- 1Key Laboratory of Crop Physiology Ecology and Production Management of Ministry of Agriculture, Nanjing Agricultural University, Nanjing, Jiangsu, China
- 2Institute of Crop Science, Nutritional Crop Physiology, University of Hohenheim, Stuttgart, Germany
The use of slow-release fertilizers and seed-fertilizers cause localized high-ammonium (NH4+) environments in agricultural fields, adversely affecting wheat growth and development and delaying its yield. Thus, it is important to investigate the physiological responses of wheat and its tolerance to NH4+ stress to improve the adaptation of wheat to high NH4+ environments. In this study, the physiological mechanisms of ammonium tolerance in wheat (Triticum aestivum) were investigated in depth by comparative analysis of two cultivars: NH4+-tolerant Xumai25 and NH4+-sensitive Yangmai20. Cultivation under hydroponic conditions with high NH4+ (5 mM NH4+, AN) and nitrate (5 mM NO3-, NN), as control, provided insights into the nuanced responses of both cultivars. Compared to Yangmai20, Xumai25 displayed a comparatively lesser sensitivity to NH4+ stress, as evident by a less pronounced reduction in dry plant biomass and a milder adverse impact on root morphology. Despite similarities in NH4+ efflux and the expression levels of TaAMT1.1 and TaAMT1.2 between the two cultivars, Xumai25 exhibited higher NH4+ influx, while maintaining a lower free NH4+ concentration in the roots. Furthermore, Xumai25 showed a more pronounced increase in the levels of free amino acids, including asparagine, glutamine, and aspartate, suggesting a superior NH4+ assimilation capacity under NH4+ stress compared to Yangmai20. Additionally, the enhanced transcriptional regulation of vacuolar glucose transporter and glucose metabolism under NH4+ stress in Xumai25 contributed to an enhanced carbon skeleton supply, particularly of 2-oxoglutarate and pyruvate. Taken together, our results demonstrate that the NH4+ tolerance of Xumai25 is intricately linked to enhanced glucose metabolism and optimized glucose transport, which contributes to the robust NH4+ assimilation capacity.
1 Introduction
Ammonium (NH4+) stress is a global challenge that severely affects crop production (Esteban et al., 2016). Accumulation of NH4+ in soils can be attributed to natural events and human activities, including atmospheric NH4+ deposition (Liu et al., 2013), soil NH4+ adsorption (Liu et al., 2008), and localized application of NH4+‐based fertilizers (Pan et al., 2016; Marino and Moran, 2019). Plants subjected to high NH4+ conditions display distinct characteristics from those grown in NO3- conditions, including external acidification, reduced cationic absorption, imbalances in carbon and nitrogen metabolisms, and oxidative damage (Bittsánszky et al., 2015; Esteban et al., 2016). Over the past two decades, several factors contributing to NH4+ tolerance have been identified, primarily via studies on NH4+-tolerant rice (Oryza sativa) and Arabidopsis thaliana. However, the specific plant traits that are responsible for NH4+ tolerance, especially in NH4+-sensitive species such as wheat (Triticum aestivum), remain unclear.
To mitigate NH4+ toxicity, plants must delicately balance NH4+ uptake, assimilation, and release (Bittsánszky et al., 2015). This balance can be achieved by either regulating transporters to reduce NH4+ uptake or developing effective detoxification mechanisms to counteract excess NH4+ accumulation (Ijato et al., 2021). In plants, the high-affinity uptake of NH4+ is primarily mediated by ammonium transporters (AMTs). Studies showed that AMT1 gene knockout significantly inhibits NH4+ uptake, while AMT1 overexpression enhances NH4+ permeability in the roots (Ranathunge et al., 2014; Li et al., 2016). In Arabidopsis, the three AMT1 proteins (AMT1;1, AMT1;2, and AMT1;3) contribute to approximately 90% of NH4+ uptake (Yuan et al., 2007). In addition, some studies have pointed to the existence of multiple NH4+ uptake channels in plants aside from AMT (Esteban et al., 2016), and the simultaneous presence of NH4+ influx and efflux has been observed in barley (Hordeum vulgare) and rice root cells under high external NH4+ conditions (Britto et al., 2001). Collectively, NH4+ uptake and efflux by plant roots is complex and needs to be assessed from multiple perspectives. The status of NH4+ uptake and efflux and the relationship of this NH4+ movement with NH4+ tolerance under NH4+ stress is still unknown.
After NH4+ is absorbed by plant cells, it is converted into glutamine (Gln) by combining with glutamate. The synthesis of glutamate from 2-oxoglutarate (2-OG) is a critical step in NH4+ assimilation and cellular defense against NH4+ stress (Bittsánszky et al., 2015). Under NH4+ stress, many plant species exhibit an increase in the activities of NH4+ assimilation enzymes, such as glutamine synthetase (GS, EC 6.3.1.2) and glutamate synthase (GOGAT, EC 1.4.7.1) (Britto and Kronzucker, 2002; Vega-Mas et al., 2019a; González-Moro et al., 2021). Notably, A. thaliana mutants lacking the GLN1;2 isoform exhibit excessive NH4+ accumulation and a high sensitivity to NH4+ stress (Hachiya et al., 2021), underscoring the significance of this pathway in the protection of plants against NH4+ toxicity. However, there remains some debate regarding the activity of GS under NH4+ stress (Jian et al., 2018). Consequently, the variability in the activities of NH4+ assimilation-related enzymes among NH4+-tolerant species/cultivars needs to be further investigated.
The principal products of NH4+ assimilation in plants are nitrogen-rich compounds, primarily amino acids, and proteins. The accumulation of these compounds reflects the capacity of plants to assimilate NH4+ and adapt to NH4+ stress (Vega-Mas et al., 2019b). Among the free amino acids, glutamate (Glu), glutamine (Gln), aspartic acid (Asp), and asparagine (Asn) consistently accumulated under NH4+ stress across various plant species (Jian et al., 2018; de la Peña et al., 2019; Vega-Mas et al., 2019a). Studies suggested that Asn and Gln, as crucial forms of nitrogen storage and transportation, reflect the nitrogen status and regulate NH4+ uptake and assimilation (Xu et al., 2012; Konishi et al., 2016). Distinctly, a previous study observed that Gln and Asn concentrations in the Arabidopsis chl1-1 mutant were lower than those in the wild type, indicating that the decline in Gln and Asn may be related to ammonium tolerance in the mutant (Jian et al., 2018). Therefore, different NH4+-tolerant cultivars might exhibit varied accumulations of NH4+ assimilates, which might be attributed to varying NH4+ assimilation capacities, however, it still needs to be validated.
Adequate carbon (C) skeleton supply is also essential to address excess NH4+ under NH4+ stress (Britto and Kronzuker, 2005). A classic hypothesis on NH4+ toxicity suggests that insufficient carbon skeletons in the root lead to NH4+ poisoning in the plant (Esteban et al., 2016). Numerous studies have reported that excess NH4+ in the root leads to a reduction in soluble sugar content and enhances the TCA cycle, to produce 2-oxoglutarate and oxaloacetate (OAA) for NH4+ assimilation (Viktor and Cramer, 2005; Ariz et al., 2013; Vega-Mas et al., 2019a). Conversely, some studies indicated that NH4+ stress increases soluble sugar content and uncouples carbon and nitrogen metabolism (Jian et al., 2018; Li et al., 2020). The complex relationship between sugar metabolism and carbon skeleton supply under NH4+ stress is responsible for varying response mechanisms and severities of NH4+ stress in plants. Improving sugar metabolism and carbon skeleton availability under NH4+ stress may enhance NH4+ tolerance in plants.
As a major global crop, wheat is essential to ensure food security for the world’s population. Notably, wheat plants exhibit high sensitivity to NH4+ stress, especially during the seedling and reproductive stages (Wang et al., 2016; Liu et al., 2021). In recent years, there has been growing evidence that NH4+ stress adversely impacts wheat seedling growth (Ijato et al., 2021; Liu et al., 2021). However, studies on the precise underlying response mechanisms in different NH4+-tolerant wheat cultivars are still scarce. In this research, we aimed to investigate the physiological and molecular processes underlying NH4+ tolerance in wheat plants. We conducted a comparative analysis of NH4+-tolerant and NH4+-sensitive cultivars under NH4+ stress, including growth responses, NH4+ uptake and assimilation, glucose metabolism, and carbon skeleton supply. The study seeks to test two hypotheses: (i) the NH4+-tolerant cultivar may have a weaker NH4+ uptake capacity than the NH4+-sensitive cultivar, and (ii) the NH4+-tolerant cultivar may have a stronger sugar metabolism, thus providing more carbon skeletons for NH4+ assimilation under NH4+ stress.
2 Materials and methods
2.1 Plant materials and experimental design
We selected two wheat cultivars (as illustrated in Supplementary Figure S1), Xumai25 (NH4+-tolerant) and Yangmai20 (NH4+-sensitive), based on the observed tolerance and sensitivity to NH4+ during pre-experiments (data not shown). The seeds of both cultivars were surface sterilized using a 10% (v/v) H2O2 solution for 15 min, followed by thorough rinsing with sterile distilled water. Subsequently, the seeds were germinated under dark conditions in Petri dishes until the seed bud was ~1 cm long. Then, the seedlings were transplanted into opaque plastic containers (45 cm × 32 cm × 25 cm, volume: 36 L) filled with water. The seedlings at the two-leaf stage were grown in a modified 50% Hoagland nutrient solution until they reached the four-leaf stage. Following this pre-treatment, the seedlings were divided into two groups. One group was treated with nitrate nitrogen (NN, 5 mM NO3−-N) nutrient solutions and the other with ammonium nitrogen (AN, 5 mM NH4+-N) nutrient solution. The concentrations and composition of macronutrients in both treatments are listed in Supplementary Table S1. The micronutrient composition in both treatments remained consistent, as previously described by Liu et al. (2021). To ensure a consistent nitrogen supply in each solution, the solutions were refreshed every three days and were continuously aerated to prevent anoxic conditions. The pH of each treatment was adjusted daily to 5.8 using 0.1 mM H2SO4 or 0.1 mM NaOH. The entire experiment was conducted in a controlled greenhouse environment with a 16 h/8 h light/dark cycle and temperature maintained at 18°C during the day and 8.5°C at night. The light intensity and relative air humidity in the greenhouse were set at 400 µmol m−2 s−1 and 60%, respectively. We adopted a completely randomized block design, and each experiment was replicated three times. Each replication consisted of three containers, and each container housed 60 plants.
The entire ammonium stress treatment was sustained for 20 days. Seedlings were collected at 0, 1, 3, 5,10, and 20 days after treatment (DAT) to assess biochemical and physiological changes. The leaves, stems, and roots of the seedlings were separated and divided into two segments. One segment was subjected to oven drying at 105°C for 20 min, followed by drying at 85°C, for dry weight and nitrogen concentration measurements. The other segment was promptly frozen in liquid nitrogen and stored at −80°C for subsequent analyses.
2.2 Root morphology analysis
After 20 days of treatment, the entire root of each wheat seedling was scanned using a V700 scanner system (Epson, Indonesia). Briefly, eight seedlings per treatment group were randomly selected and labeled before the start of the treatment. The plant roots were rinsed with water, placed in a scanning disk with a small amount of water, laid flat and evenly, and scanned. The obtained root images were analyzed using the WinRhizo Pro V700 1.0 software (Regent Instruments, Canada). The data on the length, volume, surface area, and average diameter of the roots were obtained from the software directly. Additionally, the number of lateral roots was determined by counting directly.
2.3 Measurement of root NH4+ flux
The net NH4+ influx and efflux at the root surface of two cultivars were determined using Non-invasive Micro-test Technology (NMT Physiolyzer®, Younger USA LLC, MA, USA), Xuyue (Beijing) Sci. &Tech. Co., Ltd., Beijing, China, provided the measure services. Wheat seedlings of uniform growth were selected before treatment. The measurement of root NH4+ influx according to Sun et al. (2022). The seedlings were treated with 5.0 mM NH4+ solution (mentioned above), and tested directly after 0.17, 2, 6, 24, 72, and 120 hours treatment with the high concentration NH4+ measuring solution (2.5 mM (NH4)2SO4, 0.1 mM CaCl2, pH 5.8), respectively. The measurement of root NH4+ efflux according to Di et al. (2021), wheat seedlings were treated for 0.5, 6, 24, 72, and 120 hours with the 5.0 mM NH4+ solution in advance, respectively, and then moved to a low concentration NH4+ measuring solution (0.1 mM (NH4)2SO4, 0.1 mM CaCl2, pH 5.8) for testing. Briefly, two roots were randomly selected from each plant, rinsed with distilled water, and immersed at the bottom of the Petri dish containing fresh measure solution (for the NH4+ efflux measurement, the roots were equilibrated in measure solution for 20 min). The NH4+ flux microsensor was positioned at an apex of 1600 μm on the root surface (the position with the maximum net fluxes of NH4+ selected from our preliminary experiment). Stable data was recorded for 3 min, with 8 replicates for each set of assays.
