- 1Department of Entomology, University of Georgia, Griffin, GA, United States
- 2Department of Entomology, University of Georgia, Athens, GA, United States
- 3Department of Entomology, University of Georgia, Tifton, GA, United States
- 4Department of Plant Pathology, University of Georgia, Tifton, GA, United States
- 5Department of Entomology and Plant Pathology, Auburn University, Auburn, AL, United States
Upon acquisition of persistent circulative viruses such as poleroviruses, the virus particles transcytose through membrane barriers of aphids at the midgut and salivary glands via hemolymph. Such intricate interactions can influence aphid behavior and fitness and induce associated gene expression in viruliferous aphids. Differential gene expression can be evaluated by omics approaches such as transcriptomics. Previously conducted aphid transcriptome studies used only one host species as the source of virus inoculum. Viruses typically have alternate hosts. Hence, it is not clear how alternate hosts infected with the same virus isolate alter gene expression in viruliferous vectors. To address the question, this study conducted a transcriptome analysis of viruliferous aphids that acquired the virus from different host species. A polerovirus, cotton leafroll dwarf virus (CLRDV), which induced gene expression in the cotton aphid, Aphis gossypii Glover, was assessed using four alternate hosts, viz., cotton, hibiscus, okra, and prickly sida. Among a total of 2,942 differentially expressed genes (DEGs), 750, 310, 1,193, and 689 genes were identified in A. gossypii that acquired CLRDV from infected cotton, hibiscus, okra, and prickly sida, respectively, compared with non-viruliferous aphids that developed on non-infected hosts. A higher proportion of aphid genes were overexpressed than underexpressed following CLRDV acquisition from cotton, hibiscus, and prickly sida. In contrast, more aphid genes were underexpressed than overexpressed following CLRDV acquisition from okra plants. Only four common DEGs (heat shock protein, juvenile hormone acid O-methyltransferase, and two unannotated genes) were identified among viruliferous aphids from four alternate hosts. Gene ontology (GO) enrichment analysis and Kyoto Encyclopedia of Genes and Genomes (KEGG) annotations indicated that the acquisition of CLRDV induced DEGs in aphids associated with virus infection, signal transduction, immune systems, and fitness. However, these induced changes were not consistent across four alternate hosts. These data indicate that alternate hosts could differentially influence gene expression in aphids and presumably aphid behavior and fitness despite being infected with the same virus isolate.
Introduction
Persistently transmitted single-stranded RNA phytoviruses such as poleroviruses are phloem-limited, and phloem-feeding and colonizing insects such as aphids efficiently transmit such viruses (Harris and Maramorosch, 1977; Ng and Perry, 2004). The vectors and their viruses interact intricately in these pathosystems. Ingestion of viruses occurs when aphids feed on virus-infected plants; once in the midgut, these viruses traverse into the hemocoel and then into the accessory salivary glands through transcytosis (Gray and Gildow, 2003). Also, such viruses are exclusively transmitted by specific vector species, and the specificity seems to be associated with unique receptors in vectors that mediate transcytosis (Ng and Falk, 2006; Hogenhout et al., 2008; Whitfield et al., 2015).
Persistent virus infections are known to modulate the host plant physiology and in turn alter the phenotypical traits such as leaf hue, plant growth, availability of nutrients including free amino acids and soluble carbohydrates, and profiles of volatile organic compounds (VOCs) and metabolites (Eigenbrode et al., 2002; Mauck et al., 2010). These alterations can influence vector behavior (attraction or repulsion) and performance (feeding and colonization) (Fereres and Moreno, 2009; Mauck, 2016). The host–aphid–polerovirus interactions are complex, and previous studies have reported favorable, unfavorable, and/or neutral outcomes on vector fitness (Jiménez-Martínez et al., 2004; Rajabaskar et al., 2013; Srinivasan et al., 2013; Lightle and Lee, 2014; dos Santos et al., 2016; Ghosh et al., 2016; Claudel et al., 2018; Chesnais et al., 2020, 2022; Fingu-Mabola et al., 2020; Bertasello et al., 2021; Fingu-Mabola and Francis, 2021; Jayasinghe et al., 2022). However, the majority of interactions seem to influence vector fitness and behavior positively to enhance virus transmission (Hogenhout et al., 2008).
The magnitude of the effects of virus acquisition could largely depend on virus species, vector species, host species, and their interactions. For example, the green peach aphid (Myzus persicae Sulzer) preferred and survived longer on potato leafroll virus (PLRV)-infected plants compared with non-infected plants (Eigenbrode et al., 2002; Srinivasan et al., 2006, 2008). On the contrary, viruliferous bird cherry-oat aphid (Rhopalosiphum padi L.) preferred non-infected or sham-inoculated plants compared with barley yellow dwarf virus (BYDV)-infected plants (Ingwell et al., 2012). Also, BYDV infection reduced the population growth of cereal aphids (Sitobion avenae F.) compared with non-infected plants (Fiebig et al., 2004). This shift or alteration in vector–virus interactions can be better understood by exploring their genetic and molecular bases (Brault et al., 2010; Li et al., 2019, 2020; Patton et al., 2021; Catto et al., 2022; Marmonier et al., 2022). For example, the underexpression of genes associated with immunity, hormone biosynthesis, and proteolytic pathways has been reported from transcriptome analysis in aphids (S. avenae, Schizaphis graminum Rondani, and R. padi) upon BYDV acquisition (Li et al., 2019, 2020). Similarly, the genes related to receptor activities and/or vesicular transport in M. persicae were underexpressed upon acquiring the turnip yellows virus (TuYV). However, the differentially expressed genes (DEGs) identified varied when the vector acquired the virus from an artificial medium compared with the virus-infected plant (Marmonier et al., 2022). Previous transcriptome studies have predominantly used only one host species as a source of virus acquisition. Only one study has been conducted to understand the discrepancies that may occur in vector fitness upon acquiring the same plant virus from different host species (Chesnais et al., 2022). Nonetheless, there remains a knowledge gap in understanding the impact of alternate hosts on virus–vector interactions as well as their fidelity across such hosts.
This study attempted to answer the above-stated question using another persistently transmitted polerovirus–aphid pathosystem. Cotton leafroll dwarf virus (CLRDV) is a phloem-limited, positive-sense, single-stranded RNA virus in the genus Polerovirus and belongs to the family Solemoviridae (Sõmera et al., 2021). The CLRDV genome is 5.8 kb long with seven open reading frames (ORFs) grouped into two blocks and separated by a non-coding region. The symptoms of CLRDV infection were first observed in cotton in the United States in Alabama in 2017, but the virus was identified in 2019 (Avelar et al., 2019). Subsequently, the virus has been reported in several cotton-producing states including Georgia (Aboughanem-Sabanadzovic et al., 2019; Tabassum et al., 2019; Alabi et al., 2020; Ali and Mokhtari, 2020; Ali et al., 2020; Faske et al., 2020; Iriarte et al., 2020; Price et al., 2020; Thiessen et al., 2020; Wang et al., 2020b). The CLRDV-infection symptoms include stunting; leaf rolling; vein yellowing; dark-green leaves; reddening of leaves, petioles, and stems; leaf puckering, crinkling, and deformation of leaf lamina; wilting; downward leaf drooping with V-shaped lamina folding; and small bolls (Cascardo et al., 2015; Silva et al., 2015; Brown et al., 2019; Sedhain et al., 2021; Pandey et al., 2022). Often, CLRDV also was detected in asymptomatic plants via reverse transcription–PCR (Bag et al., 2021). Further, CLRDV was detected in alternate hosts in the landscape in Georgia (Sedhain et al., 2021; Edula et al., 2023).
The cotton/melon aphid (Aphis gossypii Glover) is the only known vector of CLRDV in the United States, and it transmits the virus in a persistent and non-propagative manner (Michelotto and Busoli, 2007; Heilsnis et al., 2022, 2023). In a previous study, the aphid-mediated inoculation of CLRDV led to successful infection of hibiscus (Hibiscus acetosella Welw. Ex Hiern.), okra (Abelmoschus esculentus L.), prickly sida (Sida spinosa L.), Palmer amaranth (Amaranthus palmeri S. Wats.), and Nicotiana benthamiana Domin plants. Nevertheless, no CLRDV symptoms were observed in any of those host plants. Aphids were able to acquire the virus exclusively from CLRDV-infected hibiscus, okra, and prickly sida plants and subsequently inoculate the virus back to cotton plants. Although cotton, hibiscus, okra, and prickly sida belong to Malvaceae, there was a notable discrepancy in the amount of virus acquired by A. gossypii from those CLRDV-infected host species (Pandey et al., 2022). Also, previous studies have reported that the total fecundity and intrinsic rate of increase of A. gossypii varied among alternate hosts (Barman et al., 2018; Pandey et al., 2022).
This study explored how the acquisition of CLRDV from different host species affects the gene expression associated with behavior and/or fitness in its vector. Specifically, cotton, hibiscus, okra, and prickly sida were used as host plants to assess differential gene expression in A. gossypii post-acquisition of CLRDV by transcriptome analyses. This study hypothesized that aphid genes will be differentially expressed when they acquire the same virus isolate from different host species, and consequently aphid behavior and fitness could be differentially affected. The cDNA libraries were prepared for viruliferous and non-viruliferous A. gossypii after a 72-h acquisition access period (AAP) on CLRDV-infected or non-infected host plants. The specific objectives of this study were to i) assess the differences in gene expression in A. gossypii upon virus acquisition from four CLRDV-infected hosts and ii) locate putative hub genes and co-expressed genes (modules) in A. gossypii post-acquisition of CLRDV from specific hosts using weighted gene correlation network analysis (WGCNA).
Materials and methods
Plants and insects
The CLRDV host species identified in a previous study, viz., cotton, Gossypium hirsutum L. cv. PHY 339 WRF (Corteva, Indianapolis, IN, USA), hibiscus, H. acetosella Welw. Ex Hiern. (Johnny’s Selected Seeds, Winslow, ME, USA), okra, A. esculentus L. cv. ‘Clemson spineless 80’ (Clemson University, Clemson, SC, USA), and prickly sida, S. spinosa L. (Azlin Seed Service, Leland, MS, USA) were used as inoculum sources in this study (Pandey et al., 2022). Two to four seeds of each plant were sown per pot in Sunshine propagation mix (SunGro Horticulture Industries, Bellevue, WA, USA) in 10-cm-diameter plastic pots (depth 8 cm). The pots were kept in insect-proof cages of size 47.5 (l) × 47.5 (w) × 93 (h) cm3 (Megaview Science Co., Taichung, Taiwan) in the greenhouse. The greenhouse was maintained at 25°C, 60% relative humidity, and 14-h L:10-h D photoperiod. The seedlings were thinned post-germination, and only one plant per pot was used. Water-soluble Miracle-Gro (Scotts Miracle-Gro Products, Inc., Marysville, OH, USA) at 0.5 g/L was used for weekly fertilization. The aphids were originally collected from cotton fields in 2017 at Tifton, Georgia, and thereafter, the population was maintained in the greenhouse at the University of Georgia, Griffin Campus, under the same conditions indicated above.
Maintenance of CLRDV-infected plants
Cotton plants infected with CLRDV were originally collected from cotton fields in September 2020 at the University of Georgia, Tifton Campus, GA, USA, and maintained in the greenhouse at the above-stated conditions. Aphid-mediated CLRDV transmission to cotton seedlings was undertaken to maintain the virus inoculum source. CLRDV-infected cotton, hibiscus, okra, and prickly sida plants were obtained following the protocols described in an earlier study (Pandey et al., 2022). A. gossypii adults were provided with a 72-h AAP on CLRDV-infected cotton plants followed by a 72-h inoculation access period (IAP) on the undersurface of leaves of young seedlings at the two-true leaf stage. The CLRDV-inoculated plants were placed in aphid-proof cages under the greenhouse conditions described above. Clip cages and aphids were removed 3 days post-inoculation. The infection status of the plants was evaluated at approximately 3 weeks post-inoculation by reverse transcription–PCR as described earlier (Pandey et al., 2022).
Viruliferous and non-viruliferous aphids for RNA sequencing
The non-viruliferous A. gossypii colonies were maintained on all four host species, i.e., non-infected cotton, hibiscus, okra, and prickly sida plants, in separate insect-proof cages. Approximately 1,000 A. gossypii were collected from each colony and then introduced to non-infected or CLRDV-infected cotton, hibiscus, okra, and prickly sida plants. The aphids were allowed to feed for an AAP of 72 h. After 72 h of AAP on non-infected or CLRDV-infected plants, approximately 500 adults per treatment were collected, and total RNA was extracted from the collected aphid samples (Figure 1). Each treatment was biologically replicated four times. Another set of aphids (three pools of 10 aphids/per treatment) was used to confirm the acquisition of CLRDV in different treatments. The results indicated that 100% of pools of aphids collected from CLRDV-infected plants were positive for CLRDV, whereas aphids feeding on non-infected plants tested negative for CLRDV.
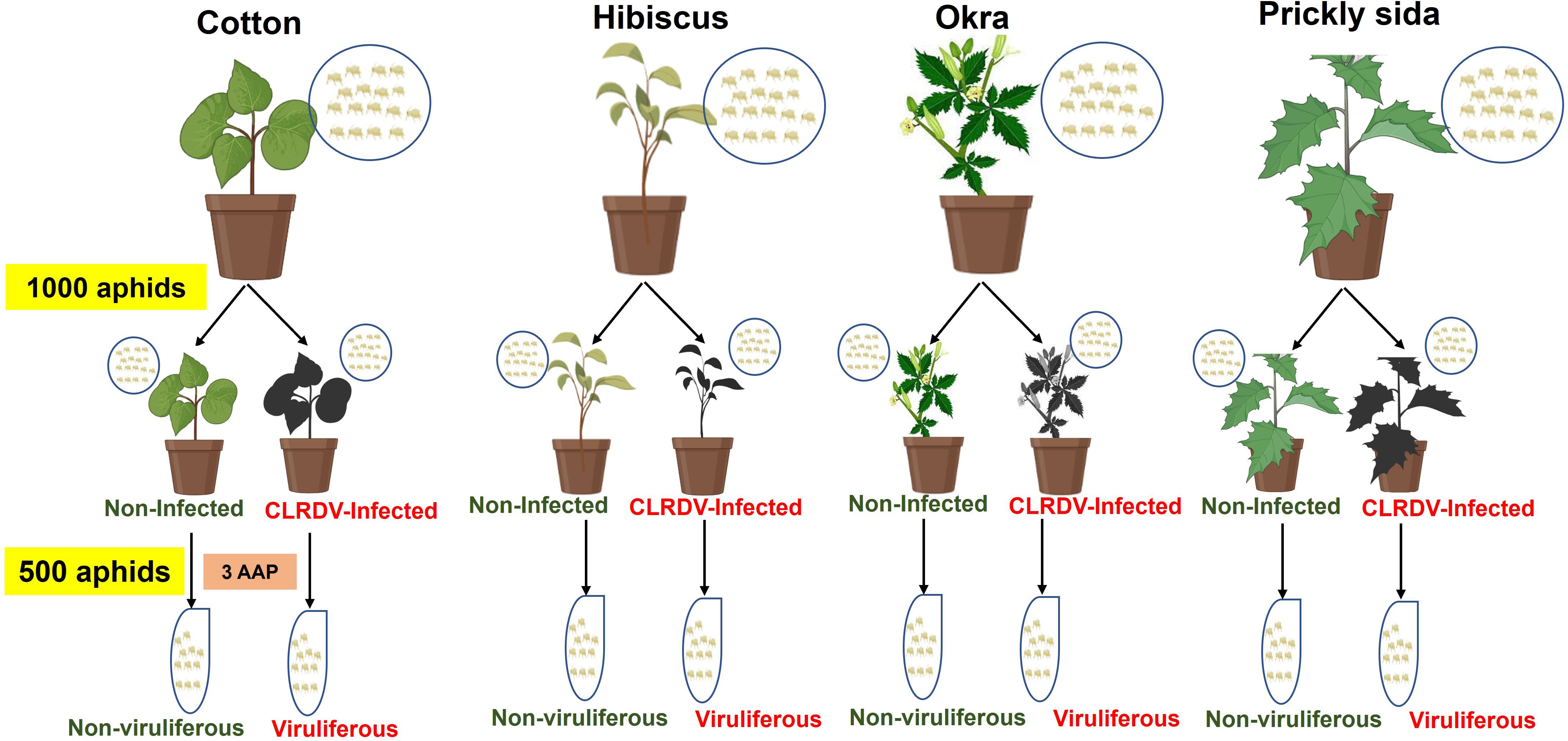
Figure 1 Schematic diagram of experimental setup for generating viruliferous and non-viruliferous aphid samples from CLRDV-infected and non-infected cotton, hibiscus, okra, and prickly sida plants. The aphid colonies were maintained on each plant species separately, and 1,000 adult aphids from each colony were collected and attached to the respective CLRDV-infected and non-infected plants. After 3 days of acquisition access period (AAP), 500 aphids from each treatment were collected for total RNA extraction. The experiment was repeated four times to obtain eight aphid samples from each host plant. CLRDV, cotton leafroll dwarf virus.
