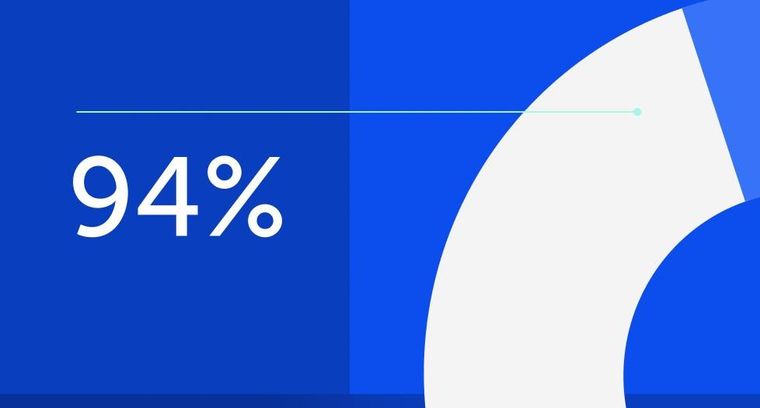
94% of researchers rate our articles as excellent or good
Learn more about the work of our research integrity team to safeguard the quality of each article we publish.
Find out more
ORIGINAL RESEARCH article
Front. Plant Sci., 17 May 2024
Sec. Plant Abiotic Stress
Volume 15 - 2024 | https://doi.org/10.3389/fpls.2024.1374142
This article is part of the Research TopicEnhancing Salinity Tolerance in Crop Plants through Agronomic, Genetic, Molecular, and Physiological ApproachesView all 18 articles
Salt stress is a well-known abiotic constraint that hampers crop productivity, affecting more than 424 million hectares of topsoil worldwide. Applying plant growth regulators externally has proven effective in enhancing crop resilience to salt stress. Previous metabolomics studies revealed an accumulation of Valine-Threonine-Isoleucine-Aspartic acid (VTID) in salt-stressed maize seedlings, suggesting its potential to assist maize adaptation to salt stress. To explore the effectiveness of VTID in enhancing salt tolerance in maize, 10 nM VTID was applied to salt-stressed maize seedlings. The results showed a remarkable 152.29% increase in plant height and a 122.40% increase in fresh weight compared to salt-stressed seedlings. Moreover, the addition of VTID enhanced the activity of antioxidant enzymes, specifically superoxide dismutase (SOD) and catalase (CAT), while reducing the level of malondialdehyde (MDA), a marker of oxidative stress. Additionally, VTID supplementation resulted in a significant increase in osmoregulatory substances such as proline. Metabolomic analysis revealed substantial changes in the metabolite profile of maize seedlings when treated with VTID during salt stress. Differential metabolites (DMs) analysis revealed that the identified DMs primarily belonged to lipids and lipid-like molecules. The receiver operating characteristic curve and linear regression analysis determined a correlation between isodolichantoside and the height of maize seedlings under salt-stress conditions. In conclusion, these findings validate that VTID effectively regulates tolerance in maize seedlings and offers valuable insights into the potential of short peptides for mitigating salt stress.
Salt stress is considered one of the most severe abiotic challenges, exerting widespread impacts on plant growth, development, and productivity worldwide (Xiao and Zhou, 2023). According to the Global Map of Salt-Affected Soils (GSASmap), a vast area of both topsoil (0-30 cm) and subsoil (30-100 cm) has been identified as salt-affected, encompassing 424 million hectares and 833 million hectares, respectively (Food and Agriculture Organization, 2023). Saline soils contain high levels of soluble salts, and pose significant threats to plants by inducing toxicity and disrupting nutrient balance, biodiversity, and water availability in the soil (Hu et al., 2022). Excessive concentrations of cations and anions (Na+ and Cl-) in the soil trigger physiological and biochemical changes in plants, resulting in reduced cellular water content and increased generation of reactive oxygen species (ROS) (Qin et al., 2022). In response to salt stress, plants employ various non-enzymatic and enzymatic mechanisms. During stress, plants accumulate osmoregulatory substances, such as proline, soluble sugars, soluble proteins, and betaine, which help to maintain physiological functions by reducing cellular osmotic potential (Ru et al., 2023). Additionally, the activities of well-known antioxidases, such as superoxide dismutase (SOD), peroxidase (POD), and catalase (CAT) are upregulated to counteract ROS induced by salt stress (Xia et al., 2014).
Besides, several exogenous plant growth regulators (PGRs), including melatonin, polyamines, brassinosteroids, and steroid hormones, have been documented to enhance stress resistance by modulating enzyme activity and metabolic levels (Gai et al., 2020). The application of 100 µM melatonin has been found to reduce salt stress by promoting root, shoot length, fresh and dry weight, increasing chlorophyll contents, and inhibiting excessive production of oxidative stress markers (Khan et al., 2024). As a type of polyamine, 0.3 mM spermidine was found to recover the Zoysiagrass seedlings’ growth by increasing antioxidant enzyme activities and decreasing H2O2 and malondialdehyde (MDA) levels (Li et al., 2016). Exogenous application of 10−9 M 24-epibrassinolide on rice variety Pusa Basmati 1 showed an improvement in plant growth, levels of protein and proline content, antioxidant enzyme activity, and expression of salt response genes (Sharma et al., 2013). Additionally, Bacillus pumilus has been found to improve rice tolerance to the combined stresses of NaCl and high boron. This is due to its ability to limit the uptake of Na+ (Khan et al., 2016). Similarly to traditional exogenous substances, poly-γ-glutamic acid, as a microbe-secreted isopeptide, increased stress tolerance in Brassica napus seedlings by activating an H2O2 burst and subsequent crosstalk between H2O2 and Ca2+ signaling (Lei et al., 2017). Furthermore, low molecular weight secreted peptides from Brassicaceae have been found to stimulate the expression of salt stress-responsive elements (Yu et al., 2020). That demonstrates the potential of exogenous plant-derived peptides to help plants resist salt stress.
Maize (Zay mays L.) holds significant economic importance globally, yet its production is increasingly challenged by climatic changes and soil salinization (Hassani et al., 2021). In response to environmental stress, plants activate their defense mechanisms, resulting in the accumulation of secondary metabolites, including lipids, terpenoids, ketones, and alkaloids (Omoto et al., 2016; Jan et al., 2021). Metabolomic technology has revolutionized the study of low molecular weight compounds in organisms or cells, facilitating the identification of diverse metabolites and metabolic pathways involved in plant cell activities and secondary network metabolism (Liu et al., 2022; Wei et al., 2023). Metabolomics studies have revealed increased levels of metabolites such as amino acids and organic acids under salt stress compared to unaffected maize (Mo et al., 2023). In the previous study, metabolomic analysis of maize seedlings under salt stress identified three up-regulated peptides, nine down-regulated peptides and amino acids with Variable Importance in the Projection (VIP) values greater than 1 and p values less than 0.05 (Supplementary Table S1) (Wang, 2020). To evaluate their impact on plant height under salt stress, three up-regulated peptides were sprayed on maize seedlings at a concentration of 1×10-7 mol/L. The results revealed that maize seedlings treated with Valine-Threonine-Isoleucine-Aspartic acid (VTID) showed improved recovery from salt treatment and displayed enhanced growth compared to untreated maize seedlings.
