- 1College of Bioscience and Biotechnology, Shenyang Agricultural University, Shenyang, China
- 2College of Horticulture, Shenyang Agricultural University, Shenyang, China
- 3Institute of Vegetable, Liaoning Academy of Agricultural Sciences, Shenyang, China
Actin depolymerizing factors (ADFs), as the important actin-binding proteins (ABPs) with depolymerizing/severing actin filaments, play a critical role in plant growth and development, and in response to biotic and abiotic stresses. However, the information and function of the ADF family in melon remains unclear. In this study, 9 melon ADF genes (CmADFs) were identified, distributed in 4 subfamilies, and located on 6 chromosomes respectively. Promoter analysis revealed that the CmADFs contained a large number of cis-acting elements related to hormones and stresses. The similarity of CmADFs with their Arabidopsis homologue AtADFs in sequence, structure, important sites and tissue expression confirmed that ADFs were conserved. Gene expression analysis showed that CmADFs responded to low and high temperature stresses, as well as ABA and SA signals. In particular, CmADF1 was significantly up-regulated under above all stress and hormone treatments, indicating that CmADF1 plays a key role in stress and hormone signaling responses, so CmADF1 was selected to further study the mechanism in plant tolerance low temperature. Under low temperature, virus-induced gene silencing (VIGS) of CmADF1 in oriental melon plants showed increased sensitivity to low temperature stress. Consistently, the stable genetic overexpression of CmADF1 in Arabidopsis improved their low temperature tolerance, possibly due to the role of CmADF1 in the depolymerization of actin filaments. Overall, our findings indicated that CmADF genes, especially CmADF1, function in response to abiotic stresses in melon.
Introduction
Melon (Cucumis melo L.), originated from tropical zone, is a worldwide fruit with high edible value and economic value, and is sensitive to low temperature. Under the influence of the environment and climate in the northern region, the oriental melon cultivated in facilities in winter and spring is often affected by low temperature, which seriously deteriorates their edible quality and commercial value, and even no harvest (Zhang et al., 2017). Low temperature has become an important limiting factor for facility cultivation of oriental melon in winter and spring.
In the process of resisting unfavorable conditions, plants have evolved strategies to protect themselves (Ding et al., 2019). The cytoskeleton is closely related to various environmental stimuli (Wang et al., 2011; Sengupta et al., 2019; Byun et al., 2021). Actin filaments are a major member of cytoskeleton and play an important role in stress responses (Zhang et al., 2010; Wang et al., 2011; Ye et al., 2013; Fan et al., 2016). Depolymerization of actin filaments in Arabidopsis plants under salt and osmotic stress can improve plant tolerance (Zhang et al., 2010; Wang et al., 2011; Ye et al., 2013). Pokorná et al. (2004) found that in 3-day-old BY-2 cells exposed at 0°C for 12 hours, actin filaments disintegrated completely, or turned into few in number, short, and sometimes branched filaments, actin bars or dots. The findings of Fan et al. (2015; 2016) suggest that actin cytoskeleton plays a key role in the tolerance of Arabidopsis seedlings to low temperature and heat stress, and specific members of actin depolymerizing factors (ADFs) may be involved in regulating plant response to low temperature and heat stress. Destabilizers of actin filaments and microtubules cause the activation of cold-inducible Brassica napus BN115 (Sangwan et al., 2001). However, the molecular mechanism of the dynamic change of actin filaments under low temperature is poorly understood.
The dynamic reorganization of intracellular actin filaments is regulated by a large number of ABPs with different functions (Hussey et al., 2006; Huang et al., 2011; Roland et al., 2008), in which ADFs are considered to be an important regulator of actin filaments changes (Maciver and Hussey, 2002; Bamburg and Bernstein, 2008). ADF is abundant and highly conserved in all eukaryotes, and plays an important role in plant growth and development as well as in response to multiple biotic and abiotic stresses (Andrianantoandro and Pollard, 2006; Dong et al., 2001, 2013). The functions of ADFs in Arabidopsis have been studied in vivo extensively. For example, AtADF1 can affect plants growth, development and morphogenesis (Dong et al., 2001), and participate in high temperature and salt stress processes (Wang et al., 2021, 2023). AtADF2 is required for normal cell growth and plant development, and its mediated actin dynamic is essential for root-knot nematode infection of Arabidopsis (Clément et al., 2009). AtADF4 relates to plants growth and development (Peng and Huang, 2006), plays a role in regulating hypocotyl growth, response to osmotic (Yao et al., 2022) and drought stresses (Zhao et al., 2016), and improves disease resistance of Arabidopsis to bacterium DC3000AvrPphB (Tian et al., 2009). AtADF5 is important for pollen germination and pollen tube growth (Zhu et al., 2017), promotes stomatal closure by regulating actin cytoskeleton remodeling under ABA and drought stresses (Qian et al., 2019), and improves the basal and acquired freezing resistance of Arabidopsis (Zhang et al., 2021). AtADF7 and AtADF10 are involved in pollen development and pollen tube growth (Daher and Geitmann, 2012; Zheng et al., 2013). Under osmotic stress, AtADF7 inhibited actin bundling protein VILLIN1 regulation of root hair formation (Bi et al., 2022). In addition, ADFs from barley and wheat have been shown to be related to plant resistance to various pathogens (Miklis et al., 2007; Fu et al., 2014; Inada et al., 2016). TaADF4 and TaADF7 from wheat play a stimulative role in resistance to the stripe rust infection (Zhang et al., 2017; Fu et al., 2014). TaADF3 negatively regulates wheat resistance against Puccinia striiformis (Tang et al., 2016). Increasing evidence has shown that ADFs play an important role in response and tolerance to various stresses (Huang et al., 2012; Xu et al., 2021). Drought resistance of OsADF3 in rice transgenic Arabidopsis is enhanced (Huang et al., 2012). AtADF5 improves the basal and acquired freezing resistance of Arabidopsis (Zhang et al., 2021). Overexpression of TaADF16 significantly improved the tolerance of transgenic plants to freezing stress (Xu et al., 2021). DaADF3 in Deschampsia antarctica enhanced the cold tolerance of transgenic rice plants (Byun et al., 2021). In the process of wheat cold acclimation, an ADF gene is induced, and the increased resistance to freezing shows that the ADF protein may be required in reorganization of the cytoskeleton under low temperatures (Ouellet et al., 2001). In short, more and more plant ADFs have been functionally characterized, while ADFs in oriental melon have not been reported.
In this study, we identified 9 CmADF genes in oriental melon and found that they were similar to homologue AtADF genes in sequence, structure, important site and tissue expression through analysis of their biological information and tissue expression. Further stress expression patterns showed that CmADFs responded to low temperature, high temperature, ABA and SA signals, especially under SA treatment, all CmADFs were dramatically up-regulated by approximately ten to hundreds of times. CmADF1 was significantly upregulated under all the above treatments, especially during 24 h of low temperature treatment, and maintained high expression, which provides the functional implication of CmADF1 in low temperature response. Further studies on the phenotype and actin filaments organization of Arabidopsis seedlings overexpressing CmADF1 under low temperature, as well as phenotype analysis and physiological identification of CmADF1 gene silenced oriental melon seedlings, indicated that CmADF1 affected the process of actin filaments depolymerization and played an important role in plant adaptation to low temperature stress.
Materials and methods
Plant materials, growth conditions, and stress treatments
The low temperature tolerant genotype Oriental melon ‘ LT-6 ‘ was provided by the Vegetable Research Institute of Liaoning Academy of Agricultural Sciences, and the CmADF1 silent plant was obtained by VIGS technology. The Oriental melon grew at 25/20°C (light 16 h/darkness 8 h) to two-leaf stage and was subjected to low temperature (4°C), high temperature (40°C), ABA (100 μM) and SA (100 μM) treatment for stress expression analysis. The second true leaves were sampled at 0, 3, 6, 12 and 24 h after treatment. Tissues of two-month-old plants, including roots, stems, young leaves, pistillate and staminate flowers, were collected for tissue expression analysis. The silenced plants of CmADF1 (TRV-A) were treated at 4°C at the two-leaf stage to observe the phenotype and analyze the physiological indexes.
The Arabidopsis thaliana plants used in this study have a Columbia background. Atadf1 (The T-DNA insertion mutant) (SALK_144459) was obtained from ABRC, and the fABD2-GFP material was donated by China Agricultural University (Wang et al., 2021). The Arabidopsis overexpression materials (CmADF1-OE and pCmADF1::GUS) were constructed by our laboratory. The seeds of WT and CmADF1-OE with 4°C vernalization for 3 days were seeded on 1/2 MS medium (Wang et al., 2023), and cultured in a 22°C incubator with a light/dark cycle of 16 h/8 h. After 14 days, the seedlings were placed in incubators at 22°C and 4°C for 0, 24 and 48h respectively, and the leaf area was counted by Image J software. WT and CmADF1-OE plants grown under normal conditions for 9 days were treated at 4°C for 12 h, and the morphology of actin filaments in leaves was observed.
Identification of the ADF gene family in oriental melon
To identify the ADF gene family members in oriental melon, amino acid sequences of 11 ADFs in Arabidopsis with ADF-H (Actin-Depolymerizing Factor Homology) domain were used as query sequences to search against the entire melon genome database (http://melonomics.net/) with the threshold E≤e-20 and default parameters by performing a BLASTP analysis. Then, the candidate sequences of ADF proteins in the melon genome were used repeatedly to search new ADFs. The longest protein sequence were selected when there were more than one predicted ADF proteins resulting from the alternative splicing by one gene. All identified ADF proteins were checked if they contained ADF-H domain by SMART (http://smart.embl-heidelberg.de/) analysis and hidden Markoy model analysis with PF00241 (http://pfam.xfam.org/family/PF00241). The protein sequences and genome sequences of CmADFs are downloaded from the Melon Genome database. CmADF paralogous genes to AtADF were named for the corresponding AtADFs. Molecular weight (kDa) and isoelectric points (pI) were calculated by the pI/Mw tool at online ProtParam (http://web.expasy.org/protparam/). Subcellular localization was predicted by WoLF PSORT (https://www.genscript.com/tools/psort). We predicted the tertiary structure of the CmADF protein using SWISS-MODEI (http://swissmodel.expasy.org/) and displayed the images using PyMOL V2.3.2 software. The genomic DNA sequence of 2000 bp upstream of gene initiation codon (ATG) was used as the promoter sequence and submitted to the promoter analysis system PlantCARE (http://bioinformatics.psb.ugent.Be/webtools/plantcare/html/) to find all potential cis-acting elements.
Sequence alignment and phylogenetic analysis
The ADF amino acid sequences of melon, Arabidopsis, cucumber, watermelon, Cucurbita maxima and Cucurbita pepo were aligned using ClustalX 2.1 (Larkin et al., 2007). ESpript3.0 (http://espript.ibcp.fr/ESPript/cgi-bin/ESPript.cgi) was used for image display. The unrooted phylogenetic tree was generated in MEGA6.0 using the neighbor-joining (NJ) method with 1000 bootstrap replicates (Tamura et al., 2013).
Chromosome localization and gene duplication
The chromosomal locations for each CmADF were determined according to melon genome information. Tandem and segmental duplications were determined using CoGe (https://genomevolution.org/CoGe/) online tool. Duplicated genes were linked using Circos software (http://circos.ca/). The non-synonymous substitution rate (Ka) and synonymous substitution rate (Ks) were calculated by DnaSp V5.0 (Librado and Rozas, 2009) software. The approximate time of each duplication event (T=Ks/2λ, λ=6.1×10-9) (Lynch and Conery, 2000) was estimated by Ks value.
Gene structure and conserved motif analysis of ADF in melon and Arabidopsis
According to structural information of ADF genes obtained from the gff3 files of melon and Arabidopsis genome databases, the intron-exon structures of each gene were drawn using GSDS (http://gsds.cbi.pku.edu.cn) online tool. MEME4.10.2 software (http://meme-suite.Org/tools/meme) was used to analyze the conserved motifs of ADF genes in melon and Arabidopsis. The maximum value of searched motif was set to 10, the length of motif was between 6 and 50, and other parameters were default values.
