- 1School of Natural Sciences, University of Tasmania, Hobart, TAS, Australia
- 2ARC Centre of Excellence for Plant Success in Nature and Agriculture, University of Tasmania, Hobart, TAS, Australia
Introduction: The seeds of wild pea (Pisum) exhibit marked physical dormancy due to impermeability of the seed coat to water, and the loss of this dormancy is thought to have been critical for domestication. Wild pea seed coats are also notably thick and rough, traits that have also reduced during domestication and are anecdotally linked to increased permeability. However, how these traits specifically interact with permeability is unclear.
Methods: To investigate this, we examined the genetic control of differences in seed coat characteristics between wild P. sativum ssp. humile and a non-dormant domesticated P. s. sativum accession in a recombinant inbred population. QTL effects were confirmed and their locations refined in segregating F4/5 populations.
Results: In this population we found a moderate correlation between testa thickness and permeability, and identified loci that affect them independently, suggesting no close functional association. However, the major loci affecting both testa thickness and permeability collocated closely with Mendel’s pigmentation locus A, suggesting flavonoid compounds under its control might contribute significantly to both traits. We also show that seed coat roughness is oligogenic in this population, with the major locus independent of both testa thickness and permeability, suggesting selection for smooth seed was unlikely to be due to effects on either of these traits.
Discussion: Results indicate loss of seed coat dormancy during domestication was not primarily driven by reduced testa thickness or smooth seededness. The close association between major permeability and thickness QTL and Mendel’s 'A' warrant further study, particularly regarding the role of flavonoids.
1 Introduction
Pea (Pisum sativum) is considered to be one of the world’s earliest-domesticated crops. Its divergence from ancestral wild forms is estimated to have occurred over 10,000 years ago in the Fertile Crescent, broadly in parallel with several other legume and cereal crops (Lev-Yadun et al., 2000; Zohary et al., 2012). Two critical steps in its domestication were the loss of pod dehiscence and seed dormancy (Ladizinsky, 1985, 1987), changes which were likely to have improved the efficiency returns for early farmers (Abbo et al., 2011).
Similar changes have occurred during the domestication of other legume crops, and the first robust insights into their genetic and molecular control has recently begun to emerge from work in species such as soybean and common bean. Genes influencing pod dehiscence (“shattering”) in these species affect pod lignification, and variously encode NAC and MYB transcription factors and a dirigent-like protein (Dong et al., 2014; Funatsuki et al., 2014; Di Vittori et al., 2021; Parker et al., 2021). In soybean, physical seed dormancy is determined by a major locus Hs-1 which has been equivocally associated with variation in distinct genes influencing either polysaccharide or calcium content of the seed coat (Jang et al., 2015; Sun et al., 2015). More recently, a single major locus that governs seed coat permeability in the Andean genepool of common bean has also been implicated in control of polysaccharide content (Soltani et al., 2021).
In pea, reduced pod dehiscence in domesticated material has been primarily attributed to the major locus DEHISCENT PODS (DPO) (Blixt, 1972; Bordat et al., 2011) which has yet to be identified at the molecular level. The genetic basis for reduction in seed dormancy is even less well understood. In wild peas physical seed dormancy is imposed by a thick, hard seed coat which may prevent water entry for many months, whereas seed coats of domesticated lines are much thinner and readily permeable to water (Smýkal et al., 2014). In addition, detailed anatomical and biochemical characterizations have revealed the upper section of macrosclereid cells (light line) to be a major barrier to water uptake in dormant seeds (Janská et al., 2019), and lower proanthocyanidin levels and less extensive cell wall deposition in seed coats of non-dormant accessions (Hradilová et al., 2017, 2019). However, systematic genetic analysis of these traits and their relationship to permeability has not been undertaken.
In addition to thickness and permeability, another seed coat trait anecdotally linked to dormancy in pea is roughness, characterized as a granular “gritty” surface texture that reflects a regular pattern of size variation within the outer layer of macrosclereid cells. This feature is absent in domesticated pea but ubiquitous in wild germplasm, and varying degrees of testa roughness are also characteristic of (or more prominent in) the wild forms of several other legumes, including lentil, sweet pea and chickpea (Lersten and Gunn, 1982; Sedláková et al., 2021). In pea, this trait has been reported as a monogenic trait under the control of the GRITTY (GTY) locus (Marx, 1969) and in view of its restriction to wild material, it is inferred to have been strongly selected against early in pea domestication.
The aim of this study was to examine the functional basis of seed physical dormancy in pea, by defining the genetic control and relationships of seed coat traits, including thickness, permeability and roughness in a wild x domesticated RIL population previously analyzed for flowering time (Williams et al., 2022).
2 Materials and methods
2.1 Plant material and growing conditions
An F8+ recombinant inbred line (RIL) population (n=137) was derived by single seed descent from the F2 of a cross between the wild P. sativum ssp. elatius line JI1794 (a representative of the northern “humile” subgroup; Hellwig et al., 2022) and the cultivar NGB5839. This population was described previously described by Weller et al. (2012) and Williams et al. (2022). This population was grown under long-day (LD) conditions (16 hours light – 8 hours dark), with 4 replicate plants per genotype. Plants were grown in a 1:1 gravel:vermiculite mixture, topped with sterilized potting mix which included controlled release fertilizer. Seeds were harvested after plants had completely senesced and dried, and were stored for at least a month to ensure they had fully matured prior to their use in permeability analyses.
2.2 Phenotypic evaluation
The harvested seeds of the RIL population and parental lines were assessed for several traits potentially related to physical dormancy (Table 1). To estimate seed coat permeability, we measured time to fully imbibe with water by submerging seed in water and retrieving, drying and weighing at regular intervals until there was no further increase in mass due to water uptake. This was measured in both young (1 month old mature dried) and old (2 year old mature dried) seed. The relative water uptake capacity of the dry seed was characterized by the relative increase in weight of the seeds at full imbibition. The thickness of the testa was initially measured on detached fragments using a micrometer, but to verify the precision of these measures, further seed coat dimensions were determined from transverse sections mounted on slides, from three representative seeds for each genotype. Images were captured at 10X and 40X magnification to allow for accurate delineation of cell layers and anatomical zones. The total thickness of the testa and the width of distinct component layers (Supplementary Figure S1) were measured using the line tool in ImageJ (Schneider et al., 2012), while roughness was measured as the ratio of the outer cuticle length over the light line length (Figure 1A, Supplementary Figure S1). Mean seed weight was recorded from ten representative seeds per genotype. Variation in seed pigmentation traits was also recorded, including for Mendel’s A locus governing flower and seed anthocyanin content and several other classical seed pigmentation loci.
