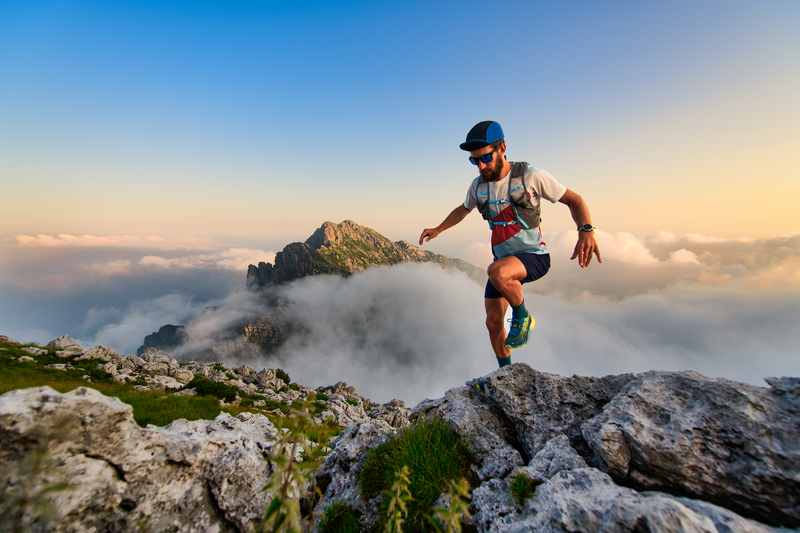
95% of researchers rate our articles as excellent or good
Learn more about the work of our research integrity team to safeguard the quality of each article we publish.
Find out more
REVIEW article
Front. Plant Sci. , 31 January 2024
Sec. Plant Abiotic Stress
Volume 15 - 2024 | https://doi.org/10.3389/fpls.2024.1310328
This article is part of the Research Topic Safe Utilization of Heavy Metals Pollution in Soils for Healthy Food View all 12 articles
Heavy metal (HM)-induced stress can lead to the enrichment of HMs in plants thereby threatening people’s lives and health via the food chain. For this reason, there is an urgent need for some reliable and practical techniques to detect and analyze the absorption, distribution, accumulation, chemical form, and transport of HMs in plants for reducing or regulating HM content. Not only does it help to explore the mechanism of plant HM response, but it also holds significant importance for cultivating plants with low levels of HMs. Even though this field has garnered significant attention recently, only minority researchers have systematically summarized the different methods of analysis. This paper outlines the detection and analysis techniques applied in recent years for determining HM concentration in plants, such as inductively coupled plasma mass spectrometry (ICP-MS), atomic absorption spectrometry (AAS), atomic fluorescence spectrometry (AFS), X-ray absorption spectroscopy (XAS), X-ray fluorescence spectrometry (XRF), laser ablation-inductively coupled plasma-mass spectrometry (LA-ICP-MS), non-invasive micro-test technology (NMT) and omics and molecular biology approaches. They can detect the chemical forms, spatial distribution, uptake and transport of HMs in plants. For this paper, the principles behind these techniques are clarified, their advantages and disadvantages are highlighted, their applications are explored, and guidance for selecting the appropriate methods to study HMs in plants is provided for later research. It is also expected to promote the innovation and development of HM-detection technologies and offer ideas for future research concerning HM accumulation in plants.
In recent years, the HM contents of soil, water, and air have enhanced manifold due to rapid development and industrialization (Vareda et al., 2019). They are classified as essential nutrients like Fe, Zn, Cu, Ni, Mn, etc.; and non-essential nutrients like As, Hg, Cr, Pb, Cd, and Ag, based on the requirements of these elements by plants to fulfill its life cycle. HMs are mainly uptaken via the roots in the soil, while HMs in the atmosphere through the leaf surface which then accumulate in plants. Cedrus deodara and Cupressus arizonica absorb HMs through their leaves (Liang et al., 2017; Cesur et al., 2021) and those of Nerium indicum, Pittosporum tobira, and Platanus acerifolia through trichomes, stomata, or the mucous layer (Liang et al., 2017). The excessive accumulation of essential elements can negatively impact plants; that of Fe can cause the buildup of reactive oxygen species (ROS), damage to lipids and proteins, impairment of cell structure, leaf chlorosis, and other adverse effects (Kobayashi et al., 2019; Ahammed et al., 2020), while that of Zn can lead to nutritional imbalance, production of ethylene, stunted growth and chlorosis (Islam et al., 2014). Non-essential elements like As, Cd, Hg, and Pb, are called “toxic HMs” as they adversely affected plants when their concentrations exceeded certain levels (Wang et al., 2020; Rahman et al., 2022). These elements affected photosynthesis and respiration, damaged the structure and function of stomata, increased oxidative stress, modulated the uptake of mineral nutrients, caused DNA breaks and enzyme inactivation, and other serious adverse effects (Jaiswal and Verma A, 2018; Genchi et al., 2020; Guo et al., 2023). The toxicological impacts of common HMs on plants are summarized in Table 1. HMs are absorbed by the body via the food chain and are accumulated in vital organs like the liver, heart, brain, and kidneys, which disrupted their normal functions and interfered with various metabolic processes (Fu and Xi, 2020). HMs are absorbed by the body via the food chain and are accumulated in vital organs like the liver, heart, brain, and kidneys, which disrupted their normal functions and interfered with various metabolic processes (Kiran et al., 2022). Excessive accumulation of As can lead to skin diseases, diabetes, neurological complications, and impaired liver and kidney function (Kiran et al., 2022). The harmful repercussions of Hg are predominantly embodied as inattention, blurred vision, unstable walking, pneumonia, pulmonary edema, lung injury, and other symptoms. It can also be toxic to fetuses, resulting in fetal blindness, intellectual disability, speech impairment, and brain damage (Kiran et al., 2022).
In plants, the roots are the main organs that absorb various nutrients and non-essential elements. Roots act as the first barrier for the entry of HMs, and most plants retain these HMs in their roots to block their transfer into the aerial parts, thereby reducing the toxic effects. Therefore, root retention is significant for plant detoxification mechanisms (Ghuge et al., 2023). Additionally, certain plant species like Arabis paniculata, Celosia argentea, and Corydalis davidii accumulate HMs to a greater extent in their aboveground parts compared to the underground parts (Li et al., 2018). Due to the robust transport capacity of certain plants, the HM content in the aboveground parts is more than ten-fold higher than that in the underground parts, and the everyday life activities are not affected, making them hyperaccumulators (Shi et al., 2023). Hyperaccumulators can eliminate, reduce, or stabilize the contents of HM pollutants included in soils thus remediating HM-polluted soil. After entering its roots, the HMs are primarily localized in the vacuoles and cell walls (Cobbett, 2000; Yin et al., 2015). The cell wall is rich in pectin, hemicellulose, cellulose, lignin, and polysaccharides (Lao et al., 2023). These negatively charged biopolymers bind to HM ions; the enzymes associated with lignin biosynthesis promote cell wall synthesis and thickening, enriching HMs in the cell wall (Keyster et al., 2020; Chen et al., 2022; Lao et al., 2023). HMs that traverse the cell wall are internalized into the vacuole through ATP-dependent transporters, where they accumulate by chelating with phytochelatins (PCs) (Keyster et al., 2020; Khan et al., 2020). Therefore, compartmentalization in cell walls and vacuoles is crucial for plant detoxification mechanisms. Plants have not developed any carriers and channels specific for non-essential elements during the evolutionary process, so these elements mainly rely on the absorption pathway of mineral elements to enter plants (Andresen and Küpper, 2013; Shahid et al., 2017). HM ions enter the plant root with the assistance of nutrient element ion channels (such as Ca2+ and K+ channels) and transport proteins present in the plasma membrane. Many transporters related to HMs, including Zn-regulated transporter, iron-regulated transporter-like proteins (ZIPs), HM-associated domain (HMA), iron-regulated transporter (IRT), natural resistance-associated macrophage protein (NRAMP), yellow stripe 1-like family (YSL), etc. have been identified (Li et al., 2017a; Leng et al., 2020; Kaur et al., 2021).
The food chain is the primary means through which humans are exposed to HMs, making the control of HM accumulation in food crops, pulses, vegetables, fruits, and medicinal plants particularly crucial. Understanding and investigating in plants the biological and physiological processing involving the transport, absorption, accumulation, and detoxification of HMs can facilitate the cultivation of edible and medicinal plants with low levels of HMs and enable the utilization of hyperaccumulators for remediation of HM-contaminated soils. To understand these mechanisms, specific instruments and technical methods are needed to obtain information regarding spatial and subcellular distribution, chemical morphology, uptake, and translocation pathways of HMs. The content of HMs can be analyzed using techniques for instance inductively coupled plasma mass spectrometry (ICP-MS) and atomic absorption spectrometry (AAS); chemical form by atomic fluorescence spectrometry (AFS) and X-ray absorption spectroscopy (XAS), respectively; spatial distribution through X-ray fluorescence spectrometry (XRF) and laser ablation-inductively coupled plasma-mass spectrometry (LA-ICP-MS); and the dynamics of uptake and transport by non-invasive micro-test technology (NMT). Moreover, “omics” and molecular biology approaches contribute to understanding the molecular mechanisms underlying plant-HM interactions, facilitating further exploration of HM-induced stress.
In recent years, the accumulation, chemical form, spatial distribution, and uptake and translocation of HMs by plants have received extensive attention. However, a complete overview of the research techniques and methods employed for gaining the information mentioned above is lacking. Therefore, this paper reviews recent advances in detecting and analyzing HMs in plants in three sections. The first section deals with the background of stress caused by HM pollution and introduces the distribution characteristics, forms of existence, and toxic effects of HMs. The second systematically discusses the principles, advantages, and applications of the frequently used detection and analytical techniques for different HMs. Finally, the current state and challenges of the various technologies are summarized, and prospects for development are projected. This review is expected to facilitate the selection of optimal research methods related to HMs in plants and provide ideas for developing and improving these technologies.
The three main origins of HMs in plants are soil, water, and air pollution (Nagajyoti et al., 2010; Rai et al., 2019). Inappropriate disposal of industrial waste, automobile exhaust emissions, mining, extraction and smelting, energy and fuel manufacturing, and other human activities can result in HM pollution (Wu et al., 2022). HM content is highest in water ecosystems and soils, with only a minuscule proportion in the atmosphere as vapor or particles. HMs are uptake by the roots via surface water and soil and then stored in the cell walls and vacuoles; additionally, they are absorbed and enriched in leaves from the atmosphere, which affects growth and development (Nadeem et al., 2010; Rai et al., 2019; Qin et al., 2021). Carrots, sweet potatoes, and other root vegetables are predominantly contaminated by HMs in the soil (Cobbett, 2000; Zhou et al., 2016; Cesur et al., 2021), while C. arizonica, Pinus sylvestris L., and Ficus elastica primarily through atmospheric pollution (Zwolak et al., 2019; Alaqouri et al., 2020; Ghoma et al., 2022).
HMs are mainly selectively absorbed or diffused from the soil into plants through roots, and H+ coupled carrier proteins or transporters are critical for the entry of HMs into the roots (DalCorso et al., 2019). For example, plants can absorb Cd through Ca2+ and K+ channels, while transporters, including ZIP, HMA, YSL, NRAMP, and IRT, are involved in the various processes of Cd absorption and transport (Andresen and Küpper, 2013; Yin et al., 2015; Shahid et al., 2017). After entry through the roots, HMs travel through the cell membrane into the cytoplasm or combine with the cell wall. Most of the absorbed HMs accumulate directly in the roots or are loaded into the xylem by transporter proteins and then, through the xylem, are translocated to the aerial part and accrue in foliage (Yang et al., 2022) (Figure 1). The accumulation levels of specific HMs are generally > 100 mg kg-1 for Cd; 1000 mg kg-1 for Pb, Co, Ni, etc.; and 10,000 mg kg-1 for Zn and Mn (Shi et al., 2023). For example, the hyperaccumulators of Pb include A. paniculata, Isachne globosa, and Pogonatherum crinitum (Kaur et al., 2021; Shi et al., 2023); of Mn include C. argentea, Polygonum lapathifolium, and Schima superba (Kaur et al., 2021; Shi et al., 2023); of Cd include Sedum alfredii Hance and Solanum nigrum L (Al-Huqail, 2023; Hu et al., 2023).; of As include Pteris vittata L (Zhang et al., 2022).; and of Zn include C. davidii, Picris divaricata, and Viola baoshanensis (in their aerial parts) (Kaur et al., 2021; Shi et al., 2023). At the subcellular level, HMs are primarily distributed in the cell walls and vacuoles, thereby compartmentalizing them, reducing their transfer to other parts, and minimizing toxicity (Corso, 2020; Yang et al., 2022). Vacuoles being non-physiologically active organelles, HMs, upon entry into the vacuoles, are isolated, passivated, and precipitated, thereby reducing their toxicity (Corso, 2020; Yang et al., 2022). Binding sites such as -COOH, -SH, and -OH provided by the cell wall can adsorb HM ions, better defining the role of the cell wall in accumulating metal to prevent their entry into plants (Ghori et al., 2019; Corso, 2020). The cell walls of Boehmeria nivea L. contain high levels of Cd (Chen et al., 2022). The cell walls and vesicles of the leaves in Leersia hexandra Swartz are the main sites of Cr(III) accumulation (Ma et al., 2022).
Figure 1 Schematic diagram of the mechanism of HM accumulation in plants. (A) Accumulation of HMs on the surface of the root system; (B) Accumulation of HMs in cell walls; (C) Root-to-shoot translocation; (D) Accumulation of HMs in leaves; (E) Endocytosis.
In plants, the chemical forms of HMs are strongly correlated with their mobility and biotoxicity (Duffus, 2002; Yang et al., 2022). As is found in distinct valence states, primarily in arsenite (AsIII) and arsenate (AsV) forms, of which AsIII is somewhat more toxic than AsV (Souri et al., 2017). Plant species, absorption mechanisms, As speciation, and soil type are critical factors that influence the speed of absorption and buildup of As through plants (Zhao et al., 2009). Moreover, the roots discriminatingly absorb particular As forms though different transporters and pathways (Gupta et al., 2011; Farooq et al., 2016). In root cells, in order to prevent As translocation to shoots, As is translocated into the vacuole in the form of AsIII or AsIII-glutathione/phytochelatin complexes or is turned into less toxic organic forms (Smita et al., 2015). In hyperaccumulator plants, AsV could be efficiently reduced to AsIII, and then mobilized from roots to aerial part (as the dominant form of As in xylem sap) (Raab et al., 2005; Su et al., 2008). Most angiosperms use a mechanism of Fe uptake based on reduction (Grillet and Schmidt, 2017). Roots can enzymatically reduce Fe by plasma membrane-bound ferric reductase/oxidase (FRO) or chemically reduce Fe by root secretion of organic compounds (Grillet and Schmidt, 2017). Using X-ray absorption near-edge spectroscopy (XANES), Cr was primarily detected as a trivalent form (less toxic than hexavalent Cr) in L. hexandra Swartz roots and leaves (Liu et al., 2014). High-performance liquid chromatography/inductively coupled plasma mass spectrometry (HPLC/ICP-MS) identified the various chemical forms of As, Hg, and Pb in lotus seeds including As(III), dimethyl arsenic acid (DMA), As(V), methyl arsenic acid (MMA), phosphorus mercury (PhHg), ethyl mercury (EtHg), methyl mercury (MeHg), Hg(II), TML, TEL, and Pb(II) (Zhang et al., 2020).
Under HM-induced stress, most metals will enter the root through general or specific pumps or ion channels, while the root also releases large amounts of secretions (e.g., malate, oxalate, citrate, etc.) that bind to the metal ions, concentrate them into the apoplast, or adsorb them to cell walls, stopping them reaching the cells (Ghori et al., 2019). When exposed to Al-induced stress, Vigna umbellata seedlings, in their late stages of growth, acclimatized to Al-related toxicity by releasing enormous amounts of citrate through their root systems (Liu et al., 2018). Using XANES, scanning electron microscopy (SEM), and synchrotron radiation (SR)-based technology, Zn in the root epidermis of Helichrysum microphyllum subsp. was identified to bind to organic molecules, like malate, histidine (His), and cysteine (Cys), thereby alleviating the toxicity related to excessive Zn, suggesting that H. microphyllum may be a potential candidate Zn-stress-tolerant plant (Boi et al., 2020). Pectin is an important constituent in cell walls and is also requisite to chelate metal ions (Ghori et al., 2019). Cd mainly existed in the pectin- and protein-bound forms in S. nigrum and Koelreuteria paniculata (Yang et al., 2018; Wang et al., 2021).
