- 1INOQ GmbH, Schnega, Germany
- 2Institute of Microbiology, Friedrich Schiller University, Jena, Germany
- 3Erfurt Research Centre for Horticultural Crops, University of Applied Sciences Erfurt, Erfurt, Germany
Arbuscular mycorrhizal fungi (AMF) play a crucial role in enhancing plant growth, but their use in agriculture is limited due to several constraints. Elevated soil phosphate levels resulting from fertilization practices strongly inhibit fungal development and reduce mycorrhizal growth response. Here, we investigated the possibility of adapting Rhizoglomus irregulare to high phosphate (Pi) levels to improve its tolerance. A fungal inoculum was produced through multiple generations in the presence of elevated Pi and used to inoculate melon plants grown under low and high phosphate conditions. Our results revealed distinct phenotypic and transcriptomic profiles between the adapted and non-adapted Rhizoglomus irregulare. The Pi adapted phenotype led to enhanced root colonization under high Pi conditions, increased vesicle abundance, and higher plant biomass at both phosphate levels. Additionally, the adaptation status influenced the expression of several genes involved in Pi uptake, Pi signaling, and mitochondrial respiration in both symbiotic partners. While the underlying mechanisms of the adaptation process require further investigation, our study raises intriguing questions. Do naturally occurring phosphate-tolerant AMF already exist? How might the production and use of artificially produced inocula bias our understanding? Our findings shed light on the adaptive capacities of Glomeromycota and challenge previous models suggesting that plants control mycorrhizal fungal growth. Moreover, our work pave the way for the development of innovative biotechnological tools to enhance the efficacy of mycorrhizal inoculum products under practical conditions with high phosphate fertilization.
1 Introduction
Arbuscular mycorrhizal fungi (AMF) are a widespread class of fungi forming mutualistic symbioses with most (72%) plant families on earth (Brundrett and Tedersoo, 2018). These microorganisms, belonging to Glomeromycota (Tedersoo et al., 2018), have been shown (i) to improve mineral plant nutrition (macro and micronutrients), (ii) to increase plant tolerance and resistance against abiotic and biotic stresses, (iii) to regulate plant development (i.e. rooting, flowering, fruit and seed formation), (iv) to improve biological and physical soil properties (Bennett and Meek, 2020; Singh et al., 2022; Shi et al., 2023). In turn, plant hosts provide a physical and physiological habitat associated with delivering of different energy sources that allows AM fungi to complete their biotrophic life cycle (Rich et al., 2017; Bedini et al., 2018).
These benefits to plants led to classify mycorrhizal fungi products as biostimulants (Rouphael et al., 2015), although they can also be considered as bioprotectants (Xavier and Boyetchko, 2002). However, despite the recognized potential of AMF as ecosystem service tools (Gianinazzi et al., 2010), challenges remain under practical conditions when considering the fertilization and/or soil chemical properties under conventional farming (Vosátka et al., 2008; Ijdo et al., 2011; Berruti et al., 2016). Mycorrhizal fungi are widespread in almost all soil ecotypes and harbor a high plasticity at species level, but their fitness is often impeded in presence of high available phosphate (Pi) concentrations. This nutrient can reduce severely mycorrhizal extra- and intra-radicular mycelial development, as well as arbuscule abundance and function (Olsson et al., 2002; Smith and Read, 2008; Kobae and Hata, 2010; Balzergue et al., 2013). The negatively correlated responses between phosphate concentration in soil and mycorrhizal colonization and functions are well investigated (Smith and Read, 2008). Physiological models were proposed that integrate hormonal signaling with plant and fungal metabolism that occur upon two different context of phosphate concentrations (Bedini et al., 2018). However, there is an urgent need to define innovative strategies to improve mycorrhizal development and performance under high phosphate levels as they appear under practice conditions in soils and substrates.
AMF face constant environmental fluctuations, including various biotic and abiotic stresses and changes in nutrient availability. Like in all organisms, environmental variation causes selection for different traits in AM fungi, which have the ability to rapidly produce variable progeny adapted to fluctuating stress conditions or host plant species (Angelard et al., 2014; Behm and Kiers, 2014; Millar and Bennett, 2016; Branco et al., 2022). It was also suggested that abiotic factors constitute potentially greater selection pressure than host plants for local adaptation of AMF (Helgason and Fitter, 2009) and that they can adapt to abiotic stress independently of their host plant (Millar and Bennett, 2016). Locally adapted traits can also be artificially induced, leading to improved mycorrhizal plant response as shown for salt or heavy metals (Sharifi et al., 2007; Oliveira et al., 2010; Bui and Franken, 2018). It seems therefore that modifying tolerance to different elements is possible, but is, in a first sense, not obvious for phosphate, given the fact that the fungal colonization in dependance on phosphate availability seems to be under the control of the host plant (Shi et al., 2021; Wang et al., 2021; Das et al., 2022). Interestingly, the concept of adaptation is commonly associated with the notion of stress, whereas phosphate is not typically considered an abiotic stressor per se, unless its concentration reaches starvation levels. Instead, it is an essential element that contributes to the cellular redox and energy status (ATP, NADP) of both plants and fungi, and is therefore closely linked to the mitochondrial respiratory pathway, which plays an important role in mycorrhizal behavior. The cytochrome oxidase (COX) and alternative oxidase (AOX) mitochondrial pathways have been shown to modulate spore germination and hyphal branching during the presymbiotic phase (Campos et al., 2015; Mercy et al., 2017). The transcription patterns of these genes have been associated with distinct intraradical mycorrhizal phenotypes in potato (Mercy et al., 2017). AOX is considered as an element of flexibility in fungal metabolism, allowing acclimation to different stress conditions and ecological fitness of fungi (Grahl et al., 2012; Thomazella et al., 2012; Xu et al., 2012; Campos et al., 2015; Del-Saz et al., 2018). This ascribed role of AOX in fungi is also true for plants (Arnholdt-Schmitt et al., 2006; Saha et al., 2016; Selinski et al., 2018), and in addition, it plays an important role in seed dormancy and germination (Mohanapriya et al., 2019; Yu et al., 2021; Racca et al., 2022). To complete this picture, adaptation to phosphate should also consider the intricate interplay of phosphate transporters (PTs) and phosphate signaling genes in both fungi and plants, which also play a role in controlling symbiotic dynamics and function. In fungi, at least 9 PT isoforms have been characterized in R. irregulare, expressed in both intra- and extra-radical mycelium (Benedetto et al., 2005; Balestrini et al., 2007; Tisserant et al., 2012; Fiorilli et al., 2013; Walder et al., 2016; Mercy et al., 2017; Zhou et al., 2021; Xie et al., 2022). Furthermore, the fungal phosphate homeostasis is regulated by the PHO pathway, and the recent expansion of sequenced Glomeromycota genomes has revealed that AMF possess many key genes involved in this pathway (Tisserant et al., 2012; Zhou et al., 2021), some of which have been shown to be significantly regulated in mycorrhizal roots in response to phosphate (Zhou et al., 2021; Zhang et al., 2023). From the plant side, host plants possess two possible Pi uptake routes: the direct uptake pathway (DUP), mediated by non-mycorrhiza-regulated Pht1 members, and the mycorrhizal uptake pathway (MUP), facilitated by AM-induced or AM-specific PTs (Bucher, 2007; Javot et al., 2007; Ferrol et al., 2019). Notably, many members of the PHT1 family are transcriptomically up-regulated in phosphate-depleted plants and repressed by higher phosphate concentrations (Nussaume et al., 2011).
Several studies pointed that if the formation and functionality of arbuscular mycorrhizal symbiosis is adversely affected by high Pi in pot experiments under controlled conditions, the influence of Pi fertilizer on AMF community structure and abundance is variable under field conditions. Some studies claimed that high soil Pi supply does not always have a negative impact on AMF diversity, fungal colonization or performance of inoculated crops (Jansa et al., 2014; Liu et al., 2016; Higo et al., 2020; Peña Venegas et al., 2021; Lutz et al., 2023). These works pointed complex influences of soil phosphate on AMF and suggested that the response to this element may be related to various factors and notably AMF identity. Liu et al. (2016) suggested that the indigenous AMF community may be selected towards specific taxa or strains that are strong competitors and less sensitive to Pi fertilizers. This raises the question if Pi-tolerant AMF lines do exist naturally or might occur in managed conventional systems. However, the demonstration that such Pi-tolerant AM species would exist in field conditions is challenging, since their isolation and testing should consider the myriad of interactions that can occur within a soil and the environment, as the variable presence of other elements like N, the microbiome, or different host plant genotypes. Therefore, as first step, it seems more reasonable to obtain proof of concept under controlled condition.
Based on these considerations, we investigated the following hypotheses: (1) the induction of artificial adaptation in Rhizoglomus irregulare (syn. Rhizophagus irregularis) can enhance tolerance to high phosphate levels, (2) such adaptation results in phenotypic changes in both the fungal symbiont and the host plant, and (3) these variations are accompanied by transcriptomic changes in both organisms.
To address these research questions, R. irregulare was cultivated in monoxenic in vitro system for five generations in the presence or absence of high phosphate concentrations. Subsequently, the fungi were cultivated under greenhouse conditions for one season, maintaining two different phosphate conditions. This approach yielded two ex vitro inocula containing R. irregulare with non-tolerant (RiQS81-Pi-) and tolerant (RiQS81-Pi+) traits towards high Pi. These inocula were then used in a greenhouse assay involving melon plants, which were grown under high Pi (HP) and low Pi (LP) conditions. We then assessed several fungal and plant phenotypic parameters, as well as the transcription of several genes involved in respiration, Pi signaling and transport, accompanied by several other symbiotic markers. We observed significant differences between the two R. irregulare inocula at both phenotypic and transcriptomic levels. The implications of these findings are discussed in terms of physiology, ecology, research models, and inoculum production.
2 Materials and methods
2.1 Fungal material
The fungal inocula used in this trial were produced by using in vitro spores obtained from two distinct cultural itineraries (Bedini, Varela et al., in preparation) as starter material. Briefly, in vitro Rhizoglomus irregulare (the homokaryotic INOQ strain QS81) cultures were set up from a single initial plate as described in Declerck et al. (1998), on normal MSR medium or on MSR medium containing 100 ppm phosphorus (P; 3,23 mM KH2PO4). Several dozen spores were transferred on each new plate, to reduce the potential variation bias a single spore could bring. This process was repeated iteratively for 5 generations, and 100 in vitro spores of the 5th generation of both culture protocols, with or without addition of phosphate, were then used to inoculate Trifolium pratensis pots in greenhouse. The plants were grown while maintaining consistent cultural conditions to the in vitro ones, with low (10 ppm P) and high (100 ppm P) phosphate concentrations, applied in the form of KH2PO4 mixed directly in the substrate (respectively 43.93 mg/L and 439.37mg/L). Fertilization was performed weekly using a standard Hoagland solution (Hoagland and Arnon, 1938) devoid of phosphate, and both fertilization and watering were carefully applied to prevent any leaching at the bottom of the pots. After 6 months, the colonized root systems were harvested, homogenized, and filtered through a 425 µm mesh sieve to obtain first ex vitro powder inocula, corresponding overall to the 6th generation. The two fungal cultures were designated as “Pi- phenotype” (RiQS81-Pi-) and “Pi+ phenotype (RiQS81-Pi+), referring to the presence or absence of high phosphate during the successive propagations. Before subsequent use in the trial, the identity of RiQS81-Pi+ and RiQS81-Pi- R. irregulare was checked by sequencing of the FLR2-LROR fragment and by RFLP on mitochondrial rDNA (Supplementary Material 1.1; Supplementary Figure S1) to confirm that the same isolate was present in both inocula.
2.2 Plant growth conditions
A greenhouse experiment was implemented using 1 L plastic pots filled with pure sterilized sand (2 x 6 h at 121°C) containing 10 ppm P (43.93 mg KH2PO4 per L substrate, also noted as LP for low Pi concentration) or 100 ppm P (439.37mg KH2PO4 per L substrate, also noted as HP for high Pi concentration). KH2PO4 was mixed in the substrate as powder form and care was taken that no leaching occurs from the bottom of the pot during subsequent waterings. Plants were inoculated or not with 100 mg ex vitro inoculum powder per L substrate, mixed in the substrate. Then, melon seeds (Cucumis melo cv Charantaise) were placed in the pot (1 seed per pot) according to the following conditions (completely randomized design): (i) non-inoculated plants, 10 ppm Pi (NM LP); (ii) plants inoculated with RiQS81-Pi- inoculum, 10 ppm Pi (RiQS81-Pi- LP); (iii) plants inoculated with RiQS81-Pi- inoculum, 100 ppm Pi (RiQS81-Pi+ HP); (iv) non inoculated plants, 100 ppm Pi (NM HP); (v) plants inoculated with RiQS81-Pi+ inoculum, 10 ppm Pi (RiQS81-Pi+ HP); (vi) plants inoculated with RiQS81-Pi+ inoculum, 100 ppm Pi (RiQS81-Pi+ HP). 8 replicates were implemented for each of the 6 conditions. Plants were fertilized (once per week, 80 ml per pot) with Hoagland solution without Pi.
Plants were harvested at day 77 after inoculation (DAI). Shoot and root fresh biomasses were assessed, and 1 g of fresh roots was immediately frozen in liquid nitrogen for molecular analyses. Remaining roots systems were stained with black ink (modified from Vierheilig et al., 1998, Supplementary Material 1.2) and fungal alkaline phosphatase (ALP) stain (modified from Guillemin et al., 1995; Supplementary Material 1.3). Fungal colonization patterns were assessed according to a modified protocol from Trouvelot et al., 1986. Two Excel spreadsheet versions were developed to facilitate the analysis, one including the classic fungal parameters F%, M%, m%, A% and a% (Mycocheck classic; Supplementary Spreadsheet 1) and one including new fungal parameters compared to the original version (Mycocheck advanced; Supplementary Spreadsheet 2). The added parameters include vesicle abundance in the root system and in colonized root fragments (V%; v%), intraradical hyphal abundance in the root system and in colonized root fragments (H%; h%), and the Arum/Paris ratio. Parameters considering the relative proportion of each intraradical structure within the roots were also included. Indeed, the evaluation of the score of each fungal structure is done independently from the other fungal parameters, which introduces a bias about the real abundance of the evaluated structures in the colonized roots. Therefore, we also calculate Ar%, Vr% and Hr%, which are the relative proportions of arbuscules, vesicles and intraradical mycelium, respectively, in the whole root system. These parameters can then be used to evaluate the abundance of arbuscules, vesicles and intraradical mycelium within the M% (ar%, vr%, hr%). The formulas are detailed in Supplementary Spreadsheet 2.
Samples from shoot parts were harvested, dried, and dried leaf material was used for analyzing the Pi content of melon leaves (g/kg DW) according to the DIN EN 15621:2017-10 norm (Landesamt für Landwirtschaft und Ländlichen Raum, Thüringen, DE).
2.3 Gene identification and expression analysis
The RNA accumulation of selected melon and R. irregulare genes were assessed in non-mycorrhizal and mycorrhizal roots inoculated with both strains (RiQS81-Pi+ and RiQS81-Pi-) and in two contrasting Pi conditions. Melon genes encoding Pi transporters of the Pht1 family, the alternative oxidase (AOX), the cytochrome C (CytC), two isoforms of the subunit 5b of the cytochrome oxidase (COX5b) which was described as the most conserved among nuclear-encoded subunits (Rizzuto et al., 1991; Grossman and Lomax, 1997), the lactate dehydrogenase (LDH), two mycorrhiza-responsive blue copper proteins (BCP1a and BCP1b), as well as proteins involved in the fatty acid metabolism and transport (FatM, RAM2, STR1 and STR2) were identified by comparison with known sequences from other plant species and retrieved from the CuGenDBv2 database for cucurbit genomics (Yu et al., 2023). Genes of interest from R. irregulare, involved in phosphate transport (PHT1 family) and signaling (PHO pathway), sugar transport (MST), as well as respiration (COX5b, CytC and AOX), were selected based on existing publications (Fiorilli et al., 2013; Mercy et al., 2017; Zhou et al., 2021; Xie et al., 2022). All accession numbers are listed in Supplementary Table S1.
Total RNA was extracted from 100 mg of melon roots using the RNeasy Plant Mini Kit (Qiagen, Hilden, Germany), according to manufacturer’s instructions. 300 ng of extracted RNA were reverse transcribed into cDNA using the RevertAid RT Kit (Thermo Fisher Scientific, Massachusetts, USA) in 20 μL following the supplier’s instruction. The cDNAs were 1:5 and 1:10 diluted (respectively for fungal and plant genes) prior to analysis. Relative expression levels of target genes were analyzed by qRT-PCR experiment using the CFX Connect Real-Time PCR Detection System (Bio-Rad, California, USA). Amplification reactions were prepared in 20 μl reaction volumes using the Blue S’Green qPCR master mix (Biozym, Hessisch Oldendorf, Germany). Eight independent biological replicates were analyzed per treatment and each sample was analyzed in triplicate. The specificity of the different amplicons was checked by a melting curve analysis at the end of the amplification protocol. Standard curves of dilution series from pooled cDNAs were used for PCR efficiency calculations for each primer pair. Several candidates were evaluated for further use as reference gene for normalization of the transcript data of target genes. After evaluation of expression stability using the applications BestKeeper, NormFinder and GeNorm (Vandesompele et al., 2002; Andersen et al., 2004; Pfaffl et al., 2004), CmACT and CmADP for the melon (Kong et al., 2014) and GiICL for R. irregulare (Lammers et al., 2001) were chosen as reference genes for our experimental conditions. Results were presented as means of normalized expression values (dCq=Cq[GOI]-Cq[HKG]). All primers are listed in Supplementary Table S1.
2.4 Data analysis
For all phenotypic and transcriptomic data (plant and fungus), statistical analyses were performed by multiple-comparison 2-ways ANOVA (post-hoc Tukey, with 2 factors as phosphate levels and mycorrhizal inoculation – respectively named phosphate and AMF). The statistical analyses were conducted with the SAS enterprise guide 9.4 (SAS Institute Inc., Cary, USA).
