- School of Life Sciences, Jiangsu University, Zhenjiang, China
The epitranscriptomic mark N6-methyladenosine (m6A) is the most common type of messenger RNA (mRNA) post-transcriptional modification in eukaryotes. With the discovery of the demethylase FTO (FAT MASS AND OBESITY-ASSOCIATED PROTEIN) in Homo Sapiens, this modification has been proven to be dynamically reversible. With technological advances, research on m6A modification in plants also rapidly developed. m6A modification is widely distributed in plants, which is usually enriched near the stop codons and 3′-UTRs, and has conserved modification sequences. The related proteins of m6A modification mainly consist of three components: methyltransferases (writers), demethylases (erasers), and reading proteins (readers). m6A modification mainly regulates the growth and development of plants by modulating the RNA metabolic processes and playing an important role in their responses to environmental signals. In this review, we briefly outline the development of m6A modification detection techniques; comparatively analyze the distribution characteristics of m6A in plants; summarize the methyltransferases, demethylases, and binding proteins related to m6A; elaborate on how m6A modification functions in plant growth, development, and response to environmental signals; and provide a summary and outlook on the research of m6A in plants.
Introduction
During gene expression, various chemical modifications occur at the levels of DNA, RNA, and proteins. These chemical modifications can preserve genetic information through mechanisms such as DNA and RNA methylation and chromatin conformation changes, all without changing the base sequence (Kumar and Mohapatra, 2021). RNA plays a crucial bridging role in gene expression, and numerous chemical modifications occur on RNA, with more than 170 types discovered so far (Ramakrishnan et al., 2022). These modifications primarily include N6-methyladenosine (m6A), 5-methylcytidine (m5C), 7-methylguanosine (m7G), 1-methyladenosine (m1A), pseudouridine (Ψ), N4-acetylcytidine (ac4C), 2′-O-methylation (2′-O-methyltransferase, Nm-MTase), and N6,2′-O-dimethyladenosine (m6Am), among others (Amos and Korn, 1958; Dunn, 1961; Desrosiers et al., 1974; Stern and Schulman, 1978; Rebane et al., 2002; Luo et al., 2022; Wu et al., 2023). Among them, m6A is one of the most abundant chemical modifications on eukaryotic messenger RNA (mRNA), found throughout fungi, animals, and plants (Fu et al., 2014; Deng et al., 2015; Sergiev et al., 2016). Furthermore, m6A modification is distributed across various cellular organelles (nucleus, chloroplasts, and the mitochondria) and RNAs (mRNA, non-coding RNA, rRNA, and tRNA) (Cohn and Volkin, 1951; Wang et al., 2017b; Murik et al., 2020). Studies have revealed that m6A modification often occurs on the specific motif RRACH (R=G/A, G>A; H=A, C, U), while m6A modification in plants also appears on the conserved motif URUAY (Y=A, G, U, or C) (Deng et al., 2018; Cheng et al., 2021). Similar to DNA and histone chemical modifications, m6A modification is also dynamically reversible and can be regulated in time and space by methyltransferases and demethylases (Jia et al., 2011). Existing studies have demonstrated that m6A modification is involved in the entire growth and development processes, from seed germination to senescence (Rudy et al., 2022; Hu et al., 2022a; Song et al., 2023; Luo et al., 2024). This article systematically reviews the advancement of m6A sequencing techniques; the distribution characteristics of m6A modification in plants; the m6A-related regulatory proteins; and the vital roles of m6A in plant growth, development, and response to environmental signals.
Development of m6A modification detection techniques
The modification of m6A was first discovered in the mRNA of mammalian cells in the 1970s (Desrosiers et al., 1974). Subsequently, it was also found in plants, such as wheat and corn (Kennedy and Lane, 1979; Nichols, 1979). However, due to technical limitations, m6A modification did not receive much attention for many years. Initially, researchers could only detect m6A in the hydrolysis products of RNA, without the ability to identify which specific RNA the m6A modification originated. Furthermore, limitations in the purification methods made it difficult to exclude the possibility of contamination by RNA types other than mRNA, leading to inaccuracies in the detection of m6A (Meyer and Jaffrey, 2017). Another challenge was that, as m6A does not affect the binding ability of adenosine to thymine or uracil, it could not be readily detected using conventional hybridization or sequencing-related methods. Instead, its detection relies on specific ribonuclease digestion and chromatographic analysis techniques (Schibler et al., 1977; Zhong et al., 2008; Meyer et al., 2012).
The study of m6A modification entered a new era following the discovery of the first RNA demethylase, the FAT MASS AND OBESITY-ASSOCIATED PROTEIN (FTO), when it became evident that the modification of m6A is dynamically reversible. The field of m6A has emerged as a prominent research focus, and the technology for its detection has been rapidly and iteratively updated (Jia et al., 2011). In 2012, two research groups independently introduced a novel m6A sequencing technique, known as m6A-seq or MeRIP-seq (methylated RNA immunoprecipitation sequencing) (Dominissini et al., 2012; Meyer et al., 2012). The primary steps involve fragmenting RNA into 100- to 200-bp segments, enriching those fragments containing m6A using a specific antibody, and subsequently performing reverse transcription sequencing to obtain the sequences of the RNAs harboring m6A. This technique revolutionized the research on m6A by enabling high-throughput sequencing, propelling the study of m6A modification into a new era of rapid development. In recent years, updated sequencing techniques have primarily focused on two areas of optimization: firstly, reducing the initial input of RNA and, secondly, enhancing the resolution of m6A detection. Here, we describe several representative methods and their brief steps (Figure 1).
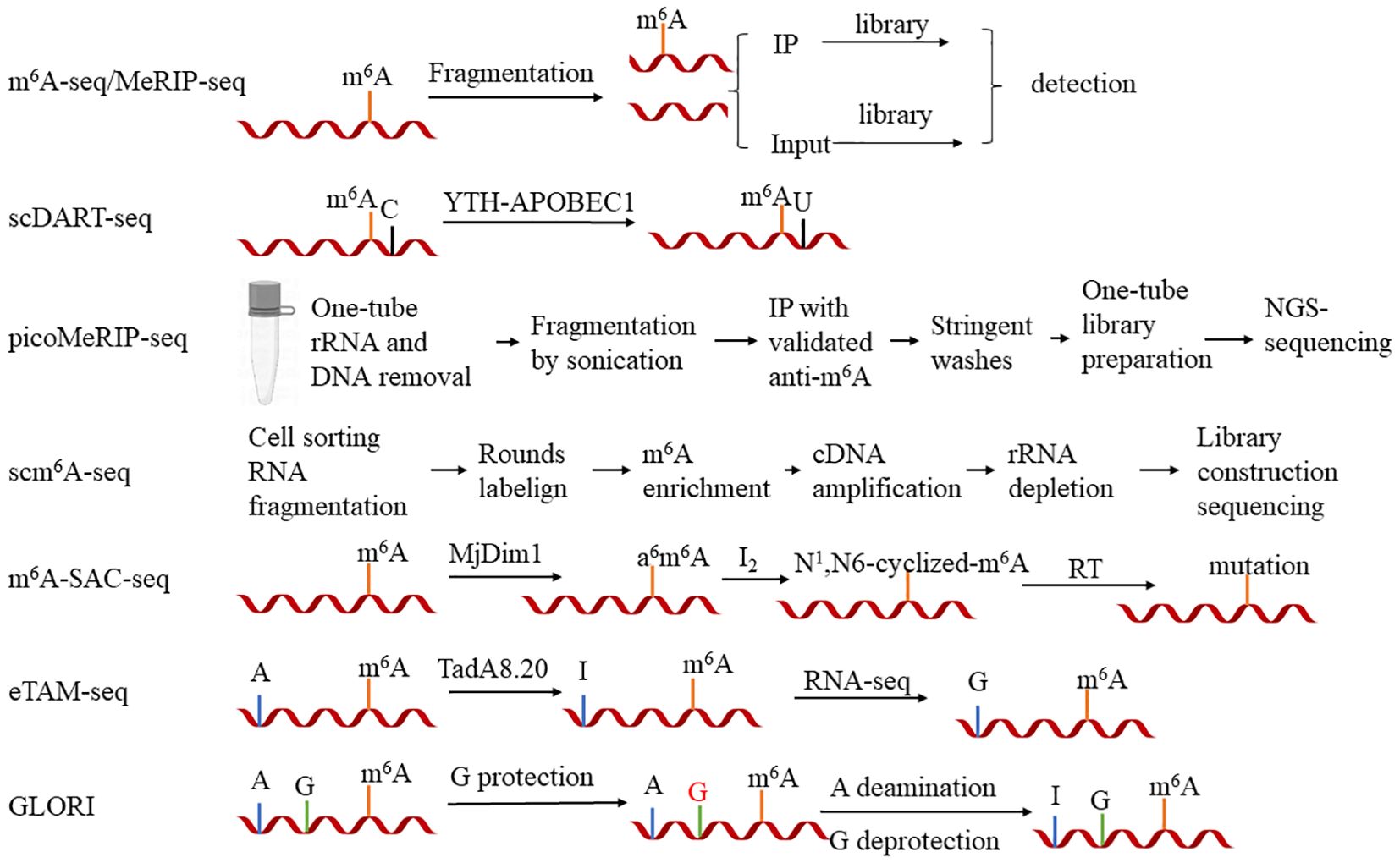
Figure 1 The primary steps of seven major high-throughput sequencing methods for N6-methyladenosine (m6A) research. 1) m6A-seq/MeRIP-seq: Initially, the RNA is fragmented, and m6A-modified RNA is enriched using antibodies (IP) and without antibodies (Input). Libraries are constructed for both groups and compared. Differences indicate the regions containing m6A modifications. 2) m6A-SAC-seq: m6A modifications are incorporated into MjDim1, which is then enzymatically converted to a6m6A. This is followed by a reaction with iodine monochloride to form N1,N6-cyclized m6A. Detection of the mutated sites through reverse transcription and sequencing can help identify the m6A sites. 3) eTAM-seq: The addition of TadA8.20 to RNA can deaminate regular adenine to inosine, while adenine modified with m6A is resistant to conversion by TadA8.20. Sequencing identifies the unconverted adenine, thus recognizing the m6A modification sites. 4) GLORI: Firstly, guanosine is protected, and then nitrous acid is used to deaminate regular adenine to inosine, with m6A remaining unreactive. Subsequently, the protection on guanosine is removed, and sequencing reads the m6A sites that did not participate in the reaction. 5) scDART-seq: The YTH-APOBEC1 protein recognizes the cytosines adjacent to the m6A sites and deaminates them to uracil. Sequencing detects the signal of cytosine to uracil conversion, identifying the m6A sites. 6) picoMeRIP-seq: A single cell is placed in a microtube to remove rRNA and DNA, and RNA fragments are generated by sonication. Subsequently, antibodies are used to enrich the m6A-modified RNA and the RNA without antibodies, followed by the elution of RNA with sodium salt. Finally, libraries are constructed in microtubes and sequencing identifies the differential peaks. 7) scm6A-seq: Cells are distributed into a 96-well plate and RNA is fragmented. This is followed by two rounds of labeling. Subsequently, the RNA is pooled into a single tube for m6A-seq.
In terms of reducing the amount of sample inputs, there are several ways to optimize the sequencing technology. scDART-seq (single-cell deamination adjacent to RNA modification target sequencing) utilizes the APOBEC1 and YTH complex to convert the cytidines adjacent to m6A into uridines. Sequencing detects the transition of cytidine to uridine, thereby pinpointing the m6A sites (Tegowski et al., 2022). Although this technique can detect m6A modification at the single-cell level, it cannot identify the m6A sites lacking adjacent cytidines. picoMeRIP-seq (picogram-scale m6A RNA immunoprecipitation and sequencing) has optimized MeRIP-seq in areas such as cell lysis strategy, RNA fragmentation method, and RNA elution conditions post-antibody binding, rendering it suitable for the detection of RNA modification with low starting amounts of RNAs/cells (Li et al., 2023b). scm6A-seq (single-cell m6A sequencing) combines a multiplex labeling approach with the MeRIP-seq principles, enabling concurrent transcriptome and m6A methylome sequencing within a single cell. This approach significantly diminishes the initial RNA input and enables sequencing at the single-cell level (Yao et al., 2023a).
In terms of enhancing the resolution of m6A detection, numerous novel techniques have emerged. The PA-m6A-seq (photo-cross-linking-assisted m6A sequencing) strategy initially treats samples with 4-thiouridine (4sU), incorporating 4sU into the RNA samples (4sU induces thymine-to-cytidine mutations at the cross-linking sites). Subsequently, the sample is incubated with an m6A antibody to bind to the full-length RNA containing 4sU. UV light at 365 nm is then utilized to induce the cross-linking between the RNA labeled with 4sU and containing m6A and the m6A antibody. Following this, RNase T1 is employed to digest the RNA into fragments of approximately 30 bp, which are subsequently sequenced (Chen et al., 2015). This method enhances the resolution of m6A-seq from 100–200 bp to approximately 30 bp. However, this technique may overlook the m6A modifications proximal to the 4sU incorporation sites. m6A-SAC-seq (m6A selective allyl chemical labeling and sequencing) utilizes an enzymatic reaction to convert m6A to a6m6A. This is further reacted with iodine and then reverse transcribed. During the reverse transcription, m6A is interpreted as a mutant base, enabling the detection of the m6A sites as mutant bases through sequencing (Hu et al., 2022c; Ge et al., 2023). This reaction employs an enzymatic method for detection and may exhibit uncertain sequence preferences. Another quantitative technique is eTAM-seq (evolved TadA-assisted N6-methyladenosine sequencing), which utilizes the deaminase TadA8.20 to convert normal adenosines into inosines. During sequencing, the inosines are misread as guanosines, whereas m6A remains unchanged and is still interpreted as adenosine. Through this process, the m6A sites can be identified. Notably, eTAM-seq exhibits reduced sensitivity to sites with low methylation levels (Xiao et al., 2023). The GLORI (glyoxal- and nitrite-mediated deamination of unmethylated adenosines) technique employs a system catalyzed by glyoxal and nitrite salts to efficiently deaminate the unmethylated adenosines into inosines (A-to-I, >98%). During sequencing, the inosines are interpreted as guanosines (G), resulting in A-to-G conversions. m6A, however, is still read as an adenosine. GLORI achieves absolute quantification of m6A at single nucleotide resolution by analyzing the proportion of adenosines in the sequence reads. One drawback of GLORI is its relatively high sequencing cost compared with that of MeRIP or m6A-seq (Liu et al., 2023).
