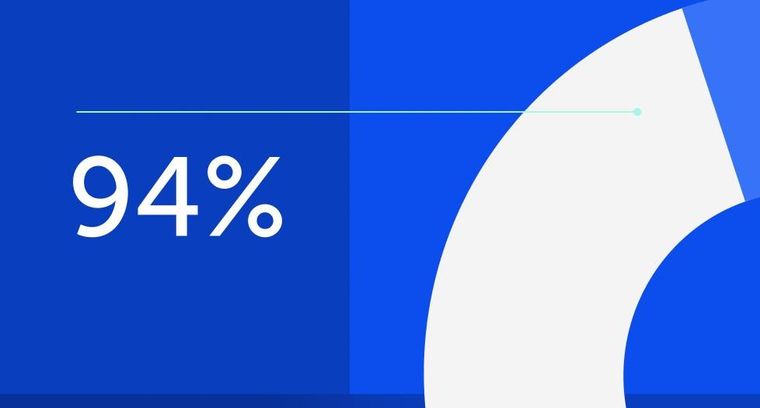
94% of researchers rate our articles as excellent or good
Learn more about the work of our research integrity team to safeguard the quality of each article we publish.
Find out more
MINI REVIEW article
Front. Plant Sci., 12 March 2024
Sec. Plant Pathogen Interactions
Volume 15 - 2024 | https://doi.org/10.3389/fpls.2024.1373801
This article is part of the Research TopicInvestigating the Elements of Plant Defense Mechanisms Within Plant Immune Responses Against PathogensView all 19 articles
The interaction between plant hosts and plant viruses is a very unique and complex process, relying on dynamically modulated intercellular redox states and the generation of reactive oxygen species (ROS). Plants strive to precisely control this state during biotic stress, as optimal redox levels enable proper induction of defense mechanisms against plant viruses. One of the crucial elements of ROS regulation and redox state is the production of metabolites, such as glutathione, or the activation of glutathione-associated enzymes. Both of these elements play a role in limiting the degree of potential oxidative damage in plant cells. While the role of glutathione and specific enzymes is well understood in other types of abiotic and biotic stresses, particularly those associated with bacteria or fungi, recent advances in research have highlighted the significance of glutathione modulation and mutations in genes encoding glutathione-associated enzymes in triggering immunity or susceptibility against plant viruses. Apparently, glutathione-associated genes are involved in precisely controlling and protecting host cells from damage caused by ROS during viral infections, playing a crucial role in the host’s response. In this review, we aim to outline the significant improvements made in research on plant viruses and glutathione, specifically in the context of their involvement in susceptible and resistant responses, as well as changes in the localization of glutathione. Analyses of essential glutathione-associated enzymes in susceptible and resistant responses have demonstrated that the levels of enzymatic activity or the absence of specific enzymes can impact the spread of the virus and activate host-induced defense mechanisms. This contributes to the complex network of the plant immune system. Although investigations of glutathione during the plant-virus interplay remain a challenge, the use of novel tools and approaches to explore its role will significantly contribute to our knowledge in the field.
The plant organisms, being generally static land organisms, are consistently exposed to a wide range of pathogens that are an ongoing danger to them. Consequently, plants have developed a sophisticated network of defense systems to protect themselves from pathogen incursions and the development of diseases (Ramirez-Prado et al., 2018; Saijo and Loo, 2020; Zechmann, 2020). The components of the plant defense system encompass physical changes in host cells, also known as constitutive defenses, such as the thickening of cell walls to stop external invaders (Martínez-González et al., 2018; Zhu and Li, 2021) or to hinder the translocation of pathogens, such as viruses, within the plant (Otulak-Kozieł et al., 2018a, b, 2020). However, physical barriers alone are often not sufficient to block pathogenesis. Therefore, plants activate internal chemical and molecular pathways to induce defense mechanisms and eliminate pathogenic invasions (Zogli and Libault, 2017; Gimenez et al., 2018; Ramirez-Prado et al., 2018; Hammerbach et al., 2019; Yang et al., 2019; Saijo and Loo, 2020; Zechmann, 2020). The speed and effectiveness of this response play a crucial role in determining the future fate of the plant host (Mandadi and Scholthof, 2013; Kozieł et al., 2021). In this context, it is important to note that plant viruses are specific pathogens that are active only inside the host cell, as they constantly rely on cellular machinery for reproduction (Pogue et al., 2002; Otulak and Garbaczewska, (2014); Kozieł et al., 2021). Thus, plant viruses generally try to keep their hosts alive for as long as possible. This characteristic makes the virus-plant interaction a prolonged one and is often highly dependent on the internal pathways of the host. During stress associated with the presence of biotic/abiotic stressors reactive oxygen species (ROS) are produced. ROS directed plant reaction and are used as signal transduction molecules that control different reaction pathways. Besides biochemical production during stress metabolism ROS are also generated by NADPH oxidases (also named respiratory burst oxidase homologs, RBOHs), peroxidases and other oxidases types (Yadav, 2010). Therefore, final level of ROS molecules could be highly dependent on involvement of many factors. Because of that, plants developed complexed ROS control system based on enzymes (like glutathione reductase-GR, glutathione S-transferase-GST, glutathione peroxidase-GPX) and scavenging non-enzymatic hydrophilic molecules like ascorbate (AsA) and glutathione (GSH) (Noctor and Foyer, 1998). Currently, we know that many (groups of) elements have established themselves as important in the defense of plants against pathogens and plant viruses such as antioxidants, lipids, ROS, jasmonic acid (JA), salicylic acid (SA), and many more (Hernández et al., 2016; Gullner et al., 2017; Koch et al., 2017; Yang et al., 2019; Ding and Ding, 2020; Van Butselaar and Van den Ackerveken, 2020). Among these elements is also glutathione (GSH), which plays a very interesting multifunctional role. As an antioxidant, glutathione enables precise direct or indirect control of ROS (such as singlet oxygen, superoxide anions, hydrogen peroxide, etc.), which often accumulate/produce at high levels during biotic stress, thereby reducing damage to the cells (Hernández et al., 2016; Gullner et al., 2017). This protection is crucial because ROS are not only engaged in signal transduction, but also can oxidize lipids, inhibit enzymes, inactivate biomolecules, and damage proteins, RNA, and DNA, causing a critical level of cell damage. GSH also activates defense pathways against pathogens by mediating between ROS, SA, JA, and ethylene (Alquéres et al., 2013; Han et al., 2013; Ghanta et al., 2014; Cheng et al., 2015; Hernández et al., 2016; Gullner et al., 2017; Künstler et al., 2019a, b; Zechmann, 2020). Moreover, many authors emphasize the high mobility of glutathione; which is systemically transported and serves as a storage form of reduced sulfur, which can be remobilized when needed by plants (Otulak-Kozieł et al., 2022a). Thus, GSH plays the role of a mediator in crucial cellular processes, such as cell cycle progression and programmed cell death (Diaz-Vivancos et al., 2010).
The GSH itself is created from amino acids, including glutamate, L-cysteine, and glycine, through two ATP-dependent enzymatic reactions mediated by γ-glutamylcysteine synthetase (γ-ECS or also known as GSH1) and GSH synthetase (GS or also named GSH2) (Noctor et al., 2002, 2012; Koramutla et al., 2021). The first and rate-controlling step, catalyzed by γ-ECS, produces γ-glutamylcysteine (γ-EC) from the amino acids L-glutamate and L-cysteine. In the second step, GSH synthetase adds glycine to γ-glutamylcysteine (γ-EC) to produce GSH (Figure 1). The reaction catalyzed by γ-ECS/GSH1 is considered the rate-controlling step of GSH synthesis, and the activity of this enzyme is regulated by cellular levels of cysteine and glutamic acid, and feedback inhibition by γ-EC and GSH (Hernández et al., 2015; Koramutla et al., 2021).
