- 1Dipartimento di Scienze della Vita, Sede Agraria, UNIMORE - Università di Modena e Reggio Emilia, Reggio Emilia, Italy
- 2Centro di Ricerca Cerealicoltura e Colture Industriali, CREA – Consiglio per la Ricerca in Agricoltura e l’Analisi dell’Economia Agraria, Vercelli, Italy
- 3Dipartimento per lo Sviluppo Sostenibile e la Transizione Ecologica, UPO – Università del Piemonte Orientale, Complesso San Giuseppe, Vercelli, Italy
- 4Centro di Ricerca Cerealicoltura e Colture Industriali, CREA – Consiglio per la Ricerca in Agricoltura e l’Analisi dell’Economia Agraria, Foggia, Italy
Climate change poses a major threat to global food security, significantly reducing crop yields as cause of abiotic stresses, and for boosting the spread of new and old pathogens and pests. Sustainable crop management as a route to mitigation poses the challenge of recruiting an array of solutions and tools for the new aims. Among these, the deployment of positive interactions between the micro-biotic components of agroecosystems and plants can play a highly significant role, as part of the agro-ecological revolution. Endophytic microorganisms have emerged as a promising solution to tackle this challenge. Among these, Arbuscular Mycorrhizal Fungi (AMF) and endophytic bacteria and fungi have demonstrated their potential to alleviate abiotic stresses such as drought and heat stress, as well as the impacts of biotic stresses. They can enhance crop yields in a sustainable way also by other mechanisms, such as improving the nutrient uptake, or by direct effects on plant physiology. In this review we summarize and update on the main types of endophytes, we highlight several studies that demonstrate their efficacy in improving sustainable yields and explore possible avenues for implementing crop-microbiota interactions. The mechanisms underlying these interactions are highly complex and require a comprehensive understanding. For this reason, omic technologies such as genomics, transcriptomics, proteomics, and metabolomics have been employed to unravel, by a higher level of information, the complex network of interactions between plants and microorganisms. Therefore, we also discuss the various omic approaches and techniques that have been used so far to study plant-endophyte interactions.
1 Introduction
The main objective of this review is to to provide a summarized update of the existing knowledge about the interactions between plants and endophytic microorganisms, focusing on their potential for improving crop production and resilience. With this aim, we reviewed their potential role for facing key challenges, such as biotic and abiotic stresses, in the context of climate change. Among the plant-microorganisms interactions, we have restricted our analysis to the endophytic ones, and among these, to the ones between endophytes and agricultural plants.
Before analysing the impact of the endophytic interactions on the stress resiliences, enclosing in a wider view the plant mineral nutrition and its relationships with quality, we have first defined the scenario of climate change in which the microbiota can have a renewed role and importance. Then, we identified the boundaries and characteristics of the endophytes within the microbiota, the general mechanisms and ontogenesis of their interactions with agricultural plants, as well as their classification in groups significant for the agricultural systems. We also explored the relevant ‘omic’ techniques as essential tools for analysing complex microbial communities and their interactions with plants. Omics are a natural choice for studying the complex symbiosis between plants and microorganisms in view of their exploitation (Plett and Martin, 2018; Sharma et al., 2020; Sandrini et al., 2022). Most papers analysed in this review are recent reviews and original papers published in the last decade, in particular the original research papers. Databases used during the research process included PubMed (https://pubmed.ncbi.nlm.nih.gov/), Litmaps (https://www.litmaps.com/), Open Knowledge Maps (https://openknowledgemaps.org/). Keywords used during the first phase of information collection comprehended: endophytes, abiotic stress, biotic stress, omics, PGPM. Several reviews have examined the interaction between plants and Plant Growth Promoting Microorganisms (PGPMs) (Vandenkoornhuyse et al., 2015; Santoyo et al., 2016; Khatoon et al., 2020), including potential benefits in biotic and abiotic stress scenarios (Miliute et al., 2015; Yan et al., 2019; Kamran et al., 2022), and have been here considered and updated. Also, the impact of climate change has been thoroughly studied and reviewed (Driga and Drigas, 2019; Lynch et al., 2021), as it has specific aspects for plant and microorganism interactions (Compant et al., 2010; Trenberth et al., 2014; Classen et al., 2015; Shahzad et al., 2021), and both these fields have been here contextualized with respect to topics treated in this review. Abiotic stressors such as drought, heat, and salinity have been included since of highly significant concern (Lipiec et al., 2013; Evelin et al., 2019; Ma et al., 2020; Angon et al., 2022). The role of microorganisms in protecting from biotic stresses (Pandey et al., 2019; Chaudhary et al., 2022), including viruses (Bao and Roossinck, 2013), nematodes (Bernard et al., 2017; Gamalero and Glick, 2020; Pulavarty et al., 2021), insects (Bradshaw et al., 2016), fungi, and bacteria (Muthu Narayanan et al., 2022) have been previously discussed and are here gathered and updated.
In this review, we combined various aspects, usually considered individually, that characterize the symbiosis between endophytes and plants: mechanisms of selection and interaction, effects on biotic and abiotic stress factors, omic techniques for the study of such complex symbioses, consequences from the nutritional point of view of the interaction between endophytes and agricultural crops. For each type of stress, we considered examples where endophytes have demonstrated beneficial effects on agricultural plants, and we critically analysed potential limits of microbe-based approach. Finally, we also briefly discussed the possible future perspectives of the use of endophytes in crop production under climate change, highlighting possible limitations and improvements.
2 The climate change scenario and plant-microorganisms interactions
Plants, in their evolutionary path outside the oceans, have established important relationships with various microorganisms, such as bacteria, fungi, protists, and viruses (Plett and Martin, 2018). These microorganisms can live associated with different plant tissues and organs and form the plant microbiota. This can be divided in different microbial communities, based on the plant parts they colonize: the phyllosphere, which includes all the aboveground plant tissues (i.e., stems and leaves), the endosphere, represented by the internal tissues, the spermosphere, i.e. the seed, and the rhizosphere, which comprehends the roots surface and the soil surrounding it, reached by the root exudates (Johnston-Monje and Raizada, 2011; Levy et al., 2018; Sharma et al., 2020). The different conditions of each of these habitats lead to diverse microbial communities, even within the same plant.
Through millions of years, pathogenic, competing, mutualistic, or symbiotic associations have been established between plants and microorganisms. Fossil records provide substantial evidence that over the past 450 million years, virtually all plants have formed symbiotic relationships with microbes since their first colonization of land. Various studies have documented microbial symbionts in fossilized plant specimens dating back to this era (Redecker et al., 2000; Krings et al., 2007; Bonfante and Genre, 2010; Kawaguchi and Minamisawa, 2010; Genre et al., 2020). Partida-Martínez and Heil (2011) suggested that the plant microbiota plays additional essential roles in phenotypic and epigenetic plasticity, as well as in the continuous evolution of plants.
The interactions between plants, soil, and microbes have played and continue to play a vital role by influencing various processes that contribute to plant health and productivity (Ahmed et al., 2019; Khatoon et al., 2020). Endophytes, the microorganisms of the endosphere, can provide benefits to the whole plant, either by promoting plant growth (Johnson et al., 2003; Rodriguez et al., 2008), eliciting the production of metabolites and useful chemicals such as antibiotics and agrochemicals (Kaul et al., 2012; Kusari et al., 2014; Wang et al., 2015), or helping plants to cope with stresses (Yan et al., 2019; Chen et al., 2021).
Since the first alerts of incoming human-driven climate change (Driga and Drigas, 2019), it became clear that the phenomenon would also impact the relationships between plants and microorganisms (Classen et al., 2015; Singh et al., 2019). Climate change manifests mainly as a global increase in temperature, dry periods, rapid changes of meteorological conditions (e.g., flash droughts), rainfall intensity and uneven distribution (Easterling et al., 2000; Hammond et al., 2012), with differences in impact linked to various geographical regions (Surjan et al., 2016). All these deviations from a previously more stable climate, particularly the rise in temperature, are shifting plant phenology and the global distribution of plants (Sykes, 2009; Geissler et al., 2023), and significantly increase the threats to survival of natural environments (Abbass et al., 2022).
Agricultural systems are both the subject of climate change impacts, more negative than positive (Shahzad et al., 2021; Yadav et al., 2021), and contributors to it, like other human activities that require energy inputs (Hatfield et al., 2020). In fact, although on a smaller scale per unit area compared to other human activities, due to the vast extent of agricultural systems, they can contribute to GreenHouse Gases (GHGs) emissions.
Some agricultural practices, such as intense tillage, irrigation and extensive fertilizers usage, in addition to the ever-increasing use of machinery operated by fossil fuels, lead to increased emissions of GHGs in the atmosphere (Lin et al., 2011; Galic et al., 2019). As for CH4 and N2O, agricultural activities are responsible for around half and three-quarters of all anthropogenic emissions, respectively (Lynch et al., 2021). Agriculture, especially in the form of livestock production and rice cultivation, is one of the main sources of CH4, which has a Global Warming Potential (GWP) much stronger than CO2 (Saunois et al., 2020). As for N2O, agriculture remains the main source of this GHG, primarily through the use of nitrogen fertilizers, both synthetic and natural. The lifetime of N2O in the atmosphere is about 120 years, and its GWP is about 210 times higher than that of CO2 (Singh, 2000).
Today, converting conventional agriculture into a sustainable, yet high-yielding system, is crucial to meet both our future food needs and the integrity of the biosphere. In fact, an increasing food demand cannot be met by simply improving current agricultural practices based on fossil carbon inputs, which are detrimental to the environment (Santoyo et al., 2017; Slepetiene et al., 2020). Indeed, the continuous use of fertilizers and pesticides derived from chemical synthesis has been shown to deteriorate agroecosystems, reduce soil biodiversity, and impair natural predators of insects (McLaughlin and Mineau, 1995; Alavaisha et al., 2019).
For this reason, it was recently proposed that the deployment of plant-microbe interactions be used as one of the strategies for converting agricultural systems from the traditional mechanistic approach to the new paradigm of agroecological intensification.
3 The plant microbiota
The metabolism and morphology of plants and their microbiota are intrinsically connected, with a dynamic interplay between both, maintaining the function of the holobiont. The holobiont concept suggests a new perspective of organisms as meta-organisms, composed of a host organism and its associated microorganisms, co-evolved as species assemblages (Berg et al., 2016). In the plant kingdom, microbiota fulfil important functions for the holobiont, promoting its growth and increasing tolerance against biotic and abiotic stressors, as well as for the ecosystem, decomposing crop residues and contributing to nutrient cycling (Chaparro et al., 2012; Mendes and Raaijmakers, 2015; Vandenkoornhuyse et al., 2015).
The composition and functional diversity of the plant microbiota is influenced by biotic factors including age or developmental stage, species or cultivar, and plant health, as well as by abiotic factors such as soil properties, nutrient status, and climatic conditions (Berg and Smalla, 2009).
Three main classes of microorganisms are reviewed as part of the plant microbiota: endophytic fungi and bacteria, residing inside the plant tissues; rhizospheric microorganisms, residing in the soil surrounding plant roots; and mycorrhizal fungi.
3.1 Endophytic bacteria and fungi
An endophyte is a microorganism that lives, at least for a portion of its life cycle, inside plant tissues without producing any symptoms of disease. Among endophytic microorganism are bacteria, fungi, actinomycetes, and viruses (Bao and Roossinck, 2013; Stępniewska and Kuźniar, 2013), and they are found in almost every plant (Partida-Martínez and Heil, 2011). Endophytes can be classified as systemic (or true endophytes) or non-systemic (or transient endophytes), depending on their life cycle and the type of relationship they establish with the plants. Indeed, there is a huge variability of symbiotic lifestyles, from mutualism to parasitism, depending on genotypic and/or environmental factors. True endophytes co-evolved with their hosts, creating mutualistic relationships, and are often vertically transmitted, while transient endophytes could shift from a pathogenic to a mutualistic behaviour, depending on external conditions (Wani et al., 2015). In any case, several factors may influence the host response to endophytic interactions: mainly host genotype, nutrient availability, environment, field management practices, and microorganism strain (Hesse et al., 2003; Malinowski and Belesky, 2006; Qawasmeh et al., 2012).
Endophytes can be found in the root tissues, where they are more abundant, but also in the aerial parts of the plant (leaf, flower) and in the seeds (Hallmann, 2001).
Endophytic bacteria could be considered as a subgroup of the rhizospheric bacteria, that acquired the ability of colonizing their host plants (Reinhold-Hurek and Hurek, 1998). In fact, rhizosphere is a highly competitive environment (Raaijmakers et al., 2002), while the internal tissue of the host may represent a protected ecological niche with minor perturbations from the external conditions of the soil or, in general, of the environment (Kasmir et al., 2011), and this could have created an evolutionary drive from the first to the second. Endophytic and rhizospheric bacteria implement very similar strategies to promote plant growth, but usually endophytic microorganisms have a higher beneficial potential.
Among bacterial endophytes, Proteobacteria are the most widely represented, including α-, β-, and γ-Proteobacteria; the latter taxon being the most diverse and widespread (Miliute et al., 2015; Santoyo et al., 2016). Other classes frequently isolated from plant tissues are Actinobacteria, Bacteroidetes, and Firmicutes (Reinhold-Hurek and Hurek, 2011). Rarer, but still present, are Acidobacteria, Planctomycetes and Verrucomicrobia (Santoyo et al., 2016). The most common bacterial genera are Bacillus (Firmicutes), and Pseudomonas (Proteobacteria). Rhizobia spp. are also included among endophytic bacteria, as they colonize internal root tissues of Fabaceae, developing the typical nodules for nitrogen fixation.
Among endophytic fungi, on the other hand, we can find the families Clavicipitaceae (associated with grasses), Cladosporiaceae, Glomerellaceae, Sebacinaceae, Pleosporaceae, and Hypocreaceae, among which the most representative genus is Trichoderma (Hardoim et al., 2015).
Key molecular and metabolic pathways at the base of host-microbe recognition and strain selection by different plant genotypes are starting to be elucidated. It is clear how different genotypes grown in different soil/environmental combinations are enriched with different endophytic strains (Granér et al., 2003; Rashid et al., 2012; Edwards et al., 2015). The role of plant genotype on strain selection and microbial population composition has been widely studied and even if it is not the main force driving microbial diversity, it is able to modulate it (Weinert et al., 2011; Bulgarelli et al., 2015; Walters et al., 2018). Interestingly, it has been shown that plant domestication, and lately the development of high-yielding genotypes, caused a reduction in the plant capacity of associating with useful microorganism (Porter and Sachs, 2020; Valente et al., 2020), since human-centered breeding neglected the traits related to microbiota association.
Considering endopythes role in promoting plant growth, especially in nutrient-deprived conditions, and in increasing plant defence against pathogen attack, either directly or indirectly, they are now considered a tool for crop management. These could sustain agricultural practices with fewer chemical inputs. However, research is still needed to further the knowledge both on the plant-side, trying to identify the genetic factors responsible for a more efficient microbial colonization, and on the microbic-side, to isolate the most promising micro-organisms and the most effective synthetic communities. Once these aspects are clarified, it will be possible to engineer plants and microbes to make their interaction more effective. It will also be possible to explore the use of root exudates or organic compounds that might serve as pre-biotics. Finally, we should be able to overcome and the bottleneck, as of the applicability of this research to open fields conditions, that remains challenging.
Another relevant application of this category of microorganisms is phytoremediation. It has been shown that some bacterial strains are tolerant to high concentrations of heavy metals, as Cd, Cu and Zn, and other pollutants. These strains favour their accumulation into the plants, promoting their growth (Ullah et al., 2015; Ma et al., 2016), even in a stressful environment.
3.2 Mycorrhizal fungi
Mycorrhizae are likely to have played a crucial role in the evolution of terrestrial plants (Bonfante and Genre, 2008; Partida-Martínez and Heil, 2011). Today, mycorrhizal fungi can be divided into ectomycorrhizae, when the hyphae colonize the root intercellular spaces, and endomycorrhizae, when they penetrate inside the plant cells. Endomycorrhizae are further divided into orchid (OM), ericoid (ERM) and arbuscular mycorrhizae (AMs) (Bonfante and Genre, 2010; Chen et al., 2021).
Ectomycorrhizae are mostly associated with woody perennial trees such as Pinaceae, Fagaceae, Dipterocarpaceae and Caesalpinoidaceae, contributing to the wellness of most forest ecosystems (van der Heijden et al., 2015). EM fungi are phylogenetically diverse and belong to Basidiomycetes, Ascomycetes and Zygomycetes, representing the orders Pezizales, Agaricales, Helotiales, Boletales, and Cantharellales. EM hyphae grow partially inside the root intercellular space and partially outside, creating a mantle covering the tip of colonized lateral roots, called the Hartig net (Shi et al., 2023).
EM fungi live in symbiosis with their hosts, but are also facultative saprotrophs, decomposing complex organic matter present in the soil and making nitrogen and phosphate available for the plants (Martin and Nehls, 2009). In turn, the EM fungi receive photosynthates from the plant. Genomic data and functional studies show the presence of specialized families of phosphate, ammonium, and nitrate transporters (Jargeat et al., 2003; Casieri et al., 2013; Becquer et al., 2014; Stuart and Plett, 2019), supporting evidence of their fundamental role in different genomes of EM fungi. The entire metabolic chain transporting N/P from the soil to the plants through EM hyphae has been fully elucidated in recent years, and many advances have been made thanks to high-quality genomic sequences available (Nehls and Plassard, 2018).
Among endomycorrhizae, Arbuscular Mycorrhiza (AM) are the most represented, as these symbioses are formed by the 70-90% of terrestrial plants species, while the fungi all belong to the monophyletic phylum Glomeromycota (Schüβler et al., 2001). AM fungi are obligate biotrophs, considered organisms with no or rare sexual reproduction, and present aseptate hyphae developing inside the plant cells, where they form the characteristic tree-shaped hyphal structure. In AM symbiosis, the fungi support the plants by supplying mainly P-based nutrients and water, while the plant supplies the fungi with carbon nutrition (Parniske, 2008). It is estimated that almost 20% of the photosynthetic products of terrestrial plants are allocated to AM (Bago, 2000). The N contribution is less pronounced in AM compared to EM, even though some publications have shown that the portion of N transported to the plant cells from AM is not negligible and depends on soil pH, moisture, and nutrient concentration (Govindarajulu et al., 2005; Jin et al., 2005; Tanaka and Yano, 2005).
Typically, a soil ecosystem characterized by mycorrhizal symbiosis features a wide variety of plant-fungi relationships, thus offering a broad functional diversity (van der Heijden et al., 1998; Burleigh et al., 2002). This diversity arises from the presence of different plant species and fungi, each with the potential to select the most cooperative partner (Werner and Kiers, 2015). Plant roots tend to be enriched with fungal species or isolates that ensure optimal growth benefits (Bever et al., 2009; Kiers et al., 2011; Verbruggen et al., 2012), while AM fungi typically select plants that can allocate the highest amount of C nutrients (Lekberg et al., 2010; Kiers et al., 2011). However, the genetic and ecological mechanisms underlying this partner selection remain unclear, and understanding them could reveal crucial insights for future agricultural applications.
The success of the mutualistic relationship depends on many factors, such as the combination of plant-fungi genotypes, their molecular and metabolic regulation, and soil characteristics (pH, structure, moisture), nutrient availability, and colonization rate. Different plant genotypes, under controlled conditions, may respond differently to AM in terms of plant growth and yield, as shown in crops such as maize (Zea mays L.) (Ramírez-Flores et al., 2019) and sorghum (Sorghum bicolor (L.) Moench) (Watts-Williams et al., 2019), or in terms of stress resistance, as demonstrated in rice (Oryza sativa L.) (Chareesri et al., 2020) and bread wheat (Triticum aestivum L.) (Lehnert et al., 2018). The results of these studies represent milestones for future breeding programs, supporting more sustainable agriculture.
Besides AM, endomycorrhizae are also represented by ericoid (ERM) and orchid (OM) mycorrhizae. As for ERM, the fungi colonize plants of the Ericaceae family, such as Calluna, Vaccinium and Erica, typically found on nutrient-poor and acidic soils (Soudzilovskaia et al., 2019).Thus, they represent an essential way to mobilize organic material in infertile soils. In OM, the fungi belong to Basidiomycetes, mainly to Rhizoctonia species, with which most orchids are associated (Favre-Godal et al., 2020). Orchids strongly depend on the nutrients coming from the fungi, especially for the initial stages of seed germination and growth.
It should be noted that there are also plants that establish different types of mycorrhiza, either spatially, temporally, or simultaneously, within the same root system. For example, this is the case with plants from Populus, Fraxinus and Eucalyptus genera (Ambriz et al., 2010; Teste et al., 2020), as well as other plants known as dual-mycorrhizal plant species. It must be noted that there are also plants establishing different types of mycorrhiza, in a spatially or temporally distinguished manner, or simultaneously, within the same root system. For example, this is the case of plants from Populus, Fraxinus and Eucalyptus genera (Ambriz et al., 2010; Teste et al., 2020) and other plants that are called dual-mycorrhizal plant species.
It is worth mentioning that often a single fungus may connect the root systems of several plants, creating what are known as common mycorrhizal networks (Figueiredo et al., 2021). This facilitates the exchange of signalling compounds and nutrients, increases pathogen resistance, and promotes plant growth.
4 Plant-endophyte interactions
Successful endophyte colonization involves compatible plant-microbe interactions (Khare et al., 2018). Several steps can be identified to accomplish the whole process, which includes attraction, recognition, and colonization (Figure 1).
