- 1HiLIFE Institute of Biotechnology and Viikki Plant Science Centre (ViPS), University of Helsinki, Helsinki, Finland
- 2Faculty of Agriculture, Food and Environment, The Robert H. Smith Institute of Plant Sciences and Genetics in Agriculture, The Hebrew University of Jerusalem, Rehovot, Israel
- 3Production Systems, Natural Resources Institute Finland (LUKE), Helsinki, Finland
Plants exhibit an array of drought responses and adaptations, where the trade-off between water loss and CO2 uptake for growth is mediated by regulation of stomatal aperture in response to soil water content (SWC), among other factors. For crop yield stability, the question is how drought timing and response patterns relate to post-drought growth resilience and vigor. We earlier identified, in a few reference varieties of barley that differed by the SWC at which transpiration was curtailed, two divergent water use strategies: water-saving (“isohydric”) and water-spending (“anisohydric”). We proposed that an isohydric strategy may reduce risk from spring droughts in climates where the probability of precipitation increases during the growing season, whereas the anisohydric is consistent with environments having terminal droughts, or with those where dry periods are short and not seasonally progressive. Here, we have examined drought response physiology in an 81-line barley (Hordeum vulgare L.) diversity set that spans 20th century European breeding and identified several lines with a third, dynamic strategy. We found a strong positive correlation between vigor and transpiration, the dynamic group being highest for both. However, these lines curtailed daily transpiration at a higher SWC than the isohydric group. While the dynamic lines, particularly cv Hydrogen and Baronesse, were not the most resilient in terms of restoring initial growth rates, their strong initial vigor and high return to initial transpiration rates meant that their growth nevertheless surpassed more resilient lines during recovery from drought. The results will be of use for defining barley physiological ideotypes suited to future climate scenarios.
1 Introduction
Drought is a ubiquitous abiotic stress that is increasing in frequency and severity as the amplitude of weather fluctuations grows due to climate change (Yin et al., 2018; Cohen et al., 2021; Seleiman et al., 2021). Plants respond to drought by reducing their evaporative water loss, concomitantly decreasing CO2 uptake for photosynthesis. The trade-off between water loss and CO2 uptake is mediated by the regulation of stomatal aperture in response to atmospheric CO2, ambient temperature, vapor pressure deficit, and light, as well as leaf hydration and soil water content (SWC) (Buckley, 2005; Kollist et al., 2014; Peters et al., 2018).
The susceptibility of an individual plant to drought stress and its ability to recover therefrom depends both on the length and intensity of the stress and on the adaptive capacity of the response mechanisms of the plant. The response mechanisms include signaling cascades that mediate stomatal closure (Merilo et al., 2015), as well as physiological and metabolic responses (Jogawat et al., 2021). Those include osmolyte accumulation for osmotic adjustment (Hildebrandt, 2018), enzymatic and non-enzymatic scavenging of excess reactive oxygen species (ROS) to mitigate dehydration and cellular damage (Das and Roychoudhury, 2014), and changes in the chloroplast proteome (Chen et al., 2021).
Under well-watered conditions, plants may differ in their water use efficiency (WUE), which is the carbon fixation or growth rate relative to the rate of transpirational water loss (Hatfield and Dold, 2019). Plants may be optimized for growth rate given non-limiting water, for water conservation, or for WUE. With the arrival of drought, two differing idealized strategies may be followed: isohydric or anisohydric (Sade et al., 2012; Moshelion et al., 2015). Isohydricity implies closing of stomata at a relatively high SWC to maintain a relatively constant water potential, thereby sacrificing carbon fixation but delaying plant dehydration, and comprises many physiological parameters (Scharwies and Dinneny, 2019). In contrast, plants with anisohydric behavior keep their stomata open to a relatively low SWC, allowing the leaf water potential to decline (Negin and Moshelion, 2016; Hoshika et al., 2020). The terms are often used, as here, loosely to refer to water use strategy, respectively water-conserving (isohydric) and non-conserving (anisohydric), rather than referring to the actual hydric status of the leaf, due to the practical difficulty in measuring leaf water potential non-destructively during the course of a drought experiment (Sade et al., 2012; De Swaef et al., 2022).
Barley is the world’s fourth most widely cultivated cereal and is grown on every continent (Lister et al., 2018; FAOSTAT, 2023). It is either cultivated as a spring crop, which is sown in the spring and harvested in the late summer autumn, or as a winter crop, which is sown in the autumn or early winter and harvested in the spring (Lister et al., 2018). Generally, spring-sown barley will experience periodic droughts at early- or mid-growth stages, whereas winter-grown barley will encounter terminal drought during grain filling and maturation (Hakala et al., 2012). Drought stress profoundly affects barley, and many other species, as a crop, leading to decreased yield and compromised quality, a problem that only increases with climate change (Moore and Lobell, 2015; Tao et al., 2017; Ray et al., 2019; Schauberger et al., 2018, 2022). Insight into the mechanisms of drought response and tolerance will enhance breeding strategies to maintain yield and quality in the face of increasing drought risks (Honsdorf et al., 2014; Gol et al., 2021; Puglisi et al., 2022).
In an earlier study of several reference varieties of barley that differed by the SWC at which transpiration declined (Paul et al., 2023), we proposed that an isohydric strategy may reduce risk from early droughts in climates where the probability of precipitation increases during the growing season, whereas an anisohydric strategy is consistent with environments having terminal droughts, or with those where dry periods are short and show little seasonal variation. In recent analyses of four high-yielding European spring barley cultivars subjected to a standardized drought treatment imposed around flowering time we found, moreover, that one variety (RGT Planet) displayed a dynamic drought response (Appiah et al., 2023). This variety displayed high transpiration under ample water supply but switched to a water-conserving phenotype upon drought.
Here, we have examined drought response in an 81-line barley diversity set, which spans (Figure 1B; Supplementary Table S1) 20th century European barley breeding. Our aim was to understand, for crop yield stability, how drought timing and response patterns relate to post-drought growth resilience and vigor. A high-precision lysimeter platform (Dalal et al., 2020) permitted highly regulated irrigation and continuous monitoring of soil and plant water relations, plant stomatal response, and biomass increase. We looked at differences in rates of transpiration and growth under well-watered conditions, transpirational responses to SWC during drought, and the degree of recovery following rewatering. We found that a dynamic transpirational response to drought is not unique to RGT Planet but represents a third strategy among barley cultivars. The results will be of use for defining barley physiological ideotypes suited to future climate scenarios.
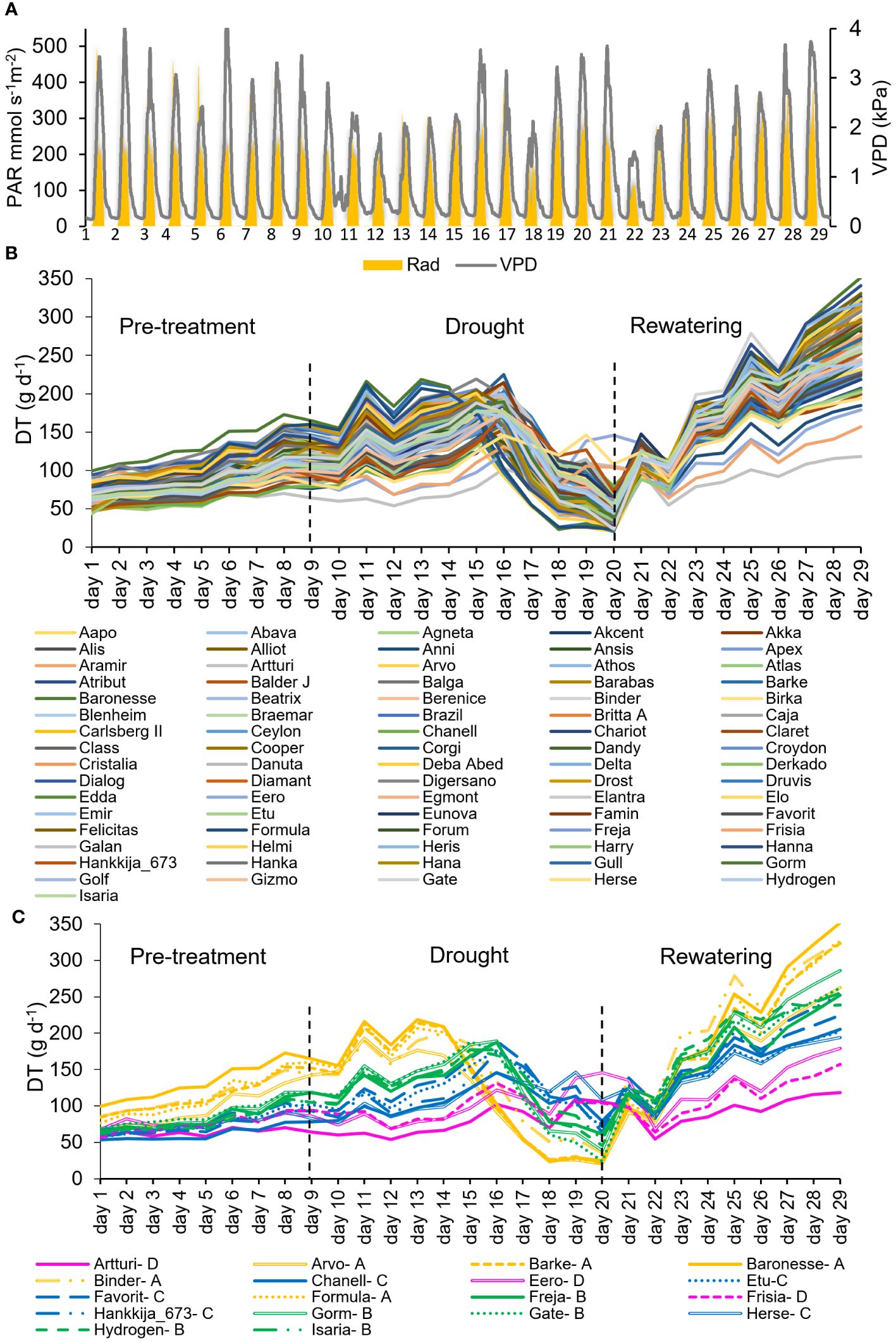
Figure 1 Daily Transpiration (DT) of the 81-line barley population grown on the lysimeter system for first screening. (A) Daily vapor pressure deficit (VPD) and Photosynthetic Active Radiation (PAR) during 29 consecutive days of the experiment. (B) DT in response to the soil-atmosphere water gradient. Each line represents a single plant for each accession. (C) DT of 18 selected lines within the 81-accession experiment during the pre-treatment, drought and rewatering shown in (B). Four groups of lines were identified in the 81-line experiment, based on their DT and stomatal closure. These are labeled as A (yellow), B (green), C (blue), D (magenta). Dashed black lines at Day 9 and Day 20 show the beginning and end of drought treatment.
2 Materials and methods
2.1 Plant material
The barley lines used in this screening experiment were from a diversity set assembled to represent the breadth of European barley breeding during the 20th century (Xu et al., 2018). The set studied here comprised 81 spring barley lines, 72 two-row and 9 six-row, from 14 countries. A subset of 18 varieties were selected from the 81 as exemplars of their water use strategy, as described below. The subset comprised 12 two-row and 6 six-row lines from 7 countries. The 18 chosen lines are distributed across the diversity space (Supplementary Figure S1), as revealed by 864 gene-based single nucleotide polymorphism (SNP) markers (Tondelli et al., 2013). Details for 81- and 18-line sets, including their release year, country of origin, pedigree and breeders, are given respectively in Supplementary Tables S1 and S2.
