- 1State Key Laboratory of Efficient Utilization of Arid and Semi-arid Arable Land in Northern China/Institute of Agricultural Resources and Regional Planning, Chinese Academy of Agricultural Sciences, Beijing, China
- 2Institute of Farmland Irrigation, Chinese Academy of Agricultural Sciences, Xinxiang, Henan, China
- 3Beijing Liangxiang Lanxin Hydraulic Engineering & Design Co., Ltd, Beijing, China
- 4Department of Soil and Land Use, Norwegian Institute of Bioeconomy Research (NIBIO), Viken, Norway
Compared to fluctuating soil water (FW) conditions, stable soil water (SW) can increase plant water use efficiency (WUE) and improve crop growth and aboveground yield. It is unknown, however, how stable and fluctuating soil water affect root vegetables. Here, the effects of SW and FW were studied on cherry radish in a pot experiment, using negative pressure irrigation and conventional irrigation, respectively. The assessed effects included agronomic parameters, physiological indices, yield, quality and WUE of cherry radish. Results showed that under similarly average soil water contents, compared with FW, SW increased plant photosynthetic rate, stomatal conductance and transpiration rate, decreased leaf proline content by 13.7–73.3% and malondialdehyde content by 12.5–40.0%, and increased soluble sugars content by 6.3–22.1%. Cherry radish had greater biomass accumulation and nutrient uptake in SW than in FW. Indeed, SW increased radish output by 34.6–94.1% with no influence on root/shoot ratio or root quality. In conclusion, soil water stability affected directly the water physiological indicators of cherry radish and indirectly its agronomic attributes and nutrient uptake, which in turn influenced the crop biomass and yield, as well as WUE. This study provides a new perspective for improving agronomy of root crops and WUE through managing soil water stability.
1 Introduction
Soil water is crucial for plant growth and development (Baier, 1965; Hey et al., 2020), and eventually affects agricultural productivity and sustainability (Leghari et al., 2021). In many growing environments ranging from arid to semi-humid, a moderate increase in soil water content (SWC) by irrigation can boost crop yield, and an optimal SWC can maximize the water use efficiency (WUE) of plants (Jahromi et al., 2023). Previous studies on soil water–plant relations have mostly focused on the SWC, however, there has been relatively limited understanding on the effects of soil water stability, i.e., stable soil water (SW) versus fluctuating soil water (FW), on crop growth and productivity (Niu et al., 2022).
In recent years, a pressure potential difference-crop initiate drawing water technique, namely as negative pressure irrigation (NPI) technology has been developed and proved as an effective irrigation method for water supply, with many potential advantages over traditional irrigation (Yang et al., 2022a). This technology uses a water potential difference caused by the negative pressure system, soil evaporation and plant transpiration to control water supply, and it can continuously and stably supply water to plants, which helps manage soil water fluctuations (Yang et al., 2022a). The technology can not only be used for studying SWC effects on crop performance, but also for assessing the effects of soil water stability (Zhang et al., 2021b; Niu et al., 2022).
Previous research on soil water stability is limited and has focused mainly on cash crops with aboveground yield. For example, Wang (2020) found that high-stability soil moisture boosted plant development and nutrient uptake of romaine lettuces (Lactuca sativa L.), with increased photosynthesis and WUE. In another study on lettuce, Gao et al. (2021) discovered that maintaining a stable water states considerably improved lettuce yield and quality, enhanced water utilization and decreased soil nitrogen loss as compared to fluctuating water conditions. On tomatoes (Solanum lycopersicum L.) and cucumbers (Cucumis sativus L.), Li et al. (2021b) confirmed that SW increased crop water productivity via maintaining soil water and nitrogen homogeneity. Elsewhere, long-term and stable irrigation during the tobacco (Nicotiana tabacum L.) growing period was found to minimize water consumption while increasing WUE, and the development and quality of flue-cured tobacco could be promoted by using appropriate SWC (Yang et al., 2022b). Similarly, studies on maize (Zea mays L.) have shown that SW could significantly promote economic factors in addition to growth and yield, compared with soil water state under wet and dry conditions (Zhang et al., 2021b; Zhang et al., 2022b). Despite these promising effects of SW conditions on the aboveground yield of the assessed crops (Niu et al., 2022; Yang et al., 2022b; Zhang et al., 2022a), their effects on root crops, particularly root vegetables, remain unknown.
Cherry radish (Raphanus sativus L. var. radculus pers) is a major root vegetable crop with highly nutritious and cherry-shaped root, a rapid growth cycle, and a short maturity period, as well as a substantial commercial value (Guo et al., 2019; Zhou et al., 2022). The growth and yield formation of radish were found to highly relate to the soil water conditions, for which proper water management increased radish yield and reduced radish root breaking (Kang and Wan, 2005; Lacerda et al., 2022). Optimizing the soil water management and planting date was found to boost radish production while reducing nitrogen loss by 40–50% (Zhang et al., 2021a), however, inappropriate water treatment was reported to have a detrimental impact on the growth and development of radish, resulting in inferior quality and yield penalties (Zomerfeld et al., 2021). Shafiq et al. (2015) found that water deficiency dramatically decreased the fresh weight of radish roots, increased the accumulation of carotenoids, malondialdehyde, glycine betaine and total soluble protein, and enhanced the antioxidant enzymes activity in radish roots. Another study has discovered that the temporal and spatial distribution of soil water, the distribution of radish root and the quality of the fruit were all strongly affected by soil water potential (Kang and Wan, 2005). Radish root development increased when the soil water potential decreased from –15 kPa to –55 kPa, although evapotranspiration decreased (Kang and Wan, 2005). Moreover, over-saturated soil decreased the rate of carbon assimilation by radish, hence limiting their shoot and root growth (Henschel et al., 2022).
Nevertheless, most of the studies focus on SWC wherein there is not much focus on soil water stability. Hence, the present study throws much insights on radish-soil water stability relations with the objectives: 1) to examine the effects of stable and fluctuating soil water on cherry radish growth and development, physiological indices, yield, quality, nutrient uptake, and WUE; 2) and to find out the optimal soil water conditions for root crops. We hypothesized: soil water stability could affect root crops morphological characteristics and physiological responses, and compared to FW, SW could sustain or improve radish growth, biomass accumulation and yield. It was expected to lay the foundation for revealing the relationship between soil water stability and root vegetables.
2 Materials and methods
2.1 Experimental site and climatic condition
The experiment was conducted in a rainproof shelter located at the Chinese Academy of Agricultural Sciences in Beijing, China (39.6°N, 116.2°E) from August through October in 2019. The study region has a typical continental climate being characterized as warm-temperate and semi-humid, with hot and rainy summers and cold and dry winters. The annual mean temperature was 10–12°C, and the annual frost-free period was 180–220 days. In this experiment, cherry radish was used as an experimental root vegetable, and it was grown in pots with dimensions of 42 cm in length, 26 cm in width, and 25 cm in height. The experimental soil particle distribution and chemical properties were listed in Table 1.
2.2 Negative pressure irrigation device
Soil water was studied under water supply from NPI devices that were developed by the Chinese Academy of Agricultural Sciences (Chinese Patents. ZL201110093923.2 and ZL201310554433.7). A NPI device (described in detail in Niu et al., 2022; Yang et al., 2022a) is consisted of a negative pressure controller, a water supply bucket (13.1 cm inner radius) and an irrigator (porous ceramic pipe, 26 cm long, 19 mm outer diameter and 10 mm internal diameter). Under the NPI system, there is a dynamic balance between soil matrix potential and irrigation pressure, thereby maintaining the stable water supply and a SW condition.
2.3 Experimental design and crop management
Six water treatments, each with six replicates, were established: NPI at –2 kPa (NPI0), –5 kPa (NPI1) and –8 kPa (NPI2) based on previous experimental results (Zhu et al., 2022), and conventional flood irrigation (CI) at 80%–90% field capacity (FC) (CI1), 70%–90% FC (CI2) and 60%–90% FC (CI3), respectively. The daily irrigation amount for NPI treatments was calculated by multiplying the water level difference by the cross-sectional area of the water supply bucket every day, and the cumulative irrigation amount was obtained by adding the daily irrigation amounts. When the SWC approached or dropped below the lower limits of CI treatments, irrigation was triggered to reach the upper limits and the irrigation amount was calculated according to the measured SWC, the set upper irrigation limits, and the soil volume in the pot (Wang, 2020).
Cherry radish was sown on August 10, 2019, for which each pot was grided to six sowing sites and two seeds per site. Each pot included 22 kg of air-dried soil with a bulk density of 1.4 g cm–3 and a FC of 29.1% (v/v). Before sowing, the same fertilizer amounts (2.8 g urea, 5.2 g superphosphate, and 5.0 g potassium sulfate) were applied to each pot and all pots were irrigated to 100% FC. On August 22, 2019, the plants were thinned to six seedlings each pot. Water treatments were started on August 29, 2019 and completed when the radish was harvested on September 20, 2019.
2.4 Sampling and measurements
2.4.1 Determination of SWC and soil water fluctuant parameters
A soil water velocity tester (AZS-100, Beijing Aozuo Ecological Instrument Co., Ltd, China) was used to measure the volumetric SWC every 2 days under NPI treatments and every day under CI treatments. The measurement was done between 17:00 and 18:00 at the center of the growth pot, where three points with an equal distance were chosen to determine SWC.
Equation (1) was used to calculate the coefficient of variation (CV) of soil water (Wang, 2020):
where SD is the standard deviation of SWC at different days, θ is the mean SWC. If CV ≤ 0.1, the soil water belongs to weak variation, if 0.1 < CV < 1, it belongs to medium variation, and if CV ≥ 1, it belongs to strong variation. The stronger the soil water fluctuation, the higher the value.
The fluctuation coefficient (δ) of soil water was computed using Equation (2):
where θi is the SWC on the ith day, θi–1 is the SWC on the (i–1)th day, and n is the number of SWC observation days. The magnitude of δ reflects the soil water stability, and the smaller the value is, the more stable the soil water.