2.4 NH4+ concentration
The determination of NH4+ concentration followed the procedure outlined by Balkos et al. (2009). Root samples were collected and subsequently desorbed in a 10 mM CaSO4 solution for 5 min to remove extracellular NH4+. Then the roots were ground to powder in liquid nitrogen, and 0.2 g of the powder was homogenized in 2 ml of pre-cooled 10 mM formic acid. The resulting mixture was subjected to centrifugation at 53,000 × g for 5 min at 2°C. The supernatant was then filtered through a 0.45 μm filter into a 2 mL polypropylene tube and assayed for NH4+ concentration using the o-phthalaldehyde (OPA) method.
2.5 Nitrogen accumulation and amino acid concentration
Fresh root, stem, and leaf samples were freeze-dried and then ground into powder for the following measurements.
For N concentration analyses, approximately 0.1g of the powder was accurately weighed and mixed with 5 ml of H2SO4. The resulting mixture was heated to 200°C until achieving a clear solution. Subsequently, the reaction was terminated by adding H2O2. The resulting solutions were then analyzed using ICP-OES (Optima 8000, Perkin Elmer). Plant nitrogen accumulation = (plant dry weight - plant dry weight before treatment) × N concentration.
The total free amino acid was determined using the ninhydrin method, following a previously described protocol with slight modifications (Yokoyama and Hiramatsu, 2003). Briefly, 0.1 g root sample powder was weighed and mixed with the extraction buffer, which consisted of acetic acid/sodium acetate (pH 5.4). Then, centrifuging the mixture and collecting the supernatant. The OD value of the supernatant was measured at 580 nm and recorded using a Pharmacia Ultra Spec Pro UV/VIS spectrophotometer (Pharmacia, Cambridge, England). The final concentration of free amino acid was calculated according to the measured simultaneously with leucine as substrate.
To determine glutamate, glutamine, aspartic acid, and asparagine concentrations, 0.1 g of the root sample powder was weighed and extracted with 3% sulfosalicylic acid (w/v) for 12 hours. Afterward, the mixture was centrifuged at 10,000 g for 10 min, and the resulting supernatant was collected. This extraction process was repeated twice, and all the supernatants were combined and then filtered through a 0.22-μm aqueous film filter. The amino acids in the filtrate were quantified using the Hitachi L-8900 automatic amino acid analyzer (L-8900; Hitachi Corp., Tokyo, Japan), following the method described by Ma et al. (2017).
2.6 Soluble sugars and carbon skeleton concentration
The sucrose and fructose concentrations were determined using the resorcinol method described by Zeng et al. (2014). Briefly, 0.1g of the root sample powder (fresh samples were freeze-dried and ground) was weighed and extracted with a sugar extraction solution. For sucrose determination, the supernatant was mixed with 2 M NaOH and incubated at 95°C for 10 min. Subsequently, 0.1 M resorcinol and 10 M HCl were added to the mixture, further incubating at 80°C for 60 min. The concentration of fructose was determined similarly to that of sucrose but without NaOH treatment before the color reaction. Both absorbances were measured at 500 nm using a Pharmacia Ultra Spec Pro UV/VIS spectrophotometer (Pharmacia, Cambridge, England). The concentration of fructose derived from hydrolyzed sucrose was subtracted to determine the free fructose concentration. The concentrations of sucrose and fructose were determined based on the corresponding standard curves.
For the quantification of glucose, pyruvate, 2-oxoglutarate (2-OG), and oxaloacetate (OAA) concentrations of roots, the HPLC method described by Georgelis et al. (2018) was used with some modifications. Approximately 0.2 g of fresh root samples were ground into a powder using liquid nitrogen and then mixed with 4 ml of the extraction solution (preheated 80% ethanol) for 5 min at 80 °C. Subsequently, the mixtures were centrifuged at 12000 g for 10 min, and the resulting supernatants were collected. After the first collection of supernatants, the pellets were resuspended in 2 ml of 50% ethanol, and the extraction procedure was repeated as described above. The supernatants were collected again, and the pellets were resuspended with 2 ml of dd-water and repeated the extraction procedure. All supernatants were collected and vigorously shaken after mixing with an equal volume of chloroform. After the extraction procedure, the aqueous phase was collected, dried under vacuum, and re-dissolved in 1 ml of 50% acetonitrile (acetonitrile: water =50: 50). Before analysis using the anion-exchange HPLC system, the samples were filtered through a 0.45 μm filter membrane. Sugar compounds were separated on a Sugar-D column (4.6×250 mm, Nacalai Tesque Inc., Japan) using a mobile phase of acetonitrile/water (75: 25, v/v) at a 1.0 ml/min flow rate. The column temperature was 40 °C, and the injection volume was 30 µl. The quantification of each sugar was performed by comparing the peak areas of the samples with those of the standard solutions.
2.7 Enzyme activity
The root GS activity was determined using a previously described method (Jiang et al., 2017). Briefly, 0.5 g of frozen root samples were weighed and extracted with 1.2 mL of extraction buffer (1 mmol L-1 EDTA, 100 mmol L-1 pH 7.6 Tris-HCl, 1 mmol L-1 MgCl2, and 10 mmol L-1 β-mercaptoethanol). This reaction mixture was then incubated at 25°C for 5 min and then transferred to a hydroxylamine hydrochloride bath at 25°C for 15 min. Subsequently, the mixture was subjected to chromatography utilizing FeCl3 solution. Then, the mixture was centrifuged at 4000 rpm for 10 min at 25°C. Finally, the optical density of the supernatant at 540 nm was measured using the Pharmacia Ultra Spec Pro UV/VIS spectrophotometer (Pharmacia, Cambridge, England).
The activity of glutamate dehydrogenase (GDH, EC 1.5.1) was determined according to the procedure outlined by Skopelitis et al. (2007). Briefly, for the assay of NADH-GDH activity, 2.6 ml of the reservoir solution (115.4 mmol L-1 pH 8.0 Tris-HCl, 23.1 mmol L-1 2-oxoglutarate, 231 mmol L-1 NH4Cl), 0.1 ml 30 mmol L-1 CaCl2, 0.1 ml 0.2 mmol L-1 NAD(P)H, and 0.1 ml deionized water were pre-added to the test tubes. The reaction was then initiated by adding 0.1 ml of root extract (same as that of GS), and the absorbance value was measured at 340 nm using a Pharmacia Ultra Spec Pro UV/VIS spectrophotometer (Pharmacia, Cambridge, England), and again after 3 min to calculate the difference. Test tubes with distilled water instead of NADH and root extracts were used as blank controls. For the analysis of NAD+-GDH activity, 2.6 ml of the reservoir solution (115.4 mmol L-1 pH 9.3 Tris-HCl, 115.4 mmol L-1 L-glutamate, 30 mmol L-1 CaCl2), 0.05 ml 30 mmol L-1 CaCl2, 0.1 ml 30 mmol L-1 NAD+, and 0.15 ml deionized water were pre-added to the test tubes. Other measurement steps were the same as for NADH-GDH. The activity of GDH was expressed as one unit of enzyme activity in terms of the amount of enzyme required to oxidize or reduce 1 μmol of NADH or NAD+ min-1 at 30 °C.
The activities of GOGAT, hexokinase (HXK, EC 2.7.1.1), phosphofructokinase activity (PFK, EC 2.7.1.11), pyruvate kinase (PK, EC 2.7.1.40), and phosphoenolpyruvate carboxylase (PEPc, 4.1.1.31) were measured using respective kits (catalog numbers: BC0070, BC1465, BC0745, BC0530, BC0540, and BC2190, respectively) purchased from Beijing Solarbio Science & Technology Co., Ltd. (Beijing, China). All enzyme activities were measured using the spectrophotometer as described previously (Chen et al., 2023). Briefly, 0.05 g of each fresh root sample was weighed and ground into powder using a freezer-mill. The powder was then treated with the respective kit reagents as per the manufacturer’s instructions. Finally, the rate of decrease in the absorbance of each reaction solution was obtained using the spectrophotometer.
2.8 RT-PCR
Total RNA from root samples was extracted using TRIzol reagent (Vazyme Bio, China). For cDNA synthesis, the HiScript III Q RT SuperMix (Vazyme Bio, China) was employed following the manufacturer’s instruction, and the cDNA samples were diluted 5× before being subjected to qPCR analysis. Real-time quantitative RT-PCR was carried out using the CFX Connect Real-Time PCR Detection System (Bio-Rad, USA) with ChamQ SYBR qPCR Master Mix (Vazyme Bio, China).
The primer sequences for TaPFK, TaHXK, and TaPK were sourced from Li et al. (2019). The primers for AMT1s (TaAMT1.1 and TaAMT1.2) were referred to by Ijato et al. (2021). The primer for TaAmt2.1 was referred to by Porras Murillo et al. (2023). The primers for tonoplast sugar transporter TaTST, tonoplast H+/glucose symporter TaERDL, and the internal reference genes ACT and ADP were listed in Supplementary Table S2. Relative expression levels were determined using the Livak and Schmittgen (2001) method.
2.9 Statistical analysis
The experiment was repeated three times during two years. Statistical analyses were performed using SPSS software version 19 (IBM Corp., Armonk, NY, USA). Analysis of variance (ANOVA) was subsequently carried out, and post hoc comparisons of means were performed using Duncan’s test. Graphs and tables were generated using Excel and Origin 2018 software (OriginLab, Northampton, MA, USA).
3 Results
3.1 Dry weight and root morphology
We first examined the growth responses of the two wheat cultivars to NH4+ stress. The AN-treated plants exhibited significantly reduced shoot, root, and total plant dry weight than the NN-treated plants, with the impact being more pronounced in Yangmai20 than in Xumai25 (Figure 1). Notably, the decrease in the root dry weight for Yangmai20 commenced at 3 DAT, while that for Xumai25 began at 5 DAT (Figure 1C).
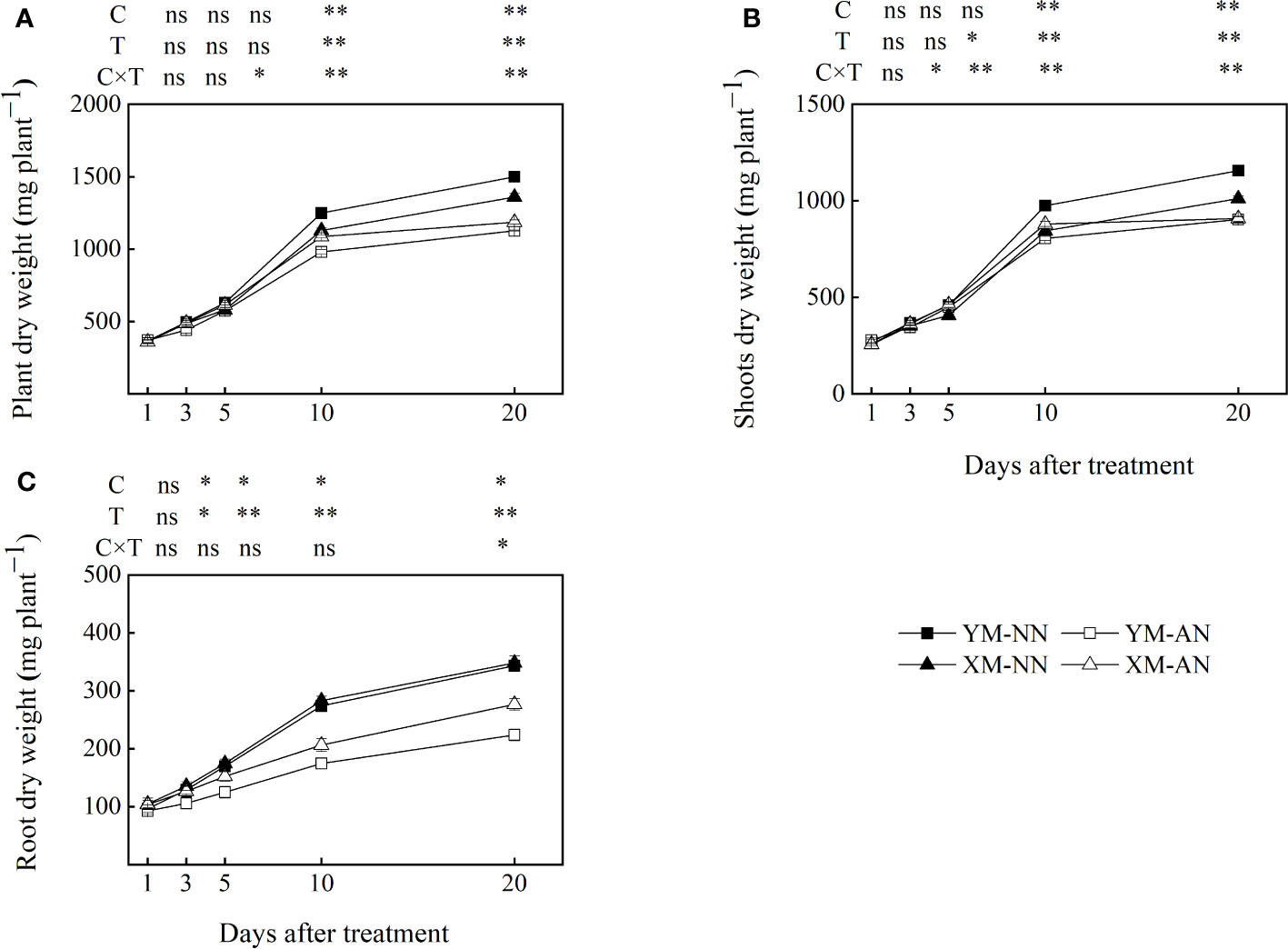
Figure 1 Effect of ammonium stress on biomass accumulation of two different ammonium-sensitive cultivars. (A) plant dry weight; (B) shoot dry weight; (C) root dry weight. Data are given as means of three biological replicates, and error bars indicate SD. NN, nitrate conditions; AN, ammonium stress conditions. YM, NH4+-sensitive cultivar Yangmai20; XM, NH4+-tolerant cultivar Xumai25. C, T, and C×T represent the F-value of cultivar, treatment, and the interaction between cultivar and treatment, respectively. The symbols * and ** indicate significant differences at the 0.05 and 0.01 levels, respectively, while ns refers to no significant difference.