Total RNA extraction and real-time fluorescence quantitative PCR analysis
All total RNA was extracted by EasyPure Plant RNA Kit (Beijing Quanshi Jin Biotechnology Co., LTD.). 18S was selected as the internal control for RT-qPCR analysis. The primer sequences are shown in Supplementary Table 3. Roche Light Cycler 480 was used to detect the relative expression level of ADFs.
Overexpression and subcellular localization of CmADF1
The full-length CDS of CmADF1 was respectively cloned into the pSuper1300-GFP and pCAMBIA 1300 vectors to generate 35S::CmADF1-GFP and 35S::CmADF1, which were then introduced into the Agrobacterium tumefaciens strain GV3101. The primer sequences are listed in Supplementary Table 3. T3 transgenic homozygous lines were screened for confocal microscope observation and low temperature study. 35S::CmADF1-GFP was used to observe subcellular localization. 35S::CmADF1was used in subsequent low temperature stress studies.
Promoter activity analysis
CmADF1 promoter fragment containing the first intron of CmADF1 was cloned in pCAMBIA1300-221 vector to generate pADF1::GUS. Homozygous lines were used for promoter activity analysis. The positive seedlings growing for 9 days were treated at 4 °C for 0, 6, and 12 h, and GUS staining was performed. The primer sequences are listed in Supplementary Table 3.
Visualization and quantitative analysis of actin filaments
As previously reported, CmADF1-OE#8 plants were hybridized with fABD2-GFP plants, and homozygous plants were selected for subsequent experiments. Confocal microscopy (Nikon A1) with a 40×objective was used to observe actin filaments in pavement cells of cotyledons with a 488-nm laser. Image J was used to measure skewness, density, length and actin cables applied to quantify actin filaments in previous reports. All experiments were repeated 3 times. 30 individual seedlings from different genotypes and treatments were screened to collect more than 200 cells from 60 images.
Western blot assays
10-day-old CmADF1-GFP overexpressing Arabidopsis seedlings were used for Western Blot. As previously reported (Liu et al., 2013; Wang et al., 2020), GFP (a labeled protein) was analyzed by SDS-PAGE. Rubisco bands were used as loading controls.
Vector construction and infection of virus-induced gene silencing
The CmADF1 gene in oriental melon were silenced by VIGS. The specific primers containing EcoRI/KpnI cleavage sites were designed to generate pTRV2-CmADF1 (Supplementary Table 3 for the sequence of primers). The method of cotyledon infection was used. The cotyledons of the germinated oriental melon seeds were fully expanded about 5 days after sowing, and they could be infected. The detailed infection process was carried out according to the method of Liao et al. (2019). About 60 infected plants were randomly divided into 3 groups, and each plant was sampled separately after treatment at 4°C for 0, 6 and 12 h. The expression of CmADF1 in the leaves of VIGS plants was detected by agarose gel electrophoresis and RT-qPCR, and the plants with transcription level lower than 50% of that of the control plants were selected for subsequent experiments.
Determination of physiological and biochemical indexes and water loss rate
Relative electrolyte leakage (REL) and water loss was measured as described previously (Xing et al., 2020). The contents of soluble protein, proline, malondiald ehyde (MDA) and the activities of SOD, CAT and POD were determined with the relevant kit of Suzhou Mengxi Biomedical Technology Co., LTD. All experiments were repeated three times.
Results
Genome-wide identification and bioinformatics analysis of ADF gene family members in oriental melon
The ADF family member from Arabidopsis with the ADF-H domain (PF00241) was used for Blast search in the entire melon genome. 11 non-redundant putative ADF proteins were found, 3 of them were produced by one gene, which was subject to differential splicing, so we selected the longest protein sequence. Finally, 9 CmADF genes were identified by SMART and Pfam analysis, and they were named with the same names as their homologue AtADF genes. CmADF proteins contain 132 (CmADF8) to 146 (CmADF6) amino acids, and their physicochemical properties are shown in Supplementary Table 1.
To clarify the phylogenetic relationships and functional divergence of CmADF gene family members, amino acid sequences of ADF from Arabidopsis, melon and other Cucurbitaceae species (cucumber, watermelon, Cucurbita maxima and Cucurbita pepo) were used to construct the phylogenetic tree (Figure 1A). Accession numbers of the ADF genes in each species were shown in Supplementary Table 1. The results showed that 9 CmADFs were distributed in 4 subfamilies and located on six of the 12 melon chromosomes (Figure 1B). Two pairs of segmentally duplicated genes ADF7/10 and ADF1/4 were detected (Figure 1B).
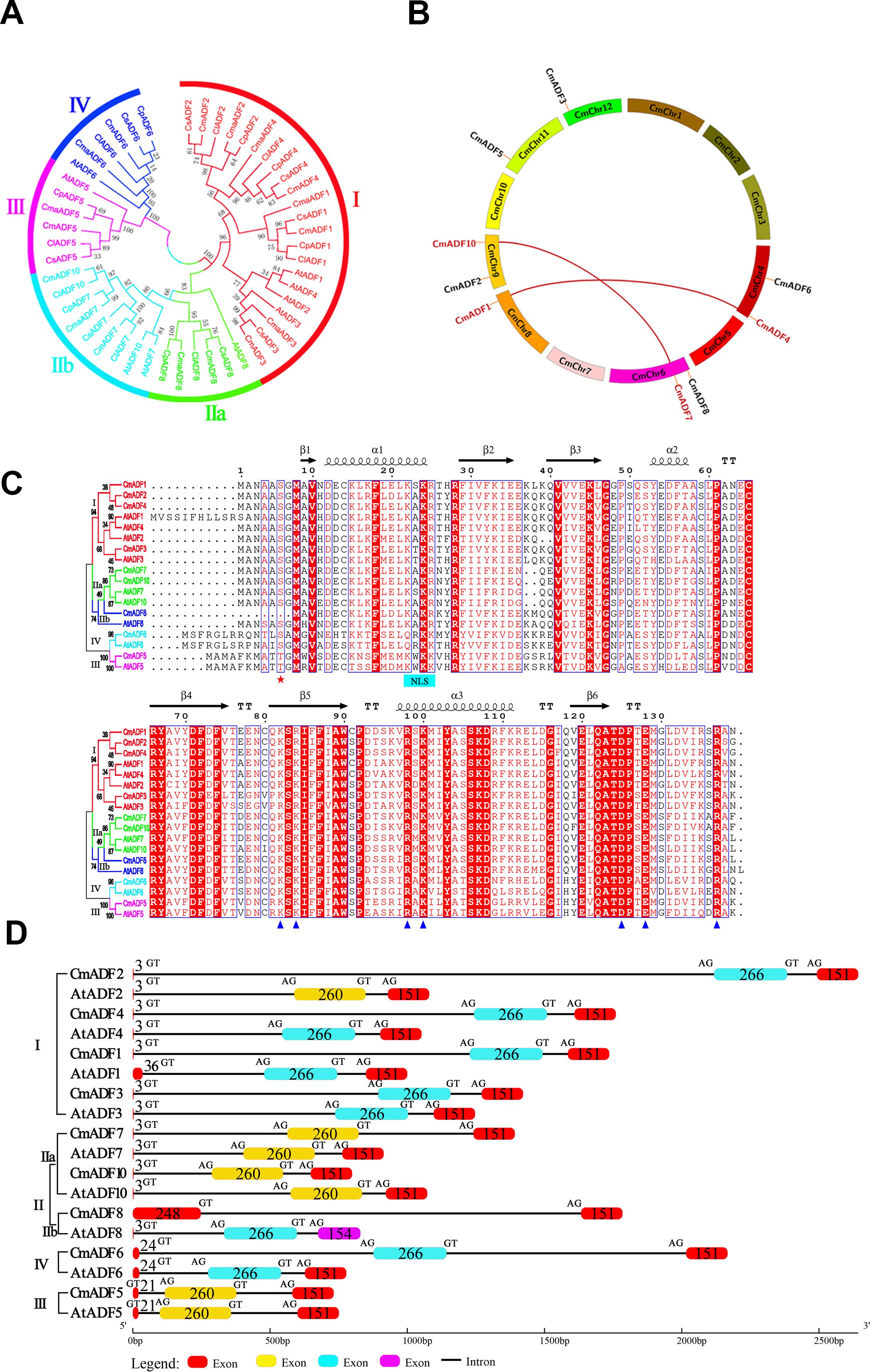
Figure 1. Phylogenetic relationship, Chromosomal location and duplication events, Sequence alignment, exon-intron structures of CmADFs. (A) Phylogenetic tree of ADF proteins from melon and other plants. Cucumis melon, Arabidopsis thaliana, Cucumis sativus, Citrullus lanatus subsp, Cucurbita maxima var, Cucurbita pepo subsp were represented by Cm, At, Cs, Cla, Cma and Cp, respectively. (B) Location and duplication events of 9 CmADF genes onto six melon chromosomes. The segmentally duplicated genes are linked by red lines. (C) Sequence alignment of CmADF and AtADF proteins. Pentagons indicate conserved sites that may bind to G-actin and F-actin, blue pentagons indicate the 6th serine site, and blue bar indicates the nuclear localization signal. (D) Exon-intron structures of ADFs in melon and Arabidopsis. The numbers indicates the length of exon. GT and AG indicate splicing sites.
As previously reported (Bowman et al., 2000), there are 5 β-strands and 3 central α-helices in the predicted tertiary structures of CmADFs (Supplementary Figure 1). A comparative analysis of the protein sequence and gene structure of the ADFs from melon and Arabidopsis showed that they contain the conserved serine-6 residue at the N-terminus (except CmADF5 and AtADF5 of which were replaced by threonine, and CmADF8 of which was deleted), conserved actin binding sites (R98/135/137 and K82/100 in AtADF1) (Dong et al., 2013) (Figure 1C), as well as conserved motifs (Supplementary Figure 2) and gene structure (Figure 1D). Exon-intron structures of ADFs in melon and Arabidopsis is very conservative (Figure 1D). The homologous ADFs of the two species were comprised three exons and two introns (except CmADF8) and same number of amino acids in the exons (except CmADF8 and AtADF1). The first exons of CmADFs and AtADFs in Subclass I and II (except CmADF8 and AtADF1) contained only 3 amino acids (start codon ATG), followed by a longer first intron (Figure 1D), while the ones of CmADFs and AtADFs in Subclass III and IV increased to 21 and 24 amino acids due to the alteration of the intron conserved clipping site (GT) after ATG (Figure 1D) and intron sliding (Nan et al., 2017).
Analysis of promoter sequences showed that in addition to many light response elements in all members, CmADF promoters contained several key defense and stress responsiveness, low-temperature responsiveness, and heat stress cis-acting elements and elements involved in the response to various hormones, such as abscisic acid (ABA), salicylic acid (SA), gibberellins (GA), auxin (IAA), ethylene, and methyl jasmonate (MeJA) (Supplementary Figure 3). It also contained DRE and MYB binding sites.
Expression pattern analysis of CmADFs in different oriental melon tissues
RT-qPCR was performed for analyzing the expression of CmADFs in different oriental melon tissues. The expression of CmADFs was clearly divided into two categories: CmADFs from subclass I, III and IV were expressed in all tissues, while CmADFs from subclass II were specifically expressed in flowers (Figure 2). Only CmADF1 was highly expressed in leaves, the other ADFs showed higher expression levels in male flowers and female flowers than that in roots, stems, and leaves. Compared with other CmADFs, CmADF2, CmADF3 and CmADF6 had extremely higher expression levels in all tissues.
