- 1Astrobiology Center, National Institutes of Natural Sciences, Osawa, Mitaka, Tokyo, Japan
- 2National Institute for Basic Biology, National Institutes of Natural Sciences, Nishigonaka, Myodaiji, Okazaki, Aichi, Japan
- 3Graduate Institute for Advanced Studies, SOKENDAI, Okazaki, Japan
Non-photochemical quenching (NPQ) is a protective mechanism used by plants to safely dissipate excess absorbed light energy as heat, minimizing photo-oxidative damage. Although the importance of NPQ as a safety valve for photosynthesis is well-known, the physiological and environmental effects of the heat produced remain unclear because the amount of heat produced by NPQ is considered negligible, and its physiological effects have not been directly observed. Here, we calculated the heat produced by NPQ and evaluated its impact on the leaf and global warming based on simplified models. Our evaluation showed that the heat produced by NPQ in a given leaf area is 63.9 W m−2 under direct sunlight. Under the standard condition, NPQ warms up the leaf at less than 0.1°C, but it could be 1°C under particular conditions with low thermal conductance. We also estimated the thermal radiation of vegetation’s NPQ to be 2.2 W m−2 par global averaged surface area. It is only 0.55% of the thermal radiation by the Earth’s surface, but still significant in the current climate change response. We further discuss the possible function of NPQ to plant physiology besides the safety valve and provide strategies with artificial modification of the NPQ mechanism to increase food production and mitigate global warming.
Introduction
Green plants operate photosynthesis to convert light energy into chemical energy to produce organic compounds that support life on Earth. However, excessive light energy can lead to the production of reactive oxygen species and other harmful byproducts, which can damage photosynthetic machinery and impair photosynthetic efficiency (Niyogi, 1999). Non-photochemical quenching (NPQ) is a protective process through which plants safely dissipate excess absorbed light energy as heat, thereby minimizing photo-oxidative damage (Demmig-Adams et al., 2014). However, despite its importance as a safety valve for photosynthesis, the effects of the heat produced by NPQ on photosynthetic reactions and surrounding environments remain unclear. Although NPQ is a complex phenomenon involving a variety of processes and is defined differently depending on the measurement method and object of observation (Lazár, 2015), it is used here as a term for regulative heat dissipation mechanisms induced under light irradiation, including the quickly reversible component and long-sustained component (Verhoeven, 2014).
Green plants utilize atmospheric carbon dioxide to produce organic carbon. Evidently, carbon dioxide has a greenhouse effect (Hansen et al., 1981), so planting trees in the desert is expected to be a mitigation strategy to reduce global warming. However, afforestation has multiple biophysical effects, such as cooling by evaporation and a warming effect due to lower albedo, therefore, it can no longer be said to mitigate global warming in general (Bonan, 2008). The effect of these plants on cooling or warming against the climate varies depending on the climate, latitude, and many unknown aspects in microhabitat and physiological response.
In this article, we estimated heat produced by NPQ and explored its significance in terms of physiological and environmental impacts. We used a simplified model to calculate the heat produced by NPQ because of its complexity, which is influenced by various environmental and biological factors, including variations in solar radiation and physiological responses. Solar radiation reaching the Earth’s surface is affected by the altitude angle and weather, and the amount of light reaching the leaf varies in complex ways depending on the canopy structure and the leaf angle. Even under the same light intensity, NPQ varies depending on the other environmental conditions, plant type, growth stage, and physiological conditions. Here, we present the simple and reliable estimation of heat produced by NPQ and its possible and maximum effects on plant physiology and the environment.
By advancing our understanding of the role of heat by NPQ, we can contribute to the development of more efficient and sustainable agricultural practices and a broader understanding of the role of photosynthesis in the Earth’s ecosystems and environment.
Energy conversion in photosynthesis and heat production by NPQ
Solar radiation reaching the Earth’s land surface, in terms of direct and circumsolar radiation, has a standard flux (ASTM G173-03) of 888 W m−2 (NREL, 2003). Visible light (400–700 nm) constitutes approximately 42.3% of this radiation and is the portion that can be absorbed by leaves (Figure 1A). In general, leaves can only capture approximately 84% of the available visible light (Björkman and Demmig, 1987). The absorbed energy is divided almost equally between the reaction centers in photosystem I (PSI) and photosystem II (PSII) (Björkman and Demmig, 1987) (Figure 1B). On the PSII side, the absorbed energy goes through three distinct pathways. First, charge separation occurs in the reaction center, facilitating the conversion of light energy into chemical energy. Second, intrinsic dissipation takes place within the chlorophyll. Finally, in the third pathway, excess energy is dissipated as heat through processes collectively referred to as NPQ, which regulates and protects the plant from potential damage (Li et al., 2009; Ruban et al., 2012).