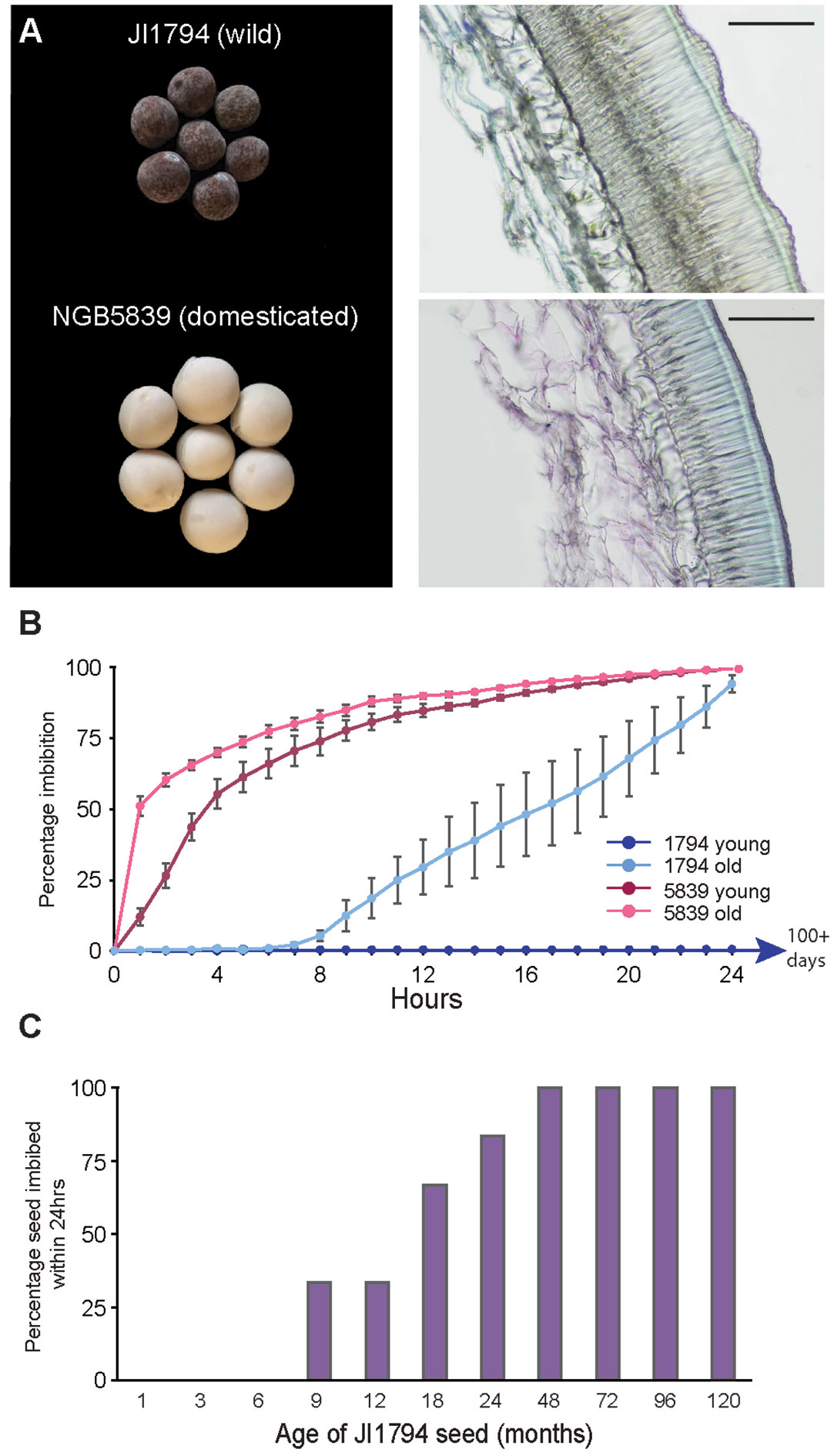
Figure 1 Comparison of seed coat traits in wild and domesticated pea. (A) Wild P. s. humile line JI1794 seed and testa section with visible roughness and domesticated P. s. sativum cultivar NGB5839 seed and testa section. Scale bars = 0.1 mm. (B) Time to fully imbibe in water for young (1 month old mature dried) and old (2 year old mature dried) seed from both wild and domesticated pea. Wild young seed had not imbibed within 100 days, after which point the experiment was concluded. (C) Effect of seed age on the ability of JI1794 seed to imbibe within 24h. All seed was mature and dry.
2.3 Genotyping and QTL analysis
Genomic DNA was extracted from young leaflets of each genotype using the CTAB extraction protocol (Doyle and Doyle, 1987), and DarTseq markers (Sansaloni et al., 2011) generated using Diversity Array Technology Pty. Ltd. (Canberra, Australia). A total of 4,599 markers (4,575 DArT and 24 gene-based markers) were used to construct a linkage map (previously described by Williams et al., 2022). The linkage map was subsequently reduced to 3073 markers for quantitative trait loci (QTL) analysis by removing every third marker, which improved computational efficiency while having minimal effect on mapping resolution, and some linkage groups inverted to maximize synteny with the Pisum genome assembly (Kreplak et al., 2019).
QTL analysis was performed using MapQTL v6 (Van Ooijen, 2009), following Williams et al. (2022). In brief, QTLs were defined by a > 3 LOD score and identified using the interval mapping (IM) function. Iterative searches for additional QTLs were performed using the restricted Multiple QTL Model (rMQM) function, which increases the power of QTL analysis by reducing residual variances attributed to previously identified QTLs (cofactors). The amount of variation explained by each QTL was estimated using the coefficient of determination (R2) which is represented as the Phenotypic Variance Explained (PVE).
2.4 Advanced generation segregating populations and fine mapping
QTL regions of interest were refined in segregating F3, F4 and F5 progeny derived from specific individuals in the original F2 population. Where possible, these progenies were selected to be homozygous (fixed) for other relevant loci influencing the focus trait. Mapping resolution within these selected QTL regions was increased through use of additional high-resolution melt (HRM) markers, either already available or newly developed from pea transcript sequences with selection guided by DArT marker positions in the RIL linkage map or the high-density consensus map of Tayeh et al. (2015). Progenies of these advanced generations were grown under the conditions described above, and phenotyped for permeability, roughness (as a binary presence/absence trait) and testa thickness (via micrometer).
3 Results
3.1 Characterisation of seed dormancy in representative wild and domesticated lines
A comparison between the wild P. s. humile line JI1794 and the domesticated P. s. sativum cultivar NGB5839 illustrates the significantly thicker testa in the wild line, and the undulating outer surface characteristic of the ‘gritty’ phenotype (Figure 1A). Recently-matured (one-month-old; “young”) dry seed of JI1794 did not imbibe or germinate even after more than 100 days of immersion in water, whereas similar seed of NGB5839 started to take up water within one hour of immersion, reached 50% imbibition within 4h (Supplementary Figure S2) and reached 100% imbibition after 24h (Figure 1B). To examine the potential influence of seed age on imbibition, we also compared JI1794 seed of various ages post-harvest. Unlike young seed, 80% of older seed that had been stored for two years in a relatively stable, cool and low-humidity indoor environment commenced imbibition within 6h of immersion and reached the fully-imbibed state by 24h (Figure 1B, failure to imbibe not shown). In contrast, this same period of storage had no effect on imbibition of NGB5839. A comparison of storage duration on permeability in JI794 (in this case detecting partial imbibition after 24h of immersion) revealed that the initial complete impermeability persisted for between 6 and 9 months, after which imbibition increased to reach 100% after 4 years of storage at relatively constant temperature and humidity (Figure 1C). This suggests that the impermeability of wild seed coats is lost as the seed ages.
3.2 Variation in seed dormancy-related traits in the RIL F8 population
We next examined variation for seed coat traits potentially related to dormancy and domestication in the JI1794 x NGB5839 RIL population. This included the thickness of the seed coat and its component layers, permeability (time to fully imbibe) and the roughness or ‘grittiness’ of the seed coat, as the main traits potentially related to dormancy. We also examined seed weight, water uptake capacity and various pigmentation features. Mean data for all traits and a correlation matrix are provided in Supplementary Table S1 and Supplementary Figure S3, with notable correlations discussed below.
Total testa thickness varied from 76 – 160 µm and exhibited a near-normal distribution in the population (Figure 2A). To examine whether specific anatomically distinct layers within the testa might vary in thickness and how this might contribute to the total thickness, we also defined specific zones within the transverse section (testa sections TT-A through TT-F; Supplementary Figure S1) and measured these individually. The largest component was section TT-D of the macrosclereid cell layer, which is the main component of the testa. This section contributed 38 – 64% of the total variation for testa thickness across the population. Testa thickness showed a positive correlation with water uptake capacity (r = 0.20, p < 0.05), along with a positive correlation with seed coat roughness (r = 0.28, p < 0.01). Testa thickness also showed a moderate negative correlation with seed weight (r = -0.26, p < 0.01), but interestingly a much stronger negative correlation with seed weight was found for the testa sections TT-A, TT-B and TT-C (r = -0.54 - -0.77, p < 0.001). Likewise, the presence of flower pigmentation had a moderately positive correlation with testa thickness (r = 0.30, p < 0.001), but this correlation was much higher when considering the thickness of testa section D (r = 0.49, p < 0.001).
