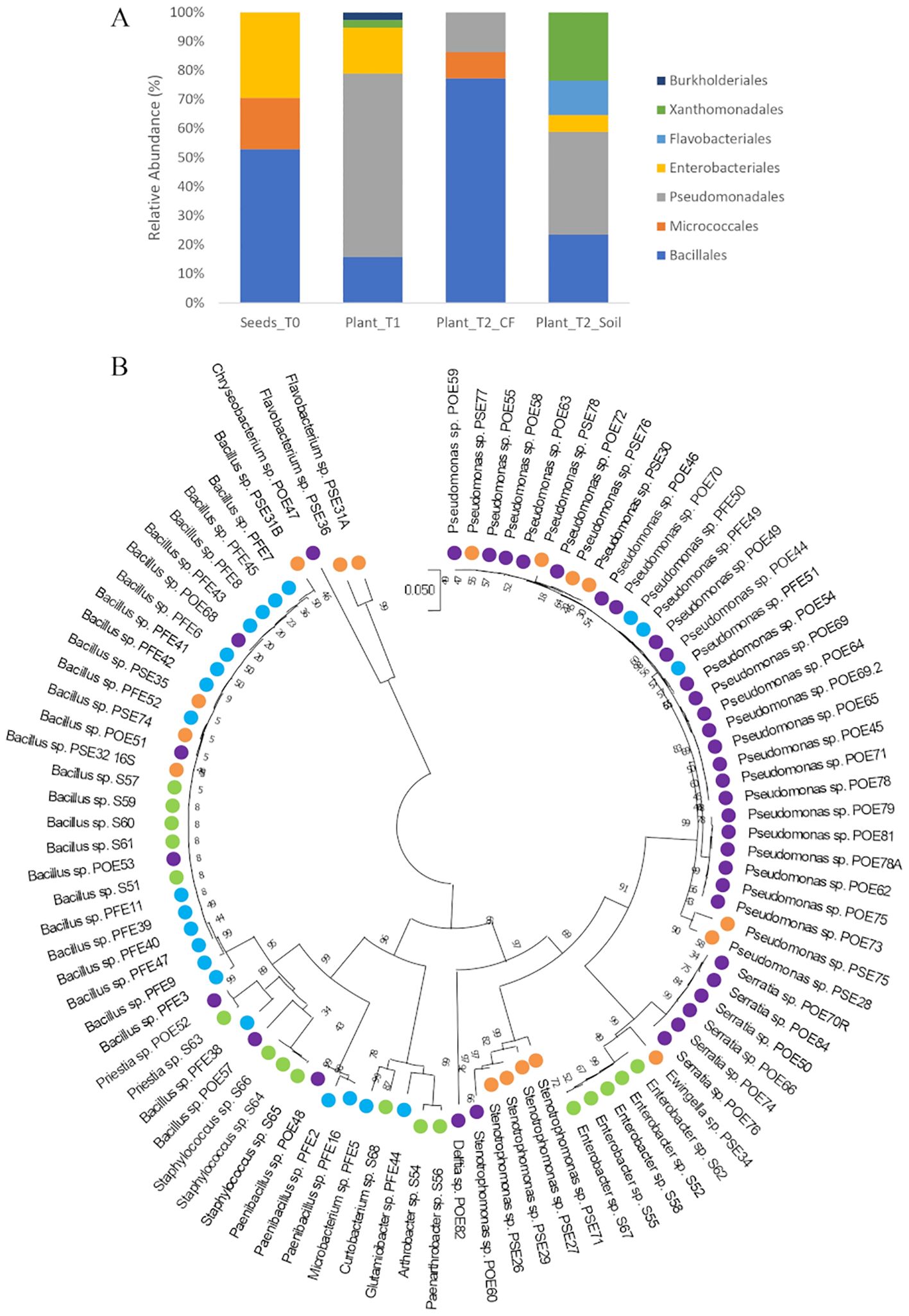
Figure 1. (A) Distribution of cultivable bacterial communities in the endosphere samples of tomato seeds (Seeds_T0) and roots (Plant_T1, Plant_T2_CF, and Plant_T2_Soil) at the taxonomic order level; (B) Phylogenetic tree based on 16S rRNA gene sequences of the 94 endophytic strains isolated in this study. The evolutionary history was inferred using the Neighbor-Joining method. The evolutionary distances were computed using the Tamura 3-parameter method. There was a total of 731 positions in the final dataset. Colors highlight the isolation source of each strain: green, Seeds_T0; violet, Plant_T1; blue, Plant_T2_CF; orange, Plant_T2_Soil.
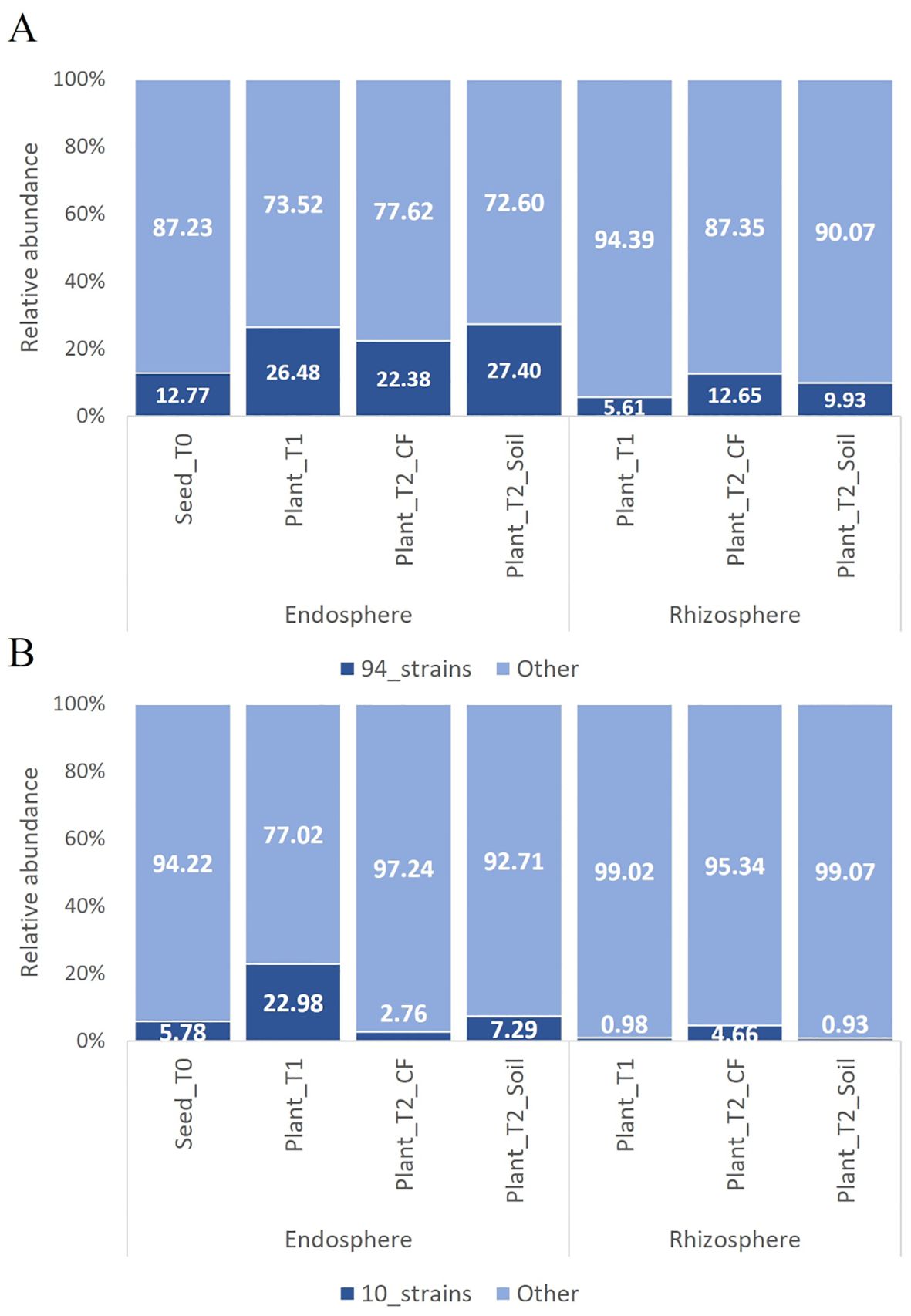
Figure 2. Relative abundance of OTUs of the bacterial communities in the tomato growth chain (Anzalone et al., 2022) matching with the 16s rRNA gene sequences of the 94 bacterial endophytic strains (A) and the ten further selected strains (B). Only sequences with ≥ 97% similarity were considered. In cases of multiple matches, the OTU with the highest identity percentage was selected from those exceeding the set threshold.
3.2 Phenotyping of beneficial bacterial traits
The bacterial strains were characterized for different beneficial properties, revealing that a high percentage of strains showed PGP traits. Approximately 87% of the bacterial strains from the tomato endospheric compartments could grow in 8% NaCl, while 51% were able to produce siderophores and solubilize insoluble organic phosphate. However, only 2% and 21% of the strains were positive for HCN and ACC deaminase production, respectively (Supplementary Figure S2A). Approximately 30% of the bacteria (28 out of 94 strains) showed antagonistic activity towards all of the tested phytopathogenic bacteria and fungi (Supplementary Figure S2B), while around 45% of the strains (41 out of 94) were antagonistic to all the bacterial pathogens. The highest antimicrobial activity (based on the number of antagonistic strains and inhibition zone radius) was observed against C. michiganensis subsp. michiganensis PVCT 156.1.1, followed by P. syringae pv. tomato PVCT 28.3.1. Roughly 54% of the endophytic strains (51 out of 94) inhibited the mycelial growth of the fungal targets Fusarium oxysporum f. sp. radicis-lycopersici PVCT 127 and Botritys cinerea Bc5 to at least some degree when compared to a non-challenged colony. Moreover, 26% of the strains achieved at least 60% growth inhibition against the former fungal pathogen, while 11% of the strains achieved the same level of inhibition against the latter (Supplementary Figure S2B).
3.3 Selection of strains from the core microbiome for further trials
To select strains suitable for various applications throughout the production chain, we determined the tomato core microbiome at the genus level by analyzing bacterial communities in a continuous experiment. We previously tracked their development from seeds to seedlings and through to mature plants under two growing conditions (Anzalone et al., 2022). Both the rhizosphere and endorhizosphere bacterial communities were included in the analysis. Twenty-seven core microbiome genera were identified across at least 75% of the 35 samples (prevalence > 75%) (Figure 3). Bacterial genera present both in the core microbiome and among the bacterial strains obtained in axenic culture included Flavobacterium, Pseudomonas, Bacillus, Enterobacter, Chryseobacterium, Arthrobacter, and Stenotrophomonas. We selected ten strains arbitrarily from these genera, reflecting a degree of subjectivity in the choice process, and excluded Flavobacterium and Stenotrophomonas strains due to their observed instability under our growth conditions (Table 1). Therefore, the selection did not take into account the in vitro potential of the strains. Based on preliminary 16S rRNA identification, we selected two and three strains of Pseudomonas and Bacillus, respectively, which could potentially represent different species according to BLASTN analysis (Supplementary Table S1). Additionally, we selected one strain each of Enterobacter and Chryseobacterium (Table 1). Three Micrococcaceae strains, identified as Arthrobacter S54, Paenarthrobacter S56, and Glutamicibacter PFE44 by 16S rRNA gene sequencing, were chosen as Gram-positive representatives of the set (Table 1), with these strains being represented by a single Arthrobacter OTU in the core microbiome (data not shown).
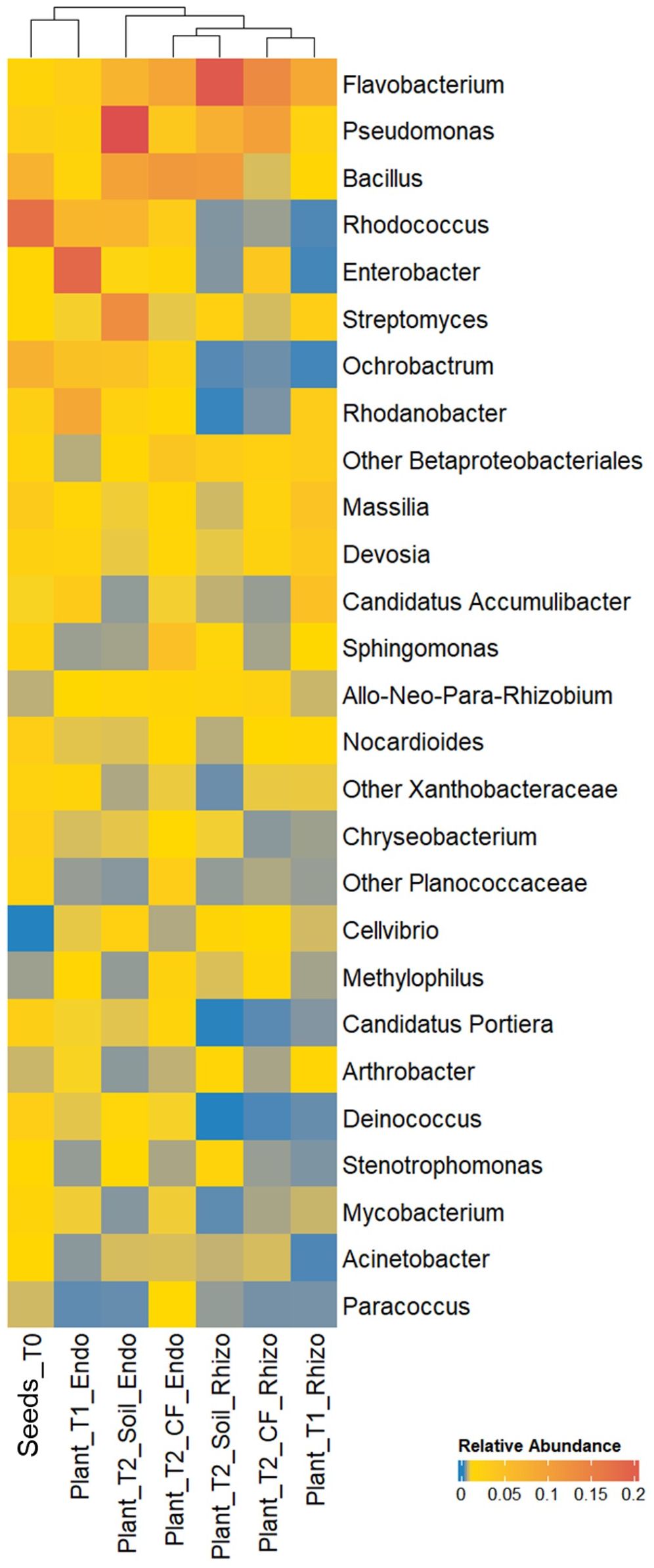
Figure 3. Relative abundance of bacterial genera of the tomato core microbiome showing a prevalence ≥ 75%, i.e. genera that were consistently present across at least the 75% of the samples of the bacterial communities in the tomato growth chain according to Anzalone et al. (2022). Bacterial genera are indicated in the right; samples are indicated on the bottom (see Material and Methods section for sample details).
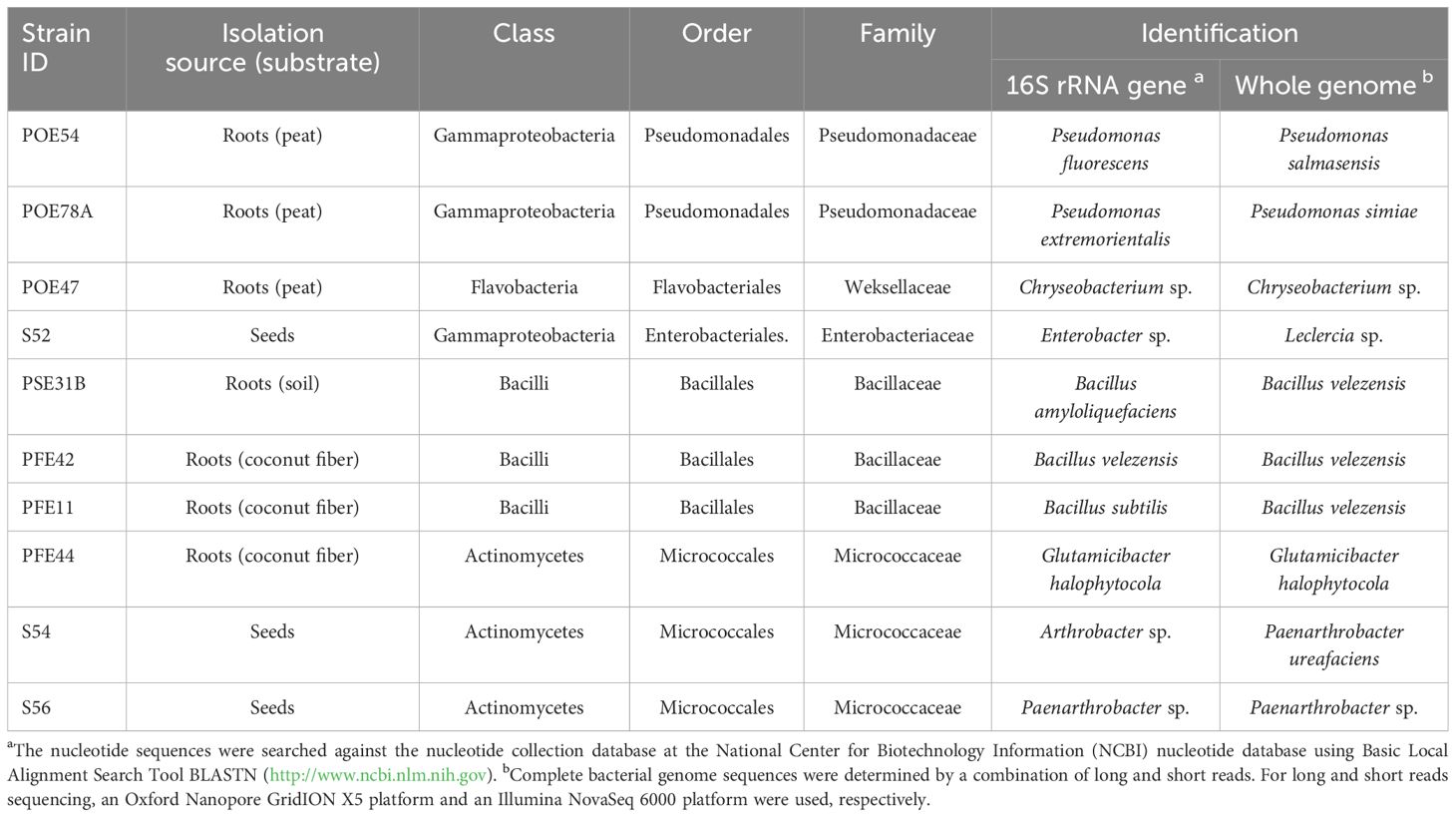
Table 1. Isolation source and identification by partial 16S rRNA gene and whole genome sequencing of the ten bacterial endophytes selected based on the core microbiome analysis.