Total RNA extraction and sequencing
The total RNA was extracted from the collected aphid samples using Qiagen RNA mini-Kit (Valencia, CA, USA) as per the manufacturer’s instructions. For each sample, 40 µL of total RNA was shipped to Novogene Corporation Inc. (Sacramento, CA, USA), and the rest of the total RNA was stored at −80°C for validation. Quality control (QC) of RNA samples was accomplished by preliminary quantitation using a NanoDrop and testing for RNA degradation and contamination via agarose gel electrophoresis. Then, RNA integrity (RIN) was assessed using Agilent 2100. Three samples failed the QC test. The samples that passed QC (RIN value >6.8 and concentration of >20 ng/μL) were used for cDNA library preparation. The library preparation began with enriching mRNA using oligo(dT) beads and removing rRNA using the Ribo-Zero kit. Then, the mRNA fragmentation was followed by first- and second-strand cDNA synthesis. Subsequently, adaptor ligation and PCR enrichment were performed for cDNA library generation. Finally, the library quality was assessed using a Qubit 2.0. NovaSeq 6000 Sequencing System (Illumina, San Diego, CA, USA) with the NovaSeq paired-end 150 sequencing platform was used for sequencing the libraries that passed QC.
Transcriptome assembly and analysis
FastQC v0.11.9 and multiQC v1.11 were used to assess the quality of raw reads before and after trimming (Andrews and FastQC, 2010; Ewels et al., 2016). The adapters were removed by using Trimmomatic v0.39 with the default setting (Bolger et al., 2014). Bowtie2 v2.4.1 was used with default mapping parameters to map the trimmed reads with the reference A. gossypii transcriptome (Langmead and Salzberg, 2012; Quan et al., 2019). RSEM v1.3.3 was used to obtain gene count estimates of the mapped reads (Li and Dewey, 2011). Fragments per kilobase million (FPKM) were determined using a custom R script with the following R libraries: dplyr, tidyverse, and stringr on R v4.1.0 (R Core Team, 2021). DESeq2 compared the gene counts from non-viruliferous aphids with viruliferous aphids to identify DEGs. Genes that had log2fold changes |LFC| ≥ 1 and a false discovery rate (FDR) ≤0.05 were identified as DEGs (Love et al., 2014). The DEGs were annotated and assigned to gene ontology (GO) classes (up to level 3) and Kyoto Encyclopedia of Genes and Genomes (KEGG) pathways (Kanehisa and Goto, 2000) using the annotated A. gossypii genome (Quan et al., 2019). TopGO (https://www.bioconductor.org/packages/release/bioc/html/topGO.html) and visualize Gene Ontology (REVIGO) web tool were used for the processing and visualization of the GO terms (Supek et al., 2011).
The WGCNA software v1.70-3 was run to create co-expression modules and identify sets of DEGs expressed in a similar pattern in A. gossypii that acquired the CLRDV from alternate hosts using the R software v4.1.0 (Langfelder and Horvath, 2008; R Core Team, 2021). The gradient-independent method with the scale-independent condition of the signed R2 set to 0.90 was used to test the soft-thresholding power modules (1 to 40). The topological overlap matrix (TOM) was constructed using the interaction relationships across the co-expression modules by using correlation expression values. A dendrogram with the parameters mergeCutHeight = 0.15 and detectCutHeight = 0.995 was set to represent the TOM. Each module was represented using randomly assigned colors. The module eigengene was calculated from the first principal component of each module. The labeledHeatmap package in WGCNA software was used to show the topological overlap of co-expression modules based on eigengenes. The network analysis of the top 30 genes from the A. gossypii turquoise was visualized using Cytoscape v3.9.0 (Shannon et al., 2003). Also, the hub genes in the most correlated clusters (magenta, pink, brown, and gray) observed in viruliferous A. gossypii that acquired the virus from each host species (cotton, hibiscus, okra, and prickly sida) were visualized using Cytoscape v3.9.0.
Validation of RNA-sequencing data by RT-qPCR
To validate transcriptomic data, 10 DEGs of A. gossypii were randomly selected for each host species (n = 40). The expression levels of the DEGs were compared between viruliferous and non-viruliferous A. gossypii that acquired CLRDV from different hosts (cotton, hibiscus, okra, and prickly sida) by RT–quantitative polymerase chain reaction (qPCR). Primer pairs designed for each DEG using Primer3web version 4.1.0 are listed in Supplementary Table S1. The GoScript™ Reverse Transcription System (Promega, Madison, WI, USA) was used to reverse-transcribe the total RNA for each sample according to the manufacturer’s instructions. Then, the cDNA was diluted 20-fold for qPCR. The 2xGoTaq® qPCR Master Mix (7.5 µL) (Promega, Madison, WI, USA), primers (0.3 µM), 1 µL of cDNA, and nuclease-free distilled water for a final volume of 15 µL were mixed. The QuantStudio™ 3 Real-Time PCR System (Applied Biosystems by Thermo Fisher Scientific, Waltham, MA, USA) was used for qPCR. The following qPCR conditions were used: an initial denaturation step at 95°C for 3 minutes followed by 40 cycles at 95°C for 15 s and 60°C for 1 minute. Three technical replicates for each sample were used, and the melting curve analysis was conducted to evaluate the specificity of the fluorescence signal. The expression level of each gene was normalized to the expression level of elongation factor 1α (EF1α)—an A. gossypii reference gene. The 2−ΔΔCt method was used to calculate the relative expression of DEGs (Ma et al., 2016).
Results
Summary of RNA sequencing
Three to four biological replicates were included per treatment on each host, resulting in seven cDNA libraries constructed for A. gossypii on each host (cotton, hibiscus, and prickly sida plants), whereas eight libraries were constructed for A. gossypii on okra. Hence, a total of 29 libraries were constructed. Raw read pairs for the generated libraries ranged from nearly 19 to 34 million. After trimming and removing the reads that aligned with the ribosomal RNA and the mitochondrial genome, 19 to 33 million reads were retained (Table 1). A. gossypii cleaned read pairs from different libraries (63 to 82%) were mapped to the A. gossypii transcriptome (Table 1).
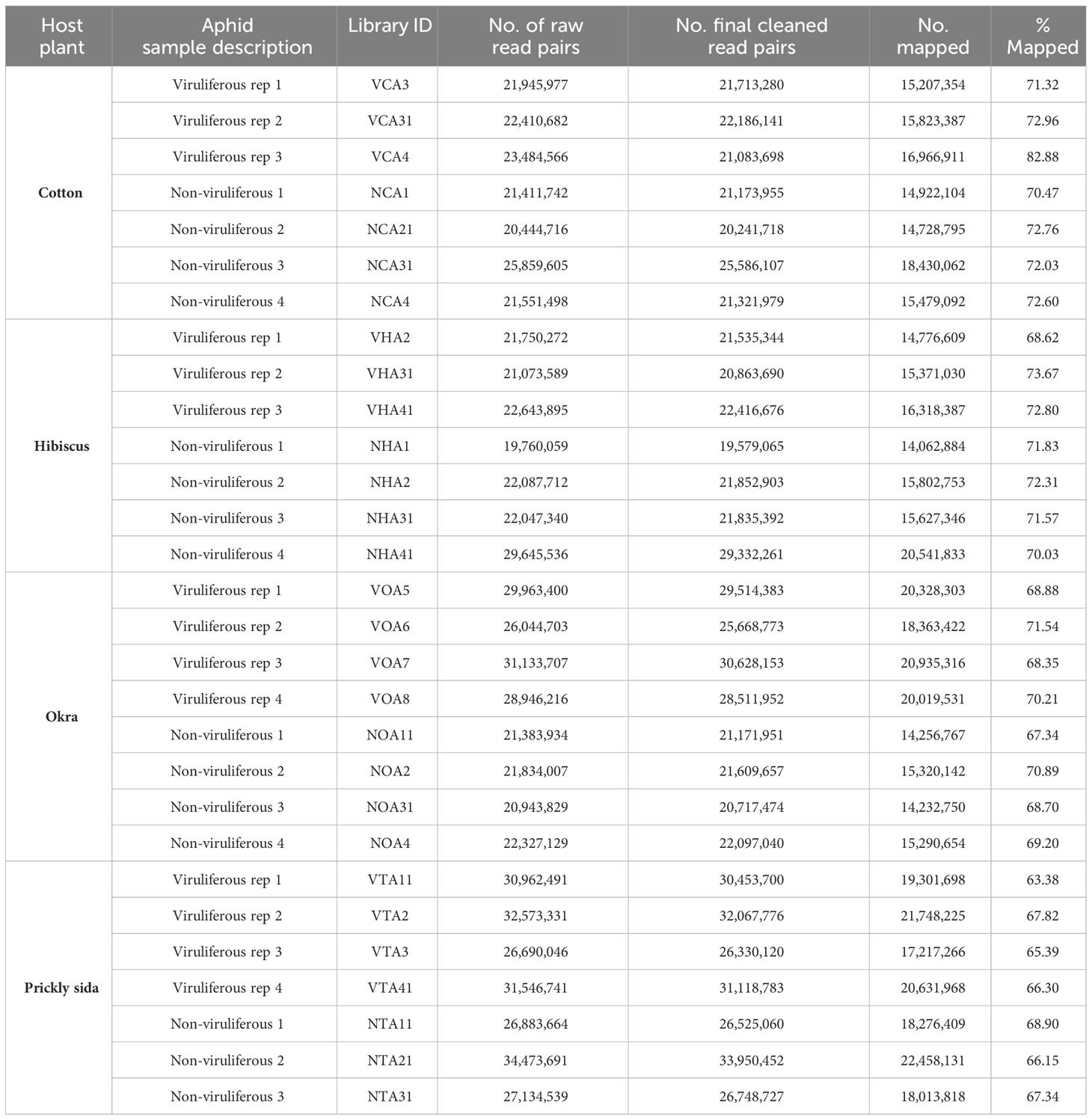
Table 1 Summary of RNA-sequencing datasets generated from Aphis gossypii adults provided with feeding access for 72 h on cotton leafroll dwarf virus-infected or non-infected cotton, hibiscus, okra, and prickly sida plants.
The reads obtained from viruliferous and non-viruliferous A. gossypii samples from different host species were normalized and clustered using FPKM and principal component analysis (PCA) for comparison. The PCA clustered viruliferous A. gossypii samples separately from the non-viruliferous samples for all four host species (Supplementary Figures S1A–D).
Overview of DEGs
Out of 14,134 annotated genes, a total of 750 (622 overexpressed and 128 underexpressed), 310 (168 overexpressed and 142 underexpressed), 1,193 (548 overexpressed and 645 underexpressed), and 689 genes (432 overexpressed and 257 underexpressed) were differentially expressed in viruliferous aphids that acquired CLRDV from infected cotton, hibiscus, okra, and prickly sida plants, respectively (Figure 2; Supplementary Figure S2).
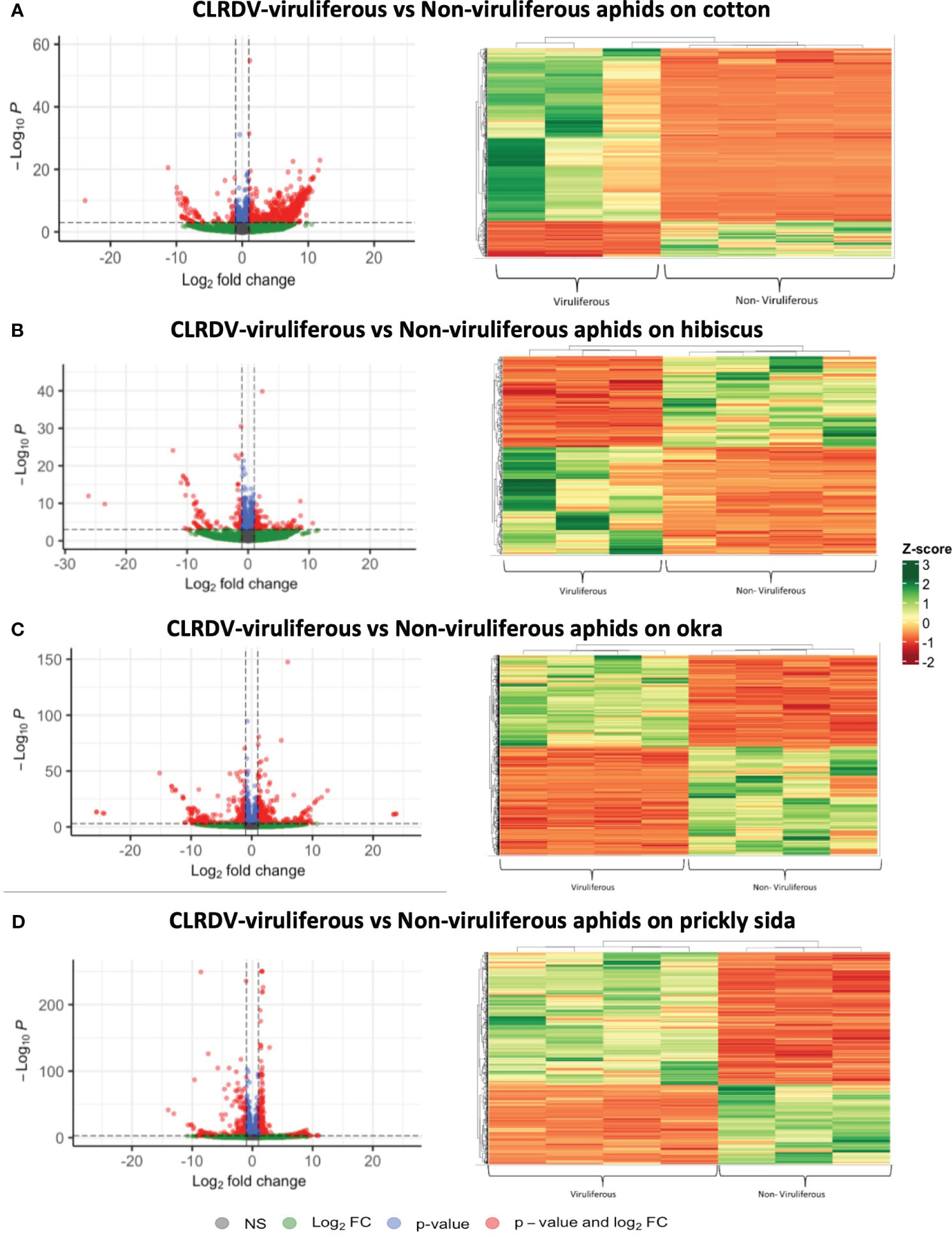
Figure 2 Left: Volcano plots detailing the differential expression profiles of CLRDV-viruliferous versus non-viruliferous Aphis gossypii. Genes with an |LFC| ≥ 1 and a false discovery rate (FDR) <0.05 are highlighted in red and were differentially expressed. Right: Hierarchical clustering analysis of normalized count data z-scores exhibited by differentially expressed genes: (A) 750 DEGs in viruliferous A. gossypii adults that acquired CLRDV from infected cotton plants, (B) 310 DEGs in viruliferous A. gossypii adults that acquired CLRDV from infected hibiscus plants, (C) 1,193 DEGs in viruliferous A. gossypii adults that acquired CLRDV from infected okra plants, and (D) 689 DEGs in viruliferous A. gossypii adults that acquired CLRDV from infected prickly sida plants. CLRDV, cotton leafroll dwarf virus; DEGs, differentially expressed genes.
RT-qPCR with 10 randomly selected DEGs per A. gossypii host (n = 40) was performed to validate RNA sequencing-based differential gene expression results (Supplementary Table S1). The expression trends of the randomly selected A. gossypii DEGs from RNA sequencing and RT-qPCR were highly consistent for all four host species (Supplementary Figures S3A–D).
Common DEGs among viruliferous A. gossypii adults feeding on different host species
Of the 2,942 DEGs in aphids that acquired CLRDV from different host species, only four genes were found to be differentially expressed in common (Figure 3). Two common DEGs were annotated as uncategorized proteins, whereas the other two were functionally annotated. Both the unknown DEGs were overexpressed in A. gossypii on all four hosts, whereas differences in the direction of expression were observed for the annotated common DEGs. The annotated common DEGs were juvenile hormone acid O-methyltransferase and heat shock protein. They were overexpressed in A. gossypii that acquired the virus from CLRDV-infected cotton and okra, whereas they were underexpressed in A. gossypii that acquired CLRDV from infected hibiscus and prickly sida (Table 2).