With the rapid development of m6A sequencing technologies, the accuracy of sequencing has continuously improved and the demand for RNA samples has also significantly decreased. Essentially, highly efficient, highly sensitive, highly specific, and unbiased single-nucleotide m6A site detection has been achieved. The progress in detection technologies has also greatly propelled the research into m6A modification. The distribution characteristics and the biological functions of m6A have been rapidly revealed.
Distribution of m6A in plants
m6A is widely found in fungi, animals, and plants (Deng et al., 2015; Sergiev et al., 2016). In plants, m6A modification is distributed across the start codon, the stop codon, the coding sequence (CDS), and the 5′-UTR and 3′-UTR of mRNAs. However, this distribution is not random, and different species exhibit distinct tendencies and patterns (Table 1). In Arabidopsis, rice, tomato, maize, tea tree, wolfberry, and sea buckthorn, m6A modifications are primarily enriched around the stop codon and 3′-UTR regions (Wan et al., 2015; Du et al., 2020; Cheng et al., 2021; Zhang et al., 2021a; Hu et al., 2022a; Zhao et al., 2023; Zhu et al., 2023). In watermelon and apple, m6A modifications are enriched in the CDS (Mao et al., 2021; Hou et al., 2022). Notably, in Arabidopsis, pear, rice, and soybean, there is a tendency for m6A modifications to be enriched around the start codon as well (Luo et al., 2014; Li et al., 2014b; Han et al., 2021; Zhang et al., 2023a). In coastal pine, m6A modifications are enriched in the 5′-UTR region (Ortigosa et al., 2022).
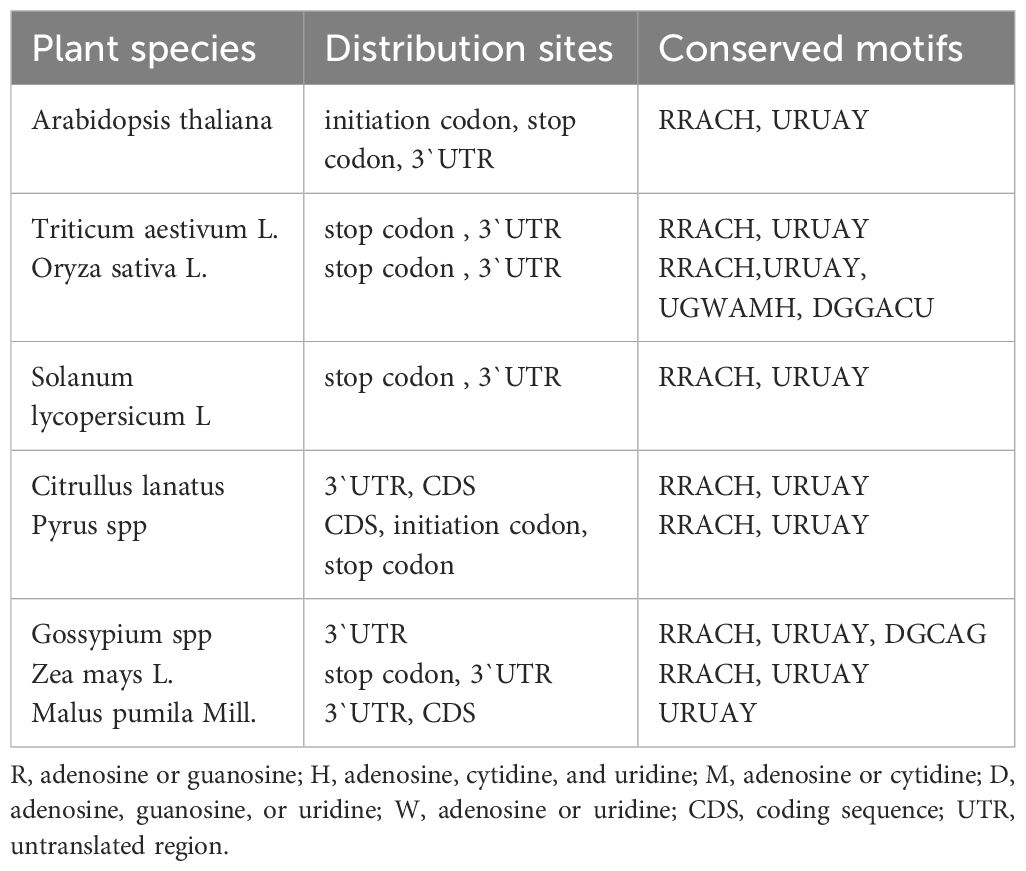
Table 1 Distribution and conserved motifs of N6-methyladenosine (m6A) modification in different plants.
The conserved m6A sequence motif in plants is identical to that in eukaryotes, being RRACH (R=G or A; H=A, C, or U) (Meyer et al., 2012). However, recent studies have identified plant-specific conserved sequences, such as URUAY (Y=A, G, U, or C), which has been detected in Arabidopsis, rice, wheat, tomato, maize, tea tree, wolfberry, and cotton (Wan et al., 2015; Zhou et al., 2019; Du et al., 2020; Zhu et al., 2021; Zhang et al., 2021b, d; Zhao et al., 2023; Li et al., 2023a). In addition, rice exhibits specific conserved sequences, including UGWAMH (W=U or A; M=C or A; H=U, A, or C), CGVCGRC (V=A/C/G; R=A/G), and DGGACU (D=A/G/U) (Zhang et al., 2019; Wang et al., 2022c). In Chlamydomonas reinhardtii mRNA, m6A modifications predominantly occur within the conserved sequence DRAC (D=G/A/U; R=A/G) (Lv et al., 2022). Furthermore, cotton has recently revealed conserved sequences such as DGCAG (D=A/G/U) and the 5′-UTR enriched sequence CAAUG (Li et al., 2023a).
m6A modification-related proteins
The modification of m6A methylation, akin to chemical modifications of DNA and histone, is also dynamic and reversible. This process involves a complex of methyltransferases, reading proteins, and demethylases, which are responsible for writing, reading, and erasing the modification, respectively (Figure 2).
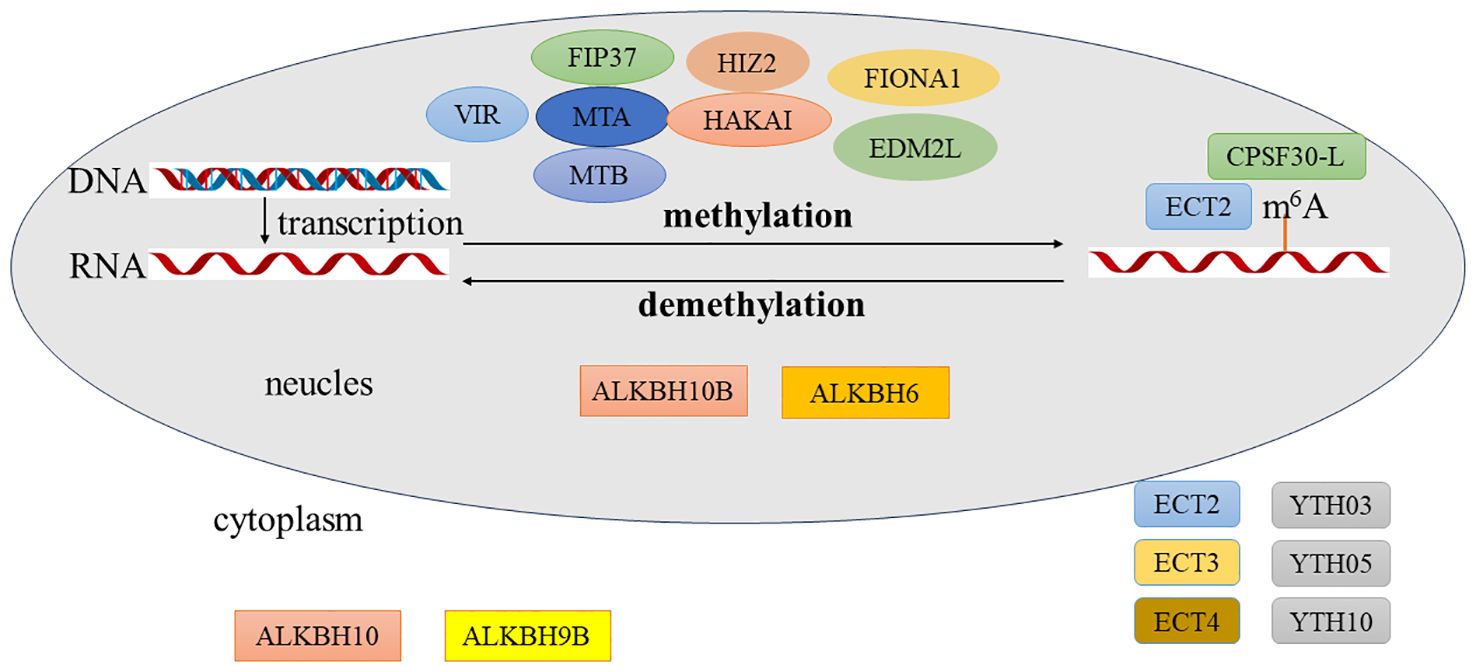
Figure 2 Process of N6-methyladenosine (m6A) modification in plants. The methyltransferases involved in m6A modification include FIP37, MTA, MTB, VIR, HIZ2, and EDM2. The demethylases include ALKBH10, ALKBH9B, and ALKBH6. The reading proteins include ECT2, ECT3, ECT4, YTH03, YTH04, YTH05, and CPSF30-L. The methyltransferases for m6A modification are all located in the cell nucleus. The demethylase ALKBH10B is found in both the cell nucleus and the cytoplasm, ALKBH6 is located in the cell nucleus, and ALKBH9B is in the cytoplasm. The reading protein ECT2 is located in both the cell nucleus and the cytoplasm, CPSF30-L is in the cell nucleus, and the rest are in the cytoplasm.
Writers
In mammals, the m6A methyltransferase complex (also known as the m6A writer) is composed of METTL3, METTL14, WTAP, and other proteins. METTL3 and METTL14 can form a heterodimer, and WTAP interacts with this dimer to achieve methylation (Liu et al., 2014; Ping et al., 2014). In addition, researchers successively discovered several enzymes involved in the methylation of m6A: METTL16, VIRMA (vir-like m6A methyltransferase associated), HAKAI (E3 ubiquitin-protein ligase Hakai), RBM15 (RNA-binding motif 15), and ZC3H13 (zinc finger CCCH domain-containing protein 13) (Horiuchi et al., 2013; Liu et al., 2014; Ping et al., 2014; Schwartz et al., 2014; Patil et al., 2016; Wen et al., 2018). These enzymes are collectively referred to as the m6A/METTL-associated complex (MACOM) (Knuckles et al., 2018).
In plants, researchers discovered the methyltransferase component MTA (methyltransferase A) in Arabidopsis. MTA is a homolog of METTL3 and participates in plant embryo development (Luo et al., 2024). They also found its interacting protein, FIP37 (FKBP12 interacting protein, 37 kDa) (Zhong et al., 2008). In fact, FIP37 was discovered in Arabidopsis as early as 2004 and was found to regulate embryo development. Subsequent research revealed that FIP37 is also a methyltransferase component and a homolog of WTAP (Vespa et al., 2004; Shen et al., 2016). In addition, MTB is the plant homolog of METTL14. VIR (virilizer) is the plant homolog of VIRMA, and the plant homolog of HAKAI is also named HAKAI (Růžička et al., 2017). HIZ2 (HAKAI-interacting zinc finger protein 2) is the plant homolog of ZC3H13, which has been found to be associated with lateral root formation in Arabidopsis (Zhang et al., 2022a). FIONA1, the plant homolog of METTL16, was discovered by several research groups to be related to flowering, chlorophyll homeostasis, and salt stress in Arabidopsis (Sun et al., 2022; Xu et al., 2022; Wang et al., 2022a; Jiang et al., 2023; Cai et al., 2024a). Currently, no homolog of RBM15 has been found in plants. Furthermore, a plant-specific m6A methyltransferase, EDM2L, was discovered in rice, which participates in the regulation of pollen development (Ma et al., 2021).
Like in animals, the m6A methyltransferases in plants exist in complex form and interact with each other. As for the five methyltransferase components in Arabidopsis—FIP37, MTA, MTB, VIR, and HAKAI—they do not significantly affect each other at the transcriptional level. However, at the protein level, these components mutually influence each other, promoting each other’s protein accumulation, and they cannot functionally substitute for one another (Shen, 2023).
Erasers
The methylation can be removed by demethylases, which are known as m6A erasers. In 2011, two mammalian m6A demethylases, i.e., FTO and ALKBH5, were discovered successively (Jia et al., 2011; Zheng et al., 2013). These enzymes belong to the divalent iron and α-ketoglutarate-dependent dioxygenase AlkB family. They initially oxidize m6A to form N6-hydroxymethyladenosine (hm6A), subsequently convert hm6A to N6-formyladenosine (f6A), and ultimately transform f6A into adenosine (A), thus completing the demethylation process (Wang et al., 2020). Nine proteins belonging to the AlkB family have been discovered in humans, including ALKBH1–8 and FTO. The identification of m6A demethylases in plants is highly significant, as it would directly demonstrate that m6A plays a dynamic and reversible regulatory role in plants. While no FTO homologs have been found in plants, there are 13 homologs of the AlkB family in Arabidopsis (Mielecki et al., 2012). Of these, AtALKBH10B, AtALKBH6, and AtALKBH9C have been identified as possessing demethylation functions (Duan et al., 2017; Huong et al., 2020; Amara et al., 2022). Furthermore, CsALKBH4 in tea, CfALKBH5 in Catalpa fargesii, SlALKBH2 and SlALKBH10B in tomato, GhALKBH10 and GhALKBH10B in cotton, OsALKBH9 in rice, PagALKBH9B and PagALKBH10B in Populus, LbALKBH10 in wolfberry, and HrALKBH10B, HrALKBH10C, and HrALKBH10D in sea buckthorn have also been identified as having demethylation functions (Zhou et al., 2019; Zhang et al., 2021a; Cui et al., 2022; Zhao et al., 2022, 2023; Zhu et al., 2023; Li et al., 2023a; Shen et al., 2023a; Zhang et al., 2023c; Tang et al., 2024). Given the numerous AlkB family homologs in plants and the diverse functions of the AlkB family proteins, the identification of more m6A demethylases requires extensive and detailed research.