To evaluate the representativeness of the ten selected strains in comparison to the total microbiome, we analyzed their 16S rRNA gene sequences against 16S rRNA gene amplicon-based metagenomic data (Anzalone et al., 2022). The selected strains accounted for 5.78% of the microbiome in the seeds and 22.98%, 2.76% and 7.29% of the microbiome in the root endosphere of nursery plantlets and plants in coconut fiber substrate or agricultural soil, respectively (Figure 2B). Except for Chryseobacterium sp. POE47, all strains exhibited more than 97% similarity with the OTUs in the core microbiome (data not shown).
3.4 Genome sequencing of beneficial endophytes
The genetic potential of the selected beneficial bacteria was explored by using a combination of long-read Oxford Nanopore and short-read Illumina sequencing to obtain their genomes in order to identify traits associated with plant growth promotion and biocontrol. The genomes were de novo assembled to create high quality, complete reference genomes, yielding individual genomes whose characteristics are detailed in Table 2. Final assembly quality was validated by estimating contamination and completeness. Overall, the assembled genomes showed over 98% completeness and <1% contamination (Table 2).
The genomic properties of all of the selected bacterial strains were similar to those of other strains belonging to the same species deposited in GenBank (data not shown).
The TYGS genome-based pipeline was used to refine the identification of the ten bacterial strains based on 16S rRNA gene sequencing (Yoon et al., 2017; Meier-Kolthoff and Göker, 2019) (Table 1; Supplementary Table S3). Strains PSE31B, PFE42 and PFE11 were all assigned to the bacterial species B. velezensis. The two Pseudomonas strains were identified as P. salmasensis strain POE54 and P. simiae strain POE78A. Two of the three bacterial strains in the Microccoccaceae family were identified at the species level as Glutamicibacter halophytocola strain PFE44 and Paenarthrobacter ureafaciens strain S54. However, strain S56 could only be identified at the genus level as Paenarthrobacter sp. with a dDDH value of 24% when compared to the closest genome reference P. ureafaciens DSM 20126T; consequently, this strain may belong to a new species. Similar results were obtained for Chryseobacterium sp. POE47 and Leclercia sp. strains S52 (Enterobacter sp. by 16S rRNA gene sequencing) when compared to the closest phylogenetic species Chryseobacterium taeanense DSM 17071T and Leclercia tamurae H6S3T, for which the corresponding dDDH values were 31.2% and 51.4%, respectively. The calculated ANI values for each strain with the closest related species supported these conclusions (Supplementary Table S3). Table 2 summarizes the general genomic characteristics of the sequenced strains.
Coding sequences were extracted from the genomes of the ten selected strains and classified using the Clusters of Orthologous Groups of proteins (COG) database, revealing four main functional gene classes that were present in all ten genomes (Supplementary Figure S3): (i) Amino acid transport and metabolism, (ii) Carbohydrate transport and metabolism, (iii) Cell wall/membrane/envelope biogenesis, and (iv) Transcription. Other highly represented gene classes included translation, ribosomal structure and biogenesis, signal transduction mechanisms, energy production and conversion, coenzyme transport and metabolism, lipid transport metabolism, and inorganic ion transport and metabolism. No genes in the chromatin structure and dynamics or nuclear structure categories were detected in any genome (Supplementary Figure S3). The “amino acid transport and metabolism” class was the most strongly represent among the COGs in the three members of the Bacillus genus, the two members of the Pseudomonas genus, and G. halophytocola strain PFE44. The most abundant gene families identified in Chryseobacterium sp. POE47 were associated with “cell wall/membrane/envelope biogenesis”, while the most strongly represented COG family for Leclercia sp. S52, P. ureafaciens S54 and Paenarthrobacter sp. S56 was “carbohydrate transport and metabolism”. Further details of the COG analysis are presented in Supplementary Figure S3.
In the KEGG analysis, genes associated with “protein families: genetic information processing” were most abundant in the Bacillus strains, Chryseobacterium sp, POE47 and Leclercia sp. S52. For the two Pseudomonas strains, the “environmental information processing” category was dominant, while “carbohydrate metabolism” was dominant in the three members of the Micrococcaceae family (Supplementary Figure S4).
At the protein level, analysis with OrthoVenn 3 (https://orthovenn3.bioinfotoolkits.net) revealed that the highest number of orthologous clusters was found in Pseudomonas species POE78A and POE54 (3874 and 3826), followed by B. velezensis PFE11, PFE42 and PSE31B (3273, 3253 and 3216). The lowest number was recorded in Chryseobacterium sp. POE47 (1679) (Supplementary Figure S5). The two pseudomonads shared 1387 clusters, while the three B. velezensis strains shared 1377. The two Paenarthrobacter strains (S54 and S56) shared 624 clusters, and 483 were also shared with the other strain belonging to the Micrococcaceae family (i.e. G. halophytocola PFE44). In total, 530 orthologous clusters were shared by all ten selected strains. A total of 329 gene clusters were specific to a single genome. Of these clusters, 101 belonged to Leclercia sp. S52 and 228 were from Chryseobacterium sp. POE47 (Supplementary Figure S5).
3.5 Genes related to potential PGP and biocontrol traits
A genome annotation analysis conducted using the PGPT-Pred function revealed genomic features associated with plant growth promotion. The genomes of all ten selected strains had similar PGPT classes, with minor differences across the diverse taxa. In all strains, the class with the highest proportion of genes was “Colonizing plant system”, followed by “Stress control and Biocontrol”, “Competitive exclusion”, “Biofertilization”, “Phytohormone and Plant Signaling”, “Bioremediation” and “Plant immune response stimulation” (Supplementary Figure S6). An insight in PGPT categories highlighted traits of interest linked to direct and indirect effect on plant growth (Figure 4). All the bacterial strains regardless of the taxonomic affiliation showed a high number of genes related to osmotic, oxidative and salinity stresses. Only G. halophytocola PFE44 showed genes encoding for stress signaling proteins related to abiotic stress defense, but in turn showed the lowest gene number related to biotic stress defense. Chryseobacterium sp. POE47 had a lower number of genes related to N acquisition, in particular, genes encoding for allantoine, trigonelline and urea usage were not detected in the genome. Notably, only the two Pseudomonas strains covered more gene categories related to biotic stress defense, including those encoding bactericidal, fungal, insecticidal and nematicidal compounds, and phytotoxin degradation (Figure 4). The three Bacillus strains distinguished for the high number of genes involved in bactericidal compound biosynthesis. Supplementary Table S4 shows the full set of genes found in the ten genomes.
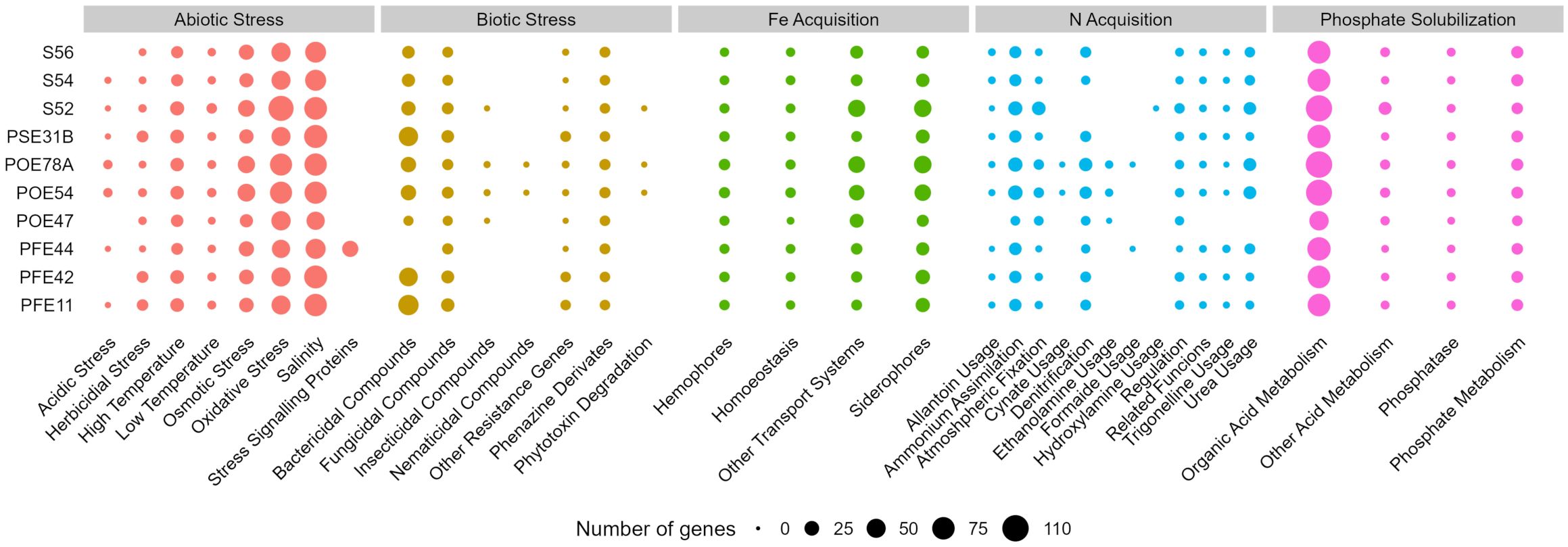
Figure 4. Number of genes of selected PGPT classes related to plant growth promotion and stress control in the genomes of the ten bacterial strains sequenced in this study. PGPTs were predicted using PGPT-Pred module of PLaBAse v1.01 (Patz et al., 2021). S56, Paenarthrobacter sp.; S54, P. ureafaciens; S52, Leclercia sp.; PSE31B, Bacillus velezensis; POE78A, Pseudomonas simiae; POE54, P. salmasensis; POE47, Chryseobacterium sp.; PFE44, Glutamicibacter halophytocola; PFE42, B. velezensis; PFE11, B. velezensis.
Annotation of the genomes against the ‘plant bacterial only interaction factors (proteins) (PIFAR)’ dataset revealed two distinct clusters among the bacterial endophytes: one comprising those exhibiting antimicrobial activity and the other comprising those that did not. Minor groups were further segregated based on their taxonomic affiliation (Figure 5). P. salmasensis POE54 and P. simiae POE78A belonged to the first group and showed the highest percentages of toxin-related factors, which accounted for 44% and 42% of the identified PIFAR, respectively (Figure 5). The three B. velezensis strains (PSE31B, PFE42 and PFE11) formed a second distinct cluster with toxin factor percentages ranging from 35 to 38% (Figure 5). Leclercia sp. strain S52 clustered more closely with, but separately, to Bacillus spp. The lowest toxin content was detected in the Paenarthrobacter strains S54 and S56 (24 and 25%), which formed a cluster with G. halophytocola PFE44 (Figure 5). These strains belonging to the Micrococcaceae family had the highest content of hormone-related factors (18%). In the remaining strains, hormone-related factors comprised only 7 to 10% of the total PIFAR (Figure 5). EPS was the second most abundant class of factors across all genomes except Chryseobacterium sp. POE47, where EPS was the most abundant class, accounting for 29% of the total detected factors (Figure 5). This strain clustered separately from the others. Detoxification related factors comprised a significant proportion of the genomes of all ten strains but were most abundant in Chryseobacterium sp. POE47 (14%) and least abundant in the B. velezensis strains (7-8%) (Figure 5). Supplementary Table S5 provides detailed information on the predicted PIFAR.
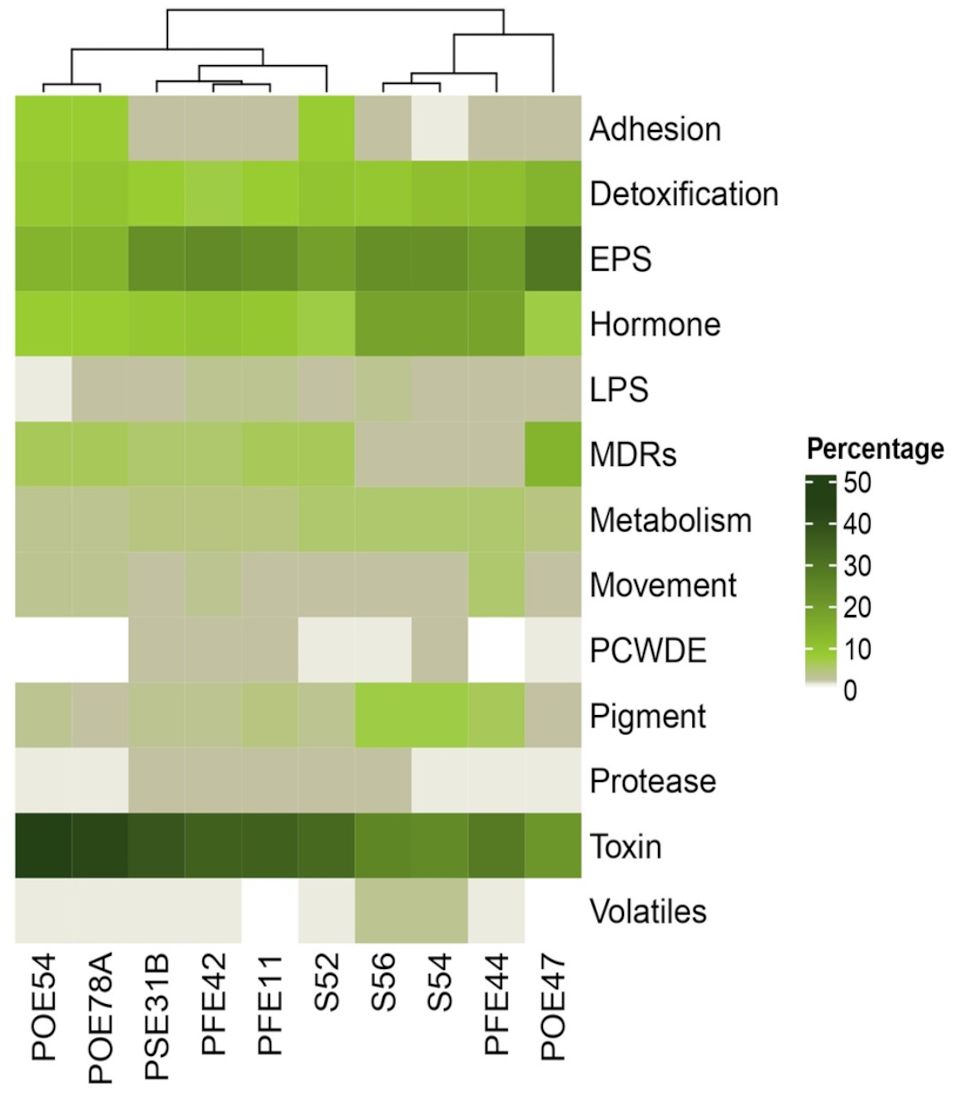
Figure 5. Percentage of genes encoding for ‘plant bacterial only interaction factors (proteins)’ predicted through PIFAR-BASE (Patz et al., 2021). Factor categories are specified on the right. Strain ID is indicated in the bottom. POE54, Pseudomonas salmasensis; POE78A, P. simiae; PSE31B, Bacillus velezensis; PFE42, B. velezensis; PFE11, B. velezensis; S52, Leclercia sp.; S56, Paenarthrobacter sp.; S54, P. ureafaciens; PFE44, Glutamicibacter halophytocola; POE47, Chryseobacterium sp.
3.6 Biosynthetic gene cluster mining
AntiSMASH 7.0 database analysis for secondary metabolites (Blin et al., 2023) based on the number of Biosynthetic Gene Clusters (BGCs) in each genome revealed a group comprising Bacillus and Pseudomonas strains with 11 to 14 BGCs, as shown in Table 3. The three members of the Micrococcaceae family (PFE44, S54 and S56) had six to eight BGCs, while Chryseobacterium sp. POE27 and Leclercia sp. S52, had the fewest BGCs (three and four, respectively).