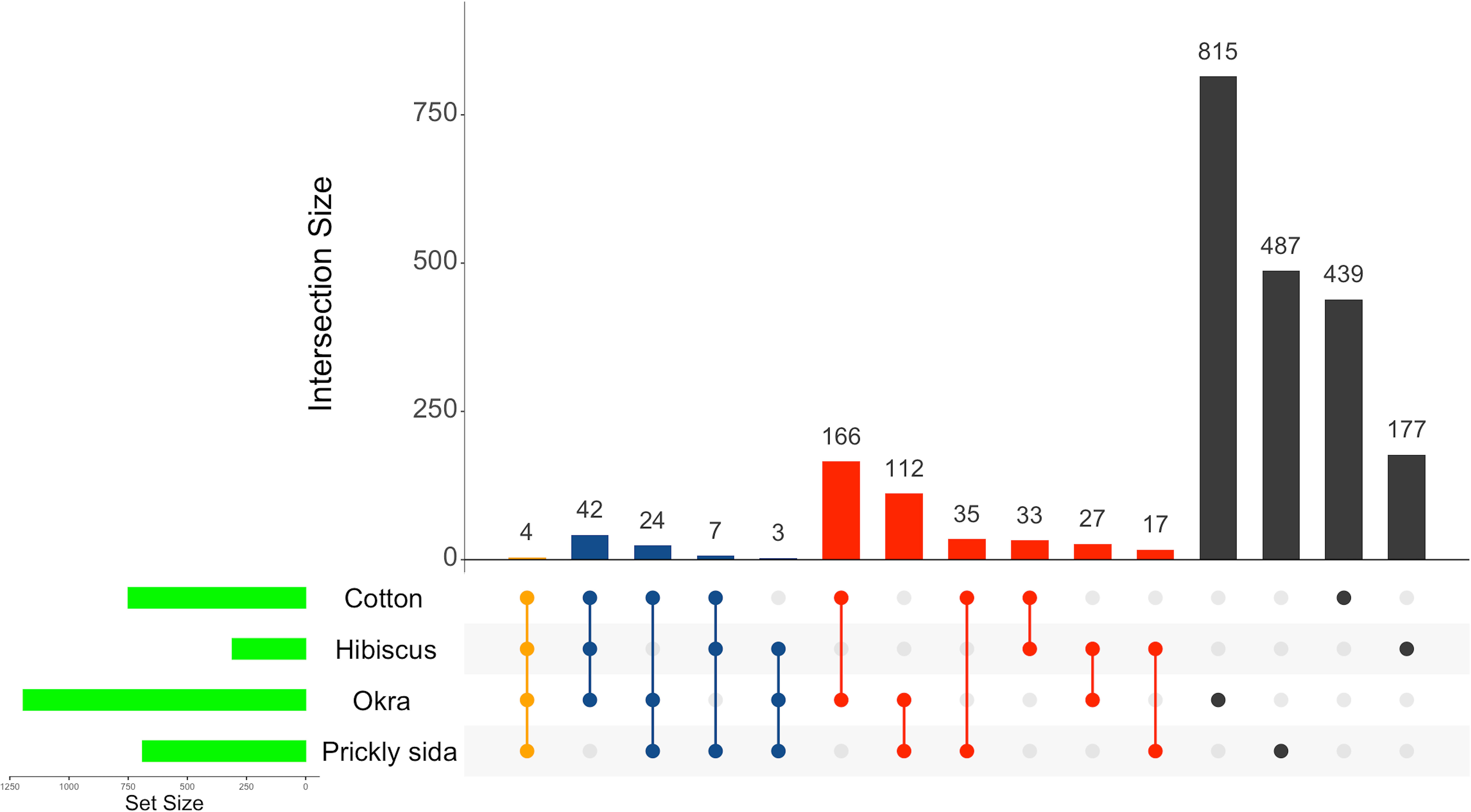
Figure 3 Normalized Venn diagram showing unique and common DEGs in viruliferous Aphis gossypii adults that acquired CLRDV from infected cotton, hibiscus, okra, and prickly sida plants. DEGs, differentially expressed genes; CLRDV, cotton leafroll dwarf virus.
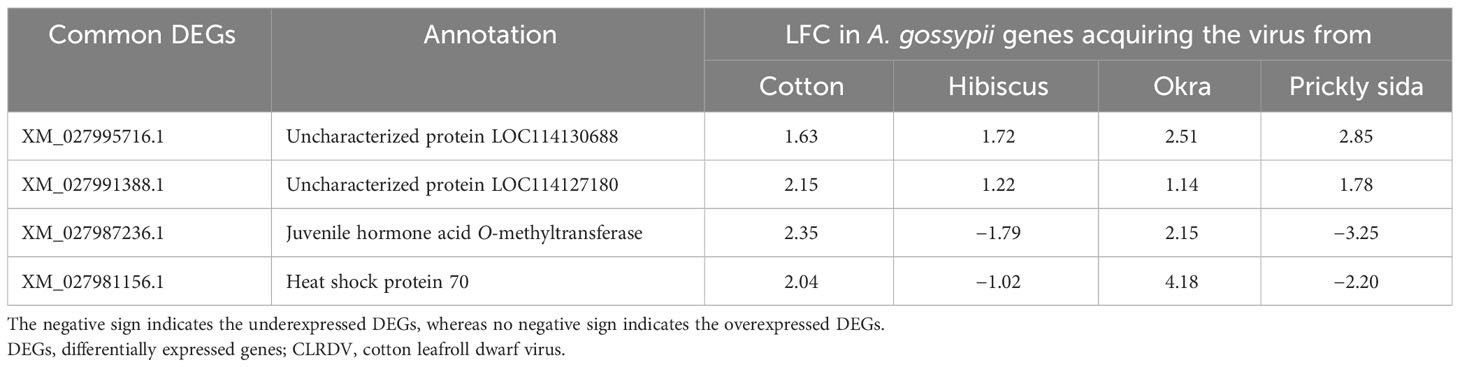
Table 2 List of common DEGs in Aphis gossypii adults that acquired CLRDV from the infected cotton, hibiscus, okra, and prickly sida plants.
Functional annotation of DEGs
Only 320 of the 750 DEGs in A. gossypii that acquired CLRDV from infected cotton were assigned functional groups under three classification systems: biological process (313 genes), molecular function (267 genes), and cellular component (287 genes). Fifty-three GO terms were assigned under the biological process category, of which only two terms (microtubule-based process and ATP metabolic process) were significant (Figure 4A; Supplementary Table S2). Thirty-five GO terms were assigned under the molecular function category, only one (a structural constituent of the cytoskeleton) of which was significant (Figure 4B; Supplementary Table S2). In the cellular component category, 30 GO terms were identified, and only one (cAMP-dependent protein kinase complex) was significant (Figure 4C; Supplementary Table S2). Similarly, the DEGs identified in aphids that acquired CLRDV from hibiscus, okra, and prickly sida were assigned functional groups under three classification systems (Supplementary Figures S4–S6; Supplementary Tables S3-S5). The categorization of these genes was used to identify the DEGs associated with virus–vector interactions.
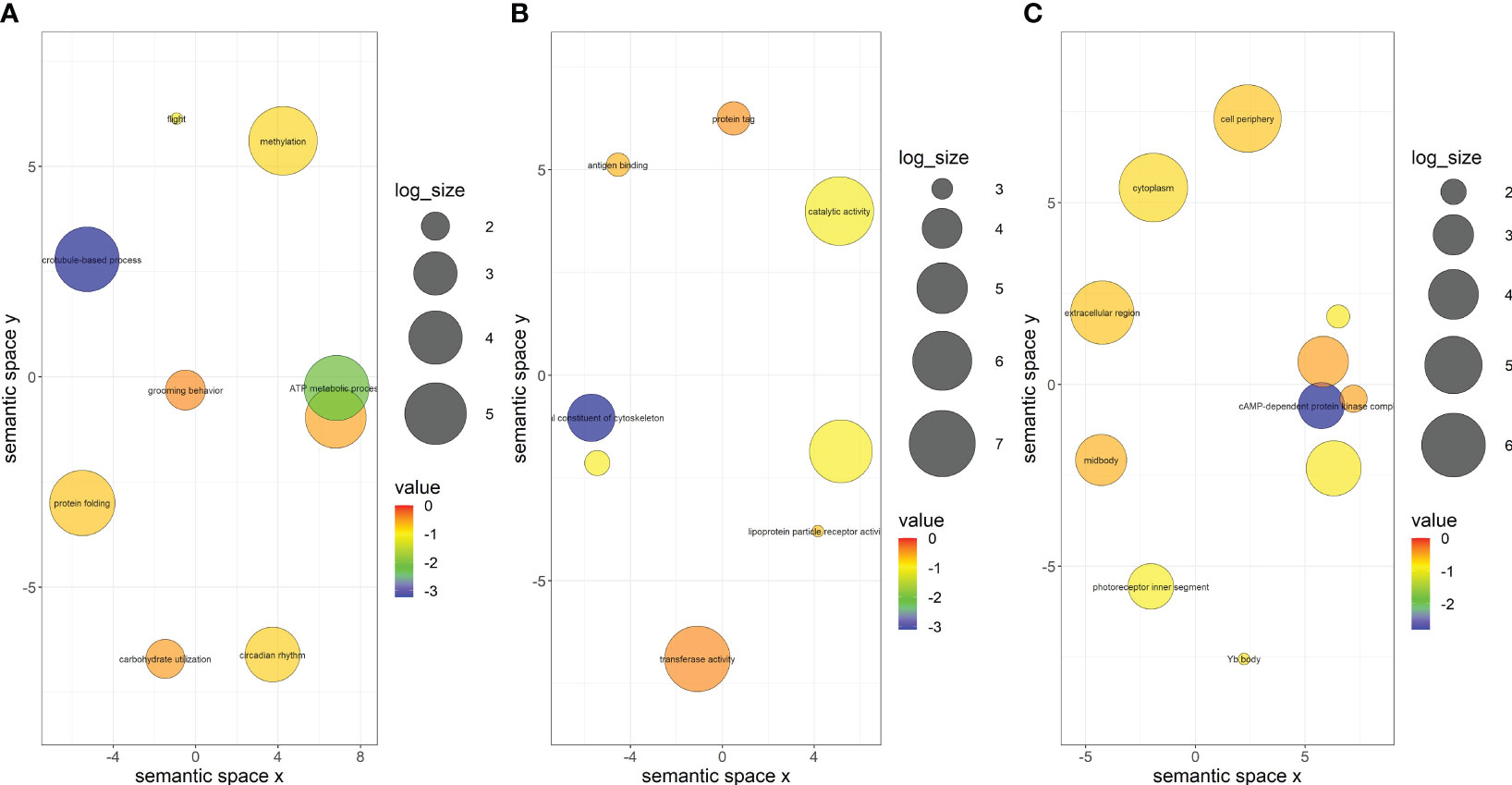
Figure 4 Scatterplots showing (A) biological process, (B) cellular component, and (C) molecular function gene ontology terms in viruliferous Aphis gossypii adults that acquired CLRDV from infected cotton plant. Cluster representatives in a two-dimensional space were derived by applying multidimensional scaling to a matrix of the semantic similarities of the gene ontology terms. The bubble color indicates the p-value, and the size indicates the frequency of the GO term in the underlying GOA database. CLRDV, cotton leafroll dwarf virus; GO, gene ontology; GOA, Gene Ontology Annotation.
Co-expression networks from A. gossypii on CLRDV hosts
The co-expression of genes from A. gossypii adults that acquired CLRDV from cotton, hibiscus, okra, and prickly sida was evaluated. WGCNA, which clusters genes into modules based on weighted gene–gene interactions, was used to evaluate co-expression. For A. gossypii, 13 modules with 19 to 180 genes in each module were identified (Figure 5A; Supplementary Table S6), and Pearson’s correlation coefficient analysis showed the connections between the four CLRDV hosts. The heatmap visualized overall patterns of co-expression of (viruliferous/non-viruliferous) aphid–host relationships (Figure 5B). A. gossypii interactions for the largest module, MEturquoise, were checked for top interacting genes among the 30 identified genes (Figure 5C). The top four most highly connected genes were XM_027993669.1 (trichohyalin-like), XM_027997289.1 (uncharacterized protein), XM_027997209.1 (glucose dehydrogenase [FAD, quinone]-like), and XM_027983368.1 (neuroendocrine convertase 1-like) with interconnectivity scores of 58.99, 57.81, 53.87, and 52.58, respectively (Supplementary Table S6).
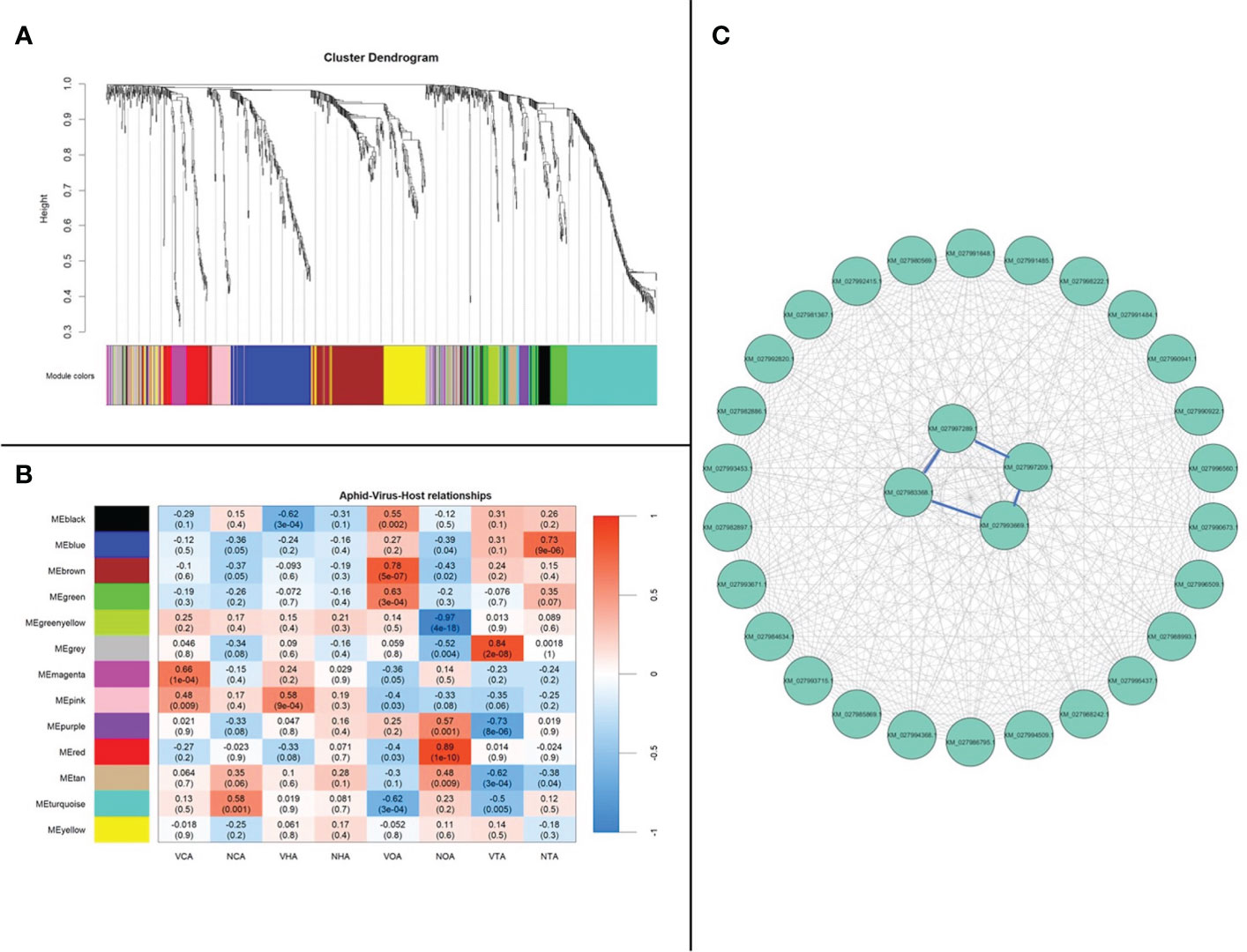
Figure 5 Aphis gossypii adults weighted gene co-expression network analysis. (A) Dendrogram clustering shows eight modules of co-expressed genes. A total of 981 genes are represented in this network, with 190 genes belonging to MEturquoise. (B) Heatmap showing the correlation of module eigengenes in relation to A. gossypii that acquired CLRDV from cotton, hibiscus, okra, and prickly sida. (C) Top 30 genes from MEturquoise with connectivity lines (blue) associated with the top 5% of the connected genes. NCA, non-viruliferous aphid from cotton; VCA, viruliferous aphid from cotton; NHA, non-viruliferous aphid from hibiscus; VHA, viruliferous aphid from hibiscus; NOA, non-viruliferous aphid from okra; VOA, viruliferous aphid from okra; NTA, non-viruliferous aphid from prickly sida; VTA, viruliferous aphid from prickly sida; CLRDV, cotton leafroll dwarf virus.
Hub genes from candidate modules
Four modules (magenta, pink, brown, and gray) were highly correlated with viruliferous A. gossypii that acquired CLRDV from infected cotton, hibiscus, okra, and prickly sida plants, respectively. The magenta module associated with viruliferous A. gossypii that acquired CLRDV from infected cotton plants contained 34 genes. Similarly, pink, brown, and gray modules associated with A. gossypii that acquired CLRDV from infected hibiscus, okra, and prickly sida plants included 40, 130, and 129 genes, respectively. The maximum connectivity in magenta, pink, and brown modules were 8.9, 8.8, and 20, respectively. However, the connectivity in the gray module was less than one. Hence, only magenta, pink, and brown modules were considered to identify candidate genes related to virus interactions and transmission (Supplementary Table S6).
Most genes in the magenta module were overexpressed only in viruliferous A. gossypii that acquired CLRDV from infected cotton plants, and 10 genes were identified as hub genes based on their high connectivity values (Figure 6A). Some of the hub genes were predicted to encode tubulin-β (XM_027988533.1 and XM_027985455.1), tubulin-α (XM_027988908.1), GPI-anchored protein (XM_027997066.1), U1 small nuclear ribonucleoprotein (XM_027985431.1), mucin-7-like (XM_027997067.1), and dynein beta chain (XM_027985730.1) (Supplementary Table S6). The tubulin-α and tubulin-β genes were associated with cellular responses following virus acquisition (Table 3).