2.2 Growth conditions
Seeds were sown into 50 ml cones filled with peat soil, on trays, one seed per cone, and the trays covered with plastic wrap and aluminum foil for two weeks at 4°C as a means to break dormancy and to enhance germination (Galkin et al., 2018). The trays were then placed in a controlled glasshouse under short-day conditions (8/16 hr light/dark, 16/10°C day/night). Following emergence, the seedlings were grown for either 8 weeks (81-line experiment) or 12 weeks (18-line experiment) in total. Seedling roots were washed and the seedlings transplanted into potting soil (Dalal et al., 2020; Paul et al., 2023) in 4L pots and placed into a semi-controlled greenhouse. After two weeks of acclimatization, the pots were mounted on the lysimeter system (Supplementary Figure S2). To maximize homogeneous exposure to the ambient conditions on the lysimeter platform, all the pots were placed in random order. Temperature and relative humidity (RH) were respectively around 24–33°C and 30–60% for the 81-line experiment, and 20–35°C and 20–80% for the 18-line experiment. The PlantArray lysimeter platform (Plant-Ditech Ltd., Israel; Dalal et al., 2019) includes a meteorological station, continuously records the physiological conditions of the experiment and is equipped with an automated irrigation system. During the 81-line and 18-line experiments, the system recorded minimum and maximum mid-day daily light intensities ranging from 116 to 493 mmol s-1 m-2 and 74 to 514 mmol s-1 m-2 respectively (Figures 1A; Supplementary Figure S3A), and vapor pressure deficits (VPD) of 1.5 to 4.3 kPa (average 2.6 kPa) and 0.6 to 4.8 kPa (average 2.2 kPa) respectively.
2.3 Experimental setup
Drought experiments were carried out as previously on the PlantArray platform, which is a high-throughput physiological diagnostic system consisting of a highly sensitive, temperature compensated multi-lysimeter array (Halperin et al., 2017; Dalal et al., 2019; Appiah et al., 2023; Paul et al., 2023) and comprised three phases: pre-treatment, drought, and rewatering. In pre-treatment, the plants were maintained on the lysimeter platform and were well watered. Under drought, the plants were exposed to dehydration by limiting the water content in each pot until they reached a specific SWC. Irrigation was carried out as described in Halperin et al. (2017). Drought was imposed as described in Paul et al. (2023), but only one drought phase. During rewatering, plants were irrigated as in the pre-treatment phase.
Altogether 81 lines were screened without replication for drought stress response and yield-related QPTs. Of this set, 18 were then chosen for more intensive investigation. For the 18 lines, 5 to 8 biological replications were made, creating a total of 139 plants. For both sets of experiments, a well-watered period, with irrigation at night until each pot reached its full capacity, was followed by a drought treatment in which irrigation was minimized. The QPTs were monitored in real time on a multi-lysimeter platform, with data collected every few minutes by SPAC analytical software throughout the experimental period. The 81-line experiment lasted a total of 29 days: 9 days of pre-treatment, 11 days of drought, and 9 days of rewatering. For the subsequent 18-line in-depth evaluation, the set was given 11 days of pre-treatment (Supplementary Figure S2B), followed by 15 days of drought (Supplementary Figure S2C) to attain a similar SWC as in the first set. The drought period was terminated for each line individually as soon as it fell below 15% SWC. All lines were then given 29 days of rewatering for recovery (Supplementary Figure S2D). Due to the second experiment’s average atmospheric VPD being lower than that of the first, the drought treatment was longer. The total elapsed time of the second experiment was 55 days.
2.4 Measurements of physiological traits
The PlantArray platform continuously collects data and sends it to a central computer for additional analysis by SPAC (soil–plant–atmosphere continuum) analytical software of soil and atmosphere data alongside plant traits (Dalal et al., 2020). The dataset included daily data (one value per day for each plant) and momentary data acquired every 3 min (480 values per day for each plant). The processed data allowed us to determine the physiological parameters of single whole plants in individual pots simultaneously for the entire experimental time, including growth rate, transpiration, stomatal conductance, and WUE (Halperin et al., 2017; Dalal et al., 2019). The physiological parameters were calculated according to Halperin et al. (2017) with data analyses made using Matlab software (MathWorks, Natick MA, USA). We chose five consecutive days during each of the pre-treatment, drought, and rewatering stages, where the VPD and PAR were most stable, to analyze the quantitative physiological traits (QPTs) of the lines in detail. Over the course of the 18-line experiment, these periods comprised days 7 to 11 during pre-treatment, 22 to 26 during drought, and days 51 to 55 during recovery. Using SPSS software, means and standard errors were determined and the one-way ANOVA Tukey Post-Hoc test at a significance level of p=0.05 was carried out for multiple comparisons of the lines. In the reported analyses, lines without significant differences share superscript letters.
3 Results
Two sets of experiments were carried out: screening of 81 lines; intensive investigation of an 18-line set representing the breadth of responses among the 81. For both sets of experiments, a well-watered period was followed by a drought treatment and then by re-watering for recovery; data were collected every few minutes by the systems analytical software.
3.1 Water use strategies during drought and recovery
Daily transpiration: The VPD and PAR during the whole experimental period is shown in Figure 1A. During the well-watered pre-treatment, daily transpiration of all 81 barley lines increased in parallel but diverged as the plants grew at different rates (Figure 1B). During the drought, the lines responded differentially regarding the day and degree to which their daily transpiration (DT) dropped. Similarly, following rewatering on day 21, though all the plants’ DT grew substantially throughout the rewatering phase, the lines responded disparately to water availability and showed varying rates and degrees of recovery, which is a measure of resilience. Initial DT was between 50 and 100 g d-1, a 2-fold spread, which during recovery enlarged to 100 to 350 g d-1, a 3.5-fold variation. Within that range, the 81 barley lines could be divided into four groups by their transpirational behavior, from which we extracted 18 lines (Groups A, five lines; B, five lines; C, five lines; D,3 lines) as representative of the range (Figure 1C) for the second set of experiments.
Physiological drought point (θc): Theta-crit (θc) is the critical SWC which becomes a limiting threshold for supporting maximal transpiration values, leading the plant to respond by reducing its transpiration rate (Halperin et al., 2017; Dalal et al., 2019), shown in Figure 2A for the 18 chosen barley lines during the 81-line experiment. The four groups (Figure 1C) define four distinct patterns for combinations of θc and the maximum transpiration rate (TRmax) under well-watered conditions. Group A and B had similar θc, at about ~30% SWC, though at different DT; Group C had a still lower DT and a very low θc, at ~20% SWC; Group D had the highest θc, ranging from 30 to 35% SWC, but the lowest DT. Comparison between the TRmax and θc under drought (Figure 2B) shows a significant difference between each group and a correlation between the two measures, TRmax declining from Group A successively to B, C, and D under well-watered conditions. The groups showed distinct rates of decline in transpiration rate vs SWC, with Groups A and B declining slowly and Groups C and D precipitously (Figure 2A). Supplementary Table S3 contains TRmax and θc for each of the 18-line subset.
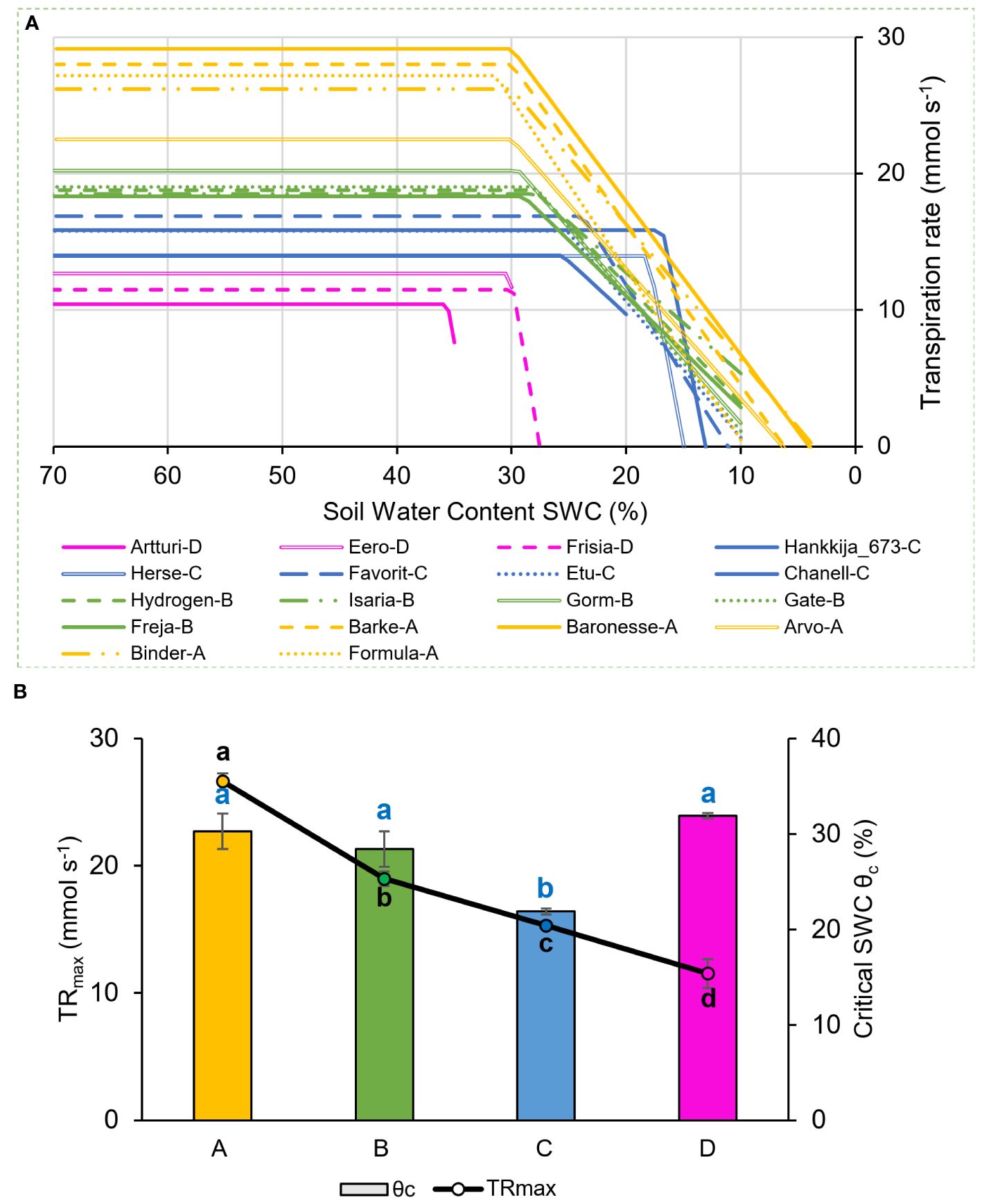
Figure 2 Transpiration rate response to drought during 81-line screening. Behavior of 18-line subset shown. (A) Piece-wise fitted line between midday whole plant transpiration rate (mmol s-1) and soil water content (SWC). The four physiological groups are as in Figure 1: A (yellow), B (green), C (blue), D (magenta). (B) Maximum transpiration rate (TRmax) and θc averaged for the four groups. Lowercase letters, significance levels for TRmax (black) and θc (blue).
