- 1Maize Research Institute, Sichuan Agricultural University, Chengdu, China
- 2Agricultural Genomics Institute at Shenzhen, Chinese Academy of Agricultural Sciences, Shenzhen, China
- 3Pingliang Academy of Agricultural Sciences, Pingliang, China
- 4Animal Feeding and Management Department, Research Base of Giant Panda Breeding, Chengdu, China
- 5Horticulture Research Institute, Sichuan Academy of Agricultural Sciences, Chengdu, China
- 6School of Urban and Rural Planning and Construction, Mianyang Teachers’ College, Mianyang, China
- 7College of Grassland Resources, Southwest Minzu University, Chengdu, China
Maize, a salt-sensitive crop, frequently suffers severe yield losses due to soil salinization. Enhancing salt tolerance in maize is crucial for maintaining yield stability. To address this, we developed an introgression line (IL76) through introgressive hybridization between maize wild relatives Zea perennis, Tripsacum dactyloides, and inbred Zheng58, utilizing the tri-species hybrid MTP as a genetic bridge. Previously, genetic variation analysis identified a polymorphic marker on Zm00001eb244520 (designated as ZmSC), which encodes a vesicle-sorting protein described as a salt-tolerant protein in the NCBI database. To characterize the identified polymorphic marker, we employed gene cloning and homologous cloning techniques. Gene cloning analysis revealed a non-synonymous mutation at the 1847th base of ZmSCIL76, where a guanine-to-cytosine substitution resulted in the mutation of serine to threonine at the 119th amino acid sequence (using ZmSCZ58 as the reference sequence). Moreover, homologous cloning demonstrated that the variation site derived from Z. perennis. Functional analyses showed that transgenic Arabidopsis lines overexpressing ZmSCZ58 exhibited significant reductions in leaf number, root length, and pod number, alongside suppression of the expression of genes in the SOS and CDPK pathways associated with Ca2+ signaling. Similarly, fission yeast strains expressing ZmSCZ58 displayed inhibited growth. In contrast, the ZmSCIL76 allele from Z. perennis alleviated these negative effects in both Arabidopsis and yeast, with the lines overexpressing ZmSCIL76 exhibiting significantly higher abscisic acid (ABA) content compared to those overexpressing ZmSCZ58. Our findings suggest that ZmSC negatively regulates salt tolerance in maize by suppressing downstream gene expression associated with Ca2+ signaling in the CDPK and SOS pathways. The ZmSCIL76 allele from Z. perennis, however, can mitigate this negative regulatory effect. These results provide valuable insights and genetic resources for future maize salt tolerance breeding programs.
1 Introduction
Salt stress is one of the major abiotic stresses, that significantly hinder agricultural production and limits the further improvement of crop yield (Zhang et al., 2023). In particular, maize is a glycophytic crop highly susceptible to salt stress. Moreover, most currently available maize germplasm resources lack salt-tolerant traits, and the underlying molecular mechanism of plant responses to salt stress remains unclear. However, the wild relatives of maize, Zea perennis, and Tripsacum dactyloides, retain beneficial traits that have been eliminated during the domestication process and represent a valuable gene pool for the genetic improvement of maize. Therefore, retrieving the lost alleles and strengthening research on the molecular mechanisms of salt stress response in maize are of great theoretical and practical significance.
Salt stress decreases soil hydraulic conductivity and increases the external osmotic pressure of plant roots (Pingle et al., 2022). Under salt stress, excessive ion uptake in plants leads to significant disruption of the dynamic ion and water balance, resulting in membrane damage and cell death (Zhu, 2016). Consequently, seed water absorption ability is significantly reduced, leading to greatly diminished germination rates, germination potential, and radicle development, making seedling establishment difficult. It has been reported that excessive uptake of Na+ in plants will compete with potassium ions, resulting in reduced K+ content, and the unbalanced Na+/K+ ratio leads to more severe damage (Rubio et al., 2020). Intensified salt stress can further cause oxidative stress, resulting in the accumulation of toxic compounds such as reactive oxygen species (ROSs). This can ultimately alter membrane functionality, impairing the cell’s ability to maintain proper ion and nutrient balances, and negatively affecting plant growth and development (Zhu, 2001; Yang and Guo, 2018).
To cope with salt stress, plants have developed various adaptive mechanisms (Yang et al., 2020). Organic osmoregulatory agents such as proline (Pro), soluble sugar, and glycine betaine (GB), play a pivotal role in preventing water loss in plants. Studies have shown a significant increase in the content of Pro and GB in maize under salt stress (Bano and Fatima, 2009). Additionally, the soluble sugar content of salt-tolerant maize lines has been reported to be higher than that of salt-sensitive lines. To enhance salt tolerance in maize, genes mediating shoot Na+ exclusion by withdrawing Na+ from the root xylem flow are known (Zhang et al., 2019, 2023).
Plant hormones play a pivotal role in the stress response, particularly abscisic acid (ABA), which is an endogenous signal molecule involved in regulating abiotic stresses in plants, such as salt stress (Sah et al., 2016; Li et al., 2024). When plants experience salt stress, the activation of the ABA signal pathway leads to the expression of downstream osmotic regulation response genes in Arabidopsis (Yu et al., 2020). Under salt stress, the osmotic potential and pre-dawn leaf water potential are reduced, leading to an increase in ABA levels, which consequently decreases the stomatal model parameters, thereby enhancing salt and drought resistance (Song et al., 2023). Furthermore, overexpression of AtLOS5 promotes ABA biosynthesis in Arabidopsis, and the transgenic plant has shown stronger salt tolerance than the wild type (Zhang et al., 2016).
In response to salt stress, plants activate signaling transduction networks. Among these networks, the salt overly sensitive (SOS), calcium-dependent protein kinase (CDPK), and mitogen-activated protein kinase (MAPK) pathways play pivotal roles in transducing environmental cues perceived by plant cell membranes to target genes (Li and Nam, 2002). CDPKs are essential factors in abiotic stress tolerance, and CDPK regulates stress tolerance by modulating ABA signaling and reducing ROS accumulation (Asano et al., 2012). The SOS pathway plays a key role in regulating ion transport under salt stress (Zhou et al., 2022). In the SOS pathway, the cascade activation of SOS3, SOS2, and SOS1 triggered by an increase in cellular Ca2+ concentration, activates SOS1, which promotes Na+ efflux, and overexpression of SOS1 significantly enhanced the salt tolerance in Arabidopsis (Shi et al., 2003).
Iqbal et al. (2019) reported a tri-species hybrid called MTP (2n = 20M+ 34T + 20P = 74; M, T, and P, stand for autotetraploid maize, T. dactyloides, and Z. perennis, respectively). MTP has exhibited excellent salt tolerance, and its hybrids with maize are fertile, allowing for the development of a series of MTP-maize introgression lines with high salt tolerance utilizing MTP as the donor and maize inbred line as the recipient (Li et al., 2023). During genetic diversity analysis of MTP-maize introgression lines, a mutated gene Zm00001eb244520 (designed as ZmSC) was detected in a salt-tolerant MTP-maize introgression line 76 (IL76), using its parent Zheng58 (Z58) as a reference. This gene was described as a salt-tolerant protein (Alexandrov et al., 2009), and a previous study showed that overexpression of TaSC, a homologous gene of ZmSC, could enhance Arabidopsis salt tolerance (Huang et al., 2012). In the current study, we aimed to elucidate the effect of the ZmSC on salt tolerance in maize under salt stress conditions and preliminarily unravel the regulatory mechanism of ZmSC in the salt response. To this end, we conducted experiments to determine the expression pattern of ZmSC under salt stress, investigate its molecular function, and identify the source of its variation. Collectively, our research provides valuable insights into the molecular mechanism of salt tolerance and provides genetic resources to facilitate the development of salt-tolerant maize varieties.
2 Materials and methods
2.1 Plant materials and growth conditions
In a previous study, a series of MTP-maize introgression lines were generated by utilizing the tri-species hybrid MTP as a donor and elite inbred line Zheng58 (Z58) as the acceptor through backcrossing and self-crossing, and IL76 (BC9F5) was one of the lines. To develop a near-isogenic line carrying the ZmSCIL76 allele from IL76(BC9F5). the line was backcrossed to Z58 for three generations and then selfed to make it homozygous, resulting in the near-isogenic line NILIL76 (BC12F6). In each generation, molecular markers were used to select for the introgressed ZmSCIL76, the molecular marker primers are listed in Supplementary Table 3. Field pollination was conducted in the experimental field of Sichuan Agriculture University (Chengdu, China) (30°26′N–31°26′N, 102°54′E–104°53′E). All the experiments of salt tolerance identification were performed in the greenhouse of Sichuan Agriculture University with 14 h of light at 28°C and 10 h of darkness at 23°C, and 75% humidity.
2.2 Cloning and bioinformatics analysis of the ZmSC
Genomic DNA and total RNA were isolated from inbred line Z58, IL76, wild relatives T. dactyloides, Z. perennis, and MTP. Electrophoresis on 1.5% agarose gels and sequencing were utilized to examine DNA and total RNA. The promoter and full-length ZmSC gene were amplified using DNA as a template, with primers designed online at NCBI (https://www.ncbi.nlm.nih.gov/tools/primer-blast/, accessed on 6 March 2023). Total RNA was reverse transcribed to cDNA, followed by amplification of CDS to detect sources of variation. The sequences were aligned using DNAMAN software. All primers were listed in Supplementary Tables 3, 4, the same below.
The Hidden Markov Model (HMM) profile of ZmSC domain UPF0220 downloaded from the Pfam database was employed to identify ZmSC genes in the maize genome (www.maizegdb.org, accessed on 6 March 2023), using the simple HMM search program of TBtools (Chen et al., 2020). To confirm the ZmSC domain, SMART and NCBI conserved Domain Data (CDD) search programs were utilized. ClustalW (Chenna et al., 2003) was utilized to carry out multiple sequence alignment analysis, and the phylogenetic tree was constructed by the neighbor-joining method of MEGA 7.0 with 1000 bootstrap replicates (Kumar et al., 2016).
