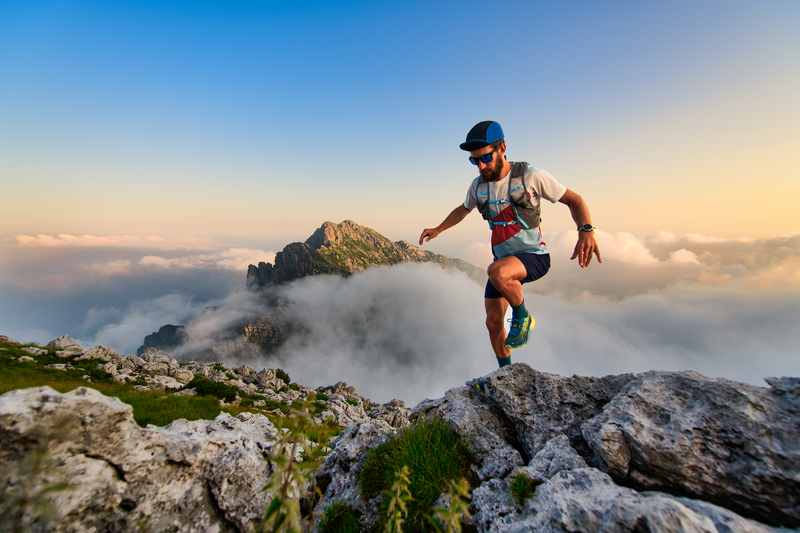
95% of researchers rate our articles as excellent or good
Learn more about the work of our research integrity team to safeguard the quality of each article we publish.
Find out more
REVIEW article
Front. Plant Sci. , 10 June 2024
Sec. Plant Genetics, Epigenetics and Chromosome Biology
Volume 15 - 2024 | https://doi.org/10.3389/fpls.2024.1398818
This article is part of the Research Topic Transcriptional and Epigenetic Landscapes of Abiotic Stress Response in Plants View all 10 articles
Abiotic and biotic stresses globally constrain plant growth and impede the optimization of crop productivity. The phytohormone auxin is involved in nearly every aspect of plant development. Auxin acts as a chemical messenger that influences gene expression through a short nuclear pathway, mediated by a family of specific DNA-binding transcription factors known as Auxin Response Factors (ARFs). ARFs thus act as effectors of auxin response and translate chemical signals into the regulation of auxin responsive genes. Since the initial discovery of the first ARF in Arabidopsis, advancements in genetics, biochemistry, genomics, and structural biology have facilitated the development of models elucidating ARF action and their contributions to generating specific auxin responses. Yet, significant gaps persist in our understanding of ARF transcription factors despite these endeavors. Unraveling the functional roles of ARFs in regulating stress response, alongside elucidating their genetic and molecular mechanisms, is still in its nascent phase. Here, we review recent research outcomes on ARFs, detailing their involvement in regulating leaf, flower, and root organogenesis and development, as well as stress responses and their corresponding regulatory mechanisms: including gene expression patterns, functional characterization, transcriptional, post-transcriptional and post- translational regulation across diverse stress conditions. Furthermore, we delineate unresolved questions and forthcoming challenges in ARF research.
Plants face numerous abiotic and biotic stresses due to their sessile nature, including water and nutrient deficiencies, high salinity, extreme temperatures, radiation, heavy metal toxicity, and biotic infections. An estimated 90% of global arable lands are exposed to one or more of the above abiotic stresses (Dos Reis et al., 2012), projected to cause up to 70% yield loss in major crops (Mantri et al., 2012). The biotic stress caused by viral, fungal, and bacterial infections cause reduction in level of photosynthesis in all major crops and is the major cause of pre- and post-harvest losses. Biotic stresses are responsible for approximately, 28.2%, 37.4%, 31.2%, 40.3%, 26.3%, and 28.8% yield losses in wheat, rice, maize, potatoes, soybeans, and cotton, respectively (Wang et al., 2013). Adaptation to such stresses is crucial for optimizing performance of plants and stability of their successive generations. Developing stress-tolerant plants remains the ultimate goal of plant breeders due to their superior yields and stability (Kambona et al., 2023). Genetic manipulation of plants remains the most prominent approach to alleviating poverty, due to its potential to increasing crop yield and mitigating nutrient deficiencies, enabling the cultivation of salt affected lands, overcoming energy crisis and production of cost-efficient biopharmaceuticals using plants as cellular factories (Ahmad and Mukhtar, 2017). Additionally, genetic modification offers the possibility of identifying candidate genes, miRNAs and transcription factors (TFs) that participate in regulating specific plant processes to improve tolerance to abiotic stresses and enhance productivity. For example, overexpression of McWRKY57 conferred tolerance to drought stress in Arabidopsis (Bai et al., 2023). miRNAq and nuclear factor YA8 enhanced salt tolerance by activating PEROSIDASE expression in response to reactive oxygen species (ROS) (Xing et al., 2021).
Plants have evolved intricate stress response mechanisms, including proper perception, signal transduction and respective physiological adjustments informed by the kind and duration of stress (Kranner et al., 2010). The perception of stress cues in plant is a complex network of input signals integrated in signal pathways that target regulators of plant growth and physiology (Scheres and van der Putten, 2017). Transcription regulation of stress-responsive genes is a pivotal biological process that confers stress tolerance in plants, and allows plants to strictly define and sustain their cellular identity and coordinates cellular activity during its life cycle (Casamassimi and Ciccodicola, 2019). Such regulations are mainly mediated by the temporal and spatial functioning of TFs that contain highly conserved DNA-binding domains (DBDs), with which they bind to specific DNA sequences in promoters of their target genes (Wang G. et al., 2015). On the other hand, TFs are either upregulated or downregulated by kinases or phosphatases and inturn binds to cis-regulatory elements in promoter of stress-inducible genes to enhance or suppress their transcription (Baillo et al., 2019). TFs also regulate stress induced responses in plants through mechanisms like posttranslational and epigenetic modifications such as variable nucleosome distribution, histone modification, DNA methylation, and synthesis of non-protein-coding RNAs (npcRNAs).
Recent studies have traced the evolutionary origins of ARFs back to early charophyte algae, where a single proto-ARF gene existed (Mutte et al., 2018). Following an initial duplication event, proto-ARFs diversified into two classes (A/B and C) during the late-divergence charophytes. In the transition to land plants, a subsequent division of class A/B into distinct classes A and B established the three evolutionary classes recognized today: A, B, and C. Further duplications within these classes expanded and diversified the ARF family in higher land plants (Mutte et al., 2018). Since the identification of the first ARF (ARF1) in Arabidopsis, 22 more ARFs have been identified and characterized from the Arabidopsis genome (Moller et al., 2017). Homology cloning and genetic approaches have since been employed ino identifying numerous homologous ARF genes in various plant species after the release of genomic data and development of bioinformatics analyses. The 23 ARFs in Arabidopsis canbe divided into three subclasses: A, B, and C (Finet et al., 2013). Most ARFs possess similar topology, with three conserved protein domains, whose properties must be understood in details. Majority of ARFs generally contain a conserved N-terminus DNA-binding domain (DBD), a variable middle region (MR) that functions as either an activator or repressor domain and a conserved C-terminal dimerization domain (CTD), which is involved in protein-protein interactions (Dinesh et al., 2015). The functions and properties of each of these domains are enumerated below.
Transcription factors are universal master regulators of gene expression that bind to unique DNA sequences in the promoter of their target genes to regulate their expression (Suter, 2020). A critical, yet unresolved in aspect of auxin biology is the mechanism by which the simple tryptophan-like indole-3-acetic acid triggers a wide range of cellular responses. During the last step of auxin signaling prior to gene regulation, the ARFs confer specificity to auxin response through selection of target genes. ARF TFs possess typical B3 DBD at their N-terminus, which allows them to bind to DNA motifs called Auxin Response Elements (AuxREs) (Boer et al., 2014; Weijers and Wagner, 2016). The first AuxRE was identified in pea (Ballas et al., 1993) and soybean (Ulmasov et al., 1995) in the promoters of auxin-responsive genes as TGTCTC (Liu et al., 1994). The identification of AuxRE is one of the most significant events that has enhanced the understanding of auxin-mediated regulation of gene expression and the creation of auxin-reporter systems (Hagen and Guilfoyle, 2002), and the identification of the first ARF protein (Ulmasov et al., 1997). The crystal structures of the DBD of ARF1 and ARF5/MONOPTEROS (MP) homodimers, as well as complex of ARF1 DBD with DNA has permitted visualization of protein-DNA interaction (Roosjen et al., 2018), and depicts how amino acids in the DBD interact with the DNA-binding motif TGTCTC (Freire-Rios et al., 2020). The higher affinity of ARFs to the TGTCGG element is because of deeper rotation of H136 into the major DNA groove, which forms additional hydrogen bonds with G5 and G6 in the TGTCGG structure (Boer et al., 2014; Freire-Rios et al., 2020). Mutations in these DNA-interacting amino acids interfered with the DNA binding properties of these ARFs and their biological functions. The TGTC serves as the invariable core element crucial for auxin response, while the final two nucleotides are variable (Boer et al., 2014). In recent years, adoption of advanced techniques has contributed to the identification of other AuxREs and the revelation that variation in the last two nucleotides of an AuxRE were permitted and could play a role in the affinity of ARFs for DNA binding. Although TGTCTC DNA-binding motif was the first to be identified, protein-binding microarrays (PBMs) has revealed that TGTCGG motif possesses relatively higher ARF binding affinity than the TGTCTC motif (Boer et al., 2014). It has been revealed through a ‘cistrome’ analysis that ARF2 and ARF5/MP have higher affinity for TGTCGG than the classical TGTCTC (O’Malley et al., 2016).
Through crystal structures, in vitro, and heterologous studies, a model in which ARF dimers bind with high affinity to distinct repeats of canonical AuxRE motifs has been unraveled. Like all TFs, ARFs bind to DNA as dimers and can homodimerize through their DBD by binding to tandem repeat motifs of TGTCNN elements. Configurations of the tandem repeat and the number of bases between the individual motifs determine their nomenclature: Inverted repeats (IR) where two AuxREs are oriented towards each other in different strands of DNA, direct repeat (DR) where two AuxREs follow each other in the same DNA strand and everted repeat (ER) where two AuxREs orient back to back in different strands of DNA (Freire-Rios et al., 2020). Yeast synthetic auxin signaling system suggest that some ARFs may activate transcription on a single AuxRE, but dimerization between the ARFs is necessary for transcription to occur (Lanctot et al., 2020). Enrichment for single AuxREs upstream of auxin-responsive genes has also been detectable (Freire-Rios et al., 2020), in affirmation to the yeast synthetic auxin signaling system. The biochemical mechanism underlying the differences in DNA-binding specificity of ARFs to single AuxRE binding sites is yet to be proven. Genome-wide DNA binding by ARFs has revealed both overlapping and distinct motif preferences for class A and B ARFs (Galli et al., 2018; Stigliani et al., 2019). DNA affinity purification and sequencing (DAP-seq) experiments performed on maize and Arabidopsis revealed that both class A and class B ARFs can bind IR7/8 motifs, while class A ARFs are additionally capable of binding to several DR and ER motifs (O’Malley et al., 2016; Galli et al., 2018; Stigliani et al., 2019). Although C-ARFs have been proven not to be involved in auxin-dependent transcriptional responses, at least in Marchantia (Mutte et al., 2018), one algal ARF related to the class C ARFs bind to the TGTCNN motifs (Carrillo-Carrasco et al., 2023).
Another element that determines the specificity of the DBD binding is the spacing between both sites of the AuxRE. The binding affinity of two ARFs differ significantly based on spacing between the AuxRE repeats, which dictates the formulation of a caliper model that determine specificity of ARFs binding sites (Boer et al., 2014). The dimerization ability of ARFs through their DBD or C-terminal PB1 domain permits strong binding to double-stranded DNA (dsDNA) carrying a pair of AuxREs with a spacer of a specific length (Boer et al., 2014; Pierre-Jerome et al., 2016). It has been reported that spacing of 7 or 8 bp in ARF1 and 5 to 9 bp in ARF5/MP is required between AuxRE repeats to enhance the interaction between these ARFs and their targeted AuxRE (Boer et al., 2014). Fluorophore or enzyme reporter genes under the control of synthetic promoters including DR5 promoter, characterized by tandem direct repeat of TGTCTC spaced at 5-bp intervals, has often been used for visualizing the distribution pattern of auxin signal in many plant species (Goldental-Cohen et al., 2017), suggesting that this repeat constellation is biologically meaningful.
The C-terminal of ARFs is a classical type -I/II PB1domain of 80-100 amino acids, which was previously named domain III/IV for ARFs and Aux/IAAs (Guilfoyle and Hagen, 2007). Besides the DBD, the PB1 domain is also an ARF interacting domain. Structural analysis on the C-terminal domain of ARFs revealed the structural basis of such heterotypic interaction of ARF5/MP (Nanao et al., 2014), ARF7 (Korasick et al., 2014), IAA17 (Han et al., 2014), and PsIAA4 (Dinesh et al., 2015). PB1 domains are also present in fungi, animals, amoeba, and in several protein families in plants. Characteristic of the type -I/II PB1 domains, the ARF PB1 domain permits for head to tail oligomerization, such that the positive face of one PB1 domain interacts with the negative face of another PB1 domain (Korasick et al., 2014). ARFs and Aux/IAAPB1s interact due to similarity in their 3D structure, such that one negative and one positive face will permit ARF-PB1 interact with AUX/IAA-PB1 in a head-to-tail manner through electrostatic interactions and hydrogen bonds (Vernoux et al., 2011; Piya et al., 2014). The positive face is characterized by an invariant lysine residue that interacts with an array of conserved aspartic and glutamic acids (Korasick et al., 2014), such that alteration in the lysine residue of the positive face hinders interactions with the negative face and preventing oligomerization (Powers et al., 2019).
The PB1 domain of ARFs contributes to their functioning in numerous ways. The PB1 domain mediates the interaction between ARFs and the AUX/IAA proteins, which is required for appropriate canonical auxin signal transduction, which will be discussed briefly. Mutation on the positive face of ARF19 that ablates oligomerization resulted in increased transcription of both auxin-responsive genes and novel targets in the absence of auxin (Powers et al., 2019), suggesting that the ARF19 PB1 mutant is acting as a constitutive auxin signaling factor probably due to its lack of interaction with its transcriptional corepressor Aux/IAAs. Further in vivo oligomerization assay revealed that ARF19 PB1 mutant did not display nuclear dimerization (Powers et al., 2019), which could be inferred that the ARF PB1 domain rather than the DBD primarily promotes ARF homodimerization. Besides the Aux/IAA-ARF interaction, the PB1 domain of ARFs is involved in transcriptional regulations. For example, ARF19 with a mutant PB1 domain that inhibits dimerization did not activate transcription of single AuxRE, but activated paired AuxRE without any hindrance. In the case of the DBD, DBD dimerization is required for both single and paired AuxRE. This data outlines the possibility that the PB1 domain confers on ARFs the ability to activate transcription of AuxREs and could stabilize ARF dimerization under less ideal AuxRE numbers.
Interestingly, the PB1 domain seems to have diverse effects on different class A ARFs, as its deletion in Marchantia polymorpha ARF1 generates a loss-of-function mutant (Kato et al., 2020), whereas in A. thaliana ARF5/MP, the mutant maintains its function and is hyperactive (Krogan et al., 2012). Although heterotypic interactions are stronger than ARF or Aux/IAA homotypic interactions, most PB1s of class A ARFs interact with Aux/IAAs. The disparity between the strength of heterotypic and homotypic interactions result from higher number of electrostatic bonds between ARF and Aux/IAA-PB1s (Parcy et al., 2016; Kim et al., 2020). However, a limited set of interactions between Aux/IAAs and Class B or C ARFs have been identified (Vernoux et al., 2011; Piya et al., 2014), which suggest that the repressor ARF proteins function independently of auxin regulation, and instead compete for DNA binding sites or heterodimerize with other ARF proteins to block transcription (Lavy et al., 2016).
Between the N-terminal DBD and C-terminal of ARFs is the middle region (MR), which is highly variable among ARF TFs. Functional characterization of the middle region thus far has been quite elusive owing to its variability. Nonetheless, the middle region provides the framework for classifying the ARF family proteins. The amino acid composition of the middle region is critical in determining an ARF’s function, with glutamine-rich ARFs acting as transcriptional activators (Wu et al., 2015), whiles those enriched in serines, prolines, and threonines functioning as transcription repressors (Tiwari et al., 2003; Guilfoyle and Hagen, 2007). The activator/repressor classification correlates with the division in subgroups A/B/C, such that those ARFs tested as activators belong to class A, while class B and C ARFs encompass those tested as repressors (Tiwari et al., 2003). The activation and repressive activity of ARFs was decoupled from auxin induction by expressing the MR alone in a synthetic transcription factor assay in carrot protoplasts (Tiwari et al., 2003).
In contrast to the ARF repressor domains, the ARF activation domain remains unknown. This occurrence is probably due in part to the intrinsic disorder in the middle region of class A ARFs. Most activation domains are not characterized by semblance in their sequence, but by sequence characteristics such as hydrophobicity and negative charge (Erijman et al., 2020). It is however worth mentioning that the intrinsic disorder predominantly found in the MR of class A ARFs does not only dictate transcription potential but extends to other cellular features. For example, the MR of ARF7 and ARF19 dictates their subcellular localization (Powers et al., 2019), which is significantly influenced by the C-terminal PB1 domain. ARF19 is differentially localized to the nucleus of young roots and cytoplasm of matured roots. This tissues specific localization of ARF19 is altered by mutation in the PB1 domain, such that more ARF19 is driven to the nucleus of matured roots compared to wild-type. This cooperative relationship between ARF MR and the PB1 is believed to drive the nucleocytoplasmic partitition of ARFs through protein condensation. The PB1 domain probably increases the local concentration of ARF19 and that the intrinsic disorder of the MR contributes to phase separation and protein condensation (Powers and Strader, 2020). Just like other transcription factors, the relationship between ARF localization and transcriptional activity provides further insight into the regulatory mechanism governing the auxin signaling cascade. It is instructive to unravel the mechanisms that drive ARF condensation and the level of participation of other ARFs in this regulatory process, which will significantly broaden our understanding of auxin signaling specificity. The MR also acts as an interaction domain for the recruitment of different types of cofactors such as chromatin remodelers that aid ARFs to carry out their functions. It however remains unknown whether class B ARFs can function as transcriptional activators at certain loci or in the presence of other unknown cofactors.
Transcription activators belonging to the class A ARFs may also induce transcription indirectly by recruiting the SWITCH/SUCROSE NONFERMENTING (SWI/SNF) chromatin-remodeling complex (Clapier and Cairns, 2009). For example, the MR of ARF5/MP increases chromatin accessibility at its binding sites by recruiting the SWI/SWF complex through interactions with BRAHMA and SPLAYED, respectively (Wu et al., 2015). This result reveals a mechanism in which ARF5/MP, and most likely other activator ARFs, alter nucleosome positioning to make more transcription factor-binding sites accessible (Wu et al., 2015; Weijers and Wagner, 2016). In contrast, Arabidopsis class B ARF harbor a conserved TPL-binding motif (RLFGV), and may additionally encode a canonical ethylene-responsive element binding factor (EAR motif), which act as repressor domains in vivo (Choi et al., 2018). For example, both the conserved RLFGV motif and the additional EAR motif are needed for ARF2 to function as a transcriptional corepressor, but only the RLFGV motif is required for TPL interactions in yeast two-hybrid experiments (Choi et al., 2018). These evidences suggest that class B ARFs act as auxin-insensitive negative regulators of auxin-responsive genes (Kato et al., 2020). Additionally, the MR of AtARF2 also harbors the EAR motif (Causier et al., 2012) which bears semblance to that found on Aux/IAAs and which permits interaction with the N-terminal part of TPL/TPRs (Ke et al., 2015). Class C ARFs possess a BRD-like domain with a slightly different sequence (VLFG).