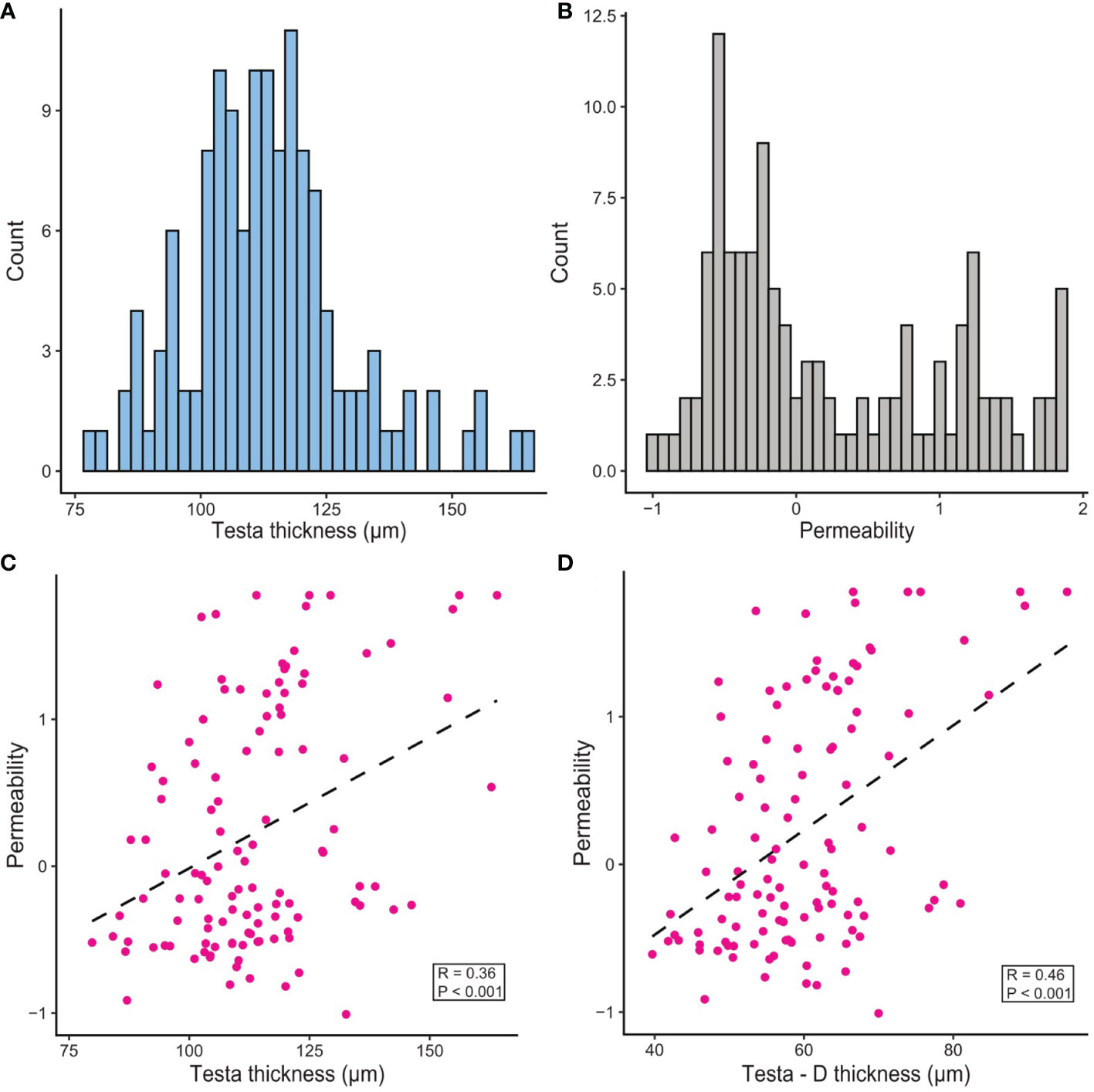
Figure 2 Distribution and correlation of dormancy-related phenotypes in the RIL F2 population. (A) Testa thickness. (B) Permeability (measured as log10[time to fully imbibe in days]). (C) Correlation of testa thickness with permeability. (D) Correlation of testa section D thickness (see Supplementary Figure 1) with permeability.
Permeability varied widely, with imbibition time ranging from 2 hours to more than 70 days, heavily skewed towards a shorter time to imbibe. To adjust this skew prior to analysis, permeability scores were log transformed, creating a bimodal distribution (Figure 2B). This measure of permeability was moderately correlated to total testa thickness, with thicker testa leading to a longer time to imbibe (r = 0.34, p < 0.001, Figure 2C). Again, this effect was more pronounced when considering the thickness of testa section D (r = 0.41, p < 0.001, Figure 2D). Permeability was not affected by seed coat roughness (r = 0.01, p > 0.05). Likewise, no correlation was detected between permeability and water uptake capacity (r = -0.04, p > 0.05), suggesting that seed water capacity was unrelated to imbibition rate. Time to fully imbibe was also positively correlated with presence of flower pigmentation (r = 0.55, p < 0.001).
3.3 QTL analysis of seed dormancy-related traits
Variability in seed permeability to water was found to be under genetic control in this population, with two QTLs discovered on chromosomes 6 (qPERM6) and 7 (qPERM7), respectively explaining 33.0% and 9.1% of the observed variation (Table 2). The qPERM6 locus was located over the region containing Mendel’s A (Figure 3), raising the possibility that A itself might influence permeability via its effects on seed-coat composition. In contrast qPERM7 was in a region distinct from any known loci likely to have an influence on seed coat properties.
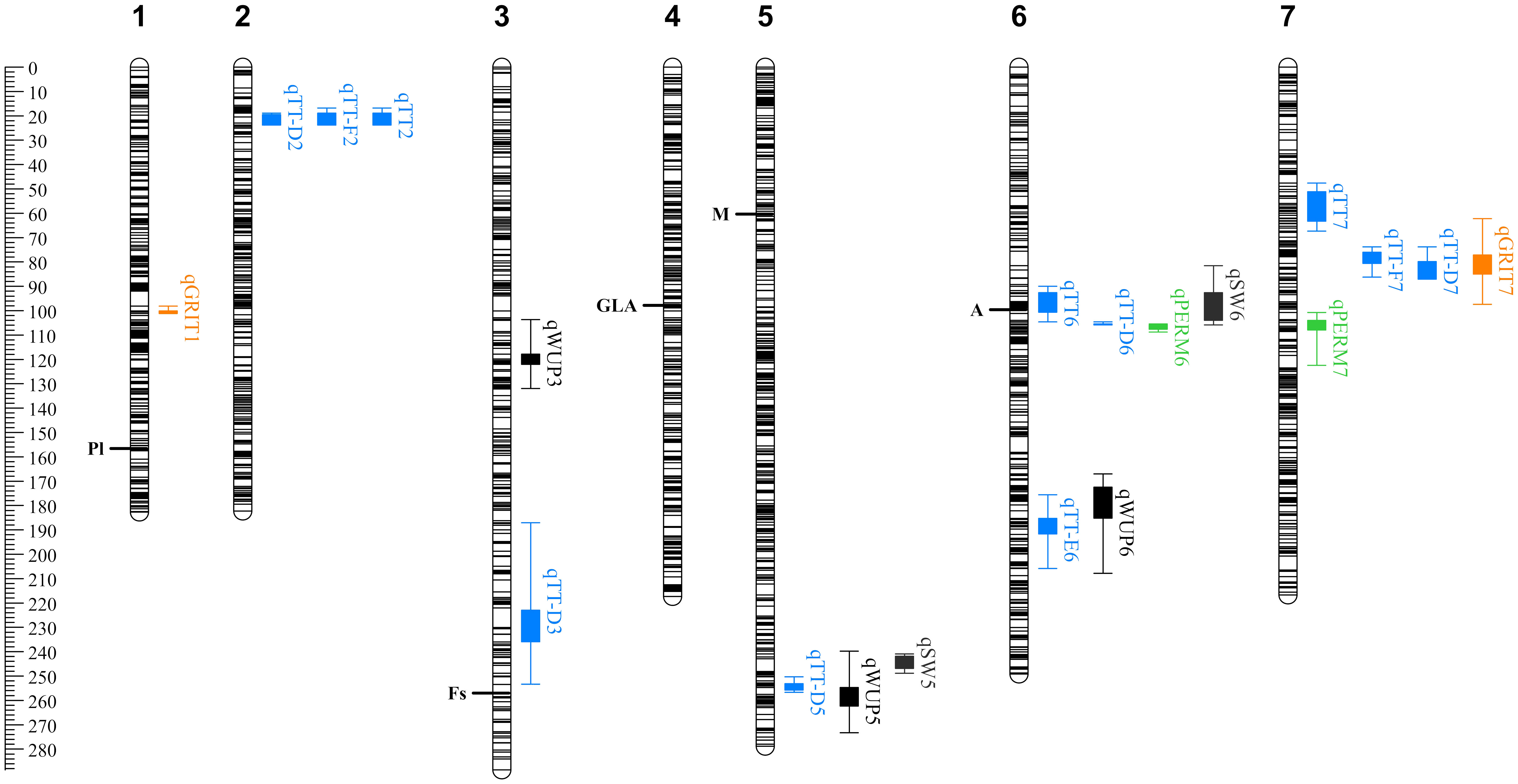
Figure 3 QTL discovered for seed physical characteristics and dormancy-related traits in the Pisum sativum RIL F8 population. Scale is in cM. QTL nomenclature follows Tables 1 and 2. Box and whiskers represent 1-LOD and 2-LOD intervals, respectively, around each QTL peak. Colors indicate broad trait categories, with permeability in green, seed coat roughness (GRITTY) in orange, testa thickness in blue and other physical characteristics in black. Previously discovered pigmentation loci (Table 1) are indicated at the position of their QTL peaks in this analysis.