2.3 ZmSC subcellular localization
The Vector pCAMBIA2300-Pro35s::eGFP and BM seamless cloning kit (Biomed) were used for the construction of recombinant vector pCAMBIA2300-Pro35s::ZmSC-eGFP. The recombinant vector was transformed into the E.coli DH5α competent cells using a heat shock protocol for propagation and validated by Sanger sequencing. Recombinant plasmids pCAMBIA2300-Pro35s::ZmSC-eGFP were transformed into the Agrobacterium strain GV3101 using the freeze-thaw method and infiltrated into four-week-old Tobacco (Nicotiana. benthamiana) leaves. For each transformation, three biological replicates were used. ER-mCherry and NLS-mCherry were used as the endoplasmic reticulum and nuclear marker, respectively. The signal was detected using a confocal microscope (Leica, Germany) 72 h after infiltration as described previously (Zou et al., 2018).
2.4 Transformation of Arabidopsis and fission yeast
The constructed vector pCAMBIA2300-Pro35s::ZmSC-eGFP was then transformed into the floral tissues of Arabidopsis thaliana via Agrobacterium-mediated floral dip method. Following transformation, plants were screened by PCR to confirm the presence of the ZmSC gene, resulting in developing three independent transgenic overexpression lines. From these lines, those exhibiting the highest ZmSC expression level, as determined by RT-qPCR, were subsequently selected for further experiments, including salt tolerance identification and physiological index measurement under NaCl stress.
Primers containing restriction sites for SalI and BamHI were used to amplify the target gene region. The digested products were recovered from the agarose gel using a DNA Recovery Kit (TIANGEN Biotech). Plasmid pREP1 was digested with restriction enzymes SalI and BamHI and connected with digested products using T4 DNA ligase. The method for transforming the recombinant vector into the E.coli DH5α competent cells, as well as validation, is the same as the method described in the 2.3 section. To transform the recombinant plasmid DNA into fission yeast (Schizosaccharomyces pombe SPQ.01), a mixture of 10 μl Carrier DNA, 1 μg recombinant plasmid DNA, 50 μl fission yeast competent cells, and 500 μl buffer was incubated in a water bath for 30 minutes in a 1.5 ml EP tube, followed by a 15-minute incubation at 42°C. The resulting product was centrifuged at 12,000 rpm for 15 seconds, and the supernatant was discarded. This process was repeated after adding 500 μl sterile ddH2O for washing. Reconstructed yeast was resuspended in 50 μl ddH2O and then daubed on MM medium. After 4–6 days of cultivation, the surviving single clones were picked up and expanded at 28°C, 200 rpm for 24h. After bacterial liquid PCR detection, the recombinant yeast was used for further identification of salt tolerance. For salt tolerance identification, positive yeast transformants and empty vector were both diluted to an OD600 of 0.8, and then further diluted to 10–2, 10–3, 10–4, and 10–5. Finally, 4 μl of the diluted cultures was cultured with MM medium containing NaCl.
2.5 RNA extraction and RT-qPCR
The total RNA of roots and leaves was extracted using the EasyPure Plant RNA Kit (TransGen Biotech, Beijing, China). RNA integrity was examined by electrophoresis in 1.5% agarose gel. Reverse transcription was immediately performed using the RevertAid First Strand cDNA Synthesis Kit. Primers were designed using an online tool from NCBI. RT-qPCR was conducted using the “SYBR” Premix Ex TaqTM kit (Takara, Japan) on a Roche LightCycler480 instrument. Glyceraldehyde 3-phosphate dehydrogenase (GAPDH) was used as an internal control. The relative abundance of transcripts was determined using the 2-ΔΔCT method (Livak and Schmittgen, 2001).
We defined the relative expression as the ratio of expression levels in the experimental group (T) to that in the control group (CK). Here, T represents conditions of salt stress or the introgression line IL76, while CK represents normal conditions or inbred Z58. Relative expression was calculated using the formula:
All experiments were performed three times to ensure accuracy.
2.6 Physiological indices measurements
Root and leaf samples were collected from 2-week-old seedlings under normal and 200 mM NaCl stress conditions for 7 d. the contents of Na+, K+, and Ca2+ were determined by atomic absorption spectrometry (Cushman et al., 2020). WinRhizo software (LC4800-II LA2400, Sainte Foy, Canada) was used to analyze the root traits, including total root length, volume, surface area, tip number, and average diameter.
The concentrations of phytohormones, including IAA (Indole acetic acid), GA (Gibberellic acid), JA (Jasmonic acid), ABA (Abscisic acid), and CTK (Cytokinin), were determined using Ruixinbio kits (Ruixin Biological Technology Co., Ltd, Quanzhou, China) by following manufacturer protocols. Each experimental procedure was conducted with three biological replicates.
2.7 Statistical analysis
Excel 2019 (Microsoft, Redmond, USA) and SPSS 27.0 (SPSS, Chicago USA) software were used for data analysis and collation, and OriginLab 2022 (Originlab, MA, USA) was used for plotting. We performed statistical analysis using t-tests and analysis of variance (ANOVA). All of the assays were performed in triplicated and repeated at least three times. Salt tolerance coefficients (STC) were calculated using the formula (Luo et al., 2017):
3 Results
3.1 Single nucleotide mutation leads to ZmSC protein variants
According to the B73 reference genome (APG_V5), ZmSC comprises a full length of 2292 bp, consisting of four exons, and encodes a UPF0220 family protein comprising 142 amino acids. Sequence alignment revealed that the CDS of ZmSCZ58 was consistent with that of the reference genome (APG_V5), and there were no mutations identified in the promoter region when comparing ZmSCZ58 with ZmSCIL76 (2 kb upstream of ZmSC of ATG). However, six SNP mutations (M1-M6) occurred in the CDS sequence of ZmSCIL76 (Figure 1A), and M5 (G to C) at the 356th base resulting in an amino acid change from serine to threonine (S to T) distinguishing ZmSCZ58 from ZmSCIL76 (Figure 1B). Homologous sequence alignment showed that maize wild relatives Zea perennis and MTP carry the C allele at M5 but not in B73 and Z58 (Figure 1C). We conducted further analysis of the frequency of M5 in 36 maize genomes, including the NAM population, and 8 wild maize relatives (5 teosinte and 3 Tripsacum dactyloides) downloaded from the maize genome database. The results indicate that the frequency of M5 is 100% in the wild relatives but only 36% in the maize population (Supplementary Figure 1). Unfortunately, we were unable to collect these germplasm resources for salt tolerance identification, however, valuation of salt tolerance demonstrated that IL76 exhibits stronger salt tolerance compared to Z58 (Supplementary Figures 2, 3). Additionally, IL76 exhibited resilience to drought stress (Supplementary Figure 4) and ABA stress (Supplementary Figure 5). These results suggest that M5 is an allele of Zea perennis, which is widely prevalent in wild maize relatives, and this allele may have been reintroduced to maize cultivation through genetic bridge MTP, and it could have been lost during maize domestication.
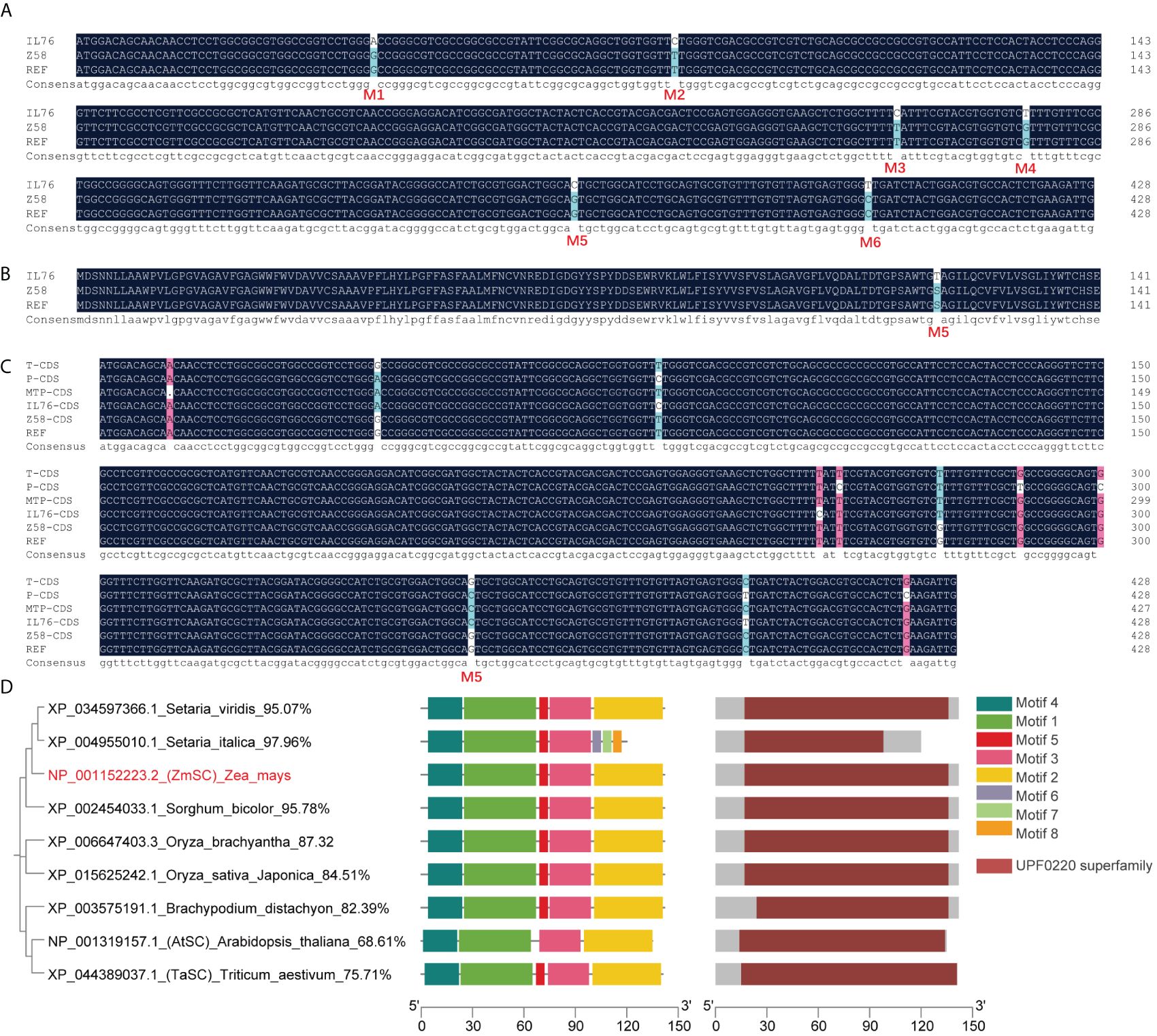
Figure 1 Mutation analysis of ZmSC coding region in IL76, and Phylogenetic tree and MEME analysis of ZmSC ortholog protein. (A) CDS sequence alignment result of ZmSC in the IL76, Z58, and B73 reference sequences; (B) The amino acid sequence alignment result of ZmSC in the IL76, Z58, and B73 reference sequences. (C) The results of homologous cloning of ZmSC CDS in wild parent T. dactyloides (T), Z. perennis (P), and MTP. (D) Phylogenetic tree, MEME analysis, and conserved domains of ZmSC ortholog protein.