3.2 Whole-plant water relations during drought and recovery
In the replicated and more extensively analyzed experiments with the 18-line subset, we regrouped the lines to take into account their behavior through drought and recovery. Group 1, with highest transpiration, had four lines (Hydrogen, Hankkija 673, Baronesse, Isaria), followed by Group 2 (Etu, Gorm, Gate, Frisia, Barke, Chanell, Favorit) and Group 3 (Arvo, Herse, Formula, Artturi, Eero, Binder, Freja) with seven lines each (Figure 3). During pre-treatment, the overall transpiration of all barley lines ranged from 73 to 207 g day-1 (Figure 3A), which dropped to 36 to 131 g day-1 (Figure 3B) during drought stress and recovered to 174 to 380 g day-1 (Figure 3C). A maximum DT of 207 g day-1 during pre-treatment was observed in Hydrogen, while the lowest DT was observed in Freja at 73 g day-1.
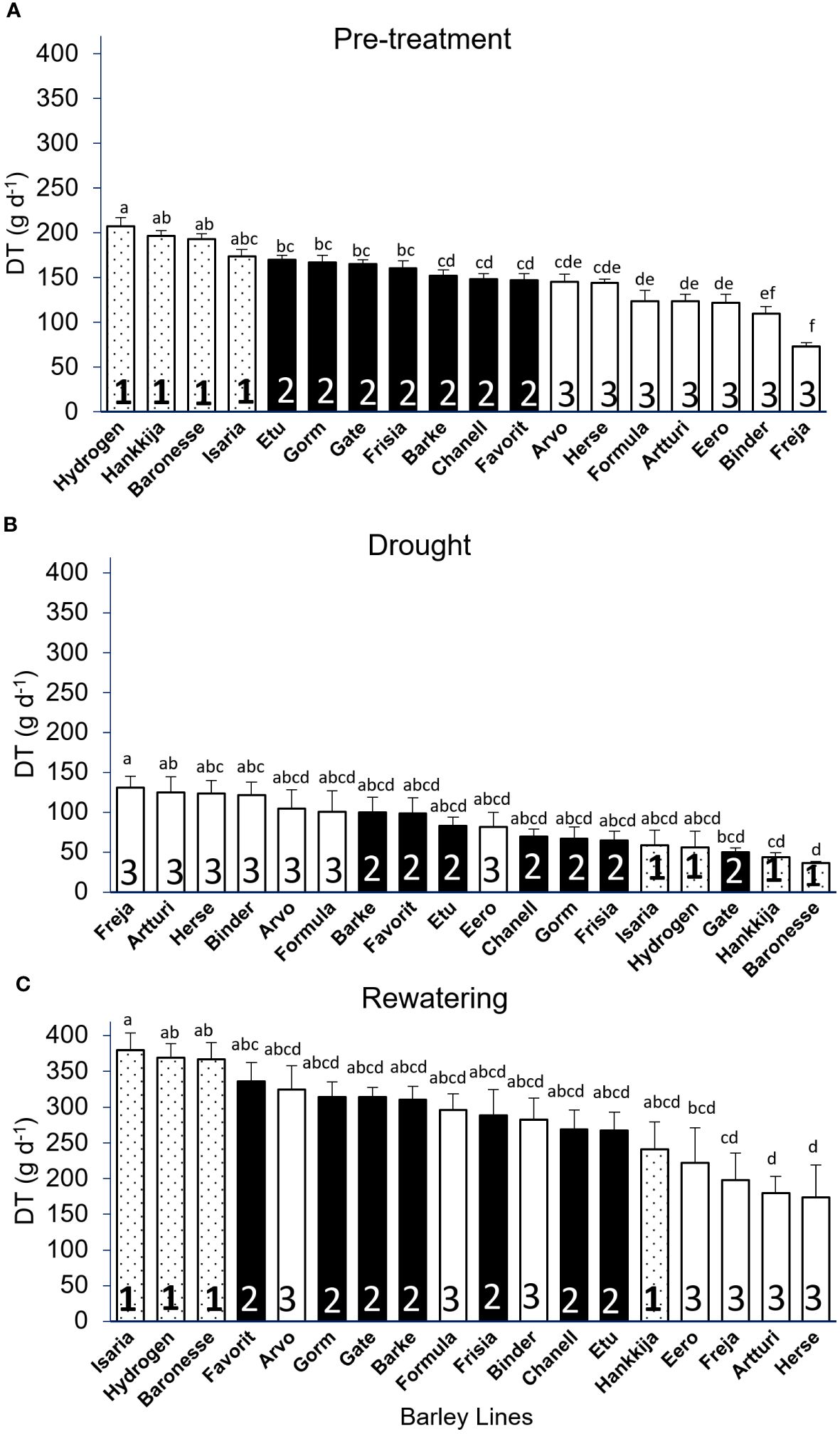
Figure 3 Daily Transpiration (DT) of 18 barley lines grouped by significance levels. Each treatment is the average of DT from 5 consecutive days when the PAR and VPD were the most identical, and for each line 5 to 8 biological replicates were measured. (A) Pre-treatment (well-watered); grouping from highest to lowest DT (average of day 7–11). (B) Drought DT, lines arranged in descending order (average of day 22–26). (C) Rewatering DT, lines arranged in descending order (average of day 51–55). Lowercase letters above the bars show significant difference between lines, those with no significant difference receiving the same letter. Group 1 has the highest DT, followed by Group 2 and Group 3. Tukey’s Post Hoc multiple comparisons test done in SPSS, with bars representing mean ± SE.
During drought, we observed Group 1 transition from the highest to the set with the lowest DT and Group 3 shift from lowest to highest, with the exception of Eero, which remained in the center of the range. The majority of the lines in all three groups reverted to their pre-treatment positions throughout the rewatering phase, even if the DT increased from a maximum of 200 g d-1 to 350 g d-1. Hankkija 673, Arvo, and Formula, on the other hand, responded differently during rewatering, remaining at a transpiration level similar to that during drought, not returning to the pre-drought level.
Except for a few outliers, most of the lines with intermediate pre-treatment DT (Group 2) remained in the center of the distribution during both drought and recovery. When the lines were compared between the treatments, we found a strong negative correlation between pre-treatment and drought, with r = -0.83 (Supplementary Figure S4A), which is driven by the opposing behaviors of Groups 1 and 3. There was a weak positive correlation, r = 0.58, between pre-treatment and rewatering (Supplementary Figure S4B), and a weak negative correlation for drought versus rewatering, r = -0.59 (Supplementary Figure S4C), likewise driven by differential behaviors by Groups 1 and 3. The daily transpiration of the 18 barley lines with all biological replicates (139 plants) for 55 consecutive days during pre-treatment, drought, and rewatering (Supplementary Figure S3B), with daily fluctuation in VPD and PAR (Supplementary Figure S3A), shows similar variations as found in the first, 81-line screening (Figures 1B, C).
Transition to drought response at θc was highly correlated (r=0.89) with whole plant transpiration levels (Emax) (Supplementary Figure S5B); three lines from Group 1 (Hydrogen, Baronesse, Hankkija 673) had both the highest SWC at θc and the highest Emax (Figure 4). Freja had both the lowest transpiration (Emax =8.8 ± 0.8 mmol s-1g-1) and θc (26.7 ± 1.2%). All the lines fell within a transpiration range of 8 to 15 mmol s-1g-1, with all θc values between 26 to 42% SWC (Figure 4; Supplementary Table S4). Hydrogen had not only the highest transpiration (Emax = 15.1 ± 1.3 mmol s-1g-1) but also the highest θc (42.6 ± 2.9%).
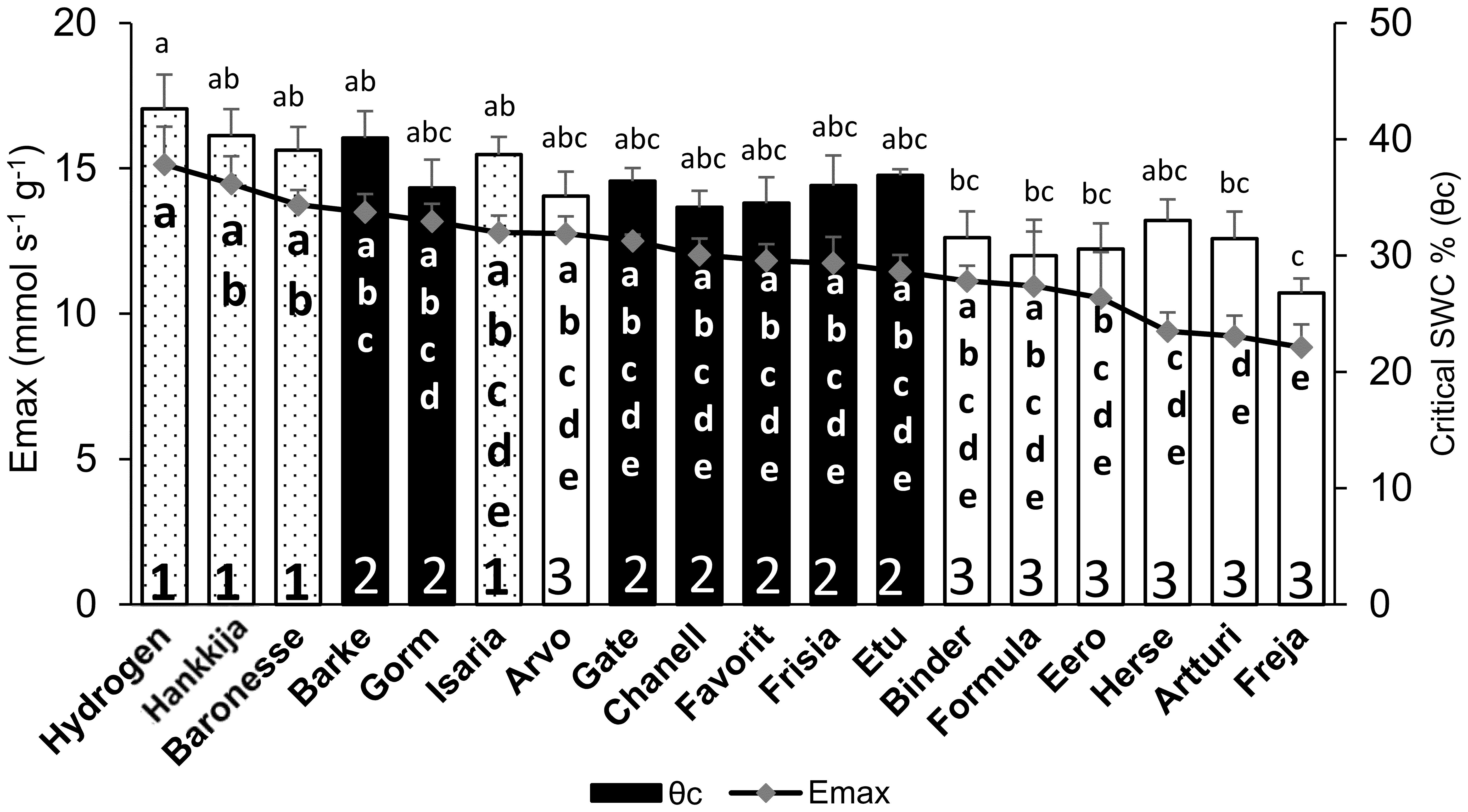
Figure 4 Comparison between midday whole plant transpiration (Emax) during pre-treatment and soil water content (SWC) at θc in 18 barley lines. Lowercase letters inside the bars are significance groups for Emax; those above the bars are for θc.