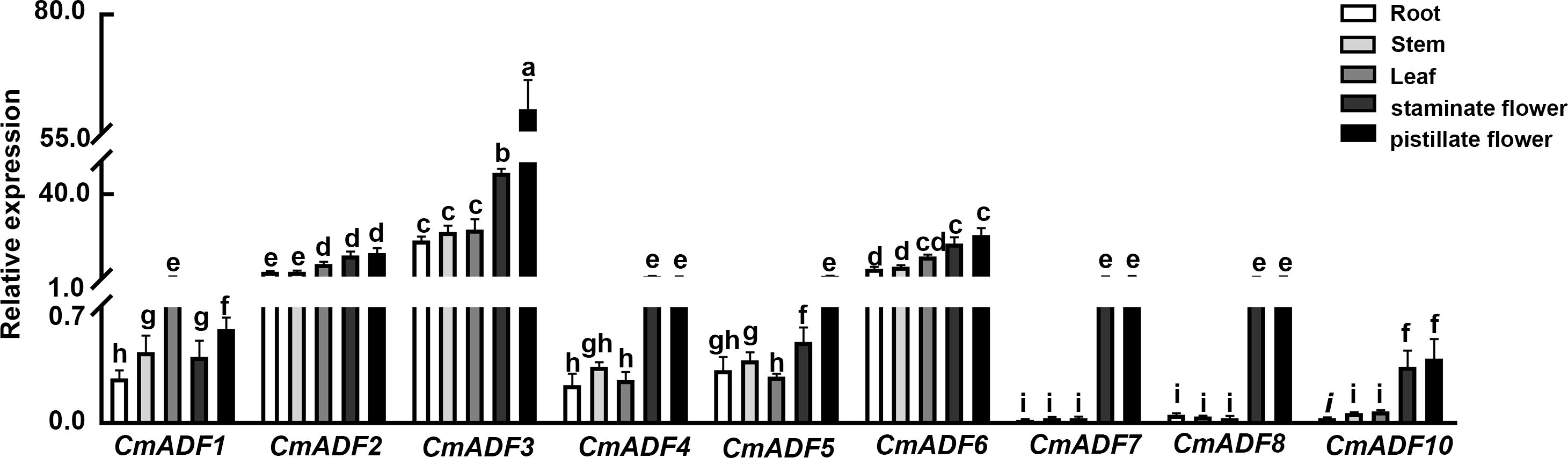
Figure 2. The expression of CmADFs in different Oriental melon tissues. The relative expression of CmADFs were determined by RT-qPCR, and 18S was used as an internal control. All values used for statistical analysis were the mean ± SD of three independently replicated experiments, and then a Tukey’s post-hoc test was performed using one-way ANOVA. Different lowercase letters indicate a significant difference.
Expression pattern analysis of CmADFs under low and high temperature stress
Temperature change is one of the main environmental stressors affecting the growth and yield of melon (Korkmaz and Dufault, 2004). In order to gain an insight into the potential function of CmADFs in unfavorable temperature conditions, we analyzed the expression of CmADFs in leaves of oriental melon seedlings at two-leaf stage under low and high temperature stress. The results showed that except for CmADF3/7/8, the expression of other CmADFs was induced by low temperature stress (Figure 3A). Among them, CmADF6 expression was the highest, and CmADF1 expression was high and stable. Under high temperature stress, the expression of CmADF1/3/4/7/8/10 was significantly induced, and the expression of CmADF10 increased the highest, while the expression of CmADF2/5/6 decreased, and CmADF2 continued to be significantly down-regulated, indicating that the expression of CmADFs was complex under high temperature stress (Figure 3B). Under low and high temperature, the expression of CmADF1/4/10 was all induced, but only CmADF1 was significantly up-regulated.
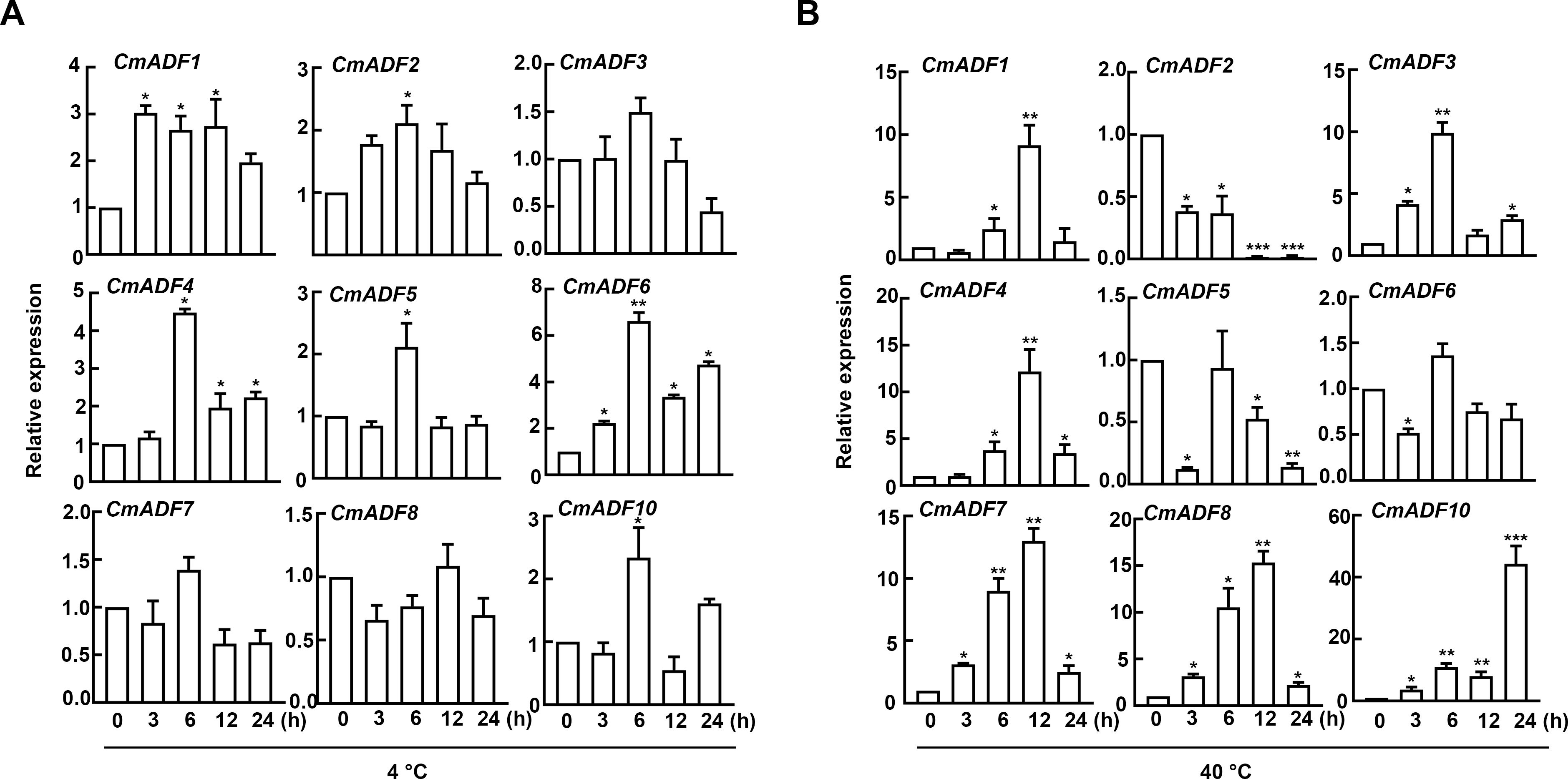
Figure 3. The expression of CmADFs under low (4°C) (A) and high (40°C) (B) temperature treatments according to RT-qPCR. 18S was used as an internal control. The values are means ± SD from three independent replicate experiments (Student’s t-test, *P < 0.05,** p < 0.01,***P < 0.001). The significant difference is represented by asterisks.
Expression pattern analysis of CmADFs in ABA and SA stress
Considering that ABA and SA are the main hormones in plant adaptation to stresses, we analyzed the expression of CmADFs in leaves under ABA and SA stress. The results showed that after ABA treatment, only CmADF2 expression was down-regulated, and all the other CmADFs were up-regulated. Other genes reached the highest expression level at 6 h after treatments, except for CmADF8, which reached the peak value at 24 h (Figure 4A). Under SA stress, all CmADFs responded sharply at the beginning, increasing their expression with approximately 13-770 folds, and continued to be highly expressed until 24 h (Figure 4B). These results reveal that CmADFs respond to SA and ABA induction. Overall, CmADF1 was significantly upregulated in response to temperature and hormonal signals. At the same time, CmADF1 expression levels was high and stable under low temperature stress, indicating that CmADF1 plays an important role in environmental and hormonal signals. Therefore, CmADF1 was selected for the study of low temperature tolerance in plants.
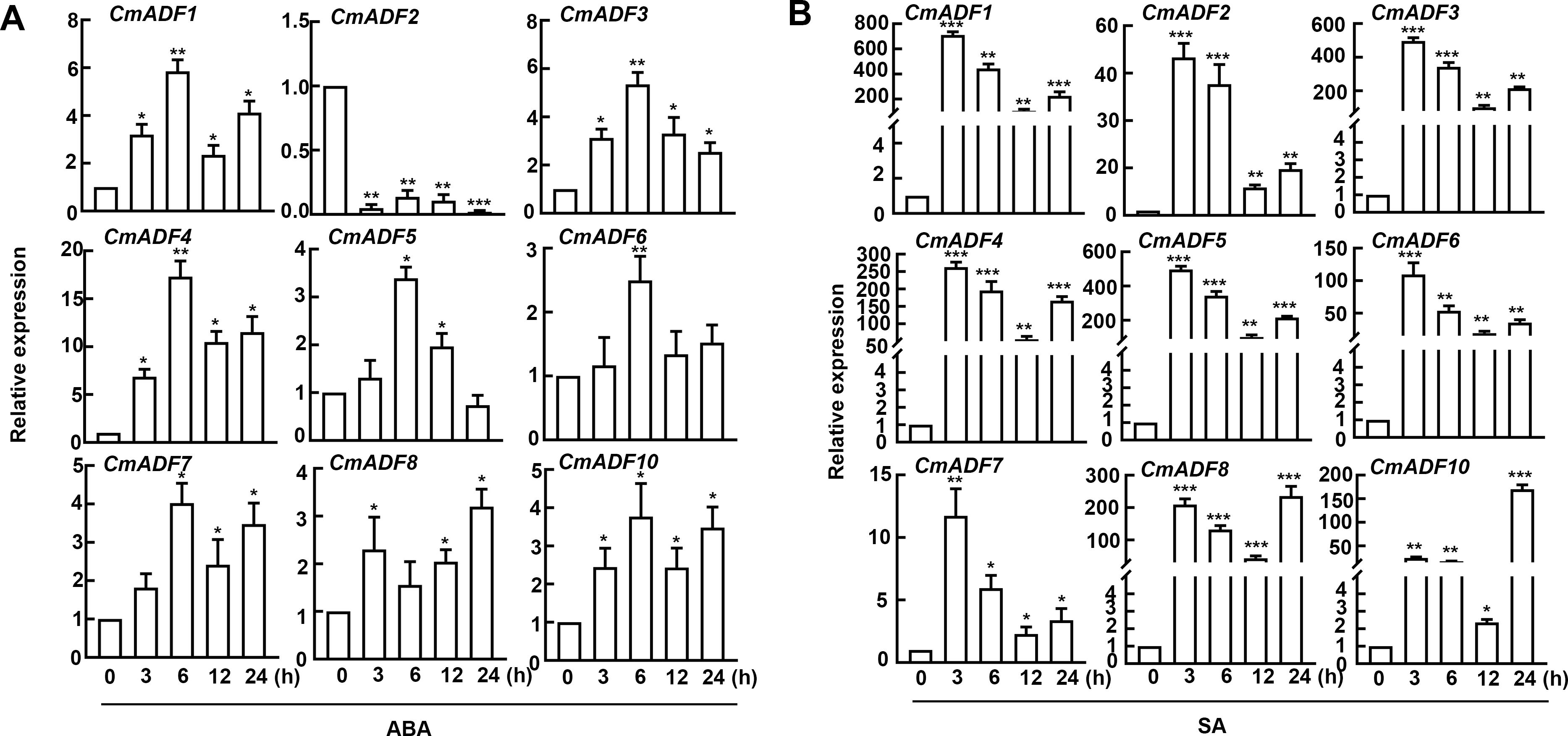
Figure 4. The expression of CmADFs under ABA (0.1 mM) (A) and SA (0.1 mM) (B) treatments according to RT-qPCR. 18S was used as an internal control. The values are means ± SD from three independent replicate experiments (Student’s t-test, *P < 0.05,** p < 0.01,***P < 0.001). The significant difference is represented by asterisks.