Based on the ortholog of amino acids, ortholog proteins of ZmSC were searched in OrthoDB (https://www.orthodb.org/, accessed on 8 March 2023) (Figure 1D), functional annotation revealed that these proteins are UPF0220 mainly transmembrane protein 50 homolog with a similar function, and five conservative motifs were detected using MEME program (https://meme-suite.org/meme/tools/meme, accessed on 8 March 2023) (Figure 1D). Among these, TaSC and AtSC have been shown to regulate salt tolerance in wheat (Huang et al., 2012). However, the function of ZmSC has not been reported. We further used the online tools (https://bioinformatics.psb.ugent.be/webtools/plantcare/html/, accessed on 8 March 2023) to predict the cis-element of 2 kb upstream of ZmSC of ATG, the promoter comprised a variety of functional elements. Including the most basic elements such as TATA-box and CAAT-box, as well as cis-element such as ABRE, AE-box, ARE, CAT-box, CGTCA-motif, G-Box, I-box, MBS, MYC, W-box, MYB, O2-site, TATC-box, TCA-element, TCCC-motif, TGACG-motif, as-1, and GC-motif, which are closely related to plant response to salt, drought, and other abiotic stress (Supplementary Table 1).
To better understand ZmSC, we searched the Zea mays genome with the UPF0220 domain and validated it in the SMART database. Only one gene (Zm00001eb100550) belonging to the ZmSC family was identified at chromosome 2. This gene encodes a transmembrane protein 50A and is involved in late endosome to vacuole transport via multivesicular body sorting pathway. Currently, only one study has reported a potential association of this gene with maize grain weight (Zhou et al., 2020), and there is no research on its stress tolerance to date. To confirm the subcellular location of ZmSC, the ZmSC sequence was fused to green fluorescent protein (GFP) and transiently expressed in tobacco (Nicotiana benthamiana) epidermal cells, this revealed that the ZmSC-eGFP protein was primarily localized in the plasma membrane and nuclear membrane (Figure 2).
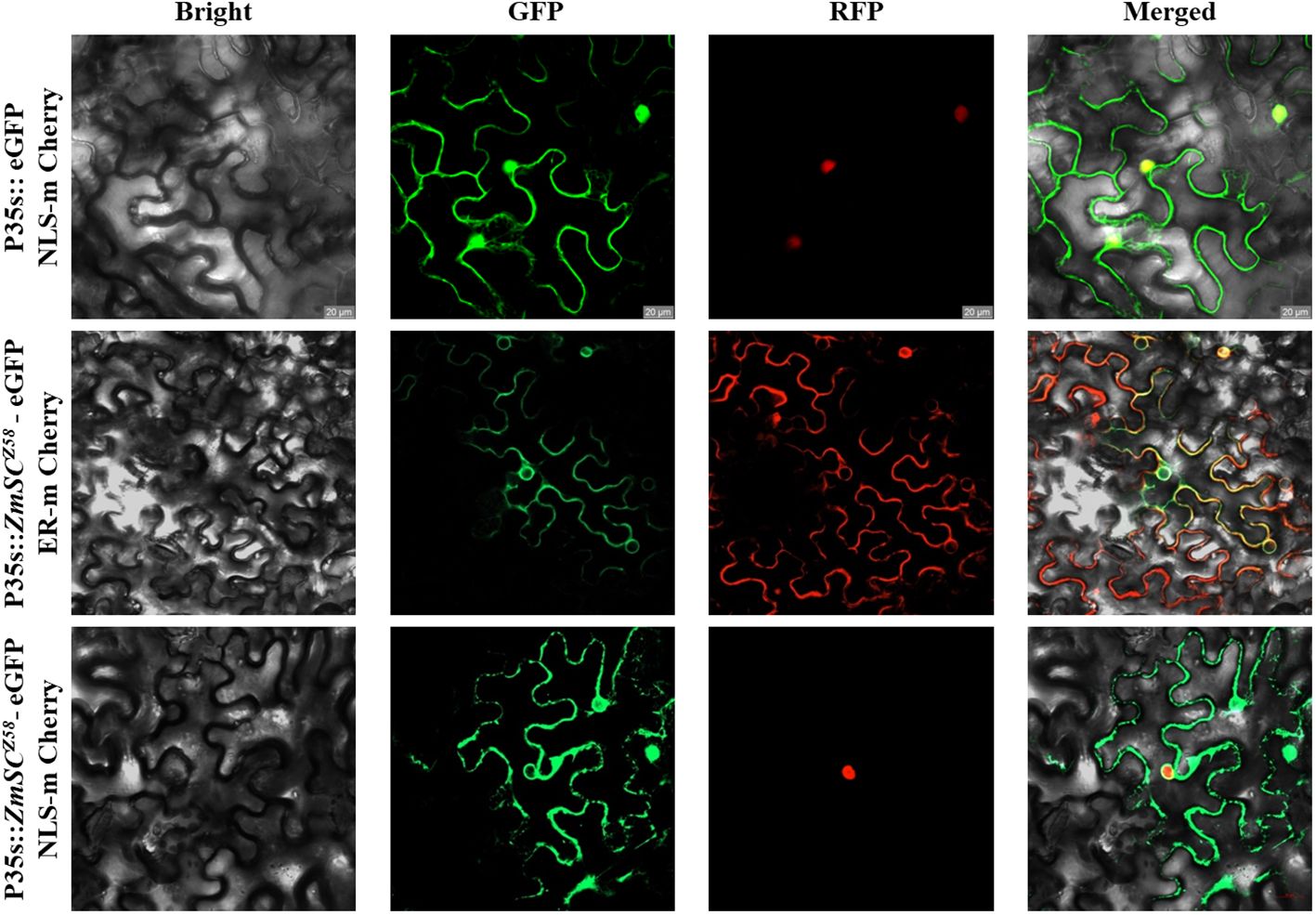
Figure 2 Subcellular localization of ZmSC-eGFP represents empty vector, NLS-mCherry (Nucleus marker chrrey), and ER-mChrrey (Endoplasmic reticulum marker chrrey) represents the nucleus and endoplasmic reticulum marker, respectively. Scale bar = 20 um.
3.2 Downregulation of ZmSCIL76 expression under salt stress
To investigate the expression patterns of ZmSC, inbred Z58, and IL76 seedlings (10 days old seedlings after germination) were exposed to salt stress (200 mM NaCl) and 100 μmol/L ABA stress for 48 hours. Leaf and root samples were collected at 0h, 3h, 6h, 9h, 12h, 24h, and 48-hour intervals for RT-qPCR analysis. The comparison of expression levels is conducted by evaluating the ratio of relative expression between ZmSCIL76 and ZmSCZ58, represented as the expression level of ZmSCIL76 to the expression level of ZmSCZ58 at each time point (Figure 3). Our results showed that, under salt stress, except for the relative expression at 24h and 48h time points in leaf tissue and 3h time point at in root tissue, all other time points exhibited a ratio less than 1.0, indicating significant differences in the response of ZmSCIL76 and ZmSCZ58 to salt stress and the expression of ZmSCIL76 was suppressed (Figure 3A). Under ABA stress conditions, the expression of ZmSCIL76 appears to be suppressed, similar to the situation under salt stress (Figure 3B). These results indicate that compared to ZmSCZ58, ZmSCIL76 exhibits lower expression levels under salt and ABA stress at most time potions.
