- 1National Key Laboratory of Water Disaster Prevention, Hohai University, Nanjing, China
- 2College of Hydrology and Water Resources, Hohai University, Nanjing, China
- 3The Key Lab of Integrated Regulation and Resource Development on Shallow Lakes, Ministry of Education, College of Environment, Hohai University, Nanjing, China
- 4Yangtze Institute for Conservation and Development, Hohai University, Nanjing, Jiangsu, China
- 5China Meteorological Administration Hydro-Meteorology Key Laboratory, Hohai University, Nanjing, Jiangsu, China
- 6Key Laboratory of Water Big Data Technology of Ministry of Water Resources, Hohai University, Nanjing, Jiangsu, China
- 7Key Laboratory of Hydrologic-Cycle and Hydrodynamic-System of Ministry of Water Resources, Hohai University, Nanjing, Jiangsu, China
- 8Botany and Microbiology Department, College of Science, King Saud University, Riyadh, Saudi Arabia
- 9Department of Botany and Microbiology, Faculty of Science, Beni Suef University, Beni-Suef, Egypt
- 10Department of Integrative Agriculture, College of Agriculture and Veterinary Medicine, United Arab Emirates University, Al Ain, Abu Dhabi, United Arab Emirates
- 11Department of Agronomy, Faculty of Agriculture, Mansoura University, Mansoura, Egypt
Introduction: Salinity negatively affects maize productivity. However, calcium lignosulfonate (CLS) could improve soil properties and maize productivity.
Methods: In this study, we evaluated the effects of CLS application on soil chemical properties, plant physiology and grain quality of maize under salinity stress. Thus, this experiment was conducted using three CLS application rates, CLS0, CLS5, and CLS10, corresponding to 0%, 5%, and 10% of soil mass, for three irrigation water salinity (WS) levels WS0.5, WS2.5, and WS5.5 corresponding to 0.5 and 2.5 and 5.5 dS/m, respectively.
Results and discussion: Results show that the WS0.5 × CLS10 combination increased potassium (K 0.167 g/kg), and calcium (Ca, 0.39 g/kg) values while reducing the sodium (Na, 0.23 g/kg) content in soil. However, the treatment WS5.5 × CLS0 decreased K (0.120 g/kg), and Ca (0.15 g/kg) values while increasing Na (0.75 g/kg) content in soil. The root activity was larger in WS0.5 × CLS10 than in WS5.5 × CLS0, as the former combination enlarged K and Ca contents in the root while the latter decreased their values. The leaf glutamine synthetase (953.9 µmol/(g.h)) and nitrate reductase (40.39 µg/(g.h)) were higher in WS0.5 × CLS10 than in WS5.5 × CLS0 at 573.4 µmol/(g.h) and 20.76 µg/(g.h), leading to the improvement in cell progression cycle, as revealed by lower malonaldehyde level (6.57 µmol/g). The K and Ca contents in the leaf (881, 278 mg/plant), stem (1314, 731 mg/plant), and grains (1330, 1117 mg/plant) were greater in WS0.5 × CLS10 than in WS5.5 × CLS0 at (146, 21 mg/plant), (201, 159 mg/plant) and (206, 157 mg/plant), respectively. Therefore, the maize was more resistance to salt stress under the CLS10 level, as a 7.34% decline in yield was noticed when salinity surpassed the threshold value (5.96 dS/m). The protein (13.6 %) and starch (89.2 %) contents were greater in WS0.5 × CLS10 than in WS5.5 × CLS0 (6.1 %) and (67.0 %), respectively. This study reveals that CLS addition can alleviate the adverse impacts of salinity on soil quality and maize productivity. Thus, CLS application could be used as an effective soil amendment when irrigating with saline water for sustainable maize production.
Introduction
Food security is threatened by salinization, water depletion, and environmental pollution. The total global water consumption in industrial, domestic, and agricultural activities is estimated to increase by 23% in 2025 and 40% by 2030 (Oumarou Abdoulaye et al., 2019; Boccaletti, 2023). Therefore, agriculture in most areas is estimated to face extreme food, water, and environmental crises over the next few decades (Kumar et al., 2022). Irrigation is the main water user, which is adopted to support agricultural production in regions where rainfall is insufficient. However, the shortage of water used for irrigation forced farmers to use low-quality water to irrigate crops (Dotaniya et al., 2023). Thus, everywhere saline irrigation is applied, the negative environmental impacts of salinization on agriculture are intensified (Mukhopadhyay et al., 2021; Sadak et al., 2024).
Salinization affects 33% of the total irrigated lands, and the amount of the world’s salt-affected soils is expected to increase yearly (Mukhopadhyay et al., 2021). This increase can be further hastened by the massive application of poor-quality water for irrigation, climate change, and excessive implementation of irrigation linked to poor drainage and exhaustive farming (do Nascimento et al., 2024). Under salt stress, crops must mainly cope with ionic and osmotic stresses. The osmotic stress is caused by reduced soil moisture potential, whereas the large salt captivation of crops causes ionic stress (Ludwiczak et al., 2021). Therefore, salinization restricts the acquisition of plant nutrients, initiates nutritious disorders, and eventually leads to crop yield losses (Alfadil et al., 2021; Ahmed et al., 2024). However, salt-resistant plants reduce sodium uptake in roots and shoots (Rady et al., 2011; Wang et al., 2022), as sodium accumulation in plant tissues is the main reason for crop deterioration under salt stress (Zhao et al., 2021; Atta et al., 2023). Therefore, the crop’s capability to eliminate the harmful sodium cations is one of the utmost tactics for reducing salinization stress.
Numerous strategies have been employed to decrease salinization effects on crops by submitting different organic materials, such as biochar, to soils (Mishra et al., 2023). Biochar is a carbonic matter generated by biomass thermal degeneration under high temperatures and low oxygen conditions (Zou et al., 2022). Biochar is of great interest due to its capability to improve soil carbon sequestration (Li et al., 2021b). Biochar can also improve the fertility of saline soils (Tang et al., 2023). Moreover, biochar enhanced soil chemical characteristics regarding soil pH, soil surface area, and soil cation exchange capacity under abiotic stresses (Yuan et al., 2023). However, applying biochar may limit crop yield by soil nutrients, causing a deficiency of nutrients available to crops (Hossain et al., 2020). Also, biochar’s frequent and long-term application causes soil compaction and degradation (Brtnicky et al., 2021). Moreover, some biochar is a potential source of soil pollution, although heavy metals are included in the biochar itself (Narayanan and Ma, 2022).
Alternatively, calcium lignosulfonate (CLS) application has great potential to regulate soil pH and enhance the fertility and quality of saline soils (Abbas et al., 2022). Calcium lignosulfonate is an amorphous material obtained during the sulfite pulping of softwood, biocompatible in soils with low cost (Liu et al., 2020). CLS is a complex organic polymer produced through lignin solubilization under alkaline conditions (Ghavidel Darestani et al., 2018; Liu et al., 2023). After completion of the pulping, the water-soluble calcium randomly lignosulfonate polymeric framework with three aromatic alcohols is separated from the cellulose, purified, acidified, evaporated, and spray dried. CLS addition has an obvious influence on the growth of plant roots, stimulates the improvement of chlorophyll, amino acids, and sugars in plants and aid in photosynthesis. Moreover, it has positively impacted shoot and root development, plant pigments, nourishing efficiency, and crop yield (Kok et al., 2021). CLS controls the stomata close and open on the leaves, thus increasing the plants’ ability under stressed conditions (Schulze et al., 2021). CLS application also improved soil health, plant–soil interactions, and rhizosphere microorganisms; moreover, the positive impacts of CLS application on crop root development were obvious (Elsawy et al., 2022). Furthermore, CLS could promote overall plant growth in maize as it has negative charges with a short-chain structure, simplifying the reaction with the salts (Ertani et al., 2019). Therefore, CLS addition could motivate root and shoot development, thereby boosting leaf growth, chlorophyll content, and crop yield (Kok et al., 2021). However, very limited information is available on the interactions between crop-soil-CLS particles and their relations to productivity and grain quality of maize with the addition of increasing CLS amounts under saline irrigation. Moreover, the physiological processes of plants and the observation of shoot development adaption during different stages are largely scarce. Therefore, soil is treated with CLS to adjust the soil properties, thereby enhancing maize productivity and quality under salinity stress is of utmost interest.
Globally, maize is the third leading food crop after rice and wheat. Salinity adversely impacts plant growth and physiology, leading to significant crop yield loss. Root–soil interactions regulate the plant growth level, and superior root development supports higher maize production (Gao et al., 2020). The maize–soil interactions are also varied by environmental variations and controlled by the availability of nutrients in the soil. Moreover, the interactions between roots and organic elements can affect overall maize physiological and biochemical attributes under salinity stress (Helaoui et al., 2023). However, the crop’s physiological attributes, nutrient uptake, and their relationships to the grain quality of maize with CLS application under salinity stress conditions are poorly understood. Therefore, the observation of maize responses to increasing rates of CLS application under salinity stress is vital to ascertain the accurate soil amendments to enhance maize productivity, and interpretations of the plant’s physiological attributes at different growth phases are essential for this objective. In addition, the water shortage has become a major constrain of agriculture production (Ingrao et al., 2023; Bakry et al., 2024). Therefore, soil quality should be enhanced by applying precise soil management tactics emphasizing irrigation approaches in crop production (Hamoud et al., 2022). To date, limited information on the effects of increasing CLS application on soil quality and maize productivity under salinity stress has been provided. Thus, to address the existing gap in knowledge, the present study hypothesized that CLS application could improve the soil chemical properties under salinity stress, which motivates the root physiological traits of maize. The study also assumed that the CLS addition could increase the availability of nutrients in the soil, increasing shoot physiological traits, nutrient uptake, and maize quality under salinity stress. To test this hypothesis mentioned above, this study evaluated the effects of CLS addition on soil chemical properties as well as the availability of nutrients in the treated soil under different levels of salinity stress. This study also determined the impact of CLS addition on the maize’s physiological performance and grain quality under varied levels of salinity stress. The guiding of this study would be of great development of sustainable agriculture, providing practical support and a theoretical base for precision soil and water management of maize. This study also identifies the mechanism by which CLS addition would improve soil quality and increase growth and yield of maize, elucidating the synergistic interaction between saline irrigation and CLS addition on grain yield and quality of maize.
Materials and methods
Study site description and soil properties
This experiment was carried out from June to November 2022 at the agricultural farm of Hohai University, Nanjing (31°57′N, 118°50′E), China. The weather of this zone is categorized as humid subtropical with four seasons. The annual mean temperature is 15.9°C, the maximum and the minimum temperatures are 43°C and −16.9°C, and the mean annual rainfall is 1,062 mm (Yang et al., 2018). The soil was collected from the topsoil layer (0 cm–15 cm), dried by sunlight, and passed through a 5-mm screen. The used soil was classified as loam-textured soil, which was characterized as follows: soil pH was 7.02 ± 0.47, the soil organic matter was 6.43 ± 0.37 g·kg−1, the total phosphorus (TP) content was 0.47 ± 0.012 g·kg−1, the available phosphorus (AP) content was 14.75 ± 1.36 mg·kg−1, the available nitrogen (AN) content was 32.41 ± 6.12 mg·kg−1, and the available potassium (AK) content was 123.46 ± 15.02 mg·kg−1.
Experimental design, treatments, and cultural practices
This study was carried out using a randomized complete block design using three repetitions (Figure 1). The first factor was saline water used for irrigation, which imposed three levels with an electrical conductivity (EC) of 0.5 dS/m as control, 2.5, and 5.5, representing WS0.5, WS2.5, and WS5, respectively. The addition made the saline irrigation of 50% sodium chloride (NaCl) and 50% calcium chloride (CaCl2) by weight in well water. NaCl was supplied to water used for irrigation to attain the proposed irrigation water salinity levels (WS2.5 and WS5.5) (melting 0.5843 g of NaCl in 1 liter of water increased the EC by 1 dS/m).
The second factor was calcium lignosulfonate (CLS) in addition to three levels: CLS0 (0%), CLS5 (5%), and CLS10 (10%) on a weight basis. A group of 27 PVC vessels were installed in an uncontrolled shelter roofed with elastic film. Every experimental vessel (depth: 40 cm, diameter: 25 cm) had tiny holes at the bottom and was occupied with 10 kg dry soil (Figure 1).
Later, CLS amounts were supplied and homogenized with the soil. The quantity of CLS at 5% (CLS5) was calculated on a weight basis, supposing the soil bulk density of 1 g/cm3. The quantity of CLS at the level of 10% (CLS10) enlarged twice to accomplish the rate of 10% (CLS10), whereas no CLS was added to the rate of 0% (CLS0).
After filling the pots with the soil mixed with the corresponding rates of CLS, the superphosphate (12%, P2O5), urea (46% N), and potassium chloride (60% K2O) as chemical fertilizers were added to the mixture according to the soil test. The pot moisture holding capacity was measured for each rate of CLS. Then, the maize variety, so-called Xianyu 335, was used. The seeds were sown in soil peat moss at three seeds per plug tray cell and thinned to one plant per hole 3 weeks after the germination. Then, the seedlings were transplanted to the pots and irrigated to 100% of pot water-holding capacity every other day for a week. Afterward, irrigation water salinity treatments were implemented every other day, and water amounts were determined based on the water quantity needed to increase the moisture content to 100% of the pot water-holding capacity.
Soil analysis
For chemical investigation, the soil samples were obtained from the topsoil layer (0 cm–20 cm). A pH meter estimated soil acidity (pH) in soil/water extract (1/2.5). The EC of soil was assessed by measuring the extract through an EC meter. Some samples were placed in a fridge at 4°C to estimate the dissolved organic carbon (DOC). Additional samples were also saved to measure soil easily oxidative carbon (EOC) and soil total organic carbon (TOC). The TOC amount was computed by the titration of ferrous ammonium sulfate and the potassium dichromate oxidation (Lu, 1999). The soil DOC level was computed in the soil/water extract at 25.5°C after shaking for 1 h (Jiang et al., 2006) and rotating at 4,500 r·min−1 for 15 min (Bankó et al., 2021). The occasioning supernatant was passed through a 0.45-mm screen, and the attained blend was measured by the titration of ferrous ammonium sulfate and the oxidation of potassium dichromate. The EOC amount was estimated by reacting finely dried samples with 333 mmol·L−1 KMnO4 through shaking at 60 r·min−1 for 60 min (Blair et al., 1995). After that, the resultant was centrifuged for 5 min at 2,000 r·min−1. The obtained mixture was diluted, and its absorbance was noted at 565 nm using a spectrophotometer. Subsamples were added to a blend of salicylic acid and selenium sulfate to measure the ion contents of potassium (K+), calcium (Ca++), and sodium (Na+) in the soil. The mixture was transferred to the ingestion cylinders, which were heated for 30 min at 100°C. The temperature was then raised to 380°C for 180 min using a hotplate (YKM-36, Shanghai, China) (Jackson, 2005). Soil K+, Ca++, and Na+ contents were identified by the flame photometer method (FB640N, Wincom, Hunan, China) (Ghosh, 1993).
