- 1State Key Laboratory of Biocatalysis and Enzyme Engineering, College of Life Sciences, Hubei University, Wuhan, China
- 2Tobacco Research Institute of Hubei Province, Wuhan, China
Fusarium is a soil-borne pathogen that poses a serious threat to the quality and yield of hundreds of crops worldwide, particularly tobacco production. Using metabolomics technology, we investigated natural metabolites from disease-conducting soil (DCS) and disease-suppressing soil (DSS) of tobacco rhizosphere as fungicides to control tobacco Fusarium wilt (TFW), which is mainly caused by Fusarium oxysporum. Furthermore, the antifungal mechanisms of these natural metabolites were preliminarily elucidated through various assessments, including antifungal activity determination, chemotaxis effect tests, PI staining experiments, and measurements of extracellular conductivity and protein content. Metabolomics results showed that the DCS with three different disease grades (G1, G5 and G9 groups) had significantly higher levels of 15, 14 and 233 differential rhizosphere metabolites (DRMs) and significantly lower levels of 72, 152 and 170 DRMs compared to the DSS (G0 group). According to KEGG pathway analysis, these DRMs were found to be enriched in the caffeine metabolism, biosynthesis of phenylpropanoids, galactose metabolism and tyrosine metabolism, etc. Linustatin, scopoletin and phenylpropiolic acid were picked out from these DRMs and found to have suppressive activity against F. oxysporum through correlation analysis and antifungal experiments. The three DRMs showed strong inhibitory effects on the growth and spore germination of F. oxysporum at concentrations of 0.5 mM or higher in each test period. Furthermore, F. oxysporum showed a phobotaxis effect against these three DRMs at concentrations as low as 0.25 mM. Finally, we found that the three DRMs had an inhibitory effect on F. oxysporum by destroying the integrity of the cell membrane and increasing the membrane permeability of F. oxysporum. This study firstly reports the inhibition activity of phenylpropiolic acid and linustatin on F. oxysporum, providing a practical and environmentally friendly method for biocontrol of TFW by using natural fungicides.
1 Introduction
Fusarium is a widespread soil-borne fungal pathogen that can infect hundreds of crops as diverse as tobacco, tomato, cotton, rice and banana, causing root rot, stem rot, or ear rot (Ma et al., 2013). It is commonly found in tobacco growing areas such as Hubei, Yunnan, Henan, Guizhou and Anhui Province in China and causes tobacco fusarium wilt (TFW) (Zhao et al., 2023). Infected tobacco plants show yellowing and wilting of the leaves, epidermal root rot, susceptibility to breakage and abscission, and browning of the vascular bundles. In severe cases, rot and necrosis occur from the stem base to the main root, with the xylem turning brown and black, and even leading to the death of the entire plant, which has a major impact on the yield and quality of the tobacco, becoming an inhibiting factor for the development of tobacco production.
To date, eleven species of TFW pathogens have been reported, including Fusarium brachygibbosum, Fusarium commune, Fusarium equiseti, Fusarium falciforme, Fusarium fujikuroi, Fusarium kyushuense, Fusarium meridionale, Fusarium oxysporum, Fusarium sinensis, Fusarium solani, and Fusarium verticillioides, in which F. oxysporum and F. solani were the main perpetrators in China (Fall et al., 2019; Gai et al., 2023; Li et al., 2023a; Rodríguez-Molina et al., 2007; Wang et al., 2013b; Zhong et al., 2020; Qiu et al., 2021a, b, 2022, 2023; Shen et al., 2023; Zhao et al., 2023). Currently, studies on the pathogenic mechanism of TFW mainly focus on F. oxysporum. It is well-known that F. oxysporum secretes various cell wall degrading enzymes and effectors to penetrate the roots and damage host plant defense systems, and then invade the root cortex and colonizes in the xylem vessels. The massively proliferating mycelium and small conidia in the vessels as well as the degradation products of the inner wall of the host vessel can block the vascular bundle, and disrupt water absorption and nutrient transport (Michielse and Rep, 2009; Gordon, 2017). On the other hand, F. oxysporum can produce various toxins such as fusaric acid, fumonisins, trichothecenes, zearalenone and ergosterol, which disrupt the normal physiological metabolism of host plants, and ultimately lead to plant wilting and death (Fernandes and Ghag, 2022).
The use of a TFW-resistant variety has been shown to be an effective and cost-effective method for controlling TFW (Shull et al., 2020). García (2015) developed a TFW-resistant variety named “Burley Pinar 2010” through five generations of self-pollination and lineage selection. In soils infected with Fusarium wilt, the yield of “Burley Pinar 2010” exceeded that of a normal variety. However, breeding a new TFW-resistant variety requires significant investment and time, which complicates the breeding work. Chemical management is currently the main TFW control method due to its high efficiency, convenience and economy. The use of chemical fungicides such as phenamacril (Zheng et al., 2022), prochloraz (Peng et al., 2022), and chloropicrin (LaMondia, 2015), leads to a significant reduction in Fusarium wilt, however, long-term use of these fungicides leads to the development of fungicide resistance of the pathogen and increases this difficulty to control (Zheng et al., 2022). In addition, inappropriate and excessive application can cause chemical residues, soil infertility and environmental pollution (Zhao et al., 2023).
Biocontrol of TFW using microorganisms and fungicides derived from plants or produced by microorganisms has become a hot topic due to its advantages in terms of effectiveness, biodegradability and environmental sustainability (Abbas and Yli-Mattila, 2022). For example, Bacillus velezensis Ba-0321 had an inhibitory effect of 75% on F. oxysporum and resulted in a high control effect on TFW of 81% (Li et al., 2023b). The natural product osthol separated from Cnidium monnieri (L.) Cusson can significantly disrupt the cell wall integrity and dynamic balance of F. oxysporum and has significant potential as an agent to control TFW (Zheng et al., 2021). The volatile organic compounds 2-methoxy-4-vinylphenol and 3,4-dimethoxystyrene, produced by the endophytic fungi Sarocladium brachiariae HND5, cause cell death by damaging the plasma membranes of F. oxysporum f. sp. cubense (Yang et al., 2021). These results indicate the potential of natural products as biocontrol agents against TFW. However, biopesticides are rarely registered for use against TFW, especially in China (Zhao et al., 2023). The main reason for this is that no biopesticides as effective as chemical fungicides have been developed to control TFW. Otherwise, they are more expensive and their application is more complicated than that of chemicals, especially in large-scale field cultivation (Egbuna et al., 2020). Therefore, it is crucial to find an effective and cost-effective biopesticide to control TFW.
The plant rhizosphere is a soil microecosystem composed of plants, soils, microbiomes and pathogens surrounding plant roots, and the essence of disease suppressing soil (DSS) is the balance of the soil microecosystem (Lv et al., 2023). Many studies focused on converting disease-conducting soil (DCS) into DSS by screening and employing beneficial microbes such as Pseudomonas (Raaijmakers and Weller, 1998), Streptomycete (Cha et al., 2016), Trichoderma (Tao et al., 2023), and obligate phages of pathogenic bacteria (Wang et al., 2019), etc. In particular, the discovery and the assembly of core microorganisms has become a focus [33], while the utilization of natural products with antipathogenic effects in the rhizosphere has been neglected. The aim of this study is to identify new metabolites negatively associated with TWB by comparing the different metabolites between DSS and DCS using metabolomics to provide a new source for the development of biopesticides to control TFW.
2 Materials and methods
2.1 Strain and chemicals
The pathogen F. oxysporum was provided by the Hubei Academy of Tobacco Sciences and cultured on PDA medium (200 g/L potato peels, 20 g/L dextrose, 15 g/L agar, pH 7.0) at 28°C. Alternariol, dibenzoyl thiamine, phenylpropiolic acid, ouabain, rosmarinic acid, linustatin, N-acetyl-Asp-Glu, estrone sulfate, scopoletin, D-(+)-glucose, acetaminophen glucuronide, pantothenic acid, 3-hydroxy-3-methylpentanedioic acid and propidium iodide were purchased from Aladdin Co. Ltd., China. All reagents were of analytical quality.
2.2 Rhizosphere soil collection
Tobacco cultivar Chuxue 26 was planted in a tobacco field continuously cultivated for 5 years in Xijiadian County (111.18354°E, 32.748336°N), Danjiangkou City, Hubei Province, China. At harvest time, tobacco plants were examined for disease severity and classified into grades 0-9 according to the GB/T23222-2008 “Classification and Investigation Methods for Tobacco Diseases and Pests” standard (Ren et al., 2008). Briefly, “G0” represents the plants without symptoms; “G1” represents the plant growth is generally normal or slightly stunted, with a few roots necrotic and appearing black, and the middle and lower leaves are chlorotic (or discoloration); “G5” represents the height of the diseased plant is one-third to one-half shorter than that of the healthy plant. Most of the roots are necrotic and black, and more than two-thirds of the leaves are wilted, with obvious dry tips and edges; and “G9” represents the dead plant. The TFW grade 0 rhizosphere soil was defined as DSS, and TFW grades 1, 5, and 9 were defined as DCS. Total rhizosphere soil was collected by five-point sampling methods. At least six plants were collected for each TFW class. All samples were transported to the laboratory immediately after collection and stored at −80°C for further analysis.