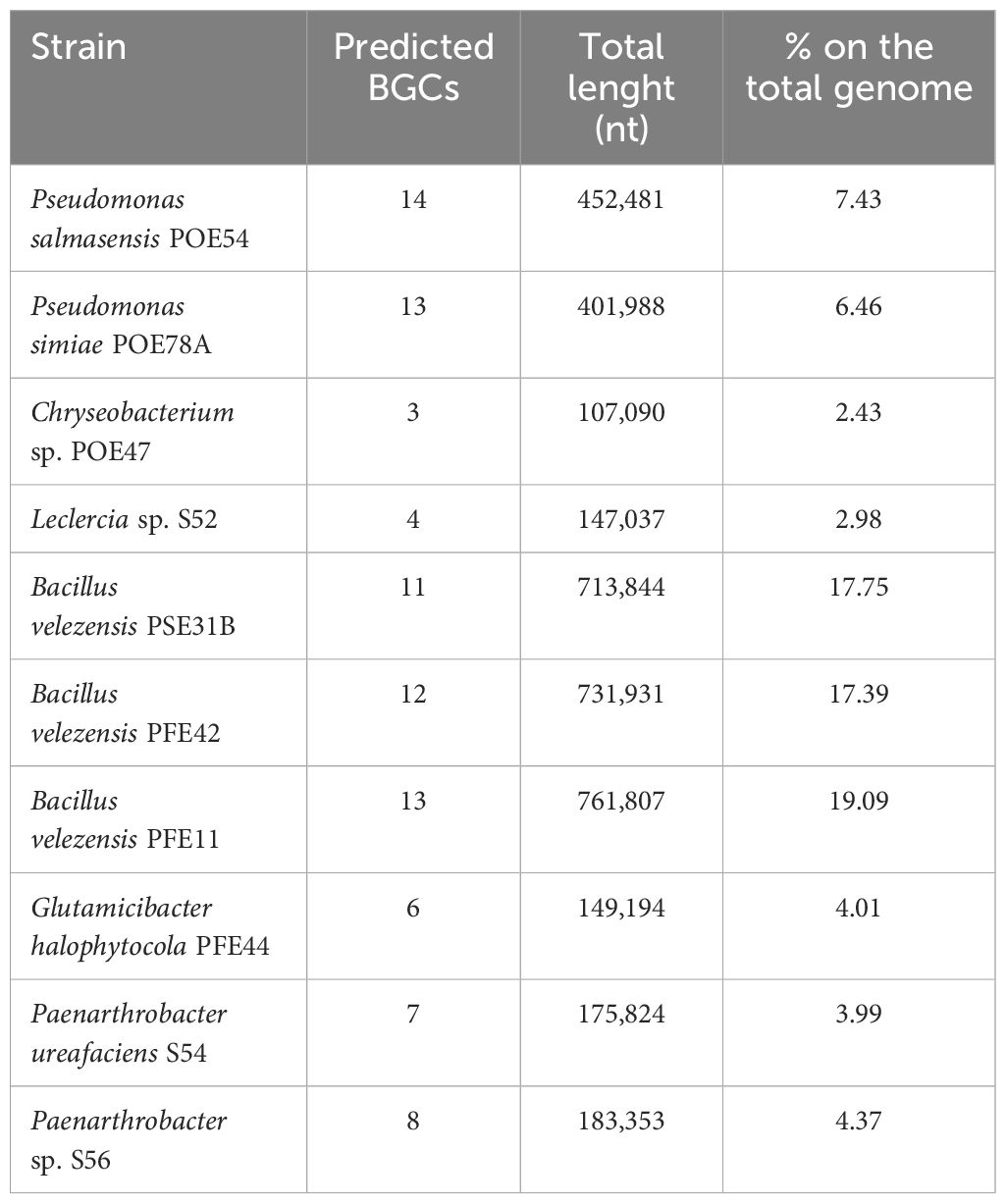
Table 3. Bacterial Biosynthetic Gene Clusters (BGCs) for secondary metabolites predicted by AntiSMASH 7.0.
The percentage of the genome encoding BGCs was highest in Bacillus strains (17-19%), followed by Pseudomonas strains (6-7%). The lowest BGC percentages were observed for Chryseobacterium sp. POE47 (Table 3). P. salmasensis POE54 had the most BGCs (14), with nine types being represented including NRPS (3), NRPS-like (1), RiPP-like (3), arylpolyene (1), betalactone (1), NI-siderophore (1), NAGGN (1), RRE-containing and hybrid (2) (Supplementary Table S6). Moreover, region 13 of this strain’s genome exhibited 100% similarity with the BGC (GenBank: KX931446.1) responsible for obafluorin biosynthesis. No putative metabolites could be identified for the other clusters by MIBiG database comparisons. BGCs associated with surfactin, fengycin, and bacilysin were detected in all Bacillus strains. Other identified BGCs were associated with various polyketides including difficidin, bacillaene, and macrolactin, and with the siderophore bacillibactin. Bacillus and Pseudomonas had higher numbers of PKS and hybrid clusters, and RiPP-like clusters, respectively, than the other strains (Supplementary Table S6). BGCs encoding the siderophores desferioxamine E and enterobactin were found in the three strains belonging to the family Micrococcaceae and Leclercia, respectively. A full list of the antiSMASH results can be found in Supplementary Table S6.
3.7 In vitro biocontrol activity against bacterial and fungal tomato pathogens
P. salmasensis strain POE54 and P. simiae strain POE78A, the three B. velezenzis strains PSE31B, PFE42 and PFE11 and Leclercia sp. strain S52 showed broad antagonistic activity against the three bacterial and two fungal tomato pathogens used in the antimicrobial assays (Table 4; Figures 6, 7). Chryseobacterium sp. strain POE47 only showed antagonistic activity against Forl PVCT 127. The three bacterial strains belonging to the genus Micrococcales, i.e. G. halophytocola PFE44, P. ureafaciens S54 and Paenarthrobacter sp. S56, generally showed no antagonistic activity although Paenarthrobacter sp. S56 displayed minor activity against Forl PVCT 127 (PGI, 21.1%) (Table 4; Figures 6, 7).
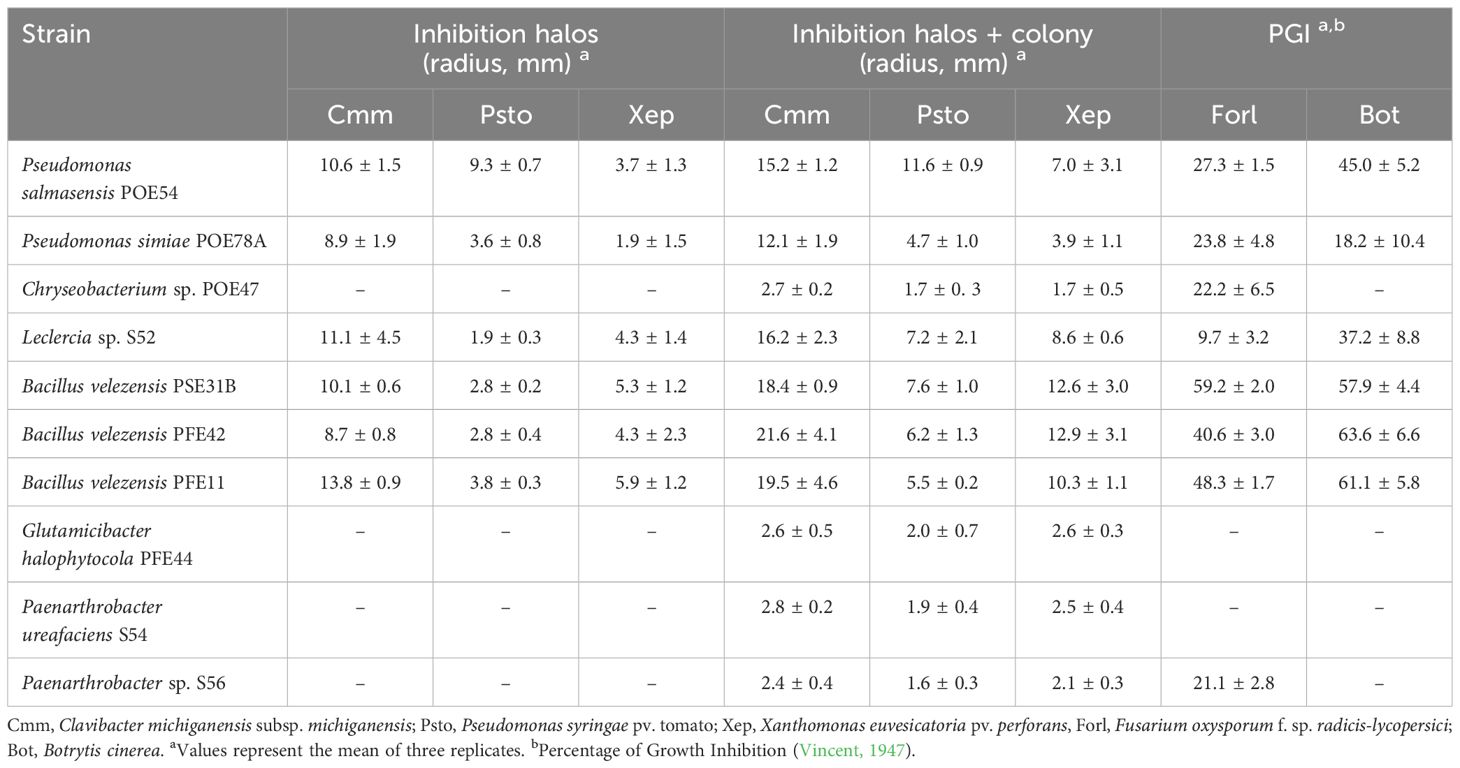
Table 4. In vitro antimicrobial activity of the selected bacterial strains against bacterial and fungal tomato pathogens.
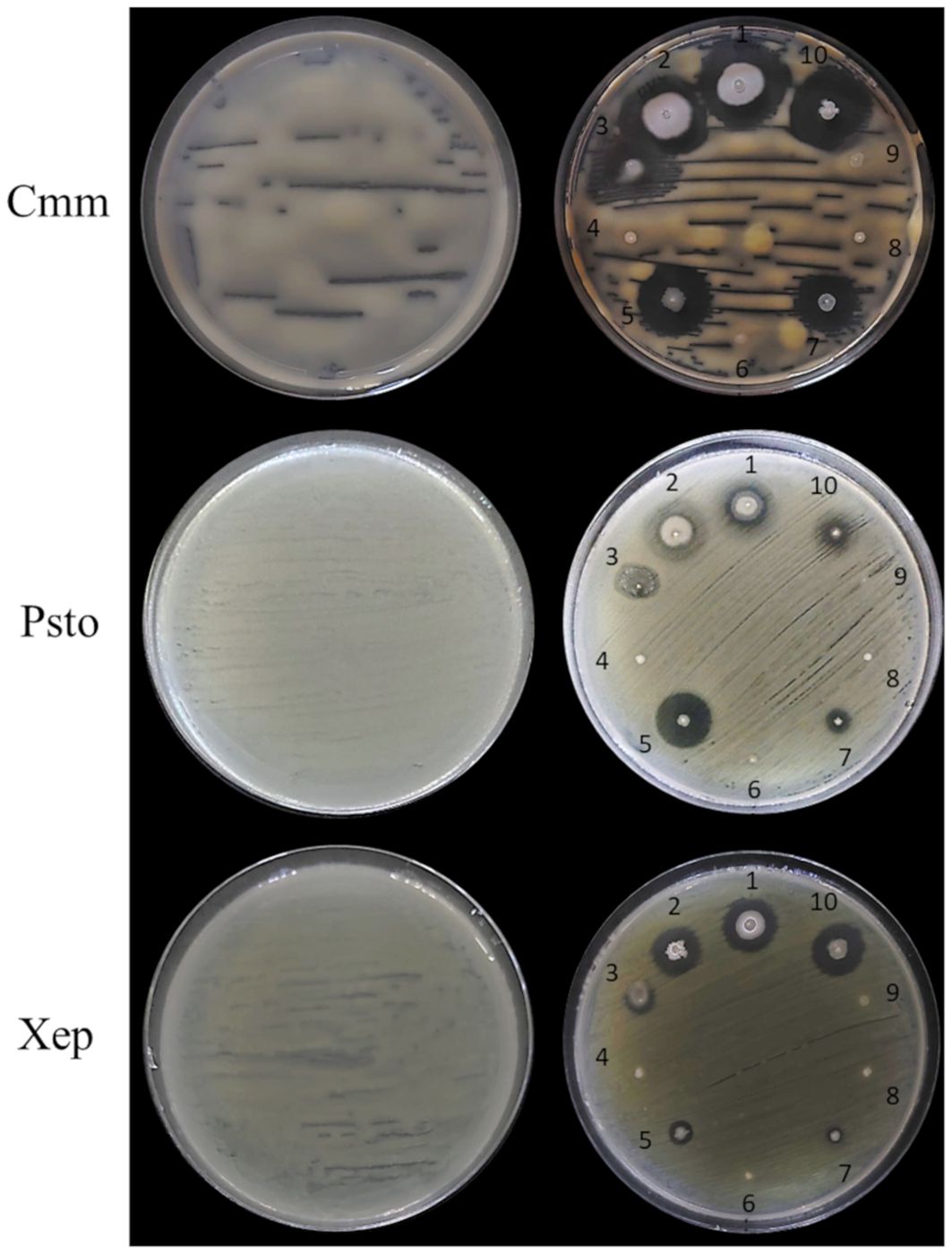
Figure 6. In vitro antimicrobial activity of the endophytic bacterial strains against tomato bacterial pathogens. Endophytic bacterial strains: 1, Bacillus velezensis PSE31B; 2, B. velezensis PFE42; 3, Leclercia sp. S52; 4, Paenarthrobacter ureafaciens S54; 5, Pseudomonas salmasensis POE54; 6, Chryseobacterium sp. POE47; 7, Pseudomonas simiae POE78A; 8, Paenarthrobacter sp. S56; 9, Glutamicibacter halophytocola PFE44; 10, B. velezensis PFE11. Bacterial pathogens: Cmm, Clavibacter michiganensis subsp. michiganensis strain PVCT 156.1.1; Psto, Pseudomonas syringae pv. tomato strain PVCT 28.3.1; Xep, Xanthomonas euvesicatoria pv. perforans strain NCPPB 4321.
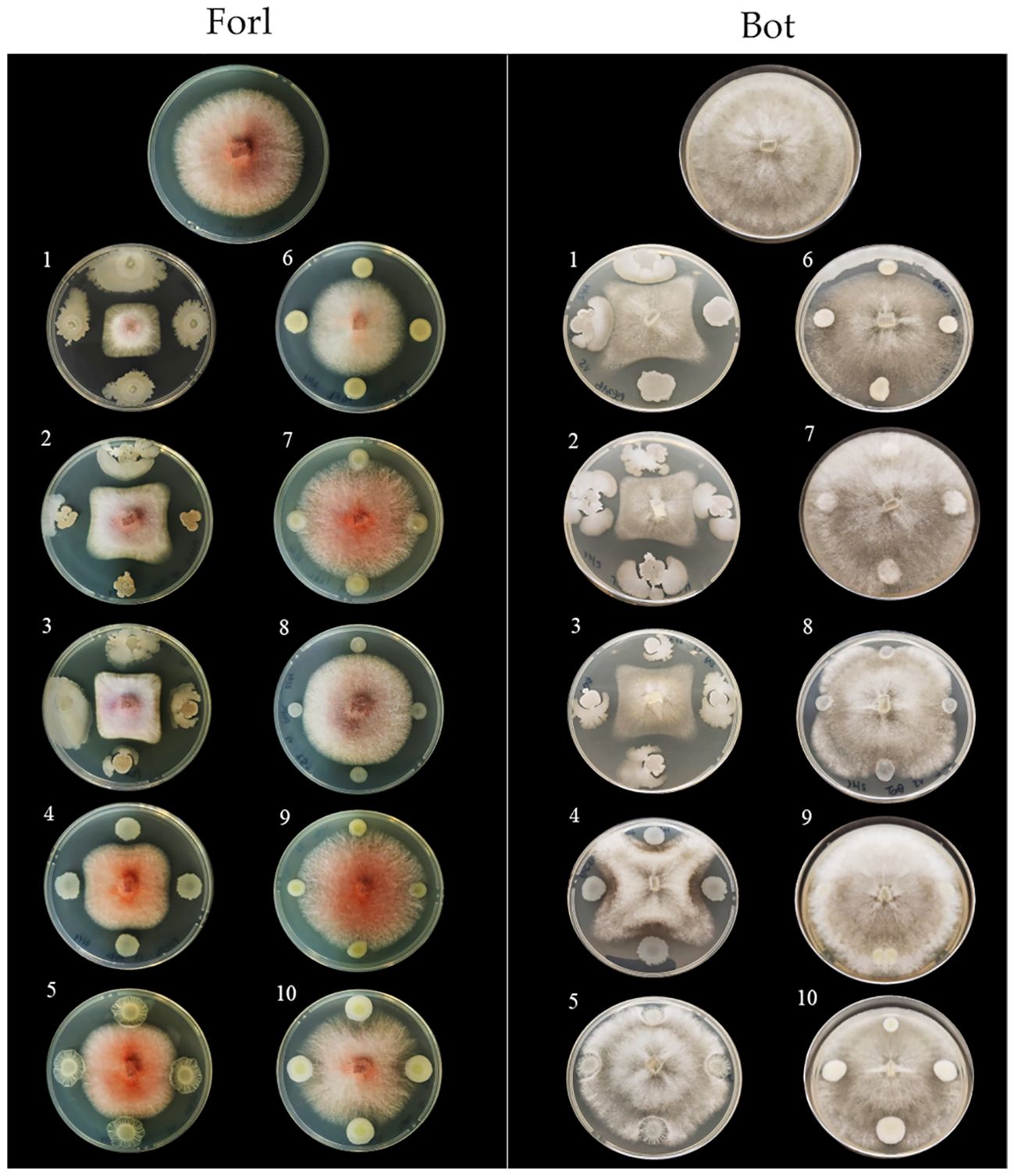
Figure 7. In vitro antimicrobial activity of the endophytic bacterial strains against tomato fungal pathogens. Endophytic bacterial strains: 1, Bacillus velezensis PSE31B; 2, B. velezensis PFE42; 3, B. velezensis PFE11; 4, Pseudomonas salmasensis POE54; 5, P. simiae POE78A; 6, Chryseobacterium sp. POE47; 7, Glutamicicbacter halophytocola PFE44; 8, Leclercia sp. S52; 9, Paenarthrobacter ureafaciens S54; 10, Paenarthrobacter sp. S56. Fungal pathogens: Forl, Fusarium oxysporum f. sp. radicis-lycopersici strain PVCT 127; Bot, Botrytis cinerea strain Bc5.