Auxin regulates multiple outputs in plants primarily by controlling the activity of thousands of genes through the nuclear auxin pathway. The canonical auxin transcriptional response system was originally characterized in flowering plants. The nuclear auxin signaling pathway consists of a small number of core components which are represented by a large gene family. Changes in cellular auxin concentrations trigger transcriptional responses of numerous genes, mediated by ARF transcription factors (Weijers and Wagner, 2016). Significant advancement in understanding the auxin signaling machinery has been achieved in recent years (Weijers and Friml, 2009). The core components of the auxin signaling pathway comprises the F-box-containing Transport Inhibitor Response 1 (TIR1) and its homologous Auxin-signaling F Box Proteins (AFBs) proteins, the transcriptional co-repressors AUXIN/INDOLE-3-ACETIC ACID (Aux/IAA), and the ARF transcription factors (Wright and Nemhauser, 2015; Kong et al., 2016). Activation of gene expression as a result of IAA-mediated assembly of TIR1/AFB proteins with AUX/IAA transcriptional regulators has been accepted as the canonical auxin signalling pathway (Figure 1). During auxin limitation, Aux/IAA protein binds to the C-terminal domain of ARFs and its co-repressor TOPLESS (TPL) to repress transcription. TPL recruits chromatin remodeling enzymes such as Histone Deacetylase 19 (HDA19) (Figure 1A) and also interacts with Mediator multiprotein complex (Figure 1B) to prevent ARF transcriptional output. For one case, the HDA19 acts as a physical impediment to maintain chromatin closure at the promoters of ARF-regulated auxin responsive genes (Szemenyei et al., 2008; Qiao et al., 2018) (Figure 1A). For another, ARFs interacts with the Mediator complex via its MR region and Aux/IAA via their PB1domains. The recruited TPL by the domain I of Aux/IAA inturn interacts with the CDK8 of the Mediator complex. Under high auxin concentration, TIR1/AFB forms SCFTIR1/AFBs ubiquitin complex and triggers Aux/IAAs polyubiquitylation and degradation via the 26S proteasome, resulting in the dissociation of ARFs to TPL-HDA19 and Mediator complex. The eviction of TPL facilitates a permissive chromatin conformation and an increase in the accessibility of transcription factors on the promoters of auxin responsive genes (Wang and Estelle, 2014; Jing et al., 2015) (Figure 1A), and permits the ARFs-Mediator complex to recruit RNA polymerase II and leading to the initiation of gene expression (Figure 1B). Comparison of TIR1, AUX/IAA and ARF orthologues across land plants and charophycean algae indicate that the assembly of the canonical auxin transcriptional response pathway is a land plant innovation.
Figure 1 The canonical nuclear auxin signaling pathway. (A) Auxin signaling involving chromatin remodeling. (B) Auxin signaling involving the Mediator complex. In the absence of auxin, ARFs are bound by Aux/IAA repressor proteins, which recruit the TOPLESS (TPL) corepressor to constitute a repressor complex that repress transcription of auxin-responsive genes. ARFs, through their DBD domain, bind to the AuxRE of auxin-responsive genes and repress their transcriptional activity through interaction between the ARF Phox and Bem1 (PB1) domain and the Aux/IAA PB1 domain. TPL recruits histone deacetylases (A) and also interacts with Mediator (B) to prevent ARF transcriptional output. Auxin increases the affinity between the SKP1-CULLIN1-F-BOX (SCF) TIR1/AFB auxin receptor complex and Aux/IAAs, which stimulates Aux/IAA polyubiquitylation and degradation via the proteasome. Once free from TPL and Aux/IAA repression, ARFs then activates the expression of auxin-responsive genes.
The auxin-related developmental defects of ett mutants suggested that ETT/ARF3 could regulate auxin signaling independently of the canonical pathway. A fundamental difference between the ETT/ARF3-mediated and the canonical models of auxin signaling is that the former does not primarily require protein degradation to activate gene expression. It was suggested that ETT/ARF3 translates local auxin concentrations to developmental outputs in the gynoecium, although the molecular mechanisms governing this occurrence had not yet been discovered (Simonini et al., 2016). ETT/ARF3 has been reported to participate in auxin dependent protein-protein interactions with several transcription factors belonging to different families, and that these interactions are relevant for auxin responsiveness of specific tissues or cell types during development (Simonini et al., 2016). In the absence of auxin, ETT/ARF3 recruits TPL to its target loci via its ES domain. TPL, in turn, recruits HDA19 to promote deacetylation of histones and repress target gene expression (Figure 2A). In the presence of auxin, ETT/ARF3 can directly interact with the auxin molecule via the ES domain, suggesting that binding of auxin disrupts the interaction between ETT/ARF3 and its corepressor TPL (Kuhn et al., 2020) (Figure 2A), which permits the regulation of auxin-responsive genes.
Figure 2 Mechanism of the non-canonical auxin-dependent signaling pathway. (A) The ETT-mediated non-canonical auxin signaling pathway. In the absence of auxin, the ETT-specific (ES) domain recruits the co-repressor TPL, which in turn, recruits HDA19 to deacetylate histones and repress target gene expression. Under elevated auxin levels, auxin binds to the ES domain of ETT and triggers the dissociation of the repressive complex, which releasing the repression of HDA19 and triggers histone acetylation and initiates gene expression. (B) The regulation of noncanonical Aux/IAAs. Certain ARFs heterodimerize with noncanonical Aux/IAAs, under no or low auxin concentrations leading to their polyubiquitylation and degradation. Auxin availability triggers phosphorylation of Aux/IAAs 4, leading to their stabilization and accumulation. These nondegradable Aux/IAAs will maintain the repression of their interactor ARFs, inhibiting the transcriptional regulation of auxin-responsive genes.
Another non-canonical auxin-dependent signaling mechanism involves the Trans-Membrane Protein Kinases (TMPKs) pathway. The TMPK subfamily was first linked to auxin signal transduction when the phenotypes of double, triple, and quadruple tmpk mutants showed cell expansion and proliferation defects, miniaturized organs, infertility, and a reduced sensitivity to exogenously applied auxin (Dai et al., 2013). Kinase cascades are rapid and could be involved in rapid, non-canonical signalling. At high auxin concentration, TMPK phosphorylates AUX/IAA at its domain II, which interferes with the poly-ubiquitination and degradation of the AUX/IAA-TPL repressor complex by the SCFTIR1/AFBs ubiquitin complex, thus inhibiting the transcriptional regulation of auxin-responsive genes (Figure 2B).
Plant growth and development are physiological processes coordinated by phytohormones. Physiological mechanisms regulating growth and development in plants are coordinated by hormonal signals, among which auxin has been implicated in virtually every aspect. Growth and development are intrinsic processes sustained by coordinated cell division, cell expansion, and cell differentiation. Auxin promotes cell division and meristem maintenance, and also plays an important role in the establishment of cellular patterning (Perrot-Rechenmann, 2010). Transcription factors are key regulators of cellular processes, both intrinsic, such as development and differentiation (Spitz and Furlong, 2012), as well as extrinsic, such as response to external signals (Lambert et al., 2018), through hormonal signaling pathways. At the molecular level, ARF TFs transduce auxin response signals by binding to the AuxRE in promoters of early auxin response genes (Wan et al., 2014). The ARFs are key components of the auxin signaling pathway known to regulate cellular processes of growth and development under normal cellular conditions (Guilfoyle and Hagen, 2007; Chandler, 2016). Several ARF genes have been reported to regulate various auxin-induced developmental processes in several plant species. ARFs are predominantly expressed during through all the periods of plant growth and development, and in different plant organs (Table 1), indicating its intricate role in plants.
Plant root system plays crucial role in regulating and optimizing plant growth and development. They are important plant organs that absorb water and nutrients from soils and translocate them to the shoot (Stone et al., 2001; Sainju et al., 2005), as well as providing a means to monitor the soil for a range of environmental conditions (Overvoorde et al., 2010). Moreover, roots provide mechanical support to plants and distribute hormones that regulate numerous physiological and biochemical processes associated with growth and development of plants. Seed plants have evolved a complex root system consisting of at least three root types, i.e., the primary root, lateral roots, and adventitious roots. Since the discovery of auxins, they have been characterized to be closely related to root development. Root phenotypes associated with auxin signaling are dosage dependent, and include the length of epidermal-derived root hairs, primary root length, number and length of lateral roots and response to gravity (Ishida et al., 2008; Peret et al., 2009). ARFs have been reported to regulate various aspects of root morphogenesis and architecture in several plant species (Table 1).
Primary roots develop from an embryonically formed meristem (De Smet et al., 2010) and is the first organ to emerge from a germinating seed in the form of a radicle. Among the five genes encoding Arabidopsis clade A ARFs, ARF5/MP is essentially involved in primary root organogenesis (Aida et al., 2002). During embryogenesis, the hypophysis acts as the primary root founder cell in Arabidopsis (Petricka et al., 2012) and requires the auxin-dependent release of MP transcription factor from its inhibition by the Aux/IAA protein BODENLOS (BDL)/IAA12 (Herud et al., 2016). MP binds directly to the AuxRE in promoter of miR390 to regulates its expression in the A. thaliana primary root meristem (Dastidar et al., 2019), and also controls embryonic root initiation by regulating genes that mediate signaling from embryo to hypophysis. ARF5/MP, TARGET OF MP 5 (TMO5) and TMO7 encode basic helix–loop–helix (bHLH) TFs, that are expressed in the hypophysis-adjacent embryo cells, and are required and partially sufficient for MP-dependent root initiation (Schlereth et al., 2010). Both Wuschel-related Homeobox 9 (WOX9) and ARF5/MP are required for hypophysis specification and primary root formation, with mutations in either WOX9 or ARF5/MP resulting in defective stem cell niche establishment of the primary root (Breuninger et al., 2008). The WOX9-ARF5/MP complex initiates primary root formation by activating RGF1 INSENSITIVEs (RGIs) in the primary root founder cell (Zhang et al., 2023). Root cap formation in Arabidopsis is regulated by miRNA160, which targets ARF10 and ARF16. The Pro(35S):MIR160 and arf10-2 arf16-2 double mutants displayed the same root tip defect, with uncontrolled cell division and blocked cell differentiation in the root distal region and showed a tumor-like root apex and loss of gravity-sensing (Wang et al., 2005). Moreover, ARF2 acted as an ABA positive responsive regulator that functions in both seed germination and primary root growth by directly regulating the expression of a homeodomain gene HB33, with ABA treatment reducing cell division and altering auxin distribution more in arf2 mutant than in WT (Wang et al., 2011).
Lateral roots (LR) are post-embryonic roots that arise from existing roots (Atkinson et al., 2014). LRs increase the volume of soil reached by roots, provide anchorage, and participate in water and nutrient uptake and transport (Dubrovsky and Laskowski, 2017). Auxin is a crucial hormone for lateral root formation, while ARFs act as key components of auxin biosynthesis, transport, signaling, and play important roles in lateral root initiation and lateral root primordium development (Jing and Strader, 2019). The de novo formation of lateral root organs requires tightly coordinated asymmetric cell division of a limited number of pericycle cells located at the xylem pole. This typically involves the formation of founder cells, followed by a number of cellular changes until the cells divide and give rise to two unequally sized daughter cells. During LR initiation, a pair of xylem pole pericycle cells are primed by auxin signaling and specified as founder cells that undergo asymmetric cell division to develop as a stage I LR primordium. This process is activated by an AUX/IAA–ARF-dependent auxin signaling cascade (Luo L. et al., 2022). The module regulating founder cell formation involves the perception of auxin signaling by the auxin receptor TIR1, which acts in the basal meristem (Figure 3). Several Aux/IAA-ARF modules have been implicated in driving lateral root formation (Stoeckle et al., 2018). The IAA28-ARF5/6/7/19 module is specific for priming cell specification (De Smet et al., 2007; De Rybel et al., 2010), and positioning new lateral root primodia (LRP) and for specifying lateral root founder cell (LRFC) identity (Du and Scheres, 2018). Auxin-regulated GATA23 TF, considered as the first molecular marker for LRFCs, is regulated in XPP cells that leave the basal meristem by the IAA28-ARF5/6/7/19 auxin signaling cascade in the basal meristem (De Rybel et al., 2010), to regulate the process of lateral root founder cell identity (Figure 3). Prohibitin 3-Nitric oxide (PHB3–NO) signaling module regulates LR initiation through modulation of the canonical AUX/IAA-mediated auxin signaling cascade. PHB3 accumulates NO in pericycle cells and LRPs, and NO in turn triggers the degradation of AUX/IAA28 and IAA14 and the activation of ARFs, thereby inducing the expression of transcription factor genes GATA23 and Lateral organ boundaries domain 16 (LBD16) to promote LR initiation and LRP development (Luo L. et al., 2022). The SLR/IAA14–ARF7–ARF19 module regulates LR initiation by activating several auxin-responsive genes (Okushima et al., 2007). ARF7 and ARF19 directly regulate the auxin-mediated transcription of LBD16/ASL18 and/or LBD29/ASL16 in roots (Okushima et al., 2007), and contributes to asymmetric breakage of root cell wall (Figure 3). Auxin-dependent cell wall remodeling also has an important patterning function during LRP formation. ARF7/19 regulates the expression of Mustache (MUS) and Mustache-like (MUL) genes during LRP initiation. MUS and MUL encoding inactive LRR-RLKs, are expressed in early-stage LRPs via regulating cell wall biosynthesis and remodeling genes such as Xyloglucan Endotransglycosylase6 (XTR6), Expansin1 (EXP1), EXP17, and Polygalacturonase Abscission Zone A. Thaliana (PGAZAT) (Xun et al., 2020) (Figure 3). ARF7/19 also regulates HAESA-LIKE 2 (HSL2) which is known to affect the expression of cell wall modifying and defense related genes (Niederhuth et al., 2013) (Figure 3). ARF7/19 module regulates the expression of LBD16/18/29, which inturn regulate the expression of downstream genes PUCHI (Goh et al., 2019), ERF2A (Berckmans et al., 2011), and CDKA1 (Feng et al., 2012), which have been implicated in lateral root initiation (Figure 3). ARF7/19 also regulates Lateral Root Primordium1 (LRP1) (Figure 3), whose expression has been shown to be induced during lateral root initiation in Arabidopsis (Singh et al., 2020). Two callose-degrading enzymes plasmodesmal-localized β-1,3 glucanase1 (PdBG1) and PdBG2, are both transcriptionally regulated by auxin in an IAA14-ARF7/19-dependent manner, which control callose deposition in LRPs during lateral root morphogenesis (Figure 3). ARF7/19 and ARF5/MP regulate Plethora 5 (PLT5), which interacts with Wuschel-related Homeobox 5 (WOX5) to regulate lateral root morphogenesis.
Figure 3 Lateral root regulation by ARFs. The IAA28-ARF5/6/7/8/19 module regulates positioning of new LRP and specification of LRFC identity by controlling the expression of GATA23 TF. PHB3 accumulates NO in pericycle cells and LRPs, which in turn triggers the degradation of AUX/IAA28/14 and the activation of ARFs and induction of GATA23 to promote LR initiation and LRP development. ARF7 and ARF19 directly regulate the auxin-mediated transcription of LBD16/ASL18 and/or LBD29/ASL16 in roots and contribute to asymmetric breakage of root cell wall. Moreover, ARF7/19 regulates the expression of HSL2, MUS and MUL genes to modulate LRP initiation via regulating cell wall biosynthesis and remodeling genes such as XTR6, EXP1/17, and PGAZAT. ARF7/19 module regulates the expression of LRP1, LBD16/18/29, which inturn regulate the expression of downstream genes PUCHI, ERF2A, and CDKA1, which have been implicated in lateral root initiation. The IAA14-ARF7/19 module regulates callose deposition in LRPs during lateral root morphogenesis. ARF7/19 and ARF5/MP regulate PLETHORA 5 (PLT5), which interacts with Wuschel-related Homeobox 5 (WOX5) to regulate lateral root morphogenesis.
Adventitious roots are those secondary roots that arrive from non-root tissues (Atkinson et al., 2014) whose initiation is controlled by precise balance of activator and repressor ARF transcripts, which is maintained by a complex regulatory network (Gutierrez et al., 2009). ARF6/8 are among the five genes encoding Arabidopsis clade A ARFs, and are required for adventitious root formation from hypocotyls (Gutierrez et al., 2009). ARF6 and ARF8 regulate adventitious root formation with the involvement of miRNA160 and miRNA167, such that, ARF6 positively controls the development of adventitious roots (Kou et al., 2022). The WOX11-ARF6/8 complex activates RGIs and LBD16 to initiate the adventitious root primordium (Zhang et al., 2023). The auxin signaling module, ARF7/ARF19-LBD16/LBD18 via AUXIN1(AUX1)/LIKE-AUXIN3 (LAX3) auxin influx carriers, is involved in adventitious root formation in Arabidopsis: single mutants aux1, lax3, arf7, arf19, lbd16 and lbd18 recorded reduced numbers of adventitious roots than in the WT (Lee et al., 2019). At the same time, double and triple mutants exhibited further decrease in adventitious root numbers compared with the corresponding single or double mutants, respectively, and the aux1 lax3 lbd16 lbd18 quadruple mutant lacked adventitious roots.
OsARF are large multigene family that plays essential roles in different tissues of the rice plant. OsARFs play crucial roles in modulating root developmental processes and optimal architecture of root system (RSA) essential for normal growth and development (Table 1). For example, OsARF1 regulates auxin-dependent differential growth in the crown roots of rice coleoptiles, and that, OsARF1 transcript abundance was stimulated by gravitropism in the lower fast-growing flank (Waller et al., 2002). Knockout of OSARF12 resulted in decreased primary root length, with osarf12 and osarf12/25 mutants displaying shorter root elongation zone compared to WT: This was occasioned by decreased expression of auxin synthesis genes OsYUCCAs and auxin efflux carriers OsPINs and OsPGPs (Qi et al., 2012). OsNAC2 functions as an upstream integrator of auxin and cytokinin signals by binding directly to the promoters of OsARF25 and a cytokinin oxidase gene (OsCKX4) to regulate primary root length and the number of crown roots in rice (Mao et al., 2020). OsARF23/24 heterodimers binds to the promoter of an actin-binding protein (RMD) and promote its expression in the auxin signaling pathway to trigger changes in F-actin organization that controls root elongation in rice (Li et al., 2014). AUX/IAA-ARF-dependent auxin signaling controls aerenchyma and lateral root development: LR number and constitutive aerenchyma formation were reduced by the dominant-negative effect of a mutated AUX/IAA protein in the iaa13 mutant. It was further revealed that ARF19 interacted with IAA13, and that LBD1-8 acted as a downstream target of ARF19; IAA13, ARF19, and LBD1-8 were highly expressed in the cortex and LR primordia, suggesting that these genes function in the initiation of constitutive aerenchyma and LR formation (Yamauchi et al., 2019). Rice stems develop adventitious root primordia at each node but mature slowly and eventually emerge only when the plant gets flooded (Lin and Sauter, 2018) to provide water, nutrients, and anchorage. In rice, OsARF16 regulates the initiation of adventitious crown root primordia by activating the expression of Crow Rootless1/Adventitious Rootless1 (CRL1/ARL1), which encodes an LBD protein (Liu et al., 2005; Wang et al., 2007).