2.3 Untargeted metabolomics and data processing
Five grams of soil sample were resuspended with 100 mL 80% methanol by well vortexing for 10 min and then sonicated at 40 kHz for 30 min at 4°C. The samples were incubated on ice for 5 min and then centrifuged at 15,000 g at 4°C for 20 min. The supernatant was concentrated to 10 mL and 20 μL of which was injected into the LC-MS/MS system. UHPLC-MS/MS analyses were performed using a Vanquish UHPLC system (ThermoFisher, USA) coupled with an Orbitrap Q ExactiveTM HF-X mass spectrometer (ThermoFisher, USA) in Novogene Co., Ltd. (Beijing, China). The samples were injected onto a Hypersil Gold column (100 × 2.1 mm, 1.9 μm) with a 12-minute linear gradient and a flow rate of 0.2 mL/min. The mobile phases consisted of water with 0.1% formic acid (solvent A) and methanol (solvent B) for the positive polarity mode, and 5 mM ammonium acetate (pH 9.0, solvent A) and methanol (solvent B) for the mode with negative polarity. The solvent gradient was adjusted as follows: 2% B, 1.5 min; 2-85% B, 3 min; 85-100% B, 10 min; 100-2% B, 10.1 min; 2% B, 12 min. The Q Exactive™ HF-X mass spectrometer was operated in positive/negative polarity mode with scan range of 100-1500 (m/z), a spray voltage of 3.5 kV, a capillary temperature of 320°C, a sheath gas flow rate of 35 psi and auxiliary gas flow rate of 10 L/min as well as an S-lens operated RF level of 60, temperature of the additional gas heater of 350°C, and full scan with data-dependent MS/MS.
The raw data files generated by UHPLC-MS/MS were processed using the Compound Discoverer 3.3 (CD3.3, ThermoFisher) to perform peak alignment, peak picking, and quantitation for each metabolite. The main parameters were set as follows: peak area was corrected with the first QC, actual mass tolerance, 5ppm; retention time tolerance, 0.2 min; signal intensity tolerance, 30%; signal-to-noise ratio, 3; and minimum intensity, 10,0000. After that, peak intensities were normalized to the total spectral intensity. The normalized data was used to predict the molecular formula based on additive ions, molecular ion peaks and fragment ions. And then peaks were matched with the mzCloud, mzVault and MassList database to obtain the accurate qualitative and relative quantitative results. Statistical analyses were performed using the statistical software R (R version R-3.4.3), Python (Python 2.7.6 version) and CentOS (CentOS release 6.6), When data were not normally distributed, standardize according to the formula: sample raw quantitation value/(The sum of sample metabolite quantitation value/The sum of QC1 sample metabolite quantitation value) to obtain relative peak areas; and compounds whose CVs of relative peak areas in QC samples were greater than 30% were removed, and finally the metabolites’ identification and relative quantification results were obtained.
The identified metabolites were annotated using the KEGG database (https://www.genome.jp/kegg/pathway.html), the HMDB database (https://hmdb.ca/metabolites) and the LIPID Maps database (http://www.lipidmaps.org/). Partial least squares discriminant analysis (PLS-DA) was performed on metaX. Significantly differential rhizosphere metabolites (DRMs) were determined by a combination of univariate and multivariate analysis (fold change≥ 2 or ≤ 0.5, P< 0.05, VIP > 1) using R statistical software (version 3.4.3). The Venn diagram was created using Venny2.1 (https://bioinfogp.cnb.csic.es/tools/venny/). Volcano plots and heatmaps of DRMs were plotted by ggplot2 and pheatmap package in the R language. The functions of these metabolites and metabolic pathways were investigated using the KEGG database.
2.4 In vitro assay for antimicrobial effect of DRMs
The inhibitory effect of DRMs on the growth of F. oxysporum mycelium was determined using the method of Stringlis et al. (2018). Briefly, a 5-mm mycelial plug of a freshly produced fungal strain was inoculated into the center of the PDA plate containing 0.5 mM DRMs for 6 d in the dark at 28 °C. PDA plates supplemented with DMSO and 0.2 g·L-1 Delvocid were used as negative and positive controls, respectively. A microplate assay was used to quantify the effect of DRMs on the growth of F. oxysporum. First, 96-well plates (Corning, #3599) were filled with 100 μL of liquid potato dextrose broth (PDB) containing 5000 fungal spores for each well. Then 100 μL of PDB with different concentrations of DRM dissolved in 80% methanol was added to the wells and equal amounts of methanol was added as a blank control. The antibiotic Delvocid (0.3 g·L-1; DSM) was used as a positive control. The 96-well plates were incubated at 28°C for 6 d and fungal growth (OD600) was measured every 2 d.
2.5 Chemotaxis assay
The quantitative measurement of the chemotaxis of the response of F. oxysporum to the DRMs was performed based on the Turrà’s method (Turrà et al., 2015). Four milliliters of water agar (0.5%, w/v) containing freshly produced microconidia (2.5 × 106 mL-1) were spread in a 90-mm diameter Petri dish. Three parallel lines, 5 mm apart, were marked in the center of the bottom of the Petri dish. Two square wells were cut in agar along the two marked lines, and then 50 μL of DRMs (0.25, 0.5 and 1 mM) or the solvent DMSO were added to each well. Pectin (1%, w/v) and its solvent H2O were used as positive and negative controls, respectively. The plates were incubated in the dark at 28°C for 12 h. The number of hyphal tips of germinating conidia pointing to the test compound, to the control, or to neither (neutral) was counted with a Zeiss microscope (200 × magnification, Axioimager). Chemotaxis was calculated as the percentage of the number of hyphae growing toward the test compound or towards the solvent. A total of 500 hyphal tips were counted for each test.
2.6 Determination of mycelial cell membrane integrity and permeability
Propidium iodide (PI) stains were used to determine cell membrane integrity of F. oxysporum according to Hu (Hu et al., 2023) with some modifications. A fresh mycelia plug was collected from the plate edge of a 7-day-old F. oxysporum, inoculated into PDB medium with different DRM concentrations, and cultured for 12 h (28°C, 180 r/min). The mycelia were centrifuged 5 000×g, 4°C for 5 min and washed three times with PBS buffer (50 mM, pH 7.4), followed by staining with 7.5 μM PI at 37°C for 20 min. The mycelium was then washed three times with PBS buffer, mounted on a slide, and observed under a fluorescence microscope (Zeiss LSM710, Germany) at an excitation wavelength of 535 nm and an emission wavelength of 590 nm.
The degree of damage to cell membrane permeability of F. oxysporum after DRM treatment was assessed by measuring extracellular relative conductivity and changes in protein content in the supernatant, as described by Hu (Hu et al., 2023). Fresh mycelia plugs were cultured in PDB medium. After 2 d, the mycelia were filtered with sterile gauze and rinsed with PBS, and then 0.5 g of mycelia were incubated in sterile water containing different concentrations of DRM (0.25, 0.5, 1 mM) for 24, 48 and 72 h, respectively. The supernatant obtained by centrifugation at 12,000 rpm for 10 min at 4°C was used to determine the conductivity with a DDSJ-318 conductivity meter (INESA, China) and the final conductivity was determined after 72 h by treating the mycelia in boiling water bath for 10 min. The relative conductivity was calculated using the following equation:
Where R0: the initial conductivity at 0 h, R1: the conductivity at 24, 48, and 72 h, R2: the conductivity of dead treatment at 72 h.
In addition, the mycelium was treated as described above, and 5 mL of supernatant was used to measure protein concentrations using a BCA protein assay kit (ThermoFisher, USA).
2.7 Statistical analysis
All data were analyzed using the one-way analysis of variance (ANOVA) method with SPSS 22.0 software (SPSS Inc., Chicago, USA) and Duncan’s multiple range test was used to test the significance of the difference between treatments (P<0.05). The correlation between the relative abundance of DRMs and TFW grade were analyzed by cor () in R language (method=spearman). All the experiments were repeated at least three times.
3 Results
3.1 Changes in the metabolome and functional analysis of DRMs
Different rhizosphere metabolites (DRMs) between DSS and DCS were analyzed in three comparison groups, G1 vs. G0, G5 vs. G0, and G9 vs. G0, in an effort to find new biopesticide to control TFW. There was little difference in the metabolites between the G0 and G1 groups, according to partial least squares discrimination analysis (PLS-DA), which showed a significant separation of metabolites between the G0 group and other groups with the exception of the G1 group (Figure 1A). Figures 1C–F displays the expression and distribution of DRMs among the four groups (Supplementary Table S1). From the G1 vs. G0, G5 vs. G0, and G9 vs. G0 comparison groups, a total of 87, 166, and 403 DRMs were obtained; 14 DRMs were the overlapping metabolites (Figure 1B; Supplementary Table S2). These 14 DRMs were choline bitartrate, N-acetyl-L-leucine, 4-hydroxyisoleucine, 1-(4-bromophenyl)-2-phenylethan-1-one, syringic acid, pantothenic acid, 3-(4-fluorophenoxy)-1-(1,4-thiazinan-4-yl)propan-1-one, L-adrenaline, DL-stachydrine, pyridoxine O-glucoside, acetaminophen glucuronide, linustatin, neosaxitoxin, and N-acetyl-L-glutamine, all of which were significantly downregulated in three comparison groups.