Three QTL were detected for total testa thickness (TT) as measured by sectioning, on chromosomes 2, 6 and 7. Of these, the qTT2 locus explained 25.5% of the observed variation and was located near the top of chromosome 2. qTT7 was located in a region distinct from qPERM7, but qTT6 was closely collocated with qPERM6. Collectively, these comparisons indicate that the genetic control of physical dormancy is at least in part independent of testa thickness, but also imply the possibility of a relationship between these traits. Additional QTL were detected when testa thickness was measured by micrometer, but the presence of collocated QTL for other traits at these additional loci suggests a measurement precision issue (see below).
In order to examine whether variation for testa thickness might reflect a specific contribution from certain cell layers or structural features we also looked at the genetic control of the thickness of the six distinct testa layers described above (Supplementary Figure S1). Significant loci were found only for testa layers D, E and F, with five QTL for qTT-D2 explaining 78.1% of the variation. The three strongest qTT-D QTL collocated with the qTT2, qTT6 and qTT7 loci, and explained 53% of the variation. Two weaker TT-D loci were detected on chromosomes 5 and 3, together explaining another 15% of the variation. The only locus detected for layer E was in a position on chromosome 6 distinct from that of qTT6 and qTT-D6, while the two loci detected for layer F co-located with the qTT2/qTT-D2 and qTT7/qTT-D7 loci. Overall, these results indicated that the observed variation in total testa thickness was primarily due to variation in layer D, and revealed the contribution of three minor loci on chromosomes 3, 5 and 6.
In addition to Mendel’s A, the four seed pigmentation traits measured all related to well-known classical loci, and major QTL were detected in the expected genomic locations when analyzed as qualitative traits (Figure 3, shown as peak position). These included the hilum pigmentation locus Pl on chromosome 1 (Lamprecht, 1948; Balarynová et al., 2022), the seed-coat “marbling” locus M on chromosome 5 (Lamprecht, 1948; Ellis et al., 2023), the seed coat speckling locus Fs on chromosome 3 (Lamprecht, 1961; Ellis et al., 2023) and the seed coat ground color locus GLA on chromosome 4 (Lamprecht, 1959; Blixt, 1962). Each of these also had a secondary QTL which collocated with Mendel’s A, reflecting their likely dependence on the action of A. However, with the exception of the A locus itself, none of these were collocated with effects on testa thickness or permeability.
Seed coat roughness has long been noted as a key distinguishing feature of wild and domesticated pea seeds; a difference attributed to a major Mendelian locus GRITTY located on chromosome 1. Assessment of this trait has typically been made somewhat subjectively based on the degree of friction encountered when rubbing seeds together (Ellis et al., 2023). We quantified it more objectively, examining seed coat sections under the light microscope and taking quantitative measurements of the surface undulations. A major QTL explaining 45% of the variation (qGRIT1) was detected on chromosome 1, in the expected position of the GTY locus. A second, minor locus (qGRIT7) explaining a further 8.8%, was located near the cluster of TT loci on chromosome 7. These results suggest that the GRITTY trait has no major genetic association with testa permeability, and only a minor association with testa thickness. Interestingly, an additional QTL for testa thickness was discovered tightly linked to qGRIT1 when measuring testa thickness using a micrometer. Given this QTL was not present when using the more accurate sectioning measurement, this likely reflects the additional apparent thickness conferred by the undulations of the seed coat when roughness is present, highlighting the need for precision in measurements when working at this scale.
Genetic control of seed water uptake and seed weight was also detected in this cross, with QTL for these traits often collocated with testa thickness QTL (Figure 3). A common locus for both was found on chromosome 5, with an additional seed weight QTL present in the QTL cluster on chromosome 6, and an additional water uptake QTL collocating with the testa thickness section E QTL on chromosome 6 (in a distinct location). There was also an independent QTL for water uptake on chromosome 3, suggesting additional physiological phenomena beyond testa thickness affecting this trait.
3.4 Validation of permeability and roughness QTL in advanced generations
As the largest permeability QTL was located on chromosome 6 in a region also featuring Mendel’s A locus and QTL for physical seed characteristics, we attempted to refine the position and determine whether it might be distinct from the A gene. We also further refined the position of the main seed roughness QTL (GRITTY) as a next step in status as a key domestication trait.
The presence of the wild allele at QTL6 conferred significantly increased testa thickness (t129 = -3.49, p < 0.001) and lower permeability (t112 = -6.75, p < 0.001) compared to the domesticated, conforming to findings in the RIL population for this QTL (Figure 4A). To validate this QTL, further advanced segregation populations were developed using single seed descent from an F2 line which was segregating for the qPERM6 locus (markers LF and A) and fixed at all other loci of interest (specifically fixed for the domesticated allele at qPERM7), thereby allowing the analysis of this QTL as a near Mendelian trait in the F4 and F5 populations developed. For greater resolution around qPERM6 region (spanning between 38.4cM and the A_1 marker) eight additional markers (Supplementary Table S2) were genotyped across these populations (Figure 4B). Markers were ordered based on recombinant frequency or (if there was no recombination) by order in the Medicago (v4.0, Tang et al., 2014) or P. sativum (v1a, Kreplak et al., 2019) reference genomes.