Figures 6 and 7 show the results of the antimicrobial activity assays against bacterial and fungal tomato pathogens. It is particularly notable that the Bacillus colonies expanded in the medium, especially when challenged with the fungal pathogens (Figure 7) and the Gram-positive bacterium Cmm strain PVCT 156.1.1 (Figure 6). Based on the radius of the inhibition halo, i.e. the region where microbial growth is absent, P. salmasensis strain POE54 showed the greatest inhibitory activity against Psto strain PVCT 28.3.1 (Table 4; Figure 6).
3.8 In planta biocontrol of bacterial and fungal diseases
Tomato plants treated with each of the ten selected endophytic bacterial strains were challenged with the fungal pathogen F. oxysporum f.sp. radicis lycopersici strain PVCT 127 by inoculation in the soil near the plant at the plant base (i.e. the crown) or with the bacterium X. euvesicatoria pv. perforans strain NCPPB 4321 by spray inoculation on the epigeal plant portion.
Forty-five days after inoculation, fusarium crown and root rot symptoms were evaluated in longitudinal sections of tomato plants running through the stem to the taproot. All control plants showed dark brown discoloration of the vascular tissues at the stem base extending about 3 cm above soil level and abundant production of adventitious roots (Table 5; Figure 8A). With the exception of the P. ureafaciens S54 treatment, all bacterial treatments reduced the percentage of infected plants and significantly reduced disease severity based on the length of discoloration at the crown base (p<0.001) (Table 5). The highest biocontrol efficacy (93.81%) was achieved by using B. velezensis PSE31B; only 33% of the plants treated with this strain had vascular discolorations and where discoloration was observed it extended for less than 0.2 cm (meaning that browning was observed only around the inoculation wounds) (Table 5; Figure 8B). Notably, even bacterial strains that showed no antagonistic activity in vitro, namely G. halophytocola PFE44 and P. ureafaciens S54, exerted significant biocontrol over fusarium crown and root rot.
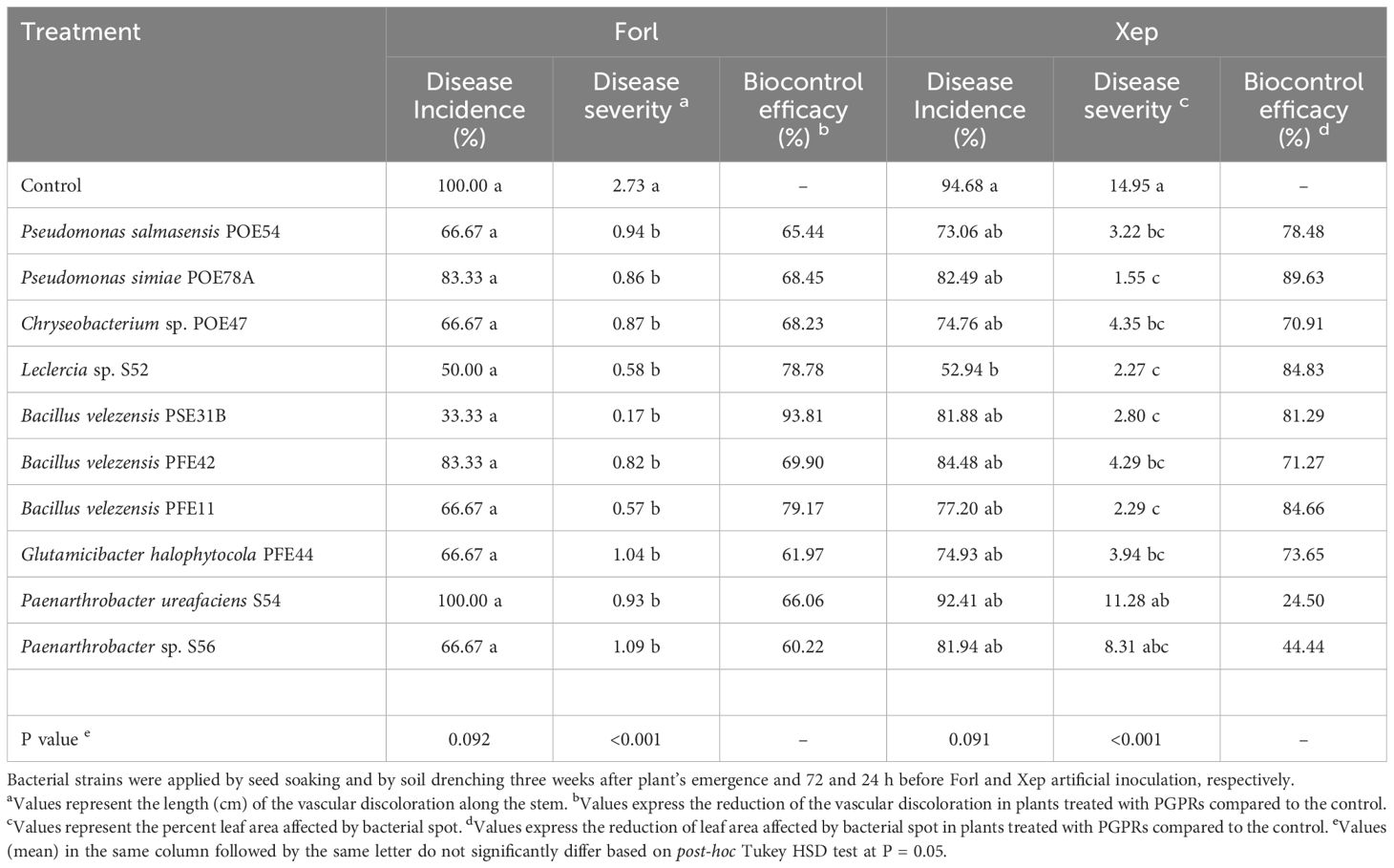
Table 5. Biocontrol efficacy of the endophytic bacterial strains against Fusarium oxysporum f. sp. radicis-lycopersici PVCT127 (Forl) and Xanthomonas euvesicatoria pv. perforans NCPPB4321 (Xep) in tomato plants in growth chamber.
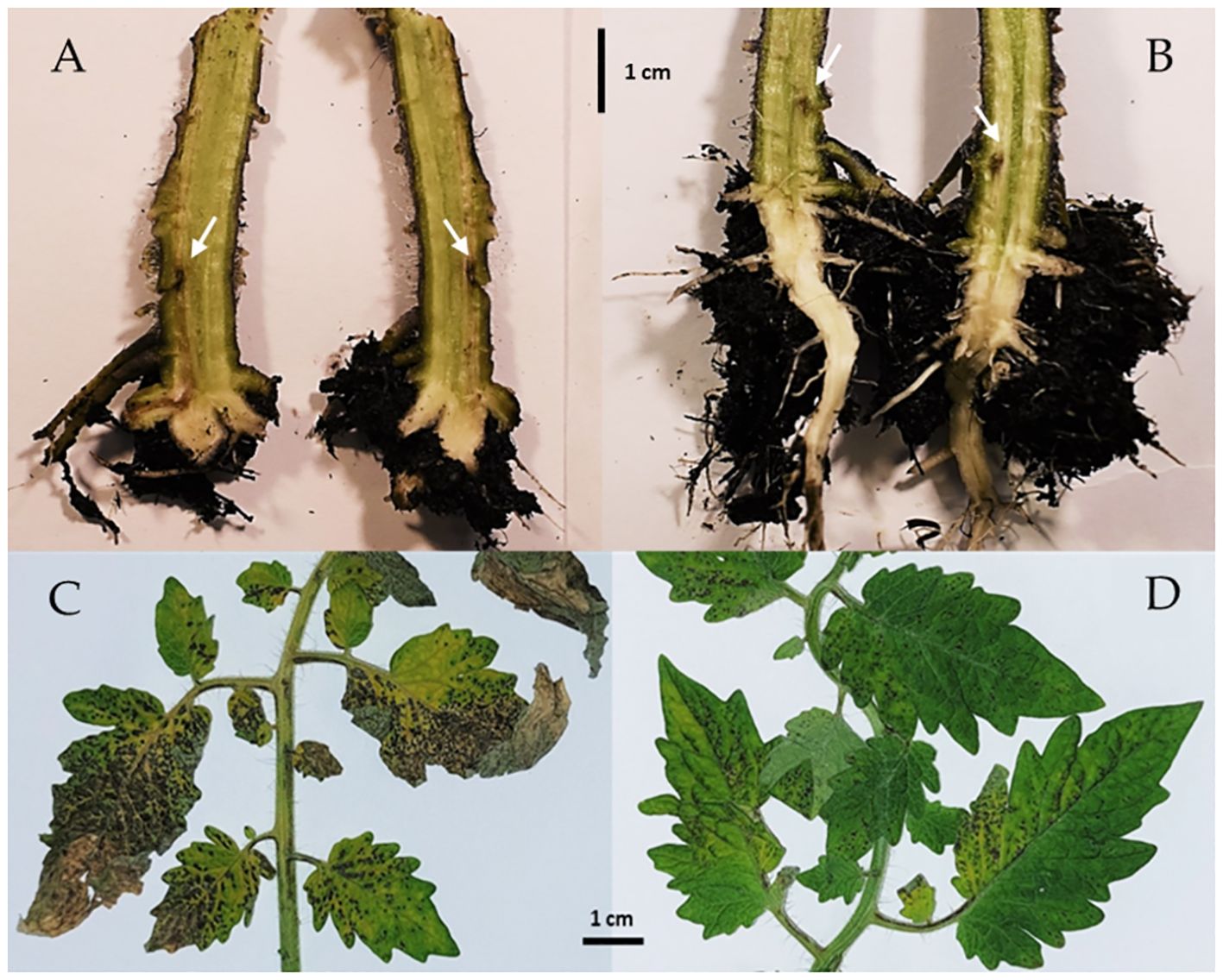
Figure 8. Symptoms of vascular discoloration in tomato plants artificially inoculated with Fusarium oxysporum f. sp. radicis-lycopersici PVCT127 (A) and in Bacillus velezensis PSE31B treated plants (B). Arrows highlight the artificial wound to assist pathogen penetration. Bacterial spot symptoms in tomato plants artificially inoculated with Xanthomonas euvesicatoria pv. perforans strain NCPPB 4321 (C) and in Pseudomonas simiae POE78A treated plants (D). In both trials bacterial endophytes were applied by seed soaking and by soil drenching three weeks after plant’s emergence and 72 and 24 h before pathogens inoculation (Fusarium and Xanthomonas, respectively).
Symptoms of bacterial spot observed six days after bacterial inoculation included both pinpoint necrotic spots and/or larger irregular spots that in some cases converged into larger lesions surrounded by chlorotic areas (Figure 8C). Disease incidence (as measured by the percentage of symptomatic leaflets) was not affected by treatment with any endophytic strain except for Leclercia sp. S52 (Table 5). However, disease severity (i.e., the percentage of the leaf area containing bacterial spots) was reduced by every bacterial treatment other than those using P. ureafaciens strain S54 or Paenarthrobacter sp. strain S56 (Table 5). The highest biocontrol efficacy (89.63%) was obtained using P. simiae POE78A (Table 5; Figure 8D).
3.9 Growth promotion in tomato nursery plantlets
All of the endophytic bacterial treatments other than those using Leclercia sp. strain S52 resulted in growth-promoting activity, although the effects were not always statistically significant. In particular, treatment with B. velezensis strains PSE31B, PFE42 and PFE11, P. salmasensis POE54 and Paenarthrobacter sp. S56 significantly enhanced the height of nursery plantlets at all monitoring time points (p<0.0001) (Figure 9). These bacterial strains also positively influenced the fresh and dry biomasses of roots and shoots (Table 6). The shoot fresh weight was significantly higher in plants treated with B. velezensis strains, P. salmasensis POE54, and Paenarthrobacter sp. S56 (p<0.001), although the treatments did not significantly increase the shoot dry weight in all cases (Table 6). Plants treated with the strains B. velezensis PSE31B and PFE11 and Paenarthrobacter sp. S56 also had significantly increased fresh and/or dry root weights (p=0.001) (Table 6).
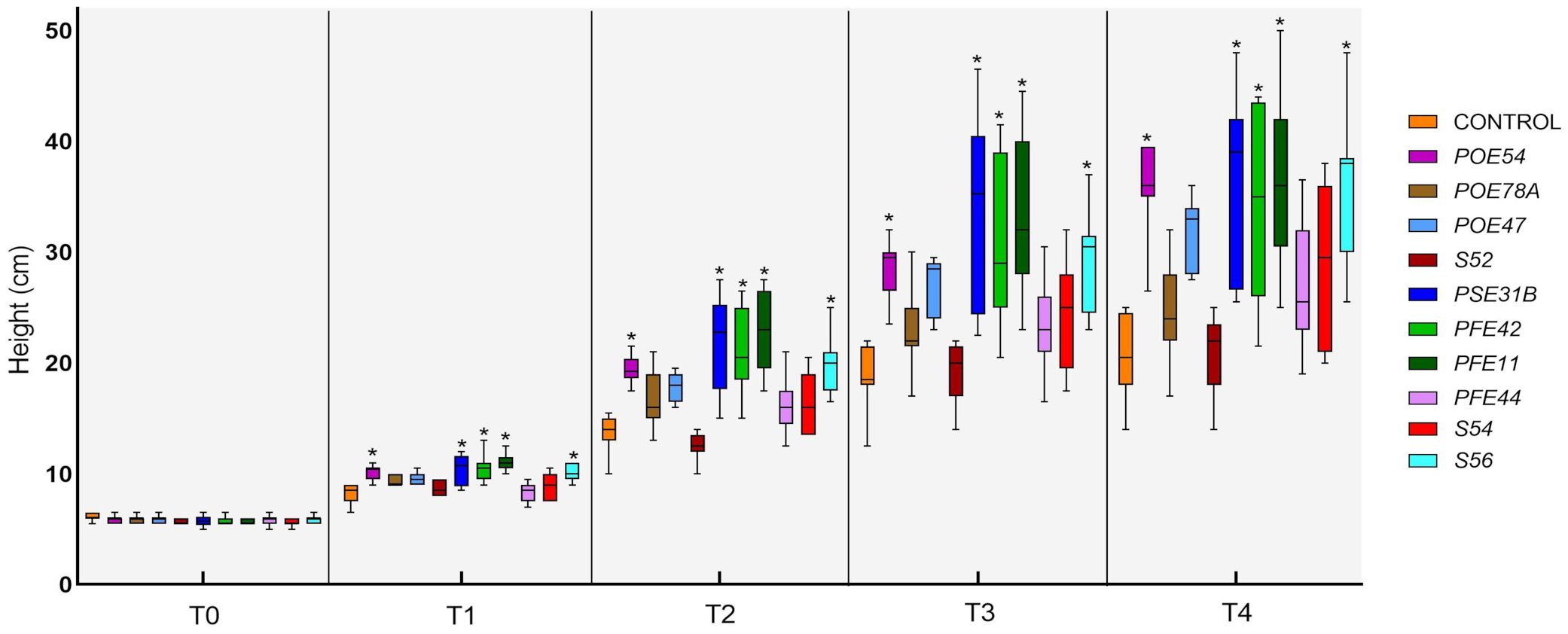
Figure 9. Time-course evaluation of tomato plant height in the PGP trial in growth chamber: T0 (before the treatments), T1-4 (1-4 weeks after the treatments). Plants were treated by soil drenching immediately after transplant. Asterisks denote statistical significance compared to the not treated plants (Control) based on post-hoc Tukey HSD test at P = 0.05. POE54, Pseudomonas salmasensis; POE78A, P. simiae; POE47, Chryseobacterium sp.; S52, Leclercia sp.; PSE31B, Bacillus velezensis; PFE42, B. velezensis; PFE11, B. velezensis; PFE44, Glutamicibacter halophytocola; S54, Paenarthrobacter ureafaciens; S56, Paenarthrobacter sp.
