- 1Department of Field Crops, Ataturk University, Erzurum, Türkiye
- 2Department of Horticulture, Ataturk University, Erzurum, Türkiye
- 3Faculty of Economy and Administrative Sciences, Department of Agricultural Trade and Management, Yeditepe University, Istanbul, Türkiye
Studies are being conducted to develop strategies to reduce the adverse effects of salinity stress. In the present study, it was aimed to determine the interactive effects of salinity stress with biochar on plant growth—the physiological and biochemical attributes of forage peas (Pisum sativum ssp. arvense L.). Salt applications were carried out with irrigation water at concentrations of 0, 25, 50, 75, and 100 mM NaCl. The experiment was conducted using a randomized complete block design with three applications [control: 0 (B0), 2.5% biochar (B1), and 5% biochar (B2)], five salt doses [0 (S0), 25 (S1), 50 (S2), 75 (S3), and 100 (S4) mM NaCl], and three replications, arranged in a 3 × 5 factorial arrangement. In the salt-stressed environment, the highest plant height (18.75 cm) and stem diameter (1.71 mm) in forage pea seedlings were obtained with the application of B1. The root fresh (0.59 g/plant) and dry weight (0.36 g/plant) were determined to be the highest in the B1 application, both in non-saline and saline environments. A decrease in plant chlorophyll content in forage pea plants was observed parallel to the increasing salt levels. Specifically, lower H2O2, MDA, and proline content were determined at all salt levels with biochar applications, while in the B0 application these values were recorded at the highest levels. Furthermore, in the study, it was observed that the CAT, POD, and SOD enzyme activities were at their lowest levels at all salt levels with the biochar application, while in the B0 application, these values were determined to be at the highest levels. There was a significant decrease in plant mineral content, excluding Cl and Na, parallel to the increasing salt levels. The findings of the study indicate that biochar amendment can enhance forage peas’ growth by modulating the plant physiology and biochemistry under salt stress. Considering the plant growth parameters, no significant difference was detected between 2.5% and 5% biochar application. Therefore, application of 2.5 biochar may be recommended.
1 Introduction
The provision of healthy food, a necessity for the rapidly growing world population, will be one of the foremost challenges in the future. To meet the nutritional needs of the increasing population, both plant and animal production capacities need to be doubled (Howell et al., 2001). However, salinity, referred to as “white death,” stands among the most critical issues affecting global soils (Taha et al., 2020; Dustgeer et al., 2021; Ahmad et al., 2023a). Millions of hectares of land are damaged every year due to excessive salinity, and significant agricultural losses occur worldwide (Singh, 2022). Dry and semi-arid areas worldwide constitute approximately 46% of the total land area, with observed salinity issues in about 50% of irrigated lands in these regions. Saline soils cover around 7% of the Earth’s total land area (Ruiz-Lozano et al., 2001), and about 5% of the cultivated land, equivalent to around 77 million hectares out of the approximately 15 billion hectares processed, is affected by excessive salinity (Sheng et al., 2008). Salinity, a limiting factor in increasing plant productivity and agricultural production in areas dedicated to agricultural activities, is predominantly prevalent in arid and semi-arid regions with low rainfall, high temperatures, and various influencing factors such as low precipitation, high evapotranspiration, elevated groundwater levels, saline irrigation water, and improper irrigation practices (Tietel et al., 2019). This situation poses a significant threat to food security, particularly for communities whose livelihoods depend on agricultural and livestock production (Cowiea et al., 2018).
Soil salinity significantly reduces crop yield as it diminishes the plant’s transpiration, respiration, water uptake, and root development (Alkharabsheh et al., 2021; Bhattacharya, 2021; Abdelaal et al., 2022). Salinity stress, a significant constraining environmental factor, imposes a restrictive effect of 20% or more on plant growth and development (Porcel et al., 2012). Many economically important crops are sensitive to salinity, struggling to thrive under salty conditions and experiencing substantial decreases in yield (Wang et al., 2023; Ahmad et al., 2023b). In soils where many cultivated forage crops are grown, the presence of salt at levels limiting their growth and development leads to significant reductions in productivity. Particularly in areas where forage crops are cultivated in arid and semi-arid regions, saline soils impose limitations on yield (Ates and Tekeli, 2007).
Forage legumes, which are among the primary sources of roughage meeting the nutritional needs of animals and are rich in crude protein, vitamins, and minerals, are generally highly sensitive to salinity (Yavas and Unay, 2018). Salinity affects nodule formation in the roots of forage legume, either directly or indirectly, by reducing nodulation (Hanumantha et al., 2016). In the classification based on the salt tolerance of forage crops, the common forage pea (Pisum sativum ssp. arvense L.) is classified among salt-sensitive forage crops (Kayin et al., 2022, 2024).
Recently, biochar (BC) has gained significant interest in soil improvement. This carbon-rich material is produced by heating waste organic matter, like crop leftovers, in a low-oxygen environment. Biochar’s benefits for soil are numerous, including increased crop yields, better nutrient retention, improved soil structure, and contributions to waste management and carbon capture (Zhang et al., 2020). Produced from agricultural waste, biochar shows promise for environmental cleanup. This is due to its beneficial properties, including high heat resistance, a large surface area, varied pore sizes, and the presence of various functional groups. Additionally, biochar derived from agricultural waste can improve soil fertility and effectively capture contaminants from gas, during composting, and in other processes like anaerobic digestion and catalysis. Agricultural biomass stands out as a perfect choice for creating porous carbon materials. Its advantages are plentiful: readily available, cost-effective, renewable, rich in carbon, and environmentally friendly (Khedulkar et al., 2023).
Turkey yields most of the world’s olive oil production with an annual amount of 420,000 tons in the recent years. Olive pomace is a by-product consisting of the oil, pit, peel, and pulp remaining after the oil is extracted from olives. The pulp remaining after the oil is extracted, called “pomace”, is used in different sectors. Pomace is a residue of olive oil factories and is an important type of biomass seen in Mediterranean countries. Pomace can be obtained in quite large quantities at low cost. The olive oil pomace can provide a very important agricultural product, although it is currently wastefully incinerated for heating or left on the ground for slow decay. Alternatively, olive oil pomace represents a valuable source of carbon and can be manipulated to furnish the soil with high-quality organic matter in the form of biochar (Ainane et al., 2023; Kavdir et al., 2023). The ingredients in a plant’s growing environment significantly impact how well seeds germinate and the overall health of the seedlings. The medium directly affects germination, growth, and development, all the way down to influencing the future health of the root system. A good growing medium provides the necessary support for the plant to stand upright, stores nutrients and water for the plant to use, allows oxygen to reach the roots, and enables the roots to exchange gases with the air around them. Biochar has gained considerable importance in the improvement of degraded agricultural areas, waste management, and renewable energy production (Enaime and Lubken, 2021). Biochar is a carbon-rich organic material formed by the pyrolysis of plant wastes in an oxygen-free or low-oxygen environment (Gupta et al., 2022). It is suggested that biochar, which is widely used as a soil amendment to alleviate these negative effects and is actively employed in many parts of the world, with its extensive surface area, could be utilized under salt stress conditions (Ortas, 2016; Xiao et al., 2016; Ali et al., 2017). In conclusion, the use of biochar can reduce the impact of soil salinity on plants, promoting healthy plant development. Additionally, biochar application has the potential to enhance plant tolerance to salt stress by increasing photosynthetic productivity and overall plant growth (Kul et al., 2021a; Yildirim et al., 2022). Quality seedlings are a high-value product that can improve the early establishment of crops; increase finish crop quality, uniformity, and yield; and decrease production time. To our best knowledge, there is limited information found in the literature in terms of examining the effect of biochar obtained from olive oil pomace on seedling growth in forage peas grown under salt stress. In this context, the aim of this study was to enhance the development, growth, nutrient uptake, and salt tolerance of salt-sensitive forage peas (Pisum sativum ssp. arvense L.) through the application of biochar derived from olive oil pomace under salt stress conditions.
2 Materials and methods
The study was conducted in the greenhouses of Atatürk University, Plant Production Application and Research Center, as well as in the laboratories of the Department of Field Crops and the Department of Horticulture at the Faculty of Agriculture. Forage pea (Pisum sativum ssp. arvense L. cv Taşkent) seeds were used as the experimental material in the research. In the study, there were three biochar [B0: without biochar (control), B1: 2.5% biochar, and B2: 5% biochar] and five salinity doses (S0: 0, S1: 25 mM, S2: 50 mM, S3: 75 mM, and S4: 100 mM NaCl) in 15 different combinations (T0: B0S0, T1: B0S1, T2: B0S2, T3: B0S3, T4: B0S4, T5: B1S0, T6: B1S1, T7: B1S2, T8: B1S3, T9: B1S4, T10: B2S0, T11: B2S1, T12: B2S2, T13: B2S3, and T14: B2S4) in a 3 × 5 factorial design with three replications. The properties of the soil used in the experiment are given in Table 1.
The properties of the biochar used in the present study are provided in Table 2. In this research, the biochar was originated from olive oil pomace. To create the biochar, a special reactor was used to slowly pyrolyze the shells at a temperature of 550°C. The biochar used in the study was produced using the thermal conversion process. This process consists of three stages: first stage reactor (depolymerization), second stage reactor (hydrolysis), and third stage reactor (cracker). The concenter solid intermediate transported to this reactor was broken down into short hydrocarbon chains at a high temperature (550°C), from which energy products (renewable natural gas and renewable crude oil) and biochar were obtained.
In the study, biochar application was carried out in containers (dimensions: 720 × 195 × 155 mm) with a soil mixture of soil, sand, and peat (3:1:1, v/v/v). Three doses were applied: 0% (control), 2.5%, and 5% of the soil weight.
In the prepared pots, 40 seeds were planted and salt applications were carried out with irrigation water at concentrations of 0, 25, 50, 75, and 100 mM NaCl after seed sowing. To prevent immediate salt stress damage to the seeds, the salt concentration started at 25 mM and was gradually increased, finally reaching the predetermined maximum doses. Salt application was performed by controlling the soil moisture and EC values in the form of irrigation water.
Plants were harvested after 50 days from sowing, where chlorophyll reading value, plant height, stem diameter, and fresh weights of plant and root were determined. Dry weights were determined after drying of fresh plant material in an oven at 67°C for 48 h. Fresh leaf samples were kept at −80°C for analyses.
Leaf area was determined with a CI-202 portable area meter (CID, Inc., USA).
To determine the leaf chlorophyll concentration of plants, samples were cut at 10-mm diameter from the middle leaves and put into 2-mL Eppendorf tubes. These discs were then placed in tubes containing cold acetone and shaken for 3 min. Next, the mixture was spun in a centrifuge to separate the chlorophyll extract. Finally, a device called a spectrophotometer (Thermo Scientific™ Multiskan™ FC Microplate Photometer) was used to measure the intensity of light absorbed by the chlorophyll extract at specific wavelengths (663 and 645 nm). Chlorophyll a, chlorophyll b, and total chlorophyll (mg g−1) were determined according to Lichtenthaler and Wellburm (1983).
The contents of P, K, Ca, Mg, S, Mn, Fe, Zn, B, Cl, Na, and Cu in the leaf and root samples were determined by using Optima 2100 DV, ICP/OES, Perkin-Elmer, Shelton, CT spectrophotometer. The nitrogen (N) content was determined according to the Micro Kjeldahl wet combustion method (Bremner, 1996; Dursun et al., 2010). The properties of the starting soil sample (pH, organic matter, EC, CaCO3, and mineral content) analyzed were determined by McLean (1982), Nelson and Sommers (1982), and Rhoades (1982).
The extraction and purification procedures for hormone analysis were conducted according to the method of Battal and Tileklioglu (2001). Indoleacetic acid (IAA), abscisic acid (ABA), gibberellic acid (GA), salicylic acid (SA), cytokinin, zeatin, and jasmonic acid were analyzed by using high-performance liquid chromatography (HPLC) through a Zorbax Eclipse-AAA C-18 column (Agilent 1200 HPLC) coupled with a UV detector of absorbance at 265 nm.
Lipid peroxidation was estimated as the malondialdehyde (MDA) content of leaves according to Heath and Packer (1968). H2O2 was determined according to Ohkawa et al. (1979).