Auxin synthesis, transport and signal transduction have been proven to be involved in regulating maize root growth and development (Nestler et al., 2016). The unique roles of ARF genes in maize growth and development are emerging from molecular genetic studies (Table 1). Auxin signal transduction is mainly controlled by ARF and Aux/IAA genes. Multiple AUX/IAA-ARF-mediated signaling plays an important role in regulating plant root formation (Goh et al., 2012). ZmIAA5 interacts with ZmARF5 to regulate maize root growth and development. Primary root length and the number of lateral roots at the seedling stage, and total number of roots and the dry root weight at the matured stage of maize overexpressing ZmIAA5 increased compared to the WT, while those of mutant zmiaa5 was significantly reduced (Yang et al., 2022). Auxin has also been implicated as the starting signal that induces crown root formation in maize. Auxin induces the degradation of AUX/IAA proteins so that ZmARF34 activate the expression of downstream target Rootless Concerning Crown and Seminal Roots (RTCS), an LOB domain protein regulating shoot-borne root initiation in maize. The induced RTCS proteins bind to the promoter of ZmARF34 and activate its transcription, which inturn promotes RTCs expression, representing an amplified mutual feedback loop that regulates ZmARF34 and RTCS transcription during coleoptilar node development and crown root formation in maize (Majer et al., 2012; Xu et al., 2015). The Rootless with Undetectable Meristems 1 (RUM1) gene encodes ZmIAA10 which is required for the initiation of embryonic seminal and post-embryonic lateral root initiation in primary roots of maize (Wang et al., 2010). RUM1 could interact with, and form complexes with transcriptional activators ZmARF25 and ZmARF34 to regulate initiation of embryonic seminal and post-embryonic lateral root initiation in primary roots of maize (von Behrens et al., 2011). ZmARF23 bound to the promoter of a known causal gene for embryonic callus induction, ZmSAUR15, and positively regulated its expression at the transcription level to promote embryonic callus formation and primary root development (Liang et al., 2023).
Photosynthesis is crucial for the existence of the vast majority of life on earth. Plants are primary producers that form the base of every ecosystem and fuel the next tropic level by utilizing photosynthesis to transform water, sunlight and carbon dioxide into oxygen and simple energy for utilization. The photosynthetic process is the principal energy source for all organisms on earth. Leaf anatomy, such as mesophyll thickness and chloroplast abundance and distribution, influences the photosynthetic capacity of plants (Oguchi et al., 2003). Moreover, the shape, size, and chlorophyll content of plant leave influence its photosynthetic capability and efficiency (Guan et al., 2017). Auxin has been proven to play central roles in leaf developmental processes such as leaf initiation, blade formation, compound leaf patterning and leaf inclination (Xiong and Jiao, 2019), with active participation of ARFs in numerous plant species (Schuetz et al., 2019), as outlined in Table 1.
The flattening of leaves to form broad blades with wider surface area is a pronounced adaptation by plants to maximize photosynthetic ability and efficiency. Adaxial-expressed ARF5/MP directly binds to the promoters of WOX1 and Pressed Flower (PRS) and activate their expression in the leaf marginal domain to enable leaf flattening, while redundant abaxial-enriched ARF2/ARF3/ARF4 repressors suppress WOX1 and PRS expression to maintain the abaxial identity (Guan et al., 2017). While arf3, arf5 and arf7 single mutants formed normal leaves in Arabidopsis, mp/arf3 or mp/arf7 displayed a breakdown in leaf formation with novel leaf structure not present in any of the single mutants, suggesting that ARF3 and ARF7 regulates rosette leaf formation and that their functions overlap and act parallel with those of ARF5/MP (Schuetz et al., 2019). ARF6 and ARF8 activate the expression of DWARF4 (DWF4), a pivotal enzyme in brassinosteroids (BR) synthesis. BRs, in turn, facilitate the demethylation of cell wall pectin, resulting in isotropic in-plane cell wall loosening, which ultimately gives rise to leaves with diverse shapes and overseeing the proximal-distal growth of leaf reproductive organs (Xiong et al., 2021). ARF2 and ARF7, with the help of IAA14, suppressed the expression of chlorophyll biosynthesis gene Protochlorophyllide Oxidoreductase A (PORA) and Genomes Uncoupled 5 (GUN5) in matured leaves, resulting in reduced chloroplast number and structure in mesophyll cells and eventual reduction in photosynthetic efficiency (Luo et al., 2023).
Leaf inclination/angle is a component of crop architecture and fundamental property of plant canopy structure, which is required for light interception, canopy photosynthesis, and energy balance. Leaf inclination of rice results mainly from the asymmetric cell division and elongation of adaxial and abaxial cells at the lamina joint (Zhou et al., 2017), which is regulated by the biosynthesis or signaling of auxin. In rice, OsARF4 participates in leaf inclination regulation via auxin and brassinosteroid (BR) signaling pathways: osarf4 mutants displayed increase in cell differentiation on the adaxial side, resulting in increased leaf inclination; however, OsARF4-overexpressing lines manifested a decrease in leaf inclination, resulting in erect leaves (Qiao et al., 2022). In another experiment, OsIAA6 interacts with OsARF1 to suppress auxin signaling and regulates leaf inclination, with rice brassinazole resistant (OsBZR1), the key transcription factor in BR signaling, binding directly to the promoter of OsIAA6 to stimulate its transcription (Xing et al., 2022), suggesting that OsIAA6–OsARF1 module regulates rice leaf inclination through synergistic action of auxin and BR. The mutant ds1 showed reduced BR sensitivity and leaf angle through a mechanism involving DS1’s interaction with OsARF11 to regulate OsBRI1 expression (Liu X. et al., 2018). Loss-of-function mutant of OsARF11, osarf11-1, displayed phenotypes with reduced plant height and leaf angle of flag leaves compared to WT in rice (Sakamoto et al., 2013). OsARF19 controls rice leaf angles by positively regulating OsGH3-5 and OsBRI1. OsARF19-overexpression rice lines showed an enlarged lamina inclination compared to WT due to its increased adaxial cell division in an auxin and brassinosteroid-dependent manner, resulting from direct activation of the early auxin responsive gene OsGH3-1 and Brassinosteroid Insensitive 1 (OsBRI1) (Zhang et al., 2015). Auxin induces OsARF6 and OsARF17 to independently and synergistically bind directly to the Increased Leaf Angle1 (ILA1) promoter and activate its expression to control secondary cell wall composition of the lamina joint to determine flag leaf angle (Zhang et al., 2015).
Mutation in maize leafbladeless1 (lbl1), that disrupt ta-siRNA biogenesis, give rise to plants with thread-like leaves that have lost top/bottom polarity. Misregulation of tasiR-ARFs target, ETT/ARF3, has emerged as the basis for the lbl1 leaf polarity defects, with plants expressing arf3a transcripts displaying insensitivity to tasiR-ARF-directed cleavage and recapitulating the phenotypes observed in lbl1 (Dotto et al., 2014). Auxin plays important roles in regulating both age-dependent and dark-induced senescence through the actions of several auxin-related genes, such as YUCCA6, Small Auxin Upregulated RNA36 (SAUR36), and Indole-3-acetic Acid Inducible 29 (IAA29) (Kim et al., 2011; Hou et al., 2013; Jiang et al., 2014). ZmbHLH112 can repress the expression of Aux/IAA related genes, and promote the binding of ARF to AUXRE in the promoter of their target genes to regulate the elongation of leaf angle cells (Zhang et al., 2022).
Flowers constitute the reproductive structures in plants and lead to formation of fruit and seed after fertilization. Unlike leaves and roots that appear as single organs, flowers have evolved into a stable plant reproductive composite structure, composed of multiple organs arranged in an orderly pattern (Endress, 2010). ARFs have been reported to modulate auxin-dependent regulation of floral organ organization mostly in Arabidopsis (Table 1). The ett/arf3mutant displayed phenotypes with abnormal floral meristem patterning and gynoecium development in Arabidopsis (Sessions et al., 1997), whiles arf1 and arf2 loss-of-function mutants illustrated abnormal abscission of floral organs (Ellis et al., 2005). Mutation analyses revealed that ARF1 and ARF2 regulated plant leaf senescence and floral organ exfoliation, and the ETT/ARF3 gene influenced defect in pistil and flower meristem formation in Arabidopsis thaliana (Nishimura et al., 2005; Quint and Gray, 2006). ARF3 has been functionally characterized to participate in regulatory pathway that modulate gynoecium morphogenesis, self-incompatibility, de novo organ-regeneration, and organ polarity (Tantikanjana and Nasrallah, 2012). ARF6 and ARF8 regulated JA biosynthesis and floral organ development via suppression of class I KNOX genes KNAT2 and KNAT6, with arf6arf8 plants displaying defective phenotypes such as aberrant vascular patterning and lack of epidermal cell differentiation in petals, which were partially suppressed by mutations in KNAT2 or KNAT6 (Tabata et al., 2010).
Floral organ development significantly influences plant reproduction and seed quality, yet its underlying regulatory mechanisms are still largely unknown, especially in crop plants. Disruption of OsARF19 regulates floral organ development and plant architecture in rice. ARF6, ARF12, ARF17, and ARF25, manifested overlapping functions in flower opening and stigma size: Single mutant, arf12, showed a reduced plant height and aborted apical spikelets, while mutation in ARF12 together with mutation in either ARF6, ARF17, or ARF25 led to the same defective phenotypes including the failed elongation of stamen filaments, increased stigma size, and morphological alteration of lodicule (Zhao et al., 2022). AUX/IAA-ZmARF complexes have been reported to predominantly affect maize reproductive growth (Ori, 2019). ZmIAA29 can influence maize florescence by interacting with ZmARF2, ZmARF7, and ZmARF25 (Ma et al., 2023). AUX/IAA proteins Barren Inflorescence 1 and Barren Inflorescence4 and ARFs forms multiple BIF1/BIF4-ARFs transcriptional repression modules involved in the regulation of the boundary basic helix-loop-helix transcription factor Barren Stalk1 (BA1), during the initial stages of reproductive organogenesis in maize and influence its inflorescence architecture (Galli et al., 2015).
Most of the gains made towards functional characterization of ARF family proteins have focused largely on their role in plant growth and development. On the contrary, the role of auxin in regulating stress responses in plants has not received much attention. However, recent molecular approaches such as expression profiling have hinted that auxin might exert some regulatory role on plant responses to environmental stress conditions (Ha et al., 2013). It is suggested that auxin might either be acting alone or together with other key phytohormones in regulating plant response to abiotic stresses such as drought, cold, temperature extremities and salinity (Zahir et al., 2010; Lee et al., 2012). These abiotic stresses affect plant viability and development, which may result in changes in plant growth and crop yield, as well as, disturbance of physiological processes such as photosynthetic or mineral uptake rates (Kou et al., 2022). Genomic studies and expression analysis revealed that, numerous ARF family proteins were differentially expressed in various species in response to key abiotic stress such as drought, salinity or cold (Jain and Khurana, 2009), suggesting that these ARFs are active participants in abiotic stress response in plant species (Table 1).
Nutrient deficiencies are major abiotic stresses that impact the growth, development and productivity of plants. Macronutrients are the building blocks of crucial cellular components like proteins and nucleic acids. Macronutrient deficiencies have far reaching consequence for optimum crop growth and yield optimization. Some ARFs have been implicated to participate in regulating macronutrient deficiency responses in plants. The framework of molecular components composing a cascade of auxin synthesis, transport, and signaling that triggers root hair (RH) elongation in response to low N has been proposed (Jia et al., 2023). Low N upregulates Tryptophan Aminotransferase of Arabidopsis 1 (TAA1) and YUCCA8 activities, which increase auxin accumulation in the root apex. Auxin is then translocated from the root apex to the RH differentiation zone by the auxin transport machinery comprising Auxin Transporter Protein 1 (AUX1) and Pin-formed 2 (PIN2). At the RH differentiation zone, auxin activates the transcription of ARF6/8 to stimulate epidermal and auxin-inducible transcriptional module Root Hair Defective 6 (RHD6)-Lotus Japonica Root Hairless-like 3 (LRL3) to steer RH elongation in response to low N (Jia et al., 2023) (Figure 4).
Figure 4 ARF is involved in abiotic stress response in Arabidopsis. (A) Low N upregulates TAA1 and YUCCA8 activity to regulate downstream genes ending with LRL3 to confer low N stress response. (B) IAA14-ARF7/19 modulates LBD16/29 and PHR1 to regulate cell wall loosening EXPs to promote lateral root development. IAA14-ARF7/19-PHR1 or IAA14-ARF7/19-MYB modulates expression of PSI genes to confer tolerance to low Pi stress. (C) CLSY1 mediates the transcriptional repression of IAA27, an upstream regulator of ARF2, which inturn modulate the expression of the K+ transporter gene HAK5 and confer tolerance to low K stress. (D) Drought signal perception activates DREB2A/B, which directly promote transcription of IAA genes in response to drought, through a mechanism mediated by ARF2, ANT and COR15A in an ABA-dependent manner.
IAA14-ARF7/19 module has been reported to modulate LR development and confer low P stress tolerance. ARF7 and ARF19, which are transcriptional activators of early auxin response genes, acts downstream of IAA14 and regulates LR formation in Arabidopsis by directly regulating the auxin-mediated transcription of LBD16/29 in roots (Okushima et al., 2007) as shown in Figure 4. Auxin-responsive LBD18 acts as a specific DNA-binding transcriptional activator that directly regulates expression of Expansin (EXP) genes (Figure 4), which encode cell wall-loosening factor that promotes lateral root emergence in Arabidopsis thaliana (Lee et al., 2013). PHOSPHATE STARVATION RESPONSE1 (PHR1)/MYB are recognized as key regulatory component of the response to Pi starvation by directly regulating various P starvation-induced (PSI) genes, which consequently affects P uptake and transport, and modulates RSA (Puga et al., 2017; Huang et al., 2018). ARF7 and ARF19, are the upstream regulators of the genes encoding PHR1/MYB family members (Figure 4).
CLSY1, a key component of the RNA-directed DNA-methylation machinery, mechanistically mediates the transcriptional repression of a negative regulator of root branching, IAA27, and promotes lateral root development under K deficiency (Shahzad et al., 2020) (Figure 4). IAA27 interacts with ARF2, which inturn modulates the expression of the K+ transporter gene HAK5 (High Affinity K+ transporter 5), with arf2 mutant plants displaying a tolerant phenotype similar to the HAK5-overexpressing lines on low-K+ medium (Zhao et al., 2016) (Figure 4), and suggests that ARF2 acts as a negative regulator of low K stress response in Arabidopsis.
The molecular link that integrates plant abscisic acid (ABA) responses to drought stress in plants has been demonstrated (Meng et al., 2015). Drought signal perception leads to activation of dehydration-responsive element-binding protein (DREB2A/B) TFs which directly promote transcription of IAA genes in response to drought stress (Figure 4). The molecular and genetic evidence presented indicate that ARF2, ANT and Cold-regulated15A (COR15A) form an ABA-mediated signaling pathway that modulates drought stress response, with ARF2 serving as a molecular link that integrates plant ABA responses to drought stress (Meng et al., 2015) (Figure 4).
Expression of seven ARF TFs, OsARF1, OsARF5, OsARF6, OsARF17, OsARF19, OsARF24 and OsARF25, is upregulated in dnr1 but downregulated in pAct : DNR1‐Flag overexpression line relative to WT. Upregulation of these ARF TFs mediates auxin-dependent activation of NO3− transporter and N-metabolism genes, resulting in improved NUE and grain yield in rice (Zhang S. et al., 2021). The osarf12 and osarf12/25 mutants with P-intoxicated phenotypes recorded higher P concentrations, up-regulation of Pi transporter encoding genes (OsIPS1, OsIPS2, OsSPX1), OsSQD2, OsMYB2P-1 and OsTIR1) and increased APase activity under Pi-sufficient/-deficient (+Pi/-Pi, 0.32/0 mM NaH2PO4) conditions compared to WT, suggesting that OsARF12 is a negative regulator of Pi homeostasis in rice (Wang et al., 2014). Knockout of OsARF16 led to loss of sensitivity of primary roots, lateral roots and root hairs to auxin and Pi response, with osarf16 mutant displaying slightly reduced shoot biomass, inhibited root growth, and reduced induction of phosphate starvation-induced genes (Shen et al., 2013). Compared to WT, osarf16 mutant displayed compromised cytokinin-induced inhibition of Pi uptake and higher Pi content under cytokinin treatment, which was occasioned by higher expression of Phosphate Transporter1 (PHT1) genes, PSI genes and purple PAPase genes (Shen et al., 2014), suggesting that OsARF16 participates in cytokinin mediated inhibition of phosphate transport and phosphate signaling in rice. Besides regulating adaptation mechanisms to macronutrient deficiencies, OsARFs have also been reported to modulate iron deficiency response adaptation in rice. OSARF16 has been reported to regulate iron deficiency response in rice by regulating auxin redistribution: Expression of OsARF16 is induced by Fe limitation in root and shoot, which inturn upregulates Fe-deficiency response genes; Consequently, in the auxin insensitive mutant, osarf16, most Fe‐deficiency symptoms were partially restored, including dwarfing, decreased photosynthesis, reduced iron content and the regulation of RSA (Shen et al., 2015). An OsARF12 knockout mutant, osarf12, displayed short primary root length, altered abundance of mitochondrial iron-regulated (OsMIR), iron (Fe)-regulated transporter 1 (OsIRT1) and short postembryonic root (OsSPR1) in roots of rice, and resulted in limited Fe content (Qi et al., 2012).
OsARF21 directly binds to the promoter of the early auxin responsive genes, Deep rooting 1 (DRO1), and regulates its expression in the auxin signaling pathway to modulate cell elongation in the root tip, causing asymmetric root growth and downward bending of the root in response to gravity to maintain high yield performance under drought conditions (Uga et al., 2013). The rice auxin response factors, OsARF11 and OsARF15, have both been reported to show differential expression under salt stress condition, suggesting that they might participate in response to salt stress response in rice (Jain and Khurana, 2009). Evaluation of changes in endogenous indole-3-acetic acid (IAA) and jasmonic acid (JA) levels and their responsive genes in rice under various abiotic stress condition revealed that OsARF4/14/18/19 were induced by cold stress, whiles OsARF11/13/16 were induced by heat stress (Du et al., 2013).
Functional characterization of ZmARFs in stress response in maize remains largely limited. Nonetheless, a few ZmARF TFs have been reported to participate in stress response and adaptation. Cytonuclear localized ZmARF2 interacts with promoter of the maize high-affinity K transporter (ZmHAK1) to promote K+ uptake and homeostasis (Sheng et al., 2020). Nucleotide diversity and favorable alleles of ZmARF31 were found to be significantly associated with low P responses traits and root architecture in maize. Thirty, fourteen, and nine natural variations were identified in ZmARF31 that were associated with P-deficiency-tolerance traits in maize (Wu et al., 2016). Overexpression of the maize ARF, ZmARF4, in Arabidopsis conferred low phosphate (Pi) stress tolerance; transgenic Arabidopsis overexpressing ZmARF4 displayed better root development, increased Pi mobilization, up-regulation of low Pi stress inducible gene (AtRNS1) and down-regulation of anthocyanin biosynthesis genes (AtDER and AtANS), under low Pi stress compared to WT (Li et al., 2022).