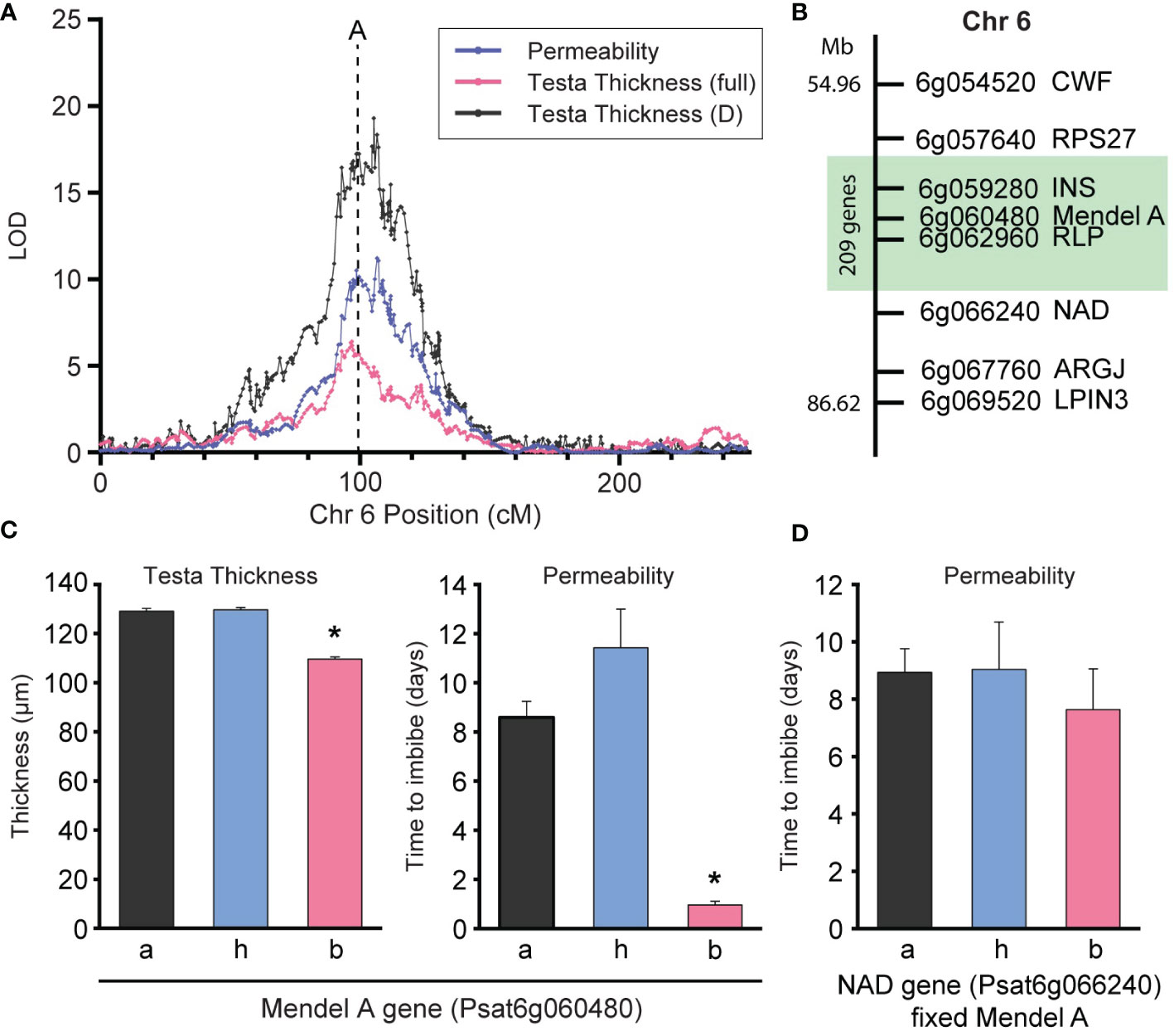
Figure 4 QTL6 impacts on testa thickness and permeability in advanced populations. (A) LOD profile of permeability, testa thickness (full) and testa section D thickness QTL near the Mendel’s A locus, which is marked with a dashed line. (B) Fine mapping of the Mendel’s A region on chromosome 6, showing the region (green) where there is significant effect of the genes on permeability and testa thickness (C) Effect of genotype at the Mendel’s A locus on testa thickness and permeability in an advanced F4 & F5 populations respectively. The * indicates significant differences between genotypes a/h and b (P < 0.01). (D) Effect of genotype of the NAD gene on permeability in advanced F5 population when the region of Mendel’s A is fixed. Data in C & D populations were fixed for the wild allele at RPS27 and CWF. Full segregation data is available in Supplementary Figure S4.
Results showed all individuals in these populations were fixed for wild alleles between RPS27 and CWF and segregating at all other loci. Phenotyping these populations (separately, due to the destructive nature of the phenotyping) confirmed the segregation of testa thickness in the F4 and permeability in the F5 (Figure 4C). Homozygosity for the domesticated allele at the Mendel’s A gene resulted in significantly thinner testa (t47 = 13.1, p < 0.001) and higher permeability (t47 = 3.4, p = 0.001) compared to the heterozygous and homozygous wild individuals, indicating RPS27 as the upper boundary for this QTL effect. Further, when only individuals fixed for wild Mendel’s A were analyzed, genotype at NAD had no significant effect on permeability in the F5 (Figure 4D), indicating NAD as the lower boundary for this QTL effect.
Of the additional markers previously genotyped in the RIL population (Williams et al., 2022), the markers FULa, AGO1 and CABB were found to span the peak of the seed coat roughness QTL (Figure 5A). To confirm the validity of this QTL, advanced F4 populations (and a subsequent F5 population) were generated by single seed descent from lines in the F2 cross which were segregating for the region between CABB and FULa on chromosome 1 but fixed for the wild allele at qPERM6 and the domesticated allele at qPERM7. Phenotyping these populations showed segregation of seed coat roughness, with the QTL effect able to be localized between FULa and CABB, a region that spans 37 MB and contains 331 genes (Figure 5B, Supplementary Table S3). Notably, genotype at AGO1 had no effect on seed permeability (Figure 5C), confirming that seed coat roughness had no detectable impact on the permeability of the seed coat to water.
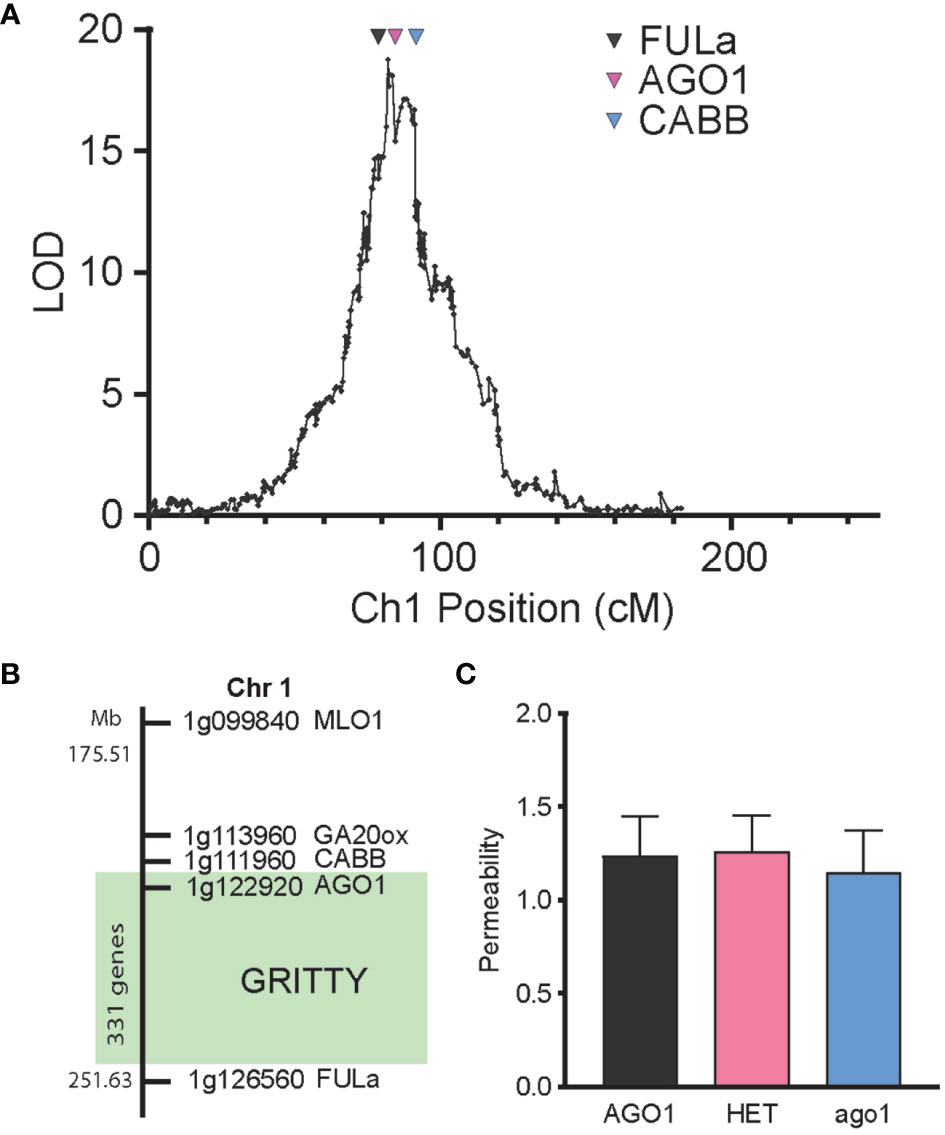
Figure 5 Genetic separation of seed coat roughness from permeability. (A) LOD profile of seed coat roughness QTL on chromosome 1 and the position of three markers FULa, AGO1 and CABB. (B) Mapping of the GRITTY region, showing the position of key markers on chromosome 1 and the region (in green) within which GRITTY has been mapped. (C) Effect of genotype at the AGO1 locus on permeability in an advanced F4 population.