Canopy Stomatal conductance (GSc): During pre-treatment, Hydrogen (816 mmol s-1g-1) had the highest GSc, whereas Freja (428 mmol s-1g-1) had the lowest (Figure 5A). The DT and GSc are highly (r=0.94) correlated, with Groups 1,2, and 3 showing differential response (Supplementary Figure S5A). When compared by DT group during pre-treatment (Figure 3A), the three lines from Group 1 with the greatest GSc (Hydrogen, Hankkija 673, Baronesse) also had the highest DT, except Isaria, which was in the middle. Similarly, the six lines from Group 3 (Freja, Artturi, Binder, Herse, Eero, and Formula) had the lowest GSc (Figure 5A) and DT (Figure 3A) values, apart from Arvo in the middle. Nevertheless, the majority of the lines in GSc pre-treatment Group 2 (filled bars) are in the same location as the DT pre-treatment.
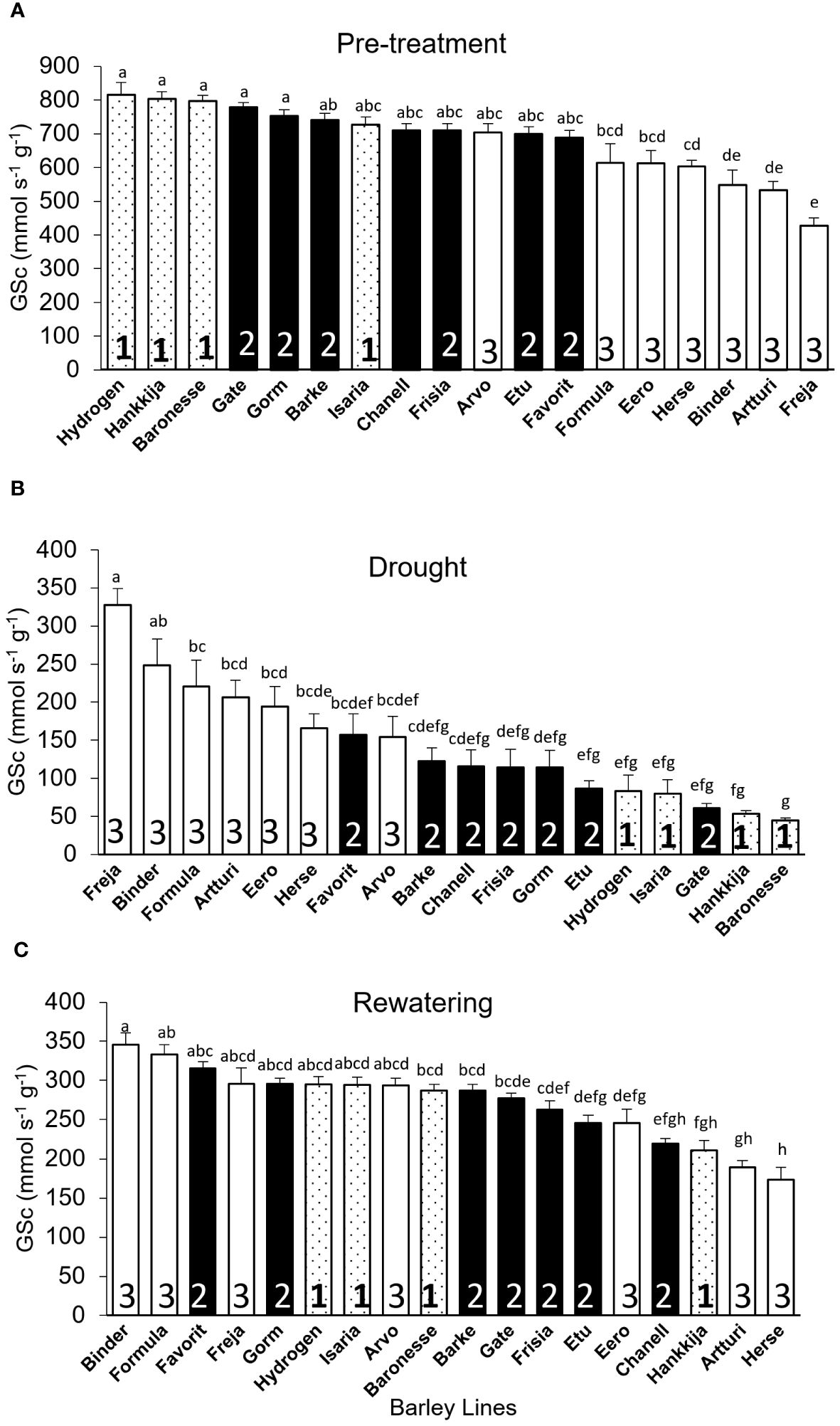
Figure 5 Canopy Stomatal Conductance (GSc) of 18 barley lines grouped by DT during pre-treatment. Each treatment is the average of GSc from 5 consecutive days when the PAR and VPD were most uniform; each line comprises 5 to 8 biological replicates. (A) Pre-treatment; lines arranged by descending GSc (average for days 7–11). (B) Drought; lines arranged by descending GSc (average for days 22–26). (C) Rewatering; lines arranged by descending GSc (average for days 51–55). Lowercase letters indicate significance groups. Bars represent mean ± SE.
During drought, however, Freja (highest) had 7.2X greater GSc than Baronesse (lowest) (Figure 5B), although the difference between the highest and lowest GSc was only 2X during pre-treatment and rewatering (Figures 5A, C). Like for DT, we observed a shift in Group 3 from lowest to highest GSc under drought, and a flip from highest to lowest GSc in Group 1 (Figure 5B). This was also evident when GSc during pre -treatment was compared to GSc during drought using Pearson correlation (r), which revealed a negative correlation of 0.95 (Supplementary Figure S6A).
During rewatering (Figure 5C), we saw a distinctive GSc pattern in which only three lines (Herse, Artturi, and Eero) out of seven in Group 3 returned to their pre-treatment behavior, while the other three (Binder, Formula, and Freja) did not shift, showing no resilient behavior. Interestingly, Arvo remained in the middle group through all three experimental phases. From Group 1, three lines (Hydrogen, Isaria, and Baronesse) moderately recovered GSc, but not Hankkija 673, which recovered poorly, as it did for DT. Correlation analysis also supports the GSc pattern, where only 2% (r = 0.02) of the lines recovered from stress after 29 days of rewatering (Supplementary Figure S6B), whereas 58% of the lines showed recovery in DT (Supplementary Figure S4B).
Calculated Plant Weight Gain (CPW): Once rewatering relieves drought-induced desiccation and biomass production begins again, calculated plant weight (CPW) gain per day indicates resumption of growth and recovery rather than simply rehydration. Like for DT and GSc, initial CPW for Hydrogen (22 g d-1) is significantly higher than for Freja (6 g d-1; Figure 6A). Group shifts between experimental phases (Figures 6A–C) are similar to those for DT (Figures 3A–C). Pre-treatment and drought CPWs are negatively correlated (r =-0.68; Supplementary Figure S7A), whereas pre-treatment and recovery show a positive correlation (r = 0.68; Supplementary Figure S7B). Altogether 68% of the 18 lines recovered from stress. Among Group 1, only Hankkija 673 did not regain high CPW, as likewise seen with GSc. Comparing drought and recovery, we found that line ranking shifted in a similar way for DT and CPW with respect to GSc.
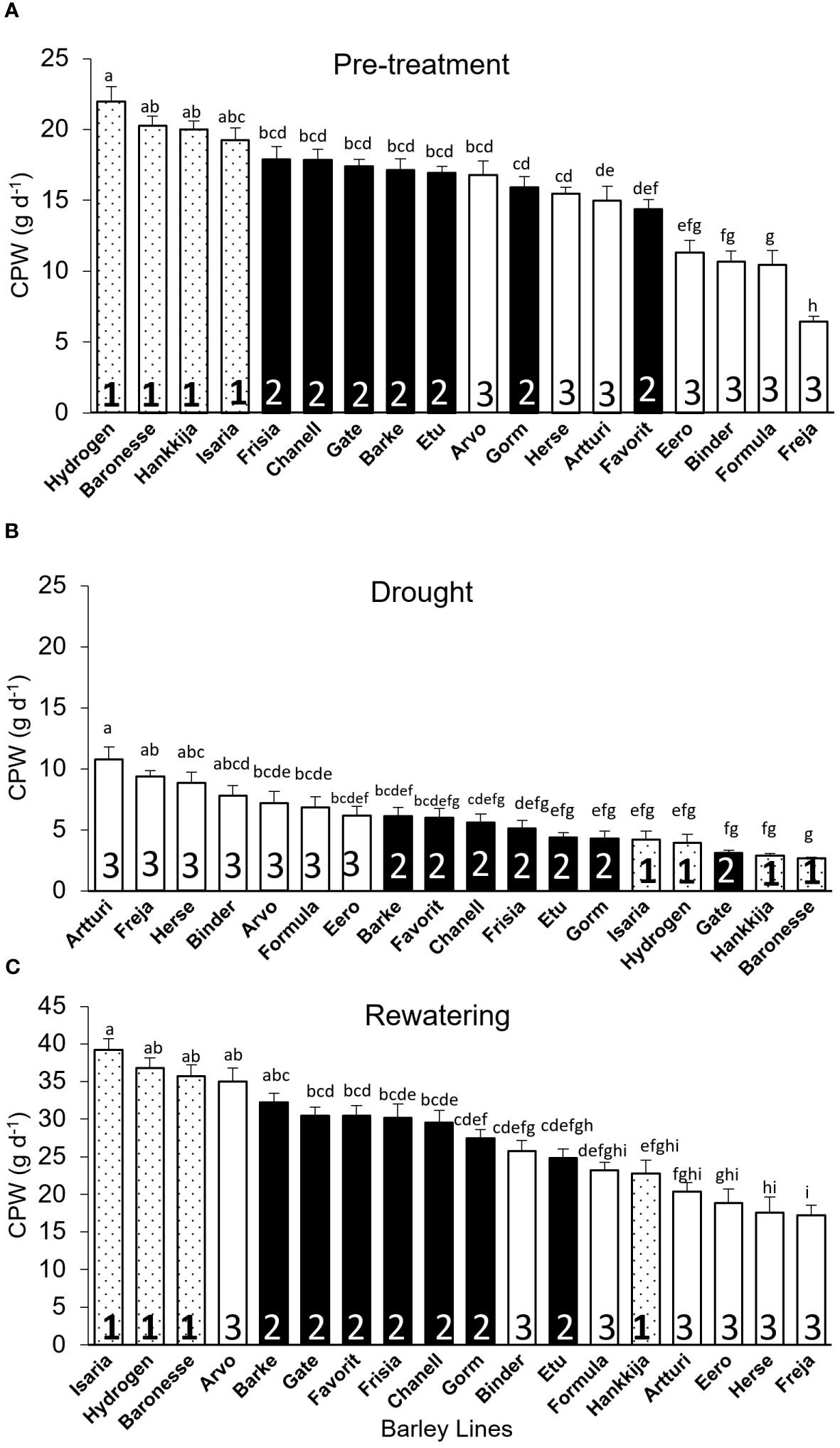
Figure 6 Calculated Plant Weight (CPW) gain per day for the 18 barley lines grouped based on significant levels during DT-pre-treatment. Each treatment is the average of CPW from 5 consecutive days when the PAR and VPD are the most identical, and each line is from of 5 to 8 biological replicates. (A) Pre-treatment (average of day 7–11). (B) Drought (average of day 22–26). (C) Recovery (average of day 51–55). For each phase, lines are arranged in descending CPW order. Lowercase letters indicate significance groups. Bars represent mean ± SE.