Measurements of root growth traits
At physiological maturity (R6), plants were collected, partitioned into grains, stems, and leaves, positioned in the oven for 3 days at 75°C, and balanced to get dry weights. Plant samples for each plant part were milled, sieved using a 1-mm mesh, and kept in the paper bags for further analysis. The soil/root tubes for each treatment were collected, and roots were isolated by carefully washing the soils. The plant roots were dried with paper tissues, and the fresh root mass was determined. The methylene blue dyeing method estimated the root active adsorption area (RAAA) using fresh root samples (Zhang et al., 1994). The root dry weight (RDW) was measured from the fresh root biomass and the water amount of the dried root.
Measurements of shoot growth and physiological traits
During the vegetative (tassel, VT) and reproductive (dent, R5) growth stages, data regarding plant height (PH, cm), stem diameter (SD, cm), leaf number per plant (NL), and leaf length (LL, cm) were recorded in triplicate. The PH was identified from the soil surface unit at the shoot’s top. The SD was identified through a digital caliper (CD 7303V, China). The NL per plant was counted, and LL was measured using a meter tap.
The photosynthesis level (Pn, µmolCO2 m2/S) of the leaf was measured through an opened-flow gas exchange apparatus. The Pn rate was measured in three replications of leaves between 08:40 and 10:50 on a sunshiny day through a moveable photosynthesis apparatus (LI-6400XT, LI-COR, USA). The leaf deoxyribonucleic acid (DNA) content was measured at the reproductive (milk, R3) growth stage and detected by flow cytometry analysis. Leaf subsamples were separated into tiny slashes and placed in 50 mM KCl, 5 mM HEPES, 1 mg/mL dithiothreitol (Sigma, St. Louis, MI, USA), 0.2% Triton X-100 (nucleus isolation buffer), and 10 mM MgSO4. The samples were passed over a 33-mm net. The nuclei were positioned in paraformaldehyde (4%) for half an hour, sedimented (200 g, 10 min, 4u C), and then suspended in a separation buffer (Sheteiwy et al., 2021a).
Measurements of the leading enzymes and antioxidant activity in crop
At physiological maturity (R6), the root glutamine synthetase [RGS, μmol/(g·h)] ability was assessed using a standard method by Chen et al. (2021). The nitrate reductase [RNR, µg/(g·h)] activity of the root was assessed by the investigation blend, including 1-g fresh roots sited in 25 mM K3PO4 buffer, 10 mM KNO3, 0.2 mM NADH, 5 mM NaHCO3, and 5-μL extract in a final quantity of 0.5 mL. The attained reaction was suspended by delivering 50 μL of 0.5 M Zn(CH3COO)2. Then, 50 μL of 0.15 mM phenazine methosulphate was delivered to oxidize the excessive NADH. The mixture was centrifuged for 5 min at 10.000× g. The quantity of NO2− was detected by blending 500 μL of supernatant with 250 μL of 1% sulfanilamide obtained in 1.5 N HCl and 250 μL of 0.02% N-(1-naphthyl) ethylene-diamine dihydrochloride. The absorbance was logged at a 540-nm wavelength using the spectrophotometric method (Ogawa et al., 1999).
At the vegetative (tassel, VT) and reproductive (dent, R5) growth stages, subsamples (0.5 g) of the ground flag were frozen in liquid N. They were then placed in a mixture of 15% glycerol, 1 mM EDTA, 0.1% Triton X-100, 1 mL Tris–HCl (pH 7.8), and 14 mM 2-mercaptoethanol. The consequent blend samples were rotated for 12,000× g at 4°C for 10 min. The glutamine synthetase [LGS, µmol/(g·h)] activity of the leaf was identified by identifying glutamyl hydroxamate formation in the mixture at 540 nm after reaction with FeCl3 (10%): TCA (24%): HCl (6 mol/L) at 1:1:1 ratio. The leaf nitrate reductase [LNR, µg/(g·h)] activity was identified through a standard method by Baki et al. (2000). Briefly, 0.2 g of the crushed leaf was kept for 30 min at 30°C in a dark place with 0.2 M KNO3, 9 mL of medium consisting of 25% isopropanol, and 0.1 M sodium phosphate buffer (acidity 7.2). The spectrophotometer detected the discharged nitrite at a wavelength of 540 nm.
The leaf’s malonaldehyde (MDA) amount was identified by a standard method of Zhou and Leul (1999). Briefly, a flag tissue sample (0.25 g) was added to liquid N by supplying 10% (w/v) TCA (5 ml). The resulting mixture was rotated at 4,000× g for 15 min at 4°C. Later, the supernatant (2 mL) was submitted to 0.67% (w/v) thiobarbituric acid (2 mL), persisted at 100°C for 30 min, and finally transmitted to an ice bath. The subsequent samples were rotated at 4°C at 2,000× g for 5 min. The spectrophotometer recorded the supernatant absorbance at 532-nm, 600-nm, and 450 nm wavelengths. The hydrogen peroxide (H2O2) level of the supernatant was logged at 390 nm by the spectrophotometer. The leaf’s relative water content (RWC, %) was estimated by drying samples at 75°C for 2 days in the oven, where dry mass was achieved afterward by sealing the targeted leaf for 2 days in deionized water. Then, the leaves were dried before reaching the turgid mass (Sheteiwy et al., 2019).
Measurements of nutrient crop removal
The wet digestive tubes for the seed, stem, leaf, and root were arranged to measure plant tissues’ K, Ca, and Na nutrient concentrations (%). The digestive tubes were supplied with 0.5 g of each plant part powder. A 1-g selenium reagent mixture was placed in the tube and supplied with 10 mL of concentrated H2SO4 (Jackson, 2005). The K, Ca, and Na percentages in the tissues of root, stem, leaf, and grain were measured by the flame photometry technique (Tandon, 1993). The amounts of K, Ca, and Na nutrients in crop removal were estimated by the following equations (Mosier and Syers, 2004; Bandaogo et al., 2015):
where NAG is the nutrient accretion in grain (mg/plant), NCG is the nutrient concentration in the grain (%), GDW is the grain dry weight (g/plant), NCL is the nutrient concentration in the leaf (%), LDW is the leaf dry weight (g/plant), NAS is the nutrient accretion in the stem (mg/plant), NCS is the nutrient concentration in the stem (%), SDW is the dry weight (g/plant), NAL is the nutrient accretion in leaf (mg/plant), NAR is the nutrient accretion in the root (g/plant), NCR is the nutrient concentration in root tissues (%), and RDW is the root dry weight (g/plant).
Approval of salt resistance model to corn
As proposed by Maas and Hoffman (1977), the model of salt tolerance was employed in corn yield as a development factor to standardize the level of decline of the factor with increasing salt stress, as indicated by its ECe (excluding the threshold value for assumed salinity and soil amendment). With yield used as a development factor, the salt resistance model (Maas and Hoffman, 1977) is given by the following equation:
where Ya is the average achieved corn yield for a given CLS addition and saline irrigation treatment (g); Ym is the average highest corn production (grain yield) achieved by the CLS0 control treatment (g) for the relevant CLS addition; ECe is the soil salinization threshold value (dS m−1); ECe is the soil salinization excluding the threshold value (dS m−1); and b is the slope value denoting the degree of decreasing yield with rising ECe excluding the threshold value.
Evaluation of seed embryo ultramorphology, yield, grain chemical structure, and quality
To identify differences in corn embryo ultramorphology for the different treatments, a scanning electron microscope was employed following a standard protocol (Wei et al., 2009). The sonication in dehydrated alcohol dehulled a grain sample (0.1 g) for 5 min. Then, grains were sliced by carpet tape to get cross sections sputter-coated to a thinness of 30 nm with a gold object in a vacuum-coating device. Silver dye was enclosed to the bottom of the seed cuts to avoid the charge on its surface. The seed endosperm ultramorphology engaged to the scanning electron microscope (JEOL JSM-1300) was checked on the core endosperm of a transverse unit at a 15-kV accumulating voltage, and the pictures for each sample were skimmed. The seed chemical structure was identified by calculating the percentages of protein, fat, and starch, according to Pan et al. (2013), using an infrared grain analyzer (FOSS, Hilleroed, Denmark). The seed’s digestive tubes were used where nutrient contents in the grains were determined using the spectrophotometer through the indophenol-blue protocol for N (Novozamsky et al., 1974) and the Barton protocol for P (Jones, 2001). Silicon level (%) was measured using a standard protocol by Zhao et al. (2019).
Data statistical analysis
The collected data were processed by the IBM-SPSS-19 statistical package, employing the analysis of variance (two-way ANOVA) and performing the process of the general linear model. Once the P values were significant, the obtained mean values were compared at the 0.05 significance level using Duncan’s multiple range test. All collected values are the averages of three replications.
Results
Calcium lignosulfonate addition enhanced soil chemical properties under salinity stress
ANOVA results exhibited that under the similar calcium lignosulfonate (CLS) addition rate, increasing the water salinity (WS) stress during WS0.5, WS2.5, and WS5.5 considerably enlarged the soil EC, pH, and Na contents while decreasing EOC, DOC, TOC, K, and Ca contents (Table 1). Under similar salinity stress, CLS0, CLS5, and CLS10 led to a large decline in the soil EC, pH, and Na+ content and a gradual enlargement in the DOC, EOC, TOC, K+, and Ca++ levels in the soil. Due to the interactive effect, treatment WS0.5 × CLS10 exhibited the lowest values of EC (3.85 and 3.00), pH (7.28 and 7.11), and Na+ (0.23 and 0.08, g/kg) and the highest EOC (6.30 and 4.23 g/kg), DOC (368.1 and 284.1 mg/kg), TOC (15.64 and 13.58 g/kg), K+ (0.167 and 0.139 g/kg), and Ca++ (0.39 and 0.19 g/kg) contents of the soil at the layers of 10 cm and 20 cm, respectively. However, treatment WS5.5 × CLS0 showed the greatest values of EC (15.03 and 14.37), pH (8.56 and 8.02), and Na+ (1.01 g/kg and 0.67 g/kg) and the lowest values of EOC (1.82 g/kg and 1.02 g/kg), DOC (172.3 mg/kg and 88.6 mg/kg), TOC (10.67 g/kg and 8.85 g/kg), K+ (0.120 g/kg and 0.101 g/kg), and Ca++ (0.15 g/kg and 0.08 g/kg) contents of the soil at the depths of 10 and 20 cm, respectively.
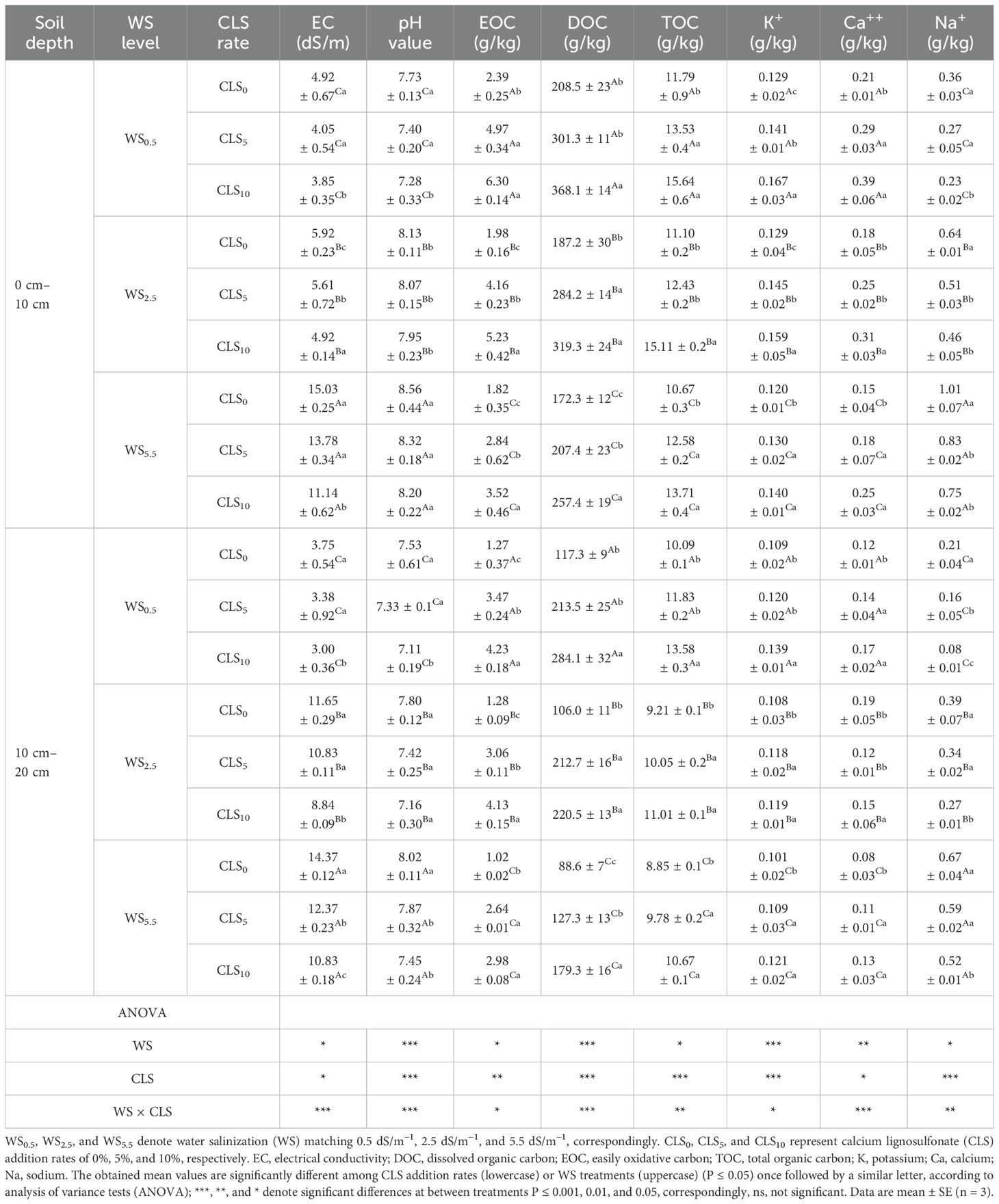
Table 1. Soil properties for different treatments, and a summary of the analysis of variance on the major impacts of salt-stress and calcium lignosulfonate addition rate on soil chemical properties.
Calcium lignosulfonate addition encouraged physiological traits and K concentration in root under salt-stress
At physiological maturity (R6), the RAAA, RDW, RNR, and RGS of roots were reduced by increasing the salinity stress during WS0.5, WS2.5, and WS55.5 whereas the RAAA, RDW, RNR, and RGS of roots enlarged with increasing CLS addition rate during CLS0, CLS5, and CLS10, respectively. In addition, the highest RAAA (6.54 m2/plant), RDW (2.61 g/plant), RNR [14.07 µg/(g·h)], and RGS (3.58 mg/g/FW/h) values in CLS10 were detected under WS0.5. However, the lowest RAAA (2.38 m2/plant), RDW (1.74 g/plant), RNR [5.87 µg/(g·h)], and RGS (1.38 mg/g/FW/h) values in CLS0 were detected under WS5.5 (Table 2).