Subcellular localization of CmADF1
Transgenic Arabidopsis plants in T3 were observed by laser scanning confocal microscope for the localization and actin filaments binding of CmADF1. As shown in Figure 5, a large number of actin filaments bundle structures formed by CmADF1-GFP green fluorescent protein in the paver cells in the control group (Figures 5A, B). These actin filaments in the paver cells were shortened or disappeared after 50 nM LatB (microfilament depolymerization drug) treatment (Figure 5A). Moreover, the filamentous structure did not change after 50 nM Oryzalin (a microtubule depolymerization drug) treatment (Figure 5B), further indicating that CmADF1 specifically binds to actin filaments rather than microtubules in Arabidopsis.
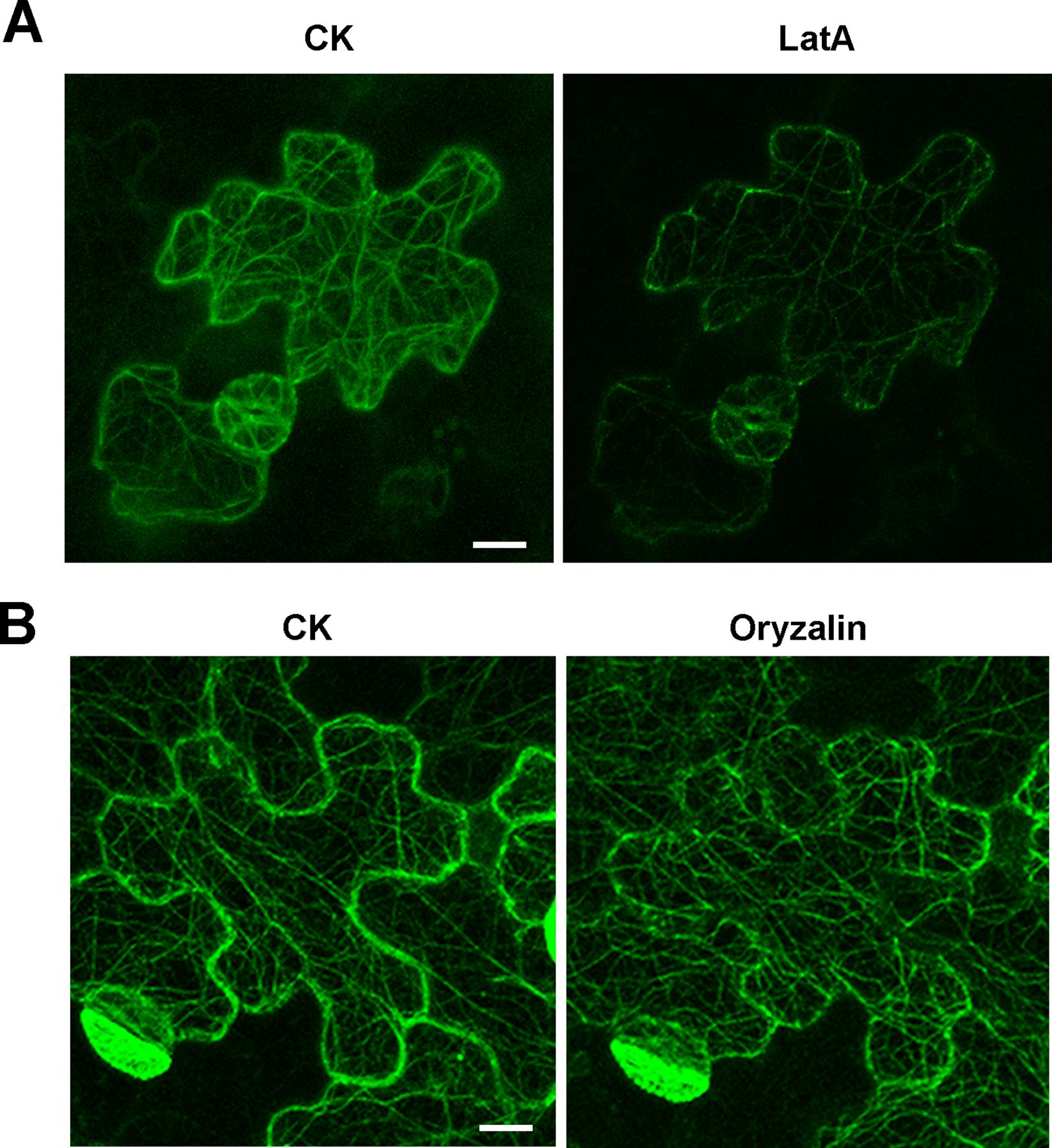
Figure 5. CmADF1 localization on actin filaments in melon. The leaves of 5-day transgenic Arabidopsis homozygous T3 plants were used for observation. (A) The actin filaments skeleton formed by CmADF1-GFP before (CK) and after Lat B treatment (50 nM). (B) The actin filaments skeleton formed by CmADF1-GFP before (CK) and after oryzalin treatment (50 nM). Scale bar = 25 μm.
Promoter activity analysis of CmADF1
It has been reported that AtADF1 plays an important role in stresses such as salt and high temperature stress (Wang et al., 2021, 2023). Our results showed that CmADF1 maintained a high expression in melon under low temperature conditions. Therefore, we investigated the mechanism of CmADF1 in melon adaptation to low temperature stress. Firstly, GUS staining was used to detect the effect of low temperature on the activity of CmADF1 promoter, and to further verify the expression pattern of CmADF1 gene under low temperature stress. After 4°C treatment, the color of pCmADF1::GUS plants deepened with the prolongation of treatment time, and the expression of CmADF1 was significantly up-regulated (Figure 6), demonstrating that low temperature promoted the activity of CmADF1 promoter.
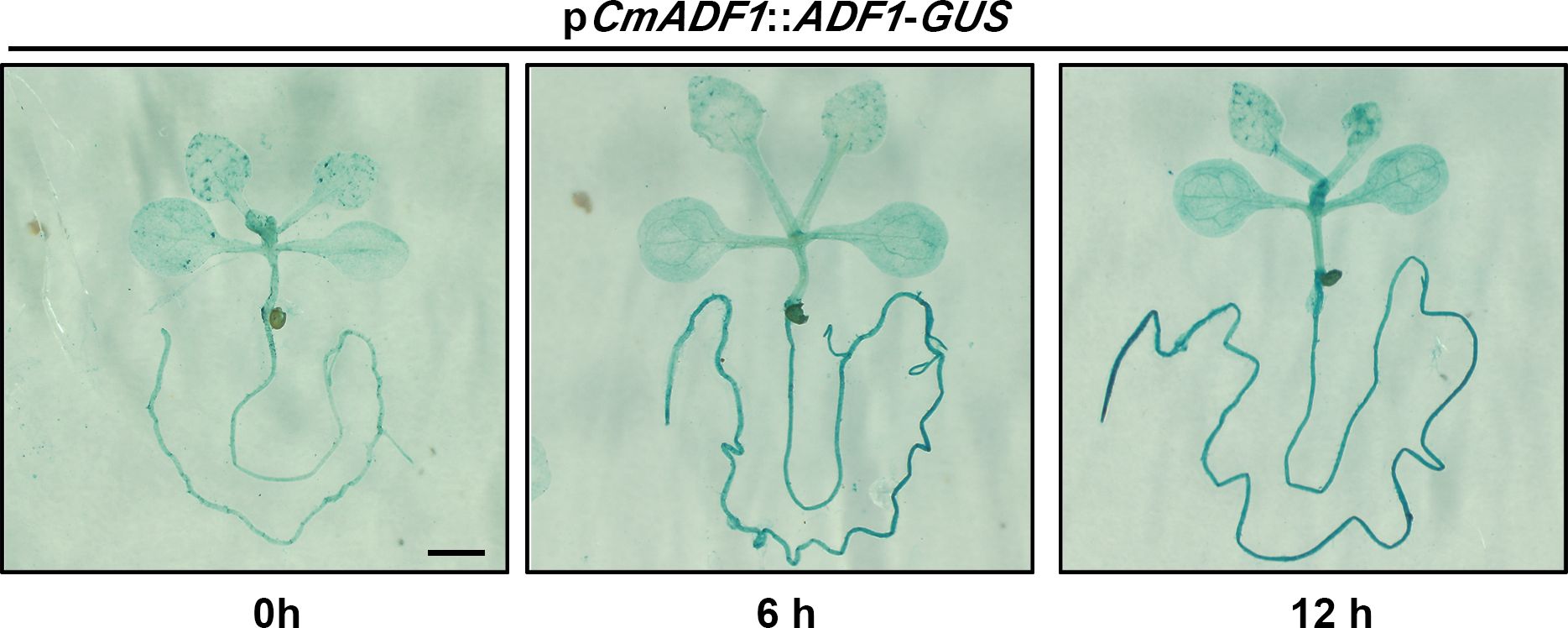
Figure 6. CmADF1 promoter activity analysis under low temperature treatment. Staining of pCmADF1::GUS transgenic Arabidopsis plants under low temperature for 0 h, 6 h and 12 h. Scale bar = 1 mm.
CmADF1 overexpression affects actin filaments stability under low temperature stress
ADF1 is an actin filament depolymerizing protein. To explore whether CmADF1 can regulate actin filaments under low temperatures, we constructed transgenic Arabidopsis overexpressed T3 homozygous lines (CmADF1-OE#6 and CmADF1-OE#8) (Supplementary Figure 4). The homozygous offsprings (CmADF1-OE#8) of CmADF1-OE#8 × fABD2-GFP were selected to observe actin filaments. Compared with WT, CmADF1-OE#8 seedlings had fewer actin filaments bundles, and more short filaments under both normal and low temperatures (Figures 7A, B). Consistent with the morphology of actin filaments, quantitative analysis of actin filament organization showed that the skewness value, bunting rate, fluorescence density and length of actin filaments in CmADF1-OE#8 were significantly lower than those in WT (Figure 7B), indicating that overexpression of CmADF1 caused the instability of actin filaments. Compared with normal conditions, the actin filaments in WT were shorter and finer under low temperature, indicating that low temperature induced the instability of intracellular actin filaments.