Next, we assessed the root morphology of the cultivars to analyze the differential root responses under NH4+ stress. At 20 DAT, for both cultivars, we observed significantly reduced length, surface area, and volume of both primary and lateral roots for AN-treated plants than NN-treated plants (Table 1). After AN treatment, Yangmai20 exhibited more prominent reductions in the length, surface area, and volume of the primary root than Xumai25 (64%, 60%, and 64% vs. 50%, 49%, and 47%, respectively). Similarly, Yangmai20 exhibited more prominent reductions in the length, surface area, and volume of the lateral roots than Xumai25 post-AN treatment (36%, 43%, and 48% vs. 22%, 27%, and 29%, respectively). Moreover, the average diameter of the primary roots of AN-treated plants was comparable to that of NN-treated plants. However, the AN-treated Yangmai20 and Xumai25 exhibited a 23% and 25% increase in the average diameter of the lateral roots and a 33% and 23% reduction in the number of lateral roots, respectively, than their NN-treated counterparts (Table 1).
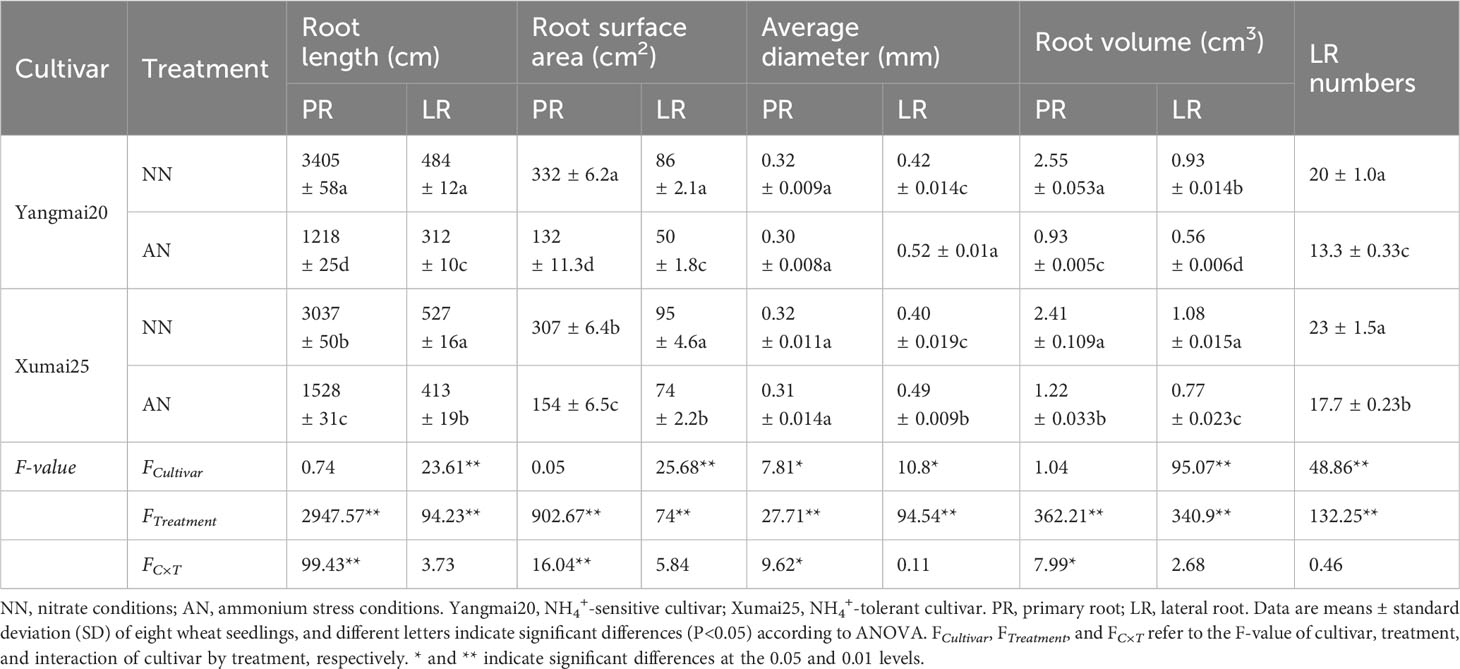
Table 1 Effects of ammonium stress on the root morphology of wheat seedlings after 20 days of treatment.
3.2 Free NH4+ concentration
Accumulation of free NH4+ in the root contributes to NH4+ toxicity in plants. Hence, we next compared free NH4+ accumulation in the roots of the two wheat cultivars. The free NH4+ concentration in the roots of both cultivars did not differ significantly after NN treatment. However, AN treatment led to an increase in the free NH4+ concentration of the roots of both cultivars, with a more prominent increase in Yangmai20 than Xumai25 (Figure 2).
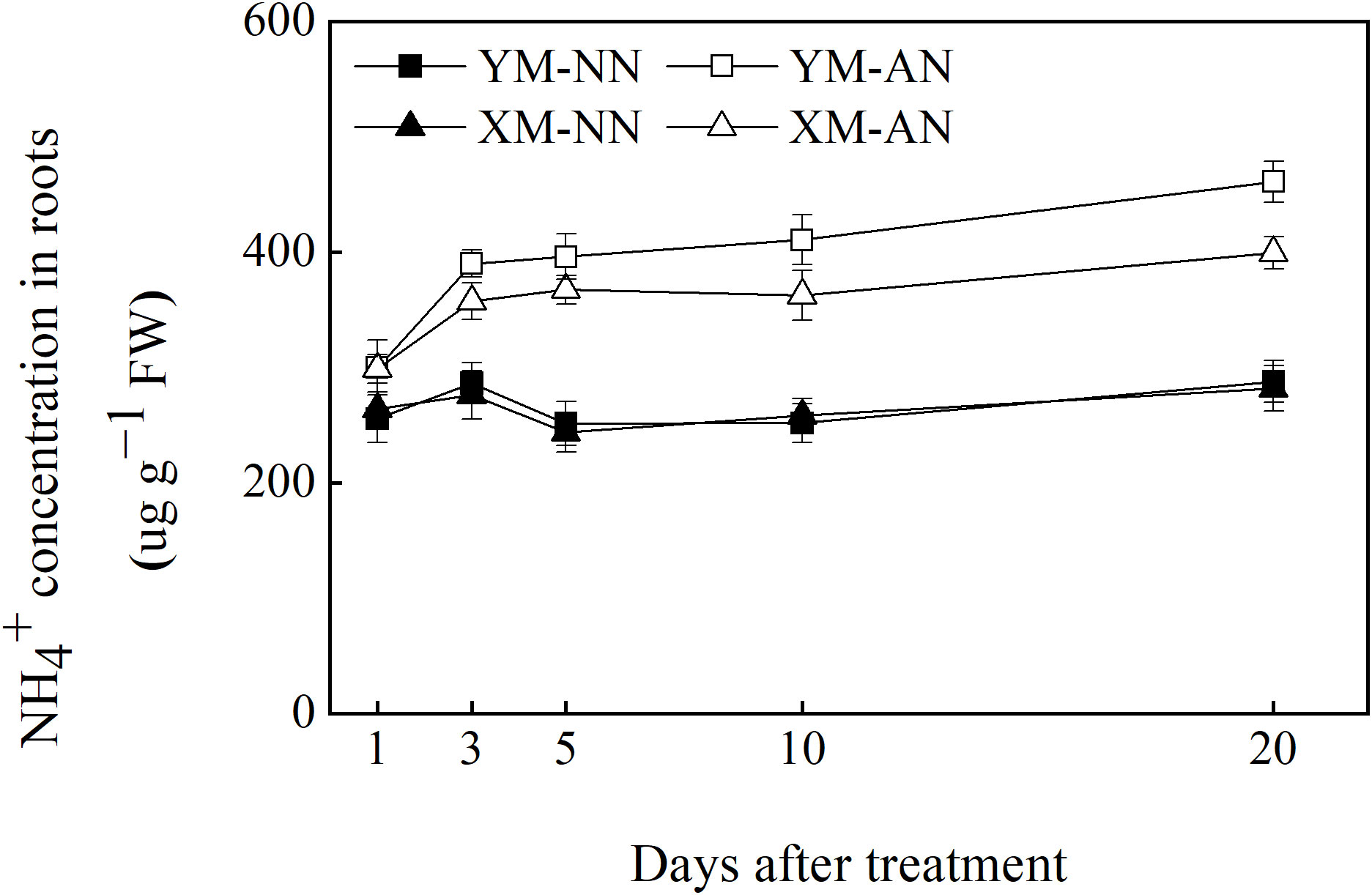
Figure 2 Effects of ammonium stress on root free NH4+ concentration of wheat seedlings after 1, 3, 5, 10, and 20 days. Data are given as means of three biological replicates, and error bars indicate SD. NN, nitrate conditions; AN, ammonium stress conditions. YM, NH4+-sensitive cultivar Yangmai20; XM, NH4+-tolerant cultivar Xumai25.
3.3 NH4+ influx and efflux
Changes in influx and efflux of NH4+ are closely related to NH4+ concentration in the plant and the severity of NH4+ toxicity. Here, we employed non-invasive micro-test technology (NMT) to dynamically measure changes in net NH4+ influx and efflux, thus revealing the differences in root NH4+ uptake between the two cultivars under NH4+ stress. The results revealed that treatment with 5 mM NH4+ stimulated NH4+ influx in the root of both cultivars, peaking at 6 h after treatment. Notably, NH4+-tolerant Xumai25 exhibited a more pronounced NH4+ influx (Figure 3A) despite a lower free NH4+ concentration in the root than NH4+-sensitive Yangmai20 (Figure 2).
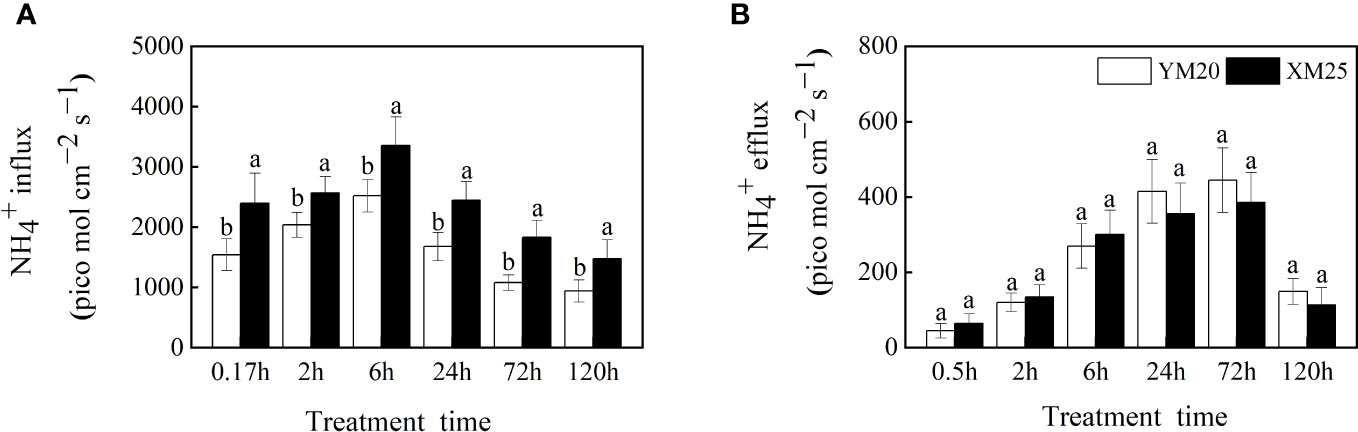
Figure 3 Effects of ammonium stress on root NH4+ influx and efflux of wheat seedlings. (A) NH4+ influx; (B) NH4+ efflux. Data are given as means of 8 replicates. Error bar labels with different letters indicate significant differences (P < 0.05) between cultivars. YM20, NH4+-sensitive cultivar Yangmai20; XM25, NH4+-tolerant cultivar Xumai25.