Readers
Methylation can be recognized by m6A-binding proteins, known as m6A readers. Knocking out or overexpressing methyltransferases and demethylases results in various phenotypes caused by changes in the m6A levels, providing proof that m6A plays an important role in biological growth and development processes. However, to understand the specific molecular mechanism by which m6A functions, it is crucial to determine how the m6A reader proteins operate. Most of the currently discovered m6A reading proteins contain the YTH (YT512-B homology) structural domain. Initially, the YTH structural domain was only regarded as an ordinary RNA-binding structural domain (Zhang et al., 2010). Subsequently, it was discovered that this structural domain recognizes m6A modifications (Wang et al., 2014a). The YTH structural domain contains a hydrophobic functional domain composed of aromatic amino acid residues, which enhances the affinity of the reading protein for m6A, allowing the protein to recognize m6A modifications (Luo and Tong, 2014; Arribas-Hernández et al., 2020).
The YTH structural domain family proteins constitute a highly conserved protein family in eukaryotic cells. Bioinformatics analysis has revealed the existence of YTH family proteins in humans, mice, fruit flies, yeast, Arabidopsis, and rice, with plants being particularly abundant in them. In Arabidopsis, the YTH structural domain is called the evolutionarily conserved C-terminal region (ECT) domain, encompassing a total of 13 members, named ECT1–ECT12 and CPSF30 (Ok et al., 2005; Li et al., 2014a). Of these, ECT2 was the first m6A reader protein discovered in plants that possesses the YTH structural domain. ECT2 binding sites are strongly enriched in the 3′-UTRs of target genes, and their function is tied to trichome morphology (Wei et al., 2018). Subsequent studies conducted by the same laboratory revealed that, in Arabidopsis, ECT2, ECT3, and ECT4 directly interact with each other in the cytoplasm and perform genetically redundant functions in the regulation of abscisic acid (ABA) response during seed germination and post-germination growth (Song et al., 2023). In addition, ECT2, ECT3, and ECT4 are also involved in normal leaf morphogenesis and the rate of leaf formation (Arribas-Hernández et al., 2020). ECT8 serves as a crucial checkpoint for the negative feedback regulation of ABA signaling by sequestering the m6A-modified ABA receptor gene PYRABACTIN RESISTANCE 1-LIKE 7 (PYL7) through phase-separated ECT8 condensates in stress granules in response to ABA (Wu et al., 2024). In Arabidopsis, the AtCPSF30 (30-kDa cleavage and polyadenylation specificity factor 30) gene encodes two differently sized proteins, CPSF30-S and CPSF30-L, via alternative polyadenylation (APA) regulation after transcription. CPSF30-L comprises CPSF30-S and an m6A-binding YTH domain (Hou et al., 2021). CPSF30-L, as an Arabidopsis m6A reader, requires its m6A-binding function for floral transition and ABA response (Song et al., 2021). Moreover, ECT1 can be recruited to ECT9 condensates and plays a negative role in plant immunity (Wang et al., 2023a). ECT12 binds to m6A-modified stress-responsive transcripts and plays a crucial role in the response to salt or dehydration stress (Amara et al., 2024). In rice, YTH03, YTH05, and YTH10 specifically bind to m6A-containing RNAs and regulate the plant height of rice in a functionally redundant manner. Furthermore, YTH07 can physically interact with EHD6, and it triggers the relocation of a portion of YTH07 from the cytoplasm into RNP granules through phase-separated condensation, leading to accelerated flowering (Cui et al., 2024). In apple, the YTH domain-containing RNA-binding protein 1 (MhYTP1) and MhYTP2 have functions in leaf senescence and fruit ripening and confer tolerance to multiple abiotic stresses (Wang et al., 2017a).
In addition, the insulin-like growth factor 2 mRNA-binding proteins (IGF2BPs), which contain four KH domains but lack YTH domains, have been demonstrated to form a distinct family of m6A readers that recognize the consensus motif GG(m6A)C (Huang et al., 2018). Studies have found that IGF2BPs are associated with mRNA stability and tumorigenesis (Ying et al., 2021). Based on amino acid sequence similarity, FLK was identified as an Arabidopsis homolog of IGF2BP, regulating floral transition by repressing the levels of a key floral repressor, FLOWERING LOCUS C (FLC), in Arabidopsis. FLK directly binds to the FLC mRNA and regulates the expression of FLC in an m6A-dependent manner (Amara et al., 2023).
Biological functions of m6A
As the most abundant internal modification on mRNA in eukaryotes, m6A can affect various RNA metabolic processes, including mRNA stability, precursor RNA splicing, polyadenylation, mRNA transport, and translation initiation (Zaccara et al., 2019). In human and animal cells, m6A modification participates in important physiological processes, such as tumor and cardiovascular disease development and osteocyte differentiation (Huang et al., 2021). Although research on the modification of m6A in plants started relatively late, numerous studies in recent years have demonstrated that it plays a crucial role in plant growth and development, biotic and abiotic stress responses, and crop trait improvement (Shao et al., 2021; Shen et al., 2023b). In particular, when plants are subjected to external environmental stresses, the dynamic and reversible changes in m6A modification can rapidly regulate gene expression, thereby conferring strong environmental adaptability to plants (Hu et al., 2022b).
m6A affects mRNA metabolism
In mammalian cells, numerous studies have demonstrated that m6A modification is linked to mRNA metabolism (Wang et al., 2014b). With the increasing research interest in plant m6A in recent years, it has also been confirmed that m6A is linked to RNA metabolism in plants. Studies have found that following the mutation of AtMTA, the level of m6A decreases, and the rate of degradation of the mRNA encoding the core component of the molecular oscillator circadian clock associated 1 (CCA1) accelerates (Wang et al., 2021). Research has also shown that the disruption of ALKBH10B elevates the m6A modification levels of FT, SPL3, and SPL9 mRNAs, accelerating their degradation (Duan et al., 2017). Moreover, the CPSF30-L protein primarily recognizes the m6A-modified far-upstream elements to control the choice of the polyadenylation site, lengthens the 3′-UTRs of transcripts, and thereby accelerates their mRNA degradation (Hou et al., 2021; Song et al., 2021). Another study discovered that R-loops are structures formed by the hybridization of RNA and DNA and that m6A modification in Arabidopsis can affect the strength of R-loops and promote gene transcription (Thomas et al., 1976; Zhang et al., 2021c). Furthermore, m6A modification can stabilize mRNA by inhibiting the cleavage action of local ribonucleases (Anderson et al., 2018). In Arabidopsis, the reader protein ECT12 plays a crucial role in modulating the stability of the m6A-marked RNA transcripts, thereby enhancing the ability of plants to cope with abiotic stresses, such as salt and drought (Amara et al., 2024). The regulation of mRNA stability by FIONA1-mediated m6A methylation also influences the expression of the genes involved in salt stress response (Cai et al., 2024a). On the other hand, as an “eraser,” ALKBH10B contributes to drought resistance by promoting the stability of transcripts, and the impact of OsALKBH9 on pollen development is also linked to its mediation of mRNA stability (Han et al., 2023a; Tang et al., 2024).
Current research indicates that there is also an important connection between the location of the m6A modification sites on mRNA and mRNA stability. Generally, m6A in the 3′-UTR tends to be negatively correlated with the gene expression levels, while m6A at the 5′ end is positively correlated with gene expression. VIR affects the elongation of 3′-UTR through alternative polyadenylation, thereby negatively regulating the mRNA stability of several salt stress-negative regulators and modulating the homeostasis of reactive oxygen species (ROS) (Hu et al., 2021). In strawberry, m6A modification in CDS regions appears to be ripening-specific and tends to stabilize the mRNAs, whereas m6A around the stop codons and within the 3′-UTRs is generally negatively correlated with the abundance of associated mRNAs. FLK, as an m6A reader protein, directly binds to a site in the 3′-UTR of FLC transcripts, repressing the FLC levels by reducing its stability and splicing (Amara et al., 2023).
m6A modification is also involved in mRNA translation and alternative splicing. In wheat, the m6A in the CDS and 3′-UTR inhibits mRNA translation, while that in the 5′-UTR and start codon can promote mRNA translation (Huang et al., 2022a). In Arabidopsis, FIONA1 regulates the accuracy and efficiency of U6 snRNA splicing (Parker et al., 2022). Genes with methylation in the 3′-UTR in soybean have higher expression levels and are more prone to alternative splicing (Zhang et al., 2023a). FLK directly binds to the m6A sites in the 3′-UTR of FLC transcripts, suppressing the levels of FLC by decreasing the transcript stability and splicing (Guo et al., 2022; Amara et al., 2023). The rice EDM2L altered the transcriptomic m6A landscape and caused a distinct m6A modification of the EAT1 transcript, leading to the dysregulation of its alternative splicing and to polyadenylation. This, in turn, affects anther development in rice (Ma et al., 2021).
Role of m6A in plant growth and development
m6A plays a crucial role throughout the plant life cycle, encompassing seed germination, embryo development, and root, stem, and leaf growth. This is in addition to its involvement in flowering and fruit maturation, all of which rely on precise regulation through m6A modification (Figure 3).
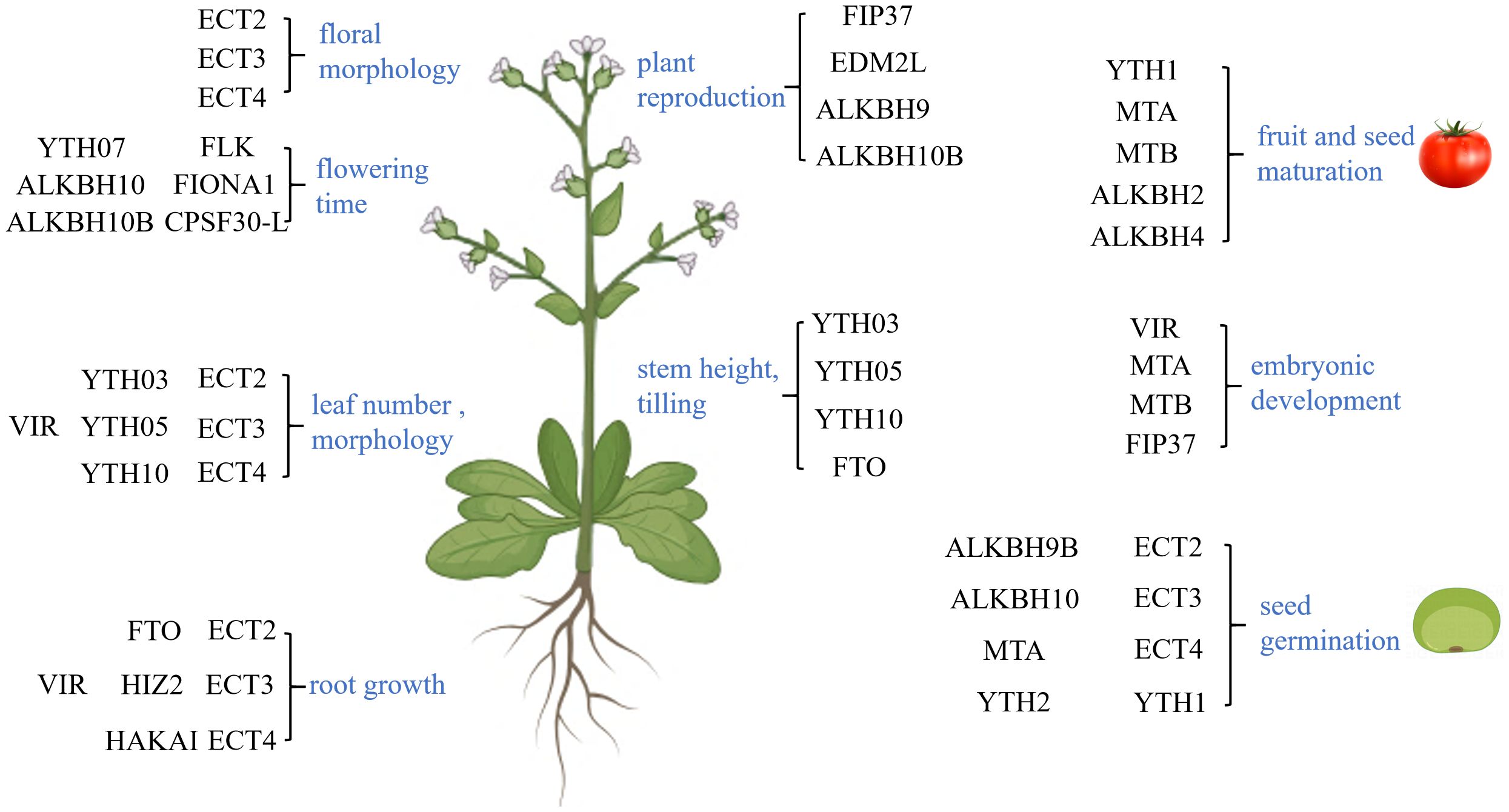
Figure 3 Function of N6-methyladenosine (m6A) in plant growth and development. m6A primarily regulates the flower morphology, flowering time, leaf number and morphology, root growth, plant reproduction, stem height, tilling, fruit and seed maturation, embryonic development, and seed germination, which are marked in blue. These functions are primarily achieved through its associated proteins.
m6A modification can influence floral morphology. Research has demonstrated that ect2/ect3/ect4 mutants exhibit a slower flower formation and an aberrant flower morphology (Arribas-Hernández et al., 2020). Furthermore, m6A modification regulates the flowering time. FIONA1 regulates floral transition by influencing the splicing of FLC and the stability of the floral activators SPL3 and SEP3 (Sun et al., 2022; Xu et al., 2022; Wang et al., 2022a; Cai et al., 2023a). In addition, the ALKBH10B-mediated mRNA demethylation enhances the mRNA stability of FLOWERING LOCUS T (FT), SPL3, and SPL9, thereby regulating flowering (Duan et al., 2017; Liu et al., 2024b). Research has found that FLK directly binds to the m6A modification site in the 3′-UTR of FLC, suppressing the levels of FLC by decreasing its stability and splicing, thus regulating flowering (Amara et al., 2023). The m6A-binding function of CPSF30-L selects the proximal poly(A) site and generates a short 3′-UTR at SOC1, RPN10, and FYVE1, thereby preventing mRNA degradation and regulating the floral transition and ABA response (Song et al., 2021). Moreover, YTH07 can physically interact with EHD6, which enhances the binding of an m6A-modified RNA and triggers the relocation of a portion of YTH07 from the cytoplasm into the RNP granules through phase-separated condensation. This leads to the sequestration of the mRNA of the rice flowering repressor OsCOL4, resulting in a reduction in its protein abundance and, thus, in accelerated flowering in rice (Cui et al., 2024).
m6A modification also plays an important role in plant reproductive regulation, primarily by regulating pollen development and affecting the plant reproductive process. OsFIP37 mediates the modification of m6A on an auxin biosynthesis gene, OsYUCCA3, during microsporogenesis, which is essential for meiotic division and subsequent pollen development in rice (Cheng et al., 2022). Moreover, OsEDM2L regulates EAT1 transcription by interacting with bHLH142 and TDR, and it further mediates m6A modification, alternative splicing, and polyadenylation of the EAT1 transcripts. This controls the expression of tapetal programmed cell death (PCD)-related genes and, subsequently, male reproduction (Ma et al., 2021). OsALKBH9 reduces the m6A modifications in the TDR and GAMYB transcripts and affects their stability to regulate pollen development (Tang et al., 2024). Furthermore, m6A RNA methylation impairs the gene expression variability and reproductive thermotolerance in Arabidopsis. Disruption of AtALKBH10B leads to a lower gene expression variability, the suppression of heat-activated genes, and a strong reduction in plant fertility (Wang et al., 2022b).
m6A modification is also closely related to embryo development. After the inactivation of AtMTA, the reduced level of m6A modification leads to the inability of developing embryos to pass through the globular stage (Zhong et al., 2008). ZmMTA dysfunction leads to severe arrest in maize embryogenesis and endosperm development (Cai et al., 2024a). Knocking out the AtFIP37 gene leads to a strong delay in endosperm development and embryo arrest, resulting in embryo lethality (Vespa et al., 2004). MiR408 activates nucleotide metabolism by inhibiting DlNUDT23, thereby regulating m6A modification and ultimately promoting early embryogenesis in longan (Xu et al., 2023b). FIP37-mediated m6A modification accelerates the degradation of WUSCHEL (WUS) and SHOOTMERISTEMLESS (STM), limiting their transcript levels to prevent excessive shoot apical meristem (SAM) proliferation (Shen et al., 2016). The inactivation of MTC, MTA, MTB, and FIP37 in moss leads to the loss of m6A, resulting in delayed gametophyte bud formation and in defective spore development (Garcias-Morales et al., 2023).
