- 1Departamento de Ciências Biológicas (DCB), Centro de Biotecnologia e Genética (CBG), Universidade Estadual de Santa Cruz (UESC), Ilhéus, Bahia, Brazil
- 2Molecular Plant Pathology Laboratory, Centro de Pesquisa do Cacau (CEPEC/CEPLAC), Ilhéus, Bahia, Brazil
Witches’ broom disease (WBD) affects cocoa trees (Theobroma cacao L.) and is caused by the fungus Moniliophthora perniciosa that grows in the apoplast in its biotrophic phase and later progresses into the tissues, causing serious losses in the production of cocoa beans. Therefore, the apoplast of T. cacao can provide important defense responses during the interaction with M. perniciosa. In this work, the protein profile of the apoplast of the T. cacao genotypes Catongo, susceptible to WBD, and CCN-51, resistant one, was evaluated. The leaves of T. cacao were collected from asymptomatic plants grown in a greenhouse (GH) and from green witches’ brooms grown under field (FD) conditions for extraction of apoplastic washing fluid (AWF). AWF was used in proteomic and enzymatic analysis. A total of 14 proteins were identified in Catongo GH and six in Catongo FD, with two proteins being common, one up-accumulated, and one down-accumulated. In CCN-51, 19 proteins were identified in the GH condition and 13 in FD, with seven proteins being common, one up-accumulated, and six down-accumulated. Most proteins are related to defense and stress in both genotypes, with emphasis on pathogenesis-related proteins (PR): PR-2 (β-1,3-glucanases), PR-3 and PR-4 (chitinases), PR-5 (thaumatine), PR-9 (peroxidases), and PR-14 (lipid transfer proteins). Furthermore, proteins from microorganisms were detected in the AWF. The enzymatic activities of PR-3 showed a significant increase (p < 0.05) in Catongo GH and PR-2 activity (p < 0.01) in CCN-51 FD. The protein profile of the T. cacao apoplastome offers insight into the defense dynamics that occur in the interaction with the fungus M. perniciosa and offers new insights in exploring future WBD control strategies.
1 Introduction
The species Theobroma cacao originated in South America (Motamayor et al., 2013). This species produces cocoa, which plays a very important role in the global economy, especially in the chocolate industry, because its beans are the main raw material for chocolate and its derivatives (Beg et al., 2017). According to reports from the International Cocoa Organization (ICCO, 2022), global cocoa bean production was approximately 5.2 million tons in 2021. The African continent stood out by contributing the largest share, 77% of the total production. In this scenario, Brazil is one of the main producers in the Americas, totaling almost 300 thousand tons in 2023 (IBGE, 2024).
Cocoa farming is threatened by one of the most serious agricultural diseases, witches’ broom disease (WBD), caused by the hemibiotrophic fungus Moniliophthora perniciosa (Aime and Phillips-Mora, 2005; Meinhardt et al., 2008; Evans, 2016; Santos et al., 2023). WBD can cause substantial losses to cocoa farming, with particular impact on plantations in Brazil, specifically in the state of Bahia, which has been facing challenges since the arrival of the fungus in 1989 (Santos et al., 2023). During the biotrophic phase, the fungus M. perniciosa grows in the apoplast of T. cacao, which represents a key environment in the interaction between plant molecules and molecules released by M. perniciosa (Ceita et al., 2007; Meinhardt et al., 2008; Santos et al., 2023).
The apoplast is an essential compartment in various physiological processes and in the plant communication with the environment. Its relevance is evident because it influences important responses to environmental stresses and plays a crucial role in plant defense against pathogens (Sakurai, 1998; Agrawal et al., 2010). Communication between apoplast with the environment is fundamental for the activation of defense mechanisms, contributing to the plant ability to quickly recognize and respond to pathogenic threats, thus strengthening resistance against invasive molecules (Agrawal et al., 2010; Doehlemann and Hemetsberger, 2013).
The apoplastic protein content of the plant is modified during plant–pathogen interaction (Martínez-González et al., 2018). Many studies have aimed to understand this modulation in order to gain better knowledge of the behavior of both the pathogen, by interfering in the plant’s defense, and of the plant whose normal development is impaired (Martínez-González et al., 2018; Wang and Wang, 2018; Hassett et al., 2020). The advent of omics technologies, particularly proteomics, has helped to elucidate cellular responses involved in different biological conditions (Mares et al., 2016, Mares et al., 2017, Mares et al., 2020; de Almeida et al., 2023; de Oliveira et al., 2024; Zugaib et al., 2023).
Understanding the molecular processes involved in the apoplast during a plant–pathogen interaction remains a challenge. Apoplastic proteomic studies, even with their challenges, have contributed to the understanding of molecular processes in pathogen–host interactions. Many defense proteins, such as pathogenesis-related proteins (PR proteins), have been identified in the apoplast (Floerl et al., 2008; Guerra-Guimarães et al., 2015; Li et al., 2016). Recently, the first apoplastic proteomic profile of contrasting genotypes regarding Theobroma cacao resistance to the WBD was reported (de Oliveira et al., 2024).
The term apoplastome described by de Oliveira et al. (2024) refers to the set of proteins identified in the apoplast of contrasting T. cacao genotypes regarding resistance to WBD. The proteins identified in this study are mainly involved in defensive processes of T. cacao, such as peroxidases, chitinases, and osmotin. Furthermore, the efficiency of the apoplastic washing fluid (AWF) from T. cacao genotypes in inhibiting the germination of M. perniciosa spores was demonstrated. The inhibition reached almost 90% for the resistant genotype (CCN-51) and 82% for the susceptible one (Catongo), as well as inducing morphological alterations in basidiospores (de Oliveira et al., 2024). These results reveal that the T. cacao apoplast represents a fundamental compartment in the complex defense network of plants.
Considering this, the present work aims to characterize the protein profile of the AWF obtained from asymptomatic plants grown in a greenhouse (GH) and from broom branches collected from field (FD)–grown plants with WBD, in two contrasting genotypes for WBD resistance (Catongo-susceptible and CCN-51–resistant). Our intention is to deepen the understanding of T. cacao defense responses, thus comprehending the role of the apoplast in the T. cacao–M. perniciosa interaction, to contribute to disease control efforts in the future.
2 Materials and methods
2.1 Collection of biological material and extraction of apoplastic fluid
Fully expanded mature leaves of adult T.cacao plants of the genotypes Catongo (susceptible to M. perniciosa) and CCN-51 (resistant to M. perniciosa) (Gramacho et al., 1992) cultivated under FD and GH conditions were collected and used to extract AWF. The collection of mature leaves on branches infected by M. perniciosa (green witches’ broom) were carried out randomly at the Active Germplasm Bank (BAG) of CEPLAC/CEPEC in Ilhéus, Bahia, Brazil (14°45′40.2″S, 39°14′03.9″W) on plants under FD conditions. Leaves from asymptomatic plants, grown in a GH at CEPLAC/CEPEC, were also used. AWF extraction was performed by vacuum infiltration followed by centrifugation, as previously described (Pirovani et al., 2008; de Oliveira et al., 2024). A total of approximately 200 μL/g fresh weight of T. cacao leaf was collected. For proteomic analysis, approximately 1,200 g of leaves of the Catongo GH genotype, 715 g of Catongo FD leaves, 800 g of CCN-51 GH leaves, and 750 g of CCN-51 FD leaves were collected. For the analysis of chitinase, β-1,3-glucanase, and protease activity, three biological replicates were performed for each sample using a total of five leaves in each AWF extraction.
2.2 Protein extraction from apoplastic fluid and SDS-PAGE
The collected AWF was lyophilized in aliquots with a volume of 15 mL and used in protein extraction according to Wang et al. (2003), with modifications according to Pirovani et al. (2008). After protein recovery, the precipitate was resuspended in urea (8 mol L−1) and quantified using the 2-D Quant Kit (Cytiva), according to the manufacturer’s recommendations, using BSA (bovine serum albumin) as standard.
After quantification, the proteins were resolved in SDS-PAGE (sodium dodecyl sulfate–polyacrylamide gel electrophoresis) in mini vats electrophoresis (Hoefer), with 8 cm × 10 cm gels at 12.5% acrylamide (Laemmli, 1970). A total of 30 μg of AWF protein from T. cacao genotypes was applied onto gels, along with 30 μg of total leaf protein extract (TE) from CCN-51 FD extracted according to Pirovani et al. (2008), to verify the protein band profiles of each sample (AWF and TE) and their differences.
2.3 Mass spectrometry and database analysis
A mass of 100 µg of AWF proteins from T. cacao genotype samples was treated and subjected to tryptic digestion according to Villén and Gygi (2008) with modifications described by de Almeida et al. (2023). The peptides were then analyzed in technical triplicates on a liquid chromatography system (Agilent 1290 Infinity II HPLC) coupled to a quadrupole/Time-of-Flight mass spectrometer (Agilent 6545 LC/QTOF) with C18 columns (100 mm × 100 mm) (de Almeida et al., 2023).
To analyze the spectral data generated for the identification of peptides, we used the software Spectrum Mill from the Broad Institute (Rev BI.07.08.214). Raw data files were converted to a peak list format (mgf) without summing the scans by the Mascot Distiller software v.2.6.2.0, 2009 (Matrix Science Ldt.). After extracting the mass spectrometry (MS)/MS spectra, a database search of T. cacao and fungi was performed. All databases were downloaded from UniProt (https://www.uniprot.org). A database of the theoretical T. cacao apoplastome (de Oliveira et al., 2024) was also used to identify predicted proteins for the apoplast. The research was carried out according to the following configurations: carbamidomethylation with fixed modifications, methionine oxidation with variable modification, trypsin for cleavage, and 0.1-Da tolerance for precursor and fragment ions. Statistical analyses were performed using the Mass Profiler Professional 15.1 software (MPP; Agilent), determined through analyses in which the inclusion criteria were defined as p ≤ 0.05 and a fold change ≥ 1.5.
The identified proteins were submitted for functional annotation and categorization according to function and cellular processes using the BLAST2GO software (Conesa et al., 2005) available at the OmicsBox platform (https://www.biobam.com/blast2go/).
2.4 Comparison of methods for characterizing the T. cacao apoplastome
The extraction efficiency of AWF from T. cacao leaves for recovery of apoplastic proteins was compared and evaluated with the efficiency of total extract (TE) of T. cacao leaves for identification of apoplastic proteins.
Initially, proteins were recovered from the AWF of T. cacao leaves carried out in the present study obtained as described in the section “Protein extraction from apoplastic fluid and SDS-PAGE.” Then, files containing raw peptide data from TE samples from T. cacao leaves carried out in previous work (de Almeida et al., 2023) were used for comparison. In terms of methodology comparison, only the CCN-51 variety was used. The raw peptide data were analyzed against the T. cacao database and the database of predicted proteins for the apoplast (de Oliveira et al., 2024) as per the previous section (“Mass spectrometry and database analysis”). For this analysis, differences in accumulation among the identified proteins were not taken into account. The data obtained did not need to pass statistical inclusion criteria (p ≤ 0.05 and fold change ≥ 1.5). Verification was based on the number of proteins obtained in each sample rather than on protein abundance.
2.5 Transcript profile of predicted apoplast proteins
The transcript pattern for the predicted apoplast proteins was verified from reference files containing transcripts from Theobroma cacao cv. ‘Comum’ (Forastero). The files in FASTA format (GCA_000208745.2_Criollo_cocoa_genome_V2/and GCA_000403535.1_Theobroma_cacaoMatina) were obtained from the GenBank database (https://ftp.ncbi.nlm.nih.gov/genomes/genbank/plant/Theobroma_cacao/latest_assembly_versions/). Relative quantification was performed for the six transcripts exclusive to the Catongo genotype and 19 exclusive to CCN-51, with 14 being common, totaling 39 transcripts, which correspond to the diversity of proteins identified in the apoplast of T. cacao in this study. Therefore, proteins that appeared repeatedly in the samples of both Catongo and CCN-51, regardless of the growth condition, were excluded to prevent duplication. Public data were used from10 T. Cacao RNA-Seq libraries (Teixeira et al., 2014), available at NCBI’s Sequence Read Archive (SRA) (https://www.ncbi.nlm.nih.gov/sra) under accession number SRA066232, with five libraries of the control condition and five of the M. perniciosa–infected condition. The relative quantification of T. cacao transcripts and ranking in descending order of expression was performed with the Galaxy platform (https://rna.usegalaxy.eu), using the Salmon extension (Patro et al., 2017). The corresponding proteins of each analyzed transcript were found with the BlastX search tool (https://blast.ncbi.nlm.nih.gov/).
To visualize the expression profile of the transcripts, a heatmap was plotted using the Complex Heatmap packages in the statistical software R (R Core Team, 2022).
2.6 System biology analysis
The protein–protein interaction network was created with the proteins identified in the apoplast of the Catongo and CCN-51 genotypes of T. cacao using the homologous sequences of Arabidopsis thaliana as reference. The network was built according to the STRING 11.5 database (https://string-db.org/) based on the following parameters: high level of confidence (0.7), no more than 50 interactions, and addition of nodes until network saturation.
The results obtained were analyzed using the Cytoscape software version 3.9.1 (https://cytoscape.org/) (Shannon et al., 2003). The Igraph package of the statistical tool R Studio was used to group the proteins and calculate the centrality (betweenness) and nodes (degree) parameters. To enrich genetic ontology terms and categories, the Biological Networks Gene Ontology v.3.0.3 (BiNGO) tool was used (Maere et al., 2005) with a Cytoscape plugin (https://apps.cytoscape.org/apps/bingo).
2.7 Western blot
A mass of 30 μg of AWF protein from cacao genotypes and 7 μL of molecular weight marker (Kaleidoscope, pre-stained, 0.45 μm pore, Bio-Rad, USA) were applied to SDS-PAGE in electrophoresis minivats (Hoefer), with 8 cm × 10 cm gels containing 12.5% acrylamide and run at a constant amperage of 30 mA as described by Oliveira et al. (2020). The membranes were incubated with PR-3 primary antibodies (8:20,000) for 1 h. Subsequently, the membrane was washed three times, for 15 min each wash, with 1× Tris-buffered saline (TBS-T) buffer followed by incubation with the secondary antibody (anti-rabbit Immunoglobulin G (IgG)) conjugated to alkaline phosphatase (7.5 μ:2000 of 1× TBS-T) for 2 h. After incubation, the membrane was washed again three times and finally incubated for 15 min with development buffer [MgCl2, 5 mmol L−1; NaCl, 100 mmol L−1; Tris-HCl (pH 9.8), 100 mmol L−1; and H2O]. The development was carried out in the absence of light with Nitroblue tetrazolium/5-bromo-4-chloro-3-indolyl phosphate (NBT/BCIP) substrates for approximately 5 min. Finally, the apparent accumulation was determined from images of the nitrocellulose membrane, using the Gel Quant Net 1.8.2 program (http://biochemlabsolutions.com/GelQuantNET.html).
2.8 Enzyme activities
For enzyme activity analysis, AWF from Catongo and CCN-51 GH and FD genotypes was collected by infiltration in ice-cold distilled water as described by de Oliveira et al. (2024). A 30-μL aliquot of each sample was separated for quantification by the Bradford method (Bradford, 1976) using BSA as standard.
2.8.1 Chitinase activity
For the analysis of chitinase activity, the Chitinase Assay Kit, Fluorimetric (SIGMA-ALDRICH), was used according to the manufacturer’s recommendations. The assay was based on the enzymatic hydrolysis of chitinase substrates that releases 4-methylumbelliferone (4MU). The fluorescence released in basic medium was measured at an excitation wavelength of 360 nm and an emission wavelength of 450 nm. Samples were pipetted in duplicates into 96-well microplates and analyzed on a SpectraMax Paradigm (Molecular Devices). Two substrates were used in each test: the substrate 4-methylumbelliferyl beta-D-N,N′,N″-triacetylchitotriose (for detection of endochitinase activity) and methylumbelliferyl beta-D-N,N′-dediacetylchitobioside hydrate (for detection of exochitinase activity). In each assay, chitinase from Trichoderma viride was used as positive control and the blank (only the substrate) as negative control. The calculation of chitinase activity was performed according to the standard curve. One unit of chitinase activity was defined as the amount of enzyme to release 1 μmol of 4-metilumbeliferona per minute.