NH4+ efflux was observed in the roots of both cultivars at 0.5 h after treatment, gradually increasing with time and peaking at 72 h after treatment. While Yangmai20 tended to exhibit higher NH4+ efflux, the efflux did not differ significantly between the two cultivars (Figure 3B).
3.4 Nitrogen status
Nitrogen accumulation intuitively reflects the plant’s ability to assimilate NH4+. Hence, we measured and compared the nitrogen status of the two wheat cultivars. Compared to the NN-treated plants, the AN-treated plants exhibited significantly enhanced nitrogen accumulation in the leaves, with more prominent accumulation in Xumai25 than in Yangmai20 (Figure 4). Differently, the stem nitrogen accumulation decreased in Yangmai20, while no significant difference was observed in Xumai25. Furthermore, compared to the NN-treated plants, the AN-treated plants exhibited significantly reduced nitrogen accumulation in the roots, with a more prominent reduction in Yangmai20 than Xumai25 (Figure 4A).
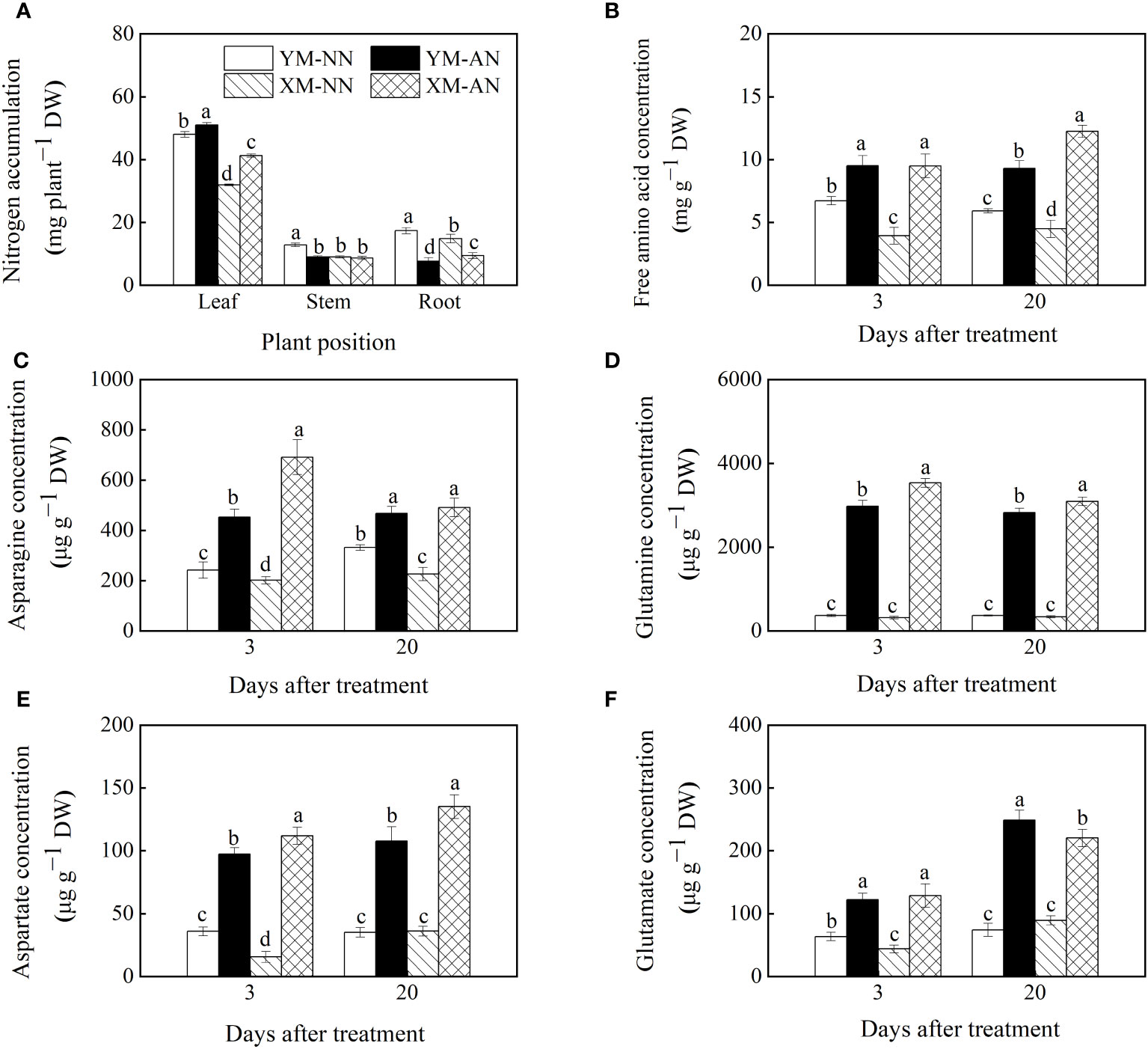
Figure 4 Effects of ammonium stress on nitrogen status of wheat seedlings at 3 and 20 days after treatment. (A) Plant nitrogen accumulation; (B) Root free amino acid concentration; (C) Root asparagine concentration; (D) Root glutamine concentration; (E) Root aspartate concentration; (F) Root glutamate concentration. Data are provided as means of three biological replicates and error bar labels with different letters indicate significant differences (P < 0.05) between cultivars and treatment. NN, nitrate conditions; AN, ammonium stress conditions. YM, NH4+-sensitive cultivar Yangmai20; XM, NH4+-tolerant cultivar Xumai25.
To elucidate the reasons underlying the varying nitrogen accumulation patterns in the two cultivars, we further measured the concentration of NH4+ assimilates in the plants. Compared to the NN-treated plants, the AN-treated plants exhibited significantly increased total free amino acid levels in the roots at 3 and 20 DAT, with substantially higher levels in Xumai25 roots than in Yangmai20 roots at 20 DAT (Figure 4B). Furthermore, the AN-treated plants exhibited significantly higher Asn, Gln, Asp, and Glu levels than the NN-treated plants (Figures 4C–F). Among the AN-treated plants, Xumai25 exhibited a higher increase in Asp, Asn, and Gln levels, but a lower increase in Glu levels than Yangmai20 (Figures 4C–F).
3.5 Root carbon skeleton supply
To investigate the effects of NH4+ stress on the carbon distribution and supply, we measured the concentrations of sucrose, fructose, glucose, pyruvate, 2-OG acid, and OAA acid in the roots of both wheat cultivars. We observed substantially decreased sucrose levels in the AN-treated plants than the NN-treated plants at 3 and 20 DAT, with higher sucrose levels in Xumai25 than Yangmai20 at 20 DAT (Figure 5A). Furthermore, we observed lower fructose concentrations in AN-treated plants than the NN-treated plants at 20 DAT; however, the fructose levels did not differ significantly between the two cultivars at any point in time (Figure 5B). Conversely, the glucose concentration steadily increased in both cultivars at 3 and 20 DAT after AN treatment (Figure 5C), with 87% and 81% increases in Yangmai20 and 58% and 43% increases in Xumai25, respectively.
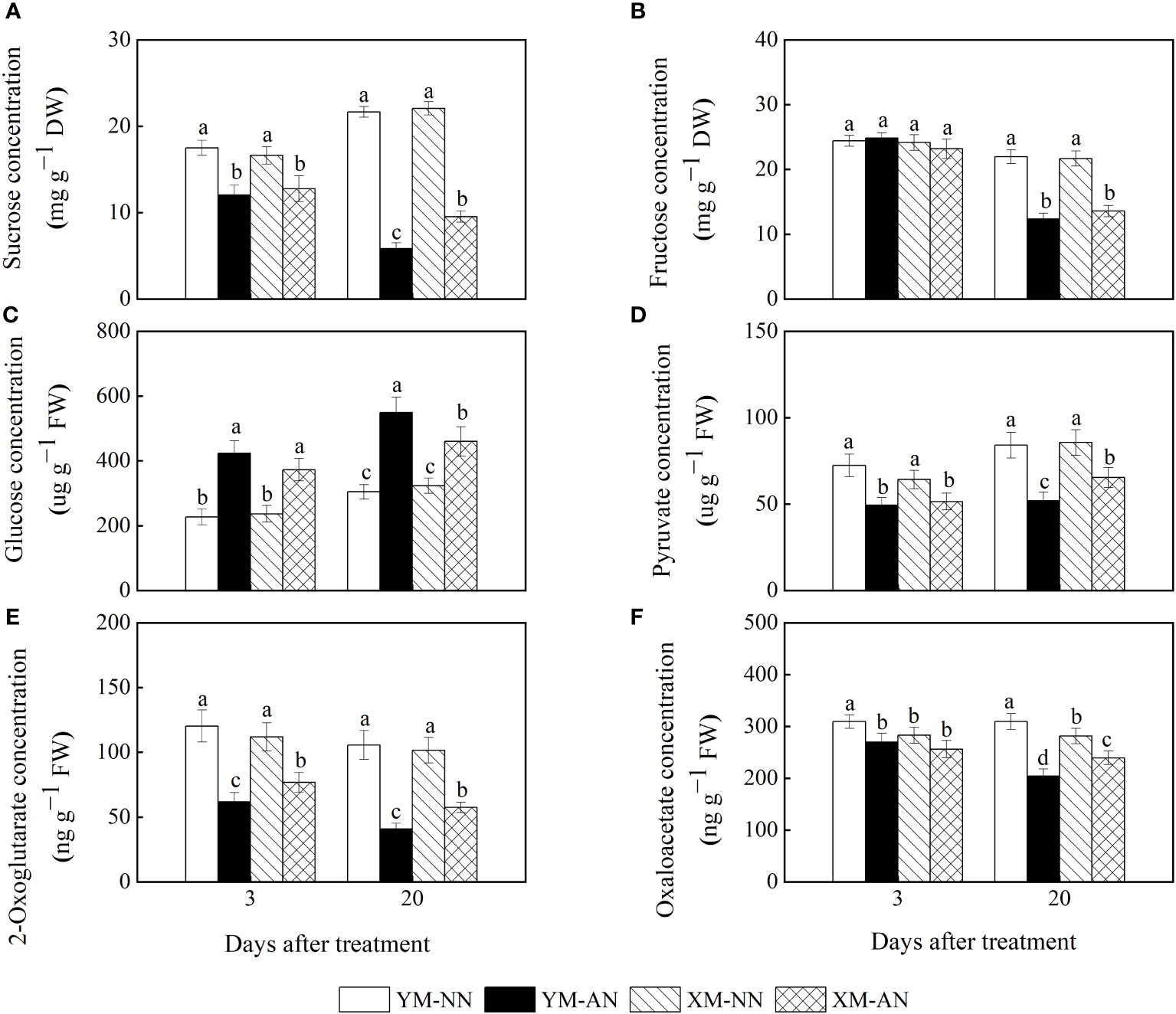
Figure 5 Effects of ammonium stress on root carbon skeleton supply of wheat seedlings at 3 and 20 days after treatment (DAT). (A) Sucrose concentration; (B) Fructose concentration; (C) Glucose concentration; (D) Pyruvate concentration; (E) 2-Oxoglutarate concentration; (F) Oxaloacetate concentration. Data are supplied as means of six biological replicates. Error bar labels with different letters indicate significant differences (P < 0.05) between cultivars and treatment. NN, nitrate conditions; AN, ammonium stress conditions. YM, NH4+-sensitive cultivar Yangmai20; XM, NH4+-tolerant cultivar Xumai25.
Furthermore, the AN-treated plants exhibited significantly reduced pyruvate and 2-OG concentrations than the NN-treated plants (Figures 5D, E), with more prominent reductions in Yangmai20 than Xumai25 (Figures 5D, E). Similarly, the AN-treated plants exhibited reduced OAA concentrations in the roots than the NN-treated plants at 20 DAT, with more prominent reductions in Yangmai20 than Xumai25 (Figure 5F).
3.6 Activities of NH4+-assimilating and sugar-metabolizing enzymes
To explore the mechanisms underlying lower glucose accumulation in Xumai25, we measured the activities of enzymes related to glucose metabolism. We observed significantly increased activities of HXK, PEPc, PFK, and PK in the AN-treated plants than the NN-treated plants (Figures 6A–D). Notably, Xumai25 exhibited a more substantial increase in HXK and PFK activities (294% and 169%, respectively) than Yangmai20 (154% and 64%, respectively) at 20 DAT (Figures 6A, B).