The objective of this study was to assess the effects of VTID supplementation on salt-stressed maize seedlings. To achieve this, we examined the activity of antioxidant enzymes, levels of phytohormones, and osmoregulators. These factors play a crucial role in mitigating the harmful effects of reactive oxygen species (ROS) during salt stress. Furthermore, metabolomics and correlation analysis were conducted to identify key metabolites produced by VTID supplementation in salt-stressed maize seedlings. Taken together, our findings indicate that supplementation with VTID has the potential to promote the growth of salt-stressed maize seedlings. This effect is achieved by enhancing the activity of antioxidant enzymes and facilitating the production of isodolichantoside, a monoterpene indole alkaloid.
VTID compound used in this study was synthesized by Bioengineering Co. Ltd (Shanghai, China). It has a relative molecular weight of 446.25 g/mol and a purity of 95%. To prepare a stock solution with a concentration of 1.12 mM, 0.5 mg of VTID was dissolved in 1 mL of double-distilled H2O (ddH2O). The maize seeds (Ningdan 33) used in this experiment were purchased from the local market in Ningxia, China.
Maize seeds were surface disinfected using 0.01% HgCl2 for 40 s, followed by rinsing thrice with sterile water (Kohli et al., 2019). The disinfected seeds were then sown on 0.8% agar medium containing Hoagland modified nutrient salts solution (NS10205-50mL, Beijing Coolaber Technology Co., Ltd, Beijing, China) and placed at 28°C with a relative humidity of 75% in an environmental chamber (Ji et al., 2022). Once the seedlings’ roots reached a length of 3 cm, they were transferred to plastic hydroponic bottles embedded in the sterile stones. For the control group, maize seedlings were irrigated with 1/2 Hoagland nutrient solution. In contrast, 1/2 Hoagland nutrient solution containing 100 mM NaCl was used for the salt stress group as described by Zhao et al. (2010).
Initially, various concentrations of VTID (i.e., 0.1 nM, 1 nM, 10 nM, 100 nM, 1 μM, 10 μM, and 100 μM) were supplemented with 1/2 Hoagland nutrient solution to determine the optimal concentration for plant growth under salt stress. It was observed that the addition of 10 nM VTID partially restored the growth of seedlings, particularly in terms of plant height and fresh weight (Figure 1). Subsequently, four treatments were implemented as follows: (i) CK0, ddH2O addition and non-salt stress treatment; (ii) T0, 10 nM VTID addition and non-salt stress treatment; (iii) CK1, ddH2O addition and salt stress treatment; (iv) T1, 10 nM VTID addition and salt stress treatment. After 12 days’ treatment, the aboveground part of seedlings were collected and stored at -80°C for subsequent analysis. The maize seedlings were cultivated at 28°C with a light-dark cycle of 16 hours of light and 8 hours of darkness, maintaining a relative humidity of 75%.
Figure 1 Plant height and fresh weight of maize seedlings under different treatments. CK0, maize seeds immersed in ddH2O and grew under non-salt stress conditions; CK1, maize seeds immersed in ddH2O and grew under salt stress conditions; 0.1 nM, 1 nM, 10 nM, 100 nM, 1 μM, 10 μM, and 100 μM indicated maize seeds immersed into different concentrations of VTID and grew under salt stress conditions.
After 12 days of treatment, the second leaf of the maize seedlings was collected to determine their physiological and biochemical indices. The activity of antioxidant enzymes, namely SOD, CAT, and POD, was determined using spectrophotometry according to the methodology outlined by Tian et al. (2024). In addition, the levels of MDA, soluble sugar, and proline were measured. The evaluation followed the instructions provided by Solarbio Science & Technology Co., Ltd. (Beijing, China), employing specific assay kits: SOD activity assay kit (BC0170), CAT activity assay kit (BC0200), POD activity assay kit (BC0090), MDA content assay kit (BC0020), plant soluble sugar content assay kit (BC0030), and proline content assay kit (BC0290).
To measure phytohormone levels, the collected leaves were pretreated to extract IAA, abscisic acid (ABA), and gibberellic acid (GA). Subsequently, enzyme-linked immunosorbent assay (ELISA) was performed using the IAA ELISA kit (MM-0953O2), ABA ELISA kit (MM-1185O2), and GA ELISA kit (MM-0125O2) following the instructions provided by Meimian Industrial Co., Ltd. (Jiangsu, China). This methodology was previously described by Ji et al. (2022).
A 50 mg sample of the second leaf was collected and placed in a 2 mL Eppendorf tube containing 6 mm diameter grinding beads. Subsequently, 400 μL extraction buffer (methanol: water = 4:1, v/v) containing an internal standard (L-2-chlorophenylalanine at a concentration of 0.02 mg/mL) was added to the tube. The samples were ground using a Wonbio-96c frozen tissue grinder (Shanghai Wanbo Biotechnology Co., Ltd, Shanghai, China) for 6 min at -10°C and 50 Hz. Following grinding, low-temperature ultrasonic extraction was performed for 30 min at 5°C and 40 kHz. Afterward, the samples were stored at -20 °C for 30 min and subsequently centrifuged at 4°C at a speed of 13000 g for 15 min. The supernatant was then transferred into a new tube for further analysis.
Ultra-high performance liquid chromatography equipped with quadruple exactive mass spectrometer (UHPLC-QE-MS) was used for metabolomic profiling according to the methods described by Xiong et al. (2023). Separation was achieved using an ACQUITY HSS T3 column (100 mm×2.1 mm i.d., 1.8 μm; Waters, USA). The UPLC system was connected to a Thermo UHPLC-Q Exactive Mass Spectrometer with electrospray ionization (ESI) operating in both positive (3500 V) and negative (-2800 V) modes (He et al., 2023). As described by del Mar Gómez-Ramos et al. (2015), full scan data was obtained at a resolution of 70,000, and MS/MS data at a resolution of 17,500. Data acquisition utilized the Data Dependent Acquisition (DDA) mode over a mass range of 70-1050 m/z. The metabolomics data presented in the study are deposited in the MetaboLights repository, accession number MTBLS9653.