2.8.2 β-1,3-glucanase activity
The enzymatic activity of glucanase was determined in accordance with the 3,5-dinitrosalicylic acid (DNS) method for measuring the released reducing sugar, as reported by Miller (1959). A reaction solution containing laminarin as substrate (2 mg mL−1) and crude AWF samples from T. cacao genotypes, in a 1:1 ratio, was incubated at 40°C under shaking for 18 h. The samples were then centrifuged at 1,792 g for 5 min and the supernatant was collected. Next, 200 μL of the reaction solution and 100 μL of the DNS solution were added to new microtubes, which were vortexed and incubated in a water bath at 100°C for 5 min. Then, the reaction was stopped by cooling on ice for about 2 min. The final volume was adjusted to 1,000 μL by adding water, and, finally, the samples were pipetted in quadruplicate into 96-well microplates and analyzed in a SpectraMax Paradigm multi-mode microplate reader (Molecular Devices), with absorbance read at a wavelength of 550 nm. Controls of each sample containing laminarin, AWF sample, and DNS were also read. A glucose standard curve was plotted as a reference. One unit (U) of enzyme activity was defined as the amount of enzyme required to release 1 μmol of glucose per minute.
2.8.3 Protease activity
The AWF of T. cacao genotypes was suspended in non-reducing loading buffer [50% glycerol, Tris-HCl (pH 6.8), and 1% bromophenol blue], and the samples were applied to 10% SDS-PAGE (Michaud et al., 1996; Pirovani et al., 2010). After the run, the qualitative analysis of the proteases present in the samples was carried out using 0.1% gelatin gel and stained in colloidal Coomassie G-250 (0.08%) according to Neuhoff et al. (1988).
3 Results
3.1 Identification of AWF proteins from T. cacao genotypes
The AWF proteins were resolved on SDS-PAGE for qualitative analysis alongside the total leaf protein extract (TE) samples to confirm differential bands between AWF and TE protein samples (Supplementary Figure 1). The full identification of T. cacao AWF peptides was initially carried out using the general T. cacao database (Supplementary Figure 2). Next, the AWF peptides were also identified against the library of proteins predicted for the apoplast proposed by de Oliveira et al. (2024) considering differentially abundant proteins (p ≤ 0.05 and a fold change ≥ 1.5) and identified exclusively in both genotypes (Figures 1, 2). For the Catongo genotype, 14 proteins were identified in the GH condition and six in the FD condition. Catongo presented 12 exclusive proteins in the GH condition and four exclusive ones in the FD condition, with two proteins in common, one up-accumulated (PR-4), and the other down-accumulated (Peroxidase A2) (Figure 1). For the CCN-51 genotype, 19 proteins were identified in the GH condition and 13 in FD. CCN-51 GH presented 12 unique proteins and six in the FD condition. There were seven proteins in common for both conditions, with one up-accumulated (PR-4) and six down-accumulated [Peroxidase A2, CO(2)-response secreted protease isoform X2, Laccase-14, Cysteine-rich repeat secretory protein, Beta-xylosidase/alpha-L-arabinofuranosidase 2, and Uclacyanin-3] (Figure 1).
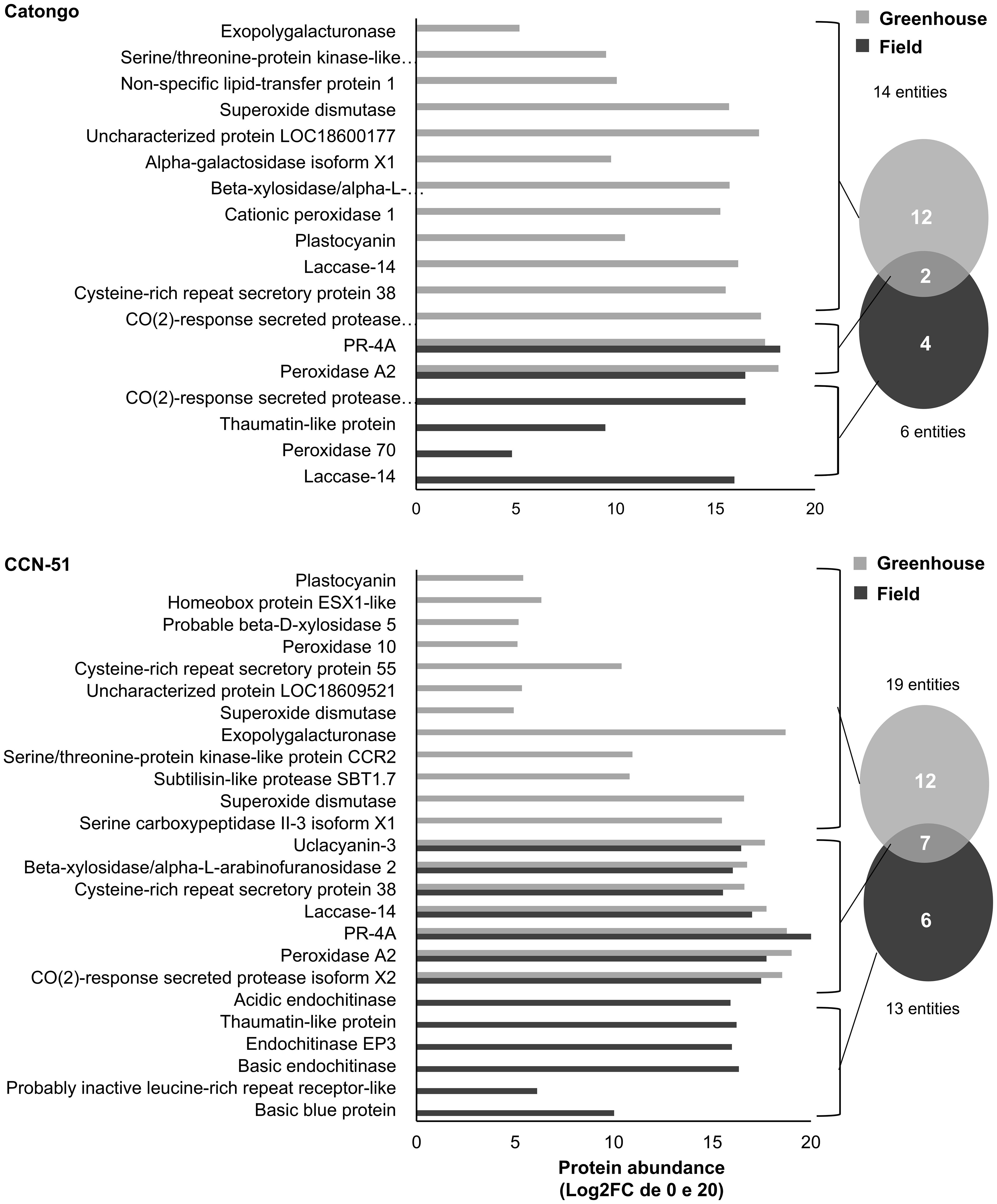
Figure 1 Proteins identified in the apoplastic washing fluid within T. cacao genotypes under different growth conditions. Unique and differentially abundant proteins (p < 0.05 and fold change ≥ 1.5) identified in the apoplastic fluid of the T. cacao Catongo (susceptible) and CCN-51 (resistant) genotypes of asymptomatic plants grown in a greenhouse and plants at the stage of green witch’s broom grown in field conditions.
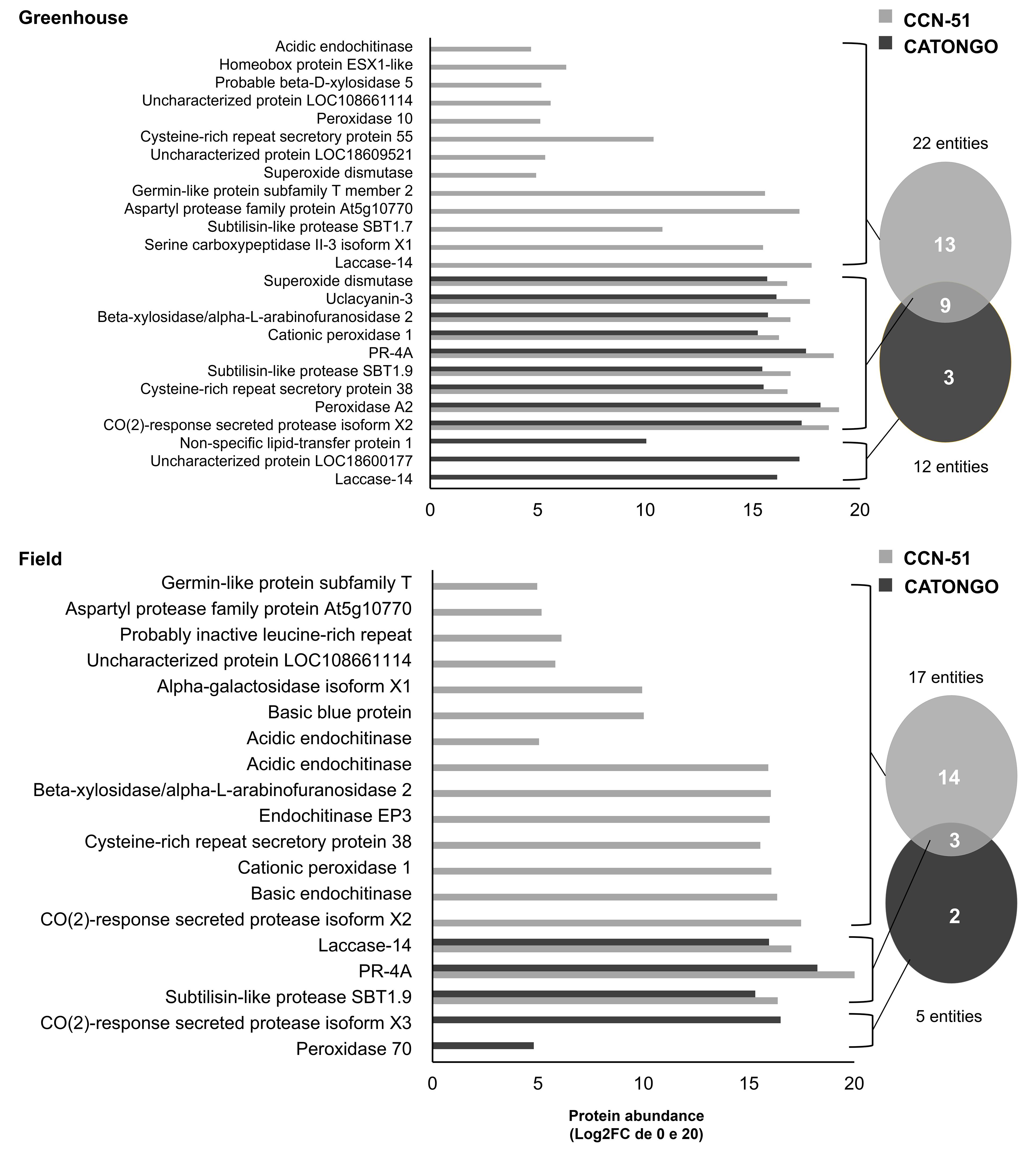
Figure 2 Proteins identified in the apoplastic washing fluid between T. cacao genotypes under different growth conditions. Unique and differentially abundant proteins (p < 0.05 and fold change ≥ 1.5) identified in the apoplastic fluid of the T. cacao Catongo (susceptible) and CCN-51 (resistant) genotypes of asymptomatic plants grown in a greenhouse and plants at the stage of green witch’s broom grown in field conditions.
In the comparison between the two genotypes in the GH condition, 22 proteins were identified in the resistant genotype (CCN-51) and 12 proteins in the susceptible genotype (Catongo), presenting 13 exclusive proteins in CCN-51 and three exclusive in Catongo. Nine proteins were in common, all being up-accumulated for the CCN-51 genotype [CO(2)-response secreted protease isoform X2, Peroxidase A2, Cysteine-rich repeat secretory protein, Subtilisin-like protease SBT1.9, PR-4, Cationic peroxidase, Beta-xylosidase/alpha-L-arabinofuranosidase 2, Uclacyanin-3, and Superoxide dismutase (SOD)] (Figure 2). In the FD condition, 17 proteins were identified in CCN-51 and five proteins in Catongo, with 14 exclusive to the CC51 genotype and two to the Catongo genotype. Three proteins were found in common to be up-accumulated in the resistant genotype (Subtilisin-like protease SBT1.9, PR-4, and Laccase-14) (Figure 2).
3.2 Efficiency of AWF extraction for apoplastome characterization
Raw peptide data from AWF samples (performed in the present study) and TE samples performed in previous work (de Almeida et al., 2023) of the CCN-51 genotype were initially analyzed against the T. cacao database to evaluate the efficiency of the methods used in the identification of apoplastic proteins. A total of 62 proteins were identified from AWF extraction and 151 proteins from TE (Figure 3). Next, the raw peptide data were analyzed against the database of predicted proteins for the T. cacao apoplast (de Oliveira et al., 2024). A total of 27 proteins (44%) predicted for the apoplast through AWF extraction and 19 proteins (13%) through leaf TE sampling (Figure 3).
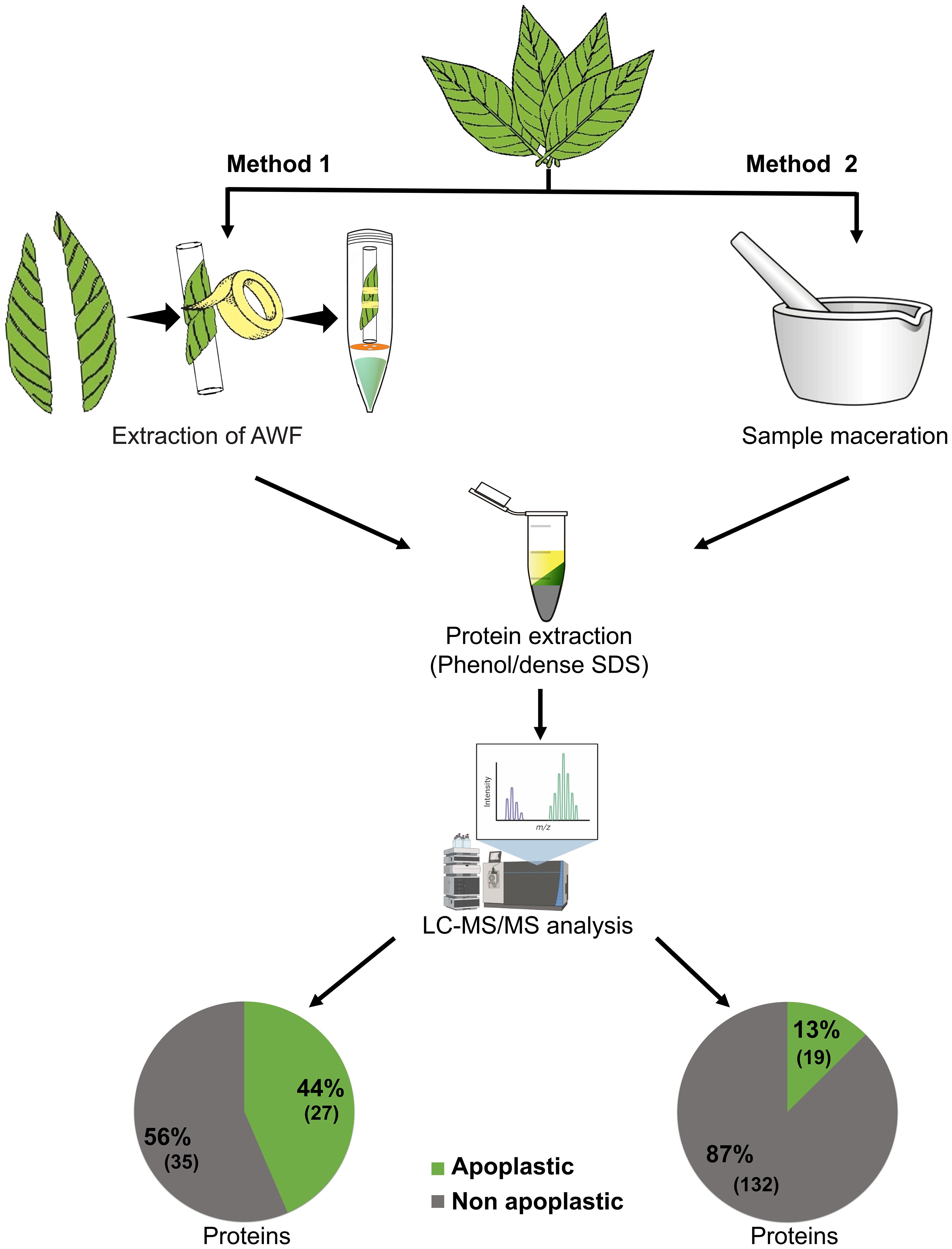
Figure 3 Efficiency of extracting apoplastic washing fluid from T. cacao leaves for characterization of the apoplastome compared to the total extract of T. cacao leaves. Method 1: Recovery of apoplastic proteins from the extraction of apoplastic washing fluid from leaves of the CCN-51 genotype (resistant), carried out in the present study. Method 2: Recovery of apoplastic proteins from the extraction of total extract from leaves of the CCN-51 genotype (resistant), carried out in a previous work (de Almeida et al., 2023). The raw peptide data were analyzed against the database of predicted proteins for the T. cacao apoplast (de Oliveira et al., 2024).