2.4.2 Determination of growth and leaf physiological indicators
Cherry radish plant height, maximum leaf length and maximum leaf width were measured on the 11th and 21th days after treatment (DAT). Plant height was measured from the ground surface to plant’s tip and a ruler was used to measure the leaf’s length and width of maximum leaf.
Gas exchange parameters, such as photosynthetic rate (Photo), stomatal conductance (Cond) and transpiration rate (Trmmol), were recorded using a portable photosynthesis system (Li-6400XT, LI-Cor, NE, USA) at 8:30–11:30 am on the 11th and 21th DAT. The photosynthetic active radiation was set to 1000 µmol m−2 s−1, the flow rate was set to 500 µmol s−1, and an open gas circuit was used. Leaf water use efficiency (WUEL) was calculated according to Sun et al. (2018), as shown in Equation (3):
where WUEL is the leaf water use efficiency (μmol CO2 mmol–1 H2O), Photo is the photosynthetic rate (µmol CO2 m–2 s–1), and Trmmol is the transpiration rate (mmol H2O m–2 s–1).
For the purpose of estimating the physiological response of plants to water treatment, the contents of proline, malondialdehyde, and soluble sugars were measured at the National Energy R&D Center for Non-food Biomass, China Agricultural University, Beijing, China. The leaves of cherry radish were collected, immersed in liquid nitrogen, frozen for 30 min, and then stored in a freezer at –80°C. Thereafter the analyzes were done using high performance liquid chromatography method for free proline contents, a thiobarbituric acid method for malondialdehyde contents, and anthrone colorimetry method for soluble sugars contents as given by Zhang et al. (2022b).
2.4.3 Determination of radish biomass, yield and quality
At harvest, the cherry radish was harvested and immediately weighed, and the diameter of the radish bulb was measured using a vernier caliper. The yield of cherry radish was considered as the fresh weight of radish bulb. Thereafter, they were dried in an oven at 105°C for 30 min before being dried at 75°C to a constant weight to determine the biomass (Li et al., 2021a; Guo et al., 2022).
Various quality parameters were determined on the radish root. The nitrate content was determined following the methodology described by GB5009.33-2016. The vitamin C content was determined using the high-performance liquid chromatography method, reducing sugar content was determined using the colorimetric method, and soluble protein content was determined using the Coomassie brilliant blue method (Ouyang et al., 2021; Zhang et al., 2022b).
2.4.4 Determination of plant nutrient uptake
After measuring biomass, the aboveground (stems and leaves) and roots were ground, and a subsample was digested for the determination of plant nutrient content. Total nitrogen content was determined in plant samples using the Kjeldahl method, as described by Bao (2000). Total phosphorus and total potassium contents were determined using the inductively coupled plasma-optical emission spectrometry with microwave digestion (Wang, 2020). The uptake of nitrogen, phosphorus and potassium was calculated according to (Yang et al., 2020), as shown in Equation (4):
where Nutrient uptake is the uptake of nitrogen, phosphorus or potassium (mg plant–1), Nutrient content is the content of nitrogen, phosphorus or potassium (g kg–1), and DM is the dry matter mass (g plant–1).
2.4.5 Evapotranspiration and WUE
The evapotranspiration of cherry radish during the experiment was calculated using the water balance method (Ertek et al., 2006; Qu et al., 2020), as expressed in Equation (5):
where ET is the evapotranspiration (L), I is the irrigation amount (L), Δs is the change in soil water storage (L) between the beginning and the end of the experiment, θ0 and θ refer to the observed SWC (%) at the beginning and the end of the experiment, respectively, and V is the soil volume in the pot (L).
Water use efficiency was calculated according to (Sun et al., 2018; Liao et al., 2022), as shown in Equations (6, 7):
where WUEY is the yield water use efficiency (g kg–1), Y is the fresh yield of cherry radish bulbs (g plant–1), ET is the evapotranspiration (L plant–1), WUEB is the biomass water use efficiency (g kg–1), B is the biomass i.e. the dry matter mass of cherry radish, including both the above- and underground parts (g plant–1).
2.5 Statistical analysis
The data were processed with Microsoft Excel 2010 (Microsoft Crop, USA) and presented as mean ± standard deviation. SPSS 22.0 (SPSS, Chicago, USA) was used to perform analysis of variance (ANOVA) among the water management treatments, following the Duncan’s multiple-range test. Significant differences between treatments at the P < 0.05 level were indicated with different letters. The software Origin Pro 2021 (OriginLab Corporation, Northampton, MA, USA) was used for making graphs. Person’s correlation and principal component analysis (PCA) were used to evaluate the relationship between soil water and plant parameters of cherry radish. To further investigate the relationships between agronomic traits, physiological indices, yield and WUE, a partial least squares path model (PLS-PM) was constructed using the “plspm” package in R language (4.1.1) (Sanchez et al., 2015). The quality of the PLS-PM was evaluated by examining the goodness-of-fit index (GOF), in which a value > 0.7 indicates the acceptable overall prediction performance of the model (Chen et al., 2022).
3 Results
3.1 Soil water parameters
The SWC under the NPI0, NPI1, and NPI2 treatments changed only slightly over time, and the coefficients of variation and fluctuation were relatively small, indicating a stable moisture status (Table 2 and Figure 1). The SWC in the CI1, CI2, and CI3 treatments varied greatly as time progressed, exhibiting a “sawtooth” shape, and the soil water fluctuant parameters were significant, indicating fluctuating moisture conditions. Mean SWC values were similar between NPI1 (86% FC) and CI1 (85% FC) treatments and between NPI2 (82% FC) and CI2 (80% FC) treatments, which suggested that the SW and FW comparison was well established with similar overall SWC conditions.
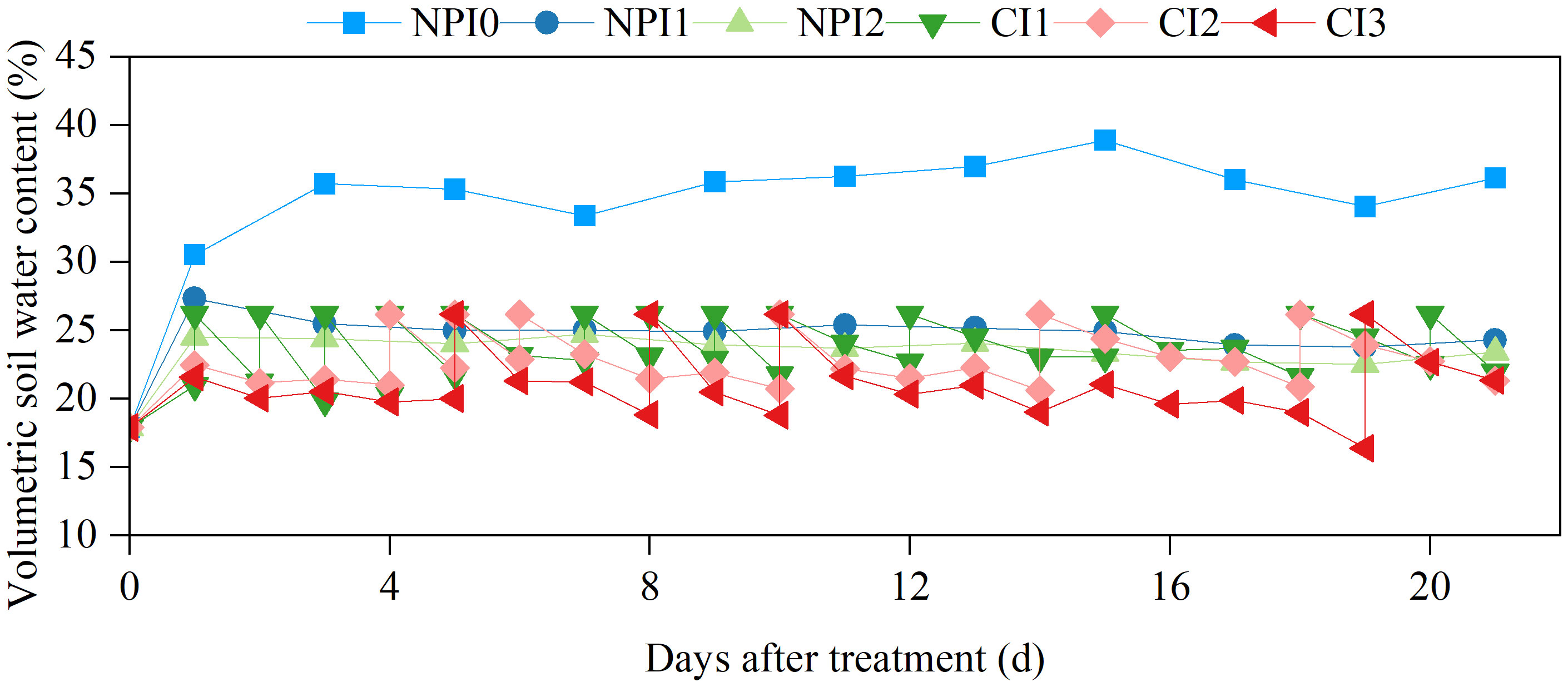
Figure 1 Temporal dynamics of volumetric soil water content under different treatments. NPI, negative pressure irrigation; CI, conventional irrigation.
3.2 Variation of agronomic traits
Agronomic traits of plants dynamically reflect their growth and development processes, which are closely linked to crop yield (Liu et al., 2019; He et al., 2020). The NPI treatments increased the radish plant height, maximum leaf length and maximum leaf width as compared to CI under similar SWC conditions (Figure 2). Specifically, NPI1 treatment raised plant height by 26.5%, maximum leaf length by 13.2%, and maximum leaf width by 10.5% on 21 DAT, compared to CI1 treatment; NPI2 treatment increased plant height by 39.7%, maximum leaf length by 36.3%, and maximum leaf width by 30.0%, compared to CI2 treatment. There was no significant difference in agronomic traits between SWC treatments under NPI or CI conditions. The results showed that under similar SWC conditions SW improved the growth and development of cherry radish compared to FW.