The development of plant roots, stems, and leaves is also regulated by m6A modification. In terms of root growth, research has found that by treating Moso bamboo with the RNA methylation inhibitor DZnepA, reducing its m6A modification level can increase the number of its lateral roots (Liufu et al., 2023). At the same time, studies have also found that the overexpression of the HIZ1 gene in Arabidopsis leads to an increase in the m6A modification levels, thereby reducing lateral roots (Zhang et al., 2022a). In addition, the ect2/ect3/ect4 mutants exhibit slow root growth and defects in root growth directionality (Arribas-Hernández et al., 2020). The silencing of GhVIR reduces the level of m6A modification, affecting the cell size, shape, and total cell number of cotton leaves, thereby affecting their morphogenesis (Huang et al., 2022b). In rice, YTH03/05/10 are cytoplasmic proteins that are highly expressed in the stem and leaf sheath (Cai et al., 2023b). Studies have found that loss of the function of YTH03/05/10 leads to dwarfism in rice. Moreover, the heterologous expression of FTO can increase the removal of m6A modification in rice to stimulate the proliferation of root meristem cells and the formation of tiller buds (Yu et al., 2021).
Seed germination is influenced by many factors, with m6A modification emerging as a crucial regulator. This modification primarily impacts seed germination by modulating the ABA response. ECT2 directly interacts with the PAB2 and PAB4 proteins, maintaining the stability of DWA1, DWA2, SDIRIP1, and CPN20 mRNAs. This process promotes the accumulation of ABI5, thereby regulating the ABA-mediated seed germination and the subsequent growth (Song et al., 2023). On the other hand, ALKBH9B negatively regulates the ABA response by reducing m6A modification, which leads to the increased mRNA stability of ABA INSENSITIVE 1 (ABI1) and BRI1-EMS-SUPPRESSOR 1 (BES1) during seed germination (Tang et al., 2021, 2022).
m6A modification can also regulate the development of fruits. Taking tomato fruit as an example, the fruit gradually increases in size during the transition from immature green to mature red. During this process, the overall m6A level and mRNA abundance also increase (Hu et al., 2022a). Studies have shown that, once the m6A modification site is recognized, SlYTH1 enhances the stability of the gibberellin (GA)-related genes, ultimately elevating the seed germination rate and promoting fruit development (Yin et al., 2022). SlALKBH2 has the ability to bind the transcript of the DNA demethylase gene SlDML2, which is required for tomato fruit ripening, and positively affects fruit ripening by regulating its stability through the demethylation of m6A (Zhou et al., 2019). MTA can also affect the ABA response by increasing the m6A modification level, enhancing the stability of NCED5 and AREB1 mRNA, or promoting the translation efficiency of ABAR, thereby promoting the maturation of strawberry fruits (Zhou et al., 2021). Furthermore, m6A affects the stability of the mRNAs related to fiber elongation, ultimately influencing cotton fiber elongation (Xing et al., 2023). The modification of m6A is closely related to the accumulation of substances during fruit maturation. Studies have shown that m6A modification significantly increases in the late stage of wheat seed development, particularly enriched in pathways related to protein and starch synthesis, indicating its close association with the accumulation of substances during wheat seed maturation (Li et al., 2022). In addition, not only the maturation of fruits but also the accumulation of substances in plant leaves is related to m6A modification. In tea plants, CsALKBH4 affects the stability and the abundance of the transcripts related to terpene biosynthesis by removing m6A modification, directly affecting the accumulation of volatile terpene compounds and the aroma of tea leaves. At the same time, by activating selective polyadenylation during sunlight withering, it indirectly regulates the content of flavonoids, catechins, and theaflavins, as well as the formation of substances related to tea flavor (Zhu et al., 2023).
m6A is involved in the plant response to environmental signals
Due to their immobility, plants have evolved a set of mechanisms through long-term natural selection to withstand the surrounding environment. In the process of plants responding to different environments, m6A modification also plays an important role.
Abiotic environmental signals
Light is an important signal for regulating plant growth and morphological development. Light can affect the morphology of plants through m6A modification as well. In Arabidopsis, the blue light receptor CRY1 interacts with FIP37, modulating m6A on the photomorphogenesis-related genes PIF3, PIF4, and PIF5, thereby accelerating the decay of their transcripts and repressing the elongation of the hypocotyl (Yang et al., 2023). Light can also regulate the circadian rhythm of plants through m6A modification. The blue light receptor CRY2 interacts with the mRNA m6A methyltransferase complex (MTA/MTB/FIP37), and by increasing the m6A modification level, it alters the degradation rate of the mRNA of the core circadian gene CCA1, thereby affecting the circadian clock in plants (Wang et al., 2021). Furthermore, the blue light-excited CRY2 undergoes liquid–liquid phase separation (LLPS) to form photobodies that recruit the m6A “writer” complex, regulates the methylation of the transcriptome, and is involved in the regulation of chlorophyll homeostasis (Jiang et al., 2023). Seagrass exhibits a peak of m6A modification during the dark period under the same photoperiod. The methylation of m6A could widely contribute to circadian regulation in seagrass, potentially affecting the photobiological behavior of these plants (Ruocco et al., 2020).
Salt stress is a major abiotic stress during plant growth. Mutants of mta, mtb, vir, and hakai in Arabidopsis exhibit m6A-dependent salt sensitivity. VIR-mediated m6A methylation modulates ROS homeostasis by negatively regulating the mRNA stability of several salt stress-negative regulators, including ATAF1, GI, and GSTU17, through affecting the 3′-UTR lengthening linked to alternative polyadenylation (Hu et al., 2021). AtECT12 promotes greater stabilization of NHX1, a positive regulator of salt stress, and decreases the stability of BGLU22 and GSTU17, which are negative regulators of salt stress, thereby positively regulating salt stress response (Lee et al., 2024). Increased AtECT8 leads to the enhanced binding of m6A-modified mRNAs, thereby accelerating the degradation of the negative regulators of salt stress response to enhance salt tolerance (Cai et al., 2024b). PagFIP37 regulates the mRNA stability of the salt-responsive transcripts in an m6A manner and plays a positive role in the response of poplar to salt stress (Zhao et al., 2024b). In rice, transcripts encoding the transcription factors, antioxidants, and auxin response-related genes exhibit changes in the m6A methylation levels in shoots or roots under salt stress, implying that m6A may mediate salt tolerance by regulating transcription, ROS homeostasis, and auxin signaling in a tissue-specific manner (Wang et al., 2022c). AtFIONA1-mediated m6A methylation regulates the production of ROS and affects the transcription levels of the salt stress-responsive genes by regulating their mRNA stability (Cai et al., 2024a). Silencing of the GhALKBH10 gene in cotton can increase the m6A modification level, enhance the antioxidant capacity, and reduce the Na+ concentration in the cytoplasm, thereby improving the plant’s tolerance to salinity (Cui et al., 2022). SlALKBH10B negatively regulates cell damage in salt stress, thereby rendering plants salt-intolerant (Shen et al., 2023a).
Drought is an environmental condition that plants often face. m6A modification primarily affects plant drought resistance in three ways. Firstly, it enhances plant drought tolerance by regulating the root system. Studies have found that PtrMTA in poplar increases the level of m6A modification, promoting root hair density and root growth, thereby improving tolerance to drought stress (Lu et al., 2020). PagALKBH9B and PagALKBH10B in poplar reduce the number of adventitious roots and the accumulation of biomass by decreasing the m6A level, leading to the decreased adaptability of plants to drought stress (Zhao et al., 2022). Secondly, m6A modification affects the expression of the drought-related genes under drought conditions (Mao et al., 2021). The overexpression of ClMTB in tobacco plants increased drought tolerance by enhancing the ROS scavenging system and alleviating photosynthesis inhibition under drought stress through increasing the m6A level (He et al., 2021). Studies have also found that SiYTH1 can stabilize SiARDP and the ROS removal-related transcripts SiAPX1, SiGRXC7, and SiGULLO4, thereby promoting stomatal closure and ROS clearance and enhancing drought resistance in Setaria italica (Luo et al., 2023). Furthermore, ECT12 and ALKBH10B positively regulate drought resistance by affecting the stability of the mRNAs involved in drought stress response in Arabidopsis (Amara et al., 2024). Thirdly, m6A modification affects drought resistance by regulating the ABA response. In sea buckthorn, m6A modification can regulate the expression levels of the ABA-related genes to enhance resistance to drought stress (Zhang et al., 2021a). GhALKBH10B was found to reduce the level of m6A in cotton, leading to the degradation of the mRNAs of the ABA signal-related genes and the Ca2+ signal-related genes, which is unfavorable for plant drought resistance (Li et al., 2023a).
Under low-temperature conditions, m6A modification can affect the translation efficiency and photosynthetic efficiency. Research has shown that the downregulation of FIP37 has no particular effect on photosynthesis under standard conditions, but is crucial for efficient photosynthesis and other chloroplast functions related to plant growth during cold acclimation (Vicente et al., 2023). Furthermore, m6A modification also affects pollen formation under low temperatures. Low-temperature stress leads to a decrease in the overall m6A level in tomato anthers, but increases the m6A modification of the ATP-binding cassette G31 (SlABCG31) in the ATP-binding pathway, leading to the decreased expression of this gene, thereby increasing the ABA content in tomato anthers and disrupting the formation of the pollen wall, resulting in pollen abortion (Yang et al., 2021).
m6A modification is also involved in the response of plants to heavy metal stress. When soybean plants are exposed to lead, the root growth is inhibited, while the transcriptome range of the m6A peaks increases (Zhang et al., 2023b). Exposure of rice to cadmium leads to abnormal root development and altered m6A modification profiles (Cheng et al., 2021). Cadmium stress also leads to an increase in the level of m6A modification across the soybean transcriptome (Han et al., 2023b). A recent study has shown that m6A modification is also involved in the copper stress response in Arabidopsis thaliana (Sharma et al., 2024).
Biotic environmental signals
m6A modification has both negative and positive impacts on the responses of plants to external biological signals. It can affect the invasion of other organisms into plants. In Arabidopsis, the m6A demethylase ALKBH9B accumulates in the cytoplasmic granules and interacts with the coat protein of the Alfalfa mosaic virus (AMV), thereby positively regulating AMV infection. The inactivation of AtALKBH9B does not affect the stability of AMV particles, but blocks the virus from infecting plants through the epidermis. Inactivating ECT2/ECT3/ECT5 can restore the infectivity of AMV in partially resistant alkbh9b mutants (Martínez-Pérez et al., 2023). Moreover, ECT1 antagonizes the salicylic acid (SA)-mediated plant responses and can be recruited to ECT9 condensates, playing a negative role in plant immunity (Wang et al., 2023a; Lee et al., 2024). The wheat gene TaMTB is a disease susceptibility gene localized in the nucleus. TaMTB can bind to wheat yellow mosaic virus (WYMV) and upregulate its m6A levels, stabilizing the viral RNA and facilitating its transport to cytoplasmic bodies, thereby positively promoting viral infection (Zhang et al., 2021d, 2022b). Deficiency of MTA1 in the rice pathogen Magnaporthe oryzae reduced the appressorial penetration and invasive growth of M. oryzae and disrupted autophagy processes (Ren et al., 2022).
m6A modification can also regulate the immune capacity of plants. During viral infection of rice, different m6A peak distributions were detected on the same gene, which may contribute to different antiviral modes between different virus infections. In apples, overexpressing the reader gene MhYTP2 can degrade the disease susceptibility genes MdMLO19 and MdMLO19-X1, increase the translation efficiency of the antioxidant gene MdGDH1L, and enhance the resistance of apples to powdery mildew (Guo et al., 2022). MhYTP2 negatively modulates the resistance of apples to Glomerella leaf spot by binding to and degrading the MdRGA2L mRNA (Guo et al., 2023). Studies have shown that HAKAI and MTA increase the m6A modification of the Pepino mosaic virus (PepMV) RNA in Nicotiana benthamiana and tomato, suppressing viral invasion. In addition, the nonsense-mediated mRNA decay (NMD) factors UPF3/SMG7 can recognize the m6A-modified viral RNA complexes and limit plant viral infection by degrading viral RNA (He et al., 2023). In N. benthamiana, the overexpression of the METTL homologs NbMETTL1 and NbMETTL2 led to increased m6A modification levels and reduced tobacco mosaic virus infectivity (Yue et al., 2023). It is interesting that plant viruses could act as inducers to disrupt m6A methylation. The virus encodes the AlkB protein to promote virus infection (Yue et al., 2022). Moreover,AhALKBH15 led to a reduction in m6A and the upregulation of the level of the resistance gene AhCQ2G6Y, promoting bacterial wilt (BW) resistance in peanut (Zhao et al., 2024a).
m6A modification is also involved in the regulation of plant resistance to herbivores. Studies have shown that the overall m6A methylation levels are elevated in soybean under Meloidogyne incognita infection (Han et al., 2022). m6A modification also acts as the main regulatory strategy for the expression of the genes involved in plant–insect interactions, which is attributed to responses to rice stem borer (RSB) infestation (Li et al., 2024) (Figure 4).