3.3 Transcriptional profile of proteins predicted for the apoplast of T. cacao genotypes
The majority transcripts were up-accumulated, such as the transcripts of the 70 peroxidase proteins identified in Catongo FD (lcl_NC_030853.1_mrna_XM_007033525.2_15125 with 89% coverage and 100% identity) and acidic proteins endochitinase and endochitinase EP3 identified in CCN-51 FD (lcl_NC _030850.1_mrna_XM_007051131.2_3841, with 74% coverage and 100% identity and lcl_NC_030853.1_mrna_XM_007033739.2_15288 with 78% coverage and 100% identity, respectively). A protein transcript common in both genotypes, thaumatin-like protein, also showed increased accumulation (lcl_NC_030852.1_mrna_XM_007040102.2_12731 with 69% coverage and 100% identity). On the other hand, transcripts referring to SOD proteins (lcl_NC_030854.1_mrna_XM_018122149.1_19942 with 54% coverage and 100% identity) identified in CCN-51 and CO(2)-response secreted protease isoform X2 identified in both genotypes (lcl_NC_030857.1_mrna_XM_007017808.2_26401 with 82% coverage and 100% identity) had reduced accumulation (Figure 4). All proteins showed coverage above 40% and 100% identity (Supplementary Table 1).
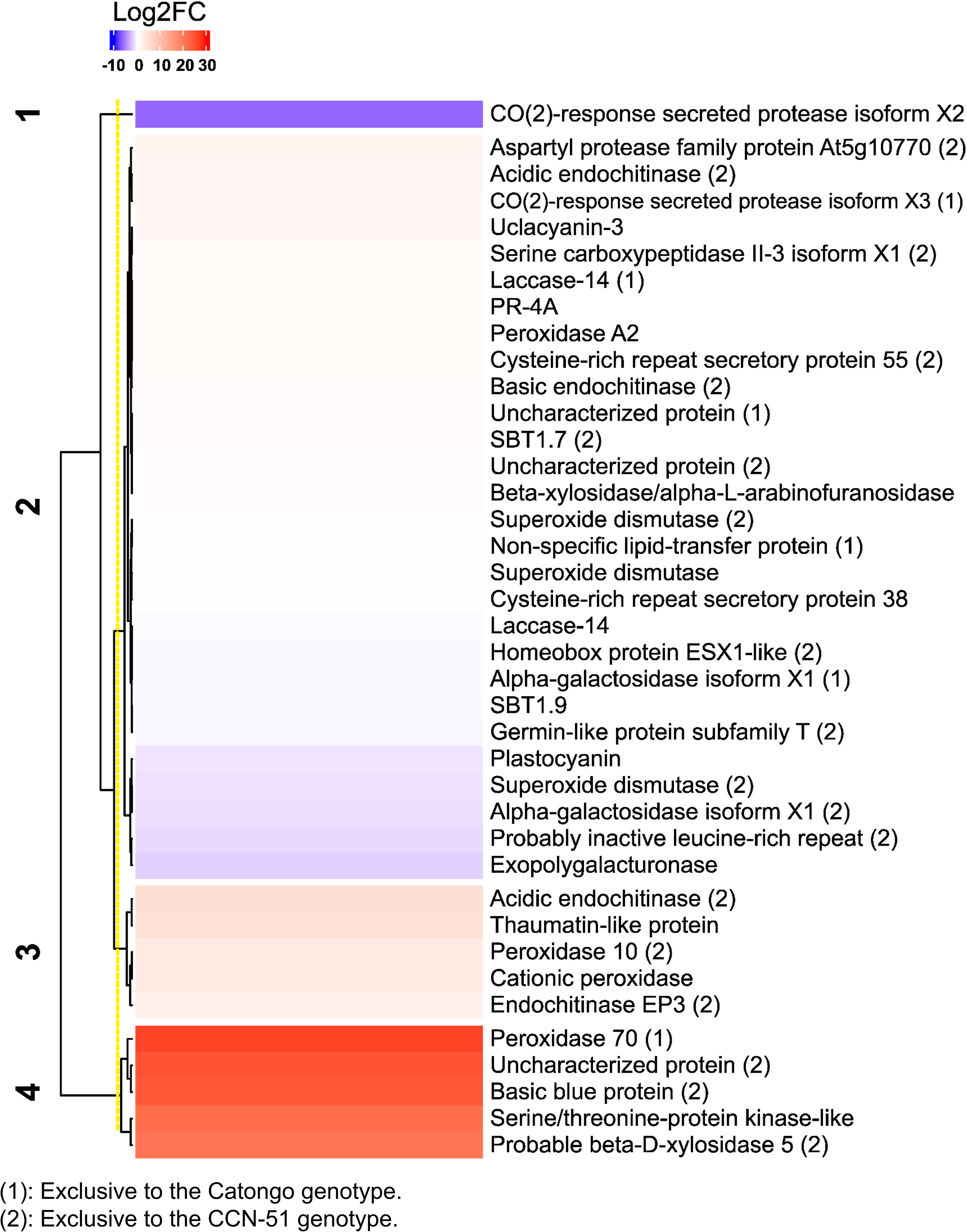
Figure 4 Transcriptional profile of apoplastic washing fluid proteins from T. cacao genotypes. Heatmap based on Pearson’s correlation coefficient, representing the differential accumulation of transcripts corresponding to apoplastic proteins of T. cacao genotypes in the database of Teixeira et al. (2014) (SRA066232). In blue, transcripts with reduced accumulation; in red, transcripts with relative increase in accumulation, related to the Log2FC scales of −10 and 30, respectively. The full name of the protein is described in Supplementary Table 1.
3.4 Functional classification of T. cacao apoplastome proteins
According to the functional classification, most of the proteins identified from both genotypes, regardless of the plant’s condition, were related to the defense and stress response and metabolic processes. For the Catongo genotype, 10 proteins (53%) were related to defense and 11 proteins (42%) were related to the CCN-51 genotype (Figure 5). A total of five proteins (26%) were involved in metabolic processes in Catongo and 10 proteins (39%) in CCN-51. Other categories were assigned in the functional classification, where one protein (4%) in the CCN-51 genotype was involved in redox processes and two (11%) proteins in the Catongo genotype.
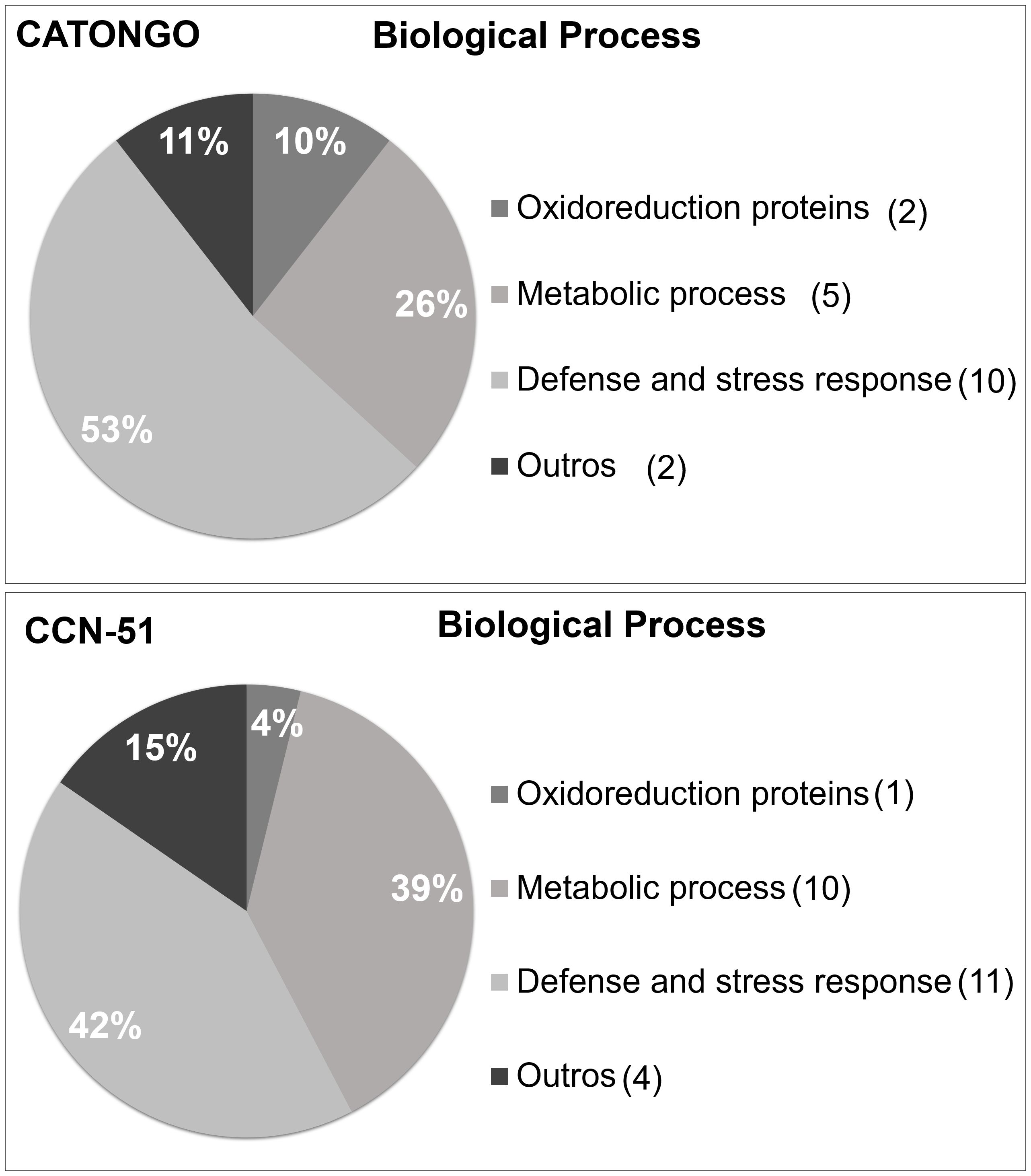
Figure 5 Functional annotation of proteins identified in apoplastic washing fluid from T. cacao genotypes. Characterization by biological processes of proteins identified in the apoplastic washing fluid of the T. cacao Catongo (susceptible) and CCN-51 (resistant) genotypes of asymptomatic plants grown in a greenhouse and plants at the stage of green witch’s broom grown in field conditions.
3.5 Interaction network of T. cacao apoplastome proteins
The protein interaction network of the Catongo genotype samples (GH and FD) showed 722 nodes, which corresponded to each specific protein, and 5,718 connectors, which corresponded to physical or functional interactions between proteins. The CCN-51 genotype (GH and FD) resulted in 941 nodes and 7,251 connectors. The larger nodes correspond to proteins found within the network (Figure 6). Of the total of 20 orthologous protein sequences in the Catongo sample, 14 were found in the network. In CCN-51, out of 35 sequences, 23 were located within the network.
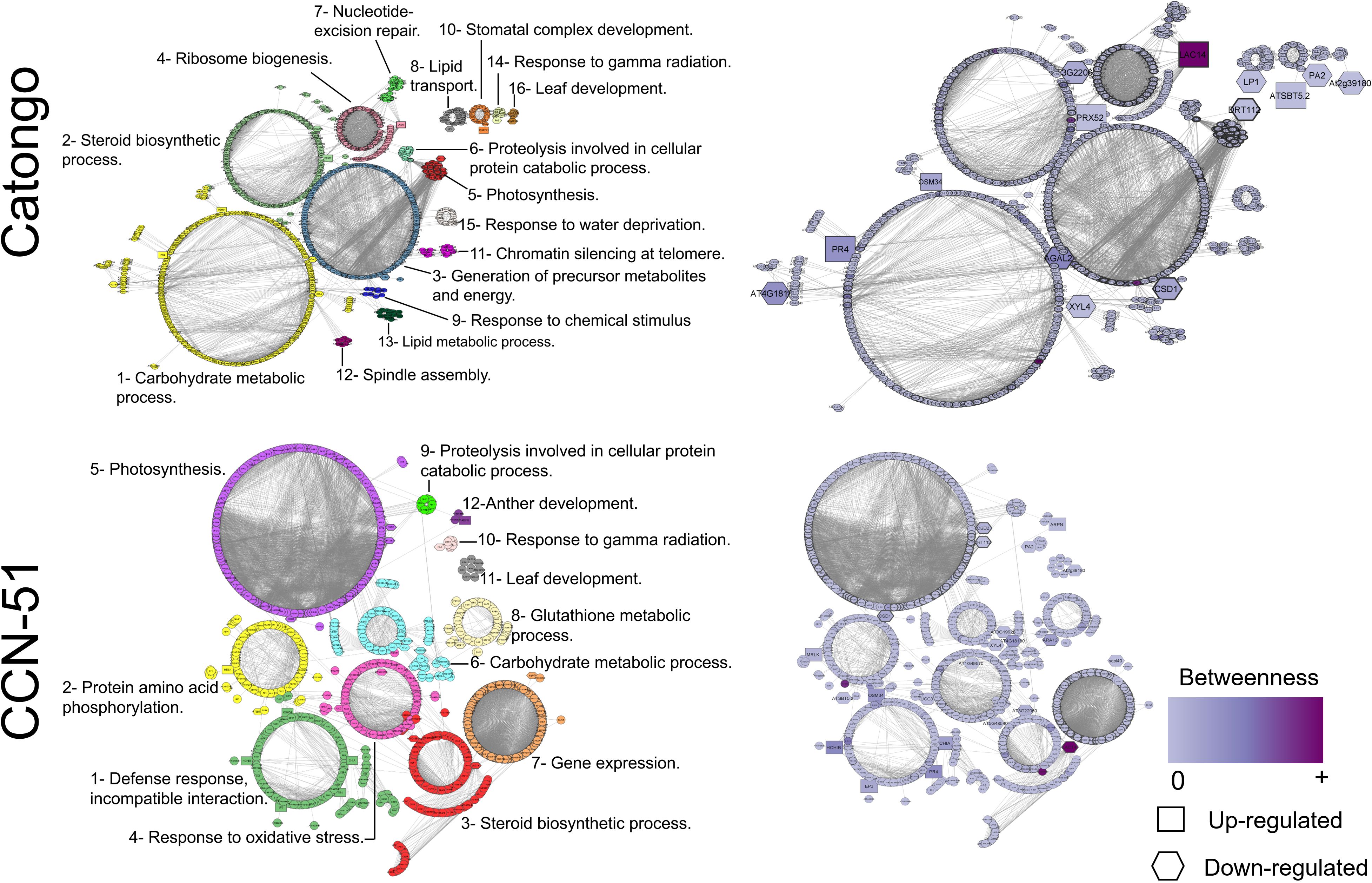
Figure 6 Protein–protein interaction network. Interaction network of apoplastic washing fluid proteins of the T. cacao Catongo (susceptible) and CCN-51 (resistant) genotypes according to homology with Arabidopsis thaliana. Each cluster is represented by a different color. The purple color scale represents the betweenness of a node and the thickness of each node represents the degree centrality. Interactions were generated and visualized in software STRING 10.5 and reconstructed by Cytoscape software.