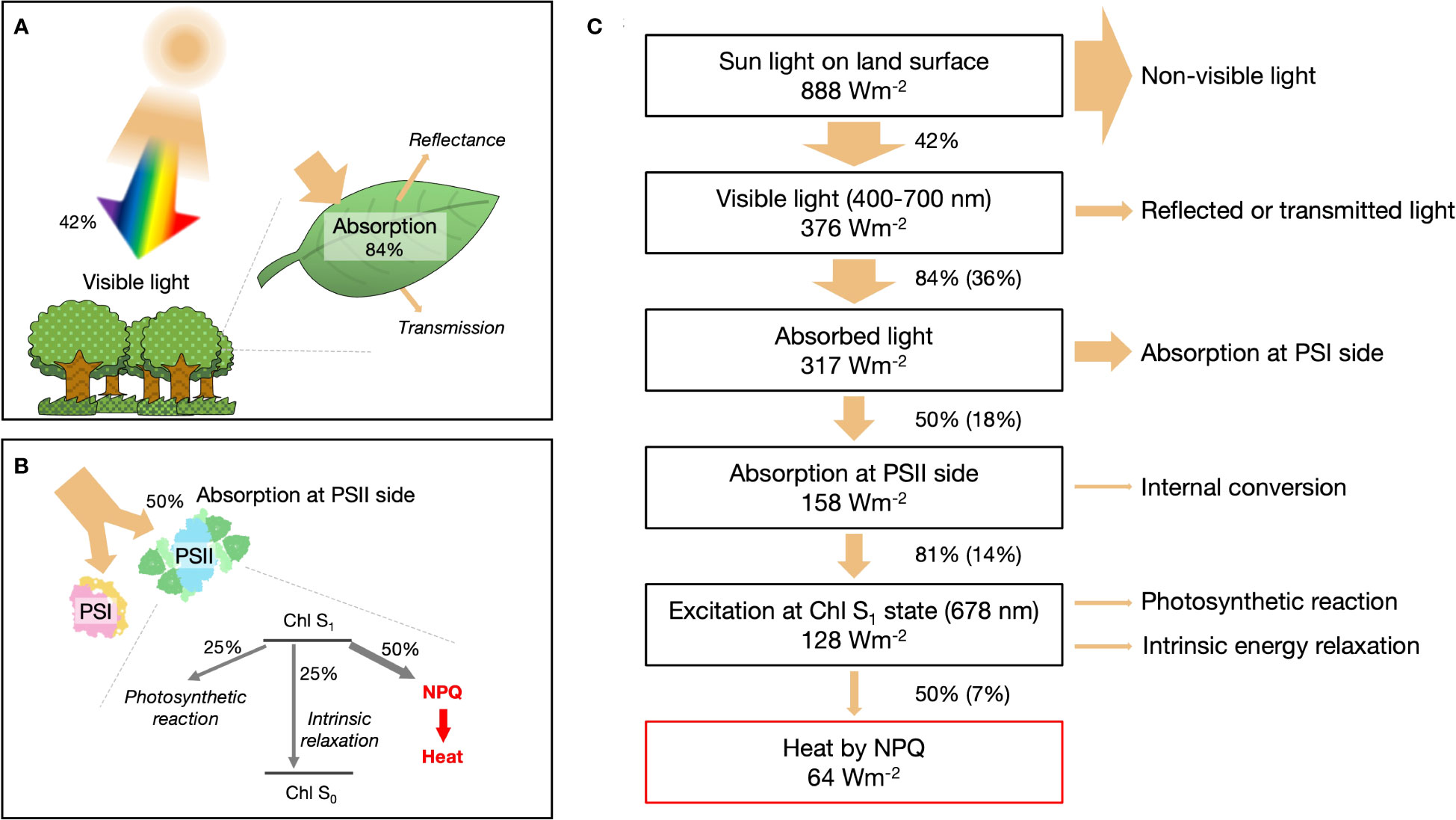
Figure 1 Quantitative assessment of heat generation via non-photochemical quenching (NPQ) on Earth. (A) Proportion of light energy absorbed by leaves. (B) Detailed quantification of light energy dissipation as thermal energy mediated by NPQ, emphasizing the involvement of photosystem I (PSI) and photosystem II (PSII) supercomplexes. (C) Schematic representation of the photonic energy conversion into thermal energy through NPQ processes on Earth.
Most of the absorbed light energy (317 Wm-2 in Figure 1C) besides NPQ is converted to heat. A small fraction of energy (≈35% at the maximum) of the charge separation in PSI and PSII is ultimately fixed as sugar; the rest is released as heat during the intermediate process. The intrinsic energy dissipation is also mostly thermal except for a few percent of fluorescence emission (Lazár, 2015). The amount of heat released by NPQ is less than that of the other processes combined, so it has not received much attention. We dare to focus on NPQ and examine in the subsequent sections whether its heat generation effect is negligible.