To assess the impact of VTID addition under salt stress and non-salt stress conditions, differences in maize height, fresh weight, SOD, CAT, and POD activities, MDA, IAA, GA, ABA, soluble sugar, and proline contents were analyzed. Analysis of variances (ANOVA) was employed to determine the significance (p < 0.05), followed by Tukey’s multiple comparison tests to assess the significance of the observed differences. Statistical analysis was performed using the IBM SPSS statistics (SPSS Inc., Chicago, IL, USA) and graphical visualization was performed using GraphPad Prism 8.0 (GraphPad Software, Boston, MA, USA).
The data obtained from UHPLC-QE-MS was processed using Progenesis QI software (Waters Corporation Milford, USA). The resulting metabolites were identified using HMDB (http://www.hmdb.ca/) and Majorbio database (Ren et al., 2022). Pairwise comparisons revealed differential metabolites (DMs) between the experimental groups. Statistical analysis including principal component analysis (PCA), and orthogonal partial least squares discriminant analysis (OPLS-DA) was performed by R package (Version 1.6.2). Significant DMs were determined based on a VIP score greater than 1 and an adjusted p-value below 0.05. To understand the biochemical pathways associated with DMs, metabolite pathway enrichment analysis (MPEA) was conducted using the Kyoto Encyclopedia of Genes and Genomes (KEGG) database (http://www.genome.jp/kegg/). ROC (Receiver Operating Characteristic) and linear regression analysis were performed through the free online platform of Majorbio cloud platform (cloud.majorbio.com).
To determine the optimal concentration of VTID supplementation, different concentrations of VTID were applied to salt-stressed maize seedlings. Initially, there was an increase in both plant height and fresh weight, followed by a subsequent decrease (Figure 1). Following extensive testing, it was discovered that the application of 10 nM VTID yielded the most remarkable results. This concentration led to a significant increase in plant height by 152.29% compared to salt-stressed seedlings (Figure 1). Furthermore, only the treatment with 10 nM VTID restored the fresh weight of maize seedlings to 2.24 g under salt stress. Conversely, the differences in plant height and fresh weight with and without VTID addition were not significant under non-salt stress. As a result, it can be concluded that the optimal concentration of 10 nM VTID significantly enhances the growth of maize seedlings under salt stress.
Environmental stresses led to the accumulation of ROS within plant cells, resulting in lipid peroxidation as indicated by MDA content. Under normal growth conditions without salt stress, the treatments with or without 10 nM VTID showed no significant changes in the activities of SOD, CAT, and POD, or the level of MDA (Figures 2A–D). Compared to salt stress without VTID, the exogenous application of VTID increased SOD and CAT activities by 10.9% and 17.3%, respectively, and significantly reduced MDA content by 32.7% (Figures 2A, B, D). There were no significant changes observed in POD activity among the four treatments (Figure 2C).
Figure 2 SOD (A), CAT (B), and POD (C) activities, MDA (D), IAA (E), GA (F), ABA. (G), Soluble sugar (H) and Proline (I) contents of maize seedlings under the different treatments (CK0, T0, CK1, and T1). CK0, ddH2O addition and non-salt stress treatment; CK1, ddH2O addition and salt stress treatment; T0, 10 nM VTID addition and non-salt stress treatment; T1, 10 nM VTID addition and salt stress treatment. Data are presented as the mean ± SEM. Bars labeled with different letters indicate a significant difference between treatments.
Phytohormones play a crucial role in plant growth adaptation under salt stress. To investigate the effect of VTID on maize seedlings under salt stress, the levels of IAA, GA, and ABA were tested. The exogenous VTID treatment resulted in an increase in the IAA content in maize seedlings under non-salt stress, but no significant difference was observed under salt stress (Figure 2E). The addition of VTID (T0 group), salt stress treatment (CK1 group), together with VTID addition and salt stress treatment (T1 group), showed an increase in the GA content in maize seedlings compared to those of blank treatment (CK0 group) (Figure 2F). However, VTID had no significant effect on ABA levels in maize seedlings under any growth conditions (Figure 2G).
Osmoregulatory substances such as soluble sugars and proline play a crucial role in reducing osmotic potential and enhancing water uptake or retention capacity in cells during salt stress (Choudhary et al., 2023). Under non-salt stressed conditions (CK0 and T0 groups), VTID had no significant effect on soluble sugars and proline contents of maize seedlings (Figures 2H, I). However, under salt stress conditions, proline level increased 1.25-fold in maize seedlings treated with VTID (T1 group) compared to those without VTID addition (CK1 group) (Figure 2I). VTID did not significantly impact soluble sugars under salt stress in maize seedlings. In conclusion, VTID supplementation increased the activity of antioxidant enzymes (SOD and CAT) while reducing the level of MDA in maize seedlings under salt stress.
Metabolomic analysis was performed to gain insights into the responses of maize to salt stress with VTID supplementation. Principal component analysis (PCA) was conducted to visualize the distribution of metabolomics responses in the different treatments: CK0, T0, CK1, and T1. To ensure good reproducibility, any outliers with significant deviations were excluded from each treatment, and each treatment was performed with five biological replicates. PCA revealed distinct separation along the PC1 axis, accounting for 27.00% of the total variation between the salt-treated groups (CK1 and T1) and the non-stressed groups (CK0 and T0) (Figure 3A). Nevertheless, the PCA plot did not exhibit clear separations between the CK0 and T0 groups, as well as between the CK1 and T1 groups (Figure 3A). To enhance the detection of intergroup differences, we utilized the OPLS-DA statistical method, which has been proven to be more sensitive in cases where variables have low correlation (Xiao et al., 2022; Zheng et al., 2023). The OPLS-DA model revealed distinct separations between CK0 and T0 (R2X = 0.489, R2Y = 0.992, Q2 = 0.563, Figures 3B, D), as well as CK1 and T1 (R2X = 0.682, R2Y = 0.999, Q2 = 0.593, Figures 3C, E), ensuring the reliability of these results.
Figure 3 PCA model and OPLS-DA with corresponding values of R2X, R2Y, and Q2. (A) PCA score plot of CK0 (red circle), CK1 (yellow circle), T0 (green circle), T1 (blue circle), and QC samples (purple circle). (B) OPLS-DA score plot of CK0 (red circle) vs T0 (green circle). (C) OPLS-DA score plot of CK1 (yellow circle) vs T1 (blue circle). (D, E) Validation plot of the CK0 vs T0 (R2X = 0.489, R2Y = 0.992, and Q2 = 0.563) and CK1 vs T1 groups (R2X = 0.682, R2Y = 0.999, and Q2 = 0.593) obtained from 200 tests, respectively. CK0, ddH2O addition and non-salt stress treatment; CK1, ddH2O addition and salt stress treatment; T0, 10 nM VTID addition and non-salt stress treatment; T1, 10 nM VTID addition and salt stress treatment.