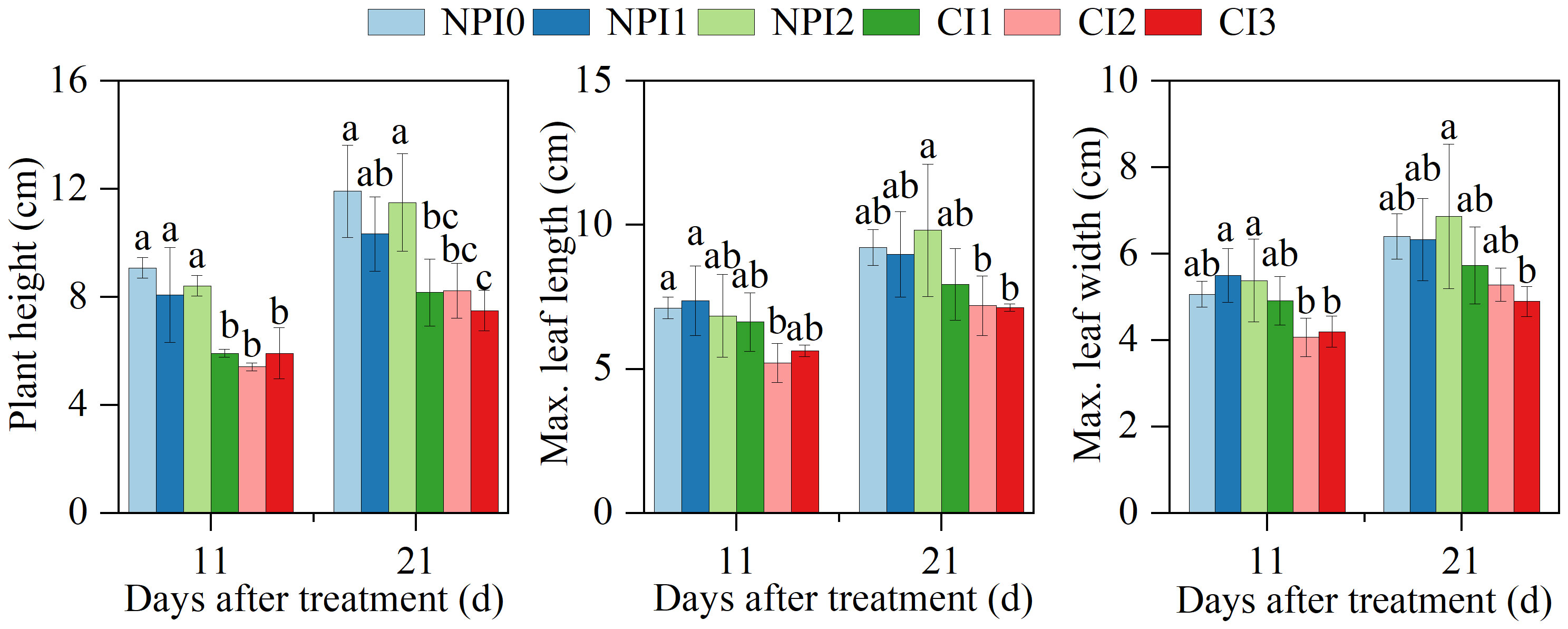
Figure 2 Variations of plant height, leaf length and leaf width under different treatments during cherry radish growing seasons. NPI, negative pressure irrigation; CI, conventional irrigation. Different lowercase letters above the columns indicate significant differences among treatments at the P < 0.05 level within the same day.
3.3 Leaf photosynthetic parameters
Photosynthesis is the fundamental physiological process for producing plant material. Gas exchange characteristics, including Photo, Cond, and Trmmol were affected by different water scheduling. The Photo of plants under NPI treatments on 21 DAT increased by 44.0% when SWC rose from 82% FC to 122% FC, and the leaf Photo under CI treatments increased by 27.8% when SWC rose from 75% FC to 85% FC (Figure 3). In 11–21 DAT, NPI1 treatment increased the Photo of cherry radish by 13.1–37.7%, Cond by 28.4–84.0%, and Trmmol by 11.3–16.9%, compared with CI1 treatment; NPI2 treatment could enhance the Photo by 9.9–33.4%, Cond by 33.2–68.3%, and Trmmol by 17.1–21.4%, compared to CI2 treatment. The results exhibited that SW was more favorable to improving the Photo, Cond, and Trmmol of cherry radish than FW under similar SWC circumstances; however, there was no discernible difference between SW and FW in terms of WUEL.
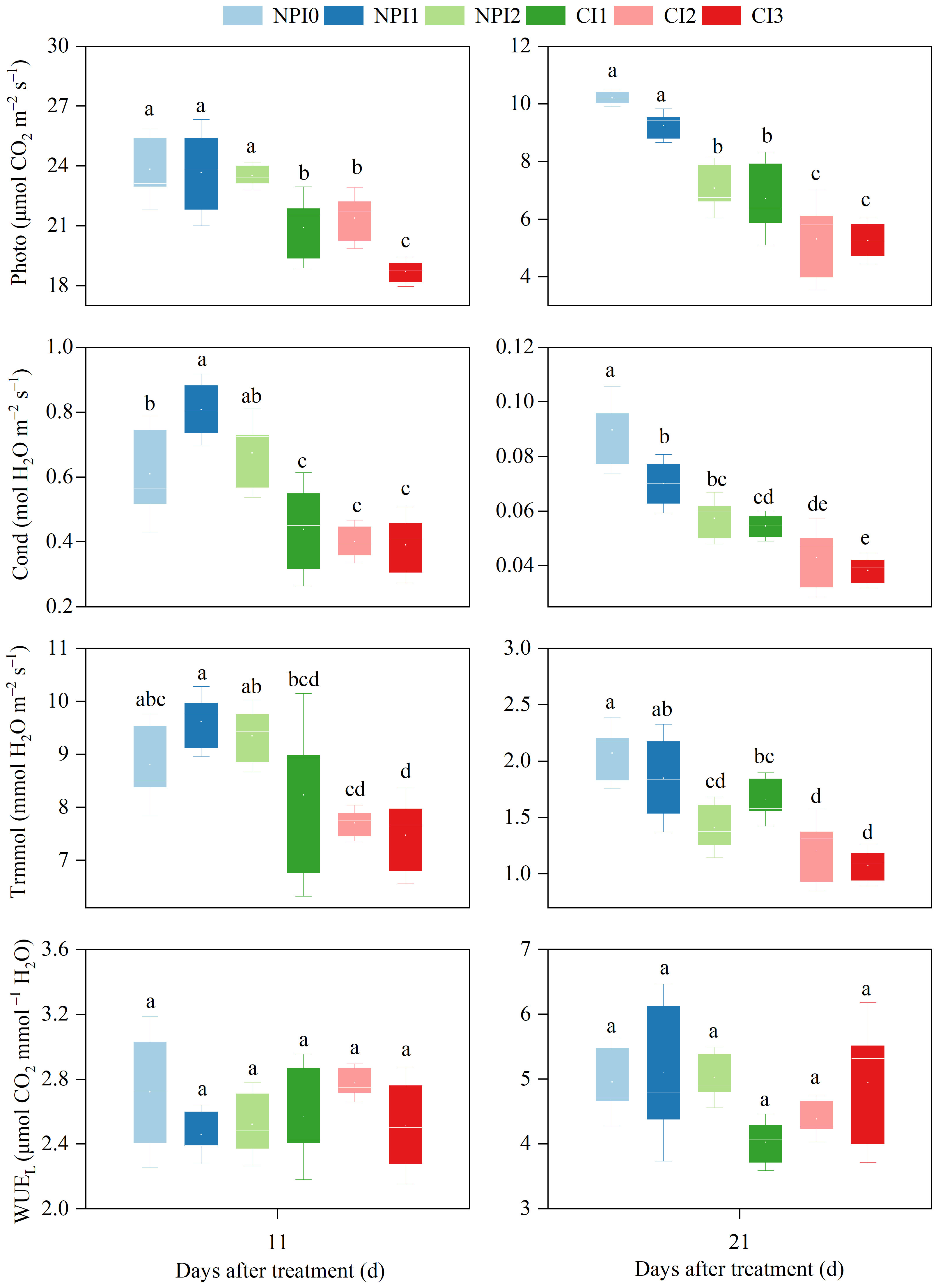
Figure 3 Variations of gas exchange parameters and WUEL under different treatments during cherry radish growing seasons. NPI, negative pressure irrigation; CI, conventional irrigation; Photo, photosynthetic rate; Cond, stomatal conductance; Trmmol, transpiration rate; WUEL, leaf level water use efficiency. Different lowercase letters above the boxes indicate significant differences among treatments at the P < 0.05 level within the same day.
3.4 Leaf proline, malondialdehyde and soluble sugars
Plants under water stress accumulate free proline and soluble sugars to regulate their osmotic potential, thus improving their growth characteristics and tolerance to water deficit (Silva and Arrabaça, 2004; Yooyongwech et al., 2016). An increase in the malondialdehyde content is a common response of stressed plants, water stress could lead to the overproduction of malondialdehyde, which causes cell membrane damage and, ultimately, plant cell death (Abbasi et al., 2020). The contents of free proline and malondialdehyde in leaves treated with NPI treatments were generally lower than those treated with CI treatments, while the content of soluble sugars was greater than that of CI treatments (Table 3). When SWC was approximately 85% FC, NPI treatments decreased the free proline contents by 13.7% and malondialdehyde contents by 12.5%, while increasing the soluble sugars content by 22.1% compared to CI treatments. When SWC was approximately 80% FC, compared to CI treatments, NPI treatments decreased free proline contents by 73.3% and malondialdehyde contents by 40.0%, but increased soluble sugars content by 6.29%. The CI2 treatment showed the highest free proline and malondialdehyde contents compared to other treatments. These results indicated that the water physiological indices of cherry radish leaves were moderately influenced by the soil water stability, with cherry radish leaves grown under SW having lower free proline and malondialdehyde contents and higher soluble sugars content than those grown under FW when SWC was essentially the same.