Under moderate direct sunlight, photosynthetic activity is saturated, and more than half of the excitation energy around PSII flows into the NPQ pathway (Supplementary Figure S1). In addition to light intensity, NPQ varies with factors such as temperature, CO2 concentration, and canopy structure. However, the general trend of previous experiments is for NPQ energy partitioning to be 40-60% when PSII quantum yield is 20-30% (Kramer et al., 2004; Melkonian et al., 2004; Guadagno et al., 2010; Chang et al., 2022). In this calculation, we assumed the yield of NPQ at PSII as 50% and the solar radiation corresponds to the midday in the average latitude for the contiguous USA. According to the simplified energy conversion model shown in Figure 1C, the amount of heat produced by NPQ is 63.9 W m−2, equivalent to 20.3% of absorbed light energy and 7.2% of solar radiation on the land surface.
Increase in leaf temperature by NPQ
Plant leaves are known to keep their temperature appropriate for photosynthesis even in direct sunlight (Michaletz et al., 2016) through transpiration, convection, and radiation. The heating effect of NPQ has been considered negligible compared to the cooling effects. When the stomata is closed and there is no transpiration, leaf surface temperature significantly increase under illumination. The previous thermoimaging analysis of tobacco leaves showed the leaf temperature increase by 1-5°C, and the enhancing NPQ increased leaf temperature increase by 10% (Kana and Vass, 2008). Under these conditions, the inside leaf temperature could be higher than the surface temperature. However, the amount of heat accumulated in leaves has not been fully evaluated because of the lack of effective probes for sensing the temperature inside the leaf tissues and in a single cell.
We assumed leaf condition to form a maximum temperature difference between the outside, closed stomata, no transpiration, and no convection in the intercellular spaces. In this case, the heat transfer is evaluated on the 1D-geometry model, as shown in Figure 2A, using the simplified Fourier’s heat transfer equation:
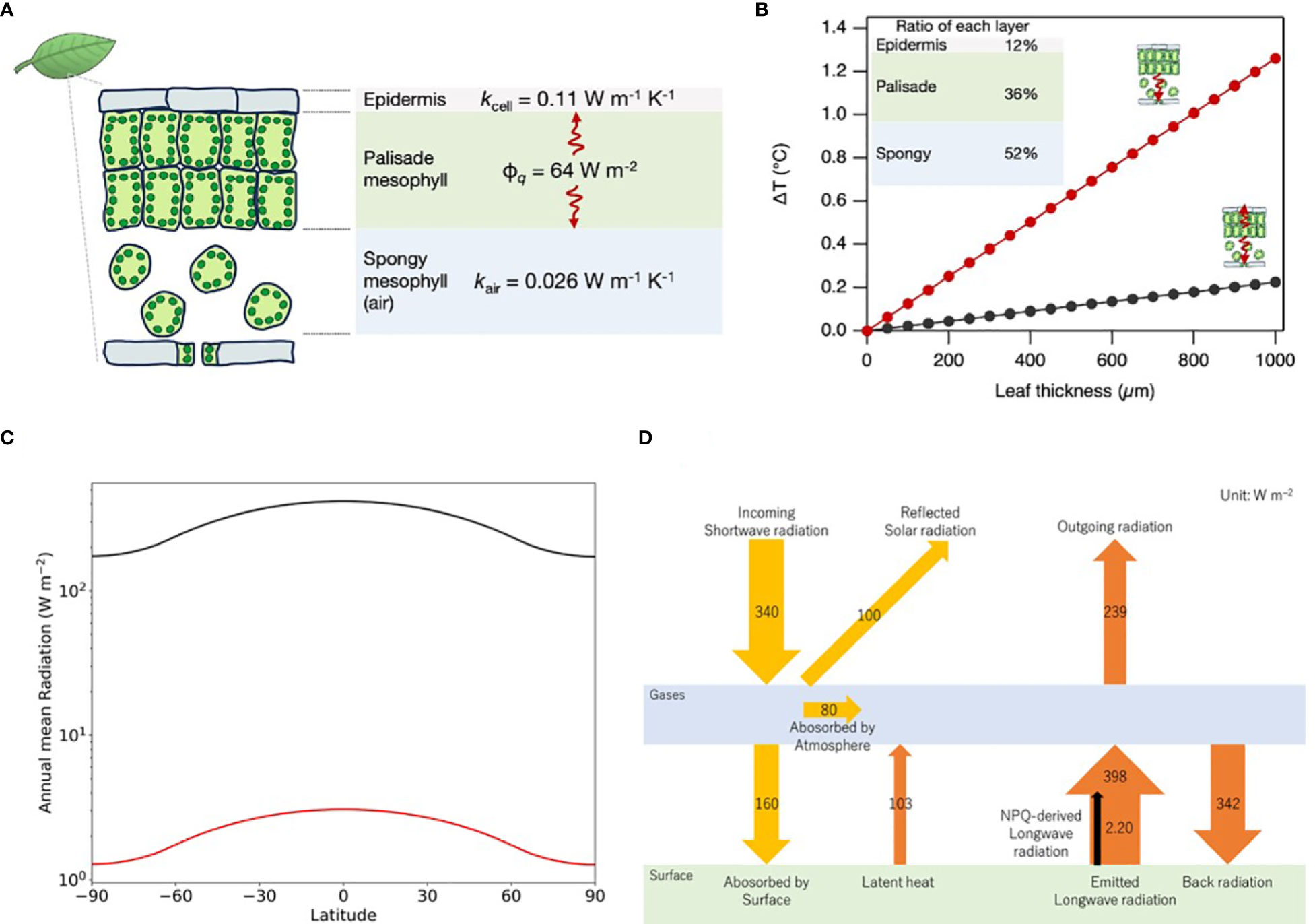
Figure 2 Thermal dynamics in leaf structures mediated by NPQ. (A) A one-dimensional geometrical model for the calculation of temperature variations induced by NPQ, incorporating the heat flux (ϕq) and the thermal conductivities of the epidermal and spongy mesophyll (kcell and kair, respectively). (B) Modeled temperature variations as a function of the leaf thickness, incorporating empirical layer proportion data derived from the Arabidopsis study (Gorsuch et al., 2010). Black and red lines represent two situations respectively, (i) the heat transfers to both abaxial (spongy layer) and adaxial (epidermis layer) sides; (ii) the heat transfers only to the abaxial side when the adaxial side is hotter than the abaxial side due to direct sunlight irradiation. (C) Variation of annual mean radiation at different latitudes. The black line is SWR and the red line is NPQ-induced LWR. (D) Schematic of Earth’s global average energy budget incorporating NPQ-induced LWR with reference to IPCC’s AR6.