3 Results
3.1 The adaption process affected fungal colonization parameters and the plant growth
3.1.1 The two R. irregulare Pi phenotypes impacted differentially the plant biomass
The plant phenotypic parameter data are shown Figure 1. The impact of phosphate as factor was significant overall, and the total fresh biomass, as well as shoot and root fresh weight were all increased at high Pi, as compared to low Pi. A significantly higher biomass (total fresh weight and shoot fresh weight) is observed for plants inoculated with RiQS81-Pi+ inoculum compared to those inoculated with the RiQS81-Pi- one. The highest values of total biomass were obtained under high Pi with non-inoculated plants and plants inoculated with RiQS81-Pi+. Under high Pi, application of RiQS81-Pi- R. irregulare significantly reduced the plant growth parameters compared to the non-inoculated plants. Under low P, and as compared to the non-inoculated plants, inoculation with RiQS81-Pi- strain significantly reduced shoot biomass, but without significant effect on root and total biomass, whereas the RiQS81-Pi+ significantly promoted shoot and total biomass. In addition, shoot Pi content (Supplementary Figure S2) was only affected by Pi concentration, and not by mycorrhizal status, indicating that the nutrient use efficiency of the plant was not affected by either fungal inoculum.
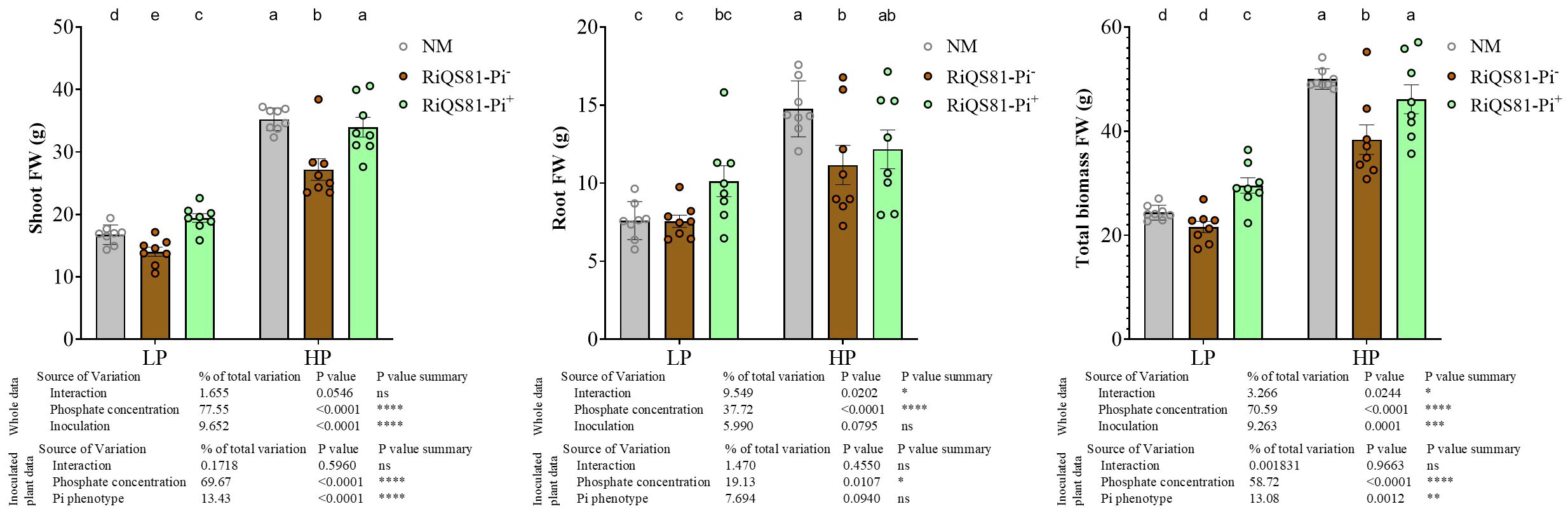
Figure 1 Effects of phosphate and inoculation on melon plant growth parameters. Melon plants (Cucumis melo) inoculated or not with RiQS81-Pi-/RiQS81-Pi+ Rhizoglomus irregulare inoculum, growing under two levels of phosphate (Pi). Plants were harvested 77 DAI. Data show means (n = 8) ± s.e.. Treatments sharing the same letter are not significantly different (p < 0.05 Tukey multiple-comparison ANOVA 2 ways, with assessment on the whole design and when considering inoculated plants alone). The tables provide statistical details per factor; significant differences are indicated by stars (ns: non-significant; * P < 0.05; ** P < 0.005;*** P < 0.0005; **** P < 0.00005). NM, Non-inoculated plants; HP, high Pi; LP, low Pi.
3.1.2 Fungal phenotype after black ink staining
Data obtained after the non-vital black ink staining are shown Figure 2 and Supplementary Figure S3. The impact of phosphate was significant overall, with a decrease of most of fungal parameter values under high Pi conditions. The impact of AMF inoculum type was also significant overall, with differences due to the Pi levels. Under low Pi, plants inoculated with RiQS81-Pi+ presented a (i) significant increase of the arbuscule parameters (Ar%, ar% and Ar%/Vr% ratio); (ii) significant reduction of the vesicle parameters (v%, V%, Vr% and vr%) and (iii) no significant influence for all other investigated fungal parameters. Under high Pi, as compared to the RiQS81-Pi- inoculum, the RiQS81-Pi+ (i) significantly increased F%, M%, m%, A%, V%, H%, Ar%, Vr%, Hr%, ar%, hr%, and Arum/Paris ratio, (ii) significantly decreased the h% and (iii) had no significant effect on all other fungal parameters studied.
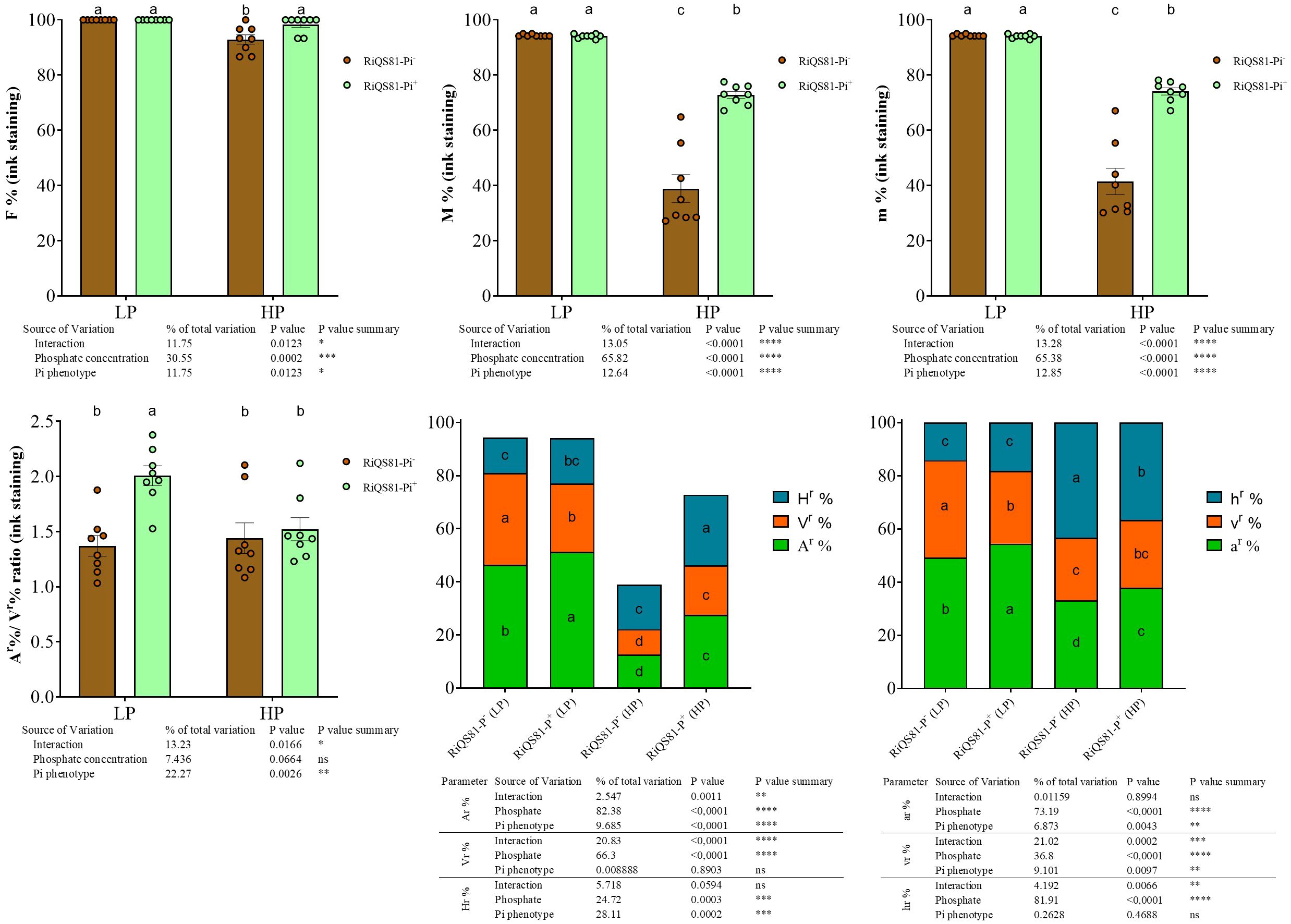
Figure 2 Fungal phenotypic parameters after black ink staining of RiQS81-Pi- and RiQS81-Pi+ R. irregulare growing within melon (Cucumis melo) roots under two levels of Pi. Roots were harvested 77 DAI, stained and fungal parameter were evaluated. Data show means (n = 8) ± s.e. Treatments sharing the same letter are not significantly different (p < 0.05; Tukey multiple-comparison ANOVA 2 ways), the non-inoculated plants were considered as outgroup to control data normality. The tables provide statistical details per factor; significant differences are indicated by stars (ns, non-significant; * P < 0.05; ** P < 0.005;*** P < 0.0005;**** P < 0.00005). Data analyses were performed separately for each parameter. NM, Non-inoculated plants; HP, high phosphate; LP, low phosphate; F%, frequency of colonized root fragments; M%, intensity of mycorrhizal colonization in the whole root system; m%, intensity of the mycorrhizal colonization in the colonized root fragments; Ar%, Vr% and Hrr%: relative proportion of arbuscules, vesicles and intraradical mycelium respectively, in the whole root system; ar%, vr% and hr%, relative proportion of arbuscules, vesicles and intraradical mycelium respectively, within the M%.
3.1.3 Fungal phenotype after ALP staining
The data obtained from the vital alkaline phosphatase (ALP) staining are shown in Figure 3 and Supplementary Figure S3. The effect of phosphate as factor was not significant overall, but with specific fungal patterns between the two R. irregulare Pi phenotypes. The F%, M%, m% and A% values were significantly higher under high Pi than under low Pi for the RiQS81-Pi-. For the RiQS81-Pi+ fungi, the M%, m% and A% values were significantly lower under high Pi than under low Pi. The impact of Pi phenotypes was significant with two contrasting situations. Under low Pi, inoculation with RiQS81-Pi+ inoculum was associated with significantly higher colonization for all fungal parameters assessed. Under high Pi, RiQS81-Pi+ inoculation (i) led to a significantly higher F% value, and (ii) has no significant influence for all other fungal parameters investigated. Furthermore, we noticed that vesicles were dark stained under high Pi but not, or less so under low Pi (Supplementary Figure S4), and their abundance was significantly higher within the Pi+ phenotype under low Pi conditions. Finally, the vesicle abundance increased significantly with increasing Pi concentration for the Pi- phenotype, but not for the Pi+ one. The percentages of fungal structure stained by ALP within the ink-stained part (Supplementary Figure S5) showed similar profiles for all fungal parameters except for F%: the highest values were obtained under high Pi RiQS81-Pi- (strongly and significantly higher than under low Pi), while the values obtained from RiQS81- Pi+ were intermediate and without significant differences between low and high Pi. Significant differences were observed between RiQS81-Pi- and RiQS81-Pi+, the latter being associated with significantly higher values under low Pi for F%, M% and v%, but significantly lower values under high Pi for M%, m%, V% and A%).
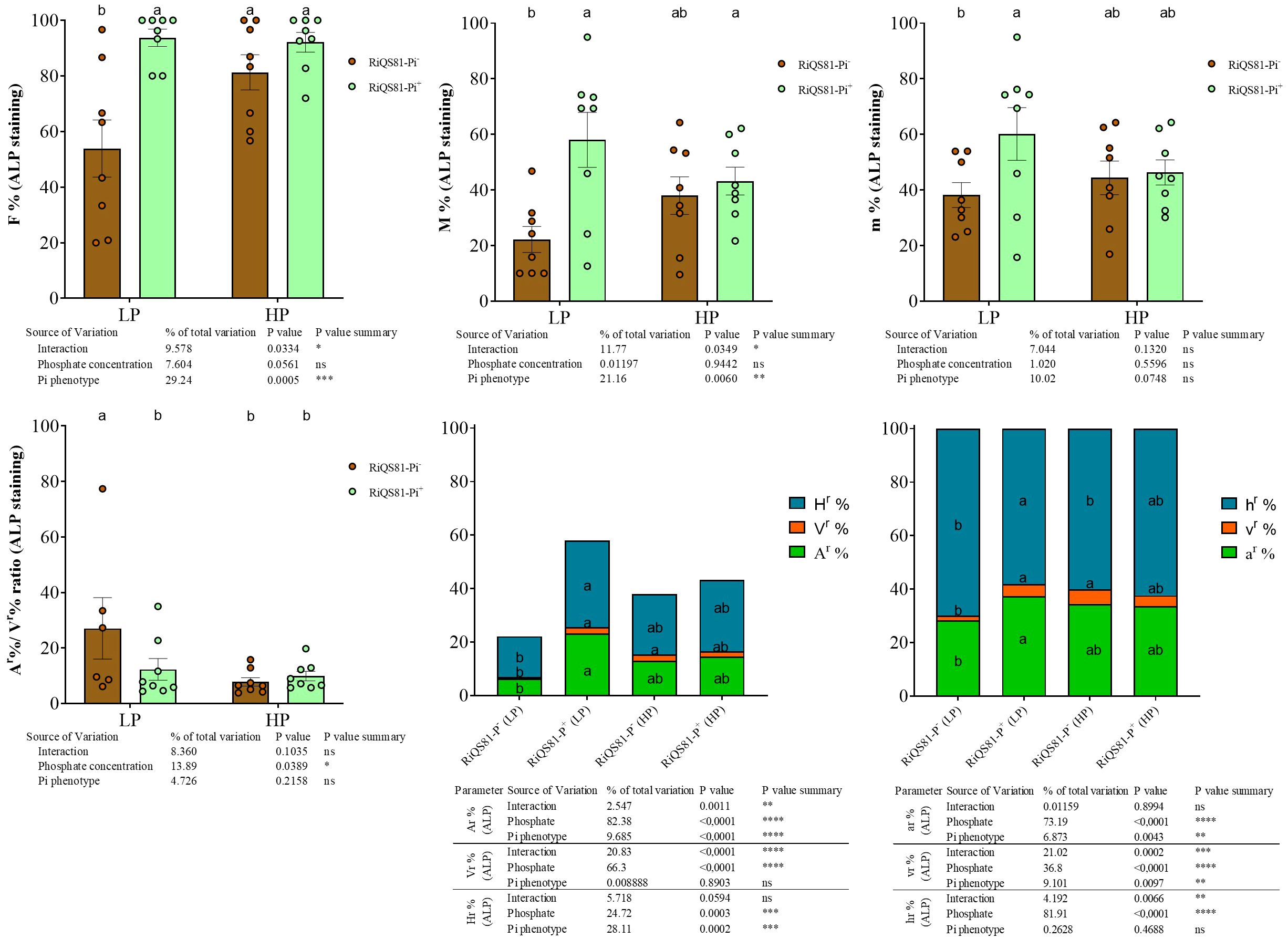
Figure 3 Fungal phenotypic parameters after ALP staining of RiQS81-Pi- and RiQS81-Pi+ R. irregulare growing within melon (Cucumis melo) roots under two levels of Pi. Roots were harvested 77 DAI and stained for alkaline phosphatase activity. Data show means (n = 8) ± s.e. Treatments sharing the same letter are not significantly different (P < 0.05; Tukey multiple-comparison ANOVA 2 ways), the non-inoculated plants were considered as outgroup to control data normality. The tables provide statistical details per factor; significant differences are indicated by stars (ns: non-significant; * P < 0.05; ** P < 0.005;*** P < 0.0005; **** P < 0.00005). Data analyses were performed separately for each parameter. NM, Non-inoculated plants; HP, high phosphate; LP, low phosphate; F%, frequency of colonized root fragments; M%, intensity of mycorrhizal colonization in the whole root system; m%, intensity of the mycorrhizal colonization in the colonized root fragments; Ar%, Vr% and Hrr%: relative proportion of arbuscules, vesicles and intraradical mycelium respectively, in the whole root system; ar%, vr% and hr%: relative proportion of arbuscules, vesicles and intraradical mycelium respectively, within the M%.
3.2 The adaption process modulated the transcription of fungal and plant genes
3.2.1 Plant genes
The transcript levels of melon genes involved in respiratory and fermentative pathways were investigated (Figure 4). The gene encoding the melon alternative oxidase CmAOX was repressed by Pi, while the genes involved in the COX pathway (CmCOX5b1, CmCOX5b2 and CmCytC) were induced by Pi. CmCytC and CmCOX5b2 showed a similar expression profile, with a higher expression in the inoculated samples under low Pi, as well as a higher transcription level in the plants inoculated with the RiQS81-Pi+ strain under this condition. The fungal Pi phenotype status of the inoculum played no role in the transcript accumulation of these genes in high Pi conditions. The lactate dehydrogenase CmLDH was constitutively expressed in our experimental conditions.