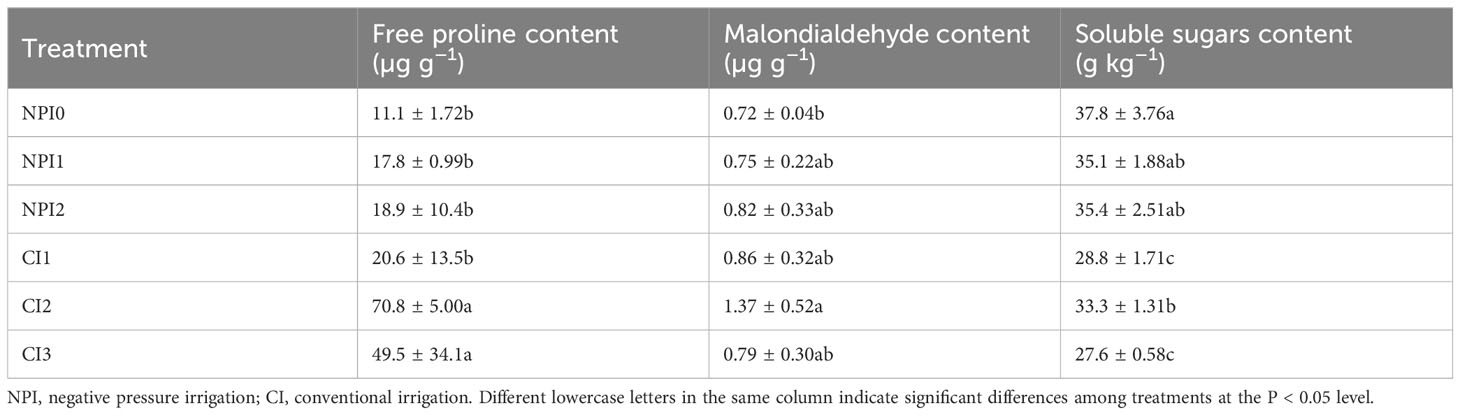
Table 3 Effects of different treatments on free proline, malondialdehyde and soluble sugars contents in cherry radish leaves.
3.5 Yield, biomass and radish quality
The yield and root transverse diameter of cherry radish in the NPI treatments declined as SWC fell, but there was no significant difference between treatments (Table 4). The yield, root transverse diameter, root longitudinal diameter, biomass, and root/shoot ratio of cherry radish under CI treatments reduced with decreasing SWC, but only 85% FC improved yield and root transverse diameter significantly compared to 80% FC and 75% FC. Comparing NPI1 treatment to CI1 treatment, there was a significant increase in cherry radish yield (34.6%), root longitudinal diameter (18.1%), and biomass (42.2%). Comparing NPI2 treatment to CI2 treatment, there was a substantial increase in yield significantly of 94.1%, root transverse diameter of 27.1%, root longitudinal diameter of 27.6% and biomass of 63.5%. SW was conducive to the accumulation of biomass, whereas soil water stability had no significant effect on the root/shoot ratio.
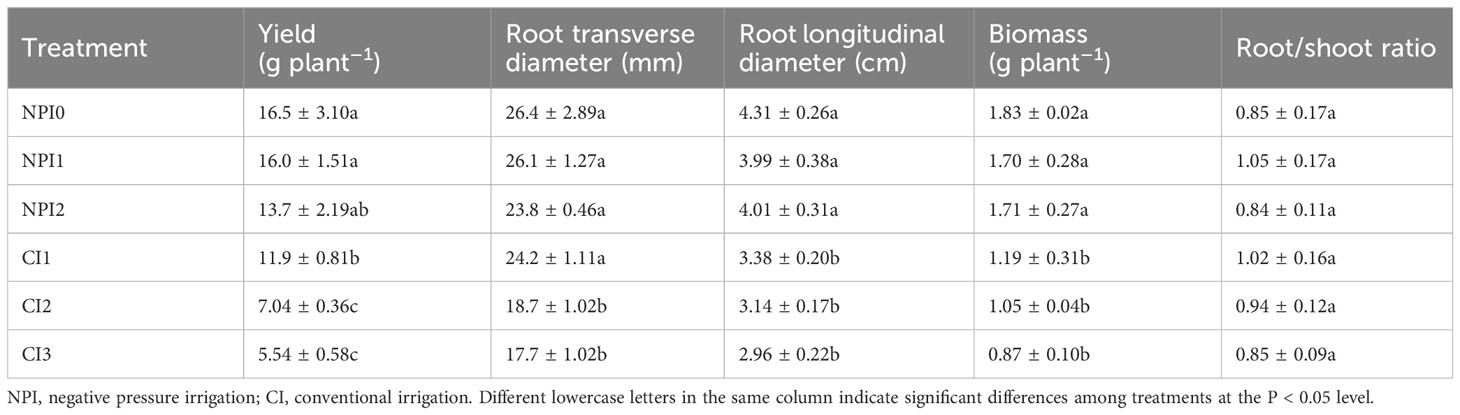
Table 4 Yield, root morphology, biomass and root/shoot ratio of cherry radish under different treatments.
Nitrate, vitamin C, reducing sugar, and soluble protein contents are quality parameters of radish (Yousaf et al., 2021; Huang et al., 2023). As shown in Table 5, there was no significant difference between NPI1 and CI1 treatments in terms of radish quality. NPI2 treatment significantly reduced soluble protein content by 28.6%, compared to CI2 treatment, but there were no significant differences in contents of nitrate, vitamin C, or reducing sugar. The highest values of nitrate, vitamin C and reducing sugar were observed in the CI2 treatment.
3.6 Content and uptake of nutrient
In the crop growth environment, the release, transformation, movement, and absorption of soil nutrients by plants were inseparable from soil water, and optimal soil water conditions were conducive to the uptake and utilization of nutrients by crops (Qu et al., 2020; Gao et al., 2021). Under similar SWC conditions, the nitrogen content of radish in the NPI treatments were significantly lower than those in the CI treatments. When SWC was about 85% FC, NPI treatments decreased nitrogen content by 10.8–11.0%, phosphorus content by 0.0–4.33% and potassium content by 10.5–20.3% compared with CI treatments. When SWC was close to 80% FC, in comparison to CI treatments, NPI treatments increased phosphorus content by 4.94–16.0%, but decreased nitrogen content by 6.32–18.2%.
In comparison to CI treatments, the uptake of total nitrogen, phosphorus, and potassium by cherry radish under NPI treatments increased by 50.8%, 72.2%, and 48.8%, respectively (Table 6). When SWC was about 85% FC, NPI treatments increased total nitrogen uptake by 25.9%, total phosphorus uptake by 40.3% and total potassium uptake by 24.9% compared with CI treatments; specifically, NPI increased nitrogen uptake by 23.6%, phosphorus uptake by 32.8%, and potassium uptake by 6.52% in stems and leaves; increased nitrogen uptake by 29.7%, phosphorus uptake by 46.7%, and potassium uptake by 29.9% in roots. When SWC was close to 80% FC, in comparison to CI treatments, NPI treatments increased total nitrogen uptake by 47.6%, total phosphorus uptake by 78.6%, and total potassium uptake by 48.2%; specifically, NPI increased nitrogen uptake by 60.9%, phosphorus uptake by 80.1% and potassium uptake by 26.1% in stems and leaves; increased nitrogen uptake by 25.7%, phosphorus uptake by 77.3%, and potassium uptake by 55.7% in roots. These results showed that SW enhanced the nutrients uptake of cherry radish compared to FW.
3.7 Evapotranspiration and WUE
Under similar SWC conditions, radish WUEY and WUEB in the NPI treatments were generally higher than those in the CI treatments (Table 7). Under NPI treatments, the evapotranspiration of cherry radish reduced as SWC decreased, the WUEY exhibited a tendency of initially increasing and then dropping with the decreasing SWC, and the WUEB increased as SWC fell. Under CI treatments, evapotranspiration and WUEY decreased as SWC decreased, whereas WUEB increased initially and then decreased as SWC decreased. The lowest value of evapotranspiration was observed in the CI3 treatment, while the NPI1 treatment showed the highest value of WUEY and the NPI2 treatment showed the highest value of WUEB from all treatments. NPI1 treatment significantly improved WUEY by 45.9% and WUEB by 53.8% compared to CI1 treatment, and NPI2 treatment remarkably increased WUEY by 74.2% and WUEB by 46.7% compared to CI2 treatment.
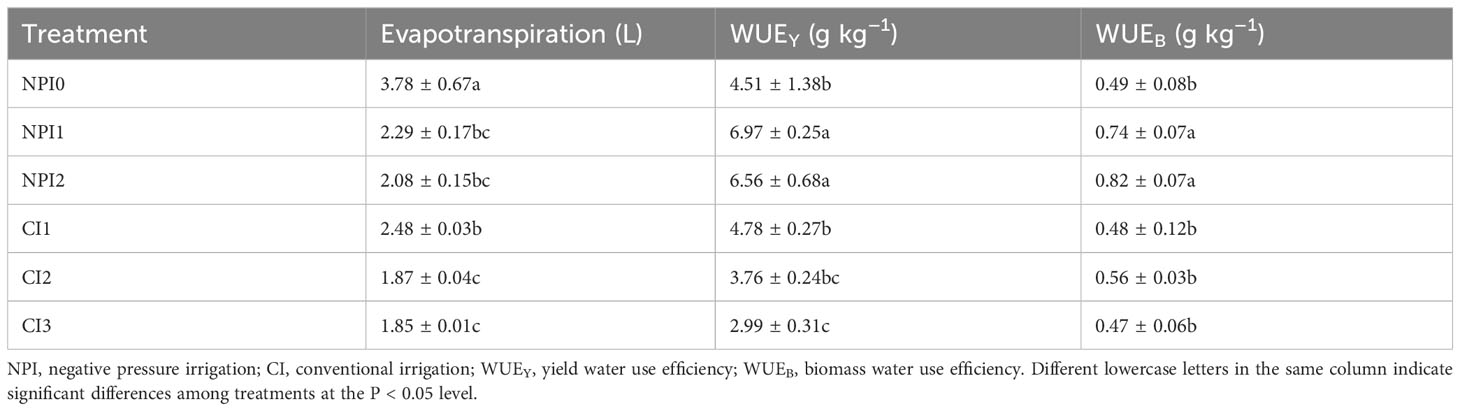
Table 7 Effects of different treatments on evapotranspiration and water use efficiency of cherry radish.