Based on the gene ontology for biological processes, 16 functional clusters for the Catongo genotype and 12 for the CCN-51 genotype were predicted. (Figure 6). According to the network analysis of the Catongo samples, the proteins predicted for cluster 1 stood out for being involved in the carbohydrate metabolic process. In cluster 1, there were 200 proteins, five of which were orthologous: Hevein-like preproprotein (PR4) and Osmotin-like (OSM34) that were up-accumulated and Alpha-galactosidase 2 (AGAL2) and Beta-D-xylosidase 4 (XYL4) down-accumulated. In cluster 2, the predicted proteins were involved in the steroid biosynthetic process, containing 117 proteins, two of which were orthologous, one peroxidase and one kinase (PRX52, up-accumulated, and AT3G22060, down-accumulated, respectively). Cluster 3 contained 119 proteins that may be involved in the generation of precursor metabolites and energy, with emphasis on the superoxide dismutase (CSD1) protein. In addition, cluster 4 contained 71 proteins possibly involved in ribosome biogenesis, with the only up-accumulated laccase (LAC14) orthologous protein (Figure 6).
The main clusters resulting from proteins of the CCN-51 genotype were cluster 1, where the proteins were involved in the incompatible interaction defense response. With 141 proteins, five of which were orthologous and were up-accumulated, the proteins basic endochitinase (HCHIB), osmotin (OSM34), chitinases (EP3 and CHIA), and hevein-like preproprotein (PR4). Cluster 3 with 130 proteins involved in the steroid biosynthetic process, having three orthologous proteins with reduced accumulation, the laccase proteins (LAC14) and two kinases (AT3G22060 and AT5G48540). Cluster 4 proteins may be involved in the response to oxidative stress, where it contains 89 proteins, two of which are up-accumulated orthologs, the uclacyanin protein (UCC3), and peroxidase (AT1G49570) (Figure 6).
The Laccase protein (LAC14), identified in both genotypes, presented a higher betweenness value (Figure 6), indicating a high interaction with other proteins (Nithya et al., 2023). The superoxide dismutase protein (CSD1) identified in both genotypes in the GH condition presented degree values above the average (Figure 6), being considered a protein that has an important regulatory role within the network (Nithya et al., 2023).
3.6 Chitinase immunodetection
The accumulation of chitinase (PR-3) identified in the apoplast of T. cacao genotypes was visualized by Western blot (Supplementary Figure 3; Figure 7). The presence of PR-3 was mainly detected in samples from plants of both genotypes under FD conditions (green witches’ broom stage) in both genotypes, with an increase of approximately 15 times in relation to samples in GH condition (asymptomatic grown in a GH) (Figure 7).
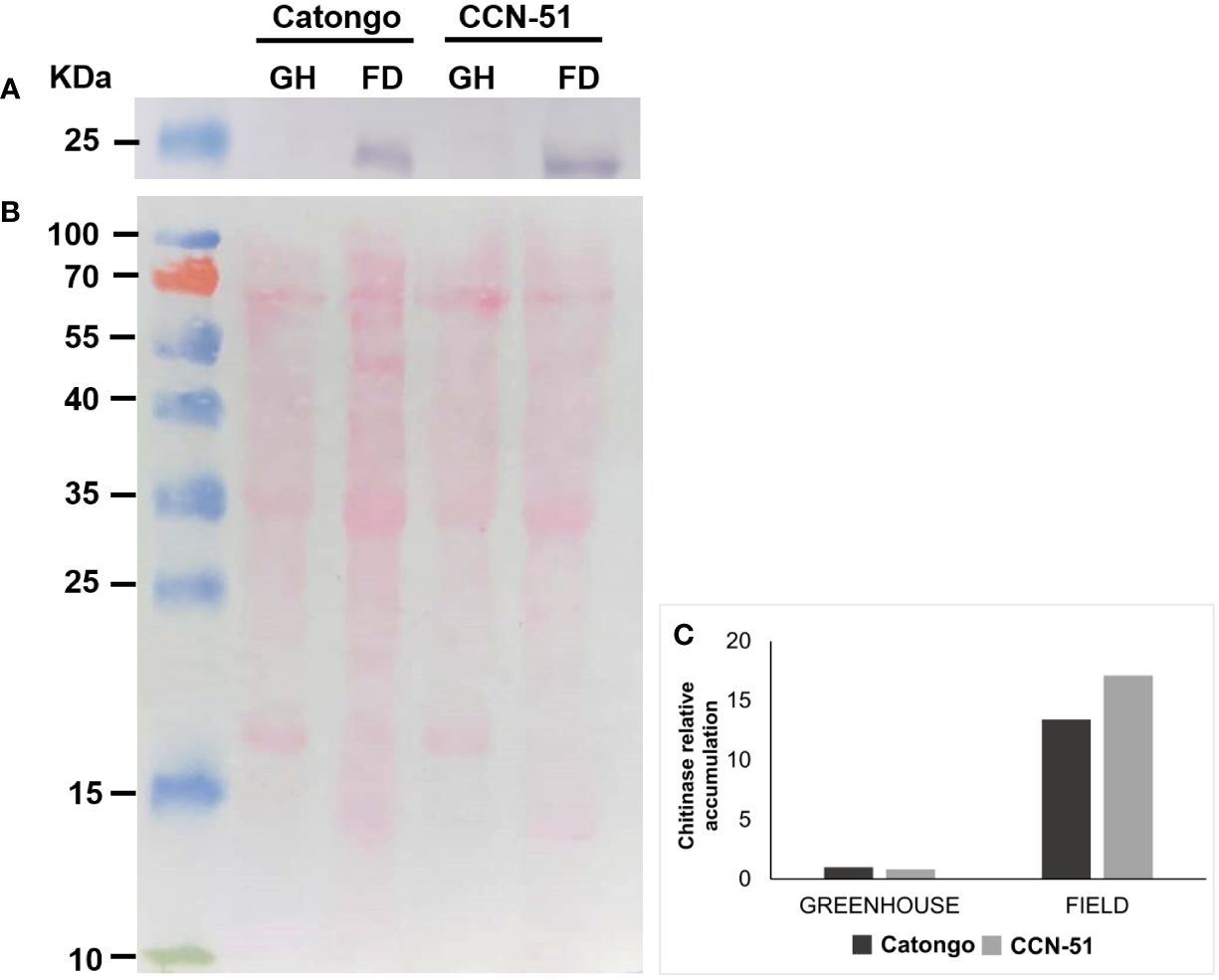
Figure 7 Immunodetection of PR-3 in apoplastic washing fluid from T. cacao genotypes. Western blot using an antibody against PR-3 in a protein sample from apoplastic washing fluid of T. cacao genotypes Catongo (susceptible) and CCN-51 (resistant) from asymptomatic plants grown in a greenhouse (GH) and of green witches’ broom stage plants grown under field (FD) conditions. (A) Protein accumulation in samples of apoplastic washing fluid from T. cacao genotypes. (B) Mirror gel. (C) Protein accumulation in T. cacao genotypes estimated by the Gel Quant Net 1.7.8 program. kDa, molecular weight.
3.7 Enzymatic activity of chitinase, β-1,3-glucanase, and proteases in AWF of T. cacao
Chitinase activity maintained similar behavior for both substrates in all samples, with higher activity detected in Catongo GH and lower activity in Catongo FD. A statistically significant difference (p < 0.05) was observed between these samples when evaluated with the substrate for endochitinase detection. Catongo GH showed activity approximately two times greater than Catongo FD (Figure 8).
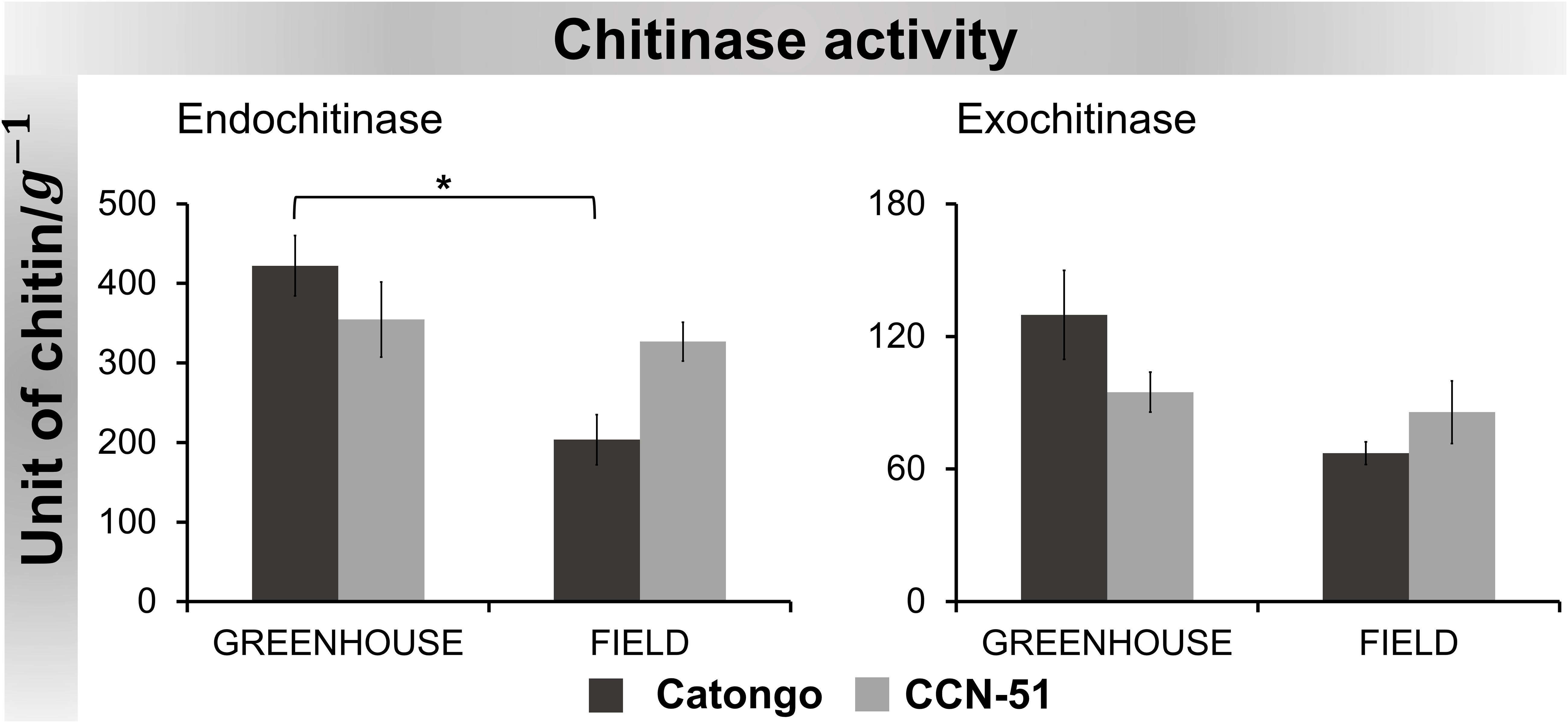
Figure 8 Chitinase activity in apoplastic washing fluid from T. cacao genotypes. The points represent the means of the triplicates of the analyses of each variety. * Significance of p < 0.05 by ANOVA followed by comparison using the Tukey test (p < 0.01 and p < 0.05). Bars correspond to standard errors of the means.
The activity of the enzyme β-1,3-glucanase was higher in CCN-51 FD compared to the other samples. Activity was significantly (p < 0.01) higher in CCN-51 FD, both compared to the GH condition and the Catongo FD sample (Figure 9).
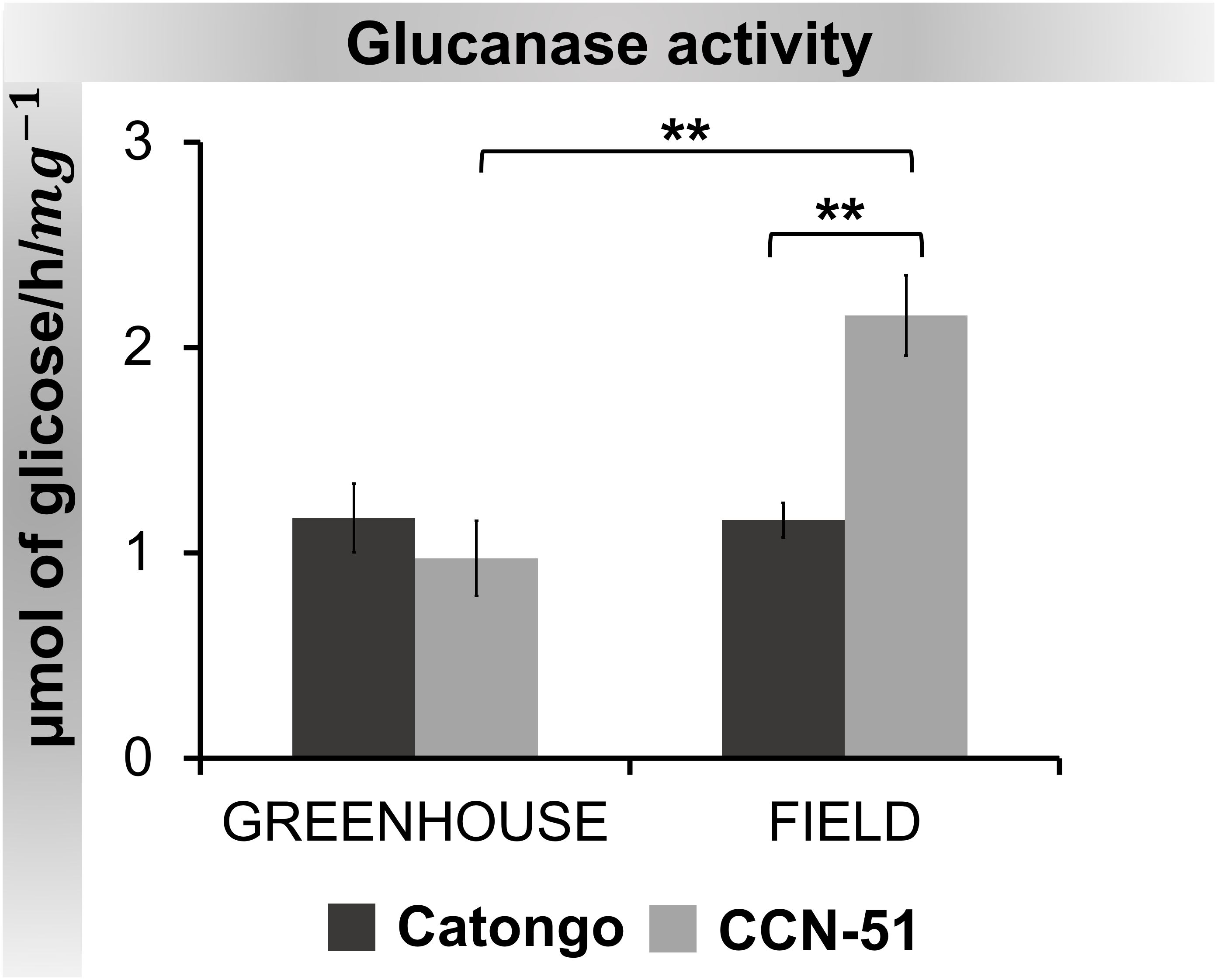
Figure 9 β-1,3-Glucanase activity in apoplastic washing fluid from T. cacao genotypes. The points represent the means of the triplicates of the analyzes of each variety. ** Significance of p < 0,01 by ANOVA followed by comparison using the Tukey test (p < 0.01 and p < 0.05). Bars correspond to standard errors of the means.
To evaluate protease activity in the crude AWF extract of T. cacao genotypes, zymography was performed on a polyacrylamide gel. The gel image (Figure 10) shows clear regions (highlighted with white outlines) where the proteases present in AWF have degraded the substrates used in the packaging gel, confirming their activity and location in the gel. The clear and continuous regions throughout the gel can be noted mainly above 30 kDa.
