- 1Centro de Biotecnología y Genómica de Plantas, Universidad Politécnica de Madrid (UPM) - Instituto Nacional de Investigación y Tecnología Agraria y Alimentaria (INIA/CSIC), Madrid, Spain
- 2Departamento de Biotecnología-Biología Vegetal, Escuela Técnica Superior de Ingeniería Agronómica, Alimentaría y de Biosistemas, UPM, Madrid, Spain
- 3Center of Excellence for Plant Environment Interactions (CEPEI), Madrid, Spain
Arabidopsis thaliana Mitogen-activated protein Kinase Phosphatase 1 (MKP1) negatively balances production of reactive oxygen species (ROS) triggered by Microbe-Associated Molecular Patterns (MAMPs) through uncharacterized mechanisms. Accordingly, ROS production is enhanced in mkp1 mutant after MAMP treatment. Moreover, mkp1 plants show a constitutive activation of immune responses and enhanced disease resistance to pathogens with distinct colonization styles, like the bacterium Pseudomonas syringae pv. tomato DC3000, the oomycete Hyaloperonospora arabidopsidis Noco2 and the necrotrophic fungus Plectosphaerella cucumerina BMM. The molecular basis of this ROS production and broad-spectrum disease resistance controlled by MKP1 have not been determined. Here, we show that the enhanced ROS production in mkp1 is not due to a direct interaction of MKP1 with the NADPH oxidase RBOHD, nor is it the result of the catalytic activity of MKP1 on RBHOD phosphorylation sites targeted by BOTRYTIS INDUCED KINASE 1 (BIK1) protein, a positive regulator of RBOHD-dependent ROS production. The analysis of bik1 mkp1 double mutant phenotypes suggested that MKP1 and BIK1 targets are different. Additionally, we showed that phosphorylation residues stabilizing MKP1 are essential for its functionality in immunity. To further decipher the molecular basis of disease resistance responses controlled by MKP1, we generated combinatory lines of mkp1-1 with plants impaired in defensive pathways required for disease resistance to pathogen: cyp79B2 cyp79B3 double mutant defective in synthesis of tryptophan-derived metabolites, NahG transgenic plant that does not accumulate salicylic acid, aba1-6 mutant impaired in abscisic acid (ABA) biosynthesis, and abi1 abi2 hab1 triple mutant impaired in proteins described as ROS sensors and that is hypersensitive to ABA. The analysis of these lines revealed that the enhanced resistance displayed by mkp1-1 is altered in distinct mutant combinations: mkp1-1 cyp79B2 cyp79B3 fully blocked mkp1-1 resistance to P. cucumerina, whereas mkp1-1 NahG displays partial susceptibility to H. arabidopsidis, and mkp1-1 NahG, mkp1-1 aba1-6 and mkp1-1 cyp79B2 cyp79B3 showed compromised resistance to P. syringae. These results suggest that MKP1 is a component of immune responses that does not directly interact with RBOHD but rather regulates the status of distinct defensive pathways required for disease resistance to pathogens with different lifestyles.
1 Introduction
Plant defense responses are orchestrated through complex signaling networks that involve both early and sustained response mechanisms, collectively contributing to the activation of different defense layers (Yuan et al., 2021). At the forefront of this defense arsenal are Pattern Recognition Receptors (PRRs) located at the plasma membrane of plant cells. These PRRs, mainly Receptor Kinases (RKs) and Receptor-Like Proteins (RLPs), sense the presence of invading pathogens in two ways: i) through the direct recognition of conserved molecules present in the pathogens, called Microbe-Associated Molecular Patterns (MAMPs; Bender and Zipfel, 2023); ii) through the recognition of plant derived-molecules, called Damage-Associated Molecular Patterns (DAMPs), released or synthesized after pathogen attack (De Lorenzo and Cervone, 2022). The engagement of PRRs initiates Pattern Triggered Immunity (PTI) responses that includes a cascade of events: apoplast alkalinization, cytoplasmic calcium influxes, reactive oxygen species (ROS) production by NADPH oxidases, phosphorylation cascades triggered by Mitogen-Activated Protein Kinases (MPKs) and Calcium Dependent protein Kinase (CPKs), and transcriptional reprogramming (DeFalco and Zipfel, 2021). The coordination of these signaling events leads to the synthesis of antimicrobial compounds, like antimicrobial peptides and metabolites (e.g. Tryptophan (Trp)-derived indol-glucosinolates), reinforcement of the cell wall, and other defense-related processes that collectively contribute to restrict pathogen colonization. Additionally, intracellular nucleotide-binding domain and leucine-rich repeat-containing receptors (NLRs) recognize pathogen derived effectors, further promoting the activation of these defenses through a different layer of disease resistance mechanism that is termed Effector-Triggered Immunity (ETI; Ngou et al., 2022).
Despite the necessity of robust activation of defense responses for effective plant disease resistance, there is a delicate control of the intensity and long-lasting of these responses to avoid an over activation of PTI/ETI. Induction of defense responses can compromise the normal development of the plant, impacting growth and reproduction (Huot et al., 2014). Excessive or prolonged activation of defense mechanisms can lead to resource allocation away from normal physiological processes, potentially hindering the plant’s ability to thrive (Monson et al., 2022). Therefore, it is essential to limit the induction of defenses to prevent detrimental effects on the overall fitness of the plant. Different mechanisms have been described to contribute to limit the induction of defensive response, like dephosphorylation of activated proteins by phosphatases, endocytosis of PRRs, or ubiquitination and degradation of activated proteins (Beck et al., 2012; Couto et al., 2016; Zhang and Zeng, 2020).
One critical aspect of the regulation of defense responses is the control of ROS production. ROS are produced during early PTI responses and are potentiated through ETI to a second wave with more sustained ROS accumulation (Castro et al., 2021; Wu et al., 2023). NADPH oxidases, called in plants RESPIRATORY BUST OXIDASE HOMOLOGS (RBOHs), are the main enzymes that account for most of these pathogen dependent ROS production (Torres and Dangl, 2005). They are plasma membrane proteins that transfer electrons from cytosolic NADPH to apoplastic oxygen, resulting in the production of superoxide (O2-), which rapidly dismutates to hydrogen peroxide (H2O2). H2O2, a more stable ROS, modulates downstream cellular targets, largely by oxidizing redox-active cysteines and other amino acids, and by travelling through the apoplast, spreading the stress signal to various regions of the plant (Bleau and Spoel, 2021; Castro et al., 2021). While ROS play a vital role in signaling, their overproduction can lead to oxidative damage, negatively impacting cellular structures and functions (Mittler, 2017). Therefore, plants possess a battery of antioxidant and detoxifying enzymes that limit ROS accumulation (Das and Roychoudhury, 2014). Moreover, ROS production is tightly controlled making its accumulation transitory, not exceeding the necessary threshold for ROS effective regulatory function and avoiding ROS excessive accumulation due to their potentially damaging effects.
In the model plant Arabidopsis thaliana the NADPH oxidase RBOHD is the key oxidase responsible for most pathogen-induced ROS production (Torres et al., 2002). The activation of this oxidase has been well characterized and involves multiple posttranslational modifications mainly acting at the cytosolic N-terminal domain of the protein, which contains Ca2+ binding EF hands (Castro et al., 2021). Upon pathogen recognition, several kinases act in concert at this N-terminal domain to regulate RBOHD activation (Kadota et al., 2015; Castro et al., 2021; Wu et al., 2023). These kinases that phosphorylate RBOHD N-terminal include: i) receptor-like cytoplasmic kinases (RLCKs) like BOTRYTIS INDUCED KINASE 1 (BIK1; Kadota et al., 2014), which plays a preeminent role in RBOHD regulation, and the RESISTANCE TO PSEUDOMONAS SYRINGAE PV. MACULICOLA 1-INDUCED PROTEIN KINASE (RIPK; Li et al., 2021); ii) RKs, like DOES NOT RESPOND TO NUCLEOTIDES 1, (DORN1; Wang et al., 2018); iii) MPKs like SERINE/THREONINE KINASE 1 (SIK1; Zhang et al., 2018); iv) and the CALCIUM-DEPENDENT PROTEIN KINASE 5 (CPK5; Dubiella et al., 2013). The precise orchestration of RBOHD activation involves convergent phosphorylation events at some specific RBOHD Ser/Tyr residues (e.g. Ser343 and Ser347) by these kinases, contributing to the fine-tuning of ROS production in response to different stimuli (Wu et al., 2023). Furthermore, phosphorylation of C-terminal residues by receptors like CYSTEINE-RICH RECEPTOR KINASE 2 (CRK2) and persulfidation of specific Cys in the C-terminus also contribute to the activation of AtRBOHD-dependent ROS production, emphasizing the complexity and versatility of regulatory mechanisms governing plant immunity mediated by RBOHD (Kimura et al., 2020; Shen et al., 2020).
Contrary to activation, fewer mechanisms are known to negatively regulate RBOHD-activity and its de-phosphorylation. Prior pathogen elicitation, transcriptional and translational control could limit RBOHD protein level to restrict ROS production (Morales et al., 2016; George et al., 2023). Also, the ubiquitination mechanism mediated by the RLCK AvrPphB SUSCEPTIBLE1-LIKE 13 (PBL13) contributes to maintain the appropriate RBOHD levels at the plasma membrane at the resting state (Lee et al., 2020). PBL13 phosphorylation of the C-term of the protein drives its ubiquitination (Lee et al., 2020), leading to RBOHD degradation in the vacuole with the contribution of XYLEM CYSTEINE PEPTIDASE 1 (XCP1; Liu et al., 2023). Once defense signaling is engaged, two mechanisms could contribute to deactivate the active RBOHD and prevent excessive ROS production. Over-accumulation of ROS in the cytosol is sensed by QUIESCIN SULFHYDRYL OXIDASE HOMOLOG 1 (QSOX1), which interacts with and oxidizes S-nitrosoglutathione reductase AtGSNOR, elevating intracellular S-nitrosoglutathione (GSNO) levels (Chae et al., 2021). High GSNO levels can promote S-nitrosylation of Cys890 in RBOHD, which inactivates the oxidase (Yun et al., 2011). Also, a recent work documents the interaction of PHAGOCYTOSIS OXIDASE/BEM1P (PB1) DOMAIN-CONTAINING PROTEIN (PB1CP) with RBOHD to negative regulates MAMP-induced ROS production. PB1CP could negatively regulate the active oxidase by competing for binding with activating kinases, such as BIK1, and by promoting endocytosis, which could lead to the degradation of the oxidase (Goto et al., 2023).
Arabidopsis thaliana Mitogen-activated protein Kinase Phosphatase 1 (MKP1) regulates various cellular processes, including growth, development, and stress responses (Ulm et al., 2002; Bartels et al., 2009; Tamnanloo et al., 2018). This phosphatase appears to function by dephosphorylating and inactivating MPKs, showing a strong interaction with MPK3 and MPK6 (Ulm et al., 2002; Bartels et al., 2009) that are two positive regulators of immune responses, like PTI (Ren et al., 2002). In the context of Arabidopsis thaliana immunity, MKP1 has emerged as an important negative regulator of PTI and disease resistance (Anderson et al., 2011; Escudero et al., 2019). Consequently, Arabidopsis thaliana mkp1 mutant alleles display broad spectrum disease resistance, but also some detrimental chlorosis and necrosis in their leaves at later stages of plant development or under some stress conditions (Bartels et al., 2009; Jiang et al., 2017; Escudero et al., 2019). The mechanism explaining how MKP1 exerts these multifaced functions is unclear. MKP1 has been proposed to function as a repressor of salicylic acid (SA) synthesis and signaling (Bartels et al., 2009). We identified an mkp1-2 allele in a mutant suppressors screening of the highly susceptible agb1-2 plants, that are impaired in β subunit (AGB1) of the heterotrimeric G protein, which is a key regulator of immune responses in Arabidopsis thaliana (Trusov et al., 2010; Torres et al., 2013; Escudero et al., 2019). Notably, we found that MKP1 was a negative regulator of ROS production, since mkp1 mutants displayed enhanced ROS accumulation in response to the MAMPs flg22 and chitin. These results highlighted MKP1 importance in fine-tuning the balance of ROS production and immune responses activation. Therefore, we aimed to investigate if MKP1 has a direct role in the inactivation of RBOHD during the immune response. Moreover, to further characterize the distinct facets of plant defense regulated by MKP1, we set to characterize genetically the contribution of different signaling pathways to the immune function of MKP1 during Arabidopsis thaliana disease resistance responses to pathogens with different lifestyles.
2 Methods and materials
2.1 Plant material and growth conditions
All Arabidopsis thaliana lines used were in Columbia-0 (Col-0) background. mkp1-1 allele and line NahG mkp1-1 were obtained by R. Ulm (Bartels et al., 2009). The allele mkp1-2, that has a weaker phenotype in disease resistance than mkp1-1 allele, was described previously (Escudero et al, 2019). Lines expressing RBOHD under its own promoter (pRBOHD, abreviated pD), pD::FLAG::RBOHD, pD::FLAG::RBOHDS39A/S339A/S343A and pD::FLAG::RBOHDS343/S347 (all 4 in rbohD background) were obtained from Y. Kadota (Kadota et al., 2014, Kadota et al., 2019). Lines 35S::MYC::MKP1 and 35S::MYC::MKP14A (with the 4 putative regulatory phosphosites mutated to Ala) in mkp1-1 background were obtained from S. Peck (Jiang et al., 2017). bik1 mutant and pBIK::BIK1::HA line were provided by Cyril Zipfel (Kadota et al., 2014). Other lines used in this work were: agb1-2 (Ullah et al., 2003), mpk3-1 and mpk6-2 (Beckers et al., 2009), NahG (Delaney et al., 1994), cyp79B2 cyp79B3 (abbreviated in figures cyp79B2/B3), aba1-6 and abi1-2 abi2-2 hab1-1 (abbreviated abi1/2 hab1; Sánchez-Vallet et al., 2012).