3.8 Correlation analysis and PCA
The data of plant indices response to soil water were presented in Figure 4A. The SWC was significantly positively correlated with gas exchange parameters, nutrient uptake, biomass, evapotranspiration, yield and root morphology of cherry radish, and significantly negatively correlated with the proline content. The soil water fluctuant parameters were significantly negatively correlated with the agronomic traits, Photo, Cond, leaf soluble sugars content, nutrient uptake, biomass, yield, root morphology, WUEY and WUEB of cherry radish. Particularly, there was a significant negative correlation between photosynthetic parameters and the soil water fluctuant parameters (except for Trmmol and δ), and the correlation coefficient was between –0.50 and –0.65 (Figure 4A). Besides, the proline content of cherry radish leaves was significantly negatively correlated with SWC (r=–0.48) and positively correlated with the variation coefficient of soil water (r=0.57); the soluble sugars content of leaves had a significant positive correlation with SWC (r=0.59), and a significant negative correlation with the coefficients of variation and fluctuation of soil water (r=–0.72 and r=–0.76).
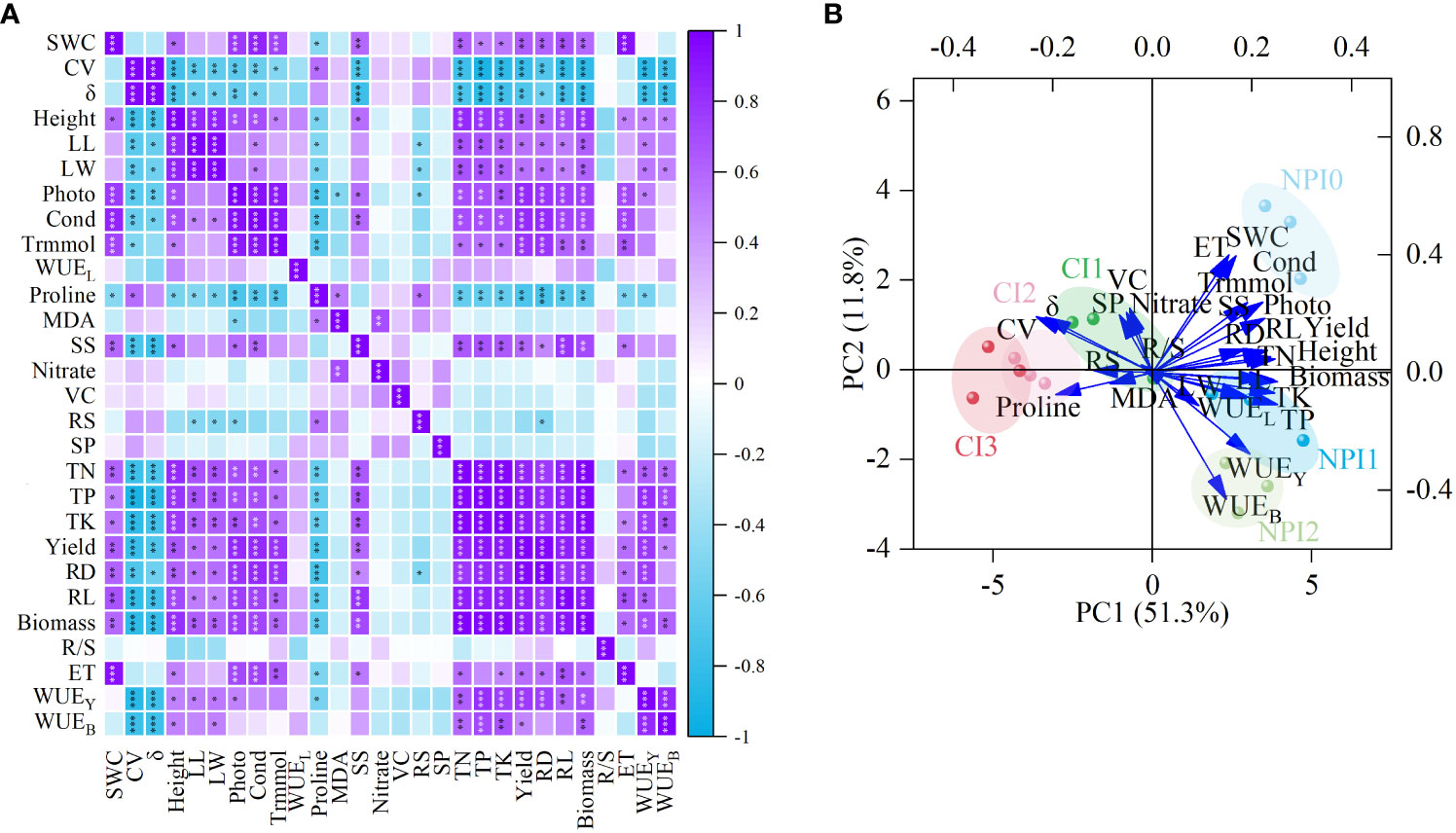
Figure 4 Correlation analysis (A) between soil water and plant indices, and biplot (B) of principal component analysis (PCA) of cherry radish indices under different treatments. The data used for agronomic traits and gas exchange parameters were the data at harvest time. SWC, soil water content; CV, variation coefficient; δ, fluctuation coefficient; Height, plant height; LL, leaf length; LW, leaf width; Photo, photosynthetic rate; Cond, stomatal conductance; Trmmol, transpiration rate; WUEL, leaf water use efficiency; Proline, free proline content; MDA, malondialdehyde content; SS, soluble sugars content; Nitrate, nitrate content; VC, vitamin C content; RS, reducing sugars content; SP, soluble protein content; TN, total nitrogen uptake; TP, total phosphorus uptake; TK, total potassium uptake; Yield, root yield; RD, root transverse diameter; RL, root longitudinal diameter; Biomass, total biomass; R/S, root/shoot ratio; ET, evapotranspiration; WUEY, yield water use efficiency; WUEB, biomass water use efficiency; NPI, negative pressure irrigation; CI, conventional irrigation. *, ** and *** indicate significant differences at P < 0.05, P < 0.01 and P < 0.001 levels, respectively. PCn indicated the extracted principal component.
Principal component analysis exhibited clear distinctions between various water treatments (Figure 4B). Six principal components were extracted, of which PC1 (primarily correlated to CV, δ, Height, soluble sugars, nutrient uptake, biomass, yield, root longitudinal diameter, WUEY and WUEB) could explain 51.3% of the variables, PC2 (primarily correlated to SWC, evapotranspiration, gas exchange parameters, proline and root transverse diameter) could explain 11.8%, PC3 (primarily correlated to malondialdehyde, nitrate and vitamin C) could explain 9.1%, PC4 (primarily correlated to leaf length, leaf width, and root/shoot ratio) could explain 8.1%, PC5 (primarily correlated to reducing sugar) could explain 5.4%, and PC6 (primarily correlated to WUEL and soluble protein) could explain 4.7%, with an 90.3% cumulative contribution rate (Supplementary Tables 1, 2). The CV, δ, SWC, and evapotranspiration (mainly composed of soil evaporation) were extrinsic environmental variables for cherry radish, and the results of the PCA indicated that the four environmental variables belonged to two categories of environmental variables, with CV and δ included in PC1, indicating soil water stability, and SWC and evapotranspiration contained in PC2, representing water content properties (because SWC was the basis of soil evaporation, with a high correlation). The FW conditions (CI1, CI2 and CI3) and SW conditions (NPI0, NPI1 and NPI2) were separated by PC1, where FW treatments were generally clustered more to the left and SW treatments more to the right on the plot. The preceding results indicated that soil water influenced the growth and development of cherry radish in two ways: soil water stability and SWC level.
3.9 Partial least squares path model between soil water and plant indices
Using PLS-PM analysis, the relationships between soil water fluctuant parameters, SWC, physiological characteristics, agronomic traits, biomass and yield, nutrient uptake, WUEY and WUEB were integrated (Figure 5). Results revealed that soil water fluctuant parameters had significant adverse effects on the photosynthetic characteristics of cherry radish with a path coefficient of –0.37, whereas SWC had a significant positive effect with a path coefficient of 0.70. The soil water fluctuant parameters had significant positive effects on the water physiological indicators of cherry radish with a path coefficient of 0.62, which demonstrated that leaf water physiological indicators of cherry radish were highly influenced by soil water stability; whereas SWC had a significant adverse effect with a path coefficient of –0.46. The proline and soluble sugars had a significant adverse effect on agronomic traits and nutrient uptake with a path coefficient of –0.64, and the agronomic traits and nutrient uptake had a significant positive effect on the cherry radish biomass and yield with a path coefficient of 0.91. The biomass and yield had a significant positive effect on the WUEY and WUEB, with a path coefficient was 1.20; whereas evapotranspiration had a significant negative effect, with a path coefficient of –0.87. The PLS-PM exhibited that soil water stability and SWC had an impact on cherry radish’s proline and soluble sugars directly, as well as indirectly on its agronomic traits and nutrient uptake, which in turn influenced the biomass and yield of cherry radish, finally influenced the WUEY and WUEB. Particularly, soil water stability had an indirect effect on the agronomic traits and nutrient uptake of cherry radish by affecting its water physiological indicators, thereby affecting the final biomass and yield.