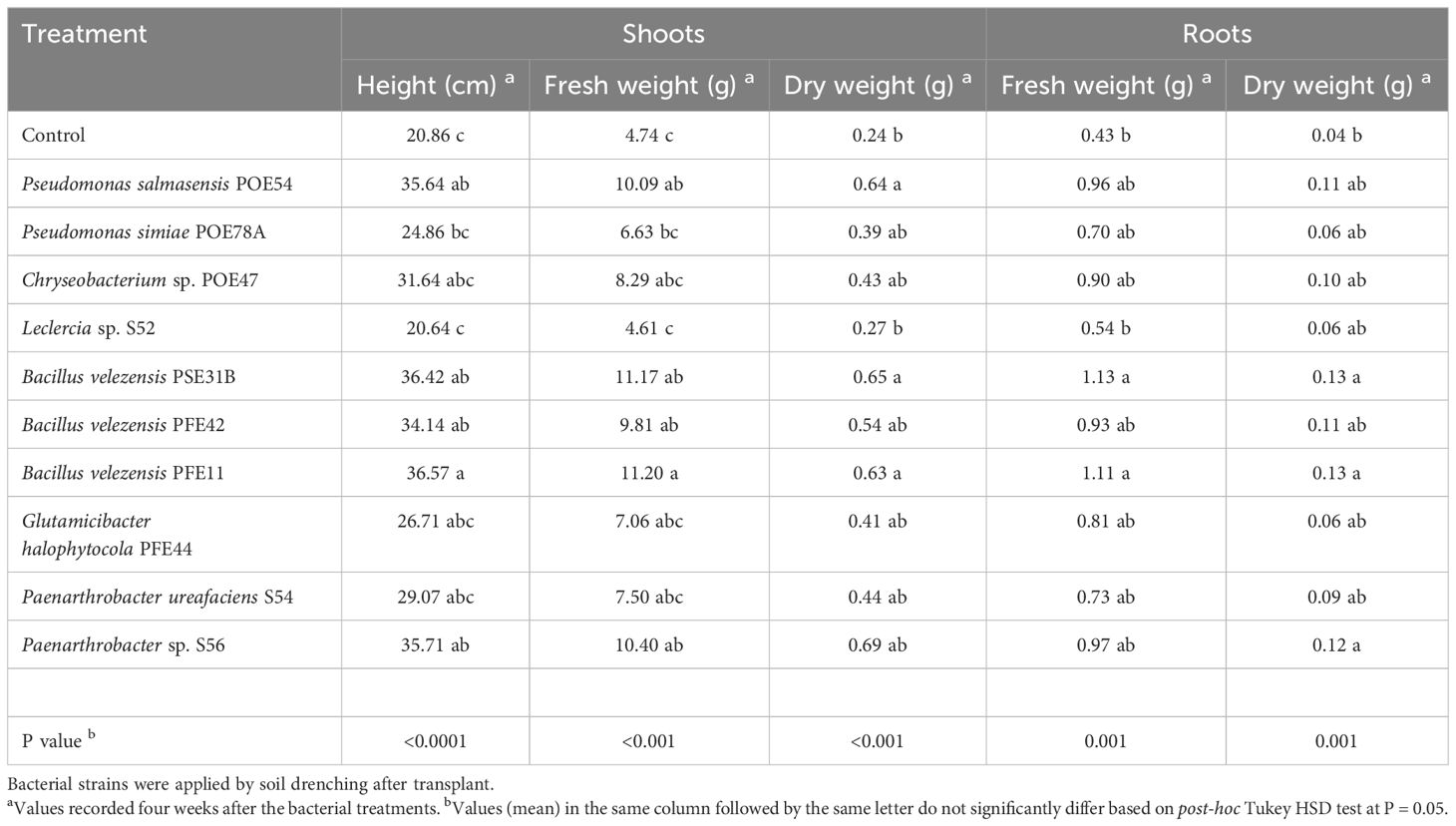
Table 6. Plant growth promotion efficacy of the endophytic bacterial strains in tomato plants in growth chamber.
4 Discussion
To identify potential bacterial bioinoculants, a microbiome-guided top-down approach was used to select ten bacterial strains belonging to different taxa from the core microbiome of tomato plants at different stages in the production chain. Bacterial endophytes isolated from tomato seeds and roots were thus not selected on the basis of in vitro analyses of phenotypic characteristics associated with PGP activity or antagonism against phytopathogenic microorganisms. This approach resulted in the selection of taxa from the comparatively understudied genera Leclercia, Chryseobacterium, Glutamicibacter and Paenarthorbacter alongside taxa more commonly used as biofertilizers and biocontrol agents such as Pseudomonas and Bacillus species. Complete genome sequencing of the strains led to the revision of identifications based on 16S rRNA gene sequences in some cases and made it possible to dissect the strains’ genetic makeup, focusing particularly on phyto-beneficial traits. The resulting information provides valuable insights into the potential biotechnological applications of the strains as efficient bioinoculants for tomato growth and protection in different stages of production.
Microbiome-guided approaches for selecting bacteria beneficial to tomato growth have been used in several studies, only a few of which have focused on the core microbiome (Tian et al., 2017; Bergna et al., 2018). The plant core microbiome is defined as any set of microbial taxa, along with their genomic and functional attributes, that is distinctive to a specific host or environment (Lundberg et al., 2012; Neu et al., 2021). Core microbes create cores of interactions that can be used to optimize microbial functions at the individual plant and ecosystem levels (Toju et al., 2018). Generally, the association between the bacteria in the core microbiome and the potential PGPRs is preceded by a preliminary in vitro screening to select strains with the greatest potential as bioinoculants (Tian et al., 2017; Bergna et al., 2018). However, such bottom-up approaches may fail to detect bacteria that do not express the desired characteristics in vitro but are nevertheless important representatives of the core microbiome in nature (Compant et al., 2019).
In this study, the core bacterial genera of the tomato microbiome were considered on the whole to support the selection of strains which application could be foreseen irrespective of the application modality (e.g. seed dressing, soil drenching) and the phenological stage. This is supported by the key concepts of vertical and horizontal transfer of the microbiota, and also apply to the core microbiota (Zeng et al., 2023). Indeed, endophytes can be transferred both vertically from the seeds of the previous generation’s plants or acquired horizontally by the surrounding environment (i.e. the rhizosphere) (Kong et al., 2019; Li et al., 2019). Anzalone et al. (2022) had previously studied the composition and shaping of the microbial community in the rhizosphere in tomato plants cultivated with and without soil from nursery growth to the greenhouse, revealing that these bacterial communities were mainly shaped by the substrate or soil in which the plants were grown and differed significantly between plant compartments (rhizosphere and endorhizosphere) and plant growth stages. Further selection allowed to set up a collection of 94 bacterial endophytes. As ubiquitous colonizers of plants, endophytes are known to strongly influence plant health and productivity; they can have a variety of desirable effects: plant growth promotion, biotic and abiotic stress resilience and resistance (Compant et al., 2005; Bulgarelli et al., 2013; Hardoim et al., 2015; Santoyo et al., 2016)
A high proportion of the collected strains showed tolerance to salt stress in vitro. This relatively high abundance of salt-tolerant bacterial strains suggests that the typically high salinity of the water and soil in the sampling area enriched the fraction of root endophytes with such tolerance, as previously demonstrated by Flemer et al. (2022). Other beneficial features observed in the collection included siderophore production and solubilization of insoluble organic phosphate; in addition, a few strains were able to produce hydrogen cyanide and ACC deaminase. Moreover, around 30% of the strains showed broad antagonistic activity against five tomato pathogens. Partial 16S rRNA gene sequencing made it possible to provisionally assign most of the strains of the collection to Bacillus and Pseudomonas spp. These genera are the most highly represented among cultivable endophytic bacteria isolated from different plant species including tomato (Tian et al., 2017; Bergna et al., 2018; Anzalone et al., 2021; Cochard et al., 2022) and from different plant compartments, as reviewed by Riva et al. (2022). Bacteria belonging to the Gram-positive genera Arthrobacter, Curtobacterium, Glutamicibacter, Microbacterium, Paenarthrobacter, Paenibacillus, Priestia and Staphylococcus and to the Gram-negative genera Chryseobacterium, Delftia, Enterobacter, Ewingella, Flavobacterium, Serratia and Stenotrophomonas were also part of our collection. Some of these genera have been isolated in other studies on beneficial bacteria (Abbamondi et al., 2016; Tian et al., 2017; Anzalone et al., 2021; Cochard et al., 2022).
Instead of limiting our search for potential beneficial properties to strains well-known for high antagonistic activity in vitro, i.e. Bacillus and Pseudomonas (Tian et al., 2017; Anzalone et al., 2021), we broadened our selection to include less frequently studied genera belonging to the core microbiome. After constructing high-quality genomes for ten strains from the core microbiome that had been assigned to diverse taxa based on preliminary 16S rRNA gene sequencing, we obtained more conclusive species- or genus-level identifications. Specifically, we discovered that the ten selected core microbiome strains belonged to the following taxa: B. velezensis (strains PSE31B, PFE42, PFE11), P. salmasensis POE54 and P. simiae POE78A. Strains Enterobacter sp. S52 and Arthrobacter sp. S54 were reassigned to Leclercia sp. and P. ureafaciens, respectively. The taxonomic affiliations of G. halophytocola PFE44, Paenarthrobacter sp. S56, and Chryseobacterium sp. POE47 were confirmed. The last wo strains could only be classified at the genus level by TYGS according to dDDH and ANI values below 70% and 95%, respectively, suggesting that they might represent new bacterial species (Goris et al., 2007; Auch et al., 2010; Meier-Kolthoff et al., 2013)
The ten bacterial strains from the tomato endosphere, although to a different extent, exhibited biocontrol and/or PGP properties, protecting tomato plants against biotic stress and stimulating the growth of tomato seedlings regardless of their activity in vitro. These results highlight the limits of in vitro screening methods, which often fail to capture the complex interactions that occur in the rhizosphere (Bulgarelli et al., 2013; Mendes et al., 2013). Nutrient availability, soil pH, microbial competition, and plant exudates all influence the efficacy of Plant Growth-Promoting Bacteria (PGPB). Therefore, in vitro assays may not accurately predict how bacteria will perform under field conditions (Berg and Smalla, 2009). Furthermore, our strains underwent testing in two distinct pathosystems featuring both belowground and aboveground pathogens. In the trials, the endophytic bacteria were applied to tomato plants by soil drenching and to seeds by seed coating. Importantly, given the diverse biocontrol mechanisms employed by bacteria, which include competing for resources, niche exclusion, and the production of antimicrobial compounds, it is possible that our bacteria may have the capability to induce systemic resistance in the tomato plants (Compant et al., 2005; Lugtenberg and Kamilova, 2009; Singh et al., 2011; Pieterse et al., 2014; Hardoim et al., 2015; Santoyo et al., 2016; Hanifah et al., 2023; Kelbessa et al., 2023).
The selected Bacillus and Pseudomonas strains, and Leclercia sp. strain S52, exhibited broad-spectrum antimicrobial activity against five tomato pathogens in vitro. The Bacillus endophytes produced the largest inhibition halos when tested against bacterial pathogens and imposed the most significant radial growth inhibition on phytopathogenic fungi. In accordance with this result, five different Bacillus species (B. subtilis, B. velezensis, B. amyloliquefaciens, B. pumilus, B. brevis and B. cereus) were previously shown to exhibit strong antagonism towards diverse phytopathogens (Dimkić et al., 2022; Etesami et al., 2023). This fact together with their high growth rates and tolerance of unfavourable environmental conditions has made them popular biocontrol agents (Fischer et al., 2013). The B. velezensis strains (and especially PSE31B) also demonstrated effective control over both tomato fusarium crown and root rot and bacterial spot in planta, reaffirming previous reports of this species’ significant biocontrol potential (Felipe et al., 2021; Chen et al., 2022). Notably, B. velezensis belongs to the B. subtilis group, which contains strains known for their ability to form beneficial associations with plant roots and exert beneficial effects including plant growth promotion and biocontrol of pathogens in several economically important crops, including tomato (Balderas-Ruíz et al., 2021; Felipe et al., 2021; Kashyap et al., 2021, 2022; Chen et al., 2022; Mosela et al., 2022). These strains also substantially promoted tomato plant growth in the growth chamber, leading to significantly increased shoot height and fresh and dry weight. These results are consistent with previous reports by Dhouib et al. (2019) and Balderas-Ruíz et al. (2021).
Our genomic findings for the Bacillus endophytes are consistent with previous results and align well with the characteristic traits of these species. Their antimicrobial activity may be partly due to their production of diverse bioactive secondary metabolites, which is driven by genes that are located within large genomic islands (BGCs) encoding mega-enzymes including non-ribosomal peptide synthetases and polyketide synthases (Ongena et al., 2007; Rabbee et al., 2019). These metabolites include surfactin and fengycin lipopeptides, which are known to exert antimicrobial activity against various fungal and bacterial pathogens, and to induce systemic resistance and promote biofilm formation (Ongena et al., 2007; Zhao et al., 2017; Penha et al., 2020). A BGC encoding enzymes producing the siderophore bacillibactin, which is highly conserved in the B. subtilis group, was also identified (Miethke et al., 2006). This siderophore enables efficient acquisition of Fe3+ and other metals in iron-deficient environments, depriving plant pathogens of essential elements (Niehus et al., 2017). Another significant group of BGCs encoded enzymes producing polyketides (e.g. difficidin, bacillaene, and macrolactin) that also play a role in antimicrobial activity (Caulier et al., 2019), and the cluster for the synthesis of bacilysin, a common broad-spectrum antimicrobial dipeptide (Nannan et al., 2021).
Both P. salmasensis POE54 and P. simiae POE78A displayed effective biocontrol of tomato diseases as well as plant growth promoting activity, with P. simiae POE78A exerting the most effective biocontrol activity against Xanthomonas euvesicatoria pv. perforans in this study. Both P. salmasensis and P. simiae were identified in a refined taxonomy of the larger P. fluorescens complex (Vela et al., 2006; Girard et al., 2021). Pseudomonas spp. have multiple traits that make them valuable biocontrol agents, including rapid in vitro growth, effective utilization of root exudates, and robust colonization and proliferation in the rhizosphere as well as the production of diverse bioactive metabolites including antibiotics, siderophores, volatiles, and extracellular enzymes, enhancing their competitive edge against other microorganisms (Haas and Défago, 2005; Mercado-Blanco and Bakker, 2007; Loper et al., 2012; Raaijmakers and Mazzola, 2012; Fischer et al., 2013; Höfte, 2021). Many root-associated pseudomonads possess BGCs for the production of antimicrobial compounds such as cyclic lipopeptide biosurfactants, 2,4-diacetylphloroglucinol, phenazines, hydrogen cyanide, and pyrrolnitrin (Loper et al., 2012). Pseudomonas strains have also been shown to effectively control Fusarium oxysporum f. sp. radicis-lycopersici and Xanthomonas euvesicatoria pv. perforans (Zhang et al., 2015; Anzalone et al., 2021; Felipe et al., 2021).
At least two widely studied P. fluorescens biocontrol strains have been reclassified as P. simiae, namely WCS417 (Berendsen et al., 2015; Pieterse et al., 2021) and PICF7 (Martínez-García et al., 2015). The phenotypic and genomic determinants of these strains were dissected and they were shown to exhibit biocontrol activity against fungal, bacterial pathogens and nematodes in a wide range of plant species, and to induce systemic resistance in the host and improve tolerance against abiotic stresses (Pieterse et al., 2021). The BGC for obafluorin, a broad-spectrum antibiotic commonly associated with P. fluorescens, was found in the genome of POE54 (Wells et al., 1984; Scott et al., 2017). In addition to BGCs responsible for siderophores, the genome of this strain contained many clusters that display no apparent similarity to known BGCs in the MIBiG database and thus warrant further investigation.
Leclercia sp. strain S52, which was isolated from tomato seeds, showed good in vitro antimicrobial activity, and also efficiently reduced the damage caused by Fusarium oxysporum f. sp. radicis-lycopersici and Xanthomonas euvesicatoria pv. perforans during in vivo trials but did not positively influence plant growth. Although new rhizosphere-associated Leclercia species and related genera have recently been described (Maddock et al., 2022), we were unable to assign our strain to any known species, suggesting that it may represent a new taxonomic entity. Despite ongoing concerns about the use of Enterobacteriaceae species as PGPRs given the existence of human pathogens within this taxon, several studies have investigated this topic (Berg et al., 2005; Erlacher et al., 2015). Many studies have confirmed that Enterobacteriaceae, including L. adecarboxylata, are indigenous components of the plant microbiome in different species (Kelemu et al., 2011; Erlacher et al., 2014, 2015; Verma et al., 2015; Shahzad et al., 2017; Tian et al., 2017; Anzalone et al., 2021). In particular, Anzalone et al. (2021) found that 40% of the endophytic bacteria isolated from the tomato endorhizosphere in four tomato farms within the area from which our samples were taken belonged to the Enterobacteriaceae family. L. adecarboxylata has previously been shown to promote plant growth (Sarma et al., 2004; Shahzad et al., 2017) and to mitigate both abiotic (Kang et al., 2019, 2021; Ahmed et al., 2021) and biotic (Lee et al., 2023) stresses. AntiSMASH analysis revealed that the genome of the selected Leclercia strain contained a BGC for the production of enterobactin, a well-known siderophore with an extraordinary iron affinity (Raymond et al., 2003).