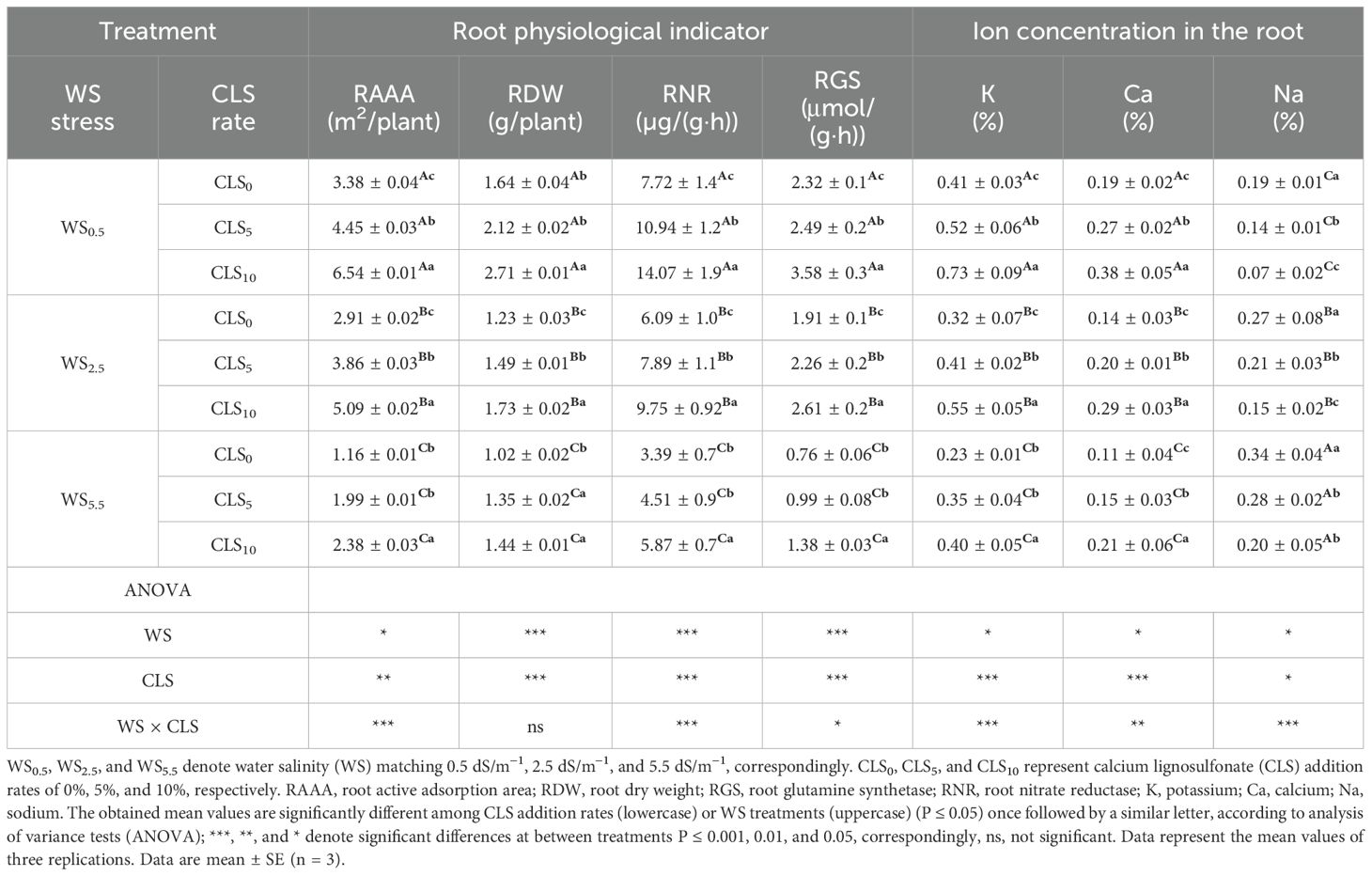
Table 2. Root physiological indicators for different treatments and a summary of the analysis of variance on the major impacts of salt stress and calcium lignosulfonate addition rate on root physiological indicators.
Significant variations in K, Ca, and Na contents in the root tissues were detected among salinity treatments. Under a similar CLS application rate, the root tissues’ K and Ca contents decreased gradually, whereas Na content increased during WS0.5, WS2.5, and WS55.5, respectively. Under the similar salinity treatment, K and Ca root contents increased gradually while decreasing Na root content during CLS0, CLS5, and CLS10. The highest K (0.73%) and Ca (0.38%) contents and the lowest Na (0.07%) content in the root tissues were recorded by the treatment WS5.5 × CLS10. However, the lowest K (0.23%) and Ca (0.11%) contents and the highest Na (0.34%) content in the root tissues were recorded by the treatment WS0.5 × CLS0 (Table 2).
Calcium lignosulfonate addition encouraged cell cycle progression of the leaf under salinity stress
The results of the flow cytometry investigation presented that CLS addition and salinity stress considerably alerted the cell cycle’s progress at exact stages (Figures 2A–I). The findings revealed that each treatment influenced the plant cell progression differently. Maximum values of side-scattered (SSC) light and forward-scattered (FSC) light signals were discovered in maize grown in the CLS10 exposed to minor salinity stress under WS0.5, signifying superior cell division, size, and granularity (Figures 2A–C).
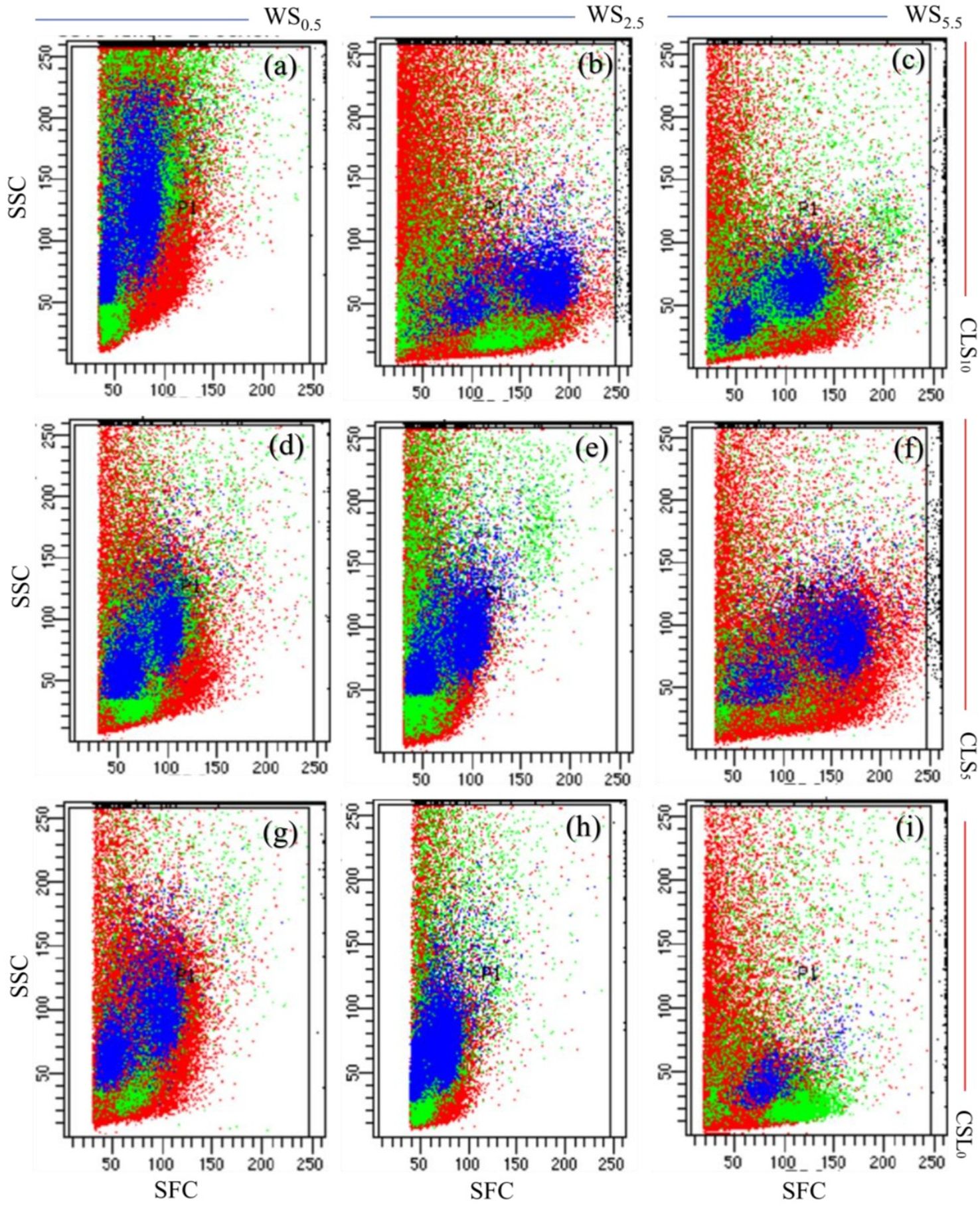
Figure 2. Maize’s leaf cell flow cytometric investigation for different treatments. (A–C) represents flow cytometric analysis for plants in soil treated with calcium lignosulfonate (CLS) addition rate of 10% under water salinization (WS) stress of WS0.5, WS2.5, and WS5.5, corresponding to 0.5, 2.5, and 5.5 dS/m, respectively; (D–F) represents flow cytometric analysis for plants in soil treated with CLS addition rate of 5% under WS0.5, WS2.5, and WS5.5, respectively; (G–I) represents flow cytometric analysis for plants grown in soil treated with CLS addition rate of 0% under WS0.5, WS2.5, and WS5.5, respectively. SSC and FSC donate side scattered and forward scattered.
Enhanced values of SSC and FSC were recognized in maize grown in CLS5, specifying an enhanced cell division, size, and granularity, with lessening salinity stress during the WS2.5 treatments. Thus, the cells’ development was slightly motivated by the variations in the cell’s progress when growing maize in CLS5 with decreasing salinity stress during the WS2.5 (Figures 2D–F). Minimum values of SSC and FSC were noted in maize grown in CLS10, demonstrating a negligible developed cell size division and granularity while maximizing salinity stress during the WS5.5. Thus, cell progression was noticeably reduced, parallel to changes in cell development when growing maize in CLS10 with lightning salinity stress during WS0.5 (Figures 2G–I).
Calcium lignosulfonate application enhanced shoot physiological traits under salt stress
During the vegetative (tassel, VT) and reproductive (dent, R5) growth stages, CLS addition significantly enhanced the RWC and Pn in all salinity stress treatments. This impact improved as the amount of CLS added increased. Under the same CLS application level, the salinity stress treatments impacted the RWC and Pn differently, where their values were increased by decreasing salt-stress during the WS5.5, WS2.5, and WS0.5 treatments. Regarding the interaction of the factors, the greatest Pn (13.13 µmol m−2 s−1 and 8.63 µmol m−2 s−1) and RWC (48.93% and 36.73%) were recorded by WS0.5 × CLS0 at vegetative (tassel, VT) and reproductive (dent, R5) growth stages, respectively. However, the lowest Pn (7.65 µmol m−2 s−1 and 4.90 µmol m−2 s−1) and RWC (10.86% and 7.99%) values were observed with WS5.5 × CLS10 (Figures 3A, B).
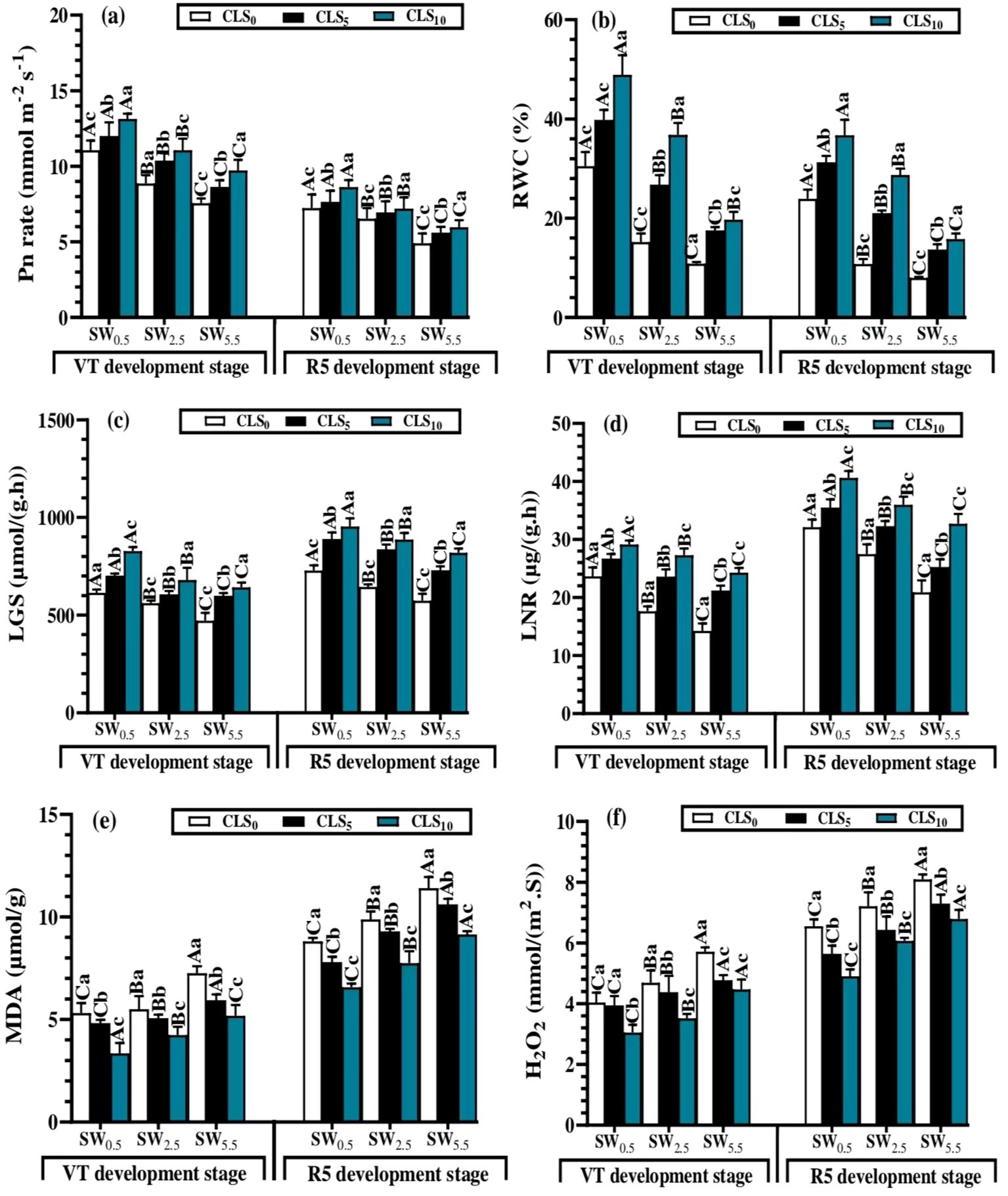
Figure 3. Photosynthesis (Pn) (A) and relative water content of leaf (RWC) (B), leaf’s glutamine synthetase (LGS) (c), leaf’s nitrate reductase (LNR) (D), malonaldehyde (MDA) (E) and hydrogen peroxide (H2O2) (F) mean values as affected by different calcium lignosulfonate addition under salinity stress for different growth stages. WS5.5, WS2.5, and WS0.5 denote water salinization (WS) stress corresponding to 5.5, 2.5, and 0.5 dS/m, respectively. CLS0, CLS5, and CLS10 represent calcium lignosulfonate (CLS) addition rates of 0%, 5%, and 10%, respectively. For the same growth stage, the means are significantly different among CLS addition rates (lowercase) or WS treatments (uppercase) (P ≤ 0.05) once followed by a similar letter, according to the analysis of variance tests. Data are mean ± SE (n = 3). VT represents the tasselling of the vegetative growth stage, and R5 represents the denting of the reproductive growth stage.