In addition, crucial ligands like PCs, glutathione (GSH), as well as metallothioneins (MTs) can chelate with HMs, activating the HM detoxification mechanisms in plants (Thakur et al., 2022). PCs are a family of proteins induced to synthesize Cys-polypeptides and are rich in them due to the presence of various metals (Ghori et al., 2019). They are common in plants, fungi, algae, and cyanobacteria (Gupta et al., 2013). PCs can utilize the thiol groups in the Cys residues to bind with Cd and sequester these complexes in vesicles through ABC transporter proteins (Ghori et al., 2019). Next, Cd can also form complexes with other proteins or compounds and be transported elsewhere through the xylem and phloem to mitigate Cd-related toxicity effectively (Ghori et al., 2019). Arabidopsis plants exhibited extreme sensitivity to Cd-induced toxicity when GSH and PC were deficient (Howden et al., 1995b; Howden et al., 1995a). The AtABCC1 and 2 transporter proteins from Arabidopsis are crucial for isolating the PC-Cd complexes within vacuoles (Park et al., 2012). Since the OsPCS15 and OsPCS5 genes may be associated with Cd reduction, in Park et al.’s study of rice subjected to both Cd and As stress, it was found that OsPCS5 and OsPCS15 were specific to Cd and As (Park et al., 2019). GSH exists in both oxidized and reduced forms, mediates the biosynthesis of PCs, its thiol groups form S-based bonds with HMs thereby sequestering them, and the complexes are transported to vacuoles for detoxification (DalCorso et al., 2008; Ghori et al., 2019). The OsCLT1 transporter protein in rice facilitated the efflux of GSH and γ-glutamyl-cysteine (γ-Glu-Cys) from plastids to the cytoplasm, mediating the biosynthesis of PCs; the mutants lacking OsCLT1 exhibited hypersensitivity to Cd and other HMs (Yang et al., 2016). MTs are Cys-rich proteins that can bind to various HM ions such as Cd2+, Cu2+, and Zn2+, maintaining metal homeostasis in plants and alleviating the undesirable toxic impacts of excessive metal ions (Saeed Ur et al., 2020; Ettiyagounder et al., 2021). PaMT3-1 from Phytolacca americana, overexpressed in tobacco, encoded a protein that bound with Cd2+ and enhanced tolerance to Cd (Zhi et al., 2020). Results from studies on tobacco indicate that overexpression of EhMT1 can enhance Cu buildup and tolerance in root cytoplasm as well as reduce hydrogen peroxide (H2O2) production (Xia et al., 2012).
Biomonitoring refers to using biomaterials or organisms to analyze specific characteristics of the biosphere (Decou et al., 2019). The application of biomarkers for environmental biomonitoring can detect the harmful effects of pollutants at an early stage and play the role of an early warning system. Morphology, physiology, and biochemistry alter when plants are subjected to HM-induced stress as well as can be used as biomarkers (Decou et al., 2019; Krayem et al., 2021). These alterations include suppression of root development, leaf discoloration and necrosis, suppression of photosynthesis and respiration, and induction of oxidative stress (Costa et al., 2018; Decou et al., 2019; Krayem et al., 2021). Substantial research has explored the biomarkers or bioindicators of HMs in plants. An analysis of biomarkers in Myriophyllum alterniflorum indicated a direct correlation between malondialdehyde (MDA), α-tocopherol, and glucose-6-phosphate dehydrogenase (G6PDH) with Cu and Cd, which suggested that these three biomarkers were effective for metal exposure analyses (Decou et al., 2019). A comparison of the selected biomarkers, MT and PC in Cystoseria indica, revealed that PC reacted to HMs to a lesser extent than MT, limiting its use in HM biomonitoring (Sinaei et al., 2018). Three types of markers (molecular markers, resistance markers, and damage markers) appeared at different frequencies when the HM accumulating plant Tillandsia ionantha Planch. was exposed to Cd (Zhang et al., 2023). Most of the markers mentioned above are really consequences of HM toxicity and are usually visible after severe toxicity, Only PCs and MTs are related to metals and the others are general stress markers. In conclusion, elucidating the mode of action and potential molecular mechanisms of biomarkers for detecting HMs in plants could improve their applicability in environmental quality assessment and facilitate the development of effective biomonitoring and phytoremediation technologies (Krayem et al., 2021; Saroop and Tamchos, 2021; Esmaeilzadeh et al., 2023).
Robust detection tools and analytical technologies enable a holistic understanding of the mechanisms involved in the uptake, transport, accumulation and detoxification of HMs in plants. In recent years, HM-detection technology has concentrated on precision, comprehensiveness, and automation, which are crucial for regulating and decreasing the levels of HMs in plants. ICP-MS, AAS, and AFS are used to detect the contents of HMs in plants. AFS and XAS can identify the chemical forms of HMs. XRF and LA-ICP-MS can elaborate the distribution of HMs in sub-cells and tissues. NMT enables measuring the flow rate of HM ions. Omics and molecular biology techniques can analyze the molecular mechanisms through which plants interact with HMs and discover more genes related to HM resistance. The principles, advantages, and applications of these analytical techniques for detecting different HMs in plants are systematically discussed subsequently.
ICP spectroscopy is a method used to analyze trace metals by measuring light emission with characteristic wavelengths when the metal is exposed to an electric current. ICP-MS includes a sample introduction system, ion source, interface, ion lens, mass filter, and an ion detector (Yu et al., 2020). The details of how it works are as follows: First, the sample is drawn into the nebulizer by a peristaltic pump or self-priming to generate an aerosol of fine liquid droplets. Next, they are introduced into the central zone of the high-temperature plasma that was created by combining the energy supplied to the coil by the ICP radio frequency (RF) emitter and Ar gas. As their absorption increases, electrons are released, and cations are formed. The ion lens then focuses them through the interface, separates them based on their mass-to-nucleus ratio using a mass filter, and transports them to the detector for analysis resulting in a mass spectrum (Wilschefski and Baxter, 2019) (Figure 2). ICP-MS provides the advantages of being capable of identifying multiple elements, fast, sensitive, and capable of detecting minute levels. It also has a wide linear range, allows for easy control of interferences, and provides information on isotopes (Wilschefski and Baxter, 2019; Theiner et al., 2020; Penanes et al., 2022).
ICP-MS is a powerful technology with excellent capability for analyzing elements and detecting the concentration of HMs in plants at trace levels (Huang et al., 2006; Wilschefski and Baxter, 2019). Using ICP-MS, the content of Cd in the aromatic medicinal plant Alkana trichophila was detected to exceed the World Health Organization (WHO) standards; however, the content of other trace elements, like Al, V, and Cr, were within the acceptable range, suggesting that A. trichophila contains excessive amounts of Cd (Izol et al., 2023). It would depend on the concentration available in the soil (Emamverdian et al., 2018; Izol et al., 2023). The levels of four HMs: Hg, As, Cd, and Pb, in 50 medicine food homology plants were detected by inductively coupled plasma-tandem mass spectrometry (ICP-MS-MS) (Fu et al., 2018). Moreover, ICP-MS is considered the preferred way for detecting HM contents because of its advantages, like high accuracy, sensitivity, selectivity, and low detection limits. It is often used in combination with other analytical methods, for example, LA-ICP-MS, single-particle- inductively coupled plasma-mass spectrometry (SP-ICP-MS), liquid chromatography- inductively coupled plasma-mass spectrometry (LC-ICP-MS), etc., for the determination of the content and the form of multiple trace elements and isotope analysis in different types of samples and various matrix samples and simplifying the sample preparing process. Utilizing HPLC-ICP-MS, the As content in quinoa and rice was detected to exceed the food pollutant standards set by the European Commission, indicating a severe overaccumulation of As (D’Amore et al., 2023). LC-ICP-MS and ICP-MS were used to detect the As content of ten species of algae, and it was found that Cystoseira species and Cystoseira fragile had the highest total As content (Pell et al., 2013). I In addition to Cystoseiraz, inorganic As was dominant in the other nine algal species, indicating that ICP-MS and LC-ICP-MS provide reasonable technical support for further exploration of the As content in marine plants (Pell et al., 2013). LA-ICP-MS detected that, except Ginkgo biloba, Pb and Cd accumulated rapidly in four tree species including Pinus densiflora, Chamaecyparis obtuse, Salix koreensis, and Platanus occidentalis, while the distribution of Fe, Cr, Mn, and other elements exhibited changes in tree-ring patterns (Kim et al., 2020). This indicates that LA-ICP-MS can be employed to determine changes in levels of certain HMs in tree rings, providing valuable information and contributing to research in environmental pollution monitoring (Kim et al., 2020). The application of SP-ICP-MS offers a distinct opportunity to detect and study the concentration of metal particles, particle-particle and particle-matrix interactions, solubility, etc. Additionally, it allows for elemental analysis of single-celled organisms and provides isotope-related information over an extensive dynamic range of individual cells. SP-ICP-MS was utilized to detect the rapid absorption of Zn by Lacuca sativa L. plants grown in a medium containing ZnO nanoparticles (Wojcieszek et al., 2019). Nicotinamide formed a complex with 70% of the Zn in leaves as detected by ICP-MS and electrospray ionization-tandem mass spectrometry (ESI-MS-MS) (Wojcieszek et al., 2019). In summary, with the update and progress of technology and methods, ICP-MS has become a multifunctional and powerful platform that can determine the total elemental concentration and expand the scope of application with other technologies, providing a multidimensional perspective for solving problems related to HMs.
AAS is one of the earliest commercially developed methods for the elemental analysis of HMs (Jin et al., 2020). AAS is an approach for quantitative analysis based on the absorption of characteristic spectral lines by atomic vapor produced from a substance. It comprises five main components: a light source, an atomization system, a spectroscopy system, a detection system, and a display unit. The operating principle is as follows: First, an atomizer turns the sample to be measured into an atomic vapor under high temperature. Next, when the atomic vapor is irradiated with a light source, it can absorb light radiation of a certain wavelength. After this, the spectroscopic system distinguishes between different spectral lines. Finally, the content of the element to be measured in the sample is determined according to the degree of attenuation of the light when it is absorbed (Figure 3). AAS possesses the advantages of high selectivity, accuracy, sensitivity, and low interferences ability. The equipment is easy to operate, enables fast analysis, and provides an extensive range of analyses (Eskina et al., 2020). Depending on the atomization device used, AAS can be categorized into cold vapor generation atomic absorption spectrometry (CVAAS), hydride generation atomic absorption spectrometry (HGAAS), graphite furnace atomic absorption spectrometry (GFAAS), and flame atomic absorption spectrometry (FAAS) (Butler et al., 2006; Yuan et al., 2011; Guo et al., 2023).
AAS is one of the very popular methods for the qualitative and quantitative analysis of HMs in plants and can be employed to measure directly the content of metal elements in the sample (Adolfo et al., 2019). For instance, 12 elements, including Ag, Cr, Cu, Ca, and Fe, were detected in the leaves of Andrographis paniculata using AAS (Kartha. and PP, 2021). Similar results were observed with eight HMs (e.g., Cd, Cr, and Hg) in cereals, tubers, and vegetables, as detected by AAS (Omeje et al., 2021). Although AAS effectively determined the composition and quantification of elements, its efficacy can be influenced by the extraction technique, the elements to be determined, and the plant tissue (Elik et al., 2020). For example, AAS detected that the Mn content of five plant samples was highest when extracted using a nitric acid-perchloric acid solution, indicating that different digestion methods used for preparing plant samples impacted the detection of HM contents (Bankaji et al., 2023). Therefore, proper digestion methods are crucial for the maximal extraction of specific metals from various samples (Bankaji et al., 2023). Although new HM detection techniques have been developed, AAS remains one of the most potent tools in plant elemental metal analysis and trace analysis due to its cost-effective advantages, low price, low detection limit, and good repeatability (Vinogradova et al., 2023).
AFS is a fast-growing technique for trace analysis and belongs to emission spectroscopy, an important branch of atomic spectroscopy. AFS consists of five parts: a light source, an atomization system, a spectroscopic system, a detection system, and a display device. The working principle of AFS is that after the sample to be analyzed is converted into atomic vapor by the atomizer, the atomic vapor absorbs light radiation at its characteristic wavelength, causing the atoms to transition from the ground state to the excited state for a duration of 8 – 10 seconds. During this process, fluorescence with either the same or different wavelength as absorption is emitted. This fluorescence is then converted into an electrical signal by a photoelectricity detector and processed into readable data by a data processing system (Costa Ferreira et al., 2019) (Figure 4). Atomic fluorescence is divided into three types: resonant fluorescence, non-resonant fluorescence, and sensitive light fluorescence. Among them, resonant fluorescence has the highest intensity and is the most commonly used. AFS by atomization can be further divided into cold vapor atomic fluorescence spectrometry (CVAFS) and hydride generation atomic fluorescence spectrometry (HGAFS). AFS offers advantages like high accuracy (similar to AAS), high sensitivity, simultaneous determination of multiple elements, low detection limits (including very low detection limits for Zn and Cd), simple spectral lines, minimal spectral interference, wide linear range, etc (Yogarajah and Tsai, 2015; Xia et al., 2019; Peng et al., 2022). It is a simple instrument with lower operating costs compared to AAS.
Studies have shown that AFS is often combined with chromatographic techniques to define trace and ultra-trace element levels in plants, as well as analyze their Chemical forms (Dai et al., 2019; Li et al., 2021; Guo et al., 2023). Cheng et al. used dual-frequency ultrasonic-assisted enzyme digestion (UDUE) combined with liquid chromatograph-hydride generation atomic fluorescence spectrometry (LC-HGAFS) technology to detect the content and form of As elements in Paeoniae Raxix rubra, Angelica Sinensis, Codonopsis pilosula, and Salvia (Cheng et al., 2020). The results indicate that inorganic As is present in both trivalent and pentavalent forms in four medicinal plants (Cheng et al., 2020). Deng et al. utilized the method of high-performance liquid chromatography and hydride generation atomic fluorescence spectrometry (HPLC-HG-AFS) to detect various forms of As in rice that include As(V), DMA, As(III), and MMA (Deng et al., 2021). Similarly, the As forms of the three aquatic plants were mainly As(III) and As(V), which were detected using liquid chromatograph-atomic fluorescence spectrophotometry (LC-AFS) by Wang et al (Wang et al., 2021). Besides, plenty of studies have shown demonstrated that As typically exists in the form of As(V) and As(III) within plants (Jedynak et al., 2010; Budzyńska et al., 2017; Budzyńska et al., 2021). Hu et al. determined the levels of inorganic Hg in various vegetable and fruit samples using hydride generation novel ultraviolet atomization atomic fluorescence spectrometry (HG-UV-AFS). They discovered that volatile methyl Hg+ hydride (MeHgH) could be converted into elemental Hg vapor through UV-based atomization and KBH4 treatment, and they also detected total Hg (Methyl Hg+ (MeHg) and Hg2+) (Hu et al., 2018). These results showed that HG-UV-AFS effectively detected gaseous organic Hg hydride, providing a reference for analyzing HMs in their gaseous state (Hu et al., 2018). Se in garlic was determined using reversed-phase high-performance liquid chromatography and hydride generation atomic fluorescence spectrometry (RP-HPLC-HG-AFS) revealing the four forms of Se: SeMeSeCys, SeMet, Se(VI), and Se(IV) (Castro Grijalba et al., 2017). Furthermore, RP-HPLC-HG-AFS method provides technical support for the Chemical forms analysis of Se in samples of complex plants by effectively separating organic and inorganic Se using different ionic liquids as mobile phases (Castro Grijalba et al., 2017). In conclusion, AFS is an effective technique for identifying the chemical forms of HMs.