Different transgenic lines and mutant combinations with mkp1-1 and mpk1-2 were generated by manual crosses and homozygous lines were identified by PCR. These include: pD::FLAG::RBOHD rbohD mkp1-1, pD::FLAG::RBOHDS39A/S339A/S343A rbohD mkp1-1, pD::FLAG::RBOHDS343/S347 rbohD mkp1-1, mkp1-2 bik1, mkp1-2 mpk3-1, mkp1-2 mpk6-2, mpk1-1 cyp79B2 cyp79B3, mkp1-1 aba1-6, mkp1-1 abi1/2 hab1-1, mkp1-1 NahG.
For soil-based plant growth, Arabidopsis thaliana seeds were sown, stratified at 4°C for 3 days in darkness, and moved to a grown chamber at 22°C, 80% relative humidity, under short day photoperiod (10-h light/14-h dark) and light intensity of 110-120 µE/m2/s. For in vitro plant growth, sterilized seeds were sown in ½ strength Murashige and Skoog (MS) medium containing 1% sucrose and subsequently stratified for 3 days in the dark at 4°C. Seeds were germinated at 22°C, and grown in a plant growth chamber under long day photoperiod (14-h light/10-h dark) and a light intensity of 150 µE/m2/s.
2.2 ROS measurement
H2O2 production was determined by a luminol-based assay. Four mm diameter disc leaves from 4-5 week-old Arabidopsis thaliana plants (n = 8) were collected in 96-wells white plates (Thermo Scientific) and incubated overnight in 100 μl of ROS Buffer (100 μg/ml peroxidase, Sigma; and 100 nM Luminol, Sigma). Luminescence was measured as RLU (relative light units) every minute over 40 minutes after induction with 1 μM flg22 or 50 μM chitohexaose (CHI6) in a Varioskan LUX luminometer (Thermo Scientific), as described in Torres et al. (2013).
2.3 Disease resistance assays
For Plectospharella cucumerina BMM (PcBMM) assays, 16-17-day-old plants were spray inoculated with a 4 × 106 fungal spores/ml suspension. For each genotype, 3 tubes with 8-10 plants were collected 4-5 days-post-inoculation (dpi) for DNA genomic extraction. PcBBM biomass was determined by qPCR, using specific primers FW 5-CAAGTACGTTCCCCGTGCCG-3 and RV 5-GAAGAGCTGGCCGAAGGGACC-3 for Pc β-TUBULIN. Samples were standardized against AtUBIQUITIN using specific primers FW 5- AAAGGACCTTCGGAGACTCCTTACG-3 and RV 5- GGTCAAGAATCGAACTTGAGGAGGTT-3. agb1-2 plants were used as a hypersusceptible control (Escudero et al., 2019).
For Pseudomonas syringae pv. tomato (Pto) DC3000 resistance assays, 21-day-old plants were spray inoculated with a bacterial suspension at a concentration of 3 x 108 colony forming units (cfu)/ml with 0.04% Silwet L-77. Four samples were collected per genotype at 4 dpi, each one containing 4 mm diameter discs. Material collected was ground and plated on Kings B media plates after serial dilution to count cfu. agb1-2 plants served as a hypersusceptible control (Torres et al., 2013).
For Hyaloperonospora arabidopsidis (Hpa) Noco2 resistance assays, 10-11-day-old seedlings were spray inoculated with a 4 × 104 spores/ml suspension. For each genotype, 3 tubes with 12-15 seedlings were collected 6 dpi for DNA genomic extraction. Hpa biomass was determined by qPCR, using specific primers FW 5-ATCTTCATCATGTAGTCGGTCAAGT-3 and RV 5-GTGTCGCACACTGTACCCATTTAT-3 for Hpa ACTIN. Samples were standardized against AtUBIQUITIN. NahG plants were used as a susceptible control (Delaney et al., 1994).
All these different pathology experiments were repeated at least three times with similar results.
2.4 Protein extraction, immunoprecipitation and immunodetection
Twelve-day-old Arabidopsis thaliana seedling (n = 15-20) growth in vitro, were treated with 500 nM flg22 or H2O for 10 minutes before fast-freezing in liquid nitrogen. Total proteins were manually ground in cold and incubated in extraction buffer [50 mM Tris-HCl pH 7.5, 200 mM NaCl, 10 mM NaF, 1 mM EDTA, 2 mM sodium orthovanadate, 1 mM sodium molybdate, 10% (v/v) glycerol, 0.1% (v/v) Tween-20, 1 mM 1,4-dithiothreitol, 1 mM phenylmethylsulfonyl fluoride and phosphatase inhibitor cocktail (Sigma-Aldrich)] for 1 hour at 4°C. Protein samples were quantified by a Bradford assay and subsequently normalized to a total protein concentration of 3-5 mg/ml.
For immunoprecipitation, samples were incubated with 20 µl of anti-MYC, anti-FLAG or anti-HA microbeads (µMACS, Miltenyi Biotec) for 2 hours. Proteins were then retained in µColumns and eluted in SDS loading buffer following Miltenyi Biotec instructions.
For co-immunoprecipitations and control loading, proteins were separated by electrophoresis using 4–15% Mini-PROTEAN TGX Gels (BIO-RAD) for 1 hour and 20 minutes at 120 V in running buffer (Laemmli). Proteins were transferred to nitrocellulose membranes using iBlot 3 Western Blot Transfer Device (Invitrogen). Membranes were blocked in TBST (Tris-buffered saline, 1% Tween-20) and 5% powder milk for 2 hours at room temperature, and subsequently incubated with anti-FLAG (from mouse; 1:2500 dilution; Merck Life Science S.L.U), anti-MYC (from mouse; 1:2500 dilution; Merck Life Science S.L.U.) or anti-HA (from rat; 1:5000 dilution; Milteny Biotec) antibodies in TBST with 3% milk at 4°C overnight. After three 10-minute washes with TBS, membranes were incubated with secondary antimouse-HRP (1:2500 dilution; Sigma Aldrich) or antirat-HRP (1:5000 dilution; Sigma Aldrich) antibodies in TBST with 3% powder milk for two hours at room temperature. After three 10-minute washes with TBS, proteins on the membranes were detected using ECL western blotting substrate (Thermo Fisher Scientific) and images were taken using iBright FL1000 Image System (Thermo Fisher Scientific). For loading controls, membranes were stained with Ponceau-S Red (Sigma Aldrich).
2.5 RNA extraction and quantification of gene expression
Collected plant material from uninfected and infected plants was fast-frozen using liquid nitrogen. After manual grinding, RNA extraction was performed using the RNeasy kit (QUIAGEN) including DNAse treatment following the supplier´s instructions. cDNA was generated using Transcriptor First Strand cDNA Synthesis kit (Roche Applied Science). For qRT-PCR analysis, reactions were performed with 40 ng of cDNA using SYBR green master mix system (Roche Applied Science). PCR conditions were as follows: 95°C for 10 min and then 45 cycles of 95°C for 15 seconds and 60°C for 1 minute. A dissociation stage was carried out at the end confirming only single products were generated. Primers used were as follows: for PR1: FW 5-CGTCTTTGTAGCTCTTGTAGGTGC-3 and RV 5-TGCCTGGTTGTGAACCCTTAG-3; for PDF1-2: FW 5-TTCTCTTTGCTGCTTTCGACG-3 and RV 5-GCATGCATTACTGTTTCCGCA-3; for PAD3: FW 5-CAACAACTCCACTCTTGCTCCC-3 and RV 5-CGACCCATCGCATAAACGTT-3; for CYP81F2: FW 5-TATTGTCCGCATGGTCACAGG-3 and RV 5-CCACTGTTGTCATTGATGTCCG-3. UBC21 (At5g25760) expression (FW 5-GCTCTTATCAAAGGACCTTCGG and RV 5- CGAACTTGAGGAGGTTGCAAAG) was used for normalizing each gene expression level using the Pfaffl method (Pfaffl, 2001).
2.6 Statical analyses
Data were analyzed using Student’s unpaired t test to calculate statistical significance of observed differences. Test results with p values less than 0.05 were considered statistically significant (*, p < 0.05; **, p < 0.005; ***, p < 0.001).
3 Results
3.1 MKP1 downregulates RBOHD activity through mechanisms independent of phosphosite targets of main RBOHD activating kinases
MKP1 was shown to function as a negative regulator of MAMP-dependent ROS production, since mkp1 mutant alleles (mkp1-1 and mkp1-2) displayed enhanced ROS accumulation in response to flg22 and chitin (Escudero et al., 2019). Considering that induction of RBOHD, the key oxidase responsible of these ROS, is mainly achieved by its phosphorylation by different kinases (Castro et al., 2021; Wu et al., 2023), we hypothesized that MKP1 could antagonize with some of these regulatory kinases and dephosphorylate RBOHD to limit ROS production. To assess this hypothesis, we introduced in mkp1-1 rbohD and rbohD genetic backgrounds constructs harboring RBOHD wild-type (WT) gene fused to a tag (FLAG) under the control of its own promoter (pD::FLAG-RBOHD line), or RBHOD carrying mutations in the phosphosites of RBOHD that are the targets of activating kinases during immunity: Ser (S) to Ala (A) mutations in three phosphosites that are the target of BIK1 during PTI responses (pD::FLAG::RBOHDS39A/S339A/S343A; Kadota et al., 2014; Li et al., 2014) and in two phosphosites that are also activated in PTI and ETI responses (pD::FLAG::RBOHDS343A/S347A; Kadota et al., 2019). We monitored H2O2 production with these transgenic lines in response to diverse MAMPs. As described previously (Escudero et al., 2019), mkp1-1 plants exhibited faster and enhanced H2O2 accumulation after bacterial MAMP flg22 treatment compared to WT Col-0 ecotype plants (Figure 1A). rbohD plants complemented with pD::FLAG-RBOHD exhibited higher ROS production than WT Col-0 plants upon flg22 treatment, that was not enhanced in mkp1-1 background (Figure 1A). As described previously (Kadota et al., 2019), rbohD plants complemented with pD::FLAG::RBOHDS39A/S339A/S343A displayed reduced ROS production and rbohD complemented with pD::FLAG::RBOHDS343A/S347A almost abolished all H2O2 production after flg22 treatment. Interestingly, under mkp1-1 background, ROS production was restored at mkp1-1 levels in these two genotypes (mkp1-1 rbohD pD::FLAG::RBOHDS39A/S339A/S343A and mkp1-1 rbohD pD::FLAG::RBOHDS343A/S347A) in comparison to plants in MKP1 WT background (Figure 1A and Supplementary Figure S1A). We also performed the same experiments after elicitation of all these genotypes with the fungal MAMP chitohexaose (CHI6), obtaining similar results, though the effect of mkp1-1 mutation on ROS levels was lower in pD::FLAG::RBOHDS343A/S347A than in pD::FLAG::RBOHDS39A/S339A/S343A (Figure 1B and Supplementary Figure S1B). The fact that the RBOHD lines with altered phosphosites displayed enhanced ROS production under mkp1-1 background compared to WT (MKP1) plants suggests that MKP1 could negatively regulate RBOHD by mediating dephosphorylation of some additional phosphosites to the ones that are targeted by BIK1 and the main regulatory kinases of RBOHD documented (Castro et al., 2021; Wu et al., 2023).