Where ΔT, ϕq, k, and dx are the temperature difference (K), heat flux (W m−2), thermal conductivity (W m−1 K–1), and length of heat transfer (m) respectively.
A typical leaf tissue structure consists of a tightly packed palisade mesophyll layer on the upper (adaxial) side and a spongy mesophyll layer on the lower (abaxial) side (Figure 2A). When we assume that the heat produced by NPQ is mainly generated in the palisade layer, the value of ΔT formed by abaxial side heat transfer via cells and adaxial side heat transfer via air (ΔTabaxial+ΔTadaxial=ΔTleaf) can be driven from the following equation:
Assuming the relative thickness of the upper epidermis, palisade, and spongy mesophyll to be 12%, 36%, and 52%, respectively, as in the case of Arabidopsis (Gorsuch et al., 2010), ΔTleaf increases with increasing leaf thickness, as shown in the graphs of Figure 2B. When the heat in palisade cells transfers to the abaxial and adaxial sides, the ΔTleaf is 0.06°C (leaf thickness ~ 250 µm in the case of Arabidopsis leaf), even when using a relatively low value of cellular thermal conductivity at 0.11 W m–1 K–1 (Sotoma et al., 2021). On the other hand, when the heat is transferred only to the abaxial side, ΔTleaf increases by 0.3°C because of the very low thermal conductivity of the air at 0.026 W m–1 K–1. Such a situation is conceivable when the ambient temperature of the abaxial side is lower than the temperature of the adaxial side, and the leaf temperature is in between either of them. In the case of thick leaves at 1 mm (e.g. evergreen trees), leaf tissue can hold a temperature gradient of about 1°C.
Although the above calculations show that NPQ could increase leaf tissue temperature by 1°C at the maximum under the extreme conditions, the temperature inside the chloroplast could be much higher. The temperature dynamics and subcellular variation inside living cells have been obscure for a long time. In mammalian cells, recently developed nanothermometry probes enable the measurement of intracellular temperature. It is revealed that the mitochondrial temperature could increase by 10°C by stimulating respiration, which is 105 times higher than the theoretical estimation (Chrétien et al., 2018; Di et al., 2022). Whereas typical animal cells with a radius of 10 µm generate 1 nW of heat per cell (Hong et al., 2020) by consuming sugars and lipids, a plant cell of the same cross-section area can continue to generate 10 times as much heat by NPQ under continuous solar illumination (314.2 µm2 × 63.9 W m–2 = 20.1 nW). Since photosynthetic electron transport occurs in the thylakoid membranes placed in chloroplast stroma, which is surrounded by a double membrane envelope, they might retain a large amount of heat locally if the membrane structures can prevent heat exchange. Indirect observation of heat emission from photosynthetic systems via photoacoustic techniques has ruled out the existence of heat barriers inside and surrounding the chloroplast (Mullineaux et al., 1994). The development of technology to directly and accurately measure inner cell temperature distribution, along with theoretical calculations considering the three-dimensional complexity of organelles and cellular arrangements within leaves is awaited.
Increase in global temperature by NPQ
The Earth’s thermal equilibrium is determined by the balance between the incoming shortwave (300-3,000 nm) radiation (SWR) from the sun and the outgoing longwave (5-25 μm) radiations (LWR) from the land surface and the atmosphere. The development of land vegetation not only has a cooling effect as it absorbs atmospheric CO2 and latent heat by transpiration but also has a warming impact due to the absorption of light energy and its conversion to heat. In this section, we calculated the share of NPQ in the global heat budget and estimated the effect on global temperature.