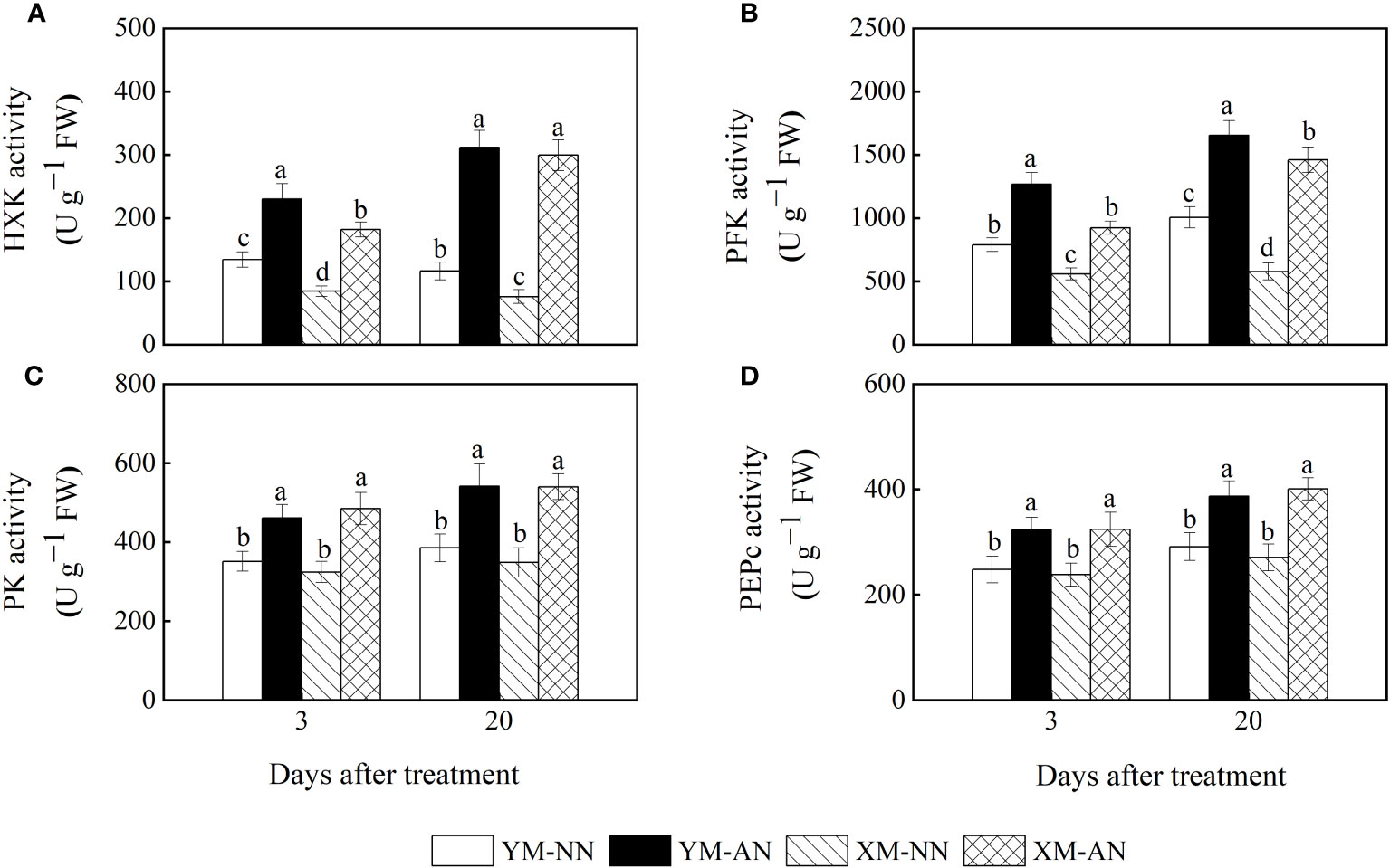
Figure 6 Effects of ammonium stress on the activity of sugar metabolizing enzyme in wheat seedlings at 3 and 20 days after treatment (DAT). (A) Hexokinase (HXK) activity; (B) Phosphofructokinase (PFK) activity; (C) Pyruvate kinase (PK) activity; (D) Phosphoenolpyruvate carboxylase (PEPc) activity. Data are expressed as means of three biological replicates. Error bar labels with different letters indicate significant differences (P < 0.05) between cultivars and treatment. NN, nitrate conditions; AN, ammonium stress conditions. YM, NH4+-sensitive cultivar Yangmai20; XM, NH4+-tolerant cultivar Xumai25.
The activities of NH4+ assimilation–related enzymes are closely related to the NH4+ assimilation capacity and NH4+ tolerance of plants. In this study, the AN-treated plants exhibited higher activities of GS, ferredoxin-dependent glutamate synthase (Fe-GOGAT), NADH-GDH, and NAD+-GDH than the NN-treated plants (Figures 7A–D). The activities of these enzymes were mildly higher in Xumai25 than in Yangmai20.
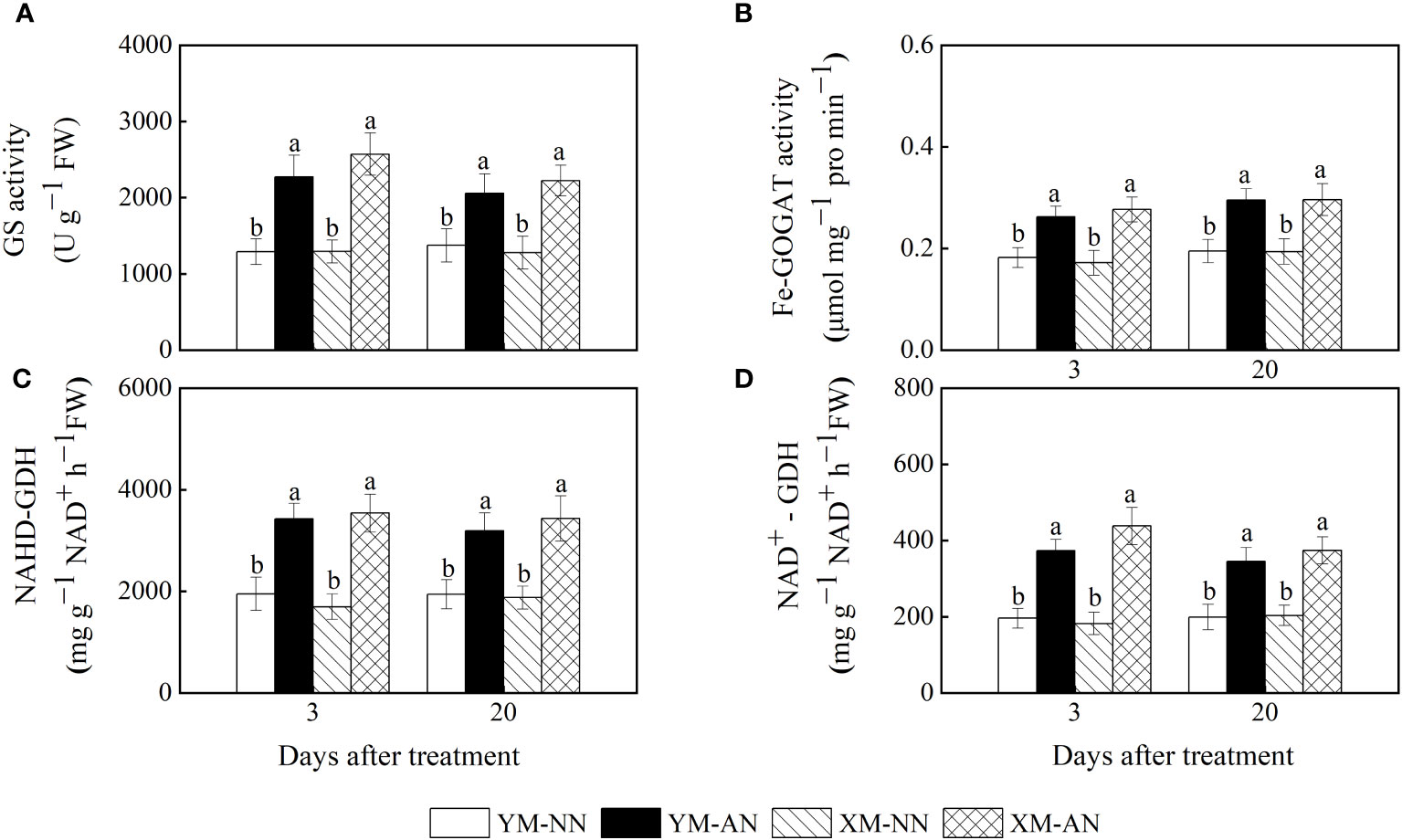
Figure 7 Effects of ammonium stress on the activity of ammonium metalizing in wheat seedlings at 3 and 20 days after treatment (DAT). (A) Glutamine synthetase activity; (B) Glutamate synthase activity; (C) NADH-GDH activity; (D) NAD+-GDH activity. Data are expressed as means of three biological replicates. Error bar labels with different letters indicate significant differences (P < 0.05) between cultivars and treatment. NN, nitrate conditions; AN, ammonium stress conditions. YM, NH4+-sensitive cultivar Yangmai20; XM, NH4+-tolerant cultivar Xumai25.
3.7 Relative gene expression correlates to NH4+ uptake and carbon supply in roots
Unlike the NN-treated plants, the AN-treated plants exhibited a rapid upregulation of TaAMT1.1 and TaAMT1.2 at 6 and 120 h post-treatment (Figures 8A–C), with no significant differences between the two cultivars. Furthermore, the AN-treated plants also exhibited TaAMT2.1 upregulation in the roots. Moreover, the NN-treated Xumai25 exhibited higher TaAMT2.1 expression than NN-treated Yangmai20 (Figure 8C).
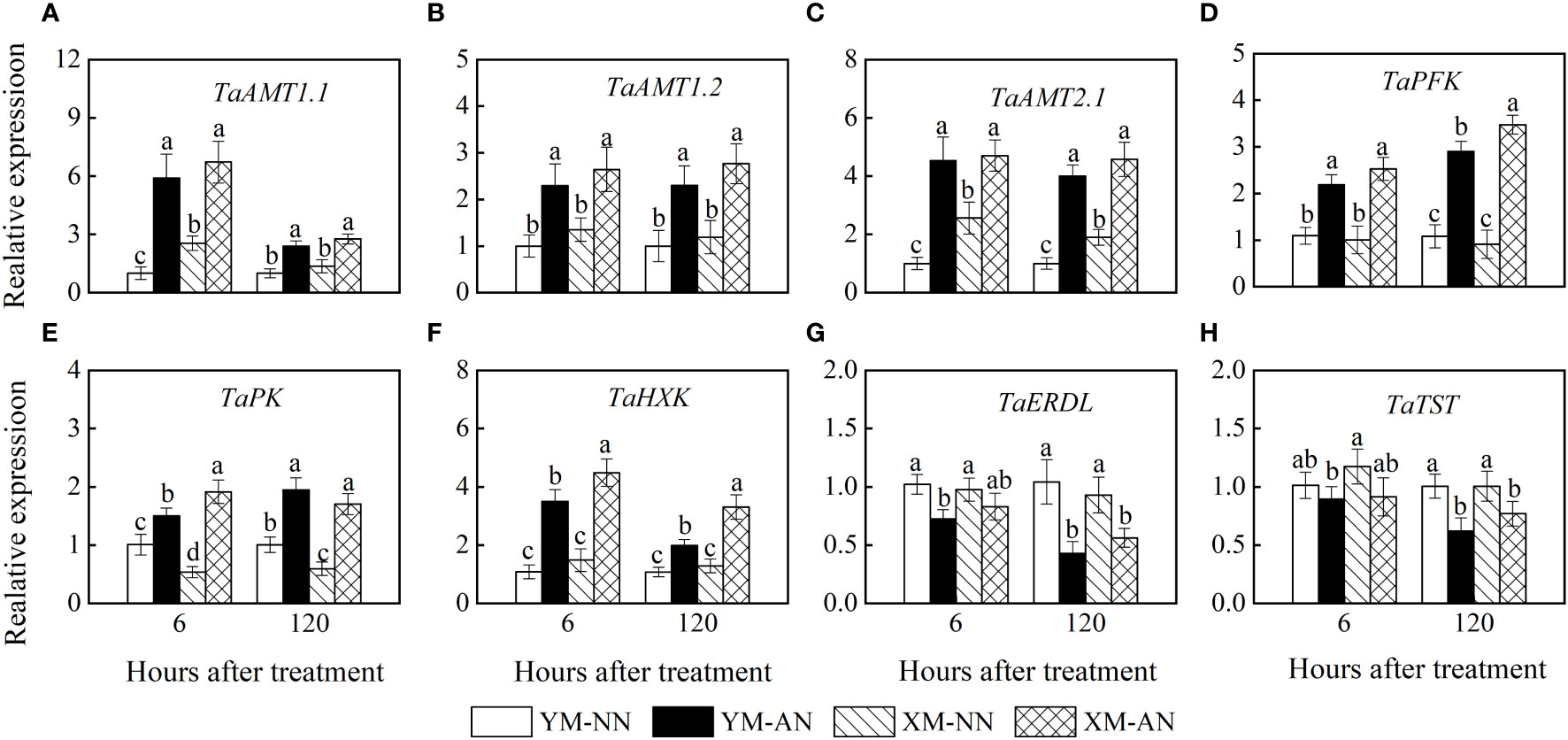
Figure 8 Effects of ammonium stress on relative gene expression in the root of wheat seedlings at 6 and 120 hours after treatment. (A) TaAMT1.1; (B) TaAMT1.2; (C) TaAMT2.1; (D) TaPFK; (E) TaPK; (F) TaHXK; (G) TaERDL; (H) TaTST. Data are expressed as means of three biological replicates. Error bar labels with different letters indicate significant differences (P < 0.05) between cultivars. NN, nitrate conditions; AN, ammonium stress conditions. YM, NH4+-sensitive cultivar Yangmai20; XM, NH4+-tolerant cultivar Xumai25.