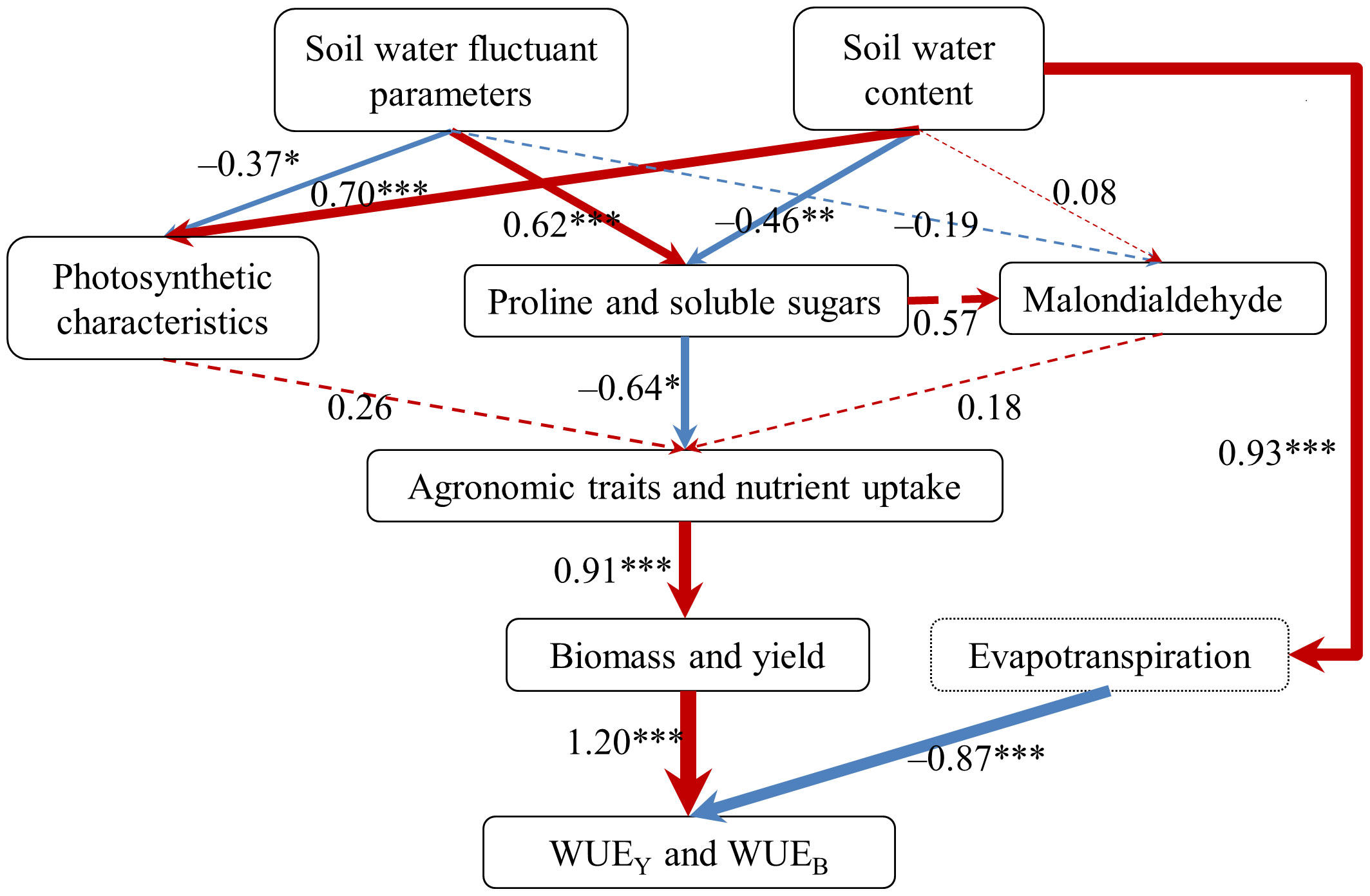
Figure 5 The partial least squares path analysis model (PLS-PM) was used to analyze how soil water affected the yield and water use efficiency of cherry radish. The path coefficients were represented by the width of the arrows. The red color indicated a positive effect, and the blue color indicated a negative effect. The solid arrows indicated significant effects (*P < 0.05; **P < 0.01; ***P < 0.001), and the dotted line indicated no significant effect (P > 0.05). WUEY, yield water use efficiency; WUEB, biomass water use efficiency. The quality of the PLS-PM was evaluated by examining the goodness-of-fit index (GOF), and the GOF of this model was 0.79. The data used in the model were the data from the harvest periods.
4 Discussion
4.1 Stable soil water enhances plant photosynthesis and avoids suffering physiological damage
Gas exchange is the foundation of plant biomass production, and the regulation of soil water on photosynthetic activity can greatly affect crop biomass and yield (Ali et al., 2019; Liu et al., 2019). According to Wang (2020), SW improved the photosynthesis of romaine lettuces and a similar phenomenon was observed in our findings. In addition, studies have found that under steady water supply, significant changes were observed in cell components linked to maize photosynthesis, including chloroplasts, plastids, and thylakoids, and genes such as psbE, psbF, psbA, and psbD related to photosystems were highly regulated (Zhang et al., 2021b). This may explain why SW increased the crop Photo. In contrast to Li et al. (2023), the results of this study demonstrated that SW improved the Photo of cherry radish compared to FW, but had no significant impact on WUEL. Besides, our findings demonstrated that photosynthetic parameters of cherry radish were highly influenced by soil water stability, and that SW was better suited to improving crop gas exchange parameters (Figure 4A), which facilitated the accumulation of photosynthetic products and increased yield.
Previous studies have shown that in respond to water stress, plants simultaneously accumulate more proline, malondialdehyde, and soluble sugars (Al-Yasi et al., 2020; Wahab et al., 2022). It has been reported that alternating soil water treatment was found to considerably increase the contents of proline, malondialdehyde and soluble sugars in maize roots when compared to the SW status under NPI treatments (Zhang et al., 2022a; Zhang et al., 2022b). Furthermore, studies have shown that stabilizing soil moisture alleviated maize’s short-term water stress (Niu et al., 2022). Importantly, proline and malondialdehyde contents in cherry radish in FW were higher than those in SW under similar SWC. It was speculated that the fluctuation of soil water caused some damage to cherry radish. Additionally, our results suggesting that the lower SWC and the stronger the soil moisture fluctuation, the more proline was accumulated in plants (Table 3 and Figure 4A). However, our study found that the higher SWC and the stronger the soil water stability, the greater the soluble sugars content was accumulated in plants (Figure 4A), which was dissimilar to what was observed in the literature of Wahab et al. (2022). This could be as a result of a greater Cond being supported by a suitable and stable water supply, which raised the concentration of sugar that entered the phloem and increased the soluble sugars content (Gao et al., 2021). Besides, the PLS-PM exhibited that the proline and soluble sugars of cherry radish were significantly positively impacted by soil water fluctuant parameters (Figure 5), which demonstrated that soil water stability had a major impact on the leaf water physiological indicators of cherry radish. Prior research has found that, in response to variations in soil water, the proline content of maize roots and leaves did not differ significantly, whereas malondialdehyde content in maize roots was significantly greater than that in maize leaves, indicating that the relationship between plant physiological indicators and soil moisture status was related to crop organs (Niu et al., 2022). However, only the leaves physiological indices of cherry radish were investigated in this study; further research is needed to determine how the various organs of cherry radish respond to soil water stability.
4.2 Stable soil water motivates the assimilation of underground parts in root vegetables
The results of this study likely demonstrated that the root yield of cherry radishes decreased as SWC decreased, which was consistent with the findings of a previous study (Wu et al., 2021). Studies have demonstrated that under SW conditions, agronomic traits such as plant height, stem thickness, and leaf area of maize, romaine lettuces, flue-cured tobacco, and Chinese cabbage were enhanced, resulting in increased crop yield (Li et al., 2017; Wang, 2020; Yang et al., 2021; Yang et al., 2022b). Interestingly, studies have showed that SW inhibited the redundant growth of maize roots (Zhang et al., 2022b), and that SW could reduce root/shoot ratio of romaine lettuces in comparison to FW (Wang, 2020). Nevertheless, our findings indicated that SW and FW had similar effects on the root/shoot ratio of cherry radish, and did not significantly inhibit the growth and development of the radish underground portion, but increased root biomass (Table 4). The results were supported by plant growth metrics, which showed that SW resulted in greater overall plant growth in comparison to FW. By comparing the results of this study with those of previous research (Albacete et al., 2014; Stagnari et al., 2018; Zhang et al., 2022b), it was found that soil water stability regulated source-library relationships, and that SW promoted the distribution of assimilate to the economic organs of plants and improved crop yield than FW. Additionally, our study revealed that the more stable the soil water, the more conducive it was to the root crop growth and development (Figure 4).
4.3 Stable soil water promotes the nutrient uptake of root vegetables via producing biomass
Plant nutrient uptake, use, and transfer are impacted by soil water (Qu et al., 2020). Studies have shown that SW promoted the uptake and utilization of nitrogen, phosphorus and potassium by crown daisy and romaine lettuces (Wang, 2020; Yang et al., 2020). The results of this study proved that compared to FW, SW increased the nitrogen uptake of cherry radish by 23.6–60.9%, phosphorus uptake by 32.8–80.1%, and potassium uptake by 6.52–55.7%, which was consistent with the previous results (Yang et al., 2022b). Notably, our study found that the increase in nutrient uptake by plants was more attributable to the increase in biomass than the improvement in nutrient content (Table 6), indicating that SW promoted the radish nutrient uptake via producing biomass, which was supported by the Figures 4, 5. In addition, the results showed that soil water stability had no significant effect on nutrient use efficiency of radish, but was affected by the level of SWC (Supplementary Table 3). When SWC was about 85% FC, SW increased the nutrient use efficiency by 3.80–14.7% compared with FW; whereas when SWC was close to 80% FC, SW enhanced the nitrogen use efficiency by 4.23% and the potassium use efficiency by 3.75%, but decreased the phosphorus use efficiency by 13.9%. Studies have revealed that phosphorus uptake was directly proportional to the volume of soil almost with the field water holding capacity and the length of time it remained moist (Simpson and Pinkerton, 1989). Moreover, studies have demonstrated that NPI treatments could transport water and nutrients directly to crop roots in line with plant requirements (Yang et al., 2022a), and that SW may promote nutrient distribution uniformity and reduce nutrient loss (Gao et al., 2021; Li et al., 2021b). Furthermore, the PLS-PM results revealed that soil water stability had an indirect effect on the agronomic traits and nutrient uptake of cherry radish by affecting its water physiological indicators, thereby affecting the final biomass and yield (Figure 5), which also confirmed our hypothesis.