XAS is a spectroscopic analysis approach that utilizes synchrotron X-ray sources and varies the photon energy to determine the absorption coefficient of a sample. The XAS consists of an absorption edge and a series of oscillating structures, mainly divided into two regions: the extended X-ray absorption fine structure (EXAFS) located in the 50 – 11000 eV region of the absorption edge, and the XANES located near the 50 eV region of the absorption edge (Sarret et al., 2013; Tang et al., 2023) (Figure 5). The XAS works by exciting the sample with X-rays, causing its core electrons to transition to either the continuum or empty orbitals (Figures 6, 7). This leads to the formation of waves that scatter with the surrounding atoms (Figure 7). EXAFS can identify local information structures, such as bond lengths, coordination numbers, and disorder of central and coordination atoms, with a spatial resolution ranging from 0.1 – 1 pm; moreover, it is sensitive to steric structures. XANES can provide a wealth of chemical information, including near-neighbor atomic positions and chemical valence states. Meng et al. discovered that methylmercury constituted the primary form of Hg in rice (Meng et al., 2014). EXAFS can also detect the Chemical forms information of metal elements by oscillating in the EXAFS region (Hu et al., 2020). Compared to EXAFS, XANES exhibits characteristics such as subtle structural changes, a shorter acquisition time, high sensitivity to information like valence states, and faster identification of chemical types and forms of elements (Hara et al., 2023; Ren et al., 2023). XAS requires low sample amounts, does not damage the sample, and provides information on coordination atom types, coordination numbers, and atomic spacing. This facilitates the study of dynamic processes in the in situ electrochemical reactions and enables the accurate establishment of conformational relationships (Newville, 2014; Mastelaro and Zanotto, 2018; Tang et al., 2023).
XAS is a local structure detection technique that can be utilized to determine the distribution and morphological structural information of metal elements in plants. Sikhumbuzo et al. detected that the photon energy position of Cd overlapped with CdO in rice crops through XANES spectroscopy, and EXAFS spectroscopy revealed that CdO formed clusters in rice with a CD-O coordination number of 4.2 and a bond distance of 2.83, indicating that the main form of Cd in rice was Cd(II)-O (Kunene et al., 2020). These results indicated that XAS could function as a fundamental tool for analyzing the local atomic structure and oxidation state of Cd in rice, which helps study the mechanism of the interactions between rice plants and Cd (Kunene et al., 2020). Huang et al. detected Cr in Coptis chinensis Franch. using XANES and observed that Cr(VI) was reverted to Cr(III) after treatment, suggesting that toxic hexavalent Cr can be transformed into non-toxic trivalent Cr in plants (Huang et al., 2018). XANES detected the main form of Pb in Oenanthe javanica as Pb-ferrihydrite, which was absorbed and transported as Pb(Ac)2-3H2O (Liu and Luo, 2019). From the above studies, it can be observed that XAS enables the detection of HMs’ forms in plants, facilitating the analysis of HMs’ absorption and transport mechanisms in plants. This provides a direction for studying plant HM tolerance and offers possibilities for investigating the valency, ligand and dynamic metabolism of HMs in plants.
XRF is an analytical technique based on the characteristic X-ray emission spectrum produced by the sample (Byers et al., 2019). It is primarily composed of an X-ray generator, a spectroscopic detection system, and a counting recording system. XRF is divided into energy-dispersive X-ray fluorescence spectroscopy (EDXRF/EDX) and wavelength-dispersive X-ray fluorescence spectroscopy (WDXRF/WDX) according to the description of X-rays (Figure 8) (Feng et al., 2021). XRF works by using an X-ray tube to excite and irradiate the sample, causing the elements in the sample to emit characteristic X-rays. These X-rays are then collimated by a filter and detected by a probe, such as a scintillation counter, which converts them into electrical signals. After signal processing, analytical results of the elemental composition of the samples are got (Figure 8). XRF is a physical analysis method, primarily used for surface scanning. XRF technology can be utilized to the direct analysis of solid samples and in situ analysis of plant samples (Marguí et al., 2009). To ground into a fine powder plant materials for high-pressure granulation, after is the preparation of plant samples by XRF technique carries on the analysis of the most common method (Rudman, 1999). It offers advantages such as high sensitivity, fast and accurate analysis, non-destructive sampling, and simple operability (Bamrah et al., 2019). SR is an advanced light source that emits pulsed light ranging from infrared to hard X-rays, with a pulse duration ≤ one nanosecond, which is many orders of magnitude higher than traditional light sources (Hu et al., 2020). Through the interaction of synchrotron radiation with matter, technologies such as XRF and X-ray photoelectron spectroscopy (XPS) have been developed (Li et al., 2015a; Li et al., 2015b). Synchrotron radiation X-ray fluorescence spectroscopy (SRXRF) can improve the sensitivity of Zn detection to about one part per billion (ppb) (Li et al., 2015a; Li et al., 2015b).
Figure 8 The operating principle schematic diagram of XRF. (A) Energy chromatograph (B) Wavelength chromatograph.
The XRF technique can generate a distinctive set of characteristics for each element in the sample, based on the concentration and intensity of the X-ray source (McGladdery et al., 2018). Fluorescence X-ray analysis is a reliable approach for both qualitative and quantitative assessment (McGladdery et al., 2018). Real-time analysis of XRF can be utilized to chart the distribution in plant tissues of HM elements (Jones et al., 2020; Ramakrishna, 2023). Studies have shown that XRF has become the preferred approach for studying the distribution of HMs in plants (Kopittke et al., 2018; van der Ent et al., 2022) The aboveground part of Origanum sipyleum L were prepared into powdered samples and aqueous extracts which were detected by XRF and found to be higher in nutrients such as K, Na, and Ca (Durmuşkahya et al., 2016). Sometimes, it can also be used in combination with other technologies to enhance the predictability of XRF (Feng et al., 2021). Vigani et al. utilized XRF and ICP-MS techniques to analyze cucumber leaves, revealing that Zn was primarily distributed in chloroplasts and mitochondria, and found that the Zn content significantly increased in chloroplasts and mitochondria when Fe was deficient, indicating that iron deficiency affected the distribution of Zn in leaves (Vigani et al., 2018). SR technology offers significant advantages in terms of spatial resolution, distribution, and chemical state-related information. Microscopic X-ray fluorescence (μ-XRF) and XAS technologies, based on SR imaging technology, can be used to study the distribution of metals and metal-like elements in plants while providing valence states of elements with femtosecond sensitivity and micro/nano range spatial resolution (Vijayan et al., 2015). Zn and Cd in leaves of Arabidopsis halleri plants were analyzed by using μ-XRF, and the results shown that they were mainly distributed in leaf trichomes, while after treatment with 100 µM Zn and 20 µM Cd, they were primarily found in mesophyll tissues (Fukuda et al., 2020). At the cellular/tissue level, Zn is mainly distributed in mesophyll tissue, while Cd is primarily found in the vascular bundles of leaf veins (Fukuda et al., 2020). The results of XAS and synchrotron radiation microscopic X-ray fluorescence (SR-μXRF) showed that the main form of La in cucumber (Cucumis sativus) plants was phosphate or carboxyl complex, while the main form of Ce was CeO, and a small portion of CeO2 was converted into a Ce(III)-carboxyl complex (Ma et al., 2015). The accumulation of Zn in leaf tips and vacuoles, as well as the dynamic complexation of histidine with Zn in edible plants, were discovered using μXRF and XAS techniques, providing new support for understanding the dynamic characteristics of Zn Chemical forms in plants (Mishra et al., 2020). C. chinensis was analyzed using SR-μXRF and LA-ICP-MS techniques to identify the distribution of Cr in root and the result revealed that Cr was largely accumulated in the vascular column, and part of the cortex (Huang et al., 2018). XRF is considered a powerful technical means for qualitative and quantitative detection of element distribution in single cells of plants or algae, making it highly suitable for detecting HMs in plants. It can aid in explaining the mechanisms behind the accumulation, absorption, and detoxification of HM elements in plants.
LA-ICP-MS is a technique which enables rapid analysis of the distribution and content of elements in a sample at a micro-regional level, both for elemental and isotopic analysis (Pan et al., 2022). The LA-ICP-MS system is composed of three components: a laser exfoliation device (LXD), an ICP source, and a mass spectrometry detector. Among these, the LXD is composed of a laser, a beam delivery system, a sample cell, and an observation system. When a laser scans a sample, the excitation beam consisting of high-energy photons causes a photo-thermal effect that volatilizes atoms or molecules on the sample surface into particles and subsequently forms a small burrow hole, a process known as laser exfoliation (Trejos et al., 2003; Trejos and Almirall, 2005; López-Fernández et al., 2016). LA-ICP-MS works by melting and vaporizing the sample through laser ablation, and the carrier gas transports the ablated particles into the plasma (Figure 9). In the plasma, the particulates are ionized into positively charged ions, then analyzed by a mass spectrometry detector to identify the content and distribution of each element (Figure 9). During the laser scanning process, the changes in ionic strength of each element are recorded over time. This raw data is then reconstructed as an image, which is ultimately calibrated and visualized. The resulting image can be further processed using algorithms to visually represent the distribution of the elements. LA-ICP-MS can perform in situ analyses rapidly and in real-time on a micro-scale. It offers several advantages, including easy sample preparation, minimal contamination levels, high spatial resolution, sensitivity, low detection limits, provides relatively simple spectra while allowing for simultaneous determination of multiple elements and information about isotope ratio (Trejos and Almirall, 2005; López-Fernández et al., 2016; Nunes et al., 2016; Davison et al., 2023; Janovszky et al., 2023).
LA-ICP-MS is a robust technique that combines not only the high sensitivity and multi-element capability of ICP-MS but also good spatial resolution and laser radiation (Nunes et al., 2016). It is commonly used to directly analyze plant tissues and generate the multi-element bio-imaging of plant tissues to facilitate assess the ecological and toxicological risks of HMs to plants, and has been successfully used to analyze elemental distributions in samples such as leaves, roots, seeds, and bark (Narewski et al., 2000; Meharg et al., 2008; Shi et al., 2009; Wu et al., 2009; Yamaji and Ma, 2019). Using LA-ICP-MS imaging to detect Pb accumulation in wheat grain at maturation, it was identified the main distribution of Pb in wheat peels and seed coating, while nutrients are primarily distributed in the inner seed coating region; it was hypothesized that removing bran may be an effective way to reduce Pb levels in wheat while retaining the nutrients (Zhang et al., 2022). LA-ICP-MS detected that Fe and Zn in Phyllostachys edulis seeds were mainly distributed and enriched in the aleurone layer and embryo and were significantly higher than rice, asserting their suitability as a natural food enhancer (Hu et al., 2023). Thus, LA-ICP-MS provided technical support for enhancing the preservation of nutrients in P. edulis seeds during food processing (Hu et al., 2023). Yamaji et al. utilized LA-ICP-MS technology to establish a bioimaging scheme for various elements at the cell level of rice nodes and identified the distribution characteristics of mineral elements between the wild-type and OsHMA2 mutant rice plants (Yamaji and Ma, 2019). K, P, Mg, and other nutrients were mainly distributed in the phloem, while Zn, Fe, and other trace elements in the vascular tissues (Yamaji and Ma, 2019). The distribution of Zn in the nodal tissues of the OsHMA2 mutant was not markedly distinct from that of the wild type. However, the contents of Zn were lower in the nodal vascular tissue I but elevated in III compared to the wild type (Yamaji and Ma, 2019). This suggests that LA-ICP-MS is a robust and powerful method for the qualitative and quantitative mapping of mineral elements in rice node tissues, especially when combined with the functional characterization of transporter proteins (Yamaji and Ma, 2019).
Non-invasive micro-test technology (NMT) is a microelectrode technology for the real-time, label-free detection of the flow rate and the influx or efflux of molecules and ions into the organisms (Yuan et al., 2021). NMT consists of a selective microelectrode system (SMS), signal amplification, microscopic imaging, 3D motion, and data processing systems. The SMS comprises a glass microsensor, Ag/AgCl electrode, electrolyte, and liquid ion exchanger (LIX). The selectivity is achieved through the specific recognition of ions by organic compounds in the large neutral molecular carriers within the LIX, this is essential for NMT. The selective microelectrode is moved back and forth between high and low electrochemical potentials carried by charged particles, measuring the potential value at each point obtaining the potential difference (AV) between the two points. The potential concentration calibration curve of the electrode was employed to measure the differences in the ion concentrations between these two points. The rate of ion movement was computed using Fick’s First Law of Diffusion and Nernst’s Equation (Figure 10). NMT can perform 3D measurements of the samples in situ with high sensitivity and spatial resolution without causing damage or requiring marker sampling. It is simple to operate and can detect the dynamic indicators of bioactivities of plants (Yuan et al., 2021; Wang et al., 2023). It can detect 50 types of metallic molecules and ions, including H+, Ca2+, Na+, K+, Cl-, Cd2+, Cu2+, and NH4+ (Mugnai et al., 2012; Mardones et al., 2018; Wu et al., 2019; Lan et al., 2020; Zhang et al., 2020; Di et al., 2021; Hou et al., 2021). As the rate of NMT-based determination is robust, it enables the investigation of the flow characteristics of metal ions (mainly in roots); it can be used to screen species as low or hyperaccumulators and explore the enrichment characteristics of plants; and it provides technical support for hyperaccumulator-based remediation of contaminated soils, plant nutrition regulation, and plant physiology research (Han et al., 2022).
NMT is a handy tool for studying ion flow, signal transduction, and functional genes in plants under HM-induced stress. It can monitor the dynamic flow of HM ions in and out of plants in real-time, which is conducive to investigating the mechanism of tolerance to HMs, the toxic effects of HMs, and the mechanism by which hyperaccumulators absorb and transport HM ions (Liu et al., 2020). The scanning ion selective electrode technology (SIET) and microelectrode ion flux estimation technology (MIFE) are commonly used for measuring ion currents in plants (Mardones et al., 2018; Hou et al., 2021; Sun et al., 2021; Sun et al., 2021; Deng et al., 2022). The inflow of Cd2+ at 300 µm around the root tips of Bidens pilosa L. plants was reduced when treated with 16 mM Ca2+, 8 mM Mg2+, 8 mM SO42-, or 18 mM K+ as detected by NMT, indicating that treatment at a large concentration of nutrient ions inhibited the absorption of Cd2+ (Wang et al., 2023). Using the NMT method, a peak in the net Cd2+ flux was observed at a distance of 100 µm from the surface of the root tips of Amaranthus hypochondriacus L., a plant famous for its ability to accumulate large quantities of this metal ion (Han et al., 2023). Furthermore, fluorescent labeling revealed that Ca2+ channel blockers conspicuously suppressed Cd2+ influx compared to other blockers, suggesting that Ca2+ channels serve as primary pathways for Cd entry through the roots (Han et al., 2023). In a similar observation, Cd primarily entered Sedum plumbizincicola through Ca2+ channels; the net flux of Cd2+ altered from inflow to outflow as the concentration of Ca2+ increased (Li et al., 2017b). NMT detected a significant reduction in the flow of Cd2+ in the root hair area of rice plants when NH4+ was added, indicating that it reduced the influx of Cd and, therefore, is beneficial for cultivating rice with lower levels of pollutants (Wu et al., 2018). The influx and efflux of ions from plant cells were detected by NMT, providing the most intuitive and accurate data for studying plant stress resistance mechanisms and understanding the relationship between ion/molecular flow and specific cellular functions (Han et al., 2022).
Plant reactions to HM toxicity are dependent on regulation by molecular factors (Raza et al., 2022). Deciphering HM toxicity and subsequent molecular, cellular and physiological responses is regarded to be a challenging, arduous and complex work (Mondal et al., 2022). A diverse range of biotechnologies are being applied to better understand and explore the pathways and mechanisms involved in plant responses and tolerance for HM toxicity (Raza et al., 2022). With the advancement of science and technology, the emergence of omics has provided a valuable terrace to investigate the physiological, biochemical, metabolical and molecular activities of HM-treated plants and cells. Well-established genomics technologies like genomics, transcriptomics, proteomics, metabolomics, ionomics, and other high-throughput technologies are valuable in elucidating plant stress responses and tolerance to HM. Through a comprehensive analysis of a large amount of omics data, the fundamental state of genes, mRNAs, proteins, metabolites, and elemental compositions in plants could be ascertained to fully explain the molecular mechanisms underlying the interaction between plants and HMs. The data gained will contribute to the enhanced research on plant stress tolerance and can be used in breeding and engineering schemes to breed plants with new and desirable agronomic traits and hyperaccumulators (Singh et al., 2016).