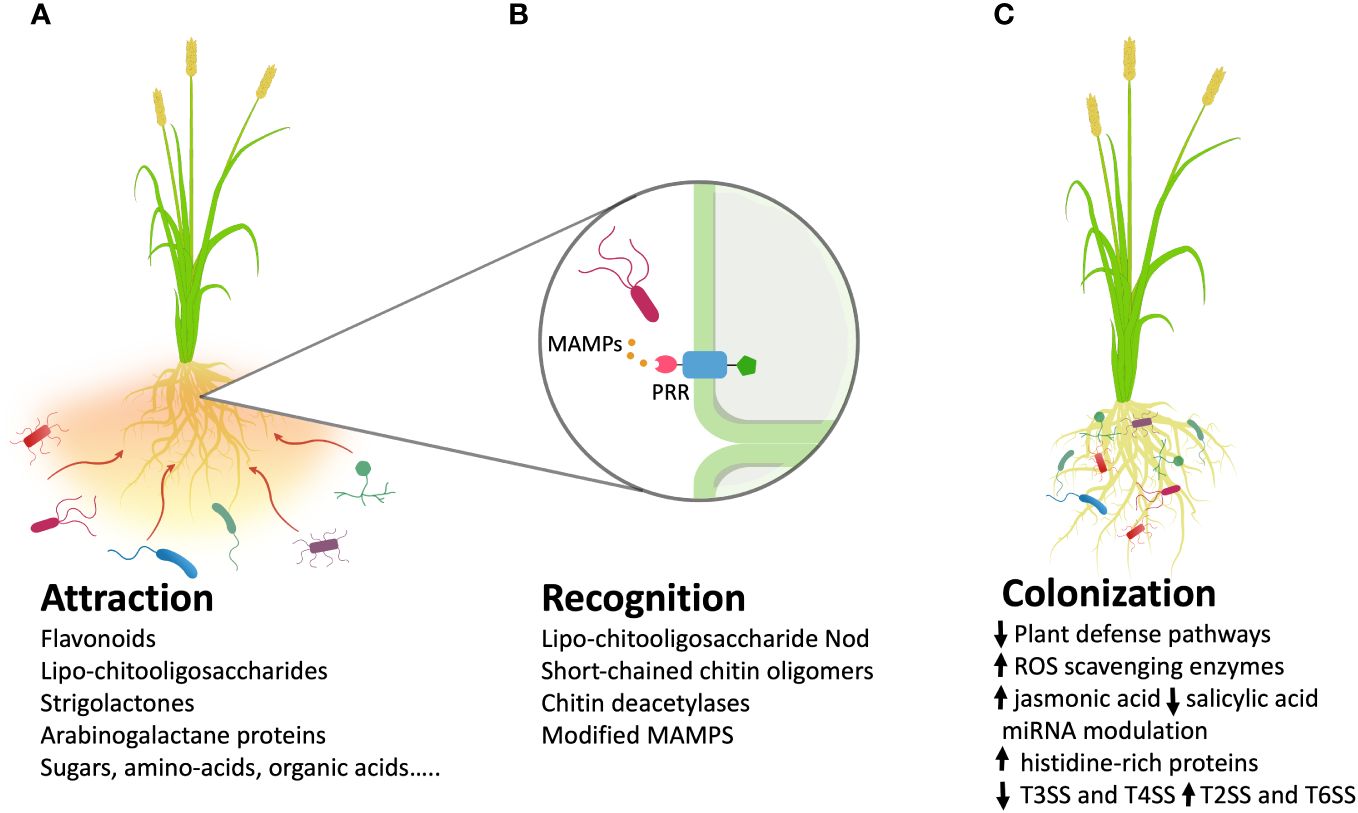
Figure 1 Metabolites and processes involved in three crucial steps of plant roots colonization by bacterial endophytes. (A) Release of molecular exudates from the roots favors the chemotactic response by the endophytes presents in the surrounding soil; (B) The recognition step is a complex phase in which plant receptors recognize microbial molecules that trigger molecular pathways. A typical recognition mechanism acts through MAMPs (Microbe-Associated Molecular Patterns) and plant PRRs (Pattern Recognition Receptors). (C) Once inside the plant, endophytes can influence many processes, for example modulating the levels of phytohormones or increasing ROS (Reactive Oxygen Species)-scavenging enzymes. (MAMPS, Microbe-Associated Molecular Patterns; ROS, Reactive Oxygen Species; T3SS, type III secretion system; T4SS, type IV secretion system; T2SS, type II secretion system; T6SS, type VI secretion system; arrow up, up regulated metabolites/processes; arrow down, down regulated metabolites/processes).
4.1 Attraction
Some endophytes are seed-borne and are present in germinated plants, thus representing a bridge across host plant generations (Coombs and Franco, 2003). Also, plants with vegetative propagation can transmit their endophytic microbiota to the next generation (Kamran et al., 2022). In others, a chemotactic response of endophytes to host plant root exudates has been observed. These exudates are rich in biomolecules (including nutrients and water), and thus attract or are recognized by friendly endophytic microbes (Compant et al., 2010; Brader et al., 2014). Flavonoids are one such group of metabolites secreted by several plants and categorized as chemo-attractants, playing an important role in endophytic interaction with the root hair. Flavonoids are used in bioformulations to affect successful infection of legume roots by rhizobia (Arora and Mishra, 2016). They are also reported to play a role with non-rhizobial endophytes, and it has been proven that in the presence of these metabolites, the colonization of roots in rice and wheat by the endophytic Serratia sp. EDA2 and Azorhizobium caulinodans ORS571 is far more effective (Webster et al., 1998; Balachandar et al., 2006). Lipo-ChitoOligosaccharides (LCO), also called Nod factors, are well-characterized signal molecules activating the Common Symbiotic Pathway (CSP) in rhizobia-legume and arbuscular mycorrhizal associations (Gough and Cullimore, 2011). Recently, StrigoLactone (SL) secreted by roots of Arabidopsis thaliana was found to act as a signal molecule for colonization of endophytic Mucor sp (Rozpądek et al., 2018). SL treatment may also activate the synthesis and release of short-chain chitin oligomers, whose perception by the plant can stimulate the symbiotic signalling pathway during early stages of host colonization (López-Ráez et al., 2017). Additionally, ArabinoGalactan Proteins (AGPs), which are highly glycosylated members of the Hydroxyproline-Rich GlycoProteins (HRGPs) superfamily of plant cell wall proteins, play a crucial role in establishing the interaction of plant with microbes (including endophyte) at several stages (Nguema-Ona et al., 2013). Several other root exudates, including sugars, amino acids, organic acids, phenolic compounds, and other secondary metabolites, are now known to be secreted by plant roots, which selectively invite the mutualistic microbes, particularly the endophytes (Chagas et al., 2017). A bacterial endophyte can also utilize the hyphae of a fungal pathogen to gain access from the soil to plant roots, thereby protecting the host from infection (Palmieri et al., 2020). showed that the endophytic rhizobacterium Rahnella aquatilis utilizes hyphae of the fungal pathogen Fusarium oxysporum to access and colonize plant roots. Metabolomic and multi-omics approaches, as those described below, would most likely increase the knowledge about metabolites released by plant seeds and roots involved in attracting favourable endophytic microorganisms. This information is necessary to address the realization of bioformulations or genetic engineering approaches to increase the production of chemo-attractants and thus the colonization of plant tissues by bacterial endophytes under normal and stressful plant growth conditions (Arif et al., 2020).
4.2 Recognition
The strategies that plants use to distinguish beneficial microbes, such as endophytes, from pathogens, are still a matter of research and not completely understood. Plants possess various PRRs (Pattern Recognition Receptors) that recognize M/PAMP (Microbe/Pathogen-Associated Molecular Patterns) ligands and initiate immune reactions (Hacquard et al., 2017). The most characterized MAMPs include flagellin, elongation factor Tu, peptidoglycan, lipopolysaccharides, bacterial cold shock proteins, bacterial superoxide dismutase, Beta-Glycan, β-glucans from oomycetes, and chitin (Newman et al., 2013). These MAMPs are recognized on the surface of plant cells by PRRs, which include receptor-like kinases and receptor-like proteins (Tang et al., 2017). Both pathogens and symbionts can be recognized by PRRs, because the M/PAMPs are not specific to pathogens. To avoid recognition by the host plant and the subsequent immune response, pathogens and symbionts have evolved complex extracellular invasion strategies. Due to the similarity of pathogen and symbiont genomes (Reinhardt et al., 2021), common extracellular strategies exist between them. They can be divided into three categories: avoiding accumulation of MAMP precursors, reducing hydrolytic MAMP release, and preventing MAMP perception (Buscaill and van der Hoorn, 2021). These strategies can involve different microbial effectors. Symbionts have developed various strategies to allow their potential hosts to better distinguish them from pathogens during the recognition phase. For example, LCO Nod Factors are perceived by legumes, activating the symbiotic pathway (Radutoiu et al., 2003; Bozsoki et al., 2020). In rice, short-length chitooligosaccharide (CO4) triggers symbiotic signal transduction with the symbiotic complex receptor MYR1–CERK1.This suppresses the formation of the CEBiP-CERK1 heteromer that would mount the immune response, while long-chain chitooligosaccharide (CO8) induces immune signalling through CEBiP-CERK1 (Chiu and Paszkowski, 2021; Zhang et al., 2021). It has also been observed that fungal endophytes produce chitin deacetylases, which deacetylate chitosan oligomers that are thus not perceived by plant receptors (Cord-Landwehr et al., 2016). There is also evidence where endophytic bacteria are known to produce their own MAMPs, which are either not recognized by PRRs of plants or trigger in plants a comparatively weak and transient defence reaction compared to pathogenic interactions (Vandenkoornhuyse et al., 2015). Along this line, it was shown that in grapevine (Vitis vinifera L.), thanks to an alteration in sequence, the perception of flagellin from an endophytic Burkholderia phytofirmans by LRR-RLK (Leucine-Rich Repeat-Receptor-Like Kinase) FLAGELLIN-SENSITIVE 2 (FLS 2) PRR was different from the perception of those of bacterial pathogens, such as Pseudomonas aeruginosa or Xanthomonas campestris (Trdá et al., 2015). Still, there are knowledge gaps about the genetic mechanisms that differentiate recognition strategies deployed by beneficial with respect to pathogenic microbes, that need to be filled through comparative genomic studies between the two microbial categories, complemented with functional analyses. Availability of complete information on gene functions involved in the endophytic recognition would allow targeted modifications of favourable strains through gene editing and/or over-expressing approaches that would improve the plants capability in recognizing microbial symbionts and protecting them from the immune response.
4.3 Colonization
Potential entry points for endophytes are cracks formed at the emergence of lateral roots, zones of root elongation, root hair cells, and wounds. Other sources include stomata, particularly of young stems and leaves, lenticels, and germinating radicles. For successful colonization, some bacteria must find their way to these apertures. Klebsiella pneumoniae 342 (Kp342) can colonize the lateral root junctions in wheat and alfalfa (Medicago sativa L.) (Dong et al., 2003). Similarly, Herbaspirillum seropedicae and Gluconacetobacter diazotrophicus dominate colonization at lateral root junctions (James et al., 1997; Luna et al., 2012). Some endophytes enter through infection colonization, where cellulolytic and pectinolytic enzymes produced by endophytes come into play (Miliute et al., 2015), such as pectate lyase, which has been implicated in the colonization of Klebsiella strains (Kovtunovych et al., 1999). Symbionts can colonize hosts while overcoming the response to Damage-Associated Molecular Patterns (DAMPs) and MAMPs, while a response against pathogens is still possible in the presence of non-pathogenic microbes (Zhou et al., 2020). Several studies have proven that there is a downregulation of plant defence pathways during the colonization of plants by mutualistic partners, such as rhizobia or AMF (Fouad et al., 2014; Benhiba et al., 2015). In the case of an oxidative burst or generation of Reactive Oxygen Species (ROS) as plant defence system, endophytes protect themselves by producing enzymes such as superoxide dismutases, catalases, peroxidases, alkyl hydroperoxide reductases, and glutathione-S-transferases (Zeidler et al., 2004). The root endophytic fungus Serendipita indica secretes a histidine-rich protein to improve its access to micronutrients and to influence oxidative stress and reactive oxygen homeostasis to facilitate the colonization of the host plant (Nostadt et al., 2020). Also, symbionts could induce Jasmonic Acid (JA) and suppress Salicylic Acid (SA) formation to Induced Systemic Resistance (ISR), whereas pathogens typically enhance the SA biosynthesis to mediate Systemic Acquired Resistance (SAR) in plants (Martínez-Medina et al., 2017). Moreover, during mutualistic interactions, late induction of SA/JA/ET signalling pathways prevents the microbe from ‘overstepping’ and ‘overpowering’ the plant (Plett and Martin, 2018). It is reported that most miRNAs induced in the host during the establishment of endophytes also target hormone-response pathways (Formey et al., 2014). During AMF infection, the miRNA E4D3Z3Y01BW0TQ is upregulated and disrupts Gibberellic Acid (GA) signalling pathway, known for repressive action against mutualistic associations (Formey et al., 2014; Martín-Rodríguez et al., 2015; Wu et al., 2016). The plant may also induce the expression of different groups of genes during colonization by diverse sets of microbes. For example, during the establishment of symbiosis, the majority of pathways targeted by miRNAs for plant defence system are turned off, thus preventing the obstacle to the proliferation of endophytes (Plett and Martin, 2018). For AMF, two receptor-like kinases called Arbuscular Receptor-like Kinase 1 (ARK1) and ARK2 are required for the sustenance of the symbiotic interaction in several plant species (Montero et al., 2021). Moreover, AMF are separated from the plant cytoplasm by a specialized host-derived membrane, which represents the main interface facilitating the bidirectional exchange of nutrients and information and protects the microbial symbionts from the immune response (Huang et al., 2021). The biosynthesis of this peri-arbuscular membrane is controlled by a gene called GLUCOSAMINE INOSITOL PHOSPHORYLCERAMIDE TRANSFERASE1 (GINT1) (Moore et al., 2021). Protein Secretion Systems (SSs) in bacteria also modulate the plant immune system. Among all known SSs, Type III Secretion System (T3SS) and Type IV Secretion System (T4SS) are essential for delivering Effector Proteins (EFs) by the pathogenic bacteria into the plant, but these are either absent or present in low abundance in mutualistic endophytic bacteria (Green and Mecsas, 2016; Liu et al., 2017). Notable exceptions can be seen in some rhizobial strains where T3SS is important for nodulation of some legumes (Ausmees et al., 2004; Okazaki et al., 2016, 2013). The T3SS is also a determinant for rice endophyte colonization by non-photosynthetic Bradyrhizobium spp (Piromyou et al., 2015). Furthermore, the Type 2 Secretion System (T2SS) was demonstrated to be required for suppressing MAMP-triggered immunity in efficient root colonizer bacteria and, notably, enhanced the colonization capacity of other tested commensal bacteria in Arabidopsis (Teixeira et al., 2021). On the other hand, in mutualistic proteobacterial endophytes, Type VI Secretion Systems (T6SSs) are present, and are also commonly found in commensal and pathogenic plant-associated bacteria. However, they are associated with important functions, which are apart from virulence, usually such as competition against other bacteria (Reinhold-Hurek and Hurek, 2011; Bernal et al., 2018). From this picture, it emerges that colonization involves a plethora of traits from both, plants and microorganisms and available data most likely shed light only on a small fraction of the involved processes. Considering the plant side, in addition to the information provided above, recent investigations highlighted that plant genes can shape the microbiota composition (Zhang et al., 2019; Deng et al., 2021; Escudero-Martinez et al., 2022; Oyserman et al., 2022; Escudero-Martinez and Bulgarelli, 2023) and that wild germplasm is supposed to support higher microbiome diversity than domesticated counterparts (e.g (Pérez-Jaramillo et al., 2018; Nerva et al., 2022). Taken together, these results indicate that there is room for genetics interventions addressed to increase both plant and beneficial microbial aptitude in establishing favourable interactions and that further functional and multi-omics investigations can increase the available targets for improving endophytic colonization by plant growth promoting microorganisms. Once plants and microbial effective targets are identified, these could be modified/introgressed/engineered into their respective genomes (Arif et al., 2020; Nerva et al., 2022; Escudero-Martinez and Bulgarelli, 2023).
5 Omics for the study of plant-endophyte interactions
The intricate network of interactions among the various actors of the microbiota requires the use of advanced techniques with higher likelihood of obtaining global information from the organisms. This is to decipher a complex system and attempt to clarify the role of each organism at the genetic, transcriptional, metabolic, and physiological/phenotypic level.
The microbiota consists of several microorganisms inhabiting soil layers and distinct plant tissues (Bulgarelli et al., 2013; Compant et al., 2019), among which different relationships can be established, depending on environmental factors. A multi-layer communication web organizes the connections among the microorganisms, between the different plants growing in the same soil, and between plants and microorganisms (Hassani et al., 2018; Xiong et al., 2021). Much has been learned about these mechanisms in recent years, thanks to the advent of Next Generation Sequencing (NGS) and, more broadly speaking, to the “omic” technologies, i.e. genomics, transcriptomics, proteomics, and metabolomics. The plant-microbe scientific community has greatly benefited from them.
5.1 Genomics and metagenomics
The development of NGS technologies has allowed to perform whole genome sequences of numerous fungi and bacteria. Overcoming the limit of traditional culture-dependent identification approaches, it has enabled the identification of as much microbial diversity as possible. Meta-genomic approaches nowadays almost routinely make use of DNA extraction from the whole soil/tissue microbial population, allowing the analysis of its gene/taxa content using next generation sequencing (Allan, 2014). The sequencing can involve the whole genome, which is then tentatively assembled and annotated, or only the 16S rRNAs. These data can be used to study the microbial diversity and to evaluate the absolute abundance of different bacterial strains, taking into account the different copy number of 16S rRNA genes in distinct bacterial genomes (Case et al., 2007; Wang et al., 2020).
It is worth mentioning that the availability of several AM fungi genomes has allowed for the study of the evolution of these organisms, which are considered as living fossils and ancient asexuals (Parniske, 2008). Their genome size is highly variable, from the 39.6 Mb of Paraglomus occultum (Malar C et al., 2022) to 784 Mb of Gigaspora margarita (Venice et al., 2020), with large genomes hosting a higher number of genes and a high proportion of transposable and active elements (Venice et al., 2020; Dallaire et al., 2021); differences that could explain their intra-specific variability.
In parallel, the study of the epigenome variability is emerging as a tool to understand a hidden layer of variability (Chaturvedi et al., 2021). Another interesting example of recent scientific advances given by the most recent sequencing technologies concerns the use of long-read sequencing and chromatin conformation capture techniques that made it possible to understand the genomic organization of multi-nucleate coenocytic hyphae of AM, demonstrating their heterokaryotic nature and supporting rare sexual reproduction events (Yildirir et al., 2022; Sperschneider et al., 2023).
5.2 Transcriptomics
This approach, coupled to advanced bioinformatic pipelines, for example using algorithms of artificial intelligence, could be considered as the most useful omic science for understanding the network of interactions. It has largely benefited from NGS technologies, whose recent advances have significantly increased the sensitivity to catch the rarest transcripts. Moreover, long-read sequencing technologies in the Iso-Seq approach, among others, allow to cover the entire transcript length thereby distinguishing rare isoform resulting from alternative splicing events (Li et al., 2017; Zhang et al., 2019).
Transcriptomics has been successfully applied to uncover the plant molecular strategies used to recruit the most favourable microbial organisms in response to diverse abiotic and biotic stresses, and to understand the microbial molecular networks used to successfully establish the symbiotic relationships (Sheibani-Tezerji et al., 2015). Furthermore, transcriptomic studies applied to bacterial cells may help decipher which strains and cells, among the endophytic or rhizospheric population, are transcriptionally active (Sharma et al., 2004; Knauth et al., 2005; Sheibani-Tezerji et al., 2015), surmounting DNA-based technologies that cannot distinguish non-viable cells. The completion of whole genome sequencing of new microbial species and strains will be crucial allowing the identification of the microbial response to different soil characteristics and plant genotypes.
RNA-seq has also been applied to the population of small RNAs, to identify and characterize plant miRNAs involved in host-microbiota communications. These small non-coding RNAs are important key regulators of different plant biological pathways, from organ development to stress response. It has been shown that microorganisms might stimulate the expression of plant miRNAs, modulating drought tolerance response, nutrient uptake, or facilitating symbiosis establishment (Mohsenifard et al., 2017; Pentimone et al., 2018; Kord et al., 2019; Tao et al., 2023). Besides plant miRNAs, other small RNA-like molecules are coded by fungi and bacteria, that could be involved in an intriguing system of cross-kingdom RNAi-mediated regulation, for example during mycorrhizal colonization (Silvestri et al., 2019), or nodule formation (Ren et al., 2019). The intriguing hypothesis of small RNAs as mobile cell-to-cell signalling molecules (Huang et al., 2019) has been explored in detail thanks to the possibility to purify Extracellular Vesicles (EV). In fact, EVs have been shown to transport small RNAs between plant host and microorganisms in both pathogenic and mutualistic interactions (Cai et al., 2019), thus their further analysis will deepen our understanding of below-ground inter- and intra-kingdom communications. Single cell transcriptomics, coupled with enhanced microscopy techniques will greatly improve our understanding of endophyte bacteria and AM fungi lifestyle inside the plants (Yin et al., 2023) and of root cells regulatory network.
5.3 Proteomics and metabolomics
In parallel with next-generation and third-generation (or single molecule) (Schadt et al., 2010) sequencing technologies, which have been successfully applied to the study of all the DNA/RNA populations present in a tissue, the analyses aimed at characterizing the entire set of proteins, with their post-translational modifications, and metabolites have evolved. This evolution is to comprehensively study all the molecules in a microbe/plant biological system, thereby increasing their sensitivity and throughput. Proteomics has been applied to plant tissues to understand how the presence of an endophyte, for example, may modulate the synthesis of different plant proteins (Lery et al., 2011), revealing their role in cellular recognition. The analysis of the protein-protein interactions, also called an interactome, is essential to unveil molecular mechanisms at the base of symbiotic relationships.
Metabolomics and proteomics have been used to analyse root exudates, containing both primary and secondary metabolites, to understand how biotic and abiotic factors might modulate their composition, and as a result, attract and associate with different microorganisms. However, analysing either the metabolites or the proteins present in a colonized plant tissue, or both, is challenging. This is because it is difficult to distinguish between molecules produced by either the plants or the fungi/bacteria. Recently, to resolve this issue, several techniques have been developed to narrow the analyses to the single-cell level, such as Mass Spectrometry Imaging (Boughton and Thinagaran, 2018), Laser ablation electrospray ionization (Bhattacharjee et al., 2020), live single-cell mass spectrometry (Masuda et al., 2018), and the spatial metabolomics pipeline (Geier et al., 2020).
In addition to soluble metabolites, plants can diffuse Volatile Organic Compounds (VOCs).Metabolomics is essential to uncover the role of these signalling molecules and their modulation in response to environmental stimuli and genotype interactions. However, their role in soil matrices could be less abundant and relevant than in aerial open-air environments.
Metabolomic analyses have shown that plants can influence their microbiota by secreting various metabolites. In turn, the microbiome can influence the metabolome of the host plant (Haichar et al., 2008).
It is now clear that to acquire global information on the interconnections existing among plants and the microbiota, single omics technologies should be integrated into a multi-omics approach (Chen et al., 2021). To this end, the development of bioinformatic tools and networking models that can integrate and visualize information is essential. This will provide a comprehensive view of the regulatory network connecting all the molecular levels from the genome to the metabolic pathways. By employing a multi-omics approach, it will be possible to deepen our knowledge on the complex interactions between plants and their growth-promoting microbial counterparts. This will be fundamental to understand how to engineer microbial communities and plants for a more sustainable agriculture. In this scenario it is fundamental to develop high-throughput phenotyping platforms, to measure and analyze qualitative and quantitative traits on a large scale, developing suitable phenomics approaches, that could be non -invasive and able to work in the field as well, in order to fill the gap with other omics techniques (Großkinsky et al., 2015).