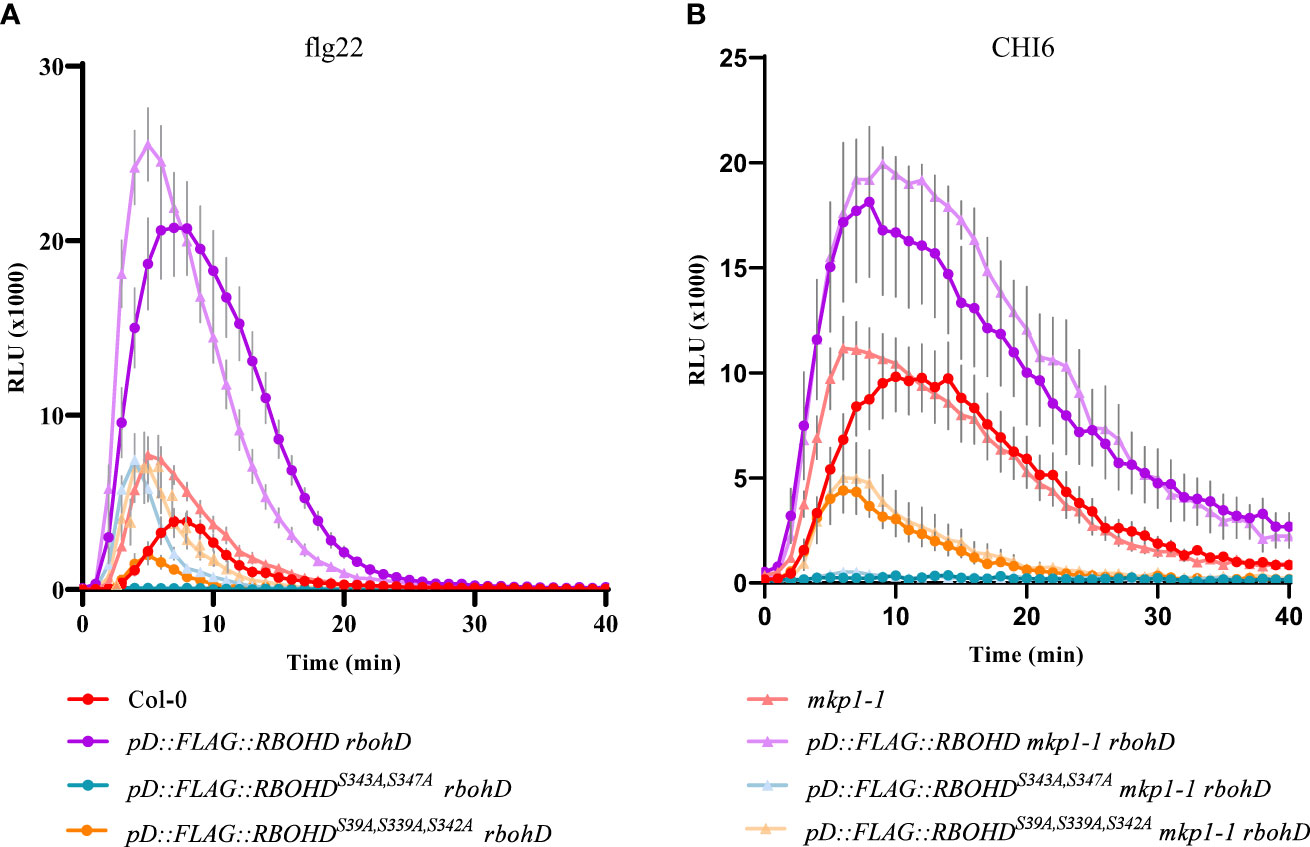
Figure 1 ROS production is increased in defective phosphosite RBOHD mutant alleles in mkp1-1 background. H2O2 production after 1 μM flg22 (A) or 50 μM Chitohexaose-CHI6 (B) measured in a luminol-based assay using leaf discs from 4-week-old plants of the listed genotypes. Arabidopsis thaliana genotypes tested include Col-0 (WT), and rbohD lines complemented with pD::FLAG::RBOHD, pD::FLAG::RBOHDS39A/S339A/S343A, and pD::FLAG::RBOHDS343/S347, under both WT (MKP1) and mkp1-1 mutant backgrounds. Relative light units (RLU) were measured over a period of 40 minutes. Values are average ± SE (n = 8). Data from one of three experiments performed that gave similar results. See Supplementary Figure S1 for additional information.
3.2 MKP1 does not directly interact and dephosphorylate RBOHD
It was previously shown that expression of 35S::MYC::MKP1 construct in mkp1-1 plants restored the susceptible phenotype against the bacterium P. syringae pv. tomato (Pto) DC3000 observed in WT plants (Jiang et al., 2017). Moreover, the use of 35S::MYC::MKP14A mkp1-1 plants, with the 4 putative regulatory phosphosites of MKP1 mutated to Ala (MKP14A), showed that these modifications are essential to restore the susceptible phenotype against Pto DC3000, suggesting that MKP1 gets stabilized through phosphorylation following treatment with MAMPs (Jiang et al., 2017). To evaluate the extension of this requirement to other patho-systems, we examined the growth of the necrotrophic fungus PcBMM on mkp1-1 lines complemented with 35S::MYC::MKP1 and 35S::MYC::MKP14A (Jiang et al., 2017). Quantification of PcBMM growth at 5 dpi revealed that MKP1 overexpression lines (35S::MYC::MKP1 mkp1-1) exhibited increased enhanced fungal growth compared to the WT, further confirming MKP1 as a negative regulator of disease resistance (Figure 2). MKP1 overexpression lines with mutated phosphosites (35S::MYC::MKP14A mkp1-1) displayed comparable resistance to mkp1-1, showing lower fungal growth than WT plants, and indicating that phosphorylation of MKP1 is required for its functional activity as negative modulator of the immune responses and disease resistance against the necrotrophic PcBMM, as shown previously for P. syringae (Jiang et al., 2017).
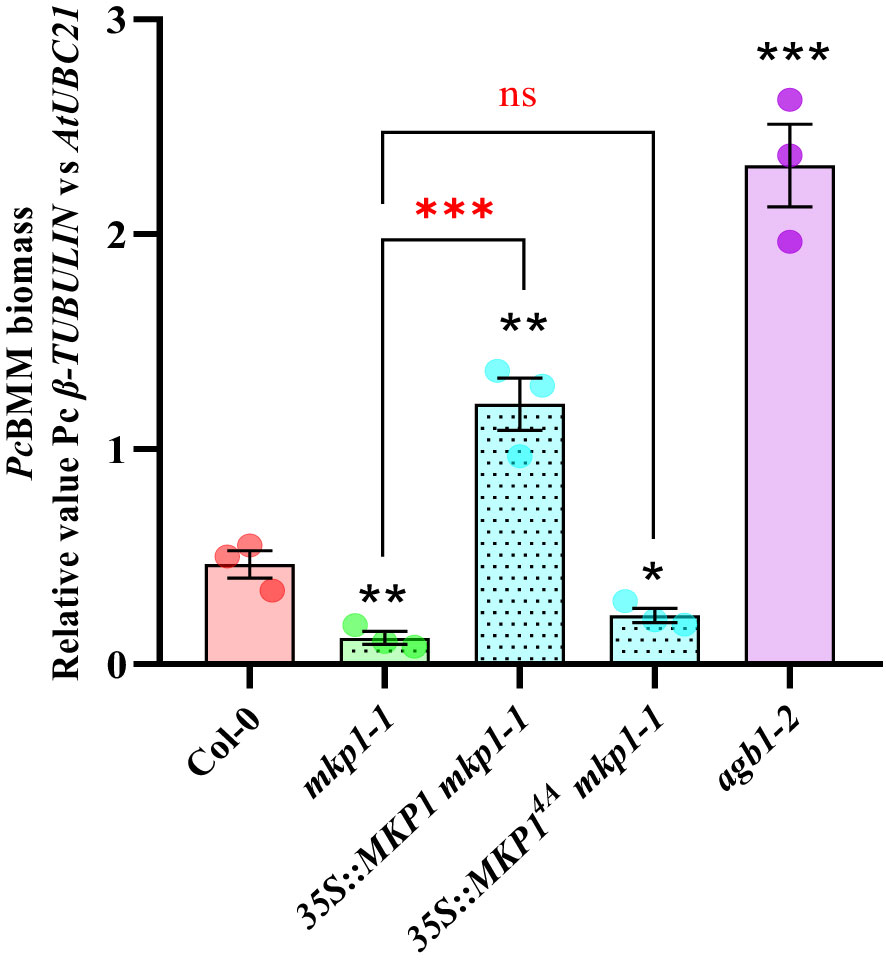
Figure 2 Phosphorylation sites of MKP1 are needed to complement mkp1-1 defense phenotype. Seventeen-day-old plants of the listed genotypes were inoculated with a suspension of 4 x 106 spores/ml of the fungus P. cucumerina (PcBMM), and fungal biomass was quantified by qPCR at 5 days-post-inoculation (dpi). Quantitative PCR was performed with specific primers (PcBMM β-TUBULIN and Arabidopsis UBC21 genes) on gDNA extracted from inoculated plants (see Materials and Methods). Values are represented as average ± SE (n = 3) and are compared to Col-0 plants values. agb1-2 was included in the experiments as a highly susceptible genotype for comparison. Horizontal keys compare the mkp1-1 with the rest of the mutants. Black and red asterisks indicate values statistically different than Col-0 wild-type plants and mkp1-1, respectively according to Student’s t-test (*p < 0.05; **p < 0.01; ***p < 0.001; ns, no significant). Experiment was performed three times with similar results.
Since we hypothesized that MKP1 could bind and dephosphorylate RBOHD to inactivate this oxidase, we crossed 35S::MYC::MKP1 plants to pD::FLAG::RBOHD and obtained double homozygous plants expressing both transgenes in double mkp1-1 rbohD mutant background to study putative interactions between MKP1 and RBOHD. We collected tissue from seedlings before and after elicitation with flg22, extracted proteins and performed co-immunoprecipitation studies. Immunodetection showed that anti-MYC antibody identified MYC-MKP1 only in samples immunoprecipitated with anti-MYC and independently of flg22/mock treatments. Conversely, anti-FLAG antibody only identified FLAG-RBOHD in samples immunoprecipitated with anti-FLAG (Figure 3). Therefore, lack of detection of FLAG-RBOHD and MYC-MKP1 in immunoprecipitated samples with anti-MYC and anti-FLAG, respectively, suggest that MKP1 and RBOHD do not interact directly, under the immunoprecipitation conditions tested. These experiments suggest that MKP1 would not directly mediate dephosphorylation and deactivation of RBOHD.
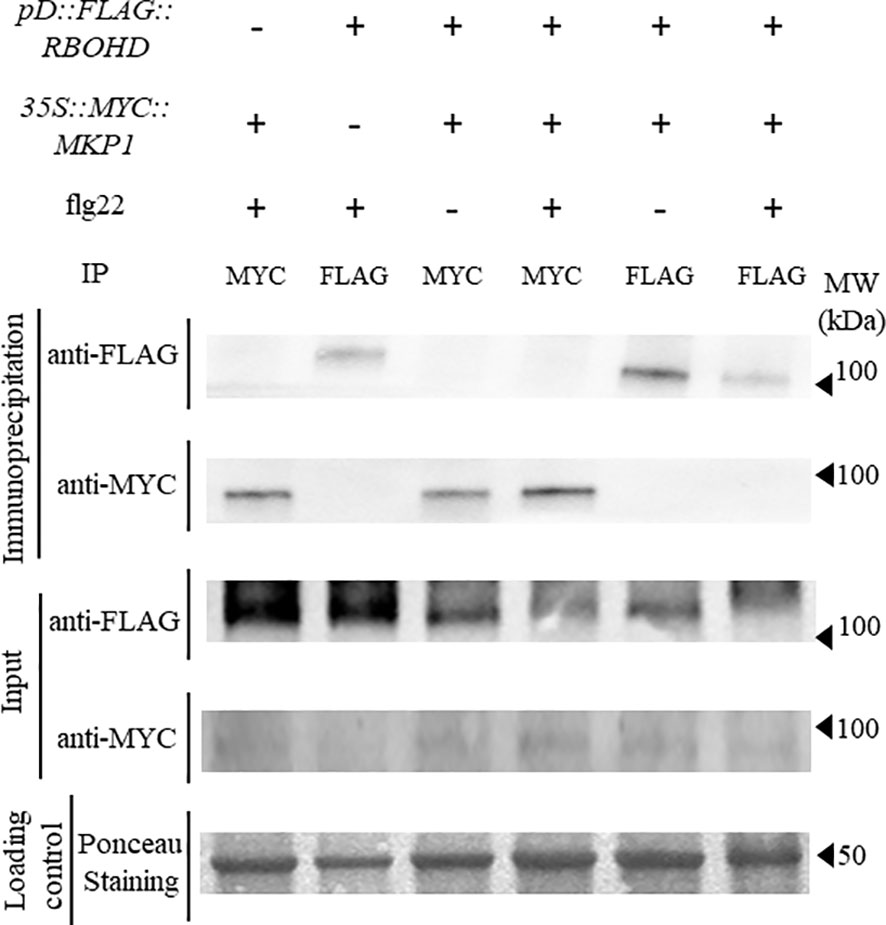
Figure 3 FLAG-RBOHD and MYC-MKP1 proteins do not interact in co-immunoprecipitation experiments. FLAG-RBOHD and MYC-MKP1 constructs were co-expressed in Arabidopsis thaliana by crossing pD::FLAG::RBOHD to 35S::MYC::MKP1 plants, and selecting double homozygous plants in rbohD mkp1-1 background. Total protein was extracted after elicitation of 12-day-old seedlings for 10 minutes with 500 nM flg22 (+) or H2O (-), and immunoprecipitated using monoclonal anti-FLAG or anti-MYC antibodies (on top of the gels). FLAG-RBOHD and MYC-MKP1 proteins were detected by western blot using anti-FLAG and anti-MYC antibodies in immunoprecipitated and control crude protein extracts (left of the gels). Loading control of crude extracts stained with red ponceau are shown to validate that the same concentration of protein was subjected to immunoprecipitation. Protein band corresponding to RUBISCO protein is shown in ponceau control. Molecular weight is indicated in kDa on the right. Experiment was performed twice with similar results.