The MDA content was assessed as the extent of lipid peroxidation in frozen leaves. The process involved grinding a 0.2-g leaf sample into powder using liquid nitrogen. The resulting mixture was centrifuged at 12,000 g for 20 min to obtain a clearer solution. To determine the MDA levels, a specific solution containing trichloroacetic acid (TCA), thiobarbituric acid (TBA), and an antioxidant (butylated hydroxytoluene) was mixed with a portion of the extract and heated. The reaction was then rapidly stopped by cooling the mixture in an ice bath. After centrifugation, the amount of light absorbed by the solution was measured at three wavelengths (400, 500, and 600 nm). Higher absorbance indicates more MDA, a breakdown product of lipids caused by peroxidation. A standard conversion factor (extinction coefficient of 155 mmol/L*cm) was used to calculate the final MDA concentration (Shams et al., 2019).
Superoxide dismutase (SOD) activity was determined by the spectrophotometric measurement of photochemical reduction of nitro blue tetrazolium (NBT). Peroxidase (POD) activity determination was based on the observation of absorbance increase at 470 nm caused by the colored compound, which is the product of the reaction in which guaiacol and H2O2 are substrates. The catalase (CAT) activity was measured based on the rate of H2O2 decomposition. The method applied by Liu et al. (2014) was used to determine the CAT, POD, and SOD activity, respectively.
A 50-mg frozen leaf sample was powdered with liquid nitrogen and extracted with a pestle and mortar with 4.5 mL of 5-sulfosalicylic acid 3% in an ice bath. The homogenates were filtered with a filter paper (#2). Then, 2 mL of filtrate was reacted with 2 mL acid-ninhydrin and 2 mL of glacial acetic acid in a test tube for 1 h at 100°C, and the reaction was terminated in an ice bath. The filtrates were used for the analysis. Proline concentration was assayed spectrophotometrically at 520 nm (Bates et al., 1973).
The experiment was established by using three replications at five pots in each replication, with two factors (salinity and biochar levels), in completely random design (CRD). For statistical analysis, an ANOVA (two-way ANOVA) technique for completely randomized design was carried out using SPSS 20.0 (SPSS 140 Inc.), and the mean differences were compared by using Duncan’s multiple-range test.
3 Results
3.1 Growth parameters
The results obtained in the study, as shown in Figures 1, 2, indicate that the application of biochar to forage peas reduces the adverse effects of salt stress on the plants.
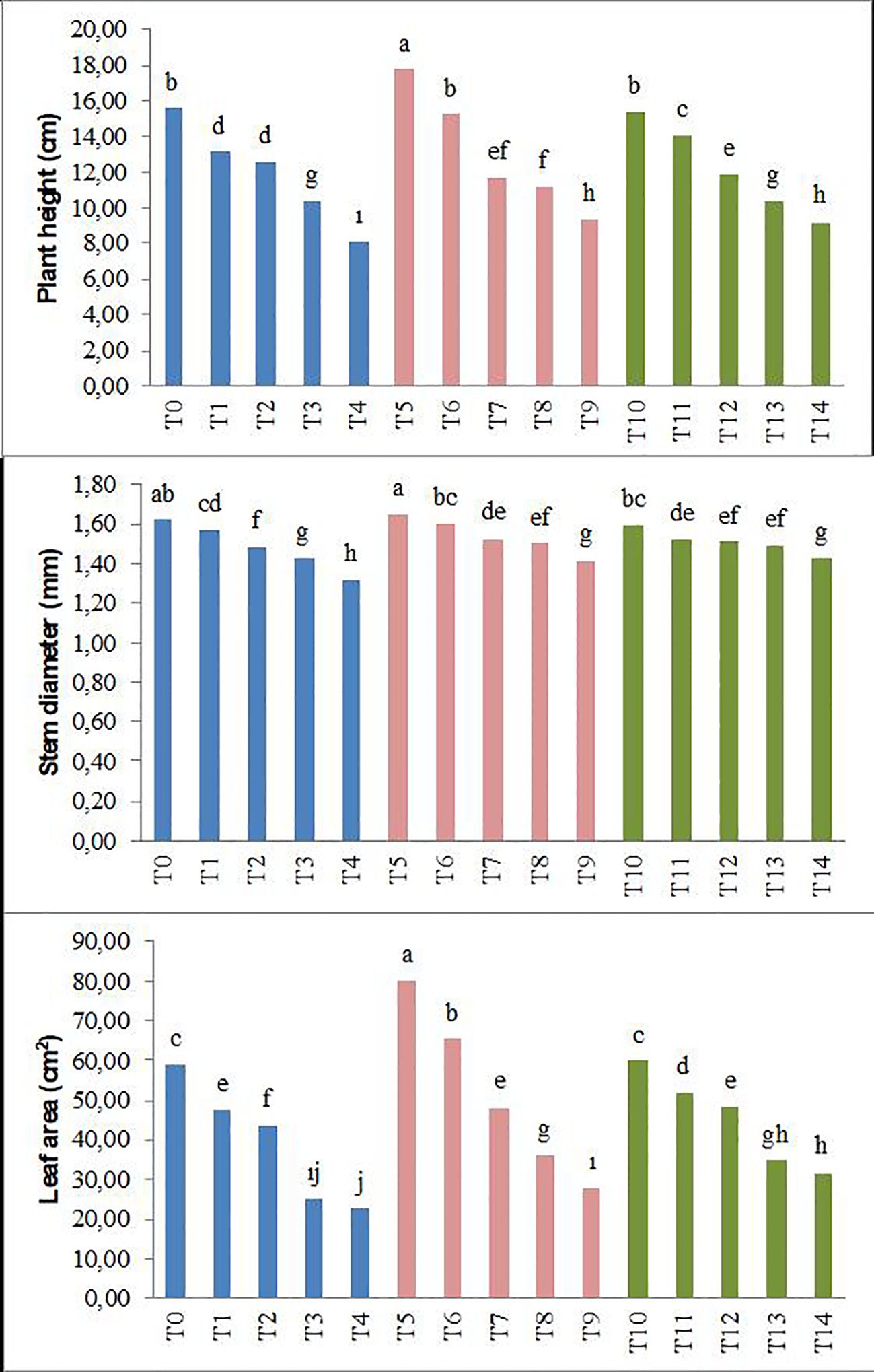
Figure 1. Effect of biochar on the plant height, stem diameter, and leaf area of forage pea seedlings under saline conditions. The different-lettered averages in each bar are significantly different according to Duncan’s multiple-range test (p ≤ 0.001). B0, without biochar (control); B1, 2.5% biochar; B2, 5% biochar; S0, 0 mM NaCl; S1, 25 mM NaCl; S2, 50 mM NaCl; S3, 75 mM NaCl; S4, 100 mM NaCl; T0, B0S0; T1, B0S1; T2, B0S2; T3, B0S3; T4, B0S4; T5, B1S0; T6, B1S1; T7, B1S2; T8, B1S3; T9, B1S4; T10, B2S0; T11, B2S1; T12, B2S2; T13, B2S3; T14, B2S4..
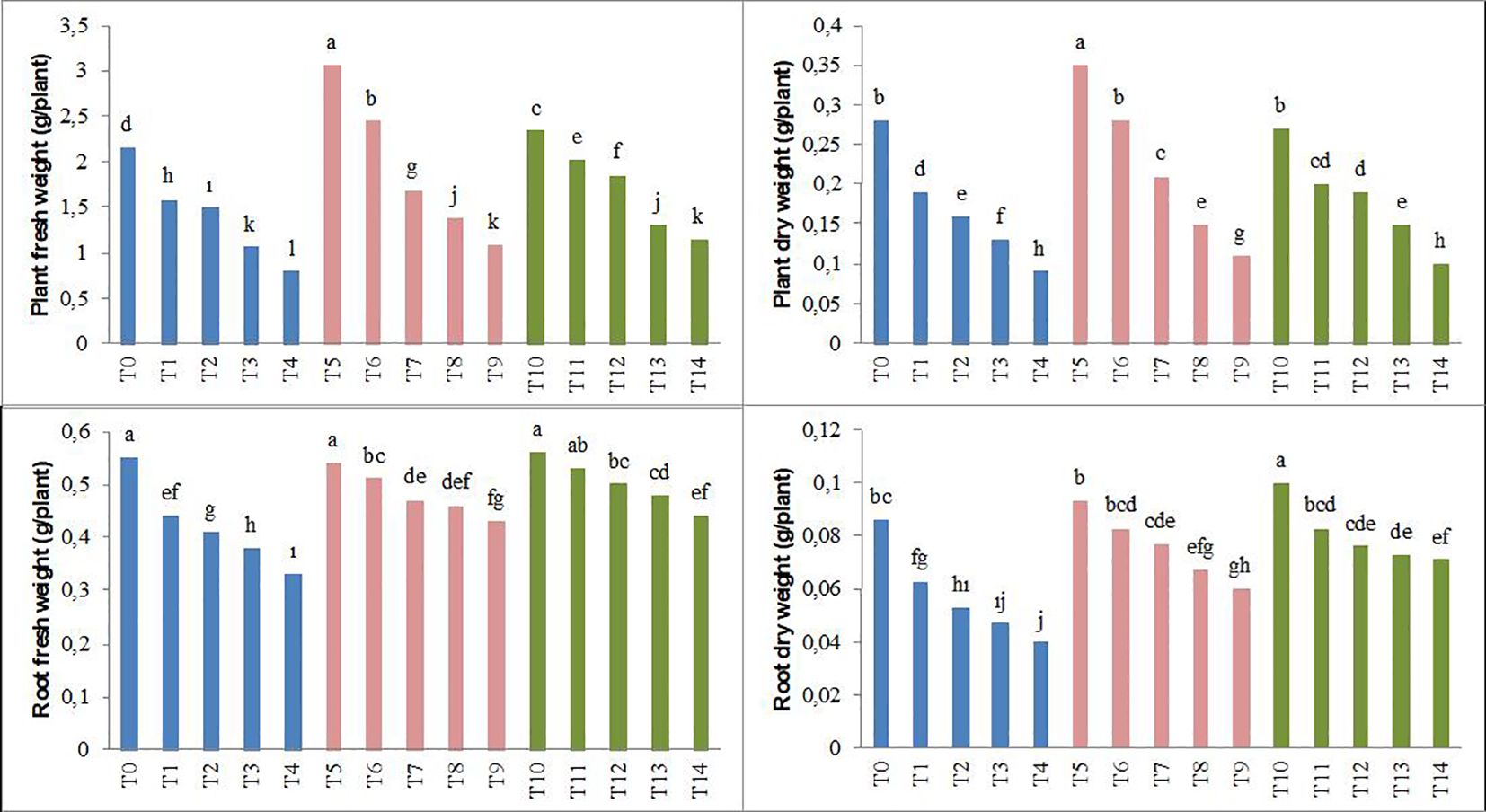
Figure 2. Effect of biochar on the plant fresh weight, plant dry weight, root fresh weight, and root dry weight of forage pea seedlings under saline conditions. The different-lettered averages in each bar are significantly different according to Duncan’s multiple-range test (p ≤ 0.001). B0, without biochar (control); B1, 2.5% biochar; B2, 5% biochar; S0, 0 mM NaCl; S1, 25 mM NaCl; S2, 50 mM NaCl; S3, 75 mM NaCl; S4, 100 mM NaCl; T0, B0S0; T1, B0S1; T2, B0S2; T3, B0S3; T4, B0S4; T5, B1S0; T6, B1S1; T7, B1S2; T8, B1S3; T9, B1S4; T10, B2S0; T11, B2S1; T12, B2S2; T13, B2S3; T14, B2S4..
Parameters such as plant height, stem diameter, leaf area, plant fresh weight, root fresh weight, plant dry weight, and root dry weight showed significant decreases in forage pea seedlings with the increase in salinity levels compared to the control groups. This decrease was 48% (plant height), 19% (stem diameter), 62% (leaf area), 63% (plant fresh weight), 40% (root fresh weight), 68% (plant dry weight), and 53% (root dry weight), respectively. However, the application of biochar derived from olive oil pomace has positively mitigated the adverse effects of salinity stress on the examined parameters. In the study, particularly in the saline environment, the highest plant height and stem diameter were achieved with the biochar applications (Figures 1, 2).