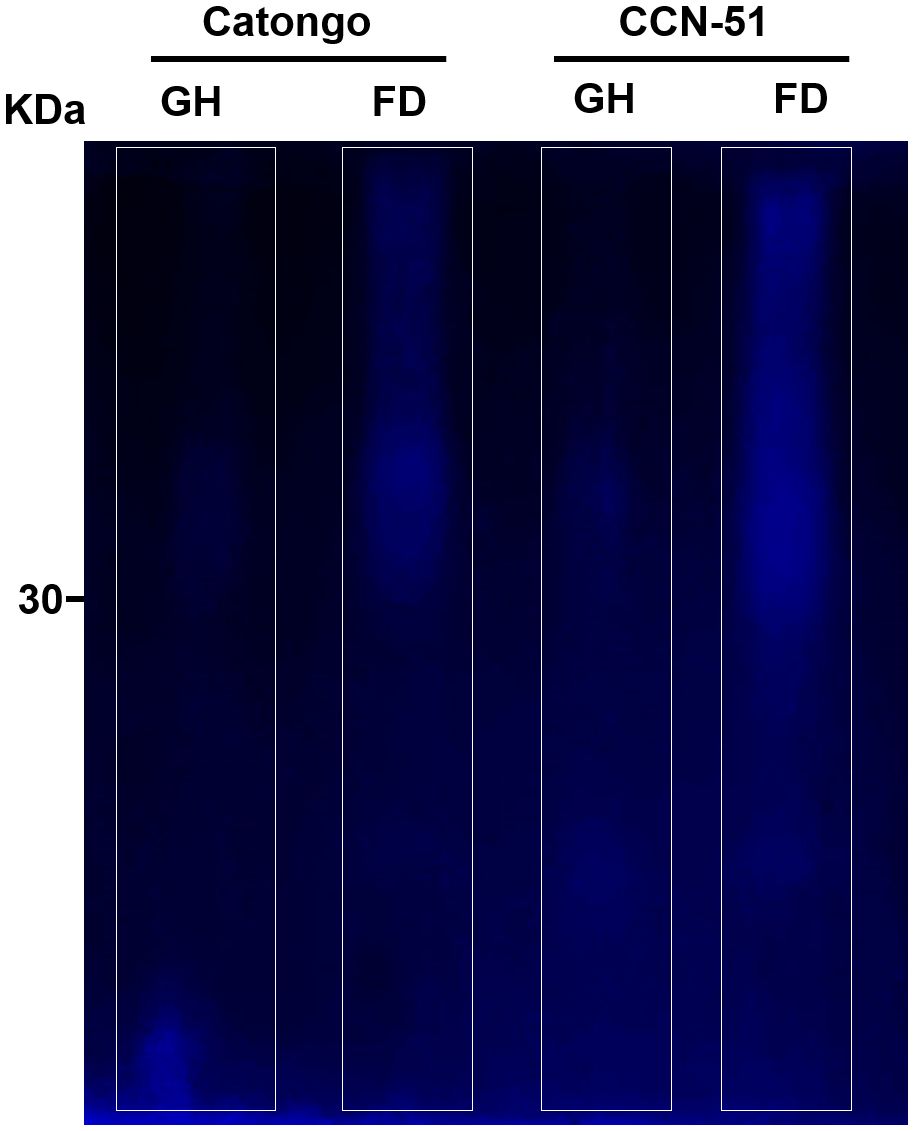
Figure 10 Proteases activity in apoplastic washing fluid from T. cacao genotypes. Zymogram with protease activity in apoplastic washing fluid of T. cacao of the Catongo (susceptible) and CCN-51 (resistant) genotypes of asymptomatic plants grown in a greenhouse (GH) and plants at the green witch’s broom stage grown under conditions of field (FD). kDa, Molecular weight.
3.8 Microorganism proteins in the AWF of T. cacao
Microorganism proteins were identified in the AWF of the Catongo and CCN-51 genotypes of T. cacao. Most of the proteins belong to the fungus M. perniciosa. Twenty-three proteins were identified in the Catongo and CCN-51 FD genotypes, with eight proteins being exclusive to the susceptible genotype (Catongo) and 10 being exclusive to the resistant genotype (CCN-51). Five M. perniciosa proteins were common to both genotypes (Tables 1, 2).
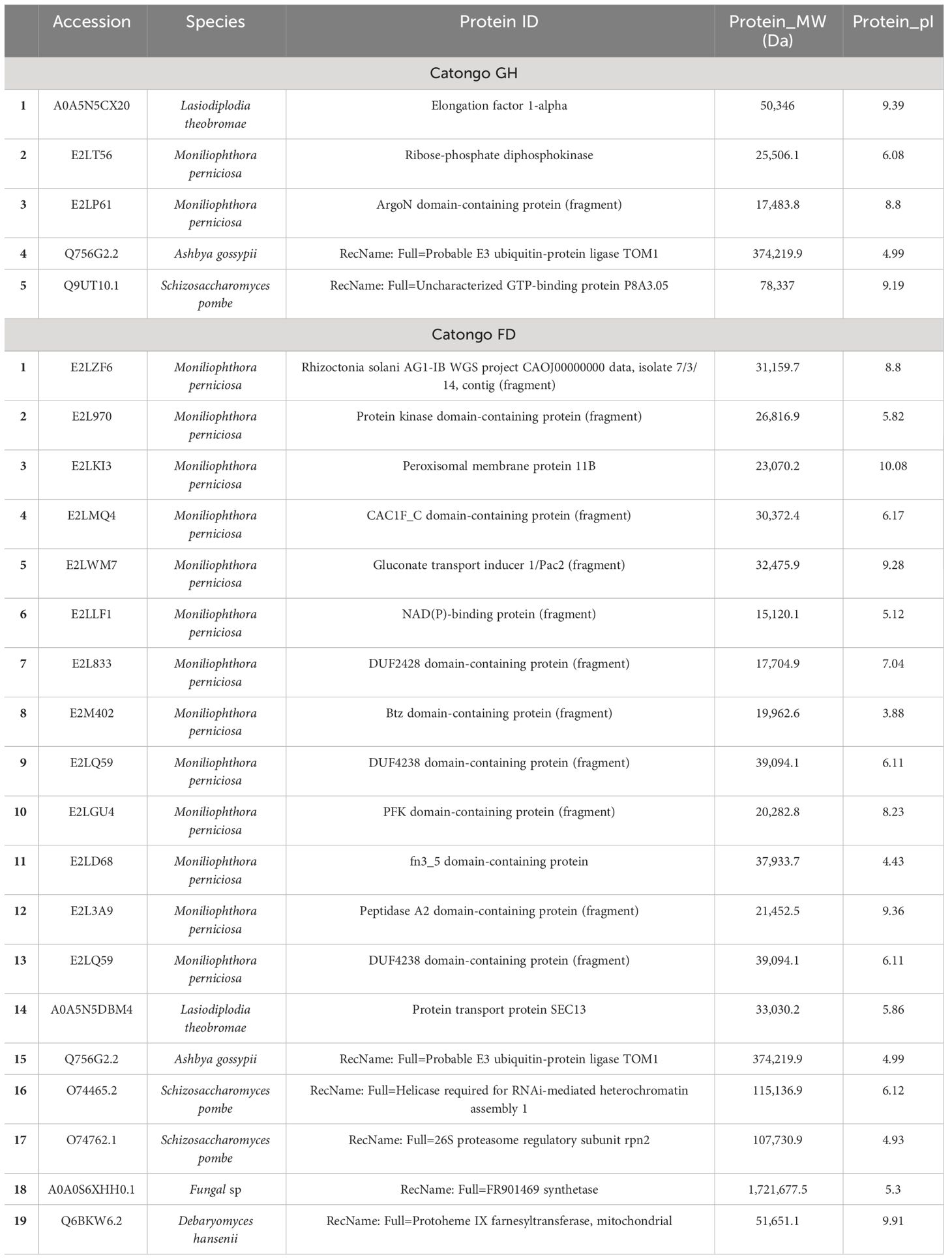
Table 1 Proteins from microorganisms identified in apoplastic washing fluid of the Catongo GH and FD genotype.
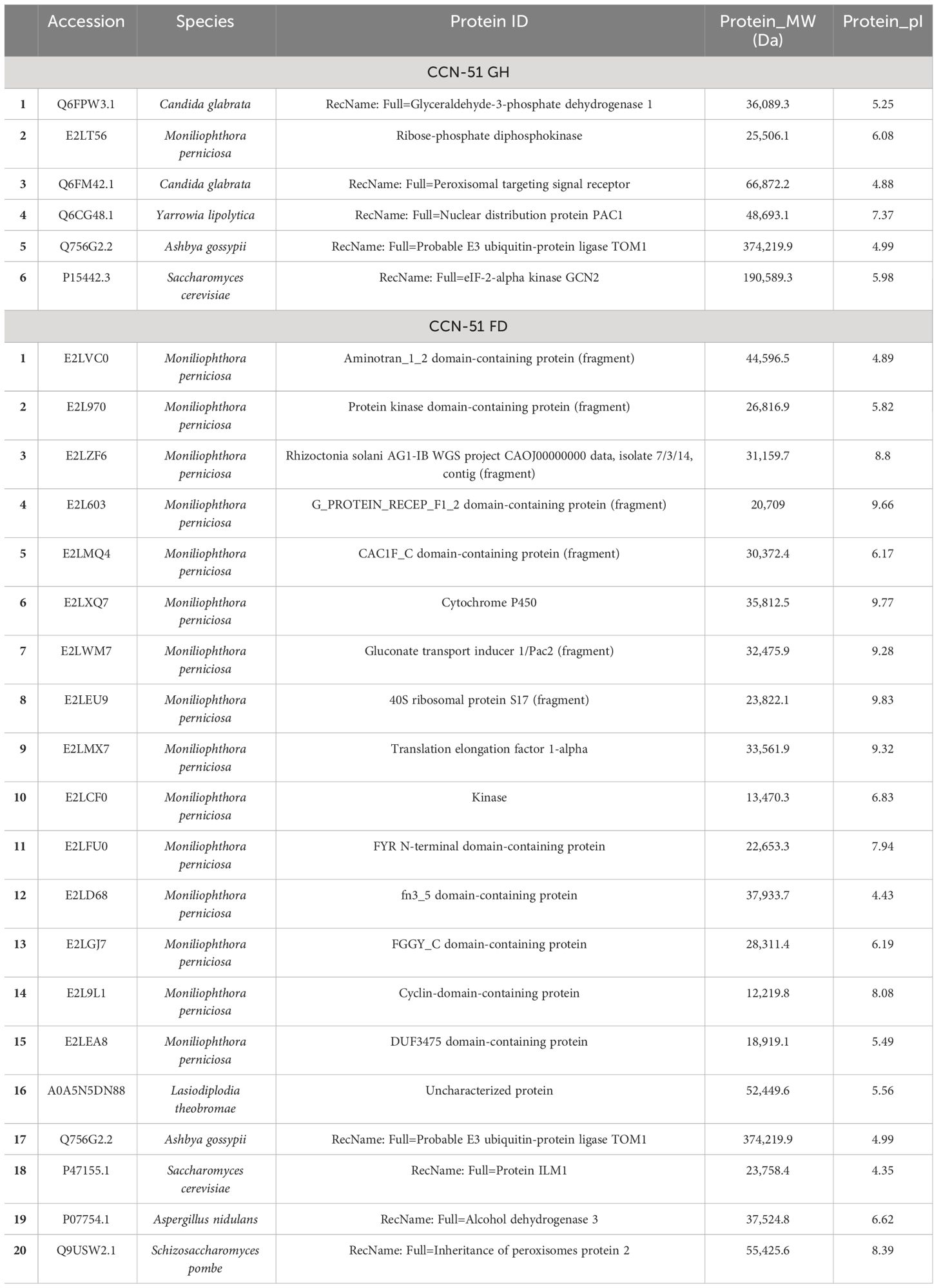
Table 2 Proteins from microorganisms identified in apoplastic washing fluid of the CCN-51 GH and FD genotypes.
Interestingly, in Catongo GH, proteins from the filamentous fungus M. perniciosa were found, as well as from the fungi Lasiodiplodia theobromae and Ashbya gossypi and the yeast Schizosaccharomyces pombe. In the CCN-51 GH genotype, a protein from M. perniciosa was also found, in addition to proteins from Candida glabrata, Yarrowia lipolytica, A. gossypi and Saccharomyces cerevisiae. In Catongo FD, proteins from M. perniciosa, L. theobromae, A. gossypi, S. pombe, and Debaryomyces hansenii were found. Proteins from M. perniciosa, L. theobromae, A. gossypi, S. pombe, as well as Aspergillus nidulans and S. cerevisiae were also found in CCN-51 FD (Tables 1, 2).
4 Discussion
4.1 The AWF extraction step improves the identification of proteins secreted in T. cacao leaves
Obtaining a good yield of apoplast proteins, which can be soluble in AWF or linked to the cell wall, is still a challenge, due to the difficulties of avoiding disruption of the cell wall and membrane. As a result, they are still poorly characterized (Delaunois et al., 2014).
The technique for obtaining apoplastic proteins, which initially involves the extraction of AWF, demonstrated a high protein yield in samples of T. cacao leaves, despite presenting cytoplasmic contamination of 56% (Figure 3). The number of apoplastic proteins identified (27) was greater from AWF extraction compared to obtaining apoplastic proteins from TE (19). For the recovery of apoplastic proteins from the TE of T. cacao leaves (Figure 3), cytoplasmic contamination of 87% was observed, corresponding to 132 proteins out of a total of 151, due to the leaf maceration process.
The total number of proteins recovered is reduced using the optimized AWF extraction technique from T. cacao leaves (62 proteins), compared to the number of proteins in the TE sample (151 proteins). However, it is important to highlight that the concentration of proteins in the apoplast is lower compared to those present in the internal space of the cells (Delaunois et al., 2014), additionally obtaining AWF also reduces cytoplasmic contamination compared to TE samples. The centrifugal force used in AWF extraction is essential to reduce this contamination, and the centrifugation-infiltration method that was used in this work has already been adopted in several works (Soares et al., 2007; Delannoy et al., 2008; Pirovani et al., 2008; Pechanova et al., 2010; de Oliveira et al., 2024), being adapted for each species and enabling better investigation within the area of subcellular proteomics.
4.2 The apoplast is rich in defense and stress proteins
Most of the proteins identified in the AWF of T. cacao genotypes are related to defense and stress, with emphasis on pathogenesis-related proteins (PR-proteins).
PR-proteins make up an important class of proteins that play a crucial role in the plant defense response against pathogens. The production of these proteins is increased when the plant is under stress, and they perform several functions, ranging from directly inhibiting the growth of pathogens to strengthening the plant’s defenses (Sels et al., 2008; Sudisha et al., 2012; dos Santos and Franco, 2023).
Currently, 19 families of PRs have been described (dos Santos and Franco, 2023), among which we identified representatives of the families PR-3, PR-4, PR-5, PR-9, and PR-14. Based on the analysis of cacao transcripts using public data carried out in a previous work (Teixeira et al., 2014), some transcripts belonging to apoplastic PR-proteins were up-accumulated in infected plants compared to healthy T. cacao (Forastero) plants used by Teixeira et al. (2014), as members of PR-3, PR-4, PR-5, PR-9, and PR-14 (Figure 4). This corroborates findings in the present work, due to the importance that PR-proteins have in host defense.
Proteins from the PR-3 family (chitinases) were detected only in the resistant genotype (CCN-51), with most representatives showing a greater accumulation in green brooms (FD) (Figure 1). Recently, chitinases (EC 3.2.1.14) were identified in the AWF of T. cacao only in the FD-grown CCN-51 genotype (de Oliveira et al., 2024). This corroborates the data revealed on PR-3 accumulation in CCN-51 FD (Figure 7). Although not identified by proteomic analysis, the protein was also accumulated in the Catongo GH and FD genotypes (susceptible) and verified by Western blot (Figure 7). A previous study demonstrated that the Catongo genotype contained a greater amount of upregulated defense and stress-related proteins, such as an acid chitinase isoform, at 45 days after inoculation with M. perniciosa, compared to the downregulated proteins (dos Santos et al., 2020). The genotype of T. cacao resistant to WBD (TSH1188) showed upregulated chitinase isoforms 42 h after inoculation and 45 days after inoculation with M. perniciosa (dos Santos et al., 2020).