3.3 BIK1 and MKP1 mediate independent mechanisms of disease resistance
We therefore hypothesized that MKP1 would exert its negative regulation of RBOHD-dependent ROS production by targeting some of the kinases modulating activation of RBOHD. We focused our studies in BIK1, with a prominent role in the activation of RBOHD (Kadota et al., 2014; Li et al., 2014). BIK1 is activated through direct phosphorylation upon MAMP perception by PRR receptors, like FLS2 (receptor for flagellin peptide flg22; Gómez-Gómez et al., 1999) or EFR (receptor for elf18 peptide; Zipfel et al., 2006). Activated BIK1 triggers ROS production by phosphorylation of RBOHD residues Serine39, Serine339 and Serine343 (Kadota et al., 2014). Lack of BIK1 results in a significant reduction of ROS production after flg22 treatment (Li et al., 2014). We crossed 35S::MYC::MKP1 to pBIK1::BIK1::HA (Kadota et al., 2014), and performed protein extraction and co-immunoprecipitation studies in the F1 population before and after elicitation with flg22. Immunodetection showed that anti-MYC antibody identified MYC-MKP1 only in samples immunoprecipitated with anti-MYC and independently of the flg22 treatment. Similarly, anti-HA antibody only identified BIK1-HA protein in samples immunoprecipitated with anti-HA (Figure 4A). Therefore, these data indicate that MKP1 and BIK1 do not directly interact, under the immunoprecipitation condition tested. To further assess if there is a genetic interaction between MKP1 and BIK1, we crossed the bik1 mutant line to mkp1-1 and mkp1-2 and identified double mutants. mkp1-1 harbors a T-DNA insertion in the Tyr-phosphatase dual specific domain and displays stronger abnormal growth than mkp1-2, which carries a W252 to stop codon mutation in the same domain (Escudero et al., 2019). Interestingly, both double mutants displayed enhanced abnormal phenotypes than the individual mutants, with the bik1 mkp1-1 mutant exhibiting further heightened aberrant growth phenotype than bik1 mkp1-2 (Supplementary Figure S2). We assessed pathogen resistance only in the double mutant bik1 mkp1-2, since the seeds obtained from bik1 mkp1-1 were scarce. Both bik1 and mkp1-2 supported less PcBMM growth than WT Col-0, and the double bik1 mkp1-2 displayed a similar resistance phenotype than the individual mutants (Figure 4B). All these data suggest that BIK1 and MKP1 targets are different, and they modulate independent pathways.
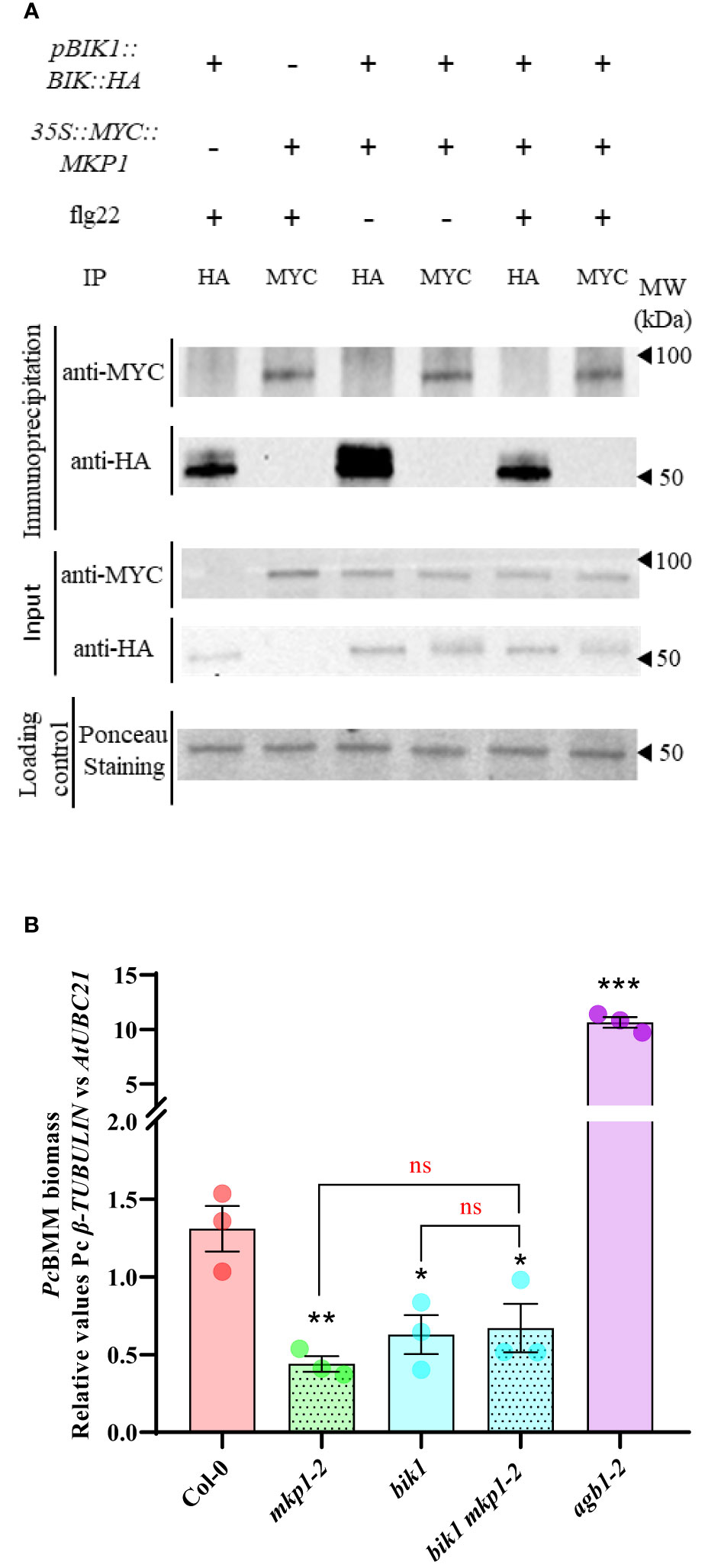
Figure 4 BIK1 and MKP1 mediate independent signaling pathways in immunity. (A) BIK1-HA and MYC-MKP1 did not interact in co-immunoprecipitation experiments. BIK1-HA and MYC-MKP1 construct were co-expressed in Arabidopsis thaliana by crossing pBIK::BIK1::HA to 35S::MYC::MKP1 plants, and total protein was extracted after elicitation for 10 minutes with 500 nM flg22 (+) or H2O(-), and immunoprecipitated using monoclonal anti-MYC or anti-HA antibodies (on top of the gels). MYC-MKP1 and BIK1-HA proteins were detected by western blot using anti-MYC and anti-HA antibodies in immunoprecipitated and control crude protein extracts (left of the gels). Loading control of crude extracts stained with red ponceau are shown to validate that the same concentration of proteins was subjected to immunoprecipitation. Protein band corresponding to RUBISCO protein is shown in ponceau control. Molecular weight is indicated in kDa on the right. Experiment was performed twice with similar results. (B) bik1 mkp1-2 double mutant displays the same enhanced disease resistance to P. cucumerina BMM (PcBMM) than single mutants. Seventeen-day-old plants of the listed genotypes were inoculated with a suspension of 4 x 106 spores/ml of the fungus PcBMM and fungal biomass was quantified by qPCR at 5 days-post-inoculation. Quantitative PCR was performed with specific primers (PcBMM β-TUBULIN and Arabidopsis UBC21 genes) on gDNA extracted from inoculated plants. agb1-2 was included in the experiments as a highly susceptible genotype for comparison. Values represented are average ± SE (n = 3) and compared to Col-0 plants. Black asterisks above each mutant indicate values statistically different than Col-0 according to Student’s t-test (*, p < 0.05; **, p < 0.005; ***, p < 0.001). Horizontal keys compare the double bik1 mkp1-2 with the individual mutants according to Student’s t-test (ns, no significant). The experiment was performed three times with similar results.
3.4 MKP1 is a repressor of MPK3 signaling in response to a necrotrophic fungus
To further characterize the defense signaling elements downregulated by MKP1, we generated combinatory mutants with mkp1 lines and mutations affecting diverse elements regulating disease resistance downstream RBOHD and BIK1. Null mutations in MPK3 and MPK6 were shown to suppress some mkp1 phenotypes. Developmental defects displayed by mkp1-1 were partially suppressed in mpk3 mkp1-1 and mpk6 mkp1-1 double mutants (Bartels et al., 2009). Even though, mpk3 and mpk6 contributed differently to this suppression since each mutant was able to suppress different mkp1-1 developmental defects (Bartels et al., 2009). Interestingly, the mkp1-1 enhanced resistance to P. syringae required only MPK6 (as mpk6 mkp1-1 were less resistant than mkp1-1) but not MPK3 (Bartels et al., 2009; Anderson et al., 2011). We wanted to expand these previous analyses with P. syringae to other pathogens with different colonization styles. Therefore, we generated double mutants mpk3-1 mkp1-2 and mkp6-2 mkp1-2 with a different mkp1 allele and assessed the growth of the fungal pathogen PcBMM on these lines. mpk3-1 supported more fungal growth at 4 dpi than WT Col-0, whereas PcBMM growth was unaltered in mpk6-2 compared to Col-0 (Figure 5). The analysis of the double mutants revealed that mpk3-1 mutation partially suppressed the mkp1-2 resistance phenotype in mpk3-1 mkp1-2, whereas mpk6-2 did not interfere with mkp1-2 resistance since mkp6-2 mkp1-2 resistance to PcBMM was similar to that of mkp1-2 (Figure 5). Thus, contrary to what happens in the defensive response against P. syringae, enhanced mkp1 disease resistance to PcBMM seems to be partially dependent on MPK3 but not on MPK6, suggesting some interaction between MKP1- and MPK3-mediated signaling pathways in response to PcBMM infection.
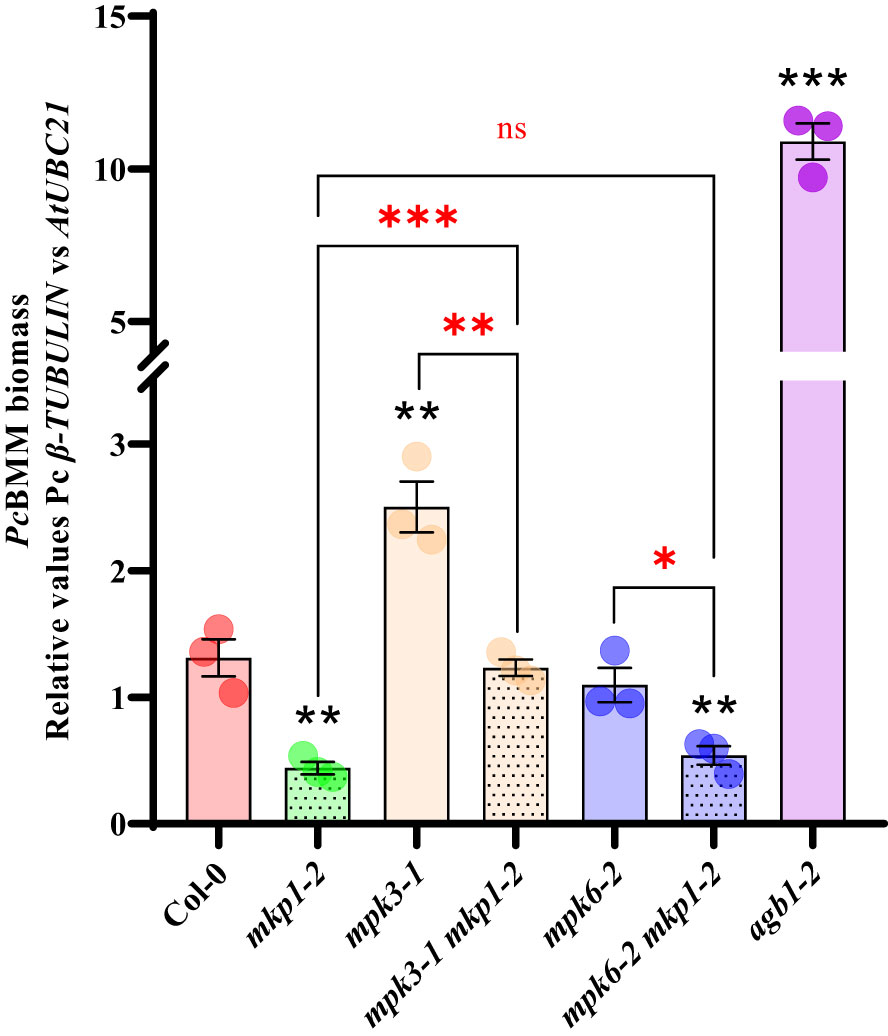
Figure 5 Enhanced disease resistance of mkp1 to the necrotrophic fungus P. cucumerina is dependent of MPK3 function. P. cucumerina BMM (PcBMM) biomass quantification in sixteen-day-old plants of the listed genotypes at 5 days-post-inoculation with a suspension of 4 x 106 spores/ml of the fungus. Quantitative PCR was performed with specific primers (PcBMM β-TUBULIN and Arabidopsis UBC21 genes) on gDNA extracted from inoculated plants (see Material and Methods). agb1-2 was included in the experiments as a highly susceptible genotype for comparison. Values represented are average ± SE (n = 3) and compared to Col-0 plants. Black asterisks above each mutant indicate values statistically different than Col-0 according to Student’s t-test (*p < 0.05; **p < 0.005; ***p < 0.001). Red marks above the keys indicate statistical differences according to Student’s t-test between genotypes (*, p < 0.05; **, p < 0.005; ***, p < 0.001; ns, no significant). The experiment was performed three times with similar results.
3.5 MKP1 regulates distinct defensive pathways in response to pathogens with different lifestyles
A metabolomic analysis performed on mkp1-2 revealed the constitutive accumulation of metabolites related to SA signaling and Trp-derived secondary metabolites, and, in a lower extent, to elements related with abscisic acid (ABA) signaling (Escudero et al., 2019). To further decipher the molecular basis of mkp1-mediated resistance phenotypes, we generated combinatory mutants with the stronger mutant allele mkp1-1 and lines disrupted in canonical signaling pathways potentially up-regulated in mkp1-1 and required for disease resistance to different pathogens. We combined mkp1-1 with: i) cyp79B2 cyp79B3 double mutant (impaired in the Trp-derived secondary metabolites pathway needed for the biosynthesis of indol-glucosinolates like camalexin and indol-3-carboxylic acid; Bednarek et al., 2009); ii) NahG line (transgenic plant over-expressing a SA hydroxylase gene that degrades this hormone to catechol; Delaney et al., 1994); iii) aba1-6 mutant (impaired in ABA biosynthesis; Niyogi et al., 1998); and abi1 abi2 hab1 (abbreviated abi1/2 hab1) triple mutant that is hypersensitive to ABA since it is defective in negative regulators of ABA signaling, which have been described as ROS sensors (Bi et al., 2022). With these mutants we assessed the effect that alteration of different signaling pathways have on the disease resistance to pathogens with different lifestyle displayed by mkp1-1 (Figure 6).