Additionally, according to the average data, the T5 application of biochar resulted in the highest plant fresh and dry weight, while the T10 application led to the highest root fresh and dry weight. The decrease caused by the highest salt level in plant fresh and dry weight and root fresh and dry weight was lower in the B2 application (T14). In conclusion, the study determined significant differences between salinity and applied biochar for all of the growth parameters examined. Considering the plant growth parameters, no significant difference was detected between 2.5% and 5% biochar application.
3.2 Chlorophyll content and SPAD value
The results of the study revealed that, compared to the control conditions, under saline conditions (25, 50, 75, and 100 mM NaCl), SPAD, chlorophyll a, chlorophyll b, and total chlorophyll values in forage pea seedlings decreased by 18%, 25%, 28%, and 30%; 7%, 11%, 13%, and 16%; 13%, 20%, 36%, and 41%; and 9%, 14%, 22%, and 25%, respectively. The T5–T9 and T10–T14 biochar applications showed an increasing trend in the parameters that tended to decrease under increasing salinity conditions (Figure 3).
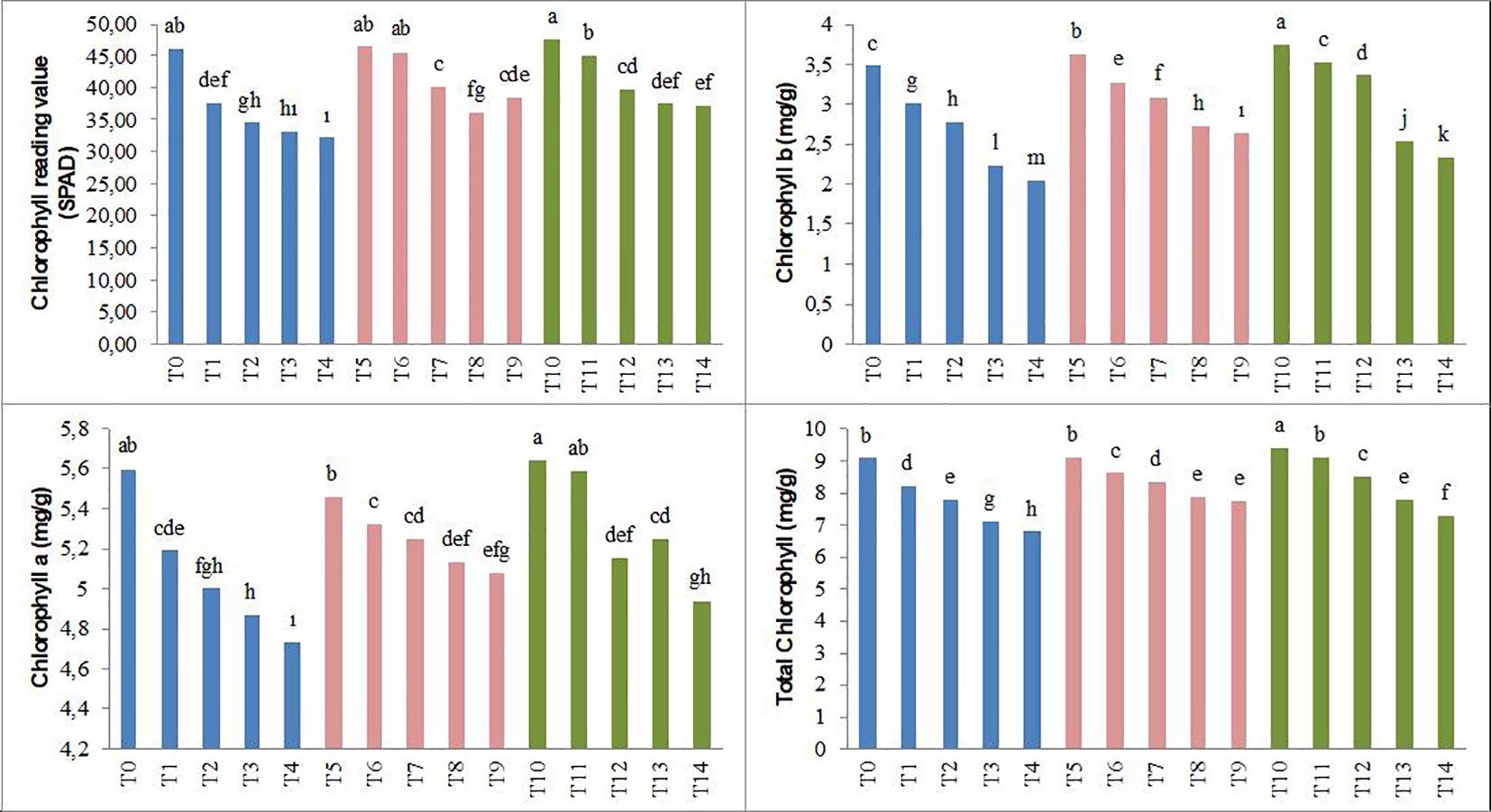
Figure 3. Effect of biochar on the chlorophyll reading value and chlorophyll content of forage pea seedlings under saline conditions. The different-lettered averages in each bar are significantly different according to Duncan’s multiple-range test (p ≤ 0.001). B0, without biochar (control); B1, 2.5% biochar; B2, 5% biochar; S0, 0 mM NaCl; S1, 25 mM NaCl; S2, 50 mM NaCl; S3, 75 mM NaCl; S4, 100 mM NaCl; T0, B0S0; T1, B0S1; T2, B0S2; T3, B0S3; T4, B0S4; T5, B1S0; T6, B1S1; T7, B1S2; T8, B1S3; T9, B1S4; T10, B2S0; T11, B2S1; T12, B2S2; T13, B2S3; T14, B2S4..
3.3 H2O2, MDA, and proline contents
It has been observed that the levels of hydrogen peroxide (H2O2), malondialdehyde (MDA), and proline in the plant significantly increased under different salinity conditions (25, 50, 75, and 100 mM NaCl) compared to the control. However, both levels of biochar application (T5–T9 and T10–T14) have been recorded to reduce the H2O2, MDA, and proline content in forage pea seedlings under salinity stress. Especially the T10–T14 application resulted in lower H2O2, MDA, and proline content at all salt levels, while in the T0–T4 application these values were determined to be the highest (Figure 4).
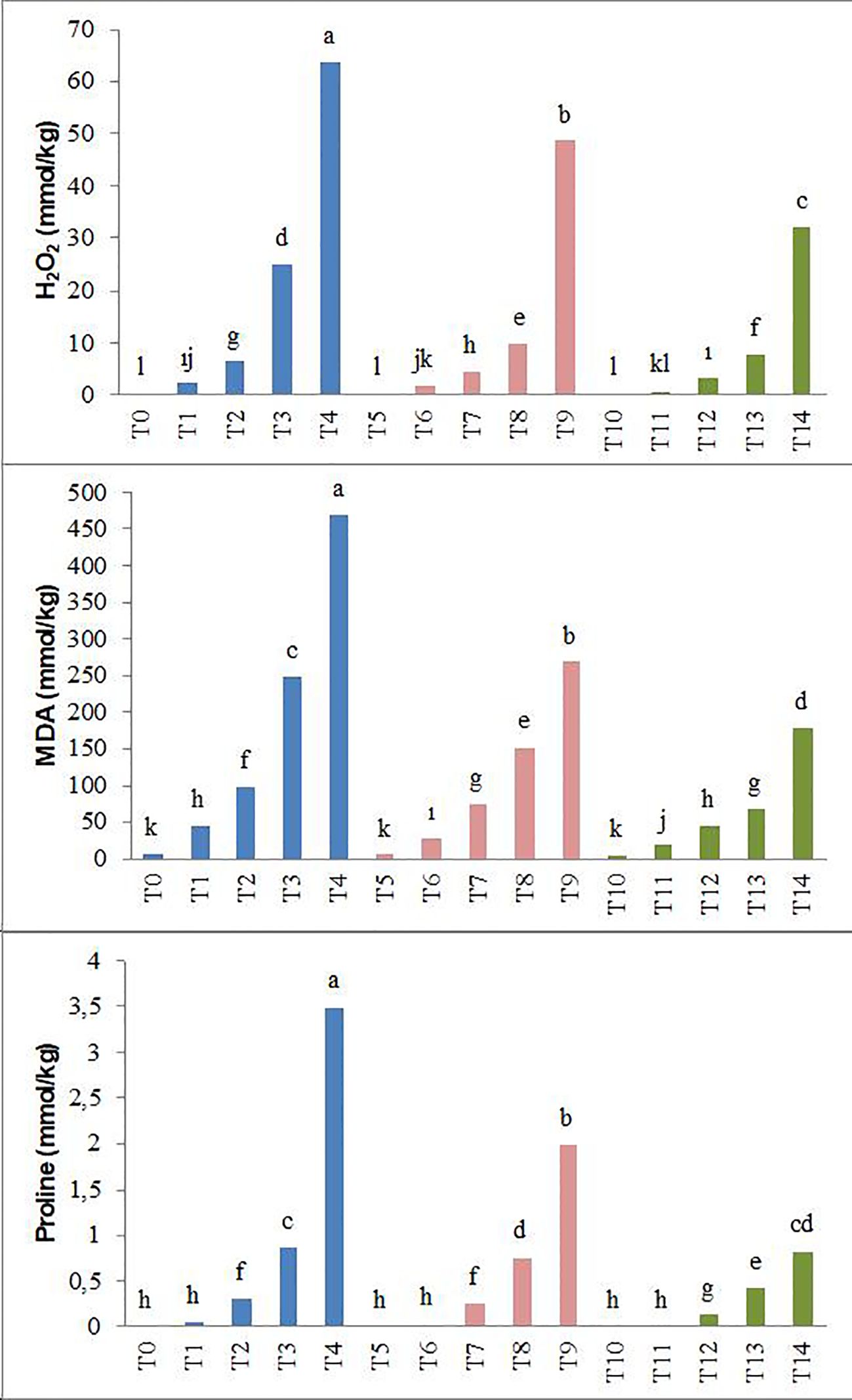
Figure 4. Effect of biochar on H2O2, MDA, and proline content of forage pea seedlings under saline conditions. The different-lettered averages in each bar are significantly different according to Duncan’s multiple-range test (p ≤ 0.001). B0, without biochar (control); B1, 2.5% biochar; B2, 5% biochar; S0, 0 mM NaCl; S1, 25 mM NaCl; S2, 50 mM NaCl; S3, 75 mM NaCl; S4, 100 mM NaCl; T0, B0S0; T1, B0S1; T2, B0S2; T3, B0S3; T4, B0S4; T5, B1S0; T6, B1S1; T7, B1S2; T8, B1S3; T9, B1S4; T10, B2S0; T11, B2S1; T12, B2S2; T13, B2S3; T14, B2S4..
3.4 Antioxidant enzyme activity
In parallel with the increasing salinity, statistically significant increases have occurred in the enzyme activities of CAT, POD, and SOD in pea seedlings. The increase caused by salinity in these parameters was lower with biochar applications. Specifically, the T10–T14 application resulted in the lowest CAT, POD, and SOD enzyme activities at all salt levels, while the T0–T4 application showed the highest levels (Figure 5).