Figure 1 GSH cellular synthesis and usage. γ-glutamylcysteine synthetase (GSH1) active in plastids; GSH synthetase (GSH2) active in plastids and cytoplasm; reduced glutathione form (GSH); oxidized glutathione form (GSSG); glutathione-S-transferase (GST); glutathione peroxidase-like (GPXL), glutaredoxin (GRX); glutathione reductase (GR); S-nitrosoglutathione (GSNO).
GSH1 (EC 6.3.2.2) is exclusively present in plastids, while GSH2 (EC 6.3.2.2) has dual localization in plastids and the cytosol, both encoded by a single gene (Wachter et al., 2005). According to the subcellular localization of GSH1, the GSH synthesis initiates in the plastids, but the predominant transcript, especially in the case of multiple GSH2 transcript populations, encodes a cytosolic GSH2, suggesting the second step occurs in the cytosol (Noctor et al., 2012). After synthesis, GSH can actively move to other cellular compartments, predominantly in its reduced or conjugated forms (Noctor et al., 2012). The reduced GSH form can readily transform into its oxidized form, GSSG, in various biochemical reactions. The cellular homeostasis between the GSH and GSSG ratio controls the cell’s redox level, maintained by reactions performed by glutathione reductases (GR) and glutathione peroxidases (GPX) (Mahmood et al., 2010). The GRs (EC 1.8.1.7) are integral to plant antioxidant defense systems against pathogens, participating in both enzymatic and nonenzymatic oxidation-reduction processes of the cell (Clarke et al., 2022). GRs depend on NADPH levels to transform GSSG to GSH, and through it, they maintain a high ratio of GSH/GSSG in the cell and contribute to the response against plant viruses (Otulak-Kozieł et al., 2023). The plant glutathione peroxidase-like enzymes (GPXL) family consists of multiple isoenzymes with distinct subcellular locations, exhibiting different tissue-specific expression patterns and involvement in various types of stress. Plant GPXLs, containing cysteine in their active site domain, may have dual roles, acting as glutathione peroxidase and thioredoxin peroxidase functions (Bela et al., 2015). The thiol-dependent activities of plant GPXL isoenzymes indicate their role in detoxifying H2O2 and organic hydroperoxides, as well as their involvement in regulating cellular redox homeostasis by maintaining thiol/disulfide or NADPH/NADP+ ratios (Bela et al., 2015). In this context, GPXL can modulate the levels of NADPH needed for GR activity in the recreation of GSH from GSSG.
Deficiency in the activity of either GSH1 or GSH2 impairs GSH production, negatively impacting plant growth and development. On the other hand, overproduction of glutathione in tobacco mutants, as reported by Künstler et al. (2019a), enhances resistance to tobacco mosaic virus (TMV) infections. Moreover, several factors including the concentrations of cysteine and glycine, the availability of ATP, photosynthetically active photon flux, and enzymes that consume GSH, also regulate GSH biosynthesis (Ogawa et al., 2004; Noctor et al., 2012). Many of these factors undergo changes during viral infections and may influence the redox state of the cell. Once produced, GSH can undergo conjugation with toxic substances (Noctor et al., 2012; Shu et al., 2016) or participate in the modulation of viral infection (Otulak-Kozieł et al., 2022a) through the action of glutathione S-transferase-GST (EC 2.5.1.18) (Figure 1). GSH can also serve as a substrate for S-glutathionylation of proteins in the presence of small redox enzyme glutaredoxins (GRX), which also utilizes GSH as a cofactor (Figure 1). Additionally, GSH can react with the NO free radical to produce GSNO, which nitrosylates target proteins. The role of S-glutathionylation or S-nitrosylation in plant-virus interaction is not well understood. Sarkar et al. (2011) suggested the involvement of GSNO in the compatible interaction of mesta yellow vein mosaic virus (MeYVMV) with Hibiscus cannabinus. In the case of GSTs, these multifunctional and essential enzymes are involved in many processes, such as detoxification, signaling, redox homeostasis, plant metabolism, growth regulation, and adaptation to biotic and abiotic stress (Chronopopulou et al., 2017). GSTs catalyze the conjugation of GSH to various hydrophobic compounds and also perform noncatalytic functions as transporters (Chronopopulou et al., 2017). They also act as signaling markers for infection by various pathogens (Dixon and Edwards, 2010; Sabetta et al., 2017). Deep sequencing investigations have revealed that the glutathione cycle and the expression profiles of GST are regulated by various plant-virus interactions involving Tobamovirus (Li et al., 2017), Geminivirus (Góngora-Castillo et al., 2012), and Tenuivirus (Sun et al., 2016).
A relatively low number of studies have focused on the importance of the glutathione cycle and glutathione-associated enzymes in plant cell responses, both compatible and incompatible, or their potential role in developing resistance to plant viruses. The complete array of plant virus-associated elements involved in the glutathione cycle remains unknown. Hence, this review presents the current understanding of the role of glutathione and glutathione-associated enzymes in the susceptible and resistant responses of plants to viruses. Additionally, it seeks to summarize potential avenues for future research, exploring various aspects of plant-pathogen interactions.
The resistance and tolerance of plants against plant viruses is directly connected with the controlled generation of ROS during virus recognition. This process facilitates signal transduction to inform the plant about infection and enables the initiation of a well-directed defense response (in resistance) or partially directed (in tolerance). Therefore, maintaining a precisely controlled level of ROS is crucial, as overproduction could disrupt plant defense/tolerance responses and lead to direct and serious damage to cells. Elements crucial for ROS control, cell protection, and known antiviral responses include the glutathione cycle, especially the levels of GSH and GSSG forms, along with glutathione-associated enzymes such as glutathione transferases (GSTs), glutathione reductases (GRs), and glutathione peroxidases (GPXs).The involvement of glutathione or glutathione treatment in resistance reactions to plant viruses has been reported for various virus types, including potato virus Y-PVY (Otulak-Kozieł et al., 2022a) on different cultivar of potato, tobacco mosaic virus—TMV on tobacco (Gullner et al., 1999; Király et al., 2012; Künstler et al., 2019a, b; Zhu et al., 2021), turnip mosaic virus-TuMV (Otulak-Kozieł et al., 2022b, 2023) on Arabidopsis and obuda pepper virus- pepper interaction (Kalapos et al., 2021). Generally, it is suggested that elevated glutathione or its external supplementation improves resistance or tolerance against plant viruses. Gullner et al. (1999) reported that the use of the cysteine precursor L-2-oxo-thazidine-carboxylic acid (OTC also known as GSH activator) on tobacco leaf discs resulted in the accumulation of glutathione and a significant reduction in TMV levels. A similar situation was reported during direct treatment by use of sulfur which inhibited the development of symptoms and limited virus levels in zucchini yellow mosaic virus (ZYMV)-infected pumpkin through an artificial increase in glutathione (Zechmann et al., 2005; Zechmann et al., 2007). Király et al. (2012) indicated that TMV-resistant tobacco plants with adequate sulfate availability showed fewer necrotic symptoms compared to those with a sulfate deficiency. These authors also postulated that virus resistance correlated with an elevated content of glutathione and Cys and the induction of glutathione. Furthermore, they observed that elevated levels of subcellular GSH in interspecific tobacco hybrid plants (Nicotiana edwardsonii var. Columbia, NEC) in response to TMV and TNV infection suggest that, in addition to SA, GSH may also contribute to the elevated virus resistance of NEC (Király et al., 2024). On the other hand the increased tolerance reaction against TMV was also confirmed by GSH and OTC treatment of tobacco GSH biosynthesis genes NbECS and NbGS mutants (Zhu et al., 2021). The results of Clemente-Moreno et al. (2010, 2013) indicated that pea and peach plants treated with OTC characterized tolerant/partially resistant response against plum pox virus (PPV) with lower level of symptoms occurrence. Moreover, after OTC treatment Clemente-Moreno et al. (2013) reported increased plant growth, increased protection to the photosynthetic machinery and the metabolism of chloroplast in PPV-infected in case of peach plants. Clemente-Moreno et al. (2013) suggested that this could be an effect of induction of non-expressor of pathogenesis-related genes 1 (NPR1) by OTC treatment. This directly indicated increased tolerance to virus infection stress could be an effect of co-involvement of GSH and NPR1 which was observed in tolerant interaction of tobacco with other types of pathogens (Ghanta et al., 2011). The GSH-related modulation of virus infection was also reported in the case of tolerant pumpkin and ZYMV (Zechmann and Müller, 2008). The exact mechanism of modulation of tolerance by GSH is still not entirely known, although Zhu et al. (2021) suggested that GSH could cooperate with