In previous sections, we calculated that the heat produced by NPQ is 63.9 W m–2 at noon in the mid-latitude, where the solar zenith angle is 48.19 degrees. This section evaluates thermal radiation by NPQ globally by considering changing solar zenith angle by diurnal cycle, annual cycle, and latitude. Time-averaged SWR at each latitude of the Earth (Q(ϕ)) is calculated using Python’s climlab package (Rose, 2018), as explained in the Supplementary Material and shown in Figure 2C. Given the Q(ϕ), NPQ-derived fraction of LWR (INPQ(ϕ)) can be obtained from the following equation:
Where ta, rland, rveg, rNPQ, and εveg are atmospheric transmittance, the ratio of land area to global area, the ratio of vegetation area to the land area, the ratio of NPQ thermal energy to solar radiation on the land surface, and emissivity of vegetation respectively. ta is estimated to be 0.47 from the ratio of the surface irradiance at 160 W m-2 to the incoming solar irradiance at 340 W m-2 (IPCC, 2021). rland is 0.30, as generally known. rveg is estimated at 0.74 from Supplementary Table S1 (FAO, 2023). rNPQ was calculated to be 0.07 in the previous section. Emissivity values change depending on the material. Typically, the emissivity for water is between 0.98 and 0.99 (Konda et al., 1994), soil is between 0.96 and 0.97, and vegetation is between 0.96 and 0.98 (Qin et al., 2006). For simplicity, we used the same values at 0.98 for vegetation and global average. The result of this calculation is shown in Figure 2C.
A weighted average of INPQ (ϕ) for each latitude (ϕ i) from 90 degrees north to 90 degrees south gives a global average value (INPQglobal).
The calculation results in 2.20 W m–2 for INPQglobal. The global average of Q (ϕ) calculated from the weighted average is at 341 W m-2, consistent with the latest estimation by IPCC at 340 W m-2 (IPCC, 2021). Our estimation of NPQ-induced LWR at 2.20 W m–2 occupies 0.55% of the LWR from the land surface at 398 W m-2 estimated by IPCC (see Figure 2D).
Finally, the relationship between the thermal radiation by NPQ and the Earth’s surface temperature is calculated from Stefan-Boltzmann’s law in the following equation:
Where I, ε, σ, and T are radiant emittance (W m–2), emissivity (0.98), Stefan–Boltzmann constant (5.67×10–8 W m–2 K–4), and temperature (K), respectively. Considering that LWR from the land surface at 398 W m–2, the surface temperature is 290.9 K (17.8°C). If the radiant emittance corresponding to NPQ (2.2 W m-2) is removed, the surface temperature decreases by 0.4 K to 290.5 K. The result indicates that NPQ contributes to an increase in the Earth’s surface temperature of 0.4°C.
Discussion
Heat dissipation by NPQ is a primary feedback regulation mechanism in photosynthesis under excessive light, and its mechanism has been intensely studied for decades (Bassi and Dall’Osto, 2021). NPQ is also a direct target for crop engineering to increase food and energy production since it is considered wasted energy. However, simply suppressing NPQ will only substitute excess energy for constitutive non-photochemical quenching (light-independent) heat dissipation, not carbon fixation (see Supplementary Figure S1). Mutational analyses revealed that NPQ-less plants and algae could grow well under low light but were less adaptive to high light and changing light conditions (Khuong et al., 2019; Perin et al., 2023). A faster off-switching of unnecessary NPQ can significantly improve crop production only under fluctuating light (Kromdijk et al., 2016; De Souza et al., 2022). Constant suppression of NPQ and improvement of photosynthetic productivity has not been successful so far. To increase the photosynthetic productivity of plants under natural conditions, NPQ suppression must be accompanied by improved energy utilization. It would require extensive genetic modification to regulate multiple targets downstream of the light reactions including dark reactions, gas exchange, carbon translocation, and sink capacity.
Conversely, we cannot ignore the possibility that thermal energy, including NPQ, is involved in the mechanisms to optimize photosynthetic functions. Illumination of dark-adapted plants induces a pronounced thermal energy dissipation and activates the light-harvesting antenna complex, transferring excitations toward the reaction centers (Janik et al., 2017). Moreover, the direct use of thermal energy via the up-conversion of excitation energy has been demonstrated experimentally (Zubik et al., 2020).
If NPQ significantly raises the intracellular temperature, it could affect biochemical and physiological reactions in photosynthesis. Heat production should be beneficial for plants to protect them from chilling stress. Snow algae and some cold-tolerant plants can perform photosynthesis under sub-freezing temperatures. It could be possible that these plants maintain local temperatures around photosynthetic reaction centers. Evergreen plants from high latitudes can sustain high rates of NPQ during winter (Verhoeven, 2014). The Antarctic psychrophile green algae temporally induce a high NPQ during the induction of photosynthesis (Takizawa et al., 2009). Heat production by NPQ might warm up the photosynthetic machinery to enable quick induction of photosynthesis at low temperatures.