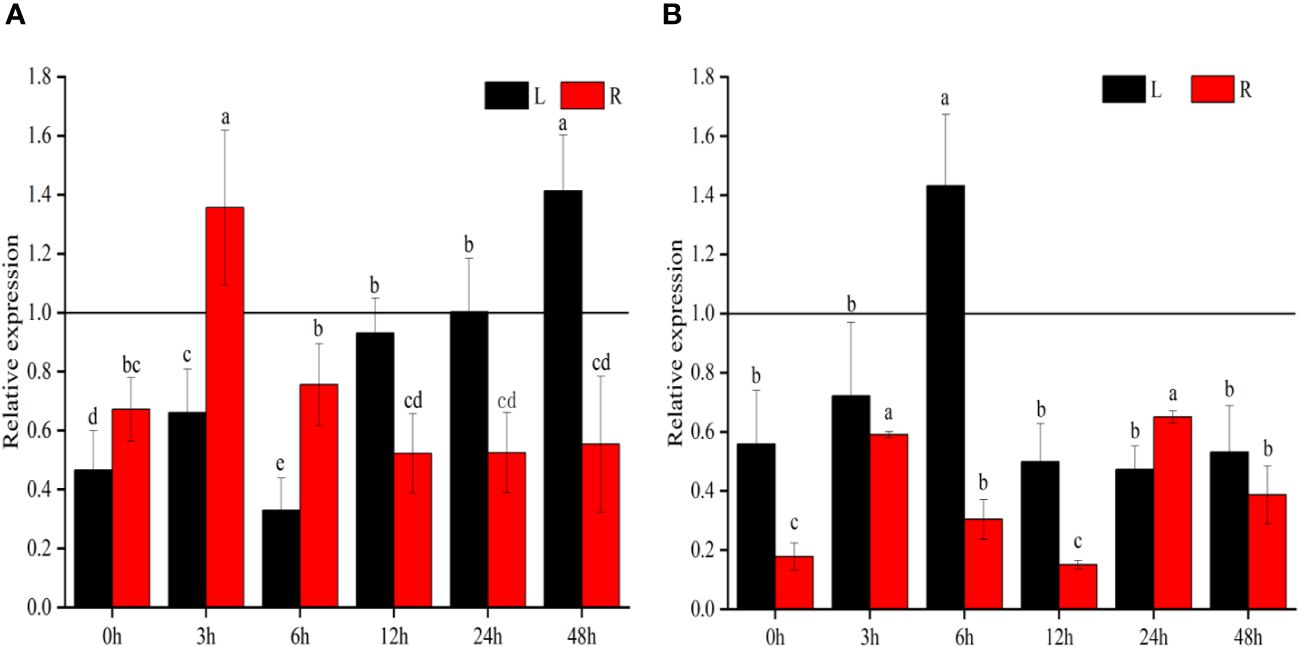
Figure 3 The relative expression of ZmSC at different time points under salt (A) and ABA (B) stress. L and R represent leaf and root tissues, respectively, the same as below. Different letters denote significant differences in the relative expression levels of ZmSC at different time points with the same tissues at the P < 0.05 level (n = 3; error bar = SD).
3.3 ZmSC negatively regulated salt tolerance
To investigate the role of ZmSC in stress responses, we constructed the expression vector pREP1-ZmSCZ58 and pREP1-ZmSCIL76 in fission yeast, while the empty vector pREP1 served as the control. All strains grew normally on MM medium; however, their growth on MM medium supplemented with gradient NaCl was inhibited to varying degrees. Notably, the growth of pREP1-ZmSCZ58 strain was severely affected when the NaCl concentration was above 300 mM, followed by pREP1-ZmSCIL76, while pREP1 exhibited the least inhibitory effect (Figure 4A). Under mannitol stress, no significant difference was observed among the three strains (Figure 4B). These results indicate that ZmSCZ58 and ZmSCIL76 play a negative regulatory role in the salt tolerance of yeast, with ZmSCZ58 exhibiting a stronger negative effect.
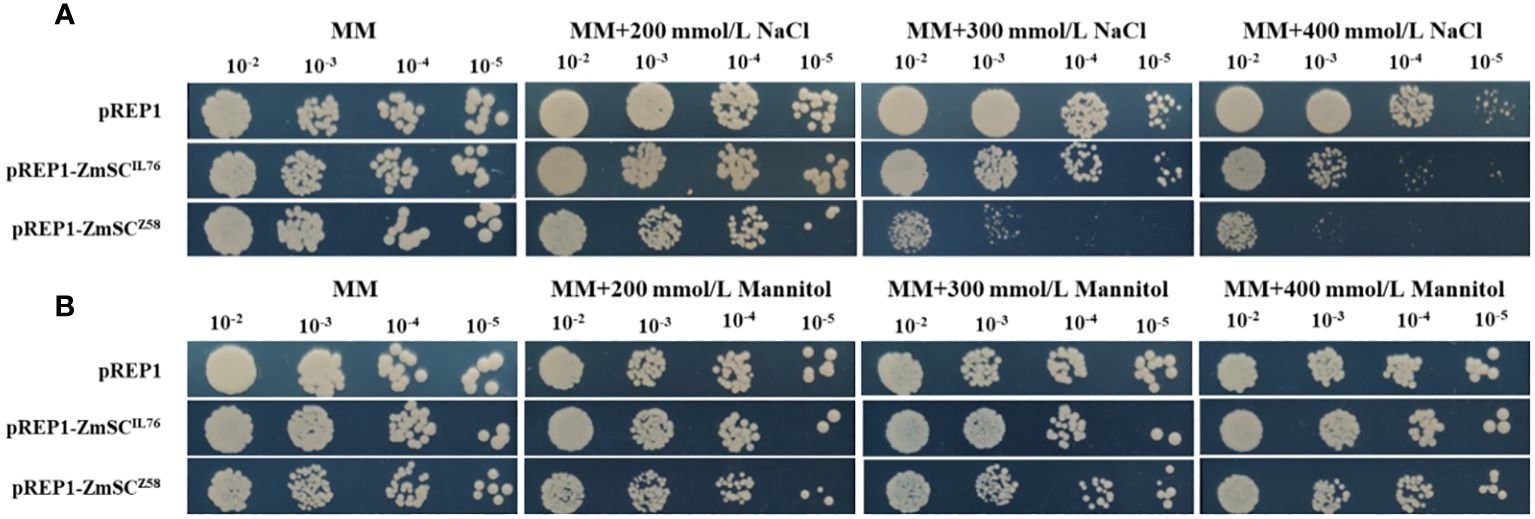
Figure 4 Expression of fission yeast transformants on different concentrations of NaCl (A) and Mannitol (B) MM medium.
To investigate the role of ZmSCZ58 and ZmSCIL76 in salt stress responses in detail, Two overexpression vectors, pCAMBIA2300-Pro35S::ZmSCZ58-eGFP and pCAMBIA2300-Pro35S::ZmSCIL76-eGFP, were constructed. Subsequently, they were transferred into Arabidopsis thaliana (WT). As a result, homozygous T3 lines were developed, and lines OE-IL76–1 (OE#ZmSCIL76) and OE-Z58–13 (OE#ZmSCZ58) showed a high expression level of ZmSC (Supplementary Figure 6) compared with WT. Therefore, these two lines were selected for identification of salt tolerance, while the WT was used as a control. After 2 weeks of growth, there were no significant differences among the three plants under normal conditions (1/2 MS medium). However, under salt stress conditions, there were noticeable differences, with OE#ZmSCZ58 showing more pronounced salt damage at both the seeding stage (Figure 5A) and mature stage (Figure 5B) than OE#ZmSCIL76. Comparative analysis of leaf number (Figure 5C), root length (Figure 5D), and the number of mature pods (Figures 5B, E) has all confirmed this phenomenon. Salt tolerance analysis of the near-isogenic lines NILIL76 (BC12F6), which were constructed through backcrossing with Z58 and self-crossing (see methods 2.1), also showed that NILIL76 had a stronger growth trend compared to Z58 (Supplementary Figure 7). Based on these results, it appears that ZmSC has a negative impact on the early growth and development of plants under salt stress, while the ZmSC mutation appears to alleviates this negative regulatory impact.
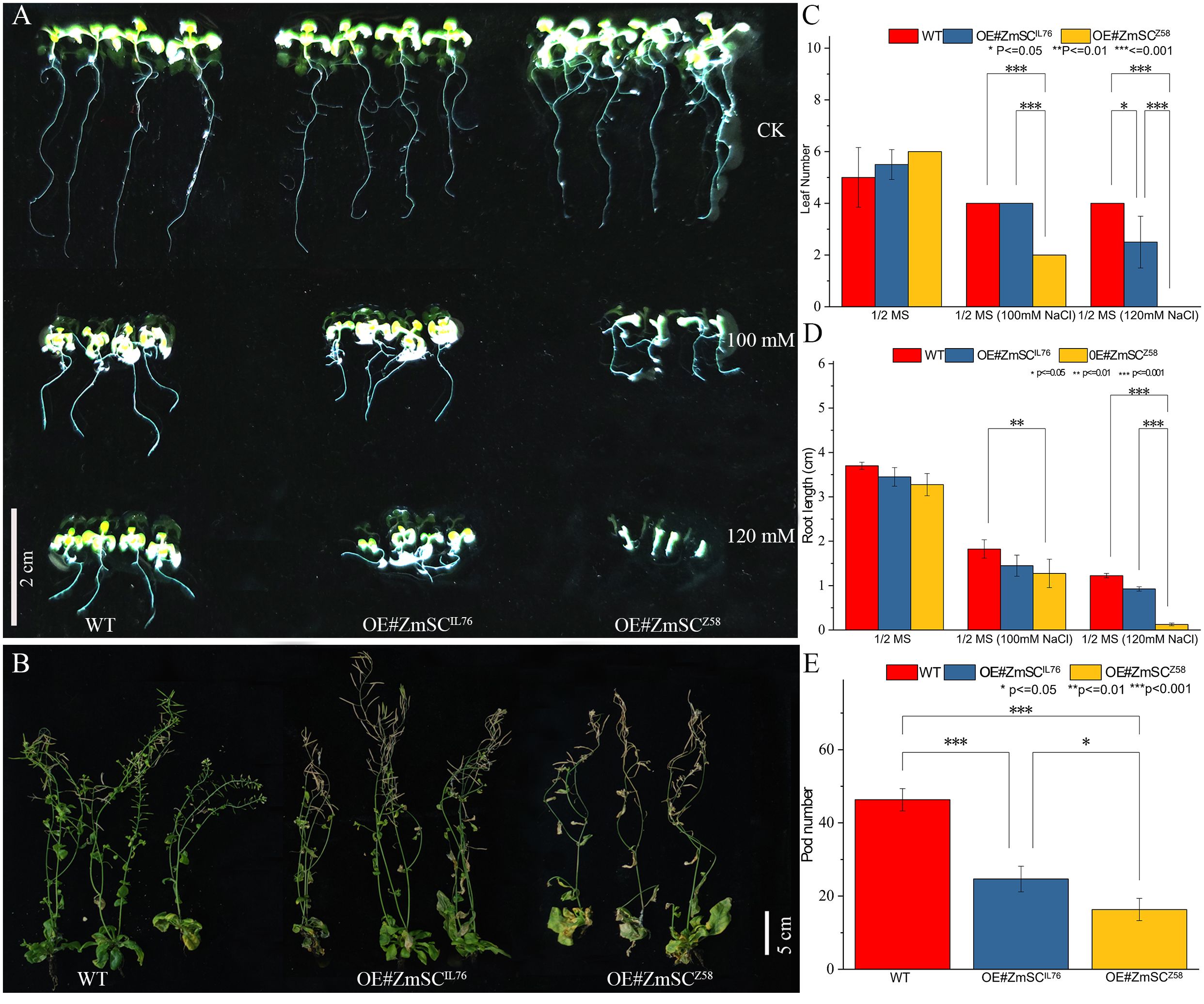
Figure 5 Phenotypes and indicators of wild-type (WT) and ZmSC-overexpressing (OE) Arabidopsis lines under stress conditions. (A) Growth of WT and OE seedlings under different stress conditions: 1/2 MS medium (control, CK), 1/2 MS supplemented with 100 mM NaCl, and 120 mM NaCl, Scale bar = 2 cm. Comparative analysis of leaf number and root length is presented in (C) and (D), respectively. (B) Growth of three-week-old WT and OE plants after four weeks of treatment with 150 mM NaCl. Scale bar = 5 cm. Comparative analysis of pods is shown in (E). Asterisks (*, **, and ***) indicate significant differences at P < 0.05, P < 0.01, and P < 0.001 levels, respectively, (n = 3; error bars = SD).