SA in modulation of that process in case TMV in tobacco (Nicotiana tabacum) and in constitutive GSH synthesis during potato virus X (PVX) accumulation control in Nicotiana benthamiana (De et al., 2018; Künstler et al., 2019b). However, glutathione levels are not only important during external induction/delivery but also during natural internal production during viral infection. Otulak-Kozieł et al. (2022a) and Otulak-Kozieł et al. (2022b; 2023) detected modulation of glutathione levels during investigations of infections caused by PVYNTN and TuMV on susceptible and resistant (with hypersensitive response or hypersensitive-like, HR or HR-like) respectively on potato and rbohF and rbohD/f mutants of Arabidopsis (Figure 2). Resistant potato plants infected by PVYNTN exhibited a dynamic increase in the content of glutathione during both resistance and, to some extent, susceptible reactions. However, the increase of glutathione (GSH+GSSG and separate GSH and GSSG forms) during HR was more dynamic and stable. This increase correlated with a significant reduction in the amount and expression of PVYNTN and the induction of the HR response. A similar pattern of stable increase in levels of GSH and GSSG was observed in Arabidopsis mutant plants with increased resistance (rbohF) and HR-like reaction (rbohD/F) infected by TuMV (Otulak-Kozieł et al., 2023), which was also associated with decreased expression of the virus. Singh et al. (2020) reported that resistant cultivars of Vigna mungo inoculated with yellow mosaic virus (YMV) also showed an induction of glutathione production, suggesting that plants with viral resistance can potentially elevate the production of glutathione during infection. Fodor et al. (1997) indicated that resistant tobacco Xanthi, in reaction to TMV, exhibited an elevation in GSH, corresponding to results with PVYNTN, YMV, and TuMV. However, GSSG levels were slightly decreased in leaves after TMV inoculation, which differed from the observations in resistant potatoes infected by PVYNTN. Király et al. (2002) and Künstler et al. (2019b) explained that higher GSSG levels indicated the importance of glutathione in the restoration of TMV resistance, suggesting the suppression of oxidative stress HR in virus-infected cells and downstream defense responses. Otulak-Kozieł et al. (2022a; 2022b; 2023) also reported changes in cellular levels of glutathione content. PVYNTN and TuMV infections significantly elevated the glutathione content in cells of resistant potato and Arabidopsis plants and their mobility to specific cell components. Ultrastructural distribution of glutathione demonstrated by Otulak-Kozieł et al. (2022a; 2022b; 2023) in resistant plants showed that glutathione was mostly deposited in the chloroplast, cytoplasm, and nucleus during PVYNTN and TuMV infections. However, both interactions differed in the case of mitochondria, where in resistant plants against PVYNTN, deposition generally remained unchanged, while Arabidopsis plants resistant to TuMV exhibited induced deposition in this organelle. Similar results were reported by Höller et al. (2010) and Zechmann (2020) in resistant tobacco interactions during TMV infection. As postulated by Clemente-Moreno et al. (2015), ROS accumulation is a common feature in plant virus infection. Therefore, not only increased production but also active redistribution of glutathione during the resistant reaction could actively protect vital organelles during infection. Elevated glutathione concentration in the chloroplast is also an important factor for ROS control and symptom development. The breakdown of the oxidative system in the chloroplast is often correlated with necrotic alterations. In the case of mitochondria, Király et al. (2012) indicated that, during incompatible TMV tobacco infection, glutathione depletion induced in the mitochondria correlated with the induction of necrotic lesions in hypersensitive responses. Data from TuMV and TMV suggest that deposition in mitochondria could vary in specific interactions with the host. Nevertheless, Zechmann (2020) and Otulak-Kozieł et al. (2022a) suggested that glutathione plays a very important role in specific cell compartments, activating plant defense and contributing to the development of resistance. Data presented in the case of TuMV infection in Arabidopsis suggest that not only the cell interior but also the apoplast could be a site of modulation of glutathione levels important for resistance (Otulak-Kozieł et al., 2023). The resistant mutants rbohF and rbohD/F of Arabidopsis exhibited the induction of GSH form deposition and summary glutathione (GSH+GSSG pool) changed the activity of apoplastic GGT (γ-glutamyl transferase) in the apoplast, with the active rerouting of GSSG from the cell wall to the symplast during TuMV infection (Figure 2). This movement enables an increased pool of GSSG in the cell for potential use by specific glutathione enzymes like GST, emphasizing the importance of glutathione-associated enzymes as key molecules in the resistant response. In the context of resistance to PVYNTN infection, potato cv. Neptun showed an increased expression of glutathione transferase StGSTF2 and a general activity of GST, corresponding with an increase in the GSSG form and indicating involvement in the resistance reaction. So increased levels of GSSG in cells that differed from the data reported by Fodor et al. (1997), were the result of a global increase in GST activity in resistant plants. Works by Gullner et al. (1995) and Wu et al. (2013) on sugarcane mosaic virus (ScMV) reported a significant increase in GST activity in resistant sorghum cultivars. Moreover, the importance of GST was also postulated by Chronopopulou et al. (2017), not only as enzymes involved in detoxification and ROS homeostasis but also as signaling molecules and adaptors in biotic stress (Chronopopulou et al., 2017; Gallé et al., 2019, 2021). Additionally, Fodor et al. (1997), indicated that GST plays a pivotal function in controlling HR and necrotization during plant-virus interaction. The importance of GST in resistance as suggested by Otulak-Kozieł et al. (2022a) and Fodor et al. (1997) was confirmed during the investigation of resistant tobacco infected by TMV (Király et al., 2012). During TMV investigations, Király et al. (2012) observed the induction of NtGSTU1 (from the tau group) expression between 3 and 6 h after virus inoculation, which manifested as enhanced HR, causing a reduction in TMV replication in plants with sufficient sulfate. Transcriptomic analyses revealed that the GST expression profile can be differentially regulated in plant-virus interactions. Generally, most GSTs are upregulated rather than downregulated during the resistance reaction, as confirmed in pepper leaves infected with Obuda pepper virus—ObPV (Kalapos et al., 2021), rice stripe virus-RSV during infection in Arabidopsis thaliana (Sun et al., 2016), Beta vulgaris and beet necrotic yellow vein virus-BNYVV interactions (Decroës et al., 2022), and the response of watermelon to cucumber green mottle mosaic virus-CGMMV (Li et al., 2017). Furthermore, the expression of specific GST genes was significantly activated in the presence of BNYVV and rice tungro spherical virus (RTSV) in resistance reactions (Larson et al., 2008; Satoh et al., 2013). Satoh et al. (2013) also postulated that rice plants’ resistance to RTSV infection induced not only GST but also the expression of genes encoding GRX, suggesting that s-gluthationylation, with the engagement of GSH, could be important in the resistance reaction. However, depletion of specific GST was shown to influence the induction of a resistant reaction. Rodriguez-Peña et al. (2021) showed that GSTU4 downregulation caused a significant reduction in the accumulation of barley mosaic virus (BMV) and PVX in a specific host. A study exploring the response of Atgstu19 and Atgstu24 mutants to TuMV infection showed significant differences in specific AtGSTU gene expression, virus concentration, ultrastructural alterations, glutathione content, and glutathione transferase and reductase activities compared with Col-0 (wild-type) and mock-inoculated plants (Otulak-Kozieł et al., 2022b). Authors reported that Atgstu24 mutants had a resistance-like reaction to TuMV (with a high decrease in virus gene expression and movement) compared to susceptible Col-0 plants, suggesting that GSTU24 may suppress plant resistance. Moreover, this mutant had upregulated expression of GSTU19 and GSTU13 highly correlated with virus limitation in the resistance-like reaction (Otulak-Kozieł et al., 2022b). Moreover, resistant Atgstu24 mutants also characterized the upregulated activity of GR. Similarly, Otulak-Kozieł et al. (2023) reported that resistant rbohF and rbohD/F mutants infected by TuMV had increased activity of GST and GR, strongly downregulated GPXL, and highly reduced levels of lipid peroxidation. The same situation was also reported by Kalapos et al. (2021), showing high suppression of GPXL based on the results of transcriptome profiling during ObPV–C. annuum in HR. On the other hand, the more tolerant of tobacco plants to pepper mild mottle virus (PMMoV-I) infection characterized decreased activity GR whereas OTC treated tolerant pea characterized GR upregulation (Clemente-Moreno et al., 2010; Hakmaoui et al., 2012).