6 The role of endophytes in protection from abiotic stresses
Plants, as sessile organisms, face continuous exposure to environmental stresses. These include both biotic factors, such as pathogens, pests and herbivores, and abiotic factors, such as heat, cold, drought, salinity, waterlogging, heavy metal toxicity, nutrient deficiency, and oxidative stresses (Cramer et al., 2011). Climate change has been increasing the negative effects of these abiotic stresses, leading to both faster events of severe stresses (e.g., flash droughts (Pendergrass et al., 2020)), and to more prolonged periods of stress, with several negative impacts on plant growth and productivity, up to more than -50% (Mahajan and Tuteja, 2005; Lohani et al., 2020; Sandrini et al., 2022).
Abiotic stress like heat or cold extremes can cause changes in membrane fluidity and protein structure, while the presence of salt or heavy metals in the soil can alter the physiological processes of enzymes and molecular interactions (Zhang et al., 2022). Salinity can also negatively impact the photosynthetic components, reducing the assimilation of CO2 and the absorption of light. This, in turn, can lead to an increase of ROS and oxidative stress (Ma et al., 2020). It is important to consider that heat, drought, and salt stress are commonly present together, exacerbating the detrimental effects on plants. To sense and respond to abiotic stresses, plants have evolved multiple complex mechanisms, which have been extensively reviewed in the last decades (Zhang et al., 2022).
Considering this negative scenario, plant-associated microorganisms appear to be promising allies for modern agriculture to face climate change (Figure 2; Table 1). For example, many rhizobacteria produce osmoprotectants in the presence of stress conditions, while other bacteria, like Pseudomonas spp., produce ExoPolySaccharides (EPS) to increase water retention in case of drought stress (Rathinasabapathi, 2000; Grover et al., 2011). The mechanisms of both interactions and potential advantages exploitable in agriculture are reviewed for each stress type.
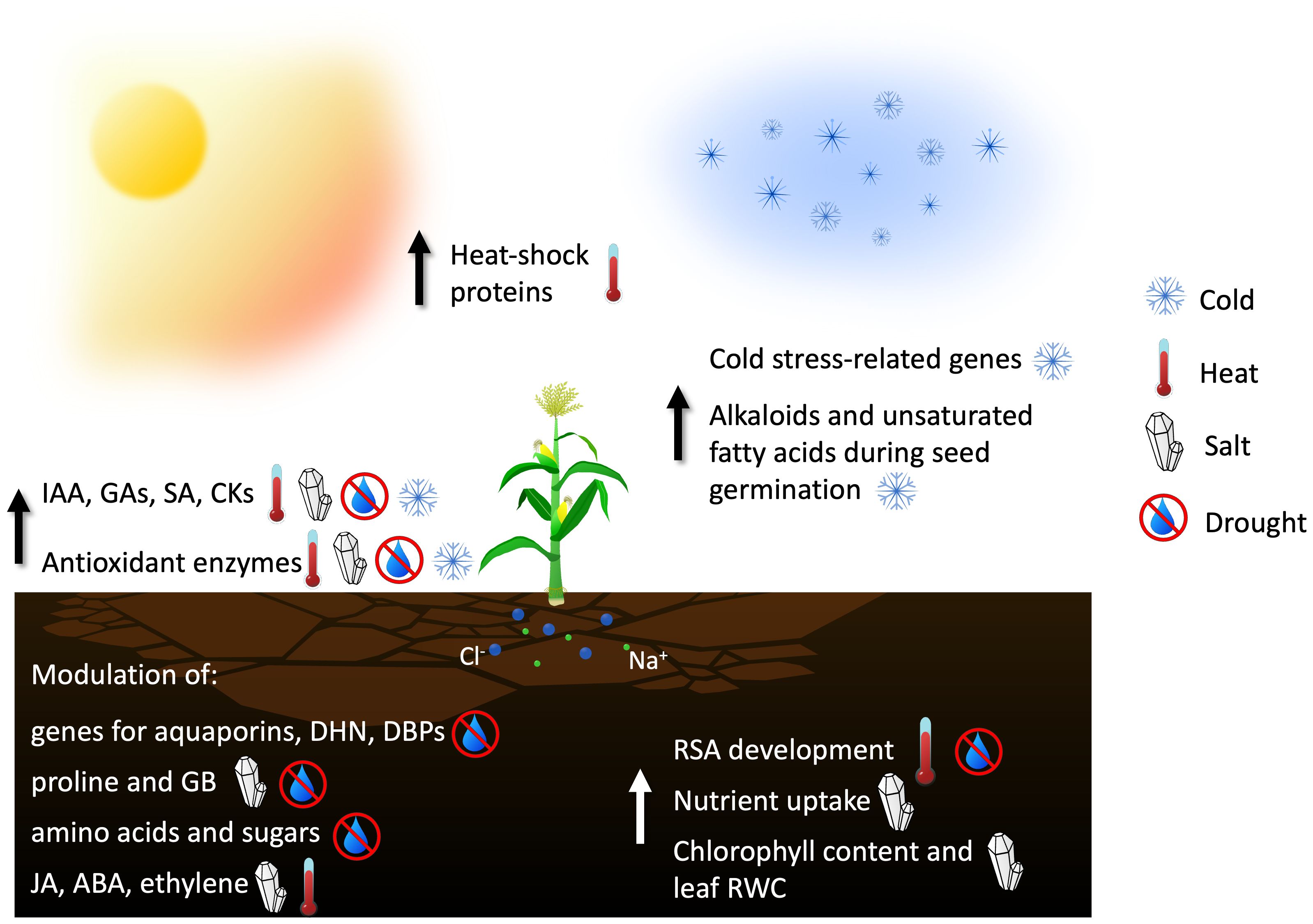
Figure 2 Main contributions of endophyte microorganisms in enhancing plant tolerance to abiotic stresses through increasing the synthesis of antioxidant molecules and heat-shock proteins, modulating the amount of phytohormones, or stimulating the development of the root structure (IAA, Indole-3-Acetic Acid, indicating auxins in general; GAs, Gibberellins; SA, Salicilic Acid; CKs, Citokinins; DHN, Dehydrin family of proteins; DBPs, Dehydration responsive element Binding Proteins; GB, Glycine Betaine; JA, Jasmonic Acid; ABA, Abscissic Acid; RSA, Root Structure Architecture; RWC, Relative Water Content).
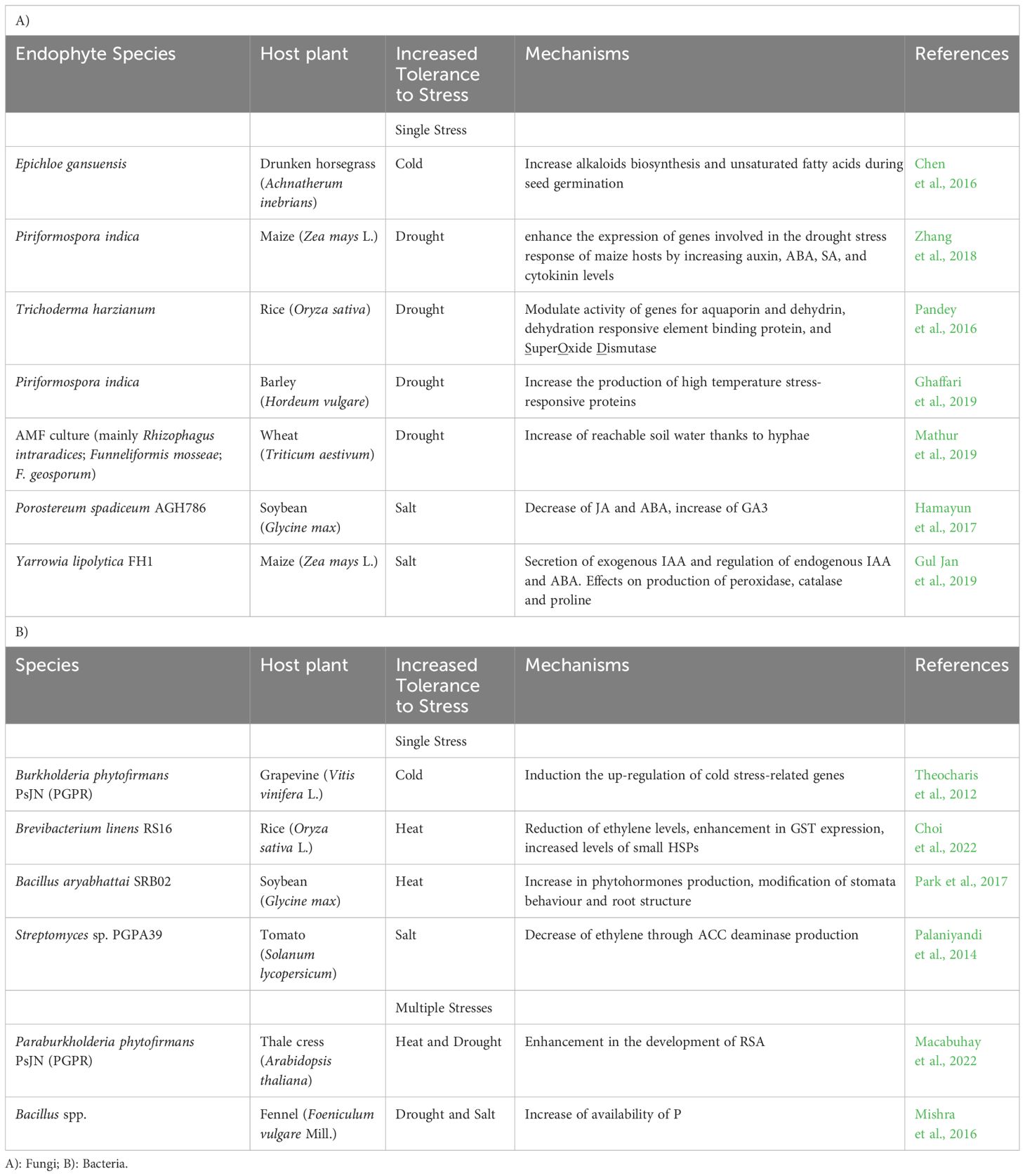
Table 1 Overview of mechanisms of protection of plants from abiotic stresses by beneficial endophytes.
B).
6.1 Temperature
Temperature changes are not necessarily harmful to plants; they can play a crucial physiological role in regulating internal clocks and controlling processes like the opening and closing of flower corollas. Some species require exposure to low temperatures to initiate important developmental processes, such as vernalization for flowering or germination (Ruelland and Zachowski, 2010). However, temperatures that are too low can induce a range of physiological responses that can be detrimental to their survival. Chilling, intended as a few degrees above 0°C air temperature, can cause reductions in enzymatic activity, rigidification of membranes, destabilization of protein complexes, and stabilization of RNA secondary structure, while also promoting the accumulation of ROS. Additionally, chilling can impair photosynthesis and increase membrane permeability, resulting in the leakage of cellular contents. Freezing (below 0°C) stress, on the other hand, can cause more severe damage, as ice formation within cells leads to mechanical disruption and cell/tissue/plant death (Ruelland and Zachowski, 2010).
There are not many reports available in literature about the protective effects of endophytes against the low temperature stresses, perhaps because such conditions also limit the growth and multiplication of microorganisms. As an example of protective effects towards cold stress, the endophytic fungus Epichloe gansuensis increases the biosynthesis of alkaloids and unsaturated fatty acids during the seed germination of Drunken horsegrass (Achnatherum inebrians), thereby increasing tolerance to cold stress (Chen et al., 2016). It was also reported that the endophytic rhizobacterium Parabulkholderia. phytofirmans PsJN induced the upregulation of some cold stress-related genes in grapevine (Theocharis et al., 2012; Chen et al., 2021).
Heat stress is defined as a temperature rise of 10-15°C above ambient, with the optimal range for plant growth about 15-24°C. Among all the different abiotic stress factors, it has the most detrimental effects on plants, reducing flower fertility and modifying crop growth with a detrimental final effect on yield (Shaffique et al., 2022). Its effects include an increase in membrane fluidity, the formation of ROS, and alterations to photosynthesis and respiration processes (Banerjee and Roychoudhury, 2018; Shaffique et al., 2022). Heat stress triggers a cascade of physiological responses that result in the release of Heat Shock Proteins (HSPs), a class of molecular chaperones that facilitate protein folding and prevent aggregation under conditions of cellular stress. These proteins can assist unfolded proteins in refolding into their proper conformation or direct them towards degradation through ubiquitination processes (Ruelland and Zachowski, 2010; Banerjee and Roychoudhury, 2018). The often simultaneous presence of heat and drought stress exhibits holistic features, as the combined effects are greater than those caused by each stress individually (Lipiec et al., 2013).
The inoculation of the endophytic bacterium Brevibacterium linens RS16 in rice plants reduced the emission of the plant stress hormone ethylene due to its 1-aminocyclopropane-1-carboxylate (ACC) deaminase activity. Plants inoculated with B. Linens RS16 also showed increased levels of small HSPs (Choi et al., 2022).
A recent report demonstrates that the application of the plant growth-promoting root endophyte Paraburkholderia phytofirmans PsJN enhances the development of the Root System Architecture (RSA) in Arabidopsis thaliana, under both normal and high-temperature conditions. This allows the plant to access a larger soil area, thereby better managing abiotic stresses such as heat and drought (Macabuhay et al., 2022).
The simultaneous presence of heat and salinity stress can greatly impact crops. The inoculation of the endophytic fungus Trichoderma virens SB10, along with Glycine Betaine (GB) treatments, conferred significant tolerance in soybean (Glycine max L.) plants against these two stresses. In presence of GB, T. virens SB10 enhanced the production of hormones like gibberellins, IAA, and SA. The co-treatment with the fungus and GB also led to a reduction in proline accumulation and Na+ uptake and an increase in macronutrient (N, Ca, K) uptake. Effects on GmHKT1 and GmSOS1 gene expression, two major genes involved in salt tolerance (Singh and Roychoudhury, 2021), were also recorded, leading to the maintenance of a high K+/Na+ ratio. Treated plants exhibited higher growth rates and an increase in antioxidant activities due to the upregulation of Ascorbate PeroXidases (APX), SuperOxide Dismutases (SOD), PerOXidases (POD) and reduced Glutathione (GSH) enzymes (Bilal et al., 2023).
6.2 Drought and salinity
These two stresses represent the main abiotic stress factors that limit crop production globally (Trenberth et al., 2014; Rodriguez and Durán, 2020).
Drought is defined as a period when the available water is insufficient for an organism or environment to function at its best (Kamran et al., 2022). Drought stress represents one of the most critical threats to plant productivity, and thus to global food production, affecting all the stages of plant growth. It leads to a reduction in turgor pressure, affecting cell division, enlargement, and differentiation (Farooq et al., 2009, 2009).
Drought thus affects plants in many ways: typical symptoms include stunted plants, scorching, rolling, and yellowing of leaves, and permanent wilting (Seleiman et al., 2021). Moderate drought stress can induce modifications in the RSA and in the allocation of resources by the plant. In the case of severe drought stress, the roots shrink, and alterations occur at PhotoSystem II (PS II) (Ma et al., 2020).
Soil salinity is defined as the increased amount of sodium (Na+) and especially chloride (Cl-) ions in soils, resulting primarily from natural events such as weathering of parent rocks, seawater, or atmospheric deposition. Other than that, anthropogenic processes, such as poor drainage facilities, irrigation with brackish groundwater, unsuitable water management, and ‘cultural’ errors in irrigated agriculture, can increase soil salinity (Evelin et al., 2019). Salinity causes ionic imbalance and alters metabolic pathways in plant cells, like the synthesis of proteins and the function of some enzymes and ribosomes. Besides, Na+ competes with other essential nutrients like phosphate, nitrate and potassium (Angon et al., 2022).
From several reports, it became evident that the plant microbiome can play a role in protecting against high salinity and drought (Yang et al., 2009; Rolli et al., 2015; Berg et al., 2016).
The endophytic fungus Piriformospora indica enhances the expression of genes involved in the drought stress response of maize hosts by increasing auxin, ABA (ABscissic Acid), SA, and cytokinin levels (Zhang et al., 2018). Also, Trichoderma harzianum was shown to improve drought tolerance in rice, by modulating the activity of genes for aquaporin and dehydrin, Dehydration responsive element-Binding Protein (DBP), and SOD (Pandey et al., 2016).
Symbiotic relationships between plants and endophytic fungi such as Piriformospora indica can enhance the adaptation of plants to drought stress by regulating amino acid and soluble sugar metabolism. For instance, P. indica was found to improve the adaptation of barley (Hordeum vulgare L.) to drought stress (Ghaffari et al., 2019). Soybean inoculated with the endophytic fungus Porostereum spadiceum AGH786 under saline conditions showed reduced effects of salinity. The endophyte caused decreasing levels of JA and ABA and increasing levels of GA3, leading to improved plant growth (Hamayun et al., 2017). Similarly, researchers observed a positive effect of the interaction between the endophytic fungus strain Yarrowia lipolytica and maize under saline conditions, which improved plant growth attributes such as leaf relative water content, levels of oxidative enzymes and chlorophyll content through the enhancement of metabolism and hormones (ABA and IAA) secretions (Gul Jan et al., 2019).
Endophytic microbes can alleviate the salt-generated oxidative stress in plants by activating genes for ion transporters, ROS scavenging, and by activating the production and signalling of phytohormones such as auxin, JA, and Ethylene (ET) (Brotman et al., 2013; Ghaffari et al., 2016; Qin et al., 2018; Eida et al., 2019).
Finally, seed bio-priming is a novel beneficial technique aiming to employ bio-stimulating agents like growth-promoting microorganisms to improve the physiological functioning of seeds and their stress resilience (Chakraborti et al., 2022). Two salt-tolerant endophytic fungi, Paecilomyces lilacinus KUCC-244 and Trichoderma hamatum Th-16 were used for bio-priming wheat and mung bean (Vigna radiata L.) seeds. Results showed that both endophytes, in particular T. hamatum Th-16, increased the growth and chlorophyll content of wheat and mung bean plants under extreme salinity conditions. The primed plants also exhibited increased activities of antioxidant enzymes and enhanced photosynthetic attributes (Irshad et al., 2023).
7 The role of endophytes in biotic stresses tolerance
Biotic stress occurs when the plant is damaged by phytopathogens, such as bacteria, fungi, viruses, insects, or herbivores that feed and thrive at the plant’s expense. It is the primary cause of harvest losses, especially those caused by bacterial and fungal phytopathogens (Chaudhary et al., 2022). It is estimated that biotic stresses cause approximately 17-30% of global crop production loss (Muthu Narayanan et al., 2022).
Until now, the standard procedure to combat plant pathogens infection has been the use of chemicals. This mechanistic approach often does not consider any ecosystemic interaction. However, pesticides can be hazardous, and the occurrence of pesticide resistance is another significant factor to consider (Hawkins et al., 2019).
Against these types of stresses, a great deal of research activity and farm-scale applications of beneficial organisms are reported, much more than against other crop limitations, such as abiotic stresses. Biological control represents a promising strategy to manage plant pathogens sustainably. The first uses of insect parasites date back to the end of the 19th century (Hajek et al., 2007). Biological control involves applying either beneficial organisms, or substances produced by them, such as enzymes, phytohormones, and secondary metabolites, to alleviate the negative effects caused by pathogens and stimulate favourable reactions in the plant (Muthu Narayanan et al., 2022).
Endophytes can use direct mechanisms to exert their biocontrol effects against phytopathogens, including the production of siderophores, to limit the availability of metal ions to pathogens, or the synthesis of antifungal compounds, or competition for a biological niche. They can also counteract pathogens through indirect mechanisms, by inducing SAR and ISR in the host plant (Pandey et al., 2019; Kamle et al., 2020; Figure 3; Table 2).
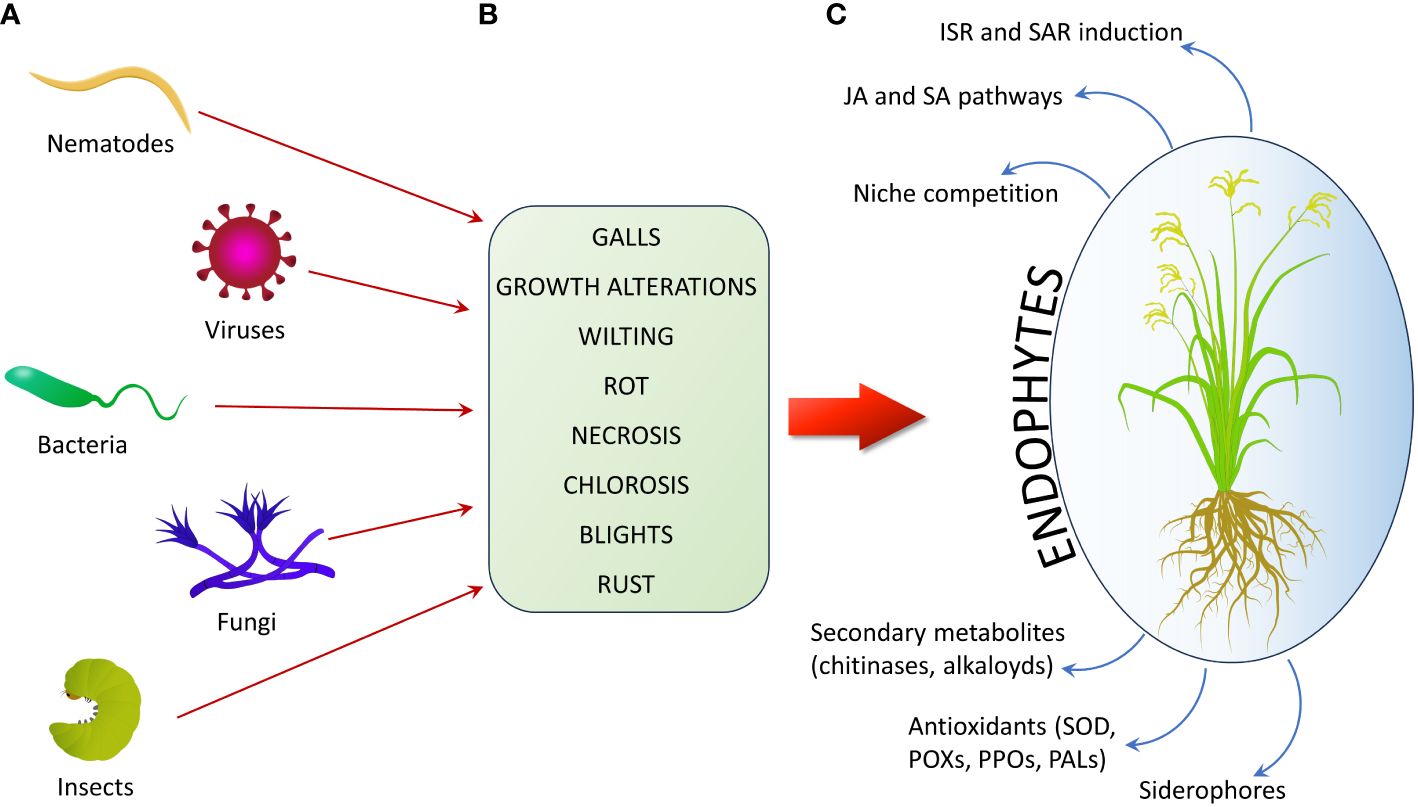
Figure 3 Schematic example of plant damages caused by phytopathogens and relative mechanisms by which endophytes can help crops contrasting pests. (A) Main groups of plant pathogens. (B) Principal types of damages caused by phytopathogens. (C) Main plant defense responses enhanced by endophytes to contrast plant parasites (ISR, Induced Systemic Resistance; SAR, Systemic Acquired Resistance; JA, Jasmonic Acid; SA, Salicylic Acid; SOD, Super-Oxide Dismutase; POX, PerOXidase; PPO, PolyPhenol Oxidase; PAL, Phenylalanine Ammonia Lyase).