Differential metabolites were defined as significantly altered metabolites with a VIP threshold (VIP > 1) based on the OPLS-DA model. Generally, fold change (FC) > 1.5 and FC < 0.667 indicates the up- or down-regulated metabolites, respectively. In the CK0 vs T0 group, eight differential metabolites were screened, including three upregulated metabolites and five downregulated metabolites (Figure 4A; Table 1). These DMs are categorized into phenylpropanoids and polyketides, organic acids and derivatives, and organoheterocyclic compounds according to HMDB annotation (Figure 4C).
Figure 4 Volcano plot of significantly differential metabolites in CK0 vs T0 group (A) and CK1 vs T1 group (B). Classification of differential metabolites in the CK0 vs T0 group (C) and CK1 vs T1 group (D) using HMDB categories, respectively. CK0 vs T0 group, ddH2O addition under non-salt stress and salt stress treatment; CK1 vs T1 group, 10 nM VTID addition under non-salt stress and salt stress treatment.
In the CK1 vs T1 group, 14 metabolites showed significant differences, with seven upregulated metabolites and seven downregulated (Figure 4B; Table 2). According to HMDB database analysis, nine differential metabolites in the CK1 vs T1 group were divided into five known categories. Among these, five metabolites were identified and annotated as lipids and lipid-like molecules (Figure 4D). Therefore, it can be concluded that lipids and lipid-like molecules are the most distinct metabolites according to the HMDB database analysis with the addition of VTID under salt stress.
In the CK0 vs T0 group, 6,7-dihydroxy-3-(2-methylbut-3-en-2-yl)-2H-chromen-2-one was the most significantly upregulated metabolite, showing a remarkable FC of 26.489. Downregulated metabolites exhibited less variation in FC, as indicated in Table 1. The addition of VTID under salt stress conditions resulted in the change of more metabolites. Among the upregulated metabolites, 3-hydroxytetradecanedioic acid showed the highest FC, reaching 4.016 (Table 2). On the other hand, 4-hydroxyphenytoin glucuronide has the lowest FC among the downregulated metabolites, with a value of only 0.154.
To evaluate the predictive performance of the differential metabolites in VTID-treated maize seedlings, the ROC curve was employed for subsequent analysis. Previous studies showed that differential metabolites with an Area Under the Curve (AUC) value greater than 0.9 possess a higher potential to be utilized as biomarkers (Xia et al., 2013). In line with this, these results showed that four metabolites including astin I, 6,7-dihydroxy-3-(2-methylbut-3-en-2-yl)-2H-chromen-2-one, (1R)-glutathionyl-(2R)-hydroxy-1,2-dihydronaphthalene, and euphodendroidin V showed better performance in VTID-treated maize seedlings without salt stress (Figure 5A). Furthermore, under salt stress conditions, the AUC values of six out of 14 differential metabolites after VTID treatment were greater than 0.9, including 3-Deoxy-D-manno-octulosonate 8-phosphate, isodolichantoside, 4-hydroxyphenytoin glucuronide, retrorsine, P-coumaroylputrescine, and succinyladenosine. These findings suggest that these metabolites have the potential to serve as biomarkers for evaluating the impact of VTID on maize seedlings under salt stress conditions (Figure 5B) (Jiang et al., 2023).
Figure 5 ROC curves of differential metabolites in the CK0 vs T0 group (A) and CK1 vs T1 group (B) with AUC > 0.9. CK0 vs T0 group, ddH2O addition under non-salt stress and salt stress treatment; CK1 vs T1 group, 10 nM VTID addition under non-salt stress and salt stress treatment.
To identify key metabolites that could predict sample differentiation after VTID treatment, ROC curve analysis was performed on the differential metabolites in the salt stress and non-salt stress groups, respectively. DMs with an AUC value greater than 0.9 were considered to have better predictive power. In the CK0 vs T0 group, four DMs with an AUC > 0.9 were identified, of which astin I and 6,7-dihydroxy-3-(2-methylbut-3-en-2-yl)-2H-chromen-2-one were upregulated by the addition of VTID. In the T1 vs CK1 group, six DMs with an AUC > 0.9 were found, including two upregulated metabolites, 3-deoxy-d-manno-octulosonate 8-phosphate and isodolichantoside.
Plant height and fresh weight are commonly used to evaluate plant growth under salt stress. Interestingly, in this experiment, VTID treatment did not significantly affect the plant height and fresh weight of maize under non-salt stress conditions. This suggests that astin I and 6,7-dihydroxy-3-(2-methylbut-3-en-2-yl)-2H-chromen-2-one may have less correlation with these growth parameters in the T0 vs CK0 group. However, under salt stress, VTID resulted in a significant increase in plant height and fresh weight (Figure 1). Linear regression analysis revealed a strong relationship (R2 = 0.8678) between isodolichantoside and plant height, suggesting that isodolichantoside can account for the variation in plant height under salt stress conditions (Figure 6B). This suggests that VTID treatment may stimulate the accumulation of isodolichantoside, thereby promoting maize growth under salt stress conditions. However, the relationship between 3-deoxy-D-manno-octulosonate 8-phosphate and plant height was below 0.8, indicating that it could not effectively explain the variation in plant height under salt stress conditions (Figure 6A). For fresh weight, the goodness of fit between these two metabolites and fresh weight was below 0.8, indicating that they could not effectively predict the change in fresh weight (Figures 6C, D). In conclusion, the significant increase in maize plant height under salt stress caused by VTID treatment may be attributed to the accumulation of isodolichotoside.
Figure 6 Linear regression analysis of 3-deoxy-D-manno-octulosonate 8-phosphate and isodolichantoside with plant height and fresh weight. (A, B) Linear regression of 3-deoxy-D-manno-octulosonate 8-phosphate (A) and isodolichantoside (B) with plant height, respectively. (C, D) Linear regression of 3-deoxy-D-manno-octulosonate 8-phosphate (C) and isodolichantoside (D) with fresh weight, respectively.
Salt stress is a major abiotic stress that adversely affects plant growth and development. It exerts a multifaceted impact on plants, disturbing critical processes including protein synthesis, enzyme activity, photosynthesis, leaf senescence, leaf growth, new leaf production, root growth, cell elongation, water uptake, seed germination, and overall yield reduction (Muchate et al., 2016; Xue et al., 2023). In response to this challenge, plants have evolved intricate biochemical mechanisms to mitigate the adverse effects. These biochemical mechanisms include activating antioxidant defense systems, developing osmotic tolerance, regulating hormonal levels, and more (Noreen and Ashraf, 2009; Yang and Guo, 2018). However, the excessive generation of ROS poses a significant threat, potentially leading to oxidative stress if the plant’s mechanisms for ROS detoxification are overwhelmed (Borges et al., 2023).