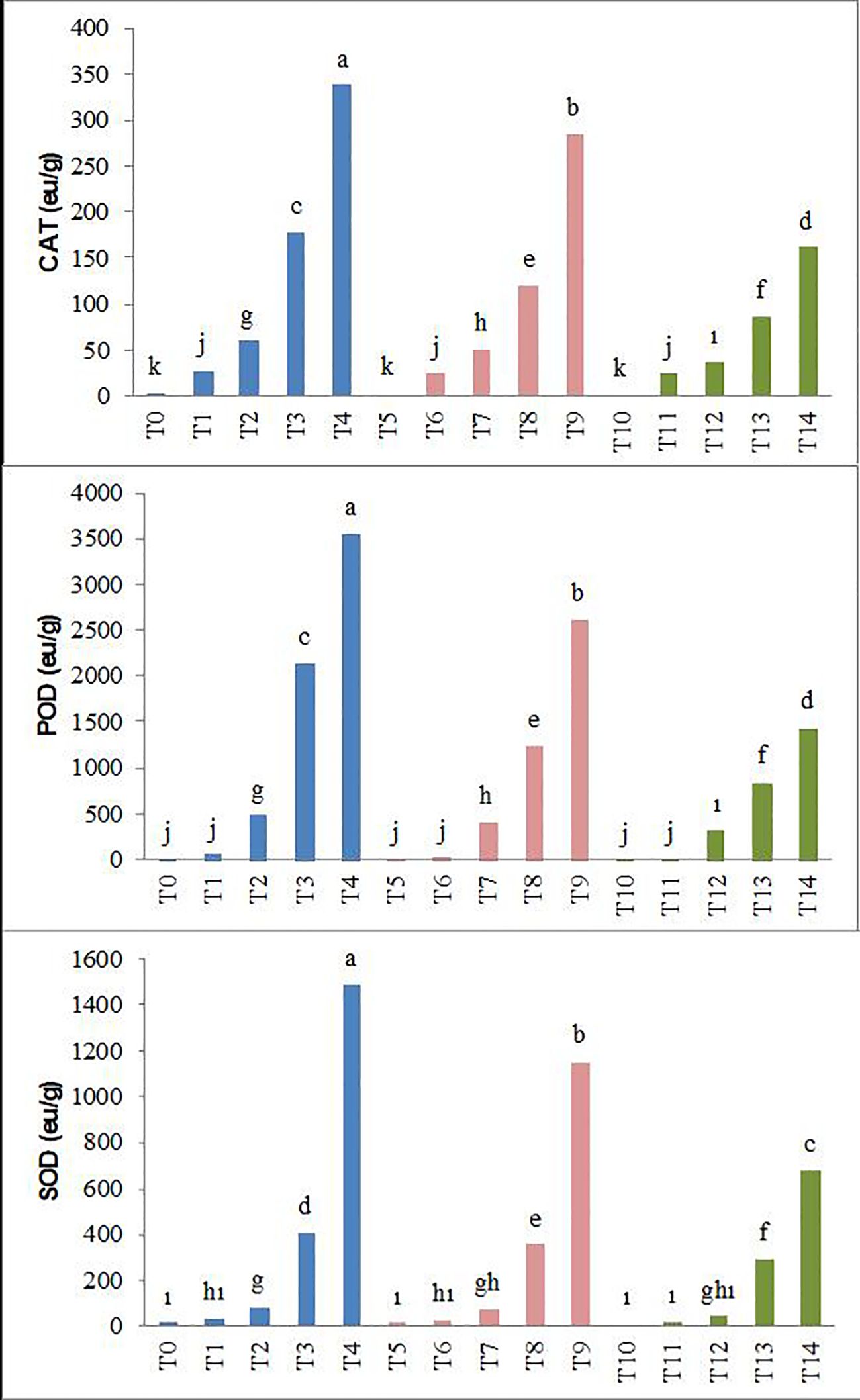
Figure 5. Effect of biochar on CAT, POD, and SOD enzyme activity of forage pea seedlings under saline conditions. The different-lettered averages in each bar are significantly different according to Duncan’s multiple-range test (p ≤ 0.001). B0, without biochar (control); B1, 2.5% biochar; B2, 5% biochar; S0, 0 mM NaCl; S1, 25 mM NaCl; S2, 50 mM NaCl; S3, 75 mM NaCl; S4, 100 mM NaCl; T0, B0S0; T1, B0S1; T2, B0S2; T3, B0S3; T4, B0S4; T5, B1S0; T6, B1S1; T7, B1S2; T8, B1S3; T9, B1S4; T10, B2S0; T11, B2S1; T12, B2S2; T13, B2S3; T14, B2S4.
3.5 Hormones
The content of indole-3-acetic acid (IAA), gibberellic acid (GA), salicylic acid (SA), cytokinin, zeatin, and jasmonic acid in forage pea seedlings, except for abscisic acid (ABA), has shown a significant decrease in response to the increased salinity levels. In general, biochar applications have reduced the content of IAA, GA, SA, cytokinin, zeatin, and jasmonic acid hormones in forage pea seedlings below normal conditions compared to the control, excluding ABA content (Table 3).
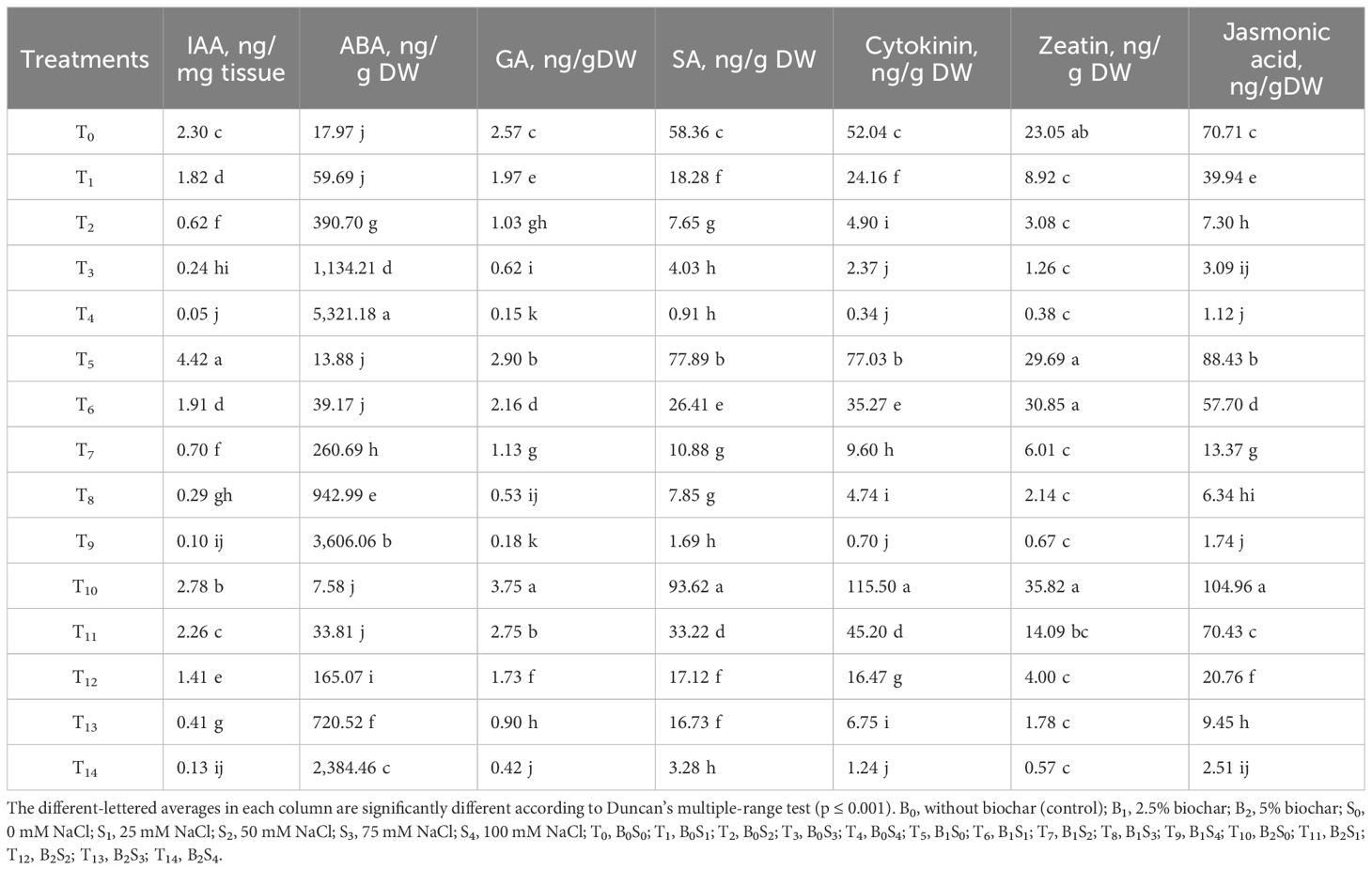
Table 3. Effect of biochar on the content of IAA, ABA, GA, SA, cytokinin, zeatin, and jasmonic acid in forage pea seedlings under saline conditions.
3.6 Leaf and root mineral content
As can be observed in Tables 4, 5, the study indicates that both biochar and salt applications significantly influenced the leaf and root mineral content of pea seedlings. The mineral content in the leaves and roots of forage pea seedlings decreased, except for Na and Cl concentrations, at 25, 50, 75, and 100 mM NaCl. However, the reduction in plant mineral content under salinity stress was comparatively lower in biochar applications.
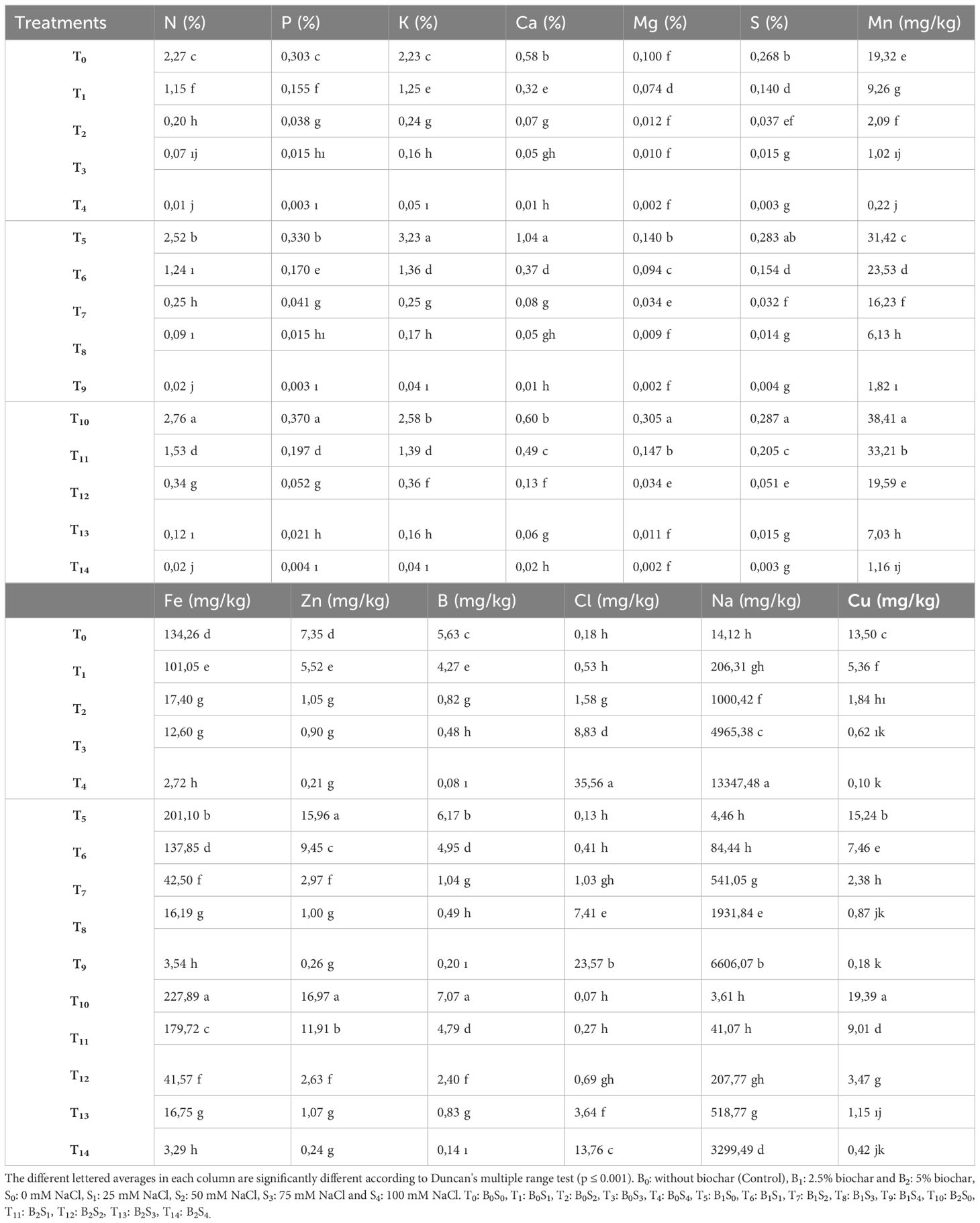
Table 4. The effect of biochar on the leaf mineral content of forage pea seedlings under saline conditions.