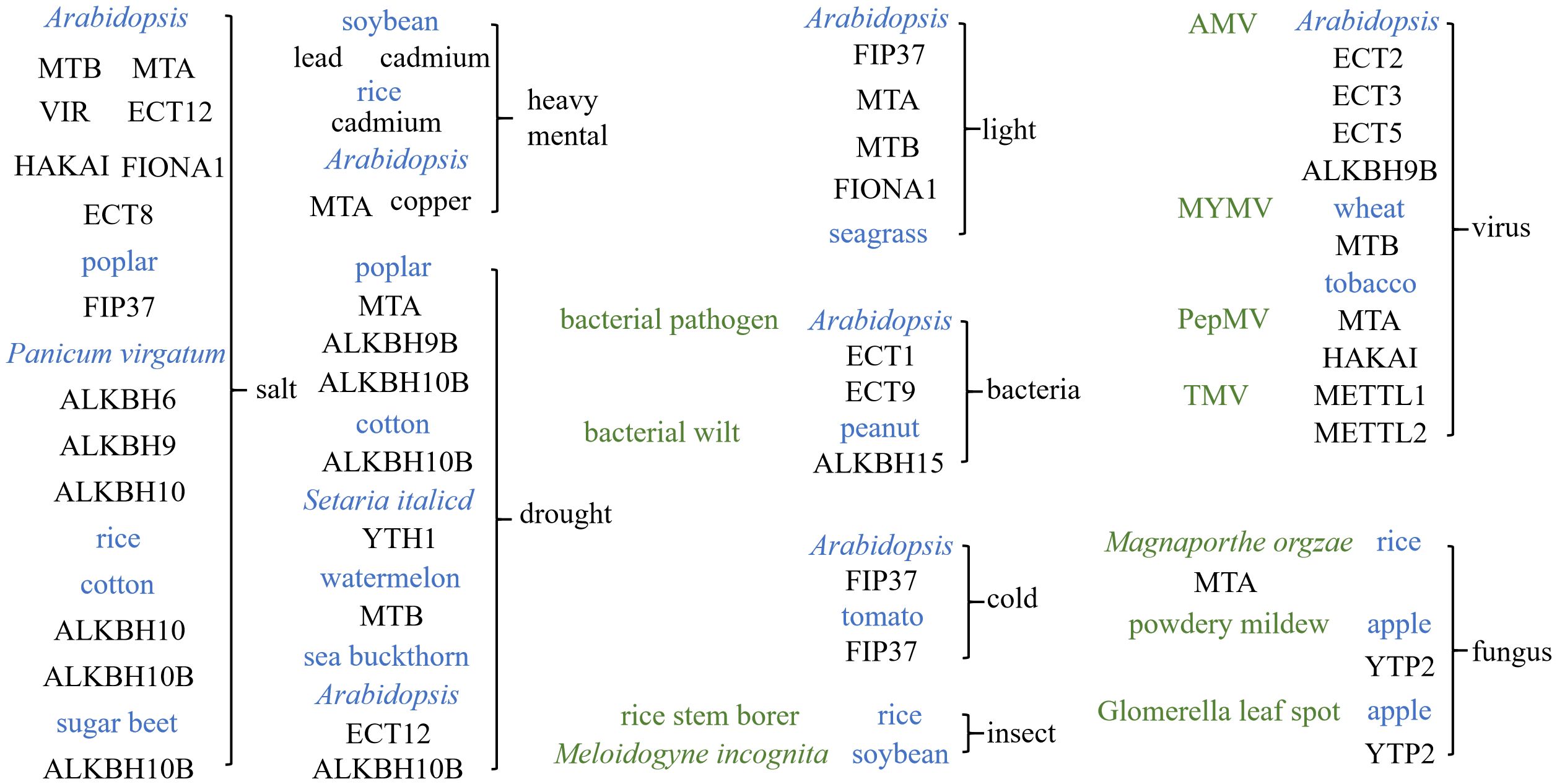
Figure 4 Function of N6-methyladenosine (m6A) in the plant response to environmental signals. The effects of environmental signaling in various species under m6A regulation in plants include: salt signaling, heavy metal signaling, drought signaling, light signaling, cold signaling, bacterial signaling, insect signaling, viral signaling, and fungal signaling. The blue color represents the species of the plant, while the green color represents the specific biological stresses.
Discussion and prospects of m6A research
In recent years, there has been a great deal of research on the modification of m6A in animals, with research in plants following closely. With the continuous development of sequencing technologies and in-depth studies, researchers have revealed the important roles of m6A modification in plant RNA metabolism. However, there are still many unknown functions waiting to be explored. This article systematically reviews the sequencing techniques for m6A modification, its distribution characteristics in plants, the related components, and its functions, helping researchers gain a deeper understanding of how m6A plays a role in RNA epigenetic regulation in plants and providing new perspectives for future research.
With advances in technology, the elucidation of the mechanism of m6A modification will become clearer in future studies. The initial m6A-seq technique had issues with high sample demand, low resolution, and inability to quantify. However, the more recent techniques such as m6A-SAC-seq, GLORI, scDART-seq, and scm6A-seq have been optimized in terms of resolution and quantification. Although most of the current studies still utilize the m6A-seq technique, better alternatives are certain to emerge as technology continues to progress. m6A modification is widespread at different locations in RNA, dynamically regulating RNA metabolism by adding or removing modifications and exerting special functions (Shi et al., 2019). In current research, m6A modification generally functions by affecting mRNA metabolism. m6A modification influences seed germination, root growth, floral morphogenesis, plant height, fruit ripening, and senescence during plant growth and development (Lu et al., 2020; Tang et al., 2021; Rudy et al., 2022; Xu et al., 2022; Hu et al., 2022a; Xu et al., 2023; Cai et al., 2023ba; Sheikh et al., 2024). In addition, it plays a role in the responses of plants to abiotic environmental signals such as drought, cold, and heavy metal stress (Lu et al., 2020; Cheng et al., 2021; Vicente et al., 2023; Zhang et al., 2024). Furthermore, during plant viral infection, m6A modification may exhibit positive or negative functions (Cheng et al., 2021; Martínez-Pérez et al., 2023; Wang et al., 2023b; Yao et al., 2023b).
LLPS plays a role in many aspects of organisms, such as gene expression regulation, cell division, and stress response. In recent years, the field of LLPS has become a hot topic in the field of life sciences, and a series of important progresses has been made in plants (Jung et al., 2020; Liu et al., 2024). LLPS also plays a key regulatory role in the biological functions involving m6A, such as blue light signal transduction, chlorophyll homeostasis, and mRNA stability (Song et al., 2021; Wang et al., 2021; Lee et al., 2022; Jiang et al., 2023; Cai et al., 2024b). Follow-up studies can focus on the upstream regulation mechanisms of m6A in relation to LLPS.
The current findings suggest that m6A plays a regulatory role in the response of plants to environmental signals; however, the precise mechanisms remain elusive, leaving numerous unexplored territories. By elucidating the functions of m6A modification, we discover that it significantly impacts plant growth and development. This implies that by studying the modification of m6A in plants, we can anticipate its potential to enhance crop yield and stress resistance traits, thus providing valuable insights for future molecular breeding endeavors.
Author contributions
YX: Writing – original draft. DZ: Writing – review & editing. LL: Writing – review & editing. Y-XX: Writing – review & editing. C-YZ: Writing – review & editing. Q-FM: Writing – review & editing. JW: Writing – review & editing. X-LT: Writing – review & editing. Y-LL: Funding acquisition, Supervision, Writing – review & editing.
Funding
The author(s) declare financial support was received for the research, authorship, and/or publication of this article. This work was supported by the National Natural Science Foundation of China (grant no. 32001582), the General Project of Natural Science Research in Colleges and Universities of Jiangsu Province (Grant No. 20KJB210002) and the Advanced Talents Scientific Research Startup Fund of Jiangsu University.
Conflict of interest
The authors declare that the research was conducted in the absence of any commercial or financial relationships that could be construed as a potential conflict of interest.
Publisher’s note
All claims expressed in this article are solely those of the authors and do not necessarily represent those of their affiliated organizations, or those of the publisher, the editors and the reviewers. Any product that may be evaluated in this article, or claim that may be made by its manufacturer, is not guaranteed or endorsed by the publisher.
References
Amara, U., Hu, J., Cai, J., Kang, H. (2023). FLK is an mRNA m6A reader that regulates floral transition by modulating the stability and splicing of FLC in Arabidopsis. Mol. Plant 16, 919–929. doi: 10.1016/j.molp.2023.04.005
Amara, U., Hu, J., Park, S. J., Kang, H. (2024). ECT12, an YTH-domain protein, is a potential mRNA m6A reader that affects abiotic stress responses by modulating mRNA stability in Arabidopsis. Plant Physiol. Biochem. 206, 108255. doi: 10.1016/j.plaphy.2023.108255
Amara, U., Shoaib, Y., Kang, H. (2022). ALKBH9C, a potential RNA m6A demethylase, regulates the response of Arabidopsis to abiotic stresses and abscisic acid. Plant Cell Environ. 45 (12), 3566–3581. doi: 10.1111/pce.14447
Amos, H., Korn, M. (1958). 5-Methyl cytosine in the RNA of Escherichia coli. Biochim. Biophys. Acta 29, 444–445. doi: 10.1016/0006-3002(58)90214-2
Anderson, S. J., Kramer, M. C., Gosai, S. J., Yu, X., Vandivier, L. E., Nelson, A. D. L., et al. (2018). N6-methyladenosine inhibits local ribonucleolytic cleavage to stabilize mRNAs in arabidopsis. Cell Rep. 25, 1146–1157.e1143. doi: 10.1016/j.celrep.2018.10.020
Arribas-Hernández, L., Simonini, S., Hansen, M. H., Paredes, E. B., Bressendorff, S., Dong, Y., et al. (2020). Recurrent requirement for the m6A-ECT2/ECT3/ECT4 axis in the control of cell proliferation during plant organogenesis. Development, 147(14). doi: 10.1242/dev.189134
Cai, J., Hu, J., Amara, U., Park, S. J., Li, Y., Jeong, D., et al. (2023a). Arabidopsis N6-methyladenosine methyltransferase FIONA1 regulates floral transition by affecting the splicing of FLC and the stability of floral activators SPL3 and SEP3. J. Exp. Bot. 74, 864–877. doi: 10.1093/jxb/erac461
Cai, J., Hu, J., Xu, T., Kang, H. (2024a). FIONA1-mediated mRNA m6A methylation regulates the response of Arabidopsis to salt stress. Plant Cell Environ. 47, 900–912. doi: 10.1111/pce.14807
Cai, L., Cui, S., Jin, T., Huang, X., Hou, H., Hao, B., et al. (2023b). The N6-methyladenosine binding proteins YTH03/05/10 coordinately regulate rice plant height. Plant Sci. 329, 111546. doi: 10.1016/j.plantsci.2022.111546
Cai, Z., Tang, Q., Song, P., Tian, E., Yang, J., Jia, G. (2024b). The m6A reader ECT8 is an abiotic stress sensor that accelerates mRNA decay in Arabidopsis. Plant Cell. doi: 10.1093/plcell/koae149
Chen, K., Lu, Z., Wang, X., Fu, Y., Luo, G. Z., Liu, N., et al. (2015). High-resolution N6 -methyladenosine (m6A) map using photo-crosslinking-assisted m6A sequencing. Angew Chem. Int. Ed Engl. 54, 1587–1590. doi: 10.1002/anie.201410647
Cheng, P., Bao, S., Li, C., Tong, J., Shen, L., Yu, H. (2022). RNA N6-methyladenosine modification promotes auxin biosynthesis required for male meiosis in rice. Dev. Cell 57, 246–259.e244. doi: 10.1016/j.devcel.2021.12.014
Cheng, Q., Wang, P., Wu, G., Wang, Y., Tan, J., Li, C., et al. (2021). Coordination of m6A mRNA methylation and gene transcriptome in rice response to cadmium stress. Rice (N Y) 14, 62. doi: 10.1186/s12284-021-00502-y
Cohn, W. E., Volkin, E. (1951). Nucleoside-5′-phosphates from ribonucleic acid. Nature 167, 483–484. doi: 10.1038/167483a0
Cui, C., Ma, Z., Wan, H., Gao, J., Zhou, B. (2022). GhALKBH10 negatively regulates salt tolerance in cotton. Plant Physiol. Biochem. 192, 87–100. doi: 10.1016/j.plaphy.2022.09.029
Cui, S., Song, P., Wang, C., Chen, S., Hao, B., Xu, Z., et al. (2024). The RNA binding protein EHD6 recruits the m6A reader YTH07 and sequesters OsCOL4 mRNA into phase-separated ribonucleoprotein condensates to promote rice flowering. Mol. Plant. doi: 10.1016/j.molp.2024.05.002
Deng, H., Cheema, J., Zhang, H., Woolfenden, H., Norris, M., Liu, Z., et al. (2018). Rice in vivo RNA structurome reveals RNA secondary structure conservation and divergence in plants. Mol. Plant 11, 607–622. doi: 10.1016/j.molp.2018.01.008
Deng, X., Chen, K., Luo, G. Z., Weng, X., Ji, Q., Zhou, T., et al. (2015). Widespread occurrence of N6-methyladenosine in bacterial mRNA. Nucleic Acids Res. 43, 6557–6567. doi: 10.1093/nar/gkv596
Desrosiers, R., Friderici, K., Rottman, F. (1974). Identification of methylated nucleosides in messenger RNA from Novikoff hepatoma cells. Proc. Natl. Acad. Sci. U.S.A. 71, 3971–3975. doi: 10.1073/pnas.71.10.3971
Dominissini, D., Moshitch-Moshkovitz, S., Schwartz, S., Salmon-Divon, M., Ungar, L., Osenberg, S., et al. (2012). Topology of the human and mouse m6A RNA methylomes revealed by m6A-seq. Nature 485, 201–206. doi: 10.1038/nature11112
Du, X., Fang, T., Liu, Y., Wang, M., Zang, M., Huang, L., et al. (2020). Global profiling of N6 -methyladenosine methylation in maize callus induction. Plant Genome 13, e20018. doi: 10.1002/tpg2.20018
Duan, H. C., Wei, L. H., Zhang, C., Wang, Y., Chen, L., Lu, Z., et al. (2017). ALKBH10B is an RNA N6-methyladenosine demethylase affecting arabidopsis floral transition. Plant Cell 29, 2995–3011. doi: 10.1105/tpc.16.00912
Dunn, D. B. (1961). The occurrence of 1-methyladenine in ribonucleic acid. Biochim. Biophys. Acta 46, 198–200. doi: 10.1016/0006-3002(61)90668-0
Fu, Y., Dominissini, D., Rechavi, G., He, C. (2014). Gene expression regulation mediated through reversible m6A RNA methylation. Nat. Rev. Genet. 15, 293–306. doi: 10.1038/nrg3724
Garcias-Morales, D., Palomar, V. M., Charlot, F., Nogué, F., Covarrubias, A. A., Reyes, J. L. (2023). N6 -Methyladenosine modification of mRNA contributes to the transition from 2D to 3D growth in the moss Physcomitrium patens. Plant J. 114, 7–22. doi: 10.1111/tpj.16173
Ge, R., Ye, C., Peng, Y., Dai, Q., Zhao, Y., Liu, S., et al. (2023). m6A-SAC-seq for quantitative whole transcriptome m6A profiling. Nat. Protoc. 18, 626–657. doi: 10.1038/s41596-022-00765-9
Guo, T., Bao, R., Yang, Z., Fu, X., Hu, L., Wang, N., et al. (2023). The m6A reader MhYTP2 negatively modulates apple Glomerella leaf spot resistance by binding to and degrading MdRGA2L mRNA. Mol. Plant Pathol. 24, 1287–1299. doi: 10.1111/mpp.13370