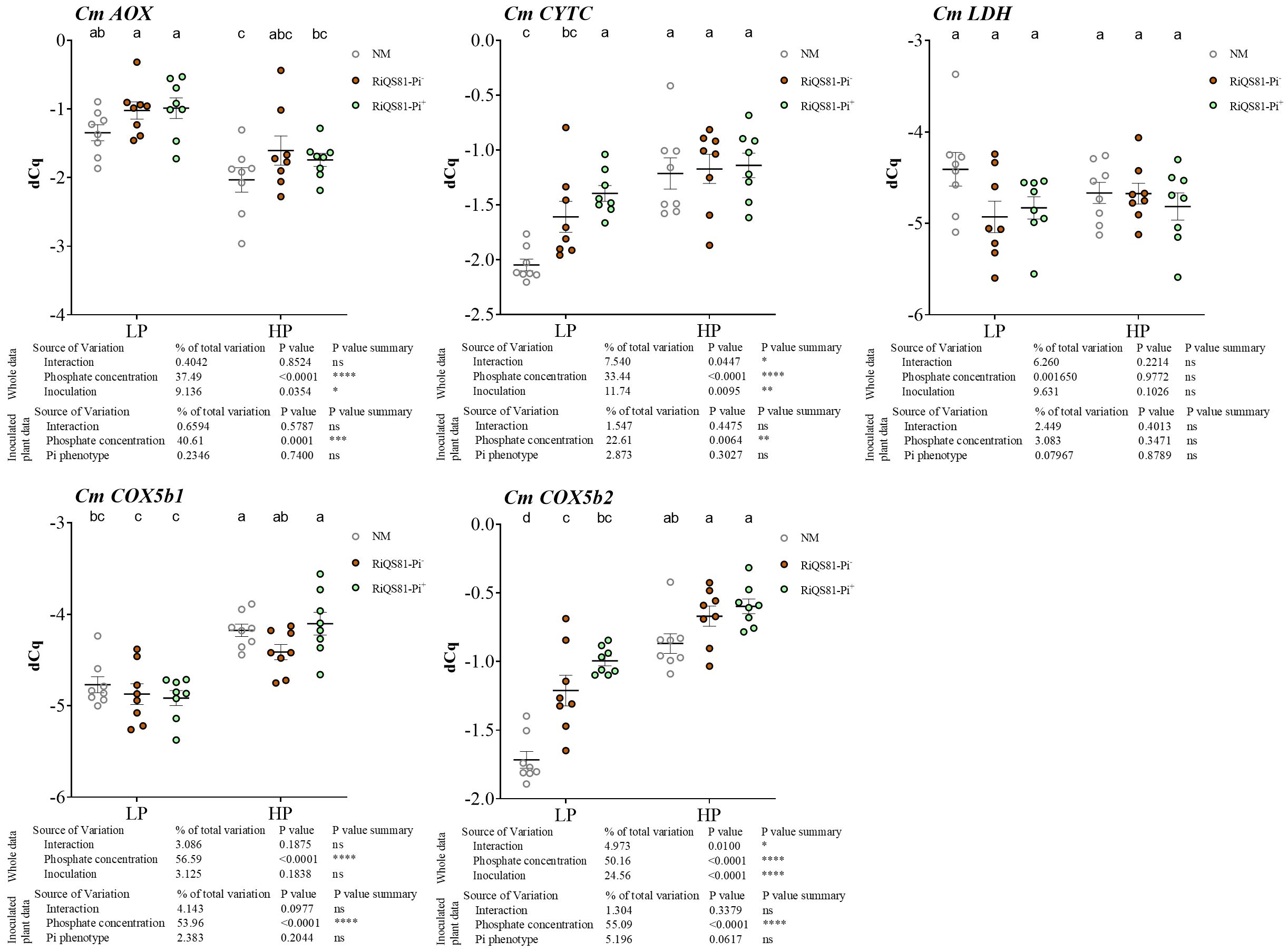
Figure 4 Expression patterns of melon genes involved in respiratory pathways and fermentation in mycorrhizal and non-mycorrhizal roots at different Pi fertilizer levels. Plants were inoculated with RiQS81-Pi- or RiQS81-Pi+ inocula. Roots were harvested at 77 DAI, RNA extracted, and expression measured by qRT-PCR of the genes encoding alternative oxidase (AOX), cytochrome C (CytC), two isoforms of the subunit 5b of the cytochrome oxidase (COX5b) and lactate dehydrogenase (LDH). Genes encoding β-actin (ACT) and ADP-ribosylation factor-1 (ADP) were used for normalization. Data show mean expression level (n = 8) ± s.e. Treatments sharing the same letter are not significantly different (p < 0.05; Tukey multiple-comparison ANOVA 2 ways, with assessment on the whole design and when considering inoculated plants alone). The tables provide statistical details per factor; significant differences are indicated by stars (ns: non-significant; * P < 0.05; ** P < 0.005;*** P < 0.0005; **** P < 0.00005). NM, Non-inoculated plants; HP, high phosphate; LP, low phosphate.
Based on the genome sequence of the melon, we identified seven Pi transporter genes belonging to the Pht1 family in melon, designated CmPT1;1 to CmPT1;7, respectively. Phylogenetic analysis revealed that the CmPT1;5 gene clustered with the AM-specific Pi transporters previously characterized in numerous plant species (Supplementary Figure S6). The expression levels of Pht1 genes were studied in melon roots under high or low Pi availability, either in the absence of AMF or colonized by RiQS81-Pi- or RiQS81-Pi+ R. irregulare (Figure 5; Supplementary Figure S7). CmPT1;2 and CmPT1;7 were very weakly expressed in the root samples, but strongly expressed in other parts of the plant (Supplementary Figure S8) and were not further considered in the analysis. All other Pi transporters were repressed by high Pi and most of them were significantly upregulated by inoculation, except CmPT1;6. CmPT1;5 was strongly upregulated in the presence of AMF, as expected from its similarity to previously characterized AMF-inducible Pi transporter genes. CmPT1;1, CmPT1;3 and CmPT1;4 showed a similar profile: in LP conditions, all three were induced by AMF, and in HP, the repression by the Pi was less strong in the presence of the RiQS81-Pi+ strain. Similar to all known AMF-inducible Pi transporters, the expression levels were correlated with the mycorrhizal colonization of the roots, suggesting that the difference between RiQS81-Pi+ and RiQS81-Pi- is most likely due to the different colonization of the roots.
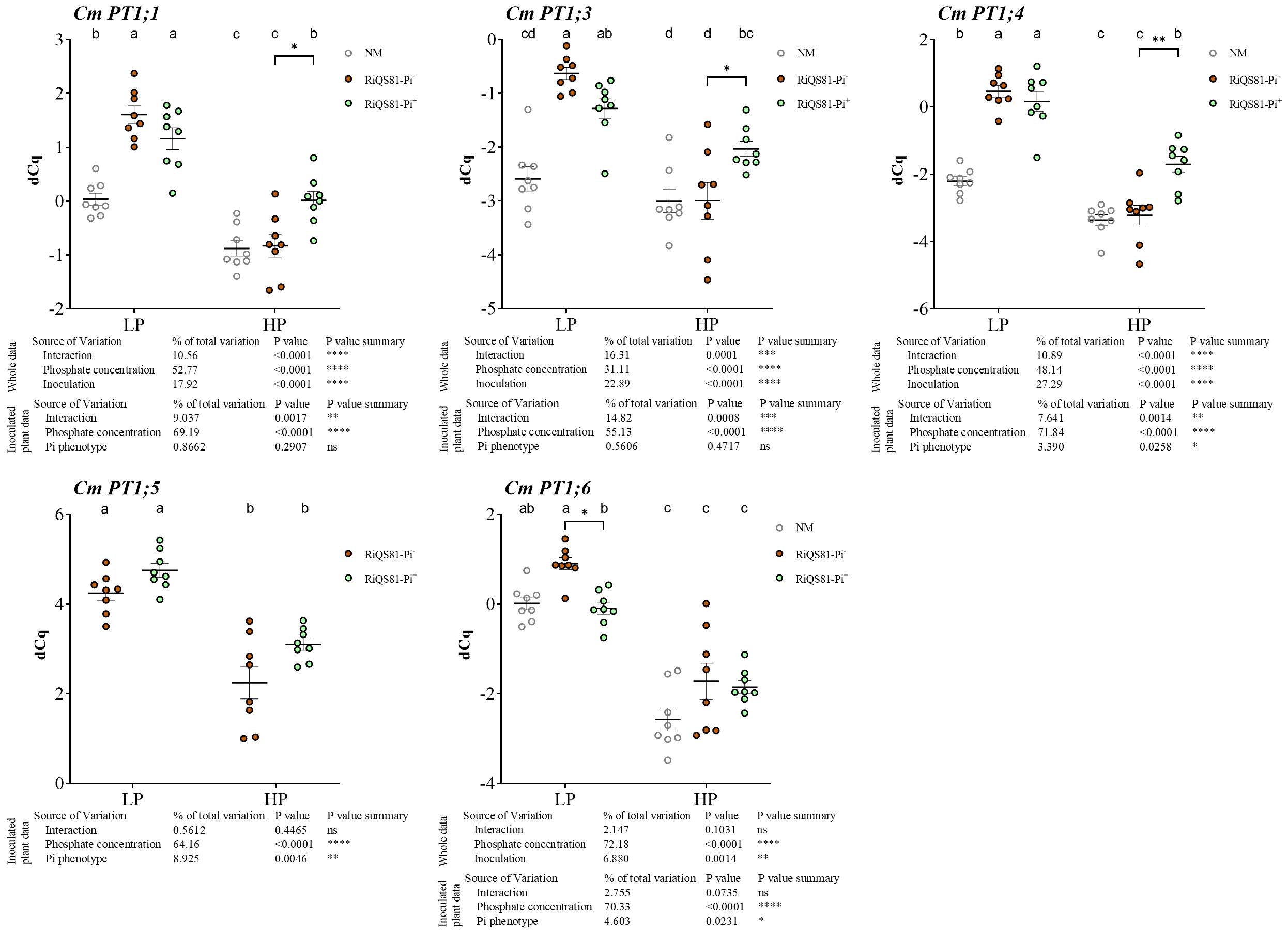
Figure 5 Expression patterns of melon Pi transporter genes belonging to the PHT1 family in mycorrhizal and non-mycorrhizal roots at Pi fertilizer levels. Plants were inoculated with RiQS81-Pi- or RiQS81-Pi+ inocula. Roots were harvested at 77 DAI, RNA extracted, and expression measured by qRT-PCR of the genes encoding the transporters CmPT1;1, CmPT1;3, CmPT1;4, CmPT1;5 and CmPT1;7. Genes encoding β-actin (ACT) and ADP-ribosylation factor-1 (ADP) were used for normalization. Data show mean expression level (n = 8) ± s.e. Treatments sharing the same letter are not significantly different (p < 0.05; Tukey multiple-comparison ANOVA 2 ways, with assessment on the whole design and when considering inoculated plants alone). The tables provide statistical details per factor; significant differences are indicated by stars (ns, non-significant; * P < 0.05; ** P < 0.005;*** P < 0.0005; **** P < 0.00005). Note that for Cm PT1;5, the non-inoculated plants data are not represented due to inexistent expression. NM, Non-inoculated plants; HP, high phosphate; LP, low phosphate.
Melon orthologs of genes involved in fatty acid biosynthesis and transport previously described in other species were identified and named as follows: CmFatM (XM_008468942.3) encoding a thioesterase; CmRAM2 (XM_008445223.3) encoding a glycerol-3-P acyltransferase; CmSTR1 (XM_008447787.3) and CmSTR2 (XM_051088815.1) encoding two half size ATP-binding cassette transporters of subfamily ABCG (Supplementary Figures S9, S10). All these genes were strongly upregulated by the presence of AM fungi in roots. In mycorrhizal plants, they were all upregulated in RiQS81-Pi+ under both low and high Pi conditions (Figure 6). For CmSTR1 and CmSTR2, the fungal Pi+ phenotype allowed to restore the same induction level in HP as in LP, although the mycorrhizal colonization was lower.
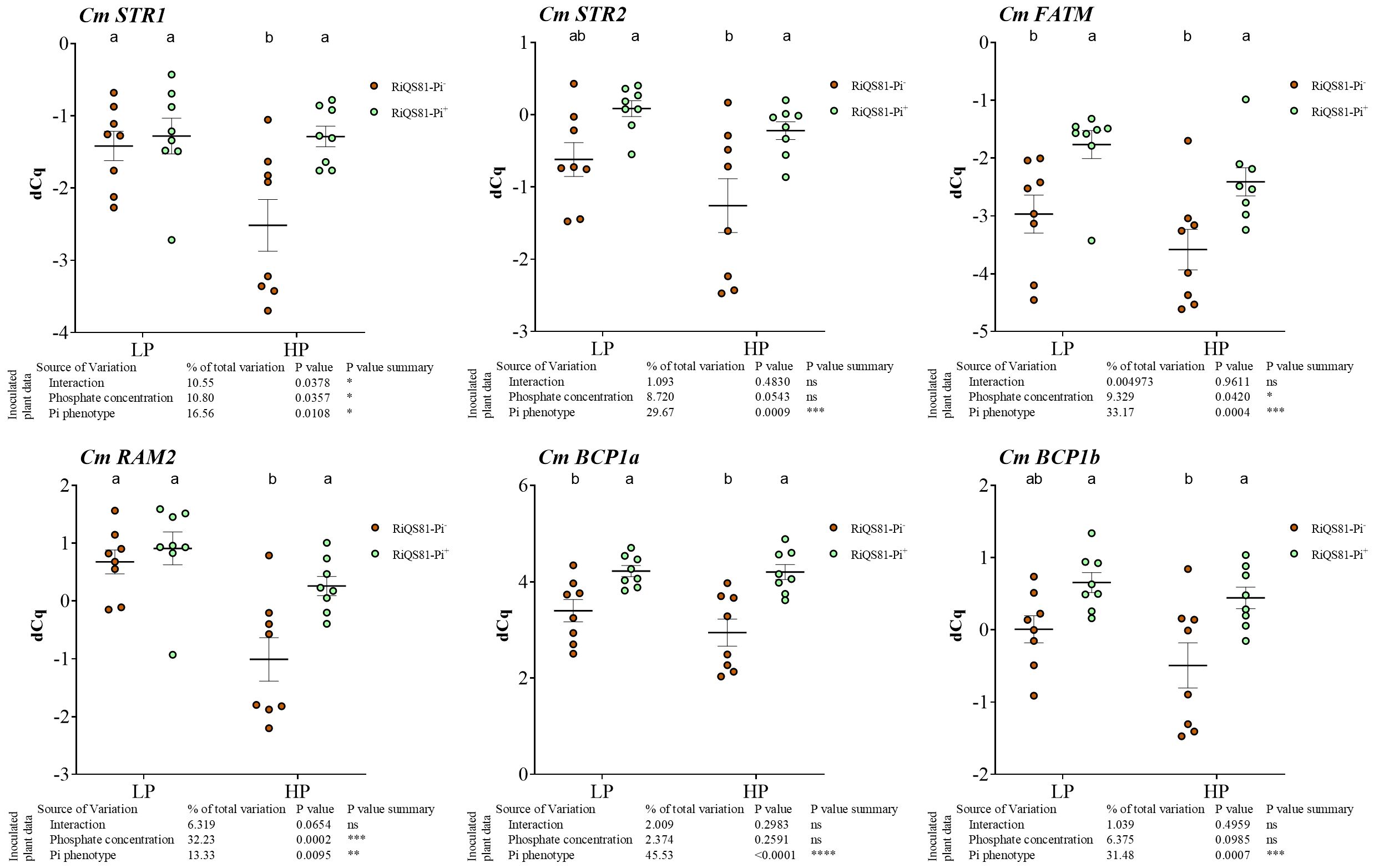
Figure 6 Expression patterns of melon genes involved in fatty acid biosynthesis and transport, and blue copper protein genes in mycorrhizal and non-mycorrhizal roots at Pi fertilizer levels. Plants were inoculated with RiQS81-Pi- or RiQS81-Pi+ inocula. Roots were harvested at 77 DAI, RNA extracted, and expression measured by qRT-PCR of the genes encoding two half size ATP-binding cassette transporters of subfamily ABCG (STR1 and STR2), a thioesterase (FatM), a glycerol-3-P acyltransferase (RAM2), and two Blue Copper-Binding Proteins (BCP1a and βCP1b). Genes encoding β-actin (ACT) and ADP-ribosylation factor-1 (ADP) were used for normalization. Data show mean expression level (n = 8) ± s.e. Treatments sharing the same letter are not significantly different (p < 0.05; Tukey multiple-comparison ANOVA 2 ways, with assessment on the whole design and when considering inoculated plants alone). The tables provide statistical details per factor; significant differences are indicated by stars (ns, non-significant; * P < 0.05; ** P < 0.005; *** P < 0.0005; **** P < 0.00005). NM, Non-inoculated plants; HP, high phosphate; LP, low phosphate.
Certain Blue Copper-Binding Proteins (BCP) were previously identified as being encoded by AMF-inducible genes (Parádi et al., 2010). Their role remains unknown, but melon, like Medicago, possesses several AMF-specific BCPs. In this study, CmBCP1a (XM_008438947.3) and CmBCP1b (XM_008439498.1) were identified and investigated. Both were strongly induced by AMF, and as for the genes involved in fatty acid metabolism, the fungal Pi+ phenotype restored the induction levels of LP in HP (Figure 6).
3.2.2 Fungal genes
As for the host plant, fungal genes involved in respiration and nutrient uptake were studied, as well as some previously described genes involved in the Pi signaling pathway.
Genes encoding the alternative oxidase, RiAOX, and the lactate deshydrogenase gene, RiLDH, were both down regulated by high Pi fertilization, but no impact of the R. irregulare Pi phenotype was observed (Figure 7). For the COX pathway, RiCytC was induced by Pi for the RiQS81-Pi- fungus, but also by the fungal Pi phenotype under LP conditions. The expression of the sugar transporter gene RiMST showed a slight induction in the LP RiQS81-Pi+ condition, but the expression was mainly constitutive.