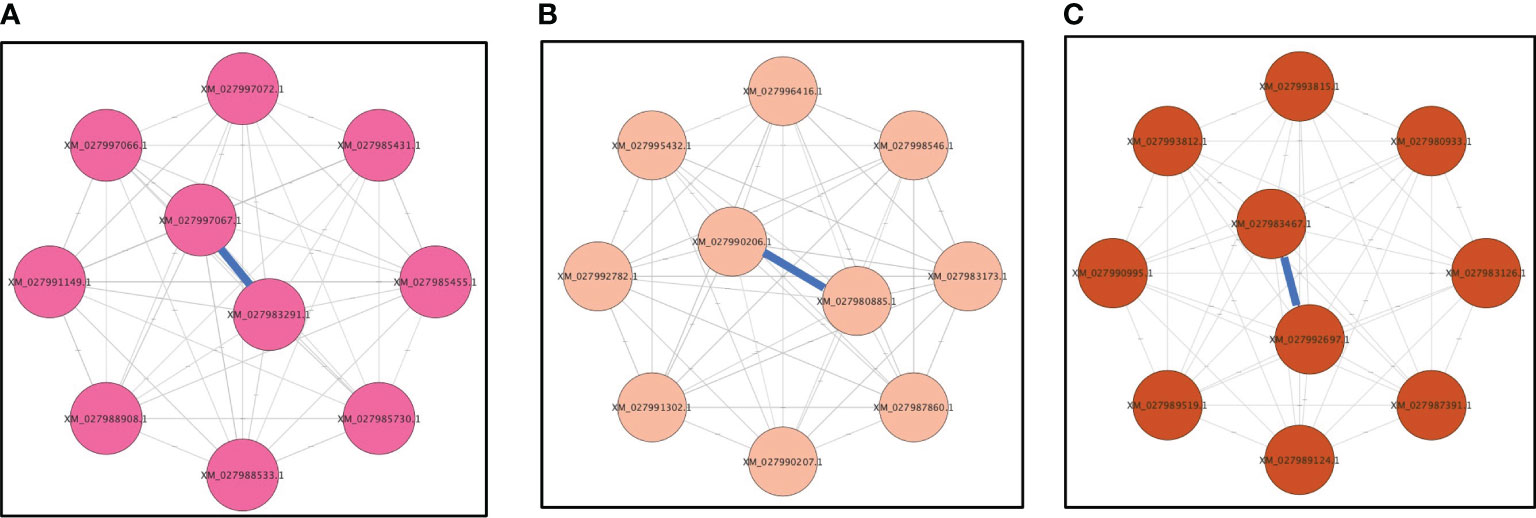
Figure 6 Top 10 genes from (A) magenta, (B) pink, and (C) brown modules with connectivity lines (blue) associated with viruliferous Aphis gossypii adults that acquired CLRDV from infected cotton, hibiscus, and okra plants, respectively. CLRDV, cotton leafroll dwarf virus.
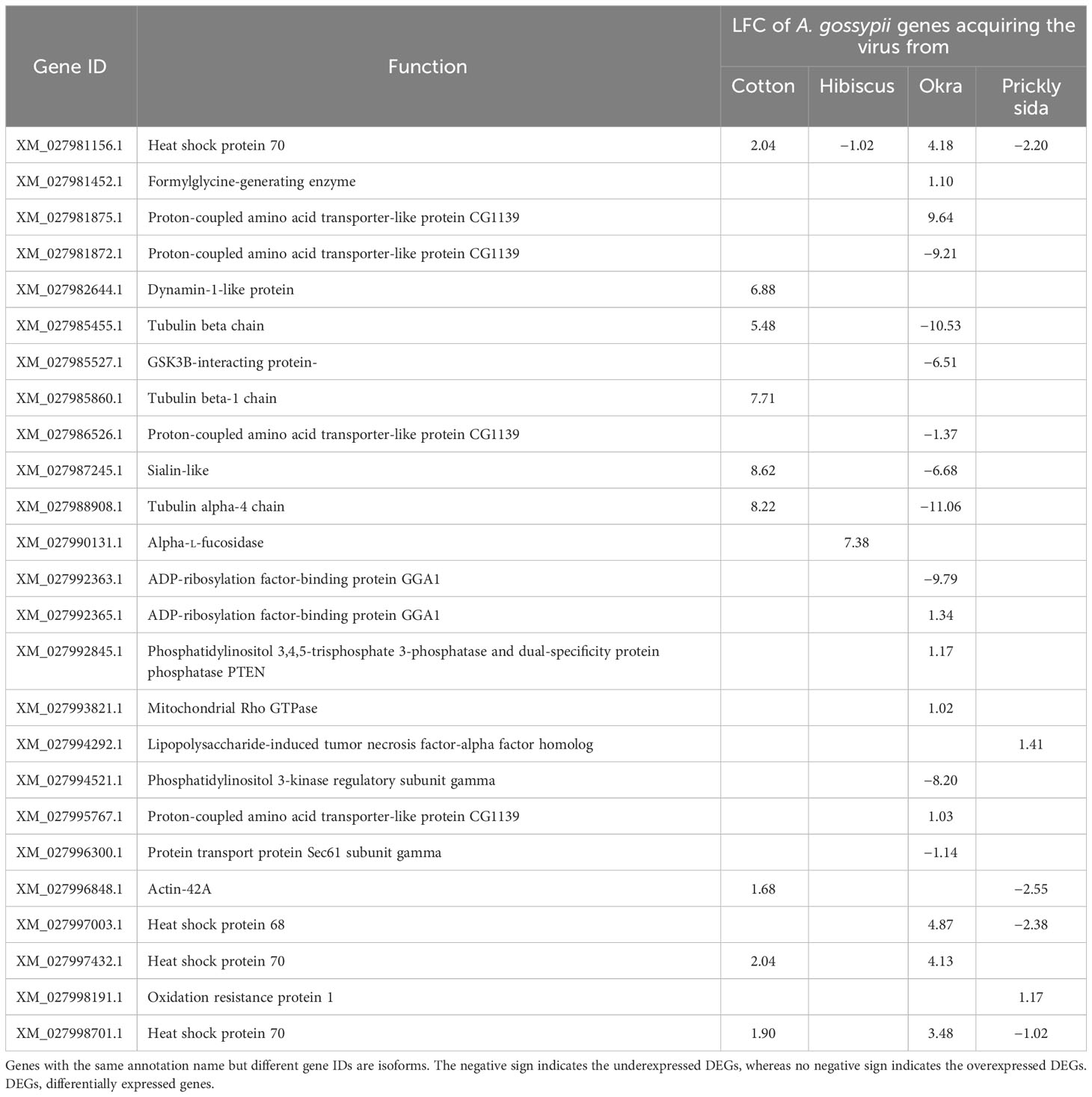
Table 3 Differential expression of genes associated with cellular responses (endocytosis, apoptosis, lysosome, and phagosome) in viruliferous Aphis gossypii adults compared with non-viruliferous adults.
Similarly, most genes in the pink module were underexpressed in viruliferous A. gossypii that acquired CLRDV from infected hibiscus plants, and 10 genes were identified as hub genes based on their high connectivity values (Figure 6B). Some of these hub genes were predicted to encode proteasome subunit-β (XM_027980885.1), trehalose transporter Tret1 (XM_027996416.1), and M-phase inducer phosphatase (XM_027998546.1) (Supplementary Table S6).
Most genes in the brown module were underexpressed in viruliferous A. gossypii that acquired CLRDV from infected okra plants, and 10 genes were identified as hub genes based on their high connectivity values (Figure 6C). These hub genes were predicted to encode ubiquitin carboxyl-terminal hydrolase 7 (XM_027983467.1), dynein heavy chain (XM_027987391.1), serine/threonine-protein kinase WNK1 (XM_027980933.1), and eukaryotic translation initiation factor 4 gamma (XM_027992697.1) (Supplementary Table S6). The serine/threonine-protein kinase genes were associated with signal transduction following virus acquisition (Table 4).
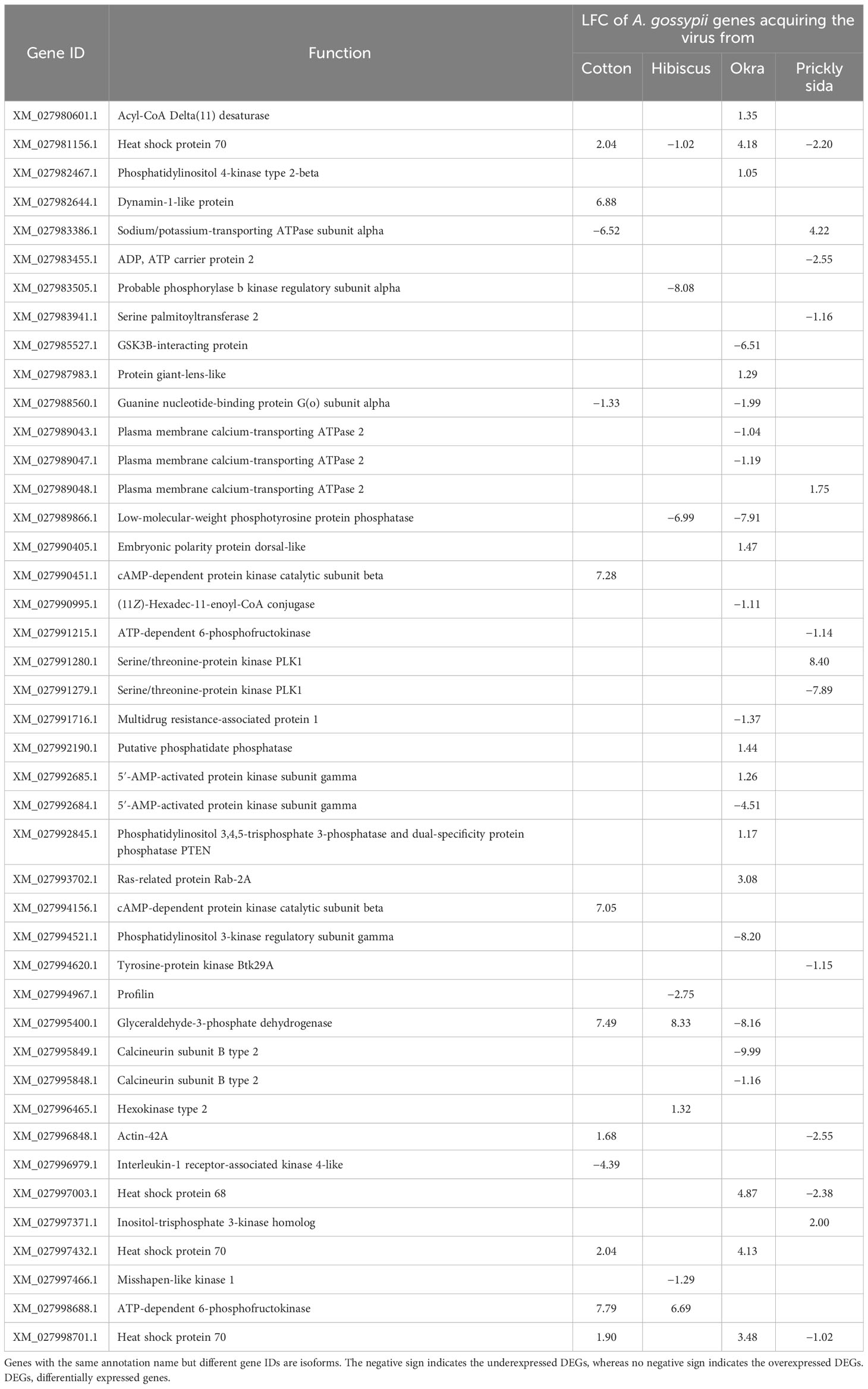
Table 4 Differential expression of genes associated with signal transduction in viruliferous Aphis gossypii adults compared with non-viruliferous adults.
DEGs among A. gossypii adults associated with virus–vector interactions
Virus infection
In A. gossypii adults, DEGs associated with different viruses, including measles virus, coronavirus, human cytomegalovirus, human immunodeficiency virus 1, herpes simplex virus 1, human T-cell leukemia virus 1, human papillomavirus, hepatitis B and C, virus, influenza A virus, and Epstein–Barr virus were identified upon CLRDV acquisition from different host species. Eleven, four, 12, and seven genes associated with virus infection in A. gossypii that acquired CLRDV from infected cotton, hibiscus, okra, and prickly sida plants, respectively, were identified. The expression of these DEGs ranged from −26.18- to 7.60-fold (Table 5).
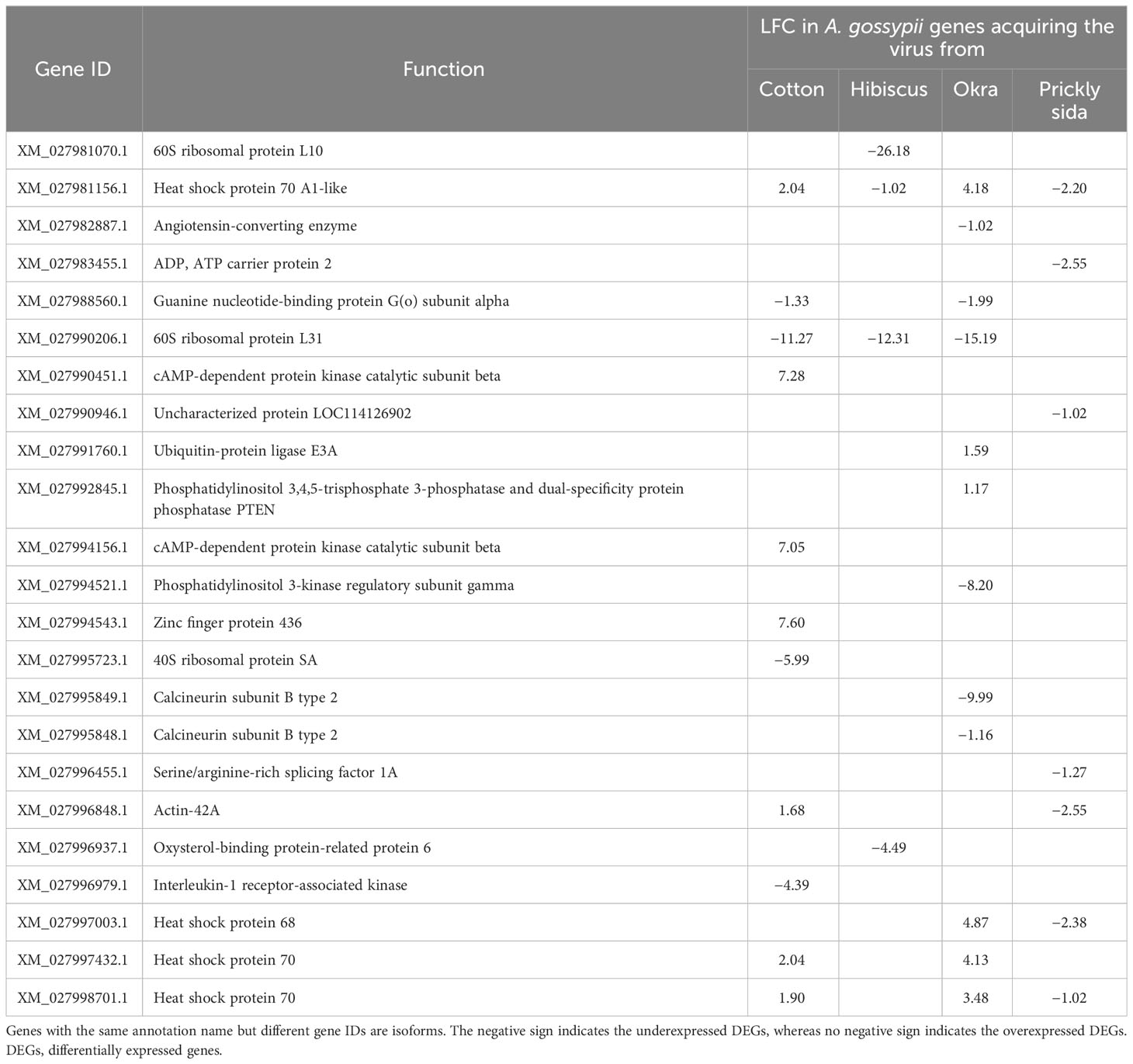
Table 5 Differential expression of genes associated with virus infection in viruliferous Aphis gossypii adults compared with non-viruliferous adults.
Signal transduction
Twelve DEGs were associated with 14 signal transduction pathways in A. gossypii that acquired CLRDV from infected cotton plants. Similarly, eight, 24, and 13 DEGs associated with different signal transduction pathways in A. gossypii that acquired CLRDV from infected hibiscus, okra, and prickly sida plants, respectively, were identified (Figure 7). The expression of these DEGs ranged from −8.08- to 8.40-fold (Table 4).