Guo, T., Liu, C., Meng, F., Hu, L., Fu, X., Yang, Z., et al. (2022). The m6A reader MhYTP2 regulates MdMLO19 mRNA stability and antioxidant genes translation efficiency conferring powdery mildew resistance in apple. Plant Biotechnol. J. 20, 511–525. doi: 10.1111/pbi.13733
Han, X., Shi, Q., He, Z., Song, W., Chen, Q., Qi, Z. (2022). Transcriptome-wide N6-methyladenosine (m6A) methylation in soybean under Meloidogyne incognita infection. aBIOTECH 3, 197–211. doi: 10.1007/s42994-022-00077-2
Han, R. P., Shoaib, Y., Cai, J., Kang, H. S. (2023a). ALKBH10B-mediated m6A demethylation is crucial for drought tolerance by affecting mRNA stability in Arabidopsis. Environ. Exp. Bot. 209. doi: 10.1016/j.envexpbot.2023.105306
Han, X., Wang, J., Zhang, Y., Kong, Y., Dong, H., Feng, X., et al. (2023b). Changes in the m6A RNA methylome accompany the promotion of soybean root growth by rhizobia under cadmium stress. J. Hazard Mater 441, 129843. doi: 10.1016/j.jhazmat.2022.129843
Han, C., Zhang, F., Qiao, X., Zhao, Y., Qiao, Q., Huang, X., et al. (2021). Multi-Omics Analysis Reveals the Dynamic Changes of RNA N6-Methyladenosine in Pear (Pyrus bretschneideri) Defense Responses to Erwinia amylovora Pathogen Infection. Front. Microbiol. 12, 803512. doi: 10.3389/fmicb.2021.803512
He, H., Ge, L., Chen, Y., Zhao, S., Li, Z., Zhou, X., et al. (2023). m6A modification of plant virus enables host recognition by NMD factors in plants. Sci. China Life Sci. doi: 10.1007/s11427-022-2377-1
He, Y., Li, Y., Yao, Y., Zhang, H., Wang, Y., Gao, J., et al. (2021). Overexpression of watermelon m6A methyltransferase ClMTB enhances drought tolerance in tobacco by mitigating oxidative stress and photosynthesis inhibition and modulating stress-responsive gene expression. Plant Physiol. Biochem. 168, 340–352. doi: 10.1016/j.plaphy.2021.10.007
Horiuchi, K., Kawamura, T., Iwanari, H., Ohashi, R., Naito, M., Kodama, T., et al. (2013). Identification of Wilms' tumor 1-associating protein complex and its role in alternative splicing and the cell cycle. J. Biol. Chem. 288, 33292–33302. doi: 10.1074/jbc.M113.500397
Hou, N., Li, C., He, J., Liu, Y., Yu, S., Malnoy, M., et al. (2022). MdMTA-mediated m6A modification enhances drought tolerance by promoting mRNA stability and translation efficiency of genes involved in lignin deposition and oxidative stress. New Phytol. 234, 1294–1314. doi: 10.1111/nph.18069
Hou, Y., Sun, J., Wu, B., Gao, Y., Nie, H., Nie, Z., et al. (2021). CPSF30-L-mediated recognition of mRNA m6A modification controls alternative polyadenylation of nitrate signaling-related gene transcripts in Arabidopsis. Mol. Plant 14, 688–699. doi: 10.1016/j.molp.2021.01.013
Hu, J., Cai, J., Park, S. J., Lee, K., Li, Y., Chen, Y., et al. (2021). N6 -Methyladenosine mRNA methylation is important for salt stress tolerance in Arabidopsis. Plant J. 106, 1759–1775. doi: 10.1111/tpj.15270
Hu, J., Cai, J., Umme, A., Chen, Y., Xu, T., Kang, H. (2022a). Unique features of mRNA m6A methylomes during expansion of tomato (Solanum lycopersicum) fruits. Plant Physiol. 188, 2215–2227. doi: 10.1093/plphys/kiab509
Hu, J., Cai, J., Xu, T., Kang, H. (2022b). Epitranscriptomic mRNA modifications governing plant stress responses: underlying mechanism and potential application. Plant Biotechnol. J. 20, 2245–2257. doi: 10.1111/pbi.13913
Hu, L., Liu, S., Peng, Y., Ge, R., Su, R., Senevirathne, C., et al. (2022c). m6A RNA modifications are measured at single-base resolution across the mammalian transcriptome. Nat. Biotechnol. 40, 1210–1219. doi: 10.1038/s41587-022-01243-z
Huang, X., Abuduwaili, N., Wang, X., Tao, M., Wang, X., Huang, G. (2022b). Cotton (Gossypium hirsutum) VIRMA as an N6-methyladenosine RNA methylation regulator participates in controlling chloroplast-dependent and independent leaf development. Int. J. Mol. Sci. 23. doi: 10.3390/ijms23179887
Huang, T., He, W. J., Li, C., Zhang, J. B., Liao, Y. C., Song, B., et al. (2022a). Transcriptome-wide analyses of RNA m6A methylation in hexaploid wheat reveal its roles in mRNA translation regulation. Front. Plant Sci. 13, 917335. doi: 10.3389/fpls.2022.917335
Huang, H., Weng, H., Sun, W., Qin, X., Shi, H., Wu, H., et al. (2018). Recognition of RNA N6-methyladenosine by IGF2BP proteins enhances mRNA stability and translation. Nat. Cell Biol. 20, 285–295. doi: 10.1038/s41556-018-0045-z
Huang, M., Xu, S., Liu, L., Zhang, M., Guo, J., Yuan, Y., et al. (2021). m6A methylation regulates osteoblastic differentiation and bone remodeling. Front. Cell Dev. Biol. 9, 783322. doi: 10.3389/fcell.2021.783322
Huong, T. T., Ngoc, L. N. T., Kang, H. (2020). Functional characterization of a putative RNA Demethylase ALKBH6 in arabidopsis growth and abiotic stress responses. Int. J. Mol. Sci. 21 (18), 6707. doi: 10.3390/ijms21186707
Jia, G., Fu, Y., Zhao, X., Dai, Q., Zheng, G., Yang, Y., et al. (2011). N6-methyladenosine in nuclear RNA is a major substrate of the obesity-associated FTO. Nat. Chem. Biol. 7, 885–887. doi: 10.1038/nchembio.687
Jiang, B., Zhong, Z., Gu, L., Zhang, X., Wei, J., Ye, C., et al. (2023). Light-induced LLPS of the CRY2/SPA1/FIO1 complex regulating mRNA methylation and chlorophyll homeostasis in Arabidopsis. Nat. Plants 9, 2042–2058. doi: 10.1038/s41477-023-01580-0
Jung, J. H., Barbosa, A. D., Hutin, S., Kumita, J. R., Gao, M., Derwort, D., et al. (2020). A prion-like domain in ELF3 functions as a thermosensor in Arabidopsis. Nature 585, 256–260. doi: 10.1038/s41586-020-2644-7
Kennedy, T. D., Lane, B. G. (1979). Wheat embryo ribonucleates. XIII. Methyl-substituted nucleoside constituents and 5'-terminal dinucleotide sequences in bulk poly(AR)-rich RNA from imbibing wheat embryos. Can. J. Biochem. 57, 927–931. doi: 10.1139/o79-112
Knuckles, P., Lence, T., Haussmann, I. U., Jacob, D., Kreim, N., Carl, S. H., et al. (2018). Zc3h13/Flacc is required for adenosine methylation by bridging the mRNA-binding factor Rbm15/Spenito to the m6A machinery component Wtap/Fl(2)d. Genes Dev. 32, 415–429. doi: 10.1101/gad.309146.117
Kumar, S., Mohapatra, T. (2021). Deciphering epitranscriptome: modification of mRNA bases provides a new perspective for post-transcriptional regulation of gene expression. Front. Cell Dev. Biol. 9, 628415. doi: 10.3389/fcell.2021.628415
Lee, H. G., Kim, J., Seo, P. J. (2022). N6-methyladenosine-modified RNA acts as a molecular glue that drives liquid-liquid phase separation in plants. Plant Signal Behav. 17, 2079308. doi: 10.1080/15592324.2022.2079308
Lee, K. P., Liu, K., Kim, E. Y., Medina-Puche, L., Dong, H., Di, M., et al. (2024). The m6A reader ECT1 drives mRNA sequestration to dampen salicylic acid-dependent stress responses in Arabidopsis. Plant Cell 36, 746–763. doi: 10.1093/plcell/koad300
Li, S., Tan, X. Y., He, Z., Shen, C., Li, Y. L., Qin, L., et al. (2024). The dynamics of N6-methyladenine RNA modification in resistant and susceptible rice varieties responding to rice stem borer damage. Insect Sci. doi: 10.1111/1744-7917.13401
Li, Y., Wang, X., Li, C., Hu, S., Yu, J., Song, S. (2014b). Transcriptome-wide N6-methyladenosine profiling of rice callus and leaf reveals the presence of tissue-specific competitors involved in selective mRNA modification. RNA Biol. 11, 1180–1188. doi: 10.4161/rna.36281
Li, Y., Wang, Y., Vera-Rodriguez, M., Lindeman, L. C., Skuggen, L. E., Rasmussen, E. M. K., et al. (2023b). Single-cell m6A mapping in vivo using picoMeRIP-seq. Nat. Biotechnol.
Li, W., Yu, Y., Chen, X., Fang, Q., Yang, A., Chen, X., et al. (2022). N6-Methyladenosine dynamic changes and differential methylation in wheat grain development. Planta 255, 125. doi: 10.1007/s00425-022-03893-4
Li, D., Zhang, H., Hong, Y., Huang, L., Li, X., Zhang, Y., et al. (2014a). Genome-wide identification, biochemical characterization, and expression analyses of the YTH domain-containing RNA-binding protein family in arabidopsis and rice. Plant Mol. Biol. Rep. 32, 1169–1186. doi: 10.1007/s11105-014-0724-2
Li, B., Zhang, M., Sun, W., Yue, D., Ma, Y., Zhang, B., et al. (2023a). N6-methyladenosine RNA modification regulates cotton drought response in a Ca2+ and ABA-dependent manner. Plant Biotechnol. J. 21, 1270–1285. doi: 10.1111/pbi.14036
Liu, Q., Liu, W., Niu, Y., Wang, T., Dong, J. (2024). Liquid-liquid phase separation in plants: Advances and perspectives from model species to crops. Plant Commun. 5, 100663. doi: 10.1016/j.xplc.2023.100663
Liu, C., Sun, H., Yi, Y., Shen, W., Li, K., Xiao, Y., et al. (2023). Absolute quantification of single-base m6A methylation in the mammalian transcriptome using GLORI. Nat. Biotechnol. 41, 355–366. doi: 10.1038/s41587-022-01487-9
Liu, J., Yue, Y., Han, D., Wang, X., Fu, Y., Zhang, L., et al. (2014). A METTL3-METTL14 complex mediates mammalian nuclear RNA N6-adenosine methylation. Nat. Chem. Biol. 10, 93–95. doi: 10.1038/nchembio.1432
Liufu, Y., Xi, F., Wu, L., Zhang, Z., Wang, H., Wang, H., et al. (2023). Inhibition of DNA and RNA methylation disturbs root development of moso bamboo. Tree Physiol. doi: 10.1093/treephys/tpad074
Lu, L., Zhang, Y., He, Q., Qi, Z., Zhang, G., Xu, W., et al. (2020). MTA, an RNA m6A methyltransferase, enhances drought tolerance by regulating the development of trichomes and roots in poplar. Int. J. Mol. Sci. 21. doi: 10.3390/ijms21072462
Luo, J. H., Guo, T., Wang, M., Liu, J. H., Zheng, L. M., He, Y. (2024). RNA m6A modification facilitates DNA methylation during maize kernel development. Plant Physiol. 194, 2165–2182. doi: 10.1093/plphys/kiad625
Luo, G. Z., Macqueen, A., Zheng, G., Duan, H., Dore, L. C., Lu, Z., et al. (2014). Unique features of the m6A methylome in Arabidopsis thaliana. Nat. Commun. 5, 5630. doi: 10.1038/ncomms6630
Luo, W., Tang, Y., Li, S., Zhang, L., Liu, Y., Zhang, R., et al. (2023). The m6A reader SiYTH1 enhances drought tolerance by affecting the mRNA stability of genes related to stomatal closure and ROS scavenging in Setaria italica. J. Integr. Plant Biol. doi: 10.1111/jipb.13575
Luo, S., Tong, L. (2014). Molecular basis for the recognition of methylated adenines in RNA by the eukaryotic YTH domain. Proc. Natl. Acad. Sci. U.S.A. 111, 13834–13839. doi: 10.1073/pnas.1412742111
Luo, Y., Yao, Y., Wu, P., Zi, X., Sun, N., He, J. (2022). The potential role of N7-methylguanosine (m7G) in cancer. J. Hematol. Oncol. 15, 63. doi: 10.1186/s13045-022-01285-5
Lv, Y., Han, F., Liu, M., Zhang, T., Cui, G., Wang, J., et al. (2022). Characteristics of N6-methyladenosine modification during sexual reproduction of Chlamydomonas reinhardtii. Genomics Proteomics Bioinf. doi: 10.1101/2022.03.26.485907
Ma, K., Han, J., Zhang, Z., Li, H., Zhao, Y., Zhu, Q., et al. (2021). OsEDM2L mediates m6A of EAT1 transcript for proper alternative splicing and polyadenylation regulating rice tapetal degradation. J. Integr. Plant Biol. 63, 1982–1994. doi: 10.1111/jipb.13167
Mao, X., Hou, N., Liu, Z., He, J. (2021). Profiling of N6-methyladenosine (m6A) modification landscape in response to drought stress in apple (Malus prunifolia (Willd.) borkh). Plants (Basel) 11. doi: 10.3390/plants11010103
Martínez-Pérez, M., Aparicio, F., Arribas-Hernández, L., Tankmar, M. D., Rennie, S., Von Bülow, S., et al. (2023). Plant YTHDF proteins are direct effectors of antiviral immunity against an N6-methyladenosine-containing RNA virus. EMBO J., e113378.