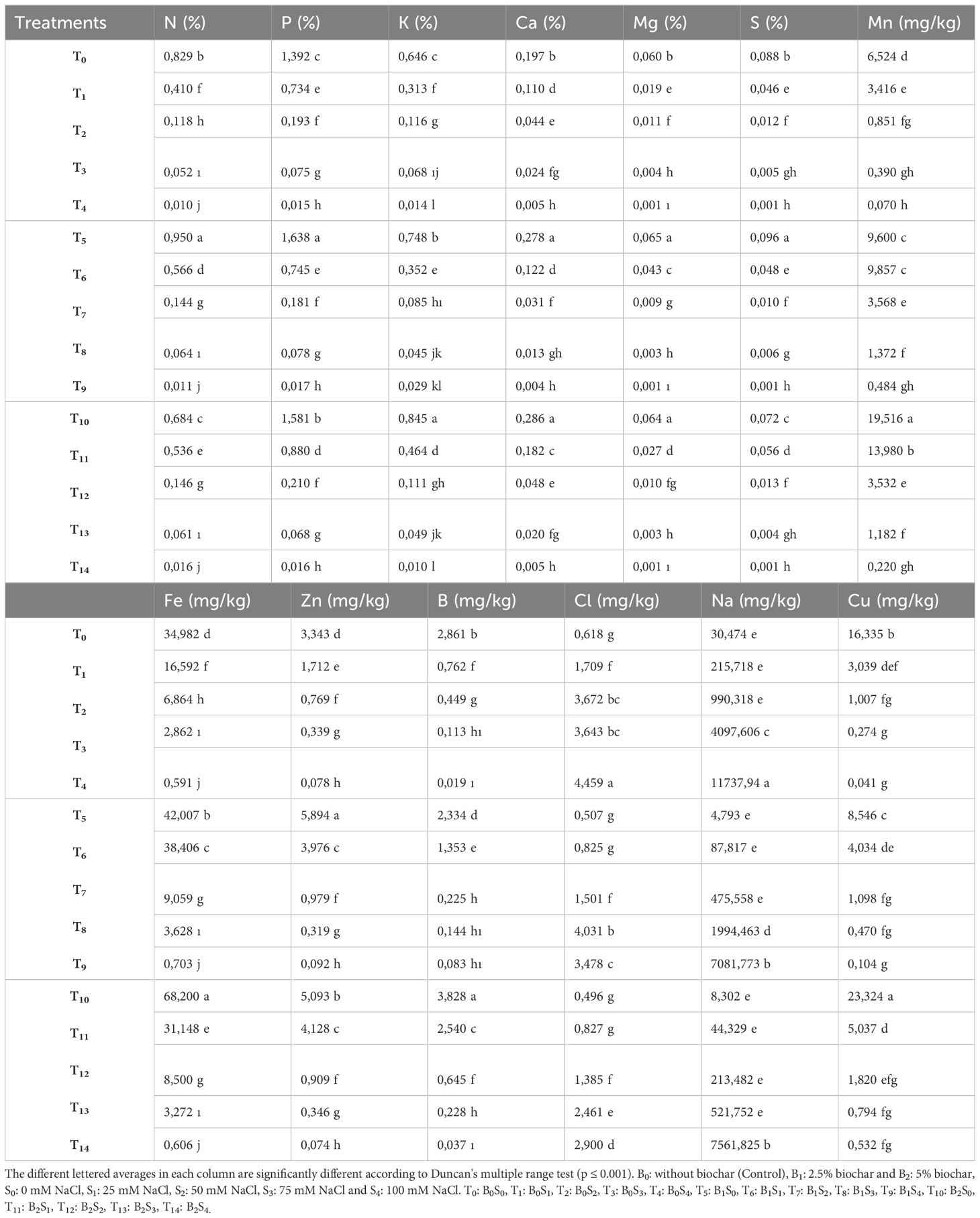
Table 5. The effect of biochar on the root mineral content of forage pea seedlings under saline conditions.
4 Discussion
Salinity, one of the major problems in arid and semi-arid areas, adversely affects not only the morphological and physiological structure of plants but also their developmental processes (Hussain et al., 2019). Consequently, salinity significantly limits plant productivity and quality in agricultural production (Koca et al., 2007). Indeed, as observed in our study, the growth of pea seedlings was negatively affected by changes in salinity levels (Figures 1–5; Tables 3–5). The adverse impact of salinity on the growth of pea seedlings has been documented in numerous studies as well (Senturk and Sivritepe, 2015; Demirkol et al., 2019; Tetiktabanlar et al., 2020). The limitations observed in the growth of pea seedlings under salinity stress conditions may be attributed not only to the negative impact on water and osmotic pressure potential but also to a reduced carbon influx into the plant. According to the research results obtained, significant decreases were observed in SPAD, chlorophyll a, chlorophyll b, and total chlorophyll values in pea seedlings. These findings are in parallel with many studies (Yılmaz et al., 2011; Kanwal et al., 2018; Sahin et al., 2018; Zambi and Ascı, 2020).
As an abiotic factor, salinity has been found to increase the formation of reactive oxygen species (ROS) (Yan et al., 2018; Kul et al., 2021b), resulting in significant increases in H2O2 and MDA values compared to the control in our results (Figure 4). While ROS is a normal byproduct of plant cellular metabolism under normal conditions and serves important functions for plant growth, environmental factors such as salinity stress lead to an increase in ROS production, creating oxidative stress in plants. This, in turn, causes molecular and cellular damage resulting in cell death, significantly damaging nucleic acids and proteins and leading to lipid peroxidation in plants (Pang and Wang, 2008; Hasanuzzaman et al., 2021; Ekinci et al., 2022).
In plants, the synthesis of soluble sugars, primarily glucose and sucrose, and particularly proline, which serves as a physiological indicator of the plant’s resistance to salt stress (Liang et al., 2018), was observed to generally increase with the escalation of salinity stress in pea seedlings. Additionally, it was observed that the application of biochar led to a decrease in proline content (Figure 4). This situation is similar to many studies (Parida and Das, 2005; Koca et al., 2007; Mohamed et al., 2007).
When exposed to abiotic factors such as salt stress, a plant becomes highly sensitive, and significant changes occur in the hydrogen peroxide (H2O2) levels (Stone and Yang, 2006). Hydrogen peroxide, a crucial type of ROS, serves as an important signaling molecule for plant defense (Hasanuzzaman et al., 2021). In the presence of increased salt levels, even with the existence of antioxidant enzymes such as CAT, SOD, and POD in the plant, it was observed in the study that the H2O2 levels increased. This phenomenon in pea seedlings under salt stress can be explained by the plant’s antioxidant enzyme systems. An increase in salinity can enhance the activities of antioxidant enzymes such as SOD, POD, and CAT, leading to an increase in the amount of H2O2 (Duman et al., 2016).
Abscisic acid (ABA), responsible for the stimulation of genes under saline conditions, reduces the inhibitory effect of NaCl. The increase in ABA concentration in leaves under salt stress conditions, a significant abiotic factor (Zhang et al., 2006), is consistent with the results obtained in our study. In plants under salt stress, it has been determined that the levels of IAA, cytokinin, and gibberellic acid decrease, while the concentration of ABA increases (Ma et al., 2021; Ji et al., 2022; Yildirim et al., 2023). This not only supports our results but also indicates that salt stress affects water relations and membrane permeability in plant cells (Karmoker and Van Steveninck, 1979). Additionally, zeatin, an important defense mechanism of plants against drought and salinity stress (Bari and Jones, 2009; Pieterse et al., 2009), has decreased in response to the increased salinity levels (Table 3).
The levels of JA in pea seedlings were found to undergo changes during salt stress applications. Jasmonic acid stimulates the genes associated with protein structures that protect plants against adverse conditions, whether biotic or abiotic, enabling the activation of enzymes (Gross and Pathier, 1994). In our study, it was observed that JA decreased in the pea seedlings used due to their sensitivity to salinity (Yavas and Unay, 2018). Although salicylic acid is known to play a crucial role in the formation of systemic resistance (SAR) in plants (Metraux, 2001) and is reported to have a protective effect on plants under abiotic stress conditions, positively influencing plant growth and development in many studies conducted under salty conditions (Hao et al., 2011; Simaei et al., 2011), this positive effect can vary depending on the salt concentrations in the environment, as observed in our study (Khodary, 2004; Baran and Dogan, 2014; Souri and Tohidloo, 2019).
In plants, salinity stress can adversely affect the relationship between mineral nutrients and nutrients due to its impact on both the availability of nutrients and their translocation within the plant (Hu and Schmidhalter, 2005; Evelin et al., 2019). In this study conducted with pea seedlings, particularly the application of 100 mM NaCl resulted in lower levels of mineral nutrient elements such as N, P, K, Ca, Mg, and Fe in both leaves and roots compared to the control conditions. Additionally, it was observed that the Na and Cl levels increased more significantly compared to the control conditions (Tables 4, 5). This imbalance among mineral nutrients may arise due to the competition of salinity stress with nutrient elements such as K, Ca, and NO3 (Hu and Schmidhalter, 2005).
Biochar is a carbon-rich material that contains organic matter and inorganic salts (humic and fulvic substances). Apart from its use for energy purposes, biochar also possesses the characteristic of being a material that can be utilized to improve soil fertility and enhance the organic carbon content of soils. Additionally, it can serve the purpose of removing heavy metals from water and soil. This dual functionality makes biochar a versatile material with applications not only in energy production but also in the enhancement of soil quality and the mitigation of heavy metal contamination in water and soil (Winsley, 2007; Lliffe, 2009). Soybean, hazelnut, tree bark, and wood pellets, including forest and agricultural residues, are used as biochar. Biochar, used to alleviate soil toxicity and reduce the adverse effects of climate change (Ennis et al., 2012; Stewart et al., 2013; Yildirim et al., 2021; Gullap et al., 2024), is also utilized in reducing the adsorption rate of sodium (Farhangi-Abriz and Ghassemi-Golezani, 2021) and mitigating the oxidative effect of NaCl (Akhtar et al., 2015). As a result of the findings obtained in our study, it has been determined that the application of biochar positively affected the growth parameters of pea seedlings (Figures 1–3). This is consistent with numerous studies (Zhang et al., 2019; Kul et al., 2021b; Ekinci et al., 2022), where biochar has been commonly used to alleviate salinity stress in plant seedlings. The application of biochar has been found to influence soil physical properties such as texture, structure, porosity, pore size distribution, available water content, and soil drainage characteristics (Glaser et al., 2002; Herath et al., 2013). These effects can further influence plant growth and development by mediating water uptake and root respiration processes. In this study, it was observed that SPAD values increased with the application of biochar. This finding may be attributed to the stimulation of plant growth, as indicated by Oktem and Oktem (2020) and Wu et al. (2023), involving plant growth-regulating effects, cellular oxidant activity, and increases in cell division and elongation. The results of this study, indicating an increase in antioxidant enzymes such as CAT, POD, and SOD, along with elevated H2O2 and MDA levels in pea seedlings due to salt stress, show similarities with previous studies (Kamran et al., 2020; Naveed et al., 2020). Consistent with our current study, it has been noted that the organic amendments used in our study were effective in alleviating the adverse effects of salt stress (Tartoura et al., 2014; Kusvuran et al., 2021). Similar to our study, the addition of biochar to the soil has been found to enhance the antioxidant enzymes and reduce the MDA content in wheat and corn plants under saline conditions (Kanwal et al., 2017; Onder and Ucar, 2021; Alamer et al., 2022; Bziouech et al., 2022).