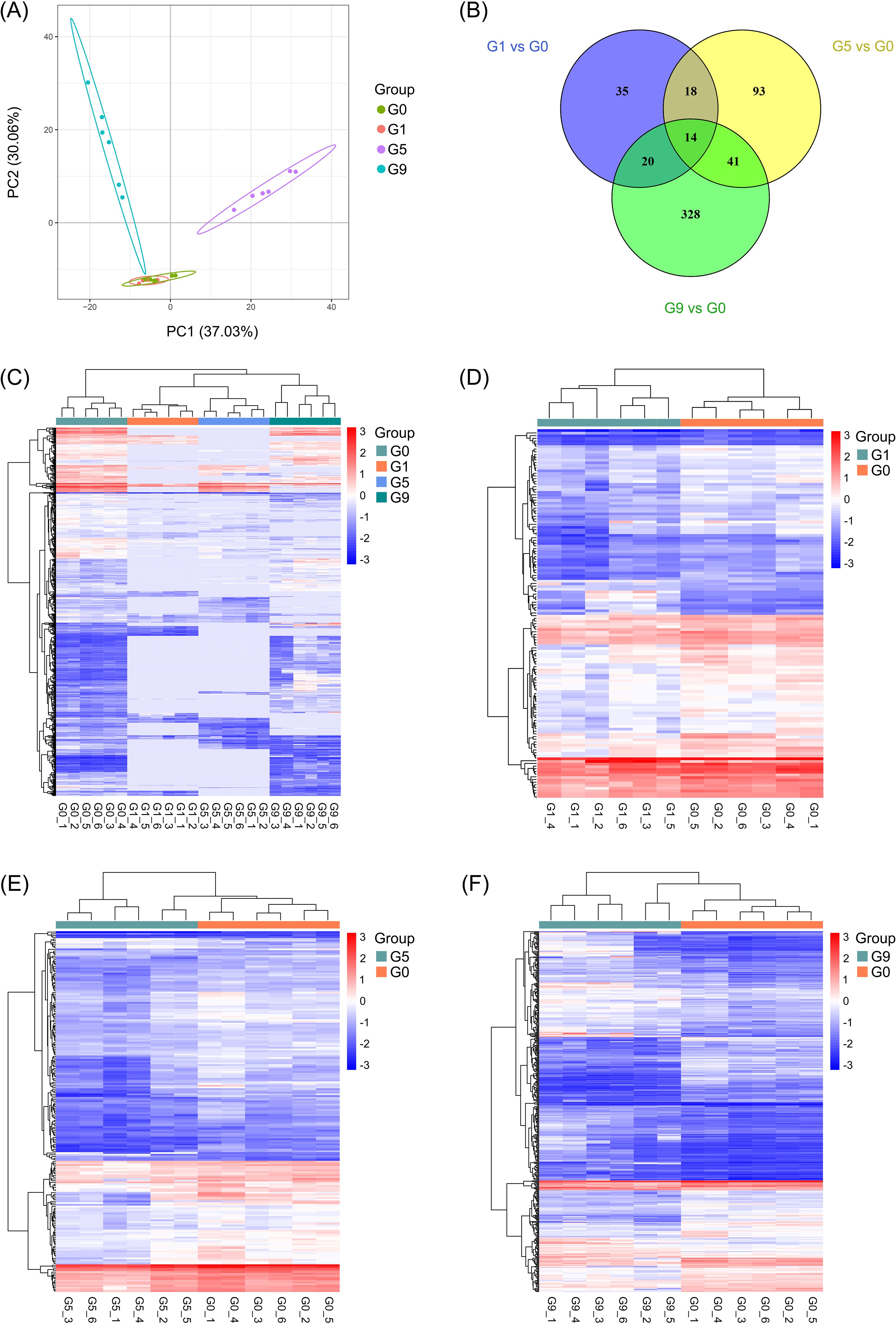
Figure 1. Metabolic profiling results of the DSS and DCS. (A) PLS-DA score plot. (B) Pairwise compare the DRMs in groups on the Venn diagram. (C–F) Hierarchical clustering analysis for the metabolites between different groups.
As illustrated in Figures 2A–C, G1, G5 and G9 exhibited significantly lower metabolites (72, 152, and 170) and significantly higher metabolites (15, 14, and 233) in comparison to G0. The top 20 DRMs in each of the three comparison groups were determined by ranking the absolute values of log2(fold change) (Figures 2D–F; Supplementary Table S2). In G1 vs. G0 group, the number of up- and down-regulated DRMs in the top 20 DRMs is equal, while the G5 vs. G0 and G9 vs. G0 groups had an uneven and opposite trend in up- and down-regulated DRMs.
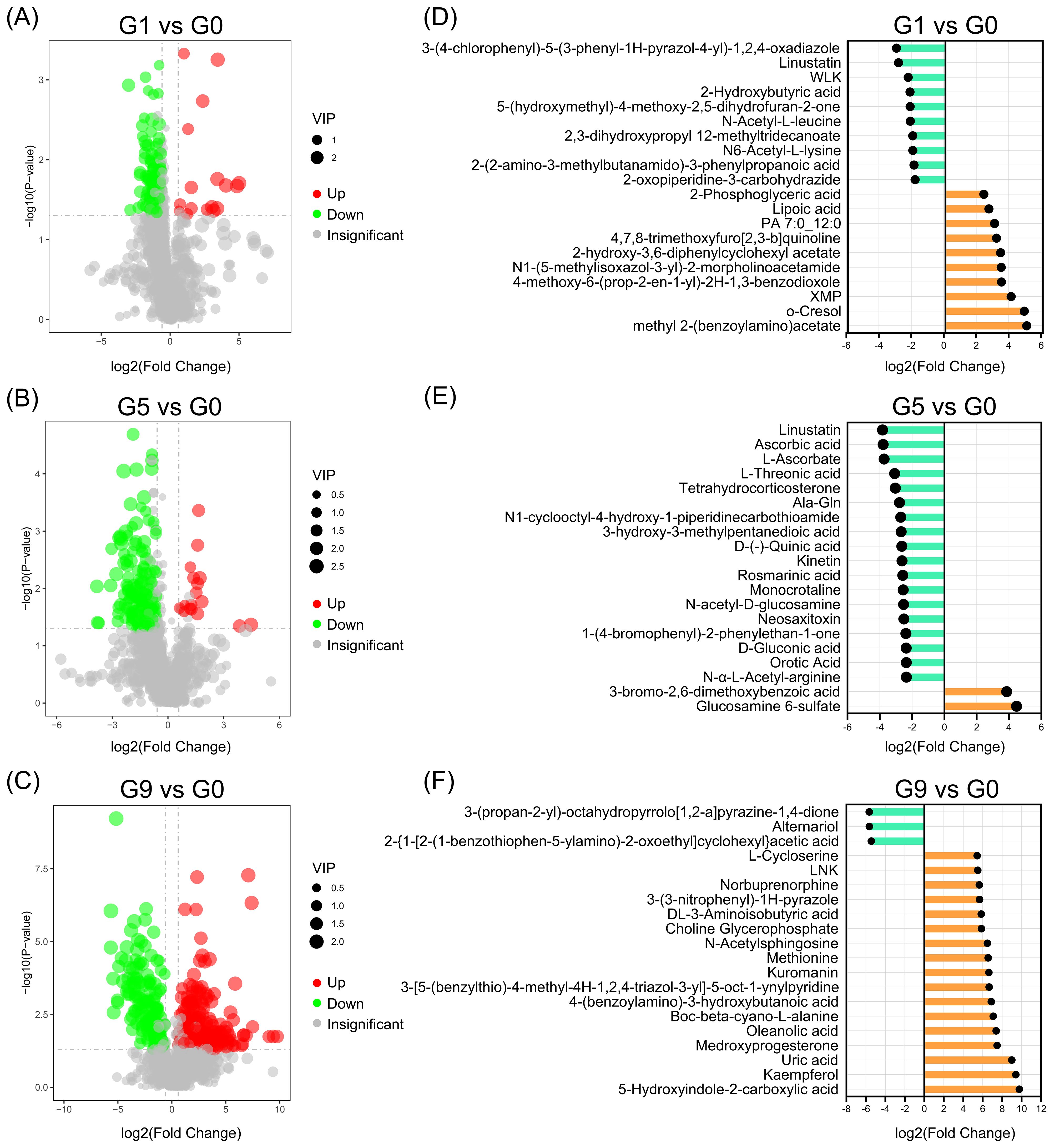
Figure 2. Up- and down-regulated DRMs in various groups. (A–C). Volcano plots show the important discriminatory metabolites. (D–F). The top twenty up- and down-regulated DRMs.
Analyzing the KEGG annotation of metabolites allowed for a deeper understanding of the variations in metabolic pathways that DRM engaged in amongst the comparison groups (Figure 3; Supplementary Table S3). Nicotinic acid, NAD+, fumaric acid, and nicotinuric acid are the four metabolites that are significantly enriched in oxidative phosphorylation and nicotinate and nicotinamide metabolism in the G1 vs. G0 group (Figure 3A). The HIF-1 signaling pathway, carbon fixation pathways in prokaryotes, two-component systems, glyoxylate and dicarboxylate metabolism, biosynthesis of alkaloids derived from terpenoid and polyketide, biosynthesis of alkaloids derived from ornithine, lysine and nicotinic acid were all significantly enriched in the G5 compared to the G0 group (Figure 3B). The metabolic pathways that were most enriched in the G9 group as compared to the G0 group were tyrosine metabolism, ABC transporters, and arginine and proline metabolism (Figure 3C). The three comparison groups shared common metabolic pathways, including phosphotransferase system (PTS), tyrosine metabolism, galactose metabolism, biosynthesis of secondary metabolites, biosynthesis of phenylpropanoids, and caffeine metabolism (Figure 3D).