3.3 Plant vigor and the impact of stress
The line groupings (1, 2, 3) based on DT proved meaningful also for vigor, defined as average gain in CPW per day during pre-treatment, with the Group 1 lines showing the highest vigor (Figure 7A). Most of the lines in Group 3 had the lowest vigor, Freja being the poorest. However, during drought, Group 3 had the highest daily CPW (Figure 6B). If resilience is considered a return to a pre-drought CPW rate, then three Group 3 lines show the highest resilience, but three within Group 3 the lowest (Figure 7B). However, Group 3 lines, which have a high (or non-responsive) CPW during drought (Figure 6B), return to a low daily CPW in absolute terms (Figure 6C). The lines displayed as well differential recovery of DT during rewatering compared with drought conditions (Figure 7C). The high recovery set included: Hydrogen and Baronesse, comprising half of Group 1; Gorm, the only line from Group 2; Freja, Formula, and Binder of the seven lines in Group 3.
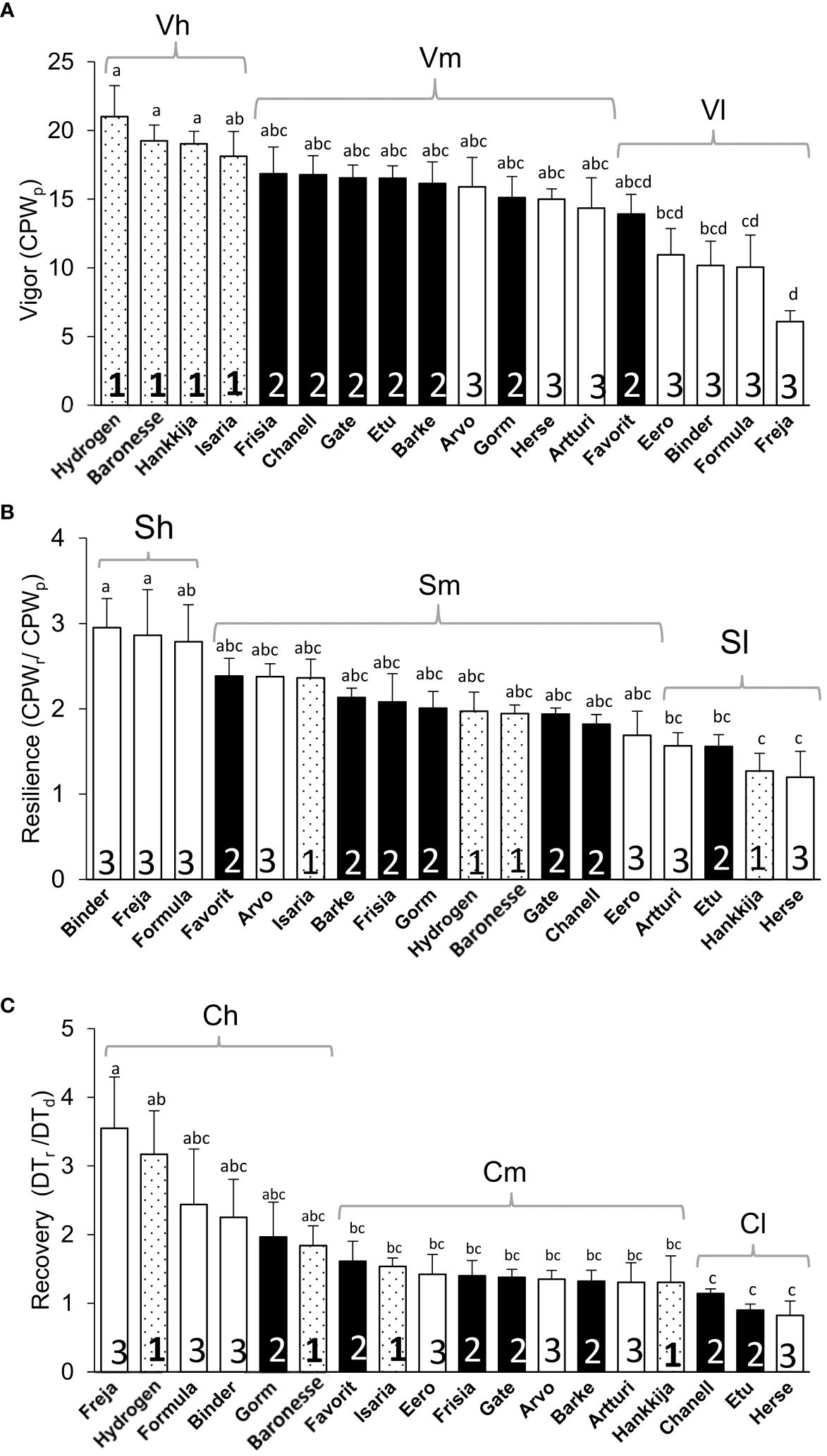
Figure 7 Vigor, Resilience, and Recovery of 18 barley lines. (A) Vigor, the average CPW from day 7 to 11 during pre-treatment (CPWp). (B) Resilience, the ratio between average CPW from day 51 to 55 during rewatering (CPWr) and pre-treatment CPW (CPWp), day 7 to 11. (C) Recovery, the ratio between average DT during rewatering, day 51 to 54 (DTr) and drought, day 18 to 26 (DTd). Means ± SE are displayed; lowercase letters indicate significance groups. Sets in brackets based on significance (lowercase letters) are high (h) medium (m), and low (l) Vigor (V), Resilience (S), and Recovery (C). The lines under high, medium and low vigor, resilience and recovery are presented in Supplementary Table S5.
3.4 Water use efficiency and yield
The quantity of biomass or grain produced per unit of water transpired gives an overall idea of the tradeoffs between carbon fixation and water transpiration made during the life cycle of the plant. First, we calculated the mean total biomass (combined dry weight of husk, seed, and shoot; Supplementary Figure S8A), yield (Supplementary Figure S8B), and seed number (Supplementary Figure S8C) from each biological replicate for all barley lines. Biomass, yield, and seed number varied respectively from 31.7 to 78.3 g, 0.4 to 14.3 g, and 9.6 to 380.4 per replicate. Isaria (78.3 ± 3.8 g) produced the highest biomass but one of the lowest yields (0.61 ± 0.06 g) and seed numbers (39 ± 5.9), hence, lowest harvest index (0.69 ± 0.08%). Eero had the lowest biomass (31.7 ± 5.1 g), a low yield (1.78 ± 0.5 g) and moderate seed number (54.8 ± 20.2). Hydrogen had high total biomass (72.2 ± 3.5 g) but poor yield (1.3 ± 0.2 g) following drought; Freja produced low biomass (41.3 ± 5.6 g) and yield (0.4 ± 0.1 g) as well as the lowest seed number (9.6 ± 2.7). Notably, Hankkija 673, which is a six-row spring barley from Finland released in 1973, had a modest biomass output (60.3 ± 3.9 g) but one of the highest yields (10.2 ± 1.4 g) and seed numbers (380.4 ± 37.1), despite its poor resilience (which measures overall biomass production) and recovery. As a six-row variety, Hankkija had only a middling seed weight (TGW; Supplementary Figure S9). The high yield of Herse (Supplementary Figure S8B) appears to be a product of its high seed number (Supplementary Figure S8C), high harvest index (Supplementary Figure S8D), and relatively high TGW (Supplementary Figure S9). The cumulative transpiration, taken as the sum of the daily transpiration of each plant throughout the experiment, enabled us to calculate the water use efficiency (WUE) for the 18 lines (Figure 8), which ranged from 0.004 to 0.009 g g-1, with Eero having the lowest (0.0045 ± 0.00032 g g-1) and Chanell having the highest (0.009 ± 0.0003 g g-1), twice that of Eero. The WUE of Freja and Hydrogen, which displayed during pre-treatment respectively the lowest and highest values for DT (Figure 3A), GSc (Figure 5A), and CPW (Figure 6A), are however, in the mid-range, with Hydrogen being more efficient (0.007 ± 0.0003 g g-1) than Freja (0.006 ± 0.0003 g g-1). Thus Baronesse, in addition to its dynamic transportational drought response, most efficiently makes the tradeoff between water lost and carbon gained.
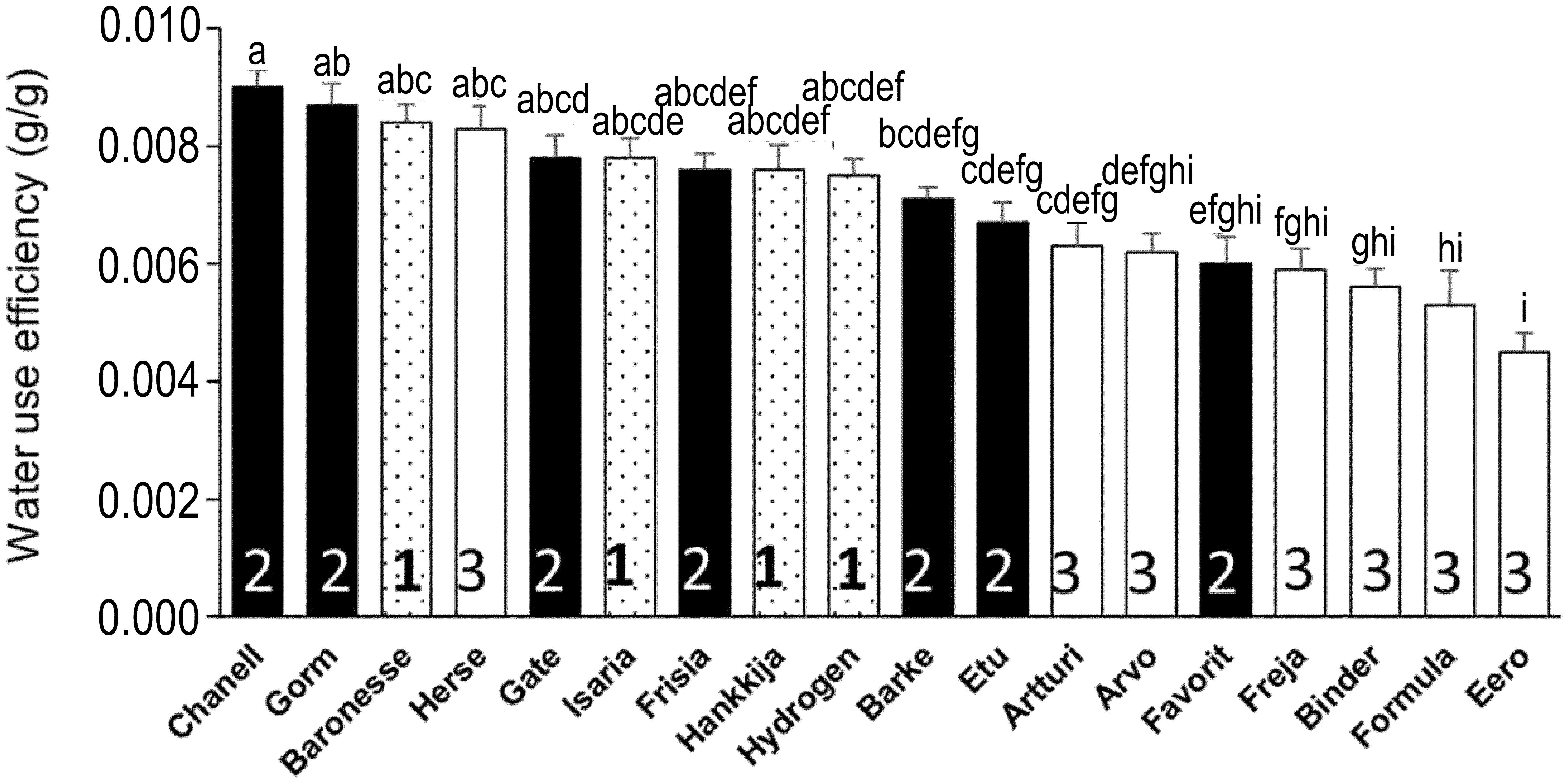
Figure 8 Water use efficiency calculated from dry biomass and cumulative transpiration. Mean ± SE; lowercase letters above the bars are significance groups.