Analysis of genomics-based data aids in identifying genes, regulators, enzymes, signaling and other molecular factors involved in the HM uptake, transportation, resistance, and tolerance mechanisms of plants (Anjitha et al., 2023). DNA mismatch repair (MMR), targeted induced local lesions in genomes (TILLING), genome-wide association studies (GWAS), and clustered regularly interspaced short palindromic repeats (CRISPR/Cas9) are some of the genomic methods that recognize genes are correlated with the interactions between plant and metal (Al-Khayri et al., 2023). GWAS study in Medicago sativa subjected to Cd stress reveals that response features are polygenic, involving several quantitative loci, and that stress-related UDP-glycosyltransferases, genes ATP-binding cassette transporters, P-type ATP transporters genes, and oxidative stress response genes are associated with Cd tolerance (Paape et al., 2022). In barley, under Cd stress, the genetic structure and genetic regulation study by QTL analysis revealed that antioxidant enzymes, stress signaling proteins and metal ion transporters showed different categories of InDels and SNPs in the QTL regions between the parents, which probably be critical for responding to Cd stress (Derakhshani et al., 2020). The ABC transporters detected are potential candidate genes that can be explored for raising of Cd-tolerant barley varieties (Derakhshani et al., 2020).
Transcriptomic profile can reflect the transcriptome differences among different cultivars, developmental stages, and tissues of plants under different environmental conditions, which provides us with further understanding and analysis of the responses under specific physiological states or stress conditions (Jamla et al., 2021). Transcriptomic analysis of Calotropis gigantea on exposure to Cd demonstrated that the leaves mitigated Cd stress by activating a variety of Cd detoxification processes such as cell wall remodeling, antioxidant systems, metal chelation, and the upregulation of genes related to the metal transporters (Yang et al., 2022). Using a gene co-expression network, the researchers was identified that BnaCn.ABCC3 and BnaC3.ABCC4 in Brassica are a pivotal factors participating in the storage of PC-Cd complexes in vacuolar (Zhang et al., 2019). The amount of genes participating in survival mechanisms and metabolic pathways would have been regulated by HM stress. Regulatory genes (e.g., TF) together with these functional genes are critical for the regulation of many stress-responsive genes, and will ultimately form gene networks (Jamla et al., 2021). In Arabidopsis, MYB4 TF modulates Cd tolerance by ameliorating the anti-oxidant defense system and enhancing the expression of the promoter regions of phytochelatin synthase 1 (PCS1) and metallothionein 1C (MT1C) (Agarwal et al., 2020).
The new insights provided by proteomics into stress-induced proteins and their involvement in mitigating HM toxicity is a powerful tool (Raza et al., 2022). It has also been used to compare changes in proteins at the organelle, cell, tissue and organ levels, and to provide an understanding of protein behavior under diverse stress situations, including HM stress (Singh et al., 2016). By 2-DE analysis, 18 proteins involved in Cd tolerance were discovered in the leaves of two tobacco genotypes, Gui Smoke 1 (Cd-sensitive) and Yunnan Smoke 2 (Cd-tolerant) (Ghatak et al., 2017). Proteomic analysis (LC-MS/MS) of Se-stressed pepper seedlings showed that 172 proteins were up-regulated and 28 proteins were down-regulated, and several heat shock proteins (HSPs), which help to cope with Se stress, were identified in the Se stress-induced differentially expressed proteins (DEPs) of pepper (Zhang et al., 2019).
Under stress conditions, plants become more focused on coping with adversity stress and survival, and regulate their growth and developmental processes. This leads to changes in the pattern of gene expression (or transcriptional reprogramming), which then causes changes in the concentration, type, and number of metabolites produced (Jamla et al., 2021). Metabolomics helps to understand the complex metabolic activities and differences in regulated metabolites produced by plants under different conditions including HM stress (Raza, 2022). In Cd-stressed tobacco plants, metabolomics investigation indicated that 76 metabolites in the leaves and 150 metabolites were in the roots individually. It is found that metabolites were in considerable abundance in the biosynthesis of amino acids, arginine and proline, flavone and flavonol, and nicotinate and nicotinamide (Zou et al., 2022). In Calendula officinalis plant exposed to Cd stress, the phenomena of the contest between specialized metabolites (triterpenoids) and general metabolites (sterols) were noted in the culture of plant roots and hairy root (Rogowska et al., 2022).
Plants need a suitable amount of minerals to maintain normal vital activities. The application of ionomics enables an in-depth understanding of the nutrient and trace element makeup of plants, explores the activities and mechanisms of plant absorption, storage, and assimilation, and provides new ideas for illuminating the response of plants to HMs (Raza et al., 2022). It has been shown that plants subjected to HM-induced stress can be adapted to unfavorable conditions via modulating elemental composition and content in the ionome (Mleczek et al., 2018). Researchers used transcriptomic and ionomic mapping to analyze the mechanisms of mitigating As(III) force in rice and found that ionome was significantly correlated with transcriptome in the aerial part of rice (Xiao et al., 2021). Upregulation of transporter genes associated with transmembrane transport and ion binding associated with nutrient elements was found, showing that plants tend to be more inclined to transport more nutrients, like ammonium, potassium and phosphate, in response to As(III) toxicity (Xiao et al., 2021).
The accurate selection of HM detection and analysis methods is crucial for explaining the stress caused by HMs in plants. By conducting a comprehensive analysis of the different, commonly used methods for HMs in plants, the application criteria and characteristics of each method were obtained, systematically compared, and summarized (Table 2).
With rapid urbanization and industrialization, HM pollution has become a major environmental problem. HMs released in the environment are absorbed and accumulated from plants, which are transmitted to humans along the food chain, causing grave adverse impacts on the life and health of plants and humans. Therefore, it is crucial to propose solutions that effectively control the content of HMs by studying the mechanisms of translocation, uptake, distribution, and storage of HMs in plants and identifying their chemical forms. This paper reviews the technical methods applied to study HMs in plants during recent years and summarizes the principles, advantages, disadvantages, and applications for these approaches.
ICP-MS and AAS are widely used to detect HM content in plants, both of which have the advantages of high sensitivity, a low detection limit, and high accuracy. Compared to AAS, ICP-MS has higher operating costs; however, it offers convenient and fast detection, lesser interference, simple sample preparation, and the ability to analyze multiple elements and their isotopes (Pei et al., 2023). These advantages meet the needs of large-scale detection, necessitating researchers with an expectation of obtaining additional information to choose ICP-MS more frequently. Therefore, designing and developing portable instruments based on ICP-MS and AAS-based multi-element analyses have become a priority. AFS and XAS are primarily used to study the chemical form of HMs in plants. AFS has a good selectivity, but its limited range of detecting elements severely restricts its application. XAS is preferred more because of its low maintenance cost and ability to provide more information, such as the type of coordinating atoms, coordination number, atomic spacing, which aligns with daily laboratory use. In recent years, XRF and LA-ICP-MS have become the primary methods often applied to the spatial distribution of HMs in plants. LA-ICP-MS, with its simple operability and instrumentation, has been widely used for studying the tissue distribution of HMs in plants. This technology can provide a basis for studying the mechanisms of HM transport and the function and localization of transport proteins by analyzing the distribution of HMs. Although SR-XRF requires large-scale instrumentation, the combined use of SR-XRF and XAS is often chosen for analyzing the chemical for and tissue distribution of HMs in plants (Bai et al., 2019; Li et al., 2020). NMT has demonstrated outstanding performance in monitoring the flow speed and direction of small molecules and ions in organisms and has rapidly developed in recent years. The difficulty in fabricating and preserving selective microelectrodes has limited their application to HM-related studies (Han et al., 2022). The rise and application of molecular biology and omics technologies have opened a new chapter in studying HMs in plants. Omics can determine the interactions between plants and HMs by obtaining metabolomics, transcriptomics, genomics, ionomics, and proteomics information on plants subjected to HM-induced stress. Molecular biology techniques, such as gene cloning and editing, investigate the function of proteins and genes and the response mechanisms of plants to HMs and provide theoretical guidance for improving the tolerance and resistance of plants to HMs.
In recent years, ICP-MS, XAS, LA-ICP-MS, NMT, omics, and molecular biology techniques have been increasingly applied in HM-related research in plants, providing technical support for studying the uptake, transport and distribution, accumulation and storage, and detoxification of HMs in plants. Using omics and molecular biology methods, the mechanisms of plant response to HM-induced stress were analyzed, and the plant varieties with HM resistance or low HM accumulation were screened. Furthermore, regarding environmental concerns, future research may focus on screening and breeding hyperaccumulating plant cultivars to remediate contaminated soils. The use of traditional methods for detecting HMs is maturing. Regarding HM detection technology, low-cost operability, and portability have become the focus of attention and application in laboratory research. Therefore, simplifying the operability of instruments, expanding their detection range, and reducing the costs of different detection technologies remain the main directions of progress. The detection technology of HMs in plants should be continuously refined and improved to develop it towards integration, efficiency, and high adaptability.
SH: Writing – original draft. YN: Writing – original draft. LX: Writing – original draft. ZL: Writing – review & editing. XS: Writing – review & editing. MD: Writing – review & editing. WH: Writing – review & editing.
The author(s) declare financial support was received for the research, authorship, and/or publication of this article. This work was supported by the National Natural Science Foundation of China (No.81903751); Research and Development Program of Shaanxi university of Chinese medicine (2023GP26), and Sci-Tech Innovative Talent System Construction Program of Shaanxi University of Chinese medicine (No. 2023-CXTD-05).
Thanks to the High-Level Key Discipline of Chemistry of Chinese Medicine of National Administration of Traditional Chinese Medicine for all aspects of support for this paper. We express our gratitude to Shengli Wu (Xi’an Ande Pharmaceutical Co., Ltd) for assistance with sample collection.
Author MD was employed by the company Xi’an Ande Pharmaceutical Co; Ltd.
The remaining authors declare that the research was conducted in the absence of any commercial or financial relationships that could be construed as a potential conflict of interest.
All claims expressed in this article are solely those of the authors and do not necessarily represent those of their affiliated organizations, or those of the publisher, the editors and the reviewers. Any product that may be evaluated in this article, or claim that may be made by its manufacturer, is not guaranteed or endorsed by the publisher.
The Supplementary Material for this article can be found online at: https://www.frontiersin.org/articles/10.3389/fpls.2024.1310328/full#supplementary-material
Adolfo, F. R., do Nascimento, P. C., Leal, G. C., Bohrer, D., Viana, C., de Carvalho, L. M., et al. (2019). Simultaneous determination of iron and nickel as contaminants in multimineral and multivitamin supplements by solid sampling HR-CS GF AAS. Talanta 195, 745–751. doi: 10.1016/j.talanta.2018.12.010
Agarwal, P., Mitra, M., Banerjee, S., Roy, S. (2020). MYB4 transcription factor, a member of R2R3-subfamily of MYB domain protein, regulates cadmium tolerance via enhanced protection against oxidative damage and increases expression of PCS1 and MT1C in Arabidopsis. Plant Sci. 297, 110501. doi: 10.1016/j.plantsci.2020.110501
Ahammed, G. J., Wu, M.-J., Wang, Y.-Q., Yan, Y.-R., Mao, Q., Ren, J.-J., et al. (2020). Melatonin alleviates iron stress by improving iron homeostasis, antioxidant defense and secondary metabolism in cucumber. Sci. Hortic. 265, 109205. doi: 10.1016/j.scienta.2020.109205
Ahmed, S., Sameen, Sardar, R. (2023). Improvement in growth and physiochemical attributes of Raphanus sativus L. through exogenous application of 28-Homobrassinolide under nickel stress. Sci. Hortic. 311, 111791. doi: 10.1016/j.scienta.2022.111791