Previous studies have emphasized the effectiveness of exogenous substances including hormones, polyamines, nutrients, amino acids, sugars, and others, in aiding plants to alleviate damage caused by abiotic stress (Zhang et al., 2021). Among these, biological peptides, classified as amino acids, have emerged as pivotal players in alleviating abiotic stress in plants (Colla et al., 2015). Glutathione serves as a notable example in this context, as it functions to inhibit oxidative stress, stimulate enzymatic and non-enzymatic activities, and preserve cell membrane integrity (Punia et al., 2021). Similarly, systemin, a small peptide, has been found to enhance antioxidant capacity by upregulating enzymatic activity in plants subjected to salt stress (Cirillo et al., 2022). Another noteworthy compound is γ-aminobutyric acid (GABA), a non-protein amino acid that plays a crucial role in plant stress response. Under salt stress conditions, the expression of GABA-transaminase involved in GABA metabolism is significantly up-regulated in rice leaves (Kim et al., 2007). Similarly, when GABA was supplemented with salt-stressed maize, it partially restored seedling growth, even under moderate and severe stressed conditions (Wang et al., 2017). The combined application of GABA and potassium (K) to wheat has demonstrated notable benefits, including improved carbon and nitrogen assimilation, enhanced accumulation of osmotic regulators, reduced oxidative stress markers, and improved photosynthesis-related traits. These synergistic effects resulted in enhanced yield traits under salt stress compared to the application of either GABA or K alone (Kumari et al., 2023). In this study, VTID exhibited remarkable salt stress resistance by restoring the growth of maize seedlings even at a low concentration of 10 nM (Figure 1). Future investigations should explore whether VTID could yield even greater benefits when combined with other plant growth regulators, thereby enhancing stress tolerance and overall plant performance.
Salt stress induces the production of ROS, triggering membrane damage and lipid peroxidation, consequently elevating levels of MDA (Lodeyro et al., 2016). MDA concentration serves as a reliable indicator of lipid peroxidation in plants induced by environmental stress (Kong et al., 2016). To counteract the detrimental effects of ROS, plants have evolved an antioxidase system, which includes enzymes such as SOD, POD, and CAT. These enzymes play a vital role in scavenging ROS and reducing oxidative damage (Gill and Tuteja, 2010; Zhao et al., 2021). Proline, on the other hand, acts as an osmoregulator in plants, minimizing water loss and serving as a nitrogen source during plant recovery (Yi et al., 2022). It also enhances the activities of antioxidant enzymes (Ali et al., 2007; Devnarain et al., 2016). Our research findings demonstrate that under salt stress conditions, the application of exogenous VTID significantly enhances the activities of SOD and CAT, elevated proline levels, and reduced MDA accumulation. However, supplementation with VTID, with or without salt treatment, does not affect POD activity, possibly due to the concentration of NaCl used in maize (Pandey et al., 2015). These results suggest that VTID may function similarly to PGRs in alleviating salt stress-induced cell membrane damage, leading to improved salt tolerance in plants.
Phytohormones also play a crucial role in regulating plant development and enhancing tolerance to salt stress. Maintaining optimal concentrations of IAA, GA, and ABA can alleviate salt stress-induced oxidative damage to plant cells (Ryu and Cho, 2015). However, our research findings indicate that exogenous VTID does not significantly affect the accumulation of IAA, GA, and ABA in maize leaves under salt stress. This suggests that while phytohormones play a crucial role in other plant responses to stress, other metabolites in VTID might predominantly contribute to enhancing plant growth in the presence of salt stress. Metabolomic analysis of VTID-treated plants under salt stress conditions revealed 14 metabolites, of which five were unclassified and five were classified into lipids and lipid-like molecules. As an essential component of plant biological membranes, changes in membrane lipid composition in plants under salt stress can alter the activity of membrane proteins and the membrane permeability to water, ions, and metabolites (Guo et al., 2022). This explains the observed alterations in lipid and lipid-like molecules in maize seedlings in response to salinity upon supplementation with VTID.
Subsequent analysis involved a linear regression correlating plant growth indicators with predictive metabolites obtained from ROC curve analysis. Remarkably, a robust relationship (R2 = 0.8678, p < 0.0001) was observed between isodolichantoside and plant height, indicating that isodolichantoside could elucidate the variation in plant height under salt stress conditions. Isodolichantoside, categorized as a monoterpene indole alkaloid (MIA), is a natural product isolated from Psychotria correae, Cephaelis correae, and Strychnos mellodora (Achenbach et al., 1993, 1995). Interestingly, isodolichantoside was previously unidentified in maize. Isodolichantoside isolated from leaf extract of P. correae, showed activity in the brine shrimp lethality test (Achenbach et al., 1995). In addition, MIAs are plant-natural products with important pharmacological, antioxidant, anti-inflammatory, anti-tumoral, immunomodulatory, antiviral, antimicrobial, and antiprotozoal activities (Matsuura et al., 2013). ROS, particularly H2O2, triggered by abiotic stress stimulates alkaloid biosynthesis and accumulation. This process serves as part of the plant’s antioxidant defense, helping restore an appropriate oxidative balance (Matsuura et al., 2014). From this, it can be concluded that VTID stimulates the accumulation of isodolichantoside to scavenge ROS and help plants survive under salt-stress conditions.
The present study investigated the effects of applying exogenous 10 nM VTID to maize seedlings under salt stress. The results revealed that the application of VTID increased proline levels, SOD and CAT activities, while decreasing MDA levels. These changes ultimately resulted in an increase in plant height and fresh weight. Metabolomics analysis indicated that VTID-induced DMs were associated with lipids and lipid-like molecules under salt stress conditions. Notably, a key metabolite, isodolichantoside showed a strong positive correlation with the height of maize seedlings, suggesting its involvement in the regulation of maize growth under salt stress conditions. Further research on short peptide VTID will provide valuable insights into its potential and will expand our current understanding of how plants regulate resistance to salt stress.
The data presented in the study are deposited in the MetaboLights repository, accession number MTBLS9653.
KW: Investigation, Methodology, Writing – original draft. XL: Validation, Visualization, Writing – original draft. XZ: Conceptualization, Formal analysis, Funding acquisition, Methodology, Project administration, Supervision, Writing – review & editing. GY: Writing – review & editing. HW: Investigation, Writing – original draft. YX: Funding acquisition, Writing – review & editing. SI: Writing – review & editing. HJ: Methodology, Writing – review & editing. YQ: Methodology, Writing – review & editing. WG: Conceptualization, Formal analysis, Funding acquisition, Methodology, Project administration, Supervision, Writing – review & editing.