Thermal production at moderate and high temperatures does not accelerate photosynthesis but might downregulate it safely. High temperature and high light stress often co-occur, and they can induce similar photoprotective mechanisms, such as state transitions (Nellaepalli et al., 2011) and activation of cyclic electron flow (Sharkey, 2005). NPQ might enhance high temperature-induced photoprotection under moderate ambient temperature. Once it can be confirmed that heat production by NPQ does not affect photosynthetic activity and plant physiology, a large-scale gene editing project to suppress NPQ can be initiated.
The artificial control of NPQ can also be used as a strategy to modulate global temperature. The radiation from plant NPQ is about 2.2 W m–2 par surface area of the earth. It is less than 1% of the thermal radiated from the Earth’s surface, but it cannot be ignored. The climate forcing caused by the doubling of atmospheric CO2 by human activities has perturbed Earth’s energy balance about 4 W m–2 (Wilson and Gea-Banacloche, 2012; Jeevanjee et al., 2021). Theoretically, about half of this could be offset by suppressing NPQ. To cool the Earth, suppression of NPQ must be coupled with suppression of the light capture because once light is absorbed by the plant, almost all of it is finally converted to heat. Increasing leaf reflectance reduces the light available for photosynthesis, but plants with suppressed NPQ may be able to grow adequately.
The environment and living organisms have coevolved in the Earth’s long history. The evolution and expansion of terrestrial plants could have made the environment cooler and caused the Ice Age (Richey et al., 2020). However, plants with NPQ could also contribute to global warming or mitigating global cooling. Most existing plants adapt to their environment by absorbing light efficiently and letting excess energy escape as heat. If plants that reflect excess light and use less light come to occupy the planet, the global environment may become colder.
Data availability statement
The original contributions presented in the study are included in the article/Supplementary Material. Further inquiries can be directed to the corresponding author/s.
Author contributions
AM: Investigation, Writing – original draft, Writing – review & editing. EK: Investigation, Writing – original draft, Writing – review & editing. JM: Conceptualization, Writing – review & editing. KT: Investigation, Project administration, Writing – original draft, Writing – review & editing.
Funding
The author(s) declare financial support was received for the research, authorship, and/or publication of this article. This work was supported by JSPS KAKENHI Grant Number JP21H05040 to JM, JP23H04960 to EK and JM, and JP23K14216 to EK; Takeda Science Foundation to EK.
Conflict of interest
The authors declare that the research was conducted in the absence of any commercial or financial relationships that could be construed as a potential conflict of interest.
Publisher’s note
All claims expressed in this article are solely those of the authors and do not necessarily represent those of their affiliated organizations, or those of the publisher, the editors and the reviewers. Any product that may be evaluated in this article, or claim that may be made by its manufacturer, is not guaranteed or endorsed by the publisher.
Supplementary material
The Supplementary Material for this article can be found online at: https://www.frontiersin.org/articles/10.3389/fpls.2024.1367795/full#supplementary-material
References
Bassi, R., Dall’Osto, L. (2021). Dissipation of light energy absorbed in excess: The molecular mechanisms. Annu. Rev. Plant Biol. 72, 47–76. doi: 10.1146/annurev-arplant-071720-015522
Björkman, O., Demmig, B. (1987). Photon yield of O2 evolution and chlorophyll fluorescence characteristics at 77 K among vascular plants of diverse origins. Planta 170, 489–504. doi: 10.1007/BF00402983
Bonan, G. B. (2008). Forests and climate change: Forcings, feedbacks, and the climate benefits of forests. Science 320, 1444–1449. doi: 10.1126/science.1155121
Chang, C. Y., Wen, J. M., Han, J. M., Kira, O., LeVonne, J., Melkonian, J., et al. (2022). Unpacking the drivers of diurnal dynamics of sun-induced chlorophyll fluorescence (SIF): Canopy structure, plant physiology, instrument configuration and retrieval methods. Remote Sens. Environ. 265, 112672. doi: 10.1016/j.rse.2021.112765
Chrétien, D., Bénit, P., Ha, H. H., Keipert, S., El-Khoury, R., Chang, Y. T., et al. (2018). Mitochondria are physiologically maintained at close to 50 °C. PloS Biol. 16, e2003992. doi: 10.1371/journal.pbio.2003992
Demmig-Adams, B., Garab, G., Adams, W., III, Govindjee, U. (2014). Non-photochemical quenching and energy dissipation in plants, algae and cyanobacteria. Springer Netherlands. doi: 10.1007/978-94-017-9032-1
De Souza, A. P., Burgess, S. J., Doran, L., Hansen, J., Manukyan, L., Maryn, N., et al. (2022). Soybean photosynthesis and crop yield are improved by accelerating recovery from photoprotection. Science 377, 851–854. doi: 10.1126/science.adc9831
Di, X., Wang, D., Su, Q. P., Liu, Y., Liao, J., Maddahfar, M., et al. (2022). Spatiotemporally mapping temperature dynamics of lysosomes and mitochondria using cascade organelle-targeting upconversion nanoparticles. Proc. Natl. Acad. Sci. U. S. A. 119, e2207402119. doi: 10.1073/pnas.2207402119
Food and Agriculture Organization of the United Nations (FAO) (2023) ESA's climate change initiative land cover (CCI-LC). Available online at: https://www.fao.org/faostat/en/#data/LC (Accessed July 21, 2023).