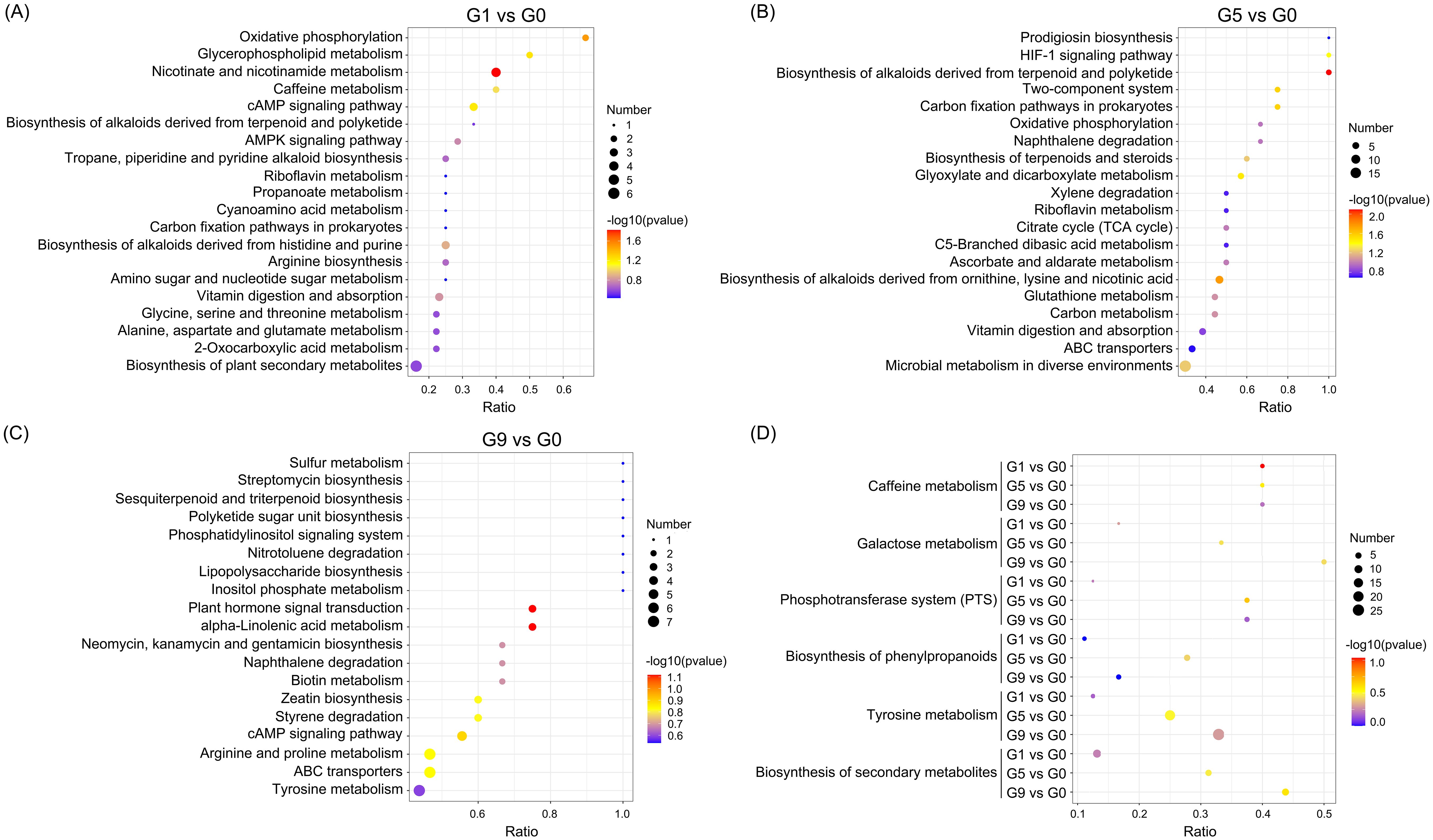
Figure 3. KEGG pathways enriched for DRMs in different comparison groups. (A–C). The scatter plots of the enrichment statistics of the KEGG pathway in G1 vs. G0, G5 vs. G0 and G9 vs. G0, respectively. (D) The common KEGG pathway in three comparison groups.
3.2 Correlation analysis
We investigated the relationship between TFW severity and DRMs content in order to pinpoint the specific DRMs that increased in DSS and decreased in DCS. We concentrated on DRMs with correlation coefficients (Corr coef) smaller than -0.7 in the G5 and G9 groups because the G1 group had less change in DRMs and milder disease symptoms. As shown in Table 1 and Supplementary Table S4, 29 DRMs were obtained, among which 3-(4-fluorophenoxy)-1-(1,4-thiazinan-4-yl)propan-1-one, alternariol, 2-Hydroxyphenylalanine, dibenzoyl thiamine, 3-[4-methyl-1-(2-methylpropanoyl)-3-oxocyclohexyl]butanoic acid, 4-(cyclohexylmethyl)-6-(2-thienyl)-2,3-dihydropyridazin-3-one hydrate and phenazine-2-carboxylic acid were significantly negatively correlated with TFW severity (Corr coef >0.8). In addition, pantothenic acid, 3-(4-fluorophenoxy)-1-(1,4-thiazinan-4-yl)propan-1-one, acetaminophen glucuronide and linustatin were all severely decreased in three comparison groups.
3.3 Screening of DRMs with anti-F. oxysporum effect
To investigate the activities of DRMs that are negatively correlated with TFW severity on the physiology and growth of F. oxysporum, 18 DRMs of alternariol, 2-hydroxyphenylalanine, dibenzoyl thiamine, phenazine-2-carboxylic acid, 2-(formylamino)benzoic acid, phenylpropiolic acid, ouabain, rosmarinic acid, linustatin, N-acetyl-Asp-Glu, 4-Methylhippuric acid, estrone sulfate, scopoletin, D-(+)-glucose, acetaminophen glucuronide, Dl-3-hydroxy-kynurenine, pantothenic acid, and 3-hydroxy-3-methylpentanedioic acid from Table 1 that we could purchase on the market were tested using the plate inhibition method. The result showed that only phenylpropiolic acid, linustatin and scopoletin showed inhibitory effect against F. oxysporum at concentrations of 0.5 mM after 6 d co-culture (Figure 4). To further dissect the inhibitory effect of these three DRMs on F. oxysporum, we examined the effects of different concentrations of three DRMs on the spore germination and spore growth of F. oxysporum. Figure 5 showed that phenylpropiolic acid, linustatin and scopoletin all significantly inhibited the growth of F. oxysporum at concentrations of 0.25 mM after 2 d, and only 0.25 mM linustatin showed strong inhibitory effects both 2 d and 4 d. At concentrations of 0.5 mM or above, the three DRMs exhibited strong inhibitory effects on F. oxysporum at all times tested.
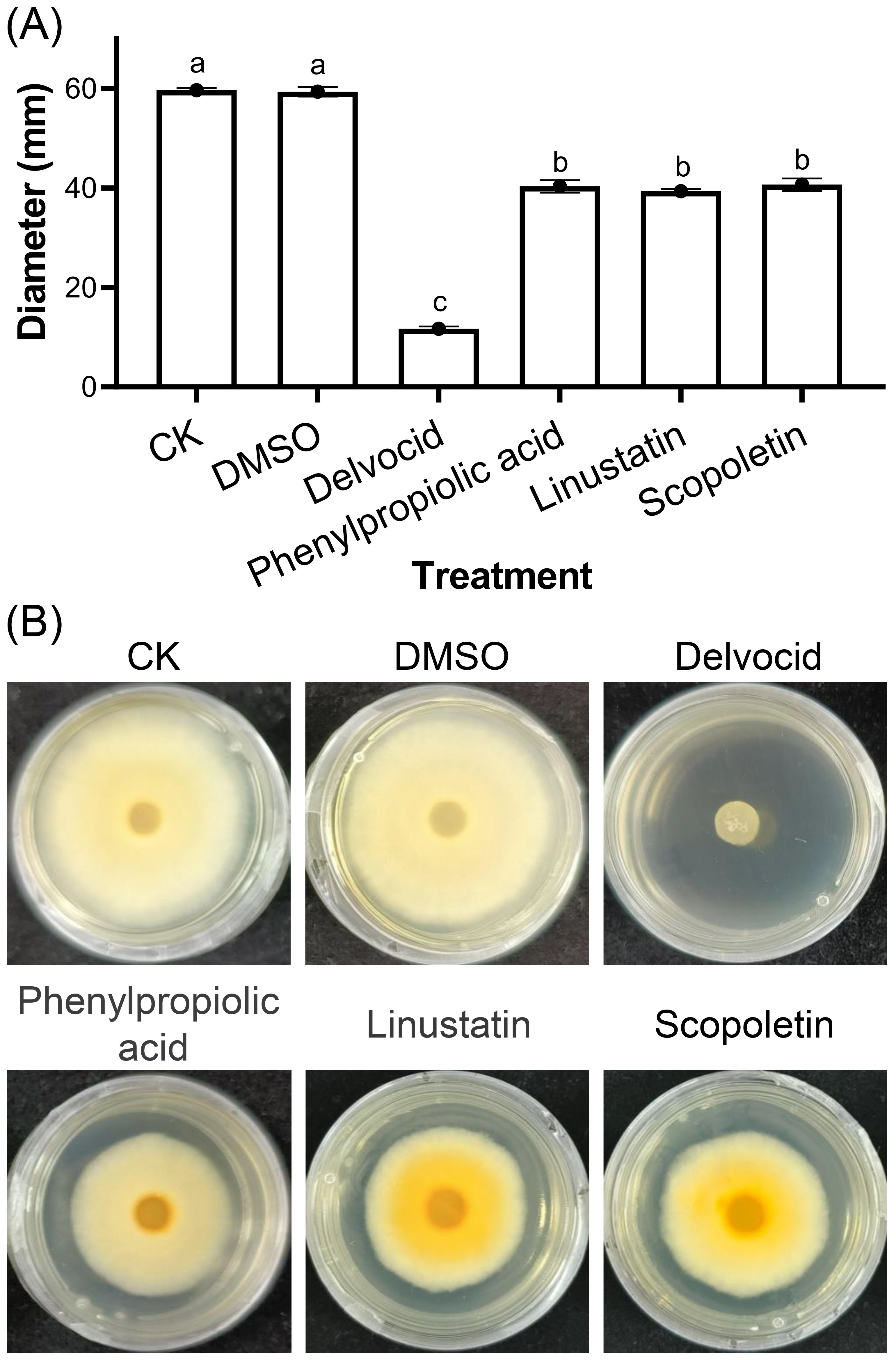
Figure 4. Effects of 0.5 mM DRMs on F oxysporum physiology (A) and representative images (B). DMSO and 0.2 g·L-1 Delvocid were used as negative and positive control, respectively. Different lowercase letters indicate significant difference (P< 0.05) according to Duncan’s multiple range test. The number of biological replicates for each experiment was n=3.