The author(s) declare financial support was received for the research, authorship, and/or publication of this article. The authors declare financial support was received for the research, authorship, and/or publication of this article This research received financial support from the National Natural Science Foundation of China (32060424 and 32072377), the Ningxia Key Research and Development Plan (2023BCF01014), Science and Technology Leading Talents of Ningxia Hui Autonomous Region (2022GKLRLX06) and National Major Agricultural Science and Technology Project.
The authors declare that the research was conducted in the absence of any commercial or financial relationships that could be construed as a potential conflict of interest.
All claims expressed in this article are solely those of the authors and do not necessarily represent those of their affiliated organizations, or those of the publisher, the editors and the reviewers. Any product that may be evaluated in this article, or claim that may be made by its manufacturer, is not guaranteed or endorsed by the publisher.
The Supplementary Material for this article can be found online at: https://www.frontiersin.org/articles/10.3389/fpls.2024.1374142/full#supplementary-material
Achenbach, H., Lottes, M., Waibel, R., Karikas, G. A., Correa, M. D., Gupta, M. P. (1995). Alkaloids and other compounds from Psychotria correae. Phytochemistry 38, 1537–1545. doi: 10.1016/0031-9422(94)00823-C
Achenbach, H., Lottes, M., Waibel, R., Karikas, G., Gupta, M. (1993). New alkaloids from the leaves of Cephaelis correae. Planta Med. 59, 619. doi: 10.1055/s-2006-959849
Ali, B., Hayat, S., Ahmad, A. (2007). 28-Homobrassinolide ameliorates the saline stress in chickpea (Cicer arietinum L.). Environ. Exp. Bot. 59, 217–223. doi: 10.1016/j.envexpbot.2005.12.002
Borges, C. V., Orsi, R. O., Maraschin, M., Lima, G. P. P. (2023). ““Chapter 27 - Oxidative stress in plants and the biochemical response mechanisms,”,” in Plant Stress Mitigators. Eds. Ghorbanpour, M., Shahid, M. A. (Academic Press, NewYork), 455–468.
Choudhary, S., Wani, K. I., Naeem, M., Khan, M. M. A., Aftab, T. (2023). Cellular responses, osmotic adjustments, and role of osmolytes in providing salt stress resilience in higher plants: polyamines and nitric oxide crosstalk. J. Plant Growth Regul. 42, 539–553. doi: 10.1007/s00344-022-10584-7
Cirillo, V., Molisso, D., Aprile, A. M., Maggio, A., Rao, R. (2022). Systemin peptide application improves tomato salt stress tolerance and reveals common adaptation mechanisms to biotic and abiotic stress in plants. Environ. Exp. Bot. 199, 104865. doi: 10.1016/j.envexpbot.2022.104865
Colla, G., Nardi, S., Cardarelli, M., Ertani, A., Lucini, L., Canaguier, R., et al. (2015). Protein hydrolysates as biostimulants in horticulture. Sci. Hortic. 196, 28–38. doi: 10.1016/j.scienta.2015.08.037
del Mar Gómez-Ramos, M., Rajski, Ł., Heinzen, H., Fernández-Alba, A. R. (2015). Liquid chromatography Orbitrap mass spectrometry with simultaneous full scan and tandem MS/MS for highly selective pesticide residue analysis. Anal. Bioanal. Chem. 407, 6317–6326. doi: 10.1007/s00216-015-8709-z
Devnarain, N., Crampton, B. G., Chikwamba, R., Becker, J. V. W., O’Kennedy, M. M. (2016). Physiological responses of selected African sorghum landraces to progressive water stress and re-watering. S. Afr. J. Bot. 103, 61–69. doi: 10.1016/j.sajb.2015.09.008
Food and Agriculture Organization. (2023). Global map of salt-affected soils (GSASmap). Available online at: https://www.fao.org/global-soil-partnership/gsasmap/en (Accessed December 5, 2023).
Gai, Z. S., Wang, Y., Ding, Y. Q., Qian, W. J., Qiu, C., Xie, H., et al. (2020). Exogenous abscisic acid induces the lipid and flavonoid metabolism of tea plants under drought stress. Sci. Rep. 10, 12275. doi: 10.1038/s41598-020-69080-1
Gill, S. S., Tuteja, N. (2010). Reactive oxygen species and antioxidant machinery in abiotic stress tolerance in crop plants. Plant Physiol. Biochem. 48, 909–930. doi: 10.1016/j.plaphy.2010.08.016
Guo, Q., Liu, L., Rupasinghe, T. W. T., Roessner, U., Barkla, B. J. (2022). Salt stress alters membrane lipid content and lipid biosynthesis pathways in the plasma membrane and tonoplast. Plant Physiol. 189, 805–826. doi: 10.1093/plphys/kiac123
Hassani, A., Azapagic, A., Shokri, N. (2021). Global predictions of primary soil salinization under changing climate in the 21st century. Nat. Commun. 12, 6663. doi: 10.1038/s41467-021-26907-3
He, M., Ren, T., Jin, Z. D., Deng, L., Liu, H., Cheng, Y., et al. (2023). Precise analysis of potassium isotopic composition in plant materials by multi-collector inductively coupled plasma mass spectrometry. Spectrochim. Acta B 209, 106781. doi: 10.1016/j.sab.2023.106781
Hu, Q., Zhao, Y., Hu, X., Qi, J., Suo, L., Pan, Y., et al. (2022). Effect of saline land reclamation by constructing the “Raised Field -Shallow Trench” pattern on agroecosystems in Yellow River Delta. Agr. Water Manage. 261, 107345. doi: 10.1016/j.agwat.2021.107345
Jan, R., Asaf, S., Numan, M., Lubna, Kim, K.-M. (2021). Plant secondary metabolite biosynthesis and transcriptional regulation in response to biotic and abiotic stress conditions. Agronomy 11, 968. doi: 10.3390/agronomy11050968
Ji, H., Yang, G., Zhang, X., Zhong, Q., Qi, Y., Wu, K., et al. (2022). Regulation of salt tolerance in the roots of Zea mays by L-histidine through transcriptome analysis. Front. Plant Sci. 13. doi: 10.3389/fpls.2022.1049954
Jiang, M., Chen, S., Lu, X., Guo, H., Chen, S., Yin, X., et al. (2023). Integrating genomics and metabolomics for the targeted discovery of new cyclopeptides with antifungal activity from a marine-derived fungus Beauveria felina. J. Agric. Food Chem. 71, 9782–9795. doi: 10.1021/acs.jafc.3c02415
Khan, Z., Jan, R., Asif, S., Farooq, M., Jang, Y. H., Kim, E. G., et al. (2024). Exogenous melatonin induces salt and drought stress tolerance in rice by promoting plant growth and defense system. Sci. Rep. 14, 1214. doi: 10.1038/s41598-024-51369-0