Given significant differences between different factors, CLS application significantly improved the LGS and LNR under salinity stress treatment. Under the same CLS rate, the salinity stress displayed a varied effect on the LGS and LNR, where their values were improved by reducing salt-stress during the WS0.5, WS2.5, and WS5.5. Given the interaction of the factors, the greatest LGS [827.7 µmol/(g·h) and 953.9 µmol/(g·h)] and LNR [29.12 µmol/(g·h) and 40.59 µg/(g·h)] were recorded by WS0.5 × CLS0 at the vegetative (tassel, VT) and reproductive (dent, R5) growth stages, respectively. However, the lowest LGS [471.6 µmol/(g·h) and 573.4 µmol/(g·h)] and LNR [14.25 µg/(g·h) and 20.86 µg/(g·h)] were recorded values were observed with WS5.5 × CLS10 (Figures 3C, D).
The MDA and H2O2 contents decreased significantly with increases in CLS addition amounts, whereas the increasing salinity stress increased MDA and H2O2 contents. Given the interaction of factors, WS5.5 × CLS0 resulted in the maximum values of MDA (7.26 µmol/g and 11.41 µmol/g) and H2O2 [5.71 mmol/(m2·S) and 7.11 mmol/(m2·S)] contents at the vegetative (tassel, VT) and reproductive (dent, R5) stages, respectively. However, the minimum MDA (3.34 and 6.57 µmol/g) and H2O2 [3.05 mmol/(m2·S) and 4.91 mmol/(m2·S)] values were recorded by WS0.5 × CLS10 (Figures 3E, F).
Calcium lignosulfonate addition stimulated crop growth indicators under salt stress
As displayed in Figures 4A–D at the vegetative growth phase, NL, LL, PH, and SD were unfavorably declined by salinity stress. WS5.5 led to lower NL, LL, PH, and SD values under all salt-stress treatments, whereas WS0.5 resulted in the greatest values of PH, SD, NL, and LL.
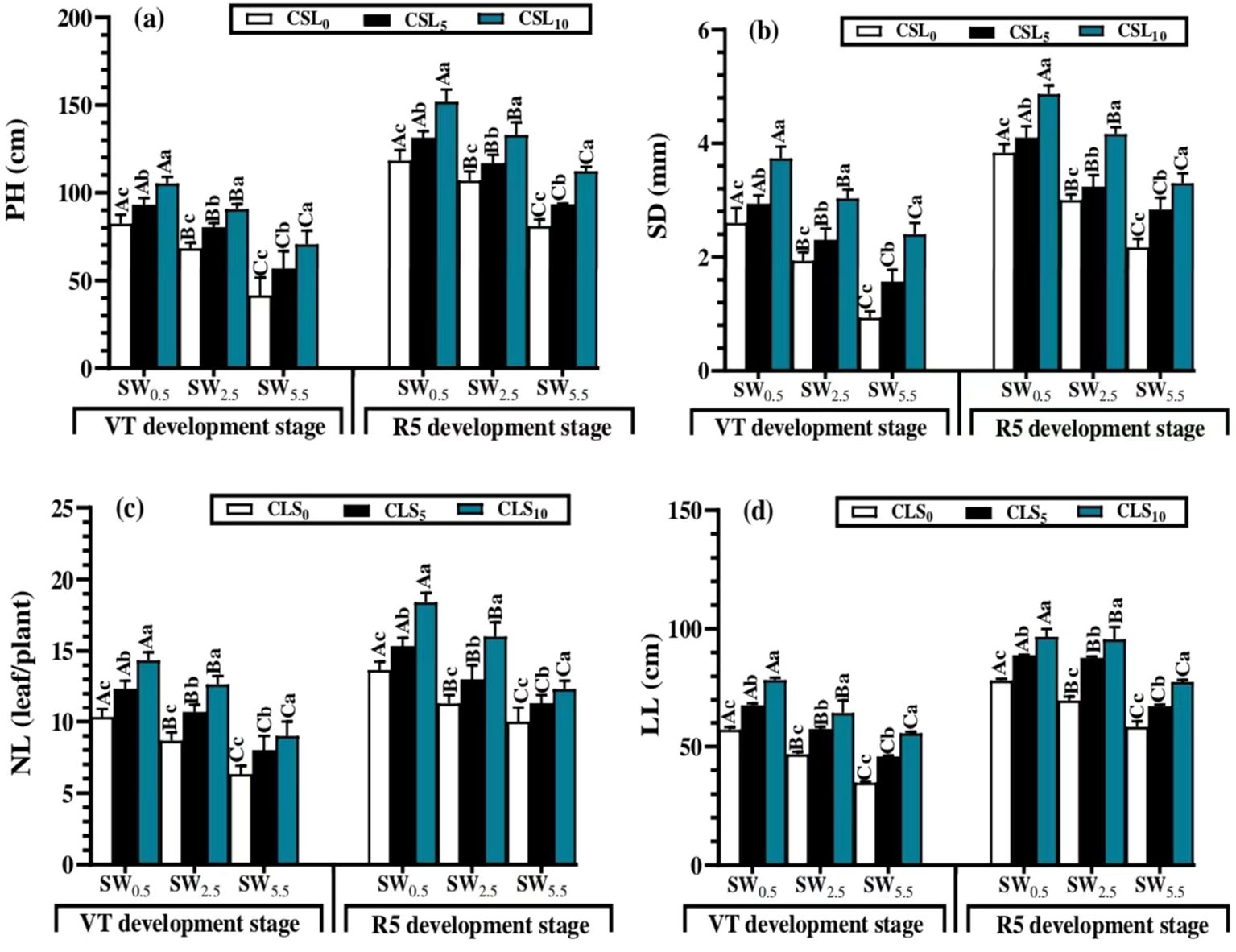
Figure 4. Plant height (PH) (A), stem diameter (SD) (B), number of leaves per plant (NL) (C) and leaf’s length (LL) (D) mean values as affected by different calcium lignosulfonate additions under salinity stress for different growth stages. WS5.5, WS2.5, and WS0.5 denote water salinization (WS) stress corresponding to 5.5, 2.5, and 0.5 dS/m, respectively. CLS0, CLS5, and CLS10 represent calcium lignosulfonate (CLS) addition rates of 0%, 5%, and 10%, respectively. For the same growth stage, the means are significantly different among CLS addition rates (lowercase) or WS treatments (uppercase) (P ≤ 0.05) once followed by a similar letter, according to the analysis of variance tests. Data are mean ± SE (n = 3). VT represents the tasselling of the vegetative growth stage, and R5 represents the denting of the reproductive growth stage.
The CLS application showed a substantial augmentation in LL, NL, PH, and SD values under the salt stress treatments. CLS10 resulted in greater PH, SD, NL, and LL values under all salt-stress treatments. Regarding interactive effects of the factors, the greatest PH, SD, NL, and LL mean values were recorded by WS0.5 × CLS10 (105.3 cm, 3.73 mm, 6 leaves/plant, and 78.3 cm), whereas the lower mean values were noticed with WS5.5 × CLS0 (42 cm, 0.93 mm, 14 leaves/plant, and 34.5 cm).
Similar to the previous phase, salinity stress reduced the growth parameters of maize during WS0.5, WS2.5, and WS5.5. However, CLS addition exhibited a major enhancement in these parameters during CLS0, CLS5, and CLS10, during the vegetative (tassel, VT) and reproductive (dent, R5) growth stages (Figures 3A–D). Therefore, the highest PH, SD, NL, and LL values were detected in WS0.5 × CLS10 (152.5 cm, 4.86 mm, 18 leaves/plant, and 96.6 cm), whereas the smallest values were noted by WS5.5 × CLS0 (81.2 cm, 2.16 mm, 10 leaves/plant, and 58.3 cm).
Calcium lignosulfonate addition increased the ion concentrations in maize under salinity stress
The variations in biomass production of corn for various CLS addition rates under salt stress were significant. The biomasses for seeds, leaves, stems, and thus shoot biomass improved with CLS rate increasing during CLS0, CLS5, and CLS10, respectively. Meanwhile, increasing salinity stress considerably reduced the masses of grains, leaves, and stems, and thus shooting. Moreover, the highest biomasses for seeds, leaves, stems, and thus shoot biomass were noticed in WS0.5 × CLS10 [51.55, 87.06, 164.21 (g/plant)]. However, the lowest values were watched in WS5.5 × CLS0 [19.31, 42.86, 71.25 (g/plant)] (Table 3).
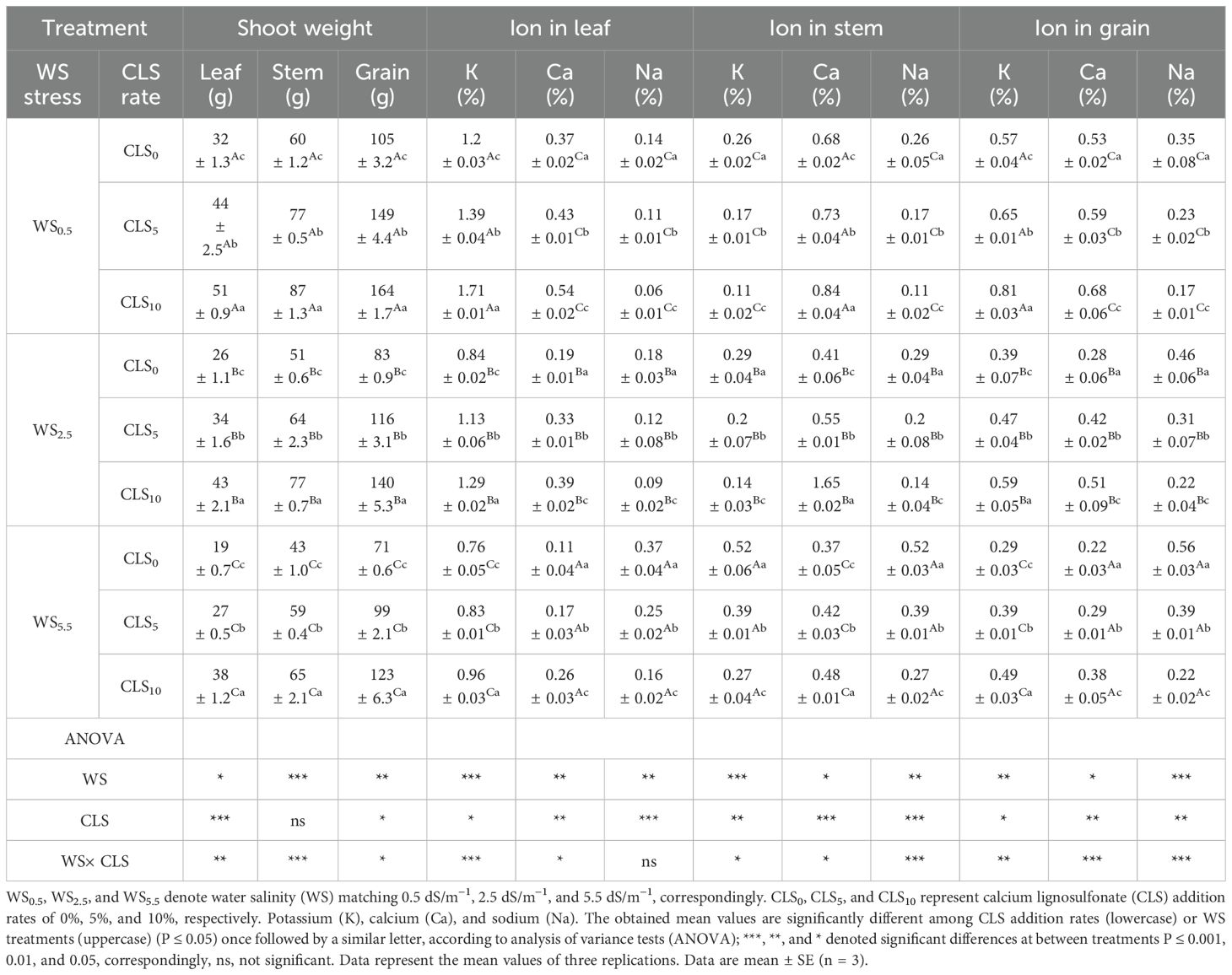
Table 3. Nutrient concentration of maize for different treatments, and a summary of the analysis of variance on the major impacts of salt stress and calcium lignosulfonate addition rate on the nutrient concentration (%) of maize.
Significant changes (P<0.05) in the ion contents in maize plants’ seeds, stems, and leaves were detected across salinity stress treatments. The maize plants were more reactive with K under WS0.5 than WS5.5 conditions through the season. The K and Ca percentages in stems, leaves, and grains enlarged when the CLS addition rate increased during CLS0, CLS5, and CLS10, respectively. Due to interaction, the maximum values of K and Ca amounts in leaves (1.71% and 0.54%), stems (1.51% and 0.84%), and grains (0.84% and 0.68%) were obtained under the WS0.5 × CLS10. However, the lowest K and Ca concentrations in leaves (0.76% and 0.11%), stems (0.52% and 0.37%), and grains (0.29% and 0.22%) were achieved under the WS5.5 × CLS0 (Table 3). Unlike the K and Ca concentrations, the Na percentages in the stems, grains, and leaves of maize were considerably increased by increases in salinity stress and reductions in CLS addition rates. Moreover, the highest Na levels in maize grains, stems, and leaves were obtained under WS5.5 × CLS0 (0.37%, 0.52%, and 0.56%). However, the lower values were witnessed in WS0.5 × CLS10 (0.06%, 0.11%, and 0.17%) (Table 3).