Figure 2 Glutathione content changes, tendency in selected glutathione metabolism- related genes expression and glutathione-associated enzymes activity in susceptible (left, AtGSTU19-TuMV, AtrbohD-TuMV interaction) as well as resistance (right, AtGSTU24-TuMV, AtrbohF-TuMV, AtrbohD/F-TuMV interaction) Arabidopsis thaliana mutants- TuMV reaction. γ-glutamyl transferase (GGT), glutathione peroxidase-like (GPXL), glutathione S-transferase (GST),glutathione S-transferase tau-class (GSTU), reduced glutathione form (GSH), oxidized glutathione form (GSSG), glutathione reductase (GR), ↑-activation or up-regulation, ↓- decrease or down-regulation.
In contrast to resistance, in susceptible plants, the generation of ROS is not well or properly controlled due to changes induced by plant virus infection in the host cell, particularly in the modulation of glutathione. In this case, the modulation is closely associated with the levels of GSH and GSSG forms. As reported in the cases of PVYNTN and TuMV, the GSH form and total glutathione levels could be upregulated in susceptible plants until the point of symptom occurrence and then highly depleted (Otulak-Kozieł et al., 2022b, 2023). In contrast to this, the GSSG form concentration decreased during infections caused by, for example, PVYNTN and TuMV (Otulak-Kozieł et al., 2022a, b). The depletion of glutathione content in susceptible reactions was also reported by Hakmaoui et al. (2012); Singh et al. (2020), and Király et al. (2012) for PMMoV in tobacco, YMV in black gram, and TMV in tobacco respectively. This data, along with reports by Hernández et al. (2017), indicates that susceptible plants, to some extent, are able to control ROS production and delay symptom development. However, without launching a resistant response, the protective potential of the GSH form (via upregulation of synthesis and specific activity of glutathione-associated enzymes) is limited, and the ability to regain GSH from GSSG is mitigated with less presence of the GSSG form. The mobility of glutathione also has an impact on in-cell and in-apoplast relocation in susceptible plants. In susceptible potatoes infected at later stages of PVYNTN infection,the localization of total glutathione decreased, even to nonstatistically significant levels in the cytoplasm and chloroplast. The mutants AtGSTU19 infected by TuMV had decreased content of glutathione in mitochondria, cytoplasm, nucleus, vacuole, and chloroplast. A similar situation was observed in rbohD mutants of Arabidopsis, also exhibiting decreased apoplastic localization (Otulak-Kozieł et al., 2023). During susceptible interactions, not only is glutathione content and distribution changed but there are also changes in the expression of genes encoding specific enzyme changes (Figure 2). In the context of transcriptomic analyses, it is generally observed that GST expression is downregulated in susceptible interactions (Sun et al., 2016; Li et al., 2017; Kalapos et al., 2021; Decroës et al., 2022). However, in specific plant-virus interactions, certain GSTs may be induced. For instance, during the infection of susceptible A. thaliana by cauliflower mosaic virus (CaMV), systemic induction of GST1 was associated with increased virus titers and the development of disease symptoms (Love et al., 2005). Pavan Kumar et al. (2017) also reported the accumulation of some GST proteins in systemically infected leaves of soybeans susceptible to mungbean yellow mosaic India virus (MYMIV) and mungbean yellow mosaic virus (MYMV). Additionally, Zhang et al. (2022) documented that Glycine max GSTU13 was associated with the development of symptoms induced by soybean mosaic virus (SMV) at both transcriptional and protein levels. Furthermore, the works of Chen et al. (2013) and Skopelitou et al. (2015) demonstrated the upregulation of NbGSTU4 and GSTU10-10 during infections caused by bamboo mosaic virus (BaMV) and SMV on susceptible hosts. Chen et al. (2013) also postulated that the NbGSTU4 protein has the ability to bind to the UTR region of (+) s virus RNA, leading to effective replication in susceptible hosts. In tomato cultivars tolerant to tomato leaf curl New Delhi virus (ToLCNDV), Sharma et al. (2021) observed significant upregulation of SlGR3, SlGST44, and SlGST96 during virus infection and different hormone treatments in the tolerant cultivar. Moreover, the virus-induced gene silencing of SlGR3 turned the tolerant cultivar into a susceptible one. Méndez-López et al. (2023), in their investigation of pepino mosaic virus (PepMV) on susceptible tomatoes, showed that SlGSTU38 acted as a susceptibility factor and outlined the dual role of the proviral SlGSTU38 protein. It was suggested that the SlGSTU38 protein interacted with PepMV capsid protein and played a role in delaying virus infection by engaging in or disturbing redox homeostasis. Otulak-Kozieł et al. (2022b) also speculated that similar viral-host protein interactions could occur in the case of GSTU19 and GSTU24 proteins during TuMV infection in different Arabidopsis mutants in various types of interactions. Based on these studies, it is suggested that not only the expression of specific GSTs but also direct interactions between GST proteins and the virus may be necessary to overcome defense mechanisms in susceptible plants. This observation aligns well with the crucial ability of viruses to interact with host proteins (for example in the Potyviridae family), leading to the induction and support of virus infection in different hosts (Chen et al., 2013; Yang et al., 2021). In the analysis of the Atgstu24/Atgstu19-TuMV pathosystem, it was found that the mutation of specific GSTs also had an effect on generating increased susceptibility in the interaction with the virus. Plants with the Atgstu19 mutation exhibited increased susceptibility compared to the already susceptible Col-0 plants, which was associated with elevated levels of TuMV expression. Additionally, in Col-0 plants, there was a general decrease in Atgstu19 expression after 7dpi, indicating that the elimination or limitation of Atgstu19 expression was crucial for the susceptibility interaction with TuMV. The same study also showed that AtGSTU1 and AtGTU24 genes were significantly altered and involved in susceptibility. Not only gene expression but also GST enzymatic activity is modulated during virus infection. Fodor et al. (1997) observed a decrease in the activity of some antioxidant enzymes, especially GST and GR, in susceptible tobacco infected by TMV. Gullner et al. (1995) and Wu et al. (2013) found decreased GST activity in susceptible sorghum cultivars during interaction with ScMV. Similar changes in reduced activity of GST and GR were reported in susceptible mutants infected with TuMV (Otulak-Kozieł et al., 2022b, 2023) after 7dpi which was associated with increased activity of GPXL in rbohD mutants (Otulak-Kozieł et al., 2023). The reduction of cellular or chloroplast GR activity was also reported in infections on compatible hosts caused by various viruses such as cocksfoot mottle virus (CfMV) on Dactylis glomerata, cucumber mosaic virus (CMV) on tomato, PPV, and prune necrotic ringspot virus (PNRSV) on apricot plants, as well as white clover mosaic virus (WCIMV) on bean plants (Li and Burritt, 2003; Amari et al., 2007; Song et al., 2009; Clemente-Moreno et al., 2015). The involvement of GR in the reduction of glutathione disulfide (GSSG) to two molecules of GSH makes this enzyme crucial for maintaining the glutathione redox potential. Therefore, the reduction of GR activity coupled with an increase in GPXL as reported in the case of YMV or TuMV (Singh et al., 2020; Otulak-Kozieł et al., 2023) creates a situation of poorly controlled and imbalanced redox hemostasis. This imbalance leads to damage of cell components such as uncontrolled lipid peroxidation and blocks the possibilities of proper initiation of defense response at the right place and time to effectively stop the infection.