Chitinases play an effective role in breaking down chitin, an essential component in the constitution of the fungal cell wall. One of the main functions of chitinases in plants is associated with the defense response against pathogenic fungi, but they can also have antibacterial and antiviral actions (dos Santos and Franco, 2023). In the T. cacao apoplast of the CCN-51 genotype (resistant), chitinases were identified, represented in cluster 1 and are involved in the defense response (Figure 6). According to the network of interactions, these proteins have a high betweenness value and can be considered bottleneck proteins. Bottleneck proteins play an important role in the regulation and control of metabolic pathways and/or cell signaling and are, therefore, essential in the communication and integration of different parts of the network (Nithya et al., 2023).
Chitinases are important in cell signaling, due to their chitinolytic activity and the formation of oligosaccharides, which act as elicitor molecules that are recognized by the host plant, favoring a cascade of signals (Kaur et al., 2022; dos Santos and Franco, 2023). Extracellular chitinases can have direct antimicrobial action by the degradation of invading hyphae (Stangarlin and Pascholati, 2000) and can be acidic or basic (Vaghela et al., 2022).
PR-4 was identified in both T. cacao genotypes, being up-accumulated in the FD condition (green witch’s broom stage) (Figure 1). When comparing protein accumulation between genotypes under the same condition, PR-4 was up-accumulated in the CCN-51 FD (resistant) genotype and down-accumulated in Catongo FD (susceptible) (Figure 2). PR-4 can act directly on the pathogen’s cell wall due to its ability to bind to chitin and may present chitinolytic activity (Ali et al., 2018; Butassi et al., 2022). Recently, this protein showed increased abundance in the apoplast of peach plants inoculated with the fungus Taphrina deformans (Butassi et al., 2022). The antifungal activity of PR-4 was proven in wheat against fungi of the genus Fusarium (Caruso et al., 1996), in transgenic grapevines in response to powdery mildew disease (Dai et al., 2016) and in response to abiotic stress in rice (Wang et al., 2011).
The PR-4 protein identified in Catongo is represented in cluster 1, involved in the carbohydrate metabolic process. In the CCN-51 genotype, it is represented in cluster 1 and has a high betweenness value and can be considered a bottleneck protein (Figure 6).
Thaumatin-like protein (PR-5) was detected in the Catongo (susceptible) and CCN-51 (resistant) genotypes exclusively in the FD condition (Figure 1). The PR-5 protein is recognized for its role in plants’ defense response against a variety of pathogens. It can accumulate in the apoplast or vacuole and act directly as an antifungal agent, inhibiting the growth and spread of invasive fungi (Stintzi et al., 1993; de Jesús-Pires et al., 2020; Manghwar and Hussain, 2021). Previous studies have demonstrated that the PR-5 protein delayed the disease caused by Phytophthora infestans in potato (Liu et al., 1994). In garlic, PR-5 genes are involved in defense against Fusarium infection (Anisimova et al., 2021). Silencing of a PR-5 gene in the wheat apoplast leads to compromised resistance against the pathogen Puccinia triticina (Zhang et al., 2018). Recently, an osmotin (PR-5) was identified in the apoplast of the WBD-resistant genotype of T. cacao (de Oliveira et al., 2024). The Catongo thaumatin-like protein is represented in cluster 1, being involved in the carbohydrate metabolic process (Figure 6). In CCN-51, it is involved in the defense response (cluster 1) representing a bottleneck protein within the network (Figure 6). Accordingly, it is suggested that this protein plays a crucial role in immune responses in T. cacao, contributing to the reinforcement and tentative resistance of T. cacao to pathogenic threats.
Peroxidases (PR-9) were detected in both genotypes under both growth conditions. Peroxidase 70 was exclusively detected in Catongo FD, peroxidase A2 was up-accumulated in Catongo and CCN-51 GH, and peroxidase 10 was exclusively detected in CCN-51 GH. The expression of two peroxidases was increased in the apoplast of barley infected by the pathogen Pyrenophora teres f. teres (Hassett et al., 2020). This protein plays an important role against pathogens in the initial stages of infection, acting as an antioxidant enzyme, and also actively participates in cell wall synthesis and remodeling (Hiraga et al., 2001; dos Santos and Franco, 2023). In CCN-51, peroxidase 10 is represented in cluster 4 (Figure 6), related to the response to oxidative stress. According to the findings in the present work, the production of peroxidases may be induced in infected FD genotypes. Because some PR-proteins can be produced constitutively (van Loon et al., 2006), it is likely that this is occurring in GH genotypes. Therefore, in normal situations, plants may express peroxidases to manage natural ROS production.
The PR-14 protein (nsLTPs) was detected exclusively in the Catongo GH genotype. PR-14 facilitates the transfer of lipids and has antifungal and antibacterial activity, by acting on the permeability of the fungal/bacterial membrane (Van Loon and Van Strien, 1999; Hoffmann-Sommergruber, 2002; Gorjanović et al., 2005; Patkar and Chattoo, 2006). This protein was represented in cluster 8, involved in lipid transport (Figure 6), but the exact biological role remains to be determined.
4.3 Down-accumulated proteins in the apoplastome in T. cacao genotypes suggest sequestration of defense pathways in FD genotypes by M. perniciosa
Asymptomatic T. cacao plants grown in the GH had a greater number of proteins identified in the apoplastome, both in comparison within and between genotypes in relation to green witches’ broom plants grown in the FD. This may be related to the modulation of the defense system (Nishad et al., 2020). It can be inferred that the defense system of FD plants was previously turned off due to more dynamic interactions with the environment and that M. perniciosa infection favors the reduction of prolonged defense.
In a previous work, it was proven that 45 days after inoculation with M. perniciosa in the Catongo (susceptible) and TSH1188 (resistant to WBD) genotypes, the accumulation of proteins related to stress and defense is reduced in Catongo, whereas, in TSH1188, it was significantly increased (dos Santos et al., 2020). The period of onset and development of WBD in the FD plants used in the present work is unknown. However, presumably more than 45 days had already passed since the onset of the disease, which may explain the reduction in protein accumulation in the genotypes of infected plants (FD).
A cysteine-rich secretory protein showed increased accumulation in the Catongo (susceptible) and CCN-51 (resistant) GH genotypes but decreased in CCN-51 FD and was not detected in Catongo FD. The cysteine-rich repeat protein is apoplastic and plays a fundamental role in plant defense against phytopathogens. A cysteine-rich repeat protein from wheat was proven to be effective against the phytopathogens Rhizoctonia cerealis and Bipolaris sorokiniana, through its proven antifungal activity in inhibiting mycelium growth, as well as regulating the expression of pathogenesis-related genes such as of β-1,3-glucanase and chitinases (Guo et al., 2020). A cotton cysteine-rich repeat protein acted on the stability of a chitinase, protecting it from cleavage by Verticillium dahliae protease. Overexpression of the protein increased resistance against the cotton fungal phytopathogen (Han et al., 2019). The Catongo cysteine-rich secretory protein is represented in cluster 2 and in CCN-51 in cluster 3, being involved in the steroid biosynthetic process in both genotypes (Figure 6). It is likely that this protein acts in the production of important compounds in the stress response of T. cacao. The reduced accumulation of cysteine-rich repeat protein in T. cacao FD (green witch broom stage) genotypes may have been mediated by the strong inhibitory activity of the fungus M. perniciosa, unlike what occurred in T. cacao GH (asymptomatic grown in a GH) genotypes.
The protein SOD was detected in both genotypes only in the GH condition. SOD plays a fundamental role in antioxidant defense in plants due to the increase in reactive oxygen species (ROS) (Mase and Tsukagoshi, 2021). SOD acts as the first line of defense against oxygen free radicals in less toxic forms, such as hydrogen peroxide (H2O2) and oxygen (O2), reducing oxidative damage (Qi et al., 2017; Wang et al., 2018). The main type of SOD found in the apoplast is copper-zinc (Cu-Zn SOD) (Alscher et al., 2002). The SOD protein identified in Catongo (susceptible) GH is represented in cluster 3 and may be involved in the generation of precursor metabolites and energy (Figure 6). This protein is classified according to the biological network as a “hub” protein, where it plays an important role in the transmission of signals, the integration of metabolic pathways, and the regulation of biological processes (Nithya et al., 2023).
Increased SOD accumulation may be associated with increased ROS, especially in response to biotic stress (Jwa and Hwang, 2017; Wang et al., 2018). The decrease in SOD in FD genotypes (green witch broom stage) in the present work may be an effect of the plant’s response to infection, where manipulation of antioxidant defenses may be a strategy used by the pathogen M. perniciosa to facilitate disease progression. Therefore, the fungus possibly interferes with the defense mechanisms of T. cacao, reducing the effectiveness of antioxidant enzymes such as SOD, which could result in greater oxidative damage and benefit the pathogen in the plant.
4.4 The activity of enzymes occurring in the T. cacao apoplast
Some PR-proteins that have enzymatic action, such as PR-3 and PR-2, were evaluated in the present study.
The chitinases evaluated, although they demonstrated a similar pattern of activity in all samples, exhibited higher endochitinase activity compared to the exochitinase activity (Figure 8). Plant chitinases are generally endochitinases with a molecular weight of 25 kDa to 36 kDa (Hoffmann-Sommergruber, 2002; Hamid et al., 2013; Vaghela et al., 2022) and are active in plant self-defense in response to phytopathogen attack (Vaghela et al., 2022).
For endochitinase (EC 3.2.1.14) activity, a statistically significant difference was observed between samples of the susceptible genotype (Catongo GH and FD) (Figure 8). The activity in the Catongo GH genotype was significantly higher than in the FD sample. Although proteomic data did not reveal the accumulation of chitinase in Catongo GH, it was possible to measure its ability to catalyze reactions in this genotype. The use of crude AWF extract with proteins in the native state may have favored its dosage in GH samples (asymptomatic plants grown in a GH).
When evaluating PR-2 activity, we observed statistically significant increases between CCN-51 FD and GH and between CCN-51 FD and Catongo FD, approximately twice as high (Figure 9). Increased PR-2 activity after infection was reported in rice infected by Magnaporthe oryzae (Wang et al., 2021), in chickpea AWF infected by Ascochyta rabiei (Hanselle and Barz, 2001), and in resistant cultivars of wheat infected by Diuraphis noxia (Van Der Westhuizen et al., 1998).
PR-2 (EC 3.2.1.39) has beta-1,3-glucanase activity, an enzyme that breaks beta-1,3-glucan bonds, an important component in the cell wall of many fungi (Hoffmann-Sommergruber, 2002). Therefore, beta-1,3-glucanases play a crucial role in the defense response of plants against fungal pathogens, because the breakdown of beta-1,3-glucan compromises the integrity of the fungal cell wall (Balasubramanian et al., 2012; dos Santos and Franco, 2023). This can lead to the death of the fungus or at least weaken its ability to invade and colonize plant tissue.
The apoplastic AWF of T. cacao genotypes also revealed a high protease activity through the zymogram gel (Figure 10), confirming the activity of proteases, such as the subtilases identified in the present work: protease secreted by CO2 response and similar proteases to subtilisin (XP_017981649.1, XP_007017870.2, XP_007018543.2 and XP_007017195.2). Subtilases are very numerous in plants and can be directed to the cell wall and contribute to wall modification, cell signaling, and response to biotic and abiotic stress (Figueiredo et al., 2014; Schaller et al., 2018). A subtilase gene in cotton is involved in the plant’s defense against the pathogen Verticillium dahliae (Duan et al., 2016). The identification of subtilases in AWF has already been reported in coffee leaves (Guerra-Guimarães et al., 2015), Arabidopsis thaliana leaves (Jiang et al., 2023) and leaves of the Catongo and CCN-51 genotypes of T. cacao (de Oliveira et al., 2024). The transcript belonging to two protein isoforms were up-accumulated (lcl_NC_030857.1_mrna_XM_018126160.1_26400 and lcl_NC_030857.1_mrna_XM_007018481.2_26945) (Figure 4). The activity of these proteases in the T. cacao apoplast, together with others identified and shown in Figures 1, 2, may be important in the infection process by modulating the plant’s defense responses.
4.5 Peptides from microorganisms are detected in the apoplast of T. cacao genotypes
A total of 40 proteins from microorganisms were identified in the AWF of T. cacao genotypes, 23 of which belonged to the fungus M. perniciosa present in the FD genotype samples (plants in the green witch’s broom stage) (Tables 1, 2). Some functions of these proteins are associated with biosynthetic processes, protein transport, and proteolysis. The FD genotypes were under a high level of stress, evident with the branches at the green witch’s broom stage used in AWF extraction, and, in this context, it was predicted that the fungus M. perniciosa would secrete molecules in the T. cacao apoplast in order to interfere with the plant defense (Fiorin et al., 2018).
It is known that the microbial community can exert a symbiotic relationship with plants, resulting from coevolution between species (Pathak et al., 2022). This relationship is possible because their genomes were shaped to survive together, so they stand out for having characteristics that prevent the recognition of microorganisms by the plants’ immune systems (Khare et al., 2018; Pathak et al., 2022).
A protein belonging to an unidentified fungal species (ID: A0A0S6XHH0.1) was found in the Catongo GH sample (Table 1). This protein’s beta-1,3-glucan inhibitory activity has already been established by in vitro and in vivo studies, showing antifungal activity against the fungi Candida albicans and Aspergillus fumigatus (Fujie et al., 2000a, Fujie et al., 2000b). We suggest that the fungus has a beneficial relationship in Catongo against possible invaders. Additional studies are essential for a more thorough understanding of its action.
In a previous work, the diversity of endophytic fungi in T. cacao (Rubini et al., 2005) was proven, including the fungus Lasiodiplodia theobromae. This corroborates our data from this study, where peptides from L. theobromae were identified. Interestingly, L. theobromae peptides were detected in both GH and FD plants. L. theobromae is a pathogenic necrotrophic fungus important to several crops, including T. cacao (Pereira et al., 2006; de Oliveira et al., 2013). Under controlled conditions, the first symptoms can be visible within 2 weeks after inoculation, such as wilting and death within 10 days (Moreira-Morrillo et al., 2021). Because the GH genotypes (asymptomatic plants grown in a GH) had no visible symptoms of any of the fungi mentioned, it is necessary to perform more comprehensive investigations, using other methods, in order to more precisely elucidate the identification of these peptides and/or functions in the plant, such as metagenome sequencing.
Yeast peptides were also found in both genotypes. Endophytic yeasts have been associated with several plant species: Saccharomyces cerevisiae, considered endophytic of Ficus carica (Ling et al., 2020); and Debaryomyces hansenii, endophytic of wheat (Wachowska et al., 2018) and rice (Tantirungkij et al., 2015), all identified in the apoplast of T. cacao. Although endophytes may play a role in increasing growth, acquiring nutrients and strengthening tolerance to stress in plants (Ling et al., 2020), additional studies are needed. Such studies would offer a more comprehensive understanding of the possible symbiotic relationship between these microorganisms and T. cacao, clarifying the benefits for the host plant.
5 Conclusion
In this study, we report the dynamics and differences of the apoplast proteome between Catongo (WBD-susceptible) and CCN-51 (WBD-resistant) T. cacao genotypes, asymptomatic, and infected by the destructive pathogen M. perniciosa. Proteins detected in the apoplast are related to the plant’s defense response; in addition to the enzymatic profile of chitinases, β-1,3-glucanases, and proteases present in AWF, we demonstrate the great importance of the T. cacao apoplast during the plant–pathogen interaction. A possible modulation and suppression of the T. cacao defense system promoted by M. perniciosa can be suggested, based on the protein profile of the FD genotypes with a significant number of down-accumulated defense proteins. A symbiotic relationship between microorganisms and T. cacao has been proposed; however, more in-depth studies should be carried out with the addition of different methodologies to confirm endophytes and their possible benefits for T. cacao. The T. cacao apoplast reveals important responses in the molecular battle against M. perniciosa and the crucial phase of the infection, biotrophic, can be understood in the light of the apoplastome. Target proteins identified here can be functionally explored in future studies with biotechnological applications in order to control WBD.