3.4 ZmSCIL76 promotes ABA accumulation
To determine whether phytohormones are involved in the regulation of ZmSC-mediated salt tolerance, we analyzed the level of five levels in Z58 and NILIL76 under salt stress, including GA, ABA, JA, IAA, and CTK. First, NILIL76 was germinated under normal and 200 mM NaCl stress, with phytohormone content measured on days 4, 6, 8, and 10, respectively, with Z58 serving as a control. The result showed that the hormone content of both NILIL76 and Z58 increased to varying degrees as the duration of salt stress increased. Phytohormones such as CTK, IAA, JA, and GA in NILIL76 were significantly lower than Z58 under salt stress, except for ABA, which was higher in NILIL76 than in Z58 under salt stress (Figure 6). And the same result was observed in the ABA content determination assays of overexpression Arabidopsis, with OE#ZmSCIL76 showing significantly higher levels than OE#ZmSCZ58 under salt stress (Supplementary Figure 10). This indicates that ZmSCIL76 may enhance the accumulation of ABA to response the salt stress.
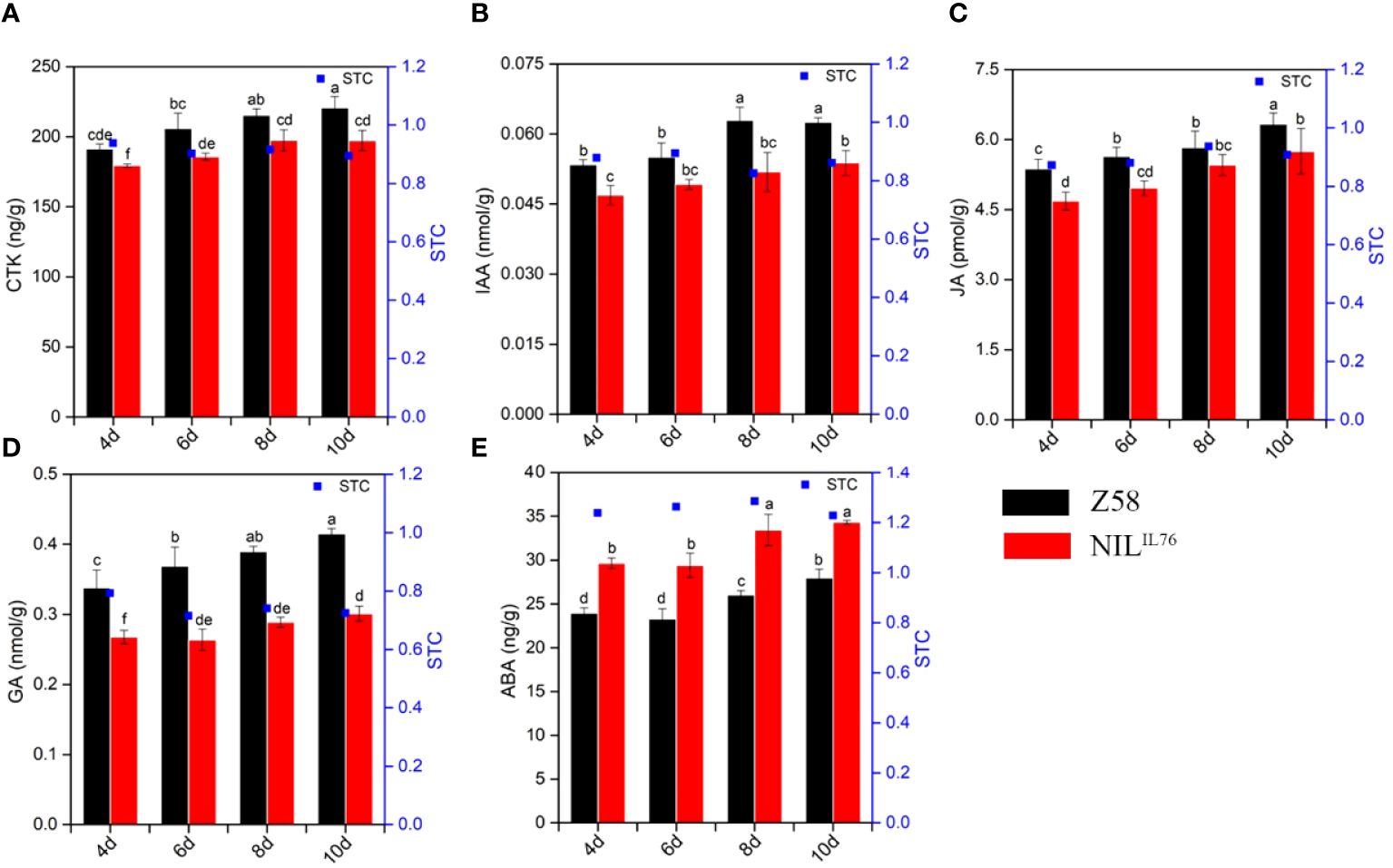
Figure 6 Phytohormone content dynamic changes under salt stress of inbred line Z58 and NILIL76. (A–E) represents the GA, ABA, JA, IAA, and CTK phytohormone content, respectively. Different letters indicate significant differences at the P < 0.05 level, the same as below.
3.5 The upstream transcription factor of ZmSC is associated with stress resistance
To explore the potential molecular regulatory mechanisms of upstream transcription factors of ZmSC, we selected the 2 kb sequence upstream of the ATG of ZmSC as the promoter sequence. Using the online tool (http://planttfdb.gao-lab.org/, accessed on 12 October 2022), we predicted 24 putative transcription factors belonging to various gene families, including NAC (11), bZIP (3), TCP (2), BBR-BPC (2), LBD (1), WOX (1), G2-like (1), C2C2-Dof (1), GRAS (1) and MADS (1) (Supplementary Table 2). From the 24 candidate transcription factors, we successfully cloned seven closely associated with abiotic stress responses for subsequent Yeast one-hybrid assay (Y1H), including ZmNAC-tf79, ZmBBR-BPC-tf4, ZmC2C2-Dof-tf29, ZmBBR-BPC-tf3, ZmMADS-tf1, ZmNAC-tf112 and ZmbZIP-obf1.
The promoter of ZmSC was inserted into the pHis2 vector, whereas the CDS of the candidate transcription factors was inserted into the pGADT7-Rec2 vector. The recombinant plasmids His-ZmSC+Rec2-target_gene were independently introduced into the genome of the AH109 yeast strain, with the empty vectors His2+Rec2 and His2-ZmSC+Rec2 serving as control. All yeast cells were grown successfully on SD/-Leu/-Trip medium, except for His2-ZmSC+Rec2-ZmNAC-tf112, whose growth was inhibited when diluted 1000-fold. For all yeast cells transformed with target genes, growth was observed when placed on the SD/-His/-Leu/-Trip/X-α-Gal medium. When grown in the presence of 100 mM 3-AT on the SD/-His/-Leu/-Trip/X-α-Gal medium, all yeasts grew normally at a concentration of 10–1 except for the control and His2-ZmSC+Rec2-ZmBBR-BPC-tf3 which failed to grow. Therefore, we conclude that all transcription factors, except ZmBBR-BPC-tf3, interacted with ZmSC (Figure 7). The RT-qPCR assays confirmed that all six transcription factors, except for ZmBBR-BPC-tf4, exhibited a significant response to salt stress (Supplementary Figure 8). However, transcription activation assays indicated that ZmNAC-tf79, ZmNAC-tf112, and ZmbZIP-obf1 possessed transcriptional activation ability (Supplementary Figure 9).
3.6 Overexpression of ZmSC in Arabidopsis suppressed the expression of the downstream genes in SOS and CDPK pathways
In a previous study, it was demonstrated that the overexpression of TaSC, a homolog of ZmSC, significantly affected the expression of a series of genes involved in CDPK and SOS pathways in Arabidopsis (Huang et al., 2012). In this study, we selected ten genes involved in CDPK and SOS pathway to investigate their response to salt stress in leaves of transgenic Arabidopsis lines overexpressing ZmSCZ58 and ZmSCIL76, with wild-type Arabidopsis used as control (Figure 8). To characterize the changes in gene expression under salt stress (150 mM NaCl) compared with normal condition (ddH2O, CK), we defined the ratio of expression levels under stress conditions (T) to those under control conditions (CK) as the relative expression level (T/CK), The results showed that in the WT line, the expression, of seven genes (AtFRY1, AtADH, AtP5CS1, AtRD29b, AtKIN2, AtCDPK1, AtSOS2) were up-regulated under salt stress compared to normal condition (relative expression > 1). However, the most of gene expression in OE#ZmSCZ58 and OE#ZmSCIL76 were down-regulated and exhibited a consistent trend (relative expression level < 1), such as AtFRY1, AtSAD1, ATCOR15a, AtRD29b, AtKIN2 and AtSOS3. Interestingly, under salt stress, the expression of genes AtP5CS1 and AtSOS2 appeared to be up-regulated in OE#ZmSCIL76, while being down-regulated in OE#ZmSCZ58. These results suggest that overexpression of ZmSCZ58 and ZmSCIL76 in Arabidopsis inhibits the expression of these genes in both the CDPK and SOS pathways.