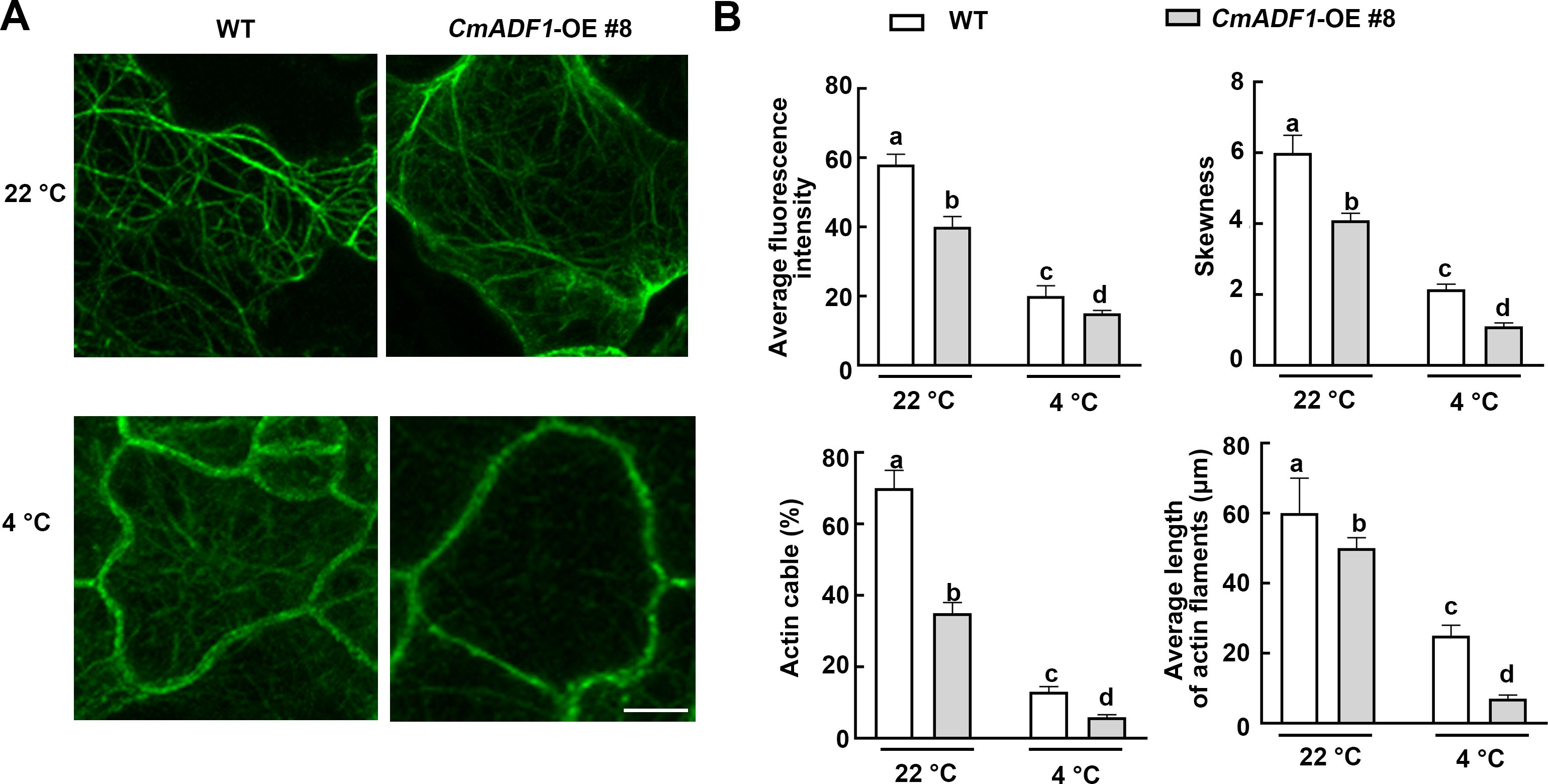
Figure 7. CmADF1 increases the instability of actin filaments under low temperatures. The organization (A), Average fluorescence density, actin cable and average length (B) of actin filaments in WT and CmADF1-OE#8 plants under low temperature treatment. Indicators in (B) are measured based on images in (A). Values are means ± SD (At least 30 individual seedlings from different genotypes and treatments were used to collect more than 300 images). One-way ANOVA followed by a Tukey’s post-hoc test is used for statistical analysis. Different lowercase letters denoted significant differences. Scale bar= 25 μm.
CmADF1 overexpression enhance the low temperature tolerance in Arabidopsis
T3 CmADF1-OE#6 and T3 CmADF1-OE#8 seedlings were used to analyze the function of CmADF1 under low temperature (Figure 8). The phenotypes of 14-day-old WT, CmADF1-OE#6 and CmADF1-OE#8 seedlings were observed under normal (22°C) and low temperature (4°C) stress for 0, 24 and 48 h. There was no significant difference between WT and overexpressed plants before low temperature treatment. After treatment for 24 and 48 h, the leaves of WT plants shrunk significantly in size (Figures 8A–C) and lost more water (Figure 8D), compared with those of overexpressed plants. WT plants were more severely damaged than overexpressed plants after 48 h of treatment, with water-soaked spots on the leaves (Figure 8C).
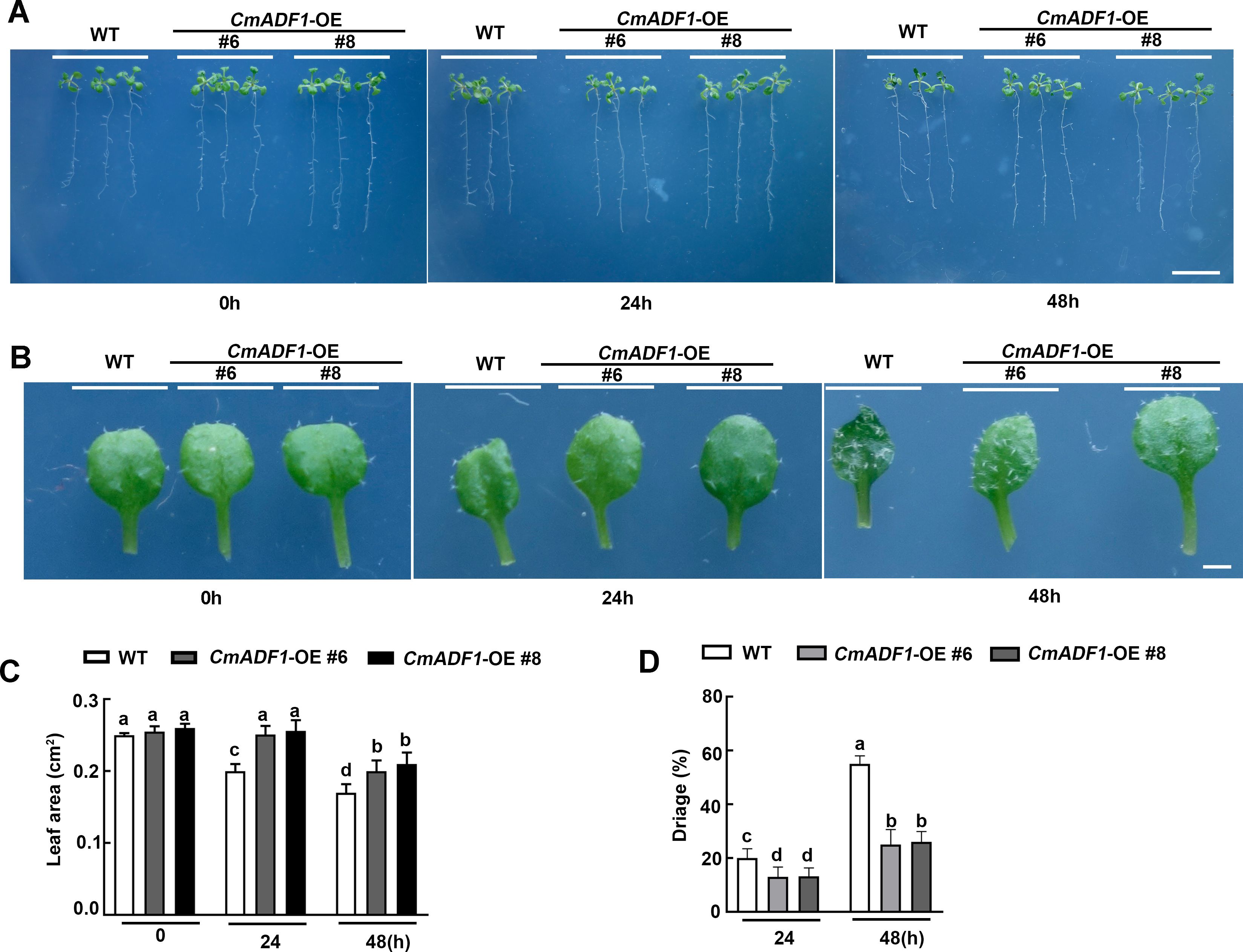
Figure 8. CmADF1-OE positively regulates low temperature tolerance of Arabidopsis. Plant Phenotype (A), Leaves Phenotype (B), Leaves Area (C), Water loss rate of plants (D) of CmADF1 transgenic seedlings under low temperature treatment. 14-day-old seedlings of WT, CmADF1-OE#6 and CmADF1-OE#8 were placed in normal temperature (22°C) and low temperature (4°C) chamber for 0, 24 and 48 h. At least 60 leaves from 30 seedlings were measured in (C) and at least 400 seedlings were measured in (D). One-way ANOVA followed by a Tukey’s post-hoc test is used for statistical analysis. Different lowercase letters denoted significant differences. Scale bar=1cm in (A), Scale bar=1mm in (B).
CmADF1-OE seedlings showed superior resistance by less damage to low temperature stress with more fine actin bundles and short filaments than WT seedlings (Figures 7A, B), which implicated that CmADF1 enhanced plant tolerance to low temperature by regulating actin filaments organization.
CmADF1-Silenced Plants are Sensitive in response to Low Temperature
The expression level of CmADF1 was detected by RT-qPCR when the CmADF1 gene silencing (TRV-A) plants obtained by VIGS technology had two leaves, and TRV-A plants with high silencing efficiency were selected for subsequent research (Figure 9A). Under optimal temperature control conditions, no significant difference in growth between TRV-A and control plants was observed (TRV-0) (Figure 9B). After treatment at 4°C for 6 and 12 h, TRV-A plants suffered more serious damage than TRV-0 plants, and their leaves shrunk more seriously and lost more water (Figures 9B–D). Compared with TRV-0 plants, the relative electrolyte leakage (REL) and malondialdehyde content of TRV-A plants were higher, while the contents of soluble protein and proline and the activities of SOD, POD and CAT were lower, reaching a significant level at 12 h (except for REL) (Figure 9E), illustrating that silencing CmADF1 reduced the low temperature tolerance of oriental melon.
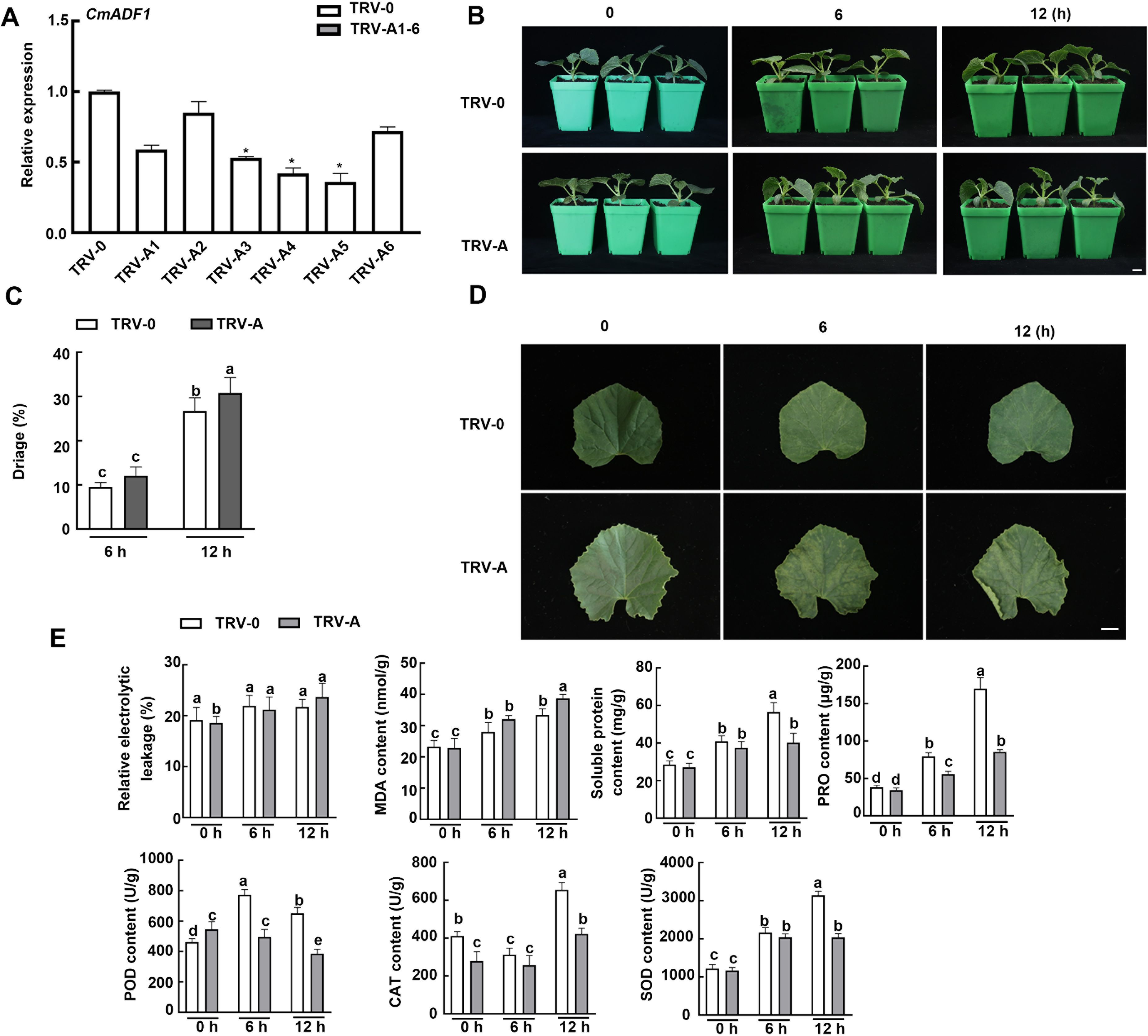
Figure 9. The silencing of CmADF1 in oriental melon seedlings by virus-induced gene silencing (VIGS) increased its sensitivity to low temperature. CmADF1 expression (A), Plant phenotype (B), Water loss rate of leaves (C), Leaves (D), the relative electrolyte leakage (REL), malondialdehyde (MDA), Soluble protein and proline content (PRO), SOD, POD and CAT activity (E) of TRV-0 (control) plants and TRV-A (CmADF1-silenced) plants under low temperature treatment. Scale bar=1 cm.