Meyer, K. D., Jaffrey, S. R. (2017). Rethinking m6A readers, writers, and erasers. Annu. Rev. Cell Dev. Biol. 33, 319–342. doi: 10.1146/annurev-cellbio-100616-060758
Meyer, K. D., Saletore, Y., Zumbo, P., Elemento, O., Mason, C. E., Jaffrey, S. R. (2012). Comprehensive analysis of mRNA methylation reveals enrichment in 3' UTRs and near stop codons. Cell 149, 1635–1646. doi: 10.1016/j.cell.2012.05.003
Mielecki, D., Zugaj, D., Muszewska, A., Piwowarski, J., Chojnacka, A., Mielecki, M., et al. (2012). Novel AlkB dioxygenases–alternative models for in silico and in vivo studies. PloS One 7, e30588. doi: 10.1371/journal.pone.0030588
Murik, O., Chandran, S. A., Nevo-Dinur, K., Sultan, L. D., Best, C., Stein, Y., et al. (2020). Topologies of N6 -adenosine methylation (m6A) in land plant mitochondria and their putative effects on organellar gene expression. Plant J. 101, 1269–1286. doi: 10.1111/tpj.14589
Nichols, J. L. (1979). N6-methyladenosine in maize poly(A)-containing RNA. Plant Sci. Lett. 15, 357–361. doi: 10.1016/0304-4211(79)90141-X
Ok, S. H., Jeong, H. J., Bae, J. M., Shin, J. S., Luan, S., Kim, K. N. (2005). Novel CIPK1-associated proteins in Arabidopsis contain an evolutionarily conserved C-terminal region that mediates nuclear localization. Plant Physiol. 139, 138–150. doi: 10.1104/pp.105.065649
Ortigosa, F., Lobato-Fernández, C., Pérez-Claros, J. A., Cantón, F. R., Ávila, C., Cánovas, F. M., et al. (2022). Epitranscriptome changes triggered by ammonium nutrition regulate the proteome response of maritime pine roots. Front. Plant Sci. 13, 1102044. doi: 10.3389/fpls.2022.1102044
Parker, M. T., Soanes, B. K., Kusakina, J., Larrieu, A., Knop, K., Joy, N., et al. (2022). m6A modification of U6 snRNA modulates usage of two major classes of pre-mRNA 5' splice site. Elife 11. doi: 10.7554/eLife.78808.sa2
Patil, D. P., Chen, C. K., Pickering, B. F., Chow, A., Jackson, C., Guttman, M., et al. (2016). m6A RNA methylation promotes XIST-mediated transcriptional repression. Nature 537, 369–373. doi: 10.1038/nature19342
Ping, X. L., Sun, B. F., Wang, L., Xiao, W., Yang, X., Wang, W. J., et al. (2014). Mammalian WTAP is a regulatory subunit of the RNA N6-methyladenosine methyltransferase. Cell Res. 24, 177–189. doi: 10.1038/cr.2014.3
Ramakrishnan, M., Rajan, K. S., Mullasseri, S., Palakkal, S., Kalpana, K., Sharma, A., et al. (2022). The plant epitranscriptome: revisiting pseudouridine and 2'-O-methyl RNA modifications. Plant Biotechnol. J. 20, 1241–1256. doi: 10.1111/pbi.13829
Rebane, A., Roomere, H., Metspalu, A. (2002). Locations of several novel 2'-O-methylated nucleotides in human 28S rRNA. BMC Mol. Biol. 3, 1. doi: 10.1186/1471-2199-3-1
Ren, Z., Tang, B., Xing, J., Liu, C., Cai, X., Hendy, A., et al. (2022). MTA1-mediated RNA m6A modification regulates autophagy and is required for infection of the rice blast fungus. New Phytol. 235, 247–262. doi: 10.1111/nph.18117
Rudy, E., Grabsztunowicz, M., Arasimowicz-Jelonek, M., Tanwar, U. K., Maciorowska, J., Sobieszczuk-Nowicka, E. (2022). N6-methyladenosine (m6A) RNA modification as a metabolic switch between plant cell survival and death in leaf senescence. Front. Plant Sci. 13, 1064131. doi: 10.3389/fpls.2022.1064131
Ruocco, M., Ambrosino, L., Jahnke, M., Chiusano, M. L., Barrote, I., Procaccini, G., et al. (2020). m6A RNA methylation in marine plants: first insights and relevance for biological rhythms. Int. J. Mol. Sci. 21. doi: 10.3390/ijms21207508
Růžička, K., Zhang, M., Campilho, A., Bodi, Z., Kashif, M., Saleh, M., et al. (2017). Identification of factors required for m6A mRNA methylation in Arabidopsis reveals a role for the conserved E3 ubiquitin ligase HAKAI. New Phytol. 215, 157–172. doi: 10.1111/nph.14586
Schibler, U., Kelley, D. E., Perry, R. P. (1977). Comparison of methylated sequences in messenger RNA and heterogeneous nuclear RNA from mouse L cells. J. Mol. Biol. 115, 695–714. doi: 10.1016/0022-2836(77)90110-3
Schwartz, S., Mumbach, M. R., Jovanovic, M., Wang, T., Maciag, K., Bushkin, G. G., et al. (2014). Perturbation of m6A writers reveals two distinct classes of mRNA methylation at internal and 5' sites. Cell Rep. 8, 284–296. doi: 10.1016/j.celrep.2014.05.048
Sergiev, P. V., Golovina, A. Y., Osterman, I. A., Nesterchuk, M. V., Sergeeva, O. V., Chugunova, A. A., et al. (2016). N6-methylated adenosine in RNA: from bacteria to humans. J. Mol. Biol. 428, 2134–2145. doi: 10.1016/j.jmb.2015.12.013
Shao, Y., Wong, C. E., Shen, L., Yu, H. (2021). N6-methyladenosine modification underlies messenger RNA metabolism and plant development. Curr. Opin. Plant Biol. 63, 102047. doi: 10.1016/j.pbi.2021.102047
Sharma, B., Govindan, G., Li, Y., Sunkar, R., Gregory, B. D. (2024). RNA N6-methyladenosine affects copper-induced oxidative stress response in arabidopsis thaliana. Noncoding RNA 10. doi: 10.3390/ncrna10010008
Sheikh, A. H., Tabassum, N., Rawat, A., Almeida Trapp, M., Nawaz, K., Hirt, H. (2024). m6A RNA methylation counteracts dark-induced leaf senescence in Arabidopsis. Plant Physiol. 194 (4), 2663–2678. doi: 10.1093/plphys/kiad660
Shen, L. (2023). Functional interdependence of N6-methyladenosine methyltransferase complex subunits in Arabidopsis. Plant Cell 35, 1901–1916. doi: 10.1093/plcell/koad070
Shen, L., Liang, Z., Gu, X., Chen, Y., Teo, Z. W., Hou, X., et al. (2016). N6-methyladenosine RNA modification regulates shoot stem cell fate in arabidopsis. Dev. Cell 38, 186–200. doi: 10.1016/j.devcel.2016.06.008
Shen, L., Ma, J., Li, P., Wu, Y., Yu, H. (2023b). Recent advances in the plant epitranscriptome. Genome Biol. 24, 43. doi: 10.1186/s13059-023-02872-6
Shen, H., Zhou, Y., Liao, C., Xie, Q., Chen, G., Hu, Z., et al. (2023a). The alkB homolog slALKBH10B negatively affects drought and salt tolerance in solanum lycopersicum. Int. J. Mol. Sci. 25. doi: 10.3390/ijms25010173
Shi, H., Wei, J., He, C. (2019). Where, when, and how: context-dependent functions of RNA methylation writers, readers, and erasers. Mol. Cell 74, 640–650. doi: 10.1016/j.molcel.2019.04.025
Song, P., Wei, L., Chen, Z., Cai, Z., Lu, Q., Wang, C., et al. (2023). m6A readers ECT2/ECT3/ECT4 enhance mRNA stability through direct recruitment of the poly(A) binding proteins in Arabidopsis. Genome Biol. 24, 103. doi: 10.1186/s13059-023-02947-4
Song, P., Yang, J., Wang, C., Lu, Q., Shi, L., Tayier, S., et al. (2021). Arabidopsis N6-methyladenosine reader CPSF30-L recognizes FUE signals to control polyadenylation site choice in liquid-like nuclear bodies. Mol. Plant 14, 571–587. doi: 10.1016/j.molp.2021.01.014
Stern, L., Schulman, L. H. (1978). The role of the minor base N4-acetylcytidine in the function of the Escherichia coli noninitiator methionine transfer RNA. J. Biol. Chem. 253, 6132–6139. doi: 10.1016/S0021-9258(17)34590-8
Sun, B., Bhati, K. K., Song, P., Edwards, A., Petri, L., Kruusvee, V., et al. (2022). FIONA1-mediated methylation of the 3'UTR of FLC affects FLC transcript levels and flowering in Arabidopsis. PloS Genet. 18, e1010386. doi: 10.1371/journal.pgen.1010386
Tang, J., Lei, D., Yang, J., Chen, S., Wang, X., Huang, X., et al. (2024). OsALKBH9-mediated m6A demethylation regulates tapetal PCD and pollen exine accumulation in rice. Plant Biotechnol. J. doi: 10.1111/pbi.14354
Tang, J., Yang, J., Duan, H., Jia, G. (2021). ALKBH10B, an mRNA m6A Demethylase, Modulates ABA Response During Seed Germination in Arabidopsis. Front. Plant Sci. 12, 712713. doi: 10.3389/fpls.2021.712713
Tang, J., Yang, J., Lu, Q., Tang, Q., Chen, S., Jia, G. (2022). The RNA N6 -methyladenosine demethylase ALKBH9B modulates ABA responses in Arabidopsis. J. Integr. Plant Biol. 64, 2361–2373. doi: 10.1111/jipb.13394
Tegowski, M., Flamand, M. N., Meyer, K. D. (2022). scDART-seq reveals distinct m6A signatures and mRNA methylation heterogeneity in single cells. Mol. Cell 82, 868–878.e810. doi: 10.1016/j.molcel.2021.12.038
Thomas, M., White, R. L., Davis, R. W. (1976). Hybridization of RNA to double-stranded DNA: formation of R-loops. Proc. Natl. Acad. Sci. U.S.A. 73, 2294–2298. doi: 10.1073/pnas.73.7.2294
Vespa, L., Vachon, G., Berger, F., Perazza, D., Faure, J. D., Herzog, M. (2004). The immunophilin-interacting protein AtFIP37 from Arabidopsis is essential for plant development and is involved in trichome endoreduplication. Plant Physiol. 134, 1283–1292. doi: 10.1104/pp.103.028050
Vicente, A. M., Manavski, N., Rohn, P. T., Schmid, L. M., Garcia-Molina, A., Leister, D., et al. (2023). The plant cytosolic m6A RNA methylome stabilizes photosynthesis in the cold. Plant Commun., 100634. doi: 10.1016/j.xplc.2023.100634
Wan, Y., Tang, K., Zhang, D., Xie, S., Zhu, X., Wang, Z., et al. (2015). Transcriptome-wide high-throughput deep m6A-seq reveals unique differential m6A methylation patterns between three organs in Arabidopsis thaliana. Genome Biol. 16, 272. doi: 10.1186/s13059-015-0839-2
Wang, Y., Du, F., Li, Y., Wang, J., Zhao, X., Li, Z., et al. (2022c). Global N6-methyladenosine profiling revealed the tissue-specific epitranscriptomic regulation of rice responses to salt stress. Int. J. Mol. Sci. 23. doi: 10.3390/ijms23042091
Wang, N., Guo, T., Wang, P., Sun, X., Shao, Y., Jia, X., et al. (2017a). MhYTP1 and mhYTP2 from apple confer tolerance to multiple abiotic stresses in arabidopsis thaliana. Front. Plant Sci. 8, 1367. doi: 10.3389/fpls.2017.01367
Wang, X., Jiang, B., Gu, L., Chen, Y., Mora, M., Zhu, M., et al. (2021). A photoregulatory mechanism of the circadian clock in Arabidopsis. Nat. Plants 7, 1397–1408. doi: 10.1038/s41477-021-01002-z
Wang, T., Kong, S., Tao, M., Ju, S. (2020). The potential role of RNA N6-methyladenosine in Cancer progression. Mol. Cancer 19, 88. doi: 10.1186/s12943-020-01204-7
Wang, Y., Li, Y., Toth, J. I., Petroski, M. D., Zhang, Z., Zhao, J. C. (2014b). N6-methyladenosine modification destabilizes developmental regulators in embryonic stem cells. Nat. Cell Biol. 16, 191–198. doi: 10.1038/ncb2902
Wang, X., Lu, Z., Gomez, A., Hon, G. C., Yue, Y., Han, D., et al. (2014a). N6-methyladenosine-dependent regulation of messenger RNA stability. Nature 505, 117–120. doi: 10.1038/nature12730
Wang, H., Niu, R., Zhou, Y., Tang, Z., Xu, G., Zhou, G. (2023a). ECT9 condensates with ECT1 and regulates plant immunity. Front. Plant Sci. 14, 1140840. doi: 10.3389/fpls.2023.1140840
Wang, Z., Tang, K., Zhang, D., Wan, Y., Wen, Y., Lu, Q., et al. (2017b). High-throughput m6A-seq reveals RNA m6A methylation patterns in the chloroplast and mitochondria transcriptomes of Arabidopsis thaliana. PloS One 12, e0185612. doi: 10.1371/journal.pone.0185612
Wang, S., Wang, H., Xu, Z., Jiang, S., Shi, Y., Xie, H., et al. (2023b). m6A mRNA modification promotes chilling tolerance and modulates gene translation efficiency in Arabidopsis. Plant Physiol. 192, 1466–1482. doi: 10.1093/plphys/kiad112