Calcium lignosulfonate application enlarged the ions uptake of corn under salinity stress
The findings specified that different CLS addition rates under salinity stress treatments affected Na, K, and Ca accumulation in the leaf, stem, root, and grain. The accumulation of K and Ca in the root, leaf, stem, and grain declined with increasing salinity stress during the WS0.5, WS2.5, and WS5.5, respectively. However, the Na accumulation in the stem, root, grain, and leaf improved during the WS0.5, WS2.5, and WS5.5 (Table 4), unlike the K and Ca accumulation trend in root, leaf, stem, and grain, which were realized during CLS0, CLS5, and CLS10. However, the Na uptake in leaf, stem, root, and grain declined with increasing CLS addition rates during CLS0, CLS5, and CLS10, respectively. Due to interaction, the highest K and Ca uptake while the lowest Na uptake in the root (19.1 mg, 10 mg, and 1.83 mg), leaf (881.5 mg, 278 mg, and 30.9 mg), stem (1,314 mg/plant, 731 mg/plant, and 95.8 mg/plant), and grain (1,330.1 mg, 1,117 mg, and 279.2 mg) were recorded by the WS0.5 × CLS10 treatment. However, the lowest K and Ca uptake while the highest Na uptake in the root (6.7 mg, 1.1 mg, and 3.81 mg), leaf (146 mg/plant, 21 mg/plant, and 71 mg/plant), stem (201 mg, 159 mg, and 230 mg), and grain (206 mg, 157 mg, and 399 mg) were recorded by the WS5.5 × CLS0 treatment (Table 4).
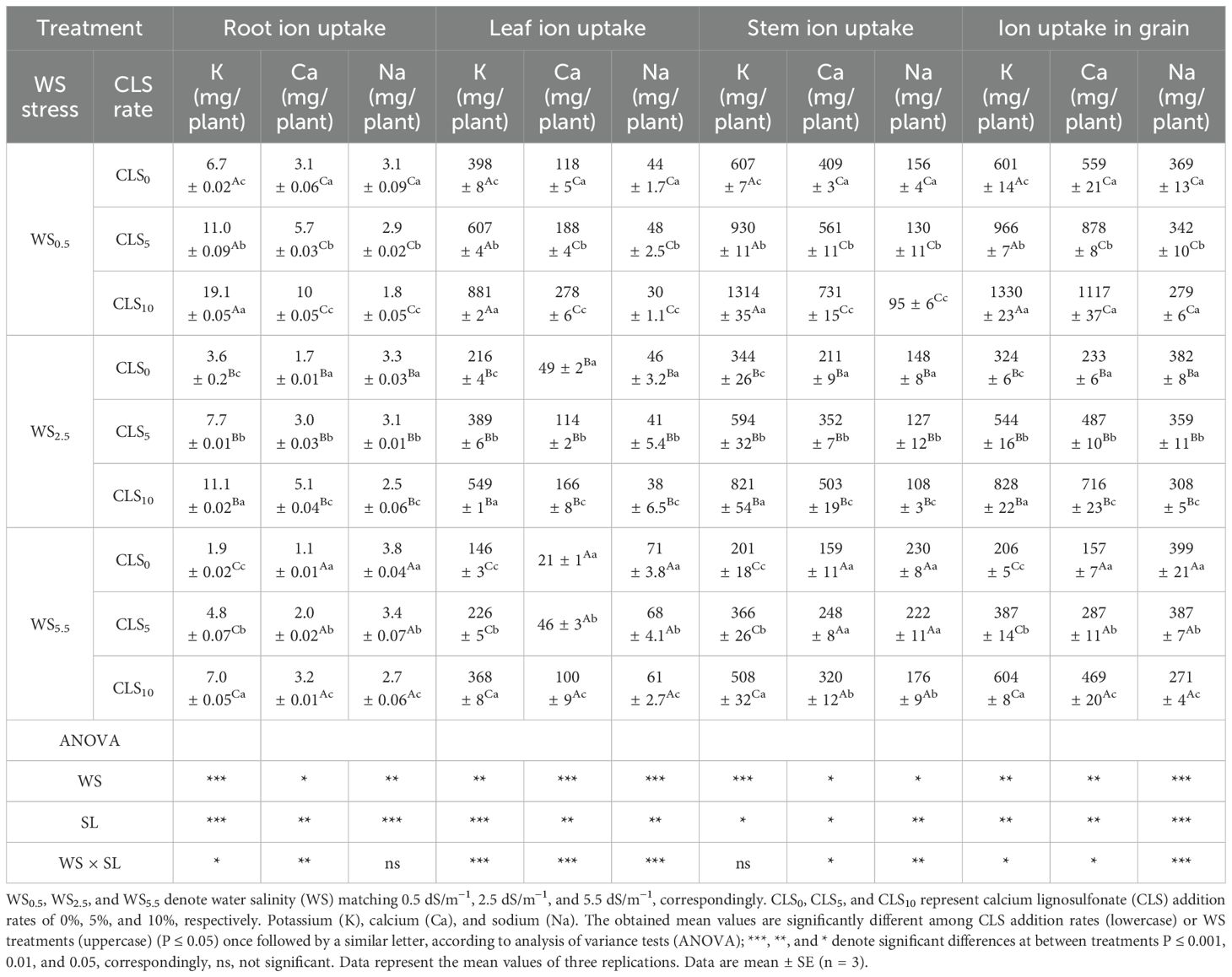
Table 4. Nutrient uptake (mg/plant) of corn for various treatments (Equations 1–4) and a summary of the analysis of variance on the major impacts of salt stress and calcium lignosulfonate addition rate on nutrient uptake of maize.
Calcium lignosulfonate addition enlarged the maize salt resistance to salinity stress
The salt resistance index for the maize plant is presented as a function of grain yield against the reached ECe mean values for every CLS addition rate (Table 5). For CLS0, no reduction until 2.09 dS/m of the threshold value was detected in the grain yield. However, surpassing the standard value, corn production declined by 27.78% for each unit enlargement in the salt-stress degree. Regarding the CLS5, a substantial rise in the ECe value to 4.02 dS/m was witnessed; however, the slope value was reduced to 14.92%. Moreover, under CLS10, a substantial enlargement in the ECe value of 5.96 dS/m was detected; however, the slope value was reduced to 7.34% (Table 5).
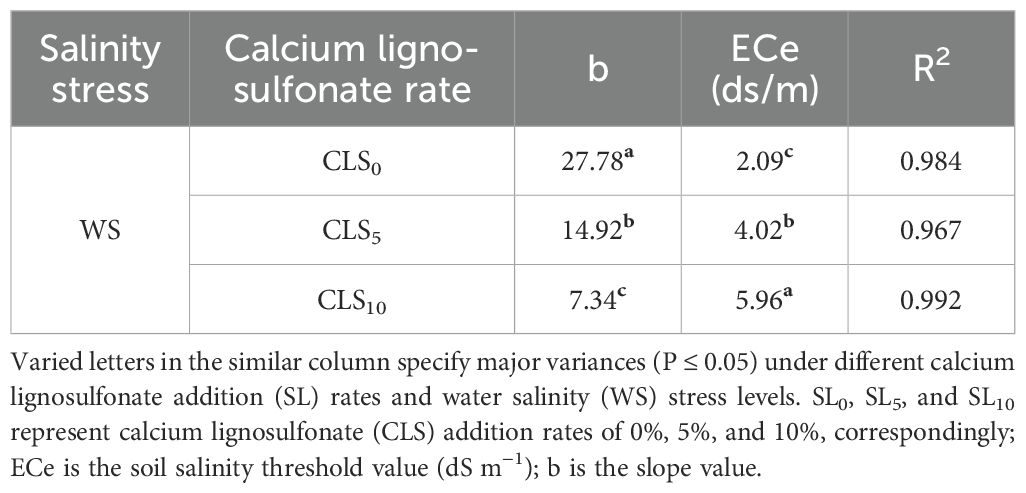
Table 5. Fitted factors of salinity resistance models projected (Equation 5) for various rates of calcium lignosulfonate addition under salinity stress.
Calcium lignosulfonate addition improved the grain quality of maize
The ultramorphology of maize seed embryo altered significantly under different treatments (Figures 5A–I). The maize seed embryo exposed to CLS0 exhibited many circular starch grains of smaller size and a plethora of unformed starch particles with untidy-edge starch granules. The ultramorphology of maize seed embryo also showed a large increase in the content of protein matrix at the periphery, increasing the injury to the seeds compared with CLS0 and WS0.5 by increasing salinity stress in the order of WS0.5 > WS2.5 > WS5.5 (Figures 5A–C).
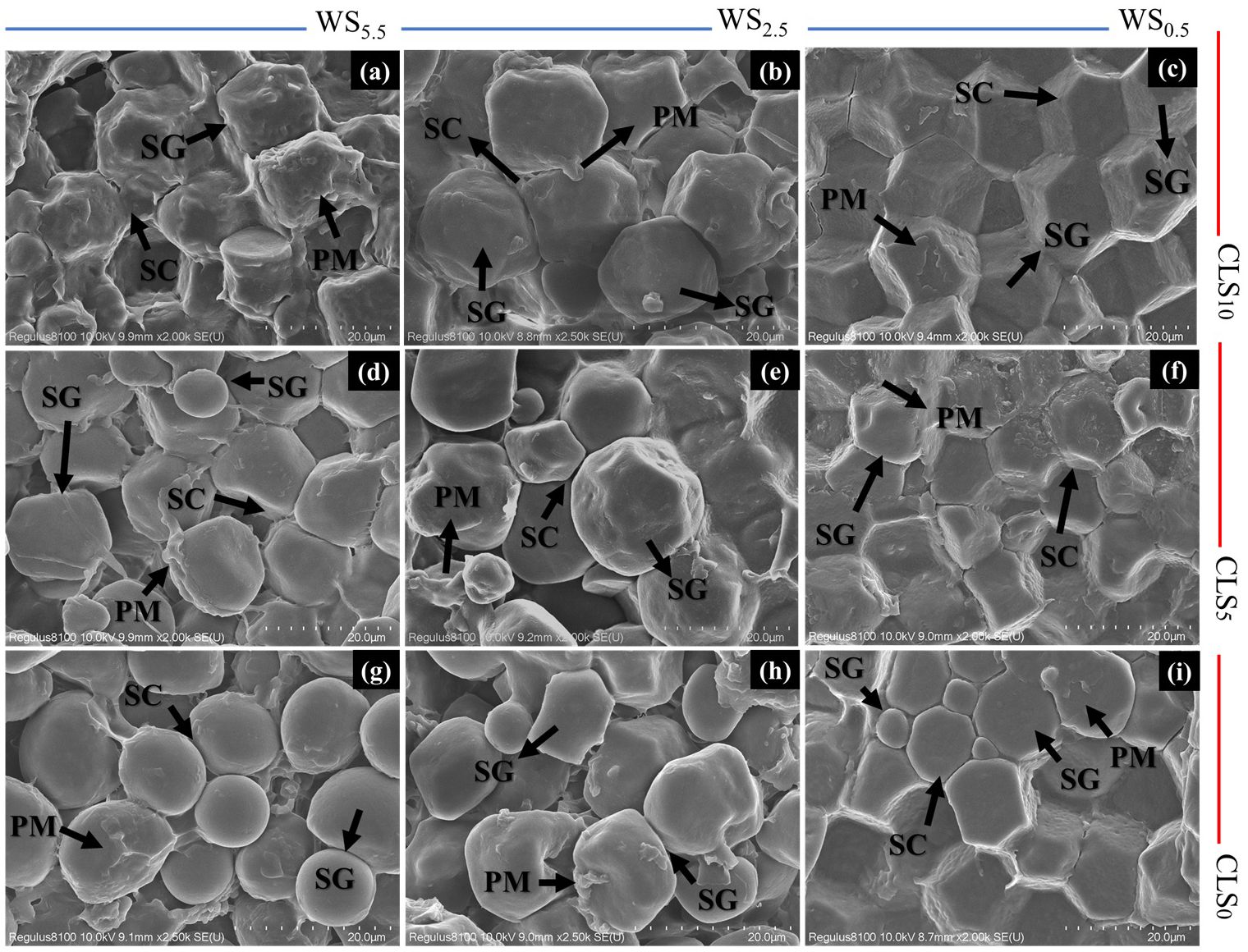
Figure 5. Scanning electron microscopy (SEM) images of the endosperm of maize seeds for different treatments. Starch granules (SG), protein matrix (PM), protein deposits (PD), and compound starch grains (CSG). (A–C) represents flow cytometric analysis for plants in soil treated with calcium lignosulfonate (CLS) addition rate of 10% under water salinization (WS) stress of WS5.5, WS2.5, and WS0.5, corresponding to 5.5, 2.5, and 0.5 dS/m, respectively; (D–F) represents flow cytometric analysis for plants in soil treated with CLS addition rate of 5% under WS5.5, WS2.5, and WS0.5, respectively; (G–I) represents flow cytometric analysis for plants grown in soil treated with CLS addition rate of 0% under WS5.5, WS2.5, and WS0.5, respectively. All images are shown at the same level of magnification, with 20-um scale bars.
However, the ultramorphology of maize grain embryo exposed to CLS5 was boosted by enhancements in crystallization and size for starch particles with an obvious drop in protein matrix over reducing salinity stress in the order of WS5.5 > WS2.5 > WS0.5 (Figures 5D–F). The positive development in the ultramorphology of maize grain embryos exposed to CLS10 developed greater with a greater size for starch grains, well-built starch particles with sharply bounded starch particles. Furthermore, the protein matrix declined, preventing the injury to maize grains compared with CLS0 and CLS10 by reducing salinity stress in WS5.5 > WS2.5 > WS0.5 (Figures 5G–I). Considerable differences in the maize grain’s quality and chemical composition signs were found through different treatments (Table 6). Under a similar calcium-sulfonated lignin addition rate, WS0.5 boosted proteins, fatty acids, starch, Si, P, and N percentages in the maize grains. During the similar salinity stress, the smallest proportions of protein, fat, starch, Si, P, and N were realized in CLS0, followed by CLS5, while the greatest percentage was detected in SL10.