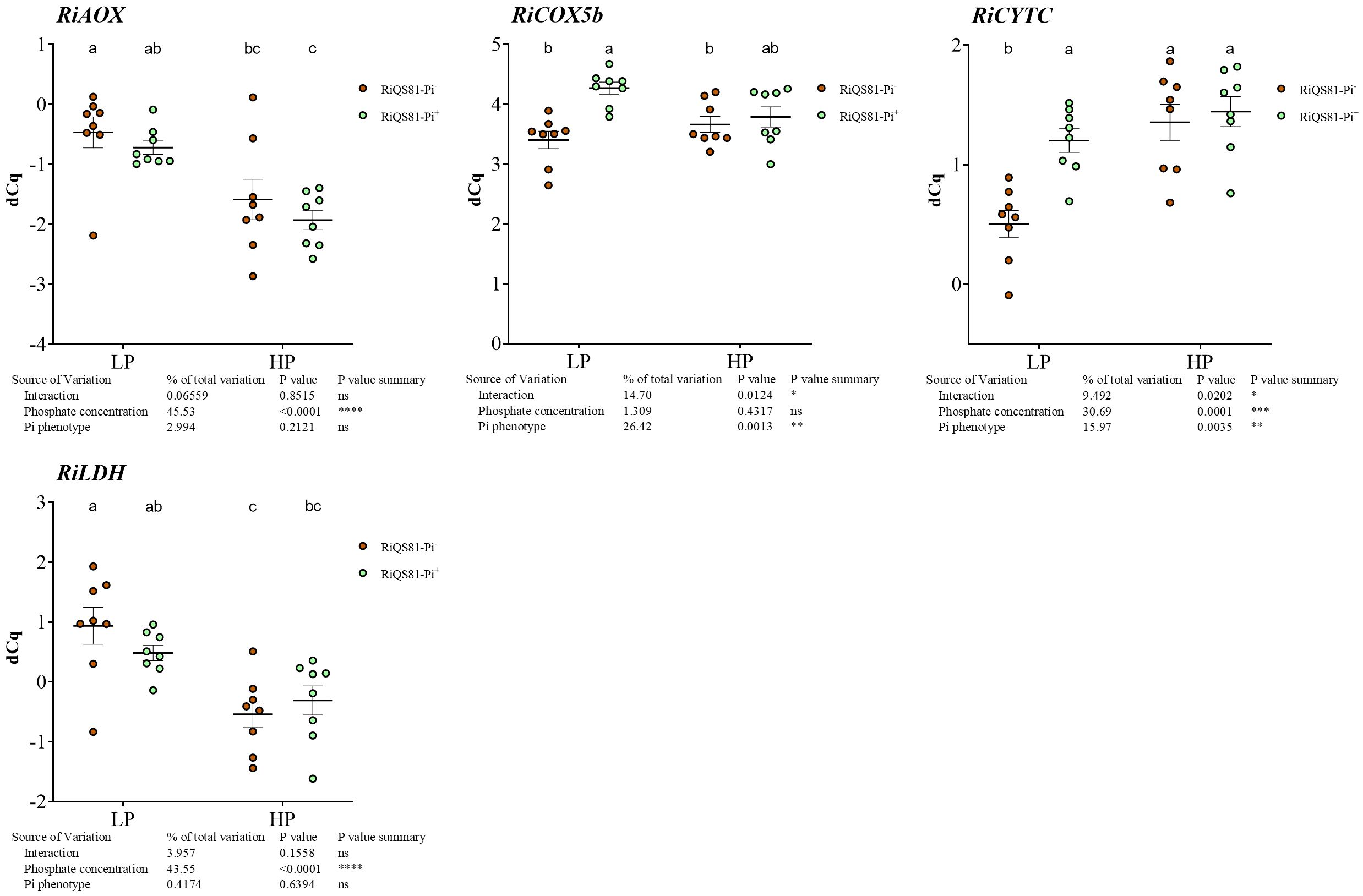
Figure 7 Expression patterns of R. irregulare genes involved in respiratory pathways and fermentation in mycorrhizal melon roots at different Pi fertilization levels. Plants were inoculated with RiQS81-Pi- or RiQS81-Pi+ inocula. Roots were harvested at 77 DAI, RNA extracted, and expression measured by qRT-PCR of the genes encoding alternative oxidase (AOX), cytochrome C (CytC), the subunit 5b of the cytochrome oxidase (COX5b) and lactate dehydrogenase (LDH). Gene encoding isocitrate lyase (ICL) was used for normalization. Data show mean expression level (n = 8) ± s.e. (p < 0.05 Tukey multiple-comparison ANOVA 2 ways, with assessment on the whole design and when considering inoculated plants alone). The tables provide statistical details per factor; significant differences are indicated by stars (ns, non-significant; * P < 0.05; ** P < 0.005; *** P < 0.0005; **** P < 0.00005). HP, high phosphate; LP, low phosphate.
To determine the role of fungal Pi transporters in the mycorrhizal association between Pi- and Pi+ phenotypes, the expression of nine previously described fungal Pi transporters (Walder et al., 2016; Xie et al., 2022) was investigated in melon roots (Figure 8; Supplementary Figure S11). RiPT3 and RiPT4 presented a too high sequence similarity to design specific primers, and the expression of these two genes was very low to undetectable in our conditions; therefore, they were not considered for further analysis. RiPT1 and RiPHO1 were the most expressed Pi transporter genes within all conditions (Supplementary Figure S11). The transcript levels of RiPT1 and RiSYG1 were not affected by Pi fertilization or the R. irregulare phenotype. RiPT6 and RiPT7 were only affected by the Pi level and were both repressed in HP conditions. RiPT2 and RiPT5 presented a similar profile, with a higher expression level only in the LP RiQS81-Pi+ condition. RiPHO1 was repressed in the LP RiQS81-Pi- condition.
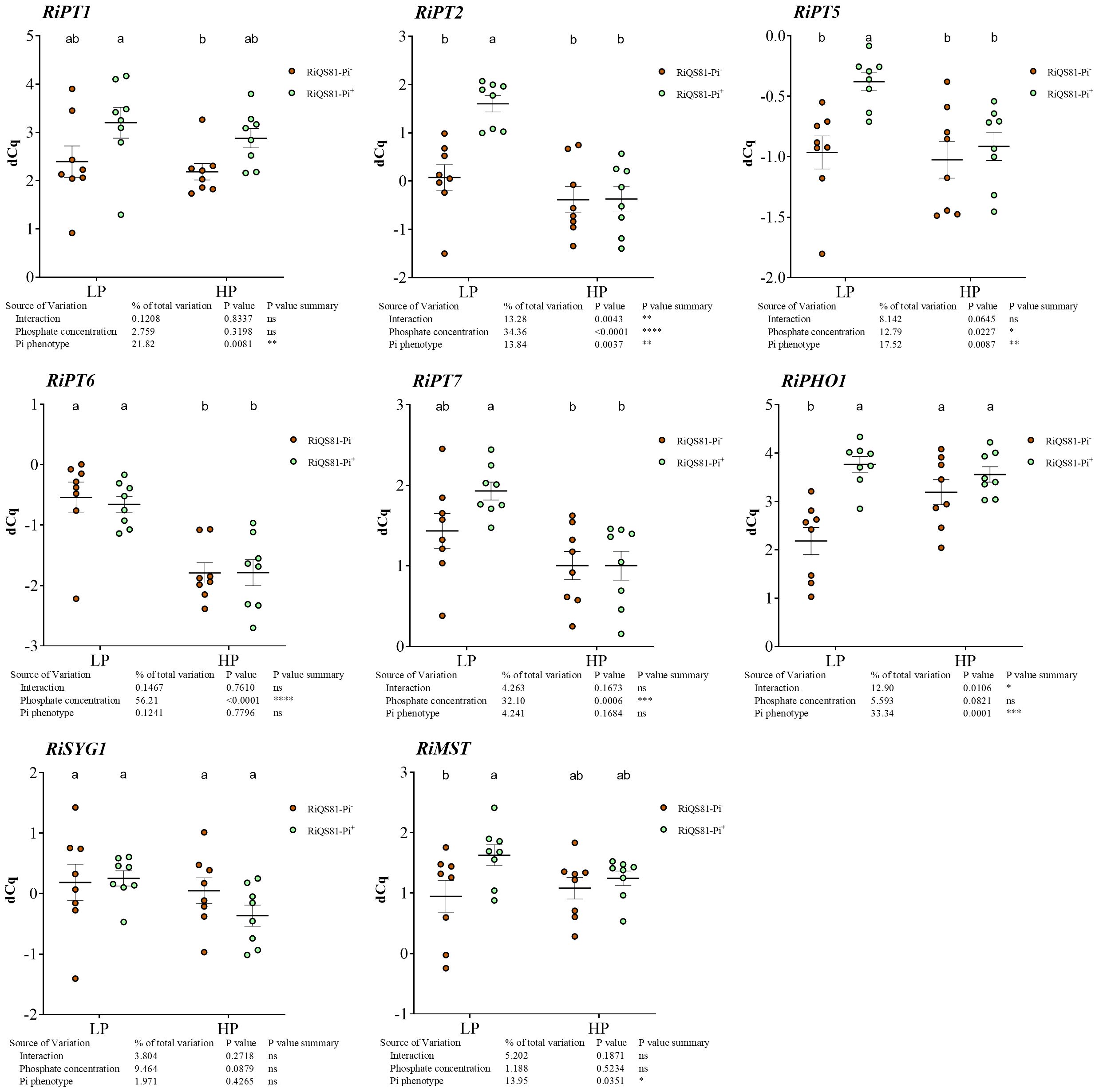
Figure 8 Expression patterns of R. irregulare Pi transporter genes belonging to the Pht1 family, and the sugar transporter gene RiMST in mycorrhizal melon roots at different Pi fertilization levels. Plants were inoculated with RiQS81-Pi- or RiQS81-Pi+ inocula. Roots were harvested at 77 DAI, RNA extracted, and expression measured by qRT-PCR of the genes encoding phosphate transporters (PT1, PT2, PT5, PT6, PT7, PHO1, SYG1) and the sugar transporter MST. Gene encoding isocitrate lyase (ICL) was used for normalization. Data show mean expression level (n = 8) ± s.e. (p < 0.05 Tukey multiple-comparison ANOVA 2 ways, with assessment on the whole design and when considering inoculated plants alone). The tables provide statistical details per factor; significant differences are indicated by stars (ns, non-significant; * P < 0.05; ** P < 0.005;*** P < 0.0005; **** P < 0.00005). HP, high phosphate; LP, low phosphate.
To investigate the effect of external phosphate concentrations and fungal Pi phenotype on the transcript levels of AM fungal genes involved in Pi uptake and metabolism, the expression profiles of six genes of the PHO pathway (Zhou et al., 2021; Xie et al., 2022) of R. irregulare in mycorrhizal C. melo roots were assessed (Figure 9). Our data show (i) a response to high Pi in Pi- phenotype in RiKCS1 (down-regulation), RiADO1, RiPHO81 (up-regulation), (ii) a response to high Pi in Pi+ phenotype in RiPHO2, RiPHO85 and RiKCS (down-regulation) and (iii) significant differences between the two R. irregulare phenotypes for RiPHO80, RiPHO81, and RiADO1 occurring only under low Pi conditions.
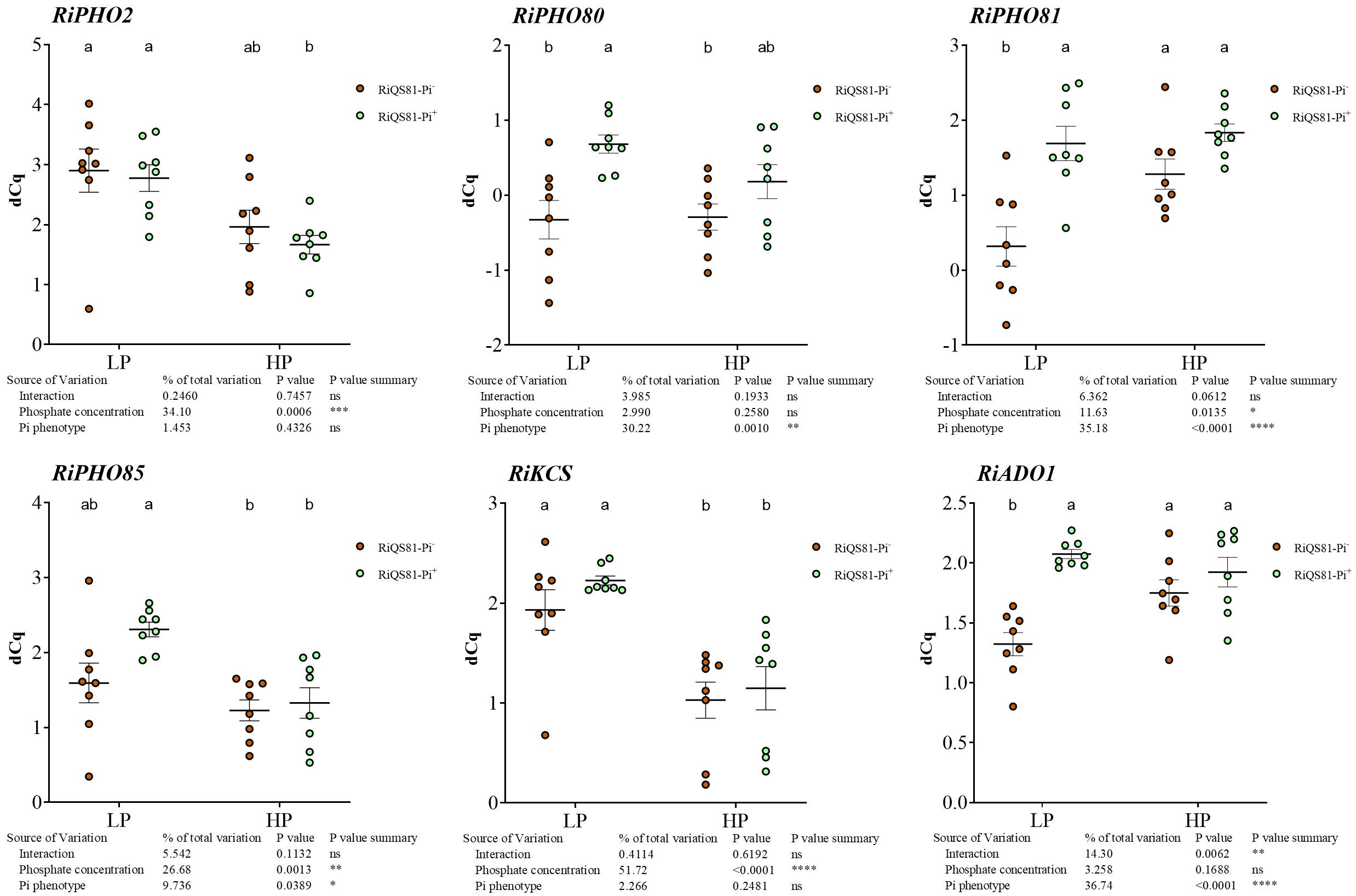
Figure 9 Expression patterns of R. irregulare genes involved in Pi-signaling in mycorrhizal melon roots at different Pi fertilization levels. Plants were inoculated with RiQS81-Pi- or RiQS81-Pi+ inocula. Roots were harvested at 77 DAI, RNA extracted, and expression measured by qRT-PCR of the genes encoding adenosine kinase (ADO1), inositol hexakiphospate kinase (KCS1) and regulators of the PHO pathway (PHO2, PHO80, PHO81, PHO85). Gene encoding isocitrate lyase (ICL) was used for normalization. Data show mean expression level (n = 8) ± s.e. (p < 0.05 Tukey multiple-comparison ANOVA 2 ways, with assessment on the whole design and when considering inoculated plants alone). The tables provide statistical details per factor; significant differences are indicated by stars (ns, non-significant; * P < 0.05; ** P < 0.005;*** P < 0.0005; **** P < 0.00005). HP, high phosphate; LP, low phosphate.
In conclusion, for each of the partners of the symbiosis, the Pi adaptation process influenced the accumulation of specific transcripts. In the fungus, we can distinguish between genes that respond only to Pi, those that are upregulated in RiQS81-Pi+ in low Pi and those whose expression is lower only in RiQS81-Pi- in low Pi (Supplementary Figure S12A). Similarly, in the plant, the expression data can be clustered into genes responding only to Pi, those that respond to inoculation with the expression following the colonization rate, and those for which inoculation with the Pi+ phenotype restored an expression similar to LP in HP, regardless of the mycorrhization rate (Supplementary Figure S12B). Several significant correlations between phenotypic patterns and transcriptomic patterns were also obtained (Supplementary Figures S13-S16).
3.3 Continuous ex vitro inoculum production under high P led to higher propagule production
In parallel to the melon pot trial, the production of R. irregulare under high Pi was pursued ex vitro following the same protocol as for the 6th generation (10 ppm Pi to produce the RiQS81-Pi-, and 100 ppm Pi to produce RiQS81-Pi+, using Plantago lanceolata). While Pi had a strong effect on reducing fungal propagules in the 6th generation, this effect tended to be buffered after 2 more generations (7th and 8th generation in 2021 and 2022, respectively), with an increased ratio of propagules between RiQS81-Pi and RiQS81-Pi+ production (Figure 10).
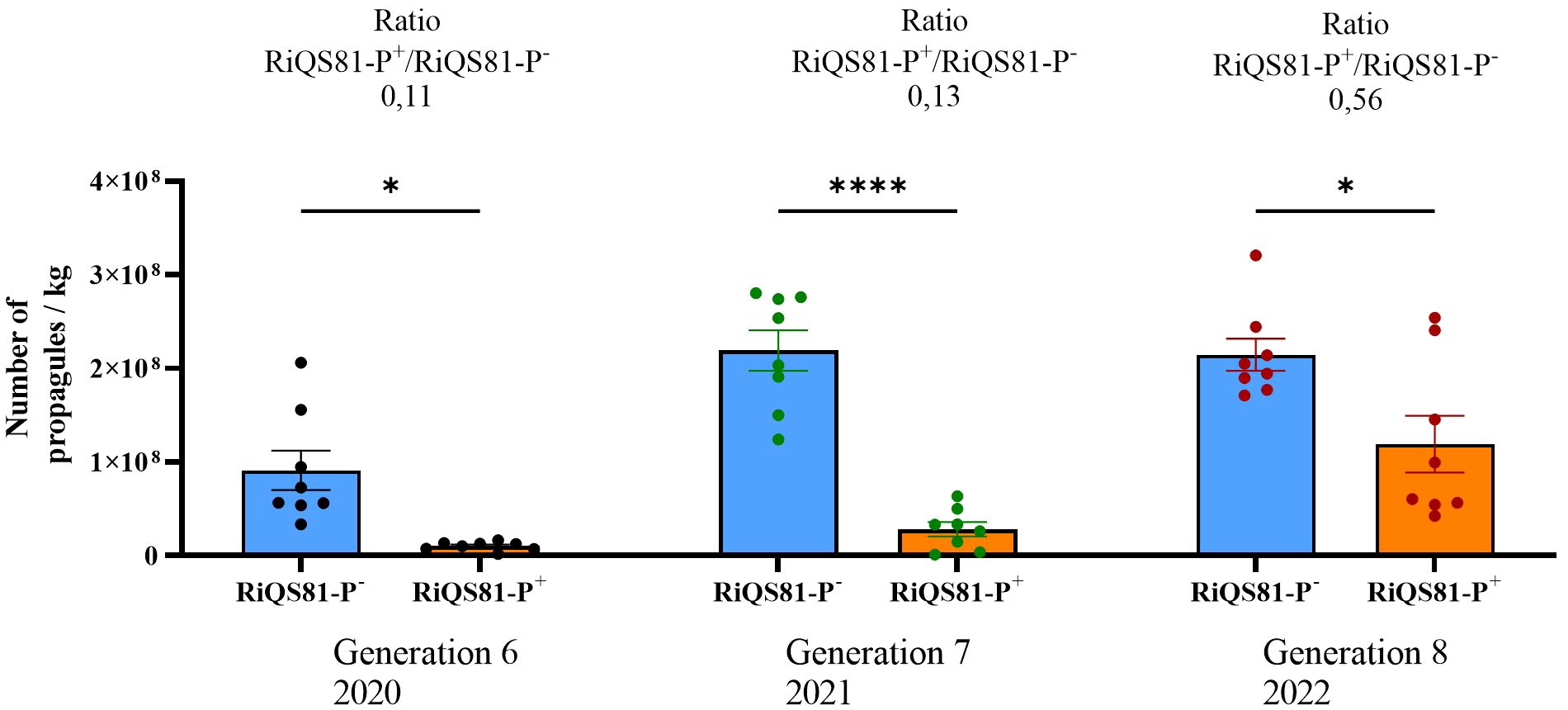
Figure 10 Number of propagules per kilo for RiQS81-Pi- and RiQS81-Pi+ inocula produced under ex vitro condition (greenhouse). Generation 6: produced in 2020, inoculation with spores from the 5th in vitro generation; generation 7: produced in 2021, inoculation with powder inoculum from 2020; generation 8: 2022, inoculation with powder inoculum from 2021. Data show mean expression level (n = 8) ± s.e. Significant differences are symbolized by stars (* P < 0.05; **** P < 0.00005; Tukey multiple-comparison ANOVA 2 ways, significant differences are represented only between RiQS81-Pi- and RiQS81-Pi+ for each production year). RiQS81-Pi-: R. irregulare produced in presence of 10 ppm phosphorus inside the substrate; RiQS81-Pi+: R. irregulare produced in presence of 100 ppm phosphorus inside the substrate.