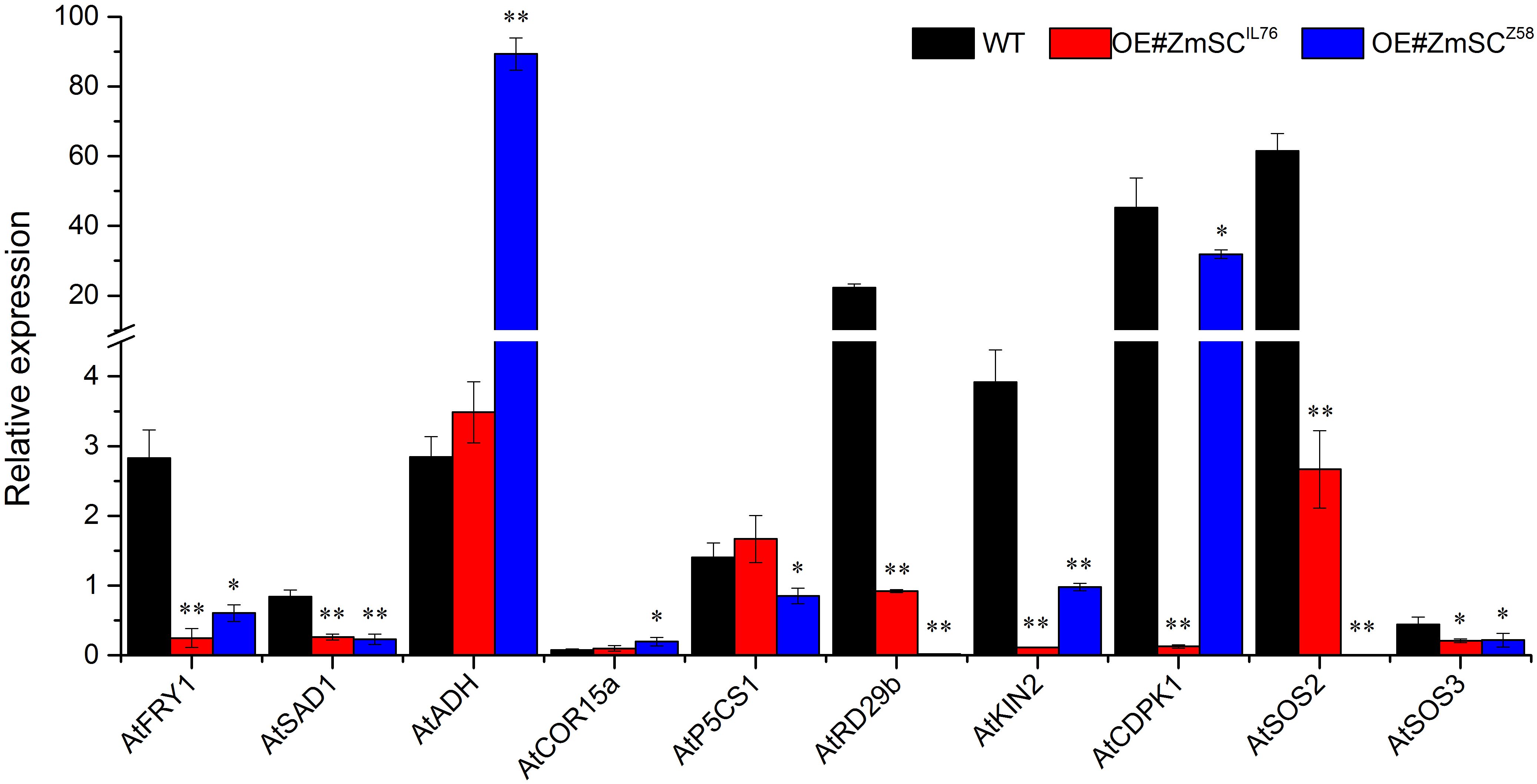
Figure 8 Expression of salt tolerance-related genes in Arabidopsis thaliana overexpressed by ZmSC under salt stress. The six-week-old wild type (WT), OE#ZmSCZ58, and OE#ZmSCIL76 plants were exposed to normal and 150 mM NaCl stress for 5 days. Leaf samples were collected for RT-qPCR analysis. The graph presents the mean ± standard deviation of three biological replicates. Asterisks (* and **) indicate significant differences at the P < 0.05, and P < 0.01 levels, respectively.
4 Discussion
4.1 MTP is a genetic bridge to retrieve superior alleles from wild species Zea perennis and Tripsacum dactyloides
Crop wild relatives (CWRs) are a valuable reservoir of genetic resources that can be used to improve the genetic traits of cultivated species. Several studies have demonstrated the potential of maize wild relatives to enhance plant architecture (Tian et al., 2019), resist gray leaf spot (Zhang et al., 2017), increase protein content (Huang et al., 2022), improve yield (Wang et al., 2022), and enhance drought (Kumar et al., 2022). However, progress has been limited in improving salt tolerance in maize using wild species, likely due to the potential salt-tolerant wild relatives of maize being polyploid, such as T. dactyloides and Z. perennis. Higher ploidy levels may result in barriers that impede the genetic exchange with maize. Fortunately, recent work by Iqbal et al. (2019) and Yan et al. (2020) have reported a new tri-species hybrid MTP, which possesses the genomes of Z. mays, T. dactyloides, and Z. perennis. Li et al. (2023) confirmed that MTP exhibited strong salt tolerance, and a series of salt tolerance introgression lines have been screened from the backcross progeny of MTP with maize, using MTP as a genetic bridge. Cold tolerance introgression lines (He et al., 2023) and novel allotetraploid maize (Iqbal et al., 2023) have also been created. The introgression line IL76 in this study is one of those introgression lines. Sequence alignment analysis of the homologs revealed that the mutant site of ZmSCIL76 leads to amino-acid changes present in both Z. perennis and MTP (Figure 1C), highlighting the potential of MTP as a gene pool for retrieving superior alleles from wild relatives.
4.2 ZmSC negatively regulates salt stress
We expressed ZmSC in both Arabidopsis and yeast and observed no significant differences in plant and yeast strain growth under normal conditions, suggesting that neither ZmSCZ58 nor ZmSCIL76 play a significant role in plant development under normal conditions. However, under salt stress, overexpression of ZmSCZ58 and ZmSCIL76 in Arabidopsis and yeast resulted in significant inhibition compared to WT (Figures 4, 5). Notably, ZmSCZ58 transgenic plants and yeasts display even greater salt sensitivity than ZmSCIL76 transgenic plants and yeasts (Figures 4, 5). These results suggest that ZmSCZ58 acts as a negative regulator of salt tolerance in both Arabidopsis and yeast and that this negative regulatory effect is impeded when the gene is mutated. This conclusion was further supported by salt stress experiments conducted on NILIL76 (Supplementary Figure 7). The intriguing aspect is the contrast between our findings, where overexpression of ZmSC negatively regulates salt tolerance in Arabidopsis, and the previous research indicating that the overexpression of the homologous gene TaSC had a positive impact on salt tolerance in Arabidopsis (Huang et al., 2012). This contrasting phenomenon may be explained by differences in the interaction of overexpressed genes. In our study, overexpression of ZmSC appears to inhibit the expression of AtFRY1, while overexpression of TaSC promotes the expression of AtFRY1. Plant domestication and selection are closely linked to environmental adaptations. Cao et al. (2019) reported that an allele conferring an amino acid variant in ZmHKT2 enhances maize salt tolerance and likely underwent positive selection during maize domestication. Similarly, the allele found in Z. perennis in our study suggests that this variant may have experienced negative selection during maize domestication, leading to its loss or reduced prevalence in the maize population (Supplementary Figure 1).
4.3 ZmSC participates in salt stress response via ABA pathway
Abscisic acid (ABA) is a crucial hormone involved in stress response, including salt stress. Previous studies in maize have established that salt stress induces ABA synthesis, leading to the activation of the ABA signaling pathway. This activation, in turn, regulates the expression of downstream osmotic stress-related genes, thereby orchestrating the plant’s response to stress (Bahrun et al., 2002; Sah et al., 2016). In our study, we conducted Y1H assays and identified significant interactions between gene ZmSC and key transcription factors, namely ZmNAC-tf79, ZmNAC-tf112, and ZmbZIP-obf1 (Figure 7). Importantly, the binding region of these transcription factors contained cis-element such as ABRE, CGTCA-motif, and TGACG-motif (Supplementary Table 1). Notably, ABRE has been previously demonstrated to play a crucial role in the ABA response (Finkelstein et al., 2005), and previous research has established that these three genes were associated with plant drought stress (Wang et al., 2020a; Cao et al. (2019)). Our RT-qPCR assays revealed that these transcription factors were induced by NaCl stress (Supplementary Figure 8). Concurrently, we observed a significantly higher accumulation of ABA in NILIL76 (Figure 6) and OE#ZmSCIL76 (Supplementary Figure 10) compared to inbred Z58 and OE#ZmSCZ58 under salt stress conditions. ZmSC significantly responds to ABA stress and shows the different expression patterns between Z58 and IL76 (Figure 3B). These findings substantiate the potential association between ZmSC and the ABA pathway in the modulation of salt tolerance.