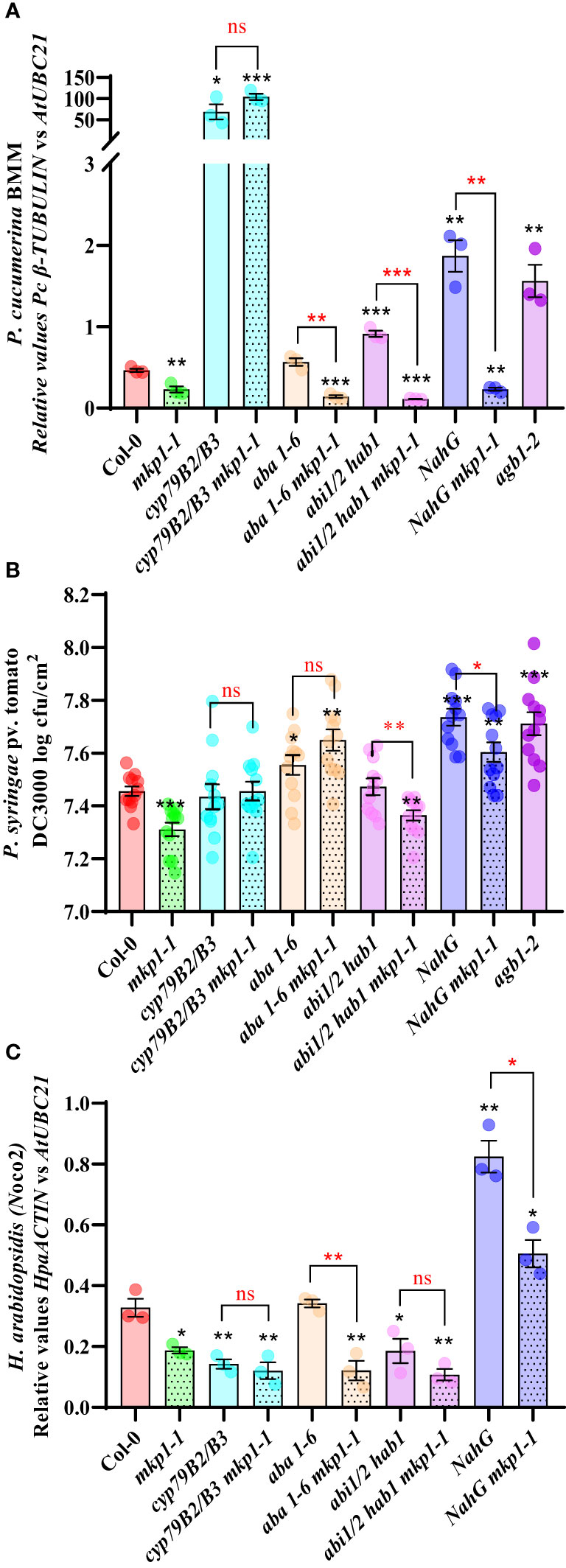
Figure 6 MKP1 regulates distinct defensive pathways in response to pathogens with different lifestyle. (A). P cucumerina BMM (PcBMM) biomass quantification in 17-day-old plants of the listed genotypes at 5 days-post-inoculation (dpi) with a suspension of 4 x 106 spores/ml of the fungus. Quantitative PCR (qPCR) was performed with specific primers (PcBMM β-TUBULIN and Arabidopsis UBC21 genes) on gDNA extracted from the inoculated plants (see Material and Methods). Results are average ± SE (n = 3). (B). Quantification of P. syringae pv. tomato DC3000 growth on 4-week-old plants of the indicated genotypes at 4 dpi after spray inoculation with a bacterial suspension (3 x 108 colony forming units (cfu)/ml). Results are average cfu ± SE (n = 4). agb1-2 was used as highly susceptible control in (A, B). (C). H. arabidopsidis (Hpa) Noco2 biomass quantification in 10-day-old seedlings of the listed genotypes at 6 dpi with 4 × 104 conidiospores/ml. Hpa growth was determined by qPCR on gDNA extracted from the inoculated plants using oligonucleotides of HpaACTIN gene, and these values were normalized to AtUBC. NahG plants were used as highly susceptible control. Results in (A–C) are average ± SE (n = 3). Black asterisks indicate statistical significance levels according to Student´s t test (*, p < 0.05; **, p < 0.01; ***, p < 0.001), compared to Col-0, wild-type plants. Red marks above black keys indicate statistical significance levels (Student´s t test) between genotype comparisons performed (*, p < 0.05; **, p < 0.005; ***, p < 0.001; ns, no significant). These experiments were performed three times with similar results.
We first examined the growth of the necrotrophic fungus PcBMM on these lines compared to Col-0 wild-type plants at 5 dpi (Figure 6A). We used agb1-2, a mutant defective in the heterotrimeric G-protein β-subunit, as hypersusceptible control (Escudero et al., 2019). Whereas NahG and abi1/2 hab1 lines were slightly more susceptible than the control, cyp79B2 cyp79B3 mutant was extremely susceptible to this pathogen compared to Col-0, as described previously (Sanchez-Vallet et al., 2010). Interestingly, cyp79B2 cyp79B3 mkp1-1 displayed the same enhanced susceptibility than cyp79B2 cyp79B3, whereas the combination of mkp1-1 with lines altered in SA signaling (NahG) or ABA signaling (aba1-6 mutant and abi1/2 hab1) did not interfere with mkp1-1 resistance (Figure 6A). These data indicates that depletion of the Trp-derived metabolites pathway in cyp79B cyp79B3 suppresses the enhanced resistance displayed by mkp1-1 to this necrotrophic fungus, whereas mutations in the other pathways have minor or no effect on mkp1-1 mediated resistance. Therefore, MKP1 seem to negatively regulate the Trp-derived metabolites pathway in response to this necrotrophic pathogen, since mkp1-1 plants constitutively accumulate Trp-derived secondary metabolites (Escudero et al., 2019) and mkp1-1 resistance to PcBMM is lost in cyp79B2 cyp79B3 background.
We also performed similar resistance analyses in response to the hemibiotrophic bacterium Pto DC3000 with the generated lines (Figure 6B). agb1-2 mutant was also used as hypersusceptible control in these experiments (Delaney et al., 1994; Torres et al., 2013). NahG and aba1-6 lines were slightly more susceptible at 4 dpi than Col-0, whereas cyp79B2 cyp79B3 supported comparable bacterial growth than the control Col-0 plants. Interestingly, cyp79B2 cyp79B3, aba1-6 and NahG combinations with mkp1-1 abolished mkp1-1 resistance (Figure 6B). In contrast, in mkp1-1 abi1/2 hab1 quadruple mutant the level of resistance to Pto DC3000 was similar to that of mkp1-1 plants (Figure 6B). These data indicate that both SA and ABA signaling and Trp-derived metabolites contribute to the disease resistance phenotype to this pathogen observed in mkp1-1 plants, suggesting that MKP1 negatively regulates all three pathways in response to Pto DC3000.
We then assessed growth of the biotrophic oomycete Hpa isolate Noco2 in these genotypes (Figure 6C). In this interaction NahG plants were the hypersusceptible control (Delaney et al., 1994). Among the different lines affected in the three signaling pathways evaluated only NahG plants showed enhanced susceptibility to this oomycete compared to Col-0, as described previously (Rairdan and Delaney, 2002). NahG was able to suppress the resistance phenotype displayed by mkp1-1, whereas all the rest of mkp1-1 combinations did not impede its enhanced resistance (Figure 6C). Notably, we found that cyp79B2 cyp79B3 supported lower Hpa growth than the control Col-0 plants, and that mkp1-1 cyp79B2 cyp79B3 lines showed a similar level of resistance to that of mkp1-1. Moreover, the aba1-6 line showed no alterations in its level of disease resistance whereas the abi1/2 hab1 lines displayed a slight lower growth of Hpa in comparison to Col-0, but these mutations did not suppress the enhanced resistance of mkp1-1 to Hpa (Figure 6C). These data indicate that, in response to this pathogen, MKP1 seem to mainly negatively regulate SA signaling, as downregulation of this pathway suppresses mkp1-1 resistance to Hpa. Also, our data point that, in addition to the previously described contribution of SA to disease resistance to this biotrophic oomycete (Lawton et al., 1995; Rairdan and Delaney, 2002), some metabolites synthesized through CYP79B2 CYP79B3 might have some negative effect on Hpa disease resistance (Figure 6C).
To further assess the signaling elements regulated by MKP1 in response to these pathogens with different lifestyles and to establish a connection between the enhanced resistance phenotypes conferred by mkp1-1 mutation in different genetic backgrounds to the expression of immune-related genes, we monitored the transcription of various marker genes at 3 dpi with the three pathogens (Figure 7). We monitored the expression of: i) PR1, marker of SA signaling (Uknes et al., 1992); ii) PDF1-2, plant defensin, marker of jasmonic acid (JA)/ethylene (ET) signaling (Penninckx et al., 1996); iii) PAD3 (encoding an enzyme of the Trp-derived metabolites pathway that catalyzes the last step of camalexin biosynthesis: Zhou et al., 1999); and iv) CYP81F2 (encoding a monooxygenase of the Trp-derived metabolites pathway that mediates the production of some indole-glucosinolates; Bednarek et al., 2009). We found that mkp1-1 plants showed higher expression levels of PDF1-2 and PAD3 than Col-0 plants upon infection with the three pathogens, and that the expression of PR1 and CYP81F2 genes was also higher in mkp1-1 than in Col-0 upon Pto and Hpa infection (Figure 7). The expression of these genes in non-inoculated mkp1-1 and Col-0 plants, and the rest of genotypes tested, was quite similar (Supplementary Figure S3), indicating that these genes exhibited enhanced up-regulated upon infection in mkp1-1 plants in comparison to Col-0, further corroborating the negative function of MKP1 in the control of disease resistance responses. The analysis of the expression of these genes in different combinatorial genetic lines and in response to infection with the three pathogens tested revealed a great complexity of transcriptional responses controlled by MKP1, and identified some patterns of expression that might explain the increased resistance of some genotypes harboring mkp1-1 mutation. For example, upon infection with PcBMM, the expression of PAD3, PDF1-2 and PR1, but not CYP81F2, was enhanced in abi1/2 hab1 mkp1-1 in comparison to abi1/2 hab1, and the expression of PAD3 and CYP81F2 was higher in aba1-6 mkp1-1 than in aba1-6 (Figure 7A). These patterns of expression could explain the enhanced resistance of these two lines harboring mkp1-1 mutation. In contrasts, the expression of these four genes was not enhanced in NahG mkp1-1 in comparison to NahG plants upon PcBMM infection (Figure 7A), indicating that the observed reduced susceptibility of NahG mkp1-1 in comparison to NahG plants (Figure 6A) was not associated to a regulatory effect of MKP1 on the pathways triggering the expression of these genes. In the infection with Pto, mkp1-1 mutation just reduced the susceptibility of NahG plants to the bacterium in NahG mkp1-1 line (Figure 6B) that showed an enhanced expression of PAD3 and CYP81F2, suggesting that regulation of MKP1 on Trp-derived metabolites pathway might explain NahG mkp1-1 reduced susceptibility phenotype (Figure 7B). In the infection of plants with Hpa, the reduction of susceptibility to this pathogen in NahG mkp1-1 and aba1-6 mkp1-1 in comparison to NahG and aba1-6 plants (Figure 6C) could just be associated to a slight increased expression of PAD3 in lines harboring mkp1-1 mutations (Figure 7C). Together these expression analyses revealed the complexity of the interactions between MKP1 and some immune pathways, which are shown here to also depend on the pathogen tested.