4 Discussion
4.1 RiQS81-Pi- and RiQS81-Pi+ differ in fungal phenotype and functionality within melon roots
We hypothesized that R. irregulare can be artificially adapted to exhibit increased tolerance to high phosphate concentrations, and that this adaptation process is accompanied by phenotypic changes in both the fungus and the host plant. Our data strongly support these hypotheses, as the adaptation conferred a new functionality with positive effects on plants under low Pi conditions and a partial alleviation of the negative MGR response typically observed under high Pi (Wang et al., 2016). Endomycorrhizal symbiosis involves a cost/benefit balance (Wipf et al., 2019), where the plant expends energy that the fungus uses to produce propagules (spores, vesicles) and mycelium, while the fungus exchanges nutrients through arbuscules that benefit plant growth. Consistent with this perspective, our results support the notion that the occurrence of the arbuscule/vesicle ratio partially modulates the plant response. Vesicles are thick-walled lipid-storing organs (Jabaji-Hare et al., 1984; Brundrett, 1991), and it is reasonable to assume that their abundance reflects the fungal energy status (resilience and propagule fitness), which may influence the benefits to the plant. Interestingly, our data demonstrate that compared to RiQS81-Pi-, the abundance of vesicles in RiQS81-Pi+ is reduced under low Pi conditions but increased under high Pi conditions, indicating a differential fitness between the two fungal phenotypes in response to Pi concentrations. This suggests that the RiQS81-Pi+ phenotype is associated with a shift in the Pi tolerance range. Furthermore, this shift implies that Pi concentration induces distinct stresses in the fungus: RiQS81-Pi- is sensitive to Pi excess, whereas RiQS81-Pi+ is sensitive to what could be perceived as Pi starvation (Huo et al., 2022). This observation suggests that the Pi phenotype is linked to changes in Pi sensing, uptake, and metabolism, which are important characteristics for distinguishing adaptation from acclimatization.
The histochemical staining of alkaline phosphatase is considered to be a marker of polyphosphate metabolic activity localized in fungal tissues within the roots, and usually coincides with plant growth stimulation. It is also known that the intensity of alkaline phosphatase decreases with the age of colonization or when soluble phosphate is added to the substrate, and that this metabolic activity is associated with mycorrhizal functionality and ability to transfer phosphate to the plant (Tisserant et al., 1993; Vivas et al., 2003; Aono et al., 2004; Funamoto et al., 2007; Zhang et al., 2023). Our data are partially consistent with these previous observations when comparing both fungal phenotypes under low Pi. However, surprisingly, the proportion of fungal structures stained by ALP under high Pi increased with RiQS81-Pi- and remained unchanged with RiQS81-Pi+, but was associated with a moderately significant correlation with plant biomass and shoot fresh weight. Interestingly, ALP staining was observed in arbuscules, intraradical hyphae, but also within vesicles, indicating the possible occurrence of active mitochondrial processes where energy is required and where polyphosphate metabolism is involved. A much smaller proportion of vesicles were stained by ALP compared to ink in the LP RiQS81-Pi condition, but the presence of intact lipid bodies would suggest that the vesicles are still alive but have probably entered a dormant, i.e. late stage. This would suggest that ALP staining is a marker of specific phosphate metabolic activity associated with physiological age.
Of note, the culture system used in this study consisted of relatively small pots filled with an inert substrate and a fertilizer containing only readily available forms of nutrients. Apart from the effect of Pi concentration, such a system can result in a neutral or occasionally negative MGR (Malcová et al., 2001; Audet and Charest, 2010; Zangaro et al., 2015; Qin et al., 2022), which was the case for RiQS81-Pi- under low Pi. However, we also observed higher transcription of the AM-inducible Pi transporter as well as higher ALP activity under this condition. This is consistent with previous work concluding that a negative or neutral MGR does not necessarily indicate a high carbon cost to the plant or a reduced supply of phosphate nutrients from the fungus to the plant (Smith et al., 2003, 2004; Nouri et al., 2014). This supports the idea that the MGR is intricately linked to the physiological state of the plant and involves complex metabolic components. Overall, we observed distinct fungal phenotypic profiles for each of the four MGR statuses obtained, suggesting that the MGR corresponds to a function of mycorrhizal activity that is unrelated to fungal fitness. Therefore, when interpreting the adaptation traits, it is important to primarily consider and compare the fungal parameters between the two inoculation conditions at a given Pi concentration. In this respect, RiQS81-Pi+ promotes MGR as compared with RiQS81-Pi-, suggesting that the adapted R. irregulare may have an alternative Pi metabolism, possibly related to nutrient transfer.
As remark, Smith and Smith (2011) pointed out that the colonization rate would not be a relevant measure of fungal biomass, as it should also consider root length, which is affected by Pi concentration. For this reason, we emphasize the importance of considering the relative abundance of interconnected mycorrhizal structures, rather than each of them independently as classically assessed by the original Trouvelot method. The relative proportions of arbuscule, vesicle and intraradical mycelium, as well as the proportion of ALP stained within the ink, are probably better phenotypic markers of the physiological status of the fungus.
4.2 Influence of the adaptation process on transcriptional responses in plants and fungi
4.2.1 Fungal nutrient transport and Pi signaling
PT1 is the most studied Pi transporter in AMF. Its regulation by Pi remains uncertain, with conflicting results. Xie et al. (2016) and Zhang et al. (2023) reported that PT1 was repressed by Pi in Gigapora margarita and R. irregulare, whereas Fiorilli et al. (2013) found no Pi regulation of this gene in R. irregulare. In our study, RiPT1 transcript levels were not affected by Pi, but a significant and positive response to Pi adaptation was observed. RiPT1 has been proposed to be mainly responsible for the Pi uptake by the extraradical mycelium (Xie et al., 2022). However, the strong and constitutive expression of RiPT1 independent of the Pi concentration in our study highlights the importance of this transporter also at the root level.
The available data on other AMF PTs are limited, sometimes contradictory, and their role in the symbiosis remains to be defined. RiPT5 was shown by Walder et al. (2016) as the only Pi transporter gene in their study, which presented a trend of correlation between mycorrhizal Pi acquisition in plants and its level, defining it as the most promising candidate involved in intraradical Pi transfer to PAS. In more recent work, PT6, as PT1, was proposed to be mainly responsible for Pi uptake by the ERM, while the SPX domain-containing transporters, such as PT7 and PHO1, may be involved in Pi export at the symbiotic interface (Ezawa and Saito, 2018; Xie et al., 2022). The transcription of PT7 was shown to be Pi concentration-independent, but necessary for arbuscule development and symbiotic Pi delivery under medium to low Pi concentrations. Indeed, inactivation of this gene resulted in premature arbuscule degradation, and RiPT7 was hypothesized to play an important role in R. irregulare development within roots at late stage, with Pi delivered by this transporter acting as a signal for continued AMF symbiont development in roots (Xie et al., 2022). In our study, RiPT6 was the most strongly correlated with ink fungal parameters, although it was only regulated by Pi levels and was repressed by HP. Interestingly, transcripts of RiPHO1, RiCOX, RiMST and several fungal ALP parameters (especially the relative arbuscule abundance) are all positively and significantly correlated, suggesting a higher metabolic and exchange activity in the Pi+ phenotype. The sugar transporter gene RiMST2 has been shown to be expressed in the IRM and its down-regulation resulted in reduced root colonization (Helber et al., 2011), suggesting a role in sugar translocation from the plant to the fungus. These observations under our experimental conditions would be more consistent with a role for RiPHO1 in the exchange between the host and the fungal partner.
RiKCS1, RiADO1, RiPHO80, RiPHO81, RiPHO85 and RiPHO2 were selected to gain insight into the possible modulation of the fungal PHO pathway between the adapted and non-adapted fungus. Few data are available on these genes in AMF. In Gigaspora margarita, pho81 transcripts were induced under low Pi and repressed under high Pi, whereas the expression of pho80 and pho85 was not affected by ambient Pi levels, suggesting a primary sensing by Pho81 whose expression levels are largely responsive to external Pi levels (Xie et al., 2016). Our data show a different pattern of gene regulation, partially consistent with the Pi response, with differences between the two fungal Pi phenotypes. RiPHO2, RiPHO85, and RiKCS1 were the only P-responsive genes, whereas RiADO1, RiPHO81, and RiPHO80 responded to the Pi adaptation process under LP conditions. These observations again question the physiological status of the LP RiQS81-Pi- plants and point to possibly less active fungal structures in this condition or a modulation of Pi sensing in the RiQS81-Pi+ strain, linked to a higher capacity to colonize the plants.
4.2.2 Plant P transport
A number of studies have shown that AM colonization of plants down-regulates the expression of the DPU Pi transporters (Rausch et al., 2001; Burleigh, 2002; Glassop et al., 2005; Requena, 2005; Nagy et al., 2006) while other studies demonstrated different results. Chen et al. (2007) showed a mycorrhiza-induced down-regulation of Pht1;1 and Pht1;2 expression under low-Pi conditions, but Pi-regulation of Pht1;2 under high-Pi conditions for pepper, eggplant, and tobacco. In tomato, Nagy et al. (2009) found no change in the transcript abundance for SlPT1 and SlPT2 upon root colonization. Our data are consistent with these latter observations, as presence of AMF did not induce downregulation of melon Pht1 transporters in any of our conditions. All melon Pht1 transporters expressed in the roots were downregulated by Pi, and all except CmPT1;6 were significantly upregulated by the presence of AMF, with CmPT1;5 expressed exclusively in AM roots. CmPT1;1, CmPT1;3, CmPT1;4 and CmPT1;5 were up-regulated in plants inoculated with RiQS81-Pi+ in HP conditions, in direct correlation with the mycorrhizal colonization parameters (Supplementary Figure S15).
4.2.3 Respiration
Although one cannot necessarily deduce the enzymatic activities from RNA accumulation for the genes involved in respiratory pathways (Sluse and Jarmuszkiewicz, 1998), the results show, both in the plant and the fungus, a down regulation of genes involved in the AOX pathway associated with an upregulation of genes involved in the COX pathway in high Pi conditions, which was expected (Bedini et al., 2018). Consistently, strong correlations were obtained between the mitochondrial respiration pathway components with plant biomass (Supplementary Figures S12, S13). In agreement with previous reports (Del-Saz et al., 2017), upregulation of plant genes involved in the COX pathway (CmCytC and CmCOX5b2) were induced by the presence of AMF in low Pi conditions, with prominent response with the Pi+ phenotype. This upregulation is reliable with positive MGR obtained with RiQS81-Pi+, but not with the negative MGR observed with RiQS81-Pi-. This would be coherent with Smith and Smith (2011), who argued that a negative MGR does not translate to a parasitic fungal behavior since a transfer of Pi and carbon still occur.
In the fungus, significant differences were also observed between the two Pi phenotypes, mainly under low Pi condition (RiCOX5b and RiCytC). This could indicate, in relation with the ALP straining results, a higher metabolic activity of Pi+ phenotype. We observed a positive correlation between the ALP stained fungal structures and the transcript abundance of fungal genes as RiCOX, RiMST and RiPHO1 (Supplementary Figure S12), reinforcing the hypothesis that the ALP activity could be related with fungal metabolic process linking sugar uptake, Pi transport and mitochondrial activity (Kizawa et al., 2016; Ortíz et al., 2020). A link between ALP parameters and RiCOX transcripts makes sense, since it was reported that the inhibition of the COX pathway by KCN suppress also alkaline phosphatase activity (Van Belle, 1972; Ezawa et al., 1995). On the other side, plant parameters are positively correlated with RiCytC transcripts. This would be consistent with a nutrient delivery from fungus to plant, as an active process requiring ATP provided by the COX pathway. In conclusion, mitochondria are major actors in mycorrhizal association and play a role in fungal tolerance to Pi.
It was previously hypothesized that the LDH could possibly be related to AOX and fermentation in AMF, to sustain energy demand (Bedini et al., 2018). This could be partially comforted by our results where the expressions patterns of RiAOX and RiLDH were similar, as well as CmAOX and RiLDH, but no correlation was found between CmAOX and CmLDH. Moreover, we noticed that both RiAOX and RiLDH were significantly and positively correlated with fungal ink parameters, and notably with the relative arbuscule and vesicle abundance, but negatively correlated with the proportion of ALP-stained structures within ink. It would therefore indicate that the fungal structures which were not stained by ALP were also viable, with a metabolism associated with the AOX pathway. This might translate to a state of dormancy notably for the vesicles. Therefore, the divergent proportion of ALP under low Pi condition between both R. irregulare phenotypes would be related to a differential physiological time, Pi- being more advanced in the life stage than Pi+ and inversely under high Pi.
4.2.4 Lipid metabolism and transport
Beyond phosphate transport and signaling, other genes related to symbiotic function have been investigated, such as those involved in fatty acid biosynthesis and transport. Arbuscular mycorrhizal fungi (AMF) lack the ability to synthesize fatty acids due to the absence of cytosolic type I fatty acid synthase genes, suggesting their reliance on the plant for fatty acid delivery. This dependence is further supported by the discovery of an AM-specific lipid biosynthetic pathway, and the upregulation of lipid biosynthesis and transporter genes in colonized cortical cells during symbiosis (Zhang et al., 2010; Gutjahr et al., 2012; Tisserant et al., 2013; Bravo et al., 2017; Jiang et al., 2017; Keymer et al., 2017; Luginbuehl et al., 2017; Morin et al., 2019; Malar C et al., 2021). The melon orthologs of the gene encoding the thioesterase FatM, the GPAT RAM2, and a pair of ABCG half-transporters unique to mycorrhizal plants and necessary to support arbuscule formation, named STR1 and STR2 (Zhang et al., 2010; Gutjahr et al., 2012; Bravo et al., 2017; Jiang et al., 2017), were examined. All of these AM-inducible plant genes, and particularly CmRAM2, were positively correlated with several fungal (ink) parameters, especially arbuscle abundance (Supplementary Figure S12). The transcript levels of some of these plant genes were significantly repressed by high Pi in the presence of the R. irregulare Pi- phenotype, which could be consistent with lower colonization; however, this is not the case for the Pi+ phenotype. Remarkably, all these plant genes are significantly differentially regulated between the two R. irregulare phenotypes under high Pi condition, as well as CmFATM under low Pi condition.
While a link between fungal colonization (ALP), RiMST and RiCOX was observed, indicating a consistent flow from sugar uptake and assimilation from arbuscule to the mitochondria, the fate of lipid seems to correlate modestly to this metabolic path. It would be interesting to see in a future trial if a stronger correlation exists with the extraradical spore density.
4.2.5 Blue copper proteins
Blue copper proteins (BCPs), characterized by their involvement in electron transfer reactions, have also been investigated in the context of AM roots. The AM-induced BCP family was shown as specifically and strongly Pi-regulated in arbuscule-containing regions of mycorrhizal roots, in the plasma membrane and the periarbuscular membrane around the arbuscule trunk (Pumplin and Harrison, 2009; Parádi et al., 2010). Although their precise role remains unclear, gene promoter activity studies suggest that BCPs may serve as mediators of electron transfer processes in arbuscule-containing cells and their vicinity (Hohnjec et al., 2005). We monitored the accumulation of transcripts encoding two melon orthologs of the mycorrhiza-responsive genes MtBCP1 (Parádi et al., 2010). Similar to the transcript levels found for genes involved in lipid metabolism and transport, the expression patterns of CmBCP1a and CmBCP2b cannot be interpreted easily, but the significant and higher levels of transcript in RiQS81-Pi+ as compared to RiQS81-Pi- is a clear result from the adaptation process.
Taken together, the molecular data suggests that the Pi tolerance status of the fungus had an influence on the molecular dialogue between the two partners of the symbiosis. It is interesting to note that most of the transcriptional differences between the two Pi phenotypes are observed under low Pi condition on the fungal side but are observed under high Pi on the plant side. We noticed fewer significant changes and larger standard errors for the fungal transcript dataset. One of the probable biases is that we used RNA extracted from whole mycorrhized roots, which means a mixture of different structures (vesicles, hyphae, arbuscules), each of which probably has different regulatory profiles. For a clearer answer about the exchanges with the plant, it would be necessary to perform the transcriptional analyses in arbuscocytes only. It would also be interesting to see how the genes related to signaling are regulated in the ERM, and in kinetics through the lifespan of the symbiosis.
4.3 Acclimatization or adaptation?
Micro-organisms, including AMF, are constantly evolving to thrive in specific environmental conditions and the complex genetics of AMF reflects their ability to adapt to a wide range of environmental variation. The genetic of AMF is complex due to the presence of multiple nuclei within a single isolate (Malbreil et al., 2014), and a high intra-isolate variation was observed at genome level, for rDNA as well as for protein-coding sequences (Corradi et al., 2007; Gibson and Reed, 2008; Savary et al., 2018). This variation was further confirmed also at transcript level, showing that the gene variants are indeed transcribed (Boon et al., 2010), and it was suggested that the different functional variants of a gene could be expressed in different environments (Oliveira et al., 2010).