In recent years, the importance of controlling redox homeostasis, particularly through glutathione, has been increasingly recognized as crucial for inducing a resistant response. However, our understanding of the exact mechanisms and the significance of glutathione, as well as the involvement of specific glutathione-associated enzymes, remains limited in the context of plant-virus interactions. This limitation is particularly evident in understanding the roles of GRX, GSNO, S-glutatylation, and S-nitrosylation processes. This is mainly due to the fact that research has traditionally focused on well-known stress molecules like SA or JA, or simply measured the activity of selected redox enzymes. The new findings highlight the importance of glutathione mobility within the cell and the direct interaction of glutathione-associated enzymes with viral factors or vRNA essential for the full-fledged development or initiation of viral infection (particularly through interactions with UTR sites in vRNA). This opens up a unique and promising new field of research. To advance our understanding, investigating the relocation of glutathione, both between different cell regions and its dynamic changes, along with gathering transcriptional data specifically focused on glutathione metabolism, will be crucial. Additionally, exploring the direct interactome of glutathione-associated proteins can help greatly in the identification of crucial elements in host-plant virus interplay. The generation of mutants for selected genes based on transcriptomic data, using advanced techniques such as CRISPR/Cas9, will further open new horizons for developing resistance to viruses or other multifactorial stresses.
EK: Writing – review & editing, Writing – original draft, Project administration, Funding acquisition, Formal analysis. KO-K: Writing – review & editing, Writing – original draft, Supervision, Formal analysis, Conceptualization. PR: Writing – review & editing, Visualization, Software.
The author(s) declare that financial support was received for the research, authorship, and/or publication of this article. This research was funded by the Polish National Science Center, NCN 2021/43/D/NZ3/00428.
The authors declare that the research was conducted in the absence of any commercial or financial relationships that could be construed as a potential conflict of interest.
All claims expressed in this article are solely those of the authors and do not necessarily represent those of their affiliated organizations, or those of the publisher, the editors and the reviewers. Any product that may be evaluated in this article, or claim that may be made by its manufacturer, is not guaranteed or endorsed by the publisher.
Alquéres, S., Meneses, C. H. S. G., Rouws, L. F. M., Rothballer, M., Baldani, I., Schmid, M., et al. (2013). The bacterial superoxide dismutase and glutathione reductase are crucial for endophytic colonization of rice roots by Gluconacetobacter diazotrophicus PAL5. Mol. Plant Microbe Interact. 26, 937–945. doi: 10.1094/MPMI-12-12-0286-R
Amari, K., Díaz-Vivancos, P., Pallás, V., Sánchez-Pina, M. A., Hernández, J. A. (2007). Oxidative stress induction by Prunus necrotic ringspot virus infection in apricot seeds. Physiol. Plant 131, 302–310. doi: 10.1111/j.1399-3054.2007.00961.x
Bela, K., Horváth, E., Gallé, Á., Szabados, L., Tari, I., Csiszár, J. (2015). Plant glutathione peroxidases: Emerging role of the antioxidant enzymes in plant development and stress responses. J. Plant Physiol. 176, 192–201. doi: 10.1016/j.jplph.2014.12.014
Chen, I. H., Chiu, M. H., Cheng, S. F., Hsu, Y. H., Tsai, C. H. (2013). The glutathione transferase of Nicotiana benthamiana NbGSTU4 plays a role in regulating the early replication of Bamboo mosaic virus. New Phytol. 199, 749–757. doi: 10.1111/nph.12304
Cheng, M.-C., Ko, K., Chang, W.-L., Kuo, W.-C., Chen, G.-H., Lin, T.-P. (2015). Increased glutathione contributes to stress tolerance and global translational changes in Arabidopsis. Plant J. 83, 926–939. doi: 10.1111/tpj.12940
Chronopopulou, E., Ataya, F. S., Pauliou, F., Perperopoulou, F., Georgakis, N., Nianiou-Obeidat, I., et al. (2017). ““Structure, evolution and functional roles of plant glutathione transferases.”,” in Glutathione in plant growth, development, and stress tolerance. Eds. Hossain, M. A., Mustafa, M. G., Diaz-Vivancos, P., Burritt, D. J. (Springer International Publishing AG, Cham, Switzerland), 195–213.
Clarke, S. F., Guy, P. L., Burritt, D. J., Jameson, P. E. (2002). Changes in the activities of antioxidant enzymes in response to virus infection and hormone treatment. Physiol. Plant 114, 157–164. doi: 10.1034/j.1399-3054.2002.1140201.x
Clemente-Moreno, M. J., Diaz-Vivancos, P., Barba-Espín., G., Hernández, J. A. (2010). Benzothiadiazole and L-2-oxothiazolidine-4-carboxylic acid reduced the severity of Sharka symptoms in pea leaves: effect on the antioxidative metabolism at subcellular level. Plant Biol. 12, 88–97. doi: 10.1111/(ISSN)1438-8677
Clemente-Moreno, M. J., Diaz-Vivancos, P., Rubio, M., Fernandez, N., Hernández, J. A. (2013). Chloroplastprotection in plum pox virus-infected peach plants by L-2-oxo-4-thiazolidine-carboxylic acidtreatments: effect in the proteome. Plant Cell Environ. 36, 640–654. doi: 10.1111/pce.12003
Clemente-Moreno, M. J., Hernández, J. A., Diaz-Vivancos, P. (2015). Sharka: How do plants respond to Plum pox virus infection? J. Exp. Bot. 66, 25–35. doi: 10.1093/jxb/eru428
De, S., Chavez-Calvillo, G., Wahlsten, M., Mäkinen, K. (2018). Disruption of the methionine cycle and reduced cellular gluthathione levels underlie potex-potyvirus synergism in Nicotiana benthamiana. Mol. Plant Pathol. 19, 1820–1835. doi: 10.1111/mpp.12661
Decroës, A., Mahillon, M., Genard, M., Lienard, C., Lima-Mendez, G., Gilmer, D., et al. (2022). Rhizomania: hide and seek of Polymyxa betae and the Beet necrotic yellow vein virus with Beta vulgaris. Mol. Plant Microbe Interact. 35, 989–1005. doi: 10.1094/MPMI-03-22-0063-R