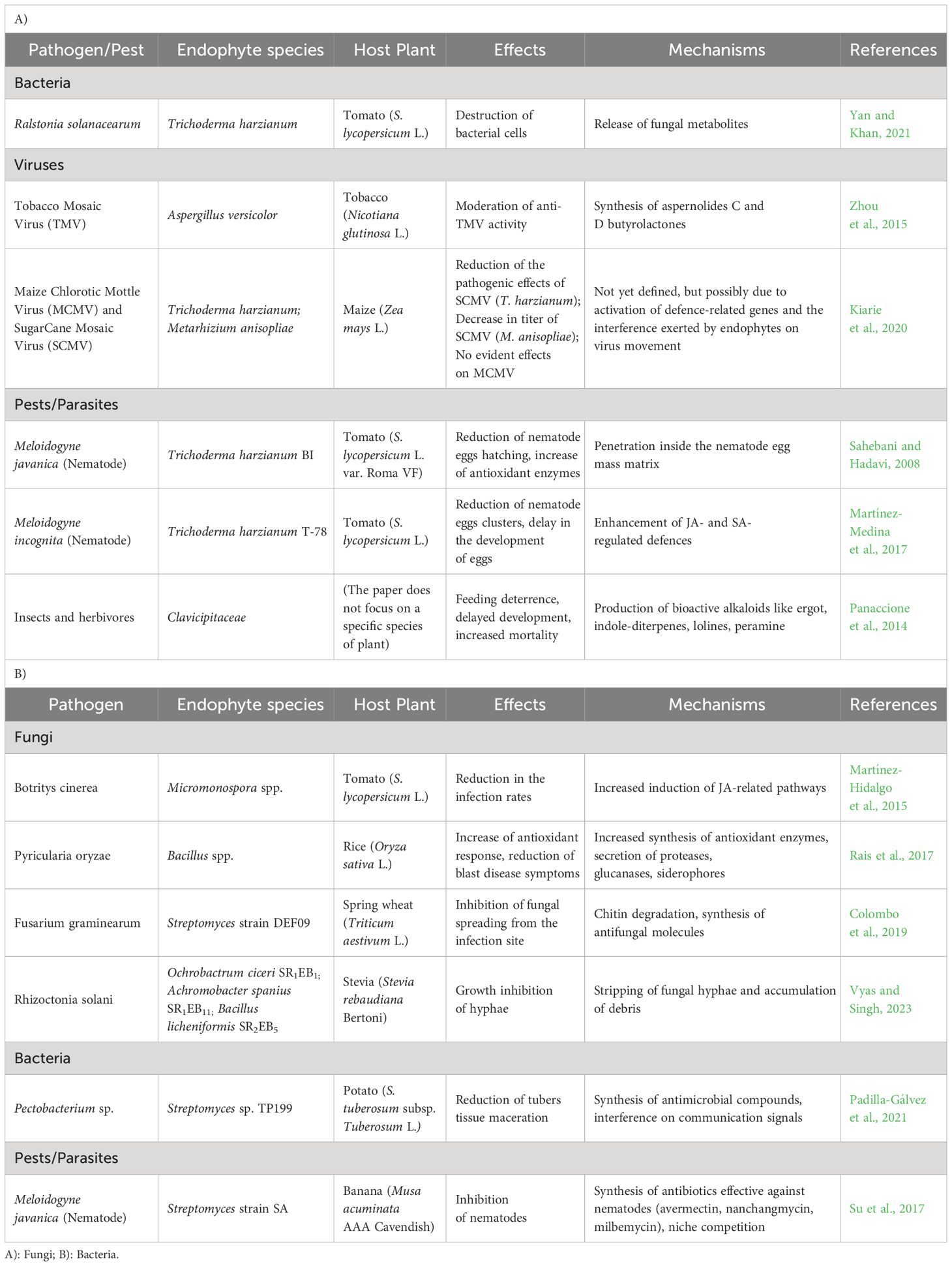
Table 2 Overview of mechanisms of protection of plants from biotic stresses (plant pathogens, pests, and parasites) by beneficial endophytes.
7.1 Bacterial pathogens
Phytopathogenic bacteria are predominantly represented by the genera Agrobacterium, Bacillus, Burkholderia, Clavibacter, Erwinia, Pantoea, Pseudomonas, Ralstonia, Streptomyces, Xanthomonas, and Xylella (Muthu Narayanan et al., 2022). Bacterial diseases can be systemic or localized, with the most common symptoms in plants being galls and overgrowth, wilting, rot, scabs, necrosis, chlorosis, and blights (Nazarov et al., 2020).
Fungal metabolites produced by Trichoderma harzianum have shown strong antibacterial activity against Ralstonia solanacearum, a phytopathogenic bacterium that causes bacterial wilt disease in tomato (Solanum lycopersicum L.) plants (Yan and Khan, 2021).
Endophytic actinobacteria were isolated from Chilean native potatoes (Solanum tuberosum subsp. tuberosum L.) and they were demonstrated to act against Pectobacterium carotovorum subsp. Carotovorum and P. atrosepticum, bacterial pathogens that cause tissue maceration symptoms in potato tubers. One of the isolates, Streptomyces sp. TP199, was found to inhibit the growth of Pectobacterium sp., reducing tissue maceration symptoms. Moreover, strain TP199 showed metal-dependent Acyl Homoserine Lactones (AHL) quorum quenching activity, which can inhibit communication between bacterial cells (Padilla-Gálvez et al., 2021).
7.2 Fungal pathogens
The most common plant pathogenic fungi are Alternaria spp., Aspergillus spp., Colletotrichum spp., Fusarium spp., Phytophthora spp., Pythium, and Pyricularia spp., while anthracnose, dieback, gall, powdery mildew, blight, rust, rot, wilt, and smut are examples of diseases caused by these fungal phytopathogens (Muthu Narayanan et al., 2022).
Some Bacillus strains significantly increased antioxidant enzymes like SOD, PerOXidase, PolyPhenol Oxidase and Phenylalanine Ammonia Lyase in leaves and roots of the rice plant, contrasting the fungus Pyricularia oryzae. They also secreted different biocontrol molecules such as proteases, glucanases, and siderophores in the rice rhizosphere (Rais et al., 2017). In wheat, Fusarium graminearum is the cause of Fusarium head blight as well as Fusarium foot and root rot. Colombo and colleagues (Colombo et al., 2019) studied the biocontrol activity of Streptomyces spp. on F. graminearum in spring wheat and one strain, DEF09, effectively inhibited FHB under controlled and field conditions by blocking the spread of the pathogen at the infection site.
Endophytes with biocontrol potential against Rhizoctonia solani, a fungal pathogen causing sheath blight disease in maize, were isolated from Stevia rebaudiana plants. Three bacterial isolates, identified as Ochrobactrum ciceri SR1EB1, Achromobacter spanius SR1EB11 and Bacillus licheniformis SR2EB5, showed growth inhibition effects against R. solani (Vyas and Singh, 2023).
Furthermore, the gram-positive bacterium Micromonospora, isolated from nitrogen-fixing nodules of leguminous plants, showed biocontrol effects on fungal pathogens and stimulation of plant immunity in tomato. Root inoculation with Micromonospora strains showed reduced infection from the fungal pathogen Botrytis cinerea, and investigations on defence mechanisms highlighted an increased induction in JA-related defence pathways (Martínez-Hidalgo et al., 2015).
7.3 Viral pathogens
Plant viruses are globally diffused plant pathogens, obligatory parasites due to their need for replication within plant cells. Plant viruses are primarily RNA viruses, and the ones considered most important are Tobacco Mosaic Virus (TMV), belonging to the family Virgaviridae, Tomato Spotted Wilt Virus (TSWV) (Tospoviridae), Tomato Yellow Leaf Curl Virus (TYLCV) (Geminiviridae), Cucumber Mosaic Virus (CMV) (Bromoviridae), Potato Virus Y (PVY) (Potyviridae), Cauliflower Mosaic Virus (CaMV) (Caulimoviridae), African Cassava Mosaic Virus (ACMV) (Geminiviridae), Plum Pox Virus (PPV) (Potyviridae), Brome Mosaic Virus (BMV) (Bromoviridae), Potato Virus X (PVX) (Alphaflexiviridae) (Scholthof et al., 2011). Symptoms of viral diseases in plants include growth suppression, deformation, discoloration, necrosis, and impaired reproduction (Nazarov et al., 2020). Investigating the contribution of microbiota towards viral infection (Zhou et al., 2015), identified two new butyrolactones (aspernolides C and D) along with two previously known butyrolactones (aspernolides A and B) from a culture of the endophytic fungus Aspergillus versicolor. When tested against viruses, both aspernolides C and D exhibited moderate anti-TMV activity. Similarly, Kiarie et al. (2020) tested the ability of fungal endophytes to contrast Maize Lethal Necrosis (MLN), a serious disease affecting maize crops in eastern Africa. This disease is caused by the co-infection of maize plants with Maize Chlorotic Mottle Virus (MCMV) (Tombusviridae) and Sugarcane Mosaic Virus (SCMV) (Potyviridae). Maize plants inoculated with T. harzianum and Metarhizium anisopliae showed reduced severity and titer of SCMV, respectively, indicating their potential to induce resistance against SCMV. However, no significant effect was observed on the MCMV.
7.4 Insects and herbivores
Invasive insects cause at least $70 billion in crop losses every year (Bradshaw et al., 2016). Panaccione et al. (2014) summarized a series of studies on the role of bioactive alkaloids produced by endophytic fungi in protecting plants against herbivores. Four major types of bioactive alkaloids (ergot alkaloids, indole-diterpenes, lolines and peramine) are produced by fungi from the Clavicipitaceae family. Symbioses between plants and these endophytes have significant effects on both insects and mammalian herbivores, largely due to the production of these bioactive alkaloids. Ergot alkaloids contribute to herbivore resistance, also affecting nematodes. They also act through feeding deterrence, delayed development, and increased mortality of insects.
Loline alkaloids exhibit insecticidal and feeding-deterrent activity. Lolines are often present in grasses with fungal endophytes of the genera Epichloë and Neotyphodium (Wilkinson et al., 2000). Genetic analyses to determine whether the production of lolines in plants is active against aphids highlighted that the endophyte Epichloë festucae showed heritable variation in the expression of loline alkaloids. Analyses on Lol+ (alkaloid expression) and Lol- (no expression) linked alkaloid expression to activity against aphids, and the levels of alkaloids in the plants were correlated with the level of anti-aphid activity (Wilkinson et al., 2000).
Peramine is the most widely distributed of the four classes of epichloae-derived secondary metabolites. It is another alkaloid that acts as a strong feeding deterrent for different insects. Peramine is water-soluble and is dispersed throughout the plant (Rowan, 1993; Panaccione et al., 2014).
Additional information about the role of Endophytic EntomoPathogenic Fungi (EEPFs) was recently made available by (Samal et al., 2023).
On the other hand, different endophytes could be used not only to prevent herbivore damage in plants but, in some cases, to favour this phenomenon for domestic herbivores, reducing undesirable molecules present in plants for livestock nutrition (Bluett et al., 2005a, 2005b).
7.5 Nematodes
Nematodes are small, non-segmented invertebrates. They are the most abundant animals on Earth and are fundamental to the soil-food web (Gamalero and Glick, 2020). The phylum Nematoda comprises more than 30,000 species (Bernard et al., 2017), classified into five groups: bacterivores, fungivores, herbivores, omnivores, and predators (van den Hoogen et al., 2020).
Nematodes include the so-called Plant-Parasitic Nematodes (PPN), among which some of the most important are root-knot nematodes (Meloidogyne spp.), cyst nematodes (Heterodera spp. and Globodera spp.), and root-lesion nematodes (Pratylenchus spp.) (Kumar and Dara, 2021). Over 4,100 species of PPNs have been identified, causing an estimated $80–$118 billion dollars per year of damage to crops (Bernard et al., 2017). PPNs can damage the host plant through a needle-like oral structure called stylet, used to release specific enzymes inside plant tissues (Pulavarty et al., 2021, p. 202), and the group of root-knot nematodes develop root knots by forming a complex of multinucleate hypertrophied giant cells, which cause visible knots or galls at the root level (Martínez-Medina et al., 2017). More detailed information about nematodes, their characteristics, and modes of action can be found in Jones et al. (2011).
The main way to fight PPNs is to use chemical nematocides, but these are expensive and harmful to the environment, and EU regulations are constantly reducing the nematocides available for agriculture (Poveda et al., 2020). Therefore, the biocontrol of nematode infection is becoming a promising possibility.
Endophytes isolated from banana (Musa acuminata AAA Cavendish) plant roots infected with Meloidogyne spp. were tested against Meloidogyne javanica, and one strain, named SA and identified as Streptomyces spp., showed an inhibition rate of more than 50% in vitro and a biocontrol efficiency of more than 70% in sterile soil against the nematode (Su et al., 2017).
Fungi could also represent a valuable source of biocontrol agents against plant-infecting nematodes. The fungus Trichoderma harzianum strain BI was used against M. javanica (Sahebani and Hadavi, 2008), reducing the infection rates of the nematode through penetration inside the nematode egg mass matrix, leading to reduced hatch levels. T. harzianum BI also increased the activity of resistance-related enzymes in plants, such as POX, PPO, and PAL. Further investigation showed that chitinase activity in T. harzianum BI culture filtrates increased in the presence of colloidal chitin and nematode eggs, implying its potential for degradation of chitin present in nematode eggs.
The root endophyte strain T. harzianum T-78 was used to study the protective effects on tomato plants against the root-knot species M. incognita (Martínez-Medina et al., 2017) using a split-root system, in which the two halves of the plant roots were allowed to grow in two different pots, one for the treatment and the second as a control. T-78 inoculation decreased the amount of root galls, and a significant reduction in the number of nematode egg clusters was observed in systemic tests. Moreover, the expression profile of the SA-responsive marker genes Pathogenesis-Related protein 1a (PR1a) and Pathogenesis-Related protein P6 (PR-P6) was higher in T-78-pretreated plants infected with the nematode and SA concentrations were higher compared with the non-pretreated ones. Finally, the expression analysis of the JA-responsive genes Proteinase Inhibitors II (PI II) and MultiCystatin (MC) after M. incognita infection showed that JA signalling was down-regulated in plants not inoculated with T-78, while in tomato plants pre-inoculated with T-78, the inhibition in genes PI II and MC showed a significant reduction.
More specific reviews are available to deepen the knowledge about the use of endophytes as biocontrol agents against nematodes (e.g., Gamalero and Glick, 2020; Kumar and Dara, 2021).
8 Role of endophytes in nutrition and quality of the final products
Plant–microbe interactions play a crucial role in improving soil nutrition and enriching micronutrients through metal solubilization, mobilization, and translocation to different parts of the plant. Micronutrient deficiency, also known as “hidden hunger”, threatens the health of billions of people worldwide, particularly in developing countries. Additionally, the intensification of crop production is causing a gradual depletion of micronutrients in agricultural soils, compromising the nutritional value of food. Iron and zinc deficiencies are widespread in the human diet, leading to several malnutrition symptoms. To overcome these deficiencies, biofortification, the process of increasing the bioavailable concentrations of essential elements in the edible portion of crop plants, is commonly achieved through plant breeding and agronomic practices. Microbial communities can be exploited as a valid alternative due to their ability to increase metal solubilization in the soil and enhance their mobilization to the plant parts. This is achieved through the production of siderophores or other chelating factors, upregulation of Zn and Fe transporters, acidification of the rhizosphere through organic acid secretion and proton extrusion, reduction of anti-nutritional factors (e.g. phytic acids), and secretion of phenolics or phytohormones like signalling molecules (Singh and Prasanna, 2020). Both bacteria and fungi have demonstrated a positive effect in improving mineral contents in the edible parts of plants, although a major representation of mycorrhizal fungi underlines the importance of this category of endophytes for supporting plant nutrition.
Bacterial endophytes have proven to be effective in the biofortification of wheat grains with Zn (Ramesh et al., 2014; Abaid Ullah et al., 2015; Singh et al., 2017). Wang et al. (2014) found a positive influence of rice inoculation with an endophyte recovered from Zn hyper-accumulator Sedum alfredii on the bioavailability of Zn in the soil and its accumulation in rice grains. Vaid et al. (2014) tested the effect of zinc-solubilizing bacteria on growth promotion and zinc nutrition of rice: bacterial inoculations significantly enhanced the total Zn uptake as well as grain methionine concentration, besides increasing the mean of many agronomic traits, like dry matter yield, productive tillers/plant, number of panicles/plants, number of grains/panicle, grain and straw yield. The screening of 129 strains of endophytic bacteria from maize stem and leaves showed that 24.5% of these isolates were siderophore producers, 14% could solubilize insoluble Zn compounds and 33% of them had phytase activity (Verma et al., 2022). Rana et al. (2012) reported a significant increase in Fe, Mn, and Cu content in wheat grains upon inoculation with the bacterial strain Providencia spp. isolated from the wheat rhizosphere. The screening of a set of 213 endophytes from several wheat genotypes allowed Singh et al. (2018) to identify promising endophytes for Zn and Fe accumulation in wheat grains. At the same time, in grains after endophyte inoculations, phytic acid, an anti-nutritional factor, was significantly decreased.
Subramanian et al. (2013) reported that the inoculation of maize plants with AM fungi improved the availability of micronutrients in soils, particularly Zn, as a consequence of rhizospheric acidification and siderophore production, and produced grains with 10-15% higher Fe and Zn contents, while the anti-nutritional factor phytic acid decreased. In wheat, the application of a consortium of AMF resulted in an increase of micronutrients (Cu, Fe, Zn, Mn) and macronutrients (N, P, K) content in the grain (Mäder et al., 2011). AMF were also able to improve selenium (Se) level in the grain, alone or in association with selenobacteria (Duran et al., 2013). Tang et al. (2022) focused on the effects of endophytic fungus Phomopsis liquidambaris on the absorption and distribution of 14 essential mineral elements in the vegetative organs and in grains of rice: the results indicated that P. liquidambaris significantly increased the accumulation of N, P, Fe, Mn, Zn, Mo, and Se in rice grains, accompanied by a significant increase in yield and protein content. AM fungal inoculation was also effective in improving the nutritional value of chickpea (Cicer arietinum L.) grain by protein, Fe and Zn grain biofortification (Pellegrino and Bedini, 2014).
The interactions of endophytic fungi with plant tissues can also boost secondary metabolite production, resulting in the development of several bioactive compounds. In lettuce (Lactuca sativa L.), AM fungi, in addition to increasing fresh weight, improved the ascorbate level (Baslam et al., 2011). In spinach (Spinacia oleracea L.), they augmented the concentration of total phenolic compounds, flavonoids and phenolic acids (Khalid et al., 2017). In tomato, AMF inoculation, in addition to increasing fruit N, P, and Cu concentration, allowed for higher antioxidant concentration and carotenoid contents (Hart et al., 2015), while in strawberry (Fragaria x ananassa var. Selva) increased concentrations of anthocyanins (Lingua et al., 2013) and of sucrose, glucose, and two vitamins, ascorbic and folic acid (Bona et al., 2015).
Heavy metals contamination of agricultural soils is an important issue all around the world, posing serious risks to food safety. Indeed, although they are not essential elements for a plant’s life, crops uptake heavy metals in soils by root systems, they transport them to aerial parts through the xylem and the phloem, and accumulate them in edible parts, threatening the food chain, and ultimately human health. Under natural conditions, heavy metals in soils originate geologically; however, their amounts are continually increased in soils by anthropogenic sources, i.e., atmospheric deposition of Particulate Matter (PM) from industrial activity and transportation, agricultural activity, such as wastewater irrigation, the application of pesticides and fertilizers (Shi et al., 2018). Among heavy metals, cadmium (Cd) and arsenic (As) are the major contaminant in agricultural soils. Cadmium, with a biological half-life of 10-30 years, has been classified as a potent human carcinogen. Endophytes and AM fungi are involved in alleviating metal toxicity to the host plant. Bacteria evolved various mechanisms to avoid heavy metal stress including: (a) transport of metals across the cytoplasmic membrane; (b) biosorption and bioaccumulation to the cell walls; (c) metal entrapment in the extracellular capsules; (d) heavy metals precipitation; and (e) metal detoxification via oxidation–reduction (Zubair et al., 2016). Several mechanisms have been hypothesized for AMF-mediated detoxification of heavy metals, including (i) bound to cell wall and deposit in the vacuoles of AMF, (ii) sequestration by the help of siderophores in the soil or into root apoplasm, (iii) bound to metallothioneins or phytochelatins inside the fungal or plant cells, and (iv) transporters at the tonoplast of both plants and fungi catalyse the transport of metals from the cytoplasm and allow their compartmentalization into vacuoles (Jan and Parray, 2016).
Among cereals, rice plants tend to accumulate more Cd than others, and this is of particular concern in the larger rice-growing areas, where populations are relying on rice for most of their caloric intake (Hu et al., 2016). Zhou et al. (2021) identified an endophytic bacterium from Cd‐contaminated soil capable of promoting rice growth and reducing Cd concentration in rice grain under Cd‐contaminated conditions.