4 Discussion
Loss of physical seed dormancy is widely considered to be one of the key domestication-related modifications in pea (Ladizinsky, 1987; Zohary, 1989; Abbo et al., 2011), and primarily involves an increase in permeability of the seed coat (Smýkal et al., 2014). However, little is known about the genetic or functional basis for this change. It has clearly been accompanied by a reduction in testa thickness (Figure 1, Plitmann and Kislev, 1989; Hradilová et al., 2017), an association that has raised the question of a possible relationship between these traits. Our genetic analysis of dormancy-related seed traits in a previously-generated wild x domesticated RIL population has shown only moderate correlation between testa thickness and permeability (Figure 2), and identified loci that affect them independently (Figure 3), suggesting there is no close functional association.
One major factor shaping the results of this study is the segregation of Mendel’s A gene in the population. This gene encodes the bHLH component of the well-known MYB/WD40/bHLH (MWB) complex which has a central role in regulation of flavonoid pathways (Xu et al., 2015; Wen et al., 2020), and naturally-arising a mutations result in complete loss of anthocyanin and derivatives in seed coats, stems and flowers (Hellens et al., 2010). A reduction in anthocyanins and proanthocyanidins in seed coats has been associated with increased permeability and reduced thickness in several species including pea (Werker et al., 1979; Lepiniec et al., 2006; Smýkal et al., 2014; Sedláková et al., 2023) and consistent with this, we found QTL for testa thickness and permeability closely co-located with A (Figure 3). The most straight-forward explanation of this co-location is that both qTT6 and qPERM6 represent direct effects of A itself, with compounds under its control contributing significantly to both traits. A possible direct effect of A on permeability could also be examined through isolation of induced a mutants, or through more detailed genetic dissection of the region.
However, despite this plausible functional link, loss of A function is unlikely to have been an early and critical step in reduction of physical dormancy given that functional A alleles are widespread in the domesticated pea germplasm (Hellens et al., 2010; Holdsworth et al., 2017). Apart from qPERM6, we only detected only one other permeability locus, qPERM7, with a much weaker effect (9% PVE, vs. 33% for qPERM6). In principle, this locus could have contributed to the reduction in dormancy during domestication, but it is somewhat surprising that it was the only other locus detected and explains so little of the variance. This result could imply that transition to increased permeability early in domestication was incremental and strongly polygenic, with qPERM7 representing the strongest effect, and a further substantial increase due to qPERM6 arose only later with the appearance of the a mutation.
An alternative interpretation is that, despite the proximity of qPERM6 to A, and the precedent for the function of A orthologs in testa permeability, the qPERM6 effect may not be solely or primarily due to A, but reflects variation at another gene in the QTL interval that arose earlier than the a mutation. If this were the case, a similar analysis with a landrace or other primitive accession carrying functional A alleles as the domesticated parent would be expected to reveal an effect at qPERM6 independent of A. One such study found no evidence for a dormancy QTL in the A region in a wild x domesticated (JI1794 x Slow) population monomorphic for A (Weeden, 2007). However this study employed small populations (n≈50) and low marker density, and warrants re-examination.
Genetic control of physical seed dormancy has been characterized to some extent in several grain legumes. In some species, 4-6 QTL have been detected (Isemura et al., 2010, 2012; Kongjaimun et al., 2012), while in other species such as lentil, bean and soybean, the trait has been attributed to single major QTL (Ladizinsky, 1985; Sun et al., 2015; Soltani et al., 2021). To date only one testa permeability locus has been characterized at the molecular level. In soybean, permeability of the intact seed coat in domesticated germplasm depends on a single major-effect locus (Hs-1) modified by additional quantitative variation (Sun et al., 2015). Hs-1 encodes a calcineurin-like protein expressed in the macrosclereid layer, and increased permeability of domesticated forms has been functionally associated with a specific amino acid substitution (Sun et al., 2015). This study also found no evidence for an effect of Hs-1 on testa thickness. Two other genes critical for physical dormancy have been identified by reverse genetics in the model legume Medicago truncatula, where mutations in class II KNOX gene KNOX4 and the keto-acyl synthase KCS12 reduce long-chain fatty acid content in the cuticular layer of the seed coat and increase its permeability (Chai et al., 2016, 2021). A mungbean ortholog of KNOX4 was also found to contribute to seed dormancy in a wild x cultivated Vigna radiata cross (Laosatit et al., 2022). Other genes potentially linked to physical dormancy in legumes include a truncated phospholipid sterol acyltransferase VsPAT1 in V. stipulacea (Takahashi et al., 2023), and a truncated tandem duplicate of a pectin acetylesterase PAE-8-2 in bean (Soltani et al., 2021). However, map locations of their pea orthologs on chromosomes 3, 4 and 5 indicate none of these genes are a candidate for either of the pea qPERM loci detected in this study. Similarly in pea, 14 differentially expressed candidate genes for dormancy were found between wild and cultivated P. elatius (Hradilová et al., 2017), but none were located within the confidence intervals of the present loci.
Loss of seed coat roughness is a characteristic feature of the domesticated Pisum germplasm and its strong early selection in parallel with thinness and permeability has historically suggested some association with one or both of these traits. Most earlier studies have assessed roughness somewhat subjectively and as a Mendelian trait. As expected, our more detailed quantitative analysis did identify a major QTL explaining 45% of the variation in the location of the classical GTY locus, but this was not co-located with QTL for testa thickness or permeability. This implies that the selection for the recessive gty allele during domestication is unlikely to be due to effects on either of these traits.
One general caveat of our study is that our assay for permeability used seeds stored in a stable environment and then tested at a constant temperature. This clearly did not capture the complex daily and seasonal environmental fluctuations likely to be experienced by a dormant seed in a natural environment. We therefore cannot definitively exclude a role for GTY and have likely not detected the full range of adaptive variation for seed dormancy represented by the parental lines. Nevertheless, the systematic analysis presented here does prepare the way for future more detailed studies and a comparison with related species.
Data availability statement
The original contributions presented in the study are included in the article/Supplementary Material. Further inquiries can be directed to the corresponding authors.
Author contributions
OW: Writing – original draft, Writing – review & editing, Data curation, Formal Analysis, Investigation, Methodology. JS: Data curation, Formal Analysis, Investigation, Methodology, Writing – original draft, Writing – review & editing. JB: Data curation, Formal Analysis, Writing – original draft, Writing – review & editing. VH: Formal Analysis, Investigation, Methodology, Supervision, Writing – original draft, Writing – review & editing. JW: Conceptualization, Data curation, Funding acquisition, Investigation, Methodology, Project administration, Supervision, Writing – original draft, Writing – review & editing.