Diaz-Vivancos, P., Wolff, T., Markovic, J., Pallardó, F. V., Foyer, C. H. (2010). A nuclear glutathione cycle within the cell cycle. Biochem. J. 431, 169–178. doi: 10.1042/BJ20100409
Ding, P., Ding, Y. (2020). Stories of salicylic acid: a plant defense hormone. Trends Plant Sci. 25, 549–565. doi: 10.1016/j.tplants.2020.01.004
Dixon, D. P., Edwards, R. (2010). Glutathione transferases. Arab. Book. 8, 1–15. doi: 10.1199/tab.0131
Fodor, J., Gullner, G., Ádám, A. L., Barna, B., Kömives, T., Király, Z. (1997). Local and systemic responses of antioxidants to tobacco mosaic virus infection and to salicylic acid in tobacco. Role in systemic acquired resistance. Plant Physiol. 114, 1443–1451. doi: 10.1104/pp.114.4.1443
Gallé, Á., Bela, K., Hajmal, A., Faragó, N., Horváth, E., Horváth, M., et al. (2021). Crosstalk between the redox signalling and the detoxification: GSTs under redox control? Plant Physiol. Biochem. 169, 149–159. doi: 10.1016/j.plaphy.2021.11.009
Gallé, Á., Czékus, Z., Bela, K., Horváth, E., Ördög, A., Csiszár, J., et al. (2019). Plant glutathione transferases and light. Front. Plant Sci. 9. doi: 10.3389/fpls.2018.01944
Ghanta, S., Bhattacharyya, D., Sinha, R., Banerjee, A., Chattopadhyay, S. (2011). Nicotiana tabacum overexpressing γ-ECS exhibits biotic stress tolerance likely through NPR1- dependent salicylicacid-mediated pathway. Planta 233, 895–910. doi: 10.1007/s00425-011-1349-4
Ghanta, S., Datta, R., Bhattacharyya, D., Sinha, R., Kumar, D., Hazra, S., et al. (2014). Multistep involvement of glutathione with salicylic acid and ethylene to combat environmental stress. J. Plant Physiol. 171, 940–950. doi: 10.1016/j.jplph.2014.03.002
Gimenez, E., Salinas, M., Manzano-Agugliaro, F. (2018). Worldwide research on plant defense against biotic stresses as improvement for sustainable agriculture. Sustainability 10, 391. doi: 10.3390/su10020391
Góngora-Castillo, E., Ibarra-Laclette, E., Trejo-Saavedra, D. L., Rivera-Bustamante, R. F. (2012). Transcriptome analysis of symptomatic and recovered leaves of geminivirus-infected pepper (Capsicum annuum). Virol. J. 9, 295. doi: 10.1186/1743-422X-9-295
Gullner, G., Komives, T., Gáborjányi, R. (1995). Differential alterations of glutathione S-transferase enzyme activities in three sorghum varieties following viral infection. Z. Naturforsch. C. 50, 459–460. doi: 10.1515/znc-1995-5-619
Gullner, G., Tobias, I., Fodor, J., Kömives, T. (1999). Elevation of glutathione level and activation of glutathione-related enzymes affect virus infection in tobacco. Free Radic. Res. 31, 155–161. doi: 10.1080/10715769900301451
Gullner, G., Zechmann, B., Künstler, A., Király, L. (2017). “The signaling roles of glutathione in plant disease resistance”,” in Glutathione in plant growth, development, and stress tolerance. Eds. Hossain, M. A., Mustafa, M. G., Diaz-Vivancos, P., Burritt, D. J. (Springer International Publishing AG, Cham, Switzerland), 331–357.
Hakmaoui, A., Pérez-Bueno, M. L., García-Fontana, B., Camejo, D., Jiménez, A., Sevilla, S., et al. (2012). Analysis of the antioxidant response of Nicotiana benthamiana to infection with two strains of Pepper mild mottle virus. J. Exp. Bot. 63, 5487–5496. doi: 10.1093/jxb/ers212
Hammerbach, A., Coutinho, T. A., Gershenzon, J. (2019). Roles of plant volatiles in defense against microbial pathogens and microbial exploitation of volatiles. Plant Cell Environ. 42, 2827–2843. doi: 10.1111/pce.13602
Han, Y., Chaouch, S., Mhamdi, A., Queval, G., Zechmann, B., Noctor, G. (2013). Functional analysis of Arabidopsis mutants points to novel roles for glutathione in coupling H2O2 to activation of salicylic acid accumulation and signaling. Antioxid. Redox Signal. 18, 2106–2121. doi: 10.1089/ars.2012.5052
Hernández, J. A., Barba, E., Diaz-Vivancos, P. (2017). “Glutathione-Mediated biotic stress tolerance,” in Glutathione in plant growth, development, and stress tolerance. Eds. Hossain, M. A., Mustafa, M. G., Diaz-Vivancos, P., Burritt, D. J. (Springer International Publishing AG, Cham, Switzerland), 309–329.
Hernández, J. A., Gullner, G., Clemente-Moreno, M. J., Künstler, A., Juhász, C., Díaz-Vivancos, P., et al. (2016). Oxidative stress and antioxidative responses in plant–virus interactions. Physiol. Mol. Plant Pathol. 94, 134–148. doi: 10.1016/j.pmpp.2015.09.001
Hernández, L. E., Sobrino-Plata, J., Montero-Palmero, M. B., Carrasco-Gil, S., Flores-Cáceres, M. L., Ortega-Villasante, C., et al. (2015). Contribution of glutathione to the control of cellular redox homeostasis under toxic metal and metalloid stress. J. Exp. Bot. 66, 2901–2911. doi: 10.1093/jxb/erv063
Höller, K., Király, L., Künstler, A., Müller, M., Gullner, G., Fattinger, M., et al. (2010). Enhanced glutathione metabolism is correlated with sulfur induced resistance in tobacco mosaic virus-infected genetically susceptible Nicotiana tabacum plants. Mol. Plant-Microbe Interact. 23, 1448–1459. doi: 10.1094/MPMI-05-10-0117
Kalapos, B., Juhász, C., Balogh, E., Kocsy, G., Tóbiás, I., Gullner, G. (2021). Transcriptome profiling of pepper leaves by RNA-Seq during an incompatible and a compatible pepper-tobamovirus interaction. Sci. Rep. 11, 20680. doi: 10.1038/s41598-021-00002-5
Király, Z., Barna, B., Kecskés, A., Fodor, J. (2002). Down-regulation of antioxidative capacity in a transgenic tobacco which fails to develop acquired resistance to necrotization caused by tobacco mosaic virus. Free Radic. Res. 36, 981–991. doi: 10.1080/1071576021000006581
Király, L., Künstler, A., Fattinger, M., Höller, K., Juhász, C., Müller, M., et al. (2012). Sulfate supply influences compartment specific glutathione metabolism and confers enhanced resistance to Tobacco mosaic virus (TMV) during a hypersensitive response. Plant Physiol. Biochem. 59, 44–54. doi: 10.1016/j.plaphy.2011.10.020
Király, L., Zechmann, B., Albert, R., Bacsó, R., Schwarczinger, I., Nagy, J., et al. (2024). Enhanced resistance to viruses in nicotiana edwardsonii ‘Columbia’Is dependent on salicylic acid, correlates with high glutathione levels, and extends to plant-pathogenic bacteria and abiotic stress. Plant-Microbe Interact. 37 (1), 36–50.