Alaqouri, H. A. A., Genc, C. O., Aricak, B., Kuzmina, N., Menshikov, S., Cetin, M. (2020). The possibility of using Scots pine needles as biomonitor in determination of heavy metal accumulation. Environ. Sci. pollut. R 27 (16), 20273–20280. doi: 10.1007/s11356-020-08449-1
Al-Huqail, A. A. (2023). Stimulating the efficiency of Cd-phytoremediation from contaminated soils by Solanum nigrum L.: Effect of foliar and soil application of yeast extract. S Afr J. Bot. 161, 512–518. doi: 10.1016/j.sajb.2023.08.053
Ali, S., Mir, R. A., Tyagi, A., Manzar, N., Kashyap, A. S., Mushtaq, M., et al. (2023). Chromium toxicity in plants: signaling, mitigation, and future perspectives. Plants 12 (7), 1502. doi: 10.3390/plants12071502
Al-Khayri, J. M., Banadka, A., Rashmi, R., Nagella, P., Alessa, F. M., Almaghasla, M. I. (2023). Cadmium toxicity in medicinal plants: An overview of the tolerance strategies, biotechnological and omics approaches to alleviate metal stress. Front. Plant Sci. 13. doi: 10.3389/fpls.2022.1047410
Andresen, E., Küpper, H. (2013). “Cadmium toxicity in plants,” in Cadmium: from toxicity to essentiality. Eds. Sigel, A., Sigel, H., Sigel, R. K. O. (Dordrecht, NL: Springer Netherlands), 395–413. doi: 10.1007/978-94-007-5179-8_13
Anjitha, K. S., Sarath, N. G., Sameena, P. P., Janeeshma, E., Shackira, A. M., Puthur, J. T. (2023). Plant response to heavy metal stress toxicity: the role of metabolomics and other omics tools. Funct. Plant Biol. 50 (12), 965–982. doi: 10.1071/fp23145
Bai, X., Li, Y.-Y., Liang, X.-J., Li, H., Zhao, J.-T., Li, Y.-F., et al. (2019). Botanic metallomics of mercury and selenium: current understanding of mercury-selenium antagonism in plant with the traditional and advanced technology. B Environ. Contam Tox 102 (5), 628–634. doi: 10.1007/s00128-019-02628-8
Bamrah, R. K., Vijayan, P., Karunakaran, C., Muir, D., Hallin, E., Stobbs, J., et al. (2019). Evaluation of X-ray fluorescence spectroscopy as a tool for nutrient analysis of pea seeds. Crop Sci. 59 (6), 2689–2700. doi: 10.2135/cropsci2019.01.0004
Banerjee, S., Islam, J., Mondal, S., Saha, A., Saha, B., Sen, A. (2023). Proactive attenuation of arsenic-stress by nano-priming: Zinc Oxide Nanoparticles in Vigna mungo (L.) Hepper trigger antioxidant defense response and reduce root-shoot arsenic translocation. J. Hazard Mater 446, 130735. doi: 10.1016/j.jhazmat.2023.130735
Bankaji, I., Kouki, R., Dridi, N., Ferreira, R., Hidouri, S., Duarte, B., et al. (2023). Comparison of digestion methods using atomic absorption spectrometry for the determination of metal levels in plants. Separations 10 (1), 40. doi: 10.3390/separations10010040
Boi, M. E., Medas, D., Aquilanti, G., Bacchetta, G., Birarda, G., Cappai, G., et al. (2020). Mineralogy and Zn Chemical Speciation in a Soil-Plant System from a Metal-Extreme Environment: A Study on Helichrysum microphyllum subsp. tyrrhenicum (Campo Pisano Mine, SW Sardinia, Italy). Minerals 10 (3), 259. doi: 10.3390/min10030259
Budzyńska, S., Mleczek, M., Goliński, P., Rutkowski, P., Niedzielski, P. (2017). The influence of As forms in substrate on the phytoextraction of this metalloid in Ulmus laevis Pall organs-Pot experiment. Microchem J. 132, 333–340. doi: 10.1016/j.microc.2017.01.030
Budzyńska, S., Niedzielski, P., Mleczek, M. (2021). Time-dependent changes of arsenic and its selected forms in a hydroponic experiment with Quercus robur L. J. Hazard Mater 405, 124244. doi: 10.1016/j.jhazmat.2020.124244
Butler, O. T., Cook, J. M., Harrington, C. F., Hill, S. J., Rieuwerts, J., Miles, D. L. (2006). Atomic spectrometry update. Environmental analysis. J. Anal. Atom Spectrom 21 (2), 217–243. doi: 10.1039/B516025C
Byers, H. L., McHenry, L. J., Grundl, T. J. (2019). XRF techniques to quantify heavy metals in vegetables at low detection limits. Food Chem. X 1, 100001. doi: 10.1016/j.fochx.2018.100001
Castro Grijalba, A., Fiorentini, E. F., Wuilloud, R. G. (2017). Ionic liquid-assisted separation and determination of selenium species in food and beverage samples by liquid chromatography coupled to hydride generation atomic fluorescence spectrometry. J. Chromatogr A 1491, 117–125. doi: 10.1016/j.chroma.2017.02.045
Cesur, A., Zeren Cetin, I., Abo Aisha, A. E. S., Alrabiti, O. B. M., Aljama, A. M. O., Jawed, A. A., et al. (2021). The usability of Cupressus arizonica annual rings in monitoring the changes in heavy metal concentration in air. Environ. Sci. pollut. R 28 (27), 35642–35648. doi: 10.1007/s11356-021-13166-4
Chen, M-Y-X., Zhang, X.-H., Jiang, P.-P., Liu, J., You, S.-H., Lv, Y.-R. (2022). Advances in heavy metals detoxification, tolerance, accumulation mechanisms, and properties enhancement of Leersia hexandra Swartz. J. Plant Interact. 17 (1), 766–778. doi: 10.1080/17429145.2022.2096266
Cheng, L., Yang, X.-A., Shi, M.-T., Zhang, W.-B. (2020). Rapid extraction of arsenic species from traditional Chinese herbal by dual-frequency ultrasound-assisted enzymatic digestion prior to spectral analysis. J. Chromatogr A 1619, 460915. doi: 10.1016/j.chroma.2020.460915
Chuparina, E. V., Aisueva, T. S. (2011). Determination of heavy metal levels in medicinal plant Hemerocallis minor Miller by X-ray fluorescence spectrometry. Environ. Chem. Lett. 9 (1), 19–23. doi: 10.1007/s10311-009-0240-z
Cobbett, C. S. (2000). Phytochelatins and their roles in heavy metal detoxification. Plant Physiol. 123 (3), 825–832. doi: 10.1104/pp.123.3.825
Corso, M. (2020). Garcia de la Torre VS. Biomolecular approaches to understanding metal tolerance and hyperaccumulation in plants. Metallomics 12 (6), 840–859. doi: 10.1039/d0mt00043d
Costa, M. B., Tavares, F. V., Martinez, C. B., Colares, I. G., Martins, C. (2018). Accumulation and effects of copper on aquatic macrophytes Potamogeton pectinatus L.: Potential application to environmental monitoring and phytoremediation. Ecotoxicol Environ. Saf. 155, 117–124. doi: 10.1016/j.ecoenv.2018.01.062
Costa Ferreira, S. L., dos Anjos, J. P., Assis Felix, C. S., da Silva Junior, M. M., Palacio, E., Cerda, V. (2019). Speciation analysis of antimony in environmental samples employing atomic fluorescence spectrometry-Review. TrAC-Trend Anal. Chem. 110, 335–343. doi: 10.1016/j.trac.2018.11.017
Dai, R., Hu, P.-Y., Wang, X., Wang, S.-X., Song, X.-M., Huang, K., et al. (2019). Visual/CVG-AFS/ICP-MS multi-mode and label-free detection of target nucleic acids based on a selective cation exchange reaction and enzyme-free strand displacement amplification. Analyst 144 (14), 4407–4412. doi: 10.1039/C9AN00642G
DalCorso, G., Farinati, S., Maistri, S., Furini, A. (2008). How plants cope with cadmium: staking all on metabolism and gene expression. J. Integr. Plant Biol. 50 (10), 1268–1280. doi: 10.1111/j.1744-7909.2008.00737.x
DalCorso, G., Fasani, E., Manara, A., Visioli, G., Furini, A. (2019). Heavy metal pollutions: state of the art and innovation in phytoremediation. Int. J. Mol. Sci. 20 (14), 3412. doi: 10.3390/ijms20143412
D’Amore, T., Miedico, O., Pompa, C., Preite, C., Iammarino, M., Nardelli, V. (2023). Characterization and quantification of arsenic species in foodstuffs of plant origin by HPLC/ICP-MS. Life 13 (2), 511. doi: 10.3390/life13020511
Davison, C., Beste, D., Bailey, M., Felipe Sotelo, M. (2023). Expanding the boundaries of atomic spectroscopy at the single-cell level: critical review of SP-ICP-MS, LIBS and LA-ICP-MS advances for the elemental analysis of tissues and single cells. Anal. Bioanal Chem 415, 6931–6950. doi: 10.1007/s00216-023-04721-8
Decou, R., Bigot, S., Hourdin, P., Delmail, D., Labrousse, P. (2019). Comparative in vitro/in situ approaches to three biomarker responses of Myriophyllum alterniflorum exposed to metal stress. Chemosphere 222, 29–37. doi: 10.1016/j.chemosphere.2019.01.105
Deng, X., Chen, B., Chen, Y.-X., Lu, L., Yuan, X.-Q., Yang, Y., et al. (2021). Variations in root morphological indices of rice (Oryza sativa L.) induced by seedling establishment methods and their relation to arsenic accumulation in plant tissues. Environ. pollut. 281, 116999. doi: 10.1016/j.envpol.2021.116999
Deng, B., Zhang, W.-W., Yang, H.-Q. (2022). Abscisic acid decreases cell death in malus hupehensis rehd. Under cd stress by reducing root cd2+ Influx and leaf transpiration. J. Plant Growth Regul. 41 (2), 639–646. doi: 10.1007/s00344-021-10327-0
Derakhshani, B., Jafary, H., Maleki Zanjani, B., Hasanpur, K., Mishina, K., Tanaka, T., et al. (2020). Combined QTL mapping and RNA-Seq profiling reveals candidate genes associated with cadmium tolerance in barley. PloS One 15 (4), e0230820. doi: 10.1371/journal.pone.0230820
Di, D.-W., Sun, L., Wang, M., Wu, J.-J., Kronzucker, H. J., Fang, S., et al. (2021). WRKY46 promotes ammonium tolerance in Arabidopsis by repressing NUDX9 and indole-3-acetic acid-conjugating genes and by inhibiting ammonium efflux in the root elongation zone. New Phytol. 232 (1), 190–207. doi: 10.1111/nph.17554
Duffus, J. H. (2002). “Heavy metals” a meaningless term? (IUPAC Technical Report). Pure Appl. Chem. 74 (5), 793–807. doi: 10.1351/pac200274050793
Durmuşkahya, C., Alp, H., Hortooğlu, Z. S., Toktas, Ü., Kayalar, H. (2016). X-ray fluorescence spectroscopic determination of heavy metals and trace elements in aerial parts of Origanum sipyleum L. from Turkey. Trop. J. Pharm. Res. 15 (5), 1013–1015. doi: 10.4314/tjpr.v15i5.16
Elik, A., Demirbas, A., Altunay, N. (2020). Analysis of zinc and chromium in grain samples using ionic liquid-based ultrasound-assisted microextraction followed by flame-AAS after microwave digestion. Biol. Trace Elem Res. 198 (2), 697–706. doi: 10.1007/s12011-020-02071-5
El-Jaoual, T., Cox, D. A. (1998). Manganese toxicity in plants. J. Plant Nutr. 21 (2), 353–386. doi: 10.1080/01904169809365409
Emamverdian, A., Yu-Long, D., Mokhberdoran, F., Yin-Feng, X. (2018). Antioxidant response of bamboo (Indocalamus latifolius) as affected by heavy metal stress. J. Elem 23 (1), 341–352. doi: 10.5601/jelem.2017.22.2.1410
Eskina, V. V., Dalnova, O. A., Filatova, D. G., Baranovskaya, V. B., Karpov, Y. A. (2020). Direct precise determination of Pd, Pt and Rh in spent automobile catalysts solution by high-resolution continuum source graphite furnace atomic absorption spectrometry. Spectrochim Acta B 165, 105784. doi: 10.1016/j.sab.2020.105784
Esmaeilzadeh, M., Tavakol, M., Mohseni, F., Mahmoudi, M., Nguyen, U. P., Fattahi, M. (2023). Biomarkers for monitoring heavy metal pollution in the Anzali Wetland. Mar. pollut. Bull. 196, 115599. doi: 10.1016/j.marpolbul.2023.115599
Ettiyagounder, P., Tamilselvan, I., Veeraswamy, D., Periasami, K., Selvaraj Paul, S. (2021). “Metallothioneins: Diverse Protein Family to Bind Metallic Ions,” in Heavy Metals. Eds. Mazen Khaled, N., Hongbo, Z. (London: IntechOpen), 9. doi: 10.5772/intechopen.97658
Farooq, M. A., Islam, F., Ali, B., Najeeb, U., Mao, B., Gill, R. A., et al. (2016). Arsenic toxicity in plants: Cellular and molecular mechanisms of its transport and metabolism. Environ. Exp. Bot. 132, 42–52. doi: 10.1016/j.envexpbot.2016.08.004
Feng, X., Zhang, H.-H., Yu, P.-Q. (2021). X-ray fluorescence application in food, feed, and agricultural science: a critical review. Crit. Rev. Food Sci. Nutr. 61 (14), 2340–2350. doi: 10.1080/10408398.2020.1776677
Fu, L., Shi, S.-Y., Chen, X.-Q. (2018). Accurate quantification of toxic elements in medicine food homologous plants using ICP-MS/MS. Food Chem. 245, 692–697. doi: 10.1016/j.foodchem.2017.10.136
Fu, Z.-S., Xi, S.-H. (2020). The effects of heavy metals on human metabolism. Toxicol. Mech. Method 30 (3), 167–176. doi: 10.1080/15376516.2019.1701594
Fukuda, N., Kitajima, N., Terada, Y., Abe, T., Nakai, I., Hokura, A. (2020). Visible cellular distribution of cadmium and zinc in the hyperaccumulator Arabidopsis halleri ssp. gemmifera determined by 2-D X-ray fluorescence imaging using high-energy synchrotron radiation. Metallomics 12 (2), 193–203. doi: 10.1039/c9mt00243j
Genchi, G., Sinicropi, M. S., Lauria, G., Carocci, A., Catalano, A. (2020). The effects of cadmium toxicity. Int. J. Env. Res. Pub He 17 (11), 3782. doi: 10.3390/ijerph17113782
Ghatak, A., Chaturvedi, P., Paul, P., Agrawal, G. K., Rakwal, R., Kim, S. T., et al. (2017). Proteomics survey of Solanaceae family: Current status and challenges ahead. J. Proteomics 169, 41–57. doi: 10.1016/j.jprot.2017.05.016
Ghoma, W. E. O., Sevik, H., Isinkaralar, K. (2022). Using indoor plants as biomonitors for detection of toxic metals by tobacco smoke. Air Qual Atmos Hlth 15 (3), 415–424. doi: 10.1007/s11869-021-01146-z
Ghori, N. H., Ghori, T., Hayat, M. Q., Imadi, S. R., Gul, A., Altay, V., et al. (2019). Heavy metal stress and responses in plants. Int. J. Environ. Sci. Te 16 (3), 1807–1828. doi: 10.1007/s13762-019-02215-8
Ghuge, S. A., Nikalje, G. C., Kadam, U. S., Suprasanna, P., Hong, J.-C. (2023). Comprehensive mechanisms of heavy metal toxicity in plants, detoxification, and remediation. J. Hazard Mater 450, 131039. doi: 10.1016/j.jhazmat.2023.131039
Grillet, L., Schmidt, W. (2017). The multiple facets of root iron reduction. J. Exp. Bot. 68 (18), 5021–5027. doi: 10.1093/jxb/erx320
Guo, Z.-L., Gao, Y.-H., Yuan, X.-Q., Yuan, M.-X., Huang, L., Wang, S.-C., et al. (2023). Effects of heavy metals on stomata in plants: A review. Int. J. Mol. Sci. 24 (11), 9302. doi: 10.3390/ijms24119302
Guo, C.-Y., Lv, L.-J., Liu, Y.-C., Ji, M.-Y., Zang, E.-H., Liu, Q., et al. (2023). Applied analytical methods for detecting heavy metals in medicinal plants. Crit. Rev. Anal. Chem. 53 (2), 339–359. doi: 10.1080/10408347.2021.1953371