4 Discussion
Plants exhibit a wide array of responses and adaptive mechanisms at the morphological, physiological, and molecular levels to drought or water deficit. Nevertheless, the utilization of these mechanisms varies significantly across different plant species or even within genotypes of the same species (Fang and Xiong, 2015), as we have earlier demonstrated for a few chosen varieties of barley (Appiah et al., 2023). Here, we have screened the QPTs of 81 barley genotypes derived from a diversity set spanning 20th Century barley breeding (Tondelli et al., 2013). From the 81 lines, 18 were chosen to represent the range of the QPTs and studied in more detail under well-watered conditions, drought stress, and subsequent recovery.
4.1 Performance under well-watered conditions
Our analysis indicated a strong correlation (r = 0.95) between vigor (CPW gain per day) and transpiration, those lines (Group 1) with the highest daily transpiration also having the highest vigor. Vigor is defined as the rate of gain in plant weight. Studies suggest that fractional changes in stomatal conductance lead to changes in transpiration (Drake et al., 2013; Zhang et al., 2019). We found a strong correlation (r = 0.94) between daily transpiration and stomatal conductance under well-watered conditions, confirming this (Supplementary Figure S7B). The correlation between higher transpiration and higher vigor therefore indicates that Group 1 lines have the capacity for the increased carbon fixation made possible by greater gas exchange, and moreover fix the carbon, especially Baronesse, with high WUE (Figure 8). However, exceptions such as Isaria and Arvo were observed, where the correlation was weak (Figures 3A, 4A). This discrepancy may be related to variations in stomatal density, distribution, size, and number within the cultivated species; our data indicate that Arvo has the highest stomatal density of the lines we have examined (Pereira et al., in prep).
4.2 Performance under drought
Across the analyzed lines, we saw wide and significantly different responses to drought. Group 1 lines, particularly Hydrogen, Hankkija 673 and Baronesse, which displayed the highest transpiration (Emax; Figure 7A), total daily transpiration (DT; Figure 3), canopy stomatal conductance (GSc; Figure 5), daily CPW (Figure 6), and WUE (Figure 8) when well-watered, shift as a group from a water non-conserving to a water-conserving strategy during drought. Group 1 then displays the lowest DT, GSc, and daily CPW gain. Notably, most Group 1 members reach θc at a higher SWC than do other lines (Figure 7A), between day 16 to 18 (drought began day 12), aligning with their higher transpiration rates. Thus, Group 1 displays a dynamic or plastic response to drought, shifting from high transpiration and growth to low, water-conserving levels during drought. Gate, a Group 2 member by its behavior under well-watered conditions, clustered with Group 1 in having low DT, GSc, and daily CPW gain under drought.
The lines in Group 3 displayed the opposite behavior as those in Group 1, having low DT, GSc, and daily CPW gain under well-watered conditions but comparatively high values under drought. Among Group 3, cv Freja was the most extreme regarding transpiration under non-limiting and limiting water, and likewise reached θc at the lowest SWC, on the final day of the drought (day 26). Herse and Artturi, respectively having among the lowest DT under well-water conditions, had together with Freja the highest DT and GSc as well as CPW gain under drought. The three lines also transitioned to θc at the lowest SWC among the 18 examined in detail. Group 3 can be described as anisohydric, as they reach θc late. Lines in Group 2, except for Gate as described above, took a middle road regarding transpiration parameters both before and during drought, as well as reaching θc at intermediate SWC. Compared to Group 3, they can be considered isohydric.
4.3 Recovery, resilience, and yield
Following the drought treatment, resumption of a full irrigation regime led to an increase in DT in all lines. Only two line – Hydrogen and Baronesse – displayed simultaneously high initial DT (Figure 3A) as well as high vigor and recovery and good resilience (Supplementary Table S5; Figure 7A), Only Hydrogen showed both low drought DT (Figure 3B) and high initial GSc (Figure 5A). A similar degree of resilience, which is the degree to which a plant resumes growth, could in principle be obtained with either low, medium or high vigor; the line need only return to its initial state. In fact, we observed that the most resilient lines (Supplementary Table S5; Figure 7C) were in fact all members of Group 3—Binder, Freja, and Formula—in terms or returning most fully to their initial growth rate, which was in any case low. Hence, as illustrated by Group 3, resilience per se is not necessarily a path to the highest post-drought yield. Freja, Formula and Binder also showed the best recovery of pre-drought DT, likewise initially low; among Group 1, Hydrogen and Baronesse recovered DT best. During rewatering, Isaria, Hydrogen, and Baronesse (all Group 1) returned to the highest rates of vigor (Figure 3C); Isaria showed the best resilience of Group 1.
We examined which of the physiological measures correlated with the final biomass and yield (Supplementary Figures S8, S9) in pots on the lysimeter. The top five for biomass included three from Group 1 and two from Group 2; while all seven from Group 3 (the anisohydric lines) were found at the bottom of the range. The top of the DT range (Group 1, Figure 3) during pre-treatment and rewatering, as well as for pre-treatment and rewatering CPW vigor (Figures 6A, C), showed the best correlation with harvest biomass (Hydrogen, Baronesse, and Isaria) or yield (Hankkija 673). The superior yield of Hankkija 673 despite its mediocre biomass is due to its first-rank harvest index, being a six-row variety, and above average grain weight (Supplementary Figure S9). Yields in greenhouse pot experiments, with highly constricted soil volumes and root architectures, are not expected to be closely similar to those from field experiments. Nevertheless, on the lysimeter, carbon capture as indicated by CPW and as the tradeoff for DT still correlates with final biomass.
4.4 Drought response strategy
The set of lines examined here can be categorized by the two formally contrasting drought strategies, “isohydric”, or water-conserving, and “anisohydric”, or water-non-conserving (Sade et al., 2012; Moshelion et al., 2015; Dalal et al., 2017). Isohydric plants would be expected to limit transpiration and to transition to θc at a relatively high SWC in order to maintain constant leaf water potential. Anisohydric plants trade a constant hydricity for higher gas exchange and carbon fixation, which would put them at risk in prolonged droughts. Based on their physiological responses, we classify Freja, Artturi, Herse, and Binder as the most anisohydric (all in Group 3), or least drought-responsive, displaying the highest transpiration under drought and reaching the θc at low SWC (Figure 7A) on the last days of that phase. Group 2 is more isohydric than Group 3, having higher pre-drought DT and a higher θc (Figure 7A).
In earlier experiments, a single cultivar, RGT Planet, was identified as representing a third drought response type, which may be described as dynamic or plastic (Appiah et al., 2023). This variety (released 2010) is currently the most popular malting barley in Europe, in part due to its consistent and high yields under farm conditions. On the PlantArray system, RGT Planet displayed high transpiration under well-watered conditions, followed by a moderate transpiration decrease under drought, conferring high resilience and, in the pot experiments, high yields that were not significantly reduced by drought. Here, we have established by screening 81 varieties that the dynamic drought strategy is not unique to the relatively new RGT Planet but is found in older lines as well. Group 1, including the “star” lines Hydrogen and Baronesse, displays this dynamic response to drought, transitioning from high-transpiration, water non-conserving to water-conserving behavior, with θc at high SWC (Figure 7A). While two- and six-row barley generally derive from distinct breeding programs, resulting in genetic structure (Supplementary Figure S1), the lines analyzed here did not divide categorically by row number into drought response strategies. Neither did drought strategy correlate with year of release: Group 1 spans 1924 (Isaria) to 1999 (Hydrogen).
Ultimately, from the breeder’s and farmer’s perspectives, the optimal drought response strategy is the one that produces the highest sustainable yield and a tolerable annual variation, commensurate with yearly fluctuations in the growth environment – drought, in this case – and a particular level of inputs. A high WUE may favor effective growth and ultimately yield when water is limiting; two Group 2 (isohydric) lines, Chanell and Gorm, together with Baronesse of Group 1 (dynamic), showed the highest (Figure 7B). Yield stability despite an early-season drought is expected to benefit from recovery of carbon fixation and thereby growth, as reflected in our experiments by DT and vigor (CPW). For the varieties, growth conditions, and drought treatment here, membership in Group 1, with a dynamic strategy, tended to favor higher biomass. Although they reached θc at a high SWC, Group 1 members nevertheless lost weight (i.e. dehydrated) most rapidly (Figure 6B) during the drought treatment while displaying a low DT during drought (Figure 3B). Isaria showed the highest rewatering DT and yet reached θc at a lower SWC, while Hankkija 673 responded at a high SWC, but recovered to a low DT.
We observed a correlation between transpiration (DT, Figure 3; GSc, Figure 5; Emax, Figure 7A) and SWC at θc (Figure 7A), with the dynamic lines (Group 1) having both the transpiration measures and θc high and the anisohydric both low (Group 3). This raises the questions of how and why the high DT, high vigor lines respond quickly to decreases in SWC, but the low DT lines reach θc only at low SWC, i.e. what controls stomatal aperture as SWC falls, and what the consequences of these water-use behaviors are. Enhanced GSc (such as in Group 1) is correlated with higher demand for root and stem hydraulic conductivity (Kudoyarova et al., 2011); increased demand under restricted hydraulic conductance from drying soil drives down water potential in the water column between root and leaf (Carminati and Javaux, 2020; Abdalla et al., 2022; Yang et al., 2023). Abundant evidence connects stomatal closure to drying signals from roots, with ABA and likely other factors implicated in the signaling (Saradadevi et al., 2017); ABA plays a central role in stomatal closure (Assmann and Jegla, 2016; Hsu et al., 2021). The overall picture therefore suggests that high DT, GSc, Emax lines, which are mostly found in Group 1, place high demand on hydraulic conductivity, which becomes limiting, setting off a drought signal to the stomatal guard cells at even moderately reduced SWC. Low conductivity lines, mostly in Group 3, in this model, put more limited demands on conductivity; SWC falls greatly without triggering stomatal closure, a behavior which has been characterized as non-conserving or anisohydric.