Koch, A., Kang, H.-G., Steinbrenner, J., Dempsey, D. A., Klessig, D. F., Kogel, K.-H. (2017). MORC Proteins: novel players in plant and animal health. Front. Plant Sci. 8. doi: 10.3389/fpls.2017.01720
Koramutla, M. K., Negi, M., Ayele, B. T. (2021). Roles of Glutathione in mediating abscisic acid signaling and its regulation of seed dormancy and drought tolerance. Genes 12, 1620. doi: 10.3390/genes12101620
Kozieł, E., Otulak-Kozieł, K., Bujarski, J. (2021). Plant cell wall as a key player during resistant and susceptible plant-virus interactions. Front. Microbiol. 12. doi: 10.3389/fmicb.2021.656809
Künstler, A., Kátay, G., Gullner, G., Király, L. (2019a). Artificial elevation of glutathione contents in salicylic acid-deficient tobacco (Nicotiana tabacum cv. Xanthi NahG) reduces susceptibility to the powdery mildew pathogen Euoidium longipes. Plant Boil. 22, 70–80. doi: 10.1111/plb.13030
Künstler, A., Király, L., Kátay, G., Enyedi, A. J., Gullner, G. (2019b). Glutathione can compensate for salicylic acid deficiency in tobacco to maintain resistance to. Tobacco mosaic virus. Front. Plant Sci. 10. doi: 10.3389/fpls.2019.01115
Larson, R. L., Wintermantel, W. M., Hill, A., Fortis, L., Nunez, A. (2008). Proteome changes in sugar beet in response to Beet necrotic yellow vein virus. Physiol. Mol. Plant Pathol. 72, 62–72. doi: 10.1016/j.pmpp.2008.04.003
Li, X., An, M., Xia, Z., Bai, X., Wu, Y. (2017). Transcriptome analysis of watermelon (Citrullus lanatus) fruits in response to Cucumber green mottle mosaic virus (CGMMV) infection. Sci. Rep. 7, 16747. doi: 10.1038/s41598-017-17140-4
Li, Z., Burritt, D. J. (2003). The influence of Cocksfoot mottle virus on antioxidant metabolism in the leaves of Dactylis glomerata L. Physiol. Mol. Plant Pathol. 62, 285–295. doi: 10.1016/S0885-5765(03)00075-4
Love, A. J., Yun, B. W., Laval, V., Loake, G. J., Milner, J. J. (2005). Cauliflower mosaic virus, a compatible pathogen of Arabidopsis, engages three distinct defense-signaling pathways and activates rapid systemic generation of reactive oxygen species. Plant Physiol. 139, 935–948. doi: 10.1104/pp.105.066803
Mahmood, Q., Ahmad, R., Kwak, S., Rashid, A., Anjum, N. A. (2010). ““Ascorbate and glutathione: protectors of plants in oxidative stress”,” in Ascorbate-glutathione pathway and stress tolerance in plants. Eds. Anjum, N., Umar, S., Chan, M. (Springer, Dordrecht, The Netherlands), 209–229.
Mandadi, K. K., Scholthof, K. B. G. (2013). Plant immune responses against viruses: how does a virus cause disease? Plant Cell 25, 1489–1505. doi: 10.1105/tpc.113.111658
Martínez-González, A. P., Ardila, H. D., Martínez-Peralta, S. T., Melgarejo-Muñoz, L. M., Castillejo-Sánchez, M. A., Jorrín-Novo, J. V. (2018). What proteomic analysis of the apoplast tells us about plant-pathogen interactions. Plant Pathol. 67, 1647–1668. doi: 10.1111/ppa.12893
Méndez-López, E., Donaire, L., Gosálvez, B., Díaz-Vivancos, P., Sánchez-Pina, M. A., Tilsner, J., et al. (2023). Tomato SlGSTU38 interacts with the PepMV coat protein and promotes viral infection. New Phytol. 1, 1–17. doi: 10.1111/nph.18728
Noctor, G., Foyer, C. H. (1998). Ascorbate and glutathione: Keeping active oxygen under control. Annu. Rev. Plant Physiol. Plant Mol. Biol. 49, 249–279. doi: 10.1146/annurev.arplant.49.1.249
Noctor, G., Gomez, L., Vanacker, H., Foyer, C. H. (2002). Interactions between biosynthesis, compartmentation and transport in the control of glutathione homeostasis and signalling. J. Exp. Bot. 53, 1283–1304. doi: 10.1093/jexbot/53.372.1283
Noctor, G., Mhamdi, A., Chaouch, S., Han, Y., Neukermans, J., Marquez-Garcia, B., et al. (2012). Glutathione in plants: an integrated overview. Plant Cell Environ. 35, 454–484. doi: 10.1111/j.1365-3040.2011.02400.x
Ogawa, K., Hatano-Iwasaki, A., Yanagida, M., Iwabuchi, M. (2004). Level of glutathione is regulated by ATP-dependent ligation of glutamate and cysteine through photosynthesis in Arabidopsis thaliana: mechanism of strong interaction of light intensity with flowering. Plant Cell Physiol. 45, 1–8. doi: 10.1093/pcp/pch008
Otulak, K., Garbaczewska, G. (2014). The participation of plant cell organelles in compatible and incompatible potato virus Y-tobacco and -potato plant interaction. Acta Physiol Plant 36, 85–99. doi: 10.1007/s11738-013-1389-4
Otulak-Kozieł, K., Kozieł, E., Bujarski, J. (2018b). Spatiotemporal changes in xylan-1/xyloglucan and xyloglucan xyloglucosyl transferase (XTH-Xet5) as a step-in of ultrastructural cell wall remodelling in potato–Potato virus Y (PVYNTN) hypersensitive and susceptible reaction. Int. J. Mol. Sci. 19, 2287. doi: 10.3390/ijms19082287
Otulak-Kozieł, K., Kozieł, E., Horváth, E., Csiszár, J. (2022b). AtGSTU19 and AtGSTU24 as moderators of the response of Arabidopsis thaliana to Turnip mosaic virus. Int. J. Mol. Sci. 23, 11531. doi: 10.3390/ijms231911531
Otulak-Kozieł, K., Kozieł, E., Lockhart, B. (2018a). Plant cell wall dynamics in compatible and incompatible potato response to infection caused by Potato virus Y (PVYNTN). Int. J. Mol. Sci. 19, 862. doi: 10.3390/ijms19030862
Otulak-Kozieł, K., Kozieł, E., Lockhart, B. E. L., Bujarski, J. J. (2020). The Expression of potato expansin A3 (StEXPA3) and extensin4 (StEXT4) genes with distribution of StEXPAs and HRGPs-extensin changes as an effect of cell wall rebuilding in two types of PVYNTN–Solanum tuberosum Interactions. Viruses 12, 66. doi: 10.3390/v12010066
Otulak-Kozieł, K., Kozieł, E., Przewodowski, W., Ciacka, K., Przewodowska, A. (2022a). Glutathione modulation in PVYNTN susceptible and resistant potato plant interactions. Int. J. Mol. Sci. 23, 3797. doi: 10.3390/ijms23073797
Otulak-Kozieł, K., Kozieł, E., Treder, K., Király, L. (2023). Glutathione contribution in interactions between Turnip mosaic virus and Arabidopsis thaliana mutants lacking respiratory burst oxidase homologs d and f. Int. J. Mol. Sci. 24, 7128. doi: 10.3390/ijms24087128
Pavan Kumar, B. K., Kanakala, S., Malathi, V. G., Gopal, M., Usha, R. (2017). Transcriptomic and proteomic analysis of yellow mosaic diseased soybean. J. Plant Biochem. Biotechnol. 26, 224–234. doi: 10.1007/s13562-016-0385-3
Pogue, G. P., Lindbo, J. A., Garger, S. J., Fitzmaurice, W. P. (2002). Making an ally from an enemy: plant virology and the new agriculture. Annu. Rev. Phytopathol. 40, 45–74. doi: 10.1146/annurev.phyto.40.021102.150133
Ramirez-Prado, J. S., Abulfaraj, A. A., Rayapuram, N., Benhamed, M., Hirt, H. (2018). Plant immunity: from signaling to epigenetic control of defense. Trends Plant Sci. 23, 833–844. doi: 10.1016/j.tplants.2018.06.004
Rodriguez-Peña, R., Mounadi, K. E., Garcia-Ruiz, H. (2021). Changes in subcellular localization of host proteins induced by plant viruses. Viruses 13, 677. doi: 10.3390/v13040677
Sabetta, W., Paradiso, A., Paciolla, C., de Pinto, M. C. (2017). “Chemistry, biosynthesis, and antioxidative function of glutathione in plants,” in Glutathione in plant growth, development, and stress tolerance. Eds. Hossain, M. A., Mustafa, M. G., Diaz-Vivancos, P., Burritt, D. J. (Springer International Publishing AG, Cham, Switzerland), 1–28.