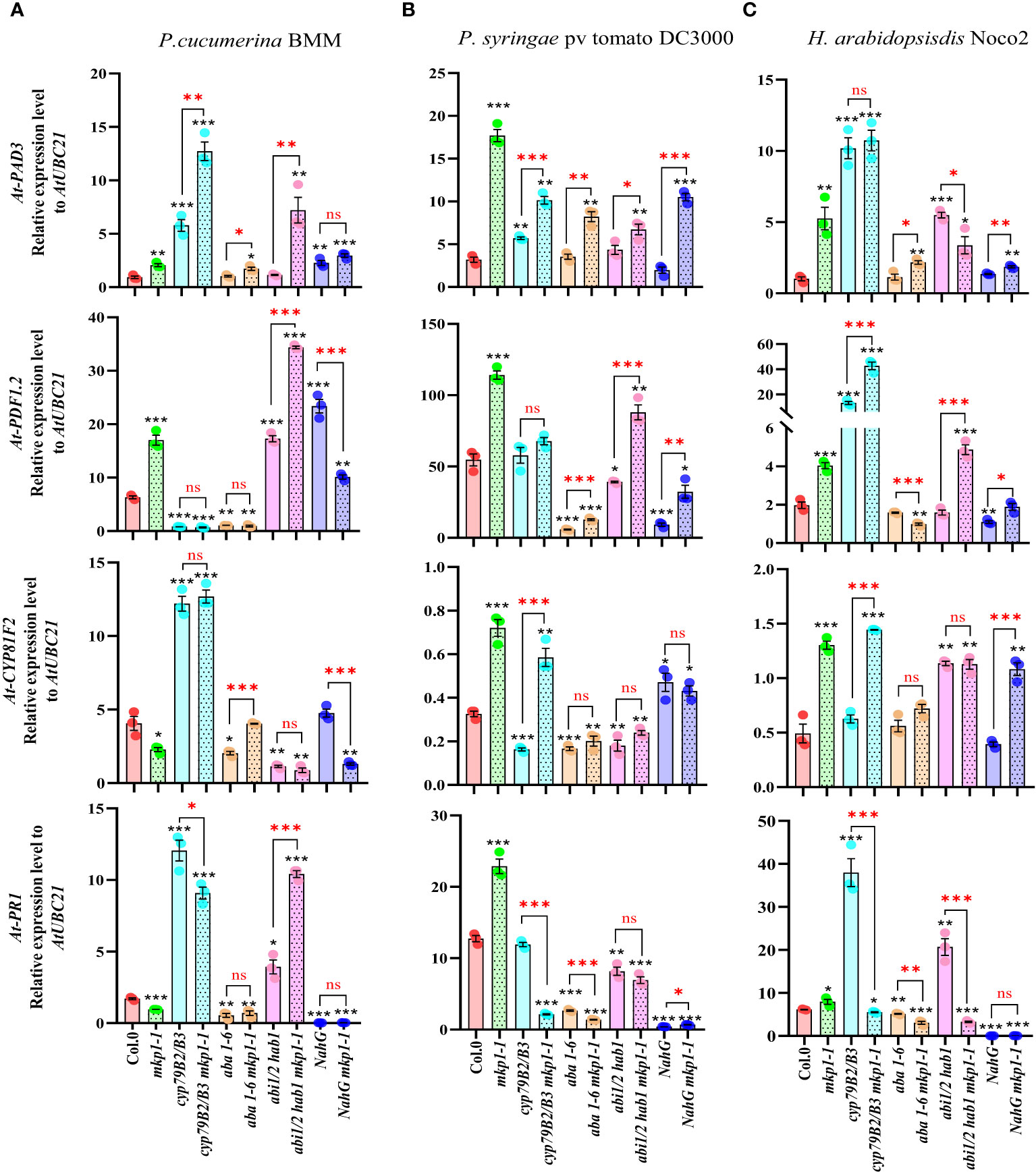
Figure 7 Expression analyses of defense marker genes in genotypes harboring mkp1-1 allele upon infection with pathogens with different lifestyle. qRT-PCR analyses of expression of defense marker genes in the indicated genotypes at 3 days-post-inoculation (dpi) with pathogens: (A) P. cucumerina BMM, performed on 17-day-old plants; (B) P. syringae pv. tomato DC3000, performed on 4-week-old plants; and (C) H. arabidopsidis Noco2, performed on 11-day-old seedlings. Expression levels of PAD3, PDF1-2, CYP81F2 and PR1 genes were quantified relative to housekeeping gene UBC21. Data represented are average ± SE of three technical replicates from 3 experimental replicates. Black asterisks indicate statistical significance levels compared to Col-0 wild type plants, according to Student´s t test (*, p < 0.05; **, p < 0.005; ***, p < 0.001). Red asterisks above black keys indicate the statistical significance levels (Student´s t test) between genotypes comparisons performed (*, p < 0.05; **, p < 0.005; ***, p < 0.001; ns, no significant). These experiments were performed three times with similar results.
4 Discussion
The activation of disease resistance requires a delicate balance between ensuring an effective defense against invading pathogens and maintaining plant development processes, that should not be compromised to guarantee plant fitness and offspring (Huot et al., 2014; Monson et al., 2022). Central to this balancing process is the regulation of ROS production, a key component of plant immune responses, but that also can be potentially harmful molecules and cause cell death in the plant (Mittler, 2017). In Arabidopsis thaliana, MKP1 has emerged as a crucial negative regulator that limits ROS production and long-lasting plant defense responses, having an impact on the control of broad-spectrum disease resistance mechanisms (Bartels et al., 2009; Escudero et al., 2019; Anderson et al., 2011; Jiang et al., 2017). The presented study delves into the molecular intricacies of MKP1-mediated immune regulation, shedding light on its involvement in diverse immune pathways.
4.1 Negative regulation of RBOHD-dependent ROS production by MKP1 is not achieved by their direct interaction
A pivotal aspect explored in this study is the negative regulation of ROS production mediated by MKP1. The NADPH oxidase RBOHD is the primary contributor to pathogen-induced ROS in Arabidopsis thaliana (Torres et al., 2002). mkp1 mutant alleles (mkp1-1 and mkp1-2) produce faster and higher level of RBOHD-dependent H2O2 accumulation than WT plants in response to MAMPs (Figure 1; Escudero et al., 2019). We and others (Jiang et al., 2018) hypothesized that MKP1 could directly dephosphorylate RBOHD and contribute to deactivate its oxidase activity to prevent excessive ROS production or to make the ROS burst transient. Several residues of RBOHD, particularly Ser343 and Ser347 in the N-terminus, are the convergent point for several activating kinases (Wu et al., 2023). Interestingly, the use of rbohD complemented lines with RBOHD alleles carrying mutations in these residues (S343A/S347A) that are the target of activating kinases during ETI and PTI, as well as in the serines targeted by the central immune regulator BIK1 (S39A/S339A/S343A), revealed that these mutated versions of RBOHD protein produced more ROS in mkp1-1 than in WT (MKP1) background (Figure 1). This is indicative that the putative MKP1 dephosphorylation targets in RBOHD would be additional residues than the ones targeted by the main regulatory kinases. However, our co-immunoprecipitation studies did not reveal a direct interaction between MYC-MKP1 and FLAG-RBOHD, even though the respective proteins were distinctly detected (Figure 3). We cannot rule out that other methodologies to determine protein/protein interaction in vivo (Bracha-Drori et al., 2004) would show interaction between RBOHD and MKP1 proteins, but we estimate that MKP1 does not achieve this regulation of ROS production through a direct interaction with RBOHD.
4.2 BIK1 and MKP1 mediate independent pathways
As an alternative, we theorized that MKP1 would target and downregulate some of the kinases that activate RBOHD in immunity. We focused our study on BIK1, an essential player in this activation under an important control mechanism (Kadota et al., 2014; Li et al., 2014). In the resting state, within the PRR complex, BIK1 undergoes ubiquitination and is subsequently directed for proteasomal degradation. Upon MAMP recognition, the PRR complex phosphorylates and releases BIK1 being further stabilized/activated by SIK1 kinase that also directly targets RBOHD (Zhang et al., 2018). However, co-immunoprecipitation experiments with MYC-MKP1 and BIK-HA did not show direct interaction between these two proteins (Figure 4A), making unlikely that MKP1 deactivates BIK1 to negative regulate RBOHD-dependent ROS production. The results obtained with plants harboring the allele RBOHDS39A/S339A/S343A with mutations in the main BIK1 targets (Figure 1) also pointed partially to this conclusion, since MKP1 contribution to ROS level produced by this allele is scarce in mpk1-1 rbohD pD::FLAG::RBOHDS39A/S339A/S343A plants. Despite the acknowledged roles of both MKP1 and BIK1 in immune regulation, the analysis of bik1 mkp1 double mutants reveals non-epistatic interactions in relation to the developmental phenotypes mediated by these genes (Supplementary Figure S2), indicating distinct targets for these proteins. This independence raises intriguing questions about the redundancy and specificity within the plant immune system, suggesting that MKP1 and BIK1 mediate diverse signaling pathways in response to different pathogens. Even though both mkp1-2 and bik1 exhibit enhanced resistance to PcBMM compared to Col-0 control, and the resistance phenotype of the double mutant is not additive and cannot be differentiated from the individual mutants (Figure 4B). These results might indicate some epistatic interaction at the level of disease resistance regulation between MKP1 and BIK1. It might be also possible that the method used to determine the level of enhanced resistance to PcBMM does not discriminate between subtle resistance differences since mkp1-2 and bik1 showed already a significant reduction of fungal growth.
Alternatively, MKP1 could exerts this negative function on ROS production acting through the repression of other PTI components such as MPK regulation. MKP1 has been shown to interact with MPK3/MPK6, among other MPKs (Ulm et al., 2002; Jiang et al., 2018), and to repress these two MPKs in stress-related signaling (Bartels et al., 2009; Anderson et al., 2011) and in cell fate decision during stomatal development (Tamnanloo et al., 2018). However, the use of a chemical-genetic conditional loss-of-function mpk3 mpk6 double mutant demonstrated that the flg22-triggered ROS burst is independent of MPK3/MPK6 activation (Xu et al., 2014), making unlikely that MKP1 repression of RBOHD-dependent ROS production is produced through these MPKs. Interestingly, we found that MKP1 mode of action involve some differential mechanism of regulation on MPK3 and MKP6 during disease resistance. In response to P. syringae MPK1 appears to repress specifically MPK6, as mpk6 mkp1-1 were less resistant than mkp1-1 (Bartels et al., 2009; Anderson et al., 2011). However, in the analysis of the resistance response to the necrotrophic fungus P. cucumerina BMM determined here, MKP1 seems to repress specifically MPK3, as mpk3-1 mkp1-2 plants were less resistant than mkp1-2 to the fungus (Figure 5). Therefore, MKP1 would target distinct MPKs in response to plant colonization by diverse pathogens, further indicating that MKP1 is able to differentially regulate distinct defensive pathways downstream PRRs in response to different pathogens.
4.3 MKP1 controls the orchestration of distinct defensive pathways in response to pathogens with different lifestyles
To broaden our understanding of how MKP1 negatively regulates immunity, we examined its impact on defensive pathways involved in disease resistance against pathogens with diverse lifestyles. Analysis of mutant combinations, involving alterations in the synthesis of Trp-derived secondary metabolites, SA and ABA signaling pathways, revealed the selective regulation exerted by MKP1 (Figure 6). This study presents compelling evidence that MKP1’s influence on disease resistance against three different pathogens varies in distinct mutant combinations, underscoring its ability to differentially regulate defensive pathways depending on the nature of the invading pathogen. Indeed, in response to the necrotrophic P. cucumerina BMM, where Trp-derived secondary metabolites play a significant role in controlling the pathogen progression (Pastorczyk et al., 2020; Bednarek et al., 2009; Sanchez-Vallet et al., 2010), MKP1 appears to predominantly negatively regulate this pathway (Figure 6A). Conversely, in the case of the interaction with the biotrophic oomycete H. arabidopsidis Noco2, where SA signaling plays a major role (Rairdan and Delaney, 2002), MKP1 is shown to primarily regulate this signaling pathway (Figure 6C). Furthermore, when responding to the hemibiotrophic bacterium P. syringae DC3000, where various signaling pathways contribute to its immunity (Rairdan and Delaney, 2002; De Torres-Zabala et al., 2007), MKP1 downregulates all three evaluated defensive mechanisms: Trp-derived metabolites, SA and ABA signaling (Figure 6B). The results showed here suggest that MKP1 negative modulating function in Arabidopsis thaliana disease resistance is quite complex and depends on the pathogen infecting the plant. Of note, a recent study by Lin et al. (2022) reveals that Arabidopsis thaliana mkp1 plants exhibit increased susceptibility to vascular-adapted bacterial pathogens such as Xanthomonas campestris pv. campestris and display compromised nonhost resistance against the rice pathogen Xanthomonas oryzae pv. oryzae. This susceptible phenotype is explained by the demonstration that MKP1 underlies a tissue-specific mechanism by positively regulating lignin biosynthesis, that appears to be a crucial aspect of vascular-specific immunity (Jiang et al., 2022; Lin et al., 2022: Molina et al., 2021).
Our analysis of defense marker gene expression further corroborates the differential MKP1 regulation of defense signaling depending on the pathogen. The upregulation of PAD3 and CYP81F2 in response to P. cucumerina BMM, and the fact that the later gene is further upregulated in mkp1-1 background, confirm the importance of Trp-derived metabolites in the interaction with this necrotrophic pathogen (Piślewska-Bednarek et al., 2018; Pastorczyk et al., 2020; Sanchez-Vallet et al., 2010) (Figure 7A). The elevated level of SA-regulated PR1 expression also shown in this interaction in the cyp79B2 cyp79B3 double mutant would be rather related to the high increase in pathogen growth supported by this mutant background (Figure 6A), since this marker gene is also induced during pathogen infection with virulent isolates (Uknes et al., 1992; Rogers and Ausubel, 1997). In line with this hypothesis, mkp1-1 cyp79B2 cyp79B3 plants showed lower PR1 expression than cyp79B2 cyp79B3 background in response to these three pathogens (Figure 7) accordingly to their reduced level of infection in comparison to cyp79B2 cyp79B3 mutants (Figure 6).