Khan, A., Zhao, X. Q., Tariq Javed, M., Khan, K. S., Bano, A., Shen, R. F., et al. (2016). Bacillus pumilus enhances tolerance in rice (Oryza sativa L.) to combined stresses of NaCl and high boron due to limited uptake of Na+. Environ. Exp. Bot. 124, 120–129. doi: 10.1016/j.envexpbot.2015.12.011
Kim, D. W., Shibato, J., Agrawal, G. K., Fujihara, S., Iwahashi, H., Kim, D. H., et al. (2007). Gene transcription in the leaves of rice undergoing salt-induced morphological changes (Oryza sativa L.). Mol. Cells 24, 45–59. doi: 10.1016/S1016-8478(23)10755-2
Kohli, S. K., Bali, S., Tejpal, R., Bhalla, V., Verma, V., Bhardwaj, R., et al. (2019). In-situ localization and biochemical analysis of bio-molecules reveals Pb-stress amelioration in Brassica juncea L. by co-application of 24-epibrassinolide and salicylic acid. Sci. Rep. 9, 3524. doi: 10.1038/s41598-019-39712-2
Kong, W., Liu, F., Zhang, C., Zhang, J., Feng, H. (2016). Non-destructive determination of malondialdehyde (MDA) distribution in oilseed rape leaves by laboratory scale NIR hyperspectral imaging. Sci. Rep. 6, 35393. doi: 10.1038/srep35393
Kumari, S., Nazir, F., Jain, K., Khan, K. M. I. (2023). GABA and potassium modulates defence systems, assimilation of nitrogen and carbon, and yield traits under salt stress in wheat. J. Plant Growth Regul. 42, 6721–6740. doi: 10.1007/s00344-023-10992-3
Lei, P., Pang, X., Feng, X., Li, S., Chi, B., Wang, R., et al. (2017). The microbe-secreted isopeptide poly-γ-glutamic acid induces stress tolerance in Brassica napus L. seedlings by activating crosstalk between H2O2 and Ca2+. Sci. Rep. 7, 41618. doi: 10.1038/srep41618
Li, S., Jin, H., Zhang, Q. (2016). The effect of exogenous spermidine concentration on polyamine metabolism and salt tolerance in zoysiagrass (zoysia japonica steud) subjected to short-term salinity stress. Front. Plant Sci. 7. doi: 10.3389/fpls.2016.01221
Liu, Y., Pan, J., Ni, S., Xing, B., Cheng, K., Peng, X. (2022). Transcriptome and metabonomics combined analysis revealed the defense mechanism involved in hydrogen-rich water-regulated cold stress response of Tetrastigma hemsleyanum. Front. Plant Sci. 13. doi: 10.3389/fpls.2022.889726
Lodeyro, A. F., Giró, M., Poli, H. O., Bettucci, G., Cortadi, A., Ferri, A. M., et al. (2016). Suppression of reactive oxygen species accumulation in chloroplasts prevents leaf damage but not growth arrest in salt-stressed tobacco plants. PloS One 11, e0159588. doi: 10.1371/journal.pone.0159588
Matsuura, H. N., Porto, D. D., Fett-Neto, A. G. (2013). ““Bioactive alkaloids from south american Psychotria and related rubiaceae,”,” in Natural Products: Phytochemistry, Botany and Metabolism of Alkaloids, Phenolics and Terpenes. Eds. Ramawat, K. G., Mérillon, J.-M. (Springer, Berlin), 119–147.
Matsuura, H. N., Rau, M. R., Fett-Neto, A. G. (2014). Oxidative stress and production of bioactive monoterpene indole alkaloids: biotechnological implications. Biotechnol. Lett. 36, 191–200. doi: 10.1007/s10529-013-1348-6
Mo, X., Zhou, M., Li, Y., Yu, L., Bai, H., Shen, P., et al. (2023). Safety assessment of a novel marine multi-stress-tolerant yeast Meyerozyma guilliermondii GXDK6 according to phenotype and whole genome-sequencing analysis. Food Sci. Hum. Well. 13. doi: 10.26599/FSHW.2022.9250170
Muchate, N. S., Nikalje, G. C., Rajurkar, N. S., Suprasanna, P., Nikam, T. D. (2016). Plant salt stress: adaptive responses, tolerance mechanism and bioengineering for salt tolerance. Bot. Rev. 82, 371–406. doi: 10.1007/s12229-016-9173-y
Noreen, Z., Ashraf, M. (2009). Assessment of variation in antioxidative defense system in salt-treated pea (Pisum sativum) cultivars and its putative use as salinity tolerance markers. J. Plant Physiol. 166, 1764–1774. doi: 10.1016/j.jplph.2009.05.005
Omoto, E., Iwasaki, Y., Miyake, H., Taniguchi, M. (2016). Salinity induces membrane structure and lipid changes in maize mesophyll and bundle sheath chloroplasts. Physiol. Plant 157, 13–23. doi: 10.1111/ppl.12404
Pandey, D. S., Patel, M., Mishra, A., Jha, B. (2015). Physio-biochemical composition and untargeted metabolomics of cumin (Cuminum cyminum L.) make it promising functional food and help in mitigating salinity stress. PloS One 10, e0144469. doi: 10.1371/journal.pone.0144469
Punia, H., Tokas, J., Malik, A., Bajguz, A., El-Sheikh, M. A., Ahmad, P. (2021). Ascorbate-glutathione oxidant scavengers, metabolome analysis and adaptation mechanisms of ion exclusion in sorghum under salt stress. Int. J. Mol. Sci. 22, 13249. doi: 10.3390/ijms222413249
Qin, X., Zhang, K., Fan, Y., Fang, H., Nie, Y., Wu, X. (2022). The bacterial MtrAB two-component system regulates the cell wall homeostasis responding to environmental alkaline stress. Microbiol. Spectr. 10, e02311–e02322. doi: 10.1128/spectrum.02311-22
Ren, Y., Yu, G., Shi, C., Liu, L., Guo, Q., Han, C., et al. (2022). Majorbio Cloud: A one-stop, comprehensive bioinformatic platform for multiomics analyses. iMeta 1, e12. doi: 10.1002/imt2.12
Ru, C., Hu, X., Chen, D., Wang, W., Zhen, J. (2023). Photosynthetic, antioxidant activities, and osmoregulatory responses in winter wheat differ during the stress and recovery periods under heat, drought, and combined stress. Plant Sci. 327, 111557. doi: 10.1016/j.plantsci.2022.111557
Ryu, H., Cho, Y.-G. (2015). Plant hormones in salt stress tolerance. J. Plant Biol. 58, 147–155. doi: 10.1007/s12374-015-0103-z
Sharma, I., Ching, E., Saini, S., Bhardwaj, R., Pati, P. K. (2013). Exogenous application of brassinosteroid offers tolerance to salinity by altering stress responses in rice variety Pusa Basmati-1. Plant Physiol. Biochem. 69, 17–26. doi: 10.1016/j.plaphy.2013.04.013
Tian, H., Fan, G., Xiong, X., Wang, H., Zhang, S., Geng, G. (2024). Characterization and transformation of the CabHLH18 gene from hot pepper to enhance waterlogging tolerance. Front. Plant Sci. 14. doi: 10.3389/fpls.2023.1285198
Wang, H. (2020). Studies on the growth promotion of maize induced by Bacillus amyloliquefaciens YM6 under salt stress and its mechanisms of salt-tolerance. North Minzu University, Ningxia (China.