Data availability statement
The datasets presented in this study can be found in online repositories. The names of the repository/repositories and accession number(s) can be found in the article/Supplementary Material.
Author contributions
IdO: Data curation, Formal analysis, Investigation, Methodology, Writing – original draft. SA: Investigation, Methodology, Writing – original draft. MF: Conceptualization, Investigation, Methodology, Software, Writing – original draft. AS: Data curation, Software, Writing – original draft. KF: Investigation, Methodology, Writing – original draft. EA: Formal analysis, Investigation, Methodology, Writing – original draft. IM-O: Conceptualization, Methodology, Software, Writing – original draft. JM: Software, Writing – original draft. EC: Software, Writing – original draft. KG: Investigation, Methodology, Supervision, Writing – original draft. CP: Conceptualization, Funding acquisition, Project administration, Resources, Supervision, Visualization, Writing – original draft.
Funding
The author(s) declare financial support was received for the research, authorship, and/or publication of this article. This work was supported by Coordenação de Aperfeiçoamento de Pessoal de Nıível Superior (CAPES) (0001), Conselho Nacional de Desenvolvimento Cientıífico e Tecnoloígico (CNPq—Processes 303765/2019-4 and 421787/2021-0), and Financier of Studies and Projects (Process 01.18.0087.00/2018).
Acknowledgments
We are grateful for the financial support granted by Coordenação de Aperfeiçoamento de Pessoal de Nível Superior (CAPES) and Financiadora de Estudos e Projetos (FINEP).
Conflict of interest
The authors declare that the research was conducted in the absence of any commercial or financial relationships that could be construed as a potential conflict of interest.
Publisher’s note
All claims expressed in this article are solely those of the authors and do not necessarily represent those of their affiliated organizations, or those of the publisher, the editors and the reviewers. Any product that may be evaluated in this article, or claim that may be made by its manufacturer, is not guaranteed or endorsed by the publisher.
Supplementary material
The Supplementary Material for this article can be found online at: https://www.frontiersin.org/articles/10.3389/fpls.2024.1387153/full#supplementary-material
References
Agrawal, G. K., Jwa, N.-S., Lebrun, M.-H., Job, D., Rakwal, R. (2010). Plant secretome: Unlocking secrets of the secreted proteins. Proteomics 10, 799–827. doi: 10.1002/pmic.200900514
Aime, M. C., Phillips-Mora, W. (2005). The causal agents of witches’ broom and frosty pod rot of cacao (chocolate, Theobroma cacao) form a new lineage of Marasmiaceae. Mycologia 97, 1012–1022. doi: 10.3852/mycologia.97.5.1012
Ali, S., Ganai, B. A., Kamili, A. N., Bhat, A. A., Mir, Z. A., Bhat, J. A., et al. (2018). Pathogenesis-related proteins and peptides as promising tools for engineering plants with multiple stress tolerance. Microbiol. Res. 212–213, 29–37. doi: 10.1016/j.micres.2018.04.008
Alscher, R. G., Erturk, N., Heath, L. S. (2002). Role of superoxide dismutases (SODs) in controlling oxidative stress in plants. J. Exp. Bot. 53, 1331–1341. doi: 10.1093/jexbot/53.372.1331
Anisimova, O. K., Shchennikova, A. V., Kochieva, E. Z., Filyushin, M. A. (2021). Pathogenesis-related genes of PR1, PR2, PR4, and PR5 families are involved in the response to fusarium infection in garlic (Allium sativum L.). Int. J. Mol. Sci. 22, 6688. doi: 10.3390/ijms22136688
Balasubramanian, V., Vashisht, D., Cletus, J., Sakthivel, N. (2012). Plant β-1,3-glucanases: their biological functions and transgenic expression against phytopathogenic fungi. Biotechnol. Lett. 34, 1983–1990. doi: 10.1007/s10529-012-1012-6
Beg, M. S., Ahmad, S., Jan, K., Bashir, K. (2017). Status, supply chain and processing of cocoa - A review. Trends Food Sci. Technol. 66, 108–116. doi: 10.1016/j.tifs.2017.06.007
Bradford, M. M. (1976). A rapid and sensitive method for the quantitation of microgram quantities of protein utilizing the principle of protein-dye binding. Anal. Biochem. 72, 248–254. doi: 10.1016/0003-2697(76)90527-3
Butassi, E., Novello, M. A., Lara, M. V. (2022). Prunus persica apoplastic proteome analysis reveals candidate proteins involved in the resistance and defense against Taphrina deformans. J. Plant Physiol. 276, 153780. doi: 10.1016/j.jplph.2022.153780
Caruso, C., Caporale, C., Chilosi, G., Vacca, F., Bertini, L., Magro, P., et al. (1996). Structural and antifungal properties of a pathogenesis-related protein from wheat kernel. J. Protein Chem. 15, 35–44. doi: 10.1007/BF01886809
Ceita, G., de, O., Macêdo, J. N. A., Santos, T. B., Alemanno, L., da Silva Gesteira, A., et al. (2007). Involvement of calcium oxalate degradation during programmed cell death in Theobroma cacao tissues triggered by the hemibiotrophic fungus Moniliophthora perniciosa. Plant Sci. 173, 106–117. doi: 10.1016/j.plantsci.2007.04.006
Conesa, A., Götz, S., García-Gómez, J. M., Terol, J., Talón, M., Robles, M. (2005). Blast2GO: a universal tool for annotation, visualization and analysis in functional genomics research. Bioinformatics 21, 3674–3676. doi: 10.1093/bioinformatics/bti610
Dai, L., Wang, D., Xie, X., Zhang, C., Wang, X., Xu, Y., et al. (2016). The Novel Gene VpPR4-1 from Vitis pseudoreticulata Increases Powdery Mildew Resistance in Transgenic Vitis vinifera L. Front. Plant Sci. 7. doi: 10.3389/fpls.2016.00695
de Almeida, N. M., de Almeida, A.-A. F., de Almeida Santos, N., Mora-Ocampo, I. Y., Pirovani, C. P. (2023). Leaf proteomic profiles in cacao scion-rootstock combinations tolerant and intolerant to cadmium toxicity. Plant Physiol. Biochem. 203, 107987. doi: 10.1016/j.plaphy.2023.107987
de Jesús-Pires, C., Ferreira-Neto, J. R. C., Pacifico Bezerra-Neto, J., Kido, E. A., de Oliveira Silva, R. L., Pandolfi, V., et al. (2020). Plant thaumatin-like proteins: Function, evolution and biotechnological applications. Curr. Protein Pept. Sci. 21, 36–51. doi: 10.2174/1389203720666190318164905
Delannoy, M., Alves, G., Vertommen, D., Ma, J., Boutry, M., Navarre, C. (2008). Identification of peptidases in Nicotiana tabacum leaf intercellular fluid. Proteomics 8, 2285–2298. doi: 10.1002/pmic.200700507
Delaunois, B., Jeandet, P., Clément, C., Baillieul, F., Dorey, S., Cordelier, S. (2014). Uncovering plant-pathogen crosstalk through apoplastic proteomic studies. Front. Plant Sci. 5. doi: 10.3389/fpls.2014.00249
de Oliveira, M. Z. A., Júnior, P. P., de Barbosa, C. J., Assmar, C. C. (2013) Fungo Lasiodiplodia theobromae: um problema para agricultura baiana. Available online at: https://www.embrapa.br/busca-de-publicacoes/-/publicacao/962191/fungo-lasiodiplodia-theobromae-um-problema-para-agricultura-baiana (Accessed January 13, 2024).
de Oliveira, I. B., Moura, I. M., Santana, J. O., Gramacho, K. P., Alves, S., dos, S., et al. (2024). Cocoa apoplastome contains defense proteins against pathogens. Phytopathology® 114 (2), 427–440. doi: 10.1094/PHYTO-03-23-0101-R
Doehlemann, G., Hemetsberger, C. (2013). Apoplastic immunity and its suppression by filamentous plant pathogens. New Phytol. 198, 1001–1016. doi: 10.1111/nph.12277
dos Santos, C., Franco, O. L. (2023). Pathogenesis-related proteins (PRs) with enzyme activity activating plant defense responses. Plants 12, 2226. doi: 10.3390/plants12112226
dos Santos, E. C., Pirovani, C. P., Correa, S. C., Micheli, F., Gramacho, K. P. (2020). The pathogen Moniliophthora perniciosa promotes differential proteomic modulation of cacao genotypes with contrasting resistance to witches´ broom disease. BMC Plant Biol. 20, 1. doi: 10.1186/s12870-019-2170-7
Duan, X., Zhang, Z., Wang, J., Zuo, K. (2016). Characterization of a novel cotton subtilase gene gbSBT1 in response to extracellular stimulations and its role in verticillium resistance. PLoS One 11, e0153988. doi: 10.1371/journal.pone.0153988
Evans, H. C. (2016). “Witches’ Broom disease (Moniliophthora perniciosa): history and biology,” in Cacao Diseases. Eds. Bailey, B. A., Meinhardt, L. W. (Springer International Publishing, Cham), 137–177. doi: 10.1007/978-3-319-24789-2_5
Figueiredo, A., Monteiro, F., Sebastiana, M. (2014). Subtilisin-like proteases in plant–pathogen recognition and immune priming: a perspective. Front. Plant Sci. 5. doi: 10.3389/fpls.2014.00739
Fiorin, G. L., Sanchéz-Vallet, A., Thomazella, D. P., de, T., do Prado, P. F. V., do Nascimento, L. C., et al. (2018). Suppression of plant immunity by fungal chitinase-like effectors. Curr. Biol. 28, 3023–3030.e5. doi: 10.1016/j.cub.2018.07.055
Floerl, S., Druebert, C., Majcherczyk, A., Karlovsky, P., Kües, U., Polle, A. (2008). Defence reactions in the apoplastic proteome of oilseed rape (Brassica napus var. napus) attenuate Verticillium longisporum growth but not disease symptoms. BMC Plant Biol. 8, 129. doi: 10.1186/1471-2229-8-129
Fujie, A., Iwamoto, T., Muramatsu, H., Okudaira, T., Nitta, K., Nakanishi, T., et al. (2000a). FR901469, a novel antifungal antibiotic from an unidentified fungus No.11243. I. Taxonomy, fermentation, isolation, physico-chemical properties and biological properties. J. Antibiot (Tokyo) 53, 912–919. doi: 10.7164/antibiotics.53.912
Fujie, A., Iwamoto, T., Muramatsu, H., Okudaira, T., Sato, I., Furuta, T., et al. (2000b). FR901469, a novel antifungal antibiotic from an unidentified fungus No.11243. II. In vitro and in vivo activities. J. Antibiot (Tokyo) 53, 920–927. doi: 10.7164/antibiotics.53.920
Gorjanović, S., Spillner, E., Beljanski, M. V., Gorjanović, R., Pavlović, M., Gojgić-Cvijanović, G. (2005). Malting barley grain non-specific lipid-transfer protein (ns-LTP): Importance for grain protection. J. Institute Brewing 111, 99–104. doi: 10.1002/jib.2005.111.issue-2
Gramacho, I. C. P., Magno, A. E. S., Mandarino, E. P., Matos, A. (1992). Cultivo e Beneficiamento do Cacau na Bahia. (Ilhéus: CEPLAC/CEDEX).
Guerra-Guimarães, L., Tenente, R., Pinheiro, C., Chaves, I., Silva, M., do, C., et al. (2015). Proteomic analysis of apoplastic fluid of Coffea arabica leaves highlights novel biomarkers for resistance against Hemileia vastatrix. Front. Plant Sci. 6. doi: 10.3389/fpls.2015.00478
Guo, F., Shan, Z., Yu, J., Xu, G., Zhang, Z. (2020). The Cysteine-Rich Repeat Protein TaCRR1 Participates in Defense against Both Rhizoctonia cerealis and Bipolaris sorokiniana in Wheat. Int. J. Mol. Sci. 21, 5698. doi: 10.3390/ijms21165698
Hamid, R., Khan, M. A., Ahmad, M., Ahmad, M. M., Abdin, M. Z., Musarrat, J., et al. (2013). Chitinases: an update. J. Pharm. Bioallied Sci. 5, 21–29. doi: 10.4103/0975-7406.106559
Han, L.-B., Li, Y.-B., Wang, F.-X., Wang, W.-Y., Liu, J., Wu, J.-H., et al. (2019). The Cotton Apoplastic Protein CRR1 Stabilizes Chitinase 28 to Facilitate Defense against the Fungal Pathogen Verticillium dahliae. Plant Cell 31, 520–536. doi: 10.1105/tpc.18.00390
Hanselle, T., Barz, W. (2001). Purification and characterisation of the extracellular PR-2b β-1,3-glucanase accumulating in different Ascochyta rabiei-infected chickpea (Cicer arietinum L.) cultivars. Plant Sci. 161, 773–781. doi: 10.1016/S0168-9452(01)00468-X
Hassett, K., Ellwood, S. R., Zulak, K. G., Muria-Gonzalez, M. J. (2020). Analysis of apoplastic proteins expressed during net form net blotch of barley. J. Plant Dis. Prot 127, 683–694. doi: 10.1007/s41348-020-00318-w
Hiraga, S., Sasaki, K., Ito, H., Ohashi, Y., Matsui, H. (2001). A large family of class III plant peroxidases. Plant Cell Physiol. 42, 462–468. doi: 10.1093/pcp/pce061
Hoffmann-Sommergruber, K. (2002). Pathogenesis-related (PR)-proteins identified as allergens. Biochem. Soc. Trans. 30, 930–935. doi: 10.1042/bst0300930
IBGE. (2024). Instituto Brasileiro de Geografia e Estatística. Available at: https://sidra.ibge.gov.br/tabela/1618 (Accessed February 14, 2024).
ICCO (2022) International Cocoa Organization - Statistics (International Cocoa Organization). Available online at: https://www.icco.org/statistics/ (Accessed January 23, 2023).