Wang, C., Yang, J., Song, P., Zhang, W., Lu, Q., Yu, Q., et al. (2022a). FIONA1 is an RNA N6-methyladenosine methyltransferase affecting Arabidopsis photomorphogenesis and flowering. Genome Biol. 23, 40. doi: 10.1186/s13059-022-02612-2
Wang, L., Zhuang, H., Fan, W., Zhang, X., Dong, H., Yang, H., et al. (2022b). m6A RNA methylation impairs gene expression variability and reproductive thermotolerance in Arabidopsis. Genome Biol. 23, 244. doi: 10.1186/s13059-022-02814-8
Wei, L. H., Song, P., Wang, Y., Lu, Z., Tang, Q., Yu, Q., et al. (2018). The m6A Reader ECT2 Controls Trichome Morphology by Affecting mRNA Stability in Arabidopsis. Plant Cell 30, 968–985. doi: 10.1105/tpc.17.00934
Wen, J., Lv, R., Ma, H., Shen, H., He, C., Wang, J., et al. (2018). Zc3h13 regulates nuclear RNA m6A methylation and mouse embryonic stem cell self-renewal. Mol. Cell 69, 1028–1038.e1026. doi: 10.1016/j.molcel.2018.02.015
Wu, Y., Pu, X., Wu, S., Zhang, Y., Fu, S., Tang, H., et al. (2023). PCIF1, the only methyltransferase of N6,2-O-dimethyladenosine. Cancer Cell Int. 23, 226. doi: 10.1186/s12935-023-03066-7
Wu, X., Su, T., Zhang, S., Zhang, Y., Wong, C. E., Ma, J., et al. (2024). N6-methyladenosine-mediated feedback regulation of abscisic acid perception via phase-separated ECT8 condensates in Arabidopsis. Nat. Plants 10, 469–482. doi: 10.1038/s41477-024-01638-7
Xiao, Y. L., Liu, S., Ge, R., Wu, Y., He, C., Chen, M., et al. (2023). Transcriptome-wide profiling and quantification of N6-methyladenosine by enzyme-assisted adenosine deamination. Nat. Biotechnol. 41, 993–1003. doi: 10.1038/s41587-022-01587-6
Xing, K., Liu, Z., Liu, L., Zhang, J., Qanmber, G., Wang, Y., et al. (2023). N6-Methyladenosine mRNA modification regulates transcripts stability associated with cotton fiber elongation. Plant J. 115, 967–985. doi: 10.1111/tpj.16274
Xu, T., Wu, X., Wong, C. E., Fan, S., Zhang, Y., Zhang, S., et al. (2022). FIONA1-mediated m6A modification regulates the floral transition in arabidopsis. Adv. Sci. (Weinh) 9, e2103628. doi: 10.1002/advs.202103628
Xu, X., Zhang, C., Xu, X., Cai, R., Guan, Q., Chen, X., et al. (2023). Riboflavin mediates m6A modification targeted by miR408, promoting early somatic embryogenesis in longan. Plant Physiol. 192, 1799–1820. doi: 10.1093/plphys/kiad139
Yang, J., Li, L., Li, X., Zhong, M., Li, X., Qu, L., et al. (2023). The blue light receptor CRY1 interacts with FIP37 to promote N6 -methyladenosine RNA modification and photomorphogenesis in Arabidopsis. New Phytol. 237, 840–854. doi: 10.1111/nph.18583
Yang, D., Xu, H., Liu, Y., Li, M., Ali, M., Xu, X., et al. (2021). RNA N6-methyladenosine responds to low-temperature stress in tomato anthers. Front. Plant Sci. 12, 687826. doi: 10.3389/fpls.2021.687826
Yao, H., Gao, C. C., Zhang, D., Xu, J., Song, G., Fan, X., et al. (2023a). scm6A-seq reveals single-cell landscapes of the dynamic m6A during oocyte maturation and early embryonic development. Nat. Commun. 14, 315. doi: 10.1038/s41467-023-35958-7
Yao, S., Zhang, J., Cheng, X., Wang, D., Yu, W., Ji, K., et al. (2023b). Genome-Wide Identification and Characterization of the YTH Domain-Containing RNA-Binding Protein Family in Liriodendron chinense. Int. J. Mol. Sci. 24 (20), 15189. doi: 10.3390/ijms242015189
Yin, S., Ao, Q., Qiu, T., Tan, C., Tu, Y., Kuang, T., et al. (2022). Tomato SlYTH1 encoding a putative RNA m6A reader affects plant growth and fruit shape. Plant Sci. 323, 111417. doi: 10.1016/j.plantsci.2022.111417
Ying, Y., Ma, X., Fang, J., Chen, S., Wang, W., Li, J., et al. (2021). EGR2-mediated regulation of m6A reader IGF2BP proteins drive RCC tumorigenesis and metastasis via enhancing S1PR3 mRNA stabilization. Cell Death Dis. 12, 750. doi: 10.1038/s41419-021-04038-3
Yu, Q., Liu, S., Yu, L., Xiao, Y., Zhang, S., Wang, X., et al. (2021). RNA demethylation increases the yield and biomass of rice and potato plants in field trials. Nat. Biotechnol. 39, 1581–1588. doi: 10.1038/s41587-021-00982-9
Yue, J., Lu, Y., Sun, Z., Guo, Y., San León, D., Pasin, F., et al. (2023). Methyltransferase-like (METTL) homologues participate in Nicotiana benthamiana antiviral responses. Plant Signal Behav. 18, 2214760. doi: 10.1080/15592324.2023.2214760
Yue, J., Wei, Y., Sun, Z., Chen, Y., Wei, X., Wang, H., et al. (2022). AlkB RNA demethylase homologues and N6 -methyladenosine are involved in Potyvirus infection. Mol. Plant Pathol. 23, 1555–1564. doi: 10.1111/mpp.13239
Zaccara, S., Ries, R. J., Jaffrey, S. R. (2019). Reading, writing and erasing mRNA methylation. Nat. Rev. Mol. Cell Biol. 20, 608–624. doi: 10.1038/s41580-019-0168-5
Zhang, M., Bodi, Z., Mackinnon, K., Zhong, S., Archer, N., Mongan, N. P., et al. (2022a). Two zinc finger proteins with functions in m6A writing interact with HAKAI. Nat. Commun. 13, 1127. doi: 10.1038/s41467-022-28753-3
Zhang, P., Gao, J., Li, X., Feng, Y., Shi, M., Shi, Y., et al. (2021c). Interplay of DNA and RNA N6-methyladenine with R-loops in regulating gene transcription in Arabidopsis. Physiol. Mol. Biol. Plants 27, 1163–1171. doi: 10.1007/s12298-021-01010-5
Zhang, J., Yao, S., Cheng, X., Zhao, Y., Yu, W., Ren, X., et al. (2024). Genome-Wide Identification and Expression Analysis of the YTH Domain-Containing RNA-Binding Protein Family in Cinnamomum camphora. Int. J. Mol. Sci. 25 (11), 5960. doi: 10.3390/ijms25115960
Zhang, Y., Han, X., Su, D., Liu, C., Chen, Q., Qi, Z. (2023b). An analysis of differentially expressed and differentially m6A-modified transcripts in soybean roots treated with lead. J. Hazard Mater 453, 131370. doi: 10.1016/j.jhazmat.2023.131370
Zhang, G., Lv, Z., Diao, S., Liu, H., Duan, A., He, C., et al. (2021a). Unique features of the m6A methylome and its response to drought stress in sea buckthorn (Hippophae rhamnoides Linn.). RNA Biol. 18, 794–803. doi: 10.1080/15476286.2021.1992996
Zhang, T., Shi, C., Hu, H., Zhang, Z., Wang, Z., Chen, Z., et al. (2022b). N6-methyladenosine RNA modification promotes viral genomic RNA stability and infection. Nat. Commun. 13, 6576. doi: 10.1038/s41467-022-34362-x
Zhang, Z., Theler, D., Kaminska, K. H., Hiller, M., de la Grange, P., Pudimat, R., et al. (2010). The YTH domain is a novel RNA binding domain. J. Biol. Chem. 285, 14701–14710. doi: 10.1074/jbc.M110.104711
Zhang, T. Y., Wang, Z. Q., Hu, H. C., Chen, Z. Q., Liu, P., Gao, S. Q., et al. (2021d). Transcriptome-wide N6-methyladenosine (m6A) profiling of susceptible and resistant wheat varieties reveals the involvement of variety-specific m6A modification involved in virus-host interaction pathways. Front. Microbiol. 12, 656302. doi: 10.3389/fmicb.2021.656302
Zhang, Y., Wang, J., Ma, W., Lu, N., Fu, P., Yang, Y., et al. (2023c). Transcriptome-wide m6A methylation in natural yellow leaf of Catalpa fargesii. Front. Plant Sci. 14, 1167789. doi: 10.3389/fpls.2023.1167789
Zhang, F., Zhang, Y. C., Liao, J. Y., Yu, Y., Zhou, Y. F., Feng, Y. Z., et al. (2019). The subunit of RNA N6-methyladenosine methyltransferase OsFIP regulates early degeneration of microspores in rice. PloS Genet. 15, e1008120. doi: 10.1371/journal.pgen.1008120
Zhang, L., Zhang, Y., Liu, J., Li, H., Liu, B., Zhao, T. (2023a). N6-methyladenosine mRNA methylation is important for the light response in soybean. Front. Plant Sci. 14, 1153840. doi: 10.3389/fpls.2023.1153840
Zhang, K., Zhuang, X., Dong, Z., Xu, K., Chen, X., Liu, F., et al. (2021b). The dynamics of N6-methyladenine RNA modification in interactions between rice and plant viruses. Genome Biol. 22, 189. doi: 10.1186/s13059-021-02410-2
Zhao, Y., Guo, Q., Cao, S., Tian, Y., Han, K., Sun, Y., et al. (2022). Genome-wide identification of the AlkB homologs gene family, PagALKBH9B and PagALKBH10B regulated salt stress response in Populus. Front. Plant Sci. 13, 994154. doi: 10.3389/fpls.2022.994154
Zhao, Y., Han, K. J., Tian, Y. T., Jia, K. H., El-Kassaby, Y. A., Wu, Y., et al. (2024b). N6-methyladenosine mRNA methylation positively regulated the response of poplar to salt stress. Plant Cell Environ. 47, 1797–1812. doi: 10.1111/pce.14844
Zhao, K., Li, Z., Ke, Y., Ren, R., Cao, Z., Li, Z., et al. (2024a). Dynamic N6-methyladenosine RNA modification regulates peanut resistance to bacterial wilt. New Phytol. 242, 231–246. doi: 10.1111/nph.19568
Zhao, J., Zhang, C., Li, S., Yuan, M., Mu, W., Yang, J., et al. (2023). Changes in m6A RNA methylation are associated with male sterility in wolfberry. BMC Plant Biol. 23, 456. doi: 10.1186/s12870-023-04458-7
Zheng, G., Dahl, J. A., Niu, Y., Fedorcsak, P., Huang, C. M., Li, C. J., et al. (2013). ALKBH5 is a mammalian RNA demethylase that impacts RNA metabolism and mouse fertility. Mol. Cell 49, 18–29. doi: 10.1016/j.molcel.2012.10.015
Zhong, S., Li, H., Bodi, Z., Button, J., Vespa, L., Herzog, M., et al. (2008). MTA is an Arabidopsis messenger RNA adenosine methylase and interacts with a homolog of a sex-specific splicing factor. Plant Cell 20, 1278–1288. doi: 10.1105/tpc.108.058883
Zhou, L., Tang, R., Li, X., Tian, S., Li, B., Qin, G. (2021). N6-methyladenosine RNA modification regulates strawberry fruit ripening in an ABA-dependent manner. Genome Biol. 22, 168. doi: 10.1186/s13059-021-02385-0
Zhou, L., Tian, S., Qin, G. (2019). RNA methylomes reveal the m6A-mediated regulation of DNA demethylase gene SlDML2 in tomato fruit ripening. Genome Biol. 20, 156. doi: 10.1186/s13059-019-1771-7
Zhu, C., Zhang, S., Zhou, C., Tian, C., Shi, B., Xu, K., et al. (2023). RNA Methylome Reveals the m6A-mediated Regulation of Flavor Metabolites in Tea Leaves under Solar-withering. Genomics Proteomics Bioinf. doi: 10.1016/j.gpb.2023.02.003
Keywords: N6-methyladenosine (m6A), m6A sequencing, conserved m6A motifs, m6A-related proteins, m6A functions
Citation: Xiang Y, Zhang D, Li L, Xue Y-X, Zhang C-Y, Meng Q-F, Wang J, Tan X-L and Li Y-L (2024) Detection, distribution, and functions of RNA N6-methyladenosine (m6A) in plant development and environmental signal responses. Front. Plant Sci. 15:1429011. doi: 10.3389/fpls.2024.1429011
Received: 07 May 2024; Accepted: 24 June 2024;
Published: 16 July 2024.
Edited by:
Wilfried Rozhon, Anhalt University of Applied Sciences, GermanyCopyright © 2024 Xiang, Zhang, Li, Xue, Zhang, Meng, Wang, Tan and Li. This is an open-access article distributed under the terms of the Creative Commons Attribution License (CC BY). The use, distribution or reproduction in other forums is permitted, provided the original author(s) and the copyright owner(s) are credited and that the original publication in this journal is cited, in accordance with accepted academic practice. No use, distribution or reproduction is permitted which does not comply with these terms.
*Correspondence: Yu-Long Li, bGl5dWxvbmdjYXNAMTYzLmNvbQ==
†ORCID: Yu-Long Li, orcid.org/0000-0003-4836-843X