The rapid response to falling SWC among Group 1 was associated, particularly for Hydrogen and Baronesse, with the highest degree of recovery. Even though their weight fell rapidly during drought, Isaria, Hydrogen, and Baronesse were the quickest to gain weight during rewatering (Figure 6C; Supplementary Table S4), while displaying a high DT (Figure 3). We earlier analyzed the gene networks upregulated and downregulated by drought and recovery in the anisohydric Golden Promise (Paul et al., 2023); autophagy was downregulated during recovery and thereby implicated in drought response. The connection of autophagy to differing degrees of recovery requires further investigation. The results presented here suggest that a dynamic drought response combined with rapid recovery, such as displayed by Group 1 here and by RGT Planet (Appiah et al., 2023), may offer a good ideotype by which to achieve yield stability under droughts of up to two weeks (conditions here). There have been many efforts to model both stomatal conductance (Buckley, 2005; Damour et al., 2010; Buckley and Mott, 2013) and the optimization of conductance vs. carbon gain (Sperry et al., 2017; Joshi et al., 2022).
While the literature touching on drought in barley is extensive (over 600 articles in PubMed), there has been a paucity of studies examining germplasm sets (10s or 100s of lines), rather than pairs of lines, with precision phenotyping such as is possible on the PlantArray platform. GWA, quantitative trait (QTL), and genomic prediction (GP) studies for yield and biomass or their components in pot- or field-grown barley populations under drought (Moualeu-Ngangué et al., 2020; Li et al., 2022; Ogrodowicz et al., 2023; Abdelghany et al., 2024), or on traits connected to drought tolerance or yield (Honsdorf et al., 2014; Dhanagond et al., 2019; Luo et al., 2020; Fusi et al., 2022; Puglisi et al., 2022) are more extensive, however. We anticipate that current collaborative work applying nested association-mapping populations, field and precision phenotyping, ideotyping, crop modeling, GWA, and multi-omic approaches will allow us to integrate the results reported here, based on the aforementioned background, into a practical framework for breeding improved drought tolerance and resilience into barley and other crops.
5 Conclusions
For food and nutritional security, the optimal drought response strategy for a crop produces the highest sustainable yield given variable stressors such as drought. We have identified barley lines displaying a dynamic transpirational response to drought, in addition to the classic isohydric (water-conserving) and anisohydric (non-conserving) response types, among an 81-line diversity set that spans 20th century breeding. Vigor and transpiration were strongly correlated and highest under well-watered conditions in the dynamic lines. Yield stability despite an early-season drought is expected to benefit from recovery of carbon fixation and thereby growth. While the isohydric lines were more resilient than the dynamic ones, the latter’s strong vigor and transpiration rates, combined with their good water use efficiency, gave them higher growth rates after drought. The results will be of use for defining barley physiological ideotypes suited to future climate scenarios and for understanding their biological basis (Paul et al., 2023).
Data availability statement
The original contributions presented in the study are included in the article/Supplementary Material. Further inquiries can be directed to the corresponding author.
Author contributions
MP: Writing – review & editing, Data curation, Formal analysis, Methodology, Writing – original draft, Investigation, Visualization. AD: Formal analysis, Investigation, Methodology, Visualization, Writing – original draft. MJ: Conceptualization, Data curation, Methodology, Writing – original draft. MM: Conceptualization, Methodology, Project administration, Resources, Software, Supervision, Writing – review & editing. AS: Conceptualization, Funding acquisition, Project administration, Supervision, Writing – review & editing.
Funding
The author(s) declare financial support was received for the research, authorship, and/or publication of this article. Funding was provided by the Academy of Finland, Decision 284897, within the ERA-NET SusCrop ClimBar project and by the Finnish Ministry of Agriculture and Forestry within the ERA-NET SusCrop BARISTA project.
Acknowledgments
We acknowledge and thank Anne-Mari Narvanto for excellent technical assistance and Triin Vahisalu for useful discussions on the physiology and molecular biology of drought response.
Conflict of interest
The authors declare that the research was conducted in the absence of any commercial or financial relationships that could be construed as a potential conflict of interest.
Publisher’s note
All claims expressed in this article are solely those of the authors and do not necessarily represent those of their affiliated organizations, or those of the publisher, the editors and the reviewers. Any product that may be evaluated in this article, or claim that may be made by its manufacturer, is not guaranteed or endorsed by the publisher.
Supplementary material
The Supplementary Material for this article can be found online at: https://www.frontiersin.org/articles/10.3389/fpls.2024.1393991/full#supplementary-material
Supplementary Figure 1 | Principal Components Analysis (PCA) plot of barley diversity set.
Supplementary Figure 2 | Plants on the lysimeter platform during 18-line experiment.
Supplementary Figure 3 | Daily Transpiration (DT) of the 18 barley lines.
Supplementary Figure 4 | Daily transpiration (DT) correlations (r) between experimental phases.
Supplementary Figure 5 | Correlations among transpirational measures.
Supplementary Figure 6 | Canopy Stomatal conductance (GSc) correlations (r) between of the treatments.
Supplementary Figure 7 | Correlations for Calculated plant weight (CPW) gain per day between treatments.
Supplementary Figure 8 | Yield estimates for the 18 barley lines from the second screening.
Supplementary Figure 9 | Thousand Grain Weight for the 18 barley lines.
References
Abdalla, M., Ahmed, M. A., Cai, G., Wankmuller, F., Schwartz, N., Litig, O., et al. (2022). Stomatal closure during water deficit is controlled by below-ground hydraulics. Ann. Bot. 129, 161–170. doi: 10.1093/aob/mcab141
Abdelghany, A. M., Lamlom, S. F., Naser, M. (2024). Dissecting the resilience of barley genotypes under multiple adverse environmental conditions. BMC Plant Biol. 24, 16. doi: 10.1186/s12870-023-04704-y
Appiah, M., Abdulai, I., Schulman, A. H., Moshelion, M., Dewi, E. S., Daszkowska-Golec, A., et al. (2023). Drought response of water-conserving and non-conserving spring barley cultivars. Front. Plant Sci. 14, 1247853. doi: 10.3389/fpls.2023.1247853
Assmann, S. M., Jegla, T. (2016). Guard cell sensory systems: recent insights on stomatal responses to light, abscisic acid, and CO(2). Curr. Opin. Plant Biol. 33, 157–167. doi: 10.1016/j.pbi.2016.07.003
Buckley, T. N. (2005). The control of stomata by water balance. New Phytol. 168, 275–292. doi: 10.1111/j.1469-8137.2005.01543.x
Buckley, T. N., Mott, K. A. (2013). Modelling stomatal conductance in response to environmental factors. Plant Cell Environ. 36, 1691–1699. doi: 10.1111/pce.12140
Carminati, A., Javaux, M. (2020). Soil rather than xylem vulnerability controls stomatal response to drought. Trends Plant Sci. 25, 868–880. doi: 10.1016/j.tplants.2020.04.003
Chen, S., Li, P., Tan, S., Pu, X., Zhou, Y., Hu, K., et al. (2021). Combined proteomic and physiological analysis of chloroplasts reveals drought and recovery response mechanisms in Nicotiana benthamiana. Plants (Basel) 10, 1127. doi: 10.3390/plants10061127
Cohen, I., Zandalinas, S. I., Huck, C., Fritschi, F. B., Mittler, R. (2021). Meta-analysis of drought and heat stress combination impact on crop yield and yield components. Physiol. Plant 171, 66–76. doi: 10.1111/ppl.13203
Dalal, A., Attia, Z., Moshelion, M. (2017). To produce or to survive: how plastic is your crop stress physiology? Front. Plant Sci. 8. doi: 10.3389/fpls.2017.02067
Dalal, A., Bourstein, R., Haish, N., Shenhar, I., Wallach, R., Moshelion, M. (2019). Dynamic physiological phenotyping of drought-stressed pepper plants treated with “Productivity-enhancing” and “Survivability-enhancing” Biostimulants. Front. Plant Sci. 10, 905. doi: 10.3389/fpls.2019.00905
Dalal, A., Shenhar, I., Bourstein, R., Mayo, A., Grunwald, Y., Averbuch, N., et al. (2020). A telemetric, gravimetric platform for real-time physiological phenotyping of plant environment interactions. Jove J. Visual. Exp. 5, 162. doi: 10.3791/61280
Damour, G., Simonneau, T., Cochard, H., Urban, L. (2010). An overview of models of stomatal conductance at the leaf level. Plant Cell Environ. 33, 1419–1438. doi: 10.1111/j.1365-3040.2010.02181.x
Das, K., Roychoudhury, A. (2014). Reactive oxygen species (ROS) and response of antioxidants as ROS-scavengers during environmental stress in plants. Front. Environ. Sci. 2. doi: 10.3389/fenvs.2014.00053
De Swaef, T., Pieters, O., Appeltans, S., Borra-Serrano, I., Coudron, W., Couvreur, V., et al. (2022). On the pivotal role of water potential to model plant physiological processes. In Silico Plants 4. doi: 10.1093/insilicoplants/diab038
Dhanagond, S., Liu, G., Zhao, Y., Chen, D., Grieco, M., Reif, J., et al. (2019). Non-invasive phenotyping reveals genomic regions involved in pre-anthesis drought tolerance and recovery in spring barley. Front. Plant Sci. 10, 1307. doi: 10.3389/fpls.2019.01307
Drake, P. L., Froend, R. H., Franks, P. J. (2013). Smaller, faster stomata: scaling of stomatal size, rate of response, and stomatal conductance. J. Exp. Bot. 64, 495–505. doi: 10.1093/jxb/ers347
Fang, Y., Xiong, L. (2015). General mechanisms of drought response and their application in drought resistance improvement in plants. Cell Mol. Life Sci. 72, 673–689. doi: 10.1007/s00018-014-1767-0
Fusi, R., Rosignoli, S., Lou, H., Sangiorgi, G., Bovina, R., Pattem, J. K., et al. (2022). Root angle is controlled by EGT1 in cereal crops employing an antigravitropic mechanism. Proc. Natl. Acad. Sci. U.S.A. 119, e2201350119. doi: 10.1073/pnas.2201350119