Biotic stresses are those adverse conditions that normally affectplant growth due to their interaction with deleterious microorganisms such as fungi, bacteria, viruses, viroids, phytoplasmas and nematodes. These microorganisms mainly growth either on or inside plant tissues and inflict varied damages leading to symptoms like chlorosis, stunting, rotting, or local lesions formation. Compared to the role of ARF TFs in regulating responses to abiotic stresses, the role of theses TFs in biotic stress response regulation has not received much research attention. The role of auxin and its signaling pathway on plan- pathogen association has long been reported (Bari and Jones, 2009).
In Arabidopsis, the transcript of ARF1 and ARF2 were repressed by F. oxysporum, whiles arf2, arf1 and arf2/arf1 displayed phenotypes with increased resistance to F. oxysporum relative to WT, these outcomes suggest that ARF1 and ARF2 promote susceptibility to F. oxysporum infestation (Lyons et al., 2015). Misregulation of ARF8 results in developmental abnormalities manifested by viral suppressors of RNA (VSR) transgenic plants and also for the phenotypes displayed during normal viral infection caused by the HcPro-encoding Turnip mosaic virus (TuMV) (Jay et al., 2011). Some OsARFs also play crucial roles in host antiviral immune defense. OsARF12 and OsARF16 interacted with OsIAA10 to positively regulate rice antiviral defense against rice dwarf virus (RDV) through a mechanism involving binding of OsARF12 to the AuxRE in promoter of OsWRK13 to activate its transcription (Qin et al., 2020). Overexpression of OsARF17 reduced accumulation of the black-streaked dwarf virus (BSDV) and rice black-streaked dwarf virus (RBSDV), whiles the accumulation of these virus and severity of their symptoms increased in osarf17 knockout mutant rice lines (Zhang et al., 2020). In maize, expression of ZmARF6 and ZmARF18 genes increased significantly in response to Colletotrichum graminicola and F. verticillioides (Saidi and Hajibarat, 2020), suggesting that these ARFs could act as positive regulators to stresses induced by Colletotrichum graminicola and F. verticillioides.
ARFs have been proven to be regulated by other TFs to mediate biological process of growth and development, as well as, stress responses (Wang and Estelle, 2014). Yeast two-hybrid and in vitro pull down assays revealed heterodimerization between the III/IV domain of ARF5/MP and the Arabidopsis BREVIS RADIX (BRX) transcription co-regulator, which promotes the transactivation potential of ARF5/MP (Guilfoyle and Hagen, 2007) (Figure 5A), which control root meristem growth (Scacchi et al., 2010). LBD18 interacts with ARFs (Figure 5A) such as ARF7 and ARF19 via the Phox and BemI domains to promote the transcriptional activity of ARF7 on the AuxRE, inhibiting the negative feedback loop exerted by AUX/IAA repressor, to constitute a double positive feedback, that ensures continued lateral root growth in response to auxin in Arabidopsis (Pandey et al., 2018). A recent study showed that Dull Nitrogen Response TF (DNR1) regulates auxin homeostasis and induction of ARFs (Figure 5A) to promote ARF-mediated activation of NPF/NRT1 and NRT2 to regulate NO3- uptake in roots, resulting in enhanced NUE and grain yield (Xing et al., 2023). Other regulatory models have been proposed to inhibit transcription of ARFs during growth and stress responses. For example, induction of Agamous (AG) represses ARF3 expression indirectly through Giant Killer (GIK) (Figure 5A) which harbors an AT-hook DNA binding motif, and is crucial for floral meristem development (Zhang et al., 2018). The Apetala2 (APT2), encoding a putative TF characterized by a novel DNA binding motif referred to as AP2 domain, directly represses ARF3 transcription (Figure 5A) during floral meristem determination (Liu et al., 2014). The rice P8 proteins have been reported to interact with the C-terminus domain of OsARF17 to prevent its dimerization with other proteins, leading to suppression of its role in conferring resistance to RBSDV and RBSD (Zhang et al., 2020). Several post-transcriptional events contribute to the cell-specific expression patterns and functions of genes. Majority of the post-transcriptional regulations of gene expression are occasioned by activities of RNA binding proteins and processing factors that are closely related with RNAs, spanning from transcription initiation to eventual death of the RNA in the cytoplasm (Dassi, 2017). MicroRNA (miRNA)-mediated regulation of auxin signaling pathway during plant development and stress responses has been reported (Luo P. et al., 2022). Numerous miRNAs have been characterized to target ARFs, leading to regulation of the downstream auxin responsive genes related to both development and stress response in plants. Two conserved miRNAs, miRNA160 and miRNA167, constitutes a complex feedback loop that regulates processes in the auxin signaling pathway by modulating the expression of ARFs (Singh and Singh, 2021)(Figure 5B). The miRNA160 and miRNA167 actively regulate mRNA abundance of ARFs in Arabidopsis, miRNA160 targets and cleaves ARF10/16/17, while miRNA167 targets and cleaves ARF6/8 (Mallory et al., 2005; Wu et al., 2006) (Figure 5B). The miRNA160/miRNA167 and their associated targets ARF6/8/17 form a regulatory network that modulates adventitious root development. Whiles miRNA167 targets ARF6 and ARF8, which functions as positive regulators of adventitious root development, miRNA160 targets ARF17, which acts as a negative regulator of adventitious root development (Gutierrez et al., 2009). However, ARF6/8/17 control their own expression at both transcriptional and posttranscriptional level by regulating the abundance of miRNA160 and miRNA167, which completes the miRNA160/miRNA167-AtARF6/8/17 feedback loop that regulates adventitious root development (Gutierrez et al., 2009). OsmiRi167a targets OsARF12, OsARF17 and OsARF25 to control tiller angle in rice, with repression of OsARF12, OsARF17 and OsARF25 in transgenic plants overexpressing OsmiRi167a, which displayed phenotypes with larger tiller angle similar to osarf12/osarf17 and osarf12/osarf25 plants (Li Y. et al., 2020). The miRNA167a positively regulates grain length and weight by dictating OsARF6 mRNA silencing to mediate OsAUX3 expression in a novel miRNA167a-OsARF6-OsAUX3 regulatory model (Qiao et al., 2021). The miRNA160 has also been reported to target ARF10 and ARF16, which act as transcription repressors, and regulate the expression of their downstream responsive genes to mediate the regulation of developmental processes in plants (Huang et al., 2016; Liu et al., 2016).
Figure 5 Regulation of ARFs by transcriptional and post-transcriptional events. BRX transcription co-regulator, DNR1 and LBD18, directly induce ARF expression to promote several aspects of plant growth and development. AP2/ERF, BOB1 and ELO3 directly repress expression of ARFs. AG indirectly represses ARF3 expression through GIK. AS1-AS2 complex indirectly activates miR390-and RDR6-dependent gene silencing to negatively regulate both ARF3 and ARF4 activities. The TAS3 genes encode tasiR-ARF species which target the mRNA of three ARF TFs, ARF2, ARF3/ETT and ARF4, for subsequent degradation. TAS3 harbors two miR390 target sites that are cleaved by miR138 to trigger the production of tasiR-ARF from the cleaved fragments. miRNA160 targets and cleaves ARF10/16/17, while miRNA167 targets and cleaves ARF6/8 in a regulatory network that modulates adventitious root development.
The most well studied Trans-acting SIRNA (TAS)-derived short interfering RNAs (siRNAs) are tasiR-ARFs, which are widely conserved across plant species and target several ARF genes (Allen et al., 2005). The TAS3 genes encode tasiR-ARF species which target the mRNA of three ARF TFs, ARF2, ETT/ARF3 and ARF4, for subsequent degradation (Ozerova et al., 2013) (Figure 5B). miRNA can trigger the biogenesis of secondary siRNAs in phase (phasiRNAs) such as the TAS by targeting their transcripts for cleavage (Liu et al., 2020). The cleaved TAS transcripts is bound to and converted to double-stranded RNAs (dsRNAs) by RNA-binding protein SUPPRSSOR OF GENE SILENCING 3 (SGS3), through RNA-dependent RNA polymerase (RDR6), and undergoes further processing to generate phasiRNAs such as tasiR-ARF (Zhang et al., 2019) (Figure 5B). In another mechanism, TAS RNA precursor TAS3 transcript bears two targets sites of miR390, cleavage at these sites trigger the production of phasiRNAs such as tasiR-ARF from the cleaved fragments (Axtell et al., 2006) (Figure 5B). ARF2, ARF3 and ARF4 have been demonstrated to be targeted and regulated by TAS3 ta-siRNA (tasiRNA-ARF) (Hunter et al., 2006) (Figure 5B), which affects developmental timing and patterning in Arabidopsis (Fahlgren et al., 2006). Assymetric leaves 1 (AS1)-AS2 also indirectly activates miR390-and RDR6-dependent post-transcriptional gene silencing to negatively regulate both ARF3 and ARF4 activities (Iwasaki et al., 2013) (Figure 5B).
miR167 positively regulates nodulation and lateral root development in Glycine max by targeting and inhibiting its target genes GmARF8a and GmARF8b (Wang Y. et al., 2015). miR167 has also been reported to positively regulate plant development and root plasticity by targetingARF6 and Indole acetic acid alanine resistant3 (IAR3) (Kinoshita et al., 2012). Digital gene expression profile revealed that microRNA response element, miRNA167, targets TcARF6 to constitute a tch-miRNA167-TcARF6 negative response module that downregulates the expression of TcARF6 in roots of Tamarix chinensis in response to salt stress (Ye et al., 2020). The expression of miRNA160a/b was strongly upregulated whiles their target ARF10 was downregulated in two cowpea genotypes under drought stress treatment (Barrera-Figueroa et al., 2011). Analysis of ta-siRNA synthesis mutants and mutated ARF3-overexpressing plants that escape tasiRNA-ARF targeting indicated that, self-pollination was hampered by short stamens in plants under drought and high salinity stress, suggesting that tasiRNA-ARF is involved in maintaining the normal morphogenesis of flowers in plants under drought and high salinity stress conditions (Matsui et al., 2014). Salt stress treatment (100 mM NaCl) induced expression of miR390, increased cleavage of TAS3, produced higher levels of tasiARFs, and subsequently enhanced cleavage of ARF3/4 (Wen et al., 2020). A miRNA160-ARF regulatory network modulates male sterility caused by long exposure to high temperature stress: overexpression of miRNA160 increased sensitivity of cotton to high temperature stress, with a reduction in ARF10/17 mRNA, leading to activation of the auxin response at the sporogenous cell proliferation stage (Ding et al., 2017; Chen et al., 2020). NtmiRNA167a transcriptionally regulates NtARF6 and NtARF8 to mediate drastic plant Pi-starvation response via modulation of various biological processes in a miRNA167a-ARF6-ARF8 negative response regulatory module, where NtmiRNA167a overexpression and NtARF6 knockdown mutant displayed reduced plant growth, biomass and increased ROS accumulation under Pi-starvation condition compared to WT (Chen et al., 2018). Interaction between miRNA160 and miRNA165/166 modulates numerous downstream responsive biological processes, in which ARFs and HD-ZIP IIIs play opposite roles in regulating leaf development and drought stress response (Yang et al., 2019). The miRNA167-ARF8 regulatory module has been revealed to regulate cell type-specific response to available nitrogen status and plastic development of lateral roots in Arabidopsis (Gifford et al., 2008).
Analysis of differentially expressed miRNA target genes revealed that, miRNA160 was induced by bacterial and fungal pathogen infection, whiles its ARF target genes were downregulated in a miRNA160-ARF module, which regulated defense response in Arabidopsis against Botrytis cinerea (Xue and Yi, 2018), in banana against Fusarium oxysporum (Cheng et al., 2019) and in cassava against Colletotrichum gloeosporioides (Pinweha et al., 2015). AtmiRNA167a targets the transcription of ARF6 and ARF8 to regulate the closure of leaf stomata to prevent entry of Pseudomonas syringae, with P35S:MIRNA167a overexpression and arf6-2 arf8-3 plants displaying extreme resistant phenotypes compared to WT (Caruana et al., 2020). The miRNA390-tasiRNA-ARF regulatory module regulates lateral root development under salt stress, with significant inhibition in expression of ARF3.1, ARF3.2 and ARF4 in miRNA390-overexpressing line under salt stress but increased in the miRNA390-knockout line (He et al., 2018).
Epigenetic mechanisms play crucial roles during the life cycle of living organisms (Duan et al., 2018), which help cells to control gene activity without changing the DNA sequence. These mechanisms help determine whether specific genes are tuned on or off, and ensure that each cell produces only the proteins that are necessary for its function (Gayon, 2016). The three most prominent epigenetic mechanisms are histone modification, DNA methylation, and noncoding RNA (ncRNA) regulation (Fessele and Wright, 2018). ARF-dependent induction of downstream auxin-responsive genes is regulated by multiple epigenetic factors, including histone modifications and the chromatin remodeling factor PICKLE (PKL) (Weiste and Droge-Laser, 2014).
Histone acetylation is a key histone modification mechanism that appears to be a dynamic reversible switch for inter-conversion between permissive and repressive transcriptional states of chromatin domains (Zhou and Hu, 2010). The co-repressor TOPLESS (TPL) recruits HDA19 to the auxin signaling repressor, AUX/IAA, in an EAR motif-dependent manner, and that the function of GCN5/HAG1 histone acetylase is directly opposed to the function of IAA12/BDL-TPL-HDA19 repressor complex in the ARF-dependent expression of auxin responsive genes (Long et al., 2006; Szemenyei et al., 2008). ARF18-HISTONEDEACETYLASE6 (HDA6) module regulates floral organ identity in Rosa hybrid: Silencing of RhHAD6 increases H3K9/K14 acetylation levels at the site adjacent to the RhARF18-binding site in the promoter of its downstream target, RhAG, and reduces petal number (Chen J. et al., 2021), which indicates that RhARF18 probably recruit RhHDA6 to the RhAG promoter to repress RhAG transcription.
DNA methylation is one of the prominent epigenetic modifications that occur extensively in living organisms (Wang et al., 2009). DNA methylation causes changes in chromatin state in plant cells undergoing dedifferentiation (Koukalova et al., 2005), and can also help to establish or maintain the undifferentiated cell state in plants (Berdasco et al., 2008). In plants, DNA demethylation depends on four bifunctional 5-methylcytosine glycosylases: Repressor of silencing 1 (ROS1), Demeter (DME), DME-like 2 (DML2), and DML3, which remove methylated bases and cleave the DNA backbone at abasic sites. The increased expression of AUXIN RESPONSE FACTOR3 (ARF3) in met1 indeed was due to DNA demethylation, suggesting that DNA methylation regulates de novo shoot regeneration by modulating auxin signaling (Li et al., 2011). BOBBERY1 (BOB1), an Arabidopsis orthologue of eukaryotic NudC domain proteins, and ELONGATA3 (ELO3), the catalytic subunit of the hioghly conserved elongator complex in Arabidopsis, has been revealed through genetic analysis to repress expression of ARF3 and ARF4, along with AS1-AS2 (Takahashi et al., 2013). BIN2 has been reported to phosphorylate ARF7 and ARF19, and in contrast to reducing activity of ARF2, ARF7, and ARF19 phosphorylation enhanced their transactivation activity, which is attributed to reduced ARF7 and ARF19 interactions with the Aux/IAA repressors.
Post-translational regulation refers to those cellular events that regulate the abundance of active proteins. It predominantly occurs either by means of reversible events as evident through post-translational modifications (PTMs) or through irreversible events such as proteolysis. PTMs are covalent processing activities that modify the properties of active proteins via proteolytic cleavage and addition of modifying group such as acetyl, phosphoryl, glycosyl and methyl, to one or multiple amino acids (Ramazi et al., 2020).
Protein phosphorylation is the most prominent PTM that acts as a crucial cellular regulatory mechanism to either activate or deactivate enzymes and receptors by phosphorylation or dephosphorylation events, which are respectively catalyzed by kinases and phosphatases at serine, threonine, or tyrosine residues (Ardito et al., 2017). The mitogen-activated protein kinases (MAPK) cascades are conserved signaling mechanism comprising reversible phosphorylation through a cascade of ATP-dependent protein kinases, which regulates multiple aspects of plant growth and development. It has been proposed that auxin signal transduction is mediated by the conserved MAPK signaling cascade (Mizoguchi et al., 1994). Auxin-induced MPK14 phosphorylated and stabilized non-canonical IAA33 and enhanced its competitiveness over canonical repressor IAA5 for binding site on promoters of ARF10 and ARF16, which mitigated inhibition of ARF10 and ARF16 by IAA5 and promoted the identity of root distal stem cell (DSC) and negatively regulated auxin signaling (Lv et al., 2020). In the canonical NAP, drought-induced MPK3/MPK6 phosphorylates and stabilizes IAA15 by inhibiting TIR1-mediated ubiquitination of IAA15, which inturn represses the transcriptional activation of LBD genes by ARF7 and ARF19, leading to limited lateral root development under drought stress in Arabidopsis (Kim et al., 2022). In the non-canonical NAP, some TMK1 family members function as PM-resident receptors or part of a receptor complex, perceiving extracellular auxin and transducing these signals into various phosphorylation events (Tan et al., 2021). The cytosolic and nucleus-translocated C terminus of TMK1 specifically interacts with and phosphorylates non-canonical IAA32 and IAA34 repressors of auxin signaling, thereby regulating ARF transcription factors to dictate differential growth of the apical hook (Cao et al., 2019). Other phosphorylation events have been reported to regulate ARF protein functions. For example, ARF2 mostly represses the expression of the HAK5 potassium transporter gene, meanwhile ARF2 is phosphorylated under low potassium stress to abolish its ability to bind to the promoter of HAK5 and diminishes its repressive effects on HAK5 (Zhao et al., 2016). The BRASSINOSTEROID-INSENSITIVE 2 (BIN2) has been implicated to target and phosphorylate ARF7 and ARF19: Phosphorylation of ARF7 and ARF19 suppresses their interaction the AUX/IAA repressor, which eventually enhances the transcription activity of ARF7 and ARF19 to regulate their downstream target gene LBD16 and LBD29 to promote lateral root organogenesis (Cho et al., 2014).
Previous studies on the role of proteolytic regulation in auxin signaling have focused on degradation of their interacting partner, the Aux/IAA proteins, as described above. Although ARF proteins have been shown to be degraded through the 26S mediated ubiquitination, and the degradation process occurs independent of IAAs (Salmon et al., 2008), not much data has been generated regarding degradation of ARFs. Degradation analysis in ARFs show that 37°C treatment increased the protein levels of HA-ARF5/MP, HA-ARF6, and HA-ARF10. On the contrary, there was a pronounced reduction in protein levels of these HA-ARFs by ABA, 4°C and salt treatments, whiles MG132 inhibited the reduction of HA-ARF6 level by ABA and 4°C treatments, suggesting that the ARF protein levels are regulated by multiple factors and that these treatments decrease HA-ARF6 level through 26S proteasome-mediated protein degradation (Li K. et al., 2020). MG132 suppressed the ethylene-dependent decrease in ARF2 protein levels during apical hook development, which strongly suggests that the ethylene-mediated degradation of ARF2 protein is via 26S proteasome degradation pathway (Li et al., 2004). Functional characterization of F-box protein AUXIN RESPONSE FACTOR F-BOX1 (ARF1) SCFARF1) revealed that this E3 ubiquitin ligase directly interacts with ARF7 and ARF19 to promote their degradation, and regulate their accumulation, condensation, and nucleo-cytoplasmic partitioning, which triggers downstream auxin responses (Jing et al., 2022).