Discussion
Highly conservative CmADF genes in oriental melon
Since the ADF gene was discovered in the early 1980s, more and more ADFs had been found gradually in different species. In eukaryotic cells, ADFs were encoded by polygene families, which were abundant in plants (Maciver and Hussey, 2002). The reported plant ADF families includes 11 ADFs in Arabidopsis (Ruzicka et al., 2007), rice (Feng et al., 2006) and tobacco (Khatun et al., 2016), 13 ADFs in maize (Huang et al., 2020), 25 ADFs in wheat (Xu et al., 2021) and 18 ADFs in soybean (Sun et al., 2023). The ADF gene family is considered to be structurally and functionally conserved in plants (McCurdy et al., 2001). In our study, 9 ADF genes were identified in oriental melon for the first time, and they were distributed to four subclasses as in Arabidopsis. Moreover, they were almost the same as their homologue AtADFs in intron-exon structure, number of amino acids contained in exons, as well as type and number of motifs. There were also some important conserved sites in CmADFs, such as the serine at position 6 of N-terminal (S6), actin binding sites (Figure 1C). The activity of ADF protein was regulated by phosphorylation of N-terminal conserved serine or threonine. The S6 mutant of ZmADF3 lost its ability to bind to G-actin and F-actin (Smertenko et al., 1998). The S6 activity of LlADF1 decreased after phosphorylation, and the binding and disassembly F-actin activities were lost (Allwood et al., 2002). The base residues (K82, R135, R137) on β-chain and α-helix form actin binding sites, which is important for G-and F-actin binding (Dong et al., 2013). These results indicate that ADF family genes are structurally conserved.
The ADFs in flowering plants probably evolved from a common ancestor (Nan et al., 2017). Fragments and tandem gene duplication are considered to be the major driving forces in the evolution of large gene families (Cannon et al., 2004). Duplicate gene pairs in Arabidopsis and wheat are likely caused by tandem duplication, while two pairs of segmentally duplicated genes ADF7/10 and ADF1/4 were observed (Figure 1B; Supplementary Table 4) in melon as in tomato (Khatun et al., 2016), maize (Huang et al., 2020), and soybean (Xu et al., 2021). The ADF gene family has been differentiated in expression pattern and function during a long evolutionary process (Kijima et al., 2016). The angiosperm ADF gene family consists of four very conserved subfamilies, which are divided into two classes: reproductive or constitutive/vegetative (Ruzicka et al., 2007). In our study, tissue expression patterns of ADFs in melon were similar to those in Arabidopsis. CmADFs in Subclass I, III, and IV are expressed in all tissues examined and may play a critical role in growth and development (Figure 2). CmADFs in Subclass II specifically expressed in flowers may contribute to reproductive development (Figure 2). Unlike AtADF8, which is mainly expressed in roots and root hairs, CmADF8 is specifically expressed in flowers, indicating that different species are relatively independent in the subsequent evolutionary process. In general, ADF family genes were quite conserved in the long-term evolution of plants.
A large number of CmADFs respond to temperature stress and hormone signals
Expression analysis of ADF gene families in tomato, maize, wheat and soybean revealed that the expression of ADFs would change significantly under abiotic stresses such as heat, cold, drought, high salt, abscisic acid (ABA), jasmonic acid (JA) and injury (Khatun et al., 2016; Huang et al., 2020; Xu et al., 2021; Sun et al., 2023). Many ADFs in tomatoes were induced by cold, heat, drought, NaCl, ABA, JA, and injury treatment (Khatun et al., 2016). In maize, ZmADF1 was significantly up-regulated under all abiotic stresses, and ZmADF2 and ZmADF3 were significantly induced under high temperature, drought and ABA treatment (Huang et al., 2020). The expression of GmADFs in soybean changed under high temperature, low temperature, drought and salt stresses, and GmADF2/5/9/12/13/16/18 were significantly induced by heat stress (Sun et al., 2023). TaADF16/17/18 in wheat promoted the freezing resistance of wheat plants acclimated to the cold (Xu et al., 2021). ADF1 in Arabidopsis has been shown to participate in high temperature and salt stresses, and is also the most important member of Arabidopsis ADF family involved in stress.
In present study, CmADF1 was significantly induced in all treatments (Figures 3, 4), suggesting that, similar to Arabidopsis, ADF1 in melon may have an important effect on stress tolerance. Under low temperature, CmADF1 was stably and highly expressed (Figure 3), while AtADF1 was not induced, but AtADF5 and AtADF9 in Subclass III were significantly up-regulated (Fan et al., 2016). This indicates that ADF genes in different plants are functionally differentiated. Members of a gene family from the same group may have similar functions (Huang et al., 2020). CmADF1/3/4 from Subclass I and CmADF7/8/10 from Subclass II were both significantly induced under high temperature, ABA, and SA treatments (Figures 3, 4), suggesting that they may confer plants tolerance to these stresses. Studies have shown that actin depolymerization can increase plant resistance to pathogens, and that SA is crucial to this process (Leontovyčová et al., 2019). The expression of CmADFs was increased several hundred-fold under SA treatments, which may enhance resistance to biotic stress by depolymerizing actin filaments dependent on SA signaling pathway. CmADF2 was sensitive to high temperature and ABA treatments, indicating the diversity and complexity of functions among family members in resistance to stress. A large number of ADFs respond to different abiotic stresses, and the function of some of them in stresses has been proven, so it is necessary for us to further study ADFs.
CmADF1 plays an important role in plant low temperature tolerance
Low temperature is an important factor affecting the yield of Oriental melon. The detail molecular mechanism of dynamic changes of actin filaments under low temperature is still uncovered, the only thing we know is that low temperature treatment leads to the depolymerization of actin filaments like the other abiotic stresses do, such as salt, high temperature, and osmotic stress (Pokorná et al., 2004; Wang et al., 2011; Fan et al., 2015). Byun et al. (2021) found that DaADF3 functions to depolymerize F-actin into G-actin in transgenic rice plants overexpressing DaADF3, and observed cytoskeleton structural changes in D. antarctica seedlings in response to cold stress treatment, which imply that DaADF3 regulates the cytoskeleton structure to adapt to changing environmental conditions, especially cold stress in D. antarctica. ADF members in Subclass I have functions in resisting biotic/abiotic stresses (Huang et al., 2020). ADF1 in Subclass I is highly expressed in all tissues and is most closely related to salt stress and high temperature stress (Wang et al., 2021, 2023).
Our study found that CmADF1 is the only gene in the melon ADF family with highly stable up-regulated under low temperatures (Figure 3A), and GUS staining (Figure 6) confirmed this result. Therefore, CmADF1 may be a primary protein responding to low temperature stress in the CmADF family. Previous studies have found that some ADFs are involved in responding to low temperature, however there is still a lack of in-depth study on actin filament dynamics. Our studies revealed that low temperature stress induced actin filaments instability (Figure 8), which is consistent with the ADF family that is functionally characterized by depolymerization and cutting actin filaments. Our results further indicated that CmADF1-OE transgenic seedlings with low temperature-promoted the depolymerization of actin filaments showed more resistant to low temperature (Figure 7A). These suggests that the actin filaments morphology of CmADF1-OE under low temperature is directly caused by the function of CmADF1 to depolymerize and cut single actin filament, and CmADF1 regulates remodeling of the actin cytoskeleton to adapt to low temperature stress in melon.
Actin filaments depolymerization have been proved to play a positive regulatory role in salt, osmotic, high temperature and drought stress (Wang et al., 2011; Fan et al., 2015). Xu et al. (2021) found that TaADF16-OE transgenic Arabidopsis plants suffered less freezing damage in comparison with WT, and had higher POD and SOD activities and more soluble sugar accumulation after a 24h incubation at 4°C. They believed that overexpression of TaADF16 may contributes to the positive effects on ROS scavenging and osmotic regulation, and enhances the freezing resistance of Arabidopsis plants. In our experiment, CmADF1-OE conferred Arabidopsis better growth status under low temperature compared with WT and Atadf1, demonstrating that CmADF1 enhanced the low temperature tolerance of seedlings and promoted seedlings growth. Meanwhile, CmADF1-silenced oriental melon seedlings showed that the contents of soluble protein and proline, and the activities of superoxide dismutase (SOD), peroxidase (POD) and Catalase (CAT) were significantly lower than those in the control. Thus, CmADF1 is a key protein that triggers low-temperature induced actin filament depolymerization in melon, which improves the plant’s low-temperature tolerance. Zhang et al. (2021) found that AtADF5, as a downstream target gene of C-repeat binding factor (CBF) signaling pathway, is involved in plant response and resistance to low temperature stress by regulating the dynamics of actin filaments. Overexpression of TaADF16 induces the expression of cold-responsive genes, which may regulate cold tolerance through interaction with ICE (inducer of CBF expression)-CBF-related genes (Xu et al., 2021). Synthetic nucleotides designed based on the DRE element contained in the DaADF3 promoter have a high binding affinity with DaCBF7 (Byun et al., 2015, 2021). We also found the DRE binding site in the CmADF1 promoter (Supplementary Figure 3). Whether the CBF protein is an important factor affecting the transcription level of CmADF1 in oriental melon with low temperature tolerance will be our next work. Together, our results demonstrate that CmADF1 plays an important role in plant adaptation to low temperature by leading to depolymerization of actin filaments, providing breakthrough insights into the molecular basis for melon adaptation to low temperature stress.