Gorsuch, P. A., Pandey, S., Atkin, O. K. (2010). Temporal heterogeneity of cold acclimation phenotypes in Arabidopsis leaves. Plant Cell Environ. 33, 244–258. doi: 10.1111/j.1365-3040.2009.02074.x
Guadagno, C. R., De Santo, A. V., D'Ambrosio, N. (2010). A revised energy partitioning approach to assess the yields of non-photochemical quenching components. Biochim. Et Biophys. Acta-Bioenergetics 1797, 525–530. doi: 10.1016/j.bbabio.2010.01.016
Hansen, J., Johnson, D., Lacis, A., Lebedeff, S., Lee, P., Rind, D., et al. (1981). Climate impact of increasing atmospheric carbon dioxide. Science 213, 957–966. doi: 10.1126/science.213.4511.957
Hong, S., Dechaumphai, E., Green, C. R., Lal, R., Murphy, A. N., Metallo, C. M., et al. (2020). Sub-nanowatt microfluidic single-cell calorimetry. Nat. Commun. 11, 2982. doi: 10.1038/s41467-020-16697-5
Intergovernmental Panel on Climate Change (IPCC) (2021)Sixth Assessment Report Chapter 7. In: The Earth’s Energy Budget, Climate Feedbacks, and Climate Sensitivity. Available online at: https://www.ipcc.ch/report/ar6/wg1/chapter/chapter-7/ (Accessed January 30, 2024).
Janik, E., Bednarska, J., Zubik, M., Luchowski, R., Mazur, R., Sowinski, K., et al. (2017). A chloroplast "wake up" mechanism: Illumination with weak light activates the photosynthetic antenna function in dark-adapted plants. J. Plant Physiol. 210, 1–8. doi: 10.1016/j.jplph.2016.12.006
Jeevanjee, N., Seeley, J. T., Paynter, D., Fueglistaler, S. (2021). An analytical model for spatially varying clear-sky CO2 forcing. J. Clim. 34, 1–55. doi: 10.1175/JCLI-D-19-0756.1
Kana, R., Vass, I. (2008). Thermoimaging as a tool for studying light-induced heating of leaves Correlation of heat dissipation with the efficiency of photosystem II photochemistry and non-photochemical quenching. Environ. Exp. Bot. 64, 90–96. doi: 10.1016/j.envexpbot.2008.02.006
Khuong, T. T. H., Robaglia, C., Caffarri, S. (2019). Photoprotection and growth under different lights of Arabidopsis single and double mutants for energy dissipation (npq4) and state transitions (pph1). Plant Cell Rep. 38, 741–753. doi: 10.1007/s00299-019-02403-3
Konda, M., Imasato, N., Nishi, K., Toda, T. (1994). Measurement of the sea surface emissivity. J. Oceanography 50, 17–30. doi: 10.1007/BF02233853
Kramer, D. M., Johnson, G., Kiirats, O., Edwards, G. E. (2004). New fluorescence parameters for the determination of QA redox state and excitation energy fluxes. Photosynthesis Res. 79, 209–218. doi: 10.1023/B:PRES.0000015391.99477.0d
Kromdijk, J., Głowacka, K., Leonelli, L., Gabilly, S. T., Iwai, M., Niyogi, K. K., et al. (2016). Improving photosynthesis and crop productivity by accelerating recovery from photoprotection. Science 354, 857–861. doi: 10.1126/science.aai8878
Lazár, D. (2015). Parameters of photosynthetic energy partitioning. J. Plant Physiol. 175, 131–147. doi: 10.1016/j.jplph.2014.10.021
Li, Z., Wakao, S., Fischer, B. B., Niyogi, K. K. (2009). Sensing and responding to excess light. Annu. Rev. Plant Biol. 60, 239–260. doi: 10.1146/annurev.arplant.58.032806.103844
Melkonian, J., Owens, T. G., Wolfe, D. W. (2004). Gas exchange and co-regulation of photochemical and nonphotochemical quenching in bean during chilling at ambient and elevated carbon dioxide. Photosynthesis Res. 79, 71–82. doi: 10.1023/B:PRES.0000011921.64567.b2
Michaletz, S. T., Weiser, M. D., McDowell, N. G., Zhou, J., Kaspari, M., Helliker, B. R., et al. (2016). The energetic and carbon economic origins of leaf thermoregulation. Nat. Plants 2, 16129. doi: 10.1038/nplants.2016.129
Mullineaux, C. W., Ruban, A. V., Horton, P. (1994). Prompt heat release associated with delta-ph-dependent quenching in spinach thylakoid membranes. Biochim. Et Biophys. Acta-Bioenergetics 1185, 119–123. doi: 10.1016/0005-2728(94)90202-X
National Renewable Energy Laboratory (NREL) (2003) Reference air mass 1.5 spectra. Available online at: https://www.nrel.gov/grid/solar-resource/assets/data/astmg173.xls (Accessed July 28, 2023).