The expression of ABA, which plays a crucial role in regulating responses to abiotic conditions such as salinity, drought, and cold stress in different plant growth processes, has been reported to increase under salt stress (Zhu, 2002; Hadiarto and Tran, 2011). This supports the results obtained in our study, as indicated by previous research (Kalifa et al., 2004; Xue and Loveridge, 2004). In the current study, it was observed that biochar, used as an organic amendment to alleviate the negative effects of salt stress, reduced the Na content in both the leaves and roots of pea seedlings. This finding suggests that the reduction in Na levels may contribute to mitigating the adverse effects of salt stress. Consequently, this reduction may lead to a decrease in the ABA hormone in pea seedlings under salt stress. Similar studies to ours have also found that biochar applications in plants have a reducing effect on Na and ABA content (Farhangi-Abriz and Torabian, 2018; Ekinci et al., 2022).
In our study, it was observed that the levels of growth hormones such as IAA, GA, SA, cytokinin, zeatin, and jasmonic acid in the leaves of pea seedlings grown under salt stress were reduced compared to the control treatment, while biochar application led to an increase in these hormones in plants (Table 3). Similar to the ABA enzyme, the difference observed among treatments may stem from the stress-mitigating effects of biochar applications on plants. This finding is consistent with previous studies indicating the mitigating effect of biochar on NaCl adverse impact (Thomas et al., 2013; Kim et al., 2016; Abriz and Torabian, 2018; Torabian et al., 2018).
In this study, which aimed to reduce the negative effects of salinity through biochar applications, it was observed that biochar applied at different doses reduced the levels of Na and Cl while increasing other mineral elements in the leaves and roots of pea seedlings (Tables 4, 5). Biochar, which improves the physical and chemical properties of the soil and, consequently, can enhance plant productivity (Farrell et al., 2013), acts as a good nutrient carrier, reserving macro (N, P, K, Ca, etc.) and micro-nutrients (Mg, Zn, etc.) for plants (Wang et al., 2012). With a wide surface area of approximately 500 m2 g-1, biochar has been reported to increase the availability of essential nutrients in the soil, such as Ca, Mg, and K, due to its high water retention and cation exchange capacity (Lorenz and Lal, 2014). In a similar study, it was mentioned that the application of biochar, with its high adsorption capacity, reduced sodium uptake and allowed plant nutrient elements such as K, Ca, and Mg present in the soil solution to become more available due to the increase in soil water content (Akhtar et al., 2015).
In our study, when the effect of biochar obtained from olive oil pomace, which is a waste, is taken into consideration, the results obtained showed that it may have positive effects on plant development in abiotic stresses such as salinity stress. Olive oil pomace is effective in the formation of microporous structure for biochar. Moreover, the high cellulose content of olive oil pomace waste, which occurs in significant amounts in oil production, is important in the conversion of biochar by pyrolysis, which has economic value (Aissaoui et al., 2023). The use of biochar obtained from olive oil pomace production can improve the soil aggregate stability and carbon content (Kavdir et al., 2023) and increase the soil pH and organic matter content (Pellera and Gidarakos, 2015).
Re-evaluation of various wastes, especially agricultural wastes, is emphasized in order to prevent environmental pollution problems. Agricultural wastes, especially as activated carbon precursors, are an important renewable and inexpensive resource. The olive oil pomace used as biochar in this study will be important in this context. This waste as a good carbon source and amendment rich in organic matter is unfortunately not recycled in Turkey. It can be concluded from the findings of the study that biochar treatments derived from olive oil pomace improved the growth of the pea seedlings under salinity stress. Biochar, as amendment, can be used to mitigate salinity stress in agricultural lands. Olive oil pomace can be used as a source in the production of biochar.
5 Conclusion
Overall, pea seedlings exhibited a stress response as salinity levels rose. This included increases in hydrogen peroxide (H2O2), malondialdehyde (MDA), proline, IAA, and antioxidant enzyme activity (CAT, POD, and SOD). Conversely, there were decreases in chlorophyll content (SPAD), plant growth hormones (IAA, GA, SA, cytokinin, zeatin, and jasmonic acid), plant growth parameters, and nutrient uptake (excluding sodium and chloride). However, the study also found that applying biochar at various dosages helped to mitigate some of the negative effects of salinity stress. The findings of the study indicate that biochar amendment can enhance forage peas’ growth by modulating the plant physiology and biochemistry under salt stress. Especially 2.5% biochar application can be used to mitigate some of the negative effects of salinity stress. This suggests a potential twofold benefit: utilizing waste materials like olive oil residue for sustainable biochar production and employing the resulting biochar as a source of organic matter to enhance plant resilience against environmental stresses. Furthermore, field studies should be conducted to get information about yield.
Data availability statement
The original contributions presented in the study are included in the article/supplementary material. Further inquiries can be directed to the corresponding author.
Author contributions
MG: Writing – original draft, Project administration, Validation. SS: Conceptualization, Investigation, Writing – original draft. TK: Investigation, Methodology, Writing – review & editing. AK: Investigation, Methodology, Writing – original draft. ME: Methodology, Formal Analysis, Writing – original draft. MT: Writing – original draft. HA: Methodology, Resources, Writing – review & editing. EY: Writing – original draft, Writing – review & editing.
Funding
The author(s) declare financial support was received for the research, authorship, and/or publication of this article. This project, conducted with the number FBA-2021-9384, is carried out at the Ataturk University Plant Production Application and Research Center. The project has received support from the Ataturk University Scientific Research Projects Coordination Unit.
Conflict of interest
The authors declare that the research was conducted in the absence of any commercial or financial relationships that could be construed as a potential conflict of interest.
The author(s) declared that they were an editorial board member of Frontiers, at the time of submission. This had no impact on the peer review process and the final decision.
Publisher’s note
All claims expressed in this article are solely those of the authors and do not necessarily represent those of their affiliated organizations, or those of the publisher, the editors and the reviewers. Any product that may be evaluated in this article, or claim that may be made by its manufacturer, is not guaranteed or endorsed by the publisher.
References
Abdelaal, K., Alsubeie, M. S., Hafez, Y., Emeran, A., Moghanm, F., Okasha, S., et al. (2022). Physiological and biochemical changes in vegetable and field crops under drought, salinity and weeds stresses: control strategies and management. Agriculture 12, 2084. doi: 10.3390/agriculture12122084
Abriz, S. A., Torabian, S. (2018). Biochar increased plant growth-promoting hormones and helped to alleviates salt stress in common bean seedlings. J. Plant Growth Regul. 37, 591–601. doi: 10.1007/s00344-017-9756-9
Ahmad, A., Tola, E., Alshahrani, T. S., Seleiman, M. F. (2023b). Enhancement of morphological and physiological performance of Zea mays L. under saline stress using ZnO nanoparticles and 24-epibrassinolide seed priming. Agronomy 13, 771. doi: 10.3390/agronomy13030771
Ahmad, I., Zhu, G., Zhou, G., Younas, M. U., Suliman, M. S. E., Liu, J., et al. (2023a). Integrated approaches for increasing plant yield under salt stress. Front. Plant Sci. 14, 1215343. doi: 10.3389/fpls.2023.1215343
Ainane, T., Mohamed Abdoul-Latif, F., Ainane, A., Kornaros, M., Abbi, R., Achira, M., et al. (2023). Optimization by design of experiments of the preparation of biochar from olive pomace and its physico-chemical characterizations. JASAB 5 (1), 1–10. doi: 10.48402/IMIST.PRSM/jasab-v5i1.39156
Aissaoui, M. H., Trabelsi, A. B. H., Abidi, S., Zaafouri, K., Haddad, K., Jamaaoui, F., et al. (2023). Sustainable biofuels and biochar production from olive mill wastes via co-pyrolysis process. Biomass Convers. Bior. 13, 8877–8890. doi: 10.1007/s13399-021-01735-z
Akhtar, S. S., Andersen, M. N., Liu, F. (2015). Residual effects of biochar on improving growth, physiology and yield of wheat under salt stress. Agric. Water Manage. 158, 61–68. doi: 10.1016/j.agwat.2015.04.010
Alamer, K. H., Perveen, S., Khaliq, A., Zia Ul Haq, M., Ibrahim, M. U., Ijaz, B. (2022). Mitigation of salinity stress in maize seedlings by the application of vermicomipost and sorghum water extracts. Plants 11, 2548. doi: 10.3390/plants11192548
Ali, S., Rizwan, M., Qayyum, M. F., Ok, Y. S., Ibrahim, M., Riaz, M., et al. (2017). Biochar soil amendment on alleviation of drought and salt stress in plants: A Critical Review. Environ. Sci. pollut. Res. 24, 12700–12712. doi: 10.1007/s11356-017-8904-x
Alkharabsheh, H. M., Seleiman, M. F., Hewedy, O. A., Battaglia, M. L., Jalal, R. S., Alhammad, B. A., et al. (2021). Field crop responses and management strategies to mitigate soil salinity in modern agriculture: A review. Agronomy 11, 2299. doi: 10.3390/agronomy11112299
Ates, E., Tekeli, A. S. (2007). Salinity tolerance of Persian clover (Trifolium resupinatum var.majus Boiss.) lines at germinaton and seedling stages. World J. Agric. Sci. 3, 71–79.
Baran, A., Dogan, M. (2014). The effect of salicylic acid on physiological in soybean (Glycine max L.) under salt stress. SDU J. Nat. Appl. Sci. 18, 78–84.
Bari, R., Jones, J. D. G. (2009). Role of hormones in plant defense responses. Plant Mol. Biol. 69, 473–488. doi: 10.1007/s11103-008-9435-0
Bates, L. S., Waldren, R. P., Teare, I. D. (1973). Rapid determination of free proline for water-stress studies. Plant Soil. 39, 205–207. doi: 10.1007/BF00018060
Battal, P., Tileklioglu, B. (2001). The effects of different mineral nutrients on the levels of cytokinins in maize (Zea mays L). Turk. J. Bot. 25, 123–130.
Bhattacharya, A. (2021). “Effect of soil water deficit on growth and development of plants: A review,” in Soil water deficit and physiological issues in plants (Springer, Singapore). doi: 10.1007/978-981-33-6276-5_5
Bremner, J. M. (1996). “Nitrogen-total,” in Methods of soil analysis part 3 chemical methods (Soil Science Society of America, Madison (WI).
Bziouech, S. A., Dhen, N., Helaoui, S., Ammar, I. B., Dridi, B. A. M. (2022). Effect of vermicompost soil additive on growth performance, physiological and biochemical responses of tomato plants (Solanum lycopersicum L. var. Firenze) to salt stress. Emir. J. Food Agric. 34, 316–328. doi: 10.9755/ejfa.2022.v34.i4.2844
Cowiea, A. L., Orrb, B. J., Sanchezc, V. M. C., Chasekd, P., Crossmane, N. D., Erleweinf, A., et al. (2018). Land in balance: The scientific conceptual framework for land degradation neutrality. Environ. Sci. Policy. 79, 25–35. doi: 10.1016/j.envsci.2017.10.011
Demirkol, G., Yılmaz, N., Ascı., O. O. (2019). The effect of salt stress on the germination and seedling growth parameters of a selected forage pea (Pisum sativum ssp. arvense L.) genotype. KSU J. Agric. Nat. 22, 354–359. doi: 10.18016/ksutarimdoga.vi.455439
Duman, Y. A., Acemi, A., Toygra, H., Yuzugullu, Y., Ozen, F. (2016). Investigation of antioxidant enzymes of Amsonia orientalis in the Presence of Salt Stress and BAP. CBU J. Sci. 12, 543–551.