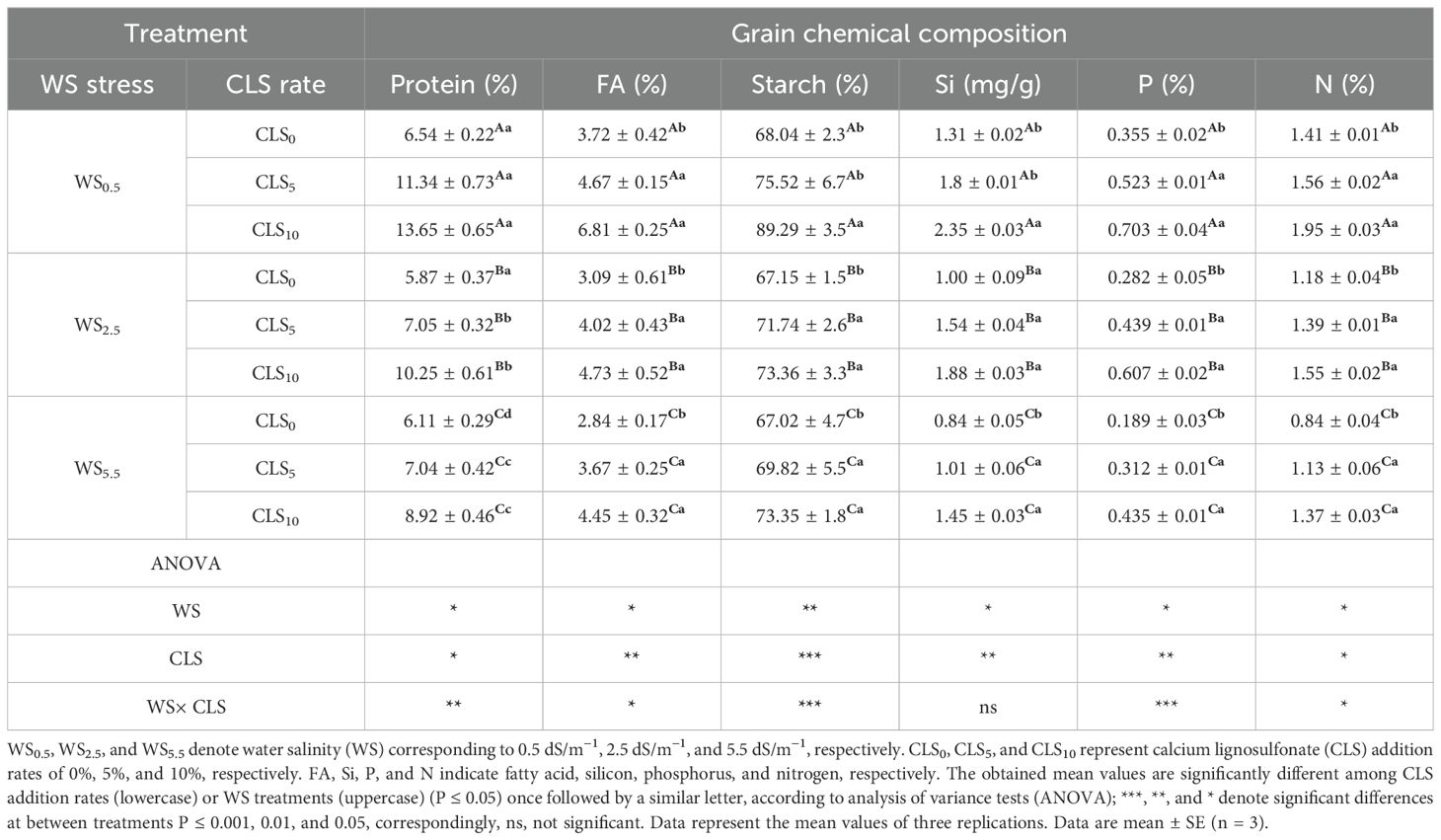
Table 6. Grain quality indicators of corn for various treatments and a summary of the analysis of variance on the major impacts of salinity stress and calcium lignosulfonate addition rate on grain’s quality.
Due to the combination, the WS0.5 × CLS10 treatment showed the greatest proportions of protein, fat, starch, Si, P, and N in the maize grains. Conversely, the WS5.5 × CLS0 treatment exhibited the smallest proportions of protein, fat, starch, Si, P, and N in the maize grains.
Discussion
This study indicates that the maize plant obviously displayed much resistance to salinity stress levels when soil was amended with calcium lignosulfonate (CLS), as revealed by increased root and shoot lengths, grain yield, and higher protein, starch, and fat contents of grain as well as lower cell damage due to lower reactive oxygen species production. This study also reveals that CLS addition could improve the physiological traits, nutrient uptake, and grain quality of maize and soil chemical characteristics under salinity stress. Accumulation of sodium (Na+) in soil markedly causes ion imbalance and toxicity to plants by limiting the competitive absorption of nutrients such as potassium Ca++ and K+ (Shen et al., 2016). Two potential working mechanisms could be attributed to Na+ mitigation using the CLS application. The first and maim mechanism is attributed to Na+ adsorption by amble hydrophilic carboxyl and hydroxyl groups on the lignin surface by complexing (Liu et al., 2020). The second mechanism is Na+ adsorption by ion exchange with the Ca++ existing on the CLS surface (Figure 6A). Therefore, the reduction in soil pH and EC with increasing CLS application was due to the soluble nutrients, in particular Ca++ and K+, entering the soil solution (Table 1). Moreover, the increases in EOC, DOC, and TOC with increasing CLS application ascertains that the CLS degeneration improved the quantities of released Ca++ and K+ in the soil. This finding is in line with the achieved results of an earlier investigation (Elsawy et al., 2022). The reduction in EOC, DOC, and TOC under salt stress specifies that high salt stress decreased the CLS degradation, thus lessening the level of released K+ and Ca++ in soil solution (Elsawy et al., 2022). Moreover, increasing the salinity stress level increased Na+ within the soil, thus increasing the soil pH. However, increasing CLS application decreased Na+ in the soil, thus alleviating the undesirable influence of salinity stress on the maize crop.
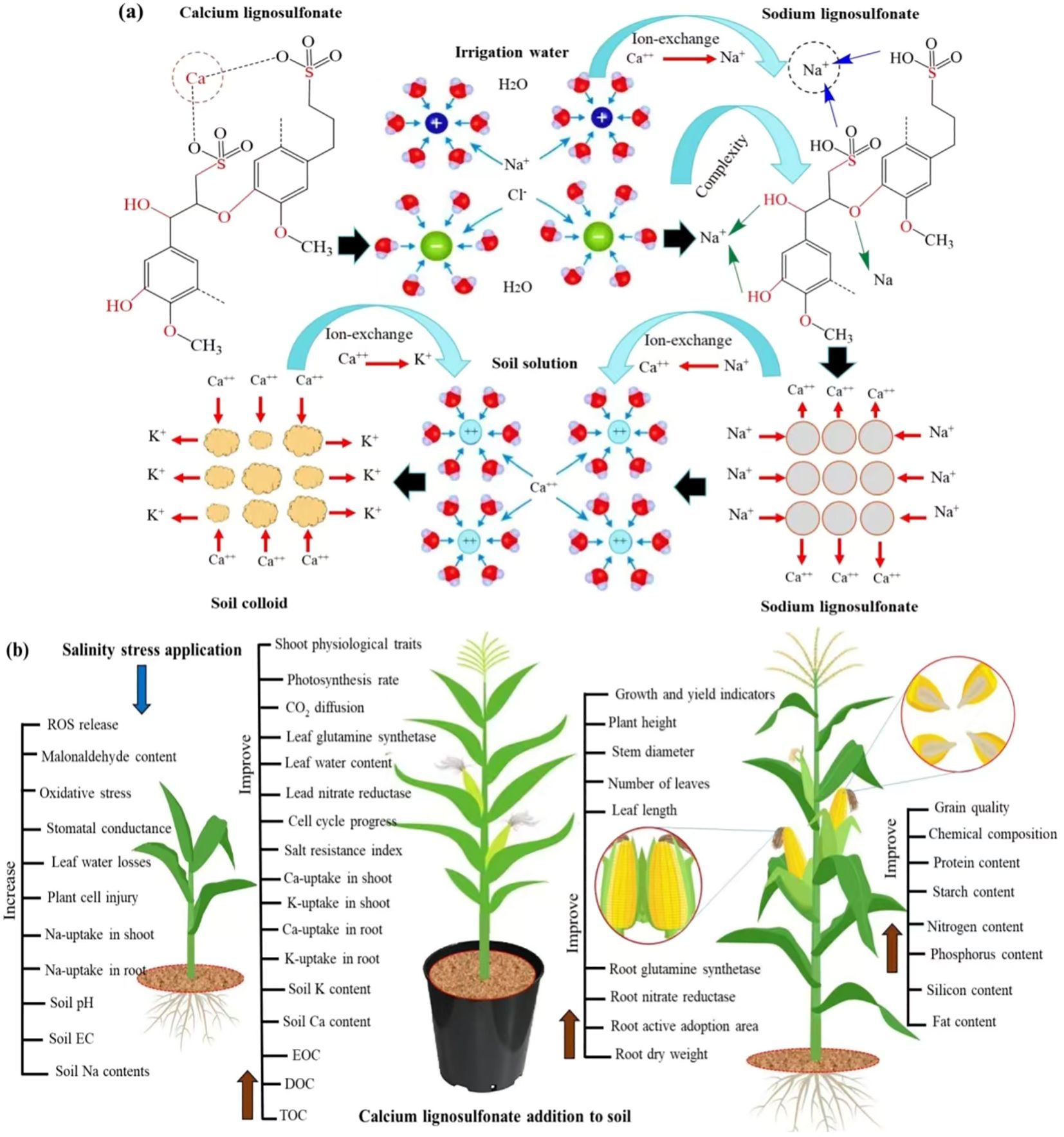
Figure 6. (A) Comprehensive model showing the working mechanisms of calcium lignosulfonate (CLS) in salt stress mitigation. (B) Comprehensive model presenting the mechanism by which CLS application improves physiological traits and grain quality of maize under salinity stress. K+, potassium ion; Ca++, calcium ion; Na+, sodium ion, and Cl-, chloride ion; EOC, easily oxidative carbon; DOC, dissolved oxidative carbon; and TOC total organic carbon.
Root physiology was enhanced under WS0.5 × CLS10 by generating heavier roots connecting to the soil through superior RAAA, indicating abundant K+ and Ca++ to roots. Conversely, the deficiency of K+ and Ca++ with salinity stress under WS5.5 × CLS0 could limit the root’s growth and, thus, RAAA. Equally, the maize root growth physiology relied on the variations in nutrient obtainability in soil (Vetterlein et al., 2022; Sadak and Dawood, 2023). Also, many crops exhibited considerable tolerance to salinity stress when soil amended with CLS, as shown by increased root growth traits (Kok et al., 2021; Elsawy et al., 2022).
A larger RNR and RGS identify sufficient root-nutrient intake compared with a smaller RNR and RGS (Li et al., 2021a). Therefore, the high RNR and RGS under WS0.5 × CLS10 show an improved K and Ca nutrient availability while reducing the accumulation of Na in the root. In contrast, the low RNR and RGS define decreased K and Ca nutrient availability and increased accumulation of Na in the root, thus reducing root activity under WS5.5 × CLS0. In this study, increasing the CLS addition rate under salinity stress increased the soil’s ability to fix Na+ ions while increasing the released K+ and Ca++ ions, letting the plant’s root maximize K and Ca uptake while minimizing Na uptake. Consistently, enzymatic activity and K and Ca uptake of maize roots in the CLS-treated soil were higher than in non-treated soil (Elsawy et al., 2022).
The flow cytometry results under the WS5.5 treatment are ascribed to the reduction of plant growth under extreme salinity stress, which reduces plant cell size and progression due to limited cell division (Sheteiwy et al., 2021b). The improvements in rice development regarding cell division and size under WS0.5 are due to minor salinity stress, which encourages plant cell size, progression, and division (Sheteiwy et al., 2019). The leaf cells’ progression, size, and division under CLS10 were significantly motivated compared with the cells’ progress, size, and division under CLS0. This enhancement in the cells’ progress, size, and division is due to the optimal growth conditions under CLS10 treatment.
The decrease in Pn and RWC of maize grown under WS5.5 × CLS0 could result from stomatal conductance started by the larger impact on the guard cells under high salinity stress. Thus, the reduction in CO2 diffusion and the RWC under WS5.5 × CLS0 conditions was also caused by the severe drop in the photosynthetic process due to the over-creation of radical oxidative species (ROS) in particular H2O2 formation. Consistently, it has been reported that crops must modify to these extreme conditions by eliminating ROS and upregulating enzymes of antioxidation (Hasanuzzaman et al., 2020; Sadak et al., 2022). In addition, Ca++ ions could enhance the photosynthesis process by regulating stomata opening and closure (Schulze et al., 2021). Conversely, under WS0.5 × SL10, the Pn and RWC of the leaf are regulated by increasing the CO2 assimilation and the RWC due to a minor impact on the guard cells under minor salinity stress.
The findings of the LNR and LGS enzymes are ascribed to the available nutrients under minor salinity stress of WS0.5 × CLS10, inhibiting the damage to plant cells by boosting the scavenging sequences, decreasing H2O2 formation, and minimizing the MDA production. However, the deficiency in available nutrients under high-salinity stress of WS5.5 × CLS0 motivated the injury to plant cells by disturbing scavenging sequences, boosting the release of H2O2 formation, and maximizing the MDA production. Consequently, the improvement of the antioxidation defense system led to the mitigation of oxidative cell injury by lessening the H2O2 and MDA contents in the leaf cell, which is consistent with the results of a pervious study (Abd El-Hameid and Sadak, 2020; Dumanović et al., 2021; Ragaey et al., 2022). Therefore, an improved ROS scavenging system can efficiently alleviate the accretion of H2O2 and retain a negligible level of ROS for plant development. Hence, CLS encourages the shoot growth of maize over the mitigation of ROS release.
As findings displayed, the PH, SD, NL, and LL were favorably influenced by the improved in the amount of CLS supplied and adversely by the increase in the salinity stress. These results are ascribed to the fact that CLS had a large ability to release Ca, K, and other nutrients, specifically at a high application level by which more nutrients were available to the plant, which was revealed by larger plant development at the different growth stages. These findings are consistent with the findings of (Elsawy et al., 2022), who indicated large enhancements in barley growth and productivity with CLS application. In contrast, the increase in salinity stress could unfavorably affect PH, SD, NL, and LL, certainly in the high salinity stress level. Therefore, all indicators of shoot physiology, including PH, SD, NL, and LL, were lower under WS5.5 than those under WS2.5 and WS0.5 salinity stress levels throughout the different growth stages.
Adding calcium lignosulfonate improved the physiology of root and shoot, demonstrating a favorable impact on the uptake of Ca and K while reducing Na accumulation in maize under salt-stress conditions. These findings are because CLS application could mitigate salt stress in maize by its high transient Na cation binding owing to large acquisition aptitude, so lowering osmotic stress through discharging K and Ca into the soil solution. Recent studies have shown positive responses of crops to CLS application under conditions of low soil quality and plant-nutrient lack (Savy and Cozzolino, 2022; Kang et al., 2024).
Our findings indicated that the seed yield of corn was reduced under high salinity stress. The reduced intake of nutrients, ion toxicity, and ion balance disorder were the fundamental reasons for corn production deterioration under salt stress (El-Bassiouny et al., 2020; Balasubramaniam et al., 2023). However, the CLS addition improved the grain yield of maize under salt stress (Elsawy et al., 2022; Savy and Cozzolino, 2022). Therefore, our findings are ascribed to the capability of CLS to enhance the discharge of K and Ca nutrients into the soil solution while reducing the availability of Na ions.