In our research, we aimed to generate a directed selection of advantageous phenotypes of R. irregulare progeny with high Pi tolerance. This selection process refers to the ability of a genotype to produce different phenotypes induced by the changing environment (Lorant et al., 2020). Successful domestication of the R. irregulare QS81 isolate was achieved after several generations in which high Pi pressure was maintained. It is worth noting that the adaptation process resulted in distinct fungal phenotypes in high Pi, but also in low Pi conditions. Therefore, the terms ‘acclimatization’ or ‘acclimation’ are not relevant in this context, as they imply the reversible adjustment of phenotype in response to environmental conditions over a lifetime (Pinsky et al., 2013; Collier et al., 2018). We have observed phenotypic changes associated with transcriptomic variations indicating an adaptive response to high Pi (stressful) environments (Ghalambor et al., 2007). This suggests the presence of cryptic genetic variation, indicating the existence of genotypic and phenotypic variance in the R. irregulare isolate used in this study (Paaby and Rockman, 2014). Initial investigations on nuclear and mitochondrial rDNA sequences did not allow the identification of specific genetic variations associated with the induced high Pi+ phenotype. On the other hand, these methods covered only a very small part of the genome and may not be relevant for the differentiation of the adapted R. irregulare. Therefore, further in-depth work is needed to unravel the possible genetic mechanisms that contributed to the phenotypic variation, such as nuclear selection/filtration or epigenetic changes (Ehinger et al., 2012; Boon et al., 2013; Angelard et al., 2014; Kokkoris et al., 2020; Peña et al., 2020; Savary et al., 2020; Chaturvedi et al., 2021; Mojica and Kültz, 2022). This may allow the identification of markers that can discriminate between the two R. irregulare Pi phenotypes.
Based on our first results, we adopt the term ‘short-term adaptation’ to describe the high Pi tolerance observed in our study, following the definition proposed by (Brooks et al., 2011). Our work provides an initial proof of concept and raises further questions for future investigation: How many generations are required to observe the first phenotypic signs of high Pi tolerance? How many generations are required to achieve a positive mycorrhizal growth response under high Pi conditions after application of RiQS81Pi+?
4.4 Open questions and perspectives
4.4.1 Do high Pi-tolerant AMF lines already exist in managed crop systems?
The possibility to induce R. irregulare Pi+ phenotype after a short number of generations raises the question of whether high Pi-tolerant AMF lines exist naturally. High Pi levels resulting from long-term high Pi fertilization are common in many conventional field soils (Cheng et al., 2013). The number of propagules in such soils may be low or even absent in some cases (Mäder et al., 2000; Oehl et al., 2003; Roy et al., 2017). However, other practices challenge the sole effect of Pi in inhibiting the mycorrhizal colonization and soil richness, such as tillage (Jansa et al., 2002; Castillo et al., 2006; Avio et al., 2013; Verzeaux et al., 2017), lack of cover crops (Soti et al., 2016), selection of low mycorrhizal plant species (Martín-Robles et al., 2018), or application of pesticides (Jin et al., 2013; Riedo et al., 2021; Edlinger et al., 2022). Moreover, a lack of Pi inhibition on the fungal community under field conditions has also been observed in long- or short-term fertilization approaches (Peña Venegas et al., 2021), but to the best of our knowledge, investigations of possible high Pi-tolerant AMF species have never been carried out. Perhaps the elaboration of high-resolution markers specific to the Pi+ phenotype could help in the detection of Pi-tolerant lines in natural and managed ecosystems, and could improve our knowledge of AMF ecology. At least, our results could suggest that adaptation to higher Pi concentrations of R. irregulare could occur in managed conventional systems, and, if true, would give more weight to the negative influence of other factors.
4.4.2 Mycorrhizal production strategies: a step backwards in the definition of a good inoculum?
Our data suggest that propagule abundance may not be the sole determinant for achieving beneficial effects on crops. Traditionally, the production of propagule-rich inocula has been pursued to increase the capacity for inoculating larger areas for commercial purposes and to meet quality criteria for scientific usage. An inoculum with fewer propagules would require higher doses to maintain a minimal but efficient number of propagules applied per plant. As a result, larger quantities would need to be produced, resulting in higher costs for customers and growers. However, the application of elevated Pi concentration during the first generation of ex vitro production of RiQS81-Pi+ significantly reduced its ability to produce propagules compared to the classical production route of RiQS81-Pi-. Interestingly, the difference between the adapted and non-adapted R. irregulare gradually decreased over subsequent generations. This suggests that in the future, it may be possible to achieve a similar number of propagules between the two R. irregulare phenotypes during production under contrasting Pi fertilization, providing definitive evidence of short-term Pi adaptation.
Conversely, all other things being equal, it is possible that production systems that typically involve specific fertilization, especially low phosphate fertilization, may adapt mycorrhizal species to produce high amounts of propagules, but could potentially lead to reduced response or specific loss of native adaptive traits to a given soil ecosystem. Furthermore, previous studies have shown that the application of exogenous mycorrhizal strains can lead to the genetic replacement of native fungal species and result in limited plant responses (Calvente et al., 2004; Schwartz et al., 2006; Rowe et al., 2007; Estrada et al., 2013; Emam, 2016). It is possible that this limitation is due to the fertilization used during mycorrhizal production, which would make mycorrhizal strains or isolates less able to thrive in certain soil conditions or lead to incompatibilities between exogenous and native species (Bencherif et al., 2023). Our results open the possibility of producing innovative and generic mycorrhizal inocula that could be better adapted to specific fertilization regimes or elemental concentrations.
4.4.3 Consequences of fertilization during inoculum production: what is the right fungal model for research purposes?
Building on the points raised in the previous paragraph, it is logical to address a potential concern about the design of inoculum production and its consequence for scientific purposes. The effects of high phosphate concentrations on mycorrhizal development and response have been extensively studied for decades. Numerous publications conducted under controlled conditions have consistently demonstrated the inhibition of mycorrhizal colonization by phosphate, often associated with negative MGR (Mosse, 1973; Thomson et al., 1986; Smith and Read, 2008; Nagy et al., 2009; Breuillin et al., 2010; Balzergue et al., 2011). Over time, these studies have delved deeper into the topic, using increasingly sophisticated tools such as transcriptomics, genetics, and mutant plants to gain a better understanding of the role of phosphate in endomycorrhizal symbioses. However, it is worth questioning whether we have generated artificial knowledge by using artificially domesticated fungal inocula. Our data challenge the notion that the phenomenon of phosphate inhibition is solely the result of a top-down mechanism in which the plant controls the fungus. Instead, we emphasize the importance of fungal respiration and phosphate metabolism, which play a role in the fungus’ own phenotypic behavior and may also have consequences for the plant response. It is important to note that the mycorrhizal inocula used in previous studies were primarily derived from pot culture systems typically produced by laboratories, companies, or glomeromycotan banks. These systems often include specific phosphate fertilization regimes as a widely used standard for optimal fungal propagation (Ijdo et al., 2011). The production of mycorrhizal fungi under such specific fertilization regimes over multiple generations could have profound effects on fungal physiology, the extent of which is largely unknown when these inocula are used for research purposes. This could potentially explain or contribute to discrepancies in the interpretation of the effects of compounds that influence mycorrhizal behavior, such as hormones or nutrients. Therefore, it will become critical to consider how a fungal inoculum used in research is produced, as it may have important implications for the results and interpretation.
5 Conclusion
We provide the first evidence that it is possible to artificially induce short-term adaptation of R. irregulare strain QS81 to high phosphate levels. After five in vitro and one ex vitro generation, this process resulted in differences in phenotypic development, plant responses, and fungal and plant transcriptomic patterns. Our study demonstrates another level of the extreme plasticity that AMF can harbor. The fungal material can be used to provide another basis for the concept of adaptive evolution in Glomeromycota and to unravel its mechanisms under controlled conditions. Moreover, such short-adapted AMF strains could provide a new strategy for the application of specific mycorrhizal inocula in plant production systems and pave the way to elaborate a biotechnological response in crops to associate mycorrhizal fungi where high phosphate fertilization is the standard.
Data availability statement
The original contributions presented in the study are included in the article/Supplementary Material. Further inquiries can be directed to the corresponding authors.
Author contributions
EL-M: Data curation, Formal analysis, Investigation, Validation, Writing – original draft. LM: Data curation, Formal analysis, Investigation, Validation, Writing – original draft. AJ: Investigation, Writing – review & editing. CS: Funding acquisition, Project administration, Resources, Writing – review & editing. PF: Conceptualization, Funding acquisition, Project administration, Resources, Writing – review & editing.
Funding
The author(s) declare financial support was received for the research, authorship, and/or publication of this article. This project has received funding from the European Union’s Horizon 2020 Research and Innovation Program under the Marie Skłodowska-Curie Grant Agreement No. 676480 and from the Thuringian Ministry for Economic Affairs, Science, and Digital Society.
Acknowledgments
The authors would like to thank Alberico Bedini for providing the 5th generation of in vitro fungal material; the INOQ greenhouse team for the help in trial implementation, and Alicia Varela Alonso & Katja Burow for the assistance with the material in FGK Erfurt. We are thankful for the reviewer’s overall comments on the manuscript and their suggestions for improving it.
Conflict of interest
Authors LM, EL-M, AJ, and CS were employed by the company INOQ GmbH.
The remaining author declares that the research was conducted in the absence of any commercial or financial relationships that could be construed as a potential conflict of interest.
Publisher’s note
All claims expressed in this article are solely those of the authors and do not necessarily represent those of their affiliated organizations, or those of the publisher, the editors and the reviewers. Any product that may be evaluated in this article, or claim that may be made by its manufacturer, is not guaranteed or endorsed by the publisher.
Supplementary material
The Supplementary Material for this article can be found online at: https://www.frontiersin.org/articles/10.3389/fpls.2024.1385245/full#supplementary-material
References
Andersen, C. L., Jensen, J. L., Ørntoft, T. F. (2004). Normalization of real-time quantitative reverse transcription-PCR data: A model-based variance estimation approach to identify genes suited for normalization, applied to bladder and colon cancer data sets. Cancer Res. 64, 5245–5250. doi: 10.1158/0008-5472.CAN-04-0496
Angelard, C., Tanner, C. J., Fontanillas, P., Niculita-Hirzel, H., Masclaux, F., Sanders, I. R. (2014). Rapid genotypic change and plasticity in arbuscular mycorrhizal fungi is caused by a host shift and enhanced by segregation. ISME J. 8, 284–294. doi: 10.1038/ismej.2013.154
Aono, T., Maldonado-Mendoza, I. E., Dewbre, G. R., Harrison, M. J., Saito, M. (2004). Expression of alkaline phosphatase genes in arbuscular mycorrhizas. New Phytol. 162, 525–534. doi: 10.1111/j.1469-8137.2004.01041.x
Arnholdt-Schmitt, B., Costa, J. H., de Melo, D. F. (2006). AOX–a functional marker for efficient cell reprogramming under stress? Trends Plant Sci. 11, 281–287. doi: 10.1016/j.tplants.2006.05.001
Audet, P., Charest, C. (2010). Identification of constraining experimental-design factors in mycorrhizal pot-growth studies. J. Bot. 2010, e718013. doi: 10.1155/2010/718013
Avio, L., Castaldini, M., Fabiani, A., Bedini, S., Sbrana, C., Turrini, A., et al. (2013). Impact of nitrogen fertilization and soil tillage on arbuscular mycorrhizal fungal communities in a Mediterranean agroecosystem. Soil Biol. Biochem. 67, 285–294. doi: 10.1016/j.soilbio.2013.09.005
Balestrini, R., Gómez-Ariza, J., Lanfranco, L., Bonfante, P. (2007). Laser microdissection reveals that transcripts for five plant and one fungal phosphate transporter genes are contemporaneously present in arbusculated cells. Mol. Plant-Microbe Interact. MPMI 20, 1055–1062. doi: 10.1094/MPMI-20-9-1055
Balzergue, C., Chabaud, M., Barker, D. G., Bécard, G., Rochange, S. F. (2013). High phosphate reduces host ability to develop arbuscular mycorrhizal symbiosis without affecting root calcium spiking responses to the fungus. Front. Plant Sci. 4. doi: 10.3389/fpls.2013.00426
Balzergue, C., Puech-Pagès, V., Bécard, G., Rochange, S. F. (2011). The regulation of arbuscular mycorrhizal symbiosis by phosphate in pea involves early and systemic signalling events. J. Exp. Bot. 62, 1049–1060. doi: 10.1093/jxb/erq335
Bedini, A., Mercy, L., Schneider, C., Franken, P., Lucic-Mercy, E. (2018). Unraveling the initial plant hormone signaling, metabolic mechanisms and plant defense triggering the endomycorrhizal symbiosis behavior. Front. Plant Sci. 9, 1800. doi: 10.3389/fpls.2018.01800
Behm, J. E., Kiers, E. T. (2014). A phenotypic plasticity framework for assessing intraspecific variation in arbuscular mycorrhizal fungal traits. J. Ecol. 102, 315–327. doi: 10.1111/1365-2745.12194
Bencherif, K., Laruelle, F., Tisserant, B., Dalpé, Y., Lounés-Hadj Sahraoui, A. (2023). Engineering approach for production of arbuscular mycorrhizal inoculum adapted to saline soil management. Stresses 3, 404–423. doi: 10.3390/stresses3020030
Benedetto, A., Magurno, F., Bonfante, P., Lanfranco, L. (2005). Expression profiles of a phosphate transporter gene (GmosPT) from the endomycorrhizal fungus Glomus mosseae. Mycorrhiza 15, 620–627. doi: 10.1007/s00572-005-0006-9
Bennett, A. E., Meek, H. C. (2020). The influence of arbuscular mycorrhizal fungi on plant reproduction. J. Chem. Ecol. 46, 707–721. doi: 10.1007/s10886-020-01192-4
Berruti, A., Lumini, E., Balestrini, R., Bianciotto, V. (2016). Arbuscular mycorrhizal fungi as natural biofertilizers: let’s benefit from past successes. Front. Microbiol. 6. doi: 10.3389/fmicb.2015.01559
Boon, E., Zimmerman, E., Lang, B. F., Hijri, M. (2010). Intra-isolate genome variation in arbuscular mycorrhizal fungi persists in the transcriptome. J. Evol. Biol. 23, 1519–1527. doi: 10.1111/j.1420-9101.2010.02019.x
Boon, E., Zimmerman, E., St-Arnaud, M., Hijri, M. (2013). Allelic Differences within and among Sister Spores of the Arbuscular Mycorrhizal Fungus Glomus etunicatum Suggest Segregation at Sporulation. PloS One 8, e83301. doi: 10.1371/journal.pone.0083301
Branco, S., Schauster, A., Liao, H.-L., Ruytinx, J. (2022). Mechanisms of stress tolerance and their effects on the ecology and evolution of mycorrhizal fungi. New Phytol. 235, 2158–2175. doi: 10.1111/nph.18308
Bravo, A., Brands, M., Wewer, V., Dörmann, P., Harrison, M. J. (2017). Arbuscular mycorrhiza-specific enzymes FatM and RAM2 fine-tune lipid biosynthesis to promote development of arbuscular mycorrhiza. New Phytol. 214, 1631–1645. doi: 10.1111/nph.14533
Breuillin, F., Schramm, J., Hajirezaei, M., Ahkami, A., Favre, P., Druege, U., et al. (2010). Phosphate systemically inhibits development of arbuscular mycorrhiza in Petunia hybrida and represses genes involved in mycorrhizal functioning. Plant J. 64, 1002–1017. doi: 10.1111/j.1365-313X.2010.04385.x