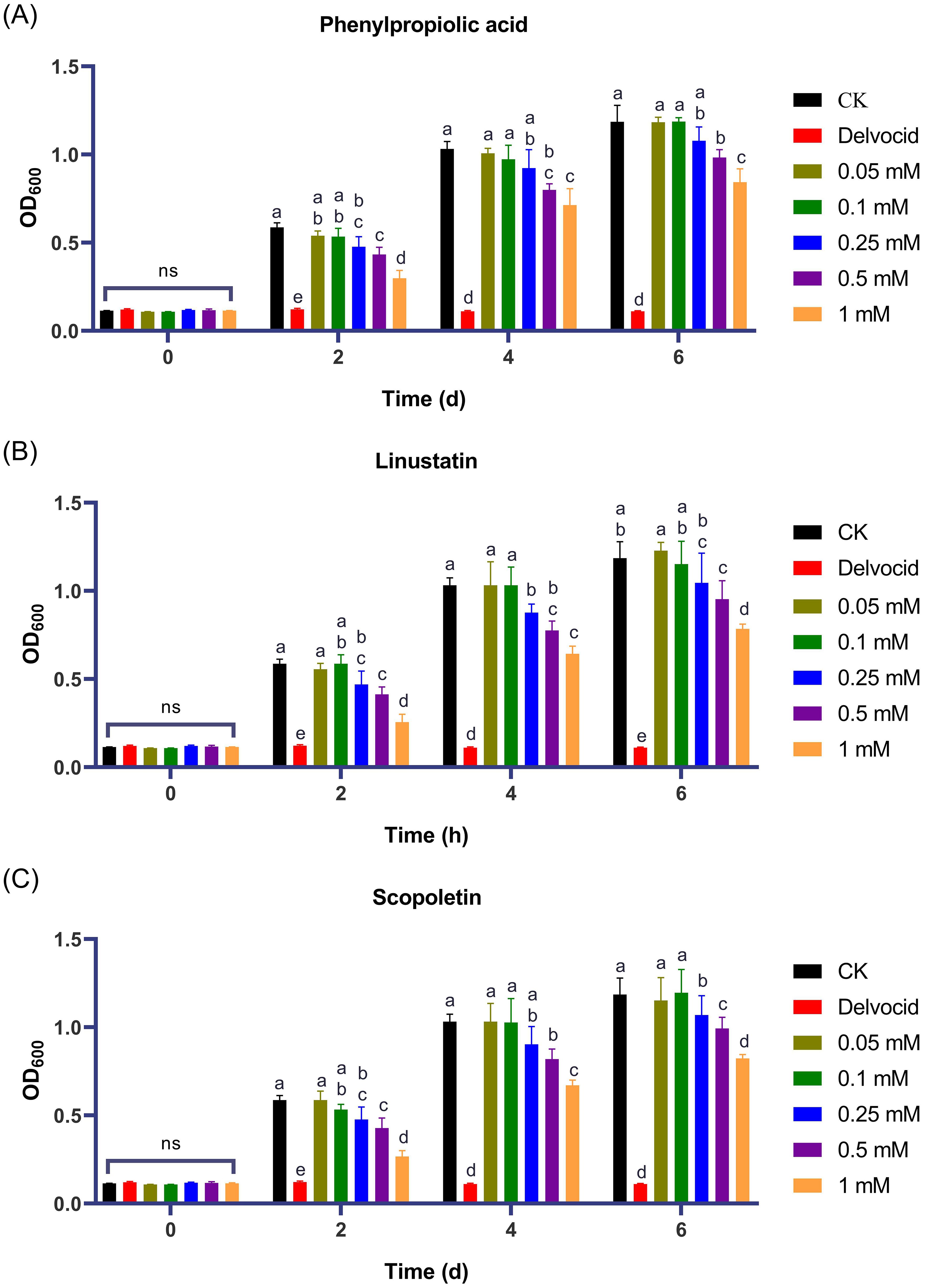
Figure 5. Effects of phenylpropiolic acid (A), linustatin (B) and scopoletin (C) on the growth of F oxysporum. Delvocid (0.3 g·L-1) were used as positive control. Different lowercase letters indicate significant difference (P< 0.05) according to Duncan’s multiple range test; ns, not significant. The number of biological replicates for each experiment was n=3.
3.4 Chemotaxis of DRMs to F. oxysporum
To investigate the effect of three DRMs on hyphal growth of F. oxysporum, we performed a chemotaxis assay. Our results showed that F. oxysporum could be significantly attracted by the positive control pectin, while it showed phobotaxis effect toward phenylpropiolic acid, linustatin and scopoletin at all three concentrations. In particular, linustatin and scopoletin had highly significant detrimental effects on fungal hyphae even at a low concentration of 0.25 mM (Figure 6).
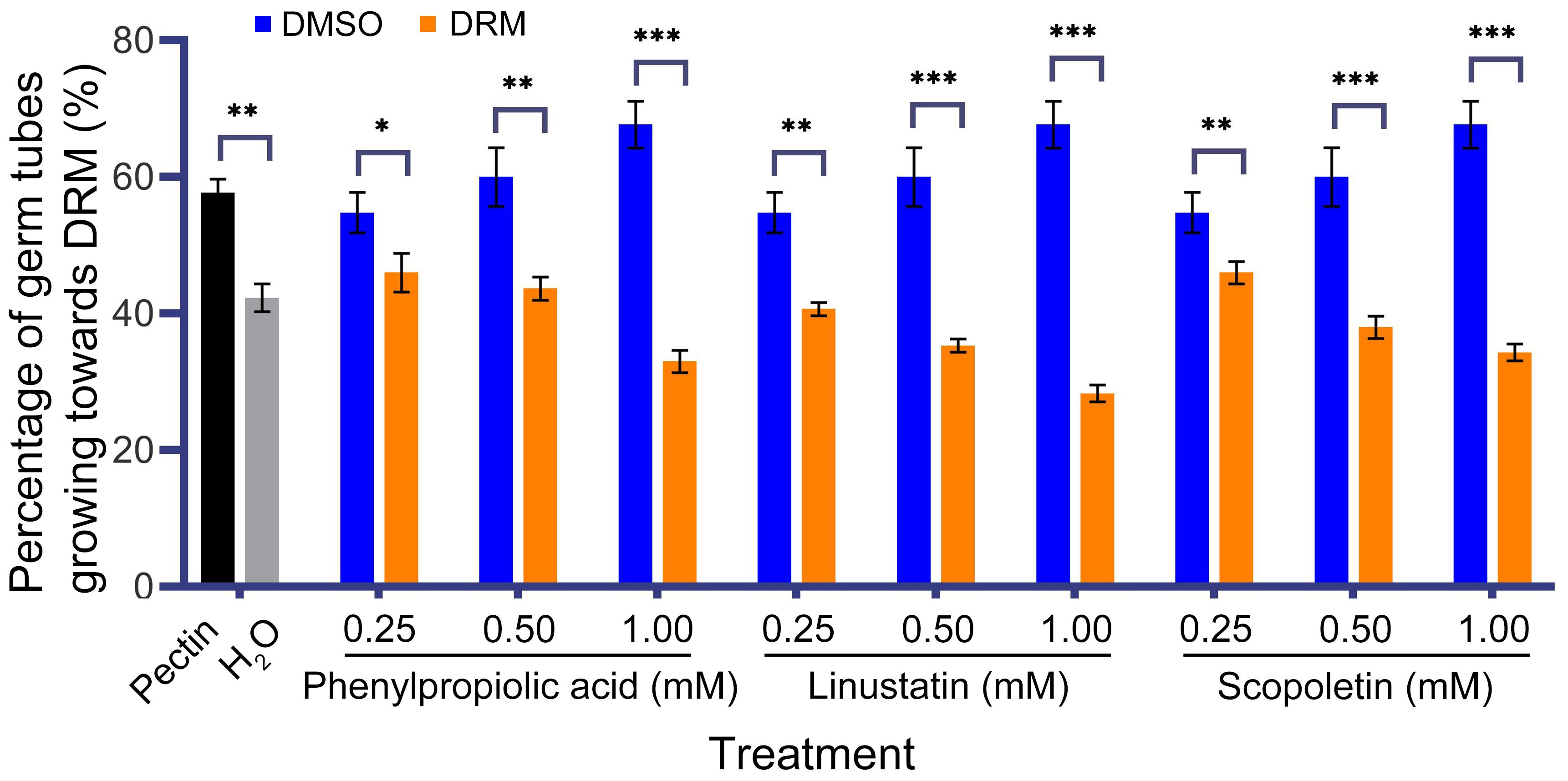
Figure 6. Chemotactic effect of F. oxysporum to DRMs. 1% Pectin was used as a positive control. Asterisks indicate significant differences between DRMs and its solvent: *P< 0.05, **P< 0.01, ***P< 0.001, student’s t test.