Small ubiquitin-like modifier (SUMO) is emerging as an important posttranslational modification that regulates plant development and defense pathways (Orosa-Puente et al., 2018; Niu et al., 2019). SUMO is covalently attached to the lysine residues of target proteins, which could modulate protein activity, stability, localization, and protein-protein interactions of target proteins (Vierstra, 2009), converse to protein degradation as witnessed during ubiquitination. SUMOylation is a crucial PTM that has significantly affected various plant responses to stress and environmental changes (Benlloch and Lois, 2018). MdARF8 is directly SUMOylated by apple SUMO E3 ligase MdSIZ1, which enhances protein stability of MdARF8, and facilitates LR formation in apple (Zhang C. L. et al., 2021). The uneven distribution of water in the soil has a direct influence on plant growth and root architecture, which are regulated by the SUMOylation of ARF7 (Bao et al., 2014; Orosa-Puente et al., 2018). SUMOylated ARF7 enhances its binding capacity to IAA3 and negatively regulates ARF7 activity, thereby inhibiting the expression of LBD16 (Orosa-Puente et al., 2018). Conversely, nonSUMOylated ARF7 cannot recruit IAA3 on the moisture side, which leads to an increased expression of LBD16 and promoted LR development (Orosa-Puente et al., 2018).
Over the past decades, the auxin signaling pathway has emerged as a complex regulatory system that modulates plant growth, development, and stresses response. ARF transcription factors serve as effectors of auxin response that transduce and translate auxin signals into the regulation of auxin responsive genes. Both forward and reverse genetic approaches have deepened our understanding of the influence of ARFs on plant development and stress responses. The differential expression of various ARFs in response to various abiotic and biotic stresses suggests that ARFs might exhibit overlapping regulatory roles in response to these stresses. We have also reviewed the modulation of ARF expression by other molecular regulators and how these transcriptional regulations influence the role of ARFs in stress response in plants.
So far, studies on ARF TFs have primarily emphasized on gene cloning and functional characterization, with majority of them focusing transcriptional levels where ARFs bind to cis-acting elements in promoter of their target genes to regulate their expression. Comparably, research on post-translational modification of ARF TFs, including mRNA precursor splicing, editing, stability, nuclear transport, and siRNA-mediated modification—critical for stress response regulation in plants—remains very limited. We propose that future analyses of ARF TFs should emphasize the synergy between transcription regulatory factors, post-transcriptional and post-translational modifications, with a strong focus on the mechanisms of action governing the post-translational modifications of ARF TFs.
Moreover, studies on ARFs have predominantly concentrated on the function of individual ARF TFs or their interaction with other proteins. However, the mechanism governing ARFs function is highly complex due to the larger number of the ARF TF family members and the scattered nature of recent research. Consequently, the regulatory network of ARF TFs remains poorly understood. Further exploration and investigation are needed to understand the role of ARF TFs in perception and transduction of internal and external signals and the interaction among various ARF TFs on physiological and biochemical processes.
It is also important to note that, although plants often encounter multiple stresses, most ARF research has focused on the function of ARFs under single stress conditions. Future functional characterization of ARF TFs should include analyses in response to multiple stresses, followed by comparison of the differences and similarities between single and multiple stresses conditions. This approach is expected to identify key nodes in the complex regulatory network of ARFs. It is also worth highlighting that many datasets related to ARF TFs are scattered and requires integration into a specific online database, which will enable researchers to access relevant ARF TF information quickly.
Research findings have revealed the potential of ARFs in regulating multiple stress conditions, highlighting the functional complexity of ARFs and emphasizing the need to address all aspects of their functioning. Recent studies have shown the existence of crosstalk in some ARF TFs, and ARFs exert their function through various signaling pathways, which can be influenced by both crosstalk and mutual coordination mechanisms.
The authors declare that the research was conducted in the absence of any commercial or financial relationships that could be construed as a potential conflict of interest.
LL: Funding acquisition, Writing – review & editing, Conceptualization, Data curation, Investigation, Writing – original draft. BY: Writing – original draft, Writing – review & editing, Data curation, Investigation. JL: Writing – review & editing, Formal analysis. FW: Conceptualization, Funding acquisition, Supervision, Writing – review & editing.
The author(s) declare financial support was received for the research, authorship, and/or publication of this article. This research was supported by the National Natural Science Foundation of China (32101785), the General Project of Natural Science Foundation of Sichuan Province (2022NSFSC0147 and 24NSFSC0766), and the Sailing Project Plan of Yibin University (2021QH09).
The authors declare that the research was conducted in the absence of any commercial or financial relationships that could be construed as a potential conflict of interest.
All claims expressed in this article are solely those of the authors and do not necessarily represent those of their affiliated organizations, or those of the publisher, the editors and the reviewers. Any product that may be evaluated in this article, or claim that may be made by its manufacturer, is not guaranteed or endorsed by the publisher.
Ahmad, N., Mukhtar, Z. (2017). Genetic manipulations in crops: Challenges and opportunities. Genomics 109, 494–505. doi: 10.1016/j.ygeno.2017.07.007
Aida, M., Vernoux, T., Furutani, M., Traas, J., Tasaka, M. (2002). Roles of PIN-FORMED1 and MONOPTEROS in pattern formation of the apical region of the Arabidopsis embryo. Development 129, 3965–3974. doi: 10.1242/dev.129.17.3965
Allen, E., Xie, Z., Gustafson, A. M., Carrington, J. C. (2005). microRNA-directed phasing during trans-acting siRNA biogenesis in plants. Cell 121, 207–221. doi: 10.1016/j.cell.2005.04.004
Ardito, F., Giuliani, M., Perrone, D., Troiano, G., Lo Muzio, L. (2017). The crucial role of protein phosphorylation in cell signaling and its use as targeted therapy (Review). Int. J. Mol. Med. 40, 271–280. doi: 10.3892/ijmm.2017.3036
Atkinson, J. A., Rasmussen, A., Traini, R., Voss, U., Sturrock, C., Mooney, S. J., et al. (2014). Branching out in roots: uncovering form, function, and regulation. Plant Physiol. 166, 538–550. doi: 10.1104/pp.114.245423
Attia, K. A., Abdelkhalik, A. F., Ammar, M. H., Wei, C., Yang, J., Lightfoot, D. A., et al. (2009). Antisense phenotypes reveal a functional expression of OsARF1, an auxin response factor, in transgenic rice. Curr. Issues Mol. Biol. 11 Suppl 1, i29–i34. doi: 10.21775/9781912530069
Axtell, M. J., Jan, C., Rajagopalan, R., Bartel, D. P. (2006). A two-hit trigger for siRNA biogenesis in plants. Cell 127, 565–577. doi: 10.1016/j.cell.2006.09.032
Bai, Y., Zhang, T., Zheng, X., Li, B., Qi, X., Xu, Y., et al. (2023). Overexpression of a WRKY transcription factor McWRKY57-like from Mentha canadensis L. enhances drought tolerance in transgenic Arabidopsis. BMC Plant Biol. 23, 216. doi: 10.1186/s12870-023-04213-y
Baillo, E. H., Kimotho, R. N., Zhang, Z., Xu, P. (2019). Transcription factors associated with abiotic and biotic stress tolerance and their potential for crops improvement. Genes (Basel) 10, 771. doi: 10.3390/genes10100771
Ballas, N., Wong, L. M., Theologis, A. (1993). Identification of the auxin-responsive element, AuxRE, in the primary indoleacetic acid-inducible gene, PS-IAA4/5, of pea (Pisum sativum). J. Mol. Biol. 233, 580–596. doi: 10.1006/jmbi.1993.1537
Bao, Y., Aggarwal, P., Robbins, N. E., 2nd, Sturrock, C. J., Thompson, M. C., Tan, H. Q., et al. (2014). Plant roots use a patterning mechanism to position lateral root branches toward available water. Proc Natl Acad Sci U S A, 111(25), 9319-9324. https://doi.org/10.1073/pnas.1400966111
Bari, R., Jones, J. D. (2009). Role of plant hormones in plant defence responses. Plant Mol. Biol. 69, 473–488. doi: 10.1007/s11103-008-9435-0
Barrera-Figueroa, B. E., Gao, L., Diop, N. N., Wu, Z., Ehlers, J. D., Roberts, P. A., et al. (2011). Identification and comparative analysis of drought-associated microRNAs in two cowpea genotypes. BMC Plant Biol. 11, 127. doi: 10.1186/1471-2229-11-127
Benlloch, R., Lois, L. M. (2018). Sumoylation in plants: mechanistic insights and its role in drought stress. J. Exp. Bot. 69, 4539–4554. doi: 10.1093/jxb/ery233
Berckmans, B., Vassileva, V., Schmid, S. P., Maes, S., Parizot, B., Naramoto, S., et al. (2011). Auxin-dependent cell cycle reactivation through transcriptional regulation of Arabidopsis E2Fa by lateral organ boundary proteins. Plant Cell 23, 3671–3683. doi: 10.1105/tpc.111.088377
Berdasco, M., Alcázar, R., García-Ortiz, M. V., Ballestar, E., Fernández, A. F., Roldán-Arjona, T., et al. (2008). Promoter DNA hypermethylation and gene repression in undifferentiated arabidopsis cells. PloS One 3, e3306. doi: 10.1371/journal.pone.0003306
Boer, D. R., Freire-Rios, A., van den Berg, W. A., Saaki, T., Manfield, I. W., Kepinski, S., et al. (2014). Structural basis for DNA binding specificity by the auxin-dependent ARF transcription factors. Cell 156, 577–589. doi: 10.1016/j.cell.2013.12.027
Bouzroud, S., Gasparini, K., Hu, G., Barbosa, M. A. M., Rosa, B. L., Fahr, M., et al. (2020). Down regulation and loss of auxin response factor 4 function using CRISPR/cas9 alters plant growth, stomatal function and improves tomato tolerance to salinity and osmotic stress. Genes (Basel) 11, 272. doi: 10.3390/genes11030272
Breuninger, H., Rikirsch, E., Hermann, M., Ueda, M., Laux, T. (2008). Differential expression of WOX genes mediates apical-basal axis formation in the Arabidopsis embryo. Dev. Cell 14, 867–876. doi: 10.1016/j.devcel.2008.03.008
Cao, M., Chen, R., Li, P., Yu, Y., Zheng, R., Ge, D., et al. (2019). TMK1-mediated auxin signalling regulates differential growth of the apical hook. Nature 568, 240–243. doi: 10.1038/s41586-019-1069-7
Carrillo-Carrasco, V. P., Hernandez-Garcia, J., Mutte, S. K., Weijers, D. (2023). The birth of a giant: evolutionary insights into the origin of auxin responses in plants. EMBO J. 42, e113018. doi: 10.15252/embj.2022113018
Caruana, J. C., Dhar, N., Raina, R. (2020). Overexpression of Arabidopsis microRNA167 induces salicylic acid-dependent defense against Pseudomonas syringae through the regulation of its targets ARF6 and ARF8. Plant Direct 4, e00270. doi: 10.1002/pld3.270
Casamassimi, A., Ciccodicola, A. (2019). Transcriptional regulation: molecules, involved mechanisms, and misregulation. Int. J. Mol. Sci. 20, 1281. doi: 10.3390/ijms20061281
Causier, B., Ashworth, M., Guo, W., Davies, B. (2012). The TOPLESS interactome: a framework for gene repression in Arabidopsis. Plant Physiol. 158, 423–438. doi: 10.1104/pp.111.186999
Chandler, J. W. (2016). Auxin response factors. Plant Cell Environ. 39, 1014–1028. doi: 10.1111/pce.12662
Chen, J., Li, Y., Li, Y., Li, Y., Wang, Y., Jiang, C., et al. (2021). AUXIN RESPONSE FACTOR 18-HISTONE DEACETYLASE 6 module regulates floral organ identity in rose (Rosa hybrida). Plant Physiol. 186, 1074–1087. doi: 10.1093/plphys/kiab130
Chen, J., Pan, A., He, S., Su, P., Yuan, X., Zhu, S., et al. (2020). Different MicroRNA Families Involved in Regulating High Temperature Stress Response during Cotton (Gossypium hirsutum L.) Anther Development. Int. J. Mol. Sci. 21, 1280. doi: 10.3390/ijms21041280
Chen, M., Zhu, X., Liu, X., Wu, C., Yu, C., Hu, G., et al. (2021). Knockout of auxin response factor slARF4 improves tomato resistance to water deficit. Int. J. Mol. Sci. 22, 3347. doi: 10.3390/ijms22073347
Chen, X., Liu, Z., Shi, G., Bai, Q., Guo, C., Xiao, K. (2018). MIR167a transcriptionally regulates ARF6 and ARF8 and mediates drastically plant Pi-starvation response via modulation of various biological processes. Plant Cell Tissue Organ Culture 133, 177–191. doi: 10.1007/s11240-017-1371-8
Cheng, C., Liu, F., Sun, X., Tian, N., Mensah, R. A., Li, D., et al. (2019). Identification of Fusarium oxysporum f. sp. cubense tropical race 4 (Foc TR4) responsive miRNAs in banana root. Sci. Rep. 9, 13682. doi: 10.1038/s41598-019-50130-2
Cho, H., Ryu, H., Rho, S., Hill, K., Smith, S., Audenaert, D., et al. (2014). A secreted peptide acts on BIN2-mediated phosphorylation of ARFs to potentiate auxin response during lateral root development. Nat. Cell Biol. 16, 66–76. doi: 10.1038/ncb2893
Choi, H. S., Seo, M., Cho, H. T. (2018). Two TPL-binding motifs of ARF2 are involved in repression of auxin responses. Front. Plant Sci. 9. doi: 10.3389/fpls.2018.00372
Clapier, C. R., Cairns, B. R. (2009). The biology of chromatin remodeling complexes. Annu. Rev. Biochem. 78, 273–304. doi: 10.1146/annurev.biochem.77.062706.153223
Dai, N., Wang, W., Patterson, S. E., Bleecker, A. B. (2013). The TMK subfamily of receptor-like kinases in Arabidopsis display an essential role in growth and a reduced sensitivity to auxin. PloS One 8, e60990. doi: 10.1371/journal.pone.0060990
Dassi, E. (2017). Handshakes and fights: the regulatory interplay of RNA-binding proteins. Front. Mol. Biosci. 4. doi: 10.3389/fmolb.2017.00067
Dastidar, M. G., Scarpa, A., Magele, I., Ruiz-Duarte, P., von Born, P., Bald, L., et al. (2019). ARF5/MONOPTEROS directly regulates miR390 expression in the Arabidopsis thaliana primary root meristem. Plant Direct 3, e00116. doi: 10.1002/pld3.116
De Rybel, B., Vassileva, V., Parizot, B., Demeulenaere, M., Grunewald, W., Audenaert, D., et al. (2010). A novel aux/IAA28 signaling cascade activates GATA23-dependent specification of lateral root founder cell identity. Curr. Biol. 20, 1697–1706. doi: 10.1016/j.cub.2010.09.007
De Smet, I., Lau, S., Mayer, U., Jurgens, G. (2010). Embryogenesis - the humble beginnings of plant life. Plant J. 61, 959–970. doi: 10.1111/j.1365-313X.2010.04143.x
De Smet, I., Tetsumura, T., De Rybel, B., Frei dit Frey, N., Laplaze, L., Casimiro, I., et al. (2007). Auxin-dependent regulation of lateral root positioning in the basal meristem of Arabidopsis. Development 134, 681–690. doi: 10.1242/dev.02753
Dinesh, D. C., Kovermann, M., Gopalswamy, M., Hellmuth, A., Calderon Villalobos, L. I., Lilie, H., et al. (2015). Solution structure of the PsIAA4 oligomerization domain reveals interaction modes for transcription factors in early auxin response. Proc. Natl. Acad. Sci. U.S.A. 112, 6230–6235. doi: 10.1073/pnas.1424077112
Ding, Y., Ma, Y., Liu, N., Xu, J., Hu, Q., Li, Y., et al. (2017). microRNAs involved in auxin signalling modulate male sterility under high-temperature stress in cotton (Gossypium hirsutum). Plant J. 91, 977–994. doi: 10.1111/tpj.13620
Dos Reis, S. P., Lima, A. M., De Souza, C. R. B. (2012). Recent molecular advances on downstream plant responses to abiotic stress. Int. J. Mol. Sci. 13, 8628–8647. doi: 10.3390/ijms13078628
Dotto, M. C., Petsch, K. A., Aukerman, M. J., Beatty, M., Hammell, M., Timmermans, M. C. (2014). Genome-wide analysis of leafbladeless1-regulated and phased small RNAs underscores the importance of the TAS3 ta-siRNA pathway to maize development. PloS Genet. 10, e1004826. doi: 10.1371/journal.pgen.1004826
Du, H., Liu, H., Xiong, L. (2013). Endogenous auxin and jasmonic acid levels are differentially modulated by abiotic stresses in rice. Front. Plant Sci. 4. doi: 10.3389/fpls.2013.00397
Du, Y., Scheres, B. (2018). Lateral root formation and the multiple roles of auxin. J. Exp. Bot. 69, 155–167. doi: 10.1093/jxb/erx223
Duan, C. G., Zhu, J. K., Cao, X. (2018). Retrospective and perspective of plant epigenetics in China. J. Genet. Genomics 45, 621–638. doi: 10.1016/j.jgg.2018.09.004