Moreover, recent research has highlighted the role of Sorting Nexin 2 proteins in modulating the trafficking and protein levels of the ABA exporter ABCG25, impacting cellular ABA levels (Liang et al., 2022), and multivesicular body pathway modulate the turnover and activity of ABA receptors and downstream regulators (Wang et al., 2020b). Given that ZmSC encodes for a transmembrane 50A-like protein involved in late endosome to vacuole transport via multivesicular body sorting pathway, suggesting a potential essential role for ZmSC in regulating salt tolerance by modulating the levels of ABA. Compared to ZmSCZ58, the mutation in ZmSCIL76 may result in reduced ABA transport, decreased ABA degradation, and regulated salt tolerance.
4.4 ZmSC is involved in the Ca2+-mediated response of salt stress
Ca2+ serves the function as a second messenger by interacting with Ca2+-sensing domains of CDPK (Wan et al., 2007). Cellular exposure to salt and other stresses increases Ca2+ levels, activating the CDPK and SOS signaling pathway to regulate downstream gene expression in response to stress (Shi et al., 2000; Ludwig et al., 2004). In a previous study, overexpression of TaSC enhanced the salt tolerance and increased Ca2+ content in Arabidopsis, likely due to the Ca2+ accumulation in transgenic Arabidopsis under salt stress, further activates CDPK pathway gene expression (Huang et al., 2012). The lack of activation in plasma membrane ion-binding channel activity, leading to a failure to release Ca2+ (Edel and Kudla, 2016), results in the down-regulation of genes, including downstream genes in the CDPK and SOS pathways.
In our study, under salt stress, the Ca2+ content significantly decreased in both IL76 and Z58, with IL76 exhibiting a higher level compared to Z58 (Supplementary Figure 3E). RT-qPCR assays revealed that the expression of genes AtFRY1, AtSAD1, AtRD29b, AtKIN2, AtCDPK1 in the CDPK pathway and AtSOS2, AtSOS3 in the SOS pathway was significantly down-regulated in overexpression ZmSCZ58 and ZmSCIL76 Arabidopsis compared with WT (Figure 8). This finding contrasts with the result reported by Huang et al. (2012), suggesting that the overexpression of ZmSCZ58 and ZmSCIL76 suppresses the expression of downstream salt tolerance-related genes in the CDPK and SOS pathways in Arabidopsis.
Given that, we speculate that ZmSC expression is activated upon recognition of the ABA signal, followed by transport of ABA to the vacuole through the ZmSC-mediated vacuolar sorting pathway, leading to its degradation. Ultimately, gene function in the Ca2+-mediated CDPK and SOS pathways becomes inhibited, leading to the disruption of ion homeostasis, osmotic balance, and oxidative regulation, consequently negatively regulating salt tolerance in plants (Figure 9).
5 Conclusion
In conclusion, our study demonstrated that ZmSC is expressed under salt and ABA stresses, functioning as a negative regulator of salt stress by suppressing the activation of genes in CDPK and SOS pathways under salt stress. Additionally, we observed that an allele derived from Z. perennis can alleviate this negative regulatory effect and enhance salt tolerance in maize. Therefore, our research establishes a robust foundation and provides valuable material for the molecular design breeding for salt tolerance in maize.
Data availability statement
The original contributions presented in the study are included in the article/Supplementary Material. Further inquiries can be directed to the corresponding author.
Author contributions
XFL: Conceptualization, Writing – original draft, Writing – review & editing, Data curation, Investigation, Visualization. QQM: Conceptualization, Data curation, Software, Visualization, Writing – original draft, Writing – review & editing. XYW: Data curation, Investigation, Writing – review & editing. YFZ: Investigation, Writing – review & editing. YBZ: Writing – review & editing. PZ: Writing – review & editing. YYD: Writing – review & editing. HYL: Writing – review & editing. YC: Writing – review & editing, Investigation. XYL: Writing – review & editing. YZL: Writing – review & editing. RYH: Writing – review & editing. YZ: Writing – review & editing, Investigation. YL: Writing – review & editing. MJC: Writing – review & editing. JMH: Writing – review & editing, Supervision. TZR: Writing – review & editing, Conceptualization. QLT: Writing – review & editing, Conceptualization, Funding acquisition, Project administration, Supervision, Writing – original draft.
Funding
The author(s) declare financial support was received for the research, authorship, and/or publication of this article. This study was supported by the National Natural Science Foundation of China (32272035), the Department of Science and Technology of Sichuan Province (2022NSFSC0167), the Department of Science and Technology of Sichuan Province (2021NZZJ0009), the National Natural Science Foundation of China (31371640), the Department of Science and Technology of Sichuan Province (2020YJ0466) and China Postdoctoral Science Foundation (2023M743850).
Conflict of interest
The authors declare that the research was conducted in the absence of any commercial or financial relationships that could be construed as a potential conflict of interest.
Publisher’s note
All claims expressed in this article are solely those of the authors and do not necessarily represent those of their affiliated organizations, or those of the publisher, the editors and the reviewers. Any product that may be evaluated in this article, or claim that may be made by its manufacturer, is not guaranteed or endorsed by the publisher.
Supplementary material
The Supplementary Material for this article can be found online at: https://www.frontiersin.org/articles/10.3389/fpls.2024.1361422/full#supplementary-material
References
Alexandrov, N. N., Brover, V. V., Freidin, S., Troukhan, M. E., Tatarinova, T. V., Zhang, H., et al. (2009). Insights into corn genes derived from large-scale cDNA sequencing. Plant Mol. Biol. 69, 179–194. doi: 10.1007/s11103-008-9415-4
Asano, T., Hayashi, N., Kikuchi, S., Ohsugi, R. (2012). CDPK-mediated abiotic stress signaling. Plant Signaling Behav. 7, 817–821. doi: 10.4161/psb.20351
Bano, A., Fatima, M. (2009). Salt tolerance in Zea mays (L). following inoculation with Rhizobium and Pseudomonas. Biol. Fertility Soils 45, 405–413. doi: 10.1007/s00374-008-0344-9
Bahrun, A., Jensen, C. R., Asch, F., Mogensen, V. O. (2002). Drought-induced changes in xylem pH, ionic composition, and ABA concentration act as early signals in field-grown maize (Zea mays L.). Journal of experimental botany 53 (367), 251–263.
Cao, L., Lu, X., Zhang, P., Wang, G., Wei, L., Wang, T. (2019). Systematic analysis of differentially expressed maize ZmbZIP genes between drought and rewatering transcriptome reveals bZIP family members involved in abiotic stress responses. Int. J. Mol. Sci. 20 (17), 4103. doi: 10.3390/ijms20174103
Cao, Y., Liang, X., Yin, P., Zhang, M., Jiang, C. (2019). A domestication-associated reduction in K+-preferring HKT transporter activity underlies maize shoot K+ accumulation and salt tolerance. New Phytol. 222, 301–317. doi: 10.1111/nph.15605
Chen, C., Chen, H., Zhang, Y., Thomas, H. R., Frank, M. H., He, Y., et al. (2020). TBtools: an integrative toolkit developed for interactive analyses of big biological data. Mol. Plant 13, 1194–1202. doi: 10.1016/j.molp.2020.06.009
Chenna, R., Sugawara, H., Koike, T., Lopez, R., Gibson, T. J., Higgins, D. G., et al. (2003). Multiple sequence alignment with the Clustal series of programs. Nucleic Acids Res. 31, 3497–3500. doi: 10.1093/nar/gkg500
Cushman, K. R., Pabuayon, I. C., Hinze, L. L., Sweeney, M. E., de Los Reyes, B. G. (2020). Networks of physiological adjustments and defenses, and their synergy with sodium (Na+) homeostasis explain the hidden variation for salinity tolerance across the cultivated Gossypium hirsutum germplasm. Front. Plant Sci. 11, 588854. doi: 10.3389/fpls.2020.588854
Edel, K. H., Kudla, J. (2016). Integration of calcium and ABA signaling. Curr. Opin. Plant Biol. 33, 83–91. doi: 10.1016/j.pbi.2016.06.010
Finkelstein, R., Gampala, S. S., Lynch, T. J., Thomas, T. L., Rock, C. D. (2005). Redundant and distinct functions of the ABA response loci ABA-INSENSITIVE(ABI)5 and ABRE-BINDING FACTOR(ABF)3. Plant Mol. Biol. 59, 253–267. doi: 10.1007/s11103-005-8767-2
He, R. Y., Yang, T., Zheng, J. J., Pan, Z. Y., Chen, Y., Zhou, Y., et al. (2023). QTL mapping and a transcriptome integrative analysis uncover the candidate genes that control the cold tolerance of maize introgression lines at the seedling stage. Int. J. Mol. Sci. 24, 2629. doi: 10.3390/ijms24032629
Huang, X., Zhang, Y., Jiao, B., Chen, G., Huang, S., Guo, F., et al. (2012). Overexpression of the wheat salt tolerance-related gene TaSC enhances salt tolerance in Arabidopsis. J. Exp. Bot. 63, 5463–5473. doi: 10.1093/jxb/ers198
Huang, Y., Wang, H., Zhu, Y., Huang, X., Li, X., Wu, Y., et al. (2022). THP9 enhances seed protein content and nitrogen-use efficiency in maize. Nature 612 (7939), 292–300.
Iqbal, M. Z., Cheng, M., Su, Y., Li, Y., Jiang, W., Li, H., et al. (2019). Allopolyploidization facilitates gene flow and speciation among corn, Zea perennis and Tripsacum dactyloides. Planta 249, 1949–1962.