Galkin, E., Dalal, A., Evenko, A., Fridman, E., Kan, I., Wallach, R., et al. (2018). Risk-management strategies and transpiration rates of wild barley in uncertain environments. Physiol. Plant 164, 412–428. doi: 10.1111/ppl.12814
Gol, L., Haraldsson, E. B., von Korff, M. (2021). Ppd-H1 integrates drought stress signals to control spike development and flowering time in barley. J. Exp. Bot. 72, 122–136. doi: 10.1093/jxb/eraa261
Hakala, K., Jauhiainen, L., Himanen, S. J., Rotter, R., Salo, T., Kahiluoto, H. (2012). Sensitivity of barley varieties to weather in Finland. J. Agric. Sci. 150, 145–160. doi: 10.1017/S0021859611000694
Halperin, O., Gebremedhin, A., Wallach, R., Moshelion, M. (2017). High-throughput physiological phenotyping and screening system for the characterization of plant-environment interactions. Plant J. 89, 839–850. doi: 10.1111/tpj.13425
Hatfield, J. L., Dold, C. (2019). Water-use efficiency: advances and challenges in a changing climate. Front. Plant Sci. 10, 103. doi: 10.3389/fpls.2019.00103
Hildebrandt, T. M. (2018). Synthesis versus degradation: directions of amino acid metabolism during Arabidopsis abiotic stress response. Plant Mol. Biol. 98, 121–135. doi: 10.1007/s11103-018-0767-0
Honsdorf, N., March, T. J., Berger, B., Tester, M., Pillen, K. (2014). High-throughput phenotyping to detect drought tolerance QTL in wild barley introgression lines. PloS One 9, e97047. doi: 10.1371/journal.pone.0097047
Hoshika, Y., Fares, S., Pellegrini, E., Conte, A., Paoletti, E. (2020). Water use strategy affects avoidance of ozone stress by stomatal closure in Mediterranean trees-A modelling analysis. Plant Cell Environ. 43, 611–623. doi: 10.1111/pce.13700
Hsu, P. K., Dubeaux, G., Takahashi, Y., Schroeder, J. I. (2021). Signaling mechanisms in abscisic acid-mediated stomatal closure. Plant J. 105, 307–321. doi: 10.1111/tpj.15067
Jogawat, A., Yadav, B., Chhaya, Lakra, N., Singh, A. K., Narayan, O. P. (2021). Crosstalk between phytohormones and secondary metabolites in the drought stress tolerance of crop plants: A review. Physiol. Plant 172, 1106–1132. doi: 10.1111/ppl.13328
Joshi, J., Stocker, B. D., Hofhansl, F., Zhou, S., Dieckmann, U., Prentice, I. C. (2022). Towards a unified theory of plant photosynthesis and hydraulics. Nat. Plants 8, 1304–1316. doi: 10.1038/s41477-022-01244-5
Kollist, H., Nuhkat, M., Roelfsema, M. R. G. (2014). Closing gaps: linking elements that control stomatal movement. New Phytol. 203, 44–62. doi: 10.1111/nph.12832
Kudoyarova, G., Veselova, S., Hartung, W., Farhutdinov, R., Veselov, D., Sharipova, G. (2011). Involvement of root ABA and hydraulic conductivity in the control of water relations in wheat plants exposed to increased evaporative demand. Planta 233, 87–94. doi: 10.1007/s00425-010-1286-7
Li, J., Yao, X., Yao, Y., An, L., Feng, Z., Wu, K. (2022). Genome-wide association mapping of hulless barely phenotypes in drought environment. Front. Plant Sci. 13, 924892. doi: 10.3389/fpls.2022.924892
Lister, D. L., Jones, H., Oliveira, H. R., Petrie, C. A., Liu, X., Cockram, J., et al. (2018). Barley heads east: Genetic analyses reveal routes of spread through diverse Eurasian landscapes. PloS One 13, e0196652. doi: 10.1371/journal.pone.0196652
Luo, H., Hill, C. B., Zhou, G., Zhang, X. Q., Li, C. (2020). Genome-wide association mapping reveals novel genes associated with coleoptile length in a worldwide collection of barley. BMC Plant Biol. 20, 346. doi: 10.1186/s12870-020-02547-5
Merilo, E., Jalakas, P., Laanemets, K., Mohammadi, O., Horak, H., Kollist, H., et al. (2015). Abscisic acid transport and homeostasis in the context of stomatal regulation. Mol. Plant 8, 1321–1333. doi: 10.1016/j.molp.2015.06.006
Moore, F. C., Lobell, D. B. (2015). The fingerprint of climate trends on European crop yields. Proc. Natl. Acad. Sci. U.S.A. 112, 2670–2675. doi: 10.1073/pnas.1409606112
Moshelion, M., Halperin, O., Wallach, R., Oren, R., Way, D. A. (2015). Role of aquaporins in determining transpiration and photosynthesis in water-stressed plants: crop water-use efficiency, growth and yield. Plant Cell Environ. 38, 1785–1793. doi: 10.1111/pce.12410
Moualeu-Ngangué, D., Dolch, C., Schneider, M., Leon, J., Uptmoor, R., Stutzel, H. (2020). Physiological and morphological responses of different spring barley genotypes to water deficit and associated QTLs. PloS One 15, e0237834. doi: 10.1371/journal.pone.0237834
Negin, B., Moshelion, M. (2016). The advantages of functional phenotyping in pre-field screening for drought-tolerant crops. Funct. Plant Biol. 44, 07–118. doi: 10.1071/FP16156
Ogrodowicz, P., Mikolajczak, K., Kempa, M., Mokrzycka, M., Krajewski, P., Kuczynska, A. (2023). Genome-wide association study of agronomical and root-related traits in spring barley collection grown under field conditions. Front. Plant Sci. 14, 1077631. doi: 10.3389/fpls.2023.1077631
Paul, M., Tanskanen, J., Jaaskelainen, M., Chang, W., Dalal, A., Moshelion, M., et al. (2023). Drought and recovery in barley: key gene networks and retrotransposon response. Front. Plant Sci. 14, 1193284. doi: 10.3389/fpls.2023.1193284
Peters, W., van der Velde, I. R., Van Schaik, E., Miller, J. B., Ciais, P., Duarte, H. F., et al. (2018). Increased water-use efficiency and reduced CO(2) uptake by plants during droughts at a continental-scale. Nat. Geosci. 11, 744–748. doi: 10.1038/s41561-018-0212-7
Puglisi, D., Visioni, A., Ozkan, H., Kara, I., Lo Piero, A. R., Rachdad, F. E., et al. (2022). High accuracy of genome-enabled prediction of belowground and physiological traits in barley seedlings. G3 (Bethesda) 12, jkac022. doi: 10.1093/g3journal/jkac022
Ray, D. K., West, P. C., Clark, M., Gerber, J. S., Prishchepov, A. V., Chatterjee, S. (2019). Climate change has likely already affected global food production. PloS One 14, e0217148. doi: 10.1371/journal.pone.0217148
Sade, N., Gebremedhin, A., Moshelion, M. (2012). Risk-taking plants: anisohydric behavior as a stress-resistance trait. Plant Signal Behav. 7, 767–770. doi: 10.4161/psb.20505
Saradadevi, R., Palta, J. A., Siddique, K. H. M. (2017). ABA-mediated stomatal response in regulating water use during the development of terminal drought in wheat. Front. Plant Sci. 8, 1251. doi: 10.3389/fpls.2017.01251
Scharwies, J. D., Dinneny, J. R. (2019). Water transport, perception, and response in plants. J. Plant Res. 132, 311–324. doi: 10.1007/s10265-019-01089-8
Schauberger, B., Kato, H., Kato, T., Watanabe, D., Ciais, P. (2022). French crop yield, area and production data for ten staple crops from 1900 to 2018 at county resolution. Sci. Data 9, 38. doi: 10.1038/s41597-022-01145-4
Seleiman, M. F., Al-Suhaibani, N., Ali, N., Akmal, M., Alotaibi, M., Refay, Y., et al. (2021). Drought stress impacts on plants and different approaches to alleviate its adverse effects. Plants (Basel) 10, 259. doi: 10.3390/plants10020259
Sperry, J. S., Venturas, M. D., Anderegg, W. R. L., Mencuccini, M., Mackay, D. S., Wang, Y., et al. (2017). Predicting stomatal responses to the environment from the optimization of photosynthetic gain and hydraulic cost. Plant Cell Environ. 40, 816–830. doi: 10.1111/pce.12852
Tao, F. L., Rotter, R. P., Palosuo, T., Diaz-Ambrona, C. G. H., Minguez, M. I., Semenov, M. A., et al. (2017). Designing future barley ideotypes using a crop model ensemble. Eur. J. Agron. 82, 144–162. doi: 10.1016/j.eja.2016.10.012
Tao, F., Rotter, R. P., Palosuo, T., Gregorio Hernandez Diaz-Ambrona, C., Minguez, M. I., Semenov, M. A., et al. (2018). Contribution of crop model structure, parameters and climate projections to uncertainty in climate change impact assessments. Glob. Chang. Biol. 24, 1291–1307. doi: 10.1111/gcb.14019
Tondelli, A., Xu, X., Moragues, M., Sharma, R., Schnaithmann, F., Ingvardsen, C., et al. (2013). Structural and temporal variation in genetic diversity of European spring two-row barley cultivars and association mapping of quantitative traits. Plant Genome 6, 1–14. doi: 10.3835/plantgenome2013.03.0007
Xu, X., Sharma, R., Tondelli, A., Russell, J., Comadran, J., Schnaithmann, F., et al. (2018). Genome-wide association analysis of grain yield-associated traits in a Pan-European barley cultivar collection. Plant Genome 11, 1–11. doi: 10.3835/plantgenome2017.08.0073
Yang, Y., Ma, X., Yan, L., Li, Y., Wei, S., Teng, Z., et al. (2023). Soil-root interface hydraulic conductance determines responses of photosynthesis to drought in rice and wheat. Plant Physiol. 194, 376–390. doi: 10.1093/plphys/kiad498
Yin, J., Gentine, P., Zhou, S., Sullivan, S. C., Wang, R., Zhang, Y., et al. (2018). Large increase in global storm runoff extremes driven by climate and anthropogenic changes. Nat. Commun. 9, 4389. doi: 10.1038/s41467-018-06765-2
Keywords: barley, Hordeum vulgare, drought response, biotic stress, climate change, vigor, transpiration
Citation: Paul M, Dalal A, Jääskeläinen M, Moshelion M and Schulman AH (2024) Precision phenotyping of a barley diversity set reveals distinct drought response strategies. Front. Plant Sci. 15:1393991. doi: 10.3389/fpls.2024.1393991
Received: 29 February 2024; Accepted: 30 May 2024;
Published: 24 June 2024.
Edited by:
Balpreet Kaur Dhatt, Bayer Crop Science, United StatesReviewed by:
Amit Kumar Mishra, Mizoram University, IndiaSimone Landi, University of Naples Federico II, Italy
Copyright © 2024 Paul, Dalal, Jääskeläinen, Moshelion and Schulman. This is an open-access article distributed under the terms of the Creative Commons Attribution License (CC BY). The use, distribution or reproduction in other forums is permitted, provided the original author(s) and the copyright owner(s) are credited and that the original publication in this journal is cited, in accordance with accepted academic practice. No use, distribution or reproduction is permitted which does not comply with these terms.
*Correspondence: Alan H. Schulman, YWxhbi5zY2h1bG1hbkBoZWxzaW5raS5maQ==
†Present address: Ahan Dalal, Institute for Molecular Physiology, Heinrich Heine University, Düsseldorf, Germany
‡These authors have contributed equally to this work
§ORCID: Maitry Paul, orcid.org/0000-0002-3558-4654
Ahan Dalal, orcid.org/0000-0003-1876-1484
Marko Jääskeläinen, orcid.org/0000-0002-6387-5539
Menachem Moshelion, orcid.org/0000-0003-0156-2884
Alan H. Schulman, orcid.org/0000-0002-4126-6177