Similarly, we observed upregulation of TaPK, TaHXK, and TaPFK in AN-treated plants at 6 h and 120 h after treatment (Figures 8D–F), with more prominent upregulation in Xumai25 than Yangmai25 at 120 h (Figures 8D–F).
Tonoplast sugar transporter (TST) and H+/glucose symporter (ERDL) mediate glucose transport across the vacuolar membrane. In this study, the AN-treated Yangmai20 exhibited a lower TaERDL expression than its NN-treated counterpart at 6 h; however, no significant differences were observed between AN- and NN-treated Xumai25. Moreover, at 120 h, all AN-treated plants exhibited significantly more TaERDL downregulation than the NN-treated plants (Figure 8G). In addition, the TaTST expression did not differ significantly between AN- and NN-treated plants at 6 h; however, this gene was significantly inhibited in the AN-treated plants than the NN-treated plants at 120 h (Figure 8H).
4 Discussion
It is well known that wheat is sensitive to NH4+ (Liu and von Wirén, 2017). In the present study, we assessed the responses of two wheat cultivars, NH4+-sensitive Yangmai20 and NH4+-tolerant Xumai25, to NH4+ stress to elucidate the mechanism of NH4+tolerance in wheat. Our results showed that NH4+ stress had a significant adverse impact on the growth of wheat seedlings (Table 1, Figure 1), which is consistent with similar observations in other plant species (Chen et al., 2020; Guo et al., 2021; Tian et al., 2021). Notably, the NH4+-tolerant cultivar, Xumai25, exhibited a less reduction in root growth and an enhanced NH4+ accumulation capacity than the NH4+-sensitive cultivar, Yangmai20, resulting in a superior overall growth of Xumai25 under NH4+ stress.
4.1 Superior root development and stronger NH4+ uptake enhances NH4+ tolerance in Xumai25
The phytotoxicity of NH4+ on root growth, even at moderate concentrations, is a well-known phenomenon across several plant species (Li et al., 2014; Di et al., 2021). The present study showed that NH4+ stress markedly impacted wheat root morphology and root dry matter accumulation, with a more pronounced effect on the primary root (Figure 1C, Table 1). This effect was manifested as suppression of root length, surface area, and volume of both cultivars (Table 1), aligning with previous observations in Arabidopsis (Liu and von Wirén, 2017). The primary root of the NH4+-sensitive cultivar, Yangmai20, was more substantially affected by NH4+ stress. Furthermore, lateral roots are known to be highly responsive to nutrient availability (Giehl and von Wiren, 2014). In this study, we observed that the plasticity of lateral roots was adversely affected by NH4+ stress as evidenced by an increase in root average diameter and a decrease in length, surface area, and volume in both cultivars, with more pronounced effects in Yangmai20 (Table 1). Concurrently, NH4+ stress led to a reduction in the number of lateral roots in both cultivars (Table 1), consistent with prior observations in Arabidopsis (Li et al., 2013), implying that NH4+ stress inhibits the germination of lateral roots in wheat (Liu and von Wirén, 2017). Taken together, our findings suggest that 5 mM NH4+ stress inhibits the growth of primary and lateral roots in the wheat seedlings, resulting in a decrease in root dry matter. The better NH4+ stress acclimatization capacity of Xumai25, compared with Yangmai20, might contribute to the superior root development in the former.
NH4+ uptake and transport in plant tissues are predominantly mediated by AMTs. The expression of AMTs is influenced by plant species as well as NH4+ concentration (Li et al., 2017). Previous studies have identified persistent NH4+ absorption via AMTs as a major contributor to the excessive free NH4+ accumulation in Arabidopsis (Li et al., 2020). Furthermore, exposure to high NH4+ concentrations tends to suppress the expressions of AMTs (Loqué et al., 2006). In the present study, both wheat cultivars exhibited an upregulation of TaAMT1.1, TaAMT1.2, and TaAMT2.1 under NH4+ stress (Figures 8A–C), which promoted NH4+ entry into the root. These findings align with the previous studies on wheat (Li et al., 2017; Ijato et al., 2021), demonstrating that, unlike Arabidopsis, wheat did not suppress the expression of AMTs under NH4+ stress to reduce NH4+ uptake. In addition, the enhanced expression of TaAMT2.1 in Xumai25 correlated with its superior NH4+ influx capacity (Figures 3A, 8C).
In addition, previous studies have shown the existence of other NH4+ uptake pathways in plant roots (Bittsánszky et al., 2015; Esteban et al., 2016). Therefore, to precisely measure NH4+ influx and efflux from wheat root epidermis, we employed the non-invasive micro-test technology (NMT) (Kühtreiber and Jaffe, 1990), which helps to exclude the influence of other NH4+ uptake channels on the results. In this study, we observed a higher NH4+ influx in Xumai25 than in Yangmai20 (Figure 3A), consistent with trends observed in 15NH4+ uptake (Supplementary Figure S2). Such NH4+ influx patterns have also been reported among different bamboo species (Zou et al., 2020). In addition, several studies have reported that despite the high net influx of NH4+ via plant roots, there might be a substantial NH4+ efflux from the roots to the outside (Britto et al., 2001; Di et al., 2021). Indeed, in the present study, both wheat cultivars exhibited NH4+ efflux from the root, but there was no significant difference in the amount of NH4+ efflux between them (Figure 3B). Taken together, the higher NH4+ influx and lower root free NH4+ concentration in Xumai25 (Figure 2), compared with that of Yangmai20, indicates its stronger NH4+ assimilation capacity, which is related to its higher ammonium tolerance. In addition, these data suggest that the NH4+-tolerant cultivar has a stronger NH4+ uptake capacity than the sensitive cultivar, disproving our first research hypothesis.
4.2 Superior NH4+ assimilation capacity positively impacts NH4+ tolerance in Xumai25
After entering plant cells, NH4+ is rapidly converted to glutamine and glutamate via the GS-GOGAT-GDH cycle (Xiao et al., 2023). Our study observed a significant increase in the activities of GS/Fe-GOGAT, NADH-GDH, and NAD+-GDH in the roots of both wheat cultivars under NH4+ stress (Figures 7A–D), further evidencing the activation of NH4+ assimilation-associated enzymes in wheat seedlings by NH4+ stress (Setién et al., 2013; González-Moro et al., 2021). In addition, some studies have suggested that GDH activity is linked to NH4+ tolerance (Cruz et al., 2006). Indeed, the current study observed a higher NADH-GDH and NAD+-GDH activities in Xumai25 than in Yangmai20, indicating a stronger NH4+ assimilation capacity of Xumai25.
A well-documented strategy for maintaining intracellular NH4+ levels in various plant species, including wheat, is to enhance NH4+ assimilation into organic molecules (Setién et al., 2013). In the present study, both cultivars exhibited a significant increase in total free amino acid levels under NH4+ stress, with Xumai25 having higher free amino acid levels than Yangmai20 (Figures 2, 4B), further evidencing the superior NH4+ assimilation capacity of Xumai25. A previous study suggested that the metabolic adaptation to NH4+ in different species is associated with their preference for synthesizing amino acid (González-Moro et al., 2021). In line with a prior study on wheat (Vega-Mas et al., 2019b), the current study observed a substantial accumulation of Asn and Gln in the root under NH4+ stress (Figures 4B, C), highlighting Asn and Gln as major storage amino acids in wheat plants. Additionally, the higher concentration of Asn in the roots of Xumai 25, compared to Yangmai 20, indicates the potential role of Asn in reducing NH4+ accumulation as well as the better adaptation of Xumai25 to NH4+ stress (Figure 4C).
Contrary to the findings in tomatoes, where NH4+ stress did not significantly alter Glu concentration (Xun et al., 2020), our study revealed a significant increase in Glu concentration in both wheat cultivars under NH4+ stress (Figure 4F), aligning with other studies on wheat (Setién et al., 2013; Vega-Mas et al., 2019a). Notably, in this present study, the Glu accumulation was lower in Xumai25 than in Yangmai20 (Figure 4F), indicating that Xumai25 was able to convert Glu to other amino acids or nitrogenous compounds more efficiently. This efficiency might also contribute to the higher tolerance of Xumai25 to NH4+ stress (Wang et al., 2020).
4.3 More efficient glucose metabolism and transport contribute to the stronger NH4+ assimilation in Xumai25
NH4+ assimilation is closely dependent on large amounts of pyruvate entering the tricarboxylic acid cycle to meet the high demand for carbon skeletons for ammonium detoxification (Vega-Mas et al., 2019b). In plants, sucrose is transported from photosynthetic leaves to the roots via the phloem and then hydrolyzed to hexoses (Glc and Fru) (Zhu et al., 2021), followed by further catabolism to provide a carbon skeleton for NH4+ assimilation. Under NH4+ stress, the sucrose and fructose levels substantially declined in the roots of both cultivars (Figures 5A, B), with a more prominent decline in Yangmai20 roots. According to Cruz et al. (2006), this decline is associated with the depletion of the carbon skeleton by root NH4+ assimilation. On the other hand, we speculate that it is also related to the inhibition of photosynthesis, which was reported to vary between the two cultivars in our previous study (Hu et al., 2024). In addition, we observed a remarkable increase in glucose concentration under NH4+ stress (Figure 5C), aligning with similar observations in Arabidopsis (Jian et al., 2018; Li et al., 2020). This result implies that NH4+ stress induces glucose accumulation in the root, and the sugar supply status from the shoot is independent of NH4+ toxicity, in agreement with Jian et al. (2018). Moreover, we observed that Xumai25 exhibited lower glucose accumulation (Figure 5C) but higher levels of pyruvate, 2-OG, and OAA at 20 DAT (Figures 5C–F) compared to Yangmai20, indicating a superior glucose metabolism in Xumai25.
In plants, glucose is metabolized to pyruvate via glycolysis (Li et al., 2019), and hexokinase, pyruvate kinase, and phosphofructokinase are key enzymes that regulate the process. A study on the transcriptome of duckweed indicated that genes associated with glycolysis are upregulated under NH4+ stress, thereby regulating carbon metabolism for ammonium detoxification (Tian et al., 2021). Consistently, our results showed that the activities of key glycolysis-related enzymes, such as HXK, PK, and PFK, were significantly increased under NH4+ stress in both cultivars (Figures 6A, B). Moreover, we observed an upregulation of the genes encoding these enzymes (Figures 8D, F), further suggesting that glycolysis is enhanced under NH4+ stress. Importantly, Xumai25 exhibited higher HXK and PFK activities and expression of genes encoding these enzymes than Yangmai20, demonstrating the former has a superior glycolytic capacity. This higher glycolytic capacity of Xumai25 can generate more pyruvate compared to Yangmai20, which explains its lower glucose accumulation and superior NH4+ assimilation.
Additionally, sugar transport into vacuoles, a crucial aspect of sugar homeostasis, is predominantly facilitated by various classes of sugar transporters in the tonoplast (Zhu et al., 2021). This phenomenon includes H+/sugar antiporters (TST) and H+/sugar symporters (ERDL), responsible for sugar influx into and efflux from vacuoles, respectively (Klemens et al., 2014). Notably, our study observed that both TaTST and TaERDL were down-regulated under NH4+ stress, with the down-regulation being more pronounced in Yangmai20 (Figures 8G, H). These results imply that NH4+ stress inhibits glucose transport, which is associated with glucose accumulation, and Xumai25 had a stronger glucose transport capacity than Yangmai20. Given that NH4+ stress encompasses osmotic stress (Bittsánszky et al., 2015; Esteban et al., 2016), it is reasonable to hypothesize that the inhibition of glucose transport under NH4+ stress might be a plant response mechanism aimed at maintaining cellular osmotic potential and mitigating oxidative stress (Xu et al., 2012). Further research is needed to decipher the molecular mechanisms underlying the role of glucose transport under NH4+ stress.
5 Conclusion
In conclusion, our investigation highlights the substantial impact of NH4+ stress on root growth, NH4+ uptake and assimilation, and glucose metabolism in different NH4+ tolerant wheat cultivars. The growth of both wheat cultivars was significantly inhibited under NH4+ stress. The NH4+-tolerant cultivar, Xumai25, showed a more robust glucose metabolism and enhanced glucose transport, which provided more carbon skeleton for NH4+ assimilation and reduced the accumulation of free NH4+ in the root, thereby exhibiting a stronger NH4+ assimilation capacity and a better root growth performance (Figure 9). This study uncovers the relationship between glucose metabolism, carbon skeleton supply induced by NH4+ stress, and NH4+ tolerance of wheat, and will provide a basis for the cultivation and breeding of new NH4+-tolerant cultivar.