Gupta, D. K., Srivastava, S., Huang, H.-G., Romero-Puertas, M. C., Sandalio, L. M. (2011). “Arsenic tolerance and Detoxification Mechanisms in Plants,” in Detoxification of Heavy Metals, vol. . p . Eds. Sherameti, I., Varma, A. (Berlin, Heidelberg: Springer Berlin Heidelberg), 169–179. doi: 10.1007/978-3-642-21408-0_9
Gupta, D. K., Vandenhove, H., Inouhe, M. (2013). “Role of Phytochelatins in Heavy Metal Stress and Detoxification Mechanisms in Plants,” in Heavy Metal Stress in Plants. Eds. Gupta, D. K., Corpas, F. J., Palma, J. M. (Berlin, Heidelberg: Springer Berlin Heidelberg), 73–94. doi: 10.1007/978-3-642-38469-1_4
Han, M.-X., Ullah, H., Yang, H., Yu, G., You, S.-H., Liu, J., et al. (2023). Cadmium uptake and membrane transport in roots of hyperaccumulator Amaranthus hypochondriacus L. Environ. pollut. 331, 121846. doi: 10.1016/j.envpol.2023.121846
Han, M.-X., Yang, H., Yu, G., Jiang, P.-P., You, S.-H., Zhang, L., et al. (2022). Application of Non-invasive Micro-test Technology (NMT) in environmental fields: A comprehensive review. Ecotoxicol Environ. Saf. 240, 113706. doi: 10.1016/j.ecoenv.2022.113706
Hara, N., Tchoudinov, G., Filipponi, A., Di Cicco, A. (2023). Local structure of solid and liquid Au as a function of temperature by x-ray absorption spectroscopy. Phys. Rev. B 107 (18), 184107. doi: 10.1103/PhysRevB.107.184107
Hou, P.-C., Wang, F.-F., Luo, B., Li, A.-X., Wang, C., Shabala, L., et al. (2021). Antioxidant enzymatic activity and osmotic adjustment as components of the drought tolerance mechanism in carex duriuscula. Plants 10 (3), 436. doi: 10.3390/plants10030436
Howden, R., Andersen, C. R., Goldsbrough, P. B., Cobbett, C. S. (1995a). A Cadmium-Sensitive, Glutathione-deficient mutant of arabidopsis thaliana. Plant Physiol. 107 (4), 1067–1073. doi: 10.1104/pp.107.4.1067
Howden, R., Goldsbrough, P. B., Andersen, C. R., Cobbett, C. S. (1995b). Cadmium-Sensitive, cad1 Mutants of Arabidopsis thaliana Are Phytochelatin Deficient. Plant Physiol. 107 (4), 1059–1066. doi: 10.1104/pp.107.4.1059
Hu, Q.-F., Wang, R., Hu, L., Chen, R., Yu, X.-J., Shao, J.-F. (2023). The potential of bamboo seeds for natural biofortification of dietary zinc and iron. NPJ Sci. Food 7 (1), 15. doi: 10.1038/s41538-023-00192-4
Hu, P.-Y., Wang, X., Yang, L., Yang, H.-Y., Tang, Y.-Y., Luo, H., et al. (2018). Speciation of mercury by hydride generation ultraviolet atomization-atomic fluorescence spectrometry without chromatographic separation. Microchem J. 143, 228–233. doi: 10.1016/j.microc.2018.08.013
Hu, H.-Q., Zhao, J.-T., Wang, L.-M., Shang, L.-H., Cui, L.-W., Gao, Y.-X., et al. (2020). Synchrotron-based techniques for studying the environmental health effects of heavy metals: Current status and future perspectives. TrAC-Trend Anal. Chem. 122, 115721. doi: 10.1016/j.trac.2019.115721
Hu, Y., Zhou, X.-Q., Shi, A., Yu, Y.-S., Rensing, C., Zhang, T.-X., et al. (2023). Exogenous silicon promotes cadmium (Cd) accumulation in Sedum alfredii Hance by enhancing Cd uptake and alleviating Cd toxicity. Front. Plant Sci. 14. doi: 10.3389/fpls.2023.1134370
Huang, J.-Q., Hu, X., Zhang, J.-R., Li, K.-X., Yan, Y., Xu, X.-B. (2006). The application of inductively coupled plasma mass spectrometry in pharmaceutical and biomedical analysis. J. Pharm. BioMed. Anal. 40 (2), 227–234. doi: 10.1016/j.jpba.2005.11.014
Huang, W.-L., Jiao, J., Ru, M., Bai, Z.-Q., Yuan, H.-L., Bao, Z.-A., et al. (2018). Localization and speciation of chromium in coptis chinensis franch. using Synchrotron Radiat. X-ray Technol. Laser Ablation ICP-MS. Sci. Rep-UK 8 (1), 8603. doi: 10.1038/s41598-018-26774-x
Islam, F., Yasmeen, T., Ali, Q., Ali, S., Arif, M. S., Hussain, S., et al. (2014). Influence of Pseudomonas aeruginosa as PGPR on oxidative stress tolerance in wheat under Zn stress. Ecotoxicol Environ. Saf. 104, 285–293. doi: 10.1016/j.ecoenv.2014.03.008
Izol, E., Çiçek, I., BehÇEt, L., Kaya, E., Tarhan, A. (2023). Trace element analysis of some medicinal and aromatic plant taxa by ICP-MS. Türk Doğa ve Fen Dergisi 12 (1), 21–29. doi: 10.46810/tdfd.1113610
Jaiswal, A., Verma A, P. J. (2018). Detrimental effects of heavy metals in soil, plants, and aquatic ecosystems and in humans. J. Environ. Pathol. Tox 37 (3), 183–197. doi: 10.1615/JEnvironPatholToxicolOncol.2018025348
Jamla, M., Khare, T., Joshi, S., Patil, S., Penna, S., Kumar, V. (2021). Omics approaches for understanding heavy metal responses and tolerance in plants. Curr. Plant Biol. 27, 100213. doi: 10.1016/j.cpb.2021.100213
Janovszky, P., Kéri, A., Palásti, D. J., Brunnbauer, L., Domoki, F., Limbeck, A., et al. (2023). Quantitative elemental mapping of biological tissues by laser-induced breakdown spectroscopy using matrix recognition. Sci. Rep-UK 13 (1), 10089. doi: 10.1038/s41598-023-37258-y
Jedynak, L., Kowalska, J., Kossykowska, M., Golimowski, J. (2010). Studies on the uptake of different arsenic forms and the influence of sample pretreatment on arsenic speciation in White mustard (Sinapis alba). Microchem J. 94 (2), 125–129. doi: 10.1016/j.microc.2009.10.001
Jin, M.-T., Yuan, H., Liu, B., Peng, J.-J., Xu, L.-P., Yang, D.-Z. (2020). Review of the distribution and detection methods of heavy metals in the environment. Anal. Methods-UK 12 (48), 5747–5766. doi: 10.1039/D0AY01577F
Jones, M. W. M., Kopittke, P. M., Casey, L., Reinhardt, J., Blamey, F. P. C., van der Ent, A. (2020). Assessing radiation dose limits for X-ray fluorescence microscopy analysis of plant specimens. Ann. Bot. 125 (4), 599–610. doi: 10.1093/aob/mcz195
Kartha., A. J. A., PP, S. (2021). Elemental analysis of Andrographis echioides (L.)Nees. leaves, a potential pharmaceutical plant using atomic absorption spectroscopy (AAS). Asian J. Pharm. Res. Dev. 9 (3), 43–47. doi: 10.22270/ajprd.v9i3.974
Kaur, R., Das, S., Bansal, S., Singh, G., Sardar, S., Dhar, H., et al. (2021). Heavy metal stress in rice: Uptake, transport, signaling, and tolerance mechanisms. Physiol. Plantarum 173 (1), 430–448. doi: 10.1111/ppl.13491
Keyster, M., Niekerk, L.-A., Basson, G., Carelse, M., Bakare, O., Ludidi, N., et al. (2020). Decoding heavy metal stress signalling in plants: towards improved food security and safety. Plants 9 (12), 1781. doi: 10.3390/plants9121781
Khan, M., Samrana, S., Zhang, Y., Malik, Z., Khan, M. D., Zhu, S.-J. (2020). Reduced glutathione protects subcellular compartments from pb-induced ROS injury in leaves and roots of upland cotton (Gossypium hirsutum L.). Front. Plant Sci. 11. doi: 10.3389/fpls.2020.00412
Kim, J. Y., Park, J., Choi, J., Kim, J. (2020). Determination of metal concentration in road-side trees from an industrial area using laser ablation inductively coupled plasma mass spectrometry. Minerals 10 (2), 175. doi: 10.3390/min10020175
Kiran, Bharti, R., Sharma, R. (2022). Effect of heavy metals: An overview. Materials Today: Proc. 51, 880–885. doi: 10.1016/j.matpr.2021.06.278
Kobayashi, T., Nozoye, T., Nishizawa, N. K. (2019). Iron transport and its regulation in plants. Free Radical Bio Med. 133, 11–20. doi: 10.1016/j.freeradbiomed.2018.10.439
Kohatsu, M. Y., Lange, C. N., Pelegrino, M. T., Pieretti, J. C., Tortella, G., Rubilar, O., et al. (2021). Foliar spraying of biogenic CuO nanoparticles protects the defence system and photosynthetic pigments of lettuce (Lactuca sativa). J. Clean Prod 324, 129264. doi: 10.1016/j.jclepro.2021.129264
Kopittke, P. M., Punshon, T., Paterson, D. J., Tappero, R. V., Wang, P., Blamey, F. P. C., et al. (2018). Synchrotron-based X-ray fluorescence microscopy as a technique for imaging of elements in plants. Plant Physiol. 178 (2), 507–523. doi: 10.1104/pp.18.00759
Krayem, M., Khatib, S. E., Hassan, Y., Deluchat, V., Labrousse, P. (2021). In search for potential biomarkers of copper stress in aquatic plants. Aquat. Toxicol. 239, 105952. doi: 10.1016/j.aquatox.2021.105952
Kunene, S. C., Lin, K.-S., Mdlovu, N. V., Lin, Y.-S., Mdlovu, N. B. (2020). Speciation and fate of toxic cadmium in contaminated paddy soils and rice using XANES/EXAFS spectroscopy. J. Hazard Mater 383, 121167. doi: 10.1016/j.jhazmat.2019.121167
Lan, X.-Y., He, Q.-S., Yang, B., Yan, Y.-Y., Li, X.-Y., Xu, F.-L. (2020). Influence of Cd exposure on H+ and Cd2+ fluxes in the leaf, stem and root of a novel aquatic hyperaccumulator-Microsorum pteropus. Chemosphere 249, 126552. doi: 10.1016/j.chemosphere.2020.126552
Lancaster, S. T., Brombach, C.-C., Corns, W. T., Feldmann, J., Krupp, E. M. (2019). Determination of methylmercury using liquid chromatography-photochemical vapour generation-atomic fluorescence spectroscopy (LC-PVG-AFS): a simple, green analytical method. J. Anal. Atom Spectrom 34 (6), 1166–1172. doi: 10.1039/C9JA00037B
Lao, R.-M., Guo, Y.-Y., Hao, W.-X., Fang, W.-J., Li, H.-Y., Zhao, Z.-W., et al. (2023). The role of lignin in the compartmentalization of cadmium in maize roots is enhanced by mycorrhiza. J. Fungi 9 (8), 852. doi: 10.3390/jof9080852
Leng, Y., Li, Y., Wen, Y., Zhao, H., Wang, Q., Li, S.-W. (2020). Transcriptome analysis provides molecular evidences for growth and adaptation of plant roots in cadimium-contaminated environments. Ecotoxicol Environ. Saf. 204, 111098. doi: 10.1016/j.ecoenv.2020.111098
Li, J.-T., Gurajala, H. K., Wu, L.-H., van der Ent, A., Qiu, R.-L., Baker, A. J. M., et al. (2018). Hyperaccumulator plants from China: A synthesis of the current state of knowledge. Environ. Sci. Technol. 52 (21), 11980–11994. doi: 10.1021/acs.est.8b01060
Li, L.-Z., Tu, C., Peijnenburg, W. J. G. M., Luo, Y.-M. (2017a). Characteristics of cadmium uptake and membrane transport in roots of intact wheat (Triticum aestivum L.) seedlings. Environ. pollut. 221, 351–358. doi: 10.1016/j.envpol.2016.11.085
Li, L.-Z., Tu, C., Wu, L.-H., Peijnenburg, W. J. G. M., Ebbs, S., Luo, Y.-M. (2017b). Pathways of root uptake and membrane transport of Cd2+ in the zinc/cadmium hyperaccumulating plant Sedum plumbizincicola. Environ. Toxicol. Chem. 36 (4), 1038–1046. doi: 10.1002/etc.3625
Li, X., Yang, D.-Z., Yuan, H., Liang, J.-P., Xu, T., Zhao, Z.-L., et al. (2019). Detection of trace heavy metals using atmospheric pressure glow discharge by optical emission spectra. High Volt 4 (3), 228–233. doi: 10.1049/hve.2019.0084
Li, K.-J., Yang, H.-Y., Yuan, X., Zhang, M. (2021). Recent developments of heavy metals detection in traditional Chinese medicine by atomic spectrometry. Microchem J. 160, 105726. doi: 10.1016/j.microc.2020.105726
Li, Y.-F., Zhao, J.-T., Li, Y.-Y., Xu, X.-H., Cui, L.-W., Zhang, B.-W., et al. (2015a). Studies on the environmental health effects and ecotoxicology of mercury by synchrotron radiation-based techniques. Scientia Sin. Chimica 45 (6), 597. doi: 10.1360/N032014-00314
Li, Y.-F., Zhao, J.-T., Qu, Y., Gao, Y.-X., Guo, Z.-H., Liu, Z.-L., et al. (2015b). Synchrotron radiation techniques for nanotoxicology. Nanomed-Nanotechnol 11 (6), 1531–1549. doi: 10.1016/j.nano.2015.04.008
Li, Y.-X., Zhu, N.-L., Liang, X.-J., Zheng, L.-R., Zhang, C.-X., Li, Y.-F., et al. (2020). A comparative study on the accumulation, translocation and transformation of selenite, selenate, and SeNPs in a hydroponic-plant system. Ecotoxicol Environ. Saf. 189, 109955. doi: 10.1016/j.ecoenv.2019.109955
Liang, J., Fang, H.-L., Zhang, T.-L., Wang, X.-X., Liu, Y.-D. (2017). Heavy metal in leaves of twelve plant species from seven different areas in Shanghai, China. Urban For Urban Gree 27, 390–398. doi: 10.1016/j.ufug.2017.03.006
Liu, M.-Y., Lou, H.-Q., Chen, W.-W., Piñeros, M. A., Xu, J.-M., Fan, W., et al. (2018). Two citrate transporters coordinately regulate citrate secretion from rice bean root tip under aluminum stress. Plant Cell Environ. 41 (4), 809–822. doi: 10.1111/pce.13150
Liu, J., Luo, L.-Q. (2019). Uptake and transport of Pb across the iron plaque of waterlogged dropwort (Oenanthe javanica DC.) based on micro-XRF and XANES. Plant Soil 441 (1-2), 191–205. doi: 10.1007/s11104-019-04106-w
Liu, Y.-H., Xue, D.-S., Li, W.-J., Li, C.-F., Wan, B. (2020). A simple method for the precise determination of multi-elements in pyrite and magnetite by ICP-MS and ICP-OES with matrix removal. Microchem J. 158, 105221. doi: 10.1016/j.microc.2020.105221
Liu, J., Yu, G., Jiang, P.-P., Zhang, X.-F., Meng, D.-J., Chen, Z., et al. (2020). Interaction of Mn and Cd during their uptake in Celosia argentea differs between hydroponic and soil systems. Plant Soil 450 (1), 323–336. doi: 10.1007/s11104-020-04514-3
Liu, J., Zhang, X.-H., You, S.-H., Wu, Q.-X., Chen, S.-M., Zhou, K.-N. (2014). Cr(VI) removal and detoxification in constructed wetlands planted with Leersia hexandra Swartz. Ecol. Eng. 71, 36–40. doi: 10.1016/j.ecoleng.2014.07.047
López-Fernández, H., de, S., Pessôa, G., Arruda, M. A. Z., Capelo-Martínez, J. L., Fdez-Riverola, F., et al. (2016). LA-iMageS: a software for elemental distribution bioimaging using LA-ICP-MS data. J. Cheminformatics 8 (1), 65. doi: 10.1186/s13321-016-0178-7
Ma, Y.-S., Jie, H.-D., Tang, Y.-Y., Xing, H.-C., Jie, Y.-C. (2022). The role of hemicellulose in cadmium tolerance in ramie (Boehmeria nivea (L.) gaud.). Plants 11 (15), 1941. doi: 10.3390/plants11151941
Ma, Y.-H., Zhang, P., Zhang, Z.-Y., He, X., Li, Y.-Y., Zhang, J., et al. (2015). Origin of the different phytotoxicity and biotransformation of cerium and lanthanum oxide nanoparticles in cucumber. Nanotoxicology 9 (2), 262–270. doi: 10.3109/17435390.2014.921344
Mardones, J. I., Shabala, L., Shabala, S., Dorantes-Aranda, J. J., Seger, A., Hallegraeff, G. M. (2018). Fish gill damage by harmful microalgae newly explored by microelectrode ion flux estimation techniques. Harmful Algae 80, 55–63. doi: 10.1016/j.hal.2018.09.004
Marguí, E., Queralt, I., Hidalgo, M. (2009). Application of X-ray fluorescence spectrometry to determination and quantitation of metals in vegetal material. TrAC-Trend Anal. Chem. 28 (3), 362–372. doi: 10.1016/j.trac.2008.11.011