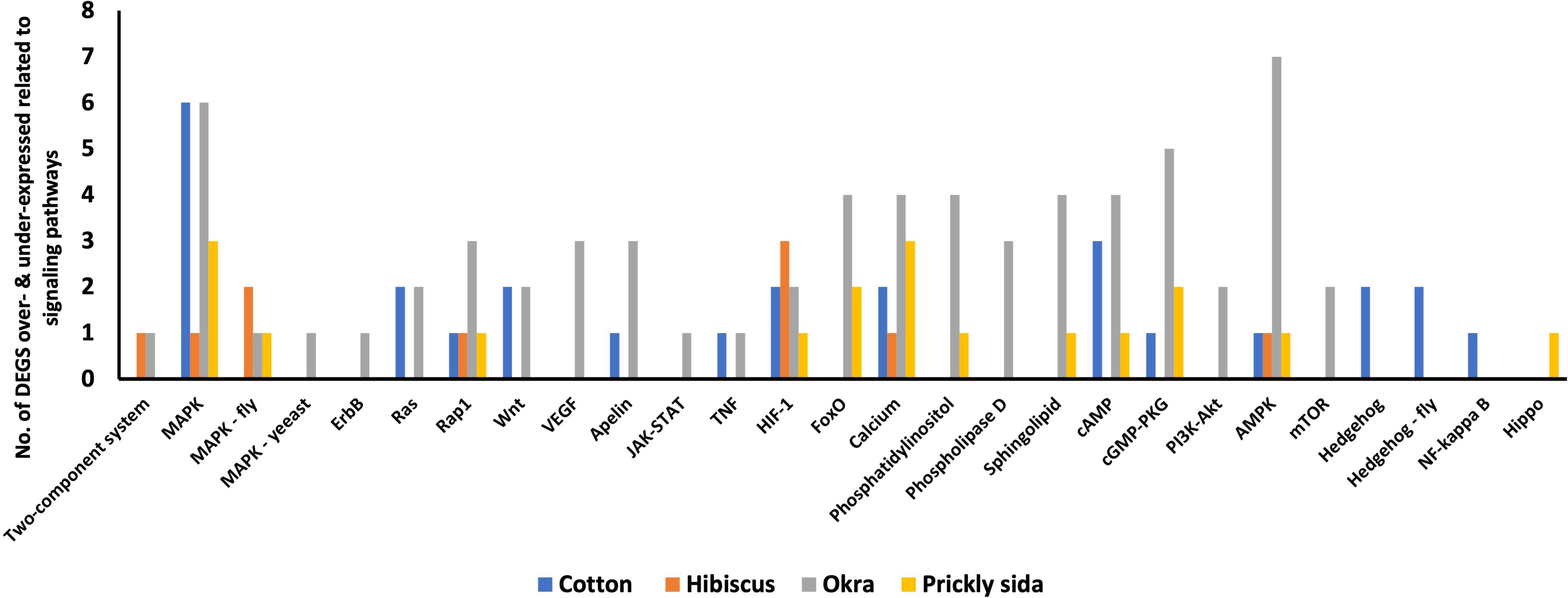
Figure 7 Number of differentially expressed genes (DEGs) related to different signaling pathways in viruliferous Aphis gossypii adults that acquired CLRDV from infected cotton, hibiscus, okra, and prickly sida plants. CLRDV, cotton leafroll dwarf virus.
Signaling molecules and virus interaction
Using KEGG annotation, one putative receptor gene was identified in A. gossypii that acquired CLRDV from infected cotton (XM_027997734.1, cardioacceleratory peptide receptor) and one from prickly sida (XM_027980613.1, neuropeptide SIFamide receptor). Putative receptor genes were not identified in A. gossypii adults that acquired CLRDV from infected hibiscus and okra plants (Table 6).
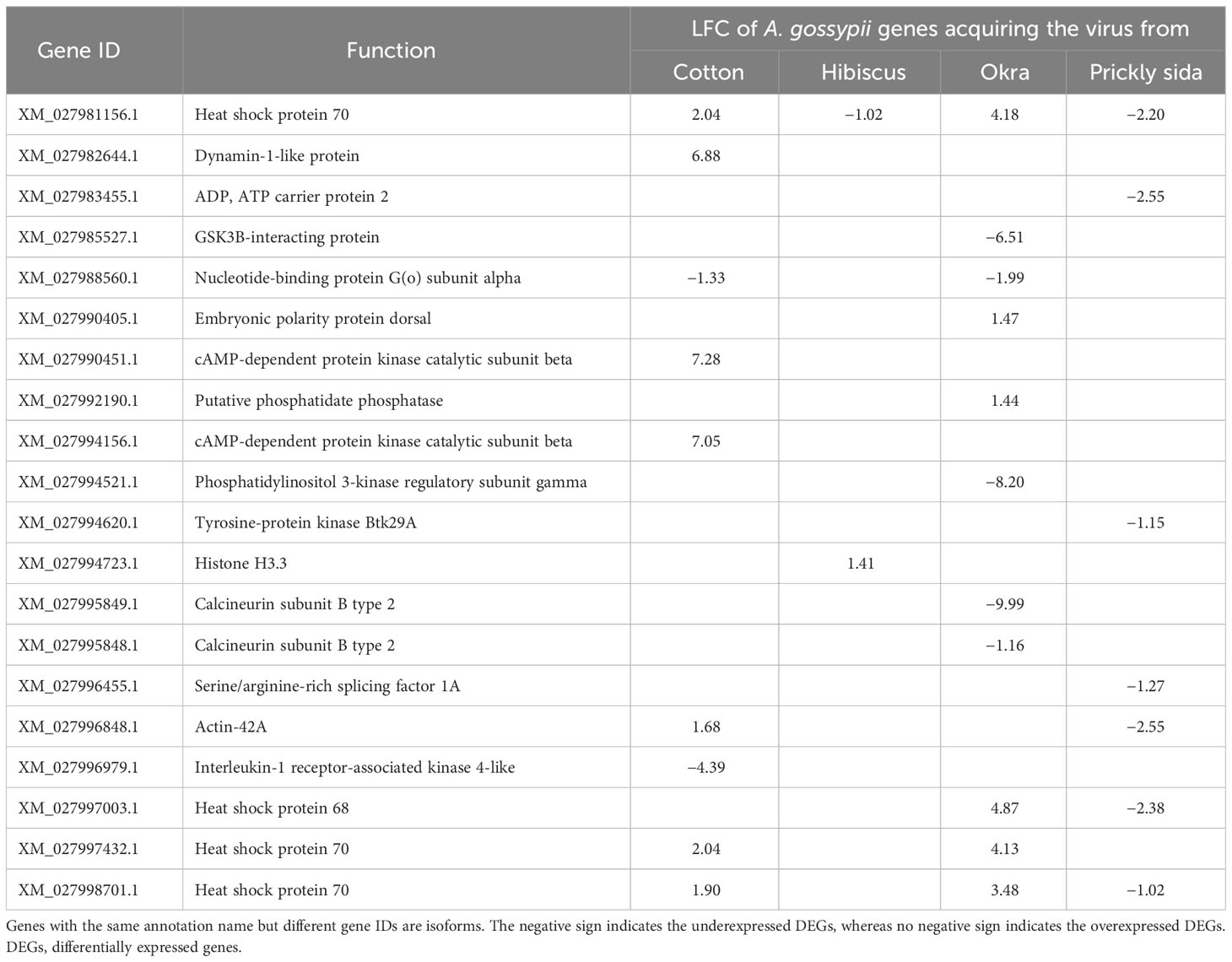
Table 6 Differential expression of genes associated with immune systems in viruliferous Aphis gossypii adults compared with non-viruliferous adults.
Immune systems
A total of nine DEGs were annotated using KEGG analysis and associated with six immune system pathways in A. gossypii adults that acquired CLRDV from infected cotton plants. Similarly, 11 DEGs with 15 immune system pathways were identified for A. gossypii adults that acquired CLRDV from infected okra plants. Only two DEGs from two immune system pathways and seven DEGs from six immune system pathways were identified in A. gossypii that acquired CLRDV from infected hibiscus and prickly sida plants, respectively. The expression of these DEGs ranged from 9.99- to 7.28-fold (Table 6; Supplementary Table S7).
Cellular processes (apoptosis, lysosome, and phagosome)
A total of six, one, 15, and three DEGs were associated with cellular processes in A. gossypii that acquired CLRDV from infected cotton, hibiscus, okra, and prickly sida plants, respectively. The expression of these DEGs ranged from −11.06- to 9.64-fold (Table 3).
In the cellular process category, heat shock proteins 70 and 68 were identified as genes playing a role in endocytosis. The heat shock proteins were overexpressed in A. gossypii acquired CLRDV from infected cotton and okra plants but underexpressed when acquired from infected hibiscus and prickly sida plants. Another gene related to endocytosis was underexpressed in A. gossypii that acquired CLRDV from infected okra plants (Table 7).
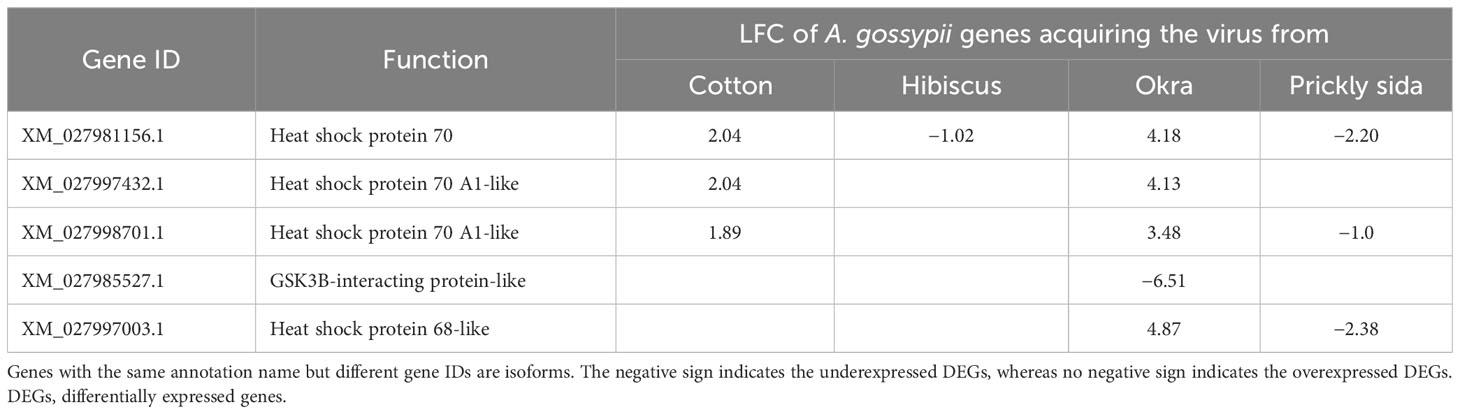
Table 7 Differential expression of genes associated with cellular responses (endocytosis) in viruliferous Aphis gossypii adults compared with non-viruliferous adults.
DEGs among A. gossypii adults associated with aphid fitness
Longevity
Based on KEGG pathway annotation, five DEGs were associated with two aging pathways in A. gossypii that acquired CLRDV from infected cotton plants (Table 8). Similarly, one, 11, and three DEGs associated with different aging pathways in A. gossypii that acquired CLRDV from infected hibiscus, okra, and prickly sida plants, respectively, were identified (Figure 7). The expression of these DEGs ranged from −8.49- to 7.28-fold (Table 8).
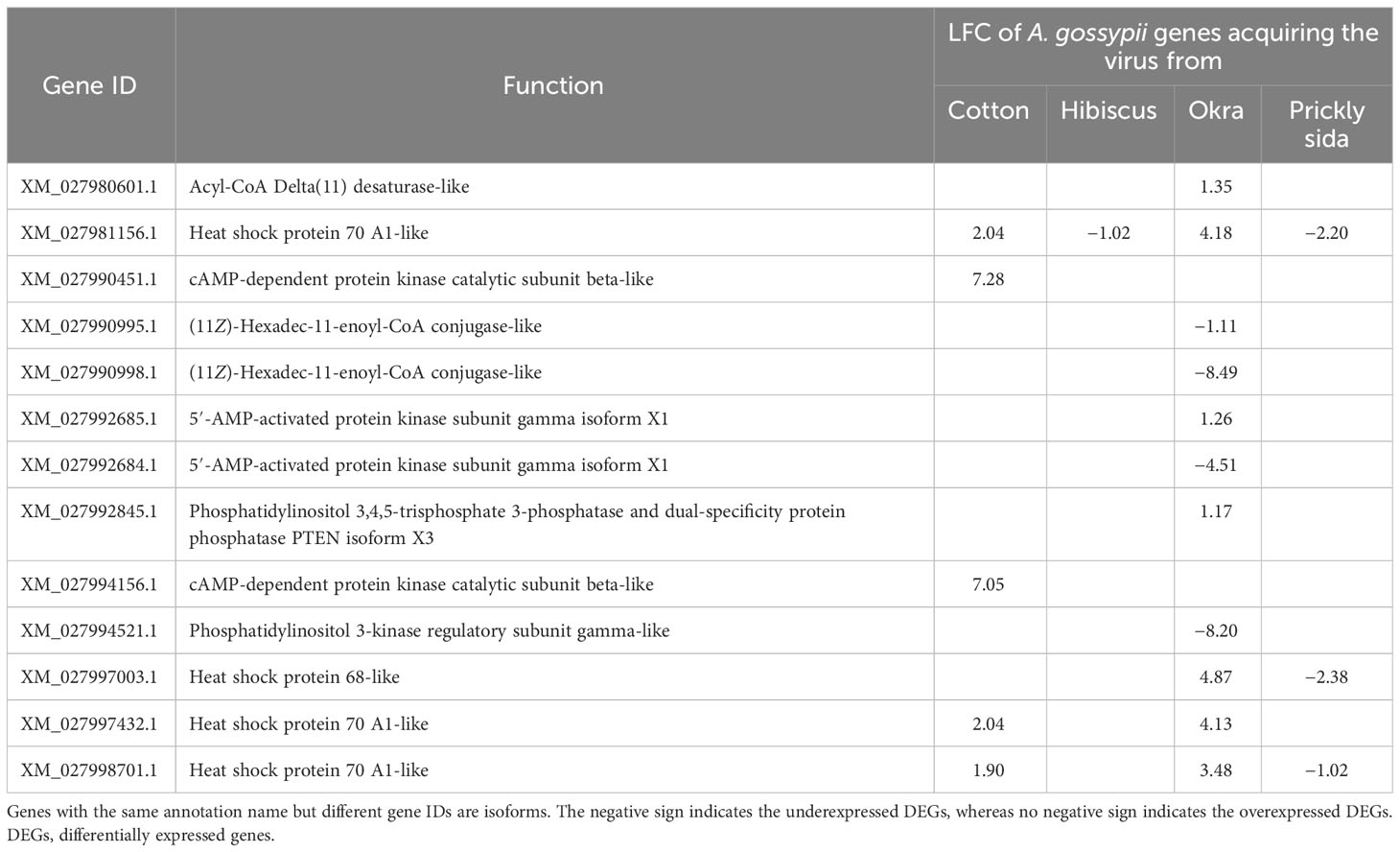
Table 8 Differential expression of genes associated with aging in viruliferous Aphis gossypii adults compared with non-viruliferous adults.
Reproduction
Using GO annotation, several genes associated with reproduction were identified in A. gossypii adults. The number of DEGs was the highest in A. gossypii that acquired CLRDV from infected okra, followed by cotton, prickly sida, and hibiscus plants (Figure 8). Unlike that in A. gossypii that acquired CLRDV from infected cotton, hibiscus, and prickly sida plants, the number of underexpressed genes in A. gossypii that acquired CLRDV from infected okra plants was higher than the overexpressed genes (Figure 8). One of the common genes identified in this GO annotation was juvenile hormone acid O-methyltransferase (Supplementary Table S8).
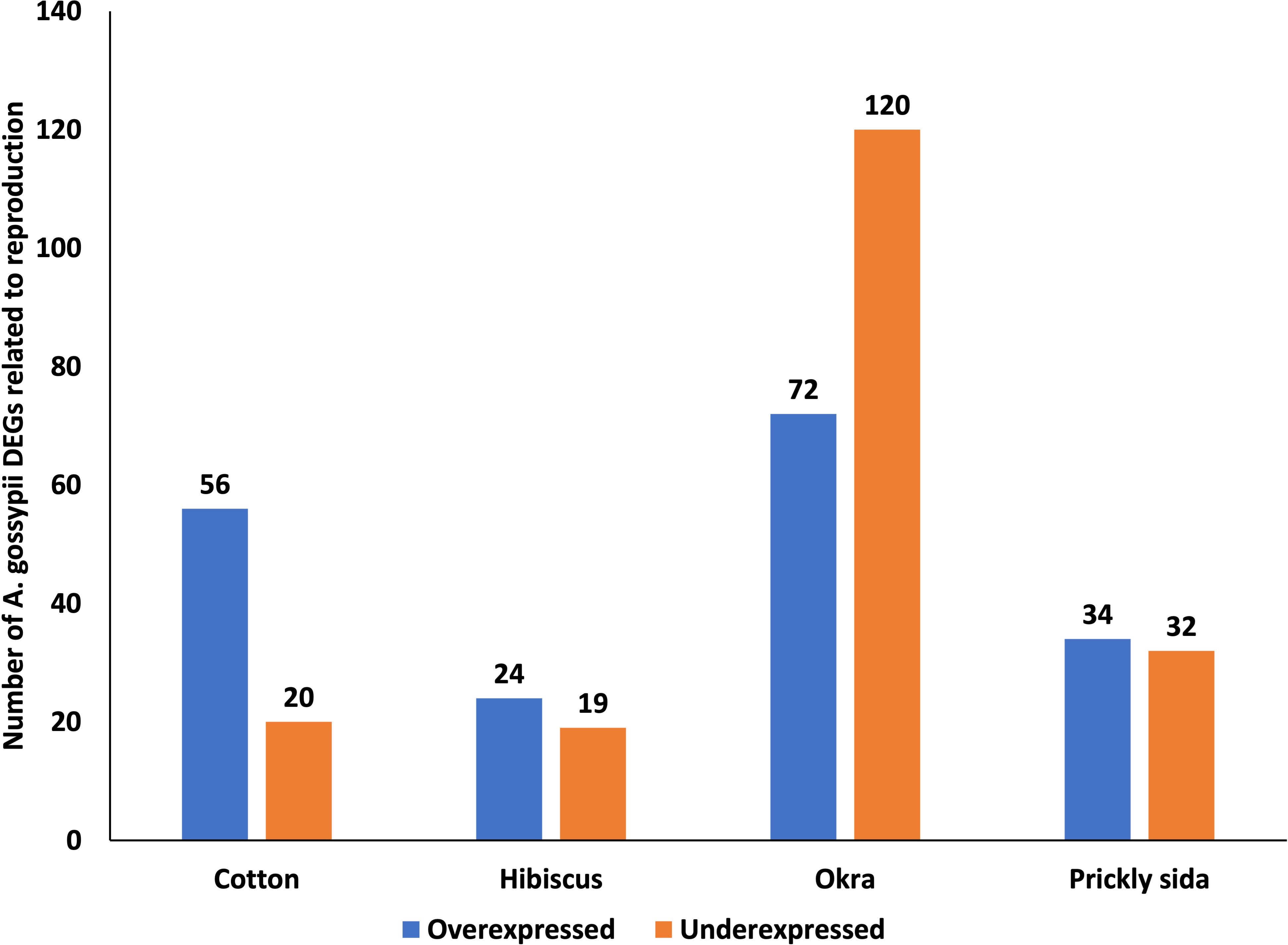
Figure 8 Number of differentially expressed genes (DEGs) related to reproduction in viruliferous Aphis gossypii adults that acquired CLRDV from infected cotton, hibiscus, okra, and prickly sida plants. CLRDV, cotton leafroll dwarf virus.