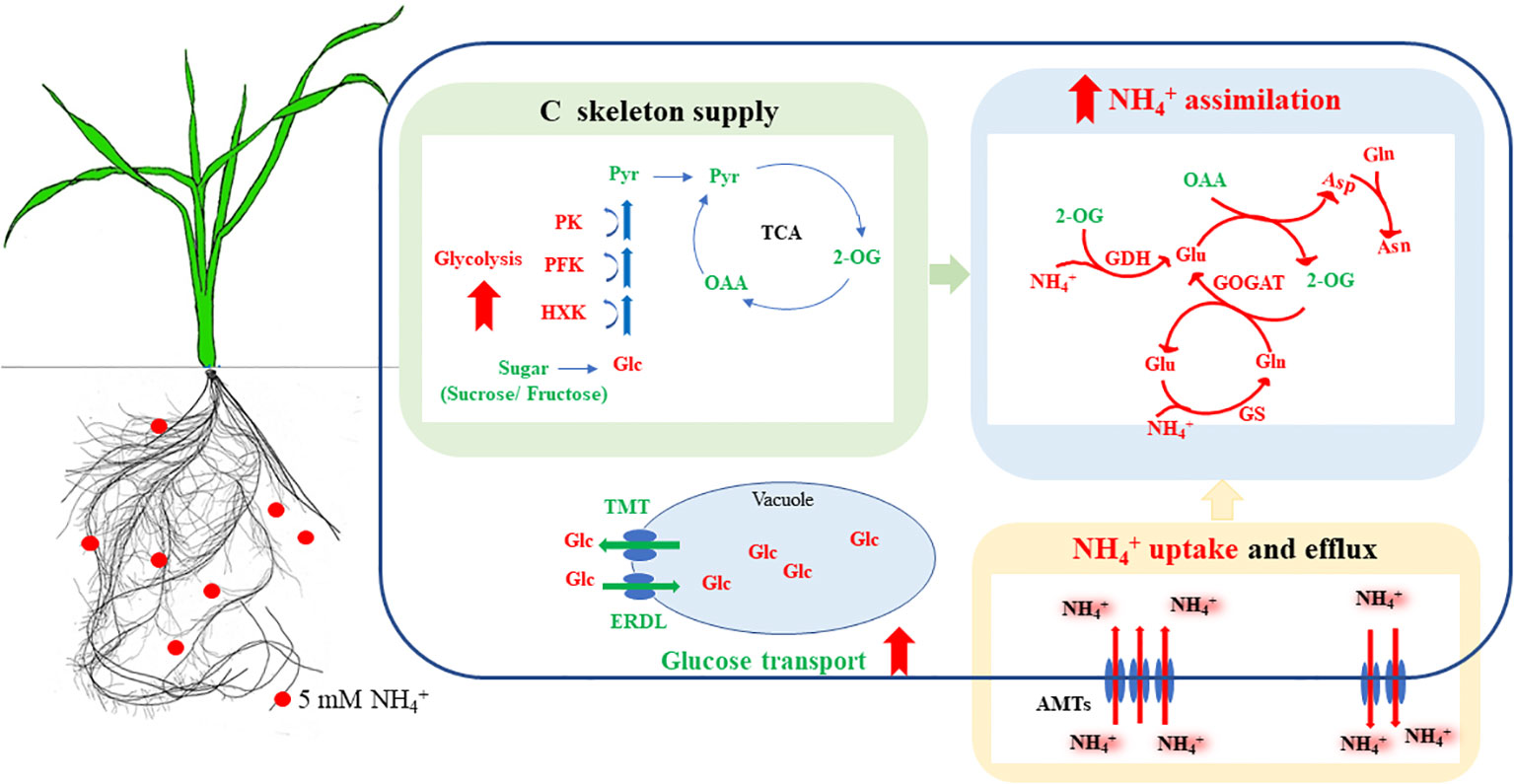
Figure 9 Physiological mechanisms of the enhanced NH4+ assimilation in NH4+-tolerant wheat cultivar under NH4+ stress. Under NH4+ stress, the NH4+-tolerant cultivar has an increased NH4+ uptake, its superior glucose metabolism and transport capacity contributed to the acquisition of more C skeletons, which improved NH4+ assimilation and reduced the accumulation of free NH4+ in the root, thus effectively alleviating the inhibitory effects of NH4+ stress. Red and green, respectively, indicate inhibition/reduction, and activation/increase under NH4+ stress. Solid red arrows indicate enhanced metabolism of the NH4+-tolerant cultivar, compared to the NH4+-sensitive cultivar. AMTs, ammonium transporters; Asn, asparagine; Asp, aspartate; ERDL, tonoplast H+/glucose symporter; GDH, glutamate dehydrogenase; GS, glutamine synthetase; GOGAT, glutamate synthase; Gln, glutamine; Glu, glutamate; HXK, hexokinase; OAA, oxaloacetate; PEPc, phosphoenolpyruvate carboxylase; PFK, phosphofructokinase; PK, pyruvate kinase; TST, tonoplast sugar transporter; 2-OG, 2-oxoglutarate.
Data availability statement
The raw data supporting the conclusions of this article will be made available by the authors, without undue reservation.
Author contributions
JH: Data curation, Formal analysis, Methodology, Writing – original draft. QZ: Writing – review & editing. BN: Writing – review & editing. CD: Writing – review & editing. ZT: Writing – review & editing. TD: Supervision, Writing – review & editing.
Funding
The author(s) declare financial support was received for the research, authorship, and/or publication of this article. We gratefully acknowledge the financial support received from the National Natural Science Foundation of China (Grant No. 32272215) and Jiangsu Provincial Key Research and Development Program (BE2021361-1) for conducting this study.
Acknowledgments
We are sincerely grateful to the other researchers and staff members involved in this project for their valuable contributions, expertise, and assistance in project management.
Conflict of interest
The authors declare that the research was conducted in the absence of any commercial or financial relationships that could be construed as a potential conflict of interest.
Publisher’s note
All claims expressed in this article are solely those of the authors and do not necessarily represent those of their affiliated organizations, or those of the publisher, the editors and the reviewers. Any product that may be evaluated in this article, or claim that may be made by its manufacturer, is not guaranteed or endorsed by the publisher.
Supplementary material
The Supplementary Material for this article can be found online at: https://www.frontiersin.org/articles/10.3389/fpls.2024.1339105/full#supplementary-material
Abbreviations
AMTs, ammonium transporters; Asn, asparagine; Asp, aspartate; DAT, days after treatment; ERDL, tonoplast H+/glucose symporter; GDH, glutamate dehydrogenase; GS, glutamine synthetase; GOGAT, glutamate synthase; Gln, glutamine; Glu, glutamate; HXK, hexokinase; LR, lateral root; OAA, oxaloacetate; PEPc, phosphoenolpyruvate carboxylase; PFK, phosphofructokinase; PK, pyruvate kinase; PR, primary root; TST, tonoplast sugar transporter; 2-OG, 2-oxoglutarate.
References
Ariz, I., Asensio, A. C., Zamarreño, A. M., García-Mina, J. M., Aparicio-Tejo, P. M., Moran, J. F. (2013). Changes in the C/N balance caused by increasing external ammonium concentrations are driven by carbon and energy availabilities during ammonium nutrition in pea plants: the key roles of asparagine synthetase and anaplerotic enzymes. Physiologia Planta. 4, 522–537. doi: 10.1111/j.1399-3054.2012.01712.x
Balkos, K. D., Britto, D. T., Kronzuceker, H. J. (2009). Optimization of ammonium acquisition and metabolism by potassium in rice (Oryza sativa L. cv. IR-72). Plant Cell Environ. 33, 23–32. doi: 10.1111/j.1365-3040.2009.02046.x
Bittsánszky, A., Pilinszky, K., Gyulai, G., Komives, T. (2015). Overcoming ammonium toxicity. Plant Sci. 231, 184–190. doi: 10.1016/j.plantsci.2014.12.005
Britto, D. T., Kronzucker, H. J. (2002). NH4+ toxicity in higher plants: a critical review. J. Plant Physiol. 6, 567–584. doi: 10.1078/0176-1617-0774
Britto, D. T., Kronzuker, H. J. (2005). Nitrogen acquisition, PEP carboxylase, and cellular pH homeostasis: new views on old paradigms. Plant Cell Environ. 11, 1396–1409. doi: 10.1111/j.1365-3040.2005.01372.x
Britto, D. T., Siddiqi, M. Y., Anthony, D. M. G., Kronzucker, H. J. (2001). Futile transmembrane NH4+ Cycling: A cellular hypothesis to explain ammonium toxicity in plants. Proc. Natl. Acad. Sci. 7, 4255–4258. doi: 10.1073/pnas.061034698
Chen, H., Jia, Y., Xu, H., Wang, Y., Zhou, Y., Huang, Z., et al. (2020). Ammonium nutrition inhibits plant growth and nitrogen uptake in citrus seedlings. Scientia Hortic. 272, 109526. doi: 10.1016/j.scienta.2020.109526
Chen, T., Zhao, M., Tang, X., Wei, W, Wen, X, Zhou, S, et al. (2023). The tigecycline resistance gene tetX has an expensive fitness cost based on increased outer membrane permeability and metabolic burden in Escherichia coli. J. Hazard. Mater. 458, 131889. doi: 10.1016/j.jhazmat.2023.131889
Cruz, C., Bio, A. F. M., Domínguez-Valdivia, M. D., Aparicio-Tejo, P. M., Lamsfus, C., Martins-Loução, M. A. (2006). How does glutamine synthetase activity determine plant tolerance to ammonium? Planta. 5, 1068–1080. doi: 10.1007/s00425-005-0155-2
de la Peña, M., González-Moro, M. B., Marino, D. (2019). Providing carbon skeletons to sustain amide synthesis in roots underlines the suitability of Brachypodium distachyon for the study of ammonium stress in cereals. AoB Plants 3. doi: 10.1093/aobpla/plz029
Di, D. W., Sun, L., Wang, M., Wu, J., Kronzucker, H. J., Fang, S., et al. (2021). WRKY46 promotes ammonium tolerance in Arabidopsis by repressing NUDX9 and indole-3-acetic acid-conjugating genes and by inhibiting ammonium efflux in the root elongation zone. New Phytologist. 1, 190–207. doi: 10.1111/nph.17554
Esteban, R., Ariz, I., Cruz, C., Moran, J. F. (2016). Review: Mechanisms of ammonium toxicity and the quest for tolerance. Plant Sci. 248, 92–101. doi: 10.1016/j.plantsci.2016.04.008
Georgelis, N., Fencil, K., Richael, C. M. (2018). Validation of a rapid and sensitive HPLC/MS method for measuring sucrose, fructose and glucose in plant tissues. Food Chem. 262, 191–198. doi: 10.1016/j.foodchem.2018.04.051
Giehl, R. F. H., von Wiren, N. (2014). Root nutrient foraging. Plant Physiol. 2, 509–517. doi: 10.1104/pp.114.245225
González-Moro, M. B., González-Moro, I., de la Peña, M., Estavillo, J. M., Aparicio-Tejo, P. M., Marino, D., et al. (2021). A multi-species analysis defines anaplerotic enzymes and amides as metabolic markers for ammonium nutrition. Front. Plant Sci. 11. doi: 10.3389/fpls.2020.632285
Guo, K., An, G., Wang, N., Pang, B., Shi, Z., Bai, H., et al. (2021). Thymol ameliorates ammonium toxicity via repressing polyamine oxidase-derived hydrogen peroxide and modulating ammonium transporters in rice root. Food Prod. Process. Nutr. 1. doi: 10.1186/s43014-021-00053-1
Hachiya, T., Inaba, J., Wakazaki, M., Sato, M., Toyooka, K., Miyagi, A., et al. (2021). Excessive ammonium assimilation by plastidic glutamine synthetase causes ammonium toxicity in Arabidopsis thaliana. Nat. Commun. 1. doi: 10.1038/s41467-021-25238-7
Hu, J., Zheng, Q., Dong, C., Liang, Z., Tian, Z., Dai, T. (2024). Enhanced stomatal conductance supports photosynthesis in wheat to improved NH4+ Tolerance. Plants 13, (1). doi: 10.3390/plants13010086
Ijato, T., Porras Murillo, R., Ganz, P., Ludewig, U., Neuhäuser, B. (2021). Concentration - dependent physiological and transcriptional adaptations of wheat seedlings to ammonium. Physiologia Planta. 3, 328–342. doi: 10.1111/ppl.13113
Jian, S., Liao, Q., Song, H., Liu, Q., Lepo, J. E., Guan, C., et al. (2018). NRT1.1-related NH4+ Toxicity is associated with a disturbed balance between NH4+ Uptake and assimilation. Plant Physiol. 4, 1473–1488. doi: 10.1104/pp.18.00410
Jiang, S., Sun, J., Tian, Z., Hu, H., Michel, E. J. S., Gao, J., et al. (2017). Root extension and nitrate transporter up-regulation induced by nitrogen deficiency improves nitrogen status and plant growth at the seedling stage of winter wheat (Triticum aestivum L.). Environ. Exp. Bot. 141, 28–40. doi: 10.1016/j.envexpbot.2017.06.006
Klemens, P. A. W., Patzke, K., Trentmann, O., Poschet, G., Büttner, M., Schulz, A., et al. (2014). Overexpression of a proton-coupled vacuolar glucose exporter impairs freezing tolerance and seed germination. New Phytologist. 1, 188–197. doi: 10.1111/nph.12642
Konishi, N., Ishiyama, K., Beier, M. P., Inoue, E., Kanno, K., Yamaya, T., et al. (2016). Contributions of two cytosolic glutamine synthetase isozymes to ammonium assimilation in Arabidopsis roots. J. Exp. Bot., w454. doi: 10.1093/jxb/erw454
Kühtreiber, W. M., Jaffe, L. F. (1990). Detection of extracellular calcium gradients with a calcium-specific vibrating electrode. J. Cell Biol. 5, 1565–1573. doi: 10.1083/jcb.110.5.1565
Li, G., Li, B., Dong, G., Feng, X., Kronzucker, H. J., Shi, W. (2013). Ammonium-induced shoot ethylene production is associated with the inhibition of lateral root formation in Arabidopsis. J. Exp. Botany. 5, 1413–1425. doi: 10.1093/jxb/ert019