Iqbal, M. Z., Wen, X., Lulu, X., Zhao, Y., Jing, L., Weiming, J., et al. (2023). Multispecies polyploidization, chromosome shuffling, and genome extraction in Zea/Tripsacum hybrids. Genetics 223, iyad029. doi: 10.1093/genetics/iyad029
Kumar, A., Singh, V. K., Saran, B., Al-Ansari, N., Singh, V. P., Adhikari, S., et al. (2022). Development of novel hybrid models for prediction of drought-and stress-tolerance indices in teosinte introgressed maize lines using artificial intelligence techniques. Sustainability 14, 2287. doi: 10.3390/su14042287
Kumar, S., Stecher, G., Tamura, K. (2016). MEGA7: molecular evolutionary genetics analysis version 7.0 for bigger datasets. Mol. Biol. Evol. 33, 1870–1874. doi: 10.1093/molbev/msw054
Li, C., He, Y. -Q., Yu, J., Kong, J. -R., Ruan, C. -C., Yang, Z. -K., et al. (2024). The rice LATE ELONGATED HYPOCOTYL enhances salt tolerance by regulating Na+/K+ homeostasis and ABA signalling. Plant Cell Environ. 47, 1625–1639. doi: 10.1111/pce.14835
Li, J., Nam, K. H. (2002). Regulation of brassinosteroid signaling by a GSK3/SHAGGY-like kinase. Science 295, 1299–1301. doi: 10.1126/science.1065769
Li, X., Wang, X., Ma, Q., Zhong, Y., Zhang, Y., Zhang, P., et al. (2023). Integrated single-molecule real-time sequencing and RNA sequencing reveal the molecular mechanisms of salt tolerance in a novel synthesized polyploid genetic bridge between maize and its wild relatives. BMC Genomics 24, 1–21. doi: 10.1186/s12864-023-09148-0
Liang, C., Li, C., Wu, J., Zhao, M., Chen, D., Liu, C., et al. (2022). SORTING NEXIN2 proteins mediate stomatal movement and the response to drought stress by modulating trafficking and protein levels of the ABA exporter ABCG25. Plant J. 110, 1603–1618. doi: 10.1111/tpj.15758
Livak, K. J., Schmittgen, T. D. (2001). Analysis of relative gene expression data using real-time quantitative PCR and the 2-ΔΔCT method. methods 25, 402–408. doi: 10.1006/meth.2001.1262
Ludwig, A. A., Romeis, T., Jones, J. D. (2004). CDPK-mediated signalling pathways: specificity and cross-talk. J. Exp. Bot. 55, 181–188. doi: 10.1093/jxb/erh008
Luo, M., Zhao, Y., Zhang, R., Xing, J., Duan, M., Li, J., et al. (2017). Mapping of a major QTL for salt tolerance of mature field-grown maize plants based on SNP markers. BMC Plant Biol. 17, 1–10. doi: 10.1186/s12870-017-1090-7
Pingle, S. N., Suryawanshi, S. T., Pawar, K. R., Harke, S. N. (2022). The effect of salt stress on proline content in maize (Zea mays). Environ. Sci. Proc. 16, 64. doi: 10.3390/environsciproc2022016064
Rubio, F., Nieves-Cordones, M., Horie, T., Shabala, S. (2020). Doing ‘business as usual’ comes with a cost: evaluating energy cost of maintaining plant intracellular K+ homeostasis under saline conditions. New Phytol. 225, 1097–1104. doi: 10.1111/nph.15852
Sah, S. K., Reddy, K. R., Li, J. (2016). Abscisic acid and abiotic stress tolerance in crop plants. Front. Plant Sci. 7, 571–597. doi: 10.3389/fpls.2016.00571
Shi, H., Ishitani, M., Kim, C., Zhu, J. K. (2000). The Arabidopsis thaliana salt tolerance gene SOS1 encodes a putative Na+/H+ antiporter. Proc. Natl. Acad. Sci. 97, 6896–6901. doi: 10.1073/pnas.120170197
Shi, H., Lee, B. H., Wu, S. J., Zhu, J. K. (2003). Overexpression of a plasma membrane Na+/H+ antiporter gene improves salt tolerance in Arabidopsis thaliana. Nat. Biotechnol. 21, 81–85. doi: 10.1038/nbt766
Song, L., Ding, R., Du, T., Kang, S., Tong, L., Xue, F., et al. (2023). Stomatal conductance parameters of tomatoes are regulated by reducing osmotic potential and pre-dawn leaf water potential via increasing ABA under salt stress. Environ. Exp. Bot. 206, 105176. doi: 10.1016/j.envexpbot.2022.105176
Tian, J., Wang, C., Xia, J., Wu, L., Xu, G., Wu, W., et al. (2019). Teosinte ligule allele narrows plant architecture and enhances high-density maize yields. Science 365, 658–664. doi: 10.1126/science.aax5482
Wan, B., Lin, Y., Mou, T. (2007). Expression of rice Ca2+-dependent protein kinases (CDPKs) genes under different environmental stresses. FEBS Lett. 581, 1179–1189. doi: 10.1016/j.febslet.2007.02.030
Wang, G., Yuan, Z., Zhang, P., Liu, Z., Wang, T., Wei, L. (2020a). Genome-wide analysis of NAC transcription factor family in maize under drought stress and rewatering. Physiol. Mol. Biol. Plants 26, 705–717. doi: 10.1007/s12298-020-00770-w
Wang, M., Li, X., Luo, S., Fan, B., Zhu, C., Chen, Z. (2020b). Coordination and crosstalk between autophagosome and multivesicular body pathways in plant stress responses. Cells 9, 119. doi: 10.3390/cells9010119
Wang, Q., Liao, Z., Zhu, C., Gou, X., Liu, Y., Xie, W., et al. (2022). Teosinte confers specific alleles and yield potential to maize improvement. Theor. Appl. Genet. 135, 3545–3562. doi: 10.1007/s00122-022-04199-5
Yan, X., Cheng, M., Li, Y., Wu, Z., Li, Y., Li, X., et al. (2020). Tripsazea, a Novel Trihybrid of Zea mays, Tripsacum dactyloides, and Zea perennis. G3: Genes Genomes Genet. 10, 839–848. doi: 10.1534/g3.119.400942
Yang, Y., Guo, Y. (2018). Unraveling salt stress signaling in plants. J. Integr. Plant Biol. 60, 796–804. doi: 10.1111/jipb.12689
Yang, Z., Li, J. L., Liu, L. N., Xie, Q., Sui, N. (2020). Photosynthetic regulation under salt stress and salt-tolerance mechanism of sweet sorghum. Front. Plant Sci. 10, 1722. doi: 10.3389/fpls.2019.01722
Yu, Z., Duan, X., Luo, L., Dai, S., Ding, Z., Xia, G. (2020). How plant hormones mediate salt stress responses. Trends Plant Sci. 25, 1117–1130. doi: 10.1016/j.tplants.2020.06.008
Zhang, J., Yu, H., Zhang, Y., Wang, Y., Li, M., Zhang, J., et al. (2016). Increased abscisic acid levels in transgenic maize overexpressing AtLOS5 mediated root ion fluxes and leaf water status under salt stress. J. Exp. Bot. 67, 1339–1355. doi: 10.1093/jxb/erv528
Zhang, M., Li, Y., Liang, X., Lu, M., Lai, J., Song, W., et al. (2023). A teosinte-derived allele of an HKT1 family sodium transporter improves salt tolerance in maize. Plant Biotechnol. J. 21, 97–108. doi: 10.1111/pbi.13927
Zhang, M., Liang, X., Wang, L., Cao, Y., Song, W., Shi, J., et al. (2019). A HAK family Na+ transporter confers natural variation of salt tolerance in maize. Nat. Plants 5, 1297–1308. doi: 10.1038/s41477-019-0565-y
Zhang, X., Yang, Q., Rucker, E., Thomason, W., Balint-Kurti, P. (2017). Fine mapping of a quantitative resistance gene for gray leaf spot of maize (Zea mays L.) derived from teosinte (Z. mays ssp. parviglumis). Theor. Appl. Genet. 130, 1285–1295. doi: 10.1007/s00122-017-2888-2
Zhou, Z., Li, G., Tan, S., Li, D., Weiß, T. M., Wang, X., et al. (2020). A QTL atlas for grain yield and its component traits in maize (Zea mays). Plant Breed. 139, 562–574. doi: 10.1111/pbr.12809
Zhou, X., Li, J., Wang, Y., Liang, X., Zhang, M., Lu, M., et al. (2022). The classical SOS pathway confers natural variation of salt tolerance in maize. New Phytol. 236, 479–494. doi: 10.1111/nph.18278
Zhu, J. K. (2001). Plant salt tolerance. Trends Plant Sci. 6, 66–71. doi: 10.1016/S1360-1385(00)01838-0
Zhu, J. K. (2016). Abiotic stress signaling and responses in plants. Cell 167, 313–324. doi: 10.1016/j.cell.2016.08.029
Keywords: salt stress, maize, wild relatives, transgenic Arabidopsis, CDPK
Citation: Li X, Ma Q, Wang X, Zhong Y, Zhang Y, Zhang P, Du Y, Luo H, Chen Y, Li X, Li Y, He R, Zhou Y, Li Y, Cheng M, He J, Rong T and Tang Q (2024) A teosinte-derived allele of ZmSC improves salt tolerance in maize. Front. Plant Sci. 15:1361422. doi: 10.3389/fpls.2024.1361422
Received: 26 December 2023; Accepted: 29 April 2024;
Published: 05 June 2024.
Edited by:
Zulfiqar Ali Sahito, Zhejiang University, ChinaReviewed by:
Qi Zheng, Henan Agricultural University, ChinaMuhammad Anwar, Hainan University, China
Faheeda Soomro, The Graduate School, Chinese Academy of Agricultural Sciences, China
Habiba Habiba, Lehman College, United States
Copyright © 2024 Li, Ma, Wang, Zhong, Zhang, Zhang, Du, Luo, Chen, Li, Li, He, Zhou, Li, Cheng, He, Rong and Tang. This is an open-access article distributed under the terms of the Creative Commons Attribution License (CC BY). The use, distribution or reproduction in other forums is permitted, provided the original author(s) and the copyright owner(s) are credited and that the original publication in this journal is cited, in accordance with accepted academic practice. No use, distribution or reproduction is permitted which does not comply with these terms.
*Correspondence: Qilin Tang, dGFuZ3FpbGluNzFAMTYzLmNvbQ==
†These authors have contributed equally to this work