Funding
The author(s) declare financial support was received for the research, authorship, and/or publication of this article. This work was funded by the Australian Research Council (grants FT120100048 and DP 160100793) and supported with an Australian Government Research Training Program Scholarship.
Acknowledgments
We acknowledge the contribution of Michelle Lang and Tracey Winterbottom to the care of plants, and the contribution of Laura James, Xintian Lee, Nicola Potter and Belinda Warren to measurement of phenotypes.
Conflict of interest
The authors declare that the research was conducted in the absence of any commercial or financial relationships that could be construed as a potential conflict of interest.
Publisher’s note
All claims expressed in this article are solely those of the authors and do not necessarily represent those of their affiliated organizations, or those of the publisher, the editors and the reviewers. Any product that may be evaluated in this article, or claim that may be made by its manufacturer, is not guaranteed or endorsed by the publisher.
Supplementary material
The Supplementary Material for this article can be found online at: https://www.frontiersin.org/articles/10.3389/fpls.2024.1359226/full#supplementary-material
References
Abbo, S., Rachamim, E., Zehavi, Y., Zezak, I., Lev-Yadun, S., Gopher, A. (2011). Experimental growing of wild pea in Israel and its bearing on Near Eastern plant domestication. Ann. Bot. 107, 1399–1404. doi: 10.1093/aob/mcr081
Balarynová, J., Klčová, B., Sekaninová, J., Kobrlová, L., Cechová, M. Z., Krejčí, P., et al. (2022). The loss of polyphenol oxidase function is associated with hilum pigmentation and has been selected during pea domestication. New Phytol. 235, 1807–1821. doi: 10.1111/nph.18256
Bordat, A., Savois, V., Nicolas, M., Salse, J., Chauveau, A., Bourgeois, M., et al. (2011). Translational genomics in legumes allowed placing in silico 5460 unigenes on the pea functional map and identified candidate genes in Pisum sativum L. G3: Genes| Genomes| Genet. 1, 93–103. doi: 10.1534/g3.111.000349
Chai, M., Queralta Castillo, I., Sonntag, A., Wang, S., Zhao, Z., Liu, W., et al. (2021). A seed coat-specific β-ketoacyl-CoA synthase, KCS12, is critical for preserving seed physical dormancy. Plant Physiol. 186, 1606–1615. doi: 10.1093/plphys/kiab152
Chai, M., Zhou, C., Molina, I., Fu, C., Nakashima, J., Li, G., et al. (2016). A class II KNOX gene, KNOX4, controls seed physical dormancy. Proc. Natl. Acad. Sci. 113, 6997–7002. doi: 10.1073/pnas.1601256113
Di Vittori, V., Bitocchi, E., Rodriguez, M., Alseekh, S., Bellucci, E., Nanni, L., et al. (2021). Pod indehiscence in common bean is associated with the fine regulation of PvMYB26. J. Exp. Bot. 72, 1617–1633. doi: 10.1093/jxb/eraa553
Dong, Y., Yang, X., Liu, J., Wang, B.-H., Liu, B.-L., Wang, Y.-Z. (2014). Pod shattering resistance associated with domestication is mediated by a NAC gene in soybean. Nat. Commun. 5, 3352. doi: 10.1038/ncomms4352
Doyle, J., Doyle, J. (1987). A rapid DNA isolation procedure for small quantities of fresh leaf tissue. Phytochemical Bulletin 19, 11–15.
Ellis, N., Hofer, J., Sizer-Coverdale, E., Lloyd, D., Aubert, G., Kreplak, J., et al. (2023). Recombinant inbred lines derived from wide crosses in Pisum. Sci. Rep. 13, 20408. doi: 10.1038/s41598-023-47329-9
Funatsuki, H., Suzuki, M., Hirose, A., Inaba, H., Yamada, T., Hajika, M., et al. (2014). Molecular basis of a shattering resistance boosting global dissemination of soybean. Proc. Natl. Acad. Sci. 111, 17797–17802. doi: 10.1073/pnas.1417282111
Hellens, R. P., Moreau, C., Lin-Wang, K., Schwinn, K. E., Thomson, S. J., Fiers, M. W., et al. (2010). Identification of Mendel's white flower character. PloS One 5, e13230. doi: 10.1371/journal.pone.0013230
Hellwig, T., Abbo, S., Ophir, R. (2022). Phylogeny and disparate selection signatures suggest two genetically independent domestication events in pea (Pisum L.). Plant J. 110, 419–439. doi: 10.1111/tpj.15678
Holdsworth, W. L., Gazave, E., Cheng, P., Myers, J. R., Gore, M. A., Coyne, C. J., et al. (2017). A community resource for exploring and utilizing genetic diversity in the USDA pea single plant plus collection. Hortic Res. 4, 17017. doi: 10.1038/hortres.2017.17
Hradilová, I., Duchoslav, M., Brus, J., Pechanec, V., Hýbl, M., Kopecký, P., et al. (2019). Variation in wild pea (Pisum sativum subsp. elatius) seed dormancy and its relationship to the environment and seed coat traits. PeerJ 7, e6263. doi: 10.7717/peerj.6263
Hradilová, I., Trněný, O., Valkova, M., Cechová, M., Janská, A., Prokešová, L., et al. (2017). A combined comparative transcriptomic, metabolomic, and anatomical analyses of two key domestication traits: pod dehiscence and seed dormancy in pea (Pisum sp.). Front. Plant Sci. 8, 542. doi: 10.3389/fpls.2017.00542
Isemura, T., Kaga, A., Tabata, S., Somta, P., Srinives, P., Shimizu, T., et al. (2012). Construction of a genetic linkage map and genetic analysis of domestication related traits in mungbean (Vigna radiata). PloS One 7, e41304. doi: 10.1371/journal.pone.0041304
Isemura, T., Kaga, A., Tomooka, N., Shimizu, T., Vaughan, D. A. (2010). The genetics of domestication of rice bean, Vigna umbellata. Ann. Bot. 106, 927–944. doi: 10.1093/aob/mcq188
Jang, S.-J., Sato, M., Sato, K., Jitsuyama, Y., Fujino, K., Mori, H., et al. (2015). A single-nucleotide polymorphism in an endo-1, 4-β-glucanase gene controls seed coat permeability in soybean. PloS One 10, e0128527. doi: 10.1371/journal.pone.0128527
Janská, A., Pecková, E., Sczepaniak, B., Smýkal, P., Soukup, A. (2019). The role of the testa during the establishment of physical dormancy in the pea seed. Ann. Bot. 123, 815–829. doi: 10.1093/aob/mcy213
Kongjaimun, A., Kaga, A., Tomooka, N., Somta, P., Vaughan, D. A., Srinives, P. (2012). The genetics of domestication of yardlong bean, Vigna unguiculata (L.) Walp. ssp. unguiculata cv.-gr. sesquipedalis. Ann. Bot. 109, 1185–1200. doi: 10.1093/aob/mcs048
Kreplak, J., Madoui, M.-A., Cápal, P., Novák, P., Labadie, K., Aubert, G., et al. (2019). A reference genome for pea provides insight into legume genome evolution. Nat. Genet. 51, 1411–1422. doi: 10.1038/s41588-019-0480-1
Ladizinsky, G. (1985). The genetics of hard seed coat in the genus Lens. Euphytica 34, 539–543. doi: 10.1007/BF00022952
Ladizinsky, G. (1987). Pulse domestication before cultivation. Econ Bot. 41, 60–65. doi: 10.1007/BF02859349
Lamprecht, H. (1948). The variation of linkage and the course of crossing over. Agri Hortique Genetica 6, 10–48. doi: 10.1371/journal.pcbi.1002462
Lamprecht, H. (1959). The inheritance of colors of a seeds of Pisum. Agri Hort Genet. Landskrona 17, 1–18.