Wang, Y., Gu, W., Meng, Y., Xie, T., Li, L., Li, J., et al. (2017). γ-aminobutyric acid imparts partial protection from salt stress injury to maize seedlings by improving photosynthesis and upregulating osmoprotectants and antioxidants. Sci. Rep. 7, 43609. doi: 10.1038/srep43609
Wei, X., Gao, C., Chang, C., Tang, Z., Li, D. (2023). Metabonomics reveals the mechanism of trehalose protecting Catharanthus roseus against low-temperature. J. Plant Growth Regul. 42, 3730–3742. doi: 10.1007/s00344-022-10833-9
Xia, J., Broadhurst, D. I., Wilson, M., Wishart, D. S. (2013). Translational biomarker discovery in clinical metabolomics: an introductory tutorial. Metabolomics 9, 280–299. doi: 10.1007/s11306-012-0482-9
Xia, X. J., Gao, C. J., Song, L. X., Zhou, Y. H., Shi, K., Yu, J. Q. (2014). Role of H2O2 dynamics in brassinosteroid-induced stomatal closure and opening in Solanum lycopersicum. Plant Cell Environ. 37, 2036–2050. doi: 10.1111/pce.12275
Xiao, Q., Mu, X., Liu, J., Li, B., Liu, H., Zhang, B., et al. (2022). Plant metabolomics: a new strategy and tool for quality evaluation of Chinese medicinal materials. Chin. Med. 17, 45. doi: 10.1186/s13020-022-00601-y
Xiao, F., Zhou, H. (2023). Plant salt response: Perception, signaling, and tolerance. Front. Plant Sci. 13. doi: 10.3389/fpls.2022.1053699
Xiong, Q., Zhang, J., Sun, C., Wang, R., Wei, H., He, H., et al. (2023). Metabolomics revealed metabolite biomarkers of antioxidant properties and flavonoid metabolite accumulation in purple rice after grain filling. Food Chem. X 18, 100720. doi: 10.1016/j.fochx.2023.100720
Xue, Y., Bai, X., Zhao, C., Tan, Q., Li, Y., Yun, L., et al. (2023). Spring photosynthetic phenology of Chinese vegetation in response to climate change and its impact on net primary productivity. Agr. For. Meteorol. 342, 109734. doi: 10.1016/j.agrformet.2023.109734
Yang, Y., Guo, Y. (2018). Elucidating the molecular mechanisms mediating plant salt-stress responses. New Phytol. 217, 523–539. doi: 10.1111/nph.14920
Yi, J., Li, H., Zhao, Y., Shao, M., Zhang, H., Liu, M. (2022). Assessing soil water balance to optimize irrigation schedules of flood-irrigated maize fields with different cultivation histories in the arid region. Agr. Water Manage. 265, 107543. doi: 10.1016/j.agwat.2022.107543
Yu, Z., Xu, Y., Zhu, L., Zhang, L., Liu, L., Zhang, D., et al. (2020). The Brassicaceae-specific secreted peptides, STMPs, function in plant growth and pathogen defense. J. Integr. Plant Biol. 62, 403–420. doi: 10.1111/jipb.12817
Zhang, H., Sun, X., Dai, M. (2021). Improving crop drought resistance with plant growth regulators and rhizobacteria: Mechanisms, applications, and perspectives. Plant Commun. 3, 100228. doi: 10.1016/j.xplc.2021.100228
Zhao, K.-F., Song, J., Fan, H., Zhou, S., Zhao, M. (2010). Growth response to ionic and osmotic stress of nacl in salt-tolerant and salt-sensitive maize. J. Integr. Plant Biol. 52, 468–475. doi: 10.1111/j.1744-7909.2010.00947.x
Zhao, S., Zhang, Q., Liu, M., Zhou, H., Ma, C., Wang, P. (2021). Regulation of plant responses to salt stress. Int. J. Mol. Sci. 22, 4609. doi: 10.3390/ijms22094609
Keywords: exogenous, maize, metabolomics, salt stress, short peptide
Citation: Wu K, Liang X, Zhang X, Yang G, Wang H, Xia Y, Ishfaq S, Ji H, Qi Y and Guo W (2024) Metabolomics analysis reveals enhanced salt tolerance in maize through exogenous Valine-Threonine-Isoleucine-Aspartic acid application. Front. Plant Sci. 15:1374142. doi: 10.3389/fpls.2024.1374142
Received: 21 January 2024; Accepted: 25 April 2024;
Published: 17 May 2024.
Edited by:
Sajid Masood, Bahauddin Zakariya University, PakistanReviewed by:
Muhammad Saqlain Zaheer, Khwaja Fareed University of Engineering and Information Technology (KFUEIT), PakistanCopyright © 2024 Wu, Liang, Zhang, Yang, Wang, Xia, Ishfaq, Ji, Qi and Guo. This is an open-access article distributed under the terms of the Creative Commons Attribution License (CC BY). The use, distribution or reproduction in other forums is permitted, provided the original author(s) and the copyright owner(s) are credited and that the original publication in this journal is cited, in accordance with accepted academic practice. No use, distribution or reproduction is permitted which does not comply with these terms.
*Correspondence: Xiu Zhang, emhhbmd4aXUxMDFAbm11LmVkdS5jbg==; Wei Guo, Z3Vvd2VpMDFAY2Fhcy5jbg==
†These authors have contributed equally to this work and share first authorship
Disclaimer: All claims expressed in this article are solely those of the authors and do not necessarily represent those of their affiliated organizations, or those of the publisher, the editors and the reviewers. Any product that may be evaluated in this article or claim that may be made by its manufacturer is not guaranteed or endorsed by the publisher.
Research integrity at Frontiers
Learn more about the work of our research integrity team to safeguard the quality of each article we publish.