Mastelaro, V. R., Zanotto, E. D. (2018). X-ray absorption fine structure (XAFS) studies of oxide glasses-A 45-year overview. Materials 11 (2), 204. doi: 10.3390/ma11020204
McGladdery, C., Weindorf, D. C., Chakraborty, S., Li, B., Paulette, L., Podar, D., et al. (2018). Elemental assessment of vegetation via portable X-ray fluorescence (PXRF) spectrometry. J. Environ. Manage 210, 210–225. doi: 10.1016/j.jenvman.2018.01.003
Meharg, A. A., Lombi, E., Williams, P. N., Scheckel, K. G., Feldmann, J., Raab, A., et al. (2008). Speciation and localization of arsenic in white and brown rice grains. Environ. Sci. Technol. 42 (4), 1051–1057. doi: 10.1021/es702212p
Meng, B., Feng, X.-B., Qiu, G.-L., Anderson, C. W. N., Wang, J.-X., Zhao, L. (2014). Localization and Speciation of mercury in brown rice with implications for pan-asian public health. Environ. Sci. Technol. 48 (14), 7974–7981. doi: 10.1021/es502000d
Mishra, B., McDonald, L. M., Roy, M., Lanzirotti, A., Myneni, S. C. B. (2020). Uptake and speciation of zinc in edible plants grown in smelter contaminated soils. PloS One 15 (4), e0226180. doi: 10.1371/journal.pone.0226180
Mleczek, M., Gąsecka, M., Waliszewska, B., Magdziak, Z., Szostek, M., Rutkowski, P., et al. (2018). Salix viminalis L.-A highly effective plant in phytoextraction of elements. Chemosphere 212, 67–78. doi: 10.1016/j.chemosphere.2018.08.055
Mondal, R., Kumar, A., Shabnam, A. A., Chaturvedi, A. K. (2022). Elucidation of molecular and physiological mechanisms addressing integrated omic approaches for heavy metal stress tolerance in crops. Crop Pasture Sci. 73 (8), 927–942. doi: 10.1071/cp21467
Mugnai, S., Azzarello, E., Baluška, F., Mancuso, S. (2012). Local root apex hypoxia induces NO-mediated hypoxic acclimation of the entire root. Plant Cell Physiol. 53 (5), 912–920. doi: 10.1093/pcp/pcs034
Nadeem, S., Saifullah, Malhi, S. S., Zia, M. H., Naeem, A., Bibi, S., et al. (2010). Role of mineral nutrition in minimizing cadmium accumulation by plants. J. Sci. Food Agric. 90 (6), 925–937. doi: 10.1002/jsfa.3916
Nagajyoti, P. C., Lee, K. D., Sreekanth, T. V. M. (2010). Heavy metals, occurrence and toxicity for plants: a review. Environ. Chem. Lett. 8 (3), 199–216. doi: 10.1007/s10311-010-0297-8
Narewski, U., Werner, G., Schulz, H., Vogt, C. (2000). Application of laser ablation inductively coupled mass spectrometry (LA-ICP-MS) for the determination of major, minor, and trace elements in bark samples. Fresenius J. Anal. Chem. 366 (2), 167–170. doi: 10.1007/s002160050032
Newville, M. (2014). Fundamentals of XAFS. Rev. Mineral Geochem 78 (1), 33–74. doi: 10.2138/rmg.2014.78.2
Nunes, M. A. G., Voss, M., Corazza, G., Flores, E. M. M., Dressler, V. L. (2016). External calibration strategy for trace element quantification in botanical samples by LA-ICP-MS using filter paper. Anal. Chim. Acta 905, 51–57. doi: 10.1016/j.aca.2015.11.049
Omeje, K. O., Ezema, B. O., Okonkwo, F., Onyishi, N. C., Ozioko, J., Rasaq, W. A., et al. (2021). Quantification of heavy metals and pesticide residues in widely consumed Nigerian food crops using atomic absorption spectroscopy (AAS) and gas chromatography (GC). Toxins 13 (12), 870. doi: 10.3390/toxins13120870
Paape, T., Heiniger, B., Santo Domingo, M., Clear, M. R., Lucas, M. M., Pueyo, J. J. (2022). Genome-wide association study reveals complex genetic architecture of cadmium and mercury accumulation and tolerance traits in medicago truncatula. Front. Plant Sci. 12. doi: 10.3389/fpls.2021.806949
Pan, H.-J., Feng, L.-X., Lu, Y.-L., Han, Y.-C., Xiong, J.-P., Li, H.-M. (2022). Calibration strategies for laser ablation ICP-MS in biological studies: A review. TrAC-Trend Anal. Chem. 156, 116710. doi: 10.1016/j.trac.2022.116710
Park, H. C., Hwang, J. E., Jiang, Y., Kim, Y. J., Kim, S. H., Nguyen, X. C., et al. (2019). Functional characterisation of two phytochelatin synthases in rice (Oryza sativa cv. Milyang 117) that respond to cadmium stress. Plant Biol. 21 (5), 854–861. doi: 10.1111/plb.12991
Park, J., Song, W.-Y., Ko, D., Eom, Y., Hansen, T. H., Schiller, M., et al. (2012). The phytochelatin transporters AtABCC1 and AtABCC2 mediate tolerance to cadmium and mercury. Plant J. 69 (2), 278–288. doi: 10.1111/j.1365-313X.2011.04789.x
Pei, J., Pan, X.-Y., Wei, G.-H., Hua, Y. (2023). Research progress of glutathione peroxidase family (GPX) in redoxidation. Front. Pharmacol. 14. doi: 10.3389/fphar.2023.1147414
Pell, A., Kokkinis, G., Malea, P., Pergantis, S. A., Rubio, R., López-Sánchez, J. F. (2013). LC-ICP-MS analysis of arsenic compounds in dominant seaweeds from the Thermaikos Gulf (Northern Aegean Sea, Greece). Chemosphere 93 (9), 2187–2194. doi: 10.1016/j.chemosphere.2013.08.003
Penanes, P. A., Galán, A. R., Huelga-Suarez, G., Rodríguez-Castrillón, J Á., Moldovan, M., Garcia Alonso, J. I. (2022). Isotopic measurements using ICP-MS: a tutorial review. J. Anal. Atom Spectrom 37 (4), 701–726. doi: 10.1039/D2JA00018K
Peng, C.-N., Zhou, J.-T., Li, C., Chen, Y.-X., Huo, Q., Xie, F. (2022). Research progress on speciation analysis of arsenic in traditional Chinese medicine. Open Chem. 20 (1), 23–39. doi: 10.1515/chem-2022-0123
Qin, G.-W., Niu, Z.-D., Yu, J.-D., Li, Z.-H., Ma, J.-Y., Xiang, P. (2021). Soil heavy metal pollution and food safety in China: Effects, sources and removing technology. Chemosphere 267, 129205. doi: 10.1016/j.chemosphere.2020.129205
Raab, A., Schat, H., Meharg, A. A., Feldmann, J. (2005). Uptake, translocation and transformation of arsenate and arsenite in sunflower (Helianthus annuus): formation of arsenic-phytochelatin complexes during exposure to high arsenic concentrations. New Phytol. 168 (3), 551–558. doi: 10.1111/j.1469-8137.2005.01519.x
Rahman, S. U., Nawaz, M. F., Gul, S., Yasin, G., Hussain, B., Li, Y.-L., et al. (2022). State-of-the-art OMICS strategies against toxic effects of heavy metals in plants: A review. Ecotoxicol Environ. Saf. 242, 113952. doi: 10.1016/j.ecoenv.2022.113952
Rai, P. K., Lee, S. S., Zhang, M., Tsang, Y. F., Kim, K.-H. (2019). Heavy metals in food crops: Health risks, fate, mechanisms, and management. Environ. Int. 125, 365–385. doi: 10.1016/j.envint.2019.01.067
Ramakrishna, P. (2023). Grain scans: fast X-ray fluorescence microscopy for high-throughput elemental mapping of rice seeds. Plant Physiol. 191 (3), 1465–1467. doi: 10.1093/plphys/kiac598
Raza, A. (2022). Metabolomics: a systems biology approach for enhancing heat stress tolerance in plants. Plant Cell Rep. 41 (3), 741–763. doi: 10.1007/s00299-020-02635-8
Raza, A., Tabassum, J., Zahid, Z., Charagh, S., Bashir, S., Barmukh, R., et al. (2022). Advances in “Omics” Approaches for improving toxic metals/metalloids tolerance in plants. Front. Plant Sci. 12. doi: 10.3389/fpls.2021.794373
Ren, J., Chi, H.-Y., Tan, L., Peng, Y.-K., Li, G.-C., Meng-Jung Li, M., et al. (2023). Semi-quantitative determination of active sites in heterogeneous catalysts for photo/electrocatalysis. J. Mater Chem. A 11 (6), 2528–2543. doi: 10.1039/d2ta09033c
Rogowska, A., Pączkowski, C., Szakiel, A. (2022). Modulation of steroid and triterpenoid metabolism in calendula officinalis plants and hairy root cultures exposed to cadmium stress. Int. J. Mol. Sci. 23 (10), 5640. doi: 10.3390/ijms23105640
Rudman, R. (1999). A practical guide for the preparation of specimens for X-ray fluorescence and X-ray diffraction analysis. J. Chem. Educ. 76 (6), 762. doi: 10.1021/ed076p762
Rukhsar Ul, H., Kausar, A., Hussain, S., Javed, T., Zafar, S., Anwar, S., et al. (2023). Zinc oxide nanoparticles as potential hallmarks for enhancing drought stress tolerance in wheat seedlings. Plant Physiol. Biochem. 195, 341–350. doi: 10.1016/j.plaphy.2023.01.014
Saeed Ur, R., Khalid, M., Hui, N., Kayani, S.-I., Tang, K. (2020). Diversity and versatile functions of metallothioneins produced by plants: A review. Pedosphere 30 (5), 577–588. doi: 10.1016/S1002-0160(20)60022-4
Saroop, S., Tamchos, S. (2021). “4-Monitoring and impact assessment approaches for heavy metals,” in Heavy Metals in the Environment. Eds. Kumar, V., Sharma, A., Cerdà, A. (Amsterdam, Netherlands, NL: Elsevier), 57–86. doi: 10.1016/B978-0-12-821656-9.00004-3
Sarret, G., Smits, E. A. H. P., Michel, H. C., Isaure, M. P., Zhao, F.-J., Tappero, R. (2013). “Use of synchrotron-Based Techniques to Elucidate Metal Uptake and Metabolism in Plants,” in Advances in Agronomy. Ed. Sparks, D. L. (Amsterdam, Netherlands (NL: Elsevier), 1–82. doi: 10.1016/b978-0-12-407247-3.00001-9
Shahid, M., Dumat, C., Khalid, S., Schreck, E., Xiong, T.-T., Niazi, N. K. (2017). Foliar heavy metal uptake, toxicity and detoxification in plants: A comparison of foliar and root metal uptake. J. Hazard Mater 325, 36–58. doi: 10.1016/j.jhazmat.2016.11.063
Shetty, R., Vidya, C. S.-N., Prakash, N. B., Lux, A., Vaculík, M. (2021). Aluminum toxicity in plants and its possible mitigation in acid soils by biochar: A review. Sci. Total Environ. 765, 142744. doi: 10.1016/j.scitotenv.2020.142744
Shi, J.-Y., Gras, M. A., Silk, W. K. (2009). Laser ablation ICP-MS reveals patterns of copper differing from zinc in growth zones of cucumber roots. Planta 229 (4), 945–954. doi: 10.1007/s00425-008-0861-7
Shi, L., Li, J., Palansooriya, K. N., Chen, Y.-H., Hou, D.-Y., Meers, E., et al. (2023). Modeling phytoremediation of heavy metal contaminated soils through machine learning. J. Hazard Mater 441, 129904. doi: 10.1016/j.jhazmat.2022.129904
Sinaei, M., Loghmani, M., Bolouki, M. (2018). Application of biomarkers in brown algae (Cystoseria indica) to assess heavy metals (Cd, Cu, Zn, Pb, Hg, Ni, Cr) pollution in the northern coasts of the Gulf of Oman. Ecotoxicol Environ. Saf. 164, 675–680. doi: 10.1016/j.ecoenv.2018.08.074
Singh, S., Parihar, P., Singh, R., Singh, V. P., Prasad, S. M. (2016). Heavy metal tolerance in plants: role of transcriptomics, proteomics, metabolomics, and ionomics. Front. Plant Sci. 6. doi: 10.3389/fpls.2015.01143
Singh, S., Tripathi, D. K., Singh, S., Sharma, S., Dubey, N. K., Chauhan, D. K., et al. (2017). Toxicity of aluminium on various levels of plant cells and organism: A review. Environ. Exp. Bot. 137, 177–193. doi: 10.1016/j.envexpbot.2017.01.005
Smita, K., Dubey, R. S., Tripathi, R. D., Debasis, C., Trivedi, P. K. (2015). Omics and biotechnology of arsenic stress and detoxification in plants: current updates and prospective. Environ. Int. 74, 221–230. doi: 10.1016/j.envint.2014.10.019
Souri, Z., Karimi, N., Norouzi, L., Ma, X. (2020). Elucidating the physiological mechanisms underlying enhanced arsenic hyperaccumulation by glutathione modified superparamagnetic iron oxide nanoparticles in Isatis cappadocica. Ecotoxicol Environ. Saf. 206, 111336. doi: 10.1016/j.ecoenv.2020.111336
Souri, Z., Karimi, N., Sandalio, L. M. (2017). Arsenic hyperaccumulation strategies: an overview. Front. Cell Dev. Biol. 5. doi: 10.3389/fcell.2017.00067
Su, Y.-H., McGrath, S. P., Zhu, Y.-G., Zhao, F.-J. (2008). Highly efficient xylem transport of arsenite in the arsenic hyperaccumulator Pteris vittata. New Phytol. 180 (2), 434–441. doi: 10.1111/j.1469-8137.2008.02584.x
Sun, D.-D., Fang, X.-M., Xiao, C.-B., Ma, Z., Huang, X.-M., Su, J.-R., et al. (2021). Kinase SnRK1.1 regulates nitrate channel SLAH3 engaged in nitrate-dependent alleviation of ammonium toxicity. Plant Physiol. 186 (1), 731–749. doi: 10.1093/plphys/kiab057
Sun, H.-W., Guo, X.-L., Qi, X.-J., Feng, F., Xie, X.-N., Zhang, Y.-L., et al. (2021). SPL14/17 act downstream of strigolactone signalling to modulate rice root elongation in response to nitrate supply. Plant J. 106 (3), 649–660. doi: 10.1111/tpj.15188
Suquila, F. A. C., Scheel, G. L., de Oliveira, F. M., Tarley, C. R. T. (2019). Assessment of ultrasound-assisted extraction combined with supramolecular solvent-based microextraction for highly sensitive cadmium determination in medicinal plant sample by TS-FF-AAS. Microchem J. 145, 1071–1077. doi: 10.1016/j.microc.2018.12.011
Tang, P., Tan, W.-Y., Deng, G.-Y., Zhang, Y.-T., Xu, S., Wang, Q.-J., et al. (2023). Understanding pseudocapacitance mechanisms by synchrotron X-ray analytical techniques. Energy Environ. Mater 6 (4), e1261. doi: 10.1002/eem2.12619
Tang, Y.-L., Zhang, J.-J., Wang, L.-J., Wang, H., Long, H.-C., Yang, L.-Y., et al. (2023). Water deficit aggravated the inhibition of photosynthetic performance of maize under mercury stress but is alleviated by brassinosteroids. J. Hazard Mater 443, 130365. doi: 10.1016/j.jhazmat.2022.130365
Thakur, M., Praveen, S., Divte, P. R., Mitra, R., Kumar, M., Gupta, C. K., et al. (2022). Metal tolerance in plants: Molecular and physicochemical interface determines the “not so heavy effect” of heavy metals. Chemosphere 287, 131957. doi: 10.1016/j.chemosphere.2021.131957
Theiner, S., Loehr, K., Koellensperger, G., Mueller, L., Jakubowski, N. (2020). Single-cell analysis by use of ICP-MS. J. Anal. Atom Spectrom 35 (9), 1784–1813. doi: 10.1039/D0JA00194E
Trejos, T., Almirall, J. R. (2005). Sampling strategies for the analysis of glass fragments by LA-ICP-MS: Part I. Micro-homogeneity study of glass and its application to the interpretation of forensic evidence. Talanta 67 (2), 388–395. doi: 10.1016/j.talanta.2005.01.042
Trejos, T., Montero, S., Almirall, J. R. (2003). Analysis and comparison of glass fragments by laser ablation inductively coupled plasma mass spectrometry (LA-ICP-MS) and ICP-MS. Anal. Bioanal Chem. 376 (8), 1255–1264. doi: 10.1007/s00216-003-1968-0
Tunçtürk, M., Rezaee Danesh, Y., Tunçtürk, R., Oral, E., Najafi, S., Nohutçu, L., et al. (2023). Safflower (Carthamus tinctorius L.) response to cadmium stress: morpho-physiological traits and mineral concentrations. Life 13 (1), 135. doi: 10.3390/life13010135