4.4 Stable soil water improves the WUE of cherry radish plants by increasing biomass
Water use efficiency reflects the energy conversion efficiency in plant production, which is a crucial indicator for determining the relationship between crop yield and water usage (Sun et al., 2018). Studies have shown that NPI treatments reduced water consumption by 53.4–67.8% and enhanced the WUE by 12.7–124.7% of Capsicum annuum L. when compared to watering treatments (Li et al., 2017). In our study, compared to CI treatments, NPI treatments increased the WUEY by 45.9–74.2% and WUEB by 46.7–53.8%, respectively, in cherry radishes grown under similar SWC conditions. However, the WUEY and WUEB calculations were based on evapotranspiration (Sun et al., 2018; Wang, 2020; Liao et al., 2022), which actually referred to the WUE in the field of irrigation technology. Since the soil evaporation component of evapotranspiration was not directly related to plant growth and development, WUEY and WUEB are unable to fully reflect the effect of soil water stability on plant WUE. Nevertheless, the measured data in our study revealed that SW increased radish yield by 34.6–94.1%, biomass by 42.2–63.5%, and leaf Trmmol by 11.3–31.9%, when compared to FW. Importantly, the rate of increase in biomass and yield exceeded that of evapotranspiration, and this was further supported by the PLS-PM results (Figure 5), indicating that soil water stability increased cherry radish WUE by promoting its biomass and yield. Moreover, the results confirmed that soil water fluctuant parameters had a negative relationship with the WUEY and WUEB of cherry radish (Figure 4). This also demonstrated that SW could improve soil water conditions to allow cherry radish to use water more effectively. It is necessary to use transcriptome or metabolomics to further study the molecular mechanism of increasing WUE in root vegetables by stable water supply mode.
5 Conclusion
In this study, NPI and CI were used to create stable and fluctuating soil water, respectively, while maintaining similar average SWC conditions between them. A pot experiment was conducted to examine the agronomic traits, gas exchange, proline, malondialdehyde, soluble sugars, biomass, yield, quality, nutrient uptake and water use of cherry radish under different soil water states.
(i) Under similar SWC conditions, as compared to FW, SW improved the Photo, Cond and Trmmol of cherry radish, decreased the proline and malondialdehyde contents of the leaves, and increased the soluble sugars content, resulting in improved growth and development of cherry radish.
(ii) Under similar SWC conditions, SW was more conducive to the accumulation of cherry radish biomass and nutrient uptake than FW. Stable soil water significantly increased the radish yield by 35–94%, with a similar effect as FW on radish quality and root/shoot ratio.
(iii) Soil water stability affected directly the root vegetable water physiological indicators, and indirectly its agronomic traits and nutrient uptake, which in turn influenced the crop biomass and yield, as well as WUE.
When SWC was comparable, SW was more conducive to the growth and development of crops than FW. Stable soil water could promote the distribution of assimilate to the economic parts of plants and increase crop yield, resulting in a great potential for enhancing WUE. This study fills the gap where soil water stability affects root crop growth, and provides a new perspective for improving agronomy of root crops and WUE through managing soil water stability.
Data availability statement
The original contributions presented in the study are included in the article/Supplementary Material. Further inquiries can be directed to the corresponding author.
Author contributions
GL: Conceptualization, Data curation, Writing – original draft, Writing – review & editing. GZ: Data curation, Formal analysis, Investigation, Writing – review & editing. JL: Supervision, Writing – review & editing. ZW: Methodology, Writing – review & editing. HL: Conceptualization, Supervision, Writing – review & editing. RZ: Supervision, Writing – review & editing. KY: Project administration, Writing – review & editing.
Funding
The author(s) declare financial support was received for the research, authorship, and/or publication of this article. This work was supported by the Third Xinjiang Scientific Expedition Program (2021xjkk0200) and the National Key Research and Development Program of China (2018YFE0112300).
Conflict of interest
Author GZ was employed by the company Beijing Liangxiang Lanxin Hydraulic Engineering & Design Co., Ltd.
The remaining authors declare that the research was conducted in the absence of any commercial or financial relationships that could be construed as a potential conflict of interest.
Publisher’s note
All claims expressed in this article are solely those of the authors and do not necessarily represent those of their affiliated organizations, or those of the publisher, the editors and the reviewers. Any product that may be evaluated in this article, or claim that may be made by its manufacturer, is not guaranteed or endorsed by the publisher.
Supplementary material
The Supplementary Material for this article can be found online at: https://www.frontiersin.org/articles/10.3389/fpls.2024.1325078/full#supplementary-material
References
Abbasi, S., Sadeghi, A., Safaie, N. (2020). Streptomyces alleviate drought stress in tomato plants and modulate the expression of transcription factors ERF1 and WRKY70 genes. Sci. Hortic. (Amsterdam). 265, 109206. doi: 10.1016/j.scienta.2020.109206
Albacete, A. A., Martínez-Andújar, C., Pérez-Alfocea, F. (2014). Hormonal and metabolic regulation of source–sink relations under salinity and drought: From plant survival to crop yield stability. Biotechnol. Adv. 32, 12–30. doi: 10.1016/j.bioteChadv.2013.10.005
Ali, S., Xu, Y., Ma, X., Henchiri, M., Cai, T., Ren, X., et al. (2019). Cultivation modes and deficit irrigation strategies to improve 13C carbon isotope, photosynthesis, and winter wheat productivity in semi-arid regions. Environ. Sci. pollut. Res. 26, 5539–5553. doi: 10.1007/s11356-018-4036-1
Al-Yasi, H., Attia, H., Alamer, K., Hassan, F., Ali, E., Elshazly, S., et al. (2020). Impact of drought on growth, photosynthesis, osmotic adjustment, and cell wall elasticity in Damask rose. Plant Physiol. Biochem. 150, 133–139. doi: 10.1016/j.plaphy.2020.02.038
Baier, W. (1965). The interrelationship of meteorological factors, soil moisture and plant growth. Int. J. Biometeorol. 9, 5–20. doi: 10.1007/BF02187304
Chen, J., Song, D., Luan, H., Liu, D., Wang, X., Sun, J., et al. (2022). Living and dead microorganisms in mediating soil carbon stocks under long-term fertilization in a rice-wheat rotation. Front. Microbiol. 13, 1–13. doi: 10.3389/fmicb.2022.854216
Ertek, A., Şensoy, S., Gedik, İ., Küçükyumuk, C. (2006). Irrigation scheduling based on pan evaporation values for cucumber (Cucumis sativus L.) grown under field conditions. Agric. Water Manage. 81, 159–172. doi: 10.1016/j.agwat.2005.03.008
Gao, X., Zhang, S., Song, Y., Long, H. (2021). Negative pressure irrigation system reduces soil nitrogen loss for Lettuce during greenhouse production. Agronomy 11, 2380. doi: 10.3390/agronomy11122380
Guo, J., Fan, J., Xiang, Y., Zhang, F., Yan, S., Zhang, X., et al. (2022). Maize leaf functional responses to blending urea and slow-release nitrogen fertilizer under various drip irrigation regimes. Agric. Water Manage. 262, 107396. doi: 10.1016/j.agwat.2021.107396
Guo, R., Li, W., Wang, X., Chen, B., Huang, Z., Liu, T., et al. (2019). Effect of photoperiod on the formation of cherry radish root. Sci. Hortic. (Amsterdam). 244, 193–199. doi: 10.1016/j.scienta.2018.09.044
He, F., Long, R., Zhang, T., Zhang, F., Wang, Z., Yang, X., et al. (2020). Quantitative trait locus mapping of yield and plant height in autotetraploid alfalfa (Medicago sativa L.). Crop J. 8, 812–818. doi: 10.1016/j.cj.2020.05.003
Henschel, J. M., de Azevedo Soares, V., Figueiredo, M. C., dos Santos, S. K., Dias, T. J., Batista, D. S. (2022). Radish (Raphanus sativus L.) growth and gas exchange responses to exogenous ascorbic acid and irrigation levels. Vegetos 36, 566–574. doi: 10.1007/s42535-022-00422-2
Hey, M. H., DiBiase, E., Roach, D. A., Carr, D. E., Haynes, K. J. (2020). Interactions between artificial light at night, soil moisture, and plant density affect the growth of a perennial wildflower. Oecologia 193, 503–510. doi: 10.1007/s00442-020-04679-9
Huang, S., Yu, K., Xiao, Q., Song, B., Yuan, W., Long, X., et al. (2023). Effect of bio-nano-selenium on yield, nutritional quality and selenium content of radish. J. Food Compos. Anal. 115, 104927. doi: 10.1016/j.jfca.2022.104927
Jahromi, M. N., Razzaghi, F., Zand-Parsa, S. (2023). Strategies to increase barley production and water use efficiency by combining deficit irrigation and nitrogen fertilizer. Irrig. Sci. 41, 261–275. doi: 10.1007/s00271-022-00811-0