Several hormones contribute uniquely to the plant’s ability to mount an effective defense against pathogens with diverse lifestyles. An antagonistic relationship between the SA and JA/ET pathways have been documented to mediate defense strategies based on the nature of the pathogen (Pieterse et al., 2012). SA plays a central role in inducing resistance against biotrophic pathogens by activating defense-related genes (Durrant and Dong, 2004). Conversely, JA and ET are primarily associated with defense against necrotrophic pathogens (Lorenzo et al., 2003). In contrast to SA, JA and ET, ABA is generally considered a negative regulator of plant defense, interfering with the activation of the main signaling hormones mediating defenses against these different pathogens (Adie et al., 2007). Even though ABA can enhance plant resistance against certain pathogens by promoting stomatal closure, thereby restricting pathogen entry (Cao et al., 2011). Our resistance analysis together with the defense gene expression studies point to a preeminent role of MKP1 in regulating the production of Trp-derived secondary metabolites that would in turn regulate other signaling elements. In fact, auxins are metabolites derived from Trp that, besides having some effects in disease resistance, also promote susceptibility by antagonizing with other hormones (Navarro et al., 2006; Robert-Seilaniantz et al., 2011). Thus, MKP1 primary regulation of Trp-derived metabolites could interfere with other hormone signaling contributing to broaden the effect mediated by MKP1 on immunity.
4.4 Conclusion
In conclusion, this study reveals the multifaceted role of MKP1 in orchestrating diverse immune responses in Arabidopsis thaliana against pathogens with different lifestyles. Beyond just understanding MKP1’s role in immunity, we also learn how it negatively regulates ROS production and various signaling pathways, providing a basis for strengthening our understanding of plant defenses mechanisms. Manipulating the expression or activity of MKP1 might be a way to generate crop protection against different pathogens. Moreover, deciphering the specificity of MKP1 in modulating different defensive pathways might open avenues for generating crops varieties (e.g. using genome editing technologies) with broad spectrum disease tailored to specific agricultural environments. However, a better understanding of the impact of inactivating MKP1 function on plant fitness would be essential to design enhanced resistance crops that do not show detrimental effect on yield. Notably, various crop species (e.g. tomato) contain several orthologs of MKP1 in their genomes and therefore it might be possible to increase crops broad spectrum disease resistance without compromising yield by impairing just one of MKP1 orthologs. Indeed, a recent article shows that mutations in MKP1 in wheat produce plants that are not only more resistant to two devastating fungal pathogens but also exhibit a higher yield compared to wild-type control plants without infection (Liu et al., 2024).
Data availability statement
The original contributions presented in the study are included in the article/Supplementary Material. Further inquiries can be directed to the corresponding authors.
Author contributions
MT: Conceptualization, Formal analysis, Investigation, Methodology, Project administration, Supervision, Writing – original draft, Writing – review & editing. DB: Formal analysis, Investigation, Methodology, Writing – original draft. AM: Conceptualization, Funding acquisition, Project administration, Resources, Supervision, Writing – original draft, Writing – review & editing.
Funding
The author(s) declare that financial support was received for the research, authorship, and/or publication of this article. This work was supported by grant PID2021-126006OB-I00 to AM funded by MCIN/AEI/10.13039/501100011033 and by “ERDF A way of making Europe”. DB was supported by PRE2019-091276 fellowship of the “Severo Ochoa Programme for Centres of Excellence in R&D (grants SEV-2016-0672 and CEX-2020-000999-S) funded by MCIN/AEI/10.13039/501100011033. 10.13039/501100011033 and by “ESF Investing in your future”.
Acknowledgments
We are grateful to R. Ulm for providing mkp1-1 NahG seeds, S. Peck for lines 35S::MYC::MKP1 and 35S::MYC::MKP14A, Y. Kadota for pD::FLAG::RBOHD, pD::FLAG::RBOHDS39A/S339A/S343A and pD::FLAG::RBOHDS343/S347 lines and C. Zipfel for bik1 and pBIK::BIK1::HA seeds. We also thank Lucia Jordá for the critical reading of the manuscript and suggestions provided.
Conflict of interest
The authors declare that the research was conducted in the absence of any commercial or financial relationships that could be construed as a potential conflict of interest.
Publisher’s note
All claims expressed in this article are solely those of the authors and do not necessarily represent those of their affiliated organizations, or those of the publisher, the editors and the reviewers. Any product that may be evaluated in this article, or claim that may be made by its manufacturer, is not guaranteed or endorsed by the publisher.
Supplementary material
The Supplementary Material for this article can be found online at: https://www.frontiersin.org/articles/10.3389/fpls.2024.1374194/full#supplementary-material
References
Adie, B. A., Perez-Perez, J., Perez-Perez, M. M., Godoy, M., Sanchez-Serrano, J. J., Schmelz, E. A., et al. (2007). ABA is an essential signal for plant resistance to pathogens affecting JA biosynthesis and the activation of defenses in Arabidopsis. Plant Cell 19, 1665–1681. doi: 10.1105/tpc.106.048041
Anderson, J. C., Bartels, S., Besteiro, M. A. G., Shahollari, B., Ulm, R., Peck, S. C. (2011). Arabidopsis MAP Kinase Phosphatase 1 (AtMKP1) negatively regulates MPK6-mediated PAMP responses and resistance against bacteria. Plant J. 67, 258–268. doi: 10.1111/j.1365-313X.2011.04588.x
Bartels, S., Anderson, J. C., González Besteiro, M. A., Carreri, A., Hirt, H., Buchala, A., et al. (2009). MAP kinase phosphatase1 and protein tyrosine phosphatase1 are repressors of salicylic acid synthesis and SNC1-mediated responses in Arabidopsis. Plant Cell 21, 2884–2897. doi: 10.1105/tpc.109.067678
Beck, M., Heard, W., Mbengue, M., Robatzek, S. (2012). The INs and OUTs of pattern recognition receptors at the cell surface. Curr. Opin. Plant Biol. 15, 367–374. doi: 10.1016/j.pbi.2012.05.004
Beckers, G. J., Jaskiewicz, M., Liu, Y., Underwood, W. R., He, S. Y., Zhang, S., et al. (2009). Mitogen-activated protein kinases 3 and 6 are required for full priming of stress responses in Arabidopsis thaliana. Plant Cell 21, 944–953. doi: 10.1105/tpc.108.062158
Bednarek, P., Pislewska-Bednarek, M., Svatoš, A., Schneider, B., Doubský, J., Mansurova, M., et al. (2009). A glucosinolate metabolism pathway in living plant cells mediates broad-spectrum antifungal defense. Science 323, 101–106. doi: 10.1126/science.1163732
Bender, K. W., Zipfel, C. (2023). Paradigms of receptor kinase signaling in plants. Biochem. J. 480, 835–854. doi: 10.1042/BCJ20220372
Bi, G., Hu, M., Fu, L., Zhang, X., Zuo, J., Li, J., et al. (2022). The cytosolic thiol peroxidase PRXIIB is an intracellular sensor for H2O2 that regulates plant immunity through a redox relay. Nat. Plants 8, 1160–1175. doi: 10.1038/s41477-022-01252-5
Bleau, J. R., Spoel, S. H. (2021). Selective redox signaling shapes plant–pathogen interactions. Plant Physiol. 186, 53–65. doi: 10.1093/plphys/kiaa088
Bracha-Drori, K., Shichrur, K., Katz, A., Oliva, M., Angelovici, R., Yalovsky, S., et al. (2004). Detection of protein–protein interactions in plants using bimolecular fluorescence complementation. Plant J. 40, 419–427. doi: 10.1111/j.1365-313X.2004.02206.x
Cao, F. Y., Yoshioka, K., Desveaux, D. (2011). The roles of ABA in plant–pathogen interactions. J. Plant Res. 124, 489–499. doi: 10.1007/s10265-011-0409-y
Castro, B., Citterico, M., Kimura, S., Stevens, D. M., Wrzaczek, M., Coaker, G. (2021). Stress-induced reactive oxygen species compartmentalization, perception and signalling. Nat. Plants 7, 403–412. doi: 10.1038/s41477-021-00887-0
Chae, H. B., Kim, M. G., Kang, C. H., Park, J. H., Lee, E. S., Lee, S. U., et al. (2021). Redox sensor QSOX1 regulates plant immunity by targeting GSNOR to modulate ROS generation. Mol. Plant 14, 1312–1327. doi: 10.1016/j.molp.2021.05.004
Couto, D., Niebergall, R., Liang, X., Bücherl, C. A., Sklenar, J., Macho, A. P., et al. (2016). The Arabidopsis protein phosphatase PP2C38 negatively regulates the central immune kinase BIK1. PloS Pathog. 12, e1005811. doi: 10.1371/journal.ppat.1005811
Das, K., Roychoudhury, A. (2014). Reactive oxygen species (ROS) and response of antioxidants as ROS-scavengers during environmental stress in plants. Front. Environ. Sci. 2, 53. doi: 10.3389/fenvs.2014.00053
DeFalco, T. A., Zipfel, C. (2021). Molecular mechanisms of early plant pattern-triggered immune signaling. Mol. Cell 81, 3449–3467. doi: 10.1016/j.molcel.2021.07.029
Delaney, T. P., Uknes, S., Vernooij, B., Friedrich, L., Weymann, K., Negrotto, D., et al. (1994). A central role of salicylic acid in plant disease resistance. Science 266, 1247–1250. doi: 10.1126/science.266.5188.1247
De Lorenzo, G., Cervone, F. (2022). Plant immunity by damage-associated molecular patterns (DAMPs). Essays Biochem. 66, 459–469. doi: 10.1042/EBC20210087
De Torres-Zabala, M., Truman, W., Bennett, M. H., Lafforgue, G., Mansfield, J. W., Rodriguez Egea, P., et al. (2007). Pseudomonas syringae pv. tomato hijacks the Arabidopsis abscisic acid signalling pathway to cause disease. EMBO J. 26, 1434–1443. doi: 10.1038/sj.emboj.7601575
Dubiella, U., Seybold, H., Durian, G., Komander, E., Lassig, R., Witte, C. P., et al. (2013). Calcium-dependent protein kinase/NADPH oxidase activation circuit is required for rapid defense signal propagation. Proc. Natl. Acad. Sci. 110, 8744–8749. doi: 10.1073/pnas.1221294110
Durrant, W. E., Dong, X. (2004). Systemic acquired resistance. Annu. Rev. Phytopathol. 42, 185–209. doi: 10.1146/annurev.phyto.42.040803.140421
Escudero, V., Torres, M.Á., Delgado, M., Sopeña-Torres, S., Swami, S., Morales, J., et al. (2019). Mitogen-activated protein kinase phosphatase 1 (MKP1) negatively regulates the production of reactive oxygen species during Arabidopsis immune responses. Mol. Plant-Microbe Interact. 32, 464–478. doi: 10.1094/MPMI-08-18-0217-FI
George, J., Stegmann, M., Monaghan, J., Bailey-Serres, J., Zipfel, C. (2023). Arabidopsis translation initiation factor binding protein CBE1 negatively regulates accumulation of the NADPH oxidase respiratory burst oxidase homolog D. J. Biol. Chem. 299, 105018. doi: 10.1016/j.jbc.2023.105018
Gómez-Gómez, L., Felix, G., Boller, T. (1999). A single locus determines sensitivity to bacterial flagellin in Arabidopsis thaliana. Plant J. 18, 277–284. doi: 10.1046/j.1365-313X.1999.00451.x
Goto, Y., Maki, N., Sklenar, J., Derbyshire, P., Menke, F. L., Zipfel, C., et al. (2023). The phagocytosis oxidase/Bem1p domain-containing protein PB1CP negatively regulates the NADPH oxidase RBOHD in plant immunity. New Phytol. 241, 1763–1779. doi: 10.1111/nph.19302
Huot, B., Yao, J., Montgomery, B. L., He, S. Y. (2014). Growth–defense tradeoffs in plants: a balancing act to optimize fitness. Mol. Plant 7, 1267–1287. doi: 10.1093/mp/ssu049
Jiang, L., Anderson, J. C., Besteiro, M. A. G., Peck, S. C. (2017). Phosphorylation of Arabidopsis MAP kinase phosphatase 1 (MKP1) is required for PAMP responses and resistance against bacteria. Plant Physiol. 175, 1839–1852. doi: 10.1104/pp.17.01152
Jiang, L., Chen, Y., Luo, L., Peck, S. C. (2018). Central roles and regulatory mechanisms of dual-specificity MAPK phosphatases in developmental and stress signaling. Front. Plant Sci. 9, 1697. doi: 10.3389/fpls.2018.01697
Jiang, L., Ji, Q., Liu, Y. (2022). Change of wind: MKP1 positively regulates vascular immunity. Trends Plant Sci. 27, 1193–1195. doi: 10.1016/j.tplants.2022.08.009