Conclusion
In this study, 9 ADF genes were identified in Oriental melon, which were clustered into four subfamilies and their proteins contain one conserved ADF-H domain specific to ADF family genes by phylogenetic tree and conserved domain analysis (Figures 1A, C). The comparative analysis of ADFs in Arabidopsis and melon showed that ADFs of these two species were highly similar in phylogenetic evolution, tertiary structure, conserved motifs and key conserved sites binding to actin, indicating that plant ADF genes are very conserved in the long-term evolution process (Figures 1A, C, D; Supplementary Figures 1, 2). Various CmADFs displayed specific tissue expression patterns (Figure 2), some were induced by temperature and hormone signals (Figures 3, 4). CmADF1/2/4/5/6/10 and CmADF1/3/4/7/8/10 were induced under low/high temperature stress, respectively (Figure 3). All CmADFs responded to SA and ABA signals (Figure 4). These results suggested that CmADFs may be involved in melon response to stress. CmADF1 had high and stable expression levels under low temperature stress (Figure 3A). CmADF1 overexpressing plants promoted the instability of actin filaments and enhanced the resistance growth to low temperature treatments (Figures 7, 8), suggesting that CmADF1 plays an important role in low temperature stress in melon. Because the expression of CmADF1 gene was significant induced by low temperature, and the CmADF1 gene promoter contained the binding sites of MYB and CBF (Supplementary Figure 3), the key transcription factors in plants tolerance low temperature stress, we speculate the role of CmADF1 in low temperature may be regulated by MYB and/or CBF class transcription factors. We will look for upstream transcription factors of the CmADF1 gene to explore the molecular mechanisms of CmADF1 in low temperature in future research.
Data availability statement
The original contributions presented in the study are included in the article/Supplementary Material. Further inquiries can be directed to the corresponding authors.
Author contributions
YL: Data curation, Formal analysis, Investigation, Writing – original draft, Writing – review & editing, Conceptualization, Methodology, Project administration, Validation. SHL: Conceptualization, Investigation, Data curation, Formal analysis, Writing – review & editing. JZ: Resources, Supervision, Validation, Formal analysis, Writing – review & editing. JC: Data curation, Formal analysis, Validation, Writing – review & editing. JW: Formal analysis, Validation, Visualization, Writing – review & editing. LiW: Data curation, Validation, Visualization, Writing – review & editing. ML: Formal analysis, Validation, Writing – review & editing. LuW: Formal analysis, Visualization, Writing – review & editing. SB: Validation, Visualization, Writing – review & editing. WL: Formal analysis, Writing – review & editing. LZ: Validation, Writing – review & editing. SLL: Investigation, Writing – review & editing. DY: Formal analysis, Writing – review & editing. CD: Writing – review & editing, Investigation. SZ: Conceptualization, Project administration, Supervision, Writing – review & editing. MH: Conceptualization, Funding acquisition, Project administration, Supervision, Writing – review & editing. YG: Funding acquisition, Project administration, Supervision, Writing – review & editing. CW: Conceptualization, Funding acquisition, Project administration, Supervision, Writing – review & editing.
Funding
The author(s) declare financial support was received for the research, authorship, and/or publication of this article. This research was supported by the National Key Research and Development Program of China (2022YFE0108200), Liaoning Provincial Natural Science Foundation of China (Grant No. 2022-BS-165), the National Natural Science Foundation of China (31970183), Comprehensive Experimental Station Project of National Watermelon and Melon Industry Technology System (CARS-25), Seed Industry Innovation Project of Shenyang (22-318-2-14), Liaoning Revitalization Talents Program (XLYC2002065), and Graduate Innovation Cultivation Fund of Shenyang Agricultural University (Nos. 2021YCXB09 and 2022YCXS19). Partly supported by the open funds of the State Key Laboratory of Plant Physiology and Biochemistry (SKLPPBKF1905), the PhD Start-up Fund of Liaoning Province (2022-BS-045), and President’s Fund of Liaoning Academy of Agricultural Sciences (2022BS0702).
Acknowledgments
The authors thank Researcher Wen Changlong and his team from Beijing Academy of Agriculture and Forestry Sciences for their guidance and opinions on the experiment.
Conflict of interest
The authors declare that the research was conducted in the absence of any commercial or financial relationships that could be construed as a potential conflict of interest.
Publisher’s note
All claims expressed in this article are solely those of the authors and do not necessarily represent those of their affiliated organizations, or those of the publisher, the editors and the reviewers. Any product that may be evaluated in this article, or claim that may be made by its manufacturer, is not guaranteed or endorsed by the publisher.
Supplementary material
The Supplementary Material for this article can be found online at: https://www.frontiersin.org/articles/10.3389/fpls.2024.1419719/full#supplementary-material
References
Allwood, E. G., Anthony, R. G., Smertenko, A. P., Reichelt, S., Drobak, B. K., Doonan, J. H., et al. (2002). Regulation of the pollen-specific actin depolymerizing factor LlADF1. Plant Cell 14, 2915–2927. doi: 10.1105/tpc.005363
Andrianantoandro, E., Pollard, T. D. (2006). Mechanism of actin filament turnover by severing and nucleation at different concentrations of ADF/cofilin. Molecular cell 24(1), 13–23. doi: 10.1016/j.molcel.2006.08.006
Bamburg, J. R., Bernstein, B. W. (2008). ADF/cofilin. Curr. Biol. CB 18, R273–R275. doi: 10.1016/j.cub.2008.02.002
Bi, S., Li, M., Liu, C., Liu, X., Cheng, J., Wang, L., et al. (2022). Actin depolymerizing factor ADF7 inhibits actin bundling protein VILLIN1 to regulate root hair formation in response to osmotic stress in Arabidopsis. PloS Genet. 18, e1010338. doi: 10.1371/journal.pgen.1010338
Bowman, G. D., Nodelman, I. M., Hong, Y., Chua, N. H., Lindberg, U., Schutt, C. E., et al. (2000). A comparative structural analysis of the ADF/cofilin family. Proteins. 41, 374–384. doi: 10.1002/1097-0134(20001115)41:3<374::aid-prot90>3.0.co;2-f
Byun, M. Y., Cui, L. H., Lee, A., Oh, H. G., Yoo, Y. H., Lee, J., et al. (2021). Abiotic stress-induced actin-depolymerizing factor 3 from deschampsia Antarctica enhanced cold tolerance when constitutively expressed in rice. Front. Plant Sci. 12. doi: 10.3389/fpls.2021.734500
Byun, M. Y., Lee, J., Cui, L. H., Kang, Y., Oh, T. K., Park, H., et al. (2015). Constitutive expression of DaCBF7, an Antarctic vascular plant Deschampsia Antarctica CBF homolog, resulted in improved cold tolerance in transgenic rice plants. Plant Sci. 236, 61–74. doi: 10.1016/j.plantsci.2015.03.020
Cannon, S. B., Mitra, A., Baumgarten, A., Young, N. D., May, G. (2004). The roles of segmental and tandem gene duplication in the evolution of large gene families in Arabidopsis thaliana. BMC Plant Biol. 4, 10. doi: 10.1186/1471-2229-4-10
Clément, M., Ketelaar, T., Rodiuc, N., Banora, M. Y., Smertenko, A., Engler, G., et al. (2009). Actin-depolymerizing factor2-mediated actin dynamics are essential for root-knot nematode infection of Arabidopsis. Plant Cell 21, 2963–2979. doi: 10.1105/tpc.109.069104
Daher, F. B., Geitmann, A. (2012). Actin depolymerizing factors ADF7 and ADF10 play distinct roles during pollen development and pollen tube growth. Plant Signal. Behav. 7, 879–881. doi: 10.4161/psb.20436
Ding, Y., Shi, Y., Yang, S. (2019). Advances and challenges in uncovering cold tolerance regulatory mechanisms in plants. New Phytol. 222, 1690–1704. doi: 10.1111/nph.15696
Dong, C. H., Tang, W. P., Liu, J. Y. (2013). Arabidopsis AtADF1 is functionally affected by mutations on actin binding sites. J. Integr. Plant Biol. 55, 250–261. doi: 10.1111/jipb.12015
Dong, C. H., Xia, G. X., Hong, Y., Ramachandran, S., Kost, B., Chua, N. H. (2001). ADF proteins are involved in the control of flowering and regulate F-actin organization, cell expansion, and organ growth in Arabidopsis. Plant Cell 13, 1333–1346. doi: 10.1105/tpc.13.6.1333
Fan, T. T., Ni, J. J., Dong, W. C., An, L. Z., Xiang, Y., Cao, S. Q. (2015). Effect of low temperature on profilins and ADFs transcription and actin cytoskeleton reorganization in Arabidopsis. Biol. Plant 59, 793–796. doi: 10.1007/s10535-015-0546-6
Fan, T., Wang, R., Xiang, Y., An, L., Cao, S. (2016). Heat stress induces actin cytoskeletal reorganization and transcript profiles of vegetative profilins and actin depolymerizing factors (ADFs) in Arabidopsis. Acta Physiol. Plant 38, 37. doi: 10.1007/s11738-016-2061-6
Feng, Y., Liu, Q., Xue, Q. (2006). Comparative study of rice and Arabidopsis actin-depolymerizing factors gene families. J. Plant Physiol. 163, 69–79. doi: 10.1016/j.jplph.2005.01.015
Fu, Y., Duan, X., Tang, C., Li, X., Voegele, R. T., Wang, X., et al. (2014). TaADF7, an actin-depolymerizing factor, contributes to wheat resistance against Puccinia striiformis f. sp. tritici. Plant Journal: Cell Molecu Lar Biol. 78, 16–30. doi: 10.1111/tpj.12457
Huang, Y. C., Huang, W. L., Hong, C. Y., Lur, H. S., Chang, M. C. (2012). Comprehensive analysis of differentially expressed rice actin depolymerizing factor gene family and heterologous overexpression of OsADF3 confers Arabido psis Thaliana drought tolerance. Rice (New York N.Y.) 5, 33. doi: 10.1186/1939-8433-5-33
Huang, J., Sun, W., Ren, J., Yang, R., Fan, J., Li, Y., et al. (2020). Genome-wide identification and characterization of actin-depolymerizing factor (ADF) family genes and expression analysis of responses to various stresses in zea mays L. Int. J. Mol. Sci. 21, 1751. doi: 10.3390/ijms21051751
Huang, S., Xiang, Y., Ren, H. (2011). “Actin-binding proteins and actin dynamics in plant cells,” in The Plant Cytoskeleton, vol. 2 . Ed. Liu, B. (Springer-Verlag New York Inc: Springer, New York, NY), 57–80. doi: 10.1007/978-1-4419-0987-9_3
Hussey, P. J., Ketelaar, T., Deeks, M. J. (2006). Control of the actin cytoskeleton in plant cell growth. Annu. Rev. Plant Biol. 57, 109–125. doi: 10.1146/annurev.arplant.57.032905.105206
Inada, N., Higaki, T., Hasezawa, S. (2016). Nuclear function of subclass I actin-depolymerizing factor contributes to susceptibility in arabidopsis to an adapted powdery mildew fungus. Plant Physiol. 170, 1420–1434. doi: 10.1104/pp.15.01265
Khatun, K., Robin, A. H., Park, J. I., Kim, C. K., Lim, K. B., Kim, M. B., et al. (2016). Genome-Wide Identification, Characteriz- ation and Expression Profiling of ADF Family Genes in Solanum lycopersicum L. Genes 7, 79. doi: 10.3390/genes7100079
Kijima, S. T., Hirose, K., Kong, S. G., Wada, M., Uyeda, T.Q. (2016). Distinct biochemical properties of arabidopsis thaliana actin isoforms. Plant & cell physiology 57 (1), 47–56. doi: 10.1093/pcp/pcv176
Korkmaz, A., Dufault, R. (2004). Differential cold stress duration and frequency treatment effects on muskmelon seedling and field growth and yield. Eur. J. Hortic. Sci. 69, 12–20.