Brooks, A. N., Turkarslan, S., Beer, K. D., Yin Lo, F., Baliga, N. S. (2011). Adaptation of cells to new environments. WIREs Syst. Biol. Med. 3, 544–561. doi: 10.1002/wsbm.136
Brundrett, M. (1991). “Mycorrhizas in Natural Ecosystems,” in Advances in Ecological Research. 21, 171–313. doi: 10.1016/S0065-2504(08)60099-9
Brundrett, M. C., Tedersoo, L. (2018). Evolutionary history of mycorrhizal symbioses and global host plant diversity. New Phytol. 220, 1108–1115. doi: 10.1111/nph.14976
Bucher, M. (2007). Functional biology of plant phosphate uptake at root and mycorrhiza interfaces. New Phytol. 173, 11–26. doi: 10.1111/j.1469-8137.2006.01935.x
Bui, V. C., Franken, P. (2018). Acclimatization of rhizophagus irregularis enhances zn tolerance of the fungus and the mycorrhizal plant partner. Front. Microbiol. 9. doi: 10.3389/fmicb.2018.03156
Burleigh, S. H. (2002). Functional diversity of arbuscular mycorrhizas extends to the expression of plant genes involved in P nutrition. J. Exp. Bot. 53, 1593–1601. doi: 10.1093/jxb/erf013
Calvente, R., Cano, C., Ferrol, N., Azcón-Aguilar, C., Barea, J. M. (2004). Analysing natural diversity of arbuscular mycorrhizal fungi in olive tree (Olea europaea L.) plantations and assessment of the effectiveness of native fungal isolates as inoculants for commercial cultivars of olive plantlets. Appl. Soil Ecol. 26, 11–19. doi: 10.1016/j.apsoil.2003.10.009
Campos, C., Cardoso, H., Nogales, A., Svensson, J., Lopez-Ráez, J. A., Pozo, M. J., et al. (2015). Intra and inter-spore variability in rhizophagus irregularis AOX gene. PloS One 10, e0142339. doi: 10.1371/journal.pone.0142339
Castillo, C. G., Rubio, R., Rouanet, J. L., Borie, F. (2006). Early effects of tillage and crop rotation on arbuscular mycorrhizal fungal propagules in an Ultisol. Biol. Fertil. Soils 43, 83–92. doi: 10.1007/s00374-005-0067-0
Chaturvedi, A., Cruz Corella, J., Robbins, C., Loha, A., Menin, L., Gasilova, N., et al. (2021). The methylome of the model arbuscular mycorrhizal fungus, Rhizophagus irregularis, shares characteristics with early diverging fungi and Dikarya. Commun. Biol. 4, 1–11. doi: 10.1038/s42003-021-02414-5
Chen, A., Hu, J., Sun, S., Xu, G. (2007). Conservation and divergence of both phosphate- and mycorrhiza-regulated physiological responses and expression patterns of phosphate transporters in solanaceous species. New Phytol. 173, 817–831. doi: 10.1111/j.1469-8137.2006.01962.x
Cheng, Y., Ishimoto, K., Kuriyama, Y., Osaki, M., Ezawa, T. (2013). Ninety-year-, but not single, application of phosphorus fertilizer has a major impact on arbuscular mycorrhizal fungal communities. Plant Soil 365, 397–407. doi: 10.1007/s11104-012-1398-x
Collier, R. J., Baumgard, L. H., Zimbelman, R. B., Xiao, Y. (2018). Heat stress: physiology of acclimation and adaptation. Anim. Front. Rev. Mag. Anim. Agric. 9, 12–19. doi: 10.1093/af/vfy031
Corradi, N., Croll, D., Colard, A., Kuhn, G., Ehinger, M., Sanders, I. R. (2007). Gene copy number polymorphisms in an arbuscular mycorrhizal fungal population. Appl. Environ. Microbiol. 73, 366–369. doi: 10.1128/AEM.01574-06
Das, D., Paries, M., Hobecker, K., Gigl, M., Dawid, C., Lam, H.-M., et al. (2022). PHOSPHATE STARVATION RESPONSE transcription factors enable arbuscular mycorrhiza symbiosis. Nat. Commun. 13, 477. doi: 10.1038/s41467-022-27976-8
Declerck, S., Strullu, D. G., Plenchette, C. (1998). Monoxenic culture of the intraradical forms of Glomus sp. isolated from a tropical ecosystem: a proposed methodology for germplasm collection. Mycologia 90, 579–585. doi: 10.1080/00275514.1998.12026946
Del-Saz, N. F., Ribas-Carbo, M., McDonald, A. E., Lambers, H., Fernie, A. R., Florez-Sarasa, I. (2018). An in vivo perspective of the role(s) of the alternative oxidase pathway. Trends Plant Sci. 23, 206–219. doi: 10.1016/j.tplants.2017.11.006
Del-Saz, N. F., Romero-Munar, A., Alonso, D., Aroca, R., Baraza, E., Flexas, J., et al. (2017). Respiratory ATP cost and benefit of arbuscular mycorrhizal symbiosis with Nicotiana tabacum at different growth stages and under salinity. J. Plant Physiol. 218, 243–248. doi: 10.1016/j.jplph.2017.08.012
Edlinger, A., Garland, G., Hartman, K., Banerjee, S., Degrune, F., García-Palacios, P., et al. (2022). Agricultural management and pesticide use reduce the functioning of beneficial plant symbionts. Nat. Ecol. Evol. 6, 1145–1154. doi: 10.1038/s41559-022-01799-8
Ehinger, M. O., Croll, D., Koch, A. M., Sanders, I. R. (2012). Significant genetic and phenotypic changes arising from clonal growth of a single spore of an arbuscular mycorrhizal fungus over multiple generations. New Phytol. 196, 853–861. doi: 10.1111/j.1469-8137.2012.04278.x
Emam, T. (2016). Local soil, but not commercial AMF inoculum, increases native and non-native grass growth at a mine restoration site. Restor. Ecol. 24, 35–44. doi: 10.1111/rec.12287
Estrada, B., Aroca, R., Maathuis, F. J. M., Barea, J. M., Ruiz-Lozano, J. M. (2013). Arbuscular mycorrhizal fungi native from a Mediterranean saline area enhance maize tolerance to salinity through improved ion homeostasis. Plant Cell Environ. 36, 1771–1782. doi: 10.1111/pce.12082
Ezawa, T., Saito, K. (2018). How do arbuscular mycorrhizal fungi handle phosphate? New insight into fine-tuning of phosphate metabolism. New Phytol. 220, 1116–1121. doi: 10.1111/nph.15187
Ezawa, T., Saito, M., Yoshida, T. (1995). Comparison of phosphatase localization in the intraradical hyphae of arbuscular mycorrhizal fungi, Glomus spp. and Gigaspora spp. Plant Soil 176, 57–63. doi: 10.1007/BF00017675
Ferrol, N., Azcón-Aguilar, C., Pérez-Tienda, J. (2019). Review: Arbuscular mycorrhizas as key players in sustainable plant phosphorus acquisition: An overview on the mechanisms involved. Plant Sci. 280, 441–447. doi: 10.1016/j.plantsci.2018.11.011
Fiorilli, V., Lanfranco, L., Bonfante, P. (2013). The expression of GintPT, the phosphate transporter of Rhizophagus irregularis, depends on the symbiotic status and phosphate availability. Planta 237, 1267–1277. doi: 10.1007/s00425-013-1842-z
Funamoto, R., Saito, K., Oyaizu, H., Saito, M., Aono, T., Funamoto, R., et al. (2007). Simultaneous in situ detection of alkaline phosphatase activity and polyphosphate in arbuscules within arbuscular mycorrhizal roots. Funct. Plant Biol. 34, 803–810. doi: 10.1071/FP06326
Ghalambor, C. K., McKay, J. K., Carroll, S. P., Reznick, D. N. (2007). Adaptive versus non-adaptive phenotypic plasticity and the potential for contemporary adaptation in new environments. Funct. Ecol. 21, 394–407. doi: 10.1111/j.1365-2435.2007.01283.x
Gianinazzi, S., Gollotte, A., Binet, M.-N., van Tuinen, D., Redecker, D., Wipf, D. (2010). Agroecology: the key role of arbuscular mycorrhizas in ecosystem services. Mycorrhiza 20, 519–530. doi: 10.1007/s00572-010-0333-3
Gibson, G., Reed, L. K. (2008). Cryptic genetic variation. Curr. Biol. 18, R989–R990. doi: 10.1016/j.cub.2008.08.011
Glassop, D., Smith, S. E., Smith, F. W. (2005). Cereal phosphate transporters associated with the mycorrhizal pathway of phosphate uptake into roots. Planta 222, 688–698. doi: 10.1007/s00425-005-0015-0
Grahl, N., Dinamarco, T. M., Willger, S. D., Goldman, G. H., Cramer, R. A. (2012). Aspergillus fumigatus mitochondrial electron transport chain mediates oxidative stress homeostasis, hypoxia responses and fungal pathogenesis: Mitochondrial respiration and Aspergillus pathogenesis. Mol. Microbiol. 84, 383–399. doi: 10.1111/j.1365-2958.2012.08034.x
Grossman, L. I., Lomax, M. I. (1997). Nuclear genes for cytochrome c oxidase. Biochim. Biophys. Acta 1352, 174–192. doi: 10.1016/s0167-4781(97)00025-0
Guillemin, J. P., Orozco, M. O., Gianinazzi-Pearson, V., Gianinazzi, S. (1995). Influence of phosphate fertilization on fungal alkaline phosphatase and succinate dehydrogenase activities in arbuscular mycorrhiza of soybean and pineapple. Agric. Ecosyst. Environ. 53, 63–69. doi: 10.1016/0167-8809(94)00555-S
Gutjahr, C., Radovanovic, D., Geoffroy, J., Zhang, Q., Siegler, H., Chiapello, M., et al. (2012). The half-size ABC transporters STR1 and STR2 are indispensable for mycorrhizal arbuscule formation in rice. Plant J. Cell Mol. Biol. 69, 906–920. doi: 10.1111/j.1365-313X.2011.04842.x
Helber, N., Wippel, K., Sauer, N., Schaarschmidt, S., Hause, B., Requena, N. (2011). A versatile monosaccharide transporter that operates in the arbuscular mycorrhizal fungus glomus sp is crucial for the symbiotic relationship with plants. Plant Cell 23, 3812–3823. doi: 10.1105/tpc.111.089813
Helgason, T., Fitter, A. H. (2009). Natural selection and the evolutionary ecology of the arbuscular mycorrhizal fungi (Phylum Glomeromycota). J. Exp. Bot. 60, 2465–2480. doi: 10.1093/jxb/erp144
Higo, M., Azuma, M., Kamiyoshihara, Y., Kanda, A., Tatewaki, Y., Isobe, K. (2020). Impact of phosphorus fertilization on tomato growth and arbuscular mycorrhizal fungal communities. Microorganisms 8, 178. doi: 10.3390/microorganisms8020178
Hoagland, D. R., Arnon, D. I. (1938). The water-culture method for growing plants without soil. Berkeley, Calif.: University of California, College of Agriculture, Agricultural Experiment Station. Circular, 347.
Hohnjec, N., Vieweg, M. F., Pühler, A., Becker, A., Küster, H. (2005). Overlaps in the transcriptional profiles of Medicago truncatula roots inoculated with two different Glomus fungi provide insights into the genetic program activated during arbuscular mycorrhiza. Plant Physiol. 137, 1283–1301. doi: 10.1104/pp.104.056572
Huo, W., Chai, X., Wang, X., Batchelor, W. D., Kafle, A., Feng, G. (2022). Indigenous arbuscular mycorrhizal fungi play a role in phosphorus depletion in organic manure amended high fertility soil. J. Integr. Agric. 21, 3051–3066. doi: 10.1016/j.jia.2022.07.045
Ijdo, M., Cranenbrouck, S., Declerck, S. (2011). Methods for large-scale production of AM fungi: past, present, and future. Mycorrhiza 21, 1–16. doi: 10.1007/s00572-010-0337-z
Jabaji-Hare, S., Deschene, A., Kendrick, B. (1984). Lipid content and composition of vesicles of a vesicular-arbuscular mycorrhizal fungus. Mycologia 76, 1024–1030. doi: 10.1080/00275514.1984.12023946
Jansa, J., Erb, A., Oberholzer, H.-R., Šmilauer, P., Egli, S. (2014). Soil and geography are more important determinants of indigenous arbuscular mycorrhizal communities than management practices in Swiss agricultural soils. Mol. Ecol. 23, 2118–2135. doi: 10.1111/mec.12706
Jansa, J., Mozafar, A., Anken, T., Ruh, R., Sanders, I. R., Frossard, E. (2002). Diversity and structure of AMF communities as affected by tillage in a temperate soil. Mycorrhiza 12, 225–234. doi: 10.1007/s00572-002-0163-z
Javot, H., Pumplin, N., Harrison, M. J. (2007). Phosphate in the arbuscular mycorrhizal symbiosis: transport properties and regulatory roles. Plant Cell Environ. 30, 310–322. doi: 10.1111/j.1365-3040.2006.01617.x
Jiang, Y., Wang, W., Xie, Q., Liu, N., Liu, L., Wang, D., et al. (2017). Plants transfer lipids to sustain colonization by mutualistic mycorrhizal and parasitic fungi. Science 356, 1172–1175. doi: 10.1126/science.aam9970
Jin, H., Germida, J. J., Walley, F. L. (2013). Suppressive effects of seed-applied fungicides on arbuscular mycorrhizal fungi (AMF) differ with fungicide mode of action and AMF species. Appl. Soil Ecol. 72, 22–30. doi: 10.1016/j.apsoil.2013.05.013
Keymer, A., Pimprikar, P., Wewer, V., Huber, C., Brands, M., Bucerius, S. L., et al. (2017). Lipid transfer from plants to arbuscular mycorrhiza fungi. eLife 6, e29107. doi: 10.7554/eLife.29107
Kizawa, K., Aono, T., Ohtomo, R. (2016). PHO8 gene coding alkaline phosphatase of Saccharomyces cerevisiae is involved in polyphosphate metabolism. J. Gen. Appl. Microbiol. 62, 297–302. doi: 10.2323/jgam.2016.05.006
Kobae, Y., Hata, S. (2010). Dynamics of periarbuscular membranes visualized with a fluorescent phosphate transporter in arbuscular mycorrhizal roots of rice. Plant Cell Physiol. 51, 341–353. doi: 10.1093/pcp/pcq013
Kokkoris, V., Stefani, F., Dalpé, Y., Dettman, J., Corradi, N. (2020). Nuclear dynamics in the arbuscular mycorrhizal fungi. Trends Plant Sci. 25, 765–778. doi: 10.1016/j.tplants.2020.05.002
Kong, Q., Yuan, J., Niu, P., Xie, J., Jiang, W., Huang, Y., et al. (2014). Screening suitable reference genes for normalization in reverse transcription quantitative real-time PCR analysis in melon. PloS One 9, e87197. doi: 10.1371/journal.pone.0087197
Lammers, P. J., Jun, J., Abubaker, J., Arreola, R., Gopalan, A., Bago, B., et al. (2001). The glyoxylate cycle in an arbuscular mycorrhizal fungus. Carbon flux and gene expression. Plant Physiol. 127, 1287–1298. doi: 10.1104/pp.010375
Liu, W., Zhang, Y., Jiang, S., Deng, Y., Christie, P., Murray, P. J., et al. (2016). Arbuscular mycorrhizal fungi in soil and roots respond differently to phosphorus inputs in an intensively managed calcareous agricultural soil. Sci. Rep. 6, 24902. doi: 10.1038/srep24902
Lorant, A., Ross-Ibarra, J., Tenaillon, M. (2020). “Genomics of Long- and Short-Term Adaptation in Maize and Teosintes,” in Statistical Population Genomics. Ed. Dutheil, J. Y. (Springer US, New York, NY), 289–311. doi: 10.1007/978-1-0716-0199-0_12
Luginbuehl, L. H., Menard, G. N., Kurup, S., Van Erp, H., Radhakrishnan, G. V., Breakspear, A., et al. (2017). Fatty acids in arbuscular mycorrhizal fungi are synthesized by the host plant. Science 356, 1175–1178. doi: 10.1126/science.aan0081
Lutz, S., Bodenhausen, N., Hess, J., Valzano-Held, A., Waelchli, J., Deslandes-Hérold, G., et al. (2023). Soil microbiome indicators can predict crop growth response to large-scale inoculation with arbuscular mycorrhizal fungi. Nat. Microbiol. 8, 2277–2289. doi: 10.1038/s41564-023-01520-w
Mäder, P., Edenhofer, S., Boller, T., Wiemken, A., Niggli, U. (2000). Arbuscular mycorrhizae in a long-term field trial comparing low-input (organic, biological) and high-input (conventional) farming systems in a crop rotation. Biol. Fertil. Soils 31, 150–156. doi: 10.1007/s003740050638
Malar C, M., Krüger, M., Krüger, C., Wang, Y., Stajich, J. E., Keller, J., et al. (2021). The genome of Geosiphon pyriformis reveals ancestral traits linked to the emergence of the arbuscular mycorrhizal symbiosis. Curr. Biol. CB 31, 1570–1577.e4. doi: 10.1016/j.cub.2021.01.058
Malbreil, M., Tisserant, E., Martin, F., Roux, C. (2014). “Chapter Nine - Genomics of Arbuscular Mycorrhizal Fungi: out of the Shadows,” in Advances in Botanical Research. 70, 259–290. doi: 10.1016/B978-0-12-397940-7.00009-4
Malcová, R., Albrechtová, J., Vosátka, M. (2001). The role of the extraradical mycelium network of arbuscular mycorrhizal fungi on the establishment and growth of Calamagrostis epigejos in industrial waste substrates. Appl. Soil Ecol. 18, 129–142. doi: 10.1016/S0929-1393(01)00156-1
Martín-Robles, N., Lehmann, A., Seco, E., Aroca, R., Rillig, M. C., Milla, R. (2018). Impacts of domestication on the arbuscular mycorrhizal symbiosis of 27 crop species. New Phytol. 218, 322–334. doi: 10.1111/nph.14962
Mercy, L., Lucic-Mercy, E., Nogales, A., Poghosyan, A., Schneider, C., Arnholdt-Schmitt, B. (2017). A functional approach towards understanding the role of the mitochondrial respiratory chain in an endomycorrhizal symbiosis. Front. Plant Sci. 8. doi: 10.3389/fpls.2017.00417
Millar, N. S., Bennett, A. E. (2016). Stressed out symbiotes: hypotheses for the influence of abiotic stress on arbuscular mycorrhizal fungi. Oecologia 182, 625–641. doi: 10.1007/s00442-016-3673-7
Mohanapriya, G., Bharadwaj, R., Noceda, C., Costa, J. H., Kumar, S. R., Sathishkumar, R., et al. (2019). Alternative oxidase (AOX) senses stress levels to coordinate auxin-induced reprogramming from seed germination to somatic embryogenesis—A role relevant for seed vigor prediction and plant robustness. Front. Plant Sci. 10. doi: 10.3389/fpls.2019.01134
Mojica, E. A., Kültz, D. (2022). Physiological mechanisms of stress-induced evolution. J. Exp. Biol. 225, jeb243264. doi: 10.1242/jeb.243264
Morin, E., Miyauchi, S., San Clemente, H., Chen, E. C. H., Pelin, A., de la Providencia, I., et al. (2019). Comparative genomics of Rhizophagus irregularis, R. cerebriforme, R. diaphanus and Gigaspora rosea highlights specific genetic features in Glomeromycotina. New Phytol. 222, 1584–1598. doi: 10.1111/nph.15687
Mosse, B. (1973). Plant growth responses to vesicular-arbuscular mycorrhiza. New Phytol. 72, 127–136. doi: 10.1111/j.1469-8137.1973.tb02017.x
Nagy, R., Drissner, D., Amrhein, N., Jakobsen, I., Bucher, M. (2009). Mycorrhizal phosphate uptake pathway in tomato is phosphorus-repressible and transcriptionally regulated. New Phytol. 181, 950–959. doi: 10.1111/j.1469-8137.2008.02721.x
Nagy, R., Vasconcelos, M. J. V., Zhao, S., McElver, J., Bruce, W., Amrhein, N., et al. (2006). Differential regulation of five pht1 phosphate transporters from maize (Zea mays L.). Plant Biol. 8, 186–197. doi: 10.1055/s-2005-873052
Nouri, E., Breuillin-Sessoms, F., Feller, U., Reinhardt, D. (2014). Phosphorus and nitrogen regulate arbuscular mycorrhizal symbiosis in petunia hybrida. PloS One 9, e90841. doi: 10.1371/journal.pone.0090841