3.5 Effects of DRMs on the integrity and permeability of the mycelial cell membrane of F. oxysporum
We examined the effects of DRMs on cell membrane integrity of F. oxysporum using PI staining. Microscopic observations showed that hyphae of the untreated control were barely stained with PI, whereas cells treated with phenylpropiolic acid, linustatin, and scopoletin all emitted red fluorescence, indicating extensive cell death and membrane permeation (Figure 7). Furthermore, as the DRM dose increases, the proportion of PI-stained cells in the total number of cells gradually increases in a dose-dependent manner.
To further confirm the damaging effect of DRMs on the cell membrane, we measured the REC and protein content changes in the supernatant after treatment with DRMs. The REC of all treated groups as well as the CK group increased over time and in a dose-dependent manner (Figures 8A–C). The groups treated with the three DRMs at a concentration of 0.5 mM or higher showed a significant increase in REC compared to the CK group at all times tested, and only linustatin increased the REC of F. oxysporum at concentrations as low as 0.25 mM. In addition, the REC of the linustatin-treated group was much higher than that of the other two groups after 24, 48, and 72 h of incubation, indicating that linustatin had a stronger damaging effect on the cell membranes of F. oxysporum than the other two DRMs. As shown in Figures 8D–F, the extracellular protein content of F. oxysporum also increased significantly after treatment with three DRMs above 0.25 mM for 24 h, 48 h, and 72 h. These results collectively suggest that the three DRMs inhibited F. oxysporum by disrupting the cell membrane and increasing the membrane permeability of F. oxysporum.
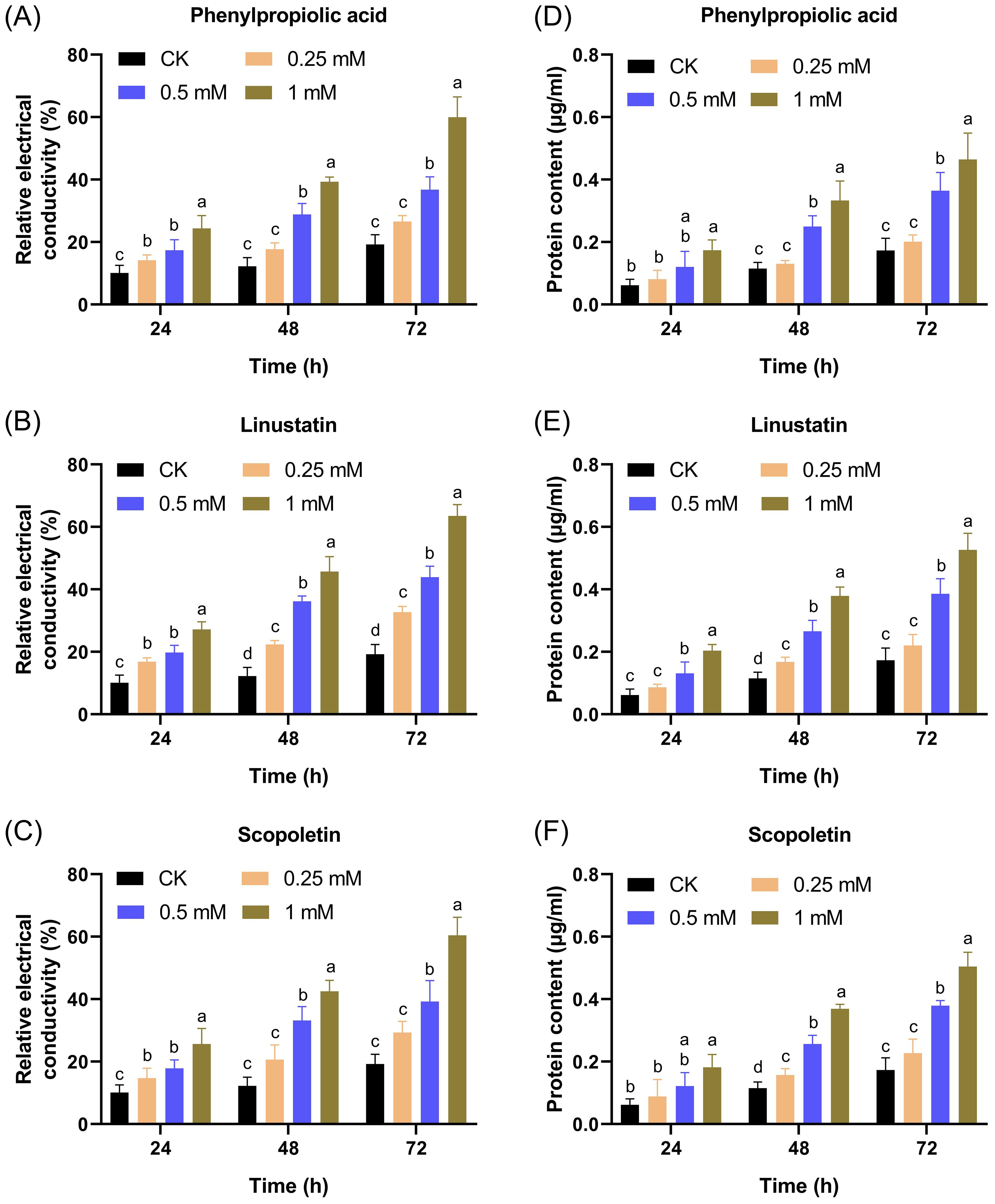
Figure 8. Effects of different concentrations of DRMs on the extracellular relative conductivity (A–C) and extracellular protein content (D–F) of F. oxysporum. Different lowercase letters indicate significant difference (P< 0.05) according to Duncan’s multiple range test; ns, not significant. The number of biological replicates for each experiment was n=3.
4 Discussion
F. oxysporum is a soil-borne pathogen that poses a serious threat to the cultivation of hundreds of crops worldwide. Numerous studies have shown that plant roots and beneficial microorganisms residing in the rhizosphere secrete a variety of secondary metabolites to combat soil-borne pathogens (Wu, 2008; Mazurier et al., 2009; Yuan et al., 2012; Wang et al., 2013a; Yan et al., 2023). Therefore, it is an effective, environmentally friendly, and safe method to control soil-borne disease by studying and applying anti-microbial metabolites from root exudates and probiotics present in the rhizosphere. In the present study, we discovered three natural metabolites with antifungal activity through comparative metabolomic analysis of DSS and DCS, of which phenylpropiolic acid and linustatin were reported for the first time. These three metabolites all have potent inhibitory effects on the spore germination and mycelium growth of F. oxysporum.
Through metabolomic analysis of DSS and DCS, we found that the relative contents of 298 DRMs, including phenylpropiolic acid, linustatin and scopoletin, were greatly reduced in DCS compared to DSS. These down-regulated metabolites were mainly phenolic acids, phenylpropanes, dipeptides and glycosides, which were enriched in metabolic pathways such as phenylpropanoids biosynthesis, tyrosine metabolism, and caffeine metabolism. The phenylpropanoid pathway is a classic metabolic pathway associated with plant growth and stress responses that can inhibit or eliminate ROS in cells under biotic or abiotic stress through the synthesis of antioxidants such as phenols and flavonoids, thereby protecting DNA, proteins, membrane lipids and other organ components from severe damage (Fu et al., 2023). On the other hand, the lignin synthesized in this pathway can promote cell lignification, thicken the cell wall and form a physical barrier to prevent the invasion of pathogens and insects (Lu et al., 2022). In the present study, the content of fumaric acid, scopoletin, L-phenylalanine and citric acid, which are involved in the biosynthesis of phenylpropanoids, deceased in DCS compared to DSS, suggesting that these metabolites were depleted to protect the plants from infection with F. oxysporum. This result was consistent with the previous study by Fu et al. (2023), in which phenylalanine, caffeic acid, trans-ferulic acid, and mustard acid, which are associated with phenylpropanoid biosynthesis of ramie growth were significantly decreased in the obstacle group compared to the CK group.
In the subsequent correlation analysis and antifungal experiment, it was confirmed that scopoletin had an inhibitory effect on F. oxysporum. Scopoletin is a type of coumarin phytoalexin that is widely distributed throughout the plant kingdom (Gnonlonfin et al., 2012). Scopoletin is known to have antibacterial and antifungal effects. Scopoletin isolated from Ixora javanica and Greenea montana was reported to have inhibitory activity against Enterococcus faecium UCLA 192 with a minimum inhibitory concentration (MIC) value of 128 μg/mL (Buathong et al., 2019). Stringlis et al. (2018) reported that scopoletin exhibits a selective inhibitory effect on the soil-borne fungal pathogens F. oxysporum and Verticillium dahlia. In addition, they found that scopoletin not only reduced the growth of F. oxysporum and deterred the fungal hyphae, but also hindered the germination of F. oxysporum spores and inhibited the pigmentation of F. oxysporum mycelium, which is consistent with our results.
In addition to scopoletin, two other DRMs phenylpropiolic acid and linustatin with anti-F. oxysporum activity were also picked out. Phenylpropiolic acid was found to be a potent inhibitor of Sporosarcina pasteurii urease with an inhibition constant of 37.1 μM (Macegoniuk et al., 2017), and also possessed antibacterial activity against Brucella abortus 544 with an MIC value of 250 μg/mL (Kazemizadeh et al., 2016). However, the antifungal effect of phenylpropiolic acid has not yet been documented. This study reports for the first time that phenylpropiolic acid has an inhibitory effect on F. oxysporum, expanding the function and application potential of phenylpropiolic acid.