Kadota, Y., Liebrand, T. W., Goto, Y., Sklenar, J., Derbyshire, P., Menke, F. L., et al. (2019). Quantitative phosphoproteomic analysis reveals common regulatory mechanisms between effector-and PAMP-triggered immunity in plants. New Phytol. 221, 2160–2175. doi: 10.1111/nph.15523
Kadota, Y., Shirasu, K., Zipfel, C. (2015). Regulation of the NADPH oxidase RBOHD during plant immunity. Plant Cell Physiol. 56, 1472–1480. doi: 10.1093/pcp/pcv063
Kadota, Y., Sklenar, J., Derbyshire, P., Stransfeld, L., Asai, S., Ntoukakis, V., et al. (2014). Direct regulation of the NADPH oxidase RBOHD by the PRR-associated kinase BIK1 during plant immunity. Mol. Cell 54, 43–55. doi: 10.1016/j.molcel.2014.02.021
Kimura, S., Hunter, K., Vaahtera, L., Tran, H. C., Citterico, M., Vaattovaara, A., et al. (2020). CRK2 and C-terminal phosphorylation of NADPH oxidase RBOHD regulate reactive oxygen species production in Arabidopsis. Plant Cell 32, 1063–1080. doi: 10.1105/tpc.19.00525
Lawton, K., Weymann, K., Friedrich, L., Vernooij, B., Uknes, S., Ryals, J. (1995). Systemic acquired resistance in Arabidopsis requires salicylic acid but not ethylene. Mol. Plant Microbe Interact. 8, 863–870. doi: 10.1094/MPMI-8-0863
Lee, D., Lal, N. K., Lin, Z. J. D., Ma, S., Liu, J., Castro, B., et al. (2020). Regulation of reactive oxygen species during plant immunity through phosphorylation and ubiquitination of RBOHD. Nat. Commun. 11, 1838. doi: 10.1038/s41467-020-15601-5
Li, L., Li, M., Yu, L., Zhou, Z., Liang, X., Liu, Z., et al. (2014). The FLS2-associated kinase BIK1 directly phosphorylates the NADPH oxidase RbohD to control plant immunity. Cell Host Microbe 15, 329–338. doi: 10.1016/j.chom.2014.02.009
Li, P., Zhao, L., Qi, F., Htwe, N. M. P. S., Li, Q., Zhang, D., et al. (2021). The receptor-like cytoplasmic kinase RIPK regulates broad-spectrum ROS signaling in multiple layers of plant immune system. Mol. Plant 14, 1652–1667. doi: 10.1016/j.molp.2021.06.010
Lin, H., Wang, M., Chen, Y., Nomura, K., Hui, S., Gui, J., et al. (2022). An MKP-MAPK protein phosphorylation cascade controls vascular immunity in plants. Sci. Adv. 8, eabg8723. doi: 10.1126/sciadv.abg8723
Liu, Y., Gong, T., Kong, X., Sun, J., Liu, L. (2023). XYLEM CYSTEINE PEPTIDASE 1 and its inhibitor CYSTATIN 6 regulate pattern-triggered immunity by modulating the stability of the NADPH oxidase RESPIRATORY BURST OXIDASE HOMOLOG D. Plant Cell 36, 471–488. doi: 10.1093/plcell/koad262
Liu, S., Zhang, F., Su, J., Fang, A., Tian, B., Yu, Y., et al. (2024). CRISPR-targeted mutagenesis of mitogen-activated protein kinase phosphatase 1 improves both immunity and yield in wheat. Plant Biotechnol. J. doi: 10.1111/pbi.14312
Lorenzo, O., Piqueras, R., Sánchez-Serrano, J. J., Solano, R. (2003). ETHYLENE RESPONSE FACTOR1 integrates signals from ethylene and jasmonate pathways in plant defense. Plant Cell 15, 165–178. doi: 10.1105/tpc.007468
Molina, A., Miedes, E., Bacete, L., Rodríguez, T., Mélida, H., Denancé, N. (2021). Arabidopsis cell wall composition determines disease resistance specificity and fitness. Proc. Natl. Acad. Sci. 118, e2010243118. doi: 10.1073/pnas.2010243118
Monson, R. K., Trowbridge, A. M., Lindroth, R. L., Lerdau, M. T. (2022). Coordinated resource allocation to plant growth–defense tradeoffs. New Phytol. 233, 1051–1066. doi: 10.1111/nph.17773
Morales, J., Kadota, Y., Zipfel, C., Molina, A., Torres, M. A. (2016). The Arabidopsis NADPH oxidases RbohD and RbohF display differential expression patterns and contributions during plant immunity. J. Exp. Bot. 67, 1663–1676. doi: 10.1093/jxb/erv558
Navarro, L., Dunoyer, P., Jay, F., Arnold, B., Dharmasiri, N., Estelle, M., et al. (2006). A plant miRNA contributes to antibacterial resistance by repressing auxin signaling. Science 312, 436–439. doi: 10.1126/science.1126088
Ngou, B. P. M., Ding, P., Jones, J. D. (2022). Thirty years of resistance: Zig-zag through the plant immune system. Plant Cell 34, 1447–1478. doi: 10.1093/plcell/koac041
Niyogi, K. K., Grossman, A. R., Björkman, O. (1998). Arabidopsis mutants define a central role for the xanthophyll cycle in the regulation of photosynthetic energy conversion. Plant Cell 10, 1121–1134. doi: 10.1105/tpc.10.7.1121
Pastorczyk, M., Kosaka, A., Piślewska-Bednarek, M., López, G., Frerigmann, H., Kułak, K., et al. (2020). The role of CYP 71A12 monooxygenase in pathogen-triggered tryptophan metabolism and Arabidopsis immunity. New Phytol. 225, 400–412. doi: 10.1111/nph.16118
Penninckx, I. A., Eggermont, K., Terras, F. R., Thomma, B. P., De Samblanx, G. W., Buchala, A., et al. (1996). Pathogen-induced systemic activation of a plant defensin gene in Arabidopsis follows a salicylic acid-independent pathway. Plant Cell 8, 2309–2323. doi: 10.1105/tpc.8.12.2309
Pfaffl, M. W. (2001). A new mathematical model for relative quantification in real-time RT–PCR. Nucleic Acids Res. 29, e45–e45. doi: 10.1093/nar/29.9.e45
Piślewska-Bednarek, M., Nakano, R. T., Hiruma, K., Pastorczyk, M., Sanchez-Vallet, A., Singkaravanit-Ogawa, S., et al. (2018). Glutathione transferase U13 functions in pathogen-triggered glucosinolate metabolism. Plant Physiol. 176, 538–551. doi: 10.1104/pp.17.01455
Pieterse, C. M., van der Does, D., Zamioudis, C., Leon-Reyes, A., Van Wees, S. C. (2012). Hormonal modulation of plant immunity. Annu. Rev. Cell Dev. Biol. 28, 489–521. doi: 10.1146/annurev-cellbio-092910-154055
Rairdan, G. J., Delaney, T. P. (2002). Role of salicylic acid and NIM1/NPR1 in race-specific resistance in Arabidopsis. Genetics 161, 803–811. doi: 10.1093/genetics/161.2.803
Ren, D., Yang, H., Zhang, S. (2002). Cell death mediated by MAPK is associated with hydrogen peroxide production in Arabidopsis. J. Biol. Chem. 277, 559–565. doi: 10.1074/jbc.M109495200
Robert-Seilaniantz, A., Grant, M., Jones, J. D. (2011). Hormone crosstalk in plant disease and defense: more than just jasmonate-salicylate antagonism. Annu. Rev. Phytopathol. 49, 317–343. doi: 10.1146/annurev-phyto-073009-114447
Rogers, E. E., Ausubel, F. M. (1997). Arabidopsis enhanced disease susceptibility mutants exhibit enhanced susceptibility to several bacterial pathogens and alterations in PR-1 gene expression. Plant Cell 9, 305–316. doi: 10.1105/tpc.9.3.305
Sánchez-Vallet, A., López, G., Ramos, B., Delgado-Cerezo, M., Riviere, M. P., Llorente, F., et al. (2012). Disruption of abscisic acid signaling constitutively activates Arabidopsis resistance to the necrotrophic fungus Plectosphaerella cucumerina. Plant Physiol. 160, 2109–2124. doi: 10.1104/pp.112.200154
Sanchez-Vallet, A., Ramos, B., Bednarek, P., López, G., Piślewska-Bednarek, M., Schulze-Lefert, P., et al. (2010). Tryptophan-derived secondary metabolites in Arabidopsis thaliana confer non-host resistance to necrotrophic Plectosphaerella cucumerina fungi. Plant J. 63, 115–127. doi: 10.1111/j.1365-313X.2010.04224.x
Shen, J., Zhang, J., Zhou, M., Zhou, H., Cui, B., Gotor, C., et al. (2020). Persulfidation-based modification of cysteine desulfhydrase and the NADPH oxidase RBOHD controls guard cell abscisic acid signaling. Plant Cell 32, 1000–1017. doi: 10.1105/tpc.19.00826
Tamnanloo, F., Damen, H., Jangra, R., Lee, J. S. (2018). MAP KINASE PHOSPHATASE1 controls cell fate transition during stomatal development. Plant Physiol. 178, 247–257. doi: 10.1104/pp.18.00475
Torres, M. A., Dangl, J. L. (2005). Functions of the respiratory burst oxidase in biotic interactions, abiotic stress and development. Curr. Opin. Plant Biol. 8, 397–403. doi: 10.1016/j.pbi.2005.05.014
Torres, M. A., Dangl, J. L., Jones, J. D. (2002). Arabidopsis gp91phox homologues AtrbohD and AtrbohF are required for accumulation of reactive oxygen intermediates in the plant defense response. Proc. Natl. Acad. Sci. 99, 517–522. doi: 10.1073/pnas.012452499
Torres, M. A., Morales, J., Sánchez-Rodríguez, C., Molina, A., Dangl, J. L. (2013). Functional interplay between Arabidopsis NADPH oxidases and heterotrimeric G protein. Mol. Plant-Microbe Interact. 26, 686–694. doi: 10.1094/MPMI-10-12-0236-R
Trusov, Y., Jordá, L., Molina, A., Botella, J. R. (2010). G proteins and plant innate immunity. Integrated G Proteins Signaling Plants. (Springer, Berlin: Signaling and communication series). 221–250.
Uknes, S., Mauch-Mani, B., Moyer, M., Potter, S., Williams, S., Dincher, S., et al. (1992). Acquired resistance in arabidopsis. Plant Cell 4, 645–656. doi: 10.1105/tpc.4.6.645
Ullah, H., Chen, J. G., Temple, B., Boyes, D. C., Alonso, J. M., Davis, K. R., et al. (2003). The β-subunit of the Arabidopsis G protein negatively regulates auxin-induced cell division and affects multiple developmental processes. Plant Cell 15, 393–409. doi: 10.1105/tpc.006148
Ulm, R., Ichimura, K., Mizoguchi, T., Peck, S. C., Zhu, T., Wang, X., et al. (2002). Distinct regulation of salinity and genotoxic stress responses by Arabidopsis MAP kinase phosphatase 1. EMBO J. 21, 6483–6493. doi: 10.1093/emboj/cdf646
Wang, L., Wilkins, K. A., Davies, J. M. (2018). Arabidopsis DORN1 extracellular ATP receptor; activation of plasma membrane K+-and Ca2+-permeable conductances. New Phytol. 218, 1301–1304. doi: 10.1111/nph.15111
Wu, B., Qi, F., Liang, Y. (2023). Fuels for ROS signaling in plant immunity. Trends Plant Sci. 28, 1124–1131. doi: 10.1016/j.tplants.2023.04.007
Xu, J., Xie, J., Yan, C., Zou, X., Ren, D., Zhang, S. (2014). A chemical genetic approach demonstrates that MPK 3/MPK 6 activation and NADPH oxidase-mediated oxidative burst are two independent signaling events in plant immunity. Plant J. 77, 222–234. doi: 10.1111/tpj.12382
Yuan, M., Ngou, B. P. M., Ding, P., Xin, X. F. (2021). PTI-ETI crosstalk: an integrative view of plant immunity. Curr. Opin. Plant Biol. 62, 102030. doi: 10.1016/j.pbi.2021.102030
Yun, B. W., Feechan, A., Yin, M., Saidi, N. B., Le Bihan, T., Yu, M., et al. (2011). S-nitrosylation of NADPH oxidase regulates cell death in plant immunity. Nature 478, 264–268. doi: 10.1038/nature10427
Zhang, M., Chiang, Y. H., Toruño, T. Y., Lee, D., Ma, M., Liang, X., et al. (2018). The MAP4 kinase SIK1 ensures robust extracellular ROS burst and antibacterial immunity in plants. Cell Host Microbe 24, 379–391. doi: 10.1016/j.chom.2018.08.007
Zhang, Y., Zeng, L. (2020). Crosstalk between ubiquitination and other post-translational protein modifications in plant immunity. Plant Commun. 1, 100041. doi: 10.1016/j.xplc.2020.100041
Zhou, N., Tootle, T. L., Glazebrook, J. (1999). Arabidopsis PAD3, a gene required for camalexin biosynthesis, encodes a putative cytochrome P450 monooxygenase. Plant Cell 11, 2419–2428. doi: 10.1105/tpc.11.12.2419
Keywords: Arabidopsis thaliana, plant immunity, reactive oxygen species (ROS), MKP1, RBOHD, signaling pathways, necrotrophic fungi, Pseudomonas syringae
Citation: Berlanga DJ, Molina A and Torres MÁ (2024) Mitogen-activated protein kinase phosphatase 1 controls broad spectrum disease resistance in Arabidopsis thaliana through diverse mechanisms of immune activation. Front. Plant Sci. 15:1374194. doi: 10.3389/fpls.2024.1374194
Received: 21 January 2024; Accepted: 05 March 2024;
Published: 21 March 2024.
Edited by:
Edmund Kozieł, Warsaw University of Life Sciences, PolandReviewed by:
Jinping Zhao, Texas A and M University, United StatesClemencia Rojas, University of Arkansas, United States
Copyright © 2024 Berlanga, Molina and Torres. This is an open-access article distributed under the terms of the Creative Commons Attribution License (CC BY). The use, distribution or reproduction in other forums is permitted, provided the original author(s) and the copyright owner(s) are credited and that the original publication in this journal is cited, in accordance with accepted academic practice. No use, distribution or reproduction is permitted which does not comply with these terms.
*Correspondence: Miguel Ángel Torres, bWlndWVsYW5nZWwudG9ycmVzQHVwbS5lcw==; Antonio Molina, YW50b25pby5tb2xpbmFAdXBtLmVz
†These authors share senior authorship