Larkin, M. A., Blackshields, G., Brown, N. P., Chenna, R., McGettigan, P. A., McWilliam, H., et al. (2007). Clustal W and clustal X version 2.0. Bioinf. (Oxford England) 23, 2947–2948. doi: 10.1093/bioinformatics/btm404
Leontovyčová, H., Kalachova, T., Trdá, L., Pospíchalová, R., Lamparová, L., Dobrev, P. I., et al. (2019). Actin depolymerization is able to increase plant resistance against pathogens via activation of salicylic acid signalling pathway. Sci. Rep. 9, 10397. doi: 10.1038/s41598-019-46465-5
Liao, J. J., Wang, C. H., Xing, Q. J., Li., Y. P., Liu, X. F., Qi, H. Y. (2019). Overexpression and VIGS system for functional gene validation in oriental melon (Cucumis melo var. makuwa Makino). Plant Cell Tissue Organ Culture (PCTOC) 137, 275–284. doi: 10.1007/s11240-019-01568-9
Liu, X., Qin, T., Ma, Q., Sun, J., Liu, Z., Yuan, M., et al. (2013). Light-regulated hypocotyl elongation involves proteasome-dependent degradation of the microtubule regulatory protein WDL3 in Arabidopsis. Plant Cell 25, 1740–1755. doi: 10.1105/tpc.113.112789
Lynch, M., Conery, J. S. (2000). The evolutionary fate and consequences of duplicate genes. Sci. (New York N.Y.) 290, 1151–1155. doi: 10.1126/science.290.5494.1151
Maciver, S. K., Hussey, P. J. (2002). The ADF/cofilin family: actin-remodel ing proteins. Genome Biol. 3, 1–12. doi: 10.1186/gb-2002-3-5-reviews3007
McCurdy, D. W., Kovar, D. R., Staiger, C. J. (2001). Actin and actin-binding proteins in higher plants. Protoplasma 215, 89–104. doi: 10.1007/BF01280306
Miklis, M., Consonni, C., Bhat, R. A., Lipka, V., Schulze-Lefert, P., Panstruga, R. (2007). Barley MLO modulates actin-dependent and actin-independent antifungal defense pathways at the cell periphery. Plant Physiol. 144, 1132–1143. doi: 10.1104/pp.107.098897
Nan, Q., Qian, D., Niu, Y., He, Y., Tong, S., Niu, Z., et al. (2017). Plant actin-depolymerizing factors possess opposing biochemical properties arising from key amino acid changes throughout evolution. Plant Cell 29, 395–408. doi: 10.1105/tpc.16.00690
Ouellet, F., Carpentier, E., Cope, M. J., Monroy, A. F., Sarhan, F. (2001). Regulation of a wheat actin- depolymerizing factor during cold acclimation. Plant Physiol. 125, 360–368. doi: 10.1104/pp.125.1.360
Peng, S. Q., Huang, D. F. (2006). Expression of an Arabidopsis actin-depolymerizing factor 4 gene (AtADF4) in tobacco causes morphological change of plants. J. Plant Physiol. Mol. Biol. 32, 52–56.
Pokorná, J., Schwarzerová, K., Zelenková, S., Petrášek, J., Janotová, I., Čapková, V., et al. (2004). Sites of actin filament initiation and reorganization in cold-treated tobacco cells. Plant Cell Environ. 27, 641–653. doi: 10.1111/j.1365-3040.2004.01186.x
Qian, D., Zhang, Z., He, J., Zhang, P., Ou, X., Li, T., et al. (2019). Arabidopsis ADF5 promotes stomatal closure by regulating actin cytoskeleton remodeling in response to ABA and drought stress. J. Exp. Bot. 70, 435–446. doi: 10.1093/jxb/ery385
Roland, J., Berro, J., Michelot, A., Blanchoin, L., Martiel, J. L. (2008). Stochastic severing of actin filaments by actin depolymerizing factor/cofilin controls the emergence of a steady dynamical regime. Biophys. J. 94, 2082–2094. doi: 10.1529/biophysj.107.121988
Ruzicka, D. R., Kandasamy, M. K., McKinney, E. C., Burgos-Rivera, B., Meagher, R. B. (2007). The ancient subclasses of Arabidopsis Actin Depolymerizing Factor genes exhibit novel and differential expression. Plant Journal: Cell Mol. Biol. 52, 460–472. doi: 10.1111/j.1365-313X.2007.03257.x
Sangwan, V., Foulds, I., Singh, J., Dhindsa, R. S. (2001). Cold-activation of Brassica napus BN115 promoter is mediated by structural changes in membranes and cytoskeleton, and requires Ca2+ influx. Plant Journal: Cell Mole- Cular Biol. 27, 1–12. doi: 10.1046/j.1365-313x.2001.01052.x
Sengupta, S., Mangu, V., Sanchez, L., Bedre, R., Joshi, R., Rajasekaran, K., et al. (2019). An actin-depolymerizing factor from the halophyte smooth cordgrass, Spartina alterniflora (SaADF2), is superior to its rice homolog (OsADF2) in conferring drought and salt tolerance when constitutively overexpressed in rice. Plant Biotechnol. J. 17, 188–205. doi: 10.1111/pbi.12957
Smertenko, A. P., Jiang, C. J., Simmons, N. J., Weeds, A. G., Davies, D. R., Hussey, P. J. (1998). Ser6 in the maize actin-depolymerizing factor, ZmADF3, is phosphorylated by a calcium-stimulated protein kinase and is essential for the control of functional activity. Plant Journal: Cell Mol. Biol. 14, 187–193. doi: 10.1046/j.1365-313x.1998.00107.x
Sun, Y., Wang, D., Shi, M., Gong, Y., Yin, S., Jiao, Y., et al. (2023). Genome-wide identification of actin-depolymerizing factor gene family and their expression patterns under various abiotic stresses in soybean (Glycine max). Front. Plant Sci. 14. doi: 10.3389/fpls.2023.1236175
Tamura, K., Stecher, G., Peterson, D., Filipski, A., Kumar, S. (2013). MEGA6: molecular evolutionary genetics analysis version 6.0. Mol. Biol. Evol. 30, 2725–2729. doi: 10.1093/molbev/mst197
Tang, C., Deng, L., Chang, D., Chen, S., Wang, X., Kang, Z. (2016). TaADF3, an actin-depolymerizing factor, negatively modulates wheat resistance against puccinia striiformis. Front. Plant Sci. 6. doi: 10.3389/fpls.2015.01214
Tian, M., Chaudhry, F., Ruzicka, D. R., Meagher, R. B., Staiger, C. J., Day, B. (2009). Arabidopsis actin-depolymerizing factor AtADF4 mediates defense signal transduction triggered by the Pseudomonas syringae effector AvrPphB. Plant Physiol. 150, 815–824. doi: 10.1104/pp.109.137604
Wang, X., Bi, S., Wang, L., Li, H., Gao, B. A., Huang, S., et al. (2020). GLABRA2 regulates actin bundling protein VILLIN1 in root hair growth in response to osmotic stress. Plant Physiol. 184, 176–193. doi: 10.1104/pp.20.00480
Wang, L., Cheng, J., Bi, S., Wang, J., Cheng, X., Liu, S., et al. (2023). Actin depolymerization factor ADF1 regulated by MYB30 plays an important role in plant thermal adaptation. Int. J. Mol. Sci. 24, 5675. doi: 10.3390/ijms24065675
Wang, L., Qiu, T., Yue, J., Guo, N., He, Y., Han, X., et al. (2021). Arabidopsis ADF1 is regulated by MYB73 and is involved in response to salt stress affecting actin filament organization. Plant Cell Physiol. 62, 1387–1395. doi: 10.1093/pcp/pcab081
Wang, C., Zhang, L. J., Huang, R. D. (2011). Cytoskeleton and plant salt stress tolerance. Plant Signaling Behav. 6, 29–31. doi: 10.4161/psb.6.1.14202
Xing, Q., Liao, J., Cao, S., Li, M., Lv, T., Qi, H. (2020). CmLOX10 positively regulates drought tolerance through jasmonic acid-mediated stomatal closure in oriental melon (Cucumis melo var. makuwa Makino). Sci. Rep. 10, 17452. doi: 10.1038/s41598-020-74550-7
Xu, K., Zhao, Y., Zhao, S., Liu, H., Wang, W., Zhang, S., et al. (2021). Genome-wide identification and low temperature responsive pattern of actin depolymerizing factor (ADF) gene family in wheat (Triticum aestivum L.). Front. Plant Sci. 12. doi: 10.3389/fpls.2021.618984
Yao, H., Li, X., Peng, L., Hua, X., Zhang, Q., Li, K., et al. (2022). Binding of 14-3-3κ to ADF4 is involved in the regulation of hypocotyl growth and response to osmotic stress in Arabidopsis. Plant science: an Int. J. Exp. Plant Biol. 320, 111261. doi: 10.1016/j.plantsci.2022.111261
Ye, J., Zhang, W., Guo, Y. (2013). Arabidopsis SOS3 plays an important role in salt tolerance by mediating calcium-dependent microfilament reorganization. Plant Cell Rep. 32, 139–148. doi: 10.1007/s00299-012-1348-3
Zhang, B., Hua, Y., Wang, J., Huo, Y., Shimono, M., Day, B., et al. (2017). TaADF4, an actin- depolymerizing factor from wheat, is required for resistance to the stripe rust pathogen Puccinia striiformis f. sp. tritici. Plant Journal: Cell Mol. Biol. 89, 1210–1224. doi: 10.1111/tpj.13459
Zhang, P., Qian, D., Luo, C., Niu, Y., Li, T., Li, C., et al. (2021). Arabidopsis ADF5 acts as a downstream target gene of CBFs in response to low-temperature stress. Front. Cell Dev. Biol. 9. doi: 10.3389/fcell.2021.635533
Zhang, Y. P., Yao, X. Q., Yang, S. J., Xv, S., Chen, Y. Y. (2017). Effects of low temperature treatment and recovery on the photosynthesis and antioxidantcharacteristics in melon seedlings. Acta Agriculturae Shanghai 33, 41–49.
Zhang, L., Yuan, M., Ge, Y., Liu, Y., Fan, J., Ruan, Y., et al. (2010). The microfilament cytoskeleton plays a vital role in salt and osmotic stress tolerance in Arabidopsis. Plant Biol. (Stuttgart Germany) 12, 70–78. doi: 10.1111/j.1438-8677.2009.00201.x
Zhao, S., Jiang, Y., Zhao, Y., Huang, S., Yuan, M., Zhao, Y., et al. (2016). CASEIN KINASE1-LIKE PROTEIN2 regulates actin filament stability and stomatal closure via phosphorylation of actin depolymerizing factor. Plant Cell 28, 1422–1439. doi: 10.1105/tpc.16.00078
Zheng, Y., Xie, Y., Jiang, Y., Qu, X., Huang, S. (2013). Arabidopsis actindepolymerizing factor7 severs actin filaments and regulates actin cable turnover to promote normal pollen tube growth. Plant Cell 25, 3405–3423. doi: 10.1105/tpc.113.117820
Keywords: genome-wide identification, CmADF1, low temperature, oriental melon, Arabidopsis
Citation: Lv Y, Liu S, Zhang J, Cheng J, Wang J, Wang L, Li M, Wang L, Bi S, Liu W, Zhang L, Liu S, Yan D, Diao C, Zhang S, He M, Gao Y and Wang C (2024) Genome-wide identification of actin-depolymerizing factor family genes in melon (Cucumis melo L.) and CmADF1 plays an important role in low temperature tolerance. Front. Plant Sci. 15:1419719. doi: 10.3389/fpls.2024.1419719
Received: 18 April 2024; Accepted: 02 August 2024;
Published: 22 August 2024.
Edited by:
Shifeng Cao, Zhejiang Wanli University, ChinaCopyright © 2024 Lv, Liu, Zhang, Cheng, Wang, Wang, Li, Wang, Bi, Liu, Zhang, Liu, Yan, Diao, Zhang, He, Gao and Wang. This is an open-access article distributed under the terms of the Creative Commons Attribution License (CC BY). The use, distribution or reproduction in other forums is permitted, provided the original author(s) and the copyright owner(s) are credited and that the original publication in this journal is cited, in accordance with accepted academic practice. No use, distribution or reproduction is permitted which does not comply with these terms.
*Correspondence: Che Wang, d2FuZ3dhbmdjaGVAMTYzLmNvbQ==; Shaobin Zhang, enNiQHN5YXUuZWR1LmNu; Ming He, bG55aG1pbmdAMTYzLmNvbQ==; Yue Gao, Z3l1ZTEyMTdAc3lhdS5lZHUuY24=
†These authors have contributed equally to this work