Nellaepalli, S., Mekala, N. R., Zsiros, O., Mohanty, P., Subramanyam, R. (2011). Moderate heat stress induces state transitions in Arabidopsis thaliana. Biochim. Et Biophys. Acta-Bioenergetics 1807, 1177–1184. doi: 10.1016/j.bbabio.2011.05.016
Niyogi, K. K. (1999). Photoprotection revisited: Genetic and molecular approaches. Annu. Rev. Plant Physiol. Plant Mol. Biol. 50, 333–359. doi: 10.1146/annurev.arplant.50.1.333
Perin, G., Bellan, A., Michelberger, T., Lyska, D., Wakao, S., Niyogi, K. K., et al. (2023). Modulation of xanthophyll cycle impacts biomass productivity in the marine microalga Nannochloropsis. Proc. Natl. Acad. Sci. U. S. A. 120, e2214119120. doi: 10.1073/pnas.2214119120
Qin, Z., Li, W., Gao, M., Zhang, H. (2006). Estimation of land surface emissivity for Landsat TM6 and its application to Lingxian Region in north China. Remote Sens. Environ. monitoring GIS Applications Geology VI 6366, 292–299. doi: 10.1117/12.689310
Richey, J. D., Montañez, I. P., Goddéris, Y., Looy, C. V., Griffis, N. P., DiMichele, W. A. (2020). Influence of temporally varying weatherability on CO2-climate coupling and ecosystem change in the Late Paleozoic. Clim. Past 16, 1759–1775. doi: 10.5194/cp-16-1759-2020
Rose, B. E. (2018). CLIMLAB: a Python toolkit for interactive, process-oriented climate modeling. J. Open Source Software 3, 24. doi: 10.1111/j.1365-3040.2005.01324.x
Ruban, A. V., Johnson, M. P., Duffy, C. D. P. (2012). The photoprotective molecular switch in the photosystem II antenna. Biochim. Biophys. Acta 1817, 167–181. doi: 10.1016/j.bbabio.2011.04.007
Sharkey, T. D. (2005). Effects of moderate heat stress on photosynthesis: importance of thylakoid reactions, RuBisCO deactivation, reactive oxygen species, and thermotolerance provided by isoprene. Plant Cell Environ. 28, 269–277. doi: 10.1111/j.1365-3040.2005.01324.x
Sotoma, S., Zhong, C., Kah, J. C. Y., Yamashita, H., Plakhotnik, T., Harada, Y., et al. (2021). In situ measurements of intracellular thermal conductivity using heater-thermometer hybrid diamond nanosensors. Sci. Adv. 7, eabd7888. doi: 10.1126/sciadv.abd7888
Takizawa, K., Takahashi, S., Hüner, N. P. A., Minagawa, J. (2009). Salinity affects the photoacclimation of Chlamydomonas raudensis Ettl UWO241. Photosynth. Res. 99, 195–203. doi: 10.1007/s11120-008-9397-8
Verhoeven, A. (2014). Sustained energy dissipation in winter evergreens. New Phytol. 201, 57–65. doi: 10.1111/nph.12466
Wilson, D. J., Gea-Banacloche, J. (2012). Simple model to estimate the contribution of atmospheric CO2 to the Earth’s greenhouse effect. Am. J. Phys. 80, 306–315. doi: 10.1119/1.3681188
Keywords: photosynthesis, non-photochemical quenching (NPQ), heat transfer, heat budget, global warming
Citation: Murakami A, Kim E, Minagawa J and Takizawa K (2024) How much heat does non-photochemical quenching produce? Front. Plant Sci. 15:1367795. doi: 10.3389/fpls.2024.1367795
Received: 09 January 2024; Accepted: 08 March 2024;
Published: 20 March 2024.
Edited by:
Artur Nosalewicz, Polish Academy of Sciences, PolandReviewed by:
Dusan Lazar, Palacký University, CzechiaConrad Mullineaux, Queen Mary University of London, United Kingdom
Wieslaw Gruszecki, Maria Curie-Sklodowska University, Poland
Copyright © 2024 Murakami, Kim, Minagawa and Takizawa. This is an open-access article distributed under the terms of the Creative Commons Attribution License (CC BY). The use, distribution or reproduction in other forums is permitted, provided the original author(s) and the copyright owner(s) are credited and that the original publication in this journal is cited, in accordance with accepted academic practice. No use, distribution or reproduction is permitted which does not comply with these terms.
*Correspondence: Kenji Takizawa, a2VuamktdEBuaWJiLmFjLmpw
†These authors have equally contributed to this work and share first authorship