Linustatin is a cyanogenic glycoside that occurs in many plants as a phytoanticipin (Sohail et al., 2023). Cyanogenic glycoside exerts its defense function by releasing toxic free hydrogen cyanide (HCN) to aerobic organisms as an inhibitor of respiration and metal containing enzymes (Cressey and Reeve, 2019). Some studies depicted that high level of linustatin in foods threaten the health of human and herbivores, but little is known about its antimicrobial effects (Schrenk et al., 2019; Zuk et al., 2020). The present study addresses this limitation that linustatin has suppressive activity against F. oxysporum.
The cell membrane is a microbial barrier that can block the entry of external substances and prevent the outflow of internal substances. When the cell membrane is damaged, membrane fluidity is inhibited, membrane permeability changes, and intracellular electrolyte leakage occurs, eventually affecting microbial growth and leading to cell death (Di Pasqua et al., 2007). Our results showed that scopoletin, phenylpropiolic acid and linustatin could all inhibit F. oxysporum by impairing cell membrane integrity and increasing cell membrane permeability of F. oxysporum mycelium, which was similar to studies in which osthole and ferulic acid exerted antifungal activity by disrupting cell membrane integrity and dynamic equilibrium of F. oxysporum (Hu et al., 2023; Yan et al., 2023). In this study, the antifungal mechanism of three DRMs was discussed from the perspective of cell membrane integrity and permeability. It is necessary to comprehensively clarify the antifungal mechanisms from other aspects, such as the effect on F. oxysporum gene expression and induction of tobacco disease resistance. Furthermore, the application effects of these three DRMs still need to be further evaluated in the field.
5 Conclusions
This aim of this study was to discover natural metabolites with inhibitory effects of F. oxysporum from the tobacco rhizosphere for the control of TFW. Phenylpropiolic acid, linustatin and scopoletin were identified and confirmed to have suppressive activity against F. oxysporum through comparative metabolomics, correlation analysis and antifungal experiments. Furthermore, the antifungal mechanism of three DRMS by disrupting cell membrane integrity and permeability of F. oxysporum was preliminarily investigated.
Data availability statement
The original contributions presented in the study are included in the article/Supplementary Material. Further inquiries can be directed to the corresponding authors.
Author contributions
WY: Data curation, Formal analysis, Writing – original draft. ST: Formal analysis, Methodology, Writing – original draft. RX: Formal analysis, Writing – review & editing. LZ: Data curation, Formal analysis, Writing – original draft. ZZ: Formal analysis, Writing – review & editing. YY: Formal analysis, Writing – original draft. YL: Conceptualization, Methodology, Writing – original draft, Writing – review & editing. HX: Conceptualization, Methodology, Writing – original draft, Writing – review & editing.
Funding
The author(s) declare financial support was received for the research, authorship, and/or publication of this article. This work was supported by China National Tobacco Corporation (grant no. 110202101047 (LS-07), 110202201019(LS-03)) and key technology project of Hubei tobacco company (grant no. 027Y2022-023). The funders were not involved in the study design, collection, analysis, interpretation of data, the writing of this article or the decision to submit it for publication.
Acknowledgments
The authors would like to thank all the reviewers who participated in the review.
Conflict of interest
The authors declare that the research was conducted in the absence of any commercial or financial relationships that could be construed as a potential conflict of interest.
Publisher’s note
All claims expressed in this article are solely those of the authors and do not necessarily represent those of their affiliated organizations, or those of the publisher, the editors and the reviewers. Any product that may be evaluated in this article, or claim that may be made by its manufacturer, is not guaranteed or endorsed by the publisher.
Supplementary material
The Supplementary Material for this article can be found online at: https://www.frontiersin.org/articles/10.3389/fpls.2024.1435963/full#supplementary-material
References
Abbas, A., Yli-Mattila, T. (2022). Biocontrol of Fusarium graminearum, a causal agent of fusarium head blight of wheat, and deoxynivalenol accumulation: from in vitro to in planta. Toxins (Basel) 14, 299. doi: 10.3390/toxins14050299
Buathong, R., Chamchumroon, V., Schinnerl, J., Bacher, M., Santimaleeworagun, W., Kraichak, E., et al. (2019). Chemovariation and antibacterial activity of extracts and isolated compounds from species of Ixora and Greenea (Ixoroideae, Rubiaceae). PeerJ 7, e6893. doi: 10.7717/peerj.6893
Cha, J., Han, S., Hong, H., Cho, H., Kim, D., Kwon, Y., et al. (2016). Microbial and biochemical basis of a fusarium wilt-suppressive soil. ISME J. 10, 119–129. doi: 10.1038/ismej.2015.95
Cressey, P., Reeve, J. (2019). Metabolism of cyanogenic glycosides: a review. Food Chem. Toxicol. 125, 225–232. doi: 10.1016/j.fct.2019.01.002
Di Pasqua, R., Betts, G., Hoskins, N., Edwards, M., Ercolini, D., Mauriello, G. (2007). Membrane toxicity of antimicrobial compounds from essential oils. J. Agric. Food Chem. 55, 4863–4870. doi: 10.1021/jf0636465
Egbuna, C., Sawicka, B., Tijjani, H., Kryeziu, T. L., Ifemeje, J. C., Skiba, D., et al. (2020). Natural Remedies for Pest, Disease and Weed Control. (New York: Academic Press) 43–53. doi: 10.1016/B978-0-12-819304-4.00004-X
Fall, L. A., Salazar, M. M., Drnevich, J., Holmes, J. R., Tseng, M. C., Kolb, F. L., et al. (2019). Field pathogenomics of fusarium head blight reveals pathogen transcriptome differences due to host resistance. Mycologia 111, 563–573. doi: 10.1080/00275514.2019.1607135
Fernandes, L. B., Ghag, S. B. (2022). Molecular insights into the jasmonate signaling and associated defense responses against wilt caused by Fusarium oxysporum. Plant Physiol. Biochem. 174, 22–34. doi: 10.1016/j.plaphy.2022.01.032
Fu, Y., Liu, T., Wang, X., Wang, Y., Gong, Q., Li, G., et al. (2023). Untargeted metabolomics reveal rhizosphere metabolites mechanisms on continuous ramie cropping. Front. Plant Sci. 14. doi: 10.3389/fpls.2023.1217956
Gai, X., Jiang, N., Lu, C., Hu, Y., Ma, J., Wang, J., et al. (2023). First report of tobacco fusarium root rot caused by Fusarium meridionale in China. Plant Dis. 107, 2553 doi: 10.1094/pdis-12-22-2957-pdn
García, V. (2015). Report of new varieties ‘Burley Pinar 2010’: first cultivar of burley tobacco Cuban. resistant to fusarium wilt (Fusarium oxysporum) and other diseases of importance in the crop. Cultivos Tropicales 36, 91. Available at: https://ediciones.inca.edu.cu/index.php/ediciones/article/view/971.
Gnonlonfin, G. J. B., Sanni, A., Brimer, L. (2012). Review scopoletin–a coumarin phytoalexin with medicinal properties. Crit. Rev. Plant Sci. 31, 47–56. doi: 10.1080/07352689.2011.616039
Gordon, T. R. (2017). Fusarium oxysporum and the fusarium wilt syndrome. Annu. Rev. Phytopathol. 55, 23–39. doi: 10.1146/annurev-phyto-080615-095919
Hu, K., Li, R., Mo, F., Ding, Y., Zhou, A., Guo, X., et al. (2023). Natural product osthole can significantly disrupt cell wall integrity and dynamic balance of Fusarium oxysporum. Pestic. Biochem. Physiol. 196, 105623. doi: 10.1016/j.pestbp.2023.105623
Kazemizadeh, A. R., Shajari, N., Shapouri, R., Adibpour, N., Teimuri-Mofrad, R. (2016). Synthesis and anti-brucella activity of some new 1,3,4-oxadiazole derivatives containing a ferrocene unit. J. Iranian Chem. Soc. 13, 1349–1355. doi: 10.1007/s13738-016-0849-3
LaMondia, J. A. (2015). Fusarium wilt of tobacco. Crop Prot. 73, 73–77. doi: 10.1016/j.cropro.2015.03.003
Li, P., Gu, S., Zhu, Y., Xu, T., Yang, Y., Wang, Z., et al. (2023a). Soil microbiota plays a key regulatory role in the outbreak of tobacco root rot. Front. Microbiol. 14, 1214167. doi: 10.3389/fmicb.2023.1214167
Li, X., Yao, C., Qiu, R., Bai, J., Liu, C., Chen, Y., et al. (2023b). Isolation, identification, and evaluation of the biocontrol potential of a Bacillus velezensis strain against tobacco root rot caused by Fusarium oxysporum. J. Appl. Microbiol. 134. doi: 10.1093/jambio/lxac049
Lu, J., Chen, H., Yang, Z., Sun, S., Luo, Q., Xie, J., et al. (2022). Physiological and molecular mechanisms of the response of roots of Pinus massoniana Lamb. to low-temperature stress. Front. Plant Sci. 13. doi: 10.3389/fpls.2022.954324
Lv, C., Wang, C., Cai, A., Zhou, Z. (2023). Global magnitude of rhizosphere effects on soil microbial communities and carbon cycling in natural terrestrial ecosystems. Sci. Total Environ. 856, 158961. doi: 10.1016/j.scitotenv.2022.158961
Ma, L. J., Geiser, D. M., Proctor, R. H., Rooney, A. P., O’Donnell, K., Trail, F., et al. (2013). Fusarium pathogenomics. Annu. Rev. Microbiol. 67, 399–416. doi: 10.1146/annurev-micro-092412-155650
Macegoniuk, K., Kowalczyk, R., Rudzińska, A., Psurski, M., Wietrzyk, J., Berlicki, Ł. (2017). Potent covalent inhibitors of bacterial urease identified by activity-reactivity profiling. Bioorgan. Medicinal Chem. Lett. 27, 1346–1350. doi: 10.1016/j.bmcl.2017.02.022
Mazurier, S., Corberand, T., Lemanceau, P., Raaijmakers, J. M. (2009). Phenazine antibiotics produced by fluorescent pseudomonads contribute to natural soil suppressiveness to fusarium wilt. ISME J. 3, 977–991. doi: 10.1038/ismej.2009.33
Michielse, C. B., Rep, M. (2009). Pathogen profile update: Fusarium oxysporum. Mol. Plant Pathol. 10, 311–324. doi: 10.1111/j.1364-3703.2009.00538.x
Peng, Q., Waqas Younas, M., Yang, J., Zhu, H., Miao, J., Gu, B., et al. (2022). Characterization of prochloraz resistance in Fusarium fujikuroi from heilongjiang province in China. Plant Dis. 106, 418–424. doi: 10.1094/pdis-02-21-0372-re
Qiu, R., Fang, W., Li, C., Zhang, Y., Liu, C., Li, X., et al. (2023). First report of Fusarium tricinctum species complex causing root rot of tobacco in China. Plant Dis. 107, 2886 doi: 10.1094/pdis-12-22-2924-pdn
Qiu, R., Li, Q., Li, J., Dong, N., Li, S. J., Guan, W., et al. (2021b). First report of fusarium root rot of tobacco caused by Fusarium sinensis in Henan province China. Plant Dis. 105, 3305 doi: 10.1094/pdis-11-20-2466-pdn
Qiu, R., Li, X., Li, C., Li, C., Xue, C., Fang, W., et al. (2022). First report of tobacco root rot caused by Fusarium falciforme in China. Plant Dis 107, 960. doi: 10.1094/pdis-06-22-1394-pdn
Qiu, R., Li, J., Zheng, W., Su, X., Xing, G., Li, S., et al. (2021a). First report of root rot of tobacco caused by Fusarium brachygibbosum in China. Plant Dis. 105, 4170 doi: 10.1094/pdis-01-21-0077-pdn
Raaijmakers, J. M., Weller, D. M. (1998). Natural plant protection by 2,4-diacetylphloroglucinol-producing Pseudomonas spp. in Take-All Decline Soils. Mol. Plant-Microbe Interactions® 11, 144–152. doi: 10.1094/MPMI.1998.11.2.144
Ren, G. W., Kong, F. Y., Wang, F. L. (2008). Grade and Investigation Method of Tobacco Diseases and Insect Pests, (Beijing: China standard Press) GB/T23222-2008. ICS 65.160, X 87.