Dubrovsky, J. G., Laskowski, M. (2017). Lateral root initiation. Encyclopedia Appl. Plant Sci. 1, 256–264. doi: 10.1016/B978-0-12-394807-6.00126-X
Ellis, C. M., Nagpal, P., Young, J. C., Hagen, G., Guilfoyle, T. J., Reed, J. W. (2005). AUXIN RESPONSE FACTOR1 and AUXIN RESPONSE FACTOR2 regulate senescence and floral organ abscission in Arabidopsis thaliana. Development 132, 4563–4574. doi: 10.1242/dev.02012
El Mamoun, I., Bouzroud, S., Zouine, M., Smouni, A. (2023). The knockdown of AUXIN RESPONSE FACTOR 2 confers enhanced tolerance to salt and drought stresses in tomato (Solanum lycopersicum L.). Plants (Basel) 12, 2804. doi: 10.3390/plants12152804
Endress, P. K. (2010). The evolution of floral biology in basal angiosperms. Philos. Trans. R Soc. Lond B Biol. Sci. 365, 411–421. doi: 10.1098/rstb.2009.0228
Erijman, A., Kozlowski, L., Sohrabi-Jahromi, S., Fishburn, J., Warfield, L., Schreiber, J., et al. (2020). A high-throughput screen for transcription activation domains reveals their sequence features and permits prediction by deep learning. Mol. Cell 78, 890–902 e896. doi: 10.1016/j.molcel.2020.08.013
Fahlgren, N., Montgomery, T. A., Howell, M. D., Allen, E., Dvorak, S. K., Alexander, A. L., et al. (2006). Regulation of AUXIN RESPONSE FACTOR3 by TAS3 ta-siRNA affects developmental timing and patterning in Arabidopsis. Curr. Biol. 16, 939–944. doi: 10.1016/j.cub.2006.03.065
Feng, Z., Sun, X., Wang, G., Liu, H., Zhu, J. (2012). LBD29 regulates the cell cycle progression in response to auxin during lateral root formation in Arabidopsis thaliana. Ann. Bot. 110, 1–10. doi: 10.1093/aob/mcs019
Fessele, K. L., Wright, F. (2018). Primer in genetics and genomics, article 6: basics of epigenetic control. Biol. Res. Nurs. 20, 103–110. doi: 10.1177/1099800417742967
Finet, C., Berne-Dedieu, A., Scutt, C. P., Marletaz, F. (2013). Evolution of the ARF gene family in land plants: old domains, new tricks. Mol. Biol. Evol. 30, 45–56. doi: 10.1093/molbev/mss220
Freire-Rios, A., Tanaka, K., Crespo, I., van der Wijk, E., Sizentsova, Y., Levitsky, V., et al. (2020). Architecture of DNA elements mediating ARF transcription factor binding and auxin-responsive gene expression in Arabidopsis. Proc. Natl. Acad. Sci. 117, 24557–24566. doi: 10.1073/pnas.2009554117
Galli, M., Khakhar, A., Lu, Z., Chen, Z., Sen, S., Joshi, T., et al. (2018). The DNA binding landscape of the maize AUXIN RESPONSE FACTOR family. Nat. Commun. 9, 4526. doi: 10.1038/s41467-018-06977-6
Galli, M., Liu, Q., Moss, B. L., Malcomber, S., Li, W., Gaines, C., et al. (2015). Auxin signaling modules regulate maize inflorescence architecture. Proc. Natl. Acad. Sci. U.S.A. 112, 13372–13377. doi: 10.1073/pnas.1516473112
Gayon, J. (2016). From Mendel to epigenetics: History of genetics. C R Biol. 339, 225–230. doi: 10.1016/j.crvi.2016.05.009
Gifford, M. L., Dean, A., Gutierrez, R. A., Coruzzi, G. M., Birnbaum, K. D. (2008). Cell-specific nitrogen responses mediate developmental plasticity. Proc. Natl. Acad. Sci. U.S.A. 105, 803–808. doi: 10.1073/pnas.0709559105
Goh, T., Kasahara, H., Mimura, T., Kamiya, Y., Fukaki, H. (2012). Multiple AUX/IAA-ARF modules regulate lateral root formation: the role of Arabidopsis SHY2/IAA3-mediated auxin signalling. Philos. Trans. R Soc. Lond B Biol. Sci. 367, 1461–1468. doi: 10.1098/rstb.2011.0232
Goh, T., Toyokura, K., Yamaguchi, N., Okamoto, Y., Uehara, T., Kaneko, S., et al. (2019). Lateral root initiation requires the sequential induction of transcription factors LBD16 and PUCHI in Arabidopsis thaliana. New Phytol. 224, 749–760. doi: 10.1111/nph.16065
Goldental-Cohen, S., Israeli, A., Ori, N., Yasuor, H. (2017). Auxin response dynamics during wild-type and entire flower development in tomato. Plant Cell Physiol. 58, 1661–1672. doi: 10.1093/pcp/pcx102
Guan, C., Wu, B., Yu, T., Wang, Q., Krogan, N. T., Liu, X., et al. (2017). Spatial auxin signaling controls leaf flattening in Arabidopsis. Curr. Biol. 27, 2940–2950 e2944. doi: 10.1016/j.cub.2017.08.042
Guilfoyle, T. J., Hagen, G. (2007). Auxin response factors. Curr. Opin. Plant Biol. 10, 453–460. doi: 10.1016/j.pbi.2007.08.014
Gutierrez, L., Bussell, J. D., Pacurar, D. I., Schwambach, J., Pacurar, M., Bellini, C. (2009). Phenotypic plasticity of adventitious rooting in Arabidopsis is controlled by complex regulation of AUXIN RESPONSE FACTOR transcripts and microRNA abundance. Plant Cell 21, 3119–3132. doi: 10.1105/tpc.108.064758
Ha, C. V., Le, D. T., Nishiyama, R., Watanabe, Y., Sulieman, S., Tran, U. T., et al. (2013). The auxin response factor transcription factor family in soybean: genome-wide identification and expression analyses during development and water stress. DNA Res. 20, 511–524. doi: 10.1093/dnares/dst027
Hagen, G., Guilfoyle, T. (2002). Auxin-responsive gene expression: genes, promoters and regulatory factors. Plant Mol. Biol. 49, 373–385. doi: 10.1023/A:1015207114117
Han, M., Park, Y., Kim, I., Kim, E.-H., Yu, T.-K., Rhee, S., et al. (2014). Structural basis for the auxin-induced transcriptional regulation by Aux/IAA17. Proc. Natl. Acad. Sci. 111, 18613–18618. doi: 10.1073/pnas.1419525112
He, F., Xu, C., Fu, X., Shen, Y., Guo, L., Leng, M., et al. (2018). The MicroRNA390/TRANS-ACTING SHORT INTERFERING RNA3 Module Mediates Lateral Root Growth under Salt Stress via the Auxin Pathway. Plant Physiol. 177, 775–791. doi: 10.1104/pp.17.01559
Herud, O., Weijers, D., Lau, S., Jurgens, G. (2016). Auxin responsiveness of the MONOPTEROS-BODENLOS module in primary root initiation critically depends on the nuclear import kinetics of the Aux/IAA inhibitor BODENLOS. Plant J. 85, 269–277. doi: 10.1111/tpj.13108
Hou, K., Wu, W., Gan, S. S. (2013). SAUR36, a small auxin up RNA gene, is involved in the promotion of leaf senescence in Arabidopsis. Plant Physiol. 161, 1002–1009. doi: 10.1104/pp.112.212787
Huang, J., Li, Z., Zhao, D. (2016). Deregulation of the osmiR160 target gene osARF18 causes growth and developmental defects with an alteration of auxin signaling in rice. Sci. Rep. 6, 29938. doi: 10.1038/srep29938
Huang, K. L., Ma, G. J., Zhang, M. L., Xiong, H., Wu, H., Zhao, C. Z., et al. (2018). The ARF7 and ARF19 transcription factors positively regulate PHOSPHATE STARVATION RESPONSE1 in Arabidopsis roots. Plant Physiol. 178, 413–427. doi: 10.1104/pp.17.01713
Hunter, C., Willmann, M. R., Wu, G., Yoshikawa, M., de la Luz Gutierrez-Nava, M., Poethig, S. R. (2006). Trans-acting siRNA-mediated repression of ETTIN and ARF4 regulates heteroblasty in Arabidopsis. Development 133, 2973–2981. doi: 10.1242/dev.02491
Ishida, T., Kurata, T., Okada, K., Wada, T. (2008). A genetic regulatory network in the development of trichomes and root hairs. Annu. Rev. Plant Biol. 59, 365–386. doi: 10.1146/annurev.arplant.59.032607.092949
Iwasaki, M., Takahashi, H., Iwakawa, H., Nakagawa, A., Ishikawa, T., Tanaka, H., et al. (2013). Dual regulation of ETTIN (ARF3) gene expression by AS1-AS2, which maintains the DNA methylation level, is involved in stabilization of leaf adaxial-abaxial partitioning in Arabidopsis. Development 140, 1958–1969. doi: 10.1242/dev.085365
Jain, M., Khurana, J. P. (2009). Transcript profiling reveals diverse roles of auxin-responsive genes during reproductive development and abiotic stress in rice. FEBS J. 276, 3148–3162. doi: 10.1111/j.1742-4658.2009.07033.x
Jay, F., Wang, Y., Yu, A., Taconnat, L., Pelletier, S., Colot, V., et al. (2011). Misregulation of AUXIN RESPONSE FACTOR 8 underlies the developmental abnormalities caused by three distinct viral silencing suppressors in Arabidopsis. PloS Pathog. 7, e1002035. doi: 10.1371/journal.ppat.1002035
Jia, Z., Giehl, R. F. H., Hartmann, A., Estevez, J. M., Bennett, M. J., von Wiren, N. (2023). A spatially concerted epidermal auxin signaling framework steers the root hair foraging response under low nitrogen. Curr. Biol. 33, 3926–3941 e3925. doi: 10.1016/j.cub.2023.08.040
Jiang, Y., Liang, G., Yang, S., Yu, D. (2014). Arabidopsis WRKY57 functions as a node of convergence for jasmonic acid- and auxin-mediated signaling in jasmonic acid-induced leaf senescence. Plant Cell 26, 230–245. doi: 10.1105/tpc.113.117838
Jing, H., Korasick, D. A., Emenecker, R. J., Morffy, N., Wilkinson, E. G., Powers, S. K., et al. (2022). Regulation of AUXIN RESPONSE FACTOR condensation and nucleo-cytoplasmic partitioning. Nat. Commun. 13, 4015. doi: 10.1038/s41467-022-31628-2
Jing, H., Strader, L. C. (2019). Interplay of auxin and cytokinin in lateral root development. Int. J. Mol. Sci. 20, 486. doi: 10.3390/ijms20030486
Jing, H., Yang, X., Zhang, J., Liu, X., Zheng, H., Dong, G., et al. (2015). Peptidyl-prolyl isomerization targets rice Aux/IAAs for proteasomal degradation during auxin signalling. Nat. Commun. 6, 7395. doi: 10.1038/ncomms8395
Kalsi, H. S., Karkhanis, A. A., Natarajan, B., Bhide, A. J., Banerjee, A. K. (2022). AUXIN RESPONSE FACTOR 16 (StARF16) regulates defense gene StNPR1 upon infection with necrotrophic pathogen in potato. Plant Mol. Biol. 109, 13–28. doi: 10.1007/s11103-022-01261-0
Kambona, C. M., Koua, P. A., Leon, J., Ballvora, A. (2023). Stress memory and its regulation in plants experiencing recurrent drought conditions. Theor. Appl. Genet. 136, 26. doi: 10.1007/s00122-023-04313-1
Kato, H., Mutte, S. K., Suzuki, H., Crespo, I., Das, S., Radoeva, T., et al. (2020). Design principles of a minimal auxin response system. Nat. Plants 6, 473–482. doi: 10.1038/s41477-020-0662-y
Ke, J., Ma, H., Gu, X., Thelen, A., Brunzelle, J. S., Li, J., et al. (2015). Structural basis for recognition of diverse transcriptional repressors by the TOPLESS family of corepressors. Sci. Adv. 1, e1500107. doi: 10.1126/sciadv.1500107
Kim, S. H., Bahk, S., Nguyen, N. T., Pham, M. L. A., Kadam, U. S., Hong, J. C., et al. (2022). Phosphorylation of the auxin signaling transcriptional repressor IAA15 by MPKs is required for the suppression of root development under drought stress in Arabidopsis. Nucleic Acids Res. 50, 10544–10561. doi: 10.1093/nar/gkac798
Kim, J. I., Murphy, A. S., Baek, D., Lee, S. W., Yun, D. J., Bressan, R. A., et al. (2011). YUCCA6 over-expression demonstrates auxin function in delaying leaf senescence in Arabidopsis thaliana. J. Exp. Bot. 62, 3981–3992. doi: 10.1093/jxb/err094
Kim, Y., Park, C., Cha, S., Han, M., Ryu, K. S., Suh, J. Y. (2020). Determinants of PB1 domain interactions in auxin response factor ARF5 and repressor IAA17. J. Mol. Biol. 432, 4010–4022. doi: 10.1016/j.jmb.2020.04.007
Kinoshita, N., Wang, H., Kasahara, H., Liu, J., Macpherson, C., Machida, Y., et al. (2012). IAA-Ala Resistant3, an evolutionarily conserved target of miR167, mediates Arabidopsis root architecture changes during high osmotic stress. Plant Cell 24, 3590–3602. doi: 10.1105/tpc.112.097006
Kirolinko, C., Hobecker, K., Wen, J., Mysore, K. S., Niebel, A., Blanco, F. A., et al. (2021). Auxin response factor 2 (ARF2), ARF3, and ARF4 mediate both lateral root and nitrogen fixing nodule development in medicago truncatula. Front. Plant Sci. 12. doi: 10.3389/fpls.2021.659061
Kong, X., Zhang, L., Ding, Z. (2016). 26S proteasome: hunter and prey in auxin signaling. Trends Plant Sci. 21, 546–548. doi: 10.1016/j.tplants.2016.05.007
Korasick, D. A., Westfall, C. S., Lee, S. G., Nanao, M. H., Dumas, R., Hagen, G., et al. (2014). Molecular basis for AUXIN RESPONSE FACTOR protein interaction and the control of auxin response repression. Proc. Natl. Acad. Sci. 111, 5427–5432. doi: 10.1073/pnas.1400074111
Kou, X. Z. X., Wu, B., Wang, C. W. C., Yang, S., Zhou, J., Xue, Z. (2022). Auxin response factors are ubiquitous in plant growth and development, and involved in crosstalk between plant hormoness: A review. Appl. Sci. 22, 1360. doi: 10.3390/app12031360
Koukalova, B., Fojtova, M., Lim, K. Y., Fulnecek, J., Leitch, A. R., Kovarik, A. (2005). Dedifferentiation of tobacco cells is associated with ribosomal RNA gene hypomethylation, increased transcription, and chromatin alterations. Plant Physiol. 139, 275–286. doi: 10.1104/pp.105.061788
Kranner, I., Minibayeva, F. V., Beckett, R. P., Seal, C. E. (2010). What is stress? Concepts, definitions and applications in seed science. New Phytol. 188, 655–673. doi: 10.1111/j.1469-8137.2010.03461.x
Krogan, N. T., Ckurshumova, W., Marcos, D., Caragea, A. E., Berleth, T. (2012). Deletion of MP/ARF5 domains III and IV reveals a requirement for Aux/IAA regulation in Arabidopsis leaf vascular patterning. New Phytol. 194, 391–401. doi: 10.1111/j.1469-8137.2012.04064.x
Kuhn, A., Ramans Harborough, S., McLaughlin, H. M., Natarajan, B., Verstraeten, I., Friml, J., et al. (2020). Direct ETTIN-auxin interaction controls chromatin states in gynoecium development. Elife 9, e51787. doi: 10.7554/eLife.51787.sa2
Lambert, S. A., Jolma, A., Campitelli, L. F., Das, P. K., Yin, Y., Albu, M., et al. (2018). The human transcription factors. Cell 172, 650–665. doi: 10.1016/j.cell.2018.01.029
Lanctot, A., Taylor-Teeples, M., Oki, E. A., Nemhauser, J. L. (2020). Specificity in auxin responses is not explained by the promoter preferences of activator ARFs. Plant Physiol. 182, 1533–1536. doi: 10.1104/pp.19.01474
Lavy, M., Prigge, M. J., Tao, S., Shain, S., Kuo, A., Kirchsteiger, K., et al. (2016). Constitutive auxin response in Physcomitrella reveals complex interactions between Aux/IAA and ARF proteins. Elife 5, e13325. doi: 10.7554/eLife.13325.025
Lee, H. W., Cho, C., Pandey, S. K., Park, Y., Kim, M. J., Kim, J. (2019). LBD16 and LBD18 acting downstream of ARF7 and ARF19 are involved in adventitious root formation in Arabidopsis. BMC Plant Biol. 19, 46. doi: 10.1186/s12870-019-1659-4
Lee, M., Jung, J. H., Han, D. Y., Seo, P. J., Park, W. J., Park, C. M. (2012). Activation of a flavin monooxygenase gene YUCCA7 enhances drought resistance in Arabidopsis. Planta 235, 923–938. doi: 10.1007/s00425-011-1552-3
Lee, H. W., Kim, M. J., Kim, N. Y., Lee, S. H., Kim, J. (2013). LBD18 acts as a transcriptional activator that directly binds to the EXPANSIN14 promoter in promoting lateral root emergence of Arabidopsis. Plant J. 73, 212–224. doi: 10.1111/tpj.12013
Li, H., Johnson, P., Stepanova, A., Alonso, J. M., Ecker, J. R. (2004). Convergence of signaling pathways in the control of differential cell growth in Arabidopsis. Dev. Cell 7, 193–204. doi: 10.1016/j.devcel.2004.07.002
Li, Y., Li, J., Chen, Z., Wei, Y., Qi, Y., Wu, C. (2020). OsmiR167a-targeted auxin response factors modulate tiller angle via fine-tuning auxin distribution in rice. Plant Biotechnol. J. 18, 2015–2026. doi: 10.1111/pbi.13360
Li, G., Liang, W., Zhang, X., Ren, H., Hu, J., Bennett, M. J., et al. (2014). Rice actin-binding protein RMD is a key link in the auxin–actin regulatory loop that controls cell growth. Proc. Natl. Acad. Sci. 111, 10377–10382. doi: 10.1073/pnas.1401680111
Li, W., Liu, H., Cheng, Z. J., Su, Y. H., Han, H. N., Zhang, Y., et al. (2011). DNA methylation and histone modifications regulate de novo shoot regeneration in Arabidopsis by modulating WUSCHEL expression and auxin signaling. PloS Genet. 7, e1002243. doi: 10.1371/journal.pgen.1002243
Li, H., Liu, H., Hao, C., Li, T., Liu, Y., Wang, X., et al. (2023). The auxin response factor TaARF15-A1 negatively regulates senescence in common wheat (Triticum aestivum L.). Plant Physiol. 191, 1254–1271. doi: 10.1093/plphys/kiac497
Li, K., Wang, S., Wu, H., Wang, H. (2020). Protein levels of several Arabidopsis auxin response factors are regulated by multiple factors and ABA promotes ARF6 protein ubiquitination. Int. J. Mol. Sci. 21, 9437. doi: 10.3390/ijms21249437
Li, J., Wu, F., He, Y., He, B., Gong, Y., Yahaya, B. S., et al. (2022). Maize transcription factor zmARF4 confers phosphorus tolerance by promoting root morphological development. Int. J. Mol. Sci. 23, 2361. doi: 10.3390/ijms23042361
Li, H., Zhang, X., Tong, B., Wang, Y., Yang, C. (2020). Expression analysis of the BpARF genes in Betula platyphylla under drought stress. Plant Physiol. Biochem. 148, 273–281. doi: 10.1016/j.plaphy.2020.01.028
Liang, T., Hu, Y., Xi, N., Zhang, M., Zou, C., Ge, F., et al. (2023). GWAS across multiple environments and WGCNA suggest the involvement of ZmARF23 in embryonic callus induction from immature maize embryos. Theor. Appl. Genet. 136, 93. doi: 10.1007/s00122-023-04341-x