In the present study, correlations between salinity stress and CLS applied and grain yield of maize were detected. Due to the salinity resistance model, the grain yield of maize was sensitive to salt stress under the non-addition of CLS as the grain yield of maize was reduced by 27.78% per unit elevation in salts above the threshold value (2.09 dS m-1). Equally, barley plants presented much tolerance to salinity stress levels when treated with CLS, leading to a larger grain yield (Elsawy et al., 2022). In opposition, after increasing the CLS rate from 5% to 10%, the values elevated from 4.02 dS m−1 to 5.96 dS m−1; meanwhile, the grain yield of maize reduced by 14.92% and 7.34%. An elevation in the ECe level with the addition of CLS at 10% level could result in a slight decrease in grain yield of maize excess of the ECe threshold standards. Thus, the grain production of maize was further resistant to salinity stress under the addition of CLS at a 10% level.
The ultrastructure of grain embryos attained by SEM confirms the mitigation of injury in grains under WS0.5 × CLS10 due to the well-organized load of protein particles surrounded by well-formed starch grains in endosperm with the nonappearance of air between starch particles. However, the injury in grain under WS5.5 × CLS0 is accredited to the incompetent load of the protein bodies with many irregular starch grains, with a considerable decrease in the number of protein particles, therefore increasing the existence of protein matrix with small dimensions and uneven-edge starch grains.
The WS0.5 × CLS10 treatment could enhance the maize grain composition, essentially affecting the seed’s nutrition. Higher P and N accumulation in grains was due to maize’s efficient growth and mineral nutrition under WS0.5 × CLS10. The good acquisition of P and N is vital to increasing the corn quality (Razaq et al., 2017; Yuan et al., 2023). As P and N occur in many essential compounds, a slight lack could reduce crop growth and limit P and N accumulation in grain (Sánchez-Rodríguez et al., 2021; Wei et al., 2024). Hence, the sharp decline in the P and N uptake in grains in the WS5.5 × CLS0 was due to inefficient mineral nutrition of maize under high salinity stress. Thus, the larger the P and N acquisition, the better the nutritional facts of maize seeds, which is consistent with the results of a previous study (Zhang et al., 2023).
Furthermore, P and N are essential elements of amino acids, enzymes, and proteins. Moreover, P and N uptakes enhance maize grain quality through larger carbohydrates, fatty acids, and protein synthesis (Sánchez-Rodríguez et al., 2021; Yuan et al., 2023). Thus, WS0.5 × CLS10 treatment improved the amino acids, protein, fatty acids, and carbohydrate contents, meanwhile encouraging the silicon contents of grains that improve the filling of proteasome and amyloplast in endosperm with the presence of air among them. Equally, applying CLS on barley contributed to alleviating the salinity stress, leading to higher grain protein content (Elsawy et al., 2022). Moreover, the enhanced P and N uptake by plants improved the amino acids, fatty acids, carbohydrate, and protein proportions and increased the seed’s silicon percentage, thus improving the nutritious facts and seed quality of maize (Sánchez-Rodríguez et al., 2021; Yuan et al., 2023). The lack of P and N uptake under the WS5.5 × CLS0 enhanced the biotic process of seed filling, thereby the chemical structure and seed quality of maize, thus discouraging the synthesis of amino acids, fatty acids, carbohydrates, and protein and the deposition of seed’s silicon percentage. To support the results of this study, the mechanism by which CLS application improves physiological traits and grain quality of maize under salinity stress (Figure 6B). However, long-term experiments should be carried out to define the effect of CLS application on the physical and biological properties of soil. Moreover, open-field trials are required to ascertain the real field response and the degree of the factor’s impact on the chemical properties of soil, development, grain yield, and quality of maize.
Conclusions
The calcium lignosulfonate (CLS) application could alleviate the salinity stress by enhancing the soil chemical properties, thereby enhancing the K+ and Ca++ ions entering the soil solution, particularly at a high level of 10%. Meanwhile, the maize root’s growth traits were motivated, achieving a high intake of K+ and Ca++ ions. Also, the shoot’s physiological attributes were motivated since leaf water losses could be decreased, therefore enhancing the photosynthesis process. Moreover, the plant cell progression cycle was improved while the oxidative stress was minimized as the antioxidant enzymes could achieve the reduction of reactive oxygen species released into the plant cell. Moreover, sufficient K and Ca nutrient uptake in plant parts increased maize tolerance to salinity stress since increasing salinity stress above the threshold values led to a minor decrease in grain yield of maize with the CLS application. Furthermore, the robust glutamine synthetase and nitrate reductase activities could increase protein and starch synthesis during grain filling and were essential to enhancing the protein, fat, and starch contents and increasing the accumulation of mineral nutrients in grains. In opposition, the maize physiology was sensitive to increasing salinity stress without CLS addition. Thus, it led to a sharp reduction in the overall plant growth, K and Ca nutrient uptake, and grain quality, as the antioxidant system does not provide substantial protection against the lipid peroxidation under salinity stress. The present study proposes that CLS application can be employed as a practical approach to enhance soil chemical properties, growth, yield, and grain quality of maize under salinity stress. The present study also provides a theoretical base and practical support for precision water and soil management for sustainable maize production. However, long-term and open-field experiments needed to be conducted to determine the impact of CLS addition on soil physical and biological properties and to confirm the actual field response, and the degree of the factor’s effect on soil properties, growth, yield and grain quality of maize.
Data availability statement
The raw data supporting the conclusions of this article will be made available by the authors, without undue reservation.
Author contributions
YA: Conceptualization, Data curation, Formal analysis, Investigation, Methodology, Writing – original draft, Writing – review & editing. HS: Conceptualization, Data curation, Formal analysis, Investigation, Methodology, Writing – original draft, Writing – review & editing. KZ: Supervision, Validation, Visualization, Writing – review & editing. MO: Software, Validation, Writing – review & editing. IA: Resources, Validation, Writing – review & editing. HA: Resources, Visualization, Writing – review & editing. MS: Resources, Validation, Writing – review & editing.
Funding
The author(s) declare that financial support was received for the research, authorship, and/or publication of this article. This study was funded by National Key Research and Development Program of China (2023YFC3006500), the fund of National Key Laboratory of Water Disaster Prevention (524015222), the Jiangsu Funding Program for Excellent Postdoctoral Talent (2023ZB897 and 2023ZB869), and the Fundamental Research Funds for the Central Universities of China (B220203051, B220204014). The authors extend their appreciation to the Researchers Supporting Project number (RSP2024R176) King Saud University, Riyadh, Saud Arabia.
Conflict of interest
The authors declare that the research was conducted in the absence of any commercial or financial relationships that could be construed as a potential conflict of interest.
The author(s) declared that they were an editorial board member of Frontiers, at the time of submission. This had no impact on the peer review process and the final decision.
Publisher’s note
All claims expressed in this article are solely those of the authors and do not necessarily represent those of their affiliated organizations, or those of the publisher, the editors and the reviewers. Any product that may be evaluated in this article, or claim that may be made by its manufacturer, is not guaranteed or endorsed by the publisher.
References
Abbas, A., Wang, Z., Zhang, Y., Peng, P., She, D. (2022). Lignin-based controlled release fertilizers: A review. Int. J. Biol. Macromolecules 222, 1801–1817. doi: 10.1016/j.ijbiomac.2022.09.265
Abd El-Hameid, A. R., Sadak, M. S. (2020). Impact of glutathione on enhancing sunflower growth and biochemical aspects and yield to alleviate salinity stress. Biocatalysis Agric. Biotechnol. 29, 101744. doi: 10.1016/j.bcab.2020.101744
Ahmed, M., Tóth, Z., Decsi, K. (2024). The impact of salinity on crop yields and the confrontational behavior of transcriptional regulators, nanoparticles, and antioxidant defensive mechanisms under stressful conditions: A review. Int. J. Mol. Sci. 25, 2654. doi: 10.3390/ijms25052654
Alfadil, A. A., Xia, J., Shaghaleh, H., Alhaj Hamoud, Y., Ibrahim, J. N., Hamad, A. A. A., et al. (2021). Wheat straw biochar application improves the morphological, physiological, and yield attributes of maize and the physicochemical properties of soil under deficit irrigation and salinity stress. J. Plant Nutr. 44, 2399–2420. doi: 10.1080/01904167.2021.1918156
Atta, K., Mondal, S., Gorai, S., Singh, A. P., Kumari, A., Ghosh, T., et al. (2023). Impacts of salinity stress on crop plants: Improving salt tolerance through genetic and molecular dissection. Front. Plant Sci. 14, 1241736. doi: 10.3389/fpls.2023.1241736
Baki, G. K. A.-E., Siefritz, F., Man, H.-M., Weiner, H., Kaldenhoff, R., Kaiser, W. M. (2000). Nitrate reductase in Zea mays L. under salinity. Plant Cell Environ. 23, 515–521. doi: 10.1046/j.1365-3040.2000.00568.x
Bakry, B. A., Sabra, D. E. M., Younis, A. M., Sadak, M. S. (2024). Impact of calcium carbonate and chitosan as signal molecule on modulating the negative effects of drought stress on peanut (Arachis hypogaea L.). Egyptian J. Chem. 67, 1–12. doi: 10.21608/ejchem.2024.255562.9105
Balasubramaniam, T., Shen, G., Esmaeili, N., Zhang, H. (2023). Plants’ response mechanisms to salinity stress. Plants 12, 2253. doi: 10.3390/plants12122253
Bandaogo, A., Bidjokazo, F., Youl, S., Safo, E., Abaidoo, R., Andrews, O. (2015). Effect of fertilizer deep placement with urea supergranule on nitrogen use efficiency of irrigated rice in Sourou Valley (Burkina Faso). Nutrient cycling agroecosystems 102, 79–89. doi: 10.1007/s10705-014-9653-6
Bankó, L., Tóth, G., Marton, C. L., Hoffmann, S. (2021). Hot-water extractable C and N as indicators for 4p1000 goals in a temperate-climate long-term field experiment: A case study from Hungary. Ecol. Indic. 126, 107364. doi: 10.1016/j.ecolind.2021.107364
Blair, G., Lefroy, R., Lisle, L. (1995). Soil carbon fractions based on their degree of oxidation, and the development of a carbon management index for agricultural systems. Australian J. Agricul. Res. 46, 1459–1466. doi: 10.1071/AR9951459
Boccaletti, G. (2023). Framing water problems with global statistics is both powerful and misleading. Nat. Water 1, 660–661. doi: 10.1038/s44221-023-00115-3
Brtnicky, M., Datta, R., Holatko, J., Bielska, L., Gusiatin, Z. M., Kucerik, J., et al. (2021). A critical review of the possible adverse effects of biochar in the soil environment. Sci. Total Environ. 796, 148756. doi: 10.1016/j.scitotenv.2021.148756
Chen, Y., Fan, P., Mo, Z., Kong, L., Tian, H., Duan, M., et al. (2021). Deep placement of nitrogen fertilizer affects grain yield, nitrogen recovery efficiency, and root characteristics in direct-seeded rice in South China. J. Plant Growth Regul. 40, 379–387. doi: 10.1007/s00344-020-10107-2
do Nascimento, T. V. M., de Oliveira, R. P., de Melo, M. T. C. (2024). Impacts of large-scale irrigation and climate change on groundwater quality and the hydrological cycle: A case study of the Alqueva irrigation scheme and the Gabros de Beja aquifer system. Sci. Total Environ. 907, 168151. doi: 10.1016/j.scitotenv.2023.168151
Dotaniya, M. L., Meena, V. D., Saha, J. K., Dotaniya, C. K., Mahmoud, A. E. D., Meena, B. L., et al. (2023). Reuse of poor-quality water for sustainable crop production in the changing scenario of climate. Environment Dev. Sustainability 25, 7345–7376. doi: 10.1007/s10668-022-02365-9
Dumanović, J., Nepovimova, E., Natić, M., Kuča, K., Jaćević, V. (2021). The significance of reactive oxygen species and antioxidant defense system in plants: A concise overview. Front. Plant Sci. 11, 552969. doi: 10.3389/fpls.2020.552969
El-Bassiouny, H. M. S., Abdallah, M., El-Enany, M. A. M., Sadak, M. S. (2020). Nano-Zinc Oxide and Arbuscular mycorrhiza Effects on Physiological and Biochemical Aspects of Wheat Cultivars under Saline Conditions. Pakistan J. Biol. Sciences: PJBS 23, 478–490. doi: 10.3923/pjbs.2020.478.490
Elsawy, H. I., Alharbi, K., Mohamed, A. M., Ueda, A., AlKahtani, M., AlHusnain, L., et al. (2022). Calcium lignosulfonate can mitigate the impact of salt stress on growth, physiological, and yield characteristics of two barley cultivars (Hordeum vulgare L.). Agriculture 12, 1459-1479. doi: 10.3390/agriculture12091459
Ertani, A., Nardi, S., Francioso, O., Pizzeghello, D., Tinti, A., Schiavon, M. (2019). Metabolite-targeted analysis and physiological traits of Zea mays L. @ in response to application of a leonardite-humate and lignosulfonate-based products for their evaluation as potential biostimulants. Agronomy 9, 445. doi: 10.3390/agronomy9080445
Gao, C., El-Sawah, A. M., Ali, D. F. I., Hamoud, Y. A., Shaghaleh, H., Sheteiwy, M. S. (2020). The integration of bio and organic fertilizers improve plant growth, grain yield, quality and metabolism of hybrid maize (Zea mays L.). Agronomy 10, 319. doi: 10.3390/agronomy10030319
Ghavidel Darestani, N., Tikka, A., Fatehi, P. (2018). Sulfonated lignin-g-styrene polymer: Production and characterization. Polymers 10, 928. doi: 10.3390/polym10080928
Ghosh, K. (1993). Methods of Analysis of Soils, Plants, Waters and Fertilisers. Journal of the Indian Society of Soil Science 41, 814–815.