Among the remaining selected strains, Chryseobacterium sp. POE47 and Paenarthrobacter sp. S56 exhibited limited in vitro antagonistic activity against the phytopathogenic fungus Fusarium oxysporum f. sp. radicis-lycopersici PVCT127 but had no detectable antagonistic effects against the tested phytopathogenic bacteria. Moreover, G. halophytocola PFE44 and P. ureafaciens S54 exhibited no in vitro antagonistic activity at all. In addition, Chryseobacterium sp. strain POE47 did not promote tomato plant growth under our experimental conditions. However, in contrast to its poor antimicrobial activity in vitro it did effectively control fusarium crown and root rot and bacterial spot in planta. In previous studies, Chryseobacterium species, formerly classified under the genus Flavobacterium, were found to enhance plant growth and exhibit biocontrol activity (Ramos Solano et al., 2008; Sang et al., 2018). Flavobacterium strains directly contribute to plant growth by promoting nutrient cycling and supplying beneficial plant hormones (Dardanelli et al., 2010; Sang et al., 2018). A recent study has also explored their halotolerance, suggesting that they might be promising PGPR in saline environments (Jung et al., 2023). Genome mining revealed that these species have a high content of EPS-coding genes, which are known to enhance plant growth and drought tolerance (Naseem et al., 2018). Beneficial traits related to stress relief, biocontrol, biofertilization, and phytohormone production were also identified, in line with the findings of Jung et al. (2023). Interestingly, our genomic analysis showed that this strain had fewer BGCs than any of the other selected strains, with none of them encoding genes producing antimicrobial compounds. This likely explains its poor in vitro antagonism.
The strains examined in this work included three bacterial strains belonging to the family Micrococcaceae, a taxon that was reclassified in 2016 (Busse, 2016). This caused its genera to be renamed to Glutamicibacter, Paeniglutamicibacter, Pseudoglutamicibacter, Paenarthrobacter, and Pseudarthrobacter (Busse, 2016). While both Glutamicibacter PFE44 and the two Paenarthrobacter strains (S54 and S56) effectively reduced the severity of fusarium crown and root rot symptoms in planta, only the Glutamicibacter endophyte significantly reduced bacterial spot severity. Both Paenarthrobacter and Glutamicibacter species have recently emerged as promising PGPR – for example, strains of P. nitroguajacolicus mitigated water scarcity in tomato and increased the vigor index values of tomato seedlings (Xiong et al., 2019; Christakis et al., 2021; Fu et al., 2021; Riva et al., 2021; Vasseur-Coronado et al., 2021). Genomic analysis of the Micrococcaceae strains revealed a predominance of genes encoding toxins, exopolysaccharides (EPS), phytohormones, and detoxification products. Moreover, AntiSMASH analysis revealed a BGC encoding desferrioxamine E, a siderophore widely produced by Streptomyces and related bacteria (Horinouchi et al., 2010). Desferrioxamine E plays a crucial role in ferric transportation and indirectly acts against fungi (Horinouchi et al., 2010). Additionally, EPS produced by G. halophytocola have been shown to mitigate abiotic stresses and promote plant growth (Xiong et al., 2019; Chen et al., 2023).
COG analysis highlighted four main functional gene classes (i.e. Amino acid transport and metabolism, Carbohydrate transport and metabolism, Cell wall/membrane/envelope biogenesis, and Transcription) common in all strains. These classes are also found among the most prevalent ones in other genomes of beneficial bacteria and endophytes (Taghavi et al., 2010; Martínez-García et al., 2015; Slama et al., 2019) and are mainly related to the basal metabolic activity of bacteria. The overall PGP potential of the ten bacterial strains was evaluated in silico by using the PGPT-Pred function of PLa-BAse (Patz et al., 2021) to analyze the prevalence of genes predicting plant growth promoting traits. This tool helped to unveil functional processes responsible for a strain’s PGP activity by revealing groups of genes with direct and indirect beneficial effects on plants. A comprehensive inventory of genes related to colonization, biofertilization, phytohormones, and plant signaling, among other processes was thus obtained for each strain. As noted above, these genes included BGCs encoding antimicrobial substances, chemotaxis- and surface attachment-related genes pivotal for recruitment and colonization in the rhizosphere (Knights et al., 2021), and bacterial secretion systems that play essential roles in out-competing other rhizobacterial strains during root colonization in the host plant (Lugtenberg and Kamilova, 2009; Pieterse et al., 2014; Lucke et al., 2020). The identified genes with direct beneficial effects on plant growth included those influencing traits related to fertilization such as potassium and phosphate solubilization, nitrogen and iron acquisition, sulfur assimilation, and carbon dioxide fixation, all of which enhance nutrient availability (Vessey, 2003; Lugtenberg and Kamilova, 2009; Singh et al., 2011; Das et al., 2022). The strains also carried several functional genes putatively involved in abiotic stress alleviation by stimulating plant immune responses and managing biotic stress via fungicidal and bactericidal activities, indirect traits that foster plant health and growth (Compant et al., 2005; Kelbessa et al., 2023).
The plant interaction traits of the ten selected strains were annotated using PIFAR to support the development of plant bioinoculants that can rapidly establish in suitable environments and form beneficial interactions upon application to plants. This analysis clearly separated the strains showing in vitro antimicrobial activity from those without such activity. Pseudomonas and Bacillus strains, belonging to the first group, showed the highest percentages of toxin-related factors compared to the other strains. Both genera are known for producing linear or cyclic lipopeptides (Ongena et al., 2007; Oni et al., 2022) that can exert toxic effects and contribute to plant colonization, also in pathogenic strains (Oni et al., 2022). In turn, the strains within the Microccocaceae family, belonging to the second group, showed the highest content of hormone-related factors. It is well established that PGPRs can excrete hormone for root uptake or manipulate hormone balance in the plants to boost growth and stress response (Backer et al., 2018). Such differences between bacterial strains pave the way to a further exploitation of each strain for the development of bioinoculants with specific target activities, such as biofertilization, biostimulation, or biocontrol.
In conclusion, the studied Bacillus and Pseudomonas strains exhibited high efficacy both in promoting plant growth and in protecting against pathogens, justifying their predominance in the bioinoculant market (Backer et al., 2018). However, other strains belonging to less known and explored genera also demonstrated effective PGP and BCA activity. The ten bacterial endophytes examined in this work were selected based on the analysis of the core microbiome of tomato seeds and the rhizosphere and endorhizosphere of tomato plants at different stages within the growing chain. The strains were arbitrarily selected among the genera present in the core microbiota. Importantly, the collected samples represent a wide range of management practices, environmental conditions, nursery materials (seeds and substrate), and conditions after transplantation into either agricultural soil that had been used for tomato cultivation for several years or soilless crop cultivation media. The genomic data obtained in this work will in future be employed to plan the use of the selected strains individually or in consortia by coupling strains with different traits, effects, and mechanisms of action to obtain synergistic beneficial effects. These strains could potentially be applied as seed dressings or soil drenches at different stages of plant growth, although further research will be needed to develop reliable standardized treatment protocols with predictable effects under specific conditions.
Data availability statement
The datasets presented in this study can be found in online repositories. The names of the repository/repositories and accession number(s) can be found in the article/Supplementary Material.
Author contributions
DN: Conceptualization, Data curation, Formal analysis, Investigation, Methodology, Writing – original draft, Writing – review & editing. FG: Investigation, Methodology, Writing – review & editing, Conceptualization, Project administration, Supervision. SG: Data curation, Formal analysis, Writing – review & editing, Investigation, Methodology. AA: Investigation, Methodology, Writing – review & editing, Conceptualization. GD: Investigation, Writing – review & editing, Data curation, Formal analysis. AM: Data curation, Formal analysis, Writing – review & editing, Investigation. MM: Investigation, Writing – review & editing. RV: Conceptualization, Funding acquisition, Project administration, Resources, Supervision, Writing – review & editing, Methodology. VC: Conceptualization, Funding acquisition, Methodology, Project administration, Resources, Supervision, Writing – review & editing.
Funding
The author(s) declare that financial support was received for the research, authorship, and/or publication of this article. VC is supported by: PON “RICERCA E INNOVAZIONE” 2014–2020, Azione II—Obiettivo Specifico 1b.‐WATER4AGRIFOOD”, n. ARS01_00825, Cod. CUP: B64I20000160005 and by the European Union Next-Generation EU (Piano Nazionale di Ripresa e Resilienza (PNRR)—missione 4, componente 2, investmento 1.4—D.D. 1032 17/06/2022, CN00000022): CUP E63C22000960006, within the Agritech National Research Center. This manuscript reflects only the authors’ view and opinions, neither the European Union nor the European Commission can be considered responsible for them. RV is supported by FORMAS (2019-01316), Carl Tryggers Stiftelse för Vetenskaplig Forskning (CTS 20:464), The Swedish Research Council (2019–04270), NOVO Nordisk Foundation (0074727), SLU Centre for Biological Control and Partnerskap Alnarp. DN PhD grant was funded by the Italian Ministry of University and Research under project PON FSE-FESR R&I 2014–20, Asse IV “Istruzione e ricerca per il recupero” – Azione IV. 5 “Dottorati su tematiche Green”.
Acknowledgments
This article is based upon work from COST Action COST Action MiCropBiomes CA22158, supported by COST (European Cooperation in Science and Technology).
Conflict of interest
The authors declare that the research was conducted in the absence of any commercial or financial relationships that could be construed as a potential conflict of interest.
Publisher’s note
All claims expressed in this article are solely those of the authors and do not necessarily represent those of their affiliated organizations, or those of the publisher, the editors and the reviewers. Any product that may be evaluated in this article, or claim that may be made by its manufacturer, is not guaranteed or endorsed by the publisher.
Supplementary material
The Supplementary Material for this article can be found online at: https://www.frontiersin.org/articles/10.3389/fpls.2024.1437947/full#supplementary-material
References
Abbamondi, G. R., Tommonaro, G., Weyens, N., Thijs, S., Sillen, W., Gkorezis, P., et al. (2016). Plant growth-promoting effects of rhizospheric and endophytic bacteria associated with different tomato cultivars and new tomato hybrids. Chem. Biol. Technol. Agric. 3, 1–10. doi: 10.1186/s40538-015-0051-3
Ahmed, B., Shahid, M., Syed, A., Rajput, V. D., Elgorban, A. M., Minkina, T., et al. (2021). Drought tolerant Enterobacter sp./Leclercia adecarboxylata secretes indole-3-acetic acid and other biomolecules and enhances the biological attributes of Vigna radiata (l.) r. wilczek in water deficit conditions. Biol. (Basel) 10, 1149. doi: 10.3390/biology10111149
Anzalone, A., Di Guardo, M., Bella, P., Ghadamgahi, F., Dimaria, G., Zago, R., et al. (2021). Bioprospecting of beneficial bacteria traits associated with tomato root in greenhouse environment reveals that sampling sites impact more than the root compartment. Front. Plant Sci. 12. doi: 10.3389/fpls.2021.637582
Anzalone, A., Mosca, A., Dimaria, G., Nicotra, D., Tessitori, M., Privitera, G. F., et al. (2022). Soil and soilless tomato cultivation promote different microbial communities that provide new models for future crop interventions. Int. J. Mol. Sci. 23 (15), 882. doi: 10.3390/ijms23158820
Auch, A. F., von Jan, M., Klenk, H. P., Göker, M. (2010). Digital DNA-DNA hybridization for microbial species delineation by means of genome-to-genome sequence comparison. Stand. Genomic Sci. 2, 117–134. doi: 10.4056/sigs.531120
Ayaz, M., Li, C. H., Ali, Q., Zhao, W., Chi, Y. K., Shafiq, M., et al. (2023). Bacterial and fungal biocontrol agents for plant disease protection: journey from lab to field, current status, challenges, and global perspectives. Molecules 28 (18), 6735. doi: 10.3390/molecules28186735
Backer, R., Rokem, J. S., Ilangumaran, G., Lamont, J., Praslickova, D., Ricci, E., et al. (2018). Plant growth-promoting rhizobacteria: Context, mechanisms of action, and roadmap to commercialization of biostimulants for sustainable agriculture. Front. Plant Sci. 871. doi: 10.3389/fpls.2018.01473
Balderas-Ruíz, K. A., Gómez-Guerrero, C. I., Trujillo-Roldán, M. A., Valdez-Cruz, N. A., Aranda-Ocampo, S., Juárez, A. M., et al. (2021). Bacillus velezensis 83 increases productivity and quality of tomato (Solanum lycopersicum L.): Pre and postharvest assessment. Curr. Res. Microb. Sci. 2, 100076. doi: 10.1016/j.crmicr.2021.100076
Berendsen, R. L., van Verk, M. C., Stringlis, I. A., Zamioudis, C., Tommassen, J., Pieterse, C. M. J., et al. (2015). Unearthing the genomes of plant-beneficial Pseudomonas model strains WCS358, WCS374 and WCS417. BMC Genomics 16, 1–23. doi: 10.1186/s12864-015-1632-z
Berg, G., Eberl, L., Hartmann, A. (2005). The rhizosphere as a reservoir for opportunistic human pathogenic bacteria. Environ. Microbiol. 7 (11), 1673–1685. doi: 10.1111/j.1462-2920.2005.00891.x
Berg, G., Köberl, M., Rybakova, D., Müller, H., Grosch, R., Smalla, K. (2017). Plant microbial diversity is suggested as the key to future biocontrol and health trends. FEMS Microbiol. Ecol. 93, fix050. doi: 10.1093/femsec/fix050
Berg, G., Smalla, K. (2009). Plant species and soil type cooperatively shape the structure and function of microbial communities in the rhizosphere. FEMS Microbiol. Ecol. 68, 1–13. doi: 10.1111/j.1574-6941.2009.00654.x