9 Conclusions and future perspectives
With a focus on endophytes, we have only scratched the surface of the enormous amount of data and information that has been produced by the scientific community worldwide, regarding the complex interactions between plants and microorganisms. Despite significant progress, many challenges remain, both from the technical and the legislative sides.
From a technical point of view, a deep understanding of the biology, way of action, and main interactions of the endophytes in the complex system of microorganism-plant-environment is necessary to optimize their usage in a one-health vision. For example, it is necessary to overcome the technical limits due to the very low fraction of culturable microorganisms inhabiting the soil, the difficulty in maintaining a stable inoculated microbial symbiont in the soil, and in increasing the plant’s aptitude to associate with beneficial microorganisms. Related to this last point, it is relevant to deepen the knowledge related to processes involved in the establishment of successful associations, namely in the attraction, recognition and colonization steps, in order to allow knowledge-driven interventions. Recent investigations uncovered, from the plant side, sorghum, tomato and barley loci affecting microbiome composition (Deng et al., 2021; Escudero-Martinez et al., 2022; Oyserman et al., 2022), thus opening the way for exploiting host-genetics to manipulate and select the crop microbiota (Escudero-Martinez and Bulgarelli, 2023), while from the microbial side new approaches of microbiome engineering that boost the positive impact of the associations are emerging (Arif et al., 2020; Nerva et al., 2022). Increasing the available targets for improving endophytic colonization by plant growth promoting microorganisms rise the possibility that in the near future it will be possible to improve plant association with beneficial microbiota through plant breeding and microbiome engineering approaches. Along these concepts is the realization of knowledge-based synthetic microbial communities (SynComs) or Artificial Microbial Consortia (AMC), that generate a defined microbial system with known taxonomic and functional profiles, thus containing multiple functions for plant growth promotion (Arif et al., 2020; Nerva et al., 2022). This can potentially solve some of the drawbacks of traditional microbial biofertilizers, such a host incompatibility, ineffective competitiveness with indigenous microbes, and inadaptability to the local environment.
Field studies provide the most natural conditions for exploring the roles of endophytes. However, the strong impact of environmental factors makes them highly variable, and often take to unpredictable results. Moreover, agricultural systems and systems intensively used by humans are often characterized by a shift (often a reduction) in microbial diversity; and this may also be extended to plants raised in pot experiments, where we expect a reduced diversity or altered structure of the microbiota (Berg et al., 2016), that pose other limits to their extension to agricultural field systems.
When transitioning from controlled experiments to field applications of potential microbial formulations, the selected strain would have to interact with naturally occurring soil microbes and endophytes. The administered endophytes should be able to colonize a broad spectrum of crops and they should acquire a niche in the plant habitat, avoiding at the same time possible negative alterations of the ecosystem. Additionally, it is also crucial to consider the proper formulation to maximize the beneficial impact on crops, while reducing costs and number of applications (Verma et al., 2021).
Another element of concern is the ability of some endophyte to become a pathogen or produce toxins. For example, fungal endophytes from genera Fusarium, Alternaria, or Aspergillus, possess qualities as PGPM, but are also mycotoxins producers (Stranska et al., 2022).
From a legislative point of view, in the translation from research to application, in each area of the world, the categorizations and rules imposed by current and future legislation on the microbial compounds must be taken into great account for their deployment in the real world. For example, in Europe beneficial microorganisms are divided in two main categories, owing to the target. The microbes that act against biotic targets (e.g. pathogens, pests) are defined as Biological Control Agents (BCA), are enclosed in the plant protection products, and are ruled by the EC Regulation no. 1107/2009.The microorganisms whose target is the mitigation of an abiotic stress (e.g. freezing temperatures, salinity) are defined as Microbial Plant Biostimulants (MPB), and are ruled by EC Regulation no. 1009/2009, within the category PFC 6(A).
The rapidly changing climate presents a complex and daunting challenge that requires urgent solutions, with anthropogenic causes of pollution and environmental degradation continuing to worsen rapidly despite warnings from experts. Even though association of plant with beneficial microorganisms is demonstrated to protect plants from a changing environment, it should also be considered that diverse environmental conditions, including climate changes, can produce currently unpredictable outcomes on the interactions between host plant and endophytic microbiota.
However, there is hope for a better future. As is normal in research, big changes start from small discoveries. The approaches we have explored in this review offer potential solutions to counteract the negative consequences of environmental stressors. Fortunately, ever innovating omic techniques and the ever-expanding set of genomic technologies offer powerful tools to help researchers gain deeper insights into the complex relationships between plants and their microbial partners. By using these tools, together with beneficial endophytes, to develop more eco-friendly and efficient agronomic practices, we can work towards a more sustainable future for our planet.
Author contributions
LS: Conceptualization, Investigation, Visualization, Writing – original draft, Writing – review & editing. EM: Writing – original draft, Writing – review & editing. GV: Conceptualization, Supervision, Visualization, Writing – original draft, Writing – review & editing. PV: Writing – original draft, Writing – review & editing. NP: Conceptualization, Supervision, Writing – original draft, Writing – review & editing.
Funding
The author(s) declare that no financial support was received for the research, authorship, and/or publication of this article.
Acknowledgments
GV and EM would like to acknowledge the AGER Project “Enabling the potential of the unexplored: exploiting tailored microbial consortia to enhance environmental, societal and economic sustainability and resilience of Italian agro-ecosystems - Micro4Life” (Rif. 2022-2903). All authors wish also to thank Dr. Valerio Battaglia of CREA, Centro di Ricerca Cerealicoltura e Colture Industriali, for useful discussions about legislative aspects of the use of beneficial microorganisms in agriculture.
Conflict of interest
The authors declare that the research was conducted in the absence of any commercial or financial relationships that could be construed as a potential conflict of interest.
Publisher’s note
All claims expressed in this article are solely those of the authors and do not necessarily represent those of their affiliated organizations, or those of the publisher, the editors and the reviewers. Any product that may be evaluated in this article, or claim that may be made by its manufacturer, is not guaranteed or endorsed by the publisher.
References
Abaid Ullah, M., Hassan, M., Jamil, M., Brader, G., Shah, M., Sessitsch, A., et al. (2015). Plant growth promoting rhizobacteria: an alternate way to improve yield and quality of wheat (Triticum aestivum). Int. J. Agric. Biol. 17, 51–60.
Abbass, K., Qasim, M. Z., Song, H., Murshed, M., Mahmood, H., Younis, I. (2022). A review of the global climate change impacts, adaptation, and sustainable mitigation measures. Environ. Sci. pollut. Res. 29, 42539–42559. doi: 10.1007/s11356-022-19718-6
Ahmed, T., Shahid, M., Noman, M., Hussain, S., Khan, M. A., Zubair, M., et al. (2019). “Plant growth-promoting rhizobacteria as biological tools for nutrient management and soil sustainability,” in Plant Growth Promoting Rhizobacteria for Agricultural Sustainability : From Theory to Practices. Eds. Kumar, A., Meena, V. S. (Springer, Singapore), 95–110. doi: 10.1007/978-981-13-7553-8_5
Alavaisha, E., Manzoni, S., Lindborg, R. (2019). Different agricultural practices affect soil carbon, nitrogen and phosphorous in Kilombero -Tanzania. J. Environ. Manage. 234, 159–166. doi: 10.1016/j.jenvman.2018.12.039
Allan, E. (2014). Metagenomics: unrestricted access to microbial communities. Virulence 5, 397–398. doi: 10.4161/viru.28057
Ambriz, E., Báez-Pérez, A., Sánchez-Yáñez, J. M., Moutoglis, P., Villegas, J. (2010). Fraxinus–Glomus–Pisolithus symbiosis: Plant growth and soil aggregation effects. Pedobiologia 53, 369–373. doi: 10.1016/j.pedobi.2010.07.001
Angon, P. B., Tahjib-Ul-Arif, M., Samin, S. I., Habiba, U., Hossain, M. A., Brestic, M. (2022). How do plants respond to combined drought and salinity stress?-A systematic review. Plants Basel Switz. 11, 2884. doi: 10.3390/plants11212884
Arif, I., Batool, M., Schenk, P. M. (2020). Plant microbiome engineering: expected benefits for improved crop growth and resilience. Trends Biotechnol. 38, 1385–1396. doi: 10.1016/j.tibtech.2020.04.015
Arora, N. K., Mishra, J. (2016). Prospecting the roles of metabolites and additives in future bioformulations for sustainable agriculture. Appl. Soil Ecol. 107, 405–407. doi: 10.1016/j.apsoil.2016.05.020
Ausmees, N., Kobayashi, H., Deakin, W. J., Marie, C., Krishnan, H. B., Broughton, W. J., et al. (2004). Characterization of NopP, a type III secreted effector of rhizobium sp. Strain NGR234. J. Bacteriol. 186, 4774–4780. doi: 10.1128/JB.186.14.4774-4780.2004
Bago, B. (2000). Putative sites for nutrient uptake in arbuscular mycorrhizal fungi. Plant Soil 226, 263–274. doi: 10.1023/A:1026456818903
Balachandar, D., Sandhiya, G. S., Sugitha, T. C. K., Kumar, K. (2006). Flavonoids and growth hormones influence endophytic colonization and in planta nitrogen fixation by a Diazotrophic Serratia sp. in rice. World J. Microbiol. Biotechnol. 22, 707–712. doi: 10.1007/s11274-005-9094-0
Banerjee, A., Roychoudhury, A. (2018). “Chapter 19 - small heat shock proteins: structural assembly and functional responses against heat stress in plants,” in Plant Metabolites and Regulation Under Environmental Stress. Eds. Ahmad, P., Ahanger, M. A., Singh, V. P., Tripathi, D. K., Alam, P., AlYemeni, M. N. (Academic Press), 367–376. doi: 10.1016/B978-0-12-812689-9.00019-4
Bao, X., Roossinck, M. J. (2013). “Chapter two - multiplexed interactions: viruses of endophytic fungi,” in Advances in Virus Research, Mycoviruses. Ed. Ghabrial, S. A. (Academic Press), 37–58. doi: 10.1016/B978-0-12-394315-6.00002-7
Baslam, M., Garmendia, I., Goicoechea, N. (2011). Arbuscular mycorrhizal fungi (AMF) improved growth and nutritional quality of greenhouse-grown lettuce. J. Agric. Food Chem. 59, 5504–5515. doi: 10.1021/jf200501c
Becquer, A., Trap, J., Irshad, U., Ali, M. A., Claude, P. (2014). From soil to plant, the journey of P through trophic relationships and ectomycorrhizal association. Front. Plant Sci. 5. doi: 10.3389/fpls.2014.00548
Benhiba, L., Fouad, M. O., Essahibi, A., Ghoulam, C., Qaddoury, A. (2015). Arbuscular mycorrhizal symbiosis enhanced growth and antioxidant metabolism in date palm subjected to long-term drought. Trees 6, 1725–1733. doi: 10.1007/s00468-015-1253-9
Berg, G., Rybakova, D., Grube, M., Köberl, M. (2016). The plant microbiome explored: implications for experimental botany. J. Exp. Bot. 67, 995–1002. doi: 10.1093/jxb/erv466
Berg, G., Smalla, K. (2009). Plant species and soil type cooperatively shape the structure and function of microbial communities in the rhizosphere. FEMS Microbiol. Ecol. 68, 1–13. doi: 10.1111/fem.2009.68.issue-1
Bernal, P., Llamas, M. A., Filloux, A. (2018). Type VI secretion systems in plant-associated bacteria. Environ. Microbiol. 20, 1–15. doi: 10.1111/1462-2920.13956
Bernard, G. C., Egnin, M., Bonsi, C., Bernard, G. C., Egnin, M., Bonsi, C. (2017). “The impact of plant-parasitic nematodes on agriculture and methods of control,” in Nematology - Concepts, Diagnosis and Control (IntechOpen). doi: 10.5772/intechopen.68958
Bever, J. D., Richardson, S. C., Lawrence, B. M., Holmes, J., Watson, M. (2009). Preferential allocation to beneficial symbiont with spatial structure maintains mycorrhizal mutualism. Ecol. Lett. 12, 13–21. doi: 10.1111/j.1461-0248.2008.01254.x
Bhattacharjee, A., Velickovic, D., Wietsma, T. W., Bell, S. L., Jansson, J. K., Hofmockel, K. S., et al. (2020). Visualizing microbial community dynamics via a controllable soil environment. mSystems 5, e00645–e00619. doi: 10.1128/mSystems.00645-19
Bilal, S., Shahzad, R., Asaf, S., Imran, M., Al-Harrasi, A., Lee, I.-J. (2023). Efficacy of endophytic SB10 and glycine betaine duo in alleviating phytotoxic impact of combined heat and salinity in Glycine max L. via regulation of redox homeostasis and physiological and molecular responses. Environ. pollut. 316, 120658. doi: 10.1016/j.envpol.2022.120658
Bluett, S. J., Thom, E. R., Clark, D. A., Macdonald, K. A., Minneé, E. M. K. (2005a). Effects of perennial ryegrass infected with either AR1 or wild endophyte on dairy production in the Waikato. N. Z. J. Agric. Res. 48, 197–212. doi: 10.1080/00288233.2005.9513650
Bluett, S. J., Thom, E. R., Clark, D. A., Waugh, C. D. (2005b). Effects of a novel ryegrass endophyte on pasture production, dairy cow milk production and calf liveweight gain. Aust. J. Exp. Agric. 45, 11–19. doi: 10.1071/EA03263
Bona, E., Lingua, G., Manassero, P., Cantamessa, S., Marsano, F., Todeschini, V., et al. (2015). AM fungi and PGP pseudomonads increase flowering, fruit production, and vitamin content in strawberry grown at low nitrogen and phosphorus levels. Mycorrhiza 25, 181–193. doi: 10.1007/s00572-014-0599-y
Bonfante, P., Genre, A. (2008). Plants and arbuscular mycorrhizal fungi: an evolutionary-developmental perspective. Trends Plant Sci. 13, 492–498. doi: 10.1016/j.tplants.2008.07.001
Bonfante, P., Genre, A. (2010). Mechanisms underlying beneficial plant–fungus interactions in mycorrhizal symbiosis. Nat. Commun. 1, 48. doi: 10.1038/ncomms1046
Boughton, B. A., Thinagaran, D. (2018). Mass spectrometry imaging (MSI) for plant metabolomics. Methods Mol. Biol. Clifton NJ 1778, 241–252. doi: 10.1007/978-1-4939-7819-9_17
Bozsoki, Z., Gysel, K., Hansen, S. B., Lironi, D., Krönauer, C., Feng, F., et al. (2020). Ligand-recognizing motifs in plant LysM receptors are major determinants of specificity. Science 369, 663–670. doi: 10.1126/science.abb3377
Brader, G., Compant, S., Mitter, B., Trognitz, F., Sessitsch, A. (2014). Metabolic potential of endophytic bacteria. Curr. Opin. Biotechnol. 27, 30–37. doi: 10.1016/j.copbio.2013.09.012
Bradshaw, C. J. A., Leroy, B., Bellard, C., Roiz, D., Albert, C., Fournier, A., et al. (2016). Massive yet grossly underestimated global costs of invasive insects. Nat. Commun. 7, 12986. doi: 10.1038/ncomms12986
Brotman, Y., Landau, U., Cuadros-Inostroza, Á., Takayuki, T., Fernie, A. R., Chet, I., et al. (2013). Trichoderma-plant root colonization: escaping early plant defense responses and activation of the antioxidant machinery for saline stress tolerance. PloS Pathog. 9, e1003221. doi: 10.1371/annotation/8b818c15-3fe0-4e56-9be2-e44fd1ed3fae
Bulgarelli, D., Garrido-Oter, R., Münch, P. C., Weiman, A., Dröge, J., Pan, Y., et al. (2015). Structure and function of the bacterial root microbiota in wild and domesticated barley. Cell Host Microbe 17, 392–403. doi: 10.1016/j.chom.2015.01.011
Bulgarelli, D., Schlaeppi, K., Spaepen, S., Ver Loren van Themaat, E., Schulze-Lefert, P. (2013). Structure and functions of the bacterial microbiota of plants. Annu. Rev. Plant Biol. 64, 807–838. doi: 10.1146/annurev-arplant-050312-120106
Burleigh, S. H., Cavagnaro, T., Jakobsen, I. (2002). Functional diversity of arbuscular mycorrhizas extends to the expression of plant genes involved in P nutrition. J. Exp. Bot. 53, 1593–1601. doi: 10.1093/jxb/erf013
Buscaill, P., van der Hoorn, R. A. L. (2021). Defeated by the nines: nine extracellular strategies to avoid microbe-associated molecular patterns recognition in plants. Plant Cell 33, 2116–2130. doi: 10.1093/plcell/koab109
Cai, Q., He, B., Weiberg, A., Buck, A. H., Jin, H. (2019). Small RNAs and extracellular vesicles: New mechanisms of cross-species communication and innovative tools for disease control. PloS Pathog. 15, e1008090. doi: 10.1371/journal.ppat.1008090
Case, R. J., Boucher, Y., Dahllöf, I., Holmström, C., Doolittle, W. F., Kjelleberg, S. (2007). Use of 16S rRNA and rpoB genes as molecular markers for microbial ecology studies. Appl. Environ. Microbiol. 73, 278–288. doi: 10.1128/AEM.01177-06
Casieri, L., Ait Lahmidi, N., Doidy, J., Veneault-Fourrey, C., Migeon, A., Bonneau, L., et al. (2013). Biotrophic transportome in mutualistic plant-fungal interactions. Mycorrhiza 23, 597–625. doi: 10.1007/s00572-013-0496-9