Saijo, Y., Loo, E. P. (2020). Plant immunity in signal integration between biotic and abiotic stress responses. New Phytol. 225 (1), 87–104. doi: 10.1111/nph.15989
Sarkar, T. S., Bhattacharjee, A., Majumdar, U., Roy, A., Maiti, D., Goswamy, A. M., et al. (2011). Biochemical characterization of compatible plant-viral interaction: a case study with a begomovirus-kenaf host-pathosystem. Plant Signal. Behav. 6, 501–509. doi: 10.4161/psb.6.4.13912
Satoh, K., Kondoh, H., De Leon, T. B., Macalalad, R. J. A., Cabunagan, R. C., Cabauatan, P. Q., et al. (2013). Gene expression responses to Rice tungro spherical virus in susceptible and resistant near-isogenic rice plants. Virus Res. 171, 111–120. doi: 10.1016/j.virusres.2012.11.003
Sharma, N., Muthamilarasan, M., Dulani, P., Prasad, M. (2021). Genomic dissection of ROS detoxifying enzyme encoding genes for their role in antioxidative defense mechanism against tomato leaf curl new delhi virus infection in tomato. Genomics 113 (3), 889–899. doi: 10.1016/j.ygeno.2021.01.022
Shu, K., Liu, X. D., Xie, Q., He, Z. H. (2016). Two faces of one seed: hormonal regulation of dormancy and germination. Mol. Plant 9, 34–45. doi: 10.1016/j.molp.2015.08.010
Singh, Y. J., Grewal, S. K., Gill, R. K. (2020). Role of glutathione in methylglyoxal detoxification pathway during Yellow mosaic virus (YMV) infection in black gram (Vigna mungo (L.) Hepper). Physiol. Mol. Plant Pathol. 111, 101513. doi: 10.1016/j.pmpp.2020.101513
Skopelitou, K., Muleta, A. W., Papageorgiou, A. C., Chronopoulou, E., Labrou, N. E. (2015). Catalytic features and crystal structure of a tau class glutathione transferase from Glycine max specifically upregulated in response to soybean mosaic virus infections. Biochim. Biophys. Acta 1854, 166–177. doi: 10.1016/j.bbapap.2014.11.008
Song, X. S., Wang, Y. J., Mao, W. H., Shi, K., Zhou, Y. H. (2009). Effect of cucumber mosaic virus infection on electron transport and antioxidant system in chloroplasts and mitochondria of cucumber and tomato leaves. Physiologia Plantarum 135, 246–257. doi: 10.1111/j.1399-3054.2008.01189.x
Sun, F., Fang, P., Li, J., Du, L., Lan, Y., Zhou, T., et al. (2016). RNA-seq-based digital gene expression analysis reveals modification of host defense responses by rice stripe virus during disease symptom development in Arabidopsis. Virol. J. 13, 202. doi: 10.1186/s12985-016-0663-7
Van Butselaar, T., Van den Ackerveken, G. (2020). Salicylic acid steers the growth–immunity tradeoff. Trends Plant Sci. 25, 566–576. doi: 10.1016/j.tplants.2020.02.002
Wachter, A., Wolf, S., Steininger, H., Bogs, J., Rausch, T. (2005). Differential targeting of GSH1 and GSH2 is achieved by multiple transcription initiation: implications for the compartmentation of glutathione biosynthesis in the Brassicaceae. Plant J. 41, 15–30. doi: 10.1111/j.1365-313X.2004.02269.x
Wu, L., Han, Z., Wang, S., Wang, X., Sun, A., Zu, X., et al. (2013). Comparative proteomic analysis of the plant-virus interaction in resistant and susceptible ecotypes of maize infected with sugarcane mosaic virus. J. Proteom. 89, 124–140. doi: 10.1016/j.jprot.2013.06.005
Yadav, S. K. (2010). Heavy metals toxicity in plants: An overview on the role of glutathione and phytochelatins in heavy metal stress tolerance of plants. S. Afr. J. Bot. 76, 167–179. doi: 10.1016/j.sajb.2009.10.007
Yang, J., Duan, G., Li, C., Liu, L., Han, G., Zhang, Y., et al. (2019). The crosstalks between jasmonic acid and other plant hormone signaling highlight the involvement of jasmonic acid as a core component in plant response to biotic and abiotic stresses. Front. Plant Sci. 10. doi: 10.3389/fpls.2019.01349
Yang, X., Li, Y., Wang, A. (2021). Research advances in Potyviruses: From the laboratory bench to the field. Annu. Rev. Phytopathol. 59, 1–29. doi: 10.1146/annurev-phyto-020620-114550
Zechmann, B. (2020). Subcellular roles of glutathione in mediating plant defense during biotic stress. Plants 9, 1067. doi: 10.3390/plants9091067
Zechmann, B., Müller, M. (2008). Effects of zucchini yellow mosaic virus infection on the subcellular distribution of glutathione and its precursors in a highly tolerant Cucurbita pepo cultivar. Botany 86, 1092–1100. doi: 10.1139/B08-048
Zechmann, B., Zellnig, G., Müller, M. (2005). Changes in the subcellular distribution of glutathione during virus infection in Cucurbita pepo (L.). Plant Biol. 7, 49–57. doi: 10.1055/s-2004-830477
Zechmann, B., Zellnig, G., Urbanek-Krajnc, A., Müller, M. (2007). Artificial elevation of glutathione affects symptom development in ZYMV-infected Cucurbita pepo L. plants. Arch. Virol. 152, 747–762. doi: 10.1007/s00705-006-0880-2
Zhang, K., Shen, Y., Wang, T., Wang, Y., Xue, S., Luan, H., et al. (2022). GmGSTU13 is related to the development of mosaic symptoms in soybean plants infected with Soybean mosaic virus. Phytopathology 112, 452–459. doi: 10.1094/PHYTO-11-20-0498-R
Zhu, Y., Li, L. (2021). Multi-layered regulation of plant cell wall thickening. Plant Cell Physiol. 62, 1867–1873. doi: 10.1093/pcp/pcab152
Zhu, F., Zhang, Q. P., Che, Y. P., Zhu, P. X., Zhang, Q. Q., Ji, Z. L. (2021). Glutathione contributes to resistance responses to TMV through a differential modulation of salicylic acid and reactive oxygen species. Mol. Plant Pathol. 22, 1668–1687. doi: 10.1111/mpp.13138
Keywords: plant virus, glutathione metabolism, resistance response, plant defense, susceptible reaction
Citation: Kozieł E, Otulak-Kozieł K and Rusin P (2024) Glutathione-the “master” antioxidant in the regulation of resistant and susceptible host-plant virus-interaction. Front. Plant Sci. 15:1373801. doi: 10.3389/fpls.2024.1373801
Received: 20 January 2024; Accepted: 23 February 2024;
Published: 12 March 2024.
Edited by:
Ahmed Abdelkhalek, City of Scientific Research and Technological Applications, EgyptReviewed by:
Nuria Montes, Hospital Universitario de La Princesa, SpainCopyright © 2024 Kozieł, Otulak-Kozieł and Rusin. This is an open-access article distributed under the terms of the Creative Commons Attribution License (CC BY). The use, distribution or reproduction in other forums is permitted, provided the original author(s) and the copyright owner(s) are credited and that the original publication in this journal is cited, in accordance with accepted academic practice. No use, distribution or reproduction is permitted which does not comply with these terms.
*Correspondence: Edmund Kozieł, ZWRtdW5kX2tvemllbEBzZ2d3LmVkdS5wbA==; Katarzyna Otulak-Kozieł, a2F0YXJ6eW5hX290dWxha0BzZ2d3LmVkdS5wbA==
†These authors have contributed equally to this work and share first authorship
Disclaimer: All claims expressed in this article are solely those of the authors and do not necessarily represent those of their affiliated organizations, or those of the publisher, the editors and the reviewers. Any product that may be evaluated in this article or claim that may be made by its manufacturer is not guaranteed or endorsed by the publisher.
Research integrity at Frontiers
Learn more about the work of our research integrity team to safeguard the quality of each article we publish.