Dursun, A., Turan, M., Ekinci, M., Gunes, A., Ataoglu, N., Esringu, A., et al. (2010). Effects of boron fertilizer on tomatoes (Lycopersicon esculentum L.), pepper (Capsicum annum L.) and cucumber (Cucumis sativus L.) yield and chemical composition. Commun. Soil Sci. Plant Anal. 41, 1576–1593. doi: 10.1080/00103624.2010.485238
Dustgeer, Z., Seleiman, M. F., Imran, K. H. A. N., Chattha, M. U., Alhammad, B. A., Jalal, R. S., et al. (2021). Glycine-betaine induced salinity tolerance in maize by regulating the physiological attributes, antioxidant defense system and ionic homeostasis. Not. Bot. Horti Agrobot. Cluj-Napoca 49, 12248–12248. doi: 10.15835/nbha49112248
Ekinci, M., Turan, M., Yıldırım, E. (2022). Biochar mitigates salt stress by regulating nutrient uptake and antioxidant activity activity, alleviating the oxidative stress and abscisic acid content in cabbage seedlings. Turk. J. Agric. For. 46, 28–37. doi: 10.3906/tar-2104-81
Enaime, G., Lubken, M. (2021). Agricultural waste-based biochar for agronomic applications. Appl. Sci. 11, 8914. doi: 10.3390/app11198914
Ennis, C. J., Evans, A. G., Islam, M., Ralebitso-Senior, T. K., Senior, E. (2012). Biochar: carbon sequestration, land remediation, and impacts on soil microbiology. Crit. Rev. Environ. Sci. Technol. 42, 2311–2364. doi: 10.1080/10643389.2011.574115
Evelin, H., Devi, T. S., Gupta, S., Kapoor, R. (2019). Mitigation of salinity stress in plants by arbuscular mycorrhizal symbiosis: current understanding and new challenges. Front. Plant Sci. 10, 470. doi: 10.3389/fpls.2019.00470
Farhangi-Abriz, S., Ghassemi-Golezani, K. (2021). Changes in soil properties and salt tolerance of safflower in response to biochar-based metal oxide nanocomposites of magnesium and manganese. Ecotoxicol. Environ. Saf. 211, 111904. doi: 10.1016/j.ecoenv.2021.111904
Farhangi-Abriz, S., Torabian, S. (2018). Biochar improved nodulation and nitrogen metabolism of soybean under salt stress. Symbiosis 74, 215–223. doi: 10.1007/s13199-017-0509-0
Farrell, M., Kuhn, T. K., Macdonald, L. M., Maddern, T. M., Murphy, D. V., Hall, P. A., et al. (2013). Microbial utilisation of biochar-derived carbon. Sci. Total Environ. 465, 288–297. doi: 10.1016/j.scitotenv.2013.03.090
Glaser, B., Lehmann, J., Zech, W. (2002). Ameliorating physical and chemical properties of highly weathered soils in the tropics with biochar-a review. Biol. Fertil. Soils. 35, 219–230. doi: 10.1007/s00374-002-0466-4
Gross, D., Pathier, B. (1994). Novel natural substances acting in plant growth regulation. J. Plant Growth Regul. 13, 93–114. doi: 10.1007/BF00210953
Gullap, M. K., Severoglu, S., Karabacak, T., Yazici, A., Ekinci, M., Turan, M., et al. (2024). Biochar derived from hazelnut shells mitigates the impact of drought stress on soybean seedlings. New Zeal. J. Crop Hortic. Sci. 52, 19–37. doi: 10.1080/01140671.2022.2079680
Gupta, P., Gupta, N., Dash, S. (2022). Biochar for sustainable environmental management. BioChar: Applications for Bioremediation of Contaminated Systems, Vol. 147. Berlin/Boston: Walter de Gruyter GmbH.
Hadiarto, T., Tran, L. S. (2011). Progress studies of drought responsive genes in rice. Plant Cell Rep. 30, 297–310. doi: 10.1007/s00299-010-0956-z
Hanumantha, R. B., Nair, R. M., Nayyar, H. (2016). Salinity and high temperature tolerance in mungbean (Vigna radiata (L.) Wilczek) from a physiological perspective. Front. Plant Sci. 7, 957. doi: 10.3389/fpls.2016.00957
Hao, J. H., Wang, X. L., Dong, C. J., Zhang, Z. G., Shang, Q. M. (2011). Salicylic acid induces stomatal closure by modulating endogenous hormone levels in cucumber cotyledons. Russ. J. Plant Physiol. 58, 906–913. doi: 10.1134/S1021443711050098
Hasanuzzaman, M., Raihan, M. R. H., Masud, A. A. C., Rahman, K., Nowroz, F., Rahman, M., et al. (2021). Regulation of reactive oxygen species and antioxidant defense in plants under salinity. Int. J. Mol. Sci. 22, 9326. doi: 10.3390/ijms22179326
Heath, R. L., Packer, L. (1968). Photoperoxidation in isolated chloroplasts: I. Kinetics and stoichiometry of fatty acid peroxidation. Arch. Biochem. Biophy. 125, 189–198. doi: 10.1016/0003-9861(68)90654-1
Herath, H. M. S. K., Camps-Arbestain, M., Hedley, M. (2013). Effect of biochar on soil physical properties in two contrasting soils: an alfisol and an andisol. Geoderma 209, 188–197. doi: 10.1016/j.geoderma.2013.06.016
Howell, T. A., Evett, S. R., Tolk, J. A. (2001). Irrigation systems and management to meet future food fiber needs and to enhance water use efficiency (Texas, USA: USDA-ARS Water Management User Unit Bushland).
Hu, Y., Schmidhalter, U. (2005). Drought and salinity: A comparison of their effects on mineral nutrition of plants. J. Plant Nutr. Soil Sci. 168, 541–549. doi: 10.1002/jpln.200420516
Hussain, S., Shaukat, M., Ashraf, M., Zhu, C., Jin, Q., Zhang, J. (2019). Salinity stress in arid and semi-arid climates: Effects and management in field crops. Climate Change Agric. 13, 201–226. doi: 10.5772/intechopen.78427
Ji, X., Tang, J., Zhang, J. (2022). Effects of salt stress on the morphology, growth and physiological parameters of Juglans microcarpa L. seedlings. Plants 11, 2381. doi: 10.3390/plants11182381
Kalifa, Y., Gilad, A., Konrad, Z., Zaccai, M. (2004). The water-and salt-stress-regulated Asr1 (abscisic acid stress ripening) gene encodes a zinc-dependent DNA-binding protein. Biochem. J. 378, 373–378. doi: 10.1042/BJ20031800
Kamran, M., Parveen, A., Ahmar, S., Malik, Z., Hussain, S., Chattha, M. S., et al. (2020). An overview of hazardous impacts of soil salinity in crops, tolerance mechanisms and amelioration through selenium supplementation- A review. Int. J. Mol. Sci. 21, 148. doi: 10.3390/ijms21010148
Kanwal, S., Ilyas, N., Shabir, S., Saeed, M., Gul, R., Zahoor, M., et al. (2017). Application of biochar in mitigation of negative effects of salinity stress in wheat (Triticum aestivum l.). J. Plant Nutr. 41(4), 526–538. doi: 10.1080/01904167.2017.1392568
Karmoker, J. L., Van Steveninck, F. M. (1979). The effect of abscisic acid on the uptake and distribution of ions in intact seedlings of Phaseolus vulgaris cv. Redland Pioneer. Plant Physiol. 45, 453–459. doi: 10.1111/j.1399-3054.1979.tb02613.x
Kavdir, Y., Ilay, R., Güven, O. B., Sungur, A. (2023). “Characterization of olive pomace biochar produced at different temperatures and their temporal effects on soil aggregation and carbon content,” in Biomass convers. Bior, 1–10. doi: 10.1007/s13399-023-03900-y
Kayin, N., Borazan, A. A., Turan, F. (2024). Effect of salt stress on morphological characteristics and secondary metabolites of some forage pea cultivars. BSJ Agric. 7, 69–76. doi: 10.47115/bsagriculture.1390139
Kayin, N., Turan, F., Aydemir, E. S. (2022). Effect of different salt concentrations on germination of forage pea. Int. J. Chem. Technol. 6, 108–113. doi: 10.32571/ijct.1171249
Khedulkar, A. P., Pandit, B., Doong, R. A. (2023). Agricultural waste to real worth biochar as a sustainable material for supercapacitor. Sci. Total Environ. 869, 161441. doi: 10.1016/j.scitotenv.2023.161441
Khodary, S. E. A. (2004). Effect of salicylic acid on the growth, photosynthesis and carbohydrate metabolism in salt stressed maize plants. Int. J. Agric. Biol. 6, 5–8.
Kim, H. S., Kim, K. R., Yang, J. E., Ok, Y. S., Owens, G., Nehls, T. (2016). Effect of biochar on reclaimed tidal land soil properties and maize (Zea mays L.) response. Chemosphere 142, 153–159. doi: 10.1016/j.chemosphere.2015.06.041
Koca, H., Bor, M., Özdemir, F., Türkan, D. (2007). The effect of salt stress on lipid peroxidation, antioxidative enzymes and proline content of sesame cultivars. Environ. Exper. Bot. 60, 344–351. doi: 10.1016/j.envexpbot.2006.12.005
Kul, R., Arjumend, T., Ekinci, M., Yildirim, E., Turan, M., Argin, S. (2021b). Biochar as an organic soil conditioner for mitigating salinity stress in tomato. Soil Sci. Plant Nutr. 67, 693–706. doi: 10.1080/00380768.2021.1998924
Kul, R., Ekinci, M., Turan, M., Yildirim, E. (2021a). Impact of biochar on growth, physiology and antioxidant activity of common bean subjected to salinity stress. Glob. J. Bot. Sci. 9, 8–13. doi: 10.12974/2311-858X.2021.09.2
Kusvuran, A., Bilgici, M., Kusvuran, S., Nazli, R. I. (2021). The effect of different organic matters on plant growth regulation and nutritional components under salt stress in sweet sorghum (Sorghum bicolor (L.) Moench). Maydica 66, 1–9.
Liang, W., Ma, X., Wan, P., Liu, L. (2018). Plant salt-tolerance mechanism: A review. Biochem. Biophy. Res. Commun. 495, 286–291. doi: 10.1016/j.bbrc.2017.11.043
Lichtenthaler, H., Wellburm, A. R. (1983). Determination of total carotenoids and chlorophyll a and b of leaf extracts in different solvents. Biochem. Soci.Trans. 11, 591–593. doi: 10.1042/bst0110591
Liu, N., Lin, Z., Guan, L., Gaughan, G., Lin, G. (2014). Antioxidant enzymes regulate reactive oxygen species during pod elongation in Pisum sativum and Brassica chinensis. PloS One 9, e87588. doi: 10.1371/journal.pone.0087588
Lliffe, R. (2009). “Is the biochar produced by an Anila stove likely to be a beneficial soil additive,” in UKBRC working paper 4 (UK Biochar Research Centre, Edinburgh, UK).
Lorenz, K., Lal, R. (2014). Soil organic carbon sequestration in agroforestry systems. A review. Agron. Sustain. Dev. 34, 443–454. doi: 10.1007/s13593-014-0212-y
Ma, Y., Wei, Z., Liu, J., Liu, X., Liu, F. (2021). Growth and physiological responses of cotton plants to salt stress. J. Agron. Crop Sci. 207, 565–576. doi: 10.1111/jac.12484
McLean, E. O. (1982). “Soil pH and lime requirement,” in Methods of soil analysis. Part 2. Chemical and microbiological properties, 2nd ed. Ed. Page, A. L. (Wiley, Madison (WI), 199–224.
Metraux, J. P. (2001). Systemic acquired resistance and salicylic acid: current state of knowledge. Eur. J. Plant Pathol. 107, 13–18.
Mohamed, A. N., Rahman, M. H., Alsadon, A. A., Islam, R. (2007). Accumulation of proline in NaCl-treated callus of six tomato (Lycopersicon esculentum Mill.) cultivars. Plant Tissue Cult. Biotechnol. 17, 217–220. doi: 10.3329/ptcb.v17i2.3242
Naveed, M., Ramzan, N., Mustafa, A., Samad, A., Niamat, B., Yaseen, M., et al. (2020). Alleviation of salinity induced oxidative stress in Chenopodium quinoa by Fe biofortification and biochar-endophyte interaction. Agron 10, 168. doi: 10.3390/agronomy10020168
Nelson, D. W., Sommers, E. (1982). “Total carbon, organic carbon and organic matter,” in Methods of soil analysis part 2. Eds. Page, A. L., Miller, R. H., Keeny, D. R. (ASA and SSSA, Madison (WI), 539–579.