Chagas, M. B., de, O., Prazeres dos Santos, I., Nascimento da Silva, L. C., Correia, M. T. d. S., Magali de Araújo, J., et al. (2017). Antimicrobial activity of cultivable endophytic fungi associated with hancornia speciosa gomes bark. Open Microbiol. J. 11, 179–188. doi: 10.2174/1874285801711010179
Chakraborti, S., Bera, K., Sadhukhan, S., Dutta, P. (2022). Bio-priming of seeds: Plant stress management and its underlying cellular, biochemical and molecular mechanisms. Plant Stress 3, 100052. doi: 10.1016/j.stress.2021.100052
Chaparro, J. M., Sheflin, A. M., Manter, D. K., Vivanco, J. M. (2012). Manipulating the soil microbiome to increase soil health and plant fertility. Biol. Fertil. Soils 48, 489–499. doi: 10.1007/s00374-012-0691-4
Chareesri, A., De Deyn, G. B., Sergeeva, L., Polthanee, A., Kuyper, T. W. (2020). Increased arbuscular mycorrhizal fungal colonization reduces yield loss of rice (Oryza sativa L.) under drought. Mycorrhiza 30, 315–328. doi: 10.1007/s00572-020-00953-z
Chaturvedi, A., Cruz Corella, J., Robbins, C., Loha, A., Menin, L., Gasilova, N., et al. (2021). The methylome of the model arbuscular mycorrhizal fungus, Rhizophagus irregularis, shares characteristics with early diverging fungi and Dikarya. Commun. Biol. 4, 1–11. doi: 10.1038/s42003-021-02414-5
Chaudhary, P., Agri, U., Chaudhary, A., Kumar, A., Kumar, G. (2022). Endophytes and their potential in biotic stress management and crop production. Front. Microbiol. 13. doi: 10.3389/fmicb.2022.933017
Chen, N., He, R., Chai, Q., Li, C., Nan, Z. (2016). Transcriptomic analyses giving insights into molecular regulation mechanisms involved in cold tolerance by Epichloë endophyte in seed germination of Achnatherum inebrians. Plant Growth Regul. 80, 367–375. doi: 10.1007/s10725-016-0177-8
Chen, X.-L., Sun, M.-C., Chong, S.-L., Si, J.-P., Wu, L.-S. (2021). Transcriptomic and metabolomic approaches deepen our knowledge of plant-endophyte interactions. Front. Plant Sci. 12. doi: 10.3389/fpls.2021.700200
Chiu, C. H., Paszkowski, U. (2021). How membrane receptors tread the fine balance between symbiosis and immunity signaling. Proc. Natl. Acad. Sci. 118, e2106567118. doi: 10.1073/pnas.2106567118
Choi, J., Roy Choudhury, A., Walitang, D. I., Lee, Y., Sa, T. (2022). ACC deaminase-producing Brevibacterium linens RS16 enhances heat-stress tolerance of rice (Oryza sativa L.). Physiol. Plant 174, e13584. doi: 10.1111/ppl.13584
Classen, A. T., Sundqvist, M. K., Henning, J. A., Newman, G. S., Moore, J. A. M., Cregger, M. A., et al. (2015). Direct and indirect effects of climate change on soil microbial and soil microbial-plant interactions: What lies ahead? Ecosphere 6, art130. doi: 10.1890/ES15-00217.1
Colombo, E. M., Kunova, A., Pizzatti, C., Saracchi, M., Cortesi, P., Pasquali, M. (2019). Selection of an Endophytic Streptomyces sp. Strain DEF09 From Wheat Roots as a Biocontrol Agent Against Fusarium graminearum. Front. Microbiol. 10. doi: 10.3389/fmicb.2019.02356
Compant, S., Samad, A., Faist, H., Sessitsch, A. (2019). A review on the plant microbiome: Ecology, functions, and emerging trends in microbial application. J. Adv. Res. Special Issue Plant Microbiome 19, 29–37. doi: 10.1016/j.jare.2019.03.004
Compant, S., van der Heijden, M. G. A., Sessitsch, A. (2010). Climate change effects on beneficial plant–microorganism interactions. FEMS Microbiol. Ecol. 73, 197–214. doi: 10.1111/j.1574-6941.2010.00900.x
Coombs, J. T., Franco, C. M. M. (2003). Isolation and identification of actinobacteria from surface-sterilized wheat roots. Appl. Environ. Microbiol. 69, 5603–5608. doi: 10.1128/AEM.69.9.5603-5608.2003
Cord-Landwehr, S., Melcher, R. L. J., Kolkenbrock, S., Moerschbacher, B. M. (2016). A chitin deacetylase from the endophytic fungus Pestalotiopsis sp. efficiently inactivates the elicitor activity of chitin oligomers in rice cells. Sci. Rep. 6, 38018. doi: 10.1038/srep38018
Cramer, G. R., Urano, K., Delrot, S., Pezzotti, M., Shinozaki, K. (2011). Effects of abiotic stress on plants: a systems biology perspective. BMC Plant Biol. 11, 163. doi: 10.1186/1471-2229-11-163
Dallaire, A., Manley, B. F., Wilkens, M., Bista, I., Quan, C., Evangelisti, E., et al. (2021). Transcriptional activity and epigenetic regulation of transposable elements in the symbiotic fungus Rhizophagus irregularis. Genome Res. 31, 2290–2302. doi: 10.1101/gr.275752.121
Deng, S., Caddell, D. F., Xu, G., Dahlen, L., Washington, L., Yang, J., et al. (2021). Genome wide association study reveals plant loci controlling heritability of the rhizosphere microbiome. ISME J. 15, 3181–3194. doi: 10.1038/s41396-021-00993-z
Dong, Y., Iniguez, A. L., Triplett, E. W. (2003). Quantitative assessments of the host range and strain specificity of endophytic colonization by Klebsiella pneumoniae 342. Plant Soil 257, 49–59. doi: 10.1023/A:1026242814060
Driga, A. M., Drigas, A. S. (2019). Climate change 101: how everyday activities contribute to the ever-growing issue. Int. J. Recent Contrib. Eng. Sci. IT IJES 7, 22–31. doi: 10.3991/ijes.v7i1.10031
Duran, P., Sobarzo, J., Jorquera, M., Azcón, R., Borie, F., Cornejo, P., et al. (2013). Enhanced selenium content in wheat grain by co-inoculation of selenobacteria and arbuscular mycorrhizal fungi: A preliminary study as a potential Se biofortification strategy. J. Cereal Sci. 57, 275–280. doi: 10.1016/j.jcs.2012.11.012
Easterling, D. R., Meehl, G. A., Parmesan, C., Changnon, S. A., Karl, T. R., Mearns, L. O. (2000). Climate extremes: observations, modeling, and impacts. Science 289, 2068–2074. doi: 10.1126/science.289.5487.2068
Edwards, J., Johnson, C., Santos-Medellín, C., Lurie, E., Podishetty, N. K., Bhatnagar, S., et al. (2015). Structure, variation, and assembly of the root-associated microbiomes of rice. Proc. Natl. Acad. Sci. U.S.A. 112, E911–E920. doi: 10.1073/pnas.1414592112
Eida, A. A., Alzubaidy, H. S., de Zélicourt, A., Synek, L., Alsharif, W., Lafi, F. F., et al. (2019). Phylogenetically diverse endophytic bacteria from desert plants induce transcriptional changes of tissue-specific ion transporters and salinity stress in Arabidopsis thaliana. Plant Sci. 280, 228–240. doi: 10.1016/j.plantsci.2018.12.002
Escudero-Martinez, C., Bulgarelli, D. (2023). Engineering the crop microbiota through host genetics. Annu. Rev. Phytopathol. 61, 257–277. doi: 10.1146/annurev-phyto-021621-121447
Escudero-Martinez, C., Coulter, M., Alegria Terrazas, R., Foito, A., Kapadia, R., Pietrangelo, L., et al. (2022). Identifying plant genes shaping microbiota composition in the barley rhizosphere. Nat. Commun. 13, 3443. doi: 10.1038/s41467-022-31022-y
Evelin, H., Devi, T. S., Gupta, S., Kapoor, R. (2019). Mitigation of salinity stress in plants by Arbuscular mycorrhizal symbiosis: current understanding and new challenges. Front. Plant Sci. 10. doi: 10.3389/fpls.2019.00470
Farooq, M., Wahid, A., Kobayashi, N., Fujita, D., Basra, S. M. A. (2009). Plant drought stress: effects, mechanisms and management. Agron. Sustain. Dev. 29, 185–212. doi: 10.1051/agro:2008021
Favre-Godal, Q., Gourguillon, L., Lordel-Madeleine, S., Gindro, K., Choisy, P. (2020). Orchids and their mycorrhizal fungi: an insufficiently explored relationship. Mycorrhiza 30, 5–22. doi: 10.1007/s00572-020-00934-2
Figueiredo, A. F., Boy, J., Guggenberger, G. (2021). Common mycorrhizae network: A review of the theories and mechanisms behind underground interactions. Front. Fungal Biol. 2. doi: 10.3389/ffunb.2021.735299
Formey, D., Sallet, E., Lelandais-Brière, C., Ben, C., Bustos-Sanmamed, P., Niebel, A., et al. (2014). The small RNA diversity from Medicago truncatularoots under biotic interactions evidences the environmental plasticity of the miRNAome. Genome Biol. 15, 457. doi: 10.1186/s13059-014-0457-4
Fouad, M. O., Essahibi, A., Benhiba, L., Qaddoury, A. (2014). Effectiveness of arbuscular mycorrhizal fungi in the protection of olive plants against oxidative stress induced by drought. Span. J. Agric. Res. 12, 763–771. doi: 10.5424/sjar/2014123-4815
Galic, M., Bilandzija, D., Percin, A., Sestak, I., Mesic, M., Blazinkov, M., et al. (2019). Effects of agricultural practices on carbon emission and soil health. J. Sustain. Dev. Energy Water Environ. Syst. 7, 539–552. doi: 10.13044/j.sdewes.d7.0271
Gamalero, E., Glick, B. R. (2020). The use of plant growth-promoting bacteria to prevent nematode damage to plants. Biology 9, 381. doi: 10.3390/biology9110381
Geier, B., Sogin, E. M., Michellod, D., Janda, M., Kompauer, M., Spengler, B., et al. (2020). Spatial metabolomics of in situ host–microbe interactions at the micrometre scale. Nat. Microbiol. 5, 498–510. doi: 10.1038/s41564-019-0664-6
Geissler, C., Davidson, A., Niesenbaum, R. A. (2023). The influence of climate warming on flowering phenology in relation to historical annual and seasonal temperatures and plant functional traits. PeerJ 11, e15188. doi: 10.7717/peerj.15188
Genre, A., Lanfranco, L., Perotto, S., Bonfante, P. (2020). Unique and common traits in mycorrhizal symbioses. Nat. Rev. Microbiol. 18, 649–660. doi: 10.1038/s41579-020-0402-3
Ghaffari, M. R., Ghabooli, M., Khatabi, B., Hajirezaei, M. R., Schweizer, P., Salekdeh, G. H. (2016). Metabolic and transcriptional response of central metabolism affected by root endophytic fungus Piriformospora indica under salinity in barley. Plant Mol. Biol. 90, 699–717. doi: 10.1007/s11103-016-0461-z
Ghaffari, M. R., Mirzaei, M., Ghabooli, M., Khatabi, B., Wu, Y., Zabet-Moghaddam, M., et al. (2019). Root endophytic fungus Piriformospora indica improves drought stress adaptation in barley by metabolic and proteomic reprogramming. Environ. Exp. Bot. 157, 197–210. doi: 10.1016/j.envexpbot.2018.10.002
Gough, C., Cullimore, J. (2011). Lipo-chitooligosaccharide signaling in endosymbiotic plant-microbe interactions. Mol. Plant-Microbe Interact. MPMI 24, 867–878. doi: 10.1094/MPMI-01-11-0019
Govindarajulu, M., Pfeffer, P. E., Jin, H., Abubaker, J., Douds, D. D., Allen, J. W., et al. (2005). Nitrogen transfer in the arbuscular mycorrhizal symbiosis. Nature 435, 819–823. doi: 10.1038/nature03610
Granér, G., Persson, P., Meijer, J., Alström, S. (2003). A study on microbial diversity in different cultivars of Brassica napus in relation to its wilt pathogen, Verticillium longisporum. FEMS Microbiol. Lett. 224, 269–276. doi: 10.1016/S0378-1097(03)00449-X
Green, E. R., Mecsas, J. (2016). Bacterial secretion systems: an overview. Microbiol. Spectr. 4, 10.1128/microbiolspec.vmbf-0012–2015. doi: 10.1128/microbiolspec.VMBF-0012-2015
Großkinsky, D. K., Svensgaard, J., Christensen, S., Roitsch, T. (2015). Plant phenomics and the need for physiological phenotyping across scales to narrow the genotype-to-phenotype knowledge gap. J. Exp. Bot. 66, 5429–5440. doi: 10.1093/jxb/erv345
Grover, M., Ali, S., Sandhya, V., Rasul, A., Venkateswarlu, B. (2011). Role of microorganisms in adaptation of agriculture crops to abiotic stresses. World J. Microbiol. Biotechnol. 27, 1231–1240. doi: 10.1007/s11274-010-0572-7
Gul Jan, F., Hamayun, M., Hussain, A., Jan, G., Iqbal, A., Khan, A., et al. (2019). An endophytic isolate of the fungus Yarrowia lipolytica produces metabolites that ameliorate the negative impact of salt stress on the physiology of maize. BMC Microbiol. 19, 3. doi: 10.1186/s12866-018-1374-6
Hacquard, S., Spaepen, S., Garrido-Oter, R., Schulze-Lefert, P. (2017). Interplay between innate immunity and the plant microbiota. Annu. Rev. Phytopathol. 55, 565–589. doi: 10.1146/annurev-phyto-080516-035623
Haichar, F.el Z., Marol, C., Berge, O., Rangel-Castro, J. I., Prosser, J. I., Balesdent, J., et al. (2008). Plant host habitat and root exudates shape soil bacterial community structure. ISME J. 2, 1221–1230. doi: 10.1038/ismej.2008.80
Hajek, A. E., McManus, M. L., Delalibera, I. (2007). A review of introductions of pathogens and nematodes for classical biological control of insects and mites. Biol. Control 41, 1–13. doi: 10.1016/j.biocontrol.2006.11.003
Hallmann, J. (2001). “Plant interactions with endophytic bacteria,” in Biot. Interact. Plant-Pathog. Assoc (CABI Books), 87–119. doi: 10.1079/9780851995120.0087
Hamayun, M., Hussain, A., Khan, S. A., Kim, H.-Y., Khan, A. L., Waqas, M., et al. (2017). Gibberellins producing endophytic fungus porostereum spadiceum AGH786 rescues growth of salt affected soybean. Front. Microbiol. 8. doi: 10.3389/fmicb.2017.00686
Hammond, F. N., Booth, C. A., Lamond, J. E., Proverbs, D. G. (2012). “Introductory insights to climate change challenges,” in Solutions to Climate Change Challenges in the Built Environment (John Wiley & Sons, Ltd), 1–9. doi: 10.1002/9781444354539.ch1
Hardoim, P. R., van Overbeek, L. S., Berg, G., Pirttilä, A. M., Compant, S., Campisano, A., et al. (2015). The hidden world within plants: ecological and evolutionary considerations for defining functioning of microbial endophytes. Microbiol. Mol. Biol. Rev. 79, 293–320. doi: 10.1128/MMBR.00050-14
Hart, M., Ehret, D. L., Krumbein, A., Leung, C., Murch, S., Turi, C., et al. (2015). Inoculation with arbuscular mycorrhizal fungi improves the nutritional value of tomatoes. Mycorrhiza 25, 359–376. doi: 10.1007/s00572-014-0617-0
Hassani, M. A., Durán, P., Hacquard, S. (2018). Microbial interactions within the plant holobiont. Microbiome 6, 58. doi: 10.1186/s40168-018-0445-0
Hatfield, J. L., Antle, J., Garrett, K. A., Izaurralde, R. C., Mader, T., Marshall, E., et al. (2020). Indicators of climate change in agricultural systems. Clim. Change 163, 1719–1732. doi: 10.1007/s10584-018-2222-2
Hawkins, N. J., Bass, C., Dixon, A., Neve, P. (2019). The evolutionary origins of pesticide resistance. Biol. Rev. 94, 135–155. doi: 10.1111/brv.12440
Hesse, U., Schöberlein, W., Wittenmayer, L., Förster, K., Warnstorff, K., Diepenbrock, W., et al. (2003). Effects of Neotyphodium endophytes on growth, reproduction and drought-stress tolerance of three Lolium perenne L. genotypes. Grass Forage Sci. 58, 407–415. doi: 10.1111/j.1365-2494.2003.00393.x
Hu, Y., Cheng, H., Tao, S. (2016). The challenges and solutions for cadmium-contaminated rice in china: A critical review. Environ. Int. 92–93, 515–532. doi: 10.1016/j.envint.2016.04.042
Huang, C.-Y., Wang, H., Hu, P., Hamby, R., Jin, H. (2019). Small RNAs - big players in plant-microbe interactions. Cell Host Microbe 26, 173–182. doi: 10.1016/j.chom.2019.07.021
Huang, S., Zhu, S., Kumar, P., MacMicking, J. D. (2021). A phase-separated nuclear GBPL circuit controls immunity in plants. Nature 594, 424–429. doi: 10.1038/s41586-021-03572-6
Irshad, K., Shaheed Siddiqui, Z., Chen, J., Rao, Y., Hamna Ansari, H., Wajid, D., et al. (2023). Bio-priming with salt tolerant endophytes improved crop tolerance to salt stress via modulating photosystem II and antioxidant activities in a sub-optimal environment. Front. Plant Sci. 14. doi: 10.3389/fpls.2023.1082480
James, E. K., Olivares, F. L., Baldani, J. I., Döbereiner, J. (1997). Herbaspirillum, an endophytic diazotroph colonizing vascular tissue 3Sorghum bicolor L. Moench. J. Exp. Bot. 48, 785–798. doi: 10.1093/jxb/48.3.785
Jan, S., Parray, J. (2016). “Use of mycorrhiza as metal tolerance strategy in plants,” in Approaches to heavy metal tolerance in plants. Eds. Jan, S., Parray, J. A. (Singapore: Springer), 57–68. doi: 10.1007/978-981-10-1693-6_4
Jargeat, P., Rekangalt, D., Verner, M.-C., Gay, G., Debaud, J.-C., Marmeisse, R., et al. (2003). Characterisation and expression analysis of a nitrate transporter and nitrite reductase genes, two members of a gene cluster for nitrate assimilation from the symbiotic basidiomycete Hebeloma cylindrosporum. Curr. Genet. 43, 199–205. doi: 10.1007/s00294-003-0387-2
Jin, H., Pfeffer, P. E., Douds, D. D., Piotrowski, E., Lammers, P. J., Shachar-Hill, Y. (2005). The uptake, metabolism, transport and transfer of nitrogen in an arbuscular mycorrhizal symbiosis. New Phytol. 168, 687–696. doi: 10.1111/j.1469-8137.2005.01536.x
Johnson, L. J., Johnson, R. D., Schardl, C. L., Panaccione, D. G. (2003). Identification of differentially expressed genes in the mutualistic association of tall fescue with Neotyphodium coenophialum. Physiol. Mol. Plant Pathol. 63, 305–317. doi: 10.1016/j.pmpp.2004.04.001
Johnston-Monje, D., Raizada, M. N. (2011). Conservation and diversity of seed associated endophytes in Zea across boundaries of evolution, ethnography and ecology. PloS One 6, e20396. doi: 10.1371/journal.pone.0020396
Jones, J., Gheysen, G., Fenoll, C. (2011). Genomics and Molecular Genetics of Plant-Nematode Interactions (Dordrecht: Springer Netherlands). doi: 10.1007/978-94-007-0434-3
Kamle, M., Borah, R., Bora, H., Jaiswal, A. K., Singh, R. K., Kumar, P. (2020). “Systemic acquired resistance (SAR) and induced systemic resistance (ISR): role and mechanism of action against phytopathogens,” in Fungal Biotechnology and Bioengineering, Fungal Biology. Eds. Hesham, A. E.-L., Upadhyay, R. S., Sharma, G. D., Manoharachary, C., Gupta, V. K. (Springer International Publishing, Cham), 457–470. doi: 10.1007/978-3-030-41870-0_20
Kamran, M., Imran, Q. M., Ahmed, M. B., Falak, N., Khatoon, A., Yun, B.-W. (2022). Endophyte-mediated stress tolerance in plants: A sustainable strategy to enhance resilience and assist crop improvement. Cells 11, 3292. doi: 10.3390/cells11203292
Kasmir, J., Senthilkumar, S., Britto, S., Michael, J. (2011). Identification of fungal endophytes from Orchidaceae members based on nrITS (Internal Transcribed Spacer) region. Int. Res. J. Biotechnol. 2 (6), 139–144.