Kang, Y., Wan, S. (2005). Effect of soil water potential on radish (Raphanus sativus L.) growth and water use under drip irrigation. Sci. Hortic. (Amsterdam). 106, 275–292. doi: 10.1016/j.scienta.2005.03.012
Lacerda, V. R., Acevedo, A. F. G., Marques, I. C., da, S., Dellabiglia, W. J., Ferraz, A. K. L., et al. (2022). Silicon as a mitigator of water deficit stress in radish crop. Sci. Hortic. (Amsterdam). 291, 110600. doi: 10.1016/j.scienta.2021.110600
Leghari, S. J., Hu, K., Wei, Y., Wang, T., Bhutto, T. A., Buriro, M. (2021). Modelling water consumption, N fates and maize yield under different water-saving management practices in China and Pakistan. Agric. Water Manage. 255, 107033. doi: 10.1016/j.agwat.2021.107033
Li, G., Bai, Y., Wang, L., Lu, Y., Zhang, J., Zhang, Y. (2021a). Effects of fertilizer under different dripline spacings on summer maize in northern China. Sci. Rep. 11, 18922. doi: 10.1038/s41598-021-98016-6
Li, G., Long, H., Zhang, R., Drohan, P. J., Xu, A., Niu, L. (2023). Stable soil moisture alleviates water stress and improves morphogenesis of tomato seedlings. Horticulturae 9, 391. doi: 10.3390/horticulturae9030391
Li, D., Long, H., Zhang, S., Wu, X., Shao, H., Wang, P. (2017). Effect of continuous negative pressure water supply on the growth, development and physiological mechanism of Capsicum annuum L. J. Integr. Agric. 16, 1978–1989. doi: 10.1016/S2095-3119(16)61572-1
Li, S., Tan, D., Wu, X., Degré, A., Long, H., Zhang, S., et al. (2021b). Negative pressure irrigation increases vegetable water productivity and nitrogen use efficiency by improving soil water and NO3—N distributions. Agric. Water Manage. 251, 106853. doi: 10.1016/j.agwat.2021.106853
Liao, Q., Gu, S., Kang, S., Du, T., Tong, L., Wood, J. D., et al. (2022). Mild water and salt stress improve water use efficiency by decreasing stomatal conductance via osmotic adjustment in field maize. Sci. Total Environ. 805, 150364. doi: 10.1016/j.scitotenv.2021.150364
Liu, J., Wang, X., Rong, Z., Gao, Y., Zhang, G., Wang, W., et al. (2019). Modified non-rectangular hyperbola equation with plant height for photosynthetic light-response curves of Potentilla anserina and Elymus nutans at various growth phases in the Heihe River Basin, Northwest China. J. Arid Land 11, 764–773. doi: 10.1007/s40333-019-0003-z
Niu, L., Wang, Z., Zhu, G., Yu, K., Li, G., Long, H. (2022). Stable soil moisture improves the water use efficiency of maize by alleviating short-term soil water stress. Front. Plant Sci. 13. doi: 10.3389/fpls.2022.833041
Ouyang, Z., Tian, J., Yan, X., Shen, H. (2021). Effects of different concentrations of dissolved oxygen on the growth, photosynthesis, yield and quality of greenhouse tomatoes and changes in soil microorganisms. Agric. Water Manage. 245, 106579. doi: 10.1016/j.agwat.2020.106579
Qu, Z., Qi, X., Liu, Y., Liu, K., Li, C. (2020). Interactive effect of irrigation and polymer-coated potassium chloride on tomato production in a greenhouse. Agric. Water Manage. 235, 106149. doi: 10.1016/j.agwat.2020.106149
Sanchez, G., Trinchera, L., Russolollo, G. (2015). plspm: Tools for partial least squares path modeling (PLS-PM).
Shafiq, S., Akram, N. A., Ashraf, M. (2015). Does exogenously-applied trehalose alter oxidative defense system in the edible part of radish (Raphanus sativus L.) under water-deficit conditions? Sci. Hortic. (Amsterdam). 185, 68–75. doi: 10.1016/j.scienta.2015.01.010
Silva, J. M., Arrabaça, M. C. (2004). Contributions of soluble carbohydrates to the osmotic adjustment in the C4 grass Setaria sphacelata: A comparison between rapidly and slowly imposed water stress. J. Plant Physiol. 161, 551–555. doi: 10.1078/0176-1617-01109
Simpson, J. R., Pinkerton, A. (1989). Fluctuations in soil moisture, and plant uptake of surface applied phosphate. Fertil. Res. 20, 101–108. doi: 10.1007/BF01055434
Stagnari, F., Galieni, A., D’Egidio, S., Pagnani, G., Pisante, M. (2018). Responses of radish (Raphanus sativus) to drought stress: Radish adapted to drought through avoidance mechanisms. Ann. Appl. Biol. 172, 170–186. doi: 10.1111/aab.12409
Sun, Q., Wang, Y., Chen, G., Yang, H., Du, T. (2018). Water use efficiency was improved at leaf and yield levels of tomato plants by continuous irrigation using semipermeable membrane. Agric. Water Manage. 203, 430–437. doi: 10.1016/j.agwat.2018.02.007
Wahab, A., Abdi, G., Saleem, M. H., Ali, B., Ullah, S., Shah, W., et al. (2022). Plants’ physio-biochemical and phyto-hormonal responses to alleviate the adverse effects of drought stress: A comprehensive review. Plants 11, 1620. doi: 10.3390/plants11131620
Wang, Z. (2020). Effects of soil moisture temporal variation on growth, physiology and water use efficiency of maize and lettuces. (Beijing, China: Chinese Academy of Agricultural Sciences).
Wu, Y., Yan, S., Fan, J., Zhang, F., Xiang, Y., Zheng, J., et al. (2021). Responses of growth, fruit yield, quality and water productivity of greenhouse tomato to deficit drip irrigation. Sci. Hortic. (Amsterdam). 275, 109710. doi: 10.1016/j.scienta.2020.109710
Yang, P., Bai, J., Yang, M., Ma, E., Yan, M., Long, H., et al. (2022a). Negative pressure irrigation for greenhouse crops in China: A review. Agric. Water Manage. 264, 107497. doi: 10.1016/j.agwat.2022.107497
Yang, P., Bian, Y., Long, H., Drohan, P. J. (2020). Comparison of emitters of ceramic tube and polyvinyl formal under negative pressure irrigation on soil water use efficiency and nutrient uptake of crown daisy. Agric. Water Manage. 228, 105830. doi: 10.1016/j.agwat.2019.105830
Yang, P., Drohan, P. J., Long, H., Bian, Y., Bryant, R. B. (2021). Negative pressure irrigation on water use efficiency, yield and quality of Brassica chinensis L. J. Sci. Food Agric. 102, 1508–1513. doi: 10.1002/jsfa.11485
Yang, P., Drohan, P. J., Long, H., Yang, M., Bian, Y., Ma, E. (2022b). Water use efficiency, yield and quality of tobacco (Nicotiana tabacum L.) using negative pressure irrigation. Ind. Crops Prod. 178, 114552. doi: 10.1016/j.indcrop.2022.114552
Yooyongwech, S., Samphumphuang, T., Tisarum, R., Theerawitaya, C., Cha-um, S. (2016). Arbuscular mycorrhizal fungi (AMF) improved water deficit tolerance in two different sweet potato genotypes involves osmotic adjustments via soluble sugar and free proline. Sci. Hortic. 198, 107–117. doi: 10.1016/j.scienta.2015.11.002
Yousaf, M., Bashir, S., Raza, H., Shah, A. N., Iqbal, J., Arif, M., et al. (2021). Role of nitrogen and magnesium for growth, yield and nutritional quality of radish. Saudi J. Biol. Sci. 28, 3021–3030. doi: 10.1016/j.sjbs.2021.02.043
Zhang, J., He, W., Smith, W. N., Grant, B. B., Ding, W., Jiang, R., et al. (2021a). Exploring management strategies to improve yield and mitigate nitrate leaching in a typical radish field in northern China. J. Environ. Manage. 290, 112640. doi: 10.1016/j.jenvman.2021.112640
Zhang, J., Ji, J., Wang, P., Long, H., Wu, X. (2022a). Molecular mechanism of negative pressure irrigation inhibiting root growth and improving water use efficiency in maize. Plant Soil 472, 127–143. doi: 10.1007/s11104-021-05190-7
Zhang, J., Wang, P., Ji, J., Long, H., Wu, X. (2021b). Transcriptome analysis reveals the molecular mechanism of yield increases in maize under stable soil water supply. PloS One 16, 1–18. doi: 10.1371/journal.pone.0257756
Zhang, J., Wang, P., Long, H., Su, S., Wu, Y., Wang, H. (2022b). Metabolomics analysis reveals the physiological mechanism underlying growth restriction in maize roots under continuous negative pressure and stable water supply. Agric. Water Manage. 263, 107452. doi: 10.1016/j.agwat.2021.107452
Zhu, G., Wang, Z., Long, H., Zhang, R., Yu, K. (2022). Effect of soil moisture on growth and water use efficiency of cherry radish under negative pressure irrigation. J. Agric. Sci. Technol. 22, 127–136. doi: 10.13304/j.nykjdb.2019.0953
Keywords: cherry radish, fluctuating soil water, negative pressure irrigation, stable soil water, water use efficiency
Citation: Li G, Zhu G, Liu J, Wang Z, Long H, Zhang R and Yu K (2024) Effects of stable and fluctuating soil water on the agronomic and biological performance of root vegetables. Front. Plant Sci. 15:1325078. doi: 10.3389/fpls.2024.1325078
Received: 20 October 2023; Accepted: 29 January 2024;
Published: 14 February 2024.
Edited by:
Lorenzo Barbanti, University of Bologna, ItalyReviewed by:
Silvia Pampana, University of Pisa, ItalyAli Baghdadi, University of Bologna, Italy
Ashish K. Chaturvedi, Centre for Water Resources Development and Management, India
Copyright © 2024 Li, Zhu, Liu, Wang, Long, Zhang and Yu. This is an open-access article distributed under the terms of the Creative Commons Attribution License (CC BY). The use, distribution or reproduction in other forums is permitted, provided the original author(s) and the copyright owner(s) are credited and that the original publication in this journal is cited, in accordance with accepted academic practice. No use, distribution or reproduction is permitted which does not comply with these terms.
*Correspondence: Huaiyu Long, bG9uZ2h1YWl5dUBjYWFzLmNu
†These authors have contributed equally to this work and share first authorship