Lin, C., Sauter, M. (2018). Control of adventitious root architecture in rice by darkness, light, and gravity. Plant Physiol. 176, 1352–1364. doi: 10.1104/pp.17.01540
Liu, X., Dinh, T. T., Li, D., Shi, B., Li, Y., Cao, X., et al. (2014). AUXIN RESPONSE FACTOR 3 integrates the functions of AGAMOUS and APETALA2 in floral meristem determinacy. Plant J. 80, 629–641. doi: 10.1111/tpj.12658
Liu, X., Dong, X., Liu, Z., Shi, Z., Jiang, Y., Qi, M., et al. (2016). Repression of ARF10 by microRNA160 plays an important role in the mediation of leaf water loss. Plant Mol. Biol. 92, 313–336. doi: 10.1007/s11103-016-0514-3
Liu, Y., Teng, C., Xia, R., Meyers, B. C. (2020). PhasiRNAs in plants: their biogenesis, genic sources, and roles in stress responses, development, and reproduction. Plant Cell 32, 3059–3080. doi: 10.1105/tpc.20.00335
Liu, Z. B., Ulmasov, T., Shi, X., Hagen, G., Guilfoyle, T. J. (1994). Soybean GH3 promoter contains multiple auxin-inducible elements. Plant Cell 6, 645–657. doi: 10.1105/tpc.6.5.645
Liu, H., Wang, S., Yu, X., Yu, J., He, X., Zhang, S., et al. (2005). ARL1, a LOB-domain protein required for adventitious root formation in rice. Plant J. 43, 47–56. doi: 10.1111/j.1365-313X.2005.02434.x
Liu, X., Yang, C. Y., Miao, R., Zhou, C. L., Cao, P. H., Lan, J., et al. (2018). DS1/OsEMF1 interacts with OsARF11 to control rice architecture by regulation of brassinosteroid signaling. Rice (N Y) 11, 46. doi: 10.1186/s12284-018-0239-9
Liu, S., Zhang, Y., Feng, Q., Qin, L., Pan, C., Lamin-Samu, A. T., et al. (2018). Tomato AUXIN RESPONSE FACTOR 5 regulates fruit set and development via the mediation of auxin and gibberellin signaling. Sci. Rep. 8, 2971. doi: 10.1038/s41598-018-21315-y
Long, J. A., Ohno, C., Smith, Z. R., Meyerowitz, E. M. (2006). TOPLESS regulates apical embryonic fate in Arabidopsis. Science 312, 1520–1523. doi: 10.1126/science.1123841
Luo, P., Di, D., Wu, L., Yang, J., Lu, Y., Shi, W. (2022). MicroRNAs are involved in regulating plant development and stress response through fine-tuning of TIR1/AFB-dependent auxin signaling. Int. J. Mol. Sci. 23, 510. doi: 10.3390/ijms23010510
Luo, W. G., Liang, Q. W., Su, Y., Huang, C., Mo, B. X., Yu, Y., et al. (2023). Auxin inhibits chlorophyll accumulation through ARF7-IAA14-mediated repression of chlorophyll biosynthesis genes in Arabidopsis. Front. Plant Sci. 14, 1172059. doi: 10.3389/fpls.2023.1172059
Luo, L., Xie, Y., Xuan, W. (2022). Prohibitin 3 gives birth to a new lateral root primordium. J. Exp. Bot. 73, 3828–3830. doi: 10.1093/jxb/erac175
Lv, B., Yu, Q., Liu, J., Wen, X., Yan, Z., Hu, K., et al. (2020). Non-canonical AUX/IAA protein IAA33 competes with canonical AUX/IAA repressor IAA5 to negatively regulate auxin signaling. EMBO J. 39, e101515. doi: 10.15252/embj.2019101515
Lyons, R., Stiller, J., Powell, J., Rusu, A., Manners, J. M., Kazan, K. (2015). Fusarium oxysporum triggers tissue-specific transcriptional reprogramming in Arabidopsis thaliana. PloS One 10, e0121902. doi: 10.1371/journal.pone.0121902
Ma, C., Dang, K., Xie, Q., Sahito, J. H., Yuan, B., Wan, J., et al. (2023). Over-expression of zmIAA29, an AUX/IAA transcription factor, improved maize flowering time. Agronomy 13, 2028. doi: 10.3390/agronomy13082028
Majer, C., Xu, C., Berendzen, K. W., Hochholdinger, F. (2012). Molecular interactions of ROOTLESS CONCERNING CROWN AND SEMINAL ROOTS, a LOB domain protein regulating shoot-borne root initiation in maize (Zea mays L.). Philos. Trans. R Soc. Lond B Biol. Sci. 367, 1542–1551. doi: 10.1098/rstb.2011.0238
Mallory, A. C., Bartel, D. P., Bartel, B. (2005). MicroRNA-directed regulation of Arabidopsis AUXIN RESPONSE FACTOR17 is essential for proper development and modulates expression of early auxin response genes. Plant Cell 17, 1360–1375. doi: 10.1105/tpc.105.031716
Mantri, N., Patade, V., Penna, S., Ford, R., Pang, E. (2012). “Abiotic stress responses in plants: present and future,” in Abiotic stress responses in plants (Springer, New York), 1–19. doi: 10.1007/978-1-4614-0634-1_1
Mao, C., He, J., Liu, L., Deng, Q., Yao, X., Liu, C., et al. (2020). OsNAC2 integrates auxin and cytokinin pathways to modulate rice root development. Plant Biotechnol. J. 18, 429–442. doi: 10.1111/pbi.13209
Marin, E., Jouannet, V., Herz, A., Lokerse, A. S., Weijers, D., Vaucheret, H., et al. (2010). miR390, Arabidopsis TAS3 tasiRNAs, and their AUXIN RESPONSE FACTOR targets define an autoregulatory network quantitatively regulating lateral root growth. Plant Cell 22, 1104–1117. doi: 10.1105/tpc.109.072553
Matsui, A., Mizunashi, K., Tanaka, M., Kaminuma, E., Nguyen, A. H., Nakajima, M., et al. (2014). tasiRNA-ARF pathway moderates floral architecture in Arabidopsis Plants subjected to drought stress. BioMed. Res. Int. 2014, 1–10. doi: 10.1155/2014/303451
Meng, L. S., Wang, Z. B., Yao, S. Q., Liu, A. (2015). The ARF2-ANT-COR15A gene cascade regulates ABA-signaling-mediated resistance of large seeds to drought in Arabidopsis. J. Cell Sci. 128, 3922–3932. doi: 10.1242/jcs.171207
Mizoguchi, T., Gotoh, Y., Nishida, E., Yamaguchi-Shinozaki, K., Hayashida, N., Iwasaki, T., et al. (1994). Characterization of two cDNAs that encode MAP kinase homologues in Arabidopsis thaliana and analysis of the possible role of auxin in activating such kinase activities in cultured cells. Plant J. 5, 111–122. doi: 10.1046/j.1365-313X.1994.5010111.x
Moller, B. K., Ten Hove, C. A., Xiang, D., Williams, N., Lopez, L. G., Yoshida, S., et al. (2017). Auxin response cell-autonomously controls ground tissue initiation in the early Arabidopsis embryo. Proc. Natl. Acad. Sci. U.S.A. 114, E2533–E2539. doi: 10.1073/pnas.1616493114
Mutte, S. K., Kato, H., Rothfels, C., Melkonian, M., Wong, G. K., Weijers, D. (2018). Origin and evolution of the nuclear auxin response system. Elife 7, e33399. doi: 10.7554/eLife.33399.035
Nanao, M. H., Vinos-Poyo, T., Brunoud, G., Thevenon, E., Mazzoleni, M., Mast, D., et al. (2014). Structural basis for oligomerization of auxin transcriptional regulators. Nat. Commun. 5, 3617. doi: 10.1038/ncomms4617
Natarajan, B., Kalsi, H. S., Godbole, P., Malankar, N., Thiagarayaselvam, A., Siddappa, S., et al. (2018). MiRNA160 is associated with local defense and systemic acquired resistance against Phytophthora infestans infection in potato. J. Exp. Bot. 69, 2023–2036. doi: 10.1093/jxb/ery025
Nestler, J., Keyes, S. D., Wissuwa, M. (2016). Root hair formation in rice (Oryza sativa L.) differs between root types and is altered in artificial growth conditions. J. Exp. Bot. 67, 3699–3708. doi: 10.1093/jxb/erw115
Niederhuth, C. E., Patharkar, O. R., Walker, J. C. (2013). Transcriptional profiling of the Arabidopsis abscission mutant hae hsl2 by RNA-Seq. BMC Genomics 14, 37. doi: 10.1186/1471-2164-14-37
Nishimura, T., Wada, T., Yamamoto, K. T., Okada, K. (2005). The Arabidopsis STV1 protein, responsible for translation reinitiation, is required for auxin-mediated gynoecium patterning. Plant Cell 17, 2940–2953. doi: 10.1105/tpc.105.036533
Niu, D., Lin, X. L., Kong, X., Qu, G. P., Cai, B., Lee, J., et al. (2019). SIZ1-mediated SUMOylation of TPR1 suppresses plant immunity in Arabidopsis. Mol. Plant 12, 215–228. doi: 10.1016/j.molp.2018.12.002
Oguchi, R., Hikosaka, K., Hirose, T. (2003). Does the photosynthetic light-acclimation need change in leaf anatomy? Plant Cell Environ. 26, 505–512. doi: 10.1046/j.1365-3040.2003.00981.x
Okushima, Y., Fukaki, H., Onoda, M., Theologis, A., Tasaka, M. (2007). ARF7 and ARF19 regulate lateral root formation via direct activation of LBD/ASL genes in Arabidopsis. Plant Cell 19, 118–130. doi: 10.1105/tpc.106.047761
Okushima, Y., Overvoorde, P. J., Arima, K., Alonso, J. M., Chan, A., Chang, C., et al. (2005). Functional genomic analysis of the AUXIN RESPONSE FACTOR gene family members in Arabidopsis thaliana: unique and overlapping functions of ARF7 and ARF19. Plant Cell 17, 444–463. doi: 10.1105/tpc.104.028316
O’Malley, R. C., Huang, S. C., Song, L., Lewsey, M. G., Bartlett, A., Nery, J. R., et al. (2016). Cistrome and epicistrome features shape the regulatory DNA landscape. Cell 166, 1598. doi: 10.1016/j.cell.2016.08.063
Ori, N. (2019). Dissecting the biological functions of ARF and aux/IAA genes. Plant Cell 31, 1210–1211. doi: 10.1105/tpc.19.00330
Orosa-Puente, B., Leftley, N., von Wangenheim, D., Banda, J., Srivastava, A. K., Hill, K., et al. (2018). Root branching toward water involves posttranslational modification of transcription factor ARF7. Science 362, 1407–1410. doi: 10.1126/science.aau3956
Overvoorde, P., Fukaki, H., Beeckman, T. (2010). Auxin control of root development. Cold Spring Harb. Perspect. Biol. 2, a001537. doi: 10.1101/cshperspect.a001537
Ozerova, L. V., Krasnikova, M. S., Troitsky, A. V., Solovyev, A. G., Morozov, S. Y. (2013). TAS3 Genes for small ta-siARF RNAs in plants belonging to subtribe Senecioninae: occurrence of prematurely terminated RNA precursors. Mol. Gen. Mikrobiol Virusol 2), 33–36. doi: 10.3103/S0891416813020043
Pandey, S. K., Lee, H. W., Kim, M. J., Cho, C., Oh, E., Kim, J. (2018). LBD18 uses a dual mode of a positive feedback loop to regulate ARF expression and transcriptional activity in Arabidopsis. Plant J. 95, 233–251. doi: 10.1111/tpj.13945
Parcy, F., Vernoux, T., Dumas, R. (2016). A glimpse beyond structures in auxin-dependent transcription. Trends Plant Sci. 21, 574–583. doi: 10.1016/j.tplants.2016.02.002
Peret, B., De Rybel, B., Casimiro, I., Benkova, E., Swarup, R., Laplaze, L., et al. (2009). Arabidopsis lateral root development: an emerging story. Trends Plant Sci. 14, 399–408. doi: 10.1016/j.tplants.2009.05.002
Perrot-Rechenmann, C. (2010). Cellular responses to auxin: division versus expansion. Cold Spring Harb. Perspect. Biol. 2, a001446. doi: 10.1101/cshperspect.a001446
Petricka, J. J., Winter, C. M., Benfey, P. N. (2012). Control of Arabidopsis root development. Annu. Rev. Plant Biol. 63, 563–590. doi: 10.1146/annurev-arplant-042811-105501
Pierre-Jerome, E., Moss, B. L., Lanctot, A., Hageman, A., Nemhauser, J. L. (2016). Functional analysis of molecular interactions in synthetic auxin response circuits. Proc. Natl. Acad. Sci. U.S.A. 113, 11354–11359. doi: 10.1073/pnas.1604379113
Pinweha, N., Asvarak, T., Viboonjun, U., Narangajavana, J. (2015). Involvement of miR160/miR393 and their targets in cassava responses to anthracnose disease. J. Plant Physiol. 174, 26–35. doi: 10.1016/j.jplph.2014.09.006
Piya, S., Shrestha, S. K., Binder, B., Stewart, C. N., Jr., Hewezi, T. (2014). Protein-protein interaction and gene co-expression maps of ARFs and Aux/IAAs in Arabidopsis. Front. Plant Sci. 5. doi: 10.3389/fpls.2014.00744
Powers, S. K., Holehouse, A. S., Korasick, D. A., Schreiber, K. H., Clark, N. M., Jing, H., et al. (2019). Nucleo-cytoplasmic partitioning of ARF proteins controls auxin responses in Arabidopsis thaliana. Mol. Cell 76, 177–190 e175. doi: 10.1016/j.molcel.2019.06.044
Powers, S. K., Strader, L. C. (2020). Regulation of auxin transcriptional responses. Dev. Dyn 249, 483–495. doi: 10.1002/dvdy.139
Puga, M. I., Rojas-Triana, M., de Lorenzo, L., Leyva, A., Rubio, V., Paz-Ares, J. (2017). Novel signals in the regulation of Pi starvation responses in plants: facts and promises. Curr. Opin. Plant Biol. 39, 40–49. doi: 10.1016/j.pbi.2017.05.007
Qi, Y., Wang, S., Shen, C., Zhang, S., Chen, Y., Xu, Y., et al. (2012). OsARF12, a transcription activator on auxin response gene, regulates root elongation and affects iron accumulation in rice (Oryza sativa). New Phytol. 193, 109–120. doi: 10.1111/j.1469-8137.2011.03910.x
Qiao, J., Jiang, H., Lin, Y., Shang, L., Wang, M., Li, D., et al. (2021). A novel miR167a-OsARF6-OsAUX3 module regulates grain length and weight in rice. Mol. Plant 14, 1683–1698. doi: 10.1016/j.molp.2021.06.023
Qiao, J., Zhang, Y., Han, S., Chang, S., Gao, Z., Qi, Y., et al. (2022). OsARF4 regulates leaf inclination via auxin and brassinosteroid pathways in rice. Front. Plant Sci. 13. doi: 10.3389/fpls.2022.979033
Qiao, L., Zhang, W., Li, X., Zhang, L., Zhang, X., Li, X., et al. (2018). Characterization and expression patterns of auxin response factors in wheat. Front. Plant Sci. 9. doi: 10.3389/fpls.2018.01395
Qin, Q., Li, G., Jin, L., Huang, Y., Wang, Y., Wei, C., et al. (2020). Auxin response factors (ARFs) differentially regulate rice antiviral immune response against rice dwarf virus. PloS Pathog. 16, e1009118. doi: 10.1371/journal.ppat.1009118
Quint, M., Gray, W. M. (2006). Auxin signaling. Curr. Opin. Plant Biol. 9, 448–453. doi: 10.1016/j.pbi.2006.07.006
Ramazi, S., Allahverdi, A., Zahiri, J. (2020). Evaluation of post-translational modifications in histone proteins: A review on histone modification defects in developmental and neurological disorders. J. Biosci. 45, 135. doi: 10.1007/s12038-020-00099-2
Roosjen, M., Paque, S., Weijers, D. (2018). Auxin Response Factors: output control in auxin biology. J. Exp. Bot. 69, 179–188. doi: 10.1093/jxb/erx237
Saidi, A., Hajibarat, Z. (2020). Computational study of environmental stress-related transcription factor binding sites in the promoter regions of maize auxin response factor (ARF) gene family. Not Sci. Biol. 12, 646–657. doi: 10.15835/nsb12310823
Sainju, U. M., Singh, B. P., Whitehead, W. F. (2005). Tillage, cover crops, and nitrogen effects on cotton and sorghum root biomass, carbon, and nitrogen. Agron. J. 97, 1279–1290. doi: 10.2134/agronj2004.0213
Sakamoto, T., Morinaka, Y., Inukai, Y., Kitano, H., Fujioka, S. (2013). Auxin signal transcription factor regulates expression of the brassinosteroid receptor gene in rice. Plant J. 73, 676–688. doi: 10.1111/tpj.12071
Salmon, J., Ramos, J., Callis, J. (2008). Degradation of the auxin response factor ARF1. Plant J. 54, 118–128. doi: 10.1111/j.1365-313X.2007.03396.x
Scacchi, E., Salinas, P., Gujas, B., Santuari, L., Krogan, N., Ragni, L., et al. (2010). Spatio-temporal sequence of cross-regulatory events in root meristem growth. Proc. Natl. Acad. Sci. U.S.A. 107, 22734–22739. doi: 10.1073/pnas.1014716108
Scheres, B., van der Putten, W. H. (2017). The plant perceptron connects environment to development. Nature 543, 337–345. doi: 10.1038/nature22010
Schlereth, A., Moller, B., Liu, W., Kientz, M., Flipse, J., Rademacher, E. H., et al. (2010). MONOPTEROS controls embryonic root initiation by regulating a mobile transcription factor. Nature 464, 913–916. doi: 10.1038/nature08836
Schuetz, M., Fidanza, M., Mattsson, J. (2019). Identification of auxin response factor-encoding genes expressed in distinct phases of leaf vein development and with overlapping functions in leaf formation. Plants (Basel) 8, 242. doi: 10.3390/plants8070242
Sessions, A., Nemhauser, J. L., McColl, A., Roe, J. L., Feldmann, K. A., Zambryski, P. C. (1997). ETTIN patterns the Arabidopsis floral meristem and reproductive organs. Development 124, 4481–4491. doi: 10.1242/dev.124.22.4481
Shahzad, Z., Eaglesfield, R., Carr, C., Amtmann, A. (2020). Cryptic variation in RNA-directed DNA-methylation controls lateral root development when auxin signalling is perturbed. Nat. Commun. 11, 218. doi: 10.1038/s41467-019-13927-3
Shen, C., Wang, S., Zhang, S., Xu, Y., Qian, Q., Qi, Y., et al. (2013). OsARF16, a transcription factor, is required for auxin and phosphate starvation response in rice (Oryza sativa L.). Plant Cell Environ. 36, 607–620. doi: 10.1111/pce.12001
Shen, C., Yue, R., Sun, T., Zhang, L., Yang, Y., Wang, H. (2015). OsARF16, a transcription factor regulating auxin redistribution, is required for iron deficiency response in rice (Oryza sativa L.). Plant Sci. 231, 148–158. doi: 10.1016/j.plantsci.2014.12.003
Shen, C., Yue, R., Yang, Y., Zhang, L., Sun, T., Tie, S., et al. (2014). OsARF16 is involved in cytokinin-mediated inhibition of phosphate transport and phosphate signaling in rice (Oryza sativa L.). PloS One 9, e112906. doi: 10.1371/journal.pone.0112906
Sheng, H., Cong, D. L., Ju, H. Y. (2020). [Functional characterization of zmHAK1 promoter and its regulatory transcription factors in maize]. Mol. Biol. (Mosk) 54, 374–388. doi: 10.1134/S0026893320030152
Si, F., Yang, C., Yan, B., Yan, W., Tang, S., Yan, Y., et al. (2022). Control of OsARF3a by OsKANADI1 contributes to lemma development in rice. Plant J. 110, 1717–1730. doi: 10.1111/tpj.15766