Kaul, S., Gupta, S., Ahmed, M., Dhar, M. K. (2012). Endophytic fungi from medicinal plants: a treasure hunt for bioactive metabolites. Phytochem. Rev. 11, 487–505. doi: 10.1007/s11101-012-9260-6
Kawaguchi, M., Minamisawa, K. (2010). Plant–microbe communications for symbiosis. Plant Cell Physiol. 51, 1377–1380. doi: 10.1093/pcp/pcq125
Khalid, M., Hassani, D., Bilal, M., Asad, F., Huang, D. (2017). Influence of bio-fertilizer containing beneficial fungi and rhizospheric bacteria on health promoting compounds and antioxidant activity of Spinacia oleracea L. Bot. Stud. 58, 35. doi: 10.1186/s40529-017-0189-3
Khare, E., Mishra, J., Arora, N. K. (2018). Multifaceted interactions between endophytes and plant: developments and prospects. Front. Microbiol. 9. doi: 10.3389/fmicb.2018.02732
Khatoon, Z., Huang, S., Rafique, M., Fakhar, A., Kamran, M. A., Santoyo, G. (2020). Unlocking the potential of plant growth-promoting rhizobacteria on soil health and the sustainability of agricultural systems. J. Environ. Manage. 273, 111118. doi: 10.1016/j.jenvman.2020.111118
Kiarie, S., Nyasani, J. O., Gohole, L. S., Maniania, N. K., Subramanian, S. (2020). Impact of fungal endophyte colonization of maize (Zea mays L.) on induced resistance to thrips- and aphid-transmitted viruses. Plants 9, 416. doi: 10.3390/plants9040416
Kiers, E. T., Duhamel, M., Beesetty, Y., Mensah, J. A., Franken, O., Verbruggen, E., et al. (2011). Reciprocal rewards stabilize cooperation in the mycorrhizal symbiosis. Science 333, 880–882. doi: 10.1126/science.1208473
Knauth, S., Hurek, T., Brar, D., Reinhold-Hurek, B. (2005). Influence of different Oryza cultivars on expression of nifH gene pools in roots of rice. Environ. Microbiol. 7, 1725–1733. doi: 10.1111/j.1462-2920.2005.00841.x
Kord, H., Fakheri, B., Ghabooli, M., Solouki, M., Emamjomeh, A., Khatabi, B., et al. (2019). Salinity-associated microRNAs and their potential roles in mediating salt tolerance in rice colonized by the endophytic root fungus Piriformospora indica. Funct. Integr. Genomics 19, 659–672. doi: 10.1007/s10142-019-00671-6
Kovtunovych, G., Lar, O., Kamalova, S., Kordyum, V., Kleiner, D., Kozyrovska, N. (1999). Correlation between pectate lyase activity and ability of diazotrophic Klebsiella oxytoca VN 13 to penetrate into plant tissues. Plant Soil 215, 1–6. doi: 10.1023/A:1004790122353
Krings, M., Taylor, T. N., Hass, H., Kerp, H., Dotzler, N., Hermsen, E. J. (2007). Fungal endophytes in a 400-million-yr-old land plant: infection pathways, spatial distribution, and host responses. New Phytol. 174, 648–657. doi: 10.1111/j.1469-8137.2007.02008.x
Kumar, K. K., Dara, S. K. (2021). Fungal and bacterial endophytes as microbial control agents for plant-parasitic nematodes. Int. J. Environ. Res. Public. Health 18, 4269. doi: 10.3390/ijerph18084269
Kusari, S., Singh, S., Jayabaskaran, C. (2014). Biotechnological potential of plant-associated endophytic fungi: hope versus hype. Trends Biotechnol. 32, 297–303. doi: 10.1016/j.tibtech.2014.03.009
Lehnert, H., Serfling, A., Friedt, W., Ordon, F. (2018). Genome-wide association studies reveal genomic regions associated with the response of wheat (Triticum aestivum L.) to mycorrhizae under drought stress conditions. Front. Plant Sci. 9. doi: 10.3389/fpls.2018.01728
Lekberg, Y., Hammer, E. C., Olsson, P. A. (2010). Plants as resource islands and storage units–adopting the mycocentric view of arbuscular mycorrhizal networks. FEMS Microbiol. Ecol. 74, 336–345. doi: 10.1111/fem.2010.74.issue-2
Lery, L. M. S., Hemerly, A. S., Nogueira, E. M., von Krüger, W. M. A., Bisch, P. M. (2011). Quantitative proteomic analysis of the interaction between the endophytic plant-growth-promoting bacterium Gluconacetobacter diazotrophicus and sugarcane. Mol. Plant-Microbe Interact. MPMI 24, 562–576. doi: 10.1094/MPMI-08-10-0178
Levy, A., Conway, J. M., Dangl, J. L., Woyke, T. (2018). Elucidating bacterial gene functions in the plant microbiome. Cell Host Microbe 24, 475–485. doi: 10.1016/j.chom.2018.09.005
Li, Y., Dai, C., Hu, C., Liu, Z., Kang, C. (2017). Global identification of alternative splicing via comparative analysis of SMRT- and Illumina-based RNA-seq in strawberry. Plant J. 90, 164–176. doi: 10.1111/tpj.13462
Lin, B. B., Chappell, M. J., Vandermeer, J., Smith, G., Quintero, E., Bezner-Kerr, R., et al. (2011). Effects of industrial agriculture on climate change and the mitigation potential of small-scale agro-ecological farms. CABI Rev. 2011, 1–18. doi: 10.1079/PAVSNNR20116020
Lingua, G., Bona, E., Manassero, P., Marsano, F., Todeschini, V., Cantamessa, S., et al. (2013). Arbuscular mycorrhizal fungi and plant growth-promoting pseudomonads increases anthocyanin concentration in strawberry fruits (Fragaria x ananassa var. Selva) in conditions of reduced fertilization. Int. J. Mol. Sci. 14, 16207–16225. doi: 10.3390/ijms140816207
Lipiec, J., Doussan, C., Nosalewicz, A., Kondracka, K. (2013). Effect of drought and heat stresses on plant growth and yield: a review. Int. Agrophysics. 27, 463–477. doi: 10.2478/intag-2013-0017
Liu, H., Carvalhais, L. C., Crawford, M., Singh, E., Dennis, P. G., Pieterse, C. M. J., et al. (2017). Inner plant values: diversity, colonization and benefits from endophytic bacteria. Front. Microbiol. 8. doi: 10.3389/fmicb.2017.02552
Lohani, N., Jain, D., Singh, M. B., Bhalla, P. L. (2020). Engineering multiple abiotic stress tolerance in canola, Brassica napus. Front. Plant Sci. 11. doi: 10.3389/fpls.2020.00003
López-Ráez, J. A., Shirasu, K., Foo, E. (2017). Strigolactones in plant interactions with beneficial and detrimental organisms: the yin and yang. Trends Plant Sci. 22, 527–537. doi: 10.1016/j.tplants.2017.03.011
Luna, M. F., Aprea, J., Crespo, J. M., Boiardi, J. L. (2012). Colonization and yield promotion of tomato by Gluconacetobacter diazotrophicus. Appl. Soil Ecol. Microorganisms Sustain. Manage. Soil 61, 225–229. doi: 10.1016/j.apsoil.2011.09.002
Lynch, J., Cain, M., Frame, D., Pierrehumbert, R. (2021). Agriculture’s contribution to climate change and role in mitigation is distinct from predominantly fossil CO2-emitting sectors. Front. Sustain. Food Syst. 4. doi: 10.3389/fsufs.2020.518039
Ma, Y., Dias, M. C., Freitas, H. (2020). Drought and salinity stress responses and microbe-induced tolerance in plants. Front. Plant Sci. 11. doi: 10.3389/fpls.2020.591911
Ma, Y., Rajkumar, M., Zhang, C., Freitas, H. (2016). Beneficial role of bacterial endophytes in heavy metal phytoremediation. J. Environ. Manage. 174, 14–25. doi: 10.1016/j.jenvman.2016.02.047
Macabuhay, A., Arsova, B., Watt, M., Nagel, K. A., Lenz, H., Putz, A., et al. (2022). Plant growth promotion and heat stress amelioration in Arabidopsis inoculated with paraburkholderia phytofirmans PsJN rhizobacteria quantified with the growScreen-agar II phenotyping platform. Plants 11, 2927. doi: 10.3390/plants11212927
Mäder, P., Kaiser, F., Adholeya, A., Singh, R., Uppal, H. S., Sharma, A. K., et al. (2011). Inoculation of root microorganisms for sustainable wheat–rice and wheat–black gram rotations in India. Soil Biol. Biochem. 43, 609–619. doi: 10.1016/j.soilbio.2010.11.031
Mahajan, S., Tuteja, N. (2005). Cold, salinity and drought stresses: an overview. Arch. Biochem. Biophys. 444, 139–158. doi: 10.1016/j.abb.2005.10.018
Malar C, M., Wang, Y., Stajich, J. E., Kokkoris, V., Villeneuve-Laroche, M., Yildirir, G., et al. (2022). Early branching arbuscular mycorrhizal fungus Paraglomus occultum carries a small and repeat-poor genome compared to relatives in the Glomeromycotina. Microb. Genomics 8, 810. doi: 10.1099/mgen.0.000810
Malinowski, D. P., Belesky, D. P. (2006). Ecological importance of Neotyphodium spp. grass endophytes in agroecosystems. Grassl. Sci. 52, 1–14. doi: 10.1111/j.1744-697X.2006.00041.x
Martin, F., Nehls, U. (2009). Harnessing ectomycorrhizal genomics for ecological insights. Curr. Opin. Plant Biol. 12, 508–515. doi: 10.1016/j.pbi.2009.05.007
Martínez-Hidalgo, P., García, J. M., Pozo, M. J. (2015). Induced systemic resistance against Botrytis cinerea by Micromonospora strains isolated from root nodules. Front. Microbiol. 6. doi: 10.3389/fmicb.2015.00922
Martínez-Medina, A., Fernandez, I., Lok, G. B., Pozo, M. J., Pieterse, C. M. J., Van Wees, S. C. M. (2017). Shifting from priming of salicylic acid- to jasmonic acid-regulated defences by Trichoderma protects tomato against the root knot nematode Meloidogyne incognita. New Phytol. 213, 1363–1377. doi: 10.1111/nph.14251
Martín-Rodríguez, J.Á., Ocampo, J. A., Molinero-Rosales, N., Tarkowská, D., Ruíz-Rivero, O., García-Garrido, J. M. (2015). Role of gibberellins during arbuscular mycorrhizal formation in tomato: new insights revealed by endogenous quantification and genetic analysis of their metabolism in mycorrhizal roots. Physiol. Plant 154, 66–81. doi: 10.1111/ppl.12274
Mathur, S., Tomar, R. S., Jajoo, A. (2019). Arbuscular mycorrhizal fungi (AMF) protects photosynthetic apparatus of wheat under drought stress. Photosynth. Res. 139, 227–238. doi: 10.1007/s11120-018-0538-4
Masuda, K., Abouleila, Y., Ali, A., Yanagida, T., Masujima, T. (2018). Live single-cell mass spectrometry (LSC-MS) for plant metabolomics. Methods Mol. Biol. Clifton NJ 1778, 269–282. doi: 10.1007/978-1-4939-7819-9_19
McLaughlin, A., Mineau, P. (1995). The impact of agricultural practices on biodiversity. Agric. Ecosyst. Environ. 55, 201–212. doi: 10.1016/0167-8809(95)00609-V
Mendes, R., Raaijmakers, J. M. (2015). Cross-kingdom similarities in microbiome functions. ISME J. 9, 1905–1907. doi: 10.1038/ismej.2015.7
Miliute, I., Buzaite, O., Baniulis, D., Stanys, V. (2015). Bacterial endophytes in agricultural crops and their role in stress tolerance: A review. Zemdirb.-Agric 102, 465–478. doi: 10.13080/z-a.2015.102.060
Mishra, B. K., Meena, K. K., Dubey, P. N., Aishwath, O. P., Kant, K., Sorty, A. M., et al. (2016). Influence on yield and quality of fennel (Foeniculum vulgare mill.) grown under semi-arid saline soil, due to application of native phosphate solubilizing rhizobacterial isolates. Ecol. Eng. 97, 327–333. doi: 10.1016/j.ecoleng.2016.10.034
Mohsenifard, E., Ghabooli, M., Mehri, N., Bakhshi, B. (2017). Regulation of miR159 and miR396 mediated by Piriformospora indica confer drought tolerance in rice. J. Plant Mol. Breed. 5, 10–18. doi: 10.22058/jpmb.2017.60864.1129
Montero, H., Lee, T., Pucker, B., Ferreras-Garrucho, G., Oldroyd, G., Brockington, S. F., et al. (2021). A mycorrhiza-associated receptor-like kinase with an ancient origin in the green lineage. Proc. Natl. Acad. Sci. 118, e2105281118. doi: 10.1073/pnas.2105281118
Moore, W. M., Chan, C., Ishikawa, T., Rennie, E. A., Wipf, H. M.-L., Benites, V., et al. (2021). Reprogramming sphingolipid glycosylation is required for endosymbiont persistence in Medicago truncatula. Curr. Biol. 31, 2374–2385.e4. doi: 10.1016/j.cub.2021.03.067
Muthu Narayanan, M., Ahmad, N., Shivanand, P., Metali, F. (2022). The role of endophytes in combating fungal- and bacterial-induced stress in plants. Mol. Basel Switz. 27, 6549. doi: 10.3390/molecules27196549
Nazarov, P. A., Baleev, D. N., Ivanova, M. I., Sokolova, L. M., Karakozova, M. V. (2020). Infectious plant diseases: etiology, current status, problems and prospects in plant protection. Acta Naturae 12, 46–59. doi: 10.32607/actanaturae.11026
Nehls, U., Plassard, C. (2018). Nitrogen and phosphate metabolism in ectomycorrhizas. New Phytol. 220, 1047–1058. doi: 10.1111/nph.15257
Nerva, L., Sandrini, M., Moffa, L., Velasco, R., Balestrini, R., Chitarra, W. (2022). Breeding toward improved ecological plant-microbiome interactions. Trends Plant Sci. 27, 1134–1143. doi: 10.1016/j.tplants.2022.06.004
Newman, M.-A., Sundelin, T., Nielsen, J. T., Erbs, G. (2013). MAMP (microbe-associated molecular pattern) triggered immunity in plants. Front. Plant Sci. 4. doi: 10.3389/fpls.2013.00139
Nguema-Ona, E., Vicré-Gibouin, M., Cannesan, M.-A., Driouich, A. (2013). Arabinogalactan proteins in root-microbe interactions. Trends Plant Sci. 18, 440–449. doi: 10.1016/j.tplants.2013.03.006
Nostadt, R., Hilbert, M., Nizam, S., Rovenich, H., Wawra, S., Martin, J., et al. (2020). A secreted fungal histidine- and alanine-rich protein regulates metal ion homeostasis and oxidative stress. New Phytol. 227, 1174–1188. doi: 10.1111/nph.16606
Okazaki, S., Kaneko, T., Sato, S., Saeki, K. (2013). Hijacking of leguminous nodulation signaling by the rhizobial type III secretion system. Proc. Natl. Acad. Sci. 110, 17131–17136. doi: 10.1073/pnas.1302360110
Okazaki, S., Tittabutr, P., Teulet, A., Thouin, J., Fardoux, J., Chaintreuil, C., et al. (2016). Rhizobium–legume symbiosis in the absence of Nod factors: two possible scenarios with or without the T3SS. ISME J. 10, 64–74. doi: 10.1038/ismej.2015.103
Oyserman, B. O., Flores, S. S., Griffioen, T., Pan, X., van der Wijk, E., Pronk, L., et al. (2022). Disentangling the genetic basis of rhizosphere microbiome assembly in tomato. Nat. Commun. 13, 3228. doi: 10.1038/s41467-022-30849-9
Padilla-Gálvez, N., Luengo-Uribe, P., Mancilla, S., Maurin, A., Torres, C., Ruiz, P., et al. (2021). Antagonistic activity of endophytic actinobacteria from native potatoes (Solanum tuberosum subsp. tuberosum L.) against Pectobacterium carotovorum subsp. carotovorum and Pectobacterium atrosepticum. BMC Microbiol. 21, 335. doi: 10.1186/s12866-021-02393-x
Palaniyandi, S. A., Damodharan, K., Yang, S. H., Suh, J. W. (2014). Streptomyces sp. strain PGPA39 alleviates salt stress and promotes growth of ‘Micro tom’ tomato plants. J. Appl. Microbiol. 117, 766–773. doi: 10.1111/jam.12563
Palmieri, D., Vitale, S., Lima, G., Di Pietro, A., Turrà, D. (2020). A bacterial endophyte exploits chemotropism of a fungal pathogen for plant colonization. Nat. Commun. 11, 5264. doi: 10.1038/s41467-020-18994-5
Panaccione, D. G., Beaulieu, W. T., Cook, D. (2014). Bioactive alkaloids in vertically transmitted fungal endophytes. Funct. Ecol. 28, 299–314. doi: 10.1111/1365-2435.12076
Pandey, V., Ansari, M. W., Tula, S., Yadav, S., Sahoo, R. K., Shukla, N., et al. (2016). Dose-dependent response of Trichoderma harzianum in improving drought tolerance in rice genotypes. Planta 243, 1251–1264. doi: 10.1007/s00425-016-2482-x
Pandey, P. K., Samanta, R., Yadav, R. N. S. (2019). Inside the plant: addressing bacterial endophytes in biotic stress alleviation. Arch. Microbiol. 201, 415–429. doi: 10.1007/s00203-019-01642-y
Park, Y.-G., Mun, B.-G., Kang, S.-M., Hussain, A., Shahzad, R., Seo, C.-W., et al. (2017). Bacillus aryabhattai SRB02 tolerates oxidative and nitrosative stress and promotes the growth of soybean by modulating the production of phytohormones. PloS One 12, e0173203. doi: 10.1371/journal.pone.0173203
Parniske, M. (2008). Arbuscular mycorrhiza: the mother of plant root endosymbioses. Nat. Rev. Microbiol. 6, 763–775. doi: 10.1038/nrmicro1987
Partida-Martínez, L. P., Heil, M. (2011). The microbe-free plant: fact or artifact? Front. Plant Sci. 2. doi: 10.3389/fpls.2011.00100
Pellegrino, E., Bedini, S. (2014). Enhancing ecosystem services in sustainable agriculture: Biofertilization and biofortification of chickpea (Cicer arietinum L.) by arbuscular mycorrhizal fungi. Soil Biol. Biochem. 68, 429–439. doi: 10.1016/j.soilbio.2013.09.030
Pendergrass, A. G., Meehl, G. A., Pulwarty, R., Hobbins, M., Hoell, A., AghaKouchak, A., et al. (2020). Flash droughts present a new challenge for subseasonal-to-seasonal prediction. Nat. Clim. Change 10, 191–199. doi: 10.1038/s41558-020-0709-0
Pentimone, I., Lebrón, R., Hackenberg, M., Rosso, L. C., Colagiero, M., Nigro, F., et al. (2018). Identification of tomato miRNAs responsive to root colonization by endophytic Pochonia chlamydosporia. Appl. Microbiol. Biotechnol. 102, 907–919. doi: 10.1007/s00253-017-8608-7
Pérez-Jaramillo, J. E., Carrión, V. J., de Hollander, M., Raaijmakers, J. M. (2018). The wild side of plant microbiomes. Microbiome 6, 143. doi: 10.1186/s40168-018-0519-z
Piromyou, P., Songwattana, P., Greetatorn, T., Okubo, T., Kakizaki, K. C., Prakamhang, J., et al. (2015). The type III secretion system (T3SS) is a determinant for rice-endophyte colonization by non-photosynthetic Bradyrhizobium. Microbes Environ. 30, 291–300. doi: 10.1264/jsme2.ME15080
Plett, J. M., Martin, F. M. (2018). Know your enemy, embrace your friend: using omics to understand how plants respond differently to pathogenic and mutualistic microorganisms. Plant J. 93, 729–746. doi: 10.1111/tpj.13802
Porter, S. S., Sachs, J. L. (2020). Agriculture and the disruption of plant–microbial symbiosis. Trends Ecol. Evol. 35, 426–439. doi: 10.1016/j.tree.2020.01.006
Poveda, J., Abril-Urias, P., Escobar, C. (2020). Biological control of plant-parasitic nematodes by filamentous fungi inducers of resistance: Trichoderma, Mycorrhizal and Endophytic fungi. Front. Microbiol. 11. doi: 10.3389/fmicb.2020.00992
Pulavarty, A., Egan, A., Karpinska, A., Horgan, K., Kakouli-Duarte, T. (2021). Plant parasitic nematodes: A review on their behaviour, host interaction, management approaches and their occurrence in two sites in the republic of Ireland. Plants 10, 2352. doi: 10.3390/plants10112352
Qawasmeh, A., Obied, H. K., Raman, A., Wheatley, W. (2012). Influence of fungal endophyte infection on phenolic content and antioxidant activity in grasses: interaction between Lolium perenne and different strains of Neotyphodium lolii. J. Agric. Food Chem. 60, 3381–3388. doi: 10.1021/jf204105k
Qin, S., Feng, W.-W., Zhang, Y.-J., Wang, T.-T., Xiong, Y.-W., Xing, K. (2018). Diversity of Bacterial Microbiota of Coastal Halophyte Limonium sinense and Amelioration of Salinity Stress Damage by Symbiotic Plant Growth-Promoting Actinobacterium Glutamicibacter halophytocola KLBMP 5180. Appl. Environ. Microbiol. 84, e01533–e01518. doi: 10.1128/AEM.01533-18
Raaijmakers, J. M., Vlami, M., de Souza, J. T. (2002). Antibiotic production by bacterial biocontrol agents. Antonie Van Leeuwenhoek 81, 537–547. doi: 10.1023/A:1020501420831
Radutoiu, S., Madsen, L. H., Madsen, E. B., Felle, H. H., Umehara, Y., Grønlund, M., et al. (2003). Plant recognition of symbiotic bacteria requires two LysM receptor-like kinases. Nature 425, 585–592. doi: 10.1038/nature02039
Rais, A., Jabeen, Z., Shair, F., Hafeez, F. Y., Hassan, M. N. (2017). Bacillus spp., a bio-control agent enhances the activity of antioxidant defense enzymes in rice against Pyricularia oryzae. PloS One 12, e0187412. doi: 10.1371/journal.pone.0187412
Ramesh, A., Sharma, S. K., Sharma, M. P., Yadav, N., Joshi, O. P. (2014). Inoculation of zinc solubilizing Bacillus aryabhattai strains for improved growth, mobilization and biofortification of zinc in soybean and wheat cultivated in Vertisols of central India. Appl. Soil Ecol. 73, 87–96. doi: 10.1016/j.apsoil.2013.08.009
Ramírez-Flores, M. R., Bello-Bello, E., Rellán-Álvarez, R., Sawers, R. J. H., Olalde-Portugal, V. (2019). Inoculation with the mycorrhizal fungus Rhizophagus irregularis modulates the relationship between root growth and nutrient content in maize (Zea mays ssp. mays L.). Plant Direct 3, e00192. doi: 10.1002/pld3.192
Rana, A., Joshi, M., Prasanna, R., Shivay, Y. S., Nain, L. (2012). Biofortification of wheat through inoculation of plant growth promoting rhizobacteria and cyanobacteria. Eur. J. Soil Biol. 50, 118–126. doi: 10.1016/j.ejsobi.2012.01.005
Rashid, S., Charles, T. C., Glick, B. R. (2012). Isolation and characterization of new plant growth-promoting bacterial endophytes. Appl. Soil Ecol. Microorganisms Sustain. Manage. Soil 61, 217–224. doi: 10.1016/j.apsoil.2011.09.011
Rathinasabapathi, B. (2000). Metabolic engineering for stress tolerance: installing osmoprotectant synthesis pathways. Ann. Bot. 86, 709–716. doi: 10.1006/anbo.2000.1254
Redecker, D., Kodner, R., Graham, L. E. (2000). Glomalean fungi from the Ordovician. Science 289, 1920–1921. doi: 10.1126/science.289.5486.1920
Reinhardt, D., Roux, C., Corradi, N., Pietro, A. D. (2021). Lineage-specific genes and cryptic sex: parallels and differences between Arbuscular mycorrhizal fungi and fungal pathogens. Trends Plant Sci. 26, 111–123. doi: 10.1016/j.tplants.2020.09.006
Reinhold-Hurek, B., Hurek, T. (1998). Life in grasses: diazotrophic endophytes. Trends Microbiol. 6, 139–144. doi: 10.1016/S0966-842X(98)01229-3
Reinhold-Hurek, B., Hurek, T. (2011). Living inside plants: bacterial endophytes. Curr. Opin. Plant Biol. 14, 435–443. doi: 10.1016/j.pbi.2011.04.004
Ren, B., Wang, X., Duan, J., Ma, J. (2019). Rhizobial tRNA-derived small RNAs are signal molecules regulating plant nodulation. Science 365, 919–922. doi: 10.1126/science.aav8907
Rodriguez, R., Durán, P. (2020). Natural holobiome engineering by using native extreme microbiome to counteract the climate change effects. Front. Bioeng. Biotechnol. 8. doi: 10.3389/fbioe.2020.00568
Rodriguez, R. J., Henson, J., Van Volkenburgh, E., Hoy, M., Wright, L., Beckwith, F., et al. (2008). Stress tolerance in plants via habitat-adapted symbiosis. ISME J. 2, 404–416. doi: 10.1038/ismej.2007.106
Rolli, E., Marasco, R., Vigani, G., Ettoumi, B., Mapelli, F., Deangelis, M. L., et al. (2015). Improved plant resistance to drought is promoted by the root-associated microbiome as a water stress-dependent trait. Environ. Microbiol. 17, 316–331. doi: 10.1111/1462-2920.12439
Rowan, D. D. (1993). Lolitrems, peramine and paxilline: Mycotoxins of the ryegrass/endophyte interaction. Agric. Ecosyst. Environ. Acremonium/Grass Interact. 44, 103–122. doi: 10.1016/0167-8809(93)90041-M
Rozpądek, P., Domka, A. M., Nosek, M., Ważny, R., Jędrzejczyk, R. J., Wiciarz, M., et al. (2018). The Role of Strigolactone in the Cross-Talk Between Arabidopsis thaliana and the Endophytic Fungus Mucor sp. Front. Microbiol. 9. doi: 10.3389/fmicb.2018.00441
Ruelland, E., Zachowski, A. (2010). How plants sense temperature. Environ. Exp. Bot. 69, 225–232. doi: 10.1016/j.envexpbot.2010.05.011
Sahebani, N., Hadavi, N. (2008). Biological control of the root-knot nematode Meloidogyne javanica by Trichoderma harzianum. Soil Biol. Biochem. 40, 2016–2020. doi: 10.1016/j.soilbio.2008.03.011
Samal, I., Bhoi, T. K., Majhi, P. K., Murmu, S., Pradhan, A. K., Kumar, D., et al. (2023). Combatting insects mediated biotic stress through plant associated endophytic entomopathogenic fungi in horticultural crops. Front. Plant Sci. 13. doi: 10.3389/fpls.2022.1098673
Sandrini, M., Nerva, L., Sillo, F., Balestrini, R., Balestrini, R., Chitarra, W., et al. (2022). Abiotic stress and belowground microbiome: the potential of omics approaches. Int. J. Mol. Sci. 23, 1091–1091. doi: 10.3390/ijms23031091
Santoyo, G., Hernández-Pacheco, C., Hernández-Salmerón, J., Hernández-León, R. (2017). The role of abiotic factors modulating the plant-microbe-soil interactions: toward sustainable agriculture. A review. Span. J. Agric. Res. 15, e03R01. doi: 10.5424/sjar/2017151-9990
Santoyo, G., Moreno-Hagelsieb, G., del Carmen Orozco-Mosqueda, Ma., Glick, B. R. (2016). Plant growth-promoting bacterial endophytes. Microbiol. Res. 183, 92–99. doi: 10.1016/j.micres.2015.11.008
Saunois, M., Stavert, A. R., Poulter, B., Bousquet, P., Canadell, J. G., Jackson, R. B., et al. (2020). The global methane budget 2000–2017. Earth Syst. Sci. Data 12, 1561–1623. doi: 10.5194/essd-12-1561-2020
Schadt, E. E., Turner, S., Kasarskis, A. (2010). A window into third-generation sequencing. Hum. Mol. Genet. 19, R227–R240. doi: 10.1093/hmg/ddq416
Scholthof, K.-B. G., Adkins, S., Czosnek, H., Palukaitis, P., Jacquot, E., Hohn, T., et al. (2011). Top 10 plant viruses in molecular plant pathology. Mol. Plant Pathol. 12, 938–954. doi: 10.1111/j.1364-3703.2011.00752.x
Schüβler, A., Schwarzott, D., Walker, C. (2001). A new fungal phylum, the Glomeromycota: phylogeny and evolution* *Dedicated to Manfred Kluge (Technische Universität Darmstadt) on the occasion of his retirement. Mycol. Res. 105, 1413–1421. doi: 10.1017/S0953756201005196
Seleiman, M. F., Al-Suhaibani, N., Ali, N., Akmal, M., Alotaibi, M., Refay, Y., et al. (2021). Drought stress impacts on plants and different approaches to alleviate its adverse effects. Plants 10, 259. doi: 10.3390/plants10020259
Shaffique, S., Khan, M. A., Wani, S. H., Pande, A., Imran, M., Kang, S.-M., et al. (2022). A review on the role of endophytes and plant growth promoting rhizobacteria in mitigating heat stress in plants. Microorganisms 10, 1286. doi: 10.3390/microorganisms10071286
Shahzad, A., Ullah, S., Dar, A. A., Sardar, M. F., Mehmood, T., Tufail, M. A., et al. (2021). Nexus on climate change: agriculture and possible solution to cope future climate change stresses. Environ. Sci. pollut. Res. Int. 28, 14211–14232. doi: 10.1007/s11356-021-12649-8
Sharma, S., Aneja, M. K., Mayer, J., Schloter, M., Munch, J. C. (2004). RNA fingerprinting of microbial community in the rhizosphere soil of grain legumes. FEMS Microbiol. Lett. 240, 181–186. doi: 10.1016/j.femsle.2004.09.026
Sharma, M., Sudheer, S., Usmani, Z., Rani, R., Gupta, P. (2020). Deciphering the omics of plant-microbe interaction: perspectives and new insights. Curr. Genomics 21, 343–362. doi: 10.2174/1389202921999200515140420
Sheibani-Tezerji, R., Rattei, T., Sessitsch, A., Trognitz, F., Mitter, B. (2015). Transcriptome profiling of the endophyte burkholderia phytofirmans psJN indicates sensing of the plant environment and drought stress. mBio 6, e00621–e00615. doi: 10.1128/mBio.00621-15
Shi, T., Ma, J., Wu, X., Ju, T., Lin, X., Zhang, Y., et al. (2018). Inventories of heavy metal inputs and outputs to and from agricultural soils: A review. Ecotoxicol. Environ. Saf. 164, 118–124. doi: 10.1016/j.ecoenv.2018.08.016
Shi, J., Wang, X., Wang, E. (2023). Mycorrhizal symbiosis in plant growth and stress adaptation: from genes to ecosystems. Annu. Rev. Plant Biol. 74, 569–607. doi: 10.1146/annurev-arplant-061722-090342
Silvestri, A., Fiorilli, V., Miozzi, L., Accotto, G. P., Turina, M., Lanfranco, L. (2019). In silico analysis of fungal small RNA accumulation reveals putative plant mRNA targets in the symbiosis between an arbuscular mycorrhizal fungus and its host plant. BMC Genomics 20, 169. doi: 10.1186/s12864-019-5561-0
Singh, S. N. (Ed.) (2000). Trace Gas Emissions and Plants (Dordrecht: Springer Netherlands). doi: 10.1007/978-94-017-3571-1
Singh, D., Geat, N., Rajawat, M. V. S., Prasanna, R., Kar, A., Singh, A. M., et al. (2018). Prospecting endophytes from different Fe or Zn accumulating wheat genotypes for their influence as inoculants on plant growth, yield, and micronutrient content. Ann. Microbiol. 68, 815–833. doi: 10.1007/s13213-018-1388-1
Singh, D., Prasanna, R. (2020). Potential of microbes in the biofortification of Zn and Fe in dietary food grains. A review. Agron. Sustain. Dev. 40, 15. doi: 10.1007/s13593-020-00619-2
Singh, D., Rajawat, M. V. S., Kaushik, R., Prasanna, R., Saxena, A. K. (2017). Beneficial role of endophytes in biofortification of Zn in wheat genotypes varying in nutrient use efficiency grown in soils sufficient and deficient in Zn. Plant Soil 416, 107–116. doi: 10.1007/s11104-017-3189-x
Singh, A., Roychoudhury, A. (2021). Gene regulation at transcriptional and post-transcriptional levels to combat salt stress in plants. Physiol. Plant 173, 1556–1572. doi: 10.1111/ppl.13502
Singh, V. K., Shukla, A. K., Singh, A. K. (2019). “Chapter 7 - Impact of climate change on plant–microbe interactions under agroecosystems,” in Climate Change and Agricultural Ecosystems. Eds. Choudhary, K. K., Kumar, A., Singh, A. K. (Woodhead Publishing), 153–179. doi: 10.1016/B978-0-12-816483-9.00007-4
Slepetiene, A., Volungevicius, J., Jurgutis, L., Liaudanskiene, I., Amaleviciute-Volunge, K., Slepetys, J., et al. (2020). The potential of digestate as a biofertilizer in eroded soils of Lithuania. Waste Manage. 102, 441–451. doi: 10.1016/j.wasman.2019.11.008
Soudzilovskaia, N. A., van Bodegom, P. M., Terrer, C., Zelfde, M.van’t E., McCallum, I., Luke McCormack, M., et al. (2019). Global mycorrhizal plant distribution linked to terrestrial carbon stocks. Nat. Commun. 10, 5077. doi: 10.1038/s41467-019-13019-2
Sperschneider, J., Yildirir, G., Rizzi, Y. S., Malar C, M., Mayrand Nicol, A., Sorwar, E., et al. (2023). Arbuscular mycorrhizal fungi heterokaryons have two nuclear populations with distinct roles in host–plant interactions. Nat. Microbiol. 8, 2142–2153. doi: 10.1038/s41564-023-01495-8
Stępniewska, Z., Kuźniar, A. (2013). Endophytic microorganisms–promising applications in bioremediation of greenhouse gases. Appl. Microbiol. Biotechnol. 97, 9589–9596. doi: 10.1007/s00253-013-5235-9
Stranska, M., Dzuman, Z., Prusova, N., Behner, A., Kolouchova, I., Lovecka, P., et al. (2022). Fungal endophytes of vitis vinifera—Plant growth promoters or potentially toxinogenic agents? Toxins 14, 66. doi: 10.3390/toxins14020066
Stuart, E. K., Plett, K. L. (2019). Digging deeper: in search of the mechanisms of carbon and nitrogen exchange in ectomycorrhizal symbioses. Front. Plant Sci. 10. doi: 10.3389/fpls.2019.01658
Su, L., Shen, Z., Ruan, Y., Tao, C., Chao, Y., Li, R., et al. (2017). Isolation of Antagonistic Endophytes from Banana Roots against Meloidogyne javanica and Their Effects on Soil Nematode Community. Front. Microbiol. 8. doi: 10.3389/fmicb.2017.02070
Subramanian, K., Balakrishnan, N., Natesan, S. (2013). Mycorrhizal symbiosis to increase the grain micronutrient content in maize. Aust. J. Crop Sci. 7, 900-910.