Bergna, A., Cernava, T., Rändler, M., Grosch, R., Zachow, C., Berg, G. (2018). Tomato seeds preferably transmit plant beneficial endophytes. Phytobiomes J. 2, 183–193. doi: 10.1094/PBIOMES-06-18-0029-R
Blin, K., Shaw, S., Augustijn, H. E., Reitz, Z. L., Biermann, F., Alanjary, M., et al. (2023). AntiSMASH 7.0: New and improved predictions for detection, regulation, chemical structures and visualisation. Nucleic Acids Res. 51, W46–W50. doi: 10.1093/nar/gkad344
Bonenfant, Q., Noé, L., Touzet, H. (2022). Porechop_ABI: discovering unknown adapters in ONT sequencing reads for downstream trimming. bioRxiv. 3 (1), vbac085. doi: 10.1093/bioadv/vbac085
Bulgarelli, D., Rott, M., Schlaeppi, K., Ver Loren van Themaat, E., Ahmadinejad, N., Assenza, F., et al. (2012). Revealing structure and assembly cues for Arabidopsis root-inhabiting bacterial microbiota. Nature 488, 91–95. doi: 10.1038/nature11336
Bulgarelli, D., Schlaeppi, K., Spaepen, S., Van Themaat, E. V. L., Schulze-Lefert, P. (2013). Structure and functions of the bacterial microbiota of plants. Annu. Rev. Plant Biol. 64, 807–838. doi: 10.1146/annurev-arplant-050312-120106
Busse, H. J. (2016). Review of the taxonomy of the genus Arthrobacter, emendation of the genus arthrobacter sensu lato, proposal to reclassify selected species of the genus Arthrobacter in the novel genera Glutamicibacter gen. Nov., Paeniglutamicibacter gen. nov., Pseudoglutamicibacter gen. nov., Paenarthrobacter gen. nov. and Pseudarthrobacter gen. nov., and emended description of Arthrobacter roseus. Int. J. Syst. Evol. Microbiol. 66, 9–37. doi: 10.1099/ijsem.0.000702
Carper, D. L., Weston, D. J., Barde, A., Timm, C. M., Lu, T.-Y., Burdick, L. H., et al. (2021). Cultivating the bacterial microbiota of populus roots. mSystems 6, 10–1128. doi: 10.1128/msystems.01306-20
Caulier, S., Nannan, C., Gillis, A., Licciardi, F., Bragard, C., Mahillon, J. (2019). Overview of the antimicrobial compounds produced by members of the Bacillus subtilis group. Front. Microbiol. 10. doi: 10.3389/fmicb.2019.00302
Chaumeil, P. A., Mussig, A. J., Hugenholtz, P., Parks, D. H. (2022). GTDB-Tk v2: memory friendly classification with the genome taxonomy database. Bioinformatics 38, 5315–5316. doi: 10.1093/bioinformatics/btac672
Chen, Q., Qiu, Y., Yuan, Y., Wang, K., Wang, H. (2022). Biocontrol activity and action mechanism of Bacillus velezensis strain SDTB038 against Fusarium crown and root rot of tomato. Front. Microbiol. 13. doi: 10.3389/fmicb.2022.994716
Chen, S. M., Zhang, C. M., Peng, H., Qin, Y. Y., Li, L., Li, C. G., et al. (2023). Exopolysaccharides from endophytic Glutamicibacter halophytocola KLBMP 5180 functions as bio-stimulants to improve tomato plants growth and salt stress tolerance. Int. J. Biol. Macromol 253, 126717. doi: 10.1016/j.ijbiomac.2023.126717
Chen, S., Zhou, Y., Chen, Y., Gu, J. (2018). Fastp: An ultra-fast all-in-one FASTQ preprocessor. Bioinformatics. 34, i884–i890. doi: 10.1093/bioinformatics/bty560
Christakis, C. A., Daskalogiannis, G., Chatzaki, A., Markakis, E. A., Mermigka, G., Sagia, A., et al. (2021). Endophytic bacterial isolates from halophytes demonstrate phytopathogen biocontrol and plant growth promotion under high salinity. Front. Microbiol. 12. doi: 10.3389/fmicb.2021.681567
Cochard, B., Giroud, B., Crovadore, J., Chablais, R., Arminjon, L., Lefort, F. (2022). Endophytic PGPR from tomato roots: isolation, in vitro characterization and in vivo evaluation of treated tomatoes (Solanum lycopersicum L.). Microorganisms 10, 765. doi: 10.3390/microorganisms10040765
Compant, S., Duffy, B., Nowak, J., Clément, C., Barka, E. A. (2005). Use of plant growth-promoting bacteria for biocontrol of plant diseases: Principles, mechanisms of action, and future prospects. Appl. Environ. Microbiol. 71, 4951–4959. doi: 10.1128/AEM.71.9.4951-4959.2005
Compant, S., Samad, A., Faist, H., Sessitsch, A. (2019). A review on the plant microbiome: Ecology, functions, and emerging trends in microbial application. J. Adv. Res. 19, 29–37. doi: 10.1016/j.jare.2019.03.004
Dardanelli, M. S., Manyani, H., González-Barroso, S., Rodríguez-Carvajal, M. A., Gil-Serrano, A. M., Espuny, M. R., et al. (2010). Effect of the presence of the plant growth promoting rhizobacterium (PGPR) Chryseobacterium balustinum Aur9 and salt stress in the pattern of flavonoids exuded by soybean roots. Plant Soil 328, 483–493. doi: 10.1007/s11104-009-0127-6
Das, P. P., Singh, K. R., Nagpure, G., Mansoori, A., Singh, R. P., Ghazi, I. A., et al. (2022). Plant-soil-microbes: A tripartite interaction for nutrient acquisition and better plant growth for sustainable agricultural practices. Environ. Res. 214, 113821. doi: 10.1016/j.envres.2022.113821
De Coster, W., Rademakers, R. (2023). NanoPack2: population-scale evaluation of long-read sequencing data. Bioinformatics 39 (5), btad311. doi: 10.1093/bioinformatics/btad311
Dhouib, H., Zouari, I., Ben Abdallah, D., Belbahri, L., Taktak, W., Triki, M. A., et al. (2019). Potential of a novel endophytic Bacillus velezensis in tomato growth promotion and protection against Verticillium wilt disease. Biol. Control 139, 104092. doi: 10.1016/j.biocontrol.2019.104092
Dimkić, I., Janakiev, T., Petrović, M., Degrassi, G., Fira, D. (2022). Plant-associated Bacillus and Pseudomonas antimicrobial activities in plant disease suppression via biological control mechanisms - A review. Physiol. Mol. Plant Pathol. 117, 101754. doi: 10.1016/j.pmpp.2021.101754
Du, J., Li, Y., Yin, Z., Wang, H., Zhang, X., Ding, X. (2020). High-throughput customization of plant microbiomes for sustainable agriculture. Front. Plant Sci. 11. doi: 10.3389/fpls.2020.569742
Edwards, U., Rogall, T., Blöcker, H., Emde, M., Böttger, E. C. (1989). Isolation and direct complete nucleotide determination of entire genes. Characterization of a gene coding for 16S ribosomal RNA. Nucleic Acids Res. 17, 7843–7853. doi: 10.1093/nar/17.19.7843
Erlacher, A., Cardinale, M., Grosch, R., Grube, M., Berg, G. (2014). The impact of the pathogen Rhizoctonia solani and its beneficial counterpart Bacillus amyloliquefaciens on the indigenous lettuce microbiome. Front. Microbiol. 5, 175. doi: 10.3389/fmicb.2014.00175
Erlacher, A., Cardinale, M., Grube, M., Berg, G. (2015). Biotic stress shifted structure and abundance of enterobacteriaceae in the lettuce microbiome. PloS One 10, e0118068. doi: 10.1371/journal.pone.0118068
Etesami, H., Jeong, B. R., Glick, B. R. (2023). Biocontrol of plant diseases by Bacillus spp. Physiol. Mol. Plant Pathol. 126, 102048. doi: 10.1016/j.pmpp.2023.102048
Fan, X. X., Xu, Z. G., Liu, X. Y., Tang, C. M., Wang, L. W., Han, X.-l. (2013). Effects of light intensity on the growth and leaf development of young tomato plants grown under a combination of red and blue light. Sci. Hortic. (Amsterdam) 153, 50–55. doi: 10.1016/j.scienta.2013.01.017
Felipe, V., Bianco, M. I., Terrestre, M., Mielnichuk, N., Romero, A. M., Yaryura, P. M. (2021). Biocontrol of tomato bacterial spot by novel Bacillus and Pseudomonas strains. Eur. J. Plant Pathol. 160, 935–948. doi: 10.1007/s10658-021-02297-6
Fischer, S., Príncipe, A., Alvarez, F., Cordero, P., Castro, M., Godino, A., et al. (2013). doi: 10.1007/978-3-642-39317-4_9
Flemer, B., Gulati, S., Bergna, A., Rändler, M., Cernava, T., Witzel, K., et al. (2022). Biotic and abiotic stress factors induce microbiome shifts and enrichment of distinct beneficial bacteria in tomato roots. Phytobiomes J. 6, 276–289. doi: 10.1094/PBIOMES-10-21-0067-R
Fu, R., Feng, H., Dini-Andreote, F., Wang, Z., Bo, C., Cao, W., et al. (2021). Modulation of the tomato rhizosphere microbiome via changes in root exudation mediated by the ethylene receptor nr. Microorganisms 9. doi: 10.3390/microorganisms9122456
Ghadamgahi, F., Tarighi, S., Taheri, P., Saripella, G. V., Anzalone, A., Kalyandurg, P. B., et al. (2022). Plant Growth-Promoting Activity of Pseudomonas aeruginosa FG106 and Its Ability to Act as a Biocontrol Agent against Potato, Tomato and Taro Pathogens. Biol. (Basel) 11, 140. doi: 10.3390/biology11010140
Girard, L., Lood, C., Höfte, M., Vandamme, P., Rokni-Zadeh, H., van Noort, V., et al. (2021). The ever-expanding pseudomonas genus: Description of 43 new species and partition of the pseudomonas putida group. Microorganisms 9, 1766. doi: 10.3390/microorganisms9081766
Goris, J., Konstantinidis, K. T., Klappenbach, J. A., Coenye, T., Vandamme, P., Tiedje, J. M. (2007). DNA-DNA hybridization values and their relationship to whole-genome sequence similarities. Int. J. Syst. Evol. Microbiol. 57, 81–91. doi: 10.1099/ijs.0.64483-0
Gurevich, A., Saveliev, V., Vyahhi, N., Tesler, G. (2013). QUAST: Quality assessment tool for genome assemblies. Bioinformatics 29, 1072–1075. doi: 10.1093/bioinformatics/btt086
Haas, D., Défago, G. (2005). Biological control of soil-borne pathogens by fluorescent pseudomonads. Nat. Rev. Microbiol. 3, 307–319. doi: 10.1038/nrmicro1129
Halebian, S., Harris, B., Finegold, S. M., Rolfe, R. D. (1981). Rapid method that aids in distinguishing gram-positive from gram-negative anaerobic bacteria. J. Clin. Microbiol. 13, 444–448. doi: 10.1128/jcm.13.3.444-448.1981
Hamonts, K., Trivedi, P., Garg, A., Janitz, C., Grinyer, J., Holford, P., et al. (2018). Field study reveals core plant microbiota and relative importance of their drivers. Environ. Microbiol. 20, 124–140. doi: 10.1111/1462-2920.14031
Hanifah, N. A. S. B., Ghadamgahi, F., Ghosh, S., Ortiz, R., Whisson, S. C., Vetukuri, R. R., et al. (2023). Comparative transcriptome profiling provides insights into the growth promotion activity of Pseudomonas fluorescens strain SLU99 in tomato and potato plants. Front. Plant Sci. 14. doi: 10.3389/fpls.2023.1141692
Hardoim, P. R., van Overbeek, L. S., Berg, G., Pirttilä, A. M., Compant, S., Campisano, A., et al. (2015). The hidden world within plants: ecological and evolutionary considerations for defining functioning of microbial endophytes. Microbiol. Mol. Biol. Rev. 79, 293–320. doi: 10.1128/mmbr.00050-14
Horinouchi, S., Ueda, K., Nakayama, J., Ikeda, T. (2010). “Cell-to-cell communications among microorganisms,” in Comprehensive Natural Products II: Chemistry and Biology. (Elsevier Ltd) 4, 283–337. doi: 10.1016/b978-008045382-8.00098-8
Jacquet, F., Jeuffroy, M. H., Jouan, J., Le Cadre, E., Litrico, I., Malausa, T., et al. (2022). Pesticide-free agriculture as a new paradigm for research. Agron. Sustain. Dev. 42 (1), 8. doi: 10.1007/s13593-021-00742-8
Jung, H., Lee, D., Lee, S., Kong, H. J., Park, J., Seo, Y. S. (2023). Comparative genomic analysis of Chryseobacterium species: deep insights into plant-growth-promoting and halotolerant capacities. Microb. Genomics 9, 001108. doi: 10.1099/mgen.0.001108
Kang, S. M., Shahzad, R., Bilal, S., Khan, A. L., Park, Y. G., Lee, K. E., et al. (2019). Indole-3-acetic-acid and ACC deaminase producing Leclercia adecarboxylata MO1 improves Solanum lycopersicum L. growth and salinity stress tolerance by endogenous secondary metabolites regulation. BMC Microbiol. 19, 1–14. doi: 10.1186/s12866-019-1450-6
Kang, S. M., Shahzad, R., Khan, M. A., Hasnain, Z., Lee, K. E., Park, H. S., et al. (2021). Ameliorative effect of indole-3-acetic acid- and siderophore-producing Leclercia adecarboxylata MO1 on cucumber plants under zinc stress. J. Plant Interact. 16, 30–41. doi: 10.1080/17429145.2020.1864039
Kashyap, A. S., Manzar, N., Nebapure, S. M., Rajawat, M. V. S., Deo, M. M., Singh, J. P., et al. (2022). Unraveling Microbial Volatile Elicitors Using a Transparent Methodology for Induction of Systemic Resistance and Regulation of Antioxidant Genes at Expression Levels in Chili against Bacterial Wilt Disease. Antioxidants 11, 404. doi: 10.3390/antiox11020404
Kashyap, A. S., Manzar, N., Rajawat, M. V. S., Kesharwani, A. K., Singh, R. P., Dubey, S. C., et al. (2021). Screening and biocontrol potential of rhizobacteria native to gangetic plains and hilly regions to induce systemic resistance and promote plant growth in chilli against bacterial wilt disease. Plants 10, 2125. doi: 10.3390/plants10102125
Kelbessa, B. G., Dubey, M., Catara, V., Ghadamgahi, F., Ortiz, R., Vetukuri, R. R. (2023). Potential of plant growth-promoting rhizobacteria to improve crop productivity and adaptation to a changing climate. CAB Rev. Perspect. Agric. Vet. Sci. Nutr. Nat. Resour 2023, 1–14. doi: 10.1079/cabireviews.2023.0001
Kelbessa, B. G., Ghadamgahi, F., Kumar, P. L., Ortiz, R., Whisson, S. C., Bhattacharjee, R., et al. (2022). Antagonistic and plant growth promotion of rhizobacteria against Phytophthora colocasiae in taro. Front. Plant Sci. 13, 1–17. doi: 10.3389/fpls.2022.1035549
Kelemu, S., Fory, P., Zuleta, C., Ricaurte, J., Rao, I., Lascano, C. (2011). Detecting Bacterial Endophytes in tropical Grasses of the Brachiaria genus and determining their role in improving plant growth. Afr. J. Biotechnol. 10, 965–976. doi: 10.5897/AJB
Knief, C. (2014). Analysis of plant microbe interactions in the era of next generation sequencing technologies. Front. Plant Sci. 5. doi: 10.3389/fpls.2014.00216
Knights, H. E., Jorrin, B., Haskett, T. L., Poole, P. S. (2021). Deciphering bacterial mechanisms of root colonization. Environ. Microbiol. Rep. 13 (4), 428–444. doi: 10.1111/1758-2229.12934
Kolmogorov, M., Yuan, J., Lin, Y., Pevzner, P. A. (2019). Assembly of long, error-prone reads using repeat graphs. Nat. Biotechnol. 37, 540–546. doi: 10.1038/s41587-019-0072-8
Kong, H. G., Song, G. C., Ryu, C. M. (2019). Inheritance of seed and rhizosphere microbial communities through plant–soil feedback and soil memory. Environ. Microbiol. Rep. 11, 479–486. doi: 10.1111/1758-2229.12760
Krstić Tomić, T., Atanasković, I., Nikolić, I., Joković, N., Stević, T., Stanković, S., et al. (2023). Culture-dependent and metabarcoding characterization of the sugar beet (Beta vulgaris L.) microbiome for high-yield isolation of bacteria with plant growth-promoting traits. Microorganisms 11, 1538. doi: 10.3390/microorganisms11061538
Kumar, M., Poonam, Ahmad, S., Singh, R. P. (2022). Plant growth promoting microbes: Diverse roles for sustainable and ecofriendly agriculture. Energy Nexus 7, 100133. doi: 10.1016/j.nexus.2022.100133
Kumar, S., Stecher, G., Li, M., Knyaz, C., Tamura, K. (2018). MEGA X: Molecular evolutionary genetics analysis across computing platforms. Mol. Biol. Evol. 35, 1547–1549. doi: 10.1093/molbev/msy096
Kwak, M.-J., Kong, H. G., Choi, K., Kwon, S.-K., Song, J. Y., Lee, J., et al. (2018). Rhizosphere microbiome structure alters to enable wilt resistance in tomato. Nat. Biotechnol. 36, 1100–1116. doi: 10.1038/nbt.4232
Lane, D. (1991). “16S/23S rRNA sequencing. In: Nucleic acid techniques in bacterial systematics,” in Nucleic Acid Technique in Bacterial Systematics, eds. Stackebrandt, E., Goodfellow, M. (New York, NY: John Wiley and Sons), 115–175.