van der Ent, A., de Jonge, M. D., Echevarria, G., Aarts, M. G. M., Mesjasz-Przybyłowicz, J., Przybyłowicz, W. J., et al. (2022). Multimodal synchrotron X-ray fluorescence imaging reveals elemental distribution in seeds and seedlings of the Zn-Cd-Ni hyperaccumulator Noccaea caerulescens. Metallomics 14 (5), mfac026. doi: 10.1093/mtomcs/mfac026
Vareda, J. P., Valente, A. J. M., Durães, L. (2019). Assessment of heavy metal pollution from anthropogenic activities and remediation strategies: A review. J. Environ. Manage 246, 101–118. doi: 10.1016/j.jenvman.2019.05.126
Vigani, G., Bohic, S., Faoro, F., Vekemans, B., Vincze, L., Terzano, R. (2018). Cellular fractionation and nanoscopic X-ray fluorescence imaging analyses reveal changes of zinc distribution in leaf cells of iron-deficient plants. Front. Plant Sci. 9. doi: 10.3389/fpls.2018.01112
Vijayan, P., Willick, I. R., Lahlali, R., Karunakaran, C., Tanino, K. K. (2015). Synchrotron radiation sheds fresh light on plant research: the use of powerful techniques to probe structure and composition of plants. Plant Cell Physiol. 56 (7), 1252–1263. doi: 10.1093/pcp/pcv080
Vinogradova, N., Glukhov, A., Chaplygin, V., Kumar, P., Mandzhieva, S., Minkina, T., et al. (2023). The content of heavy metals in medicinal plants in various environmental conditions: A review. Horticulturae 9 (2), 239. doi: 10.3390/horticulturae9020239
Wang, J.-C., Chen, X.-F., Chu, S.-H., Hayat, K., Chi, Y.-W., Zhi, Y.-E., et al. (2021). Influence of Cd toxicity on subcellular distribution, chemical forms, and physiological responses of cell wall components towards short-term Cd stress in Solanum nigrum. Environ. Sci. pollut. R 28 (11), 13955–13969. doi: 10.1007/s11356-020-11505-5
Wang, H.-J., Cui, S.-P., Ma, L., Wang, Z.-Z., Wang, H.-B. (2021). Variations of arsenic forms and the role of arsenate reductase in three hydrophytes exposed to different arsenic species. Ecotoxicol Environ. Saf. 221, 112415. doi: 10.1016/j.ecoenv.2021.112415
Wang, S.-Q., Dai, H.-P., Ji, D.-D., Cui, S., Jiang, C.-Z., Skuza, L., et al. (2023). Influencing Factors of Bidens pilosa L. Hyperaccumulating Cadmium Explored by the Real-Time Uptake of Cd2+ Influx around Root Apexes under Different Exogenous Nutrient Ion Levels. Toxics 11 (3), 227. doi: 10.3390/toxics11030227
Wang, L.-Y., Peng, X.-L., Fu, H.-J., Huang, C., Li, Y.-P., Liu, Z.-M. (2020). Recent advances in the development of electrochemical aptasensors for detection of heavy metals in food. Biosens Bioelectron 147, 111777. doi: 10.1016/j.bios.2019.111777
Wilschefski, S. C., Baxter, M. R. (2019). Inductively coupled plasma mass spectrometry: introduction to analytical aspects. Clin. biochemist Rev. 40 (3), 115–133. doi: 10.33176/aacb-19-00024
Wojcieszek, J., Jiménez-Lamana, J., Bierla, K., Asztemborska, M., Ruzik, L., Jarosz, M., et al. (2019). Elucidation of the fate of zinc in model plants using single particle ICP-MS and ESI tandem MS. J. Anal. Atom Spectrom 34 (4), 683–693. doi: 10.1039/C8JA00390D
Wu, Y.-F., Li, X., Yu, L., Wang, T.-Q., Wang, J.-N., Liu, T.-T. (2022). Review of soil heavy metal pollution in China: Spatial distribution, primary sources, and remediation alternatives. Resour Conserv. Recy 181, 106261. doi: 10.1016/j.resconrec.2022.106261
Wu, H.-H., Shabala, L., Zhou, M.-X., Su, N.-N., Wu, Q., Ul-Haq, T., et al. (2019). Root vacuolar Na+ sequestration but not exclusion from uptake correlates with barley salt tolerance. Plant J. 100 (1), 55–67. doi: 10.1111/tpj.14424
Wu, Z.-C., Zhang, W.-J., Xu, S.-J., Shi, H.-Z., Wen, D., Huang, Y.-D., et al. (2018). Increasing ammonium nutrition as a strategy for inhibition of cadmium uptake and xylem transport in rice (Oryza sativa L.) exposed to cadmium stress. Environ. Exp. Bot. 155, 734–741. doi: 10.1016/j.envexpbot.2018.08.024
Wu, B., Zoriy, M., Chen, Y.-X., Becker, J. S. (2009). Imaging of nutrient elements in the leaves of Elsholtzia splendens by laser ablation inductively coupled plasma mass spectrometry (LA-ICP-MS). Talanta 78 (1), 132–137. doi: 10.1016/j.talanta.2008.10.061
Xia, S.-A., Leng, A.-Q., Lin, Y., Wu, L., Tian, Y.-F., Hou, X.-D., et al. (2019). Integration of flow injection capillary liquid electrode discharge optical emission spectrometry and microplasma-induced vapor generation: A system for detection of ultratrace hg and cd in a single drop of human whole blood. Anal. Chem. 91 (4), 2701–2709. doi: 10.1021/acs.analchem.8b04222
Xia, Y., Lv, Y.-Y., Yuan, Y.-X., Wang, G.-P., Chen, Y.-H., Zhang, H.-S., et al. (2012). Cloning and characterization of a type 1 metallothionein gene from the copper-tolerant plant Elsholtzia haichowensis. Acta Physiol. Plant 34 (5), 1819–1826. doi: 10.1007/s11738-012-0980-4
Xiao, W.-W., Liu, P., Wang, K., Yang, Z.-G., Wang, L. (2021). Relationship between ionomics and transcriptomics of rice plant in response to arsenite stress. Environ. Exp. Bot. 189, 104565. doi: 10.1016/j.envexpbot.2021.104565
Yamaji, N., Ma, J.-F. (2019). Bioimaging of multiple elements by high-resolution LA-ICP-MS reveals altered distribution of mineral elements in the nodes of rice mutants. Plant J. 99 (6), 1254–1263. doi: 10.1111/tpj.14410
Yan, G.-C., Hua, Y.-C., Jin, H., Huang, Q.-Y., Zhou, G.-F., Xu, Y.-M., et al. (2023). Sly-miR398 participates in cadmium stress acclimation by regulating antioxidant system and cadmium transport in tomato (Solanum lycopersicum). Int. J. Mol. Sci. 24 (3), 1953. doi: 10.3390/ijms24031953
Yang, J., Gao, M.-X., Hu, H., Ding, X.-M., Lin, H.-W., Wang, L., et al. (2016). OsCLT1, a CRT-like transporter 1, is required for glutathione homeostasis and arsenic tolerance in rice. New Phytol. 211 (2), 658–670. doi: 10.1111/nph.13908
Yang, J.-Y., Li, L.-X., Zhang, X., Wu, S.-B., Han, X.-H., Li, X., et al. (2022). Comparative Transcriptomics Analysis of Roots and Leaves under Cd Stress in Calotropis gigantea L. Int. J. Mol. Sci. 23 (6), 3329. doi: 10.3390/ijms23063329
Yang, J.-W., Sun, L., Shen, X., Dai, M., Ali, I., Peng, C.-S., et al. (2022). An overview of the methods for analyzing the chemical forms of metals in plants. Int. J. Phytoremediat 24 (13), 1418–1430. doi: 10.1080/15226514.2022.2033687
Yang, M., Tan, L., Xu, Y.-Y., Zhao, Y.-H., Cheng, F., Ye, S.-M., et al. (2015). Effect of low pH and aluminum toxicity on the photosynthetic characteristics of different fast-growing eucalyptus vegetatively propagated clones. PloS One 10 (6), e0130963. doi: 10.1371/journal.pone.0130963
Yang, Z., Yang, F., Liu, J.-L., Wu, H.-T., Yang, H., Shi, Y., et al. (2022). Heavy metal transporters: Functional mechanisms, regulation, and application in phytoremediation. Sci. Total Environ. 809, 151099. doi: 10.1016/j.scitotenv.2021.151099
Yang, L.-P., Zhu, J., Wang, P., Zeng, J., Tan, R., Yang, Y.-Z., et al. (2018). Effect of Cd on growth, physiological response, Cd subcellular distribution and chemical forms of Koelreuteria paniculata. Ecotoxicol Environ. Saf. 160, 10–18. doi: 10.1016/j.ecoenv.2018.05.026
Yin, Y.-C., Wang, Y.-Q., Liu, Y.-G., Zeng, G.-M., Hu, X.-J., Hu, X., et al. (2015). Cadmium accumulation and apoplastic and symplastic transport in Boehmeria nivea (L.) Gaudich on cadmium-contaminated soil with the addition of EDTA or NTA. RSC Adv. 5 (59), 47584–47591. doi: 10.1039/C5RA05717E
Yogarajah, N., Tsai, S. S. H. (2015). Detection of trace arsenic in drinking water: challenges and opportunities for microfluidics. Environ. Sci-Wat Res. 1 (4), 426–447. doi: 10.1039/c5ew00099h
Yu, X.-X., He, M., Chen, B.-B., Hu, B. (2020). Recent advances in single-cell analysis by inductively coupled plasma-mass spectrometry: A review. Anal. Chim. Acta 1137, 191–207. doi: 10.1016/j.aca.2020.07.041
Yuan, H.-Z., Cai, Y.-W., Yang, Z., Li, Q., Liu, E.-F., Yin, H.-B. (2021). Phosphorus removal from sediments by Potamogeton crispus: New high-resolution in-situ evidence for rhizosphere assimilation and oxidization-induced retention. J. Environ. Sci. 109, 181–192. doi: 10.1016/j.jes.2021.04.010
Yuan, X.-D., Chapman, R. L., Wu, Z.-Q. (2011). Analytical methods for heavy metals in herbal medicines. Phytochem. Anal. 22 (3), 189–198. doi: 10.1002/pca.1287
Yuan, H.-Y., Liu, Q.-Q., Fu, J.-H., Wang, Y.-J., Zhang, Y.-X., Sun, Y.-M., et al. (2023). Co-exposure of sulfur nanoparticles and Cu alleviate Cu stress and toxicity to oilseed rape Brassica napus L. J. Environ. Sci. 124, 319–329. doi: 10.1016/j.jes.2021.09.040
Zhang, X.-Y., Geng, L.-P., Gao, P.-P., Dong, J.-W., Zhou, C., Li, H.-B., et al. (2022). Bioimaging of Pb by LA-ICP-MS and Pb isotopic compositions reveal distributions and origins of Pb in wheat grain. Sci. Total Environ. 802, 149729. doi: 10.1016/j.scitotenv.2021.149729
Zhang, H.-H., Jiang, Z., Qin, R., Zhang, H.-N., Zou, J.-H., Jiang, W.-S., et al. (2014). Accumulation and cellular toxicity of aluminum in seedling of Pinus massoniana. BMC Plant Biol. 14 (1), 264. doi: 10.1186/s12870-014-0264-9
Zhang, D.-G., Lei, M., Wan, X.-M., Guo, G.-H., Zhao, X.-F., Liu, Y.-H. (2022). Responses of diversity and arsenic-transforming functional genes of soil microorganisms to arsenic hyperaccumulator (Pteris vittata L.)/pomegranate (Punica granatum L.) intercropping. Sci. Total Environ. 850, 157767. doi: 10.1016/j.scitotenv.2022.157767
Zhang, Y., Sun, R.-X., Wang, L., Zhu, Y., Tuyiringire, D., Yang, Y., et al. (2020). Physiological responses of Arthrobacter sp. JQ-1 cell interfaces to co-existed di-(2-ethylhexyl) phthalate (DEHP) and copper. Ecotoxicol Environ. Saf. 205, 111163. doi: 10.1016/j.ecoenv.2020.111163
Zhang, J.-Y., Tang, Z., Agathokleous, E., Zheng, G.-L., Xu, L., Li, P. (2023). Hormesis in the heavy metal accumulator plant Tillandsia ionantha under Cd exposure: Frequency and function of different biomarkers. Sci. Total Environ. 889, 164328. doi: 10.1016/j.scitotenv.2023.164328
Zhang, C.-H., Xu, B.-Y., Geng, W., Shen, Y.-D., Xuan, D.-J., Lai, Q.-X., et al. (2019). Comparative proteomic analysis of pepper (Capsicum annuum L.) seedlings under selenium stress. PeerJ 7, e8020. doi: 10.7717/peerj.8020
Zhang, D.-Y., Yang, S.-W., Ma, Q.-F., Sun, J.-N., Cheng, H.-Y., Wang, Y.-C., et al. (2020). Simultaneous multi-elemental speciation of As, Hg and Pb by inductively coupled plasma mass spectrometry interfaced with high-performance liquid chromatography. Food Chem. 313, 126119. doi: 10.1016/j.foodchem.2019.126119
Zhang, Z.-H., Zhou, T., Tang, T.-J., Song, H.-X., Guan, C.-Y., Huang, J.-Y., et al. (2019). A multiomics approach reveals the pivotal role of subcellular reallocation in determining rapeseed resistance to cadmium toxicity. J. Exp. Bot. 70 (19), 5437–5455. doi: 10.1093/jxb/erz295
Zhao, F.-J., Ma, J.-F., Meharg, A. A., McGrath, S. P. (2009). Arsenic uptake and metabolism in plants. New Phytol. 181 (4), 777–794. doi: 10.1111/j.1469-8137.2008.02716.x
Zhi, J.-K., Liu, X., Yin, P., Yang, R.-X., Liu, J.-F., Xu, J.-C. (2020). Overexpression of the metallothionein gene PaMT3-1 from Phytolacca americana enhances plant tolerance to cadmium. Plant Cell Tiss Org 143 (1), 211–218. doi: 10.1007/s11240-020-01914-2
Zhou, H., Yang, W.-T., Zhou, X., Liu, L., Gu, J.-F., Wang, W.-L., et al. (2016). Accumulation of heavy metals in vegetable species planted in contaminated soils and the health risk assessment. Int. J. Env. Res. Pub He 13 (3), 289. doi: 10.3390/ijerph13030289
Zou, C.-M., Lu, T.-Q., Wang, R.-T., Xu, P., Jing, Y.-F., Wang, R.-L., et al. (2022). Comparative physiological and metabolomic analyses reveal that Fe3O4 and ZnO nanoparticles alleviate Cd toxicity in tobacco. J. Nanobiotechnol 20 (1), 302. doi: 10.1186/s12951-022-01509-3
Zulfiqar, U., Farooq, M., Hussain, S., Maqsood, M., Hussain, M., Ishfaq, M., et al. (2019). Lead toxicity in plants: Impacts and remediation. J. Environ. Manage 250, 109557. doi: 10.1016/j.jenvman.2019.109557
Zvěřina, O., Vychytilová, M., Rieger, J., Goessler, W. (2023). Fast and simultaneous determination of zinc and iron using HR-CS GF-AAS in vegetables and plant material. Spectrochim Acta B 201, 106616. doi: 10.1016/j.sab.2023.106616
Keywords: heavy metals, plants, detection methods, analytical methods, technology
Citation: He S, Niu Y, Xing L, Liang Z, Song X, Ding M and Huang W (2024) Research progress of the detection and analysis methods of heavy metals in plants. Front. Plant Sci. 15:1310328. doi: 10.3389/fpls.2024.1310328
Received: 09 October 2023; Accepted: 15 January 2024;
Published: 31 January 2024.
Edited by:
Jiawen Wu, Yan’an University, ChinaReviewed by:
Qingwei Zhang, Southwest University, ChinaCopyright © 2024 He, Niu, Xing, Liang, Song, Ding and Huang. This is an open-access article distributed under the terms of the Creative Commons Attribution License (CC BY). The use, distribution or reproduction in other forums is permitted, provided the original author(s) and the copyright owner(s) are credited and that the original publication in this journal is cited, in accordance with accepted academic practice. No use, distribution or reproduction is permitted which does not comply with these terms.
*Correspondence: Wenli Huang, aGVuZ19sb25nNTMxQDE2My5jb20=
Disclaimer: All claims expressed in this article are solely those of the authors and do not necessarily represent those of their affiliated organizations, or those of the publisher, the editors and the reviewers. Any product that may be evaluated in this article or claim that may be made by its manufacturer is not guaranteed or endorsed by the publisher.
Research integrity at Frontiers
Learn more about the work of our research integrity team to safeguard the quality of each article we publish.