Jiang, S., Pan, L., Zhou, Q., Xu, W., He, F., Zhang, L., et al. (2023). Analysis of the apoplast fluid proteome during the induction of systemic acquired resistance in Arabidopsis thaliana. PeerJ 11, e16324. doi: 10.7717/peerj.16324
Jwa, N.-S., Hwang, B. K. (2017). Convergent evolution of pathogen effectors toward reactive oxygen species signaling networks in plants. Front. Plant Sci. 8. doi: 10.3389/fpls.2017.01687
Kaur, S., Samota, M. K., Choudhary, M., Choudhary, M., Pandey, A. K., Sharma, A., et al. (2022). How do plants defend themselves against pathogens-Biochemical mechanisms and genetic interventions. Physiol. Mol. Biol. Plants 28, 485–504. doi: 10.1007/s12298-022-01146-y
Khare, E., Mishra, J., Arora, N. K. (2018). Multifaceted interactions between endophytes and plant: Developments and prospects. Front. Microbiol. 9. doi: 10.3389/fmicb.2018.02732
Laemmli, U. K. (1970). Cleavage of structural proteins during the assembly of the head of bacteriophage T4. Nature 227, 680–685. doi: 10.1038/227680a0
Li, Y.-B., Han, L.-B., Wang, H.-Y., Zhang, J., Sun, S.-T., Feng, D.-Q., et al. (2016). The thioredoxin gbNRX1 plays a crucial role in homeostasis of apoplastic reactive oxygen species in response to verticillium dahliae infection in cotton. Plant Physiol. 170, 2392–2406. doi: 10.1104/pp.15.01930
Ling, L., Tu, Y., Ma, W., Feng, S., Yang, C., Zhao, Y., et al. (2020). A potentially important resource: endophytic yeasts. World J. Microbiol. Biotechnol. 36, 110. doi: 10.1007/s11274-020-02889-0
Liu, D., Raghothama, K. G., Hasegawa, P. M., Bressan, R. A. (1994). Osmotin overexpression in potato delays development of disease symptoms. Proc. Natl. Acad. Sci. U.S.A. 91, 1888–1892. doi: 10.1073/pnas.91.5.1888
Maere, S., Heymans, K., Kuiper, M. (2005). BiNGO: a Cytoscape plugin to assess overrepresentation of gene ontology categories in biological networks. Bioinformatics 21, 3448–3449. doi: 10.1093/bioinformatics/bti551
Manghwar, H., Hussain, A. (2021). Mechanism of tobacco osmotin gene in plant responses to biotic and abiotic stress tolerance: A brief history. biocell 46, 623–632. doi: 10.32604/biocell.2022.017316
Mares, J. H., Gramacho, K. P., dos Santos, E. C., da Silva Santiago, A., de Andrade Silva, E. M., Alvim, F. C., et al. (2016). Protein profile and protein interaction network of Moniliophthora perniciosa basidiospores. BMC Microbiol. 16, 120. doi: 10.1186/s12866-016-0753-0
Mares, J. H., Gramacho, K. P., Santana, J. O., Oliveira de Souza, A., Alvim, F. C., Pirovani, C. P. (2020). Hydrosoluble phylloplane components of Theobroma cacao modulate the metabolism of Moniliophthora perniciosa spores during germination. Fungal Biol. 124, 73–81. doi: 10.1016/j.funbio.2019.11.008
Mares, J. H., Gramacho, K. P., Santos, E. C., da Silva Santiago, A., Santana, J. O., de Sousa, A. O., et al. (2017). Proteomic analysis during of spore germination of Moniliophthora perniciosa, the causal agent of witches’ broom disease in cacao. BMC Microbiol. 17, 176. doi: 10.1186/s12866-017-1085-4
Martínez-González, A. P., Ardila, H. D., Martínez-Peralta, S. T., Melgarejo-Muñoz, L. M., Castillejo-Sánchez, M. A., Jorrín-Novo, J. V. (2018). What proteomic analysis of the apoplast tells us about plant–pathogen interactions. Plant Pathol. 67, 1647–1668. doi: 10.1111/ppa.12893
Mase, K., Tsukagoshi, H. (2021). Reactive oxygen species link gene regulatory networks during arabidopsis root development. Front. Plant Sci. 12. doi: 10.3389/fpls.2021.660274
Meinhardt, L. W., Rincones, J., Bailey, B. A., Aime, M. C., Griffith, G. W., Zhang, D., et al. (2008). Moniliophthora perniciosa, the causal agent of witches’ broom disease of cacao: what’s new from this old foe? Mol. Plant Pathol. 9, 577–588. doi: 10.1111/j.1364-3703.2008.00496.x
Michaud, D., Cantin, L., Raworth, D. A., Vrain, T. C. (1996). Assessing the stability of cystatin/cysteine proteinase complexes using mildly-denaturing gelatin-polyacrylamide gel electrophoresis. Electrophoresis 17, 74–79. doi: 10.1002/elps.1150170113
Miller, G. L. (1959). Use of dinitrosalicylic acid reagent for determination of reducing sugar. Anal. Chem. 31, 426–428. doi: 10.1021/ac60147a030
Moreira-Morrillo, A. A., Cedeño-Moreira, Á.V., Canchignia-Martínez, F., Garcés-Fiallos, F. R., Moreira-Morrillo, A. A., Cedeño-Moreira, Á.V., et al. (2021). Lasiodiplodia theobromae (Pat.) Griffon & Maul [(sin.) Botryodiplodia theobromae Pat] en el cultivo de cacao: síntomas, ciclo biológico y estrategias de manejo. Scientia Agropecuaria 12, 653–662. doi: 10.17268/sci.agropecu.2021.068
Motamayor, J. C., Mockaitis, K., Schmutz, J., Haiminen, N., Livingstone, D., Cornejo, O., et al. (2013). The genome sequence of the most widely cultivated cacao type and its use to identify candidate genes regulating pod color. Genome Biol. 14, r53. doi: 10.1186/gb-2013-14-6-r53
Neuhoff, V., Arold, N., Taube, D., Ehrhardt, W. (1988). Improved staining of proteins in polyacrylamide gels including isoelectric focusing gels with clear background at nanogram sensitivity using Coomassie Brilliant Blue G-250 and R-250. Electrophoresis 9, 255–262. doi: 10.1002/elps.1150090603
Nishad, R., Ahmed, T., Rahman, V. J., Kareem, A. (2020). Modulation of plant defense system in response to microbial interactions. Front. Microbiol. 11. doi: 10.3389/fmicb.2020.01298
Nithya, C., Kiran, M., Nagarajaram, H. A. (2023). Dissection of hubs and bottlenecks in a protein-protein interaction network. Comput. Biol. Chem. 102, 107802. doi: 10.1016/j.compbiolchem.2022.107802
Oliveira, B. R. M., de Almeida, A.-A. F., Pirovani, C. P., Barroso, J. P., de C Neto, C. H., Santos, N. A., et al. (2020). Mitigation of Cd toxicity by Mn in young plants of cacao, evaluated by the proteomic profiles of leaves and roots. Ecotoxicology 29, 340–358. doi: 10.1007/s10646-020-02178-4
Pathak, P., Rai, V. K., Can, H., Singh, S. K., Kumar, D., Bhardwaj, N., et al. (2022). Plant-endophyte interaction during biotic stress management. Plants 11, 2203. doi: 10.3390/plants11172203
Patkar, R. N., Chattoo, B. B. (2006). Transgenic indica Rice Expressing ns-LTP-Like Protein Shows Enhanced Resistance to Both Fungal and Bacterial Pathogens. Mol. Breed. 17, 159–171. doi: 10.1007/s11032-005-4736-3
Patro, R., Duggal, G., Love, M. I., Irizarry, R. A., Kingsford, C. (2017). Salmon provides fast and bias-aware quantification of transcript expression. Nat. Methods 14, 417–419. doi: 10.1038/nmeth.4197
Pechanova, O., Hsu, C.-Y., Adams, J. P., Pechan, T., Vandervelde, L., Drnevich, J., et al. (2010). Apoplast proteome reveals that extracellular matrix contributes to multistress response in poplar. BMC Genomics 11, 674. doi: 10.1186/1471-2164-11-674
Pereira, A. L., Silva, G. S., Ribeiro, V. Q. (2006). Caracterização fisiológica, cultural e patogênica de diferentes isolados de Lasiodiplodia theobromae. Fitopatol. Bras. 31, 572–578. doi: 10.1590/S0100-41582006000600006
Pirovani, C. P., Carvalho, H. A. S., MaChado, R. C. R., Gomes, D. S., Alvim, F. C., Pomella, A. W. V., et al. (2008). Protein extraction for proteome analysis from cacao leaves and meristems, organs infected by Moniliophthora perniciosa, the causal agent of the witches’ broom disease. Electrophoresis 29, 2391–2401. doi: 10.1002/elps.200700743
Pirovani, C. P., da Silva Santiago, A., dos Santos, L. S., Micheli, F., Margis, R., da Silva Gesteira, A., et al. (2010). Theobroma cacao cystatins impair Moniliophthora perniciosa mycelial growth and are involved in postponing cell death symptoms. Planta 232, 1485–1497. doi: 10.1007/s00425-010-1272-0
Qi, J., Wang, J., Gong, Z., Zhou, J.-M. (2017). Apoplastic ROS signaling in plant immunity. Curr. Opin. Plant Biol. 38, 92–100. doi: 10.1016/j.pbi.2017.04.022
R Core Team. (2022). R: A language and environment for statistical computing. Vienna, Austria: R Foundation for Statistical Computing. Available at: https://www.r-project.org/ (Accessed December 20, 2023).
Rubini, M. R., Silva-Ribeiro, R. T., Pomella, A. W. V., Maki, C. S., Araújo, W. L., dos Santos, D. R., et al. (2005). Diversity of endophytic fungal community of cacao (Theobroma cacao L.) and biological control of Crinipellis perniciosa, causal agent of Witches’ Broom Disease. Int. J. Biol. Sci. 1, 24–33. doi: 10.7150/ijbs.1.24
Sakurai, N. (1998). Dynamic function and regulation of apoplast in the plant body. J. Plant Res. 111, 133–148. doi: 10.1007/BF02507160
Santos, A. S., Mora-Ocampo, I. Y., de Novais, D. P. S., Aguiar, E. R. G. R., Pirovani, C. P. (2023). State of the art of the molecular biology of the interaction between cocoa and witches’ Broom disease: A systematic review. Int. J. Mol. Sci. 24, 5684. doi: 10.3390/ijms24065684
Schaller, A., Stintzi, A., Rivas, S., Serrano, I., Chichkova, N. V., Vartapetian, A. B., et al. (2018). From structure to function – a family portrait of plant subtilases. New Phytol. 218, 901–915. doi: 10.1111/nph.14582
Sels, J., Mathys, J., De Coninck, B. M. A., Cammue, B. P. A., De Bolle, M. F. C. (2008). Plant pathogenesis-related (PR) proteins: A focus on PR peptides. Plant Physiol. Biochem. 46, 941–950. doi: 10.1016/j.plaphy.2008.06.011
Shannon, P., Markiel, A., Ozier, O., Baliga, N. S., Wang, J. T., Ramage, D., et al. (2003). Cytoscape: a software environment for integrated models of biomolecular interaction networks. Genome Res. 13, 2498–2504. doi: 10.1101/gr.1239303
Soares, N. C., Francisco, R., Ricardo, C. P., Jackson, P. A. (2007). Proteomics of ionically bound and soluble extracellular proteins in Medicago truncatula leaves. Proteomics 7, 2070–2082. doi: 10.1002/pmic.200600953
Stangarlin, J. R., Pascholati, S. F. (2000). Activities of ribulose-1,5-bisphosphate carboxylase-oxygenase (rubisco), chlorophyllase, β-1,3 glucanase and chitinase and chlorophyll content in bean cultivars (Phaseolus vulgaris) infected with Uromyces appendiculatus. Summa Phytopathologica 26, 34–42.
Stintzi, A., Heitz, T., Prasad, V., Wiedemann-Merdinoglu, S., Kauffmann, S., Geoffroy, P., et al. (1993). Plant ‘pathogenesis-related’ proteins and their role in defense against pathogens. Biochimie 75, 687–706. doi: 10.1016/0300-9084(93)90100-7
Sudisha, J., Sharathchandra, R. G., Amruthesh, K. N., Kumar, A., Shetty, H. S. (2012). ““Pathogenesis related proteins in plant defense response,”,” in Plant Defence: Biological Control. Eds. Mérillon, J. M., Ramawat, K. G. (Springer Netherlands, Dordrecht), 379–403. doi: 10.1007/978-94-007-1933-0_17
Tantirungkij, M., Nasanit, R., Limtong, S. (2015). Assessment of endophytic yeast diversity in rice leaves by a culture-independent approach. Antonie van Leeuwenhoek 108, 633–647. doi: 10.1007/s10482-015-0519-y
Teixeira, P. J. P. L., Thomazella, D. P., de, T., Reis, O., do Prado, P. F. V., do Rio, M. C. S., et al. (2014). High-resolution transcript profiling of the atypical biotrophic interaction between Theobroma cacao and the fungal pathogen Moniliophthora perniciosa. Plant Cell 26, 4245–4269. doi: 10.1105/tpc.114.130807
Vaghela, B., Vashi, R., Rajput, K., Joshi, R. (2022). Plant chitinases and their role in plant defense: A comprehensive review. Enzyme Microbial Technol. 159, 110055. doi: 10.1016/j.enzmictec.2022.110055
Van Der Westhuizen, A. J., Qian, X.-M., Botha, A.-M. (1998). β-1,3-Glucanases in wheat and resistance to the Russian wheat aphid. Physiologia Plantarum 103, 125–131. doi: 10.1034/j.1399-3054.1998.1030115.x
van Loon, L. C., Rep, M., Pieterse, C. M. J. (2006). Significance of inducible defense-related proteins in infected plants. Annu. Rev. Phytopathol. 44, 135–162. doi: 10.1146/annurev.phyto.44.070505.143425
Van Loon, L. C., Van Strien, E. A. (1999). The families of pathogenesis-related proteins, their activities, and comparative analysis of PR-1 type proteins. Physiol. Mol. Plant Pathol. 55, 85–97. doi: 10.1006/pmpp.1999.0213
Villén, J., Gygi, S. P. (2008). The SCX/IMAC enrichment approach for global phosphorylation analysis by mass spectrometry. Nat. Protoc. 3, 1630–1638. doi: 10.1038/nprot.2008.150
Wachowska, U., Irzykowski, W., Jędryczka, M. (2018). Agrochemicals: Effect on genetic resistance in yeasts colonizing winter wheat kernels. Ecotoxicol. Environ. Saf. 162, 77–84. doi: 10.1016/j.ecoenv.2018.06.042
Wang, Y., Branicky, R., Noë, A., Hekimi, S. (2018). Superoxide dismutases: Dual roles in controlling ROS damage and regulating ROS signaling. J. Cell Biol. 217, 1915–1928. doi: 10.1083/jcb.201708007
Wang, Y., Liu, M., Wang, X., Zhong, L., Shi, G., Xu, Y., et al. (2021). A novel β-1,3-glucanase Gns6 from rice possesses antifungal activity against Magnaporthe oryzae. J. Plant Physiol. 265, 153493. doi: 10.1016/j.jplph.2021.153493
Wang, W., Scali, M., Vignani, R., Spadafora, A., Sensi, E., Mazzuca, S., et al. (2003). Protein extraction for two-dimensional electrophoresis from olive leaf, a plant tissue containing high levels of interfering compounds. Electrophoresis 24, 2369–2375. doi: 10.1002/elps.200305500
Wang, Y., Wang, Y. (2018). Trick or treat: Microbial pathogens evolved apoplastic effectors modulating plant susceptibility to infection. Mol. Plant Microbe Interact. 31, 6–12. doi: 10.1094/MPMI-07-17-0177-FI
Wang, N., Xiao, B., Xiong, L. (2011). Identification of a cluster of PR4-like genes involved in stress responses in rice. J. Plant Physiol. 168, 2212–2224. doi: 10.1016/j.jplph.2011.07.013
Zhang, J., Wang, F., Liang, F., Zhang, Y., Ma, L., Wang, H., et al. (2018). Functional analysis of a pathogenesis-related thaumatin-like protein gene TaLr35PR5 from wheat induced by leaf rust fungus. BMC Plant Biol. 18, 76. doi: 10.1186/s12870-018-1297-2
Zugaib, M., Gramacho, K. P., Mares, J. H., Camillo, L. R., Ocampo, I. Y. M., Arevalo-Gardini, E., et al. (2023). Comparative proteome profile of ungerminated spores and mycelium of the fungus Moniliophthora roreri, causal agent of frosty pod rot disease in cacao. J. Phytopathol. 171, 242–257. doi: 10.1111/jph.13177
Keywords: apoplast, defense proteins, apoplastic washing fluid, Theobroma cacao, Moniliophthora perniciosa
Citation: De Oliveira IB, Alves SdS, Ferreira MM, Santos AS, Farias KS, Assis ETCdM, Mora-Ocampo IY, Muñoz JJM, Costa EA, Gramacho KP and Pirovani CP (2024) Apoplastomes of contrasting cacao genotypes to witches’ broom disease reveals differential accumulation of PR proteins. Front. Plant Sci. 15:1387153. doi: 10.3389/fpls.2024.1387153
Received: 16 February 2024; Accepted: 23 April 2024;
Published: 16 May 2024.
Edited by:
Brigitte Mauch-Mani, Université de Neuchâtel, SwitzerlandReviewed by:
Maria Helena S. Goldman, University of São Paulo, BrazilMario Serrano, National Autonomous University of Mexico, Mexico
Copyright © 2024 De Oliveira, Alves, Ferreira, Santos, Farias, Assis, Mora-Ocampo, Muñoz, Costa, Gramacho and Pirovani. This is an open-access article distributed under the terms of the Creative Commons Attribution License (CC BY). The use, distribution or reproduction in other forums is permitted, provided the original author(s) and the copyright owner(s) are credited and that the original publication in this journal is cited, in accordance with accepted academic practice. No use, distribution or reproduction is permitted which does not comply with these terms.
*Correspondence: Carlos Priminho Pirovani, cGlyb3ZhbmlAdWVzYy5icg==