Ohkawa, H., Ohishi, N., Yagi, K. (1979). Assay for lipid peroxides in animal tissues by thiobarbituric acid reaction. Anal. Biochem. 95, 351–358. doi: 10.1016/0003-2697(79)90738-3
Oktem, A. G., Oktem, A. (2020). Effect of humic acid application methods on yield and some yield characteristics of corn plant (Zea mays L. indentata). J. Appl. Life Sci. Int. 23, 31–37. doi: 10.9734/jalsi/2020/v23i1130196
Onder, S., Ucar, O. (2021). “Effects of salt stress on beans (Phaseolus vulgaris L.) and applıcatıons to increase salt tolerance,” in 3rd International Conference on Food, Agriculture and Veterinary 19-20 June, Izmir-Turkey.
Ortas, I. (2016). “Role of mycorrhizae and biochar on plant growth and soil quality,” in Biochar, A regional supply chain approach in view of climate change mitigation. Eds. Bruckman, V. J., Varol, E. A., Uzun, B. B., Liu, J. F. (Cambridge University Press, Cambridge. UK.), 398.
Pang, C. H., Wang, B. S. (2008). “Oxidative stress and salt tolerance in plants,” in Progress in botany (Springer Berlin Heidelberg, Berlin, Heidelberg), 231–245.
Parida, A. K., Das, A. B. (2005). Salt tolerance and salinity effects on plants: A review. Ecotoxicol. Environ. Saf. 60, 324–349. doi: 10.1016/j.ecoenv.2004.06.010
Pellera, F. M., Gidarakos, E. (2015). Effect of dried olive pomace–derived biochar on the mobility of cadmium and nickel in soil. J. Environ. Chem. Eng. 3, 1163–1176. doi: 10.1016/j.jece.2015.04.005
Pieterse, C. M., Leon-Reyes, A., van der Ent, S., Van Wees, S. C. (2009). Networking by small-molecule hormones in plant immunity. Nat. Chem. Biol. 5, 308–316. doi: 10.1038/nchembio.164
Porcel, R., Aroca, R., Ruiz-Lozano, J. M. (2012). Salinity stress alleviation using arbuscular mycorrhizal fungi. A review. Agron. Sustain. Dev. 32, 181–200. doi: 10.1007/s13593-011-0029-x
Rhoades, J. D. (1986). “Soluble salts,” in Methods of soil analysis-part II. Ed. Klute, A. (Madison (WI: ASA and SSSA), 167–179.
Ruiz-Lozano, J. M., Collados, C., Barea, J. M., Azco’n, R. (2001). Arbuscular mycorrhiza lsymbiosis can alleviate drought-induced nodule senescence in soybean plants. New Phytol. 151, 493–502.
Sahin, U., Ekinci, M., Ors, S., Turan, M., Yildiz, S., Yildirim, E. (2018). Effects of individual and combined effects of salinity and drought on physiological, nutritional and biochemical properties of cabbage (Brassica oleracea var. capitata). Sci. Hortic. 240, 196–204. doi: 10.1016/j.scienta.2018.06.016
Senturk, B., Sivritepe, H. (2015). Determination of optimum protocol for NaCl priming in pea (Pisum sativum L.) seeds. J. Agric. Faculty Uludag Univ. 29, 95–105.
Shams, M., Ekinci, M., Ors, S., Turan, M., Agar, G., Kul, R., et al. (2019). Nitric oxide mitigates salt stress effects of pepper seedlings by altering nutrient uptake, enzyme activity and osmolyte accumulation. Physiol. Mol. Biol. Plants. 25, 1149–1161. doi: 10.1007/s12298-019-00692-2
Sheng, M., Tang, M., Chen, H., Yang, B., Zhang, F., Huang, Y. (2008). Influence of arbuscular mycorrhizae on photosynthesis and water status of maize plants under salt stress. Mycorrhiza 18, 287–296. doi: 10.1007/s00572-008-0180-7
Simaei, M., Khavarinejad, R. A., Saadatmand, S., Bernard, F., Fahimi, H. (2011). Interactive effects of salicylic acid and nitric oxide on soybean plants under NaCl salinity. Russ. J. Plant Physiol. 58, 783–790. doi: 10.1134/S1021443711050220
Singh, A. (2022). Soil salinity: A global threat to sustainable development. Soil Use Manage. 38, 39–67. doi: 10.1111/sum.12772
Souri, M. K., Tohidloo, G. (2019). Effectiveness of different methods of salicylic acid application on growth characteristics of tomato seedlings under salinity. Chem. Biol. Technol. Agric. 6, 1–7. doi: 10.1186/s40538-019-0169-9
Stewart, C. E., Zheng, J., Botte, J., Cotrufo, M. F. (2013). Co-generated fast pyrolysis biochar mitigates green-house gas emissions and increases carbon sequestration in temperate soils. GCB Bioenergy 5, 153–164. doi: 10.1111/gcbb.12001
Stone, J. R., Yang, S. (2006). Hydrogen peroxide: a signaling messenger. Antioxid. Redox Signal. 8, 243–270. doi: 10.1089/ars.2006.8.243
Taha, S. R., Seleiman, M. F., Alhammad, B. A., Alkahtani, J., Alwahibi, M. S., Mahdi, A. H. (2020). Activated yeast extract enhances growth, anatomical structure, and productivity of Lupinus termis L. plants under actual salinity conditions. Agron 11, 74. doi: 10.3390/agronomy11010074
Tartoura, K. A., Youssef, S. A., Tartoura, E. S. A. (2014). Compost alleviates the negative effects of salinity via up-regulation of antioxidants in Solanum lycopersicum L. plants. Plant Growth Regul. 74, 299–310. doi: 10.1007/s10725-014-9923-y
Tetiktabanlar, I., Ozturk, L., Kısa, D., Genc, N. (2020). The effect of salt stress on the phenolic compounds in pea (Pisum sativum L.) varieties. Gaziosmanpasa J. Scient. Res. 9, 85–94.
Thomas, S. C., Frye, S., Gale, N., Garmon, M., Launchbury, R., MaChado, N., et al. (2013). Biochar mitigates negative effects of salt additions on two herbaceous plant species. J. Environ. Manage. 129, 62–68. doi: 10.1016/j.jenvman.2013.05.057
Tietel, Z., Dag, A., Yermayahu, U., Zipori, I., Beiersdorf, I., Krispin, S., et al. (2019). Irrigation-induced salinity affects olive oil quality and health-promoting properties. J. Sci. Food Agric. 99, 1180–1189. doi: 10.1002/jsfa.9287
Torabian, S., Farhangi-Abriz, S., Rathjen, J. (2018). Biochar and lignite affect H+-ATPase and H+-PPase activities in root tonoplast and nutrient contents of mung bean under salt stress. Plant Physiol. Biochem. 129, 141–149. doi: 10.1016/j.plaphy.2018.05.030
Wang, J., Pan, X., Liu, Y., Zhang, X., Xiong, Z. (2012). Effects of biochar amendment in two soils on greenhouse gas emissions and crop production. Plant Soil. 360, 287–298. doi: 10.1007/s11104-012-1250-3
Wang, N., Zhao, Z., Zhang, X., Liu, S., Zhang, K., Hu, M. (2023). Plant growth, salt removal capacity, and forage nutritive value of the annual euhalophyte Suaeda salsa irrigated with saline water. Front. Plant Sci. 13, 1040520. doi: 10.3389/fpls.2022.1040520
Winsley, P. (2007). Biochar and bioenergy production for climate change mitigation. New Z. Sci. Rev. 6, 5–10.
Wu, Y., Wang, X., Zhang, L., Zheng, Y., Liu, X., Zhang, Y. (2023). The critical role of biochar to mitigate the adverse impacts of drought and salinity stress in plants. Front. Plant Sci. 14, 1163451>Kanwal. doi: 10.3389/fpls.2023.1163451
Xiao, Q., Zhu, L. X., Shen, Y. F., Li, S. Q. (2016). Sensitivity of soil water retention and availability to biochar addition in rainfed semi-arid farmland during a three-year field experiment. Field Crops Res. 196, 284–293. doi: 10.1016/j.fcr.2016.07.014
Xue, G. P., Loveridge, C. W. (2004). HvDRF1 is involved in abscisic acid-mediated gene regulation in barley and produces two forms of AP2 transcriptional activators, interacting preferably with a CT-rich element. Plant J. 37, 326–339.
Yan, Y., Pan, C., Du, Y., Li, D., Liu, W. (2018). Exogenous salicylic acid regulates reactive oxygen species metabolism and ascorbate-glutathione cycle in Nitraria tangutorum Bobr. under salinity stress. Physiol. Mol. Biol. Plants. 24, 577–589. doi: 10.1007/s12298-018-0540-5
Yavas, I., Unay, A. (2018). Impact of some climate change parameters on root, nodule formation and nitrogen fixation in legumes. Int. J. Agric. Wildlife Sci. (IJAWS). 4, 270–278. doi: 10.24180/ijaws.366386
Yildirim, E., Ekinci, M., Turan, M. (2021). Impact of biochar in mitigating the negative effect of drought stress on cabbage seedlings. J. Soil Sci. Plant Nutr. 21, 2297–2309. doi: 10.1007/s42729-021-00522-z
Yildirim, E., Ekinci, Turan, M. (2022). Biochar mitigates salt stress by regulating nutrient uptake and antioxidant activity, alleviating the oxidative stress and abscisic acid content in cabbage seedlings. Turk. J. Agric. For. 46, 28–37. doi: 10.3906/tar-2104-81
Yildirim, E., Ekinci, M., Turan, M., Ors, S., Dursun, A. (2023). Physiological, morphological and biochemical responses of exogenous hydrogen sulfide in salt-stressed tomato seedlings. Sustainability 15, 1098. doi: 10.3390/su15021098
Yılmaz, E., Tuna, M., Burun, B. (2011). Tolerance strategies developed by plants to the effects of salt stress. C.B.U. J. Sci. 7, 647–667.
Zambi, F. H., Ascı, O. O. (2020). Effect of NaCl stress on chlorophyll and mineral content of forage pea. Int. J. Agric. Wildlife Sci. (IJAWS). 6, 562–569.
Zhang, J., Bai, Z., Huang, J., Hussain, S., Zhao, F., Zhu, C., et al. (2019). Biochar alleviated the salt stress of induced saline paddy soil and improved the biochemical characteristics of rice seedlings differing in salt tolerance. Soil Tillage Res. 195, 104372. doi: 10.1016/j.still.2019.104372
Zhang, J., Jia, W., Yang, J., Ismail, A. M. (2006). Role of ABA in integrating plant responses to drought and salt stresses. Field Crops Res. 97, 111–119. doi: 10.1016/j.fcr.2005.08.018
Zhang, L., Tang, S., Guan, Y. (2020). Excellent adsorption–desorption of ammonium by a poly (acrylic acid)-grafted chitosan and biochar composite for sustainable agricultural development. ACS Sustain. Chem. Eng. 8, 16451–16462. doi: 10.1021/acssuschemeng.0c05070
Keywords: biochar, forage crops, plant growth, PEA, salinity
Citation: Gullap MK, Karabacak T, Severoglu S, Kurt AN, Ekinci M, Turan M, Aktas H and Yildirim E (2024) Biochar derived from olive oil pomace mitigates salt stress on seedling growth of forage pea. Front. Plant Sci. 15:1398846. doi: 10.3389/fpls.2024.1398846
Received: 10 March 2024; Accepted: 16 July 2024;
Published: 19 August 2024.
Edited by:
Rabia Nazir, Pakistan Council of Scientific and Industrial Research, PakistanReviewed by:
Mohammad Bagher Hassanpouraghdam, University of Maragheh, IranMahmoud F. Seleiman, King Saud University, Saudi Arabia
Zhansheng Wu, Xi’an Polytechnic University, China
Copyright © 2024 Gullap, Karabacak, Severoglu, Kurt, Ekinci, Turan, Aktas and Yildirim. This is an open-access article distributed under the terms of the Creative Commons Attribution License (CC BY). The use, distribution or reproduction in other forums is permitted, provided the original author(s) and the copyright owner(s) are credited and that the original publication in this journal is cited, in accordance with accepted academic practice. No use, distribution or reproduction is permitted which does not comply with these terms.
*Correspondence: Ertan Yildirim, ZXJ0YW55aWxAYXRhdW5pLmVkdS50cg==