Surjan, A., Kudo, S., Uitto, J. I. (2016). “Risk and vulnerability,” in Sustainable Development and Disaster Risk Reduction, Disaster Risk Reduction. Eds. Uitto, J. I., Shaw, R. (Springer Japan, Tokyo), 37–55. doi: 10.1007/978-4-431-55078-5_3
Sykes, M. T. (2009). “Climate change impacts: vegetation,” in Encyclopedia of Life Sciences (ELS). (Chichester: John Wiley & Sons, Ltd). doi: 10.1002/9780470015902.a0021227
Tanaka, Y., Yano, K. (2005). Nitrogen delivery to maize via mycorrhizal hyphae depends on the form of N supplied. Plant Cell Environ. 28, 1247–1254. doi: 10.1111/j.1365-3040.2005.01360.x
Tang, M.-J., Lu, F., Yang, Y., Sun, K., Zhu, Q., Xu, F.-J., et al. (2022). Benefits of endophytic fungus phomopsis liquidambaris inoculation for improving mineral nutrition, quality, and yield of rice grains under low nitrogen and phosphorus condition. J. Plant Growth Regul. 41, 2499–2513. doi: 10.1007/s00344-021-10462-8
Tang, D., Wang, G., Zhou, J.-M. (2017). Receptor kinases in plant-pathogen interactions: more than pattern recognition. Plant Cell 29, 618–637. doi: 10.1105/tpc.16.00891
Tao, Y., Li, C., Liu, Y., Xu, C., Okabe, S., Matsushita, N., et al. (2023). Identification of microRNAs involved in ectomycorrhizal formation in Populus tomentosa. Tree Physiol. 43, 2012–2030. doi: 10.1093/treephys/tpad102
Teixeira, P. J. P. L., Colaianni, N. R., Law, T. F., Conway, J. M., Gilbert, S., Li, H., et al. (2021). Specific modulation of the root immune system by a community of commensal bacteria. Proc. Natl. Acad. Sci. 118, e2100678118. doi: 10.1073/pnas.2100678118
Teste, F. P., Jones, M. D., Dickie, I. A. (2020). Dual-mycorrhizal plants: their ecology and relevance. New Phytol 225, 1835–1851. doi: 10.1111/nph.16190
Theocharis, A., Bordiec, S., Fernandez, O., Paquis, S., Dhondt-Cordelier, S., Baillieul, F., et al. (2012). Burkholderia phytofirmans PsJN Primes Vitis vinifera L. and Confers a Better Tolerance to Low Nonfreezing Temperatures. Mol. Plant-Microbe Interactions® 25, 241–249. doi: 10.1094/MPMI-05-11-0124
Trdá, L., Boutrot, F., Claverie, J., Brulé, D., Dorey, S., Poinssot, B. (2015). Perception of pathogenic or beneficial bacteria and their evasion of host immunity: pattern recognition receptors in the frontline. Front. Plant Sci. 6. doi: 10.3389/fpls.2015.00219
Trenberth, K. E., Dai, A., van der Schrier, G., Jones, P. D., Barichivich, J., Briffa, K. R., et al. (2014). Global warming and changes in drought. Nat. Clim. Change 4, 17–22. doi: 10.1038/nclimate2067
Ullah, A., Mushtaq, H., Ali, H., Munis, M. F. H., Javed, M. T., Chaudhary, H. J. (2015). Diazotrophs-assisted phytoremediation of heavy metals: a novel approach. Environ. Sci. pollut. Res. Int. 22, 2505–2514. doi: 10.1007/s11356-014-3699-5
Vaid, S., Kumar, B., Sharma, A., Shukla, A., Srivastava, P. (2014). Effect of zinc solubilizing bacteria on growth promotion and zinc nutrition of rice. J. Soil Sci. Plant Nutr. 14, 889–910. doi: 10.4067/S0718-95162014005000071
Valente, J., Gerin, F., Le Gouis, J., Moënne-Loccoz, Y., Prigent–Combaret, C. (2020). Ancient wheat varieties have a higher ability to interact with plant growth-promoting rhizobacteria. Plant Cell Environ. 43, 246–260. doi: 10.1111/pce.13652
van den Hoogen, J., Geisen, S., Wall, D. H., Wardle, D. A., Traunspurger, W., de Goede, R. G. M., et al. (2020). A global database of soil nematode abundance and functional group composition. Sci. Data 7, 103. doi: 10.1038/s41597-020-0437-3
Vandenkoornhuyse, P., Quaiser, A., Duhamel, M., Le Van, A., Dufresne, A. (2015). The importance of the microbiome of the plant holobiont. New Phytol. 206, 1196–1206. doi: 10.1111/nph.13312
van der Heijden, M. G. A., Klironomos, J. N., Ursic, M., Moutoglis, P., Streitwolf-Engel, R., Boller, T., et al. (1998). Mycorrhizal fungal diversity determines plant biodiversity, ecosystem variability and productivity. Nature 396, 69–72. doi: 10.1038/23932
van der Heijden, M. G. A., Martin, F. M., Selosse, M.-A., Sanders, I. R. (2015). Mycorrhizal ecology and evolution: the past, the present, and the future. New Phytol. 205, 1406–1423. doi: 10.1111/nph.13288
Venice, F., Ghignone, S., Salvioli di Fossalunga, A., Amselem, J., Novero, M., Xianan, X., et al. (2020). At the nexus of three kingdoms: the genome of the mycorrhizal fungus Gigaspora margarita provides insights into plant, endobacterial and fungal interactions. Environ. Microbiol. 22, 122–141. doi: 10.1111/1462-2920.14827
Verbruggen, E., van der Heijden, M. G. A., Weedon, J. T., Kowalchuk, G. A., Röling, W. F. M. (2012). Community assembly, species richness and nestedness of arbuscular mycorrhizal fungi in agricultural soils. Mol. Ecol. 21, 2341–2353. doi: 10.1111/j.1365-294X.2012.05534.x
Verma, H., Kumar, D., Kumar, V., Kumari, M., Singh, S. K., Sharma, V. K., et al. (2021). The potential application of endophytes in management of stress from drought and salinity in crop plants. Microorganisms 9, 1729. doi: 10.3390/microorganisms9081729
Verma, P. K., Verma, S., Pandey, N. (2022). Root system architecture in rice: impacts of genes, phytohormones and root microbiota. 3 Biotech. 12, 239. doi: 10.1007/s13205-022-03299-9
Vyas, P., Singh, B. (2023). Plant growth-promoting endophytes from Stevia rebaudiana identified to possess bio-control potential against maize sheath blight pathogen Rhizoctonia solani. Eur. J. Plant Pathol. doi: 10.1007/s10658-023-02785-x
Walters, W. A., Jin, Z., Youngblut, N., Wallace, J. G., Sutter, J., Zhang, W., et al. (2018). Large-scale replicated field study of maize rhizosphere identifies heritable microbes. Proc. Natl. Acad. Sci. U.S.A. 115, 7368–7373. doi: 10.1073/pnas.1800918115
Wang, M., Eyre, A. W., Thon, M. R., Oh, Y., Dean, R. A. (2020). Dynamic changes in the microbiome of rice during shoot and root growth derived from seeds. Front. Microbiol. 11. doi: 10.3389/fmicb.2020.559728
Wang, Y., Yang, X., Zhang, X., Dong, L., Zhang, J., Wei, Y., et al. (2014). Improved Plant Growth and Zn Accumulation in Grains of Rice (Oryza sativa L.) by Inoculation of Endophytic Microbes Isolated from a Zn Hyperaccumulator, Sedum alfredii H. J. Agric. Food Chem. 62, 1783–1791. doi: 10.1021/jf404152u
Wang, X., Zhang, X., Liu, L., Xiang, M., Wang, W., Sun, X., et al. (2015). Genomic and transcriptomic analysis of the endophytic fungus Pestalotiopsis fici reveals its lifestyle and high potential for synthesis of natural products. BMC Genomics 16, 28. doi: 10.1186/s12864-014-1190-9
Wani, Z. A., Ashraf, N., Mohiuddin, T., Riyaz-Ul-Hassan, S. (2015). Plant-endophyte symbiosis, an ecological perspective. Appl. Microbiol. Biotechnol. 99, 2955–2965. doi: 10.1007/s00253-015-6487-3
Watts-Williams, S. J., Emmett, B. D., Levesque-Tremblay, V., MacLean, A. M., Sun, X., Satterlee, J. W., et al. (2019). Diverse Sorghum bicolor accessions show marked variation in growth and transcriptional responses to arbuscular mycorrhizal fungi. Plant Cell Environ. 42, 1758–1774. doi: 10.1111/pce.13509
Webster, G., Jain, V., Davey, M. R., Gough, C., Vasse, J., Dénarié, J., et al. (1998). The flavonoid naringenin stimulates the intercellular colonization of wheat roots by Azorhizobium caulinodans. Plant Cell Environ. 21, 373–383. doi: 10.1046/j.1365-3040.1998.00278.x
Weinert, N., Piceno, Y., Ding, G.-C., Meincke, R., Heuer, H., Berg, G., et al. (2011). PhyloChip hybridization uncovered an enormous bacterial diversity in the rhizosphere of different potato cultivars: many common and few cultivar-dependent taxa. FEMS Microbiol. Ecol. 75, 497–506. doi: 10.1111/fem.2011.75.issue-3
Werner, G. D. A., Kiers, E. T. (2015). Partner selection in the mycorrhizal mutualism. New Phytol. 205, 1437–1442. doi: 10.1111/nph.13113
Wilkinson, H. H., Siegel, M. R., Blankenship, J. D., Mallory, A. C., Bush, L. P., Schardl, C. L. (2000). Contribution of fungal loline alkaloids to protection from aphids in a grass-endophyte mutualism. Mol. Plant-Microbe Interact. MPMI 13, 1027–1033. doi: 10.1094/MPMI.2000.13.10.1027
Wu, P., Wu, Y., Liu, C.-C., Liu, L.-W., Ma, F.-F., Wu, X.-Y., et al. (2016). Identification of Arbuscular mycorrhiza (AM)-responsive microRNAs in tomato. Front. Plant Sci. 7. doi: 10.3389/fpls.2016.00429
Xiong, C., Zhu, Y.-G., Wang, J.-T., Singh, B., Han, L.-L., Shen, J.-P., et al. (2021). Host selection shapes crop microbiome assembly and network complexity. New Phytol. 229, 1091–1104. doi: 10.1111/nph.16890
Yadav, P., Jaiswal, D. K., Sinha, R. K. (2021). “7 - Climate change: Impact on agricultural production and sustainable mitigation,” in Global Climate Change. Eds. Singh, S., Singh, P., Rangabhashiyam, S., Srivastava, K. K. (Elsevier), 151–174. doi: 10.1016/B978-0-12-822928-6.00010-1
Yan, L., Khan, R. A. A. (2021). Biological control of bacterial wilt in tomato through the metabolites produced by the biocontrol fungus, Trichoderma harzianum. Egypt. J. Biol. Pest Control 31, 5. doi: 10.1186/s41938-020-00351-9
Yan, L., Zhu, J., Zhao, X., Shi, J., Jiang, C., Shao, D. (2019). Beneficial effects of endophytic fungi colonization on plants. Appl. Microbiol. Biotechnol. 103, 3327–3340. doi: 10.1007/s00253-019-09713-2
Yang, J., Kloepper, J. W., Ryu, C.-M. (2009). Rhizosphere bacteria help plants tolerate abiotic stress. Trends Plant Sci. 14, 1–4. doi: 10.1016/j.tplants.2008.10.004
Yildirir, G., Sperschneider, J., Malar C, M., Chen, E. C. H., Iwasaki, W., Cornell, C., et al. (2022). Long reads and Hi-C sequencing illuminate the two-compartment genome of the model arbuscular mycorrhizal symbiont Rhizophagus irregularis. New Phytol. 233, 1097–1107. doi: 10.1111/nph.17842
Yin, R., Xia, K., Xu, X. (2023). Spatial transcriptomics drives a new era in plant research. Plant J. 116, 1571–1581. doi: 10.1111/tpj.16437
Zeidler, D., Zähringer, U., Gerber, I., Dubery, I., Hartung, T., Bors, W., et al. (2004). Innate immunity in Arabidopsis thaliana: lipopolysaccharides activate nitric oxide synthase (NOS) and induce defense genes. Proc. Natl. Acad. Sci. U.S.A. 101, 15811–15816. doi: 10.1073/pnas.0404536101
Zhang, C., He, J., Dai, H., Wang, G., Zhang, X., Wang, C., et al. (2021). Discriminating symbiosis and immunity signals by receptor competition in rice. Proc. Natl. Acad. Sci. U.S.A. 118, e2023738118. doi: 10.1073/pnas.2023738118
Zhang, J., Liu, Y.-X., Zhang, N., Hu, B., Jin, T., Xu, H., et al. (2019). NRT1.1B is associated with root microbiota composition and nitrogen use in field-grown rice. Nat. Biotechnol. 37, 676–684. doi: 10.1038/s41587-019-0104-4
Zhang, G., Sun, M., Wang, J., Lei, M., Li, C., Zhao, D., et al. (2019). PacBio full-length cDNA sequencing integrated with RNA-seq reads drastically improves the discovery of splicing transcripts in rice. Plant J. Cell Mol. Biol. 97, 296–305. doi: 10.1111/tpj.14120
Zhang, W., Wang, J., Xu, L., Wang, A., Huang, L., Du, H., et al. (2018). Drought stress responses in maize are diminished by Piriformospora indica. Plant Signal. Behav. 13, e1414121. doi: 10.1080/15592324.2017.1414121
Zhang, H., Zhu, J., Gong, Z., Zhu, J.-K. (2022). Abiotic stress responses in plants. Nat. Rev. Genet. 23, 104–119. doi: 10.1038/s41576-021-00413-0
Zhou, F., Emonet, A., Dénervaud Tendon, V., Marhavy, P., Wu, D., Lahaye, T., et al. (2020). Co-incidence of damage and microbial patterns controls localized immune responses in roots. Cell 180, 440–453.e18. doi: 10.1016/j.cell.2020.01.013
Zhou, M., Lou, J., Li, Y.-K., Wang, Y.-D., Zhou, K., Ji, B.-K., et al. (2015). Butyrolactones from the Endophytic Fungus Aspergillus versicolor and their Anti-Tobacco Mosaic Virus Activity. J. Braz. Chem. Soc 26, 545–549. doi: 10.5935/0103-5053.20150008
Zhou, X., Liu, X., Zhao, J., Guan, F., Yao, D., Wu, N., et al. (2021). The endophytic bacterium bacillus koreensis 181–22 promotes rice growth and alleviates cadmium stress under cadmium exposure. Appl. Microbiol. Biotechnol. 105, 8517–8529. doi: 10.1007/s00253-021-11613-3
Keywords: endophytes, climate change, stress tolerance, omic techniques, arbuscular mycorrhizal fungi, microbiota
Citation: Sena L, Mica E, Valè G, Vaccino P and Pecchioni N (2024) Exploring the potential of endophyte-plant interactions for improving crop sustainable yields in a changing climate. Front. Plant Sci. 15:1349401. doi: 10.3389/fpls.2024.1349401
Received: 04 December 2023; Accepted: 05 March 2024;
Published: 20 March 2024.
Edited by:
Dilfuza Egamberdieva, Leibniz Center for Agricultural Landscape Research (ZALF), GermanyReviewed by:
Malik Mobeen Ahmad, Integral University, IndiaEvans N. Nyaboga, University of Nairobi, Kenya
Copyright © 2024 Sena, Mica, Valè, Vaccino and Pecchioni. This is an open-access article distributed under the terms of the Creative Commons Attribution License (CC BY). The use, distribution or reproduction in other forums is permitted, provided the original author(s) and the copyright owner(s) are credited and that the original publication in this journal is cited, in accordance with accepted academic practice. No use, distribution or reproduction is permitted which does not comply with these terms.
*Correspondence: Lorenzo Sena, bG9yZW56by5zZW5hQHVuaW1vcmUuaXQ=; Nicola Pecchioni, bmljb2xhLnBlY2NoaW9uaUB1bmltb3JlLml0