Discussion
In some rare instances, direct effects of persistent non-propagative viruses on their vectors’ behavior and/or fitness have been documented (Bosque-Pérez and Eigenbrode, 2011; Ingwell et al., 2012; Marmonier et al., 2022). However, most effects of persistent and non-propagative phytoviruses on their vectors’ behavior and fitness seem to be modulated by the host plants due to their altered phenotypic traits following virus infection (Eigenbrode et al., 2002; Srinivasan et al., 2006; Ngumbi et al., 2007; Hodge and Powell, 2008; Medina-Ortega et al., 2009; Werner et al., 2009; Bosque-Pérez and Eigenbrode, 2011; Legarrea et al., 2020; Fingu-Mabola and Francis, 2021; Safari Murhububa et al., 2021; Hu et al., 2022). The degree of the altered host phenotype would substantially depend upon the virus susceptibility status of the host. While this phenomenon has been researched in many persistent virus pathosystems involving hemipteran vectors, it has been extremely difficult to parse apart the host effect from the direct virus-induced impacts on vectors (Eigenbrode et al., 2002; Ingwell et al., 2012; Marmonier et al., 2022). In addition, generalizations seem to originate from host and virus-modulated effects on vectors based on individual hosts and viruses that at least possess a modest or often a promiscuous host range (Jiménez-Martínez et al., 2004; Lightle and Lee, 2014; dos Santos et al., 2016; Ghosh et al., 2016; Claudel et al., 2018; Chesnais et al., 2020; Moeini et al., 2020; Bertasello et al., 2021; Fingu-Mabola and Francis, 2021; Jayasinghe et al., 2022). This also applies to vectors and their host utilization capacities when they are generalists (Castle et al., 1998; Eigenbrode et al., 2002; Ngumbi et al., 2007; Rajabaskar et al., 2013; Ren et al., 2015; Liu et al., 2019). Host-modulated virus-induced effects on the vector are realized in the form of ecological, behavioral, and fitness patterns. However, advancements in omics techniques that capture associated gene expression patterns provide greater opportunities to explore this paradigm of vector–virus interactions. This study assessed the differences in gene expression in A. gossypii adults in response to the acquisition of CLRDV from its primary host plant (cotton) and alternate host plants (hibiscus, okra, and prickly sida). The results show that transcriptional changes observed in viruliferous A. gossypii vary substantially between the host species from which it acquired the virus. The results indicate that the host plant could be a major determinant of vector–virus interaction outcomes.
Across all four host species, most transcriptional changes were observed in A. gossypii that acquired CLRDV from infected okra plants, followed by cotton, prickly sida, and hibiscus, which represented 8.11%, 5.1%, 4.6%, and 2.1% of the overall genes in the aphid genome, respectively. Similarly, the number of unique genes of A. gossypii was the highest when the virus was acquired from okra, followed by prickly sida, cotton, and hibiscus plants. These findings indicate that CLRDV-induced transcriptional changes in A. gossypii adults upon virus acquisition from different host species vary drastically. This variation in the number of transcriptional responses occurring in A. gossypii could be affected by differences in host susceptibility to the virus, host nutrient quality, physiology, and defense mechanisms (Gadhave et al., 2019; Marmonier et al., 2022). In a previous study, the percentage of adult aphids that acquired CLRDV, the amount of virus acquired, and the percentage of aphid-mediated back-transmission of the virus varied significantly between the four host plants (Pandey et al., 2022). The transcriptional differences observed when adult A. gossypii acquired CLRDV from different hosts observed in this study, in part, could explain some of the observed variations in virus acquisition and inoculation ability of adult aphids (Pandey et al., 2022).
Previous studies also have reported varying transcriptional responses in aphids upon polerovirus acquisition. For instance, 164 DEGs were identified in M. persicae adults that acquired TuYV from infected plants compared with non-viruliferous aphids, whereas 201 DEGs were identified when the aphids acquired the virus from an artificial medium compared with non-viruliferous aphids in the same study (Marmonier et al., 2022). Similarly, the number of DEGs was greater in viruliferous aphids that acquired TuYV from Arabidopsis thaliana (L.) Heynh (1,073 genes) compared with viruliferous aphids that acquired TuYV from Camelina sativa (L.) Crantz (474 genes) (Chesnais et al., 2022). Thus, the variation in the number of DEGs may be influenced by the host species from which it is acquiring the virus. The number of DEGs in the same aphid species (M. persicae) upon acquisition of another polerovirus species PLRV from infected potato plants, when compared with their non-viruliferous counterparts, was 134 (Patton et al., 2021). The acquisition of the same virus species (BYDV) from virus-infected wheat plants resulted in significant variation in the number of DEGs of two of its aphid vectors—S. graminum (1,525 genes) and S. avenae (800 genes)—in comparison with non-viruliferous vectors (Li et al., 2019, 2020). These results reiterate that different viruses and host interactions as well as the same virus–host interactions could differentially induce gene expression in the same vector or different vectors. Also, the experimental design factors such as acquisition period, gut clearing, sequencing platforms, number of libraries sequenced, and bioinformatics tools used for analysis may have contributed to the variation in the number of DEGs across virus–vector–host pathosystems in different studies. What is missing in understanding component interactions within a phytovirus–vector pathosystem is the impact of alternate hosts on virus–vector interactions. In other words, how conserved are vector–virus interactions across host species?
Common DEGs among viruliferous A. gossypii adults associated with virus–vector interactions
In this study, the acquisition of CLRDV resulted in transcriptional changes in A. gossypii, of which only four DEGs were common between the viruliferous A. gossypii adults acquiring the virus from four host species. Among four common DEGs, the direction and/or level of the expression (over or under) of common genes varied between host species. The KEGG annotation and GO enrichment analysis revealed the role of one of the common genes (XM_027981156.1, heat shock protein 70) in virus infection, signal transduction, immune responses, longevity, and endocytosis. Heat shock protein 70 was overexpressed in A. gossypii upon acquiring the virus from CLRDV-infected cotton and okra plants, whereas it was underexpressed in A. gossypii that acquired the virus from CLRDV-infected hibiscus and prickly sida plants. In this study, the expression level of heat shock protein was nearly double in A. gossypii that acquired the virus from infected okra compared with cotton. The heat shock proteins are essential chaperone proteins known to be overexpressed in response to stress conditions. One study found that heat shock protein 70 was overexpressed upon BYDV acquisition in its aphid vector (R. padi). The BYDV infection has been reported to increase the plant surface temperature and aphid heat tolerance, suggesting a protective role (Porras et al., 2020). Another study has reported the interaction of tomato yellow leaf curl virus (TYLCV) with Bemisia tabaci (Gennadius) heat shock protein 70 in the midgut using in vitro studies. The protein was suggested to play an inhibitory role in virus transmission (Götz et al., 2012). The higher expression level of heat shock protein could be one of the reasons for reduced CLRDV acquisition and inoculation from okra to cotton plants by A. gossypii reported in an earlier study (Pandey et al., 2022).
However, the other common gene (XM_027987236.1, juvenile hormone acid O-methyltransferase) was overexpressed in aphids that acquired the virus from cotton and okra but underexpressed in aphids that acquired the virus from hibiscus and prickly sida plants. In a previous study, the JHAMT (juvenile hormone-III synthase) was overexpressed in S. avenae that acquired BYDV from wheat plants (Li et al., 2019). This gene is known to play a regulatory role, as a rate-limiting enzyme in insect juvenile hormone biosynthesis, which is essential in the development, metamorphosis, and reproduction of insects (Shinoda and Itoyama, 2003; Minakuchi et al., 2008; Niwa et al., 2008). In contrast, in another study, the underexpression of juvenile hormones in aphids was linked with increased wing development and enhanced virus spread (Quan et al., 2019; Zhang et al., 2019). Variations in the expression levels of juvenile hormone acid O-methyltransferase observed in A. gossypii suggest that CLRDV acquisition may enhance or reduce the fitness of A. gossypii depending on the host species and warrants further examination.
Unique DEGs associated with virus–vector interactions
In addition to the four DEGs in common, many unique DEGs were identified in A. gossypii depending on the plant species from which the virus was acquired. The number of DEGs uniquely expressed was the highest in aphids that acquired the virus from okra plants. For example, the ras-related protein (Rab protein) associated with signaling in the circadian clock cells in Drosophila melanogaster Meigen was uniquely identified and underexpressed in aphids that acquired the virus from okra plants (Williams et al., 2001). Rab proteins also function as transporters of vesicle cargo, responsible for trafficking among several membrane compartments (Zhang et al., 2007). Hence, the underexpression of this gene could be one of the reasons for the lower virus acquisition and/or retention ability of aphids from okra plants. The tubulin beta-1 chain gene encoding a structural constituent of the cytoskeleton was uniquely overexpressed in A. gossypii that acquired CLRDV from infected cotton plants in this study. The overexpression of this gene enhances the insects’ development and reproduction (Nielsen et al., 2010). Tubulin is also a major constituent of microtubules, which is an integral part of intracellular transport (Logan and Menko, 2019). This could be one of the reasons for better fitness and acquisition of CLRDV in adult A. gossypii that acquired the virus from cotton plants compared with the other three hosts in the previous study (Pandey et al., 2022). In contrast, this gene was underexpressed in M. persicae adults that acquired TuYV from virus-infected plants and artificial medium (Marmonier et al., 2022).
The gene coding for ubiquitin-conjugating enzyme was overexpressed sixfold in A. gossypii that acquired CLRDV from virus-infected cotton plants, whereas it was overexpressed ~1.5-fold when the virus was acquired from okra and prickly sida plants in this study. The gene was not differentially expressed in A. gossypii acquiring CLRDV from virus-infected hibiscus plants (Table S3). The overexpression of ubiquitin-conjugating enzymes was previously reported in M. persicae and B. tabaci feeding on BYDV-infected and sida golden mosaic virus (SiGMV)-infected plants, respectively (Li et al., 2020; Mugerwa et al., 2022). The conjugating enzyme can transfer the ubiquitin from E1 to the substrate and is required for Notch signaling activation during Drosophila wing development (Gonen et al., 1999; Zhang et al., 2021). Since this gene is reported in the endocytic trafficking of the Notch protein, it could potentially influence the endocytic traversion of virus particles in A. gossypii. The overexpression of this gene may partially be responsible for the efficient retention and inoculation of CLRDV upon acquisition of the virus from cotton than the other three hosts (Pandey et al., 2022). This gene also is vital for insect defense against pathogens (Lemaitre and Hoffmann, 2007).
The immune system of insects helps them defend against pathogens (Wang et al., 2016). The change in the expression level of the genes related to the immune system and different signaling pathways in A. gossypii varied between the host species from which the virus was acquired. The genes related to the MAPK signaling pathway were differentially expressed in A. gossypii that acquired the virus from all hosts. In contrast, genes related to the JAK–STAT signaling pathway were only differentially expressed in A. gossypii that acquired the virus from okra plants. The JAK–STAT signaling pathway triggers insects’ innate immunity and antiviral responses (Dostert et al., 2005; Hedges and Johnson, 2008; Kingsolver et al., 2013). One of the genes associated with the immune system in aphids is Cathepsin B (Kubo et al., 2012; Quan et al., 2019). Cathepsin B is an aphid gut cysteine protease that regulates host protein activity and plays a role in the recognition and movement of viruses at the gut level (Pinheiro et al., 2017; Heck and Brault, 2018). Cathepsin B gene transcripts were overexpressed in A. gossypii acquiring CLRDV from infected cotton and okra plants alone. It was reported previously that the cathepsin B expression in aphids depends significantly on the host species (Pinheiro et al., 2017). This may partially explain the identification of the cathepsin B gene only in two host species in this study. The overexpression of the cathepsin B gene also was reported from M. persicae that acquired TuYV from infected plants (Marmonier et al., 2022). In contrast, M. persicae that acquired PLRV from infected plants had reduced expression of cathepsin B, which was associated with enhanced PLRV transmission (Pinheiro et al., 2017). The overexpression of the cathepsin B gene in A. gossypii acquiring the virus from okra plants may be one of the reasons for reduced CLRDV retention and subsequent inoculation in the previous study (Pandey et al., 2022). However, it does not explain the CLRDV retention and inoculation results obtained in A. gossypii upon acquisition from cotton despite overexpression of cathepsin B genes (Pandey et al., 2022).
For successful aphid-mediated transmission of circulative non-propagative phytoviruses such as poleroviruses, the virus capsid protein must interact with putative receptors at the midgut and accessory salivary glands (Gray and Gildow, 2003). One of the critical gene families identified in this study was serine/threonine kinase receptors. These genes were differentially expressed in both directions (over and under) in A. gossypii upon virus acquisition from four different host species in this study. This gene also was identified as one of the hub genes in the largest module (turquoise) in WGCNA in this study. The differential expression of these genes also was reported in whiteflies that acquired another group of persistent non-propagative circulative phytoviruses (begomoviruses) compared with their non-viruliferous counterparts (Mugerwa et al., 2022). Serine/threonine kinase, in mammalian cells, also has been recorded to play a vital role in clathrin-mediated endocytosis of the rabies virus (Wang et al., 2020a). The identification of these receptors in this study highlights their potential role in the circulative movement of poleroviruses in their aphid vectors. However, the role of host plants in the differential expression of these receptors’ genes cannot be explicitly established in this study.
Another important group of DEGs is associated with xenobiotics detoxification. Genes such as cytochrome P450, ATP binding cassette transporters (ABCs), and UDP-glycosyltransferases (UGTs) were differentially expressed in A. gossypii that acquired CLRDV from different host species in this study. These detoxification genes are essential for the adaption of insects to different host plants (Quan et al., 2019). Among them, cytochrome P450 genes were mainly overexpressed in A. gossypii that acquired CLRDV from infected okra and prickly sida plants. However, they were not differentially expressed in A. gossypii that acquired CLRDV from other host species. The overexpression of cytochrome P450 genes also was reported in M. persicae upon PLRV acquisition (Patton et al., 2021). Therefore, the overexpression of these genes may assist aphids enhancing the tolerance of non-desirable host plants, which could ultimately help in virus transmission and epidemics (Casteel and Jander, 2013).
Co-expression networks and hub genes from candidate modules
Gene co-expression networks attained through WGCNA also identified modules of highly correlated genes associated with virus transmission and vector performance. Three of the four most interacting hub genes were annotated: XM_027993669.1 (trichohyalin-like), XM_027997289.1 (uncharacterized protein), XM_027997209.1 (glucose dehydrogenase [FAD, quinone]-like), and XM_027983368.1 (neuroendocrine convertase 1-like). A previous study speculated the role of trichohyalin during immune defense via tissue remodeling and interaction with cuticular binding blocks that facilitate encapsulation (Takase and Hirai, 2012; Simons, 2015; Feng et al., 2022). Similarly, the FAD glucose dehydrogenase is a detoxification enzyme, the overexpression of which induces defense by reducing quinone in parasite-infected bumble bees (Stone and Yang, 2006; Giacomini et al., 2023). The neuroendocrine convertase 1, also called proprotein convertase (PC1/3), is a neuropeptide involved in regulating insect growth and development (Greenlee and Harrison, 2004; Callier and Nijhout, 2011). Another earlier study also has identified the essential role of PC1/3 in maintaining metabolic balance and nutrient-dependent fertility in adult beetles (Fritzsche and Hunnekuhl, 2021). These highly interacting hub genes could play a significant role in the development and defense mechanisms in A. gossypii following CLRDV acquisition and could be important targets for future investigation.
Conclusion
Gene expression profiles varied in substantial magnitude with hosts even within the same family and when interacting with the same virus isolate. Only four common genes were identified between the aphids acquiring the virus from four host species. Several unique genes associated with virus infection, immunity, growth, and development were identified among all DEGs analyzed. In addition, DEG families identified in this study indicate similarity with studies involving other persistent non-propagative viruses (Li et al., 2019, 2020; Patton et al., 2021; Catto et al., 2022; Chesnais et al., 2022; Marmonier et al., 2022; Mugerwa et al., 2022). Despite the same gene families that were identified in aphids from multiple hosts, the directional patterns of these DEGs varied (in some instances overexpressed and in other instances underexpressed) with acquisition hosts. These results reiterate that host plants could have an outsized role in determining vector–virus interaction outcomes. Future studies should examine this phenomenon in other virus pathosystems as well and evaluate the effects of differential gene expression patterns in vectors on their fitness parameters and functionally associate unique gene–fitness as well as gene expression directional pattern–fitness relationships.