Lamprecht, H. (1961). Die Genenkarte von Pisum beinormaler Struktur der Chromosomen. Agri Hortique Genetica 19, 360–401.
Laosatit, K., Amkul, K., Yimram, T., Chen, J., Lin, Y., Yuan, X., et al. (2022). A class II KNOX gene, KNAT7-1, regulates physical seed dormancy in mungbean [Vigna radiata (L.) Wilczek]. Front. Plant Sci. 13. doi: 10.3389/fpls.2022.852373
Lepiniec, L., Debeaujon, I., Routaboul, J.-M., Baudry, A., Pourcel, L., Nesi, N., et al. (2006). Genetics and biochemistry of seed flavonoids. Annu. Rev. Plant Biol. 57, 405–430. doi: 10.1146/annurev.arplant.57.032905.105252
Lersten, N., Gunn, C. (1982). Testa characters in tribe Vicieae, with notes about tribes Abreae, Cicereae, and Trifolieae (Fabaceae) (Washington, DC: US Dept. of Agriculture, Agricultural Research Service).
Lev-Yadun, S., Gopher, A., Abbo, S. (2000). The cradle of agriculture. Science 288, 1602–1603. doi: 10.1126/science.288.5471.1602
Parker, T. A., Lo, S., Gepts, P. (2021). Pod shattering in grain legumes: emerging genetic and environment-related patterns. Plant Cell 33, 179–199. doi: 10.1093/plcell/koaa025
Plitmann, U., Kislev, M. (1989). “Reproductive changes induced by domestication,” in Advances in Legume Biology. Eds. Stirton, C., Zarucchi, J. (St. Louis: Missouri Botanical Garden Press), 487–504.
Sansaloni, C., Petroli, C., Jaccoud, D., Carling, J., Detering, F., Grattapaglia, D., et al. (2011). Diversity Arrays Technology (DArT) and next-generation sequencing combined: genome-wide, high throughput, highly informative genotyping for molecular breeding of Eucalyptus. BMC Proc. 5, 1–2. doi: 10.1186/1753-6561-5-S7-P54
Schneider, C. A., Rasband, W. S., Eliceiri, K. W. (2012). NIH Image to ImageJ: 25 years of image analysis. Nat. Methods 9, 671–675. doi: 10.1038/nmeth.2089
Sedláková, V., Hanáček, P., Grulichová, M., Zablatzká, L., Smýkal, P. (2021). Evaluation of seed dormancy, one of the key domestication traits in chickpea. Agronomy 11, 2292. doi: 10.3390/agronomy11112292
Sedláková, V., Zeljković, S. Ć., Štefelová, N., Smýkal, P., Hanáček, P. (2023). Phenylpropanoid content of chickpea seed coats in relation to seed dormancy. Plants 12, 2687. doi: 10.3390/plants12142687
Smýkal, P., Vernoud, V., Blair, M. W., Soukup, A., Thompson, R. D. (2014). The role of the testa during development and in establishment of dormancy of the legume seed. Front. Plant Sci. 5, 351. doi: 10.3389/fpls.2014.00351
Soltani, A., Walter, K. A., Wiersma, A. T., Santiago, J. P., Quiqley, M., Chitwood, D., et al. (2021). The genetics and physiology of seed dormancy, a crucial trait in common bean domestication. BMC Plant Biol. 21, 1–17. doi: 10.1186/s12870-021-02837-6
Sun, L., Miao, Z., Cai, C., Zhang, D., Zhao, M., Wu, Y., et al. (2015). GmHs1-1, encoding a calcineurin-like protein, controls hard-seededness in soybean. Nat. Genet. 47, 939–943. doi: 10.1038/ng.3339
Takahashi, Y., Sakai, H., Ariga, H., Teramoto, S., Shimada, T. L., Eun, H., et al. (2023). Domesticating Vigna stipulacea: chromosome-level genome assembly reveals VsPSAT1 as a candidate gene decreasing hard-seededness. Front. Plant Sci. 14. doi: 10.3389/fpls.2023.1119625
Tang, H., Krishnakumar, V., Bidwell, S., Rosen, B., Chan, A., Zhou, S., et al. (2014). An improved genome release (version Mt4. 0) for the model legume Medicago truncatula. BMC Genomics 15, 1–14. doi: 10.1186/1471-2164-15-312
Tayeh, N., Aluome, C., Falque, M., Jacquin, F., Klein, A., Chauveau, A., et al. (2015). Development of two major resources for pea genomics: the GenoPea 13.2K SNP Array and a high-density, high-resolution consensus genetic map. Plant J. 84, 1257–1273. doi: 10.1111/tpj.13070
Van Ooijen, J. (2009). MapQTL 6, Software for the mapping of quantitative trait loci in experimental populations of diploid species (Wageningen, Netherlands: Kyazma BV).
Weeden, N. F. (2007). Genetic changes accompanying the domestication of Pisum sativum: is there a common genetic basis to the ‘domestication syndrome’ for legumes? Ann. Bot. 100, 1017–1025. doi: 10.1093/aob/mcm122
Weller, J. L., Liew, L. C., Hecht, V. F., Rajandran, V., Laurie, R. E., Ridge, S., et al. (2012). A conserved molecular basis for photoperiod adaptation in two temperate legumes. Proc. Natl. Acad. Sci. 109, 21158–21163. doi: 10.1073/pnas.1207943110
Wen, W., Alseekh, S., Fernie, A. R. (2020). Conservation and diversification of flavonoid metabolism in the plant kingdom. Curr. Opin. Plant Biol. 55, 100–108. doi: 10.1016/j.pbi.2020.04.004
Werker, E., Marbach, I., Mayer, A. (1979). Relation between the anatomy of the testa, water permeability and the presence of phenolics in the genus Pisum. Ann. Bot. 43, 765–771. doi: 10.1093/oxfordjournals.aob.a085691
Williams, O., Vander Schoor, J. K., Butler, J. B., Ridge, S., Sussmilch, F. C., Hecht, V. F., et al. (2022). The genetic architecture of flowering time changes in pea from wild to crop. J. Exp. Bot. 73, 3978–3990. doi: 10.1093/jxb/erac132
Xu, W., Dubos, C., Lepiniec, L. (2015). Transcriptional control of flavonoid biosynthesis by MYB–bHLH–WDR complexes. Trends Plant Sci. 20, 176–185. doi: 10.1016/j.tplants.2014.12.001
Zohary, D. (1989). Pulse domestication and cereal domestication: How different are they? Econ Bot. 43, 31–34. doi: 10.1007/BF02859322
Keywords: Pisum, seed dormancy, seed coat, domestication, permeability, gritty, flavonoid
Citation: Williams OR, Vander Schoor JK, Butler JB, Hecht VFG and Weller JL (2024) Physical seed dormancy in pea is genetically separable from seed coat thickness and roughness. Front. Plant Sci. 15:1359226. doi: 10.3389/fpls.2024.1359226
Received: 22 December 2023; Accepted: 09 February 2024;
Published: 27 February 2024.
Edited by:
Petr Smýkal, Palacký University in Olomouc, CzechiaReviewed by:
Clarice Coyne J., United States Department of Agriculture (USDA), United StatesLeif Skot, Aberystwyth University, United Kingdom
R. Varma Penmetsa, University of California, Davis, United States
Copyright © 2024 Williams, Vander Schoor, Butler, Hecht and Weller. This is an open-access article distributed under the terms of the Creative Commons Attribution License (CC BY). The use, distribution or reproduction in other forums is permitted, provided the original author(s) and the copyright owner(s) are credited and that the original publication in this journal is cited, in accordance with accepted academic practice. No use, distribution or reproduction is permitted which does not comply with these terms.
*Correspondence: James L. Weller, amltLndlbGxlckB1dGFzLmVkdS5hdQ==