Simonini, S., Deb, J., Moubayidin, L., Stephenson, P., Valluru, M., Freire-Rios, A., et al. (2016). A noncanonical auxin-sensing mechanism is required for organ morphogenesis in Arabidopsis. Genes Dev. 30, 2286–2296. doi: 10.1101/gad.285361.116
Singh, S., Singh, A. (2021). A prescient evolutionary model for genesis, duplication and differentiation of MIR160 homologs in Brassicaceae. Mol. Genet. Genomics 296, 985–1003. doi: 10.1007/s00438-021-01797-8
Singh, S., Yadav, S., Singh, A., Mahima, M., Singh, A., Gautam, V., et al. (2020). Auxin signaling modulates LATERAL ROOT PRIMORDIUM1 (LRP1) expression during lateral root development in Arabidopsis. Plant J. 101, 87–100. doi: 10.1111/tpj.14520
Spitz, F., Furlong, E. E. (2012). Transcription factors: from enhancer binding to developmental control. Nat. Rev. Genet. 13, 613–626. doi: 10.1038/nrg3207
Stigliani, A., Martin-Arevalillo, R., Lucas, J., Bessy, A., Vinos-Poyo, T., Mironova, V., et al. (2019). Capturing auxin response factors syntax using DNA binding models. Mol. Plant 12, 822–832. doi: 10.1016/j.molp.2018.09.010
Stoeckle, D., Thellmann, M., Vermeer, J. E. (2018). Breakout-lateral root emergence in Arabidopsis thaliana. Curr. Opin. Plant Biol. 41, 67–72. doi: 10.1016/j.pbi.2017.09.005
Stone, L. R., Goodrum, D. E., Jaafar, M. N., Khan, A. H. (2001). Rooting front and water depletion depths in grain sorghum and sunflower. Agron. J. 93, 1105–1110. doi: 10.2134/agronj2001.9351105x
Suter, D. M. (2020). Transcription factors and DNA play hide and seek. Trends Cell Biol. 30, 491–500. doi: 10.1016/j.tcb.2020.03.003
Szemenyei, H., Hannon, M., Long, J. A. (2008). TOPLESS mediates auxin-dependent transcriptional repression during Arabidopsis embryogenesis. Science 319, 1384–1386. doi: 10.1126/science.1151461
Tabata, R., Ikezaki, M., Fujibe, T., Aida, M., Tian, C. E., Ueno, Y., et al. (2010). Arabidopsis auxin response factor6 and 8 regulate jasmonic acid biosynthesis and floral organ development via repression of class 1 KNOX genes. Plant Cell Physiol. 51, 164–175. doi: 10.1093/pcp/pcp176
Takahashi, H., Iwakawa, H., Ishibashi, N., Kojima, S., Matsumura, Y., Prananingrum, P., et al. (2013). Meta-analyses of microarrays of Arabidopsis asymmetric leaves1 (as1), as2 and their modifying mutants reveal a critical role for the ETT pathway in stabilization of adaxial-abaxial patterning and cell division during leaf development. Plant Cell Physiol. 54, 418–431. doi: 10.1093/pcp/pct027
Tan, S., Luschnig, C., Friml, J. (2021). Pho-view of auxin: reversible protein phosphorylation in auxin biosynthesis, transport and signaling. Mol. Plant 14, 151–165. doi: 10.1016/j.molp.2020.11.004
Tantikanjana, T., Nasrallah, J. B. (2012). Non-cell-autonomous regulation of crucifer self-incompatibility by Auxin Response Factor ARF3. Proc. Natl. Acad. Sci. U.S.A. 109, 19468–19473. doi: 10.1073/pnas.1217343109
Tiwari, S. B., Hagen, G., Guilfoyle, T. (2003). The roles of auxin response factor domains in auxin-responsive transcription. Plant Cell 15, 533–543. doi: 10.1105/tpc.008417
Uga, Y., Sugimoto, K., Ogawa, S., Rane, J., Ishitani, M., Hara, N., et al. (2013). Control of root system architecture by DEEPER ROOTING 1 increases rice yield under drought conditions. Nat. Genet. 45, 1097–1102. doi: 10.1038/ng.2725
Ulmasov, T., Hagen, G., Guilfoyle, T. J. (1997). ARF1, a transcription factor that binds to auxin response elements. Science 276, 1865–1868. doi: 10.1126/science.276.5320.1865
Ulmasov, T., Liu, Z. B., Hagen, G., Guilfoyle, T. J. (1995). Composite structure of auxin response elements. Plant Cell 7, 1611–1623. doi: 10.1105/tpc.7.10.1611
Vernoux, T., Brunoud, G., Farcot, E., Morin, V., Van den Daele, H., Legrand, J., et al. (2011). The auxin signalling network translates dynamic input into robust patterning at the shoot apex. Mol. Syst. Biol. 7, 508. doi: 10.1038/msb.2011.39
Vierstra, R. D. (2009). The ubiquitin-26S proteasome system at the nexus of plant biology. Nat. Rev. Mol. Cell Biol. 10, 385–397. doi: 10.1038/nrm2688
von Behrens, I., Komatsu, M., Zhang, Y., Berendzen, K. W., Niu, X., Sakai, H., et al. (2011). Rootless with undetectable meristem 1 encodes a monocot-specific AUX/IAA protein that controls embryonic seminal and post-embryonic lateral root initiation in maize. Plant J. 66, 341–353. doi: 10.1111/j.1365-313X.2011.04495.x
Waller, F., Furuya, M., Nick, P. (2002). OsARF1, an auxin response factor from rice, is auxin-regulated and classifies as a primary auxin responsive gene. Plant Mol. Biol. 50, 415–425. doi: 10.1023/A:1019818110761
Wan, S., Li, W., Zhu, Y., Liu, Z., Huang, W., Zhan, J. (2014). Genome-wide identification, characterization and expression analysis of the auxin response factor gene family in Vitis vinifera. Plant Cell Rep. 33, 1365–1375. doi: 10.1007/s00299-014-1622-7
Wang, K., Chen, Y., Chang, E. A., Knott, J. G., Cibelli, J. B. (2009). Dynamic epigenetic regulation of the Oct4 and Nanog regulatory regions during neural differentiation in rhesus nuclear transfer embryonic stem cells. Cloning Stem Cells 11, 483–496. doi: 10.1089/clo.2009.0019
Wang, Y., Deng, D., Bian, Y., Lv, Y., Xie, Q. (2010). Genome-wide analysis of primary auxin-responsive Aux/IAA gene family in maize (Zea mays. L.). Mol. Biol. Rep. 37, 3991–4001. doi: 10.1007/s11033-010-0058-6
Wang, R., Estelle, M. (2014). Diversity and specificity: auxin perception and signaling through the TIR1/AFB pathway. Curr. Opin. Plant Biol. 21, 51–58. doi: 10.1016/j.pbi.2014.06.006
Wang, M., Zheng, Q., Shen, Q., Guo, S. (2013). The critical role of potassium in plant stress response. Int. J. Mol. Sci. 14, 7370–7390. doi: 10.3390/ijms14047370
Wang, Y. F., Hou, X. Y., Deng, J. J., Yao, Z. H., Lyu, M. M., Zhang, R. S. (2020). AUXIN RESPONSE FACTOR 1 acts as a positive regulator in the response of poplar to trichoderma asperellum inoculation in overexpressing plants. Plants (Basel) 9, 272. doi: 10.3390/plants9020272
Wang, L., Hua, D., He, J., Duan, Y., Chen, Z., Hong, X., et al. (2011). Auxin Response Factor2 (ARF2) and its regulated homeodomain gene HB33 mediate abscisic acid response in Arabidopsis. PloS Genet. 7, e1002172. doi: 10.1371/journal.pgen.1002172
Wang, Y., Li, K., Chen, L., Zou, Y., Liu, H., Tian, Y., et al. (2015). MicroRNA167-directed regulation of the auxin response factors gmARF8a and gmARF8b is required for soybean nodulation and lateral root development. Plant Physiol. 168, 984–999. doi: 10.1104/pp.15.00265
Wang, D., Pei, K., Fu, Y., Sun, Z., Li, S., Liu, H., et al. (2007). Genome-wide analysis of the auxin response factors (ARF) gene family in rice (Oryza sativa). Gene 394, 13–24. doi: 10.1016/j.gene.2007.01.006
Wang, G., Wang, F., Huang, Q., Li, Y., Liu, Y., Wang, Y. (2015). Understanding transcription factor regulation by integrating gene expression and DNase I hypersensitive sites. BioMed. Res. Int. 2015, 757530. doi: 10.1155/2015/757530
Wang, J. W., Wang, L. J., Mao, Y. B., Cai, W. J., Xue, H. W., Chen, X. Y. (2005). Control of root cap formation by MicroRNA-targeted auxin response factors in Arabidopsis. Plant Cell 17, 2204–2216. doi: 10.1105/tpc.105.033076
Wang, S., Zhang, S., Sun, C., Xu, Y., Chen, Y., Yu, C., et al. (2014). Auxin response factor (OsARF12), a novel regulator for phosphate homeostasis in rice (Oryza sativa). New Phytol. 201, 91–103. doi: 10.1111/nph.12499
Weijers, D., Friml, J. (2009). SnapShot: Auxin signaling and transport. Cell 136, 1172 e1171. doi: 10.1016/j.cell.2009.03.009
Weijers, D., Wagner, D. (2016). Transcriptional responses to the auxin hormone. Annu. Rev. Plant Biol. 67, 539–574. doi: 10.1146/annurev-arplant-043015-112122
Weiste, C., Droge-Laser, W. (2014). The Arabidopsis transcription factor bZIP11 activates auxin-mediated transcription by recruiting the histone acetylation machinery. Nat. Commun. 5, 3883. doi: 10.1038/ncomms4883
Wen, F. L., Yue, Y., He, T. F., Gao, X. M., Zhou, Z. S., Long, X. H. (2020). Identification of miR390-TAS3-ARF pathway in response to salt stress in Helianthus tuberosus L. Gene 738, 144460. doi: 10.1016/j.gene.2020.144460
Wright, R. C., Nemhauser, J. L. (2015). New tangles in the auxin signaling web. F1000Prime Rep. 7, 19. doi: 10.12703/P7-19
Wu, F., Liu, Z., Xu, J., Gao, S., Lin, H., Liu, L., et al. (2016). Molecular evolution and association of natural variation in zmARF31 with low phosphorus tolerance in maize. Front. Plant Sci. 7. doi: 10.3389/fpls.2016.01076
Wu, M. F., Tian, Q., Reed, J. W. (2006). Arabidopsis microRNA167 controls patterns of ARF6 and ARF8 expression, and regulates both female and male reproduction. Development 133, 4211–4218. doi: 10.1242/dev.02602
Wu, M. F., Yamaguchi, N., Xiao, J., Bargmann, B., Estelle, M., Sang, Y., et al. (2015). Auxin-regulated chromatin switch directs acquisition of flower primordium founder fate. Elife 4, e09269. doi: 10.7554/eLife.09269.027
Xing, J., Cao, X., Zhang, M., Wei, X., Zhang, J., Wan, X. (2023). Plant nitrogen availability and crosstalk with phytohormones signallings and their biotechnology breeding application in crops. Plant Biotechnol. J. 21, 1320–1342. doi: 10.1111/pbi.13971
Xing, M., Wang, W., Fang, X., H., X. (2022). Rice OsIAA6 interacts with OsARF1 and regulates leaf inclination. Crop J. 10, 1580–1588. doi: 10.1016/j.cj.2022.02.010
Xing, L., Zhu, M., Luan, M., Zhang, M., Jin, L., Liu, Y., et al. (2021). miR169q and NUCLEAR FACTOR YA8 enhance salt tolerance by activating PEROXIDASE1 expression in response to ROS. Plant Physiol. 188, 608–623. doi: 10.1093/plphys/kiab498
Xiong, Y., Jiao, Y. (2019). The diverse roles of auxin in regulating leaf development. Plants (Basel) 8, 243. doi: 10.3390/plants8070243
Xiong, Y., Wu, B., Du, F., Guo, X., Tian, C., Hu, J., et al. (2021). A crosstalk between auxin and brassinosteroid regulates leaf shape by modulating growth anisotropy. Mol. Plant 14, 949–962. doi: 10.1016/j.molp.2021.03.011
Xu, C., Tai, H., Saleem, M., Ludwig, Y., Majer, C., Berendzen, K. W., et al. (2015). Cooperative action of the paralogous maize lateral organ boundaries (LOB) domain proteins RTCS and RTCL in shoot-borne root formation. New Phytol. 207, 1123–1133. doi: 10.1111/nph.13420
Xue, M., Yi, H. (2018). Enhanced Arabidopsis disease resistance against Botrytis cinerea induced by sulfur dioxide. Ecotoxicol Environ. Saf. 147, 523–529. doi: 10.1016/j.ecoenv.2017.09.011
Xun, Q., Wu, Y., Li, H., Chang, J., Ou, Y., He, K., et al. (2020). Two receptor-like protein kinases, MUSTACHES and MUSTACHES-LIKE, regulate lateral root development in Arabidopsis thaliana. New Phytol. 227, 1157–1173. doi: 10.1111/nph.16599
Yamauchi, T., Tanaka, A., Inahashi, H., Nishizawa, N. K., Tsutsumi, N., Inukai, Y., et al. (2019). Fine control of aerenchyma and lateral root development through AUX/IAA- and ARF-dependent auxin signaling. Proc. Natl. Acad. Sci. U.S.A. 116, 20770–20775. doi: 10.1073/pnas.1907181116
Yang, F., Shi, Y., Zhao, M., Cheng, B., Li, X. (2022). ZmIAA5 regulates maize root growth and development by interacting with ZmARF5 under the specific binding of ZmTCP15/16/17. PeerJ 10, e13710. doi: 10.7717/peerj.13710
Yang, T., Wang, Y., Teotia, S., Wang, Z., Shi, C., Sun, H., et al. (2019). The interaction between miR160 and miR165/166 in the control of leaf development and drought tolerance in Arabidopsis. Sci. Rep. 9, 2832. doi: 10.1038/s41598-019-39397-7
Ye, Y., Wang, J., Wang, W., Xu, L. A. (2020). ARF family identification in Tamarix chinensis reveals the salt responsive expression of TcARF6 targeted by miR167. PeerJ 8, e8829. doi: 10.7717/peerj.8829
Yuan, Y., Mei, L., Wu, M., Wei, W., Shan, W., Gong, Z., et al. (2018). SlARF10, an auxin response factor, is involved in chlorophyll and sugar accumulation during tomato fruit development. J. Exp. Bot. 69, 5507–5518. doi: 10.1093/jxb/ery328
Yuan, Y., Xu, X., Gong, Z., Tang, Y., Wu, M., Yan, F., et al. (2019). Auxin response factor 6A regulates photosynthesis, sugar accumulation, and fruit development in tomato. Hortic. Res. 6, 85. doi: 10.1038/s41438-019-0167-x
Zahir, Z. A., Shah, M. K., Naveed, M., Akhter, M. J. (2010). Substrate-dependent auxin production by Rhizobium phaseoli improves the growth and yield of Vigna radiata L. under salt stress conditions. J. Microbiol. Biotechnol. 20, 1288–1294. doi: 10.4014/jmb.1002.02010
Zhang, T., Ge, Y., Cai, G., Pan, X., Xu, L. (2023). WOX-ARF modules initiate different types of roots. Cell Rep. 42, 112966. doi: 10.1016/j.celrep.2023.112966
Zhang, Y., Ji, X., Xian, J., Wang, Y., Peng, Y. (2022). Morphological characterization and transcriptome analysis of leaf angle mutant bhlh112 in maize [Zea mays L.]. Front. Plant Sci. 13. doi: 10.3389/fpls.2022.995815
Zhang, H., Li, L., He, Y., Qin, Q., Chen, C., Wei, Z., et al. (2020). Distinct modes of manipulation of rice auxin response factor OsARF17 by different plant RNA viruses for infection. Proc. Natl. Acad. Sci. U.S.A. 117, 9112–9121. doi: 10.1073/pnas.1918254117
Zhang, Z., Teotia, S., Tang, J., Tang, G. (2019). Perspectives on microRNAs and Phased Small Interfering RNAs in Maize (Zea mays L.): Functions and Big Impact on Agronomic Traits Enhancement. Plants 8, 170. doi: 10.3390/plants8060170
Zhang, S., Wang, S., Xu, Y., Yu, C., Shen, C., Qian, Q., et al. (2015). The auxin response factor, OsARF19, controls rice leaf angles through positively regulating OsGH3-5 and OsBRI1. Plant Cell Environ. 38, 638–654. doi: 10.1111/pce.12397
Zhang, C. L., Wang, G. L., Zhang, Y. L., Hu, X., Zhou, L. J., You, C. X., et al. (2021). Apple SUMO E3 ligase MdSIZ1 facilitates SUMOylation of MdARF8 to regulate lateral root formation. New Phytol. 229, 2206–2222. doi: 10.1111/nph.16978
Zhang, K., Wang, R., Zi, H., Li, Y., Cao, X., Li, D., et al. (2018). AUXIN RESPONSE FACTOR3 regulates floral meristem determinacy by repressing cytokinin biosynthesis and signaling. Plant Cell 30, 324–346. doi: 10.1105/tpc.17.00705
Zhang, S., Zhu, L., Shen, C., Ji, Z., Zhang, H., Zhang, T., et al. (2021). Natural allelic variation in a modulator of auxin homeostasis improves grain yield and nitrogen use efficiency in rice. Plant Cell 33, 566–580. doi: 10.1093/plcell/koaa037
Zhao, Z. X., Yin, X. X., Li, S., Peng, Y. T., Yan, X. L., Chen, C., et al. (2022). miR167d-ARFs module regulates flower opening and stigma size in rice. Rice (N Y) 15, 40. doi: 10.1186/s12284-022-00587-z
Zhao, S., Zhang, M.-L., Ma, T.-L., Wang, Y. (2016). Phosphorylation of ARF2 relieves its repression of transcription of the K+ Transporter gene HAK5 in response to low potassium stress. Plant Cell 28, 3005–3019. doi: 10.1105/tpc.16.00684
Zhou, D.-X., Hu, Y. (2010). Regulatory function of histone modifications in controlling rice gene expression and plant growth. Rice 3, 103–111. doi: 10.1007/s12284-010-9045-8
Keywords: ARF, Aux/IAA, growth and development, abiotic stresses, regulatory mechanisms
Citation: Liu L, Yahaya BS, Li J and Wu F (2024) Enigmatic role of auxin response factors in plant growth and stress tolerance. Front. Plant Sci. 15:1398818. doi: 10.3389/fpls.2024.1398818
Received: 10 March 2024; Accepted: 23 May 2024;
Published: 10 June 2024.
Edited by:
Aamir W. Khan, University of Missouri, United StatesReviewed by:
Mingku Zhu, Jiangsu Normal University, ChinaCopyright © 2024 Liu, Yahaya, Li and Wu. This is an open-access article distributed under the terms of the Creative Commons Attribution License (CC BY). The use, distribution or reproduction in other forums is permitted, provided the original author(s) and the copyright owner(s) are credited and that the original publication in this journal is cited, in accordance with accepted academic practice. No use, distribution or reproduction is permitted which does not comply with these terms.
*Correspondence: Fengkai Wu, d2ZrMTI0QHNpY2F1LmVkdS5jbg==
†These authors have contributed equally to this work
Disclaimer: All claims expressed in this article are solely those of the authors and do not necessarily represent those of their affiliated organizations, or those of the publisher, the editors and the reviewers. Any product that may be evaluated in this article or claim that may be made by its manufacturer is not guaranteed or endorsed by the publisher.
Research integrity at Frontiers
Learn more about the work of our research integrity team to safeguard the quality of each article we publish.