Lee, S. H., Jeon, S. H., Park, J. Y., Kim, D. S., Kim, J. A., Jeong, H. Y., et al. (2023). Isolation and Evaluation of the Antagonistic Activity of Cnidium officinale Rhizosphere Bacteria against Phytopathogenic fungi (Fusarium solani). Microorganisms 11, 1555. doi: 10.3390/microorganisms11061555
Lemanceau, P., Blouin, M., Muller, D., Moënne-Loccoz, Y. (2017). Let the core microbiota be functional. Trends Plant Sci. 22, 583–595. doi: 10.1016/j.tplants.2017.04.008
Li, H., Parmar, S., Sharma, V. K., White, J. F. (2019). “Seed endophytes and their potential applications,” in Seed Endophytes: Biology and Biotechnology, eds Verma, S. K., White, J. J. F. (Cham: Springer International Publishing), 35–54. doi: 10.1007/978-3-030-10504-4_3
Loper, J. E., Hassan, K. A., Mavrodi, D. V., Davis, E. W., Lim, C. K., Shaffer, B. T., et al. (2012). Comparative genomics of plant-associated pseudomonas spp.: Insights into diversity and inheritance of traits involved in multitrophic interactions. PloS Genet. 8, e1002784. doi: 10.1371/journal.pgen.1002784
Lucke, M., Correa, M. G., Levy, A. (2020). The role of secretion systems, effectors, and secondary metabolites of beneficial rhizobacteria in interactions with plants and microbes. Front. Plant Sci. 11. doi: 10.3389/fpls.2020.589416
Lugtenberg, B., Kamilova, F. (2009). Plant-growth-promoting rhizobacteria. Annu. Rev. Microbiol. 63, 541–556. doi: 10.1146/annurev.micro.62.081307.162918
Lundberg, D. S., Lebeis, S. L., Paredes, S. H., Yourstone, S., Gehring, J., Malfatti, S., et al. (2012). Defining the core Arabidopsis thaliana root microbiome. Nature 488, 86–90. doi: 10.1038/nature11237
Maddock, D., Arnold, D., Denman, S., Brady, C. (2022). Description of a novel species of Leclercia, Leclercia tamurae sp. nov. and proposal of a novel genus Silvania gen. nov. containing two novel species Silvania hatchlandensis sp. nov. and Silvania confinis sp. nov. isolated from the rhizosphere of oak. BMC Microbiol. 22, 289. doi: 10.1186/s12866-022-02711-x
Manzo, D., Ferriello, F., Puopolo, G., Zoina, A., D’Esposito, D., Tardella, L., et al. (2016). Fusarium oxysporum f.sp. radicis-lycopersici induces distinct transcriptome reprogramming in resistant and susceptible isogenic tomato lines. BMC Plant Biol. 16, 1–14. doi: 10.1186/s12870-016-0740-5
Martínez-García, P. M., Ruano-Rosa, D., Schilirò, E., Prieto, P., Ramos, C., Rodríguez-Palenzuela, P., et al. (2015). Complete genome sequence of Pseudomonas fluorescens strain PICF7, an indigenous root endophyte from olive (Olea europaea L.) and effective biocontrol agent against Verticillium dahliae. Stand. Genomic Sci. 10, 1–7. doi: 10.1186/1944-3277-10-10
Meier-Kolthoff, J. P., Auch, A. F., Klenk, H. P., Göker, M. (2013). Genome sequence-based species delimitation with confidence intervals and improved distance functions. BMC Bioinf. 14, 1–14. doi: 10.1186/1471-2105-14-60
Meier-Kolthoff, J. P., Göker, M. (2019). TYGS is an automated high-throughput platform for state-of-the-art genome-based taxonomy. Nat. Commun. 10, 2181. doi: 10.1038/s41467-019-10210-3
Mendes, R., Garbeva, P., Raaijmakers, J. M. (2013). The rhizosphere microbiome: Significance of plant beneficial, plant pathogenic, and human pathogenic microorganisms. FEMS Microbiol. Rev. 37, 634–663. doi: 10.1111/1574-6976.12028
Mercado-Blanco, J., Bakker, P. A. H. M. (2007). Interactions between plants and beneficial Pseudomonas spp.: Exploiting bacterial traits for crop protection. Antonie van Leeuwenhoek 92, 367–389. doi: 10.1007/s10482-007-9167-1
Miethke, M., Klotz, O., Linne, U., May, J. J., Beckering, C. L., Marahiel, M. A. (2006). Ferri-bacillibactin uptake and hydrolysis in Bacillus subtilis. Mol. Microbiol. 61, 1413–1427. doi: 10.1111/j.1365-2958.2006.05321.x
Mosela, M., Andrade, G., Massucato, L. R., de Araújo Almeida, S. R., Nogueira, A. F., de Lima Filho, R. B., et al. (2022). Bacillus velezensis strain Ag75 as a new multifunctional agent for biocontrol, phosphate solubilization and growth promotion in maize and soybean crops. Sci. Rep. 12 (1), 15284. doi: 10.1038/s41598-022-19515-8
Nannan, C., Vu, H. Q., Gillis, A., Caulier, S., Nguyen, T. T. T., Mahillon, J. (2021). Bacilysin within the Bacillus subtilis group: gene prevalence versus antagonistic activity against Gram-negative foodborne pathogens. J. Biotechnol. 327, 28–35. doi: 10.1016/j.jbiotec.2020.12.017
Naseem, H., Ahsan, M., Shahid, M. A., Khan, N. (2018). Exopolysaccharides producing rhizobacteria and their role in plant growth and drought tolerance. J. Basic Microbiol. 58, 1009–1022. doi: 10.1002/jobm.201800309
Neu, A. T., Allen, E. E., Roy, K. (2021). Defining and quantifying the core microbiome: Challenges and prospects. Proc. Natl. Acad. Sci. U. S. A. 118 (51), e2104429118. doi: 10.1073/pnas.2104429118
Niehus, R., Picot, A., Oliveira, N. M., Mitri, S., Foster, K. R. (2017). The evolution of siderophore production as a competitive trait. Evol. (N. Y) 71, 1443–1455. doi: 10.1111/evo.13230
Ongena, M., Jourdan, E., Adam, A., Paquot, M., Brans, A., Joris, B., et al. (2007). Surfactin and fengycin lipopeptides of Bacillus subtilis as elicitors of induced systemic resistance in plants. Environ. Microbiol. 9, 1084–1090. doi: 10.1111/j.1462-2920.2006.01202.x
Oni, F. E., Esmaeel, Q., Onyeka, J. T., Adeleke, R., Jacquard, C., Clement, C., et al. (2022). Pseudomonas lipopeptide-mediated biocontrol: chemotaxonomy and biological activity. Molecules 27, 372. doi: 10.3390/molecules27020372
Osdaghi, E., Jones, J. B., Sharma, A., Goss, E. M., Abrahamian, P., Newberry, E. A., et al. (2021). A centenary for bacterial spot of tomato and pepper. Mol. Plant Pathol. 22, 1500. doi: 10.1111/mpp.13125
Parks, D. H., Imelfort, M., Skennerton, C. T., Hugenholtz, P., Tyson, G. W. (2015). CheckM: Assessing the quality of microbial genomes recovered from isolates, single cells, and metagenomes. Genome Res. 25, 1043–1055. doi: 10.1101/gr.186072.114
Patz, S., Gautam, A., Becker, M., Ruppel, S., Rodríguez-Palenzuela, P., Huson, D. (2021). PLaBAse: A comprehensive web resource for analyzing the plant growth-promoting potential of plant-associated bacteria. bioRxiv. doi: 10.1101/2021.12.13.472471
Penha, R. O., Vandenberghe, L. P. S., Faulds, C., Soccol, V. T., Soccol, C. R. (2020). Bacillus lipopeptides as powerful pest control agents for a more sustainable and healthy agriculture: recent studies and innovations. Planta 251, 1–15. doi: 10.1007/s00425-020-03357-7
Penrose, D. M., Glick, B. R. (2003). Methods for isolating and characterizing ACC deaminase-containing plant growth-promoting rhizobacteria. Physiol. Plant 118, 10–15. doi: 10.1034/j.1399-3054.2003.00086.x
Penyalver, R., Roesch, L. F. W., Piquer-Salcedo, J. E., Forner-Giner, M. A., Alguacil, M. D. M. (2022). From the bacterial citrus microbiome to the selection of potentially host-beneficial microbes. N. Biotechnol. 70, 116–128. doi: 10.1016/j.nbt.2022.06.002
Pieterse, C. M. J., Berendsen, R. L., de Jonge, R., Stringlis, I. A., Van Dijken, A. J. H., Van Pelt, J. A., et al. (2021). Pseudomonas simiae WCS417: star track of a model beneficial rhizobacterium. Plant Soil 461, 245–263. doi: 10.1007/s11104-020-04786-9
Pieterse, C. M. J., Zamioudis, C., Berendsen, R. L., Weller, D. M., Van Wees, S. C. M., Bakker, P. A. H. M. (2014). Induced systemic resistance by beneficial microbes. Annu. Rev. Phytopathol. 52, 347–375. doi: 10.1146/annurev-phyto-082712-102340
Raaijmakers, J. M., Mazzola, M. (2012). Diversity and natural functions of antibiotics produced by beneficial and plant pathogenic bacteria. Annu. Rev. Phytopathol. 50, 403–424. doi: 10.1146/annurev-phyto-081211-172908
Rabbee, M. F., Sarafat Ali, M., Choi, J., Hwang, B. S., Jeong, S. C., Baek, K. H. (2019). Bacillus velezensis: A valuable member of bioactive molecules within plant microbiomes. Molecules 24, 1046. doi: 10.3390/molecules24061046
Ramos Solano, B., Barriuso Maicas, J., Pereyra de la Iglesia, M. T., Domenech, J., Gutiérrez Mañero, F. J. (2008). Systemic disease protection elicited by plant growth promoting rhizobacteria strains: Relationship between metabolic responses, systemic disease protection, and biotic elicitors. Phytopathology 98, 451–457. doi: 10.1094/PHYTO-98-4-0451
Raymond, K. N., Dertz, E. A., Kim, S. S. (2003). Enterobactin: An archetype for microbial iron transport. Proc. Natl. Acad. Sci. U. S. A 100, 3584–3588. doi: 10.1073/pnas.0630018100
Risely, A. (2020). Applying the core microbiome to understand host–microbe systems. J. Anim. Ecol. 89, 1549–1558. doi: 10.1111/1365-2656.13229
Riva, V., Mapelli, F., Bagnasco, A., Mengoni, A., Borin, S. (2022). A meta-analysis approach to defining the culturable core of plant endophytic bacterial communities. Appl. Environ. Microbiol. 88, e02537-21. doi: 10.1128/aem.02537-21
Riva, V., Mapelli, F., Dragonetti, G., Elfahl, M., Vergani, L., Crepaldi, P., et al. (2021). Bacterial inoculants mitigating water scarcity in tomato: the importance of long-term in vivo experiments. Front. Microbiol. 12. doi: 10.3389/fmicb.2021.675552
Sang, M. K., Jeong, J. J., Kim, J., Kim, K. D. (2018). Growth promotion and root colonisation in pepper plants by phosphate-solubilising Chryseobacterium sp. strain ISE14 that suppresses Phytophthora blight. Ann. Appl. Biol. 172, 208–223. doi: 10.1111/aab.12413
Santoyo, G., Moreno-Hagelsieb, G., del Carmen Orozco-Mosqueda, M., Glick, B. R. (2016). Plant growth-promoting bacterial endophytes. Microbiol. Res. 183, 92–99. doi: 10.1016/j.micres.2015.11.008
Sarma, P. M., Bhattacharya, D., Krishnan, S., Lal, B. (2004). Degradation of polycyclic aromatic hydrocarbons by a newly discovered enteric bacterium, Leclercia adecarboxylata. Appl. Environ. Microbiol. 70, 3163–3166. doi: 10.1128/AEM.70.5.3163-3166.2004
Scott, T. A., Heine, D., Qin, Z., Wilkinson, B. (2017). An L-threonine transaldolase is required for L-threo-β-hydroxy-α-amino acid assembly during obafluorin biosynthesis. Nat. Commun. 8, 15935. doi: 10.1038/ncomms15935
Seemann, T. (2014). Prokka: Rapid prokaryotic genome annotation. Bioinformatics 30, 2068–2069. doi: 10.1093/bioinformatics/btu153
Shahzad, R., Waqas, M., Khan, A. L., Al-Hosni, K., Kang, S. M., Seo, C. W., et al. (2017). Indoleacetic acid production and plant growth promoting potential of bacterial endophytes isolated from rice (Oryza sativa L.) seeds. Acta Biol. Hung 68, 175–186. doi: 10.1556/018.68.2017.2.5
Shen, W., Le, S., Li, Y., Hu, F. (2016). SeqKit: A cross-platform and ultrafast toolkit for FASTA/Q file manipulation. PloS One 11, e0163962. doi: 10.1371/journal.pone.0163962
Shetty, S. A., Lahti, L. (2019). Microbiome data science. J. Biosci. 44, 1–6. doi: 10.1007/s12038-019-9930-2
Singh, J. S., Pandey, V. C., Singh, D. P. (2011). Efficient soil microorganisms: A new dimension for sustainable agriculture and environmental development. Agric. Ecosyst. Environ. 140, 339–353. doi: 10.1016/j.agee.2011.01.017
Slama, H. B., Cherif-Silini, H., Bouket, A. C., Qader, M., Silini, A., Yahiaoui, B., et al. (2019). Screening for Fusarium antagonistic bacteria from contrasting niches designated the endophyte Bacillus halotolerans as plant warden against fusarium. Front. Microbiol. 10. doi: 10.3389/fmicb.2018.03236
Strano, C. P., Bella, P., Licciardello, G., Caruso, A., Catara, V. (2017). Role of secondary metabolites in the biocontrol activity of Pseudomonas corrugata and Pseudomonas mediterranea. Eur. J. Plant Pathol. 149, 103–115. doi: 10.1007/s10658-017-1169-x
Taghavi, S., van der Lelie, D., Hoffman, A., Zhang, Y. B., Walla, M. D., Vangronsveld, J., et al. (2010). Genome sequence of the plant growth promoting endophytic bacterium Enterobacter sp. 638. PloS Genet. 6 (5), e1000943. doi: 10.1371/journal.pgen.1000943
Tian, B., Zhang, C., Ye, Y., Wen, J., Wu, Y., Wang, H., et al. (2017). Beneficial traits of bacterial endophytes belonging to the core communities of the tomato root microbiome. Agric. Ecosyst. Environ. 247, 149–156. doi: 10.1016/j.agee.2017.06.041
Toju, H., Peay, K. G., Yamamichi, M., Narisawa, K., Hiruma, K., Naito, K., et al. (2018). Core microbiomes for sustainable agroecosystems. Nat. Plants 4, 247–257. doi: 10.1038/s41477-018-0139-4
Vasseur-Coronado, M., du Boulois, H. D., Pertot, I., Puopolo, G. (2021). Selection of plant growth promoting rhizobacteria sharing suitable features to be commercially developed as biostimulant products. Microbiol. Res. 245, 126672. doi: 10.1016/j.micres.2020.126672
Vela, A. I., Gutiérrez, M. C., Falsen, E., Rollán, E., Simarro, I., García, P., et al. (2006). Pseudomonas simiae sp. nov., isolated from clinical specimens from monkeys (Callithrix geoffroyi). Int. J. Syst. Evol. Microbiol. 56, 2671–2676. doi: 10.1099/ijs.0.64378-0
Verma, P., Yadav, A. N., Khannam, K. S., Panjiar, N., Kumar, S., Saxena, A. K., et al. (2015). Assessment of genetic diversity and plant growth promoting attributes of psychrotolerant bacteria allied with wheat (Triticum aestivum) from the northern hills zone of India. Ann. Microbiol. 65, 1885–1899. doi: 10.1007/s13213-014-1027-4
Vessey, J. K. (2003). Plant growth promoting rhizobacteria as biofertilizers. Plant Soil 255, 571–586. doi: 10.1023/A:1026037216893
Vincent, J. M. (1947). Distortion of fungal hyphæ in the presence of certain inhibitors [20]. Nature 159, 850–850. doi: 10.1038/159850b0
Vitale, A., Rocco, M., Arena, S., Giuffrida, F., Cassaniti, C., Scaloni, A., et al. (2014). Tomato susceptibility to Fusarium crown and root rot: Effect of grafting combination and proteomic analysis of tolerance expression in the rootstock. Plant Physiol. Biochem. 83, 207–216. doi: 10.1016/j.plaphy.2014.08.006
Walker, B. J., Abeel, T., Shea, T., Priest, M., Abouelliel, A., Sakthikumar, S., et al. (2014). Pilon: An integrated tool for comprehensive microbial variant detection and genome assembly improvement. PloS One 9, e112963. doi: 10.1371/journal.pone.0112963
Wang, Z., Hu, X., Solanki, M. K., Pang, F. (2023). A synthetic microbial community of plant core microbiome can be a potential biocontrol tool. J. Agric. Food Chem. 71, 5030–5041. doi: 10.1021/acs.jafc.2c08017
Wells, J. S., Trejo, W. H., Principe, P. A., Sykes, R. B. (1984). Obafluorin, a novel β-lactone produced by Pseudomonas fluorescens. taxonomy, fermentation and biological properties. J. Antibiot. (Tokyo) 37, 802–803. doi: 10.7164/antibiotics.37.802
Xiong, Y. W., Gong, Y., Li, X. W., Chen, P., Ju, X. Y., Zhang, C. M., et al. (2019). Enhancement of growth and salt tolerance of tomato seedlings by a natural halotolerant actinobacterium Glutamicibacter halophytocola KLBMP 5180 isolated from a coastal halophyte. Plant Soil 445, 307–322. doi: 10.1007/s11104-019-04310-8
Yoon, S. H., Ha, S., Lim, J., Kwon, S., Chun, J. (2017). A large-scale evaluation of algorithms to calculate average nucleotide identity. Antonie van Leeuwenhoek 110, 1281–1286. doi: 10.1007/s10482-017-0844-4
Zeng, Q., Zhao, Y., Shen, W., Han, D., Yang, M. (2023). Seed-to-seed: plant core vertically transmitted microbiota. J. Agric. Food Chem. 71, 19255–19264. doi: 10.1021/acs.jafc.3c07092
Zhang, L., Khabbaz, S. E., Wang, A., Li, H., Abbasi, P. A. (2015). Detection and characterization of broad-spectrum antipathogen activity of novel rhizobacterial isolates and suppression of Fusarium crown and root rot disease of tomato. J. Appl. Microbiol. 118. doi: 10.1111/jam.12728
Zhao, H., Shao, D., Jiang, C., Shi, J., Li, Q., Huang, Q., et al. (2017). Biological activity of lipopeptides from Bacillus. Appl. Microbiol. Biotechnol. 101, 5951–5960. doi: 10.1007/s00253-017-8396-0
Zhu, Q., Gao, S., Xiao, B., He, Z., Hu, S. (2023). Plasmer: an accurate and sensitive bacterial plasmid prediction tool based on machine learning of shared k-mers and genomic features. Microbiol. Spectr. 11 (3), e04645-22. doi: 10.1128/spectrum.04645-22
Keywords: tomato, microbiome, endophytes, rhizosphere, PGPR, BCA, genomes
Citation: Nicotra D, Ghadamgahi F, Ghosh S, Anzalone A, Dimaria G, Mosca A, Massimino ME, Vetukuri RR and Catara V (2024) Genomic insights and biocontrol potential of ten bacterial strains from the tomato core microbiome. Front. Plant Sci. 15:1437947. doi: 10.3389/fpls.2024.1437947
Received: 24 May 2024; Accepted: 05 August 2024;
Published: 26 August 2024.
Edited by:
Gianfranco Romanazzi, Marche Polytechnic University, ItalyReviewed by:
Juan Imperial, Spanish National Research Council (CSIC), SpainAbhijeet Shankar Kashyap, National Bureau of Agriculturally Important Microorganisms (ICAR), India
Swarnalee Dutta, Jeonbuk National University, Republic of Korea
Copyright © 2024 Nicotra, Ghadamgahi, Ghosh, Anzalone, Dimaria, Mosca, Massimino, Vetukuri and Catara. This is an open-access article distributed under the terms of the Creative Commons Attribution License (CC BY). The use, distribution or reproduction in other forums is permitted, provided the original author(s) and the copyright owner(s) are credited and that the original publication in this journal is cited, in accordance with accepted academic practice. No use, distribution or reproduction is permitted which does not comply with these terms.
*Correspondence: Vittoria Catara, dmNhdGFyYUB1bmljdC5pdA==; Ramesh Raju Vetukuri, cmFtZXNoLnZldHVrdXJpQHNsdS5zZQ==