Rodríguez-Molina, M. C., Palo, E., Palo, C., Espárrago, G., Verdejo, E., Torres-Vila, L. M., et al. (2007). First report of fusarium wilt of flue-cured tobacco caused by Fusarium oxysporum f. sp. batatas in Spain. Plant Dis. 91, 323. doi: 10.1094/pdis-91-3-0323c
Schrenk, D., Bignami, M., Bodin, L., Chipman, J. K., Del Mazo, J., Grasl-Kraupp, B., et al. (2019). Evaluation of the health risks related to the presence of cyanogenic glycosides in foods other than raw apricot kernels. EFSA J. 17, e05662. doi: 10.2903/j.efsa.2019.5662
Shen, H., Deng, H., Yang, Q., Pu, X., Zhang, J., Sun, D., et al. (2023). First report of fusarium root rot of tobacco caused by Fusarium fujikuroi in guangdong province, China. Plant Dis. 107, 3294 doi: 10.1094/pdis-04-23-0658-pdn
Shull, T. E., Kurepa, J., Miller, R. D., Martinez-Ochoa, N., Smalle, J. A. (2020). Inhibition of Fusarium oxysporum f. sp. nicotianae growth by phenylpropanoid pathway intermediates. Plant Pathol. J. 36, 637–642. doi: 10.5423/ppj.Nt.08.2020.0155
Sohail, M. N., O’Donnell, N. H., Kaiser, B. N., Blomstedt, C. K., Gleadow, R. M. (2023). Wounding and methyl jasmonate increase cyanogenic glucoside concentrations in Sorghum bicolor via upregulation of biosynthesis. Plant Biol. 25, 498–508. doi: 10.1111/plb.13522
Stringlis, I. A., Yu, K., Feussner, K., de Jonge, R., Van Bentum, S., Van Verk, M. C., et al. (2018). MYB72-dependent coumarin exudation shapes root microbiome assembly to promote plant health. Proc. Natl. Acad. Sci. 115, E5213–E5222. doi: 10.1073/pnas.1722335115
Tao, C., Wang, Z., Liu, S., Lv, N., Deng, X., Xiong, W., et al. (2023). Additive fungal interactions drive biocontrol of fusarium wilt disease. New Phytol. 238, 1198–1214. doi: 10.1111/nph.18793
Turrà, D., El Ghalid, M., Rossi, F., Di Pietro, A. (2015). Fungal pathogen uses sex pheromone receptor for chemotropic sensing of host plant signals. Nature 527, 521–524. doi: 10.1038/nature15516
Wang, H., Wang, M., Xia, H., Yang, S., Guo, Y., Xu, D., et al. (2013b). First report of fusarium wilt of tobacco caused by Fusarium kyushuense in China. Plant Dis. 97, 424. doi: 10.1094/pdis-09-12-0835-pdn
Wang, X., Wei, Z., Yang, K., Wang, J., Jousset, A., Xu, Y., et al. (2019). Phage combination therapies for bacterial wilt disease in tomato. Nat. Biotechnol. 37, 1513–1520. doi: 10.1038/s41587-019-0328-3
Wang, B., Yuan, J., Zhang, J., Shen, Z., Zhang, M., Li, R., et al. (2013a). Effects of novel bioorganic fertilizer produced by Bacillus amyloliquefaciens W19 on antagonism of fusarium wilt of banana. Biol. Fertil. Soils 49, 435–446. doi: 10.1007/s00374-012-0739-5
Wu, H. (2008). Effects of vanillic acid on the growth and development of Fusarium oxysporum f. sp niveum. Allelopathy J. 22, 111–121.
Yan, H., Meng, X., Lin, X., Duan, N., Wang, Z., Wu, S. (2023). Antifungal activity and inhibitory mechanisms of ferulic acid against the growth of Fusarium graminearum. Food Biosc. 52, 102414. doi: 10.1016/j.fbio.2023.102414
Yang, Y., Chen, Y., Cai, J., Liu, X., Huang, G. (2021). Antifungal activity of volatile compounds generated by endophytic fungi Sarocladium brachiariae HND5 against Fusarium oxysporum f. sp. cubense. PloS One 16, e0260747. doi: 10.1371/journal.pone.0260747
Yuan, J., Raza, W., Shen, Q., Huang, Q. (2012). Antifungal activity of Bacillus amyloliquefaciens NJN-6 volatile compounds against Fusarium oxysporum f. sp. cubense. Appl. Environ. Microbiol. 78, 5942–5944. doi: 10.1128/aem.01357-12
Zhao, Y., Li, Y., Cao, C., Yao, J., Yuan, Q., Huang, D. (2023). Research progress of tobacco fusarium root rot. China Plant Prot. 43, 20–25.
Zheng, H., Chen, Y., Guo, Q., Wei, H., Yue, J., Zhou, H., et al. (2021). Inhibitory effect of osthole from Cnidium monnieri (L.) Cusson on Fusarium oxysporum, a common fungal pathogen of potato. Molecules 26, 3818. doi: 10.3390/molecules26133818
Zheng, Z., Liu, H., Shi, Y., Liu, Z., Teng, H., Deng, S., et al. (2022). Comparative transcriptome analysis reveals the resistance regulation mechanism and fungicidal activity of the fungicide phenamacril in Fusarium oxysporum. Sci. Rep. 12, 11081. doi: 10.1038/s41598-022-15188-5
Zhong, Q., Xiao, Y., He, B., Cao, Z., Shou, Z., Zhong, J., et al. (2020). First report of fusarium commune causing stem rot of tobacco (Nicotiana tabacum) in hunan province, China. Plant Dis. 105, 1568 doi: 10.1094/pdis-07-20-1466-pdn
Keywords: Fusarium oxysporum, tobacco fusarium wilt, metabolomics, phenylpropiolic acid, linustatin, scopoletin
Citation: Yang W, Tang S, Xu R, Zhang L, Zhou Z, Yang Y, Li Y and Xiang H (2024) LC-MS based metabolomics identification of natural metabolites against Fusarium oxysporum. Front. Plant Sci. 15:1435963. doi: 10.3389/fpls.2024.1435963
Received: 21 May 2024; Accepted: 06 August 2024;
Published: 03 September 2024.
Edited by:
Brigitte Mauch-Mani, Université de Neuchâtel, SwitzerlandReviewed by:
Daniela Pontiggia, Sapienza University of Rome, ItalySantosh Watpade, Indian Agricultural Research Institute (ICAR), India
Copyright © 2024 Yang, Tang, Xu, Zhang, Zhou, Yang, Li and Xiang. This is an open-access article distributed under the terms of the Creative Commons Attribution License (CC BY). The use, distribution or reproduction in other forums is permitted, provided the original author(s) and the copyright owner(s) are credited and that the original publication in this journal is cited, in accordance with accepted academic practice. No use, distribution or reproduction is permitted which does not comply with these terms.
*Correspondence: Yanyan Li, eWFueWFubGkwMDI1QDEyNi5jb20=; Haibo Xiang, eGhiXzIwODZAMTYzLmNvbQ==
†These authors have contributed equally to this work