Nussaume, L., Kanno, S., Javot, H., Marin, E., Nakanishi, T. M., Thibaud, M.-C. (2011). Phosphate import in plants: focus on the PHT1 transporters. Front. Plant Sci. 2. doi: 10.3389/fpls.2011.00083
Oehl, F., Sieverding, E., Ineichen, K., Mäder, P., Boller, T., Wiemken, A. (2003). Impact of land use intensity on the species diversity of arbuscular mycorrhizal fungi in agroecosystems of Central Europe. Appl. Environ. Microbiol. 69, 2816–2824. doi: 10.1128/AEM.69.5.2816-2824.2003
Oliveira, R. S., Boyer, L. R., Carvalho, M. F., Jeffries, P., Vosátka, M., Castro, P. M. L., et al. (2010). Genetic, phenotypic and functional variation within a Glomus geosporum isolate cultivated with or without the stress of a highly alkaline anthropogenic sediment. Appl. Soil Ecol. 45, 39–48. doi: 10.1016/j.apsoil.2010.01.008
Olsson, P. A., van Aarle, I. M., Allaway, W. G., Ashford, A. E., Rouhier, H. (2002). Phosphorus effects on metabolic processes in monoxenic arbuscular mycorrhiza cultures. Plant Physiol. 130, 1162–1171. doi: 10.1104/pp.009639
Ortíz, J., Sanhueza, C., Romero-Munar, A., Hidalgo-Castellanos, J., Castro, C., Bascuñán-Godoy, L., et al. (2020). In vivo metabolic regulation of alternative oxidase under nutrient deficiency—Interaction with arbuscular mycorrhizal fungi and rhizobium bacteria. Int. J. Mol. Sci. 21, 4201. doi: 10.3390/ijms21124201
Paaby, A. B., Rockman, M. V. (2014). Cryptic genetic variation: evolution’s hidden substrate. Nat. Rev. Genet. 15, 247–258. doi: 10.1038/nrg3688
Parádi, I., van Tuinen, D., Morandi, D., Ochatt, S., Robert, F., Jacas, L., et al. (2010). Transcription of two blue copper-binding protein isogenes is highly correlated with arbuscular mycorrhizal development in medicago truncatula. Mol. Plant Microbe Interact. 23, 1175–1183. doi: 10.1094/MPMI-23-9-1175
Peña, R., Robbins, C., Corella, J. C., Thuita, M., Masso, C., Vanlauwe, B., et al. (2020). Genetically different isolates of the arbuscular mycorrhizal fungus rhizophagus irregularis induce differential responses to stress in cassava. Front. Plant Sci. 11. doi: 10.3389/fpls.2020.596929
Peña Venegas, R. A., Lee, S.-J., Thuita, M., Mlay, D. P., Masso, C., Vanlauwe, B., et al. (2021). The phosphate inhibition paradigm: host and fungal genotypes determine arbuscular mycorrhizal fungal colonization and responsiveness to inoculation in cassava with increasing phosphorus supply. Front. Plant Sci. 12. doi: 10.3389/fpls.2021.693037
Pfaffl, M. W., Tichopad, A., Prgomet, C., Neuvians, T. P. (2004). Determination of stable housekeeping genes, differentially regulated target genes and sample integrity: BestKeeper – Excel-based tool using pair-wise correlations. Biotechnol. Lett. 26, 509–515. doi: 10.1023/B:BILE.0000019559.84305.47
Pinsky, M. L., Kroeker, K. J., Barshis, D. J., Logan, C. A. (2013). “Marine Conservation in a Changing Climate,” in Encyclopedia of Biodiversity (Second Edition). Ed. Levin, S. A. (Academic Press, Waltham), 32–44. doi: 10.1016/B978-0-12-384719-5.00336-1
Pumplin, N., Harrison, M. J. (2009). Live-cell imaging reveals periarbuscular membrane domains and organelle location in Medicago truncatula roots during arbuscular mycorrhizal symbiosis. Plant Physiol. 151, 809–819. doi: 10.1104/pp.109.141879
Qin, M., Li, L., Miranda, J.-P., Tang, Y., Song, B., Oosthuizen, M. K., et al. (2022). Experimental duration determines the effect of arbuscular mycorrhizal fungi on plant biomass in pot experiments: A meta-analysis. Front. Plant Sci. 13. doi: 10.3389/fpls.2022.1024874
Racca, S., Gras, D. E., Canal, M. V., Ferrero, L. V., Rojas, B. E., Figueroa, C. M., et al. (2022). Cytochrome c and the transcription factor ABI4 establish a molecular link between mitochondria and ABA-dependent seed germination. New Phytol. 235, 1780–1795. doi: 10.1111/nph.18287
Rausch, C., Daram, P., Brunner, S., Jansa, J., Laloi, M., Leggewie, G., et al. (2001). A phosphate transporter expressed in arbuscule-containing cells in potato. Nature 414, 462–465. doi: 10.1038/35106601
Requena, N. (2005). Measuring quality of service: phosphate ‘à la carte’ by arbuscular mycorrhizal fungi. New Phytol. 168, 268–271. doi: 10.1111/j.1469-8137.2005.01563.x
Rich, M. K., Nouri, E., Courty, P.-E., Reinhardt, D. (2017). Diet of arbuscular mycorrhizal fungi: bread and butter? Trends Plant Sci. 22, 652–660. doi: 10.1016/j.tplants.2017.05.008
Riedo, J., Wettstein, F. E., Rösch, A., Herzog, C., Banerjee, S., Büchi, L., et al. (2021). Widespread occurrence of pesticides in organically managed agricultural soils—the ghost of a conventional agricultural past? Environ. Sci. Technol. 55, 2919–2928. doi: 10.1021/acs.est.0c06405
Rizzuto, R., Sandonà, D., Brini, M., Capaldi, R. A., Bisson, R. (1991). The most conserved nuclear-encoded polypeptide of cytochrome c oxidase is the putative zinc-binding subunit: primary structure of subunit V from the slime mold Dictyostelium discoideum. Biochim. Biophys. Acta 1129, 100–104. doi: 10.1016/0167-4781(91)90220-g
Rouphael, Y., Franken, P., Schneider, C., Schwarz, D., Giovannetti, M., Agnolucci, M., et al. (2015). Arbuscular mycorrhizal fungi act as biostimulants in horticultural crops. Sci. Hortic. 196, 91–108. doi: 10.1016/j.scienta.2015.09.002
Rowe, H. I., Brown, C. S., Claassen, V. P. (2007). Comparisons of mycorrhizal responsiveness with field soil and commercial inoculum for six native montane species and bromus tectorum. Restor. Ecol. 15, 44–52. doi: 10.1111/j.1526-100X.2006.00188.x
Roy, J., Reichel, R., Brüggemann, N., Hempel, S., Rillig, M. C. (2017). Succession of arbuscular mycorrhizal fungi along a 52-year agricultural recultivation chronosequence. FEMS Microbiol. Ecol. 93, fix102. doi: 10.1093/femsec/fix102
Saha, B., Borovskii, G., Panda, S. K. (2016). Alternative oxidase and plant stress tolerance. Plant Signal. Behav. 11, e1256530. doi: 10.1080/15592324.2016.1256530
Savary, R., Dupuis, C., Masclaux, F. G., Mateus, I. D., Rojas, E. C., Sanders, I. R. (2020). Genetic variation and evolutionary history of a mycorrhizal fungus regulate the currency of exchange in symbiosis with the food security crop cassava. ISME J. 14, 1333–1344. doi: 10.1038/s41396-020-0606-6
Savary, R., Masclaux, F. G., Wyss, T., Droh, G., Cruz Corella, J., MaChado, A. P., et al. (2018). A population genomics approach shows widespread geographical distribution of cryptic genomic forms of the symbiotic fungus Rhizophagus irregularis. ISME J. 12, 17–30. doi: 10.1038/ismej.2017.153
Schwartz, M. W., Hoeksema, J. D., Gehring, C. A., Johnson, N. C., Klironomos, J. N., Abbott, L. K., et al. (2006). The promise and the potential consequences of the global transport of mycorrhizal fungal inoculum. Ecol. Lett. 9, 501–515. doi: 10.1111/j.1461-0248.2006.00910.x
Selinski, J., Scheibe, R., Day, D. A., Whelan, J. (2018). Alternative oxidase is positive for plant performance. Trends Plant Sci. 23, 588–597. doi: 10.1016/j.tplants.2018.03.012
Sharifi, M., Ghorbanli, M., Ebrahimzadeh, H. (2007). Improved growth of salinity-stressed soybean after inoculation with salt pre-treated mycorrhizal fungi. J. Plant Physiol. 164, 1144–1151. doi: 10.1016/j.jplph.2006.06.016
Shi, J., Wang, X., Wang, E. (2023). Mycorrhizal symbiosis in plant growth and stress adaptation: from genes to ecosystems. Annu. Rev. Plant Biol. 74, 569–607. doi: 10.1146/annurev-arplant-061722-090342
Shi, J., Zhao, B., Zheng, S., Zhang, X., Wang, X., Dong, W., et al. (2021). A phosphate starvation response-centered network regulates mycorrhizal symbiosis. Cell 184, 5527–5540.e18. doi: 10.1016/j.cell.2021.09.030
Singh, A. K., Zhu, X., Chen, C., Wu, J., Yang, B., Zakari, S., et al. (2022). The role of glomalin in mitigation of multiple soil degradation problems. Crit. Rev. Environ. Sci. Technol. 52, 1604–1638. doi: 10.1080/10643389.2020.1862561
Sluse, F. E., Jarmuszkiewicz, W. (1998). Alternative oxidase in the branched mitochondrial respiratory network: an overview on structure, function, regulation, and role. Braz. J. Med. Biol. Res. Rev. Bras. Pesqui. Medicas E Biol. 31, 733–747. doi: 10.1590/s0100-879x1998000600003
Smith, F. A., Smith, S. E. (2011). What is the significance of the arbuscular mycorrhizal colonisation of many economically important crop plants? Plant Soil 348, 63–79. doi: 10.1007/s11104-011-0865-0
Smith, S. E., Smith, F. A., Jakobsen, I. (2003). Mycorrhizal fungi can dominate phosphate supply to plants irrespective of growth responses. Plant Physiol. 133, 16–20. doi: 10.1104/pp.103.024380
Smith, S. E., Smith, F. A., Jakobsen, I. (2004). Functional diversity in arbuscular mycorrhizal (AM) symbioses: the contribution of the mycorrhizal P uptake pathway is not correlated with mycorrhizal responses in growth or total P uptake. New Phytol. 162, 511–524. doi: 10.1111/j.1469-8137.2004.01039.x
Soti, P. G., Rugg, S., Racelis, A. (2016). Potential of cover crops in promoting mycorrhizal diversity and soil quality in organic farms. J. Agric. Sci. 8, 42. doi: 10.5539/jas.v8n8p42
Tedersoo, L., Sánchez-Ramírez, S., Kõljalg, U., Bahram, M., Döring, M., Schigel, D., et al. (2018). High-level classification of the Fungi and a tool for evolutionary ecological analyses. Fungal Divers. 90, 135–159. doi: 10.1007/s13225-018-0401-0
Thomazella, D. P. T., Teixeira, P. J. P. L., Oliveira, H. C., Saviani, E. E., Rincones, J., Toni, I. M., et al. (2012). The hemibiotrophic cacao pathogen Moniliophthora perniciosa depends on a mitochondrial alternative oxidase for biotrophic development. New Phytol. 194, 1025–1034. doi: 10.1111/j.1469-8137.2012.04119.x
Thomson, B. D., Robson, A. D., Abbott, L. K. (1986). Effects of phosphorus on the formation of mycorrhizas by gigaspora calospora and glomus fasciculatum in relation to root carbohydrates. New Phytol. 103, 751–765. doi: 10.1111/j.1469-8137.1986.tb00850.x
Tisserant, B., Gianinazzi-Pearson, V., Gianinazzi, S., Gollotte, A. (1993). In planta histochemical staining of fungal alkaline phosphatase activity for analysis of efficient arbuscular mycorrhizal infections. Mycol. Res. 97, 245–250. doi: 10.1016/S0953-7562(09)80248-7
Tisserant, E., Kohler, A., Dozolme-Seddas, P., Balestrini, R., Benabdellah, K., Colard, A., et al. (2012). The transcriptome of the arbuscular mycorrhizal fungus Glomus intraradices (DAOM 197198) reveals functional tradeoffs in an obligate symbiont. New Phytol. 193, 755–769. doi: 10.1111/j.1469-8137.2011.03948.x
Tisserant, E., Malbreil, M., Kuo, A., Kohler, A., Symeonidi, A., Balestrini, R., et al. (2013). Genome of an arbuscular mycorrhizal fungus provides insight into the oldest plant symbiosis. Proc. Natl. Acad. Sci. U. S. A. 110, 20117–20122. doi: 10.1073/pnas.1313452110
Trouvelot, A., Kough, J. L., Gianinazzi-Pearson, V. (1986). “Mesure du taux de mycorhization VA d’un système radiculaire. Recherche de méthode d’estimation ayant une signification fonctionnelle,” in Physiological and genetical aspects of mycorrhizae: proceedings of the 1st european symposium on mycorrhizae (Paris: INRA Press), 217–221.
Van Belle, H. (1972). Kinetics and inhibition of alkaline phosphatases from canine tissues. Biochim. Biophys. Acta 289, 158–168. doi: 10.1016/0005-2744(72)90118-0
Vandesompele, J., De Preter, K., Pattyn, F., Poppe, B., Van Roy, N., De Paepe, A., et al. (2002). Accurate normalization of real-time quantitative RT-PCR data by geometric averaging of multiple internal control genes. Genome Biol. 3, research0034.1. doi: 10.1186/gb-2002-3-7-research0034
Verzeaux, J., Nivelle, E., Roger, D., Hirel, B., Dubois, F., Tetu, T. (2017). Spore density of arbuscular mycorrhizal fungi is fostered by six years of a no-till system and is correlated with environmental parameters in a silty loam soil. Agronomy 7, 38. doi: 10.3390/agronomy7020038
Vierheilig, H., Bago, B., Albrecht, C., Poulin, M.-J., Piché, Y. (1998). “Flavonoids and Arbuscular-Mycorrhizal Fungi,” in Flavonoids in the Living System. Eds. Manthey, J. A., Buslig, B. S. (Springer US, Boston, MA), 9–33. doi: 10.1007/978-1-4615-5335-9_2
Vivas, A., Marulanda, A., Gómez, M., Barea, J. M., Azcón, R. (2003). Physiological characteristics (SDH and ALP activities) of arbuscular mycorrhizal colonization as affected by Bacillus thuringiensis inoculation under two phosphorus levels. Soil Biol. Biochem. 35, 987–996. doi: 10.1016/S0038-0717(03)00161-5
Vosátka, M., Albrechtová, J., Patten, R. (2008). “The International Market Development for Mycorrhizal Technology,” in Mycorrhiza. Ed. Varma, A. (Springer Berlin Heidelberg, Berlin, Heidelberg), 419–438. doi: 10.1007/978-3-540-78826-3_21
Walder, F., Boller, T., Wiemken, A., Courty, P.-E. (2016). Regulation of plants’ phosphate uptake in common mycorrhizal networks: Role of intraradical fungal phosphate transporters. Plant Signal. Behav. 11, e1131372. doi: 10.1080/15592324.2015.1131372
Wang, P., Snijders, R., Kohlen, W., Liu, J., Bisseling, T., Limpens, E. (2021). Medicago SPX1 and SPX3 regulate phosphate homeostasis, mycorrhizal colonization, and arbuscule degradation. Plant Cell 33, 3470–3486. doi: 10.1093/plcell/koab206
Wang, X., Zhao, S., Bücking, H. (2016). Arbuscular mycorrhizal growth responses are fungal specific but do not differ between soybean genotypes with different phosphate efficiency. Ann. Bot. 118, 11–21. doi: 10.1093/aob/mcw074
Wipf, D., Krajinski, F., van Tuinen, D., Recorbet, G., Courty, P.-E. (2019). Trading on the arbuscular mycorrhiza market: from arbuscules to common mycorrhizal networks. New Phytol. 223, 1127–1142. doi: 10.1111/nph.15775
Xavier, L. J. C., Boyetchko, S. M. (2002). “Arbuscular mycorrhizal fungi as biostimulants and bioprotectants of crops,” in Applied Mycology and Biotechnology. 2, 311–340. doi: 10.1016/S1874-5334(02)80015-6
Xie, X., Lai, W., Che, X., Wang, S., Ren, Y., Hu, W., et al. (2022). A SPX domain-containing phosphate transporter from Rhizophagus irregularis handles phosphate homeostasis at symbiotic interface of arbuscular mycorrhizas. New Phytol. 234, 650–671. doi: 10.1111/nph.17973
Xie, X., Lin, H., Peng, X., Xu, C., Sun, Z., Jiang, K., et al. (2016). Arbuscular mycorrhizal symbiosis requires a phosphate transceptor in the gigaspora margarita fungal symbiont. Mol. Plant 9, 1583–1608. doi: 10.1016/j.molp.2016.08.011
Xu, T., Yao, F., Liang, W.-S., Li, Y.-H., Li, D.-R., Wang, H., et al. (2012). Involvement of alternative oxidase in the regulation of growth, development, and resistance to oxidative stress of Sclerotinia sclerotiorum. J. Microbiol. Seoul Korea 50, 594–602. doi: 10.1007/s12275-012-2015-7
Yu, L.-L., Liu, Y., Peng, Y., Zhu, F., Xu, F. (2021). Overexpression of cyanoalanine synthase 1 improves germinability of tobacco seeds under salt stress conditions. Environ. Exp. Bot. 182, 104332. doi: 10.1016/j.envexpbot.2020.104332
Yu, J., Wu, S., Sun, H., Wang, X., Tang, X., Guo, S., et al. (2023). CuGenDBv2: an updated database for cucurbit genomics. Nucleic Acids Res. 51, D1457–D1464. doi: 10.1093/nar/gkac921
Zangaro, W., Torezan, J. M. D., Rostirola, L. V., de Souza, P. B., Nogueira, M. A. (2015). Influence of mycorrhizas, organic substrates and container volumes on the growth of Heliocarpus popayanensis Kunth. CERNE 21, 395–403. doi: 10.1590/01047760201521031335
Zhang, Q., Blaylock, L. A., Harrison, M. J. (2010). Two Medicago truncatula half-ABC transporters are essential for arbuscule development in arbuscular mycorrhizal symbiosis. Plant Cell 22, 1483–1497. doi: 10.1105/tpc.110.074955
Zhang, S., Nie, Y., Fan, X., Wei, W., Chen, H., Xie, X., et al. (2023). A transcriptional activator from Rhizophagus irregularis regulates phosphate uptake and homeostasis in AM symbiosis during phosphorous starvation. Front. Microbiol. 13. doi: 10.3389/fmicb.2022.1114089
Keywords: phosphate, mycorrhizal symbiosis, short-term adaptation, Rhizoglomus irregulare, biotechnology
Citation: Lucic-Mercy E, Mercy L, Jeschke A, Schneider C and Franken P (2024) Short-term artificial adaptation of Rhizoglomus irregulare to high phosphate levels and its implications for fungal-plant interactions: phenotypic and transcriptomic insights. Front. Plant Sci. 15:1385245. doi: 10.3389/fpls.2024.1385245
Received: 12 February 2024; Accepted: 08 April 2024;
Published: 23 April 2024.
Edited by:
Raffaella Balestrini, National Research Council (CNR), ItalyReviewed by:
Catarina Campos, University of Evora, PortugalKarin E. Groten, Max Planck Institute for Chemical Ecology, Germany
Copyright © 2024 Lucic-Mercy, Mercy, Jeschke, Schneider and Franken. This is an open-access article distributed under the terms of the Creative Commons Attribution License (CC BY). The use, distribution or reproduction in other forums is permitted, provided the original author(s) and the copyright owner(s) are credited and that the original publication in this journal is cited, in accordance with accepted academic practice. No use, distribution or reproduction is permitted which does not comply with these terms.
*Correspondence: Eva Lucic-Mercy, bHVjaWNAaW5vcS5kZQ==