Data availability statement
The data for this article can be found in the NCBI GenBank repository at https://www.ncbi.nlm.nih.gov/ under the BioProject PRJNA934319. Raw sequence data for the BioSamples: SAMN31430961-SAMN31430989 are deposited in the SRA accessions: SRR23579709-SRR23579737.
Ethics statement
The manuscript presents research on animals that do not require ethical approval for their study.
Author contributions
SP: Data curation, Formal analysis, Investigation, Methodology, Software, Validation, Writing – original draft, Writing – review & editing. MC: Data curation, Formal analysis, Methodology, Software, Validation, Writing – review & editing. PR: Funding acquisition, Resources, Supervision, Writing – review & editing. SB: Resources, Supervision, Writing – review & editing. AJ: Resources, Supervision, Writing – review & editing. RS: Conceptualization, Funding acquisition, Project administration, Resources, Supervision, Validation, Visualization, Writing – review & editing.
Funding
The author(s) declare financial support was received for the research, authorship, and/or publication of this article. This project was financially supported by the Georgia Commodity Commission for Cotton awarded to Georgia and by Agricultural Research Service, U.S. Department of Agriculture, under Agreement No. 58-6010-0-011 awarded to Alabama.
Acknowledgments
We thank Sarah Bragg for her assistance with aphid colony maintenance in the greenhouse.
Conflict of interest
The authors declare that the research was conducted in the absence of any commercial or financial relationships that could be construed as a potential conflict of interest.
Publisher’s note
All claims expressed in this article are solely those of the authors and do not necessarily represent those of their affiliated organizations, or those of the publisher, the editors and the reviewers. Any product that may be evaluated in this article, or claim that may be made by its manufacturer, is not guaranteed or endorsed by the publisher.
Supplementary material
The Supplementary Material for this article can be found online at: https://www.frontiersin.org/articles/10.3389/fpls.2024.1341781/full#supplementary-material
Supplementary Table 2 | List of DEGs associated with significant GO terms in A. gossypii that acquired CLRDV from infected cotton plant.
Supplementary Table 3 | List of DEGs associated with significant GO terms in A. gossypii that acquired CLRDV from infected hibiscus plant.
Supplementary Table 4 | List of DEGs associated with significant GO terms in A. gossypii that acquired CLRDV from infected okra plant.
Supplementary Table 5 | List of DEGs associated with significant GO terms in A. gossypii that acquired CLRDV from infected prickly sida plant.
Supplementary Table 7 | DEGs associated with immune pathways in A. gossypii that acquired CLRDV from alternate hosts.
Supplementary Table 8 | DEGs associated with reproduction in A. gossypii that acquired CLRDV from alternate hosts.
References
Aboughanem-Sabanadzovic, N., Allen, T. W., Wilkerson, T. H., Conner, K. N., Sikora, E. J., Nichols, R. L., et al. (2019). First report of cotton leafroll dwarf virus in upland cotton (Gossypium hirsutum) in Mississippi. Plant Dis. 103, 1798. doi: 10.1094/PDIS-01-19-0017-PDN
Alabi, O. J., Isakeit, T., Vaughn, R., Stelly, D., Conner, K. N., Gaytan, B. C., et al. (2020). First report of cotton leafroll dwarf virus infecting upland cotton (Gossypium hirsutum L.) in Texas. Plant Dis. Notes 104, 10–11. doi: 10.1094/PDIS-09-19-2008-PDN
Ali, A., Mokhtari, S. (2020). First report of cotton leafroll dwarf virus infecting cotton (Gossypium hirsutum) in Kansas. Plant Dis. 104, 1880. doi: 10.1094/PDIS-12-19-2589-PDN
Ali, A., Mokhtari, S., Ferguson, C. (2020). First report of cotton leafroll dwarf virus from cotton (Gossypium hirsutum) in Oklahoma. Plant Dis. Notes 104, 2531. doi: 10.1094/PDIS-03-20-0479-PDN
Andrews, S., FastQC, A. (2010). A quality control tool for high throughput sequence data. Available online at: http://www.bioinformatics.bbsrc.ac.uk/projects/fastqc/.
Avelar, S., Schrimsher, D. W., Lawrence, K., Brown, J. K. (2019). First report of cotton leafroll dwarf virus associated with cotton blue disease symptoms in Alabama. Plant Dis. Notes 103, 592. doi: 10.1094/PDIS-09-18-1550-PDN
Bag, S., Roberts, P. M., Kemerait, R. C. (2021). Cotton leafroll dwarf disease: an emerging virus disease on cotton in the U.S. Crop Soils 54, 18–22. doi: 10.1002/crso.20105
Barman, A. K., Gadhave, K. R., Dutta, B., Srinivasan, R. (2018). Plasticity in host utilization by two host-associated populations of Aphis gossypii Glover. Bull. Entomol. Res. 108, 360–369. doi: 10.1017/S0007485317000852
Bertasello, L. E. T., Carmo-sousa, M., Prado Maluta, N. K., Rossini Pinto, L., Spotti Lopes, J. R., Gonçalves, M. C. (2021). Effect of sugarcane cultivars infected with sugarcane yellow leaf virus (Scylv) on feeding behavior and biological performance of Melanaphis sacchari (Hemiptera: Aphididae). Plants 10, 2122. doi: 10.3390/plants10102122
Bolger, A. M., Lohse, M., Usadel, B. (2014). Trimmomatic: A flexible trimmer for Illumina sequence data. Bioinformatics 30, 2114–2120. doi: 10.1093/bioinformatics/btu170
Bosque-Pérez, N. A., Eigenbrode, S. D. (2011). The influence of virus-induced changes in plants on aphid vectors: Insights from luteovirus pathosystems. Virus Res. 159, 201–205. doi: 10.1016/j.virusres.2011.04.020
Brault, V., Uzest, M., Monsion, B., Jacquot, E., Blanc, S. (2010). Aphids as transport devices for plant viruses. Comptes Rendus - Biol. 333, 524–538. doi: 10.1016/j.crvi.2010.04.001
Brown, S., Conner, K., Hagan, A., Jacobson, A., Koebernick, J., Lawrence, K., et al. (2019) . Report of a research review and planning meeting on cotton leafroll dwarf virus. Available at: https://www.cottoninc.com/cotton-production/ag-research/ plant-pathology/cotton-leafroll-dwarf-virus-research/ (Accessed 2023 Oct 21).
Callier, V., Nijhout, H. F. (2011). Control of body size by oxygen supply reveals size-dependent and size-independent mechanisms of molting and metamorphosis. Proc. Natl. Acad. Sci. U. S. A. 108, 14664–14669. doi: 10.1073/pnas.1106556108
Cascardo, R. S., Arantes, I. L. G., Silva, T. F., Sachetto-Martins, G., Vaslin, M. F. S., Corrêa, R. L. (2015). Function and diversity of P0 proteins among cotton leafroll dwarf virus isolates. Virol. J. 12, 1–10. doi: 10.1186/s12985-015-0356-7
Casteel, C. L., Jander, G. (2013). New synthesis: investigating mutualisms in virus-vector interactions. J. Chem. Ecol. 39, 809. doi: 10.1007/s10886-013-0305-0
Castle, S. J., Mowry, T. M., Berger, P. H. (1998). Differential settling by Myzus persicae (Homoptera: Aphididae) on various virus infected host plants. Ann. Entomol. Soc Am. 91, 661–667. doi: 10.1093/aesa/91.5.661
Catto, M. A., Mugerwa, H., Myers, B. K., Pandey, S., Dutta, B., Srinivasan, R. (2022). A Review on Transcriptional responses of interactions between insect vectors and plant viruses. Cells 11, 693. doi: 10.3390/cells11040693
Chesnais, Q., Caballero Vidal, G., Coquelle, R., Yvon, M., Mauck, K., Brault, V., et al. (2020). Post-acquisition effects of viruses on vector behavior are important components of manipulation strategies. Oecologia 194, 429–440. doi: 10.1007/s00442-020-04763-0
Chesnais, Q., Golyaev, V., Velt, A., Rustenholz, C., Verdier, M., Brault, V., et al. (2022). Transcriptome responses of the aphid vector Myzus persicae are shaped by identities of the host plant and the virus. Peer Community J. 2, 429–440. doi: 10.24072/pcjournal.208
Claudel, P., Chesnais, Q., Fouché, Q., Krieger, C., Halter, D., Bogaert, F., et al. (2018). The aphid-transmitted turnip yellows virus differentially affects volatiles emission and subsequent vector behavior in two Brassicaceae plants. Int. J. Mol. Sci. 19, 2316. doi: 10.3390/ijms19082316
dos Santos, R. C., Peñaflor, M. F. G. V., Sanches, P. A., Nardi, C., Bento, J. M. S. (2016). The effects of Gibberella zeae, barley yellow dwarf virus, and co-infection on Rhopalosiphum padi olfactory preference and performance. Phytoparasitica 44, 47–54. doi: 10.1007/s12600-015-0493-y
Dostert, C., Jouanguy, E., Irving, P., Troxler, L., Galiana-Arnoux, D., Hetru, C., et al. (2005). The Jak-STAT signaling pathway is required but not sufficient for the antiviral response of Drosophila. Nat. Immunol. 69, 946–953. doi: 10.1038/ni1237
Edula, S. R., Bag, S., Milner, H., Kumar, M., Suassuna, N. D., Chee, P. W., et al. (2023). Cotton leafroll dwarf disease: An enigmatic viral disease in cotton. Mol. Plant Pathol. 24, 513–526. doi: 10.1111/mpp.13335
Eigenbrode, S. D., Ding, H., Shiel, P., Berger, P. H. (2002). Volatiles from potato plants infected with potato leafroll virus attract and arrest the virus vector, Myzus persicae (Homoptera: Aphididae). Proc. R. Soc Lond. B 269, 455–460. doi: 10.1098/rspb.2001.1909
Ewels, P., Magnusson, M., Lundin, S., Käller, M. (2016). MultiQC: Summarize analysis results for multiple tools and samples in a single report. Bioinformatics 32, 3047–3048. doi: 10.1093/bioinformatics/btw354
Faske, T. R., Stainton, D., Aboughanem-Sabanadzovic, N., Allen, T. W. (2020). First report of cotton leafroll dwarf virus from upland cotton (Gossypium hirsutum) in Arkansas. Plant Dis. Notes 104, 2742. doi: 10.1094/PDIS-12-19-2610-PDN
Feng, M., Swevers, L., Sun, J. (2022). Hemocyte Clusters Defined by scRNA-Seq in Bombyx mori: In Silico analysis of predicted marker genes and implications for potential functional roles. Front. Immunol. 13. doi: 10.3389/fimmu.2022.852702
Fereres, A., Moreno, A. (2009). Behavioural aspects influencing plant virus transmission by homopteran insects. Virus Res. 141, 158–168. doi: 10.1016/j.virusres.2008.10.020
Fiebig, M., Poehling, H. M., Borgemeister, C. (2004). Barley yellow dwarf virus, wheat, and Sitobion avenae: a case of trilateral interactions. Entomol. Exp. Appl. 110, 11–21. doi: 10.1111/j.0013-8703.2004.00115.x
Fingu-Mabola, J. C., Francis, F. (2021). Aphid–plant–phytovirus pathosystems: Influencing factors from vector behaviour to virus spread. Agriculture 11, 502. doi: 10.3390/agriculture11060502
Fingu-Mabola, J. C., Martin, C., Bawin, T., Verheggen, F. J., Francis, F. (2020). Does the infectious status of aphids influence their preference towards healthy, virus-infected and endophytically colonized plants? Insects 11, 1–16. doi: 10.3390/insects11070435
Fritzsche, S., Hunnekuhl, V. S. (2021). Cell-specific expression and individual function of prohormone convertase PC1/3 in Tribolium larval growth highlights major evolutionary changes between beetle and fly neuroendocrine systems. Evodevo 12, 1–20. doi: 10.1186/s13227-021-00179-w
Gadhave, K. R., Dutta, B., Coolong, T., Srinivasan, R. (2019). A non-persistent aphid-transmitted potyvirus differentially alters the vector and non-vector biology through host plant quality manipulation. Sci. Rep. 9, 1–12. doi: 10.1038/s41598-019-39256-5
Ghosh, A., Das, A., Vijayanandraj, S., Mandal, B. (2016). Cardamom bushy dwarf virus infection in large cardamom alters plant selection preference, life stages, and fecundity of aphid vector, Micromyzus kalimpongensis (hemiptera: Aphididae). Environ. Entomol. 45, 178–184. doi: 10.1093/ee/nvv161
Giacomini, J. J., Adler, L. S., Reading, B. J., Irwin, R. E. (2023). Differential bumble bee gene expression associated with pathogen infection and pollen diet. BMC Genomics 24, 157. doi: 10.1186/s12864-023-09143-5
Gonen, H., Bercovich, B., Orian, A., Carrano, A., Takizawa, C., Yamanaka, K., et al. (1999). Identification of the ubiquitin carrier proteins, E2s, involved in signal-induced conjugation and subsequent degradation of IκBα. J. Biol. Chem. 274, 14823–14830. doi: 10.1074/jbc.274.21.14823
Götz, M., Popovski, S., Kollenberg, M., Gorovits, R., Brown, J. K., Cicero, J. M., et al. (2012). Implication of Bemisia tabaci heat shock protein 70 in begomovirus-whitefly interactions. J. Virol. 86, 13241–13252. doi: 10.1128/JVI.00880-12
Gray, S., Gildow, F. E. (2003). Luteovirus-aphid interactions. Annu. Rev. Phytopathol. 41, 539–566. doi: 10.1146/annurev.phyto.41.012203.105815
Greenlee, K. J., Harrison, J. F. (2004). Development of respiratory function in the American locust Schistocerca americana: II. Within-instar effects. J. Exp. Biol. 207, 509–517. doi: 10.1242/jeb.00766
Harris, K. F., Maramorosch, K. (1977). Aphids As Virus Vectors. (Academic Press, Cambridge, MA, USA: Elsevier). doi: 10.1016/c2013-0-10831-8
Heck, M., Brault, V. (2018). Targeted disruption of aphid transmission: a vision for the management of crop diseases caused by Luteoviridae members. Curr. Opin. Virol. 33, 24–32. doi: 10.1016/j.coviro.2018.07.007
Hedges, L. M., Johnson, K. N. (2008). Induction of host defence responses by Drosophila C virus. J. Gen. Virol. 89, 1497–1501. doi: 10.1099/vir.0.83684-0
Heilsnis, B., Mahas, J. B., Conner, K., Pandey, S., Clark, W., Koebernick, J., et al. (2023). Characterizing the vector competence of Aphis gossypii, Myzus persicae and Aphis craccivora (Hemiptera: Aphididae) to transmit cotton leafroll dwarf virus to cotton in the United States. J. Econ. Entomol. 116, 719–725. doi: 10.1093/jee/toad080
Heilsnis, B., McLaughlin, A., Conner, K., Koebernick, J., Jacobson, A. L. (2022). Vector competency of Aphis gossypii and Bemisia tabaci to transmit cotton leafroll dwarf virus. J. Cotton Sci. 26, 23–30. doi: 10.56454/VBZR9427
Hodge, S., Powell, G. (2008). Do plant viruses facilitate their aphid vectors by inducing symptoms that alter behavior and performance? Environ. Entomol. 37, 1573–1581. doi: 10.1603/0046-225X-37.6.1573
Hogenhout, S. A., Ammar, E. D., Whitfield, A. E., Redinbaugh, M. G. (2008). Insect vector interactions with persistently transmitted viruses. Annu. Rev. Phytopathol. 46, 327–359. doi: 10.1146/annurev.phyto.022508.092135
Hu, Z., Chai, R., Liu, X., Dong, Y., Su, D., Desneux, N., et al. (2022). Barley yellow dwarf virus-infected wheat plant modulated selection behavior of vector aphids. J. Pest Sci. (2004). 95, 1273–1285. doi: 10.1007/s10340-021-01458-0
Ingwell, L. L., Eigenbrode, S. D., Bosque-Pérez, N. A. (2012). Plant viruses alter insect behavior to enhance their spread. Sci. Rep. 2, 578. doi: 10.1038/srep00578
Iriarte, F., Dey, K. K., Small, I. M., Conner, K., O’Brien, K., Johnson, L., et al. (2020). First report of cotton leafroll dwarf virus (CLRDV) in Florida. Plant Dis. 104, 2744. doi: 10.1094/PDIS-10-19-2150-PDN
Jayasinghe, W. H., Akhter, M. S., Nakahara, K., Maruthi, M. N. (2022). Effect of aphid biology and morphology on plant virus transmission. Pest Manage. Sci. 78, 416–427. doi: 10.1002/ps.6629
Jiménez-Martínez, E. S., Bosque-Pérez, N. A., Berger, P. H., Zemetra, R. S., Ding, H., Eigenbrode, S. D. (2004). Volatile cues influence the response of Rhopalosiphum padi (Homoptera: Aphididae) to barley yellow dwarf virus-infected transgenic and untransformed wheat. Environ. Entomol. 33, 1207–1216. doi: 10.1603/0046-225X-33.5.1207
Kanehisa, M., Goto, S. (2000). KEGG: kyoto encyclopedia of genes and genomes. Nucleic Acids Res. 28, 27–30. doi: 10.1093/nar/28.1.27