Hamoud, Y. A., Shaghaleh, H., Wang, R., Gouertoumbo, W. F., Hamad, A. A. A., Sheteiwy, M. S., et al. (2022). Wheat straw burial improves physiological traits, yield and grain quality of rice by regulating antioxidant system and nitrogen assimilation enzymes under alternate wetting and drying irrigation. Rice Sci. 29, 473–488. doi: 10.1016/j.rsci.2022.07.007
Hasanuzzaman, M., Bhuyan, M. B., Parvin, K., Bhuiyan, T. F., Anee, T. I., Nahar, K., et al. (2020). Regulation of ROS metabolism in plants under environmental stress: A review of recent experimental evidence. Int. J. Mol. Sci. 21, 8695. doi: 10.3390/ijms21228695
Helaoui, S., Boughattas, I., Mkhinini, M., Ghazouani, H., Jabnouni, H., El Kribi-Boukhris, S., et al. (2023). Biochar application mitigates salt stress on maize plant: Study of the agronomic parameters, photosynthetic activities and biochemical attributes. Plant Stress 9, 100182. doi: 10.1016/j.stress.2023.100182
Hossain, M. Z., Bahar, M. M., Sarkar, B., Donne, S. W., Ok, Y. S., Palansooriya, K. N., et al. (2020). Biochar and its importance on nutrient dynamics in soil and plant. Biochar 2, 379–420. doi: 10.1007/s42773-020-00065-z
Ingrao, C., Strippoli, R., Lagioia, G., Huisingh, D. (2023). Water scarcity in agriculture: An overview of causes, impacts and approaches for reducing the risks. Heliyon 9, e18507. doi: 10.1016/j.heliyon.2023.e18507
Jackson, M. L. (2005). Soil chemical analysis: advanced course: a manual of methods useful for instruction and research in soil chemistry, physical chemistry of soils, soil fertility, and soil genesis (USA: UW-Madison Libraries Parallel Press).
Jiang, P. K., Xu, Q. F., Xu, Z. H., Cao, Z. H. (2006). Seasonal changes in soil labile organic carbon pools within a Phyllostachys praecox stand under high rate fertilization and winter mulch in subtropical China. For. Ecol. Manage. 236, 30–36. doi: 10.1016/j.foreco.2006.06.010
Kang, F., Meng, Y., Ge, Y., Zhang, Y., Gao, H., Ren, X., et al. (2024). Calcium-based polymers for suppression of soil acidification by improving acid-buffering capacity and inhibiting nitrification. J. Environ. Sci. 139, 138–149. doi: 10.1016/j.jes.2023.05.025
Kok, A. D., Wan Abdullah, W., Tang, C. N., Low, L. Y., Yuswan, M. H., Ong-Abdullah, J., et al. (2021). Sodium lignosulfonate improves shoot growth of Oryza sativa via enhancement of photosynthetic activity and reduced accumulation of reactive oxygen species. Sci. Rep. 11, 13226. doi: 10.1038/s41598-021-92401-x
Kumar, L., Chhogyel, N., Gopalakrishnan, T., Hasan, M. K., Jayasinghe, S. L., Kariyawasam, C. S., et al. (2022). “Chapter 4 - Climate change and future of agri-food production,” in Future Foods. Ed. Bhat, R. (India: Academic Press), 49–79.
Li, L., Li, Q., Lin, Z., Zhang, Z., Tian, H., Ashraf, U., et al. (2021a). Effects of nitrogen deep placement coupled with straw incorporation on grain quality and root traits from paddy fields. Crop Sci. 61, 3675–3686. doi: 10.1002/csc2.20578
Li, S., Ma, Q., Zhou, C., Yu, W., Shangguan, Z. (2021b). Applying biochar under topsoil facilitates soil carbon sequestration: A case study in a dryland agricultural system on the Loess Plateau. Geoderma 403, 115186. doi: 10.1016/j.geoderma.2021.115186
Liu, Y., Huang, Y., Zhang, C., Li, W., Chen, C., Zhang, Z., et al. (2020). Nano-FeS incorporated into stable lignin hydrogel: A novel strategy for cadmium removal from soil. Environ. pollut. 264, 114739. doi: 10.1016/j.envpol.2020.114739
Liu, Q., Kawai, T., Inukai, Y., Aoki, D., Feng, Z., Xiao, Y., et al. (2023). A lignin-derived material improves plant nutrient bioavailability and growth through its metal chelating capacity. Nat. Commun. 14, 4866. doi: 10.1038/s41467-023-40497-2
Ludwiczak, A., Osiak, M., Cárdenas-Pérez, S., Lubińska-Mielińska, S., Piernik, A. (2021). Osmotic stress or ionic composition: which affects the early growth of crop species more? Agronomy 11, 435. doi: 10.3390/agronomy11030435
Maas, E. V., Hoffman, G. J. (1977). Crop salt tolerance - current assessment. Journal of the irrigation and drainage division 103, 115–134.
Mishra, A. K., Das, R., George Kerry, R., Biswal, B., Sinha, T., Sharma, S., et al. (2023). Promising management strategies to improve crop sustainability and to amend soil salinity. Front. Environ. Sci. 10, 962581. doi: 10.3389/fenvs.2022.962581
Mosier, A., Syers, J. K. (2004). Agriculture and the nitrogen cycle: assessing the impacts of fertilizer use on food production and the environment (x: Island Press).
Mukhopadhyay, R., Sarkar, B., Jat, H. S., Sharma, P. C., Bolan, N. S. (2021). Soil salinity under climate change: Challenges for sustainable agriculture and food security. J. Environ. Manage. 280, 111736. doi: 10.1016/j.jenvman.2020.111736
Narayanan, M., Ma, Y. (2022). Influences of biochar on bioremediation/phytoremediation potential of metal-contaminated soils. Front. Microbiol. 13, 929730. doi: 10.3389/fmicb.2022.929730
Novozamsky, I., Eck, R. V., Van Schouwenburg, J. C., Walinga, I. (1974). Total nitrogen determination in plant material by means of the indophenol-blue method. Netherlands J. Agric. Sci..
Ogawa, T., Fukuoka, H., Yano, H., Ohkawa, Y. (1999). Relationships between nitrite reductase activity and genotype-dependent callus growth in rice cell cultures. Plant Cell Rep. 18, 576–581. doi: 10.1007/s002990050625
Oumarou Abdoulaye, A., Lu, H., Zhu, Y., Alhaj Hamoud, Y., Sheteiwy, M. (2019). The global trend of the net irrigation water requirement of maize from 1960 to 2050. Climate 7, 124. doi: 10.3390/cli7100124
Pan, S., Rasul, F., Li, W., Tian, H., Mo, Z., Duan, M., et al. (2013). Roles of plant growth regulators on yield, grain qualities and antioxidant enzyme activities in super hybrid rice (Oryza sativa L.). Rice 6, 9. doi: 10.1186/1939-8433-6-9
Rady, M., Sadak, M. S., El-Bassiouny, H., El-Monem, A. (2011). ). Alleviation the adverse effects of salinity stress in sunflower cultivars using nicotinamide and α-tocopherol. Australian J. Basic Appl. Sci. 5, 342-355.
Ragaey, M. M., Sadak, M. S., Dawood, M. F., Mousa, N. H., Hanafy, R. S., Latef, A. A. H. A. (2022). Role of signaling molecules sodium nitroprusside and arginine in alleviating salt-Induced oxidative stress in wheat. Plants 11, 1786. doi: 10.3390/plants11141786
Razaq, M., Zhang, P., Shen, H. L. (2017). Influence of nitrogen and phosphorous on the growth and root morphology of Acer mono. PloS One 12, e0171321. doi: 10.1371/journal.pone.0171321
Sadak, M. S., Dawood, M. G. (2023). Biofertilizer role in alleviating the deleterious effects of salinity on wheat growth and productivity. Gesunde Pflanzen 75, 1207–1219. doi: 10.1007/s10343-022-00783-3
Sadak, M. S., Dawood, M. G., El-Awadi, M. E.-S. (2024). Changes in growth, photosynthetic pigments and antioxidant system of Hordeum vulgare plant grown under salinity stress via signal molecules application. Vegetos, 1–17. doi: 10.1007/s42535-024-00879-3
Sadak, M. S., Sekara, A., Al-Ashkar, I., Habib-ur-Rahman, M., Skalicky, M., Brestic, M., et al. (2022). Exogenous aspartic acid alleviates salt stress-induced decline in growth by enhancing antioxidants and compatible solutes while reducing reactive oxygen species in wheat. Front. Plant Sci. 13, 987641. doi: 10.3389/fpls.2022.987641
Sánchez-Rodríguez, A. R., Rey, M.-D., Nechate-Drif, H., Castillejo, M.Á., Jorrín-Novo, J. V., Torrent, J., et al. (2021). Combining P and Zn fertilization to enhance yield and grain quality in maize grown on Mediterranean soils. Sci. Rep. 11, 7427. doi: 10.1038/s41598-021-86766-2
Savy, D., Cozzolino, V. (2022). Novel fertilising products from lignin and its derivatives to enhance plant development and increase the sustainability of crop production. J. Cleaner Production 366, 132832. doi: 10.1016/j.jclepro.2022.132832
Schulze, S., Dubeaux, G., Ceciliato, P. H., Munemasa, S., Nuhkat, M., Yarmolinsky, D., et al. (2021). A role for calcium-dependent protein kinases in differential CO2-and ABA-controlled stomatal closing and low CO2-induced stomatal opening in Arabidopsis. New Phytol. 229, 2765–2779. doi: 10.1111/nph.17079
Shen, Q., Fu, L., Dai, F., Jiang, L., Zhang, G., Wu, D. (2016). Multi-omics analysis reveals molecular mechanisms of shoot adaption to salt stress in Tibetan wild barley. BMC Genomics 17, 1–15. doi: 10.1186/s12864-016-3242-9
Sheteiwy, M. S., Abd Elgawad, H., Xiong, Y.-C., Macovei, A., Brestic, M., Skalicky, M., et al. (2021a). Inoculation with Bacillus amyloliquefaciens and mycorrhiza confers tolerance to drought stress and improve seed yield and quality of soybean plant. Physiologia Plantarum 172, 2153–2169. doi: 10.1111/ppl.13454
Sheteiwy, M. S., Ali, D. F. I., Xiong, Y.-C., Brestic, M., Skalicky, M., Hamoud, Y. A., et al. (2021b). Physiological and biochemical responses of soybean plants inoculated with Arbuscular mycorrhizal fungi and Bradyrhizobium under drought stress. BMC Plant Biol. 21, 195. doi: 10.1186/s12870-021-02949-z
Sheteiwy, M. S., Shao, H., Qi, W., Hamoud, Y. A., Shaghaleh, H., Khan, N. U., et al. (2019). GABA-alleviated oxidative injury induced by salinity, osmotic stress and their combination by regulating cellular and molecular signals in rice. Int. J. Mol. Sci. 20, 5709. doi: 10.3390/ijms20225709
Tandon, H. (1993). Methods of analysis of soils, plants, waters and fertilizers, fertilizers development and consultation organization. New Delhi India 2, 58–60.
Tang, C., Yang, J., Xie, W., Yao, R., Wang, X. (2023). Effect of biochar application on soil fertility, nitrogen use efficiency and balance in coastal salt-affected soil under barley–maize rotation. Sustainability 15, 2893. doi: 10.3390/su15042893
Vetterlein, D., Phalempin, M., Lippold, E., Schlüter, S., Schreiter, S., Ahmed, M. A., et al. (2022). Root hairs matter at field scale for maize shoot growth and nutrient uptake, but root trait plasticity is primarily triggered by texture and drought. Plant Soil 478, 119–141. doi: 10.1007/s11104-022-05434-0
Wang, C.-F., Han, G.-L., Qiao, Z.-Q., Li, Y.-X., Yang, Z.-R., Wang, B.-S. (2022). Root Na+ content negatively correlated to salt tolerance determines the salt tolerance of Brassica napus L. inbred seedlings. Plants 11, 906. doi: 10.3390/plants11070906
Wei, J., Chai, Q., Yin, W., Fan, H., Guo, Y., Hu, F., et al. (2024). Grain yield and N uptake of maize in response to increased plant density under reduced water and nitrogen supply conditions. J. Integr. Agric. 23, 122–140. doi: 10.1016/j.jia.2023.05.006
Wei, K., Dai, F., Wu, F., Zhang, G. (2009). The variation of β-amylase activity and protein fractions in barley grains as affected by genotypes and post-anthesis temperatures. J. Institute Brewing 115, 208–213. doi: 10.1002/(ISSN)2050-0416
Yang, X., Yao, L., Jin, T., Peng, L. L., Jiang, Z., Hu, Z., et al. (2018). Assessing the thermal behavior of different local climate zones in the Nanjing metropolis, China. Building Environ. 137, 171–184. doi: 10.1016/j.buildenv.2018.04.009
Yuan, Y., Liu, Q., Zheng, H., Li, M., Liu, Y., Wang, X., et al. (2023). Biochar as a sustainable tool for improving the health of salt-affected soils. Soil Environ. Health 1, 100033. doi: 10.1016/j.seh.2023.100033
Zhang, X., Tan, G., Huang, Y. (1994). Experimental technology of plant physiology (Shenyang, China: Liaoning Science and Technology Press), 51–75.
Zhang, A., Wang, X.-X., Zhang, D., Dong, Z., Ji, H., Li, H. (2023). Localized nutrient supply promotes maize growth and nutrient acquisition by shaping root morphology and physiology and mycorrhizal symbiosis. Soil Tillage Res. 225, 105550. doi: 10.1016/j.still.2022.105550
Zhao, S., Zhang, Q., Liu, M., Zhou, H., Ma, C., Wang, P. (2021). Regulation of plant responses to salt stress. Int. J. Mol. Sci. 22, 4609–4625. doi: 10.3390/ijms22094609
Zhao, X., Zhou, N., Lai, S., Frei, M., Wang, Y., Yang, L. (2019). Elevated CO2 improves lodging resistance of rice by changing physicochemical properties of the basal internodes. Sci. Total Environ. 647, 223–231. doi: 10.1016/j.scitotenv.2018.07.431
Zhou, W., Leul, M. (1999). Uniconazole-induced tolerance of rape plants to heat stress in relation to changes in hormonal levels, enzyme activities and lipid peroxidation. Plant Growth Regul. 27, 99–104. doi: 10.1023/A:1006165603300
Keywords: calcium lignosulfonate, salinization, soil characteristics, grain quality, Zea mays
Citation: Alhaj Hamoud Y, Shaghaleh H, Zhang K, Okla MK, Alaraidh IA, AbdElgawad H and Sheteiwy MS (2024) Calcium lignosulfonate-induced modification of soil chemical properties improves physiological traits and grain quality of maize (Zea mays) under salinity stress. Front. Plant Sci. 15:1397552. doi: 10.3389/fpls.2024.1397552
Received: 07 March 2024; Accepted: 26 July 2024;
Published: 20 August 2024.
Edited by:
Eduardo V. Soares, Instituto Superior de Engenharia do Porto (ISEP), PortugalReviewed by:
Szilvia Veres, University of Debrecen, HungaryMervat Sadak, National Research Centre, Egypt
Copyright © 2024 Alhaj Hamoud, Shaghaleh, Zhang, Okla, Alaraidh, AbdElgawad and Sheteiwy. This is an open-access article distributed under the terms of the Creative Commons Attribution License (CC BY). The use, distribution or reproduction in other forums is permitted, provided the original author(s) and the copyright owner(s) are credited and that the original publication in this journal is cited, in accordance with accepted academic practice. No use, distribution or reproduction is permitted which does not comply with these terms.
*Correspondence: Ke Zhang, a3poYW5nQGhodS5lZHUuY24=
†These authors have contributed equally to this work