Li, B., Li, G., Kronzucker, H. J., Baluška, F., Shi, W. (2014). Ammonium stress in Arabidopsis: signaling, genetic loci, and physiological targets. Trends Plant Sci. 2, 107–114. doi: 10.1016/j.tplants.2013.09.004
Li, T., Liao, K., Xu, X., Gao, Y., Wang, Z., Zhu, X., et al. (2017). Wheat ammonium transporter (AMT) gene family: diversity and possible role in host–pathogen interaction with stem rust. Front. Plant Sci. 8. doi: 10.3389/fpls.2017.01637
Li, W., Liu, Y., Liu, M., Zheng, Q, Li, B, Li, Z, et al. (2019). Sugar accumulation is associated with leaf senescence induced by long-term high light in wheat. Plant Sci. 287, 110169. doi: 10.1016/j.plantsci.2019.110169
Li, C., Tang, Z., Wei, J., Qu, H., Xie, Y., Xu, G. (2016). The OsAMT1.1 gene function in ammonium uptake and ammonium–potassium homeostasis over low and high ammonium concentration ranges. J. Genet. Genomics 11, 639–649. doi: 10.1016/j.jgg.2016.11.001
Li, Y., Zhou, J., Hao, D., Yang, S., Su, Y. (2020). Arabidopsis under ammonium over-supply: Characteristics of ammonium toxicity in relation to the activity of ammonium transporters. Pedosphere 3, 314–325. doi: 10.1016/S1002-0160(20)60011-X
Liu, Y., Li, Y., Tian, Z., Hu, J., Adkins, S., Dai, T. (2021). Changes of oxidative metabolism in the roots of wheat (Triticum aestivum L.) seedlings in response to elevated ammonium concentrations. J. Integr. Agric. 5, 1216–1228. doi: 10.1016/S2095-3119(20)63216-6
Liu, Y., von Wirén, N. (2017). Ammonium as a signal for physiological and morphological responses in plants. J. Exp. Botany. 10, 2581–2592. doi: 10.1093/jxb/erx086
Liu, X., Zhang, Y., Han, W., Tang, A., Shen, J., Cui, Z., et al. (2013). Enhanced nitrogen deposition over China. Nature 7438, 459–462. doi: 10.1038/nature11917
Liu, Y., Zhang, B., Li, C., Hu, F., Velde, B. (2008). Long-term fertilization influences on clay mineral composition and ammonium adsorption in a rice paddy soil. Soil Sci. Soc. America J. 6, 1580–1590. doi: 10.2136/sssaj2007.0040
Livak, K. J., Schmittgen, T. D. (2001). Analysis of relative gene expression data using real-time quantitative PCR and the 2–ΔΔCT method. Methods 4, 402–408. doi: 10.1006/meth.2001.1262
Loqué, D., Yuan, L., Kojima, S., Gojon, A., Wirth, J., Gazzarrini, S., et al. (2006). Additive contribution of AMT1;1 and AMT1;3 to high-affinity ammonium uptake across the plasma membrane of nitrogen-deficient Arabidopsis roots. Plant J. 4, 522–534. doi: 10.1111/j.1365-313X.2006.02887.x
Ma, Q., Cao, X., Xie, Y., Gu, Y., Feng, Y., Mi, W., et al. (2017). Effect of pH on the uptake and metabolism of glycine in pak choi (Brassica chinensis L.). Environ. Exp. Bot. 133, 139–150. doi: 10.1016/j.envexpbot.2016.10.013
Marino, D., Moran, J. F. (2019). Can ammonium stress be positive for plant performance? Front. Plant Sci. 10. doi: 10.3389/fpls.2019.01103
Pan, W. L., Madsen, I. J., Bolton, R. P., Graves, L., Pan, T. (2016). A sistrunkmmonia/ammonium toxicity root symptoms induced by inorganic and organic fertilizers and placement. Agron. J. 6, 2485–2492. doi: 10.2134/agronj2016.02.0122
Porras Murillo, R., Zhao, Y., Hu, J., Ijato, T., Retamal, J. P., Ludewig, U., et al. (2023). The wheat AMT2 (AMmonium Transporter) family, possible functions in ammonium uptake and pathogenic/symbiotic interactions. J. Plant Nutr. Soil Sci. 186, 164–168. doi: 10.1002/jpln.202200362
Ranathunge, K., El-kereamy, A., Gidda, S., Bi, Y., Rothstein, S. J. (2014). AMT1;1 transgenic rice plants with enhanced NH4+ permeability show superior growth and higher yield under optimal and suboptimal NH4+ conditions. J. Exp. Botany. 4, 965–979. doi: 10.1093/jxb/ert458
Setién, I., Fuertes-Mendizabal, T., González, A., Aparicio-Tejo, P. M., González-Murua, C., González-Moro, M. B., et al. (2013). High irradiance improves ammonium tolerance in wheat plants by increasing N assimilation. J. Plant Physiol. 8, 758–771. doi: 10.1016/j.jplph.2012.12.015
Skopelitis, D. S., Paranychianakis, N. V., Kouvarakis, A., Spyros, A., Stephanou, E. G., Roubelakis-Angelakis, K. A. (2007). The isoenzyme 7 of tobacco NAD(H)-dependent glutamate dehydrogenase exhibits high deaminating and low aminating activities in vivo. Plant Physiol. 145, 1726–1734. doi: 10.1104/pp.107.107813
Sun, K., Lu, F., Huang, P. W., Tang, M. J., Xu, F. J., Zhang, W., et al. (2022). Root endophyte differentially regulates plant response to NO3- and NH4+ nutrition by modulating N fluxes at the plant-fungal interface. Plant Cell Environ. 6, 1813–1828. doi: 10.1111/pce.14304
Tian, X., Fang, Y., Jin, Y., Yi, Z., Li, J., Du, A., et al. (2021). Ammonium detoxification mechanism of ammonium-tolerant duckweed (Landoltia punctata) revealed by carbon and nitrogen metabolism under ammonium stress. Environ. Pollut. 277, 116834. doi: 10.1016/j.envpol.2021.116834
Vega-Mas, I., Cukier, C., Coleto, I., Gonzalez-Murua, C., Limami, A. M., Gonzalez-Moro, M. B., et al. (2019a). Isotopic labelling reveals the efficient adaptation of wheat root TCA cycle flux modes to match carbon demand under ammonium nutrition. Sci. Rep. 9, 8925. doi: 10.1038/s41598-019-45393-8
Vega-Mas, I., Rossi, M. T., Gupta, K. J., González-Murua, C., Ratcliffe, R. G., Estavillo, J. M., et al. (2019b). Tomato roots exhibit in vivo glutamate dehydrogenase aminating capacity in response to excess ammonium supply. J. Plant Physiol., 83–91. doi: 10.1016/j.jplph.2019.03.009
Viktor, A., Cramer, M. D. (2005). The influence of root assimilated inorganic carbon on nitrogen acquisition/assimilation and carbon partitioning. New Phytologist. 1, 157–169. doi: 10.1111/j.1469-8137.2004.01204.x
Wang, F., Gao, J., Tian, Z., Liu, Y., Abid, M., Jiang, D., et al. (2016). Adaptation to rhizosphere acidification is a necessary prerequisite for wheat (Triticum aestivum L.) seedling resistance to ammonium stress. Plant Physiol. Biochem. 108, 447–455. doi: 10.1016/j.plaphy.2016.08.011
Wang, F., Gao, J., Yong, J. W.H., Liu, Y., Cao, D., He, X. (2020). Glutamate over-accumulation may serve as an endogenous indicator of tricarboxylic acid (TCA) cycle suppression under NH4+ nutrition in wheat (Triticum aestivum L.) seedlings. Environ. Exp. Botany. 177, 104130. doi: 10.1016/j.envexpbot.2020.104130
Xiao, C., Fang, Y., Wang, S., He, K. (2023). The alleviation of ammonium toxicity in plants. J. Integr. Plant Biol. 65, 1362–1368. doi: 10.1111/jipb.13467
Xu, G., Fan, X., Miller, A. J. (2012). Plant nitrogen assimilation and use efficiency. Annu. Rev. Plant Biol. 1, 153–182. doi: 10.1146/annurev-arplant-042811-105532
Xun, Z., Guo, X., Li, Y., Wen, X, Wang, C, Wang, Y (2020). Quantitative proteomics analysis of tomato growth inhibition by ammonium nitrogen. Plant Physiol. Biochem. 154, 129–141. doi: 10.1016/j.plaphy.2020.05.036
Yokoyama, S., Hiramatsu, J. (2003). A modified ninhydrin reagent using ascorbic acid instead of potassium cyanide. J. Biosci. Bioeng. 2, 204–205. doi: 10.1016/S1389-1723(03)80131-7
Yuan, L., Loqué, D., Kojima, S., Rauch, S., Ishiyama, K., Inoue, E, et al. (2007). The organization of high-affinity ammonium uptake in arabidopsis roots depends on the spatial arrangement and biochemical properties of AMT1-type transporters. Plant Cell. 8, 2636–2652. doi: 10.1105/tpc.107.052134
Zeng, Y., Yu, J., Cang, J., Liu, L., Mu, Y., Wang, J., et al. (2014). Detection of Sugar Accumulation and Expression Levels of Correlative Key Enzymes in Winter Wheat (Triticum aestivum) at Low Temperatures. Biosci. Biotechnol. Biochem. 4, 681–687. doi: 10.1271/bbb.100813
Zhu, L., Li, B., Wu, L., Li, H, Wang, Z, W, X, et al. (2021). MdERDL6-mediated glucose efflux to the cytosol promotes sugar accumulation in the vacuole through up-regulating TSTs in apple and tomato. Proc. Natl. Acad. Sci. 118 (1). doi: 10.1073/pnas.2022788118
Zou, N., Shi, W., Hou, L., Kronzucker, H. J., Huang, L., Gu, H., et al. (2020). Superior growth, N uptake and NH4+ tolerance in the giant bamboo Phyllostachys edulis over the broad-leaved tree Castanopsis fargesii at elevated NH4+ may underlie community succession and favor the expansion of bamboo. Tree Physiol. 11, 1606–1622. doi: 10.1093/treephys/tpaa086
Keywords: ammonium stress, ammonium tolerance, ammonium assimilation, glucose metabolism, wheat
Citation: Hu J, Zheng Q, Neuhäuser B, Dong C, Tian Z and Dai T (2024) Superior glucose metabolism supports NH4+ assimilation in wheat to improve ammonium tolerance. Front. Plant Sci. 15:1339105. doi: 10.3389/fpls.2024.1339105
Received: 15 November 2023; Accepted: 05 January 2024;
Published: 22 January 2024.
Edited by:
Enrique Ostria-Gallardo, University of Concepcion, ChileReviewed by:
Catalina Castro, Universidad de Concepción, ChileAsif Iqbal, Hazara University, Pakistan
Copyright © 2024 Hu, Zheng, Neuhäuser, Dong, Tian and Dai. This is an open-access article distributed under the terms of the Creative Commons Attribution License (CC BY). The use, distribution or reproduction in other forums is permitted, provided the original author(s) and the copyright owner(s) are credited and that the original publication in this journal is cited, in accordance with accepted academic practice. No use, distribution or reproduction is permitted which does not comply with these terms.
*Correspondence: Tingbo Dai, dGluZ2JvZEBuamF1LmVkdS5jbg==