Kingsolver, M. B., Huang, Z., Hardy, R. W. (2013). Insect antiviral innate immunity: Pathways, effectors, and connections. J. Mol. Biol. 425, 4921–4936. doi: 10.1016/j.jmb.2013.10.006
Kubo, Y., Hayashi, H., Matsuyama, T., Sato, H., Yamamoto, N. (2012). Retrovirus entry by endocytosis and cathepsin proteases. Adv. Virol. 2012, 14. doi: 10.1155/2012/640894
Langfelder, P., Horvath, S. (2008). WGCNA: An R package for weighted correlation network analysis. BMC Bioinf. 9, 1–13. doi: 10.1186/1471-2105-9-559
Langmead, B., Salzberg, S. L. (2012). Fast gapped-read alignment with Bowtie 2. Nat. Methods 94, 357–359. doi: 10.1038/nmeth.1923
Legarrea, S., Barman, A., Diffie, S., Srinivasan, R. (2020). Virus accumulation and whitefly performance modulate the role of alternate host species as inoculum sources of tomato yellow leaf curl virus. Plant Dis. 104, 2958–2966. doi: 10.1094/PDIS-09-19-1853-RE
Lemaitre, B., Hoffmann, J. (2007). The host defense of Drosophila melanogaster. Annu. Rev. Immunol. 25, 697–743. doi: 10.1146/annurev.immunol.25.022106.141615
Li, B., Dewey, C. N. (2011). RSEM: accurate transcript quantification from RNA-Seq data with or without a reference genome. BMC Bioinformatics 12, 323. doi: 10.1186/1471-2105-12-323
Li, D., Su, D., Tong, Z., Zhang, C., Zhang, G., Zhao, H., et al. (2019). Virus-dependent and -independent responses of Sitobion avenae (Homoptera: Aphididae) feeding on wheat infected by transmitted and nontransmitted viruses at transcriptomic level. J. Econ. Entomol. 112, 2067–2076. doi: 10.1093/jee/toz162
Li, D., Zhang, C., Tong, Z., Su, D., Zhang, G., Zhang, S., et al. (2020). Transcriptome response comparison between vector and non-vector aphids after feeding on virus-infected wheat plants. BMC Genomics 21, 638. doi: 10.1186/s12864-020-07057-0
Lightle, D., Lee, J. (2014). Raspberry viruses affect the behavior and performance of Amphorophora agathonica in single and mixed infections. Entomol. Exp. Appl. 151, 57–64. doi: 10.1111/eea.12170
Liu, J., Liu, Y., Donkersley, P., Dong, Y., Chen, X., Zang, Y., et al. (2019). Preference of the aphid Myzus persicae (Hemiptera: Aphididae) for tobacco plants at specific stages of potato virus Y infection. Arch. Virol. 164, 1567–1573. doi: 10.1007/s00705-019-04231-y
Logan, C. M., Menko, A. S. (2019). Microtubules: Evolving roles and critical cellular interactions. Exp. Biol. Med. 244, 1240–1254. doi: 10.1177/1535370219867296
Love, M. I., Huber, W., Anders, S. (2014). Moderated estimation of fold change and dispersion for RNA-seq data with DESeq2. Genome Biol. 15, 1–21. doi: 10.1186/s13059-014-0550-8
Ma, K. S., Li, F., Liang, P. Z., Chen, X. W., Liu, Y., Gao, X. W. (2016). Identification and validation of reference genes for the normalization of gene expression data in qRT-PCR Analysis in Aphis gossypii (Hemiptera: Aphididae). J. Insect Sci. 16, 1–9. doi: 10.1093/jisesa/iew003
Marmonier, A., Velt, A., Villeroy, C., Rustenholz, C., Chesnais, Q., Brault, V. (2022). Differential gene expression in aphids following virus acquisition from plants or from an artificial medium. BMC Genomics 23, 1–15. doi: 10.1186/s12864-022-08545-1
Mauck, K. E. (2016). Variation in virus effects on host plant phenotypes and insect vector behavior: what can it teach us about virus evolution? Curr. Opin. Virol. 21, 114–123. doi: 10.1016/j.coviro.2016.09.002
Mauck, K. E., De Moraes, C. M., Mescher, M. C. (2010). Deceptive chemical signals induced by a plant virus attract insect vectors to inferior hosts. Proc. Natl. Acad. Sci. U. S. A. 107, 3600–3605. doi: 10.1073/pnas.0907191107
Medina-Ortega, K. J., Bosque-Perez, N. A., Ngumbi, E., Jiménez-Martínez, E. S., Eigenbrode, S. D. (2009). Rhopalosiphum padi (Hemiptera: Aphididae) responses to volatile cues from barley yellow dwarf virus-infected wheat. Environ. Entomol. 38, 836–845. doi: 10.1603/022.038.0337
Michelotto, M. D., Busoli, A. C. (2007). Characterization of the cotton vein mosaic virus by Aphis gossypii transmission with relation to persistence and time necessary for inoculation. Bragantia 66, 441–447. doi: 10.1590/S0006-87052007000300010
Minakuchi, C., Namiki, T., Yoshiyama, M., Shinoda, T. (2008). RNAi-mediated knockdown of juvenile hormone acid O-methyltransferase gene causes precocious metamorphosis in the red flour beetle Tribolium castaneum. FEBS J. 275, 2919–2931. doi: 10.1111/j.1742-4658.2008.06428.x
Moeini, P., Afsharifar, A., Homayoonzadeh, M., Hopkins, R. J. (2020). Plant virus infection modifies plant pigment and manipulates the host preference behavior of an insect vector. Entomol. Exp. Appl. 168, 599–609. doi: 10.1111/eea.12944
Mugerwa, H., Gautam, S., Catto, M. A., Dutta, B., Brown, J. K., Adkins, S., et al. (2022). Differential transcriptional responses in two old world Bemisia tabaci cryptic species post acquisition of old and new world begomoviruses. Cells 11, 2060. doi: 10.3390/cells11132060
Ng, J. C. K., Falk, B. W. (2006). Virus-vector interactions mediating nonpersistent and semipersistent transmission of plant viruses. Annu. Rev. Phytopathol. 44, 183–212. doi: 10.1146/annurev.phyto.44.070505.143325
Ng, J. C. K., Perry, K. L. (2004). Transmission of plant viruses by aphid vectors. Mol. Plant Pathol. 5, 505–511. doi: 10.1111/j.1364-3703.2004.00240.x
Ngumbi, E., Eigenbrode, S. D., Bosque-Pérez, N. A., Ding, H., Rodriguez, A. (2007). Myzus persicae is arrested more by blends than by individual compounds elevated in headspace of PLRV-infected potato. J. Chem. Ecol. 33, 1733–1747. doi: 10.1007/s10886-007-9340-z
Nielsen, M. G., Gadagkar, S. R., Gutzwiller, L. (2010). Tubulin evolution in insects: Gene duplication and subfunctionalization provide specialized isoforms in a functionally constrained gene family. BMC Evol. Biol. 10, 1–21. doi: 10.1186/1471-2148-10-113
Niwa, R., Niimi, T., Honda, N., Yoshiyama, M., Itoyama, K., Kataoka, H., et al. (2008). Juvenile hormone acid O-methyltransferase in Drosophila melanogaster. Insect Biochem. Mol. Biol. 38, 714–720. doi: 10.1016/j.ibmb.2008.04.003
Pandey, S., Bag, S., Roberts, P., Conner, K., Balkcom, K. S., Price, A. J., et al. (2022). Prospective alternate hosts of an emerging polerovirus in cotton landscapes in the southeastern United States. Viruses 14, 2249. doi: 10.3390/v14102249
Patton, M. K. F., Hansen, A. K., Casteel, C. L. (2021). Potato leafroll virus reduces Buchnera aphidocola titer and alters vector transcriptome responses. Sci. Rep. 11, 23931. doi: 10.1038/s41598-021-02673-6
Pinheiro, P. V., Ghanim, M., Alexander, M., Rebelo, A. R., Santos, R. S., Orsburn, B. C., et al. (2017). Host plants indirectly influence plant virus transmission by altering gut cysteine protease activity of aphid vectors. Mol. Cell. Proteomics 16, S230–S243. doi: 10.1074/mcp.M116.063495
Porras, M. F., Navas, C. A., Marden, J. H., Mescher, M. C., De Moraes, C. M., Pincebourde, S., et al. (2020). Enhanced heat tolerance of viral-infected aphids leads to niche expansion and reduced interspecific competition. Nat. Commun. 11, 1–9. doi: 10.1038/s41467-020-14953-2
Price, T., Valverde, R., Singh, R., Davis, J., Brown, S., Jones, H. (2020). First report of cotton leafroll dwarf virus in Louisiana. Plant Heal. Prog. 21, 142–143. doi: 10.1094/PHP-03-20-0019-BR
Quan, Q., Hu, X., Pan, B., Zeng, B., Wu, N., Fang, G., et al. (2019). Draft genome of the cotton aphid Aphis gossypii. Insect Biochem. Mol. Biol. 105, 25–32. doi: 10.1016/j.ibmb.2018.12.007
Rajabaskar, D., Wu, Y., Bosque-Pérez, N. A., Eigenbrode, S. D. (2013). Dynamics of Myzus persicae arrestment by volatiles from potato leafroll virus-infected potato plants during disease progression. Entomol. Exp. Appl. 148, 172–181. doi: 10.1111/eea.12087
R Core Team. (2021). “R: A language and environment for statistical computing. r foundation for statistical computing,” in R foundation for statistical computing (R Found. Stat. Comput, Vienna, Austria). Available at: https://www.r-project.org/.
Ren, G.w., Wang, X.f., Chen, D., Wang, X.w., Fan, X.j., liu, X.d. (2015). Potato virus Y-infected tobacco affects the growth, reproduction, and feeding behavior of a vector aphid, Myzus persicae (Hemiptera: Aphididae). Appl. Entomol. Zool. 50, 239–243. doi: 10.1007/s13355-015-0328-9
Safari Murhububa, I., Tougeron, K., Bragard, C., Fauconnier, M. L., Bisimwa Basengere, E., Walangululu Masamba, J., et al. (2021). Banana tree infected with banana bunchy top virus attracts Pentalonia nigronervosa aphids through increased volatile organic compounds emission. J. Chem. Ecol. 47, 755–767. doi: 10.1007/s10886-021-01298-3
Sedhain, N. P., Bag, S., Morgan, K., Carter, R., Triana, P., Whitaker, J., et al. (2021). Natural host range, incidence on overwintering cotton and diversity of cotton leafroll dwarf virus in Georgia USA. Crop Prot. 144, 105604. doi: 10.1016/j.cropro.2021.105604
Shannon, P., Markiel, A., Ozier, O., Baliga, N. S., Wang, J. T., Ramage, D., et al. (2003). Cytoscape: a software environment for integrated models of biomolecular interaction networks. Genome Res. 13, 2498–2504. doi: 10.1101/gr.1239303
Shinoda, T., Itoyama, K. (2003). Juvenile hormone acid methyltransferase: A key regulatory enzyme for insect metamorphosis. Proc. Natl. Acad. Sci. U. S. A. 100, 11986–11991. doi: 10.1073/pnas.2134232100
Silva, A. K. F., Romanel, E., Silva, T. F., Castilhos, Y., Schrago, C. G., Galbieri, R., et al. (2015). Complete genome sequences of two new virus isolates associated with cotton blue disease resistance breaking in Brazil. Arch. Virol. 160, 1371–1374. doi: 10.1007/s00705-015-2380-8
Simons, M. (2015). Flies with skin blisters. J. Invest. Dermatol. 135, 1944–1945. doi: 10.1038/jid.2015.193
Sõmera, M., Fargette, D., Hébrard, E., Sarmiento, C. (2021). The ICTV report on virus classification and taxon nomenclature Solemoviridae Chapter Solemoviridae. Available online at: www.ictv.global/report/solemoviridae (Accessed July 18, 2021).
Srinivasan, R., Alvarez, J. M., Bosque-Pérez, N. A., Eigenbrode, S. D., Novy, R. G. (2008). Effect of an alternate weed host, hairy nightshade, Solanum sarrachoides, on the biology of the two most important potato leafroll virus (Luteoviridae: Polerovirus) vectors, Myzus persicae and Macrosiphum euphorbiae (Aphididae: Homoptera). Environ. Entomol. 37, 592–600. doi: 10.1603/0046-225X(2008)37[592:EOAAWH]2.0.CO;2
Srinivasan, R., Alvarez, J. M., Cervantes, F. (2013). The effect of an alternate weed host, hairy nightshade, Solanum sarrachoides (Sendtner) on green peach aphid distribution and potato leafroll virus incidence in potato fields of the Pacific Northwest. Crop Prot. 46, 52–56. doi: 10.1016/j.cropro.2012.12.015
Srinivasan, R., Alvarez, J. M., Eigenbrode, S. D., Bosque-Pérez, N. A. (2006). Influence of hairy nightshade Solanum sarrachoides (Sendtner) and potato leafroll virus (Luteoviridae: Polerovirus) on the host preference of Myzus persicae (Sulzer) (Homoptera: Aphididae). Environ. Entomol. 35, 546–553. doi: 10.1603/0046-225X-35.2.546
Stone, J. R., Yang, S. (2006). Hydrogen peroxide: A signaling messenger. Antioxidants Redox Signal. 8, 243–270. doi: 10.1089/ars.2006.8.243
Supek, F., Bošnjak, M., Škunca, N., Šmuc, T. (2011). Revigo summarizes and visualizes long lists of gene ontology terms. PloS One 6, e21800. doi: 10.1371/journal.pone.0021800
Tabassum, A., Bag, S., Roberts, P., Suassuna, N., Chee, P., Whitaker, J. R., et al. (2019). First report of cotton leafroll dwarf virus infecting cotton in Georgia, U.S.A. Plant Dis. 103, 1803. doi: 10.1094/PDIS-12-18-2197-PDN
Takase, T., Hirai, Y. (2012). Identification of the C-terminal tail domain of AHF/trichohyalin as the critical site for modulation of the keratin filamentous meshwork in the keratinocyte. J. Dermatol. Sci. 65, 141–148. doi: 10.1016/j.jdermsci.2011.12.014
Thiessen, L. D., Schappe, T., Zaccaron, M., Connor, K., Kobernick, J., Jacobson, A. L., et al. (2020). First report of Cotton leafroll dwarf virus in cotton plants affected by cotton leafroll dwarf disease in North Carolina. Plant Dis. Notes 104, 3275. doi: 10.1094/PDIS-02-20-0335-PDN
Wang, H., Greene, J., Mueller, J., Conner, K., Jacobson, A. (2020b). First report of cotton leafroll dwarf virus in cotton fields of South Carolina. Plant Dis. Notes 104, 2532. doi: 10.1094/PDIS-03-20-0635-PDN
Wang, L., Tang, N., Gao, X., Guo, D., Chang, Z., Fu, Y., et al. (2016). Understanding the immune system architecture and transcriptome responses to southern rice black-streaked dwarf virus in Sogatella furcifera. Sci. Rep. 61, 1–11. doi: 10.1038/srep36254
Wang, C., Wang, J., Shuai, L., Ma, X., Zhang, H., Liu, R., et al. (2020a). The serine/threonine kinase ap2-associated kinase 1 plays an important role in rabies virus entry. Viruses 12, 45. doi: 10.3390/v12010045
Werner, B. J., Mowry, T. M., Bosque-Pérez, N. A., Ding, H., Eigenbrode, S. D. (2009). Changes in green peach aphid responses to potato leafroll virus–induced volatiles emitted during disease progression. Environ. Entomol. 38, 1429–1438. doi: 10.1603/022.038.0511
Whitfield, A. E., Falk, B. W., Rotenberg, D. (2015). Insect vector-mediated transmission of plant viruses. Virology 479–480, 278–289. doi: 10.1016/j.virol.2015.03.026
Williams, J. A., Su, H. S., Bernards, A., Field, J., Sehgal, A. (2001). A circadian output in Drosophila mediated by neurofibromatosis-1 and Ras/MAPK. Sci. (80-. ). 293, 2251–2256. doi: 10.1126/science.1063097
Zhang, C. X., Brisson, J. A., Xu, H. J. (2019). Molecular mechanisms of wing polymorphism in insects. Annu. Rev. Entomol. 64, 297–314. doi: 10.1146/annurev-ento-011118-112448
Zhang, F., Chen, Y., Shen, J., Zhang, J. (2021). The ubiquitin conjugating enzyme UbcD1 is required for notch signaling activation during Drosophila wing development. Front. Genet. 12. doi: 10.3389/fgene.2021.770853
Keywords: Aphis gossypii, cotton leafroll dwarf virus, acquisition, alternate hosts, vector-virus interactions
Citation: Pandey S, Catto M, Roberts P, Bag S, Jacobson AL and Srinivasan R (2024) Aphid gene expression following polerovirus acquisition is host species dependent. Front. Plant Sci. 15:1341781. doi: 10.3389/fpls.2024.1341781
Received: 20 November 2023; Accepted: 19 February 2024;
Published: 08 March 2024.
Edited by:
Shengli Jing, Xinyang Normal University, ChinaReviewed by:
Saumik Basu, Washington State University, United StatesQuentin Chesnais, Institut National de recherche pour l’agriculture, l’alimentation et l’environnement (INRAE), France
Copyright © 2024 Pandey, Catto, Roberts, Bag, Jacobson and Srinivasan. This is an open-access article distributed under the terms of the Creative Commons Attribution License (CC BY). The use, distribution or reproduction in other forums is permitted, provided the original author(s) and the copyright owner(s) are credited and that the original publication in this journal is cited, in accordance with accepted academic practice. No use, distribution or reproduction is permitted which does not comply with these terms.
*Correspondence: Rajagopalbabu Srinivasan